- 1Neuro-computing and Neuro-robotics Research Group, Complutense University of Madrid, Madrid, Spain
- 2Innovation Group, Institute for Health Research San Carlos Clinical Hospital (IdISSC), Madrid, Spain
- 3Department of Biomedical Engineering, Tufts University, Medford, MA, United States
- 4Center for Biomedical Technology, Universidad Politécnica de Madrid, Madrid, Spain
- 5Department of Material Science, Civil Engineering Superior School, Universidad Politécnica de Madrid, Madrid, Spain
- 6Biomedical Research Networking Center in Bioengineering, Biomaterials and Nanomedicine (CIBER-BBN), Madrid, Spain
- 7Silk Biomed SL, Madrid, Spain
The regenerative capacity of the peripheral nervous system after an injury is limited, and a complete function is not recovered, mainly due to the loss of nerve tissue after the injury that causes a separation between the nerve ends and to the disorganized and intermingled growth of sensory and motor nerve fibers that cause erroneous reinnervations. Even though the development of biomaterials is a very promising field, today no significant results have been achieved. In this work, we study not only the characteristics that should have the support that will allow the growth of nerve fibers, but also the molecular profile necessary for a specific guidance. To do this, we carried out an exhaustive study of the molecular profile present during the regeneration of the sensory and motor fibers separately, as well as of the effect obtained by the administration and inhibition of different factors involved in the regeneration. In addition, we offer a complete design of the ideal characteristics of a biomaterial, which allows the growth of the sensory and motor neurons in a differentiated way, indicating (1) size and characteristics of the material; (2) necessity to act at the microlevel, on small groups of neurons; (3) combination of molecules and specific substrates; and (4) temporal profile of those molecules expression throughout the regeneration process. The importance of the design we offer is that it respects the complexity and characteristics of the regeneration process; it indicates the appropriate temporal conditions of molecular expression, in order to obtain a synergistic effect; it takes into account the importance of considering the process at the group of neuron level; and it gives an answer to the main limitations in the current studies.
Introduction
The peripheral nervous system (PNS) is responsible for connecting the periphery with the central nervous system, transmitting sensory signals, from the sensors to the brain (afferent) and motor and from the brain to the muscles and glands (efferent). An essential feature of the PNS is the regenerative capacity it presents after suffering damage. Depending on the degree of damage, the number of damaged nerve structures, and the proximity to the nucleus, especially important for its role in the activation of the regenerative machinery (regeneration associated genes), regeneration will be more or less complete. The two main problems were (i) the distance or gap that is generated between the two ends of the damaged nerve and (ii) the intermingled growth of sensory and motor fibers that leads to erroneous reinnervation of the target tissues, sensors, and muscle fibers, respectively (Ramón y Cajal, 1959; Nerves and nerve injuries. By Sunderland, 1969, C.M.G., M.D., B.S., D.Sc., F.R.A.C.S.(Hon.), F.R.A.C.P., F.A.A. (Melbourne). 10 × 7 in. Pp. 116 + xvi, with 197 illustrations. 1968. Edinburgh: E. & S. Livingstone Ltd., £ 12 10s, 1969; Sulaiman and Gordon, 2013).
Therefore, the priority objectives of materials engineering in this specific hot research topic are (i) the development of biomaterials that allow the bridging of the two ends of the damaged nerve; (ii) the provision of these biomaterials of a substrate that guides and supports the regenerating nerves; and (iii) the achievement of a differentiated growth of the sensory and motor nerve fibers, each of them toward its original tissues. For this, it is necessary to have a thorough knowledge of the repair processes of the PNS and the molecular basis of the regeneration of each type of nerve fiber, and we have to become able to manage to incorporate these specific processes in the new biomaterials.
Acquiring this knowledge is a very difficult task because the regeneration processes are extremely complex; the literature is very broad, with different methodologies that are not comparable to each other and results that, in most cases, are very difficult to interpret and use for the development of new therapies. The first big problem is that, for reasons of complexity of the experiments, the studies are neither systematic nor complete. Each one focuses only on some of the tens or hundreds of factors that are involved, but the factors are not the same, and their same form of expression (mRNA, proteins, genes…) is not studied. The task is further complicated because the expression of these factors depends drastically on the area of the nerve being studied (neuron soma, injured tissue, proximal stump, distal stump, target tissue…) and changes over time. All this makes it very difficult to compare the results published in the different articles and even more difficult to synthesize them. However, the most serious problem is the excessive simplification of the studies: there is a huge number of articles on the influence of the different molecules on the regeneration of the peripheral nerves, but this influence is studied considering the molecules in isolation when, as will be explained throughout this article, the regeneration process is very complex and is carried out, thanks to the synergistic action of a large number of molecules, each of which has a temporal expression pattern very different from the others.
All this represents a very big obstacle for scientists who are dedicated to tissue engineering, the development of advanced biomaterials, and cell therapy for the repair of peripheral nerve injury (PNI). Almost all of up-to-day approaches are limited to choosing restricted amounts of biomolecules or cells with regenerative properties, incorporating them into scaffolds and testing to see their efficiency, but obviously, with very poor results.
In this article, (i) we offer an understandable synthesis of the regeneration processes after a PNI and the role that neurotrophic molecules play in those processes; (ii) we create a framework and rationale to be able to engineer therapeutic solutions based on biomaterials and molecules scientifically and non-empirically neurotrophic; and (iii) we propose biomimetic approaches for the development of advanced bio-hybrid devices to be used not only in severe PNI but also in nerve–machine interfaces for amputee people.
Peripheral Nerves, Nerve Injuries, and Amputations
The PNS is responsible for transmitting sensory information from the periphery and from the internal organs to the central nervous system (spinal cord and/or brain) and for transmitting motor neural activity from the brain and/or spinal cord to the muscle fibers and glands (Flores et al., 2000; Catala and Kubis, 2013).
The main architectural elements of the PNS are the axons of the neural cells. Thick ones (Ø > 1 μm), normally associated with motor and exteroceptive or proprioceptive information, are myelinated, which means surrounded by the membranes of several Schwann cells that cover the whole axon, placed one after the other from the soma to the synaptic terminals. The gaps between Schwann cells, the spaces where axons are not wrapped by the myelin sheath, are called Nodes of Ranvier. Thin axons (0.5 < Ø < 1.5 μm), normally associated with temperature and/or pain perception, are not individually myelinated, but rather a single Schwann cell wraps up to 15 or more axons together (Figure 1; Sherman and Brophy, 2005; Griffin and Thompson, 2008; Catala and Kubis, 2013). Each myelinated axon, or group of unmyelinated axons, is surrounded and protected by the endoneurium, a thin cylinder of lax connective tissue made by collagen fibrils, fibroblasts, capillaries, fixed macrophages, and perivascular mast cells; endoneuria are filled with the endoneural fluid, a liquid containing fine proteins equivalent to the cerebrospinal fluid of the central nervous system, within which the axons are suspended (Figure 1).
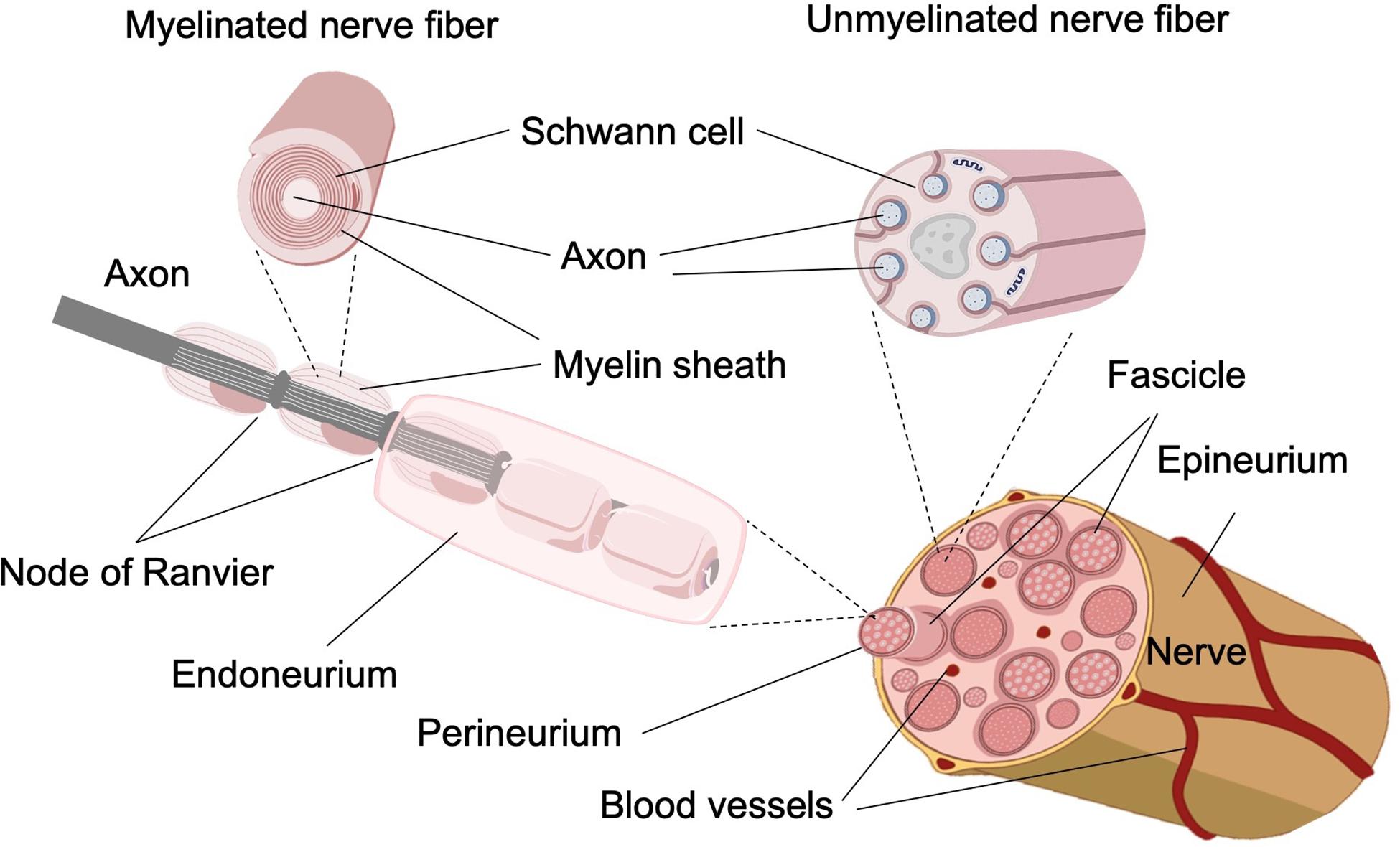
Figure 1. Representative structure of a peripheral nerve. (Top) Representation of the functional unit made up of axons wrapped by the Schwann cell. The representation of myelinated and unmyelinated fibers and their differences by the presence of myelin and by the number of axons involved by each Schwann cell can be observed. (Bottom) In the peripheral nervous system, each nerve fiber is surrounded by a Schwann cell and the endoneurium individually, consisting of loose connective tissue. In turn, the individual neurons are grouped into bundles, called fascicles, and each of these is surrounded by the perineurium. The epineurium, the outermost layer, is going to be in charge of protecting and unifying the nerve. Finally, we find the presence of the vasa nervorum.
The axons of each modality are divided in several fascicles, each of them surrounded by the perineurium, a connective tissue denser than the endoneurium, composed of several layers of flattened fibroblasts, enclosed in basal lamina (Stewart, 2003; Brushart, 2011). The basal lamina is a thin layer of extracellular tissue that underlies the epithelium and surrounds muscle fibers, adipocytes, and Schwann cells to separate them from the adjacent connective tissue on both sides and provides strength and elasticity to the peripheral nerve (Bunge et al., 1986; Court et al., 2006). Perineuria tissue is circularly oriented with respect to the direction of the axons (Figure 1; Battista and Lusskin, 1986; Flores et al., 2000).
The total numbers of fascicles are finally covered with the epineurium, a dense tissue sheet longitudinally oriented with respect to the nerve fibers, made up of connective cells, some fat cells, and collagen that holds the fascicles and irrigates the whole structure through the vasa nervorum that run along inside (Figure 1; Adams, 1942; Zochodne, 2018), which constitute the blood–nerve barrier, present at epineurium and endoneurium level where the smallest vessels are present. Formally, the epineurium is the component that defines the peripheral nerve as an organ, as a functional structure built by multifascicular anatomical structures of a high number of unidirectional channels (Figure 1). Depending on the type of information transmitted by the neurons, their soma will be found at the level of the dorsal root ganglia in the case of the sensory neurons (Krames, 2015) and on the ventral horn of the spinal cord in the case of the motor neurons of the PNS (Nógrádi et al., 2011).
The damage of any of the physical components of a peripheral nerve, caused by accidents, military activities, endogenous or exogenous toxins, metabolic diseases, etc., is included on the PNI (Dubový, 2004; Gu et al., 2011). A very special case of PNI are amputations and severe mutilations in which the distal part of a member gets lost together with a part of the nerve as well as with the target organs, sensory organs, and muscles. Amputations are due to accidents or therapeutic surgeries, mostly vascular diabetes in developed countries and war-like actions, infections, or trauma in developing and underdeveloped countries (Dillingham et al., 2002; Olasinde et al., 2002; Abou-Zamzam et al., 2003). Amputations are normally accompanied by neuropathies, by phantom limb symptoms and in the 60 to 80% of the cases also by phantom limb pain (Cuartero et al., 2012; Nikolajsen and Christensen, 2015).
In 2005, it is estimated that there were 1.6 million people suffering from limb amputations in the United States and a similar number in the EU (Mohanna et al., 2003; Ziegler-Graham et al., 2008; Marshall and Stansby, 2010). By 2050, in the United States, the number of cases is estimated to reach 3.6 million (Varma et al., 2014).
Although many of the cases of amputations are trauma-related [2879 patients between 2011 and 2012 in the United States (Low et al., 2017)], the leading cause continues to be diabetes mellitus, with more than 1.5 million lower amputations worldwide, with 1.1 million being without prosthesis (Zhang et al., 2020).
Peripheral Nerve Regeneration and Repair
In PNI, spontaneous repair occurs if the nerve, although damaged, has not been sectioned, with the outer membrane (epineurium) remaining intact regardless of the severity of the injury suffered by the internal structures, which can be damaged or even sectioned (Seddon, 1942; Sunderland, 1951; Menorca et al., 2013). Inside the nerve, the damaged Schwann cells change their phenotype, from myelinating to repairing one, and start secreting neurotropic and neurotrophic biomolecules, creating a facilitator environment that promotes the regeneration of the injured axons and guides them to their targets, sensitive or motor ones (Dubový, 2004; Eberhardt et al., 2006; Höke et al., 2006). Sensory/motor axon injury divides the neuron in two parts: the “proximal” (to the spinal cord), which comprises the soma, the part of the axon attached to the soma and the arborizations, and the “distal” (from the spinal cord), which comprises the segment of the axon detached from the soma together with their sensory/motor endings (Figure 2). The distal part of the nerve undergoes degeneration, whereas the proximal part starts growing and invading the space of the distal one, now left free. The growing fibers in the proximal part are attracted by the distal part and conveyed separately to their respective targets by the neurotropic and neurotrophic biomolecules secreted by the Schwann cells. These processes occur into the intact epineurium, which preserves the facilitator environment by retaining the secreted biomolecules close to the injured axons, and at the same time, it works as a conduit for the regenerating fibers, directing them toward the territory they have to reinnervate (see The Need of an Ordered and Differentiated Regeneration of Sensory and Motor Fibers in Amputee People for details).
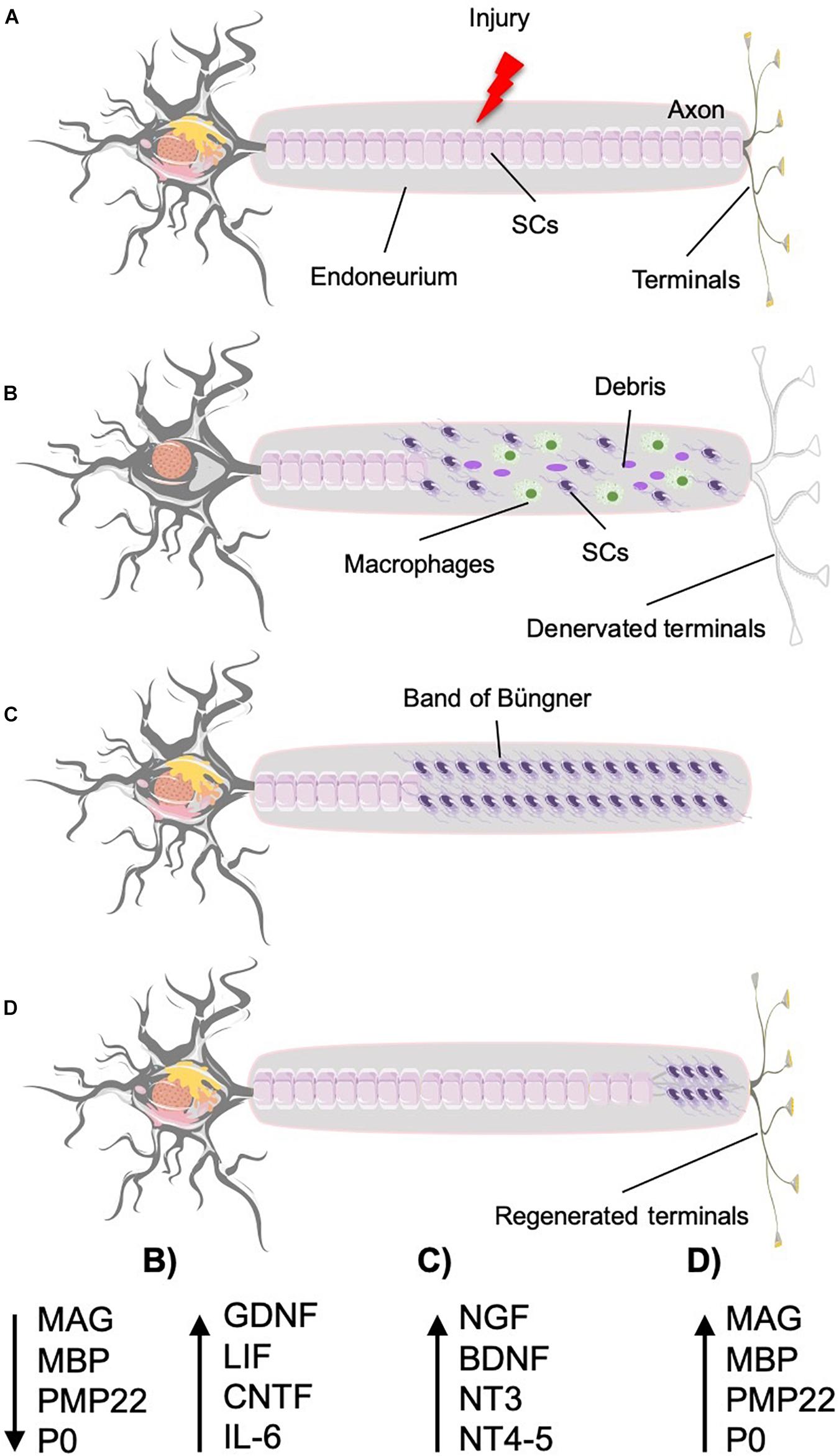
Figure 2. Nerve injury and regeneration under ideal conditions. Time course and biomolecular processes. (A) Normal neuron that suffers an injury (crush or section) that triggers the regeneration process. (B) The proximal part undergoes retrograde degeneration and chromatolysis. The distal part suffers Wallerian degeneration, provoking the denervation of the target tissue and leaving the endoneurium clean for the axon to regenerate (MAG, MBP, PMP22, and P0 levels decrease, whereas GDNF, LIF, CNTF, and IL6 production increases). (C) Schwann cells form bands of Büngner to guide the regenerated axons into the distal endoneural tube (with high levels of NGF, BDNF, NT3, and NT4–5). (D) The axon grows in contact with the surfaces of the Schwann cells toward the target organ. As the axons grow, the Schwann cells start the remyelination process, producing the myelin-associated molecules (MAG, MBP, PMP22, and P0 return to their initial levels).
Unfortunately, in the majority of the cases, PNI also implies nerve tissue losses, either due to the accident itself, or because of tissue removal during the surgical intervention. In these circumstances, to allow the neuroreparative processes, we must (artificially) restore the continuity of the epineurium and create a bridge for the intercommunication of the two ends of the sectioned nerve (Brattain, 2014; Grinsell and Keating, 2014; Faroni et al., 2015; Figure 3). State-of-the-art devices are tubular scaffolds (Pabari et al., 2014; Dalamagkas et al., 2016), grafts (Mackinnon et al., 2001; Brushart, 2011; Brattain, 2014; Dalamagkas et al., 2016) or tissue engineering bio-hybrids (Gonzalez-Perez et al., 2018).
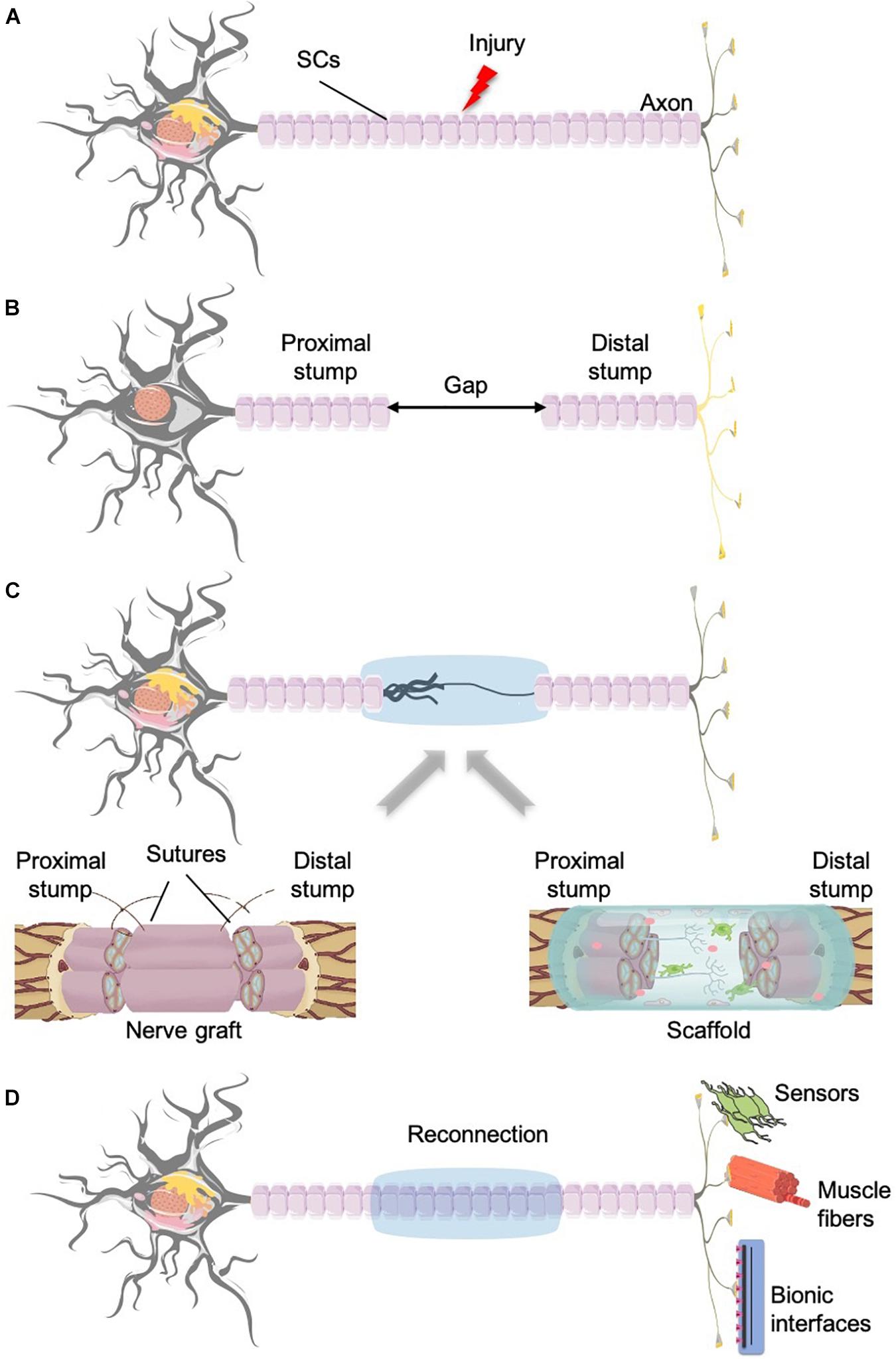
Figure 3. Nerve injury and regeneration with the aid of grafts or biomaterials scaffolds. (A) Neuron divided into proximal stump and distal part that innervates the target tissue. (B) After the damage takes place, the gap created between the two parts provokes that the regeneration cannot take place properly because of the lack of communication between the two stumps. (C) Different approaches to obtain the connection of the two stumps and the guidance of the growing axons: left, nerve graft; right, biomaterials and tissue engineering. (D) Once the reconnection of the two ends of the injured nerve is achieved, these neurons will be able to reinnervate the former target tissue, divided into sensors and muscles; or in the case that the target tissue has been lost, the reconnection with bionic interfaces.
The Need of an Ordered and Differentiated Regeneration of Sensory and Motor Fibers in Amputee People
Evidently, because of the lack of the distal end, repair is not possible in case of amputation. However, in amputees there are two very powerful reasons to achieve nerve regeneration patterns similar to those of non-amputated people, despite the lack of their (amputated) limbs. The first reason is to reduce the appearance of traumatic neuropathies and phantom limb pain, both of them caused by the aberrant regeneration of the peripheral nerves and by the formation of neuromas in the proximal stump. Indeed, aberrant regeneration and neuromas in sensory nerves could be reduced using selective electrical stimulation of the regenerating fibers (Herrera-Rincon et al., 2011; Figure 4). However, because almost all peripheral nerves are mixed, stimulation-based therapeutic approaches require the separated regeneration of sensory and motor axons. The second reason is to connect the sensory and motor nerves of the amputees to the sensors and actuators of the bionic neuroprostheses that are being available in the market, which demand well-differentiated and ordered sensory and motor axons to be separately guided and connected to the artificial sensors and actuators (Raspopovic et al., 2014; Micera, 2016; Lee et al., 2018; Günter et al., 2019; Deshmukh et al., 2020).
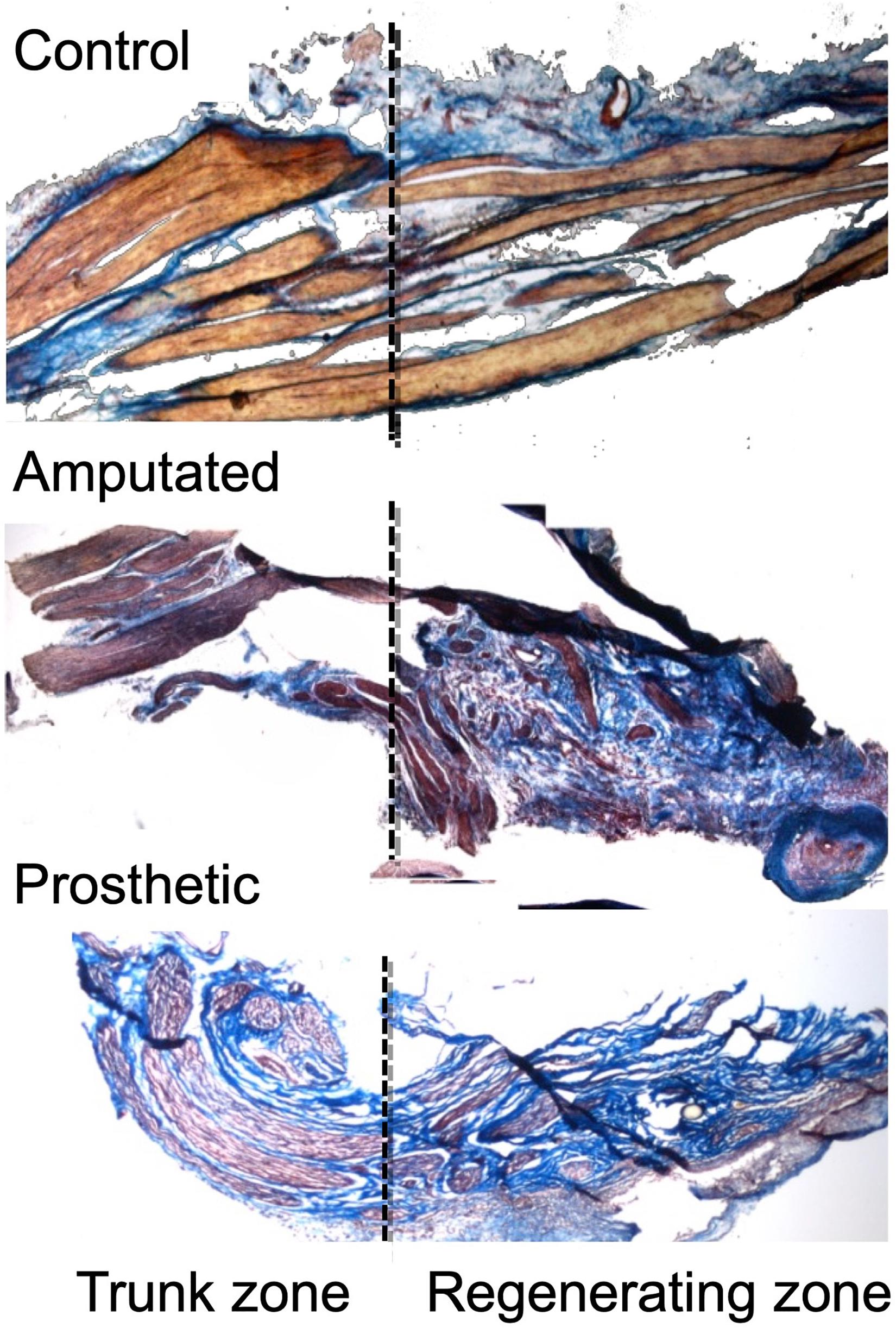
Figure 4. Effects of electric neurostimulation on the macroscopic anatomical structure of an amputated rat trigeminal peripheral nerve (Herrera-Rincon et al., 2011). Masson trichrome staining of nerve tissue from Control, Amputated, and Prosthetic animals. Nerve fibers can be detected as red-stained “bundles” crossing from left to right, surrounded by a blue-stained matrix (mostly collagen). In control nerve (top) the axons are organized in regular fascicles separated by connective tissue, whereas in the amputated nerve (middle), the regular organization of the tissue is altered. However, whereas in amputated nerves, organization is completely lost with haphazardly arranged axons dispersed inside the connective tissue, in prosthetic nerve (bottom), the fascicular organization is preserved. The importance of stimulation as a therapy to improve the regeneration of the growing fibers resides in the ability to achieve a guided and organized growth. Through Masson trichrome, we can observe the fascicular organization of the fibers, stained in brown and marked with arrow. In the left side of the images, the “trunk” is shown, the undamaged “proximal” area, which is in charge to activate the biomolecular regenerative processes. In the right, we can observe the regenerated fibers. Here we can see how when no treatment is performed, the tissue is disorganized, with a predominance of connective tissue (stained in blue, marked with arrow), whereas the treatment with electrical stimulation allows a more organized growth, maintaining the fascicular structure throughout the section (marked with arrow). Original magnification 100×. ©[2011] IEEE. Reprinted, with permission, from IEEE Proceedings.
To achieve the differentiated regeneration also in amputee persons, the most intuitive and straightforward solution would be to reproduce in the amputated nerves the processes that naturally occur in damaged nerves with preserved epineurium and target organs, which means to create in the amputated member an artificial anatomical and biomolecular environment (a bio-hybrid medium) similar to the environment that favors the natural regeneration of the peripheral nerves. Unfortunately, and despite the enormous efforts dedicated in the last 70 years to this enterprise, differential regeneration of the sensory and motor fibers has not been yet totally achieved (Johnson et al., 2015; Anand et al., 2017; del Valle et al., 2018). Among the main reasons of this failure are the high complexity of the involved biomolecular processes and the variability of the employed experimental protocols, which make experimental data difficult to interpret. However, even more important is the total absence of a scientific article describing the way these biomolecular processes should be implemented by tissue and biomedical engineers to achieve the desired regeneration of the amputated nerves. In contrast, there is an extremely high number of scientific articles analyzing the regenerative processes.
Some of the commercially available upper-limb prostheses are Michelangelo (©Otto Bock, Germany), I-Limb Ultra (©Touch Bionics, United Kingdom) Bebionic (©RLS Steeper, United Kingdom), The Taska Hand (©Taska prosthetics, New Zealand), implantable at hand level or The LUKE arm, (©Mobius Bionics, LLC, United States), the DynamicArm elbow (©Otto Bock, Germany), and the Hero Arm (©Open Bionics, United Kingdom) implanted at shoulder level and allowing mobility over the patient’s head, being the last one the first three-dimensionally (3D) printed bionic arm. Commercially available lower limb prostheses include the C-Leg (©Otto Bock, Germany), the emPOWER ankle (©BionX Medical Technologies, Inc., United States), the Proprio Foot (©Ossur, Islandia), the BiOM ankle (©BiOM, United States), and the Elan Foot (©Endolite). All them are controlled using myoelectric signals coming from residual muscles in the amputee stump.
Much of research work is focusing on obtaining prosthetic sensory signals (Graczyk et al., 2018; Cuberovic et al., 2019), necessary not only to elicit the sensory perceptions of the natural limbs (George et al., 2019; Navaraj et al., 2019), but also to increase the control and the coordination of the prosthetic device without the need of visual and/or auditory signals, to identify the type of element being manipulated and to create proprioception (Micera et al., 2011; Zecca et al., 2017; Clemente et al., 2019; D’Anna et al., 2019; Sensinger and Dosen, 2020).
Patients with either intrafascicular and intraneural electrode implants show good proprioception and object identification capabilities (Micera et al., 2008; Di Pino et al., 2012). However, disruptive neuroprosthetic solutions require individual or quasi-individual nerve–electrode connections.
With the present article, we aim at offering a comprehensive compendium describing how to create neural regeneration devices, either scaffolds or tissue-engineered bio-hybrids, and how to implement the biomolecular processes that will foster the regeneration of the amputated peripheral nerves in a well-organized and guided manner. For this endeavor, we need a deep understanding of the biomolecular processes that underlie spontaneous nerve regeneration and of the spatiotemporal patterns of the expression of these biomolecules, as well as of their dynamic interactions over time, and to be able to implement them in a bio-hybrid biomaterial environment (Schmidt and Leach, 2003; Matsumoto and Mooney, 2006; Mikos et al., 2006; Biondi et al., 2008; Wang et al., 2009; Atala et al., 2012; Gu et al., 2014; Gonzalez-Perez et al., 2018).
In the following section, we will briefly review the biomolecular processes after PNI, and then we will go in depth to the specific processes to differentiate regeneration of sensory and motor fibers in amputee people.
Spontaneous Regeneration and Repair After PNI
As mentioned previously, cellular and biomolecular processes in the proximal and the distal parts of the nerve are different. In both places, processes occur in two phases, a preparatory and a repair one. Any biomimetic device aiming at an ordered regeneration of the sensory and motor nerves has to implement both the anatomical structure of the distal part and the biomolecular processes that take place there.
Preparatory Phase
In the proximal part, both the damaged axon and the myelin sheath that surrounds it undergo retrograde degeneration, approximately up to the first node of Ranvier (Figure 2; Fu and Gordon, 1997).
In the distal part, 48 to 96 h after the lesion starts the “Wallerian degeneration,” axon and myelin decompose, whereas both Schwann cells and macrophages recruited by these cells get rid of the debris and leave empty the whole distal endoneural tube (Stoll et al., 2002; Vidal et al., 2013; Chen et al., 2015; Barton et al., 2017), during the first 7 days after the injury (Caillaud et al., 2019). The empty endoneural tubes connect the injury region to the target organs, either sensors or muscle fibers (Eva and Fawcett, 2014; Romano et al., 2015). At this stage, we observe an increase of the production of proinflammatory molecules, such as cytokines [glial cell line–derived neurotrophic factor (GDNF), leukemia inhibitory factor (LIF), ciliary neurotrophic factor receptor (CNTFR), and interleukin 6 (IL6)], accompanied by a decrease in the production of myelin-associated molecules [myelin-associated glycoprotein (MAG), myelin basic protein (MBP), peripheral myelin protein 22 (PMP22), and myelin protein 0 (P0)] (Figure 2).
The physical structure of a biomimetic regenerative device should implement the above architecture of a high number of artificial conduits and be able to support the analogous neuroregenerative biomolecular processes.
Repair Phase
In the proximal part, 7 days after the damage, small branches sprout from the extreme of the sectioned axon and form the so-called “growth cone” (Figure 5; Ramón y Cajal, 1959; Bradke et al., 2012). It is a mobile structure with specialized receptors that recognize surfaces and molecules (Ertürk et al., 2007; Koch et al., 2012) and decides to which direction should grow the regenerating axon (Figure 5; Eva and Fawcett, 2014), guiding the regeneration.
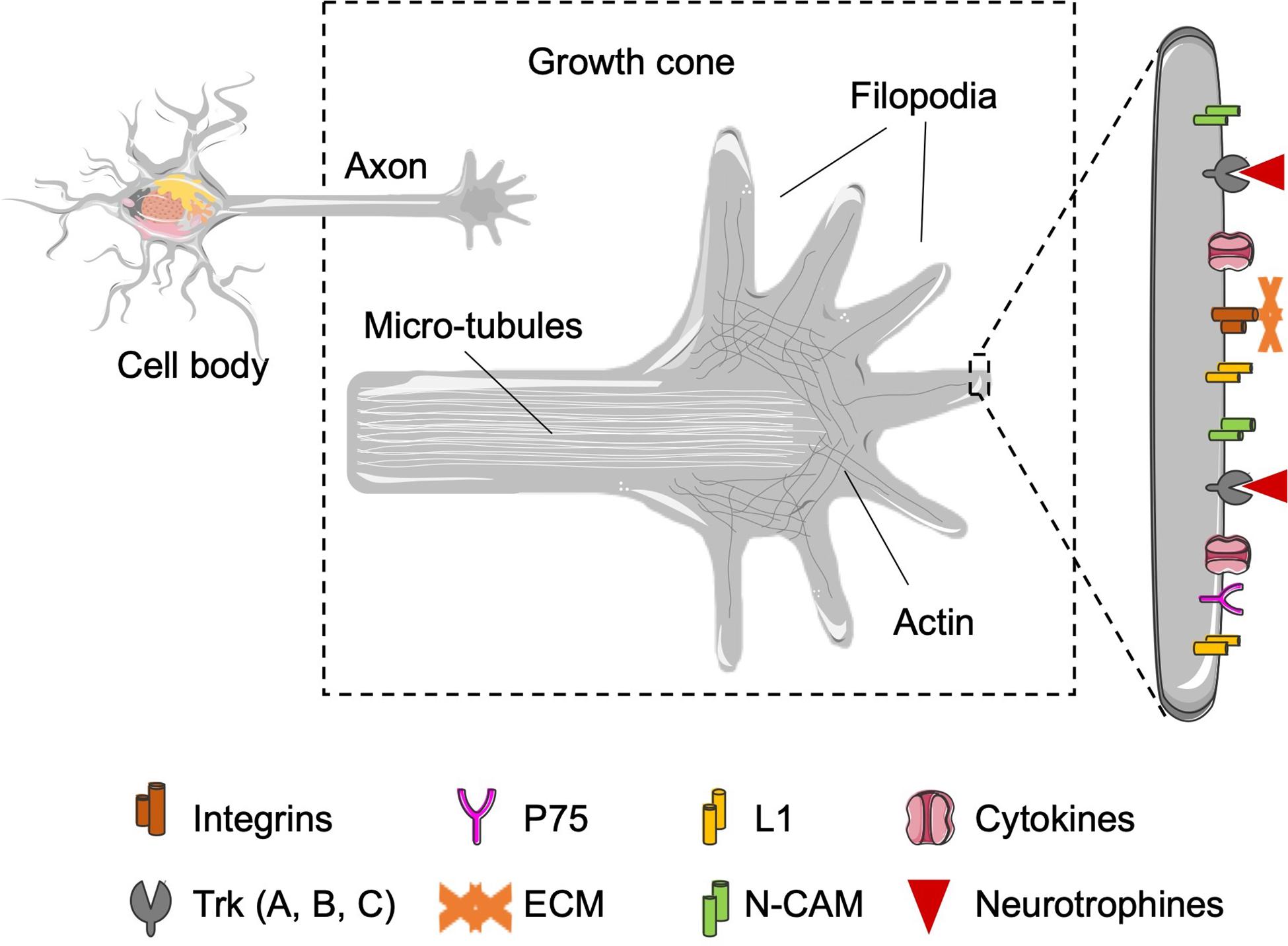
Figure 5. Representation of the growth cone in charge to interact with the Schwann cells and the ECM that surrounds the growing axons. Zoom of growth cone: representation of the growth cone in detail. The organization of the filopodia and the mobile structure in charge of interaction with the physical and chemical environment are supported by actin filaments accumulated in the proximal stump. Zoom of the filopodia: representation of the different molecules expressed in the growth cone. The molecules can be divided in three groups: (i) expression of neurotrophic factors receptors, for neurotrophins (Trk A, B, C, and p75) and neuropoietic cytokines [leukemia inhibitory factor receptor (LIFR), CNTFR…]; (ii) cell–cell junction molecules (N-CAM, L1), which will allow the interaction between the regenerating axons and the Schwann cells present in the bands of Büngner, in the distal zone, and (iii) molecules that bind to the ECM (integrins), which will act as a support for axonal guidance to its target tissue.
In the distal part, the byproducts (molecules) of the degenerated axon and myelin stimulate the denervated Schwann cells to divide inside the endoneural tube; there, they get distributed along the longitudinal axis forming a column, the so-called bands of Büngner (Figure 2C; Pfister et al., 2011). The bands of Büngner create a bridge between the regenerating axons and the target tissue, also producing the necessary neurotrophic and neurotropic biomolecules to help axons to grow. In turn, Schwann cells in the tube attract the regenerating axon to enter the distal endoneural tube (Figure 2C; Lee and Wolfe, 2000). They do it by releasing neurotrophic and neurotropic molecules (Politis et al., 1982; Politis and Spencer, 1983) recognizable by the receptors present in the surface of the growth cone (Maggi et al., 2003; Gordon, 2009; Griffin et al., 2013; Faroni et al., 2015). It is worthy to underline that each endoneural tube attracts axons of its own (sensory or motor) modality (Ramón y Cajal, 1959).
Once introduced into the endoneural tube, the axon grows in contact with the surfaces of the Schwann cells toward the target organ. Upon reinnervation, Schwann cells return back to synthesize myelin and isolate the axon again (Grinsell and Keating, 2014). Axons that do not connect with their targets or do not reach the endoneural tube (as in the case of amputation) continue to grow but in a disorganized way and form an abnormal tissue structure, the neuroma (Grinsell and Keating, 2014).
A biomimetic regenerative device should incorporate a biomolecules-generating mechanism to release the same molecules with the same concentrations in space and time as the aforementioned Schwann cells. This is a highly dimensional problem, and it is important to mimic/duplicate the cues of endogenous regenerative microenvironment, when endogenous repair systems work.
Differential Regeneration of Sensory and Motor Axons
The differential regeneration and guidance of sensory and motor axons toward their specific targets are determined by the molecules that are expressed by the Schwann cells of the distal endoneural tubes. Such molecules can be classified into two groups.
The first group includes five types of neurotrophic factors: axonal growth promoters (neurotrophins), neuropoietic cytokines, fibroblast growth factors, transforming growth factors, and insulin-like growth factors (Boyd and Gordon, 2003; Jin et al., 2009; Li et al., 2020).
The second group includes molecules integrated into the surrounding nerve tissue, mainly axonal growth promoters, such as molecules that facilitate and modulate cell–cell and cell–extracellular matrix (ECM) adhesion [glycoproteins such as neural cell adhesion molecule (N-CAM), N-cadherin, L1, integrins, etc.] (Seilheimer and Schachner, 1988; Smith et al., 1994; Franz et al., 2005; Saito et al., 2005, 2010; Gardiner et al., 2007; Tucker and Mearow, 2008; Gardiner, 2011; Anand et al., 2017) and molecules present in the ECM (fibrinogen, fibronectin, laminin, etc.) (Politis, 1989; Hammarberg et al., 2000; Zhang et al., 2003; Tucker and Mearow, 2008; Webber et al., 2008; Gardiner, 2011; Fudge and Mearow, 2013; Gonzalez-Perez et al., 2016; Table 1).
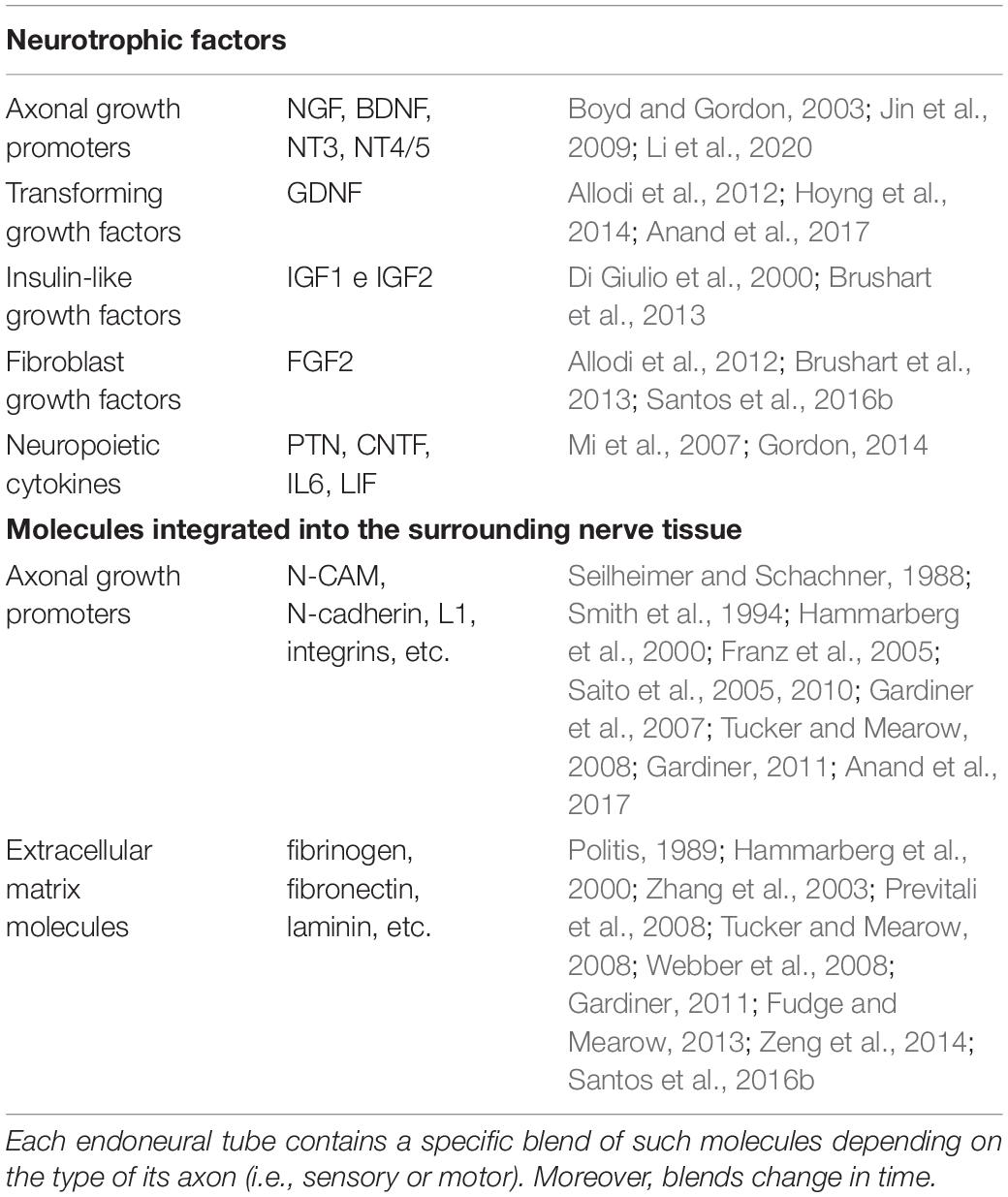
Table 1. Neurotrophic and neurotropic molecules that are expressed by the Schwann cells in the distal endoneural tubes.
With the only exception of nerve growth factor (NGF), there are no molecules favoring the growth of one type of neurons while inhibiting the growth of the other, which means favoring the growth of sensory fibers while inhibiting the growth of the motor ones, or vice versa (Wang et al., 1997; Boyd and Gordon, 2003; Pehar et al., 2004, 2006). Some of them strongly favor the regeneration of sensory fibers but have limited effect on the motor ones; others strongly favor the regeneration of the motor fibers, but they don’t display facilitatory effects on the sensory ones; finally, a third group of molecules favor the regeneration of both types of fibers, although the facilitating effect may be greater on one type of fibers than on the other (see Table 2 and references therein). Conversely, inhibition of their function through the administration of antibodies against them or against their receptors normally leads to inhibition of the regeneration process and also abnormal regeneration of the fibers (Table 2 and references inside). Several cocktails of these molecules have been designed and incorporated to scaffolds for peripheral nerve regeneration and repair with deceptive up-to-day results (Table 2; Johnson et al., 2015; Anand et al., 2017; del Valle et al., 2018). Doses of the most relevant neurotrophic factors, which have been tested to improve the molecular environment for the regenerating axons, are shown in Table 3.
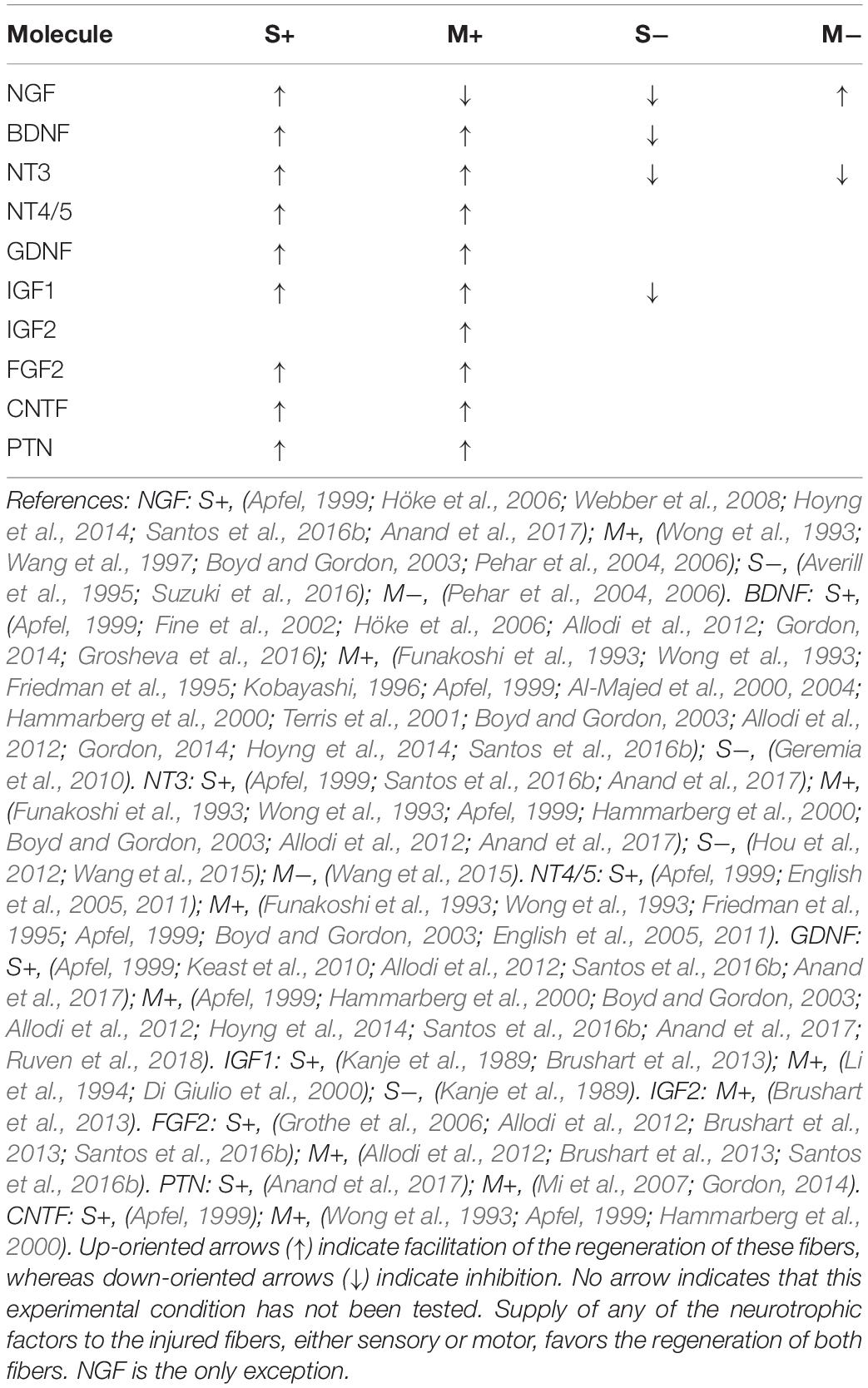
Table 2. Effects of the supply (+) or depletion (−) of axonal growth-promoting molecules in the surrounding tissue of sensory (S) or motor (M) fibers.
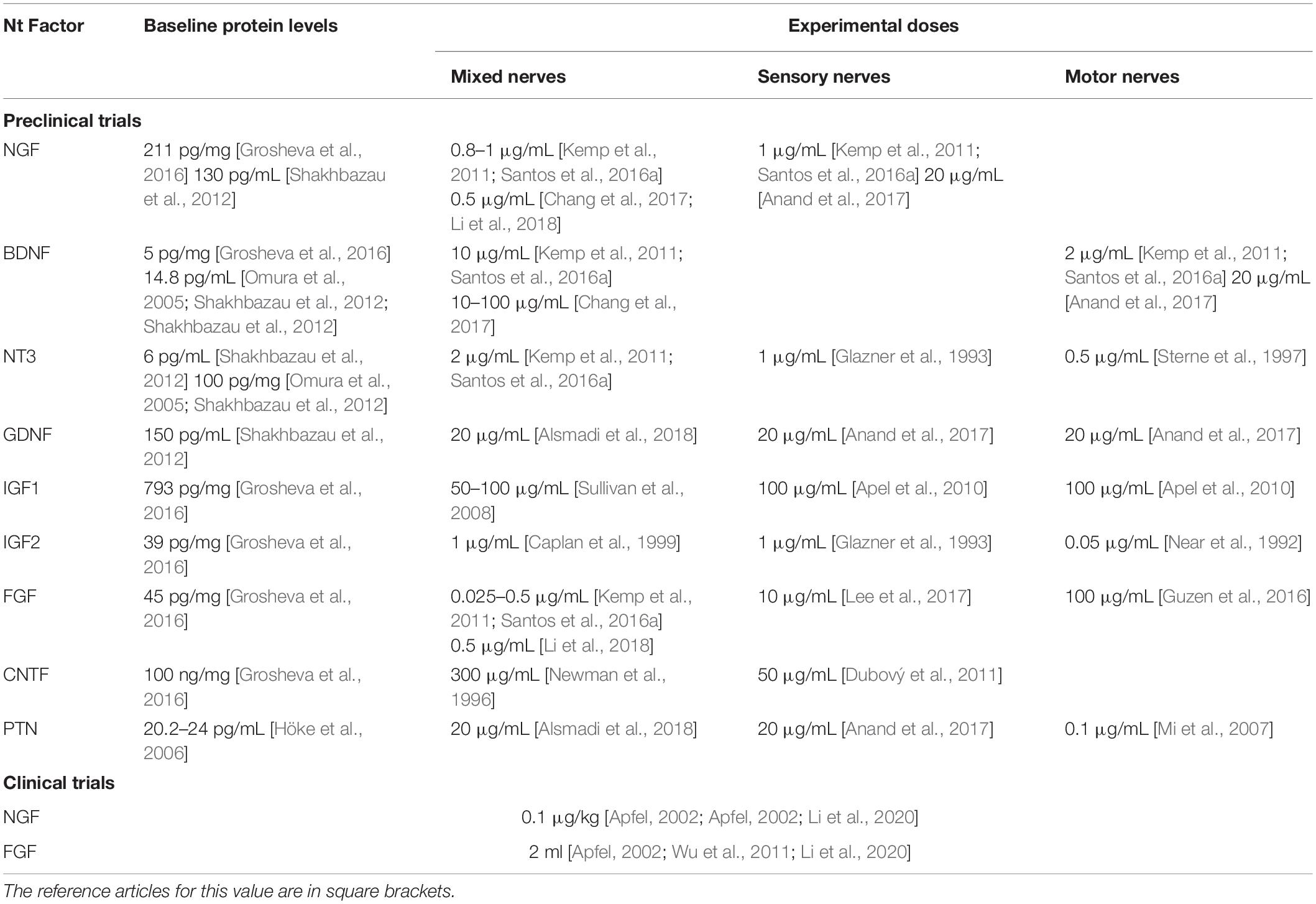
Table 3. Baseline protein levels of the most important molecules involved in the regeneration process and doses employed to obtain a favorable regenerative environment.
Although artificially delivered axonal growth-promoting molecules improve, in general terms, nerve regeneration and repair, they fail when they are used to differentiate the growth of sensory and motor fibers. This is due to the massive delivery from up-to-day devices (pumps, injections, releases from embedded biomaterials or biofunctionalized scaffolds, etc.), which neither simulate neither mimic the profile of the production of the neurotrophic factors during the regeneration. Even more important than the correct administration of the facilitator molecules is the timing of the delivery of such molecules. Timing determines if there will be an effect and which type of effect it will be. A very illustrative example is the administration of brain-derived neurotrophic factor (BDNF) and GDNF, two neurotrophic factors that, if delivered immediately after the injury, do not favor the regeneration of the nerve, while when administered after a brief period of adverse conditions for the regeneration process, their administration will have a clear favorable effect (Gordon, 2009). In PNI, what is important is timing for delivery of one or another molecule, but there is no “sensitive period” for inducing nerve regeneration after injury, typical in central nervous system injuries such as stroke (Dromerick et al., 2015; Zeiler et al., 2016; Ballester et al., 2019).
Summarizing, unsuccessful artificially guided differential regeneration of sensory and motor fibers is due to at least three main causes: (1) the delivery of neurotrophic factors acts on the whole nerve instead of on specific nerve fibers; (2) delivered doses are too high in comparison with the concentrations of the neurotrophic molecules observed in the nerve during the regeneration process; and (3) administration of the different biomolecules does not follow the time course of the concentrations of such molecules during the natural regeneration process.
Design of Devices and Scaffolds for the Differentiation of Sensory and Motor Fibers
Biological Principles
The above experimental data lead us to formulate the two biological principles that govern the regeneration of the peripheral nerves: (1) the separation of the fibers and their guidance toward their corresponding targets depend on the dynamics of the molecular gradients along the regeneration pathway (how the concentration of each molecule, at each point, varies with time), rather than on the concentration of the factors themselves; and (2) the molecules underlining these regenerative processes act at the microscopic level and not at the macroscopic one (they act at the level of individual fibers or fascicles of fibers, and not at the level of clusters of fascicles or of the whole nerve).
The design of novel approaches and devices for peripheral nerve regeneration and repair and, in particular, for the connection of amputee nerves to bionic sensory–motor prostheses should be driven by the above two principles.
Current PNIs Approaches
In case of nerve sections with <5-mm-long gaps, the ideal PNI repair approach is the neurorrhaphy of the two stumps, suturing the individual fascicles one-by-one. In >5-mm-long gaps, the treatment consists of (i) the use of autografts and allografts or (ii) the implant of tubular biomaterials that reconnect the two stumps.
The gold standard are autografts, although they have several associated side effects, such as secondary surgery, donor site morbidity, size mismatch, and limited tissue availability. Commercial allografts, such as Avance® Nerve Graft (Axogen, Inc., FL, United States), which consists of the ECM of a human nerve, without cellular or non-cellular debris (Karabekmez et al., 2009), avoid such effects; however, it is associated with the misdirection of the growing neurons and the necessity of immunosuppressant treatment.
Biomaterials to support reconnection and guidance of the regenerating axons present a series of specific characteristics that not only facilitate axon guidance but also allow acceptance of the implant by the nervous tissue like biocompatibility, biodegradability, mimetics of the host tissue, etc. Almost the totality of commercial devices for <30-mm-long PNIs consists of a hollow tubular scaffold that allows physical regeneration of the sectioned nerve through it. These scaffolds can be made by different biomaterials, such as porcine submucosa ECM, Axoguard® Nerve Connector (Axogen, Inc., FL, United States); collagen I, NeuraGen® (Integra LifeSciences Corporation, United States); polyglycolic acid, Neurotube® (Synovis Micro Companies Alliance, Inc., AL, United States); and poly-DL-lactide-co-caprolactone and polyvinyl alcohol, Neurolac® (Polyganics, Netherlands) (Tian et al., 2015; Costa Serrão de Araújo et al., 2017). Several improvements have been tested in animal models, including (i) fillings with hydrogels that favor axons regeneration; (ii) inclusion of topographic cues, like microfilaments/nanofilaments or groove patterns, to favor guidance and directionality by interacting with the growth cone; or (iii) incorporation of growth factors and supporting cells (Carvalho et al., 2019; Wang and Sakiyama-Elbert, 2019).
Guided axon regeneration in >30-mm-long gaps presents serious difficulties. In these cases, nerves lose their original topographic organization, which provoke the misdirection of the axons and the intermingled growth of the different types of neurons (Yi et al., 2019). Even though the guidance and regeneration of the neurons have been improved, axonal misdirection and innervation of the inappropriate target tissues are still unanswered clinical issues.
Approaches for Differential Regeneration
Two main approaches have been used to guide a specific type of neuron to its originally innervating tissue: (1) use of biomaterials-built devices with two separated compartments (“Y-shaped” form scaffolds) to create separate molecular environments and thus achieve a separated regeneration of the sensory and motor neurons (Anand et al., 2017; del Valle et al., 2018) and (2) replacement of the classic single lumen tube by multichanneled scaffolds, each channel resembling the endoneurium (Tran et al., 2014) and creating independent molecular environments with different neurotrophic factors (Chang et al., 2017). 3D printing is boosting the development of novel biomaterial solutions and therapeutic approaches (Dinis et al., 2015; Dixon et al., 2018; Petcu et al., 2018). However, although the aforementioned developments envision a great future for PNI therapy and restoration of the sensory and motor functions to amputee people, the correct guidance and regeneration of the peripheral neurons to their target tissues are still an open problem.
To improve axon growth control, a more complex artificial molecular environment is necessary mimicking the biomolecular processes that guide natural axon regeneration. It is essential to achieve a complete control of the spatial and temporal dynamics of the release of the appropriate molecules, taking always into account the synergies between molecules.
Design Principles for a Biomimetic Artificial Nerve
Novel devices should be biomimetic in both the structure and the biomolecular environment they create around the regenerating axon. They should be built by hundreds or thousands of scaffolding structures, such as microtubes or high-performance fibers, each of which recreating the dynamic molecular gradients of the naturally regenerating nerves (Figure 6). However, it is worthy to note that nerve rewiring is not necessary to be perfect. Brain remodeling of the sensory and motor maps will correct deficiencies in wrong peripheral nerve connections and lead to a good level of sensory and motor functions of the patients (Merzenich and Jenkins, 1993; Panetsos et al., 2008).
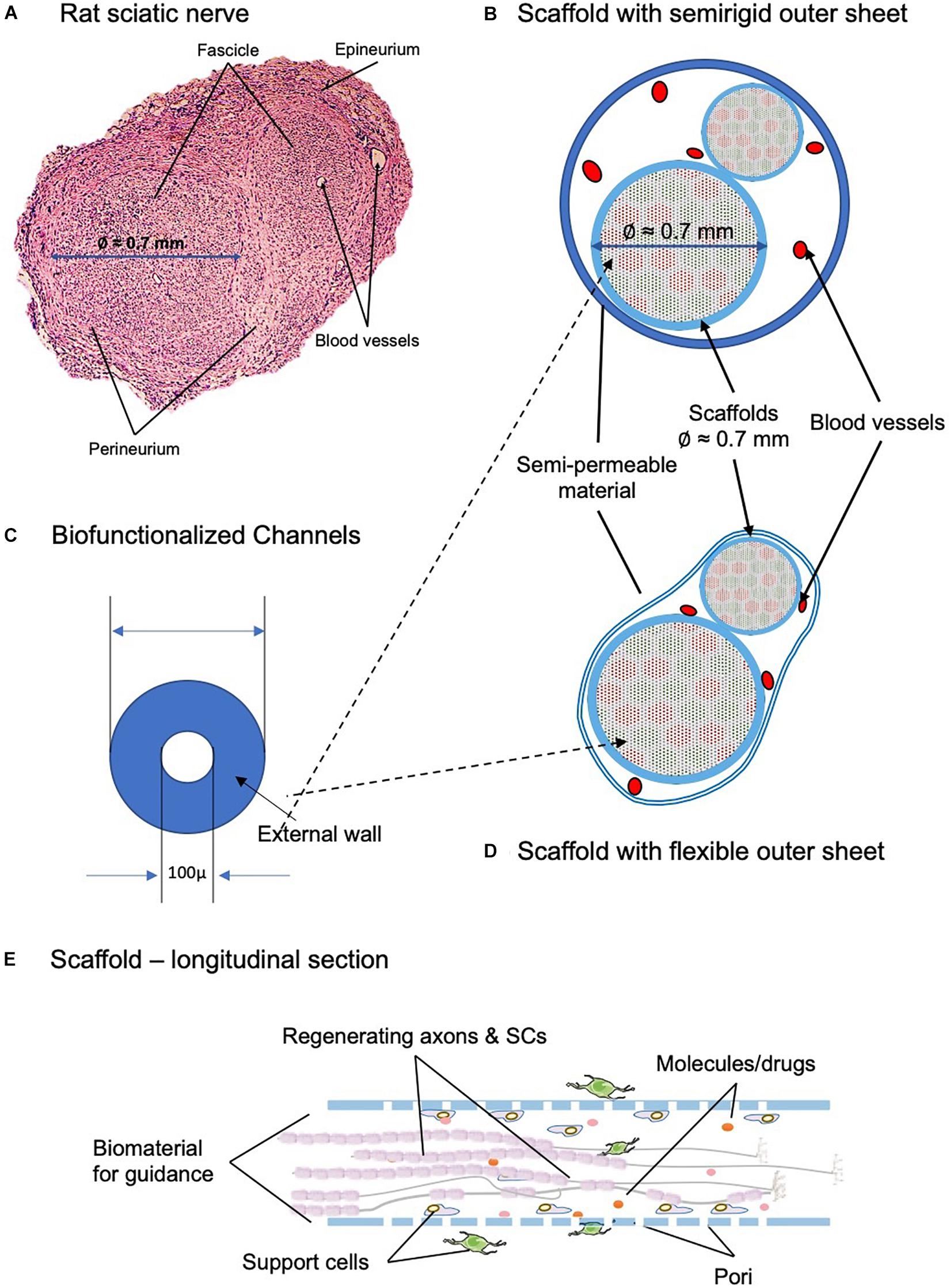
Figure 6. Desired biomimetic devices and scaffolds for neurotrophic biomolecules to be implemented in a future PNI-repair scaffold. (A) Transversal section of the rat sciatic nerve, with two fascicles and the different layers of connective tissue that organize the anatomy of the nerve. The differences on size between the fascicles can be observed. (B,D) Transversal section showing the 3D scaffold architecture, oriented to sensory–motor–guided regeneration; channels for sensory axons are in green, and motor in red. Scaffold of Ø 1.5 to 2.0 mm. The outer sheet can be both semirigid (B) or flexible (D), depending on the area of the implant and the characteristics of the surrounding tissue that defines the most adequate option. (C) Magnification of the biofunctionalized channel. Channels of Ø 100 μm for the guidance of individual axons, sensory or motor ones. (E) Longitudinal section of the scaffold representing both the physical and chemical cues that determine the regeneration of the sectioned axons. The biomaterial provides the neurons with supporting cells, such as Schwann cells (SCs), molecules, and drugs. Furthermore, it acts as a substrate for the attachment of the growing axons.
At a microscopic scale, their internal channels, the artificial endoneural guides/tubes, will guide the growth of only a few axons, ideally only one (Figure 6). Each scaffold, preferably biofunctionalized with ad hoc biomolecules, should be capable of binding to the surface receptors expressed in the growth cone, as well as of releasing facilitator biomolecules with arbitrary time profiles.
Functional connection with bionic interfaces will allow communication and control of afferent and efferent signals between nervous tissue and artificial systems. Scaffolds should be produced according to the modality of their supported nerve, because, at the distal end, we need to place the establish contact between the motor axons and the actuators of the prosthesis. Correspondingly, the scaffolds guiding the sensory axons should allow establishing contact between the axons and the artificial sensors of the prosthesis.
Implementation of Dynamic Gradients of Neurotrophic Factors for PNI Devices and Scaffolds
As stated before, in PNI without amputation, all the aforementioned biomolecules appear along the pathway of the growth cone, from the injury to the target organs. For each biomolecule, concentration values depend on the distance from the injury point and on the post-injury time (Figure 7A).
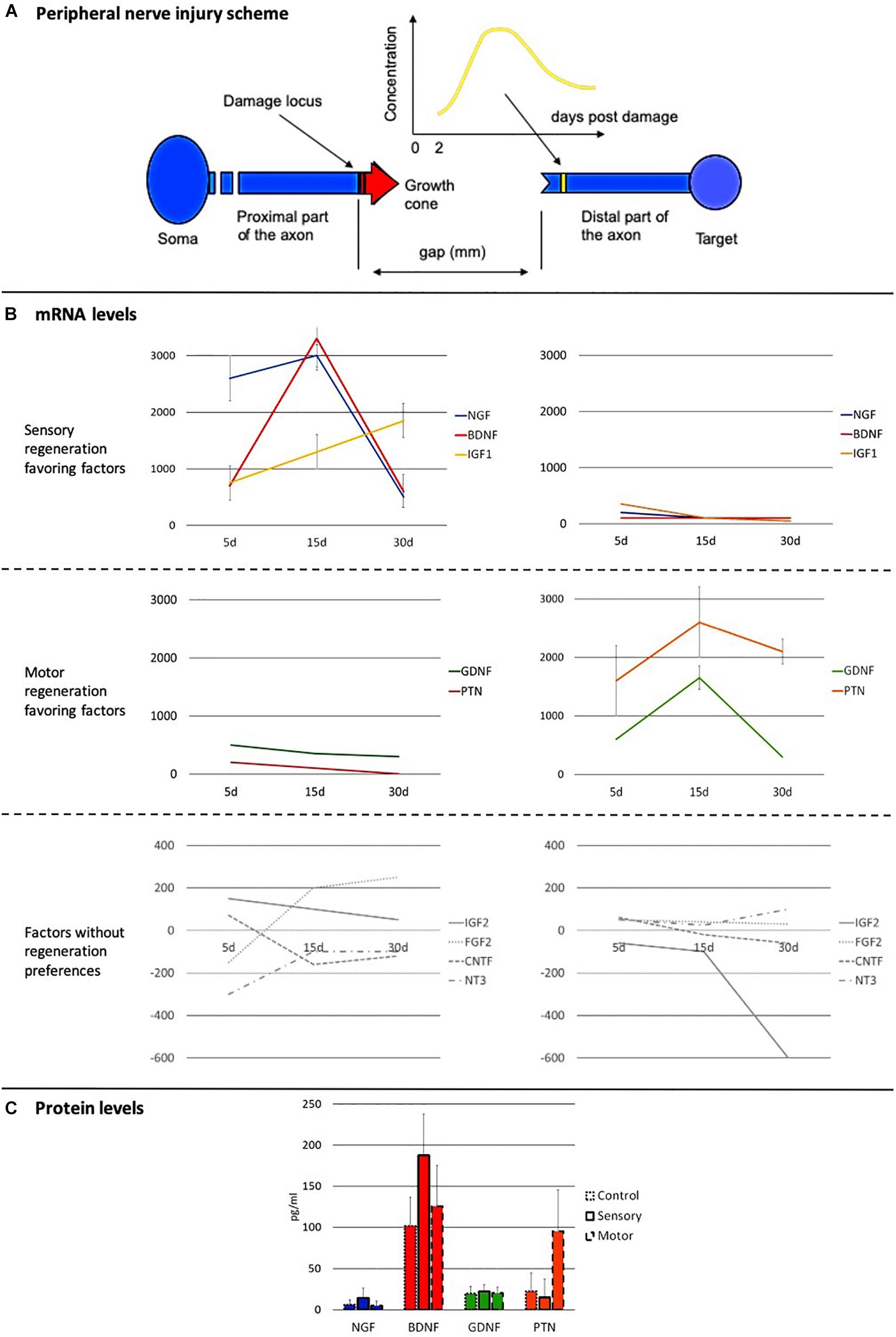
Figure 7. Dynamic behavior of the principal axon growth-promoting factors in the injured peripheral nerve. (A) Scheme of the peripheral nerve, of the damage region and of the growing process of the regenerating axons. A time-dependent expression profile of an axonal growth promoter molecule during the regeneration of the peripheral nerve at the site of the damage is also depicted. (B) mRNA expression profiles of the principal axonal growth promoter molecules in the distal stump, close to the injury locus. Left column: Expression profiles in injured sensory nerves. Right column: Expression profiles in injured motor nerves. Profiles are grouped according to their preferences in facilitating the regeneration of type of nerve: biomolecules mainly favoring the regeneration of sensory fibers (first row), biomolecules mainly favoring the regeneration of motor fibers (second row), and molecules not showing any preference for a specific type of fiber (third row). Data are represented in % change and in a logarithmic scale. (C) Protein levels (pg/mL) of the most important neurotrophic factors for the regeneration of both sensory and motor axons. Values are shown for uninjured nerves and for injured nerves 15 days after the injury [After (Höke et al., 2006)].
Molecules with strong expression during sensory axons regeneration are NGF, BDNF, and insulin-like growth factor 1 (IGF1); those with strong expression for motor fibers are pleiotrophin (PTN) and GDNF. Finally, neurotrophin 3 (NT3), ciliary neurotrophic factor (CNTF), IGF2, and fibroblast growth factor 2 (FGF2) are factors that do not show big differences in the regeneration of sensory and motor fibers.
Nerve growth factor, BDNF, and IGF1 are strongly involved in the regeneration of sensory nerves, showing very high concentrations since the beginning of the regeneration and very similar dynamics; in the motor nerve injuries, their expression is remarkably low (Figure 7B, first row). PTN and GDNF behave in a similar way but in favor of the motor axons (Figure 7B, second row). The two groups behave in similar manners, reaching their maximum concentrations 15 days after injury when promoting the regeneration, while the increase lasts only up to 5 days when the molecules are not favoring the growth of that type of fiber. All but NGF also exert a facilitating influence in the regeneration of nerves of the other sensibility, although in these cases the expression of these molecules is very limited on time and amount (Figure 7B). As for the molecules whose expression is not specific for the differential growth, such as NT3, CNTF, IGF2, and FGF2, they display neither intragroup nor intergroup similarities in their temporal behavior (Figure 7B, third row). It is important to highlight that the data represented in Figure 7 correspond to the mRNA and protein expression, which are very correlated, but not necessarily presenting the exact same profile.
Different methods have been studied for the administration of neurotrophic factors at the level of the sectioned nerve. Two main problems are the difficulty of a controlled administration for long periods of time and the diffusion of the neurotrophic factors far from the area of interest. The use of biomaterials allowed higher precision and greater control in the administration of the neurotrophic factors: osmotic pumps, hydrogels, polymeric microspheres, and the inclusion of the different molecules at the level of the tubular conduct implanted (Tajdaran et al., 2019). Factors are included within the lumen (hydrogels, nanofibers, and through the presence of cells that produce neurotrophic factors), in the conduit wall (included in the polymer or in microspheres), and at the surface (adsorbed or conjugated with other type of molecules) (Carvalho et al., 2019).
Biomaterials are important to be semipermeable, to allow the exchange of molecules between the growing axons and the environment. The size of the scaffold should be adaptable to the anatomy of each injured nerve. The presence of pores for a continuous exchange of molecules between the neural axes, the Schwann cells, and the surrounding tissue is important (Figure 6). Hydrogels seem to have the greatest future – thanks to their ability to release, the neurotrophic factors in a controlled manner, and to the presence and accumulation of high volumes of water – and to create physical conditions very similar to those of the nervous system (Li et al., 2018).
Implementation of Neurotrophic Factors–Based Separation of Sensory and Motor Fibers
To guide fibers of the two modalities (sensory and motor) toward their dedicated cylinders, it is necessary, to first identify/select the sensory and the motor ones. A reasonable way to do that is to use several neurotrophins to favor the growing of one type of fibers in one direction, while preventing fibers of the opposite modality from growing with them, and vice versa. This is not a trivial question because there is only one inhibitory neurotrophic factor (NGF), and its action is exercised against the motor fibers (Table 2). This lack of selectively inhibiting biomolecules makes the separation of the fibers by biomolecular mutual repulsion–attraction complicated.
Conveying of sensory and motor fibers to these targets using only one inhibitory neurotrophin could be achieved by an intermittent short-term release of NGF close to the scaffolds dedicated to the sensitive fibers, combined with a timely release of PTN and GDNF from the interior of the motor channels. Discrete NGF amounts should diffuse in the scaffold and attract sensory fibers while blocking the motor ones; NGF deactivation should leave motor fibers free to start growing and get attracted by the neurotrophins-releasing motor channels. NGF activation/deactivation has to operate at the microscopic level (at the level of the channels of the scaffold, see section “Repair Phase”), creating a very short-term, high-amplitude attraction gradient (biomolecules concentrations). This NGF gradient would correlate with the NGF mRNA expression, which is the fastest molecule that reaches a high level of expression.
The importance of the neurotrophic factors’ administration goes further than just guiding the growth of the regenerating neurons. The function that this type of molecules carries out is well known, in the myelination process, during development (Piirsoo et al., 2010), and in the case of remyelination, once the axons reinnervate their target (Chan et al., 2001; Acosta et al., 2015; KhorshidAhmad et al., 2016; Razavi et al., 2017, 2018). This type of molecules is involved in the modification of the Schwann cells into a myelinating phenotype, once the regeneration is completed, activation that takes place via p75 receptor (Cosgaya et al., 2002; Notterpek, 2003; Xiao et al., 2013).
Implementation of Substrate-Related and Other Facilitator Biomolecules
In the design of a biomimetic device, facilitator molecules integrated into the surrounding tissue, as well as attracting biomolecules produced by the distal end, should also been taken into account. Among the former, the most promising are fibronectin and laminin, and both can be used for the regeneration of sensory and motor fibers, although the preference of the fibronectin is slightly higher for motor, and the preference of laminin is for the sensory (Tucker and Mearow, 2008; Gonzalez-Perez et al., 2013, 2016). Factors integrated in the surrounding nerve tissue, which favor the binding of neurons to the substrate or cell–cell binding (mainly glycoproteins such as integrins, N-CAM, N-cadherins, or L1) (Seilheimer and Schachner, 1988; Smith et al., 1994; Hammarberg et al., 2000; Franz et al., 2005; Saito et al., 2005, 2010; Gardiner et al., 2007; Tucker and Mearow, 2008; Gardiner, 2011; Anand et al., 2017); ECM molecules (fibrinogen, fibronectin, or laminin) (Politis, 1989; Hammarberg et al., 2000; Zhang et al., 2003; Previtali et al., 2008; Tucker and Mearow, 2008; Webber et al., 2008; Gardiner, 2011; Fudge and Mearow, 2013; Zeng et al., 2014; Santos et al., 2016b); and molecules that act as chemorepellents or chemoattractants (semaphorins, ephrins, and netrins) (Tessier-Lavigne and Goodman, 1996; Dickson, 2002; Wang et al., 2013; Kaselis et al., 2014; Alto and Terman, 2017).
Last but not least, more molecules are produced at the distal end of the damaged nerve that serve to attract regenerating axons or to create an attractive substrate inside the distal endoneural tube (Figure 5).
In the case of biomaterial engineering and tissue engineering, these factors can serve the same functions that they perform in natural tissue, which is to facilitate the adhesion of axons and stimulate their growth, being their incorporation necessary when the artificial material is not attractive to axons.
Discussion
A well-differentiated and ordered regeneration of the sensory and motor fibers is mandatory in all PNI cases: short/non-gap injuries, large gap injuries, amputations, neuropathies of traumatic etiology, phantom limb pain, etc. Clinical problems such as incomplete regeneration, impossibility of achieving differential growth of sensory and motor nerves, etc., are all due to the fact that current therapies (biomolecules, bio-hybrid scaffolds, etc.) all act at the level of the entire nerve, and its action cannot be transferred to individual axons or fiber fascicles (Du et al., 2018; Yi et al., 2019). Any biomolecule showing affinity for one type of fiber under spontaneous PNI repair, if applied at the microscopic level (individual axons level), loses its effectivity if it is applied at macroscopic level (entire peripheral nerve). Advanced devices and novel biomaterials at the microscopic level are required for the implementation of the desired functionalities based on neurotrophic factors and facilitator biomolecules.
From the previous discussion, the basic requirements for these novel biomaterials can be inferred at three levels: geometrical, mechanical, and biochemical. The size of the axons and the fascicles implies that the cross-sectional size of the scaffold should be in the range between a few and 100 μm, whereas the longitudinal size should be in the range of millimeters and even centimeters. This geometry, in turn, possesses a heavy constraint on the mechanical performance of the biomaterial, because it must sustain the surgical process, as well as the in-service life. In addition, the material must be compatible with the neurotrophic factors and adherent facilitator biomolecules.
Most present solutions cover some, but not all of these three requirements. Both natural and artificial materials are used for repairing PNIs (Aijie et al., 2018; Boni et al., 2018). Present commercial solutions are based on poly(glycolic acid) (Neurotube) and poly(D,L-lactide-co-e-caprolactone)–based (Neurolac) (Du et al., 2018), but other solutions were explored based on polylactic acid (Zeng et al., 2014), polylactic glycolic acid (Xue et al., 2012), and polyethylene glycol (Liu et al., 2015). Other proposals based on artificial materials employ electrical conductive polymers, such as PANi (Xu et al., 2016) and indium phosphide (InP) (Gautam et al., 2017), or carbon-based materials (Boni et al., 2018), such as graphene and carbon nanotubes. The use of artificial materials faces a number of problems. Thus, their biocompatibility tends not to be optimal, and most artificial materials cannot be functionalized. Natural materials tend to be more biocompatible, and consequently, these materials were also used as scaffolds for PNIs. Among the natural materials, solutions were proposed based on collagen (Mackinnon and Lee Dellon, 1990; Archibald et al., 1995) (NeuraGen, NeuroMatrix, and NeuraWrap), gelatin (Ghasemi-Mobarakeh et al., 2008; Zhu, 2010), hyaluronic acid (Song et al., 2014; Kondyurin et al., 2017), alginate (Sitoci-Ficici et al., 2018), chitosan (Wang et al., 2006), and keratin (Apel et al., 2008). However, most of these materials are difficult to be processed in scaffolds with the geometry and mechanical properties indicated above.
The nervous system has a superior level of complexity that demands sophisticated scaffolds and architectures, as well highly tolerable biomaterial formulations. Compatibility and toxicity still are a remaining concern that has not been completely resolved for an immense number of materials. For many tissues, classical biomaterials may result in feasible strategies that in general produce good positive outcomes (Green and Elisseeff, 2016). But neurobioengineering strategies are intricate because of the restrictive conditions of the nerve microenvironment. For example, certain byproducts derived from a classic material such as the hyaluronic acid are immunogenic and may trigger inflammation (Tesar et al., 2006). Deposits of hyaluronic acid may accumulate in demyelinated lesions preventing axon remyelination and functional rewiring (Back et al., 2005).
Among the different biomaterial formats, hydrogels-based formulations have been engineered and widely used to construct porous conduits to favor axonal guidance providing suitable environments or reconnect two peripheral nerve ends (Tao et al., 2017). Hydrogel conduits have also been assessed to advance in the resolution of other clinical problems of neurological origin, e.g., reconstruction of intraspinal neural circuits in spinal cords injuries (Marchini et al., 2019) or reconstruction of neurodegenerated nigrostriatal pathways in Parkinson disease (Struzyna et al., 2018). Considering that not all biomaterials accomplish for the strict requirements of the nervous system, silk appears as a very promising solution (Zhang et al., 2012). Because of its high biocompatibility and excellent mechanical properties (Heim et al., 2009), silk is currently being explored for the development of many new therapies (Vepari and Kaplan, 2007; Fernández-García et al., 2018). Furthermore, the possibility of producing regenerated silk fibers [artificially spun fibers, from a solution of natural silk protein (Madurga et al., 2017b)] led to the generation of high-performance silk fibroin fibers with a wide range of geometries, which, in addition, can be functionalized (Madurga et al., 2017a). The development of adequate materials for scaffolds that can be used for nerve repair, bridging gaps in lesioned nerves, will require a very intensive research program. However, the present range of available materials in combination with a deeper understanding of the biology of the natural repair processes opens new and promising perspectives for the development of these therapies.
Conclusion
Current therapies are not effective and do not achieve a good regeneration because they act at the level of the entire nerve and not at the level of neuronal fiber or group of neuronal fibers and because they do not take into account the synergies between neurotrophic factors and their dynamic expressions over time and in the space. For the development of bio-hybrid materials for the correct regeneration of PNI, effective and reliable solutions must (i) work at microscopic level and (ii) take into account the molecular mechanisms of the regeneration and functional reinnervation of the sensory and motor nerves in both the proximal and the distal parts of the severed nerve.
Data Availability Statement
The original contributions presented in the study are included in the article/supplementary material, further inquiries can be directed to the corresponding author.
Author Contributions
AG-D and NJ-D: bibliographic research, information synthesis, writing the manuscript, and equal contribution. IO-Z: bibliographic research. GG, JP-R, DG-N: biomaterials aspects, critical DGN and manuscript revision. FP: manuscript design, information synthesis, supervision, and writing the manuscript. All authors contributed to the article and approved the submitted version.
Conflict of Interest
The authors declare that the research was conducted in the absence of any commercial or financial relationships that could be construed as a potential conflict of interest.
Acknowledgments
The authors gratefully acknowledge financial support received from the Spanish Ministerio de Economía y Competitividad Spain through grants MAT2016-76847-R, MAT2016-79832-R, and MAT2015-66666-C3-3-R and predoctoral FPI grant (AGD) as well as from the Comunidad de Madrid through grants Neurocentro-B2017/BMD-3760 and IND2018/BMD-9804.
Abbreviations
BDNF, brain-derived neurotrophic factor; CNTF, ciliary neurotrophic factor; CNTFR, ciliary neurotrophic factor receptor; ECM, extracellular matrix; FGF2, fibroblast growth factor 2; GDNF, glial cell line–derived neurotrophic factor; IGF1, insulin-like growth factor 1; IGF2, insulin-like growth factor 2; IL6, interleukin 6; InP, indium phosphide; LIF, leukemia inhibitory factor; LIFR, leukemia inhibitory factor receptor; MAG, myelin-associated glycoprotein; MBP, myelin basic protein; N-CAM, neural cell adhesion molecule; NGF, nerve growth factor; NT3, neurotrophin 3; NT4/5, neurotrophin 4/5; P0, myelin protein 0; p75, low-affinity nerve growth factor receptor; PANi, polyaniline; PMP22, peripheral myelin protein 22; PNI, peripheral nerve injury; PNS, peripheral nervous system; PTN, pleiotrophin; Trk, tropomyosin receptor kinase.
References
Abou-Zamzam, A. M., Teruya, T. H., Killeen, J. D., and Ballard, J. L. (2003). Major lower extremity amputation in an academic vascular center. Ann. Vasc. Sur. 17, 86–90. doi: 10.1007/s10016-001-0340-0
Acosta, C., Cortes, C., Altaweel, K., MacPhee, H., Hoogervorst, B., Bhullar, H., et al. (2015). Immune system induction of nerve growth factor in an animal model of multiple sclerosis: implications in re-myelination and myelin RepairATION AND MYELIN REPAIR. CNS Neurol. Disord. Drug Targets 14, 1069–1078. doi: 10.2174/1871527314666150317225205
Aijie, C., Xuan, L., Huimin, L., Yanli, Z., Yiyuan, K., Yuqing, L., et al. (2018). Nanoscaffolds in promoting regeneration of the peripheral nervous system. Nanomedicine 13, 1067–1085. doi: 10.2217/nnm-2017-0389
Allodi, I., Udina, E., and Navarro, X. (2012). Specificity of peripheral nerve regeneration: interactions at the axon level. Prog. Neurobiol. 98, 16–37. doi: 10.1016/j.pneurobio.2012.05.005
Al-Majed, A. A., Brushart, T. M., and Gordon, T. (2000). Electrical stimulation accelerates and increases expression of BDNF and trkB mRNA in regenerating rat femoral motoneurons. Eur. J. Neurosci. 17, 86–90. doi: 10.1046/j.1460-9568.2000.01341.x
Al-Majed, A. A., Siu, L. T., and Gordon, T. (2004). Electrical stimulation accelerates and enhances expression of regeneration-associated genes in regenerating rat femoral motoneurons. Cell. Mol. Neurobiol. 24, 379–402. doi: 10.1023/B:CEMN.0000022770.66463.f7
Alsmadi, N. Z., Bendale, G. S., Kanneganti, A., Shihabeddin, T., Nguyen, A. H., Hor, E., et al. (2018). Glial-derived growth factor and pleiotrophin synergistically promote axonal regeneration in critical nerve injuries. Acta Biomater. 78, 165–177. doi: 10.1016/j.actbio.2018.07.048
Alto, L. T., and Terman, J. R. (2017). Semaphorins and their signaling mechanisms. Methods Mol. Biol. 1493, 1–25. doi: 10.1007/978-1-4939-6448-2_1
Anand, S., Desai, V., Alsmadi, N., Kanneganti, A., Nguyen, D. H. T., Tran, M., et al. (2017). Asymmetric sensory-motor regeneration of transected peripheral nerves using molecular guidance cues. Sci. Rep. 7:14323. doi: 10.1038/s41598-017-14331-x
Apel, P. J., Garrett, J. P., Sierpinski, P., Ma, J., Atala, A., Smith, T. L., et al. (2008). Peripheral nerve regeneration using a keratin-based scaffold: long-term functional and histological outcomes in a mouse model. J. Hand Surg. 33, 1541–1547. doi: 10.1016/j.jhsa.2008.05.034
Apel, P. J., Ma, J., Callahan, M., Northam, C. N., Alton, T. B., Sonntag, W. E., et al. (2010). Effect of locally delivered IGF-1 on nerve regeneration during aging: an experimental study in rats. Muscle Nerve 41, 335–341. doi: 10.1002/mus.21485
Apfel, S. C. (1999). Neurotrophic factors in peripheral neuropathies: therapeutic implications. Brain Pathol. 9, 393–413. doi: 10.1111/j.1750-3639.1999.tb00234.x
Apfel, S. C. (2002). Nerve growth factor for the treatment of diabetic neuropathy: what went wrong, what went right, and what does the future hold? Int. Rev. Neurobiol. 50, 393–413. doi: 10.1016/s0074-7742(02)50083-0
Archibald, S. J., Shefner, J., Krarup, C., and Madison, R. D. (1995). Monkey median nerve repaired by nerve graft or collagen nerve guide tube. J. Neurosci. 15, 4109–4123. doi: 10.1523/jneurosci.15-05-04109.1995
Atala, A., Kurtis Kasper, F., and Mikos, A. G. (2012). Engineering complex tissues. Sci. Transl. Med. 4:160rv12. doi: 10.1126/scitranslmed.3004890
Averill, S., McMahon, S. B., Clary, D. O., Reichardt, L. F., and Priestley, J. V. (1995). Immunocytochemical localization of trkA receptors in chemically identified subgroups of adult rat sensory neurons. Eur. J. Neurosci. 7, 1484–1494. doi: 10.1111/j.1460-9568.1995.tb01143.x
Back, S. A., Tuohy, T. M. F., Chen, H., Wallingford, N., Craig, A., Struve, J., et al. (2005). Hyaluronan accumulates in demyelinated lesions and inhibits oligodendrocyte progenitor maturation. Nat. Med. 11, 966–972. doi: 10.1038/nm1279
Ballester, B. R., Maier, M., Duff, A., Cameirão, M., Bermúdez, S., Duarte, E., et al. (2019). A critical time window for recovery extends beyond one-year post-stroke. J. Neurophysiol. 122, 350–357. doi: 10.1152/jn.00762.2018
Barton, M. J., St John, J., Clarke, M., Wright, A., and Ekberg, J. (2017). The glia response after peripheral nerve injury: a comparison between Schwann cells and olfactory ensheathing cells and their uses for neural regenerative therapies. Int. J. Mol. Sci. 18:287. doi: 10.3390/ijms18020287
Battista, A. F., and Lusskin, R. (1986). The anatomy and physiology of the peripheral nerve. Foot Ankle 7, 65–70. doi: 10.1177/107110078600700202
Biondi, M., Ungaro, F., Quaglia, F., and Netti, P. A. (2008). Controlled drug delivery in tissue engineering. Adv. Drug Deliv. Rev. 60, 229–242. doi: 10.1016/j.addr.2007.08.038
Boni, R., Ali, A., Shavandi, A., and Clarkson, A. N. (2018). Current and novel polymeric biomaterials for neural tissue engineering. J. Biomed. Sci. 25:90. doi: 10.1186/s12929-018-0491-8
Boyd, J. G., and Gordon, T. (2003). Neurotrophic factors and their receptors in axonal regeneration and functional recovery after peripheral nerve injury. Mol. Neurobiol. 27, 277–323. doi: 10.1385/MN:27:3:277
Bradke, F., Fawcett, J. W., and Spira, M. E. (2012). Assembly of a new growth cone after axotomy: the precursor to axon regeneration. Nat. Rev. Neurosci. 13, 183–193. doi: 10.1038/nrn3176
Brattain, K. (2014). Analysis the Peripheral Nerve Repair Market in the United States. Magellan Medical Technology Consultants, Inc., 1–11.
Brushart, T. M., Aspalter, M., Griffin, J. W., Redett, R., Hameed, H., Zhou, C., et al. (2013). Schwann cell phenotype is regulated by axon modality and central-peripheral location, and persists in vitro. Exp. Neurol. 247, 272–281. doi: 10.1016/j.expneurol.2013.05.007
Bunge, R. P., Bartlett Bunge, M., and Eldridge, C. F. (1986). Linkage between axonal ensheathment and basal lamina production by Schwann cells. Annu. Rev. Neurosci. 9, 305–328. doi: 10.1146/annurev.neuro.9.1.305
Caillaud, M., Richard, L., Vallat, J. M., Desmoulière, A., and Billet, F. (2019). Peripheral nerve regeneration and intraneural revascularization. Neural Regen. Res. 14, 24–33. doi: 10.4103/1673-5374.243699
Caplan, J., Tiangco, D. A., and Terzis, J. K. (1999). Effects of IGF-II in a new end-to-side model. J. f Reconstr. Microsurg. 15, 351–358. doi: 10.1055/s-2007-1000115
Carvalho, C. R., Oliveira, J. M., and Reis, R. L. (2019). Modern trends for peripheral nerve repair and regeneration: beyond the hollow nerve guidance conduit. Front. Bioeng. Biotechnol. 7:337. doi: 10.3389/fbioe.2019.00337
Catala, M., and Kubis, N. (2013). Gross anatomy and development of the peripheral nervous system. Handb. Clin. Neurol. 115, 29–41. doi: 10.1016/B978-0-444-52902-2.00003-5
Chan, J. R., Cosgaya, J. M., Wu, Y. J., and Shooter, E. M. (2001). Neurotrophins are key mediators of the myelination program in the peripheral nervous system. Proc. Natl. Acad. Sci. U.S.A. 98, 14661–14668. doi: 10.1073/pnas.251543398
Chang, Y. C., Chen, M. H., Liao, S. Y., Wu, H. C., Kuan, C. H., Sun, J. S., et al. (2017). Multichanneled nerve guidance conduit with spatial gradients of neurotrophic factors and oriented nanotopography for repairing the peripheral nervous system. ACS Appl. Mater. Interfaces 9, 37623–37636. doi: 10.1021/acsami.7b12567
Chen, P., Piao, X., and Bonaldo, P. (2015). Role of macrophages in wallerian degeneration and axonal regeneration after peripheral nerve injury. Acta Neuropathol. 130, 605–618. doi: 10.1007/s00401-015-1482-4
Clemente, F., Valle, G., Controzzi, M., Strauss, I., Iberite, F., Stieglitz, T., et al. (2019). Intraneural sensory feedback restores grip force control and motor coordination while using a prosthetic hand. J. Neural Eng. 16:026034. doi: 10.1088/1741-2552/ab059b
Cosgaya, J. M., Chan, J. R., and Shooter, E. M. (2002). The neurotrophin receptor p75NTR as a positive modulator of myelination. Science 298, 1245–1248. doi: 10.1126/science.1076595
Costa Serrão de Araújo, G., Couto Neto, B., Harley Santos, Botelho, R., and Carpi Malta, M. (2017). Clinical evaluation after peripheral nerve repair with caprolactone neurotube. Hand 12, 168–174. doi: 10.1177/1558944716643277
Court, F. A., Wrabetz, L., and Feltri, M. L. (2006). Basal lamina: schwann cells wrap to the rhythm of space-time. Curr. Opin. Neurobiol. 16, 501–507. doi: 10.1016/j.conb.2006.08.005
Cuartero, D. M. C., García, A. M. E., and García, A. A. M. (2012). Undefined Síndrome del Miembro fantasma. Dialnet.unirioja.es. Available online at: https://dialnet.unirioja.es/servlet/articulo?codigo=6341501 (accessed September 2, 2019).
Cuberovic, I., Gill, A., Resnik, L. J., Tyler, D. J., and Graczyk, E. L. (2019). Learning of artificial sensation through long-term home use of a sensory-enabled prosthesis. Front. Neurosci. 13:853. doi: 10.3389/fnins.2019.00853
Dalamagkas, K., Tsintou, M., and Seifalian, A. (2016). Advances in peripheral nervous system regenerative therapeutic strategies: a biomaterials approach. Mater. Sci. Eng. C 65, 425–432. doi: 10.1016/j.msec.2016.04.048
D’Anna, E., Valle, G., Mazzoni, A., Strauss, I., Iberite, F., Patton, J., et al. (2019). A closed-loop hand prosthesis with simultaneous intraneural tactile and position feedback. Sci. Robot. 4:eaau8892. doi: 10.1126/scirobotics.aau8892
del Valle, J., Santos, D., Delgado-Martínez, I., de la Oliva, N., Giudetti, G., Micera, S., et al. (2018). Segregation of motor and sensory axons regenerating through bicompartmental tubes by combining extracellular matrix components with neurotrophic factors. J. Tissue Eng. Regen. Med. 12, e1991–e2000. doi: 10.1002/term.2629
Deshmukh, A., Brown, L., Barbe, M. F., Braverman, A. S., Tiwari, E., Hobson, L., et al. (2020). Fully implantable neural recording and stimulation interfaces: peripheral nerve interface applications. J. Neurosci. Methods 333:108562. doi: 10.1016/j.jneumeth.2019.108562
Di Giulio, A. M., Germani, E., Lesma, E., Muller, E., and Gorio, A. (2000). Glycosaminoglycans co-administration enhance insulin-like growth factor-I neuroprotective and neuroregenerative activity in traumatic and genetic models of motor neuron disease: a review. In. J. Dev. Neurosci. 18, 339–346. doi: 10.1016/S0736-5748(00)00015-0
Di Pino, G., Benvenuto, A., Tombini, M., Cavallo, G., Denaro, L., Denaro, V., et al. (2012). “Overview of the implant of intraneural multielectrodes in human for controlling a 5-fingered hand prosthesis, delivering sensorial feedback and producing rehabilitative neuroplasticity,” in Proceedings of the IEEE RAS and EMBS International Conference on Biomedical Robotics and Biomechatronics, Rome, 1831–1836. doi: 10.1109/BioRob.2012.6290808
Dickson, B. J. (2002). Molecular mechanisms of axon guidance. Barry J. Dickson. Sci. 298, 1959–1964.
Dillingham, T. R., Pezzin, L. E., and MacKenzie, E. J. (2002). Limb amputation and limb deficiency: epidemiology and recent trends in the United States. Southern Med. J. 95, 875–883. doi: 10.1097/00007611-200208000-00018
Dinis, T. M., Elia, R., Vidal, G., Dermigny, Q., Denoeud, C., Kaplan, D. L., et al. (2015). 3D multi-channel bi-functionalized silk electrospun conduits for peripheral nerve regeneration. J. Mech. Behav. Biomed. Mater. 41, 43–55. doi: 10.1016/j.jmbbm.2014.09.029
Dixon, A. R., Jariwala, S. H., Bilis, Z., Loverde, J. R., Pasquina, P. F., and Alvarez, L. M. (2018). Bridging the gap in peripheral nerve repair with 3D printed and bioprinted conduits. Biomaterials 186, 44–63. doi: 10.1016/j.biomaterials.2018.09.010
Dromerick, A. W., Edwardson, M. A., Edwards, D. F., Giannetti, M. L., Barth, J., Brady, K. P., et al. (2015). Critical periods after stroke study: translating animal stroke recovery experiments into a clinical trial. Front. Hum. Neurosci. 9:231. doi: 10.3389/fnhum.2015.00231
Du, J., Chen, H., Qing, L., Yang, X., and Jia, X. (2018). Biomimetic neural scaffolds: a crucial step towards optimal peripheral nerve regeneration. Biomater. Sci. 6, 1299–1311. doi: 10.1039/c8bm00260f
Dubový, P. (2004). Schwann cells and endoneurial extracellular matrix molecules as potential cues for sorting of regenerated axons: a review. Anatom. Sci. Int. 79, 198–208. doi: 10.1111/j.1447-073x.2004.00090.x
Dubový, P., Raška, O., Klusáková, I., Stejskal, L., Èelakovský, P., and Haninec, P. (2011). Ciliary neurotrophic factor promotes motor reinnervation of the musculocutaneous nerve in an experimental model of end-to-side neurorrhaphy. BMC Neurosci. 12:58. doi: 10.1186/1471-2202-12-58
Eberhardt, K. A., Irintchev, A., Al-Majed, A. A., Simova, O., Brushart, T. M., Gordon, T., et al. (2006). BDNF/TrkB signaling regulates HNK-1 carbohydrate expression in regenerating motor nerves and promotes functional recovery after peripheral nerve repair. Exp. Neurol. 198, 500–510. doi: 10.1016/j.expneurol.2005.12.018
English, A. W., Cucoranu, D., Mulligan, A., Rodriguez, J. A., and Sabatier, M. J. (2011). Neurotrophin-4/5 is implicated in the enhancement of axon regeneration produced by treadmill training following peripheral nerve injury. Eur. J. Neurosci. 33, 2265–2271. doi: 10.1111/j.1460-9568.2011.07724.x
English, A. W., Meador, W., and Carrasco, D. I. (2005). Neurotrophin-4/5 is required for the early growth of regenerating axons in peripheral nerves. Eur. J. Neurosci. 21, 2624–2634. doi: 10.1111/j.1460-9568.2005.04124.x
Ertürk, A., Hellal, F., Enes, J., and Bradke, F. (2007). Disorganized microtubules underlie the formation of retraction bulbs and the failure of axonal regeneration. J. Neurosci. 27, 9169–9180. doi: 10.1523/JNEUROSCI.0612-07.2007
Eva, R., and Fawcett, J. (2014). Integrin signalling and traffic during axon growth and regeneration. Curr. Opin. Neurobiol. 27, 179–185. doi: 10.1016/j.conb.2014.03.018
Faroni, A., Mobasseri, S. A., Kingham, P. J., and Reid, A. J. (2015). Peripheral nerve regeneration: experimental strategies and future perspectives. Adv. Drug Deliv. Rev. 82, 160–167. doi: 10.1016/j.addr.2014.11.010
Fernández-García, L., Pérez-Rigueiro, J., Martinez-Murillo, R., Panetsos, F., Ramos, M., Guinea, G. V., et al. (2018). Cortical reshaping and functional recovery induced by silk fibroin hydrogels-encapsulated stem cells implanted in stroke animals. Front. Cell. Neurosci. 12:296. doi: 10.3389/fncel.2018.00296
Fine, E. G., Decosterd, I., Papaloïzos, M., Zurn, A. D., and Aebischer, P. (2002). GDNF and NGF released by synthetic guidance channels support sciatic nerve regeneration across a long gap. Eur. J. Neurosci. 15, 589–601. doi: 10.1046/j.1460-9568.2002.01892.x
Flores, A. J., Lavernia, C. J., and Owens, P. W. (2000). Anatomy and physiology of peripheral nerve injury and repair. Am. J. Orthoped. 29, 167–173.
Franz, C. K., Rutishauser, U., and Rafuse, V. F. (2005). Polysialylated neural cell adhesion molecule is necessary for selective targeting of regenerating motor neurons. J. Neurosci. 25, 2081–2091. doi: 10.1523/JNEUROSCI.4880-04.2005
Friedman, B., Kleinfeld, D., Ip, N. Y., Verge, V. M. K., Moulton, R., Boland, P., et al. (1995). BDNF and NT-4/5 exert neurotrophic influences on injured adult spinal motor neurons. J. Neurosci. 15, 1044–1056. doi: 10.1523/jneurosci.15-02-01044.1995
Fu, S. Y., and Gordon, T. (1997). The cellular and molecular basis of peripheral nerve regeneration. Mol. Neurobiol. 14, 67–116. doi: 10.1007/BF02740621
Fudge, N. J., and Mearow, K. M. (2013). Extracellular matrix-associated gene expression in adult sensory neuron populations cultured on a laminin substrate. BMC Neurosci. 14:15. doi: 10.1186/1471-2202-14-15
Funakoshi, H., Frisén, J., Barbany, G., Timmusk, T., Zachrisson, O., Verge, V. M. K., et al. (1993). Differential expression of mRNAs for neurotrophins and their receptors after axotomy of the sciatic nerve. J. Cell Biol. 123, 455–465. doi: 10.1083/jcb.123.2.455
Gardiner, N. J. (2011). Integrins and the extracellular matrix: key mediators of development and regeneration of the sensory nervous system. Dev. Neurobiol. 71, 1054–1072. doi: 10.1002/dneu.20950
Gardiner, N. J., Moffatt, S., Fernyhough, P., Humphries, M. J., Streuli, C. H., and Tomlinson, D. R. (2007). Preconditioning injury-induced neurite outgrowth of adult rat sensory neurons on fibronectin is mediated by mobilisation of axonal α5 integrin. Mol. Cell. Neurosci. 35, 249–260. doi: 10.1016/j.mcn.2007.02.020
Gautam, V., Naureen, S., Shahid, N., Gao, Q., Wang, Y., Nisbet, D., et al. (2017). Engineering highly interconnected neuronal networks on nanowire scaffolds. Nano Lett. 17, 3369–3375. doi: 10.1021/acs.nanolett.6b05288
George, J. A., Kluger, D. T., Davis, T. S., Wendelken, S. M., Okorokova, E. V., He, Q., et al. (2019). Biomimetic sensory feedback through peripheral nerve stimulation improves dexterous use of a bionic hand. Sci. Robot. 4:eaax2352. doi: 10.1126/scirobotics.aax2352
Geremia, N. M., Pettersson, L. M. E., Hasmatali, J. C., Hryciw, T., Danielsen, N., Schreyer, D. J., et al. (2010). Endogenous BDNF regulates induction of intrinsic neuronal growth programs in injured sensory neurons. Exp. Neurol. 223, 128–142. doi: 10.1016/j.expneurol.2009.07.022
Ghasemi-Mobarakeh, L., Prabhakaran, M. P., Morshed, M., Nasr-Esfahani, M. H., and Ramakrishna, S. (2008). Electrospun poly(ε-caprolactone)/gelatin nanofibrous scaffolds for nerve tissue engineering. Biomaterials 29, 4532–4539. doi: 10.1016/j.biomaterials.2008.08.007
Glazner, G. W., Lupien, S., Miller, J. A., and Ishii, D. N. (1993). Insulin-like growth factor II increases the rate of sciatic nerve regeneration in rats. Neuroscience 54, 791–797. doi: 10.1016/0306-4522(93)90248-E
Gonzalez-Perez, F., Alé, A., Santos, D., Barwig, C., Freier, T., Navarro, X., et al. (2016). Substratum preferences of motor and sensory neurons in postnatal and adult rats. Eur. J. Neurosci. 43, 431–442. doi: 10.1111/ejn.13057
Gonzalez-Perez, F., Hernández, J., Heimann, C., Phillips, J. B., Udina, E., and Navarro, X. (2018). Schwann cells and mesenchymal stem cells in laminin-or fbronectin-aligned matrices and regeneration across a critical size defect of 15 mm in the rat sciatic nerve. J. Neurosurg. 28, 109–118. doi: 10.3171/2017.5.SPINE161100
Gonzalez-Perez, F., Udina, E., and Navarro, X. (2013). Extracellular matrix components in peripheral nerve regeneration. Int. Rev. Neurobiol. 108, 257–275. doi: 10.1016/B978-0-12-410499-0.00010-1
Gordon, T. (2009). The role of neurotrophic factors in nerve regeneration. Neurosurg. Focus 26, 1–10. doi: 10.3171/FOC.2009.26.2.E3
Gordon, T. (2014). Neurotrophic factor expression in denervated motor and sensory schwann cells: relevance to specificity of peripheral nerve regeneration. Exp. Neurol. 254, 99–108. doi: 10.1016/j.expneurol.2014.01.012
Graczyk, E. L., Resnik, L., Schiefer, M. A., Schmitt, M. S., and Tyler, D. J. (2018). Home use of a neural-connected sensory prosthesis provides the functional and psychosocial experience of having a hand again. Sci. Rep. 8:9866. doi: 10.1038/s41598-018-26952-x
Green, J. J., and Elisseeff, J. H. (2016). Mimicking biological functionality with polymers for biomedical applications. Nature 540, 386–394. doi: 10.1038/nature21005
Griffin, J. W., Hogan, M. C. V., Chhabra, A. B., and Deal, D. N. (2013). Peripheral nerve repair and reconstruction. J. Bone Joint Surg. Ser. A 95, 2144–2151. doi: 10.2106/JBJS.L.00704
Griffin, J. W., and Thompson, W. J. (2008). Biology and pathology of nonmyelinating schwann cells. Glia 56, 1518–1531. doi: 10.1002/glia.20778
Grinsell, D., and Keating, C. P. (2014). Peripheral nerve reconstruction after injury: a review of clinical and experimental therapies. BioMed Res. Int. 2014:698256. doi: 10.1155/2014/698256
Grosheva, M., Nohroudi, K., Schwarz, A., Rink, S., Bendella, H., Sarikcioglu, L., et al. (2016). Comparison of trophic factors’ expression between paralyzed and recovering muscles after facial nerve injury. A quantitative analysis in time course. Exp. Neurol. 279, 137–148. doi: 10.1016/j.expneurol.2016.02.020
Grothe, C., Haastert, K., and Jungnickel, J. (2006). Physiological function and putative therapeutic impact of the FGF-2 system in peripheral nerve regeneration-Lessons from in vivo studies in mice and rats. Brain Res. Rev. 51, 293–299. doi: 10.1016/j.brainresrev.2005.12.001
Gu, X., Ding, F., and Williams, D. F. (2014). Neural tissue engineering options for peripheral nerve regeneration. Biomaterials 35, 6143–6156. doi: 10.1016/j.biomaterials.2014.04.064
Gu, X., Ding, F., Yang, Y., and Liu, J. (2011). Construction of tissue engineered nerve grafts and their application in peripheral nerve regeneration. Prog. Neurobiol. 93, 204–230. doi: 10.1016/j.pneurobio.2010.11.002
Günter, C., Delbeke, J., and Ortiz-Catalan, M. (2019). Safety of long-term electrical peripheral nerve stimulation: review of the state of the art. J. NeuroEng. Rehabil. 16:13. doi: 10.1186/s12984-018-0474-8
Guzen, F. P., de Araújo, D. P., de Souza Lucena, E. E., de Morais, H. H. A., de Paiva Cavalcanti, J. R. L., do Nascimento, E. S., et al. (2016). Effect of FGF-2 and sciatic nerve grafting on ChAT expression in dorsal root ganglia neurons of spinal cord transected rats. Neurosc. Lett. 616, 43–48. doi: 10.1016/j.neulet.2015.08.043
Hammarberg, H., Piehl, F., Risling, M., and Cullheim, S. (2000). Differential regulation of trophic factor receptor mRNAs in spinal motoneurons after sciatic nerve transection and ventral root avulsion in the rat. J. Comp. Neurol. 426, 587–601. doi: 10.1002/1096-9861(20001030)426:4<587::AID-CNE7<3.0.CO;2-R
Heim, M., Keerl, D., and Scheibel, T. (2009). Spider silk: from soluble protein to extraordinary fiber. Angew. Chem. Int. Ed. 48, 3584–3596. doi: 10.1002/anie.200803341
Herrera-Rincon, C., Cisneros, F. J., Castellanos, J., and Panetsos, F. (2011). “Effects of neuroprosthetic stimulation on the peripheral nerves of amputees: A digital image processing study,” in 2011 5th International IEEE/EMBS Conference on Neural Engineering, NER 2011, Cancun, 634–637. doi: 10.1109/NER.2011.5910628
Höke, A., Redett, R., Hameed, H., Jari, R., Zhou, C., Li, Z. B., et al. (2006). Schwann cells express motor and sensory phenotypes that regulate axon regeneration. J. Neurosci. 26, 9646–9655. doi: 10.1523/JNEUROSCI.1620-06.2006
Hou, S., Nicholson, L., Van Niekerk, E., Motsch, M., and Blesch, A. (2012). Dependence of regenerated sensory axons on continuous neurotrophin-3 delivery. J. Neurosci. 32, 13206–13220. doi: 10.1523/JNEUROSCI.5041-11.2012
Hoyng, S. A., De Winter, F., Gnavi, S., de Boer, R., Boon, L. I., Korvers, L. M., et al. (2014). A comparative morphological, electrophysiological and functional analysis of axon regeneration through peripheral nerve autografts genetically modified to overexpress BDNF, CNTF, GDNF, NGF, NT3 or VEGF. Exp. Neurol. 261, 578–593. doi: 10.1016/j.expneurol.2014.08.002
Jin, L., Jianghai, C., Juan, L., and Hao, K. (2009). Pleiotrophin and peripheral nerve injury. Neurosurg. Rev. 32, 387–393. doi: 10.1007/s10143-009-0202-8
Johnson, B. N., Lancaster, K. Z., Zhen, G., He, J., Gupta, M. K., Kong, Y. L., et al. (2015). 3D printed anatomical nerve regeneration pathways. Adv. Funct. Mater. 25, 6205–6217. doi: 10.1002/adfm.201501760
Kanje, M., Skottner, A., Sjöberg, J., and Lundborg, G. (1989). Insulin-like growth factor I (IGF-I) stimulates regeneration of the rat sciatic nerve. Brain Res. 486, 396–398. doi: 10.1016/0006-8993(89)90531-3
Karabekmez, F. E., Duymaz, A., and Moran, S. L. (2009). Early clinical outcomes with the use of decellularized nerve allograft for repair of sensory defects within the hand. Hand 4, 245–249. doi: 10.1007/s11552-009-9195-6
Kaselis, A., Treinys, R., Vosyliute, R., and Šatkauskas, S. (2014). DRG axon elongation and growth cone collapse rate induced by Sema3A are differently dependent on NGF concentration. Cel. Mol. Neurobiol. 34, 289–296. doi: 10.1007/s10571-013-0013-x
Keast, J. R., Forrest, S. L., and Osborne, P. B. (2010). Sciatic nerve injury in adult rats causes distinct changes in the central projections of sensory neurons expressing different glial cell line-derived neurotrophic factor family receptors. J. Comp. Neurol. 518, 3024–3045. doi: 10.1002/cne.22378
Kemp, S. W. P., Webb, A. A., Dhaliwal, S., Syed, S., Walsh, S. K., and Midha, R. (2011). Dose and duration of nerve growth factor (NGF) administration determine the extent of behavioral recovery following peripheral nerve injury in the rat. Exp. Neurol. 229, 460–470. doi: 10.1016/j.expneurol.2011.03.017
KhorshidAhmad, T., Acosta, C., Cortes, C., Lakowski, T. M., Gangadaran, S., and Namaka, M. (2016). Transcriptional regulation of brain-derived neurotrophic factor (BDNF) by Methyl CpG Binding Protein 2 (MeCP2): a novel mechanism for re-myelination and/or myelin repair involved in the treatment of multiple sclerosis (MS). Mol. Neurobiol. 53, 1092–1107. doi: 10.1007/s12035-014-9074-1
Kobayashi, N. R. (1996). Increased expression of BDNF and trkB mRNA in rat facial motoneurons after axotomy. Eur. J. Neurosci. 8, 1018–1029. doi: 10.1111/j.1460-9568.1996.tb01588.x
Koch, D., Rosoff, W. J., Jiang, J., Geller, H. M., and Urbach, J. S. (2012). Strength in the periphery: growth cone biomechanics and substrate rigidity response in peripheral and central nervous system neurons. Biophys. J. 102, 452–460. doi: 10.1016/j.bpj.2011.12.025
Kondyurin, A., Tsoutas, K., Latour, Q. X., Higgins, M. J., Moulton, S. E., McKenzie, D. R., et al. (2017). Structural analysis and protein functionalization of electroconductive polypyrrole films modified by plasma immersion ion implantation. ACS Biomater. Sci. Eng. 3, 2247–2258. doi: 10.1021/acsbiomaterials.7b00369
Krames, E. S. (2015). The dorsal root ganglion in chronic pain and as a target for neuromodulation: a review. Neuromodulation 18, 24–32. doi: 10.1111/ner.12247
Lee, B., Koripalli, M. K., Jia, Y., Acosta, J., Sendi, M. S. E., Choi, Y., et al. (2018). An implantable peripheral nerve recording and stimulation system for experiments on freely moving animal subjects. Sci. Rep. 8:6115. doi: 10.1038/s41598-018-24465-1
Lee, S. H., Jin, W. P., Seo, N. R., Pang, K. M., Kim, B., Kim, S. M., et al. (2017). Recombinant human fibroblast growth factor-2 promotes nerve regeneration and functional recovery after mental nerve crush injury. Neural Regen. Res. 12, 629–636. doi: 10.4103/1673-5374.205104
Lee, S. K., and Wolfe, S. W. (2000). Peripheral nerve injury and repair. J. Am. Acad. Orthop. Surg. 8, 243–252. doi: 10.5435/00124635-200007000-00005
Li, L., Openheim, R. W., Lei, M., and Houenou, L. J. (1994). Neurotrophic agents prevent motoneuron death following sciatic nerve section in the neonatal mouse. J. Neurobiol. 25, 759–766. doi: 10.1002/neu.480250702
Li, R., Li, D. H., Zhang, H. Y., Wang, J., Li, X. K., and Xiao, J. (2020). Growth factors-based therapeutic strategies and their underlying signaling mechanisms for peripheral nerve regeneration. Acta Pharmacol. Sin. 41, 1289–1300. doi: 10.1038/s41401-019-0338-1
Li, R., Li, Y., Wu, Y., Zhao, Y., Chen, H., Yuan, Y., et al. (2018). Heparin-poloxamer thermosensitive hydrogel loaded with bFGF and NGF enhances peripheral nerve regeneration in diabetic rats. Biomaterials 168, 24–37. doi: 10.1016/j.biomaterials.2018.03.044
Liu, C., Huang, Y., Pang, M., Yang, Y., Li, S., Liu, L., et al. (2015). Tissue-engineered regeneration of completely transected spinal cord using induced neural stem cells and gelatin-electrospun poly (lactide-co-glycolide)/polyethylene glycol scaffolds. PLoS One 10:e0117709. doi: 10.1371/journal.pone.0117709
Low, E. E., Inkellis, E., and Morshed, S. (2017). Complications and revision amputation following trauma-related lower limb loss. Injury 48, 364–370. doi: 10.1016/j.injury.2016.11.019
Mackinnon, S. E., Doolabh, V. B., Novak, C. B., and Trulock, E. P. (2001). Clinical outcome following nerve allograft transplantation. Plast. Reconstr. Surg. 107, 1419–1429. doi: 10.1097/00006534-200105000-00016
Mackinnon, S. E., and Lee Dellon, A. (1990). A study of nerve regeneration across synthetic (Maxon) and biologic (collagen) nerve conduits for nerve gaps up to 5 cm in the primate. J. Reconstr. Microsurg. 6, 117–121. doi: 10.1055/s-2007-1006810
Madurga, R., Gañán-Calvo, A. M., Plaza, G. R., Guinea, G. V., Elices, M., and Pérez-Rigueiro, J. (2017a). Production of high performance bioinspired silk fibers by straining flow spinning. Biomacromolecules 18, 1127–1133. doi: 10.1021/acs.biomac.6b01757
Madurga, R., Gañán-Calvo, A. M., Plaza, G. R., Guinea, G. V., Elices, M., and Pérez-Rigueiro, J. (2017b). Straining flow spinning: production of regenerated silk fibers under a wide range of mild coagulating chemistries. Green Chem. 19, 3380–3389. doi: 10.1039/c7gc01254c
Maggi, S. P., Lowe, J. B., and Mackinnon, S. E. (2003). Pathophysiology of nerve injury. Clin. Plast. Surg. 30, 109–126. doi: 10.1016/S0094-1298(02)00101-3
Marchini, A., Raspa, A., Pugliese, R., Abd El Malek, M., Pastori, V., Lecchi, M., et al. (2019). Multifunctionalized hydrogels foster hNSC maturation in 3D cultures and neural regeneration in spinal cord injuries. Proc. Natil. Acad. Sci. U.S.A. 116, 7483–7492. doi: 10.1073/pnas.1818392116
Marshall, C., and Stansby, G. (2010). Amputation and rehabilitation. Surgery 28, 284–287. doi: 10.1016/j.mpsur.2010.01.017
Matsumoto, T., and Mooney, D. J. (2006). Cell instructive polymers. Adv. Biochem. Eng. Biotechnol. 102, 113–137. doi: 10.1007/b137207
Menorca, R. M. G., Fussell, T. S., and Elfar, J. C. (2013). Nerve physiology. Mechanisms of injury and recovery. Hand Clin. 29, 317–330. doi: 10.1016/j.hcl.2013.04.002
Merzenich, M. M., and Jenkins, W. M. (1993). Reorganization of cortical representations of the hand following alterations of skin inputs induced by nerve injury. Skin Island Transfers, and Experience. J. Hand Ther. 6, 89–104. doi: 10.1016/S0894-1130(12)80290-0
Mi, R., Chen, W., and Hoke, A. (2007). Pleiotrophin is a neurotrophic factor for spinal motor neurons. Proc. Natl. Acad. Sci. 104, 4664–4669. doi: 10.1073/pnas.0603243104
Micera, S. (2016). Staying in touch: toward the restoration of sensory feedback in hand prostheses using peripheral neural stimulation. IEEE Pulse 7, 16–19. doi: 10.1109/MPUL.2016.2539760
Micera, S., Navarro, X., Carpaneto, J., Citi, L., Tonet, O., Rossini, P. M., et al. (2008). On the use of longitudinal intrafascicular peripheral interfaces for the control of cybernetic hand prostheses in amputees. IEEE Trans. Neural Syst. Rehabil. Eng. 16, 453–472. doi: 10.1109/TNSRE.2008.2006207
Micera, S., Rossini, P. M., Rigosa, J., Citi, L., Carpaneto, J., Raspopovic, S., et al. (2011). Decoding of grasping information from neural signals recorded using peripheral intrafascicular interfaces. J. NeuroEng. Rehabil. 8:53. doi: 10.1186/1743-0003-8-53
Mikos, A. G., Herring, S. W., Ochareon, P., Elisseeff, J., Lu, H. H., Kandel, R., et al. (2006). Engineering complex tissues. Tissue Eng. 12, 3307–3339. doi: 10.1089/ten.2006.12.3307
Mohanna, P. N., Young, R. C., Wiberg, M., and Terenghi, G. (2003). A composite pol-hydroxybutyrate-glial growth factor conduit for long nerve gap repairs. J. Anat. 203, 553–565. doi: 10.1046/j.1469-7580.2003.00243.x
Navaraj, W. T., Nassar, H., and Dahiya, R. (2019). “Prosthetic hand with biomimetic tactile sensing and force feedback,” in Proceedings – IEEE International Symposium on Circuits and Systems, Sapporo, 1–4. doi: 10.1109/ISCAS.2019.8702732
Near, S. L., Whalen, L. R., Miller, J. A., and Ishii, D. N. (1992). Insulin-like growth factor II stimulates motor nerve regeneration. Proc. Natl. Acad. Sci. U.S.A. 89, 11716–11720. doi: 10.1073/pnas.89.24.11716
Newman, J. P., Verity, A. N., Hawatmeh, S., Fee, W. E., and Terris, D. J. (1996). Ciliary neurotrophic factor enhances peripheral nerve regeneration. Arch. Otolaryngol. Head Neck Surg. 122, 399–403. doi: 10.1001/archotol.1996.01890160041008
Nikolajsen, L., and Christensen, K. F. (2015). Phantom Limb Pain. Nerves Nerve Injuries 2, 23–34. doi: 10.1016/B978-0-12-802653-3.00051-8
Nógrádi, A., Pajer, K., and Márton, G. (2011). The role of embryonic motoneuron transplants to restore the lost motor function of the injured spinal cord. Ann. Anat. 193, 362–370. doi: 10.1016/j.aanat.2011.04.001
Notterpek, L. (2003). Neurotrophins in myelination: a new role for a puzzling receptor. Trends Neurosci. 26, 232–234. doi: 10.1016/S0166-2236(03)00099-7
Olasinde, A. A., Oginni, L. M., Bankole, J. O., and Adegbehingbe Oluwadiya, K. S. (2002). Indications for amputations in Ile-Ife. Nigeria. Nigerian J. Med. 11, 118–121.
Omura, T., Sano, M., Omura, K., Hasegawa, T., Doi, M., Sawada, T., et al. (2005). Different expressions of BDNF, NT3, and NT4 in muscle and nerve after various types of peripheral nerve injuries. J. Peripheral Nervous Syst. 10, 293–300. doi: 10.1111/j.1085-9489.2005.10307.x
Pabari, A., Lloyd-Hughes, H., Seifalian, A. M., and Mosahebi, A. (2014). Nerve conduits for peripheral nerve surgery. Plastic Reconstr. Surg. 133, 1420–1430. doi: 10.1097/PRS.0000000000000226
Panetsos, F., Avendano, C., Negredo, P., Castro, J., and Bonacasa, V. (2008). Neural prostheses: electrophysiological and histological evaluation of central nervous system alterations due to long-term implants of sieve electrodes to peripheral nerves in cats. IEEE Trans. Neural Syst. Rehabil. Eng. 16, 223–232. doi: 10.1109/TNSRE.2008.923707
Pehar, M., Cassina, P., Vargas, M. R., Castellanos, R., Viera, L., Beckman, J. S., et al. (2004). Astrocytic production of nerve growth factor in motor neuron apoptosis: implications for amyotrophic lateral sclerosis. J. Neurochem. 89, 464–473. doi: 10.1111/j.1471-4159.2004.02357.x
Pehar, M., Cassina, P., Vargas, M. R., Xie, Y., Beckman, J. S., Massa, S. M., et al. (2006). Modulation of p75NTR-dependent motor neuron death by a small non-peptidyl mimetic of the neurotrophin loop 1 domain. Eur. J. Neurosci. 24, 1575–1580. doi: 10.1111/j.1460-9568.2006.05040.x
Petcu, E. B., Midha, R., McColl, E., Popa-Wagner, A., Chirila, T. V., and Dalton, P. D. (2018). 3D printing strategies for peripheral nerve regeneration. Biofabrication 10:032001. doi: 10.1088/1758-5090/aaaf50
Pfister, B. J., Gordon, T., Loverde, J. R., Kochar, A. S., Mackinnon, S. E., and Kacy Cullen, D. (2011). Biomedical engineering strategies for peripheral nerve repair: surgical applications, state of the art, and future challenges. Crit. Rev. Biomed. Eng. 39, 81–124. doi: 10.1615/CritRevBiomedEng.v39.i2.20
Piirsoo, M., Kaljas, A., Tamm, K., and Timmusk, T. (2010). Expression of NGF and GDNF family members and their receptors during peripheral nerve development and differentiation of Schwann cells in vitro. Neurosci. Lett. 469, 135–140. doi: 10.1016/j.neulet.2009.11.060
Politis, M. J. (1989). Exogenous laminin induces regenerative changes in traumatized sciatic and optic nerve. Plast. Reconstr. Surg. 83, 228–235. doi: 10.1097/00006534-198902000-00004
Politis, M. J., Ederle, K., and Spencer, P. S. (1982). Tropism in nerve regeneration in vivo. Attraction of regenerating axons by diffusible factors derived from cells in distal nerve stumps of transected peripheral nerves. Brain Res. 253, 1–12. doi: 10.1016/0006-8993(82)90667-9
Politis, M. J., and Spencer, P. S. (1983). An in vivo assay of neurotropic activity. Brain Res. 278, 229–231. doi: 10.1016/0006-8993(83)90242-1
Previtali, S. C., Malaguti, M. C., Riva, N., Scarlato, M., Dacci, P., Dina, G., et al. (2008). The extracellular matrix affects axonal regeneration in peripheral neuropathies. Neurology 71, 322–331. doi: 10.1212/01.wnl.0000319736.43628.04
Ramón y Cajal, S. (1959). Degeneration & Regeneration of the Nervous System. Oxford: Oxford University Press.
Raspopovic, S., Petrini, F. M., Capogrosso, M., and Bonizzato, M. (2014). Bidirectional Limb Neuro-Prosthesis. United States Pat. Appl. Publ. Available online at: https://patents.google.com/patent/US20160331561A1/en (accessed September 2, 2019).
Razavi, S., Ghasemi, N., Mardani, M., and Salehi, H. (2017). Remyelination improvement after neurotrophic factors secreting cells transplantation in rat spinal cord injury. Iran. J. Basic Med. Sci. 20, 392–398. doi: 10.22038/ijbms.2017.8580
Razavi, S., Ghasemi, N., Mardani, M., and Salehi, H. (2018). Co-transplantation of human neurotrophic factor secreting cells and adipose-derived stem cells in rat model of multiple sclerosis. Cell J. 20, 46–52. doi: 10.22074/cellj.2018.4777
Romano, N. H., Madl, C. M., and Heilshorn, S. C. (2015). Matrix RGD ligand density and L1CAM-mediated Schwann cell interactions synergistically enhance neurite outgrowth. Acta Biomater. 11, 48–57. doi: 10.1016/j.actbio.2014.10.008
Ruven, C., Badea, S. R., Wong, W. M., and Wu, W. (2018). Combination treatment with exogenous GDNF and fetal spinal cord cells results in better motoneuron survival and functional recovery after avulsion injury with delayed root reimplantation. J. Neuropathol. Exp. Neurol. 77, 325–343. doi: 10.1093/jnen/nly009
Saito, H., Kanje, M., and Dahlin, L. B. (2010). Crossed over repair of the femoral sensory and motor branches influences N-CAM. NeuroReport 21, 841–845. doi: 10.1097/WNR.0b013e32833d40e2
Saito, H., Nakao, Y., Takayama, S., Toyama, Y., and Asou, H. (2005). Specific expression of an HNK-1 carbohydrate epitope and NCAM on femoral nerve Schwann cells in mice. Neurosci. Res. 53, 314–322. doi: 10.1016/j.neures.2005.08.006
Santos, D., Giudetti, G., Micera, S., Navarro, X., and Del Valle, J. (2016a). Focal release of neurotrophic factors by biodegradable microspheres enhance motor and sensory axonal regeneration in vitro and in vivo. Brain Res. 1636, 93–106. doi: 10.1016/j.brainres.2016.01.051
Santos, D., Gonzalez-Perez, F., Navarro, X., and Del Valle, J. (2016b). Dose-dependent differential effect of neurotrophic factors on in vitro and in vivo regeneration of motor and sensory neurons. Neural Plast. 2016:4969523. doi: 10.1155/2016/4969523
Schmidt, C. E., and Leach, J. B. (2003). Neural tissue engineering: strategies for repair and regeneration. Annu. Rev. Biomed. Eng. 5, 293–347. doi: 10.1146/annurev.bioeng.5.011303.120731
Seddon, H. J. (1942). Classification of nerve injuries. Br. Med. J. 2:438. doi: 10.1136/bmj.2.4266.438-c
Seilheimer, B., and Schachner, M. (1988). Studies of adhesion molecules mediating interactions between cells of peripheral nervous system indicate a major role for L1 in mediating sensory neuron growth on Schwann cells in culture. J. Cell Biol. 107, 341–351. doi: 10.1083/jcb.107.1.341
Sensinger, J. W., and Dosen, S. (2020). A review of sensory feedback in upper-limb prostheses from the perspective of human motor control. Front. Neurosci. 14:345. doi: 10.3389/fnins.2020.00345
Shakhbazau, A., Martinez, J. A., Xu, Q. G., Kawasoe, J., Van Minnen, J., and Midha, R. (2012). Evidence for a systemic regulation of neurotrophin synthesis in response to peripheral nerve injury. J. Neurochem. 122, 501–511. doi: 10.1111/j.1471-4159.2012.07792.x
Sherman, D. L., and Brophy, P. J. (2005). Mechanisms of axon ensheathment and myelin growth. Nat. Rev. Neurosci. 6, 683–690. doi: 10.1038/nrn1743
Sitoci-Ficici, K. H., Matyash, M., Uckermann, O., Galli, R., Leipnitz, E., Later, R., et al. (2018). Non-functionalized soft alginate hydrogel promotes locomotor recovery after spinal cord injury in a rat hemimyelonectomy model. Acta Neurochir. 160, 449–457. doi: 10.1007/s00701-017-3389-4
Smith, D. V., Klevitsky, R., Akeson, R. A., and Shipley, M. T. (1994). Expression of the neural cell adhesion molecule (NCAM) and polysialic acid during taste bud degeneration and regeneration. J. Comp. Neurol. 347, 187–196. doi: 10.1002/cne.903470204
Song, Q., Jiang, Z., Li, N., Liu, P., Liu, L., Tang, M., et al. (2014). Anti-inflammatory effects of three-dimensional graphene foams cultured with microglial cells. Biomaterials 35, 6930–6940. doi: 10.1016/j.biomaterials.2014.05.002
Sterne, G. D., Brown, R. A., Green, C. J., and Terenghi, G. (1997). Neurotrophin-3 delivered locally via fibronectin mats enhances peripheral nerve regeneration. Eur. J. Neurosci. 9, 1388–1396. doi: 10.1111/j.1460-9568.1997.tb01493.x
Stewart, J. D. (2003). Peripheral nerve fascicles: anatomy and clinical relevance. Muscle Nerve 28, 525–541. doi: 10.1002/mus.10454
Stoll, G., Jander, S., and Myers, R. R. (2002). Degeneration and regeneration of the peripheral nervous system: from Augustus Waller’s observations to neuroinflammation. J. Peripheral Nervous Syst. 7, 13–27. doi: 10.1046/j.1529-8027.2002.02002.x
Struzyna, L. A., Browne, K. D., Brodnik, Z. D., Burrell, J. C., Harris, J. P., Chen, H. I., et al. (2018). Tissue engineered nigrostriatal pathway for treatment of Parkinson’s disease. J. Tissue Eng. Regen. Med. 12, 1702–1716. doi: 10.1002/term.2698
Sulaiman, W., and Gordon, T. (2013). Neurobiology of peripheral nerve injury, regeneration, and functional recovery: from bench top research to bedside application. Ochsner J. 13, 100–108.
Sullivan, K. A., Kim, B., and Feldman, E. L. (2008). Minireview: insulin-like growth factors in the peripheral nervous system. Endocrinology 149, 5963–5971. doi: 10.1210/en.2008-1020
Sunderland, S. (1951). A classification of peripheral nerve injuries producing loss of function. Brain 74, 491–516. doi: 10.1093/brain/74.4.491
Sunderland, S. (1969). Nerves and nerve injuries. Br. J. Surg. 56, 401–401. doi: 10.1002/bjs.1800560524
Suzuki, M., Inage, K., Sakuma, Y., Orita, S., Yamauchi, K., Suzuki, T., et al. (2016). Effect of administration of antibodies against nerve growth factor in a rat model of muscle injury. Injury 47, 609–612. doi: 10.1016/j.injury.2015.11.026
Tajdaran, K., Chan, K., Gordon, T., and Borschel, G. H. (2019). Matrices, scaffolds, and carriers for protein and molecule delivery in peripheral nerve regeneration. Exp. Neurol. 319:112817. doi: 10.1016/j.expneurol.2018.08.014
Tao, J., Hu, Y., Wang, S., Zhang, J., Liu, X., Gou, Z., et al. (2017). A 3D-engineered porous conduit for peripheral nerve repair. Sci. Rep. 7:46038. doi: 10.1038/srep46038
Terris, D. J., Toft, K. M., Moir, M., Lum, J., and Wang, M. (2001). Brain-derived neurotrophic factor-enriched collagen tubule as a substitute for autologous nerve grafts. Arch. Otolaryngol. Head Neck Surg. 127, 294–298. doi: 10.1001/archotol.127.3.294
Tesar, B. M., Jiang, D., Liang, J., Palmer, S. M., Noble, P. W., and Goldstein, D. R. (2006). The role of hyaluronan degradation products as innate alloimmune agonists. Am. J. Trans. 6, 2622–2635. doi: 10.1111/j.1600-6143.2006.01537.x
Tessier-Lavigne, M., and Goodman, C. S. (1996). The molecular biology of axon guidance. Science 274, 1123–1133. doi: 10.1126/science.274.5290.1123
Tian, L., Prabhakaran, M. P., and Ramakrishna, S. (2015). Strategies for regeneration of components of nervous system: scaffolds, cells and biomolecules. Regen. Biomat. 2, 31–45. doi: 10.1093/rb/rbu017
Tran, R. T., Choy, W. M., Cao, H., Qattan, I., Chiao, J. C., Ip, W. Y., et al. (2014). Fabrication and characterization of biomimetic multichanneled crosslinked-urethane-doped polyester tissue engineered nerve guides. J. Biomed. Mater. Res. Part A 102, 2793–2804. doi: 10.1002/jbm.a.34952
Tucker, B. A., and Mearow, K. M. (2008). Peripheral sensory axon growth: from receptor binding to cellular signaling. Can. J. Neurol. Sci. 35, 551–566. doi: 10.1017/S0317167100009331
Varma, P., Stineman, M. G., and Dillingham, T. R. (2014). Epidemiology of limb loss. Phys. Med. Rehabil. Clin. North Am. 25, 1–8. doi: 10.1016/j.pmr.2013.09.001
Vepari, C., and Kaplan, D. L. (2007). Silk as a biomaterial. Prog. Polymer Sci. 32, 991–1007. doi: 10.1016/j.progpolymsci.2007.05.013
Vidal, P. M., Lemmens, E., Dooley, D., and Hendrix, S. (2013). The role of “anti-inflammatory” cytokines in axon regeneration. Cytokine Growth Factor Rev. 24, 1–12. doi: 10.1016/j.cytogfr.2012.08.008
Wang, A., Ao, Q., Cao, W., Yu, M., He, Q., Kong, L., et al. (2006). Porous chitosan tubular scaffolds with knitted outer wall and controllable inner structure for nerve tissue engineering. J. Biomed. Mater. Res. Part A 79, 36–46. doi: 10.1002/jbm.a.30683
Wang, F., Julien, D. P., and Sagasti, A. (2013). Journey to the skin: somatosensory peripheral axon guidance and morphogenesis. Cell Adh. Migr. 7, 388–394. doi: 10.4161/cam.25000
Wang, W., Salvaterra, P. M., Loera, S., and Chiu, A. Y. (1997). Brain-derived neurotrophic factor spares choline acetyltransferase mRNA following axotomy of motor neurons in vivo. J. Neurosci. Res. 47, 134–143. doi: 10.1002/(SICI)1097-4547(19970115)47:2<134::AID-JNR2<3.0.CO;2-G
Wang, X., Wenk, E., Zhang, X., Meinel, L., Vunjak-Novakovic, G., and Kaplan, D. L. (2009). Growth factor gradients via microsphere delivery in biopolymer scaffolds for osteochondral tissue engineering. J. Control. Release 134, 81–90. doi: 10.1016/j.jconrel.2008.10.021
Wang, X. Y., Gu, P. Y., Chen, S. W., Gao, W. W., Tian, H. L., Lu, X. H., et al. (2015). Endogenous neurotrophin-3 promotes neuronal sprouting from dorsal root ganglia. Neural Reg. Res. 10, 1865–1868. doi: 10.4103/1673-5374.170318
Wang, Z. Z., and Sakiyama-Elbert, S. E. (2019). Matrices, scaffolds & carriers for cell delivery in nerve regeneration. Exp. Neurol. 319:112837. doi: 10.1016/j.expneurol.2018.09.020
Webber, C. A., Xu, Y., Vanneste, K. J., Martinez, J. A., Verge, V. M. K., and Zochodne, D. W. (2008). Guiding adult mammalian sensory axons during regeneration. J. Neuropathol. Exp. Neurol. 67, 212–222. doi: 10.1097/NEN.0b013e3181654972
Wong, V., Arriaga, R., Ip, N. Y., and Lindsay, R. M. (1993). The Neurotrophins BDNF, NT-3 and NT-4/5, But Not NGF, Up-regulate the cholinergic phenotype of developing motor neurons. Eur. J. Neurosci. 5, 466–474. doi: 10.1111/j.1460-9568.1993.tb00513.x
Wu, J. C., Huang, W. C., Chen, Y. C., Tu, T. H., Tsai, Y. A., Huang, S. F., et al. (2011). Acidic fibroblast growth factor for repair of human spinal cord injury: a clinical trial – Clinical article. J. Neurosurg. 15, 216–227. doi: 10.3171/2011.4.SPINE10404
Xiao, J., Hughes, R. A., Lim, J. Y., Wong, A. W., Ivanusic, J. J., Ferner, A. H., et al. (2013). A small peptide mimetic of brain-derived neurotrophic factor promotes peripheral myelination. J. Neurochem. 125, 386–398. doi: 10.1111/jnc.12168
Xu, D., Fan, L., Gao, L., Xiong, Y., Wang, Y., Ye, Q., et al. (2016). Micro-nanostructured polyaniline assembled in cellulose matrix via interfacial polymerization for applications in nerve regeneration. ACS Appl. Mater. Interfaces 8, 17090–17097. doi: 10.1021/acsami.6b03555
Xue, C., Hu, N., Gu, Y., Yang, Y., Liu, Y., Liu, J., et al. (2012). Joint use of a chitosan/PLGA scaffold and MSCs to bridge an extra large gap in dog sciatic nerve. Neurorehabil. Neural Repair 26, 96–106. doi: 10.1177/1545968311420444
Yi, S., Xu, L., and Gu, X. (2019). Scaffolds for peripheral nerve repair and reconstruction. Exp. Neurol. 319:112761. doi: 10.1016/j.expneurol.2018.05.016
Zecca, M., Micera, S., Carrozza, M. C., and Dario, P. (2017). Control of multifunctional prosthetic hands by processing the electromyographic signal. Crit. Rev. Biomed. Eng. 45, 383–410. doi: 10.1615/CritRevBiomedEng.v45.i1-6.150
Zeiler, S. R., Hubbard, R., Gibson, E. M., Zheng, T., Ng, K., O’Brien, R., et al. (2016). Paradoxical motor recovery from a first stroke after induction of a second stroke: reopening a postischemic sensitive period. Neurorehabil. Neural Repair 30, 794–800. doi: 10.1177/1545968315624783
Zeng, C. G., Xiong, Y., Xie, G., Dong, P., and Quan, D. (2014). Fabrication and evaluation of PLLA multichannel conduits with nanofibrous microstructure for the differentiation of NSCs in vitro. Tissue Eng. Part A 20, 1038–1048. doi: 10.1089/ten.tea.2013.0277
Zhang, J., Oswald, T. M., Lineaweaver, W. C., Chen, Z., Zhang, G., Chen, Z., et al. (2003). Enhancement of rat sciatic nerve regeneration by fibronectin and laminin through a silicone chamber. J. Reconstr. Microsurg. 19, 467–472. doi: 10.1055/s-2003-44635
Zhang, Q., Zhao, Y., Yan, S., Yang, Y., Zhao, H., Li, M., et al. (2012). Preparation of uniaxial multichannel silk fibroin scaffolds for guiding primary neurons. Acta Biomater. 8, 2628–2638. doi: 10.1016/j.actbio.2012.03.033
Zhang, Y., Lazzarini, P. A., McPhail, S. M., van Netten, J. J., Armstrong, D. G., and Pacella, R. E. (2020). Global disability burdens of diabetes-related lower-extremity complications in 1990 and 2016. Diabetes Care 43, 964–974. doi: 10.2337/dc19-1614
Zhu, J. (2010). Bioactive modification of poly(ethylene glycol) hydrogels for tissue engineering. Biomaterials 31, 4639–4656. doi: 10.1016/j.biomaterials.2010.02.044
Ziegler-Graham, K., MacKenzie, E. J., Ephraim, P. L., Travison, T. G., and Brookmeyer, R. (2008). Estimating the prevalence of limb loss in the united states: 2005 to 2050. Arch. Phys. Med. Rehabil. 89, 422–429. doi: 10.1016/j.apmr.2007.11.005
Keywords: biomolecules, biomaterials, neurotrophins, prostheses, neurotrophic factors, protein, mRNA
Citation: Guedan-Duran A, Jemni-Damer N, Orueta-Zenarruzabeitia I, Guinea GV, Perez-Rigueiro J, Gonzalez-Nieto D and Panetsos F (2020) Biomimetic Approaches for Separated Regeneration of Sensory and Motor Fibers in Amputee People: Necessary Conditions for Functional Integration of Sensory–Motor Prostheses With the Peripheral Nerves. Front. Bioeng. Biotechnol. 8:584823. doi: 10.3389/fbioe.2020.584823
Received: 18 July 2020; Accepted: 25 September 2020;
Published: 03 November 2020.
Edited by:
Mingqiang Li, Sun Yat-sen University, ChinaCopyright © 2020 Guedan-Duran, Jemni-Damer, Orueta-Zenarruzabeitia, Guinea, Perez-Rigueiro, Gonzalez-Nieto and Panetsos. This is an open-access article distributed under the terms of the Creative Commons Attribution License (CC BY). The use, distribution or reproduction in other forums is permitted, provided the original author(s) and the copyright owner(s) are credited and that the original publication in this journal is cited, in accordance with accepted academic practice. No use, distribution or reproduction is permitted which does not comply with these terms.
*Correspondence: Fivos Panetsos, Zml2b3NAdWNtLmVz
†These authors have contributed equally to this work