Molecular and Functional Study of Transient Receptor Potential Vanilloid 1-4 at the Rat and Human Blood–Brain Barrier Reveals Interspecies Differences
- 1Faculté de Pharmacie, Inserm, UMRS-1144, Optimisation Thérapeutique en Neuropsychopharmacologie, Université de Paris, Paris, France
- 2Department of Chemical and Biological Engineering, University of Wisconsin-Madison, Madison, WI, United States
- 3BrainPlotting, Paris, France
- 4Service Pharmacie, Assistance Publique Hôpitaux de Paris (AP-HP), Hôpital Universitaire Necker – Enfants Malades, Paris, France
- 5Biologie du médicament et toxicologie, Assistance Publique Hôpitaux de Paris (AP-HP), Hôpital Universitaire Cochin, Paris, France
Transient receptor potential vanilloid 1-4 (TRPV1-4) expression and functionality were investigated in brain microvessel endothelial cells (BMEC) forming the blood–brain barrier (BBB) from rat and human origins. In rat, Trpv1-4 were detected by qRT-PCR in the brain cortex, brain microvessels, and in primary cultures of brain microvessel endothelial cells [rat brain microvessel endothelial cells (rPBMEC)]. A similar Trpv1-4 expression profile in isolated brain microvessels and rPBMEC was found with the following order: Trpv4 > Trpv2 > Trpv3 > Trpv1. In human, TRPV1-4 were detected in the BBB cell line human cerebral microvessel endothelial cells D3 cells (hCMEC/D3) and in primary cultures of BMEC isolated from human adult and children brain resections [human brain microvascular endothelial cells (hPBMEC)], showing a similar TRPV1-4 expression profile in both hCMEC/D3 cells and hPBMECs as follow: TRPV2 > > TRPV4 > TRPV1 > TRPV3. Western blotting and immunofluorescence experiments confirmed that TRPV2 and TRPV4 are the most expressed TRPV isoforms in hCMEC/D3 cells with a clear staining at the plasma membrane. A fluorescent dye Fluo-4 AM ester was applied to record intracellular Ca2+ levels. TRPV4 functional activity was demonstrated in mediating Ca2+ influx under stimulation with the specific agonist GSK1016790A (ranging from 3 to 1000 nM, EC50 of 16.2 ± 4.5 nM), which was inhibited by the specific TRPV4 antagonist, RN1734 (30 μM). In contrast, TRPV1 was slightly activated in hCMEC/D3 cells as shown by the weak Ca2+ influx induced by capsaicin at a high concentration (3 μM), a highly potent and specific TRPV1 agonist. Heat-induced Ca2+ influx was not altered by co-treatment with a selective potent TRPV1 antagonist capsazepine (20 μM), in agreement with the low expression of TRPV1 as assessed by qRT-PCR. Our present study reveals an interspecies difference between Rat and Human. Functional contributions of TRPV1-4 subtype expression were not identical in rat and human tissues reflective of BBB integrity. TRPV2 was predominant in the human whereas TRPV4 had a larger role in the rat. This interspecies difference from a gene expression point of view should be taken into consideration when modulators of TRPV2 or TRPV4 are investigated in rat models of brain disorders.
Introduction
During the last decade, the transient receptor potential (TRP) channels were identified as promising pharmacological targets and some drug candidates targeting TRP have even already entered clinical trials or been approved as drugs for treating neuropathic pain, epilepsia or heart failure (Moran, 2018). Once activated possibly by heat, mechanical/shear stress, various endogenous and exogenous molecules, some TRP channels expressed at the plasma membrane increase the influx of inorganic monovalent or divalent cations (Na+, K+, Ca2+, Mg2+), triggering several downstream cell functions depending on the cell type (Rodrigues et al., 2016). Indeed, the main knowledge of TRP functions and agonists come from their expression in sensory electrically excitable cells such as neurons and their role in the nociception and pain (Mickle et al., 2015). Recently, more studies have been done and evidenced the TRP expression and their ability in modulating the functionalities of non-excitable cells such as endothelial cells using various chemical agonists and antagonists, including a requirement in vasorelaxation and vascular permeability (Kassmann et al., 2013; Grace et al., 2014; Ambrus et al., 2017). TRP channels of the vanilloid subfamily (TRPV) are divided into 6 isoforms (TRPV1-6). TRPV5 and TRPV6 are mainly expressed in intestinal and renal epithelia (Peng et al., 2018) while transient receptor potential vanilloid 1-4 (TRPV1-4) are mostly expressed in diverse cell types of the CNS, exocrine organs, skin, eye, lung, heart, and blood vessels (Steinritz et al., 2018).
Transient receptor potential vanilloid 1-4 have been evidenced in peripheral endothelial cells where they modulate the vascular tone through the release of factors that induce relaxation of smooth muscle cells (Marrelli et al., 2007), play a role in modulating endothelial permeability (Villalta and Townsley, 2013), participate in angiogenesis of peripheral vascular (Smani et al., 2018), and sense hemodynamic and chemical changes (Kohler et al., 2006). Ca2+ modulation has been known to be an important factor in maintaining the function of brain microvessel endothelial cells (BMEC) forming blood–brain barrier (BBB) (Brown and Davis, 2002; De Bock et al., 2013). By modulating Ca2+ trafficking into BMEC, a growing evidence has been achieved that TRP channels may have a critical role on the BBB properties including its permeability, angiogenesis and inflammatory responses (Berrout et al., 2012; Huang et al., 2020). However, the expression or functionality of TRPV in BBB was much less studied than in non-cerebral endothelial cells. The expression or functionality of TRPV in BMEC forming the BBB was much less studied than in non-cerebral endothelial cells although there is growing evidence that TRP channels may have a critical role at the BBB by modulating Ca2+ trafficking into BMEC. Dysfunctions of the BBB are increasingly recognized as a cause or a consequence in the pathophysiology of diverse brain pathologies such as stroke, neurodegenerative and psychiatric diseases (Sweeney et al., 2019; Yang et al., 2019; Kealy et al., 2020; Yu et al., 2020). A dysfunctional BBB can thus be targeted with new drug candidates, which would be a promising way for treating brain pathologies. However, pre-clinical proof of concept studies of such drug candidates are usually developed with rodent models, thus raising the question of interspecies differences regarding the BBB equipment between rodents and human. Moreover, TRPV isoforms can be specifically activated by various physical and/or chemical stimuli such as heat, osmolality, and mechanical stress (Montell, 2001). Thus, the comparative expression levels of TRPV1-4 in the BBB of rodent pharmacological models and human are meaningful in clarifying the possible involvement of each isoform in physiological and pathological BBB. A whole transcriptomic analysis revealed a relative low Trpv2 expression in murine primary BMEC (Zhang et al., 2014) and rat Trpv2 was not immunolocalized in the brain vasculature (Nedungadi et al., 2012), whereas TRPV2 was shown highly expressed in the human cerebral microvessel endothelial cells D3 cells (hCMEC/D3) human cerebral microvessel endothelial cell line (Luo et al., 2019), suggesting interspecies differences in the expression of TRPV between rat and human BMEC.
The present work aims at determining TRPV1-4 expression in primary cultured rat BMEC, rat cortex and isolated cortex microvessels, as well as in primary cultured human BMEC isolated from patient biopsies and the hCMEC/D3 human cerebral microvessel endothelial cell line. Functional activity of human TRPV1-4 channels was subsequently studied in hCMEC/D3 cells.
Materials and Methods
Reagents and Chemicals
Cannabidiol (CBD) (1 mg/mL in methanol), tranilast (TNL), capsaicin (CAP), capsazepine (CPZ), and GSK1016790A (GSK) were all analytical grade and purchased from Sigma-Aldrich (Saint-Quentin-Fallavier, France). CaCl2, MgSO4, NaCl, NaH2PO4, NaHCO3, KH2PO4, and KCl were purchased from Merck (Fontenay-sous-Bois, France).
RNA extraction kits were bought from Qiagen (Courtaboeuf, France). Primers, RT-PCR reagents and Lipofectamine® RNAi MAX transfection reagent were obtained from Invitrogen Life Technologies (Cergy-Pontoise, France). The Power SYBR Green PCR Master Mix was purchased from Applied Biosystems (Applied BiosystemsTM, France). All other reagents and chemicals were purchased from Sigma.
Dulbecco’s modified Eagle’s medium (DMEM), DMEM/F12 + Glutamax, Hank’s buffered salt solution (HBSS), HEPES and Penicillin/Streptomycine were from Life Technologies (Cergy-Pontoise, France). fetal bovine serum (FBS) was from GE Healthcare Life Science. Liberase DL, bovine serum albumin (BSA) (cat. # A7906), Dextran (cat. # 31390), DNase I (cat. # DN25), Puromycine and b-FGF were from Sigma-Aldrich. plasma derived bovine serum (PDBS) was from First Link (Wolverhampton, United Kingdom).
Isolation of Rat Brain Microvessels
The isolation of rat brain cortex microvessels was done according to a protocol reported previously (Chaves et al., 2020). CO2 anesthetized animals were decapitated, rat brains were removed and immediately soaked in ice-cold HBSS containing 10 mmol/L HEPES. Brain cortices were isolated, minced and homogenized, and subsequent brain homogenates were centrifuged (2000 × g, 10 min), the pellets were re-suspended in 17.5% 64–76 kDa dextran (Sigma Aldrich) and centrifuged one more time (4400 × g, 15 min). The resulting pellets were suspended in HBSS containing 1% BSA and were firstly filtered through a 100 μm nylon mesh, and then through a 20 μm nylon mesh. The retained cerebral cortex microvessels were immediately collected and stored at −80°C before analysis (diameter size around 4–6 μm).
Cell Culture Conditions
Human Cerebral Microvessel Endothelial Cells D3 Cells (hCMEC/D3)
The hCMEC/D3 cell line kindly given by Cochin Institute (Paris, France). were used from passages 27 to 33 and cultured with the EndoGRO complete medium (Merck), supplemented with 5% FBS,1% streptomycin-penicillin (Gibco, Carlsbad, CA, United States), and 1 ng/mL b-FGF (Sigma) under 5% CO2 and 37°C as previously described (Luo et al., 2019). Culture flasks and plates were pre-coated with 150 μg/mL rat tail collagen type I. Assessment of intracellular calcium kinetics was carried out on confluent cultures in 24-well plates seeded at 5 × 104 cells/cm2.
HEK-293 Cells
Human embryonic kidney HEK293 cells were cultured with DMEM (Gibco) containing 10% FBS (Thermo) and 1% streptomycin-penicillin (Gibco) under 5% CO2 and 37°C.
Primary Cultures of Rat Brain Microvessel Endothelial Cells (rPBMEC)
Adult male Sprague-Dawley rats weighing 300–350 g were purchased from Charles River laboratory (L’arbresle, France). They were housed in groups of four per cage under standard 12:12-h light/dark conditions (light from 08:00 to 20:00 h) in a temperature- and humidity-controlled room. They had access to food and water ad libitum. Rats were acclimated for 7 days before experimentation. The care and treatment of animals conformed to the standards and guidelines promulgated by the European Communities Council Directive (86/609/EEC). The protocol was approved by the ethics review committee of Paris Descartes University (approval n°12-185/12-2012). Rats were deeply anesthetized by intraperitoneal administration of diazepam (5 mg/mL) and ketamine (100 mg/mL). They were perfused transcardially with Buffer 1 (HBSS, 10 mmol/L HEPES) at room temperature (RT) for 3 min. All subsequent steps were done on ice except when indicated. The cortex was dissected, cleared from adhering white matter and meninges and gently crushed in a Petri dish using a glass slide. Tissue pieces from two cortices were pooled, rinsed in Buffer 1, then centrifuged (2 min, 600 g) and resuspended in 10 mL of DMEM containing 10 mM HEPES, 0.4 WU/mL Liberase DL and 100 U/mL DNase I. Digestion was performed for 40 min at 37°C with gentle mechanical trituration with a 10 mL pipette (at 10 min), then with a P1000 pipet tip (at 20 min) and finally with a roded glass Pasteur pipette (at 30, 35, and 40 min), to obtain an homogenate with a creamy texture and almost no visible remaining piece. Digestion was stopped by adding 30 mL of DMEM + 10% FBS and the homogenate was centrifuged (5 min, 1,000 × g). The supernatant was discarded and the pellet was resuspended in 25 mL of Buffer 1 + 18% BSA and centrifuged (15 min, 2,000 × g). The compact myelin disk was eliminated and the pellet resuspended in 50 mL of Buffer 1 + 1% BSA. This suspension was filtered on a 10 μm nylon mesh (cat. # NY1004700, Millipore). Cells from the filtrate were pelleted, resuspended in DMEM + 10% FBS and counted. Cells were then seeded in 12-wells plate (1.5 × 106/well) in complete medium (DMEM/F12 + Glutamax, 20% PDBS, 10 U/mL penicillin/streptomycin, 80 μg/mL heparin, 5 ng/mL b-FGF) containing 4 μg/mL puromycin. After 3 days, medium was changed for complete medium without puromycin and cells were grown until confluency.
Primary Cultures of Human Brain Microvascular Endothelial Cells (hPBMEC)
All human samples were provided by BrainPlotting (iPEPS, Institut du Cerveau et de la Moelle épinière, Hôpital Universitaire de la Pitié-Salpêtrière, Paris, France) in partnership with Sainte-Anne Hospital, Paris (neurosurgeon Dr. Johan Pallud) and harvested during tumor scheduled resection surgery with written informed consent from the patients (authorization number CODECOH DC-2014-2229). Human brain microvessels were obtained from surgical resections of three patients: a 70-years-old female suffering from Glioblastoma, a 2-year-old child suffering from glioma and a 8-year-old child suffering from astrocytoma. Microvessels were isolated from resections of tumor, peritumoral or healthy brain tissue using an enzymatic procedure (Chaves et al., 2020) adapting methods previously published for rats (Perriere et al., 2005, 2007). Briefly, tissue samples were carefully cleaned from meninges and excess of blood; then, an enzymatic mix was used to dissociate the tissues and microvessels were isolated by retention on a 10 μM mesh. Cells were cultured in EBM-2 medium (Lonza, Basel, Switzerland) supplemented with 20% serum and growth factors (Sigma) (Perriere et al., 2005, 2007). After seeding brain capillaries in petri dishes, brain primary microvascular endothelial cells were shortly amplified and seeded (P1) on Transwell (Corning) with microporous membranes (pore size: 0.4 μm) in monoculture (CC205, CC206, and CC216) or in co-culture with the same patient’s fresh primary human cultured astrocytes (CC216). Dry cell pellets were stored at −80°C before RT-qPCR experiments.
RNA Isolation, Reverse Transcription and Quantitative Real Time PCR (qPCR)
Total RNA was purified using the RNeasy Mini kit (Qiagen GmbH, Hilden, Germany). The concentration and purity of the RNA samples were determined using the NanoDrop® ND-1000 instrument (NanoDrop Technologies, United States). Reverse transcription of total RNA was achieved in a thermocycler (PTC-100 programmable thermal controller, MJ research INC, United States) using the following conditions: 25°C for 10 min, then at 42°C for 30 min and at 99°C for 5 min, as previously reported (Dauchy et al., 2009). cDNAs were stored at −80°C. Gene expression was determined by SYBR Green fluorescence detection using an ABI Prism 7900 HT Sequence Detection System (Applied Biosystems, Foster City, CA, United States) as previously reported (Luo et al., 2019). Samples were run in duplicate. Primers (Table 1) were designed using OLIGO 6.42 software (MedProbe, Norway). The lower the cycle threshold (Ct) value the higher the amount of mRNA, RT negative controls and no-template controls had Ct values > 40. RT negative controls and no-template controls had Ct values > 40. cDNAs from HEK293 cells were used to validate primers of human genes of interest. The relative expression of each gene of interest X as compared to the expression of the housekeeping gene encoding TATA box-binding protein (TBP) was calculated as 2–(Ct(X)–Ct(TBP)). Gene expression of genes having a Ct higher than 33 was not quantified.
Western Blot
Human cerebral microvessel endothelial cells D3 cells were washed twice with cold phosphate-buffered saline (PBS) on ice, then the protein lysis buffer (150 mM NaCl, 50 mM Tris–HCl pH 7.4, 0.5% Triton X100, 0.5% sodium deoxycholate), and protease inhibitor cocktail (complete®, Sigma) was added. The Bradford assay was applied to quantify the protein concentration (BSA as a standard). Total proteins were separated on a 7.5% SDS-polyacrylamide gel and transferred to polyvinylidene difluoride (PVDF) membranes (Bio-Rad), blocked for 2 h with 5% non-fat dry milk. Membranes were incubated overnight with a mouse anti-human TRPV2 primary antibody (1/250, sc-390439, Santa Cruz Biotechnology, Dallas, TX, United States), a polyclonal rabbit anti-human TRPV4 primary antibody (1/200, ab94868, Abcam) or a monoclonal mouse anti-human β-actin primary antibody (1/3000, Millipore). A secondary anti-mouse or anti-rabbit IgG conjugated to HRP (1/2000, Santa Cruz Biotechnology) was then incubated for detection using an ECL plus Western Blot Detection System (GE Healthcare, Velizy, France).
Confocal Imaging
Human cerebral microvessel endothelial cells D3 cells were cultured on a 8-well ibidi μ-Slide (1.5 polymer coverslip, tissue culture treated from CliniSciences, Nanterre, France). At 80% confluence, cells were fixed with PBS containing 3.2% paraformaldehyde and permeabilized with 0.2% Triton-X-100 (Sigma) in PBS for 10 min. After 30 min in blocking solution (0.2% Triton-X-100, 1% BSA and 10% goat serum containing PBS) at RT, cells were incubated with the rabbit anti-human TRPV2 primary antibody (1:250, Thermo Fisher Scientific, Ref: PA1-18346), rabbit anti-human TRPV4 primary antibody (1:100, ab94868, Abcam), rabbit anti-human Claudin-5 primary antibody (1:250, Santa Cruz Biotechnology) or rabbit anti human VE-Cadherin primary antibody (1:500, Enzo Life Sciences) overnight at 4°C. After washing with PBS, μ-slides were incubated for 2 h at RT with a goat anti-rabbit-555 (1:500, Santa Cruz Biotechnology) and nuclei were stained with Hoechst 33342 (1:10000, Thermo Fisher Scientific, France). Negative control cells were incubated omitting primary antibodies. Visualization of the proteins was performed using a Zeiss LSM 510 Meta confocal microscope (Oberkochen, Germany).
Intracellular Ca2+ Signal Measurements
Fluorescence measurement of intracellular Ca2+ concentration was performed as described previously (Luo et al., 2019). hCMEC/D3 cells grown to confluency in 24-well plate were loaded with 2 μM of Fluo-4-AM (λex = 496 nm, λem = 516 nm, F14201, Thermo Fisher Scientific) for 45 min at 37°C in Hank’s buffer (5.37 mM KCl, 0.44 mM KH2PO4, 4.2 mM NaHCO3, 137.9 mM NaCl, 0.34 mM Na2HPO4, 1.5 mM CaCl2, 0.9 mM MgSO4, 5.56 mM D-Glucose at pH 7.4, all from Sigma, 500 μL/well). Cells were then washed and replaced with HBSS (500 μL/well). After 10 min of incubation at 37°C, fluorescence in the 24-well plates were read using a Victor® X2 fluorescent microplate reader (PerkinElmer, Villebon, France).
Fluorescence was determined with diverse activators of TRP channels. Cells were also pre-treated with TRP antagonists (capsazepine 10 and 20 μM, tranilast 50 μM, RN1734 30 μM) for 5 min before adding TRP agonists (GSK1016790A ranging from 3 to 1000 nM, capsaicin 1 and 3 μM, cannabidiol 15 μM) and the fluorescent signals were then recorded in the presence of fixed concentration of TRP antagonists. All TRP activators and antagonists were dissolved with 100% DMSO or ethanol as stock solutions and the final solvent concentration in treated cells did not exceed 1%, a solvent concentration that had no effect on Ca2+ entry as compared to control cells without any solvent (data not shown). Data were normalized to the control group, which is expressed as F1/F0, where F0 is the average fluorescence of the control group (no activator of TRP) and F1 is the actual fluorescence at the corresponding time for the control group (in this case the average ratio for the control group is 1 or 100%) or the treated group.
To explore heating-induced Ca2+ influx, hCMEC/D3 cells were plated in 24-wells plate and loaded with Fluo-4-AM. The plate was then placed in the detecting room of VictorTM X2 fluorescent microplate reader with automatically heating system. The plate was heating from RT to 50°C. The temperature and fluorescence were recorded at the same time by the VictorTM X2 in the presence or not of the specific TRPV antagonist. Data was expressed as ratio normalized to the average of fluorescence intensity at RT in each well.
Statistical Analysis
Data are expressed as mean value ± SEM (standard error of the mean). Statistical analysis was performed using ANOVA with Dunnett a posteriori test to compare different groups with the control. An unpaired t-test was applied between two groups. p value < 0.05 was considered statistically significant. To calculate the EC50 of the agonist (GSK1016790A), the concentration-response data were fitted to a logistic function as follows: Y = Bottom + (Top−Bottom)/(1 + 10(logEC50–X)); where X is the log of the agonist concentration. Data fitting was performed in GraphPad Prism 5.01.
Results
Thermo-Sensitive Trpv1-4 Gene Expression in Rat Cortex, Brain Microvessels and rPBMEC
We measured the relative expression of Trpv1-4 by qRT-PCR in primary cultured rat brain microvessel endothelial cells (rPBMEC) (Figure 1). Trpv1 mRNA levels were barely detectable in whole cortex, brain microvessels and rPBMEC (Figure 1A). Trpv2 expression was much higher in the whole cortex than in both brain microvessels and rPBMEC (7.99 ± 1.21, 1.19 ± 0.07, and 0.29 ± 0.04, respectively; p < 0.05 rat cortex vs. microvessels, p < 0.01 rat cortex vs. rPBMEC) and similar results were obtained for Trpv3 (0.86 ± 0.13, 0.11 ± 0.07, 0.03 ± 0.02, respectively; p < 0.05 rat cortex vs. microvessels, p < 0.05 rat cortex vs. rPBMEC). Among Trpv1-4, Trpv4 was the most highly expressed gene in brain microvessels and rPBMEC (3.13 ± 0.05 and 2.36 ± 0.16, respectively; p < 0.001 rat cortex vs. microvessels, p < 0.01 rat cortex vs. rPBMEC) while its expression in rat cortex was far below that of Trpv2. Meanwhile, no significant difference was observed for the gene expression of Trpv1 and Trpv3 between brain microvessels and rPBMEC (Figure 1A), while a significant decrease of Trpv2 and Trpv 4 gene expression was observed in rPBMEC compared with that in brain microvessels (Figure 1A, p < 0.01, p < 0.05 for Trpv2 and Trpv4, respectively, rat microvessels vs. rPBMEC). The Trpv1-4 gene expression profile expressed as a percentage of total Trpv genes expressed was similar in brain microvessels and rPBMEC with the following rank order: Trpv4 > Trpv2 > Trpv3 > > Trpv1 (Figure 1B). This is dramatically different from the Trpv1-4 gene expression profile in the whole brain cortex with the following rank order: Trpv2 > Trpv3 > Trpv4 > > Trpv1.
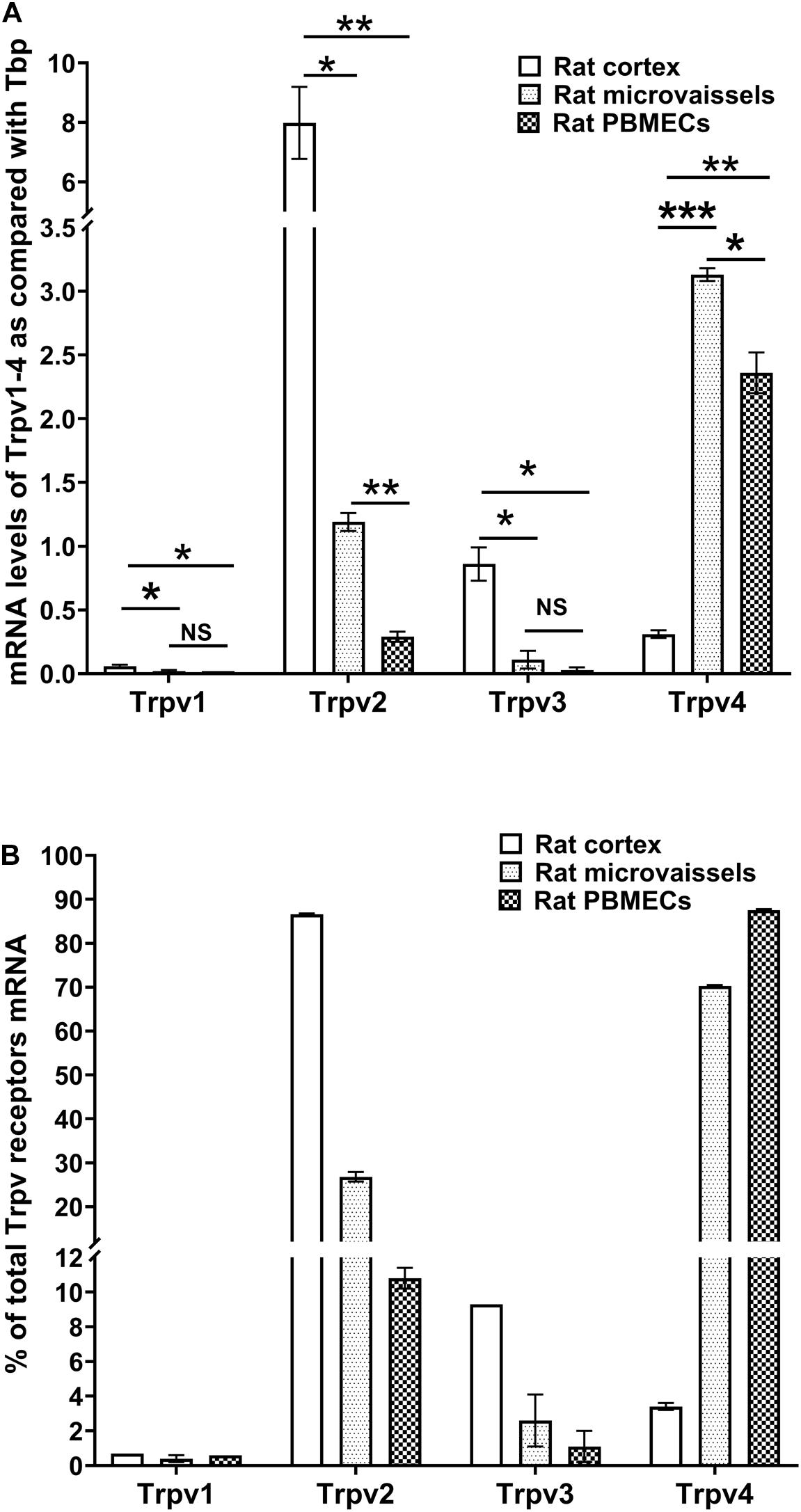
Figure 1. Expression of thermo-sensitive Trpv in rat cortex, rat microvessels and BBB endothelial cells. (A,B) mRNA levels of Trp1-4 were detected by qRT-PCR in rat brain cortex, rat brain isolated microvessels and primary cultures of rat brain microvessel endothelial cells (see section “Materials and Methods”). Data are expressed as ratio (mean ± SEM) of Trpv mRNA levels compared with those of the endogenous housekeeping gene Tbp in each sample from three different RNA extractions (A) or expressed as a percentage of total Trpv1-4 expression set at 100% (B). Inter-group comparisons were performed by ANOVA with Dunnett a posteriori test, NS not significant, *p < 0.05, **p < 0.01, ***p < 0.001.
Thermo-Sensitive TRPV1-4 Gene Expression in Human BMEC
We measured the relative expression of TRPV1-4 by qRT-PCR in hCMEC/D3 cells and primary cultured human brain microvascular endothelial cells (hPBMEC) from three different brain tumor biopsies (Figure 2A). TRPV1 mRNA levels were easily detected in hPBMEC of the adult patient culture CC216 and, albeit at a lower level, in hCMEC/D3 and hPBMEC from the two children cultures CC205 and CC206 (3.05 ± 0.26, 0.22 ± 0.01, 0.21 ± 0.01, and 0.76 ± 0.08, respectively, Figure 2A). It is noteworthy that TRPV1 expression was increased significantly by 4.8-fold (from 3.05 ± 0.26 to 14.6 ± 2.0, p < 0.01) when cells were co-cultured with isolated primary astrocytes from the same patient, while no effect was found for the other 3 TRPV isoforms (Figure 2A). TRPV2 was by far the most highly expressed gene in hCMEC/D3 and hPBMEC cells [TRPV2 data for CC205 and CC206 was also shown in Luo et al. (2019)]. TRPV4 was expressed at moderate levels in hPBMEC from the three patients (10.36 ± 2.23, 1.35 ± 0.04, and 1.79 ± 0.12) and hCMEC/D3 (1.38 ± 0.01) while TRPV3 mRNA were barely detectable in all cells. A similar TRPV1-4 gene expression profile expressed as a percentage of total TRP gene expressed was observed in hCMEC/D3 cells and hPBMEC with the following rank order: TRPV2 > > TRPV4 > TRPV1 > > TRPV3 (Figure 2B).
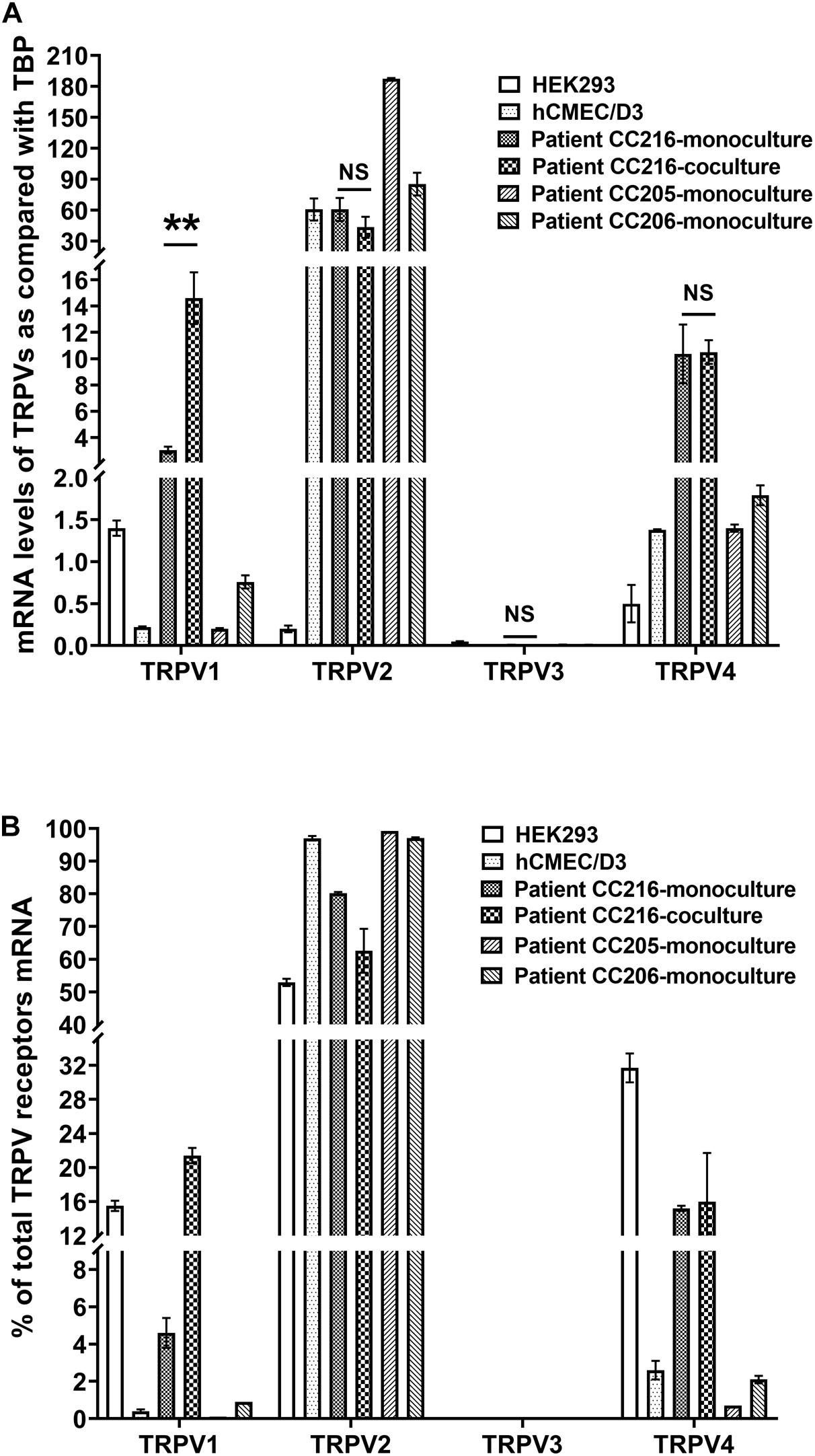
Figure 2. Expression of thermo-sensitive Trpv in human BMEC. (A,B) mRNA levels of TRPV1-4 were detected by qRT-PCR in primary cultures of brain microvessel endothelial cells obtained from patients (see section “Materials and Methods”) and in hCMEC/D3 cells. Data are expressed as ratio (mean ± SEM) of TRPV1-4 mRNA levels compared with those of the endogenous housekeeping gene TBP in each sample from three different RNA extractions (A) or expressed as a percentage of total TRPV1-4 expression set at 100% (B). When comparing TRPV1-4 expression in human BMEC culturing alone or together with astrocytes isolated from the same patient, statistical significance was determined by an unpaired t-test, NS, not significant, **p < 0.01.
Protein Expression of TRPV2 and TRPV4 in hCMEC/D3
The expression of TRPV2 and TRPV4, the two genes with the highest expression at the mRNA level in human brain endothelial cells, was then studied at the protein level. Western blot analysis showed that TRPV2 and TRPV4 proteins were expressed in hCMEC/D3 cells where they are detected in a single band at a molecular weight around 80–90 kDa, in agreement with the predicted value of TRPV2 and TRPV4 molecular weights (Figure 3A). This was confirmed by immunostaining and confocal microscopy which revealed a specific expression of both TRPV2 and TRPV4 at the plasma membrane of hCMEC/D3 cells as well as in intracellular compartments concentrated in the perinuclear region (Figure 3B). Omitting the primary antibody resulted in the absence of any detectable fluorescence signal (NEG in Figure 3B). The tight junction protein Claudine-5 was used as a positive control for brain endothelial cell phenotype as it was reported to be expressed in hCMEC/D3 cells (Weksler et al., 2005).
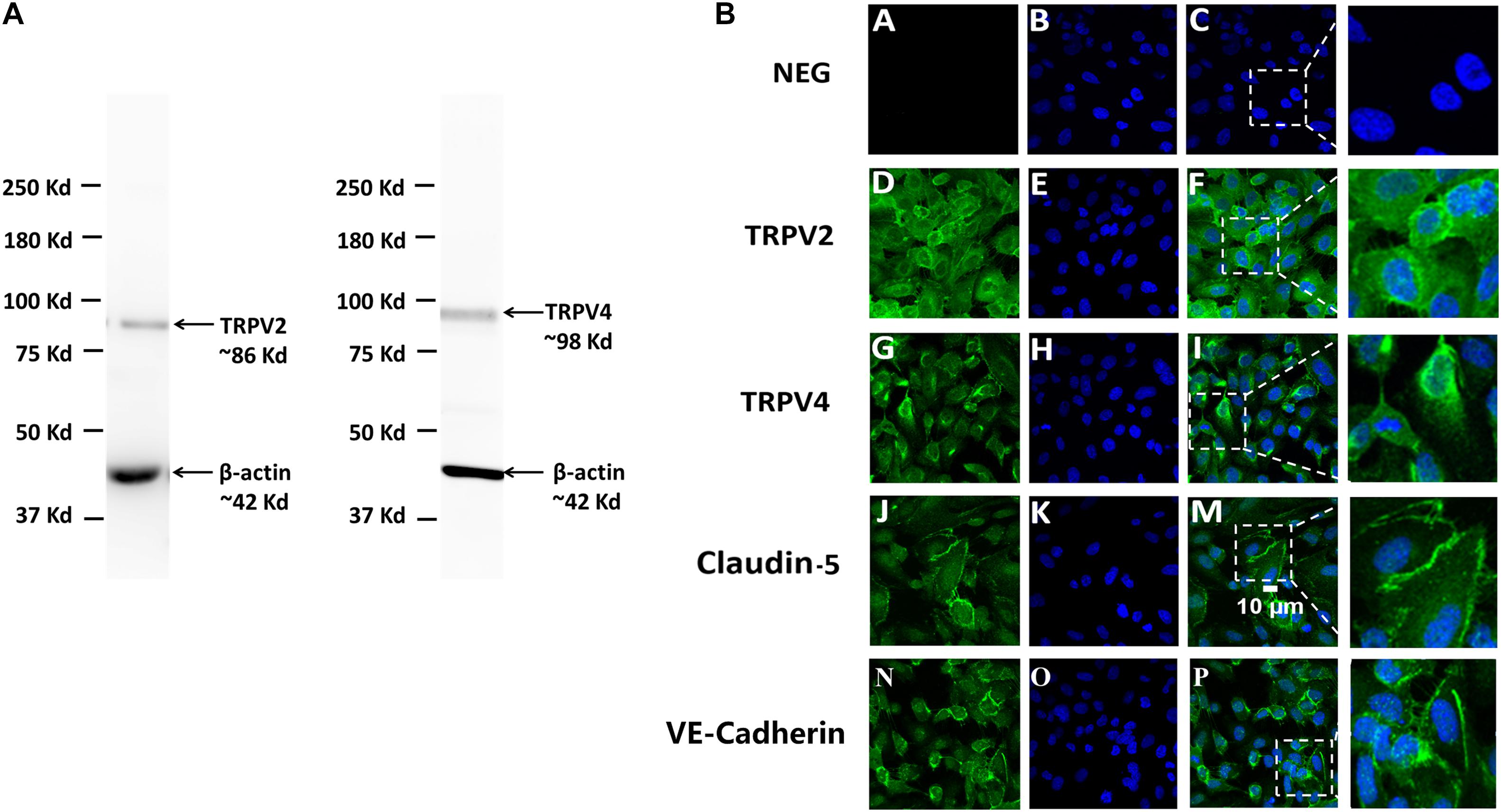
Figure 3. Protein expression of TRPV2 and TRPV4 in hCMEC/D3. (A) Expression of TRPV2 and TRPV4 determined by Western blot of total crude proteins obtained from two different protein extractions of hCMEC/D3. β-actin served as a loading control housekeeping protein. (B) Confocal immunofluorescence localization of TRPV2 and TRPV4 in hCMEC/D3. TRPV2 or TRPV4 (green) and nuclei (blue) were stained in hCMEC/D3 as described in section “Materials and Methods” (second D, E, F and third G, H, I row panels). Claudin-5 (green) and nuclei (blue) were stained in hCMEC/D3 (fourth row panel J, K, M). VE-Cadherin (green) and nuclei (blue) were stained in hCMEC/D3 (fifth row panel N, O, P). Control cells (NEG) were stained omitting the primary antibodies (first row panel A, B, C).
Functional Activity of TRPV in hCMEC/D3
Transient receptor potential vanilloid 1, TRPV2, and TRPV4 functional activity was then investigated in hCMEC/D3 cells. Ionomycin, which triggers an immediate increase of the intracellular Ca2+ concentration, was used as a positive control (2 μM ionomycin) in all Ca2+ influx experiments.
The AUC of intracellular Ca2+ levels during 20 min were not significantly affected when cells were exposed for 20 min to 1 or 3 μM CAP, a highly specific and efficient agonist of TRPV1 (Figures 4A,B). By contrast, CAP, at lower concentrations than those used in our study, were high enough to fully activate human TRPV1 (Binshtok et al., 2007). In addition to compare the AUC of intracellular Ca2+ levels during 20 min, we further compared the intracellular Ca2+ levels at specific time (Figures 4C,D). Interestingly, a significant statistical difference between the control group and 3 μM CAP group at 7 min (p = 0.019), 11 min (p = 0.041), 12 min (p = 0.049), and 16 min (p = 0.045) were found (Figures 4C,D). However, no significant difference of Ca2+ influx at any specific times was found when cells exposed to 1 μM CAP (Figures 4C,D). Ca2+ flux levels were not significantly altered when cells exposed to CAP from 1 to 3 μM (Figures 4C,D). Meanwhile, a significant 3.3-fold increase of Ca2+ influx was observed in the positive control group treated with 2 μM ionomycin (Figures 4A,B, p < 0.001 compared with the control group). TRPV1 is a known thermo-sensitive channel (Mergler et al., 2010). Therefore, we asked whether CPZ, a well-known TRPV1 antagonist, was able to block the heat-induced increase of intracellular Ca2+ concentration. As shown in Figures 4E,F, exposing cells continuously from RT to 50°C for 40 min resulted in a significant increase in intracellular Ca2+ but this Ca2+ flux was not altered by co-treatment with CPZ (10 or 20 μM; Figure 4E), in agreement with the low expression of TRPV1 assessed by qRT-PCR and the weak TRPV1 activation by CAP. Conversely, heat-induced Ca2+ influx could be blocked by TNL, a selective TRPV2 antagonist. Exposing the cells to CBD, a highly potent activator of TRPV2 and to a lesser extent of TRPV1, resulted in an increased Ca2+ influx in hCMEC/D3, an effect which was not reversed by CPZ (Figures 4G,H). Similar trends were demonstrated when comparing the Ca2+ influx at specific time (Figures 4I,J).
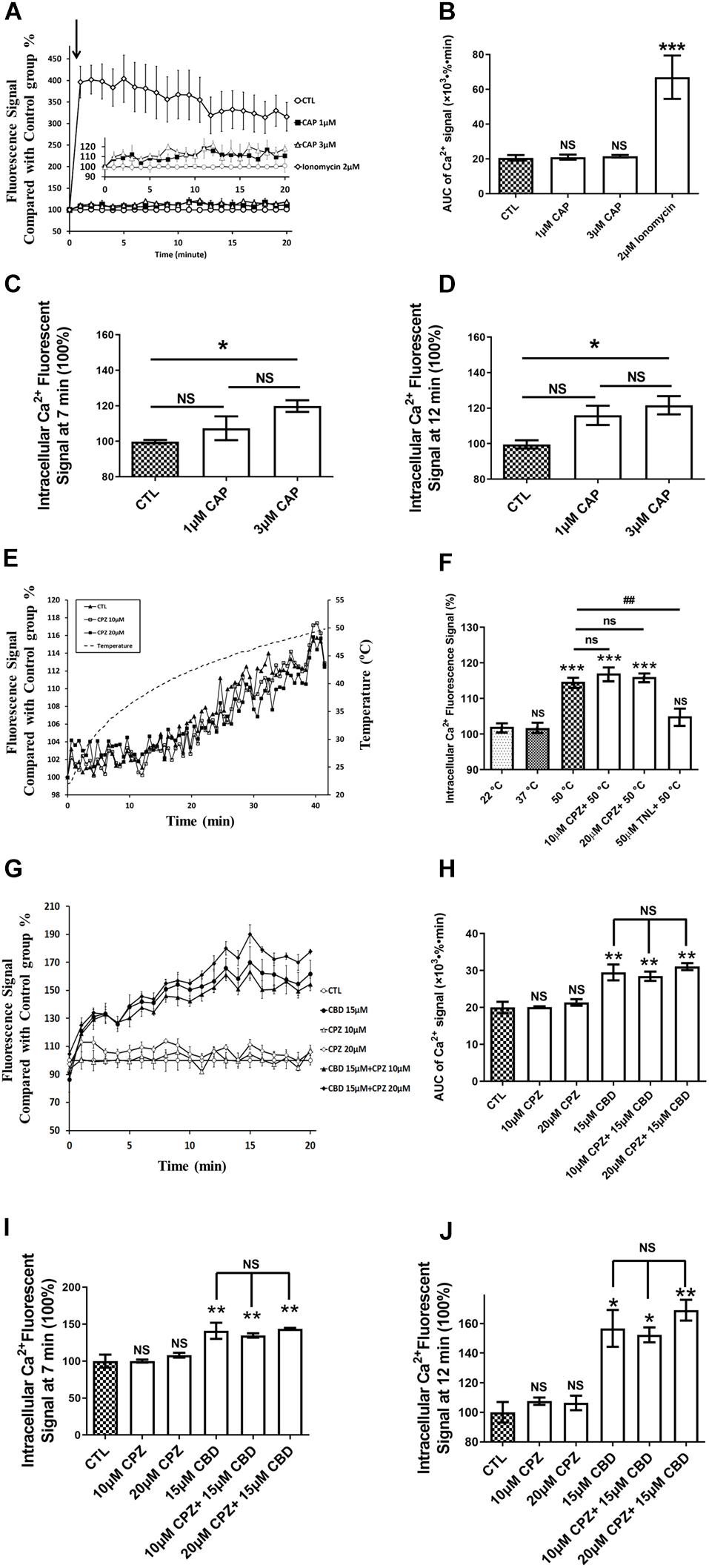
Figure 4. TRPV1 is not functional in hCMEC/D3. (A) Representative time course of [Ca2+]i in hCMEC/D3 under stimulation of TRPV1 by capsaicin (CAP). Cells were plated in 24-wells plate and loaded with Fluo-4-AM as described in section “Materials and Methods.” CAP or ionomycin (as positive control of Ca2+ entry) was added and the fluorescence was recorded. (B) Change of the area under curve (AUC) over the 20 min obtained from the experiment shown in panel (A). (C) Change in [Ca2+]i at T = 7 min obtained from the experiment shown in panel (A). (D) Change in [Ca2+]i at T = 12 min obtained from the experiment shown in panel (A). (E) Change of [Ca2+]i upon heat exposure of hCMEC/D3 for 40 min from room temperature to 50°C. Cells were plated in 24-wells plate and loaded with Fluo-4-AM. The fluorescence was recorded in the presence or not of the specific TRPV1 antagonist capsazepine (CPZ; 10 or 20 μM). (F) Changes in [Ca2+]i in hCMEC/D3 at 22, 37, or 50°C with or without CPZ or Tranilast (TNL, 50 μM) derived from panel (E). (G) Representative time course of [Ca2+]i in hCMEC/D3 stimulated by the TRPV1-2 agonist cannabidiol (CBD, 15 μM) and pre-treated or not with the TRPV1 specific antagonist CPZ. Cells were loaded with Fluo-4-AM. CBD was added and the fluorescence was recorded. When applying CPZ, cells were pre-treated with 10 or 20 μM CPZ for 5 min before incubation with 15 μM CBD and the fluorescence was recorded in the persistent presence of 10 or 20 μM CPZ. (H) Change of the area under curve (AUC) over the 20 min obtained from the experiment shown in panel (G). (I) Change in [Ca2+]i at T = 7 min obtained from the experiment shown in panel (G). (J) Change in [Ca2+]i at T = 12 min obtained from the experiment shown in panel (G). Data are expressed as mean ± SEM and inter-group comparisons were performed by ANOVA with Dunnett a posteriori test. *p < 0.05, **p < 0.01, ***p < 0.001 compared with CTL or 22°C groups or among indicated group.
To assess TRPV4 activity in hCMEC/D3, we applied GSK1016790A (GSK), a highly selective and potent agonist of TRPV4. GSK induced a dramatic and rapid concentration-dependent increase in Ca2+ levels (Figure 5A). A maximal increase in intracellular Ca2+ levels (1.6-fold) was obtained with GSK ≥100 nM (Figure 5B). An apparent concentration-effect relationship was observed with an EC50 of 16.2 ± 4.5 nM (Figure 5C). The rise in intracellular Ca2+ concentration induced by 30 nM GSK1016790A was inhibited by RN1734 pretreatment (30 μM) a well-known TRPV4 antagonist (Figures 5D,E).
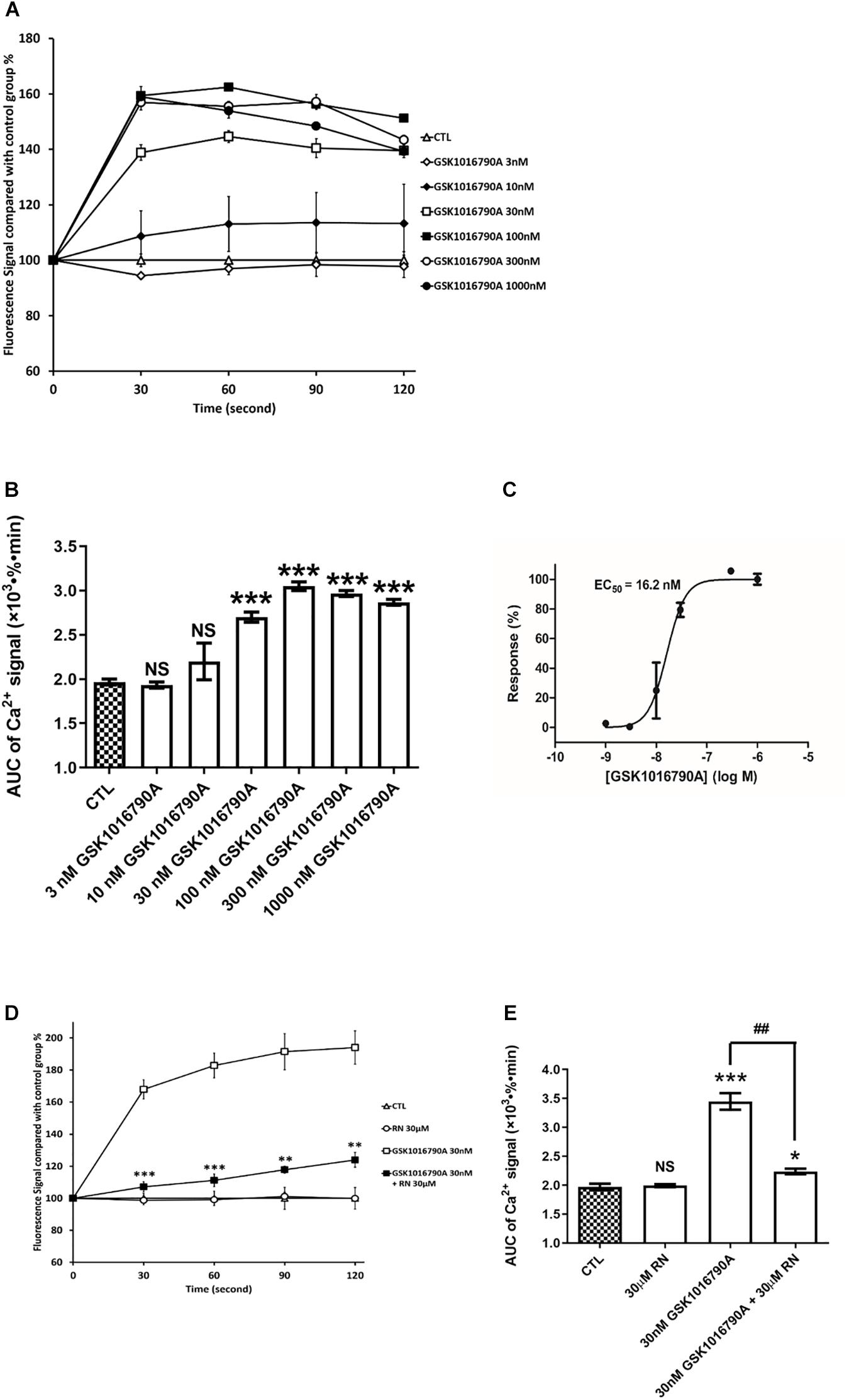
Figure 5. TRPV4 is functional in hCMEC/D3. (A) Representative time course of [Ca2+]i in hCMEC/D3 stimulated by the TRPV4 agonist GSK1016790A. Cells were plated in 24-wells plate and loaded with Fluo-4-AM as described. GSK1016790A was added and the fluorescence was recorded. (B) Change of the area under curve (AUC) over the 120 s obtained from the experiment shown in panel (A). (C) Concentration-response relationship of GSK1016790A. Measurements were obtained from panel (B). (D) Representative time course of [Ca2+]i in hCMEC/D3 stimulated by GSK1016790A and pre-treated or not with the TRPV4 specific antagonist RN1734 (RN). When applying RN, cells were pre-treated with 30 μM RN for 5 min and the fluorescence was recorded in the persistent presence of 30 μM GSK1016790A. (D) Change of the area under curve (AUC) over the 120 s obtained from the experiment shown in panel (C). Data are expressed as mean ± SEM. For panels (B,E), inter-group comparisons were performed by ANOVA with Dunnett a posteriori test, NS, not significant, *p < 0.05, ***p < 0.001 compared with CTL group, ##p < 0.01 compared with 30 nMGSK1016790A group, n = 3 in triplicate. For panel (D), inter-group comparisons were performed by ANOVA with Dunnett a posteriori test, *p < 0.01, **p < 0.01, ***p < 0.001 compared with GSK1016790A group.
Discussion
Brain microvessel endothelial cells, the main component of the BBB, are widely exposed to various stimuli from the blood and brain compartments. Among these, blood borne compounds and mechanical stress might modulate the activity of TRP channels, thus allowing Ca2+ entry into brain endothelial cells where Ca2+ concentrations need to be maintained within a certain range of concentrations through a dynamic equilibrium (Ma and Liu, 2019). Intracellular/cytosolic [Ca2+] modulates various cellular processes such as excitability, proliferation, synaptic plasticity, resistance to oxidative stress, and cell death, depending on the concentration, timing and duration of the signal (Fliniaux et al., 2018). Modifications of intracellular [Ca2+] can trigger the proliferation of BMEC and increase the permeability of the BBB by altering tight junction proteins (Ma and Liu, 2019). Furthermore, BBB dysfunctions observed in several brain pathologies are usually associated with increasing intracellular Ca2+ concentrations, suggesting that TRP-mediated Ca2+ signaling may play crucial roles in maintaining and regulating BBB functions (De Bock et al., 2013). Interestingly, the highly potent TRPV2 activator CBD increased Ca2+ entry in hCMEC/D3 cells where it induces proliferation, tubulogenesis and migration (Luo et al., 2019). Moreover, CBD has anti-inflammatory activity and is able to reduce the disruption of the BBB observed in lipopolysaccharide (LPS) (Ruiz-Valdepenas et al., 2011) and multiple sclerosis mice models (Mecha et al., 2013). CBD was demonstrated as effective in preventing disruption of an in vitro BBB model under hypoxic and glucose deprivation conditions (Hind et al., 2016). Altogether, these results suggest that activating TRPV2 might be a relevant strategy to positively modulate the BBB in the context of brain disorders including ischemic stroke (Calapai et al., 2020). Here, we looked at interspecies differences in the expression and activity of TRPV in rat and human BBB.
Transient receptor potential vanilloid 1-4 were first to be known for their expression in sensory neurons and their function in sensing nociception and pain (Mickle et al., 2015). However, the expression of TRPV1-4 in the brain is quite region-specific and heterogeneous (Kauer and Gibson, 2009; Cavanaugh et al., 2011; Nedungadi et al., 2012). For TRPV1, previous studies have believed that it can be widely expressed throughout the brain (Kauer and Gibson, 2009). However, a recent study applied a highly sensitive method using gene editing to modify the Trpv1 genetic locus in two lines of reporter mice, showing that TRPV1 is highly limitedly expressed in several specific brain regions, such as the caudal hypothalamus, the dorsal motor nucleus of the vagus, mesencephalic trigeminal nucleus, and parabrachial nucleus (Cavanaugh et al., 2011). Litter expression was found for the other brain regions (Cavanaugh et al., 2011). TRPV2 was found to be intensively expressed mainly in several brain areas including: hypothalamus, the nucleus of the solitary tract, hypoglossal nucleus, and the rostral division of the ventrolateral (Nedungadi et al., 2012). TRPV3 protein expression has not been detected in the mouse brain, although TRPV3 mRNA transcripts has been detected in the brain at low levels (Luo and Hu, 2014). TRPV3 is also evidenced to be absent in the mouse bEnd.3 brain endothelial cell line and in the freshly isolated brain capillaries and primary cultured BMECs from rat (Mickle et al., 2015). This is in accordance with the whole transcriptomic analysis demonstrating the absence of Trpv3 in murine primary BMECs (Zhang et al., 2014) and by brain vascular single-cell transcriptomics study (Vanlandewijck et al., 2018). TRPV3 is most abundantly expressed in skin keratinocytes and in cells surrounding hair follicles, where it plays an essential role in cutaneous sensation. RT-PCR or q-PCR analysis showing the absence of TRPV3 in human BMECs (Golech et al., 2004; Hatano et al., 2013) and gain-of-function mutations in human TRPV3 are associated with Olmsted syndrome, which is characterized by severe palmoplantar and periorificial keratoderma (Greco et al., 2020; Zhang et al., 2020). TRPV4 has been reported to be widely expressed throughout the brain including hippocampus, hypothalamus, cerebellum, lamina terminalis and optic chiasm, as well as a special enhanced expression in the olfactory placodes (Mangos et al., 2007; Kauer and Gibson, 2009; Shibasaki et al., 2015). Unfortunately, it is still remained largely unknown which types of cells were responsible for their expression in a specific brain region (Kauer and Gibson, 2009). Our results showed significant mRNA levels of some TRPVs in human brain endothelial cells which follow this rank of expression: TRPV2 > TRPV4 > TRPV1, while TRPV3 was barely detected. It should be noted that this rank order is based on mRNA levels and functional studies since the absolute amount of each TRPV at protein level was not investigated in this study. These observations are supported by a study showing the qualitative expression of TRPV1, 2, and 4 in human primary cultured brain endothelial cells by RT-PCR (Hatano et al., 2013). TRPV2, a thermo-sensitive TRPV channel, was by far the most highly expressed isoform in hPBMEC and hCMEC/D3 while its relative expression was much lower than that of Trpv4 in rat microvessels and rPBMEC. TRPV1 and TRPV4 were also expressed both in rPBMEC, hPBMEC and hCMEC/D3 cells, and TRPV2 was even more expressed in hPBMEC than in hCMEC/D3 cells. TRPV4 expression was further evidenced by Western blot and immunofluorescence analysis in hCMEC/D3 cells supporting previous RT-PCR and/or immunofluorescence results in human BMEC (Sullivan et al., 2012; Hatano et al., 2013). However, using specific peptides of TRPV2 and TRPV4 targeted by the primary anti-TRPV2 and antiT-RPV4 antibodies used in our study would have increased the specificity of the immunofluorescence staining. Interestingly, we detected a 4.0- and 5.8-fold higher expression of, respectively, TRPV1 and TRPV4 mRNA, in the brain endothelial cells derived from the adult patient (CC216) as compared with the two children (CC205 and CC206), suggesting that TRPV1 and TRPV4 expression might increase with age while that of TRPV2 might decrease. This is in agreement with the reported age-dependent expression and function of Trpv1 in the rodent forebrain (Koles et al., 2013), although we cannot exclude, in our case, that these differences might result from the limited numbers of patients and/or reflect the underlying pathology or the exposure of the patients to medication. Interestingly, TRPV expression in hPBMEC was modulated by co-culture with astrocytes as shown by the 4.8-fold TRPV1 increase in hPBMECs co-culture with isolated primary astrocytes obtained from the same patient. In contrast, this effect was absent for TRPV2,3,4 isoforms. Recently, Mannari et al. (2013) have reported that the astrocytic TRPV1 could directly sense the blood-borne signals in the sensory circumventricular organs in adult mouse brains. Trpv1 is regarded as a target in regulating BBB permeability (Hu et al., 2005; Beggs et al., 2010) and might be also a downstream target in the modulated BBB permeability mediated by astrocytes (Abbott, 2002; Abbott et al., 2006).
A similar expression profile was found between hPBMEC isolated from patients and the human BBB cell line hCMEC/D3, confirming previous data (Dauchy et al., 2009; Ohtsuki et al., 2013) and suggesting that hCMEC/D3 is a proper in vitro surrogate in exploring TRPV properties at the human BBB. Trpv2 gene expression was 25.8-fold more expressed in brain cortex than in rPBMEC, in accordance with a more pronounced Trpv2 immunostaining in the rat brain (Nedungadi et al., 2012). Notably, we evidenced a remarkable interspecies difference regarding the expression profile of TRPV1-4 between rPBMEC and the two human in vitro BBB models (hPBMEC and hCMEC/D3). Although TRPV2 and TRPV4 were expressed both in rat and human BMEC, Trpv4 and TRPV2 were the most expressed isoform in rPBMEC and hBMEC, respectively. Brown et al. (2008) have demonstrated Trpv1-4 expression by RT-PCR in the bEnd.3 murine BBB cell line and in isolated mouse brain microvessels, showing a higher Trpv2 expression than Trpv4, suggesting TRPV expression profile could be similar in murine and human BMEC. A whole transcriptomic analysis revealed a similar expression of Trpv2 and Trpv4 in murine primary BMEC, while Trpv1 and Trpv3 transcripts were undetectable (Zhang et al., 2014). Except for TRPV1-4, many other transporters and enzymes expressed at the BBB also demonstrated interspecies differences in expression and function levels (Deo et al., 2013). PET studies have demonstrated a significant interspecies differences for the expression and functionality of ABCB1 (i.e., P-gp), an important efflux transporter at the BBB (Syvanen et al., 2009). The brain-to-plasma concentration ratio of [11C]GR205171, a substrate of P-gp, was almost 9-times higher in humans compared with rats (Syvanen et al., 2009; Ball et al., 2013). In fact, interspecies differences in expression and/or function levels were also verified for ABCG2 (BCRP) (Ito et al., 2011; Uchida et al., 2011; Agarwal et al., 2012), MRPs (Agarwal et al., 2012), some OATPs (Ito et al., 2011; Uchida et al., 2011) and some SLCs (Ito et al., 2011; Agarwal et al., 2012).
Our current work did not indicate whether TRPV receptors are expressed at BBB in a polarized way or not. Many receptors/transporters are localized specifically at luminal or abluminal side of the brain endothelial cells in charge of different functions (Dragoni and Turowski, 2018). Very poor data were reported regarding the subcellular localization of TRPV1-3 in brain microvessels. In the rat middle cerebral arteries, TRPV4 was found to be expressed preferentially on the abluminal membrane of endothelial cells (Marrelli et al., 2007) but the observation that a selective P2Y2 receptor agonist could induce TRPV4-mediated Ca2+ entry across the luminal and abluminal face of an endothelial monolayer (Guerra et al., 2018), suggests that TRPV4 could be also expressed on the luminal face of endothelial cells. Benfenati et al. (2007) confirmed that expression of TRPV4 is abundantly localized on astrocytic endfeet area abutting pial and parenchyma blood vessels. Also, TRPV2 has been shown to be prominently localized to the umbrella apical membrane of bladder epithelium cells, while TRPV4 was identified on their abluminal surfaces (Yu et al., 2011).
Transient receptor potential vanilloid-mediated modulation of the intracellular Ca2+ concentration was further investigated using specific agonist and antagonists. The highly potent TRPV1 agonist CAP, usually effective at nanomolar levels (Flockerzi, 2007), did not increase the AUC of intracellular Ca2+ during 20 min in hCMEC/D3 at a concentration of 1 or 3 μM. Further statistical tests revealed that 3 μM CAP can stimulate the Ca2+ influx at specific time (7, 11, and 12 min post CAP stimulation), indicating a possible weak function of TRPV1 in the BBB. This in agreement with the low gene expression of TRPV1 in hCMEC/D3 and also with the recent observation that no functional activity with CAP up to 1 mM could be detected in human renal podocytes although these cells expressed TRPV1 (Ambrus et al., 2017). Notably, TRPV1-induced Ca2+ influx under CAP stimulation at specific times is not quite dose-dependently as similar Ca2+ influx levels were found in 1 or 3 μM CAP, from the view of either the AUC of Ca2+ during 20 min or the Ca2+ influx at any specific time, further identifying the quite lower TRPV1 expression at the membrane of hCMEC/D3 cells. In fact, the much lower expression of TRPV1 in BBB endothelial cells were also demonstrated by Golech et al. (2004) by RT-PCR and polyclonal antibodies. TRPV1 is also functional in responding to heat stimulation, however the heat-induced Ca2+ influx was not significantly inhibited by CPZ (10 or 20 μM), a widely used TRPV1 antagonist, suggesting that TRPV1 might not be the main functional isoform in hCMED/D3 cells. In addition, it was reported that TRPV1 could be also activated by CBD, although its agonist potency was lower than toward TRPV2 (Qin et al., 2008). Yet, no significant alteration in intracellular Ca2+ was observed when co-treating cells with CBD and CPZ, a TRPV1 antagonist, further suggesting the lack of TRPV1 activity or weak TRPV1 activity in hCMEC/D3 cells. However, we cannot exclude a functional activity of TRPV1 in hPBMECs since TRPV1 gene expression was much higher in those cells than in hCMEC/D3 cells, especially when they were co-cultured with astrocytes from the same patient. Moreover, CPZ is not a very potent antagonist of TRPV1 (Rigoni et al., 2003), that could only produce an insufficient/partial blocking of TRPV1. Therefore, the absence of functional activity of TRPV1 in hCMEC/D3 cells remains questionable.
The specific and potent TRPV4 agonist GSK triggered a rapid elevation of intracellular Ca2+ with an Emax at 100 nM (EC50 ∼16.2 nM), which can be totally inhibited by the specific TRPV4 antagonist RN1734. The EC50 for GSK in hCMEC/D3 cells was close to that reported for stimulating Ca2+ influx in another study (26.9 nM) (Sullivan et al., 2012). Previous studies have identified the expression of TRPV4 in commercial human BMECs at gene and protein levels (Hatano et al., 2013). The expression and function of TRPV4 are also verified using patch-clamp electrophysiology techniques in freshly BMECs isolated from brain capillaries of C57BL/6J mouse (Harraz et al., 2018). The specific TRPV4 agonist GSK-stimulated currents was completely abolished in TRPV4-knockout mice or when co-applying the specific TRPV4 antagonist HC-067047 in murine BMECs (Harraz et al., 2018). It is also reported that TRPV4 might play a vital role in regulating the function of blood-retina barrier, similarly to BBB, via modulating Ca2+ homeostasis in human retinal microvascular endothelial cells (Phuong et al., 2017). Due to their diverse cellular and subcellular localizations, TRP channels could mediate Ca2+ influx and generate distinct cytosolic/local intracellular Ca2+ waves that could regulate specific subcellular downstream pathways leading to different cell responses including death or survival (Fliniaux et al., 2018). In this regard, mitochondrial expression of TRPV1-2-3 has been evidenced in human odontoblasts (Wen et al., 2017), which is consistent with the intracellular staining we observed in hCMEC/D3 cells for TRPV2 and TRPV4.
Conclusion
In conclusion, our present study shows that TRPV1, TRPV2 and TRPV4 are expressed in hPBMECs. Even less expressed than TRPV2, TRPV4 is functionally expressed in the human BBB. Recently, TRPV4 has been shown to be highly expressed and functional in retinal endothelial cells (Phuong et al., 2017). The authors nicely showed by different electrophysiological and Ca2+ imaging techniques and using an in vitro retinal barrier model that TRPV4 highly contributed to Ca2+ homeostasis and barrier function. The role of TRPV4 in BBB barrier function and physiology should be evaluated in further experiments before claiming whether or not TRPV4 could be a target to be investigated for modulating the BBB. TRPV1 is much less expressed than TRPV2 and TRPV4 in the human BBB but still shows weak or limited functionality when activated by relative high concentration of selective chemical agonist. An interspecies difference between rat and human TRPV2,4 was evidenced in the BBB using a gene expression approach which may be taken into consideration for rat to human translational pharmacological approaches targeting TRPV in the BBB. This interspecies difference between rat and human TRPV should be further confirmed from a functional point of view by using for example electrophysiological techniques like patch-clamp and measuring whole-cell currents of TRPV2 and TRPV4 between human and rat BMEC.
Data Availability Statement
The datasets presented in this study can be found in online repositories. The names of the repository/repositories and accession number(s) can be found in the article/supplementary material.
Ethics Statement
The studies involving human participants were reviewed and approved by French Ministry of Higher Education and Research (CODECOH DC-2014-2229). Written informed consent to participate in this study was provided by the participants’ legal guardian/next of kin. The animal study was reviewed and approved by the Ethics Review Committee of Paris Descartes University.
Author Contributions
HL, BS, SCh, VC, MS, FG, and CC: experiments. HL and XD: writing – original draft preparation. BS, SCi, and XD: writing – review and editing. SCi and XD: supervision. All authors have read and agreed to the submitted version of the manuscript.
Conflict of Interest
NP and FG were employed by the company BrainPlotting.
The remaining authors declare that the research was conducted in the absence of any commercial or financial relationships that could be construed as a potential conflict of interest.
References
Abbott, N. J. (2002). Astrocyte-endothelial interactions and blood-brain barrier permeability. J. Anat. 200, 629–638. doi: 10.1046/j.1469-7580.2002.00064.x
Abbott, N. J., Ronnback, L., and Hansson, E. (2006). Astrocyte-endothelial interactions at the blood-brain barrier. Nat. Rev. Neurosci. 7, 41–53. doi: 10.1038/nrn1824
Agarwal, S., Uchida, Y., Mittapalli, R. K., Sane, R., Terasaki, T., and Elmquist, W. F. (2012). Quantitative proteomics of transporter expression in brain capillary endothelial cells isolated from P-glycoprotein (P-gp), breast cancer resistance protein (Bcrp), and P-gp/Bcrp knockout mice. Drug Metab. Dispos. 40, 1164–1169. doi: 10.1124/dmd.112.044719
Ambrus, L., Kelemen, B., Szabo, T., Biro, T., and Toth, B. I. (2017). Human podocytes express functional thermosensitive TRPV channels. Br. J. Pharmacol. 174, 4493–4507. doi: 10.1111/bph.14052
Ball, K., Bouzom, F., Scherrmann, J. M., Walther, B., and Decleves, X. (2013). Physiologically based pharmacokinetic modelling of drug penetration across the blood-brain barrier–towards a mechanistic IVIVE-based approach. AAPS J. 15, 913–932. doi: 10.1208/s12248-013-9496-0
Beggs, S., Liu, X. J., Kwan, C., and Salter, M. W. (2010). Peripheral nerve injury and TRPV1-expressing primary afferent C-fibers cause opening of the blood-brain barrier. Mol. Pain 6:74.
Benfenati, V., Amiry-Moghaddam, M., Caprini, M., Mylonakou, M. N., Rapisarda, C., Ottersen, O. P., et al. (2007). Expression and functional characterization of transient receptor potential vanilloid-related channel 4 (TRPV4) in rat cortical astrocytes. Neuroscience 148, 876–892. doi: 10.1016/j.neuroscience.2007.06.039
Berrout, J., Jin, M., and O’Neil, R. G. (2012). Critical role of TRPP2 and TRPC1 channels in stretch-induced injury of blood-brain barrier endothelial cells. Brain Res. 1436, 1–12. doi: 10.1016/j.brainres.2011.11.044
Binshtok, A. M., Bean, B. P., and Woolf, C. J. (2007). Inhibition of nociceptors by TRPV1-mediated entry of impermeant sodium channel blockers. Nature 449, 607–610.
Brown, R. C., and Davis, T. P. (2002). Calcium modulation of adherens and tight junction function: a potential mechanism for blood-brain barrier disruption after stroke. Stroke 33, 1706–1711. doi: 10.1161/01.str.0000016405.06729.83
Brown, R. C., Wu, L., Hicks, K., and O’Neil, R. G. (2008). Regulation of blood-brain barrier permeability by transient receptor potential type C and type v calcium-permeable channels. Microcirculation 15, 359–371. doi: 10.1080/10739680701762656
Calapai, F., Cardia, L., Sorbara, E. E., Navarra, M., Gangemi, S., Calapai, G., et al. (2020). Cannabinoids, blood-brain barrier, and brain disposition. Pharmaceutics 12:265. doi: 10.3390/pharmaceutics12030265
Cavanaugh, D. J., Chesler, A. T., Jackson, A. C., Sigal, Y. M., Yamanaka, H., Grant, R., et al. (2011). Trpv1 reporter mice reveal highly restricted brain distribution and functional expression in arteriolar smooth muscle cells. J. Neurosci. 31, 5067–5077. doi: 10.1523/jneurosci.6451-10.2011
Chaves, C., Campanelli, F., Chapy, H., Gomez-Zepeda, D., Glacial, F., Smirnova, M., et al. (2020). An interspecies molecular and functional study of organic cation transporters at the blood-brain barrier: from rodents to humans. Pharmaceutics 12:308. doi: 10.3390/pharmaceutics12040308
Dauchy, S., Miller, F., Couraud, P. O., Weaver, R. J., Weksler, B., Romero, I. A., et al. (2009). Expression and transcriptional regulation of ABC transporters and cytochromes P450 in hCMEC/D3 human cerebral microvascular endothelial cells. Biochem. Pharmacol. 77, 897–909. doi: 10.1016/j.bcp.2008.11.001
De Bock, M., Wang, N., Decrock, E., Bol, M., Gadicherla, A. K., Culot, M., et al. (2013). Endothelial calcium dynamics, connexin channels and blood-brain barrier function. Prog. Neurobiol. 108, 1–20. doi: 10.1016/j.pneurobio.2013.06.001
Deo, A. K., Theil, F. P., and Nicolas, J. M. (2013). Confounding parameters in preclinical assessment of blood-brain barrier permeation: an overview with emphasis on species differences and effect of disease states. Mol. Pharm. 10, 1581–1595. doi: 10.1021/mp300570z
Dragoni, S., and Turowski, P. (2018). Polarised VEGFA Signalling at Vascular Blood-Neural Barriers. Int. J. Mol. Sci. 19:1378. doi: 10.3390/ijms19051378
Fliniaux, I., Germain, E., Farfariello, V., and Prevarskaya, N. (2018). TRPs and Ca(2+) in cell death and survival. Cell Calc. 69, 4–18. doi: 10.1016/j.ceca.2017.07.002
Golech, S. A., McCarron, R. M., Chen, Y., Bembry, J., Lenz, F., Mechoulam, R., et al. (2004). Human brain endothelium: coexpression and function of vanilloid and endocannabinoid receptors. Brain Res. Mol. Brain Res. 132, 87–92. doi: 10.1016/j.molbrainres.2004.08.025
Grace, M. S., Baxter, M., Dubuis, E., Birrell, M. A., and Belvisi, M. G. (2014). Transient receptor potential (TRP) channels in the airway: role in airway disease. Br. J. Pharmacol. 171, 2593–2607. doi: 10.1111/bph.12538
Greco, C., Leclerc-Mercier, S., Chaumon, S., Doz, F., Hadj-Rabia, S., Molina, T., et al. (2020). Use of epidermal growth factor receptor inhibitor erlotinib to treat palmoplantar keratoderma in patients with olmsted syndrome caused by TRPV3 mutations. JAMA Dermatol. 156, 191–195. doi: 10.1001/jamadermatol.2019.4126
Guerra, G., Lucariello, A., Perna, A., Botta, L., De Luca, A., and Moccia, F. (2018). The role of endothelial Ca(2+) signaling in neurovascular coupling: a view from the lumen. Intern. J. Mol. Sci. 19:938. doi: 10.3390/ijms19040938
Harraz, O. F., Longden, T. A., Hill-Eubanks, D., and Nelson, M. T. (2018). PIP2 depletion promotes TRPV4 channel activity in mouse brain capillary endothelial cells. eLife 7:e38689.
Hatano, N., Suzuki, H., Itoh, Y., and Muraki, K. (2013). TRPV4 partially participates in proliferation of human brain capillary endothelial cells. Life Sci. 92, 317–324. doi: 10.1016/j.lfs.2013.01.002
Hind, W. H., England, T. J., and O’Sullivan, S. E. (2016). Cannabidiol protects an in vitro model of the blood-brain barrier from oxygen-glucose deprivation via PPARgamma and 5-HT1A receptors. Br. J. Pharmacol. 173, 815–825. doi: 10.1111/bph.13368
Hu, D. E., Easton, A. S., and Fraser, P. A. (2005). TRPV1 activation results in disruption of the blood-brain barrier in the rat. Br. J. Pharmacol. 146, 576–584. doi: 10.1038/sj.bjp.0706350
Huang, Q., Wang, X., Lin, X., Zhang, J., You, X., and Shao, A. (2020). The role of transient receptor potential channels in blood-brain barrier dysfunction after ischemic stroke. Biomed. Pharmacother. 131:110647. doi: 10.1016/j.biopha.2020.110647
Ito, K., Uchida, Y., Ohtsuki, S., Aizawa, S., Kawakami, H., Katsukura, Y., et al. (2011). Quantitative membrane protein expression at the blood-brain barrier of adult and younger cynomolgus monkeys. J. Pharm. Sci. 100, 3939–3950. doi: 10.1002/jps.22487
Kassmann, M., Harteneck, C., Zhu, Z., Nurnberg, B., Tepel, M., and Gollasch, M. (2013). Transient receptor potential vanilloid 1 (TRPV1), TRPV4, and the kidney. Acta Physiol. 207, 546–564. doi: 10.1111/apha.12051
Kauer, J. A., and Gibson, H. E. (2009). Hot flash: TRPV channels in the brain. Trends Neurosci. 32, 215–224. doi: 10.1016/j.tins.2008.12.006
Kealy, J., Greene, C., and Campbell, M. (2020). Blood-brain barrier regulation in psychiatric disorders. Neurosci. Lett. 726:133664. doi: 10.1016/j.neulet.2018.06.033
Kohler, R., Heyken, W. T., Heinau, P., Schubert, R., Si, H., Kacik, M., et al. (2006). Evidence for a functional role of endothelial transient receptor potential V4 in shear stress-induced vasodilatation. Arterioscler. Thromb. Vasc. Biol. 26, 1495–1502. doi: 10.1161/01.atv.0000225698.36212.6a
Koles, L., Garcao, P., Zadori, Z. S., Ferreira, S. G., Pinheiro, B. S., da Silva-Santos, C. S., et al. (2013). Presynaptic TRPV1 vanilloid receptor function is age- but not CB1 cannabinoid receptor-dependent in the rodent forebrain. Brain Res. Bull. 97, 126–135. doi: 10.1016/j.brainresbull.2013.06.007
Luo, H., Rossi, E., Saubamea, B., Chasseigneaux, S., Cochois, V., Choublier, N., et al. (2019). Cannabidiol increases proliferation, migration, tubulogenesis, and integrity of human brain endothelial cells through TRPV2 activation. Mol. Pharm. 16, 1312–1326. doi: 10.1021/acs.molpharmaceut.8b01252
Luo, J., and Hu, H. (2014). Thermally activated TRPV3 channels. Curr. Top. Membr. 74, 325–364. doi: 10.1016/b978-0-12-800181-3.00012-9
Ma, X., and Liu, W. (2019). Calcium signaling in brain microvascular endothelial cells and its roles in the function of the blood-brain barrier. Neuroreport 30, 1271–1277. doi: 10.1097/wnr.0000000000001357
Mangos, S., Liu, Y., and Drummond, I. A. (2007). Dynamic expression of the osmosensory channel trpv4 in multiple developing organs in zebrafish. Gene Expr. Patter. 7, 480–484. doi: 10.1016/j.modgep.2006.10.011
Mannari, T., Morita, S., Furube, E., Tominaga, M., and Miyata, S. (2013). Astrocytic TRPV1 ion channels detect blood-borne signals in the sensory circumventricular organs of adult mouse brains. Glia 61, 957–971. doi: 10.1002/glia.22488
Marrelli, S. P., O’Neil, R. G., Brown, R. C., and Bryan, R. M. Jr. (2007). PLA2 and TRPV4 channels regulate endothelial calcium in cerebral arteries. Am. J. Physiol. Heart Circ. Physiol. 292, H1390–H1397.
Mecha, M., Feliu, A., Inigo, P. M., Mestre, L., Carrillo-Salinas, F. J., and Guaza, C. (2013). Cannabidiol provides long-lasting protection against the deleterious effects of inflammation in a viral model of multiple sclerosis: a role for A2A receptors. Neurobiol. Dis. 59, 141–150. doi: 10.1016/j.nbd.2013.06.016
Mergler, S., Valtink, M., Coulson-Thomas, V. J., Lindemann, D., Reinach, P. S., Engelmann, K., et al. (2010). TRPV channels mediate temperature-sensing in human corneal endothelial cells. Exp. Eye Res. 90, 758–770. doi: 10.1016/j.exer.2010.03.010
Mickle, A. D., Shepherd, A. J., and Mohapatra, D. P. (2015). Sensory TRP channels: the key transducers of nociception and pain. Prog. Mol. Biol. Transl. Sci. 131, 73–118. doi: 10.1007/978-3-319-18705-1_3
Montell, C. (2001). Physiology, phylogeny, and functions of the TRP superfamily of cation channels. Sci. STKE 2001:re1. doi: 10.1126/stke.2001.90.re1
Moran, M. M. (2018). TRP channels as potential drug targets. Annu. Rev. Pharmacol. Toxicol. 58, 309–330.
Nedungadi, T. P., Dutta, M., Bathina, C. S., Caterina, M. J., and Cunningham, J. T. (2012). Expression and distribution of TRPV2 in rat brain. Exp. Neurol. 237, 223–237. doi: 10.1016/j.expneurol.2012.06.017
Ohtsuki, S., Ikeda, C., Uchida, Y., Sakamoto, Y., Miller, F., Glacial, F., et al. (2013). Quantitative targeted absolute proteomic analysis of transporters, receptors and junction proteins for validation of human cerebral microvascular endothelial cell line hCMEC/D3 as a human blood-brain barrier model. Mol. Pharm. 10, 289–296. doi: 10.1021/mp3004308
Peng, J. B., Suzuki, Y., Gyimesi, G., and Hediger, M. A. (2018). “TRPV5 and TRPV6 calcium-selective channels,” in Calcium Entry Channels in Non-Excitable Cells, eds J. A. Kozak and J. W. Putney Jr. (Boca Raton, FL: CRC Press), 241–274. doi: 10.1201/9781315152592-13
Perriere, N., Demeuse, P., Garcia, E., Regina, A., Debray, M., Andreux, J. P., et al. (2005). Puromycin-based purification of rat brain capillary endothelial cell cultures. Effect on the expression of blood-brain barrier-specific properties. J. Neurochem. 93, 279–289. doi: 10.1111/j.1471-4159.2004.03020.x
Perriere, N., Yousif, S., Cazaubon, S., Chaverot, N., Bourasset, F., Cisternino, S., et al. (2007). A functional in vitro model of rat blood-brain barrier for molecular analysis of efflux transporters. Brain Res. 1150, 1–13. doi: 10.1016/j.brainres.2007.02.091
Phuong, T. T. T., Redmon, S. N., Yarishkin, O., Winter, J. M., Li, D. Y., and Krizaj, D. (2017). Calcium influx through TRPV4 channels modulates the adherens contacts between retinal microvascular endothelial cells. J. Physiol. 595, 6869–6885. doi: 10.1113/jp275052
Qin, N., Neeper, M. P., Liu, Y., Hutchinson, T. L., Lubin, M. L., and Flores, C. M. (2008). TRPV2 is activated by cannabidiol and mediates CGRP release in cultured rat dorsal root ganglion neurons. J. Neurosci. 28, 6231–6238. doi: 10.1523/jneurosci.0504-08.2008
Rigoni, M., Trevisani, M., Gazzieri, D., Nadaletto, R., Tognetto, M., Creminon, C., et al. (2003). Neurogenic responses mediated by vanilloid receptor-1 (TRPV1) are blocked by the high affinity antagonist, iodo-resiniferatoxin. Br. J. Pharmacol. 138, 977–985. doi: 10.1038/sj.bjp.0705110
Rodrigues, T., Sieglitz, F., and Bernardes, G. J. (2016). Natural product modulators of transient receptor potential (TRP) channels as potential anti-cancer agents. Chem. Soc. Rev. 45, 6130–6137. doi: 10.1039/c5cs00916b
Ruiz-Valdepenas, L., Martinez-Orgado, J. A., Benito, C., Millan, A., Tolon, R. M., and Romero, J. (2011). Cannabidiol reduces lipopolysaccharide-induced vascular changes and inflammation in the mouse brain: an intravital microscopy study. J. Neuroinflamm. 8:5. doi: 10.1186/1742-2094-8-5
Shibasaki, K., Tominaga, M., and Ishizaki, Y. (2015). Hippocampal neuronal maturation triggers post-synaptic clustering of brain temperature-sensor TRPV4. Biochem. Biophys. Res. Commun. 458, 168–173. doi: 10.1016/j.bbrc.2015.01.087
Smani, T., Gomez, L. J., Regodon, S., Woodard, G. E., Siegfried, G., Khatib, A. M., et al. (2018). TRP channels in angiogenesis and other endothelial functions. Front. Physiol. 9:1731. doi: 10.3389/fphys.2018.01731
Steinritz, D., Stenger, B., Dietrich, A., Gudermann, T., and Popp, T. (2018). TRPs in Tox: involvement of transient receptor potential-channels in chemical-induced organ toxicity-A structured review. Cells 7:98. doi: 10.3390/cells7080098
Sullivan, M. N., Francis, M., Pitts, N. L., Taylor, M. S., and Earley, S. (2012). Optical recording reveals novel properties of GSK1016790A-induced vanilloid transient receptor potential channel TRPV4 activity in primary human endothelial cells. Mol. Pharmacol. 82, 464–472. doi: 10.1124/mol.112.078584
Sweeney, M. D., Zhao, Z., Montagne, A., Nelson, A. R., and Zlokovic, B. V. (2019). Blood-brain barrier: from physiology to disease and Back. Physiol. Rev. 99, 21–78. doi: 10.1152/physrev.00050.2017
Syvanen, S., Lindhe, O., Palner, M., Kornum, B. R., Rahman, O., Langstrom, B., et al. (2009). Species differences in blood-brain barrier transport of three positron emission tomography radioligands with emphasis on P-glycoprotein transport. Drug Metab. Dispos. 37, 635–643. doi: 10.1124/dmd.108.024745
Uchida, Y., Ohtsuki, S., Katsukura, Y., Ikeda, C., Suzuki, T., Kamiie, J., et al. (2011). Quantitative targeted absolute proteomics of human blood-brain barrier transporters and receptors. J. Neurochem. 117, 333–345. doi: 10.1111/j.1471-4159.2011.07208.x
Vanlandewijck, M., He, L., Mae, M. A., Andrae, J., Ando, K., Del Gaudio, F., et al. (2018). A molecular atlas of cell types and zonation in the brain vasculature. Nature 554, 475–480. doi: 10.1038/nature25739
Villalta, P. C., and Townsley, M. I. (2013). Transient receptor potential channels and regulation of lung endothelial permeability. Pulm. Circ. 3, 802–815. doi: 10.1086/674765
Weksler, B. B., Subileau, E. A., Perriere, N., Charneau, P., Holloway, K., Leveque, M., et al. (2005). Blood-brain barrier-specific properties of a human adult brain endothelial cell line. FASEB J. 19, 1872–1874. doi: 10.1096/fj.04-3458fje
Wen, W., Que, K., Zang, C., Wen, J., Sun, G., Zhao, Z., et al. (2017). Expression and distribution of three transient receptor potential vanilloid(TRPV) channel proteins in human odontoblast-like cells. J. Mol. Histol. 48, 367–377. doi: 10.1007/s10735-017-9735-2
Yang, C., Hawkins, K. E., Dore, S., and Candelario-Jalil, E. (2019). Neuroinflammatory mechanisms of blood-brain barrier damage in ischemic stroke. Am. J. Physiol. Cell Physiol. 316, C135–C153.
Yu, W., Hill, W. G., Apodaca, G., and Zeidel, M. L. (2011). Expression and distribution of transient receptor potential (TRP) channels in bladder epithelium. Am. J. Physiol. Ren. Physiol. 300, F49–F59.
Yu, X., Ji, C., and Shao, A. (2020). Neurovascular unit dysfunction and neurodegenerative disorders. Front. Neurosci. 14:334. doi: 10.3389/fphys.2018.00334
Zhang, A., Duchatelet, S., Lakdawala, N., Tower, R. L., Diamond, C., Marathe, K., et al. (2020). Targeted inhibition of the epidermal growth factor receptor and mammalian target of rapamycin signaling pathways in olmsted syndrome. JAMA Dermatol. 156, 196–200. doi: 10.1001/jamadermatol.2019.4141
Keywords: TRPV1, TRPV2, TRPV4, interspecies differences, blood–brain barrier, brain endothelial cell
Citation: Luo H, Saubamea B, Chasseigneaux S, Cochois V, Smirnova M, Glacial F, Perrière N, Chaves C, Cisternino S and Declèves X (2020) Molecular and Functional Study of Transient Receptor Potential Vanilloid 1-4 at the Rat and Human Blood–Brain Barrier Reveals Interspecies Differences. Front. Cell Dev. Biol. 8:578514. doi: 10.3389/fcell.2020.578514
Received: 30 June 2020; Accepted: 21 October 2020;
Published: 11 November 2020.
Edited by:
Sandra Derouiche, National Institute for Physiological Sciences (NIPS), JapanReviewed by:
Michael A. Kalwat, University of Texas Southwestern Medical Center, United StatesChandan Goswami, National Institute of Science Education and Research (NISER), India
Peter S. Reinach, Wenzhou Medical University, China
Maria A. Deli, Biological Research Center of the Hungarian Academy of Sciences, Hungary
Stefan Mergler, Charité – Universitätsmedizin Berlin, Germany
Copyright © 2020 Luo, Saubamea, Chasseigneaux, Cochois, Smirnova, Glacial, Perrière, Chaves, Cisternino and Declèves. This is an open-access article distributed under the terms of the Creative Commons Attribution License (CC BY). The use, distribution or reproduction in other forums is permitted, provided the original author(s) and the copyright owner(s) are credited and that the original publication in this journal is cited, in accordance with accepted academic practice. No use, distribution or reproduction is permitted which does not comply with these terms.
*Correspondence: Xavier Declèves, xavier.decleves@parisdescartes.fr