- 1Center for Rehabilitation Medicine, Department of Orthopedics, Zhejiang Provincial People’s Hospital (Affiliated People’s Hospital, Hangzhou Medical College), Hangzhou, China
- 2Department of Orthopedics, The Second Affiliated Hospital and Yuying Children’s Hospital of Wenzhou Medical University, Wenzhou, China
- 3Zhejiang University of Technology, Hangzhou, China
- 4Center for Operating Room, Department of Nursing, Zhejiang Provincial People’s Hospital (Affiliated People’s Hospital, Hangzhou Medical College), Hangzhou, China
The rapid development of tissue engineering makes it an effective strategy for repairing cartilage defects. The significant advantages of injectable hydrogels for cartilage injury include the properties of natural extracellular matrix (ECM), good biocompatibility, and strong plasticity to adapt to irregular cartilage defect surfaces. These inherent properties make injectable hydrogels a promising tool for cartilage tissue engineering. This paper reviews the research progress on advanced injectable hydrogels. The cross-linking method and structure of injectable hydrogels are thoroughly discussed. Furthermore, polymers, cells, and stimulators commonly used in the preparation of injectable hydrogels are thoroughly reviewed. Finally, we summarize the research progress of the latest advanced hydrogels for cartilage repair and the future challenges for injectable hydrogels.
1 Introduction
Osteoarthritis (OA) is the most common chronic disease of joints, affecting approximately 90 million adults in the United States alone (approximately 37% of the adult population) and hundreds of millions worldwide (Krishnan and Grodzinsky, 2018; Luo et al., 2021). It is characterized by degeneration and defect of articular cartilage, which can cause joint pain, reduced mobility, and stiffness (Das and Farooqi, 2008). Unlike most other organizations, cartilage is a type of special connective tissue without blood vessels, nerves, and lymph nodes, characterized by immersing chondrocytes in ECM, which consists mainly of a matrix (polysaccharides), fibrous components (fibrin), and interstitial fluid (mainly water) (Armiento et al., 2019; Sirong et al., 2020). Therefore, cartilage cannot repair itself due to insufficient nutritional support and proper progenitor cell differentiation. When cartilage defects go untreated, joints inevitably deteriorate, leading to OA and disability (Simon and Jackson, 2006; Gao et al., 2014).
Non-surgical conservative treatment and drug (painkillers and NSAIDs) therapy can effectively relieve pain in the early stages of articular cartilage lesion but cannot reverse cartilage degeneration defect (Poddar and Widstrom, 2017). Transplantation (using allogeneic or autologous cells or tissues) and stimulation (stimulating self-repair of articular cartilage) are commonly used in the late treatment of OA (Tuan et al., 2013). The former includes allograft or autologous cartilage transplantation, perichondrium and periosteum transplantation, osteochondral transplantation, ACI, and other graft repair techniques. The latter include joint cleansing and debridement, cartilage grinding and shaping, microfractures, drilling, osteotomy, and joint traction (Fuggle et al., 2020). For patients with severe OA, severe invasive total joint replacement surgery is generally considered the last resort (Katz et al., 2021).
Hydrogels are widely used in tissue engineering and are advanced cross-linked 3D hydrophilic polymer network biomaterials because of their unique properties such as high-water content, biocompatibility, porosity and biodegradation, solid elastic properties, deformability, and softness (Yang et al., 2017; Fu et al., 2018; Liu et al., 2021a). The hydrogel properties are similar to the characteristics of natural cartilage ECM and are easy to prepare. Hydrogel development is the most promising method for treating cartilage defects and cartilage regeneration (Wei et al., 2021; Huang et al., 2022). Injectable hydrogels have attracted the attention of biomaterial scientists in cartilage tissue engineering (Schaeffer et al., 2020; Wang et al., 2021a) (Figure 1). Because it can replace open implants with minimally invasive injections, it has the advantages of being less invasive, fewer complications, shorter hospital stays, and forming any desired shape in situ to match irregular defects (Liu et al., 2017a; Pascual-Garrido et al., 2018; Lin et al., 2021a). Injectable hydrogels provide hydration similar to the height of cartilage ECM. Biocompatibility and biodegradability of 3D structure and elastic properties can be controlled by improving cell metabolites and the supply of nutrients. The stimulus-response release mechanism can encapsulate cells and deliver efficient and effective bioactive molecules to the target of cartilage regeneration (Park et al., 2009; Pereira et al., 2009; Li et al., 2019). An ideal injectable hydrogel has several requirements, such as no toxic byproducts during in vivo gelation, appropriate solubility and gelation under physiological conditions, and a controlled gelation rate suitable for clinical practice (Jeznach et al., 2018).
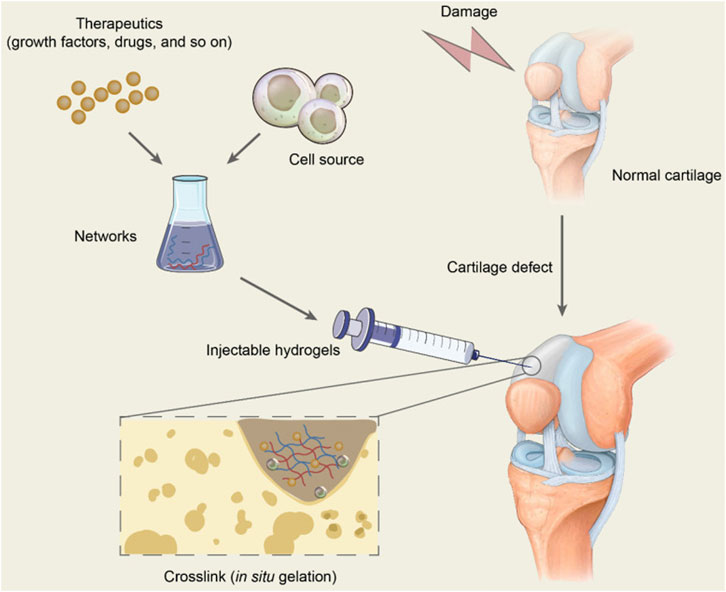
FIGURE 1. Schematic diagram of hydrogel injection in repairing cartilage defect. Adapted with permission of Wu et al. (2020).
This review aims to clarify the application of advanced injectable hydrogels in cartilage repair and regeneration. The progress and advantages of injectable hydrogels in cartilage repair and regeneration are reviewed, including the manufacturing technology (crosslinking method and structure) and suitable materials (polymers, cells and stimulators). Then, we summarize the research progress of the latest advanced injectable hydrogels in cartilage tissue engineering. Finally, the challenges in applying injectable hydrogels and their prospects in tissue engineering are also discussed.
1.1 Formation of injectable hydrogels
Gelation is a crucial step in the formation of an injectable hydrogel. According to the design structure and standard application, it is imperative to select the appropriate formation method to prepare injectable hydrogels (Li et al., 2012). There are several ways of preparing injectable hydrogels based on their reactivity or the connections they contain. The cross-linking mechanism of the hydrogel can be divided into chemical cross-linking and physical cross-linking (Liu et al., 2020) (Figure 2). One of the distinctions between them is whether or not covalent bonds are formed (Wu et al., 2020).
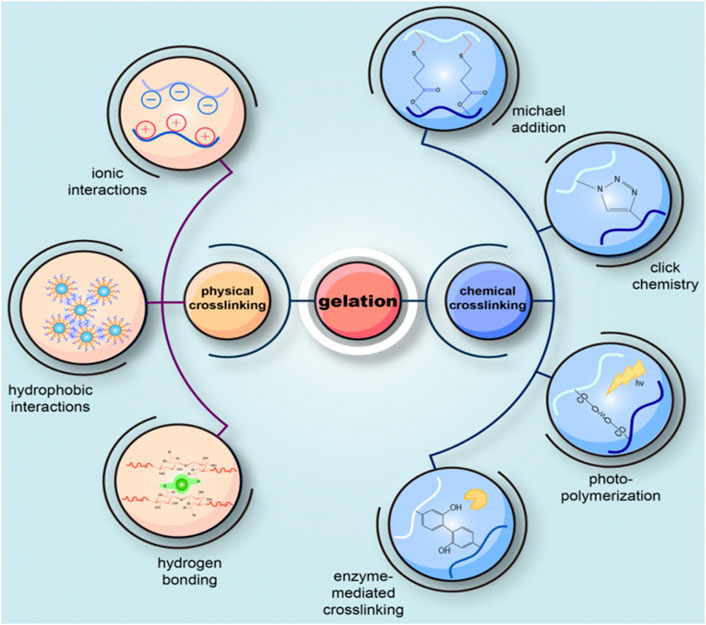
FIGURE 2. Schematic diagram of common methods for preparing injectable hydrogels. Injectable hydrogels can be roughly divided into two gel methods: physical cross-linking and chemical cross-linking reactions. The difference between them is whether or not covalent bonds are formed. Physical cross-linking is non-covalent bonding via reversible and instantaneous connections, including physicochemical or molecular entanglement interactions (hydrogen bonding, ionic or hydrophobic interactions). Chemical cross-linking forms covalent bonds in various chemical processes, including enzyme-mediated cross-linking, photopolymerization, click chemistry, Michael’s addition, Schiff base chemistry, and cross-linking agents. Adapted with permission of Wu et al. 2(2020).
1.2 Physical cross-linking
Hydrogels can be cross-linked via reversible networks or physical cross-links through physicochemical or molecular entanglement interactions, such as hydrophobic interactions, hydrogen bonds, ionic interaction, supramolecular chemistry, crystal formation, or charge condensation (Lu et al., 2018; Niemczyk et al., 2018). The mutual effects that occur in this hydrogel are fragile. However, they are numerous and lead to complex behaviors (Lynch et al., 2017; Bustamante-Torres et al., 2021; Muir and Burdick, 2021). Some injectable hydrogels by physical cross-linking are described below.
1.2.1 Hydrophobic interactions
Hydrophobic interactions (also known as hydrophobic bonding) play a significant role in the self-healing course of soft materials (Tu et al., 2019). This interaction is stronger than the van der Waals and hydrogen bond interaction. Hydrophilic and hydrophobic parts are usual in molecules that form gels through hydrophobic interactions. Hydrophobic interactions are constituted between non-polar parts to reduce their contact with water (Skopinska-Wisniewska et al., 2021).
1.2.2 Hydrogen bonding cross-linking
Hydrogen bonds can form cross-linking networks between hydrogen and electronegative atoms (He et al., 2019). Supramolecular hydrogels enhanced by multiple hydrogen bonds have good self-recovery, toughness, and recoverability as a driving force (Yu et al., 2021a).
1.2.3 Ionic interaction
The cross-linked hydrogel structure is formed when molecules with opposite electric charges interact electrostatically (Abdulghani and Morouço, 2019). Ion interactions have been widely used to physically cross-link natural polysaccharides, such as chitosan and alginate, to prepare hydrogels (Huang et al., 2017). Alginate can gelate in the presence of polyvalent cations such as Sr2+, Ca2+, Fe2+, Co2+, and Ba2+, which is related to cation binding through G blocks of alginate and the formation of so-called “egg boxes” (Lee and Mooney, 2012; Abasalizadeh et al., 2020). CaCl2 is the most commonly used ion cross-linking agent in alginate hydrogel. Due to the high solubility of CaCl2 in aqueous media, alginate gelation rates are too fast to control. In addition, reduced gel rate results in greater mechanical integrity and a more uniform structure. CaCO3 and CaSO4 can be used instead to slow down the gelling speed. In addition, a buffer containing phosphate (such as sodium hexametaphosphate) can be used because the phosphate group in the buffer competes with the carboxylic group of alginate in the reaction with calcium ions, thereby reducing gelation (Abasalizadeh et al., 2020; Piras and Smith, 2020; Hu et al., 2021a). Cai et al. (2021). successfully prepared an injectable hydrogel by in situ cross-linking sodium alginate with divalent cations released from strontium-doped bioglass. The hydrogel’s biocompatibility and mechanical properties promoted BMSC proliferation, cartilage-specific gene expression, and glycosaminoglycan secretion.
1.2.4 Supramolecular chemistry
Supramolecular chemistry hydrogels have been widely used in tissue engineering, bioelectronics, and drug delivery. It has good biocompatibility and biodegradation and contains many cell adhesion sites (Kim et al., 2011). As a key sense in supramolecular chemistry, self-assembly is mainly based on non-covalent interactions (hydrophobic/hydrophilic interactions, hydrogen bonding, van der Waals interactions, π-π stacking, and host-guest complexation) between molecules (Antoniuk and Amiel, 2016; Wang et al., 2020a). The substrate of supramolecular hydrogels, a basic molecular process, is usually non-covalent, structural, three-dimensional, responsive, dynamic, adaptive, and organized. Such molecular processes can easily interact with, interfere with, and even simulate cellular events in various biological systems (Zhou et al., 2017). Supramolecular interactions can promote physical cross-linking to form hydrogels in two ways. The first method is commonly used to create supramolecular materials, molecular gels made of small molecules with high aspect ratios, such as peptides. Once assembled, supramolecular stacks of small particles constitute long, typically rigid fibers. The second approach is that the interactions act as connections between polymer chains, including motifs based on host-guest complexation, metal-ligand ligands, and biomolecular binding (Mantooth et al., 2019; Zhou et al., 2020). Cyclodextrin-mediated host-guest interaction is an effective material for hydrogel construction, mainly because of its bioavailability, ease of chemical modification, and high reversibility and specificity of inclusion complexes composed of many hydrophobic guest molecules (Antoniuk and Amiel, 2016).
1.3 Chemical cross-linking
The convergence of years of research has led to the development of mild chemistry that can be set at temperature, physiology, osmotic pressure, and pH while avoiding using toxic reagents. Chemical cross-linking depends on the formation of covalent bonds between the reactive groups grafted to the main chain of the polymerization, and it can occur under certain conditions (Flégeau et al., 2017). These conditions include click chemistry, Michael addition, disulfide cross-linking, enzyme-mediated cross-linking, silanization, Schiff base chemistry, photopolymerization, and cross-linking agents (Radhakrishnan et al., 2014; Piantanida et al., 2019; Nguyen et al., 2021)
1.3.1 Click chemistry
Click chemistry includes copper-catalyzed alkyne-azide cycloaddition, copper-free click (strain-promoted azylene cycloaddition click, Diels-Alder click chemistry, oxime, mercaptan, and mercaptan alkyne), and pseudo click (Gopinathan and Noh, 2018; Li et al., 2021a). Click chemistry is widely used in constructing injectable hydrogels due to its mild reaction conditions, high chemical selectivity, and fast gelation time, without adding or producing cytotoxic cross-linking agents, chemical additives, and byproducts in the gelation process (Yao et al., 2020).
1.3.2 Michael addition
Michael addition reaction hydrogels are prepared by adding polymers containing thiol groups to α, β-unsaturated carbonyl polymers under standard conditions (Quadrado et al., 2021). PEG-based hydrogels based on the Michael addition reaction have been widely used in tissue engineering (Guo et al., 2021). Pupkaite et al. (Pupkaite et al., 2019) tried to overcome the shortcomings of partially injectable hydrogels, such as complex, overexpanding, potentially toxic cross-linking processes, or lack of self-healing and shear thinning. Mercaptan groups were introduced into collagen. The hydrogel was prepared by cross-linking with 8-arm polyethylene glycol maleimide. The hydrogel is cytocompatible and can be used to encapsulate and deliver cells.
1.3.3 Enzyme-mediated cross-linking
Enzyme cross-linking reactions are mild. Most enzymes catalyze reactions in water environments at neutral pH and moderate temperatures. This means that they can also be used to form hydrogels in situ, avoiding the loss of biological activity caused by natural polymers that cannot withstand the harsh chemical conditions required for crosslinking (Teixeira et al., 2012). Several enzyme-mediated injectable hydrogels are used for cartilage defect repairs, such as tyrosinase, lysyl oxidase, and transferase enzyme systems (Liu and Lin, 2019; Wang and Wang, 2021). HRP and H2O2 are the most common enzyme-mediated cross-linking agents by phenol partial carbon-oxygen/nitrogen bond or carbon-carbon bond oxidative coupling. They can easily control the physical properties of the hydrogel by changing their concentration (Ren et al., 2015). These hydrogels are formed in a matter of minutes. They showed excellent biocompatibility and supported chondrocyte proliferation and differentiation (Jin et al., 2010). Enzyme-mediated hydroxyapatite hydrogel has the advantages of injectable, non-cytotoxic, and rapid cross-linking (Jin et al., 2020). Zhang and his team proposed a biomimetic enzyme complex of ferrous glycine (Fe [Gly]2) and glucose oxidase for rapid (less than 5 s) and mild preparation of injectable tough hydrogels (Zhang et al., 2021a).
1.3.4 Schiff base chemistry
Schiff base chemistry involves the formation of dynamic covalent imine bonds by cross-linking aldehyde and amine groups. Schiff base chemistry has the advantages of being reversible, simple, pH-sensitive, and biocompatible (Xu et al., 2019; Sahajpal et al., 2022). For the formation of biopolymer hydrogels, the functions of hydrazones and imines are most commonly used to achieve dynamic cross-linking behavior (Muir and Burdick, 2021). Chen et al. (2021). prepared injectable HA hydrogels modified with methacrylate and aldehyde group through dynamic Schiff base reaction. The results showed that the hydrogel was easy to prepare quickly in situ, had good biocompatibility, promoted BMSC proliferation, and promoted the regeneration of rat cartilage.
1.3.5 Photopolymerization
Visible or near ultraviolet photopolymerization is one of the most widely studied gelation processes in the development of injectable hydrogels. Some types of hydrogels can be photopolymerized in vitro and in vivo by the interaction of photoinitiators with visible or ultraviolet light to generate free radicals and polymerize free radical chains (Meng et al., 2019; Wu et al., 2020). Photopolymerization is a fascinating method with the following characteristics: 1) It is based on chemical reactions unaffected by water, making it suitable for use in aqueous media. 2) This is usually a very rapid process, allowing the synthesis of free-standing hydrogels in minutes or seconds. 3) It allows space and time control of the cross-linking process. 4) It is very little cytotoxic under the appropriate conditions and thus does little harm to cell survival and proliferation (Nicol, 2021; Pierau and Versace, 2021). The researchers altered collagen with Methacrylamide to photo-crosslinking under ultraviolet stimulation, enabling fast in situ gelation (Zhang et al., 2020a). GelMA injectable hydrogel is formed by introducing a double bond into a gelatin polymer chain that rapidly forms a hydrogel under light initiation. The blue light initiator lithium phosphonate makes the gelation approach faster and the preparation approach easier (Yue et al., 2015; Wang et al., 2021b; Wang et al., 2021c).
1.4 Comparison of physical and chemical cross-linking
The ideal injectable hydrogel has several requirements, including: 1) no evil byproducts are produced after gelation; 2) solubility of the gelated aqueous solution under physiological conditions (pH, temperature, and ion concentration); 3) the rate of gelation is rapid enough to meet the clinical efficacy. Nevertheless, in the presence of an additional agent such as a cell or bioactive molecule, there is adequate time for appropriate mixing and injection; 4) Suitable rate of biodegradation (Salavati-Niasari and Davar, 2006; Naahidi et al., 2017; Elkhoury et al., 2021). Both physical and chemical gelation must fulfill the above requirements. However, both physical and chemical techniques have benefits and deficiencies. Therefore, the most suitable method should be selected to design injectable hydrogels.
Compared to chemical cross-linked hydrogels, physically cross-linked hydrogels typically exhibit lower mechanical properties because the physical interactions are reversible and weak, so the hydrogels that form loosen easily when physical conditions (temperature, ionic strength, electrolyte, and pH) change (Mathew et al., 2018). For example, thermosensitive cross-linked hydrogels are one of the most widely studied injectable hydrogels in tissue engineering (Xu et al., 2020; Torres-Figueroa et al., 2021). Sol-gel transformations occur during hydration and dehydration at different temperatures (Shi et al., 2021a). The CST of such hydrogels is close to the body temperature during the sol-gel transition. The polymer chain expands in a random coil conformation due to its hydrophilic interaction with water molecules. However, when the system is heated beyond CST, the polymer chains collect and collapse due to a major hydrophobic interaction between the polymer chains (Sala et al., 2017; Dethe et al., 2022). Different PEG-based polymers, Poloxam and NIPAAm, are typical examples of thermosensitive hydrogels. The polar groups in the hydrogel can form hydrogen bonds with the water molecules between the polymer chains under CST, making it soluble. Above CST, the polymer chain contracts and becomes insoluble and hydrophobic, resulting in gelation (Eslahi et al., 2016).
Furthermore, ion-sensitive injectable hydrogels for cartilage defect repair have been developed. By adding Ca2+, the alginate solution can easily constitute hydrogel through ion cross-linking through the calcium bridge between the guluronic acid residues on nearby chains (Hu et al., 2019). pH-responsive hydrogels consist of polymers with basic or acidic groups, and their mechanisms involve dissociation and binding with hydrogen ions in response to ambient pH. This hydrogel has been extensively studied in drug delivery applications because the pH curves of pathological tissues (such as infection, inflammation, and cancer) differ from those of normal tissues (Eslahi et al., 2016; Yu et al., 2020a).
These physically cross-linked injectable hydrogels can be converted from liquid to gel and organized in situ hydrogels when injected into the body without additional cross-linking agents, chemical reactions, or environmental treatments (Gao et al., 2020). Physical interactions (e.g., hydrogen bonds, electrostatic attraction) or reversible chemical bonds (e.g., imine bonds) can form cross-linked pre-gel hydrogels whose structures are reversible (Arkenberg et al., 2020; Gupta et al., 2020). Pre-gelated hydrogels are in vitro-formed hydrogels that can be injected at the target and self-heal (Oliva et al., 2017). Pre-gelated hydrogels are injectable due to their self-healing and shear thinning (Riley et al., 2019). As the shear rate increased (during injection), the stickiness of the hydrogel decreased dramatically, reflecting the shear-thinning behavior (Thambi et al., 2017; Wang et al., 2021d). Although the injection forces may interfere with the cross-linking structure and trigger the gel-sol transition, the following self-healing process can rebuild the gel after removing the strain (Liu and Hsu, 2018). Shear-thinning injectable hydrogels protect encapsulated cells from high shear forces, improving the effectiveness of cell-based therapies (Thakur et al., 2016).
On the other hand, chemically cross-linked gels typically have stronger mechanical properties because covalent bonds are permanent and rigid (Zhao et al., 2020; Ali et al., 2022). The main drawback of chemical cross-linked hydrogels is the problem of cytotoxicity, which binding reactive compounds and light radiation may cause. Fortunately, recent developments in chemical cross-linking methods have enabled good biocompatible hydrogels to be gelated under mild reaction conditions (Lee et al., 2020; Wu et al., 2020).
Advanced injectable hydrogel preparation methods need to be further developed to improve physiological stability and mechanical properties, reduce adverse effects and cytotoxicity of hydrogels in vivo, and ensure gelation occurs at a rate suitable for clinical practice. Each approach has its advantages and disadvantages. Future research will explore how to correctly select the appropriate method and improve the existing manufacturing method.
1.5 Multiscale structure of injectable hydrogels
The bearing capacity of materials is a crucial characteristic in cartilage tissue engineering. Cartilage reduces friction, shear, and compression forces between bones. Its modulus is 0.5–2 MPa (Cross et al., 2016). Hydrogel has a stiffness of two orders of magnitude lower than natural cartilage (Li et al., 2019). Poor mechanical properties and limited functionality of traditional injectable hydrogels hinder their application in cartilage (Song et al., 2015). In addition to high water content and biocompatibility, the rigid multiscale hydrogel system also has super tensile property and large fracture energy (Xin, 2022). Multiscale injectable hydrogels with high mechanical strength and stability are of particular interest in cartilage tissue engineering (Figure 3).
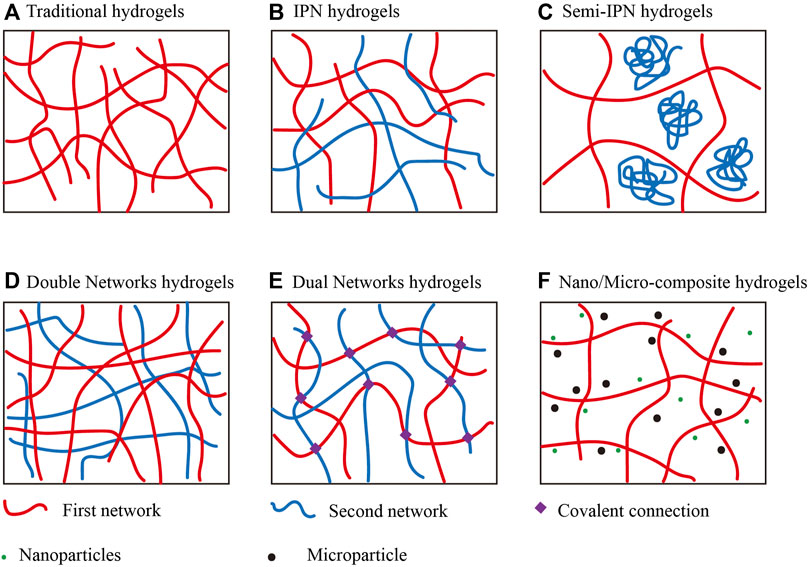
FIGURE 3. The schematic diagram depicts injectable hydrogels with different structures for cartilage regeneration engineering (A). Traditional single polymer networks. (B–F). Different multiscale structure of injectable hydrogels. Reproduced with permission of Vega et al. (2017).
1.6 Interpenetrating polymer network hydrogels
The IUPAC defines IPN as a unique polymer mixture consisting of two or more cross-linked networks whose parts are intertwined but not covalently connected and which cannot be separated unless the chemical bond breaks (Matricardi et al., 2013). IPN hydrogel was exploited to improve its mechanical properties (Zhang et al., 2015). Compared with hydrogels formed by a single polymer model, hydrogels with IPN often exhibit superior mechanical properties (Zoratto and Matricardi, 2018). Shojarazavi et al. (2021) used an IPN structure combined with silk fibroin nanofibers, alginate, and sodium cartilage ECM to enhance the mechanical properties of ECM to achieve the mechanical stiffness required for cartilage repair.
1.7 Semi-interpenetrating polymer network hydrogels
Unlike IPN, the chains of the second type of polymer in Semi-IPN are only dispersed in the network formed by the first type of polymer, rather than forming another network interpenetrating with the first type of polymer (Aminabhavi et al., 2015). IUPAC defined Semi-IPN as a polymer consisting of one or more networks and branched or linear polymers characterized by the penetration of at least one network at the molecular scale by at least some branched or linear macromolecules (Rinoldi et al., 2021). Furthermore, branched or linear polymers composed of Semi-IPN can be separated from the composed polymer network without breaking the chemical bond (Dhand et al., 2021). Thermosensitive hydrogels based on the chitosan/β-glycerophosphate system are widely used in cartilage regeneration engineering because of their good injectable properties, rapid gelation at the injection site, and ability to repair cartilage defects (Saravanan et al., 2019). However, due to their physical cross-linking network, chitosan/-glycerophosphate hydrogels exhibit high degradation rates and poor mechanical properties under physiological conditions, limiting their application (Jalani et al., 2015; Saravanan et al., 2019). Panyamao et al. (2020) used GE covalent cross-linking and pullulan Semi-IPN to improve the mechanical properties and swelling capacity of injectable thermosensitive hydrogels ground on the chitosan/β-glycerophosphate organization. Moreover, Wang et al. (2020a) prepared an injectable Semi-IPN hydrogel based on HA-SH and BPAA-AFF-OH supramolecular short peptides. The injectable hydrogel exhibits reliable mechanical strength. Moreover, compared with HA-SH hydrogel, it can enhance the expression of chondrogenesis-related genes and matrix secretion and further promote the maintenance of the hyaline cartilage phenotype.
1.8 Double networks hydrogels
Double-network hydrogels consist of two cross-linked networks with significantly different mechanical properties. The first network provides a rigid structure, and the second network is malleable. This is due to some structural parameters, namely the rate of the two hydrogel components, cross-linking density, swelling rate, and molecular weight allocation of the network polymer (Jonidi Shariatzadeh et al., 2021; Xin, 2022). Wang et al. 2(2021b) studied a double injectable hydrogel based on HAMA and GelMA. The double hydrogel combines the strong mechanical properties of HAMA with GelMA’s role in chondrocyte phenotype maintenance and ECM formation.
1.9 Dual networks hydrogels
Unlike a double network using two different mechanical properties materials, a dual network is defined as two cross-linked materials to form the same network and have a similar cross-linking mechanism. Although the dual network does not have the toughness of the double network, each material in the dual network can inject other useful properties into the hydrogel. For example, one material attracts cells and encourages migration into the injectable hydrogel, while the other effectively binds to surrounding tissue (Vega et al., 2017). Kim et al. (2021). (investigated poly(N‐isopropyl acrylamide) and PLL-based dual network injectable hydrogels encapsulating articular chondrocytes and MSCs. The model experiment of cartilage transplantation in vitro showed that dual hydrogel could promote cartilage defect repair.
1.10 Nano/micro-composite hydrogels
Mixed hydrogels integrated with nano/micron composites are networks of hydrated polymers physically or chemically cross-linked with N/MPs or other nano micron structures (Mehrali et al., 2017; Kouser et al., 2018).
N/MPs have excellent mechanical properties, surface reactivity, bioavailability, and a larger surface-to-volume ratio (Asadi et al., 2018; Zinatloo-Ajabshir et al., 2018; Piantanida et al., 2019; Ahmadian-Fard-Fini et al., 2020). The hard N/MPs enhance the soft organic polymer matrix, and the resulting nano/micron composites hydrogel can exhibit novel or enhanced biological, mechanical, conductive, optical, or magnetic properties (Motahari et al., 2015; Motealleh and Kehr, 2017; Zhao et al., 2017; Ravari et al., 2021). Inorganic materials such as clay, graphene, CNCs, hydroxyapatite, and metal nanoparticles can be used as fillers to enhance the hydrogel matrix (Davar et al., 2010; Zazakowny et al., 2016; Asadi et al., 2018; Zinatloo-Ajabshir and Salavati-Niasari, 2019). Nano-silicates with high cellular and biocompatibility could form shear-thinning hydrogels when combined with long-chain polymers (Thakur et al., 2016; Lokhande et al., 2018). POEGMA precursor polymer was physically cross-linked with CNCs, which made CNCs have excellent hydrogel dispersibility and significantly enhanced gel mechanical properties. Other gel properties, including swelling, degradation kinetics, and gelation rate, also changed significantly (De France et al., 2016). GelMA injectable hydrogel microspheres prepared by microfluidic technology are widely used in cartilage repair due to their enhanced high injectivity, structural stability, and uniform size (Han et al., 2021). Lei and his team reduced articular cartilage friction by coating the surface of injectable hydrogel microspheres with liposomes to form a self-renewing hydration layer through friction and wear. In addition, the release of an autophagy activator (rapamycin) promotes cartilage repair (Lei et al., 2022).
Moreover, the N/MPs adsorbs and retain essential stimulating factors, prolonging their release time due to the larger surface-to-volume ratio and high encapsulation efficiency of stimulating factor (Amiri et al., 2017; Nagahama et al., 2018; Wong et al., 2018; Ishihara et al., 2019; Bian et al., 2021; Zewail et al., 2021; Luu et al., 2022; Seo et al., 2022). Wang et al. (2020b) loaded the water-soluble antibiotic isoniazid into a cross-linked PEG network and encapsulated the hydrophobic antibiotic rifampicin into mesoporous silica nanoparticles. The addition of nanoparticles can significantly adjust and enhance injectable hydrogels' mechanical strength and elasticity. The release time of rifampicin was significantly longer than isoniazid and promoted cartilage repair. Lin et al. (2021b) developed PLGA microspheres loaded with TGF-β3, and injectable hydrogel-coated PLGA microspheres could sustainably release TGF-β3. This synthetic micron composite injectable hydrogel system regulates chondrocyte differentiation and biosynthesis.
The electroactive nanomaterials promote the migration, adhesion, proliferation, and differentiation of preosteoblasts and MSCs. Aniline oligomers (Penta aniline or tetra aniline) are the most commonly used conductive oligomers, with the advantages of good biocompatibility, low cost, easy synthesis, good stability, easy processing, electrochemical behavior similar to conductive polymers, and due to low MW, easy to be eliminated from the body by renal excretion (Wang et al., 2016; Hassanpour et al., 2017; Zinatloo-Ajabshir et al., 2020; Monsef and Salavati-Niasari, 2021).
2 Material of injectable hydrogels
Injectable hydrogels are generally required to have the following characteristics, including low toxicity, adequate biocompatibility, support for cell adhesion, proliferation and differentiation, biodegradability, appropriate degradation rate, and fine structure similar to the tissue or organ to be repaired, and controlled release of biomolecules (Jiang et al., 2021). Strategies for cartilage repair based on natural and synthetic injectable hydrogels and their combination were studied.
2.1 Natural polymers
Natural polymers can be broadly divided into three categories: those based on proteins, polysaccharides, and protein nucleotides. Because ECM is a complex combination of fibrin (such as laminin, collagen, and ficonin) and hydrophilic proteoglycan. A simple and effective way to simulate ECM is to prepare injectable hydrogels using natural polymers that mimic many of its characteristics (Farrell et al., 2017). Natural polymers have several advantages over synthetic polymers:1) they are more biocompatible; 2) They can contain cell-binding motifs, realize cell adhesion, proliferation, and biological activity cues, and influence cell behavior; 3) They can exhibit fibrous structures that mimic the ECM of natural tissues; 4) They can be recognized and processed metabolically by the body, allowing cells to reshape them along with cell-secreted ECM deposition (Gomez-Florit et al., 2020). On the other hand, natural polymers have low mechanical strength and vary from batch to batch and from natural source to natural source, making their molecular weight, chemical structure, and rate of degradation difficult to control (Yan et al., 2014; Coenen et al., 2018).
2.2 Protein polymer
2.2.1 Silk fibroin
SF polymers can be easily processed into various forms, including micron/nanoparticles, membranes, films, fibers, and mainly hydrogel scaffolds (Zheng et al., 2022). Chemical and physical methods can prepare SF injectable hydrogels through the β-sheet formation. Various chemical reagents, including alcohols, acids, salts, and surfactants, have been used as SF crosslinkers (Yuan et al., 2021a).
2.2.2 Gelatin
Gelatin is a commercial biomaterial whose biological properties are widely used in biomedical engineering due to its similarity to more expensive collagen as an adhesive protein (Tonda-Turo et al., 2017). Gelatin is produced by regional hydrolysis of collagen and can promote cell adhesion, proliferation, migration, and differentiation due to RGD sequence in its structure (García-Fernández et al., 2020). In addition, one of the main characteristics of this water-soluble protein is its thermal response, with reversible sol-gel transformations occurring when cooled above the critical solution temperature (25–35°C). Gelatin is therefore widely used in cartilage tissue engineering (Echave et al., 2019).
2.2.3 Collagen
Two-thirds of the dry weight of the adult joints cartilage is collagen. The fibrous network of developing cartilage is a copolymer of collagen XI, IX, and II and small amounts of other types of collagen (Eyre, 2004). Varieties of combinations of collagen with other natural polymers like inulin, combinations of fibrin, alginate, and gelatin have been transformed into formulations for injectable hydrogels, enabling in-situ formation (Rigogliuso et al., 2020).
2.3 Polysaccharide polymer
2.3.1 Alginate
Alginate is a polysaccharide extracted from brown seaweed and composed of α-l-guluronate (G block) and β-D-mannuronate (M block) copolymers linked by 1, 4-glycosidic bonds. G block of alginate is cross-linked with divalent cations such as Ba2+ and Ca2+ to form gel (Balakrishnan et al., 2014). It is widely used due to its biocompatibility, biodegradability, and ease of manufacture. However, alginate lacks the property of cell adhesion. Alginate was mixed with other polymers for cartilage repair to improve its biological properties (Jaikumar et al., 2015).
2.3.2 Chitosan
Chitosan natural polymer is a widely available polysaccharide created by completely deacetylating chitin, a structural component extracted from insect and crustacean bones. Importantly, chitosan-based materials have received much attention as hydrogels because of their good cellular compatibility, pH sensitivity, and biodegradability. In general, chitosan could dissolve in acidic solutions, and its viscosity properties can be easily adjusted by adjusting the concentration (Zheng et al., 2022). The insolubility of chitosan in water limits its use. Therefore, many studies have focused on soluble chitosan derivatives (Fattahpour et al., 2020). For example, chitosan becomes a thermosensitive polymer when mixed with polyol phosphate salts like β -glycerophosphate (BGP) (Panyamao et al., 2020).
2.3.3 Hyaluronic acid
HA is the primary component of glycosaminoglycan in ECM. It consists of repeated disaccharide units of n-acetyl-D-glucosamine and β -D-glucuronic acid, alternately linked by β-1,4 and β-1,3 glycosidic bonds (Graça et al., 2020; Li et al., 2021b). Natural HA does not affect cell adhesion or gel formation. Hence, it is necessary to chemically alter the functional groups of HA, adjusting their physical, chemical, or biological properties according to special requirements of specific applications (Zaviskova et al., 2018). In the absence of chemical cross-linking agents, hydrogels are formed by Schiff bases between the amino group of ethylene glycol-chitosan and the aldehyde group of oxidized hyaluronate. These hydrogels show good bio durability and compatibility under physiological cases, and they may be a potential injectable cell delivery system in cartilage tissue engineering (Kim et al., 2017).
2.3.4 Agar
Agar is a water-extracted cell-wall polysaccharide from Gracilariaceae and Gelidiaceae plants of seaweeds, consisting mainly of (1–3) 3, 6-hydroxy-lactose repeated units and alternating (1–4) D-galactose. Agar is solvable in water at temperatures beyond 65°C and forms a gel between 17 and 40°C (Tonda-Turo et al., 2017). Agar and gellan gum promote cartilage regeneration by inhibiting inflammatory mediators and inducing chondrogenesis and autophagy-related gene expression (Heo et al., 2020).
2.3.5 Gellan gum
Gellan gum is a bacterial polysaccharide extracted from Sphingomonas elodea. Its main glycoside chain is a repeating tetrasaccharide unit, each repeating unit contains one acetate and one L-glyceride, and one esterified substituent occurs in every two sequences (Oliveira et al., 2021). Gellan gum can form a thermally reversible injectable gel with no cytotoxicity in different test environments. It is commonly used in the food industry and has previously been used for drug delivery in the biomedical field. Gellan gum can effectively regenerate hyaline cartilage tissue in the defect (Oliveira et al., 2010). Choi et al. (2020) loaded 6-(6-amino-hexyl) amino-6-deoxy-β-cyclodextrin onto the Gellan gum chain to reduce gel temperature, enhance physicochemical properties, and improve drug delivery efficiency and release.
2.3.6 Cellulose
The physical capabilities of cellulose depend on the presence of three hydroxyl groups (OH) at the C-6, C-3, and C-2 positions. Cellulose injectable hydrogels made from carboxymethyl cellulose (CMC), methylcellulose (MC), and hydroxypropyl cellulose (HPC) have good mechanical properties and are biocompatible (Zhang et al., 2021b).
2.3.7 CS
CS is a GAG consisting of alternating units of (β-L, 4) n-acetylgalactosamine (GalNAc) and β-1,3-linked glucuronic acid (Glca). The residues of galactosamine at position 4 or 6 can be sulphated (Yuan et al., 2021b). Furthermore, CS is the most abundant GAG in the human body and the main component of chondrocyte ECM, which has attracted great attention as a biomaterial for cartilage defect repair. CS in cartilage has multifold key roles, including supporting chondrogenesis, providing resistance to stress, chondrocyte signaling, and intercellular communication (Thomas et al., 2021).
2.4 Protein nucleotide polymer
2.4.1 Deoxyribonucleic acid
DNA is a brilliant molecule because of its biocompatibility, minimal toxicity, precise molecular recognition, and easy programming (Li et al., 2020a; Li et al., 2022). Physical tangles between DNA strands or chemical connections between DNA molecules can be used to create DNA hydrogels. Chemically, polymers are held together by covalent bonds, which confer great mechanical strength and environmental stability (Kahn et al., 2017; Zhang et al., 2020b; Khajouei et al., 2020). DNA injectable hydrogels are widely used in cartilage repair engineering due to their injectable properties, adjustable mechanical properties, and good permeability (Yan et al., 2021).
2.5 Extracellular matrix
ECM hydrogel provides cells with a natural adhesion surface and superior biological activity. Preparing acellular ECM hydrogels can maximize the retention of growth factors and low molecular weight peptides present in natural ECM (Gong et al., 2021). At present, bionic and tissue-specific injectable hydrogels are prepared from various acellular ECM (amniotic membrane, cartilage, bone, heart, and lung) for cartilage regeneration engineering (Bhattacharjee et al., 2020; Gong et al., 2021; Bhattacharjee et al., 2022). Bordbar et al. (2020) developed an injectable hydrogel derived from acellular sheep chondrocyte ECM. The cells embedded in the hydrogel can differentiate into chondrocytes. Sevastianov et al. (2021) compared the effects of injectable collagen hydrogels and acellular porcine articular cartilage injectable hydrogels on rabbit BMSCs differentiation. Injectable collagen hydrogel is more beneficial in stimulating BMSCs to repair cartilage in vivo, and injectable porcine articular cartilage is an inducer for BMSCs to form chondroid tissue in vitro.
2.6 Synthetic polymer
Synthetic polymer hydrogels have been developed to meet the need for more alternative materials in tissue engineering. Synthetic polymers mainly include polymers based on PLA, PGA, PLGA, PCL, PVA, and polyester copolymers (Werkmeister et al., 2010; Yan et al., 2014). Synthetic polymers of glycopeptides mimic natural glycoproteins or glycopeptides and have great potential in biomedical applications. The extracts of glycopeptide copolymer and glycopeptide hydrogel showed good cytocompatibility in vitro. When injected subcutaneously into rats, glycopeptide hydrogels formed rapidly in situ (Ren et al., 2015). A one-component synthetic methacrylate type II collagen can be photo-crosslinked to form a firm injectable hydrogel. MSCs encapsulated in this hydrogel showed good activity and could coagulate and undergo chondrogenesis (Behan et al., 2022).
2.6.1 Poly lactic-co-glycolic acid
PLGA is a synthetic polypeptide formed by natural l-glutamic acid through an amide bond, biodegradable, avoids antigenicity or immunogenicity, and is non-toxic and hydrophilic. In addition, abundant side-chain carboxyl groups on the PLGA chain enable it to undergo chemical modification. These properties make PLGA an ideal biomedical material (Yan et al., 2014).
2.6.2 Polyethylene glycol
PEG is a non-toxic, non-immunogenic, and pollution-resistant synthetic polymer widely used as a substrate in tissue engineering, such as articular cartilage, bladder, and nerve tissue regeneration (Li et al., 2021c). A PEGDA hydrogel involved in chondroitin sulfate binder has entered clinical trials for repairing cartilage defects and has shown improved results in combination with microfractures (Qi et al., 2018; Qi et al., 20182021).
2.6.3 Polyglycolic acid
PGA is a polypeptide secreted by Bacillus subtilis natto. Many carboxylic acid groups (-COOH on the side chain of -PGA) are easily functionalized to achieve precise functions. The ultimate degradation product of PGA is glutamate, a component of collagen. Due to its excellent biocompatibility, biodegradability, and non-toxicity, PGA is used to prepare hydrogels with various functions for tissue engineering matrix, especially cartilage (Wei et al., 2022).
2.7 Natural/synthetic polymer
Synthetic polymers are easy to manufacture and replicate. However, they have poor biodegradability and biocompatibility. Naturally derived polymers are widely used in injectable hydrogels for repairing cartilage defects due to their excellent biodegradability, biocompatibility, and similar 3D microenvironment in vivo. However, rapid degradation, poor mechanical properties, and enhanced microenvironment for cell proliferation and differentiation are challenges in practical applications (Jian et al., 2012; Lee et al., 2021). The combination of natural and synthetic polymers can play to their respective strengths and compensate for their weaknesses (Peng et al., 2019; Li et al., 2021c). Yang et al. (2021) mixed injectable hydrogels of 2%DF-PEG/1.5%GCS, which significantly improved the mechanical properties and biocompatibility of hydrogels, and the hydrogels loaded with ADSCs promoted cartilage repair. Shi’s team researched and prepared an injectable hydrogel with natural antioxidant capacity. A dynamic covalent bond between PVA and phenylboronic acid grafted to HA-PBA forms hydrogels, which are further stabilized by secondary cross-linking between the acrylate portion of the HA-PBA and the free sulfhydryl group in the vulcanized gelatin (Shi et al., 2021b). The existence of a dynamic covalent bond contributes to the shear thinning of hydrogels, which makes hydrogels have suitable printing. Hydrogels protected coated chondrocytes from ROS-induced upregulation of Chondrocyte-specific catabolic genes (MMP13) and downregulation of anabolic genes (COL2 and ACAN) after incubation with H2O2.
2.8 Cells and stimulating factors integrated into injectable hydrogels
Injectable hydrogels can integrate appropriate cells and stimulating factors to stimulate damaged tissue’s original microenvironment and thus help regenerate damaged cartilage (Yang et al., 2017; Ngadimin et al., 2021). Injectable hydrogels act as the matrix to promote cell-cell interactions and cell-matrix interactions, while stimulating factors are part of signals that mediate cell adhesion and migration to scaffolds. Hence, cells and stimulating factors are important for applying injectable hydrogels in tissue engineering, as they play a vital role in cell differentiation and tissue growth (Sun et al., 2017; Cho et al., 2020; Stampoultzis et al., 2021). Living tissue cells migrate from the surrounding to the hydrogel and interact within the hydrogel to reconstruct the desired tissue at the implant site. Injectable hydrogels can also transport cells that interact with protocell populations and deliver growth factors or other therapeutic biomolecules to recapture abnormal biology (Dimatteo et al., 2018).
2.9 Cell source and cell capsulation
The requirements of injectable hydrogel-encapsulated cells for cartilage repair are as follows: 1) they can constitute cartilage tissue; 2) suitable for clinical application, that is, the source is vast, the trauma is minor, and the extraction is easy; 3) after many passages, they can obtain the required number of cells while maintaining the cartilage phenotype (Kwon et al., 2019). Embedding cells into injectable hydrogels can be achieved by embedding cells during gel formation or by inoculating cells into prefabricated porous gels (Armiento et al., 2018; Jabbari and Sepahvandi, 2022).
2.9.1 Chondrocytes
ACI has been successfully used to promote articular cartilage regeneration. Hu et al. 2(2021b). loaded chondrocytes in IPN injectable hydrogel composed of chitosan/HA/Si-HPMC. The chondrocytes proliferated well in vitro, promoting cartilage defect repair in rat models in vivo. Chiang et al. (2021) prepared an injectable HA-PAA hydrogel with magnetic navigation and glutathione release. The chondrocytes embedded in the hydrogel proliferated and differentiated at the site of cartilage damage through magnetic interaction of internal iron nanoparticles and adhesion of CD44 receptors on HA chain. The rabbit cartilage defect model produced uniform and smooth, regenerated cartilage 8 weeks after hydrogel implantation, and the columnar arrangement of chondrocytes in the deep tissue was similar to that of normal chondrocytes (Chiang et al., 2021).
However, some shortcomings still need to be addressed, such as the low number of cells that are difficult to extract and harvest. With the increase of amplification generations, chondrocytes lose their chondrogenic phenotype. Eventually, this results in lower cartilage repair (Cai et al., 2020; Zhou et al., 2022). Autologous chondrocytes are mainly derived from natural cartilage in the non-weight-bearing region of the joint, which may lead to donor site disease (Chen et al., 2018). hNCs are a clinically valuable source of cartilage tissue regeneration. hNCs are relatively easier to obtain through a marginally invasive collection program during septal surgery for nasal obstruction, with a lower incidence than chondrocytes obtained from articular cartilage (Lim et al., 2020).
2.9.2 Stem cells
Due to the insuperable limitations of chondrocytes in the treatment of damaged cartilage, a significant amount of research has focused on the research of stem cells in recent years. Stem cells are self-renewing cells that, due to their undifferentiated biology, can produce more stem cells through mitosis or can differentiate into specialized cells (Ma et al., 2018; Yin and Cao, 2021). Various types of stem cells such as ESCs, CSPCs, MSCs, and iPSCs are used to treat cartilage defects (Deng et al., 2020; Johnstone et al., 2020).
2.9.3 Embryonic stem cells
ESCs are derived from inner cell masses of blastocyst embryos and are essentially pluripotent stem cells with the ability to differentiate into all cell types in the body, potentially providing an unlimited supply of cells for cell and tissue therapy and replacement (Toh et al., 2011). However, using ESCs is linked with ethical issues, as induction of ESCs destroys embryos. In addition, ESCs will form teratoma. Because of safety concerns, it is inappropriate to use ESCs for cartilage tissue engineering at this time (Im and Shin, 2015).
CSPCs: Articular cartilage has a single cell type, chondrocytes. Although lacking intrinsic repairability, articular cartilage has been proved to contain a population of stem or progenitor cells, similar to those discovered in many other tissues, thought to be relevant to maintaining tissue homeostasis (Jiang et al., 2016). These CSPCs have been found in human, bovine, and horse articular cartilage (Jiang and Tuan, 2015). Li et al. verified the injectable hydrogels based on THA and HB-PEG multi-acrylate macromer containing CSPCs. The secretion of extracellular chondrocyte ECM was enhanced under chondrogenic conditions, and inflammatory gene expression was down-regulated (Li et al., 2020b).
MSCs are undifferentiated pluripotent stem cells characterized by the ability to self-renew when exposed to specific growth signals (Mohamed-Ahmed et al., 2018; Gonzalez-Fernandez et al., 2022). MSCs could differentiate into chondrocytes, osteoblasts, muscle cells, and adipose cells, providing great potential for cartilage tissue engineering. They can be collected from tissues such as bone marrow, umbilical cord blood, adipose, amniotic fluid, pulp, synovium, and even breast milk (Deng et al., 2014; Shao et al., 2015; Mohamed-Ahmed et al., 2018; Fu et al., 2022). MSCs also have immune-enhancing and immunosuppressive effects on the deficiency of primary histocompatibility class II antigens and the secretion of helper T cell type 2 cytokines (Ding et al., 2021).
In particular, BMSCs are considered important seed cells in the treatment of cartilage injury due to their advantages of extensive sources, easy access, strong proliferation ability, significant multidirectional differentiation potential, and the ability to regulate inflammation (Muscolino et al., 2021; Zhu et al., 2022a). Ji et al. studied a temperature-sensitive GM-HPCH injectable hydrogel loaded with BMSCs and TGF-β1. Composite hydrogels can promote the migration of BMSCs, increase the expression of migrated genes, promote the differentiation of BMSCs cartilage, and effectively repair cartilage (Ji et al., 2020). However, ADSCs showed lower levels of immunogenicity than BMSCs. ADSCs showed better stability in the treatment of osteoarthritis. This finding was supported by single-cell analysis results, which clearly showed that ADSCs were more conspecific than BMSCs (Wu et al., 2013; Mazini et al., 2019). Compared with in vitro culture conditions, the cell microenvironment in vivo can be relatively deficient in oxygen and nutrition. The failure of transplanted cells to adapt to environmental changes may be one of the reasons for the low survival rate of MSCs. Under serum deprivation and hypoxia, ADSCs were more resistant to apoptosis, implying that they may be better adapted to post-transplant conditions (Xu et al., 2017a; Zhou et al., 2019). Boyer et al. and Dehghan-Baniani et al. studied injectable hydrogels loaded with ADSCs in vivo and in vitro, promoting cartilage defect repair (Boyer et al., 2020; Dehghan-Baniani et al., 2020).
hUCMSCs are also an alternative stem cell source for cartilage tissue engineering. Compared with BMSCs, regarded as standard stem cell sources, which produced more intense type II collagen staining, the hUCMSCs produced more type I collagen and aggregative proteoglycans (Talaat et al., 2020).
iPSCs: IPSCs refer to the reprogramming of somatic cells with the potential to be self-renewing and pluripotent stem cells, similar to ESCs, but without the ethical issues and immune response that plague ESCs (Tsumaki et al., 2015; Castro-Viñuelas et al., 2018). In contrast to MSCs' limited differentiation ability after the fourth generation, IPSCs can provide abundant unlimited cell sources with low tumorigenicity (Chang et al., 2020). The potential of IPSCs to differentiate into chondrocytes and its application in cartilage defect modeling have been successfully demonstrated in several researches (Zhang et al., 2020c; Csobonyeiova et al., 2021). He et al. (He et al., 2016) successfully cultured mouse IPSCs to differentiate into chondrocytes based on the sodium alginate hydrogel platform. Xu et al. (Xu et al., 2017b) inoculated human IPSCs with PLCG hydrogel scaffolds and showed repaired chondroid tissues in rabbit cartilage defect models without teratoma.
2.10 Stimulating factor
Stimulating factors play an important role in regulating cell proliferation, migration, and differentiation (Zhong et al., 2016; Fan et al., 2020). Many studies have revealed that many cytokines are generally involved in the chondrogenic differentiation of stem cells and maintenance of chondrocyte phenotypes, IGF-1, TGF-β1 or TGF-β3, BMP-2, BMP-4 or BMP-7, and GDF-5 (Campos et al., 2019). Moreover, through genetic engineering to enhance the expression of biologically active molecules, gene therapy offers an alternative method for locally delivering the appropriate stimulus (Huang et al., 2018). Due to the fast clearance of drugs in the joint, much traditional cartilage repair drug therapy has limited efficacy. Injectable hydrogels can maintain drug release and prolong drug retention in the articular cavity. Many studies have been done on injectable hydrogels loaded with drugs (Li et al., 2019).
2.10.1 Transforming growth factor-β
The TGF-β family plays a crucial role in homeostasis and the development of various tissues. Signaling in this protein family mainly activates SMAD-dependent transcription and signaling and SMAD-independent signaling through MAPK such as TAK1 and ERK (Thielen et al., 2019). They regulate cell proliferation, migration, differentiation, and apoptosis and control the degradation and synthesis of ECM. In mammals, there are three isotypes of TGF-β. TGF-β is an inactive soluble protein complex composed of TGF-β dimer, latent TGF-β binding protein, and pre-peptide latency (Blaney Davidson et al., 2007). TGF-β1 is abundant in natural cartilage and controls cartilage ECM production by affecting the synthesis of fibronectin, proteoglycan, and collagen (Zheng et al., 2022). Zhang et al. 2(2021b) developed an injectable hydrogel system based on cross-linked thiolated chitosan and carboxymethyl cellulose as carriers for TGF-β1 in cartilage tissue engineering applications. At 8 weeks postoperatively, hydrogels loaded with TGF-β1 showed excellent repairability in a rat model of full-thickness cartilage defects of the knee. TGF-β3 is also shown to have chondrogenic properties (Martin et al., 2021). Lin et al. 2(2021b) demonstrated in vitro that injectable hydrogels loaded with TGF-β3 promoted the expression of chondrogenic genes (Col-2α and ACAN) and decreased the expression of osteogenic genes (Col-1α) in chondrocytes.
2.10.2 Bone morphogenetic protein
BMPs are protein molecules secreted by varieties of cells and are members of TGF-β superfamily. BMP plays a vital role in cartilage and bone formation and is named after its ability to induce cartilage and bone (Deng et al., 2018). BMP promotes SOX9 expression in chondrogenic MSCs. BMP acts upstream of SOX9, and SOX9 is critical for BMP-induced chondrogenesis. SOX9 and BMP participate in a positive feedback loop (Pogue and Lyons, 2006).
2.10.3 Growth/differentiation factor-5
GDF-5 is also a member of TGFβ superfamily. It is a large precursor protein consisting of two main domains: the active C-terminal domain and the N-terminal precursor domain with signal sequences and cleavage sites. GDF-5 overexpression can promote chondrogenesis, which improves MSC adhesion and chondrocyte proliferation (Sun et al., 2021). However, GDF-5 promotes osteogenesis and hypertrophy, limiting its therapeutic effect on cartilage repair. Therefore, it is better to control the anabolism and anti-catabolism of GDF-5 on chondrocytes and apply it to cartilage tissue engineering (Mang et al., 2020).
2.10.4 Insulin-like growth factor-1
IGF-1 is an anabolic growth factor that promotes cell proliferation and inhibits apoptosis and is vital in chondrogenesis and homeostasis. IGF-1 is a crucial factor promoting cartilage matrix anabolism in synovial fluid and serum. In addition to stimulating ECM production, IGF-1 can stimulate MSCs proliferation and chondrogenic differentiation (Wen et al., 2021). Many studies have demonstrated the effectiveness of IGF-1 in articular cartilage repair, and it is dose-dependent (Wei et al., 2020). High-dose IGF-1 is more conducive to the formation and integration of cartilage regeneration, while low-dose IGF-1 is more conducive to subchondral bone (Zhang et al., 2017).
2.10.5 Platelet-rich plasma
PRP is rich in various growth factors, cytokines, and proteins, and many studies have demonstrated the potential effectiveness and excellent biocompatibility of PRP for cartilage defect repair (Yan et al., 2020). PL is a natural GFs pool consisting of TGF-β1, TGF-β3, IGF-1, and VEGF and can be prepared by simple PRP thermal cycling. Due to the removal of platelet fragments by gradient centrifugation, the immunogenicity of PL is lower than that of PRP (Li et al., 2021b). Tang and his team encapsulated PL using EPL and heparin NPs into injectable hydrogels. The injectable hydrogel ameliorated early cartilage degeneration and promoted late cartilage repair in rats with knee arthritis (Tang et al., 2021).
2.10.6 Kartogenin
KGN is a stable, nonprotein small molecule with a structure of 2- [(4-phenyl) carbamoyl] benzoic acid that induces the differentiation of BMSCs into chondrocytes regulating the CBFβ-RUNX1 signaling pathway (Yuan et al., 2021b). It is more effective than growth factors in inducing cartilage regeneration and has been processed and applied in various forms in cartilage tissue engineering (Cai et al., 2019). Dehghan Baniani et al. (Dehghan-Baniani et al., 2020) incorporated KGN into a thermosensitive injectable chitosan hydrogel. KGN can be released continuously for more than 40 days and promote chondrogenic differentiation of human ADSCs in vitro (including upregulation of COL2A, SOX9, and ACAN chondrogenic genes).
2.10.7 Gene therapy
It refers to delivering nucleic acids to tissues of interest by direct (in vivo) or transduced cell-mediated (in vitro) methods using viral and non-viral vectors. In the past few decades, the strategy of expressing therapeutic transgenes at injured sites has been adopted to promote cartilage repair (Grol and Lee, 2018). However, the problems associated with non-standard procedures remain unresolved. In addition, the association of gene therapy with tissue engineering may be a promising strategy for treating cartilage and osteochondral damage (Bellavia et al., 2018). Several clinical trials of gene therapy have been conducted in patients with end-stage knee OA by intraarticular injection of human adolescent chondrocytes overexpressing cDNA encoding TGF-β1 with retroviral vectors. In the latest placebo-controlled randomized trial, clinical scores improved in the gene therapy group compared with placebo (Madry and Cucchiarini, 2016). Zhu et al. (2022b) used an injectable hydrogel to deliver miR-29b-5p (aging-associated miRNA), which was functionalized by binding to stem cell homing peptide SKPPGTSS for SMSCs recruitment contemporaneously. Sustained miR-29b-5p transport and recruitment of SMSCs, followed by chondrocyte differentiation, results in successful chondrocyte regeneration and cartilage repair. Yu et al. (2021b) implanted genetically modified ADSCs overexpressing TGF-β1 into injectable ECM hydrogels. In the rat OA model, intraarticular injection of hydrogels loaded with ADSCs overexpressing TGF-β1 significantly reduced joint inflammation, cartilage degeneration, and subchondral bone loss.
2.10.8 Drug
Until now, the conventional treatment for OA has been to reduce the main symptoms with oral or topical injections of various drugs, including NSAIDs, analgesics, and corticosteroids. The efficacy of local injection is hampered by their rapid diffusion, instability, and low retention at the target site (Mok et al., 2020). More importantly, frequent oral use of these drugs can cause serious side effects, such as increased throw of cardiovascular disease and stimulus of the gastrointestinal tract (García-Couce et al., 2022). Suitable injectable hydrogel delivery systems could sustainably release therapeutic drug concentrations in cartilage (Cao et al., 2021a; Cao et al., 2021b; Shi et al., 2021b; Khan et al., 2022). Hanafy and El-Ganainy, 2020 prepared an injectable hydrogel based on Poloxamer 407 and HA loaded with the anti-inflammatory drug DK. 40% of DK was released after 4 days. Injectable hydrogels maintain DK content and drug release percentage after 3 months of storage. Loaded DK injectable hydrogel had the greatest anti-edematous and anti-nociceptive effect compared to oral and direct injection DK. Both histomorphology and radiology showed regeneration of cartilage defects. Branco et al. (2022) prepared an injectable PVA-based hydrogel that continuously released diclofenac for cartilage regeneration.
Some of the following drugs are also used in injectable hydrogels to repair cartilage damage. Dexamethasone, a glucocorticoid, has 20–30 times the anti-inflammatory potency of natural hydrocortisone. It can reduce the loss of collagen and proteoglycan in ECM, maintain ECM synthesis, and maintain the viability of chondrocytes. In addition, it is a key reagent for inducing chondrogenesis of MSCs in vitro (García-Fernández et al., 2020; Wang et al., 2021e; García-Couce et al., 2022). GlcN is a naturally occurring amino monosaccharide, widely used to reduce joint pain and repair cartilage (Suo et al., 2020; Zhang et al., 2021c). Quercetin and naringin are flavonoids widely found in fruits and vegetables with strong anti-inflammatory and antioxidant effects. It can inhibit ECM degradation, reduce the inflammatory response and maintain chondrocyte phenotype (Yu et al., 2020b; Li et al., 2021d). Icariin can improve cartilage ECM synthesis and restrain ECM degradation, up-regulate chondrogenic specific gene expression of chondrocytes, and induce oriented chondrogenesis of BMSCs without hypertrophic differentiation (Zhu et al., 2022a).
3 Advanced injectable hydrogels for cartilage repair tissue engineering
In recent years, various injectable hydrogels with good plasticity and biological properties have been widely studied for cartilage repair tissue engineering (Table 1). Many studies have investigated the regeneration potential of injectable hydrogel cartilage in vitro and in vivo. Fattahpour et al. (2020) developed and characterized MC-CMC-Pluronic and ZnCl2 injectable hydrogels containing meloxicam. The release time of meloxicam in hydrogels containing nanoparticles was significantly longer than in hydrogels without nanoparticles. This injectable hydrogel showed good chondrocyte adhesion and proliferation. Qi et al. (2018) prepared a photo-crosslinked injectable SerMA hydrogel loaded with chondrocytes. After 8 weeks of implantation, SerMA hydrogel loaded with chondrocytes successfully formed regenerative cartilage in rabbits. Most importantly, regenerated cartilage is structurally similar to natural cartilage (Qi et al., 2018, Qi et al., 2021).
Numerous studies have investigated injectable hydrogel strategies that guide stem cell phenotypic expression and manipulate cartilage matrix properties. Liu et al. (2021b) revealed that hydrogel scaffolds with gradient distribution could better simulate the function of natural cartilage and promote stem cell differentiation than homogeneous hydrogel scaffolds. In rabbit models, injectable hydrogels containing BMSCs that sustained-release BMP-2 were more effective than microfractures alone in treating cartilage damage (Vayas et al., 2021). Zhang and his team prepared injectable hydrogels composed of hyaluronic acid-tyramine and collagen type I-tyramine-loaded BMSCs and TGF-β1 (Zhang et al., 2020d). The injectable hydrogel supports the differentiation of BMSCs into chondrocytes. In vivo experiments further demonstrated that this injectable hydrogel can achieve good repair of transparent articular cartilage. Mahajan et al. (2022) developed a silk fibroin/CMC/gelatin complex hydrogel that increases contraction and hardness over time. The contractile-mediated mechanical stimulation promotes the formation of ADSCs cartilage. The regenerated cartilage of goats is very similar to natural cartilage. The cells may have a therapeutic effect because EVs derived from them can induce stem cell differentiation and chondrocyte proliferation (Liu et al., 2017b; Saveh-Shemshaki et al., 2019; Song et al., 2021). Zhang et al. (Zhang et al., 2021d) prepared the load with BMSCs-EVs alginate/dopamine/CS/silk fibroin composite injectable hydrogel.
When the hydrogel was injected into a rat cartilage defect model, EVs released by injectable hydrogel could recruit BMSCs into the hydrogel through a chemokine signaling pathway and promote BMSCs proliferation and differentiation to promote cartilage repair. Tao et al. (2021) implanted circRNA3503 carried by SMSCS-EVS into PDLLA-PEG-PDLLA injectable hydrogel. EVs promoted chondrocyte migration and proliferation, while circRNA3503 reduced chondrocyte apoptosis and ECM degradation, and both of them combined with regenerating damaged cartilage in rats. Heirani-Tabasi et al. (2021) demonstrated that human articular chondrocytes EVs in chitosan-HA injectable hydrogels have chondrogenic differentiation effects on ADSCs. In the rabbit cartilage defect model, EVS-treated ADSCs had greater cartilage regeneration ability than untreated MSCs or ADSCs treated with EVS without gel.
In addition, injectable hydrogels have been studied to detect cartilage repair ability dynamically. According to Onofrillo et al. (Onofrillo et al., 2021), FLIH was considered a sensitive tool for monitoring the photo-crosslinked injectable hydrogels in cartilage tissue engineering structure. The generation of cartilage ECM in injectable hydrogels is related to the fluorescence loss curve, which describes the hydrogels’ degradation rate. Using FLIH can be achieved through an extensible system for sample maintenance and fluorescence recording, resulting in an analytical real-time monitoring system suitable for non-contact high-throughput evaluation of chondrogenesis.
4 Summary and perspectives
The repair of cartilage defects still faces many challenges. Injectable hydrogel is the main development direction of cartilage tissue engineering, not only because of its bionic properties similar to cartilage ECM due to its high moisture content but also because of its minimally invasive properties and strong plasticity ability to match irregular defects. First, to improve the biomechanical properties of injectable hydrogels, traditional single-network hydrogels are added with different polymer mixtures or networks, and many nano/micron-composite materials are used to alter the mechanical properties and sustained-release properties of the matrix. Integrating cells and cytokines or other stimulators into injectable hydrogels can improve the integration of hydrogels with surrounding cartilage and promote cartilage regeneration. Controlling the proliferation and differentiation of stem cells into chondrocytes is of great interest.
Despite many relatively successful preclinical studies and several advanced manufacturing methods for engineered tissues, there remain limitations that must be addressed in preparing injectable hydrogels with excellent performance for optimal regeneration of cartilage defects. First, the injectable hydrogel matrix must be able to fill the defect area with a smooth surface similar to natural cartilage without fusing with the surrounding healthy tissue. Second, rapid degradation of the hydrogel matrix before replacement by regenerative ECM may compromise its mechanical stability and therapeutic efficacy. To address this issue, appropriate exogenous cells (such as MSCs) can be added to the hydrogel matrix, or peripheral cartilage cells can be recruited to the defect area, where they generate new cartilage tissue to replace the degraded hydrogel smatrix. Therefore, the signaling pathway from stem cells to specific chondrocytes and the specific stimulation mechanism in hydrogel must be further understood. Finally, injectable hydrogels need to be further studied at the clinical level, from experimental animals to human experiments, and thoroughly evaluated factors such as biocompatibility, degradability, and comfort of hydrogel materials.
Author contributions
SZ, YL, and ZH contributed to conception and design of the study. LJ organized the database. WZ performed the statistical analysis. YT wrote the first draft of the manuscript. JL, DY, QZ, and QB wrote sections of the manuscript. All authors contributed to manuscript revision, read, and approved the submitted version.
Funding
This study was supported by Professor QB. National Natural Science Foundation of China (Grant No. 81672769); QZ. Prof. Medical Health Science and Technology Project of Zhejiang Provincial Health Commission (Grant 2021KY028) and Basic Public Welfare Research Program of Zhejiang Province (Grant LGF22H060029) and DY. Prof. Medical Health Science and Technology Project of Zhejiang Provincial Health Commission (Grant 2020KY018).
Conflict of interest
The authors declare that the research was conducted in the absence of any commercial or financial relationships that could be construed as a potential conflict of interest.
Publisher’s note
All claims expressed in this article are solely those of the authors and do not necessarily represent those of their affiliated organizations, or those of the publisher, the editors and the reviewers. Any product that may be evaluated in this article, or claim that may be made by its manufacturer, is not guaranteed or endorsed by the publisher.
References
Abasalizadeh, F., Moghaddam, S. V., Alizadeh, E., akbari, E., Kashani, E., Fazljou, S. M. B., et al. (2020). Alginate-based hydrogels as drug delivery vehicles in cancer treatment and their applications in wound dressing and 3D bioprinting. J. Biol. Eng. 14, 8. doi:10.1186/s13036-020-0227-7
Abdulghani, S., and Morouço, P. G. (2019). Biofabrication for osteochondral tissue regeneration: Bioink printability requirements. J. Mat. Sci. Mat. Med. 30 (2), 20. doi:10.1007/s10856-019-6218-x
Ahmadian-Fard-Fini, S., Ghanbari, D., Amiri, O., and Salavati-Niasari, M. (2020). Electro-spinning of cellulose acetate nanofibers/Fe/carbon dot as photoluminescence sensor for mercury (II) and lead (II) ions. Carbohydr. Polym. 229, 115428. doi:10.1016/j.carbpol.2019.115428
Ali, F., Khan, I., Chen, J., Akhtar, K., Bakhsh, E. M., and Khan, S. B. (2022). Emerging fabrication strategies of hydrogels and its applications. Gels 8 (4), 205. doi:10.3390/gels8040205
Aminabhavi, T. M., Nadagouda, M. N., More, U. A., Joshi, S. D., Kulkarni, V. H., Noolvi, M. N., et al. (2015). Controlled release of therapeutics using interpenetrating polymeric networks. Expert Opin. Drug Deliv. 12 (4), 669–688. doi:10.1517/17425247.2014.974871
Amiri, M., Salavati-Niasari, M., Pardakhty, A., Ahmadi, M., and Akbari, A. (2017). Caffeine: A novel green precursor for synthesis of magnetic CoFe2O4 nanoparticles and pH-sensitive magnetic alginate beads for drug delivery. Mat. Sci. Eng. C Mat. Biol. Appl. 76, 1085–1093. doi:10.1016/j.msec.2017.03.208
Antoniuk, I., and Amiel, C. (2016). Cyclodextrin-mediated hierarchical self-assembly and its potential in drug delivery applications. J. Pharm. Sci. 105 (9), 2570–2588. doi:10.1016/j.xphs.2016.05.010
Arkenberg, M. R., Nguyen, H. D., and Lin, C-C. (2020). Recent advances in bio-orthogonal and dynamic crosslinking of biomimetic hydrogels. J. Mat. Chem. B 8 (35), 7835–7855. doi:10.1039/d0tb01429j
Armiento, A. R., Alini, M., and Stoddart, M. J. (2019). Articular fibrocartilage - why does hyaline cartilage fail to repair? Adv. Drug Deliv. Rev. 146, 289–305. doi:10.1016/j.addr.2018.12.015
Armiento, A. R., Stoddart, M. J., Alini, M., and Eglin, D. (2018). Biomaterials for articular cartilage tissue engineering: Learning from biology. Acta Biomater. 65, 1–20. doi:10.1016/j.actbio.2017.11.021
Asadi, N., Alizadeh, E., Salehi, R., Khalandi, B., Davaran, S., and Akbarzadeh, A. (2018). Nanocomposite hydrogels for cartilage tissue engineering: A review. Artif. Cells Nanomed. Biotechnol. 46 (3), 465–471. doi:10.1080/21691401.2017.1345924
Balakrishnan, B., Joshi, N., Jayakrishnan, A., and Banerjee, R. (2014). Self-crosslinked oxidized alginate/gelatin hydrogel as injectable, adhesive biomimetic scaffolds for cartilage regeneration. Acta Biomater. 10 (8), 3650–3663. doi:10.1016/j.actbio.2014.04.031
Behan, K., Dufour, A., Garcia, O., and Kelly, D. (2022). Methacrylated cartilage ECM-based hydrogels as injectables and bioinks for cartilage tissue engineering. Biomolecules 12 (2), 216. doi:10.3390/biom12020216
Bellavia, D., Veronesi, F., Carina, V., Costa, V., Raimondi, L., De Luca, A., et al. (2018). Gene therapy for chondral and osteochondral regeneration: Is the future now? Cell. Mol. Life Sci. 75 (4), 649–667. doi:10.1007/s00018-017-2637-3
Bhattacharjee, M., Escobar Ivirico, J. L., Kan, H-M., Shah, S., Otsuka, T., Bordett, R., et al. (2022). Injectable amnion hydrogel-mediated delivery of adipose-derived stem cells for osteoarthritis treatment. Proc. Natl. Acad. Sci. U. S. A. 119 (4), e2120968119. doi:10.1073/pnas.2120968119
Bhattacharjee, M., Ivirico, J. L. E., Kan, H-M., Bordett, R., Pandey, R., Otsuka, T., et al. (2020). Preparation and characterization of amnion hydrogel and its synergistic effect with adipose derived stem cells towards IL1β activated chondrocytes. Sci. Rep. 10 (1), 18751. doi:10.1038/s41598-020-75921-w
Bian, J., Cai, F., Chen, H., Tang, Z., Xi, K., Tang, J., et al. (2021). Modulation of local overactive inflammation via injectable hydrogel microspheres. Nano Lett. 21 (6), 2690–2698. doi:10.1021/acs.nanolett.0c04713
Blaney Davidson, E. N., van der Kraan, P. M., and van den Berg, W. B. (2007). TGF-beta and osteoarthritis. Osteoarthr. Cartil. 15 (6), 597–604. doi:10.1016/j.joca.2007.02.005
Bordbar, S., Lotfi Bakhshaiesh, N., Khanmohammadi, M., Sayahpour, F. A., Alini, M., and Baghaban Eslaminejad, M. (2020). Production and evaluation of decellularized extracellular matrix hydrogel for cartilage regeneration derived from knee cartilage. J. Biomed. Mat. Res. A 108 (4), 938–946. doi:10.1002/jbm.a.36871
Boyer, C., Réthoré, G., Weiss, P., d’Arros, C., Lesoeur, J., Vinatier, C., et al. (2020). A self-setting hydrogel of silylated chitosan and cellulose for the repair of osteochondral defects: From in vitro characterization to preclinical evaluation in dogs. Front. Bioeng. Biotechnol. 8, 23. doi:10.3389/fbioe.2020.00023
Branco, A. C., Oliveira, A. S., Monteiro, I., Nolasco, P., Silva, D. C., Figueiredo-Pina, C. G., et al. (2022). PVA-based hydrogels loaded with diclofenac for cartilage replacement. Gels 8 (3), 143. doi:10.3390/gels8030143
Bustamante-Torres, M., Romero-Fierro, D., Arcentales-Vera, B., Palomino, K., Magana, H., and Bucio, E. (2021). Hydrogels classification according to the physical or chemical interactions and as stimuli-sensitive materials. Gels 7 (4), 182. doi:10.3390/gels7040182
Cai, G., Liu, W., He, Y., Huang, J., Duan, L., Xiong, J., et al. (2019). Recent advances in kartogenin for cartilage regeneration. J. Drug Target. 27 (1), 28–32. doi:10.1080/1061186X.2018.1464011
Cai, H., Wang, P., Xu, Y., Yao, Y., Liu, J., Li, T., et al. (2020). BMSCs-assisted injectable Col I hydrogel-regenerated cartilage defect by reconstructing superficial and calcified cartilage. Regen. Biomater. 7 (1), 35–45. doi:10.1093/rb/rbz028
Cai, Z., Li, Y., Song, W., He, Y., Li, H., and Liu, X. (2021). Anti-inflammatory and prochondrogenic in situ-formed injectable hydrogel crosslinked by strontium-doped bioglass for cartilage regeneration. ACS Appl. Mat. Interfaces 13 (50), 59772–59786. doi:10.1021/acsami.1c20565
Campos, Y., Almirall, A., Fuentes, G., Bloem, H. L., Kaijzel, E. L., and Cruz, L. J. (2019). Tissue engineering: An alternative to repair cartilage. Tissue Eng. Part B Rev. 25 (4), 357–373. doi:10.1089/ten.TEB.2018.0330
Cao, J., Su, J., An, M., Yang, Y., Zhang, Y., Zuo, J., et al. (2021). Correction to "novel DEK-targeting aptamer delivered by a hydrogel microneedle attenuates collagen-induced arthritis. Mol. Pharm. 18 (11), 4231. doi:10.1021/acs.molpharmaceut.1c00732
Cao, J., Su, J., An, M., Yang, Y., Zhang, Y., Zuo, J., et al. (2021). Novel DEK-targeting aptamer delivered by a hydrogel microneedle attenuates collagen-induced arthritis. Mol. Pharm. 18 (1), 305–316. doi:10.1021/acs.molpharmaceut.0c00954
Castro-Viñuelas, R., Sanjurjo-Rodríguez, C., Piñeiro-Ramil, M., Hermida-Gomez, T., Fuentes-Boquete, I., de Toro-Santos, F., et al. (2018). Induced pluripotent stem cells for cartilage repair: Current status and future perspectives. Eur. Cell. Mat. 36, 96–109. doi:10.22203/eCM.v036a08
Chang, Y-H., Wu, K-C., and Ding, D-C. (2020). Induced pluripotent stem cell-differentiated chondrocytes repair cartilage defect in a rabbit osteoarthritis model. Stem Cells Int. 2020, 1–16. doi:10.1155/2020/8867349
Chen, J., Yang, J., Wang, L., Zhang, X., Heng, B. C., Wang, D. A., et al. (2021). Modified hyaluronic acid hydrogels with chemical groups that facilitate adhesion to host tissues enhance cartilage regeneration. Bioact. Mat. 6 (6), 1689–1698. doi:10.1016/j.bioactmat.2020.11.020
Chen, W., Li, C., Peng, M., Xie, B., Zhang, L., and Tang, X. (2018). Autologous nasal chondrocytes delivered by injectable hydrogel for in vivo articular cartilage regeneration. Cell Tissue Bank. 19 (1), 35–46. doi:10.1007/s10561-017-9649-y
Chiang, M-Y., Cheng, I. Y., Chou, S-H., Tsai, J. H., Chen, Y. J., Lu, H. E., et al. (2021). A smart injectable composite hydrogel with magnetic navigation and controlled glutathione release for promoting in situ chondrocyte array and self-healing in damaged cartilage tissue. J. Mat. Chem. B 9 (45), 9370–9382. doi:10.1039/d1tb02030g
Cho, H., Kim, J., Kim, S., Jung, Y. C., Wang, Y., Kang, B. J., et al. (2020). Dual delivery of stem cells and insulin-like growth factor-1 in coacervate-embedded composite hydrogels for enhanced cartilage regeneration in osteochondral defects. J. Control. Release 327, 284–295. doi:10.1016/j.jconrel.2020.08.002
Choi, J. H., Park, A., Lee, W., Youn, J., Rim, M. A., Kim, W., et al. (2020). Preparation and characterization of an injectable dexamethasone-cyclodextrin complexes-loaded gellan gum hydrogel for cartilage tissue engineering. J. Control. Release 327, 747–765. doi:10.1016/j.jconrel.2020.08.049
Coenen, A. M. J., Bernaerts, K. V., Harings, J. A. W., Jockenhoevel, S., and Ghazanfari, S. (2018). Elastic materials for tissue engineering applications: Natural, synthetic, and hybrid polymers. Acta Biomater. 79, 60–82. doi:10.1016/j.actbio.2018.08.027
Cross, L. M., Thakur, A., Jalili, N. A., Detamore, M., and Gaharwar, A. K. (2016). Nanoengineered biomaterials for repair and regeneration of orthopedic tissue interfaces. Acta Biomater. 42, 2–17. doi:10.1016/j.actbio.2016.06.023
Csobonyeiova, M., Polak, S., Nicodemou, A., Zamborsky, R., and Danisovic, L. (2021). iPSCs in modeling and therapy of osteoarthritis. Biomedicines 9 (2), 186. doi:10.3390/biomedicines9020186
Das, S. K., and Farooqi, A. (2008). Osteoarthritis. Best. Pract. Res. Clin. Rheumatol. 22 (4), 657–675. doi:10.1016/j.berh.2008.07.002
Davar, F., Salavati-Niasari, M., and Fereshteh, Z. (2010). Synthesis and characterization of SnO2 nanoparticles by thermal decomposition of new inorganic precursor. J. Alloys Compd. 496 (1), 638–643. doi:10.1016/j.jallcom.2010.02.152
De France, K. J., Chan, K. J. W., Cranston, E. D., and Hoare, T. (2016). Enhanced mechanical properties in cellulose nanocrystal-poly(oligoethylene glycol methacrylate) injectable nanocomposite hydrogels through control of physical and chemical cross-linking. Biomacromolecules 17 (2), 649–660. doi:10.1021/acs.biomac.5b01598
Dehghan-Baniani, D., Chen, Y., Wang, D., Bagheri, R., Solouk, A., and Wu, H. (2020). Injectable in situ forming kartogenin-loaded chitosan hydrogel with tunable rheological properties for cartilage tissue engineering. Colloids Surfaces B Biointerfaces 192, 111059. doi:10.1016/j.colsurfb.2020.111059
Deng, S., Huang, R., Wang, J., Zhang, S., Chen, Z., Wu, S., et al. (2014). Miscellaneous animal models accelerate the application of mesenchymal stem cells for cartilage regeneration. Curr. Stem Cell Res. Ther. 9 (3), 223–233. doi:10.2174/1574888x09666140213201331
Deng, Z., Jin, J., Wang, S., Qi, F., Chen, X., Liu, C., et al. (2020). Narrative review of the choices of stem cell sources and hydrogels for cartilage tissue engineering. Ann. Transl. Med. 8 (23), 1598. doi:10.21037/atm-20-2342
Deng, Z. H., Li, Y. S., Gao, X., Lei, G., and Huard, J. (2018). Bone morphogenetic proteins for articular cartilage regeneration. Osteoarthr. Cartil. 26 (9), 1153–1161. doi:10.1016/j.joca.2018.03.007
Dethe, M. R., Prabakaran, A., Ahmed, H., Agrawal, M., Roy, U., and Alexander, A. (2022). PCL-PEG copolymer based injectable thermosensitive hydrogels. J. Control. Release 343, 217–236. doi:10.1016/j.jconrel.2022.01.035
Dhand, A. P., Galarraga, J. H., and Burdick, J. A. (2021). Enhancing biopolymer hydrogel functionality through interpenetrating networks. Trends Biotechnol. 39 (5), 519–538. doi:10.1016/j.tibtech.2020.08.007
Dimatteo, R., Darling, N. J., and Segura, T. (2018). In situ forming injectable hydrogels for drug delivery and wound repair. Adv. Drug Deliv. Rev. 127, 167–184. doi:10.1016/j.addr.2018.03.007
Ding, N., Li, E., Ouyang, X., Guo, J., and Wei, B. (2021). The therapeutic potential of bone marrow mesenchymal stem cells for articular cartilage regeneration in osteoarthritis. Curr. Stem Cell Res. Ther. 16 (7), 840–847. doi:10.2174/1574888X16666210127130044
Echave, M. C., Hernáez-Moya, R., Iturriaga, L., Pedraz, J. L., Lakshminarayanan, R., Dolatshahi-Pirouz, A., et al. (2019). Recent advances in gelatin-based therapeutics. Expert Opin. Biol. Ther. 19 (8), 773–779. doi:10.1080/14712598.2019.1610383
Elkhoury, K., Morsink, M., Sanchez-Gonzalez, L., Kahn, C., Tamayol, A., and Arab-Tehrany, E. (2021). Biofabrication of natural hydrogels for cardiac, neural, and bone Tissue engineering Applications. Bioact. Mat. 6 (11), 3904–3923. doi:10.1016/j.bioactmat.2021.03.040
Eslahi, N., Abdorahim, M., and Simchi, A. (2016). Smart polymeric hydrogels for cartilage tissue engineering: A review on the chemistry and biological functions. Biomacromolecules 17 (11), 3441–3463. doi:10.1021/acs.biomac.6b01235
Eyre, D. R. (2004). Collagens and cartilage matrix homeostasis. Clin. Orthop. Relat. Res. 427 (Suppl. l), S118–S122. doi:10.1097/01.blo.0000144855.48640.b9
Fan, W., Yuan, L., Li, J., Wang, Z., Chen, J., Guo, C., et al. (2020). Injectable double-crosslinked hydrogels with kartogenin-conjugated polyurethane nano-particles and transforming growth factor β3 for in-situ cartilage regeneration. Mater. Sci. Eng. C 110, 110705. doi:10.1016/j.msec.2020.110705
Farrell, K., Joshi, J., and Kothapalli, C. R. (2017). Injectable uncrosslinked biomimetic hydrogels as candidate scaffolds for neural stem cell delivery. J. Biomed. Mat. Res. A 105 (3), 790–805. doi:10.1002/jbm.a.35956
Fattahpour, S., Shamanian, M., Tavakoli, N., Fathi, M., Sadeghi-aliabadi, H., Sheykhi, S. R., et al. (2020). An injectable carboxymethyl chitosan-methylcellulose-pluronic hydrogel for the encapsulation of meloxicam loaded nanoparticles. Int. J. Biol. Macromol. 151, 220–229. doi:10.1016/j.ijbiomac.2020.02.002
Flégeau, K., Pace, R., Gautier, H., Rethore, G., Guicheux, J., Le Visage, C., et al. (2017). Toward the development of biomimetic injectable and macroporous biohydrogels for regenerative medicine. Adv. Colloid Interface Sci. 247, 589–609. doi:10.1016/j.cis.2017.07.012
Fu, L., Li, P., Zhu, J., Liao, Z., Gao, C., Li, H., et al. (2022). Tetrahedral framework nucleic acids promote the biological functions and related mechanism of synovium-derived mesenchymal stem cells and show improved articular cartilage regeneration activity in situ. Bioact. Mat. 9, 411–427. doi:10.1016/j.bioactmat.2021.07.028
Fu, N., Dong, T., Meng, A., Meng, Z., Zhu, B., and Lin, Y. (2018). Research progress of the types and preparation techniques of scaffold materials in cartilage tissue engineering. Curr. Stem Cell Res. Ther. 13 (7), 583–590. doi:10.2174/1574888X12666170718152611
Fuggle, N. R., Cooper, C., Oreffo, R. O. C., Price, A. J., Kaux, J. F., Maheu, E., et al. (2020). Alternative and complementary therapies in osteoarthritis and cartilage repair. Aging Clin. Exp. Res. 32 (4), 547–560. doi:10.1007/s40520-020-01515-1
Gao, Y., Li, Z., Huang, J., Zhao, M., and Wu, J. (2020). In situ formation of injectable hydrogels for chronic wound healing. J. Mat. Chem. B 8 (38), 8768–8780. doi:10.1039/d0tb01074j
Gao, Y., Liu, S., Huang, J., Guo, W., Chen, J., Zhang, L., et al. (2014). The ECM-cell interaction of cartilage extracellular matrix on chondrocytes. Biomed. Res. Int. 2014, 1–8. doi:10.1155/2014/648459
García-Couce, J., Tomás, M., Fuentes, G., Que, I., Almirall, A., and Cruz, L. J. (2022). Chitosan/Pluronic F127 thermosensitive hydrogel as an injectable dexamethasone delivery carrier. Gels 8 (1), 44. doi:10.3390/gels8010044
García-Fernández, L., Olmeda-Lozano, M., Benito-Garzón, L., Perez-Caballer, A., San Roman, J., and Vazquez-Lasa, B. (2020). Injectable hydrogel-based drug delivery system for cartilage regeneration. Mater. Sci. Eng. C 110, 110702. doi:10.1016/j.msec.2020.110702
Gomez-Florit, M., Pardo, A., Domingues, R. M. A., Graca, A. L., Babo, P. S., Reis, R. L., et al. (2020). Natural-based hydrogels for tissue engineering applications. Molecules 25 (24), 5858. doi:10.3390/molecules25245858
Gong, D., Yu, F., Zhou, M., Dong, W., Yan, D., Zhang, S., et al. (2021). Ex vivo and in vivo properties of an injectable hydrogel derived from acellular ear cartilage extracellular matrix. Front. Bioeng. Biotechnol. 9, 740635. doi:10.3389/fbioe.2021.740635
Gonzalez-Fernandez, P., Rodríguez-Nogales, C., Jordan, O., and Allemann, E. (2022). Combination of mesenchymal stem cells and bioactive molecules in hydrogels for osteoarthritis treatment. Eur. J. Pharm. Biopharm. 172, 41–52. doi:10.1016/j.ejpb.2022.01.003
Gopinathan, J., and Noh, I. (2018). Click chemistry-based injectable hydrogels and bioprinting inks for tissue engineering applications. Tissue Eng. Regen. Med. 15 (5), 531–546. doi:10.1007/s13770-018-0152-8
Graça, M. F. P., Miguel, S. P., Cabral, C. S. D., and Correia, I. J. (2020). Hyaluronic acid-based wound dressings: A review. Carbohydr. Polym. 241, 116364. doi:10.1016/j.carbpol.2020.116364
Grol, M. W., and Lee, B. H. (2018). Gene therapy for repair and regeneration of bone and cartilage. Curr. Opin. Pharmacol. 40, 59–66. doi:10.1016/j.coph.2018.03.005
Guo, Y., Gu, J., Jiang, Y., Zhou, Y., Zhu, Z., Ma, T., et al. (2021). Regulating the homogeneity of thiol-maleimide michael-type Addition-based hydrogels using amino biomolecules. Gels 7 (4), 206. doi:10.3390/gels7040206
Gupta, D., Gangwar, A., Jyoti, K., Sainaga Jyothi, V. G., Sodhi, R. K., Mehra, N. K., et al. (2020). Self healing hydrogels: A new paradigm immunoadjuvant for delivering peptide vaccine. Colloids Surfaces B Biointerfaces 194, 111171. doi:10.1016/j.colsurfb.2020.111171
Han, Y., Yang, J., Zhao, W., Wang, H., Sun, Y., Chen, Y., et al. (2021). Biomimetic injectable hydrogel microspheres with enhanced lubrication and controllable drug release for the treatment of osteoarthritis. Bioact. Mat. 6 (10), 3596–3607. doi:10.1016/j.bioactmat.2021.03.022
Hanafy, A. S., and El-Ganainy, S. O. (2020). Thermoresponsive Hyalomer intra-articular hydrogels improve monoiodoacetate-induced osteoarthritis in rats. Int. J. Pharm. X. 573, 118859. doi:10.1016/j.ijpharm.2019.118859
Hassanpour, M., Safardoust-Hojaghan, H., and Salavati-Niasari, M. (2017). Degradation of methylene blue and Rhodamine B as water pollutants via green synthesized Co3O4/ZnO nanocomposite. J. Mol. Liq. 229, 293–299. doi:10.1016/j.molliq.2016.12.090
He, C., Ji, H., Qian, Y., Wang, Q., Liu, X., Zhao, W., et al. (2019). Heparin-based and heparin-inspired hydrogels: Size-effect, gelation and biomedical applications. J. Mat. Chem. B 7 (8), 1186–1208. doi:10.1039/c8tb02671h
He, P., Fu, J., and Wang, D-A. (2016). Murine pluripotent stem cells derived scaffold-free cartilage grafts from a micro-cavitary hydrogel platform. Acta Biomater. 35, 87–97. doi:10.1016/j.actbio.2016.02.026
Heirani-Tabasi, A., Hosseinzadeh, S., Rabbani, S., Ahmadi Tafti, S. H., Jamshidi, K., Soufizomorrod, M., et al. (2021). Cartilage tissue engineering by co-transplantation of chondrocyte extracellular vesicles and mesenchymal stem cells, entrapped in chitosan-hyaluronic acid hydrogel. Biomed. Mat. 16 (5), 055003. doi:10.1088/1748-605X/ac0cbf
Heo, D. N., Kim, H-J., Lee, D., Lee, S. J., Lee, H. R., Kim, H., et al. (2020). Comparison of polysaccharides in articular cartilage regeneration associated with chondrogenic and autophagy-related gene expression. Int. J. Biol. Macromol. 146, 922–930. doi:10.1016/j.ijbiomac.2019.09.215
Hu, C., Lu, W., Mata, A., Nishinari, K., and Fang, Y. (2021). Ions-induced gelation of alginate: Mechanisms and applications. Int. J. Biol. Macromol. 177, 578–588. doi:10.1016/j.ijbiomac.2021.02.086
Hu, M., Guo, J., Du, J., Liu, Z., Li, P., Ren, X., et al. (2019). Development of Ca2+-based, ion-responsive superabsorbent hydrogel for cement applications: Self-healing and compressive strength. J. Colloid Interface Sci. 538, 397–403. doi:10.1016/j.jcis.2018.12.004
Hu, M., Yang, J., and Xu, J. (2021). Structural and biological investigation of chitosan/hyaluronic acid with silanized-hydroxypropyl methylcellulose as an injectable reinforced interpenetrating network hydrogel for cartilage tissue engineering. Drug Deliv. (Lond). 28 (1), 607–619. doi:10.1080/10717544.2021.1895906
Huang, J., Liu, F., Su, H., Xiong, J., Yang, L., Xia, J., et al. (2022). Advanced nanocomposite hydrogels for cartilage tissue engineering. Gels 8 (2), 138. doi:10.3390/gels8020138
Huang, K., Li, Q., Li, Y., Yao, Z., Luo, D., Rao, P., et al. (2018). Cartilage tissue regeneration: The roles of cells, stimulating factors and scaffolds. Curr. Stem Cell Res. Ther. 13 (7), 547–567. doi:10.2174/1574888X12666170608080722
Huang, Q., Zou, Y., Arno, M. C., Chen, S., Wang, T., Gao, J., et al. (2017). Hydrogel scaffolds for differentiation of adipose-derived stem cells. Chem. Soc. Rev. 46 (20), 6255–6275. doi:10.1039/c6cs00052e
Im, G-I., and Shin, K-J. (2015). Epigenetic approaches to regeneration of bone and cartilage from stem cells. Expert Opin. Biol. Ther. 15 (2), 181–193. doi:10.1517/14712598.2015.960838
Ishihara, M., Kishimoto, S., Nakamura, S., Sato, Y., and Hattori, H. (2019). Polyelectrolyte complexes of natural polymers and their biomedical applications. Polym. (Basel) 11 (4), 672. doi:10.3390/polym11040672
Jabbari, E., and Sepahvandi, A. (2022). Decellularized articular cartilage microgels as microcarriers for expansion of mesenchymal stem cells. Gels 8 (3), 148. doi:10.3390/gels8030148
Jaikumar, D., Sajesh, K. M., Soumya, S., Nimal, T., Chennazhi, K., Nair, S. V., et al. (2015). Injectable alginate-O-carboxymethyl chitosan/nano fibrin composite hydrogels for adipose tissue engineering. Int. J. Biol. Macromol. 74, 318–326. doi:10.1016/j.ijbiomac.2014.12.037
Jalani, G., Rosenzweig, D. H., Makhoul, G., Abdalla, S., Cecere, R., Vetrone, F., et al. (2015). Tough, in-situ thermogelling, injectable hydrogels for biomedical applications. Macromol. Biosci. 15 (4), 473–480. doi:10.1002/mabi.201400406
Jeznach, O., Kołbuk, D., and Sajkiewicz, P. (2018). Injectable hydrogels and nanocomposite hydrogels for cartilage regeneration. J. Biomed. Mat. Res. A 106 (10), 2762–2776. doi:10.1002/jbm.a.36449
Ji, X., Lei, Z., Yuan, M., Zhu, H., Yuan, X., Liu, W., et al. (2020). Cartilage repair mediated by thermosensitive photocrosslinkable TGFβ1-loaded GM-HPCH via immunomodulating macrophages, recruiting MSCs and promoting chondrogenesis. Theranostics 10 (6), 2872–2887. doi:10.7150/thno.41622
Jian, F., Zhang, Y., Wang, J., Ba, K., Mao, R., Lai, W., et al. (2012). Toxicity of biodegradable nanoscale preparations. Curr. Drug Metab. 13 (4), 440–446. doi:10.2174/138920012800166517
Jiang, T., Yang, T., Bao, Q., Sun, W., Yang, M., and Mao, C. (2021). Construction of tissue-customized hydrogels from cross-linkable materials for effective tissue regeneration. J. Mat. Chem. B 2021. doi:10.1039/d1tb01935j
Jiang, Y., Cai, Y., Zhang, W., Yin, Z., Hu, C., Tong, T., et al. (2016). Human cartilage-derived progenitor cells from committed chondrocytes for efficient cartilage repair and regeneration. Stem Cells Transl. Med. 5 (6), 733–744. doi:10.5966/sctm.2015-0192
Jiang, Y., and Tuan, R. S. (2015). Origin and function of cartilage stem/progenitor cells in osteoarthritis. Nat. Rev. Rheumatol. 11 (4), 206–212. doi:10.1038/nrrheum.2014.200
Jin, R., Teixeira, L. S. M., Dijkstra, P. J., van Blitterswijk, C., Karperien, M., and Feijen, J. (2010). Enzymatically-crosslinked injectable hydrogels based on biomimetic dextran-hyaluronic acid conjugates for cartilage tissue engineering. Biomaterials 31 (11), 3103–3113. doi:10.1016/j.biomaterials.2010.01.013
Jin, Y., Koh, R. H., Kim, S-H., Kim, K. M., Park, G. K., and Hwang, N. S. (2020). Injectable anti-inflammatory hyaluronic acid hydrogel for osteoarthritic cartilage repair. Mater. Sci. Eng. C 115, 111096. doi:10.1016/j.msec.2020.111096
Johnstone, B., Stoddart, M. J., and Im, G-I. (2020). Multi-disciplinary approaches for cell-based cartilage regeneration. J. Orthop. Res. 38 (3), 463–472. doi:10.1002/jor.24458
Jonidi Shariatzadeh, F., Solouk, A., Bagheri Khoulenjani, S., Bonakdar, S., and Mirzadeh, H. (2021). Injectable and reversible preformed cryogels based on chemically crosslinked gelatin methacrylate (GelMA) and physically crosslinked hyaluronic acid (HA) for soft tissue engineering. Colloids Surfaces B Biointerfaces 203, 111725. doi:10.1016/j.colsurfb.2021.111725
Kahn, J. S., Hu, Y., and Willner, I. (2017). Stimuli-responsive DNA-based hydrogels: From basic principles to applications. Acc. Chem. Res. 50 (4), 680–690. doi:10.1021/acs.accounts.6b00542
Katz, J. N., Arant, K. R., and Loeser, R. F. (2021). Diagnosis and treatment of hip and knee osteoarthritis: A review. JAMA 325 (6), 568. doi:10.1001/jama.2020.22171
Khajouei, S., Ravan, H., and Ebrahimi, A. (2020). DNA hydrogel-empowered biosensing. Adv. Colloid Interface Sci. 275, 102060. doi:10.1016/j.cis.2019.102060
Khan, F., Atif, M., Haseen, M., Kamal, S., Khan, M. S., Shahid, S., et al. (2022). Synthesis, classification and properties of hydrogels: Their applications in drug delivery and agriculture. J. Mat. Chem. B 10 (2), 170–203. doi:10.1039/d1tb01345a
Kim, D. Y., Park, H., Kim, S. W., Lee, J. W., and Lee, K. Y. (2017). Injectable hydrogels prepared from partially oxidized hyaluronate and glycol chitosan for chondrocyte encapsulation. Carbohydr. Polym. 157, 1281–1287. doi:10.1016/j.carbpol.2016.11.002
Kim, I. L., Mauck, R. L., and Burdick, J. A. (2011). Hydrogel design for cartilage tissue engineering: A case study with hyaluronic acid. Biomaterials 32 (34), 8771–8782. doi:10.1016/j.biomaterials.2011.08.073
Kim, Y. S., Mehta, S. M., Guo, J. L., Pearce, H. A., Smith, B. T., Watson, E., et al. (2021). Evaluation of tissue integration of injectable, cell-laden hydrogels of cocultures of mesenchymal stem cells and articular chondrocytes with an ex vivo cartilage explant model. Biotechnol. Bioeng. 118 (8), 2958–2966. doi:10.1002/bit.27804
Kouser, R., Vashist, A., Zafaryab, M., Rizvi, M. A., and Ahmad, S. (2018). Biocompatible and mechanically robust nanocomposite hydrogels for potential applications in tissue engineering. Mater. Sci. Eng. C 84, 168–179. doi:10.1016/j.msec.2017.11.018
Krishnan, Y., and Grodzinsky, A. J. (2018). Cartilage diseases. Matrix Biol. 71-72, 51–69. doi:10.1016/j.matbio.2018.05.005
Kwon, H., Brown, W. E., Lee, C. A., Wang, D., Paschos, N., Hu, J. C., et al. (2019). Surgical and tissue engineering strategies for articular cartilage and meniscus repair. Nat. Rev. Rheumatol. 15 (9), 550–570. doi:10.1038/s41584-019-0255-1
Lee, C., O'Connell, C. D., Onofrillo, C., Choong, P. F. M., Di Bella, C., and Duchi, S. (2020). Human articular cartilage repair: Sources and detection of cytotoxicity and genotoxicity in photo-crosslinkable hydrogel bioscaffolds. Stem Cells Transl. Med. 9 (3), 302–315. doi:10.1002/sctm.19-0192
Lee, K. Y., and Mooney, D. J. (2012). Alginate: Properties and biomedical applications. Prog. Polym. Sci. 37 (1), 106–126. doi:10.1016/j.progpolymsci.2011.06.003
Lee, S., Choi, J., Youn, J., Lee, Y., Kim, W., Choe, S., et al. (2021). Development and evaluation of gellan gum/silk fibroin/chondroitin sulfate ternary injectable hydrogel for cartilage tissue engineering. Biomolecules 11 (8), 1184. doi:10.3390/biom11081184
Lei, Y., Wang, Y., Shen, J., Cai, Z., Zhao, C., Chen, H., et al. (2022). Injectable hydrogel microspheres with self-renewable hydration layers alleviate osteoarthritis. Sci. Adv. 8 (5), eabl6449. doi:10.1126/sciadv.abl6449
Li, F., Lyu, D., Liu, S., and Guo, W. (2020). DNA hydrogels and microgels for biosensing and biomedical applications. Adv. Mat. 32 (3), e1806538. doi:10.1002/adma.201806538
Li, J., Chen, G., Xu, X., Abdou, P., Jiang, Q., Shi, D., et al. (2019). Advances of injectable hydrogel-based scaffolds for cartilage regeneration. Regen. Biomater. 6 (3), 129–140. doi:10.1093/rb/rbz022
Li, J., Lai, Y., Li, M., Chen, X., Zhou, M., Wang, W., et al. (2022). Repair of infected bone defect with Clindamycin-Tetrahedral DNA nanostructure Complex-loaded 3D bioprinted hybrid scaffold. Chem. Eng. J. 435, 134855. doi:10.1016/j.cej.2022.134855
Li, J., Liu, N., Huang, Z., Wang, W., Hou, D., and Wang, W. (2021). Intra-articular injection of loaded sPL sustained-release microspheres inhibits osteoarthritis and promotes cartilaginous repairs. J. Orthop. Surg. Res. 16 (1), 646. doi:10.1186/s13018-021-02777-9
Li, X., Sigen, A., Xu, Q., Alshehri, F., Zeng, M., Zhou, D., et al. (2020). Cartilage-derived progenitor cell-laden injectable hydrogel-an approach for cartilage tissue regeneration. ACS Appl. Bio Mat. 3 (8), 4756–4765. doi:10.1021/acsabm.0c00294
Li, X., Lu, Y., Wang, Y., Zhou, S., Li, L., and Zhao, F. (2021). Thermo-responsive injectable naringin-loaded hydrogel polymerised sodium alginate/bioglass delivery for articular cartilage. Drug Deliv. (Lond). 28 (1), 1290–1300. doi:10.1080/10717544.2021.1938752
Li, X., Xu, Q., Johnson, M., Wang, X., Lyu, J., Li, Y., et al. (2021). A chondroitin sulfate based injectable hydrogel for delivery of stem cells in cartilage regeneration. Biomater. Sci. 9 (11), 4139–4148. doi:10.1039/d1bm00482d
Li, Y., Rodrigues, J., and Tomás, H. (2012). Injectable and biodegradable hydrogels: Gelation, biodegradation and biomedical applications. Chem. Soc. Rev. 41 (6), 2193–2221. doi:10.1039/c1cs15203c
Li, Y., Wang, X., Han, Y., Sun, H. Y., Hilborn, J., and Shi, L. (2021). Click chemistry-based biopolymeric hydrogels for regenerative medicine. Biomed. Mat. 16 (2), 022003. doi:10.1088/1748-605X/abc0b3
Lim, M. H., Jeun, J. H., Kim, D. H., Park, S. H., Kim, S. J., Lee, W. S., et al. (2020). Evaluation of collagen gel-associated human nasal septum-derived chondrocytes as a clinically applicable injectable therapeutic agent for cartilage repair. Tissue Eng. Regen. Med. 17 (3), 387–399. doi:10.1007/s13770-020-00261-9
Lin, S-J., Chan, Y-C., Su, Z-C., Yeh, W., Lai, P., and Chu, I. (2021). Growth factor-loaded microspheres in mPEG-polypeptide hydrogel system for articular cartilage repair. J. Biomed. Mat. Res. A 109 (12), 2516–2526. doi:10.1002/jbm.a.37246
Lin, X., Tsao, C. T., Kyomoto, M., and Zhang, M. (2021). Injectable natural polymer hydrogels for treatment of knee osteoarthritis. Adv. Healthc. Mat. 11, e2101479. doi:10.1002/adhm.202101479
Liu, H-Y., and Lin, C-C. (2019). A diffusion-reaction model for predicting enzyme-mediated dynamic hydrogel stiffening. Gels 5 (1), 17. doi:10.3390/gels5010017
Liu, M., Zeng, X., Ma, C., Yi, H., Ali, Z., Mou, X., et al. (2017). Injectable hydrogels for cartilage and bone tissue engineering. Bone Res. 5, 17014. doi:10.1038/boneres.2017.14
Liu, R., Zhang, S., and Chen, X. (2020). Injectable hydrogels for tendon and ligament tissue engineering. J. Tissue Eng. Regen. Med. 14 (9), 1333–1348. doi:10.1002/term.3078
Liu, X., Liu, S., Yang, R., Wang, P., Zhang, W., Tan, X., et al. (2021). Gradient chondroitin sulfate/poly (γ-glutamic acid) hydrogels inducing differentiation of stem cells for cartilage tissue engineering. Carbohydr. Polym. 270, 118330. doi:10.1016/j.carbpol.2021.118330
Liu, X., Yang, Y., Li, Y., Niu, X., Zhao, B., Wang, Y., et al. (2017). Integration of stem cell-derived exosomes with in situ hydrogel glue as a promising tissue patch for articular cartilage regeneration. Nanoscale 9 (13), 4430–4438. doi:10.1039/c7nr00352h
Liu, Y., and Hsu, S-H. (2018). Synthesis and biomedical applications of self-healing hydrogels. Front. Chem. 6, 449. doi:10.3389/fchem.2018.00449
Liu, Y., Wang, M., Luo, Y., Liang, Q., Yu, Y., Chen, F., et al. (2021). Enhancing stem cell therapy for cartilage repair in osteoarthritis-A hydrogel focused approach. Gels 7 (4), 263. doi:10.3390/gels7040263
Lokhande, G., Carrow, J. K., Thakur, T., Xavier, J. R., Parani, M., Bayless, K. J., et al. (2018). Nanoengineered injectable hydrogels for wound healing application. Acta Biomater. 70, 35–47. doi:10.1016/j.actbio.2018.01.045
Lu, L., Yuan, S., Wang, J., Shen, Y., Deng, S., Xie, L., et al. (2018). the formation mechanism of hydrogels. Curr. Stem Cell Res. Ther. 13 (7), 490–496. doi:10.2174/1574888X12666170612102706
Luo, J., Zhang, Y., Zhu, S., Tong, Y., Ji, L., Zhang, W., et al. (2021). The application prospect of metal/metal oxide nanoparticles in the treatment of osteoarthritis. Schmiedeb. Arch. Pharmacol. 394 (10), 1991–2002. doi:10.1007/s00210-021-02131-0
Luu, C. H., Nguyen, G., Le, T-T., Nguyen, T. M. N., Giang Phan, V. H., Murugesan, M., et al. (2022). Graphene oxide-reinforced alginate hydrogel for controlled release of local anesthetics: Synthesis, characterization, and release studies. Gels 8 (4), 246. doi:10.3390/gels8040246
Lynch, B., Crawford, K., Baruti, O., Abdulahad, A., Webster, M., Puetzer, J., et al. (2017). The effect of hypoxia on thermosensitive poly(N-vinylcaprolactam) hydrogels with tunable mechanical integrity for cartilage tissue engineering. J. Biomed. Mat. Res. 105 (7), 1863–1873. doi:10.1002/jbm.b.33705
Ma, Q., Liao, J., and Cai, X. (2018). Different sources of stem cells and their application in cartilage tissue engineering. Curr. Stem Cell Res. Ther. 13 (7), 568–575. doi:10.2174/1574888X13666180122151909
Madry, H., and Cucchiarini, M. (2016). Gene therapy for human osteoarthritis: Principles and clinical translation. Expert Opin. Biol. Ther. 16 (3), 331–346. doi:10.1517/14712598.2016.1124084
Mahajan, A., Singh, A., Datta, D., and Katti, D. S. (2022). Bioinspired injectable hydrogels dynamically stiffen and contract to promote mechanosensing-mediated chondrogenic commitment of stem cells. ACS Appl. Mat. Interfaces 14 (6), 7531–7550. doi:10.1021/acsami.1c11840
Mang, T., Kleinschmidt-Dörr, K., Ploeger, F., Lindemann, S., and Gigout, A. (2020). The GDF-5 mutant M1673 exerts robust anabolic and anti-catabolic effects in chondrocytes. J. Cell. Mol. Med. 24 (13), 7141–7150. doi:10.1111/jcmm.15149
Mantooth, S. M., Munoz-Robles, B. G., and Webber, M. J. (2019). Dynamic hydrogels from host-guest supramolecular interactions. Macromol. Biosci. 19 (1), e1800281. doi:10.1002/mabi.201800281
Martin, A. R., Patel, J. M., Locke, R. C., Eby, M. R., Saleh, K. S., Davidson, M. D., et al. (2021). Nanofibrous hyaluronic acid scaffolds delivering TGF-β3 and SDF-1α for articular cartilage repair in a large animal model. Acta Biomater. 126, 170–182. doi:10.1016/j.actbio.2021.03.013
Mathew, A. P., Uthaman, S., Cho, K-H., Cho, C. S., and Park, I. K. (2018). Injectable hydrogels for delivering biotherapeutic molecules. Int. J. Biol. Macromol. 110, 17–29. doi:10.1016/j.ijbiomac.2017.11.113
Matricardi, P., Di Meo, C., Coviello, T., Hennink, W. E., and Alhaique, F. (2013). Interpenetrating Polymer Networks polysaccharide hydrogels for drug delivery and tissue engineering. Adv. Drug Deliv. Rev. 65 (9), 1172–1187. doi:10.1016/j.addr.2013.04.002
Mazini, L., Rochette, L., Amine, M., and Malka, G. (2019). Regenerative capacity of adipose derived stem cells (ADSCs), comparison with mesenchymal stem cells (MSCs). Int. J. Mol. Sci. 20 (10), 2523. doi:10.3390/ijms20102523
Mehrali, M., Thakur, A., Pennisi, C. P., Talebian, S., Arpanaei, A., Nikkhah, M., et al. (2017). Nanoreinforced hydrogels for tissue engineering: Biomaterials that are compatible with load-bearing and electroactive tissues. Adv. Mat. 29 (8), 1603612. doi:10.1002/adma.201603612
Meng, W., Gao, L., Venkatesan, J. K., Wang, G., Madry, H., and Cucchiarini, M. (2019). Translational applications of photopolymerizable hydrogels for cartilage repair. J. Exp. Orthop. 6 (1), 47. doi:10.1186/s40634-019-0215-3
Mohamed-Ahmed, S., Fristad, I., Lie, S. A., Suliman, S., Mustafa, K., Vindenes, H., et al. (2018). Adipose-derived and bone marrow mesenchymal stem cells: A donor-matched comparison. Stem Cell Res. Ther. 9 (1), 168. doi:10.1186/s13287-018-0914-1
Mok, S-W., Fu, S-C., Cheuk, Y-C., Chu, I. M., Chan, K. M., Qin, L., et al. (2020). Intra-articular delivery of quercetin using thermosensitive hydrogel attenuate cartilage degradation in an osteoarthritis rat model. Cartilage 11 (4), 490–499. doi:10.1177/1947603518796550
Monsef, R., and Salavati-Niasari, M. (2021). Hydrothermal architecture of Cu5V2O10 nanostructures as new electro-sensing catalysts for voltammetric quantification of mefenamic acid in pharmaceuticals and biological samples. Biosens. Bioelectron. 178, 113017. doi:10.1016/j.bios.2021.113017
Motahari, F., Mozdianfard, M. R., and Salavati-Niasari, M. (2015). Synthesis and adsorption studies of NiO nanoparticles in the presence of H2acacen ligand, for removing Rhodamine B in wastewater treatment. Process Saf. Environ. Prot. 93, 282–292. doi:10.1016/j.psep.2014.06.006
Motealleh, A., and Kehr, N. S. (2017). Nanocomposite hydrogels and their applications in tissue engineering. Adv. Healthc. Mat. 6 (1), 1600938. doi:10.1002/adhm.201600938
Muir, V. G., and Burdick, J. A. (2021). Chemically modified biopolymers for the formation of biomedical hydrogels. Chem. Rev. 121 (18), 10908–10949. doi:10.1021/acs.chemrev.0c00923
Muscolino, E., Di Stefano, A. B., Trapani, M., Sabatino, M. A., Giacomazza, D., Moschella, F., et al. (2021). Injectable xyloglucan hydrogels incorporating spheroids of adipose stem cells for bone and cartilage regeneration. Mater. Sci. Eng. C 131, 112545. doi:10.1016/j.msec.2021.112545
Naahidi, S., Jafari, M., Logan, M., Wang, Y., Yuan, Y., Bae, H., et al. (2017). Biocompatibility of hydrogel-based scaffolds for tissue engineering applications. Biotechnol. Adv. 35 (5), 530–544. doi:10.1016/j.biotechadv.2017.05.006
Nagahama, K., Oyama, N., Ono, K., Hotta, A., Kawauchi, K., and Nishikata, T. (2018). Nanocomposite injectable gels capable of self-replenishing regenerative extracellular microenvironments for in vivo tissue engineering. Biomater. Sci. 6 (3), 550–561. doi:10.1039/c7bm01167a
Ngadimin, K. D., Stokes, A., Gentile, P., and Ferreira, A. M. (2021). Biomimetic hydrogels designed for cartilage tissue engineering. Biomater. Sci. 9 (12), 4246–4259. doi:10.1039/d0bm01852j
Nguyen, T. P. T., Li, F., Shrestha, S., Tuan, R. S., Thissen, H., Forsythe, J. S., et al. (2021). Cell-laden injectable microgels: Current status and future prospects for cartilage regeneration. Biomaterials 279, 121214. doi:10.1016/j.biomaterials.2021.121214
Nicol, E. (2021). Photopolymerized porous hydrogels. Biomacromolecules 22 (4), 1325–1345. doi:10.1021/acs.biomac.0c01671
Niemczyk, B., Sajkiewicz, P., and Kolbuk, D. (2018). Injectable hydrogels as novel materials for central nervous system regeneration. J. Neural Eng. 15 (5), 051002. doi:10.1088/1741-2552/aacbab
Oliva, N., Conde, J., Wang, K., and Artzi, N. (2017). Designing hydrogels for on-demand therapy. Acc. Chem. Res. 50 (4), 669–679. doi:10.1021/acs.accounts.6b00536
Oliveira, I. M., Gonçalves, C., Shin, M. E., Lee, S., Reis, R. L., Khang, G., et al. (2021). Enzymatically crosslinked tyramine-gellan gum hydrogels as drug delivery system for rheumatoid arthritis treatment. Drug Deliv. Transl. Res. 11 (3), 1288–1300. doi:10.1007/s13346-020-00855-9
Oliveira, J. T., Gardel, L. S., Rada, T., Martins, L., Gomes, M. E., and Reis, R. L. (2010). Injectable gellan gum hydrogels with autologous cells for the treatment of rabbit articular cartilage defects. J. Orthop. Res. 28 (9), 1193–1199. doi:10.1002/jor.21114
Onofrillo, C., Duchi, S., Francis, S., O'Connell, C. D., Caballero Aguilar, L. M., Doyle, S., et al. (2021). Flash: Fluorescently LAbelled Sensitive Hydrogel to monitor bioscaffolds degradation during neocartilage generation. Biomaterials 264, 120383. doi:10.1016/j.biomaterials.2020.120383
Panyamao, P., Ruksiriwanich, W., Sirisa-Ard, P., and Charumanee, S. (2020). Injectable thermosensitive chitosan/pullulan-based hydrogels with improved mechanical properties and swelling capacity. Polym. (Basel) 12 (11), 2514. doi:10.3390/polym12112514
Park, K. M., Lee, S. Y., Joung, Y. K., Na, J. S., and Park, K. D. (2009). Thermosensitive chitosan-Pluronic hydrogel as an injectable cell delivery carrier for cartilage regeneration. Acta Biomater. 5 (6), 1956–1965. doi:10.1016/j.actbio.2009.01.040
Pascual-Garrido, C., Rodriguez-Fontan, F., Aisenbrey, E. A., Payne, K. A., Chahla, J., Goodrich, L. R., et al. (2018). Current and novel injectable hydrogels to treat focal chondral lesions: Properties and applicability. J. Orthop. Res. 36 (1), 64–75. doi:10.1002/jor.23760
Peng, L., Zhou, Y., Lu, W., Zhu, W., Li, Y., Chen, K., et al. (2019). Characterization of a novel polyvinyl alcohol/chitosan porous hydrogel combined with bone marrow mesenchymal stem cells and its application in articular cartilage repair. BMC Musculoskelet. Disord. 20 (1), 257. doi:10.1186/s12891-019-2644-7
Pereira, R. C., Scaranari, M., Castagnola, P., Grandizio, M., Azevedo, H. S., Reis, R. L., et al. (2009). Novel injectable gel (system) as a vehicle for human articular chondrocytes in cartilage tissue regeneration. J. Tissue Eng. Regen. Med. 3 (2), 97–106. doi:10.1002/term.145
Piantanida, E., Alonci, G., Bertucci, A., and De Cola, L. (2019). Design of nanocomposite injectable hydrogels for minimally invasive surgery. Acc. Chem. Res. 52 (8), 2101–2112. doi:10.1021/acs.accounts.9b00114
Pierau, L., and Versace, D-L. (2021). Light and hydrogels: A new generation of antimicrobial materials. Mater. (Basel) 14 (4), 787. doi:10.3390/ma14040787
Piras, C. C., and Smith, D. K. (2020). Multicomponent polysaccharide alginate-based bioinks. J. Mat. Chem. B 8 (36), 8171–8188. doi:10.1039/d0tb01005g
Poddar, S. K., and Widstrom, L. (2017). Nonoperative options for management of articular cartilage disease. Clin. Sports Med. 36 (3), 447–456. doi:10.1016/j.csm.2017.02.003
Pogue, R., and Lyons, K. (2006). BMP signaling in the cartilage growth plate. Curr. Top. Dev. Biol. 76, 1–48. doi:10.1016/S0070-2153(06)76001-X
Pupkaite, J., Rosenquist, J., Hilborn, J., and Samanta, A. (2019). Injectable shape-holding collagen hydrogel for cell encapsulation and delivery cross-linked using thiol-michael addition click reaction. Biomacromolecules 20 (9), 3475–3484. doi:10.1021/acs.biomac.9b00769
Qi, C., Liu, J., Jin, Y., Xu, L., Wang, G., Wang, Z., et al. (20182021). Corrigendum to “Photo-crosslinkable, injectable sericin hydrogel as 3D biomimetic extracellular matrix for minimally invasive repairing cartilage” [biomaterials 163 (2018) 89–104]. Biomaterials 278, 121134. doi:10.1016/j.biomaterials.2021.121134
Qi, C., Liu, J., Jin, Y., Xu, L., Wang, G., Wang, Z., et al. (2018). Photo-crosslinkable, injectable sericin hydrogel as 3D biomimetic extracellular matrix for minimally invasive repairing cartilage. Biomaterials 163, 89–104. doi:10.1016/j.biomaterials.2018.02.016
Quadrado, R. F. N., Macagnan, K. L., Moreira, A. S., and Fajardo, A. R. (2021). Chitosan-based hydrogel crosslinked through an aza-Michael addition catalyzed by boric acid. Int. J. Biol. Macromol. 193 (Pt B), 1032–1042. doi:10.1016/j.ijbiomac.2021.11.075
Radhakrishnan, J., Krishnan, U. M., and Sethuraman, S. (2014). Hydrogel based injectable scaffolds for cardiac tissue regeneration. Biotechnol. Adv. 32 (2), 449–461. doi:10.1016/j.biotechadv.2013.12.010
Ravari, M. K., Mashayekhan, S., Zarei, F., Sayyahpour, F. A., Taghiyar, L., and Baghban Eslaminejad, M. (2021). Fabrication and characterization of an injectable reinforced composite scaffold for cartilage tissue engineering: An in vitro study. Biomed. Mat. 16 (4), 045007. doi:10.1088/1748-605X/abed97
Ren, K., He, C., Xiao, C., Li, G., and Chen, X. (2015). Injectable glycopolypeptide hydrogels as biomimetic scaffolds for cartilage tissue engineering. Biomaterials 51, 238–249. doi:10.1016/j.biomaterials.2015.02.026
Rigogliuso, S., Salamone, M., Barbarino, E., Nicosia, A., and Ghersi, G. (2020). Production of injectable marine collagen-based hydrogel for the maintenance of differentiated chondrocytes in tissue engineering applications. Int. J. Mol. Sci. 21 (16), 5798. doi:10.3390/ijms21165798
Riley, L., Schirmer, L., and Segura, T. (2019). Granular hydrogels: emergent properties of jammed hydrogel microparticles and their applications in tissue repair and regeneration. Curr. Opin. Biotechnol. 60, 1–8. doi:10.1016/j.copbio.2018.11.001
Rinoldi, C., Lanzi, M., Fiorelli, R., Nakielski, P., Zembrzycki, K., Kowalewski, T., et al. (2021). Three-dimensional printable conductive semi-interpenetrating polymer network hydrogel for neural tissue applications. Biomacromolecules 22 (7), 3084–3098. doi:10.1021/acs.biomac.1c00524
Sahajpal, K., Shekhar, S., Kumar, A., Sharma, B., Meena, M. K., Bhagi, A. K., et al. (2022). Dynamic protein and polypeptide hydrogels based on Schiff base co-assembly for biomedicine. J. Mat. Chem. B 10 (17), 3173–3198. doi:10.1039/d2tb00077f
Sala, R. L., Kwon, M. Y., Kim, M., Gullbrand, S. E., Henning, E. A., Mauck, R. L., et al. (2017). Thermosensitive poly(N-vinylcaprolactam) injectable hydrogels for cartilage tissue engineering. Tissue Eng. Part A 23 (17-18), 935–945. doi:10.1089/ten.tea.2016.0464
Salavati-Niasari, M., and Davar, F. (2006). In situ one-pot template synthesis (IOPTS) and characterization of copper(II) complexes of 14-membered hexaaza macrocyclic ligand “3, 10-dialkyl-dibenzo-1, 3, 5, 8, 10, 12-hexaazacyclotetradecane”. Inorg. Chem. Commun. 9 (2), 175–179. doi:10.1016/j.inoche.2005.10.028
Saravanan, S., Vimalraj, S., Thanikaivelan, P., Banudevi, S., and Manivasagam, G. (2019). A review on injectable chitosan/beta glycerophosphate hydrogels for bone tissue regeneration. Int. J. Biol. Macromol. 121, 38–54. doi:10.1016/j.ijbiomac.2018.10.014
Saveh-Shemshaki, N., Nair, S. L., and Laurencin, C. T. (2019). Nanofiber-based matrices for rotator cuff regenerative engineering. Acta Biomater. 94, 64–81. doi:10.1016/j.actbio.2019.05.041
Schaeffer, C., Pfaff, B. N., Cornell, N. J., Salopek, L. S., Shan, S., Viyar, J., et al. (2020). Injectable microannealed porous scaffold for articular cartilage regeneration. Ann. Plast. Surg. 84 (6S Suppl. 5), S446–S450. doi:10.1097/SAP.0000000000002271
Seo, B-B., Kwon, Y., Kim, J., Hong, K. H., Kim, S. E., Song, H. R., et al. (2022). Injectable polymeric nanoparticle hydrogel system for long-term anti-inflammatory effect to treat osteoarthritis. Bioact. Mat. 7, 14–25. doi:10.1016/j.bioactmat.2021.05.028
Sevastianov, V. I., Basok, Y. B., Kirsanova, L. A., Grigoriev, A. M., Kirillova, A. D., Nemets, E. A., et al. (2021). A comparison of the capacity of mesenchymal stromal cells for cartilage regeneration depending on collagen-based injectable biomimetic scaffold type. Life (Basel) 11 (8), 756. doi:10.3390/life11080756
Shao, J., Zhang, W., and Yang, T. (2015). Using mesenchymal stem cells as a therapy for bone regeneration and repairing. Biol. Res. 48, 62. doi:10.1186/s40659-015-0053-4
Shi, J., Yu, L., and Ding, J. (2021). PEG-based thermosensitive and biodegradable hydrogels. Acta Biomater. 128, 42–59. doi:10.1016/j.actbio.2021.04.009
Shi, W., Fang, F., Kong, Y., Greer, S. E., Kuss, M., Liu, B., et al. (2021). Dynamic hyaluronic acid hydrogel with covalent linked gelatin as an anti-oxidative bioink for cartilage tissue engineering. Biofabrication 14 (1), 014107. doi:10.1088/1758-5090/ac42de
Shojarazavi, N., Mashayekhan, S., Pazooki, H., Mohsenifard, S., and Baniasadi, H. (2021). Alginate/cartilage extracellular matrix-based injectable interpenetrating polymer network hydrogel for cartilage tissue engineering. J. Biomater. Appl. 36 (5), 803–817. doi:10.1177/08853282211024020
Simon, T. M., and Jackson, D. W. (2006). Articular cartilage: Injury pathways and treatment options. Sports Med. Arthrosc. Rev. 14 (3), 146–154. doi:10.1097/00132585-200609000-00006
Sirong, S., Yang, C., Taoran, T., Songhang, L., Shiyu, L., Yuxin, Z., et al. (2020). Effects of tetrahedral framework nucleic acid/wogonin complexes on osteoarthritis. Bone Res. 8, 6. doi:10.1038/s41413-019-0077-4
Skopinska-Wisniewska, J., De la Flor, S., and Kozlowska, J. (2021). From supramolecular hydrogels to multifunctional carriers for biologically active substances. Int. J. Mol. Sci. 22 (14), 7402. doi:10.3390/ijms22147402
Song, F., Li, X., Wang, Q., Liao, L., and Zhang, C. (2015). Nanocomposite hydrogels and their applications in drug delivery and tissue engineering. J. Biomed. Nanotechnol. 11 (1), 40–52. doi:10.1166/jbn.2015.1962
Song, H., Zhao, J., Cheng, J., Feng, Z., Wang, J., Momtazi‐Borojeni, A. A., et al. (2021). Extracellular vesicles in chondrogenesis and cartilage regeneration. J. Cell. Mol. Med. 25 (11), 4883–4892. doi:10.1111/jcmm.16290
Stampoultzis, T., Karami, P., and Pioletti, D. P. (2021). Thoughts on cartilage tissue engineering: A 21st century perspective. Curr. Res. Transl. Med. 69 (3), 103299. doi:10.1016/j.retram.2021.103299
Sun, A. X., Lin, H., Fritch, M. R., Shen, H., Alexander, P. G., DeHart, M., et al. (2017). Chondrogenesis of human bone marrow mesenchymal stem cells in 3-dimensional, photocrosslinked hydrogel constructs: Effect of cell seeding density and material stiffness. Acta Biomater. 58, 302–311. doi:10.1016/j.actbio.2017.06.016
Sun, K., Guo, J., Yao, X., Guo, Z., and Guo, F. (2021). Growth differentiation factor 5 in cartilage and osteoarthritis: A possible therapeutic candidate. Cell Prolif. 54 (3), e12998. doi:10.1111/cpr.12998
Suo, H., Li, L., Zhang, C., Yin, J., Xu, K., Liu, J., et al. (2020). Glucosamine-grafted methacrylated gelatin hydrogels as potential biomaterials for cartilage repair. J. Biomed. Mat. Res. 108 (3), 990–999. doi:10.1002/jbm.b.34451
Talaat, W., Aryal, Ac S., Al Kawas, S., Samsudin, A. R., Kandile, N. G., Harding, D. R., et al. (2020). Nanoscale thermosensitive hydrogel scaffolds promote the chondrogenic differentiation of dental pulp stem and progenitor cells: A minimally invasive approach for cartilage regeneration. Int. J. Nanomedicine 15, 7775–7789. doi:10.2147/IJN.S274418
Tang, Q., Lim, T., Shen, L-Y., Zheng, G., Wei, X. J., Zhang, C. Q., et al. (2021). Well-dispersed platelet lysate entrapped nanoparticles incorporate with injectable PDLLA-PEG-PDLLA triblock for preferable cartilage engineering application. Biomaterials 268, 120605. doi:10.1016/j.biomaterials.2020.120605
Tao, S-C., Huang, J-Y., Gao, Y., Li, Z. X., Wei, Z. Y., Dawes, H., et al. (2021). Small extracellular vesicles in combination with sleep-related circRNA3503: A targeted therapeutic agent with injectable thermosensitive hydrogel to prevent osteoarthritis. Bioact. Mat. 6 (12), 4455–4469. doi:10.1016/j.bioactmat.2021.04.031
Teixeira, L. S. M., Feijen, J., van Blitterswijk, C. A., Dijkstra, P. J., and Karperien, M. (2012). Enzyme-catalyzed crosslinkable hydrogels: emerging strategies for tissue engineering. Biomaterials 33 (5), 1281–1290. doi:10.1016/j.biomaterials.2011.10.067
Thakur, A., Jaiswal, M. K., Peak, C. W., Carrow, J. K., Gentry, J., Dolatshahi-Pirouz, A., et al. (2016). Injectable shear-thinning nanoengineered hydrogels for stem cell delivery. Nanoscale 8 (24), 12362–12372. doi:10.1039/c6nr02299e
Thambi, T., Li, Y., and Lee, D. S. (2017). Injectable hydrogels for sustained release of therapeutic agents. J. Control. Release 267, 57–66. doi:10.1016/j.jconrel.2017.08.006
Thielen, N. G. M., van der Kraan, P. M., and van Caam, A. P. M. (2019). TGFβ/BMP signaling pathway in cartilage homeostasis. Cells 8 (9), 969. doi:10.3390/cells8090969
Thomas, J., Chopra, V., Sharma, A., Panwar, V., Kaushik, S., Rajput, S., et al. (2021). An injectable hydrogel having proteoglycan-like hierarchical structure supports chondrocytes delivery and chondrogenesis. Int. J. Biol. Macromol. 190, 474–486. doi:10.1016/j.ijbiomac.2021.08.226
Toh, W. S., Lee, E. H., and Cao, T. (2011). Potential of human embryonic stem cells in cartilage tissue engineering and regenerative medicine. Stem Cell Rev. Rep. 7 (3), 544–559. doi:10.1007/s12015-010-9222-6
Tonda-Turo, C., Gnavi, S., Ruini, F., Gambarotta, G., Gioffredi, E., Chiono, V., et al. (2017). Development and characterization of novel agar and gelatin injectable hydrogel as filler for peripheral nerve guidance channels. J. Tissue Eng. Regen. Med. 11 (1), 197–208. doi:10.1002/term.1902
Torres-Figueroa, A. V., Pérez-Martínez, C. J., Encinas, J. C., Burruel-Ibarra, S., Silvas-Garcia, M. I., Garcia Alegria, A. M., et al. (2021). Thermosensitive bioadhesive hydrogels based on poly(N-isopropylacrilamide) and poly(methyl vinyl ether-alt-maleic anhydride) for the controlled release of metronidazole in the vaginal environment. Pharmaceutics 13 (8), 1284. doi:10.3390/pharmaceutics13081284
Tsumaki, N., Okada, M., and Yamashita, A. (2015). iPS cell technologies and cartilage regeneration. Bone 70, 48–54. doi:10.1016/j.bone.2014.07.011
Tu, Y., Chen, N., Li, C., Liu, H., Zhu, R., Chen, S., et al. (2019). Advances in injectable self-healing biomedical hydrogels. Acta Biomater. 90, 1–20. doi:10.1016/j.actbio.2019.03.057
Tuan, R. S., Chen, A. F., and Klatt, B. A. (2013). Cartilage regeneration. J. Am. Acad. Orthop. Surg. 21 (5), 303–311. doi:10.5435/JAAOS-21-05-303
Vayas, R., Reyes, R., Arnau, M. R., Evora, C., and Delgado, A. (2021). Injectable scaffold for bone marrow stem cells and bone morphogenetic protein-2 to repair cartilage. Cartilage 12 (3), 293–306. doi:10.1177/1947603519841682
Vega, S. L., Kwon, M. Y., and Burdick, J. A. (2017). Recent advances in hydrogels for cartilage tissue engineering. Eur. Cell. Mat. 33, 59–75. doi:10.22203/eCM.v033a05
Wang, C., Wang, Y., Wang, C., Liu, C., Li, W., Hu, S., et al. (2021). Therapeutic application of 3B-PEG injectable hydrogel/Nell-1 composite system to temporomandibular joint osteoarthritis. Biomed. Mat. 17 (1), 015004. doi:10.1088/1748-605X/ac367f
Wang, F., Chen, J., Liu, J., and Zeng, H. (2021). Cancer theranostic platforms based on injectable polymer hydrogels. Biomater. Sci. 9 (10), 3543–3575. doi:10.1039/d0bm02149k
Wang, G., An, Y., Zhang, X., Ding, P., Bi, H., and Zhao, Z. (2021). Chondrocyte spheroids laden in GelMA/HAMA hybrid hydrogel for tissue-engineered cartilage with enhanced proliferation, better phenotype maintenance, and natural morphological structure. Gels 7 (4), 247. doi:10.3390/gels7040247
Wang, J., Li, B., Pu, X., Wang, X., Cooper, R. C., Gui, Q., et al. (2020). Injectable multicomponent biomimetic gel composed of inter-crosslinked dendrimeric and mesoporous silica nanoparticles exhibits highly tunable elasticity and dual drug release capacity. ACS Appl. Mat. Interfaces 12 (9), 10202–10210. doi:10.1021/acsami.0c01395
Wang, K-Y., Jin, X-Y., Ma, Y-H., Cai, W. J., Xiao, W. Y., Li, Z. W., et al. (2021). Injectable stress relaxation gelatin-based hydrogels with positive surface charge for adsorption of aggrecan and facile cartilage tissue regeneration. J. Nanobiotechnology 19 (1), 214. doi:10.1186/s12951-021-00950-0
Wang, Q., Li, X., Wang, P., Yao, Y., Xu, Y., Chen, Y., et al. (2020). Bionic composite hydrogel with a hybrid covalent/noncovalent network promoting phenotypic maintenance of hyaline cartilage. J. Mat. Chem. B 8 (20), 4402–4411. doi:10.1039/d0tb00253d
Wang, Q., Wang, Q., and Teng, W. (2016). Injectable, degradable, electroactive nanocomposite hydrogels containing conductive polymer nanoparticles for biomedical applications. Int. J. Nanomedicine 11, 131–144. doi:10.2147/IJN.S94777
Wang, Q-S., Xu, B-X., Fan, K-J., Fan, Y. S., Teng, H., and Wang, T. Y. (2021). Dexamethasone-loaded thermo-sensitive hydrogel attenuates osteoarthritis by protecting cartilage and providing effective pain relief. Ann. Transl. Med. 9 (14), 1120. doi:10.21037/atm-21-684
Wang, X., and Wang, Q. (2021). Enzyme-Laden bioactive hydrogel for biocatalytic monitoring and regulation. Acc. Chem. Res. 54 (5), 1274–1287. doi:10.1021/acs.accounts.0c00832
Wei, M., Hsu, Y-I., Asoh, T-A., Sung, M. H., and Uyama, H. (2022). Design of injectable poly(γ-glutamic acid)/chondroitin sulfate hydrogels with mineralization ability. ACS Appl. Bio Mat. 5 (4), 1508–1518. doi:10.1021/acsabm.1c01269
Wei, P., Xu, Y., Gu, Y., Yao, Q., Li, J., and Wang, L. (2020). IGF-1-releasing PLGA nanoparticles modified 3D printed PCL scaffolds for cartilage tissue engineering. Drug Deliv. (Lond). 27 (1), 1106–1114. doi:10.1080/10717544.2020.1797239
Wei, W., Ma, Y., Yao, X., Zhou, W., Wang, X., Li, C., et al. (2021). Advanced hydrogels for the repair of cartilage defects and regeneration. Bioact. Mat. 6 (4), 998–1011. doi:10.1016/j.bioactmat.2020.09.030
Wen, C., Xu, L., Xu, X., Wang, D., Liang, Y., and Duan, L. (2021). Insulin-like growth factor-1 in articular cartilage repair for osteoarthritis treatment. Arthritis Res. Ther. 23 (1), 277. doi:10.1186/s13075-021-02662-0
Werkmeister, J. A., Adhikari, R., White, J. F., Tebb, T., Le, T., Taing, H., et al. (2010). Biodegradable and injectable cure-on-demand polyurethane scaffolds for regeneration of articular cartilage. Acta Biomater. 6 (9), 3471–3481. doi:10.1016/j.actbio.2010.02.040
Wong, C. Y., Al-Salami, H., and Dass, C. R. (2018). Microparticles, microcapsules and microspheres: A review of recent developments and prospects for oral delivery of insulin. Int. J. Pharm. X. 537 (1-2), 223–244. doi:10.1016/j.ijpharm.2017.12.036
Wu, J., Chen, Q., Deng, C., Xu, B., Zhang, Z., Yang, Y., et al. (2020). Exquisite design of injectable hydrogels in cartilage repair. Theranostics 10 (21), 9843–9864. doi:10.7150/thno.46450
Wu, L., Cai, X., Zhang, S., Karperien, M., and Lin, Y. (2013). Regeneration of articular cartilage by adipose tissue derived mesenchymal stem cells: Perspectives from stem cell biology and molecular medicine. J. Cell. Physiol. 228 (5), 938–944. doi:10.1002/jcp.24255
Xin, H. (2022). Double-network tough hydrogels: A brief review on achievements and challenges. Gels 8 (4), 247. doi:10.3390/gels8040247
Xu, J., Liu, Y., and Hsu, S-H. (2019). Hydrogels based on Schiff base linkages for biomedical applications. Molecules 24 (16), 3005. doi:10.3390/molecules24163005
Xu, L., Liu, Y., Sun, Y., Wang, B., Xiong, Y., Lin, W., et al. (2017). Tissue source determines the differentiation potentials of mesenchymal stem cells: A comparative study of human mesenchymal stem cells from bone marrow and adipose tissue. Stem Cell Res. Ther. 8 (1), 275. doi:10.1186/s13287-017-0716-x
Xu, X., Shi, D., Liu, Y., Yao, Y., Dai, J., Xu, Z., et al. (2017). In vivo repair of full-thickness cartilage defect with human iPSC-derived mesenchymal progenitor cells in a rabbit model. Exp. Ther. Med. 14 (1), 239–245. doi:10.3892/etm.2017.4474
Xu, Y., Xu, Y., Bi, B., Hou, M., Yao, L., Du, Q., et al. (2020). A moldable thermosensitive hydroxypropyl chitin hydrogel for 3D cartilage regeneration in vitro and in vivo. Acta Biomater. 108, 87–96. doi:10.1016/j.actbio.2020.03.039
Yan, S., Wang, T., Feng, L., Zhu, J., Zhang, K., Chen, X., et al. (2014). Injectable in situ self-cross-linking hydrogels based on poly(L-glutamic acid) and alginate for cartilage tissue engineering. Biomacromolecules 15 (12), 4495–4508. doi:10.1021/bm501313t
Yan, W., Xu, X., Xu, Q., Sun, Z., Jiang, Q., and Shi, D. (2020). Platelet-rich plasma combined with injectable hyaluronic acid hydrogel for porcine cartilage regeneration: A 6-month follow-up. Regen. Biomater. 7 (1), 77–90. doi:10.1093/rb/rbz039
Yan, X., Yang, B., Chen, Y., Song, Y., Ye, J., Pan, Y., et al. (2021). Anti-friction MSCs delivery system improves the therapy for severe osteoarthritis. Adv. Mat. 33 (52), e2104758. doi:10.1002/adma.202104758
Yang, J., Jing, X., Wang, Z., Liu, X., Zhu, X., Lei, T., et al. (2021). In vitro and in vivo study on an injectable glycol chitosan/dibenzaldehyde-terminated polyethylene glycol hydrogel in repairing articular cartilage defects. Front. Bioeng. Biotechnol. 9, 607709. doi:10.3389/fbioe.2021.607709
Yang, J., Zhang, Y. S., Yue, K., and Khademhosseini, A. (2017). Cell-laden hydrogels for osteochondral and cartilage tissue engineering. Acta Biomater. 57, 1–25. doi:10.1016/j.actbio.2017.01.036
Yao, Y., Wang, P., Li, X., Xu, Y., Lu, G., Jiang, Q., et al. (2020). A di-self-crosslinking hyaluronan-based hydrogel combined with type I collagen to construct a biomimetic injectable cartilage-filling scaffold. Acta Biomater. 111, 197–207. doi:10.1016/j.actbio.2020.05.007
Yin, S., and Cao, Y. (2021). Hydrogels for large-scale expansion of stem cells. Acta Biomater. 128, 1–20. doi:10.1016/j.actbio.2021.03.026
Yu, J., Xu, K., Chen, X., Zhao, X., Yang, Y., Chu, D., et al. (2021). Highly stretchable, tough, resilient, and antifatigue hydrogels based on multiple hydrogen bonding interactions formed by phenylalanine derivatives. Biomacromolecules 22 (3), 1297–1304. doi:10.1021/acs.biomac.0c01788
Yu, R., Zhang, Y., Barboiu, M., Maumus, M., Noel, D., Jorgensen, C., et al. (2020). Biobased pH-responsive and self-healing hydrogels prepared from O-carboxymethyl chitosan and a 3-dimensional dynamer as cartilage engineering scaffold. Carbohydr. Polym. 244, 116471. doi:10.1016/j.carbpol.2020.116471
Yu, W., Hu, B., Boakye-Yiadom, K. O., Ho, W., Chen, Q., Xu, X., et al. (2021). Injectable hydrogel mediated delivery of gene-engineered adipose-derived stem cells for enhanced osteoarthritis treatment. Biomater. Sci. 9 (22), 7603–7616. doi:10.1039/d1bm01122g
Yu, W., Zhu, Y., Li, H., and He, Y. (2020). Injectable quercetin-loaded hydrogel with cartilage-protection and immunomodulatory properties for articular cartilage repair. ACS Appl. Bio Mat. 3 (2), 761–771. doi:10.1021/acsabm.9b00673
Yuan, F-Z., Wang, H-F., Guan, J., Fu, J. N., Yang, M., Zhang, J. Y., et al. (2021). Fabrication of injectable chitosan-chondroitin sulfate hydrogel embedding kartogenin-loaded microspheres as an ultrasound-triggered drug delivery system for cartilage tissue engineering. Pharmaceutics 13 (9), 1487. doi:10.3390/pharmaceutics13091487
Yuan, T., Li, Z., Zhang, Y., Shen, K., Zhang, X., Xie, R., et al. (2021). Injectable ultrasonication-induced silk fibroin hydrogel for cartilage repair and regeneration. Tissue Eng. Part A 27 (17-18), 1213–1224. doi:10.1089/ten.TEA.2020.0323
Yue, K., Trujillo-de Santiago, G., Alvarez, M. M., Tamayol, A., Annabi, N., and Khademhosseini, A. (2015). Synthesis, properties, and biomedical applications of gelatin methacryloyl (GelMA) hydrogels. Biomaterials 73, 254–271. doi:10.1016/j.biomaterials.2015.08.045
Zaviskova, K., Tukmachev, D., Dubisova, J., Vackova, I., Hejcl, A., Bystronova, J., et al. (2018). Injectable hydroxyphenyl derivative of hyaluronic acid hydrogel modified with RGD as scaffold for spinal cord injury repair. J. Biomed. Mat. Res. A 106 (4), 1129–1140. doi:10.1002/jbm.a.36311
Zazakowny, K., Lewandowska-Łańcucka, J., Mastalska-Popławska, J., Kaminski, K., Kusior, A., Radecka, M., et al. (2016). Biopolymeric hydrogels - Nanostructured TiO2 hybrid materials as potential injectable scaffolds for bone regeneration. Colloids Surf. B Biointerfaces 148, 607–614. doi:10.1016/j.colsurfb.2016.09.031
Zewail, M., Nafee, N., Helmy, M. W., and Boraie, N. (2021). Synergistic and receptor-mediated targeting of arthritic joints via intra-articular injectable smart hydrogels containing leflunomide-loaded lipid nanocarriers. Drug Deliv. Transl. Res. 11 (6), 2496–2519. doi:10.1007/s13346-021-00992-9
Zhang, F-X., Liu, P., Ding, W., Meng, Q. B., Su, D. H., Zhang, Q. C., et al. (2021). Injectable Mussel-Inspired highly adhesive hydrogel with exosomes for endogenous cell recruitment and cartilage defect regeneration. Biomaterials 278, 121169. doi:10.1016/j.biomaterials.2021.121169
Zhang, M., Shi, J., Xie, M., Wen, J., Niibe, K., Zhang, X., et al. (2020). Recapitulation of cartilage/bone formation using iPSCs via biomimetic 3D rotary culture approach for developmental engineering. Biomaterials 260, 120334. doi:10.1016/j.biomaterials.2020.120334
Zhang, Q., Xu, H., Wu, C., Shang, Y., Wu, Q., Wei, Q., et al. (2021). Tissue fluid triggered enzyme polymerization for ultrafast gelation and cartilage repair. Angew. Chem. Int. Ed. 60 (36), 19982–19987. doi:10.1002/anie.202107789
Zhang, S., Huang, D., Lin, H., Xiao, Y., and Zhang, X. (2020). Cellulose nanocrystal reinforced collagen-based nanocomposite hydrogel with self-healing and stress-relaxation properties for cell delivery. Biomacromolecules 21 (6), 2400–2408. doi:10.1021/acs.biomac.0c00345
Zhang, T., Chen, S., Dou, H., Liu, Q., Shu, G., Lin, J., et al. (2021). Novel glucosamine-loaded thermosensitive hydrogels based on poloxamers for osteoarthritis therapy by intra-articular injection. Mater. Sci. Eng. C 118, 111352. doi:10.1016/j.msec.2020.111352
Zhang, T., Tian, T., Zhou, R., Li, S., Ma, W., Zhang, Y., et al. (2020). Design, fabrication and applications of tetrahedral DNA nanostructure-based multifunctional complexes in drug delivery and biomedical treatment. Nat. Protoc. 15 (8), 2728–2757. doi:10.1038/s41596-020-0355-z
Zhang, Y., Cao, Y., Zhao, H., Zhang, L., Ni, T., Liu, Y., et al. (2020). An injectable BMSC-laden enzyme-catalyzed crosslinking collagen-hyaluronic acid hydrogel for cartilage repair and regeneration. J. Mat. Chem. B 8 (19), 4237–4244. doi:10.1039/d0tb00291g
Zhang, Y., Liu, J., Huang, L., Wang, Z., and Wang, L. (2015). Design and performance of a sericin-alginate interpenetrating network hydrogel for cell and drug delivery. Sci. Rep. 5, 12374. doi:10.1038/srep12374
Zhang, Z., Li, L., Yang, W., Cao, Y., Shi, Y., Li, X., et al. (2017). The effects of different doses of IGF-1 on cartilage and subchondral bone during the repair of full-thickness articular cartilage defects in rabbits. Osteoarthr. Cartil. 25 (2), 309–320. doi:10.1016/j.joca.2016.09.010
Zhang, Z., Lin, S., Yan, Y., You, X., and Ye, H. (2021). Enhanced efficacy of transforming growth factor-β1 loaded an injectable cross-linked thiolated chitosan and carboxymethyl cellulose-based hydrogels for cartilage tissue engineering. J. Biomaterials Sci. Polym. Ed. 32 (18), 2402–2422. doi:10.1080/09205063.2021.1971823
Zhao, H., Liu, M., Zhang, Y., Yin, J., and Pei, R. (2020). Nanocomposite hydrogels for tissue engineering applications. Nanoscale 12 (28), 14976–14995. doi:10.1039/d0nr03785k
Zhao, Z., Fang, R., Rong, Q., and Liu, M. (2017). Bioinspired nanocomposite hydrogels with highly ordered structures. Adv. Mat. 29 (45), 1703045. doi:10.1002/adma.201703045
Zheng, D., Chen, T., Han, L., Lv, S., Yin, J., Yang, K., et al. (2022). Synergetic integrations of bone marrow stem cells and transforming growth factor-β1 loaded chitosan nanoparticles blended silk fibroin injectable hydrogel to enhance repair and regeneration potential in articular cartilage tissue. Int. Wound J. 2022. doi:10.1111/iwj.13699
Zhong, J., Guo, B., Xie, J., Deng, S., Fu, N., Lin, S., et al. (2016). Crosstalk between adipose-derived stem cells and chondrocytes: When growth factors matter. Bone Res. 4, 15036. doi:10.1038/boneres.2015.36
Zhou, J., Li, J., Du, X., and Xu, B. (2017). Supramolecular biofunctional materials. Biomaterials 129, 1–27. doi:10.1016/j.biomaterials.2017.03.014
Zhou, S., Bei, Z., Wei, J., Yan, X., Wen, H., Cao, Y., et al. (2022). Mussel-inspired injectable chitosan hydrogel modified with catechol for cell adhesion and cartilage defect repair. J. Mat. Chem. B 10 (7), 1019–1030. doi:10.1039/d1tb02241e
Zhou, W., Lin, J., Zhao, K., Jin, K., He, Q., Hu, Y., et al. (2019). Single-cell profiles and clinically useful properties of human mesenchymal stem cells of adipose and bone marrow origin. Am. J. Sports Med. 47 (7), 1722–1733. doi:10.1177/0363546519848678
Zhou, Y., Zhang, Y., Dai, Z., Jiang, F., Tian, J., and Zhang, W. (2020). A super-stretchable, self-healing and injectable supramolecular hydrogel constructed by a host-guest crosslinker. Biomater. Sci. 8 (12), 3359–3369. doi:10.1039/d0bm00290a
Zhu, J., Yang, S., Qi, Y., Gong, Z., Zhang, H., Liang, K., et al. (2022). Stem cell-homing hydrogel-based miR-29b-5p delivery promotes cartilage regeneration by suppressing senescence in an osteoarthritis rat model. Sci. Adv. 8 (13), eabk0011. doi:10.1126/sciadv.abk0011
Zhu, Y., Ye, L., Cai, X., Li, Z., Fan, Y., and Yang, F. (2022). Icariin-loaded hydrogel regulates bone marrow mesenchymal stem cell chondrogenic differentiation and promotes cartilage repair in osteoarthritis. Front. Bioeng. Biotechnol. 10, 755260. doi:10.3389/fbioe.2022.755260
Zinatloo-Ajabshir, S., Morassaei, M. S., Amiri, O., Salavati-Niasari, M., and Foong, L. K. (2020). Nd2Sn2O7 nanostructures: Green synthesis and characterization using date palm extract, a potential electrochemical hydrogen storage material. Ceram. Int. 46 (11), 17186–17196. Part A). doi:10.1016/j.ceramint.2020.03.014
Zinatloo-Ajabshir, S., and Mortazavi-Derazkola, S.NdO-SiO nanocomposites (2018). Nd2O3-SiO2 nanocomposites: A simple sonochemical preparation, characterization and photocatalytic activity. Ultrason. Sonochem. 42, 171–182. doi:10.1016/j.ultsonch.2017.11.026
Zinatloo-Ajabshir, S., and Salavati-Niasari, M. (2019). Preparation of magnetically retrievable CoFe2O4@SiO2@Dy2Ce2O7 nanocomposites as novel photocatalyst for highly efficient degradation of organic contaminants. Compos. Part B Eng. 174, 106930. doi:10.1016/j.compositesb.2019.106930
Zoratto, N., and Matricardi, P. (2018). Semi-IPN- and IPN-based hydrogels. Adv. Exp. Med. Biol. 1059, 155–188. doi:10.1007/978-3-319-76735-2_7
Glossary
ECM Extracellular matrix
OA Osteoarthritis
NSAIDs Non-steroidal anti-inflammatory drugs
ACI Autologous chondrocyte implantation
HRP Horseradish peroxidase
H2O2 Hydrogen peroxide
CST Critical solution temperature
NIPAAm N-isopropyl acrylamide
IPN Interpenetrating Polymer Network
IUPAC International Union of Pure and Applied Chemistry
GE Genipin
HA-SH Thiolated hyaluronic acid
N/MPs Nano/micron particles
NPs Nanoparticles
CNCs Cellulose nanocrystals
POEGMA Poly (oligoethylene glycol methacrylate)
MW Molecular weight;
RGD Rrginine-glycine-aspartate
PLGA Poly lactic-co-glycolic acid
PLA Polylactic acid
PGA Polyglycolic acid
PCL Polycaprolactone
PVA Polyvinyl alcohol
PLL Poly l‐lysine
MPA Methoxy poly (ethylene glycol)-poly (alanine)
PL Platelet lysates
GFs Growth factors
IGF-1 Insulin-like growth factor
VEGF Vascular endothelial growth factor
TGF-β Transforming growth factor-β
TGF-β1 Transforming growth factor-β1
TGF-β3 Transforming growth factor-β3
BMP-2 Bone morphogenetic protein-2
BMP-4 Bone morphogenetic protein-4
BMP-7 Bone morphogenetic protein-7
GDF-5 Growth/differentiation factor-5
PRP Platelet-rich plasma
PDLLA Poly (D, l-lactide)
PEG Polyethylene glycol
HB-PEG Hyperbranched PEG
PEGDA PEG diacrylate
PEG-MAL PEG maleimide
GCS Glycol chitosan
DF-PEG Dibenzaldehyde-terminated PEG
PAMAM Polyamidoamine dendrimer
EGCG Epigallocatechin-3-gallate
CS Chondroitin sulfate
HA Hyaluronic acid
GAG Glycosaminoglycans
GelMA Gelatin methacrylate
HAMA Methacrylated hyaluronic acid
PBA 3-Aminophenylboronic acid
EPL ε-poly-l-lysine
PLEL Poly (d, l-lactide)-poly (ethylene glycol)-poly (d, l-lactide)
DNA Deoxyribonucleic acid
EVs Extracellular vesicles
MSCs Mesenchymal stem cells
ADSCs Adipose mesenchymal stem cells
BMSCs Bone marrow mesenchymal stem cells
SMSCs Synovium mesenchymal stem cells
CSPCs Chondrogenic stem/progenitor cells
THA Thiolfunctionalised hyaluronic acid
KGN Kartogenin
circRNA3503 Circular RNA 3503
GSH Glutathione
Dex-ox Oxidized dextran
FITC Fluorescein isothiocyanate
CMC Carboxymethyl cellulose
SF Silk fibroin
TCA Triamcinolone acetonide
Col I Type I collagen
Col 1α Type 1α collagen
Col 2α Type 2α collagen
miR-29b-5p MicroRNA-29b-5p
SAP Serum amyloid P component
ROS Reactive oxygen species
MMP13 Matrix metallopeptidase13
3D Third Dimension
COL2 Type II collagen
ACAN Aggrecan
hNCs Human nasal septal cartilage
hUCMSCs Human umbilical cord mesenchymal stem cells
CBFβ-RUNX1 Core-binding factor β-Runt-related transcription factor 1
SKPPGTSS A stem cell-homing peptide
DK Diclofenac potassium
GlcN Glucosamine
MC Methylcellulose
MC-CMC Methylcellulose-carboxymethyl chitosan
FLIH Fluorescently labeled injectable hydrogels
Si-HPMC Silanized-hydroxypropyl methylcellulose
HA–pAA Hyaluronic acid–polyacrylic acid
iPSCs Induced Pluripotent Stem Cells
ESCs Embryonic stem cells
GM-HPCH Glycidyl methacrylate-modified-hydroxypropyl chitin hydrogel
PLCG Poly(lactic-co-glycolide)
SMAD Small mothers against decapentaplegic
TAK1 Transforming growth factor beta-activated kinase1
ERK Extracellular signal-regulated kinase
SOX9 SRY-related high-mobility-group box 9
SerMA Sericin methacryloyl
Keywords: injectable hydrogels, tissue engineering, cartilage defect, osteoarthritis, advanced
Citation: Zhu S, Li Y, He Z, Ji L, Zhang W, Tong Y, Luo J, Yu D, Zhang Q and Bi Q (2022) Advanced injectable hydrogels for cartilage tissue engineering. Front. Bioeng. Biotechnol. 10:954501. doi: 10.3389/fbioe.2022.954501
Received: 27 May 2022; Accepted: 28 June 2022;
Published: 08 September 2022.
Edited by:
Yunfeng Lin, Sichuan University, ChinaReviewed by:
Xiaoru Shao, Affiliated Hospital of Jining Medical University, ChinaMasoud Salavati-Niasari, University of Kashan, Iran
Copyright © 2022 Zhu, Li, He, Ji, Zhang, Tong, Luo, Yu, Zhang and Bi. This is an open-access article distributed under the terms of the Creative Commons Attribution License (CC BY). The use, distribution or reproduction in other forums is permitted, provided the original author(s) and the copyright owner(s) are credited and that the original publication in this journal is cited, in accordance with accepted academic practice. No use, distribution or reproduction is permitted which does not comply with these terms.
*Correspondence: Qing Bi, YnF6anNybXl5QDE2My5jb20=