- 1School of Basic Medical Sciences, Wuhan University, Wuhan, China
- 2School of Life Sciences, Fudan University, Shanghai, China
Atherosclerotic cardiovascular disease is one of the leading causes of death worldwide. Establishing animal models of atherosclerosis is of great benefit for studying its complicated pathogenesis and screening and evaluating related drugs. Although researchers have generated a variety of models for atherosclerosis study in rabbits, mice and rats, the limitations of these models make it difficult to monitor the development of atherosclerosis, and these models are unsuitable for large scale screening of potential therapeutic targets. On the contrast, zebrafish can fulfill these purposes thanks to their fecundity, rapid development ex utero, embryonic transparency, and conserved lipid metabolism process. Thus, zebrafish have become a popular alternative animal model for atherosclerosis research. In this mini review, we summarize different zebrafish models used to study atherosclerosis, focusing on the latest applications of these models to the dynamic monitoring of atherosclerosis progression, mechanistic study of therapeutic intervention and drug screening, and assessment of the impacts of other risk factors.
Introduction
Atherosclerosis (AS) is the pathological basis for many cardiovascular diseases (CVDs), presenting a severe threat to human health (Lusis, 2000; Thomas et al., 2018). AS is characterized by a large amount of lipid deposition in arterial blood vessels which forms plaques and causes muscular elastic artery stenosis, resulting in lumen occlusion or ruptured hemorrhage. The pathological process of AS is very complicated (Libby et al., 2011). First, excessive low-density lipoprotein (LDL) gradually accumulates underneath the endothelium and becomes oxidized LDL (Ox-LDL), which induces inflammation and results in excessive chemokines released from endothelial cells (Di Pietro et al., 2016). Attracted by chemokines, monocytes migrate to the intima of blood vessels and become macrophages upon the stimulation of macrophage colony-stimulating factors (M-CSFs) (Shao et al., 2016). Macrophages then engulf Ox-LDL and transform into foam cells, which release a number of factors that induce smooth muscle cell (SMC) migration and the formation of a fibrous cap (Sakakura et al., 2013). Subsequently, SMCs are gradually lost from the fibrous cap, while infiltrating macrophages degrade the collagen-rich cap matrix. These two mechanisms result in thinning of the fibrous cap, which leads to plaque rupture and thrombosis (Bentzon et al., 2014; Ziegler et al., 2019).
Animal models are essential for exploring the complex molecular mechanism of AS, which are conducive to studying the occurrence and development of AS, and to assessing the therapeutic effects of diet and drug interventions on AS (Fuster et al., 2012; Lee et al., 2017a). From the early 20th century to the era of new millennium, researchers have utilized a variety of animals in AS models for over 100 years, including rabbits, mice, rats, pigs and non-human primates (Lee et al., 2017a; Vedder et al., 2020). Large animal models such as pigs and non-human primates are suitable for AS research because their vascular lesion morphology and lipid metabolism are similar to that in humans (Rapacz et al., 1986; Getz and Reardon, 2012; Laila et al., 2018). The disadvantages of these models include long modeling times, high cost, complex experimental procedures, and difficulties in obtaining large amounts of data (Lee et al., 2017a). Small mammalian animals such as rabbits and mice are cheaper to rear, and mice can be easily manipulated genetically (Mushenkova et al., 2019; Poznyak et al., 2020). However, long-term fat-fed rabbits are prone to hepatotoxicity and a severe inflammatory response, and the plasma lipid profiles differ considerably among inbred strains of mice and also among different mouse mutants, rendering large variation in their susceptibility to AS (Paigen et al., 1985; Getz and Reardon, 2012; Fan et al., 2015; Golforoush et al., 2020; Vedder et al., 2020). Thus, no single animal model is sufficient for AS research; therefore, additional novel animal models are required.
Zebrafish Models for Atherosclerosis Research
Zebrafish have proven to be an excellent animal model for developmental studies and human disease modeling (Lieschke and Currie, 2007; Gut et al., 2017). The advantages of zebrafish, including small body size, low rearing cost, large numbers of offspring, ex utero fertilization and rapid development, render it feasible and affordable to perform large scale screening of candidate targets (Gut et al., 2017). Furthermore, embryonic transparency facilitates dynamic monitoring of various cellular processes in vivo through live imaging with fluorescent reporter lines (Lieschke and Currie, 2007; Holtzman et al., 2016). Zebrafish are poikilothermic vertebrates that favor lipids as a source of energy, whereas homeothermic mammals favor carbohydrates (Stoletov et al., 2009). Although the plasma lipid profiles differ largely between zebrafish and humans, the lipid metabolism process is highly conserved which has been extensively discussed in previous reviews (Holtta-Vuori et al., 2010; Fang et al., 2014; Schlegel, 2016; Figure 1). In short, the genes involved in lipoprotein and lipid metabolism, such as apob, apoe, apoa1, ldlr, apoc2, lpl, lcat and cetp, are conserved in zebrafish (Anderson et al., 2011; Fang et al., 2014). Notably, the Apob protein in zebrafish is equivalent to APOB100, not APOB48, in humans (Otis et al., 2015). Since LDL and very-low-density lipoprotein (VLDL) containing APOB100 have a longer half-life in plasma, zebrafish are more likely to develop AS (Babin and Vernier, 1989; Stoletov et al., 2009; Fang et al., 2010). Furthermore, cholesteryl ester transfer protein (CETP), an enzyme whose net effect favors AS development in humans, is expressed in zebrafish but not in mice (Fang et al., 2014). Compared to other AS animal models, the sharp increase in oxidized lipoproteins is most significant in zebrafish fed a high cholesterol diet (HCD), making them excellent models for investigating the mechanism of lipoprotein oxidation (Stoletov et al., 2009; Fang et al., 2010). However, zebrafish models also possess their own drawbacks. Blood samples can be collected only in small quantity from zebrafish that are older than 45 days, homogenates of several larvae are used instead for studies at early developmental stages. The lipoprotein profile and LDL makeup are different and no late-stage lesion is observed in zebrafish AS models (Stoletov et al., 2009; Fang and Miller, 2012, 2013). Nevertheless, zebrafish have become a popular alternative animal model for AS research, especially for early progression of AS (Holtta-Vuori et al., 2010; Vedder et al., 2020). Thus, several zebrafish models have been established and are widely used (Figure 1).
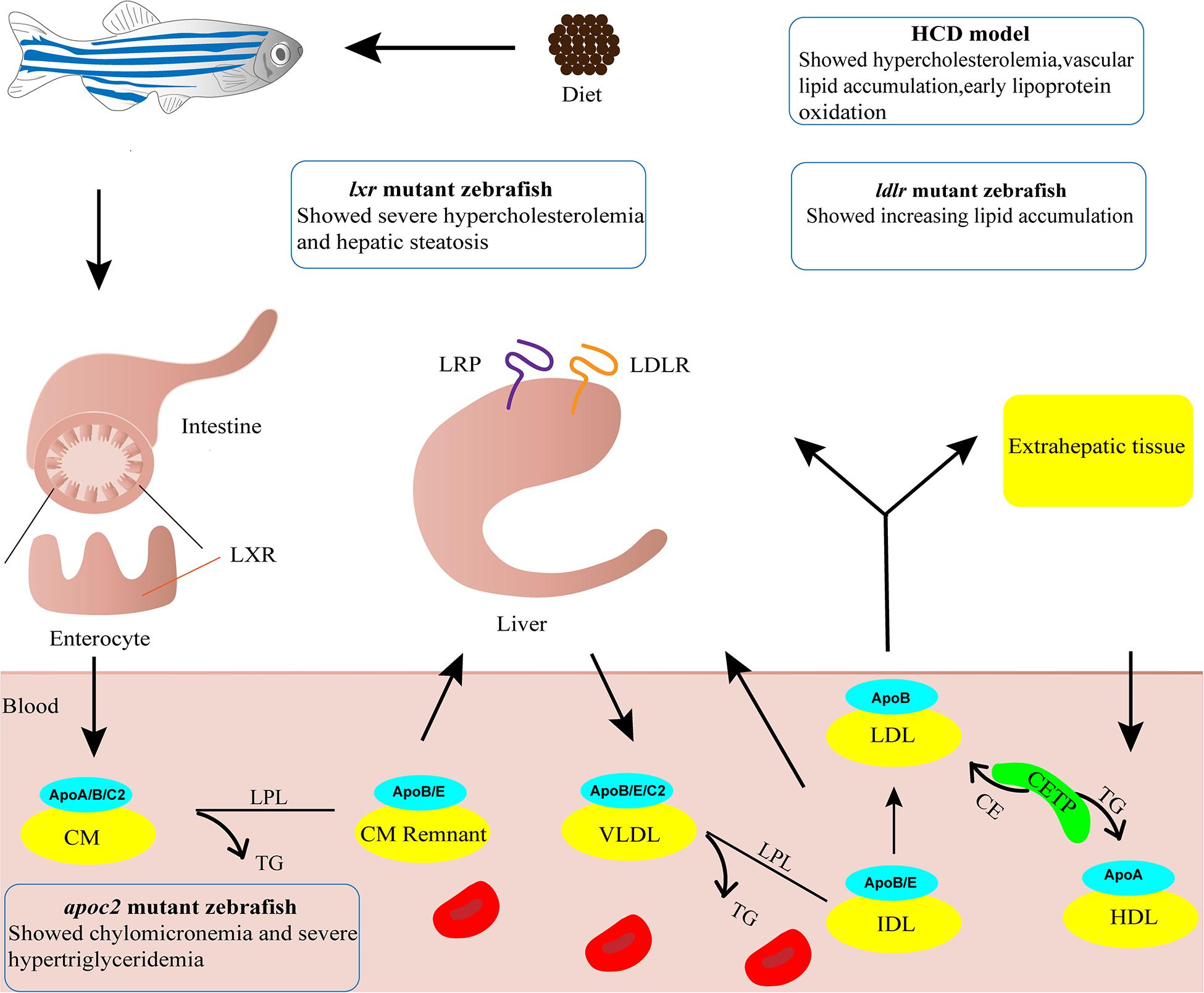
Figure 1. Zebrafish models for atherosclerosis research. Cartoon shows lipid metabolism and transport of lipoproteins in zebrafish. The phenotypes of four zebrafish models used in atherosclerosis research are indicated. ApoA/B/C2/E, Apolipoprotein A/B/C2/E; CE, cholesteryl ester; CETP, cholesteryl ester transfer protein; CM, chylomicron; CM Remnant, chylomicron remnant; HCD, high cholesterol diet; HDL, high-density lipoprotein; IDL, intermediate-density lipoprotein; LDL, low-density lipoprotein; LDLR, low-density lipoprotein receptor; LPL, lipoprotein lipase; LRP, low density lipoprotein receptor-related protein; LXR, liver x receptor; TG, triglyceride; VLDL, very-low-density lipoprotein.
High-Cholesterol Diet Model
Adult zebrafish subject to 8–12 weeks of 4% (w/w) HCD feeding are susceptible to developing hypercholesterolemia, with plasma total cholesterol level reaching 800 mg/dL, a 4-fold increase compared to normal diet (ND)-fed fish (Stoletov et al., 2009). Vascular lesions of enlarged intima with accumulation of lipid and cell infiltration can be found in sections of dorsal aorta. Such lesions are classified as fatty streaks which equal to type II AS lesions in humans, the early stage of type I-VI lesions (Stary et al., 1995; Stoletov et al., 2009). Upon a 10-day challenge with HCD, zebrafish larvae display vascular lipid accumulation, myeloid cell aggregation, and early lipoprotein oxidation (Stoletov et al., 2009; Fang et al., 2010). The vascular lipid accumulation is dose-dependent upon feeding various concentration of cholesterol (2–10%), with most reproducible results achieved at 4% cholesterol (Stoletov et al., 2009). Thus, the zebrafish HCD model is frequently used to study the early development of AS. However, a recent study reported that the 10-day challenge with HCD did not render accumulation of macrophages in the blood vessels of zebrafish larvae (Verwilligen et al., 2020). The reason for this discrepancy warrants further investigation but the difference suggests that longer HCD feeding as in adult fish may be necessary.
ldlr Mutant Zebrafish
Deficiency in the LDL receptor (LDLR) results in familial hypercholesterolemia in humans (Andersen et al., 2017). Liu et al. knocked out ldlr in zebrafish using CRISPR/Cas9 technology. The ldlr mutant zebrafish with a ND feeding showed activation of SREBP-2 pathway and developed moderate hypercholesterolemia. After being fed a HCD for only 5 days, ldlr mutant larvae showed increased lipid accumulation in blood vessels and exacerbated hypercholesterolemia, which resulted in type II AS lesions (Liu et al., 2018; Vedder et al., 2020). The reduction in diet challenge time makes this model more useful for mechanistic studies and screening of potential drugs for hypercholesterolemia.
apoc2 Mutant Zebrafish
APOC2 is an activator of lipoprotein lipase that plays an important role in lipid metabolism (Wolska et al., 2017). Liu et al. (2015) knocked out apoc2 in zebrafish using TALEN technology. The results revealed chylomicronemia and severe hypertriglyceridemia in apoc2 mutants (Liu et al., 2015), which resembled the characteristics of patients lacking APOC2 (Baggio et al., 1986). Apoc2–/– larvae fed a ND showed lipid accumulation and lipid-containing macrophages in the vasculature, similar to that observed in the development of human type II AS lesions (Liu et al., 2015; Vedder et al., 2020). As a result, apoc2–/– zebrafish without HCD feeding can serve as dyslipidemia models to investigate the molecular mechanism of LDL infiltration, oxidation and phagocytosis by vascular wall macrophages, and used as models for screening of potential drugs for hyperlipidemia.
lxr Mutant Zebrafish
Liver X receptors (LXRs) plays important roles in cholesterol catabolism (Calkin and Tontonoz, 2012). There are two subtypes of LXRs in mammals, LXRα and LXRβ, and only one lxr gene with higher homology to LXRα is present in zebrafish (Pinto et al., 2016). Zebrafish with a targeted deletion in lxr showed a substantial increase in LDL when fed a HCD or a high-fat diet (HFD), developing severe hypercholesterolemia and hepatic steatosis, with lipid deposition resembling fatty streaks in humans (Cruz-Garcia and Schlegel, 2014; Vedder et al., 2020). This model can be applied for screening intestinal-restricted LXR agonists, which can be used to suppress the development of dyslipidemia and atherosclerosis.
Dynamic Monitoring of as Progression
There are several theories of AS pathogenesis, including endothelial injury, smooth muscle cell migration and proliferation, and monocyte-derived foam cell formation (Lusis, 2000; Libby et al., 2013; Sakakura et al., 2013; Emini Veseli et al., 2017). AS is also related to oxidative stress, non-coding RNA, inflammation (Feinberg and Moore, 2016; Kattoor et al., 2017). However, no single theory clearly elaborates the progression of AS (Libby et al., 2019). It is of great importance to explore the complex and diverse pathophysiological processes of AS, especially the spatiotemporal specificity. Mammalian models, such as mice and rabbits, and human biopsy samples, can provide only endpoint results (Lee et al., 2017a,b), while zebrafish can be used to monitor the development of AS dynamically using in vivo live imaging because of their embryonic transparence and an abundance of fluorescent reporter lines (Fang and Miller, 2012; Gut et al., 2017).
Luo et al. (2019) fed Tg(lysc:EGFP), Tg(mpx:EGFP), and Tg(mpeg1:EGFP) transgenic zebrafish lines HCD to dynamically monitor myeloid cells/neutrophils in vivo during the initial stage of atherosclerosis. They also enriched endothelial cells with green fluorescence from HCD fed Tg(flk1:EGFP) fish by flow cytometry to detect gene expression (Luo et al., 2019). The results showed that endothelial cell inflammation occurred as an early pathological change, which was prior to the accumulation of myeloid cells and neutrophils in blood vessels. Lipid depositions occurred after neutrophil accumulation. Pharmacological inhibition of HCD-induced early endothelial inflammation may prevent the initial development of AS (Luo et al., 2019).
Additionally, the HCD model is used to explore the role of Ox-LDL during the early stage of AS. Oxidation events, such as malondialdehyde (MDA) formation, produce immunogenic epitopes, and specific antibodies have been used in the study of AS and cardiovascular imaging (Miller et al., 2011). Fang et al. (2011) generated a transgenic zebrafish line with a heat-shock-promoter-driven EGFP labeled single-chain monoclonal antibody, IK17, which can bind to MDA-LDL. In vivo imaging demonstrated that continuous expression of IK17-EGFP decreased HCD-induced lipid accumulation in the blood vessel wall, suggesting antioxidant antibodies may exert a therapeutic benefit. This model also provides an effective method for testing the therapeutic outcome of diets and other oxidation-specific antibodies, which may eventually be applied to human (Fang et al., 2011).
Recently, Thierer et al. (2019) developed a reporting system, LipoGlo, to monitor atherosclerotic lipoproteins sensitively and specifically. The system used a luciferase enzyme (NanoLuc) fused with ApoB to monitor the abundance, size and location of lipoprotein particles, which are decisive factors in AS (Thierer et al., 2019). The authors described the lipoprotein profiles of individual zebrafish larvae, collected images of atherosclerotic lipoprotein localization and reported multiple extravascular lipoprotein localization modes. Finally, through this system, Pla2g12b was determined to be an effective regulator of lipoprotein size (Thierer et al., 2019).
Mechanistic Study of Therapeutic Intervention and Drug Screening
Studies have shown that apolipoprotein plays important roles in the prevention and treatment of AS (Peng and Li, 2018). The overexpression of apolipoprotein A-I binding protein (AIBP) mitigates diet-induced metabolic abnormalities, reducing diet-induced lipid accumulation in zebrafish blood vessels, and showing a protective effect from AS (Schneider et al., 2018). In addition, ezetimibe promotes the expression of apolipoprotein A-II through the HNF4 and PPARα transcription factor in the HCD model (Yan et al., 2019). However, ezetimibe did not inhibit vascular lipid accumulation or macrophage recruitment induced by HCD when apolipoprotein A-II was knocked out, implying that apolipoprotein A-II plays a pivotal role in reducing AS caused by HCD (Yan et al., 2019). The effects of several drugs, including atorvastatin, fenofibrate and ezetimibe, on the cholesterol levels in HCD-fed zebrafish model have been investigated (Chen et al., 2017). Intravascular cholesterol levels were significantly increased after HCD feeding and decreased after drug treatment. Atorvastatin exerted effects in a concentration-dependent manner, while only intermediate and highest concentration of fenofibrate and ezetimibe had effects in this model (Chen et al., 2017). Another study showed combination of low doses of ezetimibe and simvastatin may have an additive effect in reducing cholesterol levels in zebrafish (Baek et al., 2012).
Drug screening is important for the discovery of novel therapeutic interventions for AS (Foks and Bot, 2017). Recent studies have identified many substances, for examples, water extracts of cinnamon, turmeric, laurel, clove, grape skin, lophatherum herb, and acai berry puree, as well as the traditional Chinese medicine monomer chrysophanol, all of which show anti-atherosclerotic activity in zebrafish models (Jin and Cho, 2011; Jin et al., 2011; Kim et al., 2012). The latest research revealed that indole-3-methanol (I3C), a cruciferous vegetable extract, inhibits lipid deposition in hyperlipidemic zebrafish larvae by activating autophagy (Jiang et al., 2019). In addition, solid-state fermented polysaccharides from king oyster mushroom had an apparent positive influence on the inhibition of the Ox-LDL induced development of foam cells from mouse macrophages and exerted a lipid-reducing effect in the lipid absorption stage of a zebrafish hyperlipidemia model (Wei et al., 2018).
Assessment of as Risk Factors
AS is a progressive chronic inflammatory disease. It is not only affected by pathogenic factors, such as diabetes, chronic kidney disease, hypertension and hyperlipidemia, but is also related to other risk factors, such as genetics, age, gender, smoking, obesity, diet and nutritional status (Hughes et al., 2013; Herrington et al., 2016). As a focus of therapeutic intervention in the future, it is of great importance to assess the effects of environmental factors, trace elements, drugs and other risk factors on AS pathogenesis.
Environmental Factors
Environmental pollution is becoming more serious worldwide, and soil cadmium pollution is one such problem (Genchi et al., 2020). Cadmium absorbed by soil can be ingested by humans through the food chain (Jaishankar et al., 2014). As a heavy metal, cadmium is harmful to human health (Edwards and Prozialeck, 2009; Lin et al., 2016), but its specific effect on lipoproteins is unknown. Researchers fed zebrafish a HCD containing cadmium chloride, and discovered that exposure to cadmium influenced the function of high-density lipoprotein (HDL), which further resulted in hyperlipidemia, inflammation, fatty liver changes and increased CETP activity in HCD zebrafish (Kim et al., 2018).
According to WHO statistics, outdoor air pollution, such as fine particulate matter with a diameter ≤2.5 μm (PM2.5), causes approximately 3.7 million deaths every year. Long-term exposure to high concentrations of PM2.5 increases the risk of cardiovascular diseases and cancers (Kloog et al., 2013; Dabass et al., 2016; Turner et al., 2020). PM2.5 has been previously reported to cause disorders in zebrafish lipid metabolism (Kim et al., 2015). Duan et al. used transcriptomics to analyze the differential expression of mRNA and microRNA. The results revealed that PM2.5 upregulates let-7b, miR-153b-3p, miR-122 and miR-24, while downregulating let-7i, miR-19a-3p, miR-19b-3p and miR-7a, indicating that PM2.5 may activate autophagy, impair vasodilation, and cause cardiovascular-related diseases, such as hypertension, atherosclerosis, and myocardial infarction (Duan et al., 2017).
Trace Elements
Trace elements play indispensable roles in metabolism. Iron is involved in various physiological processes, such as red blood cell function, oxygen transport, DNA synthesis, protein synthesis, and cell replication. Iron deficiency can lead to anemia, neurodegenerative disease, developmental delay and behavioral disorders, while excessive iron may also adversely affect health (Tsay et al., 2010; Wang and Pantopoulos, 2011; Radlowski and Johnson, 2013). Lipoprotein and iron interact with each other in the form of ferrous or ferric ions in blood (Kim et al., 2017). LDL and HDL are easily oxidized and modified by ferrous ions to produce additional atherosclerosis-related proteins (Kim et al., 2017). Studies showed that after 24 weeks of iron consumption, both ND-fed and HCD-fed zebrafish displayed significant increases in serum cholesterol and triglyceride levels, which for the first time demonstrated the influence of iron consumption on lipid homeostasis in a zebrafish model (Kim et al., 2017). It was reported that inhibition of ferroptosis alleviated AS in ApoE–/– mice, through attenuating lipid peroxidation and endothelial dysfunction in aortic endothelial cell (Bai et al., 2020). However, there is no report on ferroptosis in zebrafish AS models yet.
Drugs
Quinolones and tetracyclines are β-diketone antibiotics that are widely used to treat infectious diseases in human and animals (Aminov, 2017). Wang et al. (2018) treated zebrafish with β-diketone antibiotics and discovered that upregulation of miR-125b and miR-144 led to dyslipidemia that caused increased cholesterol content, thereby elevating the risk of zebrafish developing hyperlipidemia and atherosclerosis.
Other Risk Factors
Artificial sweeteners have been widely consumed, but problems with their safety have not been fully addressed, nor have their effects on AS progression been delineated (Gardener and Elkind, 2019). Recently, researchers revealed that zebrafish fed HCD and aspartame exhibited acute swimming deficiency and brain inflammation. Furthermore, the serum lipid profile changed with increasing CETP levels in a zebrafish AS model fed saccharin (Kim et al., 2011).
Prospects
AS is a complex and progressive disease for which the pathogenesis is not fully understood. Current clinical remedies for AS include statins, antithrombotic drugs and surgical interventions to alleviate the complications, but there are still no effective or specific drugs for AS treatment (Fukuda et al., 2015; Kobiyama and Ley, 2018). Zebrafish models may aid in understanding AS pathogenesis and the identification of novel therapeutic targets and approaches (Kobiyama and Ley, 2018). Current established zebrafish models for AS research are generally fed a short-term HCD and/or HFD, resulting in models corresponding to the early stage of AS. Furthermore, most studies utilize the optical transparency of embryonic and larval zebrafish for in vivo live imaging, which has neglected the use of adult fish model (Gut et al., 2017). Therefore, more attention should be paid in the future to generating models that can mimic middle and late stages of AS and building adult fish models, for example combination of HCD with other diets such as vitamin C-deficient diet which leads to robust oxidative stress and accelerated atherosclerotic process (Kirkwood et al., 2012), or generation of transgenic and knockout animals which render the plaques vulnerable to rupture. This will ensure the screening of new drugs or therapeutic targets in zebrafish more relevant for human AS patients.
Author Contributions
DT and FG reviewed the literature. DT, FG, CY, and RZ wrote and revised the manuscript. All authors contributed to the article and approved the submitted version.
Funding
RZ was supported by National Key R&D Program of China grant 2020YFA0803900 and 2018YFA0801000.
Conflict of Interest
The authors declare that the research was conducted in the absence of any commercial or financial relationships that could be construed as a potential conflict of interest.
Acknowledgments
We thank all lab members for in depth discussion.
Abbreviations
AIBP, A-I binding protein; AS, atherosclerosis; CETP, cholesteryl ester transfer protein; CVDs, cardiovascular diseases; HCD, high cholesterol diet; HDL, high-density lipoprotein; HFD, high fat diet; I3C, indole-3-methanol; LDL, low-density lipoprotein; LXRs, liver X receptors; MDA, malondialdehyde; M-CSF, macrophage colony-stimulating factors; ND, normal diet; Ox-LDL, oxidized LDL; VLDL, very-low-density lipoprotein.
References
Aminov, R. (2017). History of antimicrobial drug discovery: major classes and health impact. Biochem. Pharmacol. 133, 4–19. doi: 10.1016/j.bcp.2016.10.001
Andersen, L., Estrella, L., and Andersen, R. (2017). LDLR variant databases and familial hypercholesterolemia population studies. J. Am. Coll. Cardiol. 69, 754–755. doi: 10.1016/j.jacc.2016.09.988
Anderson, J. L., Carten, J. D., and Farber, S. A. (2011). Zebrafish lipid metabolism: from mediating early patterning to the metabolism of dietary fat and cholesterol. Methods Cell. Biol. 101, 111–141. doi: 10.1016/b978-0-12-387036-0.00005-0
Babin, P. J., and Vernier, J. M. (1989). Plasma lipoproteins in fish. J. Lipid Res. 30, 467–489. doi: 10.1016/s0022-2275(20)38342-5
Baek, J. S., Fang, L., Li, A. C., and Miller, Y. I. (2012). Ezetimibe and simvastatin reduce cholesterol levels in zebrafish larvae fed a high-cholesterol diet. Cholesterol 2012:564705.
Baggio, G., Manzato, E., Gabelli, C., Fellin, R., Martini, S., Enzi, G. B., et al. (1986). Apolipoprotein C-II deficiency syndrome. clinical features, lipoprotein characterization, lipase activity, and correction of hypertriglyceridemia after apolipoprotein c-ii administration in two affected patients. J. Clin. Invest. 77, 520–527. doi: 10.1172/jci112332
Bai, T., Li, M., Liu, Y., Qiao, Z., and Wang, Z. (2020). Inhibition of ferroptosis alleviates atherosclerosis through attenuating lipid peroxidation and endothelial dysfunction in mouse aortic endothelial cell. Free Radic. Biol. Med. 160, 92–102. doi: 10.1016/j.freeradbiomed.2020.07.026
Bentzon, J. F., Otsuka, F., Virmani, R., and Falk, E. (2014). Mechanisms of plaque formation and rupture. Circ. Res. 114, 1852–1866.
Calkin, A. C., and Tontonoz, P. (2012). Transcriptional integration of metabolism by the nuclear sterol-activated receptors LXR and FXR. Nat. Rev. Mol. Cell. Biol. 13, 213–224. doi: 10.1038/nrm3312
Chen, K., Wang, C. Q., Fan, Y. Q., Xie, Y. S., Yin, Z. F., Xu, Z. J., et al. (2017). Model design for screening effective Antihyperlipidemic drugs using zebrafish system. Pak. J. Pharm. Sci. 30, 1697–1707.
Cruz-Garcia, L., and Schlegel, A. (2014). Lxr-driven enterocyte lipid droplet formation delays transport of ingested lipids. J. Lipid Res. 55, 1944–1958. doi: 10.1194/jlr.m052845
Dabass, A., Talbott, E. O., Venkat, A., Rager, J., Marsh, G. M., Sharma, R. K., et al. (2016). Association of exposure to particulate matter (PM2.5) air pollution and biomarkers of cardiovascular disease risk in adult NHANES participants (2001-2008. Int. J. Hyg. Environ. Health 219, 301–310. doi: 10.1016/j.ijheh.2015.12.002
Di Pietro, N., Formoso, G., and Pandolfi, A. (2016). Physiology and pathophysiology of oxLDL uptake by vascular wall cells in atherosclerosis. Vascul. Pharmacol. 84, 1–7. doi: 10.1016/j.vph.2016.05.013
Duan, J., Yu, Y., Li, Y., Jing, L., Yang, M., Wang, J., et al. (2017). Comprehensive understanding of PM2.5 on gene and microRNA expression patterns in zebrafish (Danio rerio) model. Sci. Total Environ. 586, 666–674. doi: 10.1016/j.scitotenv.2017.02.042
Edwards, J. R., and Prozialeck, W. C. (2009). Cadmium, diabetes and chronic kidney disease. Toxicol. Appl. Pharmacol. 238, 289–293. doi: 10.1016/j.taap.2009.03.007
Emini Veseli, B., Perrotta, P., De Meyer, G. R. A., Roth, L., Van der Donckt, C., Martinet, W., et al. (2017). Animal models of atherosclerosis. Eur. J. Pharmacol. 816, 3–13.
Fan, J., Kitajima, S., Watanabe, T., Xu, J., Zhang, J., Liu, E., et al. (2015). Rabbit models for the study of human atherosclerosis: from pathophysiological mechanisms to translational medicine. Pharmacol. Ther. 146, 104–119. doi: 10.1016/j.pharmthera.2014.09.009
Fang, L., Green, S. R., Baek, J. S., Lee, S. H., Ellett, F., Deer, E., et al. (2011). In vivo visualization and attenuation of oxidized lipid accumulation in hypercholesterolemic zebrafish. J. Clin. Invest. 121, 4861–4869. doi: 10.1172/jci57755
Fang, L., Harkewicz, R., Hartvigsen, K., Wiesner, P., Choi, S. H., Almazan, F., et al. (2010). Oxidized cholesteryl esters and phospholipids in zebrafish larvae fed a high cholesterol diet: macrophage binding and activation. J. Biol. Chem. 285, 32343–32351. doi: 10.1074/jbc.m110.137257
Fang, L., Liu, C., and Miller, Y. I. (2014). Zebrafish models of dyslipidemia: relevance to atherosclerosis and angiogenesis. Transl. Res. 163, 99–108.
Fang, L., and Miller, Y. I. (2012). Emerging applications for zebrafish as a model organism to study oxidative mechanisms and their roles in inflammation and vascular accumulation of oxidized lipids. Free Radic. Biol. Med. 53, 1411–1420. doi: 10.1016/j.freeradbiomed.2012.08.004
Fang, L., and Miller, Y. I. (2013). Targeted cholesterol efflux. Cell. Cycle 12, 3345–3346. doi: 10.4161/cc.26401
Feinberg, M. W., and Moore, K. J. (2016). MicroRNA regulation of atherosclerosis. Circ. Res. 118:703–720. doi: 10.1161/circresaha.115.306300
Foks, A. C., and Bot, I. (2017). Preface: pathology and pharmacology of atherosclerosis. Eur. J. Pharmacol. 816, 1–2. doi: 10.1016/j.ejphar.2017.10.052
Fukuda, K., Matsumura, T., Senokuchi, T., Ishii, N., Kinoshita, H., Yamada, S., et al. (2015). Statins meditate anti-atherosclerotic action in smooth muscle cells by peroxisome proliferator-activated receptor-gamma activation. Biochem. Biophys. Res. Commun. 457, 23–30. doi: 10.1016/j.bbrc.2014.12.063
Fuster, J. J., Castillo, A. I., Zaragoza, C., Ibanez, B., and Andres, V. (2012). Animal models of atherosclerosis. Prog. Mol. Biol. Transl. Sci. 105, 1–23. doi: 10.1385/1-59259-073-x:1
Gardener, H., and Elkind, M. S. V. (2019). Artificial sweeteners, real risks. Stroke 50, 549–551. doi: 10.1161/strokeaha.119.024456
Genchi, G., Sinicropi, M. S., Lauria, G., Carocci, A., and Catalano, A. (2020). The effects of cadmium toxicity. Int. J. Environ. Res. Public Health 17:3782.
Getz, G. S., and Reardon, C. A. (2012). Animal models of atherosclerosis. Arterioscler. Thromb. Vasc. Biol. 32, 1104–1115.
Golforoush, P., Yellon, D. M., and Davidson, S. M. (2020). Mouse models of atherosclerosis and their suitability for the study of myocardial infarction. Basic Res. Cardiol. 115:73. doi: 10.1016/s1567-5688(02)80043-5
Gut, P., Reischauer, S., Stainier, D. Y. R., and Arnaout, R. (2017). Little fish, big data: zebrafish as a model for cardiovascular and metabolic disease. Physiol. Rev. 97, 889–938. doi: 10.1152/physrev.00038.2016
Herrington, W., Lacey, B., Sherliker, P., Armitage, J., and Lewington, S. (2016). Epidemiology of atherosclerosis and the potential to reduce the global burden of atherothrombotic disease. Circ. Res. 118, 535–546. doi: 10.1161/circresaha.115.307611
Holtta-Vuori, M., Salo, V. T., Nyberg, L., Brackmann, C., Enejder, A., Panula, P., et al. (2010). Zebrafish: gaining popularity in lipid research. Biochem. J. 429, 235–242. doi: 10.1042/bj20100293
Holtzman, N. G., Iovine, M. K., Liang, J. O., and Morris, J. (2016). Learning to fish with genetics: a primer on the vertebrate model danio rerio. Genetics 203, 1069–1089. doi: 10.1534/genetics.116.190843
Hughes, J., Kee, F., O’Flaherty, M., Critchley, J., Cupples, M., Capewell, S., et al. (2013). Modelling coronary heart disease mortality in Northern Ireland between 1987 and 2007: broader lessons for prevention. Eur. J. Prev. Cardiol. 20, 310–321. doi: 10.1177/2047487312441725
Jaishankar, M., Tseten, T., Anbalagan, N., Mathew, B. B., and Beeregowda, K. N. (2014). Toxicity, mechanism and health effects of some heavy metals. Interdiscip. Toxicol. 7, 60–72. doi: 10.2478/intox-2014-0009
Jiang, Y., Yang, G., Liao, Q., Zou, Y., Du, Y., and Huang, J. (2019). Indole-3-carbinol inhibits lipid deposition and promotes autophagy in hyperlipidemia zebrafish larvae. Environ. Toxicol. Pharmacol. 70:103205. doi: 10.1016/j.etap.2019.103205
Jin, S., and Cho, K. H. (2011). Water extracts of cinnamon and clove exhibits potent inhibition of protein glycation and anti-atherosclerotic activity in vitro and in vivo hypolipidemic activity in zebrafish. Food. Chem. Toxicol. 49, 1521–1529. doi: 10.1016/j.fct.2011.03.043
Jin, S., Hong, J. H., Jung, S. H., and Cho, K. H. (2011). Turmeric and laurel aqueous extracts exhibit in vitro anti-atherosclerotic activity and in vivo hypolipidemic effects in a zebrafish model. J. Med. Food 14, 247–256. doi: 10.1089/jmf.2009.1389
Kattoor, A. J., Pothineni, N. V. K., Palagiri, D., and Mehta, J. L. (2017). Oxidative stress in atherosclerosis. Curr. Atheroscler. Rep. 19:42.
Kim, J. Y., Hong, J. H., Jung, H. K., Jeong, Y. S., and Cho, K. H. (2012). Grape skin and loquat leaf extracts and acai puree have potent anti-atherosclerotic and anti-diabetic activity in vitro and in vivo in hypercholesterolemic zebrafish. Int. J. Mol. Med. 30, 606–614. doi: 10.3892/ijmm.2012.1045
Kim, J. Y., Kim, S. J., Bae, M. A., Kim, J. R., and Cho, K. H. (2018). Cadmium exposure exacerbates severe hyperlipidemia and fatty liver changes in zebrafish via impairment of high-density lipoproteins functionality. Toxicol. In Vitro. 47, 249–258. doi: 10.1016/j.tiv.2017.11.007
Kim, J. Y., Lee, E. Y., Choi, I., Kim, J., and Cho, K. H. (2015). Effects of the particulate matter(2).(5) (PM(2).(5)) on lipoprotein metabolism, uptake and degradation, and embryo toxicity. Mol. Cells 38, 1096–1104. doi: 10.14348/molcells.2015.0194
Kim, J. Y., Seo, J., and Cho, K. H. (2011). Aspartame-fed zebrafish exhibit acute deaths with swimming defects and saccharin-fed zebrafish have elevation of cholesteryl ester transfer protein activity in hypercholesterolemia. Food Chem. Toxicol. 49, 2899–2905. doi: 10.1016/j.fct.2011.08.001
Kim, S. H., Yadav, D., Kim, S. J., Kim, J. R., and Cho, K. H. (2017). High Consumption of iron exacerbates hyperlipidemia, atherosclerosis, and female sterility in zebrafish via acceleration of glycation and degradation of serum lipoproteins. Nutrients 9:690. doi: 10.3390/nu9070690
Kirkwood, J. S., Lebold, K. M., Miranda, C. L., Wright, C. L., Miller, G. W., Tanguay, R. L., et al. (2012). Vitamin C deficiency activates the purine nucleotide cycle in zebrafish. J. Biol. Chem. 287, 3833–3841. doi: 10.1074/jbc.m111.316018
Kloog, I., Ridgway, B., Koutrakis, P., Coull, B. A., and Schwartz, J. D. (2013). Long- and short-term exposure to PM2.5 and mortality: using novel exposure models. Epidemiology 24, 555–561. doi: 10.1097/ede.0b013e318294beaa
Laila, S. R., Astuti, D. A., Suparto, I. H., Handharyani, E., and Sajuthi, D. (2018). Metabolic and morphometric changes in Indonesian cynomolgus monkeys (Macaca fascicularis) fed an atherogenic diet composed of locally sourced ingredients. Vet. World 11, 1609–1617. doi: 10.14202/vetworld.2018.1609-1617
Lee, Y. T., Laxton, V., Lin, H. Y., Chan, Y. W. F., Fitzgerald-Smith, S., To, T. L. O., et al. (2017a). Animal models of atherosclerosis. Biomed. Rep. 6, 259–266.
Lee, Y. T., Lin, H. Y., Chan, Y. W., Li, K. H., To, O. T., Yan, B. P., et al. (2017b). Mouse models of atherosclerosis: a historical perspective and recent advances. Lipids Health Dis. 16:12.
Libby, P., Buring, J. E., Badimon, L., Hansson, G. K., Deanfield, J., Bittencourt, M. S., et al. (2019). Atherosclerosis. Nat. Rev. Dis. Primers 5:56.
Libby, P., Nahrendorf, M., and Swirski, F. K. (2013). Monocyte heterogeneity in cardiovascular disease. Semin. Immunopathol. 35, 553–562. doi: 10.1007/s00281-013-0387-3
Libby, P., Ridker, P. M., and Hansson, G. K. (2011). Progress and challenges in translating the biology of atherosclerosis. Nature 473, 317–325. doi: 10.1038/nature10146
Lieschke, G. J., and Currie, P. D. (2007). Animal models of human disease: zebrafish swim into view. Nat. Rev. Genet. 8, 353–367. doi: 10.1038/nrg2091
Lin, J., Zhang, F., and Lei, Y. (2016). Dietary intake and urinary level of cadmium and breast cancer risk: a meta-analysis. Cancer Epidemiol. 42, 101–107. doi: 10.1016/j.canep.2016.04.002
Liu, C., Gates, K. P., Fang, L., Amar, M. J., Schneider, D. A., Geng, H., et al. (2015). Apoc2 loss-of-function zebrafish mutant as a genetic model of hyperlipidemia. Dis. Model. Mech. 8, 989–998. doi: 10.1242/dmm.019836
Liu, C., Kim, Y. S., Kim, J., Pattison, J., Kamaid, A., and Miller, Y. I. (2018). Modeling hypercholesterolemia and vascular lipid accumulation in LDL receptor mutant zebrafish. J. Lipid Res. 59, 391–399. doi: 10.1194/jlr.d081521
Luo, H., Li, Q. Q., Wu, N., Shen, Y. G., Liao, W. T., Yang, Y., et al. (2019). Chronological in vivo imaging reveals endothelial inflammation prior to neutrophils accumulation and lipid deposition in HCD-fed zebrafish. Atherosclerosis 290, 125–135. doi: 10.1016/j.atherosclerosis.2019.09.017
Miller, Y. I., Choi, S. H., Wiesner, P., Fang, L., Harkewicz, R., Hartvigsen, K., et al. (2011). Oxidation-specific epitopes are danger-associated molecular patterns recognized by pattern recognition receptors of innate immunity. Circ. Res. 108, 235–248. doi: 10.1161/circresaha.110.223875
Mushenkova, N. V., Summerhill, V. I., Silaeva, Y. Y., Deykin, A. V., and Orekhov, A. N. (2019). Modelling of atherosclerosis in genetically modified animals. Am. J. Transl. Res. 11, 4614–4633.
Otis, J. P., Zeituni, E. M., Thierer, J. H., Anderson, J. L., Brown, A. C., Boehm, E. D., et al. (2015). Zebrafish as a model for apolipoprotein biology: comprehensive expression analysis and a role for ApoA-IV in regulating food intake. Dis. Model Mech. 8, 295–309. doi: 10.1242/dmm.018754
Paigen, B., Morrow, A., Brandon, C., Mitchell, D., and Holmes, P. (1985). Variation in susceptibility to atherosclerosis among inbred strains of mice. Atherosclerosis 57, 65–73. doi: 10.1016/0021-9150(85)90138-8
Peng, J., and Li, X. P. (2018). Apolipoprotein A-IV: a potential therapeutic target for atherosclerosis. Prostaglandins Other Lipid Mediat. 139, 87–92.
Pinto, C. L., Kalasekar, S. M., McCollum, C. W., Riu, A., Jonsson, P., Lopez, J., et al. (2016). Lxr regulates lipid metabolic and visual perception pathways during zebrafish development. Mol. Cell. Endocrinol. 419, 29–43. doi: 10.1016/j.mce.2015.09.030
Poznyak, A. V., Silaeva, Y. Y., Orekhov, A. N., and Deykin, A. V. (2020). Animal models of human atherosclerosis: current progress. Braz J. Med. Biol. Res. 53, e9557.
Radlowski, E. C., and Johnson, R. W. (2013). Perinatal iron deficiency and neurocognitive development. Front. Hum. Neurosci. 7:585. doi: 10.3389/fnhum.2013.00585
Rapacz, J., Hasler-Rapacz, J., Taylor, K. M., Checovich, W. J., and Attie, A. D. (1986). Lipoprotein mutations in pigs are associated with elevated plasma cholesterol and atherosclerosis. Science 234, 1573–1577. doi: 10.1126/science.3787263
Sakakura, K., Nakano, M., Otsuka, F., Ladich, E., Kolodgie, F. D., Virmani, R., et al. (2013). Pathophysiology of atherosclerosis plaque progression. Heart Lung Circ. 22, 399–411. doi: 10.1016/j.hlc.2013.03.001
Schlegel, A. (2016). Zebrafish models for dyslipidemia and atherosclerosis research. Front. Endocrinol. 7:159. doi: 10.3389/fendo.2016.00159
Schneider, D. A., Choi, S. H., Agatisa-Boyle, C., Zhu, L., Kim, J., Pattison, J., et al. (2018). AIBP protects against metabolic abnormalities and atherosclerosis. J. Lipid Res. 59, 854–863. doi: 10.1194/jlr.m083618
Shao, B. Z., Han, B. Z., Zeng, Y. X., Su, D. F., and Liu, C. (2016). The roles of macrophage autophagy in atherosclerosis. Acta Pharmacol. Sin. 37, 150–156. doi: 10.1038/aps.2015.87
Stary, H. C., Chandler, A. B., Dinsmore, R. E., Fuster, V., Glagov, S., Insull, W. Jr., et al. (1995). A definition of advanced types of atherosclerotic lesions and a histological classification of atherosclerosis. A report from the committee on vascular lesions of the council on arteriosclerosis, american heart association. Circulation 92, 1355–1374. doi: 10.1161/01.cir.92.5.1355
Stoletov, K., Fang, L., Choi, S. H., Hartvigsen, K., Hansen, L. F., Hall, C., et al. (2009). Vascular lipid accumulation, lipoprotein oxidation, and macrophage lipid uptake in hypercholesterolemic zebrafish. Circ. Res. 104, 952–960. doi: 10.1161/circresaha.108.189803
Thierer, J. H., Ekker, S. C., and Farber, S. A. (2019). The LipoGlo reporter system for sensitive and specific monitoring of atherogenic lipoproteins. Nat. Commun. 10:3426.
Thomas, H., Diamond, J., Vieco, A., Chaudhuri, S., Shinnar, E., Cromer, S., et al. (2018). Global atlas of cardiovascular disease 2000-2016: the path to prevention and control. Glob Heart 13, 143–163. doi: 10.1016/j.gheart.2018.09.511
Tsay, J., Yang, Z., Ross, F. P., Cunningham-Rundles, S., Lin, H., Coleman, R., et al. (2010). Bone loss caused by iron overload in a murine model: importance of oxidative stress. Blood 116, 2582–2589. doi: 10.1182/blood-2009-12-260083
Turner, M. C., Andersen, Z. J., Baccarelli, A., Diver, W. R., Gapstur, S. M., Pope, C. A. III, et al. (2020). Outdoor air pollution and cancer: an overview of the current evidence and public health recommendations. CA. Cancer J. Clin. 70:21632. doi: 10.3322/caac.21632
Vedder, V. L., Aherrahrou, Z., and Erdmann, J. (2020). Dare to Compare. Development of Atherosclerotic Lesions in Human, Mouse, and Zebrafish. Front. Cardiovasc. Med. 7:109. doi: 10.3389/fcvm.2020.00109
Verwilligen, R. A. F., Bussmann, J., and Van Eck, M. (2020). Zebrafish atherosclerosis: experimental definitions and difficulties. Atherosclerosis 302, 52–54. doi: 10.1016/j.atherosclerosis.2020.04.007
Wang, J., and Pantopoulos, K. (2011). Regulation of cellular iron metabolism. Biochem. J. 434, 365–381. doi: 10.1042/bj20101825
Wang, X., Zheng, Y., Ma, Y., Du, L., Chu, F., Gu, H., et al. (2018). Lipid metabolism disorder induced by up-regulation of miR-125b and miR-144 following beta-diketone antibiotic exposure to F0-zebrafish (Danio rerio). Ecotoxicol. Environ. Saf. 164, 243–252. doi: 10.1016/j.ecoenv.2018.08.027
Wei, H., Yue, S., Zhang, S., and Lu, L. (2018). Lipid-lowering effect of the Pleurotus eryngii (king oyster mushroom) polysaccharide from solid-state fermentation on both macrophage-derived foam cells and zebrafish models. Polymers (Basel) 10:50492. doi: 10.3390/polym10050492
Wolska, A., Dunbar, R. L., Freeman, L. A., Ueda, M., Amar, M. J., Sviridov, D. O., et al. (2017). Apolipoprotein C-II: New findings related to genetics, biochemistry, and role in triglyceride metabolism. Atherosclerosis 267, 49–60. doi: 10.1016/j.atherosclerosis.2017.10.025
Yan, Y., He, F., Li, Z., Xu, R., Li, T., Su, J., et al. (2019). The important role of apolipoprotein A-II in ezetimibe driven reduction of high cholesterol diet-induced atherosclerosis. Atherosclerosis 280, 99–108.
Keywords: zebrafish model, atherosclerosis, dynamic monitoring, drug screening, risk factor assessment
Citation: Tang D, Geng F, Yu C and Zhang R (2021) Recent Application of Zebrafish Models in Atherosclerosis Research. Front. Cell Dev. Biol. 9:643697. doi: 10.3389/fcell.2021.643697
Received: 18 December 2020; Accepted: 05 February 2021;
Published: 25 February 2021.
Edited by:
Vincenzo Torraca, University of London, United KingdomReviewed by:
Guido R. Y. De Meyer, University of Antwerp, BelgiumTamara Amstislavskaya, State Scientific Research Institute of Physiology and Basic Medicine, Russia
Enqi Liu, Xi’an Jiaotong University, China
Copyright © 2021 Tang, Geng, Yu and Zhang. This is an open-access article distributed under the terms of the Creative Commons Attribution License (CC BY). The use, distribution or reproduction in other forums is permitted, provided the original author(s) and the copyright owner(s) are credited and that the original publication in this journal is cited, in accordance with accepted academic practice. No use, distribution or reproduction is permitted which does not comply with these terms.
*Correspondence: Ruilin Zhang, emhhbmdydWlsaW5Ad2h1LmVkdS5jbg==
†These authors have contributed equally to this work