- Novo Nordisk Foundation Center for Biosustainability, Technical University of Denmark, Kgs Lyngby, Denmark
The human gastrointestinal tract is a complex and dynamic environment, playing a crucial role in human health. Microorganisms engineered to express a therapeutic activity have emerged as a novel modality to manage numerous diseases. Such advanced microbiome therapeutics (AMTs) must be contained within the treated individual. Hence safe and robust biocontainment strategies are required to prevent the proliferation of microbes outside the treated individual. Here we present the first biocontainment strategy for a probiotic yeast, demonstrating a multi-layered strategy combining an auxotrophic and environmental-sensitive strategy. We knocked out the genes THI6 and BTS1, causing thiamine auxotrophy and increased sensitivity to cold, respectively. The biocontained Saccharomyces boulardii showed restricted growth in the absence of thiamine above 1 ng/ml and exhibited a severe growth defect at temperatures below 20°C. The biocontained strain was well tolerated and viable in mice and demonstrated equal efficiency in peptide production as the ancestral non-biocontained strain. In combination, the data support that thi6∆ and bts1∆ enable biocontainment of S. boulardii, which could be a relevant chassis for future yeast-based AMTs.
Introduction
The therapeutic potential of the human microbiome has gained significant attention, as microbiome-host interactions play a crucial role in various diseases (Eckburg and Relman, 2007; Sears and Garrett, 2014; Itzhaki et al., 2016; Canfora et al., 2019). Accordingly, the use of engineered living microbes to treat diseases is an emerging approach in the field of synthetic biology. Advanced microbiome therapeutics (AMTs) comprise microorganisms which have been genetically modified to express a therapeutic activity while present in the human microbiota. Previous work has demonstrated the use of AMTs to deliver therapeutic biomolecules (Steidler et al., 2000; Chen et al., 2014; Arora et al., 2016) or degrade toxic compounds (Isabella et al., 2018; Kurtz et al., 2019) in the gastrointestinal tract. The probiotic yeast Saccharomyces boulardii has recently caught attention as an AMT chassis, as it allows for the biosynthesis, folding and post-translational modification of several therapeutically relevant peptides and proteins (Nielsen, 2019). S. boulardii is a safe microorganism with over 40 years of use as a human probiotic (Kelesidis and Pothoulakis, 2012). However, an essential consideration in the design of AMTs is biocontainment strategies to limit the risk of the genetically modified microorganism proliferating outside the treated individual. Therefore, strategies for biocontainment must be developed for S. boulardii to ensure its future use as an AMT.
Effective biocontainment strategies should therefore take into consideration factors including mutagenetic drift, environmental supplementation, and horizontal gene transfer (Moe-Behrens et al., 2013). Several biocontainment strategies have been explored to prevent the proliferation and survival of engineered microorganisms in undesirable environments (Wook Lee et al., 2018). These strategies include auxotrophy (Steidler et al., 2003; Bahey-El-Din et al., 2010; Isabella et al., 2018), synthetic auxotrophy (Marlière et al., 2011; Pinheiro et al., 2012; Rovner et al., 2015), multispecies consortia (Mee et al., 2014; Johns et al., 2016), synthetic gene circuits (Chan et al., 2016; Huang et al., 2016; Piraner et al., 2016; Stirling et al., 2017), CRISPR-based kill switches (Caliando and Voigt, 2015; Rottinghaus et al., 2022), or a combination of them (Gallagher et al., 2015); however, each approach carries a risk. The auxotroph can be circumvented by scavenging the essential metabolite from nearby decayed cells or cross-feeding from established ecological niches. Synthetic auxotrophy may overcome these hurdles; however, it requires the gastrointestinal tract to be supplemented with the additional survival factor. Implementing synthetic gene circuits and CRISPR-based biocontainment strategies can lead to reduced fitness of the biocontained strain (Moe-Behrens et al., 2013), causing selective pressures to escape mutants (Uribe et al., 2021). Thus, to prevent proliferation in different environments, multiple strategies must be implemented to establish a robust biocontainment system.
While methods for biocontainment of bacterial AMTs have rapidly advanced (Steidler et al., 2003; Bahey-El-Din et al., 2010; Isabella et al., 2018; Rottinghaus et al., 2022), strategies for eukaryotic AMTs are still missing. In this study, we sought to develop a biocontainment strategy for S. boulardii. Since S. boulardii is a eukaryote, the risk of horizontal gene transfer is low (Emamalipour et al., 2020), and S. boulardii is unable to mate due to its sporulation defect (McFarland, 1996; Offei et al., 2019). Together, these factors reduce the risk of introducing genetic circuits or engineered DNA into natural ecosystems. Still, the risk of engineered S. boulardii proliferating in environments outside the treated host must be addressed. To address this issue, we sought to implement a biocontainment strategy for S. boulardii by reducing the fitness of the probiotic yeast outside the human host (Figure 1). We decided to evaluate the biocontainment capacity of both cold-sensitive and auxotrophic S. boulardii strains.
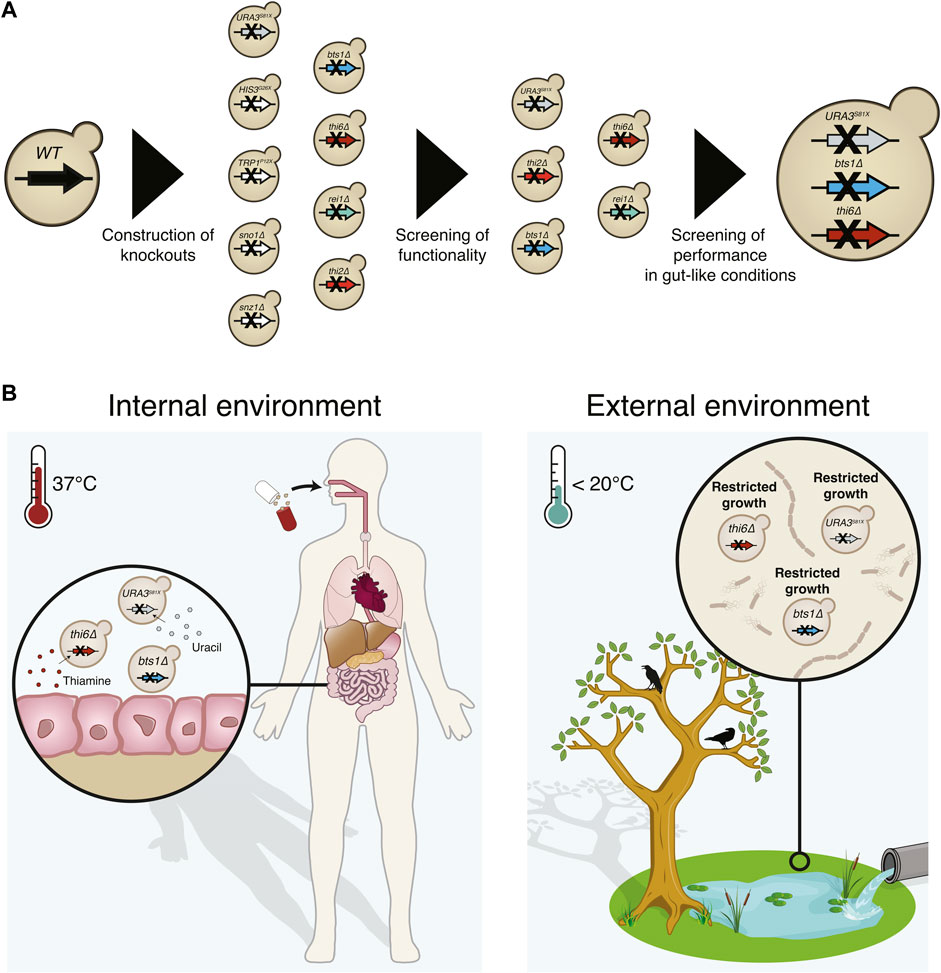
FIGURE 1. Graphical abstract of the implemented biocontainment strategy. (A) Schematic overview of the selection of the biocontainment strain. (B) Yeast cells with disruption of the genes synthesising uracil (URA3), thiamine (THI6), and geranylgeranyl diphosphate synthase (BTS1) can grow in the internal environment containing the essential nutrients and optimal temperature. However, proliferation in external environments lacking the essential nutrients and lower temperatures will be limited.
Materials and methods
Plasmids and strain construction
This study’s primers, plasmids, gBlocks and gRNA sequences are listed in Supplementary Tables S1−S4. Oligonucleotides and gBlocks were ordered from Integrated DNA Technologies (IDT). To generate the URA3S81X (SbU−), HIS3G26X (SbH−), and TRP1P12X (SbT−) disrupted strains, gBlocks with respectively gRNA were assembled with pCfB2312 (Cas9-KanMX) and transformed with their respective donor primer (Supplementary Tables S1). To generate the thi2∆, thi6∆, snz1∆, sno1∆, rei1∆, and bts1∆ strains, gBlocks from respectively gRNA were assembled with pCfB3050 (pCas9-URA3) and transformed with respectively donor primer (Supplementary Table S1). The GFP, RFP and Exendin-4 integration plasmid (Supplementary Table S2) was generated by assembly of respective gBlocks (Supplementary Table S3) with pCfB2909 (marker free) and transformed together with pCfB6920.
All plasmid assemblies were conducted with Gibson Assembly (Gibson et al., 2009) and transformed into One Shot® TOP10 Escherichia coli (Thermo Fisher Scientific). All E. coli were grown in lysogeny broth (LB) media containing 5 g/L yeast extract, 10 g/L tryptone and 10 g/L NaCl; (Sigma Aldrich) supplemented with 100 mg/L ampicillin sodium salt (Sigma Aldrich). LB agar plates contained 1% agar (Sigma Aldrich).
S. boulardii (S. cerevisiae ATCC® MYA796™) was obtained from American Type Culture Collection (ATCC). The strains created in this study are listed in Supplementary Tables S5. S. boulardii transformations were performed via high-efficiency yeast transformation using the LiAc/SS carrier DNA/PEG method (Gietz and Woods, 2006). Genomic integrations cassettes were digested with the restriction enzyme NotI (FastDgiest Enzyme, Thermo Scientific™) prior to transformation and transformed together with various helper plasmids and pre-expressed Cas9 from pCfB2312. All transformations were incubated at 30°C for 30 min and then heat-shocked in a water bath at 42°C for 60 min. All transformations followed a recovery step. The transformation tubes were microcentrifuge for 2 min at 3,000 g. Pellets were resuspended in 500 µL of YPD media containing 10 g/L yeast extract, 20 g/L casein peptone and 20 g/L glucose (Sigma Aldrich) and incubated for 2–3 h at 30 °C before being plated. All yeast transformations were plated on synthetic complete (SC) plates containing 1.7 g/L yeast nitrogen base without amino acids and ammonium sulphate (Sigma Aldrich), 1 g/L monosodium glutamate (Sigma Aldrich), 1.92 g/L Yeast Synthetic Drop-out Medium Supplements without uracil (Sigma Aldrich) and 200 mg/L geneticin (G418; Sigma Aldrich) at 37°C. Colony-PCR using OneTaq (Thermo Scientific™) confirmed the genomic integration, gene disruption and knockout. Primers flanking the null mutant gene or integration site were used to confirm successful strain modification. Genomic DNA was extracted by boiling cells at 70°C for 30 min in 20 mM NaOH. The strains were cured for pCfB2312 and helper plasmids after genome integration. One single mutant from each generated strain was cryopreserved at −80°C and used for further characterisations. All strain replicates presented are technical replicates.
Cultivation experiments
All cultivation was started from a pre-culture, inoculated with a recently streaked out colony from a −80°C cryostock, cultivated for 12–16 h. All pre-cultures were conducted in the same media as the following cultivation experiment unless other stated. Pre-culture to determine the auxotrophic strains was done in media supplemented with the required nutrition and washed three times in 1% PBS to ensure no traces of the required nutrition were transferred into the new cultures. Pre-culture to determine cold sensitivity was conducted at 37°C to ensure enough biomass.
Real-time growth monitoring
Real-time OD600 measurements were obtained every 10 min for 48–120 h with microplate reader Synergy™ H1 (BioTek) for aerobic and microaerobic conditions. Epoch 2 microplate reader (BioTek) was used for anaerobic real-timer OD600 measurements. All cultures had an initial OD600 of 0.05. The cultures were incubated into 200 µL minimal synthetic complete media (DELFT) containing 7.5 g/L (NH4)2SO4, 14.4 g/L KH2PO4, 0.5 g/L MgSO4 x 7H2O, 20 g/L glucose, 2 ml/L trace metals solution, and 1 ml/L vitamins with or without thiamine and pyridoxine (Verduyn et al., 1992), and with or without 20 mg/L uracil, 20 mg/L histidine and 20 mg/L tryptophan supplemented. CELLSTAR® 96 well cell culture plate (Greiner Bio-One) with an air-penetrable lid (Breathe-Easy, Diversified Biotech) was used for all cultivation. pH was adjusted with 1M HCl to 3, 4, 5 and 6 for the respective experiment. Cultivation was performed with continuous double orbital shaking of 548 cycles per minute (CPM) at 37 °C and 0%, 0.1%, 1% or 21% oxygen. Anaerobic conditions were obtained using a vinyl anaerobic chamber (Coy Laboratory Products Vinyl; gas mixture, 95% N2 and 5% H2), and microaerobic conditions were obtained using CO2/O2 Gas Controller (BioTek).
Thiamine dose-response experiment
The cultures were incubated into 200 µL SC media without thiamine, containing 1.7 g/L yeast nitrogen base without amino acids and thiamine (FORMEDIUM™), 1.92 g/L Yeast Synthetic Drop-out Medium Supplements without uracil, for 48 h with an initial OD600 of 0.05. The media was supplemented with either 0, 0.001, 0.01, 0.1, 1, or 400 μg/ml thiamine hydrochloride (Sigma Aldrich) and 20 mg/L uracil.
Escape rate experiment of the thiamine auxotroph
The SbU− and SbU− + thi6∆ strains were incubated in 25 ml SC with and without thiamine supplemented for 120 h. The cultures were incubated in a 250 ml shake flask with an initial OD600 of 0.05. The culture was spun down and re-suspended in 500 µL PBS. A serial dilution was generated. 100 µL of the undiluted and 5 µL from each dilution were plated on SC plates with and without thiamine supplemented (Supplementary Figures S3B, C). The plates were incubated at 37°C for 72 h. Cell mass from the undiluted 5 µL spotting was spread out on SC plates with and without thiamine supplemented (Supplementary Figures S3B, C).
Cold exposure experiment
The SbU−, SbU− + rei1∆, SbU− + bts1∆ and SbU− + bts1∆ + thi6∆ strains were incubated in 2.6 ml YPD in a 24-deep well plate (Axygen®, VWR) with a sandwich cover (Enzyscreen) and with an initial OD600 of 0.05. The plates were incubated at 15, 20, and 37°C for a maximum of 120 h. OD600 was measured at 0, 8, 24, 32, 48, 72, 96, and 120 h.
Competition experiment in the presence and absence of thiamine
Single and co-cultivation of (SbU−)-GFP and (SbU− + bts1∆ + thi6∆)-mKate were cultured in 2 ml SC media with and without thiamine in a 24-deep well plate (Axygen®, VWR) with a sandwich cover (Enzyscreen) and with an initial OD600 of 0.1. The co-culture was started with a 1:1 ratio of each strain. 20 µL of single and co-cultures were transferred into fresh media every 48th hours for a total period of 96 h (two transfers).
Competition experiments in different temperature
Single and co-cultivation of (SbU−)-GFP and (SbU− + bts1∆ + thi6∆)-mKate were cultured in 2 ml SC media YPD in a 24-deep well plate (Axygen®, VWR) with a sandwich cover (Enzyscreen) and with an initial OD600 of 0.1 at 15°C, 20°C, and 37°C. The co-culture was started with a 1:1 ratio of each strain. 20 µL of single and co-cultures were transferred into fresh media every 24th hours for a total period of 120 h (five transfers) for the 20°C and 37°C cultivation. Single and co-cultures at 15°C were transferred into fresh media every 48th hours for a total period of 144 h (three transfers). The culture was transferred every 48th hours to ensure that SbU− reached similar cell counts at all temperatures (Supplementary Figure S6).
Survival assay
Serial dilution (1,000x and 10,000x) from the 37°C co-cultivation of (SbU−)-GFP and (SbU− + bts1∆ + thi6∆)-mKate in YPD were plated on YPD plates and incubated at 15°C, 20°C and 37°C for 144, 96, and 48 h respectively. Red and green colony forming units (CFU) were counted under blue light.
Flow cytometry
All competition experiments were analysed with a NovoCyte Quanteon™ (Agilent) flow cytometry. 20 µL culture was diluted in 180 µL 1% PBS and run on the flow cytometry with a threshold of 5,000 yeast events or 60 µL sample injection. Yeast events were gated based on size events (FSC-A) < 6 × 106 and complexity events (SSC-A) < 2 × 105. Singlets were gate based on SSC (SSC-A vs. SSC-H). The (SbU-)-GFP population was quantified with the FITC channel, and (SbU− + bts1∆ + thi6∆)-mKate was quantified with PE-TexasRed. The population distribution was quantified based on FITC-H vs. PE-TexasRed-H. Absolute events were calculated.
Elisa
The Exendin-4 producing S. boulardii strains were incubated in 2 ml DELFT medium supplemented with 20 mg/L uracil in a 24-deep well plate (Axygen®, VWR) with a sandwich cover (Enzyscreen). The cultures had an initial OD600 of 0.05 and were performed with continuous shaking at 250 RPM at 37°C. All cultures were harvested after 24 and 48 h. Cell cultures were spun down at 10,000 g for 10 min at 4°C. Exendin-4 was quantified with Exendin-4 EIA (EK-070-94, Phoenix). The signals were detected by OD450 using a microplate reader Synergy™ H1 (BioTek).
Animal experiment
All animal experiments were conducted according to the Danish guidelines for experimental animal welfare, and the study protocols were approved by the Danish Animal Experiment Inspectorate (license number 2020-15-0201-00405). The study was carried out in accordance with the ARRIVE guidelines (du Sert et al., 2020). All in vivo experiments were conducted on male C57BL/6 mice (6−8 weeks old; Taconic Bioscience). Unless otherwise stated, all mice were housed at room temperature on a 12-h light/dark cycle and given ad libitum access to water and standard chow (Safe Diets, A30). All mice were randomised according to body weight and acclimated for at least 1 week prior to the first oral administration. Each animal study received a freshly prepared batch of S. boulardii. Body weight and food intake were recorded once per week. The researchers were blinded in all mouse experiments. The mice were euthanised by cervical dislocation at the end of the study.
The mice were divided into four groups (n = 4), either receiving the Sb wild-type, Sb bts1∆, Sb thi6∆ or Sb bts1∆ + thi6∆ strain. The mice were orally administered via intragastric gavage with ∼108 CFU of the S. boulardii strain in 100 µL of 1x PBS and 20% glycerol. The mice were orally administered with S. boulardii for five consecutive days, followed by a 6-day washout. The drinking water was supplemented with an antibiotic cocktail containing 0.3 g/L ampicillin sodium salt, 0.3 g/L kanamycin sulfate, 0.3 g/L metronidazole, and 0.15 g/L vancomycin hydrochloride after the washout period. After 5 days of antibiotic treatment, the mice were orally administered with S. boulardii in 100 µL of 1x PBS (containing 1.0 g/L ampicillin sodium salt, 1.0 g/L kanamycin sulfate, 1.0 g/L metronidazole, and 1.0 g/L vancomycin hydrochloride) and 10% glycerol for five consecutive days. The washout for the antibiotic-treated mice was monitored for 33 days.
The faeces were collected in pre-weighed 1.5 ml or 2.0 ml Eppendorf tubes containing 1 ml of 1x PBS and 25% glycerol and weighed again to determine the faecal weight. All sample preparation for assessing CFU numbers was kept on ice and followed the same practice. The faecal samples were homogenised by vortexed at ∼2,400 rpm for 20 min. The samples were then spun down at 100 g for 30 s, followed by a dilution series, where 5 µL of each dilution was plated in duplicates or triplicates. Under the antibiotic-treated washout period, 100 µL was plated. The faecal samples were plated on SC supplemented with 20 mg/L uracil plates containing 100 mg/L ampicillin, 50 mg/L kanamycin, and 30 mg/L chloramphenicol (Sigma Aldrich).
Statistical testing
All statistical analysing were performed in RStudio version 4.1.0 with the rstatix and DescTools packages. Unless otherwise stated, all data are presented as means +SEM. Statistical differences between groups of two were analysed with a dependent sample t-test. Bonferroni adjustments were used for multiple comparisons. Comparison of three or more groups was analysed by ANOVA with either Tukey’s HSD post hoc test or Dunnett’s post hoc test. The statistical significance level was set at p < 0.05.
Results
Selection of optimal auxotrophies for S. boulardii biocontainment
We started by investigating the impact of constraining the probiotic yeast to become dependent on the exogenous supply of metabolites for growth and survival, as it is one of the most common biocontainment strategies for genetically modified microorganisms (Wook Lee et al., 2018). Here we generated a library of auxotrophic S. boulardii strains by disrupting several genes and evaluating the burden of the gene deletion on each strain. We created one nucleoside (uracil), two amino acids (histidine and tryptophan) and two vitamins (thiamine and pyridoxine) auxotrophic strains (Figure 2A). We started by introducing a stop codon in URA3 (uracil synthesis), HIS3 (histidine synthesis) and TRP1 (tryptophan synthesis), as a foundation for selecting a biocontainment that may also be used as an auxotrophic marker for further strain engineering. Auxotrophic markers are commonly used in microbial engineering to establish an antibiotic-free platform for plasmid-based heterologous gene expression (Durmusoglu et al., 2021). These auxotrophic strains have previously been reported in S. boulardii (Liu et al., 2016). Importantly, these gene disrupted strains are suitable hosts for further genetic manipulation using existing Saccharomyces cerevisiae tools (Hudson et al., 2014; Liu et al., 2016; Durmusoglu et al., 2021; Jin et al., 2021). The strains with URA3, HIS3, and TRP1 disrupted were unable to grow unless uracil, histidine or tryptophan was supplemented to the growth medium (Figures 2B, C). The TRP1P12X (SbT−) strain also showed a metabolic burden when media was supplemented with tryptophan (Figure 2C; Supplementary Figure S1). Based on these observations, the HIS3G26X (SbH−) and URA3S81X (SbU−) strains showed to be the most promising nutritional auxotrophs compared to the SbT− strain. Combination of the URA3, HIS3, and TRP1 gene disruptions maintained their auxotrophic phenotype and showed restricted growth in media lacking their respective nutrition (Figure 2; Supplementary Figure S2). However, the triple disruption strain showed to be unstable, with growth observed after 120 h in the absence of tryptophan. The SbU− strain was selected for further gene knockouts based on a previous report showing higher gene expression from URA3-marker plasmids (Durmusoglu et al., 2021).
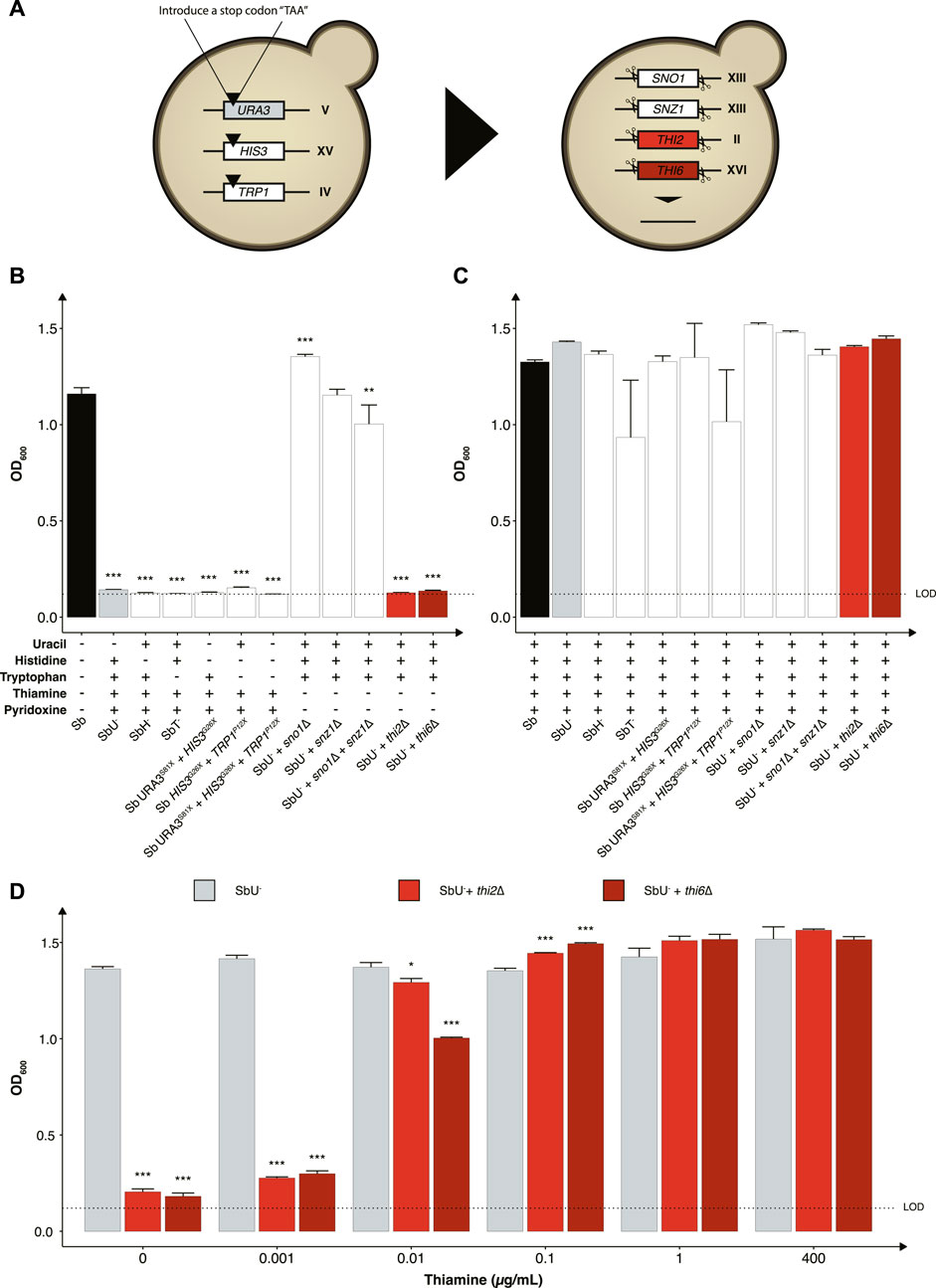
FIGURE 2. Selection of auxotrophic mutation in S. boulardii. (A) Schematic overview of the construction of the auxotrophic strains. To generate the uracil, histidine, and tryptophan auxotroph, a null mutation was introduced in the respective genes, while the genes were deleted to generate the vitamin auxotrophs. Bar plot of mean OD600 after 48 h (B) without the required nutrition supplemented and (C) with the required nutrition supplemented. (D) Bar plot of mean OD600 after 48 h under different concentrations of thiamine. Limit of detection (LOD). Data presented as mean +SEM (n = 3). * p < 0.05, ** p < 0.01 and *** p < 0.001. One-way ANOVA, Dunnett’s post hoc test with Sb (A) or SbU− (B,C) as reference.
We further investigated the phenotype of disrupting two other biosynthetic pathways in strains with uracil synthesis deficiency to determine potential additive effects. Knocking out either the THI2 or the THI6 gene in the thiamine synthesis pathway resulted in strains that were unable to grow in media lacking thiamine (Figure 2B). Knocking out the SN O 1 and SNZ1 genes of the pyridoxine synthesis pathway individually resulted in no significant growth defect, contrary to previously reported (Rodríguez-Navarro et al., 2002). Non-etheless, a significant lower final OD600 was observed when knocking out the SNO1 and SNZ1 genes in combination. To demonstrate that the growth defect resulted from the respective auxotrophies, we cultured the various strains in media containing the corresponding nutrient supplements and observed similar OD600 reaching after 48 h (Figure 2C).
To examine the robustness of the auxotrophic strains towards conditions experienced in the human gastrointestinal tract, we assessed their growth performance in minimal media with pH and oxygen conditions more closely mimicking the gastrointestinal tract. The thi2∆ strain displayed a more considerable metabolic burden compared to thi6∆, demonstrating 25%–50% increased doubling time at pH 3, 6, and in microaerobic (0.1% and 1% oxygen) and anaerobic conditions compare to thi6∆ strain (Supplementary Figure S1). The thi6∆ strain showed a slight growth defect at pH 4 and 5, demonstrating 3%–6% higher doubling time than thi2∆ (Supplementary Figure S1).
We next evaluated the thiamine sensitivity in the strains to identify the minimum thiamine concentration required for growth by the thi2∆ and thi6∆ strains to circumvent the biocontainment. Both knockouts reached a lower final OD600 at a concentration ≤0.01 μg/ml (Figure 2D). The thi6∆ strain appeared more sensitive to low thiamine concentrations than the thi2∆ strain. We also confirmed that from a 120-h culture with and without thiamine present in the media, zero escaper were identified for the thi6∆ strain (Supplementary Figure S3).
Constructing cold-sensitive S. boulardii strains
Another strategy to reduce the proliferation of genetically modified microorganisms in specific environments is to make them sensitive to temperature changes that may occur outside the targeted environment (Figure 3A). To assess this approach, we knocked out two genes (REI1 and BTS1) that were previously reported to exhibit a growth defect at a temperature lower than 25°C when knocked out in S. cerevisiae (Jiang et al., 1995; Hung and Johnson, 2006; Lebreton et al., 2006). The REI1 gene encodes a zinc finger protein part of the 60S complex, and the BTS1 gene encodes the yeast geranylgeranyl diphosphate synthase. We observed the expected growth defects at temperatures of 15°C and 20°C for both rei1∆ and bts1∆ strains (Figure 3B). While both bts1∆ and rei1∆ strains were hypersensitive at 15°C and showed limited growth after 120 h, the rei1∆ displayed a more pronounced growth defect; however, the rei1∆ strain also showed a slower growth at 37°C (Supplementary Figure S4).
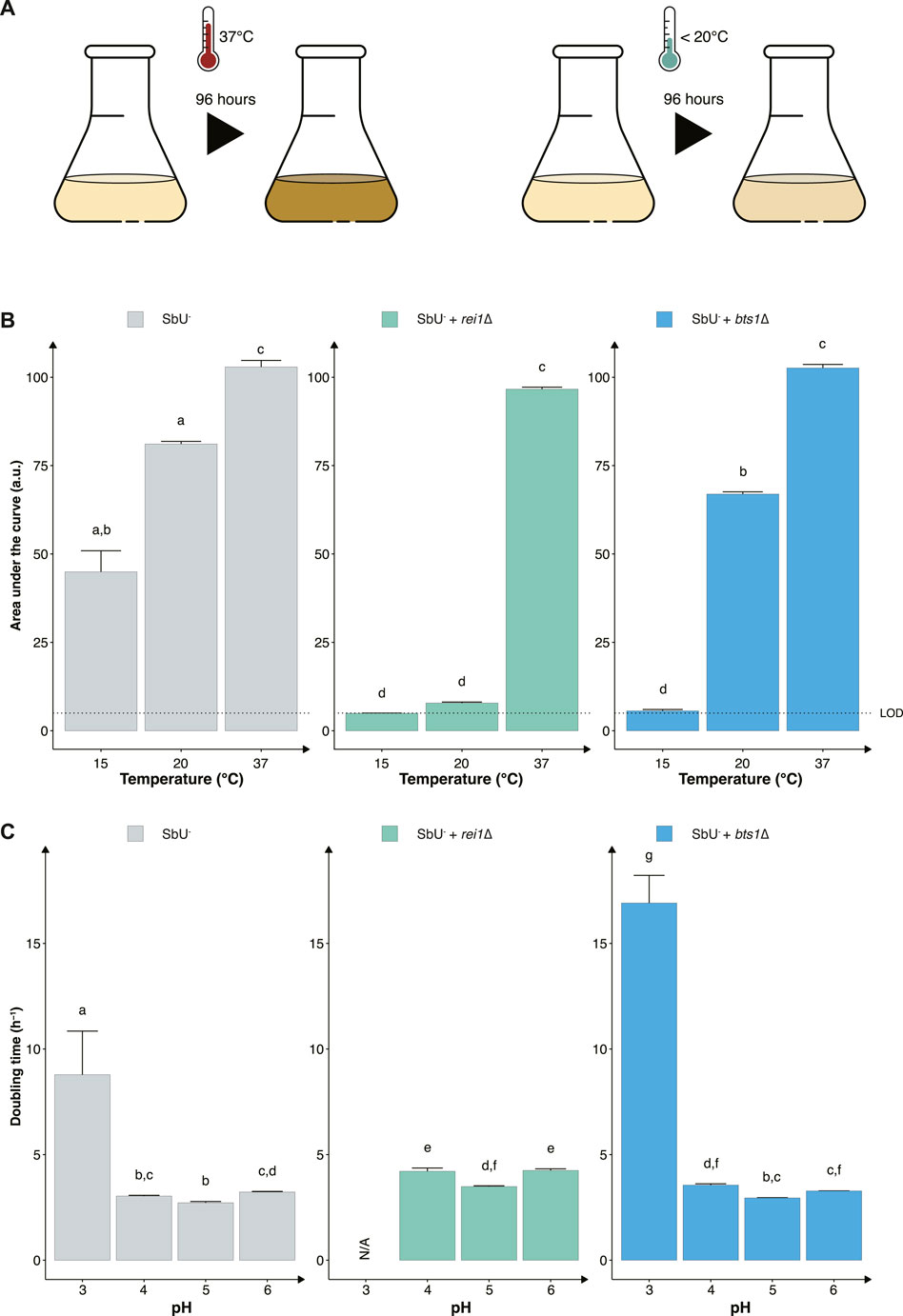
FIGURE 3. Constructing temperature-sensitive S. boulardii strains. (A) Graphical illustration of the experimental design to confirm the phenotype. (B) Bar plot of the mean area under the curve (AUC) of 96-h cultivation at 15°C, 20°C and 37°C. (C) Bar plot of the mean doubling time in pH 3, 4, 5 and 6. Limit of detection (LOD). Data presented as mean +SEM (n = 3). Two-way ANOVA, Tukey post hoc test. The different letters (a, b, c, d, e, f, g, and h) above the bars indicate statistically different groups (significance level at p < 0.05).
Furthermore, evaluating the two cold-sensitive strains in the gut-like conditions, we demonstrate that the rei1∆ strain showed a more pronounced fitness cost in minimal media at different pH (Figure 3C). The rei1∆ strain was unable to grow at a pH lower than 4 after 72 h, and the strain displayed an approximate 25% slower doubling time at pH 4, 5, and 6 compared to the parental strain (SbU−). The rei1∆ strain also showed a significantly lower growth rate in anaerobic and microaerobic conditions than SbU− (Supplementary Figure S5), while the bts1∆ demonstrated a slower growth rate in anaerobic conditions. The doubling time at anaerobic conditions was comparatively more pronounced for the bts1∆ than for the rei1∆ strain.
Combining vitamin auxotrophy with cold-sensitive mutations as a robust biocontainment strategy
To generate a more robust biocontainment strain, we combined the nutritional auxotrophy and the cold-sensitive mutant (Figure 4A). Specifically, we chose thi6∆ and bts1∆, in the SbU− background strain, based on functionality at different pH concentrations and oxygen gradients. Although the rei1∆ strain showed a more pronounced growth retardation at the lower temperature we hypothesis that additional fitness cost through genetic deletions or heterologous gene expressions would limit the strain to survive in the gastrointestinal tract. The double gene knockout of BTS1 and THI6 maintained the phenotypic effect of the individual knockouts, demonstrating the inability to grow in the absence of thiamine supplementation and slower growth in temperatures lower than 20°C (Supplementary Figure S4, S6).
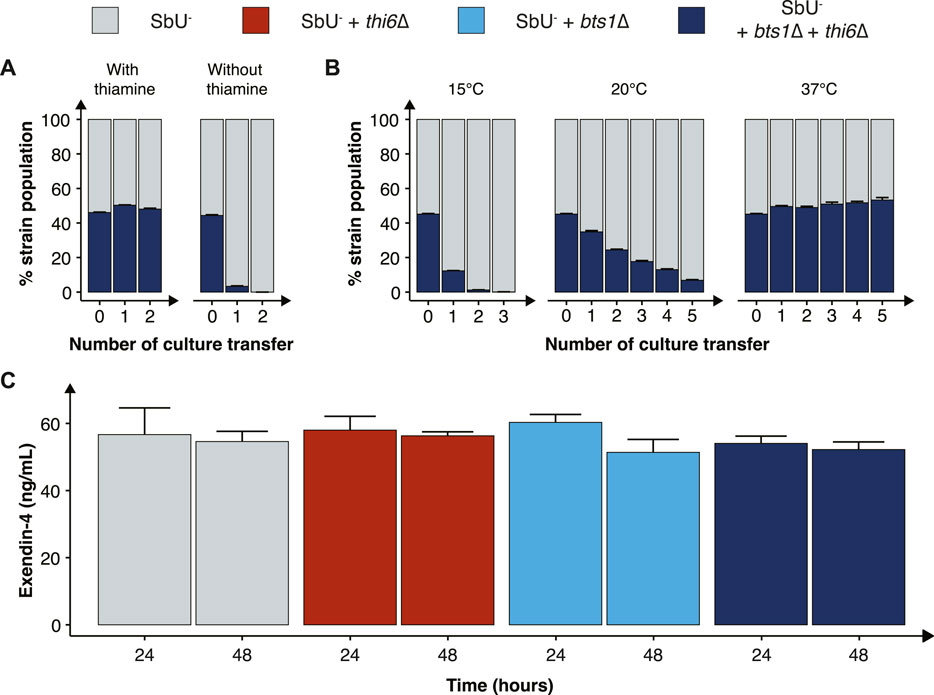
FIGURE 4. Characterisation of the combined cold-sensitive and auxotrophic strain. (A) Stacked bar plot of the percentage of SbU− and SbU− + bts1∆ + thi6∆ in a co-culture experiment with and without thiamine. The co-culture was transferred to a fresh culture every 48 h (n = 5). (B) Stacked bar plot of the % of SbU− and SbU− + bts1∆ + thi6∆ in a co-culture experiment at 15°C, 20°C, and 37°C. The co-culture was transferred to a fresh culture every 24 or 48 h (n = 5). (C) Bar plot of the mean concentration of Exendin-4 (ng/ml) quantified in the supernatant at 24 and 48 h of cultivation (n = 3). Data presented as mean +SEM. * p < 0.05, One-way ANOVA, Dunnett’s post hoc test with SbU− as reference.
Next, we evaluated the competitive fitness of the double knockout biocontainment strain in a co-culture with the parental control strain SbU− in the presence and absence of thiamine and at different temperatures. The double knockout and control strain perform similarly in the presence of thiamine; however, in the absence of thiamine, the double knockout strain was undetected after two transfers (Figure 4A; Supplementary Figure S6). In the same manner, the double knockout strain and the control strain were able to grow simultaneously at the permissive temperature conditions of 37°C; however, at 15°C and 20°C, the percentage of double knockout strains was drastically reduced incrementally with the number of culture transfers (Figure 4B). The biocontainment double knockout strain maintained ∼1% of the total yeast population after two transfers of the co-culture at 15°C and ∼5% after five transfers at 20°C.
Furthermore, we tested whether the biocontainment strain would maintain its cold-sensitive phenotype after multiple generations in co-culture with the control strain at 37°C (120 h). Here, we observed that the double knockout strain maintained its cold-sensitive phenotype, demonstrating zero colonies after plating the culture, incubating the strain at 15°C and maintaining a slower growth phenotype at 20°C (Supplementary Figure S8). The double knockout biocontainment strain also showed no synergistic growth defect at different pH concentrations and oxygen percentages (Supplementary Figure S9). We also observed an increased thiamine sensitivity by the combined biocontainment strain, showing a significantly lower OD600 in 1 μg/ml after 48 h (Supplementary Figure S10).
Finally, we sought to investigate if the double knockout biocontainment strain can produce a similar level of a recombinant protein as the parental strain SbU−. This is relevant since some gene knockouts might negatively impact protein synthesis (Puddu et al., 2019). Therefore, we engineered the biocontainment strains to produce and secrete a GLP-1 receptor agonist (Exendin-4) since in situ biosynthesis of Exendin-4 in the gastrointestinal tract with S. boulardii has previously been shown to reduce food intake and body weight in mice (Hedin et al., 2022a). We observed no significant difference in the production of Exendin-4 after 24 and 48 h (Figure 4C), suggesting that the biocontainment strain does not compromise the production of a heterologous protein.
The biocontainment strain is safe and viable in a mouse model
While we have validated the biocontainment strategy to be functional in vitro, the generated knockout strains still need to be demonstrated to be safe and viable in the gastrointestinal tract to express a therapeutic activity. To assess this, we orally administered the biocontainment strains daily for 5 days in conventional mice with an intact microbiome (Figure 5A) to study if the biocontainment strains pose any fitness cost while interacting with other microbes. We demonstrated that the wild-type, single and combined knockouts colonise equally in the mice with intact microbiome (Figure 5B). In addition, all the S. boulardii strains were washed out after 6 days, except for one mouse receiving the bts1∆ strain (Figure 5C), indicating that the knockouts did not cause any unwanted engraftment in mice.
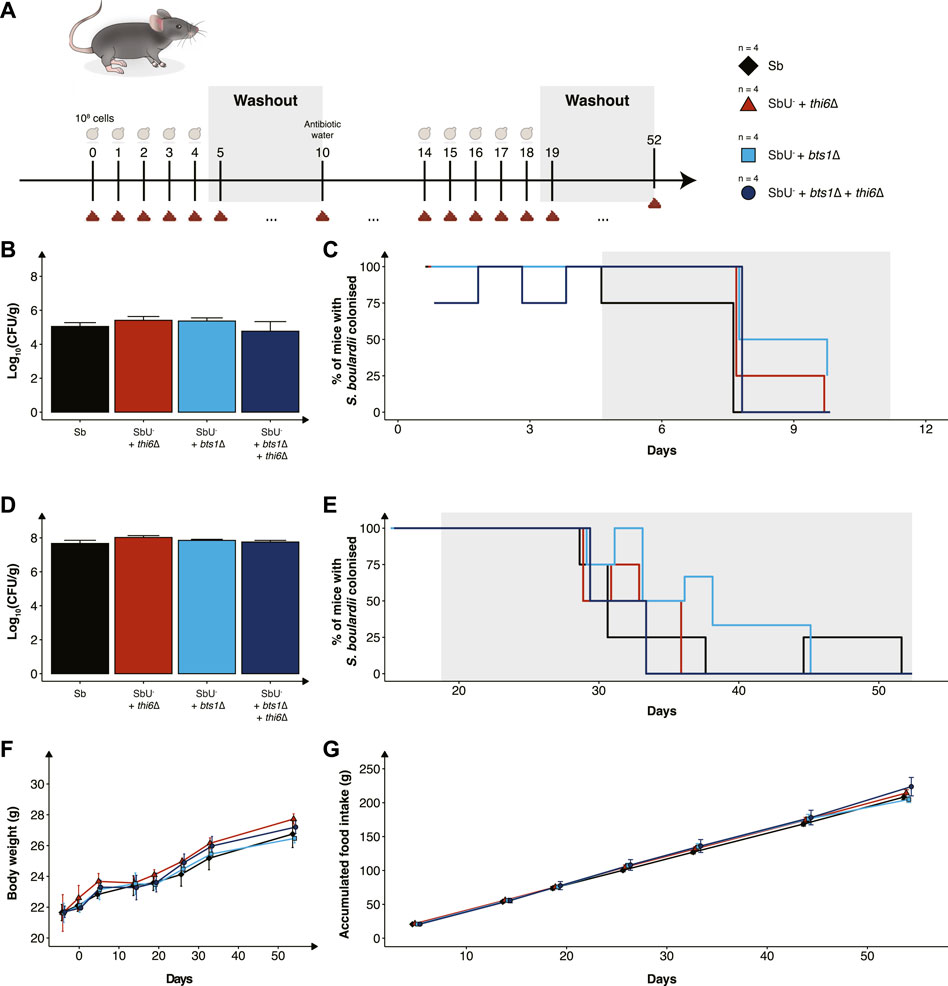
FIGURE 5. In vivo assessment of the biocontainment strains in antibiotic-treated mice. (A) Graphical scheme of the study design. Male C57BL/6 mice were orally administered with ∼108 cells of S. boulardii daily for five successive days, followed by 6 days of washout. On the 10th day was, the water supplemented with an antibiotic cocktail. The mice were again orally administered with ∼108 cells of S. boulardii daily for five successive days, followed by 34 days of washout. (B) Bar plot of S. boulardii abundance (log10 CFU per gram faeces) in conventional mice with intact microbiome. (C) Step plot of percentage of conventional mice with S. boulardii colonised (LOD ≈ 103 CFU/g). (D) Bar plot of S. boulardii abundance (log10 CFU per gram faeces) in antibiotic-treated mice. (E) Step plot of percentage of antibiotic-treated mice with S. boulardii colonised (LOD ≈50 CFU/g). (F) The body weight (grams) during the whole study. (G) Accumulated food intake (gram) throughout the whole study. Data presented as mean ± SEM (n = 4). Data were analysed with One-way ANOVA (B,D) and Two-way ANOVA (F,G), using Dunnett’s post hoc test with SbU− as reference.
Furthermore, we evaluated the biocontainment strains in an antibiotic-treated mouse model designed for pre-clinical testing of yeast-based AMTs, which allows S. boulardii strains to colonise the murine gastrointestinal tract in higher numbers and for an extended period of time (Hedin et al., 2022b). The antibiotic-treated mice were orally administered with the biocontainment strains for 5 days. Wild-type, single and combined knockouts were demonstrated to also colonise equally in the mice with a reduced microbiota (Figure 5D). Although the S. boulardii was washout slower in the antibiotic-treated mice, no mice displayed any detectable levels 33 days after the last oral administration (Figure 5E).
To investigate if the newly generated strains posed any safety concerns to the health and wellbeing of the mice, body weight, food intake and behaviour were monitored throughout the whole study. We observed no change in body weight or food intake in the mice receiving the biocontainment strains (Figures 5F, G), both during low and high colonisation of the strains. In addition, no abnormal behaviour was observed in the mice.
Discussion
Biocontainment is a crucial step in the development of AMTs, as it constrains the proliferation of genetically modified microorganisms outside the treated individual. Therefore, it limits the risk of outcompeting natural organisms and negatively affecting ecosystems and human health (Wilson, 2008). In this study, we implemented a biocontainment strategy for the probiotic yeast S. boulardii, demonstrating robust growth in the murine gastrointestinal tract and limited growth under in vitro conditions mimicking the external environments (Figure 1).
We designed a multi-layered strategy by introducing a combination of auxotrophy and temperature sensitivity. We selected the SbU− as a background strain for further genetic manipulation as it was demonstrated to have the expected phenotypic traits as auxotroph and URA3 marker plasmids have been reported to have higher gene expression than HIS3 and TRP1 marker plasmids in S. boulardii (Durmusoglu et al., 2021) and is a frequently used marker (Jensen et al., 2014; Maury et al., 2016). Next, we evaluated the effect of additional auxotrophic knockouts as potential strategies. While the thi2∆ and thi6∆ strains showed restricted growth in the functionality assay (Figure 2B), both showed a slight increase in OD600 at 0 μg/ml thiamine in the thiamine dose experiment (Figure 2D). This could be explained by the fact that a small trace amount of thiamine could be transferred from the pre-culture; alternatively, the strains are more sensitive in media lacking pyridoxine. Non-etheless, the thiamine auxotrophic strain, thi6∆, demonstrated a more pronounced and sensitive effect to lower thiamine concentrations than thi2∆. In addition, the THI2 is a transcriptional activator of thiamine biosynthetic genes (Nishimura et al., 1992), while THI6 is a gene encoding an enzyme required for thiamine synthesis (Nosakas et al., 1994); as such, circumventing the transcriptional activation might pose a higher risk of escapers than gaining back an enzymatic function. Although the thi2∆ strain demonstrated a slower doubling time in five out of seven tested conditions it showed a slightly faster growth at pH 4 and 5 (Supplementary Figure S1). This could be explained by that the S. boulardii with uracil disrupted generally grew faster at pH 4 and 5, therefore the growth defect of thi2∆ might have escalated outside the optimal pH condition.
To further contain the probiotic yeast, we investigated the potential of temperature-sensitive knockout strains. The global average temperature is calculated to be 13.9°C (Sobrino et al., 2020), although the temperature varies at different places, times of year and day we hypothesise that an AMT with reduced fitness in that temperature range would limit the proliferation on many places. We compared two gene knockouts that caused the strain to be sensitive to colder temperatures (Jiang et al., 1995; Hung and Johnson, 2006). Knocking out BTS1 and REI1 reduced the fitness of the strains at temperatures ≤20 °C (Figure 3). We prioritised the bts1∆ strain even though the rei1∆ strain showed a more pronounced growth defect at 20°C. This was based on the fact that the rei1∆ strain also displayed a slower growth at 37°C and an increased fitness cost in different pH and oxygen conditions found in the gastrointestinal tract (Figure 3; Supplementary Figure S5). Furthermore, it has been reported that overexpression of REH1 and deleting ARX1 can partially suppress the rei1∆ cold-sensitive growth phenotype (Lebreton et al., 2006; Parnell and Bass, 2009). Thus, the rei1∆ strain might increase the risk of selective pressures for escape mutants.
To build a more redundant strategy we combined the auxotrophic mutant thi6∆ and the cold-sensitive mutant bts1∆ in the background strain SbU− to further reduce the fitness in the external environments and minimise the chance of escape mutants. The final biocontainment strain exhibited similar phenotypic traits as the individual knockouts. We also demonstrated that the parental control strain drastically outcompetes the double biocontainment strain unless thiamine was supplemented and cultivated at 37°C (Figure 4). Furthermore, when thiamine was absent from the media, the double biocontainment strain was equally restricted in mono-culture as in co-culture with the control strains, indicating any potential cross-feeding of thiamine between the strains was not sufficient to maintain the growth of the biocontainment strain.
The knockout strains demonstrated a neglectable effect on peptide synthesis, indicating that the biocontainment strain could still act as an AMT for delivering therapeutic peptides (Figure 4C). Furthermore, we observed comparable phenotypic growth performance at the different pH and oxygen conditions, demonstrating the strain to be robust to potential environmental changes.
We finally determined the viability and safety profile of the single and double knockout strains in healthy mice (Figure 5). We observed no differences in the mice health and wellbeing between the different groups, demonstrating that the knockout strains did not pose a risk in healthy mice. The viability of the biocontainment strains in the faeces was demonstrated to reach equal levels compared to the wild-type, both in naïve and antibiotic-treated mice. The abundance per gram of faeces and washout of S. boulardii in mice’s gastrointestinal tract is in line with previously reported data (Hedin et al., 2022b). This supports the hypothesis that the biocontainment strain does not suffer any noticeable fitness cost in the gastrointestinal tract of a mouse. In addition, it demonstrates that the concentration of thiamine in the murine gut is sufficient for the thi6∆ strain to grow.
Moving AMTs into humans requires strategies that ensure the safety of the AMT chassis. Our study demonstrates a multi-layered biocontainment strategy validated both under laboratory growth conditions and in vivo in the gastrointestinal tract of mice. Although further combinations of strategies might be required to ensure complete containment of the yeast, we here demonstrate, to the author’s knowledge, the first biocontainment strategy as a platform for the continued development of yeast-based AMTs.
Data availability statement
The raw data supporting the conclusion of this article will be made available by the authors, without undue reservation.
Ethics statement
The animal study was reviewed and approved by Danish Animal Experiment Inspectorate (license number 2020-15-0201-00405).
Author contributions
KH and RV-U conceived the study. KH and RV-U designed the in vitro experiments, and KH, RV-U, and VK designed the in vivo experiment. KH carried out all in vitro characterisations, and KH and VK carried out all the in vivo characterisations. KH. analysed the data and wrote the manuscript. RV-U and MS supervised the study. All authors contributed to the discussion of the results. All authors read and approved the final manuscript.
Funding
This work received funding from The Novo Nordisk Foundation under NNF grant number: NNF20CC0035580, NNF Challenge programme CAMiT under Grant agreement: NNF17CO0028232 and the BestTreat project under European Union’s Horizon 2020 research and innovation programme with the Marie Skłodowska-Curie Grant agreement No 813781.
Acknowledgments
We are grateful to Anna-Maria Gutió I Vilardell for assisting in constructing the knockouts, Nicoline Munk Mikkelsen for carrying out some growth characterisations and Carmen Sands for assisting in setting up the flow cytometry experiment and providing (SbU−)-GFP. We are also grateful to Tiffany Shang Heng Mak, Carmen Sands and Troels Holger Vaaben for their feedback on the manuscript. The manuscript is currently available as a preprint on bioRxiv doi: https://doi.org/10.1101/2022.12.27.522029.
Conflict of interest
All authors are inventors on a patent filed by DTU on the Biocontainment strategy.
Publisher’s note
All claims expressed in this article are solely those of the authors and do not necessarily represent those of their affiliated organizations, or those of the publisher, the editors and the reviewers. Any product that may be evaluated in this article, or claim that may be made by its manufacturer, is not guaranteed or endorsed by the publisher.
Supplementary material
The Supplementary Material for this article can be found online at: https://www.frontiersin.org/articles/10.3389/fbioe.2023.1136095/full#supplementary-material
References
Arora, T., Wegmann, U., Bobhate, A., Lee, Y. S., Greiner, T. U., Drucker, D. J., et al. (2016). Microbially produced glucagon-like peptide 1 improves glucose tolerance in mice. Mol. Metab. 5 (8), 725–730. doi:10.1016/J.MOLMET.2016.06.006
Bahey-El-Din, M., Casey, P. G., Griffin, B. T., and Gahan, C. G. M. (2010). Efficacy of aLactococcus lactis ΔpyrGvaccine delivery platform expressing chromosomally integratedhlyfromListeria monocytogenes. Bioeng. Bugs 1 (1), 66–74. doi:10.4161/BBUG.1.1.10284
Caliando, B. J., and Voigt, C. A. (2015). Targeted DNA degradation using a CRISPR device stably carried in the host genome. Nat. Commun. 6 (11), 6989. doi:10.1038/ncomms7989
Canfora, E. E., Meex, R. C. R., Venema, K., and Blaak, E. E. (2019). Gut microbial metabolites in obesity, NAFLD and T2DM. Nat. Rev. Endocrinol. 15 (5), 5261–5273. doi:10.1038/s41574-019-0156-z
Chan, C. T. Y., Lee, J. W., Cameron, D. E., Bashor, C. J., and Collins, J. J. (2016). Deadman” and “Passcode” microbial kill switches for bacterial containment. Nat. Chem. Biol. 12 (2), 82–86. doi:10.1038/NCHEMBIO.1979
Chen, Z., Guo, L., Zhang, Y., Walzem, R. L., Pendergast, J. S., Printz, R. L., et al. (2014). Incorporation of therapeutically modified bacteria into gut microbiota inhibits obesityReporting animal research: Explanation and elaboration for the ARRIVE guidelines 2.0. J. Clin. InvestigationPLOS Biol. 12418 (87), 3391. doi:10.1172/JCI72517duSert10.1371/JOURNAL.PBIO.3000411
Durmusoglu, D., Al’Abri, I. S., Collins, S. P., Cheng, J., Eroglu, A., Beisel, C. L., et al. (2021). In situ biomanufacturing of small molecules in the mammalian gut by probiotic Saccharomyces boulardii. ACS Synth. Biol. 10 (5), 1039–1052. doi:10.1021/acssynbio.0c00562
Eckburg, P. B., and Relman, D. A. (2007). The role of microbes in Crohn’s disease. Clin. Infect. Dis. 44 (2), 256–262. doi:10.1086/510385
Emamalipour, M., Seidi, K., Zununi Vahed, S., Jahanban-Esfahlan, A., Jaymand, M., Majdi, H., et al. (2020). Horizontal gene transfer: From evolutionary flexibility to disease progression. Front. Cell Dev. Biol. 8, 229. doi:10.3389/fcell.2020.00229
Gallagher, R. R., Patel, J. R., Interiano, A. L., Rovner, A. J., and Isaacs, F. J. (2015). Multilayered genetic safeguards limit growth of microorganisms to defined environments. Nucleic Acids Res. 43 (3), 1945–1954. doi:10.1093/NAR/GKU1378
Gibson, D. G., Young, L., Chuang, R. Y., Venter, J. C., Hutchison, C. A., and Smith, H. O. (2009). Enzymatic assembly of DNA molecules up to several hundred kilobases. Nat. Methods 6 (55), 343–345. doi:10.1038/nmeth.1318
Gietz, R. D., and Woods, R. A. (2006). Yeast transformation by the LiAc/SS carrier DNA/PEG method. Methods Mol. Biol. Clift. N.J.) 313, 107–120. doi:10.1385/1-59259-958-3:107
Hedin, K. A., Zhang, H., Kruse, V., Rees, V. E., Bäckhed, F., Greiner, T. U., et al. (2022a). Oral delivery of GLP-1R agonist by an engineered probiotic yeast strain has anti-obesity effects in mice. BioRxiv. Available at: https://www.biorxiv.org/content/10.1101/2022.12.21.521368v1.
Hedin, K. A., Rees, V. E., Zhang, H., Kruse, V., Vazquez-Uribe, R., and Sommer, M. O. A. (2022b). Effects of broad-spectrum antibiotics on the colonisation of probiotic yeast Saccharomyces boulardii in the murine gastrointestinal tract. Sci. Rep. 12 (11), 8862–8869. doi:10.1038/s41598-022-12806-0
Huang, S., Lee, A. J., Tsoi, R., Wu, F., Zhang, Y., Leong, K. W., et al. (2016). Coupling spatial segregation with synthetic circuits to control bacterial survival. Mol. Syst. Biol. 12 (2), 859. doi:10.15252/MSB.20156567
Hudson, L. E., Fasken, M. B., McDermott, C. D., McBride, S. M., Kuiper, E. G., Guiliano, D. B., et al. (2014). Functional heterologous protein expression by genetically engineered probiotic yeast Saccharomyces boulardii. PLoS ONE 9 (11), 112660. doi:10.1371/JOURNAL.PONE.0112660
Hung, N.-J., and Johnson, A. W. (2006). Nuclear recycling of the pre-60S ribosomal subunit-associated factor Arx1 depends on Rei1 in Saccharomyces cerevisiae. Mol. Cell. Biol. 26 (10), 3718–3727. doi:10.1128/MCB.26.10.3718-3727.2006
Isabella, V. M., Ha, B. N., Castillo, M. J., Lubkowicz, D. J., Rowe, S. E., Millet, Y. A., et al. (2018). Development of a synthetic live bacterial therapeutic for the human metabolic disease phenylketonuria. Nat. Biotechnol. 3636 (99), 857–864. doi:10.1038/nbt.4222
Itzhaki, R. F., Lathe, R., Balin, B. J., Ball, M. J., Bearer, E. L., Braak, H., et al. (2016). Microbes and alzheimer’s disease. J. Alzheimer’s Dis. 51 (4), 979–984. doi:10.3233/JAD-160152
Jensen, N. B., Strucko, T., Kildegaard, K. R., David, F., Maury, J., Mortensen, U. H., et al. (2014). EasyClone: Method for iterative chromosomal integration of multiple genes Saccharomyces cerevisiae. Fems Yeast Res. 14 (2), 238–248. doi:10.1111/1567-1364.12118
Jiang, Y., Proteau, P., Poulter, D., and Ferro-Novick, S. (1995). BTS1 encodes a geranylgeranyl diphosphate synthase in Saccharomyces cerevisiae(*). J. Biol. Chem. 270 (37), 21793–21799. doi:10.1074/JBC.270.37.21793
Jin, Y., Yu, S., Liu, J. J., Yun, E. J., Lee, J. W., Jin, Y. S., et al. (2021). Production of neoagarooligosaccharides by probiotic yeast Saccharomyces cerevisiae var. boulardii engineered as a microbial cell factory. Microb. Cell Factories 20 (1), 160. doi:10.1186/s12934-021-01644-w
Johns, N. I., Blazejewski, T., Gomes, A. L. C., and Wang, H. H. (2016). Principles for designing synthetic microbial communities. Curr. Opin. Microbiol. 31, 146–153. doi:10.1016/J.MIB.2016.03.010
Kelesidis, T., and Pothoulakis, C. (2012). Efficacy and safety of the probiotic Saccharomyces boulardii for the prevention and therapy of gastrointestinal disorders. Ther. Adv. Gastroenterology 5 (2), 111–125. doi:10.1177/1756283X11428502
Kurtz, C. B., Millet, Y. A., Puurunen, M. K., Perreault, M., Charbonneau, M. R., Isabella, V. M., et al. (2019). An engineered E. Coli Nissle improves hyperammonemia and survival in mice and shows dose-dependent exposure in healthy humans. Sci. Transl. Med. 11 (475), 7975. doi:10.1126/scitranslmed.aau7975
Lebreton, A., Saveanu, C., Decourty, L., Rain, J. C., Jacquier, A., and Fromont-Racine, M. (2006). A functional network involved in the recycling of nucleocytoplasmic pre-60S factors. J. Cell Biol. 173 (3), 349–360. doi:10.1083/JCB.200510080
Liu, J. J., Kong, I. I., Zhang, G. C., Jayakody, L. N., Kim, H., Xia, P. F., et al. (2016). Metabolic engineering of probiotic Saccharomyces boulardii. Appl. Environ. Microbiol. 82 (8), 2280–2287. doi:10.1128/AEM.00057-16
Marlière, P., Patrouix, J., Döring, V., Herdewijn, P., Tricot, S., Cruveiller, S., et al. (2011). Chemical evolution of a bacterium’s genome. Angew. Chem. Int. Ed. 50 (31), 7109–7114. doi:10.1002/ANIE.201100535
Maury, J., Germann, S. M., Baallal Jacobsen, S. A., Jensen, N. B., Kildegaard, K. R., Herrgàrd, M. J., et al. (2016). EasyCloneMulti: A set of vectors for simultaneous and multiple genomic integrations in Saccharomyces cerevisiae. PLoS ONE 11 (3), e0150394. doi:10.1371/JOURNAL.PONE.0150394
McFarland, L. V. (1996). Saccharomyces boulardii Is Not Saccharomyces cerevisiae. Clin. Infect. Dis. 22 (1), 200–201. doi:10.1093/CLINIDS/22.1.200
Mee, M. T., Collins, J. J., Church, G. M., and Wang, H. H. (2014). Syntrophic exchange in synthetic microbial communities. Proc. Natl. Acad. Sci. U. S. A. 111 (20), E2149–E2156. doi:10.1073/pnas.1405641111
Moe-Behrens, G. H. G., Davis, R., and Haynes, K. A. (2013). Preparing synthetic biology for the world. Front. Microbiol. 4 (JAN), 5. doi:10.3389/fmicb.2013.00005
Nielsen, J. (2019). Yeast systems biology: Model organism and cell factory. Biotechnol. J. 14 (9), 1800421. doi:10.1002/biot.201800421
Nishimura, H., Kawasaki, Y., Kaneko, Y., Nosaka, K., and Iwashima, A. (1992). Cloning and characteristics of a positive regulatory gene, THI2 (PHO6), of thiamin biosynthesis in Saccharomyces cerevisiae. FEBS Lett. 297 (1–2), 155–158. doi:10.1016/0014-5793(92)80349-L
Nosakas, K., Nishimura, H., Kawasaki, Y., Tsujihara, T., and Iwashima, A. (1994). Isolation and characterization of the THI6 gene encoding a bifunctional thiamin-phosphate pyrophosphorylase/hydroxyethylthiazole kinase from Saccharomyces cerevisiae. J. Biol. Chem. 269 (48), 30510–30516. doi:10.1016/S0021-9258(18)43843-4
Offei, B., Vandecruys, P., de Graeve, S., Foulquié-Moreno, M. R., and Thevelein, J. M. (2019). Unique genetic basis of the distinct antibiotic potency of high acetic acid production in the probiotic yeast Saccharomyces cerevisiae var. Boulardii. Genome Res. 29 (9), 1478–1494. doi:10.1101/gr.243147.118
Parnell, K. M., and Bass, B. L. (2009). Functional redundancy of yeast proteins Reh1 and Rei1 in cytoplasmic 60S subunit maturation. Mol. Cell. Biol. 29 (14), 4014–4023. doi:10.1128/MCB.01582-08
Pinheiro, V. B., Taylor, A. I., Cozens, C., Abramov, M., Renders, M., Zhang, S., et al. (2012). Synthetic genetic polymers capable of heredity and evolution. Science 336 (6079), 341–344. doi:10.1126/science.1217622
Piraner, D. I., Abedi, M. H., Moser, B. A., Lee-Gosselin, A., and Shapiro, M. G. (2016). Tunable thermal bioswitches for in vivo control of microbial therapeutics. Nat. Chem. Biol. 13 (11), 75–80. doi:10.1038/nchembio.2233
Puddu, F., Herzog, M., Selivanova, A., Wang, S., Zhu, J., Klein-Lavi, S., et al. (2019). Genome architecture and stability in the Saccharomyces cerevisiae knockout collection. Nature 573 (7774), 416–420. doi:10.1038/S41586-019-1549-9
Rodríguez-Navarro, S., Llorente, B., Rodríguez-Manzaneque, M. T., Ramne, A., Uber, G., Marchesan, D., et al. (2002). Functional analysis of yeast gene families involved in metabolism of vitamins B1 and B6. Yeast 19 (14), 1261–1276. doi:10.1002/YEA.916
Rottinghaus, A. G., Ferreiro, A., Fishbein, S. R. S., Dantas, G., and Moon, T. S. (2022). Genetically stable CRISPR-based kill switches for engineered microbes. Nat. Commun. 13 (11), 672. doi:10.1038/s41467-022-28163-5
Rovner, A. J., Haimovich, A. D., Katz, S. R., Li, Z., Grome, M. W., Gassaway, B. M., et al. (2015). Recoded organisms engineered to depend on synthetic amino acids. Nature 518518 (7537), 753789–753793. doi:10.1038/nature14095
Sears, C. L., and Garrett, W. S. (2014). Microbes, microbiota, and colon cancer. Cell Host Microbe 15 (3), 317–328. doi:10.1016/J.CHOM.2014.02.007
Sobrino, J. A., Julien, Y., and García-Monteiro, S. (2020). Surface temperature of the planet earth from satellite data. Remote Sens. 1212 (2), 218. Page 218. doi:10.3390/RS12020218
Steidler, L., Hans, W., Schotte, L., Neirynck, S., Obermeier, F., Falk, W., et al. (2000). Treatment of murine colitis by Lactococcus lactis secreting interleukin-10. Science 289 (5483), 1352–1355. doi:10.1126/science.289.5483.1352
Steidler, L., Neirynck, S., Huyghebaert, N., Snoeck, V., Vermeire, A., Goddeeris, B., et al. (2003). Biological containment of genetically modified Lactococcus lactis for intestinal delivery of human interleukin 10. Nat. Biotechnol. 2121 (77), 785–789. doi:10.1038/nbt840
Stirling, F., Bitzan, L., O’Keefe, S., Redfield, E., Oliver, J. W. K., Way, J., et al. (2017). Rational design of evolutionarily stable microbial kill switches. Mol. Cell 68 (4), 686–697. doi:10.1016/J.MOLCEL.2017.10.033
Uribe, R., Rathmer, C., Jahn, L. J., Ellabaan, M. M. H., Li, S. S., and Sommer, M. O. A. (2021). Bacterial resistance to CRISPR-Cas antimicrobials. Sci. Rep. 11 (11), 17267–17269. doi:10.1038/s41598-021-96735-4
Verduyn, C., Postma, E., Scheffers, W. A., and van Dijken, J. P. (1992). Effect of benzoic acid on metabolic fluxes in yeasts: A continuous-culture study on the regulation of respiration and alcoholic fermentation. Yeast 8 (7), 501–517. doi:10.1002/YEA.320080703
Wilson, D. J. (2008). NIH guidelines for research involving recombinant DNA molecules. Acc. Res. 3 (2–3), 177–185. doi:10.1080/08989629308573848
Keywords: biocontainment, biosafety, probiotic yeast, engineered microbes, S boulardii, gut micobiome
Citation: Hedin KA, Kruse V, Vazquez-Uribe R and Sommer MOA (2023) Biocontainment strategies for in vivo applications of Saccharomyces boulardii. Front. Bioeng. Biotechnol. 11:1136095. doi: 10.3389/fbioe.2023.1136095
Received: 02 January 2023; Accepted: 06 February 2023;
Published: 20 February 2023.
Edited by:
Stephen Allen Morse, Centers for Disease Control and Prevention (CDC), United StatesReviewed by:
Kyeong Rok Choi, Korea Advanced Institute of Science and Technology (KAIST), Republic of KoreaDavid T. Stuart, University of Alberta, Canada
Copyright © 2023 Hedin, Kruse, Vazquez-Uribe and Sommer. This is an open-access article distributed under the terms of the Creative Commons Attribution License (CC BY). The use, distribution or reproduction in other forums is permitted, provided the original author(s) and the copyright owner(s) are credited and that the original publication in this journal is cited, in accordance with accepted academic practice. No use, distribution or reproduction is permitted which does not comply with these terms.
*Correspondence: Morten Otto Alexander Sommer, bXNvbUBiaW8uZHR1LmRr; Ruben Vazquez-Uribe, cnV2YXNAYmlvc3VzdGFpbi5kdHUuZGs=