- Center for Bioinformatics, School of Life Sciences, Peking-Tsinghua Center for Life Sciences, Peking University, Beijing, China
Gene drive is a genetic engineering technology that can enable super-mendelian inheritance of specific alleles, allowing them to spread through a population. New gene drive types have increased flexibility, offering options for confined modification or suppression of target populations. Among the most promising are CRISPR toxin-antidote gene drives, which disrupt essential wild-type genes by targeting them with Cas9/gRNA. This results in their removal, increasing the frequency of the drive. All these drives rely on having an effective rescue element, which consists of a recoded version of the target gene. This rescue element can be at the same site as the target gene, maximizing the chance of efficient rescue, or at a distant site, which allows useful options such as easily disrupting another essential gene or increasing confinement. Previously, we developed a homing rescue drive targeting a haplolethal gene and a toxin-antidote drive targeting a haplosufficient gene. These successful drives had functional rescue elements but suboptimal drive efficiency. Here, we attempted to construct toxin-antidote drives targeting these genes with a distant-site configuration from three loci in Drosophila melanogaster. We found that additional gRNAs increased cut rates to nearly 100%. However, all distant-site rescue elements failed for both target genes. Furthermore, one rescue element with a minimally recoded sequence was used as a template for homology-directed repair for the target gene on a different chromosomal arm, resulting in the formation of functional resistance alleles. Together, these results can inform the design of future CRISPR-based toxin-antidote gene drives.
1 Introduction
CRISPR homing gene drives have been shown to rapidly spread through laboratory populations for purposes of population suppression (Kyrou et al., 2018) or modification (Champer et al., 2020a). Such gene drives could be particularly valuable for the prevention of vector-borne disease, but they also have other applications such as the removal of invasive species and agricultural pests (Hay et al., 2021; Verkuijl et al., 2022; Wang et al., 2022). Yet, the best studied homing gene drives are “unconfined” in that a small release could lead to spread of the drive throughout the entire species, or at least all connected populations. This is undesirable when only specific populations should be targeted, such as removal of invasive species outside their native range (Scott et al., 2018; Teem et al., 2020; Ferreira-Martins et al., 2021).
Several options exist for confined gene drives (Hay et al., 2021; Wang et al., 2022), including Medea (Chen et al., 2007), haploinsufficient underdominance (Reeves et al., 2014), chromosomal rearrangements (Foster et al., 1972; Buchman et al., 2018), incompatibility underdominance (Maselko et al., 2020), and Wolbachia cytoplasmic incompatibility drive (Li and Champer, 2022). Some of the latest confined drive designs are based on CRISPR nucleases. They work by targeting and disrupting an essential gene with Cas9 (but without drive conversion as in homing drives) while also providing a recoded rescue copy of the target. These include TARE (Toxin-Antidote Recessive Embryo) (Champer et al., 2020b; Metzloff et al., 2022) and ClvR (Cleave and Rescue) (Oberhofer et al., 2019; Oberhofer et al., 2020) systems that are experimentally demonstrated and target a haplosufficient gene (in which one functioning copy is sufficient for an organism to retain high fitness). As the drive converts wild-type alleles to disrupted alleles, such alleles will be removed in disrupted allele homozygotes that lack drive alleles to provide rescue, thus increasing the frequency of the drive. Such drives only gain an introduction threshold when they have fitness costs (Champer et al., 2020d). This threshold refers to the frequency of drive individuals that must be released for drive success and serves as a measure of drive confinement. Alternate configurations of TARE drive have higher introduction thresholds, enabling flexible options for confined population modification (Champer et al., 2020c) or for self-limiting drive (Oberhofer et al., 2021b) similar to killer-rescue systems (Webster et al., 2020).
TARE drives could also support confined suppression as part of a tethered drive system (Dhole et al., 2019; Metzloff et al., 2022) if sufficiently efficient homing suppression drives can be constructed or even alone if an specialized cargo could be developed (Oberhofer et al., 2021a). Such developments would be non-trivial, so a potentially valuable option is to use a CRISPR toxin-antidote drive targeting a haplolethal gene (in which two copies are required for survival). Such TADE (Toxin-Antidote Dominant Embryo) drives are strong enough to be used for both modification and suppression (Champer et al., 2020c; Champer et al., 2020d; Pan and Champer, 2022; Zhang and Champer, 2022; Zhu and Champer, 2022). TADE is similar to TARE but has a haplolethal target gene, so any individual with even one disrupted copy is non-viable (unless there are at least two drive and wild-type target alleles present). TADE drives have not yet been constructed, although a homing rescue drive targeting a haplolethal gene was successful (Champer et al., 2020a).
One common need for all of these CRISPR toxin-antidote designs is an effective rescue element. This means that the gene is close enough to the wild-type version in terms of expression and function to avoid any significant fitness costs. The rescue element can have several possible sequence variations that could affect the chance of successful rescue. The first is its genomic location. In “same-site” rescue drives such as the experimental demonstration of TARE (Champer et al., 2020b; Metzloff et al., 2022) and most homing-based designs (DiCarlo et al., 2015; Champer et al., 2020a; Kandul et al., 2021; Terradas et al., 2021), the recoded copy is placed at the same genomic locus as the original gene and retains native upstream regulatory elements. In “distant-site” drives, the entire rescue element is located at a different genomic site as the target gene. Though such rescue elements have proven successful in CRISPR (Oberhofer et al., 2019; Oberhofer et al., 2020) and RNAi (Reeves et al., 2014) systems, upstream regulatory elements may be missed if the synthetic promoter element is too small, and genomic structure at the new site could also affect expression. Another potential variation is the degree of recoding. At a minimum, the gRNA sites themselves need to be recoded, but other coding sequence could be as well. Introns could deleted or modified, and UTRs could also be modified or changed, with greater changes reducing the chance of successful rescue, especially for haplolethal genes that may be more sensitive to level of expression.
Though potentially more difficult to generate, distant-site drives can operate at a higher efficiency when Cas9 cleavage rates are very low (Champer et al., 2020d). More importantly, such drives can be placed in a second target gene (where rescue is not desired), allowing the drive to disrupt it without additional gRNAs, simplifying construction for TADE suppression drive (Champer et al., 2020c; Champer et al., 2020d; Zhu and Champer, 2022). It can also allow targeting of genes that would otherwise be problematic due to close spacing between target sites and the drive insertion site (which could lead to formation of functional resistance alleles by undesired homology-directed repair) (Champer et al., 2020b). Finally, distant-site rescue might be required for highly confined systems in which rescue elements for two different genes must be placed at the same genomic site (Champer et al., 2020c).
In this study, we had several objectives. We make a first attempt at constructing TADE drives, with three systems using distant-site rescue in D. melanogaster, as well as two distant-site TARE drives. However, we found that rescue was inefficient for both cases. However, we were able to greatly improve Cas9 cut rates compared to previous systems (Champer et al., 2020a; Champer et al., 2020b) using additional gRNAs. When testing a minimally recoded rescue element, we found that undesired homology-directed repair formed functional resistance alleles based, despite it being located on a different chromosomal arm as the drive target site. Together, these observations provide useful lessons for the design of CRISPR toxin-antidote gene drives.
2 Materials and methods
2.1 Plasmid construction
For plasmid cloning, reagents for restriction digest, PCR, and Gibson assembly were obtained from New England Biolabs, as was 5-α competent Escherichia coli. Oligos and gBlocks were obtained from Integrated DNA Technologies. ZymoPure Midiprep kit was used to prepare injection-quality plasmids and was obtained from Zymo Research. Plasmids were confirmed by Sanger sequencing and utilized generally available methods. We provide annotated sequences of the donor plasmids and genomic target regions in ApE format (Davis and Jorgensen, 2022) (at https://github.com/jchamper/ChamperLab/tree/main/Distant-Rescue).
2.2 Generation of transgenic lines
Embryo injections were provided by Rainbow Transgenic Flies. The donor plasmid was injected into Drosophila melanogaster w1118 flies along with two helper plasmids, one providing gRNAs driven by the U6:3 promoter and one providing Cas9 driven by the hsp70 promoter (plasmid sequences for these can also be found at https://github.com/jchamper/ChamperLab/tree/main/Distant-Rescue). Flies were housed with Cornell Standard medium in a 25°C incubator on a 14/10-h day/night cycle.
2.3 Genotypes and phenotypes
Flies were anesthetized with CO2 and screened for fluorescence using the NIGHTSEA adapter SFA-GR for DsRed and SFA-RB-GO for EGFP and ECFP (it was found to provide better results than SFA-VI). Fluorescent proteins were driven by the 3xP3 promoter visualization in the white eyes of w1118 flies. DsRed was used as a marker to indicate the presence of the drive alleles, and EGFP was used to indicate the presence of the supporting nanos-Cas9 allele (Champer et al., 2019b). ECFP was used for the Rpl35A rescue-only element.
2.4 Phenotype data analysis
To calculate several statistics and rates in our study, offspring from different vials were pooled together before analysis. However, this approach did not take potential batch effects into account (different vials could be considered different batches of progeny), which could bias rate and error estimates. To account for this, we conducted an alternate analysis as in the previous studies (Champer et al., 2020a; Champer et al., 2020b; Champer S E et al., 2020; Metzloff et al., 2022; Yang et al., 2022). We fit a generalized linear mixed-effects model with a binomial distribution (by maximum likelihood, Adaptive Gauss-Hermite Quadrature, nAGQ = 25). This model allows for variance between batches, resulting in slightly different parameter estimates and slightly increased standard error estimates in most cases (any large batch effects would tend to increase these further). This analysis was performed using the R statistical computing environment (3.6.1), including packages lme4 (1.1-21, https://cran.r-project.org/web/packages/lme4/index.html) and emmeans (1.4.2, https://cran.r-project.org/web/packages/emmeans/index.html). The script is available on Github (https://github.com/jchamper/ChamperLab/tree/main/Distant-Rescue). The resulting rate and error estimates were similar to the pooled analysis (Supplementary Data Sets S1–S5).
2.5 Genotyping
To genotype flies, they were frozen, and DNA was extracted by grinding in DNAzol according to the manufacturer’s protocol (ThermoFisher Scientific). The DNA was used as a template for PCR using Q5 Hot Start DNA Polymerase from New England Biolabs. The region of interest containing gRNA target sites in RpL35A was amplified using DNA oligo primers RpL35ALeft_S_F and RpL35ARight_S2_R. This would allow amplification of wild-type sequences and sequences with resistance alleles but would not amplify recoded drive sequences because the reverse primer is outside the rescue element of all TADE drives. For all constructs, primers were used that would only produce PCR products if the genomic insertion was located where it was expected (see Supplementary Material).
3 Results
3.1 Drive constructs
In this study, several distant-site TADE drives and TARE drives were designed and constructed in Drosophila melanogaster. Cas9 and gRNAs expressed from each drive construct acted as “toxin” by cutting and disrupting target genes via end-joining repair or homology-directed repair with another disrupted allele as the template. CRISPR cleavage occurs mainly in germline cells inherited by progeny, but it can be induced in early embryos by maternally deposited Cas9 and gRNA. Each drive is located at a different genomic site than its target, but it has a recoded version of its target gene that cannot be cleaved by Cas9/gRNA. This rescue element is expected to work as an “antidote” by properly expressing the target gene. In TADE drives, flies could only survive with at least two copies of drive or wild-type alleles, which results in rapid removal of disrupted alleles because even a single disrupted allele is sufficient to render an organism non-viable in the absence of a drive allele. In TARE drive, one copy of drive or wildtype allele of target gene will always ensure the survival of flies. In both systems, drive alleles create disrupted alleles in the population, which are eventually removed by natural selection, thus increasing the drive frequency. In the distant-site TADE suppression system, the drive is placed inside a female fertility gene, disrupting it (even though it is not targeted by gRNAs). This eventually results in population suppression when the drive reaches high frequency, resulting in many sterile female drive homozygotes.
Several transgenic constructs were used in this study. One was a split homing drive targeting RpL35A with “same-site” rescue and has been described previously (Champer et al., 2020a). Another was a same-site TARE drive targeting hairy (Metzloff et al., 2022). In this study, we generally used split drives that were coupled with a split nanos-Cas9 allele with an EGFP marker (Champer et al., 2019b) (Figure 1). A similar Cas9 allele was driven by the vasa promoter, though it retained the nanos 3′UTR.
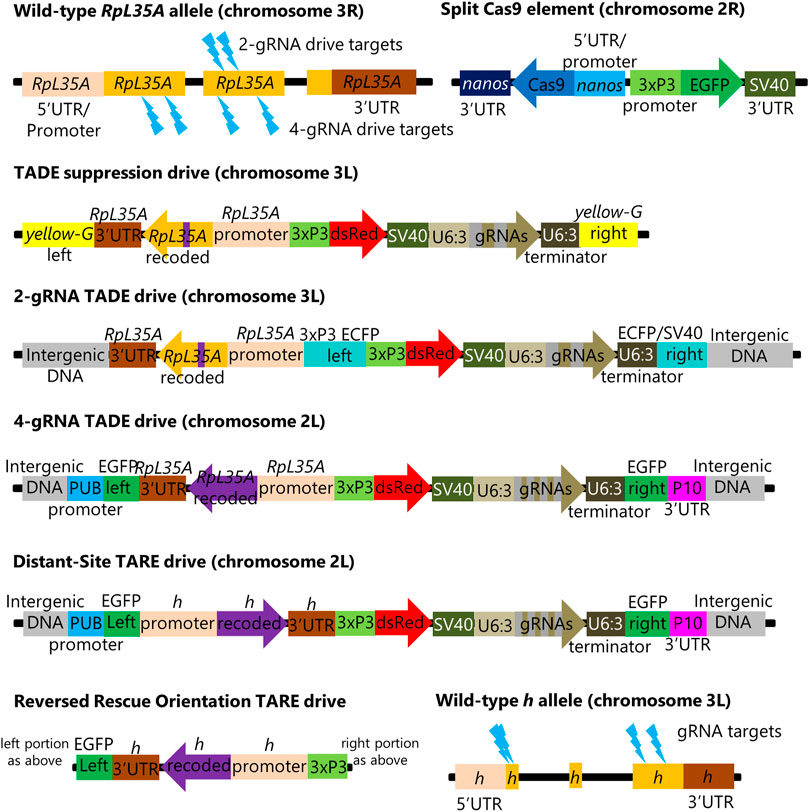
FIGURE 1. Genetic Constructs and target sites. The figure shows each element in several genetic constructs described in this study. Besides these, there is a RpL35A rescue-only construct that is similar to the 2-gRNA TADE drive but lacks the gRNAs and has an intact ECFP fluorescent protein gene instead of DsRed. Another construct is similar to nanos-Cas9, but with the nanos promoter and 5′UTR replaced by vasa elements. Lightning bolts indicate gRNA target sites. The 3xP3 Pol II promoter and the SV40 or P10 3′UTRs/terminators are used to drive the expression of DsRed and EGFP markers, while the Pol III promoter U6:3 is used to express gRNAs.
Several distant-site TADE constructs were designed, injected, and confirmed to be inserted at the correct genomic site (see Supplementary Material). One consisted of a TADE suppression drive inserted at the yellow-G locus (Figure 1), which was previously used as a target for homing suppression drives (Oberhofer et al., 2018; Yang et al., 2022) because it is a haplosufficient but essential female fertility gene. This drive had the same pair of gRNAs as the previous homing drive targeting RpL35A. It had a full rescue element that included 486 nucleotides of DNA upstream of the first exon. The next closest gene (POLDIP2) started its 5′UTR 676 nucleotides upstream of the first RpL35A exon, so the promoter likely contained all RpL35A-specific regulatory elements. Only a small portion of the rescue element was recoded (a 39-nucleotide strength in between the two gRNA target sites).
A TADE modification drive was formed by first inserting a RpL35A rescue element as above (and with an ECFP fluorescent marker) at an intergenic site on chromosome 3L that previously supported effective homing at an EGFP target site (Champer et al., 2019b). A DsRed and gRNA set as above were then inserted inside the ECFP gene (Figure 1).
A second TADE modification drive was inserted into a polyubiquitin-EGFP gene at an intergenic region in chromosome 2L that had also supported a synthetic target homing drive (Champer S E et al., 2020). However, this drive had four gRNAs that targeted different sites (Figure 1). The entire coding sequence was also recoded, and only the first two introns were retained (these introns separated exons containing the 5′UTR).
A distant-site TARE drive targeting the haplosufficient but essential gene hairy was inserted into the same spot as the 4-gRNA TADE drive. It also had four gRNAs, including two gRNAs used in earlier TARE drives (Champer et al., 2020b; Metzloff et al., 2022), an additional gRNA that was used for initial insertion of these previous same-site TARE drives (Champer et al., 2020b; Metzloff et al., 2022), and a final gRNA targeting the beginning of the coding sequence (Figure 1). The recoded rescue element was largely the same as the previous drives, but an additional 39 nucleotides of sequence was recoded after the start codon to prevent homology with the wild-type allele on both sides of the first gRNA cut site. 1718 nucleotides upstream of the 5′UTR (as measured from the isoform with the longest 5′UTR) were retained for use as possible promoter sequence for the rescue element.
A final TARE construct was as above, except that the orientation of the entire rescue element was reversed (Figure 1) to assess the potential impact of adjacent regulatory elements or transcription from the EGFP gene fragment.
3.2 TADE suppression drive has low cleavage rates
To assess the drive efficiency, female TADE suppression drive carriers (which could be heterozygous or homozygous) were crossed to male Cas9 line homozygotes. Female and male offspring with both red and green fluorescence were selected and crossed to w1118 flies. They were allowed to lay eggs for 1 week and then removed. Progeny were collected and phenotyped. The drive inheritance rate, indicated by the proportion of offspring with DsRed, was 54% for all crosses that produced viable offspring (Figure 2A; Supplementary Data Set S1), which was not significantly different than the Mendelian expectation (p = 0.1, Fisher’s exact test). This could indicate that the drive rescue element failed or that the toxin element of the drive did not function correctly (meaning that Cas9 cleavage did not occur).
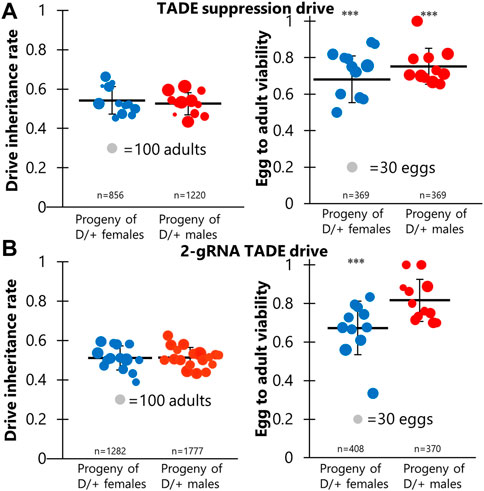
FIGURE 2. TADE drive inheritance and egg viability. Cross data are displayed for (A) the TADE suppression drive and (B) the 2-gRNA TADE drive. Drive inheritance and egg-to-adult viability was measured from crosses between drive individuals (heterozygous for the drive and for a Cas9 allele) and wild-type flies. Each dot represents offspring from one drive parent, and the size of dots is proportional to the number of total offspring for drive inheritance or the number of eggs for egg viability. Rate and standard error of the mean are displayed for all flies pooled together. No instances of drive inheritance are significantly different from the Mendelian expectation (Binomial Exact Test). *** indicates statistical significance of p < 0.0001 for egg viability compared to w1118 controls (Fisher’s Exact Test, see Supplementary Data Sets S1, S2).
To assess the latter possibility, we examined egg viability in additional crosses of the same type, comparing them to crosses between w1118 flies. Flies were allowed to lay eggs for a period of 20–24 h and were then transferred to a new vial for three consecutive days. After each transfer, the number of eggs was recorded, and all adults were later phenotyped. We found that egg viability was significantly lower in female and male drive crosses (72% and 75%) than in w1118 crosses (83%) (Figure 2A; Supplementary Data Set S1). This could potentially be explained by a ∼10% germline cut rate and a ∼2% embryo cut rate (though the embryo cut rate is even more uncertain because female and male drive crosses did not have significantly different viability) in a model where any cut inherited by offspring results in non-viability. These results are consistent with target site sequencing of six progeny from male drive crosses, which found only wild-type alleles. These low rates make it unclear if the rescue element functioned effectively. Such cut rates are substantially lower than the 82% germline and 29% embryo rates of the homing rescue drive targeting the same RpL35A gene using the same two gRNAs and Cas9 allele (Champer et al., 2020a). This difference could perhaps be explained by the different genomic location and environment of the gRNA gene in this construct. Another possibility is that some cuts resulted in homology-directed repair using the wild-type allele as the template, with was not possible in the homing drive. This would mean that actual cut rates would be higher, but the effective disruption rate of the target site would remain low unless one of the alleles were already disrupted in the germline (and thus available as a template for homology-directed repair), though such genotypes could not be recovered for testing.
3.3 Undesired homology-directed repair in a TADE modification drive
A simpler distant-site 2-gRNA TADE drive designed for modification was constructed (Figure 1), and we performed a similar set of crosses. Drive inheritance for these was 51%–52%, close to the Mendelian expectation (Figure 2B; Supplementary Data Set S2). However, the viability of eggs from drive females (66%) was significantly reduced compared to male egg viability (81%, p < 0.0001, Fisher’s exact test) and viability in w1118 crosses (83%, p < 0.0001, Fisher’s exact test) (Figure 2B; Supplementary Data Set S2). This is consistent with a 10% embryo resistance allele formation rate (in which a single resistance allele is sufficient to render the egg non-viable), or perhaps a 20% rate if only one cut site is available. However, germline rates have been generally higher than embryo cut rates in all studies, meaning that the lack of reduced viability in the progeny of males was unexplained. To assess this, we sequenced the target site of eight progeny from male drive crosses. All of these had the recoded sequence of the rescue element between the two cut sites. This means that the target on chromosome 3R was able to use the drive on chromosome 3L as a template for homology-directed repair (for which at least several hundred nucleotides would be available on either side of the cut sites), perhaps even from the same chromosome molecule. Despite apparently fairly high cut rates, this explains the full viability of progeny from male drive crosses and the relatively high viability of progeny from female drive crosses.
3.4 TADE modification drive with high cleavage rates shows failure of distant-site rescue elements
To solve the issue of low-cut rates and the use of the rescue element as a template for homology-directed repair, we constructed a distant-site 4-gRNA TADE modification drive (Figure 1). This drive has a much larger recoded region and used four completely new gRNAs. When drive and Cas9 heterozygotes were crossed to w1118, no viable progeny were obtained for both male and female crosses, despite several eggs being laid (Figure 3A; Supplementary Data Set S3). This indicates that the drive has a 100% rate of germline disruption, much higher than our TADE suppression drive. However, it also indicates that the drive was unable to provide rescue.
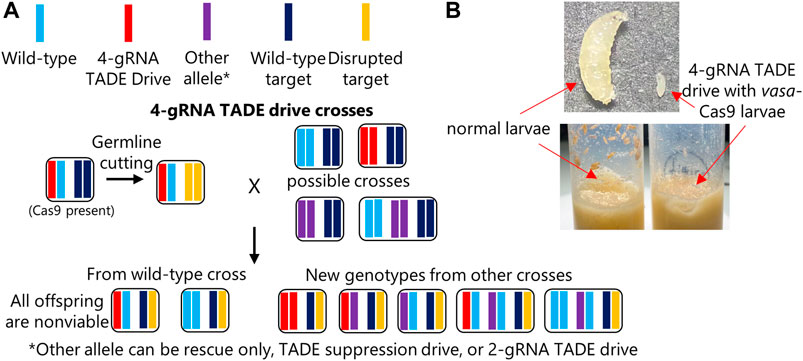
FIGURE 3. TADE rescue assessment. (A) Individuals heterozygous for the 4-gRNA TADE drive and the nanos-Cas9 allele were crossed to flies of several genotypes, including w1118 and three genotypes that could provide different distant-site rescue elements for the RpL35A target gene. However, no progeny were viable, indicating that all rescue elements failed. (B) When flies homozygous for the 4-gRNA TADE drive were crossed to flies homozygous for a vasa-Cas9 allele, offspring suffered from leaky somatic expression and failed to develop, with larvae growing more slowly and never reaching significant size (the maximum size reached is shown in the image).
An additional cross between drive/Cas9 males and drive homozygous females also failed to yield progeny, indicating the drive was unable to provide partial rescue. We also obtained no progeny from similar crosses between drive/Cas9 males and female homozygotes for the rescue-only allele, females homozygous for the TADE suppression drive, and females homozygous for the 2-gRNA TADE modification drive (Figure 3A; Supplementary Data Set S3). Thus, none of our constructs had a functioning rescue element. We also obtained no progeny when these males were crossed to proven homing rescue drive females, though this was expected because the successful same-site rescue element could likely not express RpL35A more efficiently than the wild-type allele.
3.5 Alternate Cas9 promoters and somatic expression
Because TADE drive targets a haplolethal gene, even a moderate amount of somatic expression may be sufficient to prevent success of the drive. To assess this, we generated a Cas9 allele that was nearly identical to the nanos-Cas9 construct but utilized the vasa promoter and 5′UTR elements in place of nanos upstream elements (the nanos 3′UTR was retained). In flies, vasa is known to be a strong germline promoter but also has moderate somatic expression compared to nanos, which has little to no expression in somatic cells (Gratz et al., 2014; Champer et al., 2018).
To investigate the impact of somatic expression on the 4-gRNA TADE drive, drive homozygous females were crossed to males that were homozygous for the vasa-Cas9 allele, and they were allowed to lay eggs in several vials. All offspring thus possessed both drive and Cas9 alleles. We found that eggs usually survived and hatched into larvae. However, all larvae had growth defects and never reached a substantial size (Figure 3B) before eventually expiring several days after they would normally have become pupae. This indicates that any TADE drive (or at least those with a strong toxin element) should use promoters that have little to no somatic expression.
3.6 Distant-site TARE drive assessment
Though haplolethal genes require precisely controlled expression to achieve high fitness, haplosufficient genes can tolerate a wider range of expression. Thus, we tested a distant-site TARE drive to see if distant-site rescue might function correctly. This drive used the same target gene and promoter region as previous TARE drives (Champer et al., 2020b; Metzloff et al., 2022) but used two additional gRNAs for a total of four. As expected, crosses between w1118 females and males with one copy of the drive and one copy of Cas9 had normal numbers of offspring and no biased inheritance (Figure 4A; Supplementary Data Set S4). Such progeny would still possess one wild-type hairy allele from the female parent and thus be viable. However, crosses between w1118 males and females with one copy of the drive and one copy of Cas9 produced no offspring. In these crosses, high rates of maternal Cas9 and gRNA deposition resulted in complete or nearly complete cleavage of wild-type hairy alleles in all progeny, rendering them non-viable. We can conclude that the rescue element failed because a functional rescue element would have allowed drive-carrying offspring to survive. Even when these drive/Cas9 females were crossed to drive homozygote males, no viable progeny were obtained (Supplementary Data Set S4), indicating that the distant-site TARE drive does not even provide partial rescue. In contrast, when crossed to previous functional TARE drive homozygotes (Metzloff et al., 2022), all progeny were viable (one gRNA of the new drive may have been able to cleave the old TARE drive allele, but must have done so at a low rate in the embryo).
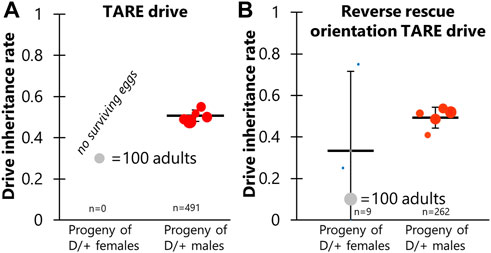
FIGURE 4. TARE drive inheritance. Cross data are displayed for (A) TARE drive and (B) Reverse rescue orientation TARE drive. Drive inheritance was measured from crosses between drive individuals (heterozygous for the drive and for a Cas9 allele) and wild-type flies. Each dot represents offspring from one drive parent, and the size of dots is proportional to the number of total offspring. Rate and standard error of the mean are displayed for all flies pooled together. No instances of drive inheritance are significantly different from the Mendelian expectation (Binomial Exact Test).
Because the polyubiquitin promoter element and EGFP fragment may have interfered with rescue element expression in the TARE drive, we constructed an identical allele in which the orientation of the rescue element was reversed (Figure 1). However, crosses involved this construct showed nearly identical results to the first TARE drive, except that a few female drive cross vials had very small numbers of surviving offspring, averaging less than one per vial (Figure 4B; Supplementary Data Set S5). Thus, the rescue element could not function in reverse orientation.
Of note, both of these 4-gRNA TARE drives showed ∼100% germline cutting and 98%–100% embryo cutting rates, as indicated by the lack of surviving offspring (Supplementary Data Set S4, S5—This estimate is based on the requirement of cutting both wild-type alleles in progeny to render the progeny non-viable, as well as many previous observations that show higher germline cut rates than embryo cut rates). This represents a substantial increase from the estimated 89% germline and 63% embryo cutting of the complete TARE drive (Metzloff et al., 2022), or the ∼100% germline cutting and ∼86% embryo cutting of the split TARE drive that used the same Cas9 element (Champer et al., 2020b). This further indicates that additional gRNAs can substantially increase total cut rates in systems where weak gRNAs may be a limiting factor.
4 Discussion
In this study, we examined the possibility of developing distant-site TADE suppression and modification drives targeting RpL35A and TARE drives targeting hairy. Though improved gRNA cassettes increased cut rates compared to previous drives targeting these genes, the drives could not function because their rescue elements were not effective. Additionally, the small recoded region in one drive could be used as a template for homology directed repair of the cleaved wild-type target allele, thus forming a functional resistance allele.
The need for effective rescue is absolutely essential for construction of any toxin-antidote drive, and the ability to perform this rescue from a site distal from the target gene would increase potential options. For example, it would preclude the need for additional gRNAs targeting the female fertility gene in TADE suppression drive (Champer et al., 2020c; Champer et al., 2020d; Zhu and Champer, 2022), which would also reduce the effects of leaky Cas9 somatic expression on female fertility (Hammond et al., 2021) (though such effects may be small compared to leaky somatic expression on the haplolethal TADE target, as demonstrated by our use of the vasa promoter). It would also enable to construction of 1-locus 2-drive systems, which are highly confined (Champer et al., 2020c). However, none of our systems was able to provide effective rescue. Distant site rescue is certainly possible, as demonstrated for several haplosufficient but essential genes in ClvR drive systems (Oberhofer et al., 2019; Oberhofer et al., 2020) and even for the haploinsufficient gene RpL14 in an RNAi system (Reeves et al., 2014). All of these ClvR drives were located at the same genomic site, so it is possible that certain genomic sites are more amenable to correct rescue expression than the ones we tested. Our own TARE drive with a haplosufficient target was only tested at one site. This site was known to support previous successful homing drives (Champer S E et al., 2020), but it is possible that the polyubiquitin promoter interfered with rescue expression (despite testing the rescue element in both possible orientations). Thus, even though our test failed, distant-site rescue for haplosufficient target should still be considered a viable option. Possible improvements could include longer promoter elements, other stronger rescue expression systems, new target genes, better genomic insertion sites for the drive, or flanking of the rescue element with sequences to protect it from local genomic structure (such as gypsy elements).
For haplolethal targets, precise expression is even more important, potentially increasing the difficulty of successful rescue. One of our TADE rescue elements was similar to the TARE element and may have failed for the same reasons. However, our other two rescue elements were at very different sites, one site a native gene and both known to support effective homing drives (Champer et al., 2019b; Yang et al., 2022). Neither were near the polyubiquitin promoter, and both had minimal recoding, meaning that potentially important introns and other sequences were preserved. Yet, both of these failed, which suggests that distant-site rescue elements for haplolethal genes may be considerably harder to construct. In this case, they likely failed through underexpression rather than overexpression, because having either one or two additional copies of the rescue element could not provide rescue for even one disrupted wild-type allele, nor did drive homozygotes show any phenotype associated with negative fitness effects when they were homozygous for wild-type RpL35A. Further underscoring the difficulty of successful rescue was our observation of homology-directed repair between the rescue element of one of the drives with minimal recoding on chromosome 3L and the target site on chromosome 3R. This indicates that recoded regions, even at distant sites (and perhaps even in different chromosomes, considering the distance between arms in chromosome 3) should probably be highly recoded, with as many mutations as possible on both sides of the target site for at least a few hundred nucleotides for any CRISPR/Cas9 toxin-antidote drives, thus minimizing the chance of using the drive’s rescue element as a template for homology-directed repair. However, this may not be the case for all genomic sites because we did not observe this phenomenon in our TADE suppression drive.
Several of our previous gene drives in Drosophila with the same nanos-Cas9 promoter had nearly 100% germline cut rates and generally high embryo cut rates when targeting the yellow (Champer et al., 2017; Champer et al., 2019a; Champer et al., 2019b), white (Champer et al., 2018), cinnabar (Champer et al., 2018), and synthetic EGFP(Champer et al., 2019b; Champer S E et al., 2020) genes. Yet, more recent successful drives targeting hairy (Champer et al., 2020b; Metzloff et al., 2022) and RpL35A (Champer et al., 2020a) had cut rates that were considerably below 100% in the germline and embryo, despite having two gRNAs. Our TADE drives using the same two 2-gRNA cassettes demonstrated further variation, with one having high germline cutting based on sequencing results and the other having fairly low cutting rates. This indicates that gRNAs, in addition to Cas9 (Champer et al., 2019b; Champer S E et al., 2020), can have varied expression based on their genomic location. For both our TARE and TADE drives, replacing the 2-gRNA cassette with a 4-gRNA cassette (and retaining the same U6:3 promoter and tRNA separation system) aiming at the same region of the target gene yielded nearly 100% cut rates. Strictly speaking, this cutting increase can only be confirmed to take place in the germline of the TADE drive and the early embryo of the TARE drive, but it was generally a successful strategy. Even if additional gRNAs are not necessarily needed to avoid functional resistance alleles, they can perhaps provide insurance against low-activity gRNAs without any significant downsides for CRISPR toxin-antidote drives (Champer et al., 2020d) [unlike homing drives (Champer S E et al., 2020)], though the risk of off-target fitness effects may be somewhat increased unless a high-fidelity nuclease is used (Langmüller et al., 2022). Additionally, caution is advised in this approach for TADE drives because if the embryo cut rate increases (as it did for our TARE drive), the confinement level of the drive will also be considerably increased (Champer et al., 2020c; Champer et al., 2020d; Zhu and Champer, 2022), which may not be desired.
In conclusion, we found that developing efficient distant-site rescue elements may be more challenging than anticipated, particularly for TADE drives. This may necessitate trying multiple sites for TARE drives using methods such as PiggyBac transformation and focusing efforts on developing same-site TADE suppression drives rather than potentially simpler distant-site systems. To ensure high germline cut rates in these drives, additional gRNAs could be added to toxin-antidote drives beyond the amount needed for functional resistance allele avoidance.
Data availability statement
The datasets presented in this study can be found in online repositories. The names of the repository/repositories and accession number(s) can be found in the article/Supplementary Material.
Author contributions
JiC and XX carried out research and analysis. JiC and JaC drafted the manuscript. All authors revised the manuscript and approved the final version.
Funding
This study was supported by laboratory startup funds from Peking University and the NSFC Overseas Youth Fund.
Conflict of interest
The authors declare that the research was conducted in the absence of any commercial or financial relationships that could be construed as a potential conflict of interest.
Publisher’s note
All claims expressed in this article are solely those of the authors and do not necessarily represent those of their affiliated organizations, or those of the publisher, the editors and the reviewers. Any product that may be evaluated in this article, or claim that may be made by its manufacturer, is not guaranteed or endorsed by the publisher.
Supplementary material
The Supplementary Material for this article can be found online at: https://www.frontiersin.org/articles/10.3389/fbioe.2023.1138702/full#supplementary-material
References
Buchman, A. B., Ivy, T., Marshall, J. M., Akbari, O. S., and Hay, B. A. (2018). Engineered reciprocal chromosome translocations drive high threshold, reversible population replacement in Drosophila. Drosoph. ACS Synth. Biol. 7 (5), 1359–1370. doi:10.1021/acssynbio.7b00451
Champer, J., Champer, S. E., and Kim, I. K. (2020c). Design and analysis of CRISPR-based underdominance toxin-antidote gene drives. Evol. Appl. 14, 1052–1069. doi:10.1111/eva.13180
Champer, J., Chung, J., Lee, Y. L., Liu, C., Yang, E., Wen, Z., et al. (2019b). Molecular safeguarding of CRISPR gene drive experiments. eLife 8, e41439. doi:10.7554/eLife.41439
Champer, J., Kim, I. K., Champer, S. E., Clark, A. G., and Messer, P. W. (2020d). Performance analysis of novel toxin-antidote CRISPR gene drive systems. BMC Biol. 18 (1), 27. doi:10.1186/s12915-020-0761-2
Champer, J., Lee, E., Yang, E., Liu, C., Clark, A. G., and Messer, P. W. (2020b). A toxin-antidote CRISPR gene drive system for regional population modification. Nat. Commun. 11 (1), 1082. doi:10.1038/s41467-020-14960-3
Champer, J., Liu, J., Oh, S. Y., Reeves, R., Luthra, A., Oakes, N., et al. (2018). Reducing resistance allele formation in CRISPR gene drive. Proc. Natl. Acad. Sci. 115 (21), 5522–5527. doi:10.1073/pnas.1720354115
Champer, J., Reeves, R., Oh, S. Y., Liu, C., Liu, J., Clark, A. G., et al. (2017). Novel CRISPR/Cas9 gene drive constructs reveal insights into mechanisms of resistance allele formation and drive efficiency in genetically diverse populations. PLoS Genet. 13 (7), e1006796. doi:10.1371/journal.pgen.1006796
Champer, J., Wen, Z., Luthra, A., Reeves, R., Chung, J., Liu, C., et al. (2019a). CRISPR gene drive efficiency and resistance rate is highly heritable with no common genetic loci of large effect. Genetics 212, 333–341. doi:10.1534/genetics.119.302037
Champer, J., Yang, E., Lee, E., Liu, J., Clark, A. G., and Messer, P. W. (2020a). A CRISPR homing gene drive targeting a haplolethal gene removes resistance alleles and successfully spreads through a cage population. Proc. Natl. Acad. Sci. 117 (39), 24377–24383. doi:10.1073/PNAS.2004373117
Champer, S. E., Oh, S. Y., Liu, C., Wen, Z., Clark, A. G., Messer, P. W., et al. (2020). Computational and experimental performance of CRISPR homing gene drive strategies with multiplexed gRNAs. Sci. Adv. 6 (10), eaaz0525. doi:10.1126/sciadv.aaz0525
Chen, C. H., Huang, H., Ward, C. M., Su, J. T., Schaeffer, L. V., Guo, M., et al. (2007). A synthetic maternal-effect selfish genetic element drives population replacement in Drosophila. Science 316 (5824), 597–600. doi:10.1126/science.1138595
Davis, M. W., and Jorgensen, E. M. (2022). ApE, a plasmid editor: A freely available DNA manipulation and visualization program. Front. Bioinforma. 0, 818619. doi:10.3389/FBINF.2022.818619
Dhole, S., Lloyd, A. L., and Gould, F. (2019). Tethered homing gene drives: A new design for spatially restricted population replacement and suppression. Evol. Appl. 12, 12827. doi:10.1111/eva.12827
DiCarlo, J. E., Chavez, A., Dietz, S. L., Esvelt, K. M., and Church, G. M. (2015). Safeguarding CRISPR-Cas9 gene drives in yeast. Nat. Biotechnol. 33 (12), 1250–1255. doi:10.1038/nbt.3412
Ferreira-Martins, D., Champer, J., McCauley, D. W., Zhang, Z., and Docker, M. F. (2021). Genetic control of invasive sea lamprey in the Great Lakes. J. Gt. Lakes. Res. 47, S764–S775. doi:10.1016/J.JGLR.2021.10.018
Foster, G. G., Whitten, M. J., Prout, T., and Gill, R. (1972). Chromosome rearrangements for the control of insect pests. Science 176 (4037), 875–880. doi:10.1126/science.176.4037.875
Gratz, S. J., Ukken, F. P., Rubinstein, C. D., Thiede, G., Donohue, L. K., Cummings, A. M., et al. (2014). Highly specific and efficient CRISPR/Cas9-catalyzed homology-directed repair in Drosophila. Genetics 196 (4), 961–971. doi:10.1534/genetics.113.160713
Hammond, A., Karlsson, X., Morianou, I., Kyrou, K., Beaghton, A., Gribble, M., et al. (2021). Regulating the expression of gene drives is key to increasing their invasive potential and the mitigation of resistance. PLOS Genet. 17, e1009321. doi:10.1371/journal.pgen.1009321
Hay, B. A., Oberhofer, G., and Guo, M. (2021). Engineering the composition and fate of wild populations with gene drive. Annu. Rev. Entomology 66, 407–434. doi:10.1146/annurev-ento-020117-043154
Kandul, N. P., Liu, J., Bennett, J. B., Marshall, J. M., and Akbari, O. S. (2021). A confinable home-and-rescue gene drive for population modification. eLife 10, e65939. doi:10.7554/elife.65939
Kyrou, K., Hammond, A. M., Galizi, R., Kranjc, N., Burt, A., Beaghton, A. K., et al. (2018). A CRISPR-Cas9 gene drive targeting doublesex causes complete population suppression in caged Anopheles gambiae mosquitoes. Nat. Biotechnol. 36, 1062–1066. doi:10.1038/nbt.4245
Langmüller, A. M., Champer, J., Lapinska, S., Xie, L., Metzloff, M., Champer, S. E., et al. (2022). Fitness effects of CRISPR endonucleases in Drosophila melanogaster populations. eLife 11, e71809. doi:10.7554/ELIFE.71809
Li, J., and Champer, J. (2023). Harnessing Wolbachia cytoplasmic incompatibility alleles for confined gene drive: A modeling study. PLoS Genet. 19, e1010591. doi:10.1371/journal.pgen.1010591
Maselko, M., Feltman, N., Upadhyay, A., Hayward, A., Das, S., Myslicki, N., et al. (2020). Engineering multiple species-like genetic incompatibilities in insects. Nat. Commun. 11 (1), 4468–4477. doi:10.1038/s41467-020-18348-1
Metzloff, M., Yang, E., Dhole, S., Clark, A. G., Messer, P. W., and Champer, J. (2022). Experimental demonstration of tethered gene drive systems for confined population modification or suppression. BMC Biol. 20 (1), 119–213. doi:10.1186/S12915-022-01292-5
Oberhofer, G., Ivy, T., and Hay, B. A. (2018). Behavior of homing endonuclease gene drives targeting genes required for viability or female fertility with multiplexed guide RNAs. Proc. Natl. Acad. Sci. 115 (40), E9343–E9352. doi:10.1073/pnas.1805278115
Oberhofer, G., Ivy, T., and Hay, B. A. (2019). Cleave and Rescue, a novel selfish genetic element and general strategy for gene drive. Proc. Natl. Acad. Sci. 116, 201816928. doi:10.1073/pnas.1816928116
Oberhofer, G., Ivy, T., and Hay, B. A. (2020). Gene drive and resilience through renewal with next generation Cleave and Rescue selfish genetic elements. Proc. Natl. Acad. Sci. U. S. A. 117 (16), 9013–9021. doi:10.1073/pnas.1921698117
Oberhofer, G., Ivy, T., and Hay, B. A. (2021a). Gene drive that results in addiction to a temperature-sensitive version of an essential gene triggers population collapse in Drosophila. Proc. Natl. Acad. Sci. U. S. A. 118 (49). doi:10.1073/PNAS.2107413118/SUPPL_FILE/PNAS.2107413118.SD01.XLSX
Oberhofer, G., Ivy, T., and Hay, B. A. (2021b). Split versions of Cleave and Rescue selfish genetic elements for measured self limiting gene drive. PLOS Genet 17, e1009385. doi:10.1371/journal.pgen.1009385
Pan, M., and Champer, J. (2022). Making waves: Comparative analysis of gene drive spread characteristics in a continuous space model. bioRxiv. doi:10.1101/2022.11.01.514650
Reeves, R. G., Bryk, J., Altrock, P. M., Denton, J. A., and Reed, F. A. (2014). First steps towards underdominant genetic transformation of insect populations. PLoS One 9 (5), e97557. doi:10.1371/journal.pone.0097557
Scott, M. J., Gould, F., Lorenzen, M., Grubbs, N., Edwards, O., and O’Brochta, D. (2018). Agricultural production: Assessment of the potential use of cas9-mediated gene drive systems for agricultural pest control. J. Responsible Innovation 5 (1), S98–S120. doi:10.1080/23299460.2017.1410343
Teem, J. L., Alphey, L., Descamps, S., Edgington, M. P., Edwards, O., Gemmell, N., et al. (2020). Genetic biocontrol for invasive species. Front. Bioeng. Biotechnol. 8, 452. doi:10.3389/fbioe.2020.00452
Terradas, G., Buchman, A. B., Bennett, J. B., Shriner, I., Marshall, J. M., Akbari, O. S., et al. (2021). Inherently confinable split-drive systems in Drosophila. Nat. Commun. 12 (1), 1480–1512. doi:10.1038/s41467-021-21771-7
Verkuijl, S. A. N., Ang, J. X. D., Alphey, L., Bonsall, M. B., and Anderson, M. A. E. (2022). The challenges in developing efficient and robust synthetic homing endonuclease gene drives. Front. Bioeng. Biotechnol. 0, 856981. doi:10.3389/FBIOE.2022.856981
Wang, G.-H., Du, J., Chu, C. Y., Madhav, M., Hughes, G. L., and Champer, J. (2022). Symbionts and gene drive: Two strategies to combat vector-borne disease. Trends Genet. 37 (7), 708–723. doi:10.1016/J.TIG.2022.02.013
Webster, S. H., Vella, M. R., and Scott, M. J. (2020). Development and testing of a novel killer–rescue self-limiting gene drive system in Drosophila melanogaster. Proc. R. Soc. B Biol. Sci. 287, 20192994. doi:10.1098/rspb.2019.2994
Yang, E., Metzloff, M., Langmuller, A. M., Xu, X., Clark, A. G., Messer, P. W., et al. (2022). A homing suppression gene drive with multiplexed gRNAs maintains high drive conversion efficiency and avoids functional resistance alleles. G3 Genes|Genomes|Genetics 12, jkac081. doi:10.1093/G3JOURNAL/JKAC081
Zhang, S., and Champer, J. (2022). Performance characteristics allow for confinement of a CRISPR toxin-antidote gene drive designed for population suppression. bioRxiv. doi:10.1101/2022.12.13.520356
Keywords: gene drive, CRISPR toxin-antidote, haplolethal gene, rescue element, multiplexed gRNAs, homology-directed repair, confined drive, population suppression
Citation: Chen J, Xu X and Champer J (2023) Assessment of distant-site rescue elements for CRISPR toxin-antidote gene drives. Front. Bioeng. Biotechnol. 11:1138702. doi: 10.3389/fbioe.2023.1138702
Received: 06 January 2023; Accepted: 03 February 2023;
Published: 13 February 2023.
Edited by:
Cheemeng Tan, University of California, Davis, United StatesReviewed by:
Robert P. Smith, Nova Southeastern University, United StatesPeng-Fei Xia, Shandong University, China
Copyright © 2023 Chen, Xu and Champer. This is an open-access article distributed under the terms of the Creative Commons Attribution License (CC BY). The use, distribution or reproduction in other forums is permitted, provided the original author(s) and the copyright owner(s) are credited and that the original publication in this journal is cited, in accordance with accepted academic practice. No use, distribution or reproduction is permitted which does not comply with these terms.
*Correspondence: Jackson Champer, amNoYW1wZXJAcGt1LmVkdS5jbg==