Cell Death and Exosomes Regulation After Myocardial Infarction and Ischemia-Reperfusion
- 1Department of Cardiovascular Surgery, The Second Xiangya Hospital, Central South University, Changsha, China
- 2Hunan Provincial Key Laboratory of Cardiovascular Research, Changsha, China
- 3Hunan Fangsheng Pharmaceutical Co., Ltd., Changsha, China
Cardiovascular disease (CVD) is the leading cause of death in the global population, accounting for about one-third of all deaths each year. Notably, with CVDs, myocardial damages result from myocardial infarction (MI) or cardiac arrhythmias caused by interrupted blood flow. Significantly, in the process of MI or myocardial ischemic-reperfusion (I/R) injury, both regulated and non-regulated cell death methods are involved. The critical factor for patients’ prognosis is the infarct area’s size, which determines the myocardial cells’ survival. Cell therapy for MI has been a research hotspot in recent years; however, exosomes secreted by cells have attracted much attention following shortcomings concerning immunogens. Exosomes are extracellular vesicles containing several biologically active substances such as lipids, nucleic acids, and proteins. New evidence suggests that exosomes play a crucial role in regulating cell death after MI as exosomes of various stem cells can participate in the cell damage process after MI. Hence, in the review herein, we focused on introducing various cell-derived exosomes to reduce cell death after MI by regulating the cell death pathway to understand myocardial repair mechanisms better and provide a reference for clinical treatment.
Introduction
Despite the considerable improvements in healthcare worldwide, acute myocardial infarction (MI) has long been the primary cause of death from coronary heart diseases (Roth et al., 2017). Endogenous cardiomyocytes have limited renewal potentials following MI, which leads to the irreversible loss of a significant number of cardiomyocytes, left ventricular remodeling, and progressive heart failure (Buja and Vela, 2008). Therefore, rescuing damaged cardiomyocytes becomes a potential strategy to preventing myocardial dysfunction and heart failure after MI.
Exosomes are small-membrane fragments with a diameter of 30–150 nm, secreted by almost all mammalian cells. Exosomes arise from a multivesicular endosomal pathway by fusion of multivesicular bodies (MVBs) with the plasma membrane, resulting in the release of vesicles as exosomes into the extracellular space. The generation of exosomes in MVBs can be divided into endosomal independent sorting complexes required for transport (ESCRT) and ESCRT-dependent mechanisms (Hurley, 2008; Stuffers et al., 2009). For example, cytoplasmic proteins’ isolation into exosomes may result from co-classification with other proteins, for which heat shock protein70 (HSP70) plays an essential role (Théry et al., 2001). RNAs may also be classified into exosomes with complex mechanisms, including binding ESCRT-II subcomplex to RNA (Irion and St Johnston, 2007) and the sumoylation of hnRNPA2B1 (Villarroya-Beltri et al., 2013). The secretion of exosomes also requires MVBs to fuse with the plasma membrane. The process may be mediated by SNARE proteins and members of the synaptotagmin family (Jahn and Scheller, 2006). Exosomes are characterized by lipid bilayers, annexin, GTPase, Rab, transmembrane, and non-membrane-bound proteins, flotillin proteins, and ESCRT components, which include Alix, HSPs, Tsg101, integrins, and transmembrane proteins (CD63, CD81, and CD82). Exosomes also express secretory cell markers. Besides, exosomes are also rich in RNA from different sources (Théry et al., 2002).
The study herein reviews the potential mechanism of cell-derived exosomes in repairing damaged cardiomyocytes and provides a reference point for future clinical treatment of patients with cardiac ischemic diseases. It should be noted that that exosome is a two-edged sword during medical therapy. Following MI, exosomes may simultaneously enhance blood vessels’ regeneration and damaged myocardium in the area around the infarcted site. The review aims to illustrate the therapeutic effect (anti-cell death) and potential molecular mechanism after myocardial ischemia.
Types of Cardiomyocyte Death and the Potential Mechanisms and Key Mediators of Cell Death in Cardiac Diseases
Following MI, cardiac I/R injury, heart failure, and other heart diseases, cell death can occur in regulated and non-regulated forms (Moe and Marín-García, 2016; Shekhar et al., 2018). Significantly, MI is caused by acute or chronic tissue deficiency of oxygen, nutrients, and growth factors. Although related imbalances following a series of MI could arise from several pathological cascades, the most common cause is acute or prolonged ischemia, with the rupture of coronary atherosclerotic plaques being the primary cause (Libby and Pasterkamp, 2015). In the 1990s, the implantation of thrombolysis or stents was considered an effective way to reduce the size of MI (Nabel and Braunwald, 2012). Although the potential risk of perfusion is still questionable after a few years, it is clear that the perfusion process will cause cell death via oxidative stress, calcium overload, and inflammation (Yellon and Hausenloy, 2007). Notwithstanding, it is difficult to assess the significant role ischemic duration and reperfusion play in the damaged myocytes. In most cases, genetic and pharmacological inhibition of cell death signaling reduces cardiomyocyte death and infarct size only in the context of myocardial ischemia-reperfusion. However, such changes are uncommon in permanent ischemia models. Remarkably, the primary reason will be the change from initially regulated cell death to unregulated cell death if the death stimulus persists (Del Re et al., 2019). Notably, extensive studies have been conducted on MI; it is still unclear which death process is dominant.
Morphological criteria consider that cell necrosis is the primary mode of myocardial cell death after MI. In contrast, apoptosis is considered to be the first regulated cell death process. Several studies have shown that the inhibition of apoptosis receptors and the reduction of mitochondrial apoptosis reduce apoptosis of cardiomyocytes and reduce the size of MII (Jeremias et al., 2000; Chen et al., 2001). Apart from apoptosis, studies show that necroptosis (Newton et al., 2016), pyroptosis (Kawaguchi et al., 2011), ferroptosis (Fang et al., 2019), parthanatos (Yang et al., 2000), autophagy-dependent cell death (Matsui et al., 2007), and mitochondrial-dependent necrosis (Baines et al., 2005) are all involved in cell death during MI. Thus, intervention is crucial as it can significantly mitigate the number of dead cardiomyocytes in peri-infarcted areas. These studies indicated that cardiomyocytes’ death during MI was not based on a specific individual death pathway.
Apoptosis
Apoptosis, a kind of programmed death, is a different way of cell death from necrosis Apoptotic cells undergo structural changes, including cell shrinkage, nuclear pyknosis, and fragmentation (Edinger and Thompson, 2004). Studies have shown that cardiomyocyte apoptosis begins after prolonged myocardial ischemia or reperfusion after short-term ischemia (Kajstura et al., 1996). Activation of pro-apoptotic factors and caspase can be detected in the absence of DNA breakage during ischemia, followed by a substantial increase during reperfusion, suggesting that the apoptotic cascade begins during ischemia but fully implemented during reperfusion (Lazou et al., 2006). Clinical studies have confirmed apoptotic cardiomyocytes in the marginal regions of an infarcted area within hours to days after acute infarction (Saraste et al., 1997).
Apoptosis is mediated by two well-defined pathways, extrinsic and intrinsic, both of which are activated in cardiomyocytes under pathophysiological conditions (Youle and Strasser, 2008). In the exogenous pathway, cell death is induced by the activation of death domain receptors on the cell membrane. It is triggered by Fas ligand or tumor necrosis factor (TNF)-α (Ashkenazi and Dixit, 1998). Increased expression of Fas and TNF-α is associated with increased apoptosis in cardiomyocytes in a model of I/R heart disease. Both Fas and TNF receptors have an intracellular death domain that recruits and activates caspase-8 in the cell membrane. The recruitment of caspase-8 then activates downstream caspases (including caspase-3) (Torre-Amione et al., 1996). Intrinsic pathways are activated by intracellular stress signals, such as hypoxia, oxidative stress, and DNA damage (Edinger and Thompson, 2004). The intrinsic pathway is regulated by the Bcl-2 protein family (Adams and Cory, 1998). Oligomerization of Bax and Bak in the Bcl-2 family results in pores formation, leading to the release of cytotoxic proteins from the mitochondria such as cytochrome C. Cytochrome C then activate procaspase-9, and the activated caspase-9 then cleaves and activates caspase-3 and caspase-7 (Adams and Cory, 1998).
Autophagy
Autophagy is an essential metabolic process where aging or damaged proteins and organelles are broken down into amino acids and fatty acids for energy generation and recycling (Cǎtanǎ et al., 2018). These metabolic processes are activated during nutrient deficiency or metabolic stress to maintain tissue function and dynamic balance (Dong et al., 2018). It has been proved that autophagy is essential for maintaining normal heart function (Zhang Q. Y. et al., 2018). Amongst scholars, it is believed that autophagy is an essential lysosome-dependent catabolic mechanism (Hewitt and Korolchuk, 2017). According to lysosomes’ transport mode and physiological function, autophagy is primarily divided into three types: macroautophagy, microautophagy, and chaperone-mediated autophagy (Zhang X. et al., 2018). Macroautophagy is the most widely studied form of autophagy. Nonetheless, over 30 autophagy-related genes (Atgs) and proteins have been found to participate in the process of autophagy (Díaz et al., 2017).
Presently, two classic signaling pathways from the entire autophagy signaling network have been described to inhibit or promote cell autophagy (Inoki et al., 2012; Shao et al., 2016). Type I PI3K-mammalian target of rapamycin (mTOR) signaling pathway is a classic inhibitory pathway. It is triggered under nutrient-rich conditions and stimulates mTOR and mTOR complex (MTORC1) via the protein kinase-B (also known as Akt) pathway, which then inhibits the formation of Atg1 complex (Kaur and Sharma, 2017). Another classic autophagy signaling pathway is induced by AMP-activated protein kinase (AMPK). AMPK is a sensor of stress and nutrient input. It activates ULK1 by inactivating mTORC1 or phosphorylating ULK1 at different residue serine kinase complexes to promote the autophagy process (Zhao et al., 2018). A study by Sciarretta et al. (2018) showed that autophagy plays a crucial role in inhibiting the occurrence and development of cardiovascular diseases such as MI, heart failure, and atherosclerosis. On the other hand, over-induction of the autophagy process may cause adverse effects to cells, the so-called “autophagy-dependent cell death” in the organism, which indicates the importance of controlling the degree of autophagy induction in disease treatment (Lambelet et al., 2018; Wang P. et al., 2018). Thus, autophagy seems to be a double-edged sword in the treatment of MI. Baseline autophagy can limit the death of cardiomyocytes, while excessive autophagy can aggravate the damage of cardiomyocytes. Demircan et al. (2018) also reported that patients with coronary heart disease or acute MI had an over-regulation of autophagy than the healthy controls. However, decreased autophagy and mitochondrial damage may lead to a weakened host’s response to hypoxic-ischemic injury, thereby adversely affecting cardiomyocytes (Ham and Raju, 2017). Laboratory research on rat models showed that autophagy could reduce the scope of acute MI after ligation of the left anterior descending artery (Aisa et al., 2017). Studies have shown that the basic autophagy process in cardiomyocytes is upregulated via the AMPK-mTOR signaling pathway, leading to reduced MI in animal models of acute MI (Foglio et al., 2017). As mentioned above, baseline autophagy or appropriately induced autophagy protects cardiomyocyte ATP production by maintaining cell homeostasis, degrading organelles or misfolded proteins, thereby protecting against ischemic injury. However, it is reported that under severe ischemia, excessive autophagy of the heart promotes cell death and worsens heart function (Li et al., 2018; Liu C. Y. et al., 2018). Interestingly, mitochondrial aldehyde dehydrogenase 2 (ALDH2) is an enzyme known to catalyze aldehyde oxidation. It can significantly promote the autophagy process during ischemia by activating AMPK and down-regulating mTOR, thereby producing cardioprotection. On the contrary, during reperfusion, ALDH2 can inhibit autophagy by activating Akt and mTOR, thereby protecting cardiomyocytes from cell death caused by hypoxia and reoxygenation (Ma et al., 2011). In summary, these studies all show that severe ischemia-induced excessive autophagy could aggravate acute MI.
Pyroptosis
Pyroptosis is a regulated form of cell death closely associated with innate immune responses, characterized by plasma membrane permeability and the extracellular release of inflammatory cytokines and rupture of the plasma membrane mediated by gasdermin-D (GSDMD) (Shi et al., 2017). Regards morphology, cell pyroptosis, combines the characteristics of necrosis and apoptosis, including the formation of necrotic cell membrane pores, cell swelling, and membrane rupture, resulting in cytoplasmic content leakage nuclear condensation, and DNA fragmentation during apoptosis. In contrast to apoptosis, pyroptosis does not involve releasing cytochromes, and the mitochondrial integrity is maintained (Fink and Cookson, 2006; Cervantes et al., 2008). In a typical pyrophosphate signaling pathway, pathogen-related molecular patterns (PAMPs) or risk-related molecular patterns (DAMPs) are detected by different inflammasomes, which are composed of nucleotide-binding oligomerization domain (NOD), NOD-like receptors (NLR) family (NLRP3, NLRP1, NLRC4, NLRP9, and NLRP6), PYHIN protein family, and pyrin protein composition. These activated inflammasomes trigger the activation of caspase-1, which eventually leads to pyroptosis (Heilig and Broz, 2018). To trigger pyrolysis, the signal domains of NLR, AIM2, and pyrin (such as PYD) bind to ASC, while ASC recruits and activates pro-caspase-1, producing active caspase-1 (Aachoui et al., 2013). Activated caspase-1 not only processes and maturates IL-1β/18 but also cleaves the GSDMD intermediate junction, releasing intramolecular inhibition of the gasdermin-N domain and inducing cell pyroptosis by forming a 10–15 nm diameter pore on the cell membrane (Shi et al., 2015; Ding et al., 2016).
In the atypical pyroptosis pathway, caspase-11 and caspase-4/5 are primarily involved and activated by cytoplasmic lipopolysaccharide (LPS), where the CARD domain recognizes the lipid part of LPS (Yang J. et al., 2015; Abu Khweek and Amer, 2020). Activated caspase-4/5/11 directly triggers pyrolysis and the release of IL-1α and HMGB1 after cutting the GSDMD-induced membrane pore formation and subsequent cell membrane rupture. Activated caspase-4/5/11 also indirectly processes IL-1β through the atypical NLRP 3/ASC/caspase-1 pathway, which is formed by GSDMD pore formation/K + efflux or through vascular wall protein-1 cleavage/ATP release/P2 × 7/K + release mediated (Kayagaki et al., 2015; Rühl and Broz, 2015).
MI is associated with a sterile inflammatory response that leads to white blood cell aggregation (Takahashi, 2010). The white blood cells that accumulate at the MI site, including neutrophils and macrophages, release inflammatory cytokines, chemokines, and proteases, further aggravating the inflammatory response after MI, promoting myocardial injury, and remodeling. This sterile inflammatory response may be mediated by Toll-like receptors (TLRs) and NLRs. NLR is an integral part of the inflammasome that mediates the release of IL-1β (Shi et al., 2015). Studies have shown that the NLRP3 inflammasome plays a vital role in MI (Toldo and Abbate, 2018). During MI, activation of NLRP3 is associated with the leakage of lysosomal cathepsin-B, induction of K + outflow, ROS production, and other mediators (Liu et al., 2017). Inflammatory bodies were observed in a mouse model of acute MI, with increased ASC, NLRP3, and Caspase-1 in scar tissue and the cytoplasm of adjacent myocardial cells in the infarction area (Mezzaroma et al., 2011). Besides, oxidative stress induced by MI can also cause cell pyroptosis. The inhibition of oxidative stress can reduce pyroptosis and decrease the activities of NF-κB and GSDMD. Inhibition of NF-κB also inhibits oxidative stress-regulated pyrogen death by reducing GSDMD (Lei et al., 2018). These results suggest that myocardial cell damage during MI is partly related to pyroptosis.
Ferroptosis
Ferroptosis is a form of regulatory cell death caused by iron-dependent lipid peroxidation (Stockwell et al., 2017). Among the members of the GPX family, Gpx4 is believed to specifically catalyze the reduction of lipid peroxides, thereby scavenging lipid reactive oxygen species (Stockwell et al., 2017). The absence of Gpx4 causes lipid peroxides and lipid ROS accumulation and in a 12/15-lipoxygenase (12/15LOX) dependent manner, leading to cell death (Seiler et al., 2008). On the other hand, the reduction of lipid peroxides by GPX4 requires the oxidation of glutathione. Glutathione is primarily synthesized by cysteine and inhibits the cystine-glutamate transporter (xCT), or cysteine deprivation will cause glutathione depletion, which will induce ferroptosis (Jiang et al., 2015). The recently discovered iron death inhibitor protein-1 (FSP1) (previously known as apoptosis-inducing factor mitochondria-2, AIFM2), an influential ferroptosis resistance factor, is believed to be a vital component of a non-mitochondrial CoQ antioxidant system, which is parallel to the typical glutathione-based GPX4 pathway anti-ferroptosis system.
Exosomes Reduce Cardiomyocyte Death After Myocardiac Injury
Recent studies have shown that the myocardial protective effect of the injected stem cells is not produced by direct differentiation itself but mediated by exosomes secreted from stem cells (Kishore and Khan, 2016; Jung et al., 2017). Thus, exosomes may be an effective alteration to overcome the shortcomings of cell therapy. Exosomes with significant cargos may merge their membrane contents into the recipient cell membrane and transport complex signal molecules into the recipient cell (Valadi et al., 2007). Exosomes play an essential role in cell-to-cell communication, regulating various cellular processes in target cells, including adjacent cells and cells in remote parts (Stoorvogel, 2012). Several studies have reported that stem cell-derived exosomes could be used to treat ischemic disease (Lai et al., 2010; Arslan et al., 2013; Ibrahim et al., 2014; Figure 1).
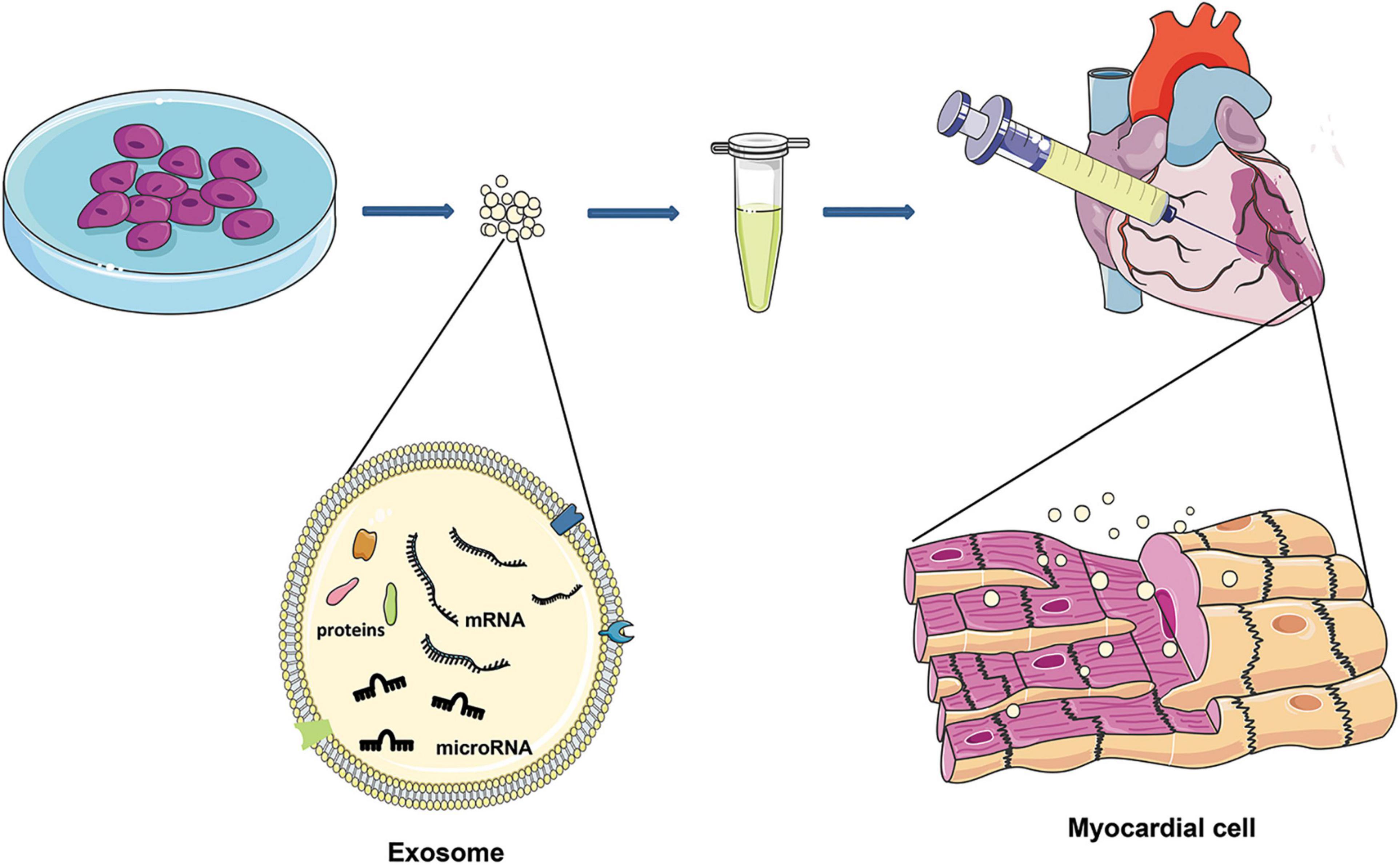
Figure 1. After MI, exosomes of different cell sources are injected around the infarction site, and exosomes can enter the damaged cardiomyocytes to reduce cardiomyocyte death. Exosomes are characterized by lipid bilayers, transmembrane and non-membrane binding proteins, and a high concentration of nucleic acids (DNA, mRNA, microRNA, and lncRNA).
Besides, exosome therapy has advantages where tumorigenesis and embolism caused by cell transplantation are significantly lower when using exosomes because they are much smaller than cells (Park K. S. et al., 2019). Also, the membrane of exosomes is primarily a lipid bilayer with fewer binding proteins. Thus, their immunogenicity is lower than that of cells and could reduce the recognition and phagocytosis of immune cells (Hood and Wickline, 2012). Moreover, with exosomes, there is (i) no self-metabolism and changes in the body temperature will not affect its biological activity where it could better exert its functional characteristics (Lai et al., 2013) and (ii) exosomes are lipid-bound nano-scale vesicles that can freely pass through the blood-brain barrier (Konala et al., 2016). Furthermore, due to their stable nature, they are thus, (i) suitable for storage and transportation as exosomes can be stored at −80°C for 4 weeks without losing their physical properties (Agrawal et al., 2017). Finally, the double-layer lipid membrane of the exosome can encapsulate and protect its contents, hence preventing the rapid degradation of cytokines and RNA and deliver therapeutic agents to the target site (Didiot et al., 2016). Generally speaking, molecular carriers with biological activity in exosomes include lipids, proteins, and nucleic acids, such as DNA, mRNA, miRNA, and lncRNA. Though exosomes have these advantages and have great potential in treating cardiovascular diseases, there are also some problems in practice. For example, the extraction of exosomes is complicated and time-consuming, and uneven purification can cause significant differences in therapeutic effects (Kishore and Khan, 2017). Potential therapeutic advances of exosomes include their role in promoting angiogenesis, anti-apoptosis, anti-immunogenicity, proliferation, and anti-fibrosis (Chaput and Théry, 2011; Wang Y. et al., 2015; Yang, 2018; Ye et al., 2019).
It is essential to note that MI and I/R injury could lead to myocardial injury. Various types of cell-derived exosomes play a crucial role in repairing cell damage after myocardial tissue injury. Here we describe several programmed death of cardiomyocytes after MI and I/R injury and the regulatory mechanisms of exosomes.
Exosomes Reduce Apoptosis
The mechanism of exosomes from different cell sources about the anti-apoptosis of cardiomyocytes was summarized in Table 1. Bone marrow mesenchymal stem cell (BMMSC)-derived exosomes play a key role in repairing myocardial injury caused by tissue reperfusion, and the exosomal miR-486-5p inhibit myocardial apoptosis by PTEN de-activation and through PI3K/Akt pathway (Sun et al., 2019). In addition, a variety of pretreatments with BMMSCs can change the composition of the secreted exosomes. In which the most common used method is hypoxic pretreatment. Studies have found that with hypoxia pretreatment of BMMSCs, the miR-214 in secreted exosomes is significantly increased, and further confirmed that the exosomal miR-214 is transferred to cardiomyocytes and inhibit the expression of CaMKII (Wang Y. et al., 2018). Another study showed that hypoxic pretreatment of mouse BMMSCs increased the expression of miR-125b-5p in their exosomes. After injected into the infarcted area, the ability of cardiomyocytes to resist apoptosis were significantly enhanced through the inhibition of p53 and BAK1 (Zhu et al., 2018). Furthermore, the increased expression of miR-24 and miR-210 in the exosomes of hypoxic BMMSCs can also increase the anti-apoptotic potency of cardiomyocytes. The specific anti-apoptotic mechanism has not been elucidated, but the expression of exosomal miR-210 depends on the amount of neutral sphingomyelinase 2 (nSMase2). It is believed that gene or microRNA modified exosomes may convert the ability of these exosomes to resist cardiomyocyte apoptosis. Studies have found that GATA binding protein-4 (GATA-4) regulates the expression of miR-15 family members in BMMSCs and improves their survival rate in an ischemic environment (Yu et al., 2013). Other methods including miR-125b overexpression in exosomes via transfecting miR-125b into BMMSCs to achieve the effect of anti-cardiomyocyte apoptosis (Chen et al., 2020). The target of miR-125b is SIRT7, which is involved in the regulation of cardiac cell apoptosis (Araki et al., 2015). All of the above are the effects of exosomes derived from BMMSCs against cardiomyocyte apoptosis after ischemia.
Exosomes derived from other stem cells have also been extensively studied. Studies have found that exosomes derived from adipose-derived mesenchymal stem cells (ADMSCs) can regulate S1P/SK1/S1PR1 signals (Deng et al., 2019). Because miR-214 is highly expressed in exosomes derived from ADMSCs, they could inhibits the expression of Bcl2L11 and SLC8a1 after delivered into the myocardiac infarcted site (Eguchi et al., 2019). The protein encoded by Bcl2L11 may induces apoptosis through bax activation or anti-apoptotic proteins neutralization (Shukla et al., 2017). The sodium/calcium exchange protein encoded by SLC8A1 causes cardiomyocyte calcium overload-related apoptosis under cardiac stress (Aurora et al., 2012). Studies have showed the anti-apoptotic ability of hypoxic cardiomyocytes by overexpressing miR-146a in ADMSCs derived exosomes. The anti-apoptotic effect of miR-146a mainly caused by the inhibition of early growth response factor 1 (EGR1) in I/R injured tissue (Yang L. et al., 2015; Pan et al., 2019). Overexpressing miR-19 in exosomes derived from umbilical cord MSCs could regulate the AKT/JNK3/caspase-3 axis via inhibiting the expression of SOX6, which may lead to the decrease of hypoxic cardiomyocyte apoptosis (Huang et al., 2020). Exosomes derived from TIMP2 overexpressed umbilical cord MSCs significantly enhance the anti-apoptotic ability of hypoxic cardiomyocytes via the inhibition of Bax and pro-caspase expression (Ni et al., 2019). Exosomes derived from iPSCs also play a key role in resisting hypoxic myocardial apoptosis. Studies have shown that exosomal miR-21 and miR-210 may be key factors in anti-cardiomyocyte apoptosis (Wang Y. et al., 2015; Adamiak et al., 2018). Previous studies reported that miR-21 inhibits apoptosis by targeting PDCD4/AP-1 in infarcted cardiomyocytes (Xiao et al., 2016). Interestingly, a study showed that exercise can rapidly increase the exosomes in plasma, which could effectively resist cardiomyocyte apoptosis via activating ERK1/2 and HSP27 in hypoxia-reoxygenated cells (Bei et al., 2017).
Exosomes Regulate Autophagy-Dependent Cell Death
Studies have shown that exosomes can reduce myocardial autophagy and death during MI or I/R injury (Figure 2). In 2018, it was discovered that exosomes derived from human MSCs reduce I/R injury by inhibiting cardiomyocyte autophagy, but the specific mechanism has not been proved (Jiang et al., 2018). Experiments have shown that miR-125b-5p is highly expressed in exosomes derived from BMMSCs. Exosomal miR-125b-5p inhibits the expression of P53 and reduces the autophagy of myocardial cells in the infarcted site when delivered to the MI heart (Xiao et al., 2018). In the mouse myocardial I/R injury model, the expression of miR-29c decreased, and the autophagy flow of myocardial cells increased. The high expression of miR-29c can reduce the excessive autophagy of hypoxic myocardium by directly inhibiting the expression of PTEN, thus inhibiting the I/R-induced excessive autophagy via the PTEN/AKT/mTOR signaling pathway (Li et al., 2020). Besides, through transfection, over expression of miR-301 in BMMSCs increases the expression of exosomal miR-301, which significantly reduces the ratio of LC3-II/LC3-I and increases P62 relative expression in the infarcted myocardial tissue. Notably, the ratio of LC3-II/LC3-I increased, and the expression level of P62 decreased when the degree of autophagy was enhanced (Li Y. et al., 2019).
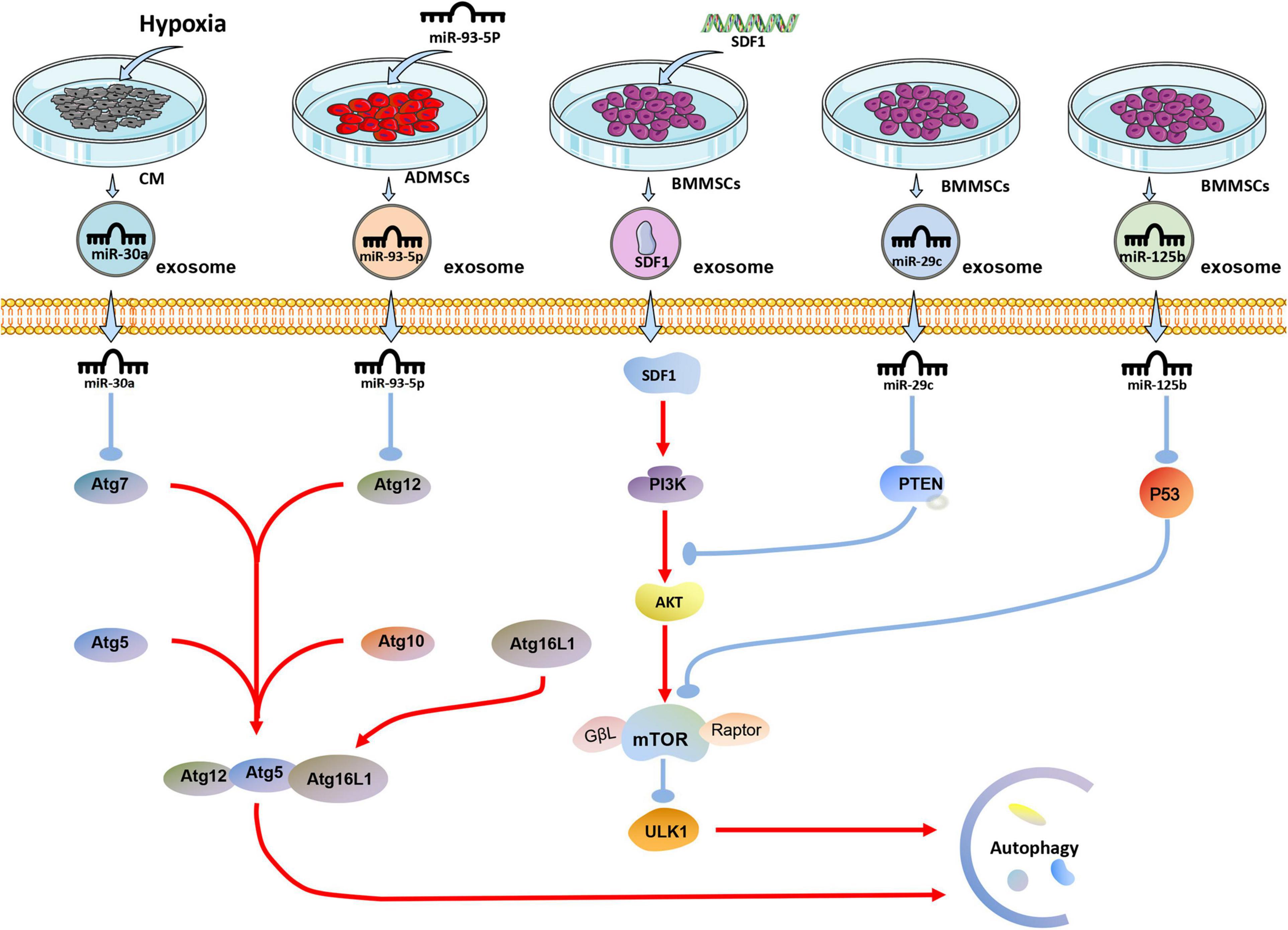
Figure 2. The effect of exosomes derived from differently treated cells on autophagy of cardiomyocytes after MI. The miR-30a in exosomes secreted by cardiomyocytes after hypoxia is targeted to Atg7 for anti-autophagy; miR-93-5P in exosomes secreted by ADMSCs transfected with miR-93-5P targeted Atg12 for anti-autophagy; SDF1 protein secreted by BMMSCs transfected with SDF gene activates PI3K/AKT pathway to resist autophagy; miR-29c and miR-125b in exosomes derived from BMMSCs target PTEN and P53, respectively, to resist autophagy.
Similarly, by overexpressing SDF1 in BMMSCs, the expression of SDF1 in exosomes was significantly increased, which significantly increased the expression of Bcl-2 in hypoxic cardiomyocytes. On the other hand, Bax, Beclin-1, LC3, and LC3II/LC3I ratio were significantly reduced, reducing excessive autophagy in cardiomyocytes (Gong et al., 2019). Studies have shown that ADMSCs overexpressing miR-93-5p can effective package miR-93-5p into exosomes. The exosomes would essentially deliver exosomal miR-93-5p to the site of MI to inhibit excessive autophagy. In vitro experiments show that miR-93-5p achieves these results by targeting Atg7 (Liu J. et al., 2018). Another interesting study found that miR-30a is highly enriched in exosomes in the serum of patients with acute MI. Exosomes are an important communication route among hypoxic cardiomyocytes, and exosomal miR-30a may inhibit autophagy in hypoxic cardiomyocytes by inhibiting Beclin-1 and Atg12 (Yang Y. et al., 2016).
Exosomes Reduce Pyroptosis
In the process of MI, NLRP3 is one of the critical molecules for myocardial cell pyroptosis. Studies have shown that exosomes derived from human MSCs can significantly reduce the expression of NLRP3 and Caspase-1 in the I/R myocardium, then reduce the myocardial pyroptosis. It has been proven that miR-320b in exosomes played a crucial role in myocardial cell pyroptosis, with NLRP3 being the target gene of miR-320b (Tang et al., 2020). Macrophage-derived exosomes occupy a large part of the circulating microcapsules in the blood (McDonald et al., 2014). Studies have found that exosomes derived from M2 macrophages can reduce myocardial damage caused by I/R injury via the high expression of exosomal miR-148a and through TXNIP-NLRP3-caspase-1 path way (McDonald et al., 2014). SIRT1 plays a central role in regulating various cellular processes related to heart development and cardiovascular diseases (Chen et al., 2010). SIRT1 expression is down-regulated in MI, and overexpression of SIRT1 can effectively reduce myocardial damage caused by MI (Mori et al., 2014).
Overexpressing LncRNA KLF3-AS1 in BMMSCs might increase the expression of LncRNA KLF3-AS1 in exosomes. LncRNA KLF3-AS1 acts as a sponge of miR-138-5p in ischemic cardiomyocytes, and the absorption of miR-138-5p increases the expression of SIRT1 in cardiomyocytes. Besides the inhibition of Caspase-1, inflammatory cytokine IL-1β, SIRT1 can also inhibit the expression of NLRP3 and Asc, thus reducing the pyroptosis of cardiomyocytes (Mao et al., 2019; Figure 3).
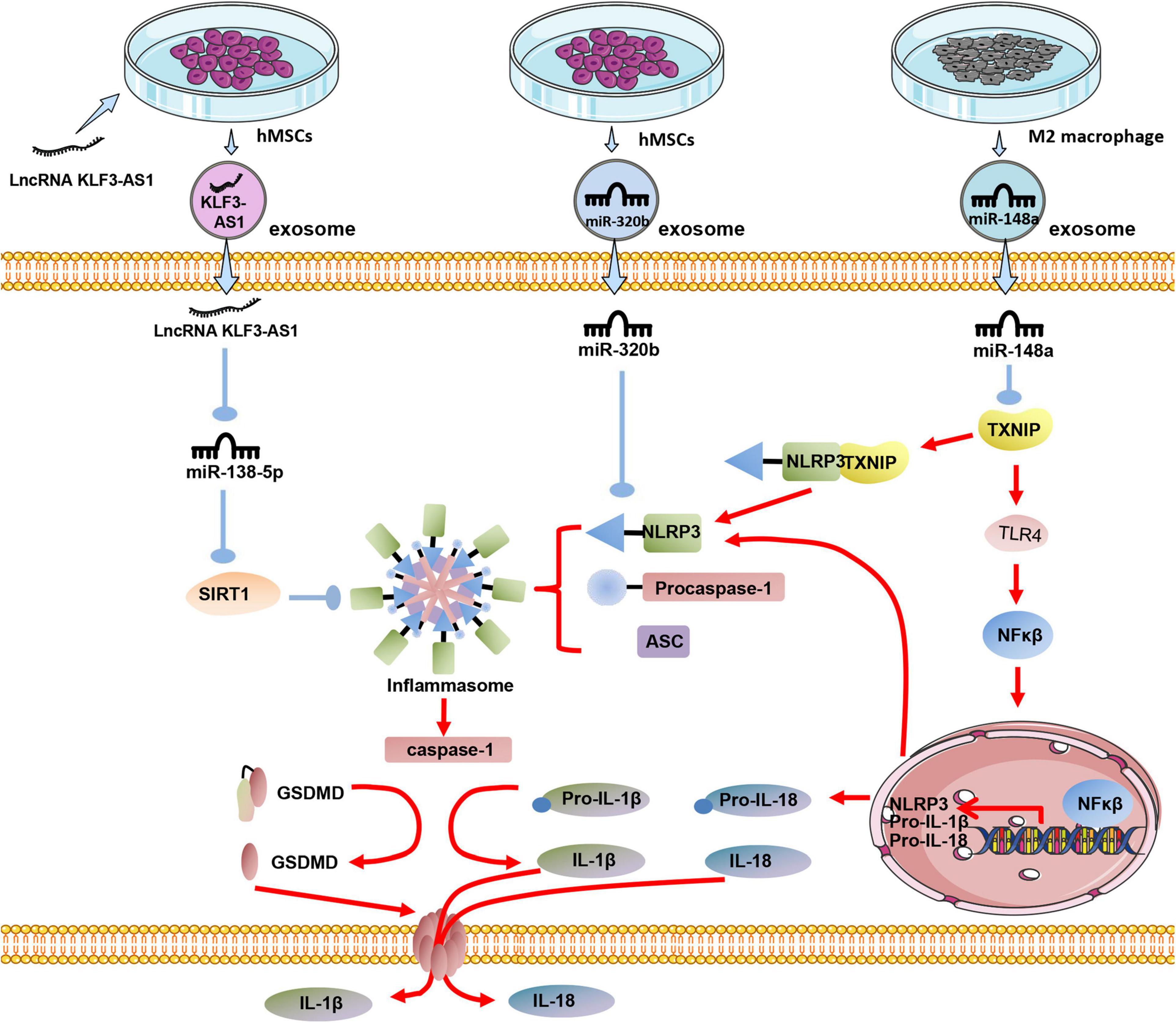
Figure 3. The effect of exosomes derived from differently treated cells on the pyrolysis of cardiomyocytes after MI. Human MSCs are transfected with LncRNA KLF3-AS1, and the secreted exosomal LncRNA KLF3-AS1 are highly expressed. LncRNA KLF3-AS1 inhibits miR-138-5p then inhibits pyroptosis; Exosomal miR-320b derived from unmodified human MSCs targets NLRP3 to inhibit cardiomyocyte pyroptosis; Exosomal miR-148a in exosomes derived from M2 macrophages inhibits TXINP expression to protect against cardiomyocyte pyroptosis.
Exosomes Reduce Ferroptosis
In a mouse model of cardiac I/R injury, both the iron chelator desferrioxamine (DFO) and the glutamine decomposition inhibitor compound-968 inhibit ferroptosis, reduce the size of MI, and improve heart function (Gao et al., 2015). Another study showed that treatment with Ferrostatin-1 or the iron chelator dexrazoxane in mice reduced the size of infarction and serum markers of myocardial injury during I/R (Fang et al., 2019). Proteomics showed that the protein level of GPX4 in myocardial cells decreased the first day and 1 week after MI in mice. The GPX4, however, slightly increased 8 weeks after infarction. RNA-seq and qRT-PCR analysis showed that the down-regulation of GPX4 occurred at the transcription level, and the down-regulation of GPX4 would cause ferroptosis of cardiomyocytes during MI (Park T. J. et al., 2019).
Significantly, Fe3+ is introduced via the transferrin receptor (TR). In the endosome, Fe3 + is first converted to Fe2+ via the Metallo-reductase six-transmembrane epithelial antigen of prostate 3 (Steap3) and later released from the endosome via the divalent metal transporter-1 (DMT1) (Xue et al., 2016; Pujol-Giménez et al., 2017). It is reported that the ferroptosis of cells is related to the up-regulation of DMT1 (Li L. B. et al., 2019; Yu et al., 2019). Notably, the expression of DMT1 in acute MI mice at 24 and 48-h was significantly higher than that in the sham operation group, while miR-23a-3p was highly expressed in exosomes derived from human MSCs (Ferguson et al., 2018). By transplanting exosomes, miR-23a-3p suppresses the iron death of myocardial cells by targeting DMT1 and improving MI’s cardiac function (Song et al., 2020; Figure 4).
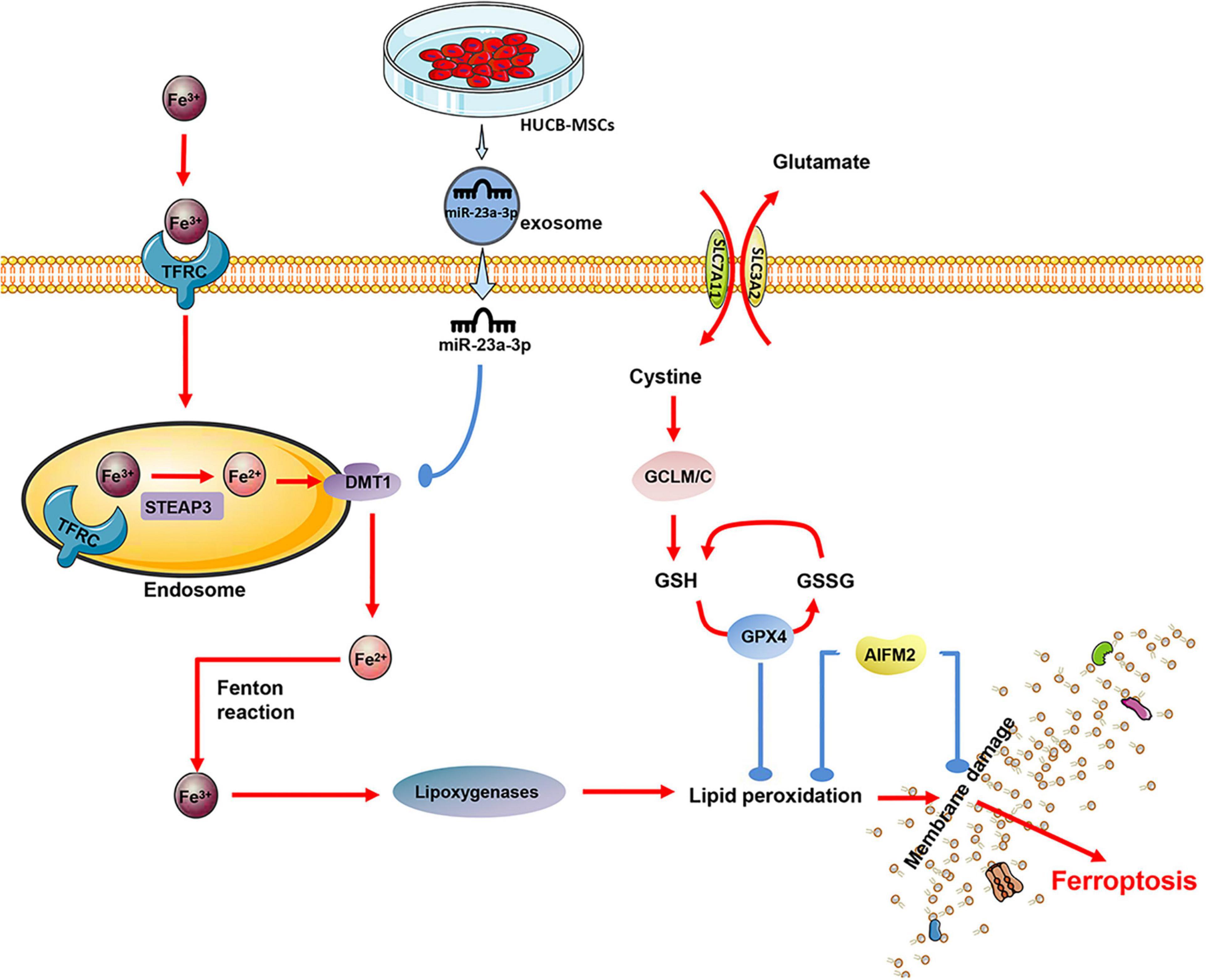
Figure 4. The exosomes derived from human umbilical cord blood (MSCs) contain a significant amount of miR-23a-3p, which inhibits the expression of DMT1 after entering the cardiomyocytes, thereby reducing intracellular lipid oxidation and inhibiting the ferroptosis of cardiomyocytes.
Conclusion
In the review herein, we discussed the anti-cell death potential of various exosomes from different cells, primarily stem cells for heart injury caused by MI or I/R. The above research shows that the same cell undergoes different treatments to change the type and proportion of the content in the exosomes from which it originates. Significantly after hypoxic treatment of stem cells, the protective substances in their exosomes increase. Studies have found that after LPS pretreated stem cells, their exosomes can enhance the M1 polarization of macrophages (Kang et al., 2018). All these indicate that modified and/or unmodified stem cells can increase the production of protective substances when they perceive danger signals, and exosomes serve as media to deliver protective substances. For the miRNAs and proteins, we believe can play a protective role, the cells are overexpressed by transfection, and these substances can also be delivered through exosomes as a medium to achieve cytoprotective effects. After modification of non-stem cells, can these cell-derived exosomes achieve the same protective effect? We believe that MSC and other stem cell-derived exosomes may be superior to other cells, because a large number of experiments have shown that stem cell-derived exosomes can play a protective role in cells, and the enhancement of protective factors can improve their ability. Besides, stem cell-derived exosomal microRNAs are potent regulators of cardiomyocytes’ survival and functional properties, cardiomyocyte progenitor cells, and endothelial cells. The exocytosis of other cells including exosomes derived from cardiac lineage-committed cells (cardiospheres, cardiac cells differentiated from pluripotent stem cells) is also worthy of attention. The exosomes naturally produced by cells have been proven to produce the desired effect. Thus, the research for engineered exosomes that can produce potent effects to optimize cardioprotection should be the next step of action. The purpose of the modification is to produce exosomes that encapsulate the desired molecule. Future research in this area should focus on identifying the role of specific molecules in exosomes. Also, exploring the mechanism of the exosome loading process will be a valuable addition. Simultaneously, it is also necessary to consider that different microRNA target combinations should be tested in different cell types and different cardiovascular environments while paying attention to safety and effectiveness.
Despite the composition of exosomal contents, keen attention should be paid to saving cardiomyocytes, particularly those involved in multiple programmed deaths. For instance, p53 participates in apoptosis and autophagy death after MI, as one of the most common natural stresses of p53 activation is hypoxia. Under hypoxic conditions, neonatal rat cardiomyocytes may lead to intranuclear cleavage of genomic DNA, accompanied by increased p53 trans-activation activity and p53 protein accumulation (Kirshenbaum and de Moissac, 1997). The heterotopic expression of the anti-apoptotic gene Bcl-2 is sufficient to antagonize p53 induced apoptosis (Ng et al., 1999). Over-activation of p53-myocardin signaling in autophagy during myocardial infarction leads to the death of myocardial cells and accelerated ischemic injury (Liu C. Y. et al., 2018). The overexpression of p53 after MI may enhance apoptosis and autophagy of cardiomyocytes, and the inhibition of this target can achieve double the result with half the effort. Therefore, for the increase of protective substances in exosomes, the typical target of cardiomyocyte hypoxia death should be significantly considered to achieve maximum effectiveness.
Author Contributions
XW and CF carried out the data collection and assembly of data, data analysis, and wrote the manuscript. XW, JP, and JG carried out the data collection and assembly. XW, CI, and JY carried out data analysis and interpretation, and manuscript revising. CF carried out the conception and design, and manuscript revising. All authors read and approved the final manuscript.
Funding
This work was supported by the Major Research Plan of the National Natural Science Foundation of China (No. 91539111 to JY) and the Key Project of Science and Technology of Hunan Province (No. 2020SK53420 to JY).
Conflict of Interest
JG was employed by the company Hunan Fangsheng Pharmaceutical Co. Ltd.
The remaining authors declare that the research was conducted in the absence of any commercial or financial relationships that could be construed as a potential conflict of interest.
Abbreviations
3 ′ UTR, 3 ′ untranslated region; ALDH2, aldehyde dehydrogenase 2; AMPK, AMP-activated protein kinase; Atgs, autophagy-related genes; CVD, Cardiovascular disease; DFO, desferrioxamine; DMT1, divalent metal transporter 1; EGR1, early growth response factor 1; ESCRT, endosomal sorting complex; GATA-4, GATA binding protein-4; GSDMD, gasdermin D; HSPs, heat shock proteins; I/R, ischemic-reperfusion; LPS, lipopolysaccharide; MI, myocardial infarction; mTOR, mammalian target of rapamycin; nSMase2, neutral sphingomyelinase 2; PAMPs, athogen-related molecular patterns; SDF1, stromal-derived factor 1; Steap3, six-transmembrane epithelial antigen of prostate 3; TIMP2, Tissue inhibitor of matrix metalloproteinase 2; NLR, nucleotide-binding oligomerization domain (NOD)-like receptor; TNF- α, tumor necrosis factor- α; TLRs, Toll-like receptors; TXNIP, thioredoxin-interacting protein.
References
Aachoui, Y., Sagulenko, V., Miao, E. A., and Stacey, K. J. (2013). Inflammasome-mediated pyroptotic and apoptotic cell death, and defense against infection. Curr. Opin. Microbiol. 16, 319–326. doi: 10.1016/j.mib.2013.04.004
Abu Khweek, A., and Amer, A. O. (2020). Pyroptotic and non-pyroptotic effector functions of caspase-11. Immunol. Rev. 297, 39–52. doi: 10.1111/imr.12910
Adamiak, M., Cheng, G., Bobis-Wozowicz, S., Zhao, L., Kedracka-Krok, S., Samanta, A., et al. (2018). Induced pluripotent stem cell (iPSC)-derived extracellular vesicles are safer and more effective for cardiac repair than iPSCs. Circ. Res. 122, 296–309. doi: 10.1161/circresaha.117.311769
Adams, J. M., and Cory, S. (1998). The Bcl-2 protein family: arbiters of cell survival. Science (New York, N.Y.) 281, 1322–1326. doi: 10.1126/science.281.5381.1322
Agrawal, A. K., Aqil, F., Jeyabalan, J., Spencer, W. A., Beck, J., Gachuki, B. W., et al. (2017). Milk-derived exosomes for oral delivery of paclitaxel. Nanomed. Nanotechnol. Biol. Med. 13, 1627–1636. doi: 10.1016/j.nano.2017.03.001
Aisa, Z., Liao, G. C., Shen, X. L., Chen, J., Li, L., and Jiang, S. B. (2017). Effect of autophagy on myocardial infarction and its mechanism. Eur. Rev. Med. Pharmacol. Sci. 21, 3705–3713.
Araki, S., Izumiya, Y., Rokutanda, T., Ianni, A., Hanatani, S., Kimura, Y., et al. (2015). Sirt7 contributes to myocardial tissue repair by maintaining transforming growth factor-β signaling pathway. Circulation 132, 1081–1093. doi: 10.1161/circulationaha.114.014821
Arslan, F., Lai, R. C., Smeets, M. B., Akeroyd, L., Choo, A., Aguor, E. N., et al. (2013). Mesenchymal stem cell-derived exosomes increase ATP levels, decrease oxidative stress and activate PI3K/Akt pathway to enhance myocardial viability and prevent adverse remodeling after myocardial ischemia/reperfusion injury. Stem Cell Res. 10, 301–312. doi: 10.1016/j.scr.2013.01.002
Ashkenazi, A., and Dixit, V. M. (1998). Death receptors: signaling and modulation. Science (New York, N.Y.) 281, 1305–1308. doi: 10.1126/science.281.5381.1305
Aurora, A. B., Mahmoud, A. I., Luo, X., Johnson, B. A., van Rooij, E., Matsuzaki, S., et al. (2012). MicroRNA-214 protects the mouse heart from ischemic injury by controlling Ca+2 overload and cell death. J. Clin. Invest. 122, 1222–1232. doi: 10.1172/jci59327
Baines, C. P., Kaiser, R. A., Purcell, N. H., Blair, N. S., Osinska, H., Hambleton, M. A., et al. (2005). Loss of cyclophilin D reveals a critical role for mitochondrial permeability transition in cell death. Nature 434, 658–662. doi: 10.1038/nature03434
Bei, Y., Xu, T., Lv, D., Yu, P., Xu, J., Che, L., et al. (2017). Exercise-induced circulating extracellular vesicles protect against cardiac ischemia-reperfusion injury. Basic Res. Cardiol. 112:38. doi: 10.1007/s00395-017-0628-z
Buja, L. M., and Vela, D. (2008). Cardiomyocyte death and renewal in the normal and diseased heart. Cardiovasc. Pathol. 17, 349–374. doi: 10.1016/j.carpath.2008.02.004
Cǎtanǎ, C. S., Atanasov, A. G., and Berindan-Neagoe, I. (2018). Natural products with anti-aging potential: affected targets and molecular mechanisms. Biotechnol. Adv. 36, 1649–1656. doi: 10.1016/j.biotechadv.2018.03.012
Cervantes, J., Nagata, T., Uchijima, M., Shibata, K., and Koide, Y. (2008). Intracytosolic Listeria monocytogenes induces cell death through caspase-1 activation in murine macrophages. Cell. Microbiol. 10, 41–52. doi: 10.1111/j.1462-5822.2007.01012.x
Chaput, N., and Théry, C. (2011). Exosomes: immune properties and potential clinical implementations. Semin. Immunopathol. 33, 419–440.
Chen, Q., Liu, Y., Ding, X., Li, Q., Qiu, F., Wang, M., et al. (2020). Bone marrow mesenchymal stem cell-secreted exosomes carrying microRNA-125b protect against myocardial ischemia reperfusion injury via targeting SIRT7. Mol. Cell. Biochem. 465, 103–114. doi: 10.1007/s11010-019-03671-z
Chen, Z., Chua, C. C., Ho, Y. S., Hamdy, R. C., and Chua, B. H. (2001). Overexpression of Bcl-2 attenuates apoptosis and protects against myocardial I/R injury in transgenic mice. Am. J. Physiol. Heart Circ. Physiol. 280, H2313–H2320. doi: 10.1152/ajpheart.2001.280.5.H2313
Chen, Z., Peng, I. C., Cui, X., Li, Y. S., Chien, S., and Shyy, J. Y. (2010). Shear stress, SIRT1, and vascular homeostasis. Proc. Natl. Acad. Sci. U.S.A. 107, 10268–10273. doi: 10.1073/pnas.1003833107
Del Re, D. P., Amgalan, D., Linkermann, A., Liu, Q., and Kitsis, R. N. (2019). Fundamental mechanisms of regulated cell death and implications for heart disease. Physiol. Rev. 99, 1765–1817. doi: 10.1152/physrev.00022.2018
Demircan, G., Kaplan, O., and Ozdas, S. B. (2018). Role of autophagy in the progress of coronary total occlusion. Bratislavske Lekarske Listy 119:103. doi: 10.4149/bll_2018_019
Deng, S., Zhou, X., Ge, Z., Song, Y., Wang, H., Liu, X., et al. (2019). Exosomes from adipose-derived mesenchymal stem cells ameliorate cardiac damage after myocardial infarction by activating S1P/SK1/S1PR1 signaling and promoting macrophage M2 polarization. Int. J. Biochem. Cell Biol. 114:105564. doi: 10.1016/j.biocel.2019.105564
Díaz, M., García, C., Sebastiani, G., de Zegher, F., López-Bermejo, A., and Ibáñez, L. (2017). Placental and cord blood methylation of genes involved in energy homeostasis: association with fetal growth and neonatal body composition. Diabetes 66, 779–784. doi: 10.2337/db16-0776
Didiot, M. C., Hall, L. M., Coles, A. H., Haraszti, R. A., Godinho, B. M., Chase, K., et al. (2016). Exosome-mediated delivery of hydrophobically modified siRNA for huntingtin mRNA silencing. Mol. Ther. 24, 1836–1847. doi: 10.1038/mt.2016.126
Ding, J., Wang, K., Liu, W., She, Y., Sun, Q., Shi, J., et al. (2016). Pore-forming activity and structural autoinhibition of the gasdermin family. Nature 535, 111–116. doi: 10.1038/nature18590
Dong, Z., Chu, G., Sima, Y., and Chen, G. (2018). Djhsp90s are crucial regulators during planarian regeneration and tissue homeostasis. Biochem. Biophys. Res. Commun. 498, 723–728. doi: 10.1016/j.bbrc.2018.03.047
Edinger, A. L., and Thompson, C. B. (2004). Death by design: apoptosis, necrosis and autophagy. Curr. Opin. Cell Biol. 16, 663–669. doi: 10.1016/j.ceb.2004.09.011
Eguchi, S., Takefuji, M., Sakaguchi, T., Ishihama, S., Mori, Y., Tsuda, T., et al. (2019). Cardiomyocytes capture stem cell-derived, anti-apoptotic microRNA-214 via clathrin-mediated endocytosis in acute myocardial infarction. J. Biol. Chem. 294, 11665–11674. doi: 10.1074/jbc.RA119.007537
Fang, X., Wang, H., Han, D., Xie, E., Yang, X., Wei, J., et al. (2019). Ferroptosis as a target for protection against cardiomyopathy. Proc. Natl. Acad. Sci. U.S.A. 116, 2672–2680. doi: 10.1073/pnas.1821022116
Ferguson, S. W., Wang, J., Lee, C. J., Liu, M., Neelamegham, S., Canty, J. M., et al. (2018). The microRNA regulatory landscape of MSC-derived exosomes: a systems view. Sci. Rep. 8:1419. doi: 10.1038/s41598-018-19581-x
Fink, S. L., and Cookson, B. T. (2006). Caspase-1-dependent pore formation during pyroptosis leads to osmotic lysis of infected host macrophages. Cell. Microbiol. 8, 1812–1825. doi: 10.1111/j.1462-5822.2006.00751.x
Foglio, E., Puddighinu, G., Germani, A., Russo, M. A., and Limana, F. (2017). HMGB1 inhibits apoptosis following MI and induces autophagy via mTORC1 inhibition. J. Cell. Physiol. 232, 1135–1143. doi: 10.1002/jcp.25576
Gao, M., Monian, P., Quadri, N., Ramasamy, R., and Jiang, X. (2015). Glutaminolysis and transferrin regulate ferroptosis. Mol. Cell 59, 298–308. doi: 10.1016/j.molcel.2015.06.011
Gong, X. H., Liu, H., Wang, S. J., Liang, S. W., and Wang, G. G. (2019). Exosomes derived from SDF1-overexpressing mesenchymal stem cells inhibit ischemic myocardial cell apoptosis and promote cardiac endothelial microvascular regeneration in mice with myocardial infarction. J. Cell. Physiol. 234, 13878–13893. doi: 10.1002/jcp.28070
Ham, P. B. III, and Raju, R. (2017). Mitochondrial function in hypoxic ischemic injury and influence of aging. Prog. Neurobiol. 157, 92–116. doi: 10.1016/j.pneurobio.2016.06.006
Heilig, R., and Broz, P. (2018). Function and mechanism of the pyrin inflammasome. Eur. J. Immunol. 48, 230–238. doi: 10.1002/eji.201746947
Hewitt, G., and Korolchuk, V. I. (2017). Repair, reuse, recycle: the expanding role of autophagy in genome maintenance. Trends Cell Biol. 27, 340–351. doi: 10.1016/j.tcb.2016.11.011
Hood, J. L., and Wickline, S. A. (2012). A systematic approach to exosome-based translational nanomedicine. Wiley Interdiscip. Rev. Nanomed. Nanobiotechnol. 4, 458–467. doi: 10.1002/wnan.1174
Huang, L., Yang, L., Ding, Y., Jiang, X., Xia, Z., and You, Z. (2020). Human umbilical cord mesenchymal stem cells-derived exosomes transfers microRNA-19a to protect cardiomyocytes from acute myocardial infarction by targeting SOX6. Cell Cycle (Georgetown, Tex.) 19, 339–353. doi: 10.1080/15384101.2019.1711305
Hurley, J. H. (2008). ESCRT complexes and the biogenesis of multivesicular bodies. Curr. Opin. Cell Biol. 20, 4–11. doi: 10.1016/j.ceb.2007.12.002
Ibrahim, A. G., Cheng, K., and Marbán, E. (2014). Exosomes as critical agents of cardiac regeneration triggered by cell therapy. Stem Cell Rep. 2, 606–619. doi: 10.1016/j.stemcr.2014.04.006
Inoki, K., Kim, J., and Guan, K. L. (2012). AMPK and mTOR in cellular energy homeostasis and drug targets. Annu. Rev. Pharmacol. Toxicol. 52, 381–400. doi: 10.1146/annurev-pharmtox-010611-134537
Irion, U., and St Johnston, D. (2007). bicoid RNA localization requires specific binding of an endosomal sorting complex. Nature 445, 554–558. doi: 10.1038/nature05503
Jahn, R., and Scheller, R. H. (2006). SNAREs–engines for membrane fusion. Nat. Rev. Mol. Cell Biol. 7, 631–643. doi: 10.1038/nrm2002
Jeremias, I., Kupatt, C., Martin-Villalba, A., Habazettl, H., Schenkel, J., Boekstegers, P., et al. (2000). Involvement of CD95/Apo1/Fas in cell death after myocardial ischemia. Circulation 102, 915–920. doi: 10.1161/01.cir.102.8.915
Jiang, L., Kon, N., Li, T., Wang, S. J., Su, T., Hibshoosh, H., et al. (2015). Ferroptosis as a p53-mediated activity during tumour suppression. Nature 520, 57–62. doi: 10.1038/nature14344
Jiang, X., Lew, K. S., Chen, Q., Richards, A. M., and Wang, P. (2018). human mesenchymal stem cell-derived exosomes reduce ischemia/reperfusion injury by the inhibitions of apoptosis and autophagy. Curr. Pharma. Design 24, 5334–5341. doi: 10.2174/1381612825666190119130441
Jung, J. H., Fu, X., and Yang, P. C. (2017). Exosomes generated from iPSC-derivatives: new direction for stem cell therapy in human heart diseases. Circ. Res. 120, 407–417. doi: 10.1161/circresaha.116.309307
Kajstura, J., Cheng, W., Reiss, K., Clark, W. A., Sonnenblick, E. H., Krajewski, S., et al. (1996). Apoptotic and necrotic myocyte cell deaths are independent contributing variables of infarct size in rats. Lab. Invest. J. Technical Methods Pathol. 74, 86–107.
Kang, H., Lee, M. J., Park, S. J., and Lee, M. S. (2018). Lipopolysaccharide-preconditioned periodontal ligament stem cells induce M1 polarization of macrophages through extracellular vesicles. Int. J. Mol. Sci. 19:3843. doi: 10.3390/ijms19123843
Kaur, A., and Sharma, S. (2017). Mammalian target of rapamycin (mTOR) as a potential therapeutic target in various diseases. Inflammopharmacology 25, 293–312. doi: 10.1007/s10787-017-0336-1
Kawaguchi, M., Takahashi, M., Hata, T., Kashima, Y., Usui, F., Morimoto, H., et al. (2011). Inflammasome activation of cardiac fibroblasts is essential for myocardial ischemia/reperfusion injury. Circulation 123, 594–604. doi: 10.1161/circulationaha.110.982777
Kayagaki, N., Stowe, I. B., Lee, B. L., O’Rourke, K., Anderson, K., Warming, S., et al. (2015). Caspase-11 cleaves gasdermin D for non-canonical inflammasome signalling. Nature 526, 666–671. doi: 10.1038/nature15541
Kirshenbaum, L. A., and de Moissac, D. (1997). The bcl-2 gene product prevents programmed cell death of ventricular myocytes. Circulation 96, 1580–1585. doi: 10.1161/01.cir.96.5.1580
Kishore, R., and Khan, M. (2016). More than tiny sacks: stem cell exosomes as cell-free modality for cardiac repair. Circ. Res. 118, 330–343. doi: 10.1161/circresaha.115.307654
Kishore, R., and Khan, M. (2017). Cardiac cell-derived exosomes: changing face of regenerative biology. Eur. Heart J. 38, 212–215. doi: 10.1093/eurheartj/ehw324
Konala, V. B., Mamidi, M. K., Bhonde, R., Das, A. K., Pochampally, R., and Pal, R. (2016). The current landscape of the mesenchymal stromal cell secretome: a new paradigm for cell-free regeneration. Cytotherapy 18, 13–24. doi: 10.1016/j.jcyt.2015.10.008
Lai, R. C., Arslan, F., Lee, M. M., Sze, N. S., Choo, A., Chen, T. S., et al. (2010). Exosome secreted by MSC reduces myocardial ischemia/reperfusion injury. Stem Cell Res. 4, 214–222. doi: 10.1016/j.scr.2009.12.003
Lai, R. C., Yeo, R. W., Tan, K. H., and Lim, S. K. (2013). Exosomes for drug delivery - a novel application for the mesenchymal stem cell. Biotechnol. Adv. 31, 543–551. doi: 10.1016/j.biotechadv.2012.08.008
Lambelet, M., Terra, L. F., Fukaya, M., Meyerovich, K., Labriola, L., Cardozo, A. K., et al. (2018). Dysfunctional autophagy following exposure to pro-inflammatory cytokines contributes to pancreatic β-cell apoptosis. Cell Death Dis. 9:96. doi: 10.1038/s41419-017-0121-5
Lazou, A., Iliodromitis, E. K., Cieslak, D., Voskarides, K., Mousikos, S., Bofilis, E., et al. (2006). Ischemic but not mechanical preconditioning attenuates ischemia/reperfusion induced myocardial apoptosis in anaesthetized rabbits: the role of Bcl-2 family proteins and ERK1/2. Apoptosis 11, 2195–2204. doi: 10.1007/s10495-006-0292-5
Lei, Q., Yi, T., and Chen, C. (2018). NF-κB-Gasdermin D (GSDMD) axis couples oxidative stress and NACHT, LRR and PYD domains-containing protein 3 (NLRP3) inflammasome-mediated cardiomyocyte pyroptosis following myocardial infarction. Med. Sci. Monit. 24, 6044–6052. doi: 10.12659/msm.908529
Li, J., Zhang, D., Wiersma, M., and Brundel, B. (2018). Role of autophagy in proteostasis: friend and foe in cardiac diseases. Cells 7:279. doi: 10.3390/cells7120279
Li, L. B., Chai, R., Zhang, S., Xu, S. F., Zhang, Y. H., Li, H. L., et al. (2019). Iron exposure and the cellular mechanisms linked to neuron degeneration in adult mice. Cells 8:198. doi: 10.3390/cells8020198
Li, T., Gu, J., Yang, O., Wang, J., Wang, Y., and Kong, J. (2020). Bone marrow mesenchymal stem cell-derived exosomal miRNA-29c decreases cardiac ischemia/reperfusion injury through inhibition of excessive autophagy via the PTEN/Akt/mTOR signaling pathway. Circ. J. 84, 1304–1311. doi: 10.1253/circj.CJ-19-1060
Li, Y., Yang, R., Guo, B., Zhang, H., Zhang, H., Liu, S., et al. (2019). Exosomal miR-301 derived from mesenchymal stem cells protects myocardial infarction by inhibiting myocardial autophagy. Biochem. Biophys. Res. Commun. 514, 323–328. doi: 10.1016/j.bbrc.2019.04.138
Libby, P., and Pasterkamp, G. (2015). Requiem for the ‘vulnerable plaque’. Eur. Heart J. 36, 2984–2987. doi: 10.1093/eurheartj/ehv349
Liu, A., Gao, X., Zhang, Q., and Cui, L. (2017). [Retracted] Cathepsin B inhibition attenuates cardiac dysfunction and remodeling following myocardial infarction by inhibiting the NLRP3 pathway. Mol. Med. Rep. 16:7873. doi: 10.3892/mmr.2017.7551
Liu, C. Y., Zhang, Y. H., Li, R. B., Zhou, L. Y., An, T., Zhang, R. C., et al. (2018). LncRNA CAIF inhibits autophagy and attenuates myocardial infarction by blocking p53-mediated myocardin transcription. Nat. Commun. 9:29. doi: 10.1038/s41467-017-02280-y
Liu, J., Jiang, M., Deng, S., Lu, J., Huang, H., Zhang, Y., et al. (2018). miR-93-5p-containing exosomes treatment attenuates acute myocardial infarction-induced myocardial damage. Mol. Ther. Nucleic Acids 11, 103–115. doi: 10.1016/j.omtn.2018.01.010
Ma, H., Guo, R., Yu, L., Zhang, Y., and Ren, J. (2011). Aldehyde dehydrogenase 2 (ALDH2) rescues myocardial ischaemia/reperfusion injury: role of autophagy paradox and toxic aldehyde. Eur. Heart J. 32, 1025–1038. doi: 10.1093/eurheartj/ehq253
Mao, Q., Liang, X. L., Zhang, C. L., Pang, Y. H., and Lu, Y. X. (2019). LncRNA KLF3-AS1 in human mesenchymal stem cell-derived exosomes ameliorates pyroptosis of cardiomyocytes and myocardial infarction through miR-138-5p/Sirt1 axis. Stem Cell Res. Ther. 10:393. doi: 10.1186/s13287-019-1522-4
Matsui, Y., Takagi, H., Qu, X., Abdellatif, M., Sakoda, H., Asano, T., et al. (2007). Distinct roles of autophagy in the heart during ischemia and reperfusion: roles of AMP-activated protein kinase and Beclin 1 in mediating autophagy. Circ. Res. 100, 914–922. doi: 10.1161/01.Res.0000261924.76669.36
McDonald, M. K., Tian, Y., Qureshi, R. A., Gormley, M., Ertel, A., Gao, R., et al. (2014). Functional significance of macrophage-derived exosomes in inflammation and pain. Pain 155, 1527–1539. doi: 10.1016/j.pain.2014.04.029
Mezzaroma, E., Toldo, S., Farkas, D., Seropian, I. M., Van Tassell, B. W., Salloum, F. N., et al. (2011). The inflammasome promotes adverse cardiac remodeling following acute myocardial infarction in the mouse. Proc. Natl. Acad. Sci. U.S.A. 108, 19725–19730. doi: 10.1073/pnas.1108586108
Moe, G. W., and Marín-García, J. (2016). Role of cell death in the progression of heart failure. Heart Fail. Rev. 21, 157–167. doi: 10.1007/s10741-016-9532-0
Mori, J., Patel, V. B., Abo Alrob, O., Basu, R., Altamimi, T., Desaulniers, J., et al. (2014). Angiotensin 1-7 ameliorates diabetic cardiomyopathy and diastolic dysfunction in db/db mice by reducing lipotoxicity and inflammation. Circ. Heart Fail. 7, 327–339. doi: 10.1161/circheartfailure.113.000672
Nabel, E. G., and Braunwald, E. (2012). A tale of coronary artery disease and myocardial infarction. N. Engl. J. Med. 366, 54–63. doi: 10.1056/NEJMra1112570
Newton, K., Dugger, D. L., Maltzman, A., Greve, J. M., Hedehus, M., Martin-McNulty, B., et al. (2016). RIPK3 deficiency or catalytically inactive RIPK1 provides greater benefit than MLKL deficiency in mouse models of inflammation and tissue injury. Cell Death Differ. 23, 1565–1576. doi: 10.1038/cdd.2016.46
Ng, I., Yeo, T. T., Soong, R., Tang, W. T., Ong, P. L., Lew, T., et al. (1999). Young Investigator’s Award: induction of apoptosis following traumatic head injury in humans. Ann. Acad. Med. Singapore 28, 363–365.
Ni, J., Liu, X., Yin, Y., Zhang, P., Xu, Y. W., and Liu, Z. (2019). Exosomes derived from TIMP2-modified human umbilical cord mesenchymal stem cells enhance the repair effect in rat model with myocardial infarction possibly by the Akt/Sfrp2 pathway. Oxid. Med. Cell. Longev. 2019:1958941. doi: 10.1155/2019/1958941
Pan, J., Alimujiang, M., Chen, Q., Shi, H., and Luo, X. (2019). Exosomes derived from miR-146a-modified adipose-derived stem cells attenuate acute myocardial infarction-induced myocardial damage via downregulation of early growth response factor 1. J. Cell. Biochem. 120, 4433–4443. doi: 10.1002/jcb.27731
Park, K. S., Bandeira, E., Shelke, G. V., Lässer, C., and Lötvall, J. (2019). Enhancement of therapeutic potential of mesenchymal stem cell-derived extracellular vesicles. Stem Cell Res. Ther. 10:288. doi: 10.1186/s13287-019-1398-3
Park, T. J., Park, J. H., Lee, G. S., Lee, J. Y., Shin, J. H., Kim, M. W., et al. (2019). Quantitative proteomic analyses reveal that GPX4 downregulation during myocardial infarction contributes to ferroptosis in cardiomyocytes. Cell Death Dis. 10:835. doi: 10.1038/s41419-019-2061-8
Pujol-Giménez, J., Hediger, M. A., and Gyimesi, G. (2017). A novel proton transfer mechanism in the SLC11 family of divalent metal ion transporters. Sci. Rep. 7:6194. doi: 10.1038/s41598-017-06446-y
Roth, G. A., Johnson, C., Abajobir, A., Abd-Allah, F., Abera, S. F., Abyu, G., et al. (2017). Global, regional, and national burden of cardiovascular diseases for 10 causes, 1990 to 2015. J. Am. Coll. Cardiol. 70, 1–25. doi: 10.1016/j.jacc.2017.04.052
Rühl, S., and Broz, P. (2015). Caspase-11 activates a canonical NLRP3 inflammasome by promoting K(+) efflux. Eur. J. Immunol. 45, 2927–2936. doi: 10.1002/eji.201545772
Saraste, A., Pulkki, K., Kallajoki, M., Henriksen, K., Parvinen, M., and Voipio-Pulkki, L. M. (1997). Apoptosis in human acute myocardial infarction. Circulation 95, 320–323. doi: 10.1161/01.cir.95.2.320
Sciarretta, S., Forte, M., Frati, G., and Sadoshima, J. (2018). New insights into the role of mTOR signaling in the cardiovascular system. Circ. Res. 122, 489–505. doi: 10.1161/circresaha.117.311147
Seiler, A., Schneider, M., Förster, H., Roth, S., Wirth, E. K., Culmsee, C., et al. (2008). Glutathione peroxidase 4 senses and translates oxidative stress into 12/15-lipoxygenase dependent- and AIF-mediated cell death. Cell Metab. 8, 237–248. doi: 10.1016/j.cmet.2008.07.005
Shao, B. Z., Han, B. Z., Zeng, Y. X., Su, D. F., and Liu, C. (2016). The roles of macrophage autophagy in atherosclerosis. Acta Pharmacol. Sin. 37, 150–156. doi: 10.1038/aps.2015.87
Shekhar, A., Heeger, P., Reutelingsperger, C., Arbustini, E., Narula, N., Hofstra, L., et al. (2018). Targeted imaging for cell death in cardiovascular disorders. JACC. Cardiovasc. Imaging 11, 476–493. doi: 10.1016/j.jcmg.2017.11.018
Shi, J., Gao, W., and Shao, F. (2017). Pyroptosis: gasdermin-mediated programmed necrotic cell death. Trends Biochem. Sci. 42, 245–254. doi: 10.1016/j.tibs.2016.10.004
Shi, J., Zhao, Y., Wang, K., Shi, X., Wang, Y., Huang, H., et al. (2015). Cleavage of GSDMD by inflammatory caspases determines pyroptotic cell death. Nature 526, 660–665. doi: 10.1038/nature15514
Shukla, S., Saxena, S., Singh, B. K., and Kakkar, P. (2017). BH3-only protein BIM: an emerging target in chemotherapy. Eur. J. Cell Biol. 96, 728–738. doi: 10.1016/j.ejcb.2017.09.002
Song, Y., Wang, B., Zhu, X., Hu, J., Sun, J., Xuan, J., et al. (2020). Human umbilical cord blood-derived MSCs exosome attenuate myocardial injury by inhibiting ferroptosis in acute myocardial infarction mice. Cell Biol. Toxicol. 37, 51–64. doi: 10.1007/s10565-020-09530-8
Stockwell, B. R., Friedmann Angeli, J. P., Bayir, H., Bush, A. I., Conrad, M., Dixon, S. J., et al. (2017). Ferroptosis: a regulated cell death nexus linking metabolism, redox biology, and disease. Cell 171, 273–285. doi: 10.1016/j.cell.2017.09.021
Stoorvogel, W. (2012). Functional transfer of microRNA by exosomes. Blood 119, 646–648. doi: 10.1182/blood-2011-11-389478
Stuffers, S., Sem Wegner, C., Stenmark, H., and Brech, A. (2009). Multivesicular endosome biogenesis in the absence of ESCRTs. Traffic (Copenhagen, Denmark) 10, 925–937. doi: 10.1111/j.1600-0854.2009.00920.x
Sun, X. H., Wang, X., Zhang, Y., and Hui, J. (2019). Exosomes of bone-marrow stromal cells inhibit cardiomyocyte apoptosis under ischemic and hypoxic conditions via miR-486-5p targeting the PTEN/PI3K/AKT signaling pathway. Thrombosis Res. 177, 23–32. doi: 10.1016/j.thromres.2019.02.002
Takahashi, M. (2010). Role of the SDF-1/CXCR4 system in myocardial infarction. Circ. J. 74, 418–423. doi: 10.1253/circj.cj-09-1021
Tang, J., Jin, L., Liu, Y., Li, L., Ma, Y., Lu, L., et al. (2020). Exosomes derived from mesenchymal stem cells protect the myocardium against ischemia/reperfusion injury through inhibiting pyroptosis. Drug Design Dev. Ther. 14, 3765–3775. doi: 10.2147/dddt.S239546
Théry, C., Boussac, M., Véron, P., Ricciardi-Castagnoli, P., Raposo, G., Garin, J., et al. (2001). Proteomic analysis of dendritic cell-derived exosomes: a secreted subcellular compartment distinct from apoptotic vesicles. J. Immunol. (Baltimore, Md. 1950) 166, 7309–7318. doi: 10.4049/jimmunol.166.12.7309
Théry, C., Zitvogel, L., and Amigorena, S. (2002). Exosomes: composition, biogenesis and function. Nat. Rev. Immunol. 2, 569–579. doi: 10.1038/nri855
Toldo, S., and Abbate, A. (2018). The NLRP3 inflammasome in acute myocardial infarction. Nat. Rev. Cardiol. 15, 203–214. doi: 10.1038/nrcardio.2017.161
Torre-Amione, G., Kapadia, S., Lee, J., Durand, J. B., Bies, R. D., Young, J. B., et al. (1996). Tumor necrosis factor-alpha and tumor necrosis factor receptors in the failing human heart. Circulation 93, 704–711. doi: 10.1161/01.cir.93.4.704
Valadi, H., Ekström, K., Bossios, A., Sjöstrand, M., Lee, J. J., and Lötvall, J. O. (2007). Exosome-mediated transfer of mRNAs and microRNAs is a novel mechanism of genetic exchange between cells. Nat. Cell Biol. 9, 654–659. doi: 10.1038/ncb1596
Villarroya-Beltri, C., Gutiérrez-Vázquez, C., Sánchez-Cabo, F., Pérez-Hernández, D., Vázquez, J., Martin-Cofreces, N., et al. (2013). Sumoylated hnRNPA2B1 controls the sorting of miRNAs into exosomes through binding to specific motifs. Nat. Commun. 4:2980. doi: 10.1038/ncomms3980
Wang, P., Shao, B. Z., Deng, Z., Chen, S., Yue, Z., and Miao, C. Y. (2018). Autophagy in ischemic stroke. Prog. Neurobiol. 163-164, 98–117. doi: 10.1016/j.pneurobio.2018.01.001
Wang, Y., Zhang, L., Li, Y., Chen, L., Wang, X., Guo, W., et al. (2015). Exosomes/microvesicles from induced pluripotent stem cells deliver cardioprotective miRNAs and prevent cardiomyocyte apoptosis in the ischemic myocardium. Int. J. Cardiol. 192, 61–69. doi: 10.1016/j.ijcard.2015.05.020
Wang, Y., Zhao, R., Liu, D., Deng, W., Xu, G., Liu, W., et al. (2018). Exosomes derived from miR-214-enriched bone marrow-derived mesenchymal stem cells regulate oxidative damage in cardiac stem cells by targeting CaMKII. Oxid. Med. Cell. Longev. 2018:4971261. doi: 10.1155/2018/4971261
Xiao, C., Wang, K., Xu, Y., Hu, H., Zhang, N., Wang, Y., et al. (2018). Transplanted mesenchymal stem cells reduce autophagic flux in infarcted hearts via the exosomal transfer of miR-125b. Circ. Res. 123, 564–578. doi: 10.1161/CIRCRESAHA.118.312758
Xiao, J., Pan, Y., Li, X. H., Yang, X. Y., Feng, Y. L., Tan, H. H., et al. (2016). Cardiac progenitor cell-derived exosomes prevent cardiomyocytes apoptosis through exosomal miR-21 by targeting PDCD4. Cell Death Dis. 7:e2277. doi: 10.1038/cddis.2016.181
Xue, X., Ramakrishnan, S. K., Weisz, K., Triner, D., Xie, L., Attili, D., et al. (2016). Iron uptake via DMT1 integrates cell cycle with JAK-STAT3 signaling to promote colorectal tumorigenesis. Cell Metab. 24, 447–461. doi: 10.1016/j.cmet.2016.07.015
Yang, J., Zhao, Y., and Shao, F. (2015). Non-canonical activation of inflammatory caspases by cytosolic LPS in innate immunity. Curr. Opin. Immunol. 32, 78–83. doi: 10.1016/j.coi.2015.01.007
Yang, L., Jiang, Y., Wen, Z., Xu, X., Xu, X., Zhu, J., et al. (2015). Over-expressed EGR1 may exaggerate ischemic injury after experimental stroke by decreasing BDNF expression. Neuroscience 290, 509–517. doi: 10.1016/j.neuroscience.2015.01.020
Yang, P. C. (2018). Induced pluripotent stem cell (iPSC)–derived exosomes for precision medicine in heart failure. Circ. Res. 122, 661–663.
Yang, Y., Li, Y., Chen, X., Cheng, X., Liao, Y., and Yu, X. (2016). Exosomal transfer of miR-30a between cardiomyocytes regulates autophagy after hypoxia. J. Mol. Med. (Berlin, Germany) 94, 711–724. doi: 10.1007/s00109-016-1387-2
Yang, Z., Zingarelli, B., and Szabó, C. (2000). Effect of genetic disruption of poly (ADP-ribose) synthetase on delayed production of inflammatory mediators and delayed necrosis during myocardial ischemia-reperfusion injury. Shock (Augusta, Ga.) 13, 60–66. doi: 10.1097/00024382-200013010-00011
Ye, M., Ni, Q., Qi, H., Qian, X., Chen, J., Guo, X., et al. (2019). Exosomes derived from human induced pluripotent stem cells-endothelia cells promotes postnatal angiogenesis in mice bearing ischemic limbs. Int. J. Biol. Sci. 15, 158–168.
Yellon, D. M., and Hausenloy, D. J. (2007). Myocardial reperfusion injury. N. Engl. J. Med. 357, 1121–1135. doi: 10.1056/NEJMra071667
Youle, R. J., and Strasser, A. (2008). The BCL-2 protein family: opposing activities that mediate cell death. Nat. Rev. Mol. Cell Biol. 9, 47–59. doi: 10.1038/nrm2308
Yu, B., Gong, M., He, Z., Wang, Y. G., Millard, R. W., Ashraf, M., et al. (2013). Enhanced mesenchymal stem cell survival induced by GATA-4 overexpression is partially mediated by regulation of the miR-15 family. Int. J. Biochem. Cell Biol. 45, 2724–2735. doi: 10.1016/j.biocel.2013.09.007
Yu, B., Kim, H. W., Gong, M., Wang, J., Millard, R. W., Wang, Y., et al. (2015). Exosomes secreted from GATA-4 overexpressing mesenchymal stem cells serve as a reservoir of anti-apoptotic microRNAs for cardioprotection. Int. J. Cardiol. 182, 349–360. doi: 10.1016/j.ijcard.2014.12.043
Yu, H., Yang, C., Jian, L., Guo, S., Chen, R., Li, K., et al. (2019). Sulfasalazine-induced ferroptosis in breast cancer cells is reduced by the inhibitory effect of estrogen receptor on the transferrin receptor. Oncol. Rep. 42, 826–838. doi: 10.3892/or.2019.7189
Zhang, Q. Y., Jin, H. F., Chen, S., Chen, Q. H., Tang, C. S., Du, J. B., et al. (2018). Hydrogen sulfide regulating myocardial structure and function by targeting cardiomyocyte autophagy. Chin. Med. J. 131, 839–844. doi: 10.4103/0366-6999.228249
Zhang, X., Evans, T. D., Jeong, S. J., and Razani, B. (2018). Classical and alternative roles for autophagy in lipid metabolism. Curr. Opin. Lipidol. 29, 203–211. doi: 10.1097/mol.0000000000000509
Zhao, X., Luo, G., Cheng, Y., Yu, W., Chen, R., Xiao, B., et al. (2018). Compound C induces protective autophagy in human cholangiocarcinoma cells via Akt/mTOR-independent pathway. J. Cell. Biochem. 119, 5538–5550. doi: 10.1002/jcb.26723
Keywords: myocardial infarction, apoptosis, autophagy-dependent death, pyroptosis, ferroptosis, exosomes, microRNA, myocardial protection
Citation: Wu X, Iroegbu CD, Peng J, Guo J, Yang J and Fan C (2021) Cell Death and Exosomes Regulation After Myocardial Infarction and Ischemia-Reperfusion. Front. Cell Dev. Biol. 9:673677. doi: 10.3389/fcell.2021.673677
Received: 28 February 2021; Accepted: 18 May 2021;
Published: 09 June 2021.
Edited by:
Xinlei Li, Nationwide Children’s Hospital, United StatesReviewed by:
Ming Dong, Guangzhou Institutes of Biomedicine and Health (CAS), ChinaPhilippe Menasché, Assistance Publique Hopitaux De Paris, France
Copyright © 2021 Wu, Iroegbu, Peng, Guo, Yang and Fan. This is an open-access article distributed under the terms of the Creative Commons Attribution License (CC BY). The use, distribution or reproduction in other forums is permitted, provided the original author(s) and the copyright owner(s) are credited and that the original publication in this journal is cited, in accordance with accepted academic practice. No use, distribution or reproduction is permitted which does not comply with these terms.
*Correspondence: Chengming Fan, fanchengming@csu.edu.cn