- 1Department of Oncology, The First Affiliated Hospital of Nanjing Medical University, Nanjing, China
- 2School of Biological and Health Systems Engineering, Arizona State University, Tempe, AZ, United States
- 3Department of Molecular and Cellular Pharmacology, University of Miami Miller School of Medicine, Miami, FL, United States
- 4Sylvester Comprehensive Cancer Center, University of Miami Miller School of Medicine, Miami, FL, United States
Biophysical cues, such as mechanical properties, play a critical role in tissue growth and homeostasis. During organ development and tissue injury repair, compressive and tensional forces generated by cell-extracellular matrix or cell-cell interaction are key factors for cell fate determination. In the vascular system, hemodynamic forces, shear stress, and cyclic stretch modulate vascular cell phenotypes and susceptibility to atherosclerosis. Despite that emerging efforts have been made to investigate how mechanotransduction is involved in tuning cell and tissue functions in various contexts, the regulatory mechanisms remain largely unknown. One of the challenges is to understand the signaling cascades that transmit mechanical cues from the plasma membrane to the cytoplasm and then to the nuclei to generate mechanoresponsive transcriptomes. YAP and its homolog TAZ, the Hippo pathway effectors, have been identified as key mechanotransducers that sense mechanical stimuli and relay the signals to control transcriptional programs for cell proliferation, differentiation, and transformation. However, the upstream mechanosensors for YAP/TAZ signaling and downstream transcriptome responses following YAP/TAZ activation or repression have not been well characterized. Moreover, the mechanoregulation of YAP/TAZ in literature is highly context-dependent. In this review, we summarize the biomechanical cues in the tissue microenvironment and provide an update on the roles of YAP/TAZ in mechanotransduction in various physiological and pathological conditions.
Introduction
The fate of individual cells is shaped and determined by both biochemical and biophysical factors in cellular microenvironments (Discher et al., 2005; Bonnans et al., 2014; Yang et al., 2014; Kumar et al., 2017). While much is known about cell signaling and functional consequences in response to biochemical cues, such as nutrients, growth factors, and hormones, the impacts of biophysical modulations on tissue growth and homeostasis in health and diseases are understudied. With developments in advanced engineering substrates and apparatus to better mimic mechanics of microenvironments in native tissues, biophysical factors, such as matrix stiffness, cell-cell contacts, and local hemodynamic forces, have been appreciated for their roles in regulating cellular functions and phenotypes (Lampi and Reinhart-King, 2018; Mohammadi and Sahai, 2018; Maurer and Lammerding, 2019; Sheetz, 2019; Yang et al., 2019). However, our knowledge is rather limited for how cells sense the changes of microenvironments and transduce such mechanical stimuli to biochemical signaling cascades governing cellular responses.
The Hippo pathway, since it was identified in Drosophila melanogaster less than two decades ago (Harvey et al., 2003; Pantalacci et al., 2003; Udan et al., 2003; Wu et al., 2003), has been extensively studied and now regarded as a master regulator of organ development, regeneration, and carcinogenesis, via integrating extrinsic and intrinsic cues that reshape cellular transcription programs (Pan, 2010; Meng et al., 2016; Moya and Halder, 2019; Dey et al., 2020). The core of the mammalian Hippo pathway is a kinase cascade consisting of the Mammalian STE20-like kinase 1/2 (MST1/2) and the Large tumor suppressor kinase 1/2 (LATS1/2). When cells are exposed to growth-inhibiting signals, MST1/2 phosphorylate LATS1/2 at their hydrophobic motif and thus activate LATS1/2, which in turn phosphorylate and inactivate two transcription factors Yes-associated protein (YAP) and Transcriptional coactivator with PDZ-binding motif (TAZ) by inducing their cytoplasmic retention and protein degradation. When the Hippo pathway is inactivated by growth-promoting signals, YAP and TAZ are dephosphorylated and thus located in the nucleus, where they bind to the TEAD family of transcription factors and drive transcription programs that promote cell proliferation, mobility, and stemness. In addition, novel Hippo kinases (e.g., MAP4Ks), accessory proteins (e.g., SAV1, NF2, and MOB1/2), and tuning machineries (e.g., the STRIPAK-PP2A complex) have been shown as indispensable components, among dozens of many other peripheral regulators that finely tune the pathway (Misra and Irvine, 2018; Ma et al., 2019; Zheng and Pan, 2019), for relaying environmental signals to the core of the Hippo pathway.
There have been many comprehensive reviews on the Hippo pathway regulation and its biological roles as cited above. In this review, we will focus on the molecular mechanisms by which the Hippo pathway is modulated by mechanical cues in microenvironments (Figure 1). Furthermore, we will summarize the recent progress on how mechanoregulation of the Hippo pathway contributes to human disease development.
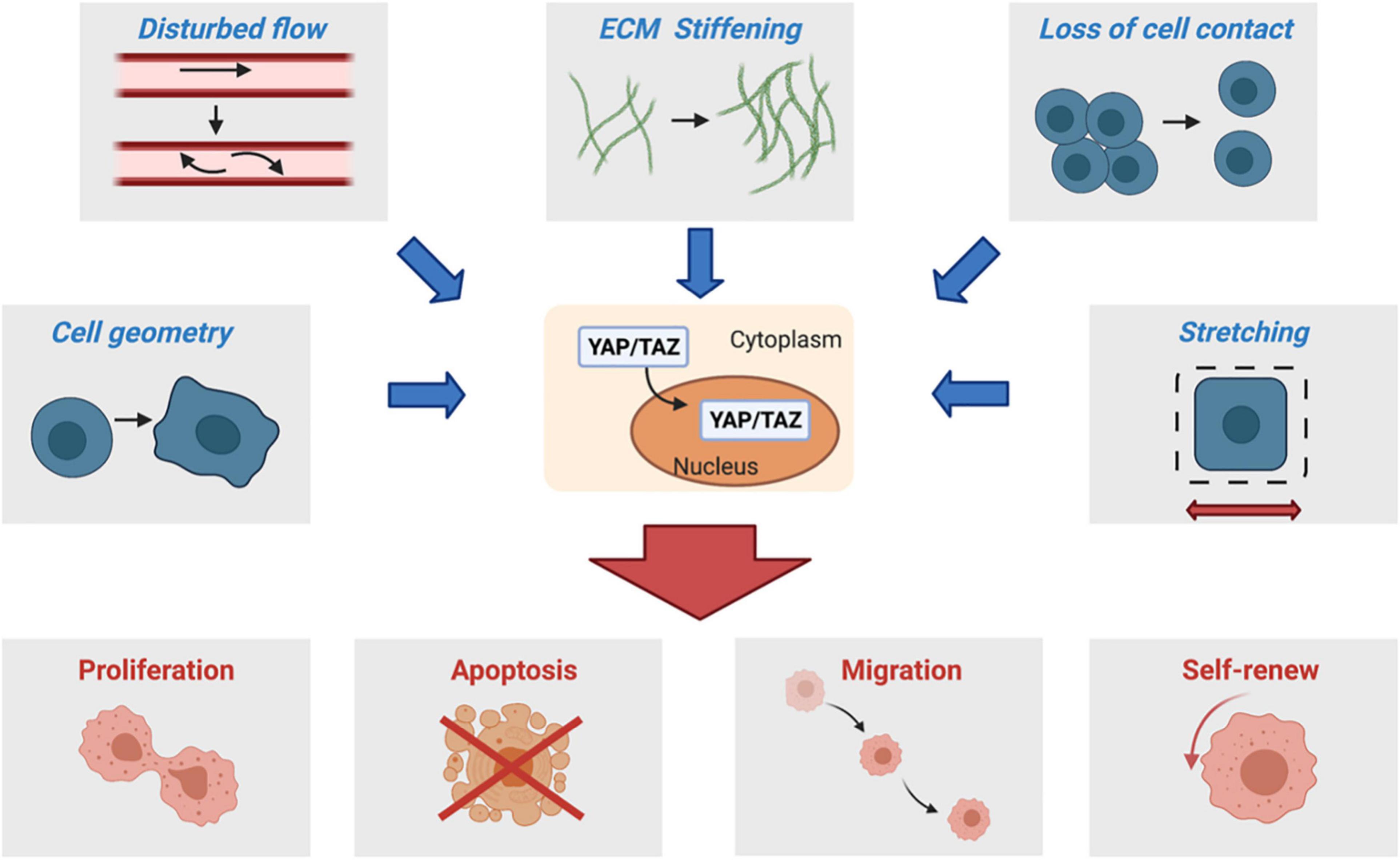
Figure 1. Changes in the biophysical environments, such as those resulting from ECM stiffening, loss of cell contact, disturbed flow, cell spreading, and stretching, promote nuclear translocation of YAP/TAZ and thus activate their transcription programs for cell proliferation, survival, migration, and self-renew, among others.
General Roles of Mechanical Signals in Cell Behaviors and Fate Decision
Cells and tissues perceive microenvironmental physical forces that are generated both internally and externally. Internal forces are mainly generated by cytoskeleton dynamics (endogenous forces), while external forces result from both environment and neighbor cells (applied forces) (Chen, 2008). The resulting mechanical cues, including tissue stiffness, stretch, and shear stress, modulate cell behaviors, such as cell proliferation, spreading, migration, and differentiation. The process through which biophysical forces are sensed and converted into biochemical signals that elicit responses is termed mechanotransduction (Ingber, 2006; Dasgupta and McCollum, 2019). This process was typically divided into mechanosensing (the act of sensing a mechanical stimulus), mechanotransmission (the act of transmitting such a stimulus to signaling events), and mechanoresponse (the functional response of cells to the mechanical stimulus) (Hoffman et al., 2011).
Mechanosensing is usually initiated at the cell surface, where plasma membrane receptors, their associated proteins, and the plasma membrane itself sense and propagate mechanical cues to trigger signaling cascades that generate mechanoresponses. Integrins, G protein-coupled receptors, Enzyme-linked Receptors (i.e., Receptor Tyrosine Kinase), and Ion Channels can be all such “mechanosensors” (Chen et al., 2017). Forces applied to these receptors or on/through their ligands change configurations of the receptors, leading to enzymatic reactions and/or cytoskeleton remodeling. Our understanding of membrane-associated mechanosensors has been greatly improved in the past decade. For example, Integrins and Piezo channels, evolutionarily conserved in mammalian cells, are mechanosensitive, characterized by the activation of corresponding downstream effectors and regulation of their biological functions in response to mechanical stimuli (Kechagia et al., 2019; Xiao, 2020). Besides the membrane-associated receptors, mechanical forces can be directly transmitted to the nucleus, the envelope of which responds to mechanical compression or stretch to alter transport of transcription factors and other nucleus-cytoplasm shuttling proteins, as well as remodel nucleoskeleton (Dahl et al., 2008; Wang et al., 2009; Enyedi et al., 2016; Elosegui-Artola et al., 2017; Hoffman et al., 2020).
It was recognized a long time ago that mechanical signals during development dynamically control gene transcription programs to determine cell fate and organ growth (Discher et al., 2005; Engler et al., 2006). However, it was then unclear how these mechano-responsive transcription programs are regulated by the mechanotransduction initiated by membrane proteins and cytoskeletons. Emerging efforts have been made to elucidate the signaling cascades that link membrane mechanosensors to the nuclear transcription machinery. One such “missing” signaling cascade that has been increasingly appreciated is the Hippo pathway, though many details of mechanoregulation of this pathway remain yet to be determined.
YAP and TAZ Are at the Center Stage of Mechanotransduction
Two landmark studies from the Piccolo group and the Sasaki group (Dupont et al., 2011; Wada et al., 2011) published in 2011 opened a new avenue for us to understand how mechanical cues modulate the activities of nuclear factors that dictate transcription programs to control cell behaviors and fate. Their studies demonstrated for the first time that YAP and TAZ, the Hippo pathway effectors, are mechanotransducers to relay cytoskeletal tension to nuclei and to regulate cell functions, and more importantly, they are functionally indispensable for the biological outputs of mechanical cues. Thereafter, new nuclear factors (i.e., β-catenin, Twist) and new mechanosensitive signaling molecules, such as RAP2 and MAP4K, have been identified (Benham-Pyle et al., 2015; Wei et al., 2015; Li et al., 2018; Meng et al., 2018), thus greatly advancing our mechanistic understanding of mechanotransduction and the Hippo pathway regulation. In the following session, we will summarize the most updated knowledge of how each type of mechanical cues regulates YAP and TAZ and the biological context(s) for the corresponding regulation (Figure 2).
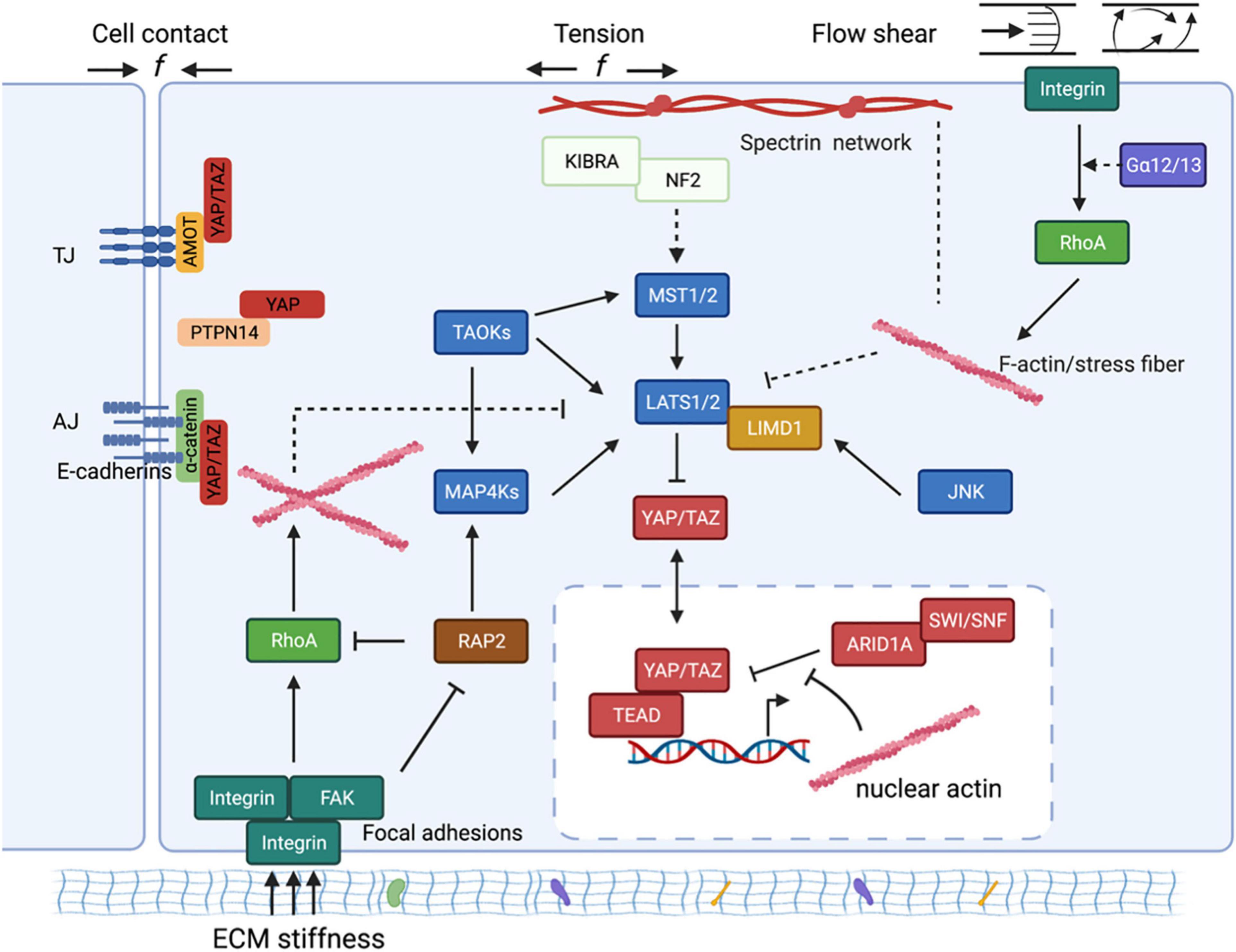
Figure 2. The schematic diagram of molecular mechanisms by which mechanical cues regulate YAP/TAZ. Mechanical cues, such as cell-cell contact, ECM stiffness, externally applied mechanical stretch, and flow shear, control YAP/TAZ activity through both Hippo-dependent and -independent pathways. The core kinase cascade consisting of MST1/2 and LATS1/2, as well as novel Hippo kinases such as MAP4Ks, respond to the mechanical cues to modulate phosphorylation and localization of YAP/TAZ. Mechanical cues can also bypass these kinases and act through both cytoplasmic and nuclear actins to modulate YAP/TAZ localization. (1) In response to cell-cell contact, AMOT directly binds to YAP and thus sequesters YAP at tight junctions regardless of YAP phosphorylation status. AMOT can simultaneously act through NF2/Merlin to activate the MST1/2-LATS1/2 kinase cascade to induce phosphorylation and inactivation of YAP. Adherens junction (AJ) protein E-cadherin, upon cell confluence, trans-dimerize and subsequently inactivate YAP/TAZ through the MST1/2-LATS1/2 kinase cascade. Cell-cell contact inhibition also promotes direct interaction between PTPN14 and YAP, and thus leads to cytoplasmic translocation of YAP. (2) ECM stiffness sensed by focal adhesion promotes actin polymerization and stress fiber formation. The Hippo kinase cascade is inactivated by actin polymerization and stress fiber formation at high ECM stiffness. On the other hand, polymerized nuclear actin, under high ECM stiffness, binds to ARID1A-SWI/SNF complex, subsequently relieving its sequestration of YAP/TAZ. In addition, the stiffness-regulated GTPase RAP2 directly activates MAP4K4/6/7 as well as inhibits Rho GTPases, leading to LATS kinase activation and YAP/TAZ inhibition. Stiffness-activated JNK can phosphorylate LIMD1, which directly binds to LATS1/2 and reduces their kinases activities, thus activating YAP/TAZ. (3) Mechanical stretch or tension also acts through actin cytoskeleton to modulate YAP/TZ activities. Spectrin, a cytoskeletal protein, serves as a key linker that connects the cellular tension-sensing system to the Hippo regulation network. (4) Flow shear patterns and speeds differentially regulate activity of the Hippo kinase cascade in endothelial cells via an integrin–Gα12/13–RhoA axis.
Extracellular Matrix (ECM) Stiffness
The mechanical microenvironment of cells is largely determined by their neighbor cells and surrounding extracellular matrix (Discher et al., 2005; Paszek et al., 2005; Engler et al., 2006; Wells, 2008). The difference in ECM composition leads to unique three-dimensional networks in various tissues and determines the rigidity or elasticity of substrates where cells habituate and grow. This physical characteristic of the microenvironment profoundly influences cell phenotypes, not only their morphology but also internal cytoskeleton organization and trafficking (Myers et al., 2011; Wong et al., 2020). To investigate the effect of ECM stiffness on cell functions, engineered hydrogels interfacing with ECM proteins, such as fibronectin and collagens, have widely been used as substrates to grow cells in vitro. As a result, ECM stiffness has been shown to regulate cell growth, migration, and differentiation, among many other important cell behaviors.
It was first shown by the Piccolo group that increased ECM stiffness promotes nuclear localization of YAP and TAZ and upregulation of their target genes (Dupont et al., 2011). Though the underlying molecular mechanisms were not fully uncovered then, they demonstrated that actin cytoskeleton tension resulting from manipulations of cell spreading and substrate rigidity constitutes the key link between ECM stiffness and YAP/TAZ activation. High ECM stiffness promotes cell spreading and subsequently leads to actin cytoskeleton tension, which in turn results in nuclear translocation of YAP/TAZ with a to-be-defined mechanism. One key player that relays stiffness signals to YAP/TAZ is RhoA GTPase, which can sense ECM stiffness through focal adhesion and in turn promote actin polymerization and stress fiber formation. Actin cytoskeleton can regulate YAP and TAZ through both Hippo-dependent and -independent pathways (Dupont et al., 2011; Wada et al., 2011). On one hand, the Hippo pathway can be inactivated by actin polymerization and stress fiber formation at high ECM stiffness, and activated by relaxed actin cytoskeletons at low ECM stiffness. Phosphorylation and localization of YAP/TAZ are thus modulated by ECM stiffness through the Hippo pathway (Codelia et al., 2014; Li et al., 2018; Meng et al., 2018). On the other hand, the ARID1A-SWI/SNF complex binds to YAP/TAZ and thus prevents the interaction between YAP/TAZ and TEAD. Polymerized nuclear actin, under high ECM stiffness, binds to ARID1A-SWI/SNF complex, subsequently relieving its sequestration of YAP/TAZ (Chang et al., 2018).
To date, our mechanistic understanding of how ECM stiffness regulates YAP/TAZ remains incomplete as emerging players have been reported. ECM stiffness can be sensed by cells through focal adhesions, as cells use integrin to pull integrin ligands in the ECM. In addition to common integrin ligands such as collagens and fibronectin, an ECM proteoglycan, Agrin, also act as an extracellular mediator of matrix mechanotransduction to regulate YAP/TAZ via integrins, its specific membrane receptor LRP4, and the actin cytoskeleton (Chakraborty et al., 2017; Chakraborty et al., 2020). Similarly, matricellular protein thrombospondin-1, acts through integrin-1 and the Hippo pathway, but not actin cytoskeleton, to activate YAP/TAZ (Yamashiro et al., 2020). Besides signaling through integrin/focal adhesion, plasma membrane protein Caveolin-1 (CAV-1) also play a critical role in mechanoregulation of YAP/TAZ (Moreno-Vicente et al., 2018). CAV1 loss promotes the interaction between YAP and the 14-3-3 protein YWHAH in an F-actin-dependent but Hippo-independent manner.
Besides membrane proteins, signaling transducers like small GTPases and protein kinases also mediate stiffness signals to the Hippo pathway. Small GTPases, such as RhoA and RAC1, have been demonstrated for their roles as molecular switches for stiffness sensing (Bae et al., 2014). Another GTPase, RAP2, also senses stiffness cues from focal adhesions (Meng et al., 2018; Yamashiro et al., 2020), as ECM stiffness modulates the GDP/GTP loading status of RAP2. Active/GTP-loading RAP2 activates non-canonical Hippo kinases MAP4K4/6/7 by direct binding to their hydrophobic motif leading to LATS activation and YAP/TAZ inhibition. JNK is also an important mediator of stiffness sensing for YAP/TAZ regulation in epithelial cells (Codelia et al., 2014). Stiffness-activated JNK can phosphorylate LIMD1, which directly binds to LATS1/2 and reduces their kinases activities, eventually leading to activation of YAP/TAZ.
Static and Cyclic Stretch
Almost all adherent cells, in vitro or in vivo, experience physical forces transmitted between cells and across tissues, and one of the most common forces in animals is tension caused by mechanical stretch. For example, when cells undergo tissue growth or disease progression, the increase in ECM stiffness generates a static tension that stretches cells. Such kind of stretch forces, as previously discussed, modulate cell behaviors via a YAP/TAZ-dependent mechanism. In addition to ECM stiffening, stretching forces from external sources, such as tissue mechanical strain and artificial cell stretching devices, are able to regulate cell functions through the interplay of the Hippo pathway (Driscoll et al., 2015; Fletcher et al., 2018). Moreover, recent research has linked YAP dysregulation to malfunction of the cardiovascular system caused by pressure overload (Byun et al., 2019), suggesting a critical regulatory role of the Hippo-YAP/TAZ pathway in cells in response to various magnitudes of stretch force.
Static stretch activates YAP/TAZ by suppressing capping and severing proteins of F-actins (Aragona et al., 2013). Additionally, the stretch force applied to the nucleus may decrease the mechanical restriction of nuclear pores, thus facilitating nuclear transport of YAP/TAZ (Elosegui-Artola et al., 2017). The linker of nucleoskeleton and cytoskeleton (LINC) complex, which transfers cytoskeletal strain to the nucleus, is a key player for mechanical stretch to activate YAP/TAZ in this process (Driscoll et al., 2015; Koushki et al., 2020).
Cyclic stretch has also been reported to activate YAP/TAZ by suppression of the Hippo pathway (Codelia et al., 2014), even in cells on soft matrices (Cui et al., 2015). In Drosophila, the physiological mechanical strain can drive activation and nuclear localization of YAP/TAZ homolog Yki in Drosophila follicular epithelium to promote cell proliferation, via inactivating the LATS1/2 homolog Warts (Fletcher et al., 2018). The novel Hippo kinase Msn plays a critical role in sensing mechanical stretch in Drosophila gut. Mechanical stretch dissociates Msn from the plasma membrane and thus prevents phosphorylation and activation of Msn by the Tao kinase, which in turn activates expansion of Drosphila gut stem cells and leads to intestinal hyperproliferation (Li et al., 2014, 2018).
In mammals, cyclic stretch is known to act through thrombospondin-1/RAP2 in blood vessel cells to activate YAP and promote vascular remodeling (Yamashiro et al., 2020). Thrombospondin-1 acts via integrin αvβ1 to form focal adhesions and promotes nuclear shuttling of YAP by inactivating the RAP2 GTPase, results in vascular remodeling in response to the pulsatile blood flow and pressure. It is worth mentioning that externally applied mechanical stretch and ECM stiffening shared many mechanosensing machineries, such as RAP2 and Msn/MAP4Ks, to regulate YAP/TAZ activities. It would be important to understand how these universal machineries work in concert with cell-specific mechanosensors (e.g., VEGFR2 in endothelial cells) to control mechanoresponses of cells in future.
Cell-Cell Contact
Cell-cell contact inhibition is the phenomenon that cells avoid proliferating as they achieve convergence in monolayers. During carcinogenesis, transformed cells slowly lose this character (Hanahan and Weinberg, 2011). Loss of contact inhibition allows the transformed cells to overcome physical restraint and in many cases contributes to cancer cell aggressiveness. Contact-dependent signaling is one of the first extracellular stimuli discovered to regulate the Hippo pathway (Zhao et al., 2007Zhao et al., 2008). Direct contact between normal cells activates the Hippo kinases, thus leading to phosphorylation and cytoplasmic retention of YAP/TAZ, triggering cell cycle arrest and/or autophagy (Pavel et al., 2018). In contrast, hypophosphorylation and nuclear localization of YAP/TAZ have been associated with loss of contact inhibition of cancer cells resulting from somatic mutations (Zhao et al., 2007; Zhang et al., 2010; Tranchant et al., 2017; Frank et al., 2018; Ouyang et al., 2020).
Various mechanisms have been proposed to explain how cell-cell contact inhibition modulates activities and localization of YAP/TAZ. These mechanisms can be roughly grouped into two types. The first one usually involves cis-interaction of cell membrane proteins at high confluence. For example, for tight junctions (TJs) form between cells, angiomotin (AMOT) complex at TJs is activated and transmit the antiproliferative signal from TJs to YAP via two independent mechanisms: AMOT can directly bind to YAP and thus sequester YAP at TJs, and/or AMOT can activate Merlin/NF2 to trigger LATS1/2-dependent YAP phosphorylation (Li et al., 2015). In addition to TJs, adherens junctions (AJs) protein E-cadherin, in confluent cells, trans-dimerize and subsequently stimulate MST1/2-LATS1/2 kinase cascade to inhibit activities of YAP/TAZ (Kim et al., 2011). PTPN14, a protein tyrosine phosphatase, can inhibit YAP transactivation activity through a direct interaction in response to cell confluence (Wang et al., 2012; Liu et al., 2013). The other type of mechanisms involves cell geometry and actin cytoskeleton remodeling. High cell density, as well as low ECM stiffness, reduces adhesive area and alters cell shape, which leads to inactivation of RhoA and subsequent reduction of stress fiber of actin cytoskeleton, which can inactivate YAP/TAZ through both Hippo kinases-dependent and -independent mechanisms (Aragona et al., 2013; Meng et al., 2018; Chang et al., 2018). Spectrin has been recognized as a key cytoskeletal protein that restricts YAP/TAZ activity in response to mechanical cues, such as cell-cell contact inhibition, through remodeling actin cytoskeleton particularly at cortical areas of cells. Loss of Spectrin proteins results in hyperactivation of YAP/TAZ, likely by elevating cortical myosin II activity, leading to cell over-proliferation even when cells confluence is reached in both mammals and Drosophila (Deng et al., 2015; Fletcher et al., 2015; Wong et al., 2015; Deng et al., 2020).
Fluid Shear Stress
Shear stress, a fluid frictional force, is another major mechanical stimulus maintaining tissue homeostasis. Indeed, one of the most studied cell types in mechanotransduction is vascular endothelial cells (ECs), lining in the innermost layer of blood vessels. ECs are constantly subjected to shear stress from blood flow, and it is well recognized that ECs are able to sense and respond to changes in flow direction, pulsatility, and magnitude of shear stress via mechanosensors and mechanosensitive signaling pathways. As a result, endothelial phenotypes are highly associated with local blood flow patterns and distinct in different regions of the vascular tree. Three recent studies independently confirmed that flow patterns modulate endothelial phenotypes through regulation of YAP/TAZ activities: unidirectional laminar flow suppresses YAP/TAZ activities to keep ECs quiescent and inert to inflammatory cells, while disturbed oscillatory flow activates YAP/TAZ to promote a pro-proliferative and -inflammatory EC phenotype (Wang K. et al., 2016; Wang L. et al., 2016; Xu et al., 2016).
Mechanistically, the integrin–Gα13–RhoA axis was first reported to mediate the flow regulation of YAP/TAZ activities in ECs (Wang L. et al., 2016) and two recent studies revealed that disturbed flow acts through integrin α5β1 to induce YAP nuclear translocation and promote the pro-atherogenic responses, via c-Abl kinase and phosphodiesterase 4D5, respectively (Li et al., 2019; Yun et al., 2019). However, the mechanisms regarding how the flow-activated integrin signaling cascades crosstalk with Hippo kinases to modulate YAP/TAZ activities remain to be studied. In addition to the integrin-mediated mechanisms, it has been shown that short-term unidirectional laminar flow (15 dyne/cm2 for 10 min) increases the nuclear localization of YAP in a LATS1/2-independent but an angiomotin-regulated manner (Nakajima et al., 2017). Last but not the least, caveolae, the plasma membrane microdomain, is known to sense shear stress signals and have been shown to relay such mechanical cues through the Hippo pathway to facilitate mechanoregulation of YAP/TAZ (Rausch et al., 2019). However, whether the caveolae-dependent mechanism mediates the flow regulation of endothelial phenotypes remains to be determined.
In addition to ECs, many other cell types, such as metastatic tumor cells and mesenchymal stem cells, are known to perceive shear stress stimuli and transduce the resulting biochemical signals in regulating cellular functions (Lee et al., 2017, 2018; Qin et al., 2019). In fact, the connection between shear stress and YAP was first reported in mesenchymal stem cells, where it was shown that exposure of mesenchymal stem cells to shear stress enhances YAP expression to promote their differentiation into chondrocytes (Zhong et al., 2013). More in vitro and in vivo studies are warranted to validate the roles of YAP/TAZ as mechanotransducers in modulating biological functions in the above-mentioned cell types.
Mechanoregulation of YAP/TAZ in Human Diseases
Mechanoregulation of YAP/TAZ plays a critical role in normal development and aging processes. For instance, it is known that physiological substrate stiffness directs human pluripotent stem cell specification by influencing cytoskeleton arrangement and intracellular tension through the YAP-TEAD complex (Pagliari et al., 2021). The behavior of limbal epithelial stem cells is strongly influenced by changes in corneal substrate stiffness, via the activation of YAP-dependent mechanotransduction pathways (Gouveia et al., 2019). Furthermore, an age-related stiffness drives YAP/TAZ-mediated pathogenic expression of ECM proteins, ultimately disrupting muscle stem cell fate (Stearns-Reider et al., 2017).
Emerging evidence connects YAP/TAZ dysregulation by mechanical cues to various human diseases. Although the underlying mechanisms remain to be defined in many cases, understanding of the Hippo pathway dysregulation by mechanical cues in human diseases potentially can provide us insights into new therapeutic strategies for these diseases (Figure 3).
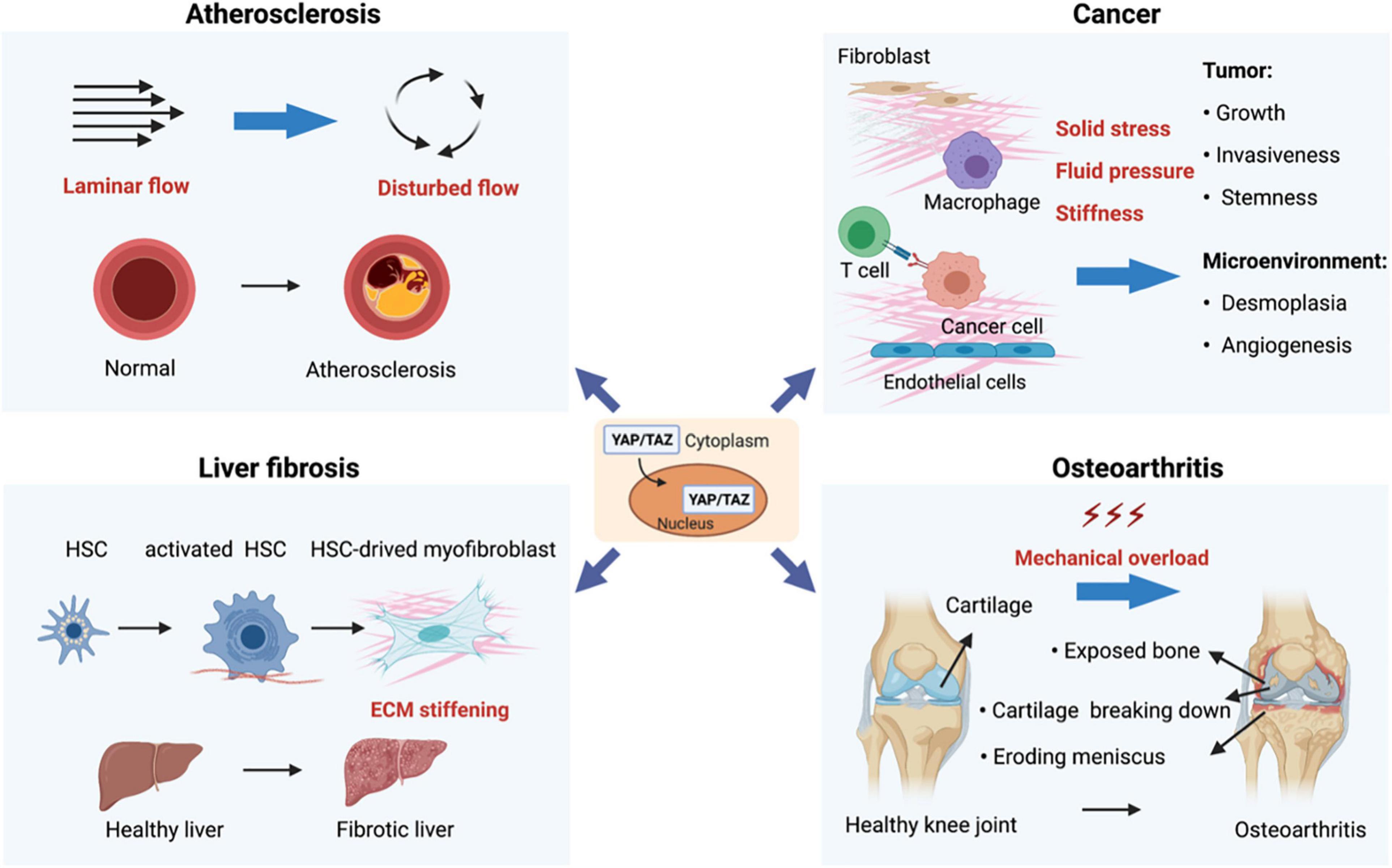
Figure 3. Mechanoregulation of YAP/TAZ in human diseases. (1) Disturbed flow-activated YAP/TAZ is a key factor that promotes atherogenesis. (2) Mechanical properties in the tumor microenvironment (such as solid stress, fluid pressure, and stiffness) act through YAP/TAZ to regulate various aspects of tumor initiation and progression. (3) YAP/TAZ-mediated mechanoresponses strongly promote organ fibrosis. For instance, hepatic injuries and subsequent inflammatory responses activate quiescent hepatic stellate cells (HSCs), leading to activation and expansion of HSCs and accumulation of extracellular. (4) Osteoarthritis (OA) is mainly caused by mechanical overload, and YAP is both necessary and adequate to preserve cartilage homeostasis in OA.
Cardiovascular Diseases (CVDs)
The cardiovascular system is subjected to continuously shifting mechanical signals, including stretch, compression, distortion, and shear. Mechanotransduction profoundly influences the development of the cardiovascular system and the regulation of physiological functions (Garoffolo and Pesce, 2019). The roles of YAP/TAZ in CVDs have been well summarized in a recent review (Yu et al., 2020). Aberrant activation of YAP/TAZ contributes to a variety of cardiovascular conditions: atherosclerosis, pulmonary hypertension, myocardial hypertrophy, angiogenesis, restenosis, and myocardial fibrosis, while hypoactivation of YAP/TAZ is associated with aortic aneurysms, aortic dissection, reperfusion of myocardial ischemia, and myocardial infarction. Our review will solely focus on YAP/TAZ dysregulation in mechanical cues resulting from/in pulmonary hypertension, atherosclerosis, and cardiac hypertrophy.
Pulmonary Hypertension (PH)
Pulmonary hypertension (PH) refers to a pathophysiologic condition of increased blood pressure within the arteries of the lungs with many possible causes (Hoeper et al., 2013).
One feature of PH is remodeling of small pulmonary arteries caused by hyperproliferation of vascular smooth muscle cells (VSMCs) and myofibroblasts, leading to aberrant deposition of collagen and elastin and vascular ECM stiffening. A number of cross-sectional studies suggest that YAP/TAZ activation downstream of ECM stiffening is a key driver of PH (Bertero et al., 2015b, 2016). Vascular remodeling and stiffening activate YAP/TAZ, which then drive a transcription program that promotes ECM deposition and crosslinking and further enhances vascular remodeling and stiffening, thus constituting a forward feedback loop. Specifically, ECM stiffening activates YAP/TAZ in myofibroblasts, endothelial cells, and VSMCs and thus facilitates proliferation of these cells. Furthermore, YAP/TAZ in these cells activate genes involved in ECM synthesis (i.e., collagens) and crosslinking (i.e., lysyl oxidase) (Bertero et al., 2015b). In addition, YAP/TAZ also link mechanical stimuli to dysregulated vascular metabolism associated with PH. ECM remodeling controls the expression of glutaminase by activating YAP/TAZ, leading to activation of glutaminolysis and anaplerosis, fostering PAECs and PASMCs proliferation and migration. In mouse models, LOX inhibitors dampened nuclear YAP/TAZ and improved end-stage manifestations of PH (Bertero et al., 2016). Increased pulsatility and shear stress have been associated with YAP/TAZ activation in pulmonary vascular ECM remodeling and pulmonary adventitial myofibroblast proliferation. It is unknown, however, whether mechanical signals from increased pulsatility and shear stress alone will be sufficient to activate YAP/TAZ in the adventitial myofibroblasts in the absence of a rigid matrix (Thenappan et al., 2018).
Atherosclerosis
Atherosclerotic plaques develop preferentially at arterial bifurcations and high curvatures but not straight segments of arteries. The site-specific manner of lesion formation suggests that local hemodynamic forces acting on vessel walls play a critical role in exerting atheroprone or atheroprotective effects on vascular cells. Indeed, as discussed previously, disturbed flow, but not steady laminar flow, activates endothelial YAP/TAZ in ECs, leading to upregulations of pro-atherogenic (e.g., VCAM1) and YAP/TAZ-target genes (e.g., CTGF and CYR61) in vitro (Wang K. et al., 2016; Wang L. et al., 2016; Xu et al., 2016). In agreement with the in vitro findings, a higher level of YAP/TAZ activation was detected in atheroprone regions than in atheroprotective regions mouse aorta, suggesting the involvement of disturbed flow-activated YAP/TAZ in atherogenesis. In ApoE–/– mice, knockdown of YAP/TAZ expression decreases the pro-atherogenic phenotypes of vascular cells and attenuates lesion development in atheroprone regions of arteries. On the other hand, atherosclerosis is encouraged by overexpression of endothelial YAP, or systemic TAZ, or a constitutively active YAP/TAZ mutation (Wang L. et al., 2016; Li et al., 2019). Taken together, these studies reassuringly uncovered the importance of YAP/TAZ as mechanotransducers in vascular cells and atherogenesis.
Besides YAP/TAZ, hemodynamic forces activate a number of signaling pathways to regulate endothelial phenotypes associated with atherosclerosis, via other transcription factors, such as KLF2, KLF4, NRF2, HIF-1α, NF-κB, AP-1, and a few others (Niu et al., 2019). These transcription factors, collectively referred to mechanosensitive transcription factors (MSTFs), crosstalk with one another to regulate ECs upon exposure to hemodynamic forces, as well as control cellular responses to oxidative stress, inflammation, and metabolic programming. Understanding how YAP/TAZ work in concert with these MSTFs in endothelium homeostasis will be important for us to obtain a panoramic view of the mechanosensing signaling network in endothelial cells.
In addition to their roles in ECs, the phenotypic switch of VSMCs from contractile to synthetic phase is regulated by YAP/TAZ activities, in response to stretch or wall stress-induced vascular remodeling (Wang et al., 2018). Stretching VSMCs activates their PI3K-PDK1 signaling, which then prevents the Hippo kinase cascade from inactivating YAP/TAZ.
Cardiac Hypertrophy
Cardiac hypertrophy is an adaptive response to hemodynamic overload. In the beginning, cardiac hypertrophy is beneficial because it increases the number of contractile units and reduces the ventricular wall pressure to a normal level according to Laplace’s law. However, as the adjustment is physically limited, cardiac hypertrophy will lead to heart failure (Nakamura and Sadoshima, 2018).
Experimental studies have supported the concept that mechanical cues, such as hemodynamic overload, predominantly affect cardiomyocytes (CMs) by stretching. Integrins and the cytoskeleton or sarcolemmal proteins (e.g., phospholipases, ion channels, and ion exchangers) are two main types of mechanosensors, by which hemodynamic overload is coupled to intracellular signals responsible for the hypertrophic response (Ruwhof and van der Laarse, 2000).
Hemodynamic overload includes two forms: pressure overload and volume overload. Endogenous YAP is a crucial mediator of hypertrophy in response to volume overload, which regulates CM growth and survival in the adult mouse myocardium in response to myocardial ischemic injury (Del Re et al., 2013). It was believed that compensatory cardiomyocyte hypertrophy is at least partially regulated by Yap1 after chronic myocardial infarction and a Yap1 deficit impairs the growth response to stress in heart, contributing to worsened operation. In response to pressure overload, endogenous YAP is triggered in CMs through a RhoA-dependent mechanism. Heterozygous YAP depletion inhibited hypertrophy, but increased fibrosis and apoptosis, and decreased cardiac functions (Byun et al., 2019). These findings highlight YAP as a potential target for myocardial infarction and hypertension.
Organ Fibrosis
Inflammation and wound-healing process following tissue injury and/or an idiopathic disease induce profibrotic responses, including abnormal ECM synthesis and deposition by fibroblasts and tissue stiffening and thickening. Such changes in structural and mechanical properties of the tissue are usually irreversible and lead to the formation of scar tissue, also known as fibrosis. The physical features of fibrotic tissues, together with the unresolved inflammation, continuously stimulate fibroblasts, resulting in pathological accumulation of ECM components and permanent tissue damage (Rockey et al., 2015; Tschumperlin et al., 2018).
YAP/TAZ serve as critical mechanotransducers in fibroblasts, coordinating profibrotic responses in various tissues, such as hepatic fibrosis, pulmonary fibrosis, kidney fibrosis, cardiovascular fibrosis, and others (Liu et al., 2015; Kim et al., 2019). The initial increase in ECM stiffness activates YAP/TAZ in fibroblasts, encouraging the development of profibrotic mediators and excessive deposition of ECM components. This results in progressive tissue stiffening, thereby forming an activation feed-forward loop to promote tissue fibrosis (Bertero et al., 2015a; Liu et al., 2015). Moreover, YAP/TAZ act as a molecular link between fibrosis and cancer. Fibrotic ECM stimulates cell proliferation and changes cell polarity, thereby promoting tumor development and growth (Noguchi et al., 2018). Various studies have assessed the efficacy of inhibiting YAP/TAZ activity as a new therapeutic strategy to reverse fibrosis (Liang et al., 2017; Haak et al., 2019; Alsamman et al., 2020; Dey et al., 2020), and the results of those have unfolded a promise of YAP/TAZ-targeting therapies for organ fibrosis.
Musculoskeletal Disorders (MSDs)
MSDs refers to diseases that affect the muscles, bones, and joints, which mainly tendinitis, carpal tunnel syndrome, osteoarthritis (OA), rheumatoid arthritis (RA), etc.
Mechanical load has been shown to activate YAP by increasing the expression of nuclear accumulation of YAP. Hyperactivation of YAP by sustained mechanical overload or YAP overexpression alone is sufficient to induce skeletal muscle hypertrophy (Goodman et al., 2015; Iyer et al., 2019; Owens et al., 2020). Besides responding to mechanical load, YAP/TAZ play a crucial role in muscle cell stemness and myogenesis (Figeac et al., 2019; Zhang L. et al., 2019). However, more studies are required as their atrophic roles of YAP/TAZ in muscle cells have also been reported (Gnimassou et al., 2017), and more details about the connection between mechanical stimuli and YAP/TAZ signaling would further improve our understanding of the roles YAP/TAZ in mechanotransduction and muscle homeostasis.
OA is mainly caused by mechanical overload (Glyn-Jones et al., 2015), and it has been reported that YAP is both necessary and adequate to preserve cartilage homeostasis in OA (Deng et al., 2018; Zhang Q. et al., 2019). A few recent studies demonstrated that suppressing YAP activity is effective in attenuating OA progression (Gong et al., 2019; Thorup et al., 2020; Zhang et al., 2020). However, research is still lacking on how the mechanical forces, especially compression and hydrostatic pressure, regulate Hippo-YAP/TAZ pathway in chondrocytes in healthy cartilage and during OA development.
Cancer
The biophysical factors in the tumor microenvironment play a pivotal role in disease progression and treatment outcomes. Common physical features that hinder cancer treatment, including solid stresses, interstitial fluid pressure, stiffness, and tumor microarchitecture, have been nicely reviewed by Nia HT, Munn LL, and Jain RK very recently (Nia et al., 2020). It has long been known that the changes in tumor microenvironment trigger signaling pathways to fuel cancer progression, immune blockade, and cancer resistance to therapy. Among the mechanosensitive pathways, the dysregulation of the Hippo-YAP signaling plays a central role in tumor development as it has been connected to most of the physical features in tumors. Emerging efforts have been made to elucidate the molecular mechanisms by which mechanical properties of various tumor types act through YAP/TAZ to promote cancer pathology.
Loss of cell-cell contact inhibition and unchecked cell growth are hallmarks of cancer, and the Hippo pathway is known to mediate contact inhibition and cell proliferation. Indeed, dysregulation of the Hippo signaling and hyperactivation of YAP/TAZ not only abolish the cell cycle regulation, as a result of losing contact inhibition, but also promote the transformation of mammary epithelial cells (Zhao et al., 2007, 2008). As the tumor grows, the changes in mechanical properties of the microenvironment continuously contribute to cancer progression and malignancy. Numerous studies have documented that stiff substrates activate YAP/TAZ to increase primary cancer cell growth, migration, and chemotherapy resistance (Lin et al., 2015; Chakraborty et al., 2017; Foster et al., 2017; Meng et al., 2018; Santinon et al., 2018; Molina et al., 2019; Ghasemi et al., 2020; Liu et al., 2020; Qin et al., 2020). Furthermore, it has been shown that Ras signaling-mediated oncogenic transformation of normal cells requires a stiff and/or fibrotic microenvironment (Meng et al., 2018; Panciera et al., 2020).
Mechanistically, the ECM-activated YAP/TAZ regulates the expression of various cytoskeletal regulators in cancer-associated fibroblasts and increases intracellular isometric tension, thus forming a forward feedback loop to enhance the tumor microenvironmental rigidity and support cancer cell growth and invasion (Calvo et al., 2013; Foster et al., 2017). It is speculated that YAP/TAZ hyperactivation is required for cancer cells to overdrive the mechanical checkpoints for growth (Aragona et al., 2013). In addition to cancer cells and cancer-associated fibroblasts, ECM stiffening during tumor progression induces vascular cell growth and allows blood vessel infiltration, potentially through the Agrin-YAP-dependent mechanism (Chakraborty et al., 2020). Indeed, it has recently been shown that the increases in tissue rigidity at metastatic sites enhance cancer angiogenesis and elevate their resistance to anti-angiogenesis therapy (Shen et al., 2020).
Cancer cells migrate and metastasize in blood and lymphatic vessels. Therefore, it would be important to know the flow regulation of YAP/TAZ in cancer cells. It has been reported that fluid shear stress induces YAP/TAZ activation to promote cancer cell migration and proliferation (Lee et al., 2017, 2018; Qin et al., 2019), suggesting a causative role of YAP/TAZ activation in cancer metastasis. In this regard, modulation of Hippo-YAP/TAZ signaling may represent a novel strategy for anti-metastasis therapies. In addition, the role of YAP/TAZ-mediated mechanosensing in tumorigenesis may be more complex and multi-functions in context-dependent manners and therefore worth further exploring. For example, a recent study in mice showed that activation of the YAP/TAZ in peritumoral normal hepatocytes suppressed liver tumor growth via cell competition mechanism against tumor cells (Moya et al., 2019). It would be important to elucidate the exact roles of YAP-dependent mechanoresponses in physical interaction between normal and tumor cells in future studies.
Conclusion
Research in the last decade has established an indispensable role of the Hippo-YAP pathway in mechanobiology of tissue growth and homeostasis, although more research is still needed to finely define biological roles and molecular mechanisms for specific pathogenesis processes. Many studies have implicated that the Hippo pathway may serve as a signaling integration hub through interpreting both mechanical and biochemical cues. One recent study worth particularly mentioning is from Barry Thompson group. They reported that mechanically induced Yki nuclear shuttling requires growth factors-activated PI3K-AKT signaling in Drosophila. This coordination of the force-regulated Yki signaling and the PI3K-AKT signaling couples cell polarity and tissue mechanics to nutritional cues in tissue growth control (Borreguero-Muñoz et al., 2019). Future investigation into the crosstalk between YAP-mediated mechanotransduction and biochemical cues in the disease microenvironment will be crucial for us to obtain a more comprehensive knowledge of pathogenesis associated with dysregulated tissue mechanics. Moreover, it will be also important to understand how the Hippo-YAP pathway works in concert with other mechanosensing mechanisms (e.g., KLFs, MRTF-SRF, TWIST, and β-catenin) to generate a singular but context-specific mechanoresponse in cells. Furthermore, as industrial and academic efforts are emerging for developing YAP-targeting agents, testing such agents in animal models of diseases associated with mechano-dysregulation will likely lead to novel therapies.
Author Contributions
XC, K-CW, and ZM worked together to conceive and draft the manuscript. All authors contributed to the article and approved the submitted version.
Funding
ZM was supported by a start-up funding from the University of Miami Miller School of Medicine and Sylvester Comprehensive Cancer Center. The study was supported in part by a NIH Grant HL135416 (to K-CW).
Conflict of Interest
The authors declare that the research was conducted in the absence of any commercial or financial relationships that could be construed as a potential conflict of interest.
Acknowledgments
We thank the colleagues who did proofreading for our manuscript.
References
Alsamman, S., Christenson, S. A., Yu, A., Ayad, N. M. E., Mooring, M. S., Segal, J. M., et al. (2020). Targeting acid ceramidase inhibits YAP/TAZ signaling to reduce fibrosis in mice. Sci. Transl. Med. 12:eaay8798.
Aragona, M., Panciera, T., Manfrin, A., Giulitti, S., Michielin, F., Elvassore, N., et al. (2013). A mechanical checkpoint controls multicellular growth through YAP/TAZ regulation by actin-processing factors. Cell 154, 1047–1059. doi: 10.1016/j.cell.2013.07.042
Bae, Y. H., Mui, K. L., Hsu, B. Y., Liu, S. L., Cretu, A., Razinia, Z., et al. (2014). A FAK-Cas-Rac-lamellipodin signaling module transduces extracellular matrix stiffness into mechanosensitive cell cycling. Sci. Signal 7:ra57. doi: 10.1126/scisignal.2004838
Benham-Pyle, B. W., Pruitt, B. L., and Nelson, W. J. (2015). Cell adhesion. Mechanical strain induces E-cadherin-dependent Yap1 and beta-catenin activation to drive cell cycle entry. Science 348, 1024–1027. doi: 10.1126/science.aaa4559
Bertero, T., Cottrill, K. A., Annis, S., Bhat, B., Gochuico, B. R., Osorio, J. C., et al. (2015a). A YAP/TAZ-miR-130/301 molecular circuit exerts systems-level control of fibrosis in a network of human diseases and physiologic conditions. Sci. Rep. 5:18277.
Bertero, T., Cottrill, K. A, Lu, Y., Haeger, C., Dieffenbach, P., Annis, S., et al. (2015b). Matrix remodeling promotes pulmonary hypertension through feedback mechanoactivation of the YAP/TAZ-miR-130/301 circuit. Cell Rep. 13, 1016–1032. doi: 10.1016/j.celrep.2015.09.049
Bertero, T., Oldham, W. M., Cottrill, K. A., Pisano, S., Vanderpool, R. R., Yu, Q., et al. (2016). Vascular stiffness mechanoactivates YAP/TAZ-dependent glutaminolysis to drive pulmonary hypertension. J. Clin. Invest. 126, 3313–3335. doi: 10.1172/jci86387
Bonnans, C., Chou, J., and Werb, Z. (2014). Remodelling the extracellular matrix in development and disease. Nat. Rev. Mol. Cell Biol. 15, 786–801. doi: 10.1038/nrm3904
Borreguero-Muñoz, N., Fletcher, G., Aguilar-Aragon, M., Elbediwy, A., Vincent-Mistiaen, Z., and Thompson, B. (2019). The hippo pathway integrates PI3K-Akt signals with mechanical and polarity cues to control tissue growth. PLoS Biol. 17:e3000509. doi: 10.1371/journal.pbio.3000509
Byun, J., Del Re, D. P., Zhai, P., Ikeda, S., Shirakabe, A., Mizushima, W., et al. (2019). Yes-associated protein (YAP) mediates adaptive cardiac hypertrophy in response to pressure overload. J. Biol. Chem. 294, 3603–3617. doi: 10.1074/jbc.ra118.006123
Calvo, F., Ege, N., Grande-Garcia, A., Hooper, S., Jenkins, R. P., Chaudhry, S. I., et al. (2013). Mechanotransduction and YAP-dependent matrix remodelling is required for the generation and maintenance of cancer-associated fibroblasts. Nat. Cell Biol. 15, 637–646. doi: 10.1038/ncb2756
Chakraborty, S., Njah, K., and Hong, W. (2020). Agrin mediates angiogenesis in the tumor microenvironment. Trends Cancer 6, 81–85. doi: 10.1016/j.trecan.2019.12.002
Chakraborty, S., Njah, K., Pobbati, A. V., Lim, Y. B., Raju, A., Lakshmanan, M., et al. (2017). Agrin as a mechanotransduction signal regulating YAP through the hippo pathway. Cell Rep. 18, 2464–2479. doi: 10.1016/j.celrep.2017.02.041
Chang, L., Azzolin, L., Di Biagio, D., Zanconato, F., Battilana, G., Lucon Xiccato, R., et al. (2018). The SWI/SNF complex is a mechanoregulated inhibitor of YAP and TAZ. Nature 563, 265–269. doi: 10.1038/s41586-018-0658-1
Chen, Y., Ju, L., Rushdi, M., Ge, C., and Zhu, C. (2017). Receptor-mediated cell mechanosensing. Mol. Biol. Cell 28, 3134–3155.
Codelia, V. A., Sun, G., and Irvine, K. D. (2014). Regulation of YAP by mechanical strain through Jnk and Hippo signaling. Curr. Biol. 24, 2012–2017. doi: 10.1016/j.cub.2014.07.034
Cui, Y., Hameed, F. M., Yang, B., Lee, K., Pan, C. Q., Park, S., et al. (2015). Cyclic stretching of soft substrates induces spreading and growth. Nat. Commun. 6:6333.
Dahl, K., Ribeiro, A., and Lammerding, J. (2008). Nuclear shape, mechanics, and mechanotransduction. Circ. Res. 102, 1307–1318. doi: 10.1161/circresaha.108.173989
Dasgupta, I., and McCollum, D. (2019). Control of cellular responses to mechanical cues through YAP/TAZ regulation. J. Biol. Chem. 294, 17693–17706.
Del Re, D. P., Yang, Y., Nakano, N., Cho, J., Zhai, P., and Yamamoto, T., et al. (2013). Yes-associated protein isoform 1 (Yap1) promotes cardiomyocyte survival and growth to protect against myocardial ischemic injury. J. Biol. Chem. 288, 3977–3988.
Deng, H., Wang, W., Yu, J., Zheng, Y., Qing, Y., and Pan, D. (2015). Spectrin regulates Hippo signaling by modulating cortical actomyosin activity. eLife 4:e06567.
Deng, H., Yang, L., Wen, P., Lei, H., Blount, P., and Pan, D. (2020). Spectrin couples cell shape, cortical tension, and Hippo signaling in retinal epithelial morphogenesis. J. Cell Biol. 219:e201907018.
Deng, Y., Lu, J., Li, W., Wu, A., Zhang, X., Tong, W., et al. (2018). Reciprocal inhibition of YAP/TAZ and NF-κB regulates osteoarthritic cartilage degradation. Nat. Commun. 9:4564.
Dey, A., Varelas, X., and Guan, K. L. (2020). Targeting the Hippo pathway in cancer, fibrosis, wound healing and regenerative medicine. Nat. Rev. Drug Discov. 19, 480–494. doi: 10.1038/s41573-020-0070-z
Discher, D. E., Janmey, P., and Wang, Y. L. (2005). Tissue cells feel and respond to the stiffness of their substrate. Science 310, 1139–1143. doi: 10.1126/science.1116995
Driscoll, T. P., Cosgrove, B. D., Heo, S. J., Shurden, Z. E., and Mauck, R. L. (2015). Cytoskeletal to nuclear strain transfer regulates YAP signaling in mesenchymal stem cells. Biophys. J. 108, 2783–2793. doi: 10.1016/j.bpj.2015.05.010
Dupont, S., Morsut, L., Aragona, M., Enzo, E., Giulitti, S., Cordenonsi, M., et al. (2011). Role of YAP/TAZ in mechanotransduction. Nature 474, 179–183.
Elosegui-Artola, A., Andreu, I., Beedle, A. E. M., Lezamiz, A., Uroz, M., Kosmalska, A. J., et al. (2017). Force triggers YAP nuclear entry by regulating transport across nuclear pores. Cell 171, 1397–1410.e14.
Engler, A. J., Sen, S., Sweeney, H. L., and Discher, D. E. (2006). Matrix elasticity directs stem cell lineage specification. Cell 126, 677–689. doi: 10.1016/j.cell.2006.06.044
Enyedi, B., Jelcic, M., and Niethammer, P. (2016). The cell nucleus serves as a mechanotransducer of tissue damage-induced inflammation. Cell 165, 1160–1170. doi: 10.1016/j.cell.2016.04.016
Figeac, N., Mohamed, A. D., Sun, C., Schonfelder, M., Matallanas, D., Garcia-Munoz, A., et al. (2019). VGLL3 operates via TEAD1, TEAD3 and TEAD4 to influence myogenesis in skeletal muscle. J. Cell Sci. 132:jcs225946.
Fletcher, G., Elbediwy, A., Khanal, I., Ribeiro, P., Tapon, N., and Thompson, B. (2015). The spectrin cytoskeleton regulates the Hippo signalling pathway. EMBO J. 34, 940–954. doi: 10.15252/embj.201489642
Fletcher, G. C., Diaz-De-La-Loza, M. D., Borreguero-Munoz, N., Holder, M., Aguilar-Aragon, M., and Thompson, B. J. (2018). Mechanical strain regulates the Hippo pathway in Drosophila. Development 145:dev159467.
Foster, C. T., Gualdrini, F., and Treisman, R. (2017). Mutual dependence of the MRTF-SRF and YAP-TEAD pathways in cancer-associated fibroblasts is indirect and mediated by cytoskeletal dynamics. Genes Dev. 31, 2361–2375. doi: 10.1101/gad.304501.117
Frank, S. R., Kollmann, C. P., Luong, P., Galli, G. G., Zou, L., Bernards, A., et al. (2018). p190 RhoGAP promotes contact inhibition in epithelial cells by repressing YAP activity. J. Cell Biol. 217, 3183–3201. doi: 10.1083/jcb.201710058
Garoffolo, G., and Pesce, M. (2019). Mechanotransduction in the cardiovascular system: from developmental origins to homeostasis and pathology. Cells 8:1607. doi: 10.3390/cells8121607
Ghasemi, H., Mousavibahar, S. H., Hashemnia, M., Karimi, J., Khodadadi, I., Mirzaei, F., et al. (2020). Tissue stiffness contributes to YAP activation in bladder cancer patients undergoing transurethral resection. Ann. N. Y. Acad. Sci. 1473, 48–61. doi: 10.1111/nyas.14358
Glyn-Jones, S., Palmer, A. J. R., Agricola, R., Price, A. J., Vincent, T. L., Weinans, H., et al. (2015). Osteoarthritis. Lancet 386, 376–387.
Gnimassou, O., Francaux, M., and Deldicque, L. (2017). Hippo pathway and skeletal muscle mass regulation in mammals: a controversial relationship. Front. Physiol. 8:190. doi: 10.3389/fphys.2017.00190
Gong, Y., Li, S. J., Liu, R., Zhan, J. F., Tan, C., Fang, Y. F., et al. (2019). Inhibition of YAP with siRNA prevents cartilage degradation and ameliorates osteoarthritis development. J. Mol. Med. 97, 103–114. doi: 10.1007/s00109-018-1705-y
Goodman, C. A., Dietz, J. M., Jacobs, B. L., Mcnally, R. M., You, J. S., and Hornberger, T. A. (2015). Yes-associated protein is up-regulated by mechanical overload and is sufficient to induce skeletal muscle hypertrophy. FEBS Lett. 589, 1491–1497. doi: 10.1016/j.febslet.2015.04.047
Gouveia, R., Lepert, G., Gupta, S., Mohan, R., Paterson, C., and Connon, C. (2019). Assessment of corneal substrate biomechanics and its effect on epithelial stem cell maintenance and differentiation. Nat. Commun. 10:1496.
Haak, A. J., Kostallari, E., Sicard, D., Ligresti, G., Choi, K. M., Caporarello, N., et al. (2019). Selective YAP/TAZ inhibition in fibroblasts via dopamine receptor D1 agonism reverses fibrosis. Sci. Transl. Med. 11:eaau6296. doi: 10.1126/scitranslmed.aau6296
Hanahan, D., and Weinberg, R. A. (2011). Hallmarks of cancer: the next generation. Cell 144, 646–674. doi: 10.1016/j.cell.2011.02.013
Harvey, K. F., Pfleger, C. M., and Hariharan, I. K. (2003). The Drosophila Mst ortholog, hippo, restricts growth and cell proliferation and promotes apoptosis. Cell 114, 457–467. doi: 10.1016/s0092-8674(03)00557-9
Hoeper, M., Bogaard, H., Condliffe, R., Frantz, R., Khanna, D., Kurzyna, M., et al. (2013). Definitions and diagnosis of pulmonary hypertension. J. Am. Coll. Cardiol. 62, D42–D50.
Hoffman, B. D., Grashoff, C., and Schwartz, M. A. (2011). Dynamic molecular processes mediate cellular mechanotransduction. Nature 475, 316–323. doi: 10.1038/nature10316
Hoffman, L. M., Smith, M. A., Jensen, C. C., Yoshigi, M., Blankman, E., Ullman, K. S., et al. (2020). Mechanical stress triggers nuclear remodeling and the formation of transmembrane actin nuclear lines with associated nuclear pore complexes. Mol. Biol. Cell 31, 1774–1787. doi: 10.1091/mbc.e19-01-0027
Ingber, D. E. (2006). Cellular mechanotransduction: putting all the pieces together again. FASEB J. 20, 811–827. doi: 10.1096/fj.05-5424rev
Iyer, S. R., Shah, S. B., Ward, C. W., Stains, J. P., Spangenburg, E. E., Folker, E. S., et al. (2019). Differential YAP nuclear signaling in healthy and dystrophic skeletal muscle. Am. J. Physiol. Cell Physiol. 317, C48–C57.
Kechagia, J., Ivaska, J., and Roca-Cusachs, P. (2019). Integrins as biomechanical sensors of the microenvironment. Nat Rev. Mol. Cell Biol. 20, 457–473.
Kim, C. L., Choi, S. H., and Mo, J. S. (2019). Role of the Hippo pathway in fibrosis and cancer. Cells 8:468.
Kim, N. G., Koh, E., Chen, X., and Gumbiner, B. M. (2011). E-cadherin mediates contact inhibition of proliferation through Hippo signaling-pathway components. Proc. Natl. Acad. Sci. U.S.A. 108, 11930–11935. doi: 10.1073/pnas.1103345108
Koushki, N., Ghagre, A., Srivastava, L. K., Sitaras, C., Yoshie, H., Molter, C., et al. (2020). Lamin A redistribution mediated by nuclear deformation determines dynamic localization of YAP. bioRxiv [Preprint]. doi: 10.1101/2020.03.19.998708
Kumar, A., Placone, J. K., and Engler, A. J. (2017). Understanding the extracellular forces that determine cell fate and maintenance. Development 144, 4261–4270. doi: 10.1242/dev.158469
Lampi, M. C., and Reinhart-King, C. A. (2018). Targeting extracellular matrix stiffness to attenuate disease: from molecular mechanisms to clinical trials. Sci. Transl. Med. 10:eaao0475. doi: 10.1126/scitranslmed.aao0475
Lee, H. J., Diaz, M. F., Price, K. M., Ozuna, J. A., Zhang, S., Sevick-Muraca, E. M., et al. (2017). Fluid shear stress activates YAP1 to promote cancer cell motility. Nat. Commun. 8:14122.
Lee, H. J., Ewere, A., Diaz, M. F., and Wenzel, P. L. (2018). TAZ responds to fluid shear stress to regulate the cell cycle. Cell Cycle 17, 147–153. doi: 10.1080/15384101.2017.1404209
Li, B., He, J., Lv, H., Liu, Y., Lv, X., Zhang, C., et al. (2019). c-Abl regulates YAPY357 phosphorylation to activate endothelial atherogenic responses to disturbed flow. J. Clin. Invest. 129, 1167–1179. doi: 10.1172/jci122440
Li, Q., Li, S., Mana-Capelli, S., Roth Flach, R., Danai, L., Amcheslavsky, A., et al. (2014). The conserved misshapen-warts-Yorkie pathway acts in enteroblasts to regulate intestinal stem cells in Drosophila. Dev. Cell 31, 291–304. doi: 10.1016/j.devcel.2014.09.012
Li, Q., Nirala, N. K., Nie, Y., Chen, H. J., Ostroff, G., Mao, J., et al. (2018). Ingestion of food particles regulates the mechanosensing misshapen-Yorkie pathway in Drosophila intestinal growth. Dev. Cell 45, 433–449.e6.
Li, Y., Zhou, H., Li, F., Chan, S. W., Lin, Z., Wei, Z., et al. (2015). Angiomotin binding-induced activation of Merlin/NF2 in the Hippo pathway. Cell Res. 25, 801–817. doi: 10.1038/cr.2015.69
Liang, M., Yu, M., Xia, R., Song, K., Wang, J., Luo, J., et al. (2017). Yap/Taz deletion in Gli(+) cell-derived myofibroblasts attenuates fibrosis. J. Am. Soc. Nephrol. 28, 3278–3290. doi: 10.1681/asn.2015121354
Lin, C. H., Pelissier, F. A., Zhang, H., Lakins, J., Weaver, V. M., Park, C., et al. (2015). Microenvironment rigidity modulates responses to the HER2 receptor tyrosine kinase inhibitor lapatinib via YAP and TAZ transcription factors. Mol. Biol. Cell 26, 3946–3953. doi: 10.1091/mbc.e15-07-0456
Liu, F., Lagares, D., Choi, K., Stopfer, L., Marinković, A., Vrbanac, V., et al. (2015). Mechanosignaling through YAP and TAZ drives fibroblast activation and fibrosis. Am. J. Physiol. Lung Cell. Mol. Physiol. 308, L344–L357.
Liu, Q. P., Luo, Q., Deng, B., Ju, Y., and Song, G. B. (2020). Stiffer matrix accelerates migration of hepatocellular carcinoma cells through enhanced aerobic glycolysis via the MAPK-YAP signaling. Cancers 12:490. doi: 10.3390/cancers12020490
Liu, X., Yang, N., Figel, S., Wilson, K., Morrison, C., Gelman, I., et al. (2013). PTPN14 interacts with and negatively regulates the oncogenic function of YAP. Oncogene 32, 1266–1273. doi: 10.1038/onc.2012.147
Ma, S., Meng, Z., Chen, R., and Guan, K. L. (2019). The Hippo pathway: biology and -pathophysiology. Annu. Rev. Biochem. 88, 577–604. doi: 10.1146/annurev-biochem-013118-111829
Maurer, M., and Lammerding, J. (2019). The driving force: nuclear mechanotransduction in cellular function, fate, and disease. Annu. Rev. Biomed. Eng. 21, 443–468. doi: 10.1146/annurev-bioeng-060418-052139
Meng, Z., Moroishi, T., and Guan, K. L. (2016). Mechanisms of Hippo pathway regulation. Genes Dev. 30, 1–17. doi: 10.1101/gad.274027.115
Meng, Z., Qiu, Y., Lin, K. C., Kumar, A., Placone, J. K., Fang, C., et al. (2018). RAP2 mediates mechanoresponses of the Hippo pathway. Nature 560, 655–660. doi: 10.1038/s41586-018-0444-0
Misra, J. R., and Irvine, K. D. (2018). The Hippo signaling network and its biological functions. Annu. Rev. Genet. 52, 65–87. doi: 10.1146/annurev-genet-120417-031621
Mohammadi, H., and Sahai, E. (2018). Mechanisms and impact of altered tumour mechanics. Nat. Cell Biol. 20, 766–774. doi: 10.1038/s41556-018-0131-2
Molina, E. R., Chim, L. K., Salazar, M. C., Mehta, S. M., Menegaz, B. A., Lamhamedi-Cherradi, S. E., et al. (2019). Mechanically tunable coaxial electrospun models of YAP/TAZ mechanoresponse and IGF-1R activation in osteosarcoma. Acta Biomater. 100, 38–51. doi: 10.1016/j.actbio.2019.09.029
Moreno-Vicente, R., Pavon, D. M., Martin-Padura, I., Catala-Montoro, M., Diez-Sanchez, A., Quilez-Alvarez, A., et al. (2018). Caveolin-1 modulates mechanotransduction responses to substrate stiffness through actin-dependent control of YAP. Cell Rep. 25, 1622–1635.e6.
Moya, I. M., Castaldo, S. A., Van Den Mooter, L., Soheily, S., Sansores-Garcia, L., Jacobs, J., et al. (2019). Peritumoral activation of the Hippo pathway effectors YAP and TAZ suppresses liver cancer in mice. Science 366, 1029–1034. doi: 10.1126/science.aaw9886
Moya, I. M., and Halder, G. (2019). Hippo-YAP/TAZ signalling in organ regeneration and regenerative medicine. Nat. Rev. Mol. Cell Biol. 20, 211–226. doi: 10.1038/s41580-018-0086-y
Myers, K. A., Applegate, K. T., Danuser, G., Fischer, R. S., and Waterman, C. M. (2011). Distinct ECM mechanosensing pathways regulate microtubule dynamics to control endothelial cell branching morphogenesis. J. Cell Biol. 192, 321–334. doi: 10.1083/jcb.201006009
Nakajima, H., Yamamoto, K., Agarwala, S., Terai, K., Fukui, H., Fukuhara, S., et al. (2017). Flow-dependent endothelial YAP regulation contributes to vessel maintenance. Dev. Cell 40, 523–536.e6.
Nakamura, M., and Sadoshima, J. (2018). Mechanisms of physiological and pathological cardiac hypertrophy. Nat. Rev. Cardiol. 15, 387–407. doi: 10.1038/s41569-018-0007-y
Nia, H., Munn, L., and Jain, R. (2020). Physical traits of cancer. Science 370:eaaz0868. doi: 10.1126/science.aaz0868
Niu, N., Xu, S., Xu, Y., Little, P., and Jin, Z. (2019). Targeting mechanosensitive transcription factors in atherosclerosis. Trends Pharmacol. Sci. 40, 253–266. doi: 10.1016/j.tips.2019.02.004
Noguchi, S., Saito, A., and Nagase, T. (2018). YAP/TAZ signaling as a molecular link between fibrosis and cancer. Int J. Mol. Sci. 19:3674. doi: 10.3390/ijms19113674
Ouyang, H., Luong, P., Frodin, M., and Hansen, S. H. (2020). p190A RhoGAP induces CDH1 expression and cooperates with E-cadherin to activate LATS kinases and suppress tumor cell growth. Oncogene 39, 5570–5587. doi: 10.1038/s41388-020-1385-2
Owens, D. J., Messeant, J., Moog, S., Viggars, M., Ferry, A., Mamchaoui, K., et al. (2020). Lamin-related congenital muscular dystrophy alters mechanical signaling and skeletal muscle growth. Int. J. Mol. Sci. 22:306. doi: 10.3390/ijms22010306
Pagliari, S., Vinarsky, V., Martino, F., Perestrelo, A., Oliver De La Cruz, J., Caluori, G., et al. (2021). YAP-TEAD1 control of cytoskeleton dynamics and intracellular tension guides human pluripotent stem cell mesoderm specification. Cell Death Differ. 28, 1193–1207. doi: 10.1038/s41418-020-00643-5
Panciera, T., Citron, A., Di Biagio, D., Battilana, G., Gandin, A., Giulitti, S., et al. (2020). Reprogramming normal cells into tumour precursors requires ECM stiffness and oncogene-mediated changes of cell mechanical properties. Nat. Mater. 19, 797–806. doi: 10.1038/s41563-020-0615-x
Pantalacci, S., Tapon, N., and Leopold, P. (2003). The salvador partner Hippo promotes apoptosis and cell-cycle exit in Drosophila. Nat. Cell Biol. 5, 921–927. doi: 10.1038/ncb1051
Paszek, M. J., Zahir, N., Johnson, K. R., Lakins, J. N., Rozenberg, G. I., Gefen, A., et al. (2005). Tensional homeostasis and the malignant phenotype. Cancer Cell 8, 241–254. doi: 10.1016/j.ccr.2005.08.010
Pavel, M., Renna, M., Park, S. J., Menzies, F. M., Ricketts, T., Fullgrabe, J., et al. (2018). Contact inhibition controls cell survival and proliferation via YAP/TAZ-autophagy axis. Nat. Commun. 9:2961.
Qin, X., Li, J., Sun, J., Liu, L., Chen, D., and Liu, Y. (2019). Low shear stress induces ERK nuclear localization and YAP activation to control the proliferation of breast cancer cells. Biochem. Biophys. Res. Commun. 510, 219–223. doi: 10.1016/j.bbrc.2019.01.065
Qin, X., Lv, X., Li, P., Yang, R., Xia, Q., Chen, Y., et al. (2020). Matrix stiffness modulates ILK-mediated YAP activation to control the drug resistance of breast cancer cells. Biochim. Biophys. Acta Mol. Basis Dis. 1866:165625. doi: 10.1016/j.bbadis.2019.165625
Rausch, V., Bostrom, J. R., Park, J., Bravo, I. R., Feng, Y., Hay, D. C., et al. (2019). The Hippo pathway regulates caveolae expression and mediates flow response via caveolae. Curr. Biol. 29, 242–255.e6.
Rockey, D. C., Bell, P. D., and Hill, J. A. (2015). Fibrosis–a common pathway to organ injury and failure. N. Engl. J. Med. 372, 1138–1149. doi: 10.1056/nejmra1300575
Ruwhof, C., and van der Laarse, A. (2000). Mechanical stress-induced cardiac hypertrophy: mechanisms and signal transduction pathways. Cardiovasc. Res. 47, 23–37. doi: 10.1016/s0008-6363(00)00076-6
Santinon, G., Brian, I., Pocaterra, A., Romani, P., Franzolin, E., Rampazzo, C., et al. (2018). dNTP metabolism links mechanical cues and YAP/TAZ to cell growth and oncogene-induced senescence. EMBO J. 37:e97780.
Sheetz, M. (2019). A tale of two states: normal and transformed, with and without rigidity sensing. Annu. Rev. Cell Dev. Biol. 35, 169–190. doi: 10.1146/annurev-cellbio-100818-125227
Shen, Y., Wang, X., Lu, J., Salfenmoser, M., Wirsik, N. M., Schleussner, N., et al. (2020). Reduction of liver metastasis stiffness improves response to bevacizumab in metastatic colorectal cancer. Cancer Cell 37, 800–817.e7.
Stearns-Reider, K., D’amore, A., Beezhold, K., Rothrauff, B., Cavalli, L., Wagner, W., et al. (2017). Aging of the skeletal muscle extracellular matrix drives a stem cell fibrogenic conversion. Aging Cell 16, 518–528. doi: 10.1111/acel.12578
Thenappan, T., Chan, S. Y., and Weir, E. K. (2018). Role of extracellular matrix in the pathogenesis of pulmonary arterial hypertension. Am. J. Physiol. Heart Circ. Physiol. 315, H1322–H1331.
Thorup, A. S., Strachan, D., Caxaria, S., Poulet, B., Thomas, B. L., Eldridge, S. E., et al. (2020). ROR2 blockade as a therapy for osteoarthritis. Sci. Transl. Med. 12:eaax3063.
Tranchant, R., Quetel, L., Tallet, A., Meiller, C., Renier, A., De Koning, L., et al. (2017). Co-occurring mutations of tumor suppressor genes, LATS2 and NF2, in malignant pleural mesothelioma. Clin. Cancer Res. 23, 3191–3202. doi: 10.1158/1078-0432.ccr-16-1971
Tschumperlin, D., Ligresti, G., Hilscher, M., and Shah, V. (2018). Mechanosensing and fibrosis. J. Clin. Invest. 128, 74–84.
Udan, R. S., Kango-Singh, M., Nolo, R., Tao, C., and Halder, G. (2003). Hippo promotes proliferation arrest and apoptosis in the Salvador/Warts pathway. Nat. Cell Biol. 5, 914–920.
Wada, K., Itoga, K., Okano, T., Yonemura, S., and Sasaki, H. (2011). Hippo pathway regulation by cell morphology and stress fibers. Development 138, 3907–3914. doi: 10.1242/dev.070987
Wang, K., Yeh, Y., Nguyen, P., Limqueco, E., Lopez, J., Thorossian, S., et al. (2016). Flow-dependent YAP/TAZ activities regulate endothelial phenotypes and atherosclerosis. Proc. Natl. Acad. Sci. U.S.A. 113, 11525–11530. doi: 10.1073/pnas.1613121113
Wang, L., Luo, J. Y., Li, B., Tian, X. Y., Chen, L. J., Huang, Y., et al. (2016). Integrin-YAP/TAZ-JNK cascade mediates atheroprotective effect of unidirectional shear flow. Nature 540, 579–582. doi: 10.1038/nature20602
Wang, N., Tytell, J. D., and Ingber, D. E. (2009). Mechanotransduction at a distance: mechanically coupling the extracellular matrix with the nucleus. Nat. Rev. Mol. Cell Biol. 10, 75–82. doi: 10.1038/nrm2594
Wang, W., Huang, J., Wang, X., Yuan, J., Li, X., Feng, L., et al. (2012). PTPN14 is required for the density-dependent control of YAP1. Genes Dev. 26, 1959–1971. doi: 10.1101/gad.192955.112
Wang, Y., Cao, W., Cui, J., Yu, Y., Zhao, Y., Shi, J., et al. (2018). Arterial wall stress induces phenotypic switching of arterial smooth muscle cells in vascular remodeling by activating the YAP/TAZ signaling pathway. Cell Physiol. Biochem. 51, 842–853. doi: 10.1159/000495376
Wei, S. C., Fattet, L., Tsai, J. H., Guo, Y., Pai, V. H., Majeski, H. E., et al. (2015). Matrix stiffness drives epithelial-mesenchymal transition and tumour metastasis through a TWIST1-G3BP2 mechanotransduction pathway. Nat. Cell Biol. 17, 678–688. doi: 10.1038/ncb3157
Wells, R. G. (2008). The role of matrix stiffness in regulating cell behavior. Hepatology 47, 1394–1400. doi: 10.1002/hep.22193
Wong, K., Li, W., An, Y., Duan, Y., Li, Z., Kang, Y., et al. (2015). β-Spectrin regulates the hippo signaling pathway and modulates the basal actin network. J. Biol. Chem. 290, 6397–6407.
Wong, S. W., Lenzini, S., Cooper, M. H., Mooney, D. J., and Shin, J. W. (2020). Soft extracellular matrix enhances inflammatory activation of mesenchymal stromal cells to induce monocyte production and trafficking. Sci. Adv. 6:eaaw0158.
Wu, S., Huang, J., Dong, J., and Pan, D. (2003). Hippo encodes a Ste-20 family protein kinase that restricts cell proliferation and promotes apoptosis in conjunction with salvador and warts. Cell 114, 445–456. doi: 10.1016/s0092-8674(03)00549-x
Xiao, B. (2020). Levering mechanically activated piezo channels for potential pharmacological intervention. Annu. Rev. Pharmacol. Toxicol. 60, 195–218.
Xu, S., Koroleva, M., Yin, M., and Jin, Z. G. (2016). Atheroprotective laminar flow inhibits Hippo pathway effector YAP in endothelial cells. Transl. Res. 176, 18–28.e2.
Yamashiro, Y., Thang, B. Q., Ramirez, K., Shin, S. J., Kohata, T., and Ohata, S. (2020). Matrix mechanotransduction mediated by thrombospondin-1/integrin/YAP in the vascular remodeling. Proc. Natl. Acad. Sci. U.S.A. 117, 9896–9905. doi: 10.1073/pnas.1919702117
Yang, B., Wolfenson, H., Chung, V. Y., Nakazawa, N., Liu, S., Hu, J., et al. (2019). Stopping transformed cancer cell growth by rigidity sensing. Nat. Mater. 19, 239–250. doi: 10.1038/s41563-019-0507-0
Yang, C., Tibbitt, M. W., Basta, L., and Anseth, K. S. (2014). Mechanical memory and dosing influence stem cell fate. Nat. Mater. 13, 645–652. doi: 10.1038/nmat3889
Yu, Y., Su, X., Qin, Q., Hou, Y., Zhang, X., Zhang, H., et al. (2020). Yes-associated protein and transcriptional coactivator with PDZ-binding motif as new targets in cardiovascular diseases. Pharmacol. Res. 159:105009.
Yun, S., Hu, R., Schwaemmle, M. E., Scherer, A. N., Zhuang, Z., and Koleske, A. J., et al. (2019). Integrin alpha5beta1 regulates PP2A complex assembly through PDE4D in atherosclerosis. J. Clin. Invest. 129, 4863–4874.
Zhang, L., Noguchi, Y. T., Nakayama, H., Kaji, T., Tsujikawa, K., Ikemoto-Uezumi, M., et al. (2019). The CalcR-PKA-Yap1 axis is critical for maintaining quiescence in muscle stem cells. Cell Rep. 29, 2154–2163.e5.
Zhang, N., Bai, H., David, K. K., Dong, J., Zheng, Y., Cai, J., et al. (2010). The Merlin/NF2 tumor suppressor functions through the YAP oncoprotein to regulate tissue homeostasis in mammals. Dev Cell 19, 27–38. doi: 10.1016/j.devcel.2010.06.015
Zhang, Q., Fang, X., Zhao, W., and Liang, Q. (2019). The transcriptional coactivator YAP1 is overexpressed in osteoarthritis and promotes its progression by interacting with Beclin-1. Gene 689, 210–219. doi: 10.1016/j.gene.2018.11.068
Zhang, X., Cai, D., Zhou, F., Yu, J., Wu, X., Yu, D., et al. (2020). Targeting downstream subcellular YAP activity as a function of matrix stiffness with Verteporfin-encapsulated chitosan microsphere attenuates osteoarthritis. Biomaterials 232:119724. doi: 10.1016/j.biomaterials.2019.119724
Zhao, B., Wei, X., Li, W., Udan, R. S., Yang, Q., Kim, J., et al. (2007). Inactivation of YAP oncoprotein by the Hippo pathway is involved in cell contact inhibition and tissue growth control. Genes Dev. 21, 2747–2761. doi: 10.1101/gad.1602907
Zhao, B., Ye, X., Yu, J., Li, L., Li, W., Li, S., et al. (2008). TEAD mediates YAP-dependent gene induction and growth control. Genes Dev. 22, 1962–1971. doi: 10.1101/gad.1664408
Zheng, Y., and Pan, D. (2019). The Hippo signaling pathway in development and disease. Dev. Cell 50, 264–282. doi: 10.1016/j.devcel.2019.06.003
Keywords: YAP, TAZ, the Hippo pathway, mechanotransduction, ECM stiffness, stretch, flow shear, contact inhibition of cells
Citation: Cai X, Wang K-C and Meng Z (2021) Mechanoregulation of YAP and TAZ in Cellular Homeostasis and Disease Progression. Front. Cell Dev. Biol. 9:673599. doi: 10.3389/fcell.2021.673599
Received: 27 February 2021; Accepted: 30 April 2021;
Published: 24 May 2021.
Edited by:
Wenqi Wang, University of California, Irvine, United StatesReviewed by:
Vijaykumar S. Meli, University of California, Irvine, United StatesJung-Soon Mo, Ajou University, South Korea
Copyright © 2021 Cai, Wang and Meng. This is an open-access article distributed under the terms of the Creative Commons Attribution License (CC BY). The use, distribution or reproduction in other forums is permitted, provided the original author(s) and the copyright owner(s) are credited and that the original publication in this journal is cited, in accordance with accepted academic practice. No use, distribution or reproduction is permitted which does not comply with these terms.
*Correspondence: Kuei-Chun Wang, a3VlaS1jaHVuLndhbmdAYXN1LmVkdQ==; Zhipeng Meng, enhtMjgyQG1pYW1pLmVkdQ==