- 1Department of Biology, Mardin Artuklu University Graduate Education Institute, Mardin, Turkey
- 2Department of Medical Services and Techniques, Vocational School of Health Services, Mardin Artuklu University, Mardin, Turkey
- 3Joint Ukrainian-Azerbaijan International Research and Education Center of Nanobiotechnology and Functional Nanosystems, Drohobych, Ukraine
- 4Department of Nutrition and Dietetics, Faculty of Health Sciences, Mardin Artuklu University, Mardin, Turkey
- 5Department of Chemistry, Faculty of Science, Dicle University, Diyarbakir, Turkey
- 6Dicle University Central Research Laboratory, , Diyarbakir, Turkey
- 7Department of Medical Biology, Dicle University Central Research Laboratory, Faculty of Medicine, Dicle University, Diyarbakir, Turkey
- 8Electricity and Energy Department, Vocational School, Mardin Artuklu University, Mardin, Turkey
- 9Department of Biophysics and Biochemistry, Baku State University, Baku, Azerbaijan
- 10Institute of Radiation Problems, National Academy of Sciences of Azerbaijan, Baku, Azerbaijan
- 11Russian Institute for Advanced Study, Moscow State Pedagogical University, Moscow, Russia
- 12Department of Botany and Plant Physiology, Baku State University, Baku, Azerbaijan
- 13Kidney Research Center, Tabriz University of Medical Sciences, Tabriz, Iran
- 14Department of Physiology, Faculty of Medical Sciences, University of Kragujevac, Kragujevac, Serbia
- 15Health Innovation & Accelerations Center, Tabriz University of Medical Sciences, Tabriz, Iran
- 16Stem Cell Research Center, Tabriz University of Medical Sciences, Tabriz, Iran
Using biological materials to synthesize metallic nanoparticles has become a frequently preferred method by researchers. This synthesis method is both fast and inexpensive. In this study, an aqueous extract obtained from chickpea (Cicer arietinum L.) (CA) leaves was used in order to synthesize silver nanoparticles (AgNPs). For specification of the synthesized AgNPs, UV-vis spectrophotometer, Fourier transform infrared spectroscopy (FT-IR), X-ray diffraction analysis (XRD), transmission electron microscopy (TEM), scanning electron microscopy (SEM), electron dispersive X-ray (EDX), and zeta potential (ZP) analyses data were used. Biologically synthesized AgNPs demonstrated a maximum surface plasmon resonance of 417.47 nm after 3 h. With the powder XRD model, the mean crystallite dimension of nanoparticles was determined as 12.17 mm with a cubic structure. According to the TEM results, the dimensions of the obtained silver nanoparticles were found to be 6.11–9.66 nm. The ZP of the electric charge on the surface of AgNPs was measured as −19.6 mV. The inhibition effect of AgNPs on food pathogen strains and yeast was determined with the minimum inhibition concentration (MIC) method. AgNPs demonstrated highly effective inhibition at low concentrations especially against the growth of B. subtilis (0.0625) and S. aureus (0.125) strains. The cytotoxic effects of silver nanoparticles on cancerous cell lines (CaCo-2, U118, Sk-ov-3) and healthy cell lines (HDF) were revealed. Despite the increase of AgNPs used against cancerous and healthy cell lines, no significant decrease in the percentage of viability was detected.
Introduction
Nanotechnology is revealing new perspectives for the diagnosis and cure of numerous deadly autoimmune and chronic disorders like cancer (Kafshdooz et al., 2018; Yadi et al., 2018). Nanoparticles have become the main subject of scientific works in the last few decades because of their diverse properties, like different catalytic behaviors, chemical stability, and electric conductivity (Patra and Baek, 2016). Nanoparticles have become an indispensable source of biological research due to their structural and dimensional similarities to biological molecules. Nanoparticles are considered antimicrobial agents because they show good antibacterial properties resulting from their extensive surface area and volume that provides desired contact with the bacterial cell (Kumar et al., 2016). These properties allow nanoparticles to be used in diagnostic, cell labeling, biomarker, drug delivery, cancer therapy, and water purification applications (Mousavi et al., 2018; Kumari et al., 2019; Kowsalya et al., 2021).
In recent years to examine the morphological properties of nanoparticles, laser CVD, physical adsorption, and emulsion polymerization techniques are commonly being used. However, these technologies require the usage of stabilizing/reducing harmful chemicals or non-biologically degradable agents (Jayaprakash et al., 2017). For this reason, it is preferred to produce nanoparticles with fast, low-cost “green synthesis” procedures that do not use toxic solvents or pollute the environment, instead of current traditional methods (Hussain et al., 2016; Jayaprakash et al., 2017; Bandeira et al., 2020). Living organisms in nature can convert metal salts into nanoparticles by reducing them. In this context, scientific studies have focused on synthesizing these nanomaterials from non-artificial sources like plants (Aktepe and Baran, 2021), bacteria (Javaid et al., 2018), fungi (Molnár et al., 2018), algae (Parial et al., 2012), seaweeds (Chellapandian et al., 2019), and viruses (Mohmed et al., 2017).
In many nanoparticle studies, gold (Au) (Hatipoğlu, 2021), silver (Ag) (Baran, 2019; Umaz et al., 2019; Baran et al., 2021b), zinc (Zn) (Jayappa et al., 2020), nickel (Ni) (Din et al., 2018), iron (Fe) (Devatha et al., 2016), platinum (Pt) (Ramkumar et al., 2017b), selenium (Se) (Abu-Elghait et al., 2021), titanium (Ti), and palladium (Pd) (Gioria et al., 2020) are frequently used metals. Especially silver (Ag) is known to be an important metal suppressing the growth of bacteria. The Ag ion can prevent cell division and DNA replication (Ramya and Subapriya, 2012). Owing to their small dimensions, silver nanoparticles (AgNPs) bind to cell membrane proteins and catalyze the formation of reactive oxygen species (ROS) in bacterial cells. Thus, they cause cell death due to oxidative stress (Hoseinnejad et al., 2018; Alkhalaf et al., 2020; Hasanzadeh et al., 2021).
The most important advantage of choosing plants as a resource in the biological synthesis of nanoparticles (NPs) is that they contain many naturally occurring reducing agents such as flavonoids, reductases, phenolic acids, and dehydrogenases, which have a key role in the synthesis of magnetic nanoparticles (MNPs) (Shumail et al., 2021).
In this study considering the properties of plants, the synthesis and stabilization of silver nanoparticles were achieved by reducing Ag metal salt by using chickpea (Cicer arietinum L.) (CA) leaf extract. Plant-based synthesized AgNPs were investigated for their effectiveness against pathogens, microorganisms, and cancerous and healthy cell lines (Figure 1).
Materials and Methods
Materials
In the study, CA leaves obtained from Büyük Çelikli Village of Sur County of Diyarbakır were used. AgNO3 (99.8% purity), colistin, vancomycin, and fluconazole were commercially purchased from Sigma Aldrich. E. coli ATCC 25922, P. aeruginosa ATCC27853, B. subtilis ATCC 11774, S. aureus ATCC 29213, and C. albicans were used to test the antimicrobial activities of AgNPs. Cytotoxicity tests (MTT) related to cell lines (CaCo-2/human colon epidermal adenocarcinoma; U118 MG/human brain glioma cells; SK-OV-3/human ovarian cancer cell line; HDF/human dermal fibroblasts) were performed in the Dicle University Central Research Laboratory.
Herbal Extraction Process
Green leaves of CA were washed with deionized distilled water to remove residues and dehydrated at 25 ± 2°C. A total of 250 g of ground plant material was mixed with deionized pure water (500 ml) and boiled in a flask. After boiling, the cooled extract was filtered with a membrane filter (0.45 μm).
Plant-Based Synthesis of Silver Nanoparticles
Firstly, an aqueous solution of 5 mM AgNO3 with solid AgNO3 was prepared. The CA extracts (500 ml) and 100 ml of AgNO3 were allowed to react in a glass vessel (1:5 ratio) at room temperature. Maximum absorbance of biologically synthesized AgNPs was determined by wavelength scanning (UV-vis spectroscopy) at various time periods (15, 30, 45, 60, 120, and 180 min) depending on the color change. At the end of the synthesis, the solution, which became a dark color depending on time, was subjected to centrifugation (6000 rpm, 20 min). The purpose of this process is to separate the synthesized nanoparticles from plant residues. The solid fraction obtained at the end of centrifugation was washed several times with distilled water and the resulting residue (AgNPs) was dried in an oven at 60°C for 72 h.
Instrumentation
The maximum absorbance of synthesized AgNPs was measured at the 300–800 nm wavelength range with a spectrophotometer (Agilent CARY 60). Size, morphology, crystal structure, surface distribution, and zeta potential (ZP) values of AgNPs were revealed by scanning electron microscopy (SEM) (EVO 40 LEQ), transmission electron microscopy (TEM) (Quanta), field emission scanning electron microscopy (FE-SEM) (Quanta FEG240), electron dispersive X-ray (EDX) (Quanta FEG 240), X-ray diffraction analysis (XRD) (Rad B-DMAX II), and Zetasizer (Malvern Ins. Ltd.). The crystal dimension of AgNPs was calculated according to the D = Kλ/(β cosθ) equation (Asadi et al., 2018; Baran et al., 2018). In addition, Fourier transform infrared spectroscopy attenuated total reflectance (FT-IR ATR) was used to identify the functional groups present in the CA extract, and the functional groups responsible for the reduction at the end of the reaction test analysis conditions of used instruments are given in Table 1.
Antimicrobial Activities of Silver Nanoparticles
Growth inhibition of plant-based AgNPs on Gram-positive (B. subtilis; S. aureus) and Gram-negative (E. coli; P. aeruginosa) strains and yeast (C. albicans) was determined using a 96-well microplate with minimum inhibition concentration (MIC) method. Mueller Hinton broth and cell culture growth medium (Roswell Park Memorial Institute medium/ RPMI) were added to the wells for the growth of bacteria and yeast. The AgNP solution was added to the wells with the culture medium and microorganisms to determine the MIC value. Firstly, 100 µL of mixed culture medium was taken from the wells each time and transferred to the next well. Then the microorganism solutions adjusted according to the 0.5 McFarland standard were added to the microplates and incubated at 37°C/24 h. The minimum concentration without growth after incubation was determined as the MIC value (Baran et al., 2020). Commercially purchased standard antibiotics (colistin, vancomycin, and fluconazole) and 1 mM AgNO3 solution were used to compare the growth inhibitory activities of AgNPs on pathogen microorganisms.
Evaluation of Viability Suppressor Activities of AgNPs by the MTT Method on Cell Line Seeding in a 96-Well Plate
The MTT method was performed to determine the plant-based AgNP ratio of cytotoxicity (viability suppressor) on cancerous and healthy cell cultures. T-75 T-flasks were used to prepare the culture medium. CaCo-2, HDF, and U118 cell lines were cultivated in DMEM solution. The human ovarian cancer cell line (SK-OV-3) was incubated in RPMI solution. Prepared cultures were incubated at 5% CO2, 37°C, and 95% air and humidity conditions. When the cells reached about 80% confluency in the hemocytometer measurement, cell cultures were suspended at different concentrations and transferred to microplates (96-well) for incubation (overnight). At the end of the period, the cultured cell lines were treated with nanoparticles at different concentrations (25, 50, 100, and 200 μg/ml) and incubated for 2 days. In the next step, the MTT solution was transferred to the microplate wells and incubated for 3 h, and then DMSO was added and kept at room temperature for 0.25 h. The absorbance (540 nm) of the microplates was measured with MultiScan Go (Thermo).
By using the below formula, the percentage viability of the cell lines was calculated.
% viability = U/C*100 (Vickers, 2017).
U: Absorbance of cells treated with AgNPs.
C: The absorbance values of control cells.
Results and Discussion
UV-Visible Spectroscopic Analysis
The color change was observed after the CA leaf extracts with AgNO3 solution were left to react in a container. The UV-vis spectrum of AgNPs appeared to change from light green to purple (Figure 2). Because of the surface plasmon resonance, AgNPs gave a peak at a specific absorbance value of about 417.47 nm. Similarly, some researchers reported that the absorption spectrum of AgNPs is between 425–461 nm (Udayasoorian et al., 2011).
Evaluation of SEM and TEM Data
SEM, FE-SEM, and TEM images of synthesized AgNPs are given in Figures 3, 4. According to these results, it was seen that the nanomaterial was mostly spherical, nano-sized, and in clusters that were not in direct contact with each other. This indicates the stabilization of the AgNPs. It was reported that AgNPs have a spherical morphology and nano-dimensions in similar studies (Ramkumar et al., 2017a; Pallela et al., 2018). The biosynthesized nanoparticles are expected to have stronger antimicrobial activity, on account of their relatively small size. In the particle measurement done with TEM, it was seen that the sizes of AgNPs were approximately between 6.11–9.66 nm and the average size was approximately 7.83 nm (Figure 4). In some studies, using different materials, the sizes of AgNPs were reported to be between 2–95 nm (Nguyen et al., 2018; Behravan et al., 2019).
Evaluation of FT-IR Analysis Data
The FT-IR spectroscopy analysis determined the functional groups involved in the plant-derived reduction. The frequency of all stretch in the range of 4500–500 cm−1 was recorded with four scans at 1 cm−1 resolution. Figure 4 shows a comparison of FT-IR spectra for the aqueous CA extract (Figure 5A) and synthesized AgNPs (Figure 5B). When the biomolecules involved in reduction during the formation of AgNPs were examined (Figures 5A,B), the absorption peak at 1635 cm−1 corresponded to the C=O stretching vibration, indicating the presence of amide. Because of the phenolic compounds in the CA leaf extract, it can be concluded that the absorption peak at 2122 cm−1 belongs to alkyne (C≡C) groups while the absorption peak at 3331 cm-1 belongs to O-H and N-H stretching (Atalar et al., 2021). Presumably, these determined functional groups are responsible for the reduction of metal ions (Mandal et al., 2015).
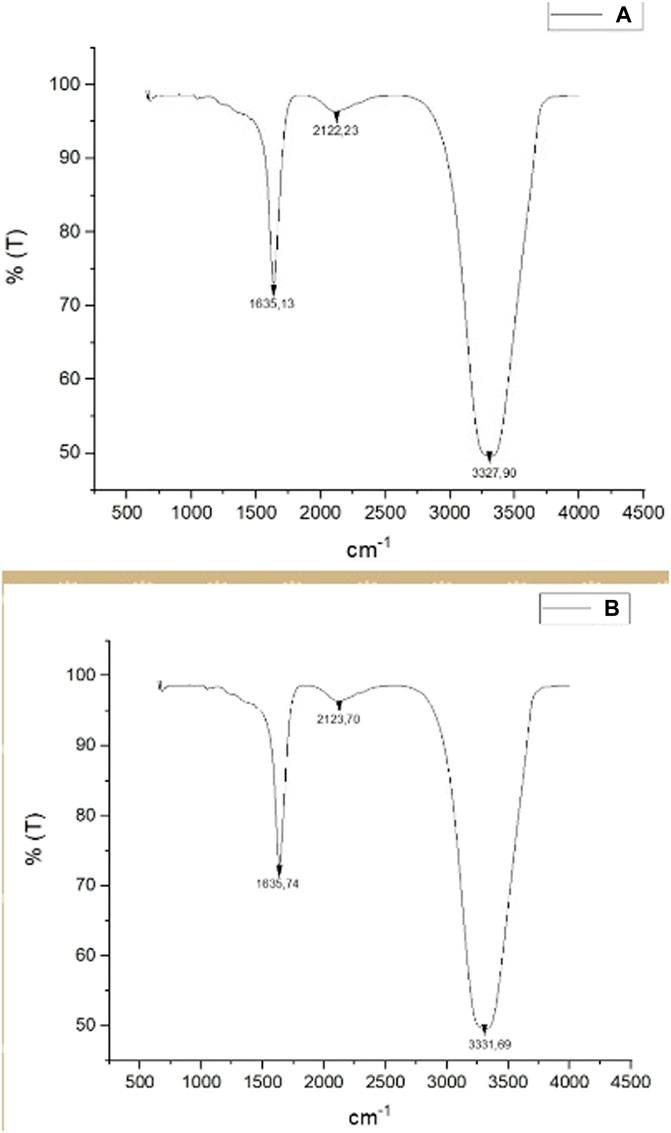
FIGURE 5. (A) FT-IR spectra data of CA leaf extract. (B) FT-IR spectra data of synthesized CA-AgNPs.
Evaluation of EDX Analysis Data
According to the EDX profile (Figure 6), it was confirmed that the biosynthesized nanoparticles had silver in their composition. It was also seen that the elemental composition of silver was high (Figure 6). AgNPs showed a typical optical absorption peak at about 3 KeV owing to the surface plasmon resonance (SPR). It can be said that the other emerging peaks are because of phytochemicals attached to the surface of AgNPs in the CA leaf pulp (Punuri et al., 2012). Khamhaengpol and Siri (2017) and Dada et al. (2019) also revealed the EDX silver peaks in their work.
XRD Analysis
The XRD spectrum model for the synthesized AgNPs is shown in Figure 7. In XRD analysis results, peaks of 111o, 200o, 220o, and 311o, which coincide with 37.96, 44.29, 64.32, and 77.33, respectively at 2θ, were sharp peaks representing the spherical crystal structure of silver. The peaks indicated that the AgNPs were cubic in structure. It has been reported in many studies that these peaks belong to silver (Huang et al., 2019; Keskin et al., 2021). The highest peak, 37.96, was taken as the peak angle. The size of the nanomaterials was calculated as approximately 12.17 nm according to the below equation ((Baran et al., 2021a).
In the equation, D = the size of the particle, K = the constant value (0.89), λ = the wavelength value of XRD (1.5418 Å), β = the FWHM value of the high peak, and cosθ = the Braggθ angle of the high peak.
Evaluation of Zeta Potential Analysis Data
The zeta potential analysis gives the electric charge on the surface of the surrounded material. The high negative value of the zeta potential prevents the particles from sticking together or clumping together. This indicates the stability of the AgNP colloid. On the other hand, nanoparticles with a significantly lower negative charge can enter the cell more easily (58–60). The zeta potential of the biosynthesized AgNPs was found to be −19.6 mV (Figure 8). This value indicated that the AgNPs were stable and uniformly distributed. The different zeta potential values of AgNPs synthesized from various materials have been reported previously (Ferreyra Maillard et al., 2018; Amer et al., 2020).
Evaluation of Antimicrobial Properties of AgNPs
The antimicrobial effects of AgNPs have become more important due to microorganisms that cause disease in humans developing resistance to conventional antibiotics. S. aureus, B. subtillis, E. coli, and P. aeruginosa strains and C. albicans yeast are pathogenic microorganisms frequently encountered in food-borne diseases (Yang et al., 2017; Mostafa et al., 2018). It was determined that the biosynthesized AgNPs significantly inhibited the growth of these microorganisms even at low concentrations (Table 2). It was observed that AgNPs strongly inhibited the growth of S. aureus and B. subtilis when compared to other microorganisms. Since silver has a strong tendency to interact with phosphorus and sulfur atoms in the bacterial cell wall, it interacts with the thiol and phosphorus groups in the bacterial cell membrane, thereby disrupting the bacterial respiration process. This causes the death of bacteria (Hamouda and Baker Jr, 2000). On the other hand, since the cell wall of Gram-positive bacteria has a hard polysaccharide layer, the transition to the Gram-positive bacterial wall is more difficult when compared to Gram-negative bacteria. Therefore, the inhibitory activity of AgNPs in Gram-positive bacteria is stronger than in Gram-negative bacteria (Tamboli and Lee, 2013). Thuc et al. (2016) reported that Gram-positive S. aureus has approximately 2–3 times higher resistance to AgNPs than Gram-negative E. coli and P. aeruginosa (Thuc et al., 2016). These effective inhibitory activities of AgNPs on different bacteria strains and yeasts were also reported by many researchers (Niknejad et al., 2015; Aygün et al., 2020).
Evaluation of Cytotoxic Activities of AgNPs
AgNPs obtained by biosynthesis of chickpea leaf extract were applied to healthy cells (HDF) and three different cancer cell lines (CaCo-2, U118, and Sk-ov 3), and the results obtained after 48 h are shown in Table 3 and Figure 9. According to these results, it was seen that there was no toxic effect in HDF with a survival rate of 79.70% at a 25 μg/ml concentration. It was determined that the most suppressed concentration of viability was on CaCo-2 cells at 200 mg/ml (Table 3). Despite the increase in the concentration of AgNPs in other cancer cell lines, the increase in the percentage of viability can be explained by the proliferative properties of AgNPs for these cells (Morais et al., 2020).
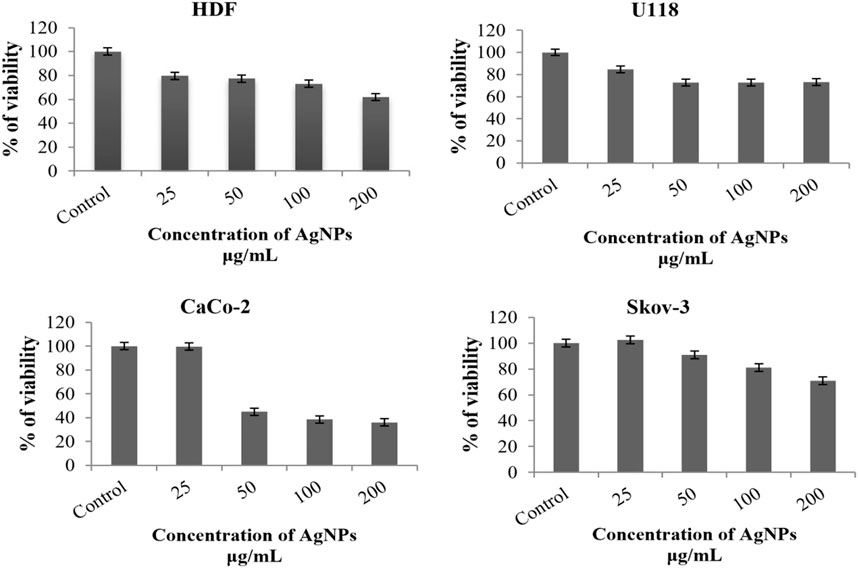
FIGURE 9. Evaluation of the percentage viability rates as a result of the cytotoxic effect of AgNPs 2 days after combining them with CaCo-2, U118, HDF, and Sk-ov-3 cell lines.
It is known that AgNPs show strong oxidative properties (Wongpreecha et al., 2018). AgNPs tend to settle in biomolecules such as cell membranes and nuclei. They exert a toxic effect by stimulating apoptosis with an increase in ROS after localization (Gliga et al., 2014; Morais et al., 2020). The concentration, exposure time, shape, size, charge, degree of deposition, and chemistry of the surface composition can have a significant impact on the toxicity of AgNPs (Swamy et al., 2015). In studies conducted to examine the toxic effects of AgNPs on CaCo-2 cells, it was reported that concentrations above 3.75–5.5 μg/ml showed toxic effects (Zein et al., 2020). Inhibitory concentrations in Sk-ov-3 cells were reported to be 9.4 μg/ml (Fahrenholtz et al., 2017) and 29.36 μg/ml (Noor et al., 2021). Zhang et al. (2010) reported that the 100 μg/ml concentration of silver nanoparticles was toxic on cell viability on HDF cell lines (Zhang et al., 2010).
Conclusion
In this study, green synthesis of silver nanoparticles (AgNPs) was carried out using Cicer arietinum leaf extract in a low-cost, environmentally friendly, simple, and fast method. No toxic or hazardous substances were used in the biosynthesis. The rapid and green synthesis of CA-AgNPs was successfully completed using the available phytochemicals in Cicer arietinum leaf extract as reducing agents. SEM and TEM images showed that spherically symmetrical plant-based AgNPs were formed due to their high stability. UV-vis absorption, XRD, and EDX analyses confirmed the synthesis of silver nanoparticles. Various microscopic analyses indicated that AgNPs had mostly spherical morphology with an average size of about 7.83 nm. The obtained analysis data showed that the smaller the size of the nanoparticles, the greater their antimicrobial activity, and the obtained AgNPs had strong antibacterial and anticandidal activity even at very low concentrations. The cytotoxic activities of CA-AgNPs were evaluated by the MTT method. A 25 μg/ml concentration of CA-AgNPs suppressed healthy cells by 20% and suppressed the viability of cancer cell lines by 1–15%. As the concentration increased, the suppression rate in cell lines other than U118 also increased. It was determined that silver nanoparticles synthesized using plant material had a high suppressive effect on the viability of CaCo-2 cells in parallel with the increase in concentration. It is known that NPs can be used in many commercial products for biological and medical applications. According to the results obtained, it is thought that CA-AgNPs can be used effectively as antimicrobial and anticancer agents in the food industry and medical applications.
Data Availability Statement
The original contributions presented in the study are included in the article/Supplementary Material, further inquiries can be directed to the corresponding authors.
Author Contributions
All authors contributed to the conception and the main idea of the work. AB, MF, CK, AH, OY, SI, MA, and MF drafted the main text, figures, and tables. CK, AE, GR, and DS supervised the work and provided the comments and additional scientific information. RK, EA, and AM also reviewed and revised the text. All authors read and approved the final version of the work to be published.
Funding
This work was funded by the Mardin Artuklu University Scientific Research Projects Coordinatorship (Project no: MAU.BAP.21. SHMYO.020).
Conflict of Interest
The authors declare that the research was conducted in the absence of any commercial or financial relationships that could be construed as a potential conflict of interest.
Publisher’s Note
All claims expressed in this article are solely those of the authors and do not necessarily represent those of their affiliated organizations, or those of the publisher, the editors and the reviewers. Any product that may be evaluated in this article, or claim that may be made by its manufacturer, is not guaranteed or endorsed by the publisher.
Acknowledgments
We are also thankful to the Tabriz University of Medical Sciences, University of Kragujevac, and Baku State University for moral support.
References
Abu-Elghait, M., Hasanin, M., Hashem, A. H., and Salem, S. S. (2021). Ecofriendly Novel Synthesis of Tertiary Composite Based on Cellulose and Myco-Synthesized Selenium Nanoparticles: Characterization, Antibiofilm and Biocompatibility. Int. J. Biol. Macromolecules 175, 294–303. doi:10.1016/j.ijbiomac.2021.02.040
Aktepe, N., and Baran, A. (2021). Fast and Low Cost Biosynthesis of AgNPs with almond Leaves: Medical Applications with Biocompatible Structures. Progr Nutr. 23, e2021271. doi:10.23751/pn.v23i3.11996
Alkhalaf, M. I., Hussein, R. H., and Hamza, A. (2020). Green Synthesis of Silver Nanoparticles by Nigella Sativa Extract Alleviates Diabetic Neuropathy through Anti-inflammatory and Antioxidant Effects. Saudi J. Biol. Sci. 27, 2410–2419. doi:10.1016/j.sjbs.2020.05.005
Amer, M. I., Moustafa, S. H., and El-Hagary, M. (2020). Enhanced Band Structure, Optoelectronic and Magnetic Properties of spray Pyrolysis Ni-Doped SnO2 Nanostructured Films. Mater. Chem. Phys. 248, 122892. doi:10.1016/j.matchemphys.2020.122892
Asadi, N., Annabi, N., Mostafavi, E., Anzabi, M., Khalilov, R., Saghfi, S., et al. (2018). Synthesis, Characterization and In Vitro Evaluation of Magnetic Nanoparticles Modified with PCL-PEG-PCL for Controlled Delivery of 5FU. Artif. Cell Nanomedicine, Biotechnol. 46, 938–945. doi:10.1080/21691401.2018.1439839
Atalar, M. N., Baran, A., Baran, M. F., Keskin, C., Aktepe, N., Yavuz, Ö., et al. (2021). Economic Fast Synthesis of Olive Leaf Extract and Silver Nanoparticles and Biomedical Applications. Particulate Sci. Tech. 1977443, 1–9. doi:10.1080/02726351.2021.1977443
Aygün, A., Özdemir, S., Gülcan, M., Cellat, K., and Şen, F. (2020). Synthesis and Characterization of Reishi Mushroom-Mediated green Synthesis of Silver Nanoparticles for the Biochemical Applications. J. Pharm. Biomed. Anal. 178, 112970. doi:10.1016/j.jpba.2019.112970
Bandeira, M., Giovanela, M., Roesch-Ely, M., and Devine, J. (2020). Green Synthesis of Zinc Oxide Nanoparticles: A Review of the Synthesis Methodology and Mechanism of Formation. Sust. Chem. Pharm. 15, 100223. doi:10.1016/j.scp.2020.100223
Baran, M. F., and Applied Ecology and Environmental Research, (2019). Synthesis, Characterization and Investigation of Antimicrobial Activity of Silver Nanoparticles from Cydonia Oblonga Leaf. Appl. Ecol. Env. Res. 17, 2583–2592. doi:10.15666/aeer/1702_25832592
Baran, M., Koç, A., and Uzan, S. J. (2018). Synthesis, Characterization and Antimicrobial Applications of Silver Nanoparticles (AgNPs) with Kenger (Gundelia Tournefortii) Leaf. EJONS Int. J Math, Eng Nat. Sci 5, 44–52.
Baran, M. F., Keskin, C., and Keskin, C. (2020). Determination of Antimicrobial and Toxic Metal Removal Activities of Plant‐Based Synthesized ( Capsicum Annuum L. Leaves), Ecofriendly, Gold Nanomaterials. Glob. Challenges 4, 1900104. doi:10.1002/gch2.201900104
Baran, A., Baran, M. F., Keskin, C., Kandemir, S. I., Valiyeva, M., Mehraliyeva, S., et al. (2021a). Ecofriendly/Rapid Synthesis of Silver Nanoparticles Using Extract of Waste Parts of Artichoke (Cynara Scolymus L.) and Evaluation of Their Cytotoxic and Antibacterial Activities. J. Nanomater. 2021, 1–10. doi:10.1155/2021/2270472
Baran, A., Keskin, C., Baran, M. F., Huseynova, I., Khalilov, R., Eftekhari, A., et al. (2021b). Ecofriendly Synthesis of Silver Nanoparticles Using Ananas Comosus Fruit Peels: Anticancer and Antimicrobial Activities. Bioinorganic Chem. Appl. 2021, 1–8. doi:10.1155/2021/2058149
Behravan, M., Panahi, A. H., Hossein Panahi, A., Ziaee, M., Mahdavi, R., and Mirzapour, A. (2019). Facile green Synthesis of Silver Nanoparticles Using Berberis Vulgaris Leaf and Root Aqueous Extract and its Antibacterial Activity. Int. J. Biol. Macromolecules 124, 148–154. doi:10.1016/j.ijbiomac.2018.11.101
Chellapandian, C., Ramkumar, B., Puja, P., Shanmuganathan, R., Pugazhendhi, A., and Kumar, P. (2019). Gold Nanoparticles Using Red Seaweed Gracilaria Verrucosa: Green Synthesis, Characterization and Biocompatibility Studies. Process Biochem. 80, 58–63. doi:10.1016/j.procbio.2019.02.009
Dada, A. O., Adekola, F. A., Dada, F. E., Adelani-Akande, A. T., Bello, M. O., and Okonkwo, C. R. (2019). Silver Nanoparticle Synthesis by Acalypha wilkesiana Extract: Phytochemical Screening, Characterization, Influence of Operational Parameters, and Preliminary Antibacterial Testing. Heliyon. 5 (10), e02517. doi:10.1016/j.heliyon.2019.e02517
Devatha, C. P., Thalla, A. K., and Katte, S. Y. (2016). Green Synthesis of Iron Nanoparticles Using Different Leaf Extracts for Treatment of Domestic Waste Water. J. Clean. Prod. 139, 1425–1435. doi:10.1016/j.jclepro.2016.09.019
Din, M. I., Rani, A., Mukhtar, M., Aihetasham, A., Mukhtar, M. J. E. N., Monitoring, , et al. (2018). Single Step green Synthesis of Stable Nickel and Nickel Oxide Nanoparticles from Calotropis Gigantea : Catalytic and Antimicrobial Potentials. Environ. Nanotechnology, Monit. Manag. 9, 29–36. doi:10.1016/j.enmm.2017.11.005
Fahrenholtz, C. D., Swanner, J., Ramirez-Perez, M., and Singh, R. (2017). Heterogeneous Responses of Ovarian Cancer Cells to Silver Nanoparticles as a Single Agent and in Combination with Cisplatin. J. Nanomater. 2017, 5107485. doi:10.1155/2017/5107485
Ferreyra Maillard, A. P. V., López de Mishima, B. A., Hollmann, A., Hollmann, A. J. C., and Biointerfaces, S. (2018). Interaction of green Silver Nanoparticles with Model Membranes: Possible Role in the Antibacterial Activity. Colloids Surf. B: Biointerfaces 171, 320–326. doi:10.1016/j.colsurfb.2018.07.044
Gioria, E., Signorini, C., Wisniewski, F., and Gutierrez, L. (2020). Green Synthesis of Time-Stable Palladium Nanoparticles Using Microfluidic Devices. J. Environ. Chem. Eng. 8, 104096. doi:10.1016/j.jece.2020.104096
Gliga, A. R., Skoglund, S., Wallinder, I. O., Fadeel, B., Karlsson, H. L. J. P., and Toxicology, F. (2014). Size-dependent Cytotoxicity of Silver Nanoparticles in Human Lung Cells: the Role of Cellular Uptake, Agglomeration and Ag Release. Part. Fibre Toxicol. 11, 1–17. doi:10.1186/1743-8977-11-11
Hamouda, T., and Baker, J. R. (2000). Antimicrobial Mechanism of Action of Surfactant Lipid Preparations in Enteric Gram-Negative Bacilli. Antimicrob. mechanism Action. surfactant lipid preparations enteric Gram-negative bacilli 89, 397–403. doi:10.1046/j.1365-2672.2000.01127.x
Hasanzadeh, A., Gholipour, B., Rostamnia, S., Eftekhari, A., Tanomand, A., Valizadeh. K, A., et al. (2021). Biosynthesis of AgNPs onto the Urea-Based Periodic Mesoporous Organosilica (AgxNPs/Ur-PMO) for Antibacterial and Cell Viability Assay. J. Colloid Interf. Sci. 585, 676–683. doi:10.1016/j.jcis.2020.10.047
Hatipoğlu, A. (2021). Green Synthesis of Gold Nanoparticles from Prunus Cerasifera Pissardii Nigra Leaf and Their Antimicrobial Activities on Some Food Pathogens. Prog. Nutr. 23, e2021241. doi:10.23751/pn.v23i3.11947
Hoseinnejad, M., Jafari, S. M., and Katouzian, I. (2018). Inorganic and Metal Nanoparticles and Their Antimicrobial Activity in Food Packaging Applications. Crit. Rev. Microbiol. 44, 161–181. doi:10.1080/1040841x.2017.1332001
Huang, X., Wang, R., Jiao, T., Zou, G., Zhan, F., Yin, J., et al. (2019). Facile Preparation of Hierarchical AgNP-Loaded MXene/Fe3O4/Polymer Nanocomposites by Electrospinning with Enhanced Catalytic Performance for Wastewater Treatment. ACS Omega 4, 1897–1906. doi:10.1021/acsomega.8b03615
Hussain, I., Singh, N. B., Singh, A., Singh, H., and Singh, S. C. (2016). Green Synthesis of Nanoparticles and its Potential Application. Biotechnol. Lett. 38, 545–560. doi:10.1007/s10529-015-2026-7
Javaid, A., Oloketuyi, S. F., Oloketuyi, M. M., and Khan, F. (2018). Diversity of Bacterial Synthesis of Silver Nanoparticles. BioNanoSci. 8, 43–59. doi:10.1007/s12668-017-0496-x
Jayappa, M. D., Kumar, M. A. P., Prabhu, A., Sheikh, S., Prabhu, A., Devasya, R. P., et al. (2020). Green Synthesis of Zinc Oxide Nanoparticles from the Leaf, Stem and In Vitro Grown Callus of Mussaenda Frondosa L.: Characterization and Their Applications. Appl. Nanosci 10, 3057–3074. doi:10.1007/s13204-020-01382-2
Jayaprakash, N., Vijaya, J. J., Vijaya, K., Kombaiah, K., Kennedy, L. J., Kennedy, R. J., et al. (2017). Green Synthesis of Ag Nanoparticles Using Tamarind Fruit Extract for the Antibacterial Studies. J. Photochem. Photobiol. B: Biol. 169, 178–185. doi:10.1016/j.jphotobiol.2017.03.013
Kafshdooz, L., Pourfathi, H., Akbarzadeh, A., Kafshdooz, T., Razban, Z., Sheervalilou, R., et al. (2018). The Role of microRNAs and Nanoparticles in Ovarian Cancer: a Review. Artif. Cell Nanomedicine, Biotechnol. 46, 241–247. doi:10.1080/21691401.2018.1454931
Keskin, C., Atalar, M. N., Firat Baran, M., and Baran, A. J. (2021). Environmentally Friendly Rapid Synthesis of Gold Nanoparticles from Artemisia Absinthium Plant Extract and Application of Antimicrobial Activities. J. Inst. Sci. Tech. 11, 365–375. doi:10.21597/jist.779169
Khamhaengpol, A., and Siri, S. (2017). Green Synthesis of Silver Nanoparticles Using Tissue Extract of Weaver Ant Larvae. Mater. Lett. 192, 72–75. doi:10.1016/j.matlet.2017.01.076
Kowsalya, E., Mosachristas, K., Balashanmugam, P., Manivasagan, V., Devasena, T., and Jaquline, C. (2021). Sustainable Use of Biowaste for Synthesis of Silver Nanoparticles and its Incorporation into Gelatin-Based Nanocomposite Films for Antimicrobial Food Packaging Applications. J. Food Process Eng. 44, e13641. doi:10.1111/jfpe.13641
Kumar, N., Biswas, K., and Gupta, R. K. (2016). Green Synthesis of Ag Nanoparticles in Large Quantity by Cryomilling. RSC Adv. 6, 111380–111388. doi:10.1039/c6ra23120a
Kumari, P., Alam, M., and Siddiqi, W. A. (2019). Usage of Nanoparticles as Adsorbents for Waste Water Treatment: An Emerging Trend. Sust. Mater. Tech. 22, e00128. doi:10.1016/j.susmat.2019.e00128
Mandal, A., Sekar, S., Chandrasekaran, N., Mukherjee, A., and Sastry, T. P. (2015). Vibrational Spectroscopic Investigation on Interaction of Sago Starch Capped Silver Nanoparticles with Collagen: a Comparative Physicochemical Study Using FT-IR and FT-Raman Techniques. RSC Adv. 5, 15763–15771. doi:10.1039/c4ra09694k
Mohmed, A. A., Saad, E., Fouda, A., Elgamal, M. S., and Salem, S. (2017). Extracellular Biosynthesis of Silver Nanoparticles Using Aspergillus Sp. And Evaluation of Their Antibacterial and Cytotoxicity. J. Appl. Life Sci. Int. 11, 1–12. doi:10.9734/jalsi/2017/33491
Molnár, Z., Bódai, V., Szakacs, G., Erdélyi, B., Fogarassy, Z., Sáfrán, G., et al. (2018). Green Synthesis of Gold Nanoparticles by Thermophilic Filamentous Fungi. Scientific Rep. 8, 1–12. doi:10.1038/s41598-018-22112-3
Morais, M., Teixeira, A. L., Teixeira, F., Machado, V., Medeiros, R., and Prior, J. A. V. (2020). Cytotoxic Effect of Silver Nanoparticles Synthesized by Green Methods in Cancer. J. Med. Chem. 63, 14308–14335. doi:10.1021/acs.jmedchem.0c01055
Mostafa, A. A., Almaary, K. S., Sholkamy, E. N., Bakri, M. M., Sholkamy, E. N., and Bakri, M. (2018). Antimicrobial Activity of Some Plant Extracts against Bacterial Strains Causing Food Poisoning Diseases. Saudi J. Biol. Sci. 25, 361–366. doi:10.1016/j.sjbs.2017.02.004
Mousavi, S. M., Ghasemi, Y., Amani, A. M., Savar Dashtaki, A., Amani, A. M., Arjmand, O., et al. (2018). Green Synthesis of Silver Nanoparticles toward Bio and Medical Applications: Review Study. Artif. Cell Nanomedicine, Biotechnol. 46, S855–S872. doi:10.1080/21691401.2018.1517769
Nguyen, T.-D., Dang, C.-H., and Mai, D.-T. (2018). Biosynthesized AgNP Capped on Novel Nanocomposite 2-Hydroxypropyl-β-Cyclodextrin/alginate as a Catalyst for Degradation of Pollutants. Carbohydr. Polym. 197, 29–37. doi:10.1016/j.carbpol.2018.05.077
Niknejad, F., Nabili, M., Daie Ghazvini, R., and Moazeni, M. (2015). Green Synthesis of Silver Nanoparticles: Another Honor for the Yeast Model Saccharomyces cerevisiae. mazu-cmm 1, 17–24. doi:10.18869/acadpub.cmm.1.3.17
Noor, F., Noor, A., Ishaq, A. R., Farzeen, I., Saleem, M. H., Ghaffar, K., et al. (2021). Recent Advances in Diagnostic and Therapeutic Approaches for Breast Cancer: A Comprehensive Review. Curr. Pharm. Des. 27, 2344–2365. doi:10.2174/1381612827666210303141416
Pallela, P. N. V. K., Ruddaraju, L. K., Ruddaraju, L. K., Yoon, S.-G., and Yoon, S.-G. (2018). Ultra Small, Mono Dispersed green Synthesized Silver Nanoparticles Using Aqueous Extract of Sida Cordifolia Plant and Investigation of Antibacterial Activity. Microb. Pathogenesis 124, 63–69. doi:10.1016/j.micpath.2018.08.026
Parial, D., Patra, H. K., Patra, A. K., and Pal, R. (2012). Screening of Different Algae for green Synthesis of Gold Nanoparticles. Eur. J. Phycology 47, 22–29. doi:10.1080/09670262.2011.653406
Patra, J. K., and Baek, K.-H. (2016). Green Synthesis of Silver Chloride Nanoparticles Using Prunus Persica L. Outer Peel Extract and Investigation of Antibacterial, Anticandidal, Antioxidant Potential. Green. Chem. Lett. Rev. 9, 132–142. doi:10.1080/17518253.2016.1192692
Punuri, J. B., Sharma, P., Sibyala, S., Tamuli, R., and Bora, U. (2012). Piper Betle-Mediated green Synthesis of Biocompatible Gold Nanoparticles. Int. Nano Lett. 2, 1–9. doi:10.1186/2228-5326-2-18
Ramkumar, V. S., Gopalakrishnan, K., Sivagurunathan, P., Saratale, G. D., Saratale, G. D., Kannapiran, E., et al. (2017a). Biofabrication and Characterization of Silver Nanoparticles Using Aqueous Extract of Seaweed Enteromorpha Compressa and its Biomedical Properties. Biotechnol. Rep. 14, 1–7. doi:10.1016/j.btre.2017.02.001
Ramkumar, V. S., Prakash, S., Ahila, N. K., Vinoj, G., Selvam, S., Kumar, G., et al. (2017b). Synthesis of Platinum Nanoparticles Using Seaweed Padina Gymnospora and Their Catalytic Activity as PVP/PtNPs Nanocomposite towards Biological Applications. Biomed. Pharmacother. 92, 479–490. doi:10.1016/j.biopha.2017.05.076
Shumail, H., Khalid, S., Ahmad, I., Khan, H., Amin, S., and Ullah, B. (2021). Review on Green Synthesis of Silver Nanoparticles through Plants. Endocr. Metab. Immune Disord. Drug Targets 21 (6), 994–1007. doi:10.2174/1871530320666200729153714
Swamy, M. K., Mohanty, S. K., Sinniah, U. R., and Spectroscopy, B. (2015). Synthesis and Characterization of Silver Nanoparticles Using Fruit Extract of Momordica Cymbalaria and Assessment of Their In Vitro Antimicrobial, Antioxidant and Cytotoxicity Activities. Spectrochimica Acta A: Mol. Biomol. Spectrosc. 151, 939–944. doi:10.1016/j.saa.2015.07.009
Tamboli, D. P., and Lee, D. S. (2013). Mechanistic Antimicrobial Approach of Extracellularly Synthesized Silver Nanoparticles against Gram Positive and Gram Negative Bacteria. J. Hazard. Mater. 260, 878–884. doi:10.1016/j.jhazmat.2013.06.003
Thuc, D. T., Huy, T. Q., Hoang, L. H., Tien, B. C., Van Chung, P., Thuy, N. T., et al. (2016). Green Synthesis of Colloidal Silver Nanoparticles through Electrochemical Method and Their Antibacterial Activity. Mater. Lett. 181, 173–177. doi:10.1016/j.matlet.2016.06.008
Udayasoorian, C., Kumar, K. V., and Jayabalakrishnan, M. (2011). Extracellular Synthesis of Silver Nanoparticles Using Leaf Extract of Cassia Auriculata. Dig. J. Nanomater. Biostructures 6, 279–283.
Umaz, A., Koç, A., Baran, M. F., Atalar, M. J., and Keski̇n, M. N. (2019). Investigation of Antimicrobial Activity and Characterization, Synthesis of Silver Nanoparticles from Hypericum Triquetrifolium Turra Plant. J. Inst. Sci. Tech. 9, 1467–1475. doi:10.21597/jist.533115
Vickers, N. J. (2017). Animal Communication: When I'm Calling You, Will You Answer Too? Curr. Biol. 27, R713–R715. doi:10.1016/j.cub.2017.05.064
Wongpreecha, J., Polpanich, D., Suteewong, T., Kaewsaneha, C., and Tangboriboonrat, P. (2018). One-pot, Large-Scale green Synthesis of Silver Nanoparticles-Chitosan with Enhanced Antibacterial Activity and Low Cytotoxicity. Carbohydr. Polym. 199, 641–648. doi:10.1016/j.carbpol.2018.07.039
Yadi, M., Mostafavi, E., Saleh, B., Davaran, S., Aliyeva, I., Khalilov, R., et al. (2018). Current Developments in green Synthesis of Metallic Nanoparticles Using Plant Extracts: a Review. Artif. Cell Nanomedicine, Biotechnol. 46, S336–S343. doi:10.1080/21691401.2018.1492931
Yang, S.-C., Lin, C.-H., Aljuffali, I. A., and Aljuffali, J.-Y. (2017). Current Pathogenic Escherichia coli Foodborne Outbreak Cases and Therapy Development. Arch. Microbiol. 199, 811–825. doi:10.1007/s00203-017-1393-y
Zein, R., Alghoraibi, I., Soukkarieh, C., Salman, A., and Alahmad, A. (2020). In-vitro Anticancer Activity against Caco-2 Cell Line of Colloidal Nano Silver Synthesized Using Aqueous Extract of Eucalyptus Camaldulensis Leaves. Heliyon 6, e04594.
Keywords: cytotoxic activity, green synthesis, nanomaterials, food pathogens, nanomedicine, SEM-EDX
Citation: Baran A, Fırat Baran M, Keskin C, Hatipoğlu A, Yavuz Ö, İrtegün Kandemir S, Adican MT, Khalilov R, Mammadova A, Ahmadian E, Rosić G, Selakovic D and Eftekhari A (2022) Investigation of Antimicrobial and Cytotoxic Properties and Specification of Silver Nanoparticles (AgNPs) Derived From Cicer arietinum L. Green Leaf Extract. Front. Bioeng. Biotechnol. 10:855136. doi: 10.3389/fbioe.2022.855136
Received: 14 January 2022; Accepted: 07 February 2022;
Published: 07 March 2022.
Edited by:
Abolfazl Heydari, Polymer Institute (SAS), SlovakiaReviewed by:
Siamak Javanbakht, University of Tabriz, IranFatma Nur Parın, Bursa Technical University, Turkey
Copyright © 2022 Baran, Fırat Baran, Keskin, Hatipoğlu, Yavuz, İrtegün Kandemir, Adican, Khalilov, Mammadova, Ahmadian, Rosić, Selakovic and Eftekhari. This is an open-access article distributed under the terms of the Creative Commons Attribution License (CC BY). The use, distribution or reproduction in other forums is permitted, provided the original author(s) and the copyright owner(s) are credited and that the original publication in this journal is cited, in accordance with accepted academic practice. No use, distribution or reproduction is permitted which does not comply with these terms.
*Correspondence: Cumali Keskin, Y2tlc2tpbm9vQGdtYWlsLmNvbQ==; Gvozden Rosić, Z3Jvc2ljQG1lZGYua2cuYWMucnM=; Dragica Selakovic, ZHJhZ2ljYTk4NEBnbWFpbC5jb20=; Aziz Eftekhari, RWZ0ZWtoYXJpYUB0YnptZWQuYWMuaXI=