- 1INSERM U1242, Proteostasis and Cancer Team, Chemistry Oncogenesis Stress Signaling, Université de Rennes 1, Rennes, France
- 2Centre Eugène Marquis, Rennes, France
- 3Institute of Biology, Medicinal Chemistry and Biotechnology, National Hellenic Research Foundation, Athens, Greece
- 4Department of Biochemistry and Biotechnology, University of Thessaly, Larissa, Greece
- 5e-NIOS Applications PC, Kallithea-Athens, Greece
- 6Rennes Brain Cancer Team (REACT), Rennes, France
CD90 is a membrane GPI-anchored protein with one Ig V-type superfamily domain that was initially described in mouse T cells. Besides the specific expression pattern and functions of CD90 that were described in normal tissues, i.e., neurons, fibroblasts and T cells, increasing evidences are currently highlighting the possible involvement of CD90 in cancer. This review first provides a brief overview on CD90 gene, mRNA and protein features and then describes the established links between CD90 and cancer. Finally, we report newly uncovered functional connections between CD90 and endoplasmic reticulum (ER) stress signaling and discuss their potential impact on cancer development.
Introduction
Thy-1/CD90 was first identified in 1964 on mouse T lymphocytes (Reif and Allen, 1964a,b) and then on rat thymocytes and neural cells (Barclay et al., 1976). Since then, more than 10, 000 publications refer to CD90 mainly in rodent and human species (Figure 1A). The CD90 gene is conserved from fish to mammal (vertebrates; Figure 1B), and homologs have been even described in some invertebrates such as squids, tunicates, and worms (Cooper and Mansour, 1989). CD90 gene organization including promoter region and methylation sites was further described and reviewed in Barclay et al. (1976); Seki et al. (1985); Cooper and Mansour (1989). Importantly, the CD90 promoter is often considered to be specifically activated in the brain. Consequently, the CD90 promoter has routinely been used to drive “brain specific” expression of proteins in mice (Feng et al., 2000). The mouse and human CD90 protein are highly similar sharing 66% identity (Figure 1C).
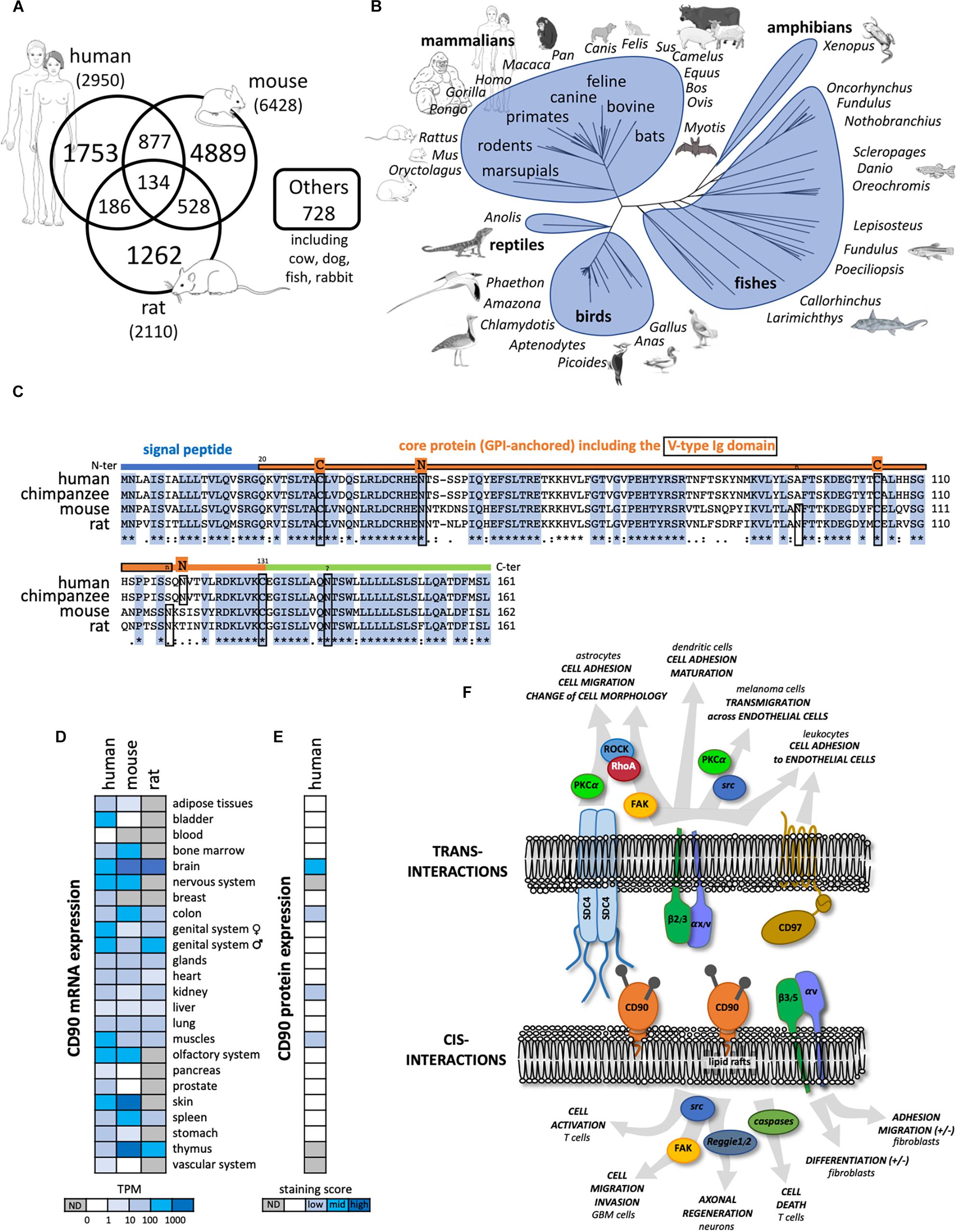
Figure 1. General features of CD90 molecule. (A) Number of publications until November 2018 referring to CD90 according to the different species collected in Pubmed (https://www.ncbi.nlm.nih.gov/pubmed). (B) Tree representing the evolution of CD90 proteins among vertebrates. (C) The CD90 protein sequences from human, chimpanzee, mouse, and rat were aligned showing a highly conserved domains. The main features of the protein including the signal peptide (blue line), the V-type Ig domain (framed orange line), the N-glycosylation sites (n in rodents and N in primates), and the cysteines involved in the di-sulfite bond (C) are represented. (D) CD90 mRNA expression patterns in normal tissues from human, mouse and rat were analyzed using the EMBL-EBI Expression Atlas (https://www.ebi.ac.uk/gxa/home). (E) CD90 protein expression patterns from human normal tissues were tested using the Human Protein Atlas (https://www.proteinatlas.org/). (F) CD90 signaling partners and ligands interacting in cis and trans were summarized including their involvement in different functions and cell types.
The CD90 protein is a small membrane glycophosphatidylinositol (GPI) anchored protein of 25 to 37 kDa, heavily N-glycosylated on two or three sites in human and mouse, respectively. One third of the CD90 molecular mass is linked to its glycosylation level (Pont, 1987; Haeryfar and Hoskin, 2004). CD90 is composed of a single V-like immunoglobulin domain anchored by a disulfide bond between Cys 28 and Cys 104. CD90 lacks an intracellular domain but is located in the outer leaflet of lipid rafts at the cell plasma membrane allowing signaling functions by cis- and trans-interactions with G inhibitory proteins, the Src family kinase (SFK) members src and c-fyn, and tubulin (Figure 1F; Rege et al., 2006; Avalos et al., 2009; Wandel et al., 2012). Interestingly, similar to what is observed for other GPI-anchored proteins such as CD55 and CD59, CD90 could be shed by specific phospholipases (i.e., PI-PLC or PLC-β) thus allowing cell to cell transfer, however, the physiological relevance of this process remains to be discovered (Haeryfar and Hoskin, 2004).
Common and distinct cellular CD90 expression patterns are observed in mouse and human. CD90 mRNA is highly expressed in nervous and olfactory systems, and skin tissues in both species. However, high CD90 mRNA expression is only found in mouse spleen and thymus (Figure 1D). In the nervous system, CD90 protein expression is observed mainly in neurons but also in some glial cells in vertebrates (Figure 1E). Recently, CD90 has been touted as a stem cell marker in various tissues such as in hematopoietic stem cells used in combination with the CD34 marker but also in hepatic, keratinocyte and mesenchymal stem cells (Kumar et al., 2016). Distinct cellular distributions of CD90 protein expression are observed in mouse (i.e., thymocytes and peripheral T cells) and human (i.e., endothelial cells and smooth muscle cells) (Rege and Hagood, 2006; Barker and Hagood, 2009; Bradley et al., 2009; Leyton and Hagood, 2014). Another important difference between the two species is the existence of two distinct murine isoforms CD90.1 and CD90.2 that differ at the residue 108 (Arg or Gln, respectively) whereas only one isoform is described in human with a histidine at position 108 (Bradley et al., 2009).
Several functions of CD90 have been described so far in physiological and pathological processes (Figure 1F). Most of these functions involve CD90 interactions with ligands such as integrins αv/β3, αx/β2, syndecan-4, CD90 itself, and CD97 (Wandel et al., 2012; Kong et al., 2013; Leyton and Hagood, 2014). CD90 plays a role in cell-cell and cell-matrix interactions, with specific implications in the regulation of axon growth and nerve regeneration, T cell activation and apoptosis, leukocytes and melanoma cell adhesion and migration, fibroblast proliferation and migration in wound healing, inflammation and fibrosis. These functions were already extensively reviewed in Rege and Hagood (2006); Barker and Hagood (2009); Bradley et al. (2009); Leyton and Hagood (2014), and will not be developed further here. Rather, we will focus on CD90 expression and functions in cancers.
Diverse Roles of CD90 in Cancers
CD90 Expression in Various Cancer Types
CD90 mRNA and protein expression was reported in several cancer types including liver, myeloid, skin, and brain (Figure 2A). According to The Cancer Genome Atlas (TCGA), CD90 transcripts were predominantly found in brain, kidney, and pancreatic tumors (Figure 2B). CD90 mRNA and protein were detected in glioma/GBM specimens (Wikstrand et al., 1985) and immortalized glioma/GBM cell lines (Kemshead et al., 1982; Seeger et al., 1982; Hurwitz et al., 1983; Wikstrand et al., 1983; Rettig et al., 1986). In the past few years, CD90 has been considered as a human GBM stem cell (GSC) marker (Liu et al., 2006; Kang and Kang, 2007; Tomuleasa et al., 2010; He et al., 2012; Nitta et al., 2015). CD90 is also expressed in GBM-associated stromal cells (GASCs) (Clavreul et al., 2012) and mesenchymal stem cell-like pericytes (Ochs et al., 2013), thereby reflecting GBM cellular heterogeneity. We recently demonstrated that CD90 expression is not only restricted to GBM stem-like cells but is also observed in more differentiated GBM cells (primary adherent lines) and in freshly dissociated GBM specimens (Avril et al., 2017a). Using the recent single-cell RNA sequencing datasets from stem-like and no-stem GBM cells and tumor migrating cells (Darmanis et al., 2017; Cook et al., 2018), we confirm herein that CD90 is expressed in tumor cells from the cancer site but also in migrating tumor cells, tumor-associated endothelial cells, and neighboring neuronal cells (Figure 2C). CD90 expression in kidney cancers is currently controversial. Primary cell lines and tumor stem cells from pediatric Wilms’ tumors and metastatic renal tumors express CD90 (Pode-Shakked et al., 2009; Royer-Pokora et al., 2010; Khan et al., 2016) as observed in renal tumor-associated endothelial cells (Mesri et al., 2013). CD90 is also highly expressed in renal cell carcinoma tumor-initiating cells characterized by CD105 expression (Bussolati et al., 2008; Khan et al., 2016). Nevertheless, CD90 expression could not be found in CSCs derived from patients with clear cell renal cell carcinoma (Galleggiante et al., 2014). The CD90 protein is expressed in almost all the pancreatic adenocarcinoma (PDAC) (n = 98) and its metastatic forms tested by tissues microarray (Zhu et al., 2014), not only in tumors cells but also in stromal cells, including fibroblasts, and vascular endothelial cells. In addition, CD90 was extensively studied in liver cancers. Almost no CD90 expressing cells are present in disease-free or in cirrhotic livers, whereas a significantly higher expression is found in hepatocellular carcinoma (HCC) cells (Yang et al., 2008; Sukowati et al., 2013). CD90 protein is also found in esophageal squamous cell carcinomas mainly in primary tumors and immortalized/primary cell lines (Tang et al., 2013). Overexpression of CD90 is also detected in prostate cancer. Indeed immunohistochemical analysis of prostate cancer samples showed distinct and differential overexpression of CD90 in cancer-associated stroma compared with non-cancer tissue stroma (True et al., 2010).
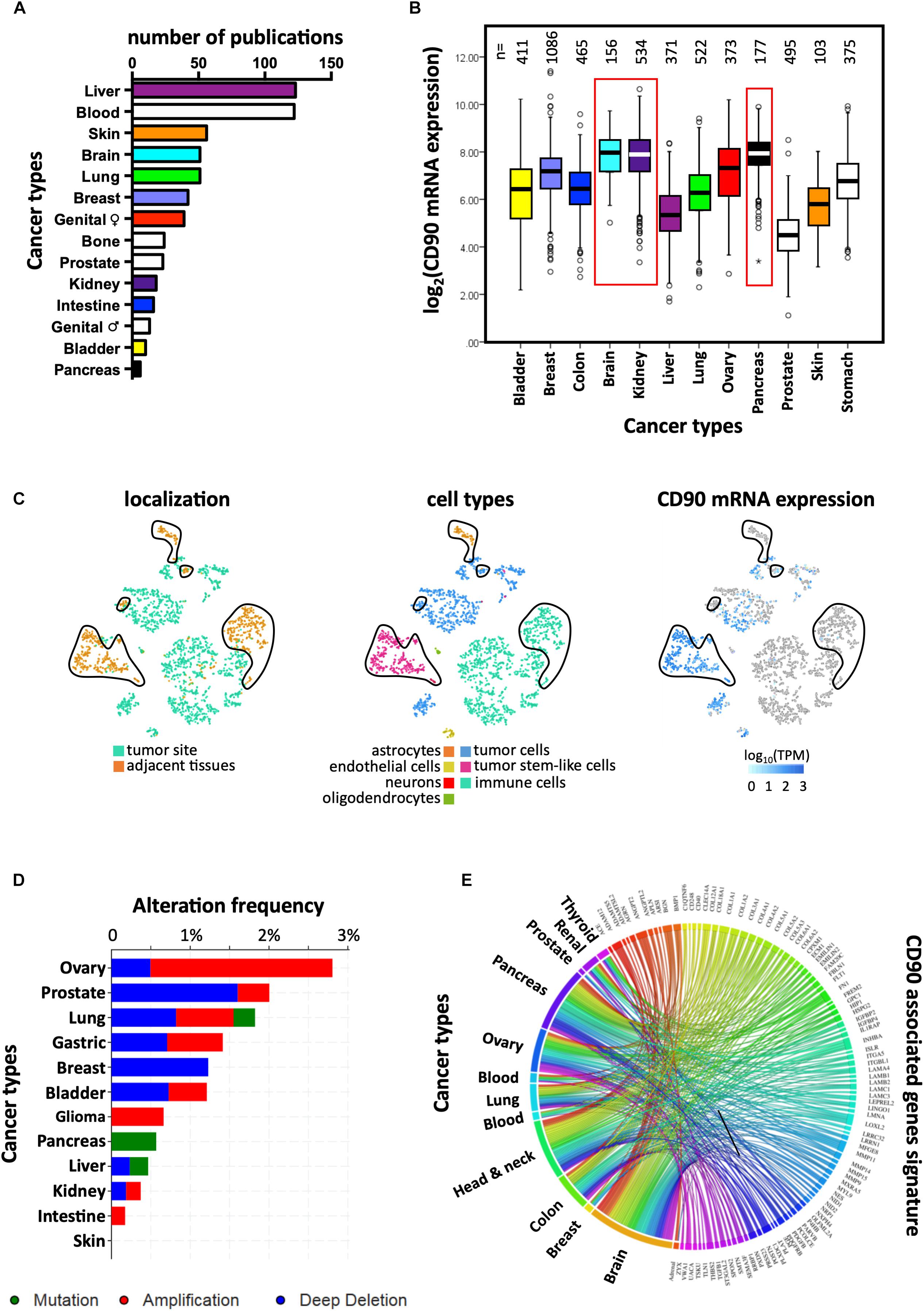
Figure 2. Links between CD90 and cancers. (A) Articles reporting CD90 in various human cancer types were collected using Pubmed and their distribution per cancer type is presented. (B) CD90 mRNA expression was analyzed among the various cancer types using the TCGA resource. The corresponding number of tumor specimens tested are indicated on the top of the graph. (C) CD90 mRNA expression was analyzed in the single cell RNA sequencing dataset from GBM specimens (Darmanis et al., 2017) using the EMBL-EBI Single Cell Expression Atlas (https://www.ebi.ac.uk/gxa/sc/home). Cell localization and cell types are also represented. (D) Frequency of CD90 gene alterations including mutations, gene amplification and deletion was analyzed among the different cancer types. (E) CD90 associated gene signature obtained from GBM specimens (Avril et al., 2017a) was tested in others cancer types using CancerMA tool (Feichtinger et al., 2012).
CD90 Somatic Mutations in Cancers
Mutagenesis is often associated with carcinogenesis. Protein expression or functions could be altered by mutations leading in turn to an oncogenic process. No study has yet reported mutations in the CD90 gene or CD90-associated related regulatory elements in any of cancer types (Kumar et al., 2016). According to the “Catalog Of Somatic Mutations In Cancer” (COSMIC; n = 47210 samples) and cBioPortal for Cancer Genomics (n = 52770 samples), the mutation frequency of CD90 in cancer is very low (0.001% with 51 and 54 mutations according to COSMIC and cBioPortal, respectively) (Cerami et al., 2012; Tate et al., 2018). Most of the CD90 mutations are missense (60.8% and 92.6% according to COSMIC and cBioPortal, respectively) and synonymous (35.3%, COSMIC) substitutions mainly found in intestine, lung, and skin cancers (Figure 2D). Only three mutations leading to sequence frameshift were detected in neuroblastoma and renal cancers. In addition, one RGS12/CD90 fusion was detected in a breast invasive lobular carcinoma. Further studies are needed to understand how these mutations could impact on CD90 functions and to clarify the potential roles of these mutations in cancers.
CD90 as a Cancer Stem Cell Marker
The cancer stem cell (CSC) concept has been proposed four decades ago, and states that tumor development is driven by a specialized cell subset, characterized by self-renewing, multi-potent, and tumor-initiating properties (Batlle and Clevers, 2017). In recent years, the role of CD90 was extensively studied in CSCs (Shaikh et al., 2016). The ability to form tumors in vivo in immunodeficient mice is considered to be one of the most important properties of CSCs. CD90+ tumor cells, considered as CSCs, from several cancers, i.e., HCC (Yang et al., 2008), gastric cancers (Jiang et al., 2012) and esophageal squamous cell carcinomas (Tang et al., 2013) were reported to form tumors in immunodeficient mice after injection of a very small amount of cells in contrast to CD90 negative counterparts. Another important feature of CSCs is their ability to grow in vitro as spheroids in serum-free medium. This feature has been recapitulated using CD90 expressing cells obtained from esophageal squamous cell carcinomas (Tang et al., 2013), gastric cancers (Jiang et al., 2012), gliomas (Kang and Kang, 2007; He et al., 2012), and lung carcinomas (Wang et al., 2013). Taken together, these studies identify CD90 as a potential CSC marker in many types of cancers. However, we have recently demonstrated in GBM that CD90 is not only a stem marker, as its expression is also observed in more differentiated GBM cells (Avril et al., 2017a).
CD90 as a Tumor Suppressive Molecule or a Prognostic Marker in Cancers
The prognostic role of CD90 is dependent on the cancer type. In GBM patients, high expression of CD90 in tumor specimens is associated with invasive features as demonstrated by imaging techniques (Avril et al., 2017a). These imaging features were previously linked to shorter patients’ survival (Colen et al., 2014). Therefore, we proposed that CD90 expression could represent a novel stratification tool for screening patients with highly invasive tumors that could be treated with dasatinib, a SFK inhibitor. Moreover, dasatinib could not only impair the adhesion/migration of CD90high differentiated tumor cells but also the proliferation of CD90high GSCs, thereby increasing its therapeutic potential in CD90high tumors (Avril et al., 2017a). In hepatoblastoma, increased expression of CD90 is significantly correlated with advanced stages of the disease, poor response to treatment and lower overall survival (Bahnassy et al., 2015). CD90 overexpression was also identified as a poor prognostic marker in acute myeloid leukemia (Buccisano et al., 2004) and HCC (Lu et al., 2011). In contrast, CD90 was also shown to exert tumor suppressor functions in several others cancers, as its downregulation is associated with poor prognosis, disease progression in ovarian adenocarcinoma (Gabra et al., 1996; Abeysinghe et al., 2003), neuroblastoma (Fiegel et al., 2008) and nasopharyngeal carcinoma predominantly observed in metastatic tumor cells in invaded lymph nodes (Lung et al., 2005). CD90 inactivation is found associated with hypermethylation of the CD90 gene promoter in CD90 negative nasopharyngeal carcinoma cell lines. Furthermore, induction of CD90 expression in nasopharyngeal carcinoma and ovarian cell lines leads to inhibition of tumor growth in vitro and in vivo, respectively (Abeysinghe et al., 2003; Lung et al., 2005). Overall, these observations illustrate the ambivalence of CD90 functions with either pro- or anti-tumoral properties depending on the cancer type.
CD90 Regulates Tumor Migration and Metastasis
Tumor invasion/migration is one of cancer hallmarks that drives to tumor dissemination leading to disease aggravation. To spread within the tissues, tumor cells use migration mechanisms that are similar to those occurring in physiological processes, including mesenchymal, amoeboid single migration or collective movements, depending on the cancer type (Friedl and Wolf, 2003; Odenthal et al., 2016). Recent studies demonstrated high invasive and metastatic capacities of CD90 expressing cells in several cancers. Indeed, NOD/SCID mice implanted subcutaneously with HCC tumor cells expressing both CD90 and CXCR4 developed distal metastatic tumors (Zhu et al., 2015). Similarly, the high metastatic capacity of CD90 and EpCAM expressing cells from primary HCC has also been observed after subcutaneously injection in immune-deficient mice (Yamashita et al., 2013). These cells have the capacity to invade surrounding tissues, to form spheroids in vitro and to exhibit high expression of TWIST1 and TWIST2, two important transcription factors involved in activation of Epithelial to Mesenchymal Transition (EMT) process. In addition, the presence of CD90 positive cells in HCC patients was also associated with a higher incidence of distant organ metastasis (usually occurring in one third of HCC patients) including lung, bone and adrenal gland; within 2 years after surgery (Yamashita et al., 2013). In a recent study, we demonstrated the critical role of CD90 in GBM migration/invasion mainly through the activation of SRC signaling. The same study demonstrated CD90 association with a cell adhesion/migration gene signature and with multifocal/multicentric MRI features in GBM patients. Importantly, this adhesion/migration profile is also found in other CD90-expressing tumors such as colonic, pancreatic and ovarian cancers (Figure 2E). Moreover, orthotopic xenografts revealed that CD90 expression induced invasive phenotypes in vivo that could be inhibited by dasatinib (Avril et al., 2017a). In melanoma, CD90 expressing endothelial cells are mainly associated with highly metastatic tumors (Ohga et al., 2012). CD90 also mediates adhesion of melanoma cells to activated human endothelial cells via its interaction with the αv/β3 integrin on the tumor cells in vitro (Saalbach et al., 2005). Furthermore, expression of αv/β3 integrin (CD51/CD61), one of the CD90 ligands, in melanoma cells is associated with tumor progression and metastases formation (Ohga et al., 2012). As VEGF and TNFα induce the expression of CD90 in endothelial cells, it has been shown that mice lacking CD90 showed markedly diminished experimental lung metastasis after injection of B16/F10 melanoma cells compared to wild-type controls (Schubert et al., 2013). Interestingly, a subpopulation of breast cancer cell line MDA-MB-231 expressing CD90 and CD105, exhibits mesenchymal stem cell-like characteristics such as high migratory capacity as compared to the parental and CD90/CD105 negative cells (Wang et al., 2015). Remarkably, in breast tumor specimens, tumor cells that express both CD90 and CD44 are confined to the periphery of the tumor, representing the tumor invasive front (Donnenberg et al., 2010).
Emerging Roles of the Unfolded Protein Response in Cancers: a Novel Link Between CD90 and ER Stress ?
During tumor invasion/migration, dramatic changes occur in cells present within the compact tumor core to become single migrating cells, these transformations are described as the EMT throughout which, cells lose their cell-cell junctions, change their morphology and modify their functions leading to cell trans/de-differentiation (Friedl and Wolf, 2003; Thiery et al., 2009). Tumor development and aggressiveness including invasion and EMT were recently linked to Endoplasmic Reticulum (ER) stress signaling (Dejeans et al., 2015; Urra et al., 2016), a topic that we will further document in the next sections. Since both the signaling response triggered to cope with ER stress [also named Unfolded Protein Response (UPR)] and CD90 expression promote tumor migration, we hypothesize that CD90 and the UPR could be somehow functionally linked to control tumor cell invasive.
An Overview on UPR and Its Sensors
Despite an elaborate network of chaperones, foldases and proteins involved in the quality control of newly synthesized proteins, the ER capacity for protein synthesis and folding can be overwhelmed upon various physiological and pathological conditions, causing an accumulation on misfolded proteins into the ER and a cellular stress called ER stress. To cope with ER stress, cells activate an adaptive signaling pathway named the UPR. The UPR activates a cascade of signals leading to the attenuation of mRNA translation and to the transcriptional increase of genes whose products are involved in ER protein folding, ER protein quality control, ER-associated degradation and protein secretion. If ER stress persists, the UPR signaling shifts from adaptive to apoptotic signals thus leading to the tumor cell death (Chevet et al., 2015; Avril et al., 2017b). The UPR activation relies on 3 ER resident proteins/sensors ATF6α, IRE1α (for Inositol-Requiring Enzyme 1 alpha; referred to as IRE1 hereafter) and PERK. Initially, it was postulated that the activation of these three sensors was controlled by the ER resident chaperone GRP78/BIP and misfolded proteins themselves, thoroughly reviewed in Chevet et al. (2015); Urra et al. (2016); Avril et al. (2017b). More recently, novel mechanisms and actors of ER stress sensors activation have been described (Rojas-Rivera et al., 2018) such as the involvement of the ATP binding pocket of BIP (Carrara et al., 2015; Kopp et al., 2018); the involvement of the chaperones ERDJ4 (Amin-Wetzel et al., 2017) and HSP47 (Sepulveda et al., 2018), the ER oxidoreductase PDIA6 (Eletto et al., 2014; Groenendyk et al., 2014), and the other protein disulfide isomerase PDIA5 (Higa et al., 2014). During cancer development, tumor cells are exposed to intrinsic challenges (related to activation of their oncogenic program or aneuploidy) and to extrinsic stresses (related to nutrient and oxygen deprivation, but also anti-cancer treatments as such irradiation or chemotherapy), which lead to an altered balance between protein folding demand and the capacity of transforming cells to cope with this, thus driving ER stress (Chevet et al., 2015; Urra et al., 2016; Avril et al., 2017b; Galmiche et al., 2017). UPR sensors have been largely studied in regard to cancer diseases (Figure 3A). A strong involvement of one of these UPR sensors, IRE1, has been recently reported in GBM biology (Drogat et al., 2007; Auf et al., 2010; Dejeans et al., 2012; Pluquet et al., 2013; Jabouille et al., 2015; Obacz et al., 2017; Lhomond et al., 2018) and will be now presented.
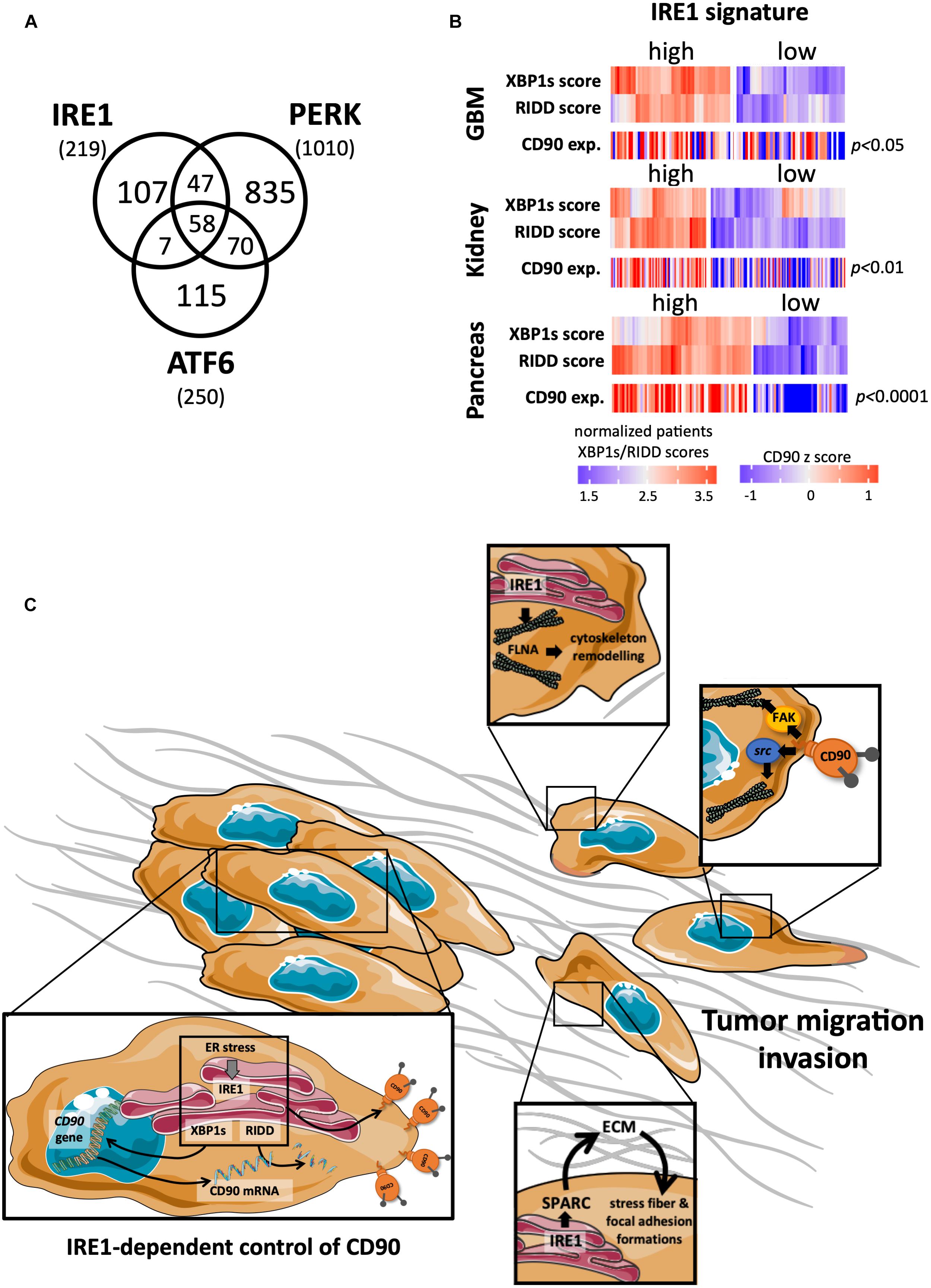
Figure 3. Links between CD90 and IRE1 activity in cancers. (A) Articles describing a role for the ER stress sensors IRE1, PERK, and ATF6 in human cancer disease were collected using Pubmed and their distribution represented per sensor type. (B) CD90 mRNA expression was analyzed according to IRE1 activity from GBM, kidney and pancreas cancers (TCGA resources). Cancer patients were classified in regard to IRE1 and XBP1s/RIDD activities as described in Lhomond et al. (2018). (C) A schematic representation of the possible CD90 regulation by IRE1 is presented.
IRE1 Activation and Down-Stream Signaling Pathways
IRE1 dimerizes/oligomerizes upon ER stress. This leads to its trans-autophosphorylation which in turn induces a conformational change to activate IRE1 endoribonuclease domain (RNase) (Chevet et al., 2015). The activation of IRE1 RNase triggers two distinct signaling pathways that lead to (i) the non-conventional splicing of the XBP1 mRNA into a novel mRNA encoding a transcription factor XBP1s; and (ii) the degradation of RNA (also called RIDD for regulated IRE1-dependent decay of RNA). XBP1s is a transcription factor that controls the expression of genes involved in protein folding, secretion, ERAD, and lipid synthesis (Chevet et al., 2015). On the other hand, RIDD targets mRNA, ribosomal RNA and microRNAs. Importantly, the selectivity of IRE1 RNase activity is highly dependent on its oligomerization state; a concept still debated as to its specificity and application (Chevet et al., 2015). IRE1 activation has also been shown to lead to c-Jun N-terminal protein kinase (JNK) phosphorylation through either the recruitment of TRAF2 (Urano et al., 2000) or the cleavage of miR17 (Lerner et al., 2012).
A Key Role of IRE1 in GBM Pathology
One of the central hallmarks of GBM is the diffuse infiltration of tumor cells into the cerebral neighboring parenchyma (Louis et al., 2007), making a complete tumor resection almost impossible (Zhong et al., 2010; Vehlow and Cordes, 2013). Interestingly, inhibition of IRE1 reduces GBM growth in vivo (Drogat et al., 2007; Auf et al., 2010) but alters tumor cell migration/invasion properties (Dejeans et al., 2012; Jabouille et al., 2015), acting for instance on SPARC expression, a molecule associated with the extracellular matrix (Dejeans et al., 2012). Furthermore IRE1, through its dual XBP1s and RIDD activities, exerts antagonistic effects on GBM aggressiveness influencing both tumor invasion, neo-angiogenesis and inflammation (Lhomond et al., 2018). Remarkably, GBM patients bearing tumors characterized by high IRE1 activity (and more precisely high XBP1s) exhibit a worse prognosis and display increased immune infiltration, angiogenesis and migration markers. This also opens interesting perspectives of connections between IRE1 activity and the growth and the invasion of GBM cells; however, further studies are required to understand how IRE1 downstream signaling impacts on these features.
Possible Links Between IRE1 and CD90 in Controlling GBM Cell Migration/Invasion
As our two recent studies independently demonstrated that CD90 and IRE1 could regulate GBM migration/invasion features, specific functional connections between these two proteins were considered herein. For instance, when tumors developed in mouse brain in our orthotopic mouse model, similar features of tumor infiltration, i.e., small but highly invasive tumors have been observed in CD90 expressing (Avril et al., 2017a) and IRE1 defective (Dominant Negative, DN; Auf et al., 2010) U87 cells. Due to its RNase activity, one could speculate that a functional regulatory link might exist between IRE1 and CD90 which in turn could therefore impact on GBM CD90-dependent migration. Ongoing studies from our laboratory aim at directly investigating the link between CD90 and IRE1 activity. Preliminary data indicate that ER stress inducers (such as tunicamycin, thapsigargin, and dithiothreitol) decrease the expression of cell surface CD90 in GBM cells. Intriguingly, transient expression of IRE1 defective form (DN) in U251 cells also decreased membrane CD90 expression, underlining a complex regulatory mechanism occurring between ER stress sensors (including IRE1) and CD90. Importantly, applying our recent classification of GBM patients according to IRE1 gene signature on the GBM TCGA cohort, we observed that tumors with high IRE1 activity expressed higher levels CD90 mRNA than tumors exhibiting low IRE1 activity. Importantly, this could be applied to others cancer types including renal and pancreas (Figure 3B). Furthermore, a CD90 associated gene signature described in Avril et al. (2017a) was also associated with IRE1 activity. Overall, these observations highlight the potential effect of IRE activity on CD90 expression and its potential role in functions linked to tumor migration/invasion. Interestingly, IRE1 has already been associated with molecules involved in cell migration, i.e., by controlling SPARC expression (Dejeans et al., 2012) and interacting directly with filamin A (Urra et al., 2018). Further functional and molecular studies are needed to better understand the connections between CD90 and IRE1 in cancer development, and in particular migration and invasion (Figure 3C).
Conclusion
Increasing evidence supports the importance of CD90 in cancer development. CD90 has been mainly considered as a useful CSC marker in various cancer types such as kidney, brain, and liver. However, even despite the absence of intracellular domain, CD90 is also able to transmit intracellular signals that lead to the activation of tumor cell migration/invasion program in liver and lung cancers, in GBM and in melanoma. In contrast, CD90 is described as a tumor suppressor molecule in nasopharyngeal carcinoma. Although further studies are required to clearly demonstrate the connections between ER stress signaling and CD90 expression, initial transcriptome analyses from cancer patients indicate that CD90 expression appears to be dependent on the activation of the UPR, a key event in various oncological clinical settings including brain, kidney, and pancreatic cancers. These different elements underline the complexity of CD90 functions in cancer, depending on both the cellular context and on the tumor microenvironment. Future studies will lead to the better understanding of CD90 regulation and functions adding to the information already available such as the CD90 mutation spectrum as seen in COSMIC/cBioPortal; the IRE1-controlled CD90 tumor expression and functions with a clarification of the involvement of the IRE1 downstream signaling pathways XBP1s and/or RIDD branches; and the relevance of cleaved CD90 released in the tumor microenvironment.
Author Contributions
CS wrote the sections of the manuscript. KV and AC organized the database and performed the statistical analysis. EC and TA contributed to conception and design of the review. TA wrote the first draft of the manuscript. All authors contributed to manuscript revision, read, and approved the submitted version.
Funding
This work was funded by la Ligue contre le Cancer (comités 35, 56 et 37) to TA; grants from INSERM, Institut National du Cancer (INCA), Région Bretagne, Rennes Métropole, Fondation pour la Recherche Médicale (FRM) to EC; EU H2020 MSCA ITN-675448 (TRAINERS) and MSCA RISE-734749 (INSPIRED) to EC and AC; PROMISE, 12CHN 204 Bilateral Greece-China Research Program of the Hellenic General Secretariat of Research and Technology and the Chinese Ministry of Science and Technology sponsored by the Program “Competitiveness and Entrepreneurship,” Priority Health of the Peripheral Entrepreneurial Program of Attiki to AC. CS was funded by a post-doctoral fellowship from the Plan Cancer.
Conflict of Interest Statement
The authors declare that the research was conducted in the absence of any commercial or financial relationships that could be construed as a potential conflict of interest.
Acknowledgments
We thank D. Doultsinos for proofreading this manuscript.
References
Abeysinghe, H. R., Cao, Q., Xu, J., Pollock, S., Veyberman, Y., Guckert, N. L., et al. (2003). THY1 expression is associated with tumor suppression of human ovarian cancer. Cancer Genet. Cytogenet. 143, 125–132. doi: 10.1016/s0165-4608(02)00855-5
Amin-Wetzel, N., Saunders, R. A., Kamphuis, M. J., Rato, C., Preissler, S., Harding, H. P., et al. (2017). A J-protein co-chaperone recruits BiP to monomerize IRE1 and repress the unfolded protein response. Cell 171, 1625.e13–1637.e13. doi: 10.1016/j.cell.2017.10.040
Auf, G., Jabouille, A., Guerit, S., Pineau, R., Delugin, M., Bouchecareilh, M., et al. (2010). Inositol-requiring enzyme 1alpha is a key regulator of angiogenesis and invasion in malignant glioma. Proc. Natl. Acad. Sci. U.S.A. 107, 15553–15558. doi: 10.1073/pnas.0914072107
Avalos, A. M., Valdivia, A. D., Munoz, N., Herrera-Molina, R., Tapia, J. C., Lavandero, S., et al. (2009). Neuronal Thy-1 induces astrocyte adhesion by engaging syndecan-4 in a cooperative interaction with alphavbeta3 integrin that activates PKCalpha and RhoA. J. Cell Sci. 122(Pt 19), 3462–3471. doi: 10.1242/jcs.034827
Avril, T., Etcheverry, A., Pineau, R., Obacz, J., Jegou, G., Jouan, F., et al. (2017a). CD90 expression controls migration and predicts dasatinib response in Glioblastoma. Clin. Cancer Res. 23, 7360–7374. doi: 10.1158/1078-0432.CCR-17-1549
Avril, T., Vauleon, E., and Chevet, E. (2017b). Endoplasmic reticulum stress signaling and chemotherapy resistance in solid cancers. Oncogenesis 6:e373. doi: 10.1038/oncsis.2017.72
Bahnassy, A. A., Fawzy, M., El-Wakil, M., Zekri, A.-R. N., Abdel-Sayed, A., and Sheta, M. (2015). Aberrant expression of cancer stem cell markers (CD44, CD90, and CD133) contributes to disease progression and reduced survival in hepatoblastoma patients: 4-year survival data. Transl. Res. 165, 396–406. doi: 10.1016/j.trsl.2014.07.009
Barclay, A. N., Letarte-Muirhead, M., Williams, A. F., and Faulkes, R. A. (1976). Chemical characterisation of the Thy-1 glycoproteins from the membranes of rat thymocytes and brain. Nature 263, 563–567. doi: 10.1038/263563a0
Barker, T. H., and Hagood, J. S. (2009). Getting a grip on Thy-1 signaling. Biochim. Biophys. Acta 1793, 921–923. doi: 10.1016/j.bbamcr.2008.10.004
Batlle, E., and Clevers, H. (2017). Cancer stem cells revisited. Nat. Med. 23, 1124–1134. doi: 10.1038/nm.4409
Bradley, J. E., Ramirez, G., and Hagood, J. S. (2009). Roles and regulation of Thy-1, a context-dependent modulator of cell phenotype. BioFactors 35, 258–265. doi: 10.1002/biof.41
Buccisano, F., Rossi, F. M., Venditti, A., Del Poeta, G., Cox, M. C., Abbruzzese, E., et al. (2004). CD90/Thy-1 is preferentially expressed on blast cells of high risk acute myeloid leukaemias. Br. J. Haematol. 125, 203–212. doi: 10.1111/j.1365-2141.2004.04883.x
Bussolati, B., Bruno, S., Grange, C., Ferrando, U., and Camussi, G. (2008). Identification of a tumor-initiating stem cell population in human renal carcinomas. FASEB J. 22, 3696–3705. doi: 10.1096/fj.08-102590
Carrara, M., Prischi, F., Nowak, P. R., Kopp, M. C., and Ali, M. M. (2015). Noncanonical binding of BiP ATPase domain to Ire1 and Perk is dissociated by unfolded protein CH1 to initiate ER stress signaling. eLife 4:e03522. doi: 10.7554/eLife.03522
Cerami, E., Gao, J., Dogrusoz, U., Gross, B. E., Sumer, S. O., Aksoy, B. A., et al. (2012). The cBio cancer genomics portal: an open platform for exploring multidimensional cancer genomics data. Cancer Discov. 2, 401–404. doi: 10.1158/2159-8290.CD-12-0095
Chevet, E., Hetz, C., and Samali, A. (2015). Endoplasmic reticulum stress-activated cell reprogramming in oncogenesis. Cancer Discov. 5, 586–597. doi: 10.1158/2159-8290.CD-14-1490
Clavreul, A., Etcheverry, A., Chassevent, A., Quillien, V., Avril, T., Jourdan, M. L., et al. (2012). Isolation of a new cell population in the glioblastoma microenvironment. J. Neurooncol. 106, 493–504. doi: 10.1007/s11060-011-0701-7
Colen, R. R., Vangel, M., Wang, J., Gutman, D. A., Hwang, S. N., Wintermark, M., et al. (2014). Imaging genomic mapping of an invasive MRI phenotype predicts patient outcome and metabolic dysfunction: a TCGA glioma phenotype research group project. BMC Med. Genomics 7:30. doi: 10.1186/1755-8794-7-30
Cook, C. E., Lopez, R., Stroe, O., Cochrane, G., Brooksbank, C., Birney, E., et al. (2018). The European bioinformatics institute in 2018: tools, infrastructure and training. Nucleic Acids Res. 47, D15–D22. doi: 10.1093/nar/gky1124
Cooper, E. L., and Mansour, M. H. (1989). Distribution of Thy-1 in invertebrates and ectothermic vertebrates. Immunol. Ser. 45, 197–219.
Darmanis, S., Sloan, S. A., Croote, D., Mignardi, M., Chernikova, S., Samghababi, P., et al. (2017). Single-Cell RNA-Seq analysis of infiltrating neoplastic cells at the migrating front of human Glioblastoma. Cell Rep. 21, 1399–1410. doi: 10.1016/j.celrep.2017.10.030
Dejeans, N., Barroso, K., Fernandez-Zapico, M. E., Samali, A., and Chevet, E. (2015). Novel roles of the unfolded protein response in the control of tumor development and aggressiveness. Semin. Cancer Biol. 33, 67–73. doi: 10.1016/j.semcancer.2015.04.007
Dejeans, N., Pluquet, O., Lhomond, S., Grise, F., Bouchecareilh, M., Juin, A., et al. (2012). Autocrine control of glioma cells adhesion and migration through IRE1alpha-mediated cleavage of SPARC mRNA. J. Cell Sci. 125(Pt 18), 4278–4287. doi: 10.1242/jcs.099291
Donnenberg, V. S., Donnenberg, A. D., Zimmerlin, L., Landreneau, R. J., Bhargava, R., Wetzel, R. A., et al. (2010). Localization of CD44 and CD90 positive cells to the invasive front of breast tumors. Cytometry B Clin. Cytom. 78, 287–301. doi: 10.1002/cyto.b.20530
Drogat, B., Auguste, P., Nguyen, D. T., Bouchecareilh, M., Pineau, R., Nalbantoglu, J., et al. (2007). IRE1 signaling is essential for ischemia-induced vascular endothelial growth factor-A expression and contributes to angiogenesis and tumor growth in vivo. Cancer Res. 67, 6700–6707. doi: 10.1158/0008-5472.can-06-3235
Eletto, D., Eletto, D., Dersh, D., Gidalevitz, T., and Argon, Y. (2014). Protein disulfide isomerase A6 controls the decay of IRE1alpha signaling via disulfide-dependent association. Mol. Cell 53, 562–576. doi: 10.1016/j.molcel.2014.01.004
Feichtinger, J., McFarlane, R. J., and Larcombe, L. D. (2012). CancerMA: a web-based tool for automatic meta-analysis of public cancer microarray data. Database 2012:bas055. doi: 10.1093/database/bas055
Feng, G., Mellor, R. H., Bernstein, M., Keller-Peck, C., Nguyen, Q. T., Wallace, M., et al. (2000). Imaging neuronal subsets in transgenic mice expressing multiple spectral variants of GFP. Neuron 28, 41–51. doi: 10.1016/s0896-6273(00)00084-2
Fiegel, H. C., Kaifi, J. T., Quaas, A., Varol, E., Krickhahn, A., Metzger, R., et al. (2008). Lack of Thy1 (CD90) expression in neuroblastomas is correlated with impaired survival. Pediatr. Surg. Int. 24, 101–105. doi: 10.1007/s00383-007-2033-4
Friedl, P., and Wolf, K. (2003). Tumour-cell invasion and migration: diversity and escape mechanisms. Nat. Rev. Cancer 3, 362–374. doi: 10.1038/nrc1075
Gabra, H., Watson, J. E., Taylor, K. J., Mackay, J., Leonard, R. C., Steel, C. M., et al. (1996). Definition and refinement of a region of loss of heterozygosity at 11q23.3-q24.3 in epithelial ovarian cancer associated with poor prognosis. Cancer Res. 56, 950–954.
Galleggiante, V., Rutigliano, M., Sallustio, F., Ribatti, D., Ditonno, P., Bettocchi, C., et al. (2014). CTR2 identifies a population of cancer cells with stem cell-like features in patients with clear cell renal cell carcinoma. J. Urol. 192, 1831–1841. doi: 10.1016/j.juro.2014.06.070
Galmiche, A., Sauzay, C., Chevet, E., and Pluquet, O. (2017). Role of the unfolded protein response in tumor cell characteristics and cancer outcome. Curr. Opin. Oncol. 29, 41–47. doi: 10.1097/cco.0000000000000339
Groenendyk, J., Peng, Z., Dudek, E., Fan, X., Mizianty, M. J., Dufey, E., et al. (2014). Interplay between the oxidoreductase PDIA6 and microRNA-322 controls the response to disrupted endoplasmic reticulum calcium homeostasis. Sci. Signal. 7:ra54. doi: 10.1126/scisignal.2004983
Haeryfar, S. M., and Hoskin, D. W. (2004). Thy-1: more than a mouse pan-T cell marker. J. Immunol. 173, 3581–3588. doi: 10.4049/jimmunol.173.6.3581
He, J., Liu, Y., Zhu, T., Zhu, J., Dimeco, F., Vescovi, A. L., et al. (2012). CD90 is identified as a candidate marker for cancer stem cells in primary high-grade gliomas using tissue microarrays. Mol. Cell. Proteomics 11:M111.010744. doi: 10.1074/mcp.M111.010744
Higa, A., Taouji, S., Lhomond, S., Jensen, D., Fernandez-Zapico, M. E., Simpson, J. C., et al. (2014). Endoplasmic reticulum stress-activated transcription factor ATF6alpha requires the disulfide isomerase PDIA5 to modulate chemoresistance. Mol. Cell. Biol. 34, 1839–1849. doi: 10.1128/MCB.01484-13
Hurwitz, E., Arnon, R., Sahar, E., and Danon, Y. (1983). A conjugate of adriamycin and monoclonal antibodies to Thy-1 antigen inhibits human neuroblastoma cells in vitro. Ann. N. Y. Acad. Sci. 417, 125–136. doi: 10.1111/j.1749-6632.1983.tb32857.x
Jabouille, A., Delugin, M., Pineau, R., Dubrac, A., Soulet, F., Lhomond, S., et al. (2015). Glioblastoma invasion and cooption depend on IRE1alpha endoribonuclease activity. Oncotarget 6, 24922–24934. doi: 10.18632/oncotarget.4679
Jiang, J., Zhang, Y., Chuai, S., Wang, Z., Zheng, D., Xu, F., et al. (2012). Trastuzumab (herceptin) targets gastric cancer stem cells characterized by CD90 phenotype. Oncogene 31, 671–682. doi: 10.1038/onc.2011.282
Kang, M.-K., and Kang, S.-K. (2007). Tumorigenesis of chemotherapeutic drug-resistant cancer stem-like cells in brain glioma. Stem Cells Dev. 16, 837–847.
Kemshead, J. T., Ritter, M. A., Cotmore, S. F., and Greaves, M. F. (1982). Human Thy-1: expression on the cell surface of neuronal and glial cells. Brain Res. 236, 451–461. doi: 10.1016/0006-8993(82)90727-2
Khan, M. I., Czarnecka, A. M., Lewicki, S., Helbrecht, I., Brodaczewska, K., Koch, I., et al. (2016). Comparative gene expression profiling of primary and metastatic renal cell carcinoma stem cell-like cancer cells. PLoS One 11:e0165718. doi: 10.1371/journal.pone.0165718
Kong, M., Munoz, N., Valdivia, A., Alvarez, A., Herrera-Molina, R., Cardenas, A., et al. (2013). Thy-1-mediated cell-cell contact induces astrocyte migration through the engagement of alphaVbeta3 integrin and syndecan-4. Biochim. Biophys. Acta 1833, 1409–1420. doi: 10.1016/j.bbamcr.2013.02.013
Kopp, M. C., Nowak, P. R., Larburu, N., Adams, C. J., and Ali, M. M. (2018). In vitro FRET analysis of IRE1 and BiP association and dissociation upon endoplasmic reticulum stress. eLife 7:e30257. doi: 10.7554/eLife.30257
Kumar, A., Bhanja, A., Bhattacharyya, J., and Jaganathan, B. G. (2016). Multiple roles of CD90 in cancer. Tumour Biol. 37, 11611–11622. doi: 10.1007/s13277-016-5112-0
Lerner, A. G., Upton, J. P., Praveen, P. V., Ghosh, R., Nakagawa, Y., Igbaria, A., et al. (2012). IRE1alpha induces thioredoxin-interacting protein to activate the NLRP3 inflammasome and promote programmed cell death under irremediable ER stress. Cell Metab. 16, 250–264. doi: 10.1016/j.cmet.2012.07.007
Leyton, L., and Hagood, J. S. (2014). Thy-1 modulates neurological cell-cell and cell-matrix interactions through multiple molecular interactions. Adv. Neurobiol. 8, 3–20. doi: 10.1007/978-1-4614-8090-7_1
Lhomond, S., Avril, T., Dejeans, N., McMahon, M., Pineau, R., Papadodima, O., et al. (2018). Antagonistic IRE1 RNase functions dictate glioblastoma tumor development. EMBO Mol. Med. 10:e7929. doi: 10.15252/emmm.201707929
Liu, G., Yuan, X., Zeng, Z., Tunici, P., Ng, H., Abdulkadir, I. R., et al. (2006). Analysis of gene expression and chemoresistance of CD133+ cancer stem cells in glioblastoma. Mol. Cancer 5:67.
Louis, D. N., Ohgaki, H., Wiestler, O. D., Cavenee, W. K., Burger, P. C., Jouvet, A., et al. (2007). The 2007 WHO classification of tumours of the central nervous system. Acta Neuropathol. 114, 97–109.
Lu, J.-W., Chang, J.-G., Yeh, K.-T., Chen, R.-M., Tsai, J. J. P., and Hu, R.-M. (2011). Overexpression of Thy1/CD90 in human hepatocellular carcinoma is associated with HBV infection and poor prognosis. Acta Histochem. 113, 833–838. doi: 10.1016/j.acthis.2011.01.001
Lung, H. L., Bangarusamy, D. K., Xie, D., Cheung, A. K. L., Cheng, Y., Kumaran, M. K., et al. (2005). THY1 is a candidate tumour suppressor gene with decreased expression in metastatic nasopharyngeal carcinoma. Oncogene 24, 6525–6532. doi: 10.1038/sj.onc.1208812
Mesri, M., Birse, C., Heidbrink, J., McKinnon, K., Brand, E., Bermingham, C. L., et al. (2013). Identification and characterization of angiogenesis targets through proteomic profiling of endothelial cells in human cancer tissues. PLoS One 8:e78885. doi: 10.1371/journal.pone.0078885
Nitta, R. T., Gholamin, S., Feroze, A. H., Agarwal, M., Cheshier, S. H., Mitra, S. S., et al. (2015). Casein kinase 2alpha regulates glioblastoma brain tumor-initiating cell growth through the beta-catenin pathway. Oncogene 34, 3688–3699. doi: 10.1038/onc.2014.299
Obacz, J., Avril, T., Le Reste, P. J., Urra, H., Quillien, V., Hetz, C., et al. (2017). Endoplasmic reticulum proteostasis in glioblastoma-From molecular mechanisms to therapeutic perspectives. Sci. Signal. 10:eaal2323. doi: 10.1126/scisignal.aal2323
Ochs, K., Sahm, F., Opitz, C. A., Lanz, T. V., Oezen, I., Couraud, P. O., et al. (2013). Immature mesenchymal stem cell-like pericytes as mediators of immunosuppression in human malignant glioma. J. Neuroimmunol. 265, 106–116. doi: 10.1016/j.jneuroim.2013.09.011
Odenthal, J., Takes, R., and Friedl, P. (2016). Plasticity of tumor cell invasion: governance by growth factors and cytokines. Carcinogenesis 37, 1117–1128.
Ohga, N., Ishikawa, S., Maishi, N., Akiyama, K., Hida, Y., Kawamoto, T., et al. (2012). Heterogeneity of tumor endothelial cells: comparison between tumor endothelial cells isolated from high- and low-metastatic tumors. Am. J. Pathol. 180, 1294–1307. doi: 10.1016/j.ajpath.2011.11.035
Pluquet, O., Dejeans, N., Bouchecareilh, M., Lhomond, S., Pineau, R., Higa, A., et al. (2013). Posttranscriptional regulation of PER1 underlies the oncogenic function of IREalpha. Cancer Res. 73, 4732–4743. doi: 10.1158/0008-5472.CAN-12-3989
Pode-Shakked, N., Metsuyanim, S., Rom-Gross, E., Mor, Y., Fridman, E., Goldstein, I., et al. (2009). Developmental tumourigenesis: NCAM as a putative marker for the malignant renal stem/progenitor cell population. J. Cell. Mol. Med. 13, 1792–1808. doi: 10.1111/j.1582-4934.2008.00607.x
Pont, S. (1987). Thy-1: a lymphoid cell subset marker capable of delivering an activation signal to mouse T lymphocytes. Biochimie 69, 315–320. doi: 10.1016/0300-9084(87)90022-8
Rege, T. A., and Hagood, J. S. (2006). Thy-1 as a regulator of cell-cell and cell-matrix interactions in axon regeneration, apoptosis, adhesion, migration, cancer, and fibrosis. FASEB J. 20, 1045–1054. doi: 10.1096/fj.05-5460rev
Rege, T. A., Pallero, M. A., Gomez, C., Grenett, H. E., Murphy-Ullrich, J. E., and Hagood, J. S. (2006). Thy-1, via its GPI anchor, modulates Src family kinase and focal adhesion kinase phosphorylation and subcellular localization, and fibroblast migration, in response to thrombospondin-1/hep I. Exp. Cell Res. 312, 3752–3767. doi: 10.1016/j.yexcr.2006.07.029
Reif, A. E., and Allen, J. M. (1964a). Immunological distinction of Akr thymocytes. Nature 203, 886–887. doi: 10.1038/203886a0
Reif, A. E., and Allen, J. M. (1964b). The Akr thymic antigen and its distribution in leukemias and nervous tissues. J. Exp. Med. 120, 413–433. doi: 10.1084/jem.120.3.413
Rettig, W. J., Chesa, P. G., Beresford, H. R., Feickert, H. J., Jennings, M. T., Cohen, J., et al. (1986). Differential expression of cell surface antigens and glial fibrillary acidic protein in human astrocytoma subsets. Cancer Res. 46(12 Pt 1), 6406–6412.
Rojas-Rivera, D., Rodriguez, D. A., Sepulveda, D., and Hetz, C. (2018). ER stress sensing mechanism: putting off the brake on UPR transducers. Oncotarget 9, 19461–19462.
Royer-Pokora, B., Busch, M., Beier, M., Duhme, C., de Torres, C., Mora, J., et al. (2010). Wilms tumor cells with WT1 mutations have characteristic features of mesenchymal stem cells and express molecular markers of paraxial mesoderm. Hum. Mol. Genet. 19, 1651–1668. doi: 10.1093/hmg/ddq042
Saalbach, A., Wetzel, A., Haustein, U.-F., Sticherling, M., Simon, J. C., and Anderegg, U. (2005). Interaction of human Thy-1 (CD 90) with the integrin alphavbeta3 (CD51/CD61): an important mechanism mediating melanoma cell adhesion to activated endothelium. Oncogene 24, 4710–4720. doi: 10.1038/sj.onc.1208559
Schubert, K., Gutknecht, D., Köberle, M., Anderegg, U., and Saalbach, A. (2013). Melanoma cells use Thy-1 (CD90) on endothelial cells for metastasis formation. Am. J. Pathol. 182, 266–276. doi: 10.1016/j.ajpath.2012.10.003
Seeger, R. C., Danon, Y. L., Rayner, S. A., and Hoover, F. (1982). Definition of a Thy-1 determinant on human neuroblastoma, glioma, sarcoma, and teratoma cells with a monoclonal antibody. J. Immunol. 128, 983–989.
Seki, T., Spurr, N., Obata, F., Goyert, S., Goodfellow, P., and Silver, J. (1985). The human Thy-1 gene: structure and chromosomal location. Proc. Natl. Acad. Sci. U.S.A. 82, 6657–6661. doi: 10.1073/pnas.82.19.6657
Sepulveda, D., Rojas-Rivera, D., Rodriguez, D. A., Groenendyk, J., Kohler, A., Lebeaupin, C., et al. (2018). Interactome screening identifies the ER luminal chaperone Hsp47 as a regulator of the unfolded protein response transducer IRE1alpha. Mol. Cell 69, 238.e7–252.e7. doi: 10.1016/j.molcel.2017.12.028
Shaikh, M. V., Kala, M., and Nivsarkar, M. (2016). CD90 a potential cancer stem cell marker and a therapeutic target. Cancer Biomark. 16, 301–307. doi: 10.3233/CBM-160590
Sukowati, C. H. C., Anfuso, B., Torre, G., Francalanci, P., Crocè, L. S., and Tiribelli, C. (2013). The expression of CD90/Thy-1 in hepatocellular carcinoma: an in vivo and in vitro study. PLoS One 8:e76830. doi: 10.1371/journal.pone.0076830
Tang, K. H., Dai, Y. D., Tong, M., Chan, Y. P., Kwan, P. S., Fu, L., et al. (2013). A CD90+ tumor-initiating cell population with an aggressive signature and metastatic capacity in esophageal cancer. Cancer Res. 73, 2322–2332. doi: 10.1158/0008-5472.CAN-12-2991
Tate, J. G., Bamford, S., Jubb, H. C., Sondka, Z., Beare, D. M., Bindal, N., et al. (2018). COSMIC: the catalogue of somatic mutations in cancer. Nucleic Acids Res. 47, D941–D947. doi: 10.1093/nar/gky1015
Thiery, J. P., Acloque, H., Huang, R. Y., and Nieto, M. A. (2009). Epithelial-mesenchymal transitions in development and disease. Cell 139, 871–890. doi: 10.1016/j.cell.2009.11.007
Tomuleasa, C., Soritau, O., Rus-Ciuca, D., Ioani, H., Susman, S., Petrescu, M., et al. (2010). Functional and molecular characterization of glioblastoma multiforme-derived cancer stem cells. J. BUON 15, 583–591.
True, L. D., Zhang, H., Ye, M., Huang, C.-Y., Nelson, P. S., Haller, P. D. V., et al. (2010). CD90/THY1 is overexpressed in prostate cancer-associated fibroblasts and could serve as a cancer biomarker. Mod. Pathol. 23, 1346–1356. doi: 10.1038/modpathol.2010.122
Urano, F., Wang, X., Bertolotti, A., Zhang, Y., Chung, P., Harding, H. P., et al. (2000). Coupling of stress in the ER to activation of JNK protein kinases by transmembrane protein kinase IRE1. Science 287, 664–666. doi: 10.1126/science.287.5453.664
Urra, H., Dufey, E., Avril, T., Chevet, E., and Hetz, C. (2016). Endoplasmic reticulum stress and the hallmarks of cancer. Trends Cancer 2, 252–262. doi: 10.1016/j.trecan.2016.03.007
Urra, H., Henriquez, D. R., Canovas, J., Villarroel-Campos, D., Carreras-Sureda, A., Pulgar, E., et al. (2018). IRE1alpha governs cytoskeleton remodelling and cell migration through a direct interaction with filamin A. Nat. Cell Biol. 20, 942–953. doi: 10.1038/s41556-018-0141-0
Vehlow, A., and Cordes, N. (2013). Invasion as target for therapy of glioblastoma multiforme. Biochim. Biophys. Acta 1836, 236–244. doi: 10.1016/j.bbcan.2013.07.001
Wandel, E., Saalbach, A., Sittig, D., Gebhardt, C., and Aust, G. (2012). Thy-1 (CD90) is an interacting partner for CD97 on activated endothelial cells. J. Immunol. 188, 1442–1450. doi: 10.4049/jimmunol.1003944
Wang, P., Gao, Q., Suo, Z., Munthe, E., Solberg, S., Ma, L., et al. (2013). Identification and characterization of cells with cancer stem cell properties in human primary lung cancer cell lines. PLoS One 8:e57020. doi: 10.1371/journal.pone.0057020
Wang, X., Liu, Y., Zhou, K., Zhang, G., Wang, F., and Ren, J. (2015). Isolation and characterization of CD105+/CD90+ subpopulation in breast cancer MDA-MB-231 cell line. Int. J. Clin. Exp. Pathol. 8, 5105–5112.
Wikstrand, C. J., Bigner, S. H., and Bigner, D. D. (1983). Demonstration of complex antigenic heterogeneity in a human glioma cell line and eight derived clones by specific monoclonal antibodies. Cancer Res. 43, 3327–3334.
Wikstrand, C. J., Grahmann, F. C., McComb, R. D., and Bigner, D. D. (1985). Antigenic heterogeneity of human anaplastic gliomas and glioma-derived cell lines defined by monoclonal antibodies. J. Neuropathol. Exp. Neurol. 44, 229–241. doi: 10.1097/00005072-198505000-00002
Yamashita, T., Honda, M., Nakamoto, Y., Baba, M., Nio, K., Hara, Y., et al. (2013). Discrete nature of EpCAM+ and CD90+ cancer stem cells in human hepatocellular carcinoma. Hepatology 57, 1484–1497. doi: 10.1002/hep.26168
Yang, Z. F., Ho, D. W., Ng, M. N., Lau, C. K., Yu, W. C., Ngai, P., et al. (2008). Significance of CD90+ cancer stem cells in human liver cancer. Cancer Cell 13, 153–166. doi: 10.1016/j.ccr.2008.01.013
Zhong, J., Paul, A., Kellie, S. J., and O’Neill, G. M. (2010). Mesenchymal migration as a therapeutic target in glioblastoma. J. Oncol. 2010:430142. doi: 10.1155/2010/430142
Zhu, J., Thakolwiboon, S., Liu, X., Zhang, M., and Lubman, D. M. (2014). Overexpression of CD90 (Thy-1) in pancreatic adenocarcinoma present in the tumor microenvironment. PLoS One 9:e115507. doi: 10.1371/journal.pone.0115507
Keywords: THY-1, CD90, cancer, invasion, migration, ER stress, IRE1
Citation: Sauzay C, Voutetakis K, Chatziioannou A, Chevet E and Avril T (2019) CD90/Thy-1, a Cancer-Associated Cell Surface Signaling Molecule. Front. Cell Dev. Biol. 7:66. doi: 10.3389/fcell.2019.00066
Received: 21 December 2018; Accepted: 09 April 2019;
Published: 26 April 2019.
Edited by:
Emanuela Felley-Bosco, University of Zurich, SwitzerlandReviewed by:
Andrew F. G. Quest, Universidad de Chile, ChileOrest William Blaschuk, McGill University, Canada
Copyright © 2019 Sauzay, Voutetakis, Chatziioannou, Chevet and Avril. This is an open-access article distributed under the terms of the Creative Commons Attribution License (CC BY). The use, distribution or reproduction in other forums is permitted, provided the original author(s) and the copyright owner(s) are credited and that the original publication in this journal is cited, in accordance with accepted academic practice. No use, distribution or reproduction is permitted which does not comply with these terms.
*Correspondence: Tony Avril, dC5hdnJpbEByZW5uZXMudW5pY2FuY2VyLmZy