Multiple GPCR Functional Assays Based on Resonance Energy Transfer Sensors
- 1Cellular Signaling Laboratory, Key Laboratory of Molecular Biophysics of Ministry of Education, College of Life Science and Technology, Huazhong University of Science and Technology, Wuhan, China
- 2Bioland Laboratory, Guangzhou Regenerative Medicine and Health Guangdong Laboratory, Guangzhou, China
G protein-coupled receptors (GPCRs) represent one of the largest membrane protein families that participate in various physiological and pathological activities. Accumulating structural evidences have revealed how GPCR activation induces conformational changes to accommodate the downstream G protein or β-arrestin. Multiple GPCR functional assays have been developed based on Förster resonance energy transfer (FRET) and bioluminescence resonance energy transfer (BRET) sensors to monitor the conformational changes in GPCRs, GPCR/G proteins, or GPCR/β-arrestin, especially over the past two decades. Here, we will summarize how these sensors have been optimized to increase the sensitivity and compatibility for application in different GPCR classes using various labeling strategies, meanwhile provide multiple solutions in functional assays for high-throughput drug screening.
Introduction
G protein-coupled receptors (GPCRs) represent one of the largest membrane receptor superfamily, which is encoded by approximately 3% of human genes and over 800 members (Klabunde and Hessler, 2002; Fredriksson et al., 2003; Pin et al., 2019). They are widely expressed in all cells and organs from brain tissue to blood vessels, and are responsible for sensing a variety of external stimuli, ranging from light and temperature to neurotransmitters, peptides, and lipids (Lagerstrom and Schioth, 2008). GPCRs are involved in diverse physiological activities and play critical roles in pathogenesis, making them important drug targets (Sriram and Insel, 2018).
Members of the GPCR superfamily share a common seven-transmembrane (7TM) topology, and are classified into classes A, B, C, and F according to sequence similarity (Fredriksson et al., 2003; Fredriksson and Schioth, 2005). Generally, class A GPCRs possess a short extracellular domain (ECD), while class C GPCRs have a large ECD called Venus Flytrap domain (VFT). Ligand binding induces conformational changes from the extracellular ligand-binding site to the intracellular side of the receptor. The outward movement of the cytoplasmic end of transmembrane domain (TM) 6 in class A GPCRs opens up an intracellular cavity to accommodate the Gα subunit and activate G protein; in class B GPCRs, TM6 shows a disruption of the helical fold and the formation of a sharp kink to bind Gα subunits (Hilger et al., 2020). In contrast to Class A and B GPCRs, which are reported to function as monomers, class C GPCRs are reported as obligatory dimers (Kniazeff et al., 2011). Ligand binding to class C GPCR leads to the closure of VFT, triggering the conformational change in the cysteine-rich domain or stalk domain, further rearranging the TMs from inactive interface to TM6/TM6 active interface, which is similar in class C GPCR homodimers such as metabotropic glutamate receptor type 2 (mGlu2) (Xue et al., 2015), mGlu5 (Koehl et al., 2019), and calcium sensing receptor (CaSR) (Liu et al., 2020), or heterodimer, like metabotropic γ-aminobutyric acid receptors (GABAB receptor) (Xue et al., 2019; Mao et al., 2020; Papasergi-Scott et al., 2020; Park et al., 2020; Shaye et al., 2020). Hence, monitoring the conformational changes of GPCRs provide a structural basis to evaluate GPCR activation.
The classical functional assays used to measure the activity of GPCRs are mainly based on downstream messengers, such as Ca2+ release, 1, 4, 5-inositol phosphate (IP3)/IP1 accumulation, cyclic adenosine monophosphate (cAMP) production, or reporter gene expression (Thomsen et al., 2005). Most of them have been successfully developed into high-throughput screening (HTS) and robust assays, and widely applied in the pharmaceutical industry and academic research (Figure 1A). Furthermore, with the discovery of G protein-independent β-arrestin signaling, functional assays have been developed by detecting β-arrestin recruitment to GPCRs or β-arrestin-induced GPCR internalization (Zhang and Xie, 2012; Figure 1B), while the first GPCR biased drug have been approved by FAD recently (Mullard, 2020). In addition to these classical assays, multiple functional assays based on resonance energy transfer (RET), which is a technology to detect the protein-protein interaction, have been developed in recent years for directly monitoring conformational changes in GPCRs, G proteins, and β-arrestins (Figure 1C). Using these sensors, the GPCR signaling profiles and GPCR activation process have been investigated at multiple scales.
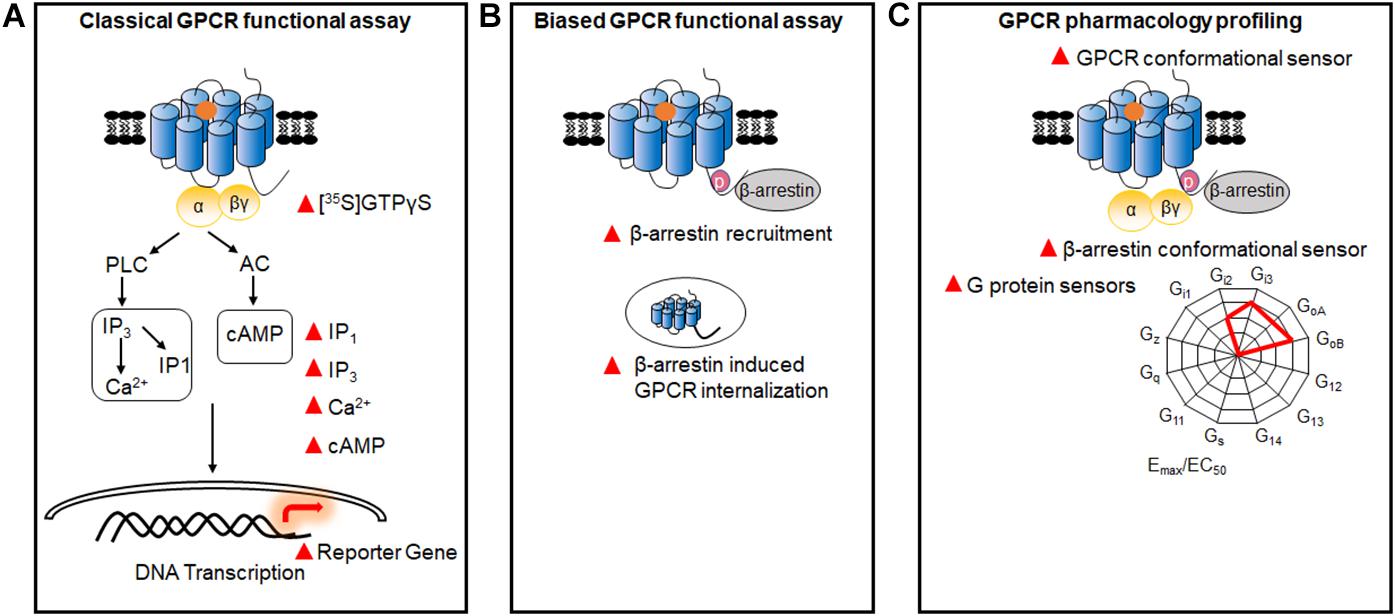
Figure 1. GPCR functional assays. (A) Classical GPCR functional assays based on the measurement of GTPγS, and Ca2+, IP1, IP3, cAMP, and reporter gene. (B) Biased GPCR functional assays. Assays were used to identify the biased signals between G proteins and β-arrestins, through detecting β-arrestin recruitment and GPCR internalization. (C) Multiple GPCR pharmacology profiling. GPCR sensors based on the conformational changes of different G protein subtypes, β-arrestins, and GPCRs.
RET sensors have been well-reviewed to illustrate GPCR activation and signaling previously (Lohse et al., 2012; Kauk and Hoffmann, 2018; El et al., 2019; Haider et al., 2019; Quast and Margeat, 2019). In this review, we have summarized the FRET and BRET sensors, which contributed to G protein and β-arrestin signaling, intra-GPCR rearrangement, and inter-GPCR movement investigations, especially in recent years. Meanwhile, we will focus on how these sensors are optimized to better investigate GPCR signaling and adapted to HTS in functional assays, as well as what new mechanism have been identified based on these sensors.
The Principles of RET
RET is a photo physical process, in which the energy of a fluorescent donor is transferred to a suitable fluorescent energy acceptor (Förster, 1948; Figure 2). The efficiency of RET depends on three parameters: (1) the emission spectrum of the donor overlaps with the excitation spectrum of the acceptor; (2) the distance between the fluorophores is within 100 Å; (3) the relative orientation of their dipole moments toward each other (the parallel dipole orientation gets highest RET) (Stryer, 1978). According to the fluorescent labels, RET sensors can be normally classified into FRET and BRET. The excitation of FRET donor fluorophores needs an extra excitation laser, while BRET is based on the use of light-emitting enzymes-luciferase and other different variations. RET is a good approach to measure the GPCR activation and signaling in a living system (Cottet et al., 2012; Lohse et al., 2012; Kauk and Hoffmann, 2018).
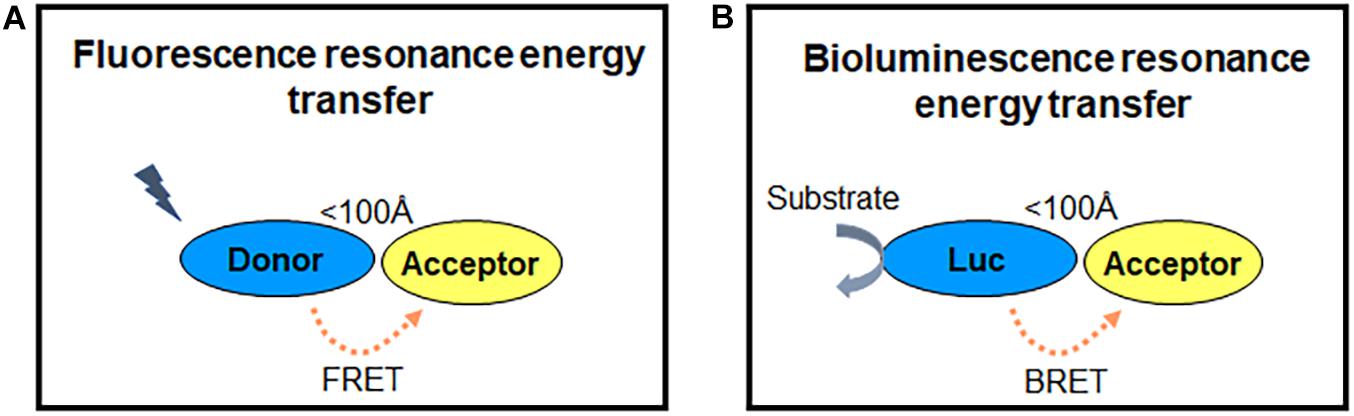
Figure 2. RET principle. (A,B) FRET and BRET detect energy transfer between two proteins within 100 Å, while one fluorophore is excited by laser as energy donor in FRET (A) and enzymes (luciferase) reacting with substrate (coelenterazine) to emit light as the energy donor in BRET (B). The energy acceptor is another fluorophore in both FRET and BRET.
Gpcr/G Protein Signaling Functional Assays
Sensors for GPCR/G Protein Interaction
G protein heterotrimer activity is initiated by exchange of GDP with GTP, when ligand binding to GPCR triggers the G protein coupling to the receptor (Neubig, 1994). Though some RET-based sensors have used to detect the dynamic interaction of G proteins to GPCRs upon stimulation (Table 1; Azpiazu and Gautam, 2004; Gales et al., 2005; Hein et al., 2005; Nobles et al., 2005; Philip et al., 2007; Audet et al., 2008), agonist-induced G protein recruitment represents high diversity and specificity among different G protein subtypes and GPCRs (Du et al., 2019) and dynamic GPCRs and G proteins interactions cannot always easily be detected. MiniG proteins are used to improve the stability of the GPCR-G protein complex (Nehme et al., 2017). They are modified by deleting membrane anchor domains and the Gβγ binding surface in wild-type Gα proteins, and mutated in many positions to increase GPCR/G protein complex stabilization (Nehme et al., 2017; Wan et al., 2018). MiniG BRET sensors used energy pair of Renilla luciferase (Rluc) and Venus fused in GPCR and miniG protein, respectively (Figure 3A), which can effectively recognize different families of GPCRs, even class F GPCRs (Wright et al., 2019). MiniG BRET sensors can be used to investigate the dynamic interactions of GPCRs with almost all G protein families, including Gαi/o, Gαs, Gα12/13, and Gαq/11 (Wan et al., 2018). And then, the miniGq sensor is successfully applied in HTS compare of 5-HT2A serotonin receptor hallucinogen agonists (Kim et al., 2020). Further optimization of miniG BRET sensors by replacing the BRET donor Rluc with NanoLuc (Nluc), which has stronger brightness and smaller size (Hall et al., 2012), or replacing the BRET donor and acceptor with NanoLuc Binary Technology (NanoBiT) pairs, which is based on the Nluc complementation system consisting of LgBiT (18 kDa) and SmBiT (1.3 kDa) proteins (Dixon et al., 2016), can further increase the sensitivity. The optimized miniG sensors can be used to detect GPCR activation in intracellular compartments, such as the Golgi apparatus (Wan et al., 2018).
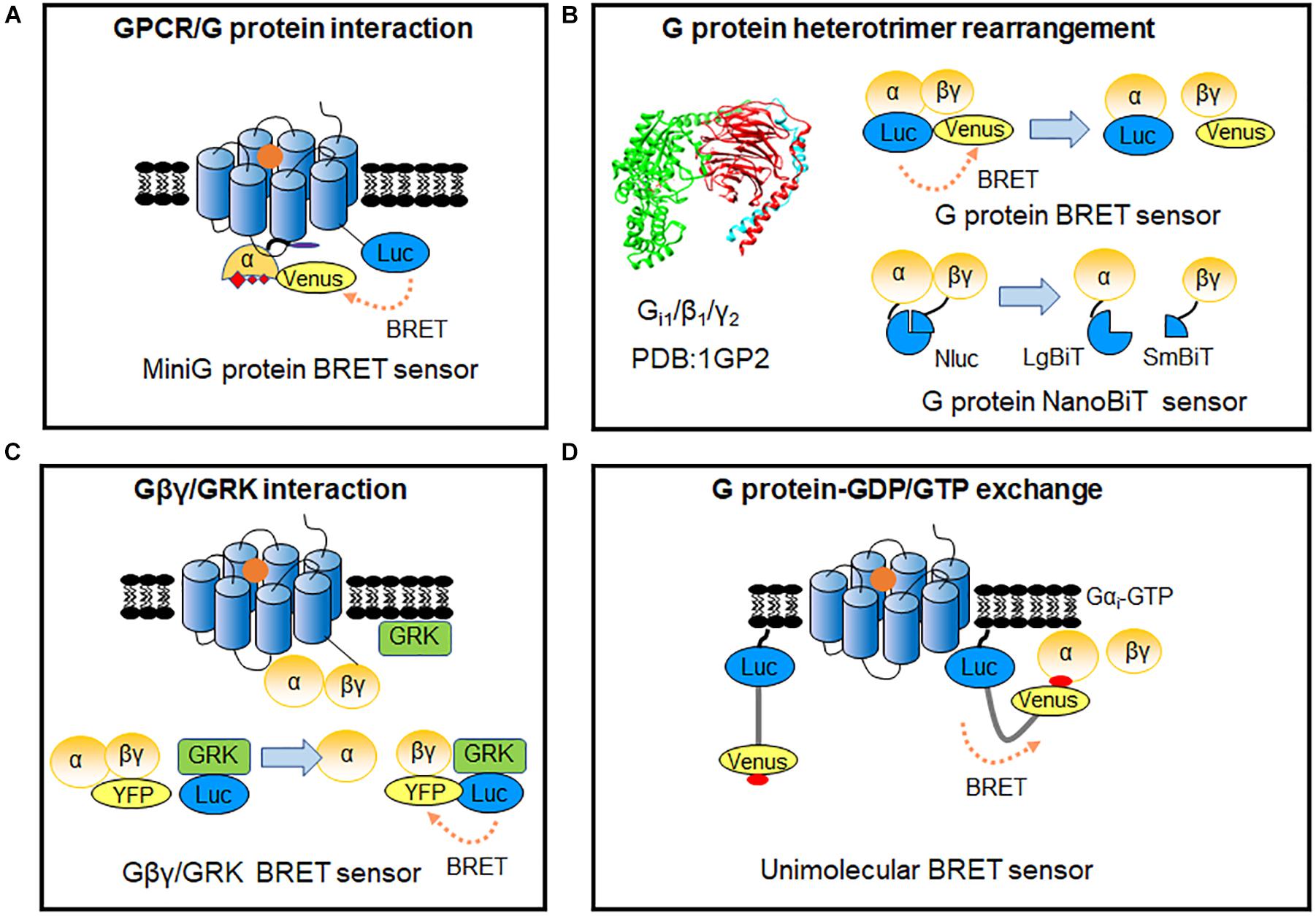
Figure 3. RET-sensors for GPCR/G protein interaction. (A) MiniG protein BRET sensor. MiniG protein was introduced into BRET sensors for G protein recruitment detection. (B) G protein heterotrimer-based sensor. Luciferase and YFP-tagged Gα-Gβγ constitute the G protein intermolecular BRET sensor for detecting the rearrangements of G protein heterotrimers (upper). G protein heterotrimer NanoBiT sensor based on Nluc complementation system was applied to monitor G protein heterotrimer rearrangements (lower). (C) Gβγ-GRK BRET sensor. The BRET signal was produced between the Gβγ and GRK after GPCR activation. (D) Unimolecular BRET sensor, BERKY biosensor (BRET biosensor with ER/K linker and YFP). After G protein activation, the detector module YFP-KB-1753 binds to active G-protein Gαi-GTP on membranes, and the BRET signal produced between N-terminus Nluc and YFP.
Sensors for G Protein Heterotrimer
As G protein can pre-associate to some GPCRs in the absence of ligand (Gales et al., 2005; Nobles et al., 2005; Philip et al., 2007; Audet et al., 2008), detecting G protein heterotrimer activity provides a more general way to evaluate GPCR activation. Measuring the proximity change between Gα and Gβγ subunits through BRET assay (Figure 3B), can reflect the G protein heterotrimer states and activation of GPCRs (Galés et al., 2006). Three flexible regions in Gαi around amino acid numbers 91, 122, and 60 were reported to be accessible for BRET sensors insertion, according to Gα subunit structure. Interestingly, the BRET ratio between Gαi1-91Rluc or Gαi1-122Rluc and green fluorescent protein (GFP) 10-Gγ decreased significantly, whereas Gαi1-60Rluc and GFP10-Gγ exhibited an increase of BRET ratio during α2A AR activation (Galés et al., 2006). This indicates that small rearrangements have occurred in the Gαβγ heterotrimer, which can be detected using BRET assay. Rluc can be replaced by Rluc8 and other enhanced luciferases (Rluc II) to increased brightness and quantum yield in BRET (Loening et al., 2006; Sauliere et al., 2012). Based on optimized BRET sensors, activation of different G protein subtypes can be measured, such as Gαi, Gαo, Gαs, Gαq, Gα12, and Gα13. GPCR ligand such as S II, which was previous known as β-arrestin-biased agonist of angiotensin II receptor type 1A (AT1R) using classical functional assays, is considered as partial agonist, as it can induce ∼20% BRET ratio change in Gi/o, Gq, and G13 compared with the full agonist Ang II (Sauliere et al., 2012). It indicated the advantage and the necessity to combine BRET sensor in GPCR functional assays and proved the HTS application of BRET sensors in G protein signaling.
The G protein BRET sensors can be useful tools to evaluate the activity of individual G protein subtypes and many sensors have been reported as summarized in Table 1. However, not all the subtypes can be detected with good sensitivity. The new BRET Gαβγ biosensors TRUPATH have systematically optimized the insertion positions of the donor in Gα and the best combination of Gβ and Gγ subtypes. 14 optimized sensors have been developed, including the first Gα15 and GαGustducin sensors (Olsen et al., 2020). TRUPATH biosensors extremely increase the sensitivity of G protein BRET functional assays, contributing to the development of a powerful platform to investigate most G proteins activation in an array of GPCRs agonists, antagonists, inverse agonists and biased ligands. Meanwhile, NanoBiT system has been used to modify the G protein sensors (Figure 3B). The advantages of the smaller size and strong signal can avoid possible steric hindrance induced by large proteins such as Rluc, GFP or Venus, and enable the stable detection in hours (Dixon et al., 2016; Inoue et al., 2019).
To further compare the activation efficacy between different G protein subtypes, Gβγ-GRK NanoBRET sensor has been developed based on the Gβγ subunit and lipid-modified reporter peptide GRK3ct (masGRK3ct) (Hollins et al., 2009; Masuho et al., 2015; Figure 3C). The NanoBRET strategy was achieved by fusing Nluc to the GRK3ct and Venus to Gβγ (Masuho et al., 2015). It can determine both the kinetics and extent of G protein activation to independently analyze the catalytic activities of GPCRs and their signaling efficacy toward various targeted Gα protein subtypes (Masuho et al., 2015; Hauser et al., 2018).
Up to now, tools that are suitable for primary cells or native tissues of endogenous GPCRs remain limited. Very recently, Maziarz et al. (2020) developed a type of BRET biosensor with ER/K linker and YFP, called BERKY biosensor to capture the GTP form of the Gα protein (Figure 3D). The membrane-anchoring sequence-fused Nluc was used as the BRET donor, and the YFP-fused synthetic peptide KB-1753 served as the acceptor (Johnston et al., 2006). This unimolecular biosensor can specifically and sensitively bind to Gαi-GTP, and causes an increased BRET signal. It allows endogenous Gα-GTP and free Gβγ to be detected in primary living cells, and record the activation of G proteins in native, physiological environments. BERKY biosensors have been developed for endogenous Gαq-GTP, Gα13-GTP, free Gβγ, and Rho-GTP in cells via a similar strategy (Maziarz et al., 2020).
GPCR/β-Arrestin Signaling Functional Assays
β-arrestins are considered to be prominent mediators of GPCR internalization, facilitating GPCR desensitization and the negative regulating G proteins (Lefkowitz, 1998). β-arrestins also act as key modulators of GPCRs to initiate G protein-independent signaling pathways (Lefkowitz and Shenoy, 2005). Based on the interaction with β-arrestins, GPCRs can be classified into class A and B. Class A GPCRs interact with β-arrestins rapidly and transiently, whereas class B GPCRs stably associate with β-arrestins with a higher affinity (Oakley et al., 2000; Hasbi et al., 2004). β-arrestins can adopt different conformational changes while interacting with phosphorylated GPCRs (Shukla et al., 2008). The dynamic of β-arrestin conformational rearrangement can be longer than GPCR/β-arrestins interaction (Nuber et al., 2016). Accordingly, there are two main types of biosensors for studying the kinetics of GPCR/β-arrestin signal based on BRET and FRET: intermolecular sensors used for monitoring GPCR/β-arrestin dynamic interactions and intramolecular sensors used for measuring β-arrestin conformational rearrangement (Table 1; Bertrand et al., 2002; Charest and Bouvier, 2003; Krasel et al., 2005).
Sensors for GPCR/β-Arrestin Interaction
BRET sensors detecting recruitment of β-arrestins to active GPCRs in living cells was firstly reported by Angers et al. (2000). They used Rluc and GFP as BRET donor and acceptor, which were fused to the C terminus of β2 Adrenergic receptor (β2AR) and β-arrestin2, respectively (Figure 4A). The results showed a large increase in BRET ratio following β2AR stimulation, and represented an agonist concentration-dependent manner. Subsequently, many similar studies investigated the recruitment of β-arrestins to GPCRs (Table 1). Furthermore, this kind of BRET sensors were successfully applied in HTS for GPCRs antagonists, which show compatibility and sensitivity as a functional assay (Hamdan et al., 2005).
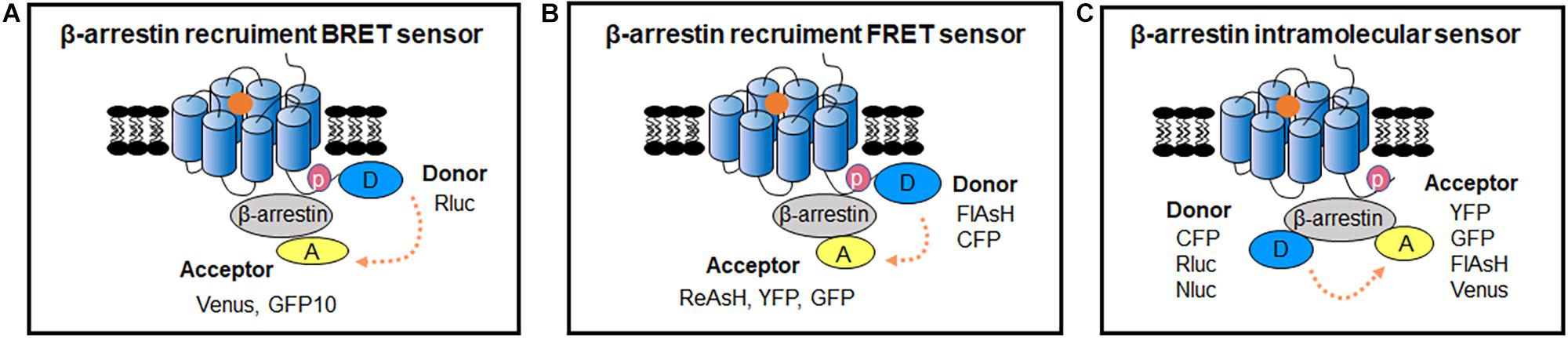
Figure 4. RET-sensors for GPCR/β-arrestin interaction and intramolecular β-arrestin conformational change. (A) Schematic representation of the BRET sensor for β-arrestin recruitment to GPCRs. Luciferase and YFP were introduced between β-arrestin and GPCRs, the BRET signal increased after GPCR activation. (B) β-arrestin recruitment FRET sensor. Different fluorophore pairs are introduced into FRET assay to better monitor β-arrestins dynamic recruitment to receptors in living cells. Donor-acceptor: FlAsH-ReAsH CFP-YFP CFP-GFP. (C) β-arrestin intramolecular sensor. The FRET-based and BRET-based intramolecular sensors are introduced in living cells to detect β-arrestins conformational changes, and multiple fluorophore pairs are applied. Donor-acceptor: CFP-FlAsH CFP-YFP CFP-GFP Rluc-YFP Rluc-GFP Rluc-Venus Nluc-Venus Nluc-YFP.
While the conventional BRET sensors use the non-natural combination of donor and acceptor from different species such as Rluc from Renilla reniformis and GFP from Aequorea Victoria to limit non-specific signals from random interaction, enhanced bystander BRET (EbBRET) sensor is composed with BRET energy pairs both from Renilla reniformis. It possesses moderate affinity, optimally transferred energy, and improved signal, which shows the advantage in investigation of compartmental and ligand-biased β-arrestin trafficking in the plasma membrane and endosomal membrane in real time (Molinari et al., 2008; Namkung et al., 2016). EbBRET sensors can work in real time image recording up to 20 min, greatly facilitating the studies of β-arrestin and GPCR trafficking at high spatial and temporal resolutions (Lan et al., 2012; Namkung et al., 2016; Cao et al., 2019).
Compared to BRET sensors, FRET sensors are more suitable for visualizing the kinetics of GPCRs and β-arrestins with high temporal resolution in cells (Lohse et al., 2012; Figure 4B). Using the FRET sensor, which consists of the CFP-labeled parathyroid hormone 1 receptor (PTH1R) and YFP-labeled β-arrestin2, has shown a time delay of β-arrestin2 recruitment to PTHR after receptor activation (Vilardaga et al., 2002, 2003). Through comparing the recruiting rate of β-arrestin2 to β2AR, repeated short-term stimulation promoted β2ARs phosphorylation and showed very rapid increase of FRET with t0.5 at 2.1 s, while the first stimulation caused fairly slow FRET change (t0.5 of 19.6 s), which indicate the GPCR phosphorylation is the rate-limiting step for β-arrestin recruitment (Krasel et al., 2005). Furthermore, β-arrestin subtypes: β-arrestin1 and β-arrestin2 can exist different recruitment manner to the same GPCR when stimulated with different ligand, such as P2Y2R (a Purine receptor subtype) agonist UTP induced both β-arrestin1 and β-arrestin2 interacted with P2Y2R, whereas ATP caused stronger interaction of β-arrestin1 than β-arrestin2 with P2Y2R (Hoffmann et al., 2008).
To reduce the size of FRET acceptor, a smaller fluorescent probe fluorescein arsenical hairpin binder (FlAsH) was introduced as FlAsH-FRET sensors (Figure 4B). A short peptide of 6 amino acids containing tetracysteine was inserted into the targeted protein, which can specifically bind to FlAsH dyes that produce FRET signal between proximate fluorophore pairs. The CFP/FlAsH FRET sensors showed almost five times the signal amplitude compared to the CFP/GFP FRET sensors (Hoffmann et al., 2005). And then, the double site-specific and orthogonal labeling FRET sensor such as FlAsH/ReAsH (a red arsenical hairpin binder) can also be introduced to investigate GPCR/β-arrestin dynamic interaction (Zurn et al., 2010).
β-Arrestin Intramolecular Sensors
β-arrestin conformational change occurs following recruitment to the receptor (Charest et al., 2005; Nuber et al., 2016). β-arrestin intramolecular BRET biosensor is based on the proximity change between the N- and C-terminus of β-arrestin (Figure 4C). Intramolecular BRET sensors (Luc-β-arrestin-YFP) indicated that β-arrestin can adopt multiple active conformations with different ligands treatment (Shukla et al., 2008). It can be optimized by using Nluc and red-shifted fluorescent protein (CyOFP1) to increase brightness and wider spectral separation (Oishi et al., 2020). This sensor can monitor the early conformational changes of β-arrestin 2 in complex with GPCRs, with a wide panel of different class A and class B GPCRs upon agonist activation, and with orphan GPCRs known to spontaneously recruit β-arrestin2. After the R170E mutation was introduced, the sensor was able to detect the partial active state of β-arrestin2. It permits the monitoring of β-arrestin change in different stage during the GPCR activation (Oishi et al., 2020). Additionally, intramolecular FlAsH-BRET sensors using Rluc and FlAsH pair or intramolecular FlAsH-FRET sensors using CFP and FlAsH pair have also been developed (Figure 4C). They confirmed distinct conformational changes in β-arrestins induced by different ligands and GPCRs (Lee et al., 2016; Nuber et al., 2016; Strungs et al., 2019).
Intramolecular Conformational GPCR Sensors
Though GPCR intramolecular RET sensors have already been developed in 1995 in purified GPCRs (Gether et al., 1995), Vilardaga et al. firstly reported a FRET sensor to detect GPCR conformational changes in living cells, which inserts CFP at the ICL3 and YFP at the C-terminus (Figure 5A) in PTHR and α2A AR (Vilardaga et al., 2003, 2005). Using this sensor, the authors presented different FRET signals induced by full agonists (a strong decrease) and partial agonists (a weak decrease) or inverse agonists (a significant increase) of α2A AR, indicating the dynamic activation process and distinct receptor conformation rearrangements specific to different ligands. Then, many similar intramolecular conformational GPCR sensors have been developed, such as β1AR (Rochais et al., 2007), β2AR (Reiner et al., 2010), A2A-adenosine receptor (A2AR) (Hoffmann et al., 2005), and B2-Bradykinin receptor (B2R) (Chachisvilis et al., 2006) as shown in Table 2. FlAsH labeling provided an alternative choice to replace CFP or YFP (Figure 5A). The FlAsH labeling (∼1 kDa) can be induced into the ICL3 of GPCRs as the energy acceptor, while inserting CFP as donor at the C-terminus. This FlAsH-FRET sensor confirmed the similar fast kinetics of GPCR activation and also showed a fivefold improvement in signal-to-noise ratio (Hoffmann et al., 2005).
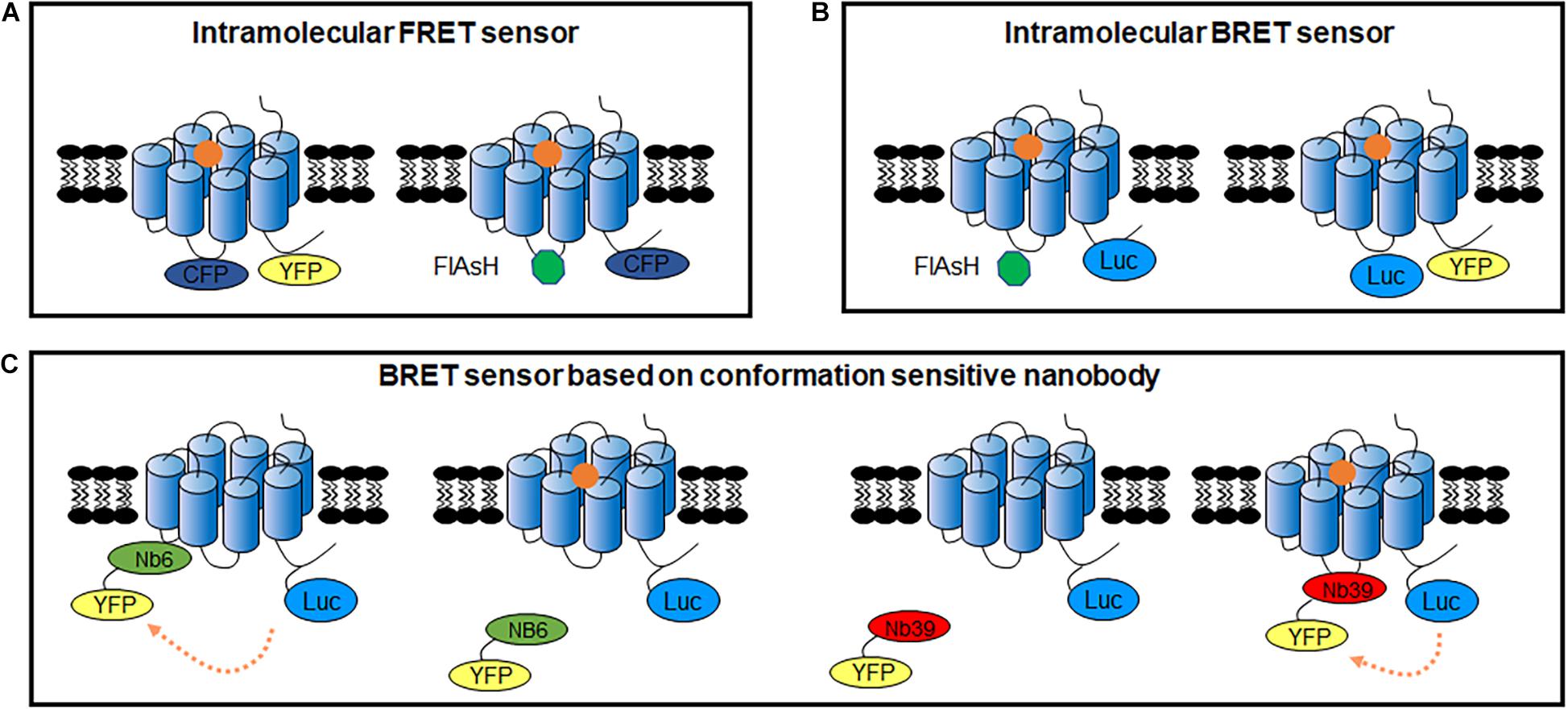
Figure 5. GPCR intracellular conformational changes sensors. (A) GPCR intracellular conformational changes sensors based on FRET between fluorescent protein (left) or FRET between CFP and FlAsH dye (right). (B) GPCR intracellular conformational changes sensors based on BRET between Rluc II and FlAsH dye (left) or BRET between Nluc and fluorescent protein (right). (C) GPCR intracellular conformational changes BRET sensors based on conformation sensitive nanobody. Nb6-YFP only binds to GPCR in inactivated state, while Nb39-YFP only binds to GPCR in activated state.
Similar intramolecular BRET sensors of GPCRs were also used to monitor the conformational rearrangement promoted by diverse ligands (Sleno et al., 2016, 2017; Bourque et al., 2017; Devost et al., 2017). Intramolecular FlAsH-BRET sensors are modified the C-tail with Rluc II, and introduced FlAsH labeling in the ICL3 (Figure 5B). In the case of the F prostanoid receptor, AT1R, and β2AR, although three FlAsH-BRET sensors were constructed in a similar way, the BRET signals exhibited receptor-specific behavior, indicating that different GPCRs have unique conformational profile (Bourque et al., 2017). Furthermore, distinct patterns of conformational changes can be observed by biased ligand. Multiple positioned FlAsH-BRET sensors of AT1R showed rapid, sustained and robust BRET signals to allow the comparison of the full agonist and biased ligands stimulation (Devost et al., 2017). Moreover, intramolecular BRET sensors can be used to investigate the allosteric interactions between two receptors, such as the heterodimeric AT1R and prostaglandin F2α receptors (Sleno et al., 2017).
Alternatively, BRET assay with Nluc and YFP or GFP10 can be used to detect the GPCR conformational rearrangements (Figure 5B), which can be induced by various compounds or mutation in the receptor as well as the impact of interacting proteins (Picard et al., 2018). Schihada et al. (2018) screened the efficacy of different fluorescent proteins or dyes as acceptors, combined with Nluc as a BRET-based α2A AR biosensor, NanoBRET 618 (Nluc and Halo Tag dye pair) showed the highest amplitude upon agonist stimulation. It is a powerful approach to distinguish slight differences induced by partial agonist in the BRET signal instead of the full agonist effect identified by classical cAMP assay. These BRET sensors are also adaptable for micro-liter plate assays with HTS formats.
A novel assay for detecting conformational changes in GPCRs, based on nanobodies recognizing specific conformations, has been reported in recent years. Several conformation-specific nanobodies for GPCRs have been developed, including κ opioid receptor (KOR) (Che et al., 2018), μ opioid receptor (Huang et al., 2015), M2-muscarinic receptors (Kruse et al., 2013), β2AR (Rasmussen et al., 2011), AT1R (Szalai et al., 2012), and mGlu2 (Scholler et al., 2017b). For example, two conformation-sensitive nanobodies of KOR, Nb39 and Nb6, recognize the active and inactive states, respectively (Che et al., 2018). Combined with the BRET approach, it can be used to detect KOR activity. For the Nb6 sensor, a strong BRET ratio decreased upon KOR activation induced by agonist, in which Nb6 dissociated from activated receptors and recovered after the antagonist addition, while the Nb39 sensor had the opposite effect (Figure 5C). Moreover, the conservative binding of Nb6 in the region provides a compatible tool for ligand-induced active conformational changes of other class A GPCRs, when replace their ICL3 by KOR ICL3 (Che et al., 2020). Nanobody-based GPCR conformational sensors also have the advantage of investigating the transactivation induced by other receptors. For example, Nb80, an active β2AR sensitive nanobody, was used to analyze the effect of vascular endothelial growth factor receptor 2 on β2AR activation (Kilpatrick et al., 2019). Nanobody application reduced the modification in GPCRs. However, as there are only a few nanobodies available for GPCRs and the intellectual property protection, the application of nanobodies in GPCR functional assays remains limited.
Regarding to the application in native cells or animals in vivo model, genetically encoded sensors based on GPCR conformational changes have incorporated circularly permuted fluorescent protein (cpGFP) to optical visualize the neurotransmitter release in brain (Sun et al., 2018; Peng et al., 2020). The cpGFP is modified from original GFP, in which the amino and carboxyl portions have been interchanged and reconnected by a short spacer between the original terminus. It is more flexible and accessible than original protein, and the fluorescence intensity of cpGFP is related to its conformation (Baird et al., 1999). Thus, cpGFP offered a suitable strategy for conformation-sensitive sensors. As similar movements between TM5 and TM6 occurred during class A GPCRs activation (Weis and Kobilka, 2018; Hilger et al., 2020), fusing cpGFP at the ICL3 would allow significant fluorescence signal changes of cpGFP following GPCR activation upon ligand binding (Doi and Yanagawa, 1999; Sun et al., 2018). Mutation to abolish the G protein coupling in these sensors is required to not change the physiological GPCR function when expressed in the animals. The advantage of genetically encoded sensors is the rapid and high resolution in two-photon imaging systems for spatial neurotransmitters detecting in living animal (Sun et al., 2018; Peng et al., 2020). However, to obtain sensors with high sensitivity, large number of screening has been done for the cpEGFP insertion and linker residues (Sun et al., 2018). The experience in developing the sensor of one neurotransmitter is not always well adapted to another. Neuromodulator sensors are available for dopamine, serotonin, norepinephrine, acetylcholine, endocannabinoid, adenosine and gastrin-releasing peptide, but for glutamate and γ-aminobutyric acid remains difficult (Labouesse and Patriarchi, 2021).
Intermolecular Conformational GPCR Dimerization Sensors
Intermolecular FRET sensors are considered as good approaches for investigating the dimerization/oligomerization of GPCRs, especially in class C GPCRs (Milligan and Bouvier, 2005; Kniazeff et al., 2011). The classical CFP/YFP FRET sensor can detect the inter-subunit conformational change. When inserting the fluorescent protein in ICL2 of mGlu1 receptor, it showed an increased FRET signal indicating the relative movement of two mGlu1 subunits (Tateyama and Kubo, 2006). Subsequently, through measuring the FRET between two mGlu1 subunits in real-time; a fast increased inter-subunit FRET signal between protomers was detected within 10 ms after glutamate application (Marcaggi et al., 2009). However, difficulties remain in these classical CFP/YFP FRET sensor applications due to low sensitivity, photobleaching and limitations of inserting position.
Time-resolved FRET (TR-FRET) use long-fluorescence lifetime fluorophore, such as lanthanide cryptate instead of fluorescent protein. The fluorescence lifetimes of these molecules are very long, ranging from 100 to 1,000 μs, which leads the efficiency of FRET is not affected by the orientation of dipole moments between donor and acceptor, and becomes truly dependent on their distance (Mathis, 1995; Selvin, 2002). Taking a fixed delay time before acquiring the signal allows the removal of most of the fluorescent background provided by biological media and instrument, which largely improves the signal-to-noise ratio, compared with classical FRET sensors (Maurel et al., 2008; Scholler et al., 2017a). TR-FRET sensors can be adapted in multi-well plates format from 96 well to 384 well for drug HTS (Scholler et al., 2017a; Liu et al., 2020).
Antibodies labeled with long-lifetime lanthanide-based cryptate fluorophores were used in the first-generation TR-FRET sensors, which target small tags fused in GPCRs, such as HA, Flag, or c-Myc (Figure 6A). It was used to prove the protein interactions and indicate the interface (Kniazeff et al., 2004; Liu et al., 2004). However, it failed to monitor the dynamic changes between the GPCR subunits, might because of the large size of the antibodies.
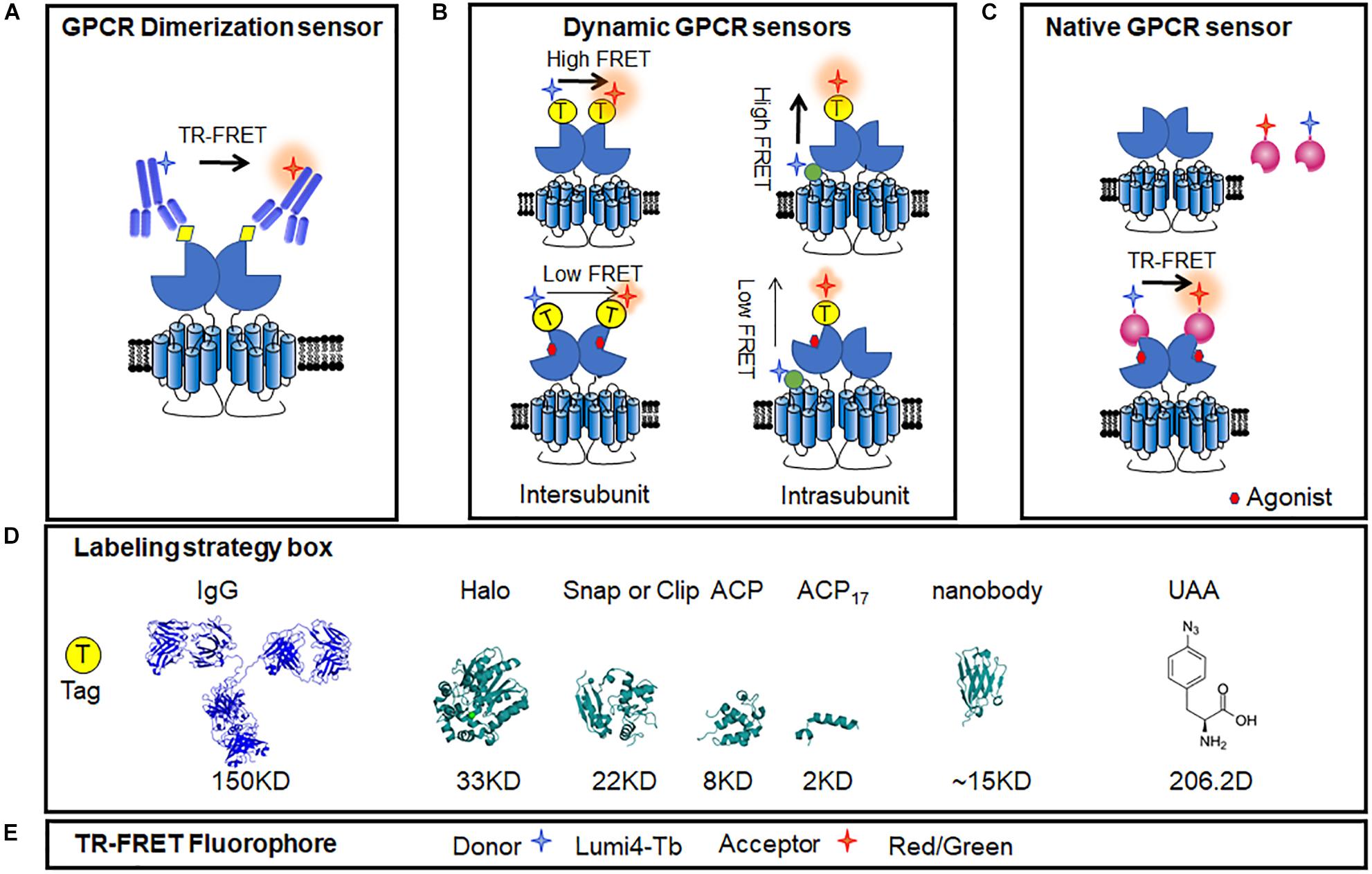
Figure 6. Intermolecular GPCR dimerization sensors. Fluorescent donors and acceptors were introduced using antibodies or tags allowing for the FRET measurement of GPCR dimerization and activation in membrane and living cells, even in the acutely isolated cells. (A) GPCR dimer TR-FRET sensor based on anti-HA/flag/c-Myc antibody. (B) Conformational changes in TR-FRET sensor of dimeric GPCR using different tags, including N-terminal labeling and the intra-subunit conformational TR-FRET sensor. (C) Conformational changes in TR-FRET sensor of GPCRs based on active conformation-binding nanobodies. (D) Ribbon model structures of the different tags used to label receptors: IgG (PDB ID: 1IGT), Halo tag (PDB ID: 5UY1), SNAP tag (PDB ID: 3KZZ), and ACP tag (PDB ID: 2MLB), UAA (4-Azido-L-phenylalanine, CAS No.: 33173-53-4). (E) Common TR-FRET compatible dyes. Lumi4-Tb was used as donor, and red or green dyes were used as acceptors.
The SNAP tag is two-thirds the size of GFP, derived from the O6-guanine nucleotide alkyltransferase that covalently reacts with benzyl-guanines (BG). The SNAP tag can be specifically and covalently labeled with any fluorophore carried by the BG benzyl group. By using non-permeant BG derivatives compatible with TR-FRET measurements, it allows to identify the dimer of GPCRs (Figure 6B). Using SNAP and CLIP labeling TR-FRET sensors, the mGluRs, which are considered strict homodimers, have been found to be heterodimers between different sub-groups (Doumazane et al., 2011). Meanwhile, SNAP labeling sensors can be used to identify oligomers, such as the GABAB receptor, which was previously considered to be heterodimers (Maurel et al., 2008; Comps-Agrar et al., 2011).
Furthermore, a N-terminal SNAP tag strategy combined with TR-FRET technology was developed to monitor the dynamic changes between two subunits in class C GPCR dimer. The mGlu receptors are dimeric entities with a large ECD, and during activation, a large conformational change results from the rotation of almost 70° of one ECD relative to the other (Huang et al., 2011). According to the available structures of mGlu receptor ECDs (Kunishima et al., 2000; Tsuchiya et al., 2002; Muto et al., 2007; Koehl et al., 2019), the distance between the N terminus varies from 2.8 nm in the inactive conformation to 3.3 nm in the active conformation. Consistently, in the TR-FRET measurement, inactive mGlu2 receptors were found to have a high FRET signal, while a low FRET signal was obtained in the active state (Figure 6B). The agonist-induced decrease in inter-subunit FRET efficiency was caused by a large change in the distance between the separated fluorophores (Doumazane et al., 2013). Such a movement is closely related to receptor activation, as agonist potencies presented by TR-FRET are perfectly correlated with those determined in cell-based functional assays (including IP1, cAMP, and Ca2+ release), indicating the efficiency and accuracy of the conformational change-sensitive TR-FRET sensor. The N-terminal SNAP strategy is feasible for most class C GPCRs, including all mGlu family members (mGlu1-8) and CaSR (Scholler et al., 2017a; Liu et al., 2020; Figure 6B). SNAP-tagged TR-FRET sensor of the mGlu5 receptor based on ECD conformation showed enhanced basal activation in the presence of D1R (Sebastianutto et al., 2020).
To increase the compatibility of SNAP-tagged TR-FRET sensor to different GPCRs, several modifications are required to obtain a large signal-to-noise ratio, including the insertion position, labeling strategies, and methods to quantify signals. For example, the N-terminal SNAP strategy does not work for the GABAB receptor because GABAB receptors do not undergo a strong conformational change similar to that of the mGlu receptors during activation (Geng et al., 2012; Lecat-Guillet et al., 2017). To detect the conformational change of this receptor, researchers kept the SNAP tag on the N-terminal of the GABAB1 subunit and introduced a short version of the acyl carrier protein (ACP)-tag (ACP17) within extracellular lobe 2 of the same subunit based on the knowledge of the activation of the GABAB receptor (Figure 6B). A high TR-FRET signal was largely decreased upon GABAB receptor activation and was suppressed by the competitive antagonist CGP54626 (Lecat-Guillet et al., 2017; Scholler et al., 2017a). Different sizes of tags, such as ACP (8 kDa) and ACP17 (2 kDa) (George et al., 2004; Yin et al., 2005) or the O6-alkylguanine-DNA alkyl transferase derivatives, SNAP (Keppler et al., 2003), CLIP (23 kDa) (Gautier et al., 2008), and Halo Tag (33 kDa) (Encell et al., 2012), combined with compatible fluorophore pairs build a toolbox for TR-FRET sensors optimization (Figures 6D,E). Another representative application of intra-subunit sensor is the luteinizing hormone (LH) receptor from the class A family, which has a large extracellular domain. After the addition of agonist, TR-FRET signals increased while the ACP17 and SNAP tags were constructed at the loop and N terminus, respectively, revealing an important conformational change within the extracellular domain of the LH receptor. The strategy of the extracellular intra-subunit sensor is feasible for these kinds of monomeric GPCRs, in which the extracellular domain undergoes a large conformational change during activation (Scholler et al., 2017a).
In addition to class C GPCR, some classes A and B GPCRs were found to form dimer or oligomer (Carrillo et al., 2003; Berthouze et al., 2005; Harding et al., 2009; Kasai et al., 2018). These heterodimers showed distinct functions and related to pathogenesis. For example, AT1R and B2R heteromerization was found to occur in human placental biopsies from pregnancies complicated by preeclampsia, and the aberrant heteromerization of AT1R-B2R was found to result in exaggerated calcium signaling and high vascular smooth muscle mechanosensitivity (Quitterer et al., 2019). TR-FRET sensors are also adaptable to other GPCR dimers, such as class A relaxin family peptide receptor 3 (RXFP3) and the LH receptor, class B PTHR, corticotropin-releasing factor receptor 1 (CRF1R), and pituitary-activating cAMP polypeptide (PACAP) receptor 1 (PAC1). The potency of a series of agonists obtained from measurements of the TR-FRET assay correlated with those obtained in functional assays (Scholler et al., 2017a). On the other side, BRET approaches using Rluc and YFP pair can be used to identify the formations of GPCR dimers (Ayoub and Pfleger, 2010; Johnstone and Pfleger, 2012; El et al., 2019). But few intermolecular BRET conformational change sensors have been reported. Though ligand-induced BRET changes have been presented in MT1/MT2 melatonin receptor heterodimers (Ayoub et al., 2002, 2004), conformational changes in other dimers such as F prostanoid receptor, were not robust enough for investigation (Sleno et al., 2016).
The unnatural amino acid (UAA) site-directed modification strategy is considered to be a potential way to build flexible RET sensors that minimize the labeling tag size into one residue. The UAA technology can be used to investigate the interaction sites between two proteins, such as β-arrestin binding to AT1R (Gagnon et al., 2019). Then, with an optimization in UAA incorporation strategy, it can measure FRET signal between two specific labeling sites of GPCR at the living cell level and the single-molecule level (Liauw et al., 2021). This UAA incorporation strategy in living cells provide a good protocol to apply UAA instead of other tags in GPCR sensors, which may have higher sensitivity to monitor more differential conformational change.
Conclusion and Perspectives
In this review, we summarized four types of conformational sensors for GPCR signaling and activation based on FRET and BRET. These sensors have identified new mechanisms of GPCRs activation process and also lead to significant breakthroughs in high-throughput drug screening toolboxes. Generally, most FRET sensors show strong intensity and microscopy compatibility, which possess better spatial and temporal resolution for imaging purposes. The TR-FRET sensor can also be applied in HTS. BRET sensors have more sustainable signals, higher signal-to-noise ratios and HTS applications. These sensors have been optimized using various labeling strategies to increase the sensitivity and compatibility, from heterogeneous systems to endogenous conditions. Using these assays, GPCR signaling and activation have been investigated on a large scale and at multiple levels. However, the introduction of BRET and FRET sensors without breaking normal expression and function remains challenging. The UAA site-directed modification strategy for FRET sensors may be a promising approach (Liauw et al., 2021). BERKY biosensors for endogenous G proteins will be a good choice to investigate endogenous GPCR activation (Maziarz et al., 2020).
Meanwhile, although smaller and smaller tags can be preferred to minimize the extra influence, a few FRET sensors based on traditional antibodies (Liu et al., 2004; Comps-Agrar et al., 2011) or labeled small molecule ligands (Albizu et al., 2010) have shown advantages in the detection of GPCR in native samples. However, due to the excessive molecular weight of antibodies or insufficient specificity of some antibodies and small molecule ligands, it is difficult to detect GPCR complexes in native tissue. Nanobodies, which have a smaller size, higher affinity, and conformation specificity, may provide breakthroughs in native GPCR functional assays and signaling (Scholler et al., 2017b; Figure 6C). As the hetero-complexes of GPCRs have received increasing attention for their connection with diseases (Prinster et al., 2005; Gomes et al., 2016), nanobody-based assays will provide useful tools for investigating roles of GPCR heteromers in physiological and pathological processes in the future.
Author Contributions
All authors listed have made a substantial, direct and intellectual contribution to the work, and approved it for publication.
Funding
JL was supported by the Ministry of Science and Technology (grant no. 2018YFA0507003), the National Natural Science Foundation of China (NSFC) (grant nos. 81720108031, 81872945, and 31721002), the Program for Introducing Talents of Discipline to the Universities of the Ministry of Education (grant no. B08029), and Key Program of Natural Science Foundation of Hubei Province (grant no. 2019ACA128) and Wuhan (2019020701011481).
Conflict of Interest
The authors declare that the research was conducted in the absence of any commercial or financial relationships that could be construed as a potential conflict of interest.
Abbreviations
β2AR, β 2-Adrenergic receptor; α2A AR, α 2A adrenergic receptor; A2AR, A2A-adenosine receptor; AT1R, angiotensin II receptor type 1A; BRET, bioluminescence resonance energy transfer; FRET, förster resonance energy transfer; GABAB receptor, metabotropic γ -aminobutyric acid receptors; GFP, green fluorescent protein; GPCRs, G protein-coupled receptors; HTS, high-throughput screening; ICL, intracellular loop; KOR, κ opioid receptor; LH, luteinizing hormone receptor; mGluR, metabotropic glutamate receptor; P2Y2R, Purinergic receptor; PTHR, Parathyroid hormone 1 receptor; Rluc, Renilla Luciferase; TM, Transmembrane helix; TR-FRET, time-resolved FRET; VFT, Venus fly-trap; YFP, yellow fluorescent protein; 7TM, seven-transmembrane.
References
Adjobo-Hermans, M. J., Goedhart, J., van Weeren, L., Nijmeijer, S., Manders, E. M., Offermanns, S., et al. (2011). Real-time visualization of heterotrimeric G protein Gq activation in living cells. BMC Biol. 9:32. doi: 10.1186/1741-7007-9-32
Albizu, L., Cottet, M., Kralikova, M., Stoev, S., Seyer, R., Brabet, I., et al. (2010). Time-resolved FRET between GPCR ligands reveals oligomers in native tissues. Nat. Chem. Biol. 6, 587–594. doi: 10.1038/nchembio.396
Angers, S., Salahpour, A., Joly, E., Hilairet, S., Chelsky, D., Dennis, M., et al. (2000). Detection of beta 2-adrenergic receptor dimerization in living cells using bioluminescence resonance energy transfer (BRET). Proc. Natl. Acad. Sci. U.S.A. 97, 3684–3689. doi: 10.1073/pnas.060590697
Audet, N., Gales, C., Archer-Lahlou, E., Vallieres, M., Schiller, P. W., Bouvier, M., et al. (2008). Bioluminescence resonance energy transfer assays revealed ligand-specific conformational changes within preformed signaling complexes containing delta-opioid receptors and heterotrimeric G proteins. J. Biol. Chem. 283, 15078–15088. doi: 10.1074/jbc.M707941200
Ayoub, M. A., and Pfleger, K. D. (2010). Recent advances in bioluminescence resonance energy transfer technologies to study GPCR heteromerization. Curr. Opin. Pharmacol. 10, 44–52. doi: 10.1016/j.coph.2009.09.012
Ayoub, M. A., Couturier, C., Lucas-Meunier, E., Angers, S., Fossier, P., Ayoub, M. A., et al. (2002). Monitoring of ligand-independent dimerization and ligand-induced conformational changes in melatonin receptors in living cells by bioluminescence resonance energy transfer. J. Biol. Chem. 277, 21522–21528. doi: 10.1074/jbc.M200729200
Ayoub, M. A., Levoye, A., Delagrange, P., and Jockers, R. (2004). Preferential formation of MT1/MT2 melatonin receptor heterodimers with distinct ligand interaction properties compared with MT2 homodimers. Mol. Pharmacol. 66, 312–321. doi: 10.1124/mol.104.000398
Azpiazu, I., and Gautam, N. (2004). A fluorescence resonance energy transfer-based sensor indicates that receptor access to a G protein is unrestricted in living mammalian cells. J. Biol. Chem. 279, 27709–27718. doi: 10.1074/jbc.M403712200
Baird, G. S., Zacharias, D. A., and Tsien, R. Y. (1999). Circular permutation and receptor insertion within green fluorescent proteins. Proc. Natl. Acad. Sci. U.S.A. 96, 11241–11246. doi: 10.1073/pnas.96.20.11241
Berthouze, M., Ayoub, M., Russo, O., Rivail, L., Sicsic, S., Fischmeister, R., et al. (2005). Constitutive dimerization of human serotonin 5-HT4 receptors in living cells. FEBS Lett. 579, 2973–2980. doi: 10.1016/j.febslet.2005.04.040
Bertrand, L., Parent, S., Caron, M., Legault, M., Joly, E., Angers, S., et al. (2002). BRET2/arrestin assay in stable recombinant cells: a platform to screen for compounds that interact with G protein-coupled receptors (GPCRS). J. Recept. Signal Transduct. Res. 22, 533–541. doi: 10.1081/rrs-120014619
Bourque, K., Petrin, D., Sleno, R., Devost, D., Zhang, A., and Hebert, T. E. (2017). Distinct conformational dynamics of three G protein-coupled receptors were measured using FlAsH-BRET biosensors. Front. Endocrinol. (Lausanne) 8:61. doi: 10.3389/fendo.2017.00061
Bunemann, M., Frank, M., and Lohse, M. J. (2003). Gi protein activation in intact cells involves subunit rearrangement rather than dissociation. Proc. Natl. Acad. Sci. U.S.A. 100, 16077–16082. doi: 10.1073/pnas.2536719100
Cao, Y., Namkung, Y., and Laporte, S. A. (2019). Methods to monitor the trafficking of beta-arrestin/G protein-coupled receptor complexes using enhanced bystander BRET. Methods Mol. Biol. 1957, 59–68. doi: 10.1007/978-1-4939-9158-7_3
Carrillo, J. J., Pediani, J., and Milligan, G. (2003). Dimers of class A G protein-coupled receptors function via agonist-mediated trans-activation of associated G proteins. J. Biol. Chem. 278, 42578–42587. doi: 10.1074/jbc.M306165200
Chachisvilis, M., Zhang, Y. L., and Frangos, J. A. (2006). G protein-coupled receptors sense fluid shear stress in endothelial cells. Proc. Natl. Acad. Sci. U.S.A. 103, 15463–15468. doi: 10.1073/pnas.0607224103
Charest, P. G., and Bouvier, M. (2003). Palmitoylation of the V2 vasopressin receptor carboxyl tail enhances beta-arrestin recruitment, leading to efficient receptor endocytosis and ERK1/2 activation. J. Biol. Chem. 278, 41541–41551. doi: 10.1074/jbc.M306589200
Charest, P. G., Terrillon, S., and Bouvier, M. (2005). Monitoring agonist-promoted conformational changes of β-arrestin in living cells by intramolecular BRET. EMBO Rep. 6, 334–340. doi: 10.1038/sj.embor.7400373
Che, T., English, J., Krumm, B. E., Kim, K., Pardon, E., Olsen, R., et al. (2020). Nanobody-enabled monitoring of the kappa opioid receptor states. Nat. Commun. 11:1145. doi: 10.1038/s41467-020-14889-7
Che, T., Majumdar, S., Zaidi, S. A., Ondachi, P., McCorvy, J. D., Wang, S., et al. (2018). Structure of the nanobody-stabilized active state of the kappa opioid receptor. Cell 172, 55–67. doi: 10.1016/j.cell.2017.12.011
Comps-Agrar, L., Kniazeff, J., Norskov-Lauritsen, L., Maurel, D., Gassmann, M., Gregor, N., et al. (2011). The oligomeric state sets the GABA(B) receptor signaling efficacy. EMBO J. 30, 2336–2349. doi: 10.1038/emboj.2011.143
Cottet, M., Faklaris, O., Maurel, D., Scholler, P., Doumazane, E., Trinquet, E., et al. (2012). BRET and Time-resolved FRET strategy to study GPCR oligomerization: from cell lines toward native tissues. Front. Endocrinol. (Lausanne) 3:92. doi: 10.3389/fendo.2012.00092
Devost, D., Sleno, R., Petrin, D., Zhang, A., Shinjo, Y., Okde, R., et al. (2017). Conformational profiling of the AT1 angiotensin II receptor reflected biased agonist, G protein coupling, and cellular context. J. Biol. Chem. 292, 5443–5456. doi: 10.1074/jbc.M116.763854
Dixon, A. S., Schwinn, M. K., Hall, M. P., Zimmerman, K., Otto, P., Lubben, T. H., et al. (2016). NanoLuc complementation reporter optimized for the accurate measurement of protein interactions in cells. ACS Chem. Biol. 11, 400–408. doi: 10.1021/acschembio.5b00753
Doi, N., and Yanagawa, H. (1999). Design of generic biosensors based on green fluorescent proteins with allosteric sites by directed evolution. FEBS Lett. 453, 305–307. doi: 10.1016/s0014-5793(99)00732-2
Doumazane, E., Scholler, P., Fabre, L., Zwier, J. M., Trinquet, E., Pin, J. P., et al. (2013). Illuminating the activation mechanisms and allosteric properties of metabotropic glutamate receptors. Proc. Natl. Acad. Sci. U.S.A. 110, E1416–E1425. doi: 10.1073/pnas.1215615110
Doumazane, E., Scholler, P., Zwier, J. M., Trinquet, E., Rondard, P., and Pin, J. P. (2011). A new approach to analyze cell surface protein complexes reveals specific heterodimeric metabotropic glutamate receptors. FASEB J. 25, 66–77. doi: 10.1096/fj.10-163147
Du, Y., Duc, N. M., Rasmussen, S., Hilger, D., Kubiak, X., Wang, L., et al. (2019). Assembly of the GPCR-G protein complex. Cell 177, 1232–1242. doi: 10.1016/j.cell.2019.04.022
El, K. C., Reverchon-Assadi, F., Hervouet-Coste, N., Blot, L., Reiter, E., and Morisset-Lopez, S. (2019). Bioluminescence resonance energy transfer as a method to study protein-protein interactions: application to G protein-coupled receptor biology. Molecules 24:537. doi: 10.3390/molecules24030537
Encell, L. P., Friedman, O. R., Zimmerman, K., Otto, P., Vidugiris, G., Wood, M. G., et al. (2012). Development of a dehalogenase-based protein fusion tag capable of rapid, selective, and covalent attachment to customizable ligands. Curr. Chem. Genom. 6, 55–71. doi: 10.2174/1875397301206010055
Förster, T. (1948). Zwischenmolekulare energiewanderung und fluoreszenz. Ann. Phys. (Leipzig) 2, 55–75.
Frank, M., Thumer, L., Lohse, M. J., and Bunemann, M. (2005). G Protein activation without subunit dissociation depends on the G{alpha}(i)-specific region. J. Biol. Chem. 280, 24584–24590. doi: 10.1074/jbc.M414630200
Fredriksson, R., and Schioth, H. B. (2005). The repertoire of G-protein-coupled receptors in fully sequenced genomes. Mol. Pharmacol. 67, 1414–1425. doi: 10.1124/mol.104.009001
Fredriksson, R., Lagerstrom, M. C., Lundin, L. G., and Schioth, H. B. (2003). G-protein-coupled receptors in the human genome form five main families. Phylogenetic analysis, paralogon groups, and fingerprints. Mol. Pharmacol. 63, 1256–1272. doi: 10.1124/mol.63.6.1256
Gagnon, L., Cao, Y., Cho, A., Sedki, D., Huber, T., Sakmar, T. P., et al. (2019). Genetic code expansion and photocross-linking identify different beta-arrestin binding modes to the angiotensin II type 1 receptor. J. Biol. Chem. 294, 17409–17420. doi: 10.1074/jbc.RA119.010324
Gales, C., Rebois, R. V., Hogue, M., Trieu, P., Breit, A., Hebert, T. E., et al. (2005). Real-time monitoring of receptor and G-protein interactions in living cells. Nat. Methods 2, 177–184. doi: 10.1038/nmeth743
Galés, C., Van Durm, J. J. J., Schaak, S., Pontier, S., Percherancier, Y., Audet, M., et al. (2006). Probing activation-promoted structural rearrangements in preassembled receptor–G protein complexes. Nat. Struct. Mol. Biol. 13, 778–786. doi: 10.1038/nsmb1134
Gautier, A., Juillerat, A., Heinis, C., Correa, I. J., Kindermann, M., Beaufils, F., et al. (2008). An engineered protein tag for multiprotein labeling in living cells. Chem Biol 15, 128–136. doi: 10.1016/j.chembiol.2008.01.007
Geng, Y., Xiong, D., Mosyak, L., Malito, D. L., Kniazeff, J., Chen, Y., et al. (2012). Structure and functional interaction of the extracellular domain of the human GABA(B) receptor GBR2. Nat. Neurosci. 15, 970–978. doi: 10.1038/nn.3133
George, N., Pick, H., Vogel, H., Johnsson, N., and Johnsson, K. (2004). Specific labeling of cell surface proteins with chemically diverse compounds. J. Am. Chem. Soc. 126, 8896–8897. doi: 10.1021/ja048396s
Gether, U., Lin, S., and Kobilka, B. K. (1995). Fluorescent labeling of purified beta 2 adrenergic receptors. Evidence for ligand-specific conformational changes. J. Biol. Chem. 270, 28268–28275. doi: 10.1074/jbc.270.47.28268
Ghanouni, P., Gryczynski, Z., Steenhuis, J. J., Lee, T. W., Farrens, D. L., Lakowicz, J. R., et al. (2001a). Functionally different agonists induce distinct conformations in the G protein coupling domain of the β2 adrenergic receptor. J. Biol. Chem. 276, 24433–24436. doi: 10.1074/jbc.C100162200
Ghanouni, P., Steenhuis, J. J., Farrens, D. L., and Kobilka, B. K. (2001b). Agonist-induced conformational changes in the G-protein-coupling domain of the β2 adrenergic receptor. Proc. Natl. Acad. Sci. USA. 98, 5997–6002. doi: 10.1073/pnas.101126198
Gomes, I., Ayoub, M. A., Fujita, W., Jaeger, W. C., Pfleger, K. D., and Devi, L. A. (2016). G protein-coupled receptor heteromers. Annu. Rev. Pharmacol. Toxicol. 56, 403–425. doi: 10.1146/annurev-pharmtox-011613-135952
Haider, R. S., Godbole, A., and Hoffmann, C. (2019). To sense or not sense new insights from GPCR-based and arrestin-based biosensors. Curr. Opin. Cell Biol. 57, 16–24. doi: 10.1016/j.ceb.2018.10.005
Hall, M. P., Unch, J., Binkowski, B. F., Valley, M. P., Butler, B. L., Wood, M. G., et al. (2012). Engineered luciferase reporter from a deep sea shrimp utilizing a novel imidazopyrazinone substrate. ACS Chem. Biol. 7, 1848–1857. doi: 10.1021/cb3002478
Hamdan, F. F., Audet, M., Garneau, P., Pelletier, J., and Bouvier, M. (2005). High-throughput screening of G protein-coupled receptor antagonists using a bioluminescence resonance energy transfer 1-based beta-arrestin2 recruitment assay. J. Biomol. Screen. 10, 463–475. doi: 10.1177/1087057105275344
Hanyaloglu, A. C., Seeber, R. M., Kohout, T. A., Lefkowitz, R. J., and Eidne, K. A. (2002). Homo- and hetero-oligomerization of thyrotropin-releasing hormone (TRH) receptor subtypes Differential regulation of β-arrestin 1 and 2. J. Biol. Chem. 277, 50422–50430. doi: 10.1074/jbc.M209340200
Harding, P. J., Attrill, H., Boehringer, J., Ross, S., Wadhams, G. H., Smith, E., et al. (2009). Constitutive dimerization of the G-protein coupled receptor, neurotensin receptor 1, reconstituted into phospholipid bilayers. Biophys. J. 96, 964–973. doi: 10.1016/j.bpj.2008.09.054
Hasbi, A., Devost, D., Laporte, S. A., and Zingg, H. H. (2004). Real-time detection of interactions between the human oxytocin receptor and G protein-coupled receptor kinase-2. Mol. Endocrinol. 18, 1277–1286. doi: 10.1210/me.2003-0440
Hauser, A. S., Chavali, S., Masuho, I., Jahn, L. J., Martemyanov, K. A., Gloriam, D. E., et al. (2018). Pharmacogenomics of GPCR drug targets. Cell 172, 41–54. doi: 10.1016/j.cell.2017.11.033
Hein, P., Frank, M., Hoffmann, C., Lohse, M. J., and Bunemann, M. (2005). Dynamics of receptor/G protein coupling in living cells. EMBO J. 24, 4106–4114. doi: 10.1038/sj.emboj.7600870
Hein, P., Rochais, F., Hoffmann, C., Dorsch, S., Nikolaev, V. O., Engelhardt, S., et al. (2006). Gs activation is time limiting in initiating receptor-mediated signaling. J. Biol. Chem. 281, 33345–33351. doi: 10.1074/jbc.M606713200
Hilger, D., Kumar, K. K., Hu, H., Pedersen, M. F., O’Brien, E. S., Giehm, L., et al. (2020). Structural insights into differences in G protein activation by family A and B GPCRs. Science 369:3373. doi: 10.1126/science.aba3373
Hoffmann, C., Gaietta, G., Bunemann, M., Adams, S. R., Oberdorff-Maass, S., Behr, B., et al. (2005). A FlAsH-based FRET approach to determine G protein-coupled receptor activation in living cells. Nat. Methods 2, 171–176. doi: 10.1038/nmeth742
Hoffmann, C., Ziegler, N., Reiner, S., Krasel, C., and Lohse, M. J. (2008). Agonist-selective, receptor-specific interactions of human P2Y receptors with beta-arrestin-1 and -2. J. Biol. Chem. 283, 30933–30941. doi: 10.1074/jbc.M801472200
Hollins, B., Kuravi, S., Digby, G. J., and Lambert, N. A. (2009). The c-terminus of GRK3 indicates rapid dissociation of G protein heterotrimers. Cell. Signal. 21, 1015–1021. doi: 10.1016/j.cellsig.2009.02.017
Huang, S., Cao, J., Jiang, M., Labesse, G., Liu, J., Pin, J. P., et al. (2011). Interdomain movement in metabotropic glutamate receptor activation. Proc. Natl. Acad. Sci. U.S.A. 108, 15480–15485. doi: 10.1073/pnas.1107775108
Huang, W., Manglik, A., Venkatakrishnan, A. J., Laeremans, T., Feinberg, E. N., Sanborn, A. L., et al. (2015). Structural insights into μ-opioid receptor activation. Nature 524, 315–321. doi: 10.1038/nature14886
Inoue, A., Raimondi, F., Kadji, F., Singh, G., Kishi, T., Uwamizu, A., et al. (2019). Illuminating G-protein-coupled selectivity of GPCRs. Cell 177, 1933–1947. doi: 10.1016/j.cell.2019.04.044
Johnston, C. A., Lobanova, E. S., Shavkunov, A. S., Low, J., Ramer, J. K., Blaesius, R., et al. (2006). Minimal determinants for binding activated Gα from the structure of a Gαi1- peptide dimer. Biochemistry U. S. 45, 11390–11400. doi: 10.1021/bi0613832
Johnstone, E. K. M., and Pfleger, K. D. G. (2012). Receptor-heteromer investigation technology and its application using BRET. Front. Endocrinol. 3:101. doi: 10.3389/fendo.2012.00101
Kasai, R. S., Ito, S. V., Awane, R. M., Fujiwara, T. K., and Kusumi, A. (2018). The Class-A GPCR dopamine D2 receptor forms transient dimers stabilized by agonists: detection by single-molecule tracking. Cell Biochem. Biophys. 76, 29–37. doi: 10.1007/s12013-017-0829-y
Kauk, M., and Hoffmann, C. (2018). Intramolecular and intermolecular FRET sensors for GPCRs monitoring conformational changes and beyond. Trends Pharmacol. Sci. 39, 123–135. doi: 10.1016/j.tips.2017.10.011
Keppler, A., Gendreizig, S., Gronemeyer, T., Pick, H., Vogel, H., and Johnsson, K. (2003). A general method for covalent labeling of fusion proteins with small molecules in vivo. Nat. Biotechnol. 21, 86–89. doi: 10.1038/nbt765
Kilpatrick, L. E., Alcobia, D. C., White, C. W., Peach, C. J., Glenn, J. R., Zimmerman, K., et al. (2019). Complex formation between VEGFR2 and beta2-Adrenoceptor. Cell Chem. Biol. 26, 830–841. doi: 10.1016/j.chembiol.2019.02.014
Kim, K., Che, T., Panova, O., DiBerto, J. F., Lyu, J., Krumm, B. E., et al. (2020). Structure of a Hallucinogen-activated Gq-coupled 5-HT2A serotonin receptor. Cell 182, 1574–1588. doi: 10.1016/j.cell.2020.08.024
Klabunde, T., and Hessler, G. (2002). Drug design strategies for targeting G-protein-coupled receptors. Chembiochem 3, 928–944. doi: 10.1002/1439-7633(20021004)3:10<928::AID-CBIC928<3.0.CO;2-5
Kniazeff, J., Bessis, A. S., Maurel, D., Ansanay, H., Prezeau, L., and Pin, J. P. (2004). The closed state of both binding domains of homodimeric mGlu receptors is required for full activity. Nat. Struct. Mol. Biol. 11, 706–713. doi: 10.1038/nsmb794
Kniazeff, J., Prezeau, L., Rondard, P., Pin, J. P., and Goudet, C. (2011). Dimers and beyond: functional puzzles of class C GPCRs. Pharmacol Ther. 130, 9–25. doi: 10.1016/j.pharmthera.2011.01.006
Koehl, A., Hu, H., Feng, D., Sun, B., Zhang, Y., Robertson, M. J., et al. (2019). Structural insights into the activation of metabotropic glutamate receptors. Nature 566, 79–84. doi: 10.1038/s41586-019-0881-4
Krasel, C., Bunemann, M., Lorenz, K., and Lohse, M. J. (2005). Beta-arrestin binding to the beta2-adrenergic receptor requires both receptor phosphorylation and activation. J. Biol. Chem. 280, 9528–9535. doi: 10.1074/jbc.M413078200
Kroeger, K. M., Hanyaloglu, A. C., Seeber, R. M., Miles, L. E., and Eidne, K. A. (2001). Constitutive and agonist-dependent homo-oligomerization of thyrotropin-releasing hormone receptor Detection in living cells using bioluminescence resonance energy transfer. J. Biol. Chem. 276, 12736–12743. doi: 10.1074/jbc.M011311200
Kruse, A. C., Ring, A. M., Manglik, A., Hu, J., Hu, K., Eitel, K., et al. (2013). Activation and allosteric modulation of the muscarinic acetylcholine receptor. Nature 504, 101–106. doi: 10.1038/nature12735
Kunishima, N., Shimada, Y., Tsuji, Y., Sato, T., Yamamoto, M., Kumasaka, T., et al. (2000). Structural basis of glutamate recognition by a dimeric metabotropic glutamate receptor. Nature 407, 971–977. doi: 10.1038/35039564
Labouesse, M. A., and Patriarchi, T. (2021). A versatile GPCR toolkit to track in vivo neuromodulation: not a one-size-fits-all sensor. Neuropsychopharmacology doi: 10.1038/s41386-021-00982-y [Epub ahead of print].
Lagerstrom, M. C., and Schioth, H. B. (2008). Structural diversity of G protein-coupled receptors and their significance in drug discovery. Nat. Rev. Drug Discov. 7, 339–357. doi: 10.1038/nrd2518
Lan, T. H., Liu, Q., Li, C., Wu, G., and Lambert, N. A. (2012). Sensitive and high-resolution localization and tracking of membrane proteins in live cells using BRET. Traffic 13, 1450–1456. doi: 10.1111/j.1600-0854.2012.01401.x
Lecat-Guillet, N., Monnier, C., Rovira, X., Kniazeff, J., Lamarque, L., Zwier, J. M., et al. (2017). FRET-based sensors reveal activation and allosteric modulation of the GABAB receptor. Cell Chem. Biol. 24, 360–370. doi: 10.1016/j.chembiol.2017.02.011
Lee, M. H., Appleton, K. M., Strungs, E. G., Kwon, J. Y., Morinelli, T. A., Peterson, Y. K., et al. (2016). The conformational signature of beta-arrestin2 predicts its trafficking and signaling functions. Nature 531, 665–668. doi: 10.1038/nature17154
Lefkowitz, R. J. (1998). G protein-coupled receptor III. New roles for receptor kinases and beta-arrestins in receptor signaling and desensitization. J. Biol. Chem. 273, 18677–18680. doi: 10.1074/jbc.273.30.18677
Lefkowitz, R. J., and Shenoy, S. K. (2005). Transduction of receptor signals by beta-arrestins. Science 308, 512–517. doi: 10.1126/science.1109237
Liauw, B. W., Afsari, H. S., and Vafabakhsh, R. (2021). Conformational rearrangement during the activation of a metabotropic glutamate receptor. Nat. Chem. Biol. 17, 291–297. doi: 10.1038/s41589-020-00702-5
Liu, H., Yi, P., Zhao, W., Wu, Y., Acher, F., Pin, J. P., et al. (2020). Illuminating allosteric modulation of calcium-sensing receptors. Proc. Natl. Acad. Sci. U.S.A. 117, 21711–21722. doi: 10.1073/pnas.1922231117
Liu, J., Maurel, D., Etzol, S., Brabet, I., Ansanay, H., Pin, J. P., et al. (2004). Molecular determinants involved in allosteric control of agonist affinity in the GABAB receptor by the GABAB2 subunit. J. Biol. Chem. 279, 15824–15830. doi: 10.1074/jbc.M313639200
Loening, A. M., Fenn, T. D., Wu, A. M., and Gambhir, S. S. (2006). Consensus-guided mutagenesis of Renilla luciferase yielded enhanced stability and light output. Protein Eng. Des. Sel. 19, 391–400. doi: 10.1093/protein/gzl023
Lohse, M. J., Nuber, S., and Hoffmann, C. (2012). Fluorescence/bioluminescence resonance energy transfer techniques to study G-protein-coupled receptor activation and signaling. Pharmacol. Rev. 64, 299–336. doi: 10.1124/pr.110.004309
Mao, C., Shen, C., Li, C., Shen, D. D., Xu, C., Zhang, S., et al. (2020). Cryo-EM structures of inactive and active GABAB receptors. Cell Res. 30, 564–573. doi: 10.1038/s41422-020-0350-5
Marcaggi, P., Mutoh, H., Dimitrov, D., Beato, M., and Knopfel, T. (2009). Optical measurement of mGluR1 conformational changes revealed fast activation, slow deactivation, and sensitization. Proc. Natl. Acad. Sci. U.S.A. 106, 11388–11393. doi: 10.1073/pnas.0901290106
Mastop, M., Reinhard, N. R., Zuconelli, C. R., Terwey, F., Gadella, T. J., van Unen, J., et al. (2018). FRET-based biosensor for measuring Galpha13 activation in single cells. PLoS One 13:e0193705. doi: 10.1371/journal.pone.0193705
Masuho, I., Ostrovskaya, O., Kramer, G. M., Jones, C. D., Xie, K., and Martemyanov, K. A. (2015). Distinct profiles of functional discrimination among G proteins determine the actions of G protein-coupled receptors. Sci. Signal. 8:ra123. doi: 10.1126/scisignal.aab4068
Mathis, G. (1995). Probing molecular interactions using homogeneous techniques based on rare-earth cryptates and fluorescence energy transfer. Clin. Chem. 41, 1391–1397.
Maurel, D., Comps-Agrar, L., Brock, C., Rives, M. L., Bourrier, E., Ayoub, M. A., et al. (2008). Cell-surface protein-protein interaction analysis with time-resolved FRET and snap-tag technologies: Application to GPCR oligomerization. Nat. Methods 5, 561–567. doi: 10.1038/nmeth.1213
Maziarz, M., Park, J., Leyme, A., Marivin, A., Garcia-Lopez, A., Patel, P. P., et al. (2020). Revealing the activity of trimeric G-proteins in live cells using a versatile biosensor design. Cell 182, 770.–785.
Milligan, G., and Bouvier, M. (2005). Methods for monitoring the quaternary structure of G protein-coupled receptors. FEBS J. 272, 2914–2925. doi: 10.1111/j.1742-4658.2005.04731.x
Molinari, P., Casella, I., and Costa, T. (2008). Functional complementation of high-efficiency resonance energy transfer: A new tool for the study of protein-binding interactions in living cells. Biochem. J. 409, 251–261. doi: 10.1042/BJ20070803
Mullard, A. (2020). FDA approves first BCMA-targeted therapeutic. Nat. Rev. Drug Discov. 19:659. doi: 10.1038/d41573-020-00157-2
Muto, T., Tsuchiya, D., Morikawa, K., and Jingami, H. (2007). Structures of the extracellular regions of group II/III metabotropic glutamate receptors. Proc. Natl. Acad. Sci. U.S.A. 104, 3759–3764. doi: 10.1073/pnas.0611577104
Namkung, Y., Le Gouill, C., Lukashova, V., Kobayashi, H., Hogue, M., Khoury, E., et al. (2016). Monitoring G protein-coupled receptor and beta-arrestin trafficking in live cells using enhanced bystander BRET. Nat. Commun. 7:12178. doi: 10.1038/ncomms12178
Nehme, R., Carpenter, B., Singhal, A., Strege, A., Edwards, P. C., White, C. F., et al. (2017). Mini-G proteins: Novel tools for studying GPCRs in their active conformation. PLoS One 12:e0175642. doi: 10.1371/journal.pone.0175642
Neubig, R. R. (1994). Membrane organization in G-protein mechanisms. FASEB J. 8, 939–946. doi: 10.1096/fasebj.8.12.8088459
Nobles, M., Benians, A., Tinker, A., and Jan, L. Y. (2005). Heterotrimeric G proteins couple with G protein-coupled receptors in living cells. Proc. Natl. Acad. Sci. U.S.A. 102, 18706–18711. doi: 10.1073/pnas.0504778102
Nuber, S., Zabel, U., Lorenz, K., Nuber, A., Milligan, G., Tobin, A. B., et al. (2016). Beta-arrestin biosensors exhibit a rapid, receptor-dependent activation/deactivation cycle. Nature 531, 661–664. doi: 10.1038/nature17198
Oakley, R. H., Laporte, S. A., Holt, J. A., Caron, M. G., and Barak, L. S. (2000). The differential affinities of visual arrestin, beta arrestin1, and beta arrestin2 for G protein-coupled receptors delineate two major classes of receptors. J. Biol. Chem. 275, 17201–17210. doi: 10.1074/jbc.M910348199
Oishi, A., Dam, J., and Jockers, R. (2020). beta-arrestin-2 BRET biosensors detect different beta-arrestin-2 conformations in interaction with GPCRs. ACS Sens 5, 57–64. doi: 10.1021/acssensors.9b01414
Olsen, R., DiBerto, J. F., English, J. G., Glaudin, A. M., Krumm, B. E., Slocum, S. T., et al. (2020). TRUPATH is an open-source biosensor platform for interrogating the GPCR transducerome. Nat. Chem. Biol. 16, 841–849. doi: 10.1038/s41589-020-0535-8
Papasergi-Scott, M. M., Robertson, M. J., Seven, A. B., Panova, O., Mathiesen, J. M., and Skiniotis, G. (2020). Structures of metabotropic GABAB receptor. Nature 584, 310–314. doi: 10.1038/s41586-020-2469-4
Park, J., Fu, Z., Frangaj, A., Liu, J., Mosyak, L., Shen, T., et al. (2020). Structure of the human GABAB receptor in an inactive state. Nature 584, 304–309. doi: 10.1038/s41586-020-2452-0
Peng, W., Wu, Z., Song, K., Zhang, S., Li, Y., and Xu, M. (2020). Regulation of the sleep homeostasis mediator adenosine by basal forebrain glutamatergic neurons. Science 369:556. doi: 10.1126/science.abb0556
Philip, F., Sengupta, P., and Scarlata, S. (2007). Signaling through a G protein-coupled receptor and its corresponding G protein follows a stoichiometrically limited model. J. Biol. Chem. 282, 19203–19216. doi: 10.1074/jbc.M701558200
Picard, L., Schönegge, A. M., Lohse, M. J., and Bouvier, M. (2018). Bioluminescence resonance energy transfer-based biosensors allow the monitoring of ligand- and transducer-mediated conformational changes in GPCRs. Commun. Biol. 1:101. doi: 10.1038/s42003-018-0101-z
Pin, J. P., Kniazeff, J., Prezeau, L., Liu, J. F., and Rondard, P. (2019). GPCR interaction is a possible method for allosteric control between receptors. Mol. Cell. Endocrinol. 486, 89–95. doi: 10.1016/j.mce.2019.02.019
Prinster, S. C., Hague, C., and Hall, R. A. (2005). Heterodimerization of G protein-coupled receptors: specificity and functional significance. Pharmacol. Rev. 57, 289–298. doi: 10.1124/pr.57.3.1
Quast, R. B., and Margeat, E. (2019). Studying GPCR conformational dynamics using single-molecule fluorescence. Mol. Cell. Endocrinol. 493:110469. doi: 10.1016/j.mce.2019.110469
Quitterer, U., Fu, X., Pohl, A., Bayoumy, K. M., Langer, A., and Abdalla, S. (2019). Beta-arrestin1 prevents preeclampsia by downregulating mechanosensitive AT1-B2 receptor heteromers. Cell 176, 318–333. doi: 10.1016/j.cell.2018.10.050
Rasmussen, S. G. F., Choi, H., Fung, J. J., Pardon, E., Casarosa, P., Chae, P. S., et al. (2011). Structure of a nanobody-stabilized active state of β2 adrenoceptor. Nature 469, 175–180. doi: 10.1038/nature09648
Reiner, S., Ambrosio, M., Hoffmann, C., and Lohse, M. J. (2010). Differential signaling of the endogenous agonists at the beta2-adrenergic receptor. J. Biol. Chem. 285, 36188–36198. doi: 10.1074/jbc.M110.175604
Rochais, F., Vilardaga, J. P., Nikolaev, V. O., Bunemann, M., Lohse, M. J., and Engelhardt, S. (2007). Real-time optical recording of beta1-adrenergic receptor activation revealed the supersensitivity of the Arg389 variant to carvedilol. J. Clin. Invest. 117, 229–235. doi: 10.1172/JCI30012
Sauliere, A., Bellot, M., Paris, H., Denis, C., Finana, F., Hansen, J. T., et al. (2012). Deciphering the complexity of biased-agonism reveals a new active AT1 receptor entity. Nat. Chem. Biol. 8, 622–630. doi: 10.1038/nchembio.961
Schihada, H., Vandenabeele, S., Zabel, U., Frank, M., Lohse, M. J., and Maiellaro, I. (2018). A universal bioluminescence resonance energy transfer sensor design enables high-sensitivity screening of the GPCR activation dynamics. Commun. Biol. 1:72. doi: 10.1038/s42003-018-0072-0
Scholler, P., Moreno-Delgado, D., Lecat-Guillet, N., Doumazane, E., Monnier, C., Charrier-Savournin, F., et al. (2017a). HTS-compatible FRET-based conformational sensors clarify membrane receptor activation. Nat. Chem. Biol. 13, 372–380. doi: 10.1038/nchembio.2286
Scholler, P., Nevoltris, D., de Bundel, D., Bossi, S., Moreno-Delgado, D., Rovira, X., et al. (2017b). Allosteric nanobodies uncover the role of hippocampal mGlu2 receptor homodimers in contextual fear consolidation. Nat. Commun. 8:1038. doi: 10.1038/s41467-017-01489-1
Sebastianutto, I., Goyet, E., Andreoli, L., Font-Ingles, J., Moreno-Delgado, D., Bouquier, N., et al. (2020). D1-mGlu5 heteromers mediate non-canonical dopamine signaling in Parkinson’s disease. J. Clin. Invest. 130, 1168–1184. doi: 10.1172/JCI126361
Selvin, P. R. (2002). Principles and biophysical applications of lanthanide-based probes. Annu. Rev. Biophys. Biomol. Struct. 31, 275–302. doi: 10.1146/annurev.biophys.31.101101.140927
Shaye, H., Ishchenko, A., Lam, J. H., Han, G. W., Xue, L., Rondard, P., et al. (2020). Structural basis for the activation of a metabotropic GABA receptor. Nature 584, 298–303. doi: 10.1038/s41586-020-2408-4
Shukla, A. K., Violin, J. D., Whalen, E. J., Gesty-Palmer, D., Shenoy, S. K., and Lefkowitz, R. J. (2008). Distinct conformational changes in beta-arrestin result in biased agonism at the seven-transmembrane receptors. Proc. Natl. Acad. Sci. U.S.A. 105, 9988–9993. doi: 10.1073/pnas.0804246105
Sleno, R., Devost, D., Pétrin, D., Zhang, A., Bourque, K., Shinjo, Y., et al. (2017). Conformational biosensors reveal allosteric interactions between the heterodimeric AT1 angiotensin and prostaglandin Fα receptors. J. Biol. Chem. 292, 12139–12152. doi: 10.1074/jbc.M117.793877
Sleno, R., Pétrin, D., Devost, D., Goupil, E., Zhang, A., and Hébert, T. E. (2016). Designing BRET-based conformational biosensors for G protein-coupled receptors. Methods 92, 11–18. doi: 10.1016/j.ymeth.2015.05.003
Sriram, K., and Insel, P. A. (2018). G Protein-coupled receptors as targets for approved drugs: How many targets and the number of drugs? Mol. Pharmacol. 93, 251–258. doi: 10.1124/mol.117.111062
Strungs, E. G., Luttrell, L. M., and Lee, M. H. (2019). Probing arrestin function using intramolecular FlAsH-BRET biosensors. Methods Mol Biol 1957, 309–322. doi: 10.1007/978-1-4939-9158-7_19
Stryer, L. (1978). Fluorescence energy transfer as a spectroscopic ruler. Annu. Rev. Biochem. 47, 819–846. doi: 10.1146/annurev.bi.47.070178.004131
Sun, F., Zeng, J., Jing, M., Zhou, J., Feng, J., Owen, S. F., et al. (2018). A genetically encoded fluorescent sensor enables rapid and specific detection of dopamine in flies, fish, and mice. Cell 174, 481–496. doi: 10.1016/j.cell.2018.06.042
Szalai, B., Barkai, L., Turu, G., Szidonya, L., Várnai, P., and Hunyady, L. (2012). Allosteric interactions within the AT1 angiotensin receptor homodimer: Role of the conserved DRY motif. Biochem. Pharmacol. 84, 477–485. doi: 10.1016/j.bcp.2012.04.014
Tateyama, M., and Kubo, Y. (2006). Dual signaling is differentially activated by different active states of the metabotropic glutamate receptor 1α. Proc. Natl. Acad. Sci. USA. 103, 1124–1128. doi: 10.1073/pnas.0505925103
Thomsen, W., Frazer, J., and Unett, D. (2005). Functional assays for screening GPCR targets. Curr. Opin. Biotechnol. 16, 655–665. doi: 10.1016/j.copbio.2005.10.008
Tsuchiya, D., Kunishima, N., Kamiya, N., Jingami, H., and Morikawa, K. (2002). Structural views of the ligand-binding cores of a metabotropic glutamate receptor complexed with an antagonist and both glutamate and Gd3+. Proc. Natl. Acad. Sci. U.S.A. 99, 2660–2665. doi: 10.1073/pnas.052708599
Vilardaga, J. P., Bunemann, M., Krasel, C., Castro, M., and Lohse, M. J. (2003). Measurement of the millisecond activation switch of G protein-coupled receptors in living cells. Nat. Biotechnol. 21, 807–812. doi: 10.1038/nbt838
Vilardaga, J. P., Krasel, C., Chauvin, S., Bambino, T., Lohse, M. J., and Nissenson, R. A. (2002). Internalization determinants of the parathyroid hormone receptor differentially regulate beta-arrestin/receptor association. J. Biol. Chem. 277, 8121–8129. doi: 10.1074/jbc.M110433200
Vilardaga, J. P., Steinmeyer, R., Harms, G. S., and Lohse, M. J. (2005). Molecular basis of inverse agonism in G protein-coupled receptors. Nat. Chem. Biol. 1, 25–28. doi: 10.1038/nchembio705
Wan, Q., Okashah, N., Inoue, A., Nehme, R., Carpenter, B., Tate, C. G., et al. (2018). Mini G protein probes for active G protein-coupled receptors (GPCRs) in live cells. J. Biol. Chem. 293, 7466–7473. doi: 10.1074/jbc.RA118.001975
Weis, W. I., and Kobilka, B. K. (2018). The molecular basis of G protein-coupled receptor activation. Annu. Rev. Biochem. 87, 897–919. doi: 10.1146/annurev-biochem-060614-033910
Wright, S. C., Kozielewicz, P., Kowalski-Jahn, M., Petersen, J., Bowin, C. F., Slodkowicz, G., et al. (2019). A conserved molecular switch in class F receptors regulates receptor activation and pathway selection. Nat. Commun. 10:667. doi: 10.1038/s41467-019-08630-2
Xue, L., Rovira, X., Scholler, P., Zhao, H., Liu, J., Pin, J. P., et al. (2015). Major ligand-induced rearrangement of the heptahelical domain interface in a GPCR dimer. Nat. Chem. Biol. 11, 134–140. doi: 10.1038/nchembio.1711
Xue, L., Sun, Q., Zhao, H., Rovira, X., Gai, S., He, Q., et al. (2019). Rearrangement of the transmembrane domain interfaces associated with the activation of a GPCR hetero-oligomer. Nat. Commun. 10:2765. doi: 10.1038/s41467-019-10834-5
Yin, J., Straight, P. D., McLoughlin, S. M., Zhou, Z., Lin, A. J., Golan, D. E., et al. (2005). Genetically encoded short peptide tag for versatile protein labeling by Sfp phosphopantetheinyl transferase. Proc. Natl. Acad. Sci. U.S.A. 102, 15815–15820. doi: 10.1073/pnas.0507705102
Zhang, R., and Xie, X. (2012). Tools for GPCR drug discovery. Acta Pharmacol. Sin. 33, 372–384. doi: 10.1038/aps.2011.173
Keywords: GPCR, functional assay, G-protein, β-arrestin, dimerization, BRET, TR-FRET
Citation: Zhou Y, Meng J, Xu C and Liu J (2021) Multiple GPCR Functional Assays Based on Resonance Energy Transfer Sensors. Front. Cell Dev. Biol. 9:611443. doi: 10.3389/fcell.2021.611443
Received: 29 September 2020; Accepted: 05 February 2021;
Published: 10 May 2021.
Edited by:
Wei Liu, Shenzhen Peking University Hong Kong University of Science and Technology Medical Center, ChinaReviewed by:
Takeaki Ozawa, The University of Tokyo, JapanMohammed Akli Ayoub, United Arab Emirates University, United Arab Emirates
Copyright © 2021 Zhou, Meng, Xu and Liu. This is an open-access article distributed under the terms of the Creative Commons Attribution License (CC BY). The use, distribution or reproduction in other forums is permitted, provided the original author(s) and the copyright owner(s) are credited and that the original publication in this journal is cited, in accordance with accepted academic practice. No use, distribution or reproduction is permitted which does not comply with these terms.
*Correspondence: Chanjuan Xu, chanjuanxu@hust.edu.cn; Jianfeng Liu, jfliu@mail.hust.edu.cn