- 1Institute of Agrobiological Sciences, National Agriculture and Food Research Organization (NARO), Tsukuba, Ibaraki, Japan
- 2Laboratory of Systems Microbiology, Data Science Center, Nara Institute of Science and Technology, Ikoma, Nara, Japan
Genetic instability of synthetic genetic devices is a key obstacle for practical use. This problem is particularly critical in kill-switches for conditional host killing. Here, we propose a genetically stable kill-switch based on a “demon and angel” expression construct of a toxic essential gene. The kill-switch conditionally overexpresses the toxic essential gene. Additionally, the identical essential gene is deleted in the genome. The essential gene is expressed at a low level to maintain host survival in the OFF state and kills the host by the overexpression in the ON state. The single expression construct is responsible for both killing the hosts and maintaining viability, reducing the emergence of loss-of-function mutants. We constructed the kill-switch using the toxic essential gene encoding tyrosyl-tRNA synthetase, tyrS, in Escherichia coli. The bacteria harboring the kill-switch were conditionally suicidal over 300 generations. Toxic overexpression of essential genes has also been found in other organisms, suggesting that the “demon and angel” kill switch is scalable to various organisms.
1 Introduction
Synthetic gene circuits for bioengineering purposes are usually not essential for host survival, but rather a burden (Boo et al., 2019; Son et al., 2021; Kumar and Hasty, 2023). Therefore, the synthetic gene circuits are genetically unstable since loss-of-function mutants have a higher fitness. This problem is a critical obstacle to the practical application of synthetic biology. Kill-switch is a genetic device that conditionally kills host cells (Moe-Behrens et al., 2013). The kill-switch is used as a core component in biological containment and density control systems (Kato, 2015; Chan et al., 2016; Kumar and Hasty, 2023). The application of genetically engineered microorganisms with biological containment in the open environments, such as live vaccines and on-site bioremediation, is considered revolutionary in the bioindustry (Piraner et al., 2017; Ishikawa et al., 2021; Sebesta et al., 2022; Nagasawa et al., 2024). In addition, highly efficient microbial production with precise growth control, particularly in multi-cell systems, is also a useful application (Miano et al., 2020; Kato, 2021). Because the kill-switches contain toxic genes, they harm the cells even in the OFF-state by leakage toxic expression and are therefore particularly prone to genetic instability, allowing loss-of-function mutants to emerge and predominate through passaging (Stirling et al., 2017). To construct a useful kill-switch, it is essential to resolve the genetic instability.
To date, several methods have been proposed to reduce the genetic instability of kill-switches. Strategies fall into two major categories. One is to reduce the frequency of mutations that cause loss-of-function. Methods include removal of transposable elements and IS elements (Umenhoffer et al., 2010; Suárez et al., 2017; Geng et al., 2019), deletion of mutagenic genes (Deatherage et al., 2018), exclusion of repetitive sequences (Hossain et al., 2020) and hosting of reduced genome cells (Chan et al., 2016). Maintenance using toxin-antitoxin systems (Fedorec et al., 2019), mobile plasmids (Lopatkin et al., 2017) and integration into the genome (Mandell et al., 2015) have been effective in preventing the loss of kill-switches encoded on plasmids. Removal of mutants by periodic renewal of the cell population is also a promising solution (Liao et al., 2019). The other is eliminating the fitness advantage of loss-of-function mutants. Minimizing unintended toxicity in the OFF state mitigates the reduced fitness of cells harboring the kill-switch (Stirling et al., 2017; Rottinghaus et al., 2022). Linking the functional expression of essential genes to kill-switches can reduce the fitness of mutants (Yang et al., 2013; Blazejewski et al., 2019). These methods assume that the emergence of loss-of-function mutations in kill-switches is inevitable and attempt only to minimize the impact of the mutations.
Here, we propose a novel principle to construct a genetically stable kill-switch, that is theoretically intolerant to mutations, using a “demon and angel” essential gene expression construct (Figure 1). In the bacterium Escherichia coli, we cloned the all ORFs of protein-coding genes in the ASKA plasmid library (Kitagawa et al., 2005). The cloned ORF can be overexpressed in the presence of IPTG by the coliphage T5 promoter with lacO operators (Bujard et al., 1987). Interestingly, overexpression of the ORFs was often toxic to the host (Kitagawa et al., 2005). These toxic ORFs include many essential genes. To construct the kill-switch, a plasmid conditionally overexpressing the toxic essential gene is transformed into the host. The ASKA plasmids meet this requirement. Meanwhile, the identical essential gene is disrupted in the genome. Therefore, the resulting bacteria must supply the essential gene product from the ASKA plasmid. The overexpression is suppressed in the absence of IPTG (OFF state), but a small amount of product is produced due to the leakage expression (Lanzer and Bujard, 1988), maintaining the bacteria in survival and growth. However, in the presence of IPTG (ON state), the essential gene is overexpressed and kills the host bacteria. In this kill-switch, a single expression construct provides not only the host-killing toxin but also the essential gene product for survival, predicting that loss-of-function mutations are largely prevented. In addition, leakage expression of toxins, which is a cause of genetic instability in conventional kill switches, is not a problem because such low-level expression is essential for survival, not poisonous. In this study, we demonstrate the principle of the “demon and angel” kill-switch using the ASKA plasmids in E. coli.
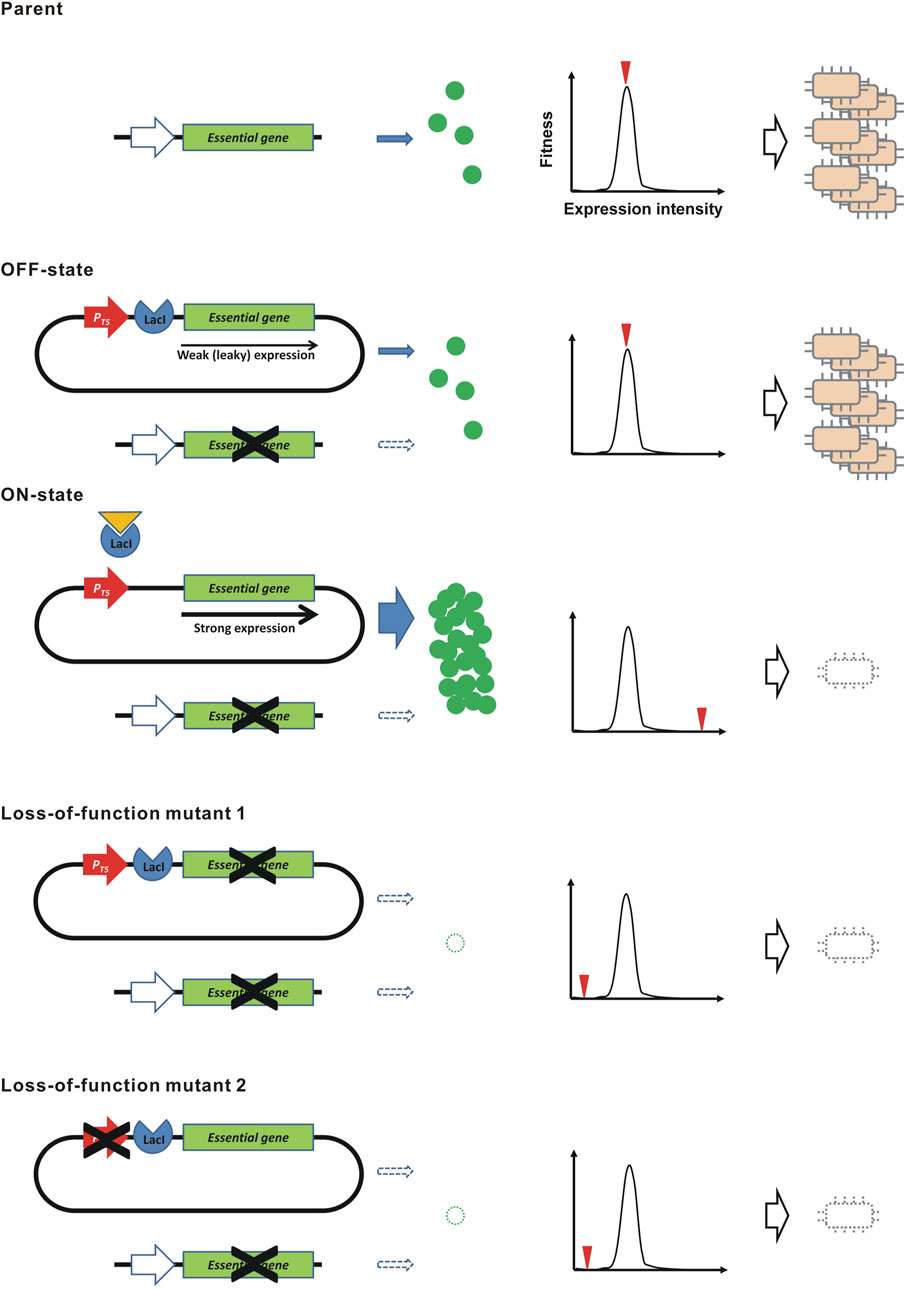
FIGURE 1. Kill-switch using the “demon and angel” expression construct of an essential gene. A schematic diagram is shown. PT5, the coliphage T5 promoter. The genes shown at the top and the bottom of each construct are the original essential genes in the genome and the synthetic overexpression constructs in the plasmid, respectively. The graph shows the relation between the expression level of the essential gene and the level of fitness. The red triangle represents the expression level by the indicated construct.
2 Materials and methods
2.1 Bacterial strains
E. coli K-12, strain AG1 [recA1 endA1 gyrA96 thi-1 hsdR17 (rKmKþ) supE44 relA1] harboring ASKA+ and ASKA-plasmid libraries, W3110, and W3110 ts 29-13 were obtained from the National BioResource Project (NIG, Japan): E. coli (https://shigen.nig.ac.jp/ecoli/strain/). BL21-AI [F−ompT hsdSB (rB− mB−)gal dcm araB::T7RNAP-tetA] was purchased commercially (ThermoFisher Scientific, MA, United States).
2.2 Bacterial culture and transformation
LB medium (1% BactoTriptone, 0.5% Bacto Yeast Extract, 1% NaCl) was used to culture all E. coli strains. Antibiotics (chloramphenicol 50 mg/L, kanamycin 25 mg/L, and tetracycline 1.25 mg/L) were added as needed. Liquid culture was performed in an air incubator with shaking (180 rpm). To prepare solid media, 2% agar was added. Bacteria were incubated at 37°C unless otherwise noted. Bacterial transformation was performed by electroporation using a Gene Pulser II™ electroporator (Bio-Rad Laboratories, CA, United States).
2.3 Evaluation for toxic overexpression
The procedure for preliminary toxicity and GFP-fluorescence evaluation for all ORFs using ASKA+ plasmid library has been described previously (Kitagawa et al., 2005). For quantitative assays, overnight cultures of E. coli harboring the ASKA-plasmid encoding the tested ORFs were used. The cultures diluted 10 to 103-fold were inoculated 250 μL onto solid medium containing 1 mM IPTG and chloramphenicol, a selection marker for ASKA-, and surviving colonies were counted. The total number of bacteria was determined by inoculating 106-fold diluted cultures onto a solid medium without IPTG. The toxicity due to overexpression was assessed by the survivor frequency (survivors/total CFU).
2.4 Preparation of BL21-AI [tyrS−; ASKA-(tyrS)]
The ASKA-clone encoding tyrS was isolated from AG1 carrying this plasmid using the Qiagen Plasmid Midi (Qiagen Inc., CA, United States) and transformed into BL21-AI. The tyrS in the genome of the resulting BL21-AI [tyrS+; ASKA-(tyrS)] strain was substituted with a knock-out cassette containing the neomycin resistance gene using λRed recombinase (Datsenko and Wanner, 2000). Among individual colonies resistant to both chloramphenicol and kanamycin, clones with successful substitution were identified by PCR using the genomic DNA as a template. Finally, the deletion of tyrS was directly confirmed by sequencing the tyrS locus using the Illumina sequencing, as detailed below.
2.5 Passage test
Plasmid loss was tested for BL21-AI [tyrS-; ASKA-(tyrS)] and the control parent strain, BL21-AI [tyrS+; ASKA-(tyrS)]. Every 24 h, the bacteria were diluted 103-fold (1 μL/1 mL fresh LB medium without selection marker antibiotics) and incubated again at 25°C. This dilution and reculturing was repeated 12 times, approximately 120 generations. On inoculation into a new medium, an aliquot of the culture was withdrawn, diluted 106-fold, and inoculated (250 μL) onto solid medium. Sixty-four colonies were selected and inoculated on solid medium containing chloramphenicol to determine plasmid maintenance.
To verify genetic stability, bacteria were cultured in the presence of chloramphenicol and kanamycin which prevent contamination by non-tested bacteria during the 1 month long serial cultures. Dilution and reculturing procedures were identical to those used in the plasmid loss test. On inoculation for reculturing, an aliquot of the cultures was withdrawn, diluted 10-fold, and inoculated (250 μL) onto solid medium containing 5 mM IPTG. Surviving colonies were counted after overnight incubation. The total number of bacteria was also measured by inoculating 106-fold diluted cultures on IPTG-free solid medium.
2.6 Genome sequencing
The procedure for genome sequencing has been described previously (Kato, 2023). Briefly, total DNA, including genomic and plasmid DNAs, was extracted using the DNeasy Blood and Tissue Kit (Qiagen). After checking the integrity of the extracted DNA, sequencing libraries were prepared using a TruSeq DNA PCR-free Sample Preparation Kit (Illumina, Inc., San Diego, CA, United States). The purified libraries were subjected to paired-end (2 × 150 bp) sequencing by Macrogen using the NovaSeq6000 platform. Raw reads were trimmed to remove adapter sequences, mapped to the E. coli BL21-AI (NZ_CP047231.1) and ASKA-(tyrS) reference sequence using BWA-MEM v0.7.17. Variants (SNPs and small INDELs) were identified using Samtools. Variant functional annotation was performed with SnpEff v.5.0e (https://pcingola.github.io/SnpEff/).
2.7 RNA-seq
Bacterial samples were homogenized using a Multi-beads Shocker® (Yasuikikai, Osaka, Japan) at 1,500 rpm for 2 min. Total RNA was extracted using the RNeasy Mini Kit and the QIAcube Connect Extraction System (Qiagen). The concentration of the total RNA samples was determined using the Synergy LX multimode reader (BioTek, VT, United States) and the QuantiFluor RNA System (Promega, WI, United States). The RNA quality was evaluated using the 5,200 Fragment Analyzer System and the Agilent HS RNA Kit (Agilent Technologies, Inc., CA, United States). After the ribosomal RNA depletion with the RiboPOOL kit (siTOOLs Biotech GmbH, Planegg, Germany), the cDNA libraries were prepared using the MGIEasy RNA Directional Library Prep Kit (MGI-Tech, Shenzhen, China). The concentration of libraries was determined as described above. A library quality check was performed using the dsDNA 915 reagent kit (Agilent Technologies) and the Fragment Analyzer. Circularized DNA and DNA nanoballs were prepared using the MGIEasy circularization kit and the DNBSEQ-G400RS high-throughput sequencing kit (MGI-Tech). The DNBs were sequenced using the DNBSEQ-G400 (MGI-Tech). The cutadapt (ver. 4.0) and sickle tools (ver. 1.33) were used to remove adapter sequences and low-quality sequence reads. The resulting reads were mapped using Bowtie 2 (ver. 2.5.0) to the E. coli BL21-AI genome (CP047231.1) and the plasmid ASKA-(tyrS). The mapped reads were quantified with FeatureCounts (ver.2.0.3). The row counts were normalized using the transcripts-per-million (TPM) method. The expression level was evaluated relative to gltA, a constitutively expressed gene (Denger et al., 2014).
3 Results
3.1 Screening of “toxic” essential genes
The ASKA plasmid is a multi-copy plasmid containing the colE1 origin (Kitagawa et al., 2005). There are two types of ASKA plasmid: ASKA+ in which gfp is fused at the 3’ terminal end of the ORF, and ASKA-which excludes the gfp. We examined growth and GFP expression of E. coli AG1 harboring ASKA+ plasmids in overexpression induction with 1 mM IPTG (Supplementary Table S1). Total 4,269 ORFs were tested. Growth inhibition was observed in 3,301 ORFs (77%), of which 2,149 (50%) were severely inhibited with almost no growth, as previously reported (Kitagawa et al., 2005) (Figure 2). Of the ORFs that severely inhibited growth, 156 were essential genes. This represents 52% of all 300 essential genes. Some of these severely growth inhibitory essential genes did not interfere with GFP expression, suggesting that the host viability is well maintained, i.e., “bacteriostatic,” presumably. By contrast, 112 of the highly growth inhibitory essential genes expressed little or no GFP, indicating a bactericidal or severely damaging effect. Thus, there are more than 102 essential genes that are strongly harmful upon overexpression.
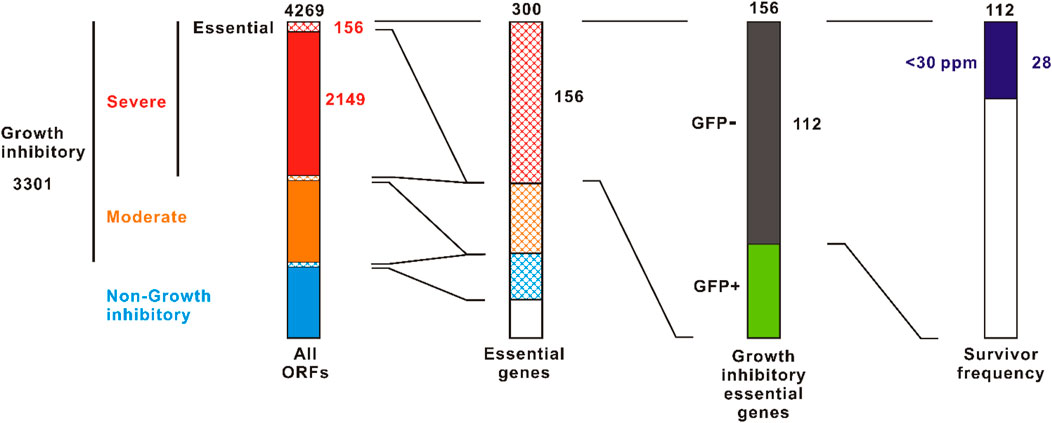
FIGURE 2. Screening for toxic essential genes in the overexpression. The ASKA+ plasmid library containing 4269 E. coli W3110 ORFs was screened. Left column is cited from Kitagawa et al., 2005 with some modifications. Survivor frequencies were evaluated using the ASKA-plasmid library to avoid the effect of GFP-tag. The value indicated at the top of each column is the total number.
For the 112 essential genes that exhibited both strong growth inhibition and suppression of GFP expression, we evaluated the survivor frequency upon overexpression (Supplementary Table S2). To avoid the effects of gfp-tag, the ASKA-plasmids were used. The survivor frequency showed significant variability. For example, with dnaX and tilS, no colonies were detected in the presence of 1 mM IPTG after inoculating around 107 CFU (Supplementary Table S2; Supplementary Figure S2). In contrast, some survivors were detected in other ORFs such as murG and ftsE. There were 28 (25%) ORFs that showed a survival frequency of <30 × 10−6. Of these, 16 were cytoplasmic proteins, 9 were integral membrane proteins, and 3 were membrane anchored proteins (Supplementary Table S2).
3.2 Realization of the “demon and angel” kill-switch
To realize the “demon and angel” kill-switch, we examined which essential gene would be a suitable model. The kill-switch must provide a small amount of functional product by leakage expression in the OFF state to maintain host viability. Membrane proteins are often difficult to express functionally and injure the host (Mathieu et al., 2019). Therefore, we decided to select a candidate essential gene among genes encoding cytoplasmic proteins. We focused on aminoacyl-tRNA synthetase (aaRS) genes. A total of 26 aaRS genes, including 22 essential genes, were found in the ASKA+ library. Of the 24 genes tested, 23 showed toxicity upon overexpression (Supplementary Table S3). Nine genes, pheT, leuS, tyrS, ileS, tilS, proS, argS, glyS and poxA, were highly growth inhibitory with no GFP expression. Among these toxic aaRS genes, we focused on the essential gene encoding the tyrosyl-tRNA synthetase tyrS (Mirande, 1991). The survivor frequency of AG1 [ASKA-(tyrS)] upon overexpression with 1 mM IPTG was only 22.5 × 10−6, indicating potent toxicity (Supplementary Tables S2, S3). After the exposure to 5 mM IPTG for 1 h or less, AG1 [ASKA-(tyrS)] rapidly and irreversibly lost the ability to form colonies, suggesting that the effect of tyrS overexpression is bactericidal and suitable for host-killing (Supplementary Figure S3). The availability of temperature-sensitive mutants and the presumed low impact of genomic gene deletion, as described below, are advantages for the application of tyrS to a proof-of-concept experiment of the kill-switch.
E. coli W3110 ts 29-13 strain is a temperature-sensitive mutant of tyrS (https://shigen.nig.ac.jp/ecoli/strain/locale/change?lang=en). This strain shows normal growth at 30°C but fails to form colonies at 42°C (Supplementary Figure S4A). We transformed the plasmid ASKA-(tyrS) into this strain. The resulting strain, W3110 ts 29-13 [ASKA-(tyrS)], recovered colony formation at 42°C in the absence of IPTG, suggesting that ASKA-(tyrS) can supply TyrS at levels required for host survival and proliferation by leakage expression (Supplementary Figure S4A). No colonies were observed in the presence of 3 mM IPTG at both 30°C and 42°C, suggesting that tyrS overexpression kills the host (Supplementary Figure S4B). These results suggest that the “demon and angel” kill-switch using tyrS is feasible to construct, as we confirmed the rescue of host survival and growth by leakage expression in the OFF state and the host-killing by overexpression in the ON state.
Next, we constructed the kill-switch in a tyrS deletion mutant strain. tyrS is part of the pdxH-tyrS-pdxY operon (Lam and Winkler, 1992; Yang et al., 1998). pdxH and pdxY are non-essential genes, suggesting that disruption of the pdxH-tyrS-pdxY operon by tyrS deletion is unlikely to impair the function of other essential genes (Tramonti et al., 2021). First, we transformed ASKA-(tyrS) into the unmodified BL21-AI to obtain BL21-AI [tyrS+; ASKA-(tyrS)] (Figure 3A) (Bhawsinghka et al., 2020). The ORF of tyrS in the genome was subsequently deleted by substituting the neomycin resistance gene. The deletion was confirmed by genome sequencing. The resulting BL21-AI [tyrS-; ASKA-(tyrS)] strain survived and grew in the absence of IPTG, presumably due to leakage expression of the plasmid tyrS (Figures 3B, C). Meanwhile, BL21-AI [tyrS-; ASKA-(tyrS)] was killed in the presence of IPTG, as well as BL21-AI [tyrS+; ASKA-(tyrS)].
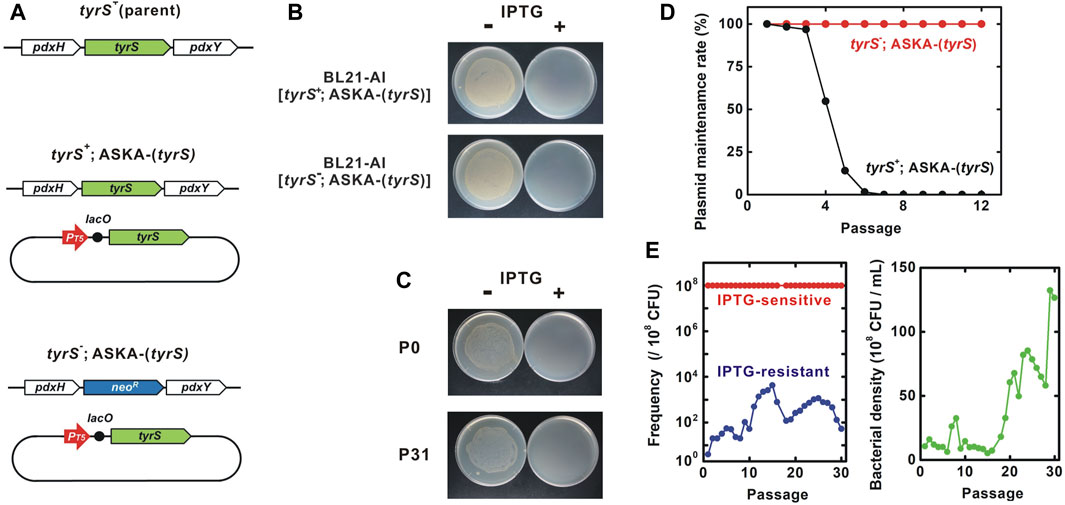
FIGURE 3. Genetic stability of the “demon and angel” kill-switch. (A) Genotype of the tested strains. BL21-AI was used as the parent strain. neoR, neomycin resistance gene. ASKA plasmids contain several other genes including lacIq and cat. (B) IPTG-sensitivity of BL21-AI carrying ASKA-(tyrS). Overnight cultures were diluted 103-fold and inoculated onto LB-agar plates with or without 5 mM IPTG. (C) IPTG-induced host killing. Bacterial viability was tested in the presence and absence of 3 mM IPTG. P0 and P31, the BL21-AI [tyrS-; ASKA-(tyrS)] population after 0 and 31 passages, respectively. (D) Passage test to evaluate the maintenance of the ASKA-plasmid encoding tyrS. Bacterial cultures were diluted 103-fold for each passage. Each data point was obtained from a sample after 24 h of incubation at 25°C. Bacteria harboring the plasmid were identified by their resistance to chloramphenicol, the selection marker for ASKA-. ASKA-(tyrS) plasmid was stably maintained in BL21-AI [tyrS-; ASKA-(tyrS)] for >12 passages whereas BL21-AI [tyrS+; ASKA-(tyrS)] lost the plasmid after six to seven passages. (E) Passage test to evaluate changes in the population frequency and bacterial density. Red and blue, IPTG-sensitive and -resistant bacteria, respectively. Green, bacterial density. Most of the bacteria remained IPTG-sensitive throughout the 30 passages. Survivor frequency showed complex changes. Bacterial density increased around the passage 18.
3.3 Genetic stability of tyrS kill-switch
Passage tests showed that BL21-AI [tyrS+; ASKA-(tyrS)] strain, which retains tyrS in the genome, lost the ASKA plasmid after six to seven passages (60–70 generations) in the absence of plasmid selection marker chloramphenicol (Figure 3D). In contrast, the ASKA plasmid was stably maintained for at least 120 generations in BL21-AI [tyrS-; ASKA-(tyrS)] in which the genomic tyrS was deleted, suggesting that the plasmid tyrS is essential for survival in this strain.
BL21-AI [tyrS-; ASKA-(tyrS)] was killed in the presence of IPTG. In a passage test, the IPTG sensitivity was preserved well through more than 30 passages, equivalent to about 300 generations (Figures 3C, E, left panel). Interestingly, the survivor frequency showed complex changes over the generations, with a range of less than 30 × 10−6. Survivors isolated from passages 14 and 30 (P14R and P30R, respectively) were IPTG-resistant, indicating the emergence of loss-of-function mutants (Figure 4A). Notably, most bacteria (>99.997% at all time points of the 30 passages) maintained the kill-switch function and no rapid replacement with the loss-of-function mutants was observed (Figure 3E, left panel).
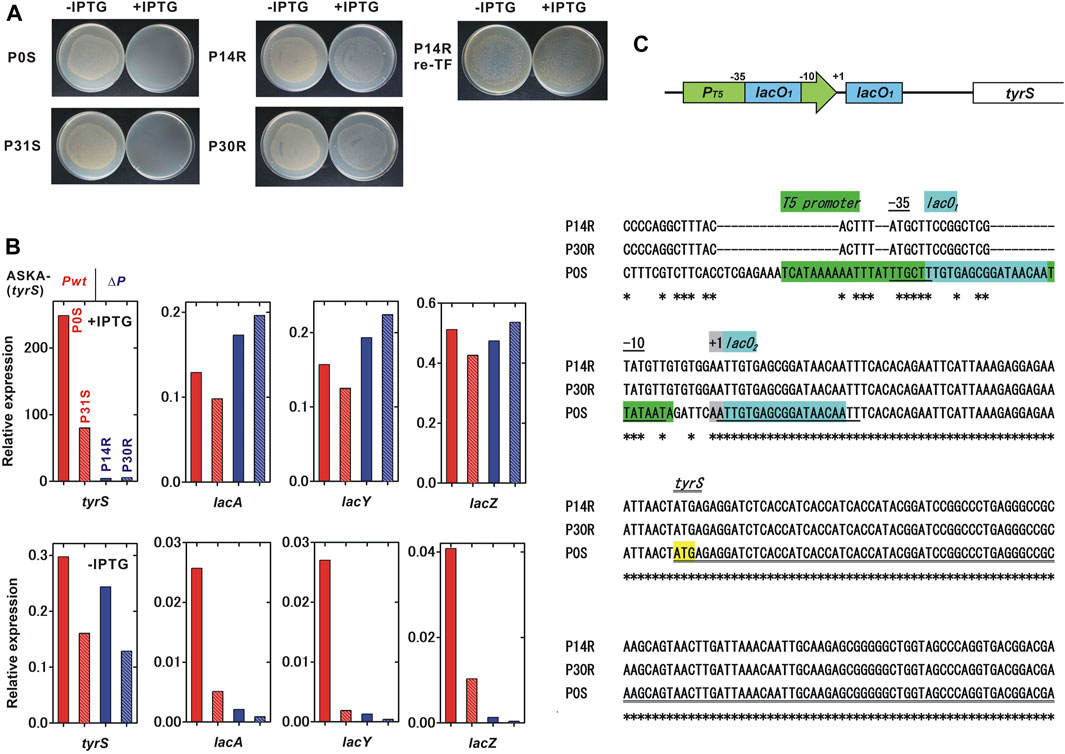
FIGURE 4. Characterization of the IPTG-resistant mutants. We characterized two IPTG-sensitive strains, P0S and P31S, and two IPTG-resistant mutants, P14R and P30R, which were isolated in the passage test shown in Figure 4. (A) IPTG sensitivity of the tested strains. P14R re-TF is the unmodified BL21-AI strain carrying the ASKA-plasmid isolated from P14R. (B) Relative expression of IPTG-inducible genes. A comprehensive analysis of gene expression was performed using RNA-seq. Red, red-striped, blue and blue-striped columns indicate P0S, P31S, P14R and P30R, respectively. The expression level was normalized to that of a constitutively expressed gene, gltA, citrate synthase. The values for each column were obtained from a single trial experiment. (C) Mutations found in the plasmid tyrS regulatory region. A schematic diagram of the tyrS regulatory region in the ASKA-plasmid is shown in the upper panel. The nucleotide sequences of the ASKA-plasmids isolated from P14R, P30R and P0S are aligned and shown in the lower panel. An asterisk indicates a conserved base.
To identify the IPTG-resistant mechanisms, the transcriptomes in the IPTG-sensitive strains isolated from passage 0 and 31, P0S and P31S, and the IPTG-resistant strains, P14R and P30R, were analyzed using RNA-seq. We found that the induction of the IPTG-regulated tyrS on the ASKA plasmid remarkably decreased in the IPTG-resistant strains although the IPTG-inducibility still remained partial (Figure 4B). The tyrS induction ratio was 836.3, 499.1, 18.1 and 42.8, for P0S, P31S, P14R and P30R, respectively. Meanwhile, the basal expression of the plasmid tyrS was similar in both the IPTG-sensitive and resistant strains. Interestingly, the IPTG-induced expression level of the native IPTG-inducible genes in the genome, lacA, lacY and lacZ, was comparable between IPTG-sensitive and resistant strains. The basal expression of those genomic genes remarkably decreased in P14R and P30R although the expression of lacI, that negatively regulates the expression of the IPTG-inducible genes, was not clearly affected (Supplementary Figure S5).
Next, we sequenced the total DNAs containing both genomic and plasmid DNA isolated from P0S, P14R and P30R, using Illumina sequencing. Severe mutations were found in the regulatory region of tyrS in the ASKA plasmid in P14R and P30R whose sequences were completely identical (Figure 4C). The coliphage T5 promoter region was largely mutated, but the −10 and −35 regions were relatively conserved. Although lacO1 sequence embedded in the promoter was almost destroyed, lacO2 located between the promoter and the translation initiation site was completely conserved. The plasmid lacI was intact. Some additional genomic mutations were also detected in P14R and P30R (Supplementary Table S4). Finally, the ASKA plasmid was extracted from P14R and transformed into unmodified BL21-AI. The resulting strain was IPTG-resistant, suggesting that the mutations on the plasmid are sufficient to cause loss of the kill-switch function (Figure 4A).
Notably, the bacterial density clearly started to increase from around the passage 18 and grew to the passage 29-30 (Figure 3E, right panel). The growth rate of P31S (doubling time = 30.3 min) was greater than those of P0S (41.5 min) and P30R (33.3 min).
4 Discussion
In this paper, we propose a novel principle for constructing kill-switches with high genetic stability. The kill-switch is based on a “demon and angel” essential gene expression construct on which both viability maintenance and host-killing depend. We constructed the kill-switch in E. coli using tyrS as a model. The viability maintenance essentiality in the OFF state and the host-killing in the ON state were maintained at least for 120 and 300 generations, respectively. Genes that are toxic upon overexpression have been reported not only in bacteria but also in various unicellular and multicellular eukaryotes (Freeman et al., 1995; Ben-Asouli et al., 2002; Sopko et al., 2006; Jiang et al., 2013). The application of the “demon and angel” kill switch should be scalable to various organisms.
CAMEOS has been proposed previously to ensure genetic stability of kill-switches by entangling the functional expression of essential and toxin genes by sequence overlapping (Blazejewski et al., 2019). However, some point mutations affect only the function of toxin and not that of the entangled essential gene. Use of bidirectional promoters to synchronize the expression of essential and toxin genes has been also reported, but similar limitation is also found (Yang et al., 2013). In the “demon and angel” kill switch, the toxin gene and the essential gene are completely identical involving the protein-coding and regulatory regions, so a mutation that affects the function of one should theoretically always affect the other, indicating the fundamental difference from the methods based on sequence overlapping.
Of the 300 essential genes in E. coli, 112 genes were associated with severely damaged phenotypes of massive growth inhibition and no GPF expression, suggesting that the essential genes with toxic overexpression phenotype are abundant. These toxic essential genes are candidate genetic parts to construct the “demon and angel” kill-switches.
Focusing on 24 aaRS genes, 23 genes were toxic, of which 9 genes exhibited the severely damaged phenotypes. The proper balance of the aaRS and the cognate tRNAs is critical in maintaining the fidelity of protein biosynthesis (Swanson et al., 1988). The production of many aaRSs is regulated via various negative autoregulatory mechanisms (Parker et al., 2020). The toxic-overexpression of some aaRS genes, including tyrS, have been previously reported (Bedouelle et al., 1990; Chalker et al., 1994; Skouloubris et al., 2003; Baick et al., 2004). In tyrS, the toxicity is directly correlated to the enzymatic activity (Bedouelle et al., 1990). This correlation is an advantage for use in the “demon and angel” kill-switch because mutations reducing toxicity always reduce essential enzymatic activity. The effect of tyrS overexpression was bactericidal, indicating its suitability for use in kill switches. Interestingly, most natural amino acid misincorporations by misacylated aminoacyl-tRNAs are tolerated (Min et al., 2003; Ruan et al., 2008). The informational disturbance caused by some aaRS overexpression could be more toxic than the misincorporation at the specific codons.
The bacteria carrying the tyrS kill-switch were killed in the presence of IPTG. For application to biological containment, we need to construct a kill-switch that causes the bacteria to be suicidal in the absence of a specific essential chemical or physical factor. Most simply, such a kill-switch could be constructed by substituting the lac system with a ligand-activated transcriptional repressor such as the Tet-off system (Schulte et al., 2019). In addition, a multilayered system containing some “demon and angel” kill-switches is a possible strategy to enhance genetic stability and reduce the survivor frequency (Torres et al., 2003; Gallagher et al., 2015; Nagasawa et al., 2024).
The “demon and angel” kill switch was designed to be highly resistant to point mutations and short insertion/deletion mutations, as described above. Surprisingly, the isolated loss-of-function mutants, P14R and P30R, acquired heavily deleted and substituted sequences in the tyrS regulatory region, suggesting that the large rearrangement of regulatory region is a serious obstacle. The following is an analysis and discussion of the underlying causes.
In the loss-of-function mutants, transcriptome analyses showed a significant decrease in the tyrS expression level upon IPTG induction although the native IPTG-inducible genes were normally upregulated. Re-transformation of the ASKA-(tyrS) plasmid isolated from the mutant reproduced the IPTG-resistant phenotype, indicating that the mutations in the plasmid were the main cause. NGS analyses detected substantial mutations in the regulatory region of the plasmidal tyrS, supporting this observation. In the mutated plasmids, the coliphage T5 promoter region was severely impaired, suggesting that the promoter activity substantially decreased. Additionally, the disruption of lacO1 embedded in the promoter sequence may mitigate the reduction in tyrS leakage expression. The sequence of the plasmidal tyrS regulatory region was completely identical between P14R and P30R, suggesting that P14R or its sister strain acquired further mutations in the genome and evolved into P30R. Based on the above observation, we propose a possible mechanism for the complex change of survivor frequency observed in the passage test. 1) Mutations in the ASKA plasmid reduced the activity of the promoter that controls the expression of tyrS, resulting in the severe decrease of tyrS expression in the ON state. The reduced level of TyrS failed to kill the host, causing the IPTG-resistance in P14R and P30R. 2) The weaker promoter activity and the lacO1 disruption also affected the tyrS leakage expression level. Overall, a similar level of tyrS expression in the OFF state was observed in both IPTG-sensitive and resistant strains, but the loss-of-function mutants had slightly higher fitness. Consequently, the proportion of the mutants gradually increased as the generation progressed. 3) Subsequently, the fraction of IPTG-resistant mutants decreased with the emergence of other mutants, such as P31S that was IPTG-sensitive and more rapidly grew, resulting in an increase in the total bacterial density as the frequency of the fast-growing mutants increased.
The loss-of-function mutants maintained tyrS expression levels in the OFF state, contributing to the higher fitness acquisition. To eliminate the fitness-advantage in the mutants, the expression level of the toxic essential gene should be adjusted to an optimal level in the bacteria harboring the functional kill-switch. This condition can be achieved by selecting and adjusting optimal genetic elements such as promoters, induction systems, RBSs, plasmid replication origins and toxic essential genes. P31S acquired a higher fitness, suggesting that evolutionary methods are also effective.
Data availability statement
The RNA-seq data presented in the study are deposited in the DDBJ/ENA/GenBank repository, accession number PRJDB17547.
Author contributions
YK: Conceptualization, Funding acquisition, Investigation, Writing–original draft. HM: Investigation, Resources, Writing–review and editing.
Funding
The author(s) declare financial support was received for the research, authorship, and/or publication of this article. This work was supported by JSPS grant number 20H03158.
Conflict of interest
The authors declare that the research was conducted in the absence of any commercial or financial relationships that could be construed as a potential conflict of interest.
The author(s) declared that they were an editorial board member of Frontiers, at the time of submission. This had no impact on the peer review process and the final decision.
Publisher’s note
All claims expressed in this article are solely those of the authors and do not necessarily represent those of their affiliated organizations, or those of the publisher, the editors and the reviewers. Any product that may be evaluated in this article, or claim that may be made by its manufacturer, is not guaranteed or endorsed by the publisher.
Supplementary material
The Supplementary Material for this article can be found online at: https://www.frontiersin.org/articles/10.3389/fbioe.2024.1365870/full#supplementary-material
References
Baick, J. W., Yoon, J. H., Suk, N., Dieter, S., Kim, S. I., Eom, S. H., et al. (2004). Growth inhibition of Escherichia coli during heterologous expression of Bacillus subtilis glutamyl-tRNA synthetase that catalyzes the formation of mischarged glutamyl-tRNA1Gln. J. Microbiol. 42, 111–116.
Bedouelle, H. U. G. U. E. S., Guez, V. A. L. E. R. I. E., Vidal-Cros, A. N. N. E., and Hermann, M. O. N. I. Q. U. E. (1990). Overproduction of tyrosyl-tRNA synthetase is toxic to Escherichia coli: a genetic analysis. J. Bacteriol. 172, 3940–3945. doi:10.1128/jb.172.7.3940-3945.1990
Ben-Asouli, Y., Banai, Y., Pel-Or, Y., Shir, A., and Kaempfer, R. (2002). Human interferon-γ mRNA autoregulates its translation through a pseudoknot that activates the interferon-inducible protein kinase PKR. Cell. 108, 221–232. doi:10.1016/s0092-8674(02)00616-5
Bhawsinghka, N., Glenn, K. F., and Schaaper, R. M. (2020). Complete genome sequence of Escherichia coli BL21-AI. Microbiol. Resour. Announc. 9, e00009–20. doi:10.1128/mra.00009-20
Blazejewski, T., Ho, H. I., and Wang, H. H. (2019). Synthetic sequence entanglement augments stability and containment of genetic information in cells. Science 365, 595–598. doi:10.1126/science.aav5477
Boo, A., Ellis, T., and Stan, G. B. (2019). Host-aware synthetic biology. Curr. Opin. Syst. Biol. 14, 66–72. doi:10.1016/j.coisb.2019.03.001
Bujard, H., Gentz, R., Lanzer, M., Stueber, D., Mueller, M., Ibrahimi, I., et al. (1987). [26] A T5 promoter-based transcription-translation system for the analysis of proteins in vitro and in vivo. Meth. Enzymol. 155, 416–433. doi:10.1016/0076-6879(87)55028-5
Chalker, A. F., Ward, J. M., Fosberry, A. P., and Hodgson, J. E. (1994). Analysis and toxic overexpression in Escherichia coli of a staphylococcal gene encoding isoleucyl-tRNA synthetase. Gene 141, 103–108. doi:10.1016/0378-1119(94)90135-x
Chan, C. T., Lee, J. W., Cameron, D. E., Bashor, C. J., and Collins, J. J. (2016). Deadman' and 'Passcode' microbial kill switches for bacterial containment. Nat. Chem. Biol. 12, 82–86. doi:10.1038/nchembio.1979
Datsenko, K. A., and Wanner, B. L. (2000). One-step inactivation of chromosomal genes in Escherichia coli K-12 using PCR products. Proc. Natl. Acad. Sci. U.S.A. 97, 6640–6645. doi:10.1073/pnas.120163297
Deatherage, D. E., Leon, D., Rodriguez, Á. E., Omar, S. K., and Barrick, J. E. (2018). Directed evolution of Escherichia coli with lower-than-natural plasmid mutation rates. Nucleic Acids Res. 46, 9236–9250. doi:10.1093/nar/gky751
Denger, K., Weiss, M., Felux, A. K., Schneider, A., Mayer, C., Spiteller, D., et al. (2014). Sulphoglycolysis in Escherichia coli K-12 closes a gap in the biogeochemical sulphur cycle. Nature 507, 114–117. doi:10.1038/nature12947
Fedorec, A. J., Ozdemir, T., Doshi, A., Ho, Y. K., Rosa, L., Rutter, J., et al. (2019). Two new plasmid post-segregational killing mechanisms for the implementation of synthetic gene networks in Escherichia coli. iScience 14, 323–334. doi:10.1016/j.isci.2019.03.019
Freeman, K., Gwadz, M., and Shore, D. (1995). Molecular and genetic analysis of the toxic effect of RAP1 overexpression in yeast. Genetics 141, 1253–1262. doi:10.1093/genetics/141.4.1253
Gallagher, R. R., Patel, J. R., Interiano, A. L., Rovner, A. J., and Isaacs, F. J. (2015). Multilayered genetic safeguards limit growth of microorganisms to defined environments. Nucl. acids Res. 43, 1945–1954. doi:10.1093/nar/gku1378
Geng, P., Leonard, S. P., Mishler, D. M., and Barrick, J. E. (2019). Synthetic genome defenses against selfish DNA elements stabilize engineered bacteria against evolutionary failure. ACS Synth. Biol. 8 (3), 521–531. doi:10.1021/acssynbio.8b00426
Hossain, A., Lopez, E., Halper, S. M., Cetnar, D. P., Reis, A. C., Strickland, D., et al. (2020). Automated design of thousands of nonrepetitive parts for engineering stable genetic systems. Nat. Biotechnol. 38, 1466–1475. doi:10.1038/s41587-020-0584-2
Ishikawa, M., Kojima, T., and Hori, K. (2021). Development of a biocontained toluene-degrading bacterium for environmental protection. Microbiol. Spectr. 9, e0025921–21. doi:10.1128/spectrum.00259-21
Jiang, J., Jing, Y., Cost, G. J., Chiang, J. C., Kolpa, H. J., Cotton, A. M., et al. (2013). Translating dosage compensation to trisomy 21. Nature 500, 296–300. doi:10.1038/nature12394
Kato, Y. (2015). An engineered bacterium auxotrophic for an unnatural amino acid: a novel biological containment system. PeerJ 3, e1247. doi:10.7717/peerj.1247
Kato, Y. (2021). An unnatural amino acid-regulated growth controller based on informational disturbance. Life 11, 920. doi:10.3390/life11090920
Kato, Y. (2023). A strategy for addicting transgene-free bacteria to synthetic modified metabolites. Front. Microbiol. 14, 1086094. doi:10.3389/fmicb.2023.1086094
Kitagawa, M., Ara, T., Arifuzzaman, M., Ioka-Nakamichi, T., Inamoto, E., Toyonaga, H., et al. (2005). Complete set of ORF clones of Escherichia coli ASKA library (A complete set of E. coli K-12 ORF archive): unique Resources for biological research. DNA Res. 12, 291–299. doi:10.1093/dnares/dsi012
Kumar, S., and Hasty, J. (2023). Stability, robustness, and containment: preparing synthetic biology for real-world deployment. Curr. Opin. Biotechnol. 79, 102880. doi:10.1016/j.copbio.2022.102880
Lam, H. M., and Winkler, M. E. (1992). Characterization of the complex pdxH-tyrS operon of Escherichia coli K-12 and pleiotropic phenotypes caused by pdxH insertion mutations. J. Bacteriol. 174, 6033–6045. doi:10.1128/jb.174.19.6033-6045.1992
Lanzer, M., and Bujard, H. (1988). Promoters largely determine the efficiency of repressor action. Proc. Natl. Acad. Sci. U.S.A. 85, 8973–8977. doi:10.1073/pnas.85.23.8973
Liao, M. J., Din, M. O., Tsimring, L., and Hasty, J. (2019). Rock-paper-scissors: engineered population dynamics increase genetic stability. Science 365, 1045–1049. doi:10.1126/science.aaw0542
Lopatkin, A. J., Meredith, H. R., Srimani, J. K., Pfeiffer, C., Durrett, R., and You, L. (2017). Persistence and reversal of plasmid-mediated antibiotic resistance. Nat. Commun. 8, 1689. doi:10.1038/s41467-017-01532-1
Mandell, D. J., Lajoie, M. J., Mee, M. T., Takeuchi, R., Kuznetsov, G., Norville, J. E., et al. (2015). Biocontainment of genetically modified organisms by synthetic protein design. Nature 518, 55–60. doi:10.1038/nature14121
Mathieu, K., Javed, W., Vallet, S., Lesterlin, C., Candusso, M. P., Ding, F., et al. (2019). Functionality of membrane proteins overexpressed and purified from E. coli is highly dependent upon the strain. Sci. Rep. 9, 2654–2715. doi:10.1038/s41598-019-39382-0
Miano, A., Liao, M. J., and Hasty, J. (2020). Inducible cell-to-cell signaling for tunable dynamics in microbial communities. Nat. Commun. 11, 1193. doi:10.1038/s41467-020-15056-8
Min, B., Kitabatake, M., Polycarpo, C., Pelaschier, J., Raczniak, G., Ruan, B., et al. (2003). Protein synthesis in Escherichia coli with mischarged tRNA. J. Bacteriol. 185, 3524–3526. doi:10.1128/jb.185.12.3524-3526.2003
Mirande, M. (1991). Aminoacyl-tRNA synthetase family from prokaryotes and eukaryotes: structural domains and their implications. Prog. Nucleic Acid. Res. Mol. Biol. 40, 95–142. doi:10.1016/s0079-6603(08)60840-5
Moe-Behrens, G. H., Davis, R., and Haynes, K. A. (2013). Preparing synthetic biology for the world. Front. Microbiol. 4, 5. doi:10.3389/fmicb.2013.00005
Nagasawa, Y., Nakayama, M., Kato, Y., Ogawa, Y., Aribam, S. D., Tsugami, H., et al. (2024). A novel vaccine strategy using quick and easy conversion of bacterial pathogens to unnatural amino acid-auxotrophic suicide derivatives. Microbiol. Spectr.
Parker, D. J., Lalanne, J.-B., Kimura, S., Johnson, G. E., Waldor, M. K., and Li, G.-W. (2020). Growth-optimized aminoacyl-tRNA synthetase levels prevent maximal tRNA charging. Cell. Syst. 11, 121–130.e6. doi:10.1016/j.cels.2020.07.005
Piraner, D. I., Abedi, M. H., Moser, B. A., Lee-Gosselin, A., and Shapiro, M. G. (2017). Tunable thermal bioswitches for in vivo control of microbial therapeutics. Nat. Chem. Biol. 13, 75–80. doi:10.1038/nchembio.2233
Rottinghaus, A. G., Ferreiro, A., Fishbein, S. R., Dantas, G., and Moon, T. S. (2022). Genetically stable CRISPR-based kill switches for engineered microbes. Nat. Commun. 13, 672. doi:10.1038/s41467-022-28163-5
Ruan, B., Palioura, S., Sabina, J., Marvin-Guy, L., Kochhar, S., LaRossa, R. A., et al. (2008). Quality control despite mistranslation caused by an ambiguous genetic code. Proc. Natl. Acad. Sci. U.S.A. 105, 16502–16507. doi:10.1073/pnas.0809179105
Schulte, M., Sterzenbach, T., Miskiewicz, K., Elpers, L., Hensel, M., and Hansmeier, N. (2019). A versatile remote control system for functional expression of bacterial virulence genes based on the tetA promoter. Int. J. .Med. Microbiol. 309, 54–65. doi:10.1016/j.ijmm.2018.11.001
Sebesta, J., Xiong, W., Guarnieri, M. T., and Yu, J. (2022). Biocontainment of genetically engineered algae. Front. Plant Sci. 13, 839446. doi:10.3389/fpls.2022.839446
Skouloubris, S., de Pouplana, L. R., De Reuse, H., and Hendrickson, T. L. (2003). A noncognate aminoacyl-tRNA synthetase that may resolve a missing link in protein evolution. Proc. Natl. Acad. Sci. U.S.A. 100, 11297–11302. doi:10.1073/pnas.1932482100
Son, H. I., Weiss, A., and You, L. (2021). Design patterns for engineering genetic stability. Curr. Opin. Biomed. Eng. 19, 100297. doi:10.1016/j.cobme.2021.100297
Sopko, R., Huang, D., Preston, N., Chua, G., Papp, B., Kafadar, K., et al. (2006). Mapping pathways and phenotypes by systematic gene overexpression. Mol. Cell. 21, 319–330. doi:10.1016/j.molcel.2005.12.011
Stirling, F., Bitzan, L., O’Keefe, S., Redfield, E., Oliver, J. W., Way, J., et al. (2017). Rational design of evolutionarily stable microbial kill switches. Mol. Cell. 68, 686–697.e3. doi:10.1016/j.molcel.2017.10.033
Suárez, G. A., Renda, B. A., Dasgupta, A., and Barrick, J. E. (2017). Reduced mutation rate and increased transformability of transposon-free Acinetobacter baylyi ADP1-ISx. Appl. Environ. Microbiol. 83, e01025–17. doi:10.1128/aem.01025-17
Swanson, R., Hoben, P., Sumner-Smith, M., Uemura, H., Watson, L., and Söll, D. (1988). Accuracy of in vivo aminoacylation requires proper balance of tRNA and aminoacyl-tRNA synthetase. Science 242, 1548–1551. doi:10.1126/science.3144042
Torres, B., Jaenecke, S., Timmis, K. N., García, J. L., and Díaz, E. (2003). A dual lethal system to enhance containment of recombinant micro-organisms. Microbiology 149, 3595–3601. doi:10.1099/mic.0.26618-0
Tramonti, A., Nardella, C., di Salvo, M. L., Barile, A., D’Alessio, F., de Crécy-Lagard, V., et al. (2021). Knowns and unknowns of vitamin B6 metabolism in Escherichia coli. EcoSal Plus. EcoSal Plus 9, eESP. doi:10.1128/ecosalplus.esp-0004-2021
Umenhoffer, K., Fehér, T., Balikó, G., Ayaydin, F., Pósfai, J., Blattner, F. R., et al. (2010). Reduced evolvability of Escherichia coli MDS42, an IS-less cellular chassis for molecular and synthetic biology applications. Microb. Cell. Factories 9, 38–12. doi:10.1186/1475-2859-9-38
Yang, S., Sleight, S. C., and Sauro, H. M. (2013). Rationally designed bidirectional promoter improves the evolutionary stability of synthetic genetic circuits. Nucleic Acids Res. 41, e33. doi:10.1093/nar/gks972
Yang, Y., Tsui, H. C. T., Man, T. K., and Winkler, M. E. (1998). Identification and function of the pdxY gene, which encodes a novel pyridoxal kinase involved in the salvage pathway of pyridoxal 5′-phosphate biosynthesis in Escherichia coli K-12. J. Bacteriol. 180, 1814–1821. doi:10.1128/jb.180.7.1814-1821.1998
Keywords: genetic stability, biological containment, toxic overexpression, genetic devices, ASKA library
Citation: Kato Y and Mori H (2024) Genetically stable kill-switch using “demon and angel” expression construct of essential genes. Front. Bioeng. Biotechnol. 12:1365870. doi: 10.3389/fbioe.2024.1365870
Received: 05 January 2024; Accepted: 13 February 2024;
Published: 28 February 2024.
Edited by:
Pasquale Stano, University of Salento, ItalyReviewed by:
Mimi Cho Yung, Lawrence Livermore National Security, United StatesDiego Bonatto, Departamento de Biologia Molecular e Biotecnologia da UFRGS, Brazil
R. Adam Thompson, LanzaTech, United States
Copyright © 2024 Kato and Mori. This is an open-access article distributed under the terms of the Creative Commons Attribution License (CC BY). The use, distribution or reproduction in other forums is permitted, provided the original author(s) and the copyright owner(s) are credited and that the original publication in this journal is cited, in accordance with accepted academic practice. No use, distribution or reproduction is permitted which does not comply with these terms.
*Correspondence: Yusuke Kato, a2F0b0BhZmZyYy5nby5qcA==
†Present address: Hirotada Mori, Innovation laboratory of Systems Microbiology and Synthetic Biology, Institute of Animal Science, Guangdong Academy of Agricultural Sciences. Guangzhou, Guangdong, China