3D in-vitro cultures of human bone marrow and Wharton’s jelly derived mesenchymal stromal cells show high chondrogenic potential
- 1Department of Medicine, Surgery and Dentistry, University of Salerno, Baronissi, SA, Italy
- 2Hematology and Transplant Center, University Hospital “San Giovanni di Dio e Ruggi D’Aragona”, Salerno, SA, Italy
- 3Guy Hilton Research Centre, School of Pharmacy and Bioengineering, Keele University, Stoke-on-Trent, Staffordshire, United Kingdom
- 4Centre for Sport and Exercise Medicine, Barts and The London School of Medicine, Queen Mary University of London, London, United Kingdom
- 5Research Centre for Biomaterials BIONAM, Università di Salerno, Fisciano, SA, Italy
In this study, chondrogenic potentials of 3D high-density cultures of Bone Marrow (BM) and Wharton’s Jelly (WJ)-derived mesenchymal stromal cells (MSCs) was investigated by chondrogenesis- and cytokine-related gene expression over a 16-day culture period supplemented with human transforming growth factor (hTGF)-β1 at 10 ng/ml. In BM-MSC 3D models, a marked upregulation of chondrogenesis-related genes, such as SOX9, COL2A1, and ACAN (all p < 0.05) and formation of spherical pellets with structured type II collagen fibers were observed. Similarly, WJ-based high-density culture appeared higher in size and more regular in shape, with a significant overexpression of COL2A1 and ACAN (all p < 0.05) at day 16. Moreover, a similar upregulation trend was documented for IL-6 and IL-10 expression in both BM and WJ 3D systems. In conclusion, MSC-based high-density cultures can be considered a promising in vitro model of cartilage regeneration and tissue engineering. Moreover, our data support the use of WJ-MSCs as a valid alternative for chondrogenic commitment of stem cells in regenerative medicine.
Introduction
Hyaline cartilage, a specialized connective tissue with the principal task to provide a regular and lubricated surface in joints, helps in preventing the wear process (Sophia Fox et al., 2009) and in minimizing subchondral bone pressure by equally distributing load forces (Bhosale and Richardson, 2008). This tissue is composed of mature cells with poor mitotic potential, termed chondrocytes, and a complex extracellular matrix (ECM) containing type II collagen and aggrecan (ACAN), the main histological cartilage markers (Coburn et al., 2013; Maldonado and Nam, 2013). Type II collagen fibers provide tensile strength, are histologically highlighted with picrosirius red staining (PSR), and are visible under polarized light because of their birefringence properties (Schmitz et al., 2010a). Conversely, fibrous cartilage contains type I collagen and shows different biomechanical behavior (Roberts et al., 2009a). Cartilage lesions represent a substantial challenge for modern orthopedics as they do not spontaneously heal due to the absence of vascularity and innervation, and untreated lesions may have an unfavorable prognosis with the development of osteoarthritis (Grässel and Muschter, 2020).
3D in vitro models of chondrogenic differentiation using human stem cells are becoming the most utilized model to study healing and regenerative events. Human mesenchymal stromal cells (MSCs) derived from bone marrow (hBM-MSCs) have a well-described chondrogenic potential (Bernardo et al., 2007). MSCs, multipotent progenitor cells with self-renewal potential, can differentiate in several tissue types, such as muscle, adipose, and trabecular bone tissue; however, MSC harvesting from BM requires invasive procedures and the mesenchymal fraction is low (Mushahary et al., 2018). For these reasons, alternative MSC sources have been identified, such as adipose and umbilical cord tissue comprised of Wharton’s Jelly and umbilical cord blood (Johnstone et al., 1998; Wagner et al., 2005). However, Wharton’s Jelly-derived mesenchymal stromal cell (hWJ-MSCs) chondrogenic potential remains poorly investigated, despite those cells have a superior proliferative capacity, making them extremely valuable in tissue engineering context (Ciardulli et al., 2020a). Moreover, MSCs could modulate microenvironment composition as described in myelodysplasia, where they can contribute to hematopoietic stem cell growth inhibition by secretion of pro-inflammatory cytokines, especially transforming growth factor-beta (TGF-β) (Patel et al., 2021). Other cytokines and growth factors are important in modulating chondrogenesis in health and disease, as pro-inflammatory cytokines, such as interleukin (IL)-1β, that induces chondrocyte-mediated ECM proteolysis, or IL-6, can significantly reduce proliferation potential of chondrocytes and favor osteoarthritis events (Zhou et al., 2018; Razmara et al., 2019; Jafri et al., 2020a; Bhogoju et al., 2022). Conversely, anti-inflammatory cytokines, such as IL-4 and IL-10, are upregulated by BM-MSC models, induce physiological cartilage turnover over osteoarthritis events, and reduce inflammatory processes of the synovia (Jafri et al., 2020b).
Interestingly, TGF-β, both hTGF-β1 and hTGF-β3, is the principal growth factor used for establishing chondrogenesis in vitro (Danišovič et al., 2012a). In particular, hTGF-β1 is the most investigated, both in vitro and in vivo experiments (Huang et al., 2004a) (Roberts et al., 2009b); however, hTGF-β1 has a short half-life when supplemented in culture medium that might be overcome using microencapsulation with biopolymeric vehicles (Wakefield et al., 1990; Palazzo et al., 2021). hTGF-β1 promotes chondrogenesis through activation of different intracellular signaling pathways, including mitogen activated protein (MAP) kinases, p38, or extracellular signal-regulated kinase-1 (ERK-1) (Tuli et al., 2003). Conventional monolayer cultures (2D) are considered unsuitable for MSC differentiation towards chondrogenic phenotype and chondrocyte expansion; furthermore, 2D culture is often reported to promote dedifferentiation processes of chondrocytes and acquisition of fibroblast phenotype (Caron et al., 2012a).
Recently, stem cell 3D cultures have been proposed for chondrogenesis studies because they better mimic cartilage microenvironment where chondrocytes reside. The two predominant strategies are either utilization of a biomaterial scaffold to support seeding and subsequent differentiation, or alternatively, scaffold-free techniques, such as 3D high-density aggregate culture with several advantages (Costa et al., 2016). Suitable biomaterials composed by natural or ECM analog components can enhance cell functions, support differentiation toward specific phenotypes, and modulate immune responses (Nii and Katayama, 2021). In the context of cartilage regeneration, synthesis of advanced biomaterials with strict biomechanical properties, such as alginate hydrogels, is important to develop new surgical adjuvants for in vivo implantation (Bidarra et al., 2014; Cao et al., 2014; Baldino et al., 2021); however, clinical application is limited because of potential calcification processes (Ma et al., 2005). Chitosan, another polysaccharide, seems less suitable as a scaffold material as chondrocytes display reduced proliferation abilities likely due to its cationic properties (Sechriest et al., 2000). Conversely, collagen hydrogels can be an appropriate support for drug delivery system and/or stem cells local implantation (Lamparelli et al., 2021; 2022b), whereas, collagen-hyaluronic acid derivatives may be proposed as implantable 3D scaffold (Lamparelli et al., 2022a).
Polylactic-co-glycolic acid (PLGA), a synthetic biocompatible material, is rapidly degraded and releases acidic components that can cause inflammatory responses. Moreover, PLGA has no natural cell recognition sites, giving poor cell affinity (Mao et al., 2021). Conversely, poly (l-lactic acid) PLA is more effective in preventing in vivo hypertrophic drift of BM-MSCs, especially in nanofibrous form and when combined with matriline-3, a non-collagenous cartilage ECM protein (Liu et al., 2018).
Among scaffold-free techniques, cell sheet technology is used to develop transplantable constructs by stimulating MSC chondrogenic differentiation by spontaneously inducing post-detachment cell contraction leading to cytoskeletal reorganization (Thorp et al., 2020; 2021b). Moreover, multilayer cell sheets can be produced to increase 3D cellular interactions, especially those mediated by N-cadherin, connexin 43, and integrin β-1, enhancing in vitro chondrogenesis (Thorp et al., 2021a).
Another effective scaffold-free technique is 3D high-density culture for the manufacture of cartilage spheroids. These 3D-systems commonly display a hypoxic core that could promote chondrogenesis of stem cells. Moreover, interactions with adjacent cells simulate those found in pre-cartilage condensations during embryonic development (Ryu et al., 2019). These aggregates can be produced using a range of methods; however, the hanging drop technique allows to control 3D-system size by modifying drop volume or cell density (Ryu et al., 2019).
Despite 3D cultures are well-recognized methods to study chondrogenic potential of various tissue derived MSCs, the ability of these stem cells in a 3D setting to modulate chondrogenic differentiation and cytokine production has not been reported yet. Therefore, we investigated 3D high-density culture performance in promoting chondrogenic commitment of human stem cells, and in modification of cytokine expression during differentiation process. Specifically, BM and WJ-MSCs were used to produce 3D culture in the presence of hTGF-β1. Chondrogenic commitment was evaluated by gene expression profiling and immunohistochemistry (IHC) analysis of specific markers, such as types I, II, III, and X collagen, SOX9 transcription factor, and ACAN. Cytokine production was also monitored by pro- and anti-inflammatory cytokine gene expression and immunoassay along the differentiation events.
Materials and methods
hMSC isolation, expansion, and characterization
hBM-MSCs were obtained from bone marrow (BM) of three healthy donors (aged between 38 and 40); whereas hWJ-MSCs were isolated from human umbilical cord of three donors (age between 23 and 31). Donors gave written informed consent to the use of samples for research purposes, with approval of local Ethic Committee (Review Board prot./SCCE n. 24988). Briefly, BM aspirate or umbilical cord were seeded in Minimum Essential Medium Alpha (α-MEM) supplemented with 1% Glutagro™, 10% Fetal Bovine Serum (FBS), and 1% Penicillin/Streptomycin, and incubated at 37°C in an atmosphere of 5% CO2 and 95% relative humidity (Giordano et al., 2014). After 72 h, non-adherent cells and other residues were aspirated, fresh media added, and the remaining adherent cells fed with fresh media twice a week. On day 14, colonies of adherent MSCs were identified, detached, and re-seeded at 4,000 cells/cm2 in the same culture conditions. Once the cell culture reached 70–80% confluence, cells were detached using 0.05% trypsin-0.53 mM EDTA and washed with 1X phosphate buffered saline (PBS) (Corning Cellgro, Manassas, VA, United States), counted using Trypan Blue (Sigma-Aldrich, Milan, IT), and subcultured at a concentration of 4 × 103 cells/cm2. At passage 2, cells were used for the experiments. Flow cytometry analysis was performed on both hBM-MSCs and hWJ-MSCs obtained at passage 2 with antibodies directed against CD90, CD105, CD73, CD14, CD34, CD45, and HLA-DR expression (Miltenyi Biotec B.V. & Co. KG, Bergisch Gladbach, Germania), as better described elsewhere (Ciardulli et al., 2020b, 2021; Scala et al., 2022).
hMSC cultures
hBM-MSCs were seeded on coverslips at a concentration of 4 × 103 cells/cm2. Once the cell cultures reached 60% confluence, cells were treated with chondrogenic medium and supplemented with either 1 ng/ml or 10 ng/ml of recombinant human TGF-β1 (PeproTech EC, Ltd., London, United Kingdom). The chondrogenic medium was composed of alpha-minimum essential medium (α-MEM) (Corning, NY, United States) with reduced FBS to 1% (Corning Cellgro) further supplemented with 1% ITS (Corning Cellgro), 1% GlutagroTM (Corning Cellgro), 50 µM of ascorbic acid phosphate, and 1% Penicillin-Streptomycin. Cells were fed twice a week with fresh medium and growth factor for up to 16 days. Untreated cells for all studied time-points were employed as control.
High density 3D cultures were obtained by the hanging drop method. Briefly, hMSCs were resuspended at a density of 5 × 105 cells/mL. Drops of cell suspension (30 µl) were dispensed on the lids of Petri dishes, that were then inverted, and hanging drop cultures were incubated. After 3 days, resulting cellular aggregates were harvested using a pipette, and transferred into an ultra-low attachment 96 multi-well plate using 100 µL/well of chondrogenic medium (supplemented with 10 ng/ml of hTGF-β1), that was changed every 2 days. 3D systems morphology was monitored with ImageJ software (rel.1.52p National Institutes of Health, United States), and diameter, area (A) and perimeter (p) were measured, and circularity was calculated using Eqn. 1:
For the evaluation of Feret’s diameter and circularity, the average value of 10 3D-systems (n = 3), were considered (De Moor et al., 2020).
RNA isolation and gene expression profiling
Type II collagen (COL2A1), SRY-Related HMG-BOX Gene 9 (SOX9), Aggrecan (ACAN), type I, III and X collagen (COL1A1, COL3A1, and COL10A1) markers were investigated, as well as pro-inflammatory cytokines Interleukin 6 (IL-6), Tumor Necrosis Factor α (TNF-α), Interleukin 12A (IL-12A), Interleukin 1β (IL-1β) and anti-inflammatory ones Interleukin 10 (IL-10), and TGF-β1. Total RNA was extracted from hBM-MSCs at each time point using QIAzol® Lysis Reagent (Qiagen, DE), chloroform (Sigma-Aldrich) and the Rneasy Mini Kit (Qiagen, DE). For each sample, 1 μg of total RNA was reverse transcribed using the iScript™ cDNA synthesis kit (Bio-Rad, Milan, IT). Relative gene expression analysis was performed in a LightCycler® 480 Instrument (Roche, IT), using the SsoAdvanced™ Universal SYBR® Green Supermix (Bio-Rad, Foster City, CA, United States) with the validated primers (Bio-Rad) and following MIQE guidelines (Bustin et al., 2009). Amplification was performed in a 10 μl final volume, including 2 ng of complementary DNA (cDNA) as template. Triplicate experiments were performed for each explored condition, and data were normalized to glyceraldehyde-3-phosphate dehydrogenase (GAPDH) expression (Hellemans et al., 2007). Fold change in gene expression was determined by the 2−ΔΔCt method and presented as relative levels vs. untreated cells at each time-point.
Immunofluorescence assays
Cells were fixed with 3.7% paraformaldehyde (PFA) for 30 min at room temperature (RT), followed by permeabilization with 0.1% Triton × −-100 for 5 min and blocked with bovine serum albumin (BSA) solution (1% w/v) for 1 h. For type II and III collagen staining, cells were incubated overnight at 4°C with a rabbit polyclonal anti-type II collagen antibody (1:100; Cat no: ab34712, Abcam, Cambridge, United Kingdom) and a mouse polyclonal anti-type III collagen antibody (1:100; Cat no: sc166316, Santa Cruz Biotech., CA, United States). Then, cells were incubated for 1 h at RT with the Alexa Fluor ™ 488 goat anti-rabbit IgG (1:400; Thermo Fisher Scientific, Waltham, MA, United States) and the DyLight 649 anti-mouse IgG (1:500; BioLegend, San Diego, CA, United States) antibody. Cell nuclei were counterstained with 4′,6-diamidino-2-phenylindole (DAPI). Images were acquired using Leica laser-scanning confocal microscope (mod. TCS SP5; Leica Microsystems, Wetzlar DE) equipped with a plan Apo 63X/1.4 NA oil immersion objective. Signal intensity, related to the proteins of interest, was quantified using ImageJ software (rel.1.52p National Institutes of Health, United States) (Suchorska et al., 2016), when reported. Five images of several fields were used for the analysis at each time point. All data were reported as fold change relative to untreated cells. Antibody specificity was assessed in our previous works (Ciardulli et al., 2020c, 2021; Lamparelli et al., 2021).
3D-spheroids were fixed in 4% PFA for 2 h at room temperature, cryo-protected in 30% sucrose (4°C, overnight), included, and sliced in 15 μm thickness samples using a cryostat (mod. CM 1950, Leica, Wetzlar, Germany). For type I and II collagen staining, samples were incubated overnight at 4°C with a mouse polyclonal anti-collagen type I antibody (1:100, Cat no: MAB3391, Abcam) and rabbit polyclonal anti-collagen type II antibody (1:100, Cat no: ab34712, Abcam). Subsequently, slices were incubated for 1 h at RT with Alexa Fluor ™ 488 goat antirabbit IgG (1:400; Thermo Fisher Scientific) and DyLight 649 anti-mouse IgG (1:500; BioLegend) antibodies. Cell nuclei were counterstained with DAPI.
Sirius red staining
For cartilage matrix histochemistry, a Sirius Red staining was performed as previously described (Hyllested et al., 2002; Greiner et al., 2021). 3D-spheroid sections were stained in hematoxylin for 8 min, washed in water for 2 min, immersed into phosphomolybdic acid for 2 min, and washed in water for 2 min. Subsequently, samples were dipped into Picrosirius Red F3BA Stain (Polysciences, Inc., Warrington, PA, United States) for 60 min, and finally into HCl 0.1 M solution for 2 min. Samples were dehydrated with an increasing ethanol gradient (70%–75%–95%–100%) and cleared in xylene for 5 min. Sections were then mounted using Eukitt (Sigma-Aldrich) mounting medium. Picrosirius red brightfield and polarized light images were acquired with a Brunel polarization microscope equipped with a Nikon D500 camera.
Safranin-O staining
Spheroids were fixed in 4% paraformaldehyde (PFA) for 24 h at room temperature, cryo-protected in 30% sucrose (4°C, overnight), included in optimal cutting temperature (OCT) compound, and cut in 10 μm-thick slices through a cryostat microtome (mod. CM 1950, Leica, Wetzlar, Germany). Safranin-O staining was used to detect acidic proteoglycans present in the ECM, according to standard methods (Schmitz et al., 2010b). In more detail, slices were stained for 10 min with Wiegert’s Iron Hematoxylin (Biognost), 5 min with 0.5 g/L Fast green (Sigma-Aldrich), and 7 min with 0.1% Safranin-O solution (Merck Millipore, United States). Samples were dehydrated with an increasing ethanol gradient (70%–75%–95%–100%) and cleared in xylene for 2 min. Sections were then mounted using Eukitt (Sigma-Aldrich) mounting medium. Safranin-O brightfield images were acquired with a microscope (BX53, Olympus, Tokyo, Japan) equipped with an Olympus SC180 camera (Tokyo, Japan) and Olympus U-TV0.5XC-3 camera adapter operating Olympus cellSens standard 3.2 software.
Live and dead staining
Cell viability within scaffolds was detected by fluorescence live/dead assay (Calcein AM solution; Cat. no C1359) and Ethidium homodimer I solution (Sigma-Aldrich). In more detail, cells were stained for 1 h at 37°C, washed in 1 × PBS, and captured by fluorescence microscope (mod. Eclipse, Nikon Corporation, Tokyo, Japan). Single images were acquired with identical light intensity, exposure time, and gain settings. Signals intensity was quantified using ImageJ software (rel.1.52p National Institutes of Health, Bethesda, MD, United States). Original images in RGB format were converted into an 8-bit (gray scale) format, and tagged areas were expressed as an average value of pixel intensity within a range from 0 (dark) to 255 (white) as better described elsewhere (Spaepen et al., 2011).
Bead-based multiplex immunoassay
For cytokine measurement in culture medium, a bead-based multiplex immunoassay was employed with two sets of beads (Beads A and Beads B) using an internal fluorescence intensity detected in APC channel. A 9-plex LEGENDplex™ Cutom Panel (BioLegend) was designed to measure IL-1β, IL-6, TNF-α, hepatocyte growth factor (HGF), IL-15, IL-10, macrophage inflammatory protein (MIP)-1α and 1β, and Dickkopf-related protein 1 (DKK1). A calibration curve was prepared for cytokine quantification following manufacturer’s instructions. Samples were diluted 1:50 with fresh complete α-MEM medium and run in duplicate. Specimens were acquired on a BD FACSVerse cytometer (BD Biosciences) equipped with two lasers (blue, 488 nm; and red lasers, 628 nm) and BD FACSuite software (BD Biosciences), and at least 3,400 beads were recorded. LEGENDplex™ Data Analysis Software Suite (BioLegend) was used for post-acquisition analysis.
Statistical analysis
For comparison between two independent groups, two-tailed independent Student’s t test was performed. One-way or two-way ANOVA was used, followed by Tukey’s multiple comparison test for comparisons between more than two groups. p values < 0.05 were accepted as significant (de Winter 2013).
Results
hTGF-β1 at 10 ng/ml efficiently induces chondrogenic differentiation of hBM-MSCs
To identify optimal conditions for chondrogenic commitment of hBM-MSC, a medium supplemented with two concentrations of hTGF-β1 (1 and 10 ng/ml) was tested in a 16-day culture system. Higher growth factor concentrations were not considered, because published literature describes inhibitory effects (Huang et al., 2004b). Unsupplemented cells harvested at matched time points were used as negative control. Culture medium with 1 ng/ml hTGF-β1 resulted in a 2.33-fold increase (p < 0.0001) of SOX9 levels at day 16, whereas 10 ng/ml hTGF-β1 stimulated a SOX9 3.94-fold up-regulation (p < 0.001). The lower hTGF-β1 supplementation induced a marked COL2A1 downregulation at day 8 followed by expression recovery to control levels at day 16. In contrast, at 10 ng/ml concentration, COL2A1 expression showed a 2-fold increase only at day 16. ACAN was not detected at each time point at both concentrations explored. COL1A1 and COL3A1 expression were always downregulated in response to hTGF-β1 supplementation (Figures 1A,B).
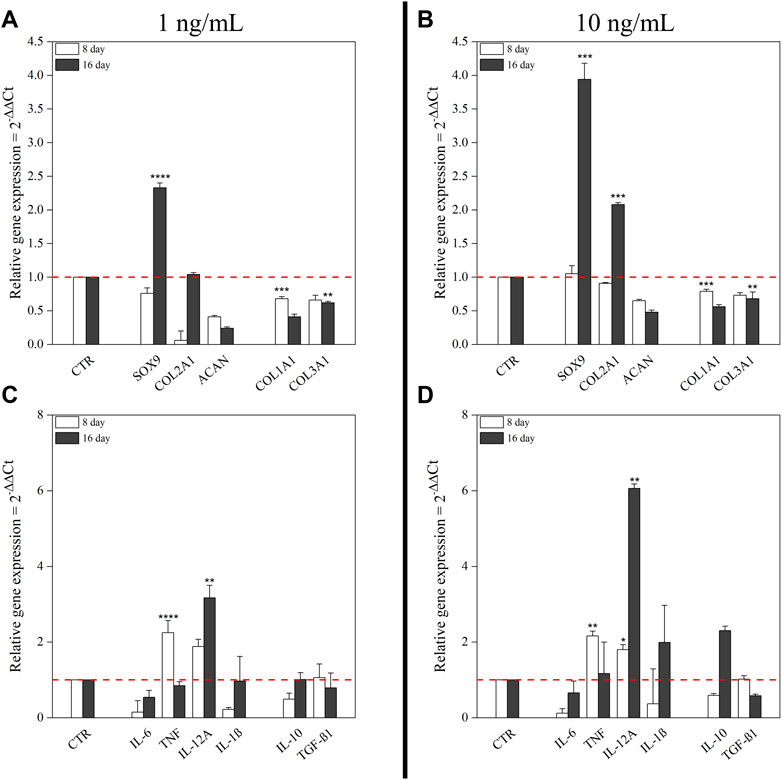
FIGURE 1. Effect of hTGF-β1 on the expression of chondrogenic markers and cytokines by hBM-MSC monolayer. Two different concentrations of hTGF-β1 (1 ng/ml and 10 ng/ml) for up to 16 days were tested. Untreated cells at matched time points were used as control. mRNA expression levels of positive and negative chondrogenic markers (COL1A1, COL2A1, COL3A1, SOX9, and ACAN) at 1 ng/ml (A) and at 10 ng/ml (B); pro- and anti-inflammatory cytokines (IL-6, TNF-α, IL-12A, IL-1β, IL-10, and TGF-β1) at 1 ng/ml (C) and at 10 ng/ml (D). All data were analyzed by two-way ANOVA, N = 3 (biological replicates); *p < 0.05, **p < 0.01, ***p < 0.001, and ****p < 0.0001 vs. control.
Cytokines expression along chondrogenic events was also monitored. Supplementation with 1 ng/ml hTGF-β1 resulted in transcriptional upregulation of pro-inflammatory cytokines, especially TNF-α and IL-12A, respectively 2.25-fold (p < 0.0001) and 1.88-fold, at day 8; while no changes in anti-inflammatory cytokines were observed. After 16 days, only IL-12A retained significant upregulation (3.17-fold; p < 0.01) (Figure 1C). At 10 ng/ml, hTGF-β1 supplementation promoted TNF-α (2.16-fold; p < 0.01) and IL-12A (1.8-fold; p < 0.05) upregulation after 8 days. Moreover, IL-12A (6.06-fold; p < 0.01) and IL-1β (1.99-fold) displayed significant changes at day 16. Among anti-inflammatory cytokines, only IL-10 was upregulated (2.3-fold) (Figure 1D).
Effects of hTGF-β1 on type II and III collagen production was monitored by semi-quantitative immunofluorescence (q-IF) assay (Figure 2A). hBM-MSCs displayed low basal expression of types II and III collagen that increased following 16 days of continuous hTGF-β1 exposure; however, type II collagen staining (green) was more intense when cells were supplemented with 10 ng/ml of growth factor at day 16. This behavior was confirmed by q-IF data, which indicated an increase (2.74-fold) of the stained protein signal at day 16 (Figure 2B). Supplementing 1 ng/ml, several spindle shaped aggregates were observed at day 16. No spindle systems were evidenced when a concentration of 10 ng/ml was used. Type III collagen signal was upregulated at day 16 in all conditions when compared to baseline (Figure 2C), while its intensity decreased following chondrogenic medium treatment compared to matched untreated cells.
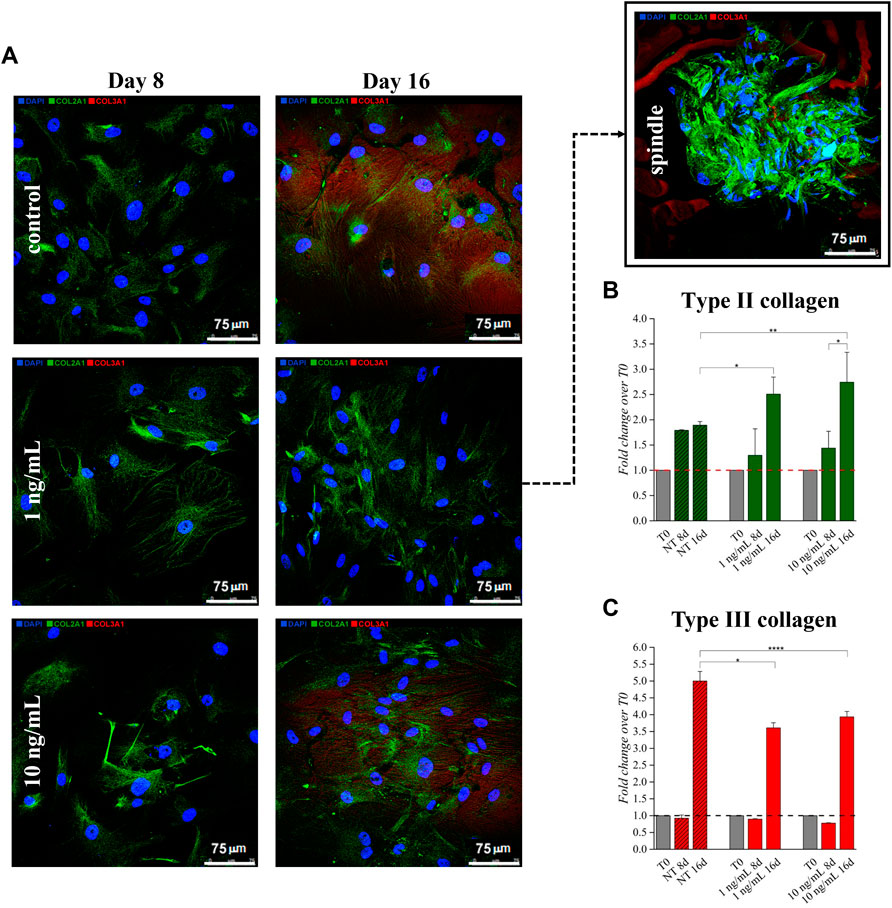
FIGURE 2. Effect of hTGF-β1 on the expression of type II (stained in green) and type III (stained in red) collagens by BM-MSC monolayer. Spindle-shaped systems were observed at Day 16 only when 1 ng/ml hTGF-β1 was supplemented (A). Higher type I collagen stain was observed when 10 ng/ml were supplemented, as indicated by signals relative quantification (B,C) *p < 0.05, **p < 0.01, ***p < 0.001, and ****p < 0.0001, one-way ANOVA. N = 3 (biological replicates). Scale bar: 75 µm.
Based on these data, 10 ng/ml hTGF-β1 was chosen as the optimal concentration for culture medium supplementation in the next 3D high-density approach.
3D high-density culture
3D high density culture were monitored for 16 days of incubation using brightfield microscopy for morphological analysis. hBM-MSC showed spherical structures with smooth borders, especially after 4 and 8 days (Figure 3A). A mean Feret’s diameter of 450 µm (±64) and circularity of 0.54 µm (±0.16) were calculated after 16 days of treatment with hTGF-β1. Similarly, control 3D culture without growth factor had a mean diameter of 460 µm (±64), with a lower circularity of roughly 0.46 µm (±0.11). hWJ-MSC 3D systems were bigger and more regular, with a mean Feret’s diameter of 1,163 µm (±69) and circularity of 0.62 µm (±0.08) at the end of chondrogenic culture (Figure 3B).
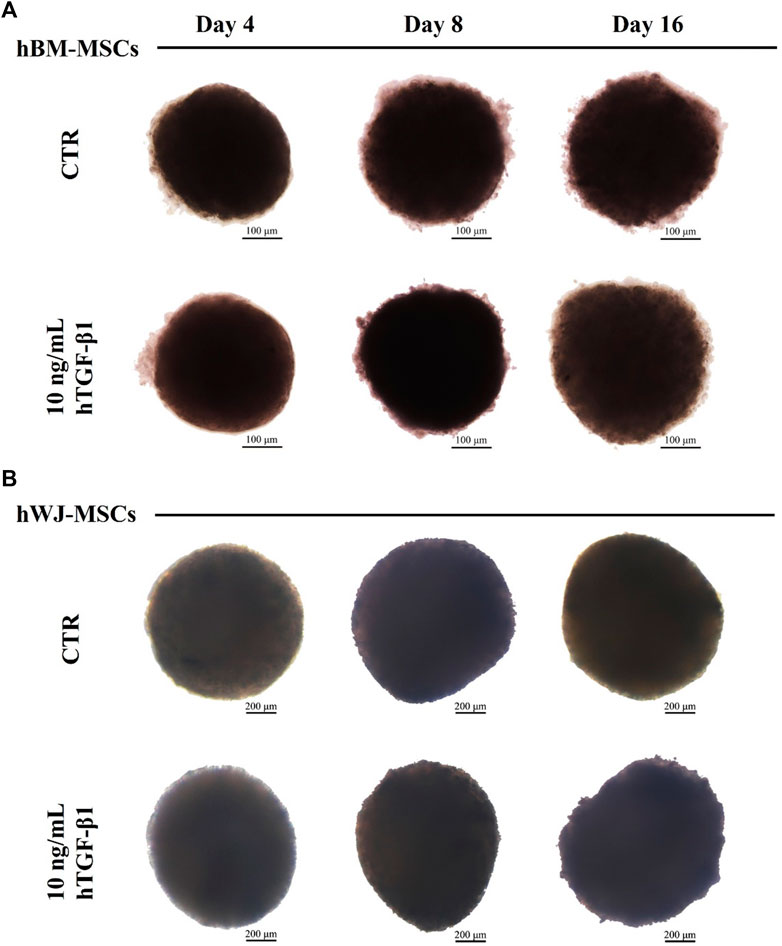
FIGURE 3. Brightfield images of hBM-MSCs (A) and hWJ-MSCs (B) 3D high-density culture supplemented with 10 ng/ml of hTGF-β1 and acquired at Days 4, 8, and 16. Untreated spheroids (CTR) at matched time-points were presented for control purposes. Scale bars were 200 micron for BM culture and 100 micron for WJ one.
Gene expression profiles of chondrogenic markers and cytokines were monitored along the experimental timeline. All chondrogenic markers were upregulated in 3D culture combined with hTGF-β1 treatment. At day 8, hBM-MSCs displayed upregulation of SOX9 (3.15-fold), COL2A1 (191.68-fold), ACAN (1.50-fold), COL3A1 (1.13-fold), and COL10A1 (242.2-fold). At day 16, increased upregulation was noted for SOX9 (8.05-fold, p < 0.001), COL2A1 (925.44-fold), ACAN (15.16-fold), and COL10A1 (3704-fold), whilst COL1A1 was downregulated and COL3A1 was unchanged compared to control (Figure 4A). On the other hand, WJ-3D systems at day 8 displayed strong upregulation of SOX9 (33.04-fold), COL2A1 (30.76-fold), COL1A1 (83.55-fold), COL3A1 (13.94-fold), and COL10A1 (231.5-fold) while ACAN underwent slight upregulation. At day 16, SOX9 (89.30-fold, p < 0.001), COL2A1 (146.60-fold), and ACAN (4.27-fold, p < 0.05) all underwent further upregulation, while COL1A1 (29.94-fold, p < 0.001), COL3A1 (6.39-fold) and COL10A1 (137.6-fold) displayed downregulation in comparison to day 8 levels (Figure 4B).
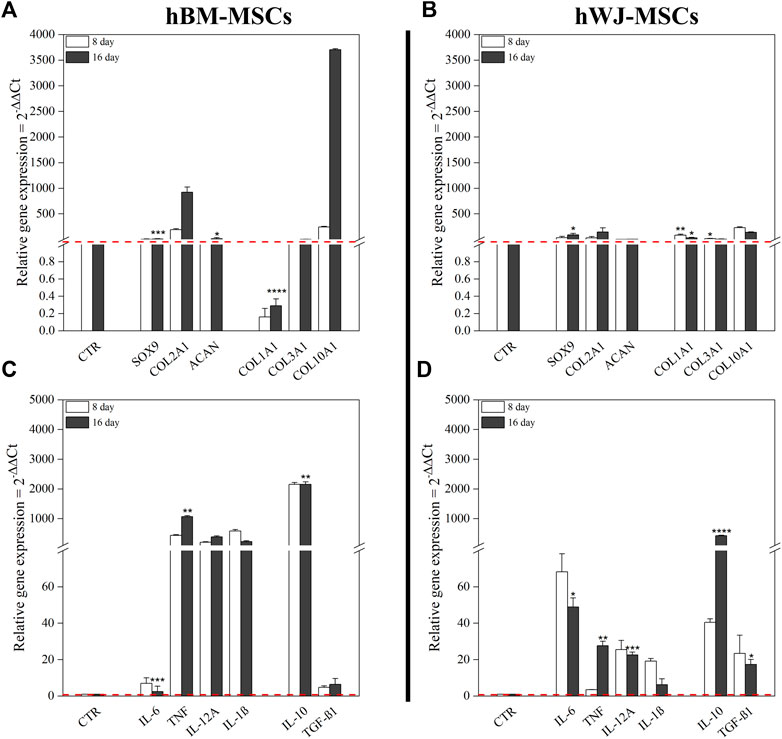
FIGURE 4. Gene expression profiles of both hBM-MSCs and hWJ-MSCs based high density culture supplemented with 10 ng/ml of hTGF-β1. mRNA expression levels of positive and negative chondrogenic markers (COL1A1, COL2A1, COL3A1, COL10A1, SOX9, and ACAN) (A,B) and cytokines (IL-6, TNF-α, IL-12A, IL-1β, IL-10, and TGF-β1) (C,D); untreated cells at time zero were used as control. *p < 0.05, **p < 0.01, ***p < 0.001, and ****p < 0.0001 (two-way ANOVA). N = 3 (Biological replicates).
hBM-MSC upregulated all pro-inflammatory cytokines genes (e.g., TNF-α and IL-12A, respectively 440.75-fold and 205-fold, at day 8). Among anti-inflammatory cytokines, IL-10 showed a strong upregulation with a 2154-fold change. After 16 days, only TNF-α underwent a further increase (1071-fold, p < 0.01), whereas the other ones retained an expression similar to that of previous time-points. Moreover, at the end of culture, IL-10 maintained its upregulated (2158-fold, p < 0.01), instead, TGF-β1 retained a slight increase compared to control (Figure 4C). However, at protein level, IL-6 tended to increase in the culture medium from day 11, as well as MIP-1α, CCL-4, and DKK1, while only HGF was significantly higher at day 11 compared to baseline (p = 0.0136) (Figure 5A). Other cytokines were not detected. In hWJ-MSCs, cytokine expression resulted in limited transcriptional upregulation of all pro-inflammatory cytokines genes, with IL-6 that reached the highest fold-value (68.24-fold) at day 8, while IL-10 showed a strong upregulation (40.47-fold). As the culture progressed, TNF-α (27.65-fold, p < 0.01) and IL-12A (22.61-fold, p < 0.001) significantly increased, while other pro-inflammatory cytokines displayed a lower shift. Moreover, IL-10 and TGF-β1 expression increased compared to control, reaching respectively 430-fold (p < 0.0001) and 17.31-fold (p < 0.05) (Figure 4D). At protein level, IL-6 tended to increase in the culture medium from day 7, as observed in BM-MSC cultures, while IL-1β was significantly reduced starting from day 7 (p = 0.0265) and DKK1 increased at day 7 (p = 0.0015) compared to baseline (Figure 5B).
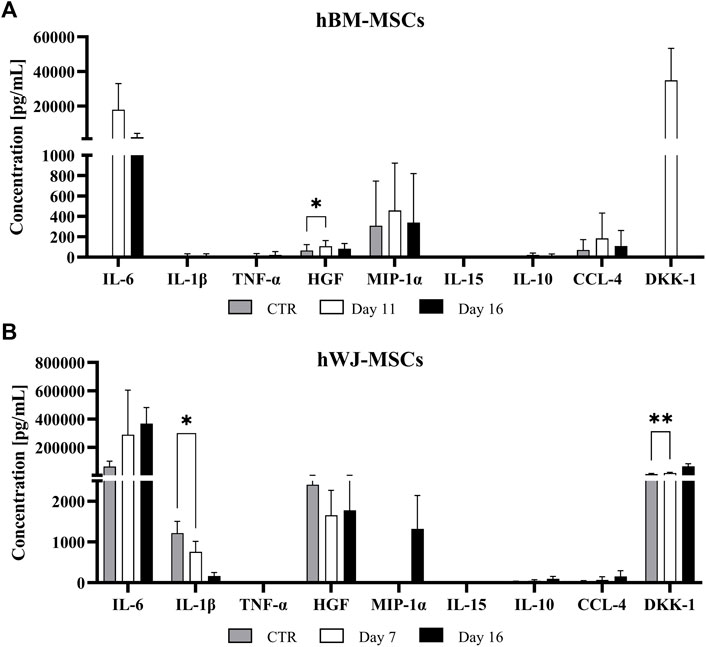
FIGURE 5. Cytokine release profiles of BM (A) and WJ (B) 3D culture in a chondrogenic medium supplemented with 10 ng/ml of TGF-β1 for up to 16 days. Cytokine levels expresses as (pg/ml) were measured in the culture medium using a multiplex bead-based immunoassay at various time points and shown as means ± SD. *p < 0.05 and **p < 0.01 (N = 2).
Cell viability inside 3D aggregate systems was performed after 8 and 16 days of culture using Live and Dead assays, and micrographs are shown in Figure 6A-B for hBM-MSCs and WJ-MSCs, respectively. The red signal, associated with dead cells, was detectable only in the core of 3D systems and varied from 5% at day 8–10% at day 16. Therefore, cultures longer than 16 days were not considered for both cells type, as also suggested by literature (Lee et al., 2017).
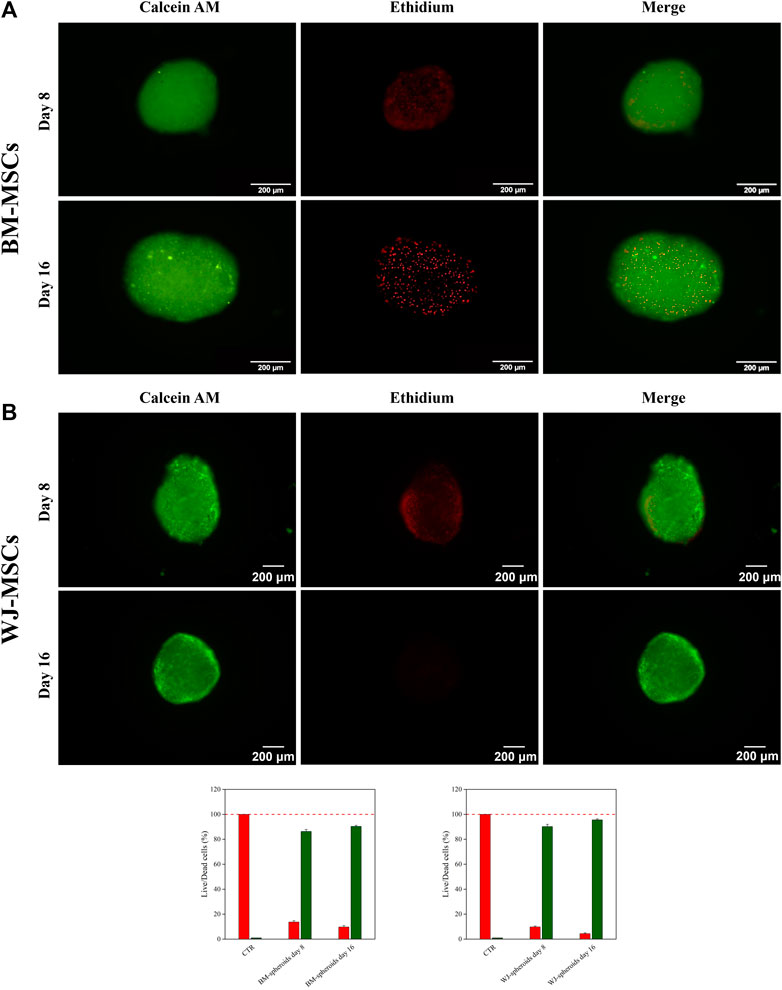
FIGURE 6. Live & Dead assay and quantify fluorescent signal of hBM-MSCs (A) and hWJ-MSCs (B) in 3D high-density cultures supplemented with 10 ng/ml of hTGF-β1 and acquired at Days 8 and 16. Viable cells appear green, non-viable cells in red. Scale bar: 200 µm.
Production of type I and II collagen (proteins) was monitored by immunofluorescence (IF) assay (see Figures 7A,B). BM-MSC 3D-system displayed a basal expression of type I collagen, that decreased following 16 days of continuous hTGF-β1 exposure; however, type II collagen staining (green) was more intense at day 16. The same trend was observed for WJ-MSC 3D system as documented by IF micrographs, where the green signal associated with type II collagen was markedly increased from 8 to 16 days. Histological evaluation by Sirius red acquired with a polarized microscope to intercept the birefringence of collagen fibers was also performed on both 3D culture. An example of the intensity of red signal, mainly associated with type II collagen, increased from 8 to 16 days, confirming previous IF data. Translucent new fibers were also clearly detected at day 16 of culture (Figure 8A). Further histological evaluation by Safranin-O for WJ-3D culture was performed and detected the accumulation of acid proteoglycans (Figure 8B).
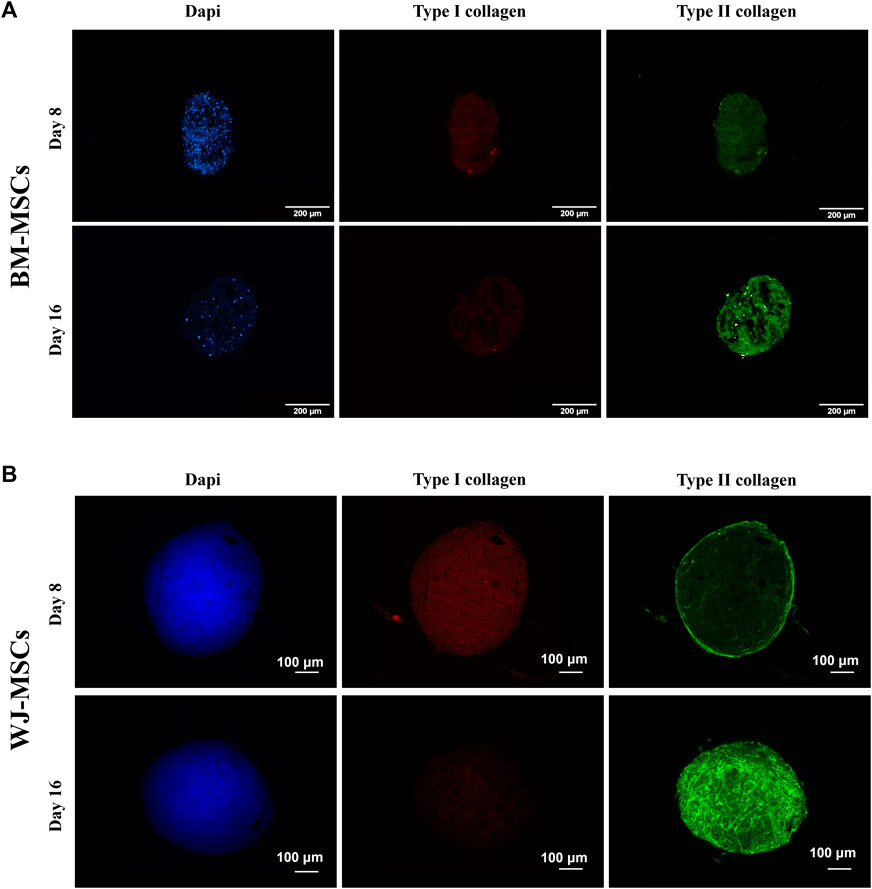
FIGURE 7. Immunofluorescence of hBM-MSCs (A) and hWJ-MSCs (B) in 3D high-density cultures supplemented with 10 ng/ml of hTGF-β1 and acquired at different time-point. Type I collagen was stained in red and type II collagen in green. DAPI was used to counterstain the nuclei (blue) Scale bars were 200 microns for BM culture and 100 microns for WJ one.
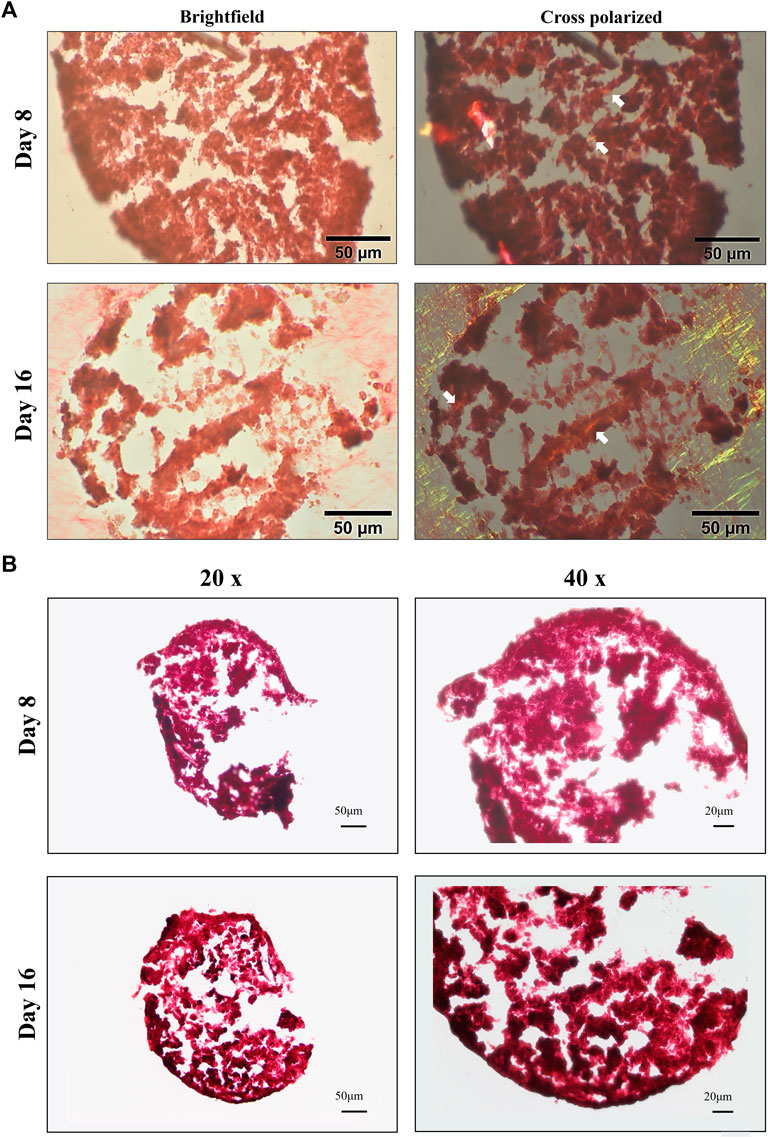
FIGURE 8. Brightfield and cross-polarized images of picrosirius red (A) and Safranin-O (B) staining on 3D high density culture at days 8 and 16. Fine birefringent collagen fibers were visible by day 8 and day 16 (see white arrows) in BM culture. Scale bar: 50 µm (A); accumulation of acid proteoglycans (stained in red) after 8 and 16 days in WJ-3D culture. Scale bar 20 µm (B).
Discussion
3D in vitro chondrogenic models using human stem cells derived from different tissues have great potential in cartilage healing and regeneration (Mobasheri et al., 2014). In this study, we investigated chondrogenic propensity of human MSCs harvested from BM and WJ using 3D high-density culture coupled with hTGF-β1 supplementation. We also observed the variations in cytokine production during differentiation by gene expression profiling for pro- and anti-inflammatory cytokine genes.
Chondrogenesis from human MSCs is a complex process, involving the activation of different genes, especially the transcription factor SOX9 that plays a key role in trigging the differentiation phase. Indeed, its upregulation promotes stem cell commitment toward chondrogenic phenotype and reduces hypertrophic drift leading to cartilage mineralization, often accompanied by apoptosis of chondrocytes and matrix ossification (Mueller and Tuan, 2008; Jiang et al., 2018). While SOX9 is rapidly upregulated along chondrogenesis, the expression of other genes emerges later. COL2A1 represents the gold standard among chondrogenic markers and is usually detectable only after several days of chondrogenic induction. In preliminary monolayer experiments, chondrogenesis-related gene expression was not affected by 1 ng/ml hTGF-β1 supplementation in culture medium, while increased when using 10 ng/ml after 16 days of culture, in agreement with previously published data (Li et al., 2011). ACAN, another chondrogenic marker, was not detected at any time point at 1 and 10 ng/ml hTGF-β1 concentrations, possibly because its expression becomes evident between 18 and 24 days of culture (Xu et al., 2008); however, some variations are described due to biological variabilities, number of culture passages, different media and TGF (β1 or β3) used, and cell types employed (e.g., adipose-, BM-, or WJ-derived MSCs) (Sacchetti et al., 2016; Huynh et al., 2019; Futrega et al., 2021). Higher concentrations of hTGF-β1 have already been reported to exert inhibitory effects on adipose-derived MSCs (Huang et al., 2004a). Therefore, we did not test higher doses in our monolayer experiments and we chose 10 ng/ml of hTGF- β1 for the 3D culture systems as the optimal condition because of a significant increase in chondrogenic-related genes. Conversely, COL1A1, COL3A1, and COL10A1 were employed as negative markers because largely reported to be dedifferentiation genes of hyaline cartilage phenotype and often associated with differentiation towards other musculoskeletal lineages. Type I collagen gene expression is related to both tenogenic commitments (Ciardulli et al., 2020b) and fibrocartilage tissue (Kovermann et al., 2019). Moreover, its expression increases in chondrocytes during progression of human osteoarthritis (Zhong et al., 2016). Type III collagen is often co-localized with type I within the same fibril (Dehne et al., 2010), for this reason, it is also considered as a negative marker. However, its exact role in chondrogenesis remains unclear because contrasting evidence on its protective or pathological functions after injuries is reported (Alcaide-Ruggiero et al., 2021). The development of a stellate or fibroblast-like phenotype during culture compromises clinical outcome of potential regenerative therapy and is a major issue for application of hBM-MSCs in cartilage defect repair (Li et al., 2019). According to published literature, these genes were downregulated in response to 1 and 10 ng/ml hTGF-β1 concentrations in our monolayer experiments confirming chondrogenic commitment of stem cells in our system culture.
In vitro chondrogenic BM-MSC differentiation in 3D high-density cultures supplemented with TGF-β1 or TGF-β3 is often accompanied by up-regulation of genes associated with chondrocyte hypertrophy, including type X collagen and its transcription factor (RUNX-2), or matrix metalloproteinases (MMPs), and by activation of alkaline phosphatase (ALP) (Agar et al., 2011). Hypertrophic processes are strongly dependent on types and concentrations of chondrogenic inducers used and also on in vitro culture time (Mueller et al., 2010). In our study, the early phase of chondrogenic differentiation, also known as pre-differentiation or commitment phase, was investigated using a short culture period (only 16 days). Even though our system could not prevent hypertrophic drift of BM-derived MSCs, we observed a high chondrogenic potential not only in the case of BM- but also with WJ-derived stem cells in 3D high-density conditions and we also documented COL10A1 downregulation over time (from 8 to 16 days) for WJ-culture, thus showing a less tendency to hypertrophic drift of these cells. WJ-MSCs are another possible source of mesenchymal stromal cells for regenerative medicine approaches; however, few or absent data are present about hWJ-MSC chondrogenic commitment efficiency. In our study, we assumed that similar events to those occurring in hBM-MSCs may be induced in WJ cells. Similar to that reported in 2D BM-MSC culture with 10 ng/ml of TGF-β1, WJ-MSCs showed SOX9 upregulation at day 16, and COL1A1 decreased expression from day 8–16. These results support the use of WJ-MSCs as a valid alternative for chondrogenic commitment of stem cells in regenerative medicine.
Monolayer culture systems have various limitations, especially in chondrogenic differentiation of human stem cells, because cells grow in adhesion at the flask bottom without mimicking the complex physiological in vivo tissue architecture. When stem cells are cultured in 2D tend to lose their stemness and differentiation potential (Lei and Schaffer, 2013), as documented by the lack of ACAN expression due to the short culture period in order to avoid de-differentiation processes on long-term 2D chondrocyte cultures (Caron et al., 2012b). Moreover, living 3D structures seem to be advantageous, especially in chondrogenesis studies, due to the presence of a hypoxic core within the structure that could stimulate differentiation. Indeed, recent studies have confirmed that hypoxia is a full-fledged chondrogenic stimulating factor, because articular cartilage is an avascular tissue with a reduced oxygen and nutrient intake. Hypoxia seems to better reproduce the natural chondrocyte environment (Lee et al., 2013; Citeroni et al., 2021). In our study, successful 3D high-density cultures were obtained when 5 × 105 cells/ml were used. BM-MSC 3D systems with a mean Feret’s diameter of 401 ± 39 µm and circularity of 0.60 ± 0.14 µm were obtained after 4 days of culture with 10 ng/ml hTGF-β1 supplemented medium. As culture moved forward, these systems became larger in size, but less regular in shape at day 16, probably due to the proliferative effect of TGF-β1 (Giudice et al., 2021). Lower cell amounts resulted in smaller and frailer 3D systems making extremely challenging further studies (data not shown).
BM-MSC 3D system was more effective than 2D cultures in driving chondrogenesis, as confirmed by the higher upregulation of markers associated with hyaline cartilage, including ACAN at day 16, not observed in monolayer experiments. Moreover, COL1A1 was significantly downregulated at day 16, confirming the capability of the 3D system to inhibit the expression of this gene (Danišovič et al., 2012b). In contrast, hWJ-MSCs high-density culture showed larger and more regular aggregates, with a mean Feret’s diameter of 1,113 ± 28 µm and circularity of 0.73 ± 0.08 at day 4 and reaching values of 1,163 ± 69 μm and 0.62 ± 0.08 µm, respectively in diameter and circularity at the end of chondrogenic culture, thus showing a rise in size trend but a slight decrease in circularity over time. Different 3D culture morphology between BM and WJ-MSCs could be caused by various patchy adhesion forces and a higher proliferative rate of WJ-MSCs compared to BM-MSCs. Indeed, cell aggregates formation can be influenced by different parameters, such as cell suspension density, manufacturing method, medium composition, or incubation time. Cells suspensions assemble into 3D pellets because of cell-to-matrix and cell-to-cell interactions via integrins and cadherins (Foty and Steinberg, 2005; Gionet-Gonzales and Leach, 2018). Despite their larger diameters, WJ-3D cultures overexpressed all chondrogenic markers, including ACAN at day 16 and COL2A1, with marked downregulation of COL1A1 and COL3A1 already starting from day 8 through day 16, suggesting that also WJ-3D system can be effective in vitro tool for cartilage tissue engineering.
3D system also displayed good cell viability, even if small mortality was observed within the core. This behavior could be due to hypoxic stress or low nutrient exchanges to which the cells within the system are subjected. Looking at immunofluorescence data of the 2D culture, up to 3-fold change of collagen II was measured by q-IF at day 16, coupled with a slight downregulation of collagen III along the culture (1-fold), especially when 10 ng were supplemented. A further observation must be done, when supplementing 1 ng/ml in BM-2D culture; i.e., in this condition spindle-shaped aggregates were detected only at Day 16; these spindle structures may be considered an early organization of cellular 3D structure in the context of tenogenic commitment (Barboni et al., 2012). Therefore, 1 ng/ml hTGF-β1 dose may stimulate alternative differentiation pathways, while 10 ng/ml hTGF-β1 could drive hBM-MSC chondrogenic commitment (Puetzer et al., 2010).
MSCs can have immunomodulatory activities as described in several hematological disorders, such as primary myelofibrosis where they can also promote marrow fibrosis, or acquired aplastic anemia where BM-MSCs maintain the physiological balance between T regulatory cells and T helper (Th) 17 lymphocytes in the BM niche (Huo et al., 2020). Moreover, cytokines are important in regulation of normal chondrogenesis and pathological drift, as during osteoarthritis (Zhou et al., 2018). However, modifications of cytokine expression during chondrogenic commitment in both 2D and 3D culture systems using both BM- and WJ-derived MSCs have not been investigated yet. In our study, we demonstrated that when supplementing 10 ng/ml of hTGF-β1 in 2D system, TNF-α and IL-12A, two pro-inflammatory cytokines, were upregulated starting from day 8 of culture. TNF-α and IL-1β might hamper chondrogenesis of mesenchymal stromal cells, through NF-κB pathway, inhibition of SOX9 expression, and increase the synthesis of degradative enzymes involved in cartilage degeneration, like matrix metalloproteinases (MMPs) (Jagielski et al., 2014). Other studies suggest that in vitro exposure to TNF-α might increase proliferation, migration, and osteogenic differentiation of MSCs (Voskamp et al., 2020). In our system, BM- or WJ-3D culture showed an upregulation of all pro-inflammatory cytokine genes explored at day 8, except for IL-6 that showed a poor upregulation followed by a decrease at day 16, as also observed at the protein levels by measuring cytokines in culture medium. IL-6 behavior is in agreement with a previous study, where its expression during chondrogenic differentiation by pellet culture decreases from day 8 to day 16 (Kondo et al., 2015). During chondrogenic commitment, the most expressed cytokine was IL-10, as we also have observed (Behrendt et al., 2018); however, this cytokine was undetectable in culture medium. Therefore, both BM- and WJ-derived MSCs can produce pro-inflammatory cytokines that might support chondrogenic processes in an autocrine manner, as proposed by more pronounced variations in chondrogenic-related genes in 3D systems. Indeed, cell-to-cell contacts and spheroid structure in 3D culture better resemble physiological tissue architecture and reproduce interactions between cells and ECM. On the other hand, despite 3D cultures can be valid in vitro systems for studying chondrogenesis, our model needs further improvements and optimization for long-term cultures of chondrocytes after the production of well-organized and functional cartilage, in order to prevent dedifferentiating and/or hypertrophic drift events. Indeed, articular cartilage structure has a complex multi-layer architecture with different compositions and functions, and chondrocytes are clustered in small areas, known as lacunae. In our 3D system, we clearly observed randomly distributed depositions of acidic proteoglycans around cells, even if still not well organized as lacunae-like structures. However, in vivo reproduction of this peculiar architecture remains the main challenge in regenerative medicine of cartilage tissue.
Conclusion
In vitro models to investigate cartilage regeneration processes are becoming increasingly important. Our results indicate that 3D high-density stem cell culture supplemented with 10 ng/ml of hTGF-β1 represents a simple and rapid 3D model of chondrogenic commitment. These 3D biomaterials are extremely versatile tools and could be used to evaluate the effects of several drugs and growth factors on cartilage regeneration and healing, as well as to explore the role of cytokines in leading chondrogenic commitment. Indeed, our 3D-culture systems highlighted a well-balanced expression of pro and anti-inflammatory cytokines suggesting their involvement in chondrogenic events. This trend was already observed for BM- but never described for WJ-3D systems and provided an important correlation between chondrogenic commitment that might be sustained by autocrine cytokine production. Our findings open interesting prospective for the use of hWJ-MSCs to develop 3D models for cartilage tissue engineering.
Data availability statement
The raw data supporting the conclusion of this article will be made available by the authors, without undue reservation.
Ethics statement
The studies involving human participants were reviewed and approved by the Review Board prot./SCCE n. 24988. The patients/participants provided their written informed consent to participate in this study.
Author contributions
EL developed experimental activity, optimized protocols and methodology, and wrote the manuscript; MC contributed to experimental activity and 3D culture organization; PS contributed to experimental activity on 3D culture; RV contributed to stem cells culture before commitment; VG isolated stem cells and characterized them with formal analysis, validated methodology, and performed immunoassay; TD performed polarized microscope acquisitions; CS provided bone marrow aspirate specimens and the methodology for hBM-MSC cultivation; NF provided contribution in data supervision and paper writing; NM helped in data interpretation, reviewed the manuscript and was responsible for funding acquisition; GDP was responsible for experimental data design, data production, curation and supervision, paper writing and editing, funding acquisition, and research project administration.
Funding
This research was developed within the Research contract between the University of Salerno and the IRCCS “Galeazzi,” Milan (IT) entitled “Design and implementation of an experimental 3D bioengineered construct for the regeneration of cartilage systems.” Scientific Advisors NM and GDP, Year 2021–24.
Acknowledgments
The authors acknowledge: MIUR within the framework of PON-RI 2014/2020. Action I.1–“Innovative PhDs with industrial characterization” Cycle XXXIV (D.D. 1090 del 04.05.2018 additional PhD fellowships). PhD project: “Development of a 3D scaffold for local growth factor delivery to improve cartilage tissue engineering”
Conflict of interest
The authors declare that the research was conducted in the absence of any commercial or financial relationships that could be construed as a potential conflict of interest.
Publisher’s note
All claims expressed in this article are solely those of the authors and do not necessarily represent those of their affiliated organizations, or those of the publisher, the editors and the reviewers. Any product that may be evaluated in this article, or claim that may be made by its manufacturer, is not guaranteed or endorsed by the publisher.
Abbreviations
hTGF-β1, human transforming growth factor β 1; hBM-MSCs, human Bone Marrow Mesenchymal Stromal Cells; hASC, human adipose stem cells; COL1A1, type I collagen; COL2A1, type II collagen; COL3A1, type III collagen; SOX9, SRY-Box transcription factor 9; ACAN, aggrecan; RT-qPCR, reverse transcription quantitative polymerase chain reaction; q-IF, semi-quantitative immunofluorescence.
References
Agar, G., Blumenstein, S., Bar-Ziv, Y., Kardosh, R., Schrift-Tzadok, M., Gal-Levy, R., et al. (2011). The chondrogenic potential of mesenchymal cells and chondrocytes from osteoarthritic subjects: A comparative analysis. CARTILAGE 2, 40–49. doi:10.1177/1947603510380899
Alcaide-Ruggiero, L., Molina-Hernández, V., Granados, M. M., and Domínguez, J. M. (2021). Main and minor types of collagens in the articular cartilage: The role of collagens in repair tissue evaluation in chondral defects. Int. J. Mol. Sci. 22, 13329. doi:10.3390/ijms222413329
Baldino, L., Concilio, S., Della Porta, G., and Tabernero, A. (2021). Editorial: Challenges and solutions in the production of advanced nanostructured biomaterials for medical applications. Front. Mat. 8, 794192. doi:10.3389/fmats.2021.794192
Barboni, B., Curini, V., Russo, V., Mauro, A., Di Giacinto, O., Marchisio, M., et al. (2012). Indirect Co-culture with tendons or tenocytes can program amniotic epithelial cells towards stepwise tenogenic differentiation. PLoS ONE 7, e30974. doi:10.1371/journal.pone.0030974
Behrendt, P., Feldheim, M., Preusse-Prange, A., Weitkamp, J. T., Haake, M., Eglin, D., et al. (2018). Chondrogenic potential of IL-10 in mechanically injured cartilage and cellularized collagen ACI grafts. Osteoarthr. Cartil. 26, 264–275. doi:10.1016/j.joca.2017.11.007
Bernardo, M. E., Emons, J. A. M., Karperien, M., Nauta, A. J., Willemze, R., Roelofs, H., et al. (2007). Human mesenchymal stem cells derived from bone marrow display a better chondrogenic differentiation compared with other sources. Connect. Tissue Res. 48, 132–140. doi:10.1080/03008200701228464
Bhogoju, S., Khan, S., and Subramanian, A. (2022). Continuous low-intensity ultrasound preserves chondrogenesis of mesenchymal stromal cells in the presence of cytokines by inhibiting NFκB activation. Biomolecules 12, 434. doi:10.3390/biom12030434
Bhosale, A. M., and Richardson, J. B. (2008). Articular cartilage: Structure, injuries and review of management. Br. Med. Bull. 87, 77–95. doi:10.1093/bmb/ldn025
Bidarra, S. J., Barrias, C. C., and Granja, P. L. (2014). Injectable alginate hydrogels for cell delivery in tissue engineering. Acta Biomater. 10, 1646–1662. doi:10.1016/j.actbio.2013.12.006
Bustin, S. A., Benes, V., Garson, J. A., Hellemans, J., Huggett, J., Kubista, M., et al. (2009). The MIQE guidelines: Minimum information for publication of quantitative real-time PCR experiments. Clin. Chem. 55, 611–622. doi:10.1373/clinchem.2008.112797
Cao, Z., Dou, C., and Dong, S. (2014). Scaffolding biomaterials for cartilage regeneration. J. Nanomater., 1–8. doi:10.1155/2014/489128
Caron, M. M. J., Emans, P. J., Coolsen, M. M. E., Voss, L., Surtel, D. A. M., Cremers, A., et al. (2012a). Redifferentiation of dedifferentiated human articular chondrocytes: Comparison of 2D and 3D cultures. Osteoarthr. Cartil. 20, 1170–1178. doi:10.1016/j.joca.2012.06.016
Caron, M. M. J., Emans, P. J., Coolsen, M. M. E., Voss, L., Surtel, D. A. M., Cremers, A., et al. (2012b). Redifferentiation of dedifferentiated human articular chondrocytes: Comparison of 2D and 3D cultures. Osteoarthr. Cartil. 20, 1170–1178. doi:10.1016/j.joca.2012.06.016
Ciardulli, M. C., Lovecchio, J., Scala, P., Lamparelli, E. P., Dale, T. P., Giudice, V., et al. (2021). 3D biomimetic scaffold for growth factor controlled delivery: An in-vitro study of tenogenic events on Wharton’s jelly mesenchymal stem cells. Pharmaceutics 13, 1448. doi:10.3390/pharmaceutics13091448
Ciardulli, M. C., Marino, L., Lamparelli, E. P., Guida, M., Forsyth, N. R., Selleri, C., et al. (2020a). Dose-response tendon-specific markers induction by growth differentiation factor-5 in human bone marrow and umbilical cord mesenchymal stem cells. Int. J. Mol. Sci. 21, 5905. doi:10.3390/ijms21165905
Ciardulli, M. C., Marino, L., Lamparelli, E. P., Guida, M., Forsyth, N. R., Selleri, C., et al. (2020b). Dose-response tendon-specific markers induction by growth differentiation factor-5 in human bone marrow and umbilical cord mesenchymal stem cells. Int. J. Mol. Sci. 21, 5905. doi:10.3390/ijms21165905
Ciardulli, M. C., Marino, L., Lovecchio, J., Giordano, E., Forsyth, N. R., Selleri, C., et al. (2020c). Tendon and cytokine marker expression by human bone marrow mesenchymal stem cells in a hyaluronate/poly-lactic-Co-glycolic acid (PLGA)/Fibrin three-dimensional (3D) scaffold. Cells 9. doi:10.3390/cells9051268
Citeroni, M. R., Mauro, A., Ciardulli, M. C., Di Mattia, M., El Khatib, M., Russo, V., et al. (2021). Amnion-derived teno-inductive secretomes: A novel approach to foster tendon differentiation and regeneration in an ovine model. Front. Bioeng. Biotechnol. 9, 649288. doi:10.3389/fbioe.2021.649288
Coburn, J. M., Bernstein, N., Bhattacharya, R., Aich, U., Yarema, K. J., and Elisseeff, J. H. (2013). Differential response of chondrocytes and chondrogenic-induced mesenchymal stem cells to C1-OH tributanoylated N-acetylhexosamines. PLoS ONE 8, e58899. doi:10.1371/journal.pone.0058899
Costa, E. C., Moreira, A. F., de Melo-Diogo, D., Gaspar, V. M., Carvalho, M. P., and Correia, I. J. (2016). 3D tumor spheroids: An overview on the tools and techniques used for their analysis. Biotechnol. Adv. 34, 1427–1441. doi:10.1016/j.biotechadv.2016.11.002
Danišovič, Ľ., Varga, I., and Polák, Š. (2012a). Growth factors and chondrogenic differentiation of mesenchymal stem cells. Tissue Cell 44, 69–73. doi:10.1016/j.tice.2011.11.005
Danišovič, Ľ., Varga, I., and Polák, Š. (2012b). Growth factors and chondrogenic differentiation of mesenchymal stem cells. Tissue Cell 44, 69–73. doi:10.1016/j.tice.2011.11.005
De Moor, L., Fernandez, S., Vercruysse, C., Tytgat, L., Asadian, M., De Geyter, N., et al. (2020). Hybrid bioprinting of chondrogenically induced human mesenchymal stem cell spheroids. Front. Bioeng. Biotechnol. 8, 484. doi:10.3389/fbioe.2020.00484
de Winter, J. C. F. (2013). Using the Student’s t-test with extremely small sample sizes. doi:10.7275/E4R6-DJ05
Dehne, T., Schenk, R., Perka, C., Morawietz, L., Pruss, A., Sittinger, M., et al. (2010). Gene expression profiling of primary human articular chondrocytes in high-density micromasses reveals patterns of recovery, maintenance, re- and dedifferentiation. Gene 462, 8–17. doi:10.1016/j.gene.2010.04.006
Foty, R. A., and Steinberg, M. S. (2005). The differential adhesion hypothesis: A direct evaluation. Dev. Biol. 278, 255–263. doi:10.1016/j.ydbio.2004.11.012
Futrega, K., Robey, P. G., Klein, T. J., Crawford, R. W., and Doran, M. R. (2021). A single day of TGF-β1 exposure activates chondrogenic and hypertrophic differentiation pathways in bone marrow-derived stromal cells. Commun. Biol. 4, 29. doi:10.1038/s42003-020-01520-0
Gionet-Gonzales, M. A., and Leach, J. K. (2018). Engineering principles for guiding spheroid function in the regeneration of bone, cartilage, and skin. Biomed. Mat. 13, 034109. doi:10.1088/1748-605X/aab0b3
Giordano, R., Canesi, M., Isalberti, M., Isaias, I., Montemurro, T., Viganò, M., et al. (2014). Autologous mesenchymal stem cell therapy for progressive supranuclear palsy: Translation into a phase I controlled, randomized clinical study. J. Transl. Med. 12, 14. doi:10.1186/1479-5876-12-14
Giudice, V., Cardamone, C., Triggiani, M., and Selleri, C. (2021). Bone marrow failure syndromes, overlapping diseases with a common cytokine signature. Int. J. Mol. Sci. 22, 705. doi:10.3390/ijms22020705
Grässel, S., and Muschter, D. (2020). Recent advances in the treatment of osteoarthritis. F1000Res. 9, 325. doi:10.12688/f1000research.22115.1
Greiner, C., Grainger, S., Farrow, S., Davis, A., Su, J. L., Saybolt, M. D., et al. (2021). Robust quantitative assessment of collagen fibers with picrosirius red stain and linearly polarized light as demonstrated on atherosclerotic plaque samples. PLoS ONE 16, e0248068. doi:10.1371/journal.pone.0248068
Hellemans, J., Mortier, G., De Paepe, A., Speleman, F., and Vandesompele, J. (2007). qBase relative quantification framework and software for management and automated analysis of real-time quantitative PCR data. Genome Biol. 8, R19. doi:10.1186/gb-2007-8-2-r19
Huang, J. I., Zuk, P. A., Jones, N. F., Zhu, M., Lorenz, H. P., Hedrick, M. H., et al. (2004a). Chondrogenic potential of multipotential cells from human adipose tissue. Plastic Reconstr. Surg. 113, 585–594. doi:10.1097/01.PRS.0000101063.27008.E1
Huang, J. I., Zuk, P. A., Jones, N. F., Zhu, M., Lorenz, H. P., Hedrick, M. H., et al. (2004b). Chondrogenic potential of multipotential cells from human adipose tissue. Plastic Reconstr. Surg. 113, 585–594. doi:10.1097/01.PRS.0000101063.27008.E1
Huo, J., Zhang, L., Ren, X., Li, C., Li, X., Dong, P., et al. (2020). Multifaceted characterization of the signatures and efficacy of mesenchymal stem/stromal cells in acquired aplastic anemia. Stem Cell Res. Ther. 11, 59. doi:10.1186/s13287-020-1577-2
Huynh, N. P. T., Zhang, B., and Guilak, F. (2019). High‐depth transcriptomic profiling reveals the temporal gene signature of human mesenchymal stem cells during chondrogenesis. FASEB J. 33, 358–372. doi:10.1096/fj.201800534R
Hyllested, J. L., Veje, K., and Ostergaard, K. (2002). Histochemical studies of the extracellular matrix of human articular cartilage—A review. Osteoarthr. Cartil. 10, 333–343. doi:10.1053/joca.2002.0519
Jafri, M. A., Kalamegam, G., Abbas, M., Al-Kaff, M., Ahmed, F., Bakhashab, S., et al. (2020a). Deciphering the association of cytokines, chemokines, and growth factors in chondrogenic differentiation of human bone marrow mesenchymal stem cells using an ex vivo osteochondral culture system. Front. Cell Dev. Biol. 7, 380. doi:10.3389/fcell.2019.00380
Jafri, M. A., Kalamegam, G., Abbas, M., Al-Kaff, M., Ahmed, F., Bakhashab, S., et al. (2020b). Deciphering the association of cytokines, chemokines, and growth factors in chondrogenic differentiation of human bone marrow mesenchymal stem cells using an ex vivo osteochondral culture system. Front. Cell Dev. Biol. 7, 380. doi:10.3389/fcell.2019.00380
Jagielski, M., Wolf, J., Marzahn, U., Völker, A., Lemke, M., Meier, C., et al. (2014). The influence of IL-10 and TNFα on chondrogenesis of human mesenchymal stromal cells in three-dimensional cultures. Int. J. Mol. Sci. 15, 15821–15844. doi:10.3390/ijms150915821
Jiang, X., Huang, X., Jiang, T., Zheng, L., Zhao, J., and Zhang, X. (2018). The role of Sox9 in collagen hydrogel-mediated chondrogenic differentiation of adult mesenchymal stem cells (MSCs). Biomater. Sci. 6, 1556–1568. doi:10.1039/C8BM00317C
Johnstone, B., Hering, T. M., Caplan, A. I., Goldberg, V. M., and Yoo, J. U. (1998). In vitro chondrogenesis of bone marrow-derived mesenchymal progenitor cells. Exp. Cell Res. 238, 265–272. doi:10.1006/excr.1997.3858
Kondo, M., Yamaoka, K., Sakata, K., Sonomoto, K., Lin, L., Nakano, K., et al. (2015). Contribution of the interleukin-6/STAT-3 signaling pathway to chondrogenic differentiation of human mesenchymal stem cells. Arthritis Rheumatol. 67, 1250–1260. doi:10.1002/art.39036
Kovermann, N., Basoli, V., Della Bella, E., Alini, M., Lischer, C., Schmal, H., et al. (2019). BMP2 and TGF-β cooperate differently during synovial-derived stem-cell chondrogenesis in a dexamethasone-dependent manner. Cells 8, 636. doi:10.3390/cells8060636
Lamparelli, E. P., Casagranda, V., Pressato, D., Maffulli, N., Della Porta, G., and Bellini, D. (2022a). Synthesis and characterization of a novel composite scaffold based on hyaluronic acid and equine type I collagen. Pharmaceutics 14, 1752. doi:10.3390/pharmaceutics14091752
Lamparelli, E. P., Ciardulli, M. C., Scala, P., Scognamiglio, M., Charlier, B., Di Pietro, P., et al. (2022b). Lipid nano-vesicles for thyroid hormone encapsulation: A comparison between different fabrication technologies, drug loading, and an in vitro delivery to human tendon stem/progenitor cells in 2D and 3D culture. Int. J. Pharm. 624, 122007. doi:10.1016/j.ijpharm.2022.122007
Lamparelli, E. P., Lovecchio, J., Ciardulli, M. C., Giudice, V., Dale, T. P., Selleri, C., et al. (2021). Chondrogenic commitment of human bone marrow mesenchymal stem cells in a perfused collagen hydrogel functionalized with hTGF-β1-Releasing PLGA microcarrier. Pharmaceutics 13, 399. doi:10.3390/pharmaceutics13030399
Lee, H.-H., Chang, C.-C., Shieh, M.-J., Wang, J.-P., Chen, Y.-T., Young, T.-H., et al. (2013). Hypoxia enhances chondrogenesis and prevents terminal differentiation through PI3K/Akt/FoxO dependent anti-apoptotic effect. Sci. Rep. 3, 2683. doi:10.1038/srep02683
Lee, S.-I., Ko, Y., and Park, J.-B. (2017). Evaluation of the shape, viability, stemness and osteogenic differentiation of cell spheroids formed from human gingiva-derived stem cells and osteoprecursor cells. Exp. Ther. Med. 13, 3467–3473. doi:10.3892/etm.2017.4388
Lei, Y., and Schaffer, D. V. (2013). A fully defined and scalable 3D culture system for human pluripotent stem cell expansion and differentiation. Proc. Natl. Acad. Sci. U. S. A. 110, E5039–E5048. doi:10.1073/pnas.1309408110
Li, H., Haudenschild, D. R., Posey, K. L., Hecht, J. T., Di Cesare, P. E., and Yik, J. H. N. (2011). Comparative analysis with collagen type II distinguishes cartilage oligomeric matrix protein as a primary TGFβ-responsive gene. Osteoarthr. Cartil. 19, 1246–1253. doi:10.1016/j.joca.2011.07.011
Li, X., Liang, Y., Xu, X., Xiong, J., Ouyang, K., Duan, L., et al. (2019). Cell-to-Cell culture inhibits dedifferentiation of chondrocytes and induces differentiation of human umbilical cord-derived mesenchymal stem cells. BioMed Res. Int., 1–11. doi:10.1155/2019/5871698
Liu, Q., Wang, J., Chen, Y., Zhang, Z., Saunders, L., Schipani, E., et al. (2018). Suppressing mesenchymal stem cell hypertrophy and endochondral ossification in 3D cartilage regeneration with nanofibrous poly(l-lactic acid) scaffold and matrilin-3. Acta Biomater. 76, 29–38. doi:10.1016/j.actbio.2018.06.027
Ma, H.-L., Chen, T.-H., Low-Tone Ho, L., and Hung, S.-C. (2005). Neocartilage from human mesenchymal stem cells in alginate: Implied timing of transplantation. J. Biomed. Mat. Res. A 74A, 439–446. doi:10.1002/jbm.a.30314
Maldonado, M., and Nam, J. (2013). The role of changes in extracellular matrix of cartilage in the presence of inflammation on the pathology of osteoarthritis. BioMed Res. Int., 1–10. doi:10.1155/2013/284873
Mao, Y., Guidoin, R., Li, Y., Brochu, G., Zhang, Z., and Wang, L. (2021). Soybean-derived phospholipids complexed poly (lactic-co-glycolic acid) nanofibrous scaffolds for tissue engineering applications. Mater. Des. 205, 109737. doi:10.1016/j.matdes.2021.109737
Mobasheri, A., Kalamegam, G., Musumeci, G., and Batt, M. E. (2014). Chondrocyte and mesenchymal stem cell-based therapies for cartilage repair in osteoarthritis and related orthopaedic conditions. Maturitas 78, 188–198. doi:10.1016/j.maturitas.2014.04.017
Mueller, M. B., Fischer, M., Zellner, J., Berner, A., Dienstknecht, T., Prantl, L., et al. (2010). Hypertrophy in mesenchymal stem cell chondrogenesis: Effect of TGF-β isoforms and chondrogenic conditioning. Cells Tissues Organs 192, 158–166. doi:10.1159/000313399
Mueller, M. B., and Tuan, R. S. (2008). Functional characterization of hypertrophy in chondrogenesis of human mesenchymal stem cells. Arthritis Rheum. 58, 1377–1388. doi:10.1002/art.23370
Mushahary, D., Spittler, A., Kasper, C., Weber, V., and Charwat, V. (2018). Isolation, cultivation, and characterization of human mesenchymal stem cells: hMSC. Cytometry 93, 19–31. doi:10.1002/cyto.a.23242
Nii, T., and Katayama, Y. (2021). Biomaterial-assisted regenerative medicine. Int. J. Mol. Sci. 22, 8657. doi:10.3390/ijms22168657
Palazzo, I., Lamparelli, E. P., Ciardulli, M. C., Scala, P., Reverchon, E., Forsyth, N., et al. (2021). Supercritical emulsion extraction fabricated PLA/PLGA micro/nano carriers for growth factor delivery: Release profiles and cytotoxicity. Int. J. Pharm. 592, 120108. doi:10.1016/j.ijpharm.2020.120108
Patel, B. A., Giudice, V., and Young, N. S. (2021). Immunologic effects on the haematopoietic stem cell in marrow failure. Best Pract. Res. Clin. Haematol. 34, 101276. doi:10.1016/j.beha.2021.101276
Puetzer, J. L., Petitte, J. N., and Loboa, E. G. (2010). Comparative review of growth factors for induction of three-dimensional in vitro chondrogenesis in human mesenchymal stem cells isolated from bone marrow and adipose tissue. Tissue Eng. Part B Rev. 16, 435–444. doi:10.1089/ten.teb.2009.0705
Razmara, E., Bitaraf, A., Yousefi, H., Nguyen, T. H., Garshasbi, M., Cho, W. C., et al. (2019). Non-coding RNAs in cartilage development: An updated review. Int. J. Mol. Sci. 20, 4475. doi:10.3390/ijms20184475
Roberts, S., Menage, J., Sandell, L. J., Evans, E. H., and Richardson, J. B. (2009a). Immunohistochemical study of collagen types I and II and procollagen IIA in human cartilage repair tissue following autologous chondrocyte implantation. Knee 16, 398–404. doi:10.1016/j.knee.2009.02.004
Roberts, S., Menage, J., Sandell, L. J., Evans, E. H., and Richardson, J. B. (2009b). Immunohistochemical study of collagen types I and II and procollagen IIA in human cartilage repair tissue following autologous chondrocyte implantation. Knee 16, 398–404. doi:10.1016/j.knee.2009.02.004
Ryu, N.-E., Lee, S.-H., and Park, H. (2019). Spheroid culture system methods and applications for mesenchymal stem cells. Cells 8, 1620. doi:10.3390/cells8121620
Sacchetti, B., Funari, A., Remoli, C., Giannicola, G., Kogler, G., Liedtke, S., et al. (2016). No identical “mesenchymal stem cells” at different times and sites: Human committed progenitors of distinct origin and differentiation potential are incorporated as adventitial cells in microvessels. Stem Cell Rep. 6, 897–913. doi:10.1016/j.stemcr.2016.05.011
Scala, P., Lovecchio, J., Lamparelli, E. P., Vitolo, R., Giudice, V., Giordano, E., et al. (2022). Myogenic commitment of human stem cells by myoblasts Co-culture: A static vs. a dynamic approach. Artif. Cells, Nanomedicine, Biotechnol. 50, 49–58. doi:10.1080/21691401.2022.2039684
Schmitz, N., Laverty, S., Kraus, V. B., and Aigner, T. (2010a). Basic methods in histopathology of joint tissues. Osteoarthr. Cartil. 18, S113–S116. doi:10.1016/j.joca.2010.05.026
Schmitz, N., Laverty, S., Kraus, V. B., and Aigner, T. (2010b). Basic methods in histopathology of joint tissues. Osteoarthr. Cartil. 18, S113–S116. doi:10.1016/j.joca.2010.05.026
Sechriest, V. F., Miao, Y. J., Niyibizi, C., Westerhausen–Larson, A., Matthew, H. W., Evans, C. H., et al. (2000). GAG‐augmented polysaccharide hydrogel: A novel biocompatible and biodegradable material to support chondrogenesis. J. Biomed. Mat. Res. 49, 5342–5541. doi:10.1002/(sici)1097-4636(20000315)49:4<534:aid-jbm12>3.0.co;2-#
Sophia Fox, A. J., Bedi, A., and Rodeo, S. A. (2009). The basic science of articular cartilage: Structure, composition, and function. Sports Health. 1, 461–468. doi:10.1177/1941738109350438
Spaepen, P., De Boodt, S., Aerts, J.-M., and Sloten, J. V. (2011). “Digital image processing of live/dead staining,” in Mammalian cell viability methods in molecular biology. Editor M. J. Stoddart (Totowa, NJ: Humana Press), 209–230. doi:10.1007/978-1-61779-108-6_21
Suchorska, W. M., Lach, M. S., Richter, M., Kaczmarczyk, J., and Trzeciak, T. (2016). Bioimaging: An useful tool to monitor differentiation of human embryonic stem cells into chondrocytes. Ann. Biomed. Eng. 44, 1845–1859. doi:10.1007/s10439-015-1443-z
Thorp, H., Kim, K., Bou-Ghannam, S., Kondo, M., Maak, T., Grainger, D. W., et al. (2021a). Enhancing chondrogenic potential via mesenchymal stem cell sheet multilayering. Regen. Ther. 18, 487–496. doi:10.1016/j.reth.2021.11.004
Thorp, H., Kim, K., Kondo, M., Grainger, D. W., and Okano, T. (2020). Fabrication of hyaline-like cartilage constructs using mesenchymal stem cell sheets. Sci. Rep. 10, 20869. doi:10.1038/s41598-020-77842-0
Thorp, H., Kim, K., Kondo, M., Maak, T., Grainger, D. W., and Okano, T. (2021b). Trends in articular cartilage tissue engineering: 3D mesenchymal stem cell sheets as candidates for engineered hyaline-like cartilage. Cells 10, 643. doi:10.3390/cells10030643
Tuli, R., Tuli, S., Nandi, S., Huang, X., Manner, P. A., Hozack, W. J., et al. (2003). Transforming growth factor-β-mediated chondrogenesis of human mesenchymal progenitor cells involves N-cadherin and mitogen-activated protein kinase and wnt signaling cross-talk. J. Biol. Chem. 278, 41227–41236. doi:10.1074/jbc.M305312200
Voskamp, C., Koevoet, W. J. L. M., Somoza, R. A., Caplan, A. I., Lefebvre, V., van Osch, G. J. V. M., et al. (2020). Enhanced chondrogenic capacity of mesenchymal stem cells after TNFα pre-treatment. Front. Bioeng. Biotechnol. 8, 658. doi:10.3389/fbioe.2020.00658
Wagner, W., Wein, F., Seckinger, A., Frankhauser, M., Wirkner, U., Krause, U., et al. (2005). Comparative characteristics of mesenchymal stem cells from human bone marrow, adipose tissue, and umbilical cord blood. Exp. Hematol. 33, 1402–1416. doi:10.1016/j.exphem.2005.07.003
Wakefield, L. M., Winokur, T. S., Hollands, R. S., Christopherson, K., Levinson, A. D., and Sporn, M. B. (1990). Recombinant latent transforming growth factor beta 1 has a longer plasma half-life in rats than active transforming growth factor beta 1, and a different tissue distribution. J. Clin. Invest. 86, 1976–1984. doi:10.1172/JCI114932
Xu, J., Wang, W., Ludeman, M., Cheng, K., Hayami, T., Lotz, J. C., et al. (2008). Chondrogenic differentiation of human mesenchymal stem cells in three-dimensional alginate gels. Tissue Eng. Part A 14, 667–680. doi:10.1089/tea.2007.0272
Zhong, L., Huang, X., Karperien, M., and Post, J. (2016). Correlation between gene expression and osteoarthritis progression in human. Int. J. Mol. Sci. 17, 1126. doi:10.3390/ijms17071126
Zhou, J.-X., Tian, Z.-G., Zhu, L.-F., Wu, W.-D., Zhou, S.-L., Zhao, Y.-T., et al. (2018). MicroRNA-615-3p promotes the osteoarthritis progression by inhibiting chondrogenic differentiation of bone marrow mesenchymal stem cells. Eur. Rev. Med. Pharmacol. Sci. 22, 6212–6220. doi:10.26355/eurrev_201810_16027
Keywords: 3D cell culture, human bone marrow mesenchymal stem cells, human Wharton’s jelly mesenchymal stem cells, hTGF-β1, chondrogenesis, cytokines
Citation: Lamparelli EP, Ciardulli MC, Giudice V, Scala P, Vitolo R, Dale TP, Selleri C, Forsyth NR, Maffulli N and Della Porta G (2022) 3D in-vitro cultures of human bone marrow and Wharton’s jelly derived mesenchymal stromal cells show high chondrogenic potential. Front. Bioeng. Biotechnol. 10:986310. doi: 10.3389/fbioe.2022.986310
Received: 04 July 2022; Accepted: 15 August 2022;
Published: 26 September 2022.
Edited by:
Ornella Parolini, Catholic University of the Sacred Heart, ItalyReviewed by:
Wanda Lattanzi, Università Cattolica del Sacro Cuore, ItalyBurhan Gharaibeh, University of Pittsburgh, United States
Copyright © 2022 Lamparelli, Ciardulli, Giudice, Scala, Vitolo, Dale, Selleri, Forsyth, Maffulli and Della Porta. This is an open-access article distributed under the terms of the Creative Commons Attribution License (CC BY). The use, distribution or reproduction in other forums is permitted, provided the original author(s) and the copyright owner(s) are credited and that the original publication in this journal is cited, in accordance with accepted academic practice. No use, distribution or reproduction is permitted which does not comply with these terms.
*Correspondence: Giovanna Della Porta, gdellaporta@unisa.it