- Shanghai Jiao Tong University Affiliated Sixth People’s Hospital, School of Biomedical Engineering, Shanghai Jiao Tong University, Shanghai, China
Astrocyte activation plays an important role during disease-induced inflammatory response in the brain. Exosomes in the brain could be released from bone marrow (BM)-derived stem cells, neuro stem cells (NSC), mesenchymal stem cells (MSC), etc. We summarized that exosomes release and transport signaling to the target cells, and then produce function. Furthermore, we discussed the pathological interactions between astrocytes and other brain cells, which are related to brain diseases such as stroke, Alzheimer’s disease (AD), Parkinson’s disease (PD), amyotrophic lateral sclerosis (ALS) disease, multiple sclerosis (MS), psychiatric, traumatic brain injury (TBI), etc. We provide up-to-date, comprehensive and valuable information on the involvement of exosomes in brain diseases, which is beneficial for basic researchers and clinical physicians.
Introduction
Astrocytes are the most abundant glial cells in the human brain. Astrocytes play key roles in the brain such as maintenance and formation of the blood-brain barrier (BBB) (Abbott et al., 2006), providing metabolic support to the nervous tissue (Benarroch, 2005; Allaman et al., 2011), regulation of regional blood flow (Attwell et al., 2010; Bazargani and Attwell, 2016), regulation of synaptic circuits (Dallerac et al., 2018), neurotransmitter recycling (Hamilton and Attwell, 2010) as well as repairing and scarring process of the brain and spinal cord following various injuries (Anderson et al., 2014; Adams and Gallo, 2018).
The diversity and essence of the functions of astrocytes make them predominant among other cells in the central nervous system. Previous studies have demonstrated various inflammatory, anti-inflammatory, neuroprotective and disease-causing effects of astrocytes in different diseases (Gupta et al., 2012; Sofroniew, 2015; Habib et al., 2020). Hundreds of researches have been conducted to increase our understanding of how astrocyte exert their effects. However, the ways astrocytes influence other cells’ functions remain largely unknown.
During the past decade, scientists found that extracellular vesicles (EVs) play important roles in both short and long-distance communications between cells within and out of the brain (Simons and Raposo, 2009; Zhuang et al., 2011; Chen et al., 2016; Paolicelli et al., 2019; Kur et al., 2020). Astrocyte-derived EVs are enriched with various biological molecules including genes, microRNAs (miRNA), and proteins. Interestingly, astrocytes-derived EVs that are secreted in normal conditions are known to be enriched with neuroprotective and neurotrophic elements. On the contrary, EVs released by astrocytes under abnormal conditions such as oxidative stress, nutrient deficiency, inflammation have been witnessed to exert neuroprotective effect and promote neurite regeneration and outgrowth (Taylor et al., 2007; Wang et al., 2011; Moidunny et al., 2012; Gosselin et al., 2013). In response to neuronal inflammation, astrocytes modify their EV component to suppress the inflammation by regulating the excitability of neurons (Chaudhuri et al., 2018). Astrocytes also modify their EV contaminations in response to extracellular stimuli. For example, upon normal extracellular stimuli, astrocyte-derived EVs contain proteins that promote neurite outgrowth, synaptic transmission, and neuronal survival. Whereas, when exposed to an inflammatory stimulus, Interleukin 1beta (IL-1β), astrocyte shed EVs that regulate peripheral immune response (Dickens et al., 2017; Chaudhuri et al., 2018; Datta Chaudhuri et al., 2020). Not only do astrocytes respond to stimulus and conditions by secreting EVs with selected cargos, but they are also influenced by EVs secreted from other cells. Researches have illustrated that when a specific neuronal exosomal miRNA is internalized into astrocyte, it can change the astrocytic function from MSCs to astrocytes and neurons regulate gene expression of astrocytes, thus improving functional recovery and neurite regeneration after stroke (Xin et al., 2013; Men et al., 2019). Unfortunately, astrocytes could also be influenced by cancer cells which can lead to devastating effects. Glioblastoma EVs alter the astrocytes to increase tumor growth and may drive astrocytes to a tumorigenic phenotype (Oushy et al., 2018). Experiments also demonstrated that astrocyte may evoke changes in glial cells’ responses that could be elicited by EVs only (Willis et al., 2020b). Overall, various EVs seem to play a key role in cell to cell communication in both healthy and disease conditions. EVs are undoubtedly one of the most important factors if not only in facilitating communication between astrocytes and other cells. In this review, we summarize the functions of astrocyte-derived EVs and other brain cell-derived EVs.
Extracellular vesicles, lipid-bound vesicles that are crucial in intracellular communication, are secreted into extracellular space. EVs exist in variety of size, content, origin and targets. EVs are mostly classified.
Into three main subtypes of microvesicles (MV), apoptotic bodies and exosomes (Borges et al., 2013; Yanez-Mo et al., 2015; Zaborowski et al., 2015) (Figure 1). EVs are loaded with different proteins, lipids, and nucleic acids (Sedgwick and D’Souza-Schorey, 2018). MVs are known as shedding vesicles, ectosomes, microparticles are more heterogenous and bigger in size (100 nm–1μm in diameter) (Raposo and Stoorvogel, 2013; Pollet et al., 2018). MVs are secreted by outward budding and splitting of plasma membrane. Apoptotic bodies are 1–5μm in diameter and are produced by blebbing of apoptotic cells (Raposo and Stoorvogel, 2013; Sedgwick and D’Souza-Schorey, 2018). Exosome was first discovered as a vesicle in the immature red blood cells in 1983 (Harding and Stahl, 1983; Pan and Johnstone, 1983), and further named “exosome” by Johnstone et al. (1987). exosomes are smaller than a bacterium and exist in various sizes from 30 to 100 nm in diameter and are formed as infusion of multivascular body with plasma membrane (Borges et al., 2013; Raposo and Stoorvogel, 2013). Exosomes are not only a way of waste disposal, but they are also released to induce an effect on their targets by making them active. Cells could utilize exosomes to send genetic signals to other cells (Nagarajah, 2016). Exosomes and other EVs mediate intracellular communication between various cells by carrying a diverse quantity of bioactive molecules (Camussi et al., 2010; Miyabe et al., 2019). The importance of the exosome-mediated intercellular communication compared with the traditional modulation factors like such as hormones, cytokines, electrical and chemical signals and etc is that communication through exosomes within the cells is highly reliant on the delivery of genetic biomaterials which can greatly and impact on cells behavior and phenotype changes (Ratajczak et al., 2006; Valadi et al., 2007; Skog et al., 2008; Mittelbrunn et al., 2011; Montecalvo et al., 2012; Zhang Y. et al., 2019), whereas Traditional modulation factors act on receptors or ligands to affect recipient cell functions (Premack and Schall, 1996; Thelen, 2001; Tran and Miller, 2003; Brooks and Waters, 2010; Camussi et al., 2010; Levin and Hammes, 2016; Miyabe et al., 2019; Van der Auwera et al., 2020). Communication through exosomes is more complex than through secretion of chemokines and cytokines because of exosome’s proper packaging of biomaterials and the study of exosomes may help in identifying disease root cells in pathophysiology (Rooj et al., 2016). Exosomes could be released into body fluids and travel to the targeting tissue. After being taken up by specific cells, they could subsequently change the function and status of the recipient cells (Salido-Guadarrama et al., 2014). Based on the genetic materials they carry, they develop a certain ability to interact with different cells (Zhang Y. et al., 2019). Exosomes play a significant role in many biological processes in both healthy and abnormal cells (Kalluri, 2016; Samanta et al., 2018; Saeedi et al., 2019; Holtzman and Lee, 2020; Ni et al., 2020; Zhou et al., 2020). The functions of exosome’s and other EVs are important in both diagnosis and therapeutics clinically due to their diverse effects on various diseases and conditions (Xu et al., 2018). In terms of therapeutic functions, Evs’ potential involvement in the treatments is promising as they could migrate toward the target cells precisely with lower adverse effects and toxicity (Schorey and Bhatnagar, 2008; Lin et al., 2019; Thery et al., 2018; Qiu et al., 2019). Many reasons make EVs predominant among other therapeutic factors in disease therapy. EVs can pass the BBB, so it is useful in the treatment of central nervous system (CNS) disease (Zhuang et al., 2011; Liu et al., 2015; Khongkow et al., 2019). EVs can be loaded with biomaterials and targeted at certain types of disease-causing cells such as tumor cells (Hao et al., 2007; Luan et al., 2017; Yang and Li, 2018; Walker et al., 2019; O’Brien et al., 2020). EVs derived from stem cells can also be useful for neuro-regeneration after CNS injuries (Luarte et al., 2016; Holm et al., 2018; Vogel et al., 2018; Branscome et al., 2020). Identification and inhibition of disease-causing EVs can also be a potential therapeutic strategy in the treatment of various diseases (EL Andaloussi et al., 2013). Scientists seek to turn the exosomes into a promising factor in the diagnosis of brain cancer to make it possible to obtain liquid samples instead of performing brain biopsy in the early and curable stages of brain cancer diagnosis (Manterola et al., 2014; Santiago-Dieppa et al., 2014). Recently, scientists also established a method by using a smartphone to detect enzymatic exosomes after brain injury in concussion diagnosis (Ko et al., 2016).
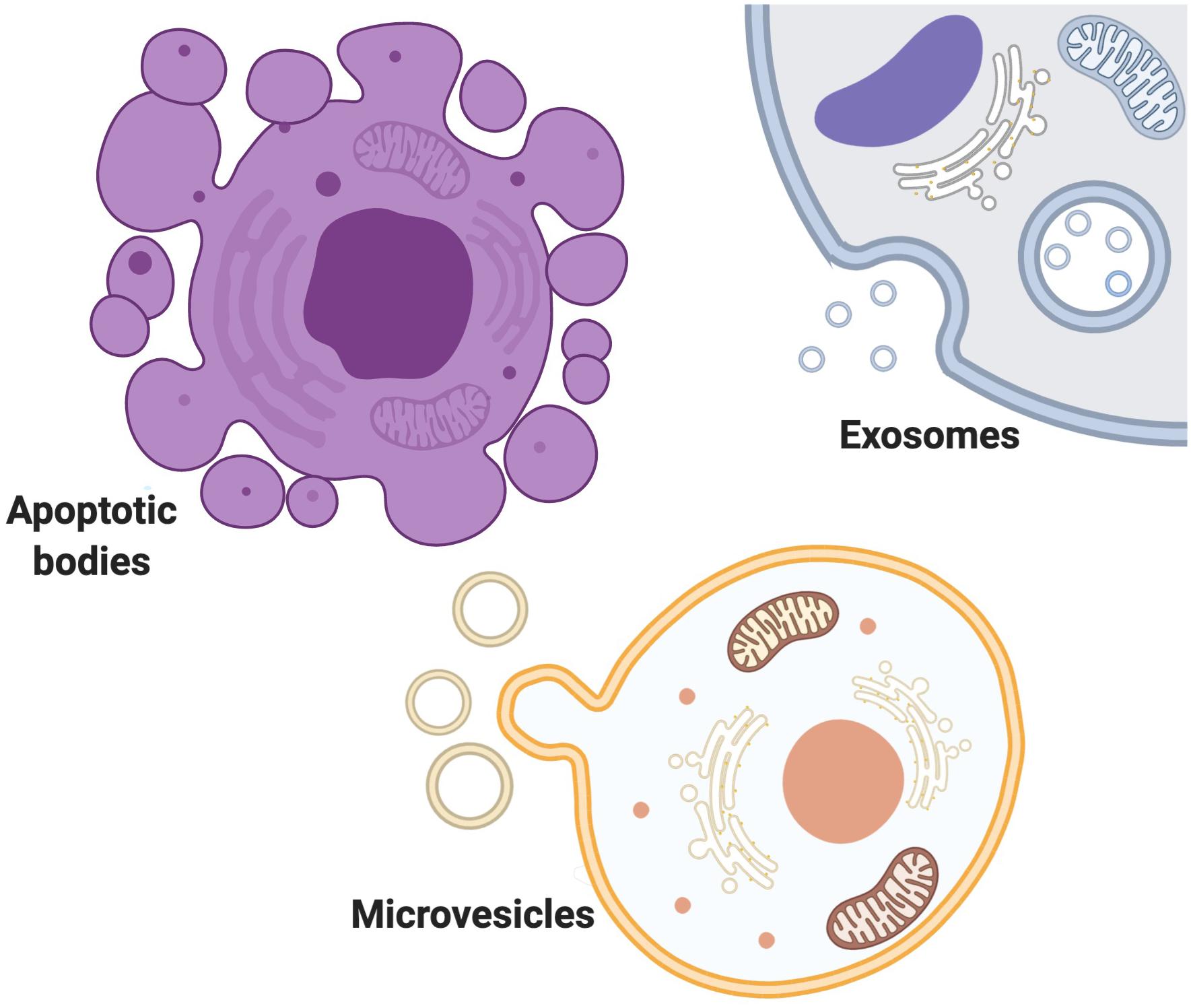
Figure 1. Extracellular vesicles exist in variety of size, content, origin and targets. EVs are mostly classified Into three main subtypes of MVs, apoptotic bodies and exosomes. MVs are secreted by outward budding and splitting of plasma membrane. Apoptotic bodies are produced by blebbing of apoptotic cells. Exosomes are formed as infusion of multivascular body with plasma membrane.
Exosomes could simply present the cell genetic information and from that, exosomes could help to distinguish the abnormal cells from normal and healthy cells (Isola and Chen, 2017). The significance of exosomes in the cell to cell communication and transportation of unique genetic materials could be considered as biomarkers in the diagnosis and therapy of various diseases. Cancer studies showed that exosomes can be considered as potential biomarkers in diagnosis and prognosis of cancer (Wong and Chen, 2019). Besides, it was also revealed that DNM3, p65, and p53 followed a dysregulated-patterns in the brain and blood exosomes in both original and recurrent stages of glioblastoma multiforme. DNM3, P65, and P53 enriched exosomes can be a potential clinical diagnostic marker for glioblastoma multiforme (Yang J.K. et al., 2017). Altogether, studies suggest that exosomes have high sensitivity and specificity in the assessment of cancer and they have the potential to be considered as biomarkers in the diagnosis and prognosis of cancers and other diseases (H Rashed et al., 2017; Abak et al., 2018).
There are also obstacles in the study of EVs in vivo. EVs are recently discovered particles in many diseases. How to study specific EV derived from a certain type of cell is still unclear and, how to prove the importance of EVs in the cell to cell communication is still an obstacle. Nevertheless, the role of astrocyte-derived EVs in both health and disease conditions have been summarized in our review.
Importance of Astrocyte-Derived EVs
Astrocytes are known to be involved in brain homeostasis regulation (Hubbard et al., 2016; Verkhratsky and Nedergaard, 2018; Jha et al., 2019). However, the underlying mechanisms of how astrocytes communicate with other cells in the brain microenvironment to regulate brain homeostasis is still unclear. The brain is capable to communicate with the rest of the body via transporting EVs to communicate healthy and disease states of the body (Gomez-Molina et al., 2019). Study of exosomes could increase our knowledge of astrocytes, their homeostasis, and metabolic regulating mechanisms since they are capable of transferring biological molecules from one cell to another (Fruhbeis et al., 2013; Silverman et al., 2019; Upadhya et al., 2020; You et al., 2020). Interestingly, miRNAs contained in the exosomes secreted by mouse astrocytes were different from the miRNAs that originally existed in mouse astrocytes. These findings suggest a mechanism that astrocytes has to select a specific miRNA for excretion via exosomes (Jovicic and Gitler, 2017). Astrocytes may also secrete EVs to affect surrounding cells in a positive or negative way. Astrocyte shedding vesicles containing miR-34a increased the sensitivity of dopaminergic neurons to neurotoxins (Mao et al., 2015). Under abnormal conditions, astrocytes are triggered to releases immune-related or anti-inflammatory related vesicles in response to stress-related stimuli. In contrast, in a normal situation, astrocyte-derived EVs promote neurite outgrowth and survival (Datta Chaudhuri et al., 2020).
Another research demonstrated that astrocytes may support oligodendrocyte differentiation by EVs, however, this support disappears with age (Willis et al., 2020a). Identification of specific stimulus that astrocytes respond to by secreting EVs may increase our understanding of CNS diseases or aged brain. Astrocyte-derived EVs contain protein biomarkers that are identified to be involved in stress-induced diseases. Besides, astrocyte-derived exosomes could maintain cell-specific markers, which allow the identification of the origin of parental cells, disease initiation and progression. Released exosomes from the cells can be isolated from the tissue and then be examined as an assessment for the identification of cellular origins of extracted exosomes from body fluids for diagnostic purposes such as blood, saliva, and urine in finding the disease-related type of cells (Venturini et al., 2019). Astrocyte-derived EVs can also be useful in drug targeted therapies as a drug or drug carrier because they can pass the BBB and be taken up by brain cells. Astrocyte-derived EVs containing Cox2 small interfering RNA was capable of restoring microglial phagocytic activity after being up-taken by microglial cells in morphine-mediate neurodegenerative mice model (Hu et al., 2018). In general, obtaining a better understanding of EVs secreted from astrocytes may improve CNS disease diagnosis as well as drug targeting therapies.
The Relationship Between Astrocyte-Derived EVs and Microenvironment
Similar to the other cells, astrocytes are surrounded by various structures and cells such as neurons, oligodendrocytes, microglia, micro-vessels, extracellular matrix (ECM), etc and they interact with each other regularly. Astrocyte-derived exosomes are one of the most significant ways of communication between astrocytes and surrounding cells. Physical or chemical microenvironmental changes affect astrocytes’ growth and behavior. Changes in astrocytes alter the type of signals they release, therefore altering the microenvironment in a positive or negative manner (Paolicelli et al., 2019; Schiera et al., 2019; You et al., 2020). A study found a novel brain penetrant inhibitor that inhibited the release of vesicles in astrocytes (Rojas et al., 2019). Recently, microenvironmental factors such as ECM, immune cells and blood were frequently studied. Microenvironmental signals play various roles in different disease initiation and progression. Changes in microenvironments could affect the communication of astrocytes with other cells. Previous studies also revealed that astrocyte secrete EVs in response to the stimuli/signals coming from the environment. The mobility of astrocyte-derived vesicles in rats increased in response to the release of ALS-IgG (Stenovec et al., 2011). Which illustrates that astrocytes derived-EVs and microenvironment influence each other regularly. For example, in response to interleukin 10 (IL-10), astrocyte-derived EVs contain proteins that lead to neuronal survival (Figure 2). Whereas, in response to IL-1β, astrocyte-derived EVs contain proteins that mediate immune response (Datta Chaudhuri et al., 2020) (Figure 2).
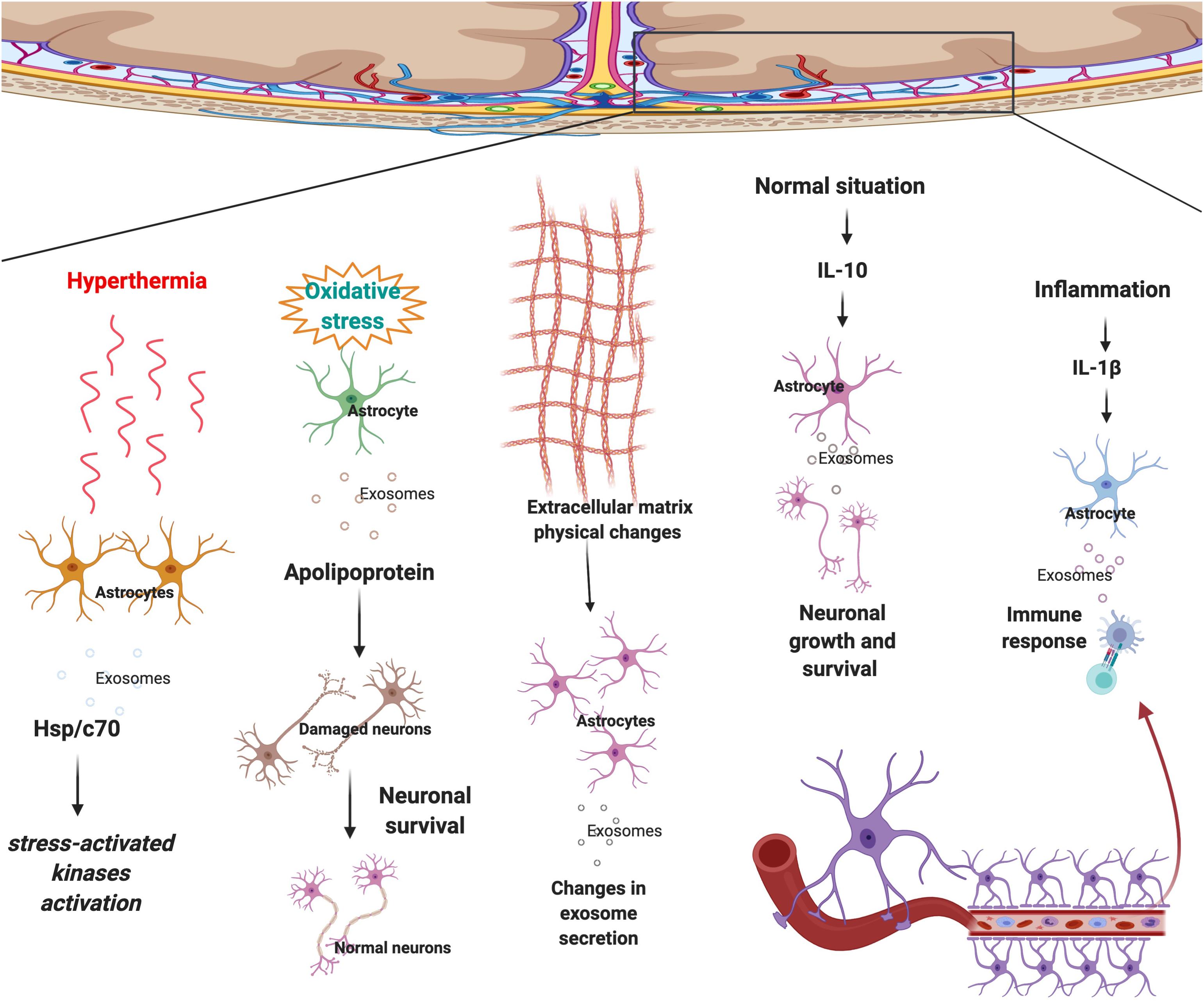
Figure 2. Astrocytes respond to surrounding signals and physical changes in the microenvironment by sending selected exosomes to maintain brain homeostasis. In response to hyperthermia astrocytes secrete exosomes that contain Hsp/c70 and further activate stress-activated kinases to lower the stress. Upon oxidative stress, EVs secreted by astrocytes transport apolipoprotein, a classical neuroprotective protein, to neurons and mediate neuronal survival. Physical changes to ECM morphology also affect astrocyte-derived EV production and possibly cell to cell communication. Following secretion of IL-10 in the normal condition, an anti-inflammatory signal, astrocyte-derived EVs contain proteins that lead to neuronal survival. Whereas, in regards to IL-1β, an inflammatory signal, astrocyte-derived EVs contain proteins that mediate immune response.
Central nervous system disease progression and initiation are not necessarily the result of genetic mutations, physical changes in the microenvironment could also cause the disease by altering astrocyte-derived EVs content and secretion. Recent research aimed to investigate the impact of scaffold morphology on the astrocyte growth and their colonization ability by mimicking ECM. As a result, it was found that physical changes in the microenvironment could affect astrocyte-derived EV production and possibly cell to cell communication (Carfi Pavia et al., 2019) (Figure 2). From this research, it is evident that astrocytes change the exosomes’ selection strategy in response to the physical changes in the micro environment. Another study demonstrated that astrocytes that are undergoing hyperthermia increased the releasing amount of heat shock protein/c70 (Hsp/c70) via exosomes into the extracellular environment following stress (Taylor et al., 2007). The study also showed that all the extracellular Hsp/c70 secreted by astrocytes were present in exosomes, implying the importance of exosome release in astrocytic function under stress conditions (Taylor et al., 2007) (Figure 2).
Stem Cell Derived Exosome Is Neuro Protective via Astrocytes
Stem cell therapies have been a famous treatment option for various CNS diseases (Lee et al., 2017; Wei et al., 2017; Sonntag et al., 2018; Sugaya and Vaidya, 2018; Zhang et al., 2018).
Recently, therapeutic abilities of stem cells in healing various CNS disease was referred to their ability of generating EVs (Yang Y. et al., 2017; Bang and Kim, 2019; Ni et al., 2019; Riazifar et al., 2019; Zhang Z.G. et al., 2019; Zhu et al., 2019; Ghosh et al., 2020). Some evidence show that stem cells may exert their effect via communication with astrocytes.
Neuro stem cells (NSCs) are known to be effective in tissue regeneration and repair in various CNS diseases (Marsh and Blurton-Jones, 2017; Ludwig et al., 2018). NSC applied its anti-inflammatory and neurotrophic effects by secreting EVs (Schindowski et al., 2008; Webb et al., 2018; Rong et al., 2019). Mouse NSC-derived exosomes have the ability to protect astrocytes by preserving their functions post-ischemic stroke (Sun et al., 2019).
Evidence demonstrated that BM-derived stem cell exosomes could affect astrocytes’ functions. BM-derived stem cells are famous for being effective in the treatment of various diseases, especially extraosseous diseases, and are known to exert their effect by releasing exosomes (Lyu et al., 2020). It was also demonstrated that EVs secreted by BM-derived stem cells could transfer selected miRNAs (Collino et al., 2010; Baglio et al., 2015). BM-derived mesenchymal stem cells (MSCs) could also be an effective therapeutic for the treatment of diabetes-induced cognitive impairment through shuttling exosomes to astrocytes and neurons that can increase the recovery of astrocytes and boost neuronal functions (Nakano et al., 2016). BM-derived stem cells miR-138-5p-overexpressing exosomes could prevent astrocytes from apoptosis after ischemic stroke by targeting lipocalin 2 (Deng et al., 2019) (Figure 3). The miR-146a overexpression in BM-derived MSC exosomes can be triggered by an enriched environment, and it could inhibit inflammation in damaged astrocytes and prevent diabetes-induced cognitive impairment (Kubota et al., 2018).
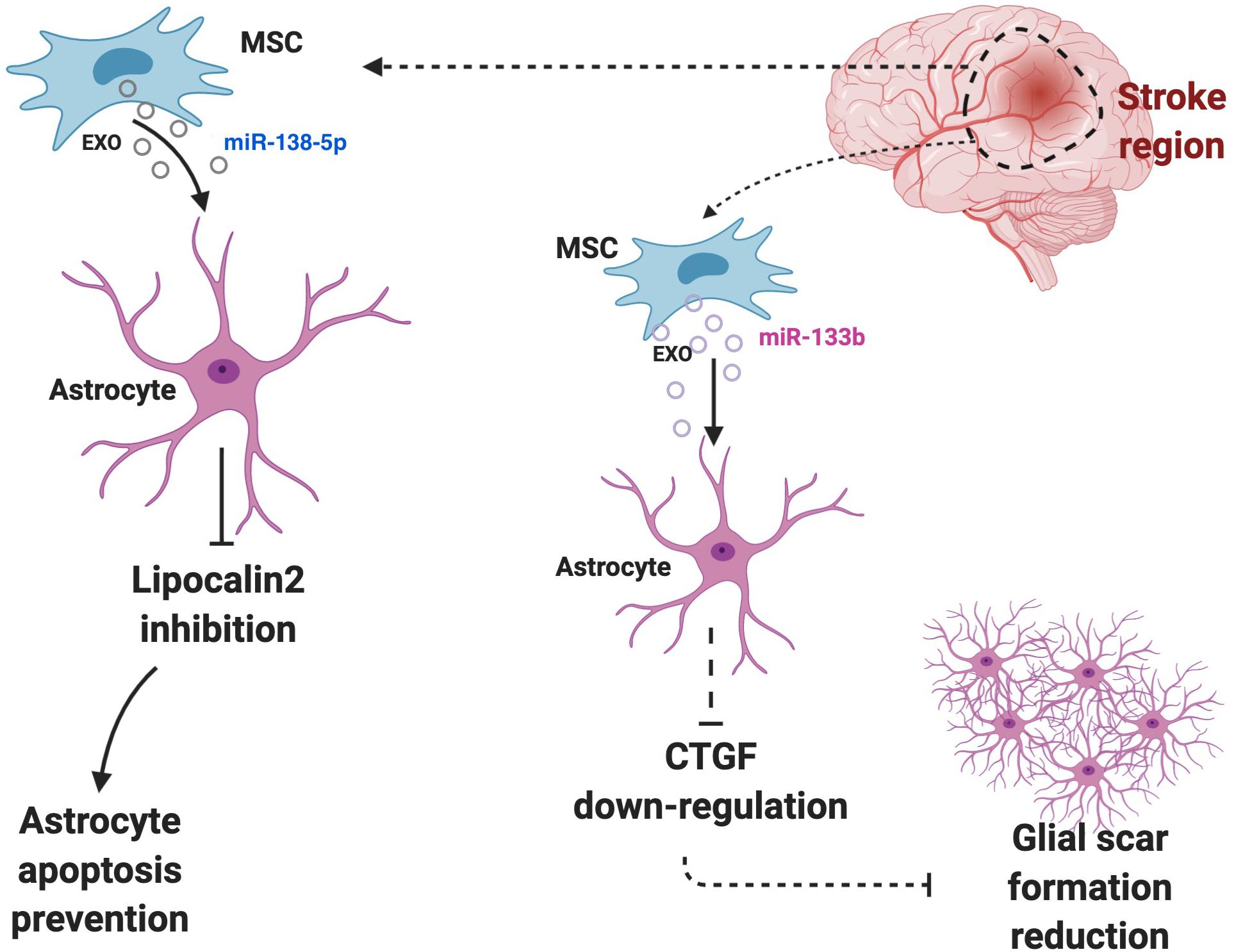
Figure 3. Astrocytes and surrounding cells in the microenvironment regularly communicate and interact with each other via exosomes. Cells may use astrocytes to exert their effects. For instance, BM-derived stem cells miR-138-5p-overexpressing exosomes prevent astrocytes from apoptosis after ischemic stroke by targeting lipocalin 2 or high level of miR-133b in astrocytes can down-regulate the expression of CTGF, which cause glial scar reduction and further increase the recovery post-ischemic stroke.
Multipotent MSCs regulate neurite outgrowth and exert other effects by releasing EVs containing key molecules (Xin et al., 2012).
MSC-derived exosomes transfer miR-133b to astrocytes and MSCs derived exosomes elevate its miR-133b level after ischemic stroke. The high level of miR-133b in astrocytes then can down-regulate the expression of connective tissue growth factor (CTGF), which could cause glial scar reduction and further increase the recovery post-ischemic stroke (Xin et al., 2013) (Figure 3). Besides, it could improve the neuronal recovery after ischemic stroke by down-regulation of rab9 Effector Protein With Kelch Motifs (RABEPK) expression in astrocytes. MSC-derived exosomes could be used in the treatment of brain disease though affecting astrocytes functions by exerting an anti-inflammatory effect. A study identified that the nuclear factor (erythroid-derived 2)-like 2 and nuclear factor-κB (Nrf2-NF-κB) signaling pathway involved in an astrocytic activation and MSC-exosomes lowered the inflammation that causes astrocytic alteration to neurotoxic astrocytes (Xian et al., 2019). The affect of MSC-derived exosomes on neurotoxic A1 astrocytes induced by an inflammatory response in post-spinal cord injured patients. Their result demonstrated that MSC-derived exosomes could exhibit an anti-inflammatory effect on spinal injury and further decrease A1 astrocytes (Wang et al., 2018).
Finding the specific exosomes involved between astrocytes and stem cells toward the specific glial cells is a promising strategy in the brain healing process in various brain diseases and help in understanding the brain development and differentiation as well as in vitro brain development. Study of astrocyte-like cell-derived EVs and their effect on inducing the differentiation of neural stem progenitor cells toward glial cells in vitro experiment may confirm the importance of exosomes in cellular communication in neuron activation during development (Stronati et al., 2019).
Astrocytes, EVs and CNS Diseases
Astrocyte-derived EVs are highly diverse in size, composition, and function. This may be evidence of why astrocytes could have both beneficial and pathological effects in various CNS diseases. Recent studies on astrocyte-derived exosomes have demonstrated the astrocytic roles in homeostasis and the possible relationship of homeostasis disruption with neurological diseases. Astrocyte-released exosomes may contain proteins with neurotrophic properties such as co-chaperone STI1. Astrocyte-derived exosomes that contain STI1 could be involved in brain injury, cancer, and neurodegenerative disorder (Hajj et al., 2013). Astrocytes may also utilize exosomes to play their role in regulating homeostasis in CNS, disruption of astrocytic balance in expressing amino acid transporters and protein kinases could relate to neurological diseases (Gosselin et al., 2013). The involvement of astrocytes and EVs in CNS diseases are summarized below. The highlighted molecules enriched EVs that are involved in disease progression and inhibition are summarized in Table 1.
Stroke and Ischemia
Astrocytes could exhibit both protective and negative effects on neurons after stroke. In the acute phase, reactive astrocytes play a neuroprotective role by decreasing the lesion extension, anti-excitotoxicity effects, and secretion of neurotrophins, whereas they may have a negative or positive effect in the chronic phase (Liu and Chopp, 2016; Pekny et al., 2019). Researches confirmed the positive effect of astrocyte-derived exosomes on neurons before and after ischemic stroke. Astrocyte-derived exosomes transfer miR-92b-3p to neurons before ischemia to protect neurons (Xu et al., 2019).
Astrocyte-derived exosomes were capable of suppressing autophagy and improving damaged neurons after ischemic stroke (Pei et al., 2019). Astrocyte-derived exosome containing prostaglandin D2 synthase lead to axonal outgrowth and increase the recovery after stroke (Hira et al., 2018). Astrocyte-derived exosome containing miR-34c protects neurons after cerebral ischemia (Wu et al., 2020). Astrocyte-derived exosome containing miR-361 protects nerve damage after stroke by mediating the AMPK/mTOR signaling pathway (Bu et al., 2020).
An in vitro study showed that oxygen-glucose depletion (OGD) in astrocytes-derived exosomes enriched miR-133b mediate neuron outgrowth and elongation after stroke (Xin et al., 2013). Astrocyte-derived exosomes also shuttle miR-190b to prevent OGD-induced autophagy and to inhibit neuronal apoptosis (Pei et al., 2020).
Under hypoxic and ischemic conditions, astrocytes release exosomes containing prion proteins that improve neuronal survival after being uptaken by neurons. Astrocyte-derived exosomes increase neuronal survival under oxidative and ischemic stress (Guitart et al., 2016). Cortical neuron-derived exosomes containing miR-181c-3p have been shown to inhibit neuroinflammation in an ischemic brain injury rat model by downregulating chemokine (C-X-C motif) ligand 1 (CXCL1) in Astrocytes (Song et al., 2019).
Extracellular vesicles secreted by astrocytes transport apolipoprotein, a classical neuroprotective protein, to neurons and mediate neuronal survival upon oxidative stress (Pascua-Maestro et al., 2018) (Figure 2).
Alzheimer’s Disease
Alzheimer’s disease is caused by amyloid plaques containing amyloid-βpeptidases and neurofibrillary tangles containing tau protein. Neuronal death and cerebral amyloid angiopathy is commonly witnessed in AD patients. Investigations of fluid biomarkers in astrocytes and neuronal cells have proved that degenerative and inflammatory factors in early and late stages of AD are different and identifying the level of degenerative and inflammatory factors in both healthy and various stages of neurodegenerative diseases may help us to evaluate our understandings toward disease-causing factors such as inflammation, cellular plasticity, neuronal injury and astrocytopathy (Elahi et al., 2019). Neuronal-derived exosomes enhance the formation of Amyloid-β fibril and the exosomes containing Amyloid–β are taken into microglial for degradation (Yuyama et al., 2012).
Amyloid peptide trigger secretion of proapoptotic exosomes and may lead to glial apoptosis in AD (Wang et al., 2012).
A recent study demonstrated that Amyloid–β exposed astrocytes overproduced the phosphor-Tau protein within exosomes, which was a biological marker of AD (Chiarini et al., 2017). Another research revealed that amyloid–β and tau released into serum were most likely from astrocytes-derived exosomes in the brain according to animal experiments (Rosas-Hernandez et al., 2019). The disease-causing mechanisms of astrocytes are still unknown. Astrocytes are transformed into A1-type astrocytes which results in neuronal cell death and damages in AD. Neurotrophic factors levels released by astrocyte exosomes were lower at the early stage of AD with no further depletion of neurotropic levels by the progression of the disease in the later stages (Goetzl et al., 2019). The number of complement proteins in plasma astrocyte-derived exosomes are usually irregular in AD and are not the same in mild cognitive impairment (Winston et al., 2019). A study explored the inflammatory roles of astrocyte-derived exosomes by quantification of their complement proteins and they revealed that the complement proteins were the product of dysregulated unknown mechanisms, which damaged neurons in the late stage of AD (Goetzl et al., 2018). Because of the diversity of exosomes and the biological molecules they carry as a signal to other cells, exosomes may also be involved in disease-preventing mechanisms. Quantification of astrocyte-derived exosome proteins in AD and frontotemporal dementia suggests that exosome is a promising target for testing inhibitory drugs in proteinopathic dementia diseases (Goetzl et al., 2016) and indicates that astrocyte-derived exosomes may participate in the pathophysiology of AD (Khongkow et al., 2019). The robust effect of astrocyte-derived EVs in AD patients can also show the usefulness of astrocyte-derived EVs in brain targeting therapies (Nogueras-Ortiz et al., 2020).
Amyotrophic Lateral Sclerosis Disease
Amyotrophic lateral sclerosis (ALS) is the most common motor neuron disease. It is an incurable disease because the pathways that lead to selective motor neuron damage are yet unknown. Astrocytes play a significant role in ALS (Basso et al., 2013). The brain astrocyte-derived EVs from ALS animals contain ALS causing proteins such as superoxide dismutase1 (SOD1), which are only found in patients with early-stage ALS (Silverman et al., 2019). Another study indicated that astrocyte-derived exosomes shuttle mutant SOD1, which was transported to the spinal neurons and attenuated neuronal cell death in ALS (Basso et al., 2013). As inflammation is one of the major factors that drive ALS, a study was designed to determine the interleukin-6 (IL-6) levels in astrocyte-derived exosomes of ALS patients. As a result, this study found a correlation between the rate of the disease progression and the IL-6 level, suggesting that astrocyte-derived exosomes in ALS patients could reveal the pathophysiology of the patients (Chen Y. et al., 2019). A study identified that neuronal-derived exosomes containing miR-124a could be up-taken by astrocytes and consequently promote glial glutamate Transporter 1 (GLT1) expression, which is involved in the pathological pathways of various CNS diseases such as ALS (Morel et al., 2013).
Parkinson’s Disease
Level of α-synuclein is crucial in the pathogenesis of Parkinson’s disease (PD). Studies indicated that α-synuclein Transferring α-synuclein via astrocytes is of the ways that cells eradicate α-synuclein. Dysfunction in α-synuclein containing cells including astrocytes could be critical in the initiation or progression of PD and resolving these pathways may be a therapeutic innovation in PD (Stefanis et al., 2019). Previous studies suggested that uptake of exosomes containing α-synuclein by astrocytes can have disease-causing effects by the propagation of pathologic α-synuclein and changing astrocyte homeostasis (Sorrentino et al., 2019). Dopaminergic neurons are fragile to oxidative stress and astrocytes may protect neurons from iron-mediated toxicity which is caused by dopamine metabolic products in PD patients. Astrocytes were able to provide necessary biological molecules via exosomes to neurons in order to increase their resistance to oxidative damage. Inhibition of astrocyte-neuron communication could be the cause of PD (Ishii et al., 2019). For example, astrocyte-derived exosome miR-200a-3p prevents MPP(+)-induced apoptotic cell death through down-regulation of mitogen-activated protein kinase kinase 4 (MKK4) pathway (Shakespear et al., 2020). Besides, a recent study demonstrated the usefulness of neuron-derived, astrocytes-derived, and oligodendrocyte-derived exosomes in human plasma as a diagnostic biomarker for PD (Ohmichi et al., 2019).
Cancer
Astrocyte-derived exosomes does not always have a positive effect on microenvironments and can be devastating. For example, astrocytes-derived exosomes enriched with miR-19a may increase brain cancer metastasis by increasing the loss of phosphatase and tensin homolog (PTEN) in tumor cells and C-C motif chemokine ligand 2 (CCL2) chemokine secretion (Zhang et al., 2015). Melanoma cells secrete EVs which can activate pro-inflammatory signals in astrocytes and promote metastasis and tumor formation (Gener Lahav et al., 2019). Glioma-derived EVs increase inhibition of TP53 and MYC signaling pathway activation in astrocytes. Those changes eventually result in pro-inflammatory development, tumor promotion, and progression (Hallal et al., 2019). Astrocytes develop tumor-like behaviors after being exposed to glioma EVs indicating that delivery of oncogenic material via EV may cause tumorigenic changes in astrocytes (Oushy et al., 2018). Previous research illustrated that drug-resistant cancers are caused by gene–gene, gene–miRNA, protein–protein signaling, and cell-to-cell interaction (Kitano, 2003, 2004). The transfer of genetic materials between cells in the tumor microenvironment, especially between tumor cells, has been demonstrated to promote tumor growth, invasion, and resistance to anti-tumor drugs (Mondal et al., 2017; Simon et al., 2018). A study found that glioblastoma patients’ blood samples showed that exosomal mRNA status could be related to treatment response (Shao et al., 2015). Other research found that miR-1238 was higher in drug-resistant glioblastoma cells and their exosomes than in drug-sensitive glioblastoma cells. This may indicate the important role of exosomal miR-1238 in mediating acquired drug resistance of glioblastoma cells (Yin et al., 2019). This is noteworthy because tumor-associated astrocyte-derived EVs contain O6-methylguanine-DNA methyltransferase (MGMT) mRNA which promotes anti-tumor resistance in glioma cells (Yu et al., 2018). Additionally, brain cancer cells communicate with astrocytes by exosomes. According to other research, it is also possible for glioblastoma cells to transfer oncogenic materials by exosomes to astrocytes in order to regulate the function of astrocytes (Rooj et al., 2016). Glioblastoma-derived exosomes could potentially convert normal astrocytes into a tumor-enhancing phenotype (Oushy et al., 2018). It was also reported that glioma cell-derived exosomes transfer long non-coding RNA, which was activated by transforming growth factor-beta (TGF-β) into astrocytes and cause miR-204-3p targeting in astrocytes and further promote the invasion of glioma cells (Bian et al., 2019). An in vitro study shown that EVs shed from U87 glioblastoma cell lines could degrade the gelatin matrix in astrocytes (Hallal et al., 2019).
As TGF-β is one of the glioblastoma biomarkers, achieving an early diagnosis of brain tumors may be possible by examining a patient’s body microfluid exosomes. Another study showed that a tumor suppressor, SAM, and SH3 domain-containing protein 1 (SASH1), was dysregulated or absent in glioma cells. Similar studies have also demonstrated the difference between the protein patterns of normal and glioma cells and the opposite effect of extracellular high-mobility group protein 1 (HMGB1) and astrocytic HMGB1 on SASH1 gene expression in one glioma cell line (Ma et al., 2019). Interaction between astrocyte-derived exosomes that contain neurotrophic induced cargoes such as co-chaperone stress-inducible protein 1 (STI1), and neuronal surface components could also enhance their pathological signaling (Hajj et al., 2013); STI1 also induced glioma through different pathways and is involved in neuronal death and neurodegenerative diseases (Erlich et al., 2007; Landemberger et al., 2018).
All these findings suggest that astrocytes may have the potential to become a therapeutic target in brain cancer patients.
Other Diseases and Inflammation
Exosomes could also help to clarify the disease-causing factors and their pathophysiology in various CNS diseases. Astrocyte-derived exosomes could target neurons and contain neuroprotective proteins such as neuroglobin, which explained the neuroprotective role of astrocytes as well as the fact that they could exert their effect by secreting exosomes (Venturini et al., 2019). Besides, miRNAs in astrocytes-derived exosomes such as miR-26a could be potentially considered as a mediator for neuronal plasticity (Lafourcade et al., 2016). Studies have demonstrated that high levels of glial fibrillary acidic protein (GFAP) in MS patients during a clinical relapse and GFAP is expressed on astrocytes. It may be evidence that suggests the involvement of astrocytes in MS.
Exosome secretion of a psychosis-altered miRNA has been shown to regulate glutamate receptor expression. A research revealed that the inhibition of astrocytic miR-223 decreased the exosome mediated reduction in the expression of glutamate Receptor, Ionotropic, N-Methyl D-Aspartate 2B (Grin2b) in neurons. As a result, they found that this psychosis-altered miRNA can regulate the expression of glutamate receptors which are antagonized by antipsychotics (Amoah et al., 2019).
Nerve growth factor (NGF) protein is hyper-expressed in reactive astrocytes during spinal cord injury (SCI). The release of NGF could be mediated by exosomes and triggered by neuronal apoptosis post SCI. The appropriate balance of the nerve growth factor by reactive astrocytes may be a potential therapy for SCI (Cheng Y.Y. et al., 2019).
Traumatic brain injury (TBI) is also a common brain disease. As complement and proteins of astrocyte-derived exosome levels are considerably higher than those in neurons. These exosome could be considered as TBI biomarkers (Goetzl et al., 2020). Also, research indicates that astrocyte-derived exosome containing gap junction protein alpha 1- 20 kDa (GJA1-20k) could protect and repair the damaged neurons in TBI. Astrocytes-derived GJA1-20k protein could attenuate the connexin43 (CX43) phosphorylation, protect the mitochondria, decrease the cell death rate, and induce neuronal recovery (Chen W. et al., 2019).
It is noted that neurons and astrocytes could regulate each other through exosomes (Chaudhuri et al., 2018; Men et al., 2019; Venturini et al., 2019). Exosomes are transferred to neurons by glial cells including astrocytes and can cause neuroinflammation and depression through miRNA dysregulation (Brites and Fernandes, 2015).
Exosomes attenuated the inflammation by inhibiting the CXCL1 in astrocytes (Song et al., 2019). Interestingly, activated human astrocyte-derived EVs modulate neuronal uptake, differentiation, and firing (You et al., 2020). Astrocytes-derived EVs enriched IL-1β lower neurite development and maturation during neuroinflammation (You et al., 2020). Astrocytes may exert their inflammatory roles by utilizing exosomes (Sofroniew, 2015). Astrocytes could also induce inflammation on other cells through toll-like receptors (TLR) signaling and by exosomes enriched inflammation-inducing factors (Ibanez et al., 2019). Up-regulation of major histocompatibility complex1 (MHCI) molecules in astrocytes affect behavioral function by immune cascades, microglial proliferation, and neuronal numbers may lead to brain dysfunction in neuroinflammation-associated diseases, astrocytes may exert those inflammatory effects by releasing exosomes to target immune or neuronal cells (Sobue et al., 2018). IL-1β enriched astrocyte-derived EVs play an important role in cellular organization, cell communication, and inflammatory responses (You et al., 2020). Astrocyte-derived exosome containing HMGB1 can also be used as a biomarker in progression of inflammation in the brain of gulf war illness with drug treatment (Madhu et al., 2019).
Conclusion and Future Perspectives
Despite of the recent efforts that have been made in understanding the role of astrocyte-derived EVs in CNS diseases, future studies are required to focus on the role of astrocyte-derived exosomes and their specific associated biological molecules as they are contained in both healthy and abnormal cells to resolve the functional effect of astrocyte secrete EVs. Identification of astrocyte-derived exosomes’ effects on both short and long-distance targets and their survival strategies may lead to the finding and development of new diagnostic and therapeutic methods. Further narrative researches on various biological molecules in astrocyte-derived exosomes or other brain cell derived-exosomes that modulate astrocytic function are required in order to further clarify their roles in the pathophysiology of various CNS diseases. Although cell-type-specific exosomes can be easily obtained in vitro, there is still difficulty in vivo study of EVs since a mix of different exosomes derived from different cells exist in extracellular space and it is not easy to distinguish them from one another. New approaches to label cell type specific exosomes are required which will be helpful for tracking cells in the study of intercellular communication in vivo. In this review, astrocyte-derived EVs have been demonstrated to carry or transfer biological molecules that are involved in both disease-causing and healing processes. Thus, astrocyte-derived EVs hold considerable potential for various clinical applications. Astrocyte-derived EVs are capable of crossing BBB and being up-taken by various brain cells, the ability of which makes them suitable to be used in drug targeted therapies a drug or drug carrier. The difference in the contents of astrocyte-secreted EVs make them suitable to be used as a biomarker for CNS disease diagnosis and progression of disease states. Study of astrocyte-derived exosomes leads to a better understanding of astrocytic functions in the brain. Despite the existence of plethora of evidence that supports the therapeutic potential of astrocyte-derived EVs, new in vitro/vivo models, more powerful imaging and tracking methods are required to track single astrocyte-derived EVs in the whole brain microenvironment in various disease and health situation in order to increase our understanding of astrocytes and their secreted EVs. Understanding the cell to cell communication via the study of astrocyte-derived exosomes and other brains cell-derived EVs may also contribute to brain development in vitro.
Author Contributions
TG conceived, designed, and wrote the manuscript. G-YY and ZZ contributed to the discussion of ideas, helped in the correction, and proofread the manuscript. All the authors contributed to the article and approved the submitted version.
Funding
This review was supported by grants from the National Key R&D Program of China (#2016YFC1300602, GYY), National Natural Science Foundation of China (NSFC) (81771251, G-YY; 81771244, ZZ; 81974179, ZZ), Shanghai Education Commission Research and Innovation Program (2019-01-07-00-02-E00064, G-YY), K. C. Wong Education Foundation (G-YY).
Conflict of Interest
The authors declare that the research was conducted in the absence of any commercial or financial relationships that could be construed as a potential conflict of interest.
Acknowledgments
The authors would like to express their gratitude to Shuyang Zhou for his kind co-operation and proofreading the manuscript. All the figures were created using BioRender.com.
Abbreviations
AD, Alzheimer’s disease; ALS, amyotrophic lateral sclerosis; AMPK/mTOR, AMP-activated protein kinase and Rapamycin; BBB, blood-brain barrier; BM, bone marrow; CCL2, C-C motif chemokine ligand 2; CNS, central nervous system; CTGF, connective tissue growth factor; CXCL1, the chemokine (C-X-C motif) ligand 1; CX43, connexin43; EV, extracellular vesicle; ECM, extracellular matrix; GFAP, glial fibrillary acidic protein; GJA1-20k, gap junction protein alpha 1- 20 kDa; GLT1, glial glutamate transporter 1; Grin2b, glutamate receptor, ionotropic, N-methyl D-aspartate 2B; Hsp/c70, heat shock protein/C-terminal70; HMGB1, high-mobility group protein 1; IL-1β, interleukin 1beta; IL-10, interleukin 10; IL-6, interleukin 6; MGMT, O6-methylguanine-DNA methyltransferase; MHC1, major histocompatibility complex1; miRNA, microRNA; MKK4, mitogen-activated protein kinase kinase 4; MSC, mesenchymal stem cell; MS, multiple sclerosis; MV, micro-vesicles; NGF, neuron growth factor; Nrf2-NF-κ B, nuclear factor (erythroid-derived 2)-like 2 and nuclear factor-κ B; NSC, neuronal stem cell; OGD, oxygen glucose deprivation; PD, Parkinson’s disease; PTEN, phosphatase and tensin homolog; TBI, traumatic brain injury; TGF-β, transforming growth factor beta; TLR, toll-like receptors; RABEPK, Rab9 effector protein with Kelch Motifs; SASH1, SAM and SH3 domain-containing protein 1; SCI, spinal cord injury; SOD1, superoxide dismutase 1;STI1, stress-inducible protein 1.
References
Abak, A., Abhari, A., and Rahimzadeh, S. (2018). Exosomes in cancer: small vesicular transporters for cancer progression and metastasis, biomarkers in cancer therapeutics. PeerJ. 6:e4763. doi: 10.7717/peerj.4763
Abbott, N. J., Ronnback, L., and Hansson, E. (2006). Astrocyte-endothelial interactions at the blood-brain barrier. Nat. Rev. Neurosci. 7, 41–53. doi: 10.1038/nrn1824
Adams, K. L., and Gallo, V. (2018). The diversity and disparity of the glial scar. Nat. Neurosci. 21, 9–15. doi: 10.1038/s41593-017-0033-9
Allaman, I., Belanger, M., and Magistretti, P. J. (2011). Astrocyte-neuron metabolic relationships: for better and for worse. Trends Neurosci. 34, 76–87. doi: 10.1016/j.tins.2010.12.001
Amoah, S. K., Rodriguez, B. A., Logothetis, C. N., Chander, P., Sellgren, C. M., Weick, J. P., et al. (2019). Exosomal secretion of a psychosis-altered miRNA that regulates glutamate receptor expression is affected by antipsychotics. Neuropsychopharmacology 45, 656–665. doi: 10.1038/s41386-019-0579-1
Anderson, M. A., Ao, Y., and Sofroniew, M. V. (2014). Heterogeneity of reactive astrocytes. Neurosci. Lett. 565, 23–29. doi: 10.1016/j.neulet.2013.12.030
Attwell, D., Buchan, A. M., Charpak, S., Lauritzen, M., Macvicar, B. A., and Newman, E. A. (2010). Glial and neuronal control of brain blood flow. Nature 468, 232–243. doi: 10.1038/nature09613
Baglio, S. R., Rooijers, K., Koppers-Lalic, D., Verweij, F. J., Perez Lanzon, M., Zini, N., et al. (2015). Human bone marrow- and adipose-mesenchymal stem cells secrete exosomes enriched in distinctive miRNA and tRNA species. Stem. Cell Res. Ther. 6:127. doi: 10.1186/s13287-015-0116-z
Bang, O. Y., and Kim, E. H. (2019). Mesenchymal stem cell-derived extracellular vesicle therapy for stroke: challenges and progress. Front. Neurol. 10:211. doi: 10.3389/fneur.2019.00211
Basso, M., Pozzi, S., Tortarolo, M., Fiordaliso, F., Bisighini, C., Pasetto, L., et al. (2013). Mutant copper-zinc superoxide dismutase (SOD1) induces protein secretion pathway alterations and exosome release in astrocytes: implications for disease spreading and motor neuron pathology in amyotrophic lateral sclerosis. J. Biol. Chem. 288, 15699–15711. doi: 10.1074/jbc.M112.425066
Bazargani, N., and Attwell, D. (2016). Astrocyte calcium signaling: the third wave. Nat. Neurosci. 19, 182–189. doi: 10.1038/nn.4201
Benarroch, E. E. (2005). Neuron-astrocyte interactions: partnership for normal function and disease in the central nervous system. Mayo Clin. Proc. 80, 1326–1338. doi: 10.4065/80.10.1326
Bian, E. B., Chen, E. F., Xu, Y. D., Yang, Z. H., Tang, F., Ma, C. C., et al. (2019). Exosomal lncRNAATB activates astrocytes that promote glioma cell invasion. Int. J. Oncol. 54, 713–721. doi: 10.3892/ijo.2018.4644
Borges, F. T., Reis, L. A., and Schor, N. (2013). Extracellular vesicles: structure, function, and potential clinical uses in renal diseases. Braz. J. Med. Biol. Res. 46, 824–830. doi: 10.1590/1414-431X20132964
Branscome, H., Paul, S., Yin, D., El-Hage, N., Agbottah, E. T., Zadeh, M. A., et al. (2020). Use of stem cell extracellular vesicles as a “Holistic” approach to CNS repair. Front. Cell Dev. Biol. 8:455. doi: 10.3389/fcell.2020.00455
Brites, D., and Fernandes, A. (2015). Neuroinflammation and depression: microglia activation, extracellular microvesicles and microRNA dysregulation. Front. Cell Neurosci. 9:476. doi: 10.3389/fncel.2015.00476
Brooks, A. J., and Waters, M. J. (2010). The growth hormone receptor: mechanism of activation and clinical implications. Nat. Rev. Endocrinol. 6, 515–525. doi: 10.1038/nrendo.2010.123
Bu, X., Li, D., Wang, F., Sun, Q., and Zhang, Z. (2020). Protective role of astrocyte-derived exosomal microRNA-361 in cerebral ischemic-reperfusion injury by regulating the AMPK/mTOR signaling pathway and targeting CTSB. Neuropsychiatr. Dis. Treat. 16, 1863–1877. doi: 10.2147/NDT.S260748
Camussi, G., Deregibus, M. C., Bruno, S., Cantaluppi, V., and Biancone, L. (2010). Exosomes/microvesicles as a mechanism of cell-to-cell communication. Kidney Int. 78, 838–848. doi: 10.1038/ki.2010.278
Carfi Pavia, F., Di Bella, M. A., Brucato, V., Blanda, V., Zummo, F., Vitrano, I., et al. (2019). A 3Dscaffold of PLLA induces the morphological differentiation and migration of primary astrocytes and promotes the production of extracellular vesicles. Mol. Med. Rep. 20, 1288–1296. doi: 10.3892/mmr.2019.10351
Chaudhuri, A. D., Dastgheyb, R. M., Yoo, S. W., Trout, A., Talbot, C. C. Jr., Hao, H., et al. (2018). TNFalpha and IL-1beta modify the miRNA cargo of astrocyte shed extracellular vesicles to regulate neurotrophic signaling in neurons. Cell Death Dis. 9:363. doi: 10.1038/s41419-018-0369-4
Chen, C. C., Liu, L., Ma, F., Wong, C. W., Guo, X. E., Chacko, J. V., et al. (2016). Elucidation of exosome migration across the blood-brain barrier model in vitro. Cell Mol. Bioeng. 9, 509–529. doi: 10.1007/s12195-016-0458-3
Chen, W., Zheng, P., Hong, T., Wang, Y., Liu, N., He, B., et al. (2019). Astrocytes-derived exosomes induce neuronal recovery after traumatic brain injury via delivering GJA1-20k. J. Tissue Eng. Regen. Med. 14, 412–423. doi: 10.1002/term.3002
Chen, Y., Xia, K., Chen, L., and Fan, D. (2019). Increased interleukin-6 levels in the astrocyte-derived exosomes of sporadic amyotrophic lateral sclerosis patients. Front. Neurosci. 13:574. doi: 10.3389/fnins.2019.00574
Cheng, Y. Y., Zhao, H. K., Chen, L. W., Yao, X. Y., Wang, Y. L., Huang, Z. W., et al. (2019). Reactive astrocytes increase expression of proNGF in the mouse model of contused spinal cord injury. Neurosci. Res. 157, 34–43. doi: 10.1016/j.neures.2019.07.007
Chiarini, A., Armato, U., Gardenal, E., Gui, L., and Dal Pra, I. (2017). Amyloid beta-exposed human astrocytes overproduce phospho-tau and overrelease it within exosomes, effects suppressed by calcilytic nps 2143-further implications for Alzheimer’s Therapy. Front. Neurosci. 11:217. doi: 10.3389/fnins.2017.00217
Collino, F., Deregibus, M. C., Bruno, S., Sterpone, L., Aghemo, G., Viltono, L., et al. (2010). Microvesicles derived from adult human bone marrow and tissue specific mesenchymal stem cells shuttle selected pattern of miRNAs. PLoS One 5:e11803. doi: 10.1371/journal.pone.0011803
Dallerac, G., Zapata, J., and Rouach, N. (2018). Versatile control of synaptic circuits by astrocytes: where, when and how? Nat. Rev. Neurosci. 19, 729–743. doi: 10.1038/s41583-018-0080-6
Datta Chaudhuri, A., Dasgheyb, R. M., DeVine, L. R., Bi, H., Cole, R. N., and Haughey, N. J. (2020). Stimulus-dependent modifications in astrocyte-derived extracellular vesicle cargo regulate neuronal excitability. Glia 68, 128–144. doi: 10.1002/glia.23708
Deng, Y., Chen, D., Gao, F., Lv, H., Zhang, G., Sun, X., et al. (2019). Exosomes derived from microRNA-138-5p-overexpressing bone marrow-derived mesenchymal stem cells confer neuroprotection to astrocytes following ischemic stroke via inhibition of LCN2. J. Biol. Eng. 13:71. doi: 10.1186/s13036-019-0193-0
Dickens, A. M., Tovar-Y-Romo, L. B., Yoo, S. W., Trout, A. L., Bae, M., Kanmogne, M., et al. (2017). Astrocyte-shed extracellular vesicles regulate the peripheral leukocyte response to inflammatory brain lesions. Sci. Signal 10:eaai7696. doi: 10.1126/scisignal.aai7696
EL Andaloussi, S., Mager, I., Breakefield, X. O., and Wood, M. J. (2013). Extracellular vesicles: biology and emerging therapeutic opportunities. Nat. Rev. Drug Discov. 12, 347–357. doi: 10.1038/nrd3978
Elahi, F. M., Casaletto, K. B., La Joie, R., Walters, S. M., Harvey, D., Wolf, A., et al. (2019). Plasma biomarkers of astrocytic and neuronal dysfunction in early- and late-onset Alzheimer’s disease. Alzheimers Dement 16, 681–695. doi: 10.1016/j.jalz.2019.09.004
Erlich, R. B., Kahn, S. A., Lima, F. R., Muras, A. G., Martins, R. A., Linden, R., et al. (2007). STI1 promotes glioma proliferation through MAPK and PI3K pathways. Glia 55, 1690–1698. doi: 10.1002/glia.20579
Fruhbeis, C., Frohlich, D., Kuo, W. P., and Kramer-Albers, E. M. (2013). Extracellular vesicles as mediators of neuron-glia communication. Front. Cell Neurosci. 7:182. doi: 10.3389/fncel.2013.00182
Gener Lahav, T., Adler, O., Zait, Y., Shani, O., Amer, M., Doron, H., et al. (2019). Melanoma-derived extracellular vesicles instigate proinflammatory signaling in the metastatic microenvironment. Int. J. Cancer 145, 2521–2534. doi: 10.1002/ijc.32521
Ghosh, S., Garg, S., and Ghosh, S. (2020). Cell-derived exosome therapy: a novel approach to treat post-traumatic brain injury mediated neural injury. ACS Chem. Neurosci. 11, 2045–2047. doi: 10.1021/acschemneuro.0c00368
Goetzl, E. J., Mustapic, M., Kapogiannis, D., Eitan, E., Lobach, I. V., Goetzl, L., et al. (2016). Cargo proteins of plasma astrocyte-derived exosomes in Alzheimer’s disease. FASEB J. 30, 3853–3859. doi: 10.1096/fj.201600756R
Goetzl, E. J., Nogueras-Ortiz, C., Mustapic, M., Mullins, R. J., Abner, E. L., Schwartz, J. B., et al. (2019). Deficient neurotrophic factors of CSPG4-type neural cell exosomes in Alzheimer disease. FASEB J. 33, 231–238. doi: 10.1096/fj.201801001
Goetzl, E. J., Schwartz, J. B., Abner, E. L., Jicha, G. A., and Kapogiannis, D. (2018). High complement levels in astrocyte-derived exosomes of Alzheimer disease. Ann. Neurol. 83, 544–552. doi: 10.1002/ana.25172
Goetzl, E. J., Yaffe, K., Peltz, C. B., Ledreux, A., Gorgens, K., Davidson, B., et al. (2020). Traumatic brain injury increases plasma astrocyte-derived exosome levels of neurotoxic complement proteins. FASEB J. 34, 3359–3366. doi: 10.1096/fj.201902842R
Gomez-Molina, C., Sandoval, M., Henzi, R., Ramirez, J. P., Varas-Godoy, M., Luarte, A., et al. (2019). Small extracellular vesicles in rat serum contain astrocyte-derived protein biomarkers of repetitive stress. Int. J. Neuropsychopharmacol. 22, 232–246. doi: 10.1093/ijnp/pyy098
Gosselin, R. D., Meylan, P., and Decosterd, I. (2013). Extracellular microvesicles from astrocytes contain functional glutamate transporters: regulation by protein kinase C and cell activation. Front. Cell Neurosci. 7:251. doi: 10.3389/fncel.2013.00251
Guitart, K., Loers, G., Buck, F., Bork, U., Schachner, M., and Kleene, R. (2016). Improvement of neuronal cell survival by astrocyte-derived exosomes under hypoxic and ischemic conditions depends on prion protein. Glia 64, 896–910. doi: 10.1002/glia.22963
Gupta, K., Patani, R., Baxter, P., Serio, A., Story, D., Tsujita, T., et al. (2012). Human embryonic stem cell derived astrocytes mediate non-cell-autonomous neuroprotection through endogenous and drug-induced mechanisms. Cell Death Differ. 19, 779–787. doi: 10.1038/cdd.2011.154
Habib, N., McCabe, C., Medina, S., Varshavsky, M., Kitsberg, D., Dvir-Szternfeld, R., et al. (2020). Disease-associated astrocytes in Alzheimer’s disease and aging. Nat. Neurosci. 23, 701–706. doi: 10.1038/s41593-020-0624-8
Hajj, G. N., Arantes, C. P., Dias, M. V., Roffe, M., Costa-Silva, B., Lopes, M. H., et al. (2013). The unconventional secretion of stress-inducible protein 1 by a heterogeneous population of extracellular vesicles. Cell Mol. Life Sci. 70, 3211–3227. doi: 10.1007/s00018-013-1328-y
Hallal, S., Mallawaaratchy, D. M., Wei, H., Ebrahimkhani, S., Stringer, B. W., Day, B. W., et al. (2019). Extracellular vesicles released by glioblastoma cells stimulate normal astrocytes to acquire a tumor-supportive phenotype Via p53 and MYC signaling pathways. Mol. Neurobiol. 56, 4566–4581. doi: 10.1007/s12035-018-1385-1
Hamilton, N. B., and Attwell, D. (2010). Do astrocytes really exocytose neurotransmitters? Nat. Rev. Neurosci. 11, 227–238. doi: 10.1038/nrn2803
Hao, S., Moyana, T., and Xiang, J. (2007). Review: cancer immunotherapy by exosome-based vaccines. Cancer Biother Radiopharm. 22, 692–703. doi: 10.1089/cbr.2007.368-R
Harding, C., and Stahl, P. (1983). Transferrin recycling in reticulocytes: pH and iron are important determinants of ligand binding and processing. Biochem. Biophys. Res. Commun. 113, 650–658. doi: 10.1016/0006-291x(83)91776-x
Hira, K., Ueno, Y., Tanaka, R., Miyamoto, N., Yamashiro, K., Inaba, T., et al. (2018). Astrocyte-derived exosomes treated with a semaphorin 3A inhibitor enhance stroke recovery via prostaglandin D2 synthase. Stroke 49, 2483–2494. doi: 10.1161/STROKEAHA.118.021272
Holm, M. M., Kaiser, J., and Schwab, M. E. (2018). Extracellular vesicles: multimodal envoys in neural maintenance and repair. Trends Neurosci. 41, 360–372. doi: 10.1016/j.tins.2018.03.006
Holtzman, J., and Lee, H. (2020). Emerging role of extracellular vesicles in the respiratory system. Exp. Mol. Med. 52, 887–895. doi: 10.1038/s12276-020-0450-9
H Rashed, M., Bayraktar, E., Abd-Ellah, M. F., Amero, P., Chavez-Reyes, A., and Rodriguez-Aguayo, C. (2017). Exosomes: from garbage bins to promising therapeutic targets. Int. J. Mol. Sci. 18:538. doi: 10.3390/ijms18030538
Hu, G., Liao, K., Niu, F., Yang, L., Dallon, B. W., Callen, S., et al. (2018). Astrocyte EV-Induced lincRNA-Cox2 Regulates Microglial Phagocytosis: implications for Morphine-Mediated Neurodegeneration. Mol. Ther. Nucleic Acids. 13, 450–463. doi: 10.1016/j.omtn.2018.09.019
Hubbard, J. A., Szu, J. I., Yonan, J. M., and Binder, D. K. (2016). Regulation of astrocyte glutamate transporter-1 (GLT1) and aquaporin-4 (AQP4) expression in a model of epilepsy. Exp. Neurol. 283(Pt A), 85–96. doi: 10.1016/j.expneurol.2016.05.003
Ibanez, F., Montesinos, J., Urena-Peralta, J. R., Guerri, C., and Pascual, M. (2019). TLR4 participates in the transmission of ethanol-induced neuroinflammation via astrocyte-derived extracellular vesicles. J. Neuroinflammation 16:136. doi: 10.1186/s12974-019-1529-x
Ishii, T., Warabi, E., and Mann, G. E. (2019). Circadian control of BDNF-mediated Nrf2 activation in astrocytes protects dopaminergic neurons from ferroptosis. Free Radic. Biol. Med. 133, 169–178. doi: 10.1016/j.freeradbiomed.2018.09.002
Isola, A. L., and Chen, S. (2017). Exosomes: the Messengers of Health and Disease. Curr. Neuropharmacol. 15, 157–165. doi: 10.2174/1570159x14666160825160421
Jha, M. K., Jo, M., Kim, J. H., and Suk, K. (2019). Microglia-astrocyte crosstalk: an intimate molecular conversation. Neuroscientist 25, 227–240. doi: 10.1177/1073858418783959
Johnstone, R. M., Adam, M., Hammond, J. R., Orr, L., and Turbide, C. (1987). Vesicle formation during reticulocyte maturation. Association of plasma membrane activities with released vesicles (exosomes). J. Biol. Chem. 262, 9412–9420.
Jovicic, A., and Gitler, A. D. (2017). Distinct repertoires of microRNAs present in mouse astrocytes compared to astrocyte-secreted exosomes. PLoS One 12:e0171418. doi: 10.1371/journal.pone.0171418
Kalluri, R. (2016). The biology and function of exosomes in cancer. J. Clin. Invest. 126, 1208–1215. doi: 10.1172/JCI81135
Khongkow, M., Yata, T., Boonrungsiman, S., Ruktanonchai, U. R., Graham, D., and Namdee, K. (2019). Surface modification of gold nanoparticles with neuron-targeted exosome for enhanced blood-brain barrier penetration. Sci. Rep. 9:8278. doi: 10.1038/s41598-019-44569-6
Kitano, H. (2004). Cancer as a robust system: implications for anticancer therapy. Nat. Rev. Cancer 4, 227–235. doi: 10.1038/nrc1300
Ko, J., Hemphill, M. A., Gabrieli, D., Wu, L., Yelleswarapu, V., Lawrence, G., et al. (2016). Smartphone-enabled optofluidic exosome diagnostic for concussion recovery. Sci. Rep. 6:31215. doi: 10.1038/srep31215
Kubota, K., Nakano, M., Kobayashi, E., Mizue, Y., Chikenji, T., Otani, M., et al. (2018). An enriched environment prevents diabetes-induced cognitive impairment in rats by enhancing exosomal miR-146a secretion from endogenous bone marrow-derived mesenchymal stem cells. PLoS One 13:e0204252. doi: 10.1371/journal.pone.0204252
Kur, I. M., Prouvot, P. H., Fu, T., Fan, W., Muller-Braun, F., Das, A., et al. (2020). Neuronal activity triggers uptake of hematopoietic extracellular vesicles in vivo. PLoS Biol. 18:e3000643. doi: 10.1371/journal.pbio.3000643
Lafourcade, C., Ramirez, J. P., Luarte, A., Fernandez, A., and Wyneken, U. (2016). MiRNAs in astrocyte-derived exosomes as possible mediators of neuronal plasticity. J. Exp. Neurosci. 10(Suppl. 1), 1–9. doi: 10.4137/JEN.S39916
Landemberger, M. C., de Oliveira, G. P., Machado, C. F., Gollob, K. J., and Martins, V. R. (2018). Loss of STI1-mediated neuronal survival and differentiation in disease-associated mutations of prion protein. J. Neurochem. 145, 409–416. doi: 10.1111/jnc.14305
Lee, J. Y., Xu, K., Nguyen, H., Guedes, V. A., Borlongan, C. V., and Acosta, S. A. (2017). Stem cell-induced biobridges as possible tools to aid neuroreconstruction after CNS injury. Front. Cell Dev. Biol. 5:51. doi: 10.3389/fcell.2017.00051
Levin, E. R., and Hammes, S. R. (2016). Nuclear receptors outside the nucleus: extranuclear signalling by steroid receptors. Nat. Rev. Mol. Cell Biol. 17, 783–797. doi: 10.1038/nrm.2016.122
Lin, Q., Qu, M., Zhou, B., Patra, H. K., Sun, Z., Luo, Q., et al. (2019). Exosome-like nanoplatform modified with targeting ligand improves anti-cancer and anti-inflammation effects of imperialine. J. Control Release 311-312, 104–116. doi: 10.1016/j.jconrel.2019.08.037
Liu, Y., Li, D., Liu, Z., Zhou, Y., Chu, D., Li, X., et al. (2015). Targeted exosome-mediated delivery of opioid receptor Mu siRNA for the treatment of morphine relapse. Sci. Rep. 5:17543. doi: 10.1038/srep17543
Liu, Z., and Chopp, M. (2016). Astrocytes, therapeutic targets for neuroprotection and neurorestoration in ischemic stroke. Prog. Neurobiol. 144, 103–120. doi: 10.1016/j.pneurobio.2015.09.008
Luan, X., Sansanaphongpricha, K., Myers, I., Chen, H., Yuan, H., and Sun, D. (2017). Engineering exosomes as refined biological nanoplatforms for drug delivery. Acta Pharmacol. Sin. 38, 754–763. doi: 10.1038/aps.2017.12
Luarte, A., Batiz, L. F., Wyneken, U., and Lafourcade, C. (2016). Potential therapies by stem cell-derived exosomes in CNS diseases: focusing on the neurogenic niche. Stem. Cells Int. 2016:5736059. doi: 10.1155/2016/5736059
Ludwig, P. E., Thankam, F. G., Patil, A. A., Chamczuk, A. J., and Agrawal, D. K. (2018). Brain injury and neural stem cells. Neural Regen. Res. 13, 7–18. doi: 10.4103/1673-5374.224361
Lyu, H., Xiao, Y., Guo, Q., Huang, Y., and Luo, X. (2020). The role of bone-derived exosomes in regulating skeletal metabolism and extraosseous Diseases. Front. Cell Dev. Biol. 8:89. doi: 10.3389/fcell.2020.00089
Ma, C., Chen, H., Zhang, S., Yan, Y., Wu, R., Wang, Y., et al. (2019). Exosomal and extracellular HMGB1 have opposite effects on SASH1 expression in rat astrocytes and glioma C6 cells. Biochem. Biophys. Res. Commun. 518, 325–330. doi: 10.1016/j.bbrc.2019.08.057
Madhu, L. N., Attaluri, S., Kodali, M., Shuai, B., Upadhya, R., Gitai, D., et al. (2019). Neuroinflammation in Gulf War llness is linked with HMGB1 and complement activation, which can be discerned from brain-derived extracellular vesicles in the blood. Brain Behav. Immun. 81, 430–443. doi: 10.1016/j.bbi.2019.06.040
Manterola, L., Guruceaga, E., Gallego Perez-Larraya, J., Gonzalez-Huarriz, M., Jauregui, P., Tejada, S., et al. (2014). A small noncoding RNA signature found in exosomes of GBM patient serum as a diagnostic tool. Neuro Oncol. 16, 520–527. doi: 10.1093/neuonc/not218
Mao, S., Sun, Q., Xiao, H., Zhang, C., and Li, L. (2015). Secreted miR-34a in astrocytic shedding vesicles enhanced the vulnerability of dopaminergic neurons to neurotoxins by targeting Bcl-2. Protein Cell 6, 529–540. doi: 10.1007/s13238-015-0168-y
Marsh, S. E., and Blurton-Jones, M. (2017). Neural stem cell therapy for neurodegenerative disorders: the role of neurotrophic support. Neurochem. Int. 106, 94–100. doi: 10.1016/j.neuint.2017.02.006
Men, Y., Yelick, J., Jin, S., Tian, Y., Chiang, M. S. R., Higashimori, H., et al. (2019). Exosome reporter mice reveal the involvement of exosomes in mediating neuron to astroglia communication in the CNS. Nat. Commun. 10:4136. doi: 10.1038/s41467-019-11534-w
Mittelbrunn, M., Gutierrez-Vazquez, C., Villarroya-Beltri, C., Gonzalez, S., Sanchez-Cabo, F., Gonzalez, M. A., et al. (2011). Unidirectional transfer of microRNA-loaded exosomes from T cells to antigen-presenting cells. Nat. Commun. 2:282. doi: 10.1038/ncomms1285
Miyabe, Y., Lian, J., Miyabe, C., and Luster, A. D. (2019). Chemokines in rheumatic diseases: pathogenic role and therapeutic implications. Nat. Rev. Rheumatol. 15, 731–746. doi: 10.1038/s41584-019-0323-6
Moidunny, S., Vinet, J., Wesseling, E., Bijzet, J., Shieh, C. H., van Ijzendoorn, S. C., et al. (2012). Adenosine A2B receptor-mediated leukemia inhibitory factor release from astrocytes protects cortical neurons against excitotoxicity. J. Neuroinflammation 9:198. doi: 10.1186/1742-2094-9-198
Mondal, A., Kumari Singh, D., Panda, S., and Shiras, A. (2017). Extracellular vesicles as modulators of tumor microenvironment and disease progression in Glioma. Front. Oncol. 7:144. doi: 10.3389/fonc.2017.00144
Montecalvo, A., Larregina, A. T., Shufesky, W. J., Stolz, D. B., Sullivan, M. L., Karlsson, J. M., et al. (2012). Mechanism of transfer of functional microRNAs between mouse dendritic cells via exosomes. Blood 119, 756–766. doi: 10.1182/blood-2011-02-338004
Morel, L., Regan, M., Higashimori, H., Ng, S. K., Esau, C., Vidensky, S., et al. (2013). Neuronal exosomal miRNA-dependent translational regulation of astroglial glutamate transporter GLT1. J. Biol. Chem. 288, 7105–7116. doi: 10.1074/jbc.M112.410944
Nagarajah, S. (2016). Exosome secretion - more than simple waste disposal? Implications for physiology, diagnostics and therapeutics. J. Circ. Biomark 5:7. doi: 10.5772/62975
Nakano, M., Nagaishi, K., Konari, N., Saito, Y., Chikenji, T., Mizue, Y., et al. (2016). Bone marrow-derived mesenchymal stem cells improve diabetes-induced cognitive impairment by exosome transfer into damaged neurons and astrocytes. Sci. Rep. 6:24805. doi: 10.1038/srep24805
Ni, H., Yang, S., Siaw-Debrah, F., Hu, J., Wu, K., He, Z., et al. (2019). Exosomes derived from bone mesenchymal stem cells ameliorate early inflammatory responses following traumatic brain injury. Front. Neurosci. 13:14. doi: 10.3389/fnins.2019.00014
Ni, Z., Zhou, S., Li, S., Kuang, L., Chen, H., Luo, X., et al. (2020). Exosomes: roles and therapeutic potential in osteoarthritis. Bone Res. 8:25. doi: 10.1038/s41413-020-0100-9
Nogueras-Ortiz, C. J., Mahairaki, V., Delgado-Peraza, F., Das, D., Avgerinos, K., Eren, E., et al. (2020). Astrocyte- and neuron-derived extracellular vesicles from alzheimer’s disease patients effect complement-mediated neurotoxicity. Cells 9:1618. doi: 10.3390/cells9071618
O’Brien, K., Breyne, K., Ughetto, S., Laurent, L. C., and Breakefield, X. O. (2020). RNA delivery by extracellular vesicles in mammalian cells and its applications. Nat. Rev. Mol. Cell Biol. 21, 585–606. doi: 10.1038/s41580-020-0251-y
Ohmichi, T., Mitsuhashi, M., Tatebe, H., Kasai, T., Ali El-Agnaf, O. M., and Tokuda, T. (2019). Quantification of brain-derived extracellular vesicles in plasma as a biomarker to diagnose Parkinson’s and related diseases. Parkinsonism Relat. Disord. 61, 82–87. doi: 10.1016/j.parkreldis.2018.11.021
Oushy, S., Hellwinkel, J. E., Wang, M., Nguyen, G. J., Gunaydin, D., Harland, T. A., et al. (2018). Glioblastoma multiforme-derived extracellular vesicles drive normal astrocytes towards a tumour-enhancing phenotype. Philos. Trans. R Soc. Lond. B Biol. Sci. 373:20160477. doi: 10.1098/rstb.2016.0477
Pan, B. T., and Johnstone, R. M. (1983). Fate of the transferrin receptor during maturation of sheep reticulocytes in vitro: selective externalization of the receptor. Cell 33, 967–978. doi: 10.1016/0092-8674(83)90040-5
Paolicelli, R. C., Bergamini, G., and Rajendran, L. (2019). Cell-to-cell communication by extracellular vesicles: focus on microglia. Neuroscience 405, 148–157. doi: 10.1016/j.neuroscience.2018.04.003
Pascua-Maestro, R., Gonzalez, E., Lillo, C., Ganfornina, M. D., Falcon-Perez, J. M., and Sanchez, D. (2018). Extracellular vesicles secreted by astroglial cells transport apolipoprotein D to neurons and mediate neuronal survival upon oxidative stress. Front. Cell Neurosci. 12:526. doi: 10.3389/fncel.2018.00526
Pei, X., Li, Y., Zhu, L., and Zhou, Z. (2019). Astrocyte-derived exosomes suppress autophagy and ameliorate neuronal damage in experimental ischemic stroke. Exp. Cell Res. 382:111474. doi: 10.1016/j.yexcr.2019.06.019
Pei, X., Li, Y., Zhu, L., and Zhou, Z. (2020). Astrocyte-derived exosomes transfer miR-190b to inhibit oxygen and glucose deprivation-induced autophagy and neuronal apoptosis. Cell Cycle 19, 906–917. doi: 10.1080/15384101.2020.1731649
Pekny, M., Wilhelmsson, U., Tatlisumak, T., and Pekna, M. (2019). Astrocyte activation and reactive gliosis-A new target in stroke? Neurosci. Lett. 689, 45–55. doi: 10.1016/j.neulet.2018.07.021
Pollet, H., Conrard, L., Cloos, A. S., and Tyteca, D. (2018). Plasma membrane lipid domains as platforms for vesicle biogenesis and shedding? Biomolecules 8:94. doi: 10.3390/biom8030094
Premack, B. A., and Schall, T. J. (1996). Chemokine receptors: gateways to inflammation and infection. Nat. Med. 2, 1174–1178. doi: 10.1038/nm1196-1174
Qiu, X., Li, Z., Han, X., Zhen, L., Luo, C., Liu, M., et al. (2019). Tumor-derived nanovesicles promote lung distribution of the therapeutic nanovector through repression of Kupffer cell-mediated phagocytosis. Theranostics 9, 2618–2636. doi: 10.7150/thno.32363
Raposo, G., and Stoorvogel, W. (2013). Extracellular vesicles: exosomes, microvesicles, and friends. J. Cell Biol. 200, 373–383. doi: 10.1083/jcb.201211138
Ratajczak, J., Wysoczynski, M., Hayek, F., Janowska-Wieczorek, A., and Ratajczak, M. Z. (2006). Membrane-derived microvesicles: important and underappreciated mediators of cell-to-cell communication. Leukemia 20, 1487–1495. doi: 10.1038/sj.leu.2404296
Riazifar, M., Mohammadi, M. R., Pone, E. J., Yeri, A., Lasser, C., Segaliny, A. I., et al. (2019). Stem cell-derived exosomes as nanotherapeutics for autoimmune and neurodegenerative disorders. ACS Nano 13, 6670–6688. doi: 10.1021/acsnano.9b01004
Rojas, C., Sala, M., Thomas, A. G., Datta Chaudhuri, A., Yoo, S. W., Li, Z., et al. (2019). A novel and potent brain penetrant inhibitor of extracellular vesicle release. Br. J. Pharmacol. 176, 3857–3870. doi: 10.1111/bph.14789
Rong, Y., Liu, W., Wang, J., Fan, J., Luo, Y., Li, L., et al. (2019). Neural stem cell-derived small extracellular vesicles attenuate apoptosis and neuroinflammation after traumatic spinal cord injury by activating autophagy. Cell Death Dis. 10:340. doi: 10.1038/s41419-019-1571-8
Rooj, A. K., Mineo, M., and Godlewski, J. (2016). MicroRNA and extracellular vesicles in glioblastoma: small but powerful. Brain Tumor. Pathol. 33, 77–88. doi: 10.1007/s10014-016-0259-3
Rosas-Hernandez, H., Cuevas, E., Raymick, J. B., Robinson, B. L., Ali, S. F., Hanig, J., et al. (2019). Characterization of serum exosomes from a transgenic mouse model of Alzheimer’s Disease. Curr. Alzheimer Res. 16, 388–395. doi: 10.2174/1567205016666190321155422
Saeedi, S., Israel, S., Nagy, C., and Turecki, G. (2019). The emerging role of exosomes in mental disorders. Transl. Psychiatry 9:122. doi: 10.1038/s41398-019-0459-9
Salido-Guadarrama, I., Romero-Cordoba, S., Peralta-Zaragoza, O., Hidalgo-Miranda, A., and Rodriguez-Dorantes, M. (2014). MicroRNAs transported by exosomes in body fluids as mediators of intercellular communication in cancer. Onco. Targets Ther. 7, 1327–1338. doi: 10.2147/OTT.S61562
Samanta, S., Rajasingh, S., Drosos, N., Zhou, Z., Dawn, B., and Rajasingh, J. (2018). Exosomes: new molecular targets of diseases. Acta Pharmacol. Sin. 39, 501–513. doi: 10.1038/aps.2017.162
Santiago-Dieppa, D. R., Steinberg, J., Gonda, D., Cheung, V. J., Carter, B. S., and Chen, C. C. (2014). Extracellular vesicles as a platform for ‘liquid biopsy’ in glioblastoma patients. Expert. Rev. Mol. Diagn. 14, 819–825. doi: 10.1586/14737159.2014.943193
Schiera, G., Di Liegro, C. M., and Di Liegro, I. (2019). Cell-to-cell communication in learning and memory: from neuro- and glio-transmission to information exchange mediated by extracellular vesicles. Int. J. Mol. Sci. 21:266. doi: 10.3390/ijms21010266
Schindowski, K., Belarbi, K., and Buee, L. (2008). Neurotrophic factors in Alzheimer’s disease: role of axonal transport. Genes Brain Behav. 7(Suppl. 1), 43–56. doi: 10.1111/j.1601-183X.2007.00378.x
Schorey, J. S., and Bhatnagar, S. (2008). Exosome function: from tumor immunology to pathogen biology. Traffic 9, 871–881. doi: 10.1111/j.1600-0854.2008.00734.x
Sedgwick, A. E., and D’Souza-Schorey, C. (2018). The biology of extracellular microvesicles. Traffic 19, 319–327. doi: 10.1111/tra.12558
Shakespear, N., Ogura, M., Yamaki, J., and Homma, Y. (2020). Astrocyte-derived exosomal microRNA miR-200a-3p prevents MPP(+)-induced apoptotic cell death through down-regulation of MKK4. Neurochem. Res. 45, 1020–1033. doi: 10.1007/s11064-020-02977-5
Shao, H., Chung, J., Lee, K., Balaj, L., Min, C., Carter, B. S., et al. (2015). Chip-based analysis of exosomal mRNA mediating drug resistance in glioblastoma. Nat. Commun. 6:6999. doi: 10.1038/ncomms7999
Silverman, J. M., Christy, D., Shyu, C. C., Moon, K. M., Fernando, S., Gidden, Z., et al. (2019). CNS-derived extracellular vesicles from superoxide dismutase 1 (SOD1)(G93A) ALS mice originate from astrocytes and neurons and carry misfolded SOD1. J. Biol. Chem. 294, 3744–3759. doi: 10.1074/jbc.RA118.004825
Simon, T., Pinioti, S., Schellenberger, P., Rajeeve, V., Wendler, F., Cutillas, P. R., et al. (2018). Shedding of bevacizumab in tumour cells-derived extracellular vesicles as a new therapeutic escape mechanism in glioblastoma. Mol. Cancer 17:132. doi: 10.1186/s12943-018-0878-x
Simons, M., and Raposo, G. (2009). Exosomes–vesicular carriers for intercellular communication. Curr. Opin. Cell Biol. 21, 575–581. doi: 10.1016/j.ceb.2009.03.007
Skog, J., Wurdinger, T., van Rijn, S., Meijer, D. H., Gainche, L., Sena-Esteves, M., et al. (2008). Glioblastoma microvesicles transport RNA and proteins that promote tumour growth and provide diagnostic biomarkers. Nat. Cell Biol. 10, 1470–1476. doi: 10.1038/ncb1800
Sobue, A., Ito, N., Nagai, T., Shan, W., Hada, K., Nakajima, A., et al. (2018). Astroglial major histocompatibility complex class I following immune activation leads to behavioral and neuropathological changes. Glia 66, 1034–1052. doi: 10.1002/glia.23299
Sofroniew, M. V. (2015). Astrocyte barriers to neurotoxic inflammation. Nat. Rev. Neurosci. 16, 249–263. doi: 10.1038/nrn3898
Song, H., Zhang, X., Chen, R., Miao, J., Wang, L., Cui, L., et al. (2019). Cortical neuron-derived exosomal MicroRNA-181c-3p inhibits neuroinflammation by downregulating CXCL1 in astrocytes of a rat model with ischemic brain injury. Neuroimmunomodulation 26, 217–233. doi: 10.1159/000502694
Sonntag, K. C., Song, B., Lee, N., Jung, J. H., Cha, Y., Leblanc, P., et al. (2018). Pluripotent stem cell-based therapy for Parkinson’s disease: current status and future prospects. Prog. Neurobiol. 168, 1–20. doi: 10.1016/j.pneurobio.2018.04.005
Sorrentino, Z. A., Giasson, B. I., and Chakrabarty, P. (2019). alpha-Synuclein and astrocytes: tracing the pathways from homeostasis to neurodegeneration in Lewy body disease. Acta Neuropathol. 138, 1–21. doi: 10.1007/s00401-019-01977-2
Stefanis, L., Emmanouilidou, E., Pantazopoulou, M., Kirik, D., Vekrellis, K., and Tofaris, G. K. (2019). How is alpha-synuclein cleared from the cell? J. Neurochem. 150, 577–590. doi: 10.1111/jnc.14704
Stenovec, M., Milosevic, M., Petrusic, V., Potokar, M., Stevic, Z., Prebil, M., et al. (2011). Amyotrophic lateral sclerosis immunoglobulins G enhance the mobility of Lysotracker-labelled vesicles in cultured rat astrocytes. Acta Physiol. 203, 457–471. doi: 10.1111/j.1748-1716.2011.02337.x
Stronati, E., Conti, R., Cacci, E., Cardarelli, S., Biagioni, S., and Poiana, G. (2019). Extracellular vesicle-induced differentiation of neural stem progenitor cells. Int. J. Mol. Sci. 20:3691. doi: 10.3390/ijms20153691
Sugaya, K., and Vaidya, M. (2018). Stem cell therapies for Neurodegenerative Diseases. Adv. Exp. Med. Biol. 1056, 61–84. doi: 10.1007/978-3-319-74470-4_5
Sun, X., Jung, J. H., Arvola, O., Santoso, M. R., Giffard, R. G., Yang, P. C., et al. (2019). Stem cell-derived exosomes protect astrocyte cultures from in vitro ischemia and decrease injury as post-stroke intravenous therapy. Front. Cell Neurosci. 13:394. doi: 10.3389/fncel.2019.00394
Taylor, A. R., Robinson, M. B., Gifondorwa, D. J., Tytell, M., and Milligan, C. E. (2007). Regulation of heat shock protein 70 release in astrocytes: role of signaling kinases. Dev. Neurobiol. 67, 1815–1829. doi: 10.1002/dneu.20559
Thery, C., Witwer, K. W., Aikawa, E., Alcaraz, M. J., Anderson, J. D., Andriantsitohaina, R., et al. (2018). Minimal information for studies of extracellular vesicles 2018 (MISEV2018): a position statement of the International Society for Extracellular Vesicles and update of the MISEV2014 guidelines. J. Extracell Vesicles 7:1535750 doi: 10.1080/20013078.2018.1535750
Tran, P. B., and Miller, R. J. (2003). Chemokine receptors: signposts to brain development and disease. Nat. Rev. Neurosci. 4, 444–455. doi: 10.1038/nrn1116
Upadhya, R., Zingg, W., Shetty, S., and Shetty, A. K. (2020). Astrocyte-derived extracellular vesicles: neuroreparative properties and role in the pathogenesis of neurodegenerative disorders. J. Control Release 323, 225–239. doi: 10.1016/j.jconrel.2020.04.017
Valadi, H., Ekstrom, K., Bossios, A., Sjostrand, M., Lee, J. J., and Lotvall, J. O. (2007). Exosome-mediated transfer of mRNAs and microRNAs is a novel mechanism of genetic exchange between cells. Nat. Cell Biol. 9, 654–659. doi: 10.1038/ncb1596
Van der Auwera, P., Frooninckx, L., Buscemi, K., Vance, R. T., Watteyne, J., Mirabeau, O., et al. (2020). RPamide neuropeptides NLP-22 and NLP-2 act through GnRH-like receptors to promote sleep and wakefulness in C. elegans. Sci. Rep. 10:9929. doi: 10.1038/s41598-020-66536-2
Venturini, A., Passalacqua, M., Pelassa, S., Pastorino, F., Tedesco, M., Cortese, K., et al. (2019). Exosomes from astrocyte processes: signaling to neurons. Front. Pharmacol. 10:1452. doi: 10.3389/fphar.2019.01452
Verkhratsky, A., and Nedergaard, M. (2018). Physiology of astroglia. Physiol. Rev. 98, 239–389. doi: 10.1152/physrev.00042.2016
Vogel, A., Upadhya, R., and Shetty, A. K. (2018). Neural stem cell derived extracellular vesicles: attributes and prospects for treating neurodegenerative disorders. EBioMedicine 38, 273–282. doi: 10.1016/j.ebiom.2018.11.026
Walker, S., Busatto, S., Pham, A., Tian, M., Suh, A., Carson, K., et al. (2019). Extracellular vesicle-based drug delivery systems for cancer treatment. Theranostics 9, 8001–8017. doi: 10.7150/thno.37097
Wang, G., Dinkins, M., He, Q., Zhu, G., Poirier, C., Campbell, A., et al. (2012). Astrocytes secrete exosomes enriched with proapoptotic ceramide and prostate apoptosis response 4 (PAR-4): potential mechanism of apoptosis induction in Alzheimer disease (AD). J. Biol. Chem. 287, 21384–21395. doi: 10.1074/jbc.M112.340513
Wang, L., Pei, S., Han, L., Guo, B., Li, Y., Duan, R., et al. (2018). Mesenchymal stem cell-derived exosomes reduce A1 astrocytes via downregulation of phosphorylated NFkappaB P65 subunit in spinal cord injury. Cell Physiol. Biochem. 50, 1535–1559. doi: 10.1159/000494652
Wang, S., Cesca, F., Loers, G., Schweizer, M., Buck, F., Benfenati, F., et al. (2011). Synapsin I is an oligomannose-carrying glycoprotein, acts as an oligomannose-binding lectin, and promotes neurite outgrowth and neuronal survival when released via glia-derived exosomes. J. Neurosci. 31, 7275–7290. doi: 10.1523/JNEUROSCI.6476-10.2011
Webb, R. L., Kaiser, E. E., Scoville, S. L., Thompson, T. A., Fatima, S., Pandya, C., et al. (2018). Human neural stem cell extracellular vesicles improve tissue and functional recovery in the murine thromboembolic stroke model. Transl. Stroke Res. 9, 530–539. doi: 10.1007/s12975-017-0599-2
Wei, L., Wei, Z. Z., Jiang, M. Q., Mohamad, O., and Yu, S. P. (2017). Stem cell transplantation therapy for multifaceted therapeutic benefits after stroke. Prog. Neurobiol. 157, 49–78. doi: 10.1016/j.pneurobio.2017.03.003
Willis, C. M., Nicaise, A. M., Bongarzone, E. R., Givogri, M., Reiter, C. R., Heintz, O., et al. (2020a). Astrocyte support for oligodendrocyte differentiation can be conveyed via extracellular vesicles but diminishes with age. Sci. Rep. 10:828. doi: 10.1038/s41598-020-57663-x
Willis, C. M., Sutter, P., Rouillard, M., and Crocker, S. J. (2020b). The effects of IL-1beta on astrocytes are conveyed by extracellular vesicles and influenced by age. Neurochem. Res. 45, 694–707. doi: 10.1007/s11064-019-02937-8
Winston, C. N., Goetzl, E. J., Schwartz, J. B., Elahi, F. M., and Rissman, R. A. (2019). Complement protein levels in plasma astrocyte-derived exosomes are abnormal in conversion from mild cognitive impairment to Alzheimer’s disease dementia. Alzheimers Dement 11, 61–66. doi: 10.1016/j.dadm.2018.11.002
Wong, C. H., and Chen, Y. C. (2019). Clinical significance of exosomes as potential biomarkers in cancer. World J. Clin. Cases 7, 171–190. doi: 10.12998/wjcc.v7.i2.171
Wu, W., Liu, J., Yang, C., Xu, Z., Huang, J., and Lin, J. (2020). Astrocyte-derived exosome-transported microRNA-34c is neuroprotective against cerebral ischemia/reperfusion injury via TLR7 and the NF-kappaB/MAPK pathways. Brain Res. Bull. 163, 84–94. doi: 10.1016/j.brainresbull.2020.07.013
Xian, P., Hei, Y., Wang, R., Wang, T., Yang, J., Li, J., et al. (2019). Mesenchymal stem cell-derived exosomes as a nanotherapeutic agent for amelioration of inflammation-induced astrocyte alterations in mice. Theranostics 9, 5956–5975. doi: 10.7150/thno.33872
Xin, H., Li, Y., Buller, B., Katakowski, M., Zhang, Y., Wang, X., et al. (2012). Exosome-mediated transfer of miR-133b from multipotent mesenchymal stromal cells to neural cells contributes to neurite outgrowth. Stem. Cells 30, 1556–1564. doi: 10.1002/stem.1129
Xin, H., Li, Y., Liu, Z., Wang, X., Shang, X., Cui, Y., et al. (2013). MiR-133b promotes neural plasticity and functional recovery after treatment of stroke with multipotent mesenchymal stromal cells in rats via transfer of exosome-enriched extracellular particles. Stem. Cells 31, 2737–2746. doi: 10.1002/stem.1409
Xu, L., Cao, H., Xie, Y., Zhang, Y., Du, M., Xu, X., et al. (2019). Exosome-shuttled miR-92b-3p from ischemic preconditioned astrocytes protects neurons against oxygen and glucose deprivation. Brain Res. 1717, 66–73. doi: 10.1016/j.brainres.2019.04.009
Xu, R., Rai, A., Chen, M., Suwakulsiri, W., Greening, D. W., and Simpson, R. J. (2018). Extracellular vesicles in cancer - implications for future improvements in cancer care. Nat. Rev. Clin. Oncol. 15, 617–638. doi: 10.1038/s41571-018-0036-9
Yanez-Mo, M., Siljander, P. R., Andreu, Z., Zavec, A. B., Borras, F. E., Buzas, E. I., et al. (2015). Biological properties of extracellular vesicles and their physiological functions. J. Extracell Vesicles 4:27066. doi: 10.3402/jev.v4.27066
Yang, J. K., Song, J., Huo, H. R., Zhao, Y. L., Zhang, G. Y., Zhao, Z. M., et al. (2017). DNM3, p65 and p53 from exosomes represent potential clinical diagnosis markers for glioblastoma multiforme. Ther. Adv. Med. Oncol. 9, 741–754. doi: 10.1177/1758834017737471
Yang, S., and Li, X. (2018). Recent advances in extracellular vesicles enriched with non-coding RNAs related to cancers. Genes Dis. 5, 36–42. doi: 10.1016/j.gendis.2017.12.001
Yang, Y., Ye, Y., Su, X., He, J., Bai, W., and He, X. (2017). MSCs-derived exosomes and neuroinflammation, neurogenesis and therapy of traumatic brain injury. Front. Cell Neurosci. 11:55. doi: 10.3389/fncel.2017.00055
Yin, J., Zeng, A., Zhang, Z., Shi, Z., Yan, W., and You, Y. (2019). Exosomal transfer of miR-1238 contributes to temozolomide-resistance in glioblastoma. EBioMedicine 42, 238–251. doi: 10.1016/j.ebiom.2019.03.016
You, Y., Borgmann, K., Edara, V. V., Stacy, S., Ghorpade, A., and Ikezu, T. (2020). Activated human astrocyte-derived extracellular vesicles modulate neuronal uptake, differentiation and firing. J. Extracell Vesicles 9:1706801. doi: 10.1080/20013078.2019.1706801
Yu, T., Wang, X., Zhi, T., Zhang, J., Wang, Y., Nie, E., et al. (2018). Delivery of MGMT mRNA to glioma cells by reactive astrocyte-derived exosomes confers a temozolomide resistance phenotype. Cancer Lett. 433, 210–220. doi: 10.1016/j.canlet.2018.06.041
Yuyama, K., Sun, H., Mitsutake, S., and Igarashi, Y. (2012). Sphingolipid-modulated exosome secretion promotes clearance of amyloid-beta by microglia. J. Biol. Chem. 287, 10977–10989. doi: 10.1074/jbc.M111.324616
Zaborowski, M. P., Balaj, L., Breakefield, X. O., and Lai, C. P. (2015). Extracellular vesicles: composition, biological relevance, and methods of study. Bioscience 65, 783–797. doi: 10.1093/biosci/biv084
Zhang, G., Khan, A. A., Wu, H., Chen, L., Gu, Y., and Gu, N. (2018). The application of nanomaterials in stem cell therapy for some neurological diseases. Curr. Drug Targets 19, 279–298. doi: 10.2174/1389450118666170328115801
Zhang, L., Zhang, S., Yao, J., Lowery, F. J., Zhang, Q., Huang, W. C., et al. (2015). Microenvironment-induced PTEN loss by exosomal microRNA primes brain metastasis outgrowth. Nature 527, 100–104. doi: 10.1038/nature15376
Zhang, Y., Liu, Y., Liu, H., and Tang, W. H. (2019). Exosomes: biogenesis, biologic function and clinical potential. Cell Biosci. 9:19. doi: 10.1186/s13578-019-0282-2
Zhang, Z. G., Buller, B., and Chopp, M. (2019). Exosomes - beyond stem cells for restorative therapy in stroke and neurological injury. Nat. Rev. Neurol. 15, 193–203. doi: 10.1038/s41582-018-0126-4
Zhou, X., Xie, F., Wang, L., Zhang, L., Zhang, S., Fang, M., et al. (2020). The function and clinical application of extracellular vesicles in innate immune regulation. Cell Mol. Immunol. 17, 323–334. doi: 10.1038/s41423-020-0391-1
Zhu, Q., Ling, X., Yang, Y., Zhang, J., Li, Q., Niu, X., et al. (2019). Embryonic stem cells-derived exosomes endowed with targeting properties as chemotherapeutics delivery vehicles for glioblastoma therapy. Adv. Sci. 6:1801899. doi: 10.1002/advs.201801899
Keywords: astrocyte, brain, extracellular vesicle, exosome, stem cell
Citation: Gharbi T, Zhang Z and Yang G-Y (2020) The Function of Astrocyte Mediated Extracellular Vesicles in Central Nervous System Diseases. Front. Cell Dev. Biol. 8:568889. doi: 10.3389/fcell.2020.568889
Received: 02 June 2020; Accepted: 24 September 2020;
Published: 15 October 2020.
Edited by:
Konstantin Glebov, University of Plymouth, United KingdomReviewed by:
Ursula Wyneken, University of the Andes, ChileJunjie Yang, University of Alabama at Birmingham, United States
Fábio G. Teixeira, University of Minho, Portugal
Copyright © 2020 Gharbi, Zhang and Yang. This is an open-access article distributed under the terms of the Creative Commons Attribution License (CC BY). The use, distribution or reproduction in other forums is permitted, provided the original author(s) and the copyright owner(s) are credited and that the original publication in this journal is cited, in accordance with accepted academic practice. No use, distribution or reproduction is permitted which does not comply with these terms.
*Correspondence: Guo-Yuan Yang, Z3l5YW5nQHNqdHUuZWR1LmNu