- 1School of Medicine, Cardiff University, Cardiff, United Kingdom
- 2School of Biosciences, Cardiff University, Cardiff, United Kingdom
- 3School of Mathematics, Cardiff University, Cardiff, United Kingdom
Egg activation at fertilization in mammalian eggs is caused by a series of transient increases in the cytosolic free Ca2+ concentration, referred to as Ca2+ oscillations. It is widely accepted that these Ca2+ oscillations are initiated by a sperm derived phospholipase C isoform, PLCζ that hydrolyses its substrate PIP2 to produce the Ca2+ releasing messenger InsP3. However, it is not clear whether PLCζ induced InsP3 formation is periodic or monotonic, and whether the PIP2 source for generating InsP3 from PLCζ is in the plasma membrane or the cytoplasm. In this study we have uncaged InsP3 at different points of the Ca2+ oscillation cycle to show that PLCζ causes Ca2+ oscillations by a mechanism which requires Ca2+ induced InsP3 formation. In contrast, incubation in Sr2+ media, which also induces Ca2+ oscillations in mouse eggs, sensitizes InsP3-induced Ca2+ release. We also show that the cytosolic level Ca2+ is a key factor in setting the frequency of Ca2+ oscillations since low concentrations of the Ca2+ pump inhibitor, thapsigargin, accelerates the frequency of PLCζ induced Ca2+ oscillations in eggs, even in Ca2+ free media. Given that Ca2+ induced InsP3 formation causes a rapid wave during each Ca2+ rise, we use a mathematical model to show that InsP3 generation, and hence PLCζ's substate PIP2, has to be finely distributed throughout the egg cytoplasm. Evidence for PIP2 distribution in vesicles throughout the egg cytoplasm is provided with a rhodamine-peptide probe, PBP10. The apparent level of PIP2 in such vesicles could be reduced by incubating eggs in the drug propranolol which also reversibly inhibited PLCζ induced, but not Sr2+ induced, Ca2+ oscillations. These data suggest that the cytosolic Ca2+ level, rather than Ca2+ store content, is a key variable in setting the pace of PLCζ induced Ca2+ oscillations in eggs, and they imply that InsP3 oscillates in synchrony with Ca2+ oscillations. Furthermore, they support the hypothesis that PLCζ and sperm induced Ca2+ oscillations in eggs requires the hydrolysis of PIP2 from finely spaced cytoplasmic vesicles.
Introduction
The fertilization of a mammalian egg involves a series of low frequency Ca2+ oscillations that last for many hours. Such Ca2+ oscillations play the key role in egg activation and the subsequent development of the embryo (Stricker, 1999). The first Ca2+ increase takes ~10 s to travel as a wave across the egg from the point of sperm entry (Miyazaki et al., 1986; Deguchi et al., 2000). However, all the subsequent Ca2+ transients have a rising phase of about 1 s which is due to a fast Ca2+ wave (>50 μm/s) that crosses the egg from apparently random points in the egg cortex (Deguchi et al., 2000). Each Ca2+ increase during the oscillations is due to release from internal Ca2+ stores via inositol 1,4,5-trisphophate receptors (IP3R) which are exclusively of type 1 IP3R in mammalian eggs (Miyazaki, 1988; Miyazaki et al., 1993). The sperm stimulates the Ca2+ oscillations via inositol 1,4,5-trisphosphate (InsP3) production, and all the reproducible studies suggest that this is principally due to the introduction of a sperm specific phospholipase Czeta (PLCζ) into the egg after gamete fusion (Saunders et al., 2002). Injection of PLCζ protein or cRNA causes prolonged Ca2+ oscillations that mimic those seen at fertilization in eggs of mice, rat, humans, cows, and pigs (Cox et al., 2002; Saunders et al., 2002; Fujimoto et al., 2004; Kouchi et al., 2004; Kurokawa et al., 2005; Bedford-Guaus et al., 2008; Ito et al., 2008; Ross et al., 2008; Yoon et al., 2012; Sato et al., 2013). PLCζ is distinctive compared to most mammalian PLC isozymes in that it is stimulated by low levels of Ca2+ such that it is maximally sensitive to Ca2+ around the resting levels in eggs (Nomikos et al., 2005). PLCζ is expected to diffuse across the egg in about 10 min following sperm-egg fusion, hence the fast Ca2+ waves seen after the initial Ca2+ transient are propagated within a cytoplasm in which PLCζ has probably dispersed throughout the egg.
There are two classes of model to explain how InsP3 causes Ca2+ oscillations in cells, both which have been proposed for fertilizing mammalian eggs (Dupont and Goldbeter, 1994; Politi et al., 2006). There are some models that propose Ca2+ dependent sensitization, and then de-sensitization, of the IP3R is necessary to generate each Ca2+ transient (Politi et al., 2006). This class of models supports the finding that mouse and hamster eggs can be stimulated to oscillate by sustained injection of InsP3, or by injection of the IP3R agonist adenophostin (Swann et al., 1989; Brind et al., 2000; Jones and Nixon, 2000). On the other hand there are other models in which Ca2+ dependent production of InsP3 generates each Ca2+ transient, and in which InsP3 is predicted to oscillate alongside Ca2+ (Politi et al., 2006). This second class of model is supported by the detection of InsP3 oscillations in mouse eggs injected with PLCζ, albeit at high levels of PLCζ (Shirakawa et al., 2006). However, it is not clear if any oscillatory changes in InsP3 oscillations are necessary for generating Ca2+ increases. Either classes of model have to incorporate the observation that the Ca2+ oscillations have a dependence upon Ca2+ influx. So for example, if fertilizing hamster or mouse eggs are incubated in Ca2+ free media the oscillations run down and stop (Igusa and Miyazaki, 1983; Lawrence and Cuthbertson, 1995; McGuinness et al., 1996). It has been suggested that the Ca2+ store content is critical in setting the timing of Ca2+ oscillations in mouse eggs. This is supported by evidence that the SERCA inhibitor thapsigargin can also be used to block sperm and PLCζ induced Ca2+ oscillations by depleting Ca2+ stores content (Kline and Kline, 1992b). However, changes in cytosolic Ca2+ may also play a role in the timing of oscillations since cytosolic Ca2+ can regulate both IP3Rs and PLCζ activity.
Sustained Ca2+ oscillations in mouse eggs can also be triggered by incubation in media containing Sr2+ instead of Ca2+ (Kline and Kline, 1992a; Bos-Mikich et al., 1995). Sr2+-induced Ca2+ oscillations resemble those seen at fertilization, and they are as effective as fertilization or PLCζ in triggering development to the blastocyst stage (Yu et al., 2008). The oscillations are dependent upon Sr2+ influx into the egg and the presence of functional IP3Rs (Zhang et al., 2005). However, it is not clear how Sr2+ causes Ca2+ oscillations. One study suggested that the effect of Sr2+ requires InsP3 production (Zhang et al., 2005). However, unlike fertilization, there is no Sr2+ induced downregulation of IP3Rs and this suggests that Sr2+ does not cause any substantial InsP3 generation (Jellerette et al., 2000). In vitro preparations of IP3Rs receptors can be stimulated to open by Sr2+ ions (Marshall and Taylor, 1994), so a direct effect of Sr2+ on IP3Rs is also likely, but any changes in InP3 sensitivity in eggs have yet to be shown.
As well as its high sensitivity to Ca2+, another unusual characteristic of PLCζ is that it does not localize to the plasma membrane (Yu et al., 2012). The substrate for PLCζ, phosphatidylinositol 4,5-bisphophate (PIP2), can be detected in the plasma membrane of mouse eggs using the PH domain of PLCδ1 (Halet et al., 2002), but the depletion of such PIP2 from the plasma membrane does not affect the generation of Ca2+ oscillations in response to PLCζ or fertilization (Yu et al., 2012). In contrast to somatic cells, mouse eggs have been shown to contain PIP2 in intracellular vesicles (Yu et al., 2012). These vesicles were detected using PIP2 antibodies and were found to be dispersed throughout the cytoplasm of mouse eggs (Yu et al., 2012). PLCζ can also be detected on small cytoplasmic vesicles using immunostaining (Yu et al., 2012). The significance of this type of intracellular localization of PLCζ and PIP2 has not been made clear.
Here we report experiments that analyse the mechanism of PLCζ induced Ca2+ oscillations in mouse eggs. We use photo-release of caged InsP3 to show that PLCζ causes Ca2+ oscillations via a positive feedback cycle of Ca2+ release and Ca2+ induced InsP3 production. In contrast the Sr2+ induced Ca2+ oscillations in mouse eggs involve a sensitization of InsP3 induced Ca2+ release. We go on to show that the cytosolic Ca2+ is more likely to be important for setting the pace of oscillations in eggs than Ca2+ store content. In addition, we present simulations to show that the restricted diffusion of InsP3 in cytoplasm implies that the source of InsP3 generation, PIP2, needs to be dispersed through the egg interior to account for PLCζ induced rapid Ca2+ waves. Finally, we provide further evidence that PIP2 is present on intracellular vesicles in eggs and that this is required for PLCζ and sperm induced Ca2+ oscillations in eggs.
Materials and Methods
Handling and Microinjection of Mouse Eggs
MF1 mice between 6 and 8 weeks of age were injected with pregnant mare's serum gonadotrophin (PMSG, Intervet) followed by human chorionic gonadotrophin (hCG, Invervet) ~50 h later (Fowler and Edwards, 1957). Eggs were collected from these mice 15 h after HCG injection, from the dissected ovaries. All animal handling and procedures were carried out under a UK Home Office License and approved by the Animal Ethics Committee at Cardiff University. Once collected, the eggs were kept at 37°C in M2 media (Sigma Aldrich). All Ca2+ dyes and intracellular probes were introduced into the cytosol of the eggs using a high pressure microinjection system with the eggs maintained in M2 media throughout (Swann, 2013). For in vitro fertilization sperm was collected from the epididymis of F1 C57/CBA hybrid male mice. The sperm were isolated in T6 media containing 16 mg/ml bovine serum albumin (BSA, Sigma Aldrich) and left to capacitate for 2–3 h before adding to eggs (Yu et al., 2012).
Measurements and Analysis of Intracellular Ca2+ and InsP3 Uncaging
In all experiments cytosolic Ca2+ was measured using fluorescent Ca2+ indictor Oregon Green BAPTA dextran (OGBD) (Life Technologies). OGBD was diluted in a KCl HEPES buffer (120 mM KCl, 20 mM HEPES at pH 7.4) so that the injection solution contained 0.33 or 0.5 mM OGBD. The OGBD mix was microinjected into eggs using high pressure pulses. In those eggs that were stimulated by adenophostin this was microinjected into eggs along with the OGBD. In this case instead of mixing the OGBD with KCL HEPES it was mixed with KCL HEPES containing 5 μM adenophostin in the same quantities. Where PLCζ cRNA was used this was microinjected alongside OGBD in the same way at a concentration of 0.02 μg/μl. For imaging, eggs were then transferred to a glass-bottomed dish, containing HKSOM media, on an epifluorescence imaging system (Nikon TiU) attached to a cooled CCD camera as described previously (Swann, 2013). Ca2+ dynamics were measured using the time-lapse imaging mode of Micromanager software (https://micro-manager.org/) where an image was captured every 10 s. Where IVF was performed, or drugs were later added to the eggs, the zona pellucidas were removed from the eggs using acid Tyrodes treatment prior to imaging. For those experiments that required InsP3 stimulation, NPE-caged-InsP3 (1 mM in the pipette) from ThermoFisher Scienific was microinjected prior to imaging at the same time as the injection of fluorescent dye (OGBD). In order to photo-release InsP3 the eggs were exposed to an electronically gated UV LED light source (365 nm, Optoled Lite, Cairn Research Ltd) that was positioned just above the dish containing the eggs. The duration of the UV pulse was controlled by a time gated TTL pulse that was delivered in between two successive fluorescence acquisitions. All data measuring Ca2+ dynamics were recorded as .tif files using the Micromanger software on the epifluorescence system.
Media, Chemicals, and Drugs
M2 media was purchased from Sigma Aldrich as a working solution. HKSOM was made up to pH 7.4, in cell culture grade water as follows: 95 mM NaCl, 0.35 mM KH2PO4, 0.2 MgSO4, 2.5 mM KCl, 4 mM NaHCO3, 20 mM HEPES, 0.01 mM EDTA, 0.2 mM Na Pyruvate, 1 mM L-glutamine, 0.2 mM glucose, 10 mM Na Lactate 1.7 mM CaCl2, 0.063 g/l Benzylpenicllin, and 10 mg/l phenol red. Ca2+ free media was made in the same way as HKSOM however CaCl2 was not added and the media was supplemented with 100 μM EGTA. Sr2+ containing media was made in the same way as HKSOM however, instead of adding 1.7 mM CaCl2, 10 mM SrCl2 was added instead.
All drugs and chemicals used, unless otherwise mentioned, were purchased from Sigma Aldrich. Propranolol was used at a working concentration of 300 μM in HKSOM media. A stock of 300 mM propranolol was made up in DMSO which was then diluted 1:100 in HKSOM media. Then 100 μl of this solution was pipetted into the imaging dish containing 900 μl of standard HKSOM. Propranolol was removed by washing out this media and replacing it with fresh HKSOM media using a perfusion system that passed 10 ml of clean HKSOM through the dish containing the eggs to ensure sufficient wash out. In a similar way a stock of 5 mM thapsigarin in DMSO was diluted 1:1,000 to a concentration of 5 μM in HKSOM and then 100 μl of this thapsigargin solution was added to the imaging dish containing 900 μl of HKSOM to give a working concentration of 500 nM of thapsigargin.
Confocal Imaging
In those eggs that were microinjected with PBP10, a solution of 1 mM PBP10 (Tocris Biosciences, UK) was made up in KCl HEPES and ~4–10 pl of this solution was microinjected into each egg. Following PBP10 microinjection, eggs were imaged on a Leica SP2 Confocal (Leica, Wetzler, Germany) microscope using a Helium-Neon laser (543 nm) at 30% intensity. Eggs were imaged in M2 media using a x63 oil objective and a pinhole aperture of 91 nm. Images were acquired with a line averaging of 8 and a resolution of 1,058 × 1,058 pixels. For each egg a single z-stack image of (1 μm depth) was captured of an equatorial slice through the egg. All images were exported as .tif files and analyzed using Image J (https://imagej.nih.gov/ij/).
Data Analysis
Quantitative data measuring the Ca2+ dynamics of the eggs on the widefield imaging system was extracted from .tif stacks using Image J (https://imagej.nih.gov/ij/). Background fluorescence was first subtracted from the egg fluorescence value. These fluorescence values were then normalized by dividing each fluorescence value in the egg by the baseline fluorescence value at the start of the imaging run to provide a relative change in fluorescence (F/F0) that could be plotted against time. These traces were produced and analyzed using SigmaPlot 12. The Confocal images were also analyzed using Image J software. PIP2 positive vesicle size and distribution was calculated using the particle analysis function on Image J and a nearest neighbor distance (Nnd) plugin in Image J. A bandpass filter function was applied to the images (large objects were filtered down to 40 pixels and small ones enlarged to 3 pixels). The threshold was altered to between 2 and 5% so only the fluorescence of the vesicles inside the image of the egg were included in the analysis. The particle analysis function was applied and configured so it recorded area, integrated intensity and coordinates for each fluorescent vesicle in the egg. These areas were used to work out the radius and diameter of the vesicles. The coordinates were fed into a nearest distance neighbor plugin (https://icme.hpc.msstate.edu/mediawiki/index.php/Nearest_Neighbor_Distances_Calculation_with_ImageJ) to give the mean distance between the vesicles. The total fluorescence of the vesicles was calculated by adding all the integrated intensity readings for a single egg which was carried out using the measure tool in ImageJ and background fluorescence values were subtracted. Statistical analysis was carried out using SigmaPlot 12. If not stated otherwise the data is presented as the mean and standard errors of the mean. Shapiro–Wilk tests for normality and tests for equal variances were conducted prior to carrying out group comparison tests. If the data passed both these tests a Student's T-test was conducted. If the data failed either or both of these tests a Mann-Whitney U-test was conducted instead.
Mathematical Method of Ca2+ Waves
The model and associated parameter values are based on the work of (Politi et al., 2006; Theodoridou et al., 2013). The reaction-diffusion equations define the interactions between free cytosolic calcium, u; stored calcium, v; and IP3, p,
The equations represent interactions in which free Ca2+ acts as a self-inhibitor but, along with InsP3 and stored Ca2+, stimulates the release of stored Ca2+, creating a system that can produce oscillations in the concentrations of calcium and InsP3. Critically, all species are able to diffuse with the same diffusion coefficient, d.
The actions of the stored Ca2+ and the InsP3 only occur in discrete regions. This spatial discreteness is controlled by the repeating function S(x, y, L). Essentially, the function S(x, y, L) creates a regular grid of squares of size Lon × Lon in which the specified kinetics are active. We are then able to alter the wavelength, or separation distance, L, between these active regions.
The equations were simulated using a finite element Runge-Kutta method on a two-dimensional disk of diameter 70 μm, which was discretised into 6,550 elements. The 2D assumption is considered valid because any dilution effects of going to three dimensions are off set equally by an increase in the third dimension production. The two-dimensional simulations can be thought of a single slice through a cell and it offers speed, clarity and insight. Finally, the boundary was specified to have a zero-flux condition, meaning that no substances were able to leak out of the domain. This is a simplification considered valid since it is known that PLCζ induced Ca2+ spikes can be generated in mouse eggs where no membrane Ca2+ fluxes occur (Miao et al., 2012). The equations are accompanied by the parameter values specified in Table 1, where all unit dimensions are chosen to make u, v, and p have units of μMol, space is in μm and time is in seconds. The initial conditions for all populations were at steady state except for a small perturbation of a two-dimensional Gaussian profile at the point (20,20), in the free Ca2+ population.
Results
PLCζ and Sr2+ Trigger Ca2+ Oscillations in Eggs via Different Mechanisms
We investigated the mechanism generating Ca2+ oscillations by using photo-release of caged InsP3 that was microinjected into mouse eggs. In initial experiments we uncaged InsP3 in unfertilized (control) mouse eggs that were not undergoing any Ca2+ oscillations. Figure 1A shows that UV pulses of light from 50 ms through to 2 s generated Ca2+ increases with the amplitudes that were larger with longer duration pulses. With the protocol we used there was adequate amounts of caged InsP3 for multiple releases of InsP3 even with longer duration pulses of UV light as illustrated by Figure 1B which shows that 3 s pulses could generate repeated large rises in Ca2+ in control eggs. We then tested the effects of triggering such pulses during Ca2+ oscillations induced by either Sr2+ media or by PLCζ injection. Figure 1C shows that when a 100 ms pulse was used in eggs injected with PLCζ the uncaging of InsP3 caused no Ca2+ increase. In contrast, Figure 1D shows Ca2+ oscillations occurring in response to Sr2+ media and in such eggs there was a rapid and large Ca2+ transient every time a pulse of just 100 ms was used to uncage InsP3. Since the response to 100 ms pulses of UV were minimal in control eggs (Figure 1A) these data show that Sr2+ media sensitizes eggs to InsP3 induced Ca2+ release and that, in contrast, IP3R are not sensitized to InsP3 by PLCζ injection.
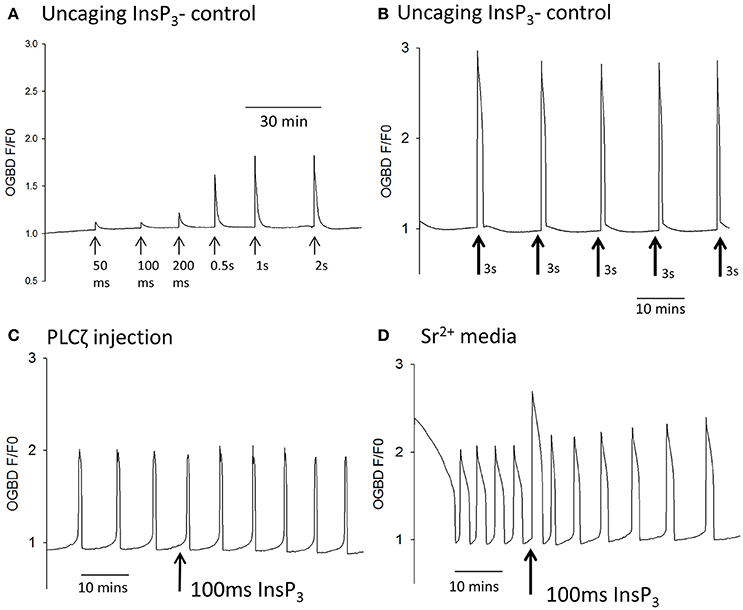
Figure 1. Ca2+ oscillations and uncaging pulses of InsP3. In (A) an example trace is shown of Ca2+ increases (as measured by OGBD fluorescence) in an egg in response to different amounts of InsP3. Eggs were injected with caged InsP3 and exposed to varying durations of UV light pulse (from 50 ms to 2 s) to photo-release the InsP3 (trace typical of n = 21 eggs). In this and all other traces shown, the pulses were applied at points indicated by the arrows. In (B) an example trace is shown of changes in cytosolic Ca2+ in an egg in response to the “uncaging” of caged InsP3 using long duration UV pulses of 3 s. Arrows indicate where pulses of UV light were applied (typical of n = 7 eggs). In (C) an example trace is shown of changes in cytosolic Ca2+ in an egg stimulated following the microinjection of mouse derived PLCζ cRNA (0.02 μg/μl) and caged InsP3. The arrow indicates where a 100 ms pulse of UV light was applied (n = 14 eggs). In all 14/14 such recordings there was no sudden increase in Ca2+ even when the pulse was applied during the pacemaker rising phase of Ca2+. In (D) an example trace is shown with changes in cytosolic Ca2+ in an egg stimulated by media containing 10 mM Sr2+. The arrow indicates where a 100 ms pulse of UV light was applied to uncage InsP3 (n = 32 eggs). In all 32/32 cases there was a rapid Ca2+ increase that started with the very next OGBD fluorescence measurement after the UV pulse (<10 s).
The two classes of model for Ca2+ oscillations, those that involve the dynamic properties of IP3Rs and those that involve InsP3 production oscillations, can be distinguished in a definitive manner by examining the response to a sudden pulse of InsP3 (Sneyd et al., 2006). Models that are dependent upon IP3R kinetics alone respond to a pulse of InsP3 by showing a transient increase in the frequency of Ca2+ oscillations (Sneyd et al., 2006). In contrast, models that depend on Ca2+ induced InsP3 production, and imply InsP3 oscillations, respond to a sudden increase in InsP3 by showing an interruption of the oscillations which leads to a resetting of the phase of oscillations (Sneyd et al., 2006). We tested the effect of using large uncaging pulses of InsP3 on Sr2+ induced, or PLCζ induced, Ca2+ oscillations in mouse eggs. Figure 2A shows that during Sr2+ induced oscillations a 3 s uncaging pulse of InsP3 caused a large increase in Ca2+ followed by a significant increase in the frequency of Ca2+ oscillations. In contrast, with PLCζ induced Ca2+ oscillations, Figure 2B shows that the same 3 s uncaging pulse of InsP3 did not cause any increase in frequency, but interrupted the periodicity of oscillations leading to a delay before the next Ca2+ increase. To confirm that this phenomenon was phase resetting, we plotted the shift in phase (PS) caused by uncaging of InsP3 against the time delay (dt) of the InsP3 pulse from the subsequent Ca2+ spike (see Figure 2C). Each of these values was divided by the time period T in order to take into account the different frequency of Ca2+ oscillations in each egg. With phase resetting this plot should give a line from 1 to 1 on each axis, and Figure 2D shows that the data from 23 PLCζ injected eggs exposed to uncaging pulses of InsP3 fit closely on such a line. These data clearly show that a pulse of InsP3 causes phase resetting of Ca2+ oscillations in mouse eggs, which is completely different from that seen with Sr2+ induced oscillations. Hence, overall the data suggest that PLCζ and Sr2+ media trigger Ca2+ oscillations in mouse eggs via fundamentally different mechanisms. Sr2+ stimulates IP3Rs to make them effectively more sensitive to InsP3, and that PLCζ induced Ca2+ oscillations involve Ca2+ stimulated InsP3 production where InsP3 acts as a dynamic variable that should oscillate in synchrony with Ca2+ oscillations.
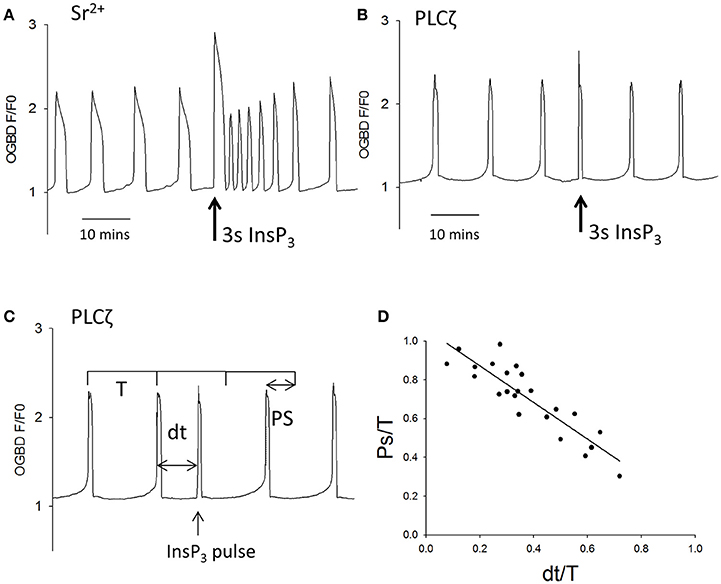
Figure 2. The effect of large pulses of InsP3 on PLCζ or Sr2+ triggered Ca2+ oscillations. (A) shows an example of the way eggs responded a large uncaging pulse of InsP3 (3 s UV light at the arrow) by an increase in the frequency of Sr2+ triggered Ca2+ oscillations (n = 20 eggs). There was a significant increase in frequency of Ca2+ spikes from 3.45 (±0.27 sem) in 20 min to 5.05 (±0.35 sem) in 20 min (p < 0.001). In (B) a similar experiment is shown but with PLCζ induced Ca2+ oscillations. The sample trace in (B) shows that a 3 s uncaging pulse of InsP3 (at the arrow) caused an immediate Ca2+ increase but no increase in frequency (n = 23 eggs). We analyzed the ability of such pulses to reset the phase of oscillations by measuring then phase shift (PS) and comparing it to time delay (dt) at which the InsP3 pulse was applied. (C) Illustrates how these values were measured on an actual sample trace. Each value was divided by the time-period (T) for the oscillations in order to normalize the values between different eggs. (D) Shows a plot of these values for all 23 eggs tested.
Cytosolic Ca2+ vs. Ca2+ Stores and the Frequency of Ca2+ Oscillations
Since Ca2+ release and InsP3 formation are predicted to form part of a positive feedback loop we decided to re-investigate some observation previously made on Ca2+ oscillations in eggs. One finding made in hamster and mouse eggs is that both sperm (and PLCζ)-triggered Ca2+ oscillations “run down” and can cease entirely in Ca2+ free media (Igusa and Miyazaki, 1983; Lawrence and Cuthbertson, 1995). This phenomena has been explained in terms of Ca2+ store depletion but the level of cytosolic Ca2+ and its effect on InsP3 production could also be important. We re-examined the role of Ca2+ stores and resting Ca2+ using the SERCA inhibitor thapsigarin. Previous studies used high concentrations (>10 μM) of thapsigargin to completely block Ca2+ oscillations in eggs (Kline and Kline, 1992b). To investigate the role of Ca2+ store content we used much lower concentrations of thapsigargin which caused only a small elevation of cytosolic Ca2+. Figures 3A,B show that the addition of 500 nM thapsigargin to mouse eggs caused a small and prolonged increase in resting cytosolic Ca2+ in normal media and Ca2+ free media, which is consistent with a slight inhibition of SERCA pumps. When the same concentration of thapsigargin was added to eggs undergoing Ca2+ oscillations in response to PLCζ there was a marked acceleration of Ca2+ oscillations, and a reduction in the amplitude of Ca2+ spikes (Figure 3C). Similar to previous reports, we found that the pattern of PLCζ induced Ca2+ oscillations show a run down in Ca2+ free media (containing EGTA). We noted that this was associated with a decline in the fluorescence of OGBD, suggesting that resting Ca2+ levels were also undergoing a decline (Figure 3D). When low concentrations of thapsigargin (500 nM) were added to PLCζ injected eggs in Ca2+ free media there was a restoration of Ca2+ oscillations (Figure 3E). It is noteworthy that in Figure 3E the eggs were in Ca2+ free media and yet the addition of thapsigargin, which would cause further Ca2+ store depletion, actually leads to a restoration of Ca2+ oscillations. Nevertheless, the restoration of Ca2+ oscillations was associated with a rise in the “basal” Ca2+ level (Figure 3E). These data are consistent with the idea that cytosolic Ca2+ plays a key role in triggering each Ca2+ rise, and that Ca2+ stores are not significantly depleted in mouse eggs by incubation in Ca2+ free media.
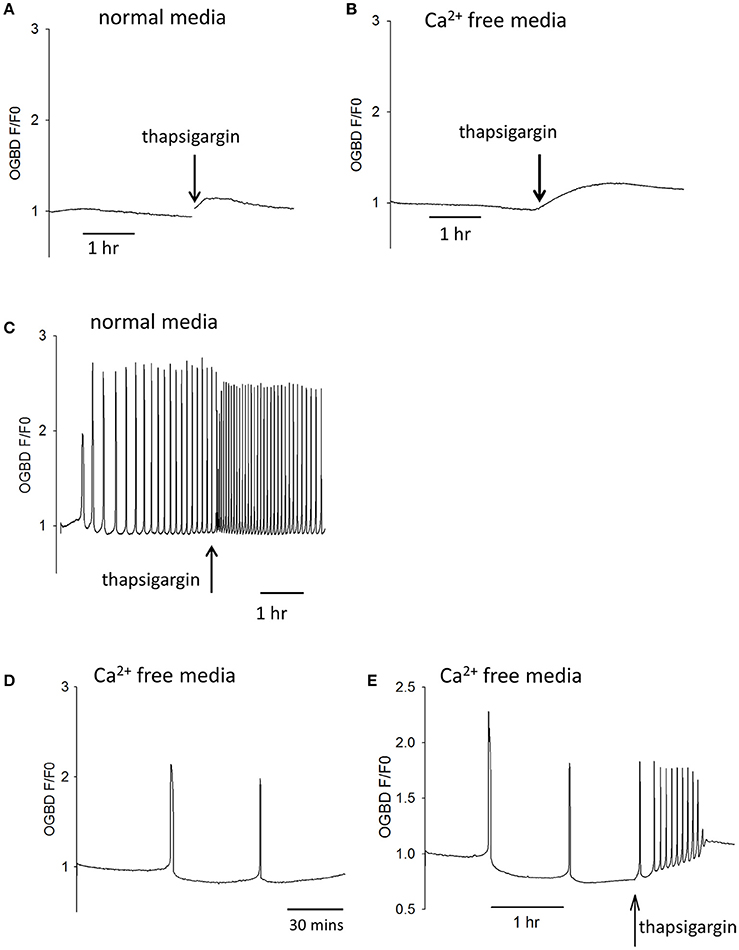
Figure 3. Cytosolic Ca2+ and the frequency of Ca2+ oscillations. In (A) an example is shown of a trace showing changes in cytosolic Ca2+ in an egg incubated in normal HKSOM media following the addition of 500 nM thapsigargin (typical of n = 12 eggs). The increase in Ca2+ was from 0.936 ± 0.013 SEM to 1.07 ± 0.0197 SEM which is a significant (P < 0.01). In (B) a similar example is shown of the addition 500 nM thapsigargin to an egg in Ca2+ free media (containing 100 μM EGTA), which was typical of n = 12 eggs. The increase in basal Ca2+ was from 0.908 ± 0.0134 SEM up to 1.29 ± 0.0168 SEM which was significant (P < 0.001). In (C) an example of one of 6 eggs is shown where the same low concentration of thapsigargin increased the frequency of Ca2+ oscillations by 1.72-fold (±0.07 SEM). In (D) a trace is shown from an egg that was injected with PLCζ RNA and then placed in Ca2+ free media. The mean number of Ca2+ spikes in such experiments was 1.56 (n = 18 eggs, ±0.31 SEM) Ca2+ spikes in 10,000 s (2 h 47 min) h. The Ca2+ levels decreased to 0.84 ± 0.029 SEM which was significantly less than the starting level (P < 0.001). In (E) is shown an example of an egg that had been injected with PLCζ RNA and then placed in Ca2+ free media as in (C). However, in these experiments 500 nM thapsigarin was added after >2 h. In 16/17 such treated eggs there was an increase in the frequency of Ca2+ oscillations. There were an average of 1.77 spikes (±0.18 SEM) before adding thapsigarin but a mean of 7.11 spikes (±1.3 SEM) after thapsigarin addition. The resting Ca2+ level increased from 0.84 ± 0.029 SEM, before adding thapsigargin to 1.076 ± 0.017 sem in eggs where it stabilized. This is a signicant increase in Ca2+ concentration (P < 0.001).
PLCζ Induced Ca2+ Oscillations and Intracellular PIP2
Previous studies of fertilizing mouse and hamster eggs show that most Ca2+ waves cross the egg in about 1 s, and propagate through the cytoplasm at speeds in excess of 50 μm/s. This matches the rising phase of (all but the initial) Ca2+ transients in mouse eggs which is ~1 s after fertilization or after PLCζ protein injection (Deguchi et al., 2000). Since data in Figure 2 implies that the upstroke of each Ca2+ rise involves an InsP3 and Ca2+ positive feedback loop, then it is necessary for both molecules to be sufficiently diffusible. The Ca2+ stores (the endoplasmic reticulum) are spread across the egg. However, this may not be the case with PIP2 that is the precursor to InsP3. In most cells PIP2 is in the plasma membrane, and if this is used in Ca2+ waves in eggs then InsP3 diffusion range might constrain the ability to generate fast Ca2+ waves. Recently, the diffusion coefficient of InsP3 in intact cells has been shown to be <10 μm2/s which means that InsP3 may only diffuse <5μm in 1 s (Dickinson et al., 2016). We have previously presented models of Ca2+ oscillations based upon Ca2+ induced InsP3 formation and InsP3 induced opening of Ca2+ release channels (Theodoridou et al., 2013). We have now simulated the Ca2+ waves in mouse eggs using a similar set of equations in a two-dimensional model of the Ca2+ wave. Figure 4 shows that with the source of Ca2+ stimulated InsP3 production at the periphery (plasma membrane) it is not possible to generate a Ca2+ wave through the egg cytoplasm, and only a concentric pattern of Ca2+ release occurs. We previously presented evidence for PIP2 being present in intracellular vesicles spread throughout the cytoplasm in mouse eggs (Yu et al., 2012). These could provide a source of InsP3 that might carry a Ca2+ wave through the cytoplasm if they are sufficiently dispersed. In Figure 4 we show simulations based upon Ca2+ induced InsP3 generation where the PIP2 is dispersed on vesicles at different distances apart (from 2 to 4 μm). Our simulations show that when the PIP2 vesicles are within 2 or 3 μm of each other a rapid Ca2+ can be generated, but that once the PIP2 is more than 3 μm the Ca2+ increase fails to occur. These results suggests that PIP2 needs to be present on vesicles spaced <3 μm apart in the cytoplasm in order to propagate a rapid Ca2+ wave of the type seen in fertilizing and PLCζ injected eggs.
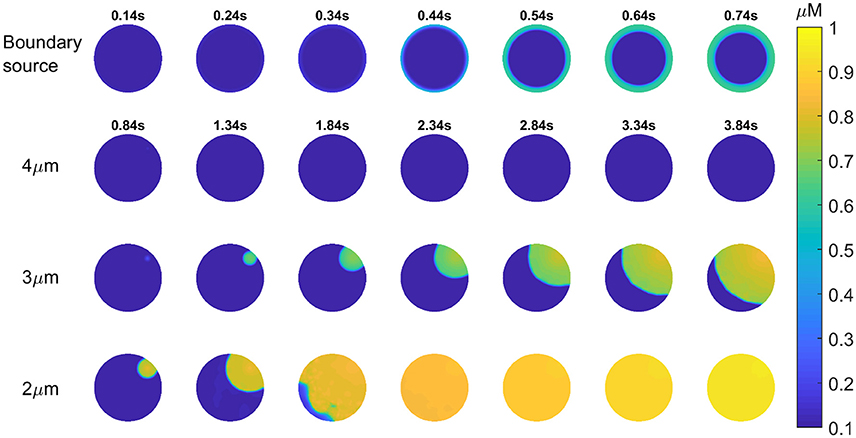
Figure 4. Simulation of InsP3 induced Ca2+ release in eggs. Images are shown for a 2-dimensional simulation of the propagation of a Ca2+ wave in a mouse egg using a mechanism based upon Ca2+ induced InsP3 formation. Images for each time series is shown in each of the rows. For the first row the only source of PIP2 for making InsP3 is at the boundary (the plasma membrane) and this does not cause a wave at all. The times for each image (in seconds) in the top row is indicated by numbers above each image. In the next three rows the source of Ca2+ induced InsP3 formation is spaced at different distances. The time intervals for each image is indicated in the second row and it is then the same for each image going down in each column. In the first row the distance for the PIP2 is 4 μm, and again no Ca2+ wave can be generated. With a PIP2 source spaced at 3 or 2 μm we found that a Ca2+ wave can be generated. With a 2 μm separation a wave occurs that crosses the “egg” in ~1 s.
Previous evidence for the existence of PIP2 within the cytoplasm of eggs came from studies using antibodies to PIP2 (Yu et al., 2012). Gelsolin is a protein that has been shown to bind to PIP2, and contains a short peptide sequence responsible for PIP2 binding (Cunningham et al., 2001). We injected mouse eggs with PBP-10, which is a probe in which rhodamine is coupled to a gelsolin peptide that binds PIP2. Figure 5A shows a mouse egg injected with PBP-10. After >1 h the fluorescence of PBP-10 could be predominantly seen in many small vesicles throughout the egg cytoplasm, with the occasional larger aggregate. This supports the hypothesis that PIP2 is localized in vesicles within mouse eggs (Yu et al., 2012). Further examination of these vesicles using particle analysis indicates that they are distributed throughout the whole egg cytoplasm. Interestingly, following nearest neighbor analysis, we found that these vesicles were ~2 μm apart (Figures 5A,D). This suggests that these PIP2 containing vesicles are within the correct distance predicted to produce the rapid rising phase of 1 s for each wave as predicted by our mathematical modeling.
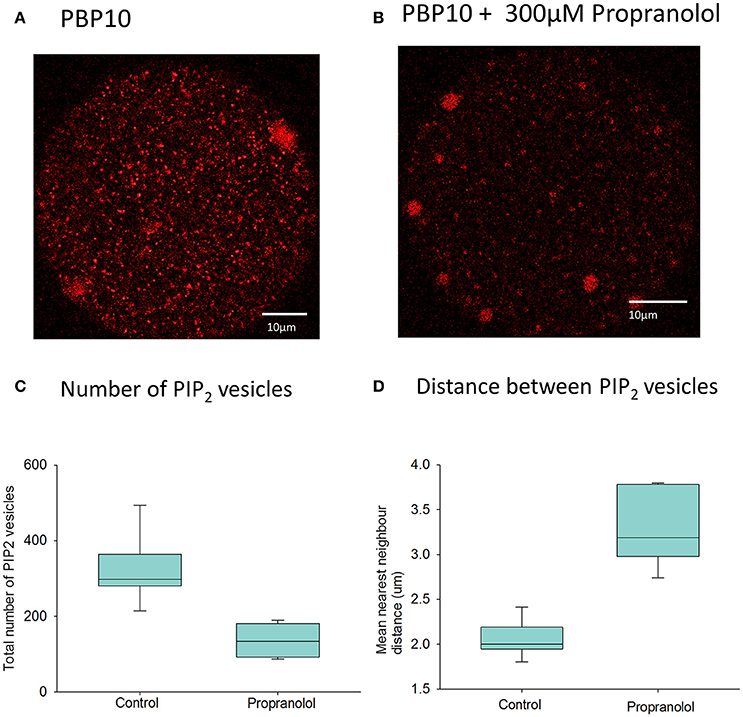
Figure 5. PIP2 distribution in mouse eggs using PBP10. In (A) an example is shown of the distribution of fluorescence of PBP10 in a mouse egg 1.5 h after injection of PBP10 (n = 21). Scale bars are 10 μm. (A) nearest neighbor analysis indicated that the mean vesicle distance for all 21 control eggs is 2.2 μm. In (B) an example is shown of an egg injected with PBP10 where and incubated in media with 300 μM propranolol (n = 13). In (C) particle analysis (n = 14 eggs) indicates that the mean vesicle diameter is 0.89 μm and the mean number of vesicles present per egg is 298.9. (C) Shows a plot of the total number of PIP2 positive vesicles present in eggs following injection of PBP10 using particle analysis. Results are shown for both eggs incubated in standard M2 media (control) (mean number of vesicles = 324, n = 7) and those incubated in M2 containing 300 μM propranolol during imaging (mean number of vesicles = 131, n = 7). There is a significant reduction in the number of PBP10 vesicles following propranolol treatment compared to control media (p = < 0.001, Student's T-test). (D) shows a plot of the mean nearest neighbor distances of PIP2 positive vesicles present in eggs. The results are shown for parallel groups of eggs incubated in standard M2 media (control) (mean distance = 2.0 μm, n = 7) and for those incubated in M2 containing 300 μM propranolol during imaging, (mean distance = 3.3 μm, n = 7). A Mann–Whitney U-test showed a significant increase in the distance between the PBP10 vesicles following propranolol treatment compared to control media (p = < 0.001).
We have previously sought to modify the level of PIP2 in mouse eggs using various phosphatases, but without success. Internal membranes in somatic cells do not in general contain much PIP2, but one organelle where PIP2 and DAG have been reported in some cells is the Golgi apparatus. In mature mammalian eggs, like mitotic cells, the Golgi is fragmented into small vesicles (Moreno et al., 2002; Axelsson and Warren, 2004). It has been shown that propranolol blocks DAG synthesis in Golgi membranes and leads to a loss of Golgi structure (Asp et al., 2009). We applied propranolol to mouse eggs injected with PBP10 and found a marked loss of staining (Figure 5B). Further particle analysis showed that the mean number of these PIP2 vesicles was significantly reduced following the addition of propranolol (Figure 5C). Furthermore, the distance of these vesicles from each other was significantly increased in those eggs treated with propranolol (Figure 5D). The overall total fluorescence of the vesicles was seen to reduce by approximately half from a mean of 5.77 × 104 RFU (n = 7) in control eggs to a mean of 2.93 × 104 RFU (n = 7) in those eggs treated with propranolol. This difference was significant following a Student's T-test (p = 0.006). This implies that propranolol is affecting PIP2 levels in cytoplasmic vesicles.
Since proproanolol appears to reduce PIP2 inside eggs, we investigated the effect of propranolol on Ca2+ oscillations. Figure 6A shows that propranolol addition to eggs undergoing Ca2+ oscillations in response to fertilization by IVF were rapidly blocked. Figure 6B shows the same effect of propranolol on those eggs stimulated by PLCζ cRNA. The inhibition by propranolol was associated with a slight decline in Ca2+ levels and the inhibition was reversed upon removal of propranolol (Figure 6C). However, whilst it blocked sperm and PLCζ induced responses, propranolol did not block Ca2+ oscillations induced in eggs by Sr2+ media, or by injection of the IP3R agonist adenophostin (Figures 6D,E). These data show that the inhibitory effects of propranolol are both reversible and specific to PLCζ and sperm induced Ca2+ oscillations. They support the proposal that PIP2 in vesicles in the cytoplasm of mouse eggs is important for the generation of PLCζ induced Ca2+ oscillations.
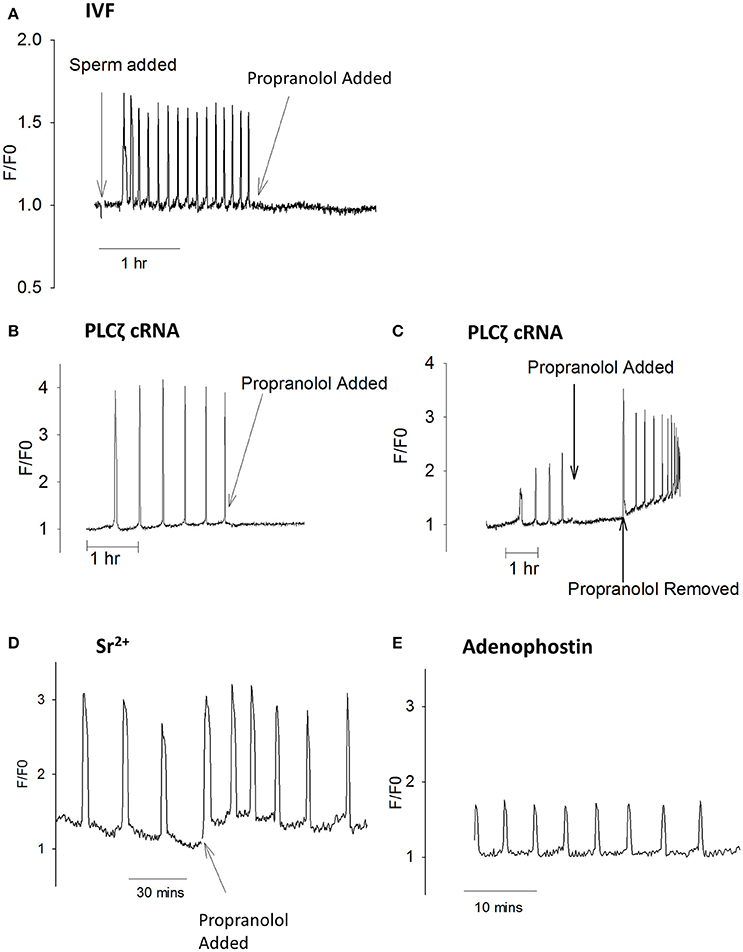
Figure 6. Ca2+ oscillations blocked by propranolol. In (A) an example is shown of a mouse egg undergoing Ca2+ oscillations at fertilization where the addition of 300 μM propranolol inhibited subsequent oscillations (n = 13 eggs). Before addition of propranolol the mean frequency was 12.2 ± 1.14 spikes/h with all eggs oscillating. After adding propranolol there were 0.8 ± 0.23 spikes /h (a significant difference from before propranolol, p = < 0.0001). 6/13 eggs stopped oscillating immediately, 4/13 eggs had one Ca2+ spike, and 3/13 has 2 spikes in an hour. (B) shows PLCζ cRNA (pipette concentration = 0.02 μg/μl) induced Ca2+ oscillations inhibited by propranolol (n = 21 eggs). Before propranolol all eggs oscillated with 4.3 ± 0.46 spikes/h. After addition of propranolol there were 0.95 ± 0.25 spikes/h (a significant difference p < 0.0001). With propranolol, 10/21 eggs stopped Ca2+ oscillations, 6/21 showed a single spike, and 5/21 had >1 Ca2+ spike. In (C) an example is shown of an egg where PLCζ induced Ca2+ oscillations were blocked by the addition of propranolol but then oscillations were restored when propranolol was washed out (typical of n = 8 eggs). Before propranolol, all eggs oscillated with 6.7 ± 1.3 spikes/h. After propranolol this decreased to 1.33 ± 0.29 spikes/h, with all oscillations stopping after 2 spikes. When propranolol was removed there were 10 ± 0.55 spikes in 30 min. Adding propranol and then removing it both caused significant changes in the number of Ca2+ spikes (p < 0.001). (D) shows an example of an egg undergoing Ca2+ oscillations in response to Sr2+ media where propranolol was subsequently added (n = 10 eggs). Before propranolol all eggs oscillated with 4.1 ± 0.29 spikes/h. After adding propranolol all eggs continues to oscillate with 3.9 ± 0.66 Ca2+ spikes/h (not significantly different). In (E) an example is shown of an egg undergoing Ca2+ oscillations in response to microinjection of 5 μM adenophostin in media that contained 300 μM propranolol form the start of the experiment (n = 8 eggs). In propranolol there were 5.5 ± 0.51 Ca2+ spikes 30 min, compared with 10.4 ± 0.71 spikes/30 min (n = 11) for eggs in media with HKSOM. This is significantly different (unpaired t-test, p = < 0.0001).
Discussion
The Ca2+ oscillations seen in mammalian eggs at fertilization have distinct characteristics compared with those seen in somatic cell types (Dupont and Goldbeter, 1994; Politi et al., 2006). The oscillations at fertilization are low frequency, and long lasting, but they have a very rapid rising phase that occurs throughout the whole cytoplasm of a very large cell, in less than a second. Considerable evidence suggests that PLCζ is the primary stimulus for these Ca2+ oscillations (Saunders et al., 2002). The current data shows that PLCζ induced Ca2+ oscillations are driven by Ca2+ induced InsP3 formation. In contrast, we show that Sr2+ media sensitizes eggs to InsP3 induced Ca2+ release. Hence, there are at least two different mechanisms for generating Ca2+ oscillations in mouse eggs. Our data also implies that the substrate of PLCζ, PIP2, needs to be localized in a finely distributed source within the egg in order to generate fast Ca2+ wave, and we present evidence that such vesicular PIP2 is required for PLCζ induced Ca2+ oscillations.
There are two fundamentally different classes of models for InsP3 induced Ca2+ oscillations in cells. One relies on the properties of InsP3 receptor and implies that stimulation involves an elevated but monotonic or constant elevation of InsP3 levels. The other involves a positive feedback model of InsP3 induced Ca2+ release and Ca2+ induced InsP3 formation. It is possible to determine which one of these two model types applies by studying the Ca2+ responses after triggering a large pulsed release of InsP3 (Sneyd et al., 2006). The IP3R based models respond to a pulse of InsP3 by temporarily increasing the frequency of Ca2+ oscillations, whereas the Ca2+-induced InsP3 formation models show an interruption in the series of Ca2+ transients with a resetting of the phase of the oscillations (Sneyd et al., 2006). We previously presented preliminary evidence for an interruption in the series transients with sperm or PLCζ induced Ca2+ oscillations responding to a pulse of InsP3 (Swann and Yu, 2008). We now show that the response of PLCζ induced Ca2+ oscillations to a sudden large pulse of InsP3 is clearly characterized by a resetting of the phase of oscillations. This means that InsP3 has to be a dynamic variable in the oscillation cycle and that it will undergo oscillations in close phase with the oscillations in Ca2+. Small oscillations in InsP3 have been recorded previously in response to high frequency Ca2+ oscillations achieved with high concentrations of PLCζ (Shirakawa et al., 2006). The sensitivity of such indicators may be limited since we can now assert that InsP3 oscillations should occur with all the PLCζ induced Ca2+ oscillations and, most significantly, that increased InsP3 production plays a causal role in generating each Ca2+ rise. We have also shown here that Sr2+ works via an entirely different mechanism in mouse eggs. The increase in frequency of Ca2+ oscillations caused by uncaging InsP3 indicates that Sr2+ induced oscillations rely on the properties of the IP3R. This is supported by the finding that Sr2+ media sensitized mouse eggs to InsP3 pulses, which is consistent with the idea that Sr2+ stimulates the opening of InsP3 receptor. These data overall show that mouse eggs have more than one mechanism for generating Ca2+ oscillations and that in some cases Ca2+ oscillations can appear to be similar in form, but be generated by different mechanisms.
It is well-established that Ca2+ free media leads to a reduction or abolishment of Ca2+ oscillations in response to fertilization or PLCζ injection in mammalian eggs (Igusa and Miyazaki, 1983; Lawrence and Cuthbertson, 1995). It has been assumed that this reflects the loss or some reduction of Ca2+ in the endoplasmic reticulum (Kline and Kline, 1992b). However, our data suggest that a reduction in resting, or interspike, cytosolic Ca2+ levels also occurs during incubation in Ca2+ free media. The reduction in cytosolic Ca2+ is apparent with the Ca2+ dye we used because it is dextran linked and hence is only within the cytosolic compartment, and because the Kd for OGBD and Ca2+ is around 250 nm. The reduction in resting Ca2+ level appears to cause the inhibition of Ca2+ oscillations, rather than a loss of Ca2+ store content, because low concentrations of thapsigargin, which will only reduce Ca2+ stores content further, actually restores Ca2+ oscillations in Ca2+ free media. The restoration of such Ca2+ oscillations by thapsigargin in our experiments was clearly associated with a rise in the basal Ca2+ level. PLCζ induced Ca2+ oscillations eventually stopped in Ca2+ free media with thapsigargin and this could be because Ca2+ stores eventually became depleted. However, the earlier rise in cytosolic Ca2+ seems to be a stimulatory factor because low concentrations of thapsigarin, which raise basal Ca2+, could also increase the frequency Ca2+ oscillations in normal media. This was associated a reduction in the amplitude of Ca2+ spikes, presumably because Ca2+ store content is reduced. Low concentrations of thapsigargin have also previously been found to stimulate Ca2+ oscillations in immature mouse oocytes (Igusa and Miyazaki, 1983; Lawrence and Cuthbertson, 1995). Hence, these data together imply that cytosolic Ca2+ level, rather than Ca2+ store content is the more significant factor setting the frequency and occurrence of physiological Ca2+ oscillations.
These data are consistent with recent studies measuring free Ca2+ inside the endoplasmic reticulum in mouse eggs (Wakai et al., 2013). It was shown that a reduction in ER Ca2+ occurs following each Ca2+ spike, but that there is no correlation between when a Ca2+ transient is initiated and the level of Ca2+ in the ER (Wakai et al., 2013). Whilst it is obvious that some Ca2+ store refilling will occur in the intervals between Ca2+ spikes, it is not likely that this sets of the pace of the low frequency Ca2+ oscillations characteristic of mammalian eggs. We suggest that the pacemaker that determines when the next Ca2+ transient occurs after PLCζ injection is more likely to be the rise in cytosolic Ca2+. A gradual rise in cytosolic Ca2+ between spikes is evident in the PLCζ induced Ca2+ oscillations in all the traces in this paper. This gradual Ca2+ increase could promote a gradual rise in InsP3 that will eventually lead to a positive feedback loop and a regenerative Ca2+ wave.
Although the Ca2+ oscillations triggered by fertilization in mammalian eggs are of low frequency, each of the waves of Ca2+ release that causes the upstroke of a Ca2+ increase crosses the egg remarkably quickly. Previous analysis of the wave dynamics of Ca2+ release in mammalian eggs have suggested that the rising phase of each Ca2+ oscillation is ~1 s. This correlates with the speed of the Ca2+ wave that crosses the egg at a speed of >50 μm/s. This is significant because the diffusion coefficient of InsP3 in intact cells has been estimated to be no more that 10 μm2/s (Dickinson et al., 2016). In models where InsP3 is elevated at a constant level during Ca2+ oscillations the restricted diffusion of InsP3 is not an issue because it will reach a steady state concentration across the egg. However, our data shows that Ca2+ and InsP3 act together in a positive feedback loop to cause each propagating Ca2+ wave. In this case the diffusion of InsP3 could be a rate limiting step. If all the InsP3 is generated in the plasma membrane then our simulations show that a Ca2+ induced InsP3 production model cannot generate Ca2+ waves through the egg cytoplasm. If we simulate the InsP3 production from discrete sites within the egg cytoplasm then rapid Ca2+ waves of some type can be generated, but full waves can only be seen when the sites of InsP3 generation are within 3 μm of each other. This suggests that in order to explain both the fast Ca2+ waves and the basic mechanism of sperm or PLCζ induced oscillations in mammalian eggs, the PIP2 substrate has to be dispersed in sites throughout the egg cytoplasm. This conclusion is similar to that previously suggested for ascidian oocyte at fertilization which also show rapid Ca2+ waves and oscillations (Dupont and Dumollard, 2004).
We previously reported evidence for a vesicular source of PIP2 in mouse eggs using immunostaining (Yu et al., 2012). The vesicular staining with PIP2 antibodies closely mimics the distribution of PLCζ also probed with antibodies (Yu et al., 2012). We now report a similar pattern of vesicular staining using another probe (PBP10) which based upon the PIP2 binding region of gelsolin (Cunningham et al., 2001). This probe has the advantage that it is microinjected into eggs that can then be imaged whilst still alive and so does not require the fixation and permeabilization procedures associated with immunostaining. It gives a very different pattern of staining from another commonly used probe for PIP2 which is the GFP-PH domain which localizes predominantly to the plasma membrane in mouse eggs (Halet et al., 2002). However, the PH domain of PLCδ1 that is used for the localization of PIP2 in such a probe may also bind cholesterol so may be influenced by factors other than PIP2 (Rissanen et al., 2017). It is entirely possible that PBP10 is also influenced by factors other than PIP2, but it is noteworthy that the PBP10 staining gives a vesicular localization pattern that closely resembles that seen with the PIP2 antibodies. The fact that two very different methods for localization PIP2 in eggs, immunostaining with a monoclonal antibody and a fluorescently tagged peptide, show such a distinctive and similar pattern of localization provides good evidence that PIP2 is indeed localized within vesicles in the cytoplasm in of mouse eggs. Using the live cell probe, PBP10, we were able to estimate that the apparently PIP2 containing vesicles we see in eggs are within about 2 μm of each other. This distance closely correlates with the estimate of how close PIP2 vesicles need to be in order to propagate a Ca2+ wave across the egg within ~1 s. Hence, our data provide a coherent view of PLCζ induced Ca2+ release in eggs in which Ca2+ induced InsP3 formation from closely spaced vesicles containing PIP2 accounts for the upstroke of each Ca2+ rise.
The precise nature of the PIP2 containing vesicles that appear to exist in mouse eggs is unclear. We have tested a number of antibodies and other probes for specific organelles in eggs and found that many either localize to the endoplasmic reticulum or else show only a limited overlap in staining with the PIP2 or PLCζ positive vesicles. The identification of PBP10 positive vesicles is further complicated by our finding that its pattern of localization does not persist after fixation and membrane permeabilization (Sanders and Swann, unpublished). In somatic cells, non-plasma membrane PIP2 has been found in the Golgi apparatus (De Matteis et al., 2005). Mature mouse egg are unusual compared with somatic cells in that they are arrested in meiosis, which is similar to the mitotic phase of the cell cycle. During mitosis the Golgi fragments to form small vesicles known as the Golgi haze (Axelsson and Warren, 2004), and the Golgi in mouse eggs has been shown to be fragmented into small vesicles (Moreno et al., 2002). The structure of the Golgi and its associated vesicles is maintained by the presence of diacyglycerol (DAG) (Asp et al., 2009). The drug propranolol disrupts Golgi resident proteins and lipids by inhibiting DAG production and as a result, it also disrupts Golgi-ER trafficking (Asp et al., 2009). Interestingly propranolol was found to block Ca2+ oscillations triggered by PLCζ and fertilization. This effect was specific in that the same concentration of propranolol did not effect oscillations when added to other Ca2+ releasing agents such as Sr2+ media which causes a pattern of oscillations most similar to fertilization. The small effect on adenosphostin induced Ca2+ oscillations is unlikely to be sufficient to explain the effects of propranolol because it was only a 2-fold decrease in oscillations compared the cessation of oscillations after propranolol in most eggs that were fertilized or injected with PLCζ. It is also noteworthy that the Ca2+ levels remained low in propranolol treated eggs, and that its effects were reversible. In mouse eggs we found that propranolol also decreased the number of the PIP2 containing vesicles and the mean distance between vesicles, therefore presumably, the availability of the vesicular PIP2 to propagate a Ca2+ wave. This effect could be because propranolol disrupts the structure of the vesicles or because trafficking between the Golgi and the ER is inhibited. Whatever the actual mechanism, the loss of PIP2 after treatment with propranolol supports our hypothesis that these vesicles are required for generating Ca2+ oscillations in eggs in response to sperm or PLCζ. Since there is evidence for intracellular PIP2 on organelles in frog and sea urchin eggs, which also show Ca2+ waves at fertilization, it is attractive to speculate that intracellular PIP2 is an important feature that allows eggs to generate the Ca2+ signal needed for egg activation.
Author Contributions
JS: Performed some of the Ca2+ measurements and the PIP2 imaging experiments, analyzed data, and co-wrote the manuscript; BA and AM: Performed Ca2+ measurement experiments on eggs and analyzed data; TW: Produced and analyzed the mathematical simulation; KS: Conceived the study, directed experiments and co-wrote the manuscript. All authors approved the final manuscript.
Conflict of Interest Statement
The authors declare that the research was conducted in the absence of any commercial or financial relationships that could be construed as a potential conflict of interest.
Acknowledgments
The authors wish to thank the School of Medicine for funding JS for her postgraduate studies, and Michail Nomikos for providing us with the PLCζ RNA used in this study.
References
Asp, L., Kartberg, F., Fernandez-Rodriguez, J., Smedh, M., Elsner, M., Laporte, F., et al. (2009). Early stages of Golgi vesicle and tubule formation require diacylglycerol. Mol. Biol. Cell 20, 780–790. doi: 10.1091/mbc.E08-03-0256
Axelsson, M. A., and Warren, G. (2004). Rapid, endoplasmic reticulum-independent diffusion of the mitotic Golgi haze. Mol. Biol. Cell 15, 1843–1852. doi: 10.1091/mbc.E03-07-0459
Bedford-Guaus, S. J., Yoon, S. Y., Fissore, R. A., Choi, Y. H., and Hinrichs, K. (2008). Microinjection of mouse phospholipase C zeta complementary RNA into mare oocytes induces long-lasting intracellular calcium oscillations and embryonic development. Reprod. Fertil. Dev 20, 875–883. doi: 10.1071/RD08115
Bos-Mikich, A., Swann, K., and Whittingham, D. G. (1995). Calcium oscillations and protein synthesis inhibition synergistically activate mouse oocytes. Mol. Reprod. Dev. 41, 84–90. doi: 10.1002/mrd.1080410113
Brind, S., Swann, K., and Carroll, J. (2000). Inositol 1,4,5-trisphosphate receptors are downregulated in mouse oocytes in response to sperm or adenophostin A but not to increases in intracellular Ca2+ or egg activation. Dev. Biol. 223, 251–265. doi: 10.1006/dbio.2000.9728
Cox, L. J., Larman, M. G., Saunders, C. M., Hashimoto, K., Swann, K., and Lai, F. A. (2002). Sperm phospholipase C zeta from humans and cynomolgus monkeys triggers Ca2+ oscillations, activation and development of mouse oocytes. Reproduction 124, 611–623. doi: 10.1530/rep.0.1240611
Cunningham, C. C., Vegners, R., Bucki, R., Funaki, M., Korde, N., Hartwig, J., et al. (2001). Cell permeant polyphosphoinositide-binding peptides that block cell motility and actin assembly. J. Biol. Chem. 276, 43390–43399. doi: 10.1074/jbc.M105289200
Deguchi, R., Shirakawa, H., Oda, S., Mohri, T., and Miyazaki, S. (2000). Spatiotemporal analysis of Ca2+ waves in relation to the sperm entry site and animal-vegetal axis during Ca2+ oscillations in fertilized mouse eggs. Dev. Biol. 218, 299–313. doi: 10.1006/dbio.1999.9573
De Matteis, M. A., Di Campli, A., and Godi, A. (2005). The role of the phosphoinositides at the Golgi complex. Biochim. Biophys. Acta 1744, 396–405. doi: 10.1016/j.bbamcr.2005.04.013
Dickinson, G. D., Ellefsen, K. L., Dawson, S. P., Pearson, J. E., and Parker, I. (2016). Hindered cytoplasmic diffusion of inositol trisphosphate restricts its cellular range of action. Sci. Signal. 9:ra108. doi: 10.1126/scisignal.aag1625
Dupont, G., and Dumollard, R. (2004). Simulation of calcium waves in ascidian eggs: insights into the origin of the pacemaker sites and the possible nature of the sperm factor. J. Cell. Sci. 117, 4313–4323. doi: 10.1242/jcs.01278
Dupont, G., and Goldbeter, A. (1994). Properties of intracellular Ca2+ waves generated by a model based on Ca2+-induced Ca2+ release. Biophys. J. 67, 2191–2204. doi: 10.1016/S0006-3495(94)80705-2
Fowler, R. E., and Edwards, R. G. (1957). Induction of super ovulation and pregnancy in mature mice by gonadotrophins. J. Endocrinol. 15, 374–384.
Fujimoto, S., Yoshida, N., Fukui, T., Amanai, M., Isobe, T., Itagaki, C., et al. (2004). Mammalian phospholipase C zeta induces oocyte activation from the sperm perinuclear matrix. Dev. Biol. 274, 370–383. doi: 10.1016/j.ydbio.2004.07.025
Halet, G., Tunwell, R., Balla, T., Swann, K., and Carroll, J. (2002). The dynamics of plasma membrane PtdIns(4,5)P(2) at fertilization of mouse eggs. J. Cell. Sci. 115, 2139–2149.
Igusa, Y., and Miyazaki, S. (1983). Effects of altered extracellular and intracellular calcium concentration on hyperpolarizing responses of the hamster egg. J. Physiol. 340, 611–632. doi: 10.1113/jphysiol.1983.sp014783
Ito, M., Shikano, T., Oda, S., Horiguchi, T., Tanimoto, S., Awaji, T., et al. (2008). Difference in Ca2+ oscillation-inducing activity and nuclear translocation ability of PLCZ1, an egg-activating sperm factor candidate, between mouse, rat, human, and medaka fish. Biol. Reprod. 78, 1081–1090. doi: 10.1095/biolreprod.108.067801
Jellerette, T., He, C. L., Wu, H., Parys, J. B., and Fissore, R. A. (2000). Down-regulation of the inositol 1,4,5-trisphosphate receptor in mouse eggs following fertilization or parthenogenetic activation. Dev. Biol. 223, 238–250. doi: 10.1006/dbio.2000.9675
Jones, K. T., and Nixon, V. L. (2000). Sperm-induced Ca2+ oscillations in mouse oocytes and eggs can be mimicked by photolysis of caged inositol 1,4,5-trisphosphate: evidence to support a continuous low level production of inositol 1,4,5-trisphosphate during mammalian fertilization. Dev. Biol. 225, 1–12. doi: 10.1006/dbio.2000.9826
Kline, D., and Kline, J. T. (1992a). Repetitive calcium transients and the role of calcium in exocytosis and cell cycle activation in the mouse egg. Dev. Biol 149, 80–89.
Kline, D., and Kline, J. T. (1992b). Thapsigargin activates a calcium influx pathway in the unfertilized mouse egg and suppresses repetitive calcium transients in the fertilized egg. J. Biol. Chem. 267, 17624–17630.
Kouchi, Z., Fukami, K., Shikano, T., Oda, S., Nakamura, Y., Takenawa, T., et al. (2004). Recombinant phospholipase Czeta has high Ca2+ sensitivity and induces Ca2+ oscillations in mouse eggs. J. Biol. Chem. 279, 10408–10412. doi: 10.1074/jbc.M313801200
Kurokawa, M., Sato, K., Wu, H., He, C., Malcuit, C., Black, S., et al. (2005). Functional, biochemical, and chromatographic characterization of the complete [Ca2+]i oscillation-inducing activity of porcine sperm. Dev. Biol. 285, 376–392. doi: 10.1016/j.ydbio.2005.06.029
Lawrence, Y. M., and Cuthbertson, K. S. (1995). Thapsigargin induces cytoplasmic free Ca2+ oscillations in mouse oocytes. Cell Calcium 17, 154–164. doi: 10.1016/0143-4160(95)90084-5
Marshall, I. C., and Taylor, C. W. (1994). Two calcium-binding sites mediate the interconversion of liver inositol 1,4,5-trisphosphate receptors between three conformational states. Biochem. J. 301(Pt 2), 591–598. doi: 10.1042/bj3010591
McGuinness, O. M., Moreton, R. B., Johnson, M. H., and Berridge, M. J. (1996). A direct measurement of increased divalent cation influx in fertilised mouse oocytes. Development 122, 2199–2206.
Miao, Y. L., Stein, P., Jefferson, W. N., Padilla-Banks, E., and Williams, C. J. (2012). Calcium influx-mediated signaling is required for complete mouse egg activation. Proc. Natl. Acad. Sci. U.S.A. 109, 4169–4174. doi: 10.1073/pnas.1112333109
Miyazaki, S. (1988). Inositol 1,4,5-trisphosphate-induced calcium release and guanine nucleotide-binding protein-mediated periodic calcium rises in golden hamster eggs. J. Cell Biol. 106, 345–353. doi: 10.1083/jcb.106.2.345
Miyazaki, S., Hashimoto, N., Yoshimoto, Y., Kishimoto, T., Igusa, Y., and Hiramoto, Y. (1986). Temporal and spatial dynamics of the periodic increase in intracellular free calcium at fertilization of golden hamster eggs. Dev. Biol. 118, 259–267. doi: 10.1016/0012-1606(86)90093-X
Miyazaki, S., Shirakawa, H., Nakada, K., and Honda, Y. (1993). Essential role of the inositol 1,4,5-trisphosphate receptor/Ca2+ release channel in Ca2+ waves and Ca2+ oscillations at fertilization of mammalian eggs. Dev. Biol. 158, 62–78. doi: 10.1006/dbio.1993.1168
Moreno, R. D., Schatten, G., and Ramalho-Santos, J. (2002). Golgi apparatus dynamics during mouse oocyte in vitro maturation: effect of the membrane trafficking inhibitor brefeldin A. Biol. Reprod. 66, 1259–1266. doi: 10.1095/biolreprod66.5.1259
Nomikos, M., Blayney, L. M., Larman, M. G., Campbell, K., Rossbach, A., Saunders, C. M., et al. (2005). Role of phospholipase C-zeta domains in Ca2+-dependent phosphatidylinositol 4,5-bisphosphate hydrolysis and cytoplasmic Ca2+ oscillations. J. Biol. Chem. 280, 31011–31018. doi: 10.1074/jbc.M500629200
Politi, A., Gaspers, L. D., Thomas, A. P., and Höfer, T. (2006). Models of IP3 and Ca2+ oscillations: frequency encoding and identification of underlying feedbacks. Biophys. J. 90, 3120–3133. doi: 10.1529/biophysj.105.072249
Rissanen, S., Salmela, L., Vattulainen, I., and Róg, T. (2017). PI(4,5)P2 binds to phospholipase C delta 1 in a cholesterol concentration dependent manner. perspective on implications to PI(4,5)P2-binding proteins. Biophys. J. 112, 137a−138a. doi: 10.1016/j.bpj.2016.11.762
Ross, P. J., Beyhan, Z., Iager, A. E., Yoon, S. Y., Malcuit, C., Schellander, K., et al. (2008). Parthenogenetic activation of bovine oocytes using bovine and murine phospholipase C zeta. BMC Dev. Biol. 8:16. doi: 10.1186/1471-213X-8-16
Sato, K., Wakai, T., Seita, Y., Takizawa, A., Fissore, R. A., Ito, J., et al. (2013). Molecular characteristics of horse phospholipase C zeta (PLCzeta). Anim. Sci. J. 84, 359–368. doi: 10.1111/asj.12044
Saunders, C. M., Larman, M. G., Parrington, J., Cox, L. J., Royse, J., Lai, F. A., et al. (2002). PLCζ: a sperm-specific trigger of Ca2+ oscillations in eggs and embryo development. Development 129, 3533–3544.
Shirakawa, H., Ito, M., Sato, M., Umezawa, Y., and Miyazaki, S. (2006). Measurement of intracellular IP3 during Ca2+ oscillations in mouse eggs with GFP-based FRET probe. Biochem. Biophys. Res. Commun. 345, 781–788. doi: 10.1016/j.bbrc.2006.04.133
Sneyd, J., Tsaneva-Atanasova, K., Reznikov, V., Bai, Y., Sanderson, M. J., and Yule, D. I. (2006). A method for determining the dependence of calcium oscillations on inositol trisphosphate oscillations. Proc. Natl. Acad. Sci. U.S.A. 103, 1675–1680. doi: 10.1073/pnas.0506135103
Stricker, S. A. (1999). Comparative biology of calcium signaling during fertilization and egg activation in animals. Dev. Biol. 211, 157–176. doi: 10.1006/dbio.1999.9340
Swann, K. (2013). Measuring Ca2+ oscillations in mammalian eggs. Methods Mol. Biol. 957, 231–248. doi: 10.1007/978-1-62703-191-2_16
Swann, K., Igusa, Y., and Miyazaki, S. (1989). Evidence for an inhibitory effect of protein kinase C on G-protein-mediated repetitive calcium transients in hamster eggs. EMBO J. 8, 3711–3718.
Swann, K., and Yu, Y. (2008). The dynamics of calcium oscillations that activate mammalian eggs. Int. J. Dev. Biol. 52, 585–594. doi: 10.1387/ijdb.072530ks
Theodoridou, M., Nomikos, M., Parthimos, D., Gonzalez-Garcia, J. R., Elgmati, K., Calver, B. L., et al. (2013). Chimeras of sperm PLCzeta reveal disparate protein domain functions in the generation of intracellular Ca2+ oscillations in mammalian eggs at fertilization. Mol. Hum. Reprod. 19, 852–864. doi: 10.1093/molehr/gat070
Wakai, T., Zhang, N., Vangheluwe, P., and Fissore, R. A. (2013). Regulation of endoplasmic reticulum Ca2+ oscillations in mammalian eggs. J. Cell Biol. 126, 5714–24. doi: 10.1242/jcs.136549
Yoon, S.-Y., Eum, J. H., Lee, J. E., Lee, H. C., Kim, Y. S., Han, J. E., et al. (2012). Recombinant human phospholipase C zeta 1 induces intracellular calcium oscillations and oocyte activation in mouse and human oocytes. Hum. Reprod. 27, 1768–80. doi: 10.1093/humrep/des092
Yu, Y., Nomikos, M., Theodoridou, M., Nounesis, G., Lai, F. A., and Swann, K. (2012). PLCζ causes Ca2+ oscillations in mouse eggs by targeting intracellular and not plasma membrane PI(4,5)P2. Mol. Biol. Cell 23, 371–80. doi: 10.1091/mbc.E11-08-0687
Yu, Y., Saunders, C. M., Lai, F. A., and Swann, K. (2008). Preimplantation development of mouse oocytes activated by different levels of human phospholipase C zeta. Hum. Reprod. 23, 365–73. doi: 10.1093/humrep/dem350
Zhang, D., Pan, L., Yang, L. H., He, X. K., Huang, X. Y., and Sun, F. Z. (2005). Strontium promotes calcium oscillations in mouse meiotic oocytes and early embryos through InsP3 receptors, and requires activation of phospholipase and the synergistic action of InsP3. Hum. Reprod. 20, 3053–3061. doi: 10.1093/humrep/dei215
Keywords: Ca2+ oscillations, phospholipase C, strontium, inositol trisphosphate, egg, phosphatidyl inositol bisphosphate
Citation: Sanders JR, Ashley B, Moon A, Woolley TE and Swann K (2018) PLCζ Induced Ca2+ Oscillations in Mouse Eggs Involve a Positive Feedback Cycle of Ca2+ Induced InsP3 Formation From Cytoplasmic PIP2. Front. Cell Dev. Biol. 6:36. doi: 10.3389/fcell.2018.00036
Received: 01 December 2017; Accepted: 15 March 2018;
Published: 03 April 2018.
Edited by:
Rafael A. Fissore, University of Massachusetts Amherst, United StatesReviewed by:
Carmen Williams, National Institute of Environmental Health Sciences (NIH), United StatesTakuya Wakai, Okayama University, Japan
Copyright © 2018 Sanders, Ashley, Moon, Woolley and Swann. This is an open-access article distributed under the terms of the Creative Commons Attribution License (CC BY). The use, distribution or reproduction in other forums is permitted, provided the original author(s) and the copyright owner are credited and that the original publication in this journal is cited, in accordance with accepted academic practice. No use, distribution or reproduction is permitted which does not comply with these terms.
*Correspondence: Karl Swann, c3dhbm5rMUBjYXJkaWZmLmFjLnVr