Human iPSCs and Genome Editing Technologies for Precision Cardiovascular Tissue Engineering
- 1Institute for Regenerative Medicine (IREM), University of Zurich, Zurich, Switzerland
- 2Wyss Zurich, University and ETH Zurich, Zurich, Switzerland
- 3Division of Pediatric Cardiology, Department of Pediatrics, Stanford School of Medicine, Stanford, CA, United States
- 4Vera Moulton Wall Center for Pulmonary Vascular Disease, Stanford School of Medicine, Stanford, CA, United States
- 5Stanford Cardiovascular Institute, Stanford School of Medicine, Stanford, CA, United States
- 6Molecular Parasitology Lab, Institute of Parasitology, University of Zurich, Zurich, Switzerland
- 7Bioscience Cardiovascular, Research and Early Development, Cardiovascular, Renal and Metabolism, R&D BioPharmaceuticals, AstraZeneca, Gothenburg, Sweden
- 8Department of Cardiovascular Surgery, Charité Universitätsmedizin Berlin, Berlin, Germany
- 9Department of Cardiothoracic and Vascular Surgery, German Heart Center Berlin, Berlin, Germany
Induced pluripotent stem cells (iPSCs) originate from the reprogramming of adult somatic cells using four Yamanaka transcription factors. Since their discovery, the stem cell (SC) field achieved significant milestones and opened several gateways in the area of disease modeling, drug discovery, and regenerative medicine. In parallel, the emergence of clustered regularly interspaced short palindromic repeats (CRISPR)-associated protein 9 (CRISPR-Cas9) revolutionized the field of genome engineering, allowing the generation of genetically modified cell lines and achieving a precise genome recombination or random insertions/deletions, usefully translated for wider applications. Cardiovascular diseases represent a constantly increasing societal concern, with limited understanding of the underlying cellular and molecular mechanisms. The ability of iPSCs to differentiate into multiple cell types combined with CRISPR-Cas9 technology could enable the systematic investigation of pathophysiological mechanisms or drug screening for potential therapeutics. Furthermore, these technologies can provide a cellular platform for cardiovascular tissue engineering (TE) approaches by modulating the expression or inhibition of targeted proteins, thereby creating the possibility to engineer new cell lines and/or fine-tune biomimetic scaffolds. This review will focus on the application of iPSCs, CRISPR-Cas9, and a combination thereof to the field of cardiovascular TE. In particular, the clinical translatability of such technologies will be discussed ranging from disease modeling to drug screening and TE applications.
Introduction
Historic Considerations: Stem Cell Research and the Foundation of Induced Pluripotent Stem Cells
Stem cells (SCs) were first described in 1961 by Drs. James A. Till and Ernest A. McCulloch at Toronto University, where they discovered that mouse bone marrow-derived SCs possessed the unique ability to differentiate toward a multitude of different cell types (Till and McCulloch, 1961), thus laying the foundation for SC research. SCs are characterized by two properties: (i) self-renewal, which allow their indefinite division, producing unaltered daughter cells and (ii) the ability to exit self-renewal and differentiate into specialized cells giving rise to the three germ layers (i.e., ectoderm, mesoderm, and endoderm) (Wobus and Boheler, 2005). Naturally occurring SCs are classified by their self-renewal and differentiation potential: (i) totipotent SCs can differentiate into any cell type and can create an entire organism, the zygote is an example of totipotent cells; (ii) pluripotent SCs have the potential to differentiate into any cell type stemming from the cell lineages (ectoderm, mesoderm, and endoderm), human embryonic SCs (hESCs) is an example of pluripotency; (iii) multipotent SCs can differentiate into cells from a specific lineage, e.g., very small embryonic-like SCs (VSELs), which are early development SCs from adult tissues; (iv) oligopotent SCs can differentiate into a small number of cell types from a specific tissue, such as adult SCs; and (v) unipotent SCs, or progenitor cells, can differentiate into a single cell type (Zakrzewski et al., 2019; Liu et al., 2020). Artificially derived SCs, or human induced pluripotent SCs (hiPSCs), are reprogrammed from a terminally differentiated cell, but carry the same potency as hESCs. Additionally, nuclear transfer SCs (NTSCs), where the nucleus of a zygote is replaced with a somatic cell, are less effective than reprogrammed iPSCs (Zakrzewski et al., 2019; Liu et al., 2020). In 1962, the technique of somatic cell nuclear transfer (SCNT) provided the first evidence that terminally differentiated cells could reprogram into a pluripotent state (Gurdon, 1962; Wilmut et al., 1997). The year 1998 marked the discovery of the first hESCs, by James Thomson (Thomson et al., 1998), and the early 2000s demonstrated the fusion between hESCs and somatic cells that confirmed the potential to revert cells potency state to enable their reprogramming (Tada et al., 2001). iPSCs were first reported in 2006 by reprogramming innate adult somatic cells using four specific genes, octamer-binding transcription factor-3/4 (Oct3/4) and sex-determining region Y-box 2 (Sox2), combined to either c-Myc or kruppel-like factor-4 (Klf4), and homeobox protein nanog (Nanog) or lin-28 homolog A (Lin28) (Takahashi and Yamanaka, 2006; Okita et al., 2007; Takahashi et al., 2007; Yu et al., 2007; Figure 1).
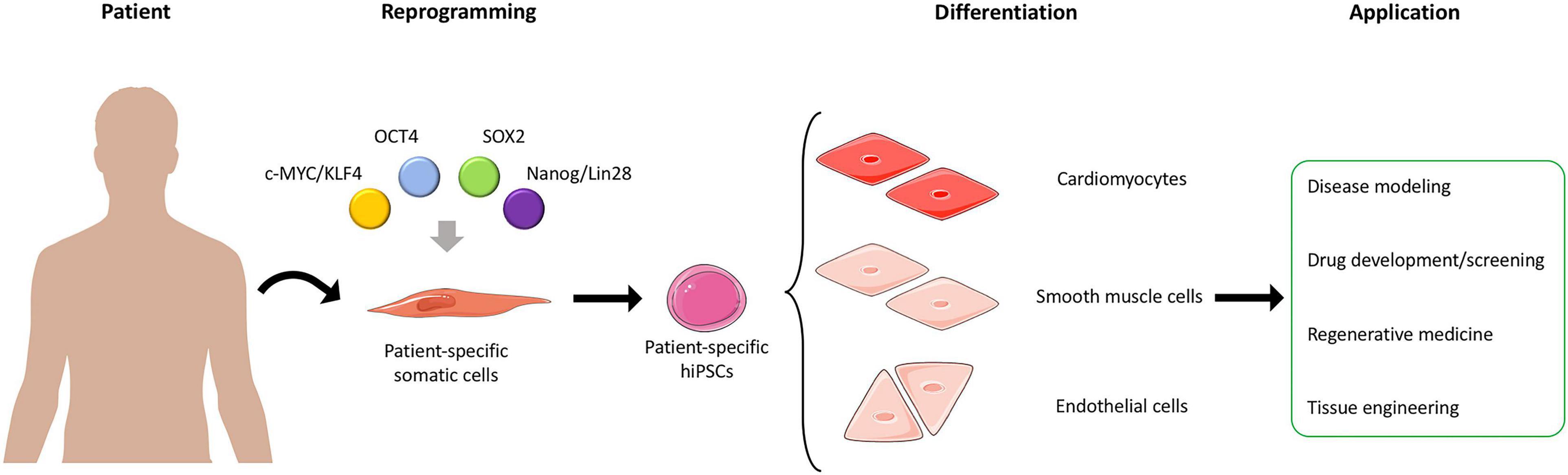
Figure 1. Generation and application of hiPSCs. Somatic cells are harvested from patients and reprogrammed into patient-specific hiPSCs. The hiPSCs can then be differentiated into different cell types, such as cardiomyocytes, smooth muscle cells, and endothelial cells, which can be used in different applications. Adapted from servier medical art, licensed under a Creative Commons Attribution 3.0 Unported License.
hiPSC Technology: Advantages and Disadvantages
The field of SCs considerably changed following the discovery of hiPSCs, and the emergence of reprogramming technology enabled the use of disease-specific hiPSCs, thereby circumventing the (ethical) limitations of hESCs (Wobus and Löser, 2011). In comparison with hESCs, the use of hiPSCs presents multiple advantages, such as reduced ethical requirements, high degree of dedifferentiation, high proliferation rate, and self-renewal ability (Wobus and Löser, 2011; Kumar et al., 2017). Such properties allow for the generation of libraries that can be used for the development of drug screening/response platforms, significantly reducing the related production costs (Wobus and Löser, 2011).
Additionally, allogenic hiPSCs can be reprogrammed from individual patients, thus retaining patient-specific properties, such as genetic information, and display no immunogenicity after transplantation (Guha et al., 2013). Hence, hiPSC-based therapies present a unique potential not only for disease modeling but also for precision medicine by establishing novel treatment strategies based on patient-specific phenotypes (Chun et al., 2011; Matsa et al., 2016). Furthermore, hiPSC’s capability to undergo almost indefinite proliferation cycles and the possibility to perform single cell clonal expansion make hiPSCs a reliable cell source for genome engineering approaches (Grobarczyk et al., 2015; Hotta and Yamanaka, 2015). Within the past decade, the application of these technologies has revolutionized several research areas, such as regenerative medicine, disease modeling, drug discovery, and human developmental biology demonstrating the reproducibility of this methodology (Kwon et al., 2018; Nikolić et al., 2018; Spitalieri et al., 2018; Cota-Coronado et al., 2019; Savoji et al., 2019; Figure 1).
Nevertheless, hiPSCs also present limitations related to the way they are produced. Former reprogramming approaches used retro- or lentiviruses as delivery system for transcription factors for somatic cell reprogramming arises safety concerns in regard to the integration of the viral system in the host genome, which can ultimately lead to genetic alteration, thus increasing tumorigenicity risks (Howe et al., 2008; Higuchi et al., 2015). More recent approaches aim at reducing the genetic alterations caused by reprogramming via non-integrating viruses (e.g., Sendai virus), episomal vectors, or through direct delivery of reprogramming factors, such as protein or mRNA to generate integration free hiPSCs (Kim et al., 2009; Okita et al., 2011; Diecke et al., 2015; Schlaeger et al., 2015; Rohani et al., 2016).
hiPSC Technology: Regulatory Considerations and Clinical Application
As previously mentioned, the differentiation potential of hiPSCs and the numerous application possibilities of hiPSC-derived products are enormous; however, their clinical translation is still considerably hampered. Lack of scalable differentiation protocols, undifferentiated cell contaminates, as well as unknown in vivo hiPSC functionality and their potential to generate teratomas still limit the broader clinical application of such technology. To foster the use of hiPSCs and their derived products into the clinics, research groups are focusing on the establishment of reliable protocols for the isolation, generation, proliferation, and differentiation of hiPSCs following GMP-compliant regulations. In addition, preclinical efficacy and safety, ethical compliance, and respect of the regulatory guidelines need to be established a priori (Haake et al., 2019).
The rapid technology translation that hiPSCs are experiencing often reveals the gaps and limitations that still need to be faced, for example, genetic instability, immunogenicity, epigenetic abnormalities (Ben-David and Benvenisty, 2011; Laurent et al., 2011; Zhao et al., 2011; Liu et al., 2017; Bragança et al., 2019; Ratajczak, 2019), as well as publication bias and late translation into clinics after their generation, which can ultimately cause misuse of the patient genetic code (Wolinetz and Collins, 2020). In this regard, guidelines that protect the cell donors’ rights must be granted in order to protect future patients. This is of great concern as several clinical trials on hiPSCs are ongoing, some of which focusing on cardiovascular diseases (CVDs) (Deinsberger et al., 2020). Given the rapid propagation of such technology, the establishment of regulatory guidelines for disease modeling, drug discovery, and clinical translation is a must and has to be enlightened by the regulatory offices and SC societies.
Rise of Genome Editing Technologies and CRISPR-Cas9
The idea of genomic information exchange via exogenous DNA homology recombination (HR) was initially demonstrated by Oliver Smithies (Smithies et al., 1985). This discovery was followed by the identification of meganucleases, which were able to introduce double strand breaks (DSBs) at specific sites in the genome, thus improving the insertion of exogenous DNA (Choulika et al., 1995; Cohen-Tannoudji et al., 1998). These findings opened the path for the development of the zinc finger nuclease (ZFN) technology in 2009, of transcription activator-like effector nuclease (TALEN) in 2011, and finally of clustered regularly interspaced short palindromic repeats (CRISPR) in 2013 (Geurts et al., 2009; Tesson et al., 2011; Gasiunas et al., 2012; Jinek et al., 2012; Cong et al., 2013; Mali et al., 2013b; Wang et al., 2013; Figure 2A). The CRISPR system originates from the defense mechanism found in archaea and bacteria (Terns and Terns, 2011). To be fully functional, the CRISPR system requires: (i) a DNA endonuclease, i.e., the CRISPR-associated protein 9 (Cas9), that cleaves the DNA specifically at the protospacer adjacent motif (PAM) and (ii) a small RNA molecule, known as the single guide RNA (sgRNA), that allows the CRISPR-Cas9 to target the specific genomic location and induce a DSB (Jinek et al., 2012; Anders et al., 2015; Figure 2A). The DSB triggers DNA repair via two different pathways, non-homologous end joining (NHEJ) or homology-directed repair (HDR) (Jinek et al., 2012; Gaj et al., 2013; Mali et al., 2013a; Figure 2A). NHEJ repair is a process that does not require a homology template and can thus introduce insertions or deletions (indel) at the cleavage site, thus causing a gene knockout if the indel occurs in an exon (Wyman and Kanaar, 2006; Hsu et al., 2014). To the contrary, HDR uses a homology DNA template to obtain high-fidelity repair, thereby allowing precise DNA insertions (Wyman and Kanaar, 2006; Hsu et al., 2014; Lin et al., 2014; Maruyama et al., 2015). In addition, further modifications of CRISPR-Cas9 allowed the development of single base editing, a fusion of cytidine or adenosine deaminase enzymes with Cas9, that enables single genetic modification without DSB (Komor et al., 2016; Porto et al., 2020). Within this technique, sgRNA targets the CRISPR-Cas9 base editor to the specific sequence of DNA. Subsequently, the cytidine deaminase induces the conversion of cytosine to uracil first, and then to thymine, with adenine as complementary base. On the other side, the adenine deaminase converts adenine into inosine, which is recognized as guanine, with cytosine as complementary base (Komor et al., 2016; Porto et al., 2020; Figure 2B). Besides the ability of CRISPR-Cas9 to permanently modify the genome, modifications of the catalytic site of the Cas9 nuclease allowed the generation of dead Cas9 (dCas9), which retains the specific binding ability to DNA, without inducing DSB (Qi et al., 2013; Hsu et al., 2014). Furthermore, fusion of dCas9 to transcription activators (e.g., VP16, VP64, p65) or transcription repressors (e.g., KRAB, SID) allowed to retarget CRISPR-Cas9 toward gene expression modulation (Dominguez et al., 2016; Mahas et al., 2018; Figure 2C). The CRISPR-Cas9 technology currently represents an established gene editing tool, which expands our understanding of genetic diseases by restoring genome integrity, and provided disease-specific cells for drug testing (Wang et al., 2014c; Zhang et al., 2014; Hinson et al., 2015).
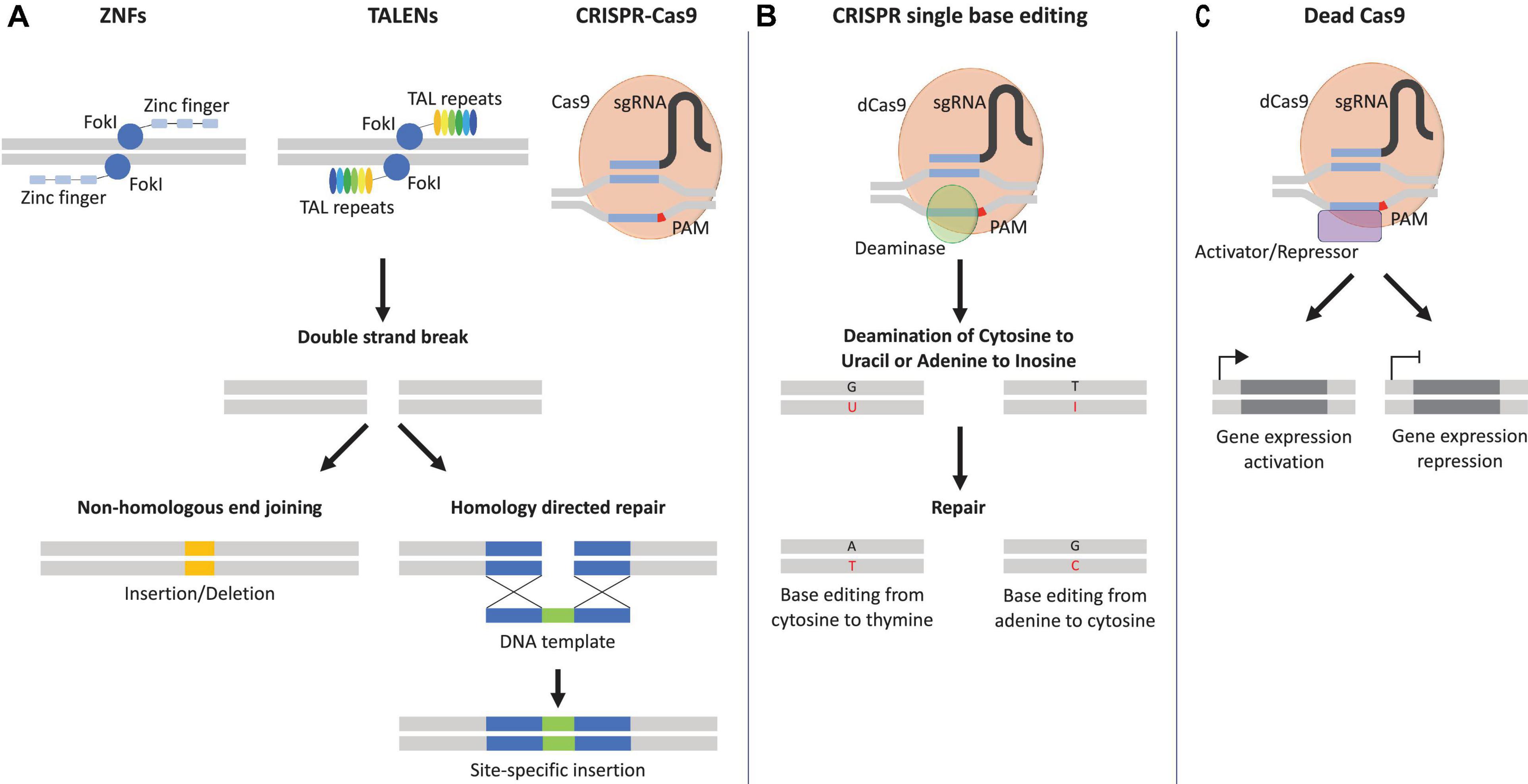
Figure 2. Genome editing tools. (A) Zinc finger nucleases (ZNFs) and transcription activator-like effector nucleases (TALENs) recognize specific genomic sites with their specific DNA-binding proteins, zinc finger and transcription activator-like repeats. Clustered regularly interspaced short palindromic repeats (CRISPR-Cas9) is directed to the specific genomic site with the help of the protospacer adjacent motif (PAM) in red and the sequence of the single guide RNA (sgRNA) in blue. Once the specific genomic site is recognized, the catalytical subunits, Flavobacterium okeanokoites type IIS restriction enzyme (FokI), or CRISPR-associated protein 9 (Cas9) induces a double strand brake (DSB) and can be repaired either by non-homologous end joining that can lead to insertion/deletion or homology-directed repair with the help of DNA template that allows the introduction of specific mutations. (B) Catalytically inactive Cas9 or dead Cas9 (dCas9) fused to a deaminase (e.g., cytidine or adenine) can result in the conversion of cytosine–guanine base pairs to thymine–adenine or vice versa, without the need of a DSB. A = adenine, T = thymine, C = cytosine, G = guanine, U = uracil, I = inosine. (C) An additional variation of dCas9 fused to a transcriptional activator/repressor can induce transcriptional activation/repression of the targeted gene.
CRISPR-Cas9 Technology: Regulatory Considerations and Clinical Application
The enthusiasm around CRISPR-Cas9 technology has garnered a great degree of attention since its first reported use in 2013 (Musunuru, 2017b). However, the ethical (moral), biomedical, safety, and legal concerns regarding the use of such application to the medical (clinical) field are gaining importance (Brokowski and Adli, 2019). In 2015, the National Academics of Sciences, Engineering, and Medicine (NASEM) compiled one of the most extensive risk analysis reports on the use of such genome editing tools on humans (Brokowski and Adli, 2019). Finally, the committee agreed on somatic genome editing experimentation, but did not allow human genome modifications nor any kind of enhancement (Memi et al., 2018; Brokowski and Adli, 2019). In this regard, CRISPR-Cas9 technology is significantly helpful for the improvement of immunotherapies, organoid engineering, drug target identification, and disease-gene modifications (Singh et al., 2019). Particularly, this system offers the great potential to progress therapies against HIV, hemophilia, cancer, and any number of yet uncurable diseases (Singh et al., 2019).
HiPSC Applications for Cardiovascular Research
The mammalian heart has limited regenerative capacity and is subjected to multiple genetic or non-genetic dysfunctions, thus resulting in heart diseases and/or failure (Skrzynia et al., 2014; Doppler et al., 2017). Currently, small and large animal models are used to study human heart diseases, yet inter-species differences as well as anatomical and physiological dissimilarities complicate the clinical translation of safe and effective therapies (Milan and MacRae, 2005; Camacho et al., 2016). Among the existing SC therapies, hiPSCs emerge as a potential cell source for CVD modeling and treatment (Park and Yoon, 2018; Sadahiro, 2019; Parrotta et al., 2020). Besides the possibility to generate hiPSCs from patients’ somatic cells and giving access to patient-specific cells, there is an added ability of hiPSCs to proliferate indefinitely, maintain the genetic information of their host, and differentiate into any cell type. This makes hiPSCs an ideal cell source to investigate CVD originating from acquired genetic or congenital defects thus establish a better understanding of the pathological mechanisms and molecular functions regulating cardiac disorders, thereby opening the path for the development of new diagnostic and therapeutic approaches (Matsa et al., 2016; Parrotta et al., 2020).
hiPSC-Derived Cardiomyocytes
Over the years, several attempts have been made to establish hiPSC differentiation protocols that imitate the signaling pathways involved in embryonic cardiovascular development to obtain functionally mature cardiac cells (Skrzynia et al., 2014). Initially, differentiation of hiPSCs into cardiomyocytes (CMs) involved single cell suspension cultures. This induced hiPSCs to spontaneously aggregate and form embryoid bodies (EBs), thus mimicking embryogenesis (Maltsev et al., 1993; Yang et al., 2008). Following EB formation, cells differentiated into the three germ layers and finally acquired CM properties (Zhang et al., 2009; Zwi et al., 2009). However, despite the promising differentiation outcomes, the presence of serum in the medium made EB-based approaches prone to variability between samples, thus compromising the reproducibility level (Osafune et al., 2008). Subsequent improvements of EB-based differentiation protocols employed cytokines and growth factors, such as Wnt proteins, bone morphogenetic proteins (BMPs), activin A, and Notch signals, combined to their respective selective inhibitors (Chau et al., 2006; Laflamme et al., 2007; Wang et al., 2011; Lian et al., 2012; Burridge et al., 2014; Bastakoty et al., 2016; Abad et al., 2017), thus increasing the efficiency of differentiation. Simplified procedures were additionally established to eliminate the EB formation process by using a monolayer-based system (Paige et al., 2010; Zhang et al., 2012; Kadari et al., 2015; Talkhabi et al., 2016). Furthermore, the development of the two-step differentiation protocols enabled the derivation of cardiac progenitor cells (CPCs) from hiPSCs, followed by a second differentiation step where either CMs, smooth muscle cells (SMCs), or endothelial cells (ECs) could be obtained (Kattman et al., 2011; Cao et al., 2013).
However, the limitations for the differentiation approaches reported so far are that hiPSC-derived cardiac cells are heterogenous and lack cellular maturity, which subsequently result in fetal CM function and morphology (Veerman et al., 2015; Machiraju and Greenway, 2019). hiPSC-derived CM immaturity impairs the proper modeling of adult CVD due to the inability to fully reiterate aging-related disease phenotypes, as well as of genetic-related pathologies, thus negatively influencing their use for drug development and screening (Veerman et al., 2015; Goversen et al., 2018; Machiraju and Greenway, 2019). To overcome the lack of mature and homogenous hiPSC-CMs, new elaborate strategies were employed to include long-term culture periods, the use of hormones in the differentiation medium, mechanical or electrical stimulation, and the use of in vivo environments (Chan et al., 2013; Kamakura et al., 2013; Yang et al., 2014; Ruan et al., 2016; Cho et al., 2017; Kadota et al., 2017; Ebert et al., 2019; Machiraju and Greenway, 2019). These latest studies resulted in increased hiPSC-CM mitochondria generation, sarcomere maturation, change of energy source to fatty acid instead of glucose, as well as an enhanced electrophysiological metabolism and response electro-, mechanical-, or pharmacological stimulation (Chan et al., 2013; Kamakura et al., 2013; Yang et al., 2014; Ruan et al., 2016; Cho et al., 2017; Kadota et al., 2017; Ebert et al., 2019; Machiraju and Greenway, 2019).
hiPSCs and CVD Modeling
An efficient reproduction of human diseases requires a relevant and precise model that recapitulates the pathophysiological mechanisms of the disease itself (Savoji et al., 2019). In vitro cell cultures and animal models can only recapitulate some physiological features of human diseases, but not the entire pathophysiological profile (Savoji et al., 2019). In fact, a broader application of existing in vitro disease models can be limited by the over simplification of in vitro approaches, the availability of patient-specific cells, and their limited proliferation potential, as in the case of CMs (Savoji et al., 2019). In this context, hiPSCs represent a promising cell source, owing to features such as human origin, unlimited proliferation capacities, and potential to differentiate into any cell type. Pioneering studies established hiPSCs from patients suffering from specific genetic conditions, thereby enabling the generation of disease models to mimic their particular molecular mechanisms (Park et al., 2008). Disease modeling based on hiPSCs generated great progress in the field of cardiovascular research, eventually providing the tools to acquire a more precise understanding of the underlying CVD mechanisms and to develop new therapeutic approaches. The ability of hiPSCs to maintain the genetic profile of the host while differentiating into cardiac-derived cells, such as CMs, SMCs, and vascular ECs, enabled the production of robust in vitro CVD models (Parrotta et al., 2020). Here, cardiac disorders including cardiomyopathies, channelopathies, and structural-based cardiac defects will be further discussed.
Cardiomyopathies
Cardiomyopathies encompass a number of disorders related to distinct genetic mutations, such as hypertrophic cardiomyopathy (HCM), dilated cardiomyopathy (DCM), arrhythmogenic right ventricular cardiomyopathy (ARVC), and left ventricular non-compaction (LVNC), and are defined by structural or functional dysfunction of the myocardium (Sisakian, 2014; Hannah-Shmouni et al., 2015).
HCM is one of the most common genetically inherited cardiomyopathies and is linked to ventricular and septum hypertrophy caused by hypertrophic CMs and fibrosis, resulting in decreased cardiac function (Wexler et al., 2009; Argulian et al., 2016). Hypertrophy of CMs is due to mutations in the genes responsible for sarcomere function, such as myosin heavy chains (MYH6, MYH7), myosin binding protein C (MYBPC3), troponin I (TNNT2, TNNT3), and tropomyosin-α-1 chain (TMP1) (Girolami et al., 2010; Sisakian, 2014). hiPSC technology allowed to better characterize HCM using patient-specific hiPSC-derived CMs harboring a MYH7 mutation (Lan et al., 2013; Dementyeva et al., 2019; Filippo Buono et al., 2020). A first study showed the potential of hiPSCs to mimic HCM phenotypes, such as enlarged cells, sarcomeric dysfunction, arrhythmia, and impaired calcium (Ca2+) handling, by using hiPSC-derived CMs from 10 patients carrying a missense mutation in the MYH7 gene (A663H) (Lan et al., 2013). Findings on Ca2+ handling abnormalities allowed the identification of specific Ca2+-channel blockers (e.g., verapamil) and their function in restoring physiological Ca2+ regulation, hence averting HCM phenotype (Lan et al., 2013). An additional study on HCM using patient-specific hiPSC-derived CMs broadened our understanding of the underlying disease mechanisms and thereby enabled the development of new therapeutic approaches (Han et al., 2014). Particularly, the study used hiPSC-derived CMs harboring a missense mutation in the MYH7 gene (R442G) in combination with genome-wide transcriptomics to investigate signaling pathways involved in the developmental process of CMs. This enabled the identification of potential therapeutic targets, such as Wnt, FGF, and Notch pathways (Han et al., 2014).
DCM represents one of the most common diagnoses in patients requiring heart transplantation and is associated with functional and structural impairment of the heart (Jacoby and McKenna, 2012). DCM relates to inherited gene mutations involved in sarcomeric protein synthesis [e.g., titin (TTN), MYH7, lamin A/C proteins (LMNA), desmin (DES)] or genes encoding ion channels [e.g., sodium channel protein type 5 subunit α (SCN5A)] (Gerull et al., 2002; Hershberger and Morales, 2007; Herman et al., 2012; Mcnally et al., 2013; Schultheiss et al., 2019). The use of patient-specific hiPSC-derived CMs again enabled to mimic the mutations causing the pathophysiological phenotype of DCM, thereby providing a better understanding of its underlying molecular and cellular mechanisms (Siu et al., 2012; Sun et al., 2012; Tse et al., 2013; Wang et al., 2014b; Hinson et al., 2015; Karakikes et al., 2015b; Lin et al., 2015; Wu et al., 2015; Wyles et al., 2016; Lee et al., 2017; Streckfuss-Bömeke et al., 2017; Yang et al., 2018; Shah et al., 2019).
Additionally, hiPSC technology allowed the modeling and the consequent discovery of specific therapeutic approaches for other cardiomyopathies, such as ARVC (Thiene et al., 2007; Caspi et al., 2013; Kim et al., 2013; Ma et al., 2013a) or LVNC (Kodo et al., 2016). In the case of ARVC, patient-specific hiPSC-derived CMs presenting the mutated PKP2 gene allowed the establishment of an ARVC in vitro model by exposing hiPSC-derived CMs to induce metabolic aging conditions (Kim et al., 2013). A subsequent study showed that ARVC PKP2 mutation resulted in the upregulation of Wnt and PPAR-γ pathways and lipid accumulation, which allowed the identification of 6-bromoindirubin-3′-oxime (BIO) as an inhibitor of glycogen synthase kinase 3β and a potential treatment to reduce lipid accumulation (Caspi et al., 2013). LVNC was successfully modeled with hiPSC-derived CMs and showed abnormal signaling of transforming growth factor β (TGF-β) due to a mutation of cardiac transcription factor T-box protein 20 (TBX20), thus impairing proper compaction of CMs (Arbustini et al., 2016). Moreover, the correction of the mutation using CRISPR-Cas9 and inhibition of TGF-β allowed to restore normal phenotype (Kodo et al., 2016).
Overall, the implementation of hiPSC-derived CMs in the development of CVD-related models provided evidence of their ability to model complex cellular phenotypes and contributed to a better understanding of a number of involved mechanisms in the disease. Moreover, the knowledge obtained from hiPSC-based CVD models uncovered novel treatment approaches. Nevertheless, there are remaining questions regarding the heterogeneity and immaturity of the hiPSC-derived CMs that need to be further investigated to be able to utilize hiPSC technology to completely model CVDs.
Channelopathies
Channelopathies are the result of mutations in genes encoding ion channels and transporters and hence lead to a cardiac electrophysiology impairment (Abriel et al., 2015; Bezzina et al., 2015; Spears and Gollob, 2015). Disorders classified as channelopathies are congenital long QT syndrome (LQTS) and catecholaminergic polymorphic ventricular tachycardia (CPVT), which induce arrhythmias, ventricular fibrillation, and seizures ending with death (Abriel et al., 2015; Bezzina et al., 2015; Spears and Gollob, 2015). The introduction of hiPSC technology allowed for extensive studies into the underlying mechanisms of such ion channel-related disorders (Sala et al., 2019).
LQTS is characterized by a delay in the cell membrane repolarization after contraction and ventricular arrhythmias, ultimately leading to heart arrest, and it is the first and most studied arrhythmic syndrome using hiPSC-based models (Abrams et al., 2010; Sala et al., 2019). LQTS exists in more than 10 different subtypes, but research has mainly focused on LQTS1, LQTS2, and LQTS3, which result from a genetic mutation of the potassium voltage-gated channel subfamily Q member 1 (KCNQ1), subfamily H member 2 (KCNH2), and sodium voltage-gated channel α-subunit 5 (SCN5A), respectively (David et al., 2014). In-depth studies of such arrhythmic syndromes paved the way for the use of hiPSCs as well as for disease modeling (Moretti et al., 2010). Several studies carried out with patient-specific hiPSCs containing a mutation in the KCNQ1, KCNH2, or SCN5A gene displayed strong similarities in the derived CMs, such as prolonged action potential, reduced potassium or sodium currents, and subsequent impairment of the ion channel’s behavior (Itzhaki et al., 2011; Matsa et al., 2011; Egashira et al., 2012; Lahti et al., 2012; Bellin et al., 2013; Mehta et al., 2014; Wang et al., 2014c; Jouni et al., 2015; Ma et al., 2015). Further understanding of channelopathy molecular mechanisms facilitated by hiPSC-derived CMs showed that specific treatments using potassium and sodium ion channel inhibitors restore proper CM function (Fatima et al., 2013; Ma et al., 2013b; Terrenoire et al., 2013; Mehta et al., 2014; Malan et al., 2016).
CPVT comprises two subtypes; CPVT1 arises from mutations in the cardiac ryanodine receptor 2 (RYR2), and CPVT2 is caused by a mutation in the calsquestrin-2 (CASQ2) gene (Leenhardt et al., 2012; Roston et al., 2017). Both phenotypes result in tachyarrhythmias triggered by stress and exercise (Swan et al., 1999; Liu et al., 2013). RYR2 is responsible for the outflow of Ca2+ from the sarcoplasmic reticulum during depolarization, whereas CASQ2 proteins bind Ca2+ in the sarcoplasmic reticulum (Leenhardt et al., 2012; Roston et al., 2017). Multiple hiPSC-based models recapitulated CPVT phenotypes, as well as showed elevated diastolic Ca2+ concentrations, reduced Ca2+ in the sarcoplasmic reticulum, and increased arrhythmias (Jung et al., 2012; Kujala et al., 2012). Furthermore, the use of hiPSC-based models identified new therapeutics, such as dantrolene, β-blockers, and flecainide (Itzhaki et al., 2012; Jung et al., 2012; Preininger et al., 2016; Sasaki et al., 2016).
Collectively, the introduction of hiPSC-derived CMs in the modeling of channelopathies allowed for the acquisition of a new knowledge into the mechanisms of cardiovascular electrophysiology. This further established novel therapeutic approaches to attenuate the observed pathophysiological conditions (Swan et al., 1999; Liu et al., 2013; Roston et al., 2017). However, the strength of the clinical translation of such technology is limited due to the lack of complete maturity of hiPSC-derived CMs, which still remains an issue that needs further investigation.
Structural Defects
Structural heart diseases typically feature an abnormality in the structure of the heart, valves, and/or vasculature and represent a rapidly growing CVD area that has been successfully modeled using hiPSC technology (Peng et al., 2019). In particular, hiPSC-derived models for bicuspid valvular and aortic calcification identified mutations in the notch homolog 1 (NOTCH1) gene (Garg et al., 2005; Theodoris et al., 2015). Additionally, the generation of hiPSC-derived SMCs allowed to recapitulate the multiple features of supravalvular aortic stenosis (SVAS) including mutations in the elastin (ELN) gene (Ge et al., 2012; Kinnear et al., 2020). Thus, hiPSC-derived models provide a great tool to investigate cellular function as well as the molecular mechanisms behind such diseases, ultimately leading to new therapeutic approaches (Ge et al., 2012; Chailangkarn and Muotri, 2017; Kinnear et al., 2020).
HiPSCs for Tissue Engineering Applications
Regenerative Medicine
hiPSC-based technology accelerated drug development and their safety evaluation by providing tools to investigate various disease mechanisms and to screen for potential treatments. Although the current therapeutic approaches provide a treatment option for specific diseases, they do not induce regeneration of the damaged cardiac tissues to this date (Altara et al., 2016). Therefore, the discovery of patient-specific hiPSCs offered a potential novel treatment option to replace damaged cardiac tissue and opened a new chapter in the field of regenerative medicine (Menasché, 2018; Wang et al., 2018; Paik et al., 2020). Furthermore, owing to the ability of potentially differentiating into any desired cell type, hiPSCs provide a means to patient-specific cardiac tissue regeneration, thus facilitating allogeneic transplantation (Šarić et al., 2008; Zhang et al., 2009; Narsinh et al., 2011; Kishino et al., 2020). The first studies using hiPSC-derived CMs to restore cardiac diseased tissue involved the direct injection of cells in the damaged area (Nelson et al., 2009; Ye et al., 2014; Shiba et al., 2016; Liu et al., 2018). However, despite the promising initial results, the implementation of hiPSC technology into the clinic faces multiple challenges (Ma et al., 2011). One of the main limitations is due to the hiPSC-derived CM purity and the risk of teratoma formation post-transplantation caused by the presence of undifferentiated cells (Ma et al., 2011). Nowadays, several methods are in place to overcome this lack of cell purity, such as the use of lactose instead of glucose supplemented medium to eliminate undifferentiated cells (Ma et al., 2011; Zhang et al., 2012). As already mentioned, another limitation is the immature phenotype of hiPSC-derived CMs (Lundy et al., 2013; Machiraju and Greenway, 2019), as well as the low engraftment efficiency of the implanted CMs after single cell transplantation (Lemcke et al., 2018; Park et al., 2019). Therefore, cardiovascular regenerative medicine shifted from single cell injections of hiPSC-derived CMs toward alternative approaches to improve engraftment. By using tissue engineering (TE) approaches, such as cell aggregates or patches, cell retention and engraftment efficiency were greatly improved (Masumoto et al., 2012; Emmert et al., 2013a, b; Wendel et al., 2015). This led the field of cardiovascular regenerative medicine toward the implementation of three-dimensional (3D)-based culture systems.
3D Cell Culture System
A major step toward the generation of cardiovascular tissue is to recapitulate the molecular environment, which is best accomplished by using 3D culture systems, such as scaffold-based, organoids, and organs-on-a-chip technologies (Zimmermann et al., 2002a, 2004; Clevers, 2016; Günter et al., 2016; Rosales and Anseth, 2016; Madl et al., 2018; Ronaldson-Bouchard and Vunjak-Novakovic, 2018). While two-dimensional culture systems are the standard approach in cardiovascular research, these fail to recapitulate the complex cellular composition and extracellular interactions existing in native tissues. In order to properly mimic cardiac function, it is therefore important to consider the 3D tissue composition to re-create the cardiovascular cellular and extracellular environments. The use of 3D culture systems presents the ability to closely mimic the in vivo structure, microenvironment, cell–cell interaction, and cell–extracellular matrix (ECM) interaction, making it an interesting technology for disease modeling, drug development/screening, and TE applications (Fong et al., 2016; Correia et al., 2018; Chaicharoenaudomrung et al., 2019). Different 3D culturing approaches are available, including non-scaffold-based systems (e.g., spheroids and organoids) and scaffold-based systems (e.g., tissue-engineered constructs), that recapitulate ECM features.
hiPSCs in Cardiac Spheroids
Cardiac spheroids are typically used to mimic the native 3D cellular environment by including multiple cell types in a self-assembly process (Polonchuk et al., 2017; Hoang et al., 2018; Yan et al., 2019). In this context, the number of cells used to generate the spheroids can have an impact on the viability of cells, especially the ones forming the core, due to reduced oxygen supply when the spheroids diameter exceeds beyond diffusion barrier limit (Tan et al., 2017). Different studies generated spheroids by combining hiPSC-derived CMs with structural heart cells, such as cardiac fibroblasts (CFs), in order to closely recapitulate the native microenvironment of the myocardium (Polonchuk et al., 2017; Tan et al., 2017; Hoang et al., 2018; Mattapally et al., 2018; Yan et al., 2019). A recent study generated cardiac spheroids by co-culturing hiPSC-derived CMs with CFs and cardiac ECs and exposed them to various Food and Drug Administration (FDA)-approved drugs to test their potential as platform for cardiotoxicity assay (Archer et al., 2018). A different study produced cardiac spheroids, representative of the morphology and biochemistry of myocardial tissue out of hiPSC-derived CMs, hiPSC-derived CFs, and cardiac ECs (Polonchuk et al., 2017). Furthermore, the hiPSC-based cardiac spheroids allowed to investigate the underlying cardiotoxicity mechanisms of doxorubicin (Polonchuk et al., 2017). Another relevant study used cardiac spheroids composed of CMs and mesenchymal stem cells (MSCs) derived from hESCs as an in vitro platform to model fibrosis (Lee M.O. et al., 2019). Treatment of the cardiac spheroids with TGF-β induced a fibrotic phenotype (Lee M.O. et al., 2019).
hiPSCs in Cardiac Organoids
Similar to spheroids, organoid culture systems are described as a 3D approach that includes a specific cellular organization and a precise architecture that ultimately relies on a process of self-assembly (Lancaster and Knoblich, 2014; Chaicharoenaudomrung et al., 2019; Hofbauer et al., 2020). Since the first in vitro creation of the murine small intestinal organoid (Sato et al., 2009), many fields have been using organoid-based culture systems to mimic and recapitulate organ-like tissue architecture and cellular composition (Sato et al., 2009; Huch et al., 2013; Lancaster et al., 2013; Chen et al., 2017). The development of hiPSC technology opened the path for the development of patient-specific hiPSC-based organoids required to re-create functional cardiac organoids (Mills et al., 2017; Richards et al., 2017). Other studies aimed at producing 3D vascular networks organoids by means of an in vitro co-culture of hiPSC-derived ECs with vascular cells, such as pericytes (Kusuma et al., 2013; Orlova et al., 2014; Chan et al., 2015). A more recent study generated organoids that recapitulated blood vessels, by differentiating hiPSCs in suspension into the mesodermal lineage prior to inducing EC differentiation. The resulting blood vessel organoid displayed morphological and functional similarities with native human blood vessels when implanted in the kidney capsule of mice (Wimmer et al., 2019). However, the self-assembly process used in most organoid procedures is still one of the limiting factors for a consistent generation of cardiovascular tissues. Specifically, this process is not yet defined, but is rather a random method resulting into heterogenous organoids in regard of cell composition, size, and shape (Brassard and Lutolf, 2019). Particularly, the size of the organoids is limited due to the manufacturing approach, thus impairing the application of organoids to regenerative medicine (Brassard and Lutolf, 2019). Nonetheless, the application of hiPSC-derived cardiac organoids to disease modeling presents multiple advantages in precision medicine by allowing the simultaneous study of a large variety of phenotypes, but also a robust technology applicable in drug development and screening (Mills et al., 2017; Voges et al., 2017; Hoang et al., 2018; Nugraha et al., 2018; Mills et al., 2019; Nugraha et al., 2019; Filippo Buono et al., 2020).
hiPSCs for Cardiovascular Tissue-Engineered Constructs
TE aims to recreate functionally native tissue by recapitulating the exact cellular composition and ECM structure by means of bioengineering methodologies. The final purpose is to replace a diseased tissue or organ and/or develop and test new therapeutics. The application of TE to the medical field is of high clinical relevance for the regeneration of tissues with limited self-regenerative potential, such as the heart, pancreas, bone, and cartilage (Risbud et al., 2001; Sepulveda et al., 2002; Niknamasl et al., 2014; Emmert et al., 2017).
The classical TE approach relies on the combination of cells and biocompatible scaffolds to engineer tissue constructs with similar properties to native tissues. In this context, the scaffold not only is responsible for the structural support of the seeded cells but also can impact different functional aspects, such as cell survival, proliferation, and differentiation (Vunjak-Novakovic et al., 2011; Buikema et al., 2013; Chun et al., 2015). The first engineered heart tissue was developed using rat CMs cultured on a scaffold (Zimmermann et al., 2002b). This pioneered the development of novel approaches using various synthetic (e.g., polylactide, polyglycolide, lactide and glycolide copolymer, polycaprolactone, and polyisopropylacrylamide) and/or natural (e.g., collagen, cellulose, chitosan, hyaluronic acid, silk fibroin, and decellularized native tissue) (Vunjak-Novakovic et al., 2011; Buikema et al., 2013; Chun et al., 2015) scaffolds in the pursuit to optimize cardiovascular tissue-engineered constructs (Murry et al., 1996; Planat-Benard et al., 2004; Ravichandran et al., 2013; Wendel et al., 2014). The advent of PSCs, more specifically hiPSCs, offered access to an unlimited source of autologous cells with the ability to theoretically differentiate into any cell type in the body. Furthermore, the combined use of a scaffold, hiPSCs, and their metabolites provided the ability to generate personalized scaffolds, thus creating a tool with tremendous application potential (Noor et al., 2019). The first engineered cardiovascular tissue construct was based on the differentiation of ESCs into CMs and displayed the potential of PSCs to recapitulate metabolic and mechanical functions of the native myocardium (Stevens et al., 2009).
One fundamental aspect of tissue-engineered constructs, which strongly impact their in vivo behavior once implanted, is their fabrication method. While cardiac patches are generated by stacking cell monolayers to produce a functional tissue, 3D cardiac tissues make use of scaffolds to optimize cell proliferation, differentiation, and survival and mimic myocardium ECM structure and composition (Eschenhagen et al., 1997; Sawa et al., 2012). Various studies produced cardiac tissues using the cell sheet approach with hiPSC-derived cardiac cell types and induced in vivo recovery of damaged heart tissue (Masumoto et al., 2014; Masumoto et al., 2016; Ishigami et al., 2018). Nonetheless, the number of layers comprising the cardiac cell sheet has to be limited to maximize oxygen and nutrients diffusion and avoid tissue necrosis, which was improved using a biodegradable biomaterial to facilitate oxygen and nutrient transfer (Matsuo et al., 2015). In the same research framework, the combination of 3D scaffolds and hiPSC technology was explored to generate cardiac tissue constructs and establish new approaches to treat cardiovascular-related defects (e.g., vascular grafts and heart valves). In this context, TE approaches were combined with hiPSC-derived ECs and hiPSC-derived SMCs to produce vessels and/or valvular constructs, which recapitulated the physiological features of their native counterparts (Nakayama et al., 2015). A recent study used hiPSC-derived vascular SMCs cultured on polyglycolic acid (PGA) scaffold to manufacture vessel substitutes, which presented similar mechanical resistance as the clinically used prosthesis (Luo et al., 2020).
Valvular heart disease (VHD) is another type of cardiac defect that may benefit from hiPSC technology. VHD remains as one of the major heart problems and requires replacement to restore proper valvular function in the majority of patients (Kheradvar et al., 2015). The currently available prostheses are either mechanical or bioprosthetic valves (Poulis et al., 2020), and although they represent the current standard of care, both substitutes still present with significant limitations (e.g., degeneration, thromboembolic risk, need for anticoagulation treatment) as reviewed elsewhere (Head et al., 2017; Fioretta et al., 2020b; Poulis et al., 2020). Particularly, TE approaches could provide a potential solution to overcome the current limitations by generating tissue-engineered heart valves (TEHVs) with the ability to grow and remodel within the patient (Schmidt and Hoerstrup, 2006; Emmert and Hoerstrup, 2017). In this context, multiple cell and SC sources have been investigated for the generation of ready-available regenerative TEHVs (Cambria et al., 2017; Emmert, 2017). At the dawn of this technology, autologous cells and tissue sources were cultured in a bioreactor system to favor cell proliferation and ECM production (Weber et al., 2012b; Fioretta et al., 2018). However, the technical and logistical challenges of this TE approach (i.e., cell isolation and expansion, donor-to-donor variability, and unknown in vivo remodeling outcomes) led to the implementation of one-step interventions (Emmert et al., 2017). In this context, bioresorbable polymer-based TEHVs were adopted preclinically in combination with pre-seeding procedures using autologous SCs [bone marrow mononuclear cells (BMMCs) and fetal cells] to modulate the early in vivo inflammatory response (Emmert et al., 2011, 2012, 2014; Weber et al., 2011, 2012a; Fioretta et al., 2020a). However, further studies need to clarify the remodeling effects that SCs can develop in combination to the valvular hemodynamic conditions in order to exclude potential deleterious effects on the implanted substitute as well as guarantee long-term functionality and adaptive remodeling (Fioretta et al., 2020a; Mela, 2020). Akin to the use of hiPSC-derived SMCs for the generation of vascular grafts, the use of hiPSCs derivatives could be of high potential in the production of autologous TEHV.
Moreover, the combination of natural or synthetic scaffolds with hiPSC technology for TE constructs demonstrated that along with providing proper cell adhesion and proliferation, scaffold vascularization was triggered and favored tissue remodeling of the TE construct once implanted (Gaballa et al., 2006; Miyagi et al., 2011; Shi et al., 2011; Geng et al., 2018; Lee A. et al., 2019). Particularly, proven the promising preclinical and clinical outcomes of decellularized tissue-engineered matrices (Schmidt et al., 2010; Driessen-Mol et al., 2014; Emmert et al., 2018; Lintas et al., 2018; Motta et al., 2018, 2019, 2020), such starting materials could provide an interesting substrate to be implemented within the hiPSCs therapies options.
Drug Development and Screening
The preclinical development of new therapeutics or drugs involves multiple processes, such as drug screening, in vitro and in vivo pharmacological and pharmacokinetic activity assessments, and safety analysis (Gromo et al., 2014). The discovery of hiPSCs provided a new platform that significantly changed preclinical drug screening and development (Paik et al., 2020). The combination of hiPSCs to next-generation sequencing, genome-wide association studies, and libraries of molecules allowed for the establishment of a powerful cell-based platform, which enabled the investigation of potential therapeutic molecules (del Álamo et al., 2016; Sharma et al., 2018; Paik et al., 2020). The application of an hiPSC-based platform to cardiovascular pharmacology facilitated the generation of patient-specific and disease-specific cell sources, such as hiPSC-derived CMs, that exhibited pathophysiological phenotypes similar to the one observed in diseased patients, thus providing a screening platform for existing and new drugs (Chun et al., 2011; del Álamo et al., 2016; Matsa et al., 2016; Sharma et al., 2018). In particular, the use of hiPSC-derived CMs as a screening tool to investigate the safety of drugs used in the treatment of HCM (such as metoprolol and verapamil) was successfully proven (Han et al., 2014). Furthermore, patient-specific hiPSC-derived CMs were employed to assess the cardiotoxicity of chemotherapeutics, such as doxorubicin and trastuzumab, showing how prolonged exposure to such drugs, induced decreased cell viability, perturbation in Ca2+ management, mitochondrial malfunction, and contraction impairment (Burridge et al., 2016; Chaudhari et al., 2016; Kitani et al., 2019). The available high-throughput assays and high-scale production of hiPSC-derived CMs enabled the simultaneous screening of multiple drugs on different lines of hiPSC-derived CMs, thus generating a faster assessment of drug-induced cardiotoxicity (del Álamo et al., 2016; Denning et al., 2016; Blinova et al., 2018; Grimm et al., 2018; Millard et al., 2018; Sharma et al., 2018; Burnett et al., 2019). Drug cardiotoxicity represents one of the main concerns in cancer treatment; thus, the development of hiPSC-based therapies provided an unprecedented advantage to evaluate and discover the cardiovascular toxicity of specific drugs, prior to clinical trials (Sharma et al., 2018).
Although 2D in vitro cell models are routinely used, such technologies lack the structural complexity, electrophysiology, and expression profile of human tissues, which can reduce the fidelity of the model and impair accurate characterization of drug effects and toxicity predictions on cells (Veerman et al., 2015; Machiraju and Greenway, 2019). In fact, the cell types constituting human organs are co-dependent for the exchange of molecules promoting growth, cell–cell interaction, and cell–ECM interaction (Paschos et al., 2015). The advent of 3D cell culture systems allowed to generate a more faithful representation of the cardiac cellular microenvironment, thus overcoming the limitations of 2D culture systems (Fong et al., 2016; Correia et al., 2018; Ronaldson-Bouchard et al., 2018). It has been suggested that the presence of 3D architecture and ECM influences the drug diffusion and dose-dependent toxicity, thereby providing a more reliable readout than 2D screening systems (Langhans, 2018; Zuppinger, 2019). The implementation of TE approaches further promoted a transition from 2D to 3D models in the drug screening processes. In this regard, several achievements have been made in the development of hiPSC-based tissue-engineered 3D cardiac platforms (Langhans, 2018; Zuppinger, 2019). As an example, hiPSC-derived CMs were used to manufacture multilayered 3D cardiac tissues and adopted to characterize drug-induced cardiotoxicity of various known drugs, such as doxorubicin, hERG-type potassium channel blockers, and isoproterenol (Takeda et al., 2018). Other approaches involve 3D printing of micro-physiological platforms simulating heart tissue, which were implemented in drug studies (Polini et al., 2014; Lind et al., 2017). To be noticed, a recent study developed an organoid-based platform and established a method to investigate drug-induced cardiotoxicity at the tissue level (Richards et al., 2020).
HiPSCs and Genome Editing Technologies for Cardiovascular Applications
The study of site-specific nucleases (SSNs) started with the findings that DNA DSBs were repaired by the cell repair machinery using the HDR or NHEJ pathway (Rouet et al., 1994a, b). Subsequently, SSNs were implemented as a tool to engineer the genome at targeted sites. The initial nucleases were found to be hybrid proteins, known as ZFNs and TALENs, and were followed by the latest, CRISPR-Cas9 (Musunuru, 2013). The discovery of these nucleases revolutionized the field of genome engineering and biomedicine (Musunuru, 2013). While ZFNs and TALENs specific targeting relies on a protein-based system with customized specificity to DNA, CRISPR-Cas9 specific DNA targeting is RNA-guided and relies on a gRNA of 20 nucleotides (Corrigan-Curay et al., 2015). CRISPR-Cas9 has quickly become the most used gene editing technology due to the simplicity and adaptability of the RNA-based targeting system, which is easily customized to target any wanted sequence in the genome (Sander and Joung, 2014; Corrigan-Curay et al., 2015). These molecular editing tools have the ability to induce a DSB at a desired location in the genome, thus leading to either NHEJ or HDR for DNA repair. This allows for the introduction of a targeted mutation related to a diseased phenotype or to correct a disease-causing mutation (Cong et al., 2013). Progress in genome engineering methods, especially using CRISPR-Cas9, led the way for the development of isogenic cell lines, which in turn allowed for the introduction or correction of a desired mutation and, thus, the generation of several disease models (Hockemeyer and Jaenisch, 2016). Furthermore, the combination of hiPSC technology and the improvement of hiPSC differentiation protocols with CRISPR-Cas9 gene editing tools have established powerful approaches for SC-related research, human disease modeling, and drug development/screening (Schwank et al., 2013; Johnson and Hockemeyer, 2015; Matano et al., 2015; Musunuru, 2017a).
Off-target effects are a limitation in the use of CRISPR-Cas9 technology and other gene editing tools (De Masi et al., 2020). Off-target effects arise from unspecific targeting of the Cas9 nuclease, due to non-specific binding of the designed sgRNA sequence (Fu et al., 2013; Tycko et al., 2016; Naeem et al., 2020). The design of sgRNA is therefore very important to properly guide the Cas9 nuclease and aims to the minimization of unspecific genome binding (off-target events). Several studies assessed the off-target occurrence in specific cell lines showing that the frequency is cell-dependent (Fu et al., 2013), and that iPSCs have a low off-target occurrence (Smith et al., 2014; Veres et al., 2014). Nevertheless, off-target effects still represent a limitation that requires further investigation to broaden the clinical use of such gene editing tools (Rincon et al., 2015; Naeem et al., 2020). An additional constraint that can affect gene editing efficiency is linked to the delivery system (e.g., electroporation, micro-injection, transfection, lipofection, or viral vector) and the format of the Cas9 nuclease components [e.g., plasmid, mRNAs, or ribonucleoprotein complex (RNP)] (Lino et al., 2018). Depending on the format of Cas9, cells and/or tissues are subjected to a prolonged exposure; thus, transient expression of the Cas9 using RNP format is preferable to limit off-target effects and avoid unwanted gene editing (Moore et al., 2015; Liang et al., 2017; Sakuma et al., 2018; Singh et al., 2018).
The implementation of gene editing technology to CVD modeling using hiPSCs produced isogenic hiPSCs, thus creating genetically matched cells containing only selected inserted mutations. This system could correlate specific mutations to the observed phenotype (Dzilic et al., 2018) as well as generate hiPSC-based disease models that recapitulated CVD (Wang et al., 2014a; Kodo et al., 2016; de la Roche et al., 2019; Garg et al., 2019; Mcdermott-roe et al., 2019). Besides introducing specific mutations, various studies showed the potential of CRISPR-Cas9 in the correction of single genetic mutations related to various diseases, such as HCM, DCM, and LQTS (Karakikes et al., 2015b; Limpitikul et al., 2017; Seeger et al., 2019). In addition to gene editing, the CRISPR-Cas9 system also possesses the ability to regulate gene expression. The development of a catalytically inactive form of the Cas9 nuclease, known as dCas9, repurposed Cas9 into a protein able to specifically bind DNA and interfere with the gene expression, when targeted to a promoter or a regulatory sequence using a gRNA (Gilbert et al., 2013; Qi et al., 2013). Studies showed that the combination of the dCas9 with a transcriptional repressor and the specific targeting of the dCas9 toward a promoter or a regulatory sequence induced a downregulation of the targeted gene (Gilbert et al., 2013; Mandegar et al., 2016). Alternatively, studies demonstrated that coupling of dCas9 to a transcriptional activator induced the recruitment of gene effectors, thus leading to an increased expression of the gene in question (Maeder et al., 2013).
Moreover, studies showed the ability of gene editing tools to repurpose terminally differentiated cells, without having to go through a pluripotent state (Gao et al., 2013). Recent investigations showed the possibility of direct reprogramming of terminally differentiated fibroblasts into skeletal myocytes by targeting dCas9 coupled to an activator toward the Myod1 gene, inducing high expression of myogenic markers, thus promoting differentiation from fibroblast directly into skeletal myocytes (Chakraborty et al., 2014). This approach could directly generate CMs and other relevant cardiac lineages in vivo, by reprogramming resident CFs to restore and regenerate damage tissue after injury.
The advances in gene editing and hiPSCs technologies triggered the research of novel therapeutic approaches including the possibility to either correct or introduce genetic mutations in patient-specific hiPSCs (Hockemeyer and Jaenisch, 2016). Furthermore, the pluripotency of hiPSCs provided massive potential to differentiate edited hiPSCs into any required cell type (e.g., CMs or cardiovascular-related cells). These autologous cells can then be transplanted back into the patient, thus circumventing the immunological response (Kishino et al., 2020). As previously mentioned, the transplantation and viability efficiency of single cells is highly dependent on the cells engraftment into the damaged tissue, which in the case of the heart has been proven very low (Lemcke et al., 2018; Park et al., 2019). In many cases, the host tissue requires the engraftment of a high number of corrected cells to overcome the diseased area. Advances in TE provided the necessary tools to overcome the limitations of single cell transplantation allowing the generation of bioengineered constructs, such as cardiac patches, vascular grafts, and heart valves (Matsuo et al., 2015; Nakayama et al., 2015; Fioretta et al., 2018; Lee A. et al., 2019; Luo et al., 2020). In this context, patient-specific somatic cells can be minimally invasively harvested, reprogrammed into hiPSCs, gene edited to correct disease-causing mutations, and then re-differentiated into the required cell types to produce autologous tissue constructs that could be implanted into the patient without stimulating an immune response. Furthermore, the CRISPR-Cas9 system has shown the ability to manipulate the immunogenicity of hiPSC-based tissue constructs by inducing the expression of immune suppressive molecules, thus reducing rejection by the host immune system (He et al., 2017; Zhao et al., 2020). Specifically, CRISPR-Cas9 has been used to generate hiPSCs lacking the human leukocyte antigen (HLA), thus reducing their immunogenicity (Han et al., 2019; Jang et al., 2019; Zhao et al., 2020).
While still in its infancy, the avenue of gene editing technology provides new opportunities to tackle the many challenges of disease modeling, regenerative medicine, and TE. Genome editing completely changed the landscape of cardiovascular research and has been demonstrated to be a powerful tool to study and manipulate genome-related molecular function.
Discussion
Therapeutic approaches to treat CVDs and regenerate severely impaired tissues are under continuous development. However, to date, except heart transplantation for advanced heart failure, no curative treatments are available. The discovery of hiPSCs has provided the researchers a novel tool to investigate the underlying mechanisms of human diseases, including CVD. The high proliferative capacity and the ability to differentiate into any cardiac cell type opened the path for the generation of in vitro disease models, recapitulating the biomolecular and structural pathologies arising from cell mutations. The parallel advances of genome engineering technologies, such as CRISPR-Cas9, further optimized disease modeling processes using hiPSCs. Furthermore, the combination of hiPSCs and CRISPR-Cas9 technologies gave a new perspective for personalized medicine, by providing the necessary tools to correlate the disease phenotype with the underlying environmental, genetic, and molecular mechanisms for each individual patient (Hsu et al., 2014; Karakikes et al., 2015a). Indeed, several studies demonstrate that not only the influence of the patient’s genetic profile but also the environmental exposure affects the development of a disease and its outcome. In other words, each human being presents discrepancies in disease initiation and progression, reinforcing the importance of personalized medicine (Loscalzo and Handy, 2014; Smith and White, 2014). In this context, hiPSCs represent a promising cell source, giving access to patient-specific hiPSC-derived cardiac cells that retain the genetic background and the environmental influence of the patient they originate from, thus allowing to monitor and recapitulate the patient’s phenotype and their response to drugs (Engle and Puppala, 2013; Liang et al., 2013; Figure 3). Furthermore, CRISPR-Cas9 technology brings another tool to investigate the impact of genetic variation against the environmental influence, by creating isogenic hiPSC lines harboring a specific mutation out of healthy donor hiPSCs and comparing the resulting phenotype with the one of hiPSCs reprogrammed from diseased patients (Hsu et al., 2014; Dzilic et al., 2018). In addition, the combination of hiPSC technology with organoid/3D cell culture systems was used to generate biobanks, which could be used in various contexts, such as drug discovery, and as proof-of-concept for genetic disease correction in combination with CRISPR-Cas9, before proceeding to clinical trials (Geurts et al., 2020; Figure 3).
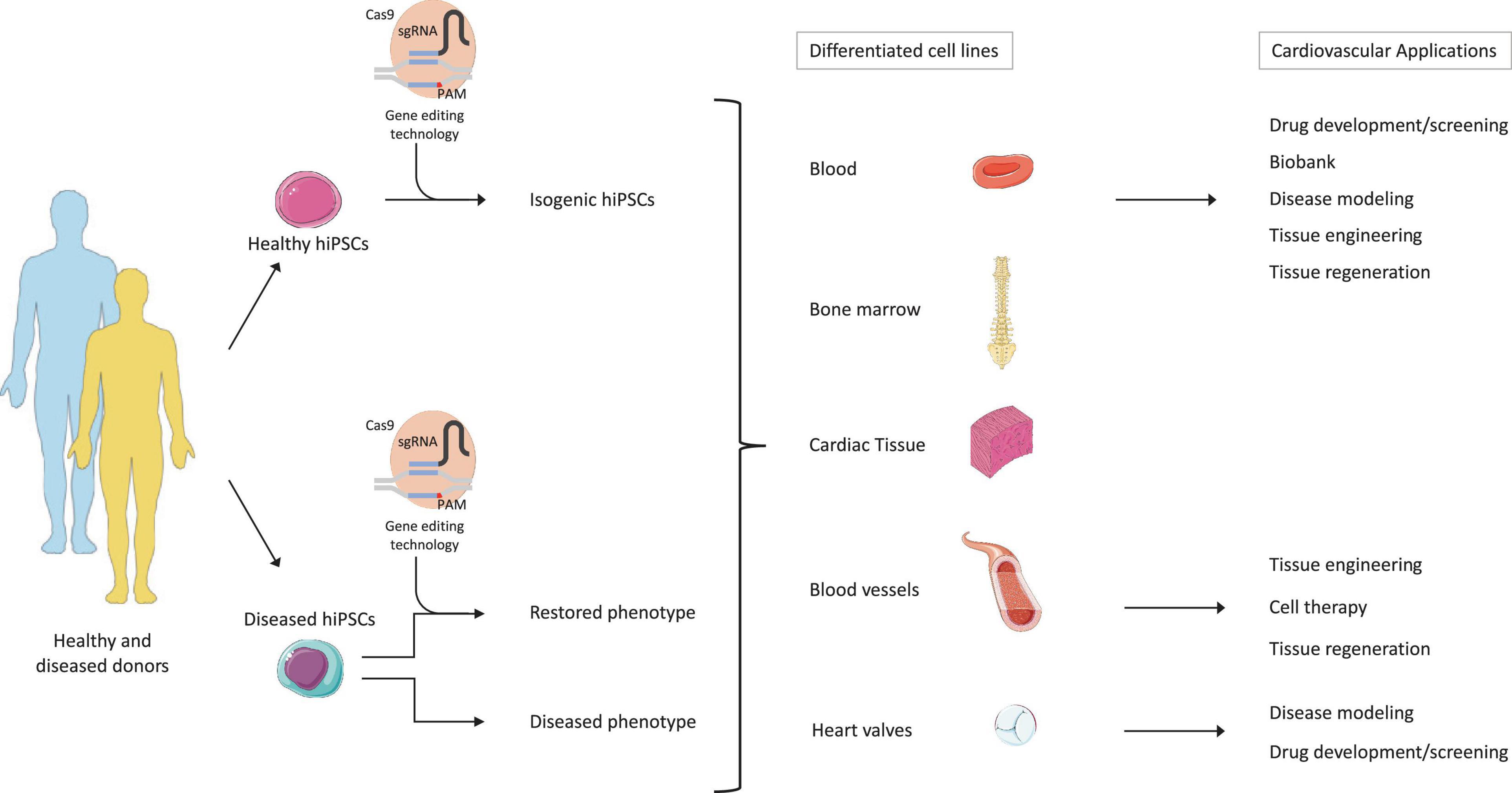
Figure 3. Therapeutic potential of hiPSC technology combined to gene editing and tissue engineering. The figure describes the potential applications of hiPSCs and gene editing. First, hiPSCs would be generated from the reprogramming of patient-specific healthy or diseased somatic cells. Second, gene editing tools, such as CRISPR-Cas9, would then generate isogenic cell lines harboring specific genetic mutations in the healthy hiPSCs, but also correct disease-causing mutations in the patient populations. The generated hiPSCs could then be re-differentiated into various cell types and/or tissue. Finally, the differentiated isogenic hiPSCs could be implemented in drug development/screening processes, biobanking, disease modeling, and tissue engineering. On the other side, the differentiated diseased hiPSCs could be further employed for disease modeling and drug development/screening. When genetic mutations are corrected, then cell-based therapy and tissue regeneration purposes can be applied. Adapted from servier medical art, licensed under a Creative Commons Attribution 3.0 Unported License.
To properly study CVD origins and their 3D environment, future treatment strategies should implement co-culture systems of hiPSC-derived CMs with other cardiac cell types and TE approaches to closely mimic in vivo pathologies. Such approaches would recapitulate cell–cell and cell–matrix interactions, but may also provide answers to CVDs that arise from cells interacting with CMs. For instance, two examples of diseases that would benefit from such an approach would be Marfan syndrome, which leads to cardiovascular defects because of dysfunctional connective tissue (Pepe et al., 2016), and the hypoplastic left heart syndrome (HLHS) (Miao et al., 2020).
Looking at translational potential, the development of new drugs is a long and tedious process that aims at the identification of potential drug-induced adverse effects (e.g., cardiotoxicity) (Ovics et al., 2020). The implementation of hiPSCs in the drug development pipeline will therefore enable to assess patient-specific drug responses and to perform early drug de-risking, thus reducing the number of “bad” lead compounds candidates progressing from the pre-clinical to clinical trials (Figure 3).
Next, the pluripotency potential of hiPSCs could also be further combined to the genetic tool CRISPR-Cas9 aiming at the generation of isogenic cell lines for regenerative medicine. These isogenic hiPSCs can then be differentiated into the desired cell types and be used as a building block to create constructs for the replacement of damaged cardiovascular tissues (Figure 3). This process can be applied to personalized tissue regeneration by using a patient-specific cell for the production of autologous tissue constructs, thereby avoiding immunogenicity issues. Furthermore, the potential of CRISPR-Cas9 to generate HLA deficient hiPSCs would grant a universal cell source with reduced immunogenicity. This will eradicate the need for autologous hiPSCs for cardiac regeneration, thereby reducing time and cost constraints associated with patient-specific cells.
However, hiPSCs still present some concerns that need to be addressed before their safe and effective translation into the clinical setting will be possible. Studies showed that hiPSCs can be subject to chromosomal aberrations, which can either be inherited from the parental cells or arise from the cellular reprogramming or prolonged culture periods, finally impacting their differentiation potential and disease modeling (Mayshar et al., 2010; Yoshihara et al., 2017). Moreover, hiPSC-derived CMs are subject to specific limitations, such as the lack of heterogenous cell population after differentiation into CMs and lack of maturity (Gherghiceanu et al., 2011; Bedada et al., 2014; Veerman et al., 2015; Koivumäki et al., 2018). These aspects considerably limit the ability of hiPSCs-derived CMs to reliably mirror the complete phenotype of mature CMs. Furthermore, the immature phenotype reduces the ability of hiPSC-derived CMs to model CVDs that manifest at a later developmental stage.
TE strategies present also some limitations that need to be addressed before their broader clinical translation, such as scaffolds biocompatibility and mechanical properties, cell–cell interactions, cell–ECM interactions, and the vascularization potential. Nonetheless, the rapid evolution in hiPSC and CRISPR-Cas9 technologies combined to TE strategies carries an enormous potential to advance the field of regenerative cardiovascular research to the next level.
Conclusion
The discovery of patient-specific hiPSCs has revolutionized the field of cardiovascular research. The differentiation potential of hiPSCs into CMs and their ability to retain the genetic background enable the generation of CVD models and investigate the underlying mechanisms responsible for pathological phenotype. On the other hand, advances in genome engineering promoted by the CRISPR-Cas9 technology enabled the generation of isogenic hiPSCs owing to specific genetic mutations but also the correction of single mutations involved in CVDs. Hence, hiPSC and CRISPR-Cas9 technologies are providing a novel treatment option for personalized medicine, and the potential combination of hiPSCs and CRISPR-Cas9 together with TE approaches could allow the generation of specific 3D disease models systems and various tissue-engineered constructs for cardiovascular regenerative purposes.
Author Contributions
EKNG, SEM, and MYE conceptualized the manuscript. EKNG and SEM drafted and edited the manuscript. SEM, MM, BN, and MYE revised and/or edited the manuscript. Supervisory and administrative tasks were handled by SEM, MYE, and SPH. All authors contributed to the manuscript with critical input.
Funding
MYE has received funding from the European Research Council (ERC) under the European Union’s Horizon 2020 Research and Innovation Program, grant agreement no. 852814 (TAVI4Life). SEM is funded through TAVI4Life.
Conflict of Interest
SPH is shareholder at Xeltis BV and LifeMatrix AG. MYE is a shareholder at LifeMatrix. BN is a AstraZeneca employee and shareholder and declares no competing interests.
The remaining authors declare that the research was conducted in the absence of any commercial or financial relationships that could be construed as a potential conflict of interest.
References
Abad, M., Hashimoto, H., Zhou, H., Morales, M. G., Chen, B., Bassel-Duby, R., et al. (2017). Notch inhibition enhances cardiac reprogramming by increasing MEF2C transcriptional activity. Stem Cell Rep. 8, 548–560. doi: 10.1016/j.stemcr.2017.01.025
Abriel, H., Rougier, J. S., and Jalife, J. (2015). Ion channel macromolecular complexes in cardiomyocytes: roles in sudden cardiac death. Circ. Res. 116, 1971–1988. doi: 10.1161/circresaha.116.305017
Altara, R., Manca, M., Sabra, R., Eid, A. A., Booz, G. W., and Zouein, F. A. (2016). Temporal cardiac remodeling post-myocardial infarction: dynamics and prognostic implications in personalized medicine. Heart Fail. Rev. 21, 25–47. doi: 10.1007/s10741-015-9513-8
Anders, C., Niewoehner, O., Duerst, A., and Jinek, M. (2015). Structural basis of PAM-dependent target DNA recognition by the Cas9 endonuclease. Nature 513, 569–573. doi: 10.1038/nature13579
Arbustini, E., Favalli, V., Narula, N., Serio, A., and Grasso, M. (2016). Left ventricular noncompaction: a distinct genetic cardiomyopathy? J. Am. Coll. Cardiol. 68, 949–966.
Archer, C. R., Sargeant, R., Basak, J., Pilling, J., Barnes, J. R., and Pointon, A. (2018). Characterization and validation of a human 3D cardiac microtissue for the assessment of changes in cardiac pathology. Sci. Rep. 8:10160.
Argulian, E., Sherrid, M. V., and Messerli, F. H. (2016). Misconceptions and facts about hypertrophic cardiomyopathy. Am. J. Med. 129, 148–152. doi: 10.1016/j.amjmed.2015.07.035
Bastakoty, D., Saraswati, S., Joshi, P., Atkinson, J., Feoktistov, I., and Liu, J. (2016). Temporary, systemic inhibition of the WNT/β-Catenin pathway promotes regenerative cardiac repair following myocardial infarct. Cell Stem Cell Regen. Med. 25, 289–313.
Bedada, F. B., Wheelwright, M., Metzger, J. M., Chan, S. S. K., Metzger, S. K., Zhang, L., et al. (2014). Maturation status of sarcomere structure and function in hiPSC-CMs. Stem Cell Rep. 1863, 594–605.
Bellin, M., Casini, S., Davis, R. P., D’Aniello, C., Haas, J., Ward-Van Oostwaard, D., et al. (2013). Isogenic human pluripotent stem cell pairs reveal the role of a KCNH2 mutation in long-QT syndrome. EMBO J. 32, 3161–3175. doi: 10.1038/emboj.2013.240
Ben-David, U., and Benvenisty, N. (2011). The tumorigenicity of human embryonic and induced pluripotent stem cells. Nat. Rev. Cancer 11, 268–277.
Bezzina, C. R., Lahrouchi, N., and Priori, S. G. (2015). Genetics of sudden cardiac death. Circ. Res. 116, 1919–1936.
Blinova, K., Dang, Q., Millard, D., Smith, G., Pierson, J., Guo, L., et al. (2018). International multisite study of human-induced pluripotent stem cell-derived cardiomyocytes for drug proarrhythmic potential assessment. Cell Rep. 24, 3582–3592. doi: 10.1016/j.celrep.2018.08.079
Bragança, J., Lopes, J. A., Mendes-Silva, L., and Santos, J. M. A. (2019). Induced pluripotent stem cells, a giant leap for mankind therapeutic applications. World J. Stem Cells 11, 421–430. doi: 10.4252/wjsc.v11.i7.421
Brassard, J. A., and Lutolf, M. P. (2019). Engineering stem cell self-organization to build better organoids. Cell Stem Cell 24, 860–876. doi: 10.1016/j.stem.2019.05.005
Brokowski, C., and Adli, M. (2019). CRISPR ethics: moral considerations for applications of a powerful tool. J. Mol. Biol. 431, 88–101. doi: 10.1016/j.jmb.2018.05.044
Buikema, J. W., Van Der Meer, P., Sluijter, J. P. G., and Domian, I. J. (2013). Concise review: engineering myocardial tissue: the convergence of stem cells biology and tissue engineering technology. Stem Cells 31, 2587–2598. doi: 10.1002/stem.1467
Burnett, S. D., Blanchette, A. D., Grimm, F. A., House, J. S., Reif, D. M., Wright, F. A., et al. (2019). Population-based toxicity screening in human induced pluripotent stem cell-derived cardiomyocytes. Toxicol. Appl. Pharmacol. 381:114711.
Burridge, P. W., Li, Y. F., Matsa, E., Wu, H., Ong, S. G., Sharma, A., et al. (2016). Human induced pluripotent stem cell-derived cardiomyocytes recapitulate the predilection of breast cancer patients to doxorubicin-induced cardiotoxicity. Nat. Med. 22, 547–556. doi: 10.1038/nm.4087
Burridge, P. W., Matsa, E., Shukla, P., Lin, Z. C., Churko, J. M., Ebert, A. D., et al. (2014). Chemically defned generation of human cardiomyocytes. Nat. Methods 11, 855–860. doi: 10.1038/nmeth.2999
Camacho, P., Fan, H., Liu, Z., and He, J. Q. (2016). Small mammalian animal models of heart disease. Am. J. Cardiovasc. Dis. 6, 70–80.
Cambria, E., Pasqualini, F. S., Wolint, P., Günter, J., Steiger, J., Bopp, A., et al. (2017). Translational cardiac stem cell therapy: advancing from first-generation to next-generation cell types. npj Regen. Med. 2:17.
Cao, N., Liang, H., Huang, J., Wang, J., Chen, Y., Chen, Z., et al. (2013). Highly efficient induction and long-term maintenance of multipotent cardiovascular progenitors from human pluripotent stem cells under defined conditions. Cell Res. 23, 1119–1132. doi: 10.1038/cr.2013.102
Caspi, O., Huber, I., Gepstein, A., Arbel, G., Maizels, L., Boulos, M., et al. (2013). Modeling of arrhythmogenic right ventricular cardiomyopathy with human induced pluripotent stem cells. Circ. Cardiovasc. Genet. 6, 557–568. doi: 10.1161/circgenetics.113.000188
Chaicharoenaudomrung, N., Kunhorm, P., and Noisa, P. (2019). Three-dimensional cell culture systems as an in vitro platform for cancer and stem cell modeling. World J. Stem Cells 11, 1065–1083. doi: 10.4252/wjsc.v11.i12.1065
Chailangkarn, T., and Muotri, A. R. (2017). Modeling Williams syndrome with induced pluripotent stem cells. Neurogenesis 4:e1283187. doi: 10.1080/23262133.2017.1283187
Chakraborty, S., Ji, H., Kabadi, A. M., Gersbach, C. A., Christoforou, N., and Leong, K. W. (2014). A CRISPR/Cas9-based system for reprogramming cell lineage specification. Stem Cell Rep. 3, 940–947. doi: 10.1016/j.stemcr.2014.09.013
Chan, X. Y., Black, R., Dickerman, K., Federico, J., Levesque, M., Mumm, J., et al. (2015). Three-dimensional vascular network assembly from diabetic patient-derived induced pluripotent stem cells. Arterioscler. Thromb. Vasc. Biol. 35, 2677–2685. doi: 10.1161/atvbaha.115.306362
Chan, Y. C., Ting, S., Lee, Y. K., Ng, K. M., Zhang, J., Chen, Z., et al. (2013). Electrical stimulation promotes maturation of cardiomyocytes derived from human embryonic stem cells. J. Cardiovasc. Transl. Res. 6, 989–999. doi: 10.1007/s12265-013-9510-z
Chau, M. D. L., Tuft, R., Fogarty, K., and Bao, Z. Z. (2006). Notch signaling plays a key role in cardiac cell differentiation. Mech. Dev. 123, 626–640. doi: 10.1016/j.mod.2006.06.003
Chaudhari, U., Nemade, H., Wagh, V., Gaspar, J. A., Ellis, J. K., Srinivasan, S. P., et al. (2016). Identification of genomic biomarkers for anthracycline-induced cardiotoxicity in human iPSC-derived cardiomyocytes: an in vitro repeated exposure toxicity approach for safety assessment. Arch. Toxicol. 90, 2763–2777. doi: 10.1007/s00204-015-1623-5
Chen, Y. W., Huang, S. X., De Carvalho, A. L. R. T., Ho, S. H., Islam, M. N., Volpi, S., et al. (2017). A three-dimensional model of human lung development and disease from pluripotent stem cells. Nat. Cell Biol. 19, 542–549.
Cho, G. S., Lee, D. I., Tampakakis, E., Murphy, S., Andersen, P., Uosaki, H., et al. (2017). Neonatal transplantation confers maturation of PSC-derived cardiomyocytes conducive to modeling cardiomyopathy. Cell Rep. 18, 571–582. doi: 10.1016/j.celrep.2016.12.040
Choulika, A., Perrin, A., Dujon, B., and Nicolas, J. F. (1995). Induction of homologous recombination in mammalian chromosomes by using the I-SceI system of Saccharomyces cerevisiae. Mol Cell Biol. 15, 1968–1973. doi: 10.1128/mcb.15.4.1968
Chun, Y. S., Byun, K., and Lee, B. (2011). Induced pluripotent stem cells and personalized medicine: current progress and future perspectives. Anat. Cell Biol. 44, 245–255. doi: 10.5115/acb.2011.44.4.245
Chun, Y. W., Balikov, D. A., Feaster, T. K., Williams, C. H., Sheng, C. C., Lee, J. B., et al. (2015). Combinatorial polymer matrices enhance in vitro maturation of human induced pluripotent stem cell-derived cardiomyocytes. Biomaterials 67, 52–64. doi: 10.1016/j.biomaterials.2015.07.004
Clevers, H. (2016). Modeling development and disease with organoids. Cell 165, 1586–1597. doi: 10.1016/j.cell.2016.05.082
Cohen-Tannoudji, M., Robine, S., Choulika, A., Pinto, D., El Marjou, F., Babinet, C., et al. (1998). I-SceI-induced gene replacement at a natural locus in embryonic stem cells. Mol. Cell Biol. 18, 1444–1448. doi: 10.1128/mcb.18.3.1444
Cong, L., Ran, F. A., Cox, D., Lin, S., Barretto, R., Hsu, P. D., et al. (2013). Multiplex genome engineering using CRISPR/Cas systems. Science 339, 819–823.
Correia, C., Koshkin, A., Duarte, P., Hu, D., Carido, M., Sebastião, M. J., et al. (2018). 3D aggregate culture improves metabolic maturation of human pluripotent stem cell derived cardiomyocytes. Biotechnol. Bioeng. 115, 630–644. doi: 10.1002/bit.26504
Corrigan-Curay, J., O’Reilly, M., Kohn, D. B., Cannon, P. M., Bao, G., Bushman, F. D., et al. (2015). Genome editing technologies: defining a path to clinic. Mol. Ther. 23, 796–806. doi: 10.1038/mt.2015.54
Cota-Coronado, A., Ramírez-Rodríguez, P. B., Padilla-Camberos, E., Díaz, éNstor, F., Flores-Fernández, J. M., et al. (2019). Implications of human induced pluripotent stem cells in metabolic disorders: from drug discovery toward precision medicine. Drug Discov. Today 24, 334–341. doi: 10.1016/j.drudis.2018.10.001
David, J., Tester, B. S., Michael, J., and Ackerman, M. D. (2014). PD. genetics of long QT syndrome. Methodist Debakey Cardiovasc. J. 10, 29–33.
de la Roche, J., Angsutararux, P., Kempf, H., Janan, M., Bolesani, E., Thiemann, S., et al. (2019). Comparing human iPSC-cardiomyocytes versus HEK293T cells unveils disease-causing effects of Brugada mutation A735V of NaV1.5 sodium channels. Sci. Rep. 9:11173.
De Masi, C., Spitalieri, P., Murdocca, M., Novelli, G., and Sangiuolo, F. (2020). Application of CRISPR/Cas9 to human-induced pluripotent stem cells: from gene editing to drug discovery. Hum. Genomics 14:25.
Deinsberger, J., Reisinger, D., and Weber, B. (2020). Global trends in clinical trials involving pluripotent stem cells: a systematic multi-database analysis. npj Regen. Med. 5:15.
del Álamo, J. C., Lemons, D., Serrano, R., Savchenko, A., and Cerignoli, F. (2016). High throughput physiological screening of iPSC-derived cardiomyocytes for drug development. Biochim. Biophys. Acta Mol. Cell Res. 1863, 1717–1727. doi: 10.1016/j.bbamcr.2016.03.003
Dementyeva, E. V., Medvedev, S. P., Kovalenko, V. R., Vyatkin, Y. V., Kretov, E. I., Slotvitsky, M. M., et al. (2019). Applying patient-specific induced pluripotent stem cells to create a model of hypertrophic cardiomyopathy. Biochemical 84, 291–298. doi: 10.1134/s0006297919030118
Denning, C., Borgdorff, V., Crutchley, J., Firth, K. S. A., George, V., Kalra, S., et al. (2016). Cardiomyocytes from human pluripotent stem cells: from laboratory curiosity to industrial biomedical platform. Biochim. Biophys. Acta Mol. Cell Res. 1863, 1728–1748. doi: 10.1016/j.bbamcr.2015.10.014
Diecke, S., Lu, J., Lee, J., Termglinchan, V., Kooreman, N. G., Burridge, P. W., et al. (2015). Novel codon-optimized mini-intronic plasmid for efficient, inexpensive, and xeno-free induction of pluripotency. Sci. Rep. 5:8081.
Dominguez, A. A., Lim, W. A., and Qi, L. S. (2016). Beyond editing: repurposing CRISPR-Cas9 for precision genome regulation and interrogation. Nat. Rev. Mol. Cell Biol. 17, 5–15. doi: 10.1038/nrm.2015.2
Doppler, S. A., Deutsch, M.-A., Serpooshan, V., Li, G., Dzilic, E., Lange, R., et al. (2017). Mammalian heart regeneration: the race to the finish line. Circ. Res. 120, 630–632. doi: 10.1161/circresaha.116.310051
Driessen-Mol, A., Emmert, M. Y., Dijkman, P. E., Frese, L., Sanders, B., Weber, B., et al. (2014). Transcatheter implantation of homologous “off-the-shelf” tissue-engineered heart valves with self-repair capacity: long-term functionality and rapid in vivo remodeling in sheep. J. Am. Coll. Cardiol. 63, 1320–1329. doi: 10.1016/j.jacc.2013.09.082
Dzilic, E., Lahm, H., Dreßen, M., Deutsch, M. A., Lange, R., Wu, S. M., et al. (2018). Genome editing redefines precision medicine in the cardiovascular field. Stem Cells Int. 2018:4136473.
Ebert, A., Joshi, A. U., Andorf, S., Dai, Y., Sampathkumar, S., Chen, H., et al. (2019). Proteasome-dependent regulation of distinct metabolic states during long-term culture of human iPSC-derived cardiomyocytes. Circ. Res. 125, 90–103. doi: 10.1161/circresaha.118.313973
Egashira, T., Yuasa, S., Suzuki, T., Aizawa, Y., Yamakawa, H., Matsuhashi, T., et al. (2012). Disease characterization using LQTS-specific induced pluripotent stem cells. Cardiovasc. Res. 95, 419–429.
Emmert, M. Y. (2017). Cell-based cardiac regeneration: current evidence, clinical controversies, translational hurdles, and future challenges for therapies. Eur. Heart J. 38, 1095–1098.
Emmert, M. Y., and Hoerstrup, S. P. (2017). Next generation heart valve substitutes: how to develop a next generation regenerative heart valve substitute? the struggle to develop the ideal valve substitute continues, 50 years after Dr D e Harken described the conditions that the valve should fulfil. Eur. Heart J. 38, 617–618. doi: 10.1093/eurheartj/ehx074
Emmert, M. Y., Fioretta, E. S., and Hoerstrup, S. P. (2017). Translational challenges in cardiovascular tissue engineering. J. Cardiovasc. Transl. Res. 10, 139–149. doi: 10.1007/s12265-017-9728-2
Emmert, M. Y., Schmitt, B. A., Loerakker, S., Sanders, B., Spriestersbach, H., Fioretta, E. S., et al. (2018). Computational modeling guides tissue-engineered heart valve design for long-term in vivo performance in a translational sheep model. Sci. Transl. Med. 10:eaan4587. doi: 10.1126/scitranslmed.aan4587
Emmert, M. Y., Weber, B., Behr, L., Frauenfelder, T., Brokopp, C. E., Grnenfelder, J., et al. (2011). Transapical aortic implantation of autologous marrow stromal cell-based tissue-engineered heart valves: first experiences in the systemic circulation. JACC Cardiovasc. Interv. 4, 822–823. doi: 10.1016/j.jcin.2011.02.020
Emmert, M. Y., Weber, B., Behr, L., Sammut, S., Frauenfelder, T., Wolint, P., et al. (2014). Transcatheter aortic valve implantation using anatomically oriented, marrow stromal cell-based, stented, tissue-engineered heart valves: technical considerations and implications for translational cell-based heart valve concepts. Eur. J. Cardiothoracic Surg. 45, 61–68. doi: 10.1093/ejcts/ezt243
Emmert, M. Y., Weber, B., Wolint, P., Behr, L., Sammut, S., Frauenfelder, T., et al. (2012). Stem cell-based transcatheter aortic valve implantation: first experiences in a pre-clinical model. JACC Cardiovasc. Interv. 5, 874–883.
Emmert, M. Y., Wolint, P., Wickboldt, N., Gemayel, G., Weber, B., Brokopp, C. E., et al. (2013a). Human stem cell-based three-dimensional microtissues for advanced cardiac cell therapies. Biomaterials 34, 6339–6354. doi: 10.1016/j.biomaterials.2013.04.034
Emmert, M. Y., Wolint, P., Winklhofer, S., Stolzmann, P., Cesarovic, N., Fleischmann, T., et al. (2013b). Transcatheter based electromechanical mapping guided intramyocardial transplantation and in vivo tracking of human stem cell based three dimensional microtissues in the porcine heart. Biomaterials 34, 2428–2441. doi: 10.1016/j.biomaterials.2012.12.021
Engle, S. J., and Puppala, D. (2013). Integrating human pluripotent stem cells into drug development. Cell Stem Cell 12, 669–677. doi: 10.1016/j.stem.2013.05.011
Eschenhagen, T., Fink, C., Remmers, U., Scholz, H., Wattchow, J., Weil, J., et al. (1997). Three-dimensional reconstitution of embryonic cardiomyocytes in a collagen matrix: a new heart muscle model system. FASEB J. 11, 683–694. doi: 10.1096/fasebj.11.8.9240969
Fatima, A., Kaifeng, S., Dittmann, S., Xu, G., Gupta, M. K., Linke, M., et al. (2013). The disease-specific phenotype in cardiomyocytes derived from induced pluripotent stem cells of two long qt syndrome type 3 patients. PLoS One 8:e83005. doi: 10.1371/journal.pone.0083005
Filippo Buono, M., von Boehmer, L., Strang, J. P., Hoerstrup, S. Y., Emmert, M., and Nugraha, B. (2020). Human cardiac organoids for modeling genetic cardiomyopathy. Cells 9:1733. doi: 10.3390/cells9071733
Fioretta, E. S., Dijkman, P. E., Emmert, M. Y., and Hoerstrup, S. P. (2018). The future of heart valve replacement: recent developments and translational challenges for heart valve tissue engineering. J. Tissue Eng. Regen. Med. 12, e323–e335.
Fioretta, E. S., Lintas, V., Mallone, A., Motta, S. E., von Boehmer, L., Dijkman, P. E., et al. (2020a). Differential leaflet remodeling of bone marrow cell pre-seeded versus nonseeded bioresorbable transcatheter pulmonary valve replacements. JACC Basic Transl. Sci. 5, 15–31. doi: 10.1016/j.jacbts.2019.09.008
Fioretta, E. S., Motta, S. E., Lintas, V., Loerakker, S., Parker, K. K., Baaijens, F. P. T., et al. (2020b). Next-generation tissue-engineered heart valves with repair, remodelling and regeneration capacity. Nat. Rev. Cardiol. 18, 92–116. doi: 10.1038/s41569-020-0422-8
Fong, A. H., Romero-López, M., Heylman, C. M., Keating, M., Tran, D., Sobrino, A., et al. (2016). Three-dimensional adult cardiac extracellular matrix promotes maturation of human induced pluripotent stem cell-derived cardiomyocytes. Tissue Eng. Part A 22, 1016–1025.
Fu, Y., Foden, J. A., Khayter, C., Maeder, M. L., Reyon, D., Joung, J. K., et al. (2013). High-frequency off-target mutagenesis induced by CRISPR-Cas nucleases in human cells. Nat. Biotechnol. 31, 822–826. doi: 10.1038/nbt.2623
Gaballa, M. A., Sunkomat, J. N. E., Thai, H., Morkin, E., Ewy, G., and Goldman, S. (2006). Grafting an acellular 3-dimensional collagen scaffold onto a non-transmural infarcted myocardium induces neo-angiogenesis and reduces cardiac remodeling. J. Heart Lung Transplant. 25, 946–954. doi: 10.1016/j.healun.2006.04.008
Gaj, T., Gersbach, C. A., and Barbas, C. F. (2013). ZFN, TALEN, and CRISPR/Cas-based methods for genome engineering. Trends Biotechnol. 31, 397–405. doi: 10.1016/j.tibtech.2013.04.004
Gao, X., Yang, J., Tsang, J. C. H., Ooi, J., Wu, D., and Liu, P. (2013). Reprogramming to pluripotency using designer TALE transcription factors targeting enhancers. Stem Cell Rep. 1, 183–197. doi: 10.1016/j.stemcr.2013.06.002
Garg, P., Oikonomopoulos, A., Chen, H., Li, Y., Lam, C. K., Sallam, K., et al. (2019). Genome editing and induced pluripotent stem cells in cardiac channelopathy. J. Am. Coll. Cardiol. 72, 62–75. doi: 10.1016/j.jacc.2018.04.041
Garg, V., Muth, A. N., Ransom, J. F., Schluterman, M. K., Barnes, R., King, I. N., et al. (2005). Mutations in NOTCH1 cause aortic valve disease. Nature 437, 270–274. doi: 10.1038/nature03940
Gasiunas, G., Barrangou, R., Horvath, P., and Siksnys, V. (2012). Cas9-crRNA ribonucleoprotein complex mediates specific DNA cleavage for adaptive immunity in bacteria. Proc. Natl. Acad. Sci. U. S. A. 109, 2579–2586.
Ge, X., Ren, Y., Bartulos, O., Lee, M. Y., Yue, Z., Kim, K. Y., et al. (2012). Modeling supravalvular aortic stenosis syndrome with human induced pluripotent stem cells. Circulation 126, 1695–1704. doi: 10.1161/circulationaha.112.116996
Geng, X., Liu, B., Liu, J., Liu, D., Lu, Y., Sun, X., et al. (2018). Interfacial tissue engineering of heart regenerative medicine based on soft cell-porous scaffolds. J. Thorac. Dis. 10(Suppl. 20), S2333–S2345.
Gerull, B., Gramlich, M., Atherton, J., McNabb, M., Trombitás, K., Sasse-Klaassen, S., et al. (2002). Mutations of TTN, encoding the giant muscle filament titin, cause familial dilated cardiomyopathy. Nat. Genet. 30, 201–204. doi: 10.1038/ng815
Geurts, A. M., Cost, G. J., Freyvert, Y., Zeitler, B., Miller, J. C., Choi, V. M., et al. (2009). Knockout rats via embryo microinjection of zinc-finger nucleases. Science 325:433. doi: 10.1126/science.1172447
Geurts, M. H., de Poel, E., Amatngalim, G. D., Oka, R., Meijers, F. M., Kruisselbrink, E., et al. (2020). CRISPR-based adenine editors correct nonsense mutations in a cystic fibrosis organoid biobank. Cell Stem Cell 26, 503–510.e7.
Gherghiceanu, M., Barad, L., Novak, A., Reiter, I., Itskovitz-Eldor, J., Binah, O., et al. (2011). Cardiomyocytes derived from human embryonic and induced pluripotent stem cells: comparative ultrastructure. J. Cell Mol. Med. 15, 2539–2551. doi: 10.1111/j.1582-4934.2011.01417.x
Gilbert, L. A., Larson, M. H., Morsut, L., Liu, Z., Brar, G. A., Torres, S. E., et al. (2013). CRISPR-mediated modular RNA-guided regulation of transcription in eukaryotes. Cell 154:442. doi: 10.1016/j.cell.2013.06.044
Girolami, F., Ho, C. Y., Semsarian, C., Baldi, M., Will, M. L., Baldini, K., et al. (2010). Clinical features and outcome of hypertrophic cardiomyopathy associated with triple sarcomere protein gene mutations. J. Am. Coll. Cardiol. 55, 1444–1453. doi: 10.1016/j.jacc.2009.11.062
Goversen, B., van der Heyden, M. A. G., van Veen, T. A. B., and de Boer, T. P. (2018). The immature electrophysiological phenotype of iPSC-CMs still hampers in vitro drug screening: special focus on IK1. Pharmacol. Ther. 183, 127–136. doi: 10.1016/j.pharmthera.2017.10.001
Grimm, F. A., Blanchette, A., House, J. S., Ferguson, K., Hsieh, N. H., Dalaijamts, C., et al. (2018). A human population-based organotypic in vitro model for cardiotoxicity screening. ALTEX 35, 441–452. doi: 10.14573/altex.1805301
Grobarczyk, B., Franco, B., Hanon, K., and Malgrange, B. (2015). Generation of isogenic human iPS cell line precisely corrected by genome editing using the CRISPR/Cas9 system. Stem Cell Rev. Rep. 11, 774–787. doi: 10.1007/s12015-015-9600-1
Gromo, G., Mann, J., and Fitzgerald, J. D. (2014). Cardiovascular drug discovery: a perspective from a research-based pharmaceutical company. Cold Spring Harb Perspect. Med. 4, 1–13.
Guha, P., Morgan, J. W., Mostoslavsky, G., Rodrigues, N. P., and Boyd, A. S. (2013). Lack of immune response to differentiated cells derived from syngeneic induced pluripotent stem cells. Stem Cell 12, 407–412. doi: 10.1016/j.stem.2013.01.006
Günter, J., Wolint, P., Bopp, A., Steiger, J., Cambria, E., Hoerstrup, S. P., et al. (2016). Microtissues in cardiovascular medicine: regenerative potential based on a 3D microenvironment. Stem Cells Int. 2016:9098523.
Gurdon, J. B. (1962). The developmental capacity of nuclei taken from intestinal epithelium cells of feeding tadpoles. J. Embryol. Exp. Morphol. 10, 622–640. doi: 10.1242/dev.10.4.622
Haake, K., Ackermann, M., and Lachmann, N. (2019). Concise review: towards the clinical translation of induced pluripotent stem cell-derived blood cells—ready for take-off. Stem Cells Transl. Med. 8, 332–339. doi: 10.1002/sctm.18-0134
Han, L., Li, Y., Tchao, J., Kaplan, A. D., Lin, B., Li, Y., et al. (2014). Study familial hypertrophic cardiomyopathy using patient-specific induced pluripotent stem cells. Cardiovasc. Res. 104, 258–269. doi: 10.1093/cvr/cvu205
Han, X., Wang, M., Duan, S., Franco, P. J., Kenty, J. H. R., Hedrick, P., et al. (2019). Generation of hypoimmunogenic human pluripotent stem cells. Proc. Natl. Acad. Sci. U. S. A. 116, 10441–10446.
Hannah-Shmouni, F., Seidelmann, S. B., Sirrs, S., Mani, A., and Jacoby, D. (2015). The genetic challenges and opportunities in advanced heart failure. Can. J. Cardiol. 31, 1338–1350. doi: 10.1016/j.cjca.2015.07.735
He, J., Rong, Z., Fu, X., and Xu, Y. (2017). A safety checkpoint to eliminate cancer risk of the immune evasive cells derived from human embryonic stem cells. Stem Cells 35, 1154–1161. doi: 10.1002/stem.2568
Head, S. J., Çelik, M., and Kappetein, A. P. (2017). Mechanical versus bioprosthetic aortic valve replacement. Eur. Heart J. 38, 2183–2191. doi: 10.1093/eurheartj/ehx141
Herman, D. S., Lam, L., Taylor, M. R. G., Wang, L., Teekakirikul, P., Christodoulou, D., et al. (2012). Truncations of titin causing dilated cardiomyopathy. N. Engl. J. Med. 366, 619–628.
Hershberger, R. E., and Morales, A. (2007). Dilated Cardiomyopathy Overview. Seattle (WA): University of Washington.
Higuchi, A., Ling, Q. D., Kumar, S. S., Munusamy, M. A., Alarfaj, A. A., Chang, Y., et al. (2015). Generation of pluripotent stem cells without the use of genetic material. Nat. Lab. Invest. 95, 26–42. doi: 10.1038/labinvest.2014.132
Hinson, J. T., Chopra, A., Nafissi, N., Polacheck, W. J., Benson, C. C., Swist, S., et al. (2015). Titin mutations in iPS cells define sarcomere insufficiency as a cause of dilated cardiomyopathy. Science 349, 982–986. doi: 10.1126/science.aaa5458
Hoang, P., Wang, J., Conklin, B. R., Healy, K. E., and Ma, Z. (2018). Generation of spatial-patterned early-developing cardiac organoids using human pluripotent stem cells. Nat. Protoc. 13, 723–737. doi: 10.1038/nprot.2018.006
Hockemeyer, D., and Jaenisch, R. (2016). Induced pluripotent stem cells meet genome editing. Cell Stem Cell 18, 573–586. doi: 10.1016/j.stem.2016.04.013
Hofbauer, P., Jahnel, S., Papai, N., Giesshammer, M., Tavernini, K., Grdseloff, N., et al. (2020). Cardioids reveal self-organizing principles of human cardiogenesis. bioRxiv [preprint] doi: 10.1101/2020.07.06.189431
Hotta, A., and Yamanaka, S. (2015). From genomics to gene therapy: induced pluripotent stem cells meet genome editing. Annu. Rev. Genet. 49, 47–70. doi: 10.1146/annurev-genet-112414-054926
Howe, S. J., Mansour, M. R., Schwarzwaelder, K., Hubank, M., Kempski, H., Brugman, M. H., et al. (2008). Insertional mutagenesis in combination with acquired somatic mutations leads to leukemogenesis following gene therapy of SCID-X1. J. Clin. Invest. 118, 3143–3150. doi: 10.1172/jci35798
Hsu, P. D., Lander, E. S., and Zhang, F. (2014). Development and applications of CRISPR-Cas9 for genome engineering. Cell 157, 1262–1278. doi: 10.1016/j.cell.2014.05.010
Huch, M., Dorrell, C., Boj, S. F., van Es, J. H., Li, V. S., van de Wetering, M., et al. (2013). In vitro expansion of single Lgr5(+) liver stem cells induced by Wnt-driven regeneration. Nature 11, 179–194.
Ishigami, M., Masumoto, H., Ikuno, T., Aoki, T., Kawatou, M., Minakata, K., et al. (2018). Human iPS cell-derived cardiac tissue sheets for functional restoration of infarcted porcine hearts. PLoS One 13:e0201650. doi: 10.1371/journal.pone.0201650
Itzhaki, I., Maizels, L., Huber, I., Gepstein, A., Arbel, G., Caspi, O., et al. (2012). Modeling of catecholaminergic polymorphic ventricular tachycardia with patient-specific human-induced pluripotent stem cells. J. Am. Coll. Cardiol. 60, 990–1000. doi: 10.1016/j.jacc.2012.02.066
Itzhaki, I., Maizels, L., Huber, I., Zwi-Dantsis, L., Caspi, O., Winterstern, A., et al. (2011). Modelling thedrome with induced pluripotent stem cells. Nature 471, 225–230.
Jacoby, D., and McKenna, W. J. (2012). Genetics of inherited cardiomyopathy. Eur. Heart J. 33, 296–304. doi: 10.1093/eurheartj/ehr260
Jang, Y., Choi, J., Park, N., Kang, J., Kim, M., Kim, Y., et al. (2019). Development of immunocompatible pluripotent stem cells via CRISPR-based human leukocyte antigen engineering. Exp. Mol. Med. 51, 1–11. doi: 10.1038/s12276-018-0190-2
Jinek, M., Chylinski, K., Fonfara, I., Hauer, M., Doudna, J. A., and Charpentier, E. (2012). A programmable dual-RNA-guided DNA endonuclease in adaptive bacterial immunity. Science 337, 816–821. doi: 10.1126/science.1225829
Johnson, J. Z., and Hockemeyer, D. (2015). Human stem cell-based disease modeling: prospects and challenges. Curr. Opin. Cell Biol. 37, 84–90. doi: 10.1016/j.ceb.2015.10.007
Jouni, M., Si-Tayeb, K., Es-Salah-Lamoureux, Z., Latypova, X., Champon, B., Caillaud, A., et al. (2015). Toward personalized medicine: using cardiomyocytes differentiated from urine-derived pluripotent stem cells to recapitulate electrophysiological characteristics of type 2 long QT syndrome. J. Am. Heart Assoc. 4:e002159.
Jung, C. B., Moretti, A., Mederos y Schnitzler, M., Iop, L., Storch, U., Bellin, M., et al. (2012). Dantrolene rescues arrhythmogenic RYR2 defect in a patient-specific stem cell model of catecholaminergic polymorphic ventricular tachycardia. EMBO Mol Med. 4, 180–191. doi: 10.1002/emmm.201100194
Kadari, A., Mekala, S., Wagner, N., Malan, D., Köth, J., Doll, K., et al. (2015). Robust generation of cardiomyocytes from human iPS cells requires precise modulation of BMP and WNT signaling. Stem Cell Rev. Rep. 11, 560–569. doi: 10.1007/s12015-014-9564-6
Kadota, S., Pabon, L., Reinecke, H., and Murry, C. E. (2017). In vivo maturation of human induced pluripotent stem cell-derived cardiomyocytes in neonatal and adult rat hearts. Stem Cell Rep. 8, 278–289. doi: 10.1016/j.stemcr.2016.10.009
Kamakura, T., Makiyama, T., Sasaki, K., Yoshida, Y., Wuriyanghai, Y., Chen, J., et al. (2013). Ultrastructural maturation of human-induced pluripotent stem cell-derived cardiomyocytes in a long-term culture. Circ. J. 77, 1307–1314. doi: 10.1253/circj.cj-12-0987
Karakikes, I., Ameen, M., Termglinchan, V., and Wu, J. C. (2015a). Human Induced pluripotent stem cell-derived cardiomyocytes: insights into molecular, cellular, and functional phenotypes. Circ. Res. 117, 80–88. doi: 10.1161/circresaha.117.305365
Karakikes, I., Stillitano, F., Nonnenmacher, M., Tzimas, C., Sanoudou, D., Termglinchan, V., et al. (2015b). Correction of human phospholamban R14del mutation associated with cardiomyopathy using targeted nucleases and combination therapy. Nat. Commun. 6:6955.
Kattman, S. J., Witty, A. D., Gagliardi, M., Dubois, N. C., Niapour, M., Hotta, A., et al. (2011). Stage-specific optimization of activin/nodal and BMP signaling promotes cardiac differentiation of mouse and human pluripotent stem cell lines. Cell Stem Cell 8, 228–240. doi: 10.1016/j.stem.2010.12.008
Kheradvar, A., Groves, E. M., Dasi, L. P., Alavi, S. H., Tranquillo, R., Grande-Allen, K. J., et al. (2015). Emerging trends in heart valve engineering: part i. solutions for future. Ann. Biomed. Eng. 43, 833–843. doi: 10.1007/s10439-014-1209-z
Kim, C., Wong, J., Wen, J., Wang, S., Wang, C., Spiering, S., et al. (2013). Studying arrhythmogenic right ventricular dysplasia with patient-specific iPSCs. Nature 494, 105–110. doi: 10.1038/nature11799
Kim, D., Kim, C. H., Moon, J., Il, Chung, Y. G., Chang, M. Y., et al. (2009). Generation of human induced pluripotent stem cells by direct delivery of reprogramming proteins. Cell Stem Cell 4, 472–476. doi: 10.1016/j.stem.2009.05.005
Kinnear, C., Agrawal, R., Loo, C., Pahnke, A., Rodrigues, D. C., Thompson, T., et al. (2020). Everolimus rescues the phenotype of elastin insufficiency in patient induced pluripotent stem cell-derived vascular smooth muscle cells. Arterioscler. Thromb. Vasc. Biol. 40, 1325–1339. doi: 10.1161/atvbaha.119.313936
Kishino, Y., Fujita, J., Tohyama, S., Okada, M., Tanosaki, S., Someya, S., et al. (2020). Toward the realization of cardiac regenerative medicine using pluripotent stem cells. BioMed. Cent Inflamm Regen. 40, 4–9.
Kitani, T., Ong, S. G., Lam, C. K., Rhee, J. W., Zhang, J. Z., Oikonomopoulos, A., et al. (2019). Human-induced pluripotent stem cell model of trastuzumab-induced cardiac dysfunction in patients with breast cancer. Circulation 139, 2451–2465. doi: 10.1161/circulationaha.118.037357
Kodo, K., Ong, S. G., Jahanbani, F., Termglinchan, V., Hirono, K., Inanloorahatloo, K., et al. (2016). iPSC-derived cardiomyocytes reveal abnormal TGF-β signalling in left ventricular non-compaction cardiomyopathy. Nat. Cell Biol. 18, 1031–1042. doi: 10.1038/ncb3411
Koivumäki, J. T., Naumenko, N., Tuomainen, T., Takalo, J., Oksanen, M., Puttonen, K. A., et al. (2018). Structural immaturity of human iPSC-derived cardiomyocytes: in silico investigation of effects on function and disease modeling. Front. Physiol. 9:80.
Komor, A. C., Kim, Y. B., Packer, M. S., Zuris, J. A., and Liu, D. R. (2016). Programmable editing of a target base in genomic DNA without double-stranded DNA cleavage. Nature 533, 420–424. doi: 10.1038/nature17946
Kujala, K., Paavola, J., Lahti, A., Larsson, K., Pekkanen-Mattila, M., Viitasalo, M., et al. (2012). Cell model of catecholaminergic polymorphic ventricular tachycardia reveals early and delayed afterdepolarizations. PLoS One 7:e44660. doi: 10.1371/journal.pone.0044660
Kumar, D., Anand, T., and Kues, K. A. (2017). Clinical potential of human-induced pluripotent stem cells: perspectives of induced pluripotent stem cells. Cell Biol. Toxicol. 33, 99–112. doi: 10.1007/s10565-016-9370-9
Kusuma, S., Shen, Y. I., Hanjaya-Putra, D., Mali, P., Cheng, L., and Gerecht, S. (2013). Self-organized vascular networks from human pluripotent stem cells in a synthetic matrix. Proc. Natl. Acad. Sci. U. S. A. 110, 12601–12606. doi: 10.1073/pnas.1306562110
Kwon, S. G., Kwon, Y. W., Lee, T. W., Park, G. T., and Kim, J. H. (2018). Recent advances in stem cell therapeutics and tissue engineering strategies. Biomater. Res. 22:36.
Laflamme, M. A., Chen, K. Y., Naumova, A. V., Muskheli, V., Fugate, J. A., Dupras, S. K., et al. (2007). Cardiomyocytes derived from human embryonic stem cells in pro-survival factors enhance function of infarcted rat hearts. Nat. Biotechnol. 25, 1015–1024. doi: 10.1038/nbt1327
Lahti, A. L., Kujala, V. J., Chapman, H., Koivisto, A. P., Pekkanen-Mattila, M., Kerkelä, E., et al. (2012). Model for long QT syndrome type 2 using human iPS cells demonstrates arrhythmogenic characteristics in cell culture. Dis. Model Mech. 5, 220–230. doi: 10.1242/dmm.008409
Lan, F., Lee, A. S., Liang, P., Sanchez-freire, V., Nguyen, P. K., Wang, L., et al. (2013). Abnormal calcium handling properties underlie familialhypertrophic cardiomyopathy pathology in patient-specificinduced pluripotent stem cells. Cell Stem Cell 12, 101–113. doi: 10.1016/j.stem.2012.10.010
Lancaster, M. A., and Knoblich, J. A. (2014). Generation of cerebral organoids from human pluripotent stem cells. Nat. Protoc. 9, 2329–2340.
Lancaster, M. A., Renner, M., Martin, C. A., Wenzel, D., Bicknell, L. S., Hurles, M. E., et al. (2013). Cerebral organoids model human brain development and microcephaly. Nature 501, 373–379.
Langhans, S. A. (2018). Three-dimensional in vitro cell culture models in drug discovery and drug repositioning. Front. Pharmacol. 9:6.
Laurent, L. C., Ulitsky, I., Slavin, I., Tran, H., Schork, A., Morey, R., et al. (2011). Dynamic changes in the copy number of pluripotency and cell proliferation genes in human ESCs and iPSCs during reprogramming and time in culture. Cell Stem Cell 8, 106–118. doi: 10.1016/j.stem.2010.12.003
Lee, A., Hudson, A. R., Shiwarski, D. J., Tashman, J. W., Hinton, T. J., Yerneni, S., et al. (2019). 3D bioprinting of collagen to rebuild components of the human heart. Science 365, 482–487. doi: 10.1126/science.aav9051
Lee, M. O., Jung, K. B., Jo, S. J., Hyun, S. A., Moon, K. S., Seo, J. W., et al. (2019). Modelling cardiac fibrosis using three-dimensional cardiac microtissues derived from human embryonic stem cells. J. Biol. Eng. 13, 1–17.
Lee, Y. K., Lau, Y. M., Cai, Z. J., Lai, W. H., Wong, L. Y., Tse, H. F., et al. (2017). Modeling treatment response for Lamin A/C related dilated cardiomyopathy in human induced pluripotent stem cells. J. Am. Heart Assoc. 6:e005677.
Leenhardt, A., Denjoy, I., and Guicheney, P. (2012). Catecholaminergic polymorphic ventricular tachycardia. Circ. Arrhythm. Electrophysiol. 5, 1044–1052.
Lemcke, H., Voronina, N., Steinhoff, G., and David, R. (2018). Recent progress in stem cell modification for cardiac regeneration. Stem Cells Int. 2018:1909346.
Lian, X., Hsiao, C., Wilson, G., Zhu, K., Hazeltine, L. B., Azarin, S. M., et al. (2012). Robust cardiomyocyte differentiation from human pluripotent stem cells via temporal modulation of canonical Wnt signaling. Proc. Natl. Acad. Sci. U. S. A. 109, E1848–E1857.
Liang, P., Lan, F., Lee, A. S., Gong, T., Sanchez-Freire, V., Wang, Y., et al. (2013). Drug screening using a library of human induced pluripotent stem cell-derived cardiomyocytes reveals disease-specific patterns of cardiotoxicity. Circulation 127, 1677–1691. doi: 10.1161/circulationaha.113.001883
Liang, Z., Chen, K., Li, T., Zhang, Y., Wang, Y., Zhao, Q., et al. (2017). Efficient DNA-free genome editing of bread wheat using CRISPR/Cas9 ribonucleoprotein complexes. Nat. Commun. 8:14261.
Limpitikul, W. B., Dick, I. E., Tester, D. J., Boczek, N. J., Limphong, P., Yang, W., et al. (2017). A precision medicine approach to the rescue of function on malignant calmodulinopathic long-QT syndrome. Circ. Res. 120, 39–48. doi: 10.1161/circresaha.116.309283
Lin, B., Li, Y., Han, L., Kaplan, A. D., Ao, Y., Kalra, S., et al. (2015). Modeling and study of the mechanism of dilated cardiomyopathy using induced pluripotent stem cells derived from individuals with duchenne muscular dystrophy. Dis. Model Mech. 8, 457–466. doi: 10.1242/dmm.019505
Lin, S., Staahl, B. T., Alla, R. K., and Doudna, J. A. (2014). Enhanced homology-directed human genome engineering by controlled timing of CRISPR/Cas9 delivery. Elife 3:e04766.
Lind, J. U., Busbee, T. A., Valentine, A. D., Pasqualini, F. S., Yuan, H., Yadid, M., et al. (2017). Instrumented cardiac microphysiological devices via multimaterial three-dimensional printing. Nat. Mater. 16, 303–308. doi: 10.1038/nmat4782
Lino, C. A., Harper, J. C., Carney, J. P., and Timlin, J. A. (2018). Delivering crispr: a review of the challenges and approaches. Drug Deliv. 25, 1234–1257. doi: 10.1080/10717544.2018.1474964
Lintas, V., Fioretta, E. S., Motta, S. E., Dijkman, P. E., Pensalfini, M., Mazza, E., et al. (2018). Development of a novel human cell-derived tissue-engineered heart valve for transcatheter aortic valve replacement: an in vitro and in vivo feasibility study. J. Cardiovasc. Transl. Res. 11, 470–482. doi: 10.1007/s12265-018-9821-1
Liu, G., David, B. T., Trawczynski, M., and Fessler, R. G. (2020). Advances in pluripotent stem cells: history, mechanisms, technologies, and applications. Stem Cell Rev. Rep. 16, 3–32. doi: 10.1007/s12015-019-09935-x
Liu, N., Napolitano, C., and Priori, S. G. (2013). Catecholaminergic polymorphic ventricular tachycardia. Prog. Cardiovasc. Dis. 51, 551–560.
Liu, X., Li, W., Fu, X., and Xu, Y. (2017). The immunogenicity and immune tolerance of pluripotent stem cell derivatives. Front. Immunol. 8:645.
Liu, Y. W., Chen, B., Yang, X., Fugate, J. A., Kalucki, F. A., Futakuchi-Tsuchida, A., et al. (2018). Human embryonic stem cell-derived cardiomyocytes restore function in infarcted hearts of non-human primates. Nat. Biotechnol. 36, 597–605.
Loscalzo, J., and Handy, D. E. (2014). Epigenetic modifications: basic mechanisms and role in cardiovascular disease (2013 grover conference series). Pulm Circ. 4, 169–174. doi: 10.1086/675979
Lundy, S. D., Zhu, W. Z., Regnier, M., and Laflamme, M. A. (2013). Structural and functional maturation of cardiomyocytes derived from human pluripotent stem cells. Stem Cells Dev. 22, 1991–2002. doi: 10.1089/scd.2012.0490
Luo, J., Qin, L., Zhao, L., Gui, L., Ellis, M. W., Huang, Y., et al. (2020). Tissue-engineered vascular grafts with advanced mechanical strength from human iPSCs. Cell Stem Cell 26, 251–261. doi: 10.1016/j.stem.2019.12.012
Ma, D., Wei, H., Lu, J., Ho, S., Zhang, G., Sun, X., et al. (2013a). Generation of patient-specific induced pluripotent stem cell-derived cardiomyocytes as a cellular model of arrhythmogenic right ventricular cardiomyopathy. Eur. Heart J. 34, 1122–1133. doi: 10.1093/eurheartj/ehs226
Ma, D., Wei, H., Lu, J., Huang, D., Liu, Z., Loh, L. J., et al. (2015). Characterization of a novel KCNQ1 mutation for type 1 long QT syndrome and assessment of the therapeutic potential of a novel IKs activator using patient-specific induced pluripotent stem cell-derived cardiomyocytes. Stem Cell Res. Ther. 6:39.
Ma, D., Wei, H., Zhao, Y., Lu, J., Li, G., Sahib, N. B. E., et al. (2013b). Modeling type 3 long QT syndrome with cardiomyocytes derived from patient-specific induced pluripotent stem cells. Int. J. Cardiol. 168, 5277–5286. doi: 10.1016/j.ijcard.2013.08.015
Ma, J., Guo, L., Fiene, S. J., Anson, B. D., Thomson, J. A., Kamp, T. J., et al. (2011). High purity human-induced pluripotent stem cell-derived cardiomyocytes: Electrophysiological properties of action potentials and ionic currents. Am. J. Physiol. Heart Circ. Physiol. 301, 2006–2017.
Machiraju, P., and Greenway, S. C. (2019). Current methods for the maturation of induced pluripotent stem cell-derived cardiomyocytes. World J. Stem Cells 11, 34–44. doi: 10.4252/wjsc.v11.i1.34
Madl, C. M., Heilshorn, S. C., and Blau, H. M. (2018). Bioengineering strategies to accelerate stem cell therapeutics. Nature 557, 335–342. doi: 10.1038/s41586-018-0089-z
Maeder, M. L., Linder, S. J., Cascio, V. M., Fu, Y., Ho, Q. H., and Joung, J. K. (2013). CRISPR RNA-guided activation of endogenous human genes. Nat. Methods 10, 977–979. doi: 10.1038/nmeth.2598
Mahas, A., Neal Stewart, C., and Mahfouz, M. M. (2018). Harnessing CRISPR/Cas systems for programmable transcriptional and post-transcriptional regulation. Biotechnol. Adv. 36, 295–310. doi: 10.1016/j.biotechadv.2017.11.008
Malan, D., Zhang, M., Stallmeyer, B., Müller, J., Fleischmann, B. K., Schulze-Bahr, E., et al. (2016). Human iPS cell model of type 3 long QT syndrome recapitulates drug-based phenotype correction. Basic Res. Cardiol. 111, 1–11.
Mali, P., Esvelt, K. M., and Church, G. M. (2013a). Cas9 as a versatile tool for engineering biology. Nat Methods 10, 957–963. doi: 10.1038/nmeth.2649
Mali, P., Yang, L., Esvelt, K. M., Aach, J., Guell, M., DiCarlo, J. E., et al. (2013b). RNA-guided human genome engineering via Cas9. Science 339, 823–826. doi: 10.1126/science.1232033
Maltsev, V. A., Rohwedel, J., Hescheler, J., and Wobus, A. M. (1993). Embryonic stem cells differentiate in vitro into cardiomyocytes representing sinusnodal, atrial and ventricular cell types. Mech. Dev. 44, 41–50. doi: 10.1016/0925-4773(93)90015-p
Mandegar, M. A., Huebsch, N., Frolov, E. B., Shin, E., Truong, A., Olvera, M. P., et al. (2016). CRISPR interference efficiently induces specific and reversible gene silencing in human iPSCs. Cell Stem Cell 18, 541–553. doi: 10.1016/j.stem.2016.01.022
Maruyama, T., Dougan, S. K., Truttmann, M. C., Bilate, A. M., Ingram, J. R., and Ploegh, H. L. (2015). Increasing the efficiency of precise genome editing with CRISPR-Cas9 by inhibition of nonhomologous end joining. Nat. Biotechnol. 33, 538–542. doi: 10.1038/nbt.3190
Masumoto, H., Ikuno, T., Takeda, M., Fukushima, H., Marui, A., Katayama, S., et al. (2014). Human iPS cell-engineered cardiac tissue sheets with cardiomyocytes and vascular cells for cardiac regeneration. Sci. Rep. 4:6716.
Masumoto, H., Matsuo, T., Yamamizu, K., Uosaki, H., Narazaki, G., Katayama, S., et al. (2012). Pluripotent stem cell-engineered cell sheets reassembled with defined cardiovascular populations ameliorate reduction in infarct heart function through cardiomyocyte-mediated neovascularization. Stem Cells 30, 1196–1205. doi: 10.1002/stem.1089
Masumoto, H., Nakane, T., Tinney, J. P., Yuan, F., Ye, F., Kowalski, W. J., et al. (2016). The myocardial regenerative potential of three-dimensional engineered cardiac tissues composed of multiple human iPS cell-derived cardiovascular cell lineages. Sci. Rep. 6:29933.
Matano, M., Date, S., Shimokawa, M., Takano, A., Fujii, M., Ohta, Y., et al. (2015). Modeling colorectal cancer using CRISPR-Cas9-mediated engineering of human intestinal organoids. Nat. Med. 21, 256–262. doi: 10.1038/nm.3802
Matsa, E., Ahrens, J. H., and Wu, J. C. (2016). Human induced pluripotent stem cells as a platform for personalized and precision cardiovascular medicine. Physiol. Rev. 96, 1093–1126. doi: 10.1152/physrev.00036.2015
Matsa, E., Rajamohan, D., Dick, E., Young, L., Mellor, I., Staniforth, A., et al. (2011). Drug evaluation in cardiomyocytes derived from human induced pluripotent stem cells carrying a long QT syndrome type 2 mutation. Eur. Heart J. 32, 952–962. doi: 10.1093/eurheartj/ehr073
Matsuo, T., Masumoto, H., Tajima, S., Ikuno, T., Katayama, S., Minakata, K., et al. (2015). Efficient long-term survival of cell grafts after myocardial infarction with thick viable cardiac tissue entirely from pluripotent stem cells. Sci. Rep. 5:16842.
Mattapally, S., Zhu, W., Fast, V. G., Gao, L., Worley, C., Kannappan, R., et al. (2018). Spheroids of cardiomyocytes derived from human-induced pluripotent stem cells improve recovery from myocardial injury in mice. Am. J. Physiol. Heart Circ. Physiol. 315, H327–H339.
Mayshar, Y., Ben-David, U., Lavon, N., Biancotti, J. C., Yakir, B., Clark, A. T., et al. (2010). Identification and classification of chromosomal aberrations in human induced pluripotent stem cells. Cell Stem Cell 7, 521–531. doi: 10.1016/j.stem.2010.07.017
Mcdermott-roe, C., Geurts, A., Musunuru, K., Mcdermott-roe, C., Lv, W., Maximova, T., et al. (2019). Investigation of a dilated cardiomyopathy – associated variant in BAG3 using genome- edited iPSC-derived cardiomyocytes. JCI insight 4:e128799.
Mcnally, E. M., Golbus, J. R., and Puckelwartz, M. J. (2013). Genetic mutations and mechanisms in dilated cardiomyopathy. J. Clin. Invest. 123, 19–26. doi: 10.1172/jci62862
Mehta, A., Sequiera, G. L., Ramachandra, C. J. A., Sudibyo, Y., Chung, Y., Sheng, J., et al. (2014). Re-trafficking of hERG reverses long QT syndrome 2 phenotype in human iPS-derived cardiomyocytes. Cardiovasc. Res. 102, 497–506. doi: 10.1093/cvr/cvu060
Mela, P. (2020). Subject- and leaflet-specific remodeling of polymeric heart valves for in situ tissue engineering: challenges towards clinical translation. JACC Basic Transl. Sci. 5, 32–34. doi: 10.1016/j.jacbts.2019.12.006
Memi, F., Ntokou, A., and Papangeli, I. (2018). CRISPR/Cas9 gene-editing: research technologies, clinical applications and ethical considerations. Semin. Perinatol. 42, 487–500. doi: 10.1053/j.semperi.2018.09.003
Menasché, P. (2018). Cell therapy trials for heart regeneration — lessons learned and future directions. Nat. Rev. Cardiol. 15, 659–671. doi: 10.1038/s41569-018-0013-0
Miao, Y., Tian, L., Martin, M., Paige, S. L., Galdos, F. X., Li, J., et al. (2020). Intrinsic endocardial defects contribute to hypoplastic left heart syndrome. Cell Stem Cell. 27, 574–589.e8.
Milan, D. J., and MacRae, C. A. (2005). Animal models for arrhythmias. Cardiovasc. Res. 67, 426–437. doi: 10.1016/j.cardiores.2005.06.012
Millard, D., Dang, Q., Shi, H., Zhang, X., Strock, C., Kraushaar, U., et al. (2018). Cross-site reliability of human induced pluripotent stem cell-derived cardiomyocyte based safety assays using microelectrode arrays: results from a blinded cipa pilot study. Toxicol. Sci. 164, 550–562. doi: 10.1093/toxsci/kfy110
Mills, R. J., Parker, B. L., Quaife-Ryan, G. A., Voges, H. K., Needham, E. J., Bornot, A., et al. (2019). Drug screening in human PSC-cardiac organoids identifies pro-proliferative compounds acting via the mevalonate pathway. Cell Stem Cell 24, 895–907.e6.
Mills, R. J., Titmarsh, D. M., Koenig, X., Parker, B. L., Ryall, J. G., Quaife-Ryan, G. A., et al. (2017). Functional screening in human cardiac organoids reveals a metabolic mechanism for cardiomyocyte cell cycle arrest. Proc. Natl. Acad. Sci. U. S. A. 114, E8372–E8381.
Miyagi, Y., Chiu, L. L. Y., Cimini, M., Weisel, R. D., Radisic, M., and Li, R. K. (2011). Biodegradable collagen patch with covalently immobilized VEGF for myocardial repair. Biomaterials 32, 1280–1290. doi: 10.1016/j.biomaterials.2010.10.007
Moore, R., Spinhirne, A., Lai, M. J., Preisser, S., Li, Y., Kang, T., et al. (2015). CRISPR-based self-cleaving mechanism for controllable gene delivery in human cells. Nucleic Acids Res. 43, 1297–1303. doi: 10.1093/nar/gku1326
Moretti, A., Bellin, M., Welling, A., Jung, C. B., Lam, J. T., and Bott-Flügel, L. (2010). Patient-specific induced pluripotent stem-cell models for long-QT syndrome. N. Engl. J. Med. 363, 1397–1409.
Motta, S. E., Fioretta, E. S., Dijkman, P. E., Lintas, V., Behr, L., Hoerstrup, S. P., et al. (2018). Development of an off-the-shelf tissue-engineered sinus valve for transcatheter pulmonary valve replacement: a proof-of-concept study. J. Cardiovasc. Transl. Res. 11, 182–191. doi: 10.1007/s12265-018-9800-6
Motta, S. E., Fioretta, E. S., Lintas, V., Dijkman, P. E., Hilbe, M., Frese, L., et al. (2020). Geometry influences inflammatory host cell response and remodeling in tissue-engineered heart valves in-vivo. Sci. Rep. 10:19882.
Motta, S. E., Lintas, V., Fioretta, E. S., Dijkman, P. E., Putti, M., Caliskan, E., et al. (2019). Human cell-derived tissue-engineered heart valve with integrated Valsalva sinuses: towards native-like transcatheter pulmonary valve replacements. npj Regen Med. 4:14.
Murry, C. E., Wiseman, R. W., Schwartz, S. M., and Hauschka, S. D. (1996). Skeletal myoblast transplantation for repair of myocardial necrosis. J. Clin. Invest. 98, 2512–2523. doi: 10.1172/jci119070
Musunuru, K. (2013). Genome editing of human pluripotent stem cells to generate human cellular disease models. Dis. Model Mech. 6, 896–904. doi: 10.1242/dmm.012054
Musunuru, K. (2017a). Genome editing: the recent history and perspective in cardiovascular diseases. J. Am. Coll. Cardiol. 70, 2808–2821.
Musunuru, K. (2017b). The hope and hype of CRISPR-Cas9 genome editing: a review. JAMA Cardiol. 2, 914–919. doi: 10.1001/jamacardio.2017.1713
Naeem, M., Majeed, S., Hoque, M. Z., and Ahmad, I. (2020). Latest developed strategies to minimize the off-target effects in CRISPR-Cas-mediated genome editing. Cells 9:1608. doi: 10.3390/cells9071608
Nakayama, K. H., Joshi, P. A., Lai, E. S., Gujar, P., Joubert, L. M., Chen, B., et al. (2015). Bilayered vascular graft derived from human induced pluripotent stem cells with biomimetic structure and function. Regen. Med. 10, 745–755. doi: 10.2217/rme.15.45
Narsinh, K., Narsinh, K. H., and Wu, J. C. (2011). Derivation of human induced pluripotent stem cells for cardiovascular disease modeling. Circ. Res. 108, 1146–1156. doi: 10.1161/circresaha.111.240374
Nelson, T. J., Martinez-Fernandez, A., Yamada, S., Perez-Terzic, C., Ikeda, Y., and Terzic, A. (2009). Repair of acute myocardial infarction with induced pluripotent stem cells induced by human stemness factors. Circulation 120, 408–416. doi: 10.1161/circulationaha.109.865154
Niknamasl, A., Ostad, S. N., Soleimani, M., Azami, M., Salmani, M. K., Lotfibakhshaiesh, N., et al. (2014). A new approach for pancreatic tissue engineering: human endometrial stem cells encapsulated in fibrin gel can differentiate to pancreatic islet beta-cell. Cell Biol. Int. 38, 1174–1182. doi: 10.1002/cbin.10314
Nikolić, M. Z., Sun, D., and Rawlins, E. L. (2018). Human lung development: recent progress and new challenges. Development 145:dev163485.
Noor, N., Shapira, A., Edri, R., Gal, I., Wertheim, L., and Dvir, T. (2019). 3D printing of personalized thick and perfusable cardiac patches and hearts. Adv. Sci. 6:1900344. doi: 10.1002/advs.201900344
Nugraha, B., Buono, M. F., and Emmert, M. Y. (2018). Modelling human cardiac diseases with 3D organoid. Eur. Heart J. 39, 4234–4237. doi: 10.1093/eurheartj/ehy765
Nugraha, B., Buono, M. F., von Boehmer, L., Hoerstrup, S. P., and Emmert, M. Y. (2019). Human cardiac organoids for disease modeling. Clin. Pharmacol. Ther. 105, 79–85. doi: 10.1002/cpt.1286
Okita, K., Ichisaka, T., and Yamanaka, S. (2007). Generation of germline-competent induced pluripotent stem cells. Nature 448, 313–317. doi: 10.1038/nature05934
Okita, K., Matsumura, Y., Sato, Y., Okada, A., Morizane, A., Okamoto, S., et al. (2011). A more efficient method to generate integration-free human iPS cells. Nat. Methods 8, 409–412. doi: 10.1038/nmeth.1591
Orlova, V. V., Van Den Hil, F. E., Petrus-Reurer, S., Drabsch, Y., Ten Dijke, P., and Mummery, C. L. (2014). Generation, expansion and functional analysis of endothelial cells and pericytes derived from human pluripotent stem cells. Nat. Protoc. 9, 1514–1531. doi: 10.1038/nprot.2014.102
Osafune, K., Caron, L., Borowiak, M., Martinez, R. J., Fitz-Gerald, C. S., Sato, Y., et al. (2008). Marked differences in differentiation propensity among human embryonic stem cell lines. Nat. Biotechnol. 26, 313–315. doi: 10.1038/nbt1383
Ovics, P., Regev, D., Baskin, P., Davidor, M., Shemer, Y., Neeman, S., et al. (2020). Drug development and the use of induced pluripotent stem cell-derived cardiomyocytes for disease modeling and drug toxicity screening. Int. J. Mol. Sci. 21, 1–42. doi: 10.7750/biodiscovery.2015.16.1
Paige, S. L., Osugi, T., Afanasiev, O. K., Pabon, L., Reinecke, H., and Murry, C. E. (2010). Endogenous wnt/β-Catenin signaling is required for cardiac differentiation in human embryonic stem cells. PLoS One 5:e11134. doi: 10.1371/journal.pone.0011134
Paik, D. T., Chandy, M., and Wu, J. C. (2020). Patient and disease–specific induced pluripotent stem cells for discovery of personalized cardiovascular drugs and therapeutics. Pharmacol. Rev. 72, 320–342. doi: 10.1124/pr.116.013003
Park, I. H., Arora, N., Huo, H., Maherali, N., Ahfeldt, T., Shimamura, A., et al. (2008). Disease-specific induced pluripotent stem cells. Cell 134, 877–886.
Park, M., and Yoon, Y. S. (2018). Cardiac regeneration with human pluripotent stem cell-derived cardiomyocytes. Korean Circ. J. 48, 974–988. doi: 10.4070/kcj.2018.0312
Park, S. J., Kim, R. Y., Park, B. W., Lee, S., Choi, S. W., Park, J. H., et al. (2019). Dual stem cell therapy synergistically improves cardiac function and vascular regeneration following myocardial infarction. Nat. Commun. 10:3123.
Parrotta, E. I., Lucchino, V., Scaramuzzino, L., Scalise, S., and Cuda, G. (2020). Modeling cardiac disease mechanisms using induced pluripotent stem cell-derived cardiomyocytes: progress, promises and challenges. Int. J. Mol. Sci. 21, 1–30.
Paschos, N. K., Brown, W. E., Eswaramoorthy, R., Hu, J. C., and Athanasiou, K. A. (2015). Advances in tissue engineering through stem cell-based co-culture. J. Tissue Eng. Regen. Med. 9, 488–503. doi: 10.1002/term.1870
Peng, G. Y., Lin, Y., Li, J. J., Wang, Y., Huang, H. Y., and Shen, Z. Y. (2019). The application of induced pluripotent stem cells in pathogenesis study and gene therapy for vascular disorders: current progress and future challenges. Stem Cells Int. 2019:9613258.
Pepe, G., Giusti, B., Sticchi, E., Abbate, R., Gensini, G. F., and Nistri, S. (2016). Marfan syndrome: current perspectives. Appl. Clin. Genet. 9, 55–65. doi: 10.2147/tacg.s96233
Planat-Benard, V., Silvestre, J. S., Cousin, B., André, M., Nibbelink, M., Tamarat, R., et al. (2004). Plasticity of human adipose lineage cells toward endothelial cells: physiological and therapeutic perspectives. Circulation 109, 656–663. doi: 10.1161/01.cir.0000114522.38265.61
Polini, A., Prodanov, L., Bhise, N. S., Manoharan, V., Dokmeci, M. R., and Khademhosseini, A. (2014). Organs-on-a-chip: a new tool for drug discovery. Expert Opin. Drug Discov. 9, 335–352. doi: 10.1517/17460441.2014.886562
Polonchuk, L., Chabria, M., Badi, L., Hoflack, J. C., Figtree, G., Davies, M. J., et al. (2017). Cardiac spheroids as promising in vitro models to study the human heart microenvironment. Sci. Rep. 7:7005.
Porto, E. M., Komor, A. C., Slaymaker, I. M., and Yeo, G. W. (2020). Base editing: advances and therapeutic opportunities. Nat. Rev. Drug Discov. 19, 839–859. doi: 10.1038/s41573-020-0084-6
Poulis, N., Zaytseva, P., Gähwiler, E. K. N., Motta, S. E., Fioretta, E. S., Cesarovic, N., et al. (2020). Tissue engineered heart valves for transcatheter aortic valve implantation: current state, challenges, and future developments. Expert Rev. Cardiovasc. Ther. 18, 681–696. doi: 10.1080/14779072.2020.1792777
Preininger, M. K., Jha, R., Maxwell, J. T., Wu, Q., Singh, M., Wang, B., et al. (2016). A human pluripotent stem cell model of catecholaminergic polymorphic ventricular tachycardia recapitulates patient-specific drug responses. Dis. Model Mech. 9, 927–939. doi: 10.1242/dmm.026823
Qi, L. S., Larson, M. H., Gilbert, L. A., Doudna, J. A., Weissman, J. S., Arkin, A. P., et al. (2013). Repurposing CRISPR as an RNA-γuided platform for sequence-specific control of gene expression. Cell 152, 1173–1183. doi: 10.1016/j.cell.2013.02.022
Ravichandran, R., Venugopal, J. R., Sundarrajan, S., Mukherjee, S., and Ramakrishna, S. (2013). Cardiogenic differentiation of mesenchymal stem cells on elastomeric poly (glycerol sebacate)/collagen core/shell fibers. World J. Cardiol. 5, 28–41. doi: 10.4330/wjc.v5.i3.28
Richards, D. J., Coyle, R. C., Tan, Y., Jia, J., Wong, K., Toomer, K., et al. (2017). Inspiration from heart development: biomimetic development of functional human cardiac organoids. Biomaterials 142, 112–123. doi: 10.1016/j.biomaterials.2017.07.021
Richards, D. J., Li, Y., Kerr, C. M., Yao, J., Beeson, G. C., Coyle, R. C., et al. (2020). Human cardiac organoids for the modelling of myocardial infarction and drug cardiotoxicity. Nat. Biomed. Eng. 4, 446–462. doi: 10.1038/s41551-020-0539-4
Rincon, M. Y., VandenDriessche, T., and Chuah, M. K. (2015). Gene therapy for cardiovascular disease: advances in vector development, targeting, and delivery for clinical translation. Cardiovasc. Res. 108, 4–20. doi: 10.1093/cvr/cvv205
Risbud, M., Ringe, J., Bhonde, R., and Sittinger, M. (2001). In vitro expression of cartilage-specific markers by chondrocytes on a biocompatible hydrogel: implications for engineering cartilage tissue. Cell Transplant. 10, 755–763. doi: 10.3727/000000001783986224
Rohani, L., Fabian, C., Holland, H., Naaldijk, Y., Dressel, R., Löffler-Wirth, H., et al. (2016). Generation of human induced pluripotent stem cells using non-synthetic mRNA. Stem Cell Res. 16, 662–672. doi: 10.1016/j.scr.2016.03.008
Ronaldson-Bouchard, K., and Vunjak-Novakovic, G. (2018). Organs-on-a-chip: a fast track for engineered human tissues in drug development. Cell Stem Cell 22, 310–324. doi: 10.1016/j.stem.2018.02.011
Ronaldson-Bouchard, K., Ma, S. P., Yeager, K., Chen, T., Song, L. J., Sirabella, D., et al. (2018). Advanced maturation of human cardiac tissue grown from pluripotent stem cells. Nature 556, 239–243. doi: 10.1038/s41586-018-0016-3
Rosales, A. M., and Anseth, K. S. (2016). The design of reversible hydrogels to capture extracellular matrix dynamics. Nat. Rev. Mater. 1:15012.
Roston, T. M., Van Petegem, F., and Sanatani, S. (2017). Catecholaminergic polymorphic ventricular tachycardia: a model for genotype-specific therapy. Curr. Opin. Cardiol. 32, 78–85. doi: 10.1097/hco.0000000000000360
Rouet, P., Smih, F., and Jasin, M. (1994a). Expression of a site-specific endonuclease stimulates homologous recombination in mammalian cells. Proc. Natl. Acad. Sci. U. S. A. 91, 6064–6068. doi: 10.1073/pnas.91.13.6064
Rouet, P., Smih, F., and Jasin, M. (1994b). Introduction of double-strand breaks into the genome of mouse cells by expression of a rare-cutting endonuclease. Mol. Cell Biol. 14, 8096–8106. doi: 10.1128/mcb.14.12.8096
Ruan, J. L., Tulloch, N. L., Razumova, M. V., Saiget, M., Muskheli, V., Pabon, L., et al. (2016). Mechanical stress conditioning and electrical stimulation promote contractility and force maturation of induced pluripotent stem cell-derived human cardiac tissue. Circulation 134, 1557–1567. doi: 10.1161/circulationaha.114.014998
Sadahiro, T. (2019). Cardiac regeneration with pluripotent stem cell-derived cardiomyocytes and direct cardiac reprogramming. Regen. Ther. 11, 95–100. doi: 10.1016/j.reth.2019.06.004
Sakuma, T., Mochida, K., Nakade, S., Ezure, T., Minagawa, S., and Yamamoto, T. (2018). Unexpected heterogeneity derived from Cas9 ribonucleoprotein-introduced clonal cells at the HPRT1 locus. Genes Cells 23, 255–263. doi: 10.1111/gtc.12569
Sala, L., Gnecchi, M., and Schwartz, P. J. (2019). Long QT syndrome modelling with cardiomyocytes derived from human-induced pluripotent stem cells. Arrhythm. Electrophysiol. Rev. 8, 105–110. doi: 10.15420/aer.2019.1.1
Sander, J. D., and Joung, J. K. (2014). CRISPR-Cas systems for editing, regulating and targeting genomes. Nat. Biotechnol. 32, 347–350. doi: 10.1038/nbt.2842
Šarić, T., Frenzel, L. P., and Hescheler, J. (2008). Immunological barriers to embryonic stem cell-derived therapies. Cells Tissues Organs 188, 78–90. doi: 10.1159/000118784
Sasaki, K., Makiyama, T., Yoshida, Y., Wuriyanghai, Y., Kamakura, T., Nishiuchi, S., et al. (2016). Patient-specific human induced pluripotent stem cell model assessed with electrical pacing validates S107 as a potential therapeutic agent for catecholaminergic polymorphic ventricular tachycardia. PLoS One 11:e0164795. doi: 10.1371/journal.pone.0164795
Sato, T., Vries, R. G., Snippert, H. J., Van De Wetering, M., Barker, N., Stange, D. E., et al. (2009). Single Lgr5 stem cells build crypt-villus structures in vitro without a mesenchymal niche. Nature 459, 262–265. doi: 10.1038/nature07935
Savoji, H., Mohammadi, M. H., Rafatian, N., Toroghi, M. K., Wang, E. Y., Zhao, Y., et al. (2019). Cardiovascular disease models: a game changing paradigm in drug discovery and screening. Biomaterials 198, 3–26. doi: 10.1016/j.biomaterials.2018.09.036
Sawa, Y., Miyagawa, S., Sakaguchi, T., Fujita, T., Matsuyama, A., Saito, A., et al. (2012). Tissue engineered myoblast sheets improved cardiac function sufficiently to discontinue LVAS in a patient with DCM: report of a case. Surg. Today 42, 181–184. doi: 10.1007/s00595-011-0106-4
Schlaeger, T. M., Daheron, L., Brickler, T. R., Entwisle, S., Chan, K., Cianci, A., et al. (2015). A comparison of non-integrating reprogramming methods. Nat. Biotechnol. 33, 58–63.
Schmidt, D., and Hoerstrup, S. P. (2006). Tissue engineered heart valves based on human cells. Swiss Med. Wkly. 136, 618–623.
Schmidt, D., Dijkman, P. E., Driessen-Mol, A., Stenger, R., Mariani, C., Puolakka, A., et al. (2010). Minimally-invasive implantation of living tissue engineered heart valves: a comprehensive approach from autologous vascular cells to stem cells. J. Am. Coll. Cardiol. 56, 510–520. doi: 10.1016/j.jacc.2010.04.024
Schultheiss, H. P., Fairweather, D. L., Caforio, A. L. P., Escher, F., Hershberger, R. E., Lipshultz, S. E., et al. (2019). Dilated cardiomyopathy. Nat. Rev. Dis. Prim. 5:32.
Schwank, G., Koo, B. K., Sasselli, V., Dekkers, J. F., Heo, I., Demircan, T., et al. (2013). Functional repair of CFTR by CRISPR/Cas9 in intestinal stem cell organoids of cystic fibrosis patients. Cell Stem Cell 13, 653–658. doi: 10.1016/j.stem.2013.11.002
Seeger, T., Shrestha, R., Lam, C. K., Chen, C., McKeithan, W. L., Lau, E., et al. (2019). A premature termination codon mutation in MYBPC3 causes hypertrophic cardiomyopathy via chronic activation of nonsense-mediated decay. Circulation 139, 799–811. doi: 10.1161/circulationaha.118.034624
Sepulveda, P., Jones, J. R., and Hench, L. L. (2002). Bioactive sol-gel foams for tissue repair. J. Biomed. Mater. Res. 59, 340–348. doi: 10.1002/jbm.1250
Shah, D., Virtanen, L., Prajapati, C., Kiamehr, M., Gullmets, J., West, G., et al. (2019). Modeling of LMNA-related dilated cardiomyopathy using human induced pluripotent stem cells. Cells 8:594. doi: 10.3390/cells8060594
Sharma, A., McKeithan, W. L., Serrano, R., Kitani, T., Burridge, P. W., and del, et al. (2018). Use of human induced pluripotent stem cell–derived cardiomyocytes to assess drug cardiotoxicity. Nat. Protoc. 13, 3018–3041.
Shi, C., Li, Q., Zhao, Y., Chen, W., Chen, B., Xiao, Z., et al. (2011). Stem-cell-capturing collagen scaffold promotes cardiac tissue regeneration. Biomaterials 32, 2508–2515. doi: 10.1016/j.biomaterials.2010.12.026
Shiba, Y., Gomibuchi, T., Seto, T., Wada, Y., Ichimura, H., Tanaka, Y., et al. (2016). Allogeneic transplantation of iPS cell-derived cardiomyocytes regenerates primate hearts. Nature 538, 388–391. doi: 10.1038/nature19815
Singh, D. D., Hawkins, R. D., Lahesmaa, R., and Tripathi, S. K. (2019). CRISPR/Cas9 guided genome and epigenome engineering and its therapeutic applications in immune mediated diseases. Semin. Cell Dev. Biol. 96, 32–43. doi: 10.1016/j.semcdb.2019.05.007
Singh, K., Evens, H., Nair, N., Rincón, M. Y., Sarcar, S., Samara-Kuko, E., et al. (2018). Efficient in vivo liver-directed gene editing using CRISPR/Cas9. Mol. Ther. 26, 1241–1254.
Sisakian, H. (2014). Cardiomyopathies: evolution of pathogenesis concepts and potential for new therapies. World J. Cardiol. 6:478. doi: 10.4330/wjc.v6.i6.478
Siu, C. W., Lee, Y. K., Ho, J. C. Y., Lai, W. H., Chan, Y. C., Ng, K. M., et al. (2012). Modeling of lamin A/C mutation premature cardiac aging using patient-specific induced pluripotent stem cells. Aging 4, 803–822. doi: 10.18632/aging.100503
Skrzynia, C., Berg, J., Willis, M., and Jensen, B. (2014). Genetics and heart failure: a concise guide for the clinician. Curr. Cardiol. Rev. 11, 10–17. doi: 10.2174/1573403x09666131117170446
Smith, C., Gore, A., Yan, W., Abalde-Atristain, L., Li, Z., He, C., et al. (2014). Whole-genome sequencing analysis reveals high specificity of CRISPR/Cas9 and TALEN-based genome editing in human iPSCs. Cell Stem Cell 15, 12–13. doi: 10.1016/j.stem.2014.06.011
Smith, L. E., and White, M. Y. (2014). The role of post-translational modifications in acute and chronic cardiovascular disease. Proteomics Clin. Appl. 8, 506–521. doi: 10.1002/prca.201400052
Smithies, O., Gregg, R. G., Boggst, S. S., Koralewski, M. A., and Kucherlapati, R. S. (1985). Insertion of DNA sequences into the locus by homologous recombination. Nature 317, 230–236. doi: 10.1038/317230a0
Spears, D. A., and Gollob, M. H. (2015). Genetics of inherited primary arrhythmia disorders. Appl. Clin. Genet. 8, 215–233. doi: 10.2147/tacg.s55762
Spitalieri, P., Talarico, R. V., Caioli, S., Murdocca, M., Serafino, A., Girasole, M., et al. (2018). Modelling the pathogenesis of myotonic dystrophy type 1 cardiac phenotype through human iPSC-derived cardiomyocytes. J. Mol. Cell Cardiol. 118, 95–109. doi: 10.1016/j.yjmcc.2018.03.012
Stevens, K. R., Pabon, L., Muskheli, V., and Murry, C. E. (2009). Scaffold-free human cardiac tissue patch created from embryonic stem cells. Tissue Eng. 15, 1211–1222. doi: 10.1089/ten.tea.2008.0151
Streckfuss-Bömeke, K., Tiburcy, M., Fomin, A., Luo, X., Li, W., Fischer, C., et al. (2017). Severe DCM phenotype of patient harboring RBM20 mutation S635A can be modeled by patient-specific induced pluripotent stem cell-derived cardiomyocytes. J. Mol. Cell Cardiol. 113, 9–21. doi: 10.1016/j.yjmcc.2017.09.008
Sun, N., Yazawa, M., Liu, J., Han, L., Sanchez-Freire, V., Abilez, O. J., et al. (2012). Patient-specific induced pluripotent stem cells as a model for familial dilated cardiomyopathy. Sci. Transl. Med. 4:130ra47.
Swan, H., Piippo, K., Viitasalo, M., Heikkilä, P., Paavonen, T., Kainulainen, K., et al. (1999). Arrhythmic disorder mapped to chromosome 1q42-q43 causes malignant polymorphic ventricular tachycardia in structurally normal hearts. J. Am. Coll. Cardiol. 34, 2035–2042. doi: 10.1016/s0735-1097(99)00461-1
Tada, M., Takahama, Y., Abe, K., Nakatsuji, N., and Tada, T. (2001). Nuclear reprogramming of somatic cells by in vitro hybridization with ES cells. Curr. Biol. 11, 1553–1558. doi: 10.1016/s0960-9822(01)00459-6
Takahashi, K., and Yamanaka, S. (2006). Induction of pluripotent stem cells from mouse embryonic and adult fibroblast cultures by defined factors. Cell 126, 663–676. doi: 10.1016/j.cell.2006.07.024
Takahashi, K., Tanabe, K., Ohnuki, M., Narita, M., Ichisaka, T., Tomoda, K., et al. (2007). Induction of pluripotent stem cells from adult human fibroblasts by defined factors. Cell 131, 861–872. doi: 10.1016/j.cell.2007.11.019
Takeda, M., Miyagawa, S., Fukushima, S., Saito, A., Ito, E., Harada, A., et al. (2018). Development of in vitro drug-induced cardiotoxicity assay by using three-dimensional cardiac tissues derived from human induced pluripotent stem cells. Tissue Eng. Part C Methods 24, 56–67. doi: 10.1089/ten.tec.2017.0247
Talkhabi, M., Aghdami, N., and Baharvand, H. (2016). Human cardiomyocyte generation from pluripotent stem cells: a state-of-art. Life Sci. 145, 98–113. doi: 10.1016/j.lfs.2015.12.023
Tan, Y., Richards, D., Coyle, R. C., Yao, J., Xu, R., Gou, W., et al. (2017). Cell number per spheroid and electrical conductivity of nanowires influence the function of silicon nanowired human cardiac spheroids. Acta Biomater. 51, 495–504. doi: 10.1016/j.actbio.2017.01.029
Terns, M. P., and Terns, R. M. (2011). CRISPR-based adaptive immune systems. Curr. Opin. Microbiol. 14, 321–327. doi: 10.1016/j.mib.2011.03.005
Terrenoire, C., Wang, K., Chan Tung, K. W., Chung, W. K., Pass, R. H., Lu, J. T., et al. (2013). Induced pluripotent stem cells used to reveal drug actions in a long QT syndrome family with complex genetics. J. Gen. Physiol. 141, 61–72. doi: 10.1085/jgp.201210899
Tesson, L., Usal, C., Meqìnoret, S., Leung, E., Niles, B. J., Remy, S., et al. (2011). Knockout rats generated by embryo microinjection of TALENs. Nat. Biotechnol. 29, 695–696. doi: 10.1038/nbt.1940
Theodoris, C. V., Li, M., White, M. P., Liu, L., He, D., Pollard, K. S., et al. (2015). Human disease modeling reveals integrated transcriptional and epigenetic mechanisms of NOTCH1 haploinsufficiency. Cell 160, 1072–1086. doi: 10.1016/j.cell.2015.02.035
Thiene, G., Corrado, D., and Basso, C. (2007). Arrhythmogenic right ventricular cardiomyopathy/dysplasia. Orphanet. J. Rare Dis. 2, 1–16. doi: 10.1007/978-88-470-0490-0_1
Thomson, J. A., Itskovitz-Eldor, J., Shapiro, S. S., Waknitz, M. A., Swiergiel, J. J., and Marshall, V. S. (1998). Embryonic stem cell lines derived from human blastocysts. Science 282, 1145–1147. doi: 10.1126/science.282.5391.1145
Till, J. E., and McCulloch, E. A. (1961). A direct measurement of the radiation sensitivity of normal mouse bone marrow cells author. Radiat Res. Soc. 14, 213–222. doi: 10.2307/3570892
Tse, H. F., Ho, J. C. Y., Choi, S. W., Lee, Y. K., Butler, A. W., Ng, K. M., et al. (2013). Patient-specific induced-pluripotent stem cells-derived cardiomyocytes recapitulate the pathogenic phenotypes of dilated cardiomyopathy due to a novel DES mutation identified by whole exome sequencing. Hum. Mol. Genet. 22, 1395–1403. doi: 10.1093/hmg/dds556
Tycko, J., Myer, V. E., and Hsu, P. D. (2016). Methods for optimizing CRISPR-Cas9 genome editing specificity. Mol. Cell 63, 355–370. doi: 10.1016/j.molcel.2016.07.004
Veerman, C. C., Kosmidis, G., Mummery, C. L., Casini, S., Verkerk, A. O., and Bellin, M. (2015). Immaturity of human stem-cell-derived cardiomyocytes in culture: fatal flaw or soluble problem? Stem Cells Dev. 24, 1035–1052. doi: 10.1089/scd.2014.0533
Veres, A., Gosis, B. S., Ding, Q., Collins, R., Ragavendran, A., Brand, H., et al. (2014). Low incidence of Off-target mutations in individual CRISPR-Cas9 and TALEN targeted human stem cell clones detected by whole-genome sequencing. Cell Stem Cell 15, 27–30. doi: 10.1016/j.stem.2014.04.020
Voges, H. K., Mills, R. J., Elliott, D. A., Parton, R. G., Porrello, E. R., and Hudson, J. E. (2017). Development of a human cardiac organoid injury model reveals innate regenerative potential. Development 144, 1118–1127. doi: 10.1242/dev.143966
Vunjak-Novakovic, G., Lui, K. O., Tandon, N., and Chien, K. R. (2011). Bioengineering heart muscle: a paradigm for regenerative medicine. Annu. Rev. Biomed. Eng. 13, 245–267. doi: 10.1146/annurev-bioeng-071910-124701
Wang, G., McCain, M. L., Yang, L., He, A., Pasqualini, F. S., Agarwal, A., et al. (2014a). Modeling the mitochondrial cardiomyopathy of Barth syndrome with iPSC and heart-on-chip technologies. J. Musculoskelet. Neuronal. Interact. 15, 616–623.
Wang, G., McCain, M. L., Yang, L., He, A., Pasqualini, F. S., Agarwal, A., et al. (2014b). Modeling the mitochondrial cardiomyopathy of barth syndrome with induced pluripotent stem cell and heart-on-chip technologies. Nat. Med. 20, 616–623. doi: 10.1038/nm.3545
Wang, H., Hao, J., and Hong, C. C. (2011). Cardiac induction of embryonic stem cells by a small molecule inhibitor of Wnt/β-catenin signaling. ACS Chem. Biol. 6, 192–197. doi: 10.1021/cb100323z
Wang, H., Yang, H., Shivalila, C. S., Dawlaty, M. M., Cheng, A. W., Zhang, F., et al. (2013). One-step generation of mice carrying mutations in multiple genes by CRISPR/cas-mediated genome engineering. Cell 153, 910–918. doi: 10.1016/j.cell.2013.04.025
Wang, Y., Liang, P., Lan, F., Wu, H., Lisowski, L., Gu, M., et al. (2014c). Genome editing of isogenic human induced pluripotent stem cells recapitulates long QT phenotype for drug testing. J. Am. Coll. Cardiol. 64, 451–459. doi: 10.1016/j.jacc.2014.04.057
Wang, Z., Su, X., Ashraf, M., Kim, I. M., Weintraub, N. L., Jiang, M., et al. (2018). Regenerative therapy for cardiomyopathies. J. Cardiovasc. Transl. Res. 11, 357–365. doi: 10.1007/s12265-018-9807-z
Weber, B., Emmert, M. Y., Behr, L., Schoenauer, R., Drögemüller, C., et al. (2012a). Prenatally engineered autologous amniotic fluid stem cell-based heart valves in the fetal circulation. Biomaterials 33, 4031–4043. doi: 10.1016/j.biomaterials.2011.11.087
Weber, B., Emmert, M. Y., and Hoerstrup, S. P. (2012b). Stem cells for heart valve regeneration. Swiss Med. Wkly. 142:w13622.
Weber, B., Scherman, J., Emmert, M. Y., Gruenenfelder, J., Verbeek, R., Bracher, M., et al. (2011). Injectable living marrow stromal cell-based autologous tissue engineered heart valves: first experiences with a one-step intervention in primates. Eur. Heart J. 32, 2830–2840. doi: 10.1093/eurheartj/ehr059
Wendel, J. S., Ye, L., Tao, R., Zhang, J., Zhang, J., Kamp, T. J., et al. (2015). Functional effects of a tissue-engineered cardiac patch from human induced pluripotent stem cell-derived cardiomyocytes in a rat infarct model. Stem Cells Transl. Med. 4, 1324–1332. doi: 10.5966/sctm.2015-0044
Wendel, J. S., Ye, L., Zhang, P., Tranquillo, R. T., and Zhang, J. J. (2014). Functional consequences of a tissue-engineered myocardial patch for cardiac repair in a rat infarct model. Tissue Eng. 20, 1325–1335. doi: 10.1089/ten.tea.2013.0312
Wexler, R., Elton, T., Pleister, A., and Feldman, D. (2009). Cardiomyopathy: an overview. Am. Fam. Physician. 79, 778–784.
Wilmut, I., Schnieke, A. E., McWhir, J., Kind, A. J., and Campbell, K. H. S. (1997). Viable offspring derived from fetal and adult mammalian cells. Nature. 386, 810–813. doi: 10.1038/385810a0
Wimmer, R. A., Leopoldi, A., Aichinger, M., Wick, N., Hantusch, B., Novatchkova, M., et al. (2019). Human blood vessel organoids as a model of diabetic vasculopathy. Nature 565, 505–510. doi: 10.1038/s41586-018-0858-8
Wobus, A. M., and Boheler, K. R. (2005). Embryonic stem cells: prospects for developmental biology and cell therapy. Physiol. Rev. 85, 635–678. doi: 10.1152/physrev.00054.2003
Wobus, A. M., and Löser, P. (2011). Present state and future perspectives of using pluripotent stem cells in toxicology research. Arch. Toxicol. 85, 79–117. doi: 10.1007/s00204-010-0641-6
Wolinetz, C. D., and Collins, F. S. (2020). Recognition of research participants’ need for autonomyremembering the legacy of henrietta lacks. J. Am. Med. Assoc. 324, 1027–1028. doi: 10.1001/jama.2020.15936
Wu, H., Lee, J., Vincent, L. G., Wang, Q., Gu, M., Lan, F., et al. (2015). Epigenetic regulation of phosphodiesterases 2A and 3A underlies compromised β-adrenergic signaling in an iPSC model of dilated cardiomyopathy. Cell Stem Cell 17, 89–100. doi: 10.1016/j.stem.2015.04.020
Wyles, S. P., Li, X., Hrstka, S. C., Reyes, S., Oommen, S., Beraldi, R., et al. (2016). Modeling structural and functional deficiencies of RBM20 familial dilated cardiomyopathy using human induced pluripotent stem cells. Hum. Mol. Genet. 25, 254–265. doi: 10.1093/hmg/ddv468
Wyman, C., and Kanaar, R. (2006). DNA double-strand break repair: all’s well that ends well. Annu. Rev. Genet. 40, 363–383. doi: 10.1146/annurev.genet.40.110405.090451
Yan, Y., Bejoy, J., Xia, J., Griffin, K., Guan, J., and Li, Y. (2019). Cell population balance of cardiovascular spheroids derived from human induced pluripotent stem cells. Sci. Rep. 9, 1–1295.
Yang, K. C., Breitbart, A., De Lange, W. J., Hofsteen, P., Futakuchi-Tsuchida, A., Xu, J., et al. (2018). Novel adult-onset systolic cardiomyopathy due to MYH7 E848G mutation in patient-derived induced pluripotent stem cells. JACC Basic Transl. Sci. 3, 728–740. doi: 10.1016/j.jacbts.2018.08.008
Yang, L., Soonpaa, M. H., Adler, Roepke, T. K., Kattman, S. J., Kennedy, M., et al. (2008). Human cardiovascular progenitor cells develop from a KDR+ embryonic-stem-cell-derived population. Nature 453, 524–528. doi: 10.1038/nature06894
Yang, X., Rodriguez, M., Pabon, L., Fischer, K. A., Reinecke, H., Regnier, M., et al. (2014). Tri-iodo-l-thyronine promotes the maturation of human cardiomyocytes-derived from induced pluripotent stem cells. J. Mol. Cell Cardiol. 72, 296–304. doi: 10.1016/j.yjmcc.2014.04.005
Ye, L., Chang, Y. H., Xiong, Q., Zhang, P., Zhang, L., Somasundaram, P., et al. (2014). Cardiac repair in a porcine model of acute myocardial infarction with human induced pluripotent stem cell-derived cardiovascular cells. Cell Stem Cell 15, 750–761. doi: 10.1016/j.stem.2014.11.009
Yoshihara, M., Hayashizaki, Y., and Murakawa, Y. (2017). Genomic instability of iPSCs: challenges towards their clinical applications. Stem Cell Rev. Rep. 13, 7–16. doi: 10.1007/s12015-016-9680-6
Yu, J., Vodyanik, M. A., Smuga-Otto, K., Antosiewicz-Bourget, J., Frane, J. L., Tian, S., et al. (2007). Induced pluripotent stem cell lines derived from human somatic cells. Science 318, 1917–1920.
Zakrzewski, W., Dobrzyński, M., Szymonowicz, M., and Rybak, Z. (2019). Stem cells: past, present and future. Stem Cell Res. Ther. 128, 329–332.
Zhang, J., Klos, M., Wilson, G. F., Herman, A. M., Lian, X., Raval, K. K., et al. (2012). Extracellular matrix promotes highly efficient cardiac differentiation of human pluripotent stem cells: the matrix sandwich method. Circ. Res. 111, 1125–1136. doi: 10.1161/circresaha.112.273144
Zhang, J., Wilson, G. F., Soerens, A. G., Koonce, C. H., Yu, J., Palecek, S. P., et al. (2009). Functional cardiomyocytes derived from human induced pluripotent stem cells. Circ. Res. 104, 30–41.
Zhang, M., D’Aniello, C., Verkerk, A. O., Wrobel, E., Frank, S., Ward-Van Oostwaard, D., et al. (2014). Recessive cardiac phenotypes in induced pluripotent stem cell models of jervell and lange-nielsen syndrome: disease mechanisms and pharmacological rescue. Proc. Natl. Acad. Sci. U. S. A. 111, E5383–E5392.
Zhao, T., Zhang, Z. N., Rong, Z., and Xu, Y. (2011). Immunogenicity of induced pluripotent stem cells. Nature 474, 212–216.
Zhao, W., Lei, A., Tian, L., Wang, X., Correia, C., Weiskittel, T., et al. (2020). Strategies for genetically engineering hypoimmunogenic universal pluripotent stem cells. iScience 23:101162. doi: 10.1016/j.isci.2020.101162
Zimmermann, W. H., Didié, M., Wasmeier, G. H., Nixdorff, U., Hess, A., Melnychenko, I., et al. (2002a). Cardiac grafting of engineered heart tissue in syngenic rats. Circulation (13 Suppl.), 151–157.
Zimmermann, W. H., Melnychenko, I., and Eschenhagen, T. (2004). Engineered heart tissue for regeneration of diseased hearts. Biomaterials 25, 1639–1647. doi: 10.1016/s0142-9612(03)00521-0
Zimmermann, W. H., Schneiderbanger, K., Schubert, P., Didié, M., Münzel, F., Heubach, J. F., et al. (2002b). Tissue engineering of a differentiated cardiac muscle construct. Circ. Res. 90, 223–230. doi: 10.1161/hh0202.103644
Zuppinger, C. (2019). 3D cardiac cell culture: a critical review of current technologies and applications. Front. Cardiovasc. Med. 6:87.
Keywords: human induced pluripotent stem cells (hiPSCs), CRISPR-Cas9, cardiovascular tissue engineering, regenerative medicine, cardiovascular disease modeling, 3D cell culture systems
Citation: Gähwiler EKN, Motta SE, Martin M, Nugraha B, Hoerstrup SP and Emmert MY (2021) Human iPSCs and Genome Editing Technologies for Precision Cardiovascular Tissue Engineering. Front. Cell Dev. Biol. 9:639699. doi: 10.3389/fcell.2021.639699
Received: 09 December 2020; Accepted: 31 March 2021;
Published: 28 June 2021.
Edited by:
Delilah Hendriks, Hubrecht Institute (KNAW), NetherlandsReviewed by:
Lei Ye, National Heart Centre Singapore, SingaporeHidetoshi Masumoto, RIKEN Center for Biosystems Dynamics Research (BDR), Japan
Copyright © 2021 Gähwiler, Motta, Martin, Nugraha, Hoerstrup and Emmert. This is an open-access article distributed under the terms of the Creative Commons Attribution License (CC BY). The use, distribution or reproduction in other forums is permitted, provided the original author(s) and the copyright owner(s) are credited and that the original publication in this journal is cited, in accordance with accepted academic practice. No use, distribution or reproduction is permitted which does not comply with these terms.
*Correspondence: Maximilian Y. Emmert, maximilian.emmert@irem.uzh.ch