- 1Department of Cellular, Molecular and Developmental Neurobiology, Cajal Institute, Consejo Superior de Investigaciones Científicas, Madrid, Spain
- 2Champalimaud Research, Champalimaud Centre for the Unknown, Lisbon, Portugal
During vertebrate embryonic development, the spinal cord is formed by the neural derivatives of a neuromesodermal population that is specified at early stages of development and which develops in concert with the caudal regression of the primitive streak. Several processes related to spinal cord specification and maturation are coupled to this caudal extension including neurogenesis, ventral patterning and neural crest specification and all of them seem to be crucially regulated by Fibroblast Growth Factor (FGF) signaling, which is prominently active in the neuromesodermal region and transiently in its derivatives. Here we review the role of FGF signaling in those processes, trying to separate its different functions and highlighting the interactions with other signaling pathways. Finally, these early functions of FGF signaling in spinal cord development may underlay partly its ability to promote regeneration in the lesioned spinal cord as well as its action promoting specific fates in neural stem cell cultures that may be used for therapeutical purposes.
Introduction
The spinal cord is the most caudal part of the nervous system which is responsible for body motion, including locomotion, somatosensation and the control of basic functions of the autonomous nervous system. During development, in addition to the neurons that reside within the spinal cord, it provides neural crest cells for the formation of sensory ganglia, ganglia of the autonomous system and for the enteric nervous system. A fundamental aspect of spinal cord development is its relation to the organs and muscles it innervates. Thus, spinal cord development appears highly coordinated in space and time with the caudal extension that accompanies the development of the whole body.
The spinal cord cells of vertebrates derive from a region initially specified as neuromesodermal progenitors (NMP) with mixed neural and mesodermal characteristics (Wilson et al., 2009; Henrique et al., 2015; Row et al., 2016), with the exception of those forming the floor plate which in amniotes derive from the node. In chick and mouse, this corresponds to a region of the epiblast adjacent to the early node and the rostral primitive streak. From this population, some cells remain in the ectoderm layer and form most of the spinal cord, while others gastrulate through the primitive streak to become part of the paraxial mesoderm (Wilson et al., 2009; Henrique et al., 2015). Later, with the closure of the caudal neuropore, the NMP region remains in the tailbud from which the caudal spinal cord and mesodermal populations segregate. Overall, this constitutes an ongoing process that takes several days to generate the complete rostrocaudal axis. Different aspects of spinal cord development such as initiation of neurogenesis, ventral patterning and neural crest specification and migration are conditioned by this caudal axis elongation (Figure 1). This is a complex process involving several signaling pathways and gene networks in which the FGF signaling pathway stands as a crucial regulator, maintaining cells in an immature state until they are displaced to a region where they are no longer influenced by it.
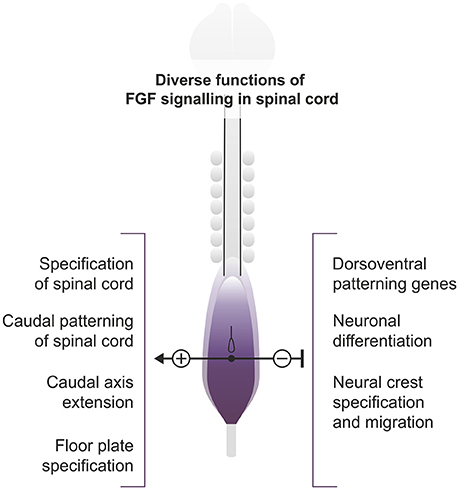
Figure 1. Roles of FGF signaling in the extending spinal cord. Diagram representing a 7-somite stage chick embryo and showing the region of active caudal FGF signaling. The different processes discussed in the review either promoted (+) or inhibited (−) by FGF signaling are shown.
FGF signaling acts in numerous stages and tissues during embryonic development and the use of experimental approaches designed to manipulate the FGF signaling pathways at specific stages and tissues has been fundamental to overcome its early roles in implantation, gastrulation, and neural induction. These include treatment of tissue explants with FGF factors or pharmacological antagonists, expression of pathway inhibitors or truncated FGFR proteins that function interfering with the normal function in the neural tube cells in ovo and the use of conditional mouse mutants specifically removing FGFs or FGFR in the NMP and its derivatives.
Here, we review the contribution of FGF signaling in the initial process of spinal cord specification and elongation and then we cover the initiation of neurogenesis, ventral patterning, and neural crest specification and migration. We make an effort to identify and separate the different steps in these highly interconnected networks governing spinal cord extension and associated events, focusing on the influence of FGF signaling on the neural tissue. In addition, we have selected some of the evidence supporting the use of FGF to promote regeneration of the lesioned adult spinal cord both acting on spinal cord cells in vivo as well as to promote expansion of neural stem cells in vitro and their differentiation toward specific neuronal fates for their use for regenerative purposes.
Note: Gene symbols are italicized in all species, but there are specie-specific differences. Thus, gene symbols for human and chick appear all in upper-case; for mouse and rat with only the first letter in upper-case and for fish, gene symbols appear with all letters in lower-case. In the case of protein symbols, they are not italicized and all letters are in upper-case, except in fishes where only the first letter is upper-case (http://www.biosciencewriters.com/Guidelines-for-Formatting-Gene-and-Protein-Names.aspx). When referring to genes from several species they have been separated by a slash.
FGF Signaling Pathway: Expression of Components in the Developing Spinal Cord
Let's first start with a brief introduction of the components of the FGF signaling pathway in the context of spinal cord development. As most signaling pathways, the FGF pathway includes ligands, receptors, modulators, intracellular transducers, and final effectors (Ornitz and Itoh, 2015). The only components exclusive for the pathway are the ligands (up to 23 FGFs have been described in vertebrates) and their receptors of the tyrosin kinase (RTK) type (FGFR1–4 in vertebrates). Other more general players, which are also used by other signaling pathways, such as the pathway inhibitors SPROUTY2, SEF, DUSP6, and the transcription factor effectors of the ETV family, are particularly associated to this pathway as the corresponding mRNAs are highly expressed in regions with high FGF activity and in particular in the caudal NMP region (Chotteau-Lelievre et al., 2001; Karabagli et al., 2002; Corson et al., 2003; Harduf et al., 2005; Lunn et al., 2007). Interestingly, they are themselves downstream targets of the pathway and are thus considered its readouts and have been the basis for the development of pathway activity reporters (Molina et al., 2007; Ekerot et al., 2008). However, as these downstream targets of the pathway are not exclusively activated by the FGF pathway, they do not constitute definitive readouts of the activity of the FGF pathway. The identification of cells where the pathway is truly active is still one of the main difficulties in the analysis of FGF function, as none of the intracellular cascades is specific for FGF signaling and the difference with other RTK pathways may be in the fine tuning of the signaling properties.
The three main intracellular cascades that can mediate the FGF signal are: the RAS-MAPK, the PI3K-AKT and the PLPCγ pathways (Figure 2). High levels of MAPK phosphorylation are detected in the NMPs and surrounding area and these depend on the activation of FGF pathway (Lunn et al., 2007). Moreover, most of the effects resulting from FGFR inhibition in this region can also be observed following the inhibition of MEK (MAPK Kinase), suggesting this is the main FGFR downstream pathway in this region (Diez del Corral et al., 2002; Delfino-Machin et al., 2005; Lunn et al., 2007; Martinez-Morales et al., 2011; Olivera-Martinez et al., 2012; Morales et al., 2016).
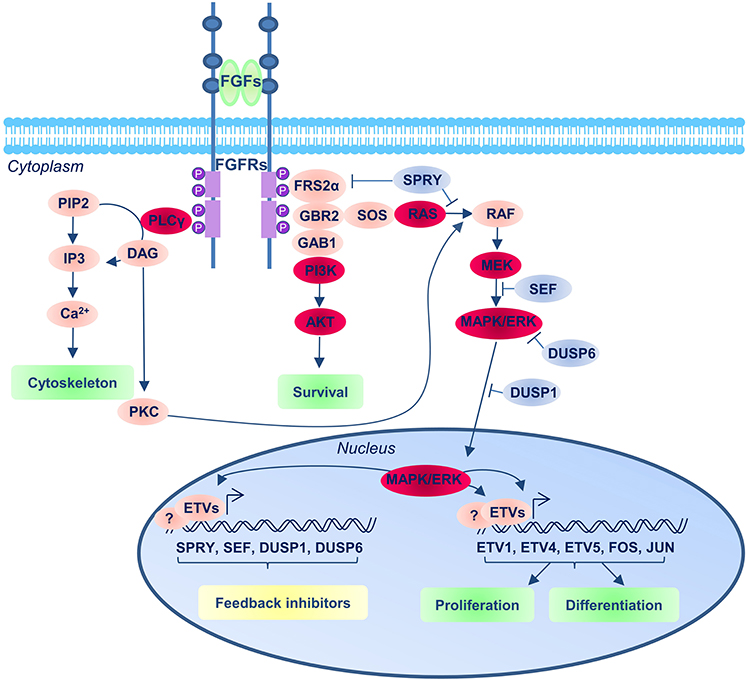
Figure 2. FGF signaling pathway. The FGFRs consist of three extracellular immunoglobulin-type domains (D1–D3; blue balls in the receptor), a single-span trans-membrane domain and an intracellular split domain. FGFs interact with the D2 and D3 domains, and promote upon binding receptor dimerization and tyrosine kinase autophosphorylation of the FGFRs that results in the recruitment and assembly of signaling complexes. The main three downstream FGF/FGFR signaling complexes operating in the context of neural development are represented (the red balloons indicate the main components of the pathway): the Ras/MEK/MAPK/ERK; the PI3K/AKT and the PLCγ pathways. The blue balloons indicate the repressor regulators of the pathways.
A gradient of AKT phosphorylation has also been described in the region surrounding the node with higher levels caudally (Dubrulle et al., 2001) but the exposure to PI3K inhibitors does not result in the same effects as blockade of FGFR signaling (Martinez-Morales et al., 2011) and thus the relevance of the AKT pathway in this context has not been addressed further.
The most comprehensive analysis of the expression patterns of FGF signaling related genes in spinal cord development has been performed in the chick. At the stages of chick spinal cord specification, several FGFs, including FGF3, FGF4, FGF8, FGF13, FGF18, are expressed in the caudal NMP region or surrounding tissues (Karabagli et al., 2002; Delfino-Machin et al., 2005). During later stages (during spinal cord elongation and including tailbud formation) FGF3, FGF4, FGF13 and FGF18 become restricted to the primitive streak while FGF8 is more broadly expressed in the streak, the adjacent NMP region and the ingressing mesoderm (Karabagli et al., 2002; Delfino-Machin et al., 2005). Expression of FGF8 is highly dynamic as those cells that progress from the NMP state to the spinal cord fate or from the presomitic mesoderm to the somitic mesoderm slowly downregulate their expression (Figures 3A–C). Expression of FGF4 and FGF8 in the NMP region (including caudal lateral epiblast and later, the tailbud) continues for several days but declines toward the final stages of somitogenesis and the cessation of axis elongation (Cunningham et al., 2011; Olivera-Martinez et al., 2012).
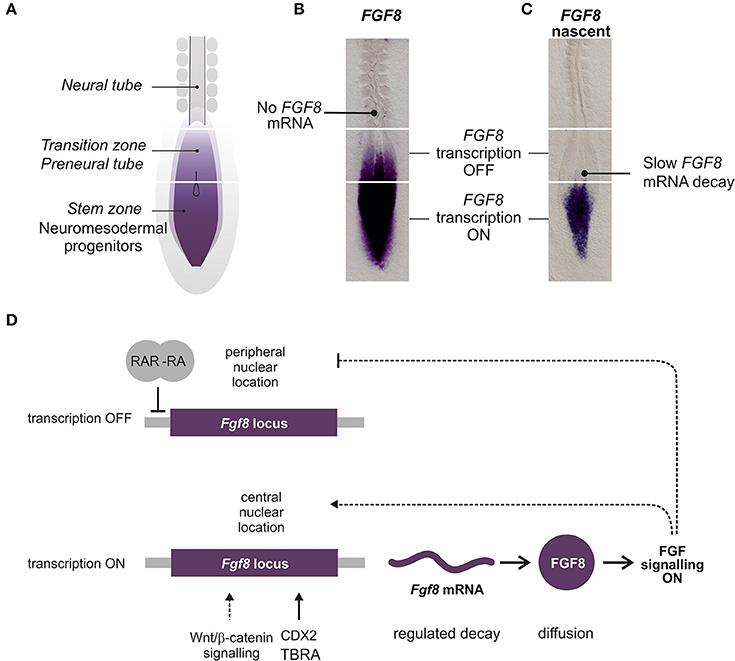
Figure 3. Regulation of caudal FGF8/Fgf8 expression during spinal cord extension. (A) Diagram representing the caudal part of a chick embryo where the different regions related to FGF8 expression are labeled. (B) Chick embryo showing FGF8 mRNA detected by in situ hybridization with a full length FGF8 probe. (C) Embryo showing the nascent FGF8 pre-mRNA detected by in situ hybridization with an intronic FGF8 probe. (D) Diagram representing the main factors contributing to the expression of Fgf8 in the caudal NMP. Lines represent possible direct interactions of transcription factors and dashed lines indirect relations.
FGFR1-3 are initially present in the NMP zone (Karabagli et al., 2002; Lunn et al., 2007; Nishita et al., 2011), but later, only FGFR1 remains throughout the neural tissue including the NMP region while FGFR2 is absent there and becomes restricted to the neural tube, rostral to Hensen's node, and FGFR3 restricts to the neural tube adjacent to somites (Karabagli et al., 2002; Lunn et al., 2007; Nishita et al., 2011).
Similar expression patterns have been described in mouse for those genes analyzed. In mouse, expression of Fgf3, Fgf4, Fgf8, Fgf17, and Fgfr1 has been reported in and around the NMP region (Gofflot et al., 1997; Wahl et al., 2007; Anderson et al., 2016a). In zebrafish, in addition to fgf4 and fgf8, fgf17, fgf17b, and fgf24 (a zebrafish exclusive gene) have also been shown in or near the tailbud (Reifers et al., 2000; Draper et al., 2003; Cao et al., 2004; Akiyama et al., 2014).
Very little is known about the regulation of expression of FGFRs and FGFs. Most work has been done with FGF8, but the control of FGF8 transcription in the spinal cord NMP region and its progressive downregulation coordinated with embryonic axial extension still constitutes an unsolved enigma (Figure 3). Three regions have been identified with respect to FGF8 expression: the most caudal region where FGF8 is actively transcribed (NMP), a more rostral region where transcription is stopped but transcripts remain (known as the transition zone or the preneural tube) and a third most rostral region, adjacent to the mesoderm ready to segment where transcripts are no longer detected (Figures 3A–C).
Several signaling pathways have been shown to influence Fgf8 expression either promoting or decreasing Fgf8 levels (Figure 3D). The WNT/β-Catenin pathway is active in the caudal region (Aulehla et al., 2003; Olivera-Martinez and Storey, 2007; Cunningham et al., 2011) and manipulation of the pathway has been shown to affect Fgf8 expression. Reduced levels of Fgf8 have been shown in the Wnt3a mouse mutant vestigial tail (Aulehla et al., 2003) and a further reduction is observed in double Wnt3a/Wnt8a mutants (Cunningham et al., 2015b). Furthermore, altering the levels of β-Catenin in the PSM promotes changes in Fgf8 expression (Aulehla et al., 2008; Dunty et al., 2008). In fact, studies in mouse craniofacial development support a direct role for WNT in Fgf8 regulation throughout a conserved Tcf/Lef site 2.8 kb upstream of Fgf8 (Wang et al., 2011). However, no upregulation of FGF8 by WNT has been observed in chick spinal cord suggesting a more indirect regulation of FGF8 by the WNT in the context of caudal neural tube (Olivera-Martinez and Storey, 2007). Evidence has also been presented for the requirement of signals from the notochord. In particular, a reduced level of FGF8 is observed in the absence of the notochord that can be rescued by SHH supplementation (Resende et al., 2010).
An autoregulatory mechanism of FGF activating FGF8 has been suggested based on the ability of FGF8 to activate the transcriptional repressor NKX1.2 (previously known as SAX1; Bertrand et al., 2000) which when overexpressed can in turn result in increased FGF8 levels (Sasai et al., 2014). However, exposure of the neural explants or embryos to FGF does not result in activation of FGF8 expression and inhibition of FGF signaling does not result in decreased FGF8 or Fgf8 levels in chick and mouse, respectively (Harrison et al., 2011; Patel et al., 2013), raising the possibility that NKX1.2 may be involved in the stabilization of FGF8/Fgf8 transcripts.
In addition, although a localized source of a regulator of FGF8 caudal to Hensen's node has been ruled out (Dubrulle and Pourquie, 2004; Harrison et al., 2011), other caudally active pathways such the HMG-CoA reductase/mevalonate pathway (mediating steroid biogenesis) could be also playing a role in FGF8 expression activation and maintenance (Olivera-Martinez et al., 2014).
Recent efforts for the characterization of the Fgf8 gene regulatory region have led to the identification of several regions driving expression around the NMP region (Beermann et al., 2006; Marinic et al., 2013) and furthermore to the identification of CDX2 and TBRA as direct transcriptional activators (Amin et al., 2016). This could explain the decreased Fgf8 levels that have been observed in Cdx2 mutants (Savory et al., 2009) but additional activators may also be acting to regulate Fgf8, such as WNT/β–Catenin.
In addition to signals maintaining FGF8/Fgf8 expression in the caudal precursor region, progressive downregulation of FGF8/Fgf8 involves cessation of transcription in cells that exit the NMP region. Retinoic acid (RA; which is produced by somites and rostral presomitic mesoderm) has been shown to downregulate FGF8/Fgf8 and reduction in RA signaling (in a vitamin A deprived quail model and in Raldh2−/− mutants) results in a rostral expansion of the FGF8/Fgf8 expression domain (Diez del Corral et al., 2003; Molotkova et al., 2005; Vermot and Pourquie, 2005; Sirbu and Duester, 2006; Olivera-Martinez and Storey, 2007; Patel et al., 2013; Kumar and Duester, 2014; Cunningham et al., 2015a). This effect of RA has recently been attributed to direct binding of RA receptor (RAR) to an RA response-element (RARE) in the regulatory region in the Fgf8 promoter (Kumar and Duester, 2014; Cunningham and Duester, 2015). This constitutes one of the few examples described where RAR bound to RA would repress gene transcription. Furthermore, additional studies show how NCOR repressors are required for RA repression of Fgf8 (Kumar et al., 2016).
However, as the forced reduction in RA signaling only promotes a limited expansion of the FGF8/Fgf8 domain, additional mechanisms of transcriptional repression must be involved. One possible theoretical mechanism proposed would involve a caudal diffusing signal transcribed in NMP cells that would repress both its own transcription as well as FGF8/Fgf8 (Harrison et al., 2011). However, to date no such signal has been identified. Moreover, failure of FGF8/Fgf8 to be expressed more anteriorly in spinal cord and somites could be due to a lack of transcriptional activators such as TBRA, CDX, and WNT expression.
Interestingly, the change in the transcriptional state of Fgf8 locus from active to inactive is associated to a change in its nuclear position from a more central location in the NMP to a more peripheral position in neural tube cells (Patel et al., 2013). This location seems to be regulated by FGF signaling per se as inhibiting FGFR signaling results in a more peripheral location of Fgf8 transcription in the caudal region. However, in spite of the change of location, transcription of Fgf8 still occurs (Harrison et al., 2011; Patel et al., 2013) suggesting that location of the Fgf8 locus to the periphery is required but is not sufficient for cessation of expression.
As mentioned above, analysis of active transcription by in situ hybridization using intronic FGF8/Fgf8 probes has shown that the actively transcribing region is rather limited and that cessation of FGF8/Fgf8 transcription seems to be abrupt (Dubrulle and Pourquie, 2004). However, the high stability of the transcript (which can perdure more than 5 h) is such that a gradient of FGF8/Fgf8 transcripts can be generated. The mechanism accounting for this high FGF8/Fgf8 stability however has not been further explored.
Final steps in the regulation of FGF8/Fgf8 expression are the decrease in FGF8/Fgf8 levels associated to the trunk to tail transition and the termination of transcription in the tailbud associated to the termination of axis elongation. Mouse embryos with mutations associated to a prolonged trunk extension (Gdf11 loss of function mutants or overexpression of OCT4; Aires et al., 2016) show an abnormal increase in Fgf8 expression in the tailbud region. In the chick, RA derived from the tailbud is also important for the correct termination of FGF8 expression (Olivera-Martinez et al., 2012) while in the mouse other mechanisms seem to be responsible (Cunningham et al., 2011), as body axis extension continues for a much longer time to form the tail in mouse.
As FGFs are secreted factors, their distribution also depends on their diffusion and transport in the extracellular medium. This has been examined in detail in zebrafish embryos mostly in the context of gastrulation but this may be extended to other situations (reviewed in Bokel and Brand, 2013). There, the binding to heparan sulfates, important constituents of the extracellular matrix, is not only relevant for the activation of the receptor by the ligands but also has an influence on the spread of Fgfs (Yu et al., 2009). The shape of the gradient is also greatly influenced by degradation of Fgf8 that may be largely due to its endocytic removal (Scholpp and Brand, 2004).
Overall, a complex gene regulatory network is in place in the NMP region involving several interconnected signaling pathways that ensures that FGF signaling components are expressed at the appropriate levels for the control of a number of processes that take place as the neural tube extends to form the spinal cord. Given the temporally controlled exposure of cells to FGFs in and around the NMP region, the measurement of the activity of the pathway at the level of the receptor as well as at the different downstream components in fixed tissue and in vivo, as the axis extends caudally, would greatly improve our understanding of the coordination of morphogenetic movements and the control of tissue differentiation.
FGFs and the Establishment of the Caudal Neuromesodermal Progenitors and of the Spinal Cord Identity
It has recently become clear that the spinal cord derives progressively from the caudal NMP region which is specified through FGF and WNT actions on sensitized epiblast cells around the primitive streak (Henrique et al., 2015). Specification of the NMP region is initiated at gastrulation stages (Muhr et al., 1999; Delfino-Machin et al., 2005; Nordstrom et al., 2006) within a region around the node and primitive streak characterized by expression of genes such as NKX1.2, CDX1, CDX2, CDX4, and HOXB8 where high MAPK signaling levels are present. This coincidence is maintained during axis extension (Delfino-Machin et al., 2005; Lunn et al., 2007) and reflects the activity of FGF in the control of the expression of those genes (Storey et al., 1998; Muhr et al., 1999; Bel-Vialar et al., 2002; Delfino-Machin et al., 2005; Nordstrom et al., 2006; Sasai et al., 2014). Thus, in the chick embryo, blockade of FGF signaling with a dominant negative form of FGFR (DN-FGFR) and with pharmacological inhibitors results in downregulation of NKX1.2 and HOXB8 in vivo and in explant cultures (Delfino-Machin et al., 2005). In double Fgf4; Fgf8 conditional mutant mice, there is a decrease in Wnt3a, Wnt5a, Cyp26a1, T-Bra in the NMP region (Naiche et al., 2011; Boulet and Capecchi, 2012). Similarly, Fgfr1 conditional mutants also show decreased levels of a number of NMP region genes, such as Gbx2 and Cyp26a1 (Wahl et al., 2007). All these results, from the current perspective that stresses the relevance of the NMP cells, suggest that FGF contributes in an important way to the specification of the NMP character in chick and mouse embryos, including genes expressed in NMP and its mesodermal derivatives (T-BRA) as well as those expressed in NMP and its neural derivatives (i.e., NKX1.2). In the same direction, in Xenopus and zebrafish embryos, expression of a dominant negative form of FGFR/Fgfr (DN-FGFR/DN-Fgfr) results in the loss of markers of NMP and its derivatives (Isaacs et al., 1994; Griffin et al., 1995; Holowacz and Sokol, 1999; Ota et al., 2009).
The NMP give rise to both spinal cord and mesodermal cells during an extended period of time and FGF levels also contribute to preserve the balance between the three cell types. For instance, double Fgf4; Fgf8 conditional mutant mice where defective signaling is restricted to the NMP and its derivatives display dramatic reduction of the presomitic mesoderm markers Tbx6 (Naiche et al., 2011; Boulet and Capecchi, 2012) and display ectopic neural tubes (Boulet and Capecchi, 2012) similar to the ones observed in Fgfr mutant chimeras (Ciruna et al., 1997). In chick, pharmacological inhibition of FGFR results in precocious and caudal expression of the neural tube specific gene SOX1 (Stavridis et al., 2010). On the other hand, situations with excessive caudal FGF8 signaling such as the Raldh2 mutant present an imbalanced NMP differentiation favoring mesodermal fate (Cunningham et al., 2015a).
This suggests a requirement of FGF signaling for the promotion of mesodermal or neuromesodermal vs. neural fates (Henrique et al., 2015). Most interestingly, FGF signaling has been shown recently to promote the expression of enzymes that drive the glycolytic metabolic state of the NMP region (Oginuma et al., 2017) that is in turn important for WNT signaling and for restraining the transition from a NMP state to a neural state (Oginuma et al., 2017). Later on, that glycolytic metabolic state in a gradient fashion also operates in presomitic mesoderm development (Bulusu et al., 2017).
In spite of FGF promotion of neuromesodermal and mesodermal fates, FGF signaling in combination with WNT signaling also appears to contribute to the activation and maintenance of the expression of the neural genes SOX2 and SOX3 through specific gene regulatory regions (Takemoto et al., 2006; Nishimura et al., 2012) and this might help to prevent the excess production of mesoderm precursors from the NMP (Yoshida et al., 2014).
The precise sequence of exposure of cells to FGF in combination with the other caudal signal WNT as well as the temporal dynamics within the cells may here determine whether cells are maintained in a NMP state, differentiate toward a mesodermal fate or toward a neural fate. This idea has been recently explored with experiments developing in vitro methods to generate a population of cells that co-express the NMP genes from mouse and human pluripotent stem cells by timed exposure to FGF2 in combination with WNTs (Gouti et al., 2014; Turner et al., 2014; reviewed in Henrique et al., 2015). This constitutes a good example of how the temporal exposure and competence to interpret FGF signals play an important role in specification of cell fates.
Once spinal cord cells leave the NMP region, FGF is not required for the maintenance of the spinal cord identity. Thus, the spinal cord specific homeobox transcription factor HOXB8, that initially requires FGF for its expression in the NMP (Delfino-Machin et al., 2005), remains actively expressed in spinal cord progenitors after the levels of FGF signaling have dropped during axis elongation.
The different mechanisms responsible for the role of FGF in specification of the NMP and then in the balance of mesodermal and neural derivatives may be related to the coactivity with other signals and/or to the temporal sequence of exposure and response of cells to FGF and other signals, as suggested by the cell culture experiments. All these crucial aspects certainly deserve now a thorough analysis within the developing embryo.
FGFs and the Control of Spinal Cord Caudal Extension
The most striking feature of embryos where FGF signaling has been diminished (once the early lethality is overcome) is the truncation of the caudal embryonic axis, observed in mouse, Xenopus and zebrafish. Mouse Fgfr1−/− embryonic chimeras cannot gastrulate properly and mutant cells tend to accumulate in the tail displaying a short axis (Ciruna et al., 1997; Ciruna and Rossant, 2001). Similarly, Fgfr1 conditional mutant mice where defective signaling is restricted to the caudal NMP and derivatives (using a TBra- driven Cre-line), result in truncated axis at the level of sacral regions (Wahl et al., 2007). An even shorter axis is observed in the double Fgf4; Fgf8 conditional knock-out (Naiche et al., 2011; Boulet and Capecchi, 2012; either using a TBra- or a Hoxb1-driven Cre-lines). A shortened tail is also apparent in the Fgf3 null mutant embryos (Anderson et al., 2016a,b). Similarly, in Xenopus and zebrafish the overexpression of DN-FGFR/DN-Fgfr versions also result in truncated embryos (Griffin et al., 1995; Holowacz and Sokol, 1999) and in chick decreased elongation rates have been observed following blockade of FGFR (Benazeraf et al., 2010).
However, in all these situations the lack of FGF signaling affects specification of both mesodermal and neural derivatives and it is therefore not possible to assess whether the defect on elongation is a consequence of an alteration in gastrulation, in the specification of NMP, spinal cord or mesoderm, the result of abnormal motility in the mesoderm (Benazeraf et al., 2010) or whether there is a more specific requirement within the spinal cord population. Support for a more localized role of FGF signaling in spinal cord caudal extension came from analysis of cell distribution after electroporating a DN-FGFR1 construct in chick NMP region (Mathis et al., 2001; therein referred to as node region). In control experiments, cells could either remain in the NMP region and continue the backward displacement or get incorporated into the neural tube. However, cells with decreased FGF signaling had an increased probability to get incorporated in the neural tube and thus would not be part of the caudally displaced NMP region suggesting some changes in cell adhesion properties of those cells, at least indirectly. In addition, a role of FGF in the maintenance of proliferating cells could also contribute to the extension of the axis (Mathis et al., 2001).
In presomitic mesoderm, axis extension has been shown to involve differential motility of cells along the rostrocaudal axis in a space constrained by lateral boundaries (possibly the lateral plate), with cells moving more in caudal presomitic mesoderm than in the rostral part. Interestingly, in that context, FGF signaling has been shown to promote cell motility (Benazeraf et al., 2010; Lawton et al., 2013). As mentioned before, FGF is required for the transcription of rate limiting enzymes responsible for the glycolytic metabolic state of the NMP that has been shown to be important for cell motility and axis elongation (Oginuma et al., 2017). The mechanism of control of cell motility is still not known but it has been proposed to be related to the ability of localized glycolytic activity to ensure rapid production of ATP for actin polymerization in the forming protrusions of motile cells (Oginuma et al., 2017). In other contexts, FGF has been shown to have chemotaxis properties (Yang et al., 2002) and this has been suggested as an additional mechanism that could in theory contribute to axis extension (Harrison et al., 2011). In any case, given that the spinal cord is composed by epithelial cells and not by mesenchymal cells (as it is the case for presomitic mesoderm) it is unlikely that the same morphogenetic mechanisms are responsible for its extension which may be a more passive process driven by mesoderm.
Recent work on the generation of a population with NMP properties by differentiation of mouse embryonic stem cells (mESCs) in adherent cell culture has shown that these cell aggregates also have the ability to elongate in vitro and that this elongation requires FGF signaling, providing an in vitro system where this function can be further examined (Turner et al., 2014). In conclusion, there are still many unknowns in relation to the cellular process of spinal cord extension. Most likely, the combination of in vitro culture systems together with imaging techniques (both in vitro and in vivo), the use of biosensors to investigate metabolism in developing embryos (such as the PYRATES mouse line, Bulusu et al., 2017) and in silico simulations will greatly contribute to the understanding of the important role of FGF signaling in spinal cord extension.
FGFs and the Control of Cell Proliferation, Cell Cycle Exit and Neuronal Differentiation
FGFs play important roles in cell survival and proliferation in many developmental contexts and in particular for neural stem cells and progenitors (Vaccarino et al., 1999; Storm et al., 2006; Maric et al., 2007). In the developing spinal cord, analysis of cell cycle exit (Sechrist and Bronner-Fraser, 1991) and of the early postmitotic marker NeuroM (Roztocil et al., 1997) revealed two regions with respect to cell proliferation. NeuroM+ cells start to appear in the region flanked by somites while no NeuroM+ cells are found in the more caudal region (the preneural tube) nor in the NMP region, coinciding with the region of influence of FGF signaling (Diez del Corral et al., 2002).
Exposure of the neural tube to FGF at a stage when some cells are already exiting the cell cycle can impair the generation of new NeuroM expressing cells (Diez del Corral et al., 2002) and by that way, the onset of neurogenesis. By following the fate of neural progenitors using time lapse imaging, it has been possible to analyze the changes in the dynamics of progenitors associated to FGF exposure (Wilcock et al., 2007). Neural progenitors and stem cells can normally experience three modes of division to give rise to neurons (N) and progenitors and stem cells (P): self-expanding, PP (i.e., giving rise to 2 progenitors or stem cells); self-replacing, PN (i.e., giving rise to a progenitor and a neuron); and self-consuming, NN (i.e., giving rise to 2 neurons). Previous studies in the developing cortex and spinal cord suggest that different modes are associated with different cell cycle duration times, with neuron generating divisions (PN or NN) characterized by a longer cell cycle than PP divisions (Takahashi et al., 1995; Calegari and Huttner, 2003; Calegari et al., 2005; Wilcock et al., 2007).
Upon exposure to FGFs, progenitors only go through PP divisions while no PN nor NN divisions could be observed (Wilcock et al., 2007). These FGF induced PP divisions exhibited the typical short PP cell cycle length while no changes in the range of cleavage plane orientation were observed. Interestingly, a subpopulation of cells was found dividing without contacting the apical membrane and with very short cell cycle times (Wilcock et al., 2007). These data support a role for FGF in the maintenance of cells characterized by a rapid cell cycle that can only generate further progenitors. Interestingly, within the embryo, shorter cell cycle lengths are observed in the region exposed to FGF with respect to the rostral neural tube (Olivera-Martinez et al., 2014) and several cell cycle genes are differentially expressed in the caudal vs. more rostral region and could be regulated by FGF (Lobjois et al., 2004; Olivera-Martinez et al., 2014). One example is CYCLIN D2, a cell cycle regulator specifically expressed in the chicken caudal neural plate that can be activated by and requires FGF signaling (Lobjois et al., 2004; Molina and Pituello, in press).
Although exposure to FGF can impede neurogenesis, blockade of FGF signal in explants is not sufficient to drive premature expression of the postmitotic and neurogenesis marker NeuroM (Diez del Corral et al., 2002). However, as discussed above, cells subject to interference with FGF signaling in the embryo tend to prematurely leave the NMP region (Mathis et al., 2001) where only proliferating cells are found and it remains to be assessed whether they have alterations in their type of division or cell cycle exit parameters.
A high level of aerobic glycolysis is known to facilitate cancer cell proliferation. Although no significant change in proliferation was observed by Oginuma et al. (2017) in embryos grown in the absence of glucose, more detailed analysis are required in order to determine a possible implication of the FGF dependent changes in metabolism in the control of proliferation during axis extension and more specifically within the spinal cord.
The contribution of FGF to the control of proliferation in the spinal cord discussed above is restricted to cells before or at the onset of neurogenesis and could be equivalent to the ability of FGF2 and FGF8 in the telencephalon to maintain the proliferative symmetrical PP divisions of neuroepithelial cells before the onset of neurogenesis (Raballo et al., 2000; Storm et al., 2006; Maric et al., 2007; Rash et al., 2013). Interestingly, the analysis of the telencephalon of mutant mouse embryos has revealed additional requirements for FGF signaling in proliferation of neurogenic lineages at different steps. At the start of telencephalon neurogenesis, neuroepithelial cells transform into radial glial cells, which divide asymmetrically to generate another radial glia and a postmitotic neuron or a basal progenitor (Gotz and Huttner, 2005) and this transition is promoted by FGF10 (Sahara and O'Leary, 2009). Finally, after neurogenesis has started, it has been demonstrated (using mutants for three FGF receptors) that FGF signaling is required to slow down the progression from radial glia to basal progenitors (Kang et al., 2009; Rash et al., 2011). Similar roles for FGF at later stages of spinal cord development remain to be explored (see below for functions during spinal cord adult neurogenesis).
In addition to a more direct action of FGF on the cell cycle, several FGF dependent pathways could mediate its influence on cell cycle exit and neuronal differentiation before the onset of neurogenesis in the spinal cord. FGF signaling is required for the expression of DELTA-1, an important component of the NOTCH signaling pathway involved in mutual inhibition in the NMP region and required to limit precocious cell cycle exit (Akai et al., 2005). Additionally, FGF signaling promotes WNT8a expression, which in turn prevents neuronal differentiation (Olivera-Martinez and Storey, 2007).
Manipulation of FGF signaling in chick embryo explants and the use of mouse mutants has shown that FGFs can reduce the levels of RA signaling, a neuronal differentiation promoter (reviewed in Diez del Corral and Morales, 2014) and this would also favor the maintenance of the progenitor state. Double Fgf4; Fgf8 conditional mutant mouse embryos exhibit increased caudal RARE-lacZ reporter expression (Naiche et al., 2011). But, at what level could FGF act on the control of RA signaling? FGF4 and FGF8 can repress the gene encoding the RA-synthesizing enzyme RALDH2 in the paraxial mesoderm (Diez del Corral et al., 2003). However, the contribution of this repression to the RA levels is probably partial since double Fgf4; Fgf8 conditional mutant mouse embryos do not exhibit increased Raldh2 expression (Boulet and Capecchi, 2012). FGF signaling is required for the caudal expression of the RA-degrading enzyme Cyp26a1 (Wahl et al., 2007) and this could also contribute to the control of RA levels similarly to what has been described in the context of the hindbrain (Gonzalez-Quevedo et al., 2010). FGF4 and FGF8 can also downregulate RARβ receptor levels in the spinal cord (Olivera-Martinez and Storey, 2007) and this would affect the sensitivity to RA levels. This receptor gene depends on RA for its activation (Olivera-Martinez and Storey, 2007) and thus its downregulation by FGF could be due to upregulation of Cyp26a1 but this has not been examined yet.
FGF signaling is also required to prevent precocious activation of PAX6 and IRX3 in chick and Pax6 in mouse (Bertrand et al., 2000; Diez del Corral et al., 2003; Patel et al., 2013), two transcription factors which promote neuronal differentiation (de la Calle-Mustienes et al., 2002; Bel-Vialar et al., 2007). Thus, FGF seems to contribute to a rather complex network that controls proliferation before the onset of neurogenesis maintaining an undifferentiated state. However, open questions still remain: does FGF signaling act differentially on the process of proliferation within NMP and then for promotion of self-renewal of neural progenitors? Does it act differently in the spinal cord than in telencephalon progenitors where it has also been involved in the appearance of intermediate progenitors? What are the cell cycle components modulated by FGF signaling in all these processes?
FGFs and Patterning of Spinal Cord Along the Rostro-Caudal Axis
Once the region of the neural plate giving rise to the spinal cord has been specified (in an FGF dependent way), FGF signaling has an additional role in the further regionalization of the spinal cord along the rostral-caudal axis. The spinal cord presents heterogeneity along the rostro-caudal axis responsible for differences in motor neuron subpopulations, interneuron distribution (Francius et al., 2013; Lai et al., 2016) or neural crest derivatives (Le Douarin et al., 2004). This regionalization, which has been mainly examined in motor neurons, is a consequence of the restricted rostro-caudal expression of Hox genes in progenitor cells and subsequently in the resulting postmitotic motor neurons (reviewed in Philippidou and Dasen, 2013).
Experiments in chick embryos have shown that exposure to FGF or electroporation of FGFs expressing constructs shifts rostrally the domain of expression of caudal HOX mRNAs (HOXB6, HOXC6, HOXB7, HOXB8, and HOXA9-B9-C9) in neural progenitors resulting in an increase in the protein levels of a subset of HOXB proteins (Bel-Vialar et al., 2002; Dasen et al., 2003). FGF signaling appears to act here by activating the transcription factor genes of the Cdx family, known to activate HOX/Hox gene expression, in particular cdx2 and cdx4 in zebrafish (Shimizu et al., 2006), CDX1 and CDX2 in the chick (Bel-Vialar et al., 2002), and Cdx1, Cdx2, and Cdx4 in mouse (van den Akker et al., 2002; Amin et al., 2016). Exposure to FGF not only has consequences in the expression of genes in progenitors but also in the resulting motor neurons (Liu et al., 2001; Dasen et al., 2003). Explants of neural tissue fated to give rise to cervical spinal cord do not express HOXC6, HOXC8, HOXC9, or HOXC10 after culture but their exposure to increasing FGF levels results in progressive activation of the production of these proteins suggesting that FGF works in a concentration dependent way. Considering that in the embryo, caudal cells are exposed to FGF for a longer period of time than rostral cells, but not necessarily to higher levels of FGF signaling, this concentration dependent effect has also been interpreted as an effect of the duration of exposure to the FGF morphogen. The mechanism to explain such concentration/time of exposure dependence is still not known but may involve the regulation of genes encoding transcription factors of the CDX family mentioned above.
The role of FGF signaling in this further caudalization, however, has not yet been ascertained by loss of function approaches and therefore, the extent of its contribution to patterning remains an open question. A possible contribution of FGF to rostro-caudal patterning of interneurons has also been suggested (Francius et al., 2013) but has not been explored yet.
FGFs and Ventral Pattern (Intermediate, Ventral and Floor Plate)
Another regionalization process where FGF signaling plays an essential role is the patterning of the spinal cord along the dorso-ventral (DV) axis which is fundamental for the assignment of neuronal subtype identities such as motor neurons and the different interneuron subtypes (reviewed in Gouti et al., 2015). Specific combinations of transcription factors of the homeodomain and bHLH families are expressed in restricted domains along the DV axis (reviewed in Le Dreau and Marti, 2012). In the ventral/intermediate neural tube this is regulated by the SHH morphogen. The graded distribution of SHH, produced in the ventral midline, results in a graded activation of the pathway and the expression of target genes (reviewed in Briscoe and Small, 2015). In addition, cross-repressive interactions between target genes occur to further delimit and ensure gene expression in the appropriate domains (Briscoe et al., 2000; Kutejova et al., 2016).
During spinal cord caudal extension, SHH is expressed in the node and along the derived notochord while in the neural tissue it is expressed in floor plate (FP) cells at the level of the somitic mesoderm. Thus, cells in the preneural tube (the transient spinal cord population derived from NMP and adjacent to presomitic mesoderm) are initially exposed to notochord derived SHH and express some SHH target genes such as GLI1, PTCH1 and PTCH2 suggesting that at least low SHH signaling is achieved (Diez del Corral et al., 2003; Morales et al., 2016). However, neural progenitors in the preneural tube do not display expression of the complete repertoire of ventral identity genes, suggesting that the pathway is being modulated in this region. A role for FGF signaling in the control of ventral patterning was first inferred from its ability to repress PAX6, a gene expressed in an intermediate domain in the neural tube (Bertrand et al., 2000). Since that observation, a more complex picture has emerged showing that FGF signaling is crucial for controlling the onset of SHH signaling and ventral patterning in the spinal cord (Diez del Corral et al., 2003; Morales et al., 2016) and for the early specification of the most ventral fate, the FP (Sasai et al., 2014; Figure 4).
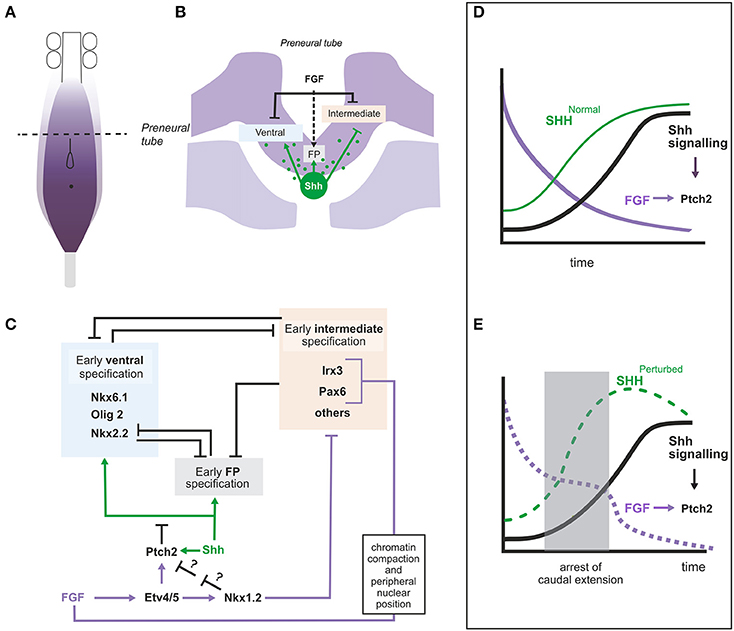
Figure 4. Role of FGF signaling in the regionalization of the intermediate and ventral neural tube. (A) Diagram representing the caudal part of an embryo showing the region with active FGF signaling and the rostro-caudal level of the transverse section represented in (B). (B) Transverse section at the level of the preneural tube showing the neural tissue and the underlying presomitic mesoderm and notochord. FGF8 (produced by neural and mesoderm tissues) and Shh (produced by notochord) are represented in purple and green respectively and their influence on intermediate, ventral and floorplate specification is shown. The dashed arrow indicates that FGF provides competence for floor plate specification. (C) Gene regulatory network relating FGF and Shh signaling in the pre-neural tube where the two signals coincide. Data from chick, mouse or both are included in this figure. (D,E) Graphs to illustrate the hypothetical role of the regulation of Ptch2 by FGF during the initial establishment of the Shh signaling levels. The graphs represent the changing levels of SHH in time at a particular position (within the dorsoventral and rostrocaudal axis). (D) In embryos continuously extending their caudal axis, the levels of FGF (purple) at a particular position would decrease constantly while the levels of SHH (green, SHHNormal) would increase until they reach their maximum. The levels of Shh signaling thus also increase progressively. (E) In embryos where elongation is arrested for some time (shaded area), FGF levels would remain constant during the arrested period, while the levels of SHH (SHHPerturbed) would accumulate more rapidly due to the decreased amount of tissue generated through which SHH could diffuse (or be transported). If Shh signaling was dependent exclusively on SHH levels, the level of the signaling would also increase to levels higher than normal and this may result in the irreversible activation of its targets. However, the ability of FGF to activate Ptch2 and thus downregulate the Shh pathway could serve to limit Shh signaling levels to normal values. FGF signaling levels (decaying in time) are shown in purple.
Forced maintenance of FGF signaling in preneural tube tissue, impairs not only PAX6 but also other ventral and intermediate patterning genes such as NKX6.1, NKX6.2, IRX3, and FOXA2 (Bertrand et al., 2000; Diez del Corral et al., 2003; Novitch et al., 2003). Conversely, interference with FGF signaling in chick embryos results in precocious caudal activation of PAX6 and IRX3 (Bertrand et al., 2000; Diez del Corral et al., 2003) and in the dorsal expansion of ventral markers such as OLIG2 and NKX6.1 (Morales et al., 2016). Reduced FGF signaling in mouse embryos results in precocious caudal Pax6 (Patel et al., 2013) and NKX6.1 expression as well as in alterations in the ventral patterning with an increase in the number of NKX6.1 expressing neural progenitor cells (Morales et al., 2016).
FGF would thus be repressing more or less indirectly the two types of SHH responding genes, ventral genes activated by SHH (FOXA2 and NKX6.1) and intermediate genes repressed by SHH (PAX6 and IRX3) (Briscoe et al., 2000). Repression of PAX6 and IRX3 and their mouse homologs seems to involve several mechanisms (Figures 4B,C). FGF signaling promotes chromatin compaction and peripheral nuclear position around the mouse Pax6 and Irx3 loci, a chromatin organization associated to transcriptionally inactive loci (Patel et al., 2013). In addition, repression of these genes appears to be mediated by transcriptional repressor NKX1.2, transcriptionally activated by FGF signaling in NMP region (Storey et al., 1998; Bertrand et al., 2000; Sasai et al., 2014; Figure 4C).
A molecular mechanism that accounts for the effect of FGF on genes relying on SHH for their expression has been identified recently (Figure 4C; Morales et al., 2016). FGF can activate the expression of PTCH2, one of the SHH receptors that also acts as an inhibitor of the SHH pathway, and can thus restrain expression of SHH targets. Experiments in chick explants have shown that PTCH2 is expressed in the preneural tube in a SHH and FGF dependent way indicating the existence of an enhanced feedback loop where SHH activates PTCH2 more efficiently in regions of high FGF signaling. This regulation also appears to be conserved in mouse as Fgfr1 conditional mutant embryos show extremely reduced Ptch2 levels (Morales et al., 2016). Surprisingly, however, the Ptch2 gene seems to be largely dispensable as no obvious phenotype has been identified yet in the mouse mutant (Holtz et al., 2013). It is possible that its function is only apparent when the development of the embryo is challenged, for example when the elongation process is altered. If elongation is arrested, the high levels of PTCH2 in the spinal cord precursor may maintain the levels of SHH signaling low and ventral patterning on standby mode until the elongation is restored (Figures 4D,E). In fact, Ptch2 is required to keep SHH signaling in check in situations of partial deficiency of the other member of the family, Ptch1, both in development and in tumorigenesis (Lee et al., 2006; Nieuwenhuis et al., 2006; Holtz et al., 2013; Zhulyn et al., 2015).
In addition, and probably as a result of its role on repressing ventral and intermediate genes, an important role for FGF on the specification of FP cells has been recently identified in chick (Sasai et al., 2014). FP territory, characterized by expression of the ARX1 protein, is induced by the highest levels of SHH that are only achieved in the cells closest to its source and also requires transient FGF exposure (Sasai et al., 2014). Here again, NKX1.2 plays an important role, providing competence to respond to high SHH levels and drive ARX1 expression. Given the repressive interactions between FP specific genes and the ventral and intermediate patterning genes (Cho et al., 2014; Kutejova et al., 2016), one important function for FGF signaling and NKX1.2 here would be to ensure that a region free of expression of non-floor plate factors such as PAX6, IRX3 and NKX2.2 is established in the future FP region (Sasai et al., 2014). Nevertheless, the details of the gene regulatory network are still not elucidated as expression of ARX1 (and other definitive floor plate markers) is only apparent well after the FGF signaling levels have decayed. Here again, the system may be highly redundant as no obvious alterations in the FP have been reported in Nkx1.2 mutant mice (Simon and Lufkin, 2003), raising the possibility that the related Nkx1.1 gene could be also playing a role.
Antagonism of the FGF signaling pathway with the RA pathway is also important in the context of ventral patterning as RA is required for expression of several intermediate and ventral genes (chick NKX6.1, IRX3, PAX6, OLIG2; Diez del Corral et al., 2003; Novitch et al., 2003; Diez del Corral and Morales, 2014 and mouse Nkx6.1, Pax6, and Olig2; Molotkova et al., 2005). However, the temporal and quantitative contributions of both FGF and RA pathways in the modulation of ventral specification require a deeper analysis.
FGFs and Neural Crest Specification
At the most dorsal part of the spinal cord, the development of a specific cell population also requires the participation of FGF signaling: the neural crest cells (NCCs). The neural crest is formed by a transient population of multipotent cells that arise from the dorsal neural tube. Once specified, NCCs undergo a process of epithelium to mesenchyme transition (EMT) that confers NCCs the ability to delaminate and migrate away from the dorsal neural tube, giving rise to NCC derivatives that include craniofacial skeleton, the peripheral nervous system (sensory neurons and glia, sympathetic neurons) and melanocytes, amongst others (Le Douarin and Kalcheim, 1999).
The process of neural crest formation implies the orchestration of a complex gene regulatory network. This involves signaling pathways and transcription factors that are responsible for the sequence of early induction of the NCC during gastrulation; the specification of the neural plate border; the expression of bona fide NCC transcription factors and the regulation of numerous downstream effectors involved in EMT, cell adhesion, and cell cycle control, amongst others (Morales et al., 2005; Sauka-Spengler and Bronner-Fraser, 2008). First, parallel to the induction and patterning of the neural plate that generates the central nervous system, at the border between the neural ectoderm and the non-neural ectoderm, the NCCs are specified through a series of steps controlled by FGF, WNT, and BMP signaling pathways (reviewed in Saint-Jeannet and Moody, 2014).
Transient exposure to FGF has been shown to allow neural tube cells to activate NCC markers in response to BMP (Sasai et al., 2014). This seems related to the ability of FGF to repress PAX6 and IRX3, two intermediate neural tube genes which can repress the NCC marker SNAIL (Sasai et al., 2014). It has been proposed that the repression of IRX3 and PAX6 by FGF, acting through activation of NKX1.2, is required for the early establishment of a territory competent to NCC specification (see the ventral patterning section for a further discussion on possible mechanisms for FGF regulation of PAX6 and IRX3). However, in FGF deficient conditions impaired NCC specification in vivo has not been reported yet. On the contrary, forced reduction of FGF signaling allowed neuroepithelial cells to prematurely initiate the expression of the early NCC specifier SNAIL2 at caudal levels (Martinez-Morales et al., 2011). This indicates that dorsal neuroepithelial progenitors in the caudal neural tube are maintained in an uncommitted non-NCC state in presence of strong FGF/MAPK signaling pathway (Martinez-Morales et al., 2011). Thus, in the elongating neural tube, as the dorsal neuroepithelial progenitors are progressively exposed to decreasing FGF signaling levels, they initiate the expression of neural crest specifier genes SNAIL2 and FOXD3.
Interestingly, upon reduction of FGF signaling, when those prematurely SNAIL2 expressing NCCs initiate the expression of other NCCs specifiers such as FOXD3, SOX5, and SOX10 they prematurely start EMT from the neural tube at mid-rostral PSM levels. Essentially, the regulated decrease in FGF signaling is primary responsible for the control of the initiation of NCC specification in the trunk, and as a consequence of that, it controls the timing of EMT and emigration. Subsequent development of trunk NCCs is highly dependent on the development of adjacent somites, which impose a segmented migration and organization to the trunk NCCs and to the derived peripheral nervous system (Sela-Donenfeld and Kalcheim, 1999). Considering that FGF signaling is important both for segmentation of the mesoderm and for the neural crest specification it would constitute an important mechanism of coordination of both tissues.
As it has been described above, FGF and RA signaling can act as opposite gradients, each one negatively regulating the activity of the other. In the context of NCC development, RA signaling produced by the somites does not appear to promote their specification but does trigger the EMT of already specified NCCs (Martinez-Morales et al., 2011). FGF and RA signaling control the timing of EMT and emigration in part through modulation of elements of the BMP and WNT signaling pathways, important signaling cascades operating in the dorsal neural tube (Sela-Donenfeld and Kalcheim, 1999; Burstyn-Cohen et al., 2004). Whereas, RA signaling triggers the initiation of WNT1 expression in the dorsal neural tube at levels where the NCCs are already specified, FGF signaling prevents the premature expression of WNT1 (Martinez-Morales et al., 2011).
Moreover, recently it has been established that another FGF ligand, FGF3, coming from the caudal presomitic mesoderm provides another level of regulation of BMP signaling in the spinal cord at tailbud stages. Fgf3 mutant embryos exhibit axis truncation, increase in neuroepithelial proliferation, delay in neural tube closure and premature neural crest formation (Anderson et al., 2016a). The removal of one copy of NOGGIN, a BMP antagonist, in Fgf3 mutants, exacerbated all the Fgf3 phenotypes including premature neural crest specification. Conversely, genetically decreasing BMP signaling in Fgf3 mutants, via loss of BMP receptor activity, ameliorates morphological defects (Anderson et al., 2016a).
In summary, the data discussed in this section show that there is a limited time window during which the onset of the NCC emigration can be modulated, once those cells have acquired the expression of the essential gene network of the NCC specification program. That window coincides with the region where FGF and RA gradients collide. This FGF function constitutes another example of a general FGF role in controlling the onset of differentiation of cell types as they are generated at the tail end, during trunk axial elongation. Again, the molecular mechanism that allows the cells to interpret and execute that temporal window imposed by FGF signaling is far from understood and remains an important open question within the developmental biology field.
FGFs and Neural Stem Maintenance in the Adult Spinal Cord
As we have discussed, during the development of the nervous system, the generation of hundreds of subtypes of neurons and glial cells relies upon the relatively fast production, amplification, specification, and differentiation of a pool of neural progenitors and neural stem cells (NSCs). Surprisingly, this strategy is retained to some extent in niches in the adult nervous system throughout lifetime under physiological conditions to generate specific subtypes of neural cells in limited numbers.
Adult NSCs are maintained into adulthood in two main niches, the ventricular-subventricular zone (V-SVZ) adjacent to the lateral ventricles and the subgranular zone (SGZ) in the hippocampus (reviewed in Fuentealba et al., 2012; Christian et al., 2014). Nevertheless, cells with neural stem cell properties can be isolated from most regions of the adult central nervous system, including, for example, the spinal cord (Weiss et al., 1996; Shihabuddin et al., 1997).
In the adult spinal cord, the cells with neural stem cell properties are the ependymal cells (Johansson et al., 1999; Meletis et al., 2008; Barnabe-Heider et al., 2010; Pfenninger et al., 2011). They rarely proliferate under physiological conditions and they mostly give rise to ependymal progeny in vivo. It is unclear which signals are responsible for maintaining this population of ependymal cells. However, in other neurogenic niches such as the SGZ of the hippocampus dentate gyrus (DG), the specific deletion of all the FGF receptors that are expressed in DG (Fgfr1, Fgfr2, and Fgfr3) in adult precursor cells has shown that, FGF signaling is required for neural stem-cell maintenance while an activated FGF receptor expressed in all precursors can increase the number of neurons produced (Kang and Hebert, 2015). The requirement for FGF receptors in maintaining stem but not progenitor cells in the adult hippocampus is reminiscent of their role in maintaining cortical radial glial stem cells during development (Kang et al., 2009).
In spite of the limited expansion of spinal cord ependymal stem cells under normal physiological conditions, their proliferation is dramatically increased after spinal cord injury, giving rise to scar-forming astrocytes as well as to a small population of remyelinating oligodendrocytes (Johansson et al., 1999; Meletis et al., 2008; Barnabe-Heider et al., 2010). More importantly, the ependymal derived astrocytes are essential for repairing the lesions because if their formation is inhibited, the lesions grow deeper over time and a higher number of axonal tracts are lost (Sabelstrom et al., 2013).
The application of FGF2 has been shown to promote functional recovery after spinal cord injury (SCI) in rodents (Lee et al., 1999; Rabchevsky et al., 1999; Yan et al., 2000; Kim et al., 2006). In SCI the recovery is thought to be due to FGF promoting the proliferation of spinal cord neural stem and progenitor cells expressing PAX6, NESTIN, and SOX2 (Shihabuddin et al., 1997; Goldshmit et al., 2014), promoting neuronal survival (Teng et al., 1998, 1999), angiogenesis (Kang et al., 2013), and causing a reduction in injury volume (Lee et al., 1999; Rabchevsky et al., 1999). In addition, FGF2 may reduce glial scar formation and astrogliosis after SCI in the mouse model (Goldshmit et al., 2014). In this situation, FGF2 influences glial cell activation, generating a proregenerative radial/progenitor-like state rather than reactive astrocytes that form scar tissue that are inhibitory to axonal regeneration. It is unclear if these proliferating astrocytes could be derived from the neural stem ependymal cells.
FGF2 also reduces the inflammatory response, as it causes the reduction in macrophage infiltration and cytokine levels (Goldshmit et al., 2014). The reduction in macrophage infiltration may be due to the ability of FGF-2 to reduce the leakiness of the blood-spinal cord barrier after SCI (Kang et al., 2010). Moreover, in combination with transplanting specific cells (Meijs et al., 2004; Kuo et al., 2011; Guzen et al., 2012; Lu et al., 2012) or with special scaffold forming hydrogels FGF1 and FGF2 can provide a proregenerative effect and may have clinical applications in the treatment of SCI (Chen et al., 2015). In fact, FGF1 is currently in clinical trials in human patients with cervical SCI (Wu et al., 2011) and more recently also in combination with special devices and rehabilitation in patients with thoracic SCI (clinical trial, NIH reference NCT02490501).
Since SCI has multiple factors that determine the progress of the injury, a combinatorial therapeutic approach including FGF will most likely be required for the most effective treatment of SCI (reviewed in Siddiqui et al., 2015; Ahuja et al., 2016).
FGFs Promoting Neurogenesis in a Dish
As a complementary approach and as a way to overcome the limited capacity for self-repair of the mammalian nervous system, efforts are being made to boost the repair process by transplanting exogenous cells into sites of injury (Rosser et al., 2007). FGFs can be used to generate, expand, and differentiate neurons in vitro and therefore have a major role to play in such cell replacement therapies.
First, FGF2 together with EGF has been extensively used to promote proliferation and self-renewal of NSCs in vitro (Kilpatrick and Bartlett, 1993; Gage et al., 1995; Gritti et al., 1996; Qian et al., 1997; Nelson and Svendsen, 2006). FGF2 converts embryonic stem cells into neural stem cells characterized by rapid self-renewing and the potential to generate neurons, astrocytes, and oligodendrocytes. This acquired tripotent neural stem cell state, which does not exist in vivo, provide high proliferative capacity and glial differentiation potential to the treated cells (Palmer et al., 1999; Laywell et al., 2000; Zhang et al., 2001; Gabay et al., 2003; Hack et al., 2004; Pollard et al., 2008). Several studies then showed that FGF2 ventralizes cultured rodent NSCs/NPCs of dorsal origin and induces oligodendrocytes from NSCs derived from regions where oligodendrocytes are not present (Gabay et al., 2003).
FGF2 has also been proved to be involved in neuronal subtype specification, as it has been shown that in-vitro-expanded human fetal forebrain-derived NSCs can generate cholinergic neurons with spinal motor neuron properties when treated with FGF2 within a specific time window (Jordan et al., 2009). Moreover, ESC-derived motor neurons, grown using a differentiation program that relies on endogenous embryoid body-derived WNTS, FGFs, and HH signaling, and then grafted isochronically into chick spinal cord, settle in appropriate columnar domains and select axonal trajectories with a fidelity that matches that of their in vivo generated counterparts (Peljto et al., 2010). Under those differentiation conditions, it is not clear if increasing FGF levels would increase motor neuron yields without sacrificing the columnar and motor pool subtype diversity achieved.
In the last few years induced pluripotent stem cells (iPSCs) have provided a platform for studying basic human development and disease mechanisms and hold great potential for future cell therapies (Murry and Keller, 2008). Nevertheless, biomedical application of iPSCs depends on the availability of robust cell expansion and differentiation protocols. A recent example is the use of FGFR inhibitor (SU5402) that promoted iPSCs to commit to a NCC cell fate that express specific genes, including PAX3, SLUG, TFAP-2α, and TWIST1 (Jaroonwitchawan et al., 2016).
FGF is also required for the specification of cell types outside the embryonic spinal cord such as the midbrain dopaminergic neurons (Ye et al., 1998). Human pluripotent stem cells have also been successfully converted into dopaminergic neurons using a novel floor plate-based strategy that involves the use of SHH and WNT agonists together with FGF8 and these are efficiently engrafted in vivo using rat, mouse and monkey models (Kriks et al., 2011). This could be promising for the development of cell-based therapies in Parkinson's disease.
Finally, it also important to consider the oncogenic risk associated to the mitogenic potential of cells treated with FGFs in transplantation experiments. As a recent example, human cord blood-derived iPSCs have been differentiated into dopaminergic neurons using either FGF2 or BMP/TGF-β inhibitor for neural induction. After transplantation in hemiparkinsonian rats in vivo, proliferation still occurred in FGF2-derived grafts (but not in BMP inhibitor treated grafts), resulting in tumor-like growth (Effenberg et al., 2015). Similarly, those effects have also been described for neurospheres derived from hIPSCs and transplanted into spinal cord injured mice (Nori et al., 2015).
Future Directions and Challenges
This review highlights the multiple steps in spinal cord development that are regulated by FGF signaling, which may be viewed as a sensor of caudal elongation serving to coordinate different aspects of spinal cord maturation to each other, to adjacent mesoderm and to axial elongation. Further analysis of FGF signaling deficiency in mouse would help ascertain the extent of its contribution to floor plate formation, early neurogenesis, rostro-caudal patterning and neural crest development.
The molecular mechanisms that link FGF signaling specifically to the different functions are still not fully identified but for most of its functions, specific transcriptional targets downstream of the pathway have been proposed. It has been shown that FGF influences transcription by changing the phosphorylation state of transcription factors such as those of the ETV family. The analysis of the regulatory regions of the proposed targets will confirm which of them are more directly regulated. FGF also has an influence on chromatin compaction and nuclear positioning of specific gene loci (Patel et al., 2013) and this may be due, at least in part to the ability of FGF to regulate chromatin modifiers such as histone deacetylase 1 (HDAC1) (Olivera-Martinez et al., 2014).
The detailed regulation of the pathway including the intracellular dynamics of the MAPK pathway with its positive and negative feedbacks (Lake et al., 2016) as well as the involvement of the other FGFR dependent cascades (AKT, PKC) in some of the processes described here also remains largely unexplored. The understanding of the mechanisms responsible for the maintenance of FGF8 and FGF4, the principal ligands in this context, in the NMP and adjacent regions and their progressive downregulation would provide a better insight into axis elongation.
So far, the majority of the literature relies on static views of the expression of ligands and pathway components at different developmental stages. However, it is clear that those are highly dynamic and thus the development of reliable biosensors to measure FGF activity in vivo would help to address fundamental questions such as the mechanisms underlying the temporal changes in the response of NMP and its derivatives to FGF.
Throughout the review, we have focused on the similarities that exist in the different vertebrate species but it would also be interesting to understand how FGF functions may have diverged to accommodate the different modes of spinal cord formation (Steventon and Martinez Arias, in press). Equally interesting would be to study the emergence, during chordate evolution, of a function of caudal FGF on development of the caudal neural tube. FGF signaling has been described in the tailbud of the amphioxus cephalochordate embryo and, although only a limited role in somitogenesis has been described (Bertrand et al., 2015), it would be interesting to assess its requirement in spinal cord development.
Some of the functions of FGF described in the development of the spinal cord may also contribute to the maintenance of the ependymal neurogenic niche present in the adult spinal cord and to the functional recovery after SCI shown in rodents and currently under study in humans. Furthermore, the role of FGFs in the maintenance and expansion of neural progenitors as well as their promotion of specific fates in vitro supports their therapeutical potential in regenerative biomedicine. The advances in understanding the detailed mechanism underlying FGF function during the development of the central nervous system, and in particular of the spinal cord, should serve to selectively potentiate some of its functions.
Author Contributions
RD and AM jointly conceived, organized and wrote the manuscript.
Conflict of Interest Statement
The authors declare that the research was conducted in the absence of any commercial or financial relationships that could be construed as a potential conflict of interest.
Acknowledgments
We thank Gil Costa (Champalimaud Research, Champalimaud Centre for the Unknown, Lisbon, Portugal) for illustrations in Figures 1, 3, 4. We acknowledge support of our work by a grant from the Spanish MINECO (BFU2014-57494-R) and from Champalimaud Foudation (to RD), and support of the publication fee by the CSIC Open Access Publication Support Initiative through its Unit of Information Resources for Research (URICI).
References
Ahuja, C. S., Martin, A. R., and Fehlings, M. (2016). Recent advances in managing a spinal cord injury secondary to trauma. F1000Res 5:1017. doi: 10.12688/f1000research.7586.1
Aires, R., Jurberg, A. D., Leal, F., Novoa, A., Cohn, M. J., and Mallo, M. (2016). Oct4 is a key regulator of vertebrate trunk length diversity. Dev. Cell 38, 262–274. doi: 10.1016/j.devcel.2016.06.021
Akai, J., Halley, P. A., and Storey, K. G. (2005). FGF-dependent Notch signaling maintains the spinal cord stem zone. Genes Dev. 19, 2877–2887. doi: 10.1101/gad.357705
Akiyama, R., Masuda, M., Tsuge, S., Bessho, Y., and Matsui, T. (2014). An anterior limit of FGF/Erk signal activity marks the earliest future somite boundary in zebrafish. Development 141, 1104–1109. doi: 10.1242/dev.098905
Amin, S., Neijts, R., Simmini, S., van Rooijen, C., Tan, S. C., Kester, L., et al. (2016). Cdx and T brachyury co-activate growth signaling in the embryonic axial progenitor niche. Cell Rep. 17, 3165–3177. doi: 10.1016/j.celrep.2016.11.069
Anderson, M. J., Schimmang, T., and Lewandoski, M. (2016a). An FGF3-BMP signaling axis regulates caudal neural tube closure, neural crest specification and anterior-posterior axis extension. PLoS Genet. 12:e1006018. doi: 10.1371/journal.pgen.1006018
Anderson, M. J., Southon, E., Tessarollo, L., and Lewandoski, M. (2016b). Fgf3-Fgf4-cis: a new mouse line for studying Fgf functions during mouse development. Genesis 54, 91–98. doi: 10.1002/dvg.22913
Aulehla, A., Wiegraebe, W., Baubet, V., Wahl, M. B., Deng, C., Taketo, M., et al. (2008). A β-catenin gradient links the clock and wavefront systems in mouse embryo segmentation. Nat. Cell Biol. 10, 186–193. doi: 10.1038/ncb1679
Aulehla, A., Wehrle, C., Brand-Saberi, B., Kemler, R., Gossler, A., Kanzler, B., et al. (2003). Wnt3a plays a major role in the segmentation clock controlling somitogenesis. Dev. Cell 4, 395–406. doi: 10.1016/S1534-5807(03)00055-8
Barnabe-Heider, F., Goritz, C., Sabelstrom, H., Takebayashi, H., Pfrieger, F. W., Meletis, K., et al. (2010). Origin of new glial cells in intact and injured adult spinal cord. Cell Stem Cell 7, 470–482. doi: 10.1016/j.stem.2010.07.014
Beermann, F., Kaloulis, K., Hofmann, D., Murisier, F., Bucher, P., and Trumpp, A. (2006). Identification of evolutionarily conserved regulatory elements in the mouse Fgf8 locus. Genesis 44, 1–6. doi: 10.1002/gene.20177
Bel-Vialar, S., Itasaki, N., and Krumlauf, R. (2002). Initiating Hox gene expression: in the early chick neural tube differential sensitivity to FGF and RA signaling subdivides the HoxB genes in two distinct groups. Development 129, 5103–5115.
Bel-Vialar, S., Medevielle, F., and Pituello, F. (2007). The on/off of Pax6 controls the tempo of neuronal differentiation in the developing spinal cord. Dev. Biol. 305, 659–673. doi: 10.1016/j.ydbio.2007.02.012
Benazeraf, B., Francois, P., Baker, R. E., Denans, N., Little, C. D., and Pourquie, O. (2010). A random cell motility gradient downstream of FGF controls elongation of an amniote embryo. Nature 466, 248–252. doi: 10.1038/nature09151
Bertrand, N., Medevielle, F., and Pituello, F. (2000). FGF signalling controls the timing of Pax6 activation in the neural tube. Development 127, 4837–4843.
Bertrand, S., Aldea, D., Oulion, S., Subirana, L., de Lera, A. R., Somorjai, I., et al. (2015). Evolution of the role of RA and FGF signals in the control of somitogenesis in chordates. PLoS ONE 10:e0136587. doi: 10.1371/journal.pone.0136587
Bokel, C., and Brand, M. (2013). Generation and interpretation of FGF morphogen gradients in vertebrates. Curr. Opin. Genet. Dev. 23, 415–422. doi: 10.1016/j.gde.2013.03.002
Boulet, A. M., and Capecchi, M. R. (2012). Signaling by FGF4 and FGF8 is required for axial elongation of the mouse embryo. Dev. Biol. 371, 235–245. doi: 10.1016/j.ydbio.2012.08.017
Briscoe, J., Pierani, A., Jessell, T. M., and Ericson, J. (2000). A homeodomain protein code specifies progenitor cell identity and neuronal fate in the ventral neural tube. Cell 101, 435–445. doi: 10.1016/S0092-8674(00)80853-3
Briscoe, J., and Small, S. (2015). Morphogen rules: design principles of gradient-mediated embryo patterning. Development 142, 3996–4009. doi: 10.1242/dev.129452
Bulusu, V., Prior, N., Snaebjornsson, M. T., Kuehne, A., Sonnen, K. F., Kress, J., et al. (2017). Spatiotemporal analysis of a glycolytic activity gradient linked to mouse embryo mesoderm development. Dev Cell 40, 331–341. e334. doi: 10.1016/j.devcel.2017.01.015
Burstyn-Cohen, T., Stanleigh, J., Sela-Donenfeld, D., and Kalcheim, C. (2004). Canonical Wnt activity regulates trunk neural crest delamination linking BMP/noggin signaling with G1/S transition. Development 131, 5327–5339. doi: 10.1242/dev.01424
Calegari, F., Haubensak, W., Haffner, C., and Huttner, W. B. (2005). Selective lengthening of the cell cycle in the neurogenic subpopulation of neural progenitor cells during mouse brain development. J. Neurosci. 25, 6533–6538. doi: 10.1523/JNEUROSCI.0778-05.2005
Calegari, F., and Huttner, W. B. (2003). An inhibition of cyclin-dependent kinases that lengthens, but does not arrest, neuroepithelial cell cycle induces premature neurogenesis. J. Cell Sci. 116(Pt 24), 4947–4955. doi: 10.1242/jcs.00825
Cao, Y., Zhao, J., Sun, Z., Zhao, Z., Postlethwait, J., and Meng, A. (2004). fgf17b, a novel member of Fgf family, helps patterning zebrafish embryos. Dev. Biol. 271, 130–143. doi: 10.1016/j.ydbio.2004.03.032
Chen, B., He, J., Yang, H., Zhang, Q., Zhang, L., Zhang, X., et al. (2015). Repair of spinal cord injury by implantation of bFGF-incorporated HEMA-MOETACL hydrogel in rats. Sci. Rep. 5:9017. doi: 10.1038/srep09017
Cho, G., Lim, Y., Cho, I. T., Simonet, J. C., and Golden, J. A. (2014). Arx together with FoxA2, regulates Shh floor plate expression. Dev. Biol. 393, 137–148. doi: 10.1016/j.ydbio.2014.06.012
Chotteau-Lelievre, A., Dolle, P., Peronne, V., Coutte, L., de Launoit, Y., and Desbiens, X. (2001). Expression patterns of the Ets transcription factors from the PEA3 group during early stages of mouse development. Mech. Dev. 108, 191–195. doi: 10.1016/S0925-4773(01)00480-4
Christian, K. M., Song, H., and Ming, G. L. (2014). Functions and dysfunctions of adult hippocampal neurogenesis. Annu. Rev. Neurosci. 37, 243–262. doi: 10.1146/annurev-neuro-071013-014134
Ciruna, B. G., Schwartz, L., Harpal, K., Yamaguchi, T. P., and Rossant, J. (1997). Chimeric analysis of fibroblast growth factor receptor-1 (Fgfr1) function: a role for FGFR1 in morphogenetic movement through the primitive streak. Development 124, 2829–2841.
Ciruna, B., and Rossant, J. (2001). FGF signaling regulates mesoderm cell fate specification and morphogenetic movement at the primitive streak. Dev. Cell 1, 37–49. doi: 10.1016/S1534-5807(01)00017-X
Corson, L. B., Yamanaka, Y., Lai, K. M., and Rossant, J. (2003). Spatial and temporal patterns of ERK signaling during mouse embryogenesis. Development 130, 4527–4537. doi: 10.1242/dev.00669
Cunningham, T. J., Brade, T., Sandell, L. L., Lewandoski, M., Trainor, P. A., Colas, A., et al. (2015a). Retinoic acid activity in undifferentiated neural progenitors is sufficient to fulfill its role in restricting Fgf8 expression for somitogenesis. PLoS ONE 10:e0137894. doi: 10.1371/journal.pone.0137894
Cunningham, T. J., and Duester, G. (2015). Mechanisms of retinoic acid signalling and its roles in organ and limb development. Nat. Rev. Mol. Cell Biol. 16, 110–123. doi: 10.1038/nrm3932
Cunningham, T. J., Kumar, S., Yamaguchi, T. P., and Duester, G. (2015b). Wnt8a and Wnt3a cooperate in the axial stem cell niche to promote mammalian body axis extension. Dev. Dyn. 244, 797–807. doi: 10.1002/dvdy.24275
Cunningham, T. J., Zhao, X., and Duester, G. (2011). Uncoupling of retinoic acid signaling from tailbud development before termination of body axis extension. Genesis 49, 776–783. doi: 10.1002/dvg.20763
Dasen, J. S., Liu, J. P., and Jessell, T. M. (2003). Motor neuron columnar fate imposed by sequential phases of Hox-c activity. Nature 425, 926–933. doi: 10.1038/nature02051
de la Calle-Mustienes, E., Glavic, A., Modolell, J., and Gomez-Skarmeta, J. L. (2002). Xiro homeoproteins coordinate cell cycle exit and primary neuron formation by upregulating neuronal-fate repressors and downregulating the cell-cycle inhibitor XGadd45-γ. Mech. Dev. 119, 69–80. doi: 10.1016/S0925-4773(02)00296-4
Delfino-Machin, M., Lunn, J. S., Breitkreuz, D. N., Akai, J., and Storey, K. G. (2005). Specification and maintenance of the spinal cord stem zone. Development 132, 4273–4283. doi: 10.1242/dev.02009
Diez del Corral, R., Breitkreuz, D. N., and Storey, K. G. (2002). Onset of neuronal differentiation is regulated by paraxial mesoderm and requires attenuation of FGF signalling. Development 129, 1681–1691.
Diez del Corral, R., and Morales, A. V. (2014). Retinoic acid signaling during early spinal cord development. J. Dev. Biol. 2, 174–197. doi: 10.3390/jdb2030174
Diez del Corral, R., Olivera-Martinez, I., Goriely, A., Gale, E., Maden, M., and Storey, K. (2003). Opposing FGF and retinoid pathways control ventral neural pattern, neuronal differentiation, and segmentation during body axis extension. Neuron 40, 65–79. doi: 10.1016/S0896-6273(03)00565-8
Draper, B. W., Stock, D. W., and Kimmel, C. B. (2003). Zebrafish fgf24 functions with fgf8 to promote posterior mesodermal development. Development 130, 4639–4654. doi: 10.1242/dev.00671
Dubrulle, J., McGrew, M. J., and Pourquie, O. (2001). FGF signaling controls somite boundary position and regulates segmentation clock control of spatiotemporal Hox gene activation. Cell 106, 219–232. doi: 10.1016/S0092-8674(01)00437-8
Dubrulle, J., and Pourquie, O. (2004). fgf8 mRNA decay establishes a gradient that couples axial elongation to patterning in the vertebrate embryo. Nature 427, 419–422. doi: 10.1038/nature02216
Dunty, W. C. Jr., Biris, K. K., Chalamalasetty, R. B., Taketo, M. M., Lewandoski, M., and Yamaguchi, T. P. (2008). Wnt3a/beta-catenin signaling controls posterior body development by coordinating mesoderm formation and segmentation. Development 135, 85–94. doi: 10.1242/dev.009266
Effenberg, A., Stanslowsky, N., Klein, A., Wesemann, M., Haase, A., Martin, U., et al. (2015). Striatal transplantation of human dopaminergic neurons differentiated from induced pluripotent stem cells derived from umbilical cord blood using lentiviral reprogramming. Cell Transplant. 24, 2099–2112. doi: 10.3727/096368914X685591
Ekerot, M., Stavridis, M. P., Delavaine, L., Mitchell, M. P., Staples, C., Owens, D. M., et al. (2008). Negative-feedback regulation of FGF signalling by DUSP6/MKP-3 is driven by ERK1/2 and mediated by Ets factor binding to a conserved site within the DUSP6/MKP-3 gene promoter. Biochem. J. 412, 287–298. doi: 10.1042/BJ20071512
Francius, C., Harris, A., Rucchin, V., Hendricks, T. J., Stam, F. J., Barber, M., et al. (2013). Identification of multiple subsets of ventral interneurons and differential distribution along the rostrocaudal axis of the developing spinal cord. PLoS ONE 8:e70325. doi: 10.1371/journal.pone.0070325
Fuentealba, L. C., Obernier, K., and Alvarez-Buylla, A. (2012). Adult neural stem cells bridge their niche. Cell Stem Cell 10, 698–708. doi: 10.1016/j.stem.2012.05.012
Gabay, L., Lowell, S., Rubin, L. L., and Anderson, D. J. (2003). Deregulation of dorsoventral patterning by FGF confers trilineage differentiation capacity on CNS stem cells in vitro. Neuron 40, 485–499. doi: 10.1016/S0896-6273(03)00637-8
Gage, F. H., Ray, J., and Fisher, L. J. (1995). Isolation, characterization, and use of stem cells from the CNS. Annu. Rev. Neurosci. 18, 159–192. doi: 10.1146/annurev.ne.18.030195.001111
Gofflot, F., Hall, M., and Morriss-Kay, G. M. (1997). Genetic patterning of the developing mouse tail at the time of posterior neuropore closure. Dev. Dyn. 210, 431–445. doi: 10.1002/(SICI)1097-0177(199712)210:4<431::AID-AJA7>3.0.CO;2-H
Goldshmit, Y., Frisca, F., Pinto, A. R., Pebay, A., Tang, J. K., Siegel, A. L., et al. (2014). Fgf2 improves functional recovery-decreasing gliosis and increasing radial glia and neural progenitor cells after spinal cord injury. Brain Behav. 4, 187–200. doi: 10.1002/brb3.172
Gonzalez-Quevedo, R., Lee, Y., Poss, K. D., and Wilkinson, D. G. (2010). Neuronal regulation of the spatial patterning of neurogenesis. Dev. Cell 18, 136–147. doi: 10.1016/j.devcel.2009.11.010
Gotz, M., and Huttner, W. B. (2005). The cell biology of neurogenesis. Nat. Rev. Mol. Cell Biol. 6, 777–788. doi: 10.1038/nrm1739
Gouti, M., Metzis, V., and Briscoe, J. (2015). The route to spinal cord cell types: a tale of signals and switches. Trends Genet. 31, 282–289. doi: 10.1016/j.tig.2015.03.001
Gouti, M., Tsakiridis, A., Wymeersch, F. J., Huang, Y., Kleinjung, J., Wilson, V., et al. (2014). In vitro generation of neuromesodermal progenitors reveals distinct roles for wnt signalling in the specification of spinal cord and paraxial mesoderm identity. PLoS Biol. 12:e1001937. doi: 10.1371/journal.pbio.1001937
Griffin, K., Patient, R., and Holder, N. (1995). Analysis of FGF function in normal and no tail zebrafish embryos reveals separate mechanisms for formation of the trunk and the tail. Development 121, 2983–2994.
Gritti, A., Parati, E. A., Cova, L., Frolichsthal, P., Galli, R., Wanke, E., et al. (1996). Multipotential stem cells from the adult mouse brain proliferate and self-renew in response to basic fibroblast growth factor. J. Neurosci. 16, 1091–1100.
Guzen, F. P., Soares, J. G., de Freitas, L. M., Cavalcanti, J. R., Oliveira, F. G., Araujo, J. F., et al. (2012). Sciatic nerve grafting and inoculation of FGF-2 promotes improvement of motor behavior and fiber regrowth in rats with spinal cord transection. Restor. Neurol. Neurosci. 30, 265–275. doi: 10.3233/RNN-2012-110184
Hack, M. A., Sugimori, M., Lundberg, C., Nakafuku, M., and Gotz, M. (2004). Regionalization and fate specification in neurospheres: the role of Olig2 and Pax6. Mol. Cell. Neurosci. 25, 664–678. doi: 10.1016/j.mcn.2003.12.012
Harduf, H., Halperin, E., Reshef, R., and Ron, D. (2005). Sef is synexpressed with FGFs during chick embryogenesis and its expression is differentially regulated by FGFs in the developing limb. Dev. Dyn. 233, 301–312. doi: 10.1002/dvdy.20364
Harrison, N. C., Diez del Corral, R., and Vasiev, B. (2011). Coordination of cell differentiation and migration in mathematical models of caudal embryonic axis extension. PLoS ONE 6:e22700. doi: 10.1371/journal.pone.0022700
Henrique, D., Abranches, E., Verrier, L., and Storey, K. G. (2015). Neuromesodermal progenitors and the making of the spinal cord. Development 142, 2864–2875. doi: 10.1242/dev.119768
Holowacz, T., and Sokol, S. (1999). FGF is required for posterior neural patterning but not for neural induction. Dev. Biol. 205, 296–308. doi: 10.1006/dbio.1998.9108
Holtz, A. M., Peterson, K. A., Nishi, Y., Morin, S., Song, J. Y., Charron, F., et al. (2013). Essential role for ligand-dependent feedback antagonism of vertebrate hedgehog signaling by PTCH1, PTCH2 and HHIP1 during neural patterning. Development 140, 3423–3434. doi: 10.1242/dev.095083
Isaacs, H. V., Pownall, M. E., and Slack, J. M. (1994). eFGF regulates Xbra expression during Xenopus gastrulation. EMBO J. 13, 4469–4481
Jaroonwitchawan, T., Muangchan, P., and Noisa, P. (2016). Inhibition of FGF signaling accelerates neural crest cell differentiation of human pluripotent stem cells. Biochem. Biophys. Res. Commun. 481, 176–181. doi: 10.1016/j.bbrc.2016.10.147
Johansson, C. B., Momma, S., Clarke, D. L., Risling, M., Lendahl, U., and Frisen, J. (1999). Identification of a neural stem cell in the adult mammalian central nervous system. Cell 96, 25–34.
Jordan, P. M., Ojeda, L. D., Thonhoff, J. R., Gao, J., Boehning, D., Yu, Y., et al. (2009). Generation of spinal motor neurons from human fetal brain-derived neural stem cells: role of basic fibroblast growth factor. J. Neurosci. Res. 87, 318–332. doi: 10.1002/jnr.21856
Kang, C. E., Baumann, M. D., Tator, C. H., and Shoichet, M. S. (2013). Localized and sustained delivery of fibroblast growth factor-2 from a nanoparticle-hydrogel composite for treatment of spinal cord injury. Cells Tissues Organs 197, 55–63. doi: 10.1159/000339589
Kang, C. E., Clarkson, R., Tator, C. H., Yeung, I. W., and Shoichet, M. S. (2010). Spinal cord blood flow and blood vessel permeability measured by dynamic computed tomography imaging in rats after localized delivery of fibroblast growth factor. J. Neurotrauma 27, 2041–2053. doi: 10.1089/neu.2010.1345
Kang, W., and Hebert, J. M. (2015). FGF signaling is necessary for neurogenesis in young mice and sufficient to reverse its decline in old mice. J. Neurosci. 35, 10217–10223. doi: 10.1523/JNEUROSCI.1469-15.2015
Kang, W., Wong, L. C., Shi, S. H., and Hebert, J. M. (2009). The transition from radial glial to intermediate progenitor cell is inhibited by FGF signaling during corticogenesis. J. Neurosci. 29, 14571–14580. doi: 10.1523/JNEUROSCI.3844-09.2009
Karabagli, H., Karabagli, P., Ladher, R. K., and Schoenwolf, G. C. (2002). Comparison of the expression patterns of several fibroblast growth factors during chick gastrulation and neurulation. Anat. Embryol. 205, 365–370. doi: 10.1007/s00429-002-0264-7
Kilpatrick, T. J., and Bartlett, P. F. (1993). Cloning and growth of multipotential neural precursors: requirements for proliferation and differentiation. Neuron 10, 255–265.
Kim, K. N., Oh, S. H., Lee, K. H., and Yoon, D. H. (2006). Effect of human mesenchymal stem cell transplantation combined with growth factor infusion in the repair of injured spinal cord. Acta Neurochir. Suppl. 99, 133–136. doi: 10.1007/978-3-211-35205-2_25
Kriks, S., Shim, J. W., Piao, J., Ganat, Y. M., Wakeman, D. R., Xie, Z., et al. (2011). Dopamine neurons derived from human ES cells efficiently engraft in animal models of Parkinson's disease. Nature 480, 547–551. doi: 10.1038/nature10648
Kumar, S., Cunningham, T. J., and Duester, G. (2016). Nuclear receptor corepressors Ncor1 and Ncor2 (Smrt) are required for retinoic acid-dependent repression of Fgf8 during somitogenesis. Dev. Biol. 418, 204–215. doi: 10.1016/j.ydbio.2016.08.005
Kumar, S., and Duester, G. (2014). Retinoic acid controls body axis extension by directly repressing Fgf8 transcription. Development 141, 2972–2977. doi: 10.1242/dev.112367
Kuo, H. S., Tsai, M. J., Huang, M. C., Chiu, C. W., Tsai, C. Y., Lee, M. J., et al. (2011). Acid fibroblast growth factor and peripheral nerve grafts regulate Th2 cytokine expression, macrophage activation, polyamine synthesis, and neurotrophin expression in transected rat spinal cords. J. Neurosci. 31, 4137–4147. doi: 10.1523/JNEUROSCI.2592-10.2011
Kutejova, E., Sasai, N., Shah, A., Gouti, M., and Briscoe, J. (2016). Neural progenitors adopt specific identities by directly repressing all alternative progenitor transcriptional programs. Dev. Cell 36, 639–653. doi: 10.1016/j.devcel.2016.02.013
Lai, H. C., Seal, R. P., and Johnson, J. E. (2016). Making sense out of spinal cord somatosensory development. Development 143, 3434–3448. doi: 10.1242/dev.139592
Lake, D., Correa, S. A., and Muller, J. (2016). Negative feedback regulation of the ERK1/2 MAPK pathway. Cell. Mol. Life Sci. 73, 4397–4413. doi: 10.1007/s00018-016-2297-8
Lawton, A. K., Nandi, A., Stulberg, M. J., Dray, N., Sneddon, M. W., Pontius, W., et al. (2013). Regulated tissue fluidity steers zebrafish body elongation. Development 140, 573–582. doi: 10.1242/dev.090381
Laywell, E. D., Rakic, P., Kukekov, V. G., Holland, E. C., and Steindler, D. A. (2000). Identification of a multipotent astrocytic stem cell in the immature and adult mouse brain. Proc. Natl. Acad. Sci. U.S.A. 97, 13883–13888. doi: 10.1073/pnas.250471697
Le Douarin, N. M., Creuzet, S., Couly, G., and Dupin, E. (2004). Neural crest cell plasticity and its limits. Development 131, 4637–4650. doi: 10.1242/dev.01350
Le Douarin, N. M., and Kalcheim, C. (1999). The Neural Crest. Cambridge, UK: Cambridge University Press.
Le Dreau, G., and Marti, E. (2012). Dorsal-ventral patterning of the neural tube: a tale of three signals. Dev. Neurobiol. 72, 1471–1481. doi: 10.1002/dneu.22015
Lee, T. T., Green, B. A., Dietrich, W. D., and Yezierski, R. P. (1999). Neuroprotective effects of basic fibroblast growth factor following spinal cord contusion injury in the rat. J. Neurotrauma 16, 347–356. doi: 10.1089/neu.1999.16.347
Lee, Y., Miller, H. L., Russell, H. R., Boyd, K., Curran, T., and McKinnon, P. J. (2006). Patched2 modulates tumorigenesis in patched1 heterozygous mice. Cancer Res. 66, 6964–6971. doi: 10.1158/0008-5472.CAN-06-0505
Liu, J.-P., Laufer, E., and Jessell, T. M. (2001). Assigning the positional identity of spinal motor neurons: rostrocaudal patterning of Hox-c expression by FGFs, Gdf11, and retinoids. Neuron 32, 997–1012. doi: 10.1016/S0896-6273(01)00544-X
Lobjois, V., Benazeraf, B., Bertrand, N., Medevielle, F., and Pituello, F. (2004). Specific regulation of cyclins D1 and D2 by FGF and Shh signaling coordinates cell cycle progression, patterning, and differentiation during early steps of spinal cord development. Dev. Biol. 273, 195–209. doi: 10.1016/j.ydbio.2004.05.031
Lu, P., Wang, Y., Graham, L., McHale, K., Gao, M., Wu, D., et al. (2012). Long-distance growth and connectivity of neural stem cells after severe spinal cord injury. Cell 150, 1264–1273. doi: 10.1016/j.cell.2012.08.020
Lunn, J. S., Fishwick, K. J., Halley, P. A., and Storey, K. G. (2007). A spatial and temporal map of FGF/Erk1/2 activity and response repertoires in the early chick embryo. Dev. Biol. 302, 536–552. doi: 10.1016/j.ydbio.2006.10.014
Maric, D., Fiorio Pla, A., Chang, Y. H., and Barker, J. L. (2007). Self-renewing and differentiating properties of cortical neural stem cells are selectively regulated by basic fibroblast growth factor (FGF) signaling via specific FGF receptors. J. Neurosci. 27, 1836–1852. doi: 10.1523/JNEUROSCI.5141-06.2007
Marinic, M., Aktas, T., Ruf, S., and Spitz, F. (2013). An integrated holo-enhancer unit defines tissue and gene specificity of the Fgf8 regulatory landscape. Dev. Cell 24, 530–542. doi: 10.1016/j.devcel.2013.01.025
Martinez-Morales, P. L., Diez del Corral, R., Olivera-Martinez, I., Quiroga, A. C., Das, R. M., Barbas, J. A., et al. (2011). FGF and retinoic acid activity gradients control the timing of neural crest cell emigration in the trunk. J. Cell Biol. 194, 489–503. doi: 10.1083/jcb.201011077
Mathis, L., Kulesa, P. M., and Fraser, S. E. (2001). FGF receptor signalling is required to maintain neural progenitors during Hensen's node progression. Nat. Cell Biol. 3, 559–566. doi: 10.1038/35078535
Meijs, M. F., Timmers, L., Pearse, D. D., Tresco, P. A., Bates, M. L., Joosten, E. A., et al. (2004). Basic fibroblast growth factor promotes neuronal survival but not behavioral recovery in the transected and Schwann cell implanted rat thoracic spinal cord. J. Neurotrauma 21, 1415–1430. doi: 10.1089/neu.2004.21.1415
Meletis, K., Barnabe-Heider, F., Carlen, M., Evergren, E., Tomilin, N., Shupliakov, O., et al. (2008). Spinal cord injury reveals multilineage differentiation of ependymal cells. PLoS Biol. 6:e182. doi: 10.1371/journal.pbio.0060182
Molina, A., and Pituello, F. (in press). Playing with the cell cycle to build the spinal cord. Dev. Biol. doi: 10.1016/j.ydbio.2016.12.022
Molina, G. A., Watkins, S. C., and Tsang, M. (2007). Generation of FGF reporter transgenic zebrafish and their utility in chemical screens. BMC Dev. Biol. 7:62. doi: 10.1186/1471-213X-7-62
Molotkova, N., Molotkov, A., Sirbu, I. O., and Duester, G. (2005). Requirement of mesodermal retinoic acid generated by Raldh2 for posterior neural transformation. Mech. Dev. 122, 145–155. doi: 10.1016/j.mod.2004.10.008
Morales, A. V., Barbas, J. A., and Nieto, M. A. (2005). How to become neural crest: from segregation to delamination. Semin. Cell Dev. Biol. 16, 655–662. doi: 10.1016/j.semcdb.2005.06.003
Morales, A. V., Espeso-Gil, S., Ocana, I., Nieto-Lopez, F., Calleja, E., Bovolenta, P., et al. (2016). FGF signaling enhances a sonic hedgehog negative feedback loop at the initiation of spinal cord ventral patterning. Dev. Neurobiol. 76, 956–971. doi: 10.1002/dneu.22368
Muhr, J., Graziano, E., Wilson, S., Jessell, T. M., and Edlund, T. (1999). Convergent inductive signals specify midbrain, hindbrain, and spinal cord identity in gastrula stage chick embryos. Neuron 23, 689–702.
Murry, C. E., and Keller, G. (2008). Differentiation of embryonic stem cells to clinically relevant populations: lessons from embryonic development. Cell 132, 661–680. doi: 10.1016/j.cell.2008.02.008
Naiche, L. A., Holder, N., and Lewandoski, M. (2011). FGF4 and FGF8 comprise the wavefront activity that controls somitogenesis. Proc. Natl. Acad. Sci. U.S.A. 108, 4018–4023. doi: 10.1073/pnas.1007417108
Nelson, A. D., and Svendsen, C. N. (2006). Low concentrations of extracellular FGF-2 are sufficient but not essential for neurogenesis from human neural progenitor cells. Mol. Cell. Neurosci. 33, 29–35. doi: 10.1016/j.mcn.2006.06.003
Nieuwenhuis, E., Motoyama, J., Barnfield, P. C., Yoshikawa, Y., Zhang, X., Mo, R., et al. (2006). Mice with a targeted mutation of patched2 are viable but develop alopecia and epidermal hyperplasia. Mol. Cell. Biol. 26, 6609–6622. doi: 10.1128/MCB.00295-06
Nishimura, N., Kamimura, Y., Ishida, Y., Takemoto, T., Kondoh, H., and Uchikawa, M. (2012). A systematic survey and characterization of enhancers that regulate Sox3 in neuro-sensory development in comparison with Sox2 enhancers. Biology 1, 714–735. doi: 10.3390/biology1030714
Nishita, J., Ohta, S., Bleyl, S. B., and Schoenwolf, G. C. (2011). Detection of isoform-specific fibroblast growth factor receptors by whole-mount in situ hybridization in early chick embryos. Dev. Dyn. 240, 1537–1547. doi: 10.1002/dvdy.22616
Nordstrom, U., Maier, E., Jessell, T. M., and Edlund, T. (2006). An early role for WNT signaling in specifying neural patterns of Cdx and Hox gene expression and motor neuron subtype identity. PLoS Biol. 4:e252. doi: 10.1371/journal.pbio.0040252
Nori, S., Okada, Y., Nishimura, S., Sasaki, T., Itakura, G., Kobayashi, Y., et al. (2015). Long-term safety issues of iPSC-based cell therapy in a spinal cord injury model: oncogenic transformation with epithelial-mesenchymal transition. Stem Cell Reports 4, 360–373. doi: 10.1016/j.stemcr.2015.01.006
Novitch, B. G., Wichterle, H., Jessell, T. M., and Sockanathan, S. (2003). A requirement for retinoic acid-mediated transcriptional activation in ventral neural patterning and motor neuron specification. Neuron 40, 81–95. doi: 10.1016/j.neuron.2003.08.006
Oginuma, M., Moncuquet, P., Xiong, F., Karoly, E., Chal, J., Guevorkian, K., et al. (2017). A gradient of glycolytic activity coordinates FGF and Wnt signaling during elongation of the body axis in amniote embryos. Dev. Cell 40, 342–353, e310. doi: 10.1016/j.devcel.2017.02.001
Olivera-Martinez, I., Harada, H., Halley, P. A., and Storey, K. G. (2012). Loss of FGF-dependent mesoderm identity and rise of endogenous retinoid signalling determine cessation of body axis elongation. PLoS Biol. 10:e1001415. doi: 10.1371/journal.pbio.1001415
Olivera-Martinez, I., Schurch, N., Li, R. A., Song, J., Halley, P. A., Das, R. M., et al. (2014). Major transcriptome re-organisation and abrupt changes in signalling, cell cycle and chromatin regulation at neural differentiation in vivo. Development 141, 3266–3276. doi: 10.1242/dev.112623
Olivera-Martinez, I., and Storey, K. G. (2007). Wnt signals provide a timing mechanism for the FGF-retinoid differentiation switch during vertebrate body axis extension. Development 134, 2125–2135. doi: 10.1242/dev.000216
Ornitz, D. M., and Itoh, N. (2015). The fibroblast growth factor signaling pathway. Wiley Interdiscip. Rev. Dev. Biol. 4, 215–266. doi: 10.1002/wdev.176
Ota, S., Tonou-Fujimori, N., and Yamasu, K. (2009). The roles of the FGF signal in zebrafish embryos analyzed using constitutive activation and dominant-negative suppression of different FGF receptors. Mech. Dev. 126, 1–17. doi: 10.1016/j.mod.2008.10.008
Palmer, T. D., Markakis, E. A., Willhoite, A. R., Safar, F., and Gage, F. H. (1999). Fibroblast growth factor-2 activates a latent neurogenic program in neural stem cells from diverse regions of the adult CNS. J. Neurosci. 19, 8487–8497.
Patel, N. S., Rhinn, M., Semprich, C. I., Halley, P. A., Dolle, P., Bickmore, W. A., et al. (2013). FGF signalling regulates chromatin organisation during neural differentiation via mechanisms that can be uncoupled from transcription. PLoS Genet. 9:e1003614. doi: 10.1371/journal.pgen.1003614
Peljto, M., Dasen, J. S., Mazzoni, E. O., Jessell, T. M., and Wichterle, H. (2010). Functional diversity of ESC-derived motor neuron subtypes revealed through intraspinal transplantation. Cell Stem Cell 7, 355–366. doi: 10.1016/j.stem.2010.07.013
Pfenninger, C. V., Steinhoff, C., Hertwig, F., and Nuber, U. A. (2011). Prospectively isolated CD133/CD24-positive ependymal cells from the adult spinal cord and lateral ventricle wall differ in their long-term in vitro self-renewal and in vivo gene expression. Glia 59, 68–81. doi: 10.1002/glia.21077
Philippidou, P., and Dasen, J. S. (2013). Hox genes: choreographers in neural development, architects of circuit organization. Neuron 80, 12–34. doi: 10.1016/j.neuron.2013.09.020
Pollard, S. M., Wallbank, R., Tomlinson, S., Grotewold, L., and Smith, A. (2008). Fibroblast growth factor induces a neural stem cell phenotype in foetal forebrain progenitors and during embryonic stem cell differentiation. Mol. Cell. Neurosci. 38, 393–403. doi: 10.1016/j.mcn.2008.03.012
Qian, X., Davis, A. A., Goderie, S. K., and Temple, S. (1997). FGF2 concentration regulates the generation of neurons and glia from multipotent cortical stem cells. Neuron 18, 81–93.
Raballo, R., Rhee, J., Lyn-Cook, R., Leckman, J. F., Schwartz, M. L., and Vaccarino, F. M. (2000). Basic fibroblast growth factor (Fgf2) is necessary for cell proliferation and neurogenesis in the developing cerebral cortex. J. Neurosci. 20, 5012–5023.
Rabchevsky, A. G., Fugaccia, I., Fletcher-Turner, A., Blades, D. A., Mattson, M. P., and Scheff, S. W. (1999). Basic fibroblast growth factor (bFGF) enhances tissue sparing and functional recovery following moderate spinal cord injury. J. Neurotrauma 16, 817–830. doi: 10.1089/neu.1999.16.817
Rash, B. G., Lim, H. D., Breunig, J. J., and Vaccarino, F. M. (2011). FGF signaling expands embryonic cortical surface area by regulating Notch-dependent neurogenesis. J. Neurosci. 31, 15604–15617. doi: 10.1523/JNEUROSCI.4439-11.2011
Rash, B. G., Tomasi, S., Lim, H. D., Suh, C. Y., and Vaccarino, F. M. (2013). Cortical gyrification induced by fibroblast growth factor 2 in the mouse brain. J. Neurosci. 33, 10802–10814. doi: 10.1523/JNEUROSCI.3621-12.2013
Reifers, F., Adams, J., Mason, I. J., Schulte-Merker, S., and Brand, M. (2000). Overlapping and distinct functions provided by fgf17, a new zebrafish member of the Fgf8/17/18 subgroup of Fgfs. Mech. Dev. 99, 39–49. doi: 10.1016/S0925-4773(00)00475-5
Resende, T. P., Ferreira, M., Teillet, M. A., Tavares, A. T., Andrade, R. P., and Palmeirim, I. (2010). Sonic hedgehog in temporal control of somite formation. Proc. Natl. Acad. Sci. U.S.A. 107, 12907–12912. doi: 10.1073/pnas.1000979107
Rosser, A. E., Zietlow, R., and Dunnett, S. B. (2007). Stem cell transplantation for neurodegenerative diseases. Curr. Opin. Neurol. 20, 688–692. doi: 10.1097/WCO.0b013e3282f132fc
Row, R. H., Tsotras, S. R., Goto, H., and Martin, B. L. (2016). The zebrafish tailbud contains two independent populations of midline progenitor cells that maintain long-term germ layer plasticity and differentiate in response to local signaling cues. Development 143, 244–254. doi: 10.1242/dev.129015
Roztocil, T., Matter-Sadzinski, L., Alliod, C., Ballivet, M., and Matter, J. M. (1997). NeuroM, a neural helix-loop-helix transcription factor, defines a new transition stage in neurogenesis. Development 124, 3263–3272.
Sabelstrom, H., Stenudd, M., Reu, P., Dias, D. O., Elfineh, M., Zdunek, S., et al. (2013). Resident neural stem cells restrict tissue damage and neuronal loss after spinal cord injury in mice. Science 342, 637–640. doi: 10.1126/science.1242576
Sahara, S., and O'Leary, D. D. (2009). Fgf10 regulates transition period of cortical stem cell differentiation to radial glia controlling generation of neurons and basal progenitors. Neuron 63, 48–62. doi: 10.1016/j.neuron.2009.06.006
Saint-Jeannet, J. P., and Moody, S. A. (2014). Establishing the pre-placodal region and breaking it into placodes with distinct identities. Dev. Biol. 389, 13–27. doi: 10.1016/j.ydbio.2014.02.011
Sasai, N., Kutejova, E., and Briscoe, J. (2014). Integration of signals along orthogonal axes of the vertebrate neural tube controls progenitor competence and increases cell diversity. PLoS Biol. 12:e1001907. doi: 10.1371/journal.pbio.1001907
Sauka-Spengler, T., and Bronner-Fraser, M. (2008). Evolution of the neural crest viewed from a gene regulatory perspective. Genesis 46, 673–682. doi: 10.1002/dvg.20436
Savory, J. G., Bouchard, N., Pierre, V., Rijli, F. M., De Repentigny, Y., Kothary, R., et al. (2009). Cdx2 regulation of posterior development through non-Hox targets. Development 136, 4099–4110. doi: 10.1242/dev.041582
Scholpp, S., and Brand, M. (2004). Endocytosis controls spreading and effective signaling range of Fgf8 protein. Curr. Biol. 14, 1834–1841. doi: 10.1016/j.cub.2004.09.084
Sechrist, J., and Bronner-Fraser, M. (1991). Birth and differentiation of reticular neurons in the chick hindbrain: ontogeny of the first neuronal population. Neuron 7, 947–963.
Sela-Donenfeld, D., and Kalcheim, C. (1999). Regulation of the onset of neural crest migration by coordinated activity of BMP4 and Noggin in the dorsal neural tube. Development 126, 4749–4762.
Shihabuddin, L. S., Ray, J., and Gage, F. H. (1997). FGF-2 is sufficient to isolate progenitors found in the adult mammalian spinal cord. Exp. Neurol. 148, 577–586. doi: 10.1006/exnr.1997.6697
Shimizu, T., Bae, Y. K., and Hibi, M. (2006). Cdx-Hox code controls competence for responding to Fgfs and retinoic acid in zebrafish neural tissue. Development 133, 4709–4719. doi: 10.1242/dev.02660
Siddiqui, A. M., Khazaei, M., and Fehlings, M. G. (2015). Translating mechanisms of neuroprotection, regeneration, and repair to treatment of spinal cord injury. Prog. Brain Res. 218, 15–54. doi: 10.1016/bs.pbr.2014.12.007
Simon, R., and Lufkin, T. (2003). Postnatal lethality in mice lacking the Sax2 homeobox gene homologous to Drosophila S59/slouch: evidence for positive and negative autoregulation. Mol. Cell Biol. 23, 9046–9060. doi: 10.1128/MCB.23.24.9046-9060.2003
Sirbu, I. O., and Duester, G. (2006). Retinoic-acid signalling in node ectoderm and posterior neural plate directs left-right patterning of somitic mesoderm. Nat. Cell Biol. 8, 271–277. doi: 10.1038/ncb1374
Stavridis, M. P., Collins, B. J., and Storey, K. G. (2010). Retinoic acid orchestrates fibroblast growth factor signalling to drive embryonic stem cell differentiation. Development 137, 881–890. doi: 10.1242/dev.043117
Steventon, B., and Martinez Arias, A. (in press). Evo-engineering the cellular molecular origins of the vertebrate spinal cord. Dev. Biol. doi: 10.1016/j.ydbio.2017.01.021
Storey, K. G., Goriely, A., Sargent, C. M., Brown, J. M., Burns, H. D., Abud, H. M., et al. (1998). Early posterior neural tissue is induced by FGF in the chick embryo. Development 125, 473–484.
Storm, E. E., Garel, S., Borello, U., Hebert, J. M., Martinez, S., McConnell, S. K., et al. (2006). Dose-dependent functions of Fgf8 in regulating telencephalic patterning centers. Development 133, 1831–1844. doi: 10.1242/dev.02324
Takahashi, T., Nowakowski, R. S., and Caviness, V. S. Jr. (1995). The cell cycle of the pseudostratified ventricular epithelium of the embryonic murine cerebral wall. J. Neurosci. 15, 6046–6057.
Takemoto, T., Uchikawa, M., Kamachi, Y., and Kondoh, H. (2006). Convergence of Wnt and FGF signals in the genesis of posterior neural plate through activation of the Sox2 enhancer N-1. Development 133, 297–306. doi: 10.1242/dev.02196
Teng, Y. D., Mocchetti, I., Taveira-DaSilva, A. M., Gillis, R. A., and Wrathall, J. R. (1999). Basic fibroblast growth factor increases long-term survival of spinal motor neurons and improves respiratory function after experimental spinal cord injury. J. Neurosci. 19, 7037–7047.
Teng, Y. D., Mocchetti, I., and Wrathall, J. R. (1998). Basic and acidic fibroblast growth factors protect spinal motor neurones in vivo after experimental spinal cord injury. Eur. J. Neurosci. 10, 798–802.
Turner, D. A., Hayward, P. C., Baillie-Johnson, P., Rue, P., Broome, R., Faunes, F., et al. (2014). Wnt/beta-catenin and FGF signalling direct the specification and maintenance of a neuromesodermal axial progenitor in ensembles of mouse embryonic stem cells. Development 141, 4243–4253. doi: 10.1242/dev.112979
Vaccarino, F. M., Schwartz, M. L., Raballo, R., Nilsen, J., Rhee, J., Zhou, M., et al. (1999). Changes in cerebral cortex size are governed by fibroblast growth factor during embryogenesis. Nat. Neurosci. 2, 246–253. doi: 10.1038/6350
van den Akker, E., Forlani, S., Chawengsaksophak, K., de Graaff, W., Beck, F., Meyer, B. I., et al. (2002). Cdx1 and Cdx2 have overlapping functions in anteroposterior patterning and posterior axis elongation. Development 129, 2181–2193.
Vermot, J., and Pourquie, O. (2005). Retinoic acid coordinates somitogenesis and left-right patterning in vertebrate embryos. Nature 435, 215–220. doi: 10.1038/nature03488
Wahl, M. B., Deng, C., Lewandoski, M., and Pourquie, O. (2007). FGF signaling acts upstream of the NOTCH and WNT signaling pathways to control segmentation clock oscillations in mouse somitogenesis. Development 134, 4033–4041. doi: 10.1242/dev.009167
Wang, Y., Song, L., and Zhou, C. J. (2011). The canonical Wnt/beta-catenin signaling pathway regulates Fgf signaling for early facial development. Dev. Biol. 349, 250–260. doi: 10.1016/j.ydbio.2010.11.004
Weiss, S., Dunne, C., Hewson, J., Wohl, C., Wheatley, M., Peterson, A. C., et al. (1996). Multipotent CNS stem cells are present in the adult mammalian spinal cord and ventricular neuroaxis. J. Neurosci. 16, 7599–7609.
Wilcock, A. C., Swedlow, J. R., and Storey, K. G. (2007). Mitotic spindle orientation distinguishes stem cell and terminal modes of neuron production in the early spinal cord. Development 134, 1943–1954. doi: 10.1242/dev.002519
Wilson, V., Olivera-Martinez, I., and Storey, K. G. (2009). Stem cells, signals and vertebrate body axis extension. Development 136, 1591–1604. doi: 10.1242/dev.021246
Wu, J. C., Huang, W. C., Chen, Y. C., Tu, T. H., Tsai, Y. A., Huang, S. F., et al. (2011). Acidic fibroblast growth factor for repair of human spinal cord injury: a clinical trial. J. Neurosurg. Spine 15, 216–227. doi: 10.3171/2011.4.SPINE10404
Yan, H. Q., Yu, J., Kline, A. E., Letart, P., Jenkins, L. W., Marion, D. W., et al. (2000). Evaluation of combined fibroblast growth factor-2 and moderate hypothermia therapy in traumatically brain injured rats. Brain Res. 887, 134–143.
Yang, X., Dormann, D., Munsterberg, A. E., and Weijer, C. J. (2002). Cell movement patterns during gastrulation in the chick are controlled by positive and negative chemotaxis mediated by FGF4 and FGF8. Dev. Cell 3, 425–437. doi: 10.1016/S1534-5807(02)00256-3
Ye, W., Shimamura, K., Rubenstein, J. L., Hynes, M. A., and Rosenthal, A. (1998). FGF and Shh signals control dopaminergic and serotonergic cell fate in the anterior neural plate. Cell 93, 755–766.
Yoshida, M., Uchikawa, M., Rizzoti, K., Lovell-Badge, R., Takemoto, T., and Kondoh, H. (2014). Regulation of mesodermal precursor production by low-level expression of B1 Sox genes in the caudal lateral epiblast. Mech. Dev. 132, 59–68. doi: 10.1016/j.mod.2014.01.003
Yu, S. R., Burkhardt, M., Nowak, M., Ries, J., Petrasek, Z., Scholpp, S., et al. (2009). Fgf8 morphogen gradient forms by a source-sink mechanism with freely diffusing molecules. Nature 461, 533–536. doi: 10.1038/nature08391
Zhang, S. C., Wernig, M., Duncan, I. D., Brustle, O., and Thomson, J. A. (2001). In vitro differentiation of transplantable neural precursors from human embryonic stem cells. Nat. Biotechnol. 19, 1129–1133. doi: 10.1038/nbt1201-1129
Keywords: spinal cord, spinal cord injury, neuromesodermal progenitors, neural stem cells, patterning, neurogenesis, caudal extension, FGF
Citation: Diez del Corral R and Morales AV (2017) The Multiple Roles of FGF Signaling in the Developing Spinal Cord. Front. Cell Dev. Biol. 5:58. doi: 10.3389/fcell.2017.00058
Received: 17 February 2017; Accepted: 11 May 2017;
Published: 02 June 2017.
Edited by:
Juan Jose Sanz-Ezquerro, Centro Nacional de Biotecnología, SpainReviewed by:
Cristina Pujades, Pompeu Fabra University, SpainGregg Duester, Sanford Burnham Prebys Medical Discovery Institute, United States
Benjamin Louis Martin, Stony Brook University, United States
Copyright © 2017 Diez del Corral and Morales. This is an open-access article distributed under the terms of the Creative Commons Attribution License (CC BY). The use, distribution or reproduction in other forums is permitted, provided the original author(s) or licensor are credited and that the original publication in this journal is cited, in accordance with accepted academic practice. No use, distribution or reproduction is permitted which does not comply with these terms.
*Correspondence: Ruth Diez del Corral, cnV0aC5kaWV6ZGVsY29ycmFsQG5ldXJvLmZjaGFtcGFsaW1hdWQub3Jn
Aixa V. Morales, YWl4YW1vcmFsZXNAY2FqYWwuY3NpYy5lcw==