- 1R&D Dept, Shanghai Proton and Heavy Ion Center (SPHIC), Shanghai, China
- 2Shanghai Key Laboratory of Radiation Oncology (20dz2261000), Shanghai, China
- 3Shanghai Engineering Research Center of Proton and Heavy Ion Radiation Therapy, Shanghai, China
- 4Department of Radiation Oncology, Shanghai Proton and Heavy Ion Center, Fudan University Cancer Hospital, Shanghai, China
- 5Albert Einstein College of Medicine, The Bronx, NY, United States
Resistance to therapy is the major hurdle in the current cancer management. Cancer cells often rewire their cellular process to alternate mechanisms to resist the deleterious effect mounted by different therapeutic approaches. The major signaling pathways involved in the developmental process, such as Notch, Hedgehog, and Wnt, play a vital role in development, tumorigenesis, and also in the resistance to the various anticancer therapies. Understanding how cancer utilizes these developmental pathways in acquiring the resistance to the multi-therapeutic approach cancer can give rise to a new insight of the anti-therapy resistance mechanisms, which can be explored for the development of a novel therapeutic approach. We present a brief overview of Notch, Hedgehog, and Wnt signaling pathways in cancer and its role in providing resistance to various cancer treatment modalities such as chemotherapy, radiotherapy, molecular targeted therapy, and immunotherapy. Understanding the importance of these molecular networks will provide a rational basis for novel and safer combined anticancer therapeutic approaches for the improvement of cancer treatment by overcoming drug resistance.
Introduction
The emergence of resistance to anticancer therapeutics is one of the major barriers that limit the efficacy of cancer therapy (Vasan et al., 2019). Resistance develops during chemotherapy, radiotherapy, molecularly targeted therapy, and immunotherapy in most cancer patients and prevents long-term survival. Resistance to therapy can be classified as intrinsic and acquired. The intrinsic resistance occurs from the sub-population of cancer cells, which already have the capability to counter a given therapy due to the pre-existing genotypic or phenotypic alterations. In contrast, the acquired resistance develops during the treatment by the therapy-induced selection of pre-existing resistant cellular state or by acquisition/adaptation to new genotype/phenotype changes required to withstand the therapy. In both these cases, major signaling pathways involved in the developmental process plays a vital role (Holohan et al., 2013; Chatterjee and Bivona, 2019).
Notch, Hedgehog, and Wnt form the major developmental signaling pathways which play a fundamental role in the dynamic transformation of a single-celled zygote into a highly complex multicellular organism (Collu et al., 2014; Siebel and Lendahl, 2017). These signaling pathways regulate the core cellular processes, including proliferation, differentiation, and migration, that collectively underlie organismal growth and development. The roles of these signaling pathways are not only restricted to the functioning of terminally differentiated somatic normal cells but also encompass adult stem cell niches, which serve to maintain the functional integrity of tissue and organs (Takebe et al., 2015; Clara et al., 2020). Due to the involvement of these signaling pathways in the basic cellular processes, its dysregulation often leads to diseases state, including cancer. Moreover, it is now widely recognized that aberrant regulation of these developmental signaling pathways plays a crucial role in providing resistance to various anticancer therapy (Takebe et al., 2015). Here, we present an overview of the role of Notch, Hedgehog, and Wnt signaling pathways in providing resistance against contemporary anticancer therapies, including molecular targeted therapy and the emerging immunotherapy. We also briefly present the current status of clinical trials that evaluate drugs targeting these signaling pathways and discuss how they can be explored for the development of novel and safer combinatorial approaches for overcoming drug resistance and enhanced efficacy.
Major Developmental Signaling in Cancer
Notch Signaling
The Notch is an evolutionarily conserved signaling pathway involved in many developmental and cellular processes, starting from the germ layer formation to the differentiation of specialized cell types in the embryo (Artavanis-Tsakonas et al., 1999). In the adult, it plays an important role in various cellular processes such as cell-fate specification, differentiation, proliferation, adhesion, apoptosis, migration, angiogenesis, epithelial-mesenchymal transition, and stem cell maintenance (Hori et al., 2013). In mammals, Notch signals through four Notch receptors (Notch 1–4) and five ligands (Jagged-1, -2, and Delta-like-1, -3, and -4), which are all type I transmembrane protein. The Notch receptor consists of an extracellular domain which contains 29–36 epidermal growth factors (EGF)-like repeats (involved in ligand-binding), the transmembrane domain, and an intracellular domain consisting of RAM domain; the Ankyrin repeats, transcriptional activator domain (TAD), and PEST domain (Gordon et al., 2007). The Notch ligands are composed of EGF-like repeats in the extracellular domain, DSL domain, and cysteine-rich region in Serrate.
Notch mediates short-range intercellular communication through interaction with ligands present on the neighboring cells. The binding of Notch ligands to the Notch receptors triggers S2 cleavage by ADAM10 and ADAM17 in the extracellular part of the receptor, leading to the shedding of the extracellular part. This is followed by the S3 cleavage in the transmembrane portion by γ-secretase. After S3 cleavage, the Intracellular domain (ICN) is released from the plasma membrane, which translocates to the nucleus. Here, it interacts with RBPJ—recombining binding protein suppressor of hairless/CBF1/suppressor of hairless/Lag-1 (RBPJK/CSL) and convert/transform the repressor complex into coactivator complex, thus promoting the transcription of the target genes (Figure 1; Kopan and Ilagan, 2009; Kopan, 2012).
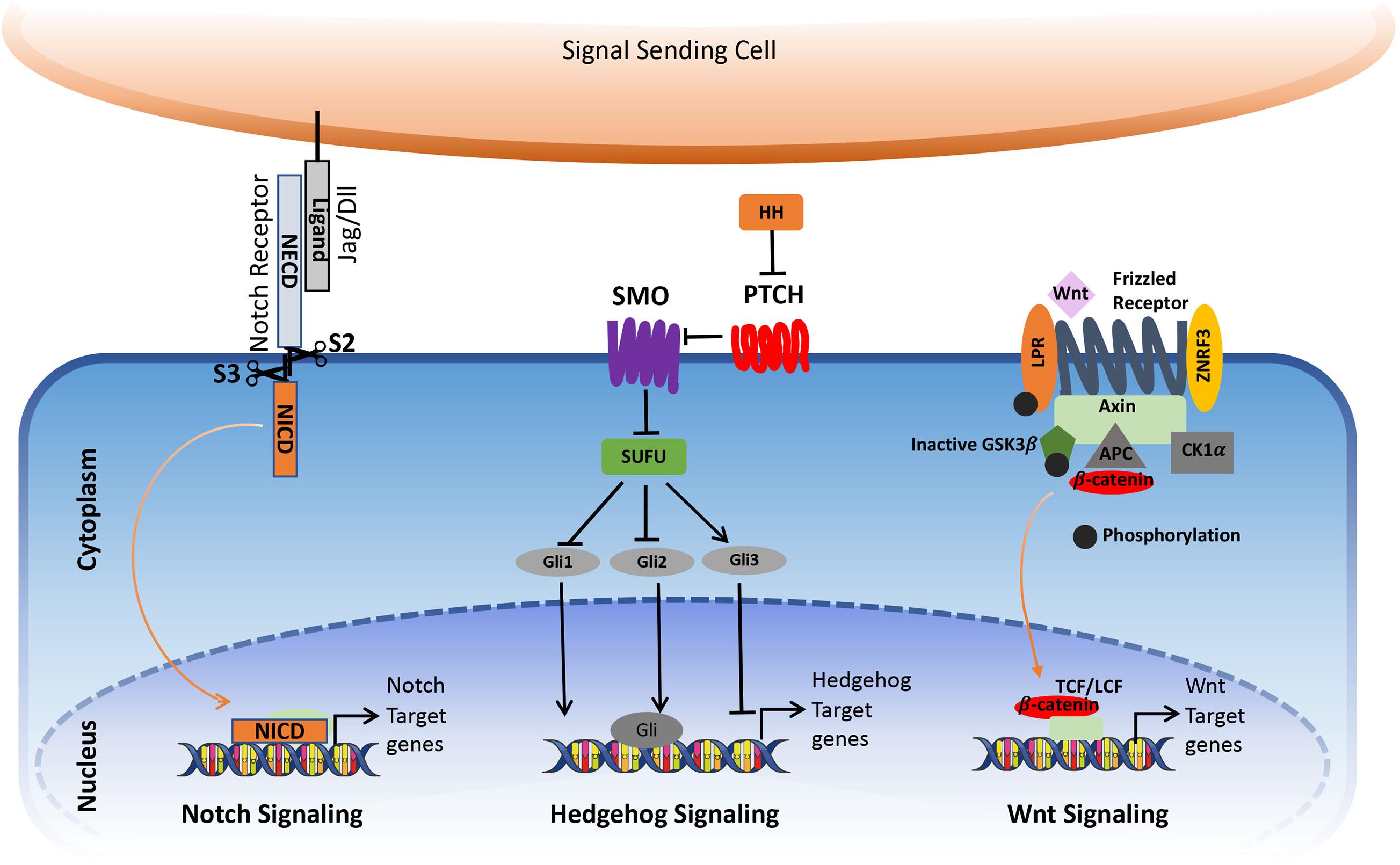
Figure 1. Simplified view of canonical Notch, Hedgehog, and Wnt signaling pathway in cancer. The figure is widely discussed in the text.
In the recent past, increasing evidences suggest the existence of non-canonical Notch signaling that is independent of canonical Notch ligand or transcription factor CSL/RBPJ (Andersen et al., 2012; Ayaz and Osborne, 2014). In this regard, other ligands that are non-canonical Notch ligands (Jagged/Dll type) have been reported to activate Notch signaling. For example, DLK1 (non-canonical Notch ligand) can directly interact with Notch and control Notch signaling. DlK1 are elevated in ovarian cancer and promotes tumorigenesis and epithelial-mesenchymal transition of high-grade ovarian carcinoma through activation of Notch signaling (Huang C. C. et al., 2019). Similarly, molecules such as Delta/Notch like EGF-related receptor (DNER) (Eiraku et al., 2002) and MB3 and contractin1 (D’Souza et al., 2008) have seen shown to have ligand activity and can activate Notch signaling independent of canonical Notch ligands. Non-canonical Notch signaling can also occur in CSL/RBPJ independent manner, where the activated Notch receptor interacts with various molecules other than CSL/RBPJ to exert its cellular process. This mode of non-canonical Notch signaling has been shown to modulate various signaling pathways such as NfKB, Pi3K, AKT, mTOR, HIF-1a, Wnt, etc., that have an important role in developmental process and cancer. For example, in T-ALL, NICD directly interacts with IKKa to maintain NfKB activity (Vacca et al., 2006). In cervical cancer, Notch activates the PI3K pathway that is independent of CSL (Veeraraghavalu et al., 2005). Moreover, in CSL-deficient mammary gland, some of the Notch mediated response was observed, confirming the existence of non-canonical Notch signaling independent of CSL. In addition, the role of non-canonical Notch signaling has also been reported in DNA damage response (DDR), where Notch1 directly interacts with ATM through FATC domain and inhibits its activation by impairing the formation of ATM-FOXO3a-KAT5/Tip60 Complex. Moreover, the negative correlation between Notch1 and ATM activation has been observed in human breast cancer, and it contributes to the survival of Notch1 driven leukemia cells upon DNA damage (Vermezovic et al., 2015; Adamowicz et al., 2016). Recently, Notch intracellular domain (NICD) was shown to interact with STING at the cyclic dinucleotide (CDN) binding domain, which resulted in inhibition of STING activation. This affected the apoptosis and necroptosis in a variety of immune cells, including T cells (Long et al., 2020). Thus the non-canonical Notch signaling represents an exciting avenue for future research, which may reveal novel strategies to block Notch signaling in diseases and chemoresistance.
As Notch signaling is involved in many crucial cellular functions, its aberrant regulation/expression often leads to pathological events ranging from developmental disorders to cancer. Indeed, many observations suggest that alterations in Notch signaling are associated with many human cancers. Moreover, Notch receptors and ligands have been found as prognostic markers in human cancers. Initially, the oncogenic role of Notch signaling was documented in T cell Acute Lymphoblastic Leukemia (T-ALL), where the activating mutations in NOTCH1 were suggested to be a major mediator for the development of malignancy (Ellisen et al., 1991). Later, its role in other hematological malignancies such as B-cell chronic lymphocytic leukemia (B-CLL) (Rosati et al., 2009), mantle cells lymphoma (MCL), Multiple Myeloma, AML, etc. has been well established (Gragnani et al., 2020). Recently, a genome-wide study in relapse cases of T-cell lymphoblastic lymphoma (T-LBL) identified Notch1 as the putative driver for relapse and malignancy (Khanam et al., 2020). Moreover, activation of Notch signaling by the T cell in the tumor microenvironment was demonstrated as key mediator of Akt-induces RT (Richter’s transformation), which is an aggressive lymphoma that occurs upon progression from chronic lymphocytic leukemia (CLL), suggesting the critical role of Notch signaling in RT transformation (Kohlhaas et al., 2021). While NOTCH receptor mutations are uncommon in other tumor forms, NOTCH is aberrantly activated via various mechanisms in several malignancies, including colorectal and pancreatic cancer, melanoma, medulloblastoma, and adenocystic carcinoma (Ranganathan et al., 2011). Conversely, NOTCH can also function as a tumor suppressor as observed in various solid tumors such as squamous cell carcinoma, liver cancer, small-cell lung cancer, etc., where the loss of function mutations in NOTCH1/2/3 have been identified (Nowell and Radtke, 2017).
Hedgehog Signaling
Hedgehog signaling is an evolutionarily conserved pathway that regulates the morphogenesis of various organs during embryogenesis and postnatal development. It regulates diverse cellular processes in the adult, including proliferation, tissue differentiation, and repair of normal tissues. In addition, it is also involved in stem cell renewal and organ homeostasis. The major components of the Hedgehog signaling pathways are the Hh ligands Sonic hedgehog (Shh), Indian Hedgehog (IHH) and Desert hedgehog (DHH), Patched (PTCH), which is a 12-transmembrane domain receptor protein, which locates in the primary cilium, the 7-transmembrane G-protein coupled receptor Smoothened (SMO), the suppressor of fused protein (SUFU) in the cytoplasm, and the glioma-associated oncogene (GLI) transcription factors.
In the absence of ligands, Ptch1 inhibits SMO accumulation in primary cilia, thereby resulting in the block of the pathway activity. The binding of the Hedgehog ligands to Ptch1 causes internalization and degradation of the Ptch receptor, thereby releasing SMO to enter the primary cilia where it promotes the dissociation of a SUFU– GLI complex. This activates GLI transcriptional activators and their translocation into the nucleus to activate the expression of Hedgehog target genes such as GLI1 and Ptch (Figure 1). In addition to the canonical activation of the Hedgehog pathway, growing evidence points toward non-canonical mechanisms through which hedgehog signaling gets activated, which can contribute to the development of several types of cancer.
Ectopic activation of the Hedgehog signaling is implicated in several cancers, including hematological malignancies and solid tumors, where it is associated with tumor development, progression, and recurrence after anticancer therapy. In hematological malignancies, the role of Hh signaling in maintaining leukemic stem cells has been well established. Thus, inhibition of HH signaling reduces the stem cell potential to initiate leukemia (Fukushima et al., 2016). This effect was observed in chronic myeloid leukemia (CML), where inhibition of HH signaling reduced the development of leukemia and enhanced the survival of the CML mouse model (Irvine et al., 2016). Similarly, in cells from patients with acute myeloid leukemia (AML), the inhibition of HH signaling in combination with 5-azacytidine showed synergistic efficacy due to reduced stem cell potential to initiate leukemia (Tibes et al., 2015). Moreover, high frequencies of somatic mutations of Ptch1 (70–90%) and a lesser extent in Smoothened (10–20%) are reported in human basal cell carcinoma (Bonilla et al., 2016).
Wnt Signaling
Wnt signaling pathway is another evolutionally conserved pathway that directs developmental processes, stem cell proliferation, and tissue homeostasis throughout the metazoans (MacDonald et al., 2009). Hence, any perturbation due to physiological stress in the Wnt signaling pathway results in pathological conditions such as birth defects, cancers, etc. (Clevers, 2006). In humans, 19 genes are encoding WNTs that bind to various receptors and stimulate different intracellular signal transduction pathways (Niehrs, 2012). Recent studies on the WNT pathway roughly divided it into either canonical (β-catenin dependent) or non-canonical (β-catenin independent) signaling pathways (Niehrs, 2012). Depending upon their potential to induce morphological transformation in a murine mammary epithelial cell line (C57MG), the Wnt family has been categorized into different types (Wong et al., 1994). Wnt1, Wnt3, Wnt3a, and Wnt7a fall under the category of highly transforming members, and Wnt2, Wnt4, Wnt5a, Wnt5b, Wnt6, Wnt7b, and Wnt11 are grouped under intermediately transforming or non-transforming members (Kikuchi et al., 2011). In general, Frizzled proteins function as common receptors for both canonical as well as non-canonical pathways (Niehrs, 2012).
The canonical Wnt signaling pathway is a well-studied pathway that is activated by the interaction of Wnt with a Frizzled (Fz) receptor and LRP5/LRP6, where LRP stands for lipoprotein receptor-related protein (which is a single-span trans-membrane receptor) (Niehrs, 2012). Followed by the ligation of Wnt and the Fz/LRP co-receptor complex, the canonical signaling pathway gets stimulated. The Fz can interact with a cytoplasmic protein called Disheveled (Dsh), which acts upstream of β-catenin GSK3β (Clevers, 2006). Another cytoplasmic protein, Axin, interacts with the intracellular domain of LRP5/6 through five phosphorylated PPPSP motifs in the cytoplasmic tail of LRP. GSK3 phosphorylates PPPSP motifs, whereas Casein kinase 1-γ (CK-1γ) phosphorylates multiple sites within LRP5/6, which in turn promotes the recruitment of Axin to LRP5/6. CK-1γ isoforms within the CK-1 family carry putative palmitoylation sites at the carboxy-terminal (Figure 1; Davidson et al., 2005).
In the inactivated/un-stimulated state, GSK-3 phosphorylates the transcriptional coactivator β-catenin that renders it in an active state. Inactivation of β-catenin is characterized by the formation of a “destruction complex” that comprises of GSK3, adenomatosis polyposis coli (APC), Axin, and casein kinase Iα (CKIα) (Niehrs, 2012) which leads to the ubiquitination of β-catenin by an E3 ubiquitin ligase called β-TrCP. This complex targets it for proteasomal degradation (Krishnamurthy and Kurzrock, 2018). This results in the absence of β-catenin to the nucleus and the repressor complex containing T-cell specific factor (TCF)/lymphoid enhancer-binding factor (LEF), and transducing-like enhancer protein (TLE)/Groucho binds and represses the activity of the target gene (Levanon et al., 1998; Brantjes et al., 2001; MacDonald et al., 2009). Following the binding of Wnt to Frizzled-Axin-LRP-5/6 complex, cytosolic GSK-3β (Glycogen synthase kinase-3 beta) is sequestered, and the phosphorylation of β-catenin is inhibited. The accumulation of hypo-phosphorylated β-catenin in the cytosol allows its migration to the nucleus, where it regulates target gene expression by interacting with the TCF/LEF family of transcription factors. This signaling is implicated in the regulation of cell differentiation and proliferation (Gordon and Nusse, 2006; Zhan et al., 2017).
Wnt signaling has a prominent role in carcinogenesis and has been widely studied in colorectal cancer. Indeed, the first evidence of dysregulated Wnt signaling came from the studies of hereditary colorectal cancer. It was found that this aberrant activation of Wnt signaling in colorectal cancer was due to the loss of function mutations in the APC gene or point mutation in the N-terminal sites of β-catenin that leads to the stabilization of β-catenin. Later, studies from several groups showed the activating mutation in Wnt signaling components in various cancer such as breast cancer, pancreatic cancer, melanoma, lung cancer, prostate, etc. (Zhang and Wang, 2020). Moreover, Aberrant WNT signaling has been reported in several hematological malignancies such as AML, CML, B-ALL, Multiple Myeloma, CLL, etc. (Frenquelli and Tonon, 2020).
Crosstalk Between Notch, Hedgehog, and Wnt Pathway in Cancer
Several seminal studies over the past decade have demonstrated that Notch, Hh, and Wnt signaling pathways crosstalk with one another, with one pathway acting as an upstream or downstream effector of another. This affects their transcriptional output in the specific cellular context in which they operate (Figure 2).
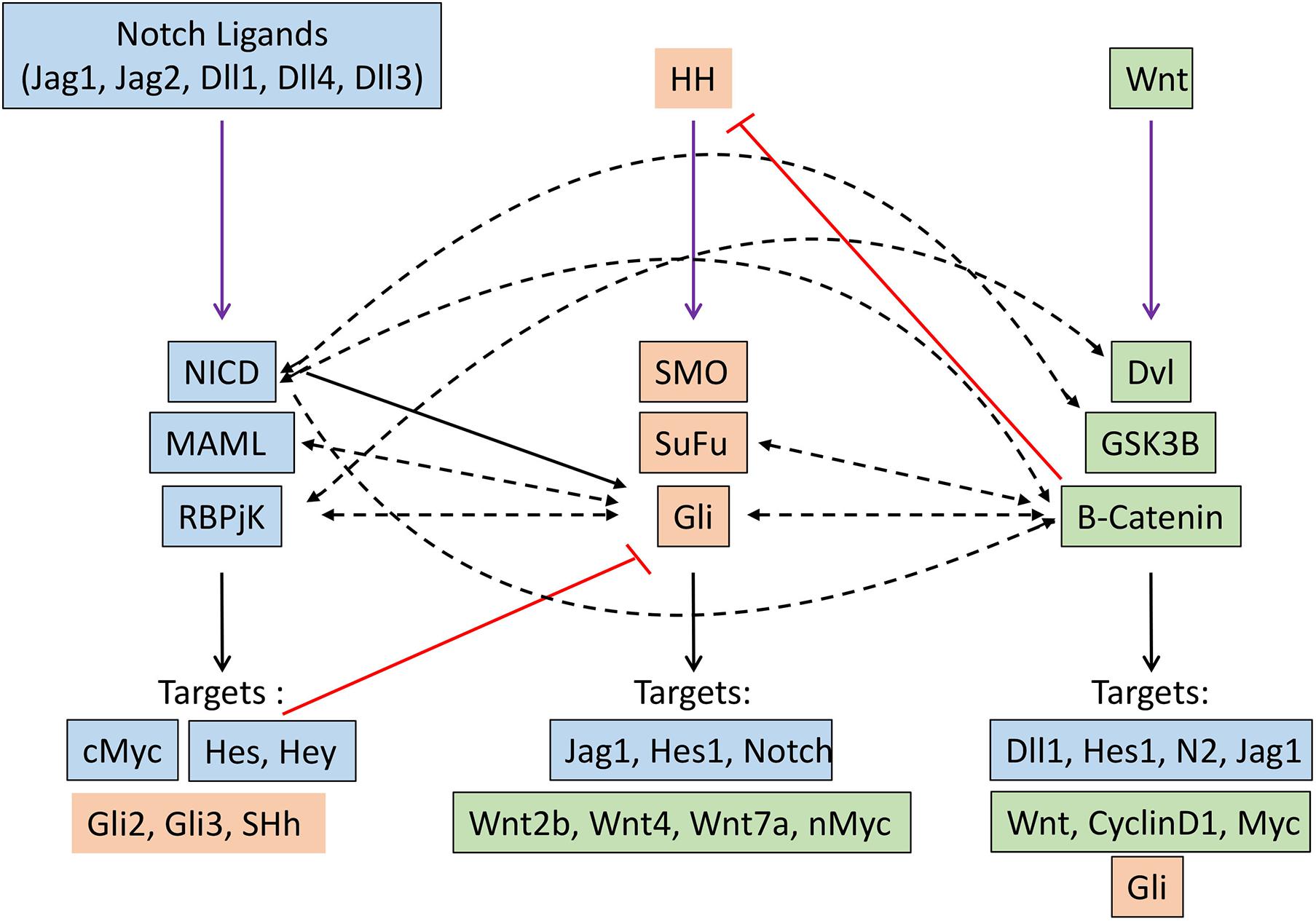
Figure 2. Crosstalk between Notch (blue), Hedgehog (orange) and Wnt (green) signaling pathways in cancer. Black arrow indicates transcriptional regulation. Dashed black arrows indicate direct interactions. Red lines indicate inhibitory regulation.
Crosstalk Between Notch and Hedgehog Pathway
A genome-wide analysis of the Notch signaling can directly regulate the expression of effector and target molecules of the hedgehog signaling pathway (Li Y. et al., 2012). Hes1, a well-known target of Notch signaling, regulate hedgehog signaling in glioblastoma and thereby therapeutic resistance by directly binding to N-boxes located within the first intron of Gli1 and repressing its expression (Schreck et al., 2010). Notch ligand Jagged1 can regulate hedgehog signaling by downregulating the expression of Gli2, thereby inducing apoptosis and reversing the taxane resistance in ovarian cancer cells both in vitro and in vivo (Steg et al., 2011). Mastermind-like1 (Maml1), which is a key component of Notch signaling, can act as the regulator of Shh signaling by directly interacting with Gli protein and working as a potent transcriptional coactivator (Quaranta et al., 2017). The indirect regulation of hedgehog signaling by Notch pathway came from the study in Neural stem cells, where the activation of Notch signaling induced the expression of Hes3 and Sonic hedgehog (Shh) through activation of serine/threonine kinase Akt, STST3, and mTOR, this, in turn, promotes the survival of the stem cells (Androutsellis-Theotokis et al., 2006). Moreover, Notch signaling regulates the dynamic localization of the key component of the hedgehog pathway at the primary cilia. This sets the overall cellular threshold for HH responsiveness. In this regard, the activation of Notch signaling results in the accumulation of Smo at the primary cilia, leading to elevated levels of HH response. Interestingly, this Notch-dependent trafficking of Smo to the primary cilia occurs without the presence of the Hh ligand, demonstrating a direct mechanism by which Notch signaling regulates the Hh pathway (Stasiulewicz et al., 2015). Similarly, HH signaling can also directly/indirectly modulate the Notch signaling by controlling the expression of key components of Notch signaling through downstream effectors. The classical Notch target, such as Hes1, can be directly induced by the Hedgehog signaling pathway in a Notch-independent manner through the binding of Gli2 to the promoter of the Hes1 gene (Wall et al., 2009).
Crosstalk Between Notch and Wnt Pathway
Crosstalk between the Notch and WNT signaling pathways has been elucidated in many developmental processes and also in tumorigenesis. These crosstalks can lead to either feedforward or feedback loop by directly/indirectly regulating the key components of each other. In skin cancer, Notch signaling has been shown to act as a tumor suppressor by inhibiting Wnt signaling. Similarly, in colorectal cancer, Notch1 signaling retained the capabilities of suppressing the expression of Wnt target genes, even when B-Catenin destruction by APC complex was disabled. In addition, Notch can tether B-catenin and thereby modulate its stability; therefore, the Notch1 loss of function leads to the activation of b-catenin (Kwon et al., 2011). Moreover, a negative correlation between Notch1 target gene Notch-regulated ankyrin repeat protein 1 (NRARP) and WNT target genes was found in human colorectal cancer (Kim et al., 2012). In contrast, NARP can act as a positive regulator of Wnt signaling by stabilizing the transcriptional factor LEF1, thereby increasing the LEF1 dependent promoter activity. Recently the role of this crosstalk has been well documented in triggering the early stage of myeloid regeneration and in myeloid malignancies (Kang et al., 2020). On the other hand, Wnt signaling can directly regulate the expression of different components of Notch signaling such as Delta-like1 (Dll1), Hes1, Notch2, Jag1, etc. (Galceran et al., 2004; Ungerback et al., 2011; Li B. et al., 2012). B-Catenin can directly interact with Notch1, resulting in reduced ubiquitination of Notch1, thereby affecting its stability and activity. Similarly, GSK3B also directly interacts with NICD-1 and phosphorylates its serine and threonine residues, thereby affecting its nuclear localization, stability, and transcriptional activities. Moreover, GSK3B can also phosphorylate NICD-2, but this results in reduced transcriptional activity. Dvl, another component of Wnt signaling, physically interacts with RBPJ, resulting in reduce the transcriptional activity of RBPJ, as observed by the promoter activity of Notch responsive reporter construct.
Crosstalk Between Wnt and Hedgehog Pathway
Gli1/2 induces expression of sFRP-1 (secreted frizzled related protein 1, which negatively regulates Wnt signaling by the subsequent cytoplasmic accumulation of B-catenin (He et al., 2006). Gli1 induces activation of Wnt2b, Wnt4, Wnt7b, which in turn promote the stability of B-catenin, thereby triggering the Wnt signaling (Li et al., 2007). N-myc, which is an important target of the Wnt signaling pathway and is related to medulloblastoma, is regulated by SHH, which promotes expression and post-transcriptional stabilization of N-Myc in mice (Kenney et al., 2003; Thomas et al., 2009). Gli3 physically interacts with the C-terminal domain of B-catenin (the region that includes the transactivation domain), thereby reducing the Wnt-mediated transcriptional activity (Ulloa et al., 2007). Similarly, SuFu is able to bind B-Catenin and export it from nucleus-thus repress B-catenin/TCF mediated transcription. Therefore loss of SuFu leads to increased risk of MB (Taylor et al., 2004). In turn Wnt signaling can modulate hedgehog signaling by directly activating the target gene of hedgehog signaling or modifying the key component of hedgehog singling by directly interacting with them. B-Catenin directly interact with Gli and leads to the proteasomal degradation of GLI, thereby decreases the proliferation of SHH dependant tumors such as MB (Zinke et al., 2015). B-catenin induces the stabilization of Gli mRNAs through upregulating CRD-BP and RNA binding protein (Noubissi et al., 2009). Moreover, it can enhance the luciferase activity of Gli-responsive elements.
Notch, Hedgehog, and Wnt Signaling in Chemo-Resistance
Chemotherapy forms one of the major therapeutic strategies in the treatment for many cancers at different stages of the disease. However, continuous exposure to the chemotherapeutic drug often leads to drug resistance, which is a major problem in cancer treatment. The mechanisms underlying chemoresistance is often complex and multifaceted, which include alteration in drug transport, detoxification of drug, increased or altered drug targets, block in apoptosis, enhanced cell survival, alteration in the cell cycle, induction in epithelial to mesenchymal transition (EMT), enrichment or induction Cancer Stem Cell (CSC) phenotype, modification of the tumor microenvironment, etc. Interestingly, Notch, Hedgehog, and Wnt are involved in modulating nearly all of these mechanisms (Figure 3). In the following section, we will limit our discussion to some of the major mechanisms involved in chemo-resistance, which are modulated by these signaling pathways (Table 1).
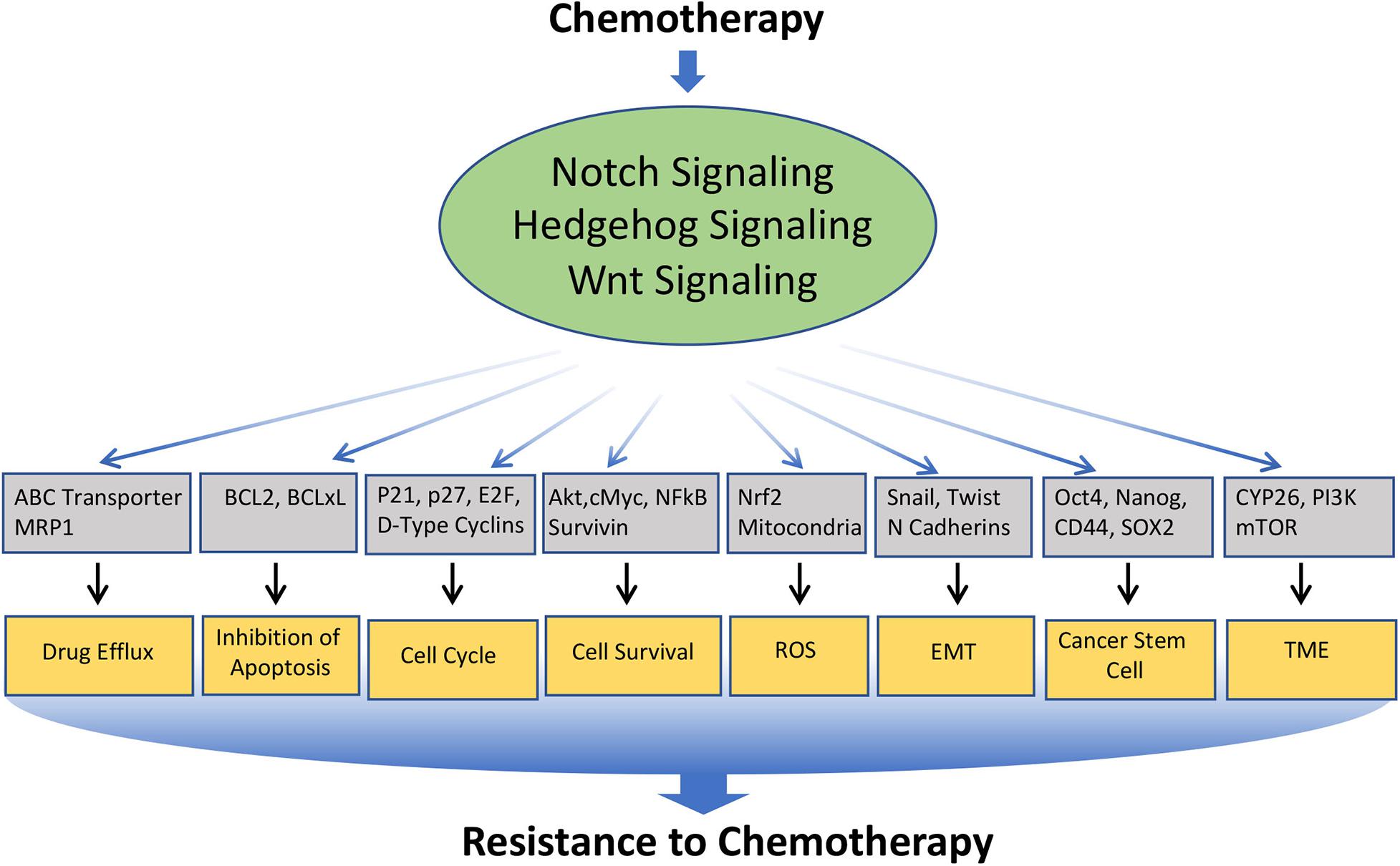
Figure 3. Involvement of Notch, Hedgehog, and Wnt signaling pathways in the resistance of cancer cells to chemotherapeutics drugs: Various anticancer drugs often upregulate Notch, Hedgehog, and Wnt signaling, which in turn regulates the key molecules involved in cellular processes such as Drug efflux, Inhibition to apoptosis, cell survival, cell-cycle, ROS, EMT, CSC, DNA damage response, TME, immune cell functions, etc. that leads to the acquisition of resistance to the chemotherapeutics drugs.
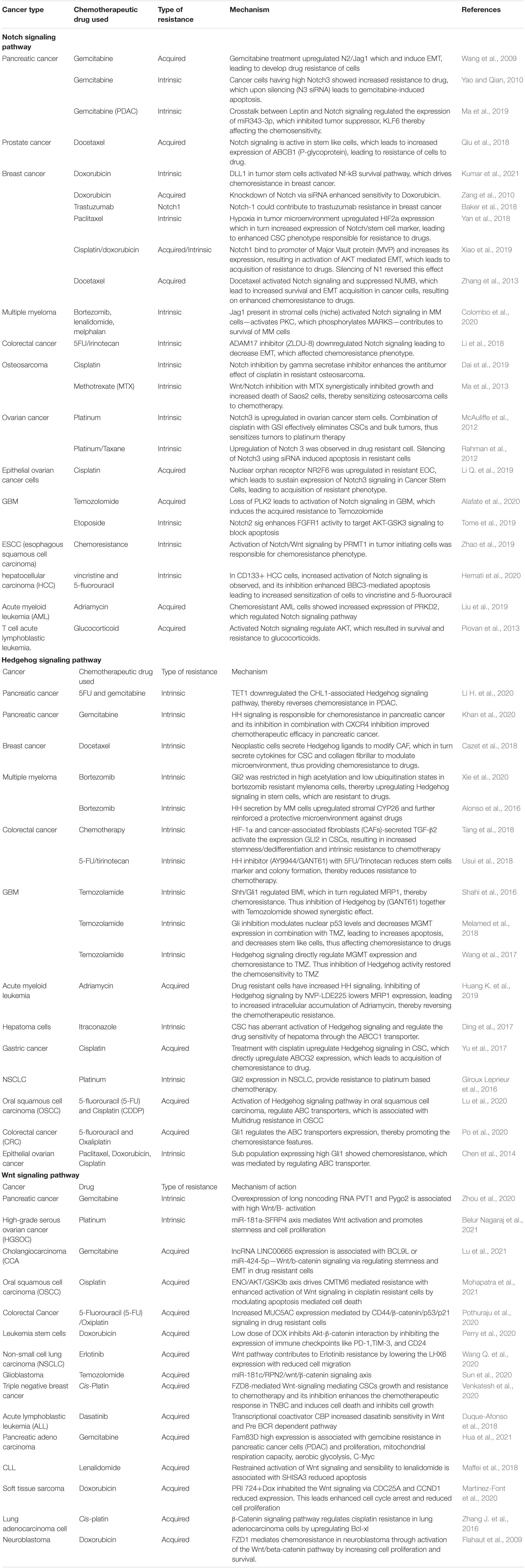
Table 1. Notch, Hedgehog, and Wnt Signaling pathway in resistance of cancer to various chemotherapies.
Drug Efflux
Drug efflux forms the primary mechanism of chemo-resistance in cancer. Emerging evidences suggest that Notch, Hedgehog, and Wnt signaling can directly modulate the drug efflux by regulating the expression of the transporter involved in drug efflux.
In prostate and breast cancer, the chemotherapeutic drug such as docetaxel or doxorubicin induces the activation of Notch signaling, which in turn increases the expression of ABCB1 and multidrug-resistant associated Protein1 (MRP1), thus increasing the drug efflux and contributing to chemo-resistance. This chemo-resistance effect is reversed by the Notch inhibitors (Zhang et al., 2013; Qiu et al., 2018).
Likewise, Hedgehog signaling directly regulates the expression of ABCB1 and ABCG2 in ovarian cancer. The inhibition of Gli1 expression decreases ABCB1 and ABCG2 gene expression levels, thus enhancing the response of ovarian cancer cells to certain chemotherapeutic drugs (Chen et al., 2014). Moreover, Hedgehog signaling transcription factor Gli1/Gli2 appears to be the primary regulator of drug response in hepatoma, Oral squamous cell carcinoma (OSCC) and Colorectal cancer (CRC) through the ABC transporter and in Acute Myleiod Leukemia and GBM through MRP1 (Shahi et al., 2016; Ding et al., 2017; Huang K. et al., 2019; Lu et al., 2020; Po et al., 2020).
Similarly, the Wnt/β-catenin pathway is known to regulate the transporters involved in drug efflux. Several TCF4/LEF binding motifs are present in the promoter region of the ABCB1 gene in humans, suggesting it as a target gene of the β-catenin/TCF4 transcriptional regulators, thus the activation of β-catenin augments ABCB1 expression. LGR5, the target molecules for the Wnt pathway in colorectal cancer cells, was found to confer resistance to chemotherapy. ABCB1 higher expression was found to associated LGR5 expression in chemoresistance to CRCs. Cancer stem cell property in CRCs is regulated by LGR5 that leads to chemoresistance to oxaliplatin and 5-fluorouracil (Liu et al., 2013). Pygopus (PYGO2) is involved in the signal transduction of the Wnt pathway and plays a critical role in the development of tumors. It is also linked with Multi-Drug Resistance in various cancers like breast, ovarian, lung, glioma, and esophageal squamous cell carcinoma. This is also one of the most up-regulated genes in chemo-resistant breast cancer. Increased expression of the PYGO2 gene upregulates the ABCB-1 gene in the resistant cells through Wnt/β-catenin pathway (Zhang Z.M. et al., 2016). CD44 is a surface protein that plays an important role in intercellular communication within the tumor microenvironment. CD44 upregulates the expression of ABC transporters, thereby inducing a form of drug resistance known as cell adhesion-mediated drug resistance (CAM-DR) (Martin-Orozco et al., 2019). CD44 has been identified to be responsible for lenalidomide resistance mediated by the Wnt cascade. Overexpression of CD44 has been observed in lenalidomide-resistant human multiple myeloma cell lines (HMCLs), with increased adhesion to bone marrow (BM) stromal cells, while inhibition of CD44 reduced the adhesion of multiple myeloma cells and reversed the resistance to lenalidomide (Spaan et al., 2018).
Inhibition of Apoptosis
Down-regulation of apoptosis induced by chemotherapeutic drugs is another mechanism that contributes to chemo-resistance in cancer. Events that lead to a block in apoptosis enhances chemo-resistance. Notch, Hedgehog, and Wnt signaling have been well known to modulate apoptosis in cancer.
Notch signaling regulates apoptosis through its interaction with the key players of apoptosis pathways (Lundell et al., 2003; Zweidler-McKay et al., 2005). Therefore, blocking Notch signaling has often been found to result in the induction of apoptosis in cancer. In platinum/Taxane resistant ovarian cancer, Notch3 has been found to be upregulated, and downregulation of Notch3 by siRNA or GSI induces apoptosis in resistant cells (Rahman et al., 2012). In pancreatic cancer, the subpopulation having high Notch3 showed increased resistance to Gemcitabine, which upon silencing (N3 siRNA) leads to induced apoptosis (Yao and Qian, 2010). Similarly, in glioblastoma, osteosarcoma and hepatocellular carcinoma (HCC) inhibition of Notch signaling induces apoptosis in the cells that are resistant to chemotherapeutic drugs like Temozolamide, Etoposide, Cisplatin, and vincristine and 5-fluorouracil (Dai et al., 2019; Tome et al., 2019; Alafate et al., 2020; Hemati et al., 2020).
Hedgehog signaling pathways has also been implicated in the regulation of apoptosis in cancer (Athar et al., 2004). Emerging evidence suggests that Hedgehog regulated apoptosis in cancer may play an essential role in the acquisition of chemo-resistance. Consequently, the treatment of glioblastoma with temozolomide together with an inhibitor of Gli induces apoptosis, thereby reducing the chemo-resistance (Melamed et al., 2018).
Several inhibitors that target apoptosis regulation by modulating Wnt signaling have been investigated. For example, Belinostat is a histone deacetylase inhibitor that induces apoptosis by decreasing the Wnt/β-catenin, CCND2, and Myc in MCF-7 cells (Lu et al., 2019). Lanatoside C inhibits Wnt/β-catenin signaling by down-regulating c-Myc in gastric cancer cells, while overexpression of c-Myc reverses the anti-tumor effect of lanatoside C, suggesting that c-Myc is a key drug target of lanatoside C (Hu et al., 2018). Wnt inhibitor FH535 has been found to markedly suppress the expression of β-catenin target genes (LEF1, CCND1, and cMYC) and potentiate imatinib-induced apoptosis (Suknuntha et al., 2017). FH535 also shows antiproliferative effects in leukemia cell lines like THP-1, Jurkat, HL60, and K562 (Suknuntha et al., 2017). Coenzyme Q0 (CoQ0; 2,3-dimethoxy-5-methyl-1,4-benzoquinone), a novel quinone derivative, showed the in vitro and in vivo anti-tumor, apoptosis, and anti-metastasis activities of CoQ0 (0–20 μM) through inhibition of Wnt/β-catenin signaling pathway.
Cell Cycle
In the recent past, it has become increasingly apparent that the cell cycle plays a critical role in chemosensitivity for the given chemotherapy, and the role of developmental pathways such as Notch, Hedgehog, and Wnt signaling in this phenomenon has been well established (Arora and Spencer, 2017).
Notch signaling regulates the cell cycle by inducing the expression of some of the critical cell-cycle related genes such as p21, p27, E2F etc. Therefore cell-cycle perturbation due to dysregulated Notch signaling in cancer forms one of the factors that impart chemoresistance to the drugs. For example, in T-cell transformation and chemoresistance, Notch1 signaling is associated with the promotion of G1-S through upregulation of CDK4 and CDK6 and downregulation of p27/KIP1 and p18/INK4C cell cycle inhibitors (Joshi et al., 2009). Therefore inhibition of Notch signaling by pharmacological or genetic approach leads to cell cycle arrest and apoptosis in cancer cells (Li et al., 2014). Similarly, in Prostate cancer stem-like cells (PCSCs), the combination of GSI with DOX promoted DOX-induced cell growth inhibition, apoptosis, cell cycle arrest, and sphere formation in PCSCs when compared to DOX only. This suggest that Notch inhibition may have clinical benefits in targeting PCSCs to enhance the anti-tumor effect of DOX in PC-3 PCSCs (Wang L. et al., 2020).
Similarly, the Hedgehog signaling pathway modulates the cell cycle progression via directly regulating the core cell cycle components such as D-type cyclins, Cip1, p57KIP2 (Neumann, 2005; Adolphe et al., 2006). Moreover, the role of cell cycle regulation by the hedgehog signaling pathway in the acquisition of chemoresistance is also well documented. Therefore, the inhibitors of the hedgehog pathway together with the chemotherapeutic drug are being explored to circumvent the chemotherapy acquired during the treatment. For example, in GBM, the combination of Hedgehog inhibitor GANT-61 with Temozolomide showed a synergistic effect, and all TMZ- resistant cell lines displayed a significant decrease in cell viability due to arrest in G2/M and increase apoptosis (Honorato et al., 2020). The combination of bortezomib with hedgehog antagonist, LDE225, increased paclitaxel sensitivity through apoptosis and G2/M arrest in ovarian cancer. Thus the inhibition of protease inhibition and Hedgehog signaling can reverse taxane-mediated chemoresistance (Steg et al., 2014).
Wnt/β−catenin signaling regulates cell proliferation by modulating the cell cycle via β -catenin, which is a well-known regulator of cell cycle progression (Nelson and Nusse, 2004). Moreover, it has been well established that a tight control of β catenin levels are required for cell cycle progression (Olmeda et al., 2003). In cancer cells, the Wnt-mediated cell cycle regulation has been implicated in imparting resistance to chemotherapy. Thus various inhibitors of Wnt signaling are being explored to attenuates the chemoresistance developed by the cancer cells. In human neuroendocrine tumor (NET) cell lines Porcupine (PORCN) inhibitor WNT974 and the β-catenin inhibitor PRI-724 resulted in the cell cycle arrest at the G1 and G2/M phases, which affected the tumor growth and viability (Jin et al., 2020).
Cell Survival
Cell survival forms an important factor that attenuates the effectiveness of conventional chemotherapy. The role of Notch, Hedgehog, and Wnt signaling in regulating cell survival in the face of chemotherapy treatment has gained much interest due to its therapeutic importance.
Report from multiple laboratories has shown the involvement of Notch signaling in the regulation of cell survival in normal development and also in cancer. Moreover, recent reports suggest that Notch signaling form the key mediator of increased cancer cell survival in the context of chemotherapy. For example, activated Notch protected T-ALL cells from glucocorticoid-mediated apoptosis, which was mediated from Notch dependent upregulation of Akt, leading to nuclear export of glucocorticoid receptor (Piovan et al., 2013). Thus the combined use of GSIs with glucocorticoids in patients with relapsed/refractory T-ALL showed a promising result in the clinical trials, where γ-Secretase inhibitors reverse glucocorticoid resistance in T cell acute lymphoblastic leukemia. In addition, the critical role of Notch signaling in tumor cell survival and apoptosis resistance was shown in B- CLL (Rosati et al., 2009). Similarly, the breast cancer cells which expressed a high level of Jagged1 shown resistance to lapatinib and increased survival with enhanced tumor-initiating potential (Shah et al., 2018). Another study reported that Notch1 mediated repression of PTEN in HER2+ breast cancer cells and was responsible for trastuzumab resistance through increased survival of breast cancer cells (Baker et al., 2018). Similarly, the Dll1, Jag1 mediated survival is responsible for chemoresistance in breast cancer and multiple myeloma.
Hh signaling also has been shown to promotes cancer cell survival, which forms a selective growth advantage to tumor cells and has been implicated in multidrug resistance. In rhabdomyosarcoma (RMS) or Ewing sarcoma (EWS), HH pathway activity and GLI1 expression contribute to cell survival and proliferation. Chemotherapeutic drugs such as Vincristine (VCR), was shown to significantly upregulate Gli1 expression in these cells. Thus treatment with small molecule inhibitor GANT61 or siRNA against GLI1 together with vincristine significantly decreased cell viability (Yoon et al., 2020). Similarly, Pemetrexed resistant NSCLC cells showed significantly increased expression of HH signaling genes (GLI1, GLI2, GLI3, PTCH1, SHH). Supporting these results, pemetrexed resistant cells treated with the HH inhibitor Gant61 showed reduced proliferation and survival compared to naïve cells. Thus, blocking the HH pathway may be a potential option to overcome resistance to various chemotherapy (Liu et al., 2020).
Similarly, Wnt signaling is involved in regulating the drug resistance of various cancer via cell survival and proliferation. Thus inhibiting Wnt signaling can sensitizes cancer cells to chemotherapy. For example, in myeloid leukemia cells, the Wnt inhibitor (FH535) sensitizes it to chemotherapeutic drug imatinib and potentiated its chemotherapeutic effect (Suknuntha et al., 2017). A similar effect of Wnt inhibitor was observed in ovarian cancer cells, where the inhibition of the Wnt pathway reversed the drug resistance by inducing apoptosis and reducing the cell survival. TNBC cells and tissues resistant to chemotherapy shows sensitivity toward Wnt mediated ST8SIA1 expression. The reduced proliferation and cell survival were observed in chemo-resistant cells with ST8SIA1 inhibition. ST8SIA1 inhibition is associated with the suppression of FAK/Akt/mTOR and Wnt/β-catenin signaling pathways (Wan et al., 2020).
Reactive Oxygen Species (ROS)
ROS plays a crucial role in cancer progression and resistance to radio-and chemotherapy (Cui et al., 2018). Their role in modulating Notch, Hedgehog, and Wnt signaling and vice-versa has been investigated in different cancer that leads to the development of resistance to therapy.
Notch and ROS can modulate each other by directly regulating their core components. Nrf2 is a well-established regulator of anti-oxidants and its overexpression cause resistance to both radio-and chemo-therapy in cancer (Tian et al., 2017). Notch can directly bind to the promoter region of Nrf2 and regulate its transcription, thereby affecting the ROS level in the cells (Wakabayashi et al., 2014). Notch signaling has also been suggested to alter the proteome of mitochondria, which results in an alteration of its function. As Mitochondria are considered as the major source of ROS in cancer (Hayes et al., 2020), the alteration of their function by Notch can have a prominent role in the acquisition of chemoresistance. Moreover, the Notch pathway was shown to be critical for controlling the ROS level in CSCs, thereby affecting the chemoresistance (Qiang et al., 2012).
Similarly, the hedgehog signaling pathway can crosstalk with ROS-induced signaling to regulate chemoresistance in various cancer. For example, in hepatocellular carcinoma HCC cells, ROS-induced NRF2 activation upregulated the expression of sonic hedgehog homolog (SHH), ultimately activating sonic hedgehog pathway. This mediated the tumor initiating function, ultimately leading to sorafenib resistance in HCC. Moreover natural compound such as, resveratrol and curcumin was shown to inhibit hypoxia-mediated activation of the Hh signaling pathway in pancreatic cancer, ultimately affecting the EMT phenotype, which has been known to play an important role in inducing chemoresistance in cancer (Cao et al., 2016; Li et al., 2016). In contrast to the activation of hedgehog in tumor cells, activation of the canonical Hh signaling pathway in stromal cells by IHH was shown to suppress tumor growth and metastases, in part, by limiting ROS activity (Kasiri et al., 2020).
The interplay between Wnt signaling and ROS has investigated in different cancer that leads to the development of resistance to therapy. Activation of Wnt canonical signaling aids the ubiquitination and proteasomal degradation of Nrf2 protein via axin1/GSK-3b complex (Tian et al., 2017). ROS/Wnt/β-catenin signaling has been shown to regulate the stemness in hepatocellular carcinoma (HCC) by targeting glutaminase 1 (GLS1) (Li B. et al., 2019). Thus, targeting GLS1 attenuates stemness properties in HCC by increasing ROS and suppressing Wnt/β-catenin pathway and hence, GLS1 served as a therapeutic target for the elimination of CSCs. Recently, mitochondrial ROS NF-kB/b catenin axis was found to play significant role in regulating gall bladder cancer. Moreover, ROS mediated alteration in Wnt signaling impacts the vascular development that constitutes changes in stem cell differentiation, angiogenesis, VEGF signaling, endothelial as well as cardiac progenitor cell recruitment, and vascular cell migration in cancer and cardiovascular diseases (Caliceti et al., 2014). Thus, targeting Wnt signaling with inhibitors that curb ROS may synergistically overcome the chemoresistance in various cancers.
Induction in Epithelial to Mesenchymal Transition (EMT)
Epithelial to mesenchymal transition (EMT) is a process where the epithelial cells lose their epithelial characteristics and acquire mesenchymal characteristics. This dramatic cell transposition process not only plays critical roles in governing embryonic development and maintaining adult tissue hemostasis (e.g., via regulating wound healing and stem cell behavior) but also contributes to pathological conditions, such as fibrosis, cancer progression, and drug resistance.
Notch signaling can directly regulate the expression of genes involved in EMT (Nieszporek et al., 2019). It is increasingly recognized that the Notch signaling is involved in the acquisition of EMT in drug-resistant cancer cells. In gemcitabine-resistant pancreatic cancer cells, Notch-2 and Jagged-1 are highly up-regulated, and downregulation of Notch signaling by siRNA leads to a partial reversal of the EMT phenotype, resulting in the mesenchymal-to-epithelial transition (MET), which is associated with decreased expression of vimentin, ZEB1, Slug, Snail, and NF-κB (Wang et al., 2009). A similar effect has also been observed in breast cancer cells resistant to cisplatin/doxorubicin/Docetaxel, where the inhibition of Notch signaling leads to a decrease in EMT, thus sensitizing them to the chemotherapeutic drugs (Zhang et al., 2013; Xiao et al., 2019). In colorectal cancer, ADAM17 inhibitor (ZLDU-8) downregulated Notch signaling leading to decrease EMT, which affected chemoresistance phenotype (Li et al., 2018).
The role of the Wnt/β-catenin pathway has also been implicated in the EMT process. Runt-related transcription factor 1 (RUNX1) plays the roles of an oncogene and an anti-oncogene in epithelial tumors, and abnormally high expression of RUNX1 is associated with metastasis and EMT. It directly interacts with catenin and targeting the promoter and enhancer regions of KIT (Li Q. et al., 2019). Further, the expression of TRIM29 (Tripartite Motif 29) has been reported to be influenced by Wnt/β-catenin. TRIM 29 can activate this pathway in colorectal cancer (CRC) cells by promoting metastasis and invasion by regulating EMT through increased expression of CD44. Rhomboid domain 1 (RHBDD1) containing has also been implicated in metastasis and invasion of CRC mediated by the Wnt/β-catenin pathway, as the expression of RHBDD1 strongly correlates with ZEB-1 in lymphatic and distal metastasis (Pothuraju et al., 2020).
Enrichment/Induction of Cancer Stem Cell Like Phenotype
Cancer Stem Cell (CSC) is a small subset of tumor cells that has the potential to self-renew and give rise to a heterogeneous population in the tumor. Many studies have demonstrated that CSC is more resistant to chemotherapy because of the higher expression of the anti-apoptotic protein, multidrug resistance gene, etc. These studies have been extensively reviewed elsewhere (Shibue and Weinberg, 2017). The role of Notch, Hedgehog, and Wnt signaling in CSC function in various cancers has been well established (Oren and Smith, 2017; Yang et al., 2020). It is now well appreciated that inhibition of these signaling pathways can reduce the CSCs/stem-like properties, thereby enhancing the chemosensitivity toward various drugs.
The Numb-/low population of castration-resistant prostate cancer has been shown to up-regulate Notch and Hedgehog signaling. This is associated with increased expression of genes linked to stem cells and greater resistance to androgen deprivation therapy (ADT). Inhibition of Notch and Hedgehog signaling pathways significantly increases apoptosis in Numb-/low cells in response to ADT (Guo et al., 2017). Consequently, overexpression of Notch3 leads to the expansion of CSCs, resulting in increased resistance against platinum in ovarian cancer. At the same time, Notch inhibition by pharmacological or genetic approaches depletes CSCs, thereby increasing tumor sensitivity to platinum (McAuliffe et al., 2012). Similarly in Epithelial Ovarian cancer and esophagous squamos cell carcinoma (ESCC) cells, sustain expression of Notch signaling in CSCs/tumor initiating cells, leads to acquisition of drug resistant phenotype (Li H. et al., 2019; Zhao et al., 2019).
In glioblastoma, CD133+ CSCs express higher levels of miR-9 and activate the SHH/PTCH1/MDR1 axis, which imparts resistance against TMZ (Munoz et al., 2015). Hedgehog pathway appears to transcriptionally regulate the expression of twist1 and snail in the acquired chemo-resistant cancer cells and chemo-sensitive squamous carcinoma (KB) cancer cells, thereby maintaining the tumor-initiating cell-like properties and consequently the chemo-resistant phenotype, which is independent of ABC transporters (Kong et al., 2015).
Likewise, the Wnt signaling also plays a prominent role in maintaining CSCs which is linked to drug resistance. In cancer such as pancreatic cancer, lung adenocarcinomas, colorectal cancer, and liver cancer, the small subpopulation of cancer cells expressing high Wnt/β-catenin signaling showed CSCs phenotype and were resistant to drug. The inhibition of Wnt signaling in these cells suppressed the stem cells phenotype, thereby affecting the chemoresistance (Taelman et al., 2010; Ilmer et al., 2015; Belur Nagaraj et al., 2021).
Modification of the Tumor Microenvironment
Increasing evidence suggests that the tumor microenvironment plays a critical role in cancer drug resistance. Apart from playing an essential role in cancer cells, Notch, Hedgehog, and Wnt signaling pathways have prominent roles in modifying tumor microenvironment, which has been implicated in providing chemo-resistance.
The Notch signaling can induce the differentiation of the neighboring cancer cells, which can adopt different cell fate, thus creating a heterogeneous population. The heterogeneity in the tumor is one of the major contributors to the acquisition of chemoresistance (Lim et al., 2017). Furthermore, Notch ligands present in the components of the tumor microenvironment, such as endothelial cells, fibroblasts, immune cells, etc., can induce Notch signaling in cancer cells leading them to acquire stem cell phenotype and becoming resistance to chemotherapy (Boelens et al., 2014; Cao et al., 2014).
In breast cancer, the hedgehog ligand secreted by neoplastic cells modifies CAF, which in-turn provides a supportive microenvironment for the acquisition of a chemo-resistant CSC phenotype via FGF5 expression and production of fibrillar collagen. Consequently, treatment with smoothened inhibitors (SMOi) down-regulates the expression of CSC markers and sensitizes tumors to chemotherapy drugs such as docetaxel (Cazet et al., 2018). Hedgehog secretion by multiple myeloma cells has been found to upregulate stromal CYP26 and further reinforce a protective microenvironment. These results suggest that crosstalk between Hedgehog and retinoid signaling can modulate the tumor microenvironment and cause resistance against anticancer therapeutics (Alonso et al., 2016). Along with other signaling pathways like Notch, TGF-b and receptor tyrosine kinase, the Wnt signaling pathway is also been known to play an important role in inducing EMT in tumor cells (Jing et al., 2011).
Likewise, the Wnt ligand or the growth factor secreted by stromal cells of TME leads to the stimulation of Wnt signaling in tumor cells, that leads to the EMT (Patel et al., 2019). For example, in colorectal cancer cells (CRCs), stimulation of hepatocyte growth factor (HGF) upregulates the β-catenin expression through the PI3-K pathway (Fodde and Brabletz, 2007). This leads to the initiation of the EMT process, which mediates the chemoresistance.
Notch, Hedgehog, and Wnt Signaling in Radio-Resistance
Radiotherapy is used in the treatment of approximately 50% of all malignancies. Resistance to radiation therapy is polymodal and is associated with several biological alterations both within the tumor and in the surrounding microenvironment. Moreover, like chemotherapy, radiotherapy also induces EMT and CSC-like phenotype in cancer cells, which contributes to the radio-resistance. As discussed earlier, Notch, Hedgehog, and Wnt have a prominent role in EMT and CSC, and inhibition of these pathways enhances radio-sensitivity through down-regulation of EMT and CSC (Yahyanejad et al., 2016; Figure 4).
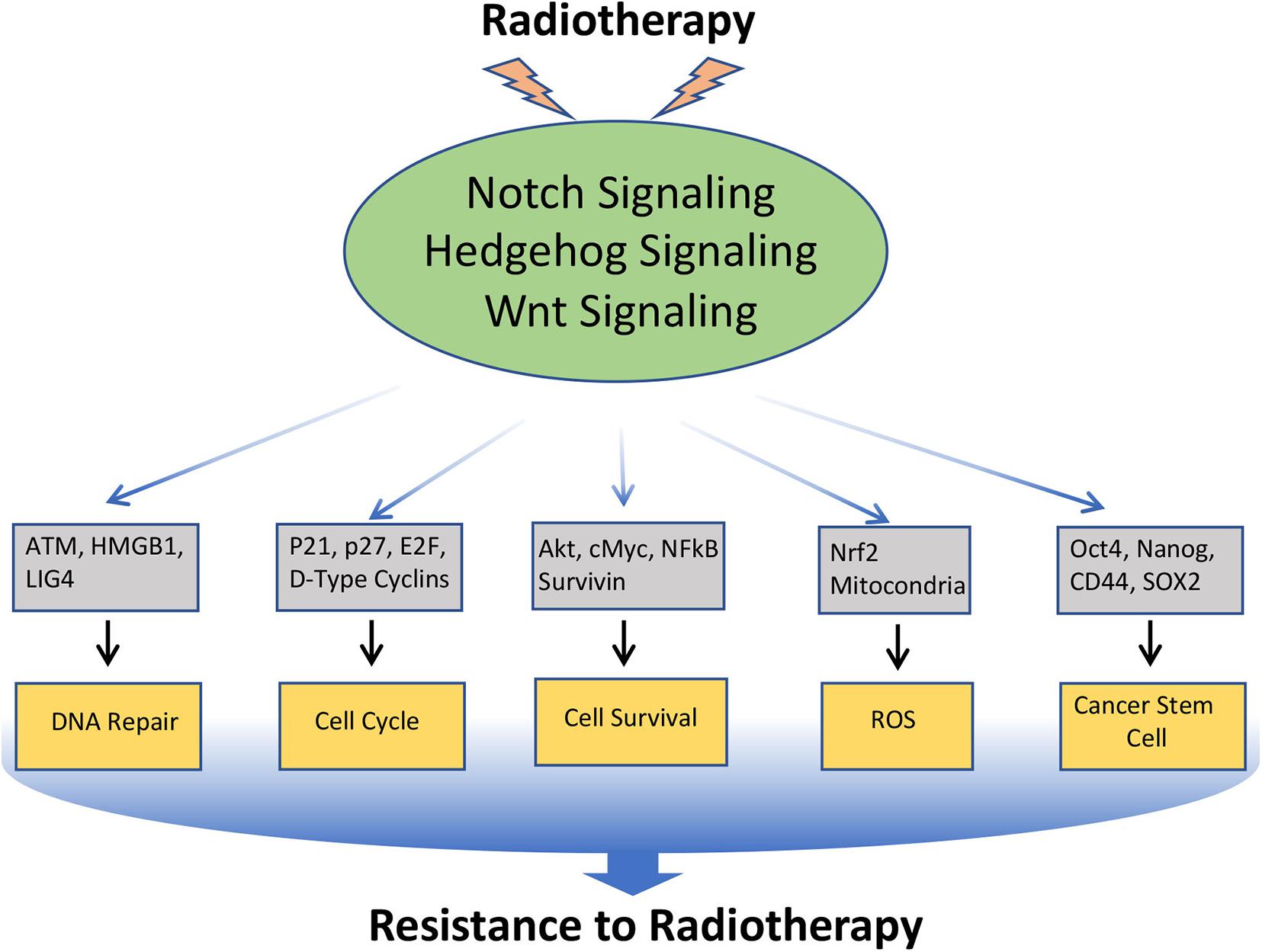
Figure 4. Involvement of Notch, Hedgehog, and Wnt signaling pathways in the resistance of cancer cells to Radiotherapy: Radiotherapy often induces Notch, Hedgehog, and Wnt signaling in cancer, which in turn regulates the key molecules involved in DNA repair, cell-cycle, cell survival, ROS generation, EMT, and CSC, that leads to the acquisition of radioresistance in cancer.
Notch Signaling in Radioresistance
It has now been well established that Notch signaling gets activated upon irradiation and plays an important role in the radio-resistance of both stem and non-stem cancer cell populations (Yahyanejad et al., 2016). Moreover, in many radio-resistant tumor and cancer cell lines, the Notch signaling is found to be upregulated. Notch signaling can regulate the DDR by directly interacting with ATM and inactivating its kinase activity (Vermezovic et al., 2015; Adamowicz et al., 2016). In line with this, an inverse correlation between Notch and pATM has been found in breast cancer, and Notch inhibition results in increased radiation sensitivity in an ATM-dependent manner. Notch signaling can also affect the radio-resistance by modulating the function/activity of other signaling molecules involved in cell survival, metabolism, cell cycle, etc. In glioma stem cells (GSCs), inhibition of Notch with γ-Secretase Inhibitors (GSIs) does not alter the DNA Damage Response of GSCs but rather suppresses the Akt activity and Mcl1 level after radiation, making GSCs more sensitive to radiation at clinically relevant doses (Wang et al., 2010). Further, ALDH positive breast cancer cells are more radio-resistant as compared to the ALDH negative counterpart. Mechanistic studies have shown that radio-resistance linked to ALDH activity stimulation is mediated through the activation of the Notch1 and AKT pathways and involves Nanog signaling. Nrf2 plays a major role in regulating the cellular antioxidant system and is activated by radiation in a dose-dependent manner. A decrease in Nrf2 expression significantly dampens Notch1 expression following ionizing radiation and potentiates IR-induced apoptosis. Nrf2-mediated Notch signaling has been an important determinant in the radio-resistance of lung cancer cells, while TRIB3 activates the Notch signaling in radio-resistant triple-negative breast cancer.
Hedgehog Signaling in Radioresistance
The role of Hedgehog signaling in the radioresistance of tumors has been well established. GLI proteins are the functional transcription activators of the Hh pathway, and the Inhibition of its activity can interfere with almost all DNA repair types in human cancer, indicating that Hh/GLI functions may play an important role in radiation-induced DNA damage (Meng et al., 2015). Indeed, Hedgehog signaling is found to be upregulated/activated following irradiation, and this has been implicated in providing radio-resistance. Consequently, the inhibition of hedgehog signaling augments the efficacy of radiation in tumors that are dependent on hedgehog signaling (Teichman et al., 2018). In HNSCC, Gli1 is often upregulated at the tumor-stroma intersection, which gets further augmented following irradiation, where it contributes to stromal-mediated radio-resistance of the tumor. Treatment with HH inhibitors has been found to enhance tumor sensitivity to radiotherapy (Gan et al., 2014). Further, intrinsic or acquired radio-resistance of tumors is often associated with up-regulated Hedgehog signaling, and the downregulation of this by either pharmacological inhibition or genetic manipulation renders them sensitive to radiation.
Wnt Signaling in Radioresistance
WNT signaling has also been implicated in the radioresistance of cancer cells. An increase in the WNT activity has been reported in radio-resistant Esophageal squamous cell carcinoma (ESCC) cell lines (Zhao et al., 2018), which upon inhibition reversed the radio-resistance phenotype of these cells (Zhao et al., 2018). Mechanistically it was shown that the Wnt signaling upregulated HMGB-1, which is chromatin-associated protein involved in the DNA repair (Zhao et al., 2018). In another study, it was shown that Wnt signaling enhances non-homologous end-joining repair in colorectal cancer, which is mediated by LIG4, a DNA ligase, transactivated by β-catenin (Jun et al., 2016). Together, these reports suggest that Wnt signaling can directly regulate the components of DNA repair machinery, thus affecting the response to the radiation. Moreover, the radiation-induced upregulation of Wnt/β-catenin pathways in squamous cell carcinoma of the head and neck (SCCHN) was shown to decrease the sensitivity of SCCHN to irradiation both in vitro and in vivo (Jing et al., 2019). Thus, targeting Wnt signaling for overcoming radio-resistance appears to be an attractive approach for cancer treatment.
Notch, Hedgehog, and Wnt Signaling in Resistance to Immunotherapy
Cancer immunotherapy has recently emerged as a new and promising option for the treatment of many malignancies. However, despite the recent successes of cancer immunotherapies, most patients are still refractory with tumors demonstrating resistance to this therapy. Notch, Hedgehog, and Wnt signaling pathways are well known to play an important role in the development, homeostasis, and function of various immune cells. Although limited studies have been carried out, available evidence suggests that these signaling pathways can mediate cancer immune evasion and resistance to immunotherapies through various mechanisms (Figure 5).
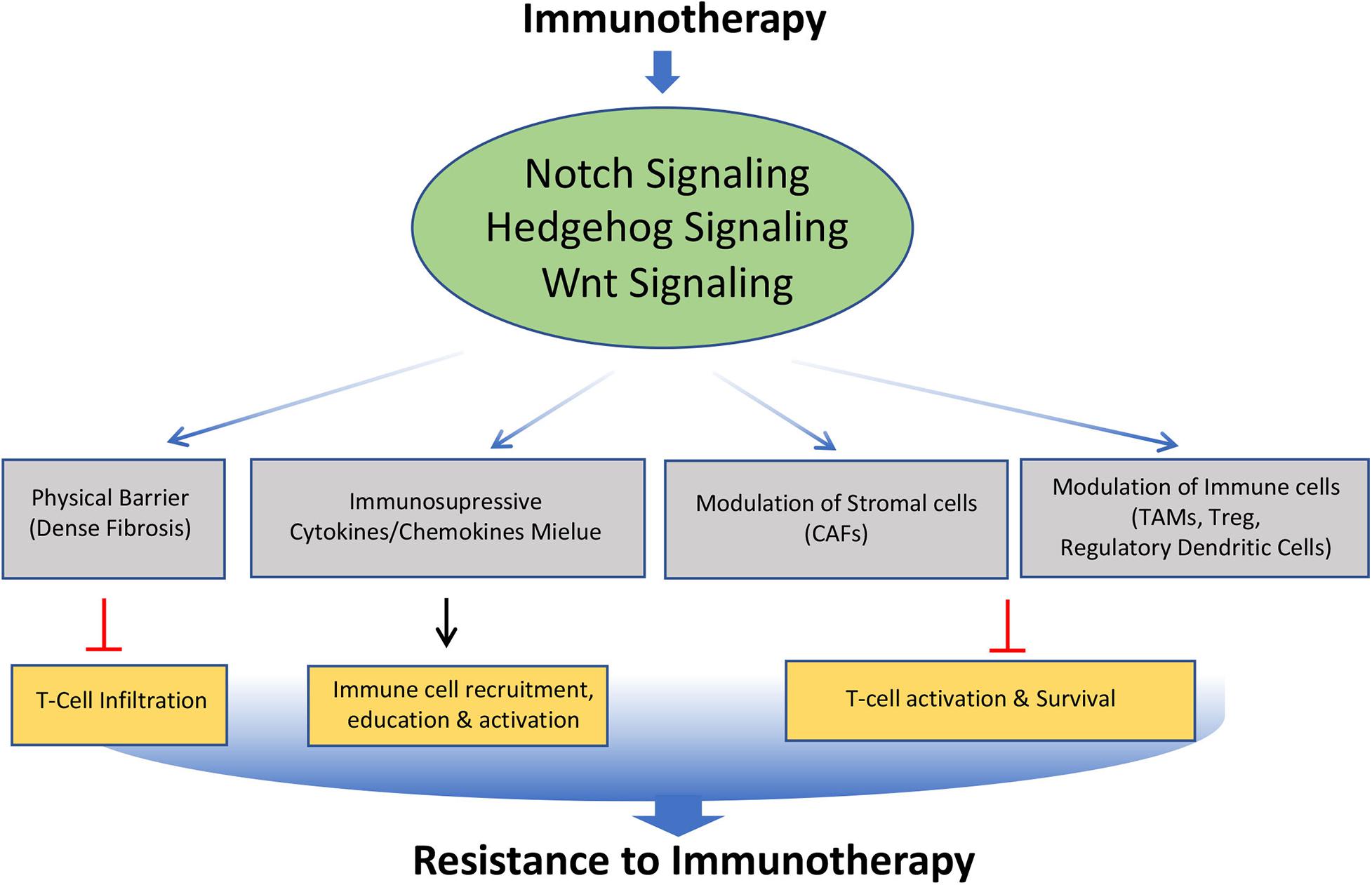
Figure 5. Role of Notch, Hedgehog, and Wnt signaling pathways involved in the acquisition of resistance to immunotherapy. Altered modulation of Notch, Hedgehog, and Wnt signaling by immunotherapy leads to modification of tumor microenvironment such as formation of dense fibrosis, secretion of immunosuppressive cytokines and chemokines, Cancer Associated Fibroblast (CAFs), Tumor Associated Macrophage (TAMs), regulatory dendritic cells. This affects the trafficking, survival and activation of T cells, leading to acquisition of immunotherapy resistance.
Notch Signaling in Resistance to Immunotherapy
Notch signaling plays multiple roles in the crosstalk between systemic inflammation, myeloid cells in the tumor microenvironment (Franklin et al., 2014), the cancer cell themselves, and multiple lymphocyte subpopulations, thereby modulating tumor immunity (Kuijk et al., 2013). Thus, mutations in the regulators of this pathway are found to be favorable for immunotherapy. In-line with this, melanoma and NSCLC patients who did not respond to immunotherapy showed seldom NOTCH1 mutation, while a marked correlation between NOTCH1/2/3 mutation and better outcome with an immune checkpoint inhibitor (ICI) were found in EGFR/ALK WT NSCLC patients (Zhang et al., 2020). Moreover, the del-NOTCH mutation, which down-regulates Notch signaling, was found to be a potential predictor of favorable ICI response in NSCLC (Zhang et al., 2020). Recent studies have shown that co-occurring mutations in Notch1–3 and homologous recombination repair (HR) genes are associated with increased immunotherapy efficacy in patients with advanced NSCLC (Mazzotta et al., 2020). Moreover, this genomic predictor is also associated with longer survival in patients with other tumor types treated with ICIs (Mazzotta et al., 2020). These observations open the possibility of personalized combination immunotherapy comprising NOTCH inhibitor and ICI in the treatment of NSCLC.
Hedgehog Signaling in Resistance to Immunotherapy
Hedgehog signaling pathway exerts complex and diverse effects on the tumor immune micro-environment. Emerging evidence suggests that the hedgehog signaling pathway has an immunosuppressive action in many cancers by modulating different components of tumor microenvironments. In Pancreatic ductal adenocarcinoma (PDAC), dense fibrosis is often observed, which acts as a barrier to immune cell infiltration into the tumor, thus making the tumor resistant to immunotherapy. As hedgehog signaling plays an important role in fibrosis, their inhibition has shown a positive effect on immunotherapy. For example, the Hedgehog signaling inhibitor, the Patched 1-interacting peptide has been found to inhibit proliferation and migration of cancer-associated fibroblasts and cancer cells and also increase the infiltration of immune cells by reducing fibrosis of PDAC, thus enhancing the effect of immunotherapy (Oyama et al., 2020). Moreover, Hedgehog (Hh) signaling in myeloid cells is critical for the functioning of Tumor-Associated Macrophages (TAM) M2 polarization and tumor growth (Petty et al., 2019). In this scenario, the sonic HedgehogHedgehog (Shh) secreted by tumor cells drives TAM M2 polarization. The TAM, in turn, suppresses the CD8+ T cells recruitment to the TME, thus mediating the immune-suppression (Petty et al., 2019).
Wnt Signaling in Resistance to Immunotherapy
Although the role of Wnt signaling in immune cell development remains controversial (Staal et al., 2008; Kabiri et al., 2015), recent studies suggest that it plays an important role in driving the primary, adaptive, and acquired resistance to anticancer immunotherapy (Luke et al., 2019). In human metastatic melanoma samples, an inverse correlation between Wnt/β-catenin pathway activation and T-cell infiltration has been found (Trujillo et al., 2018). Mechanistically, it was shown that the activation of β-catenin signaling in the tumor suppress the expression of chemokines such as CCL4, causing the failure of the dendritic cell recruitment into the tumors, thus resulting in impaired activation of the T cells (Spranger et al., 2015). Moreover, activation of β-catenin in dendritic cells up-regulates the expression of indoleamine 2,3-dioxygenase-1 (IDO) enzyme, which leads to the development of tolerogenic dendritic cells. These transformed dendritic cells favor the differentiation of regulatory T cells, which are involved in the immunotherapy resistance (Holtzhausen et al., 2015).
Notch, Hedgehog, and Wnt Signaling in Resistance to Targeted Therapy
A deep understanding of the critical molecular drivers of cancer has led to the development of targeted therapies that strike the core molecules involved in many malignancies. However, after the initial response, many cancers outsmart such efforts, and thus therapeutic resistance follows, which contributes to cancer mortality. The strategies employed by cancer cells to gain resistance to targeted therapies include activating signals upstream or downstream of oncogenes, direct target reactivation, adaptive survival mechanisms, and engagement of parallel oncogenic pathways. As many of these processes are directly regulated by the Notch, Hedgehog, and Wnt signaling pathways, cancer cells can utilize these signaling pathways to modulate the downstream effectors of the targeted molecules, thereby becoming resistant to the targeted therapies (Figure 6).
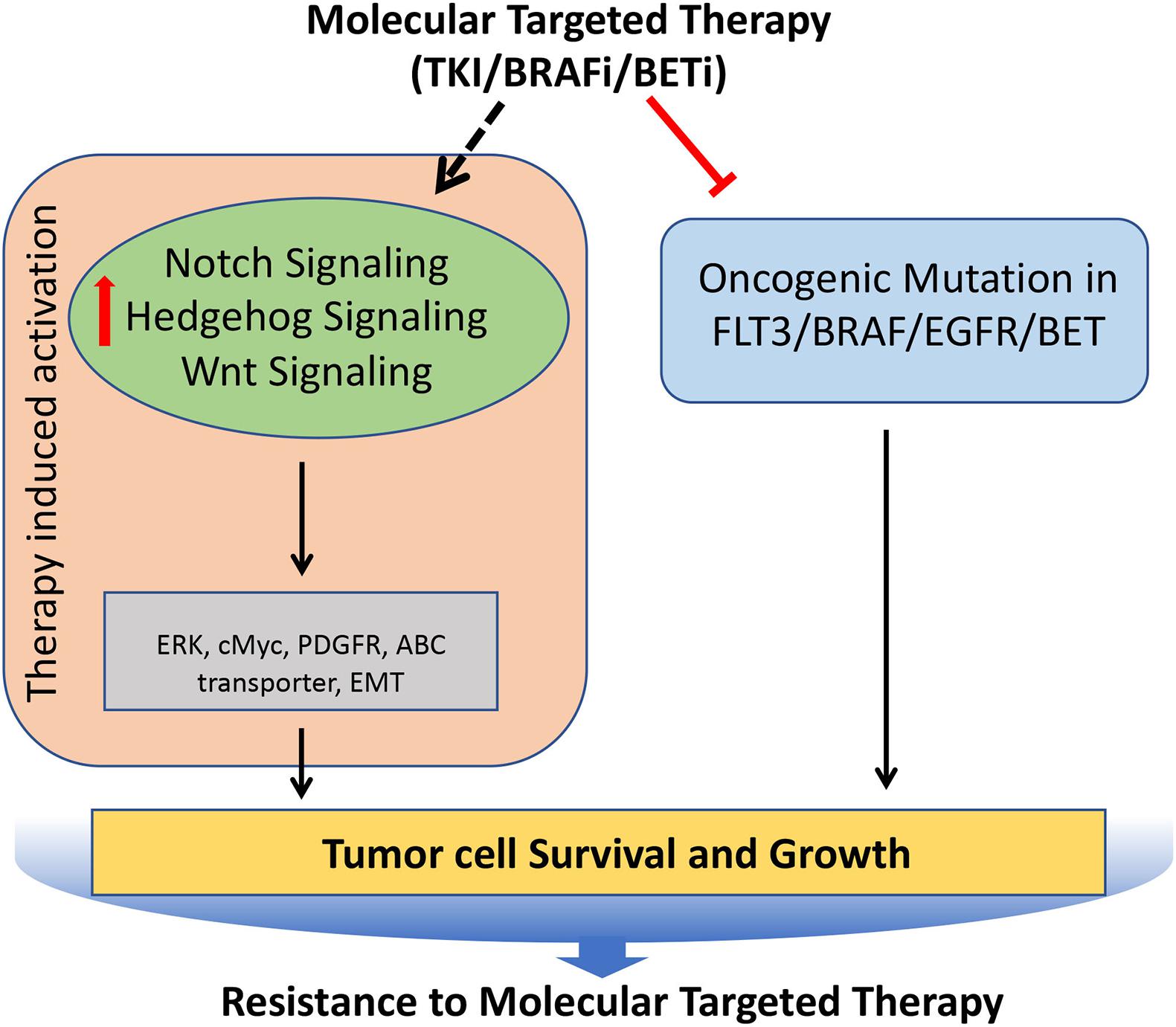
Figure 6. Role of Notch, Hedgehog, and Wnt signaling pathways involved in the acquisition of resistance to molecular targeted therapies in tumors. Molecular targeted therapies block the oncogenic mutated molecular targets in cancer, but often it leads to induced hyperactivation of Notch, Hedgehog, and Wnt signaling pathways, which regulates key molecules (such as cMyc, PDGFR, ERK etc.) involved in the tumor cell survival and growth, resulting in the development of resistance against molecular targeted therapies.
Notch Signaling in Targeted Therapy Resistance
Tumors that acquire resistance to tyrosine kinase inhibitors (TKIs), often show upregulation of the key signaling pathways. For example, genetic alternations of internal tandem duplication (ITD) and mutations of FMS-like tyrosine kinase-3 (FLT3) are most frequently observed in AML. Consequently, FLT3 TKIs are widely used in the treatment of FLT3/ITD+ AML patients. Unfortunately, most often, they acquire resistance to TK inhibitors. Mechanistically, Notch signaling was upregulated following treatment with FLT3-TKIs, which resulted in alternative ERK activation. The addition of Notch inhibitor (GSI) abrogated the alternative activation of ERK, resulting in extreme repression of ERK activity, thereby showing a synergistic antitumor effect (Li D. et al., 2020). Likewise, lung adenocarcinoma patients with activating EGFRL858R mutation show a better response to TKIs initially but subsequently develop resistance and show increased HES1 protein levels that correlate with shorter progression-free survival (Bousquet Mur et al., 2020). Administration of Notch inhibitors made these resistant tumors highly responsive to TKIs, thus implicating the role of Notch signaling in providing resistance to the TKIs (Bousquet Mur et al., 2020). BRAF is another molecular target, which is often mutated in many cancer (Davies et al., 2002). Small-molecule BRAF inhibitors (BRAFi) have been developed and have shown a significant survival advantage in patients whose tumors harbor the BRAF driver mutation (Long et al., 2018). In melanomas harboring activating BRAF mutation, the treatment with BRAFi often leads to the development of the resistance to BRAFi. Mechanistically up-regulated Notch signaling is involved in this process, and inhibition of Notch signaling can sensitize these melanomas to BRAF inhibitor (Ruggiero et al., 2019).
Hedgehog Signaling in Targeted Therapy Resistance
Hedgehog is known to co-operate with the epidermal growth factor receptor (EGFR) signaling pathway during embryogenesis. Therefore, it is not surprising that it is implicated in the resistance against TKIs. The EGFR-TKI resistance NSCLC cells show hyperactivation of hedgehog signaling. This results in EMT induction and ABCG2 up-regulation, and inhibition of hedgehog signaling increases the sensitivity to EGFR-TKIs in resistant NSCLC cells (Bora-Singhal et al., 2015; Della Corte et al., 2015). Moreover, increased expression of Gli1 has been observed in HNSCC cells when subjected to long-term EGFR inhibition. These resistant cells undergo EMT through hedgehog signaling and hedgehog inhibition with Cetuximab delay or completely block tumor recurrence (Keysar et al., 2013). BRAFi induces the activation of the Sonic Hedgehog Homolog (Shh) pathway, which in turn up-regulates the expression of PDGFRα, leading to the resistance of melanoma to BRAFi. Consequently, inhibition of Shh by LDE225 restores and increases the sensitivity of melanoma cells to BRAFi (Sabbatino et al., 2014). Likewise, Gant61 monotherapy reverses the resistance of melanoma cells to Vemurafenib. Interestingly, alternating the dosing schedules of Vemurafenib and Gant61 prevents the onset of BRAFi resistance (Faiao-Flores et al., 2017).
Wnt Signaling in Targeted Therapy Resistance
Wnt signaling has also been attributed to the development of resistance against targeted therapy. Androgen deprivation therapy (ADT) is the most widely used therapy for the advanced and metastatic prostate cancer (PCa), who cannot be cured by surgery or radiation therapy. Wnt signaling, which is known to be involved in the late stage of PCa, was shown to be activated upon the inhibition of androgen receptor (AR), resulting in therapy resistance to the ADT. Mechanistically, it was shown that the activation of Wnt signaling was not by mutation but through cross talk with other signaling pathways, growth factors, and cytokines produced in response to damaged TME followed by inhibition of androgen receptor (AR) (Yeh et al., 2019). The inhibition of Wnt/β-catenin signaling re-sensitizes the ADT resistant tumors to abiraterone acetate/prednisone (AA/P). Moreover, the reports from the preclinical studies suggest that the combination of an antiandrogen agent with a Wnt pathway inhibitor achieved enhanced growth suppression in prostate cancer (Wang et al., 2008). Wnt signaling has also been shown to be the key player in mediating the resistance to the Bromodomain and extra terminal protein (BET) inhibitors. The BET inhibitors are used as a first-in-class therapy for the treatment of acute lymphoblastic leukemia, but often the patient develops resistance to BET inhibitors. Two independent studies found that the Wnt signaling was involved in the development of primary and acquired resistance to BET inhibitors in acute lymphoblastic leukemia (Fong et al., 2015; Rathert et al., 2015). Mechanistically it was shown that BET inhibitors repress BRD4-dependant expression of MYC oncogene; however, the β-catenin maintains the expression levels of MYC in the presence of BET inhibitors, thereby mediating the resistance to BET inhibitors. Similarly, the role of Wnt signaling in promoting the resistance to BRAF inhibitors and EGFR inhibitors in colorectal cancer and lung cancer has been well documented (Scarborough et al., 2017; Chen et al., 2018).
Therapeutic Targeting of Notch, Hedgehog, and Wnt Signaling Pathways: Clinical Update and Recent Development of the Inhibitors
Inhibitors of gamma-secretase were the first to enter clinical trials and has been evaluated extensively (Cook et al., 2018). Their merit includes pan-Notch inhibitory activity, favorable tissue distribution, oral administration, and low cost (Andersson and Lendahl, 2014; Kongkavitoon et al., 2018). However, they show gastrointestinal tract toxicity, prompting cautious usage, and restraining further development for clinical applications (Takebe et al., 2014). Anti-DLL4 and DLL3 ligand inhibitors, as well as other pan Notch signaling inhibitors, have also been evaluated in clinics (Cook et al., 2018). As a monotherapy, all Notch inhibitors have been so far assessed for safety and toxicity as well as for dose optimization in Phase I/II trials, which have shown satisfactory patient compliance and toxicity (Du et al., 2019; Yang et al., 2020). Drugs targeting Notch signaling (including antibody against various components of Notch signaling) have also been evaluated as a part of combinational strategies with the standard of care therapies (like chemo- and radiotherapy) in various primary and metastatic tumors (Table 2). Phase I clinical trials have not indicated any additional toxicities induced by combining Notch inhibitors with a different standard of care chemotherapeutic drugs like gemcitabine, cisplatin/carboplatin, folate antimetabolites, taxol, temozolomide, etc. (Du et al., 2019). However, cardiotoxicity has been observed with a combination of antibody-based Notch inhibitors and carboplatin (Du et al., 2019). Phase II clinical trials with gamma-secretase inhibitor RO4929097 in metastatic melanoma and metastatic colorectal cancer have not shown a compelling clinical benefit and, therefore, has not been pursued further (Strosberg et al., 2012; Lee et al., 2015). A correlation between Notch status and response to standard of care therapies has also been not very conclusive. For example, hyperactivation of Notch1 showed no correlation with the response to a combination of methotrexate and cyclophosphamide in children with T-cell acute lymphoblastic leukemia (Clappier et al., 2010). Therefore, there is a need for identifying (or designing) drugs and/or therapeutic strategies with encouraging efficacy and acceptable toxicity, besides establishing a compelling role for targeting Notch signaling in the clinical setting.

Table 2. Overview of the clinical trials evaluating drugs targeting developmental signaling pathways.
Drugs targeting Wnt signaling that has been evaluated in clinics include porcupine inhibitors, agents targeting β-catenin/CBP, and Frizzled receptors (Table 2). Drugs targeting smoothed receptors (Smo inhibitors) viz. vismodegib, sonidegib, and glasdegib are among the therapeutics targeting hedgehog signaling, which have been so far evaluated in Phase I/II trials (Table 2). These studies have shown that, unfortunately, tumors develop resistance against Smo inhibitors and are also associated with a variety of toxicities, which limit their clinical evaluation further for efficacy (Du et al., 2019). These inhibitors have also been evaluated in combination with other chemotherapeutic drugs like cisplatin and temozolomide in the treatment of solid tumors with limited benefit (Xie et al., 2019). Arsenic trioxide is an inhibitor of Gli transcription factor and Hedgehog signaling that has also been evaluated in Phase II clinical trials for its efficacy in few solid tumors and hematological malignancies (Xie et al., 2019).
Although clinical trials of drugs to target the three developmental signaling pathways associated with the CSCs was initiated more than a decade back, it has not translated into clinical benefit both due to their inability to reduce the tumor recurrence linked to the CSCs as well as off-target toxicity (Du et al., 2019; Katoh and Katoh, 2020; Yang et al., 2020). Therefore, approaches with the potential to address both these issues have received attention in the recent past. One such approach investigated is the novel nano-drug delivery systems (NDDS) due to their efficient and targeted delivery to the CSC niche in the TME, thereby enhancing the effects on CSC and reducing the off-target effects (Du et al., 2019). For example, Notch inhibitors like DAPT, MRK-560, MK-0752, BMS-906024 with Nanoparticle (NP) carriers are under clinical development for enhanced systemic tumor delivery and limiting the side effects in breast cancer cells (Mamaeva et al., 2011; Mamaeva et al., 2016). Similarly, inhibitors of Wnt signaling like SFRP-1, Niclosamide (NIC), and cromolyn were also delivered by nanoparticle into the tumor microenvironment that blocks the Wnt5a or FRZD-7 receptors and results in Wnt signaling inhibition in TNBC, ovarian cancer, colon, colorectal cancer, and metastatic melanoma (Ghoshal et al., 2015; Liu et al., 2018). The other approach is the use of phytomedicines such as curcumin, epigallocatechin-3-gallate (EGCG), resveratrol, sulforaphane, and genistein, either as monotherapy as in combination with standard of care therapeutics. Interestingly these phytochemicals/phytomedicines show effects on the three developmental signaling pathways, which could contribute to their overall effects, besides other well-established mechanisms of action of these phytochemicals (Yang et al., 2020). Reduced toxicity associated with these phytomedicines is an added advantage that has encouraged the initiation of the Phase I/II clinical trials, which are currently in progress (Table 3; Yang et al., 2020).
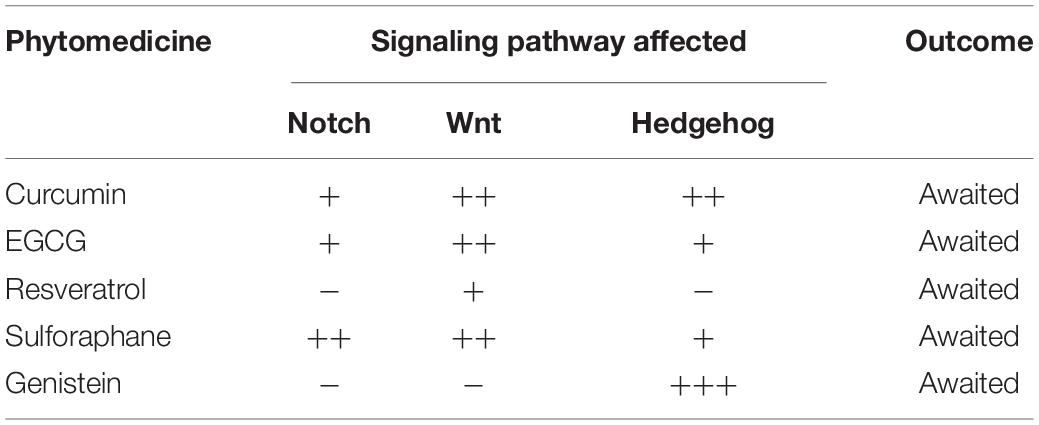
Table 3. Overview of the currently ongoing Phase I/II clinical trials using phytomedicines targeting developmental signaling pathways in solid tumors.
Conclusion
Resistance to anticancer therapy stems from complex and multifactorial processes and has still remained the main reason behind the failure of various therapies. Evolutionary conserved developmental signaling pathways such as Notch, Hedgehog, and Wnt signaling are well recognized for their role in regulating many cellular functions that play key roles in tumor development and progression. These signaling pathways are often upregulated in tumors, which often hijack these pathways to evolve continuously under the pressure induced by the therapy, thereby enabling them to become resistant to various therapies. Many inhibitors developed to target these signaling pathways have shown promising efficacy in preclinical cancer models, and some have even advanced to clinical trials with modest efficacy seen so far. However, as these signaling pathways are broadly active in normal tissue also, targeting these pathways with pan inhibitor often leads to undesirable off-target effects, particularly in the gastrointestinal tract. Therefore, one of the major challenges in the future will be to develop more selective inhibitors that will not only be more effective on tumors but have minimal normal tissue toxicity. Efforts are also required in developing synergistic drug combinations so that lower dosages can be used to improve the therapeutic index.
Author Contributions
VK and BSD conceptualized the manuscript. VK, MV, and BSD wrote the manuscript. VK and MV prepared the figures. LK, XW, CG, and JL reviewed the draft of the manuscript. All authors read and approved the final manuscript.
Funding
This work was supported by the Pudong New Area Science and Technology Development Grant (Grant No. PKJ2019-Y09).
Conflict of Interest
The authors declare that the research was conducted in the absence of any commercial or financial relationships that could be construed as a potential conflict of interest.
Acknowledgments
We thank Dr. Xiaomao Guo, Dean, Shanghai Proton and Heavy Ion Centre (SPHIC), Shanghai, China for his support and encouragement. We thank Dr. Y. Sun and Miss Zhou Ying, SPHIC, Shanghai Engineering Research Centre of Proton and Heavy Ion Radiation Therapy Shanghai, China for the administrative support and secretarial help.
References
Adamowicz, M., Vermezovic, J., and d’Adda di Fagagna, F. (2016). NOTCH1 inhibits activation of ATM by impairing the formation of an ATM-FOXO3a-KAT5/Tip60 Complex. Cell Rep. 16, 2068–2076. doi: 10.1016/j.celrep.2016.07.038
Adolphe, C., Hetherington, R., Ellis, T., and Wainwright, B. (2006). Patched1 functions as a gatekeeper by promoting cell cycle progression. Cancer Res. 66, 2081–2088. doi: 10.1158/0008-5472.can-05-2146
Alafate, W., Xu, D., Wu, W., Xiang, J., Ma, X., Xie, W., et al. (2020). Loss of PLK2 induces acquired resistance to temozolomide in GBM via activation of notch signaling. J. Exp. Clin. Cancer Res. 39:239.
Alonso, S., Hernandez, D., Chang, Y. T., Gocke, C. B., McCray, M., Varadhan, R., et al. (2016). Hedgehog and retinoid signaling alters multiple myeloma microenvironment and generates bortezomib resistance. J. Clin. Invest. 126, 4460–4468. doi: 10.1172/jci88152
Andersen, P., Uosaki, H., Shenje, L. T., and Kwon, C. (2012). Non-canonical Notch signaling: emerging role and mechanism. Trends Cell Biol. 22, 257–265. doi: 10.1016/j.tcb.2012.02.003
Andersson, E. R., and Lendahl, U. (2014). Therapeutic modulation of Notch signalling–are we there yet?”. Nat. Rev. Drug Discov. 13, 357–378. doi: 10.1038/nrd4252
Androutsellis-Theotokis, A., Leker, R. R., Soldner, F., Hoeppner, D. J., Ravin, R., Poser, S. W., et al. (2006). Notch signalling regulates stem cell numbers in vitro and in vivo. Nature 442, 823–826. doi: 10.1038/nature04940
Arora, M., and Spencer, S. L. (2017). A Cell-Cycle “Safe space” for surviving chemotherapy. Cell Syst. 5, 161–162. doi: 10.1016/j.cels.2017.09.010
Artavanis-Tsakonas, S., Rand, M. D., and Lake, R. J. (1999). Notch signaling: cell fate control and signal integration in development. Science 284, 770–776. doi: 10.1126/science.284.5415.770
Athar, M., Li, C., Tang, X., Chi, S., Zhang, X., Kim, A. L., et al. (2004). Inhibition of smoothened signaling prevents ultraviolet B-induced basal cell carcinomas through regulation of Fas expression and apoptosis. Cancer Res. 64, 7545–7552. doi: 10.1158/0008-5472.can-04-1393
Ayaz, F., and Osborne, B. A. (2014). Non-canonical notch signaling in cancer and immunity. Front. Oncol. 4:345.
Baker, A., Wyatt, D., Bocchetta, M., Li, J., Filipovic, A., Green, A., et al. (2018). Notch-1-PTEN-ERK1/2 signaling axis promotes HER2+ breast cancer cell proliferation and stem cell survival. Oncogene 37, 4489–4504. doi: 10.1038/s41388-018-0251-y
Belur Nagaraj, A., Knarr, M., Sekhar, S., Connor, R. S., Joseph, P., Kovalenko, O., et al. (2021). The miR-181a-SFRP4 axis regulates Wnt activation to drive stemness and platinum resistance in ovarian cancer. Cancer Res. doi: 10.1158/0008-5472.CAN-20-2041 Online ahead of print
Boelens, M. C., Wu, T. J., Nabet, B. Y., Xu, B., Qiu, Y., Yoon, T., et al. (2014). Exosome transfer from stromal to breast cancer cells regulates therapy resistance pathways. Cell 159, 499–513. doi: 10.1016/j.cell.2014.09.051
Bonilla, X., Parmentier, L., King, B., Bezrukov, F., Kaya, G., Zoete, V., et al. (2016). Genomic analysis identifies new drivers and progression pathways in skin basal cell carcinoma. Nat. Genet. 48, 398–406. doi: 10.1038/ng.3525
Bora-Singhal, N., Perumal, D., Nguyen, J., and Chellappan, S. (2015). Gli1-mediated regulation of Sox2 facilitates self-renewal of stem-like cells and confers resistance to EGFR inhibitors in non-small cell lung cancer. Neoplasia 17, 538–551. doi: 10.1016/j.neo.2015.07.001
Bousquet Mur, E., Bernardo, S., Papon, L., Mancini, M., Fabbrizio, E., Goussard, M., et al. (2020). Notch inhibition overcomes resistance to tyrosine kinase inhibitors in EGFR-driven lung adenocarcinoma. J. Clin. Invest. 130, 612–624.
Brantjes, H., Roose, J., van De Wetering, M., and Clevers, H. (2001). All Tcf HMG box transcription factors interact with Groucho-related co-repressors. Nucleic Acids Res. 29, 1410–1419. doi: 10.1093/nar/29.7.1410
Caliceti, C., Nigro, P., Rizzo, P., and Ferrari, R. (2014). ROS, Notch, and Wnt signaling pathways: crosstalk between three major regulators of cardiovascular biology. Biomed. Res. Int. 2014:318714.
Cao, L., Xiao, X., Lei, J., Duan, W., Ma, Q., and Li, W. (2016). Curcumin inhibits hypoxia-induced epithelialmesenchymal transition in pancreatic cancer cells via suppression of the hedgehog signaling pathway. Oncol. Rep. 35, 3728–3734. doi: 10.3892/or.2016.4709
Cao, Z., Ding, B. S., Guo, P., Lee, S. B., Butler, J. M., Casey, S. C., et al. (2014). Angiocrine factors deployed by tumor vascular niche induce B cell lymphoma invasiveness and chemoresistance. Cancer Cell 25, 350–365. doi: 10.1016/j.ccr.2014.02.005
Cazet, A. S., Hui, M. N., Elsworth, B. L., Wu, S. Z., Roden, D., Chan, C. L., et al. (2018). Targeting stromal remodeling and cancer stem cell plasticity overcomes chemoresistance in triple negative breast cancer. Nat. Commun. 9:2897.
Chatterjee, N., and Bivona, T. G. (2019). Polytherapy and targeted cancer drug resistance. Trends Cancer 5, 170–182. doi: 10.1016/j.trecan.2019.02.003
Chen, G., Gao, C., Gao, X., Zhang, D. H., Kuan, S. F., Burns, T. F., et al. (2018). Wnt/beta-catenin pathway activation mediates adaptive resistance to BRAF inhibition in colorectal cancer. Mol. Cancer Ther. 17, 806–813. doi: 10.1158/1535-7163.mct-17-0561
Chen, Y., Bieber, M. M., and Teng, N. N. (2014). Hedgehog signaling regulates drug sensitivity by targeting ABC transporters ABCB1 and ABCG2 in epithelial ovarian cancer. Mol. Carcinog. 53, 625–634.
Clappier, E., Collette, S., Grardel, N., Girard, S., Suarez, L., Brunie, G., et al. (2010). NOTCH1 and FBXW7 mutations have a favorable impact on early response to treatment, but not on outcome, in children with T-cell acute lymphoblastic leukemia (T-ALL) treated on EORTC trials 58881 and 58951. Leukemia 24, 2023–2031. doi: 10.1038/leu.2010.205
Clara, J. A., Monge, C., Yang, Y., and Takebe, N. (2020). Targeting signalling pathways and the immune microenvironment of cancer stem cells - a clinical update. Nat. Rev. Clin. Oncol. 17, 204–232. doi: 10.1038/s41571-019-0293-2
Clevers, H. (2006). Wnt/beta-catenin signaling in development and disease. Cell 127, 469–480. doi: 10.1016/j.cell.2006.10.018
Collu, G. M., Hidalgo-Sastre, A., and Brennan, K. (2014). Wnt-Notch signalling crosstalk in development and disease. Cell Mol. Life. Sci. 71, 3553–3567. doi: 10.1007/s00018-014-1644-x
Colombo, M., Garavelli, S., Mazzola, M., Platonova, N., Giannandrea, D., Colella, R., et al. (2020). Multiple myeloma exploits Jagged1 and Jagged2 to promote intrinsic and bone marrow-dependent drug resistance. Haematologica 105, 1925–1936. doi: 10.3324/haematol.2019.221077
Cook, N., Basu, B., Smith, D. M., Gopinathan, A., Evans, J., Steward, W. P., et al. (2018). A phase I trial of the gamma-secretase inhibitor MK-0752 in combination with gemcitabine in patients with pancreatic ductal adenocarcinoma. Br. J. Cancer 118, 793–801. doi: 10.1038/bjc.2017.495
Cui, Q., Wang, J. Q., Assaraf, Y. G., Ren, L., Gupta, P., Wei, L., et al. (2018). Modulating ROS to overcome multidrug resistance in cancer. Drug Resist. Updat. 41, 1–25. doi: 10.1016/j.drup.2018.11.001
Dai, G., Deng, S., Guo, W., Yu, L., Yang, J., Zhou, S., et al. (2019). Notch pathway inhibition using DAPT, a gamma-secretase inhibitor (GSI), enhances the antitumor effect of cisplatin in resistant osteosarcoma. Mol. Carcinog. 58, 3–18. doi: 10.1002/mc.22873
Davidson, G., Wu, W., Shen, J., Bilic, J., Fenger, U., Stannek, P., et al. (2005). Casein kinase 1 gamma couples Wnt receptor activation to cytoplasmic signal transduction. Nature 438, 867–872. doi: 10.1038/nature04170
Davies, H., Bignell, G. R., Cox, C., Stephens, P., Edkins, S., Clegg, S., et al. (2002). Mutations of the BRAF gene in human cancer. Nature 417, 949–954.
Della Corte, C. M., Bellevicine, C., Vicidomini, G., Vitagliano, D., Malapelle, U., Accardo, M., et al. (2015). SMO gene amplification and activation of the hedgehog pathway as novel mechanisms of resistance to anti-epidermal growth factor receptor drugs in human lung cancer. Clin. Cancer Res. 21, 4686–4697. doi: 10.1158/1078-0432.ccr-14-3319
Ding, J., Zhou, X. T., Zou, H. Y., and Wu, J. (2017). Hedgehog signaling pathway affects the sensitivity of hepatoma cells to drug therapy through the ABCC1 transporter. Lab. Invest. 97, 819–832. doi: 10.1038/labinvest.2017.34
D’Souza, B., Miyamoto, A., and Weinmaster, G. (2008). The many facets of Notch ligands. Oncogene 27, 5148–5167. doi: 10.1038/onc.2008.229
Du, F. Y., Zhou, Q. F., Sun, W. J., and Chen, G. L. (2019). Targeting cancer stem cells in drug discovery: current state and future perspectives. World J. Stem Cells 11, 398–420. doi: 10.4252/wjsc.v11.i7.398
Duque-Afonso, J., Lin, C. H., Han, K., Morgens, D. W., Jeng, E. E., Weng, Z., et al. (2018). CBP Modulates Sensitivity to dasatinib in pre-BCR+ acute lymphoblastic leukemia. Cancer Res. 78, 6497–6508. doi: 10.1158/0008-5472.can-18-1703
Eiraku, M., Hirata, Y., Takeshima, H., Hirano, T., and Kengaku, M. (2002). Delta/notch-like epidermal growth factor (EGF)-related receptor, a novel EGF-like repeat-containing protein targeted to dendrites of developing and adult central nervous system neurons. J. Biol. Chem. 277, 25400–25407. doi: 10.1074/jbc.m110793200
Ellisen, L. W., Bird, J., West, D. C., Soreng, A. L., Reynolds, T. C., Smith, S. D., et al. (1991). TAN-1, the human homolog of the Drosophila notch gene, is broken by chromosomal translocations in T lymphoblastic neoplasms. Cell 66, 649–661. doi: 10.1016/0092-8674(91)90111-b
Faiao-Flores, F., Alves-Fernandes, D. K., Pennacchi, P. C., Sandri, S., Vicente, A. L., Scapulatempo-Neto, C., et al. (2017). Targeting the hedgehog transcription factors GLI1 and GLI2 restores sensitivity to vemurafenib-resistant human melanoma cells. Oncogene 36, 1849–1861. doi: 10.1038/onc.2016.348
Flahaut, M., Meier, R., Coulon, A., Nardou, K. A., Niggli, F. K., Martinet, D., et al. (2009). The Wnt receptor FZD1 mediates chemoresistance in neuroblastoma through activation of the Wnt/beta-catenin pathway. Oncogene 28, 2245–2256. doi: 10.1038/onc.2009.80
Fodde, R., and Brabletz, T. (2007). Wnt/beta-catenin signaling in cancer stemness and malignant behavior. Curr. Opin. Cell Biol. 19, 150–158. doi: 10.1016/j.ceb.2007.02.007
Fong, C. Y., Gilan, O., Lam, E. Y., Rubin, A. F., Ftouni, S., Tyler, D., et al. (2015). BET inhibitor resistance emerges from leukaemia stem cells. Nature 525, 538–542. doi: 10.1038/nature14888
Franklin, R. A., Liao, W., Sarkar, A., Kim, M. V., Bivona, M. R., Liu, K., et al. (2014). The cellular and molecular origin of tumor-associated macrophages. Science 344, 921–925.
Frenquelli, M., and Tonon, G. (2020). WNT signaling in hematological malignancies. Front. Oncol. 10:615190.
Fukushima, N., Minami, Y., Kakiuchi, S., Kuwatsuka, Y., Hayakawa, F., Jamieson, C., et al. (2016). Small-molecule Hedgehog inhibitor attenuates the leukemia-initiation potential of acute myeloid leukemia cells. Cancer Sci. 107, 1422–1429. doi: 10.1111/cas.13019
Galceran, J., Sustmann, C., Hsu, S. C., Folberth, S., and Grosschedl, R. (2004). LEF1-mediated regulation of Delta-like1 links Wnt and notch signaling in somitogenesis. Genes Dev. 18, 2718–2723. doi: 10.1101/gad.1249504
Gan, G. N., Eagles, J., Keysar, S. B., Wang, G., Glogowska, M. J., Altunbas, C., et al. (2014). Hedgehog signaling drives radioresistance and stroma-driven tumor repopulation in head and neck squamous cancers. Cancer Res. 74, 7024–7036. doi: 10.1158/0008-5472.can-14-1346
Ghoshal, A., Goswami, U., Sahoo, A. K., Chattopadhyay, A., and Ghosh, S. S. (2015). Targeting Wnt canonical signaling by recombinant sFRP1 bound luminescent Au-nanocluster embedded nanoparticles in cancer theranostics. ACS Biomater. Sci. Eng. 1, 1256–1266. doi: 10.1021/acsbiomaterials.5b00305
Giroux Leprieur, E., Vieira, T., Antoine, M., Rozensztajn, N., Rabbe, N., Ruppert, A. M., et al. (2016). Sonic hedgehog pathway activation is associated with resistance to platinum-based chemotherapy in advanced non-small-cell lung carcinoma. Clin. Lung. Cancer 17, 301–308. doi: 10.1016/j.cllc.2015.12.007
Gordon, M. D., and Nusse, R. (2006). Wnt signaling: multiple pathways, multiple receptors, and multiple transcription factors. J. Biol. Chem. 281, 22429–22433. doi: 10.1074/jbc.r600015200
Gordon, W. R., Vardar-Ulu, D., Histen, G., Sanchez-Irizarry, C., Aster, J. C., and Blacklow, S. C. (2007). Structural basis for autoinhibition of Notch. Nat. Struct. Mol. Biol. 14, 295–300. doi: 10.1038/nsmb1227
Gragnani, L., Lorini, S., Marri, S., and Zignego, A. L. (2020). Role of notch receptors in hematologic malignancies. Cells 10:16. doi: 10.3390/cells10010016
Guo, Y., Zhang, K., Cheng, C., Ji, Z., Wang, X., Wang, M., et al. (2017). Numb(-/low) enriches a castration-resistant prostate cancer cell subpopulation associated with enhanced notch and hedgehog signaling. Clin. Cancer Res. 23, 6744–6756. doi: 10.1158/1078-0432.ccr-17-0913
Hayes, J. D., Dinkova-Kostova, A. T., and Tew, K. D. (2020). Oxidative stress in cancer. Cancer Cell 38, 167–197.
He, J., Sheng, T., Stelter, A. A., Li, C., Zhang, X., Sinha, M., et al. (2006). Suppressing Wnt signaling by the hedgehog pathway through sFRP-1. J. Biol. Chem. 281, 35598–35602. doi: 10.1074/jbc.c600200200
Hemati, H., Kaur, J., Sobti, R. C., and Trehanpati, N. (2020). Inhibition of NOTCH signaling pathway chemosensitizes HCC CD133(+) cells to vincristine and 5-fluorouracil through upregulation of BBC3. Biochem. Biophys. Res. Commun. 525, 941–947. doi: 10.1016/j.bbrc.2020.03.009
Holohan, C., Van Schaeybroeck, S., Longley, D. B., and Johnston, P. G. (2013). Cancer drug resistance: an evolving paradigm. Nat. Rev. Cancer 13, 714–726. doi: 10.1038/nrc3599
Holtzhausen, A., Zhao, F., Evans, K. S., Tsutsui, M., Orabona, C., Tyler, D. S., et al. (2015). Melanoma-derived Wnt5a promotes local dendritic-cell expression of IDO and immunotolerance: opportunities for pharmacologic enhancement of immunotherapy. Cancer Immunol. Res. 3, 1082–1095. doi: 10.1158/2326-6066.cir-14-0167
Honorato, J. R., Hauser-Davis, R. A., Saggioro, E. M., Correia, F. V., Sales-Junior, S. F., Soares, L. O. S., et al. (2020). Role of sonic hedgehog signaling in cell cycle, oxidative stress, and autophagy of temozolomide resistant glioblastoma. J. Cell. Physiol. 235, 3798–3814. doi: 10.1002/jcp.29274
Hori, K., Sen, A., and Artavanis-Tsakonas, S. (2013). Notch signaling at a glance. J. Cell Sci. 126(Pt 10), 2135–2140.
Hu, Y., Yu, K., Wang, G., Zhang, D., Shi, C., Ding, Y., et al. (2018). Lanatoside C inhibits cell proliferation and induces apoptosis through attenuating Wnt/beta-catenin/c-Myc signaling pathway in human gastric cancer cell. Biochem. Pharmacol. 150, 280–292. doi: 10.1016/j.bcp.2018.02.023
Hua, Y. Q., Zhang, K., Sheng, J., Ning, Z. Y., Li, Y., Shi, W. D., et al. (2021). Fam83D promotes tumorigenesis and gemcitabine resistance of pancreatic adenocarcinoma through the Wnt/β-catenin pathway. Life Sci. doi: 10.1016/j.lfs.2021.119205 Online ahead of print
Huang, C. C., Cheng, S. H., Wu, C. H., Li, W. Y., Wang, J. S., Kung, M. L., et al. (2019). Delta-like 1 homologue promotes tumorigenesis and epithelial-mesenchymal transition of ovarian high-grade serous carcinoma through activation of Notch signaling. Oncogene 38, 3201–3215. doi: 10.1038/s41388-018-0658-5
Huang, K., Sun, Z., Ding, B., Jiang, X., Wang, Z., Zhu, Y., et al. (2019). Suppressing hedgehog signaling reverses drug resistance of refractory acute myeloid leukemia. Onco. Targets Ther. 12, 7477–7488. doi: 10.2147/ott.s216628
Ilmer, M., Boiles, A. R., Regel, I., Yokoi, K., Michalski, C. W., Wistuba, I. I., et al. (2015). RSPO2 enhances canonical Wnt signaling to confer stemness-associated traits to susceptible pancreatic cancer cells. Cancer Res. 75, 1883–1896. doi: 10.1158/0008-5472.can-14-1327
Irvine, D. A., Zhang, B., Kinstrie, R., Tarafdar, A., Morrison, H., Campbell, V. L., et al. (2016). Deregulated hedgehog pathway signaling is inhibited by the smoothened antagonist LDE225 (Sonidegib) in chronic phase chronic myeloid leukaemia. Sci. Rep. 6:25476.
Jin, X. F., Spoettl, G., Maurer, J., Nolting, S., and Auernhammer, C. J. (2020). Inhibition of Wnt/beta-catenin signaling in neuroendocrine tumors in vitro: antitumoral effects. Cancers 12:345. doi: 10.3390/cancers12020345
Jing, Q., Li, G., Chen, X., Liu, C., Lu, S., Zheng, H., et al. (2019). Wnt3a promotes radioresistance via autophagy in squamous cell carcinoma of the head and neck. J. Cell Mol. Med. 23, 4711–4722. doi: 10.1111/jcmm.14394
Jing, Y., Han, Z., Zhang, S., Liu, Y., and Wei, L. (2011). Epithelial-Mesenchymal Transition in tumor microenvironment. Cell Biosci. 1:29.
Joshi, I., Minter, L. M., Telfer, J., Demarest, R. M., Capobianco, A. J., Aster, J. C., et al. (2009). Notch signaling mediates G1/S cell-cycle progression in T cells via cyclin D3 and its dependent kinases. Blood 113, 1689–1698. doi: 10.1182/blood-2008-03-147967
Jun, S., Jung, Y. S., Suh, H. N., Wang, W., Kim, M. J., Oh, Y. S., et al. (2016). LIG4 mediates Wnt signalling-induced radioresistance. Nat. Commun. 7:10994.
Kabiri, Z., Numata, A., Kawasaki, A., Edison, Tenen, D. G., and Virshup, D. M. (2015). Wnts are dispensable for differentiation and self-renewal of adult murine hematopoietic stem cells. Blood 126, 1086–1094. doi: 10.1182/blood-2014-09-598540
Kang, Y. A., Pietras, E. M., and Passegue, E. (2020). Deregulated notch and Wnt signaling activates early-stage myeloid regeneration pathways in leukemia. J. Exp. Med. 217:jem.20190787.
Kasiri, S., Chen, B., Wilson, A. N., Reczek, A., Mazambani, S., Gadhvi, J., et al. (2020). Stromal hedgehog pathway activation by IHH suppresses lung adenocarcinoma growth and metastasis by limiting reactive oxygen species. Oncogene 39, 3258–3275. doi: 10.1038/s41388-020-1224-5
Katoh, M., and Katoh, M. (2020). Precision medicine for human cancers with Notch signaling dysregulation (Review). Int. J. Mol. Med. 45, 279–297.
Kenney, A. M., Cole, M. D., and Rowitch, D. H. (2003). Nmyc upregulation by sonic hedgehog signaling promotes proliferation in developing cerebellar granule neuron precursors. Development 130, 15–28. doi: 10.1242/dev.00182
Keysar, S. B., Le, P. N., Anderson, R. T., Morton, J. J., Bowles, D. W., Paylor, J. J., et al. (2013). Hedgehog signaling alters reliance on EGF receptor signaling and mediates anti-EGFR therapeutic resistance in head and neck cancer. Cancer Res. 73, 3381–3392. doi: 10.1158/0008-5472.can-12-4047
Khan, M. A., Srivastava, S. K., Zubair, H., Patel, G. K., Arora, S., Khushman, M., et al. (2020). Co-targeting of CXCR4 and hedgehog pathways disrupts tumor-stromal crosstalk and improves chemotherapeutic efficacy in pancreatic cancer. J. Biol. Chem. 295, 8413–8424. doi: 10.1074/jbc.ra119.011748
Khanam, T., Sandmann, S., Seggewiss, J., Ruether, C. M., Zimmermann, M., Norvil, A. B., et al. (2020). Integrative genomic analysis of pediatric T- cell lymphoblastic lymphoma reveals candidates of clinical significance. Blood doi: 10.1182/blood.2020005381 Online ahead of print.
Kikuchi, A., Yamamoto, H., Sato, A., and Matsumoto, S. (2011). New insights into the mechanism of Wnt signaling pathway activation. Int. Rev. Cell Mol. Biol. 291, 21–71. doi: 10.1016/b978-0-12-386035-4.00002-1
Kim, H. A., Koo, B. K., Cho, J. H., Kim, Y. Y., Seong, J., Chang, H. J., et al. (2012). Notch1 counteracts WNT/beta-catenin signaling through chromatin modification in colorectal cancer. J. Clin. Invest. 122, 3248–3259. doi: 10.1172/jci61216
Kohlhaas, V., Blakemore, S. J., Al-Maarri, M., Nickel, N., Pal, M., Roth, A., et al. (2021). Active Akt signaling triggers CLL toward richter transformation via overactivation of Notch1. Blood 137, 646–660. doi: 10.1182/blood.2020005734
Kong, Y., Peng, Y., Liu, Y., Xin, H., Zhan, X., and Tan, W. (2015). Twist1 and Snail link Hedgehog signaling to tumor-initiating cell-like properties and acquired chemoresistance independently of ABC transporters. Stem Cells 33, 1063–1074. doi: 10.1002/stem.1955
Kongkavitoon, P., Butta, P., Sanpavat, A., Bhattarakosol, P., Tangtanatakul, P., Wongprom, B., et al. (2018). Regulation of periostin expression by Notch signaling in hepatocytes and liver cancer cell lines. Biochem. Biophys. Res. Commun. 506, 739–745. doi: 10.1016/j.bbrc.2018.10.144
Kopan, R., and Ilagan, M. X. (2009). The canonical Notch signaling pathway: unfolding the activation mechanism. Cell 137, 216–233. doi: 10.1016/j.cell.2009.03.045
Krishnamurthy, N., and Kurzrock, R. (2018). Targeting the Wnt/beta-catenin pathway in cancer: update on effectors and inhibitors. Cancer Treat. Rev. 62, 50–60. doi: 10.1016/j.ctrv.2017.11.002
Kuijk, L. M., Verstege, M. I., Rekers, N. V., Bruijns, S. C., Hooijberg, E., Roep, B. O., et al. (2013). Notch controls generation and function of human effector CD8+ T cells. Blood 121, 2638–2646. doi: 10.1182/blood-2012-07-442962
Kumar, S., Nandi, A., Singh, S., Regulapati, R., Li, N., Tobias, J. W., et al. (2021). Dll1 + quiescent tumor stem cells drive chemoresistance in breast cancer through NF-κB survival pathway. Nat. Commun. 12:432.
Kwon, C., Cheng, P., King, I. N., Andersen, P., Shenje, L., Nigam, V., et al. (2011). Notch post-translationally regulates beta-catenin protein in stem and progenitor cells. Nat. Cell Biol. 13, 1244–1251. doi: 10.1038/ncb2313
Lee, S. M., Moon, J., Redman, B. G., Chidiac, T., Flaherty, L. E., Zha, Y., et al. (2015). Phase 2 study of RO4929097, a gamma-secretase inhibitor, in metastatic melanoma: SWOG 0933. Cancer 121, 432–440. doi: 10.1002/cncr.29055
Levanon, D., Goldstein, R. E., Bernstein, Y., Tang, H., Goldenberg, D., Stifani, S., et al. (1998). Transcriptional repression by AML1 and LEF-1 is mediated by the TLE/Groucho corepressors. Proc. Natl. Acad. Sci. U.S.A. 95, 11590–11595. doi: 10.1073/pnas.95.20.11590
Li, B., Cao, Y., Meng, G., Qian, L., Xu, T., Yan, C., et al. (2019). Targeting glutaminase 1 attenuates stemness properties in hepatocellular carcinoma by increasing reactive oxygen species and suppressing Wnt/beta-catenin pathway. EBioMedicine 39, 239–254. doi: 10.1016/j.ebiom.2018.11.063
Li, B., Jia, Z., Wang, T., Wang, W., Zhang, C., Chen, P., et al. (2012). Interaction of Wnt/beta-catenin and notch signaling in the early stage of cardiac differentiation of P19CL6 cells. J. Cell. Biochem. 113, 629–639. doi: 10.1002/jcb.23390
Li, D., Li, T., Shang, Z., Zhao, L., Xu, Q., Tan, J., et al. (2020). Combined inhibition of Notch and FLT3 produces synergistic cytotoxic effects in FLT3/ITD(+) acute myeloid leukemia. Signal Transduct Target Ther. 5:21.
Li, D. D., Zhao, C. H., Ding, H. W., Wu, Q., Ren, T. S., Wang, J., et al. (2018). A novel inhibitor of ADAM17 sensitizes colorectal cancer cells to 5-Fluorouracil by reversing Notch and epithelial-mesenchymal transition in vitro and in vivo. Cell Prolif. 51:e12480. doi: 10.1111/cpr.12480
Li, H., Jiang, W., Liu, X. N., Yuan, L. Y., Li, T. J., Li, S., et al. (2020). TET1 downregulates epithelial-mesenchymal transition and chemoresistance in PDAC by demethylating CHL1 to inhibit the Hedgehog signaling pathway. Oncogene 39, 5825–5838. doi: 10.1038/s41388-020-01407-8
Li, H., Zhang, W., Niu, C., Lin, C., Wu, X., Jian, Y., et al. (2019). Nuclear orphan receptor NR2F6 confers cisplatin resistance in epithelial ovarian cancer cells by activating the Notch3 signaling pathway. Int. J. Cancer 145, 1921–1934.
Li, L., Zhao, F., Lu, J., Li, T., Yang, H., Wu, C., et al. (2014). Notch-1 signaling promotes the malignant features of human breast cancer through NF-kappaB activation. PLoS One 9:e95912. doi: 10.1371/journal.pone.0095912
Li, Q., Lai, Q., He, C., Fang, Y., Yan, Q., Zhang, Y., et al. (2019). RUNX1 promotes tumour metastasis by activating the Wnt/beta-catenin signalling pathway and EMT in colorectal cancer. J. Exp. Clin. Cancer Res. 38:334.
Li, W., Cao, L., Chen, X., Lei, J., and Ma, Q. (2016). Resveratrol inhibits hypoxia-driven ROS-induced invasive and migratory ability of pancreatic cancer cells via suppression of the Hedgehog signaling pathway. Oncol. Rep. 35, 1718–1726. doi: 10.3892/or.2015.4504
Li, X., Deng, W., Lobo-Ruppert, S. M., and Ruppert, J. M. (2007). Gli1 acts through Snail and E-cadherin to promote nuclear signaling by beta-catenin. Oncogene 26, 4489–4498. doi: 10.1038/sj.onc.1210241
Li, Y., Hibbs, M. A., Gard, A. L., Shylo, N. A., and Yun, K. (2012). Genome-wide analysis of N1ICD/RBPJ targets in vivo reveals direct transcriptional regulation of Wnt, SHH, and hippo pathway effectors by Notch. Stem Cells 30, 741–752. doi: 10.1002/stem.1030
Lim, J. S., Ibaseta, A., Fischer, M. M., Cancilla, B., O’Young, G., Cristea, S., et al. (2017). Intratumoural heterogeneity generated by Notch signalling promotes small-cell lung cancer. Nature 545, 360–364. doi: 10.1038/nature22323
Liu, Q., Li, W., Zhou, Y., Jian, J., Han, S., Liu, C., et al. (2019). PRKD2 Promotes Progression and Chemoresistance of AML via Regulating Notch1 Pathway. Onco. Targets Ther. 12, 10931–10941. doi: 10.2147/ott.s233234
Liu, Q., Zhu, H., Tiruthani, K., Shen, L., Chen, F., Gao, K., et al. (2018). Nanoparticle-mediated trapping of Wnt family member 5A in tumor microenvironments enhances immunotherapy for B-Raf proto-oncogene mutant melanoma. ACS Nano 12, 1250–1261. doi: 10.1021/acsnano.7b07384
Liu, Y., Huber, R. M., Kiefl, R., Tufman, A., and Kauffmann-Guerrero, D. (2020). Hedgehog pathway activation might mediate pemetrexed resistance in NSCLC cells. Anticancer. Res. 40, 1451–1458. doi: 10.21873/anticanres.14087
Liu, Y. S., Hsu, H. C., Tseng, K. C., Chen, H. C., and Chen, S. J. (2013). Lgr5 promotes cancer stemness and confers chemoresistance through ABCB1 in colorectal cancer. Biomed. Pharmacother. 67, 791–799. doi: 10.1016/j.biopha.2013.08.001
Long, G. V., Eroglu, Z., Infante, J., Patel, S., Daud, A., Johnson, D. B., et al. (2018). Long-term outcomes in patients with BRAF V600-mutant metastatic melanoma who received dabrafenib combined with trametinib. J. Clin. Oncol. 36, 667–673. doi: 10.1200/jco.2017.74.1025
Long, J., Yang, C., Zheng, Y., Loughran, P., Guang, F., Li, Y., et al. (2020). Notch signaling protects CD4 T cells from STING-mediated apoptosis during acute systemic inflammation. Sci. Adv. 6:eabc5447. doi: 10.1126/sciadv.abc5447
Lu, M., Qin, X., Zhou, Y., Li, G., Liu, Z., Geng, Z., et al. (2021). Long non-coding RNA LINC00665 promotes gemcitabine resistance of Cholangiocarcinoma cells via regulating EMT and stemness properties through miR-424-5p/BCL9L axis. Cell Death Dis. 12:72.
Lu, P., Gu, Y., Li, L., Wang, F., Yang, X., and Yang, Y. (2019). Belinostat suppresses cell proliferation by inactivating Wnt/β-catenin pathway and promotes apoptosis through regulating PKC pathway in breast cancer. Artif Cells Nan. Biotechnol. 47, 3955–3960. doi: 10.1080/21691401.2019.1671855
Lu, X., Wang, Z., Huang, H., and Wang, H. (2020). Hedgehog signaling promotes multidrug resistance by regulation of ABC transporters in oral squamous cell carcinoma. J. Oral Pathol. Med. 49, 897–906. doi: 10.1111/jop.13050
Luke, J. J., Bao, R., Sweis, R. F., Spranger, S., and Gajewski, T. F. (2019). WNT/beta-catenin pathway activation correlates with immune exclusion across human cancers. Clin. Cancer Res. 25, 3074–3083. doi: 10.1158/1078-0432.ccr-18-1942
Lundell, M. J., Lee, H. K., Perez, E., and Chadwell, L. (2003). The regulation of apoptosis by Numb/Notch signaling in the serotonin lineage of Drosophila. Development 130, 4109–4121. doi: 10.1242/dev.00593
Ma, L., Fan, Z., Du, G., and Wang, H. (2019). Leptin-elicited miRNA-342-3p potentiates gemcitabine resistance in pancreatic ductal adenocarcinoma. Biochem. Biophys. Res. Commun. 509, 845–853. doi: 10.1016/j.bbrc.2019.01.030
Ma, Y., Ren, Y., Han, E. Q., Li, H., Chen, D., Jacobs, J. J., et al. (2013). Inhibition of the Wnt-β-catenin and Notch signaling pathways sensitizes osteosarcoma cells to chemotherapy. Biochem. Biophys. Res. Commun. 431, 274–279. doi: 10.1016/j.bbrc.2012.12.118
MacDonald, B. T., Tamai, K., and He, X. (2009). Wnt/beta-catenin signaling: components, mechanisms, and diseases. Dev. Cell 17, 9–26. doi: 10.1016/j.devcel.2009.06.016
Maffei, R., Fiorcari, S., Martinelli, S., Benatti, S., Bulgarelli, J., Rizzotto, L., et al. (2018). Increased SHISA3 expression characterizes chronic lymphocytic leukemia patients sensitive to lenalidomide. Leuk Lymphoma 59, 423–433. doi: 10.1080/10428194.2017.1339872
Mamaeva, V., Niemi, R., Beck, M., Ozliseli, E., Desai, D., Landor, S., et al. (2016). Inhibiting notch activity in breast cancer stem cells by glucose functionalized nanoparticles carrying gamma-secretase inhibitors. Mol. Ther. 24, 926–936. doi: 10.1038/mt.2016.42
Mamaeva, V., Rosenholm, J. M., Bate-Eya, L. T., Bergman, L., Peuhu, E., Duchanoy, A., et al. (2011). Mesoporous silica nanoparticles as drug delivery systems for targeted inhibition of Notch signaling in cancer. Mol. Ther. 19, 1538–1546. doi: 10.1038/mt.2011.105
Martinez-Font, E., Pérez-Capó, M., Ramos, R., Felipe, I., Garcías, C., Luna, P., et al. (2020). Impact of Wnt/β-Catenin inhibition on cell proliferation through CDC25A downregulation in soft tissue sarcomas. Cancers 12:2556. doi: 10.3390/cancers12092556
Martin-Orozco, E., Sanchez-Fernandez, A., Ortiz-Parra, I., and Ayala-San Nicolas, M. (2019). WNT signaling in tumors: the way to evade drugs and immunity. Front. Immunol. 10:2854.
Mazzotta, M., Filetti, M., Occhipinti, M., Marinelli, D., Scalera, S., Terrenato, I., et al. (2020). Efficacy of immunotherapy in lung cancer with co-occurring mutations in NOTCH and homologous repair genes. J. Immunother. Cancer 8:e000946. doi: 10.1136/jitc-2020-000946
McAuliffe, S. M., Morgan, S. L., Wyant, G. A., Tran, L. T., Muto, K. W., Chen, Y. S., et al. (2012). Targeting notch, a key pathway for ovarian cancer stem cells, sensitizes tumors to platinum therapy. Proc. Natl. Acad. Sci. U.S.A. 109, E2939–E2948.
Melamed, J. R., Morgan, J. T., Ioele, S. A., Gleghorn, J. P., Sims-Mourtada, J., and Day, E. S. (2018). Investigating the role of Hedgehog/GLI1 signaling in glioblastoma cell response to temozolomide. Oncotarget 9, 27000–27015. doi: 10.18632/oncotarget.25467
Meng, E., Hanna, A., Samant, R. S., and Shevde, L. A. (2015). The impact of hedgehog signaling pathway on DNA repair mechanisms in human cancer. Cancers 7, 1333–1348. doi: 10.3390/cancers7030839
Mohapatra, P., Shriwas, O., Mohanty, S., Ghosh, A., Smita, S., and Kaushik, S. R. (2021). CMTM6 drives cisplatin resistance by regulating Wnt signaling through the ENO-1/AKT/GSK3β axis. JCI Insight 6:e143643.
Munoz, J. L., Rodriguez-Cruz, V., and Rameshwar, P. (2015). High expression of miR-9 in CD133(+) glioblastoma cells in chemoresistance to temozolomide. J. Cancer Stem Cell Res. 3:e1003.
Nelson, W. J., and Nusse, R. (2004). Convergence of Wnt, beta-catenin, and cadherin pathways. Science 303, 1483–1487. doi: 10.1126/science.1094291
Neumann, C. J. (2005). Hedgehogs as negative regulators of the cell cycle. Cell Cycle 4, 1139–1140. doi: 10.4161/cc.4.9.1999
Niehrs, C. (2012). The complex world of WNT receptor signalling. Nat. Rev. Mol. Cell Biol. 13, 767–779. doi: 10.1038/nrm3470
Nieszporek, A., Skrzypek, K., Adamek, G., and Majka, M. (2019). Molecular mechanisms of epithelial to mesenchymal transition in tumor metastasis. Acta Biochim. Pol. 66, 509–520.
Noubissi, F. K., Goswami, S., Sanek, N. A., Kawakami, K., Minamoto, T., Moser, A., et al. (2009). Wnt signaling stimulates transcriptional outcome of the Hedgehog pathway by stabilizing GLI1 mRNA. Cancer Res. 69, 8572–8578. doi: 10.1158/0008-5472.can-09-1500
Nowell, C. S., and Radtke, F. (2017). Notch as a tumour suppressor. Nat. Rev. Cancer 17, 145–159. doi: 10.1038/nrc.2016.145
Olmeda, D., Castel, S., Vilaro, S., and Cano, A. (2003). Beta-catenin regulation during the cell cycle: implications in G2/M and apoptosis. Mol. Biol. Cell 14, 2844–2860. doi: 10.1091/mbc.e03-01-0865
Oren, O., and Smith, B. D. (2017). Eliminating cancer stem cells by targeting embryonic signaling pathways. Stem Cell Rev. Rep. 13, 17–23. doi: 10.1007/s12015-016-9691-3
Oyama, Y., Onishi, H., Koga, S., Murahashi, M., Ichimiya, S., Nakayama, K., et al. (2020). Patched 1-interacting Peptide Represses Fibrosis in Pancreatic Cancer to Augment the Effectiveness of Immunotherapy. J. Immunother. 43, 121–133. doi: 10.1097/cji.0000000000000305
Patel, S., Alam, A., Pant, R., and Chattopadhyay, S. (2019). Wnt signaling and its significance within the tumor microenvironment: novel therapeutic insights. Front. Immunol. 10:2872.
Perry, J. M., Tao, F., Roy, A., Lin, T., He, X. C., Chen, S., et al. (2020). Overcoming Wnt-β-catenin dependent anticancer therapy resistance in leukaemia stem cells. Nat. Cell Biol. 22, 689–700. doi: 10.1038/s41556-020-0507-y
Petty, A. J., Li, A., Wang, X., Dai, R., Heyman, B., Hsu, D., et al. (2019). Hedgehog signaling promotes tumor-associated macrophage polarization to suppress intratumoral CD8+ T cell recruitment. J. Clin. Invest. 129, 5151–5162. doi: 10.1172/jci128644
Piovan, E., Yu, J., Tosello, V., Herranz, D., Ambesi-Impiombato, A., Da Silva, A. C., et al. (2013). Direct reversal of glucocorticoid resistance by AKT inhibition in acute lymphoblastic leukemia. Cancer Cell 24, 766–776. doi: 10.1016/j.ccr.2013.10.022
Po, A., Citarella, A., Catanzaro, G., Besharat, Z. M., Trocchianesi, S., Gianno, F., et al. (2020). Hedgehog-GLI signalling promotes chemoresistance through the regulation of ABC transporters in colorectal cancer cells. Sci. Rep. 10: 13988.
Pothuraju, R., Rachagani, S., Krishn, S. R., Chaudhary, S., Nimmakayala, R. K., Siddiqui, J. A., et al. (2020). Molecular implications of MUC5AC-CD44 axis in colorectal cancer progression and chemoresistance. Mol. Cancer 19:37.
Qiang, L., Wu, T., Zhang, H. W., Lu, N., Hu, R., Wang, Y. J., et al. (2012). HIF-1alpha is critical for hypoxia-mediated maintenance of glioblastoma stem cells by activating Notch signaling pathway. Cell Death. Differ 19, 284–294. doi: 10.1038/cdd.2011.95
Qiu, S., Deng, L., Bao, Y., Jin, K., Tu, X., Li, J., et al. (2018). Reversal of docetaxel resistance in prostate cancer by Notch signaling inhibition. Anticancer. Drugs 29, 871–879. doi: 10.1097/cad.0000000000000659
Quaranta, R., Pelullo, M., Zema, S., Nardozza, F., Checquolo, S., Lauer, D. M., et al. (2017). Maml1 acts cooperatively with Gli proteins to regulate sonic hedgehog signaling pathway. Cell Death Dis. 8:e2942. doi: 10.1038/cddis.2017.326
Rahman, M. T., Nakayama, K., Rahman, M., Katagiri, H., Katagiri, A., Ishibashi, T., et al. (2012). Notch3 overexpression as potential therapeutic target in advanced stage chemoresistant ovarian cancer. Am. J. Clin. Pathol. 138, 535–544. doi: 10.1309/ajcpkdlrq8f3ewns
Ranganathan, P., Weaver, K. L., and Capobianco, A. J. (2011). Notch signalling in solid tumours: a little bit of everything but not all the time. Nat. Rev. Cancer 11, 338–351. doi: 10.1038/nrc3035
Rathert, P., Roth, M., Neumann, T., Muerdter, F., Roe, J. S., Muhar, M., et al. (2015). Transcriptional plasticity promotes primary and acquired resistance to BET inhibition. Nature 525, 543–547. doi: 10.1038/nature14898
Rosati, E., Sabatini, R., Rampino, G., Tabilio, A., Di Ianni, M., Fettucciari, K., et al. (2009). Constitutively activated Notch signaling is involved in survival and apoptosis resistance of B-CLL cells. Blood 113, 856–865. doi: 10.1182/blood-2008-02-139725
Ruggiero, C. F., Malpicci, D., Fattore, L., Madonna, G., Vanella, V., Mallardo, D., et al. (2019). ErbB3 phosphorylation as central event in adaptive resistance to targeted therapy in metastatic melanoma: early detection in CTCs during therapy and insights into regulation by autocrine neuregulin. Cancers 11:1425. doi: 10.3390/cancers11101425
Sabbatino, F., Wang, Y., Wang, X., Flaherty, K. T., Yu, L., Pepin, D., et al. (2014). PDGFRalpha up-regulation mediated by sonic hedgehog pathway activation leads to BRAF inhibitor resistance in melanoma cells with BRAF mutation. Oncotarget 5, 1926–1941. doi: 10.18632/oncotarget.1878
Scarborough, H. A., Helfrich, B. A., Casas-Selves, M., Schuller, A. G., Grosskurth, S. E., Kim, J., et al. (2017). AZ1366: an inhibitor of tankyrase and the canonical wnt pathway that limits the persistence of non-small cell lung cancer cells following EGFR inhibition. Clin. Cancer Res. 23, 1531–1541. doi: 10.1158/1078-0432.ccr-16-1179
Schreck, K. C., Taylor, P., Marchionni, L., Gopalakrishnan, V., Bar, E. E., Gaiano, N., et al. (2010). The Notch target Hes1 directly modulates Gli1 expression and Hedgehog signaling: a potential mechanism of therapeutic resistance. Clin. Cancer Res. 16, 6060–6070. doi: 10.1158/1078-0432.ccr-10-1624
Shah, D., Wyatt, D., Baker, A. T., Simms, P., Peiffer, D. S., Fernandez, M., et al. (2018). Inhibition of HER2 Increases JAGGED1-dependent breast cancer stem cells: role for membrane JAGGED1. Clin. Cancer Res. 24, 4566–4578. doi: 10.1158/1078-0432.ccr-17-1952
Shahi, M. H., Farheen, S., Mariyath, M. P., and Castresana, J. S. (2016). Potential role of Shh-Gli1-BMI1 signaling pathway nexus in glioma chemoresistance. Tumour Biol. 37, 15107–15114. doi: 10.1007/s13277-016-5365-7
Shibue, T., and Weinberg, R. A. (2017). EMT, CSCs, and drug resistance: the mechanistic link and clinical implications. Nat. Rev. Clin. Oncol. 14, 611–629. doi: 10.1038/nrclinonc.2017.44
Siebel, C., and Lendahl, U. (2017). Notch signaling in development, tissue homeostasis, and disease. Physiol. Rev. 97, 1235–1294. doi: 10.1152/physrev.00005.2017
Spaan, I., Raymakers, R. A., van de Stolpe, A., and Peperzak, V. (2018). Wnt signaling in multiple myeloma: a central player in disease with therapeutic potential. J. Hematol. Oncol. 11:67.
Spranger, S., Bao, R., and Gajewski, T. F. (2015). Melanoma-intrinsic beta-catenin signalling prevents anti-tumour immunity. Nature 523, 231–235. doi: 10.1038/nature14404
Staal, F. J., Luis, T. C., and Tiemessen, M. M. (2008). WNT signalling in the immune system: WNT is spreading its wings. Nat. Rev. Immunol. 8, 581–593. doi: 10.1038/nri2360
Stasiulewicz, M., Gray, S. D., Mastromina, I., Silva, J. C., Bjorklund, M., Seymour, P. A., et al. (2015). A conserved role for Notch signaling in priming the cellular response to Shh through ciliary localisation of the key Shh transducer Smo. Development 142, 2291–2303. doi: 10.1242/dev.125237
Steg, A. D., Burke, M. R., Amm, H. M., Katre, A. A., Dobbin, Z. C., Jeong, D. H., et al. (2014). Proteasome inhibition reverses hedgehog inhibitor and taxane resistance in ovarian cancer. Oncotarget 5, 7065–7080. doi: 10.18632/oncotarget.2295
Steg, A. D., Katre, A. A., Goodman, B., Han, H. D., Nick, A. M., Stone, R. L., et al. (2011). Targeting the notch ligand JAGGED1 in both tumor cells and stroma in ovarian cancer. Clin. Cancer Res. 17, 5674–5685. doi: 10.1158/1078-0432.ccr-11-0432
Strosberg, J. R., Yeatman, T., Weber, J., Coppola, D., Schell, M. J., Han, G., et al. (2012). A phase II study of RO4929097 in metastatic colorectal cancer. Eur. J. Cancer 48, 997–1003. doi: 10.1016/j.ejca.2012.02.056
Suknuntha, K., Thita, T., Togarrati, P. P., Ratanachamnong, P., Wongtrakoongate, P., Srihirun, S., et al. (2017). Wnt signaling inhibitor FH535 selectively inhibits cell proliferation and potentiates imatinib-induced apoptosis in myeloid leukemia cell lines. Int. J. Hematol. 105, 196–205. doi: 10.1007/s12185-016-2116-x
Sun, J., Ma, Q., Li, B., Wang, C., Mo, L., and Zhang, X. (2020). RPN2 is targeted by miR-181c and mediates glioma progression and temozolomide sensitivity via the wnt/β-catenin signaling pathway. Cell Death Dis. 11:890.
Taelman, V. F., Dobrowolski, R., Plouhinec, J. L., Fuentealba, L. C., Vorwald, P. P., Gumper, I., et al. (2010). Wnt signaling requires sequestration of glycogen synthase kinase 3 inside multivesicular endosomes. Cell 143, 1136–1148. doi: 10.1016/j.cell.2010.11.034
Takebe, N., Miele, L., Harris, P. J., Jeong, W., Bando, H., Kahn, M., et al. (2015). Targeting Notch, Hedgehog, and Wnt pathways in cancer stem cells: clinical update. Nat. Rev. Clin. Oncol. 12, 445–464. doi: 10.1038/nrclinonc.2015.61
Takebe, N., Nguyen, D., and Yang, S. X. (2014). Targeting notch signaling pathway in cancer: clinical development advances and challenges. Pharmacol. Ther. 141, 140–149. doi: 10.1016/j.pharmthera.2013.09.005
Tang, Y. A., Chen, Y. F., Bao, Y., Mahara, S., Yatim, S. M. J. M., Oguz, G., et al. (2018). Hypoxic tumor microenvironment activates GLI2 via HIF-1α and TGF-β2 to promote chemoresistance in colorectal cancer. Proc. Natl. Acad. Sci. U.S.A. 115, E5990–E5999.
Taylor, M. D., Zhang, X., Liu, L., Hui, C. C., Mainprize, T. G., Scherer, S. W., et al. (2004). Failure of a medulloblastoma-derived mutant of SUFU to suppress WNT signaling. Oncogene 23, 4577–4583. doi: 10.1038/sj.onc.1207605
Teichman, J., Dodbiba, L., Thai, H., Fleet, A., Morey, T., Liu, L., et al. (2018). Hedgehog inhibition mediates radiation sensitivity in mouse xenograft models of human esophageal adenocarcinoma. PLoS One 13:e0194809. doi: 10.1371/journal.pone.0194809
Thomas, W. D., Chen, J., Gao, Y. R., Cheung, B., Koach, J., Sekyere, E., et al. (2009). Patched1 deletion increases N-Myc protein stability as a mechanism of medulloblastoma initiation and progression. Oncogene 28, 1605–1615. doi: 10.1038/onc.2009.3
Tian, D., Shi, Y., Chen, D., Liu, Q., and Fan, F. (2017). The Wnt inhibitor LGK-974 enhances radiosensitivity of HepG2 cells by modulating Nrf2 signaling. Int. J. Oncol. 51, 545–554. doi: 10.3892/ijo.2017.4042
Tibes, R., Al-Kali, A., Oliver, G. R., Delman, D. H., Hansen, N., Bhagavatula, K., et al. (2015). The Hedgehog pathway as targetable vulnerability with 5-azacytidine in myelodysplastic syndrome and acute myeloid leukemia. J. Hematol. Oncol. 8:114.
Tome, M., Tchorz, J., Gassmann, M., and Bettler, B. (2019). Constitutive activation of Notch2 signalling confers chemoresistance to neural stem cells via transactivation of fibroblast growth factor receptor-1. Stem Cell Res. 35:101390. doi: 10.1016/j.scr.2019.101390
Trujillo, J. A., Sweis, R. F., Bao, R., and Luke, J. J. (2018). T cell-inflamed versus Non-T Cell-inflamed tumors: a conceptual framework for cancer immunotherapy drug development and combination therapy selection. Cancer Immunol. Res. 6, 990–1000. doi: 10.1158/2326-6066.cir-18-0277
Ulloa, F., Itasaki, N., and Briscoe, J. (2007). Inhibitory Gli3 activity negatively regulates Wnt/beta-catenin signaling. Curr. Biol. 17, 545–550. doi: 10.1016/j.cub.2007.01.062
Ungerback, J., Elander, N., Grunberg, J., Sigvardsson, M., and Soderkvist, P. (2011). The Notch-2 gene is regulated by Wnt signaling in cultured colorectal cancer cells. PLoS One 6:e17957. doi: 10.1371/journal.pone.0017957
Usui, T., Sakurai, M., Umata, K., Elbadawy, M., Ohama, T., and Yamawaki, H. (2018). Hedgehog signals mediate anti-cancer drug resistance in three-dimensional primary colorectal cancer organoid culture. Int. J. Mol. Sci. 19:1098. doi: 10.3390/ijms19041098
Vacca, A., Felli, M. P., Palermo, R., Di Mario, G., Calce, A., Di Giovine, M., et al. (2006). Notch3 and pre-TCR interaction unveils distinct NF-kappaB pathways in T-cell development and leukemia. EMBO J. 25, 1000–1008. doi: 10.1038/sj.emboj.7600996
Vasan, N., Baselga, J., and Hyman, D. M. (2019). A view on drug resistance in cancer. Nature 575, 299–309.
Veeraraghavalu, K., Subbaiah, V. K., Srivastava, S., Chakrabarti, O., Syal, R., and Krishna, S. (2005). Complementation of human papillomavirus type 16 E6 and E7 by Jagged1-specific Notch1-phosphatidylinositol 3-kinase signaling involves pleiotropic oncogenic functions independent of CBF1;Su(H);Lag-1 activation. J. Virol. 79, 7889–7898. doi: 10.1128/jvi.79.12.7889-7898.2005
Venkatesh, J., Rishi, A. K., and Reddy, K. B. (2020). Novel strategies to target chemoresistant triple-negative breast cancer. Genes Cancer 11, 95–105.
Vermezovic, J., Adamowicz, M., Santarpia, L., Rustighi, A., Forcato, M., Lucano, C., et al. (2015). Notch is a direct negative regulator of the DNA-damage response. Nat. Struct. Mol. Biol. 22, 417–424. doi: 10.1038/nsmb.3013
Wakabayashi, N., Skoko, J. J., Chartoumpekis, D. V., Kimura, S., Slocum, S. L., Noda, K., et al. (2014). Notch-Nrf2 axis: regulation of Nrf2 gene expression and cytoprotection by notch signaling. Mol. Cell. Biol. 34, 653–663. doi: 10.1128/mcb.01408-13
Wall, D. S., Mears, A. J., McNeill, B., Mazerolle, C., Thurig, S., Wang, Y., et al. (2009). Progenitor cell proliferation in the retina is dependent on Notch-independent Sonic hedgehog/Hes1 activity. J. Cell Biol. 184, 101–112. doi: 10.1083/jcb.200805155
Wan, H., Li, Z., Wang, H., Cai, F., and Wang, L. (2020). ST8SIA1 inhibition sensitizes triple negative breast cancer to chemotherapy via suppressing Wnt/beta-catenin and FAK/Akt/mTOR. Clin. Transl. Oncol. 23, 902–910. doi: 10.1007/s12094-020-02484-7
Wang, G., Wang, J., and Sadar, M. D. (2008). Crosstalk between the androgen receptor and beta-catenin in castrate-resistant prostate cancer. Cancer Res. 68, 9918–9927. doi: 10.1158/0008-5472.can-08-1718
Wang, J., Wakeman, T. P., Lathia, J. D., Hjelmeland, A. B., Wang, X. F., White, R. R., et al. (2010). Notch promotes radioresistance of glioma stem cells. Stem Cells 28, 17–28.
Wang, K., Chen, D., Qian, Z., Cui, D., Gao, L., and Lou, M. (2017). Hedgehog/Gli1 signaling pathway regulates MGMT expression and chemoresistance to temozolomide in human glioblastoma. Cancer Cell Int. 17:117.
Wang, L., Zi, H., Luo, Y., Liu, T., Zheng, H., Xie, C., et al. (2020). Inhibition of Notch pathway enhances the anti-tumor effect of docetaxel in prostate cancer stem-like cells. Stem Cell Res. Ther. 11:258.
Wang, Q., Liao, J., He, Z., Su, Y., Lin, D., Xu, L., et al. (2020). LHX6 affects erlotinib resistance and migration of EGFR-mutant non-small-cell lung cancer HCC827 cells through suppressing Wnt/β-Catenin signaling. Onco Targets Ther. 13, 10983–10994. doi: 10.2147/ott.s258896
Wang, Z., Li, Y., Kong, D., Banerjee, S., Ahmad, A., Azmi, A. S., et al. (2009). Acquisition of epithelial-mesenchymal transition phenotype of gemcitabine-resistant pancreatic cancer cells is linked with activation of the notch signaling pathway. Cancer Res. 69, 2400–2407. doi: 10.1158/0008-5472.can-08-4312
Wong, G. T., Gavin, B. J., and McMahon, A. P. (1994). Differential transformation of mammary epithelial cells by Wnt genes. Mol. Cell. Biol. 14, 6278–6286. doi: 10.1128/mcb.14.9.6278
Xiao, Y. S., Zeng, D., Liang, Y. K., Wu, Y., Li, M. F., Qi, Y. Z., et al. (2019). Major vault protein is a direct target of Notch1 signaling and contributes to chemoresistance in triple-negative breast cancer cells. Cancer Lett. 440-441, 156–167. doi: 10.1016/j.canlet.2018.09.031
Xie, H., Paradise, B. D., Ma, W. W., and Fernandez-Zapico, M. E. (2019). Recent advances in the clinical targeting of hedgehog/GLI signaling in cancer. Cells 8:394. doi: 10.3390/cells8050394
Xie, Y., Liu, J., Jiang, H., Wang, J., Li, X., Wang, J., et al. (2020). Proteasome inhibitor induced SIRT1 deacetylates GLI2 to enhance hedgehog signaling activity and drug resistance in multiple myeloma. Oncogene 39, 922–934. doi: 10.1038/s41388-019-1037-6
Yahyanejad, S., Theys, J., and Vooijs, M. (2016). Targeting notch to overcome radiation resistance. Oncotarget 7, 7610–7628. doi: 10.18632/oncotarget.6714
Yan, Y., Liu, F., Han, L., Zhao, L., Chen, J., Olopade, O. I., et al. (2018). HIF-2α promotes conversion to a stem cell phenotype and induces chemoresistance in breast cancer cells by activating Wnt and Notch pathways. J. Exp. Clin. Cancer Res. 37:256.
Yang, L., Shi, P., Zhao, G., Xu, J., Peng, W., Zhang, J., et al. (2020). Targeting cancer stem cell pathways for cancer therapy. Signal Transduct. Target Ther. 5:8.
Yao, J., and Qian, C. (2010). Inhibition of Notch3 enhances sensitivity to gemcitabine in pancreatic cancer through an inactivation of PI3K/Akt-dependent pathway. Med. Oncol. 27, 1017–1022.
Yeh, Y., Guo, Q., Connelly, Z., Cheng, S., Yang, S., Prieto-Dominguez, N., et al. (2019). Wnt/Beta-catenin signaling and prostate cancer therapy resistance. Adv. Exp. Med. Biol. 1210, 351–378.
Yoon, J. W., Lamm, M., Chandler, C., Iannaccone, P., and Walterhouse, D. (2020). Up-regulation of GLI1 in vincristine-resistant rhabdomyosarcoma and ewing sarcoma. BMC Cancer 20:511.
Yu, B., Gu, D., Zhang, X., Li, J., Liu, B., and Xie, J. (2017). GLI1-mediated regulation of side population is responsible for drug resistance in gastric cancer. Oncotarget 8, 27412–27427.
Zang, S., Chen, F., Dai, J., Guo, D., Tse, W., Qu, X., et al. (2010). RNAi-mediated knockdown of Notch-1 leads to cell growth inhibition and enhanced chemosensitivity in human breast cancer. Oncol. Rep. 23, 893–899.
Zhang, C. C., Yan, Z., Zong, Q., Fang, D. D., Painter, C., Zhang, Q., et al. (2013). Synergistic effect of the gamma-secretase inhibitor PF-03084014 and docetaxel in breast cancer models. Stem Cells Transl. Med. 2, 233–242.
Zhang, J., Liu, J., Li, H., and Wang, J. (2016). β-Catenin signaling pathway regulates cisplatin resistance in lung adenocarcinoma cells by upregulating Bcl-xl. Mol. Med. Rep. 13, 2543–2551.
Zhang, K., Hong, X., Song, Z., Xu, Y., Li, C., Wang, G., et al. (2020). Identification of deleterious NOTCH mutation as novel predictor to efficacious immunotherapy in NSCLC. Clin. Cancer Res. 26, 3649–3661.
Zhang, Y., and Wang, X. (2020). Targeting the Wnt/beta-catenin signaling pathway in cancer. J. Hematol. Oncol. 13:165.
Zhang, Z. M., Wu, J. F., Luo, Q. C., Liu, Q. F., Wu, Q. W., Ye, G. D., et al. (2016). Pygo2 activates MDR1 expression and mediates chemoresistance in breast cancer via the Wnt/beta-catenin pathway. Oncogene 35, 4787–4797.
Zhao, Y., Lu, Q., Li, C., Wang, X., Jiang, L., Huang, L., et al. (2019). PRMT1 regulates the tumour-initiating properties of esophageal squamous cell carcinoma through histone H4 arginine methylation coupled with transcriptional activation. Cell Death Dis. 10:359.
Zhao, Y., Yi, J., Tao, L., Huang, G., Chu, X., Song, H., et al. (2018). Wnt signaling induces radioresistance through upregulating HMGB1 in esophageal squamous cell carcinoma. Cell Death Dis. 9:433.
Zhou, C., Yi, C., Yi, Y., Qin, W., Yan, Y., Dong, X., et al. (2020). LncRNA PVT1 promotes gemcitabine resistance of pancreatic cancer via activating Wnt/β-catenin and autophagy pathway through modulating the miR-619-5p/Pygo2 and miR-619-5p/ATG14 axes. Mol. Cancer. 19:118.
Zinke, J., Schneider, F. T., Harter, P. N., Thom, S., Ziegler, N., Toftgard, R., et al. (2015). beta-Catenin-Gli1 interaction regulates proliferation and tumor growth in medulloblastoma. Mol. Cancer 14:17.
Keywords: Notch signaling, Hedgehog signaling, Wnt signaling, tumors, resistance, anticancer, therapy
Citation: Kumar V, Vashishta M, Kong L, Wu X, Lu JJ, Guha C and Dwarakanath BS (2021) The Role of Notch, Hedgehog, and Wnt Signaling Pathways in the Resistance of Tumors to Anticancer Therapies. Front. Cell Dev. Biol. 9:650772. doi: 10.3389/fcell.2021.650772
Received: 08 January 2021; Accepted: 19 March 2021;
Published: 22 April 2021.
Edited by:
Maria Pia Felli, Sapienza University of Rome, ItalyReviewed by:
Giada Dal Collo, Erasmus Medical Center, NetherlandsMichela Colombo, University of Oxford, United Kingdom
Copyright © 2021 Kumar, Vashishta, Kong, Wu, Lu, Guha and Dwarakanath. This is an open-access article distributed under the terms of the Creative Commons Attribution License (CC BY). The use, distribution or reproduction in other forums is permitted, provided the original author(s) and the copyright owner(s) are credited and that the original publication in this journal is cited, in accordance with accepted academic practice. No use, distribution or reproduction is permitted which does not comply with these terms.
*Correspondence: Vivek Kumar, dml2ZWsua3VtYXJAc3BoaWMub3JnLmNu; Jiade J. Lu, amlhZGUubHVAc3BoaWMub3JnLmNu
†Present address: B. S. Dwarakanath, Central Research Facility, Sri Ramachandra Institute of Higher Education and Research, Chennai, India