- 1Inserm, U 1270, Paris, France
- 2Sorbonne University, UMR-S 1270, IFM, Paris, France
- 3Institut du Fer á Moulin, Paris, France
During the development of the cortex, newly generated neurons migrate long-distances in the expanding tissue to reach their final positions. Pyramidal neurons are produced from dorsal progenitors, e.g., radial glia (RGs) in the ventricular zone, and then migrate along RG processes basally toward the cortex. These neurons are hence dependent upon RG extensions to support their migration from apical to basal regions. Several studies have investigated how intracellular determinants are required for RG polarity and subsequent formation and maintenance of their processes. Fewer studies have identified the influence of the extracellular environment on this architecture. This review will focus on extracellular factors which influence RG morphology and pyramidal neuronal migration during normal development and their perturbations in pathology. During cortical development, RGs are present in different strategic positions: apical RGs (aRGs) have their cell bodies located in the ventricular zone with an apical process contacting the ventricle, while they also have a basal process extending radially to reach the pial surface of the cortex. This particular conformation allows aRGs to be exposed to long range and short range signaling cues, whereas basal RGs (bRGs, also known as outer RGs, oRGs) have their cell bodies located throughout the cortical wall, limiting their access to ventricular factors. Long range signals impacting aRGs include secreted molecules present in the embryonic cerebrospinal fluid (e.g., Neuregulin, EGF, FGF, Wnt, BMP). Secreted molecules also contribute to the extracellular matrix (fibronectin, laminin, reelin). Classical short range factors include cell to cell signaling, adhesion molecules and mechano-transduction mechanisms (e.g., TAG1, Notch, cadherins, mechanical tension). Changes in one or several of these components influencing the RG extracellular environment can disrupt the development or maintenance of RG architecture on which neuronal migration relies, leading to a range of cortical malformations. First, we will detail the known long range signaling cues impacting RG. Then, we will review how short range cell contacts are also important to instruct the RG framework. Understanding how RG processes are structured by their environment to maintain and support radial migration is a critical part of the investigation of neurodevelopmental disorders.
Introduction
The cerebral cortex is an intricate brain structure responsible for many precise functions such as thinking, decision making and long term memory, and is required for the final processing of sensory inputs and motor control. These functions rely on the way the neuronal network is precisely organized. The structure of the cortex is composed of different layers of neuronal subtypes (Taverna et al., 2014; De Juan Romero and Borrell, 2015). In the mouse, these layers are established during embryonic development in an inside-out manner via the successive migration of young neurons generated directly or indirectly from apical radial glial cells (aRGs) in the ventricular zone (VZ) to their final location in distinct superficial regions (Rakic, 1972; Kriegstein and Gotz, 2003). aRGs have a particular morphology as they grow processes that extend from the apical to the basal side of the cortex. In both rodent and primate, aRGs generate further basal intermediate neurogenic progenitors (IPs) residing in the subventricular zone (SVZ). In gyrencephalic species such as humans and other primates, neurons can also be generated from basal radial glia (bRGs), also called outer radial glia (oRG), which are distributed in an outer SVZ (Penisson et al., 2019; Matsumoto et al., 2020). bRGs can extend processes to the apical, the basal or both surfaces of the cortex (Betizeau et al., 2013). In all situations their structure provides a linear support for neuronal migration. Therefore, RGs are not only the source of neurons during embryonic cortical development but also the scaffold necessary for their proper distribution throughout the expanding cortex. The formation and maintenance of the RG scaffold is essential for the correct positioning of neurons and thus, the organization of the neuronal network.
Several cellular processes are important to consider for proper RG morphology. As they are dividing and self-renewing progenitors, RGs have been widely studied in the context of the mechanisms underlying their proliferative features (Taverna et al., 2014; Uzquiano et al., 2018). This will have an impact on the density of fibers available for supporting migration. RGs (e.g., expressing factors such as Pax6, Sox2, Hes5) can self-renew via symmetric divisions but can also carry out asymmetric divisions giving rise to different progeny including Tbr2 + IPs (Figure 1; Taverna et al., 2014; De Juan Romero and Borrell, 2015). RGs are also able to directly produce neurons. In particular, cell intrinsic mechanisms acting on mitotic spindle orientation or nucleokinesis via cytoskeletal or polarity proteins are tightly linked to daughter cell production and fate. At the structural level, how the radial processes critical to RG function are created, modulated or maintained relies on additional molecular mechanisms, which is the topic of this review.
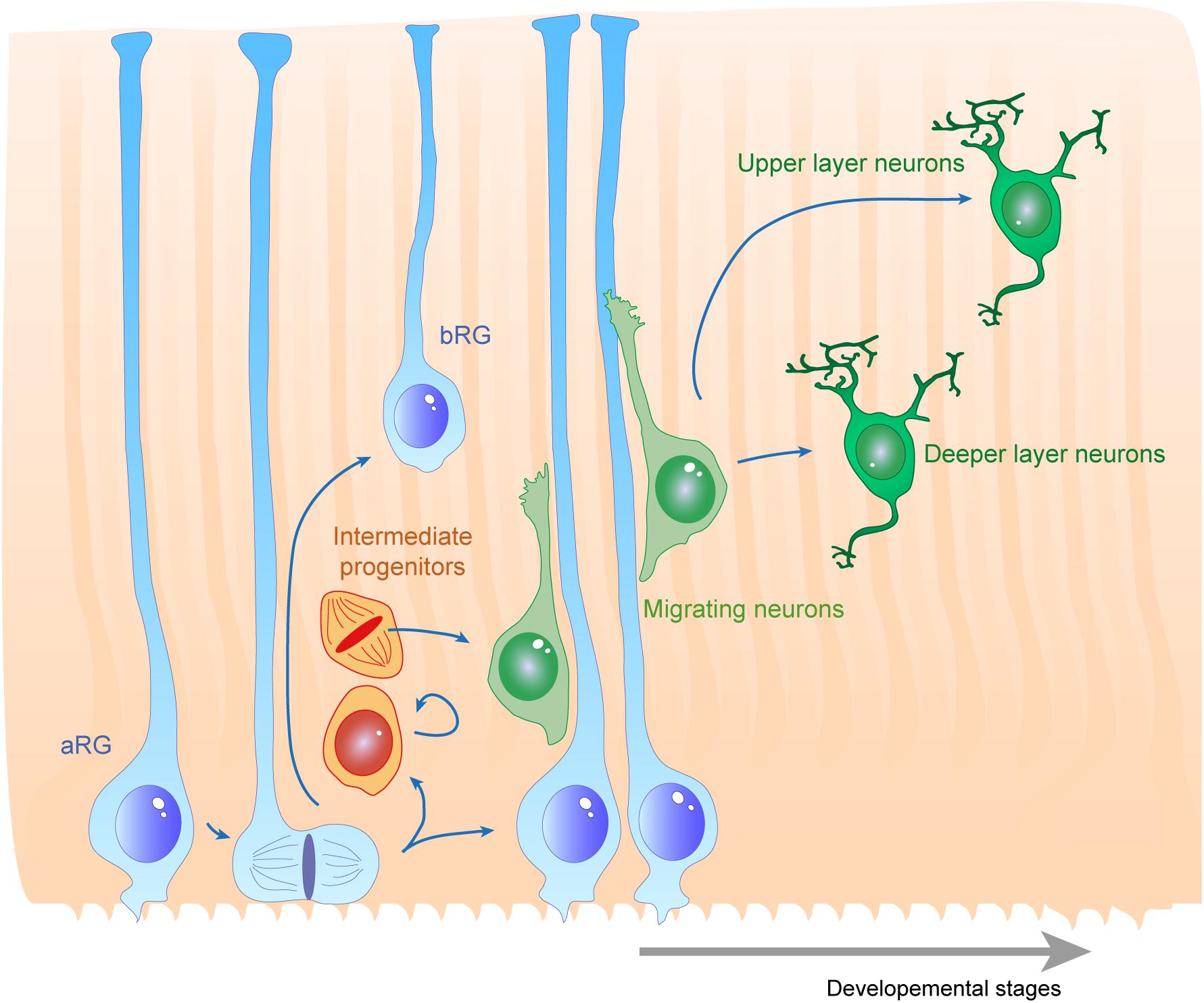
Figure 1. Radial glias function as both the source and the support of newborn neurons in the developing cortex. Apical radial glia (aRG) extend an apical process reaching the ventricular surface, where they expose their primary cilia, as well as a basal process reaching the cortical surface. Basal radial glia (bRG) have their cell bodies located in more basal areas of the cortical wall. Apical and basal processes from these cells (blue) establish the scaffold across the whole cortical wall. RGs undergo cell division, giving birth to a daughter cell which can be either another RG (apical or basal – symmetric division) or a basal progenitor (asymmetric division, intermediate progenitors are represented in orange). These cells give rise to migrating neuroblasts (green) which move along RG basal processes to reach their final position within the cortical layers. First deep layer neurons are generated, then upper layer neurons are born.
Since aRGs are structured in a very defined way, with their soma and apical processes at the border of the ventricle, they are exposed to many secreted factors from the embryonic cerebrospinal fluid (eCSF). In particular, the primary cilium extends inside the ventricle and this is a crucial signaling center for the activation of numerous molecular cascades (Sarkisian and Guadiana, 2015). At the level of their cell bodies, aRGs and bRGs are both exposed to cell−cell and cell−environment interactions. They interact with each other as well as with additional cell types such as IPs or neurons. Importantly they interact with the surrounding extracellular matrix (ECM). For example, human bRGs have been shown to produce specific proteins which interact with the ECM in their basal position (Pollen et al., 2015). Finally, their basal processes are also exposed to external signals throughout the intermediate zone (IZ), cortical plate (CP), marginal zone (MZ), and at the pial surface (Figure 2). First, we will review the different secreted molecules involved in the establishment or maintenance of RG morphology from the eCSF or in the ECM. Then, we will describe how short range interactions between cells are essential for these processes. Finally, we will detail the impact of relevant molecular players on the origin and evolution of several human neurodevelopmental diseases.
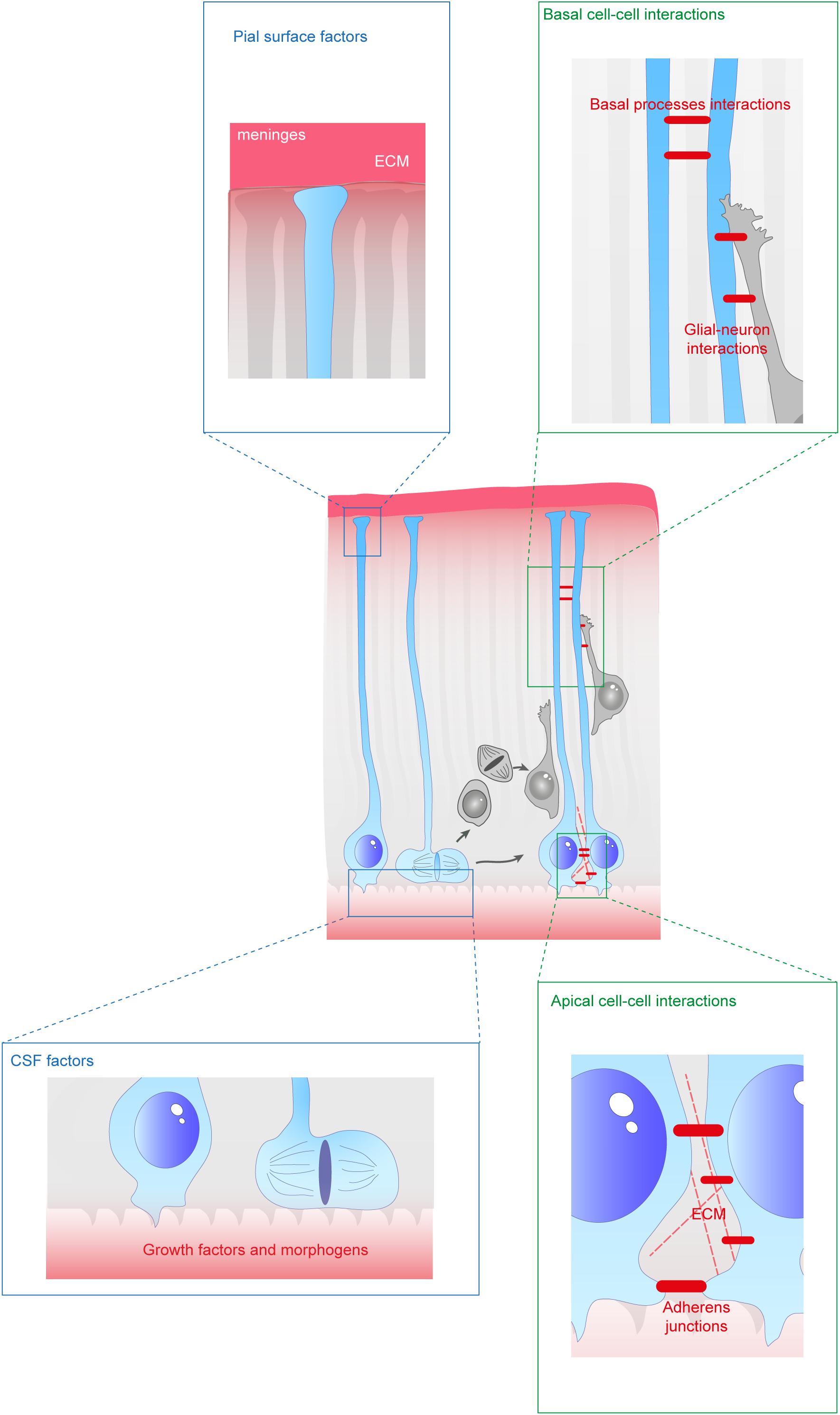
Figure 2. Extracellular factors controling the scaffolding of RGs. RGs are exposed to a variety of extracellular cues. These signals can be secreted molecules (blue boxes) or received directly from other cells (green boxes). In apical regions aRGs receive signals from the eCSF as their cell bodies and primary cilia are in contact with the ventricles. They also establish contacts between themselves and with the extracellular matrix (ECM). In basal regions, RG basal processes are exposed to secreted cues from the meninges and from already differentiated neurons. These interactions can occur while neurons are migrating along them. Basal processes also exhibit interactions between themselves.
Role of Secreted Proteins Derived From the CSF in the Formation and Maintenance of the RG Scaffold
Secreted Factors From the Embryonic Cerebrospinal Fluid (eCSF)
The cortex develops primarily from the neuroepithelium during embryonic development. Between E8.5 and E9.5 in mice, the neural tube closes, forming the ventricular cavity in which the amniotic fluid is sequestered and forms the basis for the eCSF (Lowery and Sive, 2009). Later during mouse brain development, the choroid plexus arises and secretes many factors, modifying the composition of the ventricular fluid (Chau et al., 2015). The deepest apical region of the developing brain is composed of neuroepithelial-derived aRG progenitors from E10.5. These aRGs are exposed to a variety of secreted factors from the ventricle during development. Proteomics analyses of the CSF indicates that the precise composition of secreted molecules varies during development. For instance, the concentration of Bone Morphogenic Proteins (BMPs) is higher in the amniotic fluid than in the eCSF, Sonic Hedgehog (Shh) concentration is higher in the eCSF at the beginning of aRG development (E10.5) and decreases thereafter, whereas the concentration of retinoic acid (RA) is higher at later stages (E14.5) (Chau et al., 2015). These variations in composition are required to induce the production of RGs (Sox2+) at the right time during the formation of the cortex (Chau et al., 2015). These data also suggest that certain secreted proteins or combinations of proteins in the eCSF during murine corticogenesis are required for evolving aspects of RG production and maintenance.
The composition of secreted factors in the eCSF not only changes with developmental stages but is also specific to different ventricles. Indeed, the different choroid plexus tissues present in each ventricle develop in a sequential manner (Lehtinen et al., 2011). Firstly, the choroid plexus from the fourth ventricle appears (E11 in the mouse), then the choroid plexus develops in the lateral ventricles (E12) and lastly, it develops in the third ventricle by E14. Each type of choroid plexus will express a different panel of secreted factors. For example, Shh is mainly produced in the fourth ventricle by the choroid plexus close to the hindbrain (Huang et al., 2010), whereas many other proteins are found only in the lateral ventricles (Zappaterra et al., 2007). More recently, proteomics data were integrated with RNA sequencing datasets, comparing telencephalic and hindbrain choroid plexuses (Lun et al., 2015). This spatial heterogeneity of their secretomes argue in favor of a precise and specific regulation of different brain areas. Overall, the eCSF plays multiple important roles in the formation of the nervous system (for review, Fame and Lehtinen, 2020). In this part of our review, we will describe the functional role of the main secreted factors present in the eCSF for the maintenance of RGs and therefore the formation of the RG scaffold.
Growth Factors
As mentioned in the previous section, several cytokines are found in the eCSF. Different types of molecules can be found within this family such as growth factors like Transforming Growth Factors (TGF, developed later on in this review). But not all of these cytokines have a direct effect on radial scaffolding. For example, chemokines are best known for their action on neurons (Zhu and Murakami, 2012). On the other hand growth factors are diffusible cytokines widely known to activate RG proliferation and/or sustain cell survival. Therefore, we first provide a non-exhaustive list of eCSF-derived growth factors (Figures 3, 4 and Table 1) necessary for cortical development and in particular for the integrity of the RG scaffold.
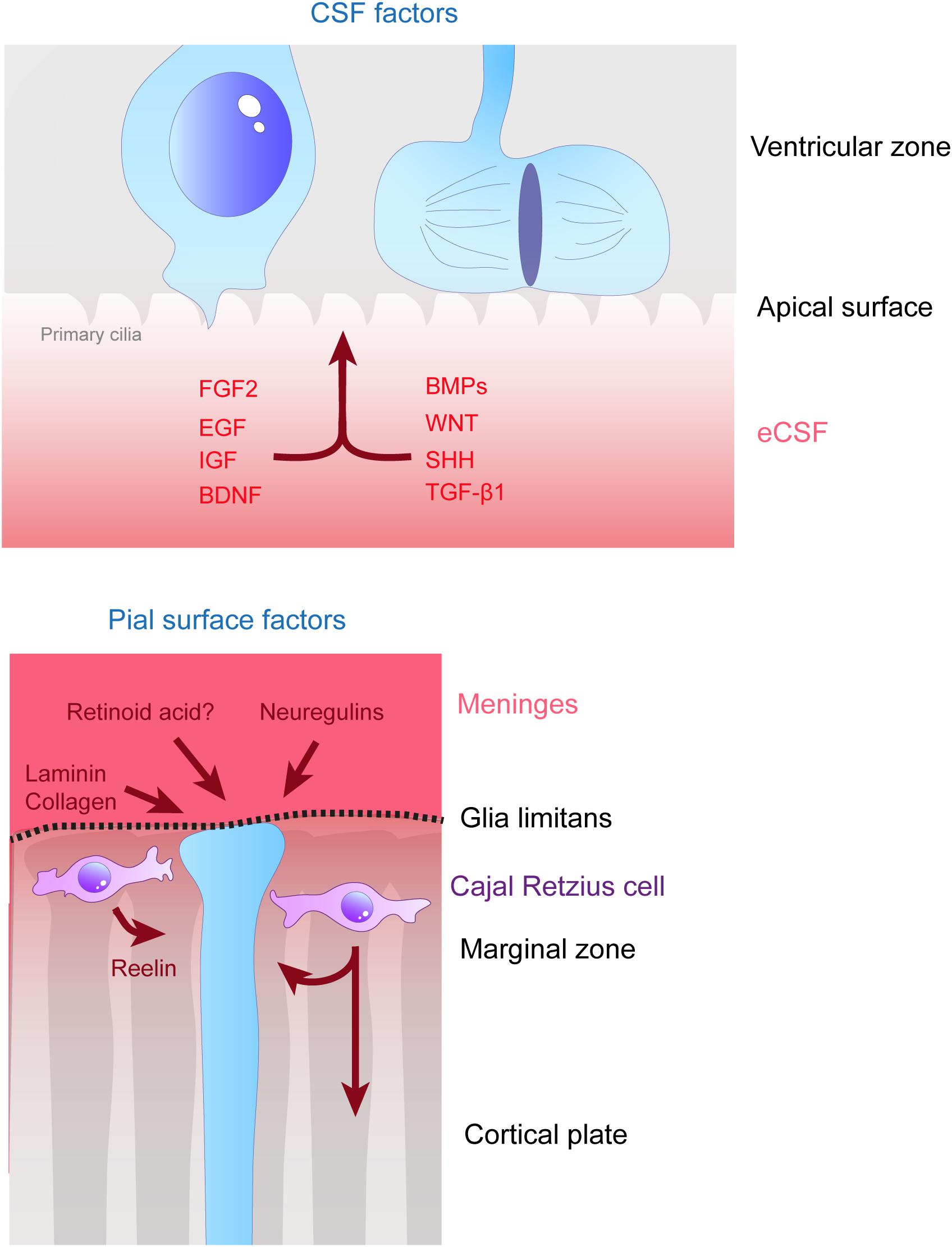
Figure 3. Remote extracellular factors controling the scaffolding of RGs. Some of the extracellular cues controlling RG development are produced and secreted from relatively remote locations. Here are represented the factors present in the CSF (upper schema) which are detailed in this review, namely FGF2, EGF, IGF, BDNF, BMPs, WNT, SHH, and TGF- β1. On the bottom schema, extracellular cues derived from the meninges and acting on the extremities of basal processes are depicted, namely, laminin, collagen, neuregulins and retinoid acid. Cajal Retzius cells (in purple) are migrating cells which in early stages of development tangentially move in the MZ of the developing cortex. These cells are a source of Reelin amongst other molecules which influence RG scaffolding.
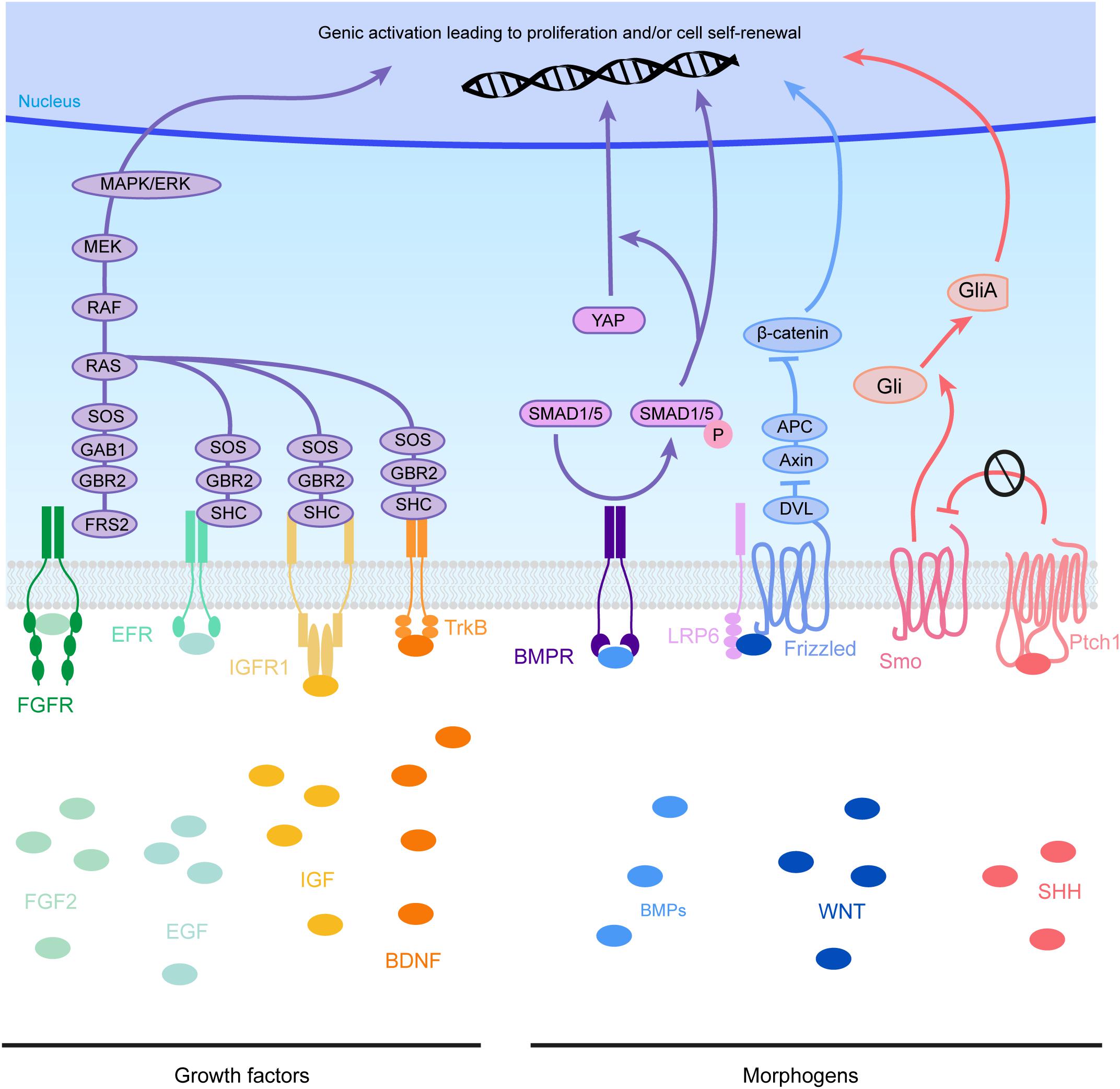
Figure 4. Molecular pathways triggered by eCSF-derived factors. The growth factors found in the eCSF are mainly known to trigger the mitogen-activated protein kinases (MAPK) pathway (also known as the RAS-RAF-MEK-ERK pathway). This molecular signaling pathway is involved in the regulation of several essential cellular processes such as proliferation, differentiation, survival and death. BMP receptors (BMPR) activate the phosphorylation of SMAD1/5, which can activate directly transcription of target genes or act via the translocation of YAP into the nucleus. WNT molecules activate the Frizzled receptors and LRP6 co-receptors which will allow Disheveled (DVL) to inhibit the Axin-APC complex. This complex is a major inhibitor of β-catenin. Therefore, upon WNT activation, β-catenin is free to be directed into the nucleus to activate its target genes. Finally SHH binds to its receptor Patched1 (Ptch1), which then releases the 7 transmembrane protein Smoothened (Smo) from its inhibition. Smo activation triggers the cleavage of Gli transcription factors into their active form (GliA). GliA is then enriched in the nucleus to allow transcription of target genes (such as Cyclin D1 or Gli itself).
Multiple Fibroblast growth factor (FGF) ligands are expressed in the developing telencephalon. At early stages (E10–E12), FGFs 8, 17, and 18 are expressed in the frontal midline area where they act as morphogens (see section “Morphogens” below). In the ventral telencephalon, FGF15 is expressed (Rash and Grove, 2006; Cholfin and Rubenstein, 2007; Hebert and Fishell, 2008), whereas in dorsal regions, FGFs 2, 9, and 10 are expressed (Vaccarino et al., 1999; Raballo et al., 2000; Sahara and O’Leary, 2009). Here, we focus on FGF2, which increases the total number of neurons in the mouse cerebral cortex and promotes self-renewal of cortical progenitor cells (Vaccarino et al., 1999; Raballo et al., 2000).
Fibroblast growth factor 2 is one of the most important growth factors for the production and maintenance of RGs during cortical development. Initially, FGF2 proteins were found present in the VZ of the murine developing cortex by immunohistochemistry (Dono et al., 1998). The source of the protein was not clearly defined but later the protein was detected in chicken eCSF (HH25) by western blot experiments (Martin and Groves, 2006), suggesting that it might be produced remotely and captured at the ventricular surface. In this study, authors show that the actual origin for FGF2 production in the chick embryo is the notochord, the mesonephros, the hepatic primordia and the brain neuroectoderm. The receptor for FGF2 (FGF2R) is expressed in the mouse VZ (E14.5) as shown by in situ hybridization (Dono et al., 1998). More recently single cell RNA-seq data shows that the Fgf2r gene is expressed in mouse RGs (Telley et al., 2019), suggesting that these cells can receive the FGF2 signal from the eCSF. The cortex of Fgf2 mutant mice is thinner and there is abnormal distribution of neurons in the cortical wall. Indeed, pulse chase analyses indicate an increase of neurons generated at E14.5 in the deep layers of the cortex (Dono et al., 1998). This suggests a defect in the ability of these cells to colonize their final target place in more superficial layers. Defects in proliferation were also identified in Fgf2 KO embryonic cortices in a separate study explaining the decrease in the size of the cortex (Raballo et al., 2000). This is in agreement with the fact that FGF2 is one of the major factors necessary for the renewal of RGs in vitro (Sun et al., 2011). The knockout (KO) of Fgfr genes in the anterior neural plate using Foxg1-Cre, inhibits the formation of the telencephalon, leaving just the midline (Paek et al., 2009). When Fgfrs are removed only from RG, their development is impaired resulting in lower numbers of Pax6 and Hes5 + cells (Kang et al., 2009). These combined data show how crucial FGF2 is for the maintenance of RGs and therefore, the formation of the cortex. Moreover, gain of function experiments performed by in utero injection of FGF2 first in the telencephalic ventricles of rat embryos at E15 (Vaccarino et al., 1999), then in mouse embryos at E11.5 (Rash et al., 2013), induces an increase in proliferation. When FGF2 signaling is overactivated locally by these manipulations, this induces the formation of gyri in the mouse cortex (Rash et al., 2013). Although gyrification can be associated with the appearance of bRGs during evolution (Penisson et al., 2019), in this case FGF2 injections did not appear to increase the proportion of bRGs in the cortical wall. This suggests that FGF2 modified the development of the architecture of the cortex via other unknown mechanisms. At the molecular level, FGF2 triggers the mitogen-activated protein kinase (MAPK) pathway to induce cell cycle and proliferation (for review, Iwata and Hevner, 2009, Figure 4). FGF2, as well as Notch signaling, can also induce calcium (Ca2+) bursting which can support communication along the RG fiber (Rash et al., 2016). Indeed, both along the RG fiber and the communication with neurons can be mediated by calcium waves, in a bidirectional manner.
Fibroblast growth factor 2 can be used in culture in combination with Epidermal growth factor (EGF). Indeed, the action of EGF on cortical progenitors has been studied for many years (Burrows et al., 1997; Lillien and Raphael, 2000). Recently, FGF2 and EGF were shown to regulate self-renewal of rat cortical progenitors in organotypic cultures in vitro (Lamus et al., 2020). These two growth factors can activate the same molecular pathways to initiate proliferation. The action of FGF2 and EGF is not a simple synergy since FGF2 can modulate the responsiveness of RGs to EGF (Lillien and Raphael, 2000). RGs are first responsive to FGF2 alone and later during cortical development, start to be also responsive to EGF (Ciccolini and Svendsen, 2001). Moreover, the effect of EGF on the proliferation of cortical progenitors is dose-dependent (Nelson et al., 2008). The combined action of EGF and FGF2 is therefore essential for the development of RGs and their maintenance during the development of the cortex. At the beginning of mouse cortical expansion (e.g., E13), the EGF receptor (EGFR) is present in the VZ and SVZ (Sun et al., 2005). At the cellular level, EGFR has been found to be localized asymmetrically in dividing RGs, controlling the fate of daughter cells. The cell inheriting EGFR is the daughter cell retaining proliferative capacity and glial markers (Sun et al., 2005). This indicates that RGs need to keep their ability to respond to EGF in order to maintain their progenitor identity. EGF signaling is therefore required for the maintenance of the RG scaffold during cortical development. Certain studies have investigated the mechanisms controlling the expression of EGFR. First the ganglioside GD3 was identified as an EGFR partner, responsible for its sustained expression in cortical progenitors in vitro (Wang and Yu, 2013). More recently, the expression of miR-129-5p, modulated by choline availability in the microenvironment, was shown to inhibit the expression of EGFR, thus impacting RG maintenance and cortical development (Trujillo-Gonzalez et al., 2019). All these data underline the essential role for EGF in the maintenance of RGs necessary for cortical development.
The Insulin-like Growth factors (IGF1 and IGF2) are a group of hormones which are present in the eCSF (Salehi et al., 2009; Zappaterra and Lehtinen, 2012; Bueno et al., 2020). IGF2 concentration in rat eCSF increases from E16 to E19 (Lehtinen et al., 2011). Gain of function experiments such as IGF1 overexpression in mouse embryo showed that this hormone induces a shortening of the cell cycle, acting in particular on S-phase. This increase in the speed of proliferation is linked to cortical hyperplasia, which is an increase in global cortical size via an increase in cell number (Hodge et al., 2004; Popken et al., 2004; Mairet-Coello et al., 2009). Both in vivo and in vitro data show that IGF2 can induce cortical growth by stimulating RG proliferation (Lehtinen et al., 2011). The reverse result is observed in Igf2 KO mice which present a neurogenesis decrease affecting the production of neurons destined for the upper cortical layers. At the apical membrane level, CSF-derived IGF2 binds to primary cilia of RGs, where IGF receptors are localized. IGF1 receptor (IGF1R) is the main receptor allowing IGFs to trigger proliferation (Zappaterra and Lehtinen, 2012). Like FGF2 and EGF, IGF can trigger the MAPK pathway but can also activate a non-canonical pathway via Gβγ signaling, which regulates the timing of the cell cycle (Yeh et al., 2013). All of these data explain why the Igf1r conditional knockout (cKO) in neural precursors leads to microcephaly (Lehtinen et al., 2011).
Brain Derived Neurotrophic Factor (BDNF) has the particularity of being expressed directly by RGs and also by Cajal-Retzius cells (Fukumitsu et al., 1998). The role of BDNF on RGs has been investigated by both injection of BDNF itself directly in ventricles at mouse E13.5 (Fukumitsu et al., 2006) and also by overexpression of Bdnf in cortical precursors in vivo via in utero electroporation (Bartkowska et al., 2007). Both strategies led to an increase in proliferation. BDNF is one of the ligands which can activate the tropomyosin receptor kinase B (TrkB) receptor. The loss of function of its gene (Ntrk2) in the mouse was achieved by different approaches such as by short hairpin RNAs (shRNAs) or by expression of a dominant-negative variant of TrkB. Blockade of TrkB signaling elicited a decrease in RG self-renewal (Bartkowska et al., 2007). TrkB receptors can be phosphorylated, which will activate the MAPK or phosphoinositide 3-kinase (PI3K) pathways (Numakawa et al., 2018). Both pathways are implicated in different functions of RG behavior during cortical development. PI3K is important for RG survival whereas MAPK activation is required for the production of neurons (Barnabe-Heider and Miller, 2003). Therefore, the pathway linking BDNF, TrkB and PI3K is essential for the maintenance of the RG scaffold, indeed activation of the BDNF-TrkB-MAPK axis can lead to premature RG differentiation into neurons via the activation of BMP7 (Ortega and Alcantara, 2010 and see below). BDNF can also activate Anoctamin 1 (ANO1), a Ca2+-activated chloride channel which is expressed in RGs (Hong et al., 2019). The growth of RG basal processes is dependent on the activity of this channel as its loss of function disrupts the extension of RG protrusions. ANO1 overexpression inversely increases this process (Hong et al., 2019). The lack of basal process growth in Ano1-deficient mice leads to disorganized cortical layers and microcephaly (Hong et al., 2019).
Transforming growth factor β 1 (TGF-β1) is a cytokine which is involved at many levels of neuronal development (Meyers and Kessler, 2017). TGF-β1 is present in the VZ of the developing cortex (Mecha et al., 2008) and its receptor, TGFRII, is highly expressed by RGs (Stipursky et al., 2014). Although its role is mainly associated with the differentiation of RGs into either neurons or glia (Stipursky et al., 2012, 2014), injection of TGF-β1 directly into the embryonic ventricles at E14 induces drastic changes in RG scaffold morphology. Basal processes seem shorter and disorganized. In fact, TGF-β1 triggers early transition of RGs into astrocytes which alters their morphology from radial to multipolar. Interestingly, these effects are similar to the action of a morphogen, as described in the next paragraph, more than a growth factor.
Thus, different growth factors play apparently critical roles influencing the formation and maintenance of RG scaffolds.
Morphogens
Contrary to growth factors which are known classically to act at the proliferation level, morphogens are instead also associated with an action at the differentiation level and to control cell fate decisions (Briscoe and Small, 2015). We review here the known roles of morphogens in the maintenance of RGs and therefore the RG scaffold (Figures 3, 4 and Table 1).
Certain members of the TGF family, e.g., the bone morphogenic proteins (BMP), have important roles in the maintenance of RG scaffolding. In vitro experiments on cultures of RGs indicated that BMP signaling is involved in the control of neurogenesis (Li et al., 1998; Mabie et al., 1999). Bmp7 has been detected in the meninges, hem and also in the eCSF (Segklia et al., 2012). When Bmp7 is removed from the mouse brain, this leads to reduced cortical thickness and number of neurons at E14.5. On the other hand, when Bmp signaling is activated by expressing a constitutively active form of its receptors (Bmpr1a or Bmpr1b), over proliferation and defects in global morphology are observed in the developing cortex (Panchision et al., 2001). In particular, folds can be seen at the brain surface, suggesting differences in RG scaffolding. More recently, the implication of Smad1/5 (canonical BMP transcription factors) was revealed by loss of function experiments in both mouse and chick (Najas et al., 2020). In these models, RG maintenance was disrupted, and premature differentiation occurred, which leads to a microcephaly phenotype. The consequences of KO were assessed on neurogenesis but not the RG scaffold per se. However, SMADs are likely to regulate neurogenesis by modulating YAP (Yes-associated protein) activity (Najas et al., 2020), since decreasing SMAD1/5 leads to a decrease in YAP translocation into RG nuclei. This is crucial for cortical development as Hippo signaling has been linked to apical RG surface integrity and adhesion (Roy et al., 2019). Moreover, KO of YAP and TAZ, a transcriptional coactivator with PDZ-binding motif, can rescue genetically driven (via a Pard3 deletion in the mouse) cortical heterotopia associated with detached RGs and higher YAP levels (Liu et al., 2018, see also section “Further Factors Identified via Human Pathology” Human pathology). Overall, these data indicate an important role of BMPs via their activation of SMADs in the control of RG behavior during cortical development.
The Wnt morphogen is implicated at many levels of neural system development and in particular in the cortex (Harrison-Uy and Pleasure, 2012). Wnt proteins are present and active in the eCSF where they are transported by lipoprotein particles (Johansson et al., 2013; Kaiser et al., 2019). Many different studies point to the role of Wnt as an essential factor in maintaining RG identity and self-renewal. A scaffolding disruption phenotype as well as proliferative defects are described in the developing hippocampus in the Lrp6 gene mouse KO, one of the most important Wnt co-receptors (Pinson et al., 2000; Zhou et al., 2004; Wang Y. et al., 2016). Concerning the intracellular signaling triggered by Wnt, the canonical pathway relying on β-catenin inhibits neurogenesis by keeping RG undifferentiated (Woodhead et al., 2006; Wrobel et al., 2007; Mutch et al., 2010; Munji et al., 2011). β-catenin can be involved in different cellular processes such as cell-cell adhesion in addition to its transcriptional role. In one study, the authors specifically abrogated β-catenin’s transcriptional role by expression of a truncated form of this molecule in the telencephalon (Draganova et al., 2015). This study showed that Wnt/β-catenin signaling regulates a network of transcription factors involved in specific stages of cortical development including Dach1, Eya2, Etv5, and also Nfix (Draganova et al., 2015). In the Wnt/β-catenin pathway, Adenomatous polyposis coli (APC) is a regulator of β-catenin driving its degradation in the absence of Wnt binding at the membrane (Nelson et al., 2015). In an APC conditional KO in mouse RGs, the scaffold of basal processes is disturbed (Nakagawa et al., 2017). It is also interesting to note that Wnt signaling has been implicated in the maintenance of basal progenitors via the regulation of N-myc (Kuwahara et al., 2010). Therefore, since several Wnt molecules are expressed at different levels of the developing cortex (i.e., Wnt7a at the apical surface and Wnt7b in the basal parenchyma), it is possible that this morphogen can regulate the RG scaffold throughout the cortex and even in superficial regions.
The presence of the Sonic Hedgehog morphogen (Shh) ligand in the developing cortex has been known for several years (Komada et al., 2008). Shh is a well-known morphogen which can control a lot of different aspects of neurodevelopment at different locations of the nervous system (for review see Ferent and Traiffort, 2015). Shh is present in the eCSF, providing a source for the VZ, as identified by the ELISA method (Huang et al., 2010; Chau et al., 2015; Lun et al., 2015). Shh production occurs in cells of the choroid plexus of the fourth ventricle of the hindbrain (Huang et al., 2010) but not from the choroid plexus from the telencephalon (Lun et al., 2015). This would suggest that ventricular derived-Shh derived from the hChP would have to travel long distances to reach the ventricular wall of the developing cortex. Very recently, Shh secretion in the eCSF was linked to the ESCRT-III system (Endosomal sorting complex required for transport). Indeed, the Chmp1a (a gene coding for the charged multivesicular body protein 1a, a subunit of the ESCRT complex) null mice present a decrease in the amount of Shh in the eCSF, correlated with a reduction in RG proliferation and the development of microcephaly (Coulter et al., 2018). This phenotype can be rescued when Shh signaling is genetically activated, showing that the ESCRT system is indeed upstream of Shh secretion. Migrating interneurons and Cajal-Retzius cells also produce Shh locally within the cortex (Dahmane et al., 2001; Flandin et al., 2011).
Several studies focused on the role of receptors or downstream signaling components of the Shh pathway during cortical development. Loss of function of the Smoothened Shh signaling activator in RG using GFAP-Cre or Nestin-Cre mice showed a decrease in proliferation, whereas activating the pathway via Patched1 receptor KO showed an increase (Dave et al., 2011; Wang L. et al., 2016). Overexpression of a constitutive form of Smo (SmoM2) increases the proportion of bRG in the developing cortex, suggesting a potential role for Shh signaling in the formation of bRGs (Wang L. et al., 2016). The role of the Patched1 co-receptor Cdon in cortical development has been highlighted by a loss-of-function study showing that deletion of Cdon leads to cortical microcephaly and reduction in RG proliferation (Zhang et al., 2006). At the molecular level, Shh controls the activity of Gli transcription factors to favor Gli2 activating forms over Gli3 repressor forms (Figure 4). Therefore, Gli2 mutant mice present a decrease in RG proliferation (Palma et al., 2004) whereas Gli3 repressor form invalidation leads to an increase in cell cycle speed (Wilson et al., 2012). Suppressor of Fused (Sufu) is an important inhibitor of Shh signaling activity. Some ectopic progenitor clusters are detected in the cortical wall, showing over proliferation, when Sufu is conditionally knocked-out in the murine cortex (using Emx1-Cre) (Yabut et al., 2015). Ultimately, this leads to major defects such as a thinner cortex and strong differentiation disruption. Very recently, Yabut et al. showed that Sufu regulation of the Shh pathway controls the expression of Fgf15 which is responsible for lineage progression of RGs (Yabut et al., 2020). This is a good example of how different extracellular cues can influence one another to modulate RG behavior.
Thus morphogens can have multiple effects but they are notable in their impact on RG structure, maintenance sand behavior.
Secreted Factors From Close Range Cells
The eCSF is not the only source of secreted factors controlling the RG scaffold. Extracellular cues can be also sent from neighboring cells throughout the tissue. For example, the formation and maintenance of the basal process is dynamic (Yokota et al., 2010), with important information received from the meninges (Radakovits et al., 2009; Siegenthaler et al., 2009). This basal communication is not well known, including the mechanisms by which the meninges provide information to basal processes for their maintenance. Here, we provide examples of proteins involved in RG scaffold maintenance in response to extracellular cues produced locally within the developing cortex or from the meninges (Figure 3).
Neuregulins (NRG) play a major role in neuronal migration and RG integrity (Anton et al., 1997; Lopez-Bendito et al., 2006). In particular, mouse KO of Nrg-1 leads to reduced cell numbers in primary cultures of embryonic progenitors (Schmid et al., 2003). NRG activates the v-Erb-a erythroblastic leukemia viral oncogene homolog (ErbB) family of tyrosine kinase receptors. ErbB2, 3, and 4 are expressed by RGs and are present along their basal processes (Schmid et al., 2003). Importantly, ErbB2 expression is specific to RGs and its loss of function in the mouse unbalances the astrocyte/RG population ratio by reducing the number of elongated RGs in the developing cortex (Schmid et al., 2003). ErbB2 interacts specifically with a redox active protein, Memo1 (Newkirk et al., 2018). Although Memo1 has been known for some time to be important for cell migration (Marone et al., 2004), its role in the branching and the maintenance of the RG scaffold was identified relatively recently (Nakagawa et al., 2019). A link has also been established between Nrg signaling and mGluR5 receptors. Indeed mGluR5 is coupled to the non-selective cation channel, canonical transient receptor potential 3 (Trpc3) (Louhivuori et al., 2015) and its loss of function in the mouse disrupts the formation of RG processes. This RG growth defect mediated by the mGluR5/Trpc3 signaling blockade can be rescued by Nrg/ErbB4 signaling showing that Nrg/ErbB4 is downstream of mGluR5/Trpc3 (Louhivuori et al., 2018).
Retinoic acid (RA) is a very well-known neurogenesis modulator. The particularity of this factor is that it is produced by different sources which could each impact cortical development. Although RA is secreted in the eCSF as described in chick (Alonso et al., 2011) and in zebrafish (Chang et al., 2016), its role on RG behavior has mainly been attributed to the meninges source (Siegenthaler et al., 2009). Indeed, when meninges are disrupted, limiting the supply of RA, or when a hypomorphic allele for the RA synthesizing enzyme Rdh10 is generated in the mouse, production of IPs is decreased (Siegenthaler et al., 2009). Nevertheless, this phenotype was not observed in Rdh10 -/- mouse embryos (Chatzi et al., 2013; Haushalter et al., 2017), nor in conditional KO embryos for the other enzyme responsible for RA synthesis, Raldh2 (Haushalter et al., 2017). Therefore, it seems that although meninges-derived RA is important, its role with respect to RGs stills needs clarifying. The role of eCSF RA has also not yet been clearly identified.
Cajal-Retzius cells, present in basal regions in the MZ of the developing cortex, secrete, amongst other factors, Reelin, a glycoprotein which interacts extracellularly with receptors on migrating neurons (Sekine et al., 2014, see also section “Further Factors Identified via Human Pathology” Human pathology). When RG basal processes reach the MZ, they branch, however this branching is impaired in the reeler mutant mouse (deficient for Reelin, Chai et al., 2015). This indicates that besides its classical role influencing migrating neurons, Reelin may also control some aspects of RG morphology and influence the scaffolding (see also Hartfuss et al., 2003; Schaefer et al., 2008). Also, Reelin was linked to maintaining hippocampal RG integrity, since reeler tissue also showed precocious conversion of RGs to astrocytes, rescued by exogenous sources of Reelin (Zhao et al., 2004). Amongst the signals secreted by the meninges, CXCL12 (chemokine (C-X-C motif) ligand 12) also called SDF1 (stromal cell-derived factor 1) can directly act on Cajal-Retzius cells and therefore indirectly modify the formation of RG scaffolding (Borrell and Marin, 2006). Briefly, CXCL12 controls tangential migration of Cajal-Retzius cells and disruption of its receptor CXCR4 leads to their displacement in deeper layers of the cortex, resulting in a dysplastic cortex (Paredes et al., 2006). Similarly in the hippocampus, CXCR4 invalidation also leads to severe phenotypes, including dentate gyrus granule neuron migration defects but also reduced proliferation of RG-like progenitors (Lu et al., 2002; Berger et al., 2007).
Bidirectional interactions between migrating neurons and RGs are essential for RG fiber growth. The glial growth factor (GGF), a soluble form of neuregulin, is expressed by migrating neurons along RG fibers and influences positively the growth of the RG fiber. In a pioneering study, Anton et al. (1997) provided evidence suggesting that the effect of GGF signaling on fiber elongation via ErbB2 is mediated through BLBP (brain lipid binding protein), an RG-expressed molecule (see also Hartfuss et al., 2003; Poluch and Juliano, 2010). It is thought that the rate of migratory neurons influences the lengthening of RG fiber, which also influences the rate of migratory neurons (Anton et al., 1997).
In the pial basement membrane (BM), a novel role for the secreted glycoprotein, Follistatin like-1 (FSTL1) was identified in RG scaffolding (Liu et al., 2015). Indeed, authors showed that in embryonic mouse cortices, RG basal processes were not parallel and their endfeet less branched. Thus, they provide data suggesting that this protein is important for the basal but not the apical process and plays its role through a unique mechanism that does not include Cdc42 and GSk3β (Liu et al., 2015). This emphasizes the fact that multiple mechanisms are involved in the formation of the RG scaffold.
Role of Cell to Cell and Cell to ECM Contacts in the Formation and Maintenance of the RG Scaffold and Proliferation
Because RGs extend across the whole cortical wall, they make numerous and various contacts. This section will focus on the impact of different contacts on their scaffold and their proliferative capacity.
Adherens Junctions (AJs)
At the onset of neurogenesis, neuroepithelial cells become RGs and lose tight junctions, but AJs are maintained (Aaku-Saraste et al., 1996). These are composed of junctional complexes including N-cadherin, β catenin, α catenin and the cytoskeleton, which connect the apical regions of RGs to each other at the apical ventricular surface facing the eCSF (Figure 5).
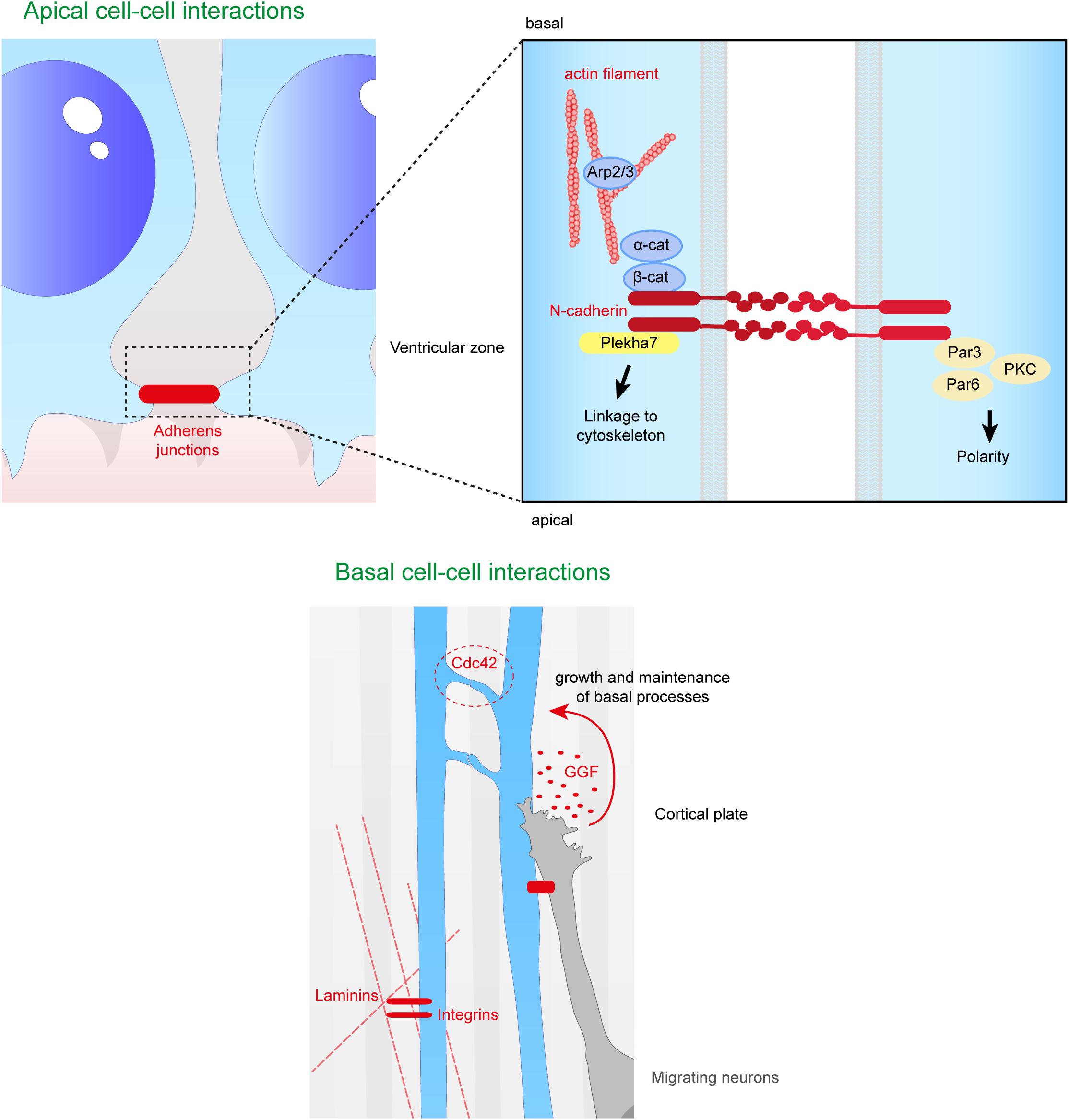
Figure 5. Close range contacts controling the scaffolding of RGs. RGs directly receive signals from neighboring cells such as other RGs or migrating neurons. On the top panel are depicted the cell–cell interactions occurring at the apical side of aRGs. Adherens junctions between aRGs are crucial for the maintenance of the scaffold. In the enlarged box is represented the binding of N-cadherins which can link extracellular contacts with the cytoskeleton (via Plekha7 or β-catenin) or with polarity proteins such as Par3, Par6, and PKC. On the bottom panel is illustrated basal cell–cell interactions. Basal processes of RGs can interact with each other inducing a Cdc42 response intracellularly. Neurons can also directly act on the glial scaffold by secreting factors such as GGF which controls growth and maintenance of basal processes. Finally, basal processes receive information from the extracellular matrix, especially via the interaction between intergins and laminins.
The extracellular domain of N-cadherin enables the anchoring of the cells to each other, while the intracellular domain is connected to β and α catenins to link the AJ to the cytoskeleton. Therefore, this complex links the actin cytoskeleton to the plasma membrane to form cadherin mediated cell-cell adhesion sites (Drees et al., 2005; Nelson, 2008; Pokutta et al., 2008; Benjamin et al., 2010; Maiden and Hardin, 2011). Many studies (Miyamoto et al., 2015; Veeraval et al., 2020) emphasize the fact that N-cadherin based AJs are key elements for the development of cortical architecture. Several proteins involved in the maintenance of AJs, including afadin, as well as N-cadherin, αE catenin, β catenin, are essential for the formation and maintenance of the RG scaffold (Table 1).
First, RG N-cadherin based AJs are Numb-dependent (Kadowaki et al., 2007; Rasin et al., 2007). Numb acts as an inhibitor of Notch signaling and is localized in apical endfeet of RGs. Numb/Numbl (homolog of Numb) interact with cadherin-catenin complexes during cortical development and are essential to maintain N-cadherin AJs. When Numb and Numbl are lacking in mouse cortices, the ventricular surface is altered, and RGs lose their radial polarity and their apical process suggesting an essential role of Numb/Numbl in apical process maintenance (Rasin et al., 2007). Moreover, N-cadherin has been shown to be required to prevent RG delamination, apical process retraction and premature differentiation (Rousso et al., 2012; Das and Storey, 2014; Wang P.S. et al., 2016). The maintenance of cadherin based AJs enables the activation of the β catenin phosphodegradation complex (Gsk3β, APC, Axin) and so reduces its level in the cytoplasm (Maher et al., 2009). As mentioned in section “Secreted Factors From Close Range Cells,” β catenin is an effector of Wnt signaling, and is stabilized in the cell, influenced by the N-cadherin and Akt pathways. Interestingly, the presence of N-cadherin also allows Akt activity, and the phosphorylation of β catenin by Akt increases its translocation to the nucleus (Zhang et al., 2010; Zhang et al., 2013). These data further suggest a role of N-cadherin in the regulation of β catenin level and distribution. N-cadherin function is also impacted by the conditional KO of afadin in the cortex, and mice exhibit a double cortex (a normotopic cortex as well as a heterotopic cortex) due to detached RGs. In these mutants Yamamoto et al. (2015) showed that major proteins (including N-cadherin) of RG AJs are not maintained at E14.5, suggesting that afadin plays an essential role in the maintenance of the AJs in the apical processes. In this case, ectopic detached Sox2 + progenitors result. RG basal processes were not altered but the apical processes were irregularly arranged in the deficient embryonic mouse cortices. Interestingly, no defects in RG proliferation and differentiation were found in these mutants (Yamamoto et al., 2015), further suggesting a crucial role of basal attachment on RG proliferation (Uzquiano et al., 2018).
It is important to mention the Arp2/3 complex that is involved in the formation and maintenance of AJs. When Arp2/3 is conditionally deleted in the mouse, RG processes are shorter and mis-oriented (Wang P.S. et al., 2016). More precisely, the ventricular surface is altered with the presence of ectopic progenitors and the speed of formation of the basal process is also reduced. The Arp2/3 complex is an effector of β catenin, and establishes a link between the formation of the RG scaffold and the cytoskeleton (Wang P.S. et al., 2016). Cdc42 and RhoA are known to be upstream regulators of the Arp2/3 complex, and control both basal process extension and apical process adhesion (Cappello et al., 2006; Yokota et al., 2010).
Also, Atypical protein kinase C λ (aPKC λ) is a protein kinase present at the level of AJs during mammalian corticogenesis, and this protein forms complexes with polarity proteins Par6 and Par3. When aPKC is conditionally deleted in mouse cortex, apical processes of RGs retract more often and RGs detach from the ventricular surface. aPKC is indispensable for neuroepithelial cells to form AJs and maintain cell polarity in the neuroepithelium (Imai et al., 2006). Also, classical polarity proteins (Par3, Llg1) are phosphorylated by aPKC during RG polarity establishment (leading to the specific bipolar RG morphology) (Yamanaka et al., 2003) emphasizing a role of classical polarity proteins in this scaffolding. As mentioned above, Pard3 conditional KO leads to detached RGs (Liu et al., 2018).
Plekha7 is another protein associated with apical AJs, and is involved in the maintenance of the RG scaffold, preventing RG delamination. Tavano et al. (2018) showed the importance of this AJ-associated protein by forcing the expression of Insulinoma-associated 1 (Insm1) in RGs. Insm1 is a transcription factor that represses Plekha7 transcription. By forced expression of Insm1, there was an increased proportion of bIPs (multipolar cells) and bRGs, suggesting an alteration of the RG scaffold across the brain. Thus, when the level of Plekha7 is reduced by Insm1 forced expression, RGs lose their apical contact and their apical processe retracts (Tavano et al., 2018). This study confirms that AJ components are crucial for the RG scaffold and more specifically for apical process integrity.
Thus, perturbing or changing AJs influences RG attachment and this is one method by which apically detached RGs can arise (Penisson et al., 2019; Kalebic and Huttner, 2020). The fate of the detached cell can be variable (e.g., aberrant RG, bRG, IP or neuron) depending often on mutant conditions. Also, as described later in this review (see section “Further Factors Identified via Human Pathology” Human pathology), certain human cortical malformation gene mutations have been identified, related to further apical adhesion complexes. These data also emphasize the importance of RG apical contacts for the intact RG scaffold and correct neuronal migration.
Extracellular Matrix (ECM) Components
The ECM is essential for corticogenesis and neural development (for review see Long and Huttner, 2019). In the embryonic cortex the ECM, composed of various proteins such as laminins, proteoglycans, dystroglycans and collagens, surrounds the cells (including RGs). Transcriptome analyses of human and mouse germinal zones in developing cortex (identified from laser microdissected material) showed that the variation of expression of ECM protein interactions and cell adhesion is likely to regulate the ability of neuronal progenitors to proliferate. Also, the expression profile of ECM proteins emphasizes differences between mouse SVZ (containing IPs and few bRGs) and human SVZ (oSVZ, iSVZ containing numerous bRGs and IPs) (Arai et al., 2011; Fietz et al., 2012). As already mentioned, further transcriptome analyses showed that an increase in the production of bRGs which no longer have contact with the eCSF, seems likely to require a modified ECM compared to ventricular aRGs (Pollen et al., 2015; Kalebic and Huttner, 2020). In a gyrencephalic model (ferret), it has been shown that inhibiting integrin (a major ECM receptor, Yamada and Sekiguchi, 2015) in the developing neocortex leads to a reduction in the number of bRGs (Fietz et al., 2010). Conversely, in the mouse increased expression of integrin increases the proliferation of basal progenitors (Stenzel et al., 2014). Recently, Kalebic et al. (2019) showed that the increased ability of bRGs to proliferate was associated with an increased number of RG processes. Indeed, increased processes allows the bRGs to multiply the reception of proliferative signals via integrin.
Radial glia basal processes extend across the cortical wall from the VZ to reach the pial surface (Rakic, 1972). These basal fibers are attached at the pial surface in part by integrin−laminin-interactions, allowing the migration of newborn neurons to reach their correct place in the CP (Graus-Porta et al., 2001; Belvindrah et al., 2007). Integrin−laminin interactions help anchor RG basal processes to the pial BM. Interestingly, laminin induces intracellular signaling via several receptors (e.g., as well as integrin, also dystroglycan, see section “Further Factors Identified via Human Pathology” Human pathology).
Related to this, TAG-1, for transient axonal glycoprotein-1, is a cell surface molecule expressed in the basal region of the cortical wall during embryonic development. This molecule also known as contactin-2 is essential for the maintenance of RG basal processes. Indeed, knockdown of TAG-1 in the mouse leads to basal process retraction and ectopic progenitors (Okamoto et al., 2013). The role of TAG-1 in basal RG process maintenance is cell autonomous, and knockdown does not affect apical surface integrity even if it increases mechanical stress in the ventricular zone. Indeed, the role of TAG-1 in the apical process is not well established. The mechanisms underlying its role in the basal RG fiber are not well defined but one hypothesis is that it is through the interaction of TAG-1 and basal lamina components such as Anoxin-1 and Laminin (Milev et al., 1996; Sittaramane et al., 2009; Okamoto et al., 2013).
Furthermore, via isolated stabilized RG clones, Li et al. (2008) showed that activated Notch promotes radial morphology, increases expression of BLBP (mentioned at the end of the section “Role of Secreted Proteins Derived From the CSF in the Formation and Maintenance of the RG Scaffold” as an actor in RG process elongation) and promotes RG adhesion on a laminin/nidogen complex. In this in vitro model, the authors observed increased expression of other adhesion proteins such as proteoglycans contributing to the brain ECM. Regarding these findings we can hypothesize that Notch action on radial morphology is likely to involve these ECM elements (Li et al., 2008). Also indirectly, Notch has been shown to be important in RG scaffolding. Indeed, the first step of the establishment of the RG scaffold is the maintenance of RG identity itself. Yoon et al. described data involving Notch signaling in the maintenance of the RG pool. By a specific deletion of mind bomb 1 in mouse embryonic neuronal progenitors, Notch activation was inhibited and premature differentiation of RGs to IPs and neurons was observed (Yoon et al., 2008).
Loulier et al. (2009) demonstrated that the integrin-laminin interaction may act also in apical processes at the ventricular surface. Using blocking antibodies delivered into the cerebral ventricle in utero, preventing the fixation of laminin to its ligand β1 integrin, they observed detachment of RG apical processes, suggesting an apical role of the integrin-laminin interaction (Loulier et al., 2009). These findings provided evidence of the ECM’s role in RG bipolar shape and proliferative ability. More recent transcriptome and proteome analyses continue to contribute information concerning the ECM and different progenitor types including RGs (Fietz et al., 2012; Pollen et al., 2015; Buchsbaum et al., 2020; Kalebic and Huttner, 2020). For example, recently, interested in periventricular heterotopia (see section “Further Factors Identified via Human Pathology” Human pathology), Buchsbaum et al. (2020) provide novel information concerning the endothelin converting enzyme-2 (ECE2) gene and RG morphology. In cerebral organoids and the developing mouse cortex, they show that knockdown of ECE2/Ece2 changes aRG morphology since these cells are less radial and bipolar. The ventricular surface was also altered, and aRG lose their apical process suggesting a role of this protein in apical RG processes. Interestingly, this ECE2-deficient phenotype is associated with ECM protein and receptor dowregulation.
Extracellular matrix components thus clearly have crucial roles in RG morphology and proliferative ability. This is a current exciting area of research which will further clarify the precise mechanisms involved.
Interactions via Basal Processes (RG−RG, RG-Neuron)
As RGs are a physical support for post-mitotic neuron migration, it is clear that neurons and RG interact. This interaction provides to neurons a mechanical support for migration but also a way to communicate with RGs that can influence the migration process. It is thus not only the integrity of the RG scaffold that is essential to allow this post-mitotic neuron migration, but also the communication between the different cell types. Coherent with this, it has been shown that connexin 43 (Cx43) and 26 (Cx26) connect migrating neurons and RG fibers via gap junction dynamic adhesive contacts (Elias et al., 2007). Importantly, when either Cx43 or Cx26 is downregulated via shRNAs injected in mouse embryonic cortex, the neuron’s ability to migrate is reduced, without however, affecting the RG scaffold and expression of other cell-cell adhesion proteins. Also, as explained in section “Role of Secreted Proteins Derived From the CSF in the Formation and Maintenance of the RG Scaffold” (see BLBP section), neuron attachments to RG fibers are important for RG process elongation. In this context, it is important to mention that N-cadherin also plays a role in the attachment of the migrating neuron to the RG fiber, with its knockdown diminishing this interaction. The correct level of N-cadherin at the neuronal cell surface is mediated via endocytic pathways dependent on Rab GTPases (Kawauchi et al., 2010; Shikanai et al., 2011).
Cdc42 is a small GTPase localized at the leading edge of basal radial fibers, where it allows the recruitment of protein complexes such as Par6-aPKC (Etienne-Manneville and Hall, 2003; Heasman and Ridley, 2008). Cdc42 plays a role in RG−RG interactions via inter-radial fibers, during the dynamic extension of the basal process (Figure 5). Indeed, Cdc42 KO in the mouse leads to shorter basal processes which do not reach the pial surface during cortical development. The number of contacts between RG fibers is also reduced. Very little is known about the role of the inter-radial fiber on the scaffold, but there is a correlation between less inter-radial fibers and shorter RG basal processes (Yokota et al., 2010). Cdc42 signaling is known to be regulated via GSK3β phosphorylation, but the phenotype of the RG scaffold when GSK3 is pharmacologically inhibited is not the same as the Cdc42 cKO phenotype. Indeed, although basal processes are shorter as in the Cdc42 cKO, they are not well oriented at the pial surface after inhibition of GSK3 and the whole scaffold shows a wavy morphology. This suggests distinct roles for these two proteins influencing RG scaffolding and basal processes (Yokota et al., 2010).
As mentioned previously, in the Wnt signaling pathway APC has a role in the maintenance and extension of the RG scaffold. APC is localized in RG tips and in the soma. When it is specifically deleted in RGs in vivo, the scaffold is mis-oriented with basal processes not directed to the pial surface (Yokota et al., 2009). Over corticogenesis, the processes appear also shorter, suggesting a role for APC in maintenance of scaffold polarity but also fiber extension. APC is involved in the response of basal process extension via neuregulin 1 signaling (see section “Role of Secreted Proteins Derived From the CSF in the Formation and Maintenance of the RG Scaffold”), but also in the stability of microtubules at cell contacts (apical AJs and in basal RG endfeet at BM sites, Yokota et al., 2009). Indeed, APC is known to interact with microtubule proteins such as EB1 and microtubules themselves, and to allow the correct localization of polarity proteins (Numb, Cdc42) in subcellular compartments. Without APC, the integrity of apical and basal cell-cell contacts may hence be altered. This may explain why in its absence, basal fibers do not respond to neuregulin 1 since the interactions are not made correctly. However, its intrinsic role in microtubule stability may also play a role in this mechanism (Yokota et al., 2009).
Further Factors Identified via Human Pathology
As previously mentioned, depletion of long range and short range factors can disrupt RG proliferation leading to microcephaly. Disruption of RG architecture on which neuronal migration relies, can also lead to other cortical malformations mentioned here, including human lissencephaly, polymicrogyria, and heterotopia. We describe the key features and genes involved in these disorders, shedding further light on external influences of neuronal migration.
Apically Disrupted RGs
When RG architecture is perturbed apically it can lead to heterotopias associated with epilepsy and sometimes intellectual disability (Bizzotto and Francis, 2015). Firstly, perturbation of RGs and neuron migration can lead to periventricular nodular heterotopia (PH) where clusters of neurons are identified close to the ventricles. In PH models, during development abnormal clusters of progenitors and neurons are found trapped at the ventricular surface (Bizzotto and Francis, 2015; Table 2). Secondly, although subcortical band heterotopia (abnormal neuron clusters found within the white matter) is usually associated with an intrinsic problem in migrating neurons, other subcortical heterotopias (SH) can arise due to perturbed and apically detached RG, which subsequently perturb migration (Kielar et al., 2014; Stouffer et al., 2016).
A number of PH genes highlighted here code for proteins regulating apical RG functions, with often as well evidence for a role in migrating neurons. PH is classically associated with mutations in Filamin A, coding for an actin cross-linking protein interacting with cell adhesion molecules such as integrins as well as other membrane proteins, enabling their anchoring to the cytoskeleton (Fox et al., 1998; Lian and Sheen, 2015; Table 2). Other PH proteins have been implicated in vesicle trafficking, e.g., ARFGEF2 required for trafficking from the Golgi apparatus (Sheen et al., 2004); and ERMARD and TMTC3, endoplasmic reticulum (ER) proteins (Conti et al., 2013; Larsen et al., 2017). ARFGEF2 mutations can perturb proliferation and have been shown to affect the localization of cadherins and β catenin at the cell surface (Sheen et al., 2004), thus disrupting AJs. Mutations in α N-catenin (CTNNA2) also give rise to severe brain malformations (complex cortical dysplasia, Schaffer et al., 2018). The apical protocadherin receptor-ligand pair DCHS1 and FAT4 also show mutations in Van Maldergem syndrome which includes PH (Cappello et al., 2013). Acute knockdown in the mouse of these genes showed accumulation of cells in the VZ, as well as migration defects. Klaus et al. (2019) went on to show defective RG morphologies and transcriptional signatures, a discontinuous apical surface and slowed migration in human in vitro organoid models (Klaus et al., 2019). RG delamination most probably due to perturbed apical adhesion or signaling was also observed in these and other models (e.g., also with EML1 mutations giving rise to SH, Kielar et al., 2014) and this can lead to subtle or severe disruption of the ventricular surface, sometimes resulting in heterotopia.
Several PH proteins (e.g., GNAI2, GSPM2 involved in G-protein signaling) are likely to be involved in the transduction of extracellular signals to intracellular effectors (Lee et al., 2006; Hamada et al., 2017). Further proteins impact intracellular signaling (e.g., NEDD4L, Broix et al., 2016). Extracellular signaling was also revealed as important for RG function in the case of ECE2 mutations (Buchsbaum et al., 2020). This enzyme localizes to the plasma membrane and its mutation in the developing mouse cortex led to RG delamination and the formation of rosettes (progenitors clustered in a circle within the tissue). Surrounding non-mutant cells also appeared to be affected (non-cell autonomous phenomenon). The ventricular surface showed morphological alterations suggesting a weakening of cell junctions and indeed proteomic analyses revealed deregulated ECM molecules, as well as cytoskeletal and cell adhesion proteins. Thus, transduction of extracellular signals as well as cell adhesion regulation are clearly important in RGs and migrating neurons, their disruption leading to PH (Lian and Sheen, 2015; Buchsbaum et al., 2020).
Breakages in the BM and Cajal-Retzius Cells
Cobblestone lissencephaly is associated with disorganized cerebral and cerebellar cortices and multiple coarse gyri, with agyric regions (Table 3, OMIM; Guerrini and Dobyns, 2014). It is often included in a broader spectrum of disorders including muscular dystrophy and eye defects, as well as sometimes agenesis of the corpus callosum, cerebellar hypoplasia and hydrocephalus (OMIM; Guerrini and Dobyns, 2014). The dysfunctional mechanisms involve an over-migration of neurons at the pial surface, due to breaks in the cortical BM (Nickolls and Bonnemann, 2018). This has been associated with RG basal process end-feet that are not well attached to the ECM (e.g., laminin, Figure 3), leading to subsequent disintegration of the RG scaffold. In mouse models, mis-localization of Cajal-Retzius cells is also observed, in some models correlated with rostro-caudal and medio-lateral gradients of the lesions and the severity of the brain phenotype (Booler et al., 2016).
As mentioned previously, receptors and glycoproteins present on RG basal membranes normally make interactions with the ECM, helping to form and maintain the BM (Nickolls and Bonnemann, 2018). Mutations in some of these molecules (see below) leads to a cobblestone lissencephaly phenotype (Table 3). In other cases, a polymicrogyria phenotype in human patients is associated with a cobblestone-like phenotype revealed in mouse models. Indeed, polymicrogyria, associated with multiple small folds at the surface of the brain (Francis et al., 2006) also often involves over-migration and BM breakages. In this disorder, regions of fused gyri within the brain parenchyma can also contain BM components (Squier and Jansen, 2014). Thus, cobblestone lissencephaly is associated with polymicrogyria and indeed, often the two are found together (Squier and Jansen, 2014). These disorders can also often be associated with other neuronal migration defects such as periventricular or subcortical heterotopia (Table 3). We examine here causes of these linked disorders, from human genetics and animal model data.
Central to cobblestone lissencephaly hypotheses is post-translational regulation of dystroglycan (coded by DAG1, dystrophin-associated glycoprotein 1), a glycoprotein present in RG basal processes, that acts as an anchor point with the ECM (Booler et al., 2016), being also important for BM structure (Henry and Campbell, 1998). It normally gets cleaved giving rise to the peripheral membrane protein α-DG and the transmembrane protein β-DG. α-DG undergoes O-linked mannosylation allowing its binding to ECM proteins such as laminin (which has α, β and γ subunits, Burgeson et al., 1994), agrin, neurexin, pikachurin, and perlecan. The ER is involved in trafficking and glycosylating secretory pathway cargo. β-DG interacts with the actin cytoskeleton via dystrophin, utrophin and plectin, and thus α-DG and β-DG link the cytoskeleton to the ECM (Booler et al., 2016).
Muscle eye brain disease (MEB), Fukuyama congenital muscular dystrophy (FCMD), and Walker Warburg syndrome (WWS) have each been associated with aberrant glycosylation of α-DG (e.g., Kobayashi et al., 1998; Yoshida et al., 2001; Michele et al., 2002). Abnormal dystroglycan−ligand interactions underlie the pathogenic mechanism of muscular dystrophy as well as brain abnormalities. Rare mutations in DAG1 (coding for dystroglycan, Hara et al., 2011; Geis et al., 2013), laminin subunit genes (e.g., LAMA2, LAMB1, and LAMC3, Barak et al., 2011; Radmanesh et al., 2013; Nelson et al., 2015; Zambonin et al., 2018) and genes coding for proteins likely to influence glycosylation and maturation of α-DG as well as potentially other proteins (ATP6V0A2, B4GAT1, B3GALNT2, FKTN, FKRP, ISPD, LARGE, POMK, POMT1, POMT2, POMGNT1, POMGNT2, SRD5A3, TMEM5, Kobayashi et al., 1998; Brockington et al., 2001; Yoshida et al., 2001; Beltran-Valero De Bernabe et al., 2002; Willer et al., 2004; Van Reeuwijk et al., 2005, 2007; Cantagrel et al., 2010; Manzini et al., 2012; Roscioli et al., 2012; Vuillaumier-Barrot et al., 2012; Buysse et al., 2013; Jae et al., 2013; Stevens et al., 2013 and see Table 3) can give rise cobblestone lissencephaly and/or polymicrogyria.
Several other genes involved in cobblestone phenotypes (TBC1D10, TMTC3, Liegel et al., 2013; Jerber et al., 2016) code for proteins which are likely to be involved in membrane trafficking and/or the ER stress response. Interestingly, TMTC3 has also been associated with 0-mannosyl glycosylation in the ER of the cell adhesion cadherins and proto-cadherins, but not α-DG (Larsen et al., 2017). As mentioned previously, cadherins are involved in RG apical cell-cell contacts, which may hence be disrupted if glycosylation does not occur correctly. Several patients with PH, already associated with abnormal RG contacts and neuronal migration (Cappello et al., 2013), exhibit mutations in TMTC3 (Larsen et al., 2017). Thus, most likely apical (periventricular) as well as basal interactions require correct glycosylation.
GPR56 mutations were identified in bilateral frontoparietal or perisylvian polymicrogyria (Piao et al., 2004; Bae et al., 2014). GPR56 is a member of an adhesion family of G protein-coupled receptors (GPRs), expressed on the basal membrane of RGs. Mouse mutants show a cobblestone-like phenotype, with abnormal basal RG processes, a disrupted BM, with neuronal over-migration and Cajal Retzius cell disruption (Li et al., 2008). Type III collagen, as well as being important in connective tissue including blood vessels, is one of the major constituents of the pial BM and a ligand for GPR56 (Luo et al., 2011). Although several disorders are associated with altered levels of the collagen COL3A1 (Kuivaniemi and Tromp, 2019), some mutations give rise to frontal predominant bilateral polymicrogyria or a cobblestone−like cortical malformation (Horn et al., 2017; Vandervore et al., 2017). COL3A1 is the α1 chain of type III collagen, an ECM protein. COL4A1, the α1 chain of type IV collagen, is also a crucial component of the BM. Cobblestone lissencephaly, polymicrogyria, schizencephaly or porencephaly were observed in certain patients with COL4A1 mutations (Labelle-Dumais et al., 2011; Cavallin et al., 2018; Zagaglia et al., 2018). Cavallin et al. (2018) discuss prenatal stroke and suggest that hydranencephaly, schizencephaly, porencephaly and polymicrogyria represent a continuum of brain injury depending on the timing and the severity of the insult. Both genetic as well as environmental factors may hence contribute to these disorders. Interestingly, COL4A2 mutations were also identified in a patient presenting heterotopia in addition to bilateral polymicrogyria suggesting perturbed neuronal migration (Cavallin et al., 2018).
By activating signaling cascades, Reelin acts at the terminal steps of neuronal migration, notably terminal translocation, without however, influencing RG-guided migration (Sekine et al., 2014). As mentioned previously however (section “Role of Secreted Proteins Derived From the CSF in the Formation and Maintenance of the RG Scaffold”), Reelin may also influence RG basal processes at least in some species. Lower concentrations of Reelin are also present in the lower IZ, in this case influencing the neuronal multipolar-bipolar transition (Sekine et al., 2014). Mutations have been identified in Reelin in autosomal recessive pachygyria associated with cerebellar hypoplasia (Hong et al., 2000). Reeler mutant mice show a similar combination of defects, including disorganized and inverted cortical lamination (D’Arcangelo et al., 1995). There are also patients which exhibit mutations in the Reelin receptor gene, very low density lipoprotein receptor, VLDLR (Valence et al., 2016). Mutations give rise to the CARMQ1 syndrome (cerebellar ataxia and mental retardation with or without quadrupedal locomotion), which can include mild simplification or thickening of cortical gyri (Valence et al., 2016). Vldlr mouse mutants show an invasion of neurons in the MZ (Hack et al., 2007) and double KO with a second Reelin receptor gene, ApoER2, phenocopies the cortical disorganization observed in reeler mutants (Trommsdorff et al., 1999). Thus, extracellular Reelin via the above-mentioned receptors, is critical for neuron migration and notably translocation in the most superficial regions of the developing cortex, as well as potentially maintenance of the RG scaffold (Weiss et al., 2003).
Conclusion and Perspectives
Overall, in this review we summarize the variety of different factors which together regulate the formation and the maintenance of RG scaffolding. The need for this variety, ranging from secreted factors in the eCSF to very local cell to cell contacts, is probably due to the unique morphology of RGs. Indeed, they spread from one side of the developing cortex to the other, and are therefore exposed to very different environments. RGs have basal endfeet in contact with secreted factors derived from the meninges and from cells migrating from remote places, such as Cajal Retzius cells or neurons, and at the same time aRGs have their cell bodies and primary cilia in contact with other RG and the eCSF (Figure 1). For the stability of the whole cortical architecture, which is based on RGs, it therefore seems to be essential that there are multiple spatial environments which signal to the different RG compartments. aRGs will also differ from bRGs in this respect and it is also clear that environments change over time further regulating these essential cell types.
It appears that there is not a single mechanism that governs the RG scaffold but instead elaborated mechanisms involving numerous actors. However, although this review is not exhaustive, it is striking that unlike what it is already known for neuronal growth and guidance, there is little research which has up till now identified specific modulators of RG growth and maintenance. We attempted here to identify and group such factors. RGs have to grow a long process to reach a remote location, crossing a relatively thick tissue, however, they are often seen as a constant scaffold acting as a support for neuronal migration. The RG scaffold is not though as static as we might imagine. Indeed, evidence provided by the work of Yokota et al. (2010), showed using videomicroscopy that RG processes, endfeet and radial fiber interactions are constantly dynamic. The function of this dynamic behavior could impact neuronal migration at the local level to finely regulate neuron positioning in the CP and hence the structure of the brain. How and why the RG scaffold is plastic still remains to be investigated. Experiments taking into account this dynamic behavior could help acquire further information to distinguish factors involved in RG growth aspects from those involved in maintenance.
Radial glia are not only defined by their morphology. They are also able to adapt their gene expression to match the changing cues in their environment and to adjust their cellular responses (Telley et al., 2019). The timing of these mechanisms is crucial as cues can be produced in waves during development (Chau et al., 2015). How these genic modulations occur still needs to be studied. However, factors influencing chromatin and RNA processing are likely to play a role (e.g., it is notable that there are several PH genes involved in these processes, including HNRNPK, INTS8, KAT6B, MED12, Clayton-Smith et al., 2011; Caro-Llopis et al., 2016; Lange et al., 2016; Oegema et al., 2017, see Table 2), although for the moment in relatively unknown ways. Further studies across corticogenesis could shed further light on such mechanisms. Transcriptomic or proteomic experiments related to these genes could further identify key targets (acting on adhesion and/or signaling) impacting progenitor and/or neuron migration function at different timepoints.
When we think of the role and formation of the RG scaffold, another aspect to consider is the evolution from lissencephalic species to gyrencephalic species, especially with respect to the appearance of bRGs. Indeed, related to this relatively new progenitor type, whose somata lie in more basal areas of the developing cortex of gyrencephalic species, the RG network is not the same as in lissencephalic species. Some questions are: what are the relationships between aRG basal processes and bRG basal processes? Do they interact? Do they respond in the same manner to extracellular cues? How is bRG maintenance initially put in place, since these cells do not receive the same extracellular cues as their apical counterparts? Are there specific extracellular cues regulating bRGs, forming a special niche in the center of the developing cortex? For instance, a study identified the specific role of PDGFD signaling in the maintenance of bRG in human cortical development compared to mouse progenitors (Lui et al., 2014). Pollen et al. found several genes specific to bRGs which are highly related to ECM interactions. For instance tenascin C (TNC) is specific to bRGs and has the ability to bind to PTPRZ1, syndecans or integrins (Pollen et al., 2015). Moreover as mentioned earlier Notch is important for RG maintenance in mouse but recently a study showed that three paralogs of human-specific NOTCH2NL are essential to control the proliferation/differentiation balance of human RGs, which can be directly linked with the expansion of the human cortex (Fiddes et al., 2018). The function of the bRG scaffold is indeed still mysterious although it is widely assumed to contribute to gyrification during evolution. These ideas and relevant mechanisms are discussed in a review on the role of bRG morphology in cortical development and evolution (Kalebic and Huttner, 2020).
Radial glia are very unique and fascinating cells: the correct orchestration of their formation, organization, and localization is crucial for correct cortical development. Since the discovery of these cells, much work has been done to understand better how these progenitors communicate and interact with their environment but still a lot remains to be investigated. This review highlights a level of complexity inherent to these cells with respect to the impact of extracellular cues on their development and maintenance. Further understanding this aspect will help clarify mechanisms involved in health and neurodevelopmental disorders.
Author Contributions
JF wrote the sections “Role of Secreted Proteins Derived From the CSF in the Formation and Maintenance of the RG Scaffold” and “Secreted Factors From Close Range Cells” and designed all the figures and parts of Table 1. DZ wrote the section “Further Factors Identified via Human Pathology” and generated Table 1. FF wrote the section “Role of Cell to Cell and Cell to ECM Contacts in the Formation and Maintenance of the RG Scaffold and Proliferation” and generated Tables 2, 3. All authors contributed to the article and approved the submitted version.
Funding
The authors were associated with the BioPsy Labex project and the Ecole des Neurosciences de Paris Ile-de-France (ENP) network. Salaries and the lab were supported by the Inserm, Centre national de la recherche scientifique (CNRS), Sorbonne University, and the French ANR under the frame of E-Rare-3 the ERA-Net for Research on Rare Diseases (ERARE18-049, HETEROMICS), the JTC 2015 Neurodevelopmental Disorders affiliated with the ANR (for NEURON8-Full- 815-006 STEM-MCD), as well as the European Cooperation on Science and Technology (COST Action CA16118 NEURO-MIG).
Conflict of Interest
The authors declare that the research was conducted in the absence of any commercial or financial relationships that could be construed as a potential conflict of interest.
Acknowledgments
We thank the NeuroMIG consortium for sharing human gene-disorder information.
References
Aaku-Saraste, E., Hellwig, A., and Huttner, W. B. (1996). Loss of occludin and functional tight junctions, but not ZO-1, during neural tube closure–remodeling of the neuroepithelium prior to neurogenesis. Dev. Biol. 180, 664–679. doi: 10.1006/dbio.1996.0336
Ackroyd, M. R., Skordis, L., Kaluarachchi, M., Godwin, J., Prior, S., Fidanboylu, M., et al. (2009). Reduced expression of fukutin related protein in mice results in a model for fukutin related protein associated muscular dystrophies. Brain 132, 439–451. doi: 10.1093/brain/awn335
Alonso, M. I., Martin, C., Carnicero, E., Bueno, D., and Gato, A. (2011). Cerebrospinal fluid control of neurogenesis induced by retinoic acid during early brain development. Dev. Dyn. 240, 1650–1659. doi: 10.1002/dvdy.22657
Anton, E. S., Marchionni, M. A., Lee, K. F., and Rakic, P. (1997). Role of GGF/neuregulin signaling in interactions between migrating neurons and radial glia in the developing cerebral cortex. Development 124, 3501–3510.
Arai, Y., Pulvers, J. N., Haffner, C., Schilling, B., Nusslein, I., Calegari, F., et al. (2011). Neural stem and progenitor cells shorten S-phase on commitment to neuron production. Nat. Commun. 2:154.
Bae, B. I., Tietjen, I., Atabay, K. D., Evrony, G. D., Johnson, M. B., Asare, E., et al. (2014). Evolutionarily dynamic alternative splicing of GPR56 regulates regional cerebral cortical patterning. Science 343, 764–768. doi: 10.1126/science.1244392
Barak, T., Kwan, K. Y., Louvi, A., Demirbilek, V., Saygi, S., Tuysuz, B., et al. (2011). Recessive LAMC3 mutations cause malformations of occipital cortical development. Nat. Genet. 43, 590–594. doi: 10.1038/ng.836
Barnabe-Heider, F., and Miller, F. D. (2003). Endogenously produced neurotrophins regulate survival and differentiation of cortical progenitors via distinct signaling pathways. J. Neurosci. 23, 5149–5160. doi: 10.1523/jneurosci.23-12-05149.2003
Bartkowska, K., Paquin, A., Gauthier, A. S., Kaplan, D. R., and Miller, F. D. (2007). Trk signaling regulates neural precursor cell proliferation and differentiation during cortical development. Development 134, 4369–4380. doi: 10.1242/dev.008227
Beltran-Valero, De Bernabe, D., Currier, S., Steinbrecher, A., Celli, J., Van Beusekom, E., et al. (2002). Mutations in the O-mannosyltransferase gene POMT1 give rise to the severe neuronal migration disorder Walker-Warburg syndrome. Am. J. Hum. Genet. 71, 1033–1043. doi: 10.1086/342975
Belvindrah, R., Graus-Porta, D., Goebbels, S., Nave, K. A., and Muller, U. (2007). Beta1 integrins in radial glia but not in migrating neurons are essential for the formation of cell layers in the cerebral cortex. J. Neurosci. 27, 13854–13865. doi: 10.1523/jneurosci.4494-07.2007
Benjamin, J. M., Kwiatkowski, A. V., Yang, C., Korobova, F., Pokutta, S., Svitkina, T., et al. (2010). AlphaE-catenin regulates actin dynamics independently of cadherin-mediated cell-cell adhesion. J. Cell Biol. 189, 339–352. doi: 10.1083/jcb.200910041
Berger, O., Li, G., Han, S. M., Paredes, M., and Pleasure, S. J. (2007). Expression of SDF-1 and CXCR4 during reorganization of the postnatal dentate gyrus. Dev. Neurosci. 29, 48–58. doi: 10.1159/000096210
Betizeau, M., Cortay, V., Patti, D., Pfister, S., Gautier, E., Bellemin-Menard, A., et al. (2013). Precursor diversity and complexity of lineage relationships in the outer subventricular zone of the primate. Neuron 80, 442–457. doi: 10.1016/j.neuron.2013.09.032
Bizzotto, S., and Francis, F. (2015). Morphological and functional aspects of progenitors perturbed in cortical malformations. Front. Cell. Neurosci. 9:30. doi: 10.3389/fncel.2015.00030
Booler, H. S., Williams, J. L., Hopkinson, M., and Brown, S. C. (2016). Degree of cajal-retzius cell mislocalization correlates with the severity of structural brain defects in mouse models of dystroglycanopathy. Brain Pathol. 26, 465–478. doi: 10.1111/bpa.12306
Borrell, V., and Marin, O. (2006). Meninges control tangential migration of hem-derived Cajal-Retzius cells via CXCL12/CXCR4 signaling. Nat. Neurosci. 9, 1284–1293. doi: 10.1038/nn1764
Bouchet, C., Gonzales, M., Vuillaumier-Barrot, S., Devisme, L., Lebizec, C., Alanio, E., et al. (2007). Molecular heterogeneity in fetal forms of type II lissencephaly. Hum. Mutat. 28, 1020–1027.
Briscoe, J., and Small, S. (2015). Morphogen rules: design principles of gradient-mediated embryo patterning. Development 142, 3996–4009. doi: 10.1242/dev.129452
Brockington, M., Blake, D. J., Prandini, P., Brown, S. C., Torelli, S., Benson, M. A., et al. (2001). Mutations in the fukutin-related protein gene (FKRP) cause a form of congenital muscular dystrophy with secondary laminin alpha2 deficiency and abnormal glycosylation of alpha-dystroglycan. Am. J. Hum. Genet. 69, 1198–1209. doi: 10.1086/324412
Broix, L., Jagline, H., Ivanova, E., Schmucker, S., Drouot, N., Clayton-Smith, J., et al. (2016). Mutations in the HECT domain of NEDD4L lead to AKT-mTOR pathway deregulation and cause periventricular nodular heterotopia. Nat. Genet. 48, 1349–1358. doi: 10.1038/ng.3676
Buchsbaum, I. Y., Kielkowski, P., Giorgio, G., O’neill, A. C., Di Giaimo, R., Kyrousi, C., et al. (2020). ECE2 regulates neurogenesis and neuronal migration during human cortical development. EMBO Rep. 21:e48204.
Bueno, D., Parvas, M., Nabiuni, M., and Miyan, J. (2020). Embryonic cerebrospinal fluid formation and regulation. Semin. Cell Dev. Biol. 102, 3–12. doi: 10.1016/j.semcdb.2019.09.006
Burgeson, R. E., Chiquet, M., Deutzmann, R., Ekblom, P., Engel, J., Kleinman, H., et al. (1994). A new nomenclature for the laminins. Matrix Biol. 14, 209–211. doi: 10.1016/b978-0-12-817068-7.00029-x
Burrows, R. C., Wancio, D., Levitt, P., and Lillien, L. (1997). Response diversity and the timing of progenitor cell maturation are regulated by developmental changes in EGFR expression in the cortex. Neuron 19, 251–267. doi: 10.1016/s0896-6273(00)80937-x
Buysse, K., Riemersma, M., Powell, G., Van Reeuwijk, J., Chitayat, D., Roscioli, T., et al. (2013). Missense mutations in beta-1,3-N-acetylglucosaminyltransferase 1 (B3GNT1) cause Walker-Warburg syndrome. Hum. Mol. Genet. 22, 1746–1754. doi: 10.1093/hmg/ddt021
Cantagrel, V., Lefeber, D. J., Ng, B. G., Guan, Z., Silhavy, J. L., Bielas, S. L., et al. (2010). SRD5A3 is required for converting polyprenol to dolichol and is mutated in a congenital glycosylation disorder. Cell 142, 203–217. doi: 10.1016/j.cell.2010.06.001
Cappello, S., Attardo, A., Wu, X., Iwasato, T., Itohara, S., Wilsch-Brauninger, M., et al. (2006). The Rho-GTPase cdc42 regulates neural progenitor fate at the apical surface. Nat. Neurosci. 9, 1099–1107. doi: 10.1038/nn1744
Cappello, S., Gray, M. J., Badouel, C., Lange, S., Einsiedler, M., Srour, M., et al. (2013). Mutations in genes encoding the cadherin receptor-ligand pair DCHS1 and FAT4 disrupt cerebral cortical development. Nat. Genet. 45, 1300–1308. doi: 10.1038/ng.2765
Caro-Llopis, A., Rosello, M., Orellana, C., Oltra, S., Monfort, S., Mayo, S., et al. (2016). De novo mutations in genes of mediator complex causing syndromic intellectual disability: mediatorpathy or transcriptomopathy? Pediatr. Res. 80, 809–815. doi: 10.1038/pr.2016.162
Cavallin, M., Mine, M., Philbert, M., Boddaert, N., Lepage, J. M., Coste, T., et al. (2018). Further refinement of COL4A1 and COL4A2 related cortical malformations. Eur. J. Med. Genet. 61, 765–772. doi: 10.1016/j.ejmg.2018.10.004
Chai, X., Fan, L., Shao, H., Lu, X., Zhang, W., Li, J., et al. (2015). Reelin Induces Branching of Neurons and Radial Glial Cells during Corticogenesis. Cereb. Cortex 25, 3640–3653. doi: 10.1093/cercor/bhu216
Chang, J. T., Lehtinen, M. K., and Sive, H. (2016). Zebrafish cerebrospinal fluid mediates cell survival through a retinoid signaling pathway. Dev. Neurobiol. 76, 75–92. doi: 10.1002/dneu.22300
Chatzi, C., Cunningham, T. J., and Duester, G. (2013). Investigation of retinoic acid function during embryonic brain development using retinaldehyde-rescued Rdh10 knockout mice. Dev. Dyn. 242, 1056–1065. doi: 10.1002/dvdy.23999
Chau, K. F., Springel, M. W., Broadbelt, K. G., Park, H. Y., Topal, S., Lun, M. P., et al. (2015). Progressive differentiation and instructive capacities of amniotic fluid and cerebrospinal fluid proteomes following neural tube closure. Dev. Cell 35, 789–802. doi: 10.1016/j.devcel.2015.11.015
Cholfin, J. A., and Rubenstein, J. L. (2007). Patterning of frontal cortex subdivisions by Fgf17. Proc. Natl. Acad. Sci. U.S.A. 104, 7652–7657. doi: 10.1073/pnas.0702225104
Ciccolini, F., and Svendsen, C. N. (2001). Neurotrophin responsiveness is differentially regulated in neurons and precursors isolated from the developing striatum. J. Mol. Neurosci. 17, 25–33. doi: 10.1385/jmn:17:1:25
Clayton-Smith, J., O’sullivan, J., Daly, S., Bhaskar, S., Day, R., Anderson, B., et al. (2011). Whole-exome-sequencing identifies mutations in histone acetyltransferase gene KAT6B in individuals with the Say-Barber-Biesecker variant of Ohdo syndrome. Am. J. Hum. Genet. 89, 675–681. doi: 10.1016/j.ajhg.2011.10.008
Conti, V., Carabalona, A., Pallesi-Pocachard, E., Parrini, E., Leventer, R. J., Buhler, E., et al. (2013). Periventricular heterotopia in 6q terminal deletion syndrome: role of the C6orf70 gene. Brain 136, 3378–3394. doi: 10.1093/brain/awt249
Cook, S. A., Bronson, R. T., Donahue, L. R., Ben-Arie, N., and Davisson, M. T. (1997). Cerebellar deficient folia (cdf): a new mutation on mouse chromosome 6. Mamm. Genome 8, 108–112. doi: 10.1007/s003359900368
Coulter, M. E., Dorobantu, C. M., Lodewijk, G. A., Delalande, F., Cianferani, S., Ganesh, V. S., et al. (2018). The ESCRT-III protein CHMP1A mediates secretion of sonic hedgehog on a distinctive subtype of extracellular vesicles. Cell Rep. 24, 973–986. doi: 10.1016/j.celrep.2018.06.100
Dahmane, N., Sanchez, P., Gitton, Y., Palma, V., Sun, T., Beyna, M., et al. (2001). The Sonic Hedgehog-Gli pathway regulates dorsal brain growth and tumorigenesis. Development 128, 5201–5212.
D’Arcangelo, G., Miao, G. G., Chen, S. C., Soares, H. D., Morgan, J. I., and Curran, T. (1995). A protein related to extracellular matrix proteins deleted in the mouse mutant reeler. Nature 374, 719–723. doi: 10.1038/374719a0
Das, R. M., and Storey, K. G. (2014). Apical abscission alters cell polarity and dismantles the primary cilium during neurogenesis. Science 343, 200–204. doi: 10.1126/science.1247521
Dave, R. K., Ellis, T., Toumpas, M. C., Robson, J. P., Julian, E., Adolphe, C., et al. (2011). Sonic hedgehog and notch signaling can cooperate to regulate neurogenic divisions of neocortical progenitors. PLoS One 6:e14680. doi: 10.1371/journal.pone.0014680
De Juan Romero, C., and Borrell, V. (2015). Coevolution of radial glial cells and the cerebral cortex. Glia 63, 1303–1319. doi: 10.1002/glia.22827
Denes, V., Witkovsky, P., Koch, M., Hunter, D. D., Pinzon-Duarte, G., and Brunken, W. J. (2007). Laminin deficits induce alterations in the development of dopaminergic neurons in the mouse retina. Vis. Neurosci. 24, 549–562. doi: 10.1017/s0952523807070514
Di Costanzo, S., Balasubramanian, A., Pond, H. L., Rozkalne, A., Pantaleoni, C., Saredi, S., et al. (2014). POMK mutations disrupt muscle development leading to a spectrum of neuromuscular presentations. Hum. Mol. Genet. 23, 5781–5792. doi: 10.1093/hmg/ddu296
Doherty, D., Chudley, A. E., Coghlan, G., Ishak, G. E., Innes, A. M., Lemire, E. G., et al. (2012). GPSM2 mutations cause the brain malformations and hearing loss in Chudley-McCullough syndrome. Am. J. Hum. Genet. 90, 1088–1093. doi: 10.1016/j.ajhg.2012.04.008
Dominov, J. A., Kravetz, A. J., Ardelt, M., Kostek, C. A., Beermann, M. L., and Miller, J. B. (2005). Muscle-specific BCL2 expression ameliorates muscle disease in laminin {alpha}2-deficient, but not in dystrophin-deficient, mice. Hum. Mol. Genet. 14, 1029–1040. doi: 10.1093/hmg/ddi095
Dono, R., Texido, G., Dussel, R., Ehmke, H., and Zeller, R. (1998). Impaired cerebral cortex development and blood pressure regulation in FGF-2-deficient mice. EMBO J. 17, 4213–4225. doi: 10.1093/emboj/17.15.4213
Draganova, K., Zemke, M., Zurkirchen, L., Valenta, T., Cantu, C., Okoniewski, M., et al. (2015). Wnt/beta-catenin signaling regulates sequential fate decisions of murine cortical precursor cells. Stem Cells 33, 170–182. doi: 10.1002/stem.1820
Drees, F., Pokutta, S., Yamada, S., Nelson, W. J., and Weis, W. I. (2005). Alpha-catenin is a molecular switch that binds E-cadherin-beta-catenin and regulates actin-filament assembly. Cell 123, 903–915. doi: 10.1016/j.cell.2005.09.021
Elias, L. A., Wang, D. D., and Kriegstein, A. R. (2007). Gap junction adhesion is necessary for radial migration in the neocortex. Nature 448, 901–907. doi: 10.1038/nature06063
Etienne-Manneville, S., and Hall, A. (2003). Cdc42 regulates GSK-3beta and adenomatous polyposis coli to control cell polarity. Nature 421, 753–756. doi: 10.1038/nature01423
Fame, R. M., and Lehtinen, M. K. (2020). Emergence and developmental roles of the cerebrospinal fluid system. Dev. Cell 52, 261–275. doi: 10.1016/j.devcel.2020.01.027
Farhan, S. M. K., Nixon, K. C. J., Everest, M., Edwards, T. N., Long, S., Segal, D., et al. (2017). Identification of a novel synaptic protein, TMTC3, involved in periventricular nodular heterotopia with intellectual disability and epilepsy. Hum. Mol. Genet. 26, 4278–4289. doi: 10.1093/hmg/ddx316
Feng, Y., Chen, M. H., Moskowitz, I. P., Mendonza, A. M., Vidali, L., Nakamura, F., et al. (2006). Filamin A (FLNA) is required for cell-cell contact in vascular development and cardiac morphogenesis. Proc. Natl. Acad. Sci. U.S.A. 103, 19836–19841. doi: 10.1073/pnas.0609628104
Ferent, J., and Traiffort, E. (2015). Hedgehog: multiple paths for multiple roles in shaping the brain and spinal cord. Neuroscientist 21, 356–371. doi: 10.1177/1073858414531457
Fiddes, I. T., Lodewijk, G. A., Mooring, M., Bosworth, C. M., Ewing, A. D., Mantalas, G. L., et al. (2018). Human-specific NOTCH2NL genes affect notch signaling and cortical neurogenesis. Cell 173, 1356–1369.e22.
Fietz, S. A., Kelava, I., Vogt, J., Wilsch-Brauninger, M., Stenzel, D., Fish, J. L., et al. (2010). OSVZ progenitors of human and ferret neocortex are epithelial-like and expand by integrin signaling. Nat. Neurosci. 13, 690–699. doi: 10.1038/nn.2553
Fietz, S. A., Lachmann, R., Brandl, H., Kircher, M., Samusik, N., Schroder, R., et al. (2012). Transcriptomes of germinal zones of human and mouse fetal neocortex suggest a role of extracellular matrix in progenitor self-renewal. Proc. Natl. Acad. Sci. U.S.A. 109, 11836–11841. doi: 10.1073/pnas.1209647109
Flandin, P., Zhao, Y., Vogt, D., Jeong, J., Long, J., Potter, G., et al. (2011). Lhx6 and Lhx8 coordinately induce neuronal expression of Shh that controls the generation of interneuron progenitors. Neuron 70, 939–950. doi: 10.1016/j.neuron.2011.04.020
Fumiyasu, I., Syu-ichi, H., Kazunori, A., Hiromichi, K., Takaki, M., Masaharu, O., et al. (2006). Inactivation of aPKCλ results in the loss of adherens junctions in neuroepithelial cells without affecting neurogenesis in mouse neocortex. Development 133, 1735–1744. doi: 10.1242/dev.02330
Fox, J. W., Lamperti, E. D., Eksioglu, Y. Z., Hong, S. E., Feng, Y., Graham, D. A., et al. (1998). Mutations in filamin 1 prevent migration of cerebral cortical neurons in human periventricular heterotopia. Neuron 21, 1315–1325. doi: 10.1016/s0896-6273(00)80651-0
Francis, F., Meyer, G., Fallet-Bianco, C., Moreno, S., Kappeler, C., Socorro, A. C., et al. (2006). Human disorders of cortical development: from past to present. Eur. J. Neurosci. 23, 877–893. doi: 10.1111/j.1460-9568.2006.04649.x
Fukumitsu, H., Furukawa, Y., Tsusaka, M., Kinukawa, H., Nitta, A., Nomoto, H., et al. (1998). Simultaneous expression of brain-derived neurotrophic factor and neurotrophin-3 in Cajal-Retzius, subplate and ventricular progenitor cells during early development stages of the rat cerebral cortex. Neuroscience 84, 115–127. doi: 10.1016/s0306-4522(97)00505-8
Fukumitsu, H., Ohtsuka, M., Murai, R., Nakamura, H., Itoh, K., and Furukawa, S. (2006). Brain-derived neurotrophic factor participates in determination of neuronal laminar fate in the developing mouse cerebral cortex. J. Neurosci. 26, 13218–13230. doi: 10.1523/jneurosci.4251-06.2006
Geis, T., Marquard, K., Rodl, T., Reihle, C., Schirmer, S., Von Kalle, T., et al. (2013). Homozygous dystroglycan mutation associated with a novel muscle-eye-brain disease-like phenotype with multicystic leucodystrophy. Neurogenetics 14, 205–213. doi: 10.1007/s10048-013-0374-9
Gould, D. B., Phalan, F. C., Breedveld, G. J., Van Mil, S. E., Smith, R. S., Schimenti, J. C., et al. (2005). Mutations in Col4a1 cause perinatal cerebral hemorrhage and porencephaly. Science 308, 1167–1171. doi: 10.1126/science.1109418
Graus-Porta, D., Blaess, S., Senften, M., Littlewood-Evans, A., Damsky, C., Huang, Z., et al. (2001). Beta1-class integrins regulate the development of laminae and folia in the cerebral and cerebellar cortex. Neuron 31, 367–379. doi: 10.1016/s0896-6273(01)00374-9
Grzmil, P., Enkhbaatar, Z., Gundsambuu, B., Oidovsambuu, O., Yalcin, S., Wolf, S., et al. (2010). Early embryonic lethality in gene trap mice with disruption of the Arfgef2 gene. Int. J. Dev. Biol. 54, 1259–1266. doi: 10.1387/ijdb.092959pg
Guerrini, R., and Dobyns, W. B. (2014). Malformations of cortical development: clinical features and genetic causes. Lancet Neurol. 13, 710–726. doi: 10.1016/s1474-4422(14)70040-7
Guo, L. T., Zhang, X. U., Kuang, W., Xu, H., Liu, L. A., Vilquin, J. T., et al. (2003). Laminin alpha2 deficiency and muscular dystrophy; genotype-phenotype correlation in mutant mice. Neuromuscul. Disord. 13, 207–215.
Hack, I., Hellwig, S., Junghans, D., Brunne, B., Bock, H. H., Zhao, S., et al. (2007). Divergent roles of ApoER2 and Vldlr in the migration of cortical neurons. Development 134, 3883–3891. doi: 10.1242/dev.005447
Hamada, N., Negishi, Y., Mizuno, M., Miya, F., Hattori, A., Okamoto, N., et al. (2017). Role of a heterotrimeric G-protein, Gi2, in the corticogenesis: possible involvement in periventricular nodular heterotopia and intellectual disability. J. Neurochem. 140, 82–95. doi: 10.1111/jnc.13878
Hara, Y., Balci-Hayta, B., Yoshida-Moriguchi, T., Kanagawa, M., Beltran-Valero, De Bernabe, D., et al. (2011). A dystroglycan mutation associated with limb-girdle muscular dystrophy. N. Engl. J. Med. 364, 939–946.
Harrison-Uy, S. J., and Pleasure, S. J. (2012). Wnt signaling and forebrain development. Cold Spring Harb. Perspect. Biol. 4:a008094. doi: 10.1101/cshperspect.a008094
Hartfuss, E., Forster, E., Bock, H. H., Hack, M. A., Leprince, P., Luque, J. M., et al. (2003). Reelin signaling directly affects radial glia morphology and biochemical maturation. Development 130, 4597–4609. doi: 10.1242/dev.00654
Haushalter, C., Asselin, L., Fraulob, V., Dolle, P., and Rhinn, M. (2017). Retinoic acid controls early neurogenesis in the developing mouse cerebral cortex. Dev. Biol. 430, 129–141. doi: 10.1016/j.ydbio.2017.08.006
Hayashi, Y. K., Ogawa, M., Tagawa, K., Noguchi, S., Ishihara, T., Nonaka, I., et al. (2001). Selective deficiency of alpha-dystroglycan in Fukuyama-type congenital muscular dystrophy. Neurology 57, 115–121. doi: 10.1212/wnl.57.1.115
Heasman, S. J., and Ridley, A. J. (2008). Mammalian Rho GTPases: new insights into their functions from in vivo studies. Nat. Rev. Mol. Cell Biol. 9, 690–701. doi: 10.1038/nrm2476
Hebert, J. M., and Fishell, G. (2008). The genetics of early telencephalon patterning: some assembly required. Nat. Rev. Neurosci. 9, 678–685. doi: 10.1038/nrn2463
Henry, M. D., and Campbell, K. P. (1998). A role for dystroglycan in basement membrane assembly. Cell 95, 859–870. doi: 10.1016/s0092-8674(00)81708-0
Hodge, R. D., D’ercole, A. J., and O’kusky, J. R. (2004). Insulin-like growth factor-I accelerates the cell cycle by decreasing G1 phase length and increases cell cycle reentry in the embryonic cerebral cortex. J. Neurosci. 24, 10201–10210. doi: 10.1523/jneurosci.3246-04.2004
Hong, G. S., Lee, S. H., Lee, B., Choi, J. H., Oh, S. J., Jang, Y., et al. (2019). ANO1/TMEM16A regulates process maturation in radial glial cells in the developing brain. Proc. Natl. Acad. Sci. U.S.A. 116, 12494–12499. doi: 10.1073/pnas.1901067116
Hong, S. E., Shugart, Y. Y., Huang, D. T., Shahwan, S. A., Grant, P. E., Hourihane, J. O., et al. (2000). Autosomal recessive lissencephaly with cerebellar hypoplasia is associated with human RELN mutations. Nat. Genet. 26, 93–96. doi: 10.1038/79246
Hong, S. K., Haldin, C. E., Lawson, N. D., Weinstein, B. M., Dawid, I. B., and Hukriede, N. A. (2005). The zebrafish kohtalo/trap230 gene is required for the development of the brain, neural crest, and pronephric kidney. Proc. Natl. Acad. Sci. U.S.A. 102, 18473–18478. doi: 10.1073/pnas.0509457102
Horn, D., Siebert, E., Seidel, U., Rost, I., Mayer, K., Abou Jamra, R., et al. (2017). Biallelic COL3A1 mutations result in a clinical spectrum of specific structural brain anomalies and connective tissue abnormalities. Am. J. Med. Genet. A 173, 2534–2538. doi: 10.1002/ajmg.a.38345
Houlihan, S. L., Lanctot, A. A., Guo, Y., and Feng, Y. (2016). Upregulation of neurovascular communication through filamin abrogation promotes ectopic periventricular neurogenesis. eLife 5:e17823.
Hu, H., Li, J., Gagen, C. S., Gray, N. W., Zhang, Z., Qi, Y., et al. (2011). Conditional knockout of protein O-mannosyltransferase 2 reveals tissue-specific roles of O-mannosyl glycosylation in brain development. J. Comp. Neurol. 519, 1320–1337. doi: 10.1002/cne.22572
Huang, X., Liu, J., Ketova, T., Fleming, J. T., Grover, V. K., Cooper, M. K., et al. (2010). Transventricular delivery of Sonic hedgehog is essential to cerebellar ventricular zone development. Proc. Natl. Acad. Sci. U.S.A. 107, 8422–8427. doi: 10.1073/pnas.0911838107
Imai, F., Hirai, S., Akimoto, K., Koyama, H., Miyata, T., Ogawa, M., et al. (2006). Inactivation of aPKClambda results in the loss of adherens junctions in neuroepithelial cells without affecting neurogenesis in mouse neocortex. Development 133, 1735–1744. doi: 10.1242/dev.02330
Iwata, T., and Hevner, R. F. (2009). Fibroblast growth factor signaling in development of the cerebral cortex. Dev. Growth Differ. 51, 299–323. doi: 10.1111/j.1440-169x.2009.01104.x
Jae, L. T., Raaben, M., Riemersma, M., Van Beusekom, E., Blomen, V. A., Velds, A., et al. (2013). Deciphering the glycosylome of dystroglycanopathies using haploid screens for lassa virus entry. Science 340, 479–483. doi: 10.1126/science.1233675
Jaiswal, M. K., Agrawal, V., Katara, G. K., Pamarthy, S., Kulshrestha, A., Chaouat, G., et al. (2015). Male fertility and apoptosis in normal spermatogenesis are regulated by vacuolar-ATPase isoform a2. J. Reprod. Immunol. 112, 38–45. doi: 10.1016/j.jri.2015.07.003
Jeong, S. J., Li, S., Luo, R., Strokes, N., and Piao, X. (2012). Loss of Col3a1, the gene for Ehlers-Danlos syndrome type IV, results in neocortical dyslamination. PLoS One 7:e29767. doi: 10.1371/journal.pone.0029767
Jerber, J., Zaki, M. S., Al-Aama, J. Y., Rosti, R. O., Ben-Omran, T., Dikoglu, E., et al. (2016). Biallelic mutations in TMTC3, encoding a transmembrane and TPR-containing protein, lead to cobblestone lissencephaly. Am. J. Hum. Genet. 99, 1181–1189. doi: 10.1016/j.ajhg.2016.09.007
Johansson, P. A., Irmler, M., Acampora, D., Beckers, J., Simeone, A., and Gotz, M. (2013). The transcription factor Otx2 regulates choroid plexus development and function. Development 140, 1055–1066. doi: 10.1242/dev.090860
Kadowaki, M., Nakamura, S., Machon, O., Krauss, S., Radice, G. L., and Takeichi, M. (2007). N-cadherin mediates cortical organization in the mouse brain. Dev. Biol. 304, 22–33. doi: 10.1016/j.ydbio.2006.12.014
Kaiser, K., Gyllborg, D., Prochazka, J., Salasova, A., Kompanikova, P., Molina, F. L., et al. (2019). WNT5A is transported via lipoprotein particles in the cerebrospinal fluid to regulate hindbrain morphogenesis. Nat. Commun. 10:1498.
Kalebic, N., Gilardi, C., Stepien, B., Wilsch-Brauninger, M., Long, K. R., Namba, T., et al. (2019). Neocortical expansion due to increased proliferation of basal progenitors is linked to changes in their morphology. Cell Stem Cell 24, 535–550.e9.
Kalebic, N., and Huttner, W. B. (2020). Basal progenitor morphology and neocortex evolution. Trends Neurosci. doi: 10.1016/j.tins.2020.07.009 [Epub ahead of print].
Kang, W., Wong, L. C., Shi, S. H., and Hebert, J. M. (2009). The transition from radial glial to intermediate progenitor cell is inhibited by FGF signaling during corticogenesis. J. Neurosci. 29, 14571–14580. doi: 10.1523/jneurosci.3844-09.2009
Kawahara, G., Guyon, J. R., Nakamura, Y., and Kunkel, L. M. (2010). Zebrafish models for human FKRP muscular dystrophies. Hum. Mol. Genet. 19, 623–633. doi: 10.1093/hmg/ddp528
Kawauchi, T., Sekine, K., Shikanai, M., Chihama, K., Tomita, K., Kubo, K., et al. (2010). Rab GTPases-dependent endocytic pathways regulate neuronal migration and maturation through N-cadherin trafficking. Neuron 67, 588–602. doi: 10.1016/j.neuron.2010.07.007
Kielar, M., Tuy, F. P., Bizzotto, S., Lebrand, C., De Juan Romero, C., Poirier, K., et al. (2014). Mutations in Eml1 lead to ectopic progenitors and neuronal heterotopia in mouse and human. Nat. Neurosci. 17, 923–933. doi: 10.1038/nn.3729
Klaus, J., Kanton, S., Kyrousi, C., Ayo-Martin, A. C., Di Giaimo, R., Riesenberg, S., et al. (2019). Altered neuronal migratory trajectories in human cerebral organoids derived from individuals with neuronal heterotopia. Nat. Med. 25, 561–568. doi: 10.1038/s41591-019-0371-0
Kobayashi, K., Nakahori, Y., Miyake, M., Matsumura, K., Kondo-Iida, E., Nomura, Y., et al. (1998). An ancient retrotransposal insertion causes Fukuyama-type congenital muscular dystrophy. Nature 394, 388–392. doi: 10.1038/28653
Komada, M., Saitsu, H., Kinboshi, M., Miura, T., Shiota, K., and Ishibashi, M. (2008). Hedgehog signaling is involved in development of the neocortex. Development 135, 2717–2727. doi: 10.1242/dev.015891
Konno, D., Shioi, G., Shitamukai, A., Mori, A., Kiyonari, H., Miyata, T., et al. (2008). Neuroepithelial progenitors undergo LGN-dependent planar divisions to maintain self-renewability during mammalian neurogenesis. Nat. Cell Biol. 10, 93–101. doi: 10.1038/ncb1673
Kornak, U., Reynders, E., Dimopoulou, A., Van Reeuwijk, J., Fischer, B., Rajab, A., et al. (2008). Impaired glycosylation and cutis laxa caused by mutations in the vesicular H+-ATPase subunit ATP6V0A2. Nat. Genet. 40, 32–34. doi: 10.1038/ng.2007.45
Kriegstein, A. R., and Gotz, M. (2003). Radial glia diversity: a matter of cell fate. Glia 43, 37–43. doi: 10.1002/glia.10250
Kuivaniemi, H., and Tromp, G. (2019). Type III collagen (COL3A1): gene and protein structure, tissue distribution, and associated diseases. Gene 707, 151–171. doi: 10.1016/j.gene.2019.05.003
Kuwahara, A., Hirabayashi, Y., Knoepfler, P. S., Taketo, M. M., Sakai, J., Kodama, T., et al. (2010). Wnt signaling and its downstream target N-myc regulate basal progenitors in the developing neocortex. Development 137, 1035–1044. doi: 10.1242/dev.046417
Labelle-Dumais, C., Dilworth, D. J., Harrington, E. P., De Leau, M., Lyons, D., Kabaeva, Z., et al. (2011). COL4A1 mutations cause ocular dysgenesis, neuronal localization defects, and myopathy in mice and Walker-Warburg syndrome in humans. PLoS Genet. 7:e1002062. doi: 10.1371/journal.pgen.1002062
Lamus, F., Martin, C., Carnicero, E., Moro, J. A., Fernandez, J. M. F., Mano, A., et al. (2020). FGF2/EGF contributes to brain neuroepithelial precursor proliferation and neurogenesis in rat embryos: the involvement of embryonic cerebrospinal fluid. Dev. Dyn. 249, 141–153. doi: 10.1002/dvdy.135
Lange, L., Pagnamenta, A. T., Lise, S., Clasper, S., Stewart, H., Akha, E. S., et al. (2016). A de novo frameshift in HNRNPK causing a Kabuki-like syndrome with nodular heterotopia. Clin. Genet. 90, 258–262. doi: 10.1111/cge.12773
Larsen, I. S. B., Narimatsu, Y., Joshi, H. J., Siukstaite, L., Harrison, O. J., Brasch, J., et al. (2017). Discovery of an O-mannosylation pathway selectively serving cadherins and protocadherins. Proc. Natl. Acad. Sci. U.S.A. 114, 11163–11168. doi: 10.1073/pnas.1708319114
Lee, C. Y., Robinson, K. J., and Doe, C. Q. (2006). Lgl, Pins and aPKC regulate neuroblast self-renewal versus differentiation. Nature 439, 594–598. doi: 10.1038/nature04299
Lehtinen, M. K., Zappaterra, M. W., Chen, X., Yang, Y. J., Hill, A. D., Lun, M., et al. (2011). The cerebrospinal fluid provides a proliferative niche for neural progenitor cells. Neuron 69, 893–905. doi: 10.1016/j.neuron.2011.01.023
Li, S., Jin, Z., Koirala, S., Bu, L., Xu, L., Hynes, R. O., et al. (2008). GPR56 regulates pial basement membrane integrity and cortical lamination. J. Neurosci. 28, 5817–5826. doi: 10.1523/jneurosci.0853-08.2008
Li, W., Cogswell, C. A., and Loturco, J. J. (1998). Neuronal differentiation of precursors in the neocortical ventricular zone is triggered by BMP. J. Neurosci. 18, 8853–8862. doi: 10.1523/jneurosci.18-21-08853.1998
Lian, G., and Sheen, V. L. (2015). Cytoskeletal proteins in cortical development and disease: actin associated proteins in periventricular heterotopia. Front. Cell. Neurosci. 9:99. doi: 10.3389/fncel.2015.00099
Liegel, R. P., Handley, M. T., Ronchetti, A., Brown, S., Langemeyer, L., Linford, A., et al. (2013). Loss-of-function mutations in TBC1D20 cause cataracts and male infertility in blind sterile mice and Warburg micro syndrome in humans. Am. J. Hum. Genet. 93, 1001–1014. doi: 10.1016/j.ajhg.2013.10.011
Lillien, L., and Raphael, H. (2000). BMP and FGF regulate the development of EGF-responsive neural progenitor cells. Development 127, 4993–5005.
Liu, J., Ball, S. L., Yang, Y., Mei, P., Zhang, L., Shi, H., et al. (2006). A genetic model for muscle-eye-brain disease in mice lacking protein O-mannose 1,2-N-acetylglucosaminyltransferase (POMGnT1). Mech. Dev. 123, 228–240. doi: 10.1016/j.mod.2005.12.003
Liu, R., Yang, Y., Shen, J., Chen, H., Zhang, Q., Ba, R., et al. (2015). Fstl1 is involved in the regulation of radial glial scaffold development. Mol. Brain 8:53.
Liu, W. A., Chen, S., Li, Z., Lee, C. H., Mirzaa, G., Dobyns, W. B., et al. (2018). PARD3 dysfunction in conjunction with dynamic HIPPO signaling drives cortical enlargement with massive heterotopia. Genes Dev. 32, 763–780. doi: 10.1101/gad.313171.118
Liu, X., Wu, H., Byrne, M., Krane, S., and Jaenisch, R. (1997). Type III collagen is crucial for collagen I fibrillogenesis and for normal cardiovascular development. Proc. Natl. Acad. Sci. U.S.A. 94, 1852–1856. doi: 10.1073/pnas.94.5.1852
Long, K. R., and Huttner, W. B. (2019). How the extracellular matrix shapes neural development. Open Biol. 9:180216. doi: 10.1098/rsob.180216
Longman, C., Brockington, M., Torelli, S., Jimenez-Mallebrera, C., Kennedy, C., Khalil, N., et al. (2003). Mutations in the human LARGE gene cause MDC1D, a novel form of congenital muscular dystrophy with severe mental retardation and abnormal glycosylation of alpha-dystroglycan. Hum. Mol. Genet. 12, 2853–2861. doi: 10.1093/hmg/ddg307
Lopez-Bendito, G., Cautinat, A., Sanchez, J. A., Bielle, F., Flames, N., Garratt, A. N., et al. (2006). Tangential neuronal migration controls axon guidance: a role for neuregulin-1 in thalamocortical axon navigation. Cell 125, 127–142. doi: 10.1016/j.cell.2006.01.042
Louhivuori, L. M., Jansson, L., Turunen, P. M., Jantti, M. H., Nordstrom, T., Louhivuori, V., et al. (2015). Transient receptor potential channels and their role in modulating radial glial-neuronal interaction: a signaling pathway involving mGluR5. Stem Cells Dev 24, 701–713. doi: 10.1089/scd.2014.0209
Louhivuori, L. M., Turunen, P. M., Louhivuori, V., Yellapragada, V., Nordstrom, T., Uhlen, P., et al. (2018). Regulation of radial glial process growth by glutamate via mGluR5/TRPC3 and neuregulin/ErbB4. Glia 66, 94–107. doi: 10.1002/glia.23230
Loulier, K., Lathia, J. D., Marthiens, V., Relucio, J., Mughal, M. R., Tang, S. C., et al. (2009). beta1 integrin maintains integrity of the embryonic neocortical stem cell niche. PLoS Biol. 7:e1000176. doi: 10.1371/journal.pbio.1000176
Lowery, L. A., and Sive, H. (2009). Totally tubular: the mystery behind function and origin of the brain ventricular system. Bioessays 31, 446–458. doi: 10.1002/bies.200800207
Lu, M., Grove, E. A., and Miller, R. J. (2002). Abnormal development of the hippocampal dentate gyrus in mice lacking the CXCR4 chemokine receptor. Proc. Natl. Acad. Sci. U.S.A. 99, 7090–7095. doi: 10.1073/pnas.092013799
Lui, J. H., Nowakowski, T. J., Pollen, A. A., Javaherian, A., Kriegstein, A. R., and Oldham, M. C. (2014). Radial glia require PDGFD-PDGFRbeta signalling in human but not mouse neocortex. Nature 515, 264–268. doi: 10.1038/nature13973
Lun, M. P., Johnson, M. B., Broadbelt, K. G., Watanabe, M., Kang, Y. J., Chau, K. F., et al. (2015). Spatially heterogeneous choroid plexus transcriptomes encode positional identity and contribute to regional CSF production. J. Neurosci. 35, 4903–4916. doi: 10.1523/jneurosci.3081-14.2015
Luo, R., Jeong, S. J., Jin, Z., Strokes, N., Li, S., and Piao, X. (2011). G protein-coupled receptor 56 and collagen III, a receptor-ligand pair, regulates cortical development and lamination. Proc. Natl. Acad. Sci. U.S.A. 108, 12925–12930. doi: 10.1073/pnas.1104821108
Mabie, P. C., Mehler, M. F., and Kessler, J. A. (1999). Multiple roles of bone morphogenetic protein signaling in the regulation of cortical cell number and phenotype. J. Neurosci. 19, 7077–7088. doi: 10.1523/jneurosci.19-16-07077.1999
Maher, M. T., Flozak, A. S., Stocker, A. M., Chenn, A., and Gottardi, C. J. (2009). Activity of the beta-catenin phosphodestruction complex at cell-cell contacts is enhanced by cadherin-based adhesion. J. Cell Biol. 186, 219–228. doi: 10.1083/jcb.200811108
Maiden, S. L., and Hardin, J. (2011). The secret life of alpha-catenin: moonlighting in morphogenesis. J. Cell Biol. 195, 543–552. doi: 10.1083/jcb.201103106
Mairet-Coello, G., Tury, A., and Dicicco-Bloom, E. (2009). Insulin-like growth factor-1 promotes G(1)/S cell cycle progression through bidirectional regulation of cyclins and cyclin-dependent kinase inhibitors via the phosphatidylinositol 3-kinase/Akt pathway in developing rat cerebral cortex. J. Neurosci. 29, 775–788. doi: 10.1523/jneurosci.1700-08.2009
Manzini, M. C., Tambunan, D. E., Hill, R. S., Yu, T. W., Maynard, T. M., Heinzen, E. L., et al. (2012). Exome sequencing and functional validation in zebrafish identify GTDC2 mutations as a cause of Walker-Warburg syndrome. Am. J. Hum. Genet. 91, 541–547. doi: 10.1016/j.ajhg.2012.07.009
Marone, R., Hess, D., Dankort, D., Muller, W. J., Hynes, N. E., and Badache, A. (2004). Memo mediates ErbB2-driven cell motility. Nat. Cell Biol. 6, 515–522. doi: 10.1038/ncb1134
Martin, K., and Groves, A. K. (2006). Competence of cranial ectoderm to respond to Fgf signaling suggests a two-step model of otic placode induction. Development 133, 877–887. doi: 10.1242/dev.02267
Matsumoto, N., Tanaka, S., Horiike, T., Shinmyo, Y., and Kawasaki, H. (2020). A discrete subtype of neural progenitor crucial for cortical folding in the gyrencephalic mammalian brain. eLife 9:e54873.
Mecha, M., Rabadan, M. A., Pena-Melian, A., Valencia, M., Mondejar, T., and Blanco, M. J. (2008). Expression of TGF-betas in the embryonic nervous system: analysis of interbalance between isoforms. Dev. Dyn. 237, 1709–1717. doi: 10.1002/dvdy.21558
Meyers, E. A., and Kessler, J. A. (2017). TGF-beta family signaling in neural and neuronal differentiation, development, and function. Cold Spring Harb. Perspect. Biol. 9:a022244. doi: 10.1101/cshperspect.a022244
Michele, D. E., Barresi, R., Kanagawa, M., Saito, F., Cohn, R. D., Satz, J. S., et al. (2002). Post-translational disruption of dystroglycan-ligand interactions in congenital muscular dystrophies. Nature 418, 417–422. doi: 10.1038/nature00837
Milev, P., Maurel, P., Haring, M., Margolis, R. K., and Margolis, R. U. (1996). TAG-1/axonin-1 is a high-affinity ligand of neurocan, phosphacan/protein-tyrosine phosphatase-zeta/beta, and N-CAM. J. Biol. Chem. 271, 15716–15723. doi: 10.1074/jbc.271.26.15716
Miyamoto, Y., Sakane, F., and Hashimoto, K. (2015). N-cadherin-based adherens junction regulates the maintenance, proliferation, and differentiation of neural progenitor cells during development. Cell Adh. Migr. 9, 183–192. doi: 10.1080/19336918.2015.1005466
Moore, S. A., Saito, F., Chen, J., Michele, D. E., Henry, M. D., Messing, A., et al. (2002). Deletion of brain dystroglycan recapitulates aspects of congenital muscular dystrophy. Nature 418, 422–425. doi: 10.1038/nature00838
Munji, R. N., Choe, Y., Li, G., Siegenthaler, J. A., and Pleasure, S. J. (2011). Wnt signaling regulates neuronal differentiation of cortical intermediate progenitors. J. Neurosci. 31, 1676–1687. doi: 10.1523/jneurosci.5404-10.2011
Mutch, C. A., Schulte, J. D., Olson, E., and Chenn, A. (2010). Beta-catenin signaling negatively regulates intermediate progenitor population numbers in the developing cortex. PLoS One 5:e12376. doi: 10.1371/journal.pone.0012376
Najas, S., Pijuan, I., Esteve-Codina, A., Usieto, S., Martinez, J. D., Zwijsen, A., et al. (2020). A SMAD1/5-YAP signaling module drives radial glia self-amplification and growth of the developing cerebral cortex. Development 147:dev187005. doi: 10.1242/dev.187005
Nakagawa, N., Li, J., Yabuno-Nakagawa, K., Eom, T. Y., Cowles, M., Mapp, T., et al. (2017). APC sets the Wnt tone necessary for cerebral cortical progenitor development. Genes Dev. 31, 1679–1692. doi: 10.1101/gad.302679.117
Nakagawa, N., Plestant, C., Yabuno-Nakagawa, K., Li, J., Lee, J., Huang, C. W., et al. (2019). Memo1-mediated tiling of radial glial cells facilitates cerebral cortical development. Neuron 103:836–852.e5.
Nelson, A. D., Suzuki, M., and Svendsen, C. N. (2008). A high concentration of epidermal growth factor increases the growth and survival of neurogenic radial glial cells within human neurosphere cultures. Stem Cells 26, 348–355. doi: 10.1634/stemcells.2007-0299
Nelson, I., Stojkovic, T., Allamand, V., Leturcq, F., Becane, H. M., Babuty, D., et al. (2015). Laminin alpha2 deficiency-related muscular dystrophy mimicking emery-dreifuss and collagen VI related diseases. J. Neuromuscul. Dis. 2, 229–240. doi: 10.3233/jnd-150093
Nelson, W. J. (2008). Regulation of cell-cell adhesion by the cadherin-catenin complex. Biochem. Soc. Trans. 36, 149–155. doi: 10.1042/bst0360149
Newkirk, M. L., Rubenstein, K. J., Kim, J. Y., Labrecque, C. L., Airas, J., Taylor, C. A., et al. (2018). Analysis of MEMO1 binding specificity for ErbB2 using fluorescence polarization and molecular dynamics simulations. Biochemistry 57, 5169–5181. doi: 10.1021/acs.biochem.8b00582
Nickolls, A. R., and Bonnemann, C. G. (2018). The roles of dystroglycan in the nervous system: insights from animal models of muscular dystrophy. Dis. Model. Mech. 11:dmm035931. doi: 10.1242/dmm.035931
Numakawa, T., Odaka, H., and Adachi, N. (2018). Actions of brain-derived neurotrophin factor in the neurogenesis and neuronal function, and its involvement in the pathophysiology of brain diseases. Int. J. Mol. Sci. 19:3650. doi: 10.3390/ijms19113650
Oegema, R., Baillat, D., Schot, R., Van Unen, L. M., Brooks, A., Kia, S. K., et al. (2017). Human mutations in integrator complex subunits link transcriptome integrity to brain development. PLoS Genet. 13:e1006809. doi: 10.1371/journal.pgen.1006809
Okamoto, M., Namba, T., Shinoda, T., Kondo, T., Watanabe, T., Inoue, Y., et al. (2013). TAG-1-assisted progenitor elongation streamlines nuclear migration to optimize subapical crowding. Nat. Neurosci. 16, 1556–1566. doi: 10.1038/nn.3525
Oliveira, J., Gruber, A., Cardoso, M., Taipa, R., Fineza, I., Goncalves, A., et al. (2018). LAMA2 gene mutation update: toward a more comprehensive picture of the laminin-alpha2 variome and its related phenotypes. Hum. Mutat. 39, 1314–1337. doi: 10.1002/humu.23599
Ortega, J. A., and Alcantara, S. (2010). BDNF/MAPK/ERK-induced BMP7 expression in the developing cerebral cortex induces premature radial glia differentiation and impairs neuronal migration. Cereb. Cortex 20, 2132–2144. doi: 10.1093/cercor/bhp275
Paavola, K. J., Stephenson, J. R., Ritter, S. L., Alter, S. P., and Hall, R. A. (2011). The N terminus of the adhesion G protein-coupled receptor GPR56 controls receptor signaling activity. J. Biol. Chem. 286, 28914–28921. doi: 10.1074/jbc.m111.247973
Paek, H., Gutin, G., and Hebert, J. M. (2009). FGF signaling is strictly required to maintain early telencephalic precursor cell survival. Development 136, 2457–2465. doi: 10.1242/dev.032656
Palma, V., Ruiz, I., and Altaba, A. (2004). Hedgehog-GLI signaling regulates the behavior of cells with stem cell properties in the developing neocortex. Development 131, 337–345. doi: 10.1242/dev.00930
Panchision, D. M., Pickel, J. M., Studer, L., Lee, S. H., Turner, P. A., Hazel, T. G., et al. (2001). Sequential actions of BMP receptors control neural precursor cell production and fate. Genes Dev. 15, 2094–2110. doi: 10.1101/gad.894701
Paredes, M. F., Li, G., Berger, O., Baraban, S. C., and Pleasure, S. J. (2006). Stromal-derived factor-1 (CXCL12) regulates laminar position of Cajal-Retzius cells in normal and dysplastic brains. J. Neurosci. 26, 9404–9412. doi: 10.1523/jneurosci.2575-06.2006
Penisson, M., Ladewig, J., Belvindrah, R., and Francis, F. (2019). Genes and mechanisms involved in the generation and amplification of basal radial glial cells. Front. Cell. Neurosci. 13:381. doi: 10.3389/fncel.2019.00381
Piao, X., Hill, R. S., Bodell, A., Chang, B. S., Basel-Vanagaite, L., Straussberg, R., et al. (2004). G protein-coupled receptor-dependent development of human frontal cortex. Science 303, 2033–2036. doi: 10.1126/science.1092780
Pinson, K. I., Brennan, J., Monkley, S., Avery, B. J., and Skarnes, W. C. (2000). An LDL-receptor-related protein mediates Wnt signalling in mice. Nature 407, 535–538. doi: 10.1038/35035124
Pokutta, S., Drees, F., Yamada, S., Nelson, W. J., and Weis, W. I. (2008). Biochemical and structural analysis of alpha-catenin in cell-cell contacts. Biochem. Soc. Trans. 36, 141–147. doi: 10.1042/bst0360141
Pollen, A. A., Nowakowski, T. J., Chen, J., Retallack, H., Sandoval-Espinosa, C., Nicholas, C. R., et al. (2015). Molecular identity of human outer radial glia during cortical development. Cell 163, 55–67. doi: 10.1016/j.cell.2015.09.004
Poluch, S., and Juliano, S. L. (2010). Populations of radial glial cells respond differently to reelin and neuregulin1 in a ferret model of cortical dysplasia. PLoS One 5:e13709. doi: 10.1371/journal.pone.0013709
Popken, G. J., Hodge, R. D., Ye, P., Zhang, J., Ng, W., O’kusky, J. R., et al. (2004). In vivo effects of insulin-like growth factor-I (IGF-I) on prenatal and early postnatal development of the central nervous system. Eur. J. Neurosci. 19, 2056–2068. doi: 10.1111/j.0953-816x.2004.03320.x
Proepper, C., Steinestel, K., Schmeisser, M. J., Heinrich, J., Steinestel, J., Bockmann, J., et al. (2011). Heterogeneous nuclear ribonucleoprotein k interacts with Abi-1 at postsynaptic sites and modulates dendritic spine morphology. PLoS One 6:e27045. doi: 10.1371/journal.pone.0027045
Raballo, R., Rhee, J., Lyn-Cook, R., Leckman, J. F., Schwartz, M. L., and Vaccarino, F. M. (2000). Basic fibroblast growth factor (Fgf2) is necessary for cell proliferation and neurogenesis in the developing cerebral cortex. J. Neurosci. 20, 5012–5023. doi: 10.1523/jneurosci.20-13-05012.2000
Racape, M., Duong Van, Huyen, J. P., Danger, R., Giral, M., Bleicher, F., et al. (2011). The involvement of SMILE/TMTC3 in endoplasmic reticulum stress response. PLoS One 6:e19321. doi: 10.1371/journal.pone.0019321
Radakovits, R., Barros, C. S., Belvindrah, R., Patton, B., and Muller, U. (2009). Regulation of radial glial survival by signals from the meninges. J. Neurosci. 29, 7694–7705. doi: 10.1523/jneurosci.5537-08.2009
Radmanesh, F., Caglayan, A. O., Silhavy, J. L., Yilmaz, C., Cantagrel, V., Omar, T., et al. (2013). Mutations in LAMB1 cause cobblestone brain malformation without muscular or ocular abnormalities. Am. J. Hum. Genet. 92, 468–474. doi: 10.1016/j.ajhg.2013.02.005
Radner, S., Banos, C., Bachay, G., Li, Y. N., Hunter, D. D., Brunken, W. J., et al. (2013). beta2 and gamma3 laminins are critical cortical basement membrane components: ablation of Lamb2 and Lamc3 genes disrupts cortical lamination and produces dysplasia. Dev. Neurobiol. 73, 209–229. doi: 10.1002/dneu.22057
Rakic, P. (1972). Mode of cell migration to the superficial layers of fetal monkey neocortex. J. Comp. Neurol. 145, 61–83. doi: 10.1002/cne.901450105
Rash, B. G., Ackman, J. B., and Rakic, P. (2016). Bidirectional radial Ca(2+) activity regulates neurogenesis and migration during early cortical column formation. Sci. Adv. 2:e1501733. doi: 10.1126/sciadv.1501733
Rash, B. G., and Grove, E. A. (2006). Area and layer patterning in the developing cerebral cortex. Curr. Opin. Neurobiol. 16, 25–34. doi: 10.1016/j.conb.2006.01.004
Rash, B. G., Tomasi, S., Lim, H. D., Suh, C. Y., and Vaccarino, F. M. (2013). Cortical gyrification induced by fibroblast growth factor 2 in the mouse brain. J. Neurosci. 33, 10802–10814. doi: 10.1523/jneurosci.3621-12.2013
Rasin, M. R., Gazula, V. R., Breunig, J. J., Kwan, K. Y., Johnson, M. B., Liu-Chen, S., et al. (2007). Numb and Numbl are required for maintenance of cadherin-based adhesion and polarity of neural progenitors. Nat. Neurosci. 10, 819–827. doi: 10.1038/nn1924
Roscioli, T., Kamsteeg, E. J., Buysse, K., Maystadt, I., Van Reeuwijk, J., Van Den Elzen, C., et al. (2012). Mutations in ISPD cause Walker-Warburg syndrome and defective glycosylation of alpha-dystroglycan. Nat. Genet. 44, 581–585.
Rousso, D. L., Pearson, C. A., Gaber, Z. B., Miquelajauregui, A., Li, S., Portera-Cailliau, C., et al. (2012). Foxp-mediated suppression of N-cadherin regulates neuroepithelial character and progenitor maintenance in the CNS. Neuron 74, 314–330. doi: 10.1016/j.neuron.2012.02.024
Roy, A., Murphy, R. M., Deng, M., Macdonald, J. W., Bammler, T. K., Aldinger, K. A., et al. (2019). PI3K-Yap activity drives cortical gyrification and hydrocephalus in mice. eLife 8:e45961.
Sahara, S., and O’Leary, D. D. (2009). Fgf10 regulates transition period of cortical stem cell differentiation to radial glia controlling generation of neurons and basal progenitors. Neuron 63, 48–62. doi: 10.1016/j.neuron.2009.06.006
Salehi, Z., Mashayekhi, F., Naji, M., and Pandamooz, S. (2009). Insulin-like growth factor-1 and insulin-like growth factor binding proteins in cerebrospinal fluid during the development of mouse embryos. J. Clin. Neurosci. 16, 950–953. doi: 10.1016/j.jocn.2008.09.018
Sarkisian, M. R., and Guadiana, S. M. (2015). Influences of primary cilia on cortical morphogenesis and neuronal subtype maturation. Neuroscientist 21, 136–151. doi: 10.1177/1073858414531074
Sasaki, J., Ishikawa, K., Kobayashi, K., Kondo-Iida, E., Fukayama, M., Mizusawa, H., et al. (2000). Neuronal expression of the fukutin gene. Hum. Mol. Genet. 9, 3083–3090. doi: 10.1093/hmg/9.20.3083
Schaefer, A., Poluch, S., and Juliano, S. (2008). Reelin is essential for neuronal migration but not for radial glial elongation in neonatal ferret cortex. Dev. Neurobiol. 68, 590–604. doi: 10.1002/dneu.20601
Schaffer, A. E., Breuss, M. W., Caglayan, A. O., Al-Sanaa, N., Al-Abdulwahed, H. Y., Kaymakcalan, H., et al. (2018). Biallelic loss of human CTNNA2, encoding alphaN-catenin, leads to ARP2/3 complex overactivity and disordered cortical neuronal migration. Nat. Genet. 50, 1093–1101. doi: 10.1038/s41588-018-0166-0
Schmid, R. S., Mcgrath, B., Berechid, B. E., Boyles, B., Marchionni, M., Sestan, N., et al. (2003). Neuregulin 1-erbB2 signaling is required for the establishment of radial glia and their transformation into astrocytes in cerebral cortex. Proc. Natl. Acad. Sci. U.S.A. 100, 4251–4256. doi: 10.1073/pnas.0630496100
Segklia, A., Seuntjens, E., Elkouris, M., Tsalavos, S., Stappers, E., Mitsiadis, T. A., et al. (2012). Bmp7 regulates the survival, proliferation, and neurogenic properties of neural progenitor cells during corticogenesis in the mouse. PLoS One 7:e34088. doi: 10.1371/journal.pone.0034088
Sekine, K., Kubo, K., and Nakajima, K. (2014). How does Reelin control neuronal migration and layer formation in the developing mammalian neocortex? Neurosci. Res. 86, 50–58. doi: 10.1016/j.neures.2014.06.004
Shaheen, R., Faqeih, E., Ansari, S., and Alkuraya, F. S. (2013). A truncating mutation in B3GNT1 causes severe Walker-Warburg syndrome. Neurogenetics 14, 243–245. doi: 10.1007/s10048-013-0367-8
Sheen, V. L., Ganesh, V. S., Topcu, M., Sebire, G., Bodell, A., Hill, R. S., et al. (2004). Mutations in ARFGEF2 implicate vesicle trafficking in neural progenitor proliferation and migration in the human cerebral cortex. Nat. Genet. 36, 69–76. doi: 10.1038/ng1276
Shikanai, M., Nakajima, K., and Kawauchi, T. (2011). N-cadherin regulates radial glial fiber-dependent migration of cortical locomoting neurons. Commun. Integr. Biol. 4, 326–330. doi: 10.4161/cib.4.3.14886
Siegenthaler, J. A., Ashique, A. M., Zarbalis, K., Patterson, K. P., Hecht, J. H., Kane, M. A., et al. (2009). Retinoic acid from the meninges regulates cortical neuron generation. Cell 139, 597–609. doi: 10.1016/j.cell.2009.10.004
Sittaramane, V., Sawant, A., Wolman, M. A., Maves, L., Halloran, M. C., and Chandrasekhar, A. (2009). The cell adhesion molecule Tag1, transmembrane protein Stbm/Vangl2, and Lamininalpha1 exhibit genetic interactions during migration of facial branchiomotor neurons in zebrafish. Dev. Biol. 325, 363–373. doi: 10.1016/j.ydbio.2008.10.030
Sklan, E. H., Serrano, R. L., Einav, S., Pfeffer, S. R., Lambright, D. G., and Glenn, J. S. (2007). TBC1D20 is a Rab1 GTPase-activating protein that mediates hepatitis C virus replication. J. Biol. Chem. 282, 36354–36361. doi: 10.1074/jbc.m705221200
Squier, W., and Jansen, A. (2014). Polymicrogyria: pathology, fetal origins and mechanisms. Acta Neuropathol. Commun. 2:80.
Stenzel, D., Wilsch-Brauninger, M., Wong, F. K., Heuer, H., and Huttner, W. B. (2014). Integrin alphavbeta3 and thyroid hormones promote expansion of progenitors in embryonic neocortex. Development 141, 795–806. doi: 10.1242/dev.101907
Stevens, E., Carss, K. J., Cirak, S., Foley, A. R., Torelli, S., Willer, T., et al. (2013). Mutations in B3GALNT2 cause congenital muscular dystrophy and hypoglycosylation of alpha-dystroglycan. Am. J. Hum. Genet. 92, 354–365. doi: 10.1016/j.ajhg.2013.01.016
Stipursky, J., Francis, D., Dezonne, R. S., Bergamo, De Araujo, A. P., Souza, L., et al. (2014). TGF-beta1 promotes cerebral cortex radial glia-astrocyte differentiation in vivo. Front. Cell. Neurosci. 8:393. doi: 10.3389/fncel.2014.00393
Stipursky, J., Francis, D., and Gomes, F. C. (2012). Activation of MAPK/PI3K/SMAD pathways by TGF-beta(1) controls differentiation of radial glia into astrocytes in vitro. Dev. Neurosci. 34, 68–81. doi: 10.1159/000338108
Stouffer, M. A., Golden, J. A., and Francis, F. (2016). Neuronal migration disorders: focus on the cytoskeleton and epilepsy. Neurobiol. Dis. 92, 18–45. doi: 10.1016/j.nbd.2015.08.003
Sun, T., Wang, X. J., Xie, S. S., Zhang, D. L., Wang, X. P., Li, B. Q., et al. (2011). A comparison of proliferative capacity and passaging potential between neural stem and progenitor cells in adherent and neurosphere cultures. Int. J. Dev. Neurosci. 29, 723–731. doi: 10.1016/j.ijdevneu.2011.05.012
Sun, Y., Goderie, S. K., and Temple, S. (2005). Asymmetric distribution of EGFR receptor during mitosis generates diverse CNS progenitor cells. Neuron 45, 873–886. doi: 10.1016/j.neuron.2005.01.045
Takeda, S., Kondo, M., Sasaki, J., Kurahashi, H., Kano, H., Arai, K., et al. (2003). Fukutin is required for maintenance of muscle integrity, cortical histiogenesis and normal eye development. Hum. Mol. Genet. 12, 1449–1459. doi: 10.1093/hmg/ddg153
Tavano, S., Taverna, E., Kalebic, N., Haffner, C., Namba, T., Dahl, A., et al. (2018). Insm1 induces neural progenitor delamination in developing neocortex via downregulation of the adherens junction belt-specific protein Plekha7. Neuron 97, 1299–1314.e8.
Taverna, E., Gotz, M., and Huttner, W. B. (2014). The cell biology of neurogenesis: toward an understanding of the development and evolution of the neocortex. Annu. Rev. Cell Dev. Biol. 30, 465–502. doi: 10.1146/annurev-cellbio-101011-155801
Telley, L., Agirman, G., Prados, J., Amberg, N., Fievre, S., Oberst, P., et al. (2019). Temporal patterning of apical progenitors and their daughter neurons in the developing neocortex. Science 364:eaav2522. doi: 10.1126/science.aav2522
Thomas, T., Voss, A. K., Chowdhury, K., and Gruss, P. (2000). Querkopf, a MYST family histone acetyltransferase, is required for normal cerebral cortex development. Development 127, 2537–2548.
Tonduti, D., Dorboz, I., Renaldo, F., Masliah-Planchon, J., Elmaleh-Berges, M., Dalens, H., et al. (2015). Cystic leukoencephalopathy with cortical dysplasia related to LAMB1 mutations. Neurology 84, 2195–2197. doi: 10.1212/wnl.0000000000001607
Trommsdorff, M., Gotthardt, M., Hiesberger, T., Shelton, J., Stockinger, W., Nimpf, J., et al. (1999). Reeler/Disabled-like disruption of neuronal migration in knockout mice lacking the VLDL receptor and ApoE receptor 2. Cell 97, 689–701. doi: 10.1016/s0092-8674(00)80782-5
Trujillo-Gonzalez, I., Wang, Y., Friday, W. B., Vickers, K. C., Toth, C. L., Molina-Torres, L., et al. (2019). MicroRNA-129-5p is regulated by choline availability and controls EGF receptor synthesis and neurogenesis in the cerebral cortex. FASEB J. 33, 3601–3612. doi: 10.1096/fj.201801094rr
Uzquiano, A., Cifuentes-Diaz, C., Jabali, A., Romero, D. M., Houllier, A., Dingli, F., et al. (2019). Mutations in the Heterotopia Gene Eml1/EML1 severely disrupt the formation of primary cilia. Cell Rep. 28, 1596–1611.e10.
Uzquiano, A., Gladwyn-Ng, I., Nguyen, L., Reiner, O., Gotz, M., Matsuzaki, F., et al. (2018). Cortical progenitor biology: key features mediating proliferation versus differentiation. J. Neurochem. 146, 500–525. doi: 10.1111/jnc.14338
Vaccarino, F. M., Schwartz, M. L., Raballo, R., Nilsen, J., Rhee, J., Zhou, M., et al. (1999). Changes in cerebral cortex size are governed by fibroblast growth factor during embryogenesis. Nat. Neurosci. 2, 246–253. doi: 10.1038/6350
Valence, S., Garel, C., Barth, M., Toutain, A., Paris, C., Amsallem, D., et al. (2016). RELN and VLDLR mutations underlie two distinguishable clinico-radiological phenotypes. Clin. Genet. 90, 545–549. doi: 10.1111/cge.12779
Van Maldergem, L., Yuksel-Apak, M., Kayserili, H., Seemanova, E., Giurgea, S., Basel-Vanagaite, L., et al. (2008). Cobblestone-like brain dysgenesis and altered glycosylation in congenital cutis laxa, Debre type. Neurology 71, 1602–1608. doi: 10.1212/01.wnl.0000327822.52212.c7
Van Reeuwijk, J., Grewal, P. K., Salih, M. A., Beltran-Valero, De Bernabe, D., Mclaughlan, J. M., et al. (2007). Intragenic deletion in the LARGE gene causes Walker-Warburg syndrome. Hum. Genet. 121, 685–690. doi: 10.1007/s00439-007-0362-y
Van Reeuwijk, J., Janssen, M., Van Den Elzen, C., Beltran-Valero, De Bernabe, D., Sabatelli, P., et al. (2005). POMT2 mutations cause alpha-dystroglycan hypoglycosylation and Walker-Warburg syndrome. J. Med. Genet. 42, 907–912. doi: 10.1136/jmg.2005.031963
Vandervore, L., Stouffs, K., Tanyalcin, I., Vanderhasselt, T., Roelens, F., Holder-Espinasse, M., et al. (2017). Bi-allelic variants in COL3A1 encoding the ligand to GPR56 are associated with cobblestone-like cortical malformation, white matter changes and cerebellar cysts. J. Med. Genet. 54, 432–440. doi: 10.1136/jmedgenet-2016-104421
Veeraval, L., O’leary, C. J., and Cooper, H. M. (2020). Adherens junctions: guardians of cortical development. Front. Cell Dev. Biol. 8:6. doi: 10.3389/fcell.2020.00006
Vogel, P., Read, R. W., Hansen, G. M., Payne, B. J., Small, D., Sands, A. T., et al. (2012). Congenital hydrocephalus in genetically engineered mice. Vet. Pathol. 49, 166–181. doi: 10.1177/0300985811415708
Von Renesse, A., Petkova, M. V., Lutzkendorf, S., Heinemeyer, J., Gill, E., Hubner, C., et al. (2014). POMK mutation in a family with congenital muscular dystrophy with merosin deficiency, hypomyelination, mild hearing deficit and intellectual disability. J. Med. Genet. 51, 275–282. doi: 10.1136/jmedgenet-2013-102236
Vuillaumier-Barrot, S., Bouchet-Seraphin, C., Chelbi, M., Devisme, L., Quentin, S., Gazal, S., et al. (2012). Identification of mutations in TMEM5 and ISPD as a cause of severe cobblestone lissencephaly. Am. J. Hum. Genet. 91, 1135–1143. doi: 10.1016/j.ajhg.2012.10.009
Wang, J., and Yu, R. K. (2013). Interaction of ganglioside GD3 with an EGF receptor sustains the self-renewal ability of mouse neural stem cells in vitro. Proc. Natl. Acad. Sci. U.S.A. 110, 19137–19142. doi: 10.1073/pnas.1307224110
Wang, L., Hou, S., and Han, Y. G. (2016). Hedgehog signaling promotes basal progenitor expansion and the growth and folding of the neocortex. Nat. Neurosci. 19, 888–896. doi: 10.1038/nn.4307
Wang, P. S., Chou, F. S., Ramachandran, S., Xia, S., Chen, H. Y., Guo, F., et al. (2016). Crucial roles of the Arp2/3 complex during mammalian corticogenesis. Development 143, 2741–2752. doi: 10.1242/dev.130542
Wang, X., Yang, N., Uno, E., Roeder, R. G., and Guo, S. (2006). A subunit of the mediator complex regulates vertebrate neuronal development. Proc. Natl. Acad. Sci. U.S.A. 103, 17284–17289. doi: 10.1073/pnas.0605414103
Wang, Y., Chang, H., Rattner, A., and Nathans, J. (2016). Frizzled receptors in development and disease. Curr. Top. Dev. Biol. 117, 113–139. doi: 10.1016/bs.ctdb.2015.11.028
Weiss, K. H., Johanssen, C., Tielsch, A., Herz, J., Deller, T., Frotscher, M., et al. (2003). Malformation of the radial glial scaffold in the dentate gyrus of reeler mice, scrambler mice, and ApoER2/VLDLR-deficient mice. J. Comp. Neurol. 460, 56–65. doi: 10.1002/cne.10644
Willer, T., Amselgruber, W., Deutzmann, R., and Strahl, S. (2002). Characterization of POMT2, a novel member of the PMT protein O-mannosyltransferase family specifically localized to the acrosome of mammalian spermatids. Glycobiology 12, 771–783. doi: 10.1093/glycob/cwf086
Willer, T., Prados, B., Falcon-Perez, J. M., Renner-Muller, I., Przemeck, G. K., Lommel, M., et al. (2004). Targeted disruption of the Walker-Warburg syndrome gene Pomt1 in mouse results in embryonic lethality. Proc. Natl. Acad. Sci. U.S.A. 101, 14126–14131. doi: 10.1073/pnas.0405899101
Williamson, R. A., Henry, M. D., Daniels, K. J., Hrstka, R. F., Lee, J. C., Sunada, Y., et al. (1997). Dystroglycan is essential for early embryonic development: disruption of Reichert’s membrane in Dag1-null mice. Hum. Mol. Genet. 6, 831–841. doi: 10.1093/hmg/6.6.831
Wilson, S. L., Wilson, J. P., Wang, C., Wang, B., and Mcconnell, S. K. (2012). Primary cilia and Gli3 activity regulate cerebral cortical size. Dev. Neurobiol. 72, 1196–1212. doi: 10.1002/dneu.20985
Woodhead, G. J., Mutch, C. A., Olson, E. C., and Chenn, A. (2006). Cell-autonomous beta-catenin signaling regulates cortical precursor proliferation. J. Neurosci. 26, 12620–12630. doi: 10.1523/jneurosci.3180-06.2006
Wong, G. K., Baudet, M. L., Norden, C., Leung, L., and Harris, W. A. (2012). Slit1b-Robo3 signaling and N-cadherin regulate apical process retraction in developing retinal ganglion cells. J. Neurosci. 32, 223–228. doi: 10.1523/JNEUROSCI.2596-11.2012
Wright, K. M., Lyon, K. A., Leung, H., Leahy, D. J., Ma, L., and Ginty, D. D. (2012). Dystroglycan organizes axon guidance cue localization and axonal pathfinding. Neuron 76, 931–944. doi: 10.1016/j.neuron.2012.10.009
Wrobel, C. N., Mutch, C. A., Swaminathan, S., Taketo, M. M., and Chenn, A. (2007). Persistent expression of stabilized beta-catenin delays maturation of radial glial cells into intermediate progenitors. Dev. Biol. 309, 285–297. doi: 10.1016/j.ydbio.2007.07.013
Yabut, O. R., Fernandez, G., Huynh, T., Yoon, K., and Pleasure, S. J. (2015). Suppressor of fused is critical for maintenance of neuronal progenitor identity during corticogenesis. Cell Rep. 12, 2021–2034. doi: 10.1016/j.celrep.2015.08.031
Yabut, O. R., Ng, H. X., Yoon, K., Arela, J. C., Ngo, T., and Pleasure, S. J. (2020). The neocortical progenitor specification program is established through combined modulation of SHH and FGF signaling. J. Neurosci. 40, 6872–6887. doi: 10.1523/jneurosci.2888-19.2020
Yagi, H., Nakagawa, N., Saito, T., Kiyonari, H., Abe, T., Toda, T., et al. (2013). AGO61-dependent GlcNAc modification primes the formation of functional glycans on alpha-dystroglycan. Sci. Rep. 3:3288.
Yamada, M., and Sekiguchi, K. (2015). Molecular basis of laminin-integrin interactions. Curr. Top. Membr. 76, 197–229. doi: 10.1016/bs.ctm.2015.07.002
Yamamoto, H., Mandai, K., Konno, D., Maruo, T., Matsuzaki, F., and Takai, Y. (2015). Impairment of radial glial scaffold-dependent neuronal migration and formation of double cortex by genetic ablation of afadin. Brain Res. 1620, 139–152. doi: 10.1016/j.brainres.2015.05.012
Yamanaka, T., Horikoshi, Y., Sugiyama, Y., Ishiyama, C., Suzuki, A., Hirose, T., et al. (2003). Mammalian Lgl forms a protein complex with PAR-6 and aPKC independently of PAR-3 to regulate epithelial cell polarity. Curr. Biol. 13, 734–743. doi: 10.1016/s0960-9822(03)00244-6
Yeh, C., Li, A., Chuang, J. Z., Saito, M., Caceres, A., and Sung, C. H. (2013). IGF-1 activates a cilium-localized noncanonical Gbetagamma signaling pathway that regulates cell-cycle progression. Dev. Cell 26, 358–368. doi: 10.1016/j.devcel.2013.07.014
Yokota, Y., Eom, T. Y., Stanco, A., Kim, W. Y., Rao, S., Snider, W. D., et al. (2010). Cdc42 and Gsk3 modulate the dynamics of radial glial growth, inter-radial glial interactions and polarity in the developing cerebral cortex. Development 137, 4101–4110. doi: 10.1242/dev.048637
Yokota, Y., Kim, W. Y., Chen, Y., Wang, X., Stanco, A., Komuro, Y., et al. (2009). The adenomatous polyposis coli protein is an essential regulator of radial glial polarity and construction of the cerebral cortex. Neuron 61, 42–56. doi: 10.1016/j.neuron.2008.10.053
Yoon, K. J., Koo, B. K., Im, S. K., Jeong, H. W., Ghim, J., Kwon, M. C., et al. (2008). Mind bomb 1-expressing intermediate progenitors generate notch signaling to maintain radial glial cells. Neuron 58, 519–531. doi: 10.1016/j.neuron.2008.03.018
Yoshida, A., Kobayashi, K., Manya, H., Taniguchi, K., Kano, H., Mizuno, M., et al. (2001). Muscular dystrophy and neuronal migration disorder caused by mutations in a glycosyltransferase, POMGnT1. Dev. Cell 1, 717–724. doi: 10.1016/s1534-5807(01)00070-3
Yoshida-Moriguchi, T., Willer, T., Anderson, M. E., Venzke, D., Whyte, T., Muntoni, F., et al. (2013). SGK196 is a glycosylation-specific O-mannose kinase required for dystroglycan function. Science 341, 896–899. doi: 10.1126/science.1239951
Yun, E. J., and Vu, T. H. (2012). mSmile is necessary for bronchial smooth muscle and alveolar myofibroblast development. Anat. Rec. 295, 167–176. doi: 10.1002/ar.21475
Zagaglia, S., Selch, C., Nisevic, J. R., Mei, D., Michalak, Z., Hernandez-Hernandez, L., et al. (2018). Neurologic phenotypes associated with COL4A1/2 mutations: expanding the spectrum of disease. Neurology 91, e2078–e2088.
Zambonin, J. L., Dyment, D. A., Xi, Y., Lamont, R. E., Hartley, T., Miller, E., et al. (2018). A novel mutation in LAMC3 associated with generalized polymicrogyria of the cortex and epilepsy. Neurogenetics 19, 61–65. doi: 10.1007/s10048-017-0534-4
Zappaterra, M. D., Lisgo, S. N., Lindsay, S., Gygi, S. P., Walsh, C. A., and Ballif, B. A. (2007). A comparative proteomic analysis of human and rat embryonic cerebrospinal fluid. J. Proteome Res. 6, 3537–3548. doi: 10.1021/pr070247w
Zappaterra, M. W., and Lehtinen, M. K. (2012). The cerebrospinal fluid: regulator of neurogenesis, behavior, and beyond. Cell. Mol. Life Sci. 69, 2863–2878. doi: 10.1007/s00018-012-0957-x
Zhang, J., Shemezis, J. R., Mcquinn, E. R., Wang, J., Sverdlov, M., and Chenn, A. (2013). AKT activation by N-cadherin regulates beta-catenin signaling and neuronal differentiation during cortical development. Neural Dev. 8:7. doi: 10.1186/1749-8104-8-7
Zhang, J., Woodhead, G. J., Swaminathan, S. K., Noles, S. R., Mcquinn, E. R., Pisarek, A. J., et al. (2010). Cortical neural precursors inhibit their own differentiation via N-cadherin maintenance of beta-catenin signaling. Dev. Cell 18, 472–479. doi: 10.1016/j.devcel.2009.12.025
Zhang, T., Zhang, S., Song, X., Zhao, X., Hou, C., Li, Z., et al. (2019). Loss of Lgl1 disrupts the radial glial fiber-guided cortical neuronal migration and causes subcortical band heterotopia in mice. Neuroscience 400, 132–145. doi: 10.1016/j.neuroscience.2018.12.039
Zhang, W., Yi, M. J., Chen, X., Cole, F., Krauss, R. S., and Kang, J. S. (2006). Cortical thinning and hydrocephalus in mice lacking the immunoglobulin superfamily member CDO. Mol. Cell. Biol. 26, 3764–3772. doi: 10.1128/mcb.26.10.3764-3772.2006
Zhao, S., Chai, X., Forster, E., and Frotscher, M. (2004). Reelin is a positional signal for the lamination of dentate granule cells. Development 131, 5117–5125. doi: 10.1242/dev.01387
Zhou, C. J., Zhao, C., and Pleasure, S. J. (2004). Wnt signaling mutants have decreased dentate granule cell production and radial glial scaffolding abnormalities. J. Neurosci. 24, 121–126. doi: 10.1523/jneurosci.4071-03.2004
Keywords: apical radial glia, cortical development, neuronal migration, scaffold, cell-cell interaction, cell signaling, extracellular matrix
Citation: Ferent J, Zaidi D and Francis F (2020) Extracellular Control of Radial Glia Proliferation and Scaffolding During Cortical Development and Pathology. Front. Cell Dev. Biol. 8:578341. doi: 10.3389/fcell.2020.578341
Received: 30 June 2020; Accepted: 08 September 2020;
Published: 16 October 2020.
Edited by:
Victor Borrell, Instituto de Neurociencias, CSIC − UMH, SpainReviewed by:
Simone Diestel, University of Bonn, GermanySamuel Pleasure, University of California, San Francisco, United States
Hidenori Tabata, Aichi Human Service Center, Japan
Copyright © 2020 Ferent, Zaidi and Francis. This is an open-access article distributed under the terms of the Creative Commons Attribution License (CC BY). The use, distribution or reproduction in other forums is permitted, provided the original author(s) and the copyright owner(s) are credited and that the original publication in this journal is cited, in accordance with accepted academic practice. No use, distribution or reproduction is permitted which does not comply with these terms.
*Correspondence: Fiona Francis, RmlvbmEuRnJhbmNpc0BpbnNlcm0uZnI=