- 1IBET, Instituto de Biologia Experimental e Tecnológica, Oeiras, Portugal
- 2ITQB NOVA, Instituto de Tecnologia Química e Biológica António Xavier, Universidade Nova de Lisboa, Oeiras, Portugal
- 3Division of Malaria Research, Proteo-Science Center, Ehime University, Matsuyama, Japan
- 4Sumitomo Pharma Co., Ltd., Osaka, Japan
- 5European Vaccine Initiative, UniversitätsKlinikum Heidelberg, Heidelberg, Germany
The malaria asexual blood-stage antigen PfRipr and its most immunogenic fragment PfRipr5 have recently risen as promising vaccine candidates against this infectious disease. Continued development of high-yielding, scalable production platforms is essential to advance the malaria vaccine research. Insect cells have supplied the production of numerous vaccine antigens in a fast and cost-effective manner; improving this platform further could prove key to its wider use. In this study, insect (Sf9 and High Five) and human (HEK293) cell hosts as well as process-optimizing strategies (new baculovirus construct designs and a culture temperature shift to hypothermic conditions) were employed to improve the production of the malaria asexual blood-stage vaccine candidate PfRipr5. Protein expression was maximized using High Five cells at CCI of 2 × 106 cell/mL and MOI of 0.1 pfu/cell (production yield = 0.49 mg/ml), with high-purity PfRipr5 binding to a conformational anti-PfRipr monoclonal antibody known to hold GIA activity and parasite PfRipr staining capacity. Further improvements in the PfRipr5 expression were achieved by designing novel expression vector sequences and performing a culture temperature shift to hypothermic culture conditions. Addition of one alanine (A) amino acid residue adjacent to the signal peptide cleavage site and a glycine-serine linker (GGSGG) between the PfRipr5 sequence and the purification tag (His6) induced a 2.2-fold increase in the expression of secreted PfRipr5 over using the expression vector with none of these additions. Performing a culture temperature shift from the standard 27–22°C at the time of infection improved the PfRipr5 expression by up to 1.7 fold. Notably, a synergistic effect was attained when combining both strategies, enabling to increase production yield post-purification by 5.2 fold, with similar protein quality (i.e., purity and binding to anti-PfRipr monoclonal antibody). This work highlights the potential of insect cells to produce the PfRipr5 malaria vaccine candidate and the importance of optimizing the expression vector and culture conditions to boost the expression of secreted proteins.
1 Introduction
Development of a highly effective malaria vaccine is urgently needed for the control and eventual eradication of this disease. Currently, only the RTS,S/AS01 vaccine against Plasmodium falciparum has been recommended by the WHO for wider use, with modest efficacy (Vogel, 2021). While the RTS,S/AS01, the novel R21/Matrix-M (Datoo et al., 2021), and whole inactivated sporozoites focus on the pre-erythrocytic stage of the parasite’s life cycle, additional asexual blood-stage vaccines are desired. However, asexual blood-stage vaccine development efforts have been significantly hampered by target antigen polymorphism (Takala et al., 2009) and a redundancy of merozoite invasion pathways into the erythrocyte (Wright and Rayner, 2014). Recently, a heterotrimeric protein complex consisting of three highly conserved proteins (PfRh5, PfRipr, and CyRPA) was identified from P. falciparum merozoites as necessary to invade erythrocytes (Volz et al., 2016; Wong et al., 2019) presenting a promising vaccine target (Ragotte et al., 2020). Vaccine development efforts have mostly advanced regarding PfRh5, showing for the first time, a significant reduction in the parasite growth rate upon a controlled human malaria infection (Minassian et al., 2021). Recently, another component of the complex, the P. falciparum merozoite Rh5 interacting protein (PfRipr), has been shown to be highly conserved (Ntege et al., 2016). In particular, its most potent fragment PfRipr_5 (aa 720-934) (hereon referred as PfRipr5) induces the generation of antibodies with the merozoite invasion inhibitory capacity similar to that obtained when vaccinating with the full length PfRipr (Nagaoka et al., 2020).
Manufacturing relevant vaccine amounts demands robust, scalable, and cost-effective expression systems. Production of malaria vaccine candidate antigens has been widely explored using different cell hosts (Crosnier et al., 2013; Jones et al., 2013). Mammalian cells are an attractive option to produce therapeutic products requiring complex processing (Dumont et al., 2016), but its use to produce recombinant protein vaccines can be more costly and yield lower expression levels than other expression systems (Cid and Bolívar, 2021). Noteworthily, insect cells allow high production yields of mammalian-like recombinant proteins at reduced costs in short-time frames (Mena and Kamen, 2011), having been successfully used to produce Rh5 (Patel et al., 2013; Hjerrild et al., 2016).
Producing recombinant protein antigens as secreted products is of upmost importance to facilitate purification. Trafficking of proteins to the secretory pathway requires the use of a signal peptide (SP) at the N-terminus of the peptide sequence. The choice of the correct SP is essential for efficient recombinant protein secretion in insect cells (Olczak and Olczak, 2006; Soejima et al., 2013). Addition or substitution of amino acid residues with different characteristics to the N- and/or C-terminal of the SP sequence is often the key to improve protein secretion (Futatsumori-Sugai and Tsumoto, 2010; Ohmuro-Matsuyama and Yamaji, 2018). The use of an SP of insect origin may be necessary to drive an efficient expression of heterologous proteins (Tessier et al., 1991). In addition, an efficient SP cleavage is required for further protein processing in the endoplasmic reticulum (ER) and through the secretory pathway; prediction of the SP cleavage site (von Heijne, 1986) can give insights on whether a protein will be efficiently secreted or not, and suggest the addition of extra residues to the SP’s C-terminus to improve the probability of cleavage and consequent secretion.
Manipulation of cell culture conditions has proven efficient in modulating the cell phenotype toward improved growth and/or recombinant protein production (Vergara et al., 2018). Adaptive laboratory evolution approaches have shown great potential to develop high-producing cell lines (Yoon et al., 2006) or cell lines with more efficient nutrient utilization (Lee and Palsson, 2010). Single or multiple time-point shifts in the process parameters such as pH (Wang et al., 2011), dissolved oxygen concentration (Dick et al., 1994), temperature (Vergara et al., 2014), or a combination thereof (Tang et al., 2009; Paul et al., 2019) have also shown to significantly improve recombinant protein production and/or activity. Specifically for insect cells, the manipulation of culture temperature has been used to modulate cell growth (Reuveny et al., 1993), glycosylation potential (Donaldson et al., 1999), baculovirus replication (Shao-Hua et al., 1998), and recombinant protein production (Rossi et al., 2012). Lowering the culture temperature reduces metabolic activity and prolongs cell viability in the culture, promoting a higher protein expression (Fernandes et al., 2020).
In this work, the production of the malaria vaccine candidate PfRipr5 using insect cells was improved by optimizing the recombinant baculovirus (rBAC) expression vector and by performing a temperature shift to hypothermic conditions (27 → 22°C) at the time-of-infection (TOI).
2 Materials and Methods
2.1 Cell Lines and Culture Media
Insect Sf9 (Invitrogen) and High Five (Invitrogen) cells were routinely sub-cultured at 0.4–1 × 106 cell/mL every 3–4 days when cell density reached 2–3 × 106 cell/mL in the Insect-XPRESSTM (Sartorius) and Sf-900TM II SFM (Thermo Fisher Scientific) medium, respectively. HEK293-E6 cells (NRC) (Durocher et al., 2002) were routinely sub-cultured at 0.5–0.6 × 106 cell/mL every 3–4 days, when cell density reached 2–3 × 106 cell/mL in the Free Style F17 (Thermo Fisher Scientific) medium supplemented with 4 mM GlutaMAX™ (Thermo Fisher Scientific), 0.1% Pluronic™ F-68 (Life Technologies) and 25 μg/ml of Geneticin (Thermo Fisher Scientific). Cells were cultured using 125–500 ml shake-flasks (Corning) with 10%–20% working volume. Insect Sf9 and High Five cells were maintained at 27°C in a shaking incubator (Inova 44R—Eppendorf) set to 100 rpm and with 2.54 cm shaking diameter. HEK 293 cells were maintained in a similar incubator at 37°C with 5% CO2 and the stirring rate set to 75 rpm.
2.2 Expression Vectors
PfRipr5 nucleotide sequence (Nagaoka et al., 2020) was codon-optimized for expression in insect or human cells, with an N-terminal gp67 SP sequence to allow secretion into the culture medium, a C-terminal His6-tag to allow purification, and a Kozak consensus sequence (GCCACT or ACC for human or insect expression plasmid, respectively) as the translation initiation site (synthetized by GenScript, Leiden, the Netherlands). Sequences were cloned into the pTT5 vector for expression in human HEK293 cells or into the pOET3 vector for expression in insect Sf9 and High Five cells. For PfRipr5 production optimization in insect cells, five additional expression vectors based on the original vector (hereon named rBAC gp67) were synthesized, comprising a different SP and/or additional amino acid residues: rBAC BVM (replace gp67 SP sequence by the bee venom melittin, BVM, SP sequence), rBAC A- (addition of one A spacer residue between the gp67 SP sequence and the PfRipr5 sequence), rBAC -GG (addition of a GG linker between the PfRipr5 sequence and the His6-tag), rBAC A-GG (addition of one A spacer residue between the gp67 SP sequence and the PfRipr5 sequence and addition of a GG linker between the PfRipr5 sequence and the His6-tag), and rBAC A-GGSGG (addition of one A spacer residue between the gp67 SP sequence and the PfRipr5 sequence and addition of a GGSGG linker between the PfRipr5 sequence and the His6-tag). Schematics of the constructs are depicted in Figure 3.
2.3 Baculovirus Generation, Amplification, and Storage
Six independent rBAC master viral stocks (MVS) were generated from the pOET3 expression vectors described previously using the flashback ULTRATM system (Oxford Expression Technologies) according to the manufacturer’s instruction. Amplification of MVS was performed as described elsewhere (Vieira et al., 2005). Briefly, the insect Sf9 cells were infected at a cell concentration at infection (CCI) of 1 × 106 cell/mL using a multiplicity of infection (MOI) of 0.1 plaque-forming units per viable cell (pfu/cell). When a cell viability of approximately 80% was reached, the supernatant was harvested by centrifugation at 200 × g and 4°C for 10 min and centrifugation at 2,000 × g and 4°C for 20 min. The clarified supernatant was aliquoted appropriately and stored at 4°C until further use.
2.4 Production of PfRipr5
2.4.1 Insect Cells as Host
PfRipr5 was produced in a 500 ml shake flask (SF) with 10% working volume and in 2 L glass-stirred tank bioreactors (STB).
In SF cultures, cells were seeded at 0.4–0.6 × 106 cell/mL and infections performed 1) at different combinations of CCI (1 and 2 × 106 cell/mL) and MOI (0.1 and 1 pfu/cell) for the initial assessment of PfRipr5 production using rBAC gp67, or 2) at CCI = 2 × 106 cell/mL and MOI = 0.1 pfu/cell for the optimization of PfRipr5 production using rBAC BVM, rBAC A-, rBAC -GG, rBAC A-GG, and rBAC A-GGSGG.
STB cultures were performed in computer-controlled BIOSTAT B-DCU 2 L vessels (Sartorius) equipped with two Rushton impellers, a sparger for gas supply, a water recirculation jacket for temperature control, and multiple ports for temperature, pH, and pO2 probes as well as for additions (e.g., culture medium) and sampling/harvesting of the cell culture. Cells were seeded at 0.4–0.6 × 106 cell/mL and infections performed at CCI = 2 × 106 cell/mL and MOI = 0.1 pfu/cell, using rBAC gp67 (initial assessment of PfRipr5 production) or rBAC A-GGSGG (optimization of PfRipr5 production). The pO2 was set to 30% of air saturation and was maintained by varying the agitation rate from 70 to 270 rpm and the percentage of O2 in the gas mixture from 0 to 100%. The gas flow rate was set to 0.01 vvm and temperature was kept at 27°C (initial assessment of PfRipr5 production) or changed to 22°C at TOI (optimization of PfRipr5 production). Bioreactors were operated with an initial working volume of 2 L.
2.4.2 Human Cells as Host
PfRipr5 was produced in 500 ml SF with 20% working volume and in 2 L glass STB.
For the productions in SF, cells were seeded at 0.5 × 106 cell/mL and transfected at ≈ 1.6 × 106 cell/mL with a mixture of pTT5-PfRipr5 plasmid (pDNA) and polyethyleneimine (PEI, Polysciences) cationic polymers prepared in 10% (v/v) of the total volume. Different combinations of the pDNA concentration ([pDNA] = 0.5 and 1 mg/L) and the pDNA:PEI ratio (1:2 and 1:1.5, w/w) were tested.
STB culture was performed in a 2 L STB set-up similar to insect cells. Cells were seeded at 0.5 × 106 cell/mL and transfected at ≈ 1.6 × 106 cell/mL with [pDNA] = 0.5 mg/L and pDNA:PEI ratio = 1:2. Culture pH was maintained at 7.4 using NaHCO3. The pO2 was set to 40% of air saturation and was maintained by varying the agitation rate from 70 to 270 rpm and the percentage of O2 in the gas mixture from 0 to 100%. The gas flow rate was set to 0.01 vvm and temperature was kept at 37°C. Bioreactors were operated with an initial working volume of 2 L.
2.5 Purification of PfRipr5
Purification of secreted PfRipr5 produced in STB was carried out on ÄKTA Explorer 100 systems (Cytiva). Cell culture bulk was harvested, filtered through 0.45 and 0.22 μm Sartopore 2 Midicap filter cartridges 10 (Sartorius), concentrated with a Sartocon disposable polyethersulfone (PES) membrane 2 × 0.1 m2 10 kDa (Sartorius), and filtered through a Nalgene cup 0.2 μm (Thermo Scientific). PfRipr5 was purified by immobilized metal ion affinity chromatography (IMAC) on a Histrap HP column (5 ml volume; Cytiva) equilibrated with 50 mM NaP, 500 mM NaCl, 5 mM DTT, and pH 8.0. After washing the column with the same buffer containing 500 mM imidazole, the protein was eluted with a linear imidazole gradient over 10 column volumes. The eluate was concentrated using AmiconUltra 15 Centrifugal filter unit 10 kDa (Merck Milipore), filtered through 0.2 μm, and loaded into a HiLoad 26/60 Superdex 75 gel permeation column (SEC) (Cytiva equilibrated with 16 mM NaP, 250 mM NaCl, 5 mM DTT, and pH 8.0). The eluate was concentrated using AmiconUltra 15 Centrifugal filter unit 10 kDa and loaded in a HiPrep desalting 26/10 column (Cytiva) equilibrated with 16 mM NaP, 250 mM NaCl, and pH 8.0 for removal of DTT. The eluate was concentrated using AmiconUltra 15 Centrifugal filter unit 10 kDa and filtered through 0.2 μm. The final sample was stored in 16 mM NaP, 250 mM NaCl, and pH 8.0 buffer formulation, aliquoted and stored at −80°C.
2.6 Analytics
2.6.1 Cell Concentration and Viability
Cell concentration and viability were assessed using a Cedex HiRes Analyzer (Roche).
2.6.2 SDS-PAGE and Western Blot
SDS-PAGE and western blot analyses were performed as reported previously (Correia et al., 2020). In particular, the reduced (R) samples were mixed with NuPAGE Sample Reducing Agent 1X and heated at 70°C for 10 min; non-reduced samples (NR) were mixed with water in replacement of the reducing agent. Both samples were run in the same gel (4%–12% Bis_Tris, NuPAGE). For PfRipr5 identification by western blot, anti-PfRipr5 antiserum generated in rabbits (Nagaoka et al., 2020) was used at a dilution 1:1,000. As the secondary antibody, an anti-rabbit IgG antibody conjugated with alkaline phosphatase was used at a dilution of 1:5,000. Protein band detection was performed with NBT/BCIP 1-Step (Thermo Scientific) for 10 min and membranes were scanned with a benchtop scanner device. The densitometry analysis of SDS-PAGE gels (to assess PfRipr5 protein purity) and western blot membranes (to assess relative productivity) was performed using FIJI software (Schindelin et al., 2012). The The PfRipr5 antigen generated by a wheat germ cell-free system (WGCFS) (Tsuboi et al., 2010; Nagaoka et al., 2020) was used in all membranes as the positive control for PfRipr5 detection and as a normalizing factor (i.e., band intensity of control = 1.0) for relative band intensity between samples in separate membranes.
2.6.3 Baculovirus Titration
Baculovirus infectious titers were determined using the MTT assay (Mena et al., 2003; Roldão et al., 2009).
2.6.4 Protein Concentration
Protein concentration was determined by spectrophotometry at 280 nm using the mySPEC equipment (VWR).
2.6.5 Dynamic Light Scattering
The size distribution of the purified PfRipr5 was analyzed by dynamic light scattering (DLS) on a Spectro Light 600 (Xtal Concepts).
2.6.6 Enzyme-Linked Immunosorbent Assay
Ninety-six well enzyme-linked immunosorbent assay (ELISA) plates (Greiner Bio-One, Kremsmünster, Austria) were coated with 50 μL of mouse anti-PfRipr mAb (clone 29B11) (produced as described in supplementary information), diluted to 2.5 μg/ml in a catcher buffer (25 mM carbonate buffer pH 9.6) and incubated at 4°C overnight. The plates were washed with phosphate-buffered saline (PBS) with 0.1% (v/v) Tween-20 (PBS-T) and then blocked with 200 μL 3% skimmed milk in PBS-T for 1 h at RT. Purified PfRipr5 was diluted in PBS, added to antibody-coated plates thrice, and incubated for 1 h at RT. After washing, the plates were incubated with 100 μL of th anti-PfRipr5 rabbit antiserum diluted 1:2,000 in PBS for 1 h at RT. After incubation, the plates were washed and incubated with HRP-conjugated anti-rabbit IgG (Cytiva) diluted 1:1,000 in PBS-T for 1 h at RT. The plates were washed and incubated for 15 min at RT with the TMB substrate solution (Thermo Scientific). The reaction was stopped with 2 M sulfuric acid, and the optical density (OD) was determined at 450 nm using a precision microplate reader (Molecular Devices, Sunnyvale, CA).
2.6.7 Surface Plasmon Resonance
Surface plasmon resonance (SPR) was performed with Biacore X100 (Cytiva). All the reagents and the sensor chip CM5 were purchased from Cytiva. Biacore X100 evaluation software was used for the analysis. A kinetic analysis was done with the mouse-antibody capture kit according to the manufacturer’s instructions. Fresh HBS-EP+ [10 mM HEPES, pH 7.4, 150 mM NaCl, 3 mM EDTA, 0.05% (v/v) surfactant P20] was used as the running buffer. The anti-PfRipr mouse mAb 29B11 (25 μg/ml) was injected into the anti-mouse antibody coated CM5. The purified PfRipr5 protein (18.8, 37.5, 75, 159, and 300 nM) was then injected. After subtracting the reference, individual association (ka) and dissociation (kd) rate constants were obtained by global fitting.
2.7 Statistical Analysis
Data are expressed as mean ± standard deviation. Differences were tested by using the unpaired t-test analysis method (p value < 0.05 was considered statistically significant, ns denotes non-statistically significant). Pearson’s correlation (r) was used to analyze the correlation between infection kinetics in SF and STB. One-way ANOVA was conducted by GraphPad Prism (Ver. 8.4.3).
2.8 Data Availability Statement
The sensitive nature of some of the reagents used in this study (e.g., cell lines, plasmids, baculoviruses, and antibodies) means that they are only readily available internally to the author’s institution staff for R&D purposes. For external researchers, the approval of reagents’ request may be obtained via email addressed to the corresponding author.
3 Results
3.1 PfRipr5 Production in Insect and Human Cells
3.1.1 Optimizing Production Conditions
Production of PfRipr5 was assessed in small-scale SF cultures using 1) the insect (High Five and Sf9) cell-baculovirus expression vector system (IC-BEVS) and 2) PEI-mediated human (HEK293) cell transfection. Aiming at finding the best production conditions, insect cells were infected at different combinations of MOI (0.1 and 1 pfu/cell) and CCI (1 and 2 × 106 cell/mL) while human cells were transfected at different combinations of PfRipr5 [pDNA] (0.5 and 1 mg/L) and pDNA:PEI ratios (1:2 and 1:1.5).
Infection kinetics in IC-BEVS were in accordance with the different conditions tested, characterized by higher cell growth and delayed onset of cell viability drop when using lower MOI (Figure 1A). Similarly, different transfection conditions resulted in distinct cell growth and viability kinetics (Figure 1B). The PfRipr5 expression varied with the cell line and condition tested, this being favored in insect cells and when using low MOIs (Supplementary Figure S1). Time-of-harvest (TOH) for each cell line and condition tested was defined based on the PfRipr5 expression and cell viability, aiming for the latter to be above 65%–70% to avoid purification issues caused by overaccumulation of cell debris and intracellular content. For insect cells, TOH was set at days 2–3 post-infection while for human cells, the TOH was set at days 4–5 post-transfection. At the selected TOH, the concentration of PfRipr5 produced in insect cells was up to 17.2-fold higher than that obtained with the best-performing transfection condition in human cells (Figure 1C). Overall, the production conditions maximizing PfRipr5 concentration at TOH in insect and human cells were CCI = 2 × 106 cell/mL and MOI = 0.1 pfu/cell, and [pDNA] = 0.5 mg/L and pDNA:PEI ratio = 1:2, respectively, and thus used in subsequent studies.
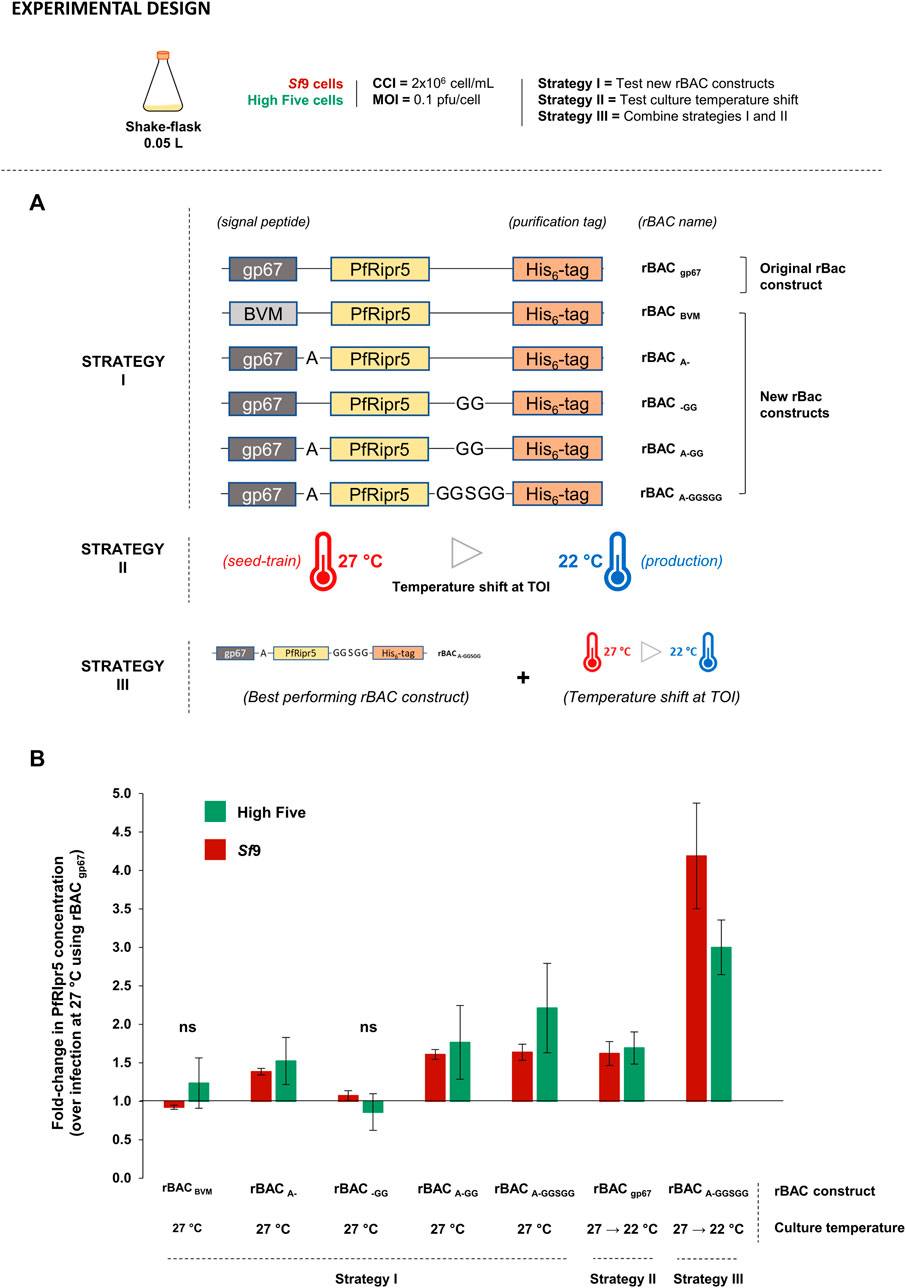
FIGURE 1. Optimization of PfRipr5 production using insect cells. (A) Optimization strategies devised. (B) Relative PfRipr5 concentration at the TOH between each optimization condition and the baseline production setup (infection with rBAC gp67 without culture temperature shift). A denotes alanine, G denotes glycine, and S denotes serine. Infections were performed using CCI = 2 × 106 cell/mL and MOI = 0.1 pfu/cell. Data are expressed as mean ± standard deviation and is relative to three biological replicates (n = 3).
3.1.2 Production at Bioreactor Scale
Production of PfRipr5 in insect (High Five and Sf9) and human (HEK293) cells was demonstrated in computer-controlled 2 L STB, using previously optimized process conditions; SF cultures were used as the control. Purification was identical for all three production runs, and the production yield and quality of purified PfRipr5 were assessed and compared.
Insect cell concentrations and viability profiles were similar between STB and SF cultures; contrary to what was observed in human cells where higher cell concentration and viability were achieved in STB when compared to SF during the initial days of transfection (Supplementary Figure S2A). PfRipr5 expression in all cell lines was confirmed by using western blot analysis (Supplementary Figure S2B), with a relative PfRipr5 concentration at TOH being maximized in insect cells (Figure 2A), consistent with SF data.
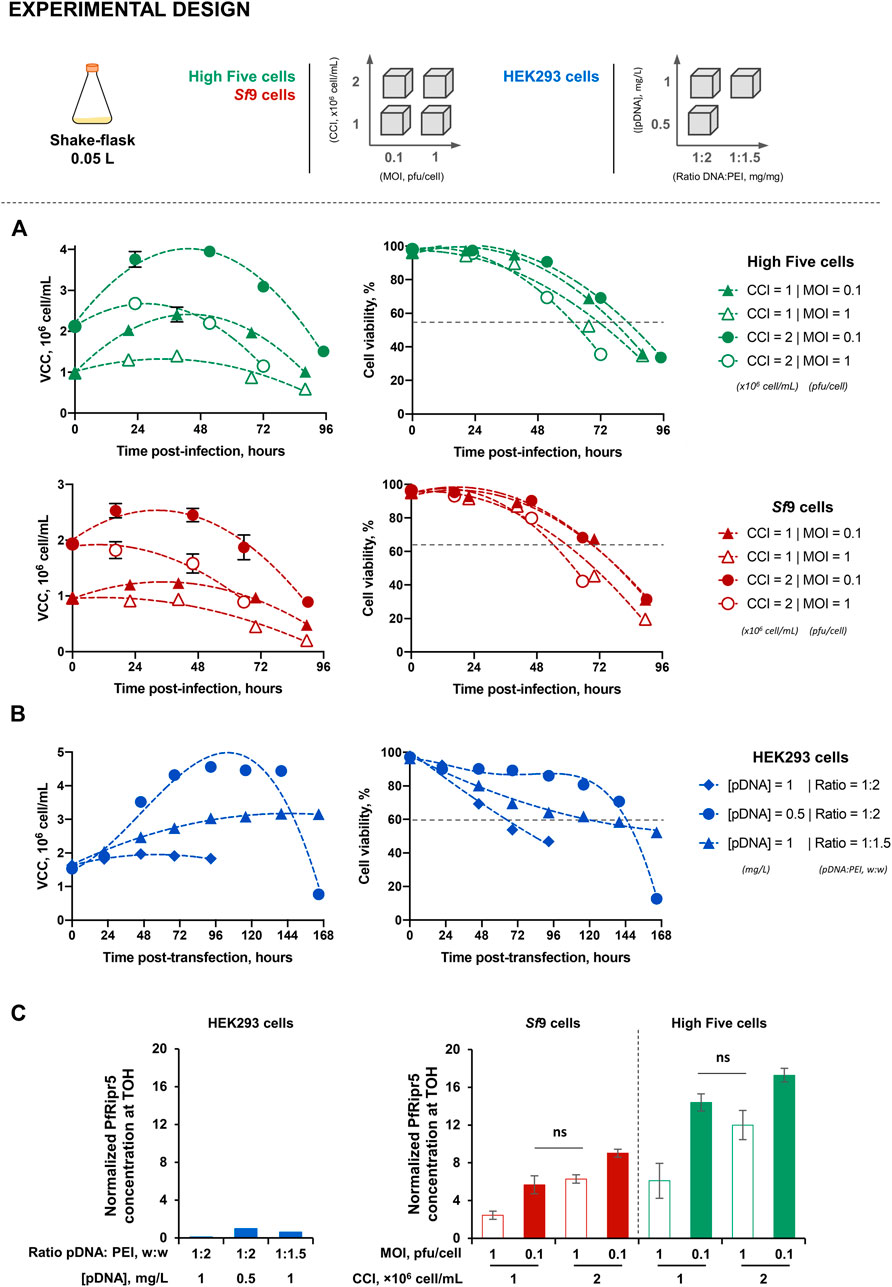
FIGURE 2. Production of the PfRipr5 recombinant protein. (A) Kinetics of cell growth and viability upon infection of insect High Five (green) and Sf9 (orange) cells at different combinations of cell concentration at infection (CCI) and multiplicity of infection (MOI). (B) Kinetics of cell growth and viability upon transfection of human HEK293 cells at different combinations of the PfRipr5 plasSmid DNA (pDNA) concentration (pDNA) and ratio pDNA:PEI. (C) Relative PfRipr5 concentration at the time-of-harvest (TOH) of each production condition. Data are expressed as mean ± standard deviation. For insect cells, data are relative to three biological replicates (n = 3). For human cells, data are relative to one biological replicate (n = 1). For figure (A,B), VCC denotes viable cell concentration. For figure (C), relative PfRipr5 expression was assessed by a densitometry analysis of western blot (Supplementary Figure S1) as described in the M&M section; graph is normalized at 1 for the best condition using human cells [i.e., (pDNA) = 0.5 mg/L and ratio pDNA:PEI = 1:2 (w:w)].
Insect and human cell-derived PfRipr5 proteins were successfully purified, all of them being identified by SDS-PAGE (Figure 2B) and western blot (Supplementary Figure S2C) analyses; nonetheless, insect cell-derived proteins presented higher purity (Table 1). Despite this difference, a similar degree of polydispersity was observed in all purified PfRipr5 proteins as assessed by dynamic light scattering (Figure 2C). Binding of purified PfRipr5 proteins to anti-PfRipr mAb 29B11, which has previously shown to hold the growth inhibition assay (GIA) activity and parasite PfRipr staining capacity (Supplementary Figures S3A–B), was assessed by ELISA and SPR as a proxy for confirming the protein´s biological activity. In ELISA, PfRipr5 produced in insect cells showed improved binding (i.e., higher absorbance at similar protein concentrations) than those produced in human cells (Figure 2D). In SPR, the KD values could not be estimated for the protein produced in human cells because of its poor fitness with the model equation (Table 1), thus corroborating its poor binding as assessed by ELISA. The KD values obtained from the PfRipr5 produced in both insect cell lines showed no statistical difference (One-way ANOVA, p = 0.3885), suggesting that they have similar functionality. Noteworthily, the production yields after purification achieved in insect cells were 4–5-fold higher than in human cells (Table 1).
Based on the aforementioned results, further production optimization was only performed in insect cells.
3.2 Optimizing PfRipr5 Production in Insect Cells: Exploring Different Baculovirus Constructs and Culture Conditions
Aiming to improve PfRipr5 production in insect cells, three different strategies were devised (Figure 3A): Strategy I—design new rBAC constructs; Strategy II—culture temperature shift at TOI; Strategy III—combine the new rBAC constructs with a culture temperature shift at TOI. The best process conditions identified before were herein used (CCI = 2 × 106 cell/mL and MOI = 0.1 pfu/cell); cultures were performed in small-scale SF. The fold-change improvement in the PfRipr5 expression is relative to non-optimized infection conditions, i.e., infection with rBAC gp67 construct at the standard culture temperature (27°C).
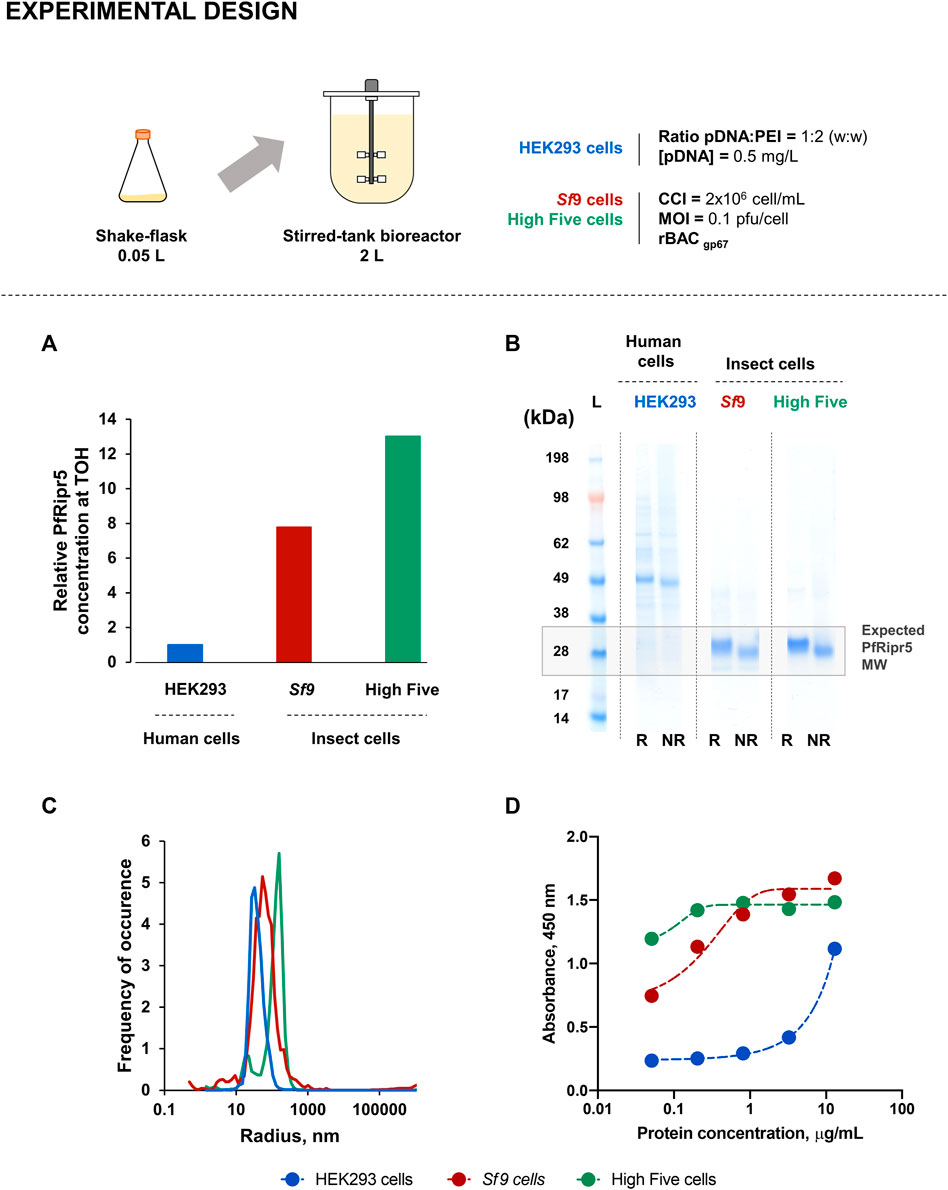
FIGURE 3. Production of PfRipr5 at 2 L stirred-tank bioreactor (STB) scale. (A) Relative PfRipr5 concentration at the TOH of each cell line. (B) SDS-PAGE of purified PfRipr5. (C) Size distribution profile of purified PfRipr5 assessed by dynamic light scattering. (D) ELISA of purified PfRipr5 using an anti-P. falciparum PfRipr mouse monoclonal antibody (mAb) 29B11. For figure (A): relative PfRipr5 expression was assessed by a densitometry analysis of western blot (Supplementary Figure S2B) as described in the M&M section. For figure (B): L denotes pre-stained protein standard SeeBlue® Plus2, R denotes reduced sample, NR denotes non-reduced sample. Data are relative to one biological replicate (n = 1).
In strategy I, rBAC constructs seem to have an impact on the PfRipr5 expression but not on the infection kinetics (Supplementary Figure S4A). Indeed, the PfRipr5 expression could be maximized using rBAC A-GGSGG (addition of the A spacer with the GGSGG linker), leading to a 2.2- and 1.6-fold improvement in the protein concentration of High Five and Sf9 cells, respectively (Figure 3B). In strategy II, lowering culture temperature at TOI reduced cell growth after infection and delayed the onset of cell viability drop ( ), and consequently TOH was set at days 4–5 post-infection; most importantly, this strategy induced a 1.7- and 1.6-fold increase in PfRipr5 concentration in High Five and Sf9 cells, respectively (Figure 3B). In strategy III, cell growth and viability kinetics mimic those observed in the previous strategy (Supplementary Figure S4C vs. Supplementary Figure S4B). Noteworthily, a synergistic effect of combining a new rBAC construct with a culture temperature shift at TOI was observed, allowing to improve the PfRipr5 expression by 3- and 4-fold in High Five and Sf9 cells, respectively (Figure 3B). Although the fold-change increase in PfRipr5 production (over non-optimized infection conditions) was higher in Sf9 cells, PfRipr5 concentration still remained superior in High Five cells. Thus, High Five cells were selected for the subsequent proof-of-concept production at a bioreactor scale.
3.3 Proof of Concept at 2 L Bioreactor Scale
PfRipr5 was produced in computer-controlled 2 L STB using the optimization strategy established previously, i.e., infection of insect High Five cells at CCI = 2 × 106 cell/mL and MOI = 0.1 pfu/cell with rBAC A-GGSGG and performing temperature shift (from 27 to 22°C) at the TOI. Purification was performed identically to previous STB runs, and the quality of the purified PfRipr5 was assessed by western blot, SDS-PAGE, DLS, ELISA, and SPR.
Cell growth and viability kinetics were identical to those previously observed in SF, allowing to harvest the culture at the expected TOH, i.e., 4 days post-infection (Figure 4A). Bands corresponding to PfRipr5 were identified by western blot both in bulk and purified materials (Figure 4B). The production yield achieved following purification was 2.55 mg/L, representing a 5.2-fold increase over the non-optimized STB production (Figure 4C; Table 1). Purity of purified PfRipr5 was >95% as assessed by SDS-PAGE, similar to that from previous STB runs performed using insect cells (Figure 4D; Table 1). Dynamic light scattering data resemble those from previous non-optimized STB runs, with the identification of an extra peak at a smaller radius corresponding to the expected size range of monomeric PfRipr5 (Figure 4E). Finally, the binding of purified PfRipr5 to anti-PfRipr mAb 29B11 was confirmed by ELISA (Figure 4F) and SPR (Table 1), with similar binding kinetics and KD values to those observed with purified PfRipr5 from previous STB runs.
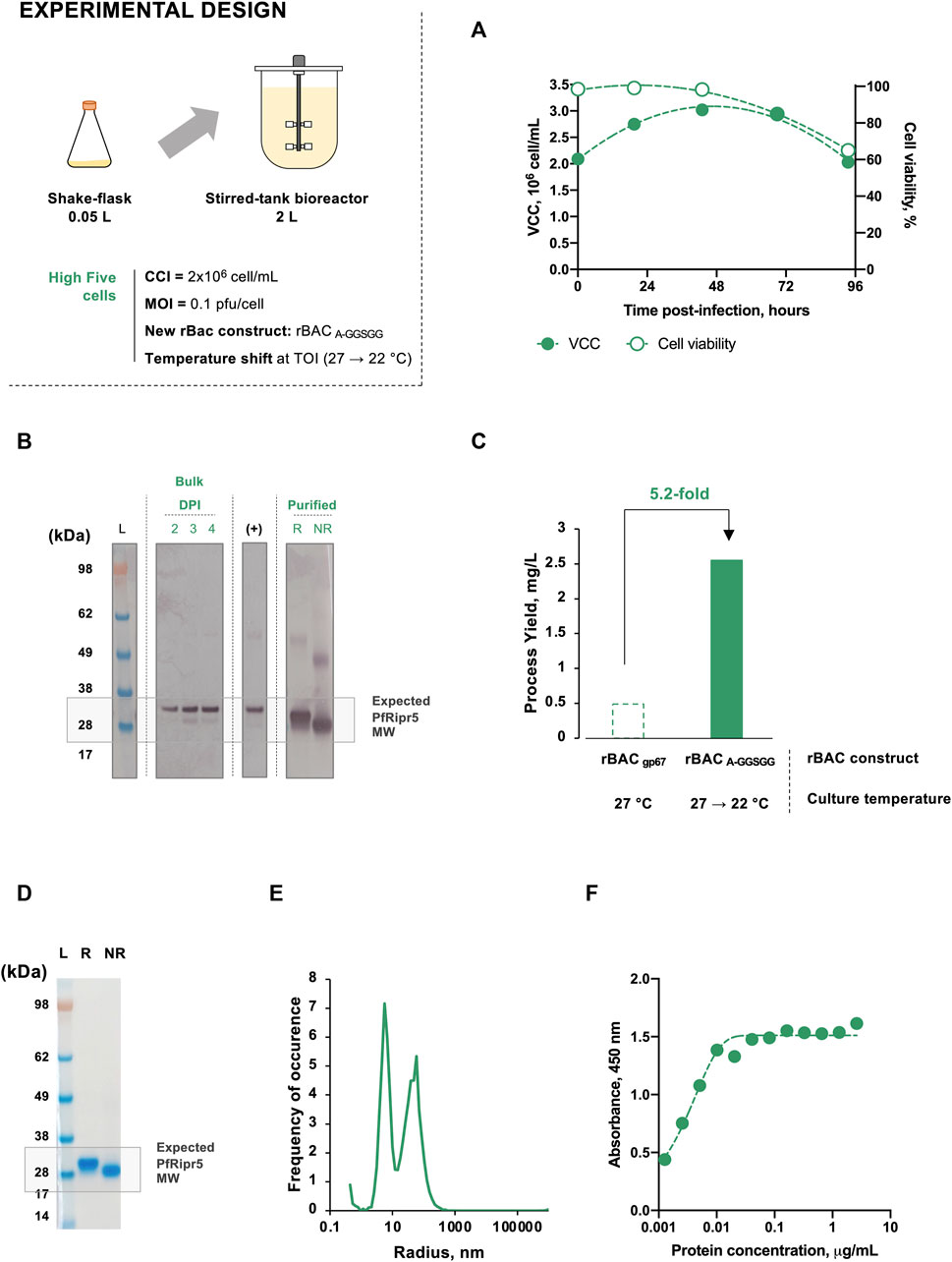
FIGURE 4. Production of PfRipr5 at 2 L STB scale using the optimized production strategy (infection of insect High Five cells with rBAC A-GGSGG and culture temperature shift from 27 to 22°C at TOI). (A) Kinetics of cell growth and viability throughout infection. (B) Western blot identification of PfRipr5 in bulk and purified samples. (C) Production yield following purification. (D) SDS-PAGE of purified PfRipr5. (E) Size distribution profile of purified PfRipr5 assessed by dynamic light scattering. (F) ELISA of purified PfRipr5 using an anti-P. falciparum PfRipr mouse mAb 29B11. For figure (A), VCC denotes viable cell concentration. For figure (B), DPI denotes day post-infection, (+) denotes the positive control (PfRipr5 produced by WGCFS). For figure (B) and figure (D), L denotes pre-stained protein standard SeeBlue® Plus2, R denotes reduced sample, and NR denotes non-reduced sample. Infection was performed using CCI = 2 × 106 cell/mL and MOI = 0.1 pfu/cell. Data are relative to one biological replicate (n = 1).
4 Discussion
In this work, we developed an optimized process for the production of PfRipr5 malaria vaccine candidate using IC-BEVS by combining new rBAC construct design with culture temperature shift (27 → 22°C) at the TOI.
Insect (High Five and Sf9) cells were identified as a better host for PfRipr5 expression than human (HEK293) cells, with High Five cells outperforming Sf9 cells as commonly seen for other recombinant proteins (Wilde et al., 2014). In addition, the expression in insect cells was highest using MOI of 0.1 virus per cell, reducing volumes being handled and wastage of commonly expensive, certified master-virus stocks in comparison to higher MOI. Purified PfRipr5 derived from insect cells presented high purity and strong binding to anti-PfRipr mAb 29B11, an antibody shown to hold GIA activity and parasite PfRipr-staining capacity and thus herein used as a proxy for confirming the protein´s biological activity. Contrastingly, HEK293 cells expressed nearly undetectable levels of PfRipr5 in the culture bulk, subsequently compromising the purification process, product purity, and ultimately its biological activity. Increase in production scale would be required to bring the human-based system close to the productivities and product quality achieved with IC-BEVS. We have also observed that in all expression systems, the percentage of PfRipr5 protein being secreted was similar (data not shown), suggesting that the lower productivity attained with HEK293 cells was a translation rather than secretion-related limitation, hence the decision of not pursuing with further strategies (e.g., using a signal peptide of mammalian origin) for improving the expression in human HEK293 cells.
Despite being reported elsewhere as capable of increasing protein secretion up to five times (Tessier et al., 1991), the use of BVM did not induce a higher PfRipr5 expression. Additional amino acid residues at the upstream positions of the SP cleavage site are commonly used as spacers; in fact, the addition of A residues at the +1 and +1/+2 positions of the SP cleavage site was shown to be essential to increase recombinant protein expression levels in human cells (Güler-Gane et al., 2016). We have explored this hypothesis in our study, adding one A residue at the +1 position of the SP cleavage site to rBAC constructs. Notably, all constructs with this modification induced a higher PfRipr5 expression. With the objective of increasing availability and/or flexibility of the purification tag (Chen et al., 2013) to aid downstream processing, linkers composed of G and S residues have been included between the PfRipr5 sequence and the His6-tag. The construct comprising both an A spacer and a GGSGG linker induced the highest PfRipr5 expression. The flexibility conferred by this longer linker may have facilitated PfRipr5 folding by reducing the interference of the purification tag in the protein’s tertiary structure during protein processing in the ER, therefore reducing the chances of impairing protein secretion. These results are in good agreement with the previous reports in which flexible G-rich linkers have been used to improve the folding and function of tagged proteins (Sabourin et al., 2007; Reddy Chichili et al., 2013).
To modulate recombinant protein production, a culture temperature shift at TOI from standard 27–22°C was performed, prolonging the infection by delaying the onset of cell viability drop and, most importantly, improving the PfRipr5 expression in both insect cell lines. Prolonging cell viability in IC-BEVS is an efficient approach to improve recombinant protein production (Steele et al., 2017). PfRipr5 is a cysteine-rich fragment of PfRipr (contains 31 cysteine residues) and thus shows high propensity to form disulfide bonds, which are part of the post-translational modifications (PTMs) that are facilitated within the secretory pathway of eukaryotic cells (Robinson and Bulleid, 2020) and are essential to maintain a tertiary structure. Strategies facilitating protein processing in the ER allowing an improved folding capacity are thus beneficial to improve protein secretion. Lowering the culture temperature in IC-BEVS can slow down the metabolic activity allowing structural protein processing (Somasundaram et al., 2016) and allow proteins to have more time for going through the ER (Donaldson et al., 1999), facilitating folding and processing of complex PTMs including disulfide bonds, and therefore may promote PfRipr5 processing and secretion.
By combining the new rBAC construct design with lowering culture temperature at TOI, a synergistic effect was observed and production yields could be improved by over 5-fold. Improved availability and/or flexibility of the purification tag as well as PfRipr5 stability and/or folding, promoted by the added spacers and linkers and by a lower culture temperature, may have facilitated the affinity (i.e., IMAC) and concentration/polishing (i.e., SEC) steps during purification while limiting protein aggregation, thus explaining the improvements observed in protein expression and quality. Importantly, the purified insect cell-derived PfRipr5 continue to show strong binding to anti-PfRipr mAb 29B11 in both ELISA and SPR analyses, thus suggesting that the biological activity was not impacted by the optimizations devised herein.
5 Conclusion
This work demonstrates the optimization of expression and purification of the asexual blood-stage malaria vaccine candidate PfRipr5 while maintaining its biological activity. To our knowledge, this is the first report of using the insect Sf9 and High Five cell lines to produce an asexual blood-stage malaria vaccine candidate based on PfRipr. These findings motivate the use of atypical culture conditions such as lower culture temperature to improve recombinant protein production using IC-BEVS and highlight the importance of optimizing the expression vector when producing secreted proteins.
Data Availability Statement
The raw data supporting the conclusion of this article will be made available by the authors, without undue reservation.
Author Contributions
Conceptualization: RC and AR. Investigation: RC, BF, RCa, HN, ET, and AF. Data curation: RC, BF, and HN. Formal Analysis: RC. Validation: AR. Supervision: AR, TT, NV, HD, and PA. Writing—original draft: RC. Writing—review and editing: RC, BF, RCa, HN, ET, TT, AF, NV, HD, PA, and AR.
Funding
iNOVA4Health–UIDB/04462/2020 and UIDP/04462/2020, a program financially supported by Fundação para a Ciência e Tecnologia (FCT)/Ministério da Ciência, Tecnologia e Ensino Superior, through national funds is acknowledged. Funding from INTERFACE Programme, through the Innovation, Technology, and Circular Economy Fund (FITEC), is gratefully acknowledged. This work was supported by FCT through the initiatives “Investigador FCT” Program (No. IF/01704/2014), Exploratory Research and Development Project (No. IF/01704/2014/CP1229/CT0001), and PhD fellowships (RC–SFRH/BD/134107/2017, BF–FRH/BD/138937/2018), and by the Japan-based Global Health Innovative Technology (GHIT) Fund grant ID: T2018-151. This work was also supported in-part by JSPS KAKENHI (Nos. JP18H02651, JP20H03481, JP21H02724, JP21K06990, and JP21KK0138) and research fund from Sumitomo Pharma Co., Ltd.
Conflict of Interest
AF was employed by Sumitomo Pharma Co., Ltd. and TT and ET are supported by a research fund from Sumitomo Dainippon Pharma Co., Ltd. AF, TT, ET, and HN are inventors on patent PCT/JP 2017/040532 on a malaria vaccine antigen, PfRipr5. These involvements did not influence the design of the study, the collection, analysis, access to, and interpretation of data, or the writing of the manuscript.
The remaining authors declare that the research was conducted in the absence of any commercial or financial relationships that could be construed as a potential conflict of interest.
Publisher’s Note
All claims expressed in this article are solely those of the authors and do not necessarily represent those of their affiliated organizations, or those of the publisher, the editors, and the reviewers. Any product that may be evaluated in this article, or claim that may be made by its manufacturer, is not guaranteed or endorsed by the publisher.
Acknowledgments
The authors wish to thank Rute Eleutério for the support in purification, Tiago Bandeiras from the Merck Satellite Lab at IBET for the support in DLS, and Júlia Costa from the Glycobiology Lab at ITQB-NOVA for the support in strategies to enhance protein secretion.
Supplementary Material
The Supplementary Material for this article can be found online at: https://www.frontiersin.org/articles/10.3389/fbioe.2022.908509/full#supplementary-material
References
Chen, X., Zaro, J. L., and Shen, W.-C. (2013). Fusion Protein Linkers: Property, Design and Functionality. Adv. Drug Deliv. Rev. 65 (10), 1357–1369. doi:10.1016/j.addr.2012.09.039
Cid, R., and Bolívar, J. (2021). Platforms for Production of Protein-Based Vaccines: From Classical to Next-Generation Strategies. Biomolecules 11 (8), 1072. doi:10.3390/biom11081072
Correia, R., Fernandes, B., Alves, P. M., Carrondo, M. J. T., and Roldão, A. (2020). Improving Influenza HA-Vlps Production in Insect High Five Cells via Adaptive Laboratory Evolution. Vaccines 8 (4), 589. doi:10.3390/vaccines8040589
Crosnier, C., Wanaguru, M., McDade, B., Osier, F. H., Marsh, K., Rayner, J. C., et al. (2013). A Library of Functional Recombinant Cell-Surface and Secreted P. Falciparum Merozoite Proteins. Mol. Cell. Proteomics 12 (12), 3976–3986. doi:10.1074/mcp.o113.028357
Datoo, M. S., Natama, M. H., Somé, A., Traoré, O., Rouamba, T., Bellamy, D., et al. (2021). Efficacy of a Low-Dose Candidate Malaria Vaccine, R21 in Adjuvant Matrix-M, with Seasonal Administration to Children in Burkina Faso: a Randomised Controlled Trial. Lancet 397 (10287), 1809–1818. doi:10.1016/s0140-6736(21)00943-0
Dick, O., Onken, U., Sattler, I., and Zeeck, A. (1994). Influence of Increased Dissolved Oxygen Concentration on Productivity and Selectivity in Cultures of a Colabomycin-Producing Strain ofStreptomyces Griseoflavus. Appl. Microbiol. Biotechnol. 41 (4), 373–377. doi:10.1007/bf00939022
Donaldson, M., Wood, H. A., Kulakosky, P. C., and Shuler, M. L. (1999). Glycosylation of a Recombinant Protein in the Tn5B1-4 Insect Cell Line: Influence of Ammonia, Time of Harvest, Temperature, and Dissolved Oxygen. Biotechnol. Bioeng. 63 (3), 255–262. doi:10.1002/(sici)1097-0290(19990505)63:3<255::aid-bit1>3.0.co;2-r
Dumont, J., Euwart, D., Mei, B., Estes, S., and Kshirsagar, R. (2016). Human Cell Lines for Biopharmaceutical Manufacturing: History, Status, and Future Perspectives. Crit. Rev. Biotechnol. 36 (6), 1110–1122. doi:10.3109/07388551.2015.1084266
Durocher, Y., Perret, S., and Kamen, A. (2002). High-level and High-Throughput Recombinant Protein Production by Transient Transfection of Suspension-Growing Human 293-EBNA1 Cells. Nucleic Acids Res. 30 (2), E9. doi:10.1093/nar/30.2.e9
Fernandes, B., Vidigal, J., Correia, R., Carrondo, M. J. T., Alves, P. M., Teixeira, A. P., et al. (2020). Adaptive Laboratory Evolution of Stable Insect Cell Lines for Improved HIV-Gag VLPs Production. J. Biotechnol. 307, 139–147. doi:10.1016/j.jbiotec.2019.10.004
Futatsumori-Sugai, M., and Tsumoto, K. (2010). Signal Peptide Design for Improving Recombinant Protein Secretion in the Baculovirus Expression Vector System. Biochem. Biophysical Res. Commun. 391 (1), 931–935. doi:10.1016/j.bbrc.2009.11.167
Güler-Gane, G., Kidd, S., Sridharan, S., Vaughan, T. J., Wilkinson, T. C. I., and Tigue, N. J. (2016). Overcoming the Refractory Expression of Secreted Recombinant Proteins in Mammalian Cells through Modification of the Signal Peptide and Adjacent Amino Acids. PloS One 11 (5), e0155340. doi:10.1371/journal.pone.0155340
Hjerrild, K. A., Jin, J., Wright, K. E., Brown, R. E., Marshall, J. M., Labbé, G. M., et al. (2016). Production of Full-Length Soluble Plasmodium Falciparum RH5 Protein Vaccine Using a Drosophila melanogaster Schneider 2 Stable Cell Line System. Sci. Rep. 6 (1), 30357. doi:10.1038/srep30357
Jones, R. M., Chichester, J. A., Mett, V., Jaje, J., Tottey, S., Manceva, S., et al. (2013). A Plant-Produced Pfs25 VLP Malaria Vaccine Candidate Induces Persistent Transmission Blocking Antibodies against Plasmodium Falciparum in Immunized Mice. PloS One 8 (11), e79538. doi:10.1371/journal.pone.0079538
Lee, D.-H., and Palsson, B. Ø. (2010). Adaptive Evolution of Escherichia coli K-12 MG1655 during Growth on a Nonnative Carbon Source, L -1,2-Propanediol. Appl. Environ. Microbiol. 76 (13), 4158–4168. doi:10.1128/aem.00373-10
Mena, J. A., and Kamen, A. A. (2011). Insect Cell Technology Is a Versatile and Robust Vaccine Manufacturing Platform. Expert Rev. Vaccines 10 (7), 1063–1081. doi:10.1586/erv.11.24
Mena, J. A., Ramírez, O. T., and Palomares, L. A. (2003). Titration of Non-occluded Baculovirus Using a Cell Viability Assay. BioTechniques 34 (2), 260–264. doi:10.2144/03342bm05
Minassian, A. M., Silk, S. E., Barrett, J. R., Nielsen, C. M., Miura, K., Diouf, A., et al. (2021). Reduced Blood-Stage Malaria Growth and Immune Correlates in Humans Following RH5 Vaccination. Med 2 (6), 701–719. e19. doi:10.1016/j.medj.2021.03.014
Nagaoka, H., Kanoi, B. N., Ntege, E. H., Aoki, M., Fukushima, A., Tsuboi, T., et al. (2020). Antibodies against a Short Region of PfRipr Inhibit Plasmodium Falciparum Merozoite Invasion and PfRipr Interaction with Rh5 and SEMA7A. Sci. Rep. 10 (1), 6573. doi:10.1038/s41598-020-63611-6
Ntege, E. H., Arisue, N., Ito, D., Hasegawa, T., Palacpac, N. M. Q., Egwang, T. G., et al. (2016). Identification of Plasmodium Falciparum Reticulocyte Binding Protein Homologue 5-interacting Protein, PfRipr, as a Highly Conserved Blood-Stage Malaria Vaccine Candidate. Vaccine 34 (46), 5612–5622. doi:10.1016/j.vaccine.2016.09.028
Ohmuro-Matsuyama, Y., and Yamaji, H. (2018). Modifications of a Signal Sequence for Antibody Secretion from Insect Cells. Cytotechnology 70 (3), 891–898. doi:10.1007/s10616-017-0109-0
Olczak, M., and Olczak, T. (2006). Comparison of Different Signal Peptides for Protein Secretion in Nonlytic Insect Cell System. Anal. Biochem. 359 (1), 45–53. doi:10.1016/j.ab.2006.09.003
Patel, S. D., Ahouidi, A. D., Bei, A. K., Dieye, T. N., Mboup, S., Harrison, S. C., et al. (2013). Plasmodium Falciparum Merozoite Surface Antigen, PfRH5, Elicits Detectable Levels of Invasion-Inhibiting Antibodies in Humans. J. Infect. Dis. 208 (10), 1679–1687. doi:10.1093/infdis/jit385
Paul, K., Rajamanickam, V., and Herwig, C. (2019). Model-based Optimization of Temperature and pH Shift to Increase Volumetric Productivity of a Chinese Hamster Ovary Fed-Batch Process. J. Biosci. Bioeng. 128 (6), 710–715. doi:10.1016/j.jbiosc.2019.06.004
Ragotte, R. J., Higgins, M. K., and Draper, S. J. (2020). The RH5-CyRPA-Ripr Complex as a Malaria Vaccine Target. Trends Parasitol. 36 (6), 545–559. doi:10.1016/j.pt.2020.04.003
Reddy Chichili, V. P., Kumar, V., and Sivaraman, J. (2013). Linkers in the Structural Biology of Protein-Protein Interactions. Protein Sci. 22 (2), 153–167. doi:10.1002/pro.2206
Reuveny, S., Kim, Y. J., Kemp, C. W., and Shiloach, J. (1993). Effect of Temperature and Oxygen on Cell Growth and Recombinant Protein Production in Insect Cell Cultures. Appl. Microbiol. Biotechnol. 38 (5), 619–623. [Internet] Available from: http://link.springer.com/10.1007/BF00182800 (cited Aug 1, 2019). doi:10.1007/bf00182800
Robinson, P. J., and Bulleid, N. J. (2020). Mechanisms of Disulfide Bond Formation in Nascent Polypeptides Entering the Secretory Pathway. Cells 9 (9), 1994. doi:10.3390/cells9091994
Roldão, A., Oliveira, R., Carrondo, M. J. T., and Alves, P. M. (2009). Error Assessment in Recombinant Baculovirus Titration: Evaluation of Different Methods. J. Virological Methods 159 (1), 69–80. doi:10.1016/j.jviromet.2009.03.007
Rossi, N., Silva, B. G., Astray, R., Swiech, K., Pereira, C. A., and Suazo, C. A. T. (2012). Effect of Hypothermic Temperatures on Production of Rabies Virus Glycoprotein by Recombinant Drosophila melanogaster S2 Cells Cultured in Suspension. J. Biotechnol. 161 (3), 328–335. doi:10.1016/j.jbiotec.2012.05.016
Sabourin, M., Tuzon, C. T., Fisher, T. S., and Zakian, V. A. (2007). A Flexible Protein Linker Improves the Function of Epitope-Tagged Proteins inSaccharomyces Cerevisiae. Yeast 24 (1), 39–45. doi:10.1002/yea.1431
Schindelin, J., Arganda-Carreras, I., Frise, E., Kaynig, V., Longair, M., Pietzsch, T., et al. (2012). Fiji: an Open-Source Platform for Biological-Image Analysis. Nat. Methods 9 (7), 676–682. doi:10.1038/nmeth.2019
Shao-Hua, C., Hong-Liang, S., and Zuo-Hu, L. (1998). Effect of Temperature Oscillation on Insect Cell Growth and Baculovirus Replication. Appl. Environ. Microbiol. 64 (6), 2237–2239. doi:10.1128/AEM.64.6.2237-2239.1998
Soejima, Y., Lee, J., Nagata, Y., Mon, H., Iiyama, K., Kitano, H., et al. (2013). Comparison of Signal Peptides for Efficient Protein Secretion in the Baculovirus-Silkworm System. Open Life Sci. 8 (1), 1–7. doi:10.2478/s11535-012-0112-6
Somasundaram, B., Chang, C., Fan, Y. Y., Lim, P.-Y., Cardosa, J., and Lua, L. (2016). Characterizing Enterovirus 71 and Coxsackievirus A16 Virus-like Particles Production in Insect Cells. Methods 95, 38–45. doi:10.1016/j.ymeth.2015.09.023
Steele, K. H., Stone, B. J., Franklin, K. M., Fath-Goodin, A., Zhang, X., Jiang, H., et al. (2017). Improving the Baculovirus Expression Vector System with Vankyrin-Enhanced Technology. Biotechnol. Prog. 33 (6), 1496–1507. doi:10.1002/btpr.2516
Takala, S. L., Coulibaly, D., Thera, M. A., Batchelor, A. H., Cummings, M. P., Escalante, A. A., et al. (2009). Extreme Polymorphism in a Vaccine Antigen and Risk of Clinical Malaria: Implications for Vaccine Development. Sci. Transl. Med. 1 (2), 2ra5. doi:10.1126/scitranslmed.3000257
Tang, Y.-J., Zhang, W., and Zhong, J.-J. (2009). Performance Analyses of a pH-Shift and DOT-Shift Integrated Fed-Batch Fermentation Process for the Production of Ganoderic Acid and Ganoderma Polysaccharides by Medicinal Mushroom Ganoderma Lucidum. Bioresour. Technol. 100 (5), 1852–1859. doi:10.1016/j.biortech.2008.10.005
Tessier, D. C., Thomas, D. Y., Khouri, H. E., Laliberié, F., and Vernet, T. (1991). Enhanced Secretion from Insect Cells of a Foreign Protein Fused to the Honeybee Melittin Signal Peptide. Gene 98 (2), 177–183. doi:10.1016/0378-1119(91)90171-7
Tsuboi, T., Takeo, S., Sawasaki, T., Torii, M., and Endo, Y. (2010). “An Efficient Approach to the Production of Vaccines against the Malaria Parasite,” in Cell-Free Protein Production [Internet]. Editors Y Endo, K Takai, and T Ueda (Totowa, NJ: Humana Press), 607, 73–83. Methods in Molecular Biology. Available from: http://link.springer.com/10.1007/978-1-60327-331-2_8 (cited Sep 27, 2021). doi:10.1007/978-1-60327-331-2_8
Vergara, M., Becerra, S., Berrios, J., Osses, N., Reyes, J., Rodríguez-Moyá, M., et al. (2014). Differential Effect of Culture Temperature and Specific Growth Rate on CHO Cell Behavior in Chemostat Culture. PLoS One., 9 (4), e93865. doi:10.1371/journal.pone.0093865
Vergara, M., Torres, M., Müller, A., Avello, V., Acevedo, C., Berrios, J., et al. (2018). High Glucose and Low Specific Cell Growth but Not Mild Hypothermia Improve Specific R-Protein Productivity in Chemostat Culture of CHO Cells. PLos One. 13 (8), e0202098. doi:10.1371/journal.pone.0202098
Vieira, H. L. A., Estêvão, C., Roldão, A., Peixoto, C. C., Sousa, M. F. Q., Cruz, P. E., et al. (2005). Triple Layered Rotavirus VLP Production: Kinetics of Vector Replication, mRNA Stability and Recombinant Protein Production. J. Biotechnol. 120 (1), 72–82. doi:10.1016/j.jbiotec.2005.03.026
Vogel, G. (2021). WHO Gives First Malaria Vaccine the Green Light. Science 374 (6565), 245–246. doi:10.1126/science.acx9344
Volz, J. C., Yap, A., Sisquella, X., Thompson, J. K., Lim, N. T. Y., Whitehead, L. W., et al. (2016). Essential Role of the PfRh5/PfRipr/CyRPA Complex during Plasmodium Falciparum Invasion of Erythrocytes. Cell. Host Microbe 20 (1), 60–71. doi:10.1016/j.chom.2016.06.004
von Heijne, G. (1986). A New Method for Predicting Signal Sequence Cleavage Sites. Nucl. Acids Res. 14 (11), 4683–4690. doi:10.1093/nar/14.11.4683
Wang, Y., Fang, X., Cheng, Y., and Zhang, X. (2011). Manipulation of pH Shift to Enhance the Growth and Antibiotic Activity ofXenorhabdus Nematophila. J. Biomed. Biotechnol. 2011, 1–9. doi:10.1155/2011/672369
Wilde, M., Klausberger, M., Palmberger, D., Ernst, W., and Grabherr, R. (2014). Tnao38, High Five and Sf9-Evaluation of Host-Virus Interactions in Three Different Insect Cell Lines: Baculovirus Production and Recombinant Protein Expression. Biotechnol. Lett. 36 (4), 743–749. doi:10.1007/s10529-013-1429-6
Wong, W., Huang, R., Menant, S., Hong, C., Sandow, J. J., Birkinshaw, R. W., et al. (2019). Structure of Plasmodium Falciparum Rh5-CyRPA-Ripr Invasion Complex. Nature 565 (7737), 118–121. doi:10.1038/s41586-018-0779-6
Wright, G. J., and Rayner, J. C. (2014). Plasmodium Falciparum Erythrocyte Invasion: Combining Function with Immune Evasion. PLoS Pathog. 10 (3), e1003943. doi:10.1371/journal.ppat.1003943
Keywords: malaria asexual blood-stage vaccine, PfRipr5, insect cells, baculovirus expression vector system, low temperature, improved production, vector design
Citation: Correia R, Fernandes B, Castro R, Nagaoka H, Takashima E, Tsuboi T, Fukushima A, Viebig NK, Depraetere H, Alves PM and Roldão A (2022) Asexual Blood-Stage Malaria Vaccine Candidate PfRipr5: Enhanced Production in Insect Cells. Front. Bioeng. Biotechnol. 10:908509. doi: 10.3389/fbioe.2022.908509
Received: 30 March 2022; Accepted: 09 May 2022;
Published: 30 June 2022.
Edited by:
Cecília Calado, Lisbon Higher Institute of Engineering (ISEL), PortugalReviewed by:
Jian Xu, East China Normal University, ChinaKisung Ko, Chung-Ang University, South Korea
Xiaodong Xu, Northwest A & F University, China
Copyright © 2022 Correia, Fernandes, Castro, Nagaoka, Takashima, Tsuboi, Fukushima, Viebig, Depraetere, Alves and Roldão. This is an open-access article distributed under the terms of the Creative Commons Attribution License (CC BY). The use, distribution or reproduction in other forums is permitted, provided the original author(s) and the copyright owner(s) are credited and that the original publication in this journal is cited, in accordance with accepted academic practice. No use, distribution or reproduction is permitted which does not comply with these terms.
*Correspondence: António Roldão, YXJvbGRhb0BpYmV0LnB0