- Department of Chemical and Biomedical Engineering, Florida A&M University-Florida State University College of Engineering, Tallhassee, FL, United States
Mesenchymal stem cell (MSC)-based therapies have demonstrated tissue repair and regeneration capacity in various preclinical models. These therapeutic effects have recently been largely attributed to the paracrine effects of the MSC secretome, including proteins and extracellular vesicles (EVs). EVs are cell-secreted nano-sized vesicles with lipid bilayer membranes that facilitate cell–cell signaling. Treatments based on MSC-derived EVs are beginning to be explored as an alternative to MSC transplantation-based therapies. However, it remains to be determined which MSC source produces EVs with the greatest therapeutic potential. This review compares the tissue regeneration capacity of EVs isolated from the two most common clinical sources of adult MSCs, bone marrow and adipose tissue, with a particular focus on their angiogenic, osteogenic, and immunomodulatory potentials. Other important issues in the development of MSC-derived EV based therapies are also discussed.
Introduction
Mesenchymal stem cell (MSC) transplantation has demonstrated great promise as a novel treatment for tissue repair and regeneration in several organ systems, including the central nervous system (CNS) (Azari et al., 2010), heart (Jeong et al., 2018), cartilage, skin, and bone (Mitxitorena et al., 2019). Displaying trophic and immunomodulatory effects upon transplantation, MSCs currently represent a critical part of clinical cell-based regenerative medicine. To date, over 950 clinical trials involving MSCs have been listed with the United States Food and Drug Administration and more than 10,000 patients have received MSC-based therapies (Pittenger et al., 2019). However, issues with MSC-based therapies, such as low cell survival rate upon transplantation, limited donor supply, donor-to-donor variability and storage issues, have prompted researchers to investigate alternative approaches. In recent years, extracellular vesicles (EVs) derived from MSCs have become the focus of much research as they exhibit many similar trophic and immunomodulatory functions. In order to translate EV-based therapies to the clinic, the relationship between MSC cell source and EV therapeutic potential needs to be clarified.
MSCs in Tissue Repair and Regeneration
Mesenchymal stem cells are a heterogeneous subset of pluripotent stromal stem cells that are easily isolated from various tissues, including adipose tissue, peripheral blood, bone marrow, synovial fluid, muscle, placenta, umbilical cord, and dental pulp (Uccelli et al., 2008). The minimal criteria for defining MSCs are: the ability to self-renew and differentiate into classical mesodermal lineage cells such as osteoblasts, adipocytes, and chondrocytes in vitro and in vivo; a CD105+, CD73+, CD90+, and CD45–, CD34–, CD11b–, CD79a–, CD19–, and HLA class II- expression profile; a fibroblast-like morphology; and, adherence to tissue culture plastic in vitro (Horwitz et al., 2005; Dominici et al., 2006). Among the various MSC sources, bone marrow (BMMSCs) and adipose (ADMSCs) are the two most commonly used in preclinical and clinical tissue regeneration applications. While, umbilical cord- derived MSCs (UCMSCs) have also been widely employed in research and clinical trials, their use in many applications is limited since they are not practical for autologous administration in adults (Kern et al., 2006). Although BMMSCs were the first MSC type to be characterized and are the most widely used (Caplan, 1991), ADMSCs are an attractive alternative as they are higher in frequency, more easily obtained and cause less donor site morbidity (Reumann et al., 2018). Furthermore, ADMSCs display a higher proliferation rate than BMMSCs in vitro and show a greater ability to maintain their stem cell characteristics, including self-renewal, proliferation, and differentiation potential, after repeated passaging (Zhu et al., 2008).
While both BMMSCs and ADMSCs have been successfully employed in preclinical tissue repair and disease models to promote angiogenesis (Jin and Lee, 2018; Zhang et al., 2019; Ryu et al., 2020), induce bone regeneration (Jin and Lee, 2018) and modulate the immune system (Tao et al., 2016; Zhao et al., 2016; Waldner et al., 2018), there appear to be several differences between cell types. In vitro studies have shown that BMMSCs exhibit significantly higher chondrogenic differentiation capacity (Noël et al., 2008; Mohamed-Ahmed et al., 2018), while ADMSCs show significantly higher adipogenic capacity in vitro (Mohamed-Ahmed et al., 2018). ADMSCs also display a higher endothelial differentiation capacity in vitro than BMMSCs (Fan et al., 2016), and superior angiogenic capacity in several preclinical ischemic injury models (Ikegame et al., 2011; El-Badawy et al., 2016). However, it remains unclear which MSC source exhibits greater osteogenic capacity or immunomodulatory potential. While some in vitro studies showed higher osteogenic differentiation in BMMSCS than ADMSCs (Park et al., 2012), others showed the opposite (Kang et al., 2012). More significantly, no significant differences in bone regeneration ability were observed in vivo between the two MSC types in rat cranial defect models (Wen et al., 2013) or canine radius defect models (Kang et al., 2012). Similarly, both MSC types showed comparable immunomodulatory potential in an immunocompetent myocardial infarction (MI) model (Paul et al., 2013), while BMMSCs displayed greater immunomodulatory potential in an endotoxic shock model (Elman et al., 2014), and ADMSCs demonstrated more effective immunosuppression of peripheral blood mononuclear cells and T-cells in vitro (Waldner et al., 2018).
EVs in Paracrine Signaling
While the therapeutic effects of transplanted MSCs were originally thought to be due to direct cell replacement (Friedenstein et al., 1968), research soon showed that intravenously administrated MSCs were largely caught in capillaries and/or cleared (Fischer et al., 2009), and that remaining MSCs contributed to short-term therapeutic effects (Caplan and Dennis, 2006). It is now widely theorized that the therapeutic effects of MSCs are mainly due to paracrine secretion of various growth factors, glycosaminoglycans, cytokines and EVs which modulate angiogenesis (Pankajakshan and Agrawal, 2014), apoptosis (Pan et al., 2012), proliferation (Di Nicola et al., 2002), differentiation (Chiossone et al., 2016), and the immune response (Dyer et al., 2014) to create a reparative microenvironment (Phinney and Pittenger, 2017). Secreted by the majority of cell types, EVs are phospholipid vesicles of different sizes, including micro-vesicles (MVs) (200 nm–1 μm) and exosomes (50–200 nm), that transport proteins, lipids, and nucleic acids (Hunter et al., 2008). Exosomes are generated in multivesicular bodies by the endosomal compartment and express endosomal markers (CD9, CD61, CD83, ALIX, TSG101) (Cosenza et al., 2017) and surface molecules that allow them to be targeted to recipient cells (Mathivanan et al., 2010). Meanwhile, MVs are the outcome of direct outward budding of the cell plasma membrane and thus carry cytoplasmic contents (Heijnen et al., 1999). EVs are recognized and internalized by recipient cells through receptor-ligand interactions (Raposo et al., 1996), endocytosis and/or phagocytosis (Morelli et al., 2004), or they can fuse with the target cell membrane and deliver their contents into the cytosol (Tkach and Théry, 2016). Recent research suggests that the paracrine efficacy of MSC-based therapies can largely be attributed to EVs. For example, conditioned MSC culture media was found to have therapeutic effects similar to direct delivery of MSCs in rodent models (Gnecchi et al., 2005; Aslam et al., 2009). Subsequently, Timmers et al. (2008) demonstrated that it was the EVs within the conditioned media that actually were effective.
Extracellular vesicles can be harvested via a variety of methods from cell culture media or clinical samples such as blood plasma, urine, and saliva. The most frequently employed isolation methods include differential ultracentrifugation and density gradient ultracentrifugation, both of which involve centrifugal forces greater than 100,000 × g and can fractionate EVs from their liquid sample of origin into subsets based on size, density, and mass (Zarovni et al., 2015; Li et al., 2017). EVs harvested from different tissues display varying content profiles, depending on their origin, age, state and environment (Tkach and Théry, 2016). For example, the microRNA (miRNA) profile of MSC-derived EVs from myoplastic syndrome patients is significantly different compared to that of EVs from disease-free patients (Muntión et al., 2016). Among EV contents, the function of bioactive lipids and proteins have been well-studied (Toh et al., 2018; Skotland et al., 2020). However, nucleic acid cargo, including mRNA, miRNAs, and other non-coding RNAs has become an increasingly hot topic in EV research. miRNAs, which are small (19–23 nucleotide) non-coding RNAs (Lau et al., 2001) that regulate gene expression via specific binding to messenger RNAs (mRNAs) (Lai, 2002), make up a large portion of the cargo within EVs (Valadi et al., 2007). miRNA transfer to recipient cells via EVs contributes significantly to paracrine signaling and has been found to be a main mediator of therapeutic effects in many preclinical studies. For example, miR-223 from BMMSCs-derived EVs contributed to cardioprotection in a surgically induced sepsis model (Wang X. et al., 2015).
The use of MSC-derived EVs in place of MSC transplantation in clinical treatments provides a number of potential advantages. EV therapies increase the accessibility of damaged tissues, since cultured MSCs are approximately 20 μm in diameter and thus tend to be caught and cleared by the circulation (Crop et al., 2010), whereas EVs are significantly smaller and have demonstrated transport through the pulmonary circulation and the blood-brain barrier (Batsali et al., 2020) (Bang and Kim, 2019). Unlike MSCS, which may undergo changes during in vitro culture that make them a clearance target of NK cells and macrophages (Eggenhofer et al., 2014), EVs are more likely to avoid immune rejection due to their low expression of membrane histocompatibility complexes (Lai et al., 2019). EVs are also more easily modified than MSCs to encapsulate desired therapeutic cargos, and are more easily stored than cells, since they are more stable when freezing and thawing (Lai et al., 2019). However, before the clinical application of MSC-derived EVs can be achieved, the optimal cell source for a given therapeutic application needs to be determined.
This review will compare the therapeutic effects of EVs isolated from BMMSCs and ADMSCs in various in vivo tissue repair and regeneration models (Figure 1). More specifically, the capacity of BMMSC- and ADMSC-derived EVs to induce angiogenesis, osteogenesis and immunomodulation will be investigated. EV cargos and any signaling pathways involved, where characterized, will also be detailed.
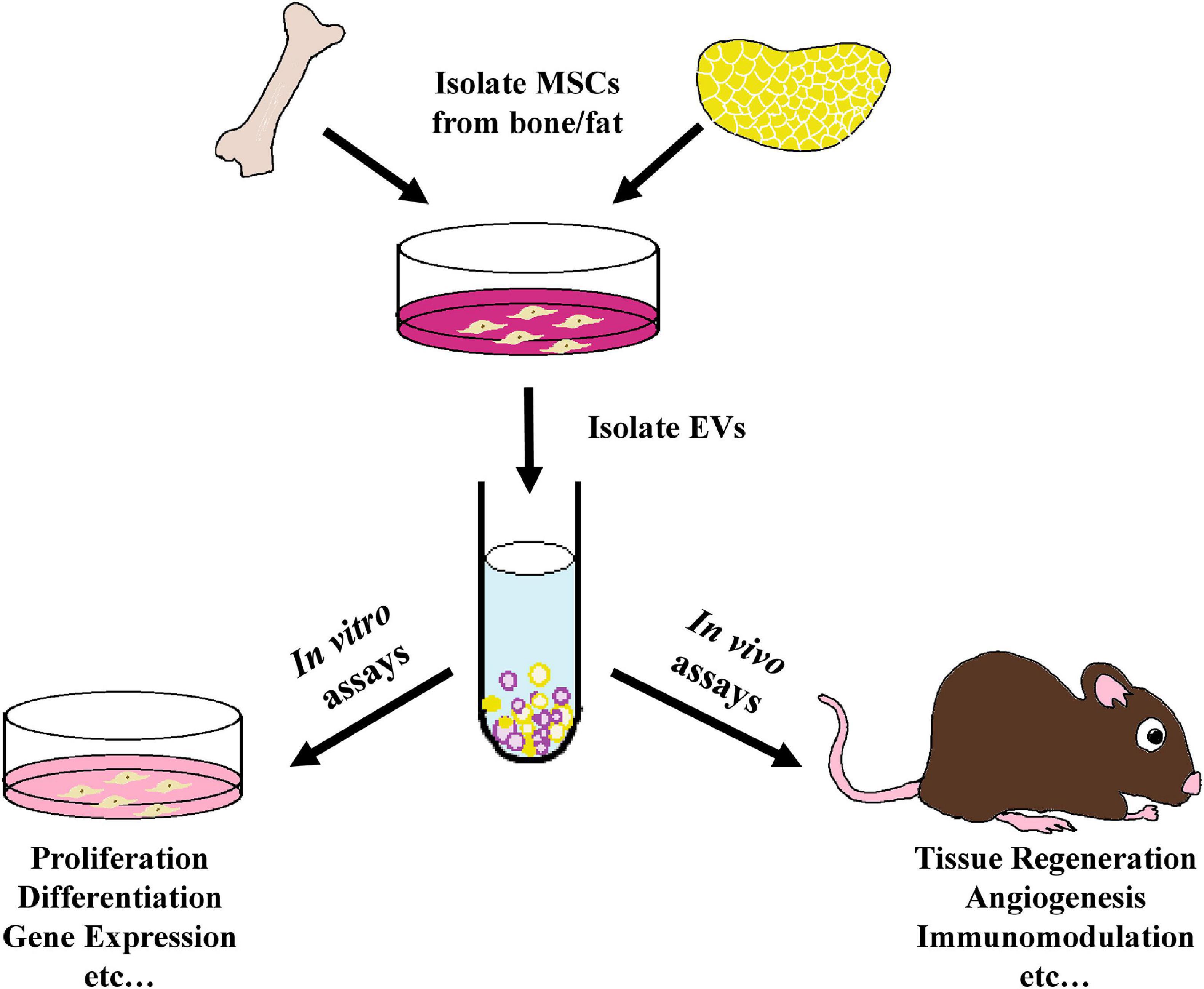
Figure 1. Schematic outline of the use of MSC- derived EVs within tissue regeneration models as analyzed in this review. EVs were isolated from the culture media of bone marrow-derived or adipose-derived MSCs and employed in various in vitro proliferation, differentiation, gene expression, and other assays, as well as within a variety of in vivo tissue regeneration studies, including preclinical animal models and human clinical trials.
Comparing the Therapeutic Efficacy of BMMSC-Derived and ADMSC-Derived EVs
Angiogenesis
Studies employing BMMSC-derived EVs in preclinical models, including calvarial defects (Liang et al., 2019), myocardial infarctions (MI) (Teng et al., 2015; Xu H. et al., 2020), random pattern dorsal skin flaps (Xie et al., 2019), intracerebral hemorrhages (ICH) (Han et al., 2019b), fracture non-unions (Zhang et al., 2020), focal cerebral ischemia models (Doeppner et al., 2015), traumatic brain injury (TBI) models (Zhang et al., 2017), STZ-induced diabetic rat models (Yu M. et al., 2020), and subcutaneous implantation models (Narayanan et al., 2016), generally demonstrated that EV treatment stimulated localized vasculogenesis and/or angiogenesis (see Table 1). Meanwhile, ADMSC- derived EVs promoted neovascularization and angiogenesis in fat grafting models (Han et al., 2019a), acute ischemic stroke models (Chen et al., 2016), acute kidney ischemia/reperfusion (I/R) models (Lin et al., 2016), and MI models (Xu H. et al., 2020) (see Table 1). With far fewer studies employing ADMSC-derived EVs than BMMSC-derived EVs, it remains unclear whether once source displays greater angiogenic potential than the other. In one study that directly compared the effects of EVs derived from both cell sources, human ADMSC-derived EVs displayed significantly increased therapeutic potential compared to BMMSC-derived EVs in a rat MI model, as indicated by improved cardiac function, reduced cardiomyocyte apoptosis and infarction area and increased microvessel density (Xu H. et al., 2020). By contrast, when comparing two separate brain ischemia model studies, BMMSC-derived EVs appeared to display increased angiogenic potential compared to ADMSCs-derived EVs, with the former exhibiting an approximately 4-fold increase in the number of endothelial cells compared to controls, while the latter showed a 1.5-fold change (Doeppner et al., 2015; Chen et al., 2016).
Few of these preclinical studies have investigated the mechanisms and signaling pathways underlying the observed increase in angiogenesis induced by MSC-derived EV therapies. In a calvarial defect model, enhanced angiogenesis due to BMMSC-derived EV treatment was coupled with endogenous MSC migration (Takeuchi et al., 2019). While, in a rat full-thickness skin wound model, human BMMSC-derived EVs accelerated angiogenesis and the cutaneous wound healing process via inhibition of the TGF-β/Smad signaling pathway, as verified by RT-qPCR and western blotting analysis (Yang et al., 2018). Similarly, in a STZ-induced diabetic rat model, BMMSC-derived EVs accelerated wound closure and increased blood vessel area and number by activating the AKT/eNOS pathway (Yu M. et al., 2020). Increased angiogenesis induced by BMMSC-derived EVs is often linked to increased VEGF signaling, as was observed in a random pattern dorsal skin flap model (Xie et al., 2019) and a subcutaneous implantation model (Narayanan et al., 2016). Similarly, HIF-1α-VEGF signaling was found to be associated with enhanced angiogenesis at the fracture site in a non-union model after treatment with BMMSC-derived EVs (Zhang et al., 2020). Meanwhile, in an acute kidney I/R model (Lin et al., 2016) and an acute ischemia stroke model (Chen et al., 2016), ADMSC-derived EVs increased expression of the angiogenesis markers CD31, vWF, VEGF, CXCR4, SDF-1α, and angiopoietin. In another study that directly compared the angiogenic capacity of BMMSC-derived and ADMSC-derived EVs, similar numbers of new blood vessels were observed in a rat MI model (Wang K. et al., 2017). However, ADMSC-EVs yielded higher numbers of CD31+ cells, while EVs derived from endometrial MSCs displayed higher angiogenic capacity than EVs from either BMMSCs or ADMSCs (Wang K. et al., 2017).
In vitro experiments further showed that EVs isolated from both BMMSCs and ADMSCs possessed great potential for inducing angiogenesis and enabled more detailed study of the pathways underlying these effects. ADMSC-derived EVs enhanced angiogenic tube formation in human brain microvessel endothelial cells via increased expression of miR-181b-5p, which, in turn, directly targeted expression of the ion channel protein TRPM7 (Yang et al., 2018). Similarly, BMMSC-derived EVs induced angiogenic tube formation in HUVECs (Teng et al., 2015; Xie et al., 2017; Kang et al., 2020; Yu M. et al., 2020; Zhang et al., 2020) and enhanced expression of the angiogenesis-related genes VEGF, ANG1, and ANG2 in hBMMSCs (Takeuchi et al., 2019) and the mRNA expression of PDGF, EGF, and ANG1 in HUVECs (Yu M. et al., 2020). In a rare study that directly compared EVs derived from the three most commonly employed clinical MSC sources, i.e., BMMSCs, ADMSCs and UCMSCs, ADMSC-derived EVs yielded the highest in vitro protein expression levels of VEGF, bFGF, and HGF in rat neonatal cardiomyocytes and also showed the strongest inhibitory effect on apoptosis (Xu H. et al., 2020).
In order to improve angiogenic therapeutic capacity, many studies isolated EVs from MSCs cultured in hypoxic conditions or in the presence of dimethyloxalylglycine (DMOG), which enhances activation of HIF-1a. In a nude mouse model of fat grafting, for example, hypoxic ADMSC-derived EVs (hyp-ADSC-EVs) dramatically promoted neovascularization and increased the protein expression of VEGF/VEGF-R compared to EVs derived in normoxic conditions (Han et al., 2019a). Similarly, in other studies hyp-ADSC-EVs were found to express significantly higher levels of VEGF and VEGF-R2/R3 and promote increased HUVEC proliferation, migration and tube-formation in vitro (Xue et al., 2018; Han et al., 2019a), compared to EVs from cells cultured in normoxia. These hyp-ADSC-EVs also dramatically changed HUVEC expression levels of the angiogenic genes Angpt1, Flk1 and Vash1, and increased activation of the PKA signaling pathway (Xue et al., 2018). Interestingly, EVs from hypoxia-preconditioned ADMSCs exhibited increased diameters (by 59 nm) compared to those derived in normoxia (Han et al., 2019a). Meanwhile, EVs derived from DMOG-stimulated hBMMSCs increased HUVEC angiogenesis in vitro and decreased expression levels of PTEN (Liang et al., 2019), a tumor suppressor gene found to promote neovascularization by inducing HUVEC migration (Zhang et al., 2016). This decreased PTEN expression was further accompanied by increased expression levels of its corresponding downstream AKT/mTOR signaling pathway members, p-AKT, mTOR, and p-mTOR (Liang et al., 2019). Increased angiogenesis in a calvarial defect model treated with EVs isolated from DMOG-stimulated hBMMSCs was also observed (Liang et al., 2019).
Osteogenesis
Although both BMMSC- and ADMSC-derived EVs have been shown to promote osteogenesis in vivo, most preclinical studies use BMMSC-derived EVs to induce bone formation and fracture healing. BMMSC-derived EVs were shown to promote bone regeneration in rat calvarial bone defect models (Qin et al., 2016; Takeuchi et al., 2019), murine femoral fracture models (Furuta et al., 2016; Xu T. et al., 2020), rat models of distraction osteogenesis (Jia et al., 2020), and subcutaneous bone formation models in nude mice (Narayanan et al., 2016; Xie et al., 2017) (see Table 2). By contrast, the bone regeneration capacity of ADMSCs-derived EVs has only been explored in two studies to date in calvarial defect models (Li et al., 2018); one of which involved EVs derived from hADMSCs engineered to overexpress miR-375 (Chen et al., 2019) (see Table 2). With so few studies of ADMSC-derived EVs, it is difficult to compare their osteogenic capacity to BMMSC-derived EVs. However, in separate rat calvarial defect studies, treatment with BMMSC-derived EVs led to a greater increase (fourfold) in bone volume [i.e., (BV)/(TV)] compared to controls (Qin et al., 2016), than that induced by ADMSC-derived EVs (approximately 1.33-fold) (Chen et al., 2019).
The mechanisms and pathways underlying the osteogenic effects of MSC-derived EVs have not been widely reported. In a non-union model, BMMSC-derived EVs enhanced osteogenesis via the activation of the BMP-2/Smad1/RUNX2 signaling pathway (Zhang et al., 2020). In a femoral fracture model, BMMSC-derived EVs from young rats yielded increased bone formation and expression of Runx2, ALP and Col I compared to those from older rats; with EV osteogenic capacity linked inversely to levels of miR-128-3p expression, which was found to negatively modulate Smad5 signaling (Xu T. et al., 2020). Significantly, additional studies also found that miRNAs played an important role in promoting EV-mediated bone regeneration, with miR-196a regulating differentiation of osteoblasts (Qin et al., 2016), while miR-375 stimulated osteogenic differentiation of MSCs by inhibiting IGFBP3 (Chen et al., 2019).
In vitro studies of MSC-derived EVs further illustrated their role in bone regeneration through promotion of MSC proliferation, migration, and osteogenic differentiation (Narayanan et al., 2016; Takeuchi et al., 2019). Many studies have observed that treatment with BMMSCs-EVs increased osteogenic differentiation and upregulation of related genes, including RUNX2, Osterix, BMP9, TGF-β1, BMP2, TGF-β, OCN, ALP, Col I, and PDGF in BMMSCs (Narayanan et al., 2016; Xu T. et al., 2020) and osteoblastic MC3T3 cells (Zhang et al., 2020). Similarly, ADMSC-derived EVs were also found to promote osteogenic differentiation of human BMMSCs in vitro by significantly increasing the expression of RUNX2, ALP, and COL1A1 (Li et al., 2018; Chen et al., 2019). Interestingly, BMMSC-derived EVs isolated from young (2-week old) SD rats promoted proliferation and enhanced the osteogenic capacity of older BMSCs, and significantly upregulated the expression of ALP, Runx2, and OCN (Jia et al., 2020). In human osteoblasts, BMMSC-derived EVs enhanced differentiation, likely due to differential expression of miR-196a (Qin et al., 2016). In another study, miR-26 was found to be crucial to the in vitro capacity of BMMSC-derived EVs to induce osteogenic differentiation via silencing experiments (Luo et al., 2019).
Immunomodulation
Effects on Macrophage Polarization
In preclinical models, EVs from MSCs have shown a variety of immunomodulatory effects. EVs from both BMMSCs and ADMSCs can change macrophage polarization from the pro-inflammatory M1 type to the anti-inflammatory M2 type in vitro and in vivo (see Table 3) (Li et al., 2020; Wang J. et al., 2020). With very few in vivo studies analyzing the effects of ADMSC-derived EVs on macrophage polarization and no direct comparison studies, it is difficult to conclude whether one EV source is more effective than the other. However, looking at separate studies that used comparable metrics, BMMSC-derived EVs induced a dramatically increased change in the expression of the M2 polarization marker CD206 (3.2-fold) in a murine acute lung injury model (Wang J. et al., 2020) compared to that induced by ADMSC-derived EVs in a murine air pouch model (1.5-fold) (Liu et al., 2020b).
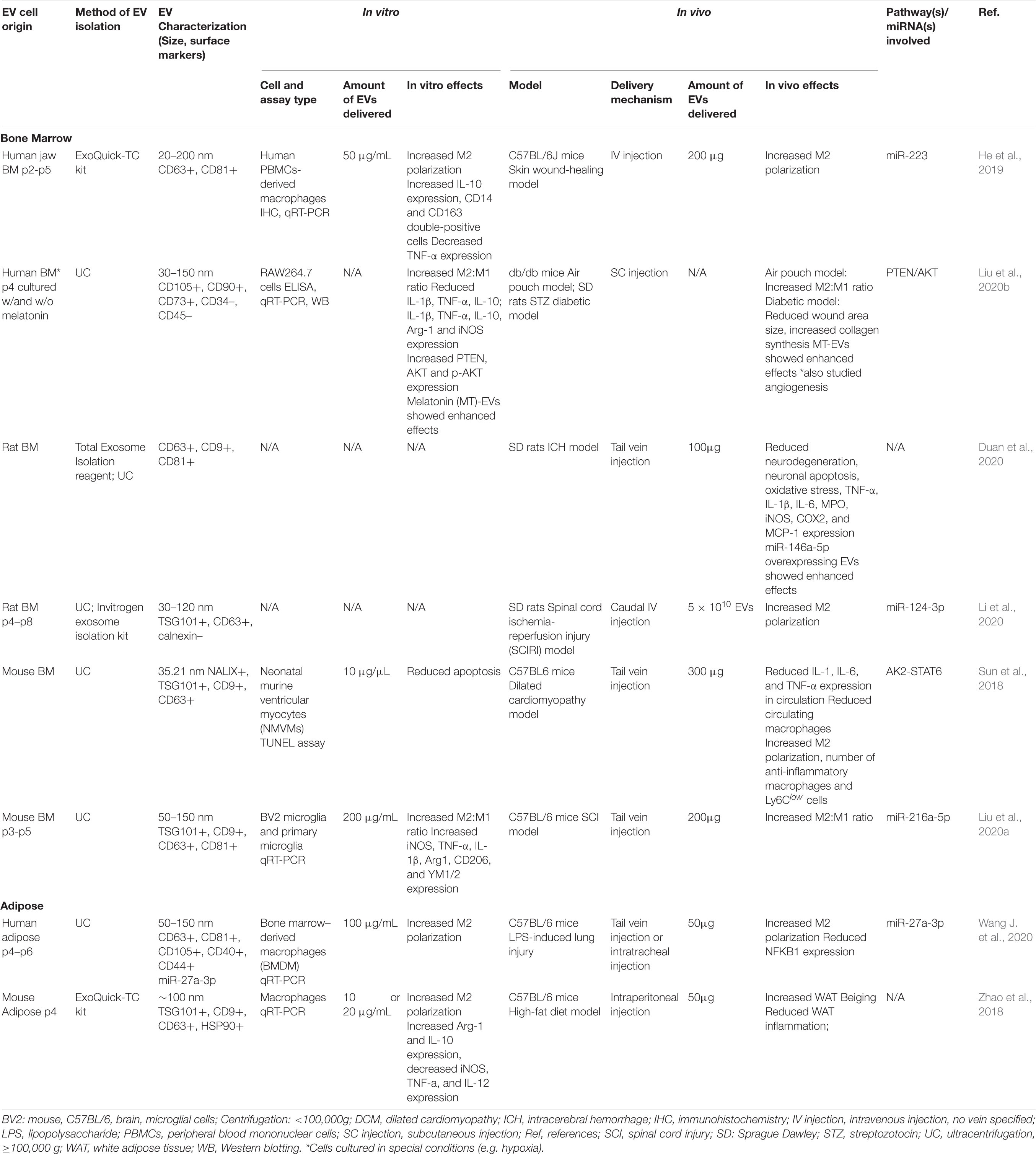
Table 3. Preclinical studies employing BMMSC- and ADMSC-derived EVs to induce macrophage polarization.
In the preclinical models reviewed here, M2 polarization stimulated by MSC-EV treatment was found to be associated with increased expression of anti-inflammatory cytokines, such as IL-10 and TGF-β (Garnier et al., 2018), and decreased secretion of IL-6 and TNF-α (Arora et al., 2018). In a murine model of acute respiratory distress syndrome BMMSC-derived EVs reduced lung damage and LPS-induced inflammation (Deng et al., 2020). As in previous studies demonstrating that metabolic reprogramming of glycolysis in macrophages contributes to M2 polarization (Zhao et al., 2020), EV-treatment in this model was associated with downregulation of glycolysis in lung macrophages and M2 polarization (Deng et al., 2020). Research in models of cutaneous wound-healing and spinal cord ischemia-reperfusion injury (SCIRI) have also shown that BMMSC-EV induced macrophage M2 polarization was associated with the AK2-STAT6 signaling pathway (Sun et al., 2018), miR-223 (He et al., 2019), and miR-124-3p/Ern1 (Li et al., 2020). BMMSC-derived EVs also inhibited M1 microglia activation and tissue neutrophil infiltration and reduced the expression of TNF-α, IL-1β, and IL-6 in a rat ICH model (Duan et al., 2020). Similarly, ADMSC-EV treatment in a murine model of LPS-induced lung injury mitigated injury, increased localized expression of miR-27a-3p, and induced M2 macrophage polarization via NFKB1 signaling (Wang J. et al., 2020).
Interestingly, hypoxic BMMSC culture conditions were found to produce EVs with enhanced capacity to induce M2 macrophage/microglia polarization. For example, in a spinal cord injury (SCI) model, treatment with EVs from hypoxia preconditioned BMMSCs resulted in increased conversion of microglia from the pro-inflammatory M1 to the anti-inflammatory M2 phenotype, compared to EVs from BMMSCs cultured in normoxia (Liu et al., 2020a). This increased shift toward M2 polarization was found to be associated with increased expression of miR-216a-5p in hypoxic EVs. In another in vitro study of the microglia M1 to M2 phenotype change, hypoxic preconditioning of BMMSCs enhanced secretion of EVs and increased the M2 polarization capacity of the BMMSC secretome as compared to normoxic conditions (Yu H. et al., 2020).
Effects on Fibrosis
Several preclinical studies have shown that MSC-derived EVs can promote tissue regeneration over fibrosis, thus reducing the formation of scar tissue and other fibrotic processes that impair normal cellular and tissue function (Mutsaers et al., 1997) (see Table 4). For example, treatment with hBMMSC-derived EVs reduced interstitial kidney fibrosis by 80% in a mouse model of STZ-induced diabetic nephropathy, while porcine ADMSC-derived EVs resulted in a 24.4% reduction in tubulointerstitial kidney fibrosis in a porcine model of metabolic syndrome and renal artery stenosis (Eirin et al., 2017; Grange et al., 2019). BMMSC-derived EVs were also studied in two different chronic kidney injury models resulting in decreased interstitial lymphocyte infiltration in a 5/6 subtotal nephrectomy model (He et al., 2012), and significantly improved renal function and histological parameters, and reduced apoptosis and fibrotic markers in a cyclosporine nephrotoxicity model (Ramírez-Bajo et al., 2020). While, the anti-fibrotic capacity of ADMSC-derived EVs was less studied in vivo, in vitro studies showed that they inhibited the proliferation of CD4+ and CD8+ T cells (Blazquez et al., 2014), increased the expression ratios of collagen III to collagen I, TGF-β3 to TGF-β1, and MMP1 and -3 to TIMP1 in dermal fibroblasts (Wang L. et al., 2017), and prevented the transformation of tubular epithelial cells to a profibrotic phenotype via activation of tubular Sox9 (Zhu et al., 2017).
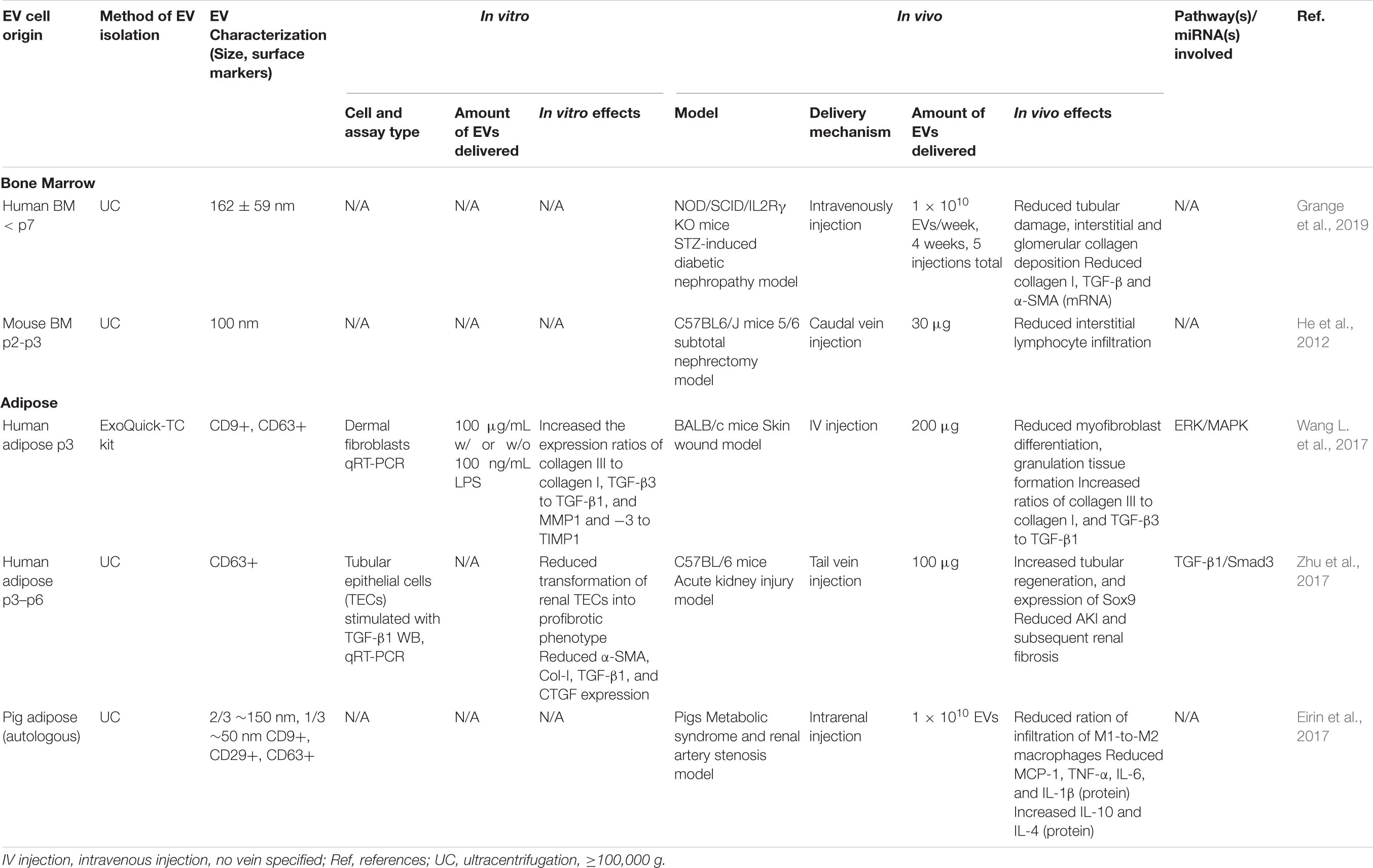
Table 4. Preclinical studies employing BMMSC- and ADMSC-derived EVs to induce anti-fibrotic effects.
Effects on Immune Cell Infiltration
Modulation of immune cell tissue infiltration is another important target of EV-based therapies. EVs derived from both MSC sources significantly influenced immune cell migration at treatment sites in various preclinical models (see Table 5); although, again, it is difficult to make efficacy comparisons. In murine models of fat grafting (di Han et al., 2018) and high-fat diet (Zhao et al., 2018), ADMSC-derived EVs decreased inflammatory cell infiltration into adipose tissue, while, in a model of type-1 diabetes mellitus, they significantly increased the number of regulatory T cells (Tregs) (Nojehdehi et al., 2018). ADMSC-derived EVs also decreased the infiltration of mast cells, CD86+ cells, and CD206+ cells in skin lesions and reduced the mRNA expression levels of various inflammatory cytokines, such as IL-4, IL-31, and TNF-α in a murine model of atopic dermatitis (Cho et al., 2018). Similarly, BMMSC-derived EVs reduced the infiltration of CD45+ immune cells in a mouse model of aristolochic acid induced nephropathy (Kholia et al., 2020), reduced the number of GFAP+ astrocytes and CD68+ cells in a TBI model (Zhang et al., 2017) and decreased infiltration of leukocytes in a murine model of focal cerebral ischemia (Wang C. et al., 2020).
Effects on Other Immune Cells and Processes
The immunomodulatory potential of both ADMSC- and BMMSC- derived EVs has also been explored in many other preclinical disease and injury models (see Table 5). For example, ADMSC-derived EVs were found to alleviate inflammation in a retinal laser injury model (Yu et al., 2016), attenuate complement levels in a SCI model (Zhao et al., 2019), and downregulate the expression of inflammatory biomarkers in an acute ischemic stroke model (Chen et al., 2016). Meanwhile, in two kidney I/R injury models ADMSC-derived EVs either upregulated the expression of tubular Sox9 (Zhu et al., 2017), or decreased the expression of inflammatory proteins, including TNF-α, NF-κB, IL-1β, MIF, PAI-1, Cox-2 (Lin et al., 2016). Similarly, ADMSC-derived EVs mitigated scar formation, inhibited granulation tissue formation, increased expression of TGF-β3 compared to TGF-β1 and increased the ratio of collagen III to collagen I in a skin wound healing model (Wang L. et al., 2017). BMMSC-derived EVs have been studied in an even wider array of preclinical injury models than those from ADMSCs (see Table 5 and below).
Extracellular vesicles from BMMSCs have been studied in a wide array of preclinical models where immunomodulation plays a key role. Localized injection of BMMSC-derived EVs decreased inflammation in both an acute MI model (Teng et al., 2015) and an ischemia/reperfusion (I/R) model (Arslan et al., 2013) of myocardial injury. In the I/R model, both local and systemic inflammation were significantly reduced via inhibition of the c-JUK signaling pathway (Arslan et al., 2013). TGF-β expression was also shown to be reduced by BMMSC-derived EVs in a muscle injury model (Iyer et al., 2020). Similarly, treatment with BMMSC-derived EVs reduced IL2 mRNA expression, increased expression of TGF-β and HGF, and increased the ratio of Treg to CD4-positive cells among NPCs in a concanavalin A-induced liver injury model (Tamura et al., 2016). Meanwhile, in an allergic airway inflammation model induced by repeated exposure to Aspergillus hyphal extract, BMMSC-derived EVs reduced lung inflammation and airway hyperreactivity, and shifted the inflammatory response of Th2 and Th17 type T-cells (Cruz et al., 2015). In an osteoarthritis model, they reduced knee joint inflammation, mainly due to EV-expressed miR-9-5p directly targeting syndecan-1 (Jin et al., 2020). Finally, BMMSC-derived EV treatment in a renal allograft rejection model resulted in higher numbers of T- and B-cells, reduced NK-cell infiltration and significantly decreased TNFα expression (Koch et al., 2015). In vitro studies illustrate additional mechanism underlying the immunomodulatory effects of MSC-derived EVs. BMMSC-derived EVs significantly inhibited proliferation of CD3-stimulated T-cells (Teng et al., 2015) and increased expression of IL-10 and TGF-β1 in blood mononuclear cells, which induced Tregs differentiation and enhanced their immunosuppressive function (mo Du et al., 2018).
Similarities and Differences in Msc-Derived Ev Signaling and Therapeutic Capacity
In the reviewed studies, treatment with both ADMSC- and BMSC- derived EVs activated several common signaling pathways related to cellular survival, proliferation and/or differentiation. For example, the VEGF pathway, which is involved in angiogenesis and thus wound healing, was observed to be modulated by EVs in fracture non-union (Zhang et al., 2020), fat grafting (Han et al., 2019a), and calvarial bone defect models (Zhu et al., 2019). Several AKT-related signaling pathways, including AKT/mTOR (Liang et al., 2019), AKT/eNOS (Yu M. et al., 2020), and PTEN/AKT (Liu et al., 2020b), were also induced by ADMSC- and BMMSC- derived EVs. This is not surprising given that AKT participates in a wide range of signaling pathways, including those involved in angiogenesis, osteogenesis, and immunomodulation. Meanwhile, signaling pathways involving Smad family proteins were also activated by BMMSC- and ADMSC- derived EVs, including those involving BMP-2/Smad1/RUNX2 (Zhang et al., 2020), TGF-β1/Smad3 (Zhu et al., 2017), and Smad5 (Xu T. et al., 2020). Observed differences between studies in activated signaling pathways are due to variations in study design and purpose, including species, in vivo model, cell type, cell passage number, cell state, and assay type. Importantly, overlapping miRNAs within EVs or in EV-activated signaling pathways were not observed between the studies reviewed here. This may be caused by real differences in miRNA expression or variations in the methods that the researchers used to study miRNAs, such as miRNA sequencing (to target wide range of differentially expressed miRNAs) vs. qRT-PCR (to target a much smaller group of miRNAs).
While EVs from both sources showed great potential in inducing angiogenesis, osteogenesis and immunomodulation in various preclinical tissue regeneration models, the therapeutic capacity of EVs derived from ADMSC has been far less widely studied than EVs from BMMSCs. This research imbalance combined with few direct comparative studies, in vivo or in vitro, makes it difficult to conclude which EV source is best for a given application. However, in vitro studies do provide insight into a few possible similarities and differences in ADMSC-derived vs. BMMSC-derived EVs. For example, although EVs from both MSC sources generally expressed CD63, CD9, and CD81, and were negative for expression of either CD45, CD34, or calnexin (Katsuda et al., 2013; Del Fattore et al., 2015; Gouveia et al., 2015; Gualerzi et al., 2017; Bari et al., 2019; Chance et al., 2019; Villatoro et al., 2019; Chance et al., 2020; Hoang et al., 2020), ADMSC-derived EVs expressed higher levels of CD63, phosphatidylserine (Chance et al., 2019), and ceramides (Gualerzi et al., 2017), while BMMSC-derived EVs displayed more protein types and a higher protein content per cell (Villatoro et al., 2019). EV cargos and resulting in vitro effects were also observed to vary significantly depending on MSC-type. For example, ADMSC-EVS expressed higher levels of HGF, whereas BMMSC-derived EVs expressed higher levels of VEGFA, FGF-2, and PDGF-BB and thus induced greater proliferation in dermal fibroblasts (Hoang et al., 2020). In direct comparison studies, ADMSC-derived EVs were shown to promote more HUVEC tube formation (Chance et al., 2020) and display higher thrombogenic activity (Chance et al., 2019) than BMMSC-derived EVs. Meanwhile, BMMSC-derived EVs increased IL-10 secretion by a factor of 1.8 in phytohemagglutinin -activated peripheral blood mononuclear cells compared to ADMSC-derived EVs (Bari et al., 2019). Interestingly, treatment with MSC-derived EVs did not induce any effects in some in vitro studies, such as those on lymphocyte (Gouveia et al., 2015) and peripheral blood mononuclear cell proliferation (Villatoro et al., 2019).
Factors and Strategies Affecting Ev Therapeutic Efficacy
The method and route of EV delivery, such as intravenous injection (IV), localized injection, subcutaneous injection (SC), intraperitoneal injection (IP), intra-arterial infusion (IA), intramuscular injection (IM), topical application, or carrier-based delivery, significantly affects EV biodistribution and thus therapeutic efficacy in vivo. For example, in one study, IV administration lead to significantly increased BMMSC-derived EV accumulation in the liver and spleen and decreased accumulation in the gastrointestinal tract compared to SC or IP delivery; whereas IP injection lead to more EVs in the pancreas compared to IV administration (Wiklander et al., 2015). Meanwhile, IP injection of BMMSC-derived EVs was more therapeutically effective in a hepatic failure model than IV injection, resulting in a better survival rate (Haga et al., 2017). However, as systemic delivery methods, such as IV or IP injection, tend to result in EV accumulation in the liver, spleen and lungs, regardless of the cell source, delivery route, or injury model being studied (Gatti et al., 2011; Wiklander et al., 2015; Eirin et al., 2017; Maudens et al., 2018), carrier-based EV delivery methods have been developed to localize and control release (Maudens et al., 2018).
Carrier-based EV delivery methods, such as hydrogel encapsulation and surface absorption on membranes or scaffolds, provide several advantages over systemic delivery methods. Localized delivery concentrates EVs in the vicinity of target tissue, potentially reducing the amount of EVs required for achieving a given therapeutic effect, and can also prolong EV release (Liu et al., 2018; Riau et al., 2019). For example, delivery of EVs isolated from ADMSCs via a pluronic F127/oxidized hyaluronic acid-poly-ε-lysine hydrogel accelerated wound healing, promoted neovascularization, and increased collagen I and III expression in a diabetic wound healing model over a 21-day period, compared to delivery of EVs alone (Wang C. et al., 2019). Similarly, EVs loaded onto poly(lactic co-glycolic acid)/polydopamine scaffolds exhibited continuous release in vitro, with 28.19 ± 9.2% EVs retained within the scaffold after 8 days, and resulted in significantly improved bone regeneration when implanted in vivo (Li et al., 2018).
EV cargo, and thus therapeutic efficacy, is strongly influenced by a variety of factors, including donor-to-donor variability (e.g., age, gender, health status), tissue and site of cell origin (e.g., vertebral vs. femoral bone marrow), cell passage number, culture microenvironment (e.g., mechanical, chemical, hypoxia vs. normoxia), and cell state (e.g., differentiation, metabolism). For example, melatonin-treated MSCs secreted smaller sized EVs that resulted in decreased inflammation and wound size and increased angiogenesis in a diabetic wound healing model compared to EVs from untreated MSCs (Liu et al., 2020b). Meanwhile, EVs isolated from rats with type 1 diabetes yielded less bone and blood vessel formation in a rat calvarial defect model than BMMSC-EVs from normal rats (Zhu et al., 2019). Further, BMSC-EVs obtained from young donors induced increased fracture healing compared to EVs from old donors (Xu T. et al., 2020).
Many studies employ specific cell culture conditions and/or pretreatments to obtain EVs with desired cargo(s), including hypoxic conditions, drug or growth factor treatments, genetic modification, and 3D culture. These treatments have been shown to increase the therapeutic potential of the resulting EVs in several models (Haque et al., 2013; Zhang et al., 2017; Zhu et al., 2018). For example, EVs isolated from 3D cultured MSCs yielded enhanced functional recovery and immunomodulation in a traumatic brain injury model compared to EVs derived from 2D culture (Zhang et al., 2017). Similarly, EVs obtained from hypoxia preconditioned MSCs exhibited increased neovascularization and repair in a myocardial injury model compared to normoxia EVs (Zhu et al., 2018). In yet another study, IL-1β treatment increased miR-146a expression in MSCs and their corresponding EVs, which in turn resulted in increased miR-146a expression and M2 polarization in EV-treated macrophages (Song et al., 2017). However, care must be taken in using such strategies to enhance EV therapeutic efficacy as cellular pretreatment can also lead to adverse effects. For example, culturing MSCs in hypoxic conditions can interfere with differentiation and mitochondria biogenesis (Ejtehadifar et al., 2015), which, in turn can affect the therapeutic efficacy of the resulting EVs, as mitochondria were shown to be transferred via MSC-derived EVs into recipient cells (Jackson et al., 2016; Konari et al., 2019).
Post-modification of EVs is also widely used in studies to modify therapeutic efficacy. EV surfaces can be modified to facilitate uptake by specific target cells. For example, MSC-derived EVs that were surface-modified with cationic pullulan displayed increased liver targeting and resulted in improved liver function compared to unmodified EVs in a rat model of liver damage (Tamura et al., 2017). Therapeutic miRNAs and other cargos can also be introduced into EVs to improve efficacy (Naseri et al., 2018). For instance, overexpressing miR-181a in MSCs-derived EVs resulted in decreased infarct size and area-at-risk in a myocardial IR injury model compared to control EVs (Wei et al., 2019). Surface modification strategies can also be used to improve EV stability within the circulation, as glycosylation of EV surface peptides was shown to EV delivery to neuroblastoma cells (Hung and Leonard, 2015). However, post-processing of EVs can also lead to adverse effects. For example, post-processing EVs via mechanical extrusion or electroporation can result in loss of EV integrity, and biological activity (Shi et al., 2018).
Obstacles to Clinical Translation of Msc-Derived Ev Therapies
There is a crucial need for studies which directly and systematically compare EVs derived from ADMSCs and BMMSCs to determine the optimal EV source for specific clinical applications. More importantly, transferring EV-based therapies to the clinic will require the development of reproducible approaches for high-yield production of EVs with well-defined properties and therapeutic potential. Standardized EV purity metrics and isolation and characterization methods will thus be critical to enable not only systematic comparison of therapeutic EV sources, also for validation of safety and efficacy. However, standardizing characterization of even a simple parameter such as EV size can be challenging. While a wide variety of methods have been used to characterize EV size, concentration, and polydispersity, including Transmission Electron Microscopy, Atomic Force Microscopy, Nanoparticle Tracking Analysis, Tunable Resistive Pulse Sensing, and Dynamic Light Scattering (Caponnetto et al., 2017), these methods can result in different size range and concentration determinations for the same EV samples (Zhu et al., 2019; Wang C. et al., 2020), even when employing different devices based on the technology (Bachurski et al., 2019; Wang C. et al., 2020). Furthermore, different EV isolation methods can also preferentially result in different EV subpopulations, exhibiting variations in EV size distribution, yield, purity, mRNA, and protein profile (Van Deun et al., 2014; Zlotogorski-Hurvitz et al., 2015).
Extracellular vesicles storage is another important issue in expanding clinical EV treatments. For example, the combination of lyophilization and cryoprotectants was found to maintain model enzyme activity within EVs to a greater extent than lyophilization alone, or storage at 4°C and –80°C (Frank et al., 2018). Further studies to systematically characterize the dynamic changes in EV content and number for varying storage periods and conditions will be required to extend EV therapeutic use.
Conclusion
The reviewed studies demonstrate that tissue regeneration therapies based on both BMMSC- and ADMSC-derived EVs show promise as alternatives to MSCs-based treatment. However, there is still limited evidence to determine which EV source is optimal for which tissue regeneration application, as there are significantly more studies which used BMMSC-derived EVs than ADMSC-derived EVs, few comparative studies, and considerable variation in overall study design. There is thus a crucial need for more studies, particularly in vivo, which directly compare the therapeutic efficacy of EVs derived from ADMSCs and BMMSCs. Optimization of donor sources, passage number, and culture conditions will also be essential to maximizing EV therapeutic capacity for specific applications. Establishment of thorough EV characterization standards, including size distribution, surface markers and cargo(s), as well as isolation and production standards will also be crucial in both systematic comparison of EV therapeutic efficacy as well as transferring EV therapies to the clinic.
Author Contributions
YL performed the literature search and wrote the manuscript. CH revised the manuscript. Both authors reviewed the manuscript and approved the final version.
Conflict of Interest
The authors declare that the research was conducted in the absence of any commercial or financial relationships that could be construed as a potential conflict of interest.
Abbreviations
MSC, mesenchymal stem cells; EV, extracellular vesicles; MI, myocardial infarction; ICH, intracerebral hemorrhages; miRNA, microRNA; BMMSC, bone marrow-derived MSC; ADMSC, adipose-derived MSC; I/R, ischemia/reperfusion; SCI, spinal cord injury; Tregs, regulatory T cells.
References
Arora, S., Dev, K., Agarwal, B., Das, P., and Syed, M. A. (2018). Macrophages: Their role, activation and polarization in pulmonary diseases. Immunobiology 223, 383–396. doi: 10.1016/j.imbio.2017.11.001
Arslan, F., Lai, R. C., Smeets, M. B., Akeroyd, L., Choo, A., Aguor, E. N. E., et al. (2013). Mesenchymal stem cell-derived exosomes increase ATP levels, decrease oxidative stress and activate PI3K/Akt pathway to enhance myocardial viability and prevent adverse remodeling after myocardial ischemia/reperfusion injury. Stem Cell Res. 10, 301–312. doi: 10.1016/j.scr.2013.01.002
Aslam, M., Baveja, R., Liang, O. D., Fernandez-Gonzalez, A., Lee, C., Mitsialis, S. A., et al. (2009). Bone marrow stromal cells attenuate lung injury in a murine model of neonatal chronic lung disease. Am. J. Respir. Crit. Care Med. 180, 1122–1130. doi: 10.1164/rccm.200902-0242OC
Azari, M. F., Mathias, L., Ozturk, E., Cram, D. S., Boyd, R. L., and Petratos, S. (2010). Mesenchymal Stem Cells for Treatment of CNS Injury. Curr. Neuropharmacol. 8, 316–323. doi: 10.2174/157015910793358204
Bachurski, D., Schuldner, M., Nguyen, P. H., Malz, A., Reiners, K. S., Grenzi, P. C., et al. (2019). Extracellular vesicle measurements with nanoparticle tracking analysis–An accuracy and repeatability comparison between NanoSight NS300 and ZetaView. J. Extracell. Vesicl. 8:6016. doi: 10.1080/20013078.2019.1596016
Bang, O. Y., and Kim, E. H. (2019). Mesenchymal Stem Cell-Derived Extracellular Vesicle Therapy for Stroke: Challenges and Progress. Front. Neurol. 10:211. doi: 10.3389/fneur.2019.00211
Bari, E., Perteghella, S., Catenacci, L., Sorlini, M., Croce, S., Mantelli, M., et al. (2019). Freeze-dried and GMP-compliant pharmaceuticals containing exosomes for acellular mesenchymal stromal cell immunomodulant therapy. Nanomedicine 14, 753–765. doi: 10.2217/nnm-2018-0240
Batsali, A. K., Georgopoulou, A., Mavroudi, I., Matheakakis, A., Pontikoglou, C. G., and Papadaki, H. A. (2020). The Role of Bone Marrow Mesenchymal Stem Cell Derived Extracellular Vesicles(MSC-EVs) in Normal and Abnormal Hematopoiesis and Their Therapeutic Potential. J. Clin. Med. 9:856. doi: 10.3390/jcm9030856
Blazquez, R., Sanchez-Margallo, F. M., de la Rosa, O., Dalemans, W., Álvarez, V., Tarazona, R., et al. (2014). Immunomodulatory potential of human adipose mesenchymal stem cells derived exosomes on in vitro stimulated T cells. Front. Immunol. 5:556. doi: 10.3389/fimmu.2014.00556
Caplan, A. I. (1991). Mesenchymal stem cells. J. Orthop. Res. 9, 641–650. doi: 10.1002/jor.1100090504
Caplan, A. I., and Dennis, J. E. (2006). Mesenchymal stem cells as trophic mediators. J. Cell. Biochem. 98, 1076–1084. doi: 10.1002/jcb.20886
Caponnetto, F., Manini, I., Skrap, M., Palmai-Pallag, T., Di Loreto, C., Beltrami, A. P., et al. (2017). Size-dependent cellular uptake of exosomes. Nanomed. Nanotechnol. Biol. Med. 13, 1011–1020. doi: 10.1016/j.nano.2016.12.009
Chance, T. C., Herzig, M. C., Christy, B. A., Delavan, C., Rathbone, C. R., Cap, A. P., et al. (2020). Human mesenchymal stromal cell source and culture conditions influence extracellular vesicle angiogenic and metabolic effects on human endothelial cells in vitro. J. Trauma Acute Care Surg. 89, S100–S108. doi: 10.1097/TA.0000000000002661
Chance, T. C., Rathbone, C. R., Kamucheka, R. M., Peltier, G. C., Cap, A. P., and Bynum, J. A. (2019). The effects of cell type and culture condition on the procoagulant activity of human mesenchymal stromal cell-derived extracellular vesicles. J. Trauma Acute Care Surg. 87, S74–S82. doi: 10.1097/TA.0000000000002225
Chen, K. H., Chen, C. H., Wallace, C. G., Yuen, C. M., Kao, G. S., Chen, Y. L., et al. (2016). Intravenous administration of xenogenic adipose-derived mesenchymal stem cells (ADMSC) and ADMSC-derived exosomes markedly reduced brain infarct volume and preserved neurological function in rat after acute ischemic stroke. Oncotarget 7, 74537–74556. doi: 10.18632/oncotarget.12902
Chen, S., Tang, Y., Liu, Y., Zhang, P., Lv, L., Zhang, X., et al. (2019). Exosomes derived from miR-375-overexpressing human adipose mesenchymal stem cells promote bone regeneration. Cell Prolif. 52:12669. doi: 10.1111/cpr.12669
Chiossone, L., Conte, R., Spaggiari, G. M., Serra, M., Romei, C., Bellora, F., et al. (2016). Mesenchymal Stromal Cells Induce Peculiar Alternatively Activated Macrophages Capable of Dampening Both Innate and Adaptive Immune Responses. Stem Cells 34, 1909–1921. doi: 10.1002/stem.2369
Cho, B. S., Kim, J. O., Ha, D. H., and Yi, Y. W. (2018). Exosomes derived from human adipose tissue-derived mesenchymal stem cells alleviate atopic dermatitis. Stem Cell Res. Ther. 9:939. doi: 10.1186/s13287-018-0939-5
Cosenza, S., Ruiz, M., Toupet, K., Jorgensen, C., and Noël, D. (2017). Mesenchymal stem cells derived exosomes and microparticles protect cartilage and bone from degradation in osteoarthritis. Sci. Rep. 7, 376–388. doi: 10.1038/s41598-017-15376-8
Crop, M. J., Baan, C. C., Korevaar, S. S., IJzermans, J. N. M., Pescatori, M., Stubbs, A. P., et al. (2010). Inflammatory conditions affect gene expression and function of human adipose tissue-derived mesenchymal stem cells. Clin. Exp. Immunol. 162, 474–486. doi: 10.1111/j.1365-2249.2010.04256.x
Cruz, F. F., Borg, Z. D., Goodwin, M., Sokocevic, D., Wagner, D. E., Coffey, A., et al. (2015). Systemic Administration of Human Bone Marrow-Derived Mesenchymal Stromal Cell Extracellular Vesicles Ameliorates Aspergillus Hyphal Extract-Induced Allergic Airway Inflammation in Immunocompetent Mice. Stem Cells Transl. Med. 4, 1302–1316. doi: 10.5966/sctm.2014-0280
Del Fattore, A., Luciano, R., Saracino, R., Battafarano, G., Rizzo, C., Pascucci, L., et al. (2015). Differential effects of extracellular vesicles secreted by mesenchymal stem cells from different sources on glioblastoma cells. Exp. Opin. Biol. Ther. 15, 495–504. doi: 10.1517/14712598.2015.997706
Deng, H., Wu, L., Liu, M., Zhu, L., Chen, Y., Zhou, H., et al. (2020). Bone Marrow Mesenchymal Stem Cell-Derived Exosomes Attenuate LPS-Induced ARDS by Modulating Macrophage Polarization Through Inhibiting Glycolysis in Macrophages. Shock Publ. Ahead Print 97:1549. doi: 10.1097/shk.0000000000001549
di Han, Y., Bai, Y., long Yan, X., Ren, J., Zeng, Q., dong Li, X., et al. (2018). Co-transplantation of exosomes derived from hypoxia-preconditioned adipose mesenchymal stem cells promotes neovascularization and graft survival in fat grafting. Biochem. Biophys. Res. Commun. 497, 305–312. doi: 10.1016/j.bbrc.2018.02.076
Di Nicola, M., Carlo-Stella, C., Magni, M., Milanesi, M., Longoni, P. D., Matteucci, P., et al. (2002). Human bone marrow stromal cells suppress T-lymphocyte proliferation induced by cellular or nonspecific mitogenic stimuli. Blood 99, 3838–3843. doi: 10.1182/blood.V99.10.3838
Doeppner, T. R., Herz, J., Görgens, A., Schlechter, J., Ludwig, K., Radtke, S., et al. (2015). Extracellular Vesicles Improve Post-Stroke Neuroregeneration and Prevent Postischemic Immunosuppression. Stem Cells Transl. Med. 4, 1131–1143. doi: 10.5966/sctm.2015-0078
Dominici, M., Le Blanc, K., Mueller, I., Slaper-Cortenbach, I., Marini, F. C., Krause, D. S., et al. (2006). Minimal criteria for defining multipotent mesenchymal stromal cells. The International Society for Cellular Therapy position statement. Cytotherapy 8, 315–317. doi: 10.1080/14653240600855905
Duan, S., Wang, F., Cao, J., and Wang, C. (2020). Exosomes derived from microRNA-146a-5p-enriched bone marrow mesenchymal stem cells alleviate intracerebral hemorrhage by inhibiting neuronal apoptosis and microglial m1 polarization. Drug Des. Devel. Ther. 14, 3143–3158. doi: 10.2147/DDDT.S255828
Dyer, D. P., Thomson, J. M., Hermant, A., Jowitt, T. A., Handel, T. M., Proudfoot, A. E. I., et al. (2014). TSG-6 Inhibits Neutrophil Migration via Direct Interaction with the Chemokine CXCL8. J. Immunol. 192, 2177–2185. doi: 10.4049/jimmunol.1300194
Eggenhofer, E., Luk, F., Dahlke, M. H., and Hoogduijn, M. J. (2014). The life and fate of mesenchymal stem cells. Front. Immunol. 5:148. doi: 10.3389/fimmu.2014.00148
Eirin, A., Zhu, X. Y., Puranik, A. S., Tang, H., McGurren, K. A., van Wijnen, A. J., et al. (2017). Mesenchymal stem cell–derived extracellular vesicles attenuate kidney inflammation. Kidney Int. 92, 114–124. doi: 10.1016/j.kint.2016.12.023
Ejtehadifar, M., Shamsasenjan, K., Movassaghpour, A., Akbarzadehlaleh, P., Dehdilani, N., Abbasi, P., et al. (2015). The effect of hypoxia on mesenchymal stem cell biology. Adv. Pharmaceut. Bull. 5, 141–149. doi: 10.15171/apb.2015.021
El-Badawy, A., Amer, M., Abdelbaset, R., Sherif, S. N., Abo-Elela, M., Ghallab, Y. H., et al. (2016). Adipose stem cells display higher regenerative capacities and more adaptable electro-kinetic properties compared to bone marrow-derived mesenchymal stromal cells. Sci. Rep. 6:36801. doi: 10.1038/srep37801
Elman, J. S., Li, M., Wang, F., Gimble, J. M., and Parekkadan, B. (2014). A comparison of adipose and bone marrow-derived mesenchymal stromal cell secreted factors in the treatment of systemic inflammation. J. Inflamm. 11, 255–261. doi: 10.1186/1476-9255-11-1
Fan, L. J., Xiao, Q. R., Lin, K. S., Wang, S. Y., Li, Z. F., Li, C. Z., et al. (2016). Comparison of endothelial differentiation capacity of adipose-derived stem cells and bone marrow mesenchymal stem cells from rats. Nan Fang Yi Ke Da Xue Xue Bao 36, 1247–1254.
Fischer, U. M., Harting, M. T., Jimenez, F., Monzon-Posadas, W. O., Xue, H., Savitz, S. I., et al. (2009). Pulmonary passage is a major obstacle for intravenous stem cell delivery: The pulmonary first-pass effect. Stem Cells Dev. 18, 683–691. doi: 10.1089/scd.2008.0253
Frank, J., Richter, M., de Rossi, C., Lehr, C. M., Fuhrmann, K., and Fuhrmann, G. (2018). Extracellular vesicles protect glucuronidase model enzymes during freeze-drying. Sci. Rep. 8:786. doi: 10.1038/s41598-018-30786-y
Friedenstein, A. J., Petrakova, K. V., Kurolesova, A. I, and Frolova, G. P. (1968). Heterotopic of bone marrow. Analysis of precursor cells for osteogenic and hematopoietic tissues. Transplantation 6, 230–247.
Furuta, T., Miyaki, S., Ishitobi, H., Ogura, T., Kato, Y., Kamei, N., et al. (2016). Mesenchymal Stem Cell-Derived Exosomes Promote Fracture Healing in a Mouse Model. Stem Cells Transl. Med. 5, 1620–1630. doi: 10.5966/sctm.2015-0285
Garnier, M., Gibelin, A., Mailleux, A. A., Leçon, V., Hurtado-Nedelec, M., Laschet, J., et al. (2018). Macrophage Polarization Favors Epithelial Repair During Acute Respiratory Distress Syndrome. Crit. Care Med. 46, 692–701e. doi: 10.1097/CCM.0000000000003150
Gatti, S., Bruno, S., Deregibus, M. C., Sordi, A., Cantaluppi, V., Tetta, C., et al. (2011). Microvesicles derived from human adult mesenchymal stem cells protect against ischaemia-reperfusion-induced acute and chronic kidney injury. Nephrol. Dial. Transpl. 26, 1474–1483. doi: 10.1093/ndt/gfr015
Gnecchi, M., He, H., Liang, O. D., Melo, L. G., Morello, F., Mu, H., et al. (2005). Paracrine action accounts for marked protection of ischemic heart by Akt-modified mesenchymal stem cells [2]. Nat. Med. 11, 367–368. doi: 10.1038/nm0405-367
Gouveia, A. V., De Andrade, Bertolino, G., Riewaldt, J., Bieback, K., Karbanová, J., et al. (2015). Extracellular vesicles secreted by bone marrow- and adipose tissue-derived mesenchymal stromal cells fail to suppress lymphocyte proliferation. Stem Cells Dev. 24, 1374–1376. doi: 10.1089/scd.2014.0563
Grange, C., Tritta, S., Tapparo, M., Cedrino, M., Tetta, C., Camussi, G., et al. (2019). Stem cell-derived extracellular vesicles inhibit and revert fibrosis progression in a mouse model of diabetic nephropathy. Sci. Rep. 9:110. doi: 10.1038/s41598-019-41100-9
Gualerzi, A., Niada, S., Giannasi, C., Picciolini, S., Morasso, C., Vanna, R., et al. (2017). Raman spectroscopy uncovers biochemical tissue-related features of extracellular vesicles from mesenchymal stromal cells. Sci. Rep. 7:10448. doi: 10.1038/s41598-017-10448-1
Haga, H., Yan, I. K., Takahashi, K., Matsuda, A., and Patel, T. (2017). Extracellular vesicles from bone marrow-derived mesenchymal stem cells improve survival from lethal hepatic failure in mice. Stem Cells Transl. Med. 6, 1262–1272. doi: 10.1002/sctm.16-0226
Han, Y., Ren, J., Bai, Y., Pei, X., and Han, Y. (2019a). Exosomes from hypoxia-treated human adipose-derived mesenchymal stem cells enhance angiogenesis through VEGF/VEGF-R. Int. J. Biochem. Cell Biol. 109, 59–68. doi: 10.1016/j.biocel.2019.01.017
Han, Y., Seyfried, D., Meng, Y., Yang, D., Schultz, L., Chopp, M., et al. (2019b). Multipotent mesenchymal stromal cell-derived exosomes improve functional recovery after experimental intracerebral hemorrhage in the rat. J. Neurosurg. 131, 290–300. doi: 10.3171/2018.2.JNS171475
Haque, N., Rahman, M. T., Abu Kasim, N. H., and Alabsi, A. M. (2013). Hypoxic culture conditions as a solution for mesenchymal stem cell based regenerative therapy. Scient. World J. 2013:2972. doi: 10.1155/2013/632972
He, J., Wang, Y., Sun, S., Yu, M., Wang, C., Pei, X., et al. (2012). Bone marrow stem cells-derived microvesicles protect against renal injury in the mouse remnant kidney model. Nephrology 17, 493–500. doi: 10.1111/j.1440-1797.2012.01589.x
He, X., Dong, Z., Cao, Y., Wang, H., Liu, S., Liao, L., et al. (2019). MSC-Derived Exosome Promotes M2 Polarization and Enhances Cutaneous Wound Healing. Stem Cells Int. 2019:2708. doi: 10.1155/2019/7132708
Heijnen, H. F. G., Schiel, A. E., Fijnheer, R., Geuze, H. J., and Sixma, J. J. (1999). Activated platelets release two types of membrane vesicles: Microvesicles by surface shedding and exosomes derived from exocytosis of multivesicular bodies and α-granules. Blood 94, 3791–3799. doi: 10.1182/blood.v94.11.3791
Hoang, D. H., Nguyen, T. D., Nguyen, H. P., Nguyen, X. H., Do, P. T. X., Dang, V. D., et al. (2020). Differential Wound Healing Capacity of Mesenchymal Stem Cell-Derived Exosomes Originated From Bone Marrow, Adipose Tissue and Umbilical Cord Under Serum- and Xeno-Free Condition. Front. Mol. Biosci. 7:119. doi: 10.3389/fmolb.2020.00119
Horwitz, E. M., Le Blanc, K., Dominici, M., Mueller, I., Slaper-Cortenbach, I., Marini, F. C., et al. (2005). Clarification of the nomenclature for MSC: The International Society for Cellular Therapy position statement. Cytotherapy 7, 393–395. doi: 10.1080/14653240500319234
Hung, M. E., and Leonard, J. N. (2015). Stabilization of exosome-targeting peptides via engineered glycosylation. J. Biol. Chem. 290, 8166–8172. doi: 10.1074/jbc.M114.621383
Hunter, M. P., Ismail, N., Zhang, X., Aguda, B. D., Lee, E. J., Yu, L., et al. (2008). Detection of microRNA expression in human peripheral blood microvesicles. PLoS One 3:e3694. doi: 10.1371/journal.pone.0003694
Ikegame, Y., Yamashita, K., Hayashi, S. I., Mizuno, H., Tawada, M., You, F., et al. (2011). Comparison of mesenchymal stem cells from adipose tissue and bone marrow for ischemic stroke therapy. Cytotherapy 13, 675–685. doi: 10.3109/14653249.2010.549122
Iyer, S. R., Scheiber, A. L., Yarowsky, P., Henn, R. F., Otsuru, S., and Lovering, R. M. (2020). Exosomes Isolated From Platelet-Rich Plasma and Mesenchymal Stem Cells Promote Recovery of Function After Muscle Injury. Am. J. Sports Med. 48, 2277–2286. doi: 10.1177/0363546520926462
Jackson, M. V., Morrison, T. J., Doherty, D. F., McAuley, D. F., Matthay, M. A., Kissenpfennig, A., et al. (2016). Mitochondrial Transfer via Tunneling Nanotubes is an Important Mechanism by Which Mesenchymal Stem Cells Enhance Macrophage Phagocytosis in the In Vitro and In Vivo Models of ARDS. Stem Cells 34, 2210–2223. doi: 10.1002/stem.2372
Jeong, H., Yim, H. W., Park, H. J., Cho, Y., Hong, H., Kim, N. J., et al. (2018). Mesenchymal stem cell therapy for ischemic heart disease: Systematic review and meta-analysis. Int. J. Stem Cells 11, 1–12. doi: 10.15283/ijsc17061
Jia, Y., Qiu, S., Xu, J., Kang, Q., and Chai, Y. (2020). Exosomes Secreted by Young Mesenchymal Stem Cells Promote New Bone Formation During Distraction Osteogenesis in Older Rats. Calcif. Tissue Int. 106, 509–517. doi: 10.1007/s00223-019-00656-4
Jin, Y. Z., and Lee, J. H. (2018). Mesenchymal stem cell therapy for bone regeneration. CiOS 10, 271–278. doi: 10.4055/cios.2018.10.3.271
Jin, Z., Ren, J., and Qi, S. (2020). Exosomal miR-9-5p secreted by bone marrow–derived mesenchymal stem cells alleviates osteoarthritis by inhibiting syndecan-1. Cell Tissue Res. 381, 99–114. doi: 10.1007/s00441-020-03193-x
Kang, B. J., Ryu, H. H., Park, S. S., Koyama, Y., Kikuchi, M., Woo, H. M., et al. (2012). Comparing the osteogenic potential of canine mesenchymal stem cells derived from adipose tissues, bone marrow, umbilical cord blood, and Wharton’s jelly for treating bone defects. J. Vet. Sci. 13, 299–310. doi: 10.4142/jvs.2012.13.3.299
Kang, I. S., Suh, J., Lee, M. N., Lee, C., Jin, J., Lee, C., et al. (2020). Characterization of human cardiac mesenchymal stromal cells and their extracellular vesicles comparing with human bone marrow derived mesenchymal stem cells. BMB Rep. 53, 118–123. doi: 10.5483/BMBRep.2020.53.2.235
Katsuda, T., Tsuchiya, R., Kosaka, N., Yoshioka, Y., Takagaki, K., Oki, K., et al. (2013). Human adipose tissue-derived mesenchymal stem cells secrete functional neprilysin-bound exosomes. Sci. Rep. 3:1197. doi: 10.1038/srep01197
Kern, S., Eichler, H., Stoeve, J., Klüter, H., and Bieback, K. (2006). Comparative Analysis of Mesenchymal Stem Cells from Bone Marrow, Umbilical Cord Blood, or Adipose Tissue. Stem Cells 24, 1294–1301. doi: 10.1634/stemcells.2005-0342
Kholia, S., Herrera Sanchez, M. B., Cedrino, M., Papadimitriou, E., Tapparo, M., Deregibus, M. C., et al. (2020). Mesenchymal Stem Cell Derived Extracellular Vesicles Ameliorate Kidney Injury in Aristolochic Acid Nephropathy. Front. Cell Dev. Biol. 8:188. doi: 10.3389/fcell.2020.00188
Koch, M., Lemke, A., and Lange, C. (2015). Extracellular Vesicles from MSC Modulate the Immune Response to Renal Allografts in a MHC Disparate Rat Model. Stem Cells Int. 2015:141. doi: 10.1155/2015/486141
Konari, N., Nagaishi, K., Kikuchi, S., and Fujimiya, M. (2019). Mitochondria transfer from mesenchymal stem cells structurally and functionally repairs renal proximal tubular epithelial cells in diabetic nephropathy in vivo. Sci. Rep. 9:40163. doi: 10.1038/s41598-019-40163-y
Lai, E. C. (2002). Micro RNAs are complementary to 3′ UTR sequence motifs that mediate negative post-transcriptional regulation. Nat. Genet. 30, 363–364. doi: 10.1038/ng865
Lai, P., Weng, J., Guo, L., Chen, X., and Du, X. (2019). Novel insights into MSC-EVs therapy for immune diseases. Biomark. Res. 7, 156–160. doi: 10.1186/s40364-019-0156-0
Lau, N. C., Lim, L. P., Weinstein, E. G., and Bartel, D. P. (2001). An abundant class of tiny RNAs with probable regulatory roles in Caenorhabditis elegans. Science 294, 858–862. doi: 10.1126/science.1065062
Li, P., Kaslan, M., Lee, S. H., Yao, J., and Gao, Z. (2017). Progress in exosome isolation techniques. Theranostics 7, 789–804. doi: 10.7150/thno.18133
Li, R., Zhao, K., Ruan, Q., Meng, C., and Yin, F. (2020). Bone marrow mesenchymal stem cell-derived exosomal microRNA-124-3p attenuates neurological damage in spinal cord ischemia-reperfusion injury by downregulating Ern1 and promoting M2 macrophage polarization. Arthritis Res. Ther. 22:2146. doi: 10.1186/s13075-020-2146-x
Li, W., Liu, Y., Zhang, P., Tang, Y., Zhou, M., Jiang, W., et al. (2018). Tissue-Engineered Bone Immobilized with Human Adipose Stem Cells-Derived Exosomes Promotes Bone Regeneration. ACS Appl. Mater. Interf. 10, 5240–5254. doi: 10.1021/acsami.7b17620
Liang, B., Liang, J. M., Ding, J. N., Xu, J., Xu, J. G., and Chai, Y. M. (2019). Dimethyloxaloylglycine-stimulated human bone marrow mesenchymal stem cell-derived exosomes enhance bone regeneration through angiogenesis by targeting the AKT/mTOR pathway. Stem Cell Res. Ther. 10:1410. doi: 10.1186/s13287-019-1410-y
Lin, K. C., Yip, H. K., Shao, P. L., Wu, S. C., Chen, K. H., Chen, Y. T., et al. (2016). Combination of adipose-derived mesenchymal stem cells (ADMSC) and ADMSC-derived exosomes for protecting kidney from acute ischemia-reperfusion injury. Int. J. Cardiol. 216, 173–185. doi: 10.1016/j.ijcard.2016.04.061
Liu, B., Lee, B. W., Nakanishi, K., Villasante, A., Williamson, R., Metz, J., et al. (2018). Cardiac recovery via extended cell-free delivery of extracellular vesicles secreted by cardiomyocytes derived from induced pluripotent stem cells. Nat. Biomed. Eng. 2, 293–303. doi: 10.1038/s41551-018-0229-7
Liu, W., Rong, Y., Wang, J., Zhou, Z., Ge, X., Ji, C., et al. (2020a). Exosome-shuttled miR-216a-5p from hypoxic preconditioned mesenchymal stem cells repair traumatic spinal cord injury by shifting microglial M1/M2 polarization. Neuroinflammation. J. 17, 1726–1737. doi: 10.1186/s12974-020-1726-7
Liu, W., Yu, M., Xie, D., Wang, L., Ye, C., Zhu, Q., et al. (2020b). Melatonin-stimulated MSC-derived exosomes improve diabetic wound healing through regulating macrophage M1 and M2 polarization by targeting the PTEN/AKT pathway. Stem Cell Res. Ther. 11:1756. doi: 10.1186/s13287-020-01756-x
Luo, Z. W., Li, F. X. Z., Liu, Y. W., Rao, S. S., Yin, H., Huang, J., et al. (2019). Aptamer-functionalized exosomes from bone marrow stromal cells target bone to promote bone regeneration. Nanoscale 11, 20884–20892. doi: 10.1039/c9nr02791b
Mathivanan, S., Ji, H., and Simpson, R. J. (2010). Exosomes: Extracellular organelles important in intercellular communication. J. Prot. 73, 1907–1920. doi: 10.1016/j.jprot.2010.06.006
Maudens, P., Seemayer, C. A., Thauvin, C., Gabay, C., Jordan, O., and Allémann, E. (2018). Nanocrystal–Polymer Particles: Extended Delivery Carriers for Osteoarthritis Treatment. Small 14:3108. doi: 10.1002/smll.201703108
Mitxitorena, I., Infante, A., Gener, B., and Rodríguez, C. I. (2019). Suitability and limitations of mesenchymal stem cells to elucidate human bone illness. World J. Stem Cells 11, 578–593. doi: 10.4252/wjsc.v11.i9.578
mo Du, Y., xun Zhuansun, Y., Chen, R., Lin, L., Lin, Y., and guo Li, J. (2018). Mesenchymal stem cell exosomes promote immunosuppression of regulatory T cells in asthma. Exp. Cell Res. 363, 114–120. doi: 10.1016/j.yexcr.2017.12.021
Mohamed-Ahmed, S., Fristad, I., Lie, S. A., Suliman, S., Mustafa, K., Vindenes, H., et al. (2018). Adipose-derived and bone marrow mesenchymal stem cells: A donor-matched comparison. Stem Cell Res. Ther. 9, 914–911. doi: 10.1186/s13287-018-0914-1
Morelli, A. E., Larregina, A. T., Shufesky, W. J., Sullivan, M. L. G., Stolz, D. B., Papworth, G. D., et al. (2004). Endocytosis, intracellular sorting, and processing of exosomes by dendritic cells. Blood 104, 3257–3266. doi: 10.1182/blood-2004-03-0824
Muntión, S., Ramos, T. L., Diez-Campelo, M., Rosón, B., Sánchez-Abarca, L. I., Misiewicz-Krzeminska, I., et al. (2016). Microvesicles from Mesenchymal Stromal Cells Are Involved in HPC-Microenvironment Crosstalk in Myelodysplastic Patients. PLoS One 11:722. doi: 10.1371/journal.pone.0146722
Mutsaers, S. E., Bishop, J. E., McGrouther, G., and Laurent, G. J. (1997). Mechanisms of tissue repair: From wound healing to fibrosis. Int. J. Biochem. Cell Biol. 29, 5–17. doi: 10.1016/S1357-2725(96)00115-X
Narayanan, R., Huang, C. C., and Ravindran, S. (2016). Hijacking the Cellular Mail: Exosome Mediated Differentiation of Mesenchymal Stem Cells. Stem Cells Int. 2016:674. doi: 10.1155/2016/3808674
Naseri, Z., Oskuee, R. K., Jaafari, M. R., and Moghadam, M. F. (2018). Exosome-mediated delivery of functionally active miRNA-142-3p inhibitor reduces tumorigenicity of breast cancer in vitro and in vivo. Int. Nanomed. J. 13, 7727–7747. doi: 10.2147/IJN.S182384
Noël, D., Caton, D., Roche, S., Bony, C., Lehmann, S., Casteilla, L., et al. (2008). Cell specific differences between human adipose-derived and mesenchymal-stromal cells despite similar differentiation potentials. Exp. Cell Res. 314, 1575–1584. doi: 10.1016/j.yexcr.2007.12.022
Nojehdehi, S., Soudi, S., Hesampour, A., Rasouli, S., Soleimani, M., and Hashemi, S. M. (2018). Immunomodulatory effects of mesenchymal stem cell–derived exosomes on experimental type-1 autoimmune diabetes. J. Cell. Biochem. 119, 9433–9443. doi: 10.1002/jcb.27260
Pan, G. Z., Yang, Y., Zhang, J., Liu, W., Wang, G. Y., Zhang, Y. C., et al. (2012). Bone marrow mesenchymal stem cells ameliorate hepatic ischemia/reperfusion injuries via inactivation of the MEK/ERK signaling pathway in rats. J. Surg. Res. 178, 935–948. doi: 10.1016/j.jss.2012.04.070
Pankajakshan, D., and Agrawal, D. K. (2014). Mesenchymal Stem Cell Paracrine Factors in Vascular Repair and Regeneration. J. Biomed. Technol. Res. 1:107. doi: 10.19104/jbtr.2014.107
Park, S. H., Sim, W. Y., Min, B. H., Yang, S. S., Khademhosseini, A., and Kaplan, D. L. (2012). Chip-Based Comparison of the Osteogenesis of Human Bone Marrow- and Adipose Tissue-Derived Mesenchymal Stem Cells under Mechanical Stimulation. PLoS One 7:e46689. doi: 10.1371/journal.pone.0046689
Paul, A., Srivastava, S., Chen, G., Shum-Tim, D., and Prakash, S. (2013). Functional Assessment of Adipose Stem Cells for Xenotransplantation Using Myocardial Infarction Immunocompetent Models: Comparison with Bone Marrow Stem Cells. Cell Biochem. Biophys. 67, 263–273. doi: 10.1007/s12013-011-9323-0
Phinney, D. G., and Pittenger, M. F. (2017). Concise Review: MSC-Derived Exosomes for Cell-Free Therapy. Stem Cells 35, 851–858. doi: 10.1002/stem.2575
Pittenger, M. F., Discher, D. E., Péault, B. M., Phinney, D. G., Hare, J. M., and Caplan, A. I (2019). Mesenchymal stem cell perspective: cell biology to clinical progress. NPJ Regener. Med. 4, 86–86. doi: 10.1038/s41536-019-0083-6
Qin, Y., Wang, L., Gao, Z., Chen, G., and Zhang, C. (2016). Bone marrow stromal/stem cell-derived extracellular vesicles regulate osteoblast activity and differentiation in vitro and promote bone regeneration in vivo. Sci. Rep. 6:961. doi: 10.1038/srep21961
Ramírez-Bajo, M. J., Martín-Ramírez, J., Bruno, S., Pasquino, C., Banon-Maneus, E., Rovira, J., et al. (2020). Nephroprotective Potential of Mesenchymal Stromal Cells and Their Extracellular Vesicles in a Murine Model of Chronic Cyclosporine Nephrotoxicity. Front. Cell Dev. Biol. 8:296. doi: 10.3389/fcell.2020.00296
Raposo, G., Nijman, H. W., Stoorvogel, W., Leijendekker, R., Harding, C. V., Melief, C. J. M., et al. (1996). B lymphocytes secrete antigen-presenting vesicles. J. Exp. Med. 183, 1161–1172. doi: 10.1084/jem.183.3.1161
Reumann, M. K., Linnemann, C., Aspera-Werz, R. H., Arnold, S., Held, M., Seeliger, C., et al. (2018). Donor site location is critical for proliferation, stem cell capacity, and osteogenic differentiation of adipose mesenchymal stem/stromal cells: Implications for bone tissue engineering. Int. J. Mol. Sci. 19:1868. doi: 10.3390/ijms19071868
Riau, A. K., Ong, H. S., Yam, G. H. F., and Mehta, J. S. (2019). Sustained delivery system for stem cell-derived exosomes. Front. Pharmacol. 10:1368. doi: 10.3389/fphar.2019.01368
Ryu, B., Sekine, H., Homma, J., Kobayashi, T., Kobayashi, E., Kawamata, T., et al. (2020). Allogeneic adipose-derived mesenchymal stem cell sheet that produces neurological improvement with angiogenesis and neurogenesis in a rat stroke model. J. Neurosurg. 132, 442–455. doi: 10.3171/2018.11.JNS182331
Shi, J., Ma, Y., Zhu, J., Chen, Y., Sun, Y., Yao, Y., et al. (2018). A review on electroporation-based intracellular delivery. Molecules 23:3044. doi: 10.3390/molecules23113044
Skotland, T., Sagini, K., Sandvig, K., and Llorente, A. (2020). An emerging focus on lipids in extracellular vesicles. Adv. Drug Deliv. Rev. 159, 308–321. doi: 10.1016/j.addr.2020.03.002
Song, Y., Dou, H., Li, X., Zhao, X., Li, Y., Liu, D., et al. (2017). Exosomal miR-146a Contributes to the Enhanced Therapeutic Efficacy of Interleukin-1β-Primed Mesenchymal Stem Cells Against Sepsis. Stem Cells 35, 1208–1221. doi: 10.1002/stem.2564
Sun, X., Shan, A., Wei, Z., and Xu, B. (2018). Intravenous mesenchymal stem cell-derived exosomes ameliorate myocardial inflammation in the dilated cardiomyopathy. Biochem. Biophys. Res. Commun. 503, 2611–2618. doi: 10.1016/j.bbrc.2018.08.012
Takeuchi, R., Katagiri, W., Endo, S., and Kobayashi, T. (2019). Exosomes from conditioned media of bone marrow-derived mesenchymal stem cells promote bone regeneration by enhancing angiogenesis. PLoS One 14:11. doi: 10.1371/journal.pone.0225472
Tamura, R., Uemoto, S., and Tabata, Y. (2016). Immunosuppressive effect of mesenchymal stem cell-derived exosomes on a concanavalin A-induced liver injury model. Inflamm. Regen. 36:30. doi: 10.1186/s41232-016-0030-5
Tamura, R., Uemoto, S., and Tabata, Y. (2017). Augmented liver targeting of exosomes by surface modification with cationized pullulan. Acta Biomater. 57, 274–284. doi: 10.1016/j.actbio.2017.05.013
Tao, H., Han, Z., Han, Z. C., and Li, Z. (2016). Proangiogenic Features of Mesenchymal Stem Cells and Their Therapeutic Applications. Stem Cells Int. 2016:14709. doi: 10.1155/2016/1314709
Teng, X., Chen, L., Chen, W., Yang, J., Yang, Z., and Shen, Z. (2015). Mesenchymal stem cell-derived exosomes improve the microenvironment of infarcted myocardium contributing to angiogenesis and anti-inflammation. Cell. Physiol. Biochem. 37, 2415–2424. doi: 10.1159/000438594
Timmers, L., Lim, S. K., Arslan, F., Armstrong, J. S., Hoefer, I. E., Doevendans, P. A., et al. (2008). Reduction of myocardial infarct size by human mesenchymal stem cell conditioned medium. Stem Cell Res. 1, 129–137. doi: 10.1016/j.scr.2008.02.002
Tkach, M., and Théry, C. (2016). Communication by Extracellular Vesicles: Where We Are and Where We Need to Go. Cell 164, 1226–1232. doi: 10.1016/j.cell.2016.01.043
Toh, W. S., Lai, R. C., Zhang, B., and Lim, S. K. (2018). MSC exosome works through a protein-based mechanism of action. Biochem. Soc. Transact. 46, 843–853. doi: 10.1042/BST20180079
Uccelli, A., Moretta, L., and Pistoia, V. (2008). Mesenchymal stem cells in health and disease. Nat. Rev. Immunol. 8, 726–736. doi: 10.1038/nri2395
Valadi, H., Ekström, K., Bossios, A., Sjöstrand, M., Lee, J. J., and Lötvall, J. O. (2007). Exosome-mediated transfer of mRNAs and microRNAs is a novel mechanism of genetic exchange between cells. Nat. Cell Biol. 9, 654–659. doi: 10.1038/ncb1596
Van Deun, J., Mestdagh, P., Sormunen, R., Cocquyt, V., Vermaelen, K., Vandesompele, J., et al. (2014). The impact of disparate isolation methods for extracellular vesicles on downstream RNA profiling. J. Extracell. Vesicles 3:858. doi: 10.3402/jev.v3.24858
Villatoro, A. J., Alcoholado, C., Martín-Astorga, M. C., Fernández, V., Cifuentes, M., and Becerra, J. (2019). Comparative analysis and characterization of soluble factors and exosomes from cultured adipose tissue and bone marrow mesenchymal stem cells in canine species. Vet. Immunol. Immunopathol. 208, 6–15. doi: 10.1016/j.vetimm.2018.12.003
Waldner, M., Zhang, W., James, I. B., Allbright, K., Havis, E., Bliley, J. M., et al. (2018). Characteristics and immunomodulating functions of adipose-derived and bone marrow-derived mesenchymal stem cells across defined human leukocyte antigen barriers. Front. Immunol. 9:1642. doi: 10.3389/fimmu.2018.01642
Wang, C., Börger, V., Sardari, M., Murke, F., Skuljec, J., Pul, R., et al. (2020). Mesenchymal Stromal Cell-Derived Small Extracellular Vesicles Induce Ischemic Neuroprotection by Modulating Leukocytes and Specifically Neutrophils. Stroke 1825–1834. doi: 10.1161/STROKEAHA.119.028012
Wang, C., Wang, M., Xu, T., Zhang, X., Lin, C., Gao, W., et al. (2019). Engineering bioactive self-healing antibacterial exosomes hydrogel for promoting chronic diabetic wound healing and complete skin regeneration. Theranostics 9, 65–76. doi: 10.7150/thno.29766
Wang, J., Huang, R., Xu, Q., Zheng, G., Qiu, G., Ge, M., et al. (2020). Mesenchymal Stem Cell-Derived Extracellular Vesicles Alleviate Acute Lung Injury Via Transfer of miR-27a-3p∗. Crit. Care Med. 1097, E599–E610. doi: 10.1097/CCM.0000000000004315
Wang, K., Jiang, Z., Webster, K. A., Chen, J., Hu, H., Zhou, Y., et al. (2017). Enhanced Cardioprotection by Human Endometrium Mesenchymal Stem Cells Driven by Exosomal MicroRNA-21. Stem Cells Transl. Med. 6, 209–222. doi: 10.5966/sctm.2015-0386
Wang, L., Hu, L., Zhou, X., Xiong, Z., Zhang, C., Shehada, H. M. A., et al. (2017). Exosomes secreted by human adipose mesenchymal stem cells promote scarless cutaneous repair by regulating extracellular matrix remodelling. Sci. Rep. 7:2919. doi: 10.1038/s41598-017-12919-x
Wang, X., Gu, H., Qin, D., Yang, L., Huang, W., Essandoh, K., et al. (2015). Exosomal MIR-223 Contributes to Mesenchymal Stem Cell-Elicited Cardioprotection in Polymicrobial Sepsis. Sci. Rep. 5:13721. doi: 10.1038/srep13721
Wei, Z., Qiao, S., Zhao, J., Yihai, L., Qiaoling, L., Zhonghai, W., et al. (2019). miRNA-181a over-expression in mesenchymal stem cell-derived exosomes influenced inflammatory response after myocardial ischemia-reperfusion injury. Life Sci. 232:6632. doi: 10.1016/j.lfs.2019.116632
Wen, Y., Jiang, B., Cui, J., Li, G., Yu, M., Wang, F., et al. (2013). Superior osteogenic capacity of different mesenchymal stem cells for bone tissue engineering. Oral Radiol. 116:24. doi: 10.1016/j.oooo.2012.02.024
Wiklander, O. P. B., Nordin, J. Z., O’Loughlin, A., Gustafsson, Y., Corso, G., Mäger, I., et al. (2015). Extracellular vesicle in vivo biodistribution is determined by cell source, route of administration and targeting. J. Extracell. Vesicles 4, 1–13. doi: 10.3402/jev.v4.26316
Xie, H., Wang, Z., Zhang, L., Lei, Q., Zhao, A., Wang, H., et al. (2017). Extracellular Vesicle-functionalized Decalcified Bone Matrix Scaffolds with Enhanced Pro-angiogenic and Pro-bone Regeneration Activities. Sci. Rep. 7:622. doi: 10.1038/srep45622
Xie, L., Wang, J., Zhang, Y., Chen, H., Lin, D., Ding, J., et al. (2019). The effects of local injection of exosomes derived from BMSCs on random skin flap in rats. Am. J. Transl. Res. 11, 7063–7073.
Xu, H., Wang, Z., Liu, L., Zhang, B., and Li, B. (2020). Exosomes derived from adipose tissue, bone marrow, and umbilical cord blood for cardioprotection after myocardial infarction. J. Cell. Biochem. 121, 2089–2102. doi: 10.1002/jcb.27399
Xu, T., Luo, Y., Wang, J., Zhang, N., Gu, C., Li, L., et al. (2020). Exosomal miRNA-128-3p from mesenchymal stem cells of aged rats regulates osteogenesis and bone fracture healing by targeting Smad5. Nanobiotechnol. J. 18:601. doi: 10.1186/s12951-020-00601-w
Xue, C., Shen, Y., Li, X., Li, B., Zhao, S., Gu, J., et al. (2018). Exosomes Derived from Hypoxia-Treated Human Adipose Mesenchymal Stem Cells Enhance Angiogenesis Through the PKA Signaling Pathway. Stem Cells Dev. 27, 456–465. doi: 10.1089/scd.2017.0296
Yang, Y., Cai, Y., Zhang, Y., Liu, J., and Xu, Z. (2018). Exosomes Secreted by Adipose-Derived Stem Cells Contribute to Angiogenesis of Brain Microvascular Endothelial Cells Following Oxygen–Glucose Deprivation In Vitro Through MicroRNA-181b/TRPM7 Axis. J. Mol. Neurosci. 65, 74–83. doi: 10.1007/s12031-018-1071-9
Yu, B., Shao, H., Su, C., Jiang, Y., Chen, X., Bai, L., et al. (2016). Exosomes derived from MSCs ameliorate retinal laser injury partially by inhibition of MCP-1. Sci. Rep. 6:562. doi: 10.1038/srep34562
Yu, H., Xu, Z., Qu, G., Wang, H., Lin, L., Li, X., et al. (2020). Hypoxic Preconditioning Enhances the Efficacy of Mesenchymal Stem Cells-Derived Conditioned Medium in Switching Microglia toward Anti-inflammatory Polarization in Ischemia/Reperfusion. Cell. Mol. Neurobiol. 1007, 868–875. doi: 10.1007/s10571-020-00868-5
Yu, M., Liu, W., Li, J., Lu, J., Lu, H., Jia, W., et al. (2020). Exosomes derived from atorvastatin-pretreated MSC accelerate diabetic wound repair by enhancing angiogenesis via AKT/eNOS pathway. Stem Cell Res. Ther. 11:350. doi: 10.1186/s13287-020-01824-2
Zarovni, N., Corrado, A., Guazzi, P., Zocco, D., Lari, E., Radano, G., et al. (2015). Integrated isolation and quantitative analysis of exosome shuttled proteins and nucleic acids using immunocapture approaches. Methods 87, 46–58. doi: 10.1016/j.ymeth.2015.05.028
Zhang, J., Guan, J., Qi, X., Ding, H., Yuan, H., Xie, Z., et al. (2016). Dimethyloxaloylglycine promotes the angiogenic activity of mesenchymal stem cells derived from iPSCs via activation of the PI3K/Akt pathway for bone regeneration. Int. J. Biol. Sci. 12, 639–652. doi: 10.7150/ijbs.14025
Zhang, L., Jiao, G., Ren, S., Zhang, X., Li, C., Wu, W., et al. (2020). Exosomes from bone marrow mesenchymal stem cells enhance fracture healing through the promotion of osteogenesis and angiogenesis in a rat model of nonunion. Stem Cell Res. Ther. 11, 1562–1569. doi: 10.1186/s13287-020-1562-9
Zhang, Y., Chopp, M., Zhang, Z. G., Katakowski, M., Xin, H., Qu, C., et al. (2017). Systemic administration of cell-free exosomes generated by human bone marrow derived mesenchymal stem cells cultured under 2D and 3D conditions improves functional recovery in rats after traumatic brain injury. Neurochem. Int. 111, 69–81. doi: 10.1016/j.neuint.2016.08.003
Zhang, Y., Yu, S., Tuazon, J., Lee, J. Y., Corey, S., Kvederis, L., et al. (2019). Neuroprotective effects of human bone marrow mesenchymal stem cells against cerebral ischemia are mediated in part by an anti-apoptotic mechanism. Neural. Regen. Res. 14, 597–604. doi: 10.4103/1673-5374.247464
Zhao, C., Zhou, X., Qiu, J., Xin, D., Li, T., Chu, X., et al. (2019). Exosomes derived from bone marrow mesenchymal stem cells inhibit complement activation in rats with spinal cord injury. Drug Des. Devel. Ther. 13, 3693–3704. doi: 10.2147/DDDT.S209636
Zhao, H., Shang, Q., Pan, Z., Bai, Y., Li, Z., Zhang, H., et al. (2018). Exosomes from adipose-derived stem cells attenuate adipose inflammation and obesity through polarizing M2 macrophages and beiging in white adipose tissue. Diabetes 67, 235–247. doi: 10.2337/db17-0356
Zhao, M., Li, F., Jian, Y., Wang, X., Yang, H., Wang, J., et al. (2020). Salvianolic acid B regulates macrophage polarization in ischemic/reperfused hearts by inhibiting mTORC1-induced glycolysis. Eur. J. Pharmacol. 871:2916. doi: 10.1016/j.ejphar.2020.172916
Zhao, Q., Ren, H., and Han, Z. (2016). Mesenchymal stem cells: Immunomodulatory capability and clinical potential in immune diseases. J. Cell. Immunother. 2, 3–20. doi: 10.1016/j.jocit.2014.12.001
Zhu, F., Shin, O., Pei, G., Hu, Z., Yang, J., Zhu, H., et al. (2017). Adipose-derived mesenchymal stem cells employed exosomes to attenuate AKI-CKD transition through tubular epithelial cell dependent Sox9 activation. Oncotarget 8, 70707–70726. doi: 10.18632/oncotarget.19979
Zhu, J., Lu, K., Zhang, N., Zhao, Y., Ma, Q., Shen, J., et al. (2018). Myocardial reparative functions of exosomes from mesenchymal stem cells are enhanced by hypoxia treatment of the cells via transferring microRNA-210 in an nSMase2-dependent way. Artif. Cells Nanomed. Biotechnol. 46, 1659–1670. doi: 10.1080/21691401.2017.1388249
Zhu, Y., Jia, Y., Wang, Y., Xu, J., and Chai, Y. (2019). Impaired Bone Regenerative Effect of Exosomes Derived from Bone Marrow Mesenchymal Stem Cells in Type 1 Diabetes. Stem Cells Transl. Med. 8, 593–605. doi: 10.1002/sctm.18-0199
Zhu, Y., Liu, T., Song, K., Fan, X., Ma, X., and Cui, Z. (2008). Adipose-derived stem cell: A better stem cell than BMSC. Cell Biochem. Funct. 26, 664–675. doi: 10.1002/cbf.1488
Keywords: mesenchymal stem cell, extracellular vesicle, bone marrow, adipose, angiogenesis, osteogenesis, immunomodulation, tissue regeneration
Citation: Liu Y and Holmes C (2021) Tissue Regeneration Capacity of Extracellular Vesicles Isolated From Bone Marrow-Derived and Adipose-Derived Mesenchymal Stromal/Stem Cells. Front. Cell Dev. Biol. 9:648098. doi: 10.3389/fcell.2021.648098
Received: 31 December 2020; Accepted: 02 February 2021;
Published: 26 February 2021.
Edited by:
Sveva Bollini, University of Genoa, ItalyReviewed by:
Michela Pozzobon, University of Padua, ItalyStefania Bruno, University of Turin, Italy
Copyright © 2021 Liu and Holmes. This is an open-access article distributed under the terms of the Creative Commons Attribution License (CC BY). The use, distribution or reproduction in other forums is permitted, provided the original author(s) and the copyright owner(s) are credited and that the original publication in this journal is cited, in accordance with accepted academic practice. No use, distribution or reproduction is permitted which does not comply with these terms.
*Correspondence: Christina Holmes, Y2Fob2xtZXNAZW5nLmZhbXUuZnN1LmVkdQ==