- 1Institute for Organic Chemistry and Biochemistry, Technical University of Darmstadt, Darmstadt, Germany
- 2Ferring Darmstadt Laboratories, Darmstadt, Germany
- 3Ferring Biologics Innovation Centre, Epalinges, Switzerland
- 4Centre for Synthetic Biology, Technical University of Darmstadt, Darmstadt, Germany
Yeast-surface display (YSD) is commonly applied to screen Fab immune or naïve libraries for binders of predefined target molecules. However, reformatting of isolated variants represents a time-intensive bottleneck. Herein, we present a novel approach to facilitate a lean transition from antibody screening using YSD Fab libraries to the production of full-length IgG antibodies in Expi293-F cells. In this study, utilizing Golden Gate Cloning (GGC) and a bidirectional promoter system, an exemplary Fab-displaying YSD library was generated based on immunised transgene rats. After subsequent screening for antigen-specific antibody candidates by fluorescence-activated cell sorting (FACS), the Fab-encoding genes were subcloned into a bidirectional mammalian expression vector, exhibiting CH2-CH3 encoding genes, in a GGC-mediated, PCR-free manner. This novel, straightforward and time-saving workflow allows the VH/VL pairing to be preserved. This study resulted in antibody variants exhibiting suitable biophysical properties and covered a broad VH diversity after two rounds of FACS screening, as revealed by NGS analysis. Ultimately, we demonstrate that the implication of such a gene transfer system streamlines antibody hit discovery efforts, allowing the faster characterisation of antibodies against a plethora of targets that may lead to new therapeutic agents.
Introduction
Monoclonal antibodies (mAbs) have shown great potential both as therapeutic and diagnostic tools, with the global monoclonal antibody market expected to reach $300 billion in revenues by 2025 (Lu et al., 2020). Today, a wide variety of display technologies are established for the identification of mAb candidates from immune, synthetic or naïve libraries, among them phage display (McCafferty et al., 1990), ribosome display (Schaffitzel et al., 1999; Lipovsek and Plückthun, 2004), mRNA display (Lipovsek and Plückthun, 2004; Josephson et al., 2014), mammalian display (Parthiban et al., 2019) and yeast display (Boder and Wittrup, 1997). However, all these technologies require laborious subcloning of isolated mAb-encoding genes into protein expression vectors. Even though this process was improved within the last years, PCR-based subcloning always bears the risk of incorporating unintended mutations. Due to the increasing interest in developing mAbs against a plethora of targets, we sought out to streamline the antibody hit discovery workflow.
Besides phage display, particularly yeast-surface display (YSD) has become widely applicable for screening of large libraries (Boder and Wittrup, 1997). The first approved therapeutic antibody generated via YSD was Sintilimab, a PD-1 blocking antibody, approved in 2018 for the treatment of relapsed or refractory classical Hodgkin’s lymphoma in China (Hoy, 2019; Valldorf et al., 2021). While advances in YSD technology have facilitated the generation of large Fab antibody libraries using streamlined approaches (Rosowski et al., 2018; Roth et al., 2018), the pitfall that follows antibody screening, namely reformation of Fabs into full-length IgG molecules, remains a tedious procedure. Reformatting into IgG molecules is required in order to fully discover the activity and function of mAbs and to assay their properties, such as Fc-mediated functions (Kapur et al., 2014; Bournazos and Ravetch, 2017). Furthermore, the handling of each antibody individually is required in order to preserve the unique VH and VL pairing.
In recent years, Cruz-Teran and others (2017) have shown that a modification of the yeast cell surface allows one to switch between cell-surface display and secretion of full-length antibodies in order to circumvent subcloning of hit candidates into a suitable expression vector for mammalian expression (Cruz-Teran et al., 2017; Krah et al., 2020). Nevertheless, the glycosylation patterns in baker’s yeast cells differ significantly from those in humans (Tanner and Lehle, 1987; Wildt and Gerngross, 2005) and the yields by application of such methods are very limited. On the contrary, two mammalian cell lines are commonly used for small- to mid-scale antibody production due to their human-like glycosylation and high titres, namely Human Embryonic Kidney 293 (HEK293) and Chinese Hamster Ovary (CHO) cells (Li et al., 2010; Vazquez-Lombardi et al., 2018; Carrara et al., 2021a). To continue the production of IgG molecules in mammalian cells and avoid the cumbersome reformatting steps, we have developed a novel two-pot, two-step cloning procedure in order to facilitate the transition of hit candidates from a YSD-display vector to a mammalian bidirectional (BiDi) expression vector. Initial studies were carried out to analyse the most suitable BiDi promoter for both κ- and λ-isotype antibodies (Carrara et al., 2021b). On top of simplifying and facilitating the transition between display on yeast cells to production in mammalian cells, VH and VL pairing is also preserved. To date, a few high-throughput platforms have been described in order to batch reformat from the scFv format to IgG molecules from phage display libraries (Renaut et al., 2012; Batonick et al., 2016; Xiao et al., 2017; Liu et al., 2018; Reader et al., 2019), but to the best of our knowledge, there have been no such reports for YSD Fab libraries.
In this study, we performed an initial proof-of-concept (PoC) experiment with the therapeutic anti-PD-L1 antibody Durvalumab to establish the reformatting workflow (Figure 1) (Alvarez-Argote and Dasanu, 2019). Subsequently, a Fab library resulting from immunised OmniRats against a TAMR was generated, screened, reformatted, and produced as well as purified using this streamlined approach. The TAM receptors (TAMR), comprising Tyro3, Axl, and MerTK, belong to the family of receptor tyrosine kinases, which have been the focus of several studies over the last decade due to their implications in a number of diseases (Alvarez-Argote and Dasanu, 2019; Reader et al., 2019). The resulting variants unveiled appropriate biophysical properties and covered the entire VH diversity, as revealed by NGS analysis. This method paves the way for facilitating hit discovery processes by expediting the transition between YSD-vectors and mammalian expression of hit candidates.
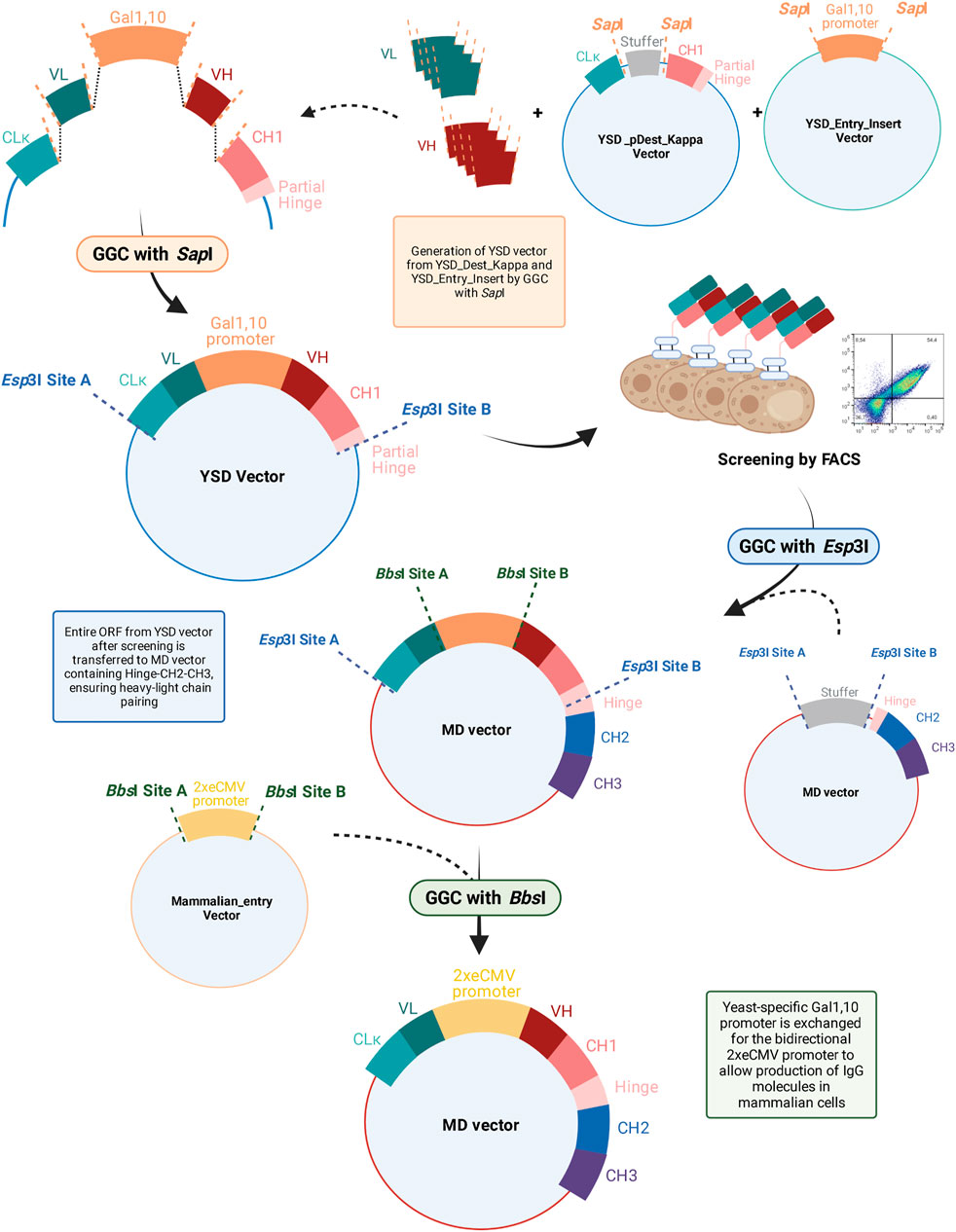
FIGURE 1. Overview of bulk reformatting workflow from YSD vector to the engineered mammalian destination (MD) vector. VH, heavy chain variable domain; VL, light chain variable domain; CLκ, light chain constant kappa domain; GGC, Golden Gate Cloning; YSD, yeast surface display; MD, mammalian destination; ORF, open reading frame; FACS, fluorescence-activated cell sorting.
Materials and Methods
Plasmids and Yeast Strains
Plasmids and yeast strains used, as well as their cultivation and media, were previously described in detail elsewhere (Bogen et al., 2020a; Bogen et al., 2020b). For library generation, the yeast destination vector, YSD_pDest_Kappa, comprising a coding sequence for a κ CL and a CH1-hinge-Aga2p fusion, as well as the yeast entry vector, YSD_Entry_Insert, encoding the Gal1,10 promoter were used. By SapI-mediated GGC, VH and VL sequences, previously amplified from cDNA (see below) were subcloned into the destination vector, similar to the approach described previously (Rosowski et al., 2018). The mammalian destination (MD) vector was recently described in detail (Carrara et al., 2021b). In brief, the MD vector encodes a stuffer sequence flanked by Esp3I sites, adjacent to coding sequences comprising partial hinge-CH2-CH3 domains. By means of Golden Gate cloning, VL-CL and VH-CH1 encoding sequences can be inserted into MD. Additionally, the bidirectional promoter is flanked by BbsI sites and can be chosen freely. The 2xeCMV promoter combination was shown to be the most suitable for recombinant antibody production and was thus used herein. The sequence of this promoter construct is available from (Carrara et al., 2021b).
Immunisation
OmniRats (Osborn et al., 2013) were immunised by genetic immunisation encoding TAMR at Aldevron (Freiburg, Germany). After adequate titres were observed, lymph nodes and spleens were extracted. All procedures were carried out in accordance with local animal welfare guidelines and protection laws.
Library Generation
From immunised OmniRats, total RNA was isolated from lymph nodes and spleens using RNeasy Plus Mini Kit (QIAGEN). Subsequently, cDNA was synthesised using SuperScript III First-Strand Kit (Thermo Fisher Scientific) from 25 µl RNA with random hexamer primers, dNTPs and water to an end volume of 35 µl. The mixture was first incubated at 65°C for 5 min followed by a 1 min incubation on ice. 35 µl containing 1x RT-Buffer, MgCl2, DTT, RNase OUT and 200 U SuperScript III RT were added. The mixture was incubated for 5 min at 25°C and 60 min at 50°C. The reaction was terminated by incubating for 5 min at 80°C. 1 µl RNase H was added to each tube and incubated for 20 min at 37°C.
With the cDNA, human antibody variable regions from OmniRats were amplified using two successive, nested PCR reactions. For the first PCR, unique forward primers annealing to the leader sequence of VH or VL kappa were combined with reverse primers annealing to rat CH1 or CL domains, respectively, using Q5-High Fidelity DNA Polymerase. After PCR Clean-Up using Wizard SV Gel and PCR Clean-Up System (Promega) and verifying successful amplification by agarose gel electrophoresis, a second PCR was performed to amplify human VH and VL domains and incorporating SapI recognition sequences for subsequent Golden Gate Cloning (GGC) into the YSD vector (primer sequences can be found in Supplementary Table S1). Therefore, the purified VH and VL PCR products were assembled with the plasmids YSD_Entry_Insert and YSD_pDest_Kappa. For comparison, an additional anti-TAMR Fab library was generated by homologous recombination as described by Benatuil et al. in EBY100 yeast cells (Benatuil et al., 2010).
Library Sorting
For library sorting, 5 × 108 induced yeast cells were washed with PBS containing 0.1% BSA (PBS-B) and incubated with 250 nM TAMR-His6 for 30 min on ice. After washing with PBS-B, cells were stained with Goat F(ab’)2-anti-human-Kappa PE-conjugate (1:75 dilution, Southern Biotech) to detect surface presentation, with anti-His6 AlexaFluor647-conjugate (1:50 dilution, Thermo Fisher Scientific) for target binding and incubated for 15 min on ice. After a final washing step, cells were analysed by FACS using a Sony Cell Sorter SH800S. Sorting rounds were performed with decreasing target antigen concentrations. For subsequent rounds, TAMR-Fc was used as soluble antigen, staining with an anti-human IgG PE-conjugate (1:50 dilution, Thermo Fisher Scientific).
Next-Generation Sequencing
Plasmids were isolated from yeast cell populations of the initial library, as well as the 1st and 2nd sorting rounds using the Zymoprep Yeast Plasmid Miniprep Kit (Zymo Research). By means of PCR utilizing Q5 High-Fidelity DNA Polymerase (New England Biolabs), the VH and VL genes were amplified and purified by the PCR Clean-Up System (Promega). Next-generation sequencing by Illumina sequencing was performed at Genewiz (Leipzig, Germany) and resulting NGS data were analysed using Geneious Prime 2020.1.2.
Reformatting
Plasmids of yeast cells, enriched during FACS, were isolated using the Zymoprep Yeast Plasmid Miniprep Kit (Zymo Research). Subsequently, plasmids were transformed into chemically competent XL1 blue E. coli cells and cultivated overnight in 50 ml dYT media with 100 μg/ml ampicillin. The next day, plasmids were isolated using the PureYield™ Plasmid Midiprep System (Promega). For reformatting into the mammalian expression vector, 75 ng of the YSD vector population, encoding the bidirectional CL-VL-Gal1,10-VH-CH1-partial hinge ORFs, was subjected to GGC with 75 ng of the Mammalian Destination (MD) vector, exhibiting the sequences encoding the partial Hinge-CH2-CH3. By Esp3I-mediated GGC, the complete bidirectional yeast-derived ORF cassette was inserted into the MD vector. While the 3’ end of the CL domain exhibited a stop codon, the CH1-partial hinge region of the yeast vector aligns with the partial-hinge-CH2-CH3 region of the MD vector, resulting in an ORF encoding the complete heavy chain. Subsequently, the Golden Gate products were transformed into chemically competent XL1 blue E. coli cells and cultivated overnight in 50 ml dYT media with 25 μg/ml chloramphenicol. Plasmids were isolated using the PureYield™ Plasmid Midiprep System (Promega) and 75 ng were subsequently subjected to GGC cloning utilizing the Mammalian_entry vector. By BbsI-mediated GGC, the Gal1,10 promoter was removed from the vector and exchanged by the bidirectional 2xeCMV promoter cassette. The Golden Gate products were transformed into chemically competent XL1 blue E. coli cells and cultivated overnight in 50 ml dYT media with 25 μg/ml chloramphenicol and subsequently isolated by using the PureYield™ Plasmid Midiprep System (Promega). Golden Gate reactions were performed according to the manufacturer’s instruction. In brief, a 20 µl Golden Gate reaction containing 75 ng of the destination vector, 75 ng of the parental vector harbouring the desired insert, 2 µl T4 ligase buffer, 1 µl of the respective type IIS restriction enzyme (Esp3I or BbsI), and 0.5 µl T4 ligase was used. All reagents for GGC reactions were supplied by New England Biolabs. Golden Gate cloning was performed in 30 cycles consisting each of 1 min at 37°C and 1 min at 16°C for restriction and ligation steps, respectively.
Transient Transfection and Purification
Expi293-F cells (Thermo Fisher Scientific) were used for transient production of antibodies. The cells were cultured in Expi293 Expression Medium (Thermo Fisher Scientific) at 37°C, 8% CO2, and 110 rpm, and sub-passaged every 3–4 days. Transfection was carried out using Expifectamine293 according to the manufacturer’s manual, using 1 µg plasmid DNA per ml culture volume. Six days post-transfection, cells were harvested by centrifugation and the cell culture supernatants were sterile filtered before being applied to a HiTrap MabSelect PrismA column (Cytiva) using an ÄKTA Pure 25 system following the manufacturer’s instructions. Subsequently, a desalting step against PBS was performed using HiTrap Desalting columns (Cytiva).
Biolayer Interferometry
For kinetics and affinity determination, an Octet Red96 (FortéBio) was utilised. Antibodies were loaded onto anti-human Fc Capture (AHC) biosensor tips (Sartorius) and associated to different concentrations of His-tagged TAMR in the range of 6.25–200 nM. Kinetics were determined using Savitzky-Golay filtering and fitted using a 1:1 Langmuir binding model.
Thermal Stability and Size-Exclusion Chromatography
To evaluate the thermal stability of the isolated and reformatted antibodies, melting points (TM) were determined utilising the Prometheus NT.48 NanoDSF Protein Stability Instrument (NanoTemper Technologies) at 0.5 mg/ml between 25–90°C with a heating rate of 1°C/min as previously described (Bogen et al., 2020a). Size exclusion chromatography was carried out using an Agilent Technologies 1260 Infinity system as previously described (Bogen et al., 2020a). In short, 22 µl of a 0.5 mg/ml protein solution were applied onto a TSKgel SuperSW3000 column (Tosoh) at a constant flow rate of 0.35 ml/min using sterile filtered PBS (pH 7.4) as mobile phase.
Results
Overview of Cloning Strategy From Yeast-Surface Display to Mammalian Destination Vector
To ease the workflow from YSD-mediated antibody discovery to their expression in a mammalian system, we developed a high-throughput, bulk cloning procedure that avoids possible PCR-mediated mutations and conserves heavy chain—light chain pairing while yielding a full-length IgG molecule and significantly reducing hands-on time. In principle, VH and VL genes amplified from virtually any origin, for example, an immunised animal, can be subcloned by SapI-mediated GGC into a YSD-vector utilizing the Gal1,10-based bidirectional promoter, similar to the previously described system (Rosowski et al., 2018). Upon transformation in Saccharomyces cerevisiae and FACS-assisted enrichment, yeast cells expressing target-specific Fabs are isolated. For subsequent characterization, expression of full-length IgG molecules in mammalian cells is crucial, as this represents the final expression system in the final format for therapeutic mAbs. However, bulk reformatting of multiple antibody candidates from an enriched pool of binders is not feasible with conventional methods as the original heavy chain—light chain pairing is lost during this process. This could be avoided by using scFvs or other single chain antibody formats. However, for most therapeutic applications, IgG antibodies are favoured as demonstrated by the number of approved IgGs in comparison to approved scFv-based drugs (Reichert, 2021). Furthermore, using Fab libraries for YSD allows for screening in an architecture most closely resembling the final IgG format and has shown to result in more promising antibody variants compared to scFv libraries (Sivelle et al., 2018).
Conventionally, the VH and VL sequences are reformatted for each single candidate separately in order to retain the heavy chain—light chain pairing. As more mAb candidates are available, this process becomes more laborious and tedious. Furthermore, PCR-based subcloning bears the risk of introducing unintended mutations, further increasing workload and slowing down the discovery process. To circumvent all these disadvantages, we engineered a mammalian destination vector (MD), encoding a partial hinge region followed by CH2- and CH3-encoding genes. By Golden Gate Cloning using Esp3I, the whole CL-VL-Gal1,10-VH-CH1-partial hinge ORF is extracted from the YSD vector and inserted into the MD vector, resulting in a full-length heavy chain ORF, enabling the transfer of a whole population of binding molecules in a one-pot reaction. Due to the physical linkage with the yeast Gal1,10 promoter, heavy chain—light chain coupling is maintained. In the next step, this yeast-specific promoter is exchanged by BbsI-mediated Golden Gate Assembly for the 2xeCMV promoter, which was recently demonstrated to be the optimal bidirectional promoter for mAb expression (Carrara et al., 2021b). An overview of the entire procedure is depicted in Figure 1. This final vector enables transient transfection of mammalian cells for mAb production and additionally circumvents the need of co-transfecting separate heavy and light chain-encoding plasmids, which is needed in conventional procedures. All vectors were designed in silico and subsequently ordered at GeneArt (Thermo Fisher Scientific).
PoC Study With Durvalumab
To verify whether this Golden Gate-assisted discovery process is feasible for subcloning of yeast-displayed Fabs into the mammalian expression vector, we performed a PoC study with the FDA-approved antibody Durvalumab, which recognizes the PD-L1 antigen and blocks the PD-1/PD-L1 axis. The respective VH and VL genes were subcloned into the yeast display vector and upon transformation of yeast cells and subsequent FACS analysis, the display of the respective Fab as well as PD-L1 recognition was verified (Figure 2A). Upon Esp3I-mediated subcloning of the antibody-encoding genes and the Gal1,10 promoter into the MD vector, followed by promoter exchange in a BbsI-assisted manner, a mammalian expression vector was generated. Transient transfection of Expi293-F cells yielded full-length Durvalumab molecules able to recognize PD-L1 in BLI experiments (Figure 2B) with an affinity of 364 pM., similar to what has been described in literature (Tan et al., 2018). Encouraged by these initial results, we planned on translating this process to an antibody hit discovery campaign.
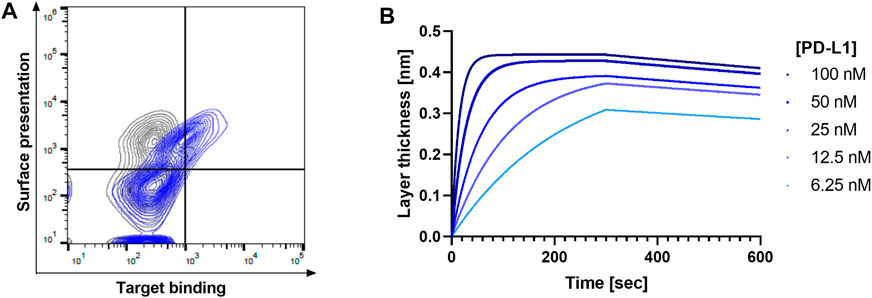
FIGURE 2. Verification of proof-of-concept study using Durvalumab. (A) FACS contour plot showing the display of the Durvalumab Fab and its ability to bind biotinylated PD-L1 (blue) compared to the negative control without antigen (grey). Surface presentation was detected using an anti-kappa AlexaFluor647 antibody, while target binding was detected using streptavidin-PE. (B) Affinity determination of Durvalumab to PD-L1 after production in Expi293-F cells. 10 μg/ml antibody was loaded and associated to different PD-L1 concentrations.
Generation of TAMR Library and Screening
We initiated genetic immunisation of transgenic OmniRats, rodents exhibiting a part of the human antibody germline loci (Osborn et al., 2013), with TAMR. Upon cDNA synthesis after total RNA extraction of spleen and lymph node cells, VH- and VL-encoding genes were amplified and subcloned into the yeast display vector using SapI-mediated GGC and the YSD_Entry_insert providing the bidirectional Gal1,10 promoter. For library generation, SapI was chosen, as this type IIS restriction enzyme does not recognize any antibody germline sequences within OmniRat-derived mAbs. A summary of the cleavage sites within the OmniRat repertoire with the herein used type IIS restriction enzymes, as well as BsaI as a reference, is represented in Supplementary Table S2.
Upon yeast transformation, the resulting library consisted of 3 x 108 clones and was screened via FACS. The first sorting round was performed using 250 nM His-tagged antigen. The subsequent screening round was performed with 250 nM Fc-tagged TAMR, and the outcome of round 2 was stained with 100 nM Fc-tagged TAMR to get higher affinity binders (Figure 3). A sufficient enrichment was observed after two sorting rounds, with 54.5% of the Fab-displaying yeast cells binding to the target antigen.
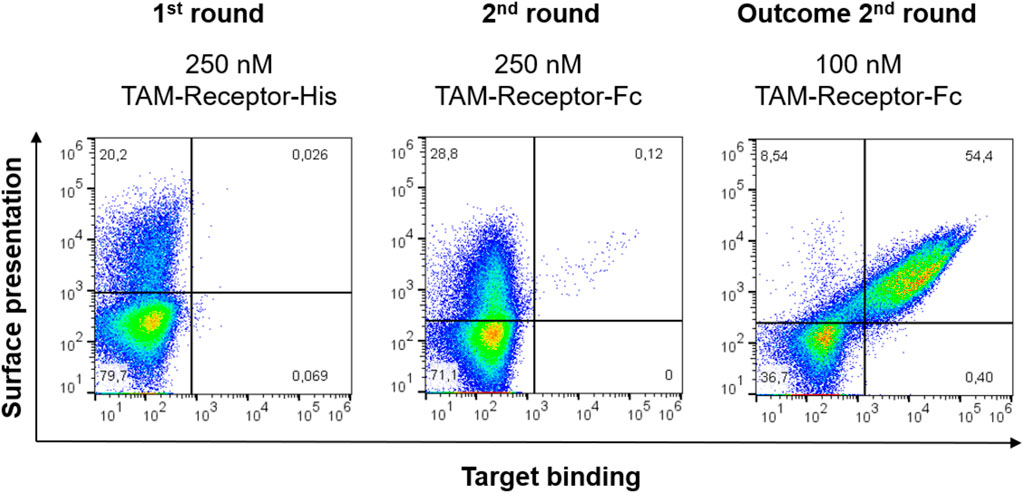
FIGURE 3. Sorting of the TAMR yeast library. Target binding to TAM-Receptor is depicted on the x-axis using either an anti-His AlexaFlour647-conjugated or anti-human IgG-PE conjugated antibody for either TAMR-His or TAMR-Fc, respectively. Surface presentation is shown on the y-axis as detected using a goat F (ab’)2 anti-human Kappa-AlexaFluor647 or -PE conjugate, respectively. 100’000 events are shown.
Reformatting of YSD-Enriched Antibodies Into the Mammalian Destination Vector
The antibody-encoding plasmids from the yeast cells after the second sorting round were isolated and antibody-encoding genes were subcloned into the MD vector. Upon promoter exchange from Gal1,10 to 2xeCMV, the obtained final mammalian expression vectors were then subjected to Sanger sequencing. Analysis of 20 single clones revealed 14 unique antibodies, which, in part, originate from multiple clonotypes (Supplementary Figure S1). By clustering the sequences based on amino acid differences, especially in the CDR3, ten candidates were chosen for further analysis. These ten clones were referred to as K9, K12, K13, K15, K17, K22, K24, K26, K27, and K28.
Next-Generation Sequencing
To confirm that no diversity is lost during the subcloning of the Fabs from the library to the expression vector, next-generation sequencing (NGS) was performed on the initial library as well as the 1st screening round outcome (Supplementary Figure S2). The library diversity from the last sorting round was compared to the individual sequences from isolated variants, and colour-coded with the respective sequences from isolated clones (Figure 4A). The entire diversity of VH sequence families obtained from library sorting was retained in the population of reformatted clones, whereas a few VL sequence families were missing. As only 20 clones were inspected, it is probable that more variability could be covered by sequencing a larger sample. Nonetheless, the VH diversity is generally sufficient to obtain highly specific antibodies (Xu and Davis, 2000) and thus is more critical. Figure 4B depicts the single clones and their respective VH and VL combinations. From this NGS analysis, one can appreciate that no large diversity was lost within the process as the entire VH repertoire was covered within the single clones.
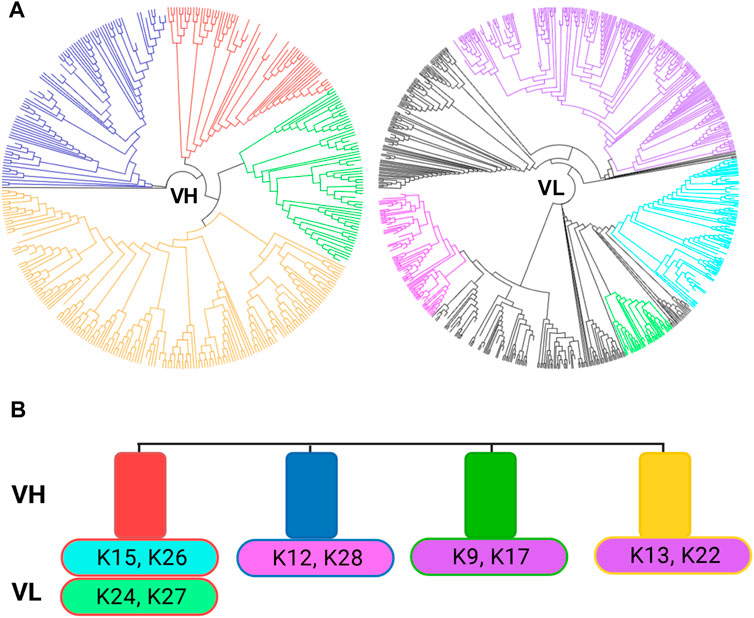
FIGURE 4. Next generation sequencing results from the 2nd round of sorting. (A) Tree view of the VH and VL sequences after the second sorting round separated by VH and VL sequences. Tree maps were generated using Geneious Prime 2020.1.2. The colour-coding corresponds to the VH/VL sequences isolated in the 10 single clones depicted in B. (B) Representation of the found VH and VL in the 10 isolated clones. The rectangles represent the VH clusters, while the ovals represent the VL sequences. Colour-coding of the corresponding VH and VL pairs.
Transient Expression in Expi293-F Cells, Purification and Characterisation
Ten single clones were produced in Expi293-F cells and purified via Protein A to characterise their biophysical properties. Size exclusion chromatography (SEC) revealed favourable aggregation behaviour for most clones, showing minimal aggregation (Figure 5A). K28 exhibited the most aggregation behaviour with 15.3% HMW. NanoDSF measurements showed melting temperatures (TM) between 65 and 70°C, with K13 and K28 exhibiting the lowest and highest TM values with 65.7 and 70.8°C, respectively (Supplementary Figure S3). The observed TMs represent a high thermal stability and are within a reasonable range for non-glycosylated human IgG1 antibodies (Garber and Demarest, 2007; Dashivets et al., 2015). SDS-PAGE analysis revealed the expected heavy and light chain bands (Figure 5B).
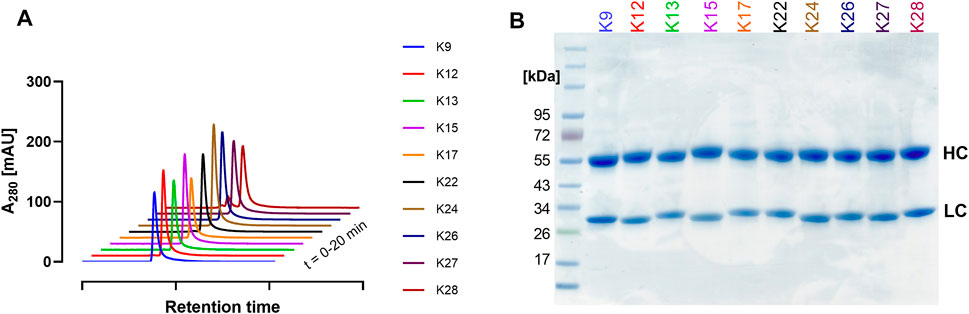
FIGURE 5. Characterisation of single clones. (A) SEC profiles and (B) SDS-PAGE analysis under reducing conditions, showing the respective heavy (HC) and light (LC) chains at their anticipated molecular weights. The curves in (A) are nudged by 1 and 10 data units in the x- and y-axis, respectively. Colour-coding represents the different single clones identified.
Furthermore, kinetics determination via BLI indicated a range of single-digit nanomolar affinities to soluble His-tagged TAMR for the vast majority of clones, namely K9, K13, K15, K17, K22, K26, K27, and K28 (Supplementary Figure S4). K27 exhibited the highest affinity with 5.85 nM, whereas K9 showed the lowest affinity with 43.1 nM (Table 1). Nevertheless, the affinities are within a notable range.
Taken together, the biophysical characterisation revealed 10 promising antibodies targeting TAMR in this proof-of-concept study using the novel approach in order to facilitate the transition from YSD to antibody production in mammalian cells.
Discussion
In this study, we developed a straightforward reformatting approach to yield mammalian expression vectors based on YSD-screened antibody libraries in a PCR-free manner, based on Golden Gate Cloning. This process allows for bulk cloning of yeast display-enriched antibodies, which was previously not possible due to the loss of the heavy chain—light chain pairing, resulting in reduced hands-on time compared to conventional approaches. Although the issue of cognate heavy and light chain pairing can be omitted by the use of scFvs or scFabs, these molecules come with certain limitations, such as their aggregation behaviour (Röthlisberger et al., 2005; Bates and Power, 2019). Furthermore, the utilization of a Fab-displaying yeast library allows for screening in the final format of the antigen binding site for most therapeutic applications.
However, during library generation, VH and VL genes, amplified from cDNA isolated from immunised animals, are shuffled and therefore their natural pairing is lost. Still, this is also true for conventional yeast libraries, as well as for phage or mammalian display systems. Our novel system could be combined with B cell cloning in the future (Tiller, 2011; Carbonetti et al., 2017; Ouisse et al., 2017), which might allow for a workflow starting from B cells, followed by yeast display screening and mammalian antibody production while maintaining the naturally occurring heavy chain—light chain pairing during the whole antibody discovery process.
Previously, Roth and others have demonstrated the successful generation of a bidirectional YSD vector system to isolate CEACAM6-specific antibodies from immunised OmniRat libraries (Roth et al., 2018). For their library generation, BsaI was utilized, which occurs frequently in antibody frameworks (Nelson and Valadon, 2017). To circumvent the risk of losing variants due to internal BsaI sites, we chose SapI for library generation, which does not occur in OmniRat-derived antibody germlines. As depicted in Supplementary Table S2, the other type IIS enzymes employed in this workflow do not have high occurrences within the OmniRat repertoire. Besides human antibodies, chicken-derived antibodies have gained traction within the scientific literature in recent years (Kim et al., 2018; Grzeschik et al., 2019; Bogen et al., 2020a; Bogen et al., 2020b; Bogen et al., 2020c; Hinz et al., 2020; Mwale et al., 2020; Elter et al., 2021). As the chicken germline does not exhibit SapI sites either, this workflow can easily be applied to immunised chickens as well, and most likely, to other immunised animals, like mice or rabbits.
Future applications could also include antibody discovery workflows containing a combination of display technologies, for example, by cross-linking phage and yeast display and the presented MD vector for subsequent mammalian production (Patel et al., 2011). Additionally, Akamatsu and others have previously developed a mammalian cell surface display vector to isolate IgG molecules directly using mammalian display (Akamatsu et al., 2007) that can be used to combine yeast surface display with subsequent mammalian display and production. Such workflows can benefit from the advantages of each display technology but are not limited by their own disadvantages. For instance, combining the larger diversity of phage display libraries with the ability to sort for high-affinity antibodies (Marks, 2020) or to perform epitope binning by yeast surface display may ultimately reduce further screening steps within the discovery workflow (e.g., functional assays, binding affinities, etc.). The herein presented convenient and time-efficient two-pot, two-step method transition from YSD to mammalian expression vector can be further optimised to yield a one-pot, two-step reaction, performing the transfer of antibody VH and VL sequences as well as the promoter exchange within the same “pot”. Furthermore, improvement of the yeast preparation can obviate the need for transformation of E. coli and lead to the direct transition from the yeast preparation into the final MD vector for mammalian antibody production. Moreover, a toolbox of different MD vectors containing different Fc variants [e.g., LALA (Lund et al., 1991), N297Q (Wang et al., 2018), RF mutation (Jendeberg et al., 1997)] could be constructed to allow the practical and time-saving exchange for a whole population. This would also pave the way for establishing the methodology for bispecific or multi-specific antibody formats.
Taken together, we designed a straightforward method to preserve heavy chain—light chain paring while allowing bulk reformatting of YSD-enriched antibody hit candidates into mammalian expression vectors with reduced hands-on time. This procedure has the potential to speed up the drug discovery process and might aid the discovery of therapeutically interesting antibody candidates in the future.
Data Availability Statement
The original contributions presented in the study are included in the article/Supplementary Material, further inquiries can be directed to the corresponding author.
Author Contributions
DF, JB, SC, and LD performed experiments. DF, JB, SC, LD, JG, and HK analysed the data. SZ, JG, and BH gave scientific advice. DF, JB, and SC wrote the article. All authors contributed to the article and approved the submitted version.
Conflict of Interest
The authors declare that the research was conducted in the absence of any commercial or financial relationships that could be construed as a potential conflict of interest.
Publisher’s Note
All claims expressed in this article are solely those of the authors and do not necessarily represent those of their affiliated organizations, or those of the publisher, the editors and the reviewers. Any product that may be evaluated in this article, or claim that may be made by its manufacturer, is not guaranteed or endorsed by the publisher.
Acknowledgments
The authors would like to thank Ferring Pharmaceuticals, especially the department of GPRD, for funding. The funders played no role in study design, data collection and analysis, or preparation of the manuscript. The authors would also like to thank Prof. Fessner for the possibility to perform NanoDSF measurements in his laboratory. Figures were created with BioRender.com. We acknowledge support by the Deutsche Forschungsgemeinschaft (DFG—German Research Foundation) and the Open Access Publishing Fund of Technical University of Darmstadt.
Supplementary Material
The Supplementary Material for this article can be found online at: https://www.frontiersin.org/articles/10.3389/fbioe.2022.794389/full#supplementary-material
References
Akamatsu, Y., Pakabunto, K., Xu, Z., Zhang, Y., and Tsurushita, N. (2007). Whole IgG Surface Display on Mammalian Cells: Application to Isolation of Neutralizing Chicken Monoclonal anti-IL-12 Antibodies. J. Immunol. Methods 327, 40–52. doi:10.1016/j.jim.2007.07.007
Alvarez-Argote, J., and Dasanu, C. A. (2019). Durvalumab in Cancer Medicine: a Comprehensive Review. Expert Opin. Biol. Ther. 19, 927–935. doi:10.1080/14712598.2019.1635115
Bates, A., and Power, C. A. (2019). David vs. Goliath: The Structure, Function, and Clinical Prospects of Antibody Fragments. Antibodies 8, 28. doi:10.3390/antib8020028
Batonick, M., Kiss, M. M., Fuller, E. P., Magadan, C. M., Holland, E. G., Zhao, Q., et al. (2016). pMINERVA: A Donor-Acceptor System for the In Vivo Recombineering of scFv into IgG Molecules. J. Immunol. Methods 431, 22–30. doi:10.1016/j.jim.2016.02.003
Benatuil, L., Perez, J. M., Belk, J., and Hsieh, C.-M. (2010). An Improved Yeast Transformation Method for the Generation of Very Large Human Antibody Libraries. Protein Eng. Des. Sel. 23, 155–159. doi:10.1093/protein/gzq002
Boder, E. T., and Wittrup, K. D. (1997). Yeast Surface Display for Screening Combinatorial Polypeptide Libraries. Nat. Biotechnol. 15, 553–557. doi:10.1038/nbt0697-553
Bogen, J. P., Carrara, S. C., Fiebig, D., Grzeschik, J., Hock, B., and Kolmar, H. (2020). Expeditious Generation of Biparatopic Common Light Chain Antibodies via Chicken Immunization and Yeast Display Screening. Front. Immunol. 11. doi:10.3389/fimmu.2020.606878
Bogen, J. P., Grzeschik, J., Krah, S., Zielonka, S., and Kolmar, H. (2020). Rapid Generation of Chicken Immune Libraries for Yeast Surface Display. Methods Mol. Biology,Genotype Phenotype Coupling 16 (3), 289–302. doi:10.1007/978-1-4939-9853-1_16
Bogen, J. P., Storka, J., Yanakieva, D., Fiebig, D., Grzeschik, J., Hock, B., et al. (2020). Isolation of Common Light Chain Antibodies from Immunized Chickens Using Yeast Biopanning and Fluorescence‐Activated Cell Sorting. Biotechnol. J. 16, 2000240. doi:10.1002/biot.202000240
Bournazos, S., and Ravetch, J. V. (2017). Diversification of IgG Effector Functions. Int. Immunol. 29, 303–310. doi:10.1093/intimm/dxx025
Carbonetti, S., Oliver, B. G., Vigdorovich, V., Dambrauskas, N., Sack, B., Bergl, E., et al. (2017). A Method for the Isolation and Characterization of Functional Murine Monoclonal Antibodies by Single B Cell Cloning. J. Immunol. Methods 448, 66–73. doi:10.1016/j.jim.2017.05.010
Carrara, S. C., Fiebig, D., Bogen, J. P., Grzeschik, J., Hock, B., and Kolmar, H. (2021). Recombinant Antibody Production Using a Dual-Promoter Single Plasmid System. Antibodies 10, 18. doi:10.3390/antib10020018
Carrara, S. C., Ulitzka, M., Grzeschik, J., Kornmann, H., Hock, B., and Kolmar, H. (2021). From Cell Line Development to the Formulated Drug Product: The Art of Manufacturing Therapeutic Monoclonal Antibodies. Int. J. Pharm. 594, 120164. doi:10.1016/j.ijpharm.2020.120164
Cruz-Teran, C. A., Tiruthani, K., Mischler, A., and Rao, B. M. (2017). Inefficient Ribosomal Skipping Enables Simultaneous Secretion and Display of Proteins in Saccharomyces cerevisiae. ACS Synth. Biol. 6, 2096–2107. doi:10.1021/acssynbio.7b00144
Dashivets, T., Thomann, M., Rueger, P., Knaupp, A., Buchner, J., and Schlothauer, T. (2015). Multi-Angle Effector Function Analysis of Human Monoclonal IgG Glycovariants. PLoS One 10, e0143520. doi:10.1371/journal.pone.0143520
Elter, A., Bogen, J. P., Hinz, S. C., Fiebig, D., Macarrón Palacios, A., Grzeschik, J., et al. (2021). Humanization of Chicken‐Derived scFv Using Yeast Surface Display and NGS Data Mining. Biotechnol. J. 16, 2000231. doi:10.1002/biot.202000231
Garber, E., and Demarest, S. J. (2007). A Broad Range of Fab Stabilities within a Host of Therapeutic IgGs. Biochem. Biophysical Res. Commun. 355, 751–757. doi:10.1016/j.bbrc.2007.02.042
Grzeschik, J., Yanakieva, D., Roth, L., Krah, S., Hinz, S. C., Elter, A., et al. (2019). Yeast Surface Display in Combination with Fluorescence‐activated Cell Sorting Enables the Rapid Isolation of Antibody Fragments Derived from Immunized Chickens. Biotechnol. J. 14, 1800466. doi:10.1002/biot.201800466
Hinz, S. C., Elter, A., Rammo, O., Schwämmle, A., Ali, A., Zielonka, S., et al. (2020). A Generic Procedure for the Isolation of pH- and Magnesium-Responsive Chicken scFvs for Downstream Purification of Human Antibodies. Front. Bioeng. Biotechnol. 8. doi:10.3389/fbioe.2020.00688
Hoy, S. M. (2019). Sintilimab: First Global Approval. Drugs 79, 341–346. doi:10.1007/s40265-019-1066-z
Jendeberg, L., Nilsson, P., Larsson, A., Denker, P., Uhlén, M., Nilsson, B., et al. (1997). Engineering of Fc1 and Fc3 from Human Immunoglobulin G to Analyse Subclass Specificity for Staphylococcal Protein A. J. Immunol. Methods 201, 25–34. doi:10.1016/S0022-1759(96)00215-3
Josephson, K., Ricardo, A., and Szostak, J. W. (2014). mRNA Display: from Basic Principles to Macrocycle Drug Discovery. Drug Discov. Today 19, 388–399. doi:10.1016/j.drudis.2013.10.011
Kapur, R., Einarsdottir, H. K., and Vidarsson, G. (2014). IgG-effector Functions: "The Good, the Bad and the Ugly". Immunol. Lett. 160, 139–144. doi:10.1016/j.imlet.2014.01.015
Kim, Y. M., Park, J. S., Kim, S. K., Jung, K. M., Hwang, Y. S., Han, M., et al. (2018). The Transgenic Chicken Derived Anti-CD20 Monoclonal Antibodies Exhibits Greater Anti-cancer Therapeutic Potential with Enhanced Fc Effector Functions. Biomaterials 167, 58–68. doi:10.1016/j.biomaterials.2018.03.021
Krah, S., Günther, R., Becker, S., Zielonka, S., and Rhiel, L. (2020). Chemical Modification of the Yeast Cell Surface Allows the Switch between Display and Soluble Secretion of Full-Length Antibodies. Methods Mol. Biology,Genotype Phenotype Coupling 2070, 335–349. doi:10.1007/978-1-4939-9853-1_19
Li, F., Vijayasankaran, N., Shen, A., Kiss, R., and Amanullah, A. (2010). Cell Culture Processes for Monoclonal Antibody Production. MAbs 2, 466–479. doi:10.4161/mabs.2.5.12720
Lipovsek, D., and Plückthun, A. (2004). In-vitro Protein Evolution by Ribosome Display and mRNA Display. J. Immunol. Methods 290, 51–67. doi:10.1016/j.jim.2004.04.008
Liu, Y., Gu, M., Wu, Y., Wang, W., Wang, R., Du, M., et al. (2018). High-throughput Reformatting of Phage-Displayed Antibody Fragments to IgGs by One-step Emulsion PCR. Protein Eng. Des. Sel. 31, 427–436. doi:10.1093/protein/gzz004
Lu, R.-M., Hwang, Y.-C., Liu, I.-J., Lee, C.-C., Tsai, H.-Z., Li, H.-J., et al. (2020). Development of Therapeutic Antibodies for the Treatment of Diseases. J. Biomed. Sci. 27, 1. doi:10.1186/s12929-019-0592-z
Lund, J., Winter, G., Jones, P. T., Pound, J. D., Tanaka, T., Walker, M. R., et al. (1991). Human Fc Gamma RI and Fc Gamma RII Interact with Distinct but Overlapping Sites on Human IgG. J. Immunol. 147, 2657–2662.
Marks, J. D. (2020). “Monoclonal Antibodies From Display Libraries,” in Molecular Biology of B Cells (Elsevier), 511–531. doi:10.1016/B978-012053641-2/50033-2
McCafferty, J., Griffiths, A. D., Winter, G., and Chiswell, D. J. (1990). Phage Antibodies: Filamentous Phage Displaying Antibody Variable Domains. Nature 348, 552–554. doi:10.1038/348552a0
Mwale, P. F., Lee, C.-H., Lin, L.-T., Leu, S.-J., Huang, Y.-J., Chiang, L.-C., et al. (2020). Expression, Purification, and Characterization of Anti-zika Virus Envelope Protein: Polyclonal and Chicken-Derived Single Chain Variable Fragment Antibodies. Ijms 21, 492. doi:10.3390/ijms21020492
Nelson, R. S., and Valadon, P. (2017). A Universal Phage Display System for the Seamless Construction of Fab Libraries. J. Immunol. Methods 450, 41–49. doi:10.1016/j.jim.2017.07.011
Osborn, M. J., Ma, B., Avis, S., Binnie, A., Dilley, J., Yang, X., et al. (2013). High-Affinity IgG Antibodies Develop Naturally in Ig-Knockout Rats Carrying Germline Human IgH/Igκ/Igλ Loci Bearing the Rat CHRegion. J. I. 190, 1481–1490. doi:10.4049/jimmunol.1203041
Ouisse, L.-H., Gautreau-Rolland, L., Devilder, M.-C., Osborn, M., Moyon, M., Visentin, J., et al. (2017). Antigen-specific Single B Cell Sorting and Expression-Cloning from Immunoglobulin Humanized Rats: a Rapid and Versatile Method for the Generation of High Affinity and Discriminative Human Monoclonal Antibodies. BMC Biotechnol. 17, 3. doi:10.1186/s12896-016-0322-5
Parthiban, K., Perera, R. L., Sattar, M., Huang, Y., Mayle, S., Masters, E., et al. (2019). A Comprehensive Search of Functional Sequence Space Using Large Mammalian Display Libraries Created by Gene Editing. MAbs 11, 884–898. doi:10.1080/19420862.2019.1618673
Patel, C. A., Wang, J., Wang, X., Dong, F., Zhong, P., Luo, P. P., et al. (2011). Parallel Selection of Antibody Libraries on Phage and Yeast Surfaces via a Cross-Species Display. Protein Eng. Des. Sel. 24, 711–719. doi:10.1093/protein/gzr034
Reader, R. H., Workman, R. G., Maddison, B. C., and Gough, K. C. (2019). Advances in the Production and Batch Reformatting of Phage Antibody Libraries. Mol. Biotechnol. 61, 801–815. doi:10.1007/s12033-019-00207-0
Reichert, J. (2021). Therapeutic Monoclonal Antibodies Approved or in Review in the European Union or the United States. The Antibody Society. https://www.antibodysociety.org/resources/approved-antibodies/.
Renaut, L., Monnet, C., Dubreuil, O., Zaki, O., Crozet, F., Bouayadi, K., et al. (2012). Affinity Maturation of Antibodies: Optimized Methods to Generate High-Quality ScFv Libraries and Isolate IgG Candidates by High-Throughput Screening. Antib. Engineering,Methods Mol. Biol. 907, 451–461. doi:10.1007/978-1-61779-974-7_26
Rosowski, S., Becker, S., Toleikis, L., Valldorf, B., Grzeschik, J., Demir, D., et al. (2018). A Novel One-step Approach for the Construction of Yeast Surface Display Fab Antibody Libraries. Microb. Cell Fact. 17, 3. doi:10.1186/s12934-017-0853-z
Roth, L., Grzeschik, J., Hinz, S. C., Becker, S., Toleikis, L., Busch, M., et al. (2018). Facile Generation of Antibody Heavy and Light Chain Diversities for Yeast Surface Display by Golden Gate Cloning. Biol. Chem. 400, 383–393. doi:10.1515/hsz-2018-0347
Röthlisberger, D., Honegger, A., and Plückthun, A. (2005). Domain Interactions in the Fab Fragment: A Comparative Evaluation of the Single-Chain Fv and Fab Format Engineered with Variable Domains of Different Stability. J. Mol. Biol. 347, 773–789. doi:10.1016/j.jmb.2005.01.053
Schaffitzel, C., Hanes, J., Jermutus, L., and Plückthun, A. (1999). Ribosome Display: an In Vitro Method for Selection and Evolution of Antibodies from Libraries. J. Immunol. Methods 231, 119–135. doi:10.1016/S0022-1759(99)00149-0
Sivelle, C., Sierocki, R., Ferreira-Pinto, K., Simon, S., Maillere, B., and Nozach, H. (2018). Fab Is the Most Efficient Format to Express Functional Antibodies by Yeast Surface Display. MAbs 10, 720–729. doi:10.1080/19420862.2018.1468952
Tan, S., Liu, K., Chai, Y., Zhang, C. W.-H., Gao, S., Gao, G. F., et al. (2018). Distinct PD-L1 Binding Characteristics of Therapeutic Monoclonal Antibody Durvalumab. Protein Cell 9, 135–139. doi:10.1007/s13238-017-0412-8
Tanner, W., and Lehle, L. (1987). Protein Glycosylation in Yeast. Biochimica Biophysica Acta (BBA) - Rev. Biomembr. 906, 81–99. doi:10.1016/0304-4157(87)90006-2
Tiller, T. (2011). Single B Cell Antibody Technologies. New Biotechnol. 28, 453–457. doi:10.1016/j.nbt.2011.03.014
Valldorf, B., Hinz, S. C., Russo, G., Pekar, L., Mohr, L., Klemm, J., et al. (2021). Antibody Display Technologies: Selecting the Cream of the Crop. Biol. Chem. 403, 455–477. doi:10.1515/hsz-2020-0377
Vazquez-Lombardi, R., Nevoltris, D., Luthra, A., Schofield, P., Zimmermann, C., and Christ, D. (2018). Transient Expression of Human Antibodies in Mammalian Cells. Nat. Protoc. 13, 99–117. doi:10.1038/nprot.2017.126
Wang, X., Mathieu, M., and Brezski, R. J. (2018). IgG Fc Engineering to Modulate Antibody Effector Functions. Protein Cell 9, 63–73. doi:10.1007/s13238-017-0473-8
Wildt, S., and Gerngross, T. U. (2005). The Humanization of N-Glycosylation Pathways in Yeast. Nat. Rev. Microbiol. 3, 119–128. doi:10.1038/nrmicro1087
Xiao, X., Douthwaite, J. A., Chen, Y., Kemp, B., Kidd, S., Percival-Alwyn, J., et al. (2017). A High-Throughput Platform for Population Reformatting and Mammalian Expression of Phage Display Libraries to Enable Functional Screening as Full-Length IgG. MAbs 9, 996–1006. doi:10.1080/19420862.2017.1337617
Keywords: antibody hit discovery, bidirectional promoter, reformatting, golden gate cloning, monoclonal antibodies, yeast surface display
Citation: Fiebig D, Bogen JP, Carrara SC, Deweid L, Zielonka S, Grzeschik J, Hock B and Kolmar H (2022) Streamlining the Transition From Yeast Surface Display of Antibody Fragment Immune Libraries to the Production as IgG Format in Mammalian Cells. Front. Bioeng. Biotechnol. 10:794389. doi: 10.3389/fbioe.2022.794389
Received: 13 October 2021; Accepted: 20 April 2022;
Published: 10 May 2022.
Edited by:
Kequan Chen, Nanjing Tech University, ChinaReviewed by:
Tae Hyeon Yoo, Ajou University, South KoreaGordana Wozniak-Knopp, University of Natural Resources and Life Sciences, Austria
Xiaoling Wu, South China University of Technology, China
Copyright © 2022 Fiebig, Bogen, Carrara, Deweid, Zielonka, Grzeschik, Hock and Kolmar. This is an open-access article distributed under the terms of the Creative Commons Attribution License (CC BY). The use, distribution or reproduction in other forums is permitted, provided the original author(s) and the copyright owner(s) are credited and that the original publication in this journal is cited, in accordance with accepted academic practice. No use, distribution or reproduction is permitted which does not comply with these terms.
*Correspondence: Harald Kolmar, a29sbWFyQGJpb2NoZW1pZS10dWQuZGU=
†These authors have contributed equally to this work and share first authorship