- 1Burke Neurological Institute, White Plains, NY, United States
- 2Feil Family Brain and Mind Research Institute, Weill Cornell Medicine, New York, NY, United States
Neural injury in mammals often leads to persistent functional deficits as spontaneous repair in the peripheral nervous system (PNS) is often incomplete, while endogenous repair mechanisms in the central nervous system (CNS) are negligible. Peripheral axotomy elicits growth-associated gene programs in sensory and motor neurons that can support reinnervation of peripheral targets given sufficient levels of debris clearance and proximity to nerve targets. In contrast, while damaged CNS circuitry can undergo a limited amount of sprouting and reorganization, this innate plasticity does not re-establish the original connectivity. The utility of novel CNS circuitry will depend on effective connectivity and appropriate training to strengthen these circuits. One method of enhancing novel circuit connectivity is through the use of electrical stimulation, which supports axon growth in both central and peripheral neurons. This review will focus on the effects of CNS and PNS electrical stimulation in activating axon growth-associated gene programs and supporting the recovery of motor and sensory circuits. Electrical stimulation-mediated neuroplasticity represents a therapeutically viable approach to support neural repair and recovery. Development of appropriate clinical strategies employing electrical stimulation will depend upon determining the underlying mechanisms of activity-dependent axon regeneration and the heterogeneity of neuronal subtype responses to stimulation.
Introduction
Following injury to the adult mammalian central nervous system (CNS), neural circuits are permanently disrupted as severed axons fail to undergo spontaneous regeneration. The limited regenerative response of injured CNS neurons is due to both intrinsic and extrinsic factors. These growth-restrictive mechanisms play a large part in the poor clinical outcomes following brain or spinal cord trauma; however, despite limited regenerative capacity, mounting evidence has shown extensive spontaneous sprouting of CNS axon collaterals in the injured adult CNS.
The mammalian nervous system has an intrinsic capacity for structural and functional reorganization in response to a variety of stimuli during development, learning, or in response to pathological insults (Cramer et al., 2011; von Bernhardi et al., 2017). This innate plasticity is a key component of the system to adapt to a highly changing environment. Injury induced disruption of connectivity in the adult nervous system triggers plastic growth mechanisms and the sprouting of collaterals from spared, intact fibers (Maier and Schwab, 2006). Lesion-induced structural plasticity correlates with a limited degree of function recovery (Chen and Zheng, 2014); however, this innate plasticity falls far short of mediating recovery of damaged CNS circuits.
Recent findings suggest that manipulation of neuronal activity can drive plasticity related growth mechanisms and augment collateral sprouting, thereby enhancing the functional effect of axonal remodeling (Carmel and Martin, 2014). Electrical stimulation has long been known to enhance regeneration of peripheral axons (Hoffman and Binet, 1952; Pockett and Gavin, 1985). More recently, electrical stimulation has been used to enhance CNS plasticity in rodent models as well as to modulate and strengthen spared circuitry in individuals with spinal cord injury. Manipulation of activity-dependent neuroplasticity has great potential for improving neurological recovery from CNS injury. Herein we will discuss the systems-level effects of augmenting activity on injured neuronal circuits.
Peripheral Nervous System Regeneration
In contrast to most CNS neurons, neurons of the peripheral nervous system (PNS) have a more robust regenerative response to injury (Ramón y Cajal, 1991; Mahar and Cavalli, 2018). This owes to the intrinsic regenerative program activated following PNS injury (McQuarrie and Grafstein, 1973). In the dorsal root ganglia (DRG), this program is characterized by a robust neuronal and non-neuronal response and regulation of a distinct transcriptional program (Tsujino et al., 2000; Costigan et al., 2002; Boeshore et al., 2004; Seijffers et al., 2007; Stam et al., 2007; Chandran et al., 2016). Within the damaged nerve, there is an activation and proliferation of Schwann cells and a robust inflammatory response, resulting in both trophic support and the clearance of myelin debris to allow for axon regeneration (Hall, 1986; Kang and Lichtman, 2013). Peripheral nerve injury has therefore proven to be an invaluable model for studying the underlying mechanisms that support axon regeneration and the structural plasticity of injured neurons.
Despite the innate regenerative potential of PNS neurons after injury, recovery of function remains limited. Several factors can hamper recovery, such as age, extent of injury and disruption of endoneurium, perineurium, or epineurium, and neuroma formation. One critical factor is the slow rate of regeneration in the adult PNS of 1–3 mm per day (Scheib and Hoke, 2013). Axons need to regrow over long distances before reaching appropriate targets, particularly in humans, and a significant delay in target-reinnervation leads to the irreversible atrophy of muscles and end-organs (Lee and Wolfe, 2000). In the absence of appropriate sensory end organs and motor endplate organization, the chances of successful restoration of function dwindle. In order to combat these effects and improve functional outcomes following PNS injury, an ideal treatment would be one that accelerates axon regeneration.
Peripheral Conditioning and Molecular Pathways That Support Regeneration
The innate regenerative ability of adult mammalian PNS neurons has been used as a model to study the intrinsic mechanisms underlying the regenerative program. Primary sensory neurons are located in the DRG and extend axons into both CNS and PNS. Each axon exhibits a distinct response to injury in the adult. As described above, the peripheral axon retains the ability to regenerate following axotomy. In contrast, the CNS axon of the same cell will fail to regenerate after spinal cord injury. Intriguingly, the regenerative program activated by peripheral nerve injury conditions DRG neurons to mount an enhanced regenerative response to a second injury, whether in the peripheral or central axon (McQuarrie and Grafstein, 1973; Richardson and Issa, 1984). The process of peripheral conditioning results in the activation of a robust signaling cascade, inflammation, and transcriptional changes of thousands of genes in the DRG (Boeshore et al., 2004; Seijffers et al., 2007; Stam et al., 2007). Peripheral conditioning can be driven by axotomy (Richardson and Issa, 1984), inflammation (Steinmetz et al., 2005), demyelination (Hollis et al., 2015b), or electrical stimulation (Senger et al., 2018).
Another important change after conditioning lesion is the transient increase of second messenger cyclic nucleotide cAMP levels. Artificial elevation of cAMP can support a limited amount of sensory axon regeneration in the injured spinal cord (Qiu et al., 2002; Blesch et al., 2012). Downstream modulators of cAMP signaling have been directly linked to the regeneration program, including protein kinase A (Cai et al., 1999, 2001), cAMP response element-binding protein (CREB) (Gao et al., 2004), CREB binding protein (CBP) (Tedeschi et al., 2009) and arginase 1 (Cai et al., 2002).
Activation of the pro-regenerative transcriptional response in the somata of regenerating neurons requires a retrograde signal from the injury site. One candidate for this rapid signal is the early influx of calcium at the injury site (Ziv and Spira, 1995; Wolf et al., 2001; Mandolesi et al., 2004; Ghosh-Roy et al., 2010; Cho et al., 2013). Rapid calcium influx is a hallmark of injury conserved across species from invertebrates to mammals (Rishal and Fainzilber, 2014). Increased calcium levels in the axoplasm act locally on calcium-dependent enzymes related to protein synthesis, cytoskeletal modification, and growth cone formation (Chierzi et al., 2005; Bradke et al., 2012).
Additionally, early axotomy-induced calcium influx rapidly propagates retrogradely to the cell soma. Axotomy of cultured cortical neurons initiates a rapid membrane depolarization at the injury site triggering a fast-retrograde spiking activity and sustained cell body depolarization (Mandolesi et al., 2004). Injury-induced depolarization stimulates the calcium entry through activation of voltage-gated calcium channels (VGCC) or inversion of the sodium-calcium exchange pump following a rise in cytosolic sodium concentration through voltage-gated sodium channels (Mandolesi et al., 2004). Intracellular calcium stores in sensory neurons are released following the initial increase in calcium concentration induced by peripheral injury (Rigaud et al., 2009). These stores are required for the propagation of the calcium wave as pre-emptive depletion of ryanodine receptor-sensitive intracellular calcium stores prevents back propagation and activation at the soma (Cho et al., 2013). It is likely that this calcium wave is a critical preliminary signal to prime neurons for regeneration as preventing it in cortical neurons by inhibiting TTX-sensitive sodium channels impairs neurite extension after in vitro injury (Mandolesi et al., 2004). Artificially elevating calcium influx in motor and sensory neurons by activation of the non-selective, light-sensitive cation channel channelrhodopsin can increase the rate of functional regeneration in mice (Ward et al., 2016, 2018). In C. elegans sensory neurons, this channelrhodopsin-mediated regeneration has been shown to depend on ryanodine receptor channel release of endoplasmic reticulum calcium stores (Sun et al., 2014). Interestingly, additional epigenetic-mediated changes by calcium increase after injury have been identified. Back propagation of calcium waves regulates epigenetic mechanisms including the release of histone deacetylase 5 (HDAC5) from the nucleus, and inactivation of HDAC3, leading to the initiation of a pro-regenerative transcriptional program (Cho et al., 2013; Hervera et al., 2019).
Electrical stimulation of peripheral nerves elicits this retrograde calcium signal. Genetically encoded calcium indicators can be used to image the calcium wave in vivo by fluorescent microscopy. Expression of the calcium indicator GCaMP6s in lumbar level 4 primary sensory neurons has been used to visualize calcium transients in response to sciatic nerve stimulation. Both large and small-diameter sensory somata show maximal calcium responses to low-frequency (20 Hz) stimulation (Chisholm et al., 2018). Short duration pulses (250 ms duration, 250 mA amplitude) preferentially activated large-diameter neurons, while longer duration (1 ms duration, 5 mA amplitude) activated most both A and C fiber cell bodies. Whether these distinct stimulation parameters elicit differential cellular responses is unknown.
The spatiotemporal regulation of calcium influx can regulate the regenerative program as calcium transients in the proximal segment after injury impair regeneration. In non-regenerating sensory neurons of drosophila, injury induces local calcium transients through the mechanosensitive cation channel dmPiezo, which inhibits axon regeneration by activating the calcium signaling regulator Ca2+/calmodulin-dependent protein kinase II (CamKII) (Song et al., 2019). Nitric oxide synthase (NOS) and the cGMP-dependent kinase PKG act downstream of Piezo-mediated calcium signaling to inhibit axon regeneration in drosophila (Song et al., 2019). Piezo-mediated inhibition of regeneration is conserved in mouse models of sensory axotomy and conditional deletion of Piezo1 enhances regeneration in vivo (Song et al., 2019). Furthermore, expression of the α2δ2 subunit of voltage gated calcium channels has been proposed to be a developmental switch underlying the decrease in axonal growth capacity that accompanies PNS maturation (Tedeschi et al., 2016). Genetic deletion of the encoding gene Cacna2d2 promotes neurite elongation from cultured primary sensory neurons, while α2δ2 overexpression inhibits elongation through Cav2-mediated influx of pre-synaptic extracellular calcium (Tedeschi et al., 2016). Blockade of α2δ2 by clinically approved gabapentinoid drugs promotes neurite elongation in vitro and sensory axon regeneration and regenerative sprouting from injured corticospinal axons in vivo (Tedeschi et al., 2016; Sun et al., 2020).
Neuronal Activity and Molecular Control Over Axon Growth
Neuronal activity can activate growth-associated molecular pathways. In an optic nerve model of CNS injury, driving activity in retinal ganglion cells (RGCs) by high-contrast visual stimulation or via chemogenetics approaches can enhance optic nerve regeneration (Lim et al., 2016). In contrast, suppressing neuronal activity with chemogenetics prevents the pro-regenerative effect of high-contrast visual stimulation (Lim et al., 2016). Furthermore, light-sensitive RGCs that express melanopsin are resistant to axotomy and exhibit high levels of phosphorylated ribosomal protein S6 (pS6) (Li et al., 2016). RGC pS6 levels decrease after axon elongation during development and this downregulation can be attenuated by deletion of the phosphatase and tensin homolog (PTEN) (Park et al., 2008). PTEN deletion from RGCs and other CNS neurons leads to elevated PI3K/mTOR (mammalian target of rapamycin) signaling, enhanced phosphorylation of S6, and an increased capacity for axonal growth after injury (Park et al., 2008; Liu et al., 2010). Alternatively, activating mTOR via over-expression of a constitutively active form of ras homolog enriched in brain 1 (cRheb1) appears to enhance RGC regeneration, though not nearly as robustly as PTEN deletion (Park et al., 2008; Lim et al., 2016).
Chronic electrical stimulation of the motor cortex over ten consecutive days leads to activation of the mTOR pathway, inactivation of PTEN, and increased phosphorylation of ribosomal protein S6 (Zareen et al., 2018). Additionally, this chronic stimulation drives increased levels of Janus kinase-signal transducer and activator of transcription (JAK/STAT) signaling (Zareen et al., 2018), a critical mediator of cytokine signaling. Enhancing JAK/STAT signaling through deletion of suppressor of cytokine signaling 3 (SOCS3) increases optic nerve regeneration (Smith et al., 2009). JAK/STAT signaling acts independently of mTOR, while SOCS3 and PTEN co-deletion deletion act synergistically to promote sustained optic nerve regeneration (Sun et al., 2011). In the motor cortex, the activation of these separate molecular pathways by chronic, daily electrical stimulation drives distinct aspects of structural remodeling in the intact corticospinal circuitry. New corticospinal collaterals sprout and form synaptic connections in the spinal cord during 10 days of stimulation. Pharmacological studies implicate mTOR signaling in collateral formation as corticospinal collateral sprouting is blocked by rapamycin; whereas, inhibiting Stat3 activation with AG490 reduced both bouton-like structures on corticospinal axons as well as levels of cFos expression in ipsilateral cervical spinal cord neurons without affecting corticospinal collateral formation (Zareen et al., 2018). These studies demonstrate that not only can electrical stimulation activate growth-promoting molecular pathways, it can strengthen the formation of novel connections needed to elicit functional recovery.
Regeneration competent PNS neurons have been extensively studied to determine the molecular pathways critical for driving axon regeneration. Regeneration-associated genes (RAGs) have been largely defined as the coordinated and complementary genes activated by peripheral conditioning paradigms in PNS neurons. These include developmental growth-associated proteins (e.g., GAP-43, CAP23, and SPRR1A), transcription factors (e.g., ATF-3, c-Jun, Sox11, Smad1, Klf family members, and Stat3), and signaling pathways (MAPK, cytokine, JAK-STAT, TGF-b, neurotrophin) (Chandran et al., 2016; Mahar and Cavalli, 2018). In much the same manner that electrical stimulation of the cortex activates pro-regenerative molecular pathways, electrical stimulation of peripheral nerves has been shown to induce identified RAG expression in both sensory and motor neurons. Direct low-frequency electrical stimulation of the intact rodent sciatic nerves at 20 Hz for 1 h induces a significant upregulation in the expression of growth associated protein-43 (GAP-43), the neurotrophin BDNF and its high-affinity receptor trkB, as well as increased phosphorylation of the transcription factor cAMP response element binding protein (CREB) in DRGs neurons (English et al., 2007; Senger et al., 2018, 2019). This gene induction is comparable to injury-induced RAG induction (Senger et al., 2018, 2019). Similarly, brief electrical stimulation of the injured rat femoral nerve rapidly activates a RAGs response in motor neurons with upregulation of GAP43 and Tα1-Tubulin, as well as an increase in BDNF and trkB expression in stimulated motor neurons (Al-Majed et al., 2000a, 2004). The effects of electrical stimulation extend to perineuronal glial cells, with increased GFAP expression in satellite glia (Senger et al., 2018). In addition to the transcriptional regulation that occurs with electrical stimulation, 1 h of low-frequency stimulation of intact rat sciatic nerves increases intracellular cAMP levels in DRG neurons similar to nerve injury (Udina et al., 2008). Despite the fact that electrical stimulation and axotomy induce similar increases in cAMP, 1 h of low-frequency electrical stimulation is not sufficient to fully recapitulate the regenerative response of peripheral conditioning of sensory neurons to a central spinal cord injury (Udina et al., 2008; Goganau et al., 2018).
While electrical stimulation activates many of the same molecular pathways as peripheral conditioning via crush injury, it does not fully recapitulate the growth-promoting effects of conditioning. Following a subsequent peripheral injury, previous exposure to low-frequency electrical stimulation enhances the initiation of regeneration, but does not increase the rate of peripheral motor or sensory axon regeneration (Al-Majed et al., 2000b; Brushart et al., 2002). The limited effectiveness of electrical conditioning extends to the CNS as well, as regeneration of the central axon of dorsal column projecting sensory neurons after a spinal cord injury is less robust in rats conditioned 1 week prior with electrical stimulation than those subjected to nerve crush injury (Goganau et al., 2018). Electrical stimulation immediately following a dorsal column spinal cord injury allows for an increased initiation of regeneration; however, these axons fail to elongate through the injury site, in contrast to more robust regenerative effects of nerve crush injury (Udina et al., 2008). Despite the limitations of electrical stimulation compared to nerve crush, stimulation is a more attractive potential therapeutic approach to enhancing axon regeneration. The use of a conditioning nerve crush injury in patients is impractical as it would increase the risk of surgical complications and nerve crush elicits neuropathic pain (Hollis et al., 2015b).
Some of the limited effectiveness of current electrical conditioning may be alleviated by further optimization of stimulation parameters. Primary sensory neurons are sensitive to the frequency of electrically stimulated action potential patterns, activating discrete transcriptional programs based upon temporal changes in bursting patterns (Lee et al., 2017). It has long been known that specific patterns of induced neural activity in primary sensory neurons regulate immediate early gene expression independent of intracellular calcium levels (Sheng et al., 1993). The duration of neural activity induces unique gene expression profiles in a mechanistically distinct manner (Tyssowski et al., 2018). Defined action potential bursts underlie a temporal specificity in calcium influx through voltage gated calcium channels, leading to a tightly regulated activation of cAMP-responsive element binding protein (CREB) and mitogen-activated protein kinase (MAPK) signaling pathways (Fields et al., 1997; Wheeler et al., 2012). These same mechanisms may regulate the regenerative responses to discrete patterns of electrical stimulation. Indeed, modulation of stimulation frequency and current appears to alter the morphological and electrical properties of regenerated rat sciatic nerve (Lu et al., 2008, 2009). Despite the activation of the regenerative program found in vivo by low frequency (20 Hz) electrical stimulation, 20 Hz trains separated by 5 min intervals arrested neurite outgrowth of primary sensory neurons in vitro (Enes et al., 2010). Calcium influx through voltage-gated Cav1.2 calcium channels was proposed to mediate the stimulation evoked arrest of axon growth, with enhanced neurite outgrowth from cultured Cav1.2 deleted sensory neurons (Enes et al., 2010). Stimulation intensity of primary sensory neurons differentially regulates signaling through Cav1 and Cav2 channels (Wheeler et al., 2012), potentially driving distinct transcriptional responses and differences in the regenerative response.
Furthermore, maintenance of the energy supply in neurons is critical to meet metabolic demands and supporting physiological homeostasis. Axonal mitochondrial transport is crucial to this end. Following injury, the bioenergetic balance is highly disrupted, with mitochondrial depolarization and ATP depletion (Zhou et al., 2016). Increasing mitochondrial transport enhances peripheral and central axon regeneration (Cartoni et al., 2016; Zhou et al., 2016; Han et al., 2020). Electrical stimulation of peripheral nerves increases mitochondrial transport in a frequency dependent fashion (Sajic et al., 2013). Low frequency stimulation (1 Hz) mobilizes mitochondria transport in both anterograde and retrograde directions, while higher frequency (50 Hz) stimulation further augmented anterograde transport only (Sajic et al., 2013). The increase in mitochondrial transport mediated by electrical stimulation may support the regenerative response following injury.
Functional Effects of Electrical Stimulation on Circuit Recovery
Electrical stimulation has been used to hasten the recovery of both peripheral and central circuits after injury. While limited in comparison to the growth-promoting effects of conditioning crush injury, post-injury electrical stimulation has proven safe and effective in both animal models and humans. Low-frequency (4 Hz) stimulation of the soleus muscle in the rabbit was used to shorten the time for recovery of soleus function after axonotmesis of the soleus motor nerve (Nix and Hopf, 1983). This more rapid recovery of function elicited by post-injury electrical stimulation has also been observed in rodent models of nerve repair. A single hour of low-frequency electrical stimulation immediately following sciatic nerve transection and repair enhances axon regeneration and accelerates recovery of motor and sensory function, as measured by electrophysiological recordings and thermal sensitivity in the reinnervated hindpaw (Figure 1; Singh et al., 2012). Similar single 20 Hz electrical stimulation has been shown to enhance recovery after femoral nerve transection and epineurial suture repair, with quadriceps function returning 6-weeks earlier in stimulated mice than in controls (Ahlborn et al., 2007). Low-frequency stimulation post-surgery has been combined with daily locomotor training to accelerate the recovery of neurophysiological function after sciatic transection and epineurial suture repair (Asensio-Pinilla et al., 2009). More recently, the use of conditioning electrical stimulation was directly compared to nerve crush conditioning in a rat model of tibial nerve repair, in which the tibial nerve was conditioned 1 week prior to a nerve transection and epineurial suture repair (Senger et al., 2019). In these studies, conditioning by electrical stimulation was found to elicit greater physiological and functional recovery than conditioning by nerve crush. This apparent discrepancy with the effects observed in vitro and in models of subsequent spinal cord injury (Goganau et al., 2018) is likely due to slowing of the regenerating axons upon reaching the disrupted cytoarchitecture, proliferative Schwann cells, and inflammation at the nerve crush site. The efficacy of both pre- and post-injury electrical stimulation in enhancing regeneration of rodent peripheral nerves and accelerating functional outcomes, together with the feasibility of its use in bedside settings makes brief low frequency electrical stimulation a clinically relevant approach to mediate PNS repair. Indeed, clinical studies have found that electrical stimulation following surgical intervention to treat either full digital nerve transection or median nerve crush (carpal tunnel syndrome) is well-tolerated and enhances the recovery of both sensory and motor function (Gordon et al., 2010; Wong et al., 2015).
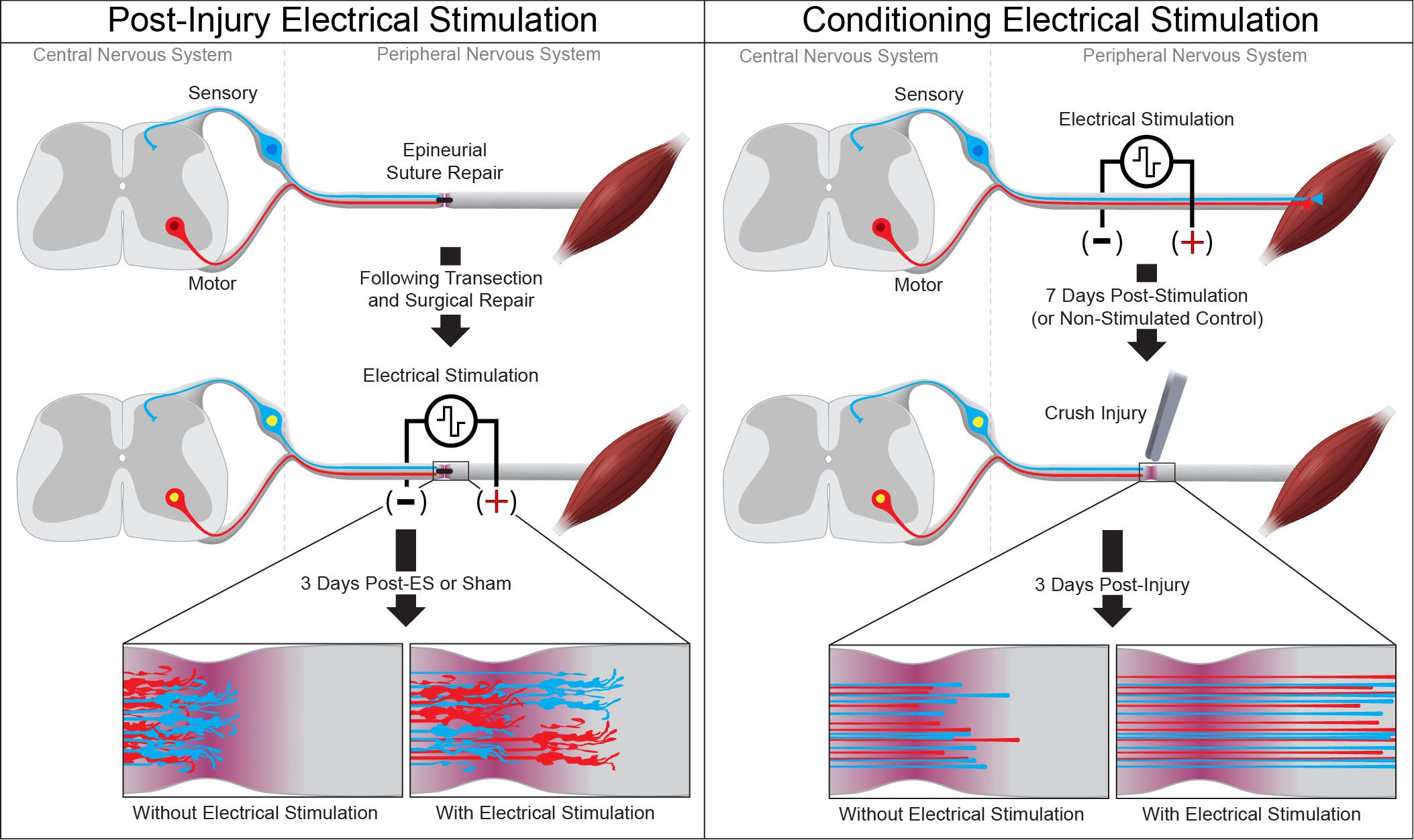
Figure 1. Electrical stimulation enhances peripheral axon regeneration. A single session of low-frequency electrical stimulation (1 h, 20 Hz) can enhance motor and sensory axon regeneration following epineurial suture repair (left) or when used as a conditioning stimulus prior to subsequent axotomy (right).
Within the CNS, chronic electrical stimulation drives collateral sprouting from intact descending corticospinal circuits (Figure 2). The corticospinal circuit is the primary motor pathway in primates and largely projects to the contralateral spinal cord. Ten days of stimulation (333 Hz, 45 ms bursts, every 2 s, for 6 h/day) of the medullary pyramid promotes sprouting of the corticospinal tract across the midline into the ipsilateral, denervated spinal cord (Brus-Ramer et al., 2007). This collateral sprouting is similar to what is observed following unilateral transection of the medullary pyramids (pyramidotomy), when the absence of corticospinal input to the spinal cord leads to cross-midline sprouting from the spared, intact corticospinal tract (Brus-Ramer et al., 2007; Lee et al., 2010). Performing chronic electrical stimulation after unilateral pyramidotomy results in an additive effect on corticospinal collateral sprouting (Brus-Ramer et al., 2007). Stimulation of the contralesional motor cortex mimics the direct stimulation of the pyramids, and this enhanced circuit plasticity drives recovery of skilled locomotor function after unilateral pyramidotomy in acute and chronic injury models (Carmel et al., 2010, 2014). Recovery is likely mediated by the formation of a novel, ipsilateral corticospinal circuit as inactivation of both ipsilateral and contralateral motor cortex impairs the behavioral recovery, while intact animals are only affected by contralateral cortical inactivation (Carmel et al., 2014). The growth-promoting effects of cortical stimulation are not restricted to intact circuits, as cortical electrical stimulation following cervical spinal cord injury drives corticospinal axon growth proximal to the injury site and enhances the recovery of forelimb function (Batty et al., 2020). In addition to the enhancement of axonal growth, the restoration of function through novel corticospinal circuits requires the synapse-promoting effects of cortical electrical stimulation. Pairing of cortical stimulation with peripheral afferents transiently strengthens the response to descending corticospinal input in acute experiments in rats (Mishra et al., 2017). Similar results have been demonstrated in healthy and spinal cord injured individuals (Bunday and Perez, 2012). In rats, repeated pairing of intermittent theta burst cortical stimulation with trans-spinal direct current stimulation after cervical spinal cord injury results in a strengthening of novel connections in the spinal cord. Furthermore, this paired stimulation paradigm can support the recovery of dextereous forelimb movements that depend on corticospinal function (Zareen et al., 2017; Yang et al., 2019). A brief summary of rodent electrical stimulation studies can be found in Table 1.
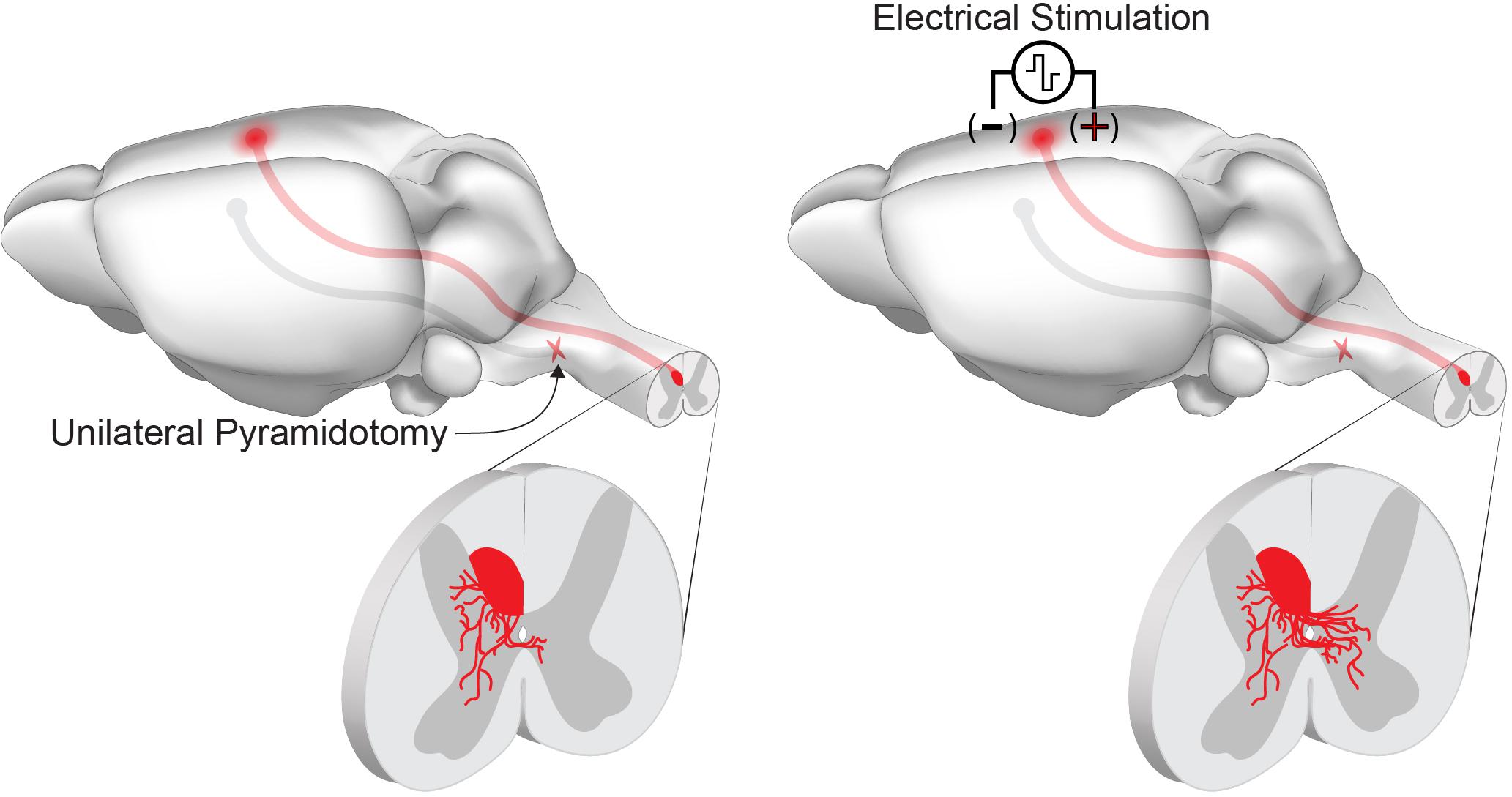
Figure 2. Chronic electrical stimulation can drive corticospinal tract sprouting following injury. Unilateral pyramidotomy activates spontaneous collateral sprouting from the contralateral, intact corticospinal tract within the spinal cord. Chronic stimulation of the motor cortex activates neuron intrinsic growth pathways and enhances collateral sprouting and connectivity of spared corticospinal tract axons.
Therapeutic Potential of Electrical Stimulation
In contrast to the robust regenerative response in rodents, injury of peripheral nerves in humans often results in incomplete or inadequate functional recovery (Scheib and Hoke, 2013). The efficacy of pre- and post-injury electrical stimulation in improving regeneration and functional outcomes has been demonstrated in multiple rodent models of peripheral nerve injury. This, combined with the feasibility of use in bedside settings, makes brief, low-frequency electrical stimulation a clinically viable approach in peripheral nerve repair as a means to mitigate the effects of slow regeneration rate on the loss of end-organs and muscle atrophy (Lee and Wolfe, 2000). To date, 1 h of low-frequency (20 Hz) electrical stimulation has been successfully applied in clinical practice (Gordon et al., 2010; Wong et al., 2015). Individuals with motor axon loss from chronic median nerve compression at the wrist underwent decompression surgery. The group treated with single low-frequency electrical stimulation for 1 h after surgery showed enhanced reinnervation of thenar muscles characterized by improved motor and sensory nerve conduction in comparison to the surgery only group (Gordon et al., 2010). Electrical stimulation was also effective in improving physiological recovery and the return of normal sensory function when applied following epineurial repair of transected digital nerves (Wong et al., 2015). The effectiveness of these clinical findings on peripheral nerve repair are consistent with the evidence obtained from animal models. The recent findings that pre-conditioning of peripheral nerves with electrical stimulation has a robust effect on axon regeneration (Senger et al., 2019) suggests that there may be a benefit to performing pre-operative electrical stimulation in cases of surgical interventions involving intact nerves, such as nerve transfer. Further clinical studies are warranted to determine if the functional benefit of low-frequency electrical stimulation translates to long-distance nerve repair surgeries, such as brachial plexus repair. Additionally, the potential for peripheral nerve stimulation to enhance the sprouting of central sensory axons after spinal cord injury could be used to restore sensory function critical for movement (Takeoka et al., 2014). Strengthening ascending mechanosensory input to only a small proportion (<5%) of dorsal column nuclei is sufficient to support some level of functional recovery (Hollis et al., 2015a), so the translation of PNS electrical stimulation may prove to be a viable approach to restore sensory function after spinal cord injury.
Despite the limited regenerative capacity of CNS axons after spinal cord injury, some spontaneous compensatory sprouting from spared and injured axons can occur in both rodent and non-human primates (Ghosh et al., 2010; Rosenzweig et al., 2010). Mounting evidence indicates that this post-lesion plasticity can be increased by artificially driving neuronal activity. Epidural electrical stimulation has been employed in both animal models and in paraplegic individuals to engage motor circuits and support locomotor recovery. The extent to which this neuromodulation can have lasting impacts on motor circuit remodeling after injury is not yet known. Following complete thoracic spinal cord injury in rats, spinal motor networks are engaged during periods of epidural electrical stimulation, which allows for locomotion in the absence of descending input (Ichiyama et al., 2005). Chronically injured paraplegic individuals have similarly shown engagement of spinal motor circuits in the presence of epidural electric stimulation. Epidural stimulation in motor complete individuals allows for volitional movement of paralyzed leg muscles, including overground walking during stimulation (Harkema et al., 2011; Angeli et al., 2014, 2018). Furthermore, repeated pairing of activity-based training with epidural stimulation in a motor complete individual over several years has supported the recovery of trained volitional motor movements, independent of stimulation (Rejc et al., 2017). Patterning of epidural stimulation over the lumbar spinal cord to sequentially activate agonist-antagonist muscle groups supports the recovery of stimulation-mediated walking in chronic paraplegic individuals when paired with intensive rehabilitation (Wagner et al., 2018). Furthermore, some of the individuals enrolled in this study regained voluntary leg movements in the absence of stimulation. The underlying activity-dependent mechanism activated by epidural electrical stimulation paired with rehabilitation remains unknown. It is likely that the initial responses are activating motor circuits below the injury and residual supraspinal circuitry is able to engage these excited motor networks either directly or indirectly through spared propriospinal circuitry. The eventual recovery of limited volitional control in several individuals may result from engaging many of the same neuroplasticity mechanisms identified in electrical stimulation studies in animal models.
In contrast to implanted epidural stimulators, transcutaneous electrical spinal cord stimulation (TESS) is a non-invasive and painless neuromodulation strategy that augments motor and sensory function after SCI (Gerasimenko et al., 2015; Hofstoetter et al., 2015; Gad et al., 2018; Benavides et al., 2020). Cervical TESS, in combination with training, has been shown to induce a long-term improvement in volitional motor control and restoration of hand sensory function individuals with chronic incomplete SCI (Inanici et al., 2018). TESS utilizes currents from 5 to 50 Hz, with a carrier frequency (Russian current) between 5 and 10 kHz (Benavides et al., 2020). Changes in spinal sensory and motor circuit excitability have been proposed to underlie TESS-mediated functional effects, with neuroplasticity mechanisms facilitating a reorganization of spinal networks during intensive, rehabilitative training (Inanici et al., 2018; Benavides et al., 2020). The extent of anatomical circuit remodeling induced by TESS remains to be determined.
Among the different approaches that have been proposed to enhance post-lesion plasticity, extrinsic manipulation of neuronal activity by electrical stimulation is an attractive therapeutic approach. Electrical stimulation has been demonstrated to engage plasticity mechanisms in several central and peripheral neural circuits and has already shown feasibility in clinical settings. Development of appropriate strategies will likely depend upon the selective activation of desired neural subtypes in a temporally and spatially organized manner. Non-targeted electrical fields have been used to trigger population responses; however, it may be that distinct electrical stimulation parameters can selectively affect neuronal subtype responses and circuit-specific functional outcomes. Whether the molecular mechanisms activated by electrical stimulation are consistent across neuronal populations is unknown. The sprouting response of corticospinal axons to electrical stimulation contrasts with the elongation observed in stimulated PNS axons. These differences may arise from disparate stimulation parameters, discrete responses of these distinct populations to electrical stimulation, or from interactions of conserved stimulation-mediated molecular pathways with the intrinsic limitations of adult CNS neurons to axon elongation. Further studies will be required to identify whether CNS-tuned parameters of electrical stimulation can drive a regenerative response in transected corticospinal axons.
In the context of SCI, preclinical and clinical studies have clearly demonstrated that the stimulation of local spinal networks can drive lasting functional improvements (Ievins and Moritz, 2017; Courtine and Sofroniew, 2019; Hutson and Di Giovanni, 2019). Cortical and spinal cord paired electrical stimulation is an attractive approach to both enhance intrinsic sprouting and strengthen residual and newly formed collateral connections within the spinal cord (Dixon et al., 2016; Mishra et al., 2017). Additionally, it is likely that targeted rehabilitation or concurrent therapies, such as constraint-induced movement therapy, will be needed to strengthen and engage novel circuitry driven by electrical stimulation. The development of novel technologies will allow for the combining of biomedical devices for stimulation with rehabilitation and molecular or genetic control over neuroplasticity to support recovery after neurological injury and improve individuals’ quality of life.
Author Contributions
JJ and EH wrote and edited the manuscript. SA created the illustrations. All authors contributed to the article and approved the submitted version.
Funding
Financial support was provided by The Winifred Masterson Burke Foundation, the NIH Common Fund (1DP2NS106663), and the New York State Department of Health Spinal Cord Injury Research Board (C33267GG and C34463GG).
Conflict of Interest
The authors declare that the research was conducted in the absence of any commercial or financial relationships that could be construed as a potential conflict of interest.
Acknowledgments
The authors would like to thank Amanda Bernstein for critical reading of the manuscript.
References
Ahlborn, P., Schachner, M., and Irintchev, A. (2007). One hour electrical stimulation accelerates functional recovery after femoral nerve repair. Exper. Neurol. 208, 137–144. doi: 10.1016/j.expneurol.2007.08.005
Al-Majed, A. A., Brushart, T. M., and Gordon, T. (2000a). Electrical stimulation accelerates and increases expression of BDNF and trkB mRNA in regenerating rat femoral motoneurons. Eur. J. Neurosci. 12, 4381–4390. doi: 10.1111/j.1460-9568.2000.01341.x
Al-Majed, A. A., Neumann, C. M., Brushart, T. M., and Gordon, T. (2000b). Brief electrical stimulation promotes the speed and accuracy of motor axonal regeneration. J. Neurosci. 20, 2602–2608. doi: 10.1523/jneurosci.20-07-02602.2000
Al-Majed, A. A., Tam, S. L., and Gordon, T. (2004). Electrical stimulation accelerates and enhances expression of regeneration-associated genes in regenerating rat femoral motoneurons. Cell. Mol. Neurobiol. 24, 379–402. doi: 10.1023/b:cemn.0000022770.66463.f7
Angeli, C. A., Boakye, M., Morton, R. A., Vogt, J., Benton, K., Chen, Y., et al. (2018). recovery of over-ground walking after chronic motor complete spinal cord injury. New Engl. J. Med. 379, 1244–1250. doi: 10.1056/nejmoa1803588
Angeli, C. A., Edgerton, V. R., Gerasimenko, Y. P., and Harkema, S. J. (2014). Altering spinal cord excitability enables voluntary movements after chronic complete paralysis in humans. Brain 137, 1394–1409. doi: 10.1093/brain/awu038
Asensio-Pinilla, E., Udina, E., Jaramillo, J., and Navarro, X. (2009). Electrical stimulation combined with exercise increase axonal regeneration after peripheral nerve injury. Exper. Neurol. 219, 258–265. doi: 10.1016/j.expneurol.2009.05.034
Batty, N. J., Torres-Espín, A., Vavrek, R., Raposo, P., and Fouad, K. (2020). Single-session cortical electrical stimulation enhances the efficacy of rehabilitative motor training after spinal cord injury in rats. Exper. Neurol. 324:113136. doi: 10.1016/j.expneurol.2019.113136
Benavides, F. D., Jo, H. J., Lundell, H., Edgerton, V. R., Gerasimenko, Y., and Perez, M. A. (2020). Cortical and subcortical effects of transcutaneous spinal cord stimulation in humans with tetraplegia. J. Neurosci. 40, 2633–2643. doi: 10.1523/jneurosci.2374-19.2020
Blesch, A., Lu, P., Tsukada, S., Alto, L. T., Roet, K., Coppola, G., et al. (2012). Conditioning lesions before or after spinal cord injury recruit broad genetic mechanisms that sustain axonal regeneration: Superiority to camp-mediated effects. Exper. Neurol. 235, 162–173. doi: 10.1016/j.expneurol.2011.12.037
Boeshore, K. L., Schreiber, R. C., Vaccariello, S. A., Sachs, H. H., Salazar, R., Lee, J., et al. (2004). Novel changes in gene expression following axotomy of a sympathetic ganglion: a microarray analysis. J. Neurobiol. 59, 216–235. doi: 10.1002/neu.10308
Bradke, F., Fawcett, J. W., and Spira, M. E. (2012). Assembly of a new growth cone after axotomy: the precursor to axon regeneration. Nat. Rev. Neurosci. 13, 183–193. doi: 10.1038/nrn3176
Brushart, T. M., Hoffman, P. N., Royall, R. M., Murinson, B. B., Witzel, C., and Gordon, T. (2002). Electrical stimulation promotes motoneuron regeneration without increasing its speed or conditioning the neuron. J. Neurosci. 22, 6631–6638. doi: 10.1523/jneurosci.22-15-06631.2002
Brus-Ramer, M., Carmel, J. B., Chakrabarty, S., and Martin, J. H. (2007). Electrical stimulation of spared corticospinal axons augments connections with ipsilateral spinal motor circuits after injury. J. Neurosci. 27, 13793–13801. doi: 10.1523/jneurosci.3489-07.2007
Bunday, K. L., and Perez, M. A. (2012). Motor recovery after spinal cord injury enhanced by strengthening corticospinal synaptic transmission. Curr. Biol. 22, 2355–2361. doi: 10.1016/j.cub.2012.10.046
Cai, D., Deng, K., Mellado, W., Lee, J., Ratan, R. R., and Filbin, M. T. (2002). Arginase I and polyamines act downstream from cyclic AMP in overcoming inhibition of axonal growth MAG and myelin in vitro. Neuron 35, 711–719. doi: 10.1016/s0896-6273(02)00826-7
Cai, D., Qiu, J., Cao, Z., Mcatee, M., Bregman, B. S., and Filbin, M. T. (2001). Neuronal cyclic AMP controls the developmental loss in ability of axons to regenerate. J. Neurosci. 21, 4731–4739. doi: 10.1523/jneurosci.21-13-04731.2001
Cai, D., Shen, Y., De Bellard, M., Tang, S., and Filbin, M. T. (1999). Prior exposure to neurotrophins blocks inhibition of axonal regeneration by MAG and Myelin via a cAMP-dependent mechanism. Neuron 22, 89–101. doi: 10.1016/s0896-6273(00)80681-9
Carmel, J. B., Berrol, L. J., Brus-Ramer, M., and Martin, J. H. (2010). Chronic electrical stimulation of the intact corticospinal system after unilateral injury restores skilled locomotor control and promotes spinal axon outgrowth. J. Neurosci. 30, 10918–10926. doi: 10.1523/jneurosci.1435-10.2010
Carmel, J. B., Kimura, H., and Martin, J. H. (2014). Electrical stimulation of motor cortex in the uninjured hemisphere after chronic unilateral injury promotes recovery of skilled locomotion through ipsilateral control. J. Neurosci. 34, 462–466. doi: 10.1523/jneurosci.3315-13.2014
Carmel, J. B., and Martin, J. H. (2014). Motor cortex electrical stimulation augments sprouting of the corticospinal tract and promotes recovery of motor function. Front. Integr. Neurosci. 8:51. doi: 10.3389/fnint.2014.00051
Cartoni, R., Norsworthy, M. W., Bei, F., Wang, C., Li, S., Zhang, Y., et al. (2016). The mammalian-specific protein armcx1 regulates mitochondrial transport during axon regeneration. Neuron 92, 1294–1307. doi: 10.1016/j.neuron.2016.10.060
Chandran, V., Coppola, G., Nawabi, H., Omura, T., Versano, R., Huebner, E. A., et al. (2016). A systems-level analysis of the peripheral nerve intrinsic axonal growth program. Neuron 89, 956–970. doi: 10.1016/j.neuron.2016.01.034
Chen, M., and Zheng, B. (2014). Axon plasticity in the mammalian central nervous system after injury. Trends Neurosci. 37, 583–593. doi: 10.1016/j.tins.2014.08.008
Chierzi, S., Ratto, G. M., Verma, P., and Fawcett, J. W. (2005). The ability of axons to regenerate their growth cones depends on axonal type and age, and is regulated by calcium, cAMP and ERK. Eur. J. Neurosci. 21, 2051–2062. doi: 10.1111/j.1460-9568.2005.04066.x
Chisholm, K. I., Khovanov, N., Lopes, D. M., La Russa, F., and Mcmahon, S. B. (2018). Large scale in vivo recording of sensory neuron activity with GCaMP6. eNeuro 5:ENEURO.0417-17.2018.
Cho, Y., Sloutsky, R., Naegle, K. M., and Cavalli, V. (2013). Injury-induced HDAC5 nuclear export is essential for axon regeneration. Cell 155, 894–908. doi: 10.1016/j.cell.2013.10.004
Costigan, M., Befort, K., Karchewski, L., Griffin, R. S., D’urso, D., Allchorne, A., et al. (2002). Replicate high-density rat genome oligonucleotide microarrays reveal hundreds of regulated genes in the dorsal root ganglion after peripheral nerve injury. BMC Neurosci. 3:16. doi: 10.1186/1471-2202-3-16
Courtine, G., and Sofroniew, M. V. (2019). Spinal cord repair: advances in biology and technology. Nat. Med. 25, 898–908. doi: 10.1038/s41591-019-0475-6
Cramer, S. C., Sur, M., Dobkin, B. H., O’brien, C., Sanger, T. D., Trojanowski, J. Q., et al. (2011). Harnessing neuroplasticity for clinical applications. Brain 134, 1591–1609.
Dixon, L., Ibrahim, M. M., Santora, D., and Knikou, M. (2016). Paired associative transspinal and transcortical stimulation produces plasticity in human cortical and spinal neuronal circuits. J. Neurophysiol. 116, 904–916. doi: 10.1152/jn.00259.2016
Enes, J., Langwieser, N., Ruschel, J., Carballosa-Gonzalez, M. M., Klug, A., Traut, M. H., et al. (2010). Electrical activity suppresses axon growth through Ca(v)1.2 channels in adult primary sensory neurons. Curr. Biol. 20, 1154–1164. doi: 10.1016/j.cub.2010.05.055
English, A. W., Schwartz, G., Meador, W., Sabatier, M. J., and Mulligan, A. (2007). Electrical stimulation promotes peripheral axon regeneration by enhanced neuronal neurotrophin signaling. Dev. Neurobiol. 67, 158–172. doi: 10.1002/dneu.20339
Fields, R. D., Eshete, F., Stevens, B., and Itoh, K. (1997). Action potential-dependent regulation of gene expression: temporal specificity in Ca2+, cAMP-responsive element binding proteins, and mitogen-activated protein kinase signaling. J. Neurosci. 17, 7252–7266. doi: 10.1523/jneurosci.17-19-07252.1997
Gad, P., Lee, S., Terrafranca, N., Zhong, H., Turner, A., Gerasimenko, Y., et al. (2018). Non-invasive activation of cervical spinal networks after severe paralysis. J. Neurotraum. 35, 2145–2158. doi: 10.1089/neu.2017.5461
Gao, Y., Deng, K., Hou, J., Bryson, J. B., Barco, A., Nikulina, E., et al. (2004). Activated CREB is sufficient to overcome inhibitors in myelin and promote spinal axon regeneration in vivo. Neuron 44, 609–621. doi: 10.1016/j.neuron.2004.10.030
Gerasimenko, Y., Gorodnichev, R., Moshonkina, T., Sayenko, D., Gad, P., and Reggie Edgerton, V. (2015). Transcutaneous electrical spinal-cord stimulation in humans. Ann. Phys. Rehabil. Med. 58, 225–231. doi: 10.1016/j.rehab.2015.05.003
Geremia, N. M., Gordon, T., Brushart, T. M., Al-Majed, A. A., and Verge, V. M. (2007). Electrical stimulation promotes sensory neuron regeneration and growth-associated gene expression. Exp. Neurol. 205, 347–359. doi: 10.1016/j.expneurol.2007.01.040
Ghosh, A., Haiss, F., Sydekum, E., Schneider, R., Gullo, M., Wyss, M. T., et al. (2010). Rewiring of hindlimb corticospinal neurons after spinal cord injury. Nat. Neurosci. 13, 97–104. doi: 10.1038/nn.2448
Ghosh-Roy, A., Wu, Z., Goncharov, A., Jin, Y., and Chisholm, A. D. (2010). Calcium and cyclic AMP promote axonal regeneration in Caenorhabditis elegans and require DLK-1 kinase. J. Neurosci. 30, 3175–3183. doi: 10.1523/jneurosci.5464-09.2010
Goganau, I., Sandner, B., Weidner, N., Fouad, K., and Blesch, A. (2018). Depolarization and electrical stimulation enhance in vitro and in vivo sensory axon growth after spinal cord injury. Exp. Neurol. 300, 247–258. doi: 10.1016/j.expneurol.2017.11.011
Gordon, T., Amirjani, N., Edwards, D. C., and Chan, K. M. (2010). Brief post-surgical electrical stimulation accelerates axon regeneration and muscle reinnervation without affecting the functional measures in carpal tunnel syndrome patients. Exp. Neurol. 223, 192–202. doi: 10.1016/j.expneurol.2009.09.020
Hall, S. M. (1986). The effect of inhibiting schwann cell mitosis on the re-innervation of acellular autografts in the peripheral nervous system of the mouse. Neuropathol. Appl. Neurobiol. 12, 401–414. doi: 10.1111/j.1365-2990.1986.tb00151.x
Han, Q., Xie, Y., Ordaz, J. D., Huh, A. J., Huang, N., Wu, W., et al. (2020). Restoring cellular energetics promotes axonal regeneration and functional recovery after spinal cord injury. Cell Metab. 31, 623–641.
Harkema, S., Gerasimenko, Y., Hodes, J., Burdick, J., Angeli, C., Chen, Y., et al. (2011). Effect of epidural stimulation of the lumbosacral spinal cord on voluntary movement, standing, and assisted stepping after motor complete paraplegia: a case study. Lancet 377, 1938–1947. doi: 10.1016/s0140-6736(11)60547-3
Hervera, A., Zhou, L., Palmisano, I., Mclachlan, E., Kong, G., Hutson, T. H., et al. (2019). PP4-dependent HDAC3 dephosphorylation discriminates between axonal regeneration and regenerative failure. EMBO J. 38:e101032.
Hoffman, H., and Binet, F. (1952). Acceleration and retardation of the process of axon-sprouting in partially denervated muscles. Austr. J. Exper. Biol. Med. Sci. 30, 541–566. doi: 10.1038/icb.1952.52
Hofstoetter, U. S., Krenn, M., Danner, S. M., Hofer, C., Kern, H., Mckay, W. B., et al. (2015). Augmentation of voluntary locomotor activity by transcutaneous spinal cord stimulation in motor-incomplete spinal cord-injured individuals. Artif. Organ. 39, E176–E186.
Hollis, E. R. II, Ishiko, N., Pessian, M., Tolentino, K., Lee-Kubli, C. A., Calcutt, N. A., et al. (2015a). Remodelling of spared proprioceptive circuit involving a small number of neurons supports functional recovery. Nat. Commun. 6:6079.
Hollis, E. R. II, Ishiko, N., Tolentino, K., Doherty, E., Rodriguez, M. J., Calcutt, N. A., et al. (2015b). A novel and robust conditioning lesion induced by ethidium bromide. Exper. Neurol. 265, 30–39. doi: 10.1016/j.expneurol.2014.12.004
Hutson, T. H., and Di Giovanni, S. (2019). The translational landscape in spinal cord injury: focus on neuroplasticity and regeneration. Nat. Rev. Neurol. 15, 732–745. doi: 10.1038/s41582-019-0280-3
Ichiyama, R. M., Gerasimenko, Y. P., Zhong, H., Roy, R. R., and Edgerton, V. R. (2005). Hindlimb stepping movements in complete spinal rats induced by epidural spinal cord stimulation. Neurosci. Lett. 383, 339–344. doi: 10.1016/j.neulet.2005.04.049
Ievins, A., and Moritz, C. T. (2017). Therapeutic stimulation for restoration of function after spinal cord injury. Physiology 32, 391–398. doi: 10.1152/physiol.00010.2017
Inanici, F., Samejima, S., Gad, P., Edgerton, V. R., Hofstetter, C. P., and Moritz, C. T. (2018). Transcutaneous electrical spinal stimulation promotes long-term recovery of upper extremity function in chronic tetraplegia. IEEE Trans. Neural Syst. Rehabil. Eng. 26, 1272–1278. doi: 10.1109/tnsre.2018.2834339
Jack, A. S., Hurd, C., Forero, J., Nataraj, A., Fenrich, K., Blesch, A. et al. (2018). Cortical electrical stimulation in female rats with a cervical spinal cord injury to promote axonal outgrowth. J. Neurosci. Res. 96, 852–862. doi: 10.1002/jnr.24209
Kang, H., and Lichtman, J. W. (2013). Motor axon regeneration and muscle reinnervation in young adult and aged animals. J. Neurosci. 33, 19480–19491. doi: 10.1523/jneurosci.4067-13.2013
Lee, J. K., Geoffroy, C. G., Chan, A. F., Tolentino, K. E., Crawford, M. J., Leal, M. A., et al. (2010). Assessing spinal axon regeneration and sprouting in Nogo-, MAG-, and OMgp-deficient mice. Neuron 66, 663–670. doi: 10.1016/j.neuron.2010.05.002
Lee, P. R., Cohen, J. E., Iacobas, D. A., Iacobas, S., and Fields, R. D. (2017). Gene networks activated by specific patterns of action potentials in dorsal root ganglia neurons. Sci. Rep. 7:43765.
Lee, S. K., and Wolfe, S. W. (2000). Peripheral nerve injury and repair. J. Am. Acad. Orthop. Surg. 8, 243–252.
Li, S., Yang, C., Zhang, L., Gao, X., Wang, X., Liu, W., et al. (2016). Promoting axon regeneration in the adult CNS by modulation of the melanopsin/GPCR signaling. Proc. Natl. Acad. Sci. U.S.A. 113, 1937–1942. doi: 10.1073/pnas.1523645113
Lim, J.-H. A., Stafford, B. K., Nguyen, P. L., Lien, B. V., Wang, C., Zukor, K., et al. (2016). Neural activity promotes long-distance, target-specific regeneration of adult retinal axons. Nat. Neurosci. 19, 1073–1084. doi: 10.1038/nn.4340
Liu, K., Lu, Y., Lee, J. K., Samara, R., Willenberg, R., Sears-Kraxberger, I., et al. (2010). PTEN deletion enhances the regenerative ability of adult corticospinal neurons. Nat. Neurosci. 13, 1075–1081. doi: 10.1038/nn.2603
Lu, M. C., Ho, C. Y., Hsu, S. F., Lee, H. C., Lin, J. H., Yao, C. H., et al. (2008). Effects of electrical stimulation at different frequencies on regeneration of transected peripheral nerve. Neurorehabil. Neural Repair. 22, 367–373. doi: 10.1177/1545968307313507
Lu, M. C., Tsai, C. C., Chen, S. C., Tsai, F. J., Yao, C. H., and Chen, Y. S. (2009). Use of electrical stimulation at different current levels to promote recovery after peripheral nerve injury in rats. J. Traum. 67, 1066–1072. doi: 10.1097/ta.0b013e318182351a
Mahar, M., and Cavalli, V. (2018). Intrinsic mechanisms of neuronal axon regeneration. Nat. Rev. Neurosci. 19, 323–337. doi: 10.1038/s41583-018-0001-8
Maier, I. C., and Schwab, M. E. (2006). Sprouting, regeneration and circuit formation in the injured spinal cord: factors and activity. Philos. Trans. R. Soc. Lond. B Biol. Sci. 361, 1611–1634. doi: 10.1098/rstb.2006.1890
Mandolesi, G., Madeddu, F., Bozzi, Y., Maffei, L., and Ratto, G. M. (2004). Acute physiological response of mammalian central neurons to axotomy: ionic regulation and electrical activity. FASEB J. 18, 1934–1936. doi: 10.1096/fj.04-1805fje
McQuarrie, I. G., and Grafstein, B. (1973). Axon outgrowth enhanced by a previous nerve injury. Archiv. Neurol. 29, 53–55. doi: 10.1001/archneur.1973.00490250071008
Mishra, A. M., Pal, A., Gupta, D., and Carmel, J. B. (2017). Paired motor cortex and cervical epidural electrical stimulation timed to converge in the spinal cord promotes lasting increases in motor responses. J. Physiol. 595, 6953–6968. doi: 10.1113/jp274663
Nix, W. A., and Hopf, H. C. (1983). Electrical stimulation of regenerating nerve and its effect on motor recovery. Brain Res. 272, 21–25. doi: 10.1016/0006-8993(83)90360-8
Park, K. K., Liu, K., Hu, Y., Smith, P. D., Wang, C., Cai, B., et al. (2008). Promoting axon regeneration in the adult CNS by modulation of the PTEN/mTOR pathway. Science 322, 963–966. doi: 10.1126/science.1161566
Pockett, S., and Gavin, R. M. (1985). Acceleration of peripheral nerve regeneration after crush injury in rat. Neurosci. Lett. 59, 221–224. doi: 10.1016/0304-3940(85)90203-4
Qiu, J., Cai, D., Dai, H., Mcatee, M., Hoffman, P. N., Bregman, B. S., et al. (2002). Spinal axon regeneration induced by elevation of cyclic AMP. Neuron 34, 895–903. doi: 10.1016/s0896-6273(02)00730-4
Ramón y Cajal, S. (1991). Cajal’s Degeneration and Regeneration of the Nervous System. New York, NY: Oxford University Press.
Rejc, E., Angeli, C. A., Atkinson, D., and Harkema, S. J. (2017). Motor recovery after activity-based training with spinal cord epidural stimulation in a chronic motor complete paraplegic. Sci. Rep. 7:13476.
Richardson, P. M., and Issa, V. M. (1984). Peripheral injury enhances central regeneration of primary sensory neurones. Nature 309, 791–793. doi: 10.1038/309791a0
Rigaud, M., Gemes, G., Weyker, P. D., Cruikshank, J. M., Kawano, T., Wu, H.-E., et al. (2009). Axotomy depletes intracellular calcium stores in primary sensory neurons. Anesthesiol. J. Am. Soc. Anesthesiol. 111, 381–392. doi: 10.1097/aln.0b013e3181ae6212
Rishal, I., and Fainzilber, M. (2014). Axon-soma communication in neuronal injury. Nat. Rev. Neurosci. 15, 32–42. doi: 10.1038/nrn3609
Rosenzweig, E. S., Courtine, G., Jindrich, D. L., Brock, J. H., Ferguson, A. R., Strand, S. C., et al. (2010). Extensive spontaneous plasticity of corticospinal projections after primate spinal cord injury. Nat. Neurosci. 13, 1505–1510. doi: 10.1038/nn.2691
Sajic, M., Mastrolia, V., Lee, C. Y., Trigo, D., Sadeghian, M., Mosley, A. J., et al. (2013). Impulse conduction increases mitochondrial transport in adult mammalian peripheral nerves in vivo. PLoS Biol. 11:e1001754. doi: 10.1371/journal.pbio.1001754
Scheib, J., and Hoke, A. (2013). Advances in peripheral nerve regeneration. Nat. Rev. Neurol. 9, 668–676.
Seijffers, R., Mills, C. D., and Woolf, C. J. (2007). ATF3 increases the intrinsic growth state of DRG neurons to enhance peripheral nerve regeneration. J. Neurosci. 27, 7911–7920. doi: 10.1523/jneurosci.5313-06.2007
Senger, J. L., Chan, K. M., Macandili, H., Chan, A. W. M., Verge, V. M. K., Jones, K. E., et al. (2019). Conditioning electrical stimulation promotes functional nerve regeneration. Exp. Neurol. 315, 60–71. doi: 10.1016/j.expneurol.2019.02.001
Senger, J. L. B., Verge, V. M. K., Macandili, H. S. J., Olson, J. L., Chan, K. M., and Webber, C. A. (2018). Electrical stimulation as a conditioning strategy for promoting and accelerating peripheral nerve regeneration. Exper. Neurol. 302, 75–84. doi: 10.1016/j.expneurol.2017.12.013
Sheng, H. Z., Fields, R. D., and Nelson, P. G. (1993). Specific regulation of immediate early genes by patterned neuronal activity. J. Neurosci. Res. 35, 459–467. doi: 10.1002/jnr.490350502
Singh, B., Xu, Q.-G., Franz, C. K., Zhang, R., Dalton, C., Gordon, T., et al. (2012). Accelerated axon outgrowth, guidance, and target reinnervation across nerve transection gaps following a brief electrical stimulation paradigm. Cell 116:498. doi: 10.3171/2011.10.jns11612
Smith, P. D., Sun, F., Park, K. K., Cai, B., Wang, C., Kuwako, K., et al. (2009). SOCS3 deletion promotes optic nerve regeneration in vivo. Neuron 64, 617–623. doi: 10.1016/j.neuron.2009.11.021
Song, Y., Li, D., Farrelly, O., Miles, L., Li, F., Kim, S. E., et al. (2019). The mechanosensitive ion channel piezo inhibits axon regeneration. Neuron 102, 373–389.
Stam, F. J., Macgillavry, H. D., Armstrong, N. J., De Gunst, M. C., Zhang, Y., Van Kesteren, R. E., et al. (2007). Identification of candidate transcriptional modulators involved in successful regeneration after nerve injury. Eur. J. Neurosci. 25, 3629–3637. doi: 10.1111/j.1460-9568.2007.05597.x
Steinmetz, M. P., Horn, K. P., Tom, V. J., Miller, J. H., Busch, S. A., Nair, D., et al. (2005). Chronic enhancement of the intrinsic growth capacity of sensory neurons combined with the degradation of inhibitory proteoglycans allows functional regeneration of sensory axons through the dorsal root entry zone in the mammalian spinal cord. J. Neurosci. 25, 8066–8076. doi: 10.1523/jneurosci.2111-05.2005
Sun, F., Park, K. K., Belin, S., Wang, D., Lu, T., Chen, G., et al. (2011). Sustained axon regeneration induced by co-deletion of PTEN and SOCS3. Nature 480, 372–375. doi: 10.1038/nature10594
Sun, L., Shay, J., Mcloed, M., Roodhouse, K., Chung, S. H., Clark, C. M., et al. (2014). Neuronal regeneration in C. elegans requires subcellular calcium release by ryanodine receptor channels and can be enhanced by optogenetic stimulation. J. Neurosci. 34, 15947–15956. doi: 10.1523/jneurosci.4238-13.2014
Sun, W., Larson, M. J., Kiyoshi, C. M., Annett, A. J., Stalker, W. A., Peng, J., et al. (2020). Gabapentinoid treatment promotes corticospinal plasticity and regeneration following murine spinal cord injury. J. Clin. Invest. 130, 345–358. doi: 10.1172/jci130391
Takeoka, A., Vollenweider, I., Courtine, G., and Arber, S. (2014). Muscle spindle feedback directs locomotor recovery and circuit reorganization after spinal cord injury. Cell 159, 1626–1639. doi: 10.1016/j.cell.2014.11.019
Tedeschi, A., Dupraz, S., Laskowski, C. J., Xue, J., Ulas, T., Beyer, M., et al. (2016). The calcium channel subunit Alpha2delta2 suppresses axon regeneration in the adult CNS. Neuron 92, 419–434. doi: 10.1016/j.neuron.2016.09.026
Tedeschi, A., Nguyen, T., Puttagunta, R., Gaub, P., and Di Giovanni, S. (2009). A p53-CBP/p300 transcription module is required for GAP-43 expression, axon outgrowth, and regeneration. Cell Death Differ. 16, 543–554. doi: 10.1038/cdd.2008.175
Tsujino, H., Kondo, E., Fukuoka, T., Dai, Y., Tokunaga, A., Miki, K., et al. (2000). Activating transcription factor 3 (ATF3) induction by axotomy in sensory and motoneurons: a novel neuronal marker of nerve injury. Mol. Cell. Neurosci. 15, 170–182. doi: 10.1006/mcne.1999.0814
Tyssowski, K. M., Destefino, N. R., Cho, J. H., Dunn, C. J., Poston, R. G., Carty, C. E., et al. (2018). Different neuronal activity patterns induce different gene expression programs. Neuron 98, 530–546.
Udina, E., Furey, M., Busch, S., Silver, J., Gordon, T., and Fouad, K. (2008). Electrical stimulation of intact peripheral sensory axons in rats promotes outgrowth of their central projections. Exper. Neurol. 210, 238–247. doi: 10.1016/j.expneurol.2007.11.007
von Bernhardi, R., Bernhardi, L. E., and Eugenin, J. (2017). What is neural plasticity? Adv. Exp. Med. Biol. 1015, 1–15.
Wagner, F. B., Mignardot, J.-B., Le Goff-Mignardot, C. G., Demesmaeker, R., Komi, S., Capogrosso, M., et al. (2018). Targeted neurotechnology restores walking in humans with spinal cord injury. Nature 563, 65–71.
Ward, P. J., Clanton, S. L. II, and English, A. W. (2018). Optogenetically enhanced axon regeneration: motor versus sensory neuron-specific stimulation. Eur. J. Neurosci. 47, 294–304. doi: 10.1111/ejn.13836
Ward, P. J., Jones, L. N., Mulligan, A., Goolsby, W., Wilhelm, J. C., and English, A. W. (2016). Optically-induced neuronal activity is sufficient to promote functional motor axon regeneration in vivo. PLoS One 11:e0154243. doi: 10.1371/journal.pbio.154243
Wheeler, D. G., Groth, R. D., Ma, H., Barrett, C. F., Owen, S. F., Safa, P., et al. (2012). Ca(V)1 and Ca(V)2 channels engage distinct modes of Ca(2+) signaling to control CREB-dependent gene expression. Cell 149, 1112–1124. doi: 10.1016/j.cell.2012.03.041
Wolf, J. A., Stys, P. K., Lusardi, T., Meaney, D., and Smith, D. H. (2001). Traumatic axonal injury induces calcium influx modulated by tetrodotoxin-sensitive sodium channels. J. Neurosci. 21, 1923–1930. doi: 10.1523/jneurosci.21-06-01923.2001
Wong, J. N., Olson, J. L., Morhart, M. J., and Chan, K. M. (2015). Electrical stimulation enhances sensory recovery: a randomized controlled trial. Ann. Neurol. 77, 996–1006. doi: 10.1002/ana.24397
Yang, Q., Ramamurthy, A., Lall, S., Santos, J., Ratnadurai-Giridharan, S., Lopane, M., et al. (2019). Independent replication of motor cortex and cervical spinal cord electrical stimulation to promote forelimb motor function after spinal cord injury in rats. Exper. Neurol. 320:112962. doi: 10.1016/j.expneurol.2019.112962
Zareen, N., Dodson, S., Armada, K., Awad, R., Sultana, N., Hara, E., et al. (2018). Stimulation-dependent remodeling of the corticospinal tract requires reactivation of growth-promoting developmental signaling pathways. Exper. Neurol. 307, 133–144. doi: 10.1016/j.expneurol.2018.05.004
Zareen, N., Shinozaki, M., Ryan, D., Alexander, H., Amer, A., Truong, D. Q., et al. (2017). Motor cortex and spinal cord neuromodulation promote corticospinal tract axonal outgrowth and motor recovery after cervical contusion spinal cord injury. Exper. Neurol. 297, 179–189. doi: 10.1016/j.expneurol.2017.08.004
Zhou, B., Yu, P., Lin, M.-Y., Sun, T., Chen, Y., and Sheng, Z.-H. (2016). Facilitation of axon regeneration by enhancing mitochondrial transport and rescuing energy deficits. J. Cell Biol. 214, 103–119. doi: 10.1083/jcb.201605101
Keywords: conditioning injury, regeneration associated genes, sprouting, plasticity, functional recovery, spinal cord injury
Citation: Jara JS, Agger S and Hollis ER II (2020) Functional Electrical Stimulation and the Modulation of the Axon Regeneration Program. Front. Cell Dev. Biol. 8:736. doi: 10.3389/fcell.2020.00736
Received: 18 April 2020; Accepted: 15 July 2020;
Published: 18 August 2020.
Edited by:
Luis B. Tovar-y-Romo, National Autonomous University of Mexico, MexicoReviewed by:
Fengquan Zhou, Johns Hopkins University, United StatesCedric G. Geoffroy, Texas A&M University, United States
Copyright © 2020 Jara, Agger and Hollis II. This is an open-access article distributed under the terms of the Creative Commons Attribution License (CC BY). The use, distribution or reproduction in other forums is permitted, provided the original author(s) and the copyright owner(s) are credited and that the original publication in this journal is cited, in accordance with accepted academic practice. No use, distribution or reproduction is permitted which does not comply with these terms.
*Correspondence: Edmund R. Hollis II, ZWRoMzAwMUBtZWQuY29ybmVsbC5lZHU=