Transcriptional Regulation of the Creatine Utilization Genes of Corynebacterium glutamicum ATCC 14067 by AmtR, a Central Nitrogen Regulator
- 1Guangdong Key Laboratory of Fermentation and Enzyme Engineering, School of Biology and Biological Engineering, South China University of Technology, Guangzhou, China
- 2Guangdong Research Center of Industrial Enzyme and Green Manufacturing Technology, School of Biology and Biological Engineering, South China University of Technology, Guangzhou, China
In the genus Corynebacterium, AmtR is a key component of the nitrogen regulatory system, and it belongs to the TetR family of transcription regulators. There has been much research on AmtR structure, functions, and regulons in the type strain C. glutamicum ATCC 13032, but little research in other C. glutamicum strains. In this study, chromatin immunoprecipitation and massively parallel DNA sequencing (ChIP-seq) was performed to identify the AmtR regulon in C. glutamicum ATCC 14067. Ten peaks were obtained in the C. glutamicum ATCC 14067 genome including two new peaks related to three operons (RS_01910-RS_01915, RS_15995, and RS_16000). The interactions between AmtR and the promoter regions of the three operons were confirmed by electrophoretic mobility shift assays (EMSAs). The RS_01910, RS_01915, RS_15995, and RS_16000 are not present in the type strain C. glutamicum ATCC 13032. Sequence analysis indicates that RS_01910, RS_01915, RS_15995, and RS_16000, are related to the degradation of creatine and creatinine; RS_01910 may encode a protein related to creatine transport. The genes RS_01910, RS_01915, RS_15995, and RS_16000 were given the names crnA, creT, cshA, and hyuB, respectively. Real-time quantitative PCR (RT-qPCR) analysis and sfGFP (superfolder green fluorescent protein) analysis reveal that AmtR directly and negatively regulates the transcription and expression of crnA, creT, cshA, and hyuB. A growth test shows that C. glutamicum ATCC 14067 can use creatine or creatinine as a sole nitrogen source. In comparison, a creT deletion mutant strain is able to grow on creatinine but loses the ability to grow on creatine. This study provides the first genome-wide captures of the dynamics of in vivo AmtR binding events and the regulatory network they define. These elements provide more options for synthetic biology by extending the scope of the AmtR regulon.
Introduction
Corynebacterium glutamicum is a Gram-positive and generally regarded as safe (GRAS) microorganism with less endotoxicity. C. glutamicum usually adapts to different growth environments and pressures in industrial applications and laboratory research and it can be widely used for the production of amino acids, organic acids, and other products related to health, cosmetics, and food (Becker et al., 2011; Binder et al., 2013). Genome sequencing (Tauch et al., 2002; Kalinowski et al., 2003; Lv et al., 2012), transcriptome sequencing (Hayashi et al., 2002; Fan et al., 2021), metabolome (Krömer Jens et al., 2004; Feith et al., 2020), and proteome (Hermann et al., 2001; Jiang et al., 2020) analyses gradually elucidated the perfected genome-scale metabolic map of C. glutamicum, expanding the scope of its application. At present, not only the type-strain C. glutamicum ATCC 13032, but many non-type strains have also been studied and applied, for example, C. glutamicum ATCC 14067 (Huang et al., 2017; Zhang et al., 2021), C. glutamicum R (Kubota et al., 2015), C. glutamicum AS 1.542 (Chen et al., 2012), C. glutamicum ATCC 13869 (Kikuchi et al., 2006; Liu et al., 2018b). These strains have apparent differences in several phenotypic characteristics (Yang and Yang, 2017).
Gene transcription and expression is regulated by transcriptional factors, including sigma factors and two-component systems that are essential for stabilizing cell homeostasis (López-Maury et al., 2008). The research on transcription factors in C. glutamicum was mainly focused on the type strain C. glutamicum ATCC 13032. But there are specific regulatory elements in other strains that give those strains different metabolic capabilities. Most C. glutamicum strains cannot use l-arabinose as a carbon source, however, the gene cluster for l-arabinose utilization and its regulation have been reported in C. glutamicum ATCC 31831 (Kuge et al., 2015). Fifteen paa (paaTK, paaABCDEGJFH, paaI, paaY, and paaZ) genes encode the phenylacetic acid degradation pathways that regulated by PaaR in C. glutamicum AS 1.542 (Chen et al., 2012). A shikimate transporter, regulated by ShiR in C. glutamicum R, was identified and a shikimic acid biosensor was constructed from ShiR for monitoring shikimate synthesis in C. glutamicum RES167 (Kubota et al., 2015; Liu et al., 2018a). The regulatory elements in such strains provide more options for synthetic biology.
C. glutamicum can use a wide range of nitrogen compounds as nitrogen sources, and AmtR is a critical component of the nitrogen regulatory system that belongs to the TetR family of transcription regulators (Jakoby et al., 2000). In the type strain C. glutamicum ATCC 13032, there has been much research on AmtR structure, function, and regulons (Jakoby et al., 2000; Bendt et al., 2004; Hasselt et al., 2011). A total of 35 genes are regulated by AmtR, including the genes that encode the ammonium transporters amtA, amtB, the urea uptake system urtABCDE, the l-glutamate uptake system gluABCD, the creatinine transporter crnT, the ABC-type transport systems Ncgl 1915-1918 involved in the transport of nitrogen sources. The ureABCEFGD, gltBD, gdh, glnA, and codA encoding proteins are involved in nitrogen metabolism (Hasselt et al., 2011). In addition, the mez gene encoding the malic enzyme involved in carbon metabolism is also regulated by AmtR (Gourdon et al., 2000). The binding of AmtR to its regulon promoter region relies on conserved sequences and no small molecule effectors have been identified. A PII-type signal transduction protein GlnK, adenylylated at Tyr51, has been proposed to derepress expression of the AmtR regulons (Strösser et al., 2004).
C. glutamicum ATCC 14067 is an important industrial workhorse employed as an l-glutamate producer and a parental strain for breeding the producers of l-lysine and l-glutamine (Li et al., 2007; Jianzhong et al., 2014; Lv et al., 2021). In this study, ChIP-seq was performed to identify the regulon of AmtR in the non-type strain C. glutamicum ATCC 14067. Four new target genes (RS_01910, RS_01915, RS_15995, and RS_16000) were determined. The proteins encoded by these new target genes may be related to creatine and creatinine degradation, and these genes were given the names crnA, creT, hyuB, and cshA, respectively. Creatine, a component of the dissolve-free amino acid (DFAA) pool, is a metabolite of metazoans. It is found in the excretions of different animals, including earthworms and birds (Wyss and Kaddurah-Daouk, 2000). In addition, some phytoplankton may also produce creatine via metabolism (Wawrik et al., 2017). It has been reported that C. glutamicum ATCC 13032 cannot utilize creatine as a carbon or nitrogen source. We investigated the abilities of C. glutamicum ATCC 14067 to degrade creatine. The result show C. glutamicum ATCC 14067 could grow in CGXII medium with creatine as a sole nitrogen source, and CreT is related to creatine transport. Real-time quantitative PCR (RT-qPCR) analysis and sfGFP (superfolder green fluorescent protein) analysis reveal that AmtR directly and negatively regulate the transcription and expression of crnA, creT, hyuB, and cshA.
Materials and Methods
Bacterial Strains, Media, and Culture Conditions
The strains and plasmids used in this study are listed in Supplementary Table S1. Escherichia coli DH5α was used for gene cloning, and BL21 (DE3) was used for protein expression. E. coli strains were grown in Luria-Bertani (LB) (10 g/L tryptone, 5 g/L yeast extract, and 10 g/L sodium chloride) medium at 37°C or 16°C. C. glutamicum strains were grown overnight in BHI medium (37 g/L brain-heart infusion) (Becton, Dickinson and Co.) and then cultured in fresh BHI medium or CGXII medium (Keilhauer et al., 1993) at 30°C. Cells grown in the CGXII medium, were centrifuged and washed twice with CGXII and then cultured in fresh CGXII medium. If necessary, CGXII medium was supplemented with 10 mM creatine or creatinine as a nitrogen and carbon source. Strain growth was monitored by measuring the optical density (OD) of the cultures at 600 nm. The medium was supplemented with antibiotics at the following concentrations: kanamycin (Kan): 50 μg/ml for E. coli and 25 μg/ml for C. glutamicum, chloramphenicol (Chl): 15 μg/ml for E. coli and 7.5 μg/ml for C. glutamicum.
Construction of the amtR, creT Deletion Mutants and amtR-3Flag Complementation in C. glutamicum ATCC 14067
Standard DNA cloning, Gibson assembly, and transformation procedures were employed (Sambrook and Russell, 2001; Gibson et al., 2009). The amtR and creT deletion mutants (△amtR and △creT) in C. glutamicum ATCC 14067 were constructed using homologous recombination (Huang et al., 2017). The DNA fragments of the upstream and downstream homology arms of amtR and creT were amplified by PCR using the primers amtR-L/amtR-L-lox71 and amtR-R/amtR-R-lox66, creT-L/creT-L-lox71 and creT-R/creT-R-lox66 overlapping PCR with a Cre-kan cassette to construct a self-excisable cassette, respectively. The self-excisable cassettes were introduced into C. glutamicum ATCC 14067 carrying the recombinase-exonuclease pairs by electroporation (Takano et al., 2008). BHI solid medium supplemented with Kan 25 μg/ml and Chl 7.5 μg/ml was used for recombinant selection. Theophylline (1 mM) was used for inducing Cre expression that mediated intermolecular excision. The DNA fragments for amtR and creT deletion strains were confirmed by DNA sequencing (Sangon Biotech, China). A 3Flag tag was added to the C-terminal of AmtR, and the amtR-3Flag fragment was amplified using the primers amtR-3Flag-S/amtR-3Flag-A. A plasmid backbone was amplified from plasmid pEC-XK99E using the primers 99E-S/99E-A. Then the plasmid backbone and the amtR-3Flag fragment were assembled by Gibson assembly to construct the plasmid pEC-XK99E-amtR-3Flag, which was introduced into the △amtR strains by electroporation. The primers used in this study are listed in Supplementary Table S2.
Western Blot Analysis
Complemented strain C. glutamicum 14067-△amtR:amtR-3Flag was grown in BHI medium, and AmtR-3Flag production was induced using 0.5 mM IPTG (isopropyl -β-d-thiogalactopyranoside) for 8 h. AmtR-3Flag production was not induced with 0.5 mM IPTG as the negative control. The cells were harvested by centrifugation and washed with PBS buffer (150 mM NaCl, 3 mM KCl, 10 mM Na2PO4, 3 mM KH2PO4, pH 7.5), suspended in PBS buffer to normalize the culture densitiy based on the OD600 value, and disintegrated with silica beads (0.1 mm) for 12 cycle of 30 s at a speed rating of 6.0 with 3 min resting intervals by Bead Ruptor 12 (OMNI International, United States). Soluble extracts were fractionated on a 12% denaturing polyacrylamide gel before being transferred to a polyvinylidene difluoride (PVDF) membrane (Millipore, Bedford, MA, United States). The membrane was blocked with 3% bovine serum albumin and incubated overnight at 4°C with a 1:2,000 dilution of Flag-specific (Sigma-Aldrich, St. Louis, MO, United States) mouse antiserum, and incubated with a 1:5,000 dilution of horseradish peroxidase-conjugated goat anti-mouse IgG (Santa Cruz Biotechnology, Dallas, TX, United States). Finally, the immunoreactive protein bands were visualized with an ECL reagent (Thermo Fisher Scientific Inc., Waltham, MA).
Overexpression and Purification of AmtR
AmtR-His6 expression plasmid constructed by PCR using the primers amtR-S/amtR-A was inserted into pET28a, which had a His6-tag at its C-terminus. The plasmid was confirmed by DNA sequencing (Sangon Biotech, China). AmtR with a C-terminal His6-tag was expressed in E. coli BL21, plasmid carrying cells were grown to an OD600 of 0.6 at 37°C in LB medium, and protein production was induced using 0.5 mM IPTG at 16°C for 16 h. Cells were harvested by centrifugation and suspended in buffer A (100 mM Tris-HCl, 100 mM NaCl, pH 7.5), then supplemented with Mini protease inhibitor cocktail tablets (Roche, Germany). Cells disrupted by sonication at 4°C, and cell debris was removed by centrifugation at 4°C (15,000 g for 20 min). The AmtR-His6 was purified by 5 ml nickel affinity chromatography using Ni-NTA agarose (Novagen, United States).
Total RNA Extraction and RT-qPCR Analysis
The C. glutamicum ATCC 14067 wild-type and △amtR strains were grown overnight in BHI medium, inoculated into fresh CGXII medium to an OD600 of 0.2, and cultured for 8 h before the extraction of RNA. If necessary, CGXII medium was supplemented with 10 mM creatine as a nitrogen source. Total RNA was extracted using an RNA extraction kit (Tiangen, Beijing, China) with on-column DNaseI treatment. The final RNA concentrations and purities were determined on a Thermo Scientific NanoDrop spectrophotometer; equal amounts of RNA (1 μg) were used to generate cDNA (Toyobo, Tsuruga, Japan) using 6-mer random primers. Primers for various genes (Supplementary Table S2) were designed using Primer Premier6 software. Three independent RT-qPCR experiments were performed, and each experiment was run in triplicate. The reactions were run on an Applied Biosystems 7500 real-time system (Applied Biosystems), and the transcript levels were normalized to the 16S rRNA level in each sample using the ∆∆CT method.
Construction of sfGFP Reporter Plasmids and Fluorescence Assay
Promoters of creT, cshA, and hyuB were amplified from genomic DNA with the primers creT-S/creT-A, cshA-S/cshA-A, and hyuB-S/hyuB-A, respectively. The plasmid backbone was amplified from plasmid pEC-XK99E with the primers 99E-sfGFP-S/99E-sfGFP-A. The promoters were then assembled to the plasmid backbone by Gibson assembly to construct the plasmids pEC-XK99E-PcreT-sfGFP, pEC-XK99E-PcshA-sfGFP, and pEC-XK99E-PhyuB-sfGFP. These plasmids were introduced into the C. glutamicum ATCC 14067 wild-type and △amtR strains by electroporation.
The wild-type C. glutamicum ATCC 14067 strain and the △amtR strain with reporter plasmids were grown overnight in BHI medium, the cells washed with CGXII medium, then inoculated into fresh CGXII medium to an OD600 of 0.2. If necessary, CGXII medium was supplemented with 10 mM creatine as a nitrogen source. After cultivation at 30°C for 8 h, the cells were washed twice with PBS buffer and resuspended in 200 μl PBS buffer, in 96-well plates for measurement of GFP fluorescence. Fluorescence was assessed at an excitation wavelength of 488 nm and an emission wavelength of 520 nm using a multifunctional microplate reader (Infinite M200, Tecan, Switzerland).
ChIP-Seq
The ChIP-seq protocol was based on previous experiments with C. glutamicum (Jungwirth et al., 2013). Strain C. glutamicum ATCC-14067-△amtR:amtR-3Flag was grown at 30°C in BHI medium, and AmtR-3Flag production was induced using 0.5 mM IPTG for 8 h. AmtR-3Flag production was not induced with 0.5 mM IPTG as the negative control. To achieve protein-DNA crosslinking in vivo, a final concentration of 1% formaldehyde was added to the cultures, which was incubated at room temperature for 15 min with gentle shaking. A final concentration of 125 mM glycine was added to stop crosslinking. Cells were harvested by centrifugation, washed twice with a complete protease inhibitor cocktail (Roche) in an ice-cold Tris buffer (20 mM Tris-HCl pH 7.5, 150 mM NaCl), and stored at −80°C. To prepare lysates, the pellets were resuspended in FA-1 buffer (HEPES-KOH at 50 mM [pH 7.5], NaCl at 140 mM, EDTA at 1 mM, Triton X-100 at 1%, and complete protease inhibitor cocktail). One ml sample of this cell suspension was mixed with 0.6 g of 0.1 mm silica beads (BioSpec Products, United States), and the cells were disrupted for 12 cycles of 30 s at a speed rating of 6.0 with 3 min resting intervals by Bead Ruptor 12 (OMNI International, United States). The cell debris was removed by centrifugation at 14,000 g for 15 min at 4°C, and the DNA in the supernatant was sheared to an average length of 200–500 bp by sonication in a water bath (Bioruptor, Diagenode). The lysates were pre-cleared with 30 μl of ChIP magnetic A + G beads (Merck Millipore). The rest of the pre-cleared lysates were incubated overnight at 4°C with monoclonal anti-Flag M2 (Sigma-Aldrich). Protein-DNA complexes were immunoprecipitated with 50 μl of ChIP magnetic A + G beads for 4 h at 4°C and subsequently washed sequentially with low-salt washing buffer (0.1% SDS, 1% Triton X-100, 2 mM EDTA, 20 mM Tris-HCl pH 8.1, 150 mM NaCl), with high-salt washing buffer (0.1% SDS, 1% Triton X-100, 2 mM EDTA, 20 mM Tris-HCl pH 8.1, 500 mM NaCl), with LiCl washing buffer (0.25 M LiCl, 1% NP-40, 1% deoxycholate, 1 mM EDTA, 10 mM Tris-HCl pH 8.1) and finally twice with TE buffer (10 mM Tris-HCl pH 8.1, 1 mM EDTA). The magnetic beads were resuspended in 200 μl of elution buffer (ChIP kit, 17-10086, Merck Millipore). Crosslinking was reverted for 8 h at 65°C. Proteinase K and RNase A were used to remove protein and RNA, respectively. The DNA was extracted with phenol-chloroform and was used for ChIP-seq library preparation. The library was constructed by Novogene Corporation (Beijing, China). Subsequently, pair-end sequencing of sample was performed on Illumina platform (Illumina, CA, United States). The ChIP-seq reads were aligned to the C. glutamicum ATCC 14067 genome using BWA mem (v 0.7.12). The enriched peaks were then identified using MACS (v 2.1.0) software (Zhang et al., 2008).
Electrophoretic Mobility Shift Assay
DNA-binding was determined by Electrophoretic Mobility Shift Assay (EMSA). The fragments PcreT-1, PcreT-2, PhyuB-1, PhyuB-2, and PhyuB-3 covering the putative AmtR binding sequence in crnT, hyuB, and cshA promotor were annealed using two complementary single-stranded oligonucleotides as described previously (Kraxner et al., 2019).
Purified AmtR was mixed with promoter fragments according to the manufacturer’s protocol (LightShift Chemiluminescent EMSA Kit, Thermo); a total volume of 20 μl contained 1 × binding buffer (10 mM Tris, 50 mM KCl, 1 mM DTT, pH 7.0), 5 mM MgCl, 10 mM EDTA, 2.5% glycerol, 0.05% NP-40, 50 ng/μl Poly (dIdC). The mixture was run on 6% Native-PAGE (polyacrylamide gels) at 100 V in 0.5 × TBE (45 mM Tris, 45 mM boric acid, 2 mM EDTA, pH 8.3). The DNA probe was detected using GelRed.
Bioinformatics Data Analysis
The gene sequence of C. glutamicum ATCC 14067 used in this study was obtained from the GenBank file for NZ_CP022614. Databank searches were performed using BLAST (https://blast.ncbi.nlm.nih.gov/Blast.cgi) (Altschul et al., 1990). The putative -35, -10 elements and transcription start site (TSS) in the promoters were predicted using an online website (http://www.fruitfly.org/seq_tools/promoter.html) (Reese, 2001). The sequence analysis tool MEME (http://meme-suite.org/) (Bailey et al., 2015) was used for the identification of AmtR binding motif in the genus Corynebacterium.
Result
Genome-Wide Identification of the AmtR Regulon by ChIP-Seq
AmtR acts as a transcriptional regulator and plays an essential role in the genus Corynebacterium. Previous studies have identified the target genes of AmtR by transcriptomics and EMSA (Hasselt et al., 2011; Chen et al., 2013). These studies mainly focused on the type strain C. glutamicum ATCC 13032. Due to the different environments and selection pressure during evolution, various strains have apparent differences in several phenotypic characteristics (Yang and Yang, 2017). To identify the direct binding loci of AmtR in C. glutamicum ATCC 14067, the pEC-XK99E-amtR-3Flag plasmid was used to express the AmtR protein fused with a C-terminal 3Flag-tag and driven by the lac promoter in the ∆amtR strain. Western blotting confirmed the expression of AmtR-3Flag (Supplementary Figure S1). AmtR-3Flag IP and negative IP DNA were pooled and subjected to Illumina sequencing. To identify the AmtR binding regions, ChIP-seq peaks obtained from the AmtR-3Flag IP samples were compared to the negative IP sample. On ChIP-seq maps, the fold change of peaks above 1.5 was fixed as the minimum cut-off value for AmtR peak calling. On this basis, ten AmtR peaks were detected (Figure 1). Nine of the peaks were located in the promoter regions, and related to 26 verified genes and 4 new target genes (Supplementary Table S3). The other one of the peaks was located in the coding region of argR, and the related sequence did not have a putative AmtR binding site. EMSA was used to detect the direct interactions of AmtR with the DNA fragment (argR-EMSA, 398 bp) that related to the peak located in the coding region of argR. The positive control is the promoter region of creT (PcreT, 444 bp), DNA fragments obtained by PCR amplification. AmtR could not bind and shift the argR-EMSA (Supplementary Figure S2).
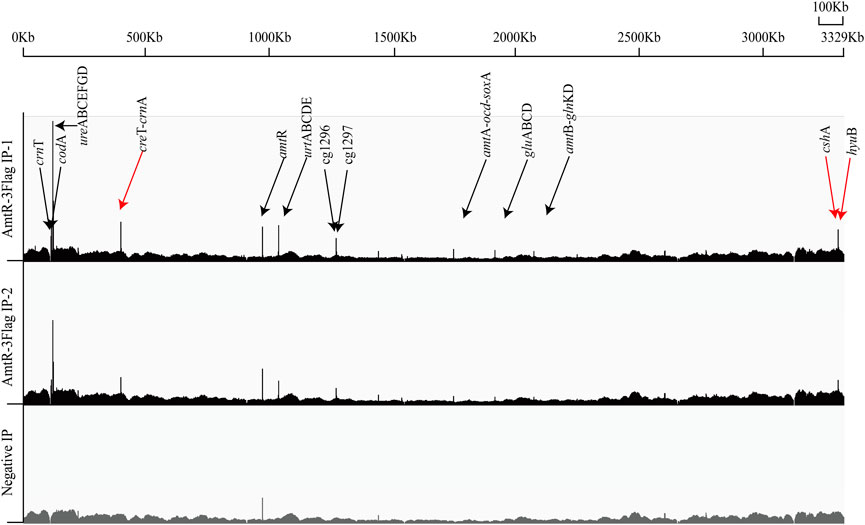
FIGURE 1. Genome-wide in vivo identification of the AmtR binding sites in C. glutamicum ATCC 14067. AmtR-3Flag IP reads were plotted against the number of reads from the Negative IP. Black arrows show the verified genes; red arrows show the new target genes. In AmtR-3Flag IP, AmtR-3Flag production was induced using 0.5 mM IPTG for 8 h; AmtR-3Flag production was not induced with 0.5 mM IPTG as the negative control.
Validation of Novel AmtR Targets
In C. glutamicum ATCC 14067, two new peaks related to three operons were identified using ChIP-seq. (Figure 2). According to a previous prediction by CoryneRegNet 7.0 (Parise et al., 2020), CEY17_RS01915 is the first gene in a two-gene operon encoding putative MFS transporter and cretininase, and CEY17_RS15995 and CEY17_RS16000 are single gene operons encoding putative N-carbamoylsarcosine amidase and hydantoin utilization protein HyuB, respectively. The RS_01910, RS_01915, RS_15995, and RS_16000 were given the names crnA, creT, cshA, and hyuB in C. glutamicum. The putative TSS (Figure 2) of these genes was predicted using the online website (http://www.fruitfly.org/seq_tools/promoter.html). In C. glutamicum, previous studies have reported that AmtR has a 14-bp palindromic binding sequence consisting of two conserved 4-bp sequences forming an inverted repeat separated by a random 6-bp spacer (CTAT-N6-ATAG) (Hasselt et al., 2011). The DNA sequence analysis revealed that the promoter of creT had two potential AmtR binding sites, PcreT-1 and PcreT-2. The intergenic region of cshA and hyuB had three potential AmtR binding sites, PhyuB-1, PhyuB-2, and PhyuB-3, respectively. PhyuB-2 and PhyuB-3 in the promoter of hyuB are not strictly conserved. To detect the binding of AmtR and potential AmtR binding site, we assayed the purified AmtR binding to the potential AmtR binding sites in vitro. AmtR with a C-terminal His6-tag was expressed in E. coli BL21 and purified by affinity nickel affinity chromatography as described in the Materials and Methods. DNA fragments contained the binding motif were annealed using two complementary single-stranded oligonucleotides (Supplementary Table S2). AmtR was able to bind and shift the DNA fragments (Figure 3). Increasing amounts of AmtR and 100 ng DNA fragments were used. As the amount of AmtR increased, the binding of AmtR with DNA fragments gradually increased. AmtR is strongly bound with PcreT-1, PcreT-2, PhyuB-1, and PhyuB-2, and weakly bound with PhyuB-3. The positive control was the protomer region of amtA previously reported, and the negative control was the protomer region of hyuB that did not contain the AmtR binding sequence (Figure 3).
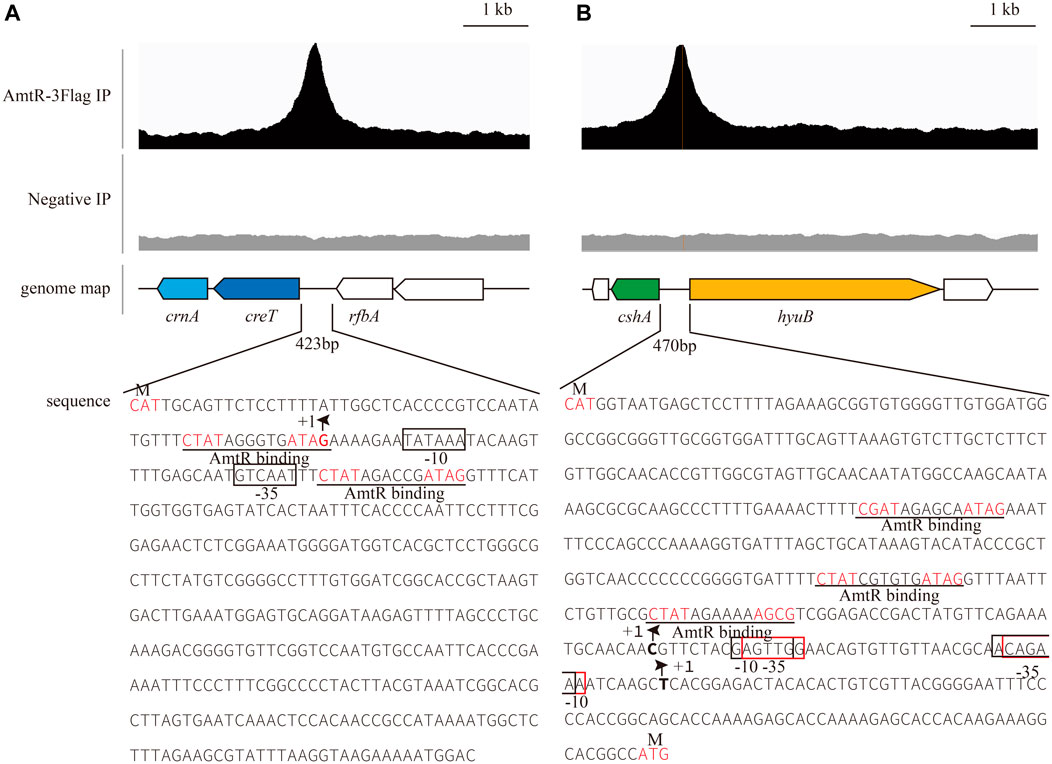
FIGURE 2. AmtR ChIP-seq peaks of selected genes. The putative palindrome sequence is shown in red; the AmtR binding sequences are predicted by MEME and underlined. +1 indicates the transcription start site (TSS), and putative -35, -10 elements and TSSs in the promoters of creT-crnA, cshA, and hyuB were predicted using the online website (http://www.fruitfly.org/seq_tools/promoter.html) (Reese, 2001). (A) Putative -35 and -10 elements in the promoter of creT-crnA enclosed by black rectangles. (B) Putative -35 and -10 elements in the promoter of cshA enclosed by black rectangles, -35 and -10 elements in the promoter of hyuB enclosed by red rectangles.
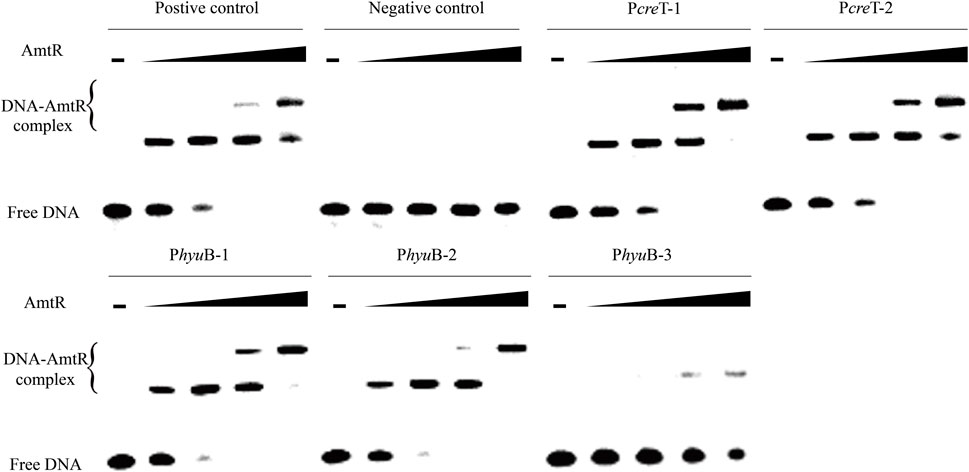
FIGURE 3. AmtR interacts in vitro with different DNA fragments. Minus represents that AmtR was not added, the lower band is the free DNA that is unbound with AmtR. Black triangle represents that AmtR was gradually increasing. The DNA fragments were annealed using two complementary single-stranded oligonucleotides. The positive control is the protomer region of amtA reported, and the negative control is the protomer region of hyuB that does not contain the AmtR binding sequence. Increasing amounts of AmtR (0, 0.1, 0.2, 0.4, and 0.8 μg) and 100 ng DNA fragments were used.
Utilization of Creatine as an Alternative Nitrogen Source in C. glutamicum ATCC 14067
A reciprocal BLASTP search of CreT, CrnA, CshA, and HyuB was done against the genome database in NCBI to identify homologs. CrnA shares 34% sequence identity with creatininase from Pseudomonas putida (Beuth et al., 2003), CshA shares 60% sequence identity with N-carbamoylsarcosine amidase from Arthrobacter sp. (Roma˜o et al., 1992), and HyuB shares 44% sequence identity with hydantoinase from Pseudomonas sp. NS671. Hydantoinase in Pseudomonas sp. NS671 is encoded by two distinctly different subunits, HyuA and HyuB (Watabe et al., 1992; Ishikawa et al., 1997). No homologous functional protein for CreT has been reported. The model for the location of the trans-membrane helices in CreT was predicted by TMHMM v. 2.0 (http://www.cbs.dtu.dk/services/TMHMM) (Supplementary Figure S3). Hydropathy analysis shows that CreT is an integral membrane protein with 11 putative membrane-spanning domains. Interestingly, CreT has 50% similarities with the MFS transporter encoded by the gene located on upstream of creatinase in P. putida NBRC 14164 (PP4_RS10800). Since clustered genes usually encode proteins that physically interact, or different enzymes in the same metabolic pathway (Bork et al., 1998; Dandekar et al., 1998), it is possible that creT encodes a protein related to creatine transport. Creatininase catalyzes both the conversion of creatine and creatinine; N-carbamoylsarcosine amidase catalyzes the hydrolysis of N-carbamoylsarcosine to sarcosine with the liberation of carbon dioxide and ammonia; hydantoinase catalyzes the hydrolysis of hydantoin to N-carbamylamino acid. We hypothesized that CreT, CrnA, CshA, and HyuB were related to creatine and creatinine degradation.
To verify whether C. glutamicum ATCC 14067 can degrade creatine and creatinine, we conducted growth assays of C. glutamicum ATCC 14067 and C. glutamicum ATCC13032 in CGXII medium with creatine or creatinine as a nitrogen source. C. glutamicum ATCC 14067 can grow on creatine or creatinine at a concentration of 10 mM (Figure 4A). While C. glutamicum ATCC 13032 is able to utilize creatinine but not creatine as a nitrogen source (Figure 4C), consistent with previous reports. To verify the functions of CreT, CrnA, CshA, and HyuB, the transcripts of creT, crnA, cshA, and hyuB in CGXII medium with creatine or ammonium and urea as nitrogen sources were analyzed by RT-qPCR. In CGXII medium with 10 mM creatine as a sole nitrogen source, the levels of the creT, crnA, cshA, and hyuB transcripts were higher than those growing on ammonium and urea (Figure 5A). To further validate the results above, PcreT-sfGFP, PcshA-sfGFP, and PhyuB-sfGFP reporter plasmids were transformed into the wild-type strain, and promoter activities of PcreT, PcshA, and PhyuB in these strains were observed with 10 mM creatine or ammonium and urea. The GFP fluorescence of PcreT, PcshA, and PhyuB was significantly higher when the strain was grown with creatine than with ammonium and urea (Figure 5B).
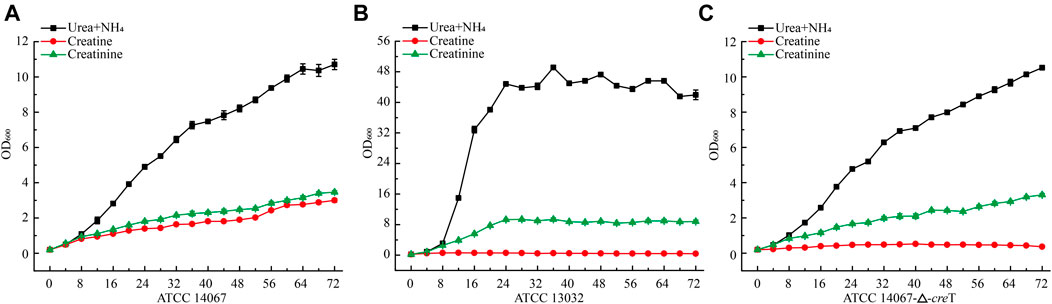
FIGURE 4. Growth curve of C. glutamicum ATCC 14067 wild-type, the △creT, and C. glutamicum ATCC 13032 strains with a different nitrogen sources. (A) C. glutamicum ATCC 14067 grows in CGXII medium with ammonium and urea, 10 mM creatine or 10 mM creatinine as nitrogen sources. (B) C. glutamicum ATCC 14067-△creT grows in CGXII medium with ammonium and urea, 10 mM creatine or 10 mM creatinine as nitrogen sources. (C) C. glutamicum ATCC 13032 grows in CGXII medium with ammonium and urea, 10 mM creatine or 10 mM creatinine as nitrogen sources.
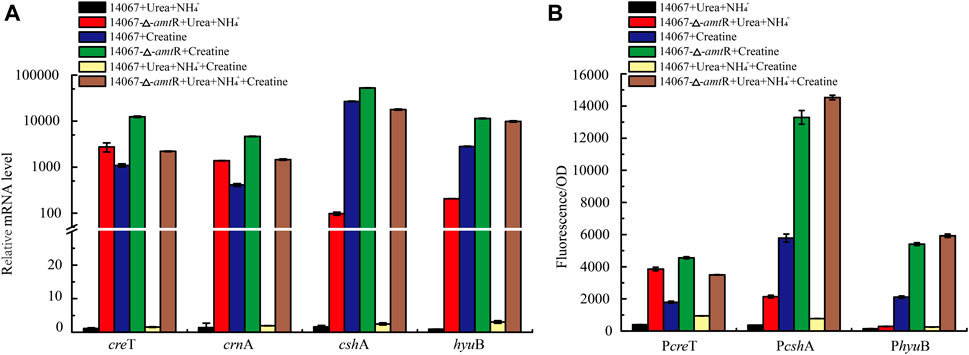
FIGURE 5. AmtR regulates creatine degradation in C. glutamicum ATCC 14067. (A) Relative expression of creT, crnA, cshA, and hyuB of C. glutamicum ATCC 14067 wild-type and ΔamtR strains grown in CGXII medium with creatine or ammonium and urea as nitrogen sources. (B) Promoter activity of creT, cshA, and hyuB of C. glutamicum ATCC 14067 wild-type and ΔamtR strains grown in CGXII medium with creatine or ammonium and urea as nitrogen sources.
In addition, we tested the growth of C. glutamicum ATCC 14067 and C. glutamicum ATCC 13032 in CGXII medium with 10 mM creatine, 10 mM creatinine, or 10 mM glucose as sole carbon source. CGXII medium without carbon source was used as a negative control. After culturing for 48 h at 30°C, the OD of cultures at 600 nm was measured. C. glutamicum ATCC 14067 and C. glutamicum ATCC 13032 could not grow in CGXII medium with creatine or creatinine as the carbon source (Supplementary Figure S4).
To further verify the function of CreT in creatine degradation in C. glutamicum ATCC 14067, creT was deleted, and the in-frame deletion mutant △creT was obtained. The ∆creT strain could grow as the C. glutamicum ATCC 14067 wild-type strain in CGXII medium with ammonium and urea or creatinine as a nitrogen source, but the ∆creT strain lost the ability to grow on creatine. These results demonstrate that creT encodes a protein related to creatine transport in C. glutamicum ATCC 14067.
AmtR Represses Target Gene Transcription and Expression
To investigate the contribution of AmtR to creT, crnA, cshA, and hyuB, the ∆amtR strain was constructed using homologous recombination. The C. glutamicum ATCC 14067 wild-type and ∆amtR strains were cultured in CGXII medium with ammonium and urea or ammonium, urea, and 10 mM creatine as nitrogen sources for 8 h. When ammonium and urea were used as nitrogen sources, compared with the wild-type strain, the creT, crnA, cshA, and hyuB transcript levels in the ∆amtR strain were increased significantly. The levels of creT, crnA, cshA, and hyuB increased by 2,427-, 998-, 61-, and 229-fold, respectively (Figure 5A). When the wild-type strain used ammonium, urea, and 10 mM creatine as nitrogen sources, the creT, crnA, cshA, and hyuB transcript levels were higher than those with ammonium and urea as nitrogen sources, but only increased by 1.5-, 1.9-, 2.5-, and 3.0-fold, respectively. The results indicate that creatine could not or very weakly induce the transcription of creT, crnA, cshA, and hyuB when ammonium and urea are abundant. The corresponding sfGFP assays confirmed this result. The PcreT-sfGFP, PcshA-sfGFP, and PhyuB-sfGFP reporter plasmids were transformed into the C. glutamicum ATCC 14067 wild-type and △amtR strains. The resulting strains were cultured in CGXII medium for 8 h, the sfGFP activities were measured (Figure 5B). Combining RT-qPCR analysis and sfGFP assays, we identified that AmtR represses the transcription and expression of the target genes.
Proteins Related to Creatine or Creatinine Degradation in the Genus Corynebacterium and Related Bacteria
CrnT and CodA have been reported related to creatinine transport and degradation, and SoxA was predicted as a sarcosine oxidase in C. glutamicum ATCC 13032 (Kalinowski et al., 2003). To identify proteins related to creatine or creatinine degradation in bacteria, a reciprocal BLASTP search of CrnT, CodA, CreT, CrnA, SoxA, CshA, and HyuB against the genome database in NCBI was carried out. These proteins were found to be present in some Corynebacterium species. In C. glutamicum, all proteins related to creatine and creatinine degradation in ATCC 14067 are also present in ATCC 15168, BE, and YI strains. CrnT, CodA, CreT, CrnA, and SoxA are present in 17 strains, including ATCC 21831, ATCC 13869, ZL-1, and so on. CrnT, CodA, and SoxA are present in the other strains. In addition, Corynebacterium callunae DSM 20147, Corynebacterium vitaeruminis DSM 20294, and Corynebacterium deserti GIMN1.010 have different proteins related to creatine and creatinine degradation (Figure 6). Analysis of the promoters’ sequence of genes that encode proteins related to creatine and creatinine degradation revealed that the promoter regions have similar sequences to the AmtR binding site (Supplementary Figure S5).
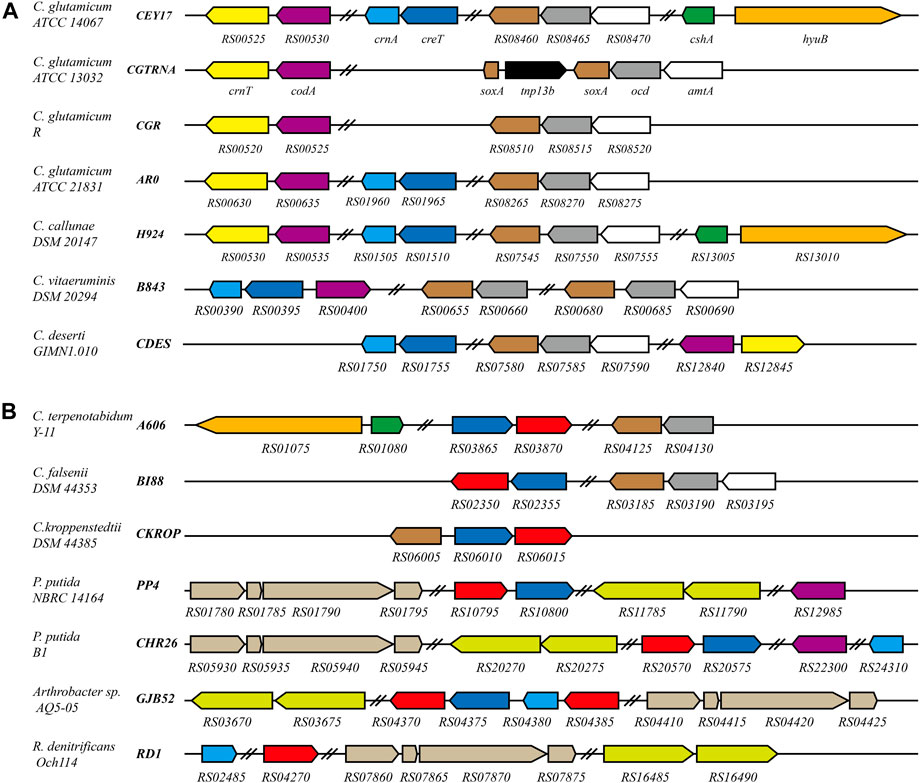
FIGURE 6. Schematic representation of the creatine and creatinine degradation gene cluster in C. glutamicum and related bacteria. Black bold italics indicate locus_tag prefix; homologous genes are presented in the same color. Red represents the gene encoding creatinase (CreA). Navy blue represents the gene encoding the protein related to creatine transport. In C. glutamicum ATCC 13032, soxA is interrupted by tnp13b. In P. putida NBRC 14164, P. putida B1, Arthrobacter sp. AQ5-05 and R. denitrificans Och114, sarcosine oxidase is a heterotetrameric; hydantoinase is a heterodimer. Double slashes indicate that the genes are not continuous. (A) The genes related to creatine and creatinine degradation are regulated by AmtR in these strains. (B) Homologs of AmtR are not present in these strains.
In Corynebacterium terpenotabidum Y-11, Corynebacterium falsenii DSM 44353, and Corynebacterium kroppenstedtii DSM 44385, A606_RS03870, BI88_RS02350, and CKROP_RS06015 encode a putative creatinase that hydrolyzes creatine to sarcosine and urea. According to the sequence alignment using Clustal Omega, those proteins share 59, 58, and 59% sequence identity with creatinase from Arthrobacter sp. TE 1826, respectively (Nishiya et al., 1998). The promoter regions of those genes encoding creatine, and creatinine degradation proteins do not have sequences similar to the AmtR binding site. Further analysis found that these strains do not contain a homolog of AmtR.
There are many bacterial species distinct from Corynebacterium that can degrade creatine or creatinine as nitrogen sources. For example, Pseudomonas (Yamada et al., 1985), Arthrobacter (Nishiya et al., 1998), and Roseobacter (Wawrik et al., 2017) strains (Figure 6B). Interestingly, the homologous transporters are ubiquitous and usually adjacent to creatinase or creatininase in these genomes (Figure 6). This supports the speculation that CreT is related to creatine transport. In addition, R. denitrificans Och114 can degrade creatine as a nitrogen source. Still, the genome does not contain CreT homologous protein, which may indicate that creatine can also enter bacterial cells through other pathways.
Discussion
In bacteria, the PII signal transduction proteins act in conjunction with various transcription factors to control nitrogen metabolism, such as nitrate assimilation through the global nitrogen control factor NtcA in all cyanobacteria characterized to date (Llácer et al., 2010). Nitrogen limitation in enteric bacteria is regulated by NtrBC (Caballero et al., 2005), which activates the expression of over 100 genes; nitrogen metabolism in Bacillus subtilis under conditions of nitrogen limitation is regulated by TnrA (Wray et al., 1996); the GlnR controls nitrogen metabolism in Streptomyces coelicolor and regulates at least 50 nitrogen response genes (Tiffert et al., 2008). The AmtR is the central nitrogen regulator in C. glutamicum, and it has been reported that AmtR in conjunction with the PII signal transduction protein GlnK triggers the dissociation of AmtR from DNA. The conservative sequence of the AmtR binding site contains two conserved 4-bp sequences forming an inverted repeat separated by a random 6-bp spacer, which is CTAT-N6-ATAG. In addition, in Streptomyces avermitilis, Rhodococcus jostii RHA1, Arthrobacter aurescens, Mycobacterium smegmatis, and Nocardia farcinica, both glnR and amtR-homologous genes are present in the genome. The GlnR is the central nitrogen regulator in those strains, and AmtR has a 22 bp consensus TAtCTGTCa-n2-cGACAGATAT sequence (Chen et al., 2013), similar to the AmtR binding site sequence in C. glutamicum. In different species, AmtR and its homologous protein have similar binding sequences.
The binding site of AmtR in the C. glutamicum ATCC 14067 genome was identified by ChIP-seq in BHI medium. Ten peaks were obtained in the C. glutamicum ATCC 14067 genome including two new peaks related to three operons. ChIP-seq detected a total of 30 genes include four new target genes, fewer than the 35 genes previously reported (Hasselt et al., 2011). There may be some genes not strictly regulated by AmtR. In a nitrogen-rich medium, the transcription and expression of gdh and gln, which encode l-glutamate dehydrogenase and l-glutamine synthetase, are only loosely controlled by AmtR (Hasselt et al., 2011).
To identify the transcription and expression of creT, crnA, cshA, and hyuB regulated by AmtR, we used 50 bp double-stranded DNA containing the potential AmtR binding sites annealed using two complementary single-stranded oligonucleotides. EMSA showed that PcreT-1, PcreT-2, PhyuB-1, and PhyuB-2 have a robust affinity when binding with AmtR, and PhyuB-3 has a very weak affinity with AmtR. PhyuB-1, PhyuB-2, PhyuB-3 are located in the intergenic region of cshA and hyuB, cshA is transcribed divergently from hyuB. RT-qPCR and other assays were employed to investigate the effect of the △amtR strain on the promoter activity of cshA and hyuB. The result of the analyses showed that the transcription and expression of cshA and hyuB in the ∆amtR strain were significantly higher than in the wild-type strain. In previous studies, urea acted as an alternative nitrogen source that could diffuse across the cytoplasmic membrane present in high concentrations in the medium; the transporter of urea is strictly and fully repressed by AmtR in C. glutamicum (Siewe et al., 1998). Creatinine acts as a membrane-impermeable nitrogen source, and the genes that code transport and degrade creatinine are limited to nitrogen starvation and strictly regulated by AmtR (Bendt et al., 2004). The ∆creT strain cannot use creatine as a nitrogen source, which indicates that creatine cannot diffuse across the cytoplasmic membrane. The operons creT-crnA, cshA, and hyuB may be limited to nitrogen starvation and be strictly regulated by AmtR.
The new regulons of AmtR in ATCC 14067 are not present in the type strain C. glutamicum ATCC 13032. The sequences of CreT, CrnA, CshA, and HyuB were analyzed in C. glutamicum strains. CreT and CrnA are present in 17 strains. CreT is related to creatine transport, and its homologous protein is ubiquitous in species that can utilize creatine. The marine bacteria Roseobacter denitrificans Och114 can grow on creatine as a nitrogen source, but it has no protein homologous to CreT (Figure 6). However, there may be other ways to take up creatine into the cell. CrnT and CodA related to creatinine transport and degradation are present in all C. glutamicum strains. All strains of C. glutamicum can use creatinine as a nitrogen source, and only some strains can use creatine as a nitrogen source. It has been reported that creatinine inhibits bacterial replication. C. glutamicum can resist creatinine stress in the environment by degrading creatinine to N-methylhydantoin and ammonia. Ammonia is used in other pathways, and N-methylhydantoin is a dead-end product accumulating in the cells (Bendt et al., 2004). Incomplete degradation of creatinine is detrimental, leading to the arrest of C. glutamicum growth when the concentration of creatinine is high. In C. glutamicum ATCC 14067, CshA and HyuB can further react with the N-methylhydantoin to yield sarcosine, and CrnA can catalyze the conversion of both creatine and creatinine (Beuth et al., 2003). When the creatinine concentration is high, it is converted into creatine that does not inhibit bacteria. If N-methylhydantoin is further degraded to sarcosine or creatinine is converted to creatine, it can improve the survival ability of C. glutamicum ATCC 14067 when the concentration of creatinine is high. Intraspecies variation of C. glutamicum is characterized by different strains with different abilities to degrade creatine and creatinine. Similar characteristics have been seen in Pseudomonas. P. putida NBRC 14164 has the genes related to the degradation of creatine, but these are not present in P. putida KT2440 (Nelson et al., 2002; Ohji et al., 2014). Possibly, these strains gain the ability to degrade creatine via horizontal gene transfer.
C. glutamicum ATCC 14067 cannot use creatine or creatinine as the sole carbon source (Supplementary Figure S4). Creatine and creatinine produce ammonia and glycine during the degradation process, but glycine cannot be re-utilized. The glycine cleavage system that degrades glycine is also lacking in C. glutamicum (Hüser Andrea et al., 2005), and thus glycine will accumulate in the cells. In some bacteria, creatine or creatinine can be used as the sole carbon source since creatine or creatinine can be degraded into glycine. Serine hydroxymethyltransferase and 10-formyltetrahydrofolate hydrolase further catalyze glycine into serine and pyruvate (Chlumsky et al., 1995; Meskys et al., 2001; Willsey and Wargo, 2015).
It has been reported that the degradation pathway of creatine and related metabolites in bacteria is regulated by transcription factors. Creatinine transport and degradation are regulated by AmtR in C. glutamicum (Bendt et al., 2004), and sarcosine catabolism is transcriptionally regulated by GbdR and SouR in Pseudomonas aeruginosa (Willsey and Wargo, 2015). The pathway degrading creatine and creatinine into glycine is regulated by AmtR in C. glutamicum ATCC 14067 (Figure 7).
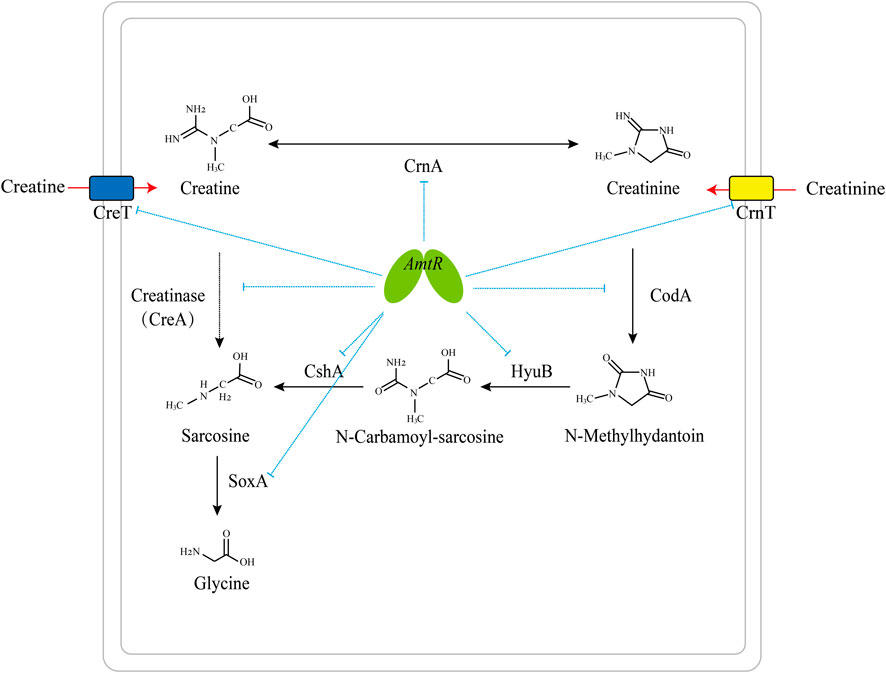
FIGURE 7. Model of the AmtR regulating creatine and creatinine degradation in C. glutamicum ATCC 14067. Blue dotted line: transcriptional repression by AmtR; black arrows: creatine and creatinine degradation pathway; red arrows: the transport of creatine and creatinine; dotted arrow: creatinase (CreA) is not present in C. glutamicum ATCC 14067.
Data Availability Statement
The data presented in the study are deposited in the National Center for Biotechnology Information repository, accession number PRJNA798895.
Author Contributions
HZ and SZ designed the experiments. HZ, ZO and NZ performed the experiments. HZ wrote the manuscript. HZ, SH and SZ conceived the project. All authors contributed to the manuscript, read and approved the final version.
Funding
This work was supported by Tianjin Synthetic Biotechnology Innovation Capacity Improvement Project (TSBICIP-KJGG-005) and the National Key R&D Program of China (Grant no. 2018YFA0901700).
Conflict of Interest
The authors declare that the research was conducted in the absence of any commercial or financial relationships that could be construed as a potential conflict of interest.
Publisher’s Note
All claims expressed in this article are solely those of the authors and do not necessarily represent those of their affiliated organizations, or those of the publisher, the editors and the reviewers. Any product that may be evaluated in this article, or claim that may be made by its manufacturer, is not guaranteed or endorsed by the publisher.
Supplementary Material
The Supplementary Material for this article can be found online at: https://www.frontiersin.org/articles/10.3389/fbioe.2022.816628/full#supplementary-material
References
Altschul, S. F., Gish, W., Miller, W., Myers, E. W., and Lipman, D. J. (1990). Basic Local Alignment Search Tool. J. Mol. Biol. 215 (3), 403–410. doi:10.1016/S0022-2836(05)80360-2
Bailey, T. L., Johnson, J., Grant, C. E., and Noble, W. S. (2015). The MEME Suite. Nucleic Acids Res. 43 (W1), W39–W49. doi:10.1093/nar/gkv416
Becker, J., Zelder, O., Häfner, S., Schröder, H., and Wittmann, C. (2011). From Zero to Hero-Design-Based Systems Metabolic Engineering of Corynebacterium Glutamicum for L-Lysine Production. Metab. Eng. 13 (2), 159–168. doi:10.1016/j.ymben.2011.01.003
Bendt, A. K., Beckers, G., Silberbach, M., Wittmann, A., and Burkovski, A. (2004). Utilization of Creatinine as an Alternative Nitrogen Source in Corynebacterium Glutamicum. Arch. Microbiol. 181 (6), 443–450. doi:10.1007/s00203-004-0679-z
Beuth, B., Niefind, K., and Schomburg, D. (2003). Crystal Structure of Creatininase from Pseudomonas Putida: A Novel Fold and a Case of Convergent Evolution. J. Mol. Biol. 332 (1), 287–301. doi:10.1016/S0022-2836(03)00860-X
Binder, S., Siedler, S., Marienhagen, J., Bott, M., and Eggeling, L. (2013). Recombineering in Corynebacterium Glutamicum Combined with Optical Nanosensors: a General Strategy for Fast Producer Strain Generation. Nucleic Acids Res. 41 (12), 6360–6369. doi:10.1093/nar/gkt312
Bork, P., Dandekar, T., Diaz-Lazcoz, Y., Eisenhaber, F., Huynen, M., and Yuan, Y. (1998). Predicting Function: from Genes to Genomes and Back 1 1Edited by P. E. Wright. J. Mol. Biol. 283 (4), 707–725. doi:10.1006/jmbi.1998.2144
Caballero, A., Esteve-Núñez, A., Zylstra, G. J., and Ramos, J. L. (2005). Assimilation of Nitrogen from Nitrite and Trinitrotoluene in Pseudomonas Putida JLR11. J. Bacteriol. 187 (1), 396–399. doi:10.1128/JB.187.1.396-399.2005
Chen, X., Kohl, T. A., Rückert, C., Rodionov, D. A., Li, L.-H., Ding, J.-Y., et al. (2012). Phenylacetic Acid Catabolism and its Transcriptional Regulation in Corynebacterium Glutamicum. Appl. Environ. Microbiol. 78 (16), 5796–5804. doi:10.1128/AEM.01588-12
Chen, Y., Zhu, H., Zheng, G., Jiang, W., and Lu, Y. (2013). Functional Analysis of TetR-Family Regulator AmtRsav in Streptomyces Avermitilis. Microbiology 159 (Pt_12), 2571–2583. doi:10.1099/mic.0.071449-0
Chlumsky, L. J., Zhang, L., and Jorns, M. S. (1995). Sequence Analysis of Sarcosine Oxidase and Nearby Genes Reveals Homologies with Key Enzymes of Folate One-Carbon Metabolism. J. Biol. Chem. 270 (31), 18252–18259. doi:10.1074/jbc.270.31.18252
Dandekar, T., Snel, B., Huynen, M., and Bork, P. (1998). Conservation of Gene Order: a Fingerprint of Proteins that Physically Interact. Trends Biochem. Sci. 23 (9), 324–328. doi:10.1016/S0968-0004(98)01274-2
Fan, L., Wang, Y., Qian, J., Gao, N., Zhang, Z., Ni, X., et al. (2021). Transcriptome Analysis Reveals the Roles of Nitrogen Metabolism and Sedoheptulose Bisphosphatase Pathway in Methanol‐dependent Growth of Corynebacterium Glutamicum. Microb. Biotechnol. 14, 1797–1808. doi:10.1111/1751-7915.13863
Feith, A., Schwentner, A., Teleki, A., Favilli, L., Blombach, B., and Takors, R. (2020). Streamlining the Analysis of Dynamic 13C-Labeling Patterns for the Metabolic Engineering of Corynebacterium Glutamicum as L-Histidine Production Host. Metabolites 10 (11), 458. doi:10.3390/metabo10110458
Gibson, D. G., Young, L., Chuang, R.-Y., Venter, J. C., Hutchison, C. A., and Smith, H. O. (2009). Enzymatic Assembly of DNA Molecules up to Several Hundred Kilobases. Nat. Methods 6 (5), 343–345. doi:10.1038/nmeth.1318
Gourdon, P., Baucher, M.-F., Lindley, N. D., and Guyonvarch, A. (2000). Cloning of the Malic Enzyme Gene from Corynebacterium Glutamicum and Role of the Enzyme in Lactate Metabolism. Appl. Environ. Microbiol. 66 (7), 2981–2987. doi:10.1128/AEM.66.7.2981-2987.2000
Hasselt, K., Rankl, S., Worsch, S., and Burkovski, A. (2011). Adaptation of AmtR-Controlled Gene Expression by Modulation of AmtR Binding Activity in Corynebacterium Glutamicum. J. Biotechnol. 154 (2), 156–162. doi:10.1016/j.jbiotec.2010.09.930
Hayashi, M., Mizoguchi, H., Shiraishi, N., Obayashi, M., Nakagawa, S., Imai, J.-i., et al. (2002). Transcriptome Analysis of Acetate Metabolism inCorynebacterium glutamicumUsing a Newly Developed Metabolic Array. Biosci. Biotechnol. Biochem. 66 (6), 1337–1344. doi:10.1271/bbb.66.1337
Hermann, T., Pfefferle, W., Baumann, C., Busker, E., Schaffer, S., Bott, M., et al. (2001). Proteome Analysis ofCorynebacterium Glutamicum. ELECTROPHORESIS 22 (9), 1712–1723. doi:10.1002/1522-2683(200105)22:9<1712:AID-ELPS1712>3.0.CO;2-G
Huang, Y., Li, L., Xie, S., Zhao, N., Han, S., Lin, Y., et al. (2017). Recombineering Using RecET in Corynebacterium Glutamicum ATCC14067 via a Self-Excisable Cassette. Sci. Rep. 7 (1), 7916. doi:10.1038/s41598-017-08352-9
Hüser, A. T., Chassagnole, C., Lindley, N. D., Merkamm, M., Guyonvarch, A., Elišáková, V., et al. (2005). Rational Design of a Corynebacterium Glutamicum Pantothenate Production Strain and its Characterization by Metabolic Flux Analysis and Genome-wide Transcriptional Profiling. Appl. Environ. Microbiol. 71 (6), 3255–3268. doi:10.1128/AEM.71.6.3255-3268.2005
Ishikawa, T., Watabe, K., Mukohara, Y., and Nakamura, H. (1997). Mechanism of Stereospecific Conversion Ofdl-5-Substituted Hydantoins to the Correspondingl-Amino Acids byPseudomonassp. Strain NS671. Biosci. Biotechnol. Biochem. 61 (1), 185–187. doi:10.1271/bbb.61.185
Jakoby, M., Nolden, L., Meier-Wagner, J., Krämer, R., and Burkovski, A. (2000). AmtR, a Global Repressor in the Nitrogen Regulation System ofCorynebacterium Glutamicum. Mol. Microbiol. 37 (4), 964–977. doi:10.1046/j.1365-2958.2000.02073.x
Jiang, Y., Huang, M.-Z., Chen, X.-L., and Zhang, B. (2020). Proteome Analysis Guided Genetic Engineering of Corynebacterium Glutamicum S9114 for Tween 40-triggered Improvement in L-Ornithine Production. Microb. Cell Fact 19 (1), 2. doi:10.1186/s12934-019-1272-0
Jungwirth, B., Sala, C., Kohl, T. A., Uplekar, S., Baumbach, J., Cole, S. T., et al. (2013). High-resolution Detection of DNA Binding Sites of the Global Transcriptional Regulator GlxR in Corynebacterium Glutamicum. Microbiology 159 (Pt_1), 12–22. doi:10.1099/mic.0.062059-0
Kalinowski, J., Bathe, B., Bartels, D., Bischoff, N., Bott, M., Burkovski, A., et al. (2003). The Complete Corynebacterium Glutamicum ATCC 13032 Genome Sequence and its Impact on the Production of L-Aspartate-Derived Amino Acids and Vitamins. J. Biotechnol. 104 (1-3), 5–25. doi:10.1016/s0168-1656(03)00154-8
Keilhauer, C., Eggeling, L., and Sahm, H. (1993). Isoleucine Synthesis in Corynebacterium Glutamicum: Molecular Analysis of the ilvB-ilvN-ilvC Operon. J. Bacteriol. 175 (17), 5595–5603. doi:10.1128/jb.175.17.5595-5603.1993
Kikuchi, Y., Date, M., Itaya, H., Matsui, K., and Wu, L.-F. (2006). Functional Analysis of the Twin-Arginine Translocation Pathway in Corynebacterium Glutamicum ATCC 13869. Appl. Environ. Microbiol. 72 (11), 7183–7192. doi:10.1128/AEM.01528-06
Kraxner, K. J., Polen, T., Baumgart, M., and Bott, M. (2019). The Conserved Actinobacterial Transcriptional Regulator FtsR Controls Expression of ftsZ and Further Target Genes and Influences Growth and Cell Division in Corynebacterium Glutamicum. BMC Microbiol. 19 (1), 179. doi:10.1186/s12866-019-1553-0
Krömer, J. O., Sorgenfrei, O., Klopprogge, K., Heinzle, E., and Wittmann, C. (2004). In-Depth Profiling of Lysine-Producing Corynebacterium Glutamicum by Combined Analysis of the Transcriptome, Metabolome, and Fluxome. J. Bacteriol. 186 (6), 1769–1784. doi:10.1128/JB.186.6.1769-1784.2004
Kubota, T., Tanaka, Y., Takemoto, N., Hiraga, K., Yukawa, H., and Inui, M. (2015). Identification and Expression Analysis of a Gene Encoding a Shikimate Transporter of Corynebacterium Glutamicum. Microbiology 161 (2), 254–263. doi:10.1099/mic.0.083733-0
Kuge, T., Teramoto, H., and Inui, M. (2015). AraR, an L -Arabinose-Responsive Transcriptional Regulator in Corynebacterium Glutamicum ATCC 31831, Exerts Different Degrees of Repression Depending on the Location of its Binding Sites within the Three Target Promoter Regions. J. Bacteriol. 197 (24), 3788–3796. doi:10.1128/JB.00314-15
Li, L., Wada, M., and Yokota, A. (2007). Cytoplasmic Proteome Reference Map for a Glutamic Acid-producingCorynebacterium Glutamicum ATCC 14067. PROTEOMICS 7 (23), 4317–4322. doi:10.1002/pmic.200700269
Liu, C., Zhang, B., Liu, Y.-M., Yang, K.-Q., and Liu, S.-J. (2018a). New Intracellular Shikimic Acid Biosensor for Monitoring Shikimate Synthesis in Corynebacterium Glutamicum. ACS Synth. Biol. 7 (2), 591–601. doi:10.1021/acssynbio.7b00339
Liu, J., Wang, Y., Lu, Y., Ni, X., Guo, X., Zhao, J., et al. (2018b). Mutations in Peptidoglycan Synthesis Gene ponA Improve Electrotransformation Efficiency of Corynebacterium Glutamicum ATCC 13869. Appl. Environ. Microbiol. 84 (24), e02225–02218. doi:10.1128/AEM.02225-18
Llacer, J. L., Espinosa, J., Castells, M. A., Contreras, A., Forchhammer, K., and Rubio, V. (2010). Structural Basis for the Regulation of NtcA-dependent Transcription by Proteins PipX and PII. Proc. Natl. Acad. Sci. 107 (35), 15397–15402. doi:10.1073/pnas.1007015107
López-Maury, L., Marguerat, S., and Bähler, J. (2008). Tuning Gene Expression to Changing Environments: from Rapid Responses to Evolutionary Adaptation. Nat. Rev. Genet. 9 (8), 583–593. doi:10.1038/nrg2398
Lv, Q., Hu, M., Tian, L., Liu, F., Wang, Q., Xu, M., et al. (2021). Enhancing L-Glutamine Production in Corynebacterium Glutamicum by Rational Metabolic Engineering Combined with a Two-Stage pH Control Strategy. Bioresour. Tech. 341, 125799. doi:10.1016/j.biortech.2021.125799
Lv, Y., Liao, J., Wu, Z., Han, S., Lin, Y., and Zheng, S. (2012). Genome Sequence of Corynebacterium Glutamicum ATCC 14067, Which Provides Insight into Amino Acid Biosynthesis in Coryneform Bacteria. J. Bacteriol. 194 (3), 742–743. doi:10.1128/JB.06514-11
Meskys, R., Harris, R. J., Casaite, V., Basran, J., and Scrutton, N. S. (2001). Organization of the Genes Involved in Dimethylglycine and Sarcosine Degradation inArthrobacterspp. Eur. J. Biochem. 268 (12), 3390–3398. doi:10.1046/j.1432-1327.2001.02239.x
Nelson, K. E., Weinel, C., Paulsen, I. T., Dodson, R. J., Hilbert, H., Martins dos Santos, V. A. P., et al. (2002). Complete Genome Sequence and Comparative Analysis of the Metabolically Versatile Pseudomonas Putida KT2440. Environ. Microbiol. 4 (12), 799–808. doi:10.1046/j.1462-2920.2002.00366.x
Nishiya, Y., Toda, A., and Imanaka, T. (1998). Gene Cluster for Creatinine Degradation in Arthrobacter Sp. TE1826. Mol. Gen. Genet. 257 (5), 581–586. doi:10.1007/s004380050685
Ohji, S., Yamazoe, A., Hosoyama, A., Tsuchikane, K., Ezaki, T., and Fujita, N. (2014). The Complete Genome Sequence of Pseudomonas Putida NBRC 14164 T Confirms High Intraspecies Variation. Genome Announc 2 (1), e00029–00014. doi:10.1128/genomeA.00029-14
Parise, M. T. D., Parise, D., Kato, R. B., Pauling, J. K., Tauch, A., Azevedo, V. A. d. C., et al. (2020). CoryneRegNet 7, the Reference Database and Analysis Platform for Corynebacterial Gene Regulatory Networks. Sci. Data 7 (1), 142. doi:10.1038/s41597-020-0484-9
Reese, M. G. (2001). Application of a Time-Delay Neural Network to Promoter Annotation in the Drosophila melanogaster Genome. Comput. Chem. 26 (1), 51–56. doi:10.1016/S0097-8485(01)00099-7
Roma˜o, M. J. o., Turk, D., Gomis-Rüth, F.-X., Huber, R., Schumacher, G., Möllering, H., et al. (1992). Crystal Structure Analysis, Refinement and Enzymatic Reaction Mechanism of N-Carbamoylsarcosine Amidohydrolase from Arthrobacter Sp. At 2·0Å resolution. J. Mol. Biol. 226 (4), 1111–1130. doi:10.1016/0022-2836(92)91056-U
Sambrook, J. F., and Russell, D. W. (2001). Molecular Cloning: A Laboratory Manual. Ney York, NY: Cold Spring Harbor Laboratory Press.
Siewe, R. M., Weil, B., Burkovski, A., Eggeling, L., Krämer, R., and Jahns, T. (1998). Urea Uptake and Urease Activity in Corynebacterium Glutamicum. Arch. Microbiol. 169 (5), 411–416. doi:10.1007/s002030050591
Strösser, J., Lüdke, A., Schaffer, S., Krämer, R., and Burkovski, A. (2004). Regulation of GlnK Activity: Modification, Membrane Sequestration and Proteolysis as Regulatory Principles in the Network of Nitrogen Control in Corynebacterium Glutamicum. Mol. Microbiol. 54 (1), 132–147. doi:10.1111/j.1365-2958.2004.04247.x
Takano, H., Shimizu, A., Shibosawa, R., Sasaki, R., Iwagaki, S., Minagawa, O., et al. (2008). Characterization of Developmental colony Formation in Corynebacterium Glutamicum. Appl. Microbiol. Biotechnol. 81 (1), 127–134. doi:10.1007/s00253-008-1622-z
Tauch, A., Homann, I., Mormann, S., Rüberg, S., Billault, A., Bathe, B., et al. (2002). Strategy to Sequence the Genome of Corynebacterium Glutamicum ATCC 13032: Use of a Cosmid and a Bacterial Artificial Chromosome Library. J. Biotechnol. 95 (1), 25–38. doi:10.1016/S0168-1656(01)00443-6
Tiffert, Y., Supra, P., Wurm, R., Wohlleben, W., Wagner, R., and Reuther, J. (2008). The Streptomyces Coelicolor GlnR Regulon: Identification of New GlnR Targets and Evidence for a central Role of GlnR in Nitrogen Metabolism in Actinomycetes. Mol. Microbiol. 67 (4), 861–880. doi:10.1111/j.1365-2958.2007.06092.x
Watabe, K., Ishikawa, T., Mukohara, Y., and Nakamura, H. (1992). Cloning and Sequencing of the Genes Involved in the Conversion of 5-substituted Hydantoins to the Corresponding L-Amino Acids from the Native Plasmid of Pseudomonas Sp. Strain NS671. J. Bacteriol. 174 (3), 962–969. doi:10.1128/jb.174.3.962-969.1992
Wawrik, B., Bronk, D., Baer, S., Chi, L., Sun, M., Cooper, J., et al. (2017). Bacterial Utilization of Creatine in Seawater. Aquat. Microb. Ecol. 80, 153–165. doi:10.3354/ame01850
Willsey, G. G., and Wargo, M. J. (2016). Sarcosine Catabolism in Pseudomonas aeruginosa Is Transcriptionally Regulated by SouR. J. Bacteriol. 198 (2), 301–310. doi:10.1128/JB.00739-15
Wray, L. V., Ferson, A. E., Rohrer, K., and Fisher, S. H. (1996). TnrA, a Transcription Factor Required for Global Nitrogen Regulation in Bacillus Subtilis. Proc. Natl. Acad. Sci. 93 (17), 8841–8845. doi:10.1073/pnas.93.17.8841
Wyss, M., and Kaddurah-Daouk, R. (2000). Creatine and Creatinine Metabolism. Physiol. Rev. 80 (3), 1107–1213. doi:10.1152/physrev.2000.80.3.1107
Xu, J., Xia, X., Zhang, J., Guo, Y., and Zhang, W. (2014). An Overlooked Effect of Glycine Betaine on Fermentation: Prevents Caramelization and Increases the L-Lysine Production. J. Microbiol. Biotechnol. 24 (10), 1368–1376. doi:10.4014/jmb.1404.04009
Yamada, H., Shimizu, S., Kim, J. M., Shinmen, Y., and Sakai, T. (1985). A Novel Metabolic Pathway for Creatinine Degradation inPseudomonas Putida77. FEMS Microbiol. Lett. 30 (3), 337–340. doi:10.1111/j.1574-6968.1985.tb01106.x
Yang, J., and Yang, S. (2017). Comparative Analysis of Corynebacterium Glutamicum Genomes: a New Perspective for the Industrial Production of Amino Acids. BMC Genomics 18 (1), 940. doi:10.1186/s12864-016-3255-4
Zhang, L., Lin, X., Wang, T., Guo, W., and Lu, Y. (2021). Development and Comparison of Cell-free Protein Synthesis Systems Derived from Typical Bacterial Chassis. Bioresour. Bioproc. 8 (1), 58. doi:10.1186/s40643-021-00413-2
Keywords: Corynebacterium glutamicum, ChIP-seq, AmtR, creatine, creatinine, MFS transporter
Citation: Zhang H, Ouyang Z, Zhao N, Han S and Zheng S (2022) Transcriptional Regulation of the Creatine Utilization Genes of Corynebacterium glutamicum ATCC 14067 by AmtR, a Central Nitrogen Regulator. Front. Bioeng. Biotechnol. 10:816628. doi: 10.3389/fbioe.2022.816628
Received: 16 November 2021; Accepted: 13 January 2022;
Published: 09 February 2022.
Edited by:
Chong Li, Agricultural Genomics Institute at Shenzhen (CAAS), ChinaReviewed by:
Yu Wang, Tianjin Institute of Industrial Biotechnology (CAS), ChinaCharles Swofford, Massachusetts Institute of Technology, United States
Copyright © 2022 Zhang, Ouyang, Zhao, Han and Zheng. This is an open-access article distributed under the terms of the Creative Commons Attribution License (CC BY). The use, distribution or reproduction in other forums is permitted, provided the original author(s) and the copyright owner(s) are credited and that the original publication in this journal is cited, in accordance with accepted academic practice. No use, distribution or reproduction is permitted which does not comply with these terms.
*Correspondence: Shuangyan Han, syhan@scut.edu.cn; Suiping Zheng, spzheng@scut.edu.cn