The Impact of miRNAs in Health and Disease of Retinal Pigment Epithelium
- 1Telethon Institute of Genetics and Medicine, Naples, Italy
- 2Department of Biology, Polytechnic and Basic Sciences School, University of Naples Federico II, Naples, Italy
MicroRNAs (miRNAs), a class of non-coding RNAs, are essential key players in the control of biological processes in both physiological and pathological conditions. miRNAs play important roles in fine tuning the expression of many genes, which often have roles in common molecular networks. miRNA dysregulation thus renders cells vulnerable to aberrant fluctuations in genes, resulting in degenerative diseases. The retinal pigment epithelium (RPE) is a monolayer of polarized pigmented epithelial cells that resides between the light-sensitive photoreceptors (PR) and the choriocapillaris. The demanding physiological functions of RPE cells require precise gene regulation for the maintenance of retinal homeostasis under stress conditions and the preservation of vision. Thus far, our understanding of how miRNAs function in the homeostasis and maintenance of the RPE has been poorly addressed, and advancing our knowledge is central to harnessing their potential as therapeutic agents to counteract visual impairment. This review focuses on the emerging roles of miRNAs in the function and health of the RPE and on the future exploration of miRNA-based therapeutic approaches to counteract blinding diseases.
Introduction
In vertebrates, the RPE originates from the dorsal portion of the optic cup, while the retina and the optic stalk develop from the distal/ventral portion (Amram et al., 2017). Once specified, the RPE cells begin to differentiate, changing in size and shape. With the folding of the optic cup, RPE progenitor cells constitute a ciliated and pseudostratified epithelium, which is committed into a cuboidal epithelium following the formation of interphotoreceptor matrix. As eye morphogenesis proceeds, the presumptive RPE tissue assumes an apical to basolateral polarity and forms both microvilli and tight junctions. On further differentiation, the RPE cells adopts a hexagonal shape, with elongated microvilli and a smooth basal surface which makes continuous contact with its basement membrane (Marmorstein et al., 1998). In adults, RPE tissue comprises a monolayer of polarized and pigmented epithelial cells that resides at the interface between the light-sensitive outer segments of the PR and vessels of choriocapillaris (Strauss, 1995, 2005). The RPE layer is a critical constituent tissue capable of absorbing the light energy focused by the lens on the retina, thus mitigating photo-oxidative stress (Bok, 1993). In addition, the RPE represents part of the tight retinal–blood barrier (Simo et al., 2010); supports the isomerization of all-trans-retinal to 11-cis-retinal, the visual chromophore required for photoreceptor excitability (Strauss, 2005); protects from oxidative stress (Strauss, 2005); secretes growth factors that help to maintain the structural integrity of photoreceptors and choriocapillaris endothelium (Strauss, 2005; Mazzoni et al., 2014); establishes ocular immune privilege by expressing immunosuppressive factors (Ao et al., 2018); and finally is critical in the continuous renewal of the photoreceptors outer segments (POS), which are regularly shed by phagocytosis, to rebuild light-sensitive outer segments from the base of the photoreceptors (Mazzoni et al., 2014). To realize these complex functions, intricate molecular networks, activated by extracellular and intracellular signals, are simultaneously employed to maintain RPE homeostasis and function. Due to the significant activity of microRNAs (miRNAs) in modulating essential biological processes by targeting networks of functionally correlated genes, it is unsurprising that miRNAs have emerged as indispensable components of these molecular networks in the RPE (Soundara Pandi et al., 2013; Greene et al., 2014). Importantly, alterations to gene regulatory networks controlling any of the above activities of the RPE can lead to degeneration of the retina and loss of visual function. This in turn gives rise to diseases including retinitis pigmentosa, age-related macular degeneration (AMD), and other blindness conditions in humans (Strauss, 2005; Amram et al., 2017).
miRNAs Biogenesis and Function
Identified in the early 1990s in Caenorhabditis elegans (Lee et al., 1993; Wightman et al., 1993), miRNAs are a class of small (typically 20–26 nucleotides) non-coding RNA molecules that regulate gene expression at the posttranscriptional level, in a well-characterized process in which the miRNAs bind to target sites in the messenger RNA. miRNA biogenesis [reviewed by Ha and Kim (2014) and Treiber et al. (2019)] is a complex process that begins with a long primary transcript comprising a stem-loop hairpin structure (pri-miRNA), which is transcribed by RNA polymerase II, capped, and polyadenylated (Treiber et al., 2019). Pri-miRNA subsequently undergoes stepwise processing to produce single hairpins (typically 60–70 nucleotides) referred to as precursor miRNAs (pre-miRNAs) by the RNase III enzyme Drosha and its cofactor DiGeorge syndrome critical region gene 8 (Dgcr8), which compose the “Drosha microprocessor” complex (Hata and Kashima, 2016). Following Drosha processing, pre-miRNA is then exported to the cytoplasm by exportin 5 (Xpo5), where maturation is completed through a second round of stepwise processing by the RNase III enzyme Dicer and its cofactor transactivation-responsive RNA-binding protein (TRBP or PACT). This gives rise to a small RNA duplex (Ha and Kim, 2014). The miRNA duplex is then handed over to a member of the Argonaute (AGO) protein family, which selects one of the strands of this duplex (the guide strand) and discards the other strand (the passenger strand) (Treiber et al., 2019). Finally, the miRNA/AGO complex and the GW182/TNRC6 family of proteins form the active miRNA-induced silencing complex (miRISC), which is recruited to target mRNAs by pairing the “seed” region of miRNA, with a partially complementary sequence in the 3′-untranslated region (3′-UTR) of target mRNAs (Ha and Kim, 2014). Thus, miRNA-guided gene silencing promotes translational inhibition and mRNA decay (Treiber et al., 2019). Most miRNAs are expressed in a highly tissue-specific manner (Wienholds et al., 2005). Importantly, miRNAs have been shown to display either protective or pathological-promoting effects in several tissues. Although the number of miRNAs known to be involved in eye development has increased significantly, a more complete understanding of how miRNAs regulate cellular processes and how their own expression is regulated is yet to be achieved. In the RPE, miRNAs were found to be key regulators of tissue differentiation, homeostasis, and function. In this review, we discuss some of the known functions of the most relevant miRNAs involved in the RPE development, maintenance, and function. A more comprehensive dissection of the roles of miRNAs in the RPE will not only improve our understanding of the molecular networks controlling RPE homeostasis and our diagnostic abilities but will also lay the foundations for studying how miRNAs could act as therapeutic tools for the treatment of ocular diseases.
miRNAs as Emerging Regulators of RPE Development and Maintenance
The gene regulatory networks involved in establishing the RPE development and differentiation have been extensively studied in a variety of model organisms reviewed in Amram et al. (2017), but the critical roles of miRNAs in these processes have only recently been explored. Recent studies have reported miRNA expression profiles during RPE development and differentiation in a variety of species, using multiple approaches including next generation sequencing (NGS) technology and in situ hybridization (ISH) analysis (Deo et al., 2006; Xu et al., 2007; Damiani et al., 2008; Decembrini et al., 2009; Arora et al., 2010; Georgi and Reh, 2010; Hackler et al., 2010; Karali et al., 2010; La Torre et al., 2013; Wohl and Reh, 2016). Importantly, NGS analyses showed dynamic miRNA expression profiles during RPE differentiation, indicating a strict correlation between miRNA expression levels and the stages of iPSC-RPE differentiation (Wang et al., 2014). To characterize the roles of miRNAs in the RPE development, depletion of the enzymes essential for their maturation and processing were examined in several animal models. Conditional knockout (cKO) mice, for Dicer1, Drosha, or Dgcr8 genes in the RPE, demonstrated that this class of proteins is relevant for RPE differentiation and maintenance. Dicer1 cKO in the ocular pigmented cell lineage around postnatal (PN) day 9.5, generated by crossing Dgcr8LoxP/LoxP animals with Dct-Cre, Tyrp2-Cre, and α-Cre mice, provided the earliest evidence of the impact of simultaneous loss of miRNAs in the generation and survival of non-retinal cell types (Davis et al., 2011). Consistent with these findings, Dicer1 cKO at the optic vesicle stage (E9.5), using Dct-Cre mice, revealed alterations to RPE differentiation. At PN11 days, Dicer1loxP/loxP/Dct-Cre mice showed RPE cells that were smaller than normal, depigmented, and failed to express enzymes required for the visual cycle (Ohana et al., 2015; Amram et al., 2017). However, RPE cell fate and hexagonal cell morphology were preserved, as was the conserved expression of transcription factors (i.e., OTX2, MITF, and SOX9), which participate in RPE specification and maintenance (Amram et al., 2017). Similar phenotypes were also observed in both RPE-specific Drosha and Dgcr8 cKO mouse models (Ohana et al., 2015; Amram et al., 2017). Importantly, the maintenance of RPE cell fate was further corroborated by the transcription profile of Dicer1-deficient RPE cells. These data gave rise to the hypothesis that RPE fate might be acquired and maintained through miRNA-independent mechanisms. Together, these findings supported the notion that miRNAs were necessary for the execution of proper differentiation programs during RPE development (Ohana et al., 2015; Amram et al., 2017). In contrast to the findings on the role of Dicer1 in miRNA biogenesis during RPE differentiation, a non-canonical Dicer1 activity was demonstrated to participate in the adult RPE from Dicer1loxP/loxP/Best1-Cre mice, with Cre recombinase expression beginning at P10 and peaking at P28 (Iacovelli et al., 2011). In differentiated RPE cells, a primary role for Dicer1 was documented in the degradation of toxic transcripts of Alu transposable elements, rather than miRNA biogenesis (Iacovelli et al., 2011). Supporting this notion, single miRNA-binding protein knockout mice, for Ago1, Ago3, Ago4, or Tarbp2, did not reveal RPE morphological alterations (Sundermeier and Palczewski, 2016). Consistent with these findings, AAV-mediated delivery of a Best1-Cre recombinase expression cassette into mice carrying conditional Drosha, Dgcr8, or Ago2 alleles did not show any defects in RPE morphology (Sundermeier and Palczewski, 2016). However, lack of miRNA biogenesis in both differentiating and adult RPE commonly affected the homeostasis and survival of the adjacent photoreceptor cells. Nevertheless, to gain deeper phenotypic understanding, additional studies should analyze alternative miRNA biogenesis mechanisms and the compensatory molecular networks that counteract lack of enzymes essential for miRNA maturation and processing in the RPE (Yang and Lai, 2011).
The Impact of miRNAs on RPE Differentiation, Proliferation, and Migration
Notably, in a few cases, the roles of individual miRNAs have been dissected in fish, frogs, and mice, demonstrating the relevance of these small non-coding RNAs in RPE development. The members of miR-204/211 family are arguably the most extensively studied miRNAs in the RPE. These miRNAs are identifiable from their seed regions and are highly enriched in the developing and differentiated RPE. Overexpression of miR-204/211 in human fetal RPE (hfRPE) cells has been shown to effectively counteract a lack of MITF, a key regulator of RPE differentiation, and has been reported to rescue RPE de-differentiation phenotype (Adijanto et al., 2012). Similarly, studies in human parthenogenetic embryonic stem cells (hPESCs) and hfRPE demonstrated that miR-204 upregulation contributes to RPE differentiation program by repressing the target gene CTNNBIP1, an inhibitor of the Wnt/β-catenin pathway (Li et al., 2012). Interestingly, although both miR-204 and miR-211 were required for RPE differentiation, deletion of only one member of this miRNA family resulted in no visible alterations to RPE differentiation, suggesting possible redundancy of function, at least during RPE development in vivo. The role of miRNAs in RPE differentiation was also confirmed through studies on miR-184 in hiPSC-RPE and zebrafish, demonstrating the role of miR-184 in controlling RPE differentiation. Downregulation of dre-miR-184 suppressed the expression levels of RPE markers, while its overexpression stimulates RPE development by inhibiting the AKT2/mTOR pathway (Jiang et al., 2016). Further supportive evidence for the role of miRNAs in RPE differentiation programs has been reported in additional studies. miR-20b/106a and miR-222/221 families have been described to regulate RPE differentiation by modulating the expression levels of several transcription factors including Sox9, Otx1, Pax6, and Meis2 (Ohana et al., 2015). Among the miRNAs downregulated during RPE differentiation, both miR-302d-3p and miR-410 are prominently repressed during RPE development. Importantly, overexpression of miR-302d-3p induced hiPSC-RPE de-differentiation and impairment of cellular phagocytosis and promoted cell-cycle progression, via repression of both TGF-βR2 and p21Waf1/Cip1, a cyclin-dependent kinase inhibitor, encoded by CDKN1A (Li et al., 2012; Jiang et al., 2018). Importantly, the inhibition of miR-410 facilitated RPE differentiation in both AESCs and umbilical cord blood-derived mesenchymal stem cells (UCB-MSCs) by derepression multiple RPE development-relevant genes, such as RPE65 and OTX2 (Choi et al., 2015, 2017). Moreover, multiple studies have reported that inhibition of RPE proliferation and migration were induced by increased expression miR-451a (Shao et al., 2019) and miR-218 (Yao et al., 2019). On the other hand, an overproliferative effect of miR-182 (Figure 1) was reported to downregulate the p-Akt pathway in RPE via repression of c-Met expression (Wang et al., 2016a). Similarly, overexpression of miR-34a in cultured subconfluent ARPE-19 cells displayed remarkable inhibition of c-Met, in turn reducing HGF/SF expression and preventing cell proliferation and migration (Hou et al., 2013). Moreover, besides these well characterized miRNAs, additional RPE-enriched miRNAs have recently emerged as regulators of RPE development (Table 1). However, their capacities to alter RPE cell differentiation, proliferation, and migration and the identification of their targets and regulatory networks remain to be solved.
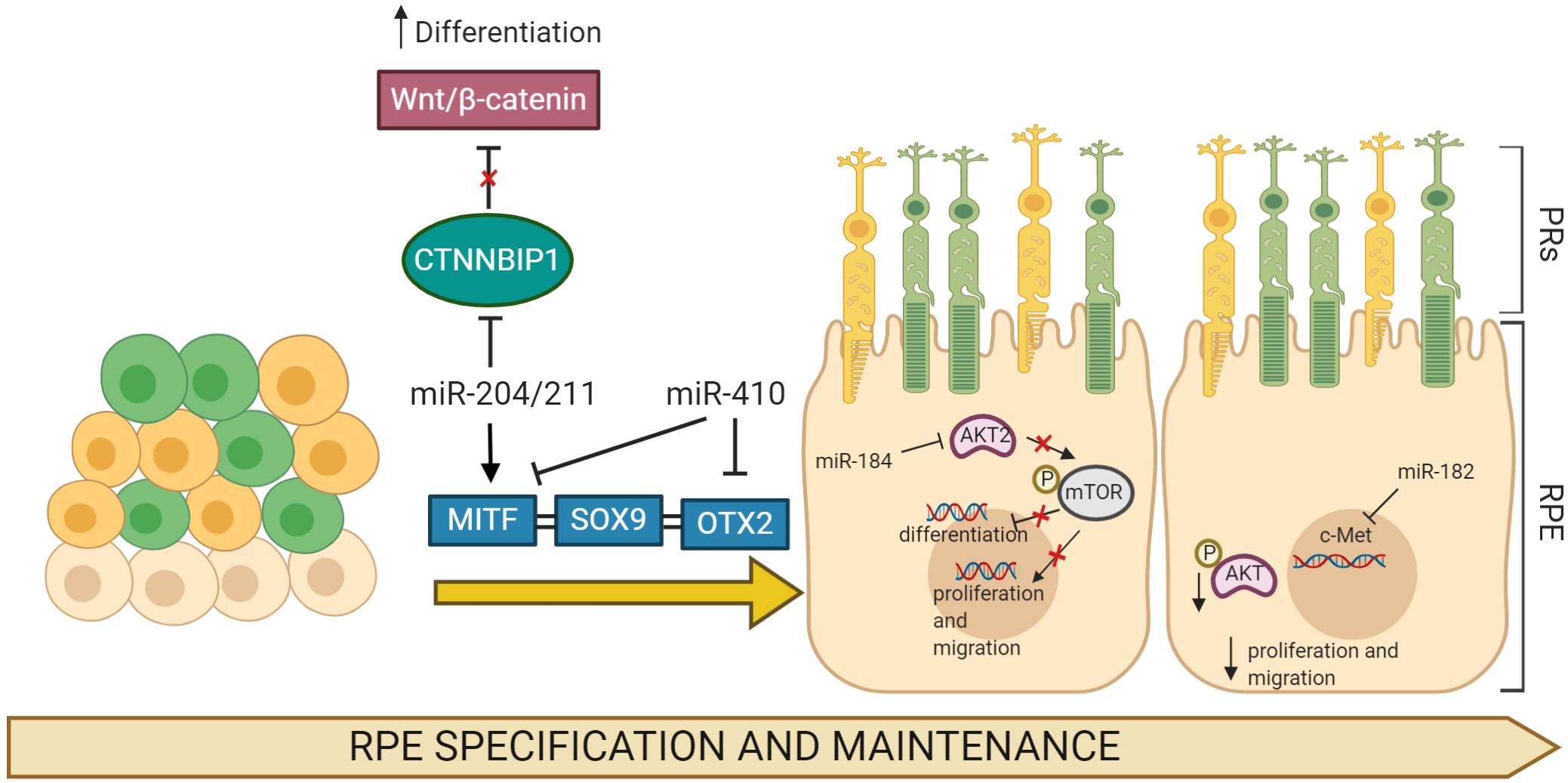
Figure 1. MicroRNA (miRNA)-regulated gene networks involved in RPE specification and maintenance. Simplified schematic of a gene network regulating development, differentiation, and migration in RPE cells. Key genes in these processes, MITF, SOX9, and OTX2, are all regulated by specific miRNAs that modulate their expression profiles. The expression of multiple miRNAs increases and/or affects the processes of RPE differentiation, proliferation, and migration through the regulation of target gene mRNAs.
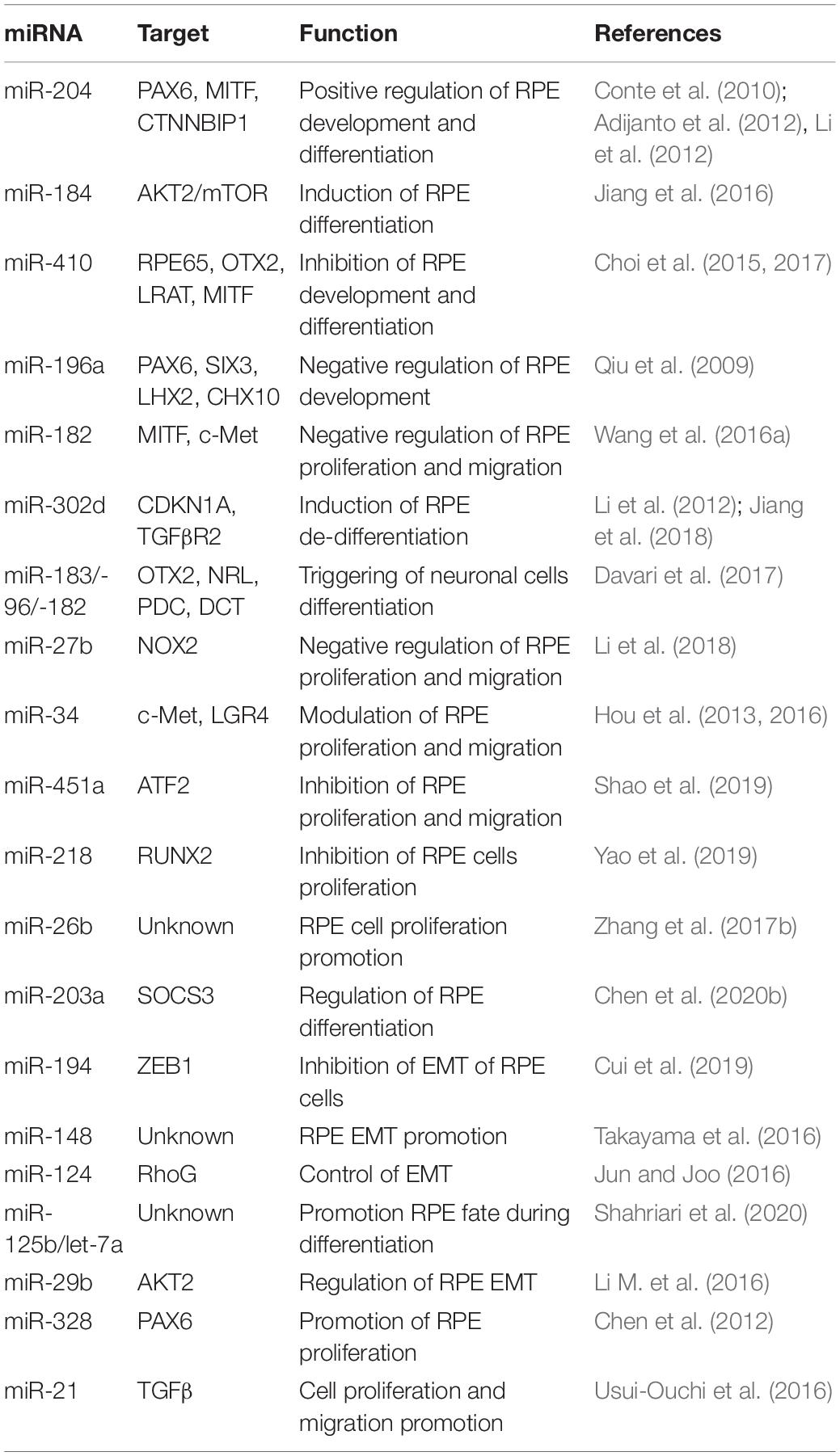
Table 1. Selected miRNAs involved in the regulation of RPE development, differentiation, and migration.
RPE Homeostasis and Function
The RPE exhibits common features of an epithelium tissue, preserving the structural and physiological integrities of adjacent tissues. The RPE plays crucial roles in retinal homeostasis, including the formation of the outer blood–retinal barrier (BRB), through junctional complexes, which prevents the entry of toxic molecules and plasma components into the retina (Rizzolo, 2007). Alterations to RPE physiology and homeostasis result in photoreceptor death and blindness. As part of the BRB, the RPE transports water, ions, and metabolic waste from the subretinal space to the choriocapillaris (Marmor, 1990; Hamann, 2002), where it takes up nutrients such as glucose or vitamin A, retinol, and fatty acids to the photoreceptors (Ban and Rizzolo, 2000; Marmorstein, 2001). The RPE secretes different growth factors according to variable physiological and pathological conditions. During eye development, growth factors released from RPE play key roles in choroidal neovascularization. To regulate transport across the RPE, numerous pumps, channels, and carriers are specifically distributed to either the apical or the basolateral membrane. Transporters and channels with roles in the selective transport to and from the choroid vasculature are located in the basal RPE. The apical side of the RPE projects long thin and sheet-like microvilli into the interphotoreceptor matrix. The long microvilli engulf the tips of the rod and cone photoreceptor outer segments; this ensures that the segments are orientated in the appropriate plane for optics and maximizes the cellular surface for efficient epithelial transport. The short microvilli mainly function in the turnover of POS through phagocytosis. Moreover, RPE cells are constantly exposed to oxidative stress due to exposure to light and the generation of reactive oxygen species (ROS) following phagocytosis of POS (Plafker et al., 2012). A robust endogenous antioxidant defense mechanism is therefore critical. This is characterized by the production of endogenous antioxidants such as glutathione, whose production decreases with age and/or incidence of degenerative diseases (Beatty et al., 2000). Oxidative stress alters RPE integrity and promotes the activation of the inflammation system, activating complementary cascades and upregulating the production of cytokines and chemokines. Notably, the mature RPE itself maintains tissue homeostasis through long-term cell survival, with little evidence of cell turnover and a stem cell compartment for de novo cell production. This static homeostasis suggests that as RPE cells age, substantial tissue changes occur that compromise RPE function and morphology, triggering causes of AMD (Fuhrmann et al., 2014). Based on its structural characteristics, the RPE plays crucial roles in retinal homeostasis, of which a primary role is the formation of the outer BRB that prevents the entrance of toxic molecules and plasma components into the retina (Rizzolo, 2007). Breakdown of the BRB can lead to visual loss in a number of ocular disorders, including diabetic retinopathy (DR).
MicroRNAs Are the “Traffic Wanders” of the RPE Homeostatic Center
Maintenance of RPE layer integrity is controlled by junctional complexes, characterized by the presence of multiple proteins including occludin, claudins, and junctional adhesion molecules. This type of cell–cell adhesion establishes a barrier between the subretinal space and choriocapillaris (Harhaj and Antonetti, 2004). The first evidence for the role of miRNAs in RPE homeostasis was reported from studies on both Dicer and Dgcr8 cKO mice, where ultrastructural analysis of RPE revealed disorganized basal infoldings with large cytoplasmic vacuoles and debris accumulation at the interface of Bruch’s membrane, resulting in atrophy and disruptions to the integrity of the RPE (Sundermeier et al., 2017; Wright et al., 2020). Furthermore, miRNA studies on miR-204 revealed its prominent functional role in the formation and maintenance of the epithelial barrier of the RPE. Wang et al. (2010) demonstrated that miR-204 significantly alters claudin 10 and 19, tight-junction proteins both highly expressed in human RPE, while miR-204/211 affects transthyretin (TTR) secretion, an important marker for epithelial barrier integrity and critical for vitamin A transport across the RPE (Cichon et al., 2014). In agreement with this, overexpression of the miR-204/211 family in primary hfRPE induced high expression levels of RPE-specific genes, triggering cell reprogramming to RPE epithelial cells characterized by hexagonal shape with junctional multiplexes (Adijanto et al., 2012). Additionally, it has also been observed that miR-148a induced reduction in ZO-1 expression and disruptions to RPE morphology (Takayama et al., 2016). Moreover, the expression of miR-20b/106a and miR-222/221 families are likely to be essential for maintaining RPE barrier properties and function and have crucial functional roles in RPE homeostasis (Ohana et al., 2015). As part of the BRB, the RPE transports water, ions, and metabolic waste from the subretinal space to the choriocapillaris (Marmor, 1990; Hamann, 2002), where it takes up nutrients such as glucose, vitamin A, retinol, and fatty acids to the photoreceptors (Ban and Rizzolo, 2000; Marmorstein, 2001). To regulate transport across the RPE, various pumps, channels, and transporters are distributed specifically to either the apical or the basolateral membrane. In particular, it has been shown that Kir7.1 is the most abundant potassium channel localized at the apical membrane in the RPE (Yang et al., 2008). Kir7.1 mediates the interactions between retinal photoreceptors and RPE following transitions between light and dark. Wang et al. (2010) also provided the earliest evidence that miR-204 indirectly suppresses Kir7.1 via TGF-βR2 repression in human RPE and thus likely limits potassium efflux across the RPE apical membrane. Additionally, the Kir7.1 channel is functionally coupled to other apical membrane potassium transporters, and the recycling capacity of this channel is maintained by high levels of miR-204 expression. In this context, supporting these observations, miR-204–/– mice showed a reduced efflux of K+ transport, which accumulates in the RPE and induces cell swelling and alteration of subretinal space (Zhang et al., 2019). Furthermore, in several studies, it has been shown that miR-204 controls the expression of its host gene, namely, TRPM3, which conducts Ca2+ and Zn2+ ions in renal cell carcinoma (Hall et al., 2014). Depletion of miR-204 may also increase TRPM3 expression, resulting in general alterations to ion transportation in the RPE. On the other hand, TRPM3 also cooperates with ZO-1 to maintain junctional permeability and barrier function at the apical membrane and to sense light-induced Ca2+ levels in the photoreceptor matrix during the visual cycle (Zhao et al., 2015). The latter suggests that the loss of miR-204 could alter the homeostasis of RPE through multiple mechanisms. Other miRNAs have also been identified as crucial regulators of ion channels. Noteworthy among them, Naso et al. (2020) demonstrated that miR-211-mediated inhibition of Ezrin releases a Ca2+ microdomain flux into cells through potentiation of TRPML1 channel sensitivity, a lysosomal cation channel implicated in lysosomal biogenesis and function (Gomez et al., 2018). miR-211–/– mice show severely compromised vision characterized by an accumulation of phagolysosomes which contain poorly processed POS in the RPE and cone dystrophy (Naso et al., 2020). Confirming the central relevance of miRNAs in RPE homeostasis, miRNAs also take part in cellular responses to environmental stresses such as hypoxia, oxidative stress, and inflammation. Remarkably, oxidative stress stimulates the production of miRNAs that play a role in connecting the antioxidant defense system with imbalances in redox state. Among those, miR-626 represses Keap1, which results in the promotion and activation of Nrf2-dependent antioxidant signaling to protect RPE against oxidative stress (Xu et al., 2019). In line with this finding, Lin et al. (2011) showed antiapoptotic effects of miR-23 against oxidative injury through regulation of Fas, suggesting the relevance of miR-23 as a key regulator of ROS-mediated cell death/survival. Finally, miR-30b has been reported to increase the cellular antioxidant defense against oxidative stress by targeting the catalase gene. Conversely, miR-144 has recently been described to exert its apoptotic function by targeting Nrf2. In response to oxidative stimuli, miR-144 directly represses Nrf2, causing reductions to endogenous levels of glutathione and leaving RPE cells susceptible to oxidative stress (Jadeja et al., 2020). These changes in miRNA expression levels suggest dual roles for miRNAs, giving rise to protective or pathological apoptotic phenotypes. Remarkably, Nrf2 also protects retinal tissues from vascular inflammation. A number of additional studies hint at miRNAs playing a role in counteracting the chronic neovascularization in RPE by regulating the expression patterns of proangiogenic factors. For example, miR-9 has been demonstrated to target Srpk1 gene, controlling the alternative splicing of angiogenic VEGF165 in human RPE cells under oxidative stress (Yoon et al., 2014). Notably, overexpression of miR-9 effectively reduces the mRNA levels of proangiogenic VEGF165a in hypoxia, while the inhibition of miR-9 decreases antiangiogenic VEGF165b mRNA levels (Figure 2). These findings further imply that alterations in specific miRNAs (listed in the Table 2) may be used as pathophysiological biomarkers and, additionally, that the precise control of their expression patterns could offer therapeutic strategies to counteract the onset and progression of diverse retinal inherited disorders.
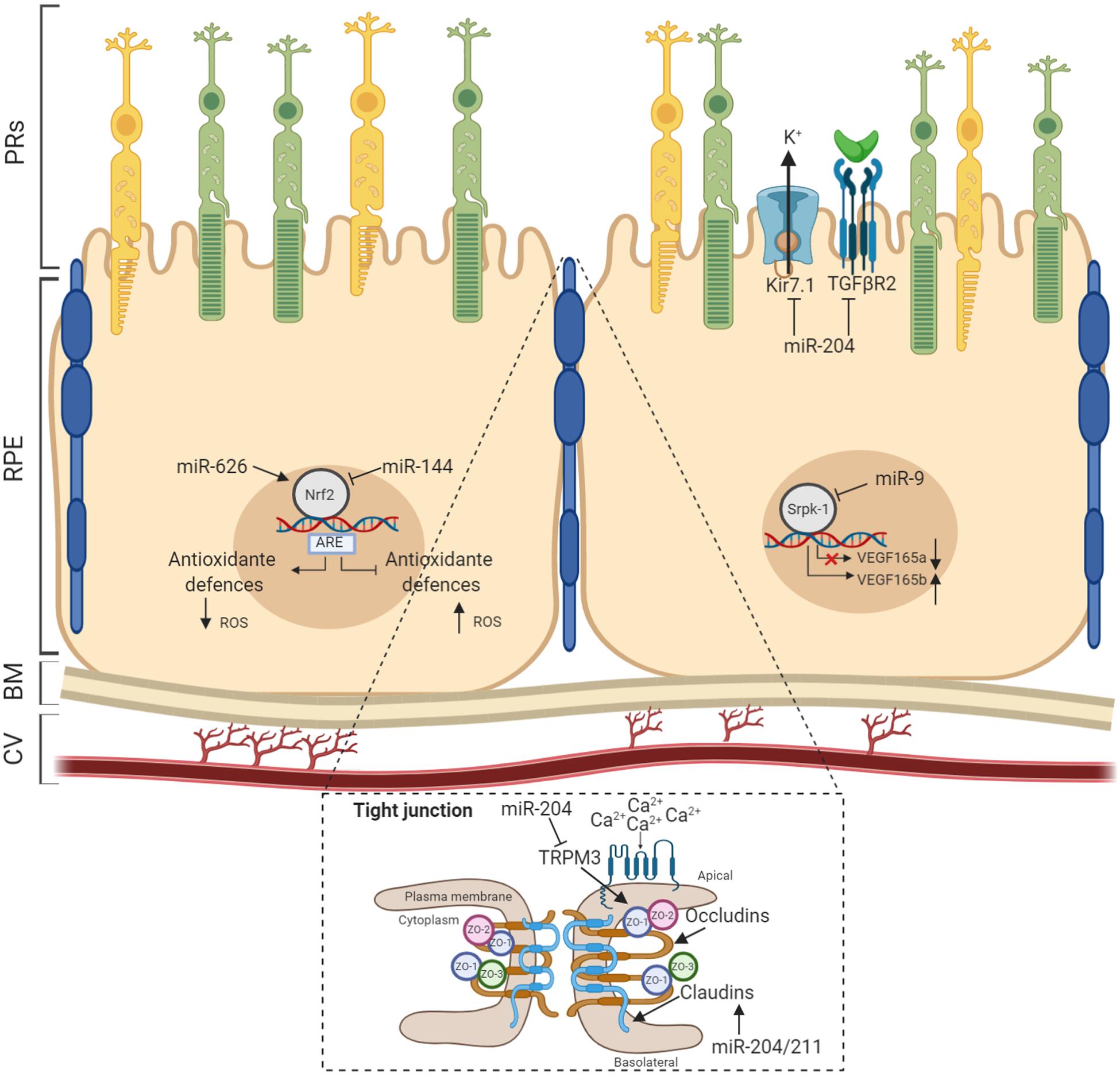
Figure 2. MicroRNAs (miRNAs) act as modulators of RPE homeostasis. Several miRNAs have been demonstrated to influence the correct functioning of the RPE homeostatic machinery. Recognized miRNAs species associated to specific RPE functionality are shown with validated targets. BM, Bruch’s membrane; CV, choroidal vasculature.
Visual Cycle in the RPE
Vision relies on the functional interaction between RPE and photoreceptors. RPE takes an active part in the visual cycle, as it expresses the enzymes required for reisomerization of the 11-cis chromophore from all-trans-retinal, which is essential for initiating the photoreceptor response to light. All-trans-retinal is reduced to all-trans-retinol within the photoreceptors and then transported to the RPE, where it is esterified by LRAT (Saari and Bredberg, 1989; Ruiz et al., 1999). The all-trans-retinyl ester product is then isomerized by RPE65 and hydrolyzed to release 11-cis-retinol (Redmond et al., 2005). Importantly, phagocytosis and autophagy are crucial cellular mechanisms required for maintaining RPE/PRs homeostasis and supporting the visual system. Light exposure to photoreceptors is accompanied by photo-oxidation of proteins and phospholipids of the outer segments (Beatty et al., 2000). To maintain their function, the POS are shed on a circadian basis (LaVail, 1976, 1980) and phagocyted by the RPE, where they fuse with lysosomes for the degradation and recycling of the ingested POS cargo (Anderson et al., 1978). Alterations to any of the above activities of the RPE is thought to be the primary cause in retinal pathologies, including AMD, because the RPE is vital for the health, survival, and function of the adjacent retinal PRs and choroid (Nandrot et al., 2004, 2006). Remarkably, oscillations in genes related to these cellular processes were observed to respond rapidly to changes in the light environment supporting combinatorial light-dependent and circadian-mediated regulation of visual POS turnover. Phagocytosis and degradation of POS occurs by a non-canonical form of autophagy termed LC3-associated phagocytosis (LAP), which exploits the effectors of autophagy in the process of phagocytosis (Kim et al., 2013; Ferguson and Green, 2014). Importantly, during LAP process, the phagocytic machinery takes advantage of autophagy components Atg5, Atg7, Atg3, Atg12, and Atg16l1 for the lipidation of LC3, resulting in LC3 recruitment to the phagosome and uses a BECN1-PIK3C3/VPS34 complex lacking Atg14. LAP is also independent of the autophagy preinitiation complex consisting of ULK1/Atg13/FIP200 (Kim et al., 2013). Engulfed POS enters the phagosome on a daily basis, the Atg12-Atg5-Atg16L1 complex is recruited, as is the lipidated form of LC3 (LC3-II). Only then does the lysosome fuse with the phagosome forming the phagolysosome leading to degradation of the POS cargo (Ferguson and Green, 2014).
The MicroRNAs as Key Players in the Phagocytosis and Cell Clearance
The relevance of miRNAs in RPE phagocytosis and cell clearance soon became evident from studies on the RPE from Dgcr8 cKO mice. Specifically, these mice displayed significant decreases in the phagocytotic process accompanied by the presence of lipid droplet-like structures and a lower recovery rate of the visual chromophore 11-cis-retinal in the RPE (Sundermeier et al., 2017). Importantly, the daily phagocytosis of POS requires flexibility and the correct orientation of the cytoskeleton in the RPE (Law et al., 2015); uptake and intracellular processing of extracellular material are mediated by both actin and myosin, as well as microtubules for the fusion of lysosomes and phagosomes (Stossel, 1977). Several studies identified Ezrin and ezrin–radixin–moesin (ERM)-binding phosphoprotein 50 (EBP50) as the complex responsible for the polarization dynamics of the RPE microvilli (Bonilha et al., 1999; Kivela et al., 2000; Nawrot et al., 2004). Notably, at the apical surface of RPE, Ezrin and EBP50 can associate with the cellular retinaldehyde-binding protein (CRALBP) in a complex necessary for the release of 11-cis-retinal or uptake of all-trans-retinol (Bonilha et al., 2004). Preliminary evidence for the role of miRNA in phagocytosis was reported by Murad et al. (2014) showing that inhibition of miR-184 results in the upregulation of Ezrin gene and causes downregulation of the phagocytosis in ARPE-19 cells, altering RPE homeostasis. Interestingly, RPE primary culture from AMD donors also showed downregulation of miR-184, consistent with an altered visual cycle (Murad et al., 2014). Moreover, recently, an interesting study showed that miR-302d-3p interrupts phagocytosis in the RPE cells. The latter was correlated to a role for miR-302d-3p in promoting RPE dedifferentiation by targeting P21Waf1/Cip1, a cyclin-dependent kinase inhibitor (Jiang et al., 2018). A number of additional miRNAs have been implicated to phagocytosis process, including miR-410 (Choi et al., 2017), miR-194 (Cui et al., 2019), and miR-25 (Zhang et al., 2017a) (Figure 3). miR-25 was the first miRNA to be well characterized in vivo. Oxidative stress promotes miR-25 expression in the RPE cells by STAT3 signaling, this in turn decreases phagocytosis through direct miR-25-mediated targeting of IGTAV and PEDF. Interestingly, subretinal injection of antago-miR-25 in a rat model of retinal degeneration, induced by sodium iodate (SI)-mediated oxidative stress, regressed the impairments of phagocytosis and also ameliorated the RPE degeneration (Zhang et al., 2017a). Similarly, in vitro studies suggest that inhibition of miR-410 is also able to induce phagocytic capabilities by increasing gene and protein expression of RPE-specific factors (Choi et al., 2017). A recent study has also reported that miR-204 is also involved in phagocytosis and in the processing of phagocyted POS by lysosomes. More specifically, miR-204–/– mice are characterized by retinal degeneration caused by the incomplete degradation of POS and lysosomal accumulation of rhodopsin (Zhang et al., 2019). The study revealed that the absence of miR-204 causes an increase in Rab22a, an inhibitor of endosomal maturation. Rab22a is essential to the maturation of early autophagosomal/endosomal intermediates that do not fuse with lysosomes. Thus, miR-204 reduction determines an accumulation of undigested POS, showing a blockage of phagolysosome activity (Zhang et al., 2019). Mice lacking miR-204 also showed inefficient transportation of 11-cis-retinal from RPE to the photoreceptors associated with significant reduction to specific gene expression levels (i.e., RPE65, LRAT, and TTR) required for visual pigment regeneration (Zhang et al., 2019). Importantly, severe retinal defects were observed in patients affected by a dominant mutation in miR-204, as the genetic cause of a retinal degeneration associated to other ocular manifestations (Conte et al., 2015). A number of additional miRNAs have been validated to modulate autophagy and recycle of visual components in the RPE (Table 3). miR-30 was also found to play an important role in autophagy by downregulating Beclin 1 and Atg5 expression (Yu et al., 2012). Moreover, Lian et al. (2019) demonstrated that miR-24 plays an important role in regulating autophagy in the RPE. This study identified chitinase-3-like protein 1 (CHI3L1) as a main target. Importantly, CHI3L1 was suggested to activate the AKT/mTOR and ERK pathways resulting in aberrant autophagy and RPE dysfunction (Lian et al., 2019). Notably, the circadian clock and light are the major regulators of POS phagocytosis and autophagy in the RPE. Several miRNAs were identified to play a role in modulating circadian timing and light-induced responses (Xu et al., 2007; Huang et al., 2008; Pegoraro and Tauber, 2008). Among these, miR-211 is of particular importance because it shows a light-dependent expression pattern (Krol et al., 2010) and it is relevant in homeostasis maintenance of retinal cells through diurnal lysosomal biogenesis in the RPE (Naso et al., 2020). miR-211–/– mice are characterized by defective lysosomal biogenesis and degradative capacities, resulting in the accumulation of POS within the RPE. While miR-211 activation induced autophagy and rescued the anomalies in lysosomal biogenesis. This study also showed that miR-211 targets and represses Ezrin; this in turn promotes a Ca2+-mediated activation of calcineurin, which leads to TFEB nuclear translocation, thus inducing the expression of lysosomal and autophagic genes (Naso et al., 2020).
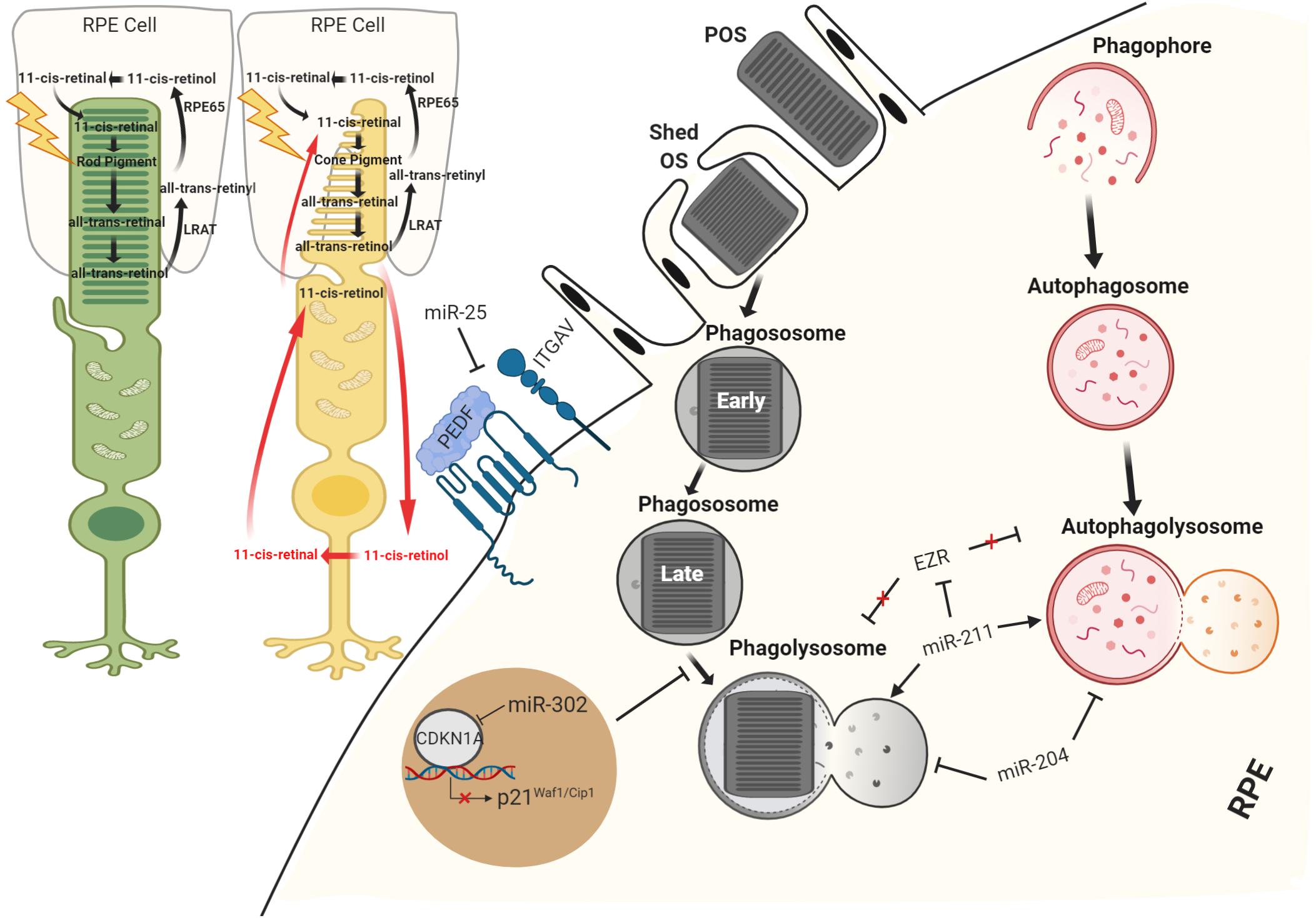
Figure 3. Schematic view of miRNA regulation in RPE recycle. miRNAs have been demonstrated to function both as supporters of visual system and as important regulators of RPE homeostasis maintaining processes, phagocytosis, and autophagy.
miRNA Functions in RPE Diseases
Age-related macular degeneration is a multifactorial, degenerative disease and the most common cause of vision impairment and blindness in the elderly population, with approximately 30–50 million people affected worldwide. The susceptibility to develop “dry” and “wet” AMD forms is dependent on a combination of genetic components and environmental factors. AMD, as well as other forms of retinal degeneration, is often similar and associated with impaired function of the RPE. AMD is characterized by deposits of lipofuscin and extracellular protein aggregates called “drusen” determining progressive dysfunction of autophagy and both RPE and photoreceptor cell death. Interestingly, studies carried out on AMD highlighted that Dicer1 mRNA was reduced in the RPE, but not into neural retina, by 65 ± 3% compared to control eyes, while there was no differences in Drosha and Dgcr8 mRNAs in AMD eyes (Kaneko et al., 2011). To define the relevance of Dicer1 reduction in the RPE, Kaneko et al. (2011) crossed Dicer1f/f mice with BEST1 Cre mice, which express Cre recombinase under the control of the RPE-cell-specific BEST1 promoter. Results showed that these mice displayed RPE cell degeneration in comparison to controls. However, Dicer1 dysregulation in these mice was associated to Dicer1 capacity of silencing toxic Alu transcripts rather than miRNA biogenesis (Kaneko et al., 2011). To verify the hypothesis that miRNA dysregulation is implicated in the pathophysiology of AMD, global expression profiles of miRNAs have been investigated. Several studies examined + circulating miRNAs as well as tissue-specific miRNA expression patterns from age-matched control and affected individuals. The results show a number of discrepancies in the number and type of miRNA identified, probably due to the procedures applied to quantify miRNAs and selection of analyzed samples. In spite of these discrepancies, it is evident that most of miRNAs participated to oxidative stress, inflammation, lipid metabolism, and angiogenesis. Remarkably, miRNA expression profiles in both AMD mouse and rat models exhibited overlapping results, suggesting that the main role of miRNAs in RPE is to protect against oscillations in gene expression and counteract environmental stress, in an effort to preserve RPE cellular homeostasis (Berber et al., 2017). Table 4 lists the most commonly reported miRNAs identified in pathological RPE conditions. Interestingly, the most frequently altered expression identified was that of miR-146a, which occurred in both “dry” and “wet” AMD patients and was found in the RPE of different AMD animal models. Remarkably, in vivo and in vitro studies of specific miR-146a functions demonstrated key roles including repressing expression of IL-6 and VEGF-A genes and inactivating the NF-κB signaling pathway in the RPE. The latter, together with the gain-of function studies for miR-146a, suggests a crucial role for this miRNA in controlling genetic pathways essential to innate immune responses, inflammation, and the microglial activation state, which are major features in the pathogenesis of AMD (Hao et al., 2016). This further suggests that miR-146a may represent a useful disease biomarker and, additionally, a valuable therapeutic target for AMD treatment. Moreover, recent findings reported the role of the epithelial–mesenchymal transition (EMT) in the RPE as a pathological feature of the early stages of AMD (Shu et al., 2020). Remarkably, transforming growth factor-beta (TGFβ) has been identified as a key cytokine orchestrating EMT, and its alteration has been largely associated with onset and progression of several ophthalmological diseases, including AMD (Wang et al., 2019). Interestingly, dynamic changes in expression levels of several miRNAs were associated with TGF-β signaling during EMT in the RPE (Li D.D. et al., 2016). Among these, miR-29b was the most significantly downregulated. The function of the miR-29b was examined in ARPE-19. Additional consequences of miR-29b downregulation included the downregulation of E-cadherin and ZO-1, upregulation of α-smooth muscle actin (α-SMA), and increased cell migration. In vitro analyses showed that overexpression of miR-29b was sufficient to reverse TGF-β-mediated EMT through targeting Akt2. In agreement with this, silencing of the Akt2 abolished miR-29b-mediated repression of EMT process (Li M. et al., 2016). Similarly, a detailed study by Jun and Joo (2016) demonstrated that miR-124 was also involved in EMT in the ARPE-19. miR-124 overexpression induced occludin (OCLN) and ZO-1 and repressed α-SMA by directly targeting Ras homology growth-related (RHOG) (Jun and Joo, 2016). Besides miR-29b and miR-124, other miRNAs have been shown to negatively control the EMT. The levels of the ZEB1 protein, a key transcription factor in EMT, in ARPE-19 cells were decreased in the presence of miR-194 duplexes and elevated on miR-194 inhibition. In agreement with these observations, the levels of expression of ZEB1 target genes were reduced in response to miR-194 overexpression as a consequence of alterations in ZEB1 repression. The miR-194 targeting of ZEB1 has also been confirmed in vivo. Exogenous administration of miR-194 ameliorated the pathogenesis of proliferative vitreoretinopathy in a rat model (Cui et al., 2019). Accordingly, a recent study also showed that both miR-302d and miR-93 exert their functions in regulating TGFB signaling in the RPE (Fuchs et al., 2020). Altogether, these data highlight a potential use for miRNA: as a promising therapeutic resource for the treatment of ocular disorders. An additional key player involved in AMD and pathophysiology of RPE is miR-211. Indeed, two independent studies based on genome-wide searches for novel AMD risk factors identified miR-211 and its host gene, namely TRPM1, as a locus-containing risk factors for AMD (Persad et al., 2017; Orozco et al., 2020). Remarkably, the RPE phenotype in the miR-211–/– mice was similar to that observed in animal models for AMD conditions. Moreover, miR-211 gene delivery via gene therapy was also explored to promote RPE and PR survival in AMD (Cunnusamy et al., 2012). These studies, together with in vitro studies demonstrating the function of miR-211 in the physiology of RPE, support that miR-211 is central to RPE homeostasis and, consequently, may serve as a precious molecular tool to counteract AMD disease. A number of additional examples of miRNAs involved in inflammation, oxidative stress, and angiogenesis have already been shown to be potential therapeutic targets for AMD. miR-23a is downregulated in RPE cells from AMD patients, and overexpression of this miRNA in ARPE-19 reduces cell apoptosis by regulating Fas (Lin et al., 2011). On the contrary, upregulation of miR-218 accelerates the apoptosis of ARPE-19 cells, negatively regulating Runx2. These finding reveal that miR-218/Runx2 axis could be a therapeutic target for retinal diseases (Yao et al., 2019) (Figure 4).
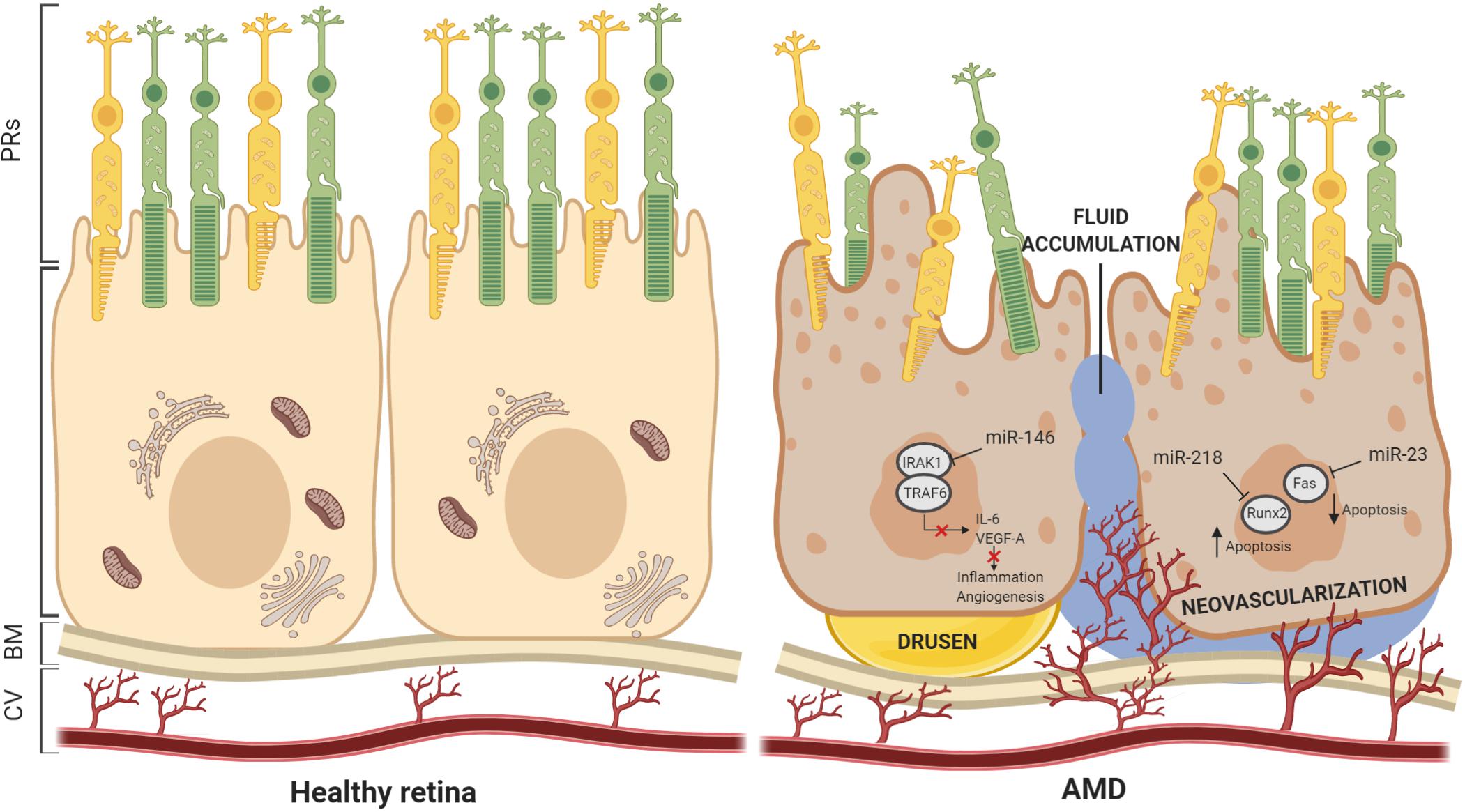
Figure 4. Schematic view of miRNAs dysfunction in AMD. miRNAs have been demonstrated to play key roles in maintaining the structure and functionality of Bruch’s membrane and RPE cells. Incidence of drusen and neovascularization, fluid accumulation, and vascular leakage are often consequences of miRNAs dysfunction. In this scheme, we report examples of miRNAs dysregulation associated with AMD pathogenesis. BM, Bruch’s membrane; CV, choroidal vasculature.
Conclusion
We are in the process of increasing our knowledge on the role of miRNAs as key regulators of RPE homeostasis, function, and survival. miRNAs play an important role in contributing to the precise control of RPE homeostasis in both physiological and pathological states. These insights are opening up avenues to a wide range of novel research areas, including gene-independent medicine, and offers an exclusive class of biomarkers for diagnostic analysis. Recent preclinical studies indicate that miRNAs-based gene therapy is nearing the transition to clinical trial stages. As a single miRNA has the potential to modulate and orchestrate entire molecular programs, future studies are required to shed new light on how they may be safely used as key factors stabilizing and/or resetting the RPE state in pathological conditions. However, recent findings suggest that they are highly promising molecular tools to treat and counteract retinal degeneration including the AMD onset and progression.
Author Contributions
DI, GG, and IC have contributed to wrote the manuscript and prepared the figures. All authors contributed to the article and approved the submitted version.
Conflict of Interest
The authors declare that the research was conducted in the absence of any commercial or financial relationships that could be construed as a potential conflict of interest.
Acknowledgments
We are grateful to Phoebe Ashley-Norman for critical reading of the manuscript. Work in the Conte group is supported by grants from the Italian Telethon Foundation and National MPS Society.
Abbreviations
TNRC6, trinucleotide repeat-containing gene 6A; OTX2, orthodenticle homeobox 2; MITF, melanocyte inducing transcription factor; SOX9, SRY-box 9; TARBP2, TAR RNA-binding protein 2; CTNNBIP1, catenin beta interacting protein 1; Wnt, wingless-type MMTV integration site family member; AKT, protein kinase B; mTOR, mammalian target of rapamycin; PAX6, paired box protein; TGF-βR2, transforming growth factor beta receptor II; CDKN1A, cyclin-dependent kinase inhibitor 1A; RPE65, retinal pigment epithelium-specific 65 kDa; HGF/SF, hepatocyte growth factor/scatter factor; TTR, transthyretin; ZO-1, zonula occludens-1; KIR7.1, inward-rectifier potassium channels; TRPM3, transient receptor potential cation channel subfamily M member 3; TRPML1, mammalian mucolipin transient receptor potential; KEAP1, Kelch-like ECH-associated protein 1; NRF2, nuclear factor erythroid 2-related factor 2; SRPK1, serine–arginine protein kinase 1; VEGF165, vascular endothelial growth factor 165; LRAT, lecithin retinol acyltransferase; ATG, autophagy-related; ATG16l1, autophagy-related 16-like 1; BECN1, Beclin 1; PIK3C3/VPS34, phosphatidylinositol 3-kinase, catalytic subunit type 3; ULK1, Unc-51-like autophagy activating kinase 1; FIP200, FAK family kinase-interacting protein of 200 kDa; STAT3, signal transducer and activator of transcription 3; IGTAV, integrin α V; PEDF, pigment epithelium-derived factor; RAB22A, Ras-related protein Rab-22A; CHI3L1, chitinase-3-like protein 1; ERK, extracellular signal-regulated kinase; TFEB, transcription factor EB; IL-6, interleukin 6; VEGF-A, vascular endothelial growth factor A; NF-κβ, nuclear factor kappa-light-chain-enhancer of activated B cells; TRPM1, transient receptor potential cation channel subfamily M member 1; RUNX2, Runt-related transcription factor 2; SIX3, six homeobox 3; LHX2, LIM/homeobox 2; CHX10, Ceh-10 homeodomain-containing homolog; NRL, neural retina leucine zipper; PDC, phosducin; DCT, dopachrome tautomerase; NOX2, NADPH oxidase 2; LGR4, leucine-rich repeat containing G protein-coupled receptor 4; ATF2, activating transcription factor 2; SOCS3, suppressor of cytokine signaling 3; ZEB, zinc finger E-box -binding homeobox; RhoG, Ras homology growth-related; TGFβ, transforming growth factor beta; ABCA1, ATP-binding cassette transporter A1; Cdc25A, cell division cycle 25 A; DAXX, death domain associated protein; PPARα, peroxisome proliferator activated receptor α; NR3C1, nuclear receptor subfamily 3 group C member 1; BCL-2, B-cell lymphoma 2; SIRT1, NAD-dependent deacetylase sirtuin-1; HSP70, heat shock protein 70 kDa; CUL3, Cullin-3; NKILA, NF-kappaB interacting LncRNA; HK2, hexokinase 2; 15-LOX, 15-lipoxygenase; SYN-2, synapsin II; KLH17, Kelch-like family member 7; RDH11, retinol dehydrogenase 11; CERK1, chitin elicitor receptor kinase 1; AIPL1, aryl hydrocarbon receptor interacting protein-like 1; USH1G, Usher syndrome type-1G; MnSOD, manganese superoxide dismutase; TRXR2, thioredoxin reductase 2; GLS1, glutaminase 1; PDGFβ, platelet-derived growth factor β; iASPP, inhibitor of apoptosis-stimulating protein of p53; HIF1A, hypoxia-inducible factor 1-alpha; ASM, acid sphingomyelinase; TLR4, Toll-like receptor 4; NLRP3, NOD–, LRR–, and pyrin domain-containing protein 3; MC5R, melanocortin 5; BACH1, BTB domain and CNC homolog 1; SHIP1, SH-2 containing inositol 5 ′ polyphosphatase 1; CEBPB, CCAAT enhancer binding protein beta; IKK, inhibitor of upstream I κ B kinase; IL-8, interleukin 8; TRAF6, TNF-receptor-associated factor 6; IRAK1, interleukin 1 receptor-associated kinase 1; SRPK1, SRSF protein kinase 1; CEBP, CCAAT enhancer binding protein; FLT1, Fms-related receptor tyrosine kinase 1; BDNF, brain-derived neurotrophic factor; LAMTOR, late endosomal/lysosomal adaptor and MAPK and mTOR activator 1; MMP2, matrix metalloproteinase-2; MMP9, matrix metalloproteinase-9; TNF-α, tumor necrosis factor-alpha; MYO7A, myosin VIIA; TREM2, triggering receptor expressed on myeloid cells 2; ITGB3, integrin subunit beta 3; CRP: C-reactive protein; PON2, paraoxonase 2; RB1, RB transcriptional corepressor 1; RPGR, retinitis pigmentosa GTPase regulator; EDNR, endothelin 3; CFH, complement factor H; VEGFR1, vascular endothelial growth factor receptor 1; CCL3, C–C motif chemokine ligand 3.
References
Adijanto, J., Castorino, J. J., Wang, Z. X., Maminishkis, A., Grunwald, G. B., and Philp, N. J. (2012). Microphthalmia-associated transcription factor (MITF) promotes differentiation of human retinal pigment epithelium (RPE) by regulating microRNAs-204/211 expression. J. Biol. Chem. 287, 20491–20503.
Amram, B., Cohen-Tayar, Y., David, A., and Ashery-Padan, R. (2017). The retinal pigmented epithelium - from basic developmental biology research to translational approaches. Int. J. Dev. Biol. 61, 225–234.
Anderson, D. H., Fisher, S. K., and Steinberg, R. H. (1978). Mammalian cones: disc shedding, phagocytosis, and renewal. Invest. Ophthalmol. Vis. Sci. 17, 117–133.
Ao, J., Wood, J. P., Chidlow, G., Gillies, M. C., and Casson, R. J. (2018). Retinal pigment epithelium in the pathogenesis of age-related macular degeneration and photobiomodulation as a potential therapy? Clin. Exp. Ophthalmol. 46, 670–686. doi: 10.1111/ceo.13121
Arora, A., Guduric-Fuchs, J., Harwood, L., Dellett, M., Cogliati, T., and Simpson, D. A. (2010). Prediction of microRNAs affecting mRNA expression during retinal development. BMC Dev. Biol. 10:1. doi: 10.1186/1471-213X-10-1
Ban, Y., and Rizzolo, L. J. (2000). Differential regulation of tight junction permeability during development of the retinal pigment epithelium. Am. J. Physiol. Cell Physiol. 279, C744–C750.
Beatty, S., Koh, H., Phil, M., Henson, D., and Boulton, M. (2000). The role of oxidative stress in the pathogenesis of age-related macular degeneration. Surv. Ophthalmol. 45, 115–134. doi: 10.1016/s0039-6257(00)00140-5
Berber, P., Grassmann, F., Kiel, C., and Weber, B. H. (2017). An eye on age-related macular degeneration: the role of MicroRNAs in disease pathology. Mol. Diagn. Ther. 21, 31–43. doi: 10.1007/s40291-016-0234-z
Bhattacharjee, S., Zhao, Y., Dua, P., Rogaev, E. I., and Lukiw, W. J. (2016). microRNA-34a-mediated down-regulation of the microglial-enriched triggering receptor and phagocytosis-sensor TREM2 in age-related macular degeneration. PLoS One 11:e0150211. doi: 10.1371/journal.pone.0150211
Bok, D. (1993). The retinal pigment epithelium: a versatile partner in vision. J. Cell. Sci. Suppl. 17, 189–195. doi: 10.1242/jcs.1993.supplement_17.27
Bonilha, V. L., Bhattacharya, S. K., West, K. A., Crabb, J. S., Sun, J., Rayborn, M. E., et al. (2004). Support for a proposed retinoid-processing protein complex in apical retinal pigment epithelium. Exp. Eye Res. 79, 419–422. doi: 10.1016/j.exer.2004.04.001
Bonilha, V. L., Finnemann, S. C., and Rodriguez-Boulan, E. (1999). Ezrin promotes morphogenesis of apical microvilli and basal infoldings in retinal pigment epithelium. J. Cell Biol. 147, 1533–1548. doi: 10.1083/jcb.147.7.1533
Cai, J., Zhang, H., Zhang, Y. F., Zhou, Z., and Wu, S. (2019). MicroRNA-29 enhances autophagy and cleanses exogenous mutant alphaB-crystallin in retinal pigment epithelial cells. Exp. Cell Res. 374, 231–248. doi: 10.1016/j.yexcr.2018.11.028
Chen, K. C., Hsi, E., Hu, C. Y., Chou, W. W., and Liang, C. L. (2012). MicroRNA-328 may influence myopia development by mediating the PAX6 gene. Invest. Ophthalmol. Vis. Sci. 53, 2732–2739. doi: 10.1167/iovs.11-9272
Chen, Q., Qiu, F., Zhou, K., Matlock, H. G., and Takahashi, Y. (2017). Pathogenic Role of microRNA-21 in Diabetic Retinopathy Through Downregulation of PPARalpha. Diabetes Metab. Res. Rev. 66, 1671–1682. doi: 10.2337/db16-1246
Chen, X., Jiang, C., Sun, R., Yang, D., and Liu, Q. (2020a). Circular noncoding RNA NR3C1 acts as a miR-382-5p sponge to protect rpe functions via regulating PTEN/AKT/mTOR signaling pathway. Mol. Ther. 28, 929–945. doi: 10.1016/j.ymthe.2020.01.010
Chen, X., Sun, R., Yang, D., Jiang, C., and Liu, Q. (2020b). LINC00167 regulates RPE differentiation by targeting the miR-203a-3p/SOCS3 axis. Mol. Ther. Nucleic Acids 19, 1015–1026. doi: 10.1016/j.omtn.2019.12.040
Chen, Z. J., Rong, L., Huang, D., and Jiang, Q. (2019). Targeting cullin 3 by miR-601 activates Nrf2 signaling to protect retinal pigment epithelium cells from hydrogen peroxide. Biochem. Biophys. Res. Commun. 515, 679–687. doi: 10.1016/j.bbrc.2019.05.171
Cheng, L. B., Li, K. R., Yi, N., Li, X. M., Wang, F., Xue, B., et al. (2017). miRNA-141 attenuates UV-induced oxidative stress via activating Keap1-Nrf2 signaling in human retinal pigment epithelium cells and retinal ganglion cells. Oncotarget 8, 13186–13194. doi: 10.18632/oncotarget.14489
Choi, S. W., Kim, J. J., Seo, M. S., Park, S. B., Kang, T. W., Lee, J. Y., et al. (2015). miR-410 Inhibition Induces RPE Differentiation of Amniotic Epithelial Stem Cells via Overexpression of OTX2 and RPE65. Stem Cell. Rev. Rep. 11, 376–386. doi: 10.1007/s12015-014-9568-2
Choi, S. W., Kim, J. J., Seo, M. S., Park, S. B., Shin, T. H., Shin, J. H., et al. (2017). Inhibition by miR-410 facilitates direct retinal pigment epithelium differentiation of umbilical cord blood-derived mesenchymal stem cells. J. Vet. Sci. 18, 59–65. doi: 10.4142/jvs.2017.18.1.59
Chung, S. H., Gillies, M., Yam, M., Wang, Y., and Shen, W. (2016). Differential expression of microRNAs in retinal vasculopathy caused by selective Muller cell disruption. Sci. Rep. 6:28993.
Cichon, C., Sabharwal, H., Ruter, C., and Schmidt, M. A. (2014). MicroRNAs regulate tight junction proteins and modulate epithelial/endothelial barrier functions. Tissue Barriers 2:e944446. doi: 10.4161/21688362.2014.944446
Conte, I., Carrella, S., Avellino, R., Karali, M., Marco-Ferreres, R., Bovolenta, P., et al. (2010). miR-204 is required for lens and retinal development via Meis2 targeting. Proc. Natl. Acad. Sci. U.S.A. 107, 15491–15496. doi: 10.1073/pnas.0914785107
Conte, I., Hadfield, K. D., Barbato, S., Carrella, S., Pizzo, M., Bhat, R. S., et al. (2015). MiR-204 is responsible for inherited retinal dystrophy associated with ocular coloboma. Proc. Natl. Acad. Sci. U.S.A. 112, E3236–E3245.
Cui, L., Lyu, Y., Jin, X., Wang, Y., Li, X., Wang, J., et al. (2019). miR-194 suppresses epithelial-mesenchymal transition of retinal pigment epithelial cells by directly targeting ZEB1. Ann. Transl. Med. 7:751. doi: 10.21037/atm.2019.11.90
Cunnusamy, K., Ufret-Vincenty, R., and Wang, S. (2012). Next-generation therapeutic solutions for age-related macular degeneration. Pharm. Pat. Anal. 1, 193–206. doi: 10.4155/ppa.12.12
Damiani, D., Alexander, J. J., O’Rourke, J. R., McManus, M., Jadhav, A. P., Cepko, C. L., et al. (2008). Dicer inactivation leads to progressive functional and structural degeneration of the mouse retina. J. Neurosci. 28, 4878–4887. doi: 10.1523/jneurosci.0828-08.2008
Davari, M., Soheili, Z. S., Samiei, S., Sharifi, Z., and Pirmardan, E. R. (2017). Overexpression of miR-183/-96/-182 triggers neuronal cell fate in Human Retinal Pigment Epithelial (hRPE) cells in culture. Biochem. Biophys. Res. Commun. 483, 745–751. doi: 10.1016/j.bbrc.2016.12.071
Davis, N., Mor, E., and Ashery-Padan, R. (2011). Roles for Dicer1 in the patterning and differentiation of the optic cup neuroepithelium. Development 138, 127–138. doi: 10.1242/dev.053637
Decembrini, S., Bressan, D., Vignali, R., Pitto, L., Mariotti, S., Rainaldi, G., et al. (2009). MicroRNAs couple cell fate and developmental timing in retina. Proc. Natl. Acad. Sci. U.S.A. 106, 21179–21184. doi: 10.1073/pnas.0909167106
Deo, M., Yu, J. Y., Chung, K. H., Tippens, M., and Turner, D. L. (2006). Detection of mammalian microRNA expression by in situ hybridization with RNA oligonucleotides. Dev. Dyn. 235, 2538–2548. doi: 10.1002/dvdy.20847
Donato, L., Bramanti, P., Scimone, C., Rinaldi, C., and Giorgianni, F. (2018). miRNAexpression profile of retinal pigment epithelial cells under oxidative stress conditions. FEBS Open Biol. 8, 219–233. doi: 10.1002/2211-5463.12360
Ferguson, T. A., and Green, D. R. (2014). Autophagy and phagocytosis converge for better vision. Autophagy 10, 165–167. doi: 10.4161/auto.26735
Fernando, N., Wong, J. H. C., Das, S., Dietrich, C., and Aggio-Bruce, R. (2020). MicroRNA-223 regulates retinal function and inflammation in the healthy and degenerating retina. Front. Cell. Dev. Biol. 8:516. doi: 10.3389/fcell.2020.00516
Fuchs, H. R., Meister, R., Lotke, R., and Framme, C. (2020). The microRNAs miR-302d and miR-93 inhibit TGFB-mediated EMT and VEGFA secretion from ARPE-19 cells. Exp. Eye Res. 201:108258. doi: 10.1016/j.exer.2020.108258
Fuhrmann, S., Zou, C., and Levine, E. M. (2014). Retinal pigment epithelium development, plasticity, and tissue homeostasis. Exp. Eye Res. 123, 141–150. doi: 10.1016/j.exer.2013.09.003
Georgi, S. A., and Reh, T. A. (2010). Dicer is required for the transition from early to late progenitor state in the developing mouse retina. J. Neurosci. 30, 4048–4061. doi: 10.1523/jneurosci.4982-09.2010
Gomez, N. M., Lu, W., Lim, J. C., Kiselyov, K., Campagno, K. E., Grishchuk, Y., et al. (2018). Robust lysosomal calcium signaling through channel TRPML1 is impaired by lysosomal lipid accumulation. FASEB J. 32, 782–794. doi: 10.1096/fj.201700220rr
Greene, W. A., Muniz, A., Plamper, M. L., Kaini, R. R., and Wang, H. C. (2014). MicroRNA expression profiles of human iPS cells, retinal pigment epithelium derived from iPS, and fetal retinal pigment epithelium. J. Vis. Exp. 2014:e51589.
Ha, M., and Kim, V. N. (2014). Regulation of microRNA biogenesis. Nat. Rev. Mol. Cell Biol. 15, 509–524.
Hackler, L. Jr., Wan, J., Swaroop, A., Qian, J., and Zack, D. J. (2010). MicroRNA profile of the developing mouse retina. Invest. Ophthalmol. Vis. Sci. 51, 1823–1831. doi: 10.1167/iovs.09-4657
Hall, D. P., Cost, N. G., Hegde, S., Kellner, E., Mikhaylova, O., Stratton, Y., et al. (2014). TRPM3 and miR-204 establish a regulatory circuit that controls oncogenic autophagy in clear cell renal cell carcinoma. Cancer Cell 26, 738–753. doi: 10.1016/j.ccell.2014.09.015
Hamann, S. (2002). Molecular mechanisms of water transport in the eye. Int. Rev. Cytol. 215, 395–431. doi: 10.1016/s0074-7696(02)15016-9
Hao, Y., Zhou, Q., Ma, J., Zhao, Y., and Wang, S. (2016). miR-146a is upregulated during retinal pigment epithelium (RPE)/choroid aging in mice and represses IL-6 and VEGF-A expression in RPE cells. J. Clin. Exp. Ophthalmol. 7:562.
Haque, R., Chun, E., Howell, J. C., Sengupta, T., and Chen, D. (2012). MicroRNA-30b-mediated regulation of catalase expression in human ARPE-19 cells. PLoS One 7:e42542. doi: 10.1371/journal.pone.0042542
Harhaj, N. S., and Antonetti, D. A. (2004). Regulation of tight junctions and loss of barrier function in pathophysiology. Int. J. Biochem. Cell Biol. 36, 1206–1237. doi: 10.1016/j.biocel.2003.08.007
Hata, A., and Kashima, R. (2016). Dysregulation of microRNA biogenesis machinery in cancer. Crit. Rev. Biochem. Mol. Biol. 51, 121–134. doi: 10.3109/10409238.2015.1117054
Hou, Q., Tang, J., Wang, Z., Wang, C., Chen, X., Hou, L., et al. (2013). Inhibitory effect of microRNA-34a on retinal pigment epithelial cell proliferation and migration. Invest. Ophthalmol. Vis. Sci. 54, 6481–6488. doi: 10.1167/iovs.13-11873
Hou, Q., Zhou, L., Tang, J., Ma, N., Xu, A., Tang, J., et al. (2016). LGR4 is a direct target of MicroRNA-34a and modulates the proliferation and migration of retinal pigment epithelial ARPE-19 cells. PLoS One 11:e0168320. doi: 10.1371/journal.pone.0168320
Howell, J. C., Chun, E., Farrell, A. N., Hur, E. Y., Caroti, C. M., Iuvone, P. M., et al. (2013). Global microRNA expression profiling: curcumin (diferuloylmethane) alters oxidative stress-responsive microRNAs in human ARPE-19 cells. Mol. Vis. 19, 544–560.
Hu, Z., Lv, X., Chen, L., Gu, X., Qian, H., Fransisca, S., et al. (2019). Protective effects of microRNA-22-3p against retinal pigment epithelial inflammatory damage by targeting NLRP3 inflammasome. J. Cell. Physiol. 234, 18849–18857. doi: 10.1002/jcp.28523
Huang, K. M., Dentchev, T., and Stambolian, D. (2008). MiRNA expression in the eye. Mamm. Genome 19, 510–516. doi: 10.1007/s00335-008-9127-8
Iacovelli, J., Zhao, C., Wolkow, N., Veldman, P., Gollomp, K., Ojha, P., et al. (2011). Generation of Cre transgenic mice with postnatal RPE-specific ocular expression. Invest. Ophthalmol. Vis. Sci. 52, 1378–1383. doi: 10.1167/iovs.10-6347
Jadeja, R. N., Jones, M. A., Abdelrahman, A. A., Powell, F. L., Thounaojam, M. C., Gutsaeva, D., et al. (2020). Inhibiting microRNA-144 potentiates Nrf2-dependent antioxidant signaling in RPE and protects against oxidative stress-induced outer retinal degeneration. Redox Biol. 28:101336. doi: 10.1016/j.redox.2019.101336
Jiang, C., Qin, B., Liu, G., Sun, X., Shi, H., Ding, S., et al. (2016). MicroRNA-184 promotes differentiation of the retinal pigment epithelium by targeting the AKT2/mTOR signaling pathway. Oncotarget 7, 52340–52353. doi: 10.18632/oncotarget.10566
Jiang, C., Xie, P., Sun, R., Sun, X., Liu, G., Ding, S., et al. (2018). c-Jun-mediated microRNA-302d-3p induces RPE dedifferentiation by targeting p21(Waf1/Cip1). Cell Death Dis. 9:451.
Jun, J. H., and Joo, C. K. (2016). MicroRNA-124 controls transforming growth factor beta1-induced epithelial-mesenchymal transition in the retinal pigment epithelium by targeting RHOG. Invest. Ophthalmol. Vis. Sci. 57, 12–22.
Kaneko, H., Dridi, S., Tarallo, V., Gelfand, B. D., Fowler, B. J., Cho, W. G., et al. (2011). DICER1 deficit induces Alu RNA toxicity in age-related macular degeneration. Nature 471, 325–330.
Karali, M., Peluso, I., Gennarino, V. A., Bilio, M., Verde, R., Lago, G., et al. (2010). miRNeye: a microRNA expression atlas of the mouse eye. BMC Genomics 11:715. doi: 10.1186/1471-2164-11-715
Kim, J. Y., Zhao, H., Martinez, J., Doggett, T. A., and Kolesnikov, A. V. (2013). Noncanonical autophagy promotes the visual cycle. Cell 154, 365–376. doi: 10.1016/j.cell.2013.06.012
Kivela, T., Jaaskelainen, J., Vaheri, A., and Carpen, O. (2000). Ezrin, a membrane-organizing protein, as a polarization marker of the retinal pigment epithelium in vertebrates. Cell Tissue Res. 301, 217–223. doi: 10.1007/s004410000225
Krol, J., Busskamp, V., Markiewicz, I., Stadler, M. B., and Ribi, S. (2010). Characterizing light-regulated retinal microRNAs reveals rapid turnover as a common property of neuronal microRNAs. Cell 141, 618–631. doi: 10.1016/j.cell.2010.03.039
Kutty, R. K., Nagineni, C. N., Samuel, W., Vijayasarathy, C., and Hooks, J. J. (2010a). Inflammatory cytokines regulate microRNA-155 expression in human retinal pigment epithelial cells by activating JAK/STAT pathway. Biochem. Biophys. Res. Commun. 402, 390–395. doi: 10.1016/j.bbrc.2010.10.042
Kutty, R. K., Nagineni, C. N., Samuel, W., Vijayasarathy, C., Jaworski, C., Duncan, T., et al. (2013). Differential regulation of microRNA-146a and microRNA-146b-5p in human retinal pigment epithelial cells by interleukin-1beta, tumor necrosis factor-alpha, and interferon-gamma. Mol. Vis. 19, 737–750.
Kutty, R. K., Samuel, W., Jaworski, C., Duncan, T., Nagineni, C. N., and Yan, P. (2010b). MicroRNA expression in human retinal pigment epithelial (ARPE-19) cells: increased expression of microRNA-9 by N-(4-hydroxyphenyl)retinamide. Mol. Vis. 16, 1475–1486.
La Torre, A., Georgi, S., and Reh, T. A. (2013). Conserved microRNA pathway regulates developmental timing of retinal neurogenesis. Proc. Natl. Acad. Sci. U.S.A. 110, E2362–E2370.
LaVail, M. M. (1976). Rod outer segment disk shedding in rat retina: relationship to cyclic lighting. Science 194, 1071–1074. doi: 10.1126/science.982063
LaVail, M. M. (1980). Circadian nature of rod outer segment disc shedding in the rat. Invest. Ophthalmol. Vis. Sci. 19, 407–411.
Law, A. L., Parinot, C., Chatagnon, J., Gravez, B., Sahel, J. A., Bhattacharya, S. S., et al. (2015). Cleavage of Mer tyrosine kinase (MerTK) from the cell surface contributes to the regulation of retinal phagocytosis. J. Biol. Chem. 290, 4941–4952. doi: 10.1074/jbc.m114.628297
Lee, R. C., Feinbaum, R. L., and Ambros, V. (1993). The C. elegans heterochronic gene lin-4 encodes small RNAs with antisense complementarity to lin-14. Cell 75, 843–854. doi: 10.1016/0092-8674(93)90529-y
Li, D. D., Zhong, B. W., Zhang, H. X., Zhou, H. Y., Luo, J., Liu, Y., et al. (2016). Inhibition of the oxidative stress-induced miR-23a protects the human retinal pigment epithelium (RPE) cells from apoptosis through the upregulation of glutaminase and glutamine uptake. Mol. Biol. Rep. 43, 1079–1087. doi: 10.1007/s11033-016-4041-8
Li, H., Gong, Y., Qian, H., Chen, T., and Liu, Z. (2015). Brain-derived neurotrophic factor is a novel target gene of the has-miR-183/96/182 cluster in retinal pigment epithelial cells following visible light exposure. Mol. Med. Rep. 12, 2793–2799. doi: 10.3892/mmr.2015.3736
Li, J., Hui, L., Kang, Q., and Li, R. (2018). Down-regulation of microRNA-27b promotes retinal pigment epithelial cell proliferation and migration by targeting Nox2. Pathol. Res. Pract. 214, 925–933. doi: 10.1016/j.prp.2018.05.025
Li, M., Li, H., Liu, X., Xu, D., and Wang, F. (2016). MicroRNA-29b regulates TGF-beta1-mediated epithelial-mesenchymal transition of retinal pigment epithelial cells by targeting AKT2. Exp. Cell Res. 345, 115–124. doi: 10.1016/j.yexcr.2014.09.026
Li, W. B., Zhang, Y. S., Lu, Z. Y., Dong, L. J., Wang, F. E., Dong, R., et al. (2012). Development of retinal pigment epithelium from human parthenogenetic embryonic stem cells and microRNA signature. Invest. Ophthalmol. Vis. Sci. 53, 5334–5343. doi: 10.1167/iovs.12-8303
Lian, C., Lou, H., Zhang, J., Tian, H., Ou, Q., Xu, J. Y., et al. (2019). MicroRNA-24 protects retina from degeneration in rats by down-regulating chitinase-3-like protein 1. Exp. Eye Res. 188, 107791. doi: 10.1016/j.exer.2019.107791
Lin, H., Qian, J., Castillo, A. C., Long, B., Keyes, K. T., Chen, G., et al. (2011). Effect of miR-23 on oxidant-induced injury in human retinal pigment epithelial cells. Invest. Ophthalmol. Vis. Sci. 52, 6308–6314. doi: 10.1167/iovs.10-6632
Liu, P., Peng, Q. H., Tong, P., and Li, W. J. (2019). Astragalus polysaccharides suppresses high glucose-induced metabolic memory in retinal pigment epithelial cells through inhibiting mitochondrial dysfunction-induced apoptosis by regulating miR-195. Mol. Med. 25:21.
Lukiw, W. J., Surjyadipta, B., Dua, P., and Alexandrov, P. N. (2012). Common micro RNAs (miRNAs) target complement factor H (CFH) regulation in Alzheimer’s disease (AD) and in age-related macular degeneration (AMD). Int. J. Biochem. Mol. Biol. 3, 105–116.
Marmor, M. F. (1990). Control of subretinal fluid: experimental and clinical studies. Eye 4(Pt 2), 340–344. doi: 10.1038/eye.1990.46
Marmorstein, A. D. (2001). The polarity of the retinal pigment epithelium. Traffic 2, 867–872. doi: 10.1034/j.1600-0854.2001.21202.x
Marmorstein, A. D., Finnemann, S. C., Bonilha, V. L., and Rodriguez-Boulan, E. (1998). Morphogenesis of the retinal pigment epithelium: toward understanding retinal degenerative diseases. Ann. N. Y. Acad. Sci. 857, 1–12. doi: 10.1111/j.1749-6632.1998.tb10102.x
Masuda, T., Wahlin, K., Wan, J., Hu, J., and Maruotti, J. (2014). Transcription factor SOX9 plays a key role in the regulation of visual cycle gene expression in the retinal pigment epithelium. J. Biol. Chem. 289, 12908–12921. doi: 10.1074/jbc.m114.556738
Mazzoni, F., Safa, H., and Finnemann, S. C. (2014). Understanding photoreceptor outer segment phagocytosis: use and utility of RPE cells in culture. Exp. Eye Res. 126, 51–60. doi: 10.1016/j.exer.2014.01.010
Morris, D. R., Bounds, S. E., Liu, H., Ding, W. Q., Chen, Y., Liu, Y., et al. (2020). Exosomal MiRNA transfer between retinal microglia and RPE. Int. J. Mol. Sci. 21:3541. doi: 10.3390/ijms21103541
Muhammad, F., Trivett, A., Wang, D., and Lee, D. J. (2019). Tissue-specific production of MicroRNA-155 inhibits melanocortin 5 receptor-dependent suppressor macrophages to promote experimental autoimmune uveitis. Eur. J. Immunol. 49, 2074–2082.
Murad, N., Kokkinaki, M., Gunawardena, N., Gunawan, M. S., Hathout, Y., Janczura, K. J., et al. (2014). miR-184 regulates ezrin, LAMP-1 expression, affects phagocytosis in human retinal pigment epithelium and is downregulated in age-related macular degeneration. FEBS J. 281, 5251–5264. doi: 10.1111/febs.13066
Nandrot, E. F., Anand, M., Sircar, M., and Finnemann, S. C. (2006). Novel role for alphavbeta5-integrin in retinal adhesion and its diurnal peak. Am. J. Physiol. Cell Physiol. 290, C1256–C1262.
Nandrot, E. F., Kim, Y., Brodie, S. E., Huang, X., Sheppard, D., and Finnemann, S. C. (2004). Loss of synchronized retinal phagocytosis and age-related blindness in mice lacking alphavbeta5 integrin. J. Exp. Med. 200, 1539–1545. doi: 10.1084/jem.20041447
Naso, F., Intartaglia, D., Falanga, D., Soldati, C., Polishchuk, E., Giamundo, G., et al. (2020). Light-responsive microRNA miR-211 targets Ezrin to modulate lysosomal biogenesis and retinal cell clearance. EMBO J. 39:e102468.
Nawrot, M., West, K., Huang, J., Possin, D. E., Bretscher, A., Crabb, J. W., et al. (2004). Cellular retinaldehyde-binding protein interacts with ERM-binding phosphoprotein 50 in retinal pigment epithelium. Invest. Ophthalmol. Vis. Sci. 45, 393–401. doi: 10.1167/iovs.03-0989
Ohana, R., Weiman-Kelman, B., Raviv, S., Tamm, E. R., Pasmanik-Chor, M., Rinon, A., et al. (2015). MicroRNAs are essential for differentiation of the retinal pigmented epithelium and maturation of adjacent photoreceptors. Development 142, 2487–2498. doi: 10.1242/dev.121533
Oltra, M., Vidal-Gil, L., Maisto, R., Oltra, S. S., Romero, F. J., Sancho-Pelluz, J., et al. (2019). miR302a and 122 are deregulated in small extracellular vesicles from ARPE-19 cells cultured with H2O2. Sci. Rep. 9:17954.
Orozco, L. D., Chen, H. H., Cox, C., Katschke, K. J. Jr., and Arceo, R. (2020). Integration of eQTL and a single-cell atlas in the human eye identifies causal genes for age-related macular degeneration. Cell. Rep. 30:e1246.
Pegoraro, M., and Tauber, E. (2008). The role of microRNAs (miRNA) in circadian rhythmicity. J. Genet. 87, 505–511. doi: 10.1007/s12041-008-0073-8
Persad, P. J., Heid, I. M., Weeks, D. E., Baird, P. N., de Jong, E. K., Haines, J. L., et al. (2017). Joint Analysis of Nuclear and Mitochondrial Variants in Age-Related Macular Degeneration Identifies Novel Loci TRPM1 and ABHD2/RLBP1. Invest. Ophthalmol. Vis. Sci. 58, 4027–4038. doi: 10.1167/iovs.17-21734
Plafker, S. M., O’Mealey, G. B., and Szweda, L. I. (2012). Mechanisms for countering oxidative stress and damage in retinal pigment epithelium. Int. Rev. Cell. Mol. Biol. 298, 135–177. doi: 10.1016/b978-0-12-394309-5.00004-3
Qian, C., Liang, S., Wan, G., Dong, Y., Lu, T., and Yan, P. (2019). Salidroside alleviates high-glucose-induced injury in retinal pigment epithelial cell line ARPE-19 by down-regulation of miR-138. RNA Biol. 16, 1461–1470. doi: 10.1080/15476286.2019.1637696
Qiu, R., Liu, Y., Wu, J. Y., Liu, K., Mo, W., and He, R. (2009). Misexpression of miR-196a induces eye anomaly in Xenopus laevis. Brain Res. Bull. 79, 26–31. doi: 10.1016/j.brainresbull.2008.12.009
Redmond, T. M., Poliakov, E., Yu, S., Tsai, J. Y., Lu, Z., and Gentleman, S. (2005). Mutation of key residues of RPE65 abolishes its enzymatic role as isomerohydrolase in the visual cycle. Proc. Natl. Acad. Sci. U.S.A. 102, 13658–13663. doi: 10.1073/pnas.0504167102
Rizzolo, L. J. (2007). Development and role of tight junctions in the retinal pigment epithelium. Int. Rev. Cytol. 258, 195–234. doi: 10.1016/s0074-7696(07)58004-6
Ruiz, A., Winston, A., Lim, Y. H., Gilbert, B. A., Rando, R. R., and Bok, D. (1999). Molecular and biochemical characterization of lecithin retinol acyltransferase. J. Biol. Chem. 274, 3834–3841. doi: 10.1074/jbc.274.6.3834
Ryhanen, T., Hyttinen, J. M., Kopitz, J., Rilla, K., Kuusisto, E., Mannermaa, E., et al. (2009). Crosstalk between Hsp70 molecular chaperone, lysosomes and proteasomes in autophagy-mediated proteolysis in human retinal pigment epithelial cells. J. Cell Mol. Med. 13, 3616–3631. doi: 10.1111/j.1582-4934.2008.00577.x
Saari, J. C., and Bredberg, D. L. (1989). Lecithin:retinol acyltransferase in retinal pigment epithelial microsomes. J. Biol. Chem. 264, 8636–8640.
Shahriari, F., Satarian, L., Moradi, S., Zarchi, A. S., Gunther, S., Kamal, A., et al. (2020). MicroRNA profiling reveals important functions of miR-125b and let-7a during human retinal pigment epithelial cell differentiation. Exp. Eye Res. 190:107883. doi: 10.1016/j.exer.2019.107883
Shao, Y., Dong, L. J., Takahashi, Y., Chen, J., Liu, X., Chen, Q., et al. (2019). miRNA-451a regulates RPE function through promoting mitochondrial function in proliferative diabetic retinopathy. Am. J. Physiol. Endocrinol. Metab. 316, E443–E452.
Shen, J., Yang, X., Xie, B., Chen, Y., Swaim, M., Hackett, S. F., et al. (2008). MicroRNAs regulate ocular neovascularization. Mol. Ther. 16, 1208–1216. doi: 10.1038/mt.2008.104
Shu, D. Y., Butcher, E., and Saint-Geniez, M. (2020). EMT and EndMT: emerging roles in age-related macular degeneration. Int. J. Mol. Sci. 21:4271. doi: 10.3390/ijms21124271
Simo, R., Villarroel, M., Corraliza, L., Hernandez, C., and Garcia-Ramirez, M. (2010). The retinal pigment epithelium: something more than a constituent of the blood-retinal barrier–implications for the pathogenesis of diabetic retinopathy. J. Biomed. Biotechnol. 2010:190724.
Smit-McBride, Z., Forward, K. I., Nguyen, A. T., Bordbari, M. H., Oltjen, S. L., and Hjelmeland, L. M. (2014). Age-dependent increase in miRNA-34a expression in the posterior pole of the mouse eye. Mol. Vis. 20, 1569–1578.
Soundara Pandi, S. P., Chen, M., Guduric-Fuchs, J., Xu, H., and Simpson, D. A. (2013). Extremely complex populations of small RNAs in the mouse retina and RPE/choroid. Invest. Ophthalmol. Vis. Sci. 54, 8140–8151. doi: 10.1167/iovs.13-12631
Strauss, O. (1995). “The Retinal Pigment Epithelium,” in Webvision: The Organization of the Retina and Visual System, eds H. Kolb, E. Fernandez, and R. Nelson (Salt Lake City, UT: National Library of Medicine).
Strauss, O. (2005). The retinal pigment epithelium in visual function. Physiol. Rev. 85, 845–881. doi: 10.1152/physrev.00021.2004
Sun, H. J., Jin, X. M., Xu, J., and Xiao, Q. (2020). Baicalin alleviates age-related macular degeneration via miR-223/NLRP3-regulated pyroptosis. Pharmacology 105, 28–38. doi: 10.1159/000502614
Sundermeier, T. R., and Palczewski, K. (2016). The impact of microRNA gene regulation on the survival and function of mature cell types in the eye. FASEB J. 30, 23–33. doi: 10.1096/fj.15-279745
Sundermeier, T. R., Sakami, S., Sahu, B., Howell, S. J., Gao, S., Dong, Z., et al. (2017). MicroRNA-processing enzymes are essential for survival and function of mature retinal pigmented epithelial cells in mice. J. Biol. Chem. 292, 3366–3378. doi: 10.1074/jbc.m116.770024
Takayama, K., Kaneko, H., Hwang, S. J., Ye, F., Higuchi, A., Tsunekawa, T., et al. (2016). Increased ocular levels of MicroRNA-148a in cases of retinal detachment promote epithelial-mesenchymal transition. Invest. Ophthalmol. Vis. Sci. 57, 2699–2705. doi: 10.1167/iovs.15-18660
Tang, X., Dai, Y., Wang, X., Zeng, J., and Li, G. (2018). MicroRNA-27a protects retinal pigment epithelial cells under high glucose conditions by targeting TLR4. Exp. Ther. Med. 16, 452–458.
Tang, Y., Lu, Q., Wei, Y., Han, L., Ji, R., Li, Q., et al. (2015). Mertk deficiency alters expression of micrornas in the retinal pigment epithelium cells. Metab. Brain Dis. 30, 943–950. doi: 10.1007/s11011-015-9653-5
Tian, B., Maidana, D. E., Dib, B., Miller, J. B., Bouzika, P., Miller, J. W., et al. (2016). miR-17-3p exacerbates oxidative damage in human retinal pigment epithelial cells. PLoS One 11:e0160887. doi: 10.1371/journal.pone.0160887
Tong, N., Jin, R., Zhou, Z., and Wu, X. (2019). Involvement of microRNA-34a in age-related susceptibility to oxidative stress in ARPE-19 cells by targeting the silent mating type information regulation 2 homolog 1/p66shc pathway: implications for age-related macular degeneration. Front. Aging Neurosci. 11:137. doi: 10.3389/fnagi.2019.00137
Treiber, T., Treiber, N., and Meister, G. (2019). Regulation of microRNA biogenesis and its crosstalk with other cellular pathways. Nat. Rev. Mol. Cell Biol. 20, 5–20. doi: 10.1038/s41580-018-0059-1
Usui-Ouchi, A., Ouchi, Y., Kiyokawa, M., Sakuma, T., Ito, R., and Ebihara, N. (2016). Upregulation of Mir-21 levels in the vitreous humor is associated with development of proliferative vitreoretinal disease. PLoS One 11:e0158043. doi: 10.1371/journal.pone.0158043
Wang, F. E., Zhang, C., Maminishkis, A., Dong, L., Zhi, C., Li, R., et al. (2010). MicroRNA-204/211 alters epithelial physiology. FASEB J. 24, 1552–1571. doi: 10.1096/fj.08-125856
Wang, H. C., Greene, W. A., Kaini, R. R., Shen-Gunther, J., Chen, H. I., Cai, H., et al. (2014). Profiling the microRNA expression in human iPS and iPS-derived retinal pigment epithelium. Cancer Inform. 13, 25–35.
Wang, K., Li, H., Sun, R., Liu, C., Luo, Y., Fu, S., et al. (2019). Emerging roles of transforming growth factor beta signaling in wet age-related macular degeneration. Acta Biochim. Biophys. Sin. 51, 1–8. doi: 10.1093/abbs/gmy145
Wang, L., Dong, F., Reinach, P. S., He, D., Zhao, X., Chen, X., et al. (2016a). MicroRNA-182 suppresses HGF/SF-induced increases in retinal pigment epithelial cell proliferation and migration through targeting c-Met. PLoS One 11:e0167684. doi: 10.1371/journal.pone.0167684
Wang, L., Lee, A. Y., Wigg, J. P., Peshavariya, H., and Liu, P. (2016b). miRNA involvement in angiogenesis in age-related macular degeneration. J. Physiol. Biochem. 72, 583–592.
Wang, Q., Navitskaya, S., Chakravarthy, H., Huang, C., Kady, N., Lydic, T. A., et al. (2016c). Dual anti-inflammatory and anti-angiogenic action of miR-15a in diabetic retinopathy. EBioMedicine 11, 138–150. doi: 10.1016/j.ebiom.2016.08.013
Wienholds, E., Kloosterman, W. P., Miska, E., Alvarez-Saavedra, E., Berezikov, E., de Bruijn, E., et al. (2005). MicroRNA expression in zebrafish embryonic development. Science 309, 310–311. doi: 10.1126/science.1114519
Wightman, B., Ha, I., and Ruvkun, G. (1993). Posttranscriptional regulation of the heterochronic gene lin-14 by lin-4 mediates temporal pattern formation in C. elegans. Cell 75, 855–862. doi: 10.1016/0092-8674(93)90530-4
Wohl, S. G., and Reh, T. A. (2016). The microRNA expression profile of mouse Muller glia in vivo and in vitro. Sci. Rep. 6:35423.
Wright, C. B., Uehara, H., Kim, Y., Yasuma, T., Yasuma, R., Hirahara, S., et al. (2020). Chronic Dicer1 deficiency promotes atrophic and neovascular outer retinal pathologies in mice. Proc. Natl. Acad. Sci. U.S.A. 117, 2579–2587. doi: 10.1073/pnas.1909761117
Xiao, H., and Liu, Z. (2019). Effects of microRNA217 on high glucoseinduced inflammation and apoptosis of human retinal pigment epithelial cells (ARPE19) and its underlying mechanism. Mol. Med. Rep. 20, 5125–5133.
Xu, S., Witmer, P. D., Lumayag, S., Kovacs, B., and Valle, D. (2007). MicroRNA (miRNA) transcriptome of mouse retina and identification of a sensory organ-specific miRNA cluster. J. Biol. Chem. 282, 25053–25066. doi: 10.1074/jbc.m700501200
Xu, X. Z., Tang, Y., Cheng, L. B., Yao, J., Jiang, Q., Li, K. R., et al. (2019). Targeting Keap1 by miR-626 protects retinal pigment epithelium cells from oxidative injury by activating Nrf2 signaling. Free Radic. Biol. Med. 143, 387–396. doi: 10.1016/j.freeradbiomed.2019.08.024
Yan, J., Qin, Y., Yu, J., Peng, Q., and Chen, X. (2018). MiR-340/iASPP axis affects UVB-mediated retinal pigment epithelium (RPE) cell damage. J. Photochem. Photobiol. B 186, 9–16. doi: 10.1016/j.jphotobiol.2018.04.005
Yang, D., Zhang, X., and Hughes, B. A. (2008). Expression of inwardly rectifying potassium channel subunits in native human retinal pigment epithelium. Exp. Eye Res. 87, 176–183. doi: 10.1016/j.exer.2008.05.010
Yang, J. S., and Lai, E. C. (2011). Alternative miRNA biogenesis pathways and the interpretation of core miRNA pathway mutants. Mol. Cell. 43, 892–903. doi: 10.1016/j.molcel.2011.07.024
Yao, R., Yao, X., Liu, R., Peng, J., and Tian, T. (2019). Glucose-induced microRNA-218 suppresses the proliferation and promotes the apoptosis of human retinal pigment epithelium cells by targeting RUNX2. Biosci. Rep. 39:BSR2019 2580.
Ye, Z., Li, Z. H., and He, S. Z. (2017). miRNA-1273g-3p involvement in development of diabetic retinopathy by modulating the autophagy-lysosome pathway. Med. Sci. Monit. 23, 5744–5751. doi: 10.12659/msm.905336
Yoon, C., Kim, D., Kim, S., Park, G. B., Hur, D. Y., Yang, J. W., et al. (2014). MiR-9 regulates the post-transcriptional level of VEGF165a by targeting SRPK-1 in ARPE-19 cells. Graefes Arch. Clin. Exp. Ophthalmol. 252, 1369–1376. doi: 10.1007/s00417-014-2698-z
Yu, Y., Yang, L., Zhao, M., Zhu, S., Kang, R., Vernon, P., et al. (2012). Targeting microRNA-30a-mediated autophagy enhances imatinib activity against human chronic myeloid leukemia cells. Leukemia 26, 1752–1760. doi: 10.1038/leu.2012.65
Zhang, C., Miyagishima, K. J., Dong, L., Rising, A., Nimmagadda, M., Liang, G., et al. (2019). Regulation of phagolysosomal activity by miR-204 critically influences structure and function of retinal pigment epithelium/retina. Hum. Mol. Genet. 28, 3355–3368. doi: 10.1093/hmg/ddz171
Zhang, J., Wang, J., Zheng, L., Wang, M., and Lu, Y. (2017). miR-25 Mediates Retinal Degeneration Via Inhibiting ITGAV and PEDF in Rat. Curr. Mol. Med. 17, 359–374.
Zhang, R., Liu, Z., Chen, B., and Zhang, J. (2017). The impact of miR-26b on retinal pigment epithelium cells in rhegmatogenous retinal detachment model. Int. J. Clin. Exp. Pathol. 10, 8141–8147.
Zhao, P. Y., Gan, G., Peng, S., Wang, S. B., Chen, B., Adelman, R. A., et al. (2015). TRP channels localize to subdomains of the apical plasma membrane in human fetal retinal pigment epithelium. Invest. Ophthalmol. Vis. Sci. 56, 1916–1923. doi: 10.1167/iovs.14-15738
Keywords: miRNAs, retinal pigment epithelium, RPE differentiation, miR-204, miR-211, AMD
Citation: Intartaglia D, Giamundo G and Conte I (2021) The Impact of miRNAs in Health and Disease of Retinal Pigment Epithelium. Front. Cell Dev. Biol. 8:589985. doi: 10.3389/fcell.2020.589985
Received: 31 July 2020; Accepted: 17 December 2020;
Published: 15 January 2021.
Edited by:
Jose Maria Carvajal-Gonzalez, University of Extremadura, SpainReviewed by:
Timothy Blenkinsop, Icahn School of Medicine at Mount Sinai, United StatesVera Lucia Bonilha, Cleveland Clinic, United States
Copyright © 2021 Intartaglia, Giamundo and Conte. This is an open-access article distributed under the terms of the Creative Commons Attribution License (CC BY). The use, distribution or reproduction in other forums is permitted, provided the original author(s) and the copyright owner(s) are credited and that the original publication in this journal is cited, in accordance with accepted academic practice. No use, distribution or reproduction is permitted which does not comply with these terms.
*Correspondence: Ivan Conte, conte@tigem.it
†These authors have contributed equally to this work