- Institute of Biochemistry and Cell Biology, National Research Council, Naples, Italy
One of the fundamental features of biomembranes is the ability to fuse or to separate. These processes called respectively membrane fusion and fission are central in the homeostasis of events such as those related to intracellular membrane traffic. Proteins that contain amphipathic helices (AHs) were suggested to mediate membrane fission via shallow insertion of these helices into the lipid bilayer. Here we analyze the AH-containing proteins that have been identified as essential for membrane fission and categorize them in few subfamilies, including small GTPases, Atg proteins, and proteins containing either the ENTH/ANTH- or the BAR-domain. AH-containing fission-inducing proteins may require cofactors such as additional proteins (e.g., lipid-modifying enzymes), or lipids (e.g., phosphatidylinositol 4,5-bisphosphate [PtdIns(4,5)P2], phosphatidic acid [PA], or cardiolipin). Both PA and cardiolipin possess a cone shape and a negative charge (−2) that favor the recruitment of the AHs of fission-inducing proteins. Instead, PtdIns(4,5)P2 is characterized by an high negative charge able to recruit basic residues of the AHs of fission-inducing proteins. Here we propose that the AHs of fission-inducing proteins contain sequence motifs that bind lipid cofactors; accordingly (K/R/H)(K/R/H)xx(K/R/H) is a PtdIns(4,5)P2-binding motif, (K/R)x6(F/Y) is a cardiolipin-binding motif, whereas KxK is a PA-binding motif. Following our analysis, we show that the AHs of many fission-inducing proteins possess five properties: (a) at least three basic residues on the hydrophilic side, (b) ability to oligomerize, (c) optimal (shallow) depth of insertion into the membrane, (d) positive cooperativity in membrane curvature generation, and (e) specific interaction with one of the lipids mentioned above. These lipid cofactors favor correct conformation, oligomeric state and optimal insertion depth. The most abundant lipid in a given organelle possessing high negative charge (more negative than −1) is usually the lipid cofactor in the fission event. Interestingly, naturally occurring mutations have been reported in AH-containing fission-inducing proteins and related to diseases such as centronuclear myopathy (amphiphysin 2), Charcot-Marie-Tooth disease (GDAP1), Parkinson’s disease (α-synuclein). These findings add to the interest of the membrane fission process whose complete understanding will be instrumental for the elucidation of the pathogenesis of diseases involving mutations in the protein AHs.
Introduction
Membrane fission (or scission), the process opposite to membrane fusion, consists in the splitting of one membrane into two separate membranes; instead, membrane fusion is a process by which two biological membranes converge into one membrane (Falanga et al., 2009; Kozlov et al., 2010; Scott and Youle, 2010; Knorr et al., 2017). Several examples of membrane fission processes can be found in the literature: cell division (Bohuszewicz et al., 2016; Caspi and Dekker, 2018; Stoten and Carlton, 2018), endocytosis (Renard et al., 2015; Ferreira and Boucrot, 2018; Kaksonen and Roux, 2018; Mettlen et al., 2018; Sandvig et al., 2018; Genet et al., 2019), caveolae biogenesis (Parton et al., 2006; Kirkham et al., 2008; Ariotti et al., 2015), budding of vesicles from endomembranes (Lee et al., 2005; Krauss et al., 2008; Hagen et al., 2015; Park et al., 2019), nuclear envelope repair (Isermann and Lammerding, 2017), neuron pruning (Loncle et al., 2015), mitochondrial division (Adachi et al., 2016; Huber et al., 2016; Pagliuso et al., 2018; Tandler et al., 2018; Tilokani et al., 2018; Agrawal and Ramachandran, 2019; Irajizad et al., 2019; Yoshida and Mogi, 2019), plastid division (Osteryoung and Pyke, 2014; Yoshida, 2018; Yoshida and Mogi, 2019), peroxisome fission (Huber et al., 2016; Schrader et al., 2016; Su et al., 2018), endosome fission (Daly and Cullen, 2018; Hoyer et al., 2018; Kitamata et al., 2019), vacuole fission (Gopaldass et al., 2017), Golgi membrane fission (Weigert et al., 1999; Hidalgo Carcedo et al., 2004; Bonazzi et al., 2005; Yang et al., 2005, 2008, 2011; Corda et al., 2006; Colanzi et al., 2007; Valente et al., 2013), macropinocytosis (Liberali et al., 2008), macroautophagy (Knorr et al., 2015; Yu and Melia, 2017; Osawa et al., 2019), microautophagy (Uttenweiler and Mayer, 2008; Li et al., 2012), thylakoid membrane remodeling (Chuartzman et al., 2008), formation of multivesicular bodies (Piper and Katzmann, 2007), sporulation in bacteria (Doan et al., 2013; Gifford and Meyer, 2015); formation of bacterial membrane vesicles (Eriksson et al., 2009; Schwechheimer and Kuehn, 2015; Bitto and Kaparakis-Liaskos, 2017), bacterial chromatophores (Bohuszewicz et al., 2016) and bacterial magnetosomes (Uebe and Schüler, 2016); formation of membrane vesicles in archaea (Ellen et al., 2010), virus budding from the cell (Rossman and Lamb, 2013; Adu-Gyamfi et al., 2015; Bigalke and Heldwein, 2015; Herneisen et al., 2017). Most of these membrane fission reactions are mediated by specialized proteins (Campelo and Malhotra, 2012; Frolov et al., 2015; Renard et al., 2015) that include the AH-containing proteins (see, e.g., Antonny et al., 1997; Farsad et al., 2001; Boucrot et al., 2012; Martyna et al., 2017).
Based on the many examples of membrane fission driven by AH insertion into the membrane bilayer, we have summarized in this review the known AH-containing proteins and related mechanisms linked to the fission event. Following a brief outline on the hemifission intermediate, we here propose to divide the membrane fission processes in four classes based on direct/indirect energy consumption and on the budding toward the cytosol or away from the cytosol. We then discuss the fission events mediated by the AH-containing proteins and underline the role of specific lipid cofactors and of the oligomeric state. We also report the naturally occurring mutations of these proteins.
Protein-Mediated Membrane Fission
Stages of Fission Process: “Neck-Hemifission” Model
Membrane fission proceeds through the following steps: membrane neck intermediate, hemifission intermediate, and two separate membrane formation (Corda et al., 2002; Kozlovsky and Kozlov, 2003; Bashkirov et al., 2008; Campelo and Malhotra, 2012; Frolov et al., 2015; Antonny et al., 2016; McDargh and Deserno, 2018; Pannuzzo et al., 2018). Hemifission is an intermediate state where the proximal monolayer (also called contacting monolayer) of the bilayer forming the neck coalesces, thus breaking the inner volume into two parts, while the distal monolayer remains continuous. Separation of distal monolayers completes the fission process. The existence of hemifission intermediate is confirmed by experimental observation that membrane fission is non-leaky (Bashkirov et al., 2008).
Neck and hemifission intermediates of the membrane fission process resemble pore and hemifusion intermediates of the opposite membrane fusion process. Membrane fusion proceeds via an intermediate named stalk, a lipidic hourglass-shaped connection between the contacting membrane leaflets. As a result of the radial expansion of the stalk, a hemifusion diaphragm forms. This intermediate is a bilayer formed by two distal leaflets of the fusing membranes. Finally, a fusion pore forms and establishes continuity between aqueous spaces of fusing membrane-enclosed compartments. The difference between membrane neck (sometimes referred to as “fission pore”) and fusion pore is that neck narrows, while fusion pore expands. By analogy with the “stalk-pore model” of membrane fusion (Chernomordik and Kozlov, 2008; Martens and McMahon, 2008; Kielian, 2014; Podbilewicz, 2014; Zhukovsky et al., 2019a; and references therein), we will call this membrane fission pathway as “neck-hemifission model.”
Classes of Membrane Fission Reactions
Renard et al. (2018) suggested to classify membrane fission mechanisms into two main categories: active fission (with the direct consumption of cellular energy by nucleoside triphosphate hydrolysis) and passive fission (without the direct use of energy). Many membrane fission processes are dependent on the interaction of fission-inducing proteins with specific lipid molecules (see below). In some cases, passive fission might be energized indirectly, via the energy used in the synthesis of these lipid cofactors (Gopaldass et al., 2017).
Moreover, membrane fission processes can be classified into two types: “normal topology fission” (membrane-enclosed compartments bud toward the cytosol) and “reverse topology fission” (membrane-enclosed compartments bud away from the cytosol) (Votteler and Sundquist, 2013; Frolov et al., 2015; Schöneberg et al., 2017; Caspi and Dekker, 2018; Snead and Stachowiak, 2018). During normal topology fission, distal monolayer of a membrane-enclosed compartment is cytoplasmic, whereas proximal monolayer is exoplasmic (Figure 1). During reverse topology fission, distal monolayer is exoplasmic, and proximal monolayer is cytoplasmic (Figure 1). We would like to point out that after “normal topology fission,” disjoint union of two membrane-enclosed compartments (as in the case of formation of post-Golgi carriers) or joint union (as in the case of endocytosis) can form. Similarly, after “reverse topology fission,” disjoint union (as in the case of cell division) or joint union [as in the case of herpesvirus primary envelopment (Bailer, 2017; Klupp et al., 2018) or formation of multivesicular bodies (Piper and Katzmann, 2007)] can form (Figure 1). Based on these considerations, we propose to divide membrane fission processes into four classes (Figure 2):
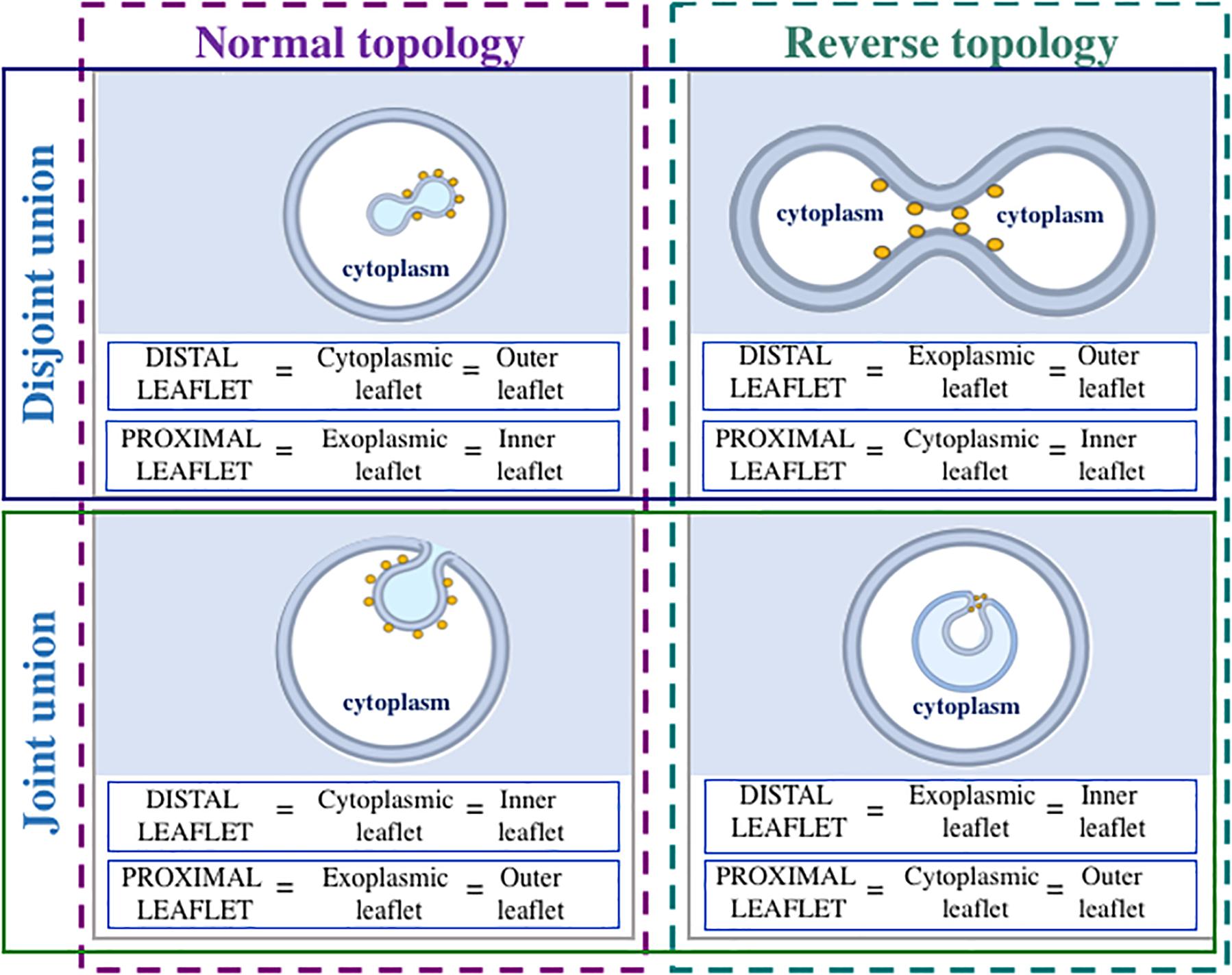
Figure 1. Nomenclature of leaflets (monolayers) during different kinds of membrane fission reaction. Only membranes decorated with fission-inducing proteins (yellow circles) are considered. When membrane fission takes place, separation of proximal leaflets is followed by separation of distal leaflets. During normal topology fission (membrane-enclosed compartment buds toward the cytoplasm), distal leaflet is cytoplasmic, whereas proximal leaflet is exoplasmic. During reverse topology fission (membrane-enclosed compartment buds away from the cytoplasm), distal leaflet is exoplasmic, and proximal leaflet is cytoplasmic. During both normal and reverse topology fission reactions, disjoint or joint union of membrane-enclosed compartments can form. If disjoint union forms, outer leaflet is distal, and inner leaflet is proximal. When joint union forms, outer leaflet is proximal, whereas inner leaflet is distal. Fission-inducing proteins are usually present on the cytoplasmic side of the membrane.
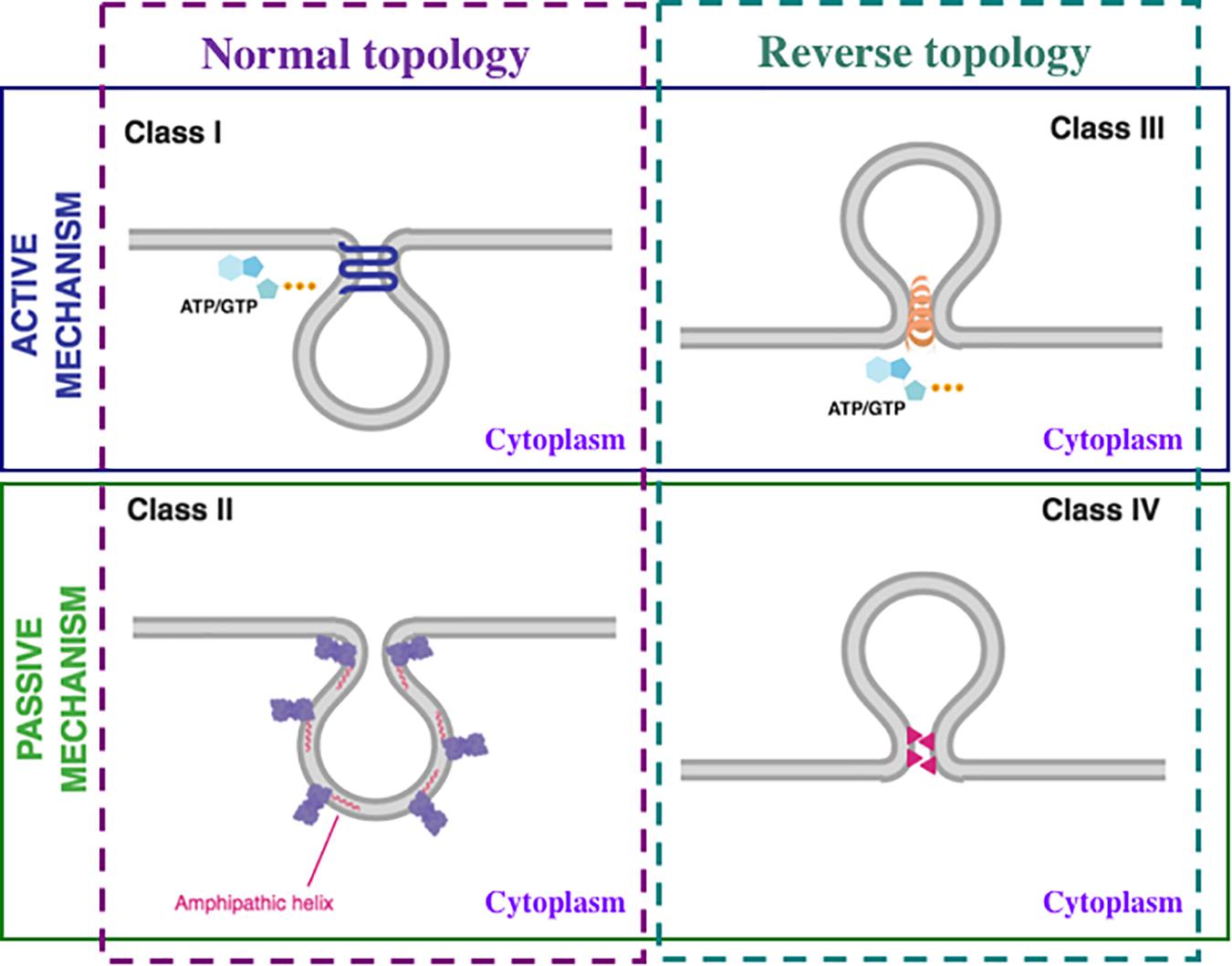
Figure 2. Mechanisms of membrane fission. Membrane fission mechanisms can be classified as active, that require consumption of cellular energy by nucleoside triphosphate (ATP or GTP) hydrolysis (upper panel; blue line) or passive that do not require the direct use of energy (lower panel; green line). Each of these above-mentioned categories can be further divided into two types: normal topology, when the vesicle buds toward the cytoplasm (left panel; purple dashed line) and reverse topology, when the vesicle buds away from the cytoplasm (right panel; dark green dashed line). Consequently, membrane fission mechanisms are divided into four classes: Class I, active mechanism with normal topology; Class II, passive mechanism with normal topology; Class III, active mechanism with reverse topology; Class IV, passive mechanism with reverse topology. The details are in the text.
Class I: Active mechanism, normal topology. Examples: fission mediated by the large GTPase dynamin and other members of the dynamin superfamily (Daumke and Praefcke, 2016; Ramachandran and Schmid, 2018; Ford and Chappie, 2019; Jimah and Hinshaw, 2019) and by the small AH-containing GTPases Arf1 (Krauss et al., 2008; Beck et al., 2011; Bottanelli et al., 2017; Dodonova et al., 2017) and Sar1 (Bielli et al., 2005; Lee et al., 2005; Bacia et al., 2011; Hariri et al., 2014; Hanna et al., 2016; Melero et al., 2018).
Class II: Passive mechanism, normal topology. Examples: fission mediated, in the absence of nucleoside triphosphate hydrolysis, by Bacillus subtilis FisB (Doan et al., 2013), C-terminal Binding Protein 1 Short form/Brefeldin A ADP-Ribosylation substrate (CtBP1-S/BARS) (Spanò et al., 1999; Weigert et al., 1999; Hidalgo Carcedo et al., 2004; Valente et al., 2005, 2012; Liberali et al., 2008; Pagliuso et al., 2016; Zhukovsky et al., 2019b), and by numerous AH-containing proteins, such as endophilins (Rostovtseva et al., 2009; Ambroso et al., 2014; Genet et al., 2019), amphiphysins (Wu and Baumgart, 2014; Snead et al., 2019), epsins (Ford et al., 2002; Boucrot et al., 2012; Brooks et al., 2015), α-synuclein (Nakamura et al., 2011; Braun et al., 2017; Pozo Devoto and Falzone, 2017; Fakhree et al., 2019), GDAP1 (Huber et al., 2016), ankyrin repeats and KH domain-containing protein 1 (ANKHD1) (Kitamata et al., 2019), Saccharomyces cerevisiae Atg18 (Gopaldass et al., 2017), Agrobacterium tumefaciens PmtA (Danne et al., 2017b), EcMurG (van den Brink-van der Laan et al., 2003), Acholeplasma laidlawii MGS (Eriksson et al., 2009; Ariöz et al., 2014; Ge et al., 2014) and DGS (Eriksson et al., 2009).
Class III: Active mechanism, reverse topology. Examples: fission mediated by the ESCRT machinery (Chiaruttini and Roux, 2017; Schöneberg et al., 2017; Stoten and Carlton, 2018; Ahmed et al., 2019; Gatta and Carlton, 2019), bacterial cell division (Bohuszewicz et al., 2016; Vedyaykin et al., 2019) and archaeal cell division (Caspi and Dekker, 2018). Of note, Vps4 ATPase (Monroe et al., 2014; Caspi and Dekker, 2018), BDLP1 containing N-terminal GTPase domain (Bohuszewicz et al., 2016), and CdvC containing ATPase subunit (Caspi and Dekker, 2018) proteins provide energy for the ESCRT pathway, bacterial cell division, and archaeal cell division, respectively. Interestingly, archaeal CdvC is the homolog of eukaryotic Vps4 (Caspi and Dekker, 2018).
Class IV: Passive mechanism, reverse topology. Examples: fission mediated by the herpesvirus fission machine (Bigalke and Heldwein, 2015), influenza A virus AH-containing protein M2 (Herneisen et al., 2017; Martyna et al., 2017; Madsen et al., 2018), Ebola virus VP40 (Soni and Stahelin, 2014; Adu-Gyamfi et al., 2015).
Although in Renard et al. (2018) CtBP1-S/BARS-mediated fission is classified as an active fission mechanism, we propose that this process should be considered as passive, because no direct consumption of energy by nucleoside triphosphate hydrolysis takes place during this process.
Any membrane fission reaction belongs to the category of active or passive fission and almost all fission processes can be classified as normal topology or reverse topology fission. Moreover, only very few fission processes (such as division of IMMs) are mediated by proteins not residing in the cytosol. Therefore, with very few exceptions, all fission reactions belong to one of the four classes described above. Moreover, many fission reactions mediated by AH-containing proteins are very diverse. However, assigning of these reactions to one of four classes I–IV will allow to find similarities in fission processes which seem to be completely different.
Fission Driven by Amphipathic Helix-Containing Proteins
Amphipathicity is the segregation of hydrophobic and hydrophilic amino acid residues between the two opposite faces of the protein α-helix, a distribution well suited for membrane binding (Drin and Antonny, 2010; Giménez-Andrés et al., 2018). A hydrophobic moment was introduced (Eisenberg et al., 1982, 1984) to estimate whether a protein sequence, when considered as helical, exhibits one polar and one hydrophobic face (Drin and Antonny, 2010). A large value of hydrophobic moment suggests that the protein sequence can be folded as AH perpendicularly to its axis (Eisenberg et al., 1982).
Various fission reactions are known to be driven by the large GTPase dynamin and other mechanoenzymes belonging to the dynamin superfamily (Daumke and Praefcke, 2016; Ramachandran and Schmid, 2018; Ford and Chappie, 2019; Jimah and Hinshaw, 2019; and references therein). In other fission processes that are not dependent on mechanoenzymes, proteins that contain AHs often play an important role (see, e.g., Antonny et al., 1997; Farsad et al., 2001; Boucrot et al., 2012; Martyna et al., 2017; and references therein).
Amphipathic helices are involved in many biological processes such as protein–protein interactions or interaction with biomembranes (Segrest et al., 1990, 1992). When an AH binds to membrane, it orients itself parallel to the membrane plane. The hydrophobic side of this helix inserts into the interior of the bilayer, while residues of the hydrophilic side interact with the lipid headgroups (Drin and Antonny, 2010; Roberts et al., 2013). The insertion of AHs into the bilayer promotes membrane curvature thus supporting the fission reaction (Campelo et al., 2008; Martyna et al., 2017).
Moreover, some protein segments (named “AH motifs”) of membrane-binding proteins are not completely helical in solution, but become AHs upon interaction with the membrane (Cornell and Taneva, 2006; Drin and Antonny, 2010; Ambroso et al., 2014; Chong et al., 2014; Horchani et al., 2014; Barneda et al., 2015; Frolov et al., 2015; and references therein). The presence of anionic lipids in the membrane often promotes the AH folding (see, for example, Davidson et al., 1998; Kweon et al., 2006; Fernandes et al., 2008; Horchani et al., 2014; Brady et al., 2015; Mizuno et al., 2017; Ryan et al., 2018).
The ability to sense membrane curvature by some proteins favors their interaction with curved membranes. This process not always leads to curvature generation. However, other AHs are able to induce curvature (Drin and Antonny, 2010; Wilz et al., 2011), an event required for proteins mediating membrane fission. The basic principles governing membrane curvature generation remain to be clarified (Khattree et al., 2013).
Amphipathic helices characterized by the presence of basic residues at the polar-non-polar interface are well suited for interaction with membranes (Mishra and Palgunachari, 1996; Davidson et al., 1998; Polozov et al., 1998; Mozsolits et al., 2004), especially with those containing negatively charged phospholipids by electrostatic attraction (Polozov et al., 1998). It has been hypothesized that interfacial positively charged residues help to anchor the AH in the lipid bilayer (Mishra and Palgunachari, 1996). We would like to emphasize that the AHs of many fission-inducing proteins contain at least two basic residues (Arg and/or Lys) each, at the polar-non-polar interface. Examples are: H0 helix of endophilin A1 contains Lys7 and Lys16 (Ambroso et al., 2014); AH of PmtA contains Lys6, Arg8, Lys12 (Danne et al., 2017b); AH of Atg18 contains Arg372 and Arg377 (Gopaldass et al., 2017). In PmtA, Arg8 and Lys12 are involved in protein attachment to anionic lipids (Danne et al., 2017b). Overall, the AHs of many fission proteins contain at least three positively charged residues. On the contrary, the AHs of proteins that sense but do not generate membrane curvature, such as ArfGAP1, GMAP-210, Kes1p, often contain not more than one basic residue (Drin and Antonny, 2010).
Campelo et al. (2008), similarly to Zemel et al. (2008), predicted that shallow (penetrating ca. 40% of the monolayer thickness) insertion of AH is particularly effective in the generation of membrane curvature. Since AHs and AH-containing protein segments are particularly suitable as membrane curvature-generating inclusions, their shallow insertions were suggested to be sufficient to mediate membrane fission (Boucrot et al., 2012).
The insertion depth of the AHs of fission-inducing proteins was studied experimentally. Using two hydrophobic quenchers of tryptophan fluorescence (shallow quencher and deep quencher), Hanna et al. (2016) detected shallow penetration of the Sar1B AH into the membrane, just below the hydrophilic headgroups. EPR measurements demonstrated shallow insertion of the AHs of endophilin A1 (Gallop et al., 2006), epsin (Lai et al., 2012), and α-synuclein (Jao et al., 2008) that embed at the level of lipid phosphate groups (Gallop et al., 2006; Lai et al., 2012) or just below this level (Jao et al., 2008). According to molecular dynamics simulations, the insertion depth of AHs from epsin and Sar1p is lower than that of Arf1, and these helices from epsin and Sar1p generate higher membrane curvature than the AH from Arf1 (Li, 2018). Interaction of the AHs of fission-inducing proteins with specific lipids might ensure the optimal insertion depth for the generation of membrane curvature and for the interaction with other fission-inducing proteins, as discussed below.
An additional mechanism foresees a cooperativity among proteins where the first insertion of an AH from a given protein able to sense membrane curvature (i.e., to bind selectively to curved membranes) favors the insertion of additional AHs (Gallop et al., 2006; Lundmark et al., 2008; Miller et al., 2015; Hanna et al., 2016; Martyna et al., 2017). In turn, these AHs induce more membrane curvature facilitating the insertion of additional AHs. Due to this positive cooperativity in membrane curvature generation, the runaway process leading to membrane fission might take place once a critical concentration of AH-containing fission-inducing proteins is reached (Miller et al., 2015).
Amphipathic helix-containing fission-inducing proteins are recognized as a separate superfamily of scission factors (Gallop et al., 2006; Boucrot et al., 2012; Martyna et al., 2017). Among these proteins there are: Arf1 (Krauss et al., 2008; Beck et al., 2011; Bottanelli et al., 2017; Dodonova et al., 2017), Sar1 (Bielli et al., 2005; Lee et al., 2005; Bacia et al., 2011; Hariri et al., 2014; Hanna et al., 2016; Melero et al., 2018), epsins (Ford et al., 2002; Boucrot et al., 2012; Brooks et al., 2015), CALM (Miller et al., 2015), endophilin A1 (Gallop et al., 2006; Ambroso et al., 2014), endophilin A2 (Boucrot et al., 2015; Renard et al., 2015; Simunovic et al., 2017; Genet et al., 2019), endophilin A3 (Boucrot et al., 2012), endophilin B1 (Rostovtseva et al., 2009; Takahashi et al., 2016), mammalian amphiphysin 1 (Snead et al., 2019) and amphiphysin 2 (Wu and Baumgart, 2014), Drosophila amphiphysin (Isas et al., 2015), PICK1 (Karlsen et al., 2015), α-synuclein (Nakamura et al., 2011; Braun et al., 2017; Pozo Devoto and Falzone, 2017; Fakhree et al., 2019), GDAP1 (Huber et al., 2016), caveolin-1 (Parton et al., 2006; Kirkham et al., 2008; Ariotti et al., 2015), ANKHD1 (Kitamata et al., 2019), peroxins Pex11B (Yoshida et al., 2015) and Pex11p (Opaliński et al., 2011; Su et al., 2018), yeast PROPPIN Atg18 (Gopaldass et al., 2017), Snf7 belonging to the ESCRT complex (Buchkovich et al., 2013), influenza A virus M2 (Rossman et al., 2010; Roberts et al., 2013; Herneisen et al., 2017; Madsen et al., 2018), and bacterial enzymes PmtA (Danne et al., 2017b), MurG (van den Brink-van der Laan et al., 2003; Lind et al., 2007; Albesa-Jové et al., 2014), MGS (Lind et al., 2007; Eriksson et al., 2009; Ge et al., 2014), and DGS (Eriksson et al., 2009). Unlike wt proteins, mutants of Arf1 (Beck et al., 2011), Sar1 (Lee et al., 2005), endophilin A1 (Farsad et al., 2001; Masuda et al., 2006; Mim et al., 2012), Drosophila amphiphysin (Yoon et al., 2012), mammalian amphiphysin 1 (Farsad et al., 2001), Atg18 (Gopaldass et al., 2017) lacking AHs were unable to generate membrane curvature.
Increasing of the hydrophobic moment favors folding of AH motif into an α-helix and binding to membrane (Drin and Antonny, 2010). Thus, it is not surprizing that mutations of hydrophobic residues, within AHs, to Ala or to hydrophilic residues (substitutions that decrease hydrophobic moment) inhibit the ability of fission proteins such as Arf1 (Krauss et al., 2008), Sar1p (Lee et al., 2005), endophilin A1 (Farsad et al., 2001; Gallop et al., 2006; Masuda et al., 2006; Suresh and Edwardson, 2010), epsin (Ford et al., 2002; Boucrot et al., 2012), Pex11p (Opaliński et al., 2011), Pex11B (Yoshida et al., 2015), yeast Atg2 (Kotani et al., 2018), mammalian Atg2A (Tamura et al., 2017), caveolin-1 (Parton et al., 2006; Kirkham et al., 2008; Ariotti et al., 2015), M2 (Rossman et al., 2010; Roberts et al., 2013), PmtA (Danne et al., 2017b), to generate membrane curvature. On the contrary, mutations of hydrophobic residues to more bulky hydrophobic Trp (substitutions that increase hydrophobic moment) promoted the ability of Arf1 (Krauss et al., 2008), epsin (Ford et al., 2002; Boucrot et al., 2012), and Pex11p (Opaliński et al., 2011) to induce membrane curvature, see Table 1.
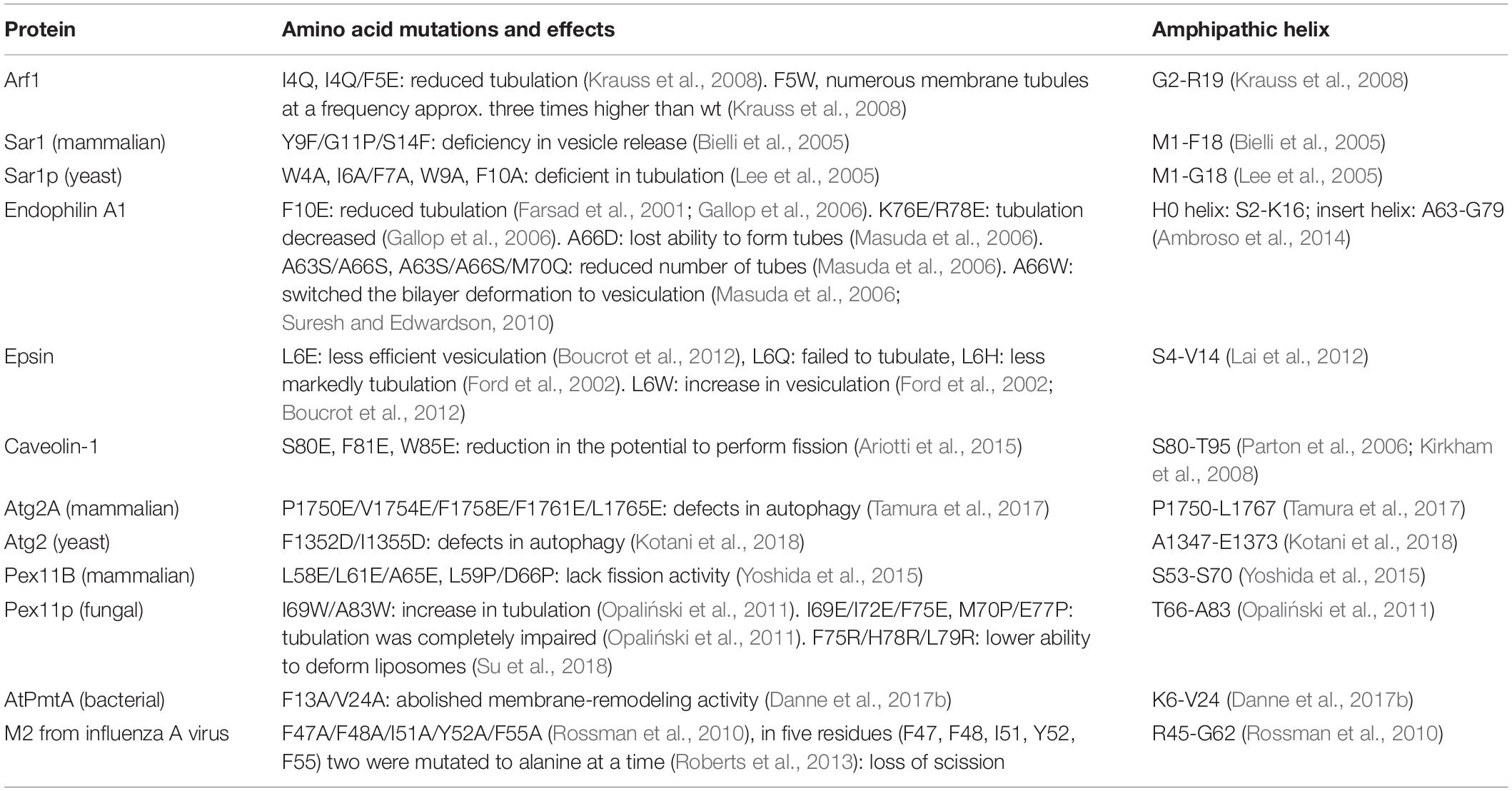
Table 1. Mutations in amphipathic helices of fission-inducing proteins that inhibit or promote the ability of these proteins to generate membrane curvature.
Substitution of positively charged residues of the AH hydrophilic face for negatively charged residues leads to repulsion from similarly charged membrane and, thus, to the reduced ability of AH to induce membrane curvature. This conclusion was confirmed experimentally. Mutations of basic Lys and Arg to negatively charged Glu within AH of endophilin A1 (Gallop et al., 2006) inhibited the ability of this fission-inducing protein to generate membrane curvature.
The influence of the mutations of AH residues on the ability of AH-containing proteins to generate membrane curvature is summarized in Table 1.
Some of AH-containing fission-inducing proteins, such as the small GTPases Arf1 and Sar1, mediate class I fission reactions. Other proteins, such as yeast Atg18 and bacterial enzymes PmtA, MurG, MGS, DGS, drive class II fission processes. Snf7 protein is involved in class III membrane fission reactions of the ESCRT pathway. Influenza virus M2 mediates class IV fission reaction. However, some AH-containing proteins, such as endophilin B1 and amphiphysin 1, could be involved, in complex with mechanoenzymes belonging to the dynamin superfamily, in class I fission reactions (Takahashi et al., 2016; Takeda et al., 2018), or, in the absence of mechanoenzymes, in class II fission reactions (Rostovtseva et al., 2009; Snead et al., 2019). Hence, belonging to a certain class is a property of the process, not a property of the protein molecule.
We should keep in mind that some membrane fission-inducing protein complexes, such as herpesvirus fission machine (Bigalke and Heldwein, 2015; Lorenz et al., 2015; Klupp et al., 2018), Ebola virus fission protein VP40 (Soni and Stahelin, 2014; Adu-Gyamfi et al., 2015), and FisB that mediates fission during sporulation in B. subtilis (Doan et al., 2013), contain neither proteins belonging to the dynamin superfamily nor AH-containing proteins.
Subfamilies of Amphipathic Helix-Containing Fission-Inducing Proteins
At least four subfamilies of proteins containing AHs able to drive membrane fission can be identified so far: small GTPases, ENTH/ANTH domain-containing proteins, BAR domain-containing proteins, and Atg proteins. Moreover, we suppose that synuclein family of proteins can be also considered as a subfamily of AH-containing fission-inducing proteins.
Small GTPases
The small GTPases Sar1 (Bielli et al., 2005; Lee et al., 2005; Bacia et al., 2011; Hariri et al., 2014; Hanna et al., 2016; Melero et al., 2018) and Arf1 (Krauss et al., 2008; Beck et al., 2011; Bottanelli et al., 2017; Dodonova et al., 2017) are involved in the intracellular trafficking of proteins and lipids in COP-coated vesicles (Graham and Kozlov, 2010; Adolf et al., 2013; Cevher-Keskin, 2013; Yorimitsu et al., 2014; and references therein). Sar1 plays a role in the COPII-mediated anterograde trafficking from the ER to the Golgi apparatus, whereas Arf1 is involved in COPI-mediated retrograde trafficking from the Golgi to the ER, as well as in the intra-Golgi transport. Sar1 and Arf1, as well as other small GTPase family proteins, share structural similarities. These two proteins are highly conserved in evolution. Yeast S. cerevisiae has one Sar1, whereas mammals have two Sar1 paralogs, denoted Sar1A and Sar1B in humans (Loftus et al., 2012). Sar1 and Arf1 function as molecular switches. They are cytosolic and inactive when bound to GDP. Upon exchange of GDP to GTP, these small GTPases undergo a conformational change into their active form. In this way, the N-terminal AH becomes exposed. This helix has one hydrophobic face, which requires engagement in a hydrophobic environment and thus it inserts into the cytoplasmic-membrane leaflet (Graham and Kozlov, 2010; Adolf et al., 2013). The AH of Arf1 is myristoylated, unlike the AH of Sar1. Membrane-bound Sar1/Arf1 recruit coat proteins and initiate the formation of COP-coated vesicles. Insertion of the AHs of Sar1 and Arf1 into the membrane drives fission and, in turn, the release of these COP-coated vesicles (Bielli et al., 2005; Lee et al., 2005; Krauss et al., 2008; Hanna et al., 2016).
ENTH/ANTH Domain-Containing Proteins
Epsins are highly conserved fission-inducing proteins that contain the N-terminal ENTH domain (Sen et al., 2012). Epsins play a role in the fission of CCVs during CME (Ford et al., 2002; Boucrot et al., 2012; Brooks et al., 2015). Four epsin paralogs are known in mammals: epsin 1 to 3 and epsin-related (EpsinR) (Takatori and Tomita, 2018). The role of epsin 1 in membrane curvature generation is well known (Ford et al., 2002; Kweon et al., 2006), although epsin 2 and epsin 3 are also involved in CME (Boucrot et al., 2012). The ENTH domain is approximately 140 residues long and has a compact globular structure of seven α-helices followed by an eighth helix that is not aligned with the other seven (Sen et al., 2012). Upon interaction with PtdIns(4,5)P2-containing membrane, ENTH domain of epsin 1 undergoes a conformational change that leads to the formation of an additional N-terminal α-helix denoted helix 0 (H0) (Ford et al., 2002) consisting of residues Met1-Tyr17 (Martyna et al., 2017), or residues Ser4–Val14, as reported by Lai et al. (2012). This is the helix inserting into the membrane. Primary sequences of the N-termini (residues 1–17) of human epsins 1, 2, 3 are very similar. It has been proposed that insertion of H0 helix into the bilayer induces membrane curvature required for fission (Ford et al., 2002; Boucrot et al., 2012), although recent study raised doubts on this mechanism and suggested that, instead, crowding of intrinsically disordered protein domains is able to drive fission (Snead et al., 2017).
Dynamin plays an important role in the fission of CCVs. Nevertheless, epsin is able to mediate CCV fission in dynamin-depleted cells. Boucrot et al. (2012) suggested that epsin molecules present at the neck of a budding vesicle might provide the force that destabilizes the neck of budding vesicle, thus leading to fission.
It should be emphasized that the role of epsins in the formation and fission of CCVs is somewhat similar to the role of Sar1 and Arf1 in the formation and fission of COP vesicles (Boucrot et al., 2012; Adolf et al., 2013). In both cases, an external trigger leads to the exposure of an AH that inserts into the cytosolic leaflet of the membrane and is assumed to drive the fission of vesicles. In case of small GTPases, exchange of GDP for GTP plays a role of such a trigger, whereas in case of epsin, AH exposure is triggered by the interaction with PtdIns(4,5)P2-containing membrane. Epsin, as well as small GTPases, might create a high-energy state at the neck of budding vesicle, and this state is relaxed by fission of this vesicle from the donor membrane (Boucrot et al., 2012; Adolf et al., 2013). Moreover, small GTPases are responsible for recruitment of COP proteins, whereas epsin is involved in clathrin coat assembly.
The CALM protein, also known as PICALM, is also involved in fission of CCVs during CME (Miller et al., 2015). CALM contains a N-terminal ANTH domain that is approximately twice as big as the ENTH domain, but has the same core of α-helices (Duncan and Payne, 2003; Legendre-Guillemin et al., 2004; Takatori and Tomita, 2018; Kaneda et al., 2019). ENTH and ANTH domains are so similar that several ANTH domains were originally designated ENTH domains, although later these two domains were subclassified as two distinct domains, ENTH and ANTH (Ford et al., 2002). Structurally, ENTH and ANTH domains are similar to the VHS domain (De Craene et al., 2012). Most ENTH and ANTH domains are lipid-binding domains, they interact specifically with phosphoinositides present in the membrane. However, the mechanisms of the interaction of ENTH and ANTH domains with PtdIns(4,5)P2 are quite different (Kaneda et al., 2019; and references therein).
ANTH domain-containing protein CALM, like ENTH domain-containing protein epsin 1, are characterized by an N-terminal AH that is supposed to insert into the membrane and to play a role in membrane fission (Miller et al., 2015). Proteins containing ENTH/ANTH domains (epsins 1, 2, 3 and CALM) are somewhat similar and can be classified as separate subfamily of AH-containing fission-inducing proteins. Possibly, the plant ANTH domain-containing protein AP180 also belongs to this subfamily (Kaneda et al., 2019). Similarly, new fission proteins belonging to this subfamily might be discovered in the future, based on the identification of AHs in their structure.
BAR Domain-Containing Proteins
The BAR domain is found in many proteins implicated in various cellular processes, most of which are related to membrane remodeling (Stanishneva-Konovalova et al., 2016; Carman and Dominguez, 2018; Nishimura et al., 2018; Simunovic et al., 2019). BAR domains typically form banana-shaped homodimers, where each protomer consists of three-helix antiparallel coiled-coil structure. Because of its curved shape, the BAR domain is able to sense membrane curvature, i.e., to bind preferentially to curved membranes. Thus, the concave shape of the BAR-domain dimer is positively charged and interacts with the negatively charged cytoplasmic monolayer of biomembranes. Most BAR domain proteins also contain auxiliary domains involved in the interactions with other proteins or with membranes (Mim et al., 2012; Carman and Dominguez, 2018). Based on their structural properties, BAR domain proteins can be classified into three main groups: a classical BAR (including N-BAR), F-BAR, and I-BAR (Ahmed et al., 2010; Stanishneva-Konovalova et al., 2016; Nishimura et al., 2018). Proteins belonging to the N-BAR subgroup contain an N-terminal sequence H0 that folds into an AH upon membrane binding. Endophilins (Kjaerulff et al., 2011) and amphiphysins (Wu et al., 2009; Prokic et al., 2014) belong to the N-BAR subgroup and thus contain these H0-AH motifs. Moreover, endophilins contain an additional AH, known as H1I (helix 1 insert). In mammals endophilins are encoded by five genes: endophilins A1, A2, A3, B1, B2, whereas amphiphysins are encoded by two genes: amphiphysin 1 and amphiphysin 2 (also known as BIN1) (Kjaerulff et al., 2011; Prokic et al., 2014).
Bin/Amphiphysin/Rvs domain protein PICK1 contains an internal AH (Karlsen et al., 2015; Herlo et al., 2018). Mammalian endophilins A1 (Gallop et al., 2006; Ambroso et al., 2014), A2 (Boucrot et al., 2015; Renard et al., 2015; Simunovic et al., 2017; Genet et al., 2019), A3 (Boucrot et al., 2012) and B1 (Rostovtseva et al., 2009; Takahashi et al., 2016), mammalian amphiphysin 1 (Snead et al., 2019) and amphiphysin 2 (Wu and Baumgart, 2014), Drosophila amphiphysin (Isas et al., 2015), as well as mammalian PICK1 (Karlsen et al., 2015) are all involved in membrane fission. The AHs were suggested to generate membrane curvature and to play a role in membrane fission mediated by endophilins (Gallop et al., 2006; Masuda et al., 2006; Boucrot et al., 2012; Yoon et al., 2012) and amphiphysins (Peter et al., 2004; Boucrot et al., 2012; Yoon et al., 2012; Wu and Baumgart, 2014). Moreover, a higher number of AHs in endophilins compared with amphiphysins was suggested to correlate with increased ability to penetrate the membrane and to drive membrane fission (Boucrot et al., 2012; Yoon et al., 2012). However, other studies question the role of these AHs in membrane curvature generation (e.g., see Chen et al., 2016). The BAR domains were suggested to restrict (Boucrot et al., 2012) or to promote membrane fission (Snead et al., 2019). Finally, Snead et al. (2019) reported that the steric pressure among disordered domains of amphiphysin 1 plays a role in fission. Based on these reports, the molecular mechanism taking place in endophilin- and amphiphysin-induced fission remains to be fully elucidated.
Like AH motif of epsin (Ford et al., 2002), AH motifs of BAR domain-containing fission-inducing proteins endophilins and amphiphysins are also disordered in solution, but become α-helical upon interaction with the membrane (Löw et al., 2008; Jao et al., 2010). In this they differ from the fission-inducing small GTPases Arf1 and Sar1, whose AHs are already formed but are buried in the GDP-bound conformation, and only become exposed upon exchange of GDP to GTP (see above).
Often, endophilins mediate or inhibit fission cooperatively with the proteins belonging to the dynamin superfamily. Endophilin A2 and dynamin contribute to the fission of Shiga-toxin-induced tubules (Renard et al., 2015). In a dynamin-dependent manner, endophilins A2 and A1 mediate clathrin-independent internalization route named FEME (Boucrot et al., 2015). Endophilin A1 inhibits dynamin-mediated membrane fission via intercalation between turns of the dynamin helix (Hohendahl et al., 2017). Endophilin B1 and dynamin 2 cooperatively induce the fission of Golgi membranes during autophagy (Takahashi et al., 2016), while the dynamic clustering of dynamin-amphiphysin 1 rings was suggested to regulate fission of large unilamellar vesicles (Takeda et al., 2018).
Interestingly, predicted curved structure of the ten C-terminal ankyrin repeats of AH-containing fission-inducing protein ANKHD1 is similar to the lipid-binding surface of BAR domain-containing proteins (Kitamata et al., 2019).
Atg Proteins
Two Atg proteins, Atg2 and Atg18, contain AHs and mediate membrane fission (Gopaldass et al., 2017; Tamura et al., 2017; Kotani et al., 2018). Macroautophagy (hereinafter called autophagy) is a degradation process highly conserved from yeast to mammals (Feng et al., 2014; Knorr et al., 2015; Yu and Melia, 2017; Osawa et al., 2019; and references therein). During autophagy, a bowl-shaped membrane vesicle called phagophore appears, engulfs a part of the cytoplasm (including cytotoxins, damaged organelles, and invasive microbes), and expands. Finally, the open end of phagophore closes, and thus, the inner and outer bilayers become separate entities, and a double-membrane vesicle called autophagosome forms. Closure of the phagophore, leading to the autophagosome formation, is a membrane fission event (Knorr et al., 2015; Yu and Melia, 2017); and, moreover, is a reverse-topology fission event (Schöneberg et al., 2017). Finally, the outer membrane of the autophagosome fuses with a lysosome or vacuole, and captured cytoplasmic contents, together with the inner membrane of the autophagosome, is degraded by hydrolases. The resulting materials, such as amino acids, are released to the cytosol and recycled.
Many Atg proteins are required for the formation of autophagosome. Atg2 is one of these proteins. Two mammalian homologs of yeast Atg2 were identified: Atg2A and Atg2B (Velikkakath et al., 2012). Yeast Atg2 (Kotani et al., 2018) as well as mammalian Atg2 proteins (Velikkakath et al., 2012; Tamura et al., 2017; Tang et al., 2017) play a role in autophagosome formation. Two mammalian Atg2 homologs, Atg2A and Atg2B, have redundant functions in autophagy (Tamura et al., 2017). In the absence of mammalian Atg2A and Atg2B, the closure of autophagosomes is impaired (Velikkakath et al., 2012; Tang et al., 2017), indicating that these proteins are involved in membrane fission event, although other molecules might also be required for this process. Both yeast Atg2 (Kotani et al., 2018) and mammalian Atg2A (Tamura et al., 2017; Chowdhury et al., 2018) contain AHs, and the AH of yeast Atg2 corresponds to the AH of mammalian Atg2A (Kotani et al., 2018). These AHs are required for autophagosome formation (Tamura et al., 2017; Kotani et al., 2018). Mutations of hydrophobic residues of AH from human Atg2A to negatively charged residues abolish membrane-binding capability (Chowdhury et al., 2018) and causes defects in autophagy (Tamura et al., 2017). Similarly, mutations of hydrophobic residues of yeast Atg2 to negatively charged Asp inhibit autophagy (Kotani et al., 2018). From these observations, it derives that yeast and mammalian homologs of Atg2 belong to the family of AH-containing membrane fission-inducing proteins.
Autophagy-related proteins are classified into six functional groups (Velikkakath et al., 2012; Osawa et al., 2019). Atg2 and Atg18 belong to the same group. Atg18 forms a complex with Atg2 and also plays a role in autophagosome formation (Kotani et al., 2018). On top of its role in autophagy, Atg18 also mediates vacuole fission (Gopaldass et al., 2017). Amphipathic α-helical character of the Atg18 segment (residues ∼Pro366-Ser383) is required for Atg18-driven vacuole fission (Gopaldass et al., 2017). Vacuole fission is a normal topology fission event, and, hence, Atg18 is an interesting kind of protein that is involved in fission processes of two opposite topologies: formation of autophagosome (reverse topology) and vacuole fission (normal topology). It is noteworthy that Atg18 displays different requirements in these two fission processes. In vacuole fission, AH is crucial, whereas its binding partner Atg2 is dispensable. In autophagy, interaction of Atg18 with Atg2 is necessary, whereas the Atg18 AH is of moderate importance (Gopaldass et al., 2017). However, the ability of Atg18 to bind phosphatidylinositol 3-phosphate (PtdIns3P) is required for both processes: autophagy (Kotani et al., 2018) and vacuole fission (Gopaldass et al., 2017).
Interestingly, the AH motif of Atg18, similar to the AH motifs of epsins, endophilins and amphiphysins, is unstructured in solution, but transforms into an AH upon membrane binding (Gopaldass et al., 2017). Whereas small GTPases Arf1 and Sar1, ENTH/ANTH domain fission-inducing proteins, endophilins and amphiphysins contain N-terminal AH (see above), AHs of Atg proteins, Atg2 and Atg18, similar to the AH of PICK1, are internal and far, in primary sequence, from the N-terminus.
Synucleins
Mitochondria are double-membrane-bound organelles that constantly undergo fusion and fission events, referred to as mitochondrial dynamics (Tilokani et al., 2018). Fission of OMM occurs at the ER contact sites. Protein adaptors, such as MFF and MiD49 and MiD51, recruit Drp1, a soluble protein belonging to the dynamin superfamily. Drp1 mediates OMM constriction, whereas final step, namely scission, is mediated by another mechanoenzyme, dynamin2 (Dnm2). Other proteins, such as INF2, Spire1C, actin, and Myosin IIA play a role in this fission process (Pagliuso et al., 2018; Tilokani et al., 2018; and references therein). Simplified model for OMM fission is shown in Figure 4 of Tilokani et al. (2018). Fission of IMM occurs independently of OMM fission (Agrawal and Ramachandran, 2019). Mechanisms regulating constriction of IMM are poorly understood.
Proteins belonging to the synuclein family (Lavedan, 1998), namely α-synuclein (Kamp et al., 2010; Nakamura et al., 2011; O’Donnell et al., 2014; Martinez et al., 2018) and β-synuclein (Nakamura et al., 2011; Taschenberger et al., 2013), are involved in mitochondrial fragmentation. α-Synuclein contains a uniquely long α-11/3 AH that forms upon binding to a lipid bilayer (George et al., 1995; Davidson et al., 1998; Drin and Antonny, 2010; Braun et al., 2017). Similar AHs are present also in β-synuclein and γ-synuclein (Ducas and Rhoades, 2012). β-Synuclein mediates less fragmentation than α-synuclein, whereas γ-synuclein causes very little if any fragmentation (Nakamura et al., 2011). Therefore, in this section we mostly discuss α-synuclein.
α-Synuclein is closely associated with the development of Parkinson’s disease. The function of this protein is not well understood. As already mentioned, α-synuclein drives fragmentation of mitochondria (Kamp et al., 2010; Nakamura et al., 2011; O’Donnell et al., 2014; Martinez et al., 2018). Uncontrolled mitochondrial fragmentation might contribute to the development of Parkinson’s disease (Varkey et al., 2010; Panchal and Tiwari, 2019) and, importantly, duplication and triplication of SNCA (α-synuclein) gene cause a severe form of this disease (Olgiati et al., 2015; Konno et al., 2016). The authors of Kamp et al. (2010) and Nakamura et al. (2011) hypothesized that fragmentation of mitochondria might be independent of Drp1, whereas Martinez et al. (2018) reported that Drp1 is required for α-synuclein-mediated mitochondrial fragmentation. α-Synuclein might drive fragmentation of mitochondria by decreasing the rate of fusion or, alternatively, by promoting fission (Nakamura, 2013). Kamp et al. (2010) hypothesized that the influence of this protein on mitochondrial dynamics is based on inhibition of fusion, whereas Nakamura et al. (2011) suppose that, quite opposite, α-synuclein acts by promoting membrane fission. The latter hypothesis is supported by the fact that α-synuclein is able to convert large vesicles (liposomes) into smaller vesicles, i.e., mediate membrane fission (Varkey et al., 2010; Nakamura et al., 2011; Fakhree et al., 2019). AH of α-synuclein contributes to the generation of membrane curvature (Braun et al., 2014, 2017; Fakhree et al., 2019). Thus, it is reasonable to hypothesize that α-synuclein and, possibly, also β-synuclein belong to the large family of AH-containing fission-inducing proteins.
In summary, in most AH-containing fission-inducing proteins AHs were shown or suggested to play an important role in various fission events. As indicated, the AH penetration in the lipid bilayer can be the starting point of the fission process, followed by the recruitment of other proteins that mediate the final fission event (Sundborger et al., 2011; Meinecke et al., 2013). Shallow insertion of AHs into the membrane was suggested to drive membrane fission (Boucrot et al., 2012). However, few recent publications of Jeanne Stachowiak’s group consider also an alternative mechanism foreseeing that the steric pressure generated by bulky disordered domains can drive membrane fission (Snead et al., 2017, 2019; Snead and Stachowiak, 2018), whereas the role of insertions, such as AHs, is to anchor proteins to the membrane (Snead et al., 2017). Whether the different mechanisms coexist or one prevails over the others remains to be defined.
The Role of Lipid Cofactors in Membrane Fission Driven by Amphipathic Helix-Containing Proteins
Lipid Cofactors: An Overview
Many AH-containing fission-inducing proteins require one or two specific lipids to generate membrane curvature and to complete the fission process (Figure 3). Most of these lipids are anionic. Such lipids could be named lipid cofactors (Ramachandran, 2018), or lipid factors (Danne et al., 2017b), or lipid ligands (Gopaldass et al., 2017). Examples of lipid cofactors of AH-containing fission proteins are: (1) PtdIns(4,5)P2 for epsin (Kweon et al., 2006; Boucrot et al., 2012; Lai et al., 2012), mammalian amphiphysin 2 (Lee et al., 2002; Wu and Baumgart, 2014), Drosophila amphiphysin (Yoon et al., 2012), and mammalian endophilin A1 (Yoon et al., 2012); (2) cardiolipin and MMPE for PmtA (Danne et al., 2017b); (3) PtdIns3P and PtdIns(3,5)P2 for Atg18 (Gopaldass et al., 2017; Scacioc et al., 2017); (4) cholesterol for M2 protein from influenza A virus (Ekanayake et al., 2016; Elkins et al., 2017, 2018; Herneisen et al., 2017; Pan et al., 2019; and references therein), see Table 2.
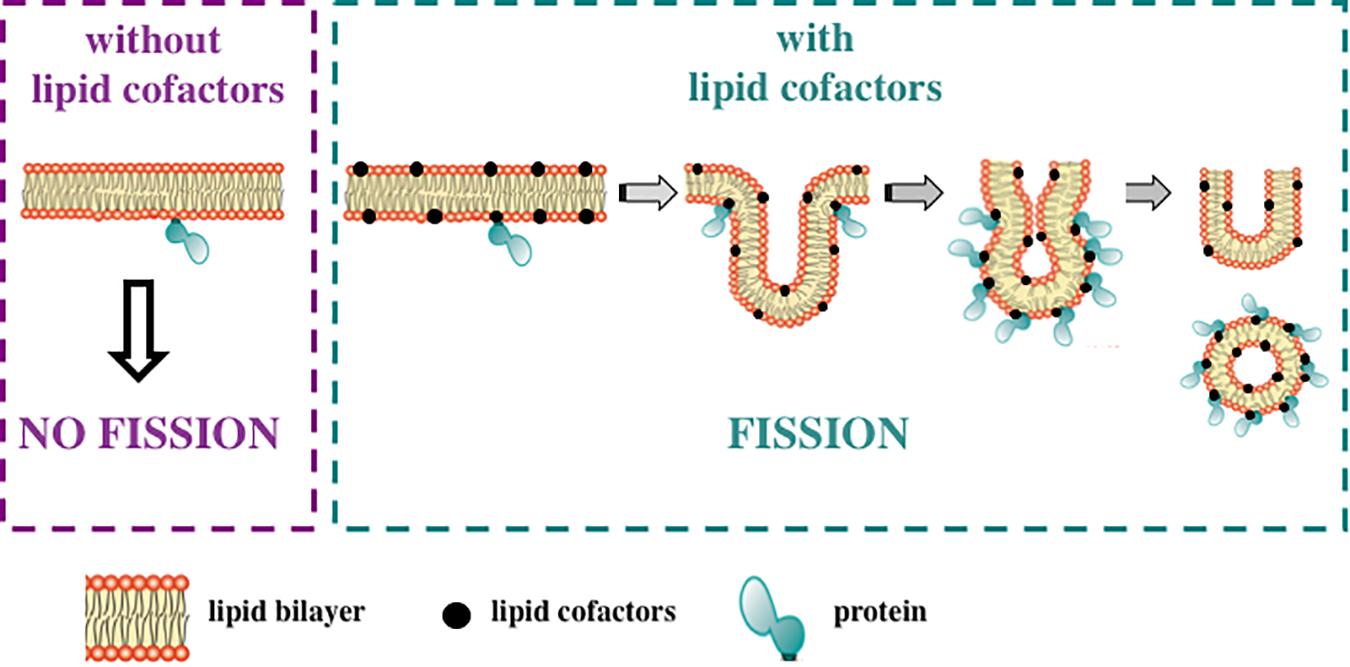
Figure 3. Lipid cofactors are required for fission-inducing proteins. Many AH-containing and AH-free fission-inducing proteins need specific lipids, named lipid cofactors, to promote and complete membrane fission. Thus, the ability of the fission-inducing proteins to support this process is strictly dependent on membrane lipid composition. Of note, proteins are not able to induce membrane fission in the absence of these lipids (left panel; purple dashed line). Conversely, in the presence of such lipid cofactors, fission occurs through the following steps: membrane neck, hemifission and formation of two separate membranes (right panel; green dashed line). Lipid cofactors include: PtdIns(4,5)P2, PA, cardiolipin, MMPE, PtdIns3P, PtdIns(3,5)P2 and cholesterol. See text for details.
The lipid cofactor-binding sites were found in the AHs of few fission-inducing proteins: PtdIns(4,5)P2-binding site in epsin (Kweon et al., 2006; Lai et al., 2012), site that binds PtdIns(3,5)P2 and PtdIns3P in Atg18 (Gopaldass et al., 2017), and cholesterol-binding site in M2 (Elkins et al., 2017, 2018). It is not surprizing that anionic lipid-binding sites contain basic residues: Arg7, Arg8, Lys11 of epsin belong to the PtdIns(4,5)P2-binding site, whereas Arg285 and Arg286 of Atg18 are involved in the interaction with PtdIns(3,5)P2 and PtdIns3P. Accordingly, other fission-inducing proteins may contain few basic residues as anionic lipid cofactor-binding sites that are located close to each other in primary sequence.
In some membrane fission reactions involving AH-containing proteins, the role of specific lipids is not yet fully established. Possibly, cardiolipin is a cofactor for membrane fission mediated by Pex11 [peroxisome fission (Opaliński et al., 2011; Su et al., 2018)]; bacterial MGS (Eriksson et al., 2009; Ariöz et al., 2013), DGS (Edman et al., 2003; Eriksson et al., 2009), and MurG (van den Brink-van der Laan et al., 2003; Lind et al., 2007) proteins; and by MinD [bacterial division (Zhou and Lutkenhaus, 2003; Renner and Weibel, 2012)]. PA might be a lipid cofactor for endophilin B1 (also known as Bif-1) (Zhang et al., 2011) that contains two AHs (Kjaerulff et al., 2011) and plays a role in membrane fission (Rostovtseva et al., 2009; Takahashi et al., 2011, 2016). Possibly, PtdIns(4,5)P2 is a lipid cofactor in membrane vesiculation mediated by PICK1 (Pan et al., 2007; Karlsen et al., 2015). PS might be a lipid cofactor in the fission reaction mediated by ANKHD1 (Kitamata et al., 2019). Arf1 binds to PtdIns(4,5)P2 and PA (Randazzo, 1997; Manifava et al., 2001; Krauss et al., 2008), but the role of these interactions in Arf1-mediated membrane fission is not completely clear.
As we already mentioned, an AH-containing protein α-synuclein induces fragmentation of mitochondria (Kamp et al., 2010; Nakamura et al., 2011; O’Donnell et al., 2014; Martinez et al., 2018) and converts large vesicles (liposomes) into smaller vesicles (Varkey et al., 2010; Nakamura et al., 2011; Fakhree et al., 2019). Moreover, AH plays a role in the α-synuclein-driven generation of membrane curvature (Braun et al., 2014, 2017; Fakhree et al., 2019). Hence, we can consider α-synuclein as an AH-containing fission-inducing protein. Interestingly, α-synuclein binds to cardiolipin (Nakamura et al., 2008; Robotta et al., 2014; Ryan et al., 2018) and PA (Perrin et al., 2000; Nakamura et al., 2011; Mizuno et al., 2017) with high affinity. Moreover, this protein prefers negatively charged lipids cardiolipin (Nakamura et al., 2008) and PA (Nakamura et al., 2008; Mizuno et al., 2017) to another negatively charged lipid PS. The cone shape of cardiolipin, whose tail is wider than its headgroup (see below), might contribute to the affinity of α-synuclein to cardiolipin-containing bilayers (Ghio et al., 2019; and references therein). PA (Mizuno et al., 2017) and cardiolipin (Ryan et al., 2018) promote the formation of the AH of α-synuclein. Importantly, α-synuclein mediates fission of liposomes that contain both PC and cardiolipin, but not liposomes that contain only PC (Nakamura et al., 2011). We propose that cardiolipin and, possibly, also PA are cofactors in membrane fission mediated by AH-containing protein α-synuclein.
To the best of our knowledge, membrane fission mediated by small GTPase Sar1 does not need any lipid cofactors. It has been stressed that Arf1 and Sar1 use the energy of GTP hydrolysis, whereas no enzymatic activity has been found or predicted for Atg18 (Gopaldass et al., 2017). However, Atg18 membrane recruitment and oligomerization needs PtdIns3P and PtdIns(3,5)P2 as lipid cofactors (see Table 2), and synthesis of these lipids is energy-consuming. Hence, this fission process can be energized indirectly, via the energy invested into the synthesis of lipid cofactors (Gopaldass et al., 2017). Possibly, such indirect driving force of the reaction can also be considered for other AH-containing fission proteins.
Anionic lipid cofactors cannot be replaced with other anionic lipids: see Table 2. Tubulation mediated by endophilin A1 requires PtdIns(4,5)P2 but not PS (Yoon et al., 2012). PmtA tubulates liposomes containing cardiolipin, but is less efficient in tubulating liposomes containing PG, PtdIns4P or PA (Danne et al., 2017b). The AH-containing fission proteins epsin (Boucrot et al., 2012), PmtA (Danne et al., 2017b), Atg18 (Gopaldass et al., 2017) mediate fission of membranes containing their lipid cofactors, but they are inefficient with membranes containing other acidic lipids.
Electrostatic interaction between positively charged residues belonging to AH and negatively charged phospholipids was proposed to overcome the energetic cost of spreading lipids apart and thus to play an important role in the generation of membrane curvature needed for the fission event (Khattree et al., 2013; Roberts et al., 2013; Brady et al., 2015). Most probably, anionic lipid cofactors are required for the recruitment of AH-containing fission proteins. Furthermore, as pointed out above, the shallow penetration of some AHs leads to the generation of local membrane curvature through the pushing of the lipid headgroups apart (the “wedge” mechanism) (McMahon and Gallop, 2005; Campelo et al., 2008; Drin and Antonny, 2010; Baumgart et al., 2011; Lai et al., 2012; McMahon and Boucrot, 2015; and references therein). At least in some cases, the depth of the AH insertion into the membrane depends on the presence of negatively charged lipids in the membrane (Sani et al., 2012; Palomares-Jerez et al., 2013). Indeed, the interaction of curvature-inducing AHs with anionic lipids might contribute to the optimal depth of AH insertion.
As pointed out above, the presence of anionic lipids in the membrane often promotes the folding of AH (Davidson et al., 1998; Kweon et al., 2006; Fernandes et al., 2008; Horchani et al., 2014; Brady et al., 2015; Mizuno et al., 2017; Ryan et al., 2018). Thus, another mode for the anionic lipid to mediate membrane curvature generation and promote membrane fission is facilitating the folding of AH motif, once the protein segment comes to close contact with the membrane.
Simple electrostatic attraction between acidic lipids and basic residues of AHs does not explain the specificity of the action of lipid cofactors on fission proteins. AH-containing fission proteins epsin (Kweon et al., 2006), endophilin A1 (Yoon et al., 2012), amphiphysin 2 (Lee et al., 2002), PmtA (Danne et al., 2017b), Atg18 (Gopaldass et al., 2017) interact specifically with the membranes containing their lipid cofactors, but poorly with the membranes containing other anionic lipids. For example, PmtA binds cardiolipin-containing liposomes, but poorly binds PA-containing liposomes (Danne et al., 2017b). Most probably, a specific interaction of fission proteins with their lipid cofactors is necessary for the correct conformation and oligomeric structure of these proteins.
Reverse-topology membrane fission-inducing protein M2 from influenza A virus contains an AH, but uses neutral cholesterol as a cofactor in the membrane fission process (Elkins et al., 2017; Herneisen et al., 2017; Pan et al., 2019; and references therein), see Table 2. However, we should keep in mind that in the case of reverse topology fission, AHs insert into the proximal leaflet of the budding membrane-enclosed compartment, and not into the distal leaflet, as in the case of the normal-topology fission. Hence, the process of membrane remodeling is fundamentally different, and the requirements for the lipid cofactor might also be different.
Cholesterol may control the insertion depth of the M2 AH in the bilayer (Kim et al., 2015; Herneisen et al., 2017), thus, contributing to the optimal depth of the AH insertion, as we have discussed above for the interaction of the AHs of other fission-inducing proteins with their anionic lipid cofactors. Moreover, cholesterol was suggested to promote a more compact conformation of the M2 AH (Herneisen et al., 2017). This protein was shown also to cluster at the phase boundary of cholesterol-rich liquid-ordered phase and cholesterol-poor liquid-disordered phase (Roberts et al., 2013). Such a localization of a fission-inducing protein at the neck of the budding virus can generate the membrane curvature required for membrane fission (Elkins et al., 2017; Martyna et al., 2017). A similar mechanism might be applicable for few other membrane fission processes mediated by AH-containing proteins interacting with specific lipid cofactors.
Some fission-inducing proteins, in which no definitely established AHs have yet been identified, also require lipid cofactors for their activity. For example, PA is a lipid cofactor for CtBP1-S/BARS (Weigert et al., 1999; Pagliuso et al., 2016); PS is a lipid cofactor for Ebola virus VP40 (Soni and Stahelin, 2014; Adu-Gyamfi et al., 2015; Del Vecchio et al., 2018); PtdIns(4,5)P2 is a putative lipid cofactor for dynamin (Jost et al., 1998); cardiolipin is a probable lipid cofactor for FisB (Doan et al., 2013) and, moreover, activates mechanoenzyme Drp1 that plays a role in mitochondrial fission (Francy et al., 2017).
Lipid cofactors should not be confused with lipids that just recruit fission-inducing proteins to the membrane, but are not required for the fission reaction per se. Unlike lipids that just recruit proteins, lipid cofactors are indispensable for fission: membrane curvature generation cannot proceed efficiently without them, see Table 2. For example, PtdIns4P was suggested to have a direct role in the anchoring of the protein complex-bound CtBP1-S/BARS (Valente et al., 2013). However, only PA, but not PtdIns4P, allowed CtBP1-S/BARS to induce liposome tubulation (Yang et al., 2008). Hence, PA, but not PtdIns4P, can be considered a lipid cofactor in CtBP1-S/BARS-mediated fission. However, often the information concerning particular lipid is incomplete. In this case, it is difficult to figure out if this lipid a true cofactor or protein-recruiting lipid.
Interestingly, two fission-inducing proteins, A. tumefaciens PmtA and mammalian CtBP1-S/BARS, are known to play a role in the synthesis of their own lipid cofactors. The difference between PmtA and CtBP1-S/BARS is that PmtA is itself an enzyme that produces its own lipid cofactor MMPE (Danne et al., 2017b), whereas CtBP1-S/BARS binds to and activates an enzyme, LPAATδ that catalyzes the synthesis of PA required for CtBP1-S/BARS-mediated membrane fission (Pagliuso et al., 2016; Zhukovsky et al., 2019b). Such production of lipid cofactors promoted by the very fission-inducing proteins and then using them guarantees availability of these lipids where and when they are needed.
Cardiolipin, PA, and PtdIns(4,5)P2 Act as Lipid Cofactors in Several Membrane Fission Reactions
We noted that the three negatively charged lipids PtdIns(4,5)P2, cardiolipin, and PA might play a role of lipid cofactors in several fission reactions mediated by different proteins. Cardiolipin is an unusual phospholipid that consists of two PA molecules connected with a glycerol backbone and thus contains two phosphates and four fatty acid tails. Cardiolipin is present in the membranes of most bacteria, whereas in mammalian and plant cells it is found mostly in the IMM and, to a lesser extent, in the OMM and in peroxisomes (Wriessnegger et al., 2007; Musatov and Sedlak, 2017; Pagliuso et al., 2018). PA containing a unique phosphomonoester headgroup is a minor phospholipid present in various organelles (Zhukovsky et al., 2019a). PtdIns(4,5)P2 is enriched in the PM of eukaryotic cells (Olivença et al., 2018; Dickson and Hille, 2019).
The role that cardiolipin and PA play in membrane fission reactions might be determined by their structure, namely combination of charge and shape (Danne et al., 2017b; Agrawal and Ramachandran, 2019; Zhukovsky et al., 2019a). Packing defects are locations on the membrane surface where the hydrophobic interior of the membrane is exposed to the solvent. AHs have a tendency to bind such defects induced by cone-shaped lipids, i.e., lipids whose tails are wider than their headgroups (Ouberai et al., 2013; Vanni et al., 2013; Lauwers et al., 2016). At physiological conditions, cardiolipin carries a charge −2 (Arnarez et al., 2016; Sathappa and Alder, 2016; Boyd et al., 2018; and references therein) and, moreover, has a cone shape (Arnarez et al., 2016; Carranza et al., 2017; Basu Ball et al., 2018; Su et al., 2018; and references therein). PA also has a cone shape (Kooijman et al., 2005). According to the electrostatic/hydrogen bond switch mechanism, the PA charge can change from −1 to −2 upon interaction with Lys or Arg (Kooijman et al., 2007). Hence, cone-shaped lipid PA, as cone-shaped lipid cardiolipin, is able to have charge −2. Combination of double negative charge and cone shape of cardiolipin and PA allows positively charged protein segments, such as AHs with basic residues on the hydrophilic face, to interact with the membranes containing these lipids. The combination of net charge and cone shape of cardiolipin seems to be responsible for the membrane-remodeling activity of PmtA (Danne et al., 2017b).
PtdIns(4,5)P2, like cardiolipin and PA, is a lipid cofactor in membrane fission reactions mediated by various proteins, such as epsin (Kweon et al., 2006; Boucrot et al., 2012; Lai et al., 2012), mammalian amphiphysin 2 (Lee et al., 2002; Wu and Baumgart, 2014), Drosophila amphiphysin (Yoon et al., 2012) and mammalian endophilin A1 (Yoon et al., 2012). Like cardiolipin and PA, PtdIns(4,5)P2 possesses a high negative charge. The charge of PtdIns(4,5)P2 depends on various factors, but at the physiological conditions, the charge of this lipid is approximately −4 (McLaughlin et al., 2002; Heo et al., 2006; Kooijman et al., 2009; and references therein). However, unlike cardiolipin and PA that have a cone shape, PtdIns(4,5)P2 and other phosphoinositides have an inverted-cone shape, i.e., their headgroups are wider than their tails (Martin, 2012; Suetsugu et al., 2014; McMahon and Boucrot, 2015; and references therein). Besides PtdIns(4,5)P2, other phosphoinositides also function as lipid cofactors in membrane fission processes, e.g., PtdIns3P and PtdIns(3,5)P2 for Atg18 (Gopaldass et al., 2017). We hypothesize that, despite of their shape, PtdIns(4,5)P2 and PtdIns(3,5)P2 are able to be lipid cofactors required for membrane fission reactions because of extremely high negative charge that recruits basic residues of the AHs of fission-inducing proteins to PBP-containing membranes due to the electrostatic attractions. If a PBP-containing membrane contains also cone-shaped lipids, membrane packing defects are present in such membrane, and some of these defects are present in the vicinity of PBP molecules. If a membrane contains cone-shaped lipids, membrane packing defects should be evenly distributed on the membrane surface (Vamparys et al., 2013). Co-localization of some of these defects with highly charged PBPs creates suitable condition for the recruitment of AHs belonging to the fission-inducing proteins.
Alternatively, we hypothesize that, while AH-containing fission-inducing proteins interacting with cardiolipin and PA play a major role in fission, AH-containing proteins that bind PtdIns(4,5)P2 often mediate membrane fission cooperatively with the proteins belonging to the dynamin superfamily (Boucrot et al., 2012, 2015; Meinecke et al., 2013). Possibly, interaction with cone-shaped lipids, such as cardiolipin and PA, is required for AH-containing proteins that mediate fission in the absence of proteins belonging to the dynamin superfamily, but is not required for AH-containing proteins that mediate fission cooperatively with the proteins belonging to this superfamily.
Of note, PS, phosphatidylinositol (PtdIns) and PG that possess net charge −1 at neutral pH (Li et al., 2015) are less frequently used as lipid cofactors in membrane fission processes, compared with PA, cardiolipin and PtdIns(4,5)P2 that possess higher negative charge.
Putative Sequence Motifs That Specifically Recognize Cardiolipin, PA, and PtdIns(4,5)P2
It is reasonable to hypothesize that AHs of proteins interacting specifically with each of three lipids cardiolipin, PA, PtdIns(4,5)P2, might contain sequence motifs that recognize these lipids. Primary sequences of the AHs of fission-inducing proteins that were shown or suggested to use cardiolipin and PtdIns(4,5)P2 as cofactors are presented in Tables 3, 4. We found that (K/R)x6(F/Y) motif is present in all but one AHs of cardiolipin-binding fission-inducing proteins we know, whereas (K/R/H)(K/R/H)xx(K/R/H) motif is present in most AHs of PtdIns(4,5)P2-binding fission-inducing proteins we know. Here K is Lys, R is Arg, F is Phe, Y is Tyr, H is His, and x is any residue. Although it is quite possible that the (K/R)x6(F/Y) and (K/R/H)(K/R/H)xx(K/R/H) motifs in some of AHs of fission-inducing proteins are not involved in the specific interaction with lipids, we suppose that in most cases these two motifs are functionally essential.
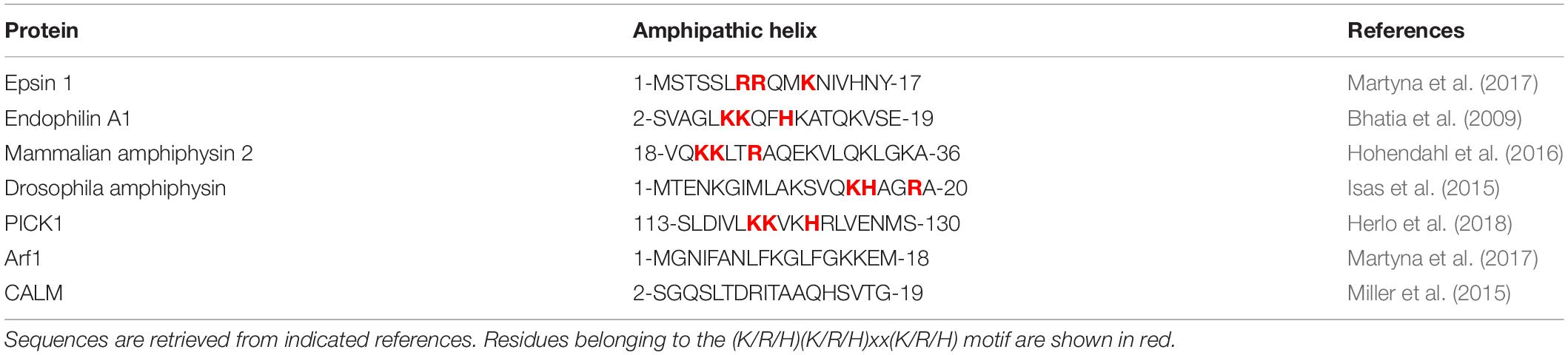
Table 4. Amphipathic helices of fission-inducing proteins that use PtdIns(4,5)P2 as a lipid cofactor.
Cardiolipin
Among proteins that are involved in membrane fission and are listed in the Tables 3, 4, the (K/R)x6(F/Y) motif is present in AHs of six out of seven cardiolipin-binding proteins, but absent in AHs of all seven PtdIns(4,5)P2-binding proteins. In general, (K/R)x6(F/Y) motifs in the AHs of various proteins are rare. Among 16 AHs shown in the Table 1 of Drin and Antonny (2010), this motif is present only in α-synuclein, a protein that binds cardiolipin (Nakamura et al., 2008; Robotta et al., 2014; Ryan et al., 2018). However, except proteins listed in the Table 3, this motif is present in the AHs of at least two proteins that interact with cardiolipin but, to the best of our knowledge, are not known to be involved in membrane fission: PmtA from Bradyrhizobium japonicum (Danne et al., 2017a) and ATG3 (Nath et al., 2014; Hervás et al., 2017). Arg14 and Phe21 belong to the (K/R)x6(F/Y) motif in the AH of PmtA from B. japonicum (Danne et al., 2017a), whereas Lys11 and Tyr18 belong to the (K/R)x6(F/Y) motif in the AH of Atg3 (Hervás et al., 2017). In the AH of cardiolipin-binding fission-inducing protein PmtA from A. tumefaciens (Danne et al., 2017b), both Lys12 and Phe19 belonging to the Kx6F motif are critical for membrane binding (Danne et al., 2015). Based on these observations, we make a conclusion that, most probably, many (K/R)x6(F/Y) pairs in the AHs of cardiolipin-binding proteins are functional cardiolipin-binding motifs.
In the protein α-helix, each amino acid residue corresponds to a 100° turn in the helix. (K/R)x6(F/Y) motif contains one basic residue (Arg or Lys) and one aromatic residue (Phe or Tyr) spaced roughly 700° in the circle, very close to 720° (two turns). Hence, basic residue and aromatic residue of the (K/R)x6(F/Y) motif are on the same face of the helix, two turns apart, see Figure 4. Such localization might be suitable for two residues to interact with the same cardiolipin molecule.
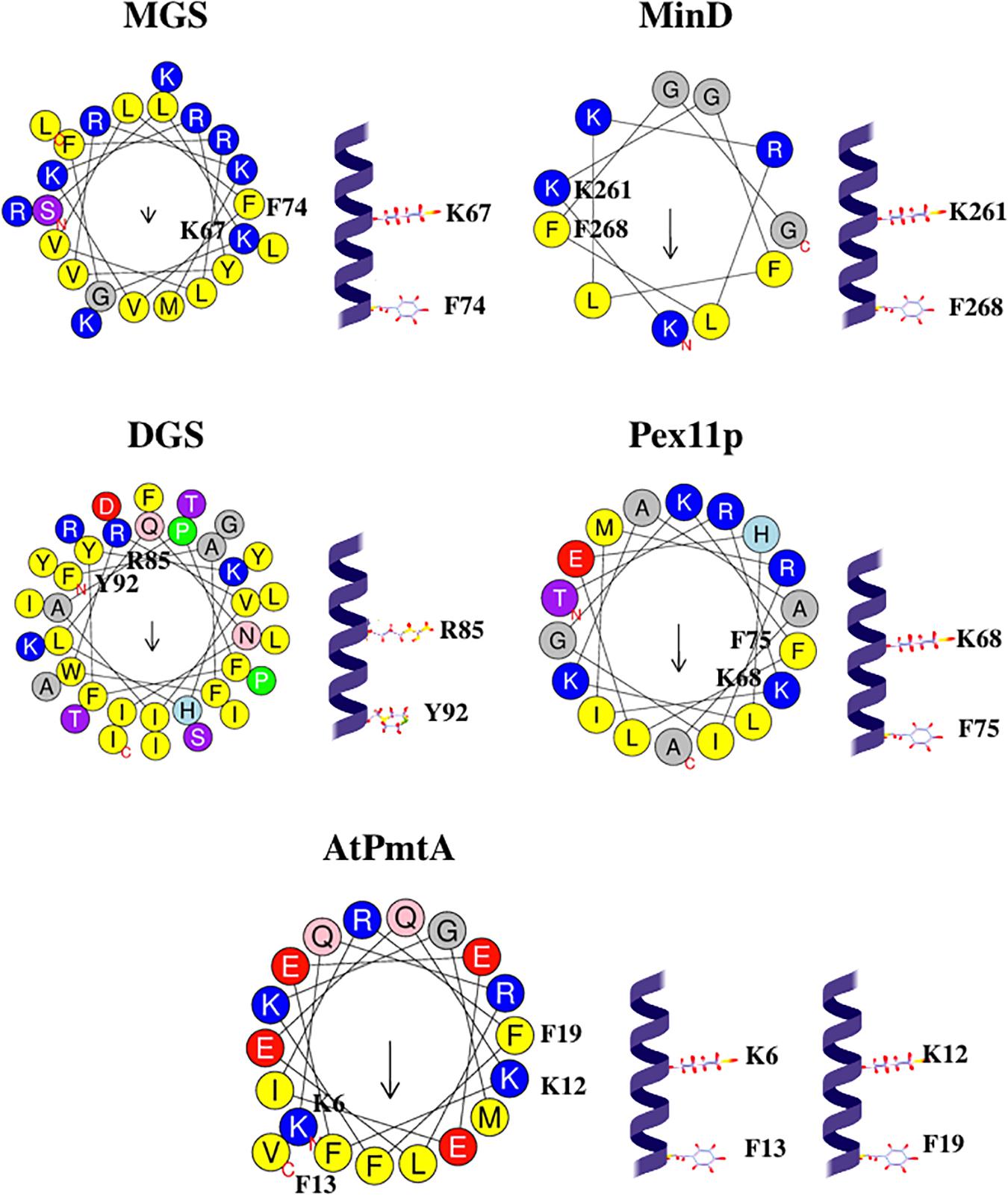
Figure 4. Configurations and helical wheel representations of some of the (K/R)x6(F/Y) motif-containing AHs belonging to the fission-inducing proteins that use cardiolipin as cofactor. Helical wheel projections were generated using Heliquest software (http://heliquest.ipmc.cnrs.fr; Gautier et al., 2008). Residues belonging to the (K/R)x6(F/Y) motifs are highlighted. Color coding for residues: yellow for hydrophobic, purple for Ser (S) and Thr (T), blue for Lys (K) and Arg (R), red for acidic, pink for Asn (N) and Gln (Q), gray for small residues (Ala, A and Gly, G), green for Pro (P), and light blue for His (H). The arrow in helical wheels corresponds to the hydrophobic moment. Species names and UniProt accession numbers: Acholeplasma laidlawii MGS, Q93P60; Escherichia coli MinD, P0AEZ3; A. laidlawii DGS, Q8KQL6; Penicillium chrysogenum Pex11p, B6GZG8; Agrobacterium tumefaciens AtPmtA, A0A2L2L7Q9.
Kx6F motifs in MGS and MinD contain positively charged Lys followed by Gly. Interestingly, a positively charged residue preceding flexibility-conferring Gly may be considered a footprint for cardiolipin binding (Planas-Iglesias et al., 2015).
In (K/R)x6(F/Y) motifs within AHs of cardiolipin-binding proteins, Gly is often present close to basic (Lys or Arg) and aromatic (Phe or Tyr) residues, see Table 3. Hence, in most (K/R)x6(F/Y) motifs, three residues are present: positively charged residue (Lys or Arg), aromatic residue (Phe or Tyr), and Gly. Such a combination of these three kinds of residues is somewhat similar to the cardiolipin-binding motif (Y/W/F)(K/R)G (Ruprecht et al., 2014; Kunji et al., 2016; Duncan et al., 2018), where W is Trp and G is Gly. We suppose that, as suggested for the (Y/W/F)(K/R)G motif, aromatic residues (Phe or Tyr) in (K/R)x6(F/Y) motif might be involved in the interaction with acyl chain (Ruprecht et al., 2014), whereas neighboring Gly might be involved in the interaction with phosphate groups (Kunji et al., 2016; Duncan et al., 2018).
PtdIns(4,5)P2
Lys, Arg, and His are basic amino acid residues. Hence, all three residues belonging to the putative PtdIns(4,5)P2-binding (K/R/H)(K/R/H)xx(K/R/H) motif are positively charged. This motif is present in five out of seven AHs of fission-inducing PtdIns(4,5)P2-binding proteins we know, but in AHs of only two out of seven cardiolipin-binding fission-inducing proteins we know, see Tables 3, 4. AH of Arf1 does not contain (K/R/H)(K/R/H)xx(K/R/H) motif: see Table 4. However, if we consider Arg19 adjacent to this AH, we find that Arf1 contains a K15K16xxR19 sequence that conforms to the (K/R/H)(K/R/H)xx(K/R/H) motif. Double mutation of two Lys belonging to this K15K16xxR19 motif to Leu affected Arf1 binding to PtdIns(4,5)P2 but not to PA (Randazzo, 1997). Moreover, (K/R/H)(K/R/H)xx(K/R/H) motifs are present in the AHs of at least three PtdIns(4,5)P2-binding proteins that, to the best of our knowledge, are not known to be involved in membrane fission: Spo20 (Horchani et al., 2014), STIM2 (Bhardwaj et al., 2013), and SH3YL1 (Hasegawa et al., 2011). His75, Lys76, and His79 belong to the (K/R/H)(K/R/H)xx(K/R/H) motif in the AH of Spo20 (Horchani et al., 2014); K732K733PSK736 and K742K743KSK746 are two (K/R/H)(K/R/H)xx(K/R/H) motifs in the AH of STIM2 (Bhardwaj et al., 2013), whereas Lys14, Lys15, and Lys18 belong to the (K/R/H)(K/R/H)xx(K/R/H) motif in the AH of SH3YL1 (Hasegawa et al., 2011). Residues belonging to the (K/R/H)(K/R/H)xx(K/R/H) motif within AHs play a role in the interaction with PtdIns(4,5)P2: Arg7, Arg8, Lys11 in epsin 1 (Ford et al., 2002; Lai et al., 2012) and Lys14, Lys15, Lys18 in SH3YL1 (Hasegawa et al., 2011). We make a conclusion that, most probably, many (K/R/H)(K/R/H)xx(K/R/H) motifs are functional PtdIns(4,5)P2-binding motifs in the AHs of various proteins that interact with this lipid.
Taking into account that each residue corresponds to a 100° turn in the protein α-helix, all three basic residues belonging to the (K/R/H)(K/R/H)xx(K/R/H) motif are located on the same face of the helix: see Figure 5. Such localization might be favorable for the interaction of these residues with the same PtdIns(4,5)P2 molecule. Possibly, in the (K/R/H)(K/R/H)xx(K/R/H) motifs of various proteins, two adjacent positively charged residues interact with 1- and 4-phosphate groups of PtdIns(4,5)P2, similar to the two Arg belonging to the R7R8xxK11 motif in epsin 1 (Lai et al., 2012).
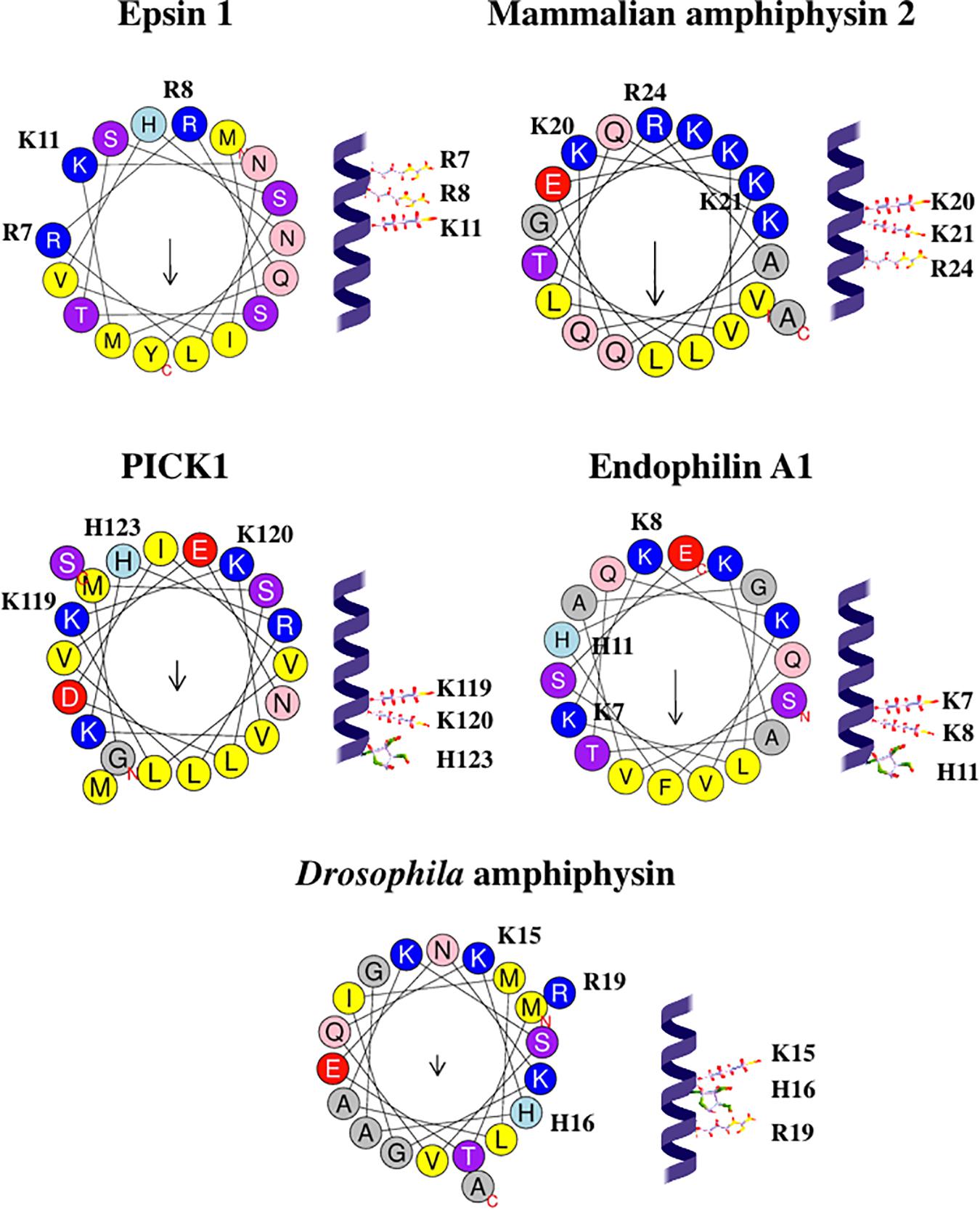
Figure 5. Configurations and helical wheel representations of the (K/R/H)(K/R/H)xx(K/R/H) motif-containing AHs belonging to the fission-inducing proteins that use PtdIns(4,5)P2 as cofactor. Helical wheel projections were generated using Heliquest software (http://heliquest.ipmc.cnrs.fr; Gautier et al., 2008). Residues belonging to the (K/R/H)(K/R/H)xx(K/R/H) motifs are highlighted. Color coding for residues: yellow for hydrophobic, purple for Ser (S) and Thr (T), blue for Lys (K) and Arg (R), red for acidic, pink for Asn (N) and Gln (Q), gray for small residues (Ala, A and Gly, G), green for Pro (P), and light blue for His (H). The arrow in helical wheels corresponds to the hydrophobic moment. Species names and UniProt accession numbers: Homo sapiens epsin 1, Q9Y6I3; Rattus norvegicus amphiphysin 2, O08839; R. norvegicus PICK1, Q9EP80; R. norvegicus endophilin A1, O35179; Drosophila melanogaster amphiphysin, Q7KLE5.
According to Nishimura et al. (2018), the BAR domain-containing proteins do not have any specific phosphoinositide-binding pockets. However, we hypothesize that (K/R/H)(K/R/H)xx(K/R/H) motif is a specific PtdIns(4,5)P2-binding motif in AHs (and, perhaps, other segments) of various proteins, including BAR domain-containing proteins. We predict that residues Lys7, Lys8, His11 in mammalian endophilin A1, residues Lys20, Lys21, Arg24 in mammalian amphiphysin 2, residues Lys15, His16, Arg19 in Drosophila amphiphysin are involved in the interaction with PtdIns(4,5)P2. We expect that mutations involving these residues will inhibit such interaction as well as membrane curvature generation and membrane fission mediated by these proteins.
Phosphatidic Acid
At least eight AHs of PA-binding proteins contain KxK (Lys – any residue – Lys) motifs, see Table 5. One of these proteins, α-synuclein, might mediate membrane fission (see above), whereas other seven proteins, Atg3 (Nath et al., 2014; Hervás et al., 2017), CDeT11-24 (Petersen et al., 2012), Opi1 (Loewen et al., 2004; Hofbauer et al., 2018), Spo20 (Horchani et al., 2014), DHN1 (Koag et al., 2003, 2009), CCT also known as Pcyt1a (Cornell, 2016), and Tam41 (Jiao et al., 2019), are not known to be involved in fission (to the best of our knowledge). In Atg3, residues Lys9 and Lys11, belonging to the KxK motif, are essential for membrane interaction (Hervás et al., 2017). In Opi1, mutation of five Lys (including Lys119 and Lys121 belonging to the KxK motif) to Arg leads to the loss of the preference for PA-containing over PS-containing liposomes (Hofbauer et al., 2018).
Amphipathic helices of few PA-binding proteins, including fission-inducing proteins endophilin B1 (Rostovtseva et al., 2009; Kjaerulff et al., 2011; Zhang et al., 2011) and Arf1 (Krauss et al., 2008; Beck et al., 2011; Martyna et al., 2017) do not contain KxK motifs. Moreover, the authors of Putta et al. (2016), Kassas et al. (2017), Zegarlińska et al. (2018), and Tanguy et al. (2019) stress that there is no known well-defined PA-binding motif or domain. However, here we hypothesize that KxK motif in AHs is involved in specific interaction with PA. It is possible that other sequence motifs recognizing PA will be discovered in the future.
If KxK motif is present in the protein α-helix (each amino acid residue corresponds to a 100° turn in the helix), two Lys belonging to the KxK motif are on two opposite faces of the helix, see Figure 6. Hence, in the AH, KxK motif usually places two Lys at the polar/non-polar interface. Such a position of basic residues is typical for AHs that interact with membranes (Mishra and Palgunachari, 1996; Davidson et al., 1998; Polozov et al., 1998; Mozsolits et al., 2004), especially with membranes that contain acidic phospholipids (Polozov et al., 1998). Most Lys residues in the AH of α-synuclein belong to the KxK motifs, and, interestingly, Perrin et al. (2000) stress that such localization of Lys along the polar/non-polar interface might be important for α-synuclein interaction with PA-containing membranes. At first sight, such localization of two Lys, at the opposite sides of an α-helix, is not optimal for the interaction with the same lipid molecule. However, Hofbauer et al. (2018) underline that KRK motif in Opi1 interacts specifically with PA. Moreover, this motif forms a “three-finger grip” that tightly binds the PA phosphate headgroup but fails to accommodate the larger PS headgroup (Hofbauer et al., 2018). We suppose that, similarly, KxK motif in the AH of other proteins might form a “two-finger grip” that binds specifically the PA phosphate headgroup, although with somewhat lower affinity compared with KRK motif, if the residue between two Lys is not positively charged.
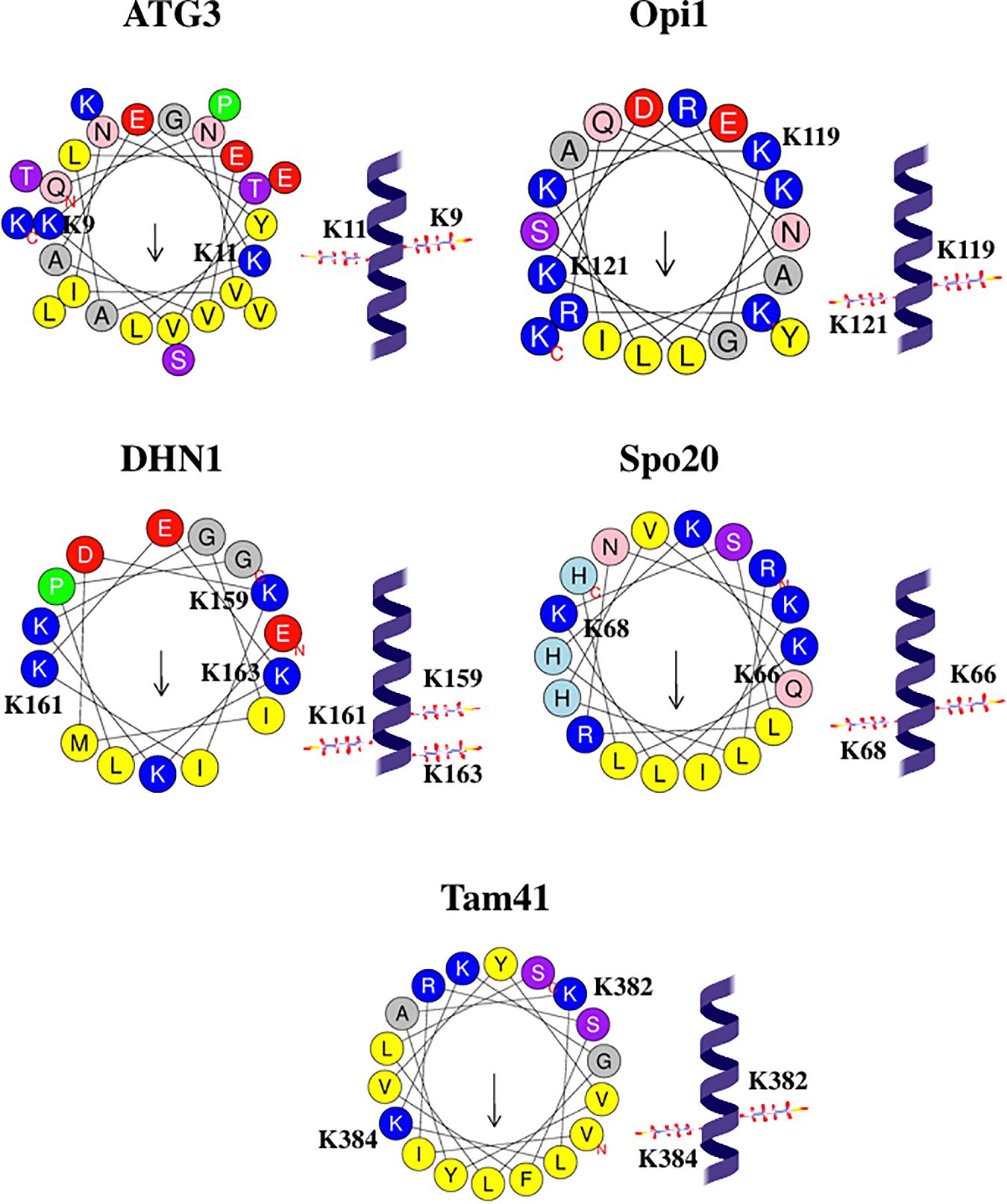
Figure 6. Configurations and helical wheel representations of some of the KxK motif-containing AHs belonging to the PA-binding proteins. Helical wheel projections were generated using Heliquest software (http://heliquest.ipmc.cnrs.fr; Gautier et al., 2008). Residues belonging to the KxK motifs are highlighted. Color coding for residues: yellow for hydrophobic, purple for Ser (S) and Thr (T), blue for Lys (K) and Arg (R), red for acidic, pink for Asn (N) and Gln (Q), gray for small residues (Ala, A and Gly, G), green for Pro (P), and light blue for His (H). The arrow in helical wheels corresponds to the hydrophobic moment. Species names and UniProt accession numbers: Homo sapiens ATG3, Q9NT62; Saccharomyces cerevisiae Opi1, P21957; Zea mays DHN1, P12950; S. cerevisiae Spo20, Q04359; Schizosaccharomyces pombe Tam41, O74339.
As we pointed out, the penetration of AHs into ca. 40% of monolayer thickness is optimal for generation of membrane curvature and driving membrane fission (Campelo et al., 2008; Zemel et al., 2008; Boucrot et al., 2012; Hanna et al., 2016). We hypothesize that the specific interaction of lipid cofactor with sequence motif ensures optimal depth of the insertion of AH into lipid bilayer. Insertion of AH approximately at the level of membrane hydrophilic-hydrophobic interface is optimal for generating membrane curvature (Li, 2018). The KxK motifs within PA-associated AH places two Lys close to this interface (see Figure 6) and, hence, is able to ensure shallow insertion of this AH into the membrane and generation of the high membrane curvature, required for driving membrane fission. Moreover, in few AHs of cardiolipin-binding proteins, such as AHs from MGS (Eriksson et al., 2009), AtPmtA (Danne et al., 2017b), Pex11p (Opaliński et al., 2011), the orientation of the (K/R)x6(F/Y) motif ensures the position of these two residues (basic Lys or Arg and aromatic Phe or Tyr) close to the hydrophilic-hydrophobic interface, so that basic residue is somewhat closer to the hydrophobic face of the helix, and aromatic residue is somewhat closer to the hydrophilic face of the helix, see Figure 4. We suggest that such orientation also ensures shallow insertion of the AH, that is optimal for driving fission.
Interestingly, α-synuclein that might use cardiolipin and, possibly, also PA as lipid cofactors in fission reaction (see above) contains, in its AH, putative cardiolipin-binding Kx6Y motif as well as five copies of putative PA-binding KxK motifs, see Tables 3, 5. Moreover, Atg3 that is not known to be involved in any membrane fission process, interacts with both cardiolipin and PA (Nath et al., 2014; Hervás et al., 2017), and contains, in its AH, putative cardiolipin-binding K11x6Y18 motif overlapping with the putative PA-binding K9xK11 motif.
We predict that mutations of residues belonging to the KxK motifs in AHs of PA-binding proteins as well as (K/R)x6(F/Y) motifs in AHs of cardiolipin-binding proteins will inhibit the binding of these proteins to the membranes containing these lipids. For proteins that possess an ability to generate membrane curvature or to mediate membrane fission, these processes will also be inhibitied in the mutants.
Interestingly, Lys in cardiolipin-binding and PA-binding AHs are much more frequent than Arg, see Tables 3, 5. Mutation of five Lys to Arg in Opi1 leads to the loss of the preference for PA-containing over PS-containing liposomes (Hofbauer et al., 2018). Due to its longer aliphatic side chain, Lys penetrate more deeply into lipid bilayer, and thus interaction of lipids with Lys might be more stable than interaction of lipids with Arg (Davidson et al., 1998). Furthermore, the increase in PA charge induced by Lys is higher than that induced by Arg (Kooijman et al., 2007). For these reasons, the presence of Lys in sequence motifs that recognize cardiolipin and PA is preferable to the presence of Arg.
Amphipathic helices of some of the fission-inducing proteins that use cardiolipin, PtdIns(4,5)P2 and PA as cofactors do not contain, correspondingly, (K/R)x6(F/Y) motif, (K/R/H)(K/R/H)xx(K/R/H) motif, and KxK motif. Other residues yet to be identified are therefore involved in the interaction with these lipids.
Characteristics of Lipid Cofactors Involved in Membrane Fission Processes in Different Organelles
We noted that in many membrane fission processes mediated by AH-containing proteins in a particular organelle or bacterium, the most abundant lipids in this organelle (or bacterium) possessing high negative charge (more negative than −1) play a role of lipid cofactors. Moreover, fission-inducing proteins often contain in their AHs sequence motifs that recognize these lipids. The following highly charged lipids are over-represented in specific organelles and hence might play the role of lipid cofactors with various fission-inducing proteins: cardiolipin in bacteria, mitochondria (Musatov and Sedlak, 2017; Pagliuso et al., 2018) and peroxisomes (Wriessnegger et al., 2007); PtdIns3P and PtdIns(3,4)P2 in the endosomes; PtdIns4P in the Golgi apparatus; PtdIns5P in the nuclear envelope; PtdIns(4,5)P2 in the PM; PtdIns(3,5)P2 in endosomes, lysosomes and vacuoles (Takatori et al., 2016; Olivença et al., 2018; Dickson and Hille, 2019).
Knowing the primary sequence of the AH of a fission-inducing proteins allows to anticipate which lipid is used by this protein as a cofactor in fission reactions, and which organelle this protein functions in, see Table 6. For example, eukaryotic fission protein containing (K/R)x6(F/Y) motif in its AH might be expected to use cardiolipin as cofactor and, hence, to mediate fission of mitochondria or peroxisomes. On the other hand, a fission-inducing protein that does not contain (K/R)x6(F/Y) motif but contains a (K/R/H)(K/R/H)xx(K/R/H) motif in its AH might use PtdIns(4,5)P2 as cofactor and, hence, might be involved in endocytosis.
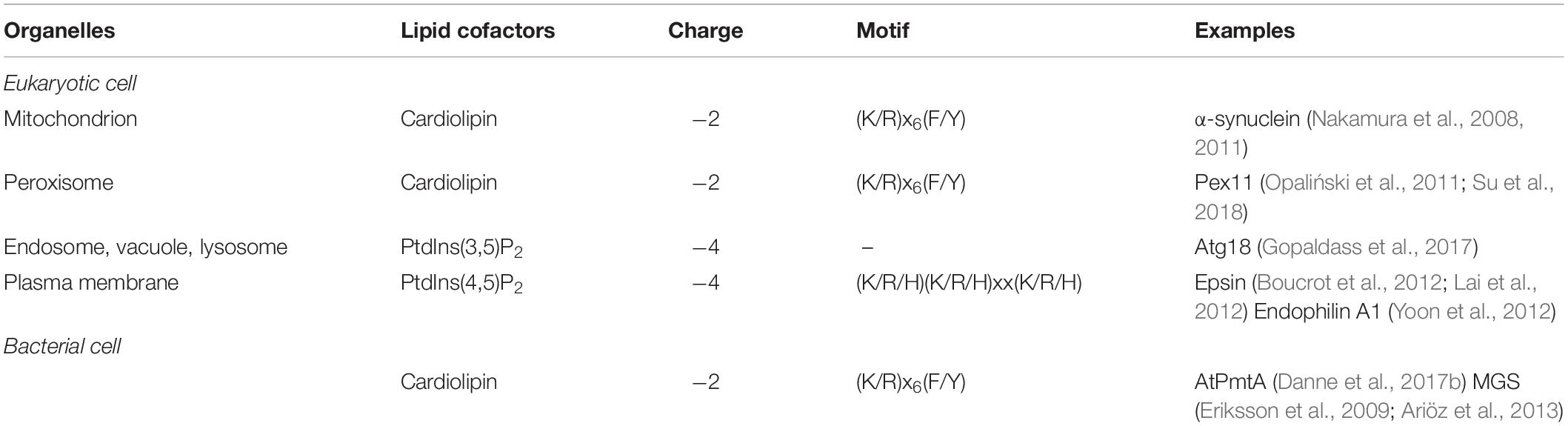
Table 6. Typical lipid cofactors that are used by fission-inducing proteins in different organelles and in bacterial cells, and putative sequence motifs within amphipathic helices of these proteins that specifically bind their lipid cofactors.
As pointed out above, we hypothesize that the most abundant organelle-specific lipid possessing high negative charge might play a role of a lipid cofactor in membrane fission processes.
We have already mentioned AH-containing proteins (such as α-synuclein, Pex11, PmtA, MGS, DGS, epsin, endophilin A1) that mediate fission in different organelles and follow this rule, see Table 6. Most probably, a similar pattern also applies to fission-inducing proteins in general. For example, cardiolipin is a putative lipid cofactor for FisB that mediates fission during sporulation in a bacterium B. subtilis (Doan et al., 2013). FisB is not known to contain any AH. Moreover, cardiolipin activates the mechanoenzyme Drp1 that plays an important role in mitochondrial fission (Francy et al., 2017). Besides being cofactor for fission mediated by AH-containing proteins such as epsin (Kweon et al., 2006; Boucrot et al., 2012; Lai et al., 2012) and endophilin A1 (Yoon et al., 2012), PtdIns(4,5)P2 is a putative cofactor for the mechanoenzyme dynamin (Jost et al., 1998) that is responsible for endocytosis, i.e., fission at PM. PtdIns4P, whose charge is between −2 and −3 at physiological pH (van Paridon et al., 1986), was suggested to play a direct role in membrane fission of Golgi membranes mediated by CtBP1-S/BARS (Yang et al., 2008; Valente et al., 2013). Moreover, besides being cofactor for vacuole fission mediated by AH-containing fission-inducing protein Atg18 (Gopaldass et al., 2017), PtdIns(3,5)P2 plays an important role in various fission processes in vacuoles and endo-lysosomes (Dove et al., 2009; Miner et al., 2019; and references therein). We are not aware of any role that PtdIns5P plays in any fission process. However, this phenomenon might be expected in an organelle such as the nuclear envelope, in which the content of this lipid is high, compared with other highly charged lipids. This type of reasoning can be applied to other phosphoinositides enriched in different organelles.
Oligomerization of Amphipathic Helix-Containing Fission-Inducing Proteins
An oligomeric structure is required for membrane fission and membrane curvature generation mediated by various AH-containing fission-inducing proteins. For example, Arf1 (Beck et al., 2008, 2011), Sar1 (Hariri et al., 2014), epsin 1 (Lai et al., 2012), endophilin A1 (Gallop et al., 2006; Mim et al., 2012; Yoon et al., 2012; Capraro et al., 2013), endophilin A2 (Gortat et al., 2012), Drosophila amphiphysin (Peter et al., 2004; Yoon et al., 2012), ANKHD1 (Kitamata et al., 2019), GDAP1 (Huber et al., 2016) form dimers. Endophilin B1 (Rostovtseva et al., 2009), PICK1 (Karlsen et al., 2015) and influenza virus M2 (Rossman et al., 2010) form tetramers (dimers of dimers). In some cases, dimers may direct the formation of higher order oligomers, such as, for example, in the case of epsin 1 (Yoon et al., 2010; Lai et al., 2012) and endophilin A1 (Gallop et al., 2006; Yoon et al., 2012). Moreover, dimers of Sar1 (Hariri et al., 2014), epsin 1 (Lai et al., 2012), and endophilin A1 (Mim et al., 2012; Capraro et al., 2013) were reported to form ordered lattices. Human Pex11B (Yoshida et al., 2015), fungal Pex11p (Su et al., 2018), Snf7 (Saksena et al., 2009), caveolin-1 (Fernandez et al., 2002), and α-synuclein (Nakamura et al., 2011) form oligomers. The protein mutations that disrupt their oligomerization inhibit the ability of Arf1 (Beck et al., 2008, 2011), epsin 1 (Yoon et al., 2010), and endophilin A2 (Gortat et al., 2012) to deform or vesiculate membranes.
Sometimes, lipid cofactors required for membrane fission events promote oligomerization of AH-containing fission-inducing proteins. Thus, PtdIns(3,4)P2 induces Atg18 oligomerization (Gopaldass et al., 2017), whereas cholesterol prevents disruption of influenza virus M2 tetramers (Ekanayake et al., 2016) and shifts these tetramers toward a more compact conformation (Herneisen et al., 2017). In general, lipid cofactors such as PtdIns(4,5)P2 for endophilin A1 and Drosophila amphiphysin (Yoon et al., 2012), can promote membrane penetration of the AHs and thus induce protein oligomerization upon membrane binding.
Formation of the oligomers of some AH-containing fission-inducing proteins, such as epsin 1 (Yoon et al., 2010), endophilin A1 (Mim et al., 2012), peroxins Pex11B (Yoshida et al., 2015) and Pex11p (Su et al., 2018), influenza virus M2 (Ekanayake et al., 2016), is driven, at least partially, by direct interaction of their AHs. Membrane curvature generated by the insertion of AH of fission-inducing protein into the membrane is amplified upon protein oligomerization due to the concerted penetration of multiple AHs, ultimately causing membrane tubulation or fission (Campelo et al., 2008; Yoon et al., 2010; Lai et al., 2012; Hariri et al., 2014; Pan et al., 2019).
Interestingly, oligomerization of endophilin B1 is induced by chaperone activity of the apoptosis regulator Bax (Rostovtseva et al., 2009). Bax does not stably associate with endophilin B1 oligomers. Despite of this, even when the concentration of Bax is 10 times lower than the concentration of endophilin B1, Bax induces endophilin B1 oligomerization, a structure required for membrane fission. As result, endophilin B1 with Bax induces massive vesiculation of liposomes, whereas endophilin B1 alone and Bax alone do not have such an effect (Rostovtseva et al., 2009). Interestingly, in normal cells, endophilin B1 and Bax do not interact. However, during apoptosis they bind transiently and, due to the membrane rearrangements mediated by oligomeric endophilin B1, they promote release of cytochrome C from mitochondria (Rostovtseva et al., 2009).
Diseases Associated With Mutations in the Amphipathic Helices of Fission-Inducing Proteins
The role that AHs play in the function of fission-inducing proteins is emphasized by the fact that few naturally occurring mutations of residues belonging to the AHs are associated with human diseases. Some of these mutants are defective in inducing membrane curvature, whereas other mutants generate membrane curvature more vigorously than wt proteins. Both kinds of mutations might contribute to the development of diseases.
Amphiphysin 2 (also known as BIN1) contains an AH (Nicot et al., 2007; Böhm et al., 2014; Hohendahl et al., 2016) and induces membrane vesiculation (Wu and Baumgart, 2014). Deletion mutation ΔLys21 and substitution mutations Arg24Cys and Lys35Asn (Nicot et al., 2007; Böhm et al., 2014) within the AH Val18-Ala36 (Hohendahl et al., 2016) of amphiphysin 2 cause CNM. Unlike wt amphiphysin 2, none of these three mutants induce membrane tubulation (Nicot et al., 2007; Böhm et al., 2014). Nicot et al. (2007) and Hohendahl et al. (2016) underlined that these mutations change the charge of the hydrophilic face of the AH, and such change might disrupt the interaction with negatively charged lipids of the membrane and, in turn, may lead to a defect in the ability to generate membrane curvature.
Expression of GDAP1 induces fission of peroxisomes and mitochondria, and this fission is critically dependent on the AH Val292-Phe309 (Huber et al., 2016). The Asn297Lys mutation into the AH is associated with Charcot-Marie-Tooth disease (Moroni et al., 2009).
α-Synuclein induces mitochondrial fragmentation (Kamp et al., 2010; Nakamura et al., 2011; O’Donnell et al., 2014; Martinez et al., 2018) possibly explained by its role in membrane fission (Varkey et al., 2010; Nakamura et al., 2011; Fakhree et al., 2019). α-Synuclein contains a long AH that forms on membrane binding (George et al., 1995; Davidson et al., 1998; Drin and Antonny, 2010; Braun et al., 2017), and the insertion of this AH into membranes contributes to membrane curvature generation (Braun et al., 2014, 2017; Fakhree et al., 2019). The role of α-synuclein in mitochondrial fission was suggested to be related to the pathogenesis of Parkinson’s disease (Nakamura et al., 2011; Pozo Devoto and Falzone, 2017). Indeed, a few naturally occurring mutations of residues belonging to the α-synuclein AH (Pozo Devoto et al., 2017) are associated with Parkinson’s disease: Ala30Pro (Krüger et al., 1998), Glu46Lys (Zarranz et al., 2004), His50Gln (Appel-Cresswell et al., 2013), Gly51Asp (Kiely et al., 2013; Lesage et al., 2013), Ala53Thr (Polymeropoulos et al., 1997), and Ala53Glu (Pasanen et al., 2014). Overexpression of the Ala53Thr α-synuclein mutant led to more significant reductions of mitochondrial size than the overexpression of wt α-synuclein (Pozo Devoto et al., 2017). Uncontrolled mitochondrial fragmentation might contribute to the development of Parkinson’s disease (Varkey et al., 2010; Panchal and Tiwari, 2019). Hence, the role of Ala53Thr mutation in the development of Parkinson’s disease might be, at least partially, explained by the extensive mitochondrial fragmentation mediated by this mutant involving residue belonging to the AH.
In the future, the discovery of new naturally occurring mutations of residues within the AHs of fission-inducing proteins will facilitate the ultimate definition of the role that the interaction of AHs with the membranes play in the pathogenesis of the related diseases.
Conclusion
In this review, we have considered fission-inducing proteins that contain AHs. Other fission-inducing proteins are known (Campelo and Malhotra, 2012; Frolov et al., 2015; Renard et al., 2015) indicating that the molecular events finally causing a bilayer to divide may follow different strategies. This would parallel the case of membrane fusion, where the structures of fusion-inducing proteins belonging to different classes are very different from each other and mechanisms of membrane rearrangements mediated by these proteins are also dissimilar (Kielian, 2014; Podbilewicz, 2014). The aspects that remain to be elucidated in both membrane fusion and fission are indeed the very last steps of these processes. While it is now relatively clear how the hemifission (or hemifusion) intermediate is obtained by the fission- or fusion-inducing proteins (see above and Chernomordik and Kozlov, 2008; Podbilewicz, 2014), the disruption of this structure and formation of the two bilayers (or their fusion) is an energy-demanding event not yet fully clarified. Protein rearrangements, lipid modifications or synthesis, GTP/GDP can all potentially contribute to this energy requirement. How they function, or if other molecules or sources of energy intervene, is still matter of debate. These are fascinating aspects in the membrane dynamics field that we are confident will be solved in the near future thanks to the continuously improved knowledge of the systems, together with the new morphological and instrumental tools being developed.
Author Contributions
MZ, AL, DC, and CV wrote the manuscript. AF and CV made the figures. MZ and AF made the tables.
Funding
The work in the authors’ laboratories was supported by the Italian Association for Cancer Research (AIRC) (DC: IG10341 and IG18776; AL: IG20786 and IG15767), the AIRC-Fondazione Cariplo TRansforming IDEas in Oncological research project (TRIDEO) (CV: IG17524), the PRONAT project, the PRIN project no. 20177XJCHX, the SATIN POR project 2014–2020, and the Italian-MIUR Cluster project Medintech (CNT01_00177_962865).
Conflict of Interest
The authors declare that the research was conducted in the absence of any commercial or financial relationships that could be construed as a potential conflict of interest.
Acknowledgments
We thank the funding agencies for supporting our research and Dr. R. Le Donne for some help in figure preparation.
Abbreviations
AH, amphipathic helix; ANKHD1, ankyrin repeat and KH domain-containing protein 1; Arf, ADP ribosylation factor; ArfGAP1, ADPribosylation factor GTPase-activating protein 1; Atg, autophagy-related protein; ATP, adenosine triphosphate; AtPmtA, Agrobacterium tumefaciens phospholipid N-methyltransferase; BAR, Bin/Amphiphysin/Rvs; BDLP1, bacterial dynaminlike protein 1; Bif-1, Bax-interacting factor 1; BIN1, bridging integrator-1; CALM, Clathrin Assembly Lymphoid-Myeloid leukemia protein; CCT, cytidine triphosphate:phosphocholine cytidylyltransferase; CCVs, clathrin-coated vesicles; CdvC, Cell division protein C; CL, cardiolipin; CME, clathrin-mediated endocytosis; CNM, centronuclear myopathy; COP, coat protein; CtBP1-S/BARS, C-terminal binding protein 1 shorter isoform/brefeldin A ADP-ribosylated substrate; DGS, diglucosyldiacylglycerol synthase; DHN1, dehydrin 1; Dnm2, dynamin2; DRP, dynamin-related protein; EcMurG, Escherichia coli MurG; EHD1, Eps15-homology domain-containing protein 1; ENTH/ANTH, Epsin N-Terminal Homology/AP180 N-Terminal Homology; EPR, electron paramagnetic resonance; ER, endoplasmic reticulum; ESCRT, endosomal sorting complexes required for transport; F-BAR, FCH-BAR; FEME, fast endophilin-mediated endocytosis; FisB, fission protein B; GDAP1, Ganglioside-induced Differentiation Associated Protein 1; GDP, guanosine diphosphate; GMAP-210, Golgi Microtubule-Associated Protein 210; GTP, guanosine triphosphate; GUVs, giant unilamellar vesicles; I-BAR, Inverse BAR; IMM, inner mitochondrial membrane; INF2, inverted formin-2; LPAAT, lysophosphatidic acid acyltransferase; MFF, mitochondrial fission factor; MGS, monoglucosyldiacylglycerol synthase; MiD49 and MiD51, mitochondrial dynamics proteins 49 and 51; MinD, septum site-determining protein MinD; MMPE, monomethyl-phosphatidylethanolamine; MurG, N-acetylmuramyl-(pentapeptide) pyrophosphoryl-undecaprenol N-acetylglucosamine transferase; N-BAR, N-terminal amphipathic helixcontaining BAR; OMM, outer mitochondrial membrane; Opi1, overproduction of inositol 1; PA, phosphatidic acid; PBP, phosphatidylinositol bisphosphate; PC, phosphatidylcholine; Pcyt1a, cytidine triphosphate:phosphocholine cytidylyltransferase alpha; Pex11, peroxisomal membrane protein 11; PG, phosphatidylglycerol; PICK1, protein interacting with C kinase 1; PM, plasma membrane; PmtA, phospholipid N-methyltransferase; POPG, 1-palmitoyl-2-oleoyl-sn-glycero-3-phosphatidylglycerol; PROPPIN, β-propellers that bind polyphosphoinositides; PS, phosphatidylserine; PtdIns, phosphatidylinositol; PtdIns3P, phosphatidylinositol 3-phosphate; PtdIns4P, phosphatidylinositol 4-phosphate; PtdIns5P, phosphatidylinositol 5-phosphate; PtdIns(3,4)P2, phosphatidylinositol 3,4-bisphosphate; PtdIns(3,5)P2, phosphatidylinositol 3,5-bisphosphate; PtdIns(3,4,5)P2, phosphatidylinositol 3,4,5-trisphosphate; PtdIns(4,5)P2, phosphatidylinositol 4,5-bisphosphate; Sar, secretion-associated Ras-related protein; SH3YL1, SRC homology 3 domain containing Ysc84-like 1; SNCA, α-synuclein; Snf7, sucrose non-fermenting protein 7; Spo20, sporulationspecific protein 20; STIM2, stromal interaction molecule 2; Tam41, translocator assembly and maintenance protein 41; VHS, Vps27, Hrs, and STAM; VP40, viral protein 40; Vps4, Vacuolar protein sorting-associated protein 4A; wt, wild type.
References
Adachi, Y., Itoh, K., Yamada, T., Cerveny, K. L., Suzuki, T. L., Macdonald, P., et al. (2016). Coincident phosphatidic acid interaction restrains Drp1 in mitochondrial division. Mol. Cell 63, 1034–1043. doi: 10.1016/j.molcel.2016.08.013
Adolf, F., Herrmann, A., Hellwig, A., Beck, R., Brügger, B., and Wieland, F. T. (2013). Scission of COPI and COPII vesicles is independent of GTP hydrolysis. Traffic 14, 922–932. doi: 10.1111/tra.12084
Adu-Gyamfi, E., Johnson, K. A., Fraser, M. E., Scott, J. L., Soni, S. P., Jones, K. R., et al. (2015). Host cell plasma membrane phosphatidylserine regulates the assembly and budding of Ebola virus. J. Virol. 89, 9440–9453. doi: 10.1128/jvi.01087-15
Agrawal, A., and Ramachandran, R. (2019). Exploring the links between lipid geometry and mitochondrial fission: emerging concepts. Mitochondrion. doi: 10.1016/j.mito.2019.07.010 [Epub ahead of print]
Ahmed, I., Akram, Z., Iqbal, H. M. N., and Munn, A. L. (2019). The regulation of Endosomal Sorting Complex Required for Transport and accessory proteins in multivesicular body sorting and enveloped viral budding - an overview. Int. J. Biol. Macromol. 127, 1–11. doi: 10.1016/j.ijbiomac.2019.01.015
Ahmed, S., Bu, W., Lee, R. T. C., Maurer-Stroh, S., and Goh, W. I. (2010). F-BAR domain proteins: families and function. Commun. Integr. Biol. 3, 116–121. doi: 10.4161/cib.3.2.10808
Albesa-Jové, D., Giganti, D., Jackson, M., Alzari, P. M., and Guerin, M. E. (2014). Structure–function relationships of membrane-associated GT-B glycosyltransferases. Glycobiology 24, 108–124. doi: 10.1093/glycob/cwt101
Ambroso, M. R., Hegde, B. G., and Langen, R. (2014). Endophilin A1 induces different membrane shapes using a conformational switch that is regulated by phosphorylation. Proc. Natl. Acad. Sci. U.S.A. 111, 6982–6987. doi: 10.1073/pnas.1402233111
Antonny, B., Beraud-Dufour, S., Chardin, P., and Chabre, M. (1997). N-terminal hydrophobic residues of the G-protein ADP-ribosylation factor-1 insert into membrane phospholipids upon GDP to GTP exchange. Biochemistry 36, 4675–4684. doi: 10.1021/bi962252b
Antonny, B., Burd, C., De Camilli, P., Chen, E., Daumke, O., Faelber, K., et al. (2016). Membrane fission by dynamin: what we know and what we need to know. EMBO J. 35, 2270–2284.
Appel-Cresswell, S., Vilarino-Guell, C., Encarnacion, M., Sherman, H., Yu, I., Shah, B., et al. (2013). α-synuclein p.H50Q, a novel pathogenic mutation for Parkinson’s disease. Mov. Disord. 28, 811–813. doi: 10.1002/mds.25421
Ariotti, N., Rae, J., Leneva, N., Ferguson, C., Loo, D., Okano, S., et al. (2015). Molecular characterization of caveolin-induced membrane curvature. J. Biol. Chem. 290, 24875–24890. doi: 10.1074/jbc.m115.644336
Ariöz, C., Gotzke, H., Lindholm, L., Eriksson, J., Edwards, K., Daley, D. O., et al. (2014). Heterologous overexpression of a monotopic glucosyltransferase (MGS) induces fatty acid remodeling in Escherichia coli membranes. Biochim. Biophys. Acta 1838, 1862–1870. doi: 10.1016/j.bbamem.2014.04.001
Ariöz, C., Ye, W., Bakali, A., Ge, C., Liebau, J., Götzke, H., et al. (2013). Anionic lipid binding to the foreign protein MGS provides a tight coupling between phospholipid synthesis and protein overexpression in Escherichia coli. Biochemistry 52, 5533–5544. doi: 10.1021/bi400616n
Arnarez, C., Marrink, S. J., and Periole, X. (2016). Molecular mechanism of cardiolipin-mediated assembly of respiratory chain supercomplexes. Chem. Sci. 7, 4435–4443. doi: 10.1039/c5sc04664e
Bacia, K., Futai, E., Prinz, S., Meister, A., Daum, S., Glatte, D., et al. (2011). Multibudded tubules formed by COPII on artificial liposomes. Sci. Rep. 1:17.
Bailer, S. M. (2017). Venture from the interior-herpesvirus pUL31 escorts capsids from nucleoplasmic replication compartments to sites of primary envelopment at the inner nuclear membrane. Cells 6:E46.
Barneda, D., Planas-Iglesias, J., Gaspar, M. L., Mohammadyani, D., Prasannan, S., Dormann, D., et al. (2015). The brown adipocyte protein CIDEA promotes lipid droplet fusion via a phosphatidic acid-binding amphipathic helix. eLife 4:e07485.
Bashkirov, P. V., Akimov, S. A., Evseev, A. I., Schmid, S. L., Zimmerberg, J., and Frolov, V. A. (2008). GTPase cycle of dynamin is coupled to membrane squeeze and release, leading to spontaneous fission. Cell 135, 1276–1286. doi: 10.1016/j.cell.2008.11.028
Basu Ball, W., Neff, J. K., and Gohil, V. M. (2018). The role of nonbilayer phospholipids in mitochondrial structure and function. FEBS Lett. 592, 1273–1290. doi: 10.1002/1873-3468.12887
Baumgart, T., Capraro, B. R., Zhu, C., and Das, S. L. (2011). Thermodynamics and mechanics of membrane curvature generation and sensing by proteins and lipids. Annu. Rev. Phys. Chem. 62, 483–506. doi: 10.1146/annurev.physchem.012809.103450
Beck, R., Prinz, S., Diestelkotter-Bachert, P., Rohling, S., Adolf, F., Hoehner, K., et al. (2011). Coatomer and dimeric ADP ribosylation factor 1 promote distinct steps in membrane scission. J. Cell Biol. 194, 765–777. doi: 10.1083/jcb.201011027
Beck, R., Sun, Z., Adolf, F., Rutz, C., Bassler, J., Wild, K., et al. (2008). Membrane curvature induced by Arf1-GTP is essential for vesicle formation. Proc. Natl. Acad. Sci. U.S.A. 105, 11731–11736. doi: 10.1073/pnas.0805182105
Bhardwaj, R., Müller, H. M., Nickel, W., and Seedorf, M. (2013). Oligomerization and Ca2 +/calmodulin control binding of the ER Ca2 +-sensors STIM1 and STIM2 to plasma membrane lipids. Biosci. Rep. 33:e00077.
Bhatia, V. K., Madsen, K. L., Bolinger, P. Y., Kunding, A., Hedegård, P., Gether, U., et al. (2009). Amphipathic motifs in BAR domains are essential for membrane curvature sensing. EMBO J. 28, 3303–3314. doi: 10.1038/emboj.2009.261
Bielli, A., Haney, C. J., Gabreski, G., Watkins, S. C., Bannykh, S. I., and Aridor, M. (2005). Regulation of Sar1 NH2 terminus by GTP binding and hydrolysis promotes membrane deformation to control COPII vesicle fission. J. Cell Biol. 171, 919–924. doi: 10.1083/jcb.200509095
Bigalke, J. M., and Heldwein, E. E. (2015). Structural basis of membrane budding by the nuclear egress complex of herpesviruses. EMBO J. 34, 2921–2936. doi: 10.15252/embj.201592359
Bitto, N. J., and Kaparakis-Liaskos, M. (2017). The therapeutic benefit of bacterial membrane vesicles. Int. J. Mol. Sci. 18:E1287.
Böhm, J., Biancalana, V., Malfatti, E., Dondaine, N., Koch, C., Vasli, N., et al. (2014). Adult-onset autosomal dominant centronuclear myopathy due to BIN1 mutations. Brain 137, 3160–3170. doi: 10.1093/brain/awu272
Bohuszewicz, O., Liu, J., and Low, H. H. (2016). Membrane remodelling in bacteria. J. Struct. Biol. 196, 3–14. doi: 10.1016/j.jsb.2016.05.010
Bonazzi, M., Spanò, S., Turacchio, G., Cericola, C., Valente, C., Colanzi, A., et al. (2005). CtBP3/BARS drives membrane fission in dynamin-independent transport pathways. Nat. Cell Biol. 7, 570–580. doi: 10.1038/ncb1260
Bottanelli, F., Kilian, N., Ernst, A. M., Rivera-Molina, F., Schroeder, L. K., Kromann, E. B., et al. (2017). A novel physiological role for ARF1 in the formation of bidirectional tubules from the Golgi. Mol. Biol. Cell 28, 1676–1687. doi: 10.1091/mbc.e16-12-0863
Boucrot, E., Ferreira, A. P. A., Almeida-Souza, L., Debard, S., Vallis, Y., Howard, G., et al. (2015). Endophilin marks and controls a clathrin-independent endocytic pathway. Nature 517, 460–465. doi: 10.1038/nature14067
Boucrot, E., Pick, A., Çamdere, G., Liska, N., Evergren, E., McMahon, H. T., et al. (2012). Membrane fission is promoted by insertion of amphipathic helices and is restricted by crescent BAR domains. Cell 149, 124–136. doi: 10.1016/j.cell.2012.01.047
Boyd, K. J., Alder, N. N., and May, E. R. (2018). Molecular dynamics analysis of cardiolipin and monolysocardiolipin on bilayer properties. Biophys. J. 114, 2116–2127. doi: 10.1016/j.bpj.2018.04.001
Brady, J. P., Claridge, J. K., Smith, P. G., and Schnell, J. R. (2015). A conserved amphipathic helix is required for membrane tubule formation by Yop1p. Proc. Natl. Acad. Sci. U.S.A. 112, E639–E648.
Braun, A. R., Lacy, M. M., Ducas, V. C., Rhoades, E., and Sachs, J. N. (2014). α-Synuclein-induced membrane remodeling is driven by binding affinity, partition depth, and interleaflet order asymmetry. J. Am. Chem. Soc. 136, 9962–9972. doi: 10.1021/ja5016958
Braun, A. R., Lacy, M. M., Ducas, V. C., Rhoades, E., and Sachs, J. N. (2017). α-Synuclein’s uniquely long amphipathic helix enhances its membrane binding and remodeling capacity. J. Membr. Biol. 250, 183–193. doi: 10.1007/s00232-017-9946-1
Brooks, A., Shoup, D., Kustigian, L., Puchalla, J., Carr, C. M., and Rye, H. S. (2015). Single particle fluorescence burst analysis of epsin induced membrane fission. PLoS One 10:e0119563. doi: 10.1371/journal.pone.0119563
Buchkovich, N. J., Henne, W. M., Tang, S., and Emr, S. D. (2013). Essential N-terminal insertion motif anchors the ESCRT-III filament during MVB vesicle formation. Dev. Cell 27, 201–214. doi: 10.1016/j.devcel.2013.09.009
Campelo, F., and Malhotra, V. (2012). Membrane fission: the biogenesis of transport carriers. Annu. Rev. Biochem. 81, 407–427. doi: 10.1146/annurev-biochem-051710-094912
Campelo, F., McMahon, H. T., and Kozlov, M. M. (2008). The hydrophobic insertion mechanism of membrane curvature generation by proteins. Biophys. J. 95, 2325–2339. doi: 10.1529/biophysj.108.133173
Capraro, B. R., Shi, Z., Wu, T., Chen, Z., Dunn, J. M., Rhoades, E., et al. (2013). Kinetics of endophilin N-BAR domain dimerization and membrane interactions. J. Biol. Chem. 288, 12533–12543. doi: 10.1074/jbc.m112.435511
Carman, P. J., and Dominguez, R. (2018). BAR domain proteins-a linkage between cellular membranes, signaling pathways, and the actin cytoskeleton. Biophys. Rev. 10, 1587–1604. doi: 10.1007/s12551-018-0467-7
Carranza, G., Angius, F., Ilioaia, O., Solgadi, A., Miroux, B., and Arechaga, I. (2017). Cardiolipin plays an essential role in the formation of intracellular membranes in Escherichia coli. Biochim. Biophys. Acta Biomembr. 1859, 1124–1132. doi: 10.1016/j.bbamem.2017.03.006
Caspi, Y., and Dekker, C. (2018). Dividing the archaeal way: the ancient Cdv cell-division machinery. Front. Microbiol. 9:174. doi: 10.3389/fmicb.2018.00174
Cevher-Keskin, B. (2013). ARF1 and SAR1 GTPases in endomembrane trafficking in plants. Int. J. Mol. Sci. 14, 18181–18199. doi: 10.3390/ijms140918181
Chen, Z., Zhu, C., Kuo, C. J., Robustelli, J., and Baumgart, T. (2016). The N-terminal amphipathic helix of endophilin does not contribute to its molecular curvature generation capacity. J. Am. Chem. Soc. 138, 14616–14622. doi: 10.1021/jacs.6b06820
Chernomordik, L. V., and Kozlov, M. M. (2008). Mechanics of membrane fusion. Nat. Struct. Mol. Biol. 15, 675–683. doi: 10.1038/nsmb.1455
Chiaruttini, N., and Roux, A. (2017). Dynamic and elastic shape transitions in curved ESCRT-III filaments. Curr. Opin. Cell Biol. 47, 126–135. doi: 10.1016/j.ceb.2017.07.002
Chong, S. S. Y., Taneva, S. G., Lee, J. M. C., and Cornell, R. B. (2014). The curvature sensitivity of a membrane-binding amphipathic helix can be modulated by the charge on a flanking region. Biochemistry 53, 450–461. doi: 10.1021/bi401457r
Chowdhury, S., Otomo, C., Leitner, A., Ohashi, K., Aebersold, R., Lander, G. C., et al. (2018). Insights into autophagosome biogenesis from structural and biochemical analyses of the ATG2A-WIPI4 complex. Proc. Natl. Acad. Sci. U.S.A. 115, E9792–E9801.
Chuartzman, S. G., Nevo, R., Shimoni, E., Charuvi, D., Kiss, V., Ohad, I., et al. (2008). Thylakoid membrane remodeling during state transitions in Arabidopsis. Plant Cell 20, 1029–1039. doi: 10.1105/tpc.107.055830
Colanzi, A., Hidalgo Carcedo, C., Persico, A., Cericola, C., Turacchio, G., Bonazzi, M., et al. (2007). The Golgi mitotic checkpoint is controlled by BARS-dependent fission of the Golgi ribbon into separate stacks in G2. EMBO J. 26, 2465–2476. doi: 10.1038/sj.emboj.7601686
Corda, D., Colanzi, A., and Luini, A. (2006). The multiple activities of CtBP/BARS proteins: the Golgi view. Trends Cell Biol. 16, 167–173. doi: 10.1016/j.tcb.2006.01.007
Corda, D., Iurisci, C., and Berrie, C. P. (2002). Biological activities and metabolism of the lysophosphoinositides and glycerophosphoinositols. Biochim. Biophys. Acta 1582, 52–69. doi: 10.1016/s1388-1981(02)00137-3
Cornell, R. B. (2016). Membrane lipid compositional sensing by the inducible amphipathic helix of CCT. Biochim. Biophys. Acta 1861, 847–861. doi: 10.1016/j.bbalip.2015.12.022
Cornell, R. B., and Taneva, S. G. (2006). Amphipathic helices as mediators of the membrane interaction of amphitropic proteins, and as modulators of bilayer physical properties. Curr. Protein Pept. Sci. 7, 539–552. doi: 10.2174/138920306779025675
Daly, J. L., and Cullen, P. J. (2018). Endoplasmic reticulum-endosome contact sites: specialized interfaces for orchestrating endosomal tubule fission? Biochemistry 57, 6738–6740. doi: 10.1021/acs.biochem.8b01176
Danne, L., Aktas, M., Gleichenhagen, J., Grund, N., Wagner, D., Schwalbe, H., et al. (2015). Membrane-binding mechanism of a bacterial phospholipid N-methyltransferase. Mol. Microbiol. 95, 313–331. doi: 10.1111/mmi.12870
Danne, L., Aktas, M., Grund, N., Bentler, T., Erdmann, R., and Narberhaus, F. (2017a). Dissection of membrane-binding and -remodeling regions in two classes of bacterial phospholipid N-methyltransferases. Biochim. Biophys. Acta Biomembr. 1859, 2279–2288. doi: 10.1016/j.bbamem.2017.09.013
Danne, L., Aktas, M., Unger, A., Linke, W. A., Erdmann, R., and Narberhaus, F. (2017b). Membrane remodeling by a bacterial phospholipid-methylating enzyme. mBio 8:e02082-16.
Daumke, O., and Praefcke, G. J. K. (2016). Invited review: mechanisms of GTP hydrolysis and conformational transitions in the dynamin superfamily. Biopolymers 105, 580–593. doi: 10.1002/bip.22855
Davidson, W. S., Jonas, A., Clayton, D. F., and George, J. M. (1998). Stabilization of α-synuclein secondary structure upon binding to synthetic membranes. J. Biol. Chem. 273, 9443–9449. doi: 10.1074/jbc.273.16.9443
De Craene, J.-O., Ripp, R., Lecompte, O., Thompson, J. D., Poch, O., and Friant, S. (2012). Evolutionary analysis of the ENTH/ANTH/VHS protein superfamily reveals a coevolution between membrane trafficking and metabolism. BMC Genomics 13:297. doi: 10.1186/1471-2164-13-297
Del Vecchio, K., Frick, C. T., Gc, J. B., Oda, S.-I., Gerstman, B. S., Saphire, E. O., et al. (2018). A cationic, C-terminal patch and structural rearrangements in Ebola virus matrix VP40 protein control its interactions with phosphatidylserine. J. Biol. Chem. 293, 3335–3349. doi: 10.1074/jbc.m117.816280
Dickson, E. J., and Hille, B. (2019). Understanding phosphoinositides: rare, dynamic, and essential membrane phospholipids. Biochem. J. 476, 1–23. doi: 10.1042/bcj20180022
Doan, T., Coleman, J., Marquis, K. A., Meeske, A. J., Burton, B. M., Karatekin, E., et al. (2013). FisB mediates membrane fission during sporulation in Bacillus subtilis. Genes Dev. 27, 322–334. doi: 10.1101/gad.209049.112
Dodonova, S. O., Aderhold, P., Kopp, J., Ganeva, I., Röhling, S., Hagen, W. J. H., et al. (2017). 9Å structure of the COPI coat reveals that the Arf1 GTPase occupies two contrasting molecular environments. eLife 6:e26691.
Dove, S. K., Dong, K., Kobayashi, T., Williams, F. K., and Michell, R. H. (2009). Phosphatidylinositol 3,5-bisphosphate and Fab1p/PIKfyve underPPIn endo-lysosome function. Biochem. J. 419, 1–13. doi: 10.1042/bj20081950
Drin, G., and Antonny, B. (2010). Amphipathic helices and membrane curvature. FEBS Lett. 584, 1840–1847. doi: 10.1016/j.febslet.2009.10.022
Ducas, V. C., and Rhoades, E. (2012). Quantifying interactions of β-synuclein and γ-synuclein with model membranes. J. Mol. Biol. 423, 528–539. doi: 10.1016/j.jmb.2012.08.008
Duncan, A. L., Ruprecht, J. J., Kunji, E. R. S., and Robinson, A. J. (2018). Cardiolipin dynamics and binding to conserved residues in the mitochondrial ADP/ATP carrier. Biochim. Biophys. Acta Biomembr. 1860, 1035–1045. doi: 10.1016/j.bbamem.2018.01.017
Duncan, M. C., and Payne, G. S. (2003). ENTH/ANTH domains expand to the Golgi. Trends Cell Biol. 13, 211–215. doi: 10.1016/s0962-8924(03)00076-x
Edman, M., Berg, S., Storm, P., Wikström, M., Vikström, S., Öhman, A., et al. (2003). Structural features of glycosyltransferases synthesizing major bilayer and nonbilayer-prone membrane lipids in Acholeplasma laidlawii and Streptococcus pneumoniae. J. Biol. Chem. 278, 8420–8428. doi: 10.1074/jbc.m211492200
Eisenberg, D., Weiss, R. M., and Terwilliger, T. C. (1982). The helical hydrophobic moment: a measure of the amphiphilicity of a helix. Nature 299, 371–374. doi: 10.1038/299371a0
Eisenberg, D., Weiss, R. M., and Terwilliger, T. C. (1984). The hydrophobic moment detects periodicity in protein hydrophobicity. Proc. Natl. Acad. Sci. U.S.A. 81, 140–144. doi: 10.1073/pnas.81.1.140
Ekanayake, E. V., Fu, R., and Cross, T. A. (2016). Structural influences: cholesterol, drug, and proton binding to full-length Influenza A M2 protein. Biophys. J. 110, 1391–1399. doi: 10.1016/j.bpj.2015.11.3529
Elkins, M. R., Sergeyev, I. V., and Hong, M. (2018). Determining cholesterol binding to membrane proteins by cholesterol 13C labeling in yeast and dynamic nuclear polarization NMR. J. Am. Chem. Soc. 140, 15437–15449. doi: 10.1021/jacs.8b09658
Elkins, M. R., Williams, J. K., Gelenter, M. D., Dai, P., Kwon, B., Sergeyev, I. V., et al. (2017). Cholesterol-binding site of the influenza M2 protein in lipid bilayers from solid-state NMR. Proc. Natl. Acad. Sci. U.S.A. 114, 12946–12951. doi: 10.1073/pnas.1715127114
Ellen, A. F., Zolghadr, B., Driessen, A. M. J., and Albers, S.-V. (2010). Shaping the archaeal cell envelope. Archaea 2010:608243.
Eriksson, H. M., Wessman, P., Ge, C., Edwards, K., and Wieslander, Å. (2009). Massive formation of intracellular membrane vesicles in Escherichia coli by a monotopic membrane-bound lipid glycosyltransferase. J. Biol. Chem. 284, 33904–33914. doi: 10.1074/jbc.m109.021618
Fakhree, M. A. A., Engelbertink, S. A. J., van Leijenhorst-Groener, K. A., Blum, C., and Claessens, M. M. A. E. (2019). Cooperation of helix insertion and lateral pressure to remodel membranes. Biomacromolecules 20, 1217–1223. doi: 10.1021/acs.biomac.8b01606
Falanga, A., Cantisani, M., Pedone, C., and Galdiero, S. (2009). Membrane fusion and fission: enveloped viruses. Protein Pept. Lett. 16, 751–759. doi: 10.2174/092986609788681760
Farsad, K., Ringstad, N., Takei, K., Floyd, S. R., Rose, K., and De Camilli, P. (2001). Generation of high curvature membranes mediated by direct endophilin bilayer interactions. J. Cell Biol. 155, 193–200. doi: 10.1083/jcb.200107075
Feng, Y., He, D., Yao, Z., and Klionsky, D. J. (2014). The machinery of macroautophagy. Cell Res. 24, 24–41. doi: 10.1038/cr.2013.168
Fernandes, F., Loura, L. M. S., Chichón, F. J., Carrascosa, J. L., Fedorov, A., and Prieto, M. (2008). Role of helix 0 of the N-BAR domain in membrane curvature generation. Biophys. J. 94, 3065–3073. doi: 10.1529/biophysj.107.113118
Fernandez, I., Ying, Y., Albanesi, J., and Anderson, R. G. W. (2002). Mechanism of caveolin filament assembly. Proc. Natl. Acad. Sci. U.S.A. 99, 11193–11198. doi: 10.1073/pnas.172196599
Ferreira, A. P. A., and Boucrot, E. (2018). Mechanisms of carrier formation during clathrin-independent endocytosis. Trends Cell Biol. 28, 188–200. doi: 10.1016/j.tcb.2017.11.004
Ford, M. G. J., Mills, I. G., Peter, B. J., Vallis, Y., Praefcke, G. J. K., Evans, P. R., et al. (2002). Curvature of clathrin-coated pits driven by epsin. Nature 419, 361–366. doi: 10.1038/nature01020
Ford, M. G. J., and Chappie, J. S. (2019). The structural biology of the dynamin-related proteins: new insights into a diverse, multitalented family. Traffic 20, 717–740. doi: 10.1111/tra.12676
Francy, C. A., Clinton, R. W., Fröhlich, C., Murphy, C., and Mears, J. A. (2017). Cryo-EM studies of Drp1 reveal cardiolipin interactions that activate the helical oligomer. Sci. Rep. 7:10744.
Frolov, V. A., Escalada, A., Akimov, S. A., and Shnyrova, A. V. (2015). Geometry of membrane fission. Chem. Phys. Lipids 185, 129–140. doi: 10.1016/j.chemphyslip.2014.07.006
Gallop, J. L., Jao, C. C., Kent, H. M., Butler, P. J. G., Evans, P. R., Langen, R., et al. (2006). Mechanism of endophilin N-BAR domain-mediated membrane curvature. EMBO J. 25, 2898–2910. doi: 10.1038/sj.emboj.7601174
Gatta, A. T., and Carlton, J. G. (2019). The ESCRT-machinery: closing holes and expanding roles. Curr. Opin. Cell Biol. 59, 121–132. doi: 10.1016/j.ceb.2019.04.005
Gautier, R., Douguet, D., Antonny, B., and Drin, G. (2008). HELIQUEST: a web server to screen sequences with specific α-helical properties. Bioinformatics 24, 2101–2102. doi: 10.1093/bioinformatics/btn392
Ge, C., Georgiev, A., Öhman, A., Wieslander, Å., and Kelly, A. A. (2011). Tryptophan residues promote membrane association for a plant lipid glycosyltransferase involved in phosphate stress. J. Biol. Chem. 286, 6669–6684. doi: 10.1074/jbc.m110.138495
Ge, C., Gómez-Llobregat, J., Skwark, M. J., Ruysschaert, J.-M., Wieslander, Å., and Lindén, M. (2014). Membrane remodeling capacity of a vesicle-inducing glycosyltransferase. FEBS J. 281, 3667–3684. doi: 10.1111/febs.12889
Genet, G., Boyé, K., Mathivet, T., Ola, R., Zhang, F., Dubrac, A., et al. (2019). Endophilin-A2 dependent VEGFR2 endocytosis promotes sprouting angiogenesis. Nat. Commun. 10:2350.
George, J. M., Jin, H., Woods, W. S., and Clayton, D. F. (1995). Characterization of a novel protein regulated during the critical period for song learning in the zebra finch. Neuron 15, 361–372. doi: 10.1016/0896-6273(95)90040-3
Ghio, S., Camilleri, A., Caruana, M., Ruf, V. C., Schmidt, F., Leonov, A., et al. (2019). Cardiolipin promotes pore-forming activity of α-synuclein oligomers in mitochondrial membranes. ACS Chem. Neurosci. 10, 3815–3829. doi: 10.1021/acschemneuro.9b00320
Gifford, S. M., and Meyer, P. (2015). Enzyme function is regulated by its localization. Comput. Biol. Chem. 59(Pt B), 113–122. doi: 10.1016/j.compbiolchem.2015.08.004
Giménez-Andrés, M., Čopič, A., and Antonny, B. (2018). The many faces of amphipathic helices. Biomolecules 8:45. doi: 10.3390/biom8030045
Gopaldass, N., Fauvet, B., Lashuel, H., Roux, A., and Mayer, A. (2017). Membrane scission driven by the PROPPIN Atg18. EMBO J. 36, 3274–3291. doi: 10.15252/embj.201796859
Gortat, A., San-Roman, M. J., Vannier, C., and Schmidt, A. A. (2012). Single point mutation in Bin/Amphiphysin/Rvs (BAR) sequence of endophilin impairs dimerization, membrane shaping, and Src homology 3 domain-mediated partnership. J. Biol. Chem. 287, 4232–4247. doi: 10.1074/jbc.m111.325837
Graham, T. R., and Kozlov, M. M. (2010). Interplay of proteins and lipids in generating membrane curvature. Curr. Opin. Cell Biol. 22, 430–436. doi: 10.1016/j.ceb.2010.05.002
Ha, S., Walker, D., Shi, Y., and Walker, S. (2000). The 1.9 Å crystal structure of Escherichia coli MurG, a membrane-associated glycosyltransferase involved in peptidoglycan biosynthesis. Protein Sci. 9, 1045–1052. doi: 10.1110/ps.9.6.1045
Hagen, C., Dent, K. C., Zeev-Ben-Mordehai, T., Grange, M., Bosse, J. B., Whittle, C., et al. (2015). Structural basis of vesicle formation at the inner nuclear membrane. Cell 163, 1692–1701. doi: 10.1016/j.cell.2015.11.029
Hanna, M. G. T., Mela, I., Wang, L., Henderson, R. M., Chapman, E. R., Edwardson, J. M., et al. (2016). Sar1 GTPase activity is regulated by membrane curvature. J. Biol. Chem. 291, 1014–1027. doi: 10.1074/jbc.m115.672287
Hariri, H., Bhattacharya, N., Johnson, K., Noble, A. J., and Stagg, S. M. (2014). Insights into the mechanisms of membrane curvature and vesicle scission by the small GTPase Sar1 in the early secretory pathway. J. Mol. Biol. 426, 3811–3826. doi: 10.1016/j.jmb.2014.08.023
Hasegawa, J., Tokuda, E., Tenno, T., Tsujita, K., Sawai, H., Hiroaki, H., et al. (2011). SH3YL1 regulates dorsal ruffle formation by a novel phosphoinositide-binding domain. J. Cell Biol. 193, 901–916. doi: 10.1083/jcb.201012161
Heo, W. D., Inoue, T., Park, W. S., Kim, M. L., Park, B. O., Wandless, T. J., et al. (2006). PI(3,4,5)P3 and PI(4,5)P2 lipids target proteins with polybasic clusters to the plasma membrane. Science 314, 1458–1461. doi: 10.1126/science.1134389
Herlo, R., Lund, V. K., Lycas, M. D., Jansen, A. M., Khelashvili, G., Andersen, R. C., et al. (2018). An amphipathic helix directs cellular membrane curvature sensing and function of the BAR domain protein PICK1. Cell Rep. 23, 2056–2069. doi: 10.1016/j.celrep.2018.04.074
Herneisen, A. L., Sahu, I. D., McCarrick, R. M., Feix, J. B., Lorigan, G. A., and Howard, K. P. (2017). A budding-defective M2 mutant exhibits reduced membrane interaction, insensitivity to cholesterol, and perturbed interdomain coupling. Biochemistry 56, 5955–5963. doi: 10.1021/acs.biochem.7b00924
Hervás, J. H., Landajuela, A., Antón, Z., Shnyrova, A. V., Goñi, F. M., and Alonso, A. (2017). Human ATG3 binding to lipid bilayers: role of lipid geometry, and electric charge. Sci. Rep. 7:15614.
Hidalgo Carcedo, C., Bonazzi, M., Spanò, S., Turacchio, G., Colanzi, A., Luini, A., et al. (2004). Mitotic Golgi partitioning is driven by the membrane-fissioning protein CtBP3/BARS. Science 305, 93–96. doi: 10.1126/science.1097775
Hofbauer, H. F., Gecht, M., Fischer, S. C., Seybert, A., Frangakis, A. S., Stelzer, E. H. K., et al. (2018). The molecular recognition of phosphatidic acid by an amphipathic helix in Opi1. J. Cell Biol. 217, 3109–3126. doi: 10.1083/jcb.201802027
Hohendahl, A., Roux, A., and Galli, V. (2016). Structural insights into the centronuclear myopathy-associated functions of BIN1 and dynamin 2. J. Struct. Biol. 196, 37–47. doi: 10.1016/j.jsb.2016.06.015
Hohendahl, A., Talledge, N., Galli, V., Shen, P. S., Humbert, F., De Camilli, P., et al. (2017). Structural inhibition of dynamin-mediated membrane fission by endophilin. eLife 6:e26856.
Horchani, H., de Saint-Jean, M., Barelli, H., and Antonny, B. (2014). Interaction of the Spo20 membrane-sensor motif with phosphatidic acid and other anionic lipids, and influence of the membrane environment. PLoS One 9:e113484. doi: 10.1371/journal.pone.0113484
Hoyer, M. J., Chitwood, P. J., Ebmeier, C. C., Striepen, J. F., Qi, R. Z., Old, W. M., et al. (2018). A novel class of ER membrane proteins regulates ER-associated endosome fission. Cell 175, 254 e14–265 e14.
Huber, N., Bieniossek, C., Wagner, K. M., Elsässer, H.-P., Suter, U., Berger, I., et al. (2016). Glutathione-conjugating and membrane-remodeling activity of GDAP1 relies on amphipathic C-terminal domain. Sci. Rep. 6:36930.
Irajizad, E., Ramachandran, R., and Agrawal, A. (2019). Geometric instability catalyzes mitochondrial fission. Mol. Biol. Cell 30, 160–168. doi: 10.1091/mbc.e18-01-0018
Isas, J. M., Ambroso, M. R., Hegde, P. B., Langen, J., and Langen, R. (2015). Tubulation by amphiphysin requires concentration-dependent switching from wedging to scaffolding. Structure 23, 873–881. doi: 10.1016/j.str.2015.02.014
Isermann, P., and Lammerding, J. (2017). Consequences of a tight squeeze: nuclear envelope rupture and repair. Nucleus 8, 268–274. doi: 10.1080/19491034.2017.1292191
Jao, C. C., Hegde, B. G., Chen, J., Haworth, I. S., and Langen, R. (2008). Structure of membrane-bound α-synuclein from site-directed spin labeling and computational refinement. Proc. Natl. Acad. Sci. U.S.A. 105, 19666–19671. doi: 10.1073/pnas.0807826105
Jao, C. C., Hegde, B. G., Gallop, J. L., Hegde, P. B., McMahon, H. T., Haworth, I. S., et al. (2010). Roles of amphipathic helices and the Bin/Amphiphysin/Rvs (BAR) domain of endophilin in membrane curvature generation. J. Biol. Chem. 285, 20164–20170. doi: 10.1074/jbc.m110.127811
Jiao, H., Yin, Y., and Liu, Z. (2019). Structures of the mitochondrial CDP-DAG synthase Tam41 suggest a potential lipid substrate pathway from membrane to the active site. Structure 27, 1258 e4–1269 e4.
Jimah, J. R., and Hinshaw, J. E. (2019). Structural insights into the mechanism of dynamin superfamily proteins. Trends Cell Biol. 29, 257–273. doi: 10.1016/j.tcb.2018.11.003
Jost, M., Simpson, F., Kavran, J. M., Lemmon, M. A., and Schmid, S. L. (1998). Phosphatidylinositol-4,5-bisphosphate is required for endocytic coated vesicle formation. Curr. Biol. 8, 1399–1402.
Kaksonen, M., and Roux, A. (2018). Mechanisms of clathrin-mediated endocytosis. Nat. Rev. Mol. Cell Biol. 19, 313–326.
Kamp, F., Exner, N., Lutz, A. K., Wender, N., Hegermann, J., Brunner, B., et al. (2010). Inhibition of mitochondrial fusion by α-synuclein is rescued by PINK1, Parkin and DJ-1. EMBO J. 29, 3571–3589. doi: 10.1038/emboj.2010.223
Kaneda, M., van Oostende-Triplet, C., Chebli, Y., Testerink, C., Bednarek, S. Y., and Geitmann, A. (2019). Plant AP180 N-terminal homolog (ANTH) proteins are involved in clathrin-dependent endocytosis during pollen tube growth in Arabidopsis thaliana. Plant Cell Physiol. 60, 1316–1330.
Karlsen, M. L., Thorsen, T. S., Johner, N., Ammendrup-Johnsen, I., Erlendsson, S., Tian, X., et al. (2015). Structure of dimeric and tetrameric complexes of the BAR domain protein PICK1 determined by small-angle X-Ray scattering. Structure 23, 1258–1270. doi: 10.1016/j.str.2015.04.020
Kassas, N., Tanguy, E., Thahouly, T., Fouillen, L., Heintz, D., Chasserot-Golaz, S., et al. (2017). Comparative characterization of phosphatidic acid sensors and their localization during frustrated phagocytosis. J. Biol. Chem. 292, 4266–4279. doi: 10.1074/jbc.m116.742346
Khattree, N., Ritter, L. M., and Goldberg, A. F. X. (2013). Membrane curvature generation by a C-terminal amphipathic helix in peripherin-2/rds, a tetraspanin required for photoreceptor sensory cilium morphogenesis. J. Cell Sci. 126, 4659–4670. doi: 10.1242/jcs.126888
Kielian, M. (2014). Mechanisms of virus membrane fusion proteins. Annu. Rev. Virol. 1, 171–189. doi: 10.1146/annurev-virology-031413-085521
Kiely, A. P., Asi, Y. T., Kara, E., Limousin, P., Ling, H., Lewis, P., et al. (2013). α-Synucleinopathy associated with G51D SNCA mutation: a link between Parkinson’s disease and multiple system atrophy? Acta Neuropathol. 125, 753–769. doi: 10.1007/s00401-013-1096-7
Kim, S. S., Upshur, M. A., Saotome, K., Sahu, I. D., McCarrick, R. M., Feix, J. B., et al. (2015). Cholesterol-dependent conformational exchange of the C-terminal domain of the Influenza A M2 protein. Biochemistry 54, 7157–7167. doi: 10.1021/acs.biochem.5b01065
Kirkham, M., Nixon, S. J., Howes, M. T., Abi-Rached, L., Wakeham, D. E., Hanzal-Bayer, M., et al. (2008). Evolutionary analysis and molecular dissection of caveola biogenesis. J. Cell Sci. 121, 2075–2086. doi: 10.1242/jcs.024588
Kitamata, M., Hanawa-Suetsugu, K., Maruyama, K., and Suetsugu, S. (2019). Membrane-deformation ability of ANKHD1 is involved in the early endosome enlargement. iScience 17, 101–118. doi: 10.1016/j.isci.2019.06.020
Kjaerulff, O., Brodin, L., and Jung, A. (2011). The structure and function of endophilin proteins. Cell Biochem. Biophys. 60, 137–154. doi: 10.1007/s12013-010-9137-5
Klupp, B. G., Hellberg, T., Rönfeldt, S., Franzke, K., Fuchs, W., and Mettenleiter, T. C. (2018). Function of the nonconserved N-Terminal domain of pseudorabies virus pUL31 in nuclear egress. J. Virol. 92:e00566-18.
Knorr, R. L., Lipowsky, R., and Dimova, R. (2015). Autophagosome closure requires membrane scission. Autophagy 11, 2134–2137. doi: 10.1080/15548627.2015.1091552
Knorr, R. L., Mizushima, N., and Dimova, R. (2017). Fusion and scission of membranes: ubiquitous topological transformations in cells. Traffic 18, 758–761. doi: 10.1111/tra.12509
Koag, M. C., Fenton, R. D., Wilkens, S., and Close, T. J. (2003). The binding of maize DHN1 to lipid vesicles. Gain of structure and lipid specificity. Plant Physiol. 131, 309–316. doi: 10.1104/pp.011171
Koag, M.-C., Wilkens, S., Fenton, R. D., Resnik, J., Vo, E., and Close, T. J. (2009). The K-segment of maize DHN1 mediates binding to anionic phospholipid vesicles and concomitant structural changes. Plant Physiol. 150, 1503–1514. doi: 10.1104/pp.109.136697
Konno, T., Ross, O. A., Puschmann, A., Dickson, D. W., and Wszolek, Z. K. (2016). Autosomal dominant Parkinson’s disease caused by SNCA duplications. Parkinson. Relat. Disord. 22(Suppl. 1), S1–S6.
Kooijman, E. E., Chupin, V., Fuller, N. L., Kozlov, M. M., de Kruijff, B., Burger, K. N. J., et al. (2005). Spontaneous curvature of phosphatidic acid and lysophosphatidic acid. Biochemistry 44, 2097–2102. doi: 10.1021/bi0478502
Kooijman, E. E., King, K. E., Gangoda, M., and Gericke, A. (2009). Ionization properties of phosphatidylinositol polyphosphates in mixed model membranes. Biochemistry 48, 9360–9371. doi: 10.1021/bi9008616
Kooijman, E. E., Tieleman, D. P., Testerink, C., Munnik, T., Rijkers, D. T. S., Burger, K. N. J., et al. (2007). An electrostatic/hydrogen bond switch as the basis for the specific interaction of phosphatidic acid with proteins. J. Biol. Chem. 282, 11356–11364. doi: 10.1074/jbc.m609737200
Kotani, T., Kirisako, H., Koizumi, M., Ohsumi, Y., and Nakatogawa, H. (2018). The Atg2-Atg18 complex tethers pre-autophagosomal membranes to the endoplasmic reticulum for autophagosome formation. Proc. Natl. Acad. Sci. U.S.A. 115, 10363–10368. doi: 10.1073/pnas.1806727115
Kozlov, M. M., McMahon, H. T., and Chernomordik, L. V. (2010). Protein-driven membrane stresses in fusion and fission. Trends Biochem. Sci. 35, 699–706. doi: 10.1016/j.tibs.2010.06.003
Kozlovsky, Y., and Kozlov, M. M. (2003). Membrane fission: model for intermediate structures. Biophys. J. 85, 85–96. doi: 10.1016/s0006-3495(03)74457-9
Krauss, M., Jia, J.-Y., Roux, A., Beck, R., Wieland, F. T., De Camilli, P., et al. (2008). Arf1-GTP-induced tubule formation suggests a function of Arf family proteins in curvature acquisition at sites of vesicle budding. J. Biol. Chem. 283, 27717–27723. doi: 10.1074/jbc.m804528200
Krüger, R., Kuhn, W., Müller, T., Woitalla, D., Graeber, M., Kösel, S., et al. (1998). Ala30Pro mutation in the gene encoding α-synuclein in Parkinson’s disease. Nat. Genet. 18, 106–108.
Kunji, E. R. S., Aleksandrova, A., King, M. S., Majd, H., Ashton, V. L., Cerson, E., et al. (2016). The transport mechanism of the mitochondrial ADP/ATP carrier. Biochim. Biophys. Acta 1863, 2379–2393.
Kweon, D.-H., Shin, Y.-K., Shin, J. Y., Lee, J.-H., Lee, J.-B., Seo, J.-H., et al. (2006). Membrane topology of helix 0 of the Epsin N-terminal homology domain. Mol. Cells 21, 428–435.
Lai, C.-L., Jao, C. C., Lyman, E., Gallop, J. L., Peter, B. J., McMahon, H. T., et al. (2012). Membrane binding and self-association of the epsin N-terminal homology domain. J. Mol. Biol. 423, 800–817. doi: 10.1016/j.jmb.2012.08.010
Lauwers, E., Goodchild, R., and Verstreken, P. (2016). Membrane lipids in presynaptic function and disease. Neuron 90, 11–25. doi: 10.1016/j.neuron.2016.02.033
Lee, E., Marcucci, M., Daniell, L., Pypaert, M., Weisz, O. A., Ochoa, G.-C., et al. (2002). Amphiphysin 2 (Bin1) and T-tubule biogenesis in muscle. Science 297, 1193–1196. doi: 10.1126/science.1071362
Lee, M. C. S., Orci, L., Hamamoto, S., Futai, E., Ravazzola, M., and Schekman, R. (2005). Sar1p N-terminal helix initiates membrane curvature and completes the fission of a COPII vesicle. Cell 122, 605–617. doi: 10.1016/j.cell.2005.07.025
Legendre-Guillemin, V., Wasiak, S., Hussain, N. K., Angers, A., and McPherson, P. S. (2004). ENTH/ANTH proteins and clathrin-mediated membrane budding. J. Cell Sci. 117, 9–18. doi: 10.1242/jcs.00928
Lesage, S., Anheim, M., Letournel, F., Bousset, L., Honoré, A., Rozas, N., et al. (2013). G51D α-synuclein mutation causes a novel parkinsonian-pyramidal syndrome. Ann. Neurol. 73, 459–471. doi: 10.1002/ana.23894
Li, J., Wang, X., Zhang, T., Wang, C., Huang, Z., and Luo, X, et al. (2015). A review on phospholipids and their main applications in drug delivery systems. Asian J. Pharmaceutic. Sci. 10, 81–98. doi: 10.1016/j.ajps.2014.09.004
Li, W.-W., Li, J., and Bao, J.-K. (2012). Microautophagy: lesser-known self-eating. Cell Mol. Life Sci. 69, 1125–1136. doi: 10.1007/s00018-011-0865-5
Li, Z. -L. (2018). Molecular dynamics simulations of membrane deformation induced by amphiphilic helices of Epsin, Sar1p, and Arf1. Chin. Phys. B 27:038703. doi: 10.1088/1674-1056/27/3/038703
Liberali, P., Kakkonen, E., Turacchio, G., Valente, C., Spaar, A., Perinetti, G., et al. (2008). The closure of Pak1-dependent macropinosomes requires the phosphorylation of CtBP1/BARS. EMBO J. 27, 970–981. doi: 10.1038/emboj.2008.59
Lind, J., Rämö, T., Klement, M. L., Bárány-Wallje, E., Epand, R. M., Epand, R. F., et al. (2007). High cationic charge and bilayer interface-binding helices in a regulatory lipid glycosyltransferase. Biochemistry 46, 5664–5677. doi: 10.1021/bi700042x
Loewen, C. J. R., Gaspar, M. L., Jesch, S. A., Delon, C., Ktistakis, N. T., Henry, S. A., et al. (2004). Phospholipid metabolism regulated by a transcription factor sensing phosphatidic acid. Science 304, 1644–1647. doi: 10.1126/science.1096083
Loftus, A. F., Hsieh, V. L., and Parthasarathy, R. (2012). Modulation of membrane rigidity by the human vesicle trafficking proteins Sar1A and Sar1B. Biochem. Biophys. Res. Commun. 426, 585–589. doi: 10.1016/j.bbrc.2012.08.131
Loncle, N., Agromayor, M., Martin-Serrano, J., and Williams, D. W. (2015). An ESCRT module is required for neuron pruning. Sci. Rep. 5:8461.
Lorenz, M., Vollmer, B., Unsay, J. D., Klupp, B. G., García-Sáez, A. J., Mettenleiter, T. C., et al. (2015). A single herpesvirus protein can mediate vesicle formation in the nuclear envelope. J. Biol. Chem. 290, 6962–6974. doi: 10.1074/jbc.m114.627521
Löw, C., Weininger, U., Lee, H., Schweimer, K., Neundorf, I., Beck-Sickinger, A. G., et al. (2008). Structure and dynamics of helix-0 of the N-BAR domain in lipid micelles and bilayers. Biophys. J. 95, 4315–4323. doi: 10.1529/biophysj.108.134155
Lundmark, R., Doherty, G. J., Vallis, Y., Peter, B. J., and McMahon, H. T. (2008). Arf family GTP loading is activated by, and generates, positive membrane curvature. Biochem J 414, 189–194. doi: 10.1042/bj20081237
Madsen, J. J., Grime, J. M. A., Rossman, J. S., and Voth, G. A. (2018). Entropic forces drive clustering and spatial localization of influenza A M2 during viral budding. Proc. Natl. Acad. Sci. U.S.A. 115, E8595–E8603.
Manifava, M., Thuring, J. W. J. F., Lim, Z.-Y., Packman, L., Holmes, A. B., and Ktistakis, N. T. (2001). Differential binding of traffic-related proteins to phosphatidic acid- or phosphatidylinositol (4,5)- bisphosphate-coupled affinity reagents. J. Biol. Chem. 276, 8987–8994. doi: 10.1074/jbc.m010308200
Martens, S., and McMahon, H. T. (2008). Mechanisms of membrane fusion: disparate players and common principles. Nat. Rev. Mol. Cell Biol. 9, 543–556. doi: 10.1038/nrm2417
Martin, T. F. J. (2012). Role of PI(4,5)P2 in vesicle exocytosis and membrane fusion. Subcell Biochem. 59, 111–130. doi: 10.1007/978-94-007-3015-1_4
Martinez, J. H., Alaimo, A., Gorojod, R. M., Porte Alcon, S., Fuentes, F., Coluccio Leskow, F., et al. (2018). Drp-1 dependent mitochondrial fragmentation and protective autophagy in dopaminergic SH-SY5Y cells overexpressing α-synuclein. Mol. Cell Neurosci. 88, 107–117. doi: 10.1016/j.mcn.2018.01.004
Martyna, A., Bahsoun, B., Badham, M. D., Srinivasan, S., Howard, M. J., and Rossman, J. S. (2017). Membrane remodeling by the M2 amphipathic helix drives influenza virus membrane scission. Sci. Rep. 7:44695.
Masuda, M., Takeda, S., Sone, M., Ohki, T., Mori, H., Kamioka, Y., et al. (2006). Endophilin BAR domain drives membrane curvature by two newly identified structure-based mechanisms. EMBO J. 25, 2889–2897. doi: 10.1038/sj.emboj.7601176
McDargh, Z. A., and Deserno, M. (2018). Dynamin’s helical geometry does not destabilize membranes during fission. Traffic 19, 328–335. doi: 10.1111/tra.12555
McLaughlin, S., Wang, J., Gambhir, A., and Murray, D. (2002). PIP2 and proteins: interactions, organization, and information flow. Annu. Rev. Biophys. Biomol. Struct. 31, 151–175. doi: 10.1146/annurev.biophys.31.082901.134259
McMahon, H. T., and Boucrot, E. (2015). Membrane curvature at a glance. J. Cell Sci. 128, 1065–1070. doi: 10.1242/jcs.114454
McMahon, H. T., and Gallop, J. L. (2005). Membrane curvature and mechanisms of dynamic cell membrane remodelling. Nature 438, 590–596. doi: 10.1038/nature04396
Meinecke, M., Boucrot, E., Camdere, G., Hon, W.-C., Mittal, R., and McMahon, H. T. (2013). Cooperative recruitment of dynamin and BIN/Amphiphysin/Rvs (BAR) domain-containing proteins leads to GTP-dependent membrane scission. J. Biol. Chem. 288, 6651–6661. doi: 10.1074/jbc.m112.444869
Melero, A., Chiaruttini, N., Karashima, T., Riezman, I., Funato, K., Barlowe, C., et al. (2018). Lysophospholipids facilitate COPII vesicle formation. Curr. Biol. 28, 1950.e6–1958 e6.
Mettlen, M., Chen, P.-H., Srinivasan, S., Danuser, G., and Schmid, S. L. (2018). Regulation of clathrin-mediated endocytosis. Annu. Rev. Biochem. 87, 871–896.
Miller, S. E., Mathiasen, S., Bright, N. A., Pierre, F., Kelly, B. T., Kladt, N., et al. (2015). CALM regulates clathrin-coated vesicle size and maturation by directly sensing and driving membrane curvature. Dev. Cell 33, 163–175. doi: 10.1016/j.devcel.2015.03.002
Mim, C., Cui, H., Gawronski-Salerno, J. A., Frost, A., Lyman, E., Voth, G. A., et al. (2012). Structural basis of membrane bending by the N-BAR protein endophilin. Cell 149, 137–145. doi: 10.1016/j.cell.2012.01.048
Miner, G. E., Sullivan, K. D., Guo, A., Jones, B. C., Hurst, L. R., Ellis, E. C., et al. (2019). Phosphatidylinositol 3,5-bisphosphate regulates the transition between trans-SNARE complex formation and vacuole membrane fusion. Mol. Biol. Cell 30, 201–208. doi: 10.1091/mbc.e18-08-0505
Mishra, V. K., and Palgunachari, M. N. (1996). Interaction of model class A1, class A2, and class Y amphipathic helical peptides with membranes. Biochemistry 35, 11210–11220. doi: 10.1021/bi960760f
Mizuno, S., Sasai, H., Kume, A., Takahashi, D., Satoh, M., Kado, S., et al. (2017). Dioleoyl-phosphatidic acid selectively binds to α-synuclein and strongly induces its aggregation. FEBS Lett. 591, 784–791. doi: 10.1002/1873-3468.12592
Monroe, N., Han, H., Gonciarz, M. D., Eckert, D. M., Karren, M. A., Whitby, F. G., et al. (2014). The oligomeric state of the active Vps4 AAA ATPase. J. Mol. Biol. 426, 510–525. doi: 10.1016/j.jmb.2013.09.043
Moroni, I., Morbin, M., Milani, M., Ciano, C., Bugiani, M., Pagliano, E., et al. (2009). Novel mutations in the GDAP1 gene in patients affected with early-onset axonal Charcot-Marie-Tooth type 4A. Neuromuscul. Disord. 19, 476–480. doi: 10.1016/j.nmd.2009.04.014
Mozsolits, H., Lee, T.-H., Clayton, A. H., Sawyer, W. H., and Aguilar, M.-I. (2004). The membrane-binding properties of a class A amphipathic peptide. Eur. Biophys. J. 33, 98–108. doi: 10.1007/s00249-003-0332-9
Musatov, A., and Sedlák, E. (2017). Role of cardiolipin in stability of integral membrane proteins. Biochimie 142, 102–111. doi: 10.1016/j.biochi.2017.08.013
Nakamura, K. (2013). α-Synuclein and mitochondria: partners in crime? Neurotherapeutics 10, 391–399. doi: 10.1007/s13311-013-0182-9
Nakamura, K., Nemani, V. M., Azarbal, F., Skibinski, G., Levy, J. M., Egami, K., et al. (2011). Direct membrane association drives mitochondrial fission by the Parkinson disease-associated protein α-synuclein. J. Biol. Chem. 286, 20710–20726. doi: 10.1074/jbc.m110.213538
Nakamura, K., Nemani, V. M., Wallender, E. K., Kaehlcke, K., Ott, M., and Edwards, R. H. (2008). Optical reporters for the conformation of α-synuclein reveal a specific interaction with mitochondria. J. Neurosci. 28, 12305–12317. doi: 10.1523/jneurosci.3088-08.2008
Nath, S., Dancourt, J., Shteyn, V., Puente, G., Fong, W. M., Nag, S., et al. (2014). Lipidation of the LC3/GABARAP family of autophagy proteins relies on a membrane-curvature-sensing domain in Atg3. Nat. Cell Biol. 16, 415–424. doi: 10.1038/ncb2940
Nicot, A.-S., Toussaint, A., Tosch, V., Kretz, C., Wallgren-Pettersson, C., Iwarsson, E., et al. (2007). Mutations in amphiphysin 2 (BIN1) disrupt interaction with dynamin 2 and cause autosomal recessive centronuclear myopathy. Nat. Genet. 39, 1134–1139. doi: 10.1038/ng2086
Nishimura, T., Morone, N., and Suetsugu, S. (2018). Membrane re-modelling by BAR domain superfamily proteins via molecular and non-molecular factors. Biochem. Soc. Trans. 46, 379–389. doi: 10.1042/bst20170322
O’Donnell, K. C., Lulla, A., Stahl, M. C., Wheat, N. D., Bronstein, J. M., and Sagasti, A. (2014). Axon degeneration and PGC-1α-mediated protection in a zebrafish model of α-synuclein toxicity. Dis. Model. Mech. 7, 571–582. doi: 10.1242/dmm.013185
Olgiati, S., Thomas, A., Quadri, M., Breedveld, G. J., Graafland, J., Eussen, H., et al. (2015). Early-onset parkinsonism caused by α-synuclein gene triplication: clinical and genetic findings in a novel family. Parkinson. Relat. Disord. 21, 981–986. doi: 10.1016/j.parkreldis.2015.06.005
Olivença, D. V., Uliyakina, I., Fonseca, L. L., Amaral, M. D., Voit, E. O., and Pinto, F. R. (2018). A mathematical model of the phosphoinositide pathway. Sci. Rep. 8:3904.
Opaliński, Ł., Kiel, J. A. K. W., Williams, C., Veenhuis, M., and van der Klei, I. J. (2011). Membrane curvature during peroxisome fission requires Pex11. EMBO J. 30, 5–16. doi: 10.1038/emboj.2010.299
Osawa, T., Alam, J. M., and Noda, N. N. (2019). Membrane-binding domains in autophagy. Chem. Phys. Lipids 218, 1–9. doi: 10.1016/j.chemphyslip.2018.11.001
Osteryoung, K. W., and Pyke, K. A. (2014). Division and dynamic morphology of plastids. Annu. Rev. Plant Biol. 65, 443–472. doi: 10.1146/annurev-arplant-050213-035748
Ouberai, M. M., Wang, J., Swann, M. J., Galvagnion, C., Guilliams, T., Dobson, C. M., et al. (2013). α-Synuclein senses lipid packing defects and induces lateral expansion of lipids leading to membrane remodeling. J. Biol. Chem. 288, 20883–20895. doi: 10.1074/jbc.m113.478297
Pagliuso, A., Cossart, P., and Stavru, F. (2018). The ever-growing complexity of the mitochondrial fission machinery. Cell Mol. Life Sci. 75, 355–374. doi: 10.1007/s00018-017-2603-0
Pagliuso, A., Valente, C., Giordano, L. L., Filograna, A., Li, G., Circolo, D., et al. (2016). Golgi membrane fission requires the CtBP1-S/BARS-induced activation of lysophosphatidic acid acyltransferase δ. Nat. Commun. 7:12148.
Palomares-Jerez, M. F., Nemesio, H., Franquelim, H. G., Castanho, M. A. R. B., and Villalaín, J. (2013). N-terminal AH2 segment of protein NS4B from hepatitis C virus. Binding to and interaction with model biomembranes. Biochim. Biophys. Acta 1828, 1938–1952. doi: 10.1016/j.bbamem.2013.04.020
Pan, J., Dalzini, A., and Song, L. (2019). Cholesterol and phosphatidylethanolamine lipids exert opposite effects on membrane modulations caused by the M2 amphipathic helix. Biochim. Biophys. Acta Biomembr. 1861, 201–209. doi: 10.1016/j.bbamem.2018.07.013
Pan, L., Wu, H., Shen, C., Shi, Y., Jin, W., Xia, J., et al. (2007). Clustering and synaptic targeting of PICK1 requires direct interaction between the PDZ domain and lipid membranes. EMBO J. 26, 4576–4587. doi: 10.1038/sj.emboj.7601860
Panchal, K., and Tiwari, A. K. (2019). Mitochondrial dynamics, a key executioner in neurodegenerative diseases. Mitochondrion 47, 151–173. doi: 10.1016/j.mito.2018.11.002
Pannuzzo, M., McDargh, Z. A., and Deserno, M. (2018). The role of scaffold reshaping and disassembly in dynamin driven membrane fission. eLife 7:e39441.
Park, S.-Y., Yang, J.-S., Li, Z., Deng, P., Zhu, X., Young, D., et al. (2019). The late stage of COPI vesicle fission requires shorter forms of phosphatidic acid and diacylglycerol. Nat. Commun. 10:3409.
Parton, R. G., Hanzal-Bayer, M., and Hancock, J. F. (2006). Biogenesis of caveolae: a structural model for caveolin-induced domain formation. J. Cell Sci. 119, 787–796. doi: 10.1242/jcs.02853
Pasanen, P., Myllykangas, L., Siitonen, M., Raunio, A., Kaakkola, S., Lyytinen, J., et al. (2014). Novel α-synuclein mutation A53E associated with atypical multiple system atrophy and Parkinson’s disease-type pathology. Neurobiol. Aging 35:2180 e1-e5.
Perrin, R. J., Woods, W. S., Clayton, D. F., and George, J. M. (2000). Interaction of human α-Synuclein and Parkinson’s disease variants with phospholipids. Structural analysis using site-directed mutagenesis. J. Biol. Chem. 275, 34393–34398. doi: 10.1074/jbc.m004851200
Peter, B. J., Kent, H. M., Mills, I. G., Vallis, Y., Butler, P. J. G., Evans, P. R., et al. (2004). BAR domains as sensors of membrane curvature: the amphiphysin BAR structure. Science 303, 495–499. doi: 10.1126/science.1092586
Petersen, J., Eriksson, S. K., Harryson, P., Pierog, S., Colby, T., Bartels, D., et al. (2012). The lysine-rich motif of intrinsically disordered stress protein CDeT11-24 from Craterostigma plantagineum is responsible for phosphatidic acid binding and protection of enzymes from damaging effects caused by desiccation. J. Exp. Bot. 63, 4919–4929. doi: 10.1093/jxb/ers173
Piper, R. C., and Katzmann, D. J. (2007). Biogenesis and function of multivesicular bodies. Annu. Rev. Cell Dev. Biol. 23, 519–547. doi: 10.1146/annurev.cellbio.23.090506.123319
Planas-Iglesias, J., Dwarakanath, H., Mohammadyani, D., Yanamala, N., Kagan, V. E., and Klein-Seetharaman, J. (2015). Cardiolipin interactions with proteins. Biophys. J. 109, 1282–1294. doi: 10.1016/j.bpj.2015.07.034
Podbilewicz, B. (2014). Virus and cell fusion mechanisms. Annu. Rev. Cell Dev. Biol. 30, 111–139. doi: 10.1146/annurev-cellbio-101512-122422
Polozov, I. V., Polozova, A. I., Mishra, V. K., Anantharamaiah, G. M., Segrest, J. P., and Epand, R. M. (1998). Studies of kinetics and equilibrium membrane binding of class A and class L model amphipathic peptides. Biochim. Biophys. Acta 1368, 343–354. doi: 10.1016/s0005-2736(97)00210-1
Polymeropoulos, M. H., Lavedan, C., Leroy, E., Ide, S. E., Dehejia, A., Dutra, A., et al. (1997). Mutation in the α-synuclein gene identified in families with Parkinson’s disease. Science 276, 2045–2047. doi: 10.1126/science.276.5321.2045
Pozo Devoto, V. M., Dimopoulos, N., Alloatti, M., Pardi, M. B., Saez, T. M., Otero, M. G., et al. (2017). αSynuclein control of mitochondrial homeostasis in human-derived neurons is disrupted by mutations associated with Parkinson’s disease. Sci. Rep. 7:5042.
Pozo Devoto, V. M., and Falzone, T. L. (2017). Mitochondrial dynamics in Parkinson’s disease: a role for α-synuclein? Dis. Model. Mech. 10, 1075–1087. doi: 10.1242/dmm.026294
Prokic, I., Cowling, B. S., and Laporte, J. (2014). Amphiphysin 2 (BIN1) in physiology and diseases. J. Mol. Med. 92, 453–463. doi: 10.1007/s00109-014-1138-1
Putta, P., Rankenberg, J., Korver, R. A., van Wijk, R., Munnik, T., Testerink, C., et al. (2016). Phosphatidic acid binding proteins display differential binding as a function of membrane curvature stress and chemical properties. Biochim. Biophys. Acta 1858, 2709–2716. doi: 10.1016/j.bbamem.2016.07.014
Ramachandran, R. (2018). Mitochondrial dynamics: the dynamin superfamily and execution by collusion. Semin. Cell Dev. Biol. 76, 201–212. doi: 10.1016/j.semcdb.2017.07.039
Ramachandran, R., and Schmid, S. L. (2018). The dynamin superfamily. Curr. Biol. 28, R411–R416. doi: 10.1016/j.semcdb.2017.07.039
Randazzo, P. A. (1997). Functional interaction of ADP-ribosylation factor 1 with phosphatidylinositol 4,5-bisphosphate. J. Biol. Chem. 272, 7688–7692.
Renard, H.-F., Johannes, L., and Morsomme, P. (2018). Increasing diversity of biological membrane fission mechanisms. Trends Cell Biol. 28, 274–286. doi: 10.1016/j.tcb.2017.12.001
Renard, H.-F., Simunovic, M., Lemière, J., Boucrot, E., Garcia-Castillo, M. D., Arumugam, S., et al. (2015). Endophilin-A2 functions in membrane scission in clathrin-independent endocytosis. Nature 517, 493–496. doi: 10.1038/nature14064
Renner, L. D., and Weibel, D. B. (2012). MinD and MinE interact with anionic phospholipids and regulate division plane formation in Escherichia coli. J. Biol. Chem. 287, 38835–38844. doi: 10.1074/jbc.m112.407817
Roberts, K. L., Leser, G. P., Ma, C., and Lamb, R. A. (2013). The amphipathic helix of influenza A virus M2 protein is required for filamentous bud formation and scission of filamentous and spherical particles. J. Virol. 87, 9973–9982. doi: 10.1128/jvi.01363-13
Robotta, M., Gerding, H. R., Vogel, A., Hauser, K., Schildknecht, S., Karreman, C., et al. (2014). α-synuclein binds to the inner membrane of mitochondria in an α-helical conformation. Chembiochem 15, 2499–2502. doi: 10.1002/cbic.201402281
Rossman, J. S., Jing, X., Leser, G. P., and Lamb, R. A. (2010). Influenza virus M2 protein mediates ESCRT-independent membrane scission. Cell 142, 902–913. doi: 10.1016/j.cell.2010.08.029
Rossman, J. S., and Lamb, R. A. (2013). Viral membrane scission. Annu. Rev. Cell Dev. Biol. 29, 551–569. doi: 10.1146/annurev-cellbio-101011-155838
Rostovtseva, T. K., Boukari, H., Antignani, A., Shiu, B., Banerjee, S., Neutzner, A., et al. (2009). Bax activates endophilin B1 oligomerization and lipid membrane vesiculation. J. Biol. Chem. 284, 34390–34399. doi: 10.1074/jbc.m109.021873
Ruprecht, J. J., Hellawell, A. M., Harding, M., Crichton, P. G., McCoy, A. J., and Kunji, E. R. S. (2014). Structures of yeast mitochondrial ADP/ATP carriers support a domain-based alternating-access transport mechanism. Proc. Natl. Acad. Sci. U.S.A. 111, E426–434.
Ryan, T., Bamm, V. V., Stykel, M. G., Coackley, C. L., Humphries, K. M., Jamieson-Williams, R., et al. (2018). Cardiolipin exposure on the outer mitochondrial membrane modulates α-synuclein. Nat. Commun. 9:817.
Saksena, S., Wahlman, J., Teis, D., Johnson, A. E., and Emr, S. D. (2009). Functional reconstitution of ESCRT-III assembly and disassembly. Cell 136, 97–109. doi: 10.1016/j.cell.2008.11.013
Sandvig, K., Kavaliauskiene, S., and Skotland, T. (2018). Clathrin-independent endocytosis: an increasing degree of complexity. Histochem. Cell Biol. 150, 107–118. doi: 10.1007/s00418-018-1678-5
Sani, M.-A., Whitwell, T. C., and Separovic, F. (2012). Lipid composition regulates the conformation and insertion of the antimicrobial peptide maculatin 1.1. Biochim. Biophys. Acta 1818, 205–211. doi: 10.1016/j.bbamem.2011.07.015
Sathappa, M., and Alder, N. N. (2016). The ionization properties of cardiolipin and its variants in model bilayers. Biochim. Biophys. Acta 1858, 1362–1372. doi: 10.1016/j.bbamem.2016.03.007
Scacioc, A., Schmidt, C., Hofmann, T., Urlaub, H., Kühnel, K., and Pérez-Lara, A. (2017). Structure based biophysical characterization of the PROPPIN Atg18 shows Atg18 oligomerization upon membrane binding. Sci. Rep. 7:14008.
Schöneberg, J., Lee, I.-H., Iwasa, J. H., and Hurley, J. H. (2017). Reverse-topology membrane scission by the ESCRT proteins. Nat. Rev. Mol. Cell Biol. 18, 5–17. doi: 10.1038/nrm.2016.121
Schrader, M., Costello, J. L., Godinho, L. F., Azadi, A. S., and Islinger, M. (2016). Proliferation and fission of peroxisomes - An update. Biochim. Biophys. Acta 1863, 971–983. doi: 10.1016/j.bbamcr.2015.09.024
Schwechheimer, C., and Kuehn, M. J. (2015). Outer-membrane vesicles from Gram-negative bacteria: biogenesis and functions. Nat. Rev. Microbiol. 13, 605–619. doi: 10.1038/nrmicro3525
Segrest, J. P., De Loof, H., Dohlman, J. G., Brouillette, C. G., and Anantharamaiah, G. M. (1990). Amphipathic helix motif: Classes and properties. Proteins 8, 103–117. doi: 10.1002/prot.340080202
Segrest, J. P., Jones, M. K., De Loof, H., Brouillette, C. G., Venkatachalapathi, Y. V., and Anantharamaiah, G. M. (1992). The amphipathic helix in the exchangeable apolipoproteins: a review of secondary structure and function. J. Lipid Res. 33, 141–166.
Sen, A., Madhivanan, K., Mukherjee, D., and Aguilar, R. C. (2012). The epsin protein family: coordinators of endocytosis and signaling. Biomol. Concepts 3, 117–126.
Simunovic, M., Evergren, E., Callan-Jones, A., and Bassereau, P. (2019). Curving cells inside and out: roles of BAR domain proteins in membrane shaping and its cellular implications. Annu. Rev. Cell Dev. Biol. 35, 111–129 doi: 10.1146/annurev-cellbio-100617-060558
Simunovic, M., Manneville, J.-B., Renard, H.-F., Evergren, E., Raghunathan, K., Bhatia, D., et al. (2017). Friction mediates scission of tubular membranes scaffolded by BAR proteins. Cell 170, 172.e11–184.e11.
Snead, W. T., Hayden, C. C., Gadok, A. K., Zhao, C., Lafer, E. M., Rangamani, P., et al. (2017). Membrane fission by protein crowding. Proc. Natl. Acad. Sci. U.S.A. 114, E3258–E3267.
Snead, W. T., and Stachowiak, J. C. (2018). Structure versus stochasticity-the role of molecular crowding and intrinsic disorder in membrane fission. J. Mol. Biol. 430, 2293–2308. doi: 10.1016/j.jmb.2018.03.024
Snead, W. T., Zeno, W. F., Kago, G., Perkins, R. W., Richter, J. B., Zhao, C., et al. (2019). BAR scaffolds drive membrane fission by crowding disordered domains. J. Cell Biol. 218, 664–682. doi: 10.1083/jcb.201807119
Soni, S. P., and Stahelin, R. V. (2014). The Ebola virus matrix protein VP40 selectively induces vesiculation from phosphatidylserine-enriched membranes. J. Biol. Chem. 289, 33590–33597. doi: 10.1074/jbc.m114.586396
Spanò, S., Silletta, M. G., Colanzi, A., Alberti, S., Fiucci, G., Valente, C., et al. (1999). Molecular cloning and functional characterization of brefeldin A-ADP-ribosylated substrate. A novel protein involved in the maintenance of the Golgi structure. J. Biol. Chem. 274, 17705–17710. doi: 10.1074/jbc.274.25.17705
Stanishneva-Konovalova, T. B., Derkacheva, N. I., Polevova, S. V., and Sokolova, O. S. (2016). The role of BAR domain proteins in the regulation of membrane dynamics. Acta Nat. 8, 60–69.
Stoten, C. L., and Carlton, J. G. (2018). ESCRT-dependent control of membrane remodelling during cell division. Semin. Cell Dev. Biol. 74, 50–65. doi: 10.1016/j.semcdb.2017.08.035
Su, J., Thomas, A. S., Grabietz, T., Landgraf, C., Volkmer, R., Marrink, S. J., et al. (2018). The N-terminal amphipathic helix of Pex11p self-interacts to induce membrane remodelling during peroxisome fission. Biochim. Biophys. Acta Biomembr. 1860, 1292–1300. doi: 10.1016/j.bbamem.2018.02.029
Suetsugu, S., Kurisu, S., and Takenawa, T. (2014). Dynamic shaping of cellular membranes by phospholipids and membrane-deforming proteins. Physiol. Rev. 94, 1219–1248. doi: 10.1152/physrev.00040.2013
Sundborger, A., Soderblom, C., Vorontsova, O., Evergren, E., Hinshaw, J. E., and Shupliakov, O. (2011). An endophilin-dynamin complex promotes budding of clathrin-coated vesicles during synaptic vesicle recycling. J. Cell Sci. 124, 133–143. doi: 10.1242/jcs.072686
Suresh, S., and Edwardson, J. M. (2010). The endophilin N-BAR domain perturbs the structure of lipid bilayers. Biochemistry 49, 5766–5771. doi: 10.1021/bi100760e
Takahashi, Y., Meyerkord, C. L., Hori, T., Runkle, K., Fox, T. E., Kester, M., et al. (2011). Bif-1 regulates Atg9 trafficking by mediating the fission of Golgi membranes during autophagy. Autophagy 7, 61–73. doi: 10.4161/auto.7.1.14015
Takahashi, Y., Tsotakos, N., Liu, Y., Young, M. M., Serfass, J., Tang, Z., et al. (2016). The Bif-1-Dynamin 2 membrane fission machinery regulates Atg9-containing vesicle generation at the Rab11-positive reservoirs. Oncotarget 7, 20855–20868.
Takatori, S., Tatematsu, T., Cheng, J., Matsumoto, J., Akano, T., and Fujimoto, T. (2016). Phosphatidylinositol 3,5-Bisphosphate-rich membrane domains in endosomes and lysosomes. Traffic 17, 154–167. doi: 10.1111/tra.12346
Takatori, S., and Tomita, T. (2018). AP180 N-Terminal Homology (ANTH) and Epsin N-Terminal Homology (ENTH) domains: physiological functions and involvement in disease. Adv. Exp. Med. Biol. doi: 10.1007/5584_2018_218 [Epub ahead of print]
Takeda, T., Kozai, T., Yang, H., Ishikuro, D., Seyama, K., Kumagai, Y., et al. (2018). Dynamic clustering of dynamin-amphiphysin helices regulates membrane constriction and fission coupled with GTP hydrolysis. eLife 7:e30246.
Tamura, N., Nishimura, T., Sakamaki, Y., Koyama-Honda, I., Yamamoto, H., and Mizushima, N. (2017). Differential requirement for ATG2A domains for localization to autophagic membranes and lipid droplets. FEBS Lett. 591, 3819–3830. doi: 10.1002/1873-3468.12901
Tandler, B., Hoppel, C. L., and Mears, J. A. (2018). Morphological Pathways of mitochondrial division. Antioxidants 7:E30.
Tang, Z., Takahashi, Y., Chen, C., Liu, Y., He, H., Tsotakos, N., et al. (2017). Atg2A/B deficiency switches cytoprotective autophagy to non-canonical caspase-8 activation and apoptosis. Cell Death Differ. 24, 2127–2138. doi: 10.1038/cdd.2017.133
Tanguy, E., Wang, Q., Moine, H., and Vitale, N. (2019). Phosphatidic Acid: from pleiotropic functions to neuronal pathology. Front. Cell Neurosci. 13:2. doi: 10.3389/fncel.2019.00002
Taschenberger, G., Toloe, J., Tereshchenko, J., Akerboom, J., Wales, P., Benz, R., et al. (2013). β-synuclein aggregates and induces neurodegeneration in dopaminergic neurons. Ann. Neurol. 74, 109–118. doi: 10.1002/ana.23905
Tilokani, L., Nagashima, S., Paupe, V., and Prudent, J. (2018). Mitochondrial dynamics: overview of molecular mechanisms. Essays Biochem. 62, 341–360. doi: 10.1042/ebc20170104
Uebe, R., and Schüler, D. (2016). Magnetosome biogenesis in magnetotactic bacteria. Nat. Rev. Microbiol. 14, 621–637. doi: 10.1038/nrmicro.2016.99
Uttenweiler, A., and Mayer, A. (2008). Microautophagy in the yeast Saccharomyces cerevisiae. Methods Mol Biol 445, 245–259. doi: 10.1007/978-1-59745-157-4_16
Valente, C., Luini, A., and Corda, D. (2013). Components of the CtBP1/BARS-dependent fission machinery. Histochem. Cell Biol. 140, 407–421. doi: 10.1007/s00418-013-1138-1
Valente, C., Spanò, S., Luini, A., and Corda, D. (2005). Purification and functional properties of the membrane fissioning protein CtBP3/BARS. Methods Enzymol. 404, 296–316. doi: 10.1016/s0076-6879(05)04027-9
Valente, C., Turacchio, G., Mariggiò, S., Pagliuso, A., Gaibisso, R., Di Tullio, G., et al. (2012). A 14-3-3γ dimer-based scaffold bridges CtBP1-S/BARS to PI(4)KIIIβ to regulate post-Golgi carrier formation. Nat. Cell Biol. 14, 343–354. doi: 10.1038/ncb2445
Vamparys, L., Gautier, R., Vanni, S., Bennett, W. F., Tieleman, D. P., Antonny, B., et al. (2013). Conical lipids in flat bilayers induce packing defects similar to that induced by positive curvature. Biophys. J. 104, 585–593. doi: 10.1016/j.bpj.2012.11.3836
van den Brink-van der Laan, E., Boots, J.-W. P., Spelbrink, R. E. J., Kool, G. M., Breukink, E., Killian, J. A., et al. (2003). Membrane interaction of the glycosyltransferase MurG: a special role for cardiolipin. J. Bacteriol. 185, 3773–3779. doi: 10.1128/jb.185.13.3773-3779.2003
van Paridon, P. A., de Kruijff, B., Ouwerkerk, R., and Wirtz, K. W. A. (1986). Polyphosphoinositides undergo charge neutralization in the physiological pH range: a 31P-NMR study. Biochim. Biophys. Acta 877, 216–219. doi: 10.1016/0005-2760(86)90137-2
Vanni, S., Vamparys, L., Gautier, R., Drin, G., Etchebest, C., Fuchs, P. F. J., et al. (2013). Amphipathic lipid packing sensor motifs: probing bilayer defects with hydrophobic residues. Biophys. J. 104, 575–584. doi: 10.1016/j.bpj.2012.11.3837
Varkey, J., Isas, J. M., Mizuno, N., Jensen, M. B., Bhatia, V. K., Jao, C. C., et al. (2010). Membrane curvature induction and tubulation are common features of synucleins and apolipoproteins. J. Biol. Chem. 285, 32486–32493. doi: 10.1074/jbc.m110.139576
Vedyaykin, A. D., Ponomareva, E. V., Khodorkovskii, M. A., Borchsenius, S. N., and Vishnjakov, I. E. (2019). Mechanisms of bacterial cell division. Microbiology 88, 245–260.
Velikkakath, A. K. G., Nishimura, T., Oita, E., Ishihara, N., and Mizushima, N. (2012). Mammalian Atg2 proteins are essential for autophagosome formation and important for regulation of size and distribution of lipid droplets. Mol. Biol. Cell 23, 896–909. doi: 10.1091/mbc.e11-09-0785
Votteler, J., and Sundquist, W. I. (2013). Virus budding and the ESCRT pathway. Cell Host Microbe 14, 232–241. doi: 10.1016/j.chom.2013.08.012
Weigert, R., Silletta, M. G., Spanò, S., Turacchio, G., Cericola, C., Colanzi, A., et al. (1999). CtBP/BARS induces fission of Golgi membranes by acylating lysophosphatidic acid. Nature 402, 429–433. doi: 10.1038/46587
Wilz, L., Fan, W., and Zhong, Q. (2011). Membrane curvature response in autophagy. Autophagy 7, 1249–1250. doi: 10.4161/auto.7.10.16738
Wriessnegger, T., Gübitz, G., Leitner, E., Ingolic, E., Cregg, J., de la Cruz, B. J., et al. (2007). Lipid composition of peroxisomes from the yeast Pichia pastoris grown on different carbon sources. Biochim. Biophys. Acta 1771, 455–461. doi: 10.1016/j.bbalip.2007.01.004
Wu, T., and Baumgart, T. (2014). BIN1 membrane curvature sensing and generation show autoinhibition regulated by downstream ligands and PI(4,5)P2. Biochemistry 53, 7297–7309. doi: 10.1021/bi501082r
Wu, Y., Matsui, H., and Tomizawa, K. (2009). Amphiphysin I and regulation of synaptic vesicle endocytosis. Acta Med. Okayama 63, 305–323.
Yang, J.-S., Gad, H., Lee, S. Y., Mironov, A., Zhang, L., Beznoussenko, G. V., et al. (2008). A role for phosphatidic acid in COPI vesicle fission yields insights into Golgi maintenance. Nat. Cell Biol. 10, 1146–1153. doi: 10.1038/ncb1774
Yang, J.-S., Lee, S. Y., Spanò, S., Gad, H., Zhang, L., Nie, Z., et al. (2005). A role for BARS at the fission step of COPI vesicle formation from Golgi membrane. EMBO J. 24, 4133–4143. doi: 10.1038/sj.emboj.7600873
Yang, J.-S., Valente, C., Polishchuk, R. S., Turacchio, G., Layre, E., Moody, D. B., et al. (2011). COPI acts in both vesicular and tubular transport. Nat. Cell Biol. 13, 996–1003. doi: 10.1038/ncb2273
Yoon, Y., Tong, J., Lee, P. J., Albanese, A., Bhardwaj, N., Källberg, M., et al. (2010). Molecular basis of the potent membrane-remodeling activity of the epsin 1 N-terminal homology domain. J. Biol. Chem. 285, 531–540. doi: 10.1074/jbc.m109.068015
Yoon, Y., Zhang, X., and Cho, W. (2012). Phosphatidylinositol 4,5-bisphosphate (PtdIns(4,5)P2) specifically induces membrane penetration and deformation by Bin/Amphiphysin/Rvs (BAR) domains. J. Biol. Chem. 287, 34078–34090. doi: 10.1074/jbc.m112.372789
Yorimitsu, T., Sato, K., and Takeuchi, M. (2014). Molecular mechanisms of Sar/Arf GTPases in vesicular trafficking in yeast and plants. Front. Plant Sci. 5:411. doi: 10.3389/fpls.2014.00411
Yoshida, Y. (2018). Insights into the mechanisms of chloroplast division. Int. J. Mol. Sci. 19:E733.
Yoshida, Y., and Mogi, Y. (2019). How do plastids and mitochondria divide? Microscopy 68, 45–56. doi: 10.1093/jmicro/dfy132
Yoshida, Y., Niwa, H., Honsho, M., Itoyama, A., and Fujiki, Y. (2015). Pex11 mediates peroxisomal proliferation by promoting deformation of the lipid membrane. Biol. Open 4, 710–721. doi: 10.1242/bio.201410801
Yu, S., and Melia, T. J. (2017). The coordination of membrane fission and fusion at the end of autophagosome maturation. Curr. Opin. Cell Biol. 47, 92–98. doi: 10.1016/j.ceb.2017.03.010
Zarranz, J. J., Alegre, J., Gómez-Esteban, J. C., Lezcano, E., Ros, R., Ampuero, I., et al. (2004). The new mutation, E46K, of α-synuclein causes Parkinson and Lewy body dementia. Ann. Neurol. 55, 164–173. doi: 10.1002/ana.10795
Zegarlińska, J., Piaścik, M., Sikorski, A. F., and Czogalla, A. (2018). Phosphatidic acid - a simple phospholipid with multiple faces. Acta Biochim. Pol. 65, 163–171. doi: 10.18388/abp.2018_2592
Zemel, A., Ben-Shaul, A., and May, S. (2008). Modulation of the spontaneous curvature and bending rigidity of lipid membranes by interfacially adsorbed amphipathic peptides. J. Phys. Chem. B 112, 6988–6996. doi: 10.1021/jp711107y
Zhang, C., Li, A., Gao, S., Zhang, X., and Xiao, H. (2011). The TIP30 protein complex, arachidonic acid and coenzyme A are required for vesicle membrane fusion. PLoS One 6:e21233. doi: 10.1371/journal.pone.0021233
Zhou, H., and Lutkenhaus, J. (2003). Membrane binding by MinD involves insertion of hydrophobic residues within the C-terminal amphipathic helix into the bilayer. J. Bacteriol. 185, 4326–4335. doi: 10.1128/jb.185.15.4326-4335.2003
Zhukovsky, M. A., Filograna, A., Luini, A., Corda, D., and Valente, C. (2019a). Phosphatidic acid in membrane rearrangements. FEBS Lett. 593, 2428–2451 doi: 10.1002/1873-3468.13563
Keywords: membrane fission, membrane scission, fission-inducing protein, amphipathic helix, shallow insertion, lipid cofactor, lipid-binding site, neck-hemifission model
Citation: Zhukovsky MA, Filograna A, Luini A, Corda D and Valente C (2019) Protein Amphipathic Helix Insertion: A Mechanism to Induce Membrane Fission. Front. Cell Dev. Biol. 7:291. doi: 10.3389/fcell.2019.00291
Received: 16 May 2019; Accepted: 06 November 2019;
Published: 10 December 2019.
Edited by:
Vladimir Lupashin, University of Arkansas for Medical Sciences, United StatesReviewed by:
Bruno Goud, Centre National de la Recherche Scientifique (CNRS), FrancePatricia Bassereau, Institut Curie, France
Copyright © 2019 Zhukovsky, Filograna, Luini, Corda and Valente. This is an open-access article distributed under the terms of the Creative Commons Attribution License (CC BY). The use, distribution or reproduction in other forums is permitted, provided the original author(s) and the copyright owner(s) are credited and that the original publication in this journal is cited, in accordance with accepted academic practice. No use, distribution or reproduction is permitted which does not comply with these terms.
*Correspondence: Mikhail A. Zhukovsky, bWlraGFpbC56aHVrb3Zza3lAaWJiYy5jbnIuaXQ=; Daniela Corda, ZGFuaWVsYS5jb3JkYUBjbnIuaXQ=; Carmen Valente, Y2FybWVuLnZhbGVudGVAaWJiYy5jbnIuaXQ=