Microbes of traditional fermentation processes as synthetic biology chassis to tackle future food challenges
- Max Planck Institute for Terrestrial Microbiology, Marburg, Germany
Microbial diversity is magnificent and essential to almost all life on Earth. Microbes are an essential part of every human, allowing us to utilize otherwise inaccessible resources. It is no surprise that humans started, initially unconsciously, domesticating microbes for food production: one may call this microbial domestication 1.0. Sourdough bread is just one of the miracles performed by microbial fermentation, allowing extraction of more nutrients from flour and at the same time creating a fluffy and delicious loaf. There are a broad range of products the production of which requires fermentation such as chocolate, cheese, coffee and vinegar. Eventually, with the rise of microscopy, humans became aware of microbial life. Today our knowledge and technological advances allow us to genetically engineer microbes - one may call this microbial domestication 2.0. Synthetic biology and microbial chassis adaptation allow us to tackle current and future food challenges. One of the most apparent challenges is the limited space on Earth available for agriculture and its major tolls on the environment through use of pesticides and the replacement of ecosystems with monocultures. Further challenges include transport and packaging, exacerbated by the 24/7 on-demand mentality of many customers. Synthetic biology already tackles multiple food challenges and will be able to tackle many future food challenges. In this perspective article, we highlight recent microbial synthetic biology research to address future food challenges. We further give a perspective on how synthetic biology tools may teach old microbes new tricks, and what standardized microbial domestication could look like.
Introduction
Synthetic biology is a discipline of biology which aims to domesticate and standardize DNA parts, modularize cellular processes on regulatory and functional level, and ultimately, aims to construct synthetic organisms from scratch serving as chassis in application based processes (Wang et al., 2018; Ostrov et al., 2019; Schindler, 2020). In recent years, many technological advances have been made, especially towards the design, synthesis and construction of synthetic DNAs up to whole genome size (Schindler et al., 2018; Ostrov et al., 2019). However, building designer organisms from scratch is still limited due to the compulsory large-scale DNA synthesis and extensive genetic engineering during the “debugging” phase, as well as the lack of understanding of what the essential components supporting a synthetic minimal life are. Minimal chassis would be outstanding tools for researchers, facilitating testing of parts and pathways within a minimal cellular metabolism while causing minimum interference. However, it will take more time until such tools for systematic analysis are available. On the other hand, cell-free biology using either cell extracts or the essential purified proteins and cofactors has become popular (Shimizu et al., 2005; Swartz, 2006). Researchers are able to produce valuable compounds in cell-free systems but the method currently lacks scalability (Dondapati et al., 2020).
Today, a broad spectrum of products are generated by microbes including engineered microbes in industrialized processes. Here, often random mutagenesis and Adaptive Laboratory Evolution (ALE) are tools employed to optimize strains, but evolution is not always beneficial, strains are constantly evolving to minimize cellular burden which may cause scale-up process to fail (Schmidt, 2005; Ellis, 2019). Bearing this in mind, the diversity of currently industrially used microbial chassis is rather low in comparison to the actual microbial biodiversity. In many cases there are unexplored wild-type microorganisms which may perform as well or even better for a given product compared to engineered model organisms. Researchers need to move away from the dichromatic view of model and non-model organisms to appreciate the whole diversity available for application. Now that most laboratories can perform whole genome sequencing and produce high quality reference genomes of microbes using combinations of short- and long-read sequencing techniques, we are not limited to the use of a handful of model organisms, and are able to expand into use of non-model microorganisms. Further advances of synthetic biology tools such as standardized DNA assembly and innovative genome manipulation tools allow the quick establishment of new microbial systems. In addition to this, many high-throughput technologies are broadly available for in-depth characterization, such as transcriptome, proteome and metabolome profiling allowing researchers to accumulate large-scale datasets with the potential to create whole-cell models (Sun et al., 2021; Lu et al., 2022). This is due to the efforts of interdisciplinary work between experimentalists, data scientists and software engineers to constantly improve software tools, many of which are now able to be used in a plug-and-play manner by any user.
All of these advances allow researchers to tackle global challenges. One of the biggest challenges humankind is facing is how to guarantee a sustainable food supply for all humans on Earth (Searchinger et al., 2019). In recent times, this has become more apparent, indicated by the collapsing supply chains due to the SARS-CoV-2 pandemic and geopolitical instability (Barman et al., 2021; Moosavi et al., 2022). Presumably such factors will not decline and will instead become more evident in the light of climate change (IFPRI, 2022). Researchers, policy makers and politicians need to work together to identify solutions to how everyone on Earth can be supplied with sustainable food. However, these challenges extend beyond food production. For example, the preserving, packaging and logistics behind each product on the shelves are challenges but also opportunities which synthetic biology can use to make future foods more sustainable (Figure 1A).
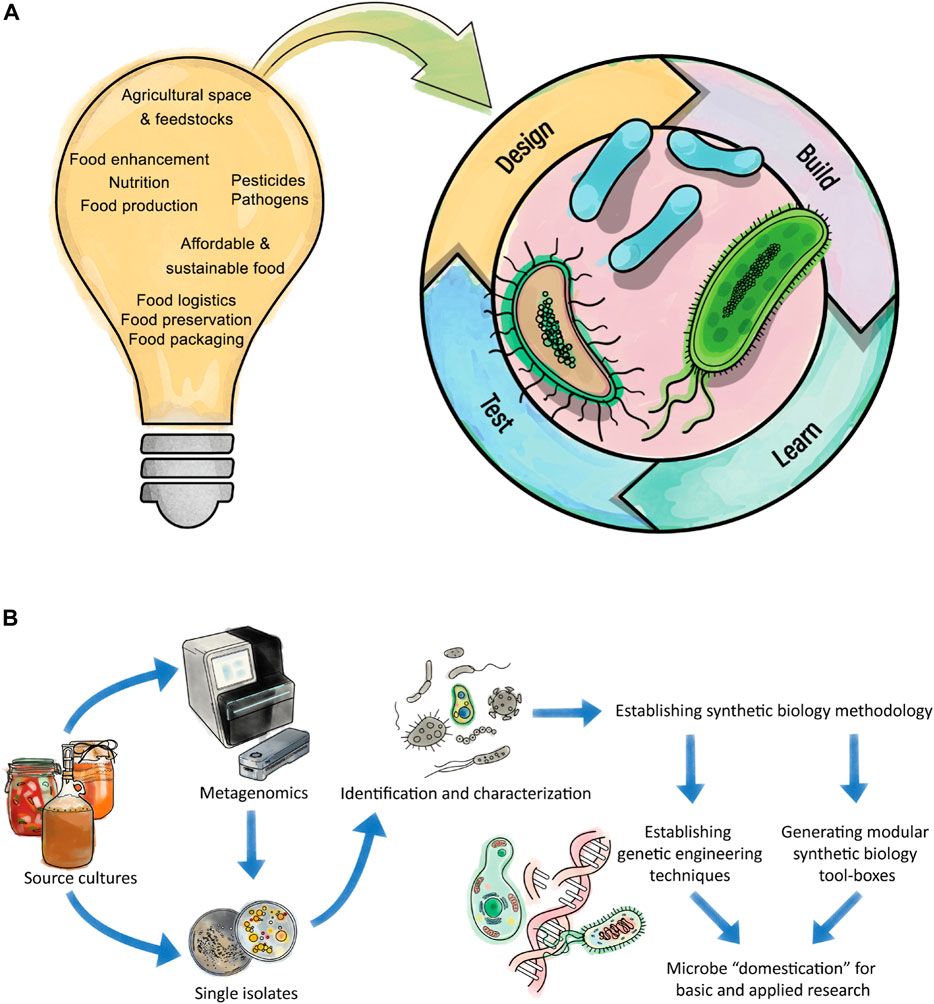
FIGURE 1. Challenges of future foods which give rise to opportunities for synthetic biology. (A) Synthetic biology tries to implement engineering principles into life. The lightbulb highlights some of the challenges for future foods. These challenges may be inspirational for experimental designs for synthetic biology methodology with the potential to improve a process or overcome related problems. Microbes can be altered through the “Design-Build-Test-Learn” cycle for a greater aim and particular microbes from traditional fermentation processes have the potential to address future food challenges. (B) Workflow showing a pipeline to domesticate microbes, for example from traditional fermentations processes. The initial source can be analyzed by traditional isolation of individual microbes or by metagenomics approaches to initially get an overview of the community before individuals are isolated. The isolated microbes need to be identified and characterized. Once the organism is known, one can start to make the organism accessible for synthetic biology approaches. Therefore, initial genetic engineering methods need to be established (i.e., transformation procedures), followed by advances in engineering tools (i.e., CRISPR/Cas-based methods) and the generation of modular tool-boxes for quick and reliable engineering of the organism. The established tools allow microbe domestication, for example by removal or addition of genes, for easier handling. Subsequently the domesticated microbe can be used for intensive engineering towards a desired goal for example the assimilation of a sustainable feedstock.
Within the scope of this perspective we highlight how synthetic biology research can adapt microorganisms from traditional fermentation processes, making them accessible for synthetic biology workflows to obtain new models to tackle future food challenges. We highlight how microorganisms can help us to improve food quality, reduce environmental impact by local production and alternative feedstocks, and how microbes can be used to build biomaterials serving as sustainable packaging material. We further give opinions where we believe there are gaps and propose future directions researchers should investigate.
Microbial organisms from traditional fermentation
Many daily products such as sourdough bread, coffee, and chocolate are produced by microorganisms in a process called fermentation (Marco et al., 2017; Dimidi et al., 2019). Since the work of van Loewenhook and Pasteur we have been aware that humans used and domesticated microbes for the preparation and preservation of food. The majority of organisms within fermentation processes are generally recognized as safe (“GRAS organisms”) and have the potential to be model systems. One disadvantage of fermented food over highly industrialized food is the rather time-consuming production process. However, it pays off with health benefits and complex flavors over highly industrialized products (Sanlier et al., 2019). Even though the procedure takes time it is scalable and one can find certain industrial products where fermentation was used to produce them for example raw apple cider vinegar, chocolate and coffee. With a rise of health awareness there is an increased interest from various companies towards traditional fermentation and products like sourdough bread and kombucha have become available to more consumers. Table 1 gives an overview of some fermented foods and the composition of the microbial community. Many of these cultures are domesticated from their original source for generations resulting in balanced communities with adaptation towards a respective condition or product. Often traditional fermentation cultures contain Saccharomyces cerevisiae strains and various lactic acid bacteria (LAB). Both are traditionally known for their roles in fermentation processes and are extensively explored in synthetic biology (Mays and Nair, 2018; De Filippis et al., 2020; Molinet and Cubillos, 2020; Schindler, 2020). However, within this perspective article, we would like to draw attention to some other, less explored microbes and their complex communities. Highly interesting microbes can be found in traditional farmhouse brewing. As an example, Kveik yeasts, which are a genetically distinct group of domesticated S. cerevisiae brewing yeasts, are highly temperature-tolerant, have an impressive fermentation speed and exceptionally high flocculation, resulting in crystal clear products within a short period of time (Krogerus et al., 2018; Preiss et al., 2018; Foster et al., 2022). At the same time, Kveik yeasts produce highly desirable flavor profiles during fermentation (Kawa-Rygielska et al., 2021; Luo et al., 2021; Kawa-Rygielska et al., 2022). Farmhouse brewing strains are often communities of various microbes (bacteria and yeasts) and not single strains which are used in industry. Microbial communities, especially from traditional fermentation processes, are treasure troves for potential new synthetic biology chassis based on their phenotypic properties. Systematic analysis of microbial communities from fermentation cultures is important to understand their composition (i.e. by metagenomics), their products (i.e. by metabolomics), and their ecology (Lavefve et al., 2019). With Next Generation Sequencing techniques being available to the majority of researchers, systematic studies of fermentative cultures are becoming more frequent (Pswarayi and Ganzle, 2019; Weckx et al., 2019; Arikan et al., 2020; Comasio et al., 2020; Fernandez-Nino et al., 2021).
Domestication and tool-box creation for non-model organisms
Databases with whole genome data are growing constantly (Kodama et al., 2012). The reason for this, besides the dropping sequencing costs, is metagenomics studies. Metagenomics is the approach to sequence complex samples, rather than single organisms, to reconstruct communities of whole ecosystems (Ghurye et al., 2016; Lui et al., 2021; Zhang et al., 2021). However, besides the growing sequence data, the number of well-established model organisms in laboratories is limited. Research would benefit by broadening our molecular understanding of various, different organisms. Microbes from fermentation cultures are of particular interest based on their abilities to produce, enhance and preserve food. Isolation of individual microbes of a community can be achieved with standard cultivation techniques in media mimicking the fermentation process. A respective example workflow for microbe isolation and its domestication is given in Figure 1B. If one aims to isolate microbes it is important to be familiar with the Nagoya protocol which is an attempt to limit biopiracy (Smith et al., 2017). Once microbes of interest are isolated, the first step is their classification based on sequencing genetic markers (i.e., 16S RNA, internal transcribed spacer (ITS)) followed by whole genome sequencing if required. The combination of short- and long-read sequencing allows sequencing and assembly of any microbial genome (Bashir et al., 2012; Moss et al., 2020). One reason for this is that computational tools have become accessible and user-friendly (Ejigu and Jung, 2020; Jung et al., 2020). Sequencing genomes and performing de novo genome assembly is now affordable and achievable within a matter of days. Genome sequencing data can be supported with transcriptome data to enable accurate genome annotation (Prasad et al., 2017). However, a transcriptome sample always represents gene expression in one particular condition, not representing all transcripts, which may be the current major bottleneck in understanding genomes. In the future, complete genome assembly and annotation from metagenomic samples will further advance, resulting in more data in sequence databases (Yang et al., 2021). This sequence space will be of great value to understand enzymes and enzyme complexes and investigate their potential application, especially in the context of larger numbers of complete genomes available (De Filippis et al., 2020). While biological and biochemical characterization remains a bottleneck, laboratory automation in the form of biofoundries helps to accelerate research in this regard (Hillson et al., 2019; Farzaneh and Freemont, 2021).
Genetic engineering of communities is challenging due to multiple factors, but more and more organisms are becoming genetically accessible (Johns et al., 2016; McCarty and Ledesma-Amaro, 2019; Tsoi et al., 2019). To engineer non-model microbes, one must understand the organism to a greater extent and there are still challenges in the engineering of non-model organisms besides the recent advances in genetic engineering tools (Yan and Fong, 2017; Ren et al., 2020). However, comparing the identified microbe with already known, related species, may give hints as to which types of techniques may be successful for genetic engineering approaches. Being able to perform efficient genetic engineering is important to make a microorganism viable for future synthetic biology applications. The initial step is the transfer and stable incorporation of DNA sequences into the microbial genome in an ideally marker-free procedure. Once an efficient transformation procedure is established researchers can make use of the power of synthetic biology. One of the advantages of synthetic biology is the use of modular tool-boxes allowing quick and reliable construction of reusable DNA part libraries based on well-characterized genetic parts (Kelwick et al., 2014). A technology which has been widely adopted to achieve this is Golden Gate cloning, which relies on the use of type IIS restriction enzymes and allows modular cloning (Engler et al., 2008; Werner et al., 2012). Individual parts can be combined into transcriptional units to build complex pathways and it seems a size limit has not yet been reached. Researchers are able to construct whole synthetic chromosomes in mega-base ranges (Schindler et al., 2018; Zumkeller et al., 2018). In combination with laboratory automation, new organisms can be domesticated and explored. Different constructs can be built and screened, and deletion or interference libraries can be constructed to engineer an organism to understand its biology and biochemistry or for its application in industry.
In recent years studies were proposed and made towards direct and indirect improvement of agriculture, food quality, and sustainability, ideally creating a circular bioeconomy, but many challenges remain (Marvik and Philp, 2020). Genetic engineering has become highly precise allowing almost any change in genomes and synthetic biology allows researchers to make opportunities out of these challenges (El Karoui et al., 2019). Today, the organism of choice to tackle a challenge no longer needs to be haploid; CRISPR-based engineering allows efficient engineering of diploid and polyploid organisms (Knott and Doudna, 2018; Lian et al., 2018). Many yeast strains underwent complex genomic variations during their industrial domestication, resulting in complex, polyploid strains. This is common for example in brewing yeasts (Gallone et al., 2016). However, such genome alterations can cause sterility and limit breeding programs. Engineering such strains with CRISPR-based tools has an advantage as microbial diploid and polyploid phenotypes might be lost if forced to become haploid, should they be viable at all. Meanwhile, a recent publication highlights a novel non-GMO technique to unlock the functional potential of polyploid yeasts allowing its application in the food industry under the current legislation (Mozzachiodi et al., 2022).
Future food generated by engineered microbes
Domesticating a microbe and generating an easy-to-use synthetic biology tool-box can facilitate the engineering of individual organisms and potentially communities, and opens new perspectives for future foods. In Table 2, we summarize a list of current products and compounds which production is based in the use of microbes engineered with synthetic biology tools. The main advantages for all these products include less consumption of water, less land as they would be produced by fermentation in bioreactors, no animal use, and some of them include enhancements in the organoleptic and nutritional properties.
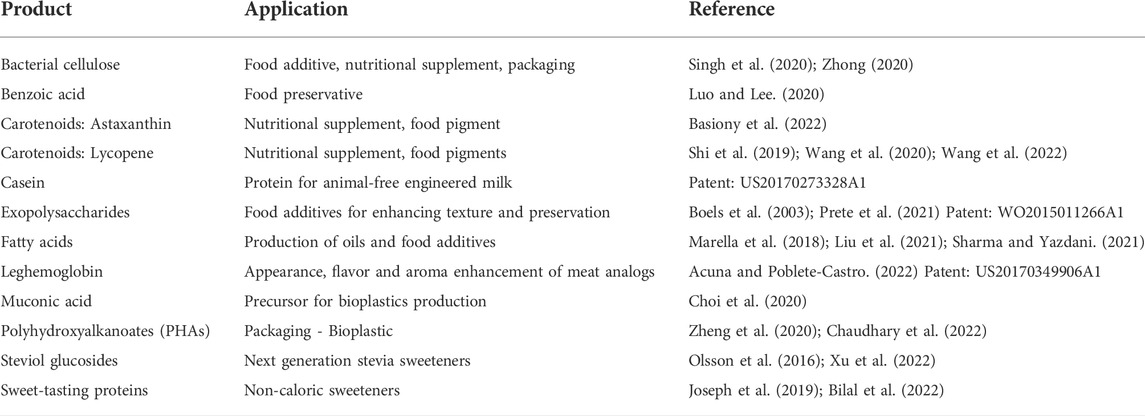
TABLE 2. Products available or expected to enter the market in the near future produced by engineered microorganisms.
Beyond engineering microbes, much research has also been done to improve food quality itself by the production of supplements and compounds. Such is the case of Golden Rice, genetically engineered to produce β-carotene with the goal of overcoming vitamin A deficiencies, which might be one of the widely known yet controversial developments of genetically modified foods as a strategy for fighting malnutrition (Ye et al., 2000; Enserink, 2008; Stokstad, 2019). Importantly, Golden Rice does not solve the cause of malnutrition, society must step in to guarantee a balanced and sustainable diet for everyone. Further, placing genetically engineered plants into the field has always been restricted and highly controversial, especially in Europe, although the UK recently announced intentions to change its policy (Ledford, 2021). However, genetically engineered crops, like all other crops, have the drawback of requiring sufficient agricultural land, fertilizers and pesticides. In addition, genetically engineered crops pose a potential threat for biodiversity. Researchers may tackle these risks and challenges by replacing crops with microbes. The reason for this is that the desired product can be produced in fermentation vessels, which can be installed in almost any location. Fermentation vessels have a reasonable footprint, are not seasonal, do not require sunlight and therefore do not compete with nature and housing space. Further, it has been shown that various microbes like Escherichia coli and S. cerevisiae can be modified to produce various carotenoids in high yields (Shi et al., 2019; Wang et al., 2020; Basiony et al., 2022). Exemplary key products for future foods which currently are, or in the near future will be, produced based on synthetic biology principles are highlighted in table 2.
An example where researchers replaced plants with microbes is in the engineering of industrial brewing yeast to produce some of the flavor molecules needed in hopped beers (Denby et al., 2018). Hops are water-intensive crops with underlying seasonal quality changes. Engineered yeast strains for brewing purposes can be simply distributed and potentially produce consistent flavor profiles. Another example is the microbial production of milk and meat replacements (Table 2). Agriculture is one of the driving factors of climate change, with the dairy and cattle industries particularly producing large quantities of methane emissions. Researchers are on the path to produce alternative milk products through fermentation of plant-based material, and synthetic biology may offer additional alternatives in the future (Tangyu et al., 2019). The same holds true for the replacement of meat (Rubio et al., 2020). Already today, mycoproteins from non-genetically engineered Fusarium venenatum, formed into various meat alternatives are sold under the trademark Quorn (Trinci, 1992). The isolated fungus originated from soil samples in the 1960s where alternative food sources were investigated to tackle “the world’s flagging supply of protein foods”. This organism has since been domesticated in industrial scale fermentations. In recent years researchers started to explore strain optimization, applying CRISPR/Cas9 genome editing rather than random mutagenesis (Wilson and Harrison, 2021). Filamentous fungi in general are promising candidates to solve future food challenges (Strong et al., 2022). In the future genetic engineering will be able to not only improve products and make them more sustainable, but also to build ideal hosts suitable for future food production based on microbes for example from traditional fermentations. There are many advantages of using microbes as food or future food producers: they do not need large spaces and they usually do not need light. Microbes have the potential to allow on-demand, decentralized food production with consistent nutritional properties.
Water is becoming a limiting resource on Earth, but there are microbes from many traditional fermentation cultures which are salt tolerant and might be ideal chassis for the production of future foods based on sea water. Synthetic biology can do much more than enhancing individual products. Synthetic biology aims to tackle problems caused by climate change and manmade pollution by seeing CO2 and plastic waste as alternative feedstocks (Bar-Even, 2016; Schwander et al., 2016; Sadler and Wallace, 2021).
Synthetic biology for alternative feedstocks and biomaterials
Future food has challenges besides producing food itself. There is a need for alternatives for preserving, sustainable packaging and the resources needed for biomass production. In order to propagate and grow microbes at an industrial scale, suitable feedstocks are mandatory. Classical feedstocks compete with food-chain supplies, and therefore researchers are investigating alternative feedstocks. Alternative feedstocks should be cheap, easy to produce and should not cause competition with the classical food-chain. Resources from waste streams, for example spent brewers grain, pomace or organic waste, may be suitable feedstocks but have high variations between batches and seasons. Alternative roads would be the implementation of synthetic pathways allowing assimilation of alternative carbon sources. In recent years researchers have looked into the possibility of C1 compound assimilation, and in particular the assimilation of CO2, methanol and formate have sparked interest (Yishai et al., 2016; Claassens et al., 2018; Cotton et al., 2020; Fabarius et al., 2021; Jiang et al., 2021; Marcellin et al., 2022). Genetic engineering generally allows the modification of microbial hosts, enabling them to assimilate alternative, sustainable carbon sources. These projects now need to prove that they are scalable. This would be a major step towards a sustainable bioeconomy. Other alternative feedstocks like plastic waste are being explored and researchers are making progress in terms of identification, characterization and engineering of PET degrading enzymes (PETase) (Tournier et al., 2020; Sonnendecker et al., 2022). Idionella sakaiensis is a microbe where the complete degradation and assimilation of PET was discovered for the first time (Yoshida et al., 2016). Recent research shows post-consumer plastic treated with PETase under specific conditions can convert PET into terephthalate (TA) which can for example be converted by genetically engineered Escherichia coli cells to produce vanillin (Sadler and Wallace, 2021). There might be a day where genetically engineered microbes can build substantial biomass on a reasonable timescale from PET degradation. It was shown that TA can be used as feedstock for bacterial production of polyhydroxyalkanoates (PHAs) which can serve as bioplastic and are a sustainable alternative to PET based on their superior degradation properties (Kenny et al., 2008). Those abilities could be transferred to other microbes with synthetic biology tools including microbes from traditional fermentation processes and use PET as feedstock.
Future food challenges extend beyond production of food itself, with transportation and packaging also representing major challenges. Currently, one of the major sources of plastic waste is the packaging material of food. Moving away from single-use packaging towards reusable packaging would be already a big step. However, this is not always feasible however, this is not always feasible, but thankfully biological degradable plastics are emerging alternatives (Chen and Patel, 2012). This makes sustainable biomaterials a very important factor for future food challenges. Synthetic biology is offering innovative solutions to tackle these challenges of future food as well. Researchers are working on various biomaterials ranging from bioplastics, to spider silk, to cellulose-based materials and many more (Le Feuvre and Scrutton, 2018). Bioplastics already have an application in real life while other materials are still under development. The potential of materials to produce, for example, alternatives to petroleum-based packaging material is enormous. Especially if consumers move away from single-use packaging, the potential of biological materials is a considerable opportunity. Cellulose, which is naturally produced by microbes, is a promising biomaterial and has many applications (Zhong, 2020). Bacterial cellulose might one day be used to produce sustainable packaging for the food industry (Nesic et al., 2019). Inventors are exploring the potential of synthetic wood generated from the cellulose material of the kombucha industry in another example of the upcycling of waste streams with origin from fermentation cultures.
Researchers are able to perform sophisticated genetic engineering in increasing numbers of microbes. While most of the time a rather limited set of model organisms is applied, nature has much more to offer. Most likely there are organisms in nature which will perform much better in given tasks compared to any engineered E. coli or S. cerevisiae strain. Microbes from traditional fermentation procedures are a treasure trove in which to identify novel organisms which may perform desired tasks with only little effort in genetic engineering. There are opportunities for the production, enhancement and preservation of food, but also for biomaterials and alternative feedstocks to build a circular bioeconomy, with synthetic biology offering a suitable tool-box with which to achieve these goals.
Conclusion and perspectives
For many reasons future food will not be produced as it is done today. The current methods of agriculture are not sustainable and are harmful to the Earth. In moving towards future food, many challenges will be addressed - some of which are already known and others which will become apparent during the transition. However, these challenges represent opportunities and synthetic biology in combination with traditional techniques might be one approach to tackle them. One of the major challenges will be the opinion of the consumer, who would have to be convinced that, at least to a certain proportion, food will be produced by engineered microorganisms. Microbes from traditional food fermentation procedures may provide the ideal chassis for synthetic biology by balancing tradition and modern technologies. This may sound futuristic but there are already many examples of products from engineered microbes used for medical purposes such as vaccines or drug precursors. So why not apply engineered microbes to create a circular bioeconomy?
An obvious challenge, but at the same time a research accelerator for future food, lies in the exploration of space. Humankind seems set to again become an explorer, and aims to install settlements on the Moon and Mars. The efforts of space exploration will greatly benefit from synthetic biology and its application. With the establishment of a settlement far away from Earth, food supplies will rely on the progress of synthetic biology to create future foods. Will these developments be beneficial for everyone, similar to the development of Teflon now essential part of non-stick pans common in many kitchens?
Why is the acceptance of products from genetically engineered microbes or crops to feed humanity low? To the knowledge of the authors, no human being has died so far because of the consumption of food originating from genetically engineered sources, but according to the United Nations every day 25,000 people, including more than 10,000 children, die from starvation and malnutrition! From the authors’ perspective, the major challenge might not be sustainable food production: it is convincing policy makers, politicians and consumers to make sustainable choices and be open towards novel technologies such as precision gene editing to optimize production and enhance products. The authors acknowledge that vigorous testing of such engineered organisms and their products is compulsory and there must be regulations, however, one of the advantages of microorganisms is that they are kept under controlled environments and may even contain engineered safety switches to ensure they do not interfere with biodiversity if exposed to the environment. Nature has been suffering from decades of traditional agriculture and industrialization. Engineered microorganisms should be explored further to tackle future food challenges and ultimately establish a circular bioeconomy.
Data availability statement
The original contributions presented in the study are included in the article/Supplementary Material, further inquiries can be directed to the corresponding author.
Author contributions
DS conceived, planned and designed the study. AR, RS and DS wrote the article. All authors approved the final manuscript.
Funding
This work was funded by the Max Planck Society in the framework of the MaxGENESYS project. Publishing costs were covered by the Max Planck Digital Library (MDPL) and Frontiers publishing agreement.
Acknowledgments
We thank the Schindler research group and MaxGENESYS biofoundry team for fruitful and inspiring discussions. We thank Sally Jones for critical reading of the manuscript and Ehmad Chehrghani Bozcheloe for preparing the artwork.
Conflict of interest
The authors declare that the research was conducted in the absence of any commercial or financial relationships that could be construed as a potential conflict of interest.
Publisher’s note
All claims expressed in this article are solely those of the authors and do not necessarily represent those of their affiliated organizations, or those of the publisher, the editors and the reviewers. Any product that may be evaluated in this article, or claim that may be made by its manufacturer, is not guaranteed or endorsed by the publisher.
References
Acuna, J. M. B., and Poblete-Castro, I. (2022). Rational engineering of natural polyhydroxyalkanoates producing microorganisms for improved synthesis and recovery. Microb. Biotechnol. doi:10.1111/1751-7915.14109
Ahnan-Winarno, A. D., Cordeiro, L., Winarno, F. G., Gibbons, J., and Xiao, H. (2021). Tempeh: A semicentennial review on its health benefits, fermentation, safety, processing, sustainability, and affordability. Compr. Rev. Food Sci. Food Saf. 20 (2), 1717–1767. [Online ahead of print]. doi:10.1111/1541-4337.12710
Allwood, J. G., Wakeling, L. T., and Bean, D. C. (2021). Fermentation and the microbial community of Japanese koji and miso: A review. J. Food Sci. 86 (6), 2194–2207. doi:10.1111/1750-3841.15773
Arikan, M., Mitchell, A. L., Finn, R. D., and Gurel, F. (2020). Microbial composition of kombucha determined using amplicon sequencing and shotgun metagenomics. J. Food Sci. 85 (2), 455–464. doi:10.1111/1750-3841.14992
Bar-Even, A. (2016). Formate assimilation: The metabolic architecture of natural and synthetic pathways. Biochemistry 55 (28), 3851–3863. doi:10.1021/acs.biochem.6b00495
Barman, A., Das, R., and De, P. K. (2021). Logistics and supply chain management of food industry during COVID-19: Disruptions and a recovery plan. Environ. Syst. Decis. 2, 1–12. doi:10.1007/s10669-021-09836-w
Bashir, A., Klammer, A., Robins, W. P., Chin, C. S., Webster, D., Paxinos, E., et al. (2012). A hybrid approach for the automated finishing of bacterial genomes. Nat. Biotechnol. 30 (7), 701–707. doi:10.1038/nbt.2288
Basiony, M., Ouyang, L., Wang, D., Yu, J., Zhou, L., Zhu, M., et al. (2022). Optimization of microbial cell factories for astaxanthin production: Biosynthesis and regulations, engineering strategies and fermentation optimization strategies. Synth. Syst. Biotechnol. 7 (2), 689–704. doi:10.1016/j.synbio.2022.01.002
Belleggia, L., Aquilanti, L., Ferrocino, I., Milanovic, V., Garofalo, C., Clementi, F., et al. (2020). Discovering microbiota and volatile compounds of surstromming, the traditional Swedish sour herring. Food Microbiol. 91, 103503. doi:10.1016/j.fm.2020.103503
Bilal, M., Ji, L., Xu, S., Zhang, Y., Iqbal, H. M. N., and Cheng, H. (2022). Bioprospecting and biotechnological insights into sweet-tasting proteins by microbial hosts-a review. Bioengineered 13 (4), 9816–9829. doi:10.1080/21655979.2022.2061147
Boels, I. C., Kleerebezem, M., and de Vos, W. M. (2003). Engineering of carbon distribution between glycolysis and sugar nucleotide biosynthesis in Lactococcus lactis. Appl. Environ. Microbiol. 69 (2), 1129–1135. doi:10.1128/AEM.69.2.1129-1135.2003
Bokulich, N. A., and Bamforth, C. W. (2013). The microbiology of malting and brewing. Microbiol. Mol. Biol. Rev. 77 (2), 157–172. doi:10.1128/MMBR.00060-12
Chaudhary, V., Punia Bangar, S., Thakur, N., and Trif, M. (2022). Recent advancements in smart biogenic packaging: Reshaping the future of the food packaging industry. Polym. (Basel) 14 (4), 829. doi:10.3390/polym14040829
Chen, G. Q., and Patel, M. K. (2012). Plastics derived from biological sources: Present and future: A technical and environmental review. Chem. Rev. 112 (4), 2082–2099. doi:10.1021/cr200162d
Choi, S., Lee, H. N., Park, E., Lee, S. J., and Kim, E. S. (2020). Recent advances in microbial production of cis, cis-muconic acid. Biomolecules 10 (9), 1238. doi:10.3390/biom10091238
Claassens, N. J., Sanchez-Andrea, I., Sousa, D. Z., and Bar-Even, A. (2018). Towards sustainable feedstocks: A guide to electron donors for microbial carbon fixation. Curr. Opin. Biotechnol. 50, 195–205. doi:10.1016/j.copbio.2018.01.019
Comasio, A., Verce, M., Van Kerrebroeck, S., and De Vuyst, L. (2020). Diverse microbial composition of sourdoughs from different origins. Front. Microbiol. 11, 1212. doi:10.3389/fmicb.2020.01212
Comitini, F., Agarbati, A., Canonico, L., and Ciani, M. (2021). Yeast interactions and molecular mechanisms in wine fermentation: A comprehensive review. Int. J. Mol. Sci. 22 (14), 7754. doi:10.3390/ijms22147754
Cotton, C. A., Claassens, N. J., Benito-Vaquerizo, S., and Bar-Even, A. (2020). Renewable methanol and formate as microbial feedstocks. Curr. Opin. Biotechnol. 62, 168–180. doi:10.1016/j.copbio.2019.10.002
Dan, T., Ren, W., Liu, Y., Tian, J., Chen, H., Li, T., et al. (2019). Volatile flavor compounds profile and fermentation characteristics of milk fermented by Lactobacillus delbrueckii subsp. bulgaricus. Front. Microbiol. 10, 2183. doi:10.3389/fmicb.2019.02183
De Filippis, F., Pasolli, E., and Ercolini, D. (2020). The food-gut axis: Lactic acid bacteria and their link to food, the gut microbiome and human health. FEMS Microbiol. Rev. 44 (4), 454–489. doi:10.1093/femsre/fuaa015
Denby, C. M., Li, R. A., Vu, V. T., Costello, Z., Lin, W., Chan, L. J. G., et al. (2018). Industrial brewing yeast engineered for the production of primary flavor determinants in hopped beer. Nat. Commun. 9 (1), 965. doi:10.1038/s41467-018-03293-x
Dimidi, E., Cox, S. R., Rossi, M., and Whelan, K. (2019). Fermented foods: Definitions and characteristics, impact on the gut microbiota and effects on gastrointestinal health and disease. Nutrients 11 (8), 1806. doi:10.3390/nu11081806
Dondapati, S. K., Stech, M., Zemella, A., and Kubick, S. (2020). Cell-free protein synthesis: A promising option for future drug development. BioDrugs 34 (3), 327–348. doi:10.1007/s40259-020-00417-y
Ejigu, G. F., and Jung, J. (2020). Review on the computational genome annotation of sequences obtained by Next-Generation Sequencing. Biol. (Basel) 9 (9), 295. doi:10.3390/biology9090295
El Karoui, M., Hoyos-Flight, M., and Fletcher, L. (2019). Future trends in synthetic biology - a report. Front. Bioeng. Biotechnol. 7, 175. doi:10.3389/fbioe.2019.00175
Ellis, T. (2019). Predicting how evolution will beat us. Microb. Biotechnol. 12 (1), 41–43. doi:10.1111/1751-7915.13327
Engler, C., Kandzia, R., and Marillonnet, S. (2008). A one pot, one step, precision cloning method with high throughput capability. PLoS One 3 (11), e3647. doi:10.1371/journal.pone.0003647
Enserink, M. (2008). Tough lessons from golden rice. Science 320 (5875), 468–471. doi:10.1126/science.320.5875.468
Fabarius, J. T., Wegat, V., Roth, A., and Sieber, V. (2021). Synthetic methylotrophy in yeasts: Towards a circular bioeconomy. Trends Biotechnol. 39 (4), 348–358. doi:10.1016/j.tibtech.2020.08.008
Farzaneh, T., and Freemont, P. S. (2021). Biofoundries are a nucleating hub for industrial translation. Synth. Biol. 6 (1), ysab013. doi:10.1093/synbio/ysab013
Fernandez-Nino, M., Rodriguez-Cubillos, M. J., Herrera-Rocha, F., Anzola, J. M., Cepeda-Hernandez, M. L., Aguirre Mejia, J. L., et al. (2021). Dissecting industrial fermentations of fine flavour cocoa through metagenomic analysis. Sci. Rep. 11 (1), 8638. doi:10.1038/s41598-021-88048-3
Foster, B., Tyrawa, C., Ozsahin, E., Lubberts, M., Krogerus, K., Preiss, R., et al. (2022). Kveik brewing yeasts demonstrate wide flexibility in beer fermentation temperature tolerance and exhibit enhanced trehalose accumulation. Front. Microbiol. 13, 747546. doi:10.3389/fmicb.2022.747546
Fukami, H., Higa, Y., Hisano, T., Asano, K., Hirata, T., and Nishibe, S. (2021). A review of red yeast rice, a traditional fermented food in Japan and east asia: Its characteristic ingredients and application in the maintenance and improvement of health in lipid metabolism and the circulatory system. Molecules 26 (6), 1619. doi:10.3390/molecules26061619
Gallone, B., Steensels, J., Prahl, T., Soriaga, L., Saels, V., Herrera-Malaver, B., et al. (2016). Domestication and divergence of Saccharomyces cerevisiae beer yeasts. Cell. 166 (6), 1397–1410. e16 e1316. doi:10.1016/j.cell.2016.08.020
Ghurye, J. S., Cepeda-Espinoza, V., and Pop, M. (2016). Metagenomic assembly: Overview, challenges and applications. Yale J. Biol. Med. 89 (3), 353–362.
Hillson, N., Caddick, M., Cai, Y., Carrasco, J. A., Chang, M. W., Curach, N. C., et al. (2019). Building a global alliance of biofoundries. Nat. Commun. 10 (1), 2040. doi:10.1038/s41467-019-10079-2
IFPRI (2022). “Global food policy report: Climate change and food systems,” in Global food policy report (Washington, DC: IFPRI), 2022. doi:10.2499/9780896294257
Jiang, W., Hernandez Villamor, D., Peng, H., Chen, J., Liu, L., Haritos, V., et al. (2021). Metabolic engineering strategies to enable microbial utilization of C1 feedstocks. Nat. Chem. Biol. 17 (8), 845–855. doi:10.1038/s41589-021-00836-0
Johns, N. I., Blazejewski, T., Gomes, A. L., and Wang, H. H. (2016). Principles for designing synthetic microbial communities. Curr. Opin. Microbiol. 31, 146–153. doi:10.1016/j.mib.2016.03.010
Joseph, J. A., Akkermans, S., Nimmegeers, P., and Van Impe, J. F. M. (2019). Bioproduction of the recombinant sweet protein thaumatin: Current state of the art and perspectives. Front. Microbiol. 10, 695. doi:10.3389/fmicb.2019.00695
Jung, H., Ventura, T., Chung, J. S., Kim, W. J., Nam, B. H., Kong, H. J., et al. (2020). Twelve quick steps for genome assembly and annotation in the classroom. PLoS Comput. Biol. 16 (11), e1008325. doi:10.1371/journal.pcbi.1008325
Kawa-Rygielska, J., Adamenko, K., Pietrzak, W., Paszkot, J., Glowacki, A., and Gasinski, A. (2022). Characteristics of New England India Pale Ale beer produced with the use of Norwegian kveik yeast. Molecules 27 (7), 2291. doi:10.3390/molecules27072291
Kawa-Rygielska, J., Adamenko, K., Pietrzak, W., Paszkot, J., Glowacki, A., Gasinski, A., et al. (2021). The potential of traditional Norwegian kveik yeast for brewing novel beer on the example of foreign extra stout. Biomolecules 11 (12), 1778. doi:10.3390/biom11121778
Kelwick, R., MacDonald, J. T., Webb, A. J., and Freemont, P. (2014). Developments in the tools and methodologies of synthetic biology. Front. Bioeng. Biotechnol. 2, 60. doi:10.3389/fbioe.2014.00060
Kenny, S. T., Runic, J. N., Kaminsky, W., Woods, T., Babu, R. P., Keely, C. M., et al. (2008). Up-cycling of PET (polyethylene terephthalate) to the biodegradable plastic PHA (polyhydroxyalkanoate). Environ. Sci. Technol. 42 (20), 7696–7701. doi:10.1021/es801010e
Kluz, M. I., Pietrzyk, K., Pastuszczak, M., Kacaniova, M., Kita, A., Kapusta, I., et al. (2022). Microbiological and physicochemical composition of various types of homemade kombucha beverages using alternative kinds of sugars. Foods 11 (10), 1523. doi:10.3390/foods11101523
Knott, G. J., and Doudna, J. A. (2018). CRISPR-Cas guides the future of genetic engineering. Science 361 (6405), 866–869. doi:10.1126/science.aat5011
Kodama, Y., Shumway, M., and Leinonen, R.International Nucleotide Sequence Database Collaboration (2012). The sequence read archive: Explosive growth of sequencing data. Nucleic Acids Res. 40, D54–D56. Database issue. doi:10.1093/nar/gkr854
Krogerus, K., Preiss, R., and Gibson, B. (2018). A Unique Saccharomyces cerevisiae x Saccharomyces uvarum hybrid isolated from Norwegian farmhouse beer: Characterization and reconstruction. Front. Microbiol. 9, 2253. doi:10.3389/fmicb.2018.02253
Kumar, P., Chatli, M. K., Verma, A. K., Mehta, N., Malav, O. P., Kumar, D., et al. (2017). Quality, functionality, and shelf life of fermented meat and meat products: A review. Crit. Rev. Food Sci. Nutr. 57 (13), 2844–2856. doi:10.1080/10408398.2015.1074533
Lavefve, L., Marasini, D., and Carbonero, F. (2019). Microbial ecology of fermented vegetables and non-alcoholic drinks and current knowledge on their impact on human health. Adv. Food Nutr. Res. 87, 147–185. doi:10.1016/bs.afnr.2018.09.001
Le Feuvre, R. A., and Scrutton, N. S. (2018). A living foundry for synthetic biological materials: A synthetic biology roadmap to new advanced materials. Synth. Syst. Biotechnol. 3 (2), 105–112. doi:10.1016/j.synbio.2018.04.002
Ledford, H. (2021). New rules will make UK gene-edited crop research easier. Nature. [Online ahead of print]. doi:10.1038/d41586-021-01572-0
Li, S., Li, P., Feng, F., and Luo, L. X. (2015). Microbial diversity and their roles in the vinegar fermentation process. Appl. Microbiol. Biotechnol. 99 (12), 4997–5024. doi:10.1007/s00253-015-6659-1
Lian, J., Bao, Z., Hu, S., and Zhao, H. (2018). Engineered CRISPR/Cas9 system for multiplex genome engineering of polyploid industrial yeast strains. Biotechnol. Bioeng. 115 (6), 1630–1635. doi:10.1002/bit.26569
Liu, Y., Benitez, M. G., Chen, J., Harrison, E., Khusnutdinova, A. N., and Mahadevan, R. (2021). Opportunities and challenges for microbial synthesis of fatty acid-derived chemicals (FACs). Front. Bioeng. Biotechnol. 9, 613322. doi:10.3389/fbioe.2021.613322
Lu, H., Kerkhoven, E. J., and Nielsen, J. (2022). Multiscale models quantifying yeast physiology: Towards a whole-cell model. Trends Biotechnol. 40 (3), 291–305. doi:10.1016/j.tibtech.2021.06.010
Lui, L. M., Nielsen, T. N., and Arkin, A. P. (2021). A method for achieving complete microbial genomes and improving bins from metagenomics data. PLoS Comput. Biol. 17 (5), e1008972. doi:10.1371/journal.pcbi.1008972
Luo, S. R., DeMarsh, T. A., deRiancho, D., Stelick, A., and Alcaine, S. D. (2021). Characterization of the fermentation and sensory profiles of novel yeast-fermented acid whey beverages. Foods 10 (6), 1204. doi:10.3390/foods10061204
Luo, Z. W., and Lee, S. Y. (2020). Metabolic engineering of Escherichia coli for the production of benzoic acid from glucose. Metab. Eng. 62, 298–311. doi:10.1016/j.ymben.2020.10.002
Marcellin, E., Angenent, L. T., Nielsen, L. K., and Molitor, B. (2022). Recycling carbon for sustainable protein production using gas fermentation. Curr. Opin. Biotechnol. 76, 102723. doi:10.1016/j.copbio.2022.102723
Marco, M. L., Heeney, D., Binda, S., Cifelli, C. J., Cotter, P. D., Foligne, B., et al. (2017). Health benefits of fermented foods: Microbiota and beyond. Curr. Opin. Biotechnol. 44, 94–102. doi:10.1016/j.copbio.2016.11.010
Marella, E. R., Holkenbrink, C., Siewers, V., and Borodina, I. (2018). Engineering microbial fatty acid metabolism for biofuels and biochemicals. Curr. Opin. Biotechnol. 50, 39–46. doi:10.1016/j.copbio.2017.10.002
Marvik, O. J., and Philp, J. (2020). The systemic challenge of the bioeconomy: A policy framework for transitioning towards a sustainable carbon cycle economy. EMBO Rep. 21 (10), e51478. doi:10.15252/embr.202051478
Mays, Z. J., and Nair, N. U. (2018). Synthetic biology in probiotic lactic acid bacteria: At the frontier of living therapeutics. Curr. Opin. Biotechnol. 53, 224–231. doi:10.1016/j.copbio.2018.01.028
McCarty, N. S., and Ledesma-Amaro, R. (2019). Synthetic biology tools to engineer microbial communities for biotechnology. Trends Biotechnol. 37 (2), 181–197. doi:10.1016/j.tibtech.2018.11.002
Molinet, J., and Cubillos, F. A. (2020). Wild yeast for the future: Exploring the use of wild strains for wine and beer fermentation. Front. Genet. 11, 589350. doi:10.3389/fgene.2020.589350
Moosavi, J., Fathollahi-Fard, A. M., and Dulebenets, M. A. (2022). Supply chain disruption during the COVID-19 pandemic: Recognizing potential disruption management strategies. Int. J. Disaster Risk Reduct. 75, 102983. doi:10.1016/j.ijdrr.2022.102983
Moss, E. L., Maghini, D. G., and Bhatt, A. S. (2020). Complete, closed bacterial genomes from microbiomes using nanopore sequencing. Nat. Biotechnol. 38 (6), 701–707. doi:10.1038/s41587-020-0422-6
Mozzachiodi, S., Krogerus, K., Gibson, B., Nicolas, A., and Liti, G. (2022). Unlocking the functional potential of polyploid yeasts. Nat. Commun. 13 (1), 2580. doi:10.1038/s41467-022-30221-x
Nesic, A., Cabrera-Barjas, G., Dimitrijevic-Brankovic, S., Davidovic, S., Radovanovic, N., and Delattre, C. (2019). Prospect of polysaccharide-based materials as advanced food packaging. Molecules 25 (1), 135. doi:10.3390/molecules25010135
Olsson, K., Carlsen, S., Semmler, A., Simon, E., Mikkelsen, M. D., and Moller, B. L. (2016). Microbial production of next-generation stevia sweeteners. Microb. Cell. Fact. 15 (1), 207. doi:10.1186/s12934-016-0609-1
Ostrov, N., Beal, J., Ellis, T., Gordon, D. B., Karas, B. J., Lee, H. H., et al. (2019). Technological challenges and milestones for writing genomes. Science 366 (6463), 310–312. doi:10.1126/science.aay0339
Prado, M. R., Blandon, L. M., Vandenberghe, L. P., Rodrigues, C., Castro, G. R., Thomaz-Soccol, V., et al. (2015). Milk kefir: Composition, microbial cultures, biological activities, and related products. Front. Microbiol. 6, 1177. doi:10.3389/fmicb.2015.01177
Prasad, T. S., Mohanty, A. K., Kumar, M., Sreenivasamurthy, S. K., Dey, G., Nirujogi, R. S., et al. (2017). Integrating transcriptomic and proteomic data for accurate assembly and annotation of genomes. Genome Res. 27 (1), 133–144. doi:10.1101/gr.201368.115
Preiss, R., Tyrawa, C., Krogerus, K., Garshol, L. M., and van der Merwe, G. (2018). Traditional Norwegian kveik are a genetically distinct group of domesticated Saccharomyces cerevisiae brewing yeasts. Front. Microbiol. 9, 2137. doi:10.3389/fmicb.2018.02137
Prete, R., Alam, M. K., Perpetuini, G., Perla, C., Pittia, P., and Corsetti, A. (2021). Lactic acid bacteria exopolysaccharides producers: A sustainable tool for functional foods. Foods 10 (7), 1653. doi:10.3390/foods10071653
Pswarayi, F., and Ganzle, M. G. (2019). Composition and origin of the fermentation microbiota of mahewu, a Zimbabwean fermented cereal beverage. Appl. Environ. Microbiol. 85 (11), e03130–18. doi:10.1128/AEM.03130-18
Ren, J., Lee, J., and Na, D. (2020). Recent advances in genetic engineering tools based on synthetic biology. J. Microbiol. 58 (1), 1–10. doi:10.1007/s12275-020-9334-x
Rubio, N. R., Xiang, N., and Kaplan, D. L. (2020). Plant-based and cell-based approaches to meat production. Nat. Commun. 11 (1), 6276. doi:10.1038/s41467-020-20061-y
Sadler, J. C., and Wallace, S. (2021). Microbial synthesis of vanillin from waste poly(ethylene terephthalate). Green Chem. 23 (13), 4665–4672. doi:10.1039/d1gc00931a
Sanlier, N., Gokcen, B. B., and Sezgin, A. C. (2019). Health benefits of fermented foods. Crit. Rev. Food Sci. Nutr. 59 (3), 506–527. doi:10.1080/10408398.2017.1383355
Schindler, D., Dai, J., and Cai, Y. (2018). Synthetic genomics: A new venture to dissect genome fundamentals and engineer new functions. Curr. Opin. Chem. Biol. 46, 56–62. doi:10.1016/j.cbpa.2018.04.002
Schindler, D. (2020). Genetic engineering and synthetic genomics in yeast to understand life and boost biotechnology. Bioeng. (Basel) 7 (4), 137. doi:10.3390/bioengineering7040137
Schmidt, F. R. (2005). Optimization and scale up of industrial fermentation processes. Appl. Microbiol. Biotechnol. 68 (4), 425–435. doi:10.1007/s00253-005-0003-0
Schwander, T., Schada von Borzyskowski, L., Burgener, S., Cortina, N. S., and Erb, T. J. (2016). A synthetic pathway for the fixation of carbon dioxide in vitro. Science 354 (6314), 900–904. doi:10.1126/science.aah5237
Searchinger, T., Waite, R., Hanson, C., ranganathan, J., and Matthews, E. (2019). World resources report: Creating a sustainable food future. World Resources Institute. Available at: https://www.wri.org/research/creating-sustainable-food-future
Sharma, A., and Yazdani, S. S. (2021). Microbial engineering to produce fatty alcohols and alkanes. J. Ind. Microbiol. Biotechnol. 48 (1-2), kuab011. doi:10.1093/jimb/kuab011
Shi, B., Ma, T., Ye, Z., Li, X., Huang, Y., Zhou, Z., et al. (2019). Systematic metabolic engineering of Saccharomyces cerevisiae for lycopene overproduction. J. Agric. Food Chem. 67 (40), 11148–11157. doi:10.1021/acs.jafc.9b04519
Shimizu, Y., Kanamori, T., and Ueda, T. (2005). Protein synthesis by pure translation systems. Methods 36 (3), 299–304. doi:10.1016/j.ymeth.2005.04.006
Singh, A., Walker, K. T., Ledesma-Amaro, R., and Ellis, T. (2020). Engineering bacterial cellulose by synthetic biology. Int. J. Mol. Sci. 21 (23), 9185. doi:10.3390/ijms21239185
Smith, D., da Silva, M., Jackson, J., and Lyal, C. (2017). Explanation of the Nagoya Protocol on access and benefit sharing and its implication for microbiology. Microbiol. Read. 163 (3), 289–296. doi:10.1099/mic.0.000425
Sonnendecker, C., Oeser, J., Richter, P. K., Hille, P., Zhao, Z., Fischer, C., et al. (2022). Low carbon footprint recycling of post-consumer PET plastic with a metagenomic polyester hydrolase. ChemSusChem 15 (9), e202101062. doi:10.1002/cssc.202101062
Stokstad, E. (2019). After 20 years, golden rice nears approval. Science 366 (6468), 934. doi:10.1126/science.366.6468.934
Strong, P. J., Self, R., Allikian, K., Szewczyk, E., Speight, R., O'Hara, I., et al. (2022). Filamentous fungi for future functional food and feed. Curr. Opin. Biotechnol. 76, 102729. doi:10.1016/j.copbio.2022.102729
Sun, G., Ahn-Horst, T. A., and Covert, M. W. (2021). The E. coli whole-cell modeling project. EcoSal Plus 9 (2), eESP00012020. doi:10.1128/ecosalplus.ESP-0001-2020
Swartz, J. (2006). Developing cell-free biology for industrial applications. J. Ind. Microbiol. Biotechnol. 33 (7), 476–485. doi:10.1007/s10295-006-0127-y
Tangyu, M., Muller, J., Bolten, C. J., and Wittmann, C. (2019). Fermentation of plant-based milk alternatives for improved flavour and nutritional value. Appl. Microbiol. Biotechnol. 103 (23-24), 9263–9275. doi:10.1007/s00253-019-10175-9
Tournier, V., Topham, C. M., Gilles, A., David, B., Folgoas, C., Moya-Leclair, E., et al. (2020). An engineered PET depolymerase to break down and recycle plastic bottles. Nature 580 (7802), 216–219. doi:10.1038/s41586-020-2149-4
Trinci, A. P. J. (1992). Myco-protein: A twenty-year overnight success story. Mycol. Res. 96(1), 1–13. doi:10.1016/S0953-7562(09)80989-1
Tsoi, R., Dai, Z., and You, L. (2019). Emerging strategies for engineering microbial communities. Biotechnol. Adv. 37 (6), 107372. doi:10.1016/j.biotechadv.2019.03.011
Wang, L., Jiang, S., Chen, C., He, W., Wu, X., Wang, F., et al. (2018). Synthetic genomics: From DNA synthesis to genome design. Angew. Chem. Int. Ed. 57 (7), 1748–1756. doi:10.1002/anie.201708741
Wang, Y. H., Zhang, R. R., Yin, Y., Tan, G. F., Wang, G. L., Liu, H., et al. (2022). Advances in engineering the production of the natural red pigment lycopene: A systematic review from a biotechnology perspective. J. Adv. Res. [Online ahead of print]. doi:10.1016/j.jare.2022.06.010
Wang, Z., Sun, J., Yang, Q., and Yang, J. (2020). Metabolic engineering Escherichia coli for the production of lycopene. Molecules 25 (14), 3136. doi:10.3390/molecules25143136
Weckx, S., Van Kerrebroeck, S., and De Vuyst, L. (2019). Omics approaches to understand sourdough fermentation processes. Int. J. Food Microbiol. 302, 90–102. doi:10.1016/j.ijfoodmicro.2018.05.029
Werner, S., Engler, C., Weber, E., Gruetzner, R., and Marillonnet, S. (2012). Fast track assembly of multigene constructs using Golden Gate cloning and the MoClo system. Bioengineered 3 (1), 38–43. doi:10.4161/bbug.3.1.18223
Wilson, F. M., and Harrison, R. J. (2021). CRISPR/Cas9 mediated editing of the Quorn fungus Fusarium venenatum A3/5 by transient expression of Cas9 and sgRNAs targeting endogenous marker gene PKS12. Fungal Biol. Biotechnol. 8 (1), 15. doi:10.1186/s40694-021-00121-8
Xu, Y., Wang, X., Zhang, C., Zhou, X., Xu, X., Han, L., et al. (2022). De novo biosynthesis of rubusoside and rebaudiosides in engineered yeasts. Nat. Commun. 13 (1), 3040. doi:10.1038/s41467-022-30826-2
Yan, Q., and Fong, S. S. (2017). Challenges and advances for genetic engineering of non-model bacteria and uses in consolidated bioprocessing. Front. Microbiol. 8, 2060. doi:10.3389/fmicb.2017.02060
Yang, C., Chowdhury, D., Zhang, Z., Cheung, W. K., Lu, A., Bian, Z., et al. (2021). A review of computational tools for generating metagenome-assembled genomes from metagenomic sequencing data. Comput. Struct. Biotechnol. J. 19, 6301–6314. doi:10.1016/j.csbj.2021.11.028
Ye, X., Al-Babili, S., Kloti, A., Zhang, J., Lucca, P., Beyer, P., et al. (2000). Engineering the provitamin A (beta-carotene) biosynthetic pathway into (carotenoid-free) rice endosperm. Science 287 (5451), 303–305. doi:10.1126/science.287.5451.303
Yishai, O., Lindner, S. N., Gonzalez de la Cruz, J., Tenenboim, H., and Bar-Even, A. (2016). The formate bio-economy. Curr. Opin. Chem. Biol. 35, 1–9. doi:10.1016/j.cbpa.2016.07.005
Yoshida, S., Hiraga, K., Takehana, T., Taniguchi, I., Yamaji, H., Maeda, Y., et al. (2016). A bacterium that degrades and assimilates poly(ethylene terephthalate). Science 351 (6278), 1196–1199. doi:10.1126/science.aad6359
Zabat, M. A., Sano, W. H., Wurster, J. I., Cabral, D. J., and Belenky, P. (2018). Microbial community analysis of Sauerkraut fermentation reveals a stable and rapidly established community. Foods 7 (5), 77. doi:10.3390/foods7050077
Zhang, L., Chen, F., Zeng, Z., Xu, M., Sun, F., Yang, L., et al. (2021). Advances in metagenomics and its application in environmental microorganisms. Front. Microbiol. 12, 766364. doi:10.3389/fmicb.2021.766364
Zheng, X., Shi, X., and Wang, B. (2021). A review on the general cheese processing technology, flavor biochemical pathways and the influence of yeasts in cheese. Front. Microbiol. 12, 703284. doi:10.3389/fmicb.2021.703284
Zheng, Y., Chen, J. C., Ma, Y. M., and Chen, G. Q. (2020). Engineering biosynthesis of polyhydroxyalkanoates (PHA) for diversity and cost reduction. Metab. Eng. 58, 82–93. doi:10.1016/j.ymben.2019.07.004
Zhong, C. (2020). Industrial-scale production and applications of bacterial cellulose. Front. Bioeng. Biotechnol. 8, 605374. doi:10.3389/fbioe.2020.605374
Keywords: synthetic biology, microbe domestication, microbial chassis, fermenation, future food, biological materials, yeast
Citation: Ramírez Rojas AA, Swidah R and Schindler D (2022) Microbes of traditional fermentation processes as synthetic biology chassis to tackle future food challenges. Front. Bioeng. Biotechnol. 10:982975. doi: 10.3389/fbioe.2022.982975
Received: 30 June 2022; Accepted: 10 August 2022;
Published: 16 September 2022.
Edited by:
Eva Garcia-Ruiz, Spanish National Research Council (CSIC), SpainReviewed by:
Eun Joong Oh, Purdue University, United StatesJingwen Zhou, Jiangnan University, China
Yanfeng Liu, Jiangnan University, China
Copyright © 2022 Ramírez Rojas, Swidah and Schindler. This is an open-access article distributed under the terms of the Creative Commons Attribution License (CC BY). The use, distribution or reproduction in other forums is permitted, provided the original author(s) and the copyright owner(s) are credited and that the original publication in this journal is cited, in accordance with accepted academic practice. No use, distribution or reproduction is permitted which does not comply with these terms.
*Correspondence: Daniel Schindler, daniel.schindler@mpi-marburg.mpg.de
†These authors have contributed equally to this work and share first authorship