- 1School of Chemical and Biotechnology, Sastra University, Thanjavur, India
- 2Institute for Stem Cell Biology and Regenerative Medicine, GKVK Campus, Bangalore, India
The extracellular matrix (ECM) is a complex network of proteins and proteoglycans secreted by keratinocytes, fibroblasts and immune cells. The function of the skin ECM has expanded from being a scaffold that provides structural integrity, to a more dynamic entity that is constantly remodeled to maintain tissue homeostasis. The ECM functions as ligands for cell surface receptors such as integrins, dystroglycans, and toll-like receptors (TLRs) and regulate cellular signaling and immune cell dynamics. The ECM also acts as a sink for growth factors and cytokines, providing critical cues during epithelial morphogenesis. Dysregulation in the organization and deposition of ECMs lead to a plethora of pathophysiological conditions that are exacerbated by aberrant ECM-immune cell interactions. In this review, we focus on the interplay between ECM and immune cells in the context of skin diseases and also discuss state of the art therapies that target the key molecular players involved.
Introduction
Homeostasis in the skin, the largest organ of the body, is critical for maintaining its structural, protective and regulatory functions. Alterations in this homeostasis, as seen in major skin disorders such as Atopic Dermatitis (AD), Psoriasis, Epidermolysis Bullosa (EB), and skin cancer, lead to impaired skin hydration, thermoregulation, and increased susceptibility to infections (Mellerio, 2010; Romanovsky, 2014; Nyström and Bruckner-Tuderman, 2018). An important role in exacerbating the disease condition is played by dysregulated interactions between two important goal keepers of skin function, the immune cells and the extracellular matrix (ECM). Immune cells play a critical role in the breakdown, synthesis, and remodeling of ECM components that are important for physiological processes such as mammary gland remodeling, lung branching morphogenesis and wound healing (Allori et al., 2008; Rozario and DeSimone, 2010). ECM components such as laminin and collagens, on the other hand, act as ligands for immune cells and facilitate their adhesion and trafficking (Simon and Bromberg, 2017). Proteolytic enzymes secreted by the immune cells generate fragmented laminin and collagen peptides that can serve as chemotactic signals for the recruitment of immune cells (Bonnans et al., 2014). Moreover, ECM components and their physical properties can drive specific activation states of immune cells such as macrophages (McWhorter et al., 2015). In certain chronic disease conditions, excess ECM deposition results in fibrosis that can later develop into cancer (Bonnans et al., 2014). Therefore, maintaining a balance between remodeling by the immune system and ECM dynamics becomes critical to maintain homeostasis.
Skin diseases are largely incurable and therefore remain a major burden; globally posing an adverse socio-economic and psychological impact on the affected populations. The prevalence estimates of psoriasis can go as high as 1% in the U.S. to 8.5% in Norway (Parisi et al., 2013). AD affects up to one-fifth of the population in developed countries (Weidinger and Novak, 2016). Skin cancers, especially non-melanoma skin cancer affects over 3 million Americans yearly with high mortality rates (Apalla et al., 2017). The prevalence of EB has risen to 11.07 per million live births globally and the numbers continue to rise (Fine, 2016). Commonly used treatment regimes for skin diseases include, the use of topical steroids and anti-inflammatory medications to control disease progression (Thomas et al., 2018; Yu et al., 2018). However, there is a paucity of curative therapies, which necessitates further understanding of the mechanisms that exacerbate the disease condition. Thus, focusing on the crosstalk between ECM and immune cells in skin diseases may help identify better targets for therapeutic interventions. In this review, we highlight the bidirectional interactions between ECM and immune cells in the major skin diseases and discuss the therapeutic strategies for dampening this “War of the Titans.”
The Skin and its ECM
Mammalian skin comprises of a stratified epithelium known as the epidermis and a connective tissue layer termed the dermis. The epidermis is made up of specialized cells containing keratin intermediate filaments known as keratinocytes and is stratified into different layers, namely, basal (stratum basale), suprabasal (stratum spinosum), granular (stratum granulosum), and cornified (stratum corneum) layers (Figure 1) (Fuchs and Raghavan, 2002; Breitkreutz et al., 2013). The basal keratinocytes comprise a pool of proliferating stem cells, which undergo terminal differentiation and eventually lose their nuclei to form the outermost cornified layer (Blanpain and Fuchs, 2006). Each of these epidermal layers is characterized by the expression of different structural proteins. The basal keratinocytes express keratin 5 and 14, which are replaced by keratin 1 and 10 as they differentiate into the spinous layer. The granular layer, as the name suggests, contains keratohyalin granules made up of lipids and precursors of proteins such as loricrin, filaggrin, and involucrin. These proteins and lipids are covalently crosslinked by transglutaminase to form the outermost layer, the stratum corneum that is 10–30 cell layers thick. The stratum corneum is composed of flattened, and enucleated cells embedded in matrix that is rich in proteins and insoluble lipids (Candi et al., 2005). Mutations in any of the above mentioned genes can lead to skin diseases, some of which are discussed later in the review (Chandran, 2013). The dermis is a highly vascularized tissue comprising of fibroblasts, adipocytes and various pools of innate and adaptive immune cells (Mueller et al., 2014). It is divided into a thin upper papillary dermis with loosely arranged collagen fibrils and a thick lower reticular dermis with dense collagen packing. Furthermore, the dermal and hypodermal compartments of the skin also comprise of triglyceride rich adipocyte depot called the dermal white adipose tissue (DWAT) and subcutaneous white adipose tissue (SWAT), respectively (Driskell et al., 2014).
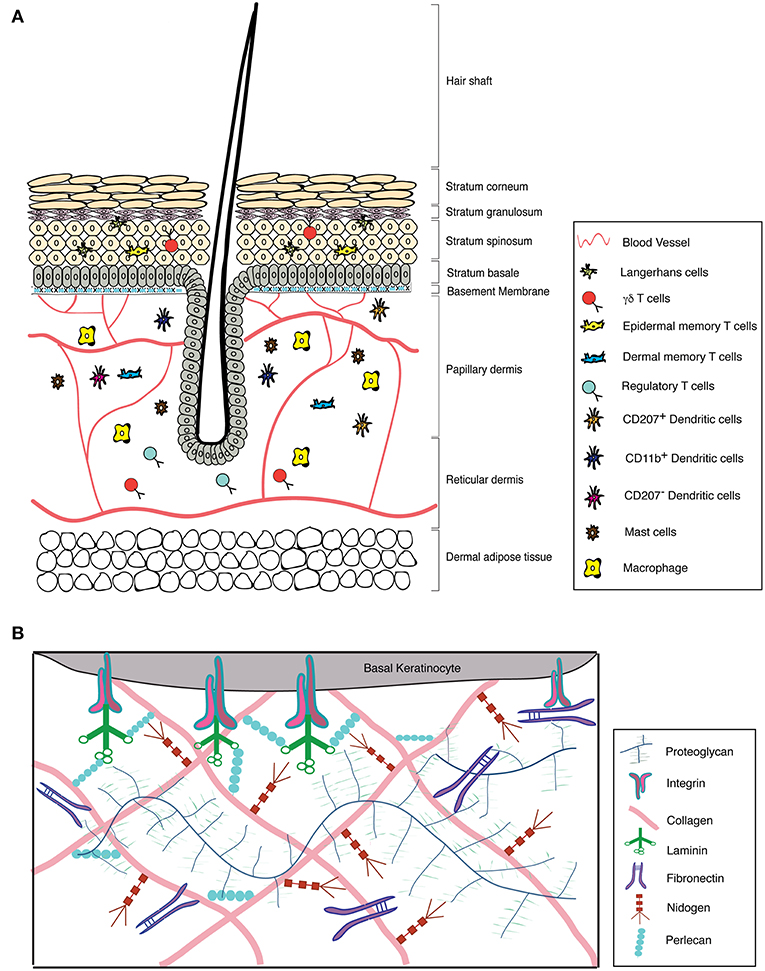
Figure 1. Structure of the skin with its associated ECM and immune cell repertoire. (A) Structure of the skin and its immune components. (B) Graphical representation of the major components of the skin ECM.
ECM components in the skin are present in the basement membrane and interstitial matrix (Frantz et al., 2010). The BM is a non-cellular array of structural ECM components that separates epidermis from dermis. It is composed of different subtypes of laminins and collagens, primarily laminin 5 (laminin-332) and collagen IV, which are non-covalently connected through perlecan and nidogen (Breitkreutz et al., 2013). This forms a highly dynamic fibrous structure that also functions as a diffusion barrier and serves as a repository of growth factors (Jayadev and Sherwood, 2017). The interstitial matrix is localized to the interstitial spaces in the dermal compartments. These primarily comprise of other collagen subtypes that also impart structural stability to the tissue (Bonnans et al., 2014). Additionally, there are non-structural ECM components called matricellular proteins that are synthesized by the epidermal and dermal cells during development and under non-homeostatic conditions. Matricellular proteins are involved in modulating cell function by interacting with structural ECM proteins as well as with various receptors, hormones and proteases. These interactions are a function of local availability of growth factors and overall composition of the ECM. Tenascin-C (TNC), thrombospondin (TSP), a secreted protein, acidic and rich in cysteine (SPARC), osteopontin (OPN), decorin, periostin (POSTN), and cysteine-rich protein 61 (CCN-1) are some of the major matricellular proteins (Bornstein, 2009). While basement membrane components are relatively stable, matricellular proteins undergo rapid turnover (Bonnans et al., 2014).
Organization of the BM components is mediated through transmembrane heterodimeric cell surface receptors called integrins that are expressed by the basal keratinocytes (Hynes, 2002; Andriani et al., 2003). Integrins are composed of α and β subunits. There are 18 α and 8 β subunits that form 24 different combinations. In the skin epidermis, α2β1, α3β1, and α6β4 are the main integrin pairs that are expressed (Watt and Fujiwara, 2011). Integrin β4 and β1 are components of multi-protein cell-substratum junctional complexes known as hemidesmosomes and focal adhesions, respectively. These structures facilitate the adherence of basal keratinocytes to its underlying BM either via intermediate filaments (in hemidesmosomes) or via actin cytoskeleton network (in focal adhesions) (Tsuruta et al., 2011). ECM-integrin signaling at the BM is indispensable for several cellular processes such as cell proliferation, differentiation, adhesion, migration, and apoptosis (Hegde and Raghavan, 2013). Any disruptions in the ECM-integrin interactions lead to adhesion defects (Nelson and Larsen, 2015). Recent work from our laboratory uncovered the importance of ECM-integrin interaction in embryonic skin homeostasis. We showed that loss of integrin β1 in embryonic skin results in ECM degradation that is exacerbated through a sterile inflammatory response (Kurbet et al., 2016).
Immunomodulatory functions of ECM
ECM molecules are potent regulators of immune cell function (Table 1). Alterations in the structure and composition of ECM in disease conditions provide immune cells with physical and biochemical cues that, in turn, affect their activation states. Several studies have shown that both enzymatically digested ECM components as well as intact ECM proteins can modulate activation, fate determination, and chemotaxis of immune cells (Lu et al., 2011; Hallmann et al., 2015; Simon and Bromberg, 2017).
Skin Immune Cell Repertoire and its Role in Maintaining ECM Dynamics
The skin contains a diverse population of immune cells that continuously surveil the organ to protect from assaults and maintain homeostasis. The epidermal and dermal compartments of the skin have distinct populations of immune cells that perform multiple functions including antigen cross-presentation to effector cells, tolerance induction, mediation of inflammation, promotion of growth and repair, regulation of stem cell quiescence, and maintenance of epidermal differentiation (Di Meglio et al., 2011). Langerhan cells and dendritic epidermal T cells are primarily located in the epidermis while, distinct populations of other dendritic cells and macrophages are present in the dermis (Heath and Carbone, 2013). Mast cells and T cell populations including αβ, γδT, Tregs, Th17, and memory T cells get recruited to both the epidermis and dermis depending on the homeostatic status of the skin (Matejuk, 2018). Details of the expression markers, location, and regulatory roles of immune cells in maintaining skin homeostasis are listed in Table 2.
Immune cells are one of the most important regulators of ECM degradation, synthesis, assembly and remodeling. Mechanisms by which immune cells accomplish this include–(i) synthesis of enzymes that remodel ECM components, (ii) synthesis of cytokines and growth factors that induce ECM synthesis or degradation, and (iii) synthesis of ECM components.
(i) Synthesis of enzymes that remodel ECM components: Metalloproteinases are enzymes secreted by the immune cells that cleave ECM proteins leading to alterations in the physical and biochemical properties of the tissue. Degradation of ECM by metalloproteinases aids in the trafficking of immune cells, generates bioactive peptides, and releases the sequestered growth factors (Mott and Werb, 2004). Metalloproteinases include MMPs, (matrix metalloproteinases) adamlysins including ADAMS (a disintegrin and metalloproteinases), ADAMTs (ADAMs containing a thrombospondin motif), Meprins as well as serine proteases such as plasmins, granzymes, and cathepsins. These enzymes cleave collagens (MMP-1, -2, -3, -7, -8, -9, -10, -11, -12, -13, -14, -16, -19, -25, -26; ADAM-10, -12 and -15; Meprin a and b), elastins (MMP-2, -3, -7, -9, -10, -11, - 12), gelatins (MMP-2, -3, -7, -9, -12, -14, -15, -16, -17, -23A, - 23B, -24, -25; ADAM-9, -10, -12, -15), aggrecans (MMP-3, -13; ADAMTs), and laminins (MMP-3, -7, -11, -12, -14, -15, -19, Meprin α and β) (Bonnans et al., 2014). Several studies have highlighted the important roles played by metalloproteinases, derived from immune cells, in remodeling the ECM thereby driving the pathophysiology and severity of various diseases. The T cell repertoire, particularly Th1 cells secrete gelatinases, MMP-2 and -9 that further stimulate the release of gelatinases from macrophages (Oviedo-Orta et al., 2008). In squamous cell carcinoma, mast cells, macrophages and neutrophils secrete MMP-9 that exacerbates the disease condition by increasing keratinocyte hyperproliferation and metastasis (Coussens et al., 2000). Dendritic and Langerhans cells in skin secrete MMP-2 and -9 to facilitate their trans-epithelial migration for antigen presentation (Ratzinger et al., 2002). Monocytes and lung resident macrophages are the primary source of MMP-8 that eventually contributes to fibrosis in idiopathic pulmonary fibrosis (Craig et al., 2014). Likewise, MMP-8 and -12 is synthesized by neutrophils and macrophages in cystic fibrosis accelerating disease progression (Wagner et al., 2016). Increased expression of MMP-9 by splenic and infiltrating T cells correlates with the growth in mammary tumors (Owen et al., 2003). Furthermore, in UVB irradiated skin, neutrophil elastase causes the degradation of ECM components by activating MMP-1, and MMP-2 (Takeuchi et al., 2010).
(ii) Synthesis of cytokines and growth factors that induce ECM synthesis or degradation: Cytokines (IL-4, IL-13, and IL-33) and growth factors (TGF-β) secreted by immune cells stimulate the synthesis of ECM components (Verrecchia et al., 2001; Fujitsu et al., 2003; Rankin et al., 2010). In mouse dermal fibroblasts, TGF-β signaling results in the activation of SMAD2/3, which, in turn, increases the expression of ECM, related genes such as Col1a1 and Col3a1 (Verrecchia et al., 2001; Li et al., 2003). IL-13 produced by innate lymphoid cells stimulates the differentiation of fibroblasts to myofibroblasts increasing collagen synthesis and its accumulation in fibrosis (Fichtner-Feigl et al., 2006). In bronchial asthma, Th2 cytokines IL-4 and IL-13 stimulate the synthesis of periostin from bronchial fibrocytes, leading to sub-epithelial fibrosis (Takayama et al., 2006; Aoudjehane et al., 2008). On the other hand, IL-6 and TNF-α produced by inflammatory immune cells can reduce the synthesis of MMP-2, which, in turn, protects liver hepatocytes from fibrosis (Bansal et al., 2005).
(iii) Synthesis of ECM components: Immune cells can themselves be a source for various ECM proteins. Macrophages and T cells present in tuberculosis associated granulomas produce OPN that aid in their chemotaxis, adhesion, and proliferation (O'regan et al., 1999). In a subset of patients with systemic stenosis, circulating CD14+ monocytes overexpress versican, which is associated with the aggressive fibrosis (Masuda et al., 2013). TAMs (tumor associated åmacrophages) modify the architecture of the tumor matrix by synthesizing proteoglycans, fibronectin, OPN, SPARC and various collagen subtypes (Liguori et al., 2011).
Taken together, these studies point to a critical role played by immune cells in modulating ECM dynamics during normal development and in disease states.
Skin Diseases
Atopic Dermatitis
Atopic dermatitis (AD) is a chronic, relapsing and TH2 cell/IgE driven inflammatory skin disorder characterized by intense pruritus and eczematous lesions (Bieber, 2008). The onset of AD is caused by barrier dysfunction, due to heritable mutations in the filaggrin (FLG) gene, and environmental triggers culminating in loss of skin hydration, enhanced sensitization to allergens and susceptibility to pathogenic agents (Weidinger and Novak, 2016). Clinical manifestations of AD occur both in children (Childhood AD) and adults (Adult AD) with similar clinical symptoms but with discrete pathophysiologies. While childhood AD is characterized by additional Th17/Th22 driven cascades along with tight junction and lipid metabolism disorders, AD in adults is associated with a Th1 specific response with aberrations in the epidermal differentiation complex (Brunner et al., 2018). AD usually precedes other allergic disorders like food allergies, allergic rhinitis, and asthma, commonly referred to as the “Atopic March,” the mechanism for which remains undefined (Dharmage et al., 2014).
Abnormalities in BM composition, suggesting ECM dysfunction, have been reported in AD. Affected skin in AD patients has decreased BM thickness, along with reduced expression of collagen-IV and integrin α6 in the BM zone (Kim et al., 2018). A report by Shin et al. (2015) also suggested that the loss of the regenerative capability of the skin correlated with lower expression of p63 protein, a putative stem cell marker, in AD affected skin (Shin et al., 2015). Several SNPs (single nucleotide polymorphisms) in LAMA3 gene correlated with increased susceptibility to AD (Stemmler et al., 2014). In acute AD, an increase in the expression of hyaluronan (HA), an extracellular polysaccharide, and hyaluronan synthase 3 (HAS3), an epidermal specific enzyme responsible for the synthesis and extracellular transport of hyaluronan, is observed (Ohtani et al., 2009). Increased expression of HA has been associated with abnormal keratinocyte differentiation, a hallmark of AD (Malaisse et al., 2014).
IL-4 and IL-13 are important cytokines known to play a critical role in AD pathogenesis. In situ hybridization studies on skin biopsy samples show a greater number of IL-13 positive cells in asymptomatic, acute and chronically affected AD patients compared to unaffected individuals (Hamid et al., 1996). Optimum expression of IL-13 is critical to maintain epidermal barrier homeostasis since both excess and insufficient levels of IL-13 provoke epidermal barrier dysfunction (Strid et al., 2016). IL-4 and IL-13 downregulate the expression of filaggrin, involucrin, loricrin, and the production of antimicrobial peptides. This exacerbates the skin barrier dysfunction and predisposes AD-affected skin to infection by microbes (Howell et al., 2007; Kim et al., 2008; Kisich et al., 2008). IL-4 is also shown to repress the expression of fibronectin in immortalized human keratinocytes (Serezani et al., 2017). In a human skin equivalent model system, IL-13 expression leads to the loss of BM structure and regenerative capability of the skin, recapitulating the AD phenotype (Shin et al., 2015). Furthermore, IL-13 treated human keratinocytes attract CD4+ CCR4+ T cells in vitro, providing a mechanism for a feed-forward loop that recruits more Th2 cells to acute AD lesions (Purwar et al., 2006). A critical correlation exists between IL-13 and MMP-9 mediated BM protein remodeling in AD. IL-13 is co-expressed with MMP-9 in skin samples from atopic eczema patients and it also increases MMP-9 synthesis in cultured keratinocytes (Purwar et al., 2008). IL-4 and IL-13 treated keratinocytes show an overexpression of TNC aggravating the inflammatory response (Ogawa et al., 2005). While mast cells and CD4+/CD8+ T cells are well known sources of IL-13 in AD affected skin, other immune cells including macrophages and dendritic cells can also be potential sources, which warrants further investigation (Obara et al., 2002). Taken together, these studies highlight the critical role played by IL-4 and IL-13 in driving AD pathogenesis.
Immune cell mediated remodeling of ECM in AD has been widely reported. BM remodeling matrix metalloproteinases (MMPs) including MMP-1, -3, -7, -8, and -9 is involved in AD pathophysiology (Harper et al., 2010). AD patients have enhanced serum levels of MMP-8 and -9 (Devillers et al., 2007). An immediate early effect in increase of MMP-9 by langerhans cells is observed upon treatment of skin with hapten, a well-known model for contact hypersensitivity (Kobayashi, 1997). The hyperplasic epidermis in AD secretes thymic stromal lymphopoeitin (TSLP), an IL-2 family cytokine, that activate DCs. DCs, in turn, produce MMP-3 and -9 that aid in their migration to lymph nodes where they activate naïve T cells (Ratzinger et al., 2002). Additionally, degranulation product of epidermal mast cells in AD-affected skin is associated with increased expression of MMP-7 (Landriscina et al., 2015). These reports indicate an indirect immune cell mediated BM remodeling in the context of AD. However, focused studies addressing this crosstalk are limited.
Monocytes and macrophages also play an important role in AD pathogenesis. An insightful review on the role of macrophages in AD has been previously published (Kasraie and Werfel, 2013). In AD skin, the epidermis overexpresses monocyte chemoattractant protein 1 (MCP-1) and, this results in the recruitment of monocytes in a CCR-2 dependent manner (Vestergaard et al., 2004). Acute and chronically inflamed AD skin show enhanced infiltration of heterogeneous pools of macrophages (Kiekens et al., 2001). Immunohistochemistry and ELISA analysis of AD skin and peripheral blood show increased CD163 expressing macrophages. Their role in aggravating disease condition is suggested by in vitro studies where the macrophages show an impaired TLR-2 signaling and increased secretion of pro-inflammatory cytokines such as IL-1β, IL-8, and IL-6 (Niebuhr et al., 2009). Given the preponderance of evidence on ECM-macrophage crosstalk in diseases, it is tempting to speculate that these heterogeneous pools of macrophages may play an important role in ECM remodeling.
Interestingly, recent studies point to the importance of ECM driven cascades in AD pathogenesis. Mitamura et al., showed that IL-13 upregulates the synthesis of POSTN which, in turn, increases IL-24 expression in the epidermal keratinocytes. IL-24, in turn, downregulates the expression of filaggrin, that contributes to the barrier dysfunction seen in atopic dermatitis (Mitamura et al., 2018). Another study showed that, the binding of TNF superfamily member 14 (TNFSF-14) to its receptor HVEM (herpes virus entry mediator) on keratinocytes resulted in the synthesis of POSTN and development of AD. Blocking this pathway by HVEM deletion and HVEM specific antibody resulted in suppression in AD symptoms (Herro et al., 2018). The importance of OPN in AD is highlighted in several studies. Its expression is elevated in the serum of patients with allergic contact dermatitis (Reduta et al., 2015). In disease conditions, immune cells such as T cells, NKT cells, and plasmacytoid dendritic cells synthesize OPN that acts as a bridging molecule between the innate and adaptive immune system (Clemente et al., 2016). OPN promotes a pro-inflammatory environment by enhancing production of IL-12 by macrophages and suppressing IL-27 production from conventional dendritic cells, thereby activating Th1 and Th17 cells (Clemente et al., 2016). OPN binds to CD44 receptors on mast cells leading to its recruitment and degranulation (Bulfone-Paus and Paus, 2008). Thus, studies on matricellular proteins POSTN and OPN have highlighted therapeutically targetable pathways that are the key drivers of AD symptoms.
Collectively, AD is characterized by a multitude of dysregulated immune responses, as well as close bidirectional interactions with matricellular and basement membrane components that exacerbate disease pathophysiology.
Psoriasis
Psoriasis is a skin disorder associated with pathological changes such as appearance of erythematous lesions, scaling of the epidermis, inflammation, and vascular abnormalities (Nestle et al., 2009). Lesions appear as red and scaly plaques in areas like the elbow, joints, lower back, and the scalp (Boehncke and Schön, 2015). It is classified into plaque, guttate, erythrodermic, and pustular types with plaque psoriasis (or psoriasis vulgaris) being the most common type associated with about 90% of the cases (Dubois Declercq and Pouliot, 2013). Based on the age of onset of the disease, psoriasis is classified as Type I (occurs in individuals below 40 years of age) and Type II (sets in after 40 years of age) (Langley et al., 2005). Type I is more common, runs in families and is associated with more severe phenotypes compared to Type II (Schmitt-Egenolf et al., 1991). The exact cause of this disease is still unknown. However, a combination of genetic and environmental factors such as infection, injury, and various administered drugs may be responsible for disease onset (Langley et al., 2005).
Cell death due to injury or trauma is thought to trigger the keratinocytes to produce antimicrobial peptides like LL-37 that activate plasmacytoid dendritic cells (pDCs) (Morizane and Gallo, 2012). The pDCs secrete IL-12 and IL-23 and mount a T cell mediated inflammatory response. The T cells, primarily Th1, Th17, and Th22, secrete the cytokines IFN-γ and TNF that promote inflammation in psoriatic skin. An increase in the infiltration of neutrophils, dendritic cells and T cells in the lesional skin of psoriatic patients evokes a crosstalk between the activated keratinocytes and immune cells (Baliwag et al., 2015; Albanesi et al., 2018). Although, T cells and dendritic cells primarily drive psoriasis, interesting roles for macrophages and neutrophils have recently emerged. Depletion of macrophages by clodronate treatment leads to alleviation of the psoriatic phenotypes in mice (Stratis et al., 2006). Neutrophil derived cytokines such as IL-1β, IL-6, IL-17, and IL-23 are associated with specific gene expression changes in keratinocytes that result in epidermal hyperproliferation and abnormal differentiation (Terui et al., 2000; Tecchio et al., 2014). In contrast, antibody mediated neutralization of neutrophils does not reduce inflammation. Interestingly, neutrophils in psoriatic skin form web-like extracellular traps, referred to as NET (Neutrophil extracellular trap) that is composed of chromatin and antimicrobial components such as LL-37, neutrophil elastase, and myeloperoxidase (Fuchs, 2007). The formation of NET is associated with NETosis, which involves killing of pathogens through the release of IL-17 and antimicrobial peptides (Hu et al., 2016).
Several studies have underscored the contribution of ECM proteins in the pathogenesis of psoriasis. A disorganized BM (laminin) in the psoriatic skin is associated with keratinocyte-derived plasminogen activating factor (McFadden et al., 2012). Laminin-332 and -511 are overexpressed in the BM of psoriatic skin lesions. Furthermore increased expression of laminin-511 in HaCat cells stimulated proliferation and inhibited apoptosis providing a mechanism for the observed epidermal hyperplasia (Natsumi et al., 2018). Additionally, alteration in laminin α1 chain along with overexpression of fibronectin in the papillary dermis and T cell lymphokines are correlated with abnormal cellular morphology (Vaccaro et al., 2002). While laminins are shown to facilitate neutrophil recruitment and enhance the phagocytic ability of dendritic cells, its role in immunomodulation in the context of psoriasis remains elusive (Wondimu et al., 2004; García-Nieto et al., 2010; Simon and Bromberg, 2017).
CCN-1, a matricellular protein is overexpressed in the lesional and non-lesional psoriatic epidermis in response to pro-inflammatory cytokines IL-17 and TNF-α. It binds to integrin α6β1 on keratinocytes and activates PI3K/Akt/NF-κB pathway and result in the upregulation of ICAM, HLA-DR, and HLA-ABC genes. These genes, in turn, increase the immune cell-keratinocyte interaction and mount an inflammatory response. Its role in promoting epidermal hyperplasia and inflammation was further demonstrated in IL-23 induced psoriatic skin lesions, where the knockdown of CCN-1 ameliorated the phenotypes (Sun et al., 2015). Other studies have shown that CCN-1 stimulates secretion of the cytokines IL-1β and IL-8 by psoriatic keratinocytes via the p38 MAPK and JNK/NF-κB signaling pathways, respectively underscoring its role in regulation of inflammation (Sun et al., 2017; Wu et al., 2017). Furthermore, CCN-1 promotes CCL-20 chemokine synthesis from hyperplasic epidermis of psoriatic skin that causes increased recruitment of immune cells (Li H. et al., 2017). Taken together, these studies highlight an important role played by CCN-1 in regulation inflammation in psoriatic skin.
OPN, expressed by epithelial cells, is shown to act as a potent pro-inflammatory stimulant and activates immune cells such as T-cells, dendritic cells, and macrophages/monocytes (Clemente et al., 2016). Interaction of OPN with integrin and CD44 leads to the promotion of Th1 response and suppression of Th2 response. In psoriasis, OPN is highly expressed in the PBMCs (peripheral blood mononuclear cell), skin and plasma. Buommino et al showed that OPN secreted by PBMCs enhances the expression pro-inflammatory cytokines such as IL-1β, TNF-α, and IFN-γ. TNF-α upregulates OPN expression while anti-TNF-α antibody treatment abrogates its synthesis (Buommino et al., 2012).
Overall, these studies suggest that ECM components play a critical role in psoriatic skin by acting as co-stimulatory factors for keratinocytes and immune cell activation leading to chronic progression of the disease.
Epidermolysis Bullosa
Epidermolysis bullosa (EB) is a group of rare heritable diseases characterized by blistering and fragile skin. It is associated with anomalies such as palmar-plantar thickening, syndactyly, dysphagia, gingival hyperkeratosis, cardiomyopathy, and osteoporosis (Maldonado-Colin et al., 2017). The blistering results from mechanical stress that eventually gives rise to chronic wound conditions (Medeiros and Riet-Correa, 2015). EB is caused by mutations in hemidesmosomal and extracellular matrix genes and the mode of inheritance can be either dominant or recessive. EB has been broadly categorized into simplex (EBS), junctional (JEB), dystrophic (DEB) types, and kindler syndrome (Shinkuma, 2015; Barna et al., 2017). The severity of disease is a function of the location of the split and the genes affected. So far mutations in at least 19 genes are reported to cause different types of EBs (Vahidnezhad et al., 2018). The site of splitting associated with various mutations is illustrated in Figure 2.
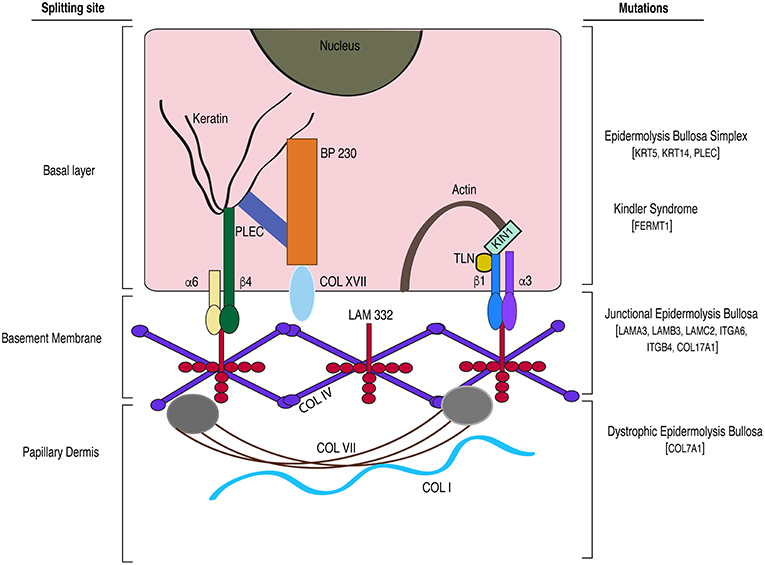
Figure 2. A model depicting the gene mutations and the site of splitting associated with the different subtypes of epidermolysis bullosa. BP, bullous pemphigoid; TLN, Talin; α6, integrin alpha 6; β4, integrin beta 4; α3, integrin alpha 3; β1, integrin beta 1; LAM 332, laminin-332 protein; PLEC, plectin; COL, collagen; KIN1 (FERMT1), kindlin-1; KRT, keratin; ITG, integrin.
EBS is caused by mutations in the genes coding for either keratin 5 (KRT5) or keratin 14 (KRT14) accounting for the four major subtypes. KRT5/14 form a stable cytoskeletal network and are involved in maintaining tissue integrity (Coulombe and Lee, 2012). Mutations in KRT5/14 result in rupture of basal keratinocytes leading to increased susceptibility to infection and dehydration. The least severe form of EBS is Weber-Cockayne type characterized by mild blistering restricted to feet and hands whereas the most severe form known as Dowling-Maera type is marked by extensive blistering, which however seems to get better with age. The other two subtypes are Koebner type and EBS with mottled pigmentation (Chung and Uitto, 2010a). Additionally, mutation in the plectin (PLEC) gene, a hemidesmosomal protein, is associated with a rare type of EBS known as Ogna type (Pfendner et al., 2005). The features common to all the subtypes are thickened palm and soles (palmoplantar keratoderma), non-scarring blisters, alopecia and dystrophic nails (Chung and Uitto, 2010a). EBS is the most prevalent and mildest type of EB, and exhibits an autosomal dominant inheritance pattern (Coulombe et al., 2009). Mouse knockouts for Krt5 and Krt14 serve as good models for studying EBS disease pathogenesis (Coulombe et al., 1991; Peters et al., 2001).
JEB is an autosomal recessive form of EB caused by mutations in the laminin-332 chains encoded by LAMA3, LAMB3, LAMC2 genes, and COL17A1 genes that code for collagen XVII/BP180 protein (Sawamura et al., 2010). Collagen-XVII is an important structural element of the hemidesmosomal complex that together with laminins in the BM, maintain the dermal-epidermal adhesion. JEB can be further classified into two subtypes; Herlitz and non-Herlitz based on its severity. Patients with the Non-Herlitz subtype display blisters primarily in the feet, elbows and knees, which are non-life threatening (Yancey and Hintner, 2010). The Herlitz subtype, on the other hand, causes severe disease, and is associated with widespread blistering of the lining of oral and digestive tracts, syndactyly and alopecia, and restricts life expectancy to 1 year after birth (Yuen et al., 2011).
Col17a1 knockout mice display the blistering pattern associated with the non-Herlitz subtype and show prolonged survival; whereas Lama3 and Lamc2 knockouts recapitulate the Herlitz type of EB, exhibiting severe skin blistering resulting in perinatal lethality (Bubier et al., 2010; Natsuga et al., 2010). Two other rare autosomal recessive forms of EB include EB with pyloric atresia (EB-PA) and EB with muscular dystrophy (EB-MD). EB-PA is caused by mutations in ITGA6 and ITGB4 genes whereas EB-MD is associated with mutations in the PLEC gene (Shimizu et al., 1999; Yancey and Hintner, 2010). Itga6 and Itgb4 knockout mouse models recapitulate the dermal-epidermal detachment leading to development of EB-PA (Georges-Labouesse et al., 1996; Mencía et al., 2016).
DEB is associated with mutations in Col7a1 gene resulting in the loss of collagen-VII protein. Collagen-VII is an anchoring fibril protein crucial for dermal-epidermal integrity and is present in the BM on the side of papillary dermis (Chung and Uitto, 2010b). The two major subtypes of DEB based on mode of inheritance and severity include autosomal dominant (DDEB) and recessive (RDEB) types. Unlike EBS and JEB, DEB is associated with extensive scarring (Shinkuma, 2015). RDEB, the more severe subtype is characterized by pseudosyndactyly, alopecia with scarring, and esophageal stenosis that leads to dysphagia. This disease is further complicated by the development of aggressive squamous cell carcinoma in juvenile patients (Shinkuma, 2015). The conditional Col7a1 KO mice recapitulate all of the phenotypes of RDEB and has proven to be an invaluable tool to understand disease progression as well as to test therapeutic interventions (Natsuga et al., 2010).
Kindler syndrome is the only skin disease known to be associated with actin cytoskeleton related abnormalities and is characterized by variable planes of cleavage in the skin (Medeiros and Riet-Correa, 2015). Keratinocytes express kindlin-1, a protein that regulates cellular processes such as proliferation, migration and cell-substratum adhesion (Rognoni et al., 2016). Mutations in the FERMT1 gene, coding for the kindlin-1 protein, lead to this rare fragile skin blistering disorder with symptoms similar to other EB subtypes (Lai-Cheong et al., 2009). Kindlin-1 deficient mice display skin atrophy without blister formation (Ussar et al., 2008). The loss of kindlin-1 from keratinocytes results in dysregulated integrin signaling and focal adhesion turnover (Margadant et al., 2013).
Another form of EB called aquisita (EBA) is caused by the production of autoantibodies against collagen-VII protein. The two subtypes of EBA are classical and the inflammatory types. The classical form is characterized by trauma induced blistering in adults whereas the inflammatory form starts in childhood and show more widespread blistering that is not restricted to the injured sites (Kasperkiewicz et al., 2016).
The null and conditional KO mouse models that have been discussed above recapitulate various aspects of disease progression of the EB class of skin disorders and are being used as platforms to develop therapeutic interventions for these devastating diseases (Georges-Labouesse et al., 1996; Cao et al., 2001; Peters et al., 2001; Bruckner-Tuderman et al., 2010).
Insights into the role of inflammation and immune cells in EB pathophysiology have come from the studies on Krt5, Itgb4, and Col7a1 knockouts that cause EBS, EB-PA, and DEB, respectively. Increased expression of chemokines and cytokines such as CCL-2, CCL-19, CCL-20, IL-6, and IL-1β is reported in Krt5 knockout skin. The cytokine expression is associated with a 2-fold increase in the recruitment of Langerhans cells to the EB affected skin (Lu et al., 2007; Roth et al., 2009). Loss of Itgb4 in mice leads to severe blistering associated with Th2 inflammatory response, which suggests involvement of immune cells in EB pathophysiology (Han et al., 2018). Knockout of Col7a1 recapitulates RDEB and creates a chronic wound condition underscoring its role in maintenance of epidermal-dermal integrity. Collagen-VII deficient wounds display suprabasal expression of α6β4 that lead to a dysregulated α6β4-laminin-332-JNK-STAT3. This, in turn, delays wound re-epithelialization and prolongs the presence of inflammatory CD11b cells in skin (Nyström et al., 2013). Interestingly, depletion of collagen-VII from immunodeficient mice (NSG mice) results in a less severe disease phenotype. Taken together, these studies emphasize the critical role played by the immune cells in exacerbating the disease condition (Esposito et al., 2016).
Blisters in the bullous diseases are usually associated with increased infiltration of cytotoxic T cells, B cells, neutrophils and other immune cells as well as their secreted cytokines (Hussein et al., 2007). Previous work has suggested that there is a substantial increase in the levels of cytokines such as IL-1, IL-6, IL-15, and TNF-α, and chemokines such as CXCL-1, CXCL-2, CXCL-3, CCL-5, CCL-27 in the blister fluids of EB patients compared to healthy controls. Moreover, the cytokine and chemokine expression profile varies between EB subtypes such that early DEB and JEB blisters expressed lower levels of cytokines and chemokines compared to that of early EBS (Alexeev et al., 2017). In EBA, several groups have shown that autoantibody production against COL7A1 is mediated through a milieu of neutrophils, macrophages, B cells and T cells and these phenotypes are ameliorated by the depletion of neutrophils and T cells (Sitaru et al., 2010; Iwata et al., 2013; Bieber et al., 2016).
MMPs secreted by immune cells can also influence the recruitment pattern of other immune cells to the injured tissue. Studies have shown that the severity of RDEB is a function of the type of MMP synthesized, its expression level and the balance between MMPs and TIMPs (Bodemer et al., 2003). Interestingly, a SNP (single nucleotide polymorphism) in the MMP1 gene has also been implicated in increasing disease severity by influencing the expression of collagen-VII (Titeux et al., 2008). Likewise the correlation between MMP-9 and blister formation was shown in a study where treatment with doxycycline derivative, an inhibitor of MMP-9, alleviated the phenotype (Lettner et al., 2013). Collectively MMPs can serve as effective therapeutic targets.
Since DEB is a disorder of the basement membrane zone (BMZ), it is not surprising that, in RDEB patients that lack collagen-VII, two major BM components collage-IV and laminin-332 display a disrupted distribution pattern and do not co-localize at the BMZ (Onetti Muda et al., 1996). The disruption precedes blister formation suggesting that it could be a direct consequence of the lack of collagen-VII. Dermal-epidermal splitting also leads to an increase in the expression of TNC at the site of the split, which could be in response to the cytokines secreted either by epidermis or the recruited immune cells (Schenk et al., 1995). These studies suggest that TNC can serve as a potential link between the immune and ECM components in DEB.
Interaction between collagen-VII and cochlin is required to mount an immune cell mediated antibacterial response. In RDEB patients, absence of this interaction leads to increased bacterial colonization that further exacerbates the disease condition (Nyström et al., 2018). Lack of collagen-VII in RDEB destabilize the BMZ and cause the release of TGF-β associated with ECM increasing its bioavailability. TGF-β mediated activation of dermal fibroblasts, in turn, cause excessive deposition of ECM components (Nyström and Bruckner-Tuderman, 2018). In a recent case study, monozygotic twins suffering from RDEB exhibited distinct phenotypes due to changes in the expression of TGF-β target genes, despite having similar loss of collagen-VII. The more affected individual displayed increased fibrosis, which was ameliorated by treatment with the anti-fibrotic the matricellular protein decorin (Odorisio et al., 2014). These data point to the complexity of managing and treating RDEB, a genetic disease that is associated with loss in ECM organization which is further exacerbated by TGF-β mediated alteration in ECM dynamics. The involvement of matricellular proteins in mounting an immune response in EB provides an additional avenue for therapeutic intervention. Taken together, while EBs remain one of the most challenging diseases to manage and treat, and future treatment paradigms will need to take into account the immune-ECM interactions.
Skin Cancers
Skin cancers are broadly classified as melanoma and non-melanoma types. Melanoma is an aggressive skin cancer that originates from melanocytes; the pigment producing cells in the skin (Villanueva and Herlyn, 2008). Non-melanoma skin cancers include Squamous cell carcinoma (SCC), Basal cell carcinoma (BCC), and Merkel cell carcinoma (MCC). SCCs originate from interfollicular stem or progenitor cell population in the epidermis and BCCs from the progenitor cells present in interfollicular epidermis and upper infundibulum (Donovan, 2009; Wang et al., 2011). MCCs, on the other hand, are the cancer of neuroendocrine (merkel) cells present in the basal layer of the epidermis (Munde et al., 2013). Other aggressive forms of skin cancer include cutaneous T and B lymphomas, a varied group of extranodal lymphoproliferative disorders associated with abnormal accumulation and proliferation of T and B lymphocytes in skin (Pandolfino et al., 2000; Siegel et al., 2012). Cutaneous T cell lymphomas are the most common with 65% incidence rate as compared to B cell lymphomas with a 25% incidence rate (Sokołowska-Wojdyło et al., 2015). All skin cancer types show a positive correlation with exposure to UV radiation as well as mutations in genes associated with tumor suppression, DNA repair, apoptosis and cell cycle regulation (D'Orazio et al., 2013; Kandoth et al., 2013).
Tumor resident and recruited immune cells play a dynamic role in regulating cancer progression. The tumor and its stroma recruit different populations of immune cells by releasing chemotactic factors and cytokines resulting in a chronic inflammatory condition (Binnewies et al., 2018). The immune cell repertoire, associated with the tumor microenvironment includes monocytes, macrophages, neutrophils, dendritic cells, natural killer cells, T and B cells (Cavallo et al., 2011). Factors such as CCL-2, CCL-5, CCL-17, CCL-22, CXCL-12, M-CSF, VEGF, and TGF-β synthesized by the tumor stroma aid in the recruitment of monocytes and subsets of T cells (Owen et al., 2003; Hiratsuka et al., 2006; Yang et al., 2008). In melanomas, the chemokines CCL-2, CCL-3, CCL-5, CXCL-9, and CXCL-10 recruit CD8+ T cells (Harlin et al., 2009). In a recent study it was shown that, tumor derived factors such as colony stimulating factor-1 (CSF-1), CCL-2, IL-34, and VEGF recruit circulating macrophages to the melanomas (Pieniazek et al., 2018). In human melanomas, CCL-2, also known as MCP-1, is associated with an increased inflammatory response and recruitment of macrophages and T cells (Ilkovitch and Lopez, 2008). Similarly in BCCs, CXCL-9, CXCL-10, and CXCL-11 recruit CXCR-3 expressing effector and memory T cells (Lo et al., 2011). In BCCs, the chemokines, CCL-17, CCL-18, and CCL-22 that recruit Tregs are overexpressed in the tumor islands and peritumoral skin. The stromal cells in turn synthesize CCL-17, which suggests a mechanism for the Treg infiltration seen in the stroma surrounding the BCC nests (Omland et al., 2016). In MCC, a recent study has shown that over expression of CCL-17 and its receptor CCR-4, aid in recruitment of CD4+ Tregs, Th2, and Th17 cells (Rasheed et al., 2018). Macrophage derived CCL-18 in cutaneous T cell lymphomas (CTCL) recruit T lymphocytes to the skin and is associated with enhanced invasion (Wu et al., 2014). Overall, the tumor and its stroma recruit a plethora of immune cells that contributes to tumor progression.
Dynamic crosstalk between the stroma and immune cells, change the tumor milieu from a tumor suppressive (or immunogenic) to tumorigenic (or immunosuppressive) state. Specifically, tumor associated macrophages (TAMs) switch from pro-inflammatory M1 macrophages, that are antigen presenting cells, to anti-inflammatory M2 macrophages, that are tissue remodeling and tumor growth promoting cells (Tariq et al., 2017). M1 macrophages phagocytose tumor cells, produce reactive oxygen (ROS) and nitrogen (RNS) species and secrete pro-inflammatory cytokines such as IL-6, IL-23, IL-12, and TNF-α that activate Th1 response (Komohara et al., 2014; Noy and Pollard, 2014). In contrast, M2 macrophages express anti-inflammatory cytokines like IL-10 and TGF-β that play a role in promoting tumor growth (Aras and Raza Zaidi, 2017). Depletion of M2 macrophages by treating with clodronate in CTCL xenograft mouse models, reduces angiogenesis and tumor growth (Wu et al., 2014). Human keratinocytes can inactivate T cell response by downregulating signaling pathways critical for T cell recruitment and cytotoxicity (Bronte and Zanovello, 2005; Bluth et al., 2009). In SCCs and BCCs, immunosuppression is mediated by preventing the recruitment of T cells to the cancer site through the activation of EGFR-Ras-MAPK signaling pathway (Pivarcsi et al., 2007). Interestingly, Tregs are known to suppress immune response by producing IL-10 and TGF-β and compete for T-cells-activating pro-inflammatory cytokines (Jarnicki et al., 2006). They are critical for inducing tolerance to self-antigens and also inhibit the antigen presenting ability of DCs (Sakaguchi et al., 2009). The transcription factor FOXP3 is important in regulating Treg function. Increased FOXP3 expressing Tregs correlates with poor disease outcome in SCCs, BCCs and melanomas (Moreira et al., 2010). In BCCs, recruited Tregs build an immunosuppressive niche by locally synthesizing TGF-β (Omland et al., 2016). In MCC, M2 macrophages and Tregs present in the tumor maintain an immunosuppressive state contributing to a pro-tumorigenic microenvironment (Gaiser et al., 2018). Interestingly, increased recruitment of CD8+ T cells to the skin is associated with better prognosis in MCCs (Touzé et al., 2011).
Taken together, there is growing evidence that tumor microenvironment in cancers develop immune suppressive mechanisms to evade the T cell mediated cytotoxicity that can promote metastasis.
The ECM in cancer is highly remodeled and disorganized due to aberrant deposition by activated fibroblasts and enzymatic remodeling by the tumor and immune cells (Karagiannis et al., 2012). Changes in ECM dynamics promote tumor growth by regulating proliferation, survival, adhesion and migration (Pickup et al., 2014). The BM in melanomas has reduced thickness compared to healthy controls due to the absence of collagen-VII (Schmoeckel et al., 1989). A study of the BM in BCC affected skin showed aberrations in bullous pemphigoid antigens possibly due to its abnormal synthesis by the tumor associated cells (Stanley et al., 1982). Similarly, the BM structure in aggressive SCCs is irregular and discontinuous (Sakr et al., 1987). Matrix metalloproteinases such as MMP-1, -2, -3, -8, -9, -10, -13, -15, -16, and -26 can facilitate tumor growth, invasion, and angiogenesis in BCC, SCC and melanomas (Pittayapruek et al., 2016). In a recent study, expression profiling of BCCs showed an increase in ECM remodeling proteins including MMP-1, -3, -8, -9, and Cathepsin-K indicating the important role played by these enzymes in cancer progression (Ciazynska et al., 2018). In organ culture models of BCC the increased expression of MMP-2 and MMP-9 correlates with degradation of collagen-I and -IV at the tumor front (Goździalska et al., 2016). Likewise, the expression of ADAM-10, -12, and -17 was specifically observed in deep invasion area of BCC biopsies and correlated with the loss of collagen-XVII (Oh et al., 2009). The TAMs in BCCs induce COX-2 expression, which, in turn, results in a COX-2 dependent release of MMP-9, VEGF, and FGF that promotes tumor invasion (Tjiu et al., 2009). Interestingly, MMP-9 producing inflammatory macrophages and neutrophils are associated with BM degradation in BCC patients (Boyd et al., 2008).
Similarly, in SCCs the γ2 chain of laminin-332 is overexpressed in the invasive front (Hamasaki et al., 2011). In a DMBA (7,12-dimethylbenz-anthracene)-TPA (12-O-tetradecanoyl-phorbol-13-acetate) induced mouse model of SCCs, overexpression of collagen-XVII, integrin α6β4 and the γ2 chain of laminin, promoted tumorigenesis (Moilanen et al., 2017). Increased laminin-332 expression with high MMP-2 and MMP-14 activity is seen in invasive SCCs with poor prognosis (Marinkovich, 2007). Reduced expression of collagen-VII in SCCs enhances invasion, dysregulates epithelial differentiation and drives epithelial to mesenchymal transition (Martins et al., 2009). Loss of collagen-VII expression (in RDEB) results in an upregulation of TGF-β signaling and increased angiogenesis in SCCs underscoring the critical role played by collagen-VII in suppressing TGF-β signaling (Martins et al., 2016). Transgenic mice lacking MMP-9 reduced the tumor invasiveness in a mouse model of SCC whereas, transplanting MMP-9 producing bone marrow cells increased tumor invasiveness (Coussens et al., 2000). The cleaved oligosaccharides generated by heparinize digestion of heparin sulfate chains is shown to play an important role in tumor progression in BCCs and SCCs (Pinhal et al., 2016). The abundance and stiffness of collagen also play an important role in melanoma progression where it stimulates proliferation and differentiation in a YAP/PAX3/MITF dependent manner (Miskolczi et al., 2018). In melanoma and BCCs, cathepsin K expression is associated with a fibromucinous stroma around the tumor nests, indicative of ECM degradation (Ishida et al., 2013). Interestingly, the matricellular protein POSTN is associated with metastatic progression in melanoma by attenuating adhesion and promoting migration (Fukuda et al., 2015). Tumor fronts of aggressive BCCs and SCCs overexpress TNC (Schalkwijk et al., 1991; Dang et al., 2006). Likewise, CCN-1 and CCN-2 are overexpressed in keratinocytes of BCCs in a YAP dependent manner promoting cell proliferation and survival (Quan et al., 2014).
The crosstalk between immune cells and ECM is thought to be critical in driving skin cancer pathogenesis. In patient samples of SSCs, an increase in CD163+ TAMs is associated with enhanced expression of MMP-9 and MMP-11, which promotes angiogenesis and tumor growth. The same study also reports the activation of IL-4 dependent STAT-6 signaling in TAMs that induces differentiation of Th2 cells, further maintaining an immune-suppressive environment (Pettersen et al., 2011). In a DMBA-TPA induced mouse model of SCC, Gr1+ neutrophils produce remodeling enzymes including myeloperoxidase (MPO) and elastase, which are associated with poor disease outcome (Gasparoto et al., 2012). TNF-α and TGF-β secreted by macrophages synergistically increase melanoma migration in a NF-κB dependent manner through the release of MMP-1 and MT1-MMPs (Li R. et al., 2017). Hyaluronic and proteoglycan link protein (HAPLN-1) is an ECM protein highly expressed in young fibroblasts compared to aged fibroblasts and is important for maintaining stability of hyaluronan and collagen (Ecker et al., 2019). Since T cells use collagen fibrils to infiltrate tumors, downregulating HAPLN-1 expression in melanoma models results in decreased CD3+ T cell infiltration associated with increased tumor cell extravasation. On the other hand treatment with recombinant HAPLN-1 increases the T cell recruitment and reduces the tumor growth. The association between collagen fiber stability and T cell migration has been suggested as one of the reasons for aggressive melanomas in aged individuals (Kaur et al., 2018). In CTCL, T lymphocytes localize toward the epidermis by expressing integrin α3β1 allowing them to bind with laminin-332 and migrate through the BM, which interestingly does not result in ECM disorganization (Wayner et al., 1993). Tumor derived OPN binds to macrophages through integrin α9β1 resulting in the overexpression of COX-2 and MMP-9 and promotes angiogenesis in melanomas (Kale et al., 2014). In melanoma models, IFN-γ induced by TSP-1 overexpression is critical for macrophage recruitment and polarization to the M1 state (Martin-Manso et al., 2008). This points to an important role played by TSP-1 in suppressing tumor growth.
The ECM-immune cell interactions have been well studied in the context of cancer since the ECM is highly remodeled by both the tumor and immune cells to facilitate the tumor growth. The tumor microenvironment can switch from being anti-tumorigenic to pro tumorigenic depending on dynamic interactions between the tumors; ECM and immune compartments and can result in aggressive cancer with poor prognosis. Understanding the signaling events regulating this process has led to the development of therapies targeting the immune system as evidenced by advent of immunotherapy in treating cancer. Figure 3 summarizes the ECM-immune interactions in all of the skin diseases discussed in this review.
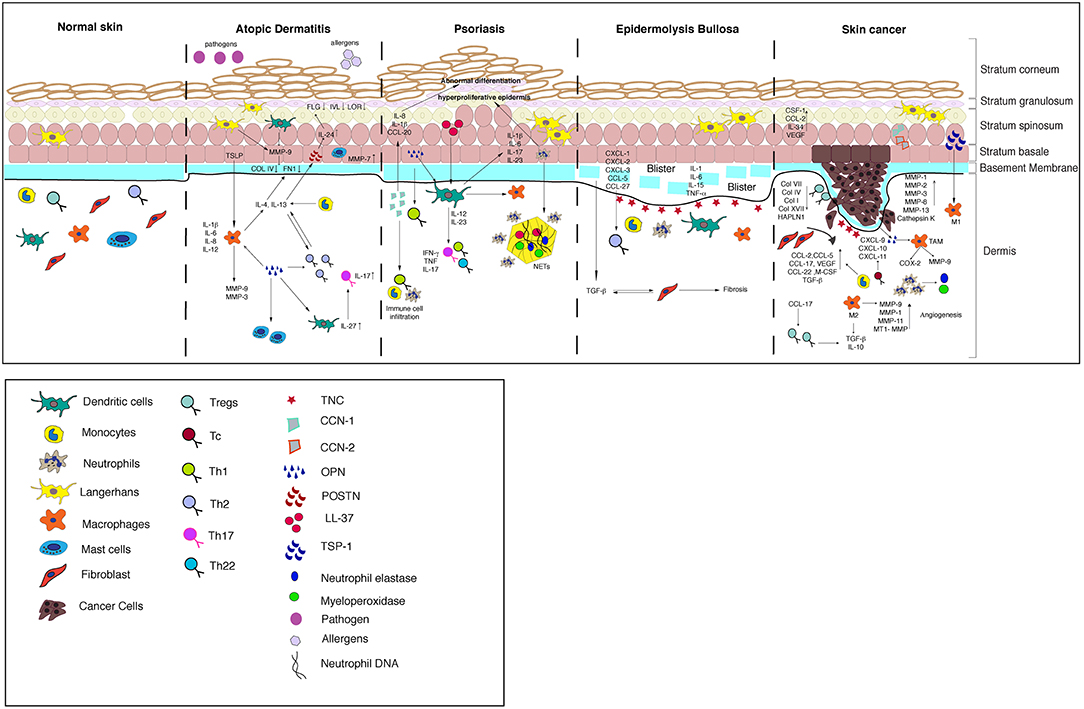
Figure 3. A model summarizing the crosstalk between the ECM and immune cells in the skin diseases discussed in this review.
Current State of the Art Therapeutic Interventions
Currently available treatment approaches for skin diseases involve treatment with anti-inflammatory drugs, analgesics, topical steroids, and anti-histamines (Grassberger et al., 2004; Abraham and Roga, 2014). Conventional therapies for the treatment of the diseases discussed in this review are listed in the Table 3. With the advent of techniques such as nanomedicine, gene therapy (genetic engineering), stem cell transplantation, and cell and protein replacement therapy, curative treatment of skin diseases seem achievable and have been discussed below.
Nanomedicine
Nanomedicine involves the use of particles within the nanometer range, for the detection and treatment of diseases. They are effective drug delivery agents for targeted therapy that enhance drug bioavailability and facilitate controlled drug release for long-term effect. Various nanoparticles as delivery agents for treatment of skin diseases include liposomes, solid lipid nanoparticles, polymeric micelles and nanospheres, dendrimers, nanotubes, quantum dots, gold particles etc. that has been reviewed previously (Dianzani et al., 2014). In mouse and rat models of AD and psoriasis, nanoparticle based delivery of drugs such as tacrolimus, corticosteroids, retinoids, methotrexate, and cyclosporine result in better outcomes (Palmer and DeLouise, 2016). Similarly, doxorubicin-loaded cationic solid lipid nanoparticles (DOX-SLN) have shown better drug penetration and inhibition of skin cancer tumor growth (Huber et al., 2015). In RDEB, localized delivery of gold nanoparticle coated with epigallocatechin-3-gallate (E3G) in SCC tumor site has been shown to abrogate the adverse effect of the drug in the liver (Manoukian et al., 2014). Additionally in EB, a novel nanocapsule based wound dressing technique has been developed. Upon infection, it releases antibiotics and undergoes a color change that indicates response to an active infection (Zhou et al., 2018). The promise of nanomedicine provides new therapeutic opportunities for treating skin disorders that have been refractory to treatment so far.
Gene and Stem Cell Therapy
Gene therapy involves the introduction of corrected copy of genes into cells for treating genetic disorders (Anguela and High, 2019). Likewise, stem cell therapy refers to stem cell transplantation, either from the same (autologous) or from a different (allogeneic) individual replacing affected cells. Alternatively, gene-corrected autologous stem cells can be engineered to generate skin substitutes followed by transplantation (Karantalis et al., 2015). Gene and stem cell therapy have proven highly beneficial for genetic skin disorders such as EB which continues to be a major health burden due to lack of effective treatment. The retroviral mediated transfer of COL7A1 into fibroblasts and keratinocytes have shown successful incorporation of collagen-VII at the dermal-epidermal junction of the engineered skin grafts (Ferrari et al., 2006; Piaceski et al., 2018). In a recent path-breaking paper, physicians were able to repair the skin of a 7-year old JEB patient who had lost 80% of his epidermis. The JEB was caused by a mutation in the LAMB3 gene. Autologous non-lesional keratinocytes were used to introduce the corrected gene copy, and these cells were used to generate epidermal skin grafts. The patient showed complete recovery 2 years after the skin was grafted (Hirsch et al., 2017).
Under homeostatic conditions, bone marrow derived mesenchymal stem cells have been shown to regulate proliferation and maturation of T cells, NK cells, B cells, and dendritic cells. However, this regulation is perturbed in AD and psoriatic patients and bone marrow transplantation has been shown to result in remission of diseases (Li et al., 2015; Shin et al., 2017). Similarly, transplantation of bone marrow derived mesenchymal stromal cells into RDEB patients showed symptomatic improvement (Uitto, 2008).
Genetically engineered bone marrow cells have been designed to carry tumor antigens for targeting skin cancers. Autologous transplantation of lymphocytes that are genetically modified to express tumor antigen MAGE-A3 has been shown to mount effective tumor killing response in melanoma (Fontana et al., 2009). Furthermore, engineering T cells with NY-ESO-1, melanoma antigen resulted in tumor regression in clinical trials (Dummer et al., 2008). Adenovirus mediated transduction of T cells with IL-2 has also shown improved prognosis in clinical trials (Puzanov and Flaherty, 2010).
While at its infancy, stem cell therapies provide the promise of a “cure” particularly for devastating skin blistering disorders. The challenge will be to reduce the costs of such treatments to make it more accessible to patients.
Cell and Protein Replacement Therapy
Protein replacement therapy involves introduction of intact proteins for supplementing its loss from the patients and is primarily used in treatment of EB. Fibroblasts that can produce intact collagen-VII protein have been intradermally injected to RDEB patients and mouse models. The recipients, in turn, showed increased production of collagen-VII at the dermal-epidermal junction and enhanced wound healing (Nyström et al., 2013). Likewise, in mice that were grafted with skin from RDEB patients, topical application of recombinant collagen-VII protein to wounds resulted in collagen-VII incorporation in the wounded regions, and efficient repair (Uitto, 2008). More work will be required in the future to test the efficacy of this treatment in restoring normal skin function.
Conclusion, Perspectives and Future Directions
In this review we have highlighted the signaling and regulatory cascades central to the interactions between ECM and immune cells in several skin diseases. Based on the extensive literature reviewed, it is conceivable that the pathways governing this crosstalk can serve in developing novel effective targets for therapeutic interventions. Therefore, an important area of future research would be to identify these cascades. While, in diseases like AD, Psoriasis, and cancer, the interaction is well understood, further investigation is required to discern the crosstalk in EB. Additionally, skin disease conditions are often accompanied by a systemic response thereby, necessitating the use of combinatorial therapies that target both the local and systemic responses. While available therapies primarily rely on mitigating inflammatory response, directly targeting of the ECM–immune cell pathways might contribute to better disease outcome. Additionally, with the emerging functions of matricellular proteins such as decorin, POSTN, OPN, and CCN-1 in driving the skin diseases, a thorough investigation of their roles could further aid in better understanding of disease pathophysiology. Ongoing state of art therapeutic interventions such as stem cell transplantation, immunotherapy, gene therapy, and nanomedicine hold the promise for treating the most intractable of these conditions.
Author Contributions
All authors listed have made a substantial, direct and intellectual contribution to the work, and approved it for publication.
Funding
This paper has been funded by the DST Grant to SR and AK (EMR/2016/003199).
Conflict of Interest Statement
The authors declare that the research was conducted in the absence of any commercial or financial relationships that could be construed as a potential conflict of interest.
Acknowledgments
We thank, Avinanda Banerjee, Sonakshi Mishra, and other members of the Raghavan lab for critical feedback on the manuscript. OB and UA are supported by DBT predoctoral fellowships.
References
Abraham, A., and Roga, G. (2014). Topical steroid-damaged skin. Indian J. Dermatol. 59, 456–459. doi: 10.4103/0019-5154.139872
Adair-Kirk, T. L., and Senior, R. M. (2008). Fragments of extracellular matrix as mediators of inflammation. Int. J. Biochem. Cell Biol. 40, 1101–1110. doi: 10.1016/j.biocel.2007.12.005
Albanesi, C., Madonna, S., Gisondi, P., and Girolomoni, G. (2018). The interplay between keratinocytes and immune cells in the pathogenesis of psoriasis. Front. Immunol. 9:1549. doi: 10.3389/fimmu.2018.01549
Alexeev, V., Salas-Alanis, J. C., Palisson, F., Mukhtarzada, L., Fortuna, G., Uitto, J., et al. (2017). Pro-inflammatory chemokines and cytokines dominate the blister fluid molecular signature in patients with epidermolysis bullosa and affect leukocyte and stem cell migration. J. Invest. Dermatol. 137, 2298–2308. doi: 10.1016/j.jid.2017.07.002
Ali, N., Zirak, B., Rodriguez, R. S., Pauli, M. L., Truong, H. A., Lai, K., et al. (2017). Regulatory T cells in skin facilitate epithelial stem cell differentiation. Cell 169, 1119–1129. doi: 10.1016/j.cell.2017.05.002
Allori, A. C., Sailon, A. M., and Warren, S. M. (2008). Biological basis of bone formation, remodeling, and repair-part II: extracellular matrix. Tissue Eng. Part B Rev. 14, 275–283. doi: 10.1089/ten.teb.2008.0083
Almutawa, F., Alnomair, N., Wang, Y., Hamzavi, I., and Lim, H. W. (2013). Systematic review of UV-based therapy for psoriasis. Am. J. Clin. Dermatol. 14, 87–109. doi: 10.1007/s40257-013-0015-y
An, B., and Brodsky, B. (2016). Collagen binding to OSCAR: the odd couple. Blood 127, 521–522. doi: 10.1182/blood-2015-12-682476
Andriani, F., Margulis, A., Lin, N., Griffey, S., and Garlick, J. A. (2003). Analysis of microenvironmental factors contributing to basement membrane assembly and normalized epidermal phenotype. J. Invest. Dermatol. 120, 923–931. doi: 10.1046/j.1523-1747.2003.12235.x
Anguela, X. M., and High, K. A. (2019). Entering the modern era of gene therapy. Annu. Rev. Med. 70, 273–288. doi: 10.1146/annurev-med-012017-043332
Aoudjehane, L., Pissaia, A., Scatton, O., Podevin, P., Massault, P. P., Chouzenoux, S., et al. (2008). Interleukin-4 induces the activation and collagen production of cultured human intrahepatic fibroblasts via the STAT-6 pathway. Lab. Investig. 88, 973–985. doi: 10.1038/labinvest.2008.61
Apalla, Z., Lallas, A., Sotiriou, E., Lazaridou, E., and Ioannides, D. (2017). Epidemiological trends in skin cancer. Dermatol. Pract. Concept. 7, 1–6. doi: 10.5826/dpc.0702a01
Aras, S., and Raza Zaidi, M. (2017). TAMeless traitors: macrophages in cancer progression and metastasis. Br. J. Cancer 117, 1583–1591. doi: 10.1038/bjc.2017.356
Bai, T., Chen, C.-C., and Lau, L. F. (2010). Matricellular protein CCN1 activates a proinflammatory genetic program in murine macrophages. J. Immunol. 184, 3223–3232. doi: 10.4049/jimmunol.0902792
Baliwag, J., Barnes, D. H., and Johnston, A. (2015). Cytokines in psoriasis. Cytokine. 73, 342–350. doi: 10.1016/j.cyto.2014.12.014
Bansal, M. B., Kovalovich, K., Gupta, R., Li, W., Agarwal, A., Radbill, B., et al. (2005). Interleukin-6 protects hepatocytes from CCl4-mediated necrosis and apoptosis in mice by reducing MMP-2 expression. J. Hepatol. 42, 548–556. doi: 10.1016/j.jhep.2004.11.043
Barna, B. K., Eördegh, G., Iván, G., Piffkó, J., Silló, P., and Antal, M. (2017). Az epidermolysis bullosa szájüregi tünetei és annak ellátása. Orv. Hetil. 158, 1577–1583. doi: 10.1556/650.2017.30844
Bieber, K., Witte, M., Sun, S., Hundt, J. E., Kalies, K., Dräger, S., et al. (2016). T cells mediate autoantibody-induced cutaneous inflammation and blistering in epidermolysis bullosa acquisita. Sci. Rep. 6:38357. doi: 10.1038/srep38357
Bieber, T. (2008). Mechanism of disease atopic dermatitis. N. Engl. J. Med. 47, 193–218. doi: 10.1056/NEJMra074081
Binnewies, M., Roberts, E. W., Kersten, K., Chan, V., Fearon, D. F., Merad, M., et al. (2018). Understanding the tumor immune microenvironment (TIME) for effective therapy. Nat. Med. 24, 541–550. doi: 10.1038/s41591-018-0014-x
Blanpain, C., and Fuchs, E. (2006). Epidermal stem cells of the skin. Annu. Rev. Cell Dev. Biol. 22, 339–373. doi: 10.1146/annurev.cellbio.22.010305.104357
Bluth, M. J., Zaba, L. C., Moussai, D., Suárez-Farias, M., Kaporis, H., Fan, L., et al. (2009). Myeloid dendritic cells from human cutaneous squamous cell carcinoma are poor stimulators of T-cell proliferation. J. Invest. Dermatol. 129, 2451–2462. doi: 10.1038/jid.2009.96
Bocian, C., Urbanowitz, A. K., Owens, R. T., Iozzo, R. V., Gotte, M., and Seidler, D. G. (2013). Decorin potentiates interferon-γ activity in a model of allergic inflammation. J. Biol. Chem. 288, 12699–12711. doi: 10.1074/jbc.M112.419366
Bodemer, C., Tchen, S. I., Ghomrasseni, S., Séguier, S., Gaultier, F., Fraitag, S., et al. (2003). Skin expression of metalloproteinases and tissue inhibitor of metalloproteinases in sibling patients with recessive dystrophic epidermolysis and intrafamilial phenotypic variation. J. Invest. Dermatol. 121, 273–279. doi: 10.1046/j.1523-1747.2003.12325.x
Boehncke, W. H., and Schön, M. P. (2015). Psoriasis. Lancet 386, 983–994. doi: 10.1016/S0140-6736(14)61909-7
Bonnans, C., Chou, J., and Werb, Z. (2014). Remodelling the extracellular matrix in development and disease. Nat. Rev. Mol. Cell Biol. 15, 786–801. doi: 10.1038/nrm3904
Bornstein, P. (2001). Thrombospondins as matricellular modulators of cell function. J. Clin. Invest. 107, 929–934. doi: 10.1172/JCI12749
Bornstein, P. (2009). Matricellular proteins: an overview. J. Cell Commun. Signal. 3, 163–165. doi: 10.1007/s12079-009-0069-z
Bos, J. D., Teunissen, M. B. M., Cairo, I., Krieg, S. R., Kapsenberg, M. L., Das, P. K., et al. (1990). T-cell receptor γδ bearing cells in normal human skin. J. Invest. Dermatol. 94, 37–42. doi: 10.1111/1523-1747.ep12873333
Boyd, S., Tolvanen, K., Virolainen, S., Kuivanen, T., Kyllönen, L., and Saarialho-Kere, U. (2008). Differential expression of stromal MMP-1, MMP-9 and TIMP-1 in basal cell carcinomas of immunosuppressed patients and controls. Virchows Arch. 452, 83–90. doi: 10.1007/s00428-007-0526-0
Breitkreutz, D., Koxholt, I., Thiemann, K., and Nischt, R. (2013). Skin basement membrane: The foundation of epidermal integrity - BM functions and diverse roles of bridging molecules nidogen and perlecan. Biomed Res. Int. 2013:179784. doi: 10.1155/2013/179784
Bronte, V., and Zanovello, P. (2005). Regulation of immune responses by L-arginine metabolism. Nat. Rev. Immunol. 5, 641–654. doi: 10.1038/nri1668
Bruckner-Tuderman, L., McGrath, J. A., Robinson, E. C., and Uitto, J. (2010). Animal models of epidermolysis bullosa: update 2010. J. Invest. Dermatol. 130, 1485–1488. doi: 10.1038/jid.2010.75
Brunner, P. M., Israel, A., Zhang, N., Leonard, A., Wen, H. C., Huynh, T., et al. (2018). Early-onset pediatric atopic dermatitis is characterized by TH2/TH17/TH22-centered inflammation and lipid alterations. J. Allergy Clin. Immunol. 141, 2094–2106. doi: 10.1016/j.jaci.2018.02.040
Buback, F., Renkl, A. C., Schulz, G., and Weiss, J. M. (2009). Osteopontin and the skin: multiple emerging roles in cutaneous biology and pathology. Exp. Dermatol. 18, 750–759. doi: 10.1111/j.1600-0625.2009.00926.x
Bubier, J. A., Sproule, T. J., Alley, L. M., Webb, C. M., Fine, J. D., Roopenian, D. C., et al. (2010). A mouse model of generalized non-herlitz junctional epidermolysis bullosa. J. Invest. Dermatol. 130, 1819–1828. doi: 10.1038/jid.2010.46
Bulfone-Paus, S., and Paus, R. (2008). Osteopontin as a new player in mast cell biology. Eur. J. Immunol. 38, 338–341. doi: 10.1002/eji.200738131
Buommino, E., De Filippis, A., Gaudiello, F., Balato, A., Balato, N., Tufano, M. A., et al. (2012). Modification of osteopontin and MMP-9 levels in patients with psoriasis on anti-TNF-α therapy. Arch. Dermatol. Res. 304, 481–485. doi: 10.1007/s00403-012-1251-3
Candi, E., Schmidt, R., and Melino, G. (2005). The cornified envelope: a model of cell death in the skin. Nat. Rev. Mol. Cell Biol. 6, 328–340. doi: 10.1038/nrm1619
Cao, T., Longley, M. A., Wang, X. J., and Roop, D. R. (2001). An inducible mouse model for epidermolysis bullosa simplex: implications for gene therapy. J. Cell Biol. 152, 651–656. doi: 10.1083/jcb.152.3.651
Cavallo, F., De Giovanni, C., Nanni, P., Forni, G., and Lollini, P. L. (2011). 2011: The immune hallmarks of cancer. Cancer Immunol. Immunother. 60, 319–326. doi: 10.1007/s00262-010-0968-0
Chandran, V. (2013). The genetics of psoriasis and psoriatic arthritis. Clin. Rev. Allergy Immunol. 44, 149–156. doi: 10.1007/s12016-012-8303-5
Cheuk, S., Schlums, H., Gallais Sérézal, I., Martini, E., Chiang, S. C., Marquardt, N., et al. (2017). CD49a expression defines tissue-resident CD8+T cells poised for cytotoxic function in human skin. Immunity 46, 287–300. doi: 10.1016/j.immuni.2017.01.009
Chu, C.-C., Di Meglio, P., and Nestle, F. O. (2011). Harnessing dendritic cells in inflammatory skin diseases. Semin. Immunol. 23, 28–41. doi: 10.1016/j.smim.2011.01.006
Chung, H. J., and Uitto, J. (2010a). Epidermolysis bullosa with Pyloric atresia. Dermatol. Clin. 28, 43–54. doi: 10.1016/j.det.2009.10.005
Chung, H. J., and Uitto, J. (2010b). Type VII collagen: the anchoring fibril protein at fault in dystrophic epidermolysis bullosa. Dermatol. Clin. 28, 93–105. doi: 10.1016/j.det.2009.10.011
Ciazynska, M., Bednarski, I. A., Wódz, K., Kolano, P., Narbutt, J., Sobjanek, M., et al. (2018). Proteins involved in cutaneous basal cell carcinoma development. Oncol. Lett. 16, 4064–4072. doi: 10.3892/ol.2018.9126
Clark, R. A. (2010). Skin-resident T cells: the ups and downs of on site immunity. J. Invest. Dermatol. 130, 362–370. doi: 10.1038/jid.2009.247
Clark, R. A., Chong, B., Mirchandani, N., Brinster, N. K., Yamanaka, K.-I., Dowgiert, R. K., et al. (2006). The vast majority of CLA+ T cells are resident in normal skin. J. Immunol. 176, 4431–4439. doi: 10.4049/jimmunol.176.7.4431
Clemente, N., Raineri, D., Cappellano, G., Boggio, E., Favero, F., Soluri, M. F., et al. (2016). Osteopontin bridging innate and adaptive immunity in autoimmune diseases. J. Immunol. Res. 2016:7675437. doi: 10.1155/2016/7675437
Coulombe, P. A., Hutton, M. E., Vassar, R., and Fuchs, E. (1991). A function for keratins and a common thread among different types of epidermolysis bullosa simplex diseases. J. Cell Biol. 115, 1661–1674. doi: 10.1083/jcb.115.6.1661
Coulombe, P. A., Kerns, M. L., and Fuchs, E. (2009). Epidermolysis bullosa simplex: a paradigm for disorders of tissue fragility. J. Clin. Invest. 119, 1784–1793. doi: 10.1172/JCI38177
Coulombe, P. A., and Lee, C. H. (2012). Defining keratin protein function in skin epithelia: epidermolysis bullosa simplex and its aftermath. J. Invest. Dermatol. 132(3 Pt 2), 763–775. doi: 10.1038/jid.2011.450
Coussens, L. M., Tinkle, C. L., Hanahan, D., and Werb, Z. (2000). MMP-9 supplied by bone marrow-derived cells contributes to skin carcinogenesis. Cell 103, 481–490. doi: 10.1016/S0092-8674(00)00139-2
Craig, V. J., Polverino, F., Laucho-Contreras, M. E., Shi, Y., Liu, Y., Osorio, J. C., et al. (2014). Mononuclear phagocytes and airway epithelial cells: novel sources of matrix metalloproteinase-8 (MMP-8) in patients with idiopathic pulmonary fibrosis. PLoS ONE 9:e97485. doi: 10.1371/journal.pone.0097485
Cruz, M. S., Diamond, A., Russell, A., and Jameson, J. M. (2018). Human αβ and γδ T cells in skin immunity and disease. Front. Immunol. 9:1304. doi: 10.3389/fimmu.2018.01304
Dang, C., Gottschling, M., Roewert, J., Forschner, T., Stockfleth, E., and Nindl, I. (2006). Tenascin-C patterns and splice variants in actinic keratosis and cutaneous squamous cell carcinoma. Br. J. Dermatol. 155, 763–770. doi: 10.1111/j.1365-2133.2006.07401.x
Devillers, A. C., van Toorenenbergena, W., Klein Heerenbrink, G. J., Muldert, P. G. H., and Oranjea, P. (2007). Elevated levels of plasma matrix metalloproteinase-9 in patients with atopic dermatitis: a pilot study. Clin. Exp. Dermatol. 32, 311–313. doi: 10.1111/j.1365-2230.2007.02378.x
Dharmage, S. C., Lowe, A. J., Matheson, M. C., Burgess, J. A., Allen, K. J., and Abramson, M. J. (2014). Atopic dermatitis and the atopic march revisited. Allergy Eur. J. Allergy Clin. Immunol. 69, 17–27. doi: 10.1111/all.12268
Di Meglio, P., Perera, G. K., and Nestle, F. O. (2011). The multitasking organ: recent insights into skin immune function. Immunity 35, 857–869. doi: 10.1016/j.immuni.2011.12.003
Dianzani, C., Zara, G. P., Maina, G., Pettazzoni, P., Pizzimenti, S., Rossi, F., et al. (2014). Drug delivery nanoparticles in skin cancers. Biomed Res. Int. 2014:895986. doi: 10.1155/2014/895986
Donovan, J. (2009). Review of the hair follicle origin hypothesis for basal cell Carcinoma. Dermatologic Surg. 35, 1311–1323. doi: 10.1111/j.1524-4725.2009.01236.x
D'Orazio, J., Jarrett, S., Amaro-Ortiz, A., and Scott, T. (2013). UV radiation and the skin. Int. J. Mol. Sci. 14, 12222–12248. doi: 10.3390/ijms140612222
Driskell, R. R., Jahoda, C. A. B., Chuong, C. M., Watt, F. M., and Horsley, V. (2014). Defining dermal adipose tissue. Exp. Dermatol. 23, 629–631. doi: 10.1111/exd.12450
Dubois Declercq, S., and Pouliot, R. (2013). Promising new treatments for psoriasis. Sci. World J. 2013:980419. doi: 10.1155/2013/980419
Dummer, R., Rochlitz, C., Velu, T., Acres, B., Limacher, J. M., Bleuzen, P., et al. (2008). Intralesional adenovirus-mediated interleukin-2 gene transfer for advanced solid cancers and melanoma. Mol. Ther. 16, 985–994. doi: 10.1038/mt.2008.32
Duvic, M., Nagpal, S., Asano, A. T., and Chandraratna, R. A. S. (1997). Molecular mechanisms of tazarotene action in psoriasis. J. Am. Acad. Dermatol. 37, S18–S24. doi: 10.1016/S0190-9622(97)70412-2
Ecker, B. L., Kaur, A., Douglass, S. M., Webster, M. R., Almeida, F. V., Marino, G. E., et al. (2019). Age-related changes in HAPLN1 increase lymphatic permeability and affect routes of melanoma metastasis. Cancer Discov. 9, 82–95. doi: 10.1158/2159-8290.CD-18-0168
Esposito, S., Guez, S., Orenti, A., Tadini, G., Scuvera, G., Corti, L., et al. (2016). Autoimmunity and cytokine imbalance in inherited epidermolysis bullosa. Int. J. Mol. Sci. 17:1625. doi: 10.3390/ijms17101625
Ferrari, S., Pellegrini, G., Matsui, T., Mavilio, F., and De, L. M. (2006). Towards a gene therapy clinical trial for epidermolysis bullosa. Rev.Recent Clin.Trials 1, 155–162. doi: 10.2174/157488706776876472
Fichtner-Feigl, S., Strober, W., Kawakami, K., Puri, R. K., and Kitani, A. (2006). IL-13 signaling through the IL-13α2receptor is involved in induction of TGF-β1production and fibrosis. Nat. Med. 12, 99–106. doi: 10.1038/nm1332
Fine, J. D. (2016). Epidemiology of inherited epidermolysis bullosa based on incidence and prevalence estimates from the national epidermolysis Bullosa registry. JAMA Dermatol. 152, 1231–1238. doi: 10.1001/jamadermatol.2016.2473
Flacher, V., Tripp, C. H., Mairhofer, D. G., Steinman, R. M., Stoitzner, P., Idoyaga, J., et al. (2014). Murine Langerin+ dermal dendritic cells prime CD8+ T cells while Langerhans cells induce cross-tolerance. EMBO Mol. Med. 6, 1191–1204. doi: 10.15252/emmm.201404707
Fontana, R., Bregni, M., Cipponi, A., Raccosta, L., Rainelli, C., Maggioni, D., et al. (2009). Peripheral blood lymphocytes genetically modified to express the self/tumor antigen MAGE-A3 induce antitumor immune responses in cancer patients. Blood 113, 1651–1660. doi: 10.1182/blood-2008-07-168666
Frantz, C., Stewart, K. M., and Weaver, V. M. (2010). The extracellular matrix at a glance. J. Cell Sci. 123(Pt 24), 4195–4200. doi: 10.1242/jcs.023820
Frey, H., Schroeder, N., Manon-Jensen, T., Iozzo, R. V., and Schaefer, L. (2013). Biological interplay between proteoglycans and their innate immune receptors in inflammation. FEBS J. 280, 2165–2179. doi: 10.1111/febs.12145
Fuchs, E. (2007). Scratching the surface of skin development. Nature 445, 834–842. doi: 10.1038/nature05659
Fuchs, E., and Raghavan, S. (2002). Getting under the skin of epidermal morphogenesis. Nat. Rev. Genet. 3, 199–209. doi: 10.1038/nrg758
Fujitsu, Y., Fukuda, K., Kumagai, N., and Nishida, T. (2003). IL-4-induced cell proliferation and production of extracellular matrix proteins in human conjunctival fibroblasts. Exp. Eye Res. 76, 107–114. doi: 10.1016/S0014-4835(02)00248-8
Fukuda, K., Sugihara, E., Ohta, S., Izuhara, K., Funakoshi, T., Amagai, M., et al. (2015). Periostin is a key niche component for wound metastasis of melanoma. PLoS ONE 10:e0129704. doi: 10.1371/journal.pone.0129704
Fulop, T., Khalil, A., and Larbi, A. (2012). The role of elastin peptides in modulating the immune response in aging and age-related diseases. Pathol. Biol. 60, 28–33. doi: 10.1016/j.patbio.2011.10.006
Gaiser, M. R., Weis, C. A., Gaiser, T., Jiang, H., Buder-Bakhaya, K., Herpel, E., et al. (2018). Merkel cell carcinoma expresses the immunoregulatory ligand CD200 and induces immunosuppressive macrophages and regulatory T cells. Oncoimmunology 7:e1426517. doi: 10.1080/2162402X.2018.1426517
García-Nieto, S., Johal, R. K., Shakesheff, K. M., Emara, M., Pierre-Joseph, R., Chau, D. Y. S., et al. (2010). Laminin and fibronectin treatment leads to generation of dendritic cells with superior endocytic capacity. PLoS ONE 5:e10123. doi: 10.1371/journal.pone.0010123
Gasparoto, T. H., De Oliveira, C. E., De Freitas, L. T., Pinheiro, C. R., Ramos, R. N., Da Silva, A. L., et al. (2012). Inflammatory events during murine squamous cell carcinoma development. J. Inflamm. 9:46. doi: 10.1186/1476-9255-9-46
Gay, D., Kwon, O., Zhang, Z., Spata, M., Plikus, M. V., Holler, P. D., et al. (2013). Fgf9 from dermal γδ T cells induces hair follicle neogenesis after wounding. Nat. Med. 19, 916–923. doi: 10.1038/nm.3181
Georges-Labouesse, E., Messaddeq, N., Yehia, G., Cadalbert, L., Dierich, A., and Le Meur, M. (1996). Absence of integrin α6 leads to epidermolysis bullosa and neonatal death in mice. Nat. Genet. 13, 370–373. doi: 10.1038/ng0796-370
Goldschneider, K. R., Good, J., Harrop, E., Liossi, C., Lynch-Jordan, A., Martinez, A. E., et al. (2014). Pain care for patients with epidermolysis bullosa: best care practice guidelines. BMC Med. 12:178. doi: 10.1186/s12916-014-0178-2
Goździalska, A., Wojas-Pelc, A., Drag, J., Brzewski, P., Jaśkiewicz, J., and Pastuszczak, M. (2016). Expression of metalloproteinases (MMP-2 and MMP-9) in basal-cell carcinoma. Mol. Biol. Rep. 43, 1027–1033. doi: 10.1007/s11033-016-4040-9
Grassberger, M., Steinhoff, M., Schneider, D., and Luger, T. A. (2004). Pimecrolimus - an anti-inflammatory drug targeting the skin. Exp. Dermatol. 13, 721–730. doi: 10.1111/j.0906-6705.2004.00269.x
Hallmann, R., Zhang, X., Di Russo, J., Li, L., Song, J., Hannocks, M. J., et al. (2015). The regulation of immune cell trafficking by the extracellular matrix. Curr. Opin. Cell Biol. 36, 54–61. doi: 10.1016/j.ceb.2015.06.006
Hamasaki, H., Koga, K., Aoki, M., Hamasaki, M., Koshikawa, N., Seiki, M., et al. (2011). Expression of laminin 5-γ2 chain in cutaneous squamous cell carcinoma and its role in tumour invasion. Br. J. Cancer. 105, 824–832. doi: 10.1038/bjc.2011.283
Hamid, Q., Naseer, T., Minshall, E. M., Song, Y. L., Boguniewicz, M., and Leung, D. Y. M. (1996). In vivo expression of IL-12 and IL-13 in atopic dermatitis. J. Allergy Clin. Immunol. 98, 225–231. doi: 10.1016/S0091-6749(96)70246-4
Han, L., Wang, L., Tang, S., Yuan, L., Wu, S., Du, X., et al. (2018). ITGB4 deficiency in bronchial epithelial cells directs airway inflammation and bipolar disorder-related behavior. J. Neuroinflammation 15:246. doi: 10.1186/s12974-018-1283-5
Harlin, H., Meng, Y., Peterson, A. C., Zha, Y., Tretiakova, M., Slingluff, C., et al. (2009). Chemokine expression in melanoma metastases associated with CD8+ T-cell recruitment. Cancer Res. 69, 3077–3085. doi: 10.1158/0008-5472.CAN-08-2281
Harper, J. I., Godwin, H., Green, A., Wilkes, L. E., Holden, N. J., Moffatt, M., et al. (2010). A study of matrix metalloproteinase expression and activity in atopic dermatitis using a novel skin wash sampling assay for functional biomarker analysis. Br. J. Dermatol. 162, 397–403. doi: 10.1111/j.1365-2133.2009.09467.x
Heath, W. R., and Carbone, F. R. (2013). The skin-resident and migratory immune system in steady state and memory: innate lymphocytes, dendritic cells and T cells. Nat. Immunol. 14, 978–985. doi: 10.1038/ni.2680
Hegde, S., and Raghavan, S. (2013). A skin-depth analysis of integrins: role of the integrin network in health and disease. Cell Commun. Adhes. 20, 155–169. doi: 10.3109/15419061.2013.854334
Henri, S., Poulin, L. F., Tamoutounour, S., Ardouin, L., Guilliams, M., de Bovis, B., et al. (2010). CD207 + CD103 + dermal dendritic cells cross-present keratinocyte-derived antigens irrespective of the presence of Langerhans cells. J. Exp. Med. 207, 189–206. doi: 10.1084/jem.20091964
Henrot, P., Truchetet, M.-E., Fisher, G., Taïeb, A., and Cario, M. (2018). CCN proteins as potential actionable targets in scleroderma. Exp. Dermatol. 28, 11–18. doi: 10.1111/exd.13806
Herro, R., Shui, J. W., Zahner, S., Sidler, D., Kawakami, Y., Kawakami, T., et al. (2018). LIGHT - HVEM signaling in keratinocytes controls development of dermatitis. J. Exp. Med. 215, 415–422. doi: 10.1084/jem.20170536
Hiratsuka, S., Watanabe, A., Aburatani, H., and Maru, Y. (2006). Tumour-mediated upregulation of chemoattractants and recruitment of myeloid cells predetermines lung metastasis. Nat. Cell Biol. 8, 1369–1375. doi: 10.1038/ncb1507
Hirsch, T., Rothoeft, T., Teig, N., Bauer, J. W., Pellegrini, G., De Rosa, L., et al. (2017). Regeneration of the entire human epidermis using transgenic stem cells. Nature 551, 327–332. doi: 10.1038/nature24487
Hoeffel, G., Wang, Y., Greter, M., See, P., Teo, P., Malleret, B., et al. (2012). Adult Langerhans cells derive predominantly from embryonic fetal liver monocytes with a minor contribution of yolk sac-derived macrophages. J. Exp. Med. 209, 1167–1181. doi: 10.1084/jem.20120340
Howell, M. D., Kim, B. E., Gao, P., Grant, A. V., Boguniewicz, M., DeBenedetto, A., et al. (2007). Cytokine modulation of atopic dermatitis filaggrin skin expression. J. Allergy Clin. Immunol. 120, 150–155. doi: 10.1016/j.jaci.2007.04.031
Hu, S. C. S., Yu, H. S., Yen, F. L., Lin, C. L., Chen, G. S., and Lan, C. C. E. (2016). Neutrophil extracellular trap formation is increased in psoriasis and induces human β-defensin-2 production in epidermal keratinocytes. Sci. Rep. 6:31119. doi: 10.1038/srep31119
Huber, L. A., Pereira, T. A., Ramos, D. N., Rezende, L. C. D., Emery, F. S., Sobral, L. M., et al. (2015). Topical skin cancer therapy using doxorubicin-loaded cationic lipid nanoparticles and iontophoresis. J. Biomed. Nanotechnol. 11, 1975–1988. doi: 10.1166/jbn.2015.2139
Hussein, M. R., Ali, F. M. N., and Omar, A. E. M. M. (2007). Immunohistological analysis of immune cells in blistering skin lesions. J. Clin. Pathol. 60, 62–71. doi: 10.1136/jcp.2006.037010
Hynes, R. O. (2002). Integrins: bidirectional, allosteric signaling machines. Cell 110, 673–687. doi: 10.1016/S0092-8674(02)00971-6
Ilkovitch, D., and Lopez, D. M. (2008). Immune modulation by melanoma-derived factors. Exp. Dermatol. 17, 977–985. doi: 10.1111/j.1600-0625.2008.00779.x
Ishida, M., Kojima, F., and Okabe, H. (2013). Cathepsin K expression in basal cell carcinoma. J. Eur. Acad. Dermatol. Venereol. 27, 128–130. doi: 10.1111/j.1468-3083.2011.04436.x
Iwata, H., Bieber, K., Tiburzy, B., Chrobok, N., Kalies, K., Shimizu, A., et al. (2013). B cells, dendritic cells, and macrophages are required to induce an autoreactive CD4 helper T cell response in experimental epidermolysis bullosa acquisita. J. Immunol. 191, 2978–2988. doi: 10.4049/jimmunol.1300310
Jacobi, A., Mayer, A., and Augustin, M. (2015). Keratolytics and emollients and their role in the therapy of psoriasis: a systematic review. Dermatol. Ther. 5, 1–18. doi: 10.1007/s13555-015-0068-3
Jarnicki, A. G., Lysaght, J., Todryk, S., and Mills, K. H. G. (2006). Suppression of antitumor immunity by IL-10 and TGF- -producing T cells infiltrating the growing tumor: influence of tumor environment on the induction of CD4+ and CD8+ regulatory T cells. J. Immunol. 177, 896–904. doi: 10.4049/jimmunol.177.2.896
Jayadev, R., and Sherwood, D. R. (2017). Basement membranes. Curr. Biol. 27, R207–R211. doi: 10.1016/j.cub.2017.02.006
Jimenez, S. A., Hitraya, E., and Varga, J. (1996). Pathogenesis of scleroderma. collagen. Rheum. Dis. Clin. North Am. 22, 647–674. doi: 10.1016/S0889-857X(05)70294-5
Johnson, D. B., and Sosman, J. A. (2015). Therapeutic advances and treatment options in metastatic melanoma. JAMA Oncol. 1, 380–386. doi: 10.1001/jamaoncol.2015.0565
Kale, S., Raja, R., Thorat, D., Soundararajan, G., Patil, T. V., and Kundu, G. C. (2014). Osteopontin signaling upregulates cyclooxygenase-2 expression in tumor-associated macrophages leading to enhanced angiogenesis and melanoma growth via α9β1 integrin. Oncogene 33, 2295–2306. doi: 10.1038/onc.2013.184
Kamata, M., Fujita, H., Hamanaka, T., Takahashi, K., Koga, H., Hashimoto, T., et al. (2013). Anti-laminin γ1 pemphigoid accompanied by autoantibodies to laminin α3 and γ2 subunits of laminin-332. JAMA Dermatol. 33, 2295–2306. doi: 10.1001/jamadermatol.2013.5358
Kandoth, C., McLellan, M. D., Vandin, F., Ye, K., Niu, B., Lu, C., et al. (2013). Mutational landscape and significance across 12 major cancer types. Nature 502, 333–339. doi: 10.1038/nature12634
Kaplan, D. H. (2017). Ontogeny and function of murine epidermal Langerhans cells. Nat. Immunol. 18, 1068–1075. doi: 10.1038/ni.3815
Karagiannis, G. S., Poutahidis, T., Erdman, S. E., Kirsch, R., Riddell, R. H., and Diamandis, E. P. (2012). Cancer-associated fibroblasts drive the progression of metastasis through both paracrine and mechanical pressure on cancer tissue. Mol. Cancer Res. 10, 1403–1418. doi: 10.1158/1541-7786.MCR-12-0307
Karantalis, V., Schulman, I. H., Balkan, W., and Hare, J. M. (2015). Allogeneic cell therapy: a new paradigm in therapeutics. Circ. Res. 116, 12–15. doi: 10.1161/CIRCRESAHA.114.305495
Kasperkiewicz, M., Sadik, C. D., Bieber, K., Ibrahim, S. M., Manz, R. A., Schmidt, E., et al. (2016). Epidermolysis bullosa acquisita: from pathophysiology to novel therapeutic options. J. Invest. Dermatol. 136, 24–33. doi: 10.1038/JID.2015.356
Kasraie, S., and Werfel, T. (2013). Role of macrophages in the pathogenesis of atopic dermatitis. Mediators Inflamm. 2013:942375. doi: 10.1155/2013/942375
Kaur, A., Ecker, B. L., Douglass, S. M., Kugel, C. H., Webster, M. R., Almeida, F. V., et al. (2018). Remodeling of the collagen matrix in aging skin promotes melanoma metastasis and affects immune cell motility. Cancer Discov. 9, 64–81. doi: 10.1158/2159-8290.CD-18-0193
Kelsh, R., You, R., Horzempa, C., Zheng, M., and McKeown-Longo, P. J. (2014). Regulation of the innate immune response by fibronectin: synergism between the III-1 and EDA domains. PLoS ONE 9:e102974. doi: 10.1371/journal.pone.0102974
Kiekens, R. C. M., Thepen, T., Oosting, A. J., Bihari, I. C., Van De Winkel, J. G. J., Bruijnzeel-Koomen, C. A. F. M., et al. (2001). Heterogeneity within tissue-specific macrophage and dendritic cell populations during cutaneous inflammation in atopic dermatitis. Br. J. Dermatol. 145, 957–965. doi: 10.1046/j.1365-2133.2001.04508.x
Kim, B. E., Leung, D. Y. M., Boguniewicz, M., and Howell, M. D. (2008). Loricrin and involucrin expression is down-regulated by Th2 cytokines through STAT-6. Clin. Immunol. 126, 332–337. doi: 10.1016/j.clim.2007.11.006
Kim, T. I., Park, H. J., Won, Y. Y., Choi, H., Jeong, K. H., Sung, J. Y., et al. (2018). Basement membrane status is intact in urticarial dermatitis vs. adult-onset atopic dermatitis. Ann. Dermatol. 30, 258–261. doi: 10.5021/ad.2018.30.2.258
Kisich, K. O., Carspecken, C. W., Fiéve, S., Boguniewicz, M., and Leung, D. Y. M. (2008). Defective killing of Staphylococcus aureus in atopic dermatitis is associated with reduced mobilization of human β-defensin-3. J. Allergy Clin. Immunol. 122, 62–68. doi: 10.1016/j.jaci.2008.04.022
Kobayashi, Y. (1997). Langerhan's cell produce type IV collagenase (MMP-9) following epicutaneous stimulation with haptens. Immunology 90, 496–501. doi: 10.1046/j.1365-2567.1997.00212.x
Koga, C., Kabashima, K., Shiraishi, N., Kobayashi, M., and Tokura, Y. (2008). Possible pathogenic role of Th17 cells for atopic dermatitis. J. Invest. Dermatol. 128, 2625–2630. doi: 10.1038/jid.2008.111
Komohara, Y., Jinushi, M., and Takeya, M. (2014). Clinical significance of macrophage heterogeneity in human malignant tumors. Cancer Sci. 105, 1–8. doi: 10.1111/cas.12314
Kurbet, A. S., Hegde, S., Bhattacharjee, O., Marepally, S., Vemula, P. K., and Raghavan, S. (2016). Sterile inflammation enhances ECM degradation in integrin β1 KO embryonic skin. Cell Rep. 16, 3334–3347. doi: 10.1016/j.celrep.2016.08.062
Lai-Cheong, J. E., Tanaka, A., Hawche, G., Emanuel, P., Maari, C., Taskesen, M., et al. (2009). Kindler syndrome: a focal adhesion genodermatosis. Br. J. Dermatol. 160, 233–242. doi: 10.1111/j.1365-2133.2008.08976.x
Landriscina, A., Rosen, J., Albanese, J., Amin, B., and Friedman, A. J. (2015). Identifying new biologic targets in atopic dermatitis (AD): A retrospective histologic analysis. J. Am. Acad. Dermatol. 73, 521–523. doi: 10.1016/j.jaad.2015.06.036
Langley, R. G. B., Krueger, G. G., and Griffiths, C. E. M. (2005). Psoriasis: epidemiology, clinical features, and quality of life. Ann. Rheum. Dis. 64(Suppl. 2): ii18–ii23. doi: 10.1136/ard.2004.033217
Lettner, T., Lang, R., Klausegger, A., Hainzl, S., Bauer, J. W., and Wally, V. (2013). MMP-9 and CXCL8/IL-8 are potential therapeutic targets in Epidermolysis bullosa simplex. PLoS ONE 8:e70123. doi: 10.1371/journal.pone.0070123
Lewin, J., and Carucci, J. (2015). Advances in the management of basal cell carcinoma. F1000Prime Rep. 7:53. doi: 10.12703/P7-53
Li, H., Li, H., Huo, R., Wu, P., Shen, Z., Xu, H., et al. (2017). Cyr61/CCN1 induces CCL20 production by keratinocyte via activating p38 and JNK/AP-1 pathway in psoriasis. J. Dermatol. Sci. 88, 46–56. doi: 10.1016/j.jdermsci.2017.05.018
Li, J. H., Huang, X. R., Zhu, H. J., Johnson, R., and Lan, H. Y. (2003). Role of TGF-β signaling in extracellular matrix production under high glucose conditions. Kidney Int. 63, 2010–2019. doi: 10.1046/j.1523-1755.2003.00016.x
Li, R., Hebert, J. D., Lee, T. A., Xing, H., Boussommier-Calleja, A., Hynes, R. O., et al. (2017). Macrophage-secreted TNFα and TGFβ1 influence migration speed and persistence of cancer cells in 3D tissue culture via independent pathways. Cancer Res. 77, 279–290. doi: 10.1158/0008-5472.CAN-16-0442
Li, X., Li, J., Wang, L., Niu, X., Hou, R., Liu, R., et al. (2015). Transmission of psoriasis by allogeneic bone marrow transplantation and blood transfusion. Blood Cancer J. 5:e288. doi: 10.1038/bcj.2015.15
Liguori, M., Solinas, G., Germano, G., Mantovani, A., and Allavena, P. (2011). Tumor-associated macrophages as incessant builders and destroyers of the cancer stroma. Cancers 3, 3740–3761. doi: 10.3390/cancers3043740
Liu, A. Y., Zheng, H., and Ouyang, G. (2014). Periostin, a multifunctional matricellular protein in inflammatory and tumor microenvironments. Matrix Biol. 37, 150–156. doi: 10.1016/j.matbio.2014.04.007
Lo, B. K. K., Jalili, R. B., Zloty, D., Ghahary, A., Cowan, B., Dutz, J. P., et al. (2011). CXCR3 ligands promote expression of functional indoleamine 2,3-dioxygenase in basal cell carcinoma keratinocytes. Br. J. Dermatol. 165, 1030–1036. doi: 10.1111/j.1365-2133.2011.10489.x
Lu, H., Chen, J., Planko, L., Zigrino, P., Klein-Hitpass, L., and Magin, T. M. (2007). Induction of inflammatory cytokines by a keratin mutation and their repression by a small molecule in a mouse model for EBS. J. Invest. Dermatol. 127, 2781–2789. doi: 10.1038/sj.jid.5700918
Lu, P., Takai, K., Weaver, V. M., and Werb, Z. (2011). Extracellular matrix degradation and remodeling in development and disease. Cold Spring Harb. Perspect. Biol. 3. doi: 10.1101/cshperspect.a005058
Macleod, A. S., Hemmers, S., Garijo, O., Chabod, M., Mowen, K., Witherden, D. A., et al. (2013). Dendritic epidermal T cells regulate skin antimicrobial barrier function. J. Clin. Invest. 123, 4364–4374. doi: 10.1172/JCI70064
Maggi, L., Santarlasci, V., Capone, M., Peired, A., Frosali, F., Crome, S. Q., et al. (2010). CD161 is a marker of all human IL-17-producing T-cell subsets and is induced by RORC. Eur. J. Immunol. 40, 2174–2181. doi: 10.1002/eji.200940257
Malaisse, J., Bourguignon, V., De Vuyst, E., Lambert De Rouvroit, C., Nikkels, A. F., Flamion, B., et al. (2014). Hyaluronan metabolism in human keratinocytes and atopic dermatitis skin is driven by a balance of hyaluronan synthases 1 and 3. J. Invest. Dermatol. 134, 2174–2182. doi: 10.1038/jid.2014.147
Maldonado-Colin, G., Hernández-Zepeda, C., Durán-McKinster, C., and García-Romero, M. (2017). Inherited epidermolysis bullosa: a multisystem disease of skin and mucosae fragility. Indian J. Paediatr. Dermatol. 88, 185–198. doi: 10.4103/ijpd.ijpd_16_17
Malissen, B., Tamoutounour, S., and Henri, S. (2014). The origins and functions of dendritic cells and macrophages in the skin. Nat. Rev. Immunol. 14, 417–428. doi: 10.1038/nri3683
Manoukian, M., Ott, S., Rajadas, J., and Inayathullah, M. (2014). Polymeric nanoparticles to combat squamous cell carcinomas in patients with dystrophic epidermolysis bullosa. Recent Patents Nanomed. 4, 15–24. doi: 10.2174/1877912304666140708184013
Margadant, C., Kreft, M., Zambruno, G., and Sonnenberg, A. (2013). Kindlin-1 regulates integrin dynamics and adhesion turnover. PLoS ONE 8:e65341. doi: 10.1371/journal.pone.0065341
Marinkovich, M. P. (2007). Tumour microenvironment: Laminin 332 in squamous-cell carcinoma. Nat. Rev. Cancer. 7, 370–380. doi: 10.1038/nrc2089
Martin-Manso, G., Galli, S., Ridnour, L. A., Tsokos, M., Wink, D. A., and Roberts, D. D. (2008). Thrombospondin 1 promotes tumor macrophage recruitment and enhances tumor cell cytotoxicity of differentiated U937 cells. Cancer Res. 68, 7090–7099. doi: 10.1158/0008-5472.CAN-08-0643
Martins, L., Caley, M. P., Moore, K., Szentpetery, Z., Marsh, S. T., Murrell, D. F., et al. (2016). Suppression of TGFβ and angiogenesis by Type VII collagen in cutaneous SCC. J. Natl. Cancer Inst. 108:djv293. doi: 10.1093/jnci/djv293
Martins, V. L., Vyas, J. J., Chen, M., Purdie, K., Mein, C. A., South, A. P., et al. (2009). Increased invasive behaviour in cutaneous squamous cell carcinoma with loss of basement-membrane type VII collagen. J. Cell Sci. 122, 1788–1799. doi: 10.1242/jcs.042895
Masuda, A., Yasuoka, H., Satoh, T., Okazaki, Y., Yamaguchi, Y., and Kuwana, M. (2013). Versican is upregulated in circulating monocytes in patients with systemic sclerosis and amplifies a CCL2-mediated pathogenic loop. Arthritis Res. Ther. 15:R74. doi: 10.1186/ar4251
Matejuk, A. (2018). Skin immunity. Arch. Immunol. Ther. Exp. 66, 45–54. doi: 10.1007/s00005-017-0477-3
Mayba, J. N., and Gooderham, M. J. (2017). Review of atopic dermatitis and topical therapies. J. Cutan. Med. Surg. 21, 227–236. doi: 10.1177/1203475416685077
McFadden, J., Fry, L., Powles, A. V., and Kimber, I. (2012). Concepts in psoriasis: psoriasis and the extracellular matrix. Br. J. Dermatol. 167, 980–986. doi: 10.1111/j.1365-2133.2012.11149.x
McFadden, J. P., Basketter, D. A., Dearman, R. J., and Kimber, I. R. (2011). Extra domain A-positive fibronectin-positive feedback loops and their association with cutaneous inflammatory disease. Clin. Dermatol. 29, 257–265. doi: 10.1016/j.clindermatol.2010.11.003
McWhorter, F. Y., Davis, C. T., and Liu, W. F. (2015). Physical and mechanical regulation of macrophage phenotype and function. Cell. Mol. Life Sci. 72, 1303–1316. doi: 10.1007/s00018-014-1796-8
Medeiros, G. X., and Riet-Correa, F. (2015). Epidermolysis bullosa in animals: a review. Vet. Dermatol. 26, 3–13. doi: 10.1111/vde.12176
Mellerio, J. E. (2010). Epidermolysis bullosa care in the United Kingdom. Dermatol. Clin. 28, 395–396. doi: 10.1016/j.det.2010.02.015
Mencía, Á., García, M., García, E., Llames, S., Charlesworth, A., de Lucas, R., et al. (2016). Identification of two rare and novel large deletions in ITGB4 gene causing epidermolysis bullosa with pyloric atresia. Exp. Dermatol. 25, 269–274. doi: 10.1111/exd.1293
Meyaard, L., Adema, G. J., Chang, C., Woollatt, E., Sutherland, G. R., Lanier, L. L., et al. (1997). LAIR-1, a novel inhibitory receptor expressed on human mononuclear leukocytes. Immunity 7, 283–290. doi: 10.1016/S1074-7613(00)80530-0
Migden, M. R., Chang, A. L. S., Dirix, L., Stratigos, A. J., and Lear, J. T. (2018). Emerging trends in the treatment of advanced basal cell carcinoma. Cancer Treat. Rev. 64, 1–10. doi: 10.1016/j.ctrv.2017.12.009
Mildner, A., and Jung, S. (2014). Development and function of dendritic cell subsets. Immunity 40, 642–656. doi: 10.1016/j.immuni.2014.04.016
Miskolczi, Z., Smith, M. P., Rowling, E. J., Ferguson, J., Barriuso, J., and Wellbrock, C. (2018). Collagen abundance controls melanoma phenotypes through lineage-specific microenvironment sensing. Oncogene 37, 3166–3182. doi: 10.1038/s41388-018-0209-0
Mitamura, Y., Nunomura, S., Nanri, Y., Ogawa, M., Yoshihara, T., Masuoka, M., et al. (2018). The IL-13/periostin/IL-24 pathway causes epidermal barrier dysfunction in allergic skin inflammation. Allergy Eur. J. Allergy Clin. Immunol. 73, 1881–1891. doi: 10.1111/all.13437
Moilanen, J. M., Löffek, S., Kokkonen, N., Salo, S., Väyrynen, J. P., Hurskainen, T., et al. (2017). Significant role of collagen XVII and integrin β4 in migration and invasion of the less aggressive squamous cell carcinoma cells. Sci. Rep. 7:45057. doi: 10.1038/srep45057
Moreira, G., Fulgêncio, L. B., de Mendonça, E. F., Leles, C. R., Batista, A. C., and da Silva, T. A. (2010). T regulatory cell markers in oral squamous cell carcinoma: relationship with survival and tumor aggressiveness. Oncol. Lett. 1, 127–132. doi: 10.3892/ol_00000023
Mori, R., Shaw, T. J., and Martin, P. (2008). Molecular mechanisms linking wound inflammation and fibrosis: knockdown of osteopontin leads to rapid repair and reduced scarring. J. Exp. Med. 205, 43–51. doi: 10.1084/jem.20071412
Morizane, S., and Gallo, R. L. (2012). Antimicrobial peptides in the pathogenesis of psoriasis. J. Dermatol. 39, 225–230. doi: 10.1111/j.1346-8138.2011.01483.x
Mott, J. D., and Werb, Z. (2004). Regulation of matrix biology by matrix metalloproteinases. Curr. Opin. Cell Biol. 16, 558–564. doi: 10.1016/j.ceb.2004.07.010
Mueller, S. N., Zaid, A., and Carbone, F. R. (2014). Tissue-resident T cells: dynamic players in skin immunity. Front. Immunol. 5:332. doi: 10.3389/fimmu.2014.00332
Munde, P., Khandekar, S., Dive, A., and Sharma, A. (2013). Pathophysiology of merkel cell. J. Oral Maxillofac. Pathol. 17, 408–412. doi: 10.4103/0973-029X.125208
Nagao, K., Ginhoux, F., Leitner, W. W., Motegi, S.-I., Bennett, C. L., Clausen, B. E., et al. (2009). Murine epidermal Langerhans cells and langerin-expressing dermal dendritic cells are unrelated and exhibit distinct functions. Proc. Natl. Acad. Sci. U.S.A. 106, 3312–3317. doi: 10.1073/pnas.0807126106
Natsuga, K., Shinkuma, S., Nishie, W., and Shimizu, H. (2010). Animal models of epidermolysis bullosa. Dermatol. Clin. 28, 137–142. doi: 10.1016/j.det.2009.10.016
Natsumi, A., Sugawara, K., Yasumizu, M., Mizukami, Y., Sano, S., Morita, A., et al. (2018). Re-investigating the basement membrane zone of psoriatic epidermal lesions: is Laminin-511 a new player in psoriasis pathogenesis? J. Histochem. Cytochem. 66, 847–862. doi: 10.1369/0022155418782693
Nelson, D. A., and Larsen, M. (2015). Heterotypic control of basement membrane dynamics during branching morphogenesis. Dev. Biol. 401, 103–109. doi: 10.1016/j.ydbio.2014.12.011
Nestle, F. O., Kaplan, D. H., and Barker, J. (2009). Mechanisms of disease psoriasis. N. Engl. J. Med. 361, 496–509. doi: 10.1056/NEJMra0804595
Niebuhr, M., Lutat, C., Sigel, S., and Werfel, T. (2009). Impaired TLR-2 expression and TLR-2-mediated cytokine secretion in macrophages from patients with atopic dermatitis. Allergy Eur. J. Allergy Clin. Immunol. 64, 1580–1587. doi: 10.1111/j.1398-9995.2009.02050.x
Noy, R., and Pollard, J. W. (2014). Tumor-associated macrophages: from mechanisms to therapy. Immunity 41, 49–61. doi: 10.1016/j.immuni.2014.09.021
Nyström, A., Bornert, O., Kühl, T., Gretzmeier, C., Thriene, K., Dengjel, J., et al. (2018). Impaired lymphoid extracellular matrix impedes antibacterial immunity in epidermolysis bullosa. Proc. Natl. Acad. Sci. U.S.A. 115, E705–E714. doi: 10.1073/pnas.1709111115
Nyström, A., and Bruckner-Tuderman, L. (2018). Injury- and inflammation-driven skin fibrosis: the paradigm of epidermolysis bullosa. Matrix Biol. 68–69, 547–560. doi: 10.1016/j.matbio.2018.01.016
Nystrom, A., Thriene, K., Mittapalli, V., Kern, J. S., Kiritsi, D., Dengjel, J., et al. (2015). Losartan ameliorates dystrophic epidermolysis bullosa and uncovers new disease mechanisms. EMBO Mol. Med. 7, 1211–1228. doi: 10.15252/emmm.201505061
Nyström, A., Velati, D., Mittapalli, V. R., Fritsch, A., Kern, J. S., and Bruckner-Tuderman, L. (2013). Collagen VII plays a dual role in wound healing. J. Clin. Invest. 123, 3498–3509. doi: 10.1172/JCI68127
Obara, W., Kawa, Y., Ra, C., Nishioka, K., Soma, Y., and Mizoguchi, M. (2002). T cells and mast cells as a major source of interleukin-13 in atopic dermatitis. Dermatology 205, 11–17. doi: 10.1159/000063145
Odorisio, T., di Salvio, M., Orecchia, A., di Zenzo, G., Piccinni, E., Cianfarani, F., et al. (2014). Monozygotic twins discordant for recessive dystrophic epidermolysis bullosa phenotype highlight the role of TGF-β signalling in modifying disease severity. Hum. Mol. Genet. 23, 3907–3922. doi: 10.1093/hmg/ddu102
Ogawa, K., Ito, M., Takeuchi, K., Nakada, A., Heishi, M., Suto, H., et al. (2005). Tenascin-C is upregulated in the skin lesions of patients with atopic dermatitis. J. Dermatol. Sci. 40, 35–41. doi: 10.1016/j.jdermsci.2005.06.001
Oh, S. T., Schramme, A., Stark, A., Tilgen, W., Gutwein, P., and Reichrath, J. (2009). The disintegrin-metalloproteinases ADAM 10, 12 and 17 are upregulated in invading peripheral tumor cells of basal cell carcinomas. J. Cutan. Pathol. 36, 395–401. doi: 10.1111/j.1600-0560.2008.01082.x
Ohtani, T., Memezawa, A., Okuyama, R., Sayo, T., Sugiyama, Y., Inoue, S., et al. (2009). Increased hyaluronan production and decreased E-cadherin expression by cytokine-stimulated keratinocytes lead to spongiosis formation. J. Invest. Dermatol. 129, 1412–1420. doi: 10.1038/jid.2008.394
Omland, S. H., Nielsen, P. S., Gjerdrum, L. M. R., and Gniadecki, R. (2016). Immunosuppressive environment in basal cell carcinoma: the role of regulatory T cells. Acta Derm. Venereol. 96, 917–921. doi: 10.2340/00015555-2440
Onetti Muda, A., Paradisi, M., Angelo, C., Puddu, P., and Faraggiana, T. (1996). Three-dimensional distribution of basement membrane components in dystrophic recessive epidermolysis bullosa. J. Pathol. 179, 427–431. doi: 10.1002/(SICI)1096-9896(199608)179:4<427::AID-PATH608>3.0.CO;2-T
O'regan, A. W., Chupp, G. L., Lowry, J. A., Goetschkes, M., Mulligan, N., and Berman, J. S. (1999). Osteopontin is associated with T cells in sarcoid granulomas and has T cell adhesive and cytokine-like properties in vitro. J. Immunol. 162, 1024–1031.
Oviedo-Orta, E., Bermudez-Fajardo, A., Karanam, S., Benbow, U., and Newby, A. C. (2008). Comparison of MMP-2 and MMP-9 secretion from T helper 0, 1 and 2 lymphocytes alone and in coculture with macrophages. Immunology 124, 42–50. doi: 10.1111/j.1365-2567.2007.02728.x
Owen, J. L., Iragavarapu-Charyulu, V., Gunja-Smith, Z., Herbert, L. M., Grosso, J. F., and Lopez, D. M. (2003). Up-regulation of matrix metalloproteinase-9 in T lymphocytes of mammary tumor bearers: role of vascular endothelial growth factor. J. Immunol. 171, 4340–4351. doi: 10.4049/jimmunol.171.8.4340
Oyama, N., Chan, I., Neill, S. M., Hamada, T., South, A. P., Wessagowit, V., et al. (2003). Autoantibodies to extracellular matrix protein 1 in lichen sclerosus. Lancet 362, 118–123. doi: 10.1016/S0140-6736(03)13863-9
Palmer, B. C., and DeLouise, L. A. (2016). Nanoparticle-enabled transdermal drug delivery systems for enhanced dose control and tissue targeting. Molecules 21:1719. doi: 10.3390/molecules21121719
Pandolfino, T. L., Siegel, R. S., Kuzel, T. M., Rosen, S. T., and Guitart, J. (2000). Primary cutaneous B-cell lymphoma: review and current concepts. J. Clin. Oncol. 18, 2152–2168. doi: 10.1200/JCO.2000.18.10.2152
Papier, A., and Strowd, L. C. (2018). Atopic dermatitis: a review of topical nonsteroid therapy. Drugs Context 7:212521. doi: 10.7573/dic.212521
Parisi, R., Symmons, D. P. M., Griffiths, C. E. M., and Ashcroft, D. M. (2013). Global epidemiology of psoriasis: a systematic review of incidence and prevalence. J. Invest. Dermatol. 133, 377–385. doi: 10.1038/jid.2012.339
Peck, A., and Mellins, E. D. (2010). Precarious balance: Th17 cells in host defense. Infect. Immun. 78, 32–38. doi: 10.1128/IAI.00929-09
Peters, B., Kirfel, J., Bü, H., Vidal, M., and Magin, T. M. (2001). Complete cytolysis and neonatal lethality in keratin 5 knockout mice reveal its fundamental role in skin integrity and in epidermolysis bullosa simplex. Mol. Biol. Cell. 12, 1775–1789. doi: 10.1091/mbc.12.6.1775
Pettersen, J. S., Fuentes-Duculan, J., Suárez-Farias, M., Pierson, K. C., Pitts-Kiefer, A., Fan, L., et al. (2011). Tumor-associated macrophages in the cutaneous SCC microenvironment are heterogeneously activated. J. Invest. Dermatol. 131, 1322–1330. doi: 10.1038/jid.2011.9
Pfendner, E. G., Sadowski, S. G., and Uitto, J. (2005). Epidermolysis bullosa simplex: recurrent and de novo mutations in the KRT5 and KRT14 genes, phenotype/genotype correlations, and implications for genetic counseling and prenatal diagnosis. J. Invest. Dermatol. 125, 239–243. doi: 10.1111/j.0022-202X.2005.23818.x
Piaceski, A. D., Larouche, D., Ghani, K., Bisson, F., Ghio, S. C., Larochelle, S., et al. (2018). Translating the combination of gene therapy and tissue engineering for treating recessive dystrophic epidermolysis bullosa. Eur. Cells Mater. 35, 73–86. doi: 10.22203/eCM.v035a06
Pickup, M. W., Mouw, J. K., and Weaver, V. M. (2014). The extracellular matrix modulates the hallmarks of cancer. EMBO Rep. 15, 1243–1253. doi: 10.15252/embr.201439246
Pieniazek, M., Matkowski, R., and Donizy, P. (2018). Macrophages in skin melanoma-the key element in melanomagenesis (Review). Oncol. Lett. 15, 5399–5404. doi: 10.3892/ol.2018.8021
Pinhal, M. A. S., Costa, A. S., Serrano, R. L., Almeida, M. C. L., Theodoro, T. R., and Machado Filho, C. D. S. (2016). Expression of heparanase in basal cell carcinoma and squamous cell carcinoma. An. Bras. Dermatol. 91, 595–600. doi: 10.1590/abd1806-4841.20164957
Pittayapruek, P., Meephansan, J., Prapapan, O., Komine, M., and Ohtsuki, M. (2016). Role of matrix metalloproteinases in photoaging and photocarcinogenesis. Int. J. Mol. Sci. 17:868. doi: 10.3390/ijms17060868
Pivarcsi, A., Müller, A., Hippe, A., Rieker, J., van Lierop, A., Steinhoff, M., et al. (2007). Tumor immune escape by the loss of homeostatic chemokine expression. Proc. Natl. Acad. Sci. U.S.A. 104, 19055–19060. doi: 10.1073/pnas.0705673104
Purwar, R., Kraus, M., Werfel, T., and Wittmann, M. (2008). Modulation of keratinocyte-derived MMP-9 by IL-13: a possible role for the pathogenesis of epidermal inflammation. J. Invest. Dermatol. 128, 59–66. doi: 10.1038/sj.jid.5700940
Purwar, R., Werfel, T., and Wittmann, M. (2006). IL-13-stimulated human keratinocytes preferentially attract CD4+CCR4+ T cells: possible role in atopic dermatitis. J. Invest. Dermatol. 126, 1043–1051. doi: 10.1038/sj.jid.5700085
Puzanov, I., and Flaherty, K. T. (2010). Targeted molecular therapy in melanoma. Semin. Cutan. Med. Surg. 29, 196–201. doi: 10.1016/j.sder.2010.06.005
Quan, T., Xu, Y., Qin, Z., Robichaud, P., Betcher, S., Calderone, K., et al. (2014). Elevated YAP and its downstream targets CCN1 and CCN2 in basal cell carcinoma: impact on keratinocyte proliferation and stromal cell activation. Am. J. Pathol. 184, 937–943. doi: 10.1016/j.ajpath.2013.12.017
Rankin, A. L., Mumm, J. B., Murphy, E., Turner, S., Yu, N., McClanahan, T. K., et al. (2010). IL-33 induces IL-13-dependent cutaneous fibrosis. J. Immunol. 184, 1526–1535. doi: 10.4049/jimmunol.0903306
Rasheed, K., Abdulsalam, I., Fismen, S., Grimstad, Ø., Sveinbjørnsson, B., Moens, U., et al. (2018). CCL17/TARC and CCR4 expression in Merkel cell carcinoma. Oncotarget 9, 31432–31447. doi: 10.18632/oncotarget.25836
Ratzinger, G., Stoitzner, P., Ebner, S., Lutz, M. B., Layton, G. T., Rainer, C., et al. (2002). Matrix metalloproteinases 9 and 2 are necessary for the migration of langerhans cells and dermal dendritic cells from human and murine skin. J. Immunol. 168, 4361–4371. doi: 10.4049/jimmunol.168.9.4361
Reduta, T., Niecinska, M., Pawłos, A., Sulkiewicz, A., and Sokołowska, M. (2015). Serum osteopontin levels in disseminated allergic contact dermatitis. Adv. Med. Sci. 60, 273–276. doi: 10.1016/j.advms.2015.05.001
Ribero, S., Stucci, L. S., Daniels, G. A., and Borradori, L. (2017). Drug therapy of advanced cutaneous squamous cell carcinoma: is there any evidence? Curr. Opin. Oncol. 29, 129–135. doi: 10.1097/CCO.0000000000000359
Rognoni, E., Ruppert, R., and Fässler, R. (2016). The kindlin family: functions, signaling properties and implications for human disease. J. Cell Sci. 129, 17–27. doi: 10.1242/jcs.161190
Romanovsky, A. A. (2014). Skin temperature: its role in thermoregulation. Acta Physiol. 210, 498–507. doi: 10.1111/apha.12231
Roth, W., Reuter, U., Wohlenberg, C., Bruckner-Tuderman, L., and Magin, T. M. (2009). Cytokines as genetic modifiers in K5-/- mice and in human epidermolysis bullosa simplex. Hum. Mutat. 30, 832–841. doi: 10.1002/humu.20981
Rozario, T., and DeSimone, D. W. (2010). The extracellular matrix in development and morphogenesis: a dynamic view. Dev. Biol. 341, 126–140. doi: 10.1016/j.ydbio.2009.10.026
Rüegg, C. R., Chiquet-Ehrismann, R., and Alkan, S. S. (1989). Tenascin, an extracellular matrix protein, exerts immunomodulatory activities. Proc. Natl. Acad. Sci. U.S.A. 86, 7437–7441.
Rygiel, T. P., Stolte, E. H., de Ruiter, T., van de Weijer, M. L., and Meyaard, L. (2011). Tumor-expressed collagens can modulate immune cell function through the inhibitory collagen receptor LAIR-1. Mol. Immunol. 49, 402–406. doi: 10.1016/j.molimm.2011.09.006
Sakaguchi, S., Miyara, M., Costantino, C. M., and Hafler, D. A. (2010). FOXP3 + regulatory T cells in the human immune system. Nat. Rev. Immunol. 10, 490–500. doi: 10.1038/nri2785
Sakaguchi, S., Wing, K., Onishi, Y., Prieto-Martin, P., and Yamaguchi, T. (2009). Regulatory T cells: How do they suppress immune responses? Int. Immunol. 21, 1105–1111. doi: 10.1093/intimm/dxp095
Sakr, W. A., Zarbo, R. J., Jacobs, J. R., and Crissman, J. D. (1987). Distribution of basement membrane in squamous cell carcinoma of the head and neck. Hum. Pathol. 18, 1043–1050. doi: 10.1016/S0046-8177(87)80221-6
Sandig, H., McDonald, J., Gilmour, J., Arno, M., Lee, T. H., and Cousins, D. J. (2009). Fibronectin is a TH1-specific molecule in human subjects. J. Allergy Clin. Immunol. 124, 528–535. doi: 10.1109/CSPA.2012.6194761
Sawamura, D., Nakano, H., and Matsuzaki, Y. (2010). Overview of epidermolysis bullosa. J. Dermatol. 37, 214–219. doi: 10.1111/j.1346-8138.2009.00800.x
Schalkwijk, J., Van Vlijmen, I., Oosterling, B., Perret, C., Koopman, R., Van den Born, J., et al. (1991). Tenascin expression in hyperproliferative skin diseases. Br. J. Dermatol. 124, 13–20. doi: 10.1111/j.1365-2133.1991.tb03276.x
Schenk, S., Bruckner-Tuderman, L., and Chiquet-Ehrismann, R. (1995). Dermo-epidermal separation is associated with induced tenascin expression in human skin. Br. J. Dermatol. 133, 13–22.
Schmitt-Egenolf, M., Boehncke, W. H., Christophers, E., Ständer, M., and Sterry, W. (1991). Type I and type II psoriasis show a similar usage of T-cell receptor variable regions. J. Invest. Dermatol. 97, 1053–1056. doi: 10.1111/1523-1747.ep12492569.
Schmoeckel, C., Stolz, W., Sakai, L. Y., Burgeson, R. E., Timpl, R., and Krieg, T. (1989). Structure of basement membranes in malignant melanoma and nevocytic nevi. J. Invest. Dermatol. 92, 663–668. doi: 10.1016/0022-202X(89)90179-6.
Sehra, S., Serezani, A. P. M., Ocaña, J. A., Travers, J. B., and Kaplan, M. H. (2016). Mast cells regulate epidermal barrier function and the development of allergic skin inflammation. J. Invest. Dermatol. 136, 1429–1437. doi: 10.1016/j.jid.2016.03.019.
Seidler, D. G., Mohamed, N. A., Bocian, C., Stadtmann, A., Hermann, S., Schafers, K., et al. (2011). The role for decorin in delayed-type hypersensitivity. J. Immunol. 187, 6108–6119. doi: 10.4049/jimmunol.1100373
Senyürek, I., Kempf, W. E., Klein, G., Maurer, A., Kalbacher, H., Schäfer, L., et al. (2014). Processing of laminin α chains generates peptides involved in wound healing and host defense. J. Innate Immun. 6, 467–484. doi: 10.1159/000357032
Serezani, A. P. M., Bozdogan, G., Sehra, S., Walsh, D., Krishnamurthy, P., Sierra Potchanant, E. A., et al. (2017). IL-4 impairs wound healing potential in the skin by repressing fibronectin expression. J. Allergy Clin. Immunol. 139, 142–151. doi: 10.1016/j.jaci.2016.07.012
Shimizu, H., Takizawa, Y., Pulkkinen, L., Murata, S., Kawai, M., Hachisuka, H., et al. (1999). Epidermolysis bullosa simplex associated with muscular dystrophy: phenotype-genotype correlations and review of the literature. J. Am. Acad. Dermatol. 41, 950–956. doi: 10.1016/S0190-9622(99)70252-5
Shin, J. W., Choi, Y. J., Choi, H. R., Na, J. I., Kim, K. H., Park, I. A., et al. (2015). Defective basement membrane in atopic dermatitis and possible role of IL-13. J. Eur. Acad. Dermatol. Venereol. 29, 2060–2062. doi: 10.1111/jdv.12596
Shin, T. H., Kim, H. S., Choi, S. W., and Kang, K. S. (2017). Mesenchymal stem cell therapy for inflammatory skin diseases: clinical potential and mode of action. Int. J. Mol. Sci. 18:244. doi: 10.3390/ijms18020244
Shinkuma, S. (2015). Dystrophic epidermolysis bullosa: a review. Clin. Cosmet. Investig. Dermatol. 8, 275–284. doi: 10.2147/CCID.S54681
Siegel, B. R. S., Pandolfino, T., Guitart, J., Rosen, S., and Kuzel, T. M. (2012). Primary cutaneous T-cell lymphoma : review and current concepts. J. Clin. Oncol. 18, 2908–2925. doi: 10.1200/JCO.2000.18.15.2908
Simon, T., and Bromberg, J. S. (2017). Regulation of the immune system by laminins. Trends Immunol. 38, 858–871. doi: 10.1016/j.it.2017.06.002
Singh, T. P., Zhang, H. H., Borek, I., Wolf, P., Hedrick, M. N., Singh, S. P., et al. (2016). Monocyte-derived inflammatory Langerhans cells and dermal dendritic cells mediate psoriasis-like inflammation. Nat. Commun. 7:13581. doi: 10.1038/ncomms13581
Sitaru, A. G., Sesarman, A., Mihai, S., Chiriac, M. T., Zillikens, D., Hultman, P., et al. (2010). T cells are required for the production of blister-inducing autoantibodies in experimental epidermolysis bullosa acquisita. J. Immunol. 184, 1596–1603. doi: 10.4049/jimmunol.0901412
Sokołowska-Wojdyło, M., Olek-Hrab, K., and Ruckemann-Dziurdzinska, K. (2015). Primary cutaneous lymphomas: diagnosis and treatment. Postep. Dermatologii i Alergol. 32, 368–383. doi: 10.5114/pdia.2015.54749
Stanley, J. R., Beckwith, J. B., Fuller, R. P., and Katz, S. I. (1982). A specific antigenic defect of the basement membrane is found in basal cell carcinoma but not in other epidermal tumors. Cancer 50, 1486–1490. doi: 10.1002/1097-0142(19821015)50:8<1486::AID-CNCR2820500807>3.0.CO;2-F
Stemmler, S., Parwez, Q., Petrasch-Parwez, E., Epplen, J. T., and Hoffjan, S. (2014). Association of variation in the LAMA3 gene, encoding the alpha-chain of laminin 5, with atopic dermatitis in a German case-control cohort. BMC Dermatol. 14:17. doi: 10.1186/1471-5945-14-17
Stratis, A., Pasparakis, M., Rupec, R. A., Markur, D., Hartmann, K., Scharffetter-Kochanek, K., et al. (2006). Pathogenic role for skin macrophages in a mouse model of keratinocyte-induced psoriasis-like skin inflammation. J. Clin. Invest. 116, 2094–2104. doi: 10.1172/JCI27179
Strid, J., McLean, W. H. I., and Irvine, A. D. (2016). Too much, too little or just enough: a goldilocks effect for IL-13 and skin barrier regulation? J. Invest. Dermatol. 136, 561–564. doi: 10.1016/j.jid.2015.12.025
Stultz, C. M., Thomas, A. H., and Edelman, E. R. (2007). Collagen fragments modulate innate immunity. Exp. Biol. Med. 232, 406–411. doi: 10.3181/00379727-232-2320406
Su, P., Chen, S., Zheng, Y. H., Zhou, H. Y., Yan, C. H., Yu, F., et al. (2016). Novel function of extracellular matrix protein 1 in suppressing Th17 cell development in experimental autoimmune encephalomyelitis. J. Immunol. 197, 1054–1064. doi: 10.4049/jimmunol.1502457.
Sumaria, N., Roediger, B., Ng, L. G., Qin, J., Pinto, R., Cavanagh, L. L., et al. (2011). Cutaneous immunosurveillance by self-renewing dermal γδ T cells. J. Exp. Med. 208, 505–518. doi: 10.1084/jem.20101824
Sun, Y., Zhang, J., Zhai, T., Li, H., Li, H., Huo, R., et al. (2017). CCN1 promotes IL-1β production in keratinocytes by activating p38 MAPK signaling in psoriasis. Sci. Rep. 7:43310. doi: 10.1038/srep43310
Sun, Y., Zhang, J., Zhou, Z., Wu, P., Huo, R., Wang, B., et al. (2015). CCN1, a pro-inflammatory factor, aggravates psoriasis skin lesions by promoting keratinocyte activation. J. Invest. Dermatol. 135, 2666–2675. doi: 10.1038/jid.2015.231
Takayama, G., Arima, K., Kanaji, T., Toda, S., Tanaka, H., Shoji, S., et al. (2006). Periostin: a novel component of subepithelial fibrosis of bronchial asthma downstream of IL-4 and IL-13 signals. J. Allergy Clin. Immunol. 118, 98–104. doi: 10.1016/j.jaci.2006.02.046
Takeuchi, H., Gomi, T., Shishido, M., Watanabe, H., and Suenobu, N. (2010). Neutrophil elastase contributes to extracellular matrix damage induced by chronic low-dose UV irradiation in a hairless mouse photoaging model. J. Dermatol. Sci. 60, 151–158. doi: 10.1016/j.jdermsci.2010.09.001
Tariq, M., Zhang, J., Liang, G., Ding, L., He, Q., and Yang, B. (2017). Macrophage polarization: anti-cancer strategies to target tumor-associated macrophage in breast cancer. J. Cell. Biochem. 118, 2484–2501. doi: 10.1002/jcb.25895
Tecchio, C., Micheletti, A., and Cassatella, M. A. (2014). Neutrophil-derived cytokines: facts beyond expression. Front. Immunol. 5:508. doi: 10.3389/fimmu.2014.00508
Tello, T. L., Coggshall, K., Yom, S. S., and Yu, S. S. (2018). Merkel cell carcinoma: an update and review: current and future therapy. J. Am. Acad. Dermatol. 78, 445–454. doi: 10.1016/j.jaad.2017.12.004
Terui, T., Ozawa, M., and Tagami, H. (2000). Role of neutrophils in induction of acute inflammation in T-cell-mediated immune dermatosis, psoriasis: a neutrophil-associated inflammation-boosting loop. Exp. Dermatol. 9, 1–10. doi: 10.1034/j.1600-0625.2000.009001001.x
Thomas, L. W., Elsensohn, A., Bergheim, T., Shiu, J., and Ganesan, A. (2018). Intramuscular steroids in the treatment of dermatologic disease: a systematic review. J. Drugs Dermatol. 17, 323–329.
Titeux, M., Pendaries, V., Tonasso, L., Décha, A., Bodemer, C., and Hovnanian, A. (2008). A frequent functional SNP in the MMP1 promoter is associated with higher disease severity in recessive dystrophic epidermolysis bullosa. Hum. Mutat. 29, 267–276. doi: 10.1002/humu.20647
Tjiu, J. W., Chen, J. S., Shun, C. T., Lin, S. J., Liao, Y. H., Chu, C. Y., et al. (2009). Tumor-associated macrophage-induced invasion and angiogenesis of human basal cell carcinoma cells by cyclooxygenase-2 induction. J. Invest. Dermatol. 129, 1016–1025. doi: 10.1038/jid.2008.310
Torsekar, R., and Gautam, M. (2017). Topical therapies in psoriasis. Indian Dermatol. Online J. 8, 235–245. doi: 10.4103/2229-5178.209622
Touzé, A., Le Bidre, E., Laude, H., Fleury, M. J. J., Cazal, R., Arnold, F., et al. (2011). High levels of antibodies against merkel cell polyomavirus identify a subset of patients with merkel cell carcinoma with better clinical outcome. J. Clin. Oncol. 29, 1612–1619. doi: 10.1200/JCO.2010.31.1704
Trémezaygues, L., and Reichrath, J. (2011). Vitamin D analogs in the treatment of psoriasis: where are we standing and where will we be going? Dermatoendocrinology 3, 180–186. doi: 10.4161/derm.17534
Tsuruta, D., Hashimoto, T., Hamill, K. J., and Jones, J. C. R. (2011). Hemidesmosomes and focal contact proteins: functions and cross-talk in keratinocytes, bullous diseases and wound healing. J. Dermatol. Sci. 62, 1–7. doi: 10.1016/j.jdermsci.2011.01.005
Uitto, J. (2008). Epidermolysis bullosa: prospects for cell-based therapies. J. Invest. Dermatol. 128, 2140–2142. doi: 10.1038/jid.2008.216
Uitto, J., and Shamban, A. (1987). Heritable skin diseases with molecular defects in collagen or elastin. Dermatol. Clin. 5, 63–84. doi: 10.1016/S0733-8635(18)30767-8
Ussar, S., Moser, M., Widmaier, M., Rognoni, E., Harrer, C., Genzel-Boroviczeny, O., et al. (2008). Loss of kindlin-1 causes skin atrophy and lethal neonatal intestinal epithelial dysfunction. PLoS Genet. 4:e1000289. doi: 10.1371/journal.pgen.1000289
Uva, L., Miguel, D., Pinheiro, C., Antunes, J., Cruz, D., Ferreira, J., et al. (2012). Mechanisms of action of topical corticosteroids in psoriasis. Int. J. Endocrinol. 2012:561018. doi: 10.1155/2012/561018
Vaccaro, M., Magaudda, L., Cutroneo, G., Trimarchi, F., Barbuzza, O., Guarneri, F., et al. (2002). Changes in the distribution of laminin α1 chain in psoriatic skin: immunohistochemical study using confocal laser scanning microscopy. Br. J. Dermatol. 146, 392–398. doi: 10.1046/j.1365-2133.2002.04637.x
Vahidnezhad, H., Youssefian, L., Saeidian, A. H., Touati, A., Sotoudeh, S., Jazayeri, A., et al. (2018). Next generation sequencing identifies double homozygous mutations in two distinct genes (EXPH5 and COL17A1) in a patient with concomitant simplex and junctional epidermolysis bullosa. Hum. Mutat. 39, 1349–1354. doi: 10.1002/humu.23592
Verrecchia, F., Chu, M. L., and Mauviel, A. (2001). Identification of novel TGF-β/Smad gene targets in dermal fibroblasts using a combined cDNA microarray/promoter transactivation approach. J. Biol. Chem. 276, 17058–17062. doi: 10.1074/jbc.M100754200
Vestergaard, C., Just, H., Baumgartner Nielsen, J., Thestrup-Pedersen, K., and Deleuran, M. (2004). Expression of CCR2 on monocytes and macrophages in chronically inflamed skin in atopic dermatitis and psoriasis. Acta Derm. Venereol. 84, 353–358. doi: 10.1080/00015550410034444
Villanueva, J., and Herlyn, M. (2008). Melanoma and the tumor microenvironment. Curr. Oncol. Rep. 10, 439–446. doi: 10.1007/s11912-008-0067-y
Wagner, C. J., Schultz, C., and Mall, M. A. (2016). Neutrophil elastase and matrix metalloproteinase 12 in cystic fibrosis lung disease. Mol. Cell. Pediatr. 3:25. doi: 10.1186/s40348-016-0053-7
Wang, G. Y., Wang, J., Mancianti, M.-L., and Epstein, E. H. Jr. (2011). Basal cell carcinomas arise from hair follicle stem cells in Ptch1(+/-) mice. Cancer Cell. 19, 114–124. doi: 10.1016/j.ccr.2010.11.007
Watt, F. M., and Fujiwara, H. (2011). Cell-extracellular matrix interactions in normal and diseased skin. Cold Spring Harb. Perspect. Biol. 3:a005124. doi: 10.1101/cshperspect.a005124
Wayner, E. A., Gil, S. G., Murphy, G. F., Wilke, M. S., and Carter, W. G. (1993). Epiligrin, a component of epithelial basement membranes, is an adhesive ligand for α3β1 positive T lymphocytes. J. Cell Biol. 121, 1141–1152. doi: 10.1083/jcb.121.5.1141
Weidinger, S., and Novak, N. (2016). Atopic dermatitis. Lancet 387, 1109–1122. doi: 10.1016/S0140-6736(15)00149-X
Wollina, U. (2012). Cutaneous Tcell lymphoma: update on treatment. Int. J. Dermatol. 51, 1019–1036. doi: 10.1111/j.1365-4632.2011.05337.x
Wondimu, Z., Geberhiwot, T., Ingerpuu, S., Juronen, E., Xie, X., Lindbom, L., et al. (2004). An endothelial laminin isoform, laminin 8 (α4β1γ1), is secreted by blood neutrophils, promotes neutrophil migration and extravasation, and protects neutrophils from apoptosis. Blood 104, 1859–1866. doi: 10.1182/blood-2004-01-0396
Wu, P., Ma, G., Zhu, X., Gu, T., Zhang, J., Sun, Y., et al. (2017). Cyr61/CCN1 is involved in the pathogenesis of psoriasis vulgaris via promoting IL-8 production by keratinocytes in a JNK/NF-κB pathway. Clin. Immunol. 174, 53–62. doi: 10.1016/j.clim.2016.11.003
Wu, X., Schulte, B. C., Zhou, Y., Haribhai, D., Mackinnon, A. C., Plaza, J. A., et al. (2014). Depletion of M2-like tumor-associated macrophages delays cutaneous T-cell lymphoma development in vivo. J. Invest. Dermatol. 134, 2814–2822. doi: 10.1038/jid.2014.206
Yamaguchi, Y. (2014). Periostin in skin tissue skin-related diseases. Allergol. Int. 63, 161–170. doi: 10.2332/allergolint.13-RAI-0685
Yamaguchi, Y., Mann, D. M., and Ruoslahti, E. (1990). Negative regulation of transforming growth factor-β by the proteoglycan decorin. Nature 346, 281–284. doi: 10.1038/346281a0
Yanagi, T., Kitamura, S., and Hata, H. (2018). Novel therapeutic targets in cutaneous squamous cell carcinoma. Front. Oncol. 8:79. doi: 10.3389/fonc.2018.00079
Yancey, K. B., and Hintner, H. (2010). Non-herlitz junctional epidermolysis bullosa. Dermatol. Clin. 28, 67–77. doi: 10.1016/j.det.2009.10.008
Yanez, D. A., Lacher, R. K., Vidyarthi, A., and Colegio, O. R. (2017). The role of macrophages in skin homeostasis. Pflugers Arch. Eur. J. Physiol. 469, 455–463. doi: 10.1007/s00424-017-1953-7
Yang, L., Huang, J., Ren, X., Gorska, A. E., Chytil, A., Aakre, M., et al. (2008). Abrogation of TGFβ signaling in mammary carcinomas recruits Gr-1+CD11b+ myeloid cells that promote metastasis. Cancer Cell. 13, 23–35. doi: 10.1016/j.ccr.2007.12.004
Yu, S. H., Drucker, A. M., Lebwohl, M., and Silverberg, J. I. (2018). A systematic review of the safety and efficacy of systemic corticosteroids in atopic dermatitis. J. Am. Acad. Dermatol. 78, 733–740.e11. doi: 10.1016/j.jaad.2017.09.074
Yuen, W. Y., Lemmink, H. H., Van Dijk-Bos, K. K., Sinke, R. J., and Jonkman, M. F. (2011). Herlitz junctional epidermolysis bullosa: diagnostic features, mutational profile, incidence and population carrier frequency in the Netherlands. Br. J. Dermatol. 165, 1314–1322. doi: 10.1111/j.1365-2133.2011.10553.x
Zaid, A., Mackay, L. K., Rahimpour, A., Braun, A., Veldhoen, M., Carbone, F. R., et al. (2014). Persistence of skin-resident memory T cells within an epidermal niche. Proc. Natl. Acad. Sci. U.S.A. 111, 5307–5312. doi: 10.1073/pnas.1322292111
Zhou, J., Yao, D., Qian, Z., Hou, S., Li, L., Jenkins, A. T. A., et al. (2018). Bacteria-responsive intelligent wound dressing: simultaneous in situ detection and inhibition of bacterial infection for accelerated wound healing. Biomaterials 161, 11–23. doi: 10.1016/j.biomaterials.2018.01.024
Keywords: extracellular matrix, skin diseases, immune cells, crosstalk, therapeutics
Citation: Bhattacharjee O, Ayyangar U, Kurbet AS, Ashok D and Raghavan S (2019) Unraveling the ECM-Immune Cell Crosstalk in Skin Diseases. Front. Cell Dev. Biol. 7:68. doi: 10.3389/fcell.2019.00068
Received: 12 January 2019; Accepted: 09 April 2019;
Published: 07 May 2019.
Edited by:
Charles D. Little, University of Kansas Medical Center, United StatesReviewed by:
Lidija Radenovic, University of Belgrade, SerbiaNagaraj Balasubramanian, Indian Institute of Science Education and Research, India
Copyright © 2019 Bhattacharjee, Ayyangar, Kurbet, Ashok and Raghavan. This is an open-access article distributed under the terms of the Creative Commons Attribution License (CC BY). The use, distribution or reproduction in other forums is permitted, provided the original author(s) and the copyright owner(s) are credited and that the original publication in this journal is cited, in accordance with accepted academic practice. No use, distribution or reproduction is permitted which does not comply with these terms.
*Correspondence: Srikala Raghavan, c3Jpa2FsYUBpbnN0ZW0ucmVzLmlu
†These authors have contributed equally to this work and are co-first authors