- 1Department of Biology, University of Central Arkansas, Conway, AR, USA
- 2Interdisciplinary Biomedical Sciences, University of Arkansas for Medical Sciences, Little Rock, AR, USA
Mitochondrial function is dependent upon mitochondrial structure which is in turn dependent upon mitochondrial dynamics, including fission, fusion, and motility. Here we examined the relationship between mitochondrial dynamics and the cytoskeleton in Dictyostelium discoideum. Using time-lapse analysis, we quantified mitochondrial fission, fusion, and motility in the presence of cytoskeleton disrupting pharmaceuticals and the absence of the potential mitochondria-cytoskeleton linker protein, CluA. Our results indicate that microtubules are essential for mitochondrial movement, as well as fission and fusion; actin plays a less significant role, perhaps selecting the mitochondria for transport. We also suggest that CluA is not a linker protein but plays an unidentified role in mitochondrial fission and fusion. The significance of our work is to gain further insight into the role the cytoskeleton plays in mitochondrial dynamics and function. By better understanding these processes we can better appreciate the underlying mitochondrial contributions to many neurological disorders characterized by altered mitochondrial dynamics, structure, and/or function.
Introduction
Mitochondria are highly specialized eukaryotic organelles responsible for producing the majority of a cell's adenosine triphosphate (ATP). They also play a vital role in many other cellular processes, such as the synthesis of heme groups and the regulation of membrane potential, calcium homeostasis, apoptosis, and cell differentiation (Mitchell, 1961; Frezza et al., 2006; Lill and Mühlenhoff, 2008; Baughman et al., 2011; De Stefani et al., 2011; Martinou and Youle, 2011; Maeda and Chida, 2013). To carry out these cellular processes the mitochondria must function properly, which is largely controlled by the organelle's morphology and distribution throughout the cell (Nunnari and Suomalainen, 2012).
For instance, in several organisms like animals, flies, and yeast, as well as specialized neuronal cells, the mitochondria exist in a reticular dynamic network, while in organisms like Dictyostelium and Arabidopsis the mitochondria exist as individual organelles (Chen, 1988; Bereiter-Hahn, 1990; Nunnari et al., 1997; Rizzuto et al., 1998; Schimmel et al., 2012; El Zawily et al., 2014). In either case, the appropriate morphologies are maintained by fission and fusion events (Nunnari et al., 1997; Bleazard et al., 1999; Gilson et al., 2003; Karbowski and Youle, 2003; Twig and Shirihai, 2011; El Zawily et al., 2014).
The cytoskeleton, in addition to affecting mitochondrial morphology plays a crucial role in maintaining mitochondrial distribution throughout the cell by facilitating organelle transport to areas with high metabolic demands (Van Gestel et al., 2002; Bereiter-Hahn et al., 2008; Kostal and Arriaga, 2011; Nekrasova et al., 2011; Wu et al., 2013). In order to rapidly respond to cellular demands, the cytoskeleton must have a communication system allowing it to influence organelle transport and position. This system usually encompasses various linker and motor proteins. The interaction between the cytoskeleton and the mitochondria is poorly understood; however several studies suggest that cytoskeletal network proteins interact with components of the fission and fusion machinery (Liesa et al., 2009), as well with the calcium sensing GTPase- Miro (Fransson et al., 2006; Frederick and Shaw, 2007).
Mitochondrial dynamics (fission, fusion, motility) also regulate the organelle's morphology and distribution. Disruption of these dynamics has been linked to a loss of metabolic function, an increase in ROS concentration, impairment of ATP synthesis, and a decrease in overall membrane potential (Margolin, 2000; Karbowski and Youle, 2003; Baloh et al., 2007; Chen and Chan, 2009; Nunnari and Suomalainen, 2012; Youle and van der Bliek, 2012; Picard et al., 2013). A variety of diseases, such as Alzheimer's, Parkinson's, Charcot-Marie-Tooth 2A, and Huntington's have also been linked to disruption in mitochondrial dynamics and morphology, though the mechanism driving these afflictions are unknown (Nunnari and Suomalainen, 2012).
Thus, to better understand mitochondrial dynamics and their role in disease we utilized D. discoideum, a lower eukaryotic model organism. In addition to lower eukaryotes sometimes being easier to tease out molecular mechanisms, D. discoideum is also a mitochondrial disease system (Barth et al., 2007; Annesley and Fisher, 2009; Annesley et al., 2014). Mitochondrial diseases caused by a specific mutation often manifest with a variety of clinical symptoms in humans. It has become apparent that unlike humans, D. discoideum cells do not exhibit this variation in symptoms, thus simplifying the study on mitochondrial diseases (Francione et al., 2011).
In D. discoideum mitochondrial distribution is maintained by the protein CluA (Zhu et al., 1997), which has homologs across a variety of organisms, including Arabidopsis thaliana, Saccharomyces cerevisiae, and Drosophila melanogaster. In all organisms studied to date, the absence of this protein results in clustered mitochondria (Zhu et al., 1997; Fields et al., 1998; Logan et al., 2003; Cox and Spradling, 2009). In plants, the organelles are found in small distinct clusters distributed throughout the cell (El Zawily et al., 2014), while larger, nuclearly centered clusters have been identified in D. discoideum. Further work has shown that these D. discoideum mitochondria are interconnected by thin membranous strands with limited movement (Fields et al., 2002). It has been hypothesized that CluA may represent a novel family of proteins which link the cytoskeleton to mitochondria. In addition to clustered mitochondria, the absence of CluA also decreases the rates of fission and fusion (Schimmel et al., 2012). Further, the nuclear localization of the mitochondrial clusters suggest they only move in an anterograde fashion (Zhu et al., 1997). Interestingly the D. discoideum Miro homolog, GemA, is not involved in mitochondrial transport along the cytoskeleton (Vlahou et al., 2011); further supporting the notion that CluA links the cytoskeleton and mitochondria. Therefore, it is conceivable that CluA has direct influence on mitochondrial dynamics by association with the cytoskeleton; however, it is presently unclear if CluA interacts with actin or microtubule motor proteins. Thus, to determine CluA's function the relationship between mitochondria and the cytoskeleton in D. discoideum must be elucidated.
It has been demonstrated that in animal cells mitochondria move along microtubules to travel long distances and use actin filaments for short distances (Wu et al., 2013). Drosophila mitochondria primarily use microtubules (Cox and Spradling, 2009), as do fission yeast (Yaffe et al., 2003), whereas actin is predominantly used by both plants and budding yeast (Van Gestel et al., 2002). There is even evidence that mitochondria use intermediate filaments in 3T3 fibroblast cells for proper motility regulation (Nekrasova et al., 2011). Thus, we analyzed D. discoideum mitochondria to establish whether microtubules or actin filaments were utilized for proper motility. Additionally, we examined whether or not cluA− mitochondrial morphology is dependent upon these cytoskeletal components and how these components affect motility of the cluA− mitochondria. Finally, due to mounting evidence linking impaired fission and/or fusion to aberrant mitochondrial motility and cellular health (Cagalinec et al., 2013), we assessed the influence of the cytoskeleton on mitochondrial fission and fusion.
To carry out these experiments, we disrupted the microtubules with nocodazole and the actin filaments with latrunculin-B. Through immunofluorescence and time-lapse imaging, we quantified mitochondrial morphology, motility, and fission and fusion rates. Taken together our results show that the cluA− clustered mitochondrial phenotype is partially dependent upon the actin and microtubule cytoskeletons, and that mitochondrial motility is not affected by loss of CluA. Therefore, we conclude that CluA does not play a significant role in connecting the mitochondria to the cytoskeleton. Further, we show that in D. discoideum, microtubules, but not actin, are important for both mitochondrial velocity as well as fission and fusion. Thus, we can infer that, as is the case with mammalian cells, microtubules play a much larger role in mitochondrial morphology and motility than actin. Finally, despite previous research, we did not find an interaction linking the mitochondria to the microtubule cytoskeleton through CluA, and conclude it may serve an unidentified function affecting mitochondrial morphology and distribution.
Methods
Strain Culture and Growth Conditions
All Dictyostelium discoideum strains described were obtained from the Dicty-Stock Center (Fey et al., 2013). Wild-type (AX4) was deposited by Bill Loomis and cluA− by Margaret Clarke. The strains were cultured axenically in liquid HL5 medium supplemented with streptomycin (final concentration of 300 ug/ml) and ampicillin (final concentration 150 ug/ml) at 22°C shaking at 125 rpm.
Preparation of D. discoideum for Experiments
AX4 and cluA− cells were diluted to 3 × 104 cells/ml in HL-5 liquid media until cells reached log phase. Log phase cells (5.0 ml) were washed by centrifuging at 500 × g for 4 min and resuspended in 5 ml of room temperature Lo-Flo (Formedium). Cells were stained with 0.1 uM MitoTracker CMXRos (Invitrogen) and incubated for 4 h at room temperature while shaking. Excess MitoTracker was removed by washing the cells twice with Lo-Flo.
Cytoskeleton Disruption
During the 4 h Lo-Flo incubation period, the drugs or their appropriate vehicle control were added to the cells to disrupt the cytoskeleton. To inhibit the actin portion of the cytoskeleton, 10 uM latrunculin-B (Sigma) or equivalent volume of vehicle control (EtOH) was added to the cells for the final 30 min of incubation in Lo-Flo media. Nocodazole (10 ug/ml) (Sigma) or a vehicle control of dimethyl sulfoxide (DMSO) (Sigma) was used to inhibit the microtubule component of the cytoskeleton and was added in the final hour of the 4-h incubation period. To inhibit both actin and microtubules, latrunculin-B (10 uM) and nocodazole (10 ug/ml) were used with the equal volume of ethanol and DMSO for a control. For washout experiments, after drug treatment cells were washed and then incubated at room temperature with shaking for 1 h prior to processing for immunofluorescence.
Following incubation, the cells were washed twice at 500 × g for 4 min to remove excess MitoTracker and resuspended in 5 ml Lo-Flo plus the appropriate drug or vehicle in preparation for live cell imaging or immunofluorescence. Drug effectiveness was confirmed with immunofluorescence (see below), in all drug treatments the cytoskeleton was significantly different from vehicle controls.
Immunofluorescence of Dictyostelium Strains
AX4 (wild-type) and cluA− strains of D. discoideum were grown to log phase (about 2–4 × 106) then pelleted at 500 × g for 4 min and resuspended in Lo-Flo liquid medium (Formedium). Cells were treated with MitoTracker, nocodazole, latrunculin-B, or both or treated with DMSO, ethanol, or both, as previously described, to disrupt the cytoskeleton and stain the mitochondria. Stained and treated cells were washed by pelleting at 500 × g for 4 min two times, and resuspended in room temperature Lo-Flo liquid media to the original volume. Drugs or control were added back to the washed and stained cells. A 22 × 22 mm coverslip was placed into a 6-well plate. About 500 ul of washed and stained cells were added to the coverslips and allowed to adhere for 30 min. The coverslips with adhered cells were then washed twice with 10 mM MES-NaOH by gently adding and removing the solution to remove any cells that did not adhere to the coverslips. The adhered cells were fixed with 1 ml of 3% paraformaldehyde diluted in 10 mM Pipes (pH 6.0) for 30 min and then quenched with 100 mM glycine (1 ml) diluted in 1xPBS for 5 min. The membranes of the adhered and fixed cells were then permeabilized by using 0.02% Triton X-100 for 5 min. The permeabilized cells were washed three times by gently adding and removing 1xPBS and then blocked with 0.045% fish gelatin, 0.5% BSA in 1xPBS (PBG) for 1 h at room temperature. These cells were prepared for either actin or tubulin visualization.
To visualize actin, the blocked cells were stained for 1 h at room temperature in the dark using 0.5 ul of 6.6 uM phalloidin (Life Tech) in 500 ul PBG per coverslip. Excess phalloidin was removed by washing with 1xPBS three times for 5 min each before mounting the coverslips with SlowFade Gold (Invitrogen) onto glass slides.
To visualize tubulin, tubulin primary antibodies (mouse anti-tubulin, DSHB 12G10) were diluted 1:150 in PBG and added to the coverslips. The primary antibodies were allowed to sit overnight at 4°C. The following day, the cells were washed with 1xPBS three times for 5 min each. The secondary antibody (AlexaFluor 488 goat α mouse IgG) (Life Tech A11001) diluted 1:250 in PBG was added and incubated in the dark for 1 h at room temperature. The coverslips with treated cells were then washed with 1xPBS three times for 5 min each and mounted to glass slides with SlowFade Gold.
Quantification of Morphology
Cells were imaged using a Zeiss laser scanning LSM 510 Pascal confocal microscope to obtain z-stack images. The images were observed and classified according to the appearance of their microtubule and actin cytoskeleton and mitochondrial distribution. The microtubule cytoskeleton is present throughout the cell, while the actin localized around the cell at the membrane. The microtubule cytoskeleton morphology was classified as either little to none, patchy, or complete. Morphological classification was assessed by whether the microtubules appeared as disjointed and not extending throughout the cell (little to none), were in the astral configuration characteristic of the microtubule origin center (patchy), or branched throughout the cell and extending to the cell membrane (complete). The actin cytoskeleton was assessed by whether actin around the periphery of the cell was either absent or mostly absent (none), present but disjointed (patchy) or present and complete at the edge of the entire cell (complete). Mitochondrial morphology was determined to be distributed, loose cluster, or tight cluster (Figure 1). Distributed mitochondria were evenly dispersed throughout the cytoplasm, while clustered mitochondria were tightly aggregated toward the center or periphery of the cell. Loose clusters were considered loose mitochondrial aggregates.
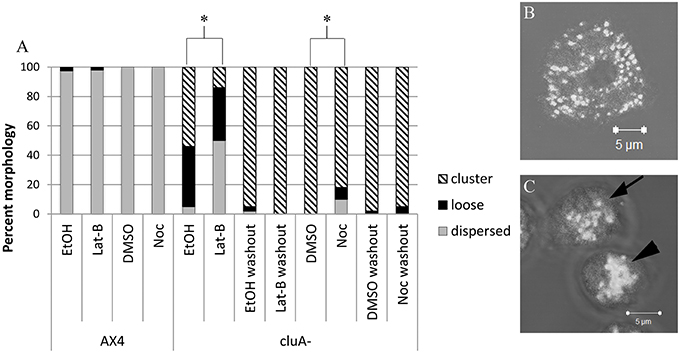
Figure 1. Analysis of mitochondria distribution in AX4 and cluA− cytoskeleton disrupted cells. (A) AX4 mitochondrial morphology remained dispersed and was unaffected by all treatments. The cluA− clustered mitochondria phenotype was significantly decreased with more loose clusters and dispersed mitochondrial when treated with latrunculin-B (p < 0.0001) or nocodazole (p < 0.0001). (B,C) Examples of mitochondrial distribution. (B) AX4 cell with dispersed mitochondria, (C) cluA− cells where top cell (with arrow) shows loosely clustered mitochondria, bottom cell (with arrowhead) shows a tight cluster. *Indicates significant differences.
Statistical Analysis-Immunofluorescence
Each experiment was repeated a minimum of three times per condition with a minimum of forty cells being quantified. Statistical analysis was performed using Prism Graph Pad 6.07. A Chi square (or Fisher when appropriate) analysis was conducted to determine statistical significance among treatments and strains. A p-value of less than 0.05 was considered statistically significant.
Quantification of Mitochondrial Fission and Fusion in Dictyostelium Strains
Washed and MitoTracker stained cells (0.5 ml) were placed in Nunc Lab-TekII 4-well chambered coverglass for imaging. A Zeiss laser scanning LSM Pascal confocal microscope with a pinhole setting of 144 um (1.36 airy units), resulting in an optical slice of 1.1 um was used to image washed, stained, and treated cells. A single plane was imaged every 677.38 ms for 100 time points, or until bleaching occurred.
To quantify fission events, mitochondria must be visible prior to the single organelle splitting into two. Fusion was quantified when two mitochondria approached and moved together for a couple of frames and then fused into a single organelle. If two organelles came together or split, then returned to their original state by the next frame, they were classified as “drive-bys” and were not quantified.
Statistical Analysis-Fission and Fusion
Statistical analysis was performed using JMP 11.0.0 (SAS Institute, Inc.) software. The rates of fission and fusion were calculated by averaging the number of events/min/cells for each strain and treatment. A minimum of 30 cells for each strain was used for quantification. Kruskal-Wallis analysis with a Steel-Dwass post-hoc was performed for statistical analysis. A p-value of less than 0.05 was considered statistically significant.
Kymograph Generation and Motility Analysis
Kymographs were generated using ImageJ from single plane confocal time lapse images (Schneider et al., 2012). A region of interest (ROI) was selected within a cell in the first image of each series. ROIs were drawn through the left, middle, and right portions of the cell in every instance where cells were visible throughout the entire series of images. The ROIs were stacked and converted to generate kymographs that depict mitochondrial movement within the region of interest over time. Kymographs were generated for a minimum of 20 cells in each treatment for AX4 and cluA− strains. To quantify motility, the speed in pixels/0.677 s was calculated and converted to micrometers/second for comparisons. Mitochondrial motility from the left, middle, and right portions were calculated and averaged for each cell. Kruskal-Wallis with Steel Dwass post-hoc analysis was conducted using JMP software for statistical comparisons of single drug vs. single control. A p-value of less than 0.05 was considered statistically significant.
The percent of mitochondria moving were counted from the kymographs created for motility analysis. Mitochondria in each kymograph were counted and classified as either moving or not moving, with stationary mitochondria being considered a straight vertical line from the top of the kymograph to the bottom. An average percent of mitochondria moving was calculated for each strain and treatment and compared; a minimum of 20 cells were analyzed for each treatment. For analysis, nonparametric Kruskal-Wallis and post-hoc Steel-Dwass statistical tests were used. A p-value of less than 0.05 was considered statistically significant.
Results
The Relationship between the Cytoskeleton and Mitochondrial Morphology in D. discoideum
We quantified mitochondrial morphology after disrupting actin with latrunculin-B (Lat-B) or microtubules with nocodazole (Noc) in wild-type (AX4) and cluA− strains. The morphology and motility rates were compared to respective controls, as well as comparisons within treatment and across strains. These comparisons allowed us to determine the effect of each component of the cytoskeleton on mitochondrial morphology in cluA− cells.
As expected, alteration of the cytoskeleton with these pharmaceuticals does not affect mitochondrial distribution in AX4 cells when compared to vehicle control cells (Noc: p = 1.0; Lat-B: p = 0.9999; Figure 1). However, disruption of the cytoskeleton did alter mitochondrial distribution in cluA− cells. Treatment with either nocodazole or latrunculin-B changed cluA− mitochondria from their characteristic clustered morphology to a higher prevalence of loose clusters (Noc: p < 0.0001; Lat-B: p < 0.0001; Figure 1). As a further control, we treated the cells with vehicle or drugs then washed the cells and quantified mitochondrial morphology. Results from washout experiments indicate that indeed all changes to mitochondrial morphology are specific to the treatments, though interestingly the vehicles themselves have some effect (Figure 1). Especially EtOH, which increases the number of loose mitochondria compared to the washout controls. To determine if there was a synergistic effect between actin and microtubules, mitochondrial morphology of both strains was analyzed when the cells were exposed to nocodazole and latrunculin-B simultaneously. Our results indicate that there is no significant synergistic effect (data not shown), thus we can conclude that both cytoskeletal filaments are involved in maintaining the tightly clustered cluA− phenotype but do not affect the wild-type dispersed mitochondrial phenotype.
The Relationship between the Cytoskeleton and Mitochondrial Motility in D. discoideum
To determine the role of actin and microtubules in mitochondrial motility, cells were treated with cytoskeletal disrupting drugs and mitochondrial velocity and the percentage of organelles moving was calculated from kymographs. Again we analyzed both AX4 wild-type cells and cluA− cells, to determine not only the role of the cytoskeleton but also the role CluA may play in mitochondrial motility.
When actin is inhibited with latrunculin-B, AX4 had an average mitochondrial speed of 0.164 ± 0.007 um/s while the ethanol control averaged a speed of 0.165 ± 0.007 um/s, with no statistical difference between treatments (p = 1.0; Figure 2A). Similarly, the cluA− latrunculin-B and control treated cells averaged mitochondrial speeds of 0.161 ± 0.007 um/s and 0.158 ± 0.007 um/s, respectively, with no statistical significance (p = 1.0; Figure 2A). A comparison of treatments across strains also proved to not be statistically significant for latrunculin-B (p = 1.0) and ethanol (p = 1.0; Figure 2A). Thus, neither actin nor CluA has a significant role in mitochondrial velocity.
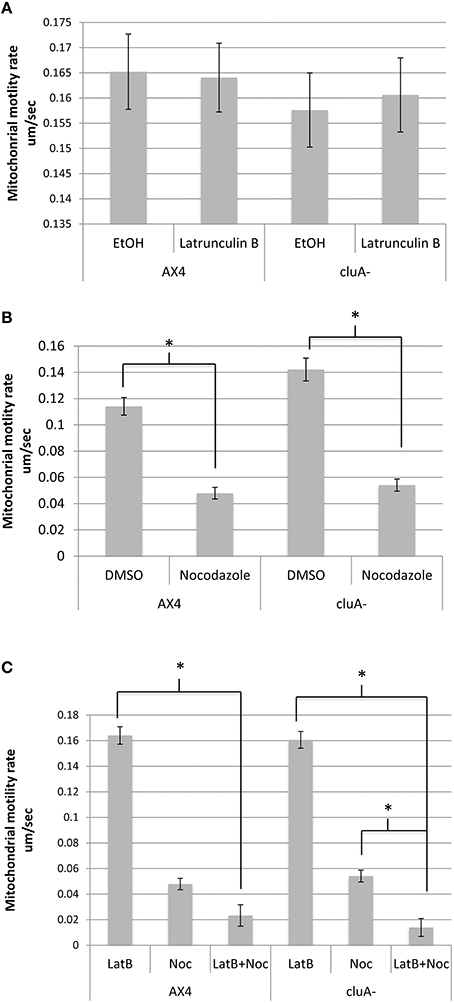
Figure 2. Average mitochondrial speed compared across strains and treatments. (A) There was no significant difference between treatments in AX4 (p = 1.0) or cluA− (p = 1.0) when treated with latrunculin-B. Comparing the treatments across strains also failed to prove statistical significance in ethanol (p = 1.0) and latrunculin-B (p = 1.0) treatments. (B) AX4 and cluA− mitochondria treated with nocodazole moved significantly slower than in vehicle treated cells (AX4, p < 0.0001; cluA−, p < 0.0001). AX4 DMSO mitochondrial speed was not significantly slower than the cluA− DMSO treatment (p = 0.57) or nocodazole treatment (p = 0.99). (C) Cells were treated with nocodazole and latrunculin-B simultaneously to determine if there was a synergistic effect between actin and microtubules. In AX4 only latrunculin-B (p < 0.0001) but not nocodazole (p = 0.29) single treatments had significantly higher mitochondrial rates compared to the double treatment. In cluA− both single treatments (Lat-B: p < 0.0001; Noc: p = 0.0031) had significantly higher motility rates compared to the double drug treated cluA− cells. CluA plays no direct role in mitochondrial motility, while microtubules determine the speed of mitochondrial movement; though in the absence of CluA, actin does play a significant role also. *Indicates significant differences.
When measuring motility for microtubule inhibited cells, there was a significant difference between the drug treated cells and their control for both strains. In AX4, the DMSO control had an average rate of 0.1141 ± 0.007 um/s while the nocodazole treated mitochondria moved 55% slower at 0.0479 ± 0.004 um/s (p < 0.0001). Similarly, in cluA−, mitochondrial speed in the control was 0.1422 ± 0.009 um/s and 0.0541 ± 0.005 um/s in the nocodazole treated cells, a 64% reduction in mitochondrial speed than the control cells (p < 0.0001; Figure 2B). Comparing treatments across strains proved that there was no significant difference in nocodazole or DMSO treated AX4 and cluA− (Noc: p = 0.99; DMSO: p = 0.57), though the DMSO vehicle AX4 cells had a 21% slower mitochondrial speed than DMSO cluA− (Figure 2B).
Again we determined if there was a synergistic effect between microtubules and actin in terms of mitochondrial velocity. In AX4, there is no statistical difference between nocodazole treatment and double drug treatment (p = 0.29; Figure 2C). Interestingly, in cluA− cells, nocodazole treated cells had a statistically higher motility rate, by 72%, than the double drug treated cells (p = 0.0031; Figure 2C). These results indicate that microtubules have the largest role in velocity, but when actin, microtubules, and CluA are disrupted, it is apparent that actin also has a contributory effect.
In addition to measuring the speed of mitochondrial movement we also quantified the percent of mitochondria moving from the kymographs. Approximately 72 and 87% of mitochondria are moving in the vehicle control EtOH and DMSO treated AX4 cells respectively (Figure 3). When treated with latrunculin-B, the percent of mitochondria moving was reduced by about 75% (p < 0.0001), while nocodazole treatment reduced the number of moving mitochondria by 39% (p < 0.0005). Further analysis showed that there is no synergism between microtubules and actin for determining how many mitochondria are moving. Thus, both actin and microtubules are necessary for determining how many mitochondria move, but actin is likely the predominant cytoskeletal element.
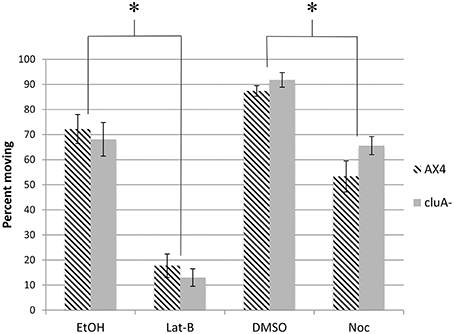
Figure 3. Percent of mitochondria moving in cytoskeleton disrupted AX4 and cluA− strains. In AX4 cells, all drug treatments significantly lowered the percent of mitochondria moving in comparison to their appropriate controls (Lat-B: p < 0.0001; Noc: p = 0.0005). This also occurred in the cluA− strain (Lat-B: p < 0.0001; Noc: p < 0.0013). Inhibiting the cytoskeleton significantly decreased the percent of mitochondria moving in both wild-type and cluA− strains. *Indicates significant differences.
For cluA− single vehicle controls, 92% of mitochondria were moving in DMSO treated cells, with 68% moving in ethanol. When microtubules were inhibited, the percent of mitochondria moving was reduced by 28% (p < 0.0013), while inhibiting actin reduced the number of moving mitochondria by 81% (p < 0.0001; Figure 3). Again there is no synergistic effect, thus these results suggest in cluA−, as in AX4, that actin plays the predominant role in the percent of moving mitochondria but both cytoskeletal elements are involved.
Overall, both actin and microtubules are necessary for mitochondrial motility in D. discoideum, while CluA seems to have no significant role. Our results indicate that microtubules play the largest role in determining velocity of movement, while actin seems to be more important for how many mitochondria are moving.
Assessing the Relationship between the Cytoskeleton and Mitochondrial Fission and Fusion in D. discoideum
Little is known about the cytoskeletal influences on mitochondrial fission and fusion but it is expected that the cytoskeleton would play a critical role. To give more insight to this potential interaction, we quantified both fission and fusion rates in the wild-type and cluA− strains when the microtubules or actin filaments were disrupted. The fission and fusion rates of drug treated cells were compared to an appropriate vehicle control, as well as across strains. Comparing these fission and fusion rates allowed us to determine the role of the cytoskeleton in D. discoideum fission and fusion.
Using laser scanning confocal microscopy, a series of single plane images of D. discoideum were captured that showed the real time mitochondrial movement in the cells. Fission and fusion events were quantified in each cell and a rate was calculated. When the actin cytoskeleton was inhibited in AX4 cells, fission (p = 1.0) and fusion (p = 1.0) rates were not significantly different compared to the control, additionally fission and fusion remained balanced within each treatment for both latrunculin-B (p = 1.0) and ethanol (p = 1.0) treated cells (Table 1).
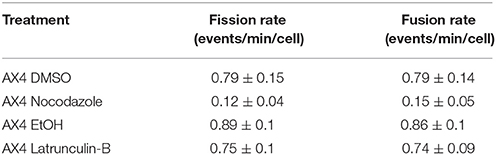
Table 1. Data table for the comparison of fission and fusion rates, with standard error, of all AX4 treatments.
The AX4 microtubule cytoskeleton was inhibited using nocodazole. In these cells the rates of fission and fusion remained balanced (Noc: p = 1.0; DMSO: p = 1.0; Table 1) though, it was apparent that inhibiting microtubules significantly lowered fission by 85% (p = 0.004) and fusion by 81% (p = 0.003; Table 1). Further analysis demonstrated there was no synergistic effect between microtubules and actin in these processes (data not shown), thus microtubules are required for mitochondrial fission and fusion while actin plays little to no role in these processes.
As the cytoskeleton is required for fission and fusion, we wondered if disrupting the cytoskeleton would also decrease the rates of fission and fusion in cells lacking CluA. Our results show that DMSO treated cluA− mitochondria fission and fusion rates were balanced (p = 1.0) and similar to the ethanol control rates, which were also balanced (p = 1.0; Table 2). cluA− strains treated with nocodazole showed significantly lower fission and fusion compared to the DMSO control, 81 and 78% respectively (fission, p < 0.0001; fusion, p < 0.0001; Table 2). Within treatment, the rates of fission and fusion remained balanced (Noc: p = 1.0; Table 2). When treated with the actin inhibiting drug latrunculin-B, there was no difference in fission (p = 1.0) or fusion (p = 1.0) when compared to the ethanol control (Table 2). Again, as in wild-type cells, further analysis demonstrated there was no synergistic effect between microtubules and actin in fission and fusion in cluA− cells (data not shown). Thus, the microtubules exert a greater influence on regulating mitochondrial fission and fusion. Moreover, actin filaments were found to have no significant effect, even in the absence of CluA.
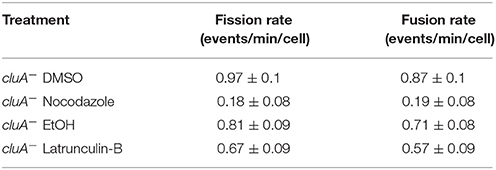
Table 2. Data table for the comparison of fission and fusion rates, with standard error, of all cluA− treatments.
Discussion
The Role of Microtubules in Mitochondrial Dynamics
Here we present data suggesting that microtubules are the predominant cytoskeletal element for moving and distributing D. discoideum mitochondria. We show an almost complete loss of motility in cells treated with nocodazole as well as the relaxed and more distributed clusters found in cluA− cells with disrupted microtubules. Additionally we and others have observed a small population of mitochondria that associate with the microtubules (Vlahou et al., 2011). Finally work by Vlahou et al demonstrates that mitochondrial distribution is dependent upon intact microtubules (Vlahou et al., 2011).
We also demonstrate that microtubules are essential for mitochondrial fission and fusion. It has not yet been teased out whether disruption of fission and fusion in these cells prevents motility or if motility must be functional for fission and fusion to take place. It has been suggested that blocks of fission and fusion will inhibit motility and distribution. Incomplete fission can result in a tangle of interconnected mitochondria and incomplete fusion can result in mitochondrial aggregates, thus motility's effect on the processes seems clear (Chen and Chan, 2009). On the other hand, it has been shown that loss of Miro, which inhibits motility, subsequently inhibits fusion (Cagalinec et al., 2013). It is logical to assume that motility facilitates fission and fusion as at least one mitochondrion must move toward another for fusion to take place and once divided the organelles must move apart to remain separate. Either way it is apparent that mitochondrial dynamics are intimately linked to motility and in D. discoideum, as suggested by our data, regulated by microtubules.
The Role of Actin in Mitochondrial Dynamics
Our results suggest that disruption of actin decreases the number of mitochondria moving, but the ones that are moving, move at the same speed and go to the same locations as in untreated cells. This suggests to us that while actin may not be a major highway for mitochondrial movement it may function as an entrance ramp, helping mitochondria get to the highway as needed. If this is the case, it is apparent that by actively targeting mitochondria, the cell can select the organelles that need to be transported to the sites of high energy needs or perhaps undergo fission and fusion to repair mitochondrial DNA preventing a buildup of damaged or older mitochondria. Microtubules can then move the selected mitochondria and regulate fission and fusion events. This model is similar to mitochondrial behavior in neurons. Neurons utilize the actin cytoskeleton to move mitochondria shorter distances and microtubules for long distance transport (Morris and Hollenbeck, 1995). The shorter distance movement is due to the neuron's need to retain mitochondria at sites of high ATP utilization (Boldogh and Pon, 2006).
The Role of CluA in Mitochondrial Dynamics
CluA is required for distribution and plays a role in fission and fusion. Our results indicate that tight cluster formation is dependent upon CluA, microtubules, and actin, but CluA is not a significant player in mitochondrial motility. Therefore, CluA is most likely not an adaptor protein linking mitochondria to the cytoskeleton. Instead we suggest that D. discoideum must have a novel adaptor protein not yet identified; perhaps an intermediate filament as indicated in 3T3 fibroblast cells (Nekrasova et al., 2011).
Interestingly while we suggest CluA is not a linker protein, the cytoskeleton does appear to play a larger role in mitochondrial distribution and motility when CluA is absent. The clustered phenotype of cluA− cells is relaxed by the disruption of the actin and microtubule cytoskeletons, and there is a synergistic decrease in motility when CluA, microtubules, and actin are all disrupted. Perhaps this is simply a result of mis-regulation of fission and fusion in cluA− cells.
In conclusion, D. discoideum mitochondria move along the microtubule cytoskeleton, similar to what is reported in animal cells, and without this movement mitochondrial fission and fusion cannot take place. Finally, we propose that the link between mitochondria and the microtubules is not CluA and that this protein plays an as yet unidentified role in mitochondrial fission and fusion.
Author Contributions
LW contributed to the acquisition, analysis and interpretation of the data, she drafted, revised, and approves the final version of the manuscript. GB contributed to the design and acquisition of the work. He revised and approves the final version of the manuscript. KN contributed to the design, acquisition, and interpretation of the work. She drafted, revised, and approves the final version of the manuscript. LW, GB, KN agree to be accountable for all aspects of the work.
Conflict of Interest Statement
The authors declare that the research was conducted in the absence of any commercial or financial relationships that could be construed as a potential conflict of interest.
Acknowledgments
The project described was supported by grants from the National Center for Research Resources (5P20RR016460-11) and the National Institute of General Medical Sciences (8 P20 GM103429-11) from the National Institutes of Health. The Zeiss LSM Pascal confocal microscope used for image acquisition was funded by NSF Award Number 0215847. The authors would like to thank the University of Central Arkansas and the Biology Department for support of this work.
References
Annesley, S., and Fisher, P. (2009). Dictyostelium discoideum—a model for many reasons. Mol. Cell. Biochem. 329, 73–91. doi: 10.1007/s11010-009-0111-8
Annesley, S. J., Chen, S., Francione, L. M., Sanislav, O., Chavan, A. J., Farah, C., et al. (2014). Dictyostelium, a microbial model for brain disease. Biochim. Biophys. Acta Gen. Sub. 1840, 1413–1432. doi: 10.1016/j.bbagen.2013.10.019
Baloh, R. H., Schmidt, R. E., Pestronk, A., and Milbrandt, J. (2007). Altered axonal mitochondrial transport in the pathogenesis of charcot-marie-tooth disease from Mitofusin 2 mutations. J. Neurosci. 27, 422–430. doi: 10.1523/JNEUROSCI.4798-06.2007
Barth, C., Le, P., and Fisher, P. R. (2007). Mitochondrial biology and disease in Dictyostelium. Int. Rev. Cytol. 263, 207–252. doi: 10.1016/S0074-7696(07)63005-8
Baughman, J. M., Perocchi, F., Girgis, H. S., Plovanich, M., Belcher-Timme, C. A., Sancak, Y., et al. (2011). Integrative genomics identifies MCU as an essential component of the mitochondrial calcium uniporter. Nature 476, 341–345. doi: 10.1038/nature10234
Bereiter-Hahn, J. (1990). “Behavior of mitochondria in the living cell,” in International Review of Cytology, Vol. 122, eds K. W. Jeon and M. Friedlander (San Diego, CA; London: Academic Press), 1–63. doi: 10.1016/S0074-7696(08)61205-X
Bereiter-Hahn, J., Vöth, M., Mai, S., and Jendrach, M. (2008). Structural implications of mitochondrial dynamics. Biotechnol. J. 3, 765–780. doi: 10.1002/biot.200800024
Bleazard, W., McCaffery, J., King, E., Bale, S., Mozdy, A., Tieu, Q., et al. (1999). The dynamin-related GTPases, Dnm1, regulates mitochondrial fission in yeast. Nat. Cell Biol. 1, 298–304. doi: 10.1038/13014
Boldogh, I. R., and Pon, L. A. (2006). Interactions of mitochondria with the actin cytoskeleton. Biochim. Biophys. Acta Mol. Cell Res. 1763, 450–462. doi: 10.1016/j.bbamcr.2006.02.014
Cagalinec, M., Safiulina, D., Liiv, M., Liiv, J., Choubey, V., Wareski, P., et al. (2013). Principles of the mitochondrial fusion and fission cycle in neurons. J. Cell Sci. 126, 2187–2197. doi: 10.1242/jcs.118844
Chen, H., and Chan, D. C. (2009). Mitochondrial dynamics–fusion, fission, movement, and mitophagy–in neurodegenerative diseases. Hum. Mol. Genet. 18, R169–R176. doi: 10.1093/hmg/ddp326
Chen, L. B. (1988). Mitochondrial membrane potential in living cells. Annu. Rev. Cell Biol. 4, 155–181. doi: 10.1146/annurev.cb.04.110188.001103
Cox, R., and Spradling, A. (2009). clueless, a conserved Drosophila gene required fro mitochondrial subcellular localization, interacts genetically with parkin. Disease Models Mechan. 2, 490–499. doi: 10.1242/dmm.002378
De Stefani, D., Raffaello, A., Teardo, E., Szabò, I., and Rizzuto, R. (2011). A 40 kDa protein of the inner membrane is the mitochondrial calcium uniporter. Nature 476, 336–340. doi: 10.1038/nature10230
El Zawily, A. M., Schwarzländer, M., Finkemeier, I., Johnston, I. G., Benamar, A., Cao, Y., et al. (2014). FRIENDLY regulates mitochondrial distribution, fusion, and quality control in Arabidopsis. Plant Physiol. 166, 808–828. doi: 10.1104/pp.114.243824
Fey, P., Dodson, R. J., Basu, S., and Chisholm, R. L. (2013). “One stop shop for everything dictyostelium: dictyBase and the dicty stock center in 2012,” in Dictyostelium Discoideum Protocols, eds L. Eichinger and F. Rivero (Totowa, NJ: Humana Press), 59–92.
Fields, S. D., Conrad, M. N., and Clarke, M. (1998). The S. cerevisiae CLU1 and D. discoideum cluA genes are functional homologues that influence mitochondrial morphology and distribution. J. Cell Sci. 111, 1717–1727.
Fields, S. D., Arana, Q., Heuser, J., and Clarke, M. (2002). Mitochondrial membrane dynamics are altered in cluA− mutants of Dictyostelium. J. Muscle Res. Cell Motil. 23, 829–838. doi: 10.1023/A:1024492031696
Francione, L. M., Annesley, S. J., Carilla-Latorre, S., Escalante, R., and Fisher, P. R. (2011). The Dictyostelium model for mitochondrial disease. Semin. Cell Dev. Biol. 22, 120–130. doi: 10.1016/j.semcdb.2010.11.004
Fransson, Å., Ruusala, A., and Aspenström, P. (2006). The atypical Rho GTPases Miro-1 and Miro-2 have essential roles in mitochondrial trafficking. Biochem. Biophys. Res. Commun. 344, 500–510. doi: 10.1016/j.bbrc.2006.03.163
Frederick, R. L., and Shaw, J. M. (2007). Moving mitochondria: establishing distribution of an essential organelle. Traffic 8, 1668–1675. doi: 10.1111/j.1600-0854.2007.00644.x
Frezza, C., Cipolat, S., Martins de Brito, O., Micaroni, M., Beznoussenko, G. V., Rudka, T., et al. (2006). OPA1 Controls apoptotic cristae remodeling independently from mitochondrial fusion. Cell 126, 177–189. doi: 10.1016/j.cell.2006.06.025
Gilson, P., Yu, X.-C., Hereld, D., Barth, C., Savage, A., Kiefel, B., et al. (2003). Two dictyostelium orthologs of the prokaryotic cell division protein FtsZ localize to mitochondria and are required for the maintenance of normal mitochondrial morphology. Eukaryotic Cell 2, 1315–1326. doi: 10.1128/EC.2.6.1315-1326.2003
Karbowski, M., and Youle, R. (2003). Dynamics of mitochondrial morphology in healthy cells and during apoptosis. Cell Death Differ. 10, 870–880. doi: 10.1038/sj.cdd.4401260
Kostal, V., and Arriaga, E. A. (2011). Capillary electrophoretic analysis reveals subcellular binding between individual mitochondria and cytoskeleton. Anal. Chem. 83, 1822–1829. doi: 10.1021/ac200068p
Liesa, M., Palacín, M., and Zorzano, A. (2009). Mitochondrial dynamics in mammalian health and disease. Physiol. Rev. 89, 799–845. doi: 10.1152/physrev.00030.2008
Lill, R., and Mühlenhoff, U. (2008). Maturation of iron-sulfur proteins in eukaryotes: mechanisms, connected processes, and diseases. Annu. Rev. Biochem. 77, 669–700. doi: 10.1146/annurev.biochem.76.052705.162653
Logan, D., Scott, I., and Tobin, A. (2003). The genetic control of plant mitochondrial morphology and dynamics. Plant J. 36, 500–509. doi: 10.1046/j.1365-313X.2003.01894.x
Maeda, Y., and Chida, J. (2013). Control of cell differentiation by mitochondria, typically evidenced in dictyostelium development. Biomolecules 3:943. doi: 10.3390/biom3040943
Margolin, W. (2000). Organelle division: self-assembling GTPases caught in the middle. Curr. Biol. 10, R328–R330. doi: 10.1016/S0960-9822(00)00458-9
Martinou, J.-C., and Youle, R. J. (2011). Mitochondria in apoptosis: Bcl-2 family members and mitochondrial dynamics. Dev. Cell 21, 92–101. doi: 10.1016/j.devcel.2011.06.017
Mitchell, P. (1961). Coupling of phosphorylation to electron and hydrogen transfer by a chemi-osmotic type of mechanism. Nature 191, 144–148. doi: 10.1038/191144a0
Morris, R. L., and Hollenbeck, P. J. (1995). Axonal transport of mitochondria along microtubules and F-actin in living vertebrate neurons. J. Cell Biol. 131, 1315–1326. doi: 10.1083/jcb.131.5.1315
Nekrasova, O. E., Mendez, M. G., Chernoivanenko, I. S., Tyurin-Kuzmin, P. A., Kuczmarski, E. R., Gelfand, V. I., et al. (2011). Vimentin intermediate filaments modulate the motility of mitochondria. Mol. Biol. Cell 22, 2282–2289. doi: 10.1091/mbc.E10-09-0766
Nunnari, J., Marshall, W., Straight, A., Murray, A., Sedat, J., and Walter, P. (1997). Mitochondrial transmission during mating in S. cerevisiae is determined by mitochondrial fusion and fission and the intramitochondrial segregation of mtDNA. Mol. Biol. Cell 8, 1233–1242. doi: 10.1091/mbc.8.7.1233
Nunnari, J., and Suomalainen, A. (2012). Mitochondria: in sickness and in health. Cell 148, 1145–1159. doi: 10.1016/j.cell.2012.02.035
Picard, M., Shirihai, O. S., Gentil, B. J., and Burelle, Y. (2013). Mitochondrial morphology transitions and functions: implications for retrograde signaling? Am. J. Physiol. Regul. Integr. Compar. Physiol. 304, R393–R406. doi: 10.1152/ajpregu.00584.2012
Rizzuto, R., Pinton, P., Carrington, W., Fay, F. S., Fogarty, K. E., Lifshitz, L. M., et al. (1998). Close contacts with the endoplasmic reticulum as determinants of mitochondrial Ca2+ responses. Science 280, 1763–1766. doi: 10.1126/science.280.5370.1763
Schimmel, B., Berbusse, G., and Naylor, K. (2012). Mitochondrial fission and fusion in Dictyostelium discoideum: a search for proteins involved in membrane dynamics. BMC Res. 5:505. doi: 10.1186/1756-0500-5-505
Schneider, C. A., Rasband, W. S., and Eliceiri, K. W. (2012). NIH Image to ImageJ: 25 years of image analysis. Nat. Meth. 9, 671–675. doi: 10.1038/nmeth.2089
Twig, G., and Shirihai, O. S. (2011). The interplay between mitochondrial dynamics and mitophagy. Antioxid. Redox Signal. 14, 1939–1951. doi: 10.1089/ars.2010.3779
Van Gestel, K., Köhler, R. H., and Verbelen, J. P. (2002). Plant mitochondria move on F−actin, but their positioning in the cortical cytoplasm depends on both F−actin and microtubules. J. Exp. Bot. 53, 659–667. doi: 10.1093/jexbot/53.369.659
Vlahou, G., Eliáš, M., von Kleist-Retzow, J.-C., Wiesner, R. J., and Rivero, F. (2011). The Ras related GTPase Miro is not required for mitochondrial transport in Dictyostelium discoideum. Eur. J. Cell Biol. 90, 342–355. doi: 10.1016/j.ejcb.2010.10.012
Wu, M., Kalyanasundaram, A., and Zhu, J. (2013). Structural and biomechanical basis of mitochondrial movement in eukaryotic cells. Int. J. Nanomed. 8, 4033–4042. doi: 10.2147/IJN.S52132
Yaffe, M. P., Stuurman, N., and Vale, R. D. (2003). Mitochondrial positioning in fission yeast is driven by association with dynamic microtubules and mitotic spindle poles. Proc. Natl. Acad. Sci. U.S.A. 100, 11424–11428. doi: 10.1073/pnas.1534703100
Youle, R. J., and van der Bliek, A. M. (2012). Mitochondrial fission, fusion, and stress. Science 337, 1062–1065. doi: 10.1126/science.1219855
Keywords: mitochondria, fission, fusion, cytoskeleton, latrunculin, nocodazole, Dictyostelium discoideum, CluA
Citation: Woods LC, Berbusse GW and Naylor K (2016) Microtubules Are Essential for Mitochondrial Dynamics–Fission, Fusion, and Motility–in Dictyostelium discoideum. Front. Cell Dev. Biol. 4:19. doi: 10.3389/fcell.2016.00019
Received: 28 January 2016; Accepted: 03 March 2016;
Published: 22 March 2016.
Edited by:
Michael Schrader, University of Exeter, UKCopyright © 2016 Woods, Berbusse and Naylor. This is an open-access article distributed under the terms of the Creative Commons Attribution License (CC BY). The use, distribution or reproduction in other forums is permitted, provided the original author(s) or licensor are credited and that the original publication in this journal is cited, in accordance with accepted academic practice. No use, distribution or reproduction is permitted which does not comply with these terms.
*Correspondence: Kari Naylor, a2tuYXlsb3JAdWNhLmVkdQ==