- 1Cancer Sciences, Faculty of Medicine, University of Southampton, Southampton, UK
- 2University Surgery, University of Southampton, Southampton General Hospital, Southampton, UK
- 3Department of Experimental Therapeutics, MD Anderson Cancer Center, Houston, TX, USA
It is well established that the tumor microenvironment (TME) contributes to cancer progression. Stromal cells can be divided into mesenchymal, vascular, and immune. Signaling molecules secreted by the tumor corrupts these cells to create “activated” stroma. Equally, the extracellular matrix (ECM) contributes to tumor development and invasion by forming a biologically active scaffold. In this review we describe the key structural, cellular and signaling components of the TME with a perspective on stromal soluble factors and microRNAs (miRNAs).
Introduction
The relationship between tumor and stroma is symbiotic. Stromal cells are corrupted by malignant epithelium, creating a permissive microenvironment which drives cancer progression (Hill et al., 2005). Unlike cancer cells which transform through a series of genetic mutations, stromal cells are mostly genetically intact (Boehm et al., 1997; Allinen et al., 2004). Targeting this component of the tumor microenvironment (TME) should therefore be considered in cancer therapy. There has been an exponential rise in research into this field (reviewed in Witz, 2009). Table 1 summarizes the important studies.
There is a difference between TME and stroma that should be defined at the outset. The stroma is a histological unit consisting of peri-tumoral cells within an extracellular scaffold. The TME is a functional ecosystem of tumor and stromal elements that interact through signaling molecules (Figure 1). This review provides a compositional description of TME components which are relevant to cancer progression. Additionally we have listed the signals provided by the stroma that effect cancer cells, including cell-extracellular matrix interactions, soluble factors such as cyto/chemokines and extracellular vesicles such as exosomes.
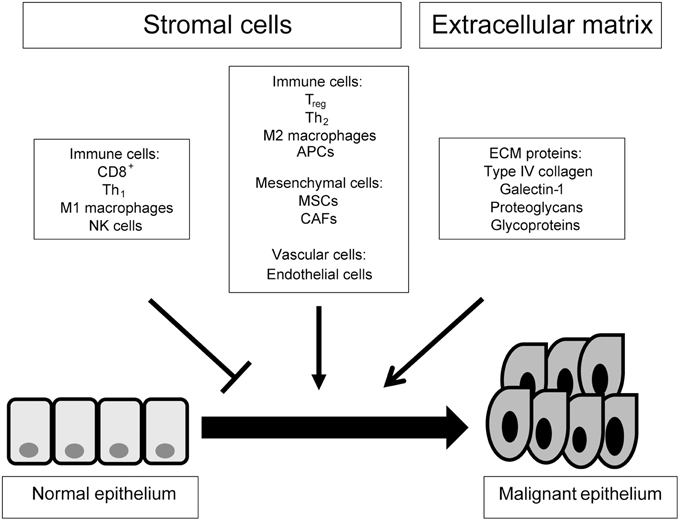
Figure 1. A top down view of the tumor microenvironment. This scheme gives an outline of the cellular and acellular components of the tumor microenvironment and their contribution to tumorigenesis. ECM, extracellular matrix; APC, antigen presenting cell; NK, natural killer; Treg, regulatory T cell; Th, helper T cell; CAF, cancer-associated fibroblast; MSC, mesenchymal stem cell.
Mesenchymal Cells
Cancer-associated Fibroblasts
Cancer-associated fibroblasts (CAFs) are the predominant cell type in the stroma, responsible for the structural architecture of the extracellular matrix (ECM; Kalluri and Zeisberg, 2006). They modulate the ECM by expressing several key proteins such as periostin (Kikuchi et al., 2008) and tenascin-C (De Wever et al., 2004). In normal physiology, α-SMA positive fibroblasts (myofibroblasts) have a contractile function to close a wound. In the cancer setting, myofibroblasts remain persistently activated, facilitating cancer progression (Marsh et al., 2013). Desmoplastic tumors characterized by a dense stromal reaction do not always contain myofibroblasts (Chang et al., 2011), suggesting that not all CAFs are myofibroblasts.
It is not clear how CAFs contribute to tumourigenesis but studies have demonstrated neoplastic transformation in their presence (Hayward et al., 2001). Olumi et al. (1999) showed that when human prostate CAFs were co-cultured with normal prostate epithelial cells, they stimulated rapid epithelial growth and altered histology. Moreover, simulation of CAF signaling by Wnt-1-transfected fibroblasts caused morphological transformation in mammary epithelial cells (Jue et al., 1992). Importantly, secretion of soluble factors such as transforming growth factor-β (TGF-β) and hepatocyte growth factor by stromal fibroblasts was shown to induce malignant transformation (Kuperwasser et al., 2004)
In early cancer, the host tissue is remodeled to accommodate the developing tumor. Microscopically this is characterized by compositional changes and stiffening of the ECM (Bonnans et al., 2014). For this to happen, collagen is cross linked with other ECM molecules such as elastin, a process catalyzed by lysyl oxidase (LOX; Erler et al., 2006). CAFs produce LOX and collagen in sufficient quantities to facilitate this process (Levental et al., 2009). Importantly, LOX inhibitors such as beta-aminopropionitrile and magnolol synergistically reduce migration and invasion of MDA231 breast cancer cells (Chen et al., 2012).
CAFs play an important role in angiogenesis. When CAF-secreted fibroblast growth factor-2 (FGF-2) is inhibited, angiogenesis is reduced (Pietras et al., 2008). Furthermore, brivanib (a dual VEGF/ FGF tyrosine kinase inhibitor) effectively blocks angiogenesis in a pancreatic neuroendocrine tumor model (Allen et al., 2011). This is of particular interest because selective inhibition of VEGF alone with bevacizumab leads to drug resistance (Lieu et al., 2013). Targeting stromal and cancer-driven angiogenic signaling in combination may present a more effective treatment option. Importantly, the angiogenic effects of CAFs are not limited to their local environment. For example, fibroblast expression of stromal cell-derived factor-1 (SDF-1/ CXCL12) acts as a systemic chemotactic signal for circulating immature endothelial cells (ECs), leading to breast cancer vascularization and metastasis (Orimo et al., 2005).
There is growing evidence suggesting that CAFs induce invasiveness and metastatic capability of cancer cells. Epithelial-mesenchymal transition (EMT) is a cellular programme that induces cancer cell metastasis (reviewed in Kalluri and Weinberg, 2009). CAFs promote this transition in multiple cancers (Yu et al., 2014; Zhou et al., 2014). Moreover, there is evidence to suggest that CAFs guide metastatic cells to prime the secondary site for colonization (Xu et al., 2010).
Mesenchymal Stem Cells
Mesenchymal stem cells (MSCs) are defined by their adherence properties, ability to differentiate into different cell types and surface markers (CD73, CD90, and CD105; Dominici et al., 2006). At least 20% of CAFs originate from MSCs and recruitment is dependent on TGF-β and SDF-1 (Quante et al., 2011). CAFs abundantly express these chemotactic signals. Additionally, cancer cells can induce differentiation of MSCs to CAFs. Indeed, the exposure of human MSCs to conditioned media from MDA231 breast cancer cells stimulated expression of myofibroblast markers such as α-SMA (Mishra et al., 2008).
Weinberg and colleagues marked the importance of MSCs for breast cancer metastasis. Once recruited to the TME, stromal MSCs secrete CCL5 (RANTES) and enhance the metastatic capability of breast cancer cells (Karnoub et al., 2007). Similarly, HS-5 human bone marrow stromal cells increased proliferation, migration, and invasion of Huh7 hepatocellular cancer cells. These functional effects were attenuated by knocking down CCL5 (Bai et al., 2014).
Immune Cells
Immune cells in the TME can have pro- or anti-tumor effects. Tumor progression can be stunted or inhibited by immunosurveillance but established tumors and metastases have the ability to modify the TME in order to escape immunity (Vesely et al., 2011). The immune response produced by M1 macrophages, T helper-1 cells, cytotoxic T cells, antigen presenting cells (APCs), and natural killer (NK) cells supports tumor rejection; whereas, M2 macrophages, regulatory T cells, and T helper-2 cells support tumor progression (Verbeke et al., 2011).
Macrophages
Macrophages are phagocytic cells that play a critical role in innate and adaptive immunity. They are classified into pro inflammatory M1 and anti-inflammatory M2 subtypes. M1 macrophages take part in immunosurveillance. Through the release of pro-inflammatory cytokines such as interleukin-1 and tumor necrosis factor alpha (TNF-α), they inhibit tumor progression (Noy and Pollard, 2014). Conversely, M2 macrophages release immunosuppressive cytokines such as interleukin-10 and allow tumor progression (Mantovani et al., 2002; Solinas et al., 2009); hence they are called tumor-associated macrophages (TAMs). TAMs have been shown to facilitate tumourigenesis and tumor progression in colonic (Liu et al., 2011a) and renal cell (Daurkin et al., 2011) carcinomas, respectively. The effects of TAMs are not limited to immune modulation. In melanoma, TAMs promote endothelial recruitment and angiogenesis via the release of adrenomedullin (Chen et al., 2011). In another study, TAMs directly enhanced the invasiveness of SKBR3 breast cancer cells by exporting a certain microRNA (miR-223) in extracellular vesicles (Yang et al., 2011).
T-lymphocytes
CD8+ cytotoxic T cells induce growth arrest, necrosis, and apoptosis in tumor cells by the release of various cytokines including interferon gamma (IFN-γ; Matsushita et al., 2015). The residual protein components of the apoptotic cells are then phagocytosed by APCs and exposed to maturing lymphocytes in lymphoid organs (Chiang et al., 2015). This potentiates tumor suppression. Conversely, regulatory T cells (Tregs) promote immune tolerance by expressing a cytokine profile that attenuates the proliferation of CD8+ cells, inhibits APCs and macrophages and reduces the lytic activity of NK cells (Facciabene et al., 2012). This potentiates tumor progression. Indeed, Tregs have been found in higher numbers in various cancers such as liver (Gao et al., 2007) and breast (Bates et al., 2006). In theory, therapies that increase the proportion of CD8+ cells to Tregs may attenuate tumor progression. For example, lympho-depletion followed by autologous transfusion of tumor-infiltrating CD8+ cells in patients with metastatic melanoma prompted clinical and radiological regression of metastases in 18 of 35 human subjects (Dudley et al., 2005). More recently, the anti-CD25 monoclonal antibody daclizumab has been shown to suppress Tregs and enhance anti-tumor response (Ohmura et al., 2008). In another study, daclizumab depleted CD25-high Tregs and allowed an enhanced IFN-γ-mediated CD4/ CD8+ response in metastatic breast cancer patients (Rech et al., 2012).
There are several classes of CD4+ helper T (Th) cells but Th1 and Th2 are functionally prominent in cancer progression (Knutson and Disis, 2005). Th1 cells are necessary for the activation and persistence of CD8+ cells. Indeed, intravenous injection of antigen-specific Th1 cells induced CD8+ cell-mediated tumor regression in a fibrosarcoma model (Surman et al., 2000). The role of Th2 cells is less clear but in patients with renal carcinoma and melanoma, circulating CD4+ cells display Th2-polarized (IL-5) responses to MAGE-6 epitopes in active disease and Th1-polarized (IFN-γ) responses in remission (Tatsumi et al., 2002). Similarly, CD4+ cells from patients with stage I renal carcinoma showed predominantly Th1-polarized responses to EphA2, whereas CD4+ cells from later stages showed progressively more Th2-polarized responses (Tatsumi et al., 2003). Overall, the presence of Th2 cells marks poor prognosis compared to Th1 cells.
Antigen-presenting Cells
APCs process and display antigens with MHC proteins to naïve T cells. MHC I-expressing cells stimulate CD8+ cells whereas MHC II-expressing cells stimulate CD4+ cells. In general, APCs are classified into professional and non- professional cells. The most important professional APCs are dendritic cells (DCs). Fibroblasts are an example of non-professional APCs which do not constitutively express MHC II but can stimulate T-cells by expressing IFN-γ (Sprent, 1995).
APCs from the TME of rat colonic carcinoma did not stimulate CD8+ cells as well as non-tumor APCs (Chaux et al., 1997). This was attributed to a lack of co-stimulatory factor B7, suggesting that cancer cells make APCs functionally deficient. Human renal and pancreatic cancer cell lines express interleukin-6 and macrophage colony stimulating factor, which alter the differentiation of APCs from CD34+ to CD14+ progenitors. CD14+ cells express little MHC II and cannot evoke a significant immune response, thereby lacking APC function and allowing tumor escape (Menetrier-Caux et al., 1998; Bharadwaj et al., 2007). Furthermore, in the presence of malignant cells, APC progenitors differentiate into immature myeloid-derived suppressors (Almand et al., 2001) and TAMs (Cortez-Retamozo et al., 2012), both of which are immunosuppressive.
Natural Killer Cells
NK cells are innate immune cells which are important in halting tumor progression (Eguizabal et al., 2014). NK cells destroy tumor cells in animal models of several human cancers (reviewed in Schiavoni et al., 2013) by detecting cell surface changes such as reduced MHC I (Karre et al., 1986). In an immunocompetent environment, NK cells select out APCs which do not express MHC I sufficiently, thereby maintaining a pool of APCs which are best equipped to present foreign antigens (Moretta, 2002). However, NK-mediated immunity is dampened in the TME by tumor-secreted cytokines such as TGF-β (Wilson et al., 2011). NK cells isolated from triple negative breast cancers exhibited less antibody-mediated cytotoxicity, a phenomenon that can be reversed by addition of the pro-inflammatory IL-2/IL-15 complex (Roberti et al., 2012). In contrast to this, microarray analysis of intra-tumoral NK cells from non-small cell lung cancer patients showed upregulation of pro-cytotoxic genes (NKp44, granzyme-A and -B) compared to extra-tumoral NK cells (Gillard-Bocquet et al., 2013). To explain this, the authors propose that NK cells are activated but functionally exhausted in the tumor setting. Thus the activity, rather than presence, of NK cells suggests good prognosis in cancer patients.
Vasculature
The stromal vasculature is made up of a capillary network of ECs surrounded by pericytes that provide structural and physiological support. It is well established that hypoxia limits tumor progression and that this drives angiogenesis (Folkman, 1971). The pathophysiology of angiogenesis has been extensively reviewed elsewhere (Otrock et al., 2007).
Endothelial Cells
ECs in the TME are microscopically different to regular ECs. They lack a pericyte covering, have leaky tight junctions and exhibit sprouting (Carmeliet and Jain, 2011). Stromal tissue hypoxia triggers the release of VEGF from pericytes, activating VEGF-2 receptors on adjacent ECs. These ECs become “tip” cells and migrate toward hypoxic tissue that has the highest VEGF concentration. ECs lagging behind the “tip” bind to each other through surface ligand-receptor complexes, slowing their own migration and allowing the chain of ECs to lengthen, or sprout (Gerhardt et al., 2003). At reduced oxygen concentration, the expression of hypoxia inducible transcription factors (HIF) -1 and -2 by ECs is upregulated (Wong et al., 2013). HIF-1 is associated with increased proliferation and migration of ECs (Tang et al., 2004), whereas HIF-2 promotes EC maturation and quiescence (Skuli et al., 2009). Deletion of both HIF-1 and -2 suppresses primary tumor invasion but whereas HIF-1 deletion reduces metastasis, HIF-2 deletion increases it (Branco-Price et al., 2012). The evidence suggests that modulation of HIFs and their downstream effects may well be a therapeutic option in parallel to established anti-VEGF treatments.
Extracellular Matrix
ECM constitutes the cellular scaffold of the TME providing structural support to tumor epithelium and stromal cells alike. ECM is produced by mesenchymal cell types including fibroblasts, chondrocytes, and osteoblasts and consists of various components including collagens, galectins, proteoglycans, and glycoproteins (Denys et al., 2009). ECM has the capacity to both initiate and channel signaling cascades within the TME (Hynes, 2009), and through bidirectional interplay with malignant cells, impact upon tumor progression and metastasis (Murphy et al., 1992; Sadej et al., 2008). Furthermore, its biomechanical properties determine to an extent the dynamics of ECM turnover, thus influencing the ability of malignant cells to invade (Egeblad et al., 2010). Equally, ECM may provide a “cancer stem-cell” niche and is implicated in angiogenesis and inflammation pathways which contribute to a pro-metastatic TME. (Reviewed by Lu et al., 2012). In the following section we will highlight the key components of ECM, their roles during disease progression and their clinical relevance in cancer.
Type IV collagen is the major component of the basement membrane (Mariyama et al., 1992), and possibly the most important protein in the ECM, that separates epithelium from stroma. In desmoplastic tumors such as pancreatic carcinoma, type IV collagen, like other ECM proteins, binds to integrin receptors on cancer cells, promoting their survival (Ohlund et al., 2013). Galectin-1 is a carbohydrate binding protein with several important effects on tumor cells namely: adhesion to the ECM, increased migration and stromal immune suppression (Rabinovich, 2005). Proteoglycans such as heparan sulfate maintain the physical connections between different ECM components (Vlodavsky and Friedmann, 2001). Indeed, salivary gland tumors expressing more heparanase are associated with poorer survival (Ben-Izhak et al., 2006). Glycoproteins such as fibronectin and laminin-1 are ligands for β-integrins, cellular proteins which mediate cell-ECM signaling (Hynes, 2002). ECM expression of fibronectin and laminin-1 correlates with poor prognostic features in breast cancer (Ioachim et al., 2002). In a 3-dimensional breast cancer model, inhibition of fibronectin/ α5β 1-integrin binding prompted apoptosis and greater radio-sensitivity (Nam et al., 2010).
The key enzymes regulating ECM turnover are matrix metalloproteinases (MMPs) and tissue inhibitors of metalloproteinases (TIMPs). MMPs are zinc-dependent endopeptidases, capable of degrading almost all ECM proteins. Increased MMP expression is associated with most tumors (McCawley and Matrisian, 2000). Traditionally, it was thought that cancer cells secreted MMPs in order to digest the ECM and permit invasion (Recklies et al., 1980). We now know that MMPs are secreted by both tumor and stromal cells and are important in other aspects of cancer progression such as angiogenesis (Gonzalez-Villasana et al., 2015) and metastasis (Che et al., 2015). TIMPs negatively regulate MMP activity. TIMP-3 is most specific to the ECM (Gu et al., 2014). When breast cancer and ocular melanoma cell lines were transfected with TIMP-3 and injected into nude mice, tumor growth was significantly reduced (Anand-Apte et al., 1996). Methylation of the TIMP-3 gene promoter is the mechanism by which TIMP-3 is inactivated in cancer (Gu et al., 2008). TIMPs are not simply MMP inhibitors. TIMP-3 for example, prevents VEGF from binding to the VEGF-2 receptor, thereby inhibiting angiogenesis (Qi et al., 2003).
New Players in Stroma-Cancer Cell Interaction: MicroRNAs
In recent years it has become evident that stroma tumor interaction is not simply composed of paracrine signaling of soluble factors and cell-matrix adhesion. Lipid membrane bound small vesicles, namely exosomes, are secreted from both cancer and stromal cells and influence gene expression of cells in the vicinity (Valadi et al., 2007). These vesicles deliver their RNA and protein cargo and alter gene expression in the recipient cells. Among their cargo miRNAs stand out as major players because they are relatively stable compared to mRNA and proteins and can therefore accumulate to a level that can exert a stable biological effect (Valadi et al., 2007). MiRNAs are non-coding RNAs that are approximately 20 nucleotides long. They undergo enzymatic activation in the cytoplasm, where they bind to the 3' untranslated region of coding mRNAs to prevent protein translation (reviewed in Mirnezami et al., 2009). MiRNAs regulate a variety of cellular processes such as proliferation, differentiation, and apoptosis (Esquela-Kerscher and Slack, 2006). Aberrant miRNAs fail to properly regulate these processes, leading to malignant transformation (Calin et al., 2002). The association between certain miRNA signatures and certain cancers (Grange et al., 2014; Wang et al., 2014), has fueled great interest in miRNAs as diagnostic and prognostic tumor markers. Additionally, stromal exosomes were shown to induce cancer cell behavior with RNA transfer (Boelens et al., 2014). Below we discuss three important stromal miRNAs that can play a role in cancer progression by different mechanisms.
MiR-21 is an oncomir and associated with aggressive colorectal cancer (CRC; Liu et al., 2011b). Importantly, recent articles robustly proved that CRC cells themselves do not express miR-21; it is produced by stromal fibroblasts (Nielsen et al., 2011; Bullock et al., 2013). It is quite likely that stromal mir-21 is transferred to CRC cells by exosomes. MiR-21 directly targets the tumor suppressor protein PDCD4 and protects cancer cells from apoptosis (Asangani et al., 2008). A secondary miR-21 target is reversion-inducing cysteine- rich protein with Kazal motifs (RECK; Gabriely et al., 2008; Reis et al., 2012). RECK is an MMP inhibitor which prevents degradation of the ECM. MiR-21-transfected fibroblasts express less RECK and more MMP-2, permitting greater invasion by CRC cells (Bullock et al., 2013).
Bronisz et al. (2012) defined an axis between the tumor suppressor protein PTEN, miR-320 and the oncogenic transcription factor ETS2. When the PTEN gene was selectively ablated from mouse mammary fibroblasts, miR-320 expression was reduced and ETS2 expression was increased. This led to activation of an oncogenic secretome from stromal fibroblasts, responsible for promoting tumor angiogenesis and invasion. To corroborate this, microarray analysis of 126 human breast carcinomas showed a significant inverse correlation between miR-320 and ETS2. Thus a stromal microRNA can induce a pro-oncogenic/inflammatory secretome to contribute to cancer progression.
There is an important stromal miRNA locus (miR-212/132 family) which regulates normal breast development (Ucar et al., 2010). When miR-212/132 is deleted, MMP-9 increases and collagen deposition around mammary ducts is altered. This corresponds with peri-ductal TGF-β activation, leading to abnormal ductal outgrowths. It will be of great interest to study whether miR-212/132 is de-regulated in breast cancer stroma by altering the ECM and activating TGF-β.
Conclusion
Taking a systemic approach we have dissected the components of the TME and their relevance to cancer progression. We have described several potential therapeutic targets in the TME besides cancer cells. Perhaps the ubiquity of these targets in normal and malignant tissue is a limiting factor. Nonetheless, a better understanding of the TME will be the key to overcoming this problem.
Author Contributions
AM conceptualized this review. RB and AM decided on the content. RB and AS drafted the work with significant contributions from MB, HS, JP. RG prepared the figure. All authors approve the final version of the manuscript and agree to be accountable for all aspects of the work.
Conflict of Interest Statement
The authors declare that the research was conducted in the absence of any commercial or financial relationships that could be construed as a potential conflict of interest.
Acknowledgments
RB is an NIHR funded Academic Clinical Fellow with project funding from the University of Southampton Research Management Committee.
References
Allen, E., Walters, I. B., and Hanahan, D. (2011). Brivanib, a dual FGF/VEGF inhibitor, is active both first and second line against mouse pancreatic neuroendocrine tumors developing adaptive/evasive resistance to VEGF inhibition. Clin. Cancer Res. 17, 5299–5310. doi: 10.1158/1078-0432.CCR-10-2847
Allinen, M., Beroukhim, R., Cai, L., Brennan, C., Lahti-Domenici, J., Huang, H., et al. (2004). Molecular characterization of the tumor microenvironment in breast cancer. Cancer Cell 6, 17–32 doi: 10.1016/j.ccr.2004.06.010
Almand, B., Clark, J. I., Nikitina, E., van Beynen, J., English, N. R., Knight, S. C., et al. (2001). Increased production of immature myeloid cells in cancer patients: a mechanism of immunosuppression in cancer. J. Immunol. 166, 678–689. doi: 10.4049/jimmunol.166.1.678
Anand-Apte, B., Bao, L., Smith, R., Iwata, K., Olsen, B. R., Zetter B., et al. (1996). A review of tissue inhibitor of metalloproteinases-3 (TIMP-3) and experimental analysis of its effect on primary tumor growth. Biochem. Cell Biol. 74, 853–862. doi: 10.1139/o96-090
Asangani, I. A., Rasheed, S. A., Nikolova, D. A., Leupold, J. H., Colburn, N. H., Post, S., et al. (2008). MicroRNA-21 (miR-21) post-transcriptionally downregulates tumor suppressor Pdcd4 and stimulates invasion, intravasation and metastasis in colorectal cancer. Oncogene 27, 2128–2136. doi: 10.1038/sj.onc.1210856
Bai, H., Weng, Y., Bai, S., Jiang, Y., Li, B., He, F., et al. (2014). CCL5 secreted from bone marrow stromal cells stimulates the migration and invasion of Huh7 hepatocellular carcinoma cells via the PI3K-Akt pathway. Int. J. Oncol. 45, 333–343. doi: 10.3892/ijo.2014.2421
Bates, G. J., Fox, S. B., Han, C., Leek, R. D., Garcia, J. F., Harris, A. L., et al. (2006). Quantification of regulatory T cells enables the identification of high-risk breast cancer patients and those at risk of late relapse. J. Clin. Oncol. 24, 5373–5380. doi: 10.1200/JCO.2006.05.9584
Ben-Izhak, O., Kaplan-Cohen, V., Ilan, N., Gan, S., Vlodavsky, I., and Nagler, R. (2006). Heparanase expression in malignant salivary gland tumors inversely correlates with long-term survival. Neoplasia 8, 879–884. doi: 10.1593/neo.06382
Bharadwaj, U., Li, M., Zhang, R., Chen, C., and Yao, Q. (2007). Elevated interleukin-6 and G-CSF in human pancreatic cancer cell conditioned medium suppress dendritic cell differentiation and activation. Cancer Res. 67, 5479–5488. doi: 10.1158/0008-5472.CAN-06-3963
Boehm, T., Folkman, J., Browder, T., and O'Reilly, M. S. (1997). Antiangiogenic therapy of experimental cancer does not induce acquired drug resistance. Nature 390, 404–407. doi: 10.1038/37126
Boelens, M. C., Wu, T. J., Nabet, B. Y., Xu, B., Qiu, Y., Yoon, T., et al. (2014). Exosome transfer from stromal to breast cancer cells regulates therapy resistance pathways. Cell 23, 499–513. doi: 10.1016/j.cell.2014.09.051
Bonnans, C., Chou, J., and Werb, Z. (2014). Remodelling the extracellular matrix in development and disease. Nat. Rev. Mol. Cell Biol. 15, 786–801. doi: 10.1038/nrm3904
Branco-Price, C., Zhang, N., Schnelle, M., Evans, C., Katschinski, D. M., Liao, D., et al. (2012). Endothelial cell HIF-1alpha and HIF-2alpha differentially regulate metastatic success. Cancer Cell 21, 52–65. doi: 10.1016/j.ccr.2011.11.017
Bronisz, A., Godlewski, J., Wallace, J. A., Merchant, A. S., Nowicki, M. O., Mathsyaraja, H., et al. (2012). Reprogramming of the tumour microenvironment by stromal PTEN-regulated miR-320. Nat. Cell Biol. 14, 159–167. doi: 10.1038/ncb2396
Bullock, M. D., Pickard, K. M., Nielsen, B. S., Sayan, A. E., Jenei, V., Mellone, M., et al. (2013). Pleiotropic actions of miR-21 highlight the critical role of deregulated stromal microRNAs during colorectal cancer progression. Cell Death Dis. 4:e684. doi: 10.1038/cddis.2013.213
Calin, G. A., Dumitru, C. D., Shimizu, M., Bichi, R., Zupo, S., Noch, E., et al. (2002). Frequent deletions and down-regulation of micro- RNA genes miR15 and miR16 at 13q14 in chronic lymphocytic leukemia. Proc. Natl. Acad. Sci. U.S.A. 99, 15524–15529. doi: 10.1073/pnas.242606799
Carmeliet, P., and Jain, R. K. (2011). Principles and mechanisms of vessel normalization for cancer and other angiogenic diseases. Nat. Rev. Drug Discov. 10, 417–427. doi: 10.1038/nrd3455
Chang, K. C., Lu, Y. C., Lin, M. J., Chen, H. Y., and Jin, Y. T. (2011). Desmoplastic tumour-associated stroma versus neural tissue in central nervous system metastasis: effects of different microenvironments on tumour growth. Histopathology 59, 31–39. doi: 10.1111/j.1365-2559.2011.03898.x
Chaux, P., Favre, N., Martin, M., and Martin, F. (1997). Tumor-infiltrating dendritic cells are defective in their antigen-presenting function and inducible B7 expression in rats. Int. J. Cancer 72, 619–624.
Che, Y. L., Luo, S. J., Li, G., Cheng, M., Gao, Y. M., Li, X. M., et al. (2015). The C3G/Rap1 pathway promotes secretion of MMP-2 and MMP-9 and is involved in serous ovarian cancer metastasis. Cancer Lett. 359, 241–249. doi: 10.1016/j.canlet.2015.01.019
Chen, L. C., Tu, S. H., Huang, C. S., Chen, C. S., Ho, C. T., Lin, H. W., et al. (2012). Human breast cancer cell metastasis is attenuated by lysyl oxidase inhibitors through down-regulation of focal adhesion kinase and the paxillin-signaling pathway. Breast Cancer Res. Treat. 134, 989–1004. doi: 10.1007/s10549-012-1986-8
Chen, P., Huang, Y., Bong, R., Ding, Y., Song, N., Wang, X., et al. (2011). Tumor-associated macrophages promote angiogenesis and melanoma growth via adrenomedullin in a paracrine and autocrine manner. Clin. Cancer Res. 17, 7230–7239. doi: 10.1158/1078-0432.CCR-11-1354
Chiang, C. L., Balint, K., Coukos, G., and Kandalaft, L. E. (2015). Potential approaches for more successful dendritic cell-based immunotherapy. Expert Opin. Biol. Ther. 1–14. doi: 10.1517/14712598.2015.1000298
Cortez-Retamozo, V., Etzrodt, M., Newton, A., Rauch, P. J., Chudnovskiy, A., Berger, C., et al. (2012). Origins of tumor-associated macrophages and neutrophils. Proc. Natl. Acad. Sci. U.S.A. 109, 2491–2496. doi: 10.1073/pnas.1113744109
Daurkin, I., Eruslanov, E., Stoffs, T., Perrin, G. Q., Algood, C., Gilbert, S. M., et al. (2011). Tumor-associated macrophages mediate immunosuppression in the renal cancer microenvironment by activating the 15-lipoxygenase-2 pathway. Cancer Res. 71, 6400–6409. doi: 10.1158/0008-5472.CAN-11-1261
Delinassios, J. G., and Kottaridis, S. D. (1984). Interactions between human fibroblasts and HeLa cells in vitro. Biol. Cell 50, 9–16. doi: 10.1111/j.1768-322X.1984.tb00250.x
Denys, H., Braems, G., Lambein, K., Pauwels, P., Hendrix, A., De Boeck, A., et al. (2009). The extracellular matrix regulates cancer progression and therapy response: implications for prognosis and treatment. Curr. Pharm. Des. 15, 1373–1384. doi: 10.2174/138161209787846711
De Wever, O., Nguyen, Q. D., Van Hoorde, L., Bracke, M., Bruyneel, E., Gespach, C., et al. (2004). Tenascin-C and SF/HGF produced by myofibroblasts in vitro provide convergent pro-invasive signals to human colon cancer cells through RhoA and Rac. FASEB J. 18, 1016–1018. doi: 10.1096/fj.03-1110fje
Dominici, M., Le Blanc, K., Mueller, I., Slaper-Cortenbach, I., Marini, F., Krause, D., et al. (2006). Minimal criteria for defining multipotent mesenchymal stromal cells. The International Society for Cellular Therapy position statement. Cytotherapy 8, 315–317. doi: 10.1080/14653240600855905
Dudley, M. E., Wunderlich, J. R., Yang, J. C., Sherry, R. M., Topalian, S. L., Restifo N. P., et al. (2005). Adoptive cell transfer therapy following non-myeloablative but lymphodepleting chemotherapy for the treatment of patients with refractory metastatic melanoma. J. Clin. Oncol. 23, 2346–2357. doi: 10.1200/JCO.2005.00.240
Egeblad, M., Rasch, M. G., and Weaver, V. M. (2010). Dynamic interplay between the collagen scaffold and tumor evolution. Curr. Opin. Cell Biol. 22, 697–706. doi: 10.1016/j.ceb.2010.08.015
Eguizabal, C., Zenarruzabeitia, O., Monge, J., Santos, S., Vesga, M. A., Maruri, N., et al. (2014). Natural killer cells for cancer immunotherapy: pluripotent stem cells-derived NK cells as an immunotherapeutic perspective. Front. Immunol. 5:439. doi: 10.3389/fimmu.2014.00439
Erler, J. T., Bennewith, K. L., Nicolau, M., Dornhofer, N., Kong, C., Le, Q. T., et al. (2006). Lysyl oxidase is essential for hypoxia-induced metastasis. Nature 440, 1222–1226. doi: 10.1038/nature04695
Esquela-Kerscher, A., and Slack, F. J. (2006). Oncomirs - microRNAs with a role in cancer. Nat. Rev. Cancer 6, 259–269. doi: 10.1038/nrc1840
Facciabene, A., Motz, G. T., and Coukos, G. (2012). T-regulatory cells: key players in tumor immune escape and angiogenesis. Cancer Res. 72, 2162–2171. doi: 10.1158/0008-5472.CAN-11-3687
Folkman, J. (1971). Tumor angiogenesis: therapeutic implications. N. Engl. J. Med. 285, 1182–1186. doi: 10.1056/NEJM197111182852108
Folkman, J., Merler, E., Abernathy, C., and Williams, G. (1971). Isolation of a tumor factor responsible for angiogenesis. J. Exp. Med. 133, 275–288. doi: 10.1084/jem.133.2.275
Gabriely, G., Wurdinger, T., Kesari, S., Esau, C. C., Burchard, J., Linsley, P. S., et al. (2008). MicroRNA 21 promotes glioma invasion by targeting matrix metalloproteinase regulators. Mol. Cell. Biol. 28, 5369–5380. doi: 10.1128/MCB.00479-08
Gao, Q., Qiu, S. J., Fan, J., Zhou, J., Wang, X. Y., Xiao, Y. S., et al. (2007). Intratumoral balance of regulatory and cytotoxic T cells is associated with prognosis of hepatocellular carcinoma after resection. J. Clin. Oncol. 25, 2586–2593. doi: 10.1200/JCO.2006.09.4565
Gerhardt, H., Golding, M., Fruttiger, M., Ruhrberg, C., Lundkvist, A., Abramsson, A., et al. (2003). VEGF guides angiogenic sprouting utilizing endothelial tip cell filopodia. J. Cell Biol. 161, 1163–1177. doi: 10.1083/jcb.200302047
Gillard-Bocquet, M., Caer, C., Cagnard, N., Crozet, L., Perez, M., Fridman, W., et al. (2013). Lung tumor microenvironment induces specific gene expression signature in intratumoral NK cells. Front. Immunol. 4:19. doi: 10.3389/fimmu.2013.00019
Gonzalez-Villasana, V., Fuentes-Mattei, E., Ivan, C., Dalton, H. J., Rodriguez-Aguayo, C., Fernandez-de Thomas, R. J., et al. (2015). Rac1/Pak1/p38/MMP-2 axis regulates angiogenesis in ovarian cancer. Clin. Cancer Res. 21, 2127–2137. doi: 10.1158/1078-0432.CCR-14-2279
Grange, C., Collino, F., Tapparo, M., and Camussi, G. (2014). Oncogenic micro-RNAs and Renal Cell Carcinoma. Front. Oncol. 4:49. doi: 10.3389/fonc.2014.00049
Gu, P., Xing, X., Tanzer, M., Rocken, C., Weichert, W., Ivanauskas, A., et al. (2008). Frequent loss of TIMP-3 expression in progression of esophageal and gastric adenocarcinomas. Neoplasia 10, 563–572. doi: 10.1593/neo.08208
Gu, X., Fu, M., Ding, Y., Ni, H., Zhang, W., Zhu, Y., et al. (2014). TIMP-3 expression associates with malignant behaviors and predicts favorable survival in HCC. PLoS ONE 9:e106161. doi: 10.1371/journal.pone.0106161
Hayward, S. W., Wang, Y., Cao, M., Hom, Y. K., Zhang, B., Grossfeld, G. D., et al. (2001). Malignant transformation in a nontumorigenic human prostatic epithelial cell line. Cancer Res. 61, 8135–8142.
Hersh, E. M., Mavligit, G. M., Gutterman, J. U., and Barsales, P. B. (1976). Mononuclear cell content of human solid tumors. Med. Pediatr. Oncol. 2, 1–9. doi: 10.1002/mpo.2950020102
Hill, R., Song, Y., Cardiff, R. D., and Van Dyke, T. (2005). Selective evolution of stromal mesenchyme with p53 loss in response to epithelial tumorigenesis. Cell 123, 1001–1011. doi: 10.1016/j.cell.2005.09.030
Hynes, R. O. (2002). Integrins: bidirectional, allosteric signaling machines. Cell 110, 673–687. doi: 10.1016/S0092-8674(02)00971-6
Hynes, R. O. (2009). The extracellular matrix: not just pretty fibrils. Sciencel 326, 1216–1219. doi: 10.1126/science.1176009
Ioachim, E., Charchanti, A., Briasoulis, E., Karavasilis, V., Tsanou, H., Arvanitis, D., et al. (2002). Immunohistochemical expression of extracellular matrix components tenascin, fibronectin, collagen type IV and laminin in breast cancer: their prognostic value and role in tumour invasion and progression. Eur. J. Cancer 38, 2362–2370. doi: 10.1016/S0959-8049(02)00210-1
Jones, P. A., and DeClerck, Y. A. (1980). Destruction of extracellular matrices containing glycoproteins, elastin, and collagen by metastatic human tumor cells. Cancer Res. 40, 3222–3227.
Jue, S. F., Bradley, R. S., Rudnicki, J. A., Varmus, H. E., and Brown, A. M. (1992). The mouse Wnt-1 gene can act via a paracrine mechanism in transformation of mammary epithelial cells. Mol. Cell. Biol. 12, 321–328.
Kalluri, R., and Weinberg, R. A. (2009). The basics of epithelial-mesenchymal transition. J. Clin. Invest. 119, 1420–1428. doi: 10.1172/JCI39104
Kalluri, R., and Zeisberg, M. (2006). Fibroblasts in cancer. Nat. Rev. Cancer 6, 392–401. doi: 10.1038/nrc1877
Karnoub, A. E., Dash, A. B., Vo, A. P., Sullivan, A., Brooks, M. W., Bell, G. W., et al. (2007). Mesenchymal stem cells within tumour stroma promote breast cancer metastasis. Nature 449, 557–563. doi: 10.1038/nature06188
Karre, K., Ljunggren, H. G., Piontek, G., and Kiessling, R. (1986). Selective rejection of H-2-deficient lymphoma variants suggests alternative immune defence strategy. Nature 319, 675–678. doi: 10.1038/319675a0
Kikuchi, Y., Kashima, T. G., Nishiyama, T., Shimazu, K., Morishita, Y., Shimazaki, M., et al. (2008). Periostin is expressed in pericryptal fibroblasts and cancer-associated fibroblasts in the colon. J. Histochem. Cytochem. 56, 753–764. doi: 10.1369/jhc.2008.951061
Knutson, K. L., and Disis, M. L. (2005). Tumor antigen-specific T helper cells in cancer immunity and immunotherapy. Cancer Immunol. Immunother. 54, 721–728. doi: 10.1007/s00262-004-0653-2
Kuperwasser, C., Chavarria, T., Wu, M., Magrane, G., Gray, J. W., Carey, L., et al. (2004). Reconstruction of functionally normal and malignant human breast tissues in mice. Proc. Natl. Acad. Sci. U.S.A. 101, 4966–4971. doi: 10.1073/pnas.0401064101
Levental, K. R., Yu, H., Kass, L., Lakins, J. N., Egeblad, M., Erler, J. T., et al. (2009). Matrix crosslinking forces tumor progression by enhancing integrin signaling. Cell 139, 891–906. doi: 10.1016/j.cell.2009.10.027
Lieu, C. H., Tran, H., Jiang, Z. Q., Mao, M., Overman, M. J., Lin, E., et al. (2013). The association of alternate VEGF ligands with resistance to anti-VEGF therapy in metastatic colorectal cancer. PLoS ONE 8:e77117. doi: 10.1371/journal.pone.0077117
Liu, J., Zhang, N., Li, Q., Zhang, W., Ke, F., Leng, Q., et al. (2011a). Tumor-associated macrophages recruit CCR6+ regulatory T cells and promote the development of colorectal cancer via enhancing CCL20 production in mice. PLoS ONE 6:e19495. doi: 10.1371/journal.pone.0019495
Liu, M., Tang, Q., Qiu, M., Lang, N., Li, M., Zheng, Y., et al. (2011b). miR-21 targets the tumor suppressor RhoB and regulates proliferation, invasion and apoptosis in colorectal cancer cells. FEBS Lett. 585, 2998–3005. doi: 10.1016/j.febslet.2011.08.014
Lu, P., Weaver, V. M., and Werb, Z. (2012). The extracellular matrix: a dynamic niche in cancer progression. J. Cell Biol. 196, 395–406. doi: 10.1083/jcb.201102147
Mantovani, A., Sozzani, S., Locati, M., Allavena, P., and Sica, A. (2002). Macrophage polarization: tumor-associated macrophages as a paradigm for polarized M2 mononuclear phagocytes. Trends Immunol. 23, 549–555. doi: 10.1016/S1471-4906(02)02302-5
Mariyama, M., Kalluri, R., Hudson, B. G., and Reeders, S. T. (1992). The alpha 4(IV) chain of basement membrane collagen. Isolation of cDNAs encoding bovine alpha 4(IV) and comparison with other type IV collagens. J. Biol. Chem. 267, 1253–1258.
Marsh, T., Pietras, K., and McAllister, S. S. (2013). Fibroblasts as architects of cancer pathogenesis. Biochim. Biophys. Acta 1832, 1070–1078. doi: 10.1016/j.bbadis.2012.10.013
Matsushita, H., Hosoi, A., Ueha, S., Abe, J., Fujieda, N., Tomura, M., et al. (2015). Cytotoxic T Lymphocytes Block Tumor Growth Both by Lytic Activity and IFNγ-Dependent Cell-Cycle Arrest. Cancer Immunol. Res. 3, 26–36. doi: 10.1158/2326-6066.CIR-14-0098
McCawley, L. J., and Matrisian, L. M. (2000). Matrix metalloproteinases: multifunctional contributors to tumor progression. Mol. Med. Today 6, 149–156. doi: 10.1016/S1357-4310(00)01686-5
Menetrier-Caux, C., Montmain, G., Dieu, M. C., Bain, C., Favrot, M. C., Caux, C., et al. (1998). Inhibition of the differentiation of dendritic cells from CD34(+) progenitors by tumor cells: role of interleukin-6 and macrophage colony-stimulating factor. Blood 92, 4778–4791.
Mirnezami, A. H., Pickard, K., Zhang, L., Primrose, J. N., and Packham, G. (2009). MicroRNAs: key players in carcinogenesis and novel therapeutic targets. Eur. J. Surg. Oncol. 35, 339–347. doi: 10.1016/j.ejso.2008.06.006
Mishra, P. J., Mishra, P. J., Humeniuk, R., Medina, D. J., Alexe, G., Mesirov, J. P., et al. (2008). Carcinoma-associated fibroblast-like differentiation of human mesenchymal stem cells. Cancer Res. 68, 4331–4339. doi: 10.1158/0008-5472.CAN-08-0943
Moretta, A. (2002). Natural killer cells and dendritic cells: rendezvous in abused tissues. Nat. Rev. Immunol. 2, 957–964. doi: 10.1038/nri956
Moses, H. L., Branum, E. L., Proper, J. A., and Robinson, R. A. (1981). Transforming growth factor production by chemically transformed cells. Cancer Res. 41, 2842–2848.
Murphy, B. C., Pienta, K. J., and Coffey, D. S. (1992). Effects of extracellular matrix components and dihydrotestosterone on the structure and function of human prostate cancer cells. Prostate 20, 29–41. doi: 10.1002/pros.2990200105
Nam, J. M., Onodera, Y., Bissell, M. J., and Park, C. C. (2010). Breast cancer cells in three-dimensional culture display an enhanced radioresponse after coordinate targeting of integrin alpha5beta1 and fibronectin. Cancer Res. 70, 5238–5248. doi: 10.1158/0008-5472.CAN-09-2319
Nickell, K. A., Halper, J., and Moses, H. L. (1983). Transforming growth factors in solid human malignant neoplasms. Cancer Res. 43, 1966–1971.
Nielsen, B. S., Jorgensen, S., Fog, J. U., Sokilde, R., Christensen, I. J., Hansen, U., et al. (2011). High levels of microRNA-21 in the stroma of colorectal cancers predict short disease-free survival in stage II colon cancer patients. Clin. Exp. Metastasis 28, 27–38. doi: 10.1007/s10585-010-9355-7
Noy, R., and Pollard, J. W. (2014). Tumor-associated macrophages: from mechanisms to therapy. Immunity 41, 49–61. doi: 10.1016/j.immuni.2014.06.010
Ohlund, D., Franklin, O., Lundberg, E., Lundin, C., and Sund, M. (2013). Type IV collagen stimulates pancreatic cancer cell proliferation, migration, and inhibits apoptosis through an autocrine loop. BMC Cancer 13:154. doi: 10.1186/1471-2407-13-154
Ohmura, Y., Yoshikawa, K., Saga, S., Ueda, R., Kazaoka, Y., and Yamada, S. (2008). Combinations of tumor-specific CD8+ CTLs and anti-CD25 mAb provide improved immunotherapy. Oncol. Rep. 19, 1265–1270. doi: 10.3892/or.19.5.1265
Olumi, A. F., Grossfeld, G. D., Hayward, S. W., Carroll, P. R., Tlsty, T. D., and Cunha, G. R. (1999). Carcinoma-associated fibroblasts direct tumor progression of initiated human prostatic epithelium. Cancer Res. 59, 5002–5011.
Orimo, A., Gupta, P. B., Sgroi, D. C., Arenzana-Seisdedos, F., Delaunay, T., Naeem, R., et al. (2005). Stromal fibroblasts present in invasive human breast carcinomas promote tumor growth and angiogenesis through elevated SDF-1/CXCL12 secretion. Cell 121, 335–348. doi: 10.1016/j.cell.2005.02.034
Otrock, Z. K., Mahfouz, R. A., Makarem, J. A., and Shamseddine, A. I. (2007). Understanding the biology of angiogenesis: review of the most important molecular mechanisms. Blood Cells Mol. Dis. 39, 212–220. doi: 10.1016/j.bcmd.2007.04.001
Paget, S. (1889). The distribution of secondary growths in cancers of the breast. Lancet 133, 571–573. doi: 10.1016/S0140-6736(00)49915-0
Pietras, K., Pahler, J., Bergers, G., and Hanahan, D. (2008). Functions of paracrine PDGF signaling in the proangiogenic tumor stroma revealed by pharmacological targeting. PLoS Med. 5:e19. doi: 10.1371/journal.pmed.0050019
Qi, J. H., Ebrahem, Q., Moore, N., Murphy, G., Claesson-Welsh, L., Bond, M., et al. (2003). A novel function for tissue inhibitor of metalloproteinases-3 (TIMP3): inhibition of angiogenesis by blockage of VEGF binding to VEGF receptor-2. Nat. Med. 9, 407–415. doi: 10.1038/nm846
Quante, M., Tu, S. P., Tomita, H., Gonda, T., Wang, S. S., Takashi, S., et al. (2011). Bone marrow-derived myofibroblasts contribute to the mesenchymal stem cell niche and promote tumor growth. Cancer Cell 19, 257–272. doi: 10.1016/j.ccr.2011.01.020
Rabinovich, G. A. (2005). Galectin-1 as a potential cancer target. Br. J. Cancer 92, 1188–1192. doi: 10.1038/sj.bjc.6602493
Rech, A. J., Mick, R., Martin, S., Recio, A., Aqui, N. A., Powell, D. J. Jr., et al. (2012). CD25 blockade depletes and selectively reprograms regulatory T cells in concert with immunotherapy in cancer patients. Sci. Transl. Med. 4:134ra162. doi: 10.1126/scitranslmed.3003330
Recklies, A. D., Tiltman, K. J., Stoker, T. A., and Poole, A. R. (1980). Secretion of proteinases from malignant and nonmalignant human breast tissue. Cancer Res. 40, 550–556.
Reis, S. T., Pontes-Junior, J., Antunes, A. A., Dall'Oglio, M. F., Dip, N., Passerotti, C., et al. (2012). miR-21 may acts as an oncomir by targeting RECK, a matrix metalloproteinase regulator, in prostate cancer. BMC Urol. 12:14. doi: 10.1186/1471-2490-12-14
Roberti, M. P., Rocca, Y. S., Amat, M., Pampena, M. B., Loza, J., Coló, F., et al. (2012). IL-2- or IL-15-activated NK cells enhance Cetuximab-mediated activity against triple-negative breast cancer in xenografts and in breast cancer patients. Breast Cancer Res. Treat. 136, 659–671. doi: 10.1007/s10549-012-2287-y
Roskelley, C. D., Desprez, P. Y., and Bissell, M. J. (1994). Extracellular matrix-dependent tissue-specific gene expression in mammary epithelial cells requires both physical and biochemical signal transduction. Proc. Natl. Acad. Sci. U.S.A. 91, 12378–12382. doi: 10.1073/pnas.91.26.12378
Russel, S. W., Doe, W. F., and Cochrane, C. G. (1976). Number of macrophages and distribution of mitotic activity in regressing and progressing Moloney sarcomas. J. Immunol. 116, 164–166.
Sadej, R., Inai, K., Rajfur, Z., Ostapkowicz, A., Kohler, J., Skladanowski, A., et al. (2008). Tenascin C interacts with ecto-5'-nucleotidase (eN) and regulates adenosine generation in cancer cells. Biochim. Biophys. Acta 1782, 35–40. doi: 10.1016/j.bbadis.2007.11.001
Schiavoni, G., Gabriele, L., and Mattei, F. (2013). The tumor microenvironment: a pitch for multiple players. Front. Oncol. 3:90. doi: 10.3389/fonc.2013.00090
Skuli, N., Liu, L., Runge, A., Wang, T., Yuan, L., Patel, S., et al. (2009). Endothelial deletion of hypoxia-inducible factor-2alpha (HIF-2alpha) alters vascular function and tumor angiogenesis. Blood 114, 469–477. doi: 10.1182/blood-2008-12-193581
Solinas, G., Germano, G., Mantovani, A., and Allavena, P. (2009). Tumor-associated macrophages (TAM) as major players of the cancer-related inflammation. J. Leukoc. Biol. 86, 1065–1073. doi: 10.1189/jlb.0609385
Sprent, J. (1995). Antigen-presenting cells. Professionals and amateurs. Curr. Biol. 5, 1095–1097. doi: 10.1016/S0960-9822(95)00219-3
Surman, D. R., Dudley, M. E., Overwijk, W. W., and Restifo, N. P. (2000). Cutting edge: CD4+ T cell control of CD8+ T cell reactivity to a model tumor antigen. J. Immunol. 164, 562–565. doi: 10.4049/jimmunol.164.2.562
Tang, N., Wang, L., Esko, J., Giordano, F. J., Huang, Y., Gerber, H. P., et al. (2004). Loss of HIF-1alpha in endothelial cells disrupts a hypoxia-driven VEGF autocrine loop necessary for tumorigenesis. Cancer Cell 6, 485–495. doi: 10.1016/j.ccr.2004.09.026
Tatsumi, T., Herrem, C. J., Olson, W. C., Finke, J. H., Bukowski, R. M., Kinch, M. S., et al. (2003). Disease stage variation in CD4+ and CD8+ T-cell reactivity to the receptor tyrosine kinase EphA2 in patients with renal cell carcinoma. Cancer Res. 63, 4481–4489.
Tatsumi, T., Kierstead, L. S., Ranieri, E., Gesualdo, L., Schena, F. P., Finke, J. H., et al. (2002). Disease-associated bias in T helper type 1 (Th1)/Th2 CD4(+) T cell responses against MAGE-6 in HLA-DRB10401(+) patients with renal cell carcinoma or melanoma. J. Exp. Med. 196, 619–628. doi: 10.1084/jem.20012142
Ucar, A., Vafaizadeh, V., Jarry, H., Fiedler, J., Klemmt, P. A., Thum, T., et al. (2010). miR-212 and miR-132 are required for epithelial stromal interactions necessary for mouse mammary gland development. Nat. Genet. 42, 1101–1108. doi: 10.1038/ng.709
Valadi, H., Ekstrom, K., Bossios, A., Sjostrand, M., Lee, J. J., and Lotvall, J. O. (2007). Exosome-mediated transfer of mRNAs and microRNAs is a novel mechanism of genetic exchange between cells. Nat. Cell Biol. 9, 654–659. doi: 10.1038/ncb1596
Verbeke, H., Struyf, S., Laureys, G., and Van Damme, J. (2011). The expression and role of CXC chemokines in colorectal cancer. Cytokine Growth Factor Rev. 22, 345–358. doi: 10.1016/j.cytogfr.2011.09.002
Vesely, M. D., Kershaw, M. H., Schreiber, R. D., and Smyth, M. J. (2011). Natural innate and adaptive immunity to cancer. Annu. Rev. Immunol. 29, 235–271. doi: 10.1146/annurev-immunol-031210-101324
Vlodavsky, I., and Friedmann, Y. (2001). Molecular properties and involvement of heparanase in cancer metastasis and angiogenesis. J. Clin. Invest. 108, 341–347. doi: 10.1172/JCI13662
Wang, Y., Kim, S., and Kim, I. M. (2014). Regulation of metastasis by microRNAs in ovarian cancer. Front. Oncol. 4:143. doi: 10.3389/fonc.2014.00143
Wilson, E. B., El-Jawhari, J. J., Neilson, A. L., Hall, G. D., Melcher, A. A., Meade, J. L., et al. (2011). Human tumour immune evasion via TGF-beta blocks NK cell activation but not survival allowing therapeutic restoration of anti-tumour activity. PLoS ONE 6:e22842. doi: 10.1371/journal.pone.0022842
Witz, I. P. (2009). The tumor microenvironment: the making of a paradigm. Cancer Microenviron. 2(Suppl. 1), 9–17. doi: 10.1007/s12307-009-0025-8
Wong, B. W., Kuchnio, A., Bruning, U., and Carmeliet, P. (2013). Emerging novel functions of the oxygen-sensing prolyl hydroxylase domain enzymes. Trends Biochem. Sci. 38, 3–11. doi: 10.1016/j.tibs.2012.10.004
Xu, Z., Vonlaufen, A., Phillips, P. A., Fiala-Beer, E., Zhang, X., Yang, L., et al. (2010). Role of pancreatic stellate cells in pancreatic cancer metastasis. Am. J. Pathol. 177, 2585–2596. doi: 10.2353/ajpath.2010.090899
Yang, M., Chen, J., Su, F., Yu, B., Su, F., Lin, L., et al. (2011). Microvesicles secreted by macrophages shuttle invasion-potentiating microRNAs into breast cancer cells. Mol. Cancer 10:117. doi: 10.1186/1476-4598-10-117
Yu, Y., Xiao, C. H., Tan, L. D., Wang, Q. S., Li, X. Q., and Feng, Y. M. (2014). Cancer-associated fibroblasts induce epithelial-mesenchymal transition of breast cancer cells through paracrine TGF-beta signalling. Br. J. Cancer 110, 724–732. doi: 10.1038/bjc.2013.768
Keywords: tumor microenvironment, cancer stroma, cancer-associated fibroblast, extracellular matrix, microRNA
Citation: Bhome R, Bullock MD, Al Saihati HA, Goh RW, Primrose JN, Sayan AE and Mirnezami AH (2015) A top-down view of the tumor microenvironment: structure, cells and signaling. Front. Cell Dev. Biol. 3:33. doi: 10.3389/fcell.2015.00033
Received: 05 March 2015; Accepted: 08 May 2015;
Published: 29 May 2015.
Edited by:
Ala-Eddin Al Moustafa, McGill University and Concordia University, CanadaReviewed by:
Santos Mañes, Consejo Superior Investigaciones Científicas, SpainClaudia D. Andl, Vanderbilt University, USA
Copyright © 2015 Bhome, Bullock, Al Saihati, Goh, Primrose, Sayan and Mirnezami. This is an open-access article distributed under the terms of the Creative Commons Attribution License (CC BY). The use, distribution or reproduction in other forums is permitted, provided the original author(s) or licensor are credited and that the original publication in this journal is cited, in accordance with accepted academic practice. No use, distribution or reproduction is permitted which does not comply with these terms.
*Correspondence: A. Emre Sayan and Alex H. Mirnezami, Cancer Sciences Division, Southampton General Hospital, Somers Building, Tremona Road, Southampton SO16 6YD, UK,YS5lLnNheWFuQHNvdG9uLmFjLnVr;YWhtQHNvdG9uLmFjLnVr