Potential Impact of Diabetes and Obesity on Alveolar Type 2 (AT2)-Lipofibroblast (LIF) Interactions After COVID-19 Infection
- 1Faculty of Sciences and Advanced Technologies in Biology, University of Science and Culture, Tehran, Iran
- 2Department of Stem Cells and Developmental Biology, Cell Science Research Center, Royan Institute for Stem Cell Biology and Technology, ACECR, Tehran, Iran
- 3Key Laboratory of Interventional Pulmonology of Zhejiang Province, Department of Pulmonary and Critical Care Medicine, The First Affiliated Hospital of Wenzhou Medical University, Wenzhou, China
- 4Excellence Cluster Cardio-Pulmonary System, Justus Liebig University Giessen, Giessen, Germany
- 5Faculty of Veterinary Medicine, University of Tehran, Tehran, Iran
- 6Reproductive Epidemiology Research Center, Royan Institute for Reproductive Biomedicine, ACECR, Tehran, Iran
- 7Department of Genetics, Reproductive Biomedicine Research Center, Royan Institute for Reproductive Biomedicine, ACECR, Tehran, Iran
Severe acute respiratory syndrome coronavirus 2 (SARS-CoV-2), a new emerging respiratory virus, caused evolving pneumonia outbreak around the world. In SARS-Cov-2 infected patients, diabetes mellitus (DM) and obesity are two metabolic diseases associated with higher severity of SARS-CoV-2 related complications, characterized by acute lung injury requiring assisted ventilation as well as fibrosis development in surviving patients. Different factors are potentially responsible for this exacerbated response to SARS-CoV-2 infection. In patients with DM, base-line increase in inflammation and oxidative stress represent preexisting risk factors for virus-induced damages. Such factors are also likely to be found in obese patients. In addition, it has been proposed that massive injury to the alveolar epithelial type 2 (AT2) cells, which express the SARS-CoV-2 receptor angiotensin-converting enzyme 2 (ACE2), leads to the activation of their stromal niches represented by the Lipofibroblasts (LIF). LIF are instrumental in maintaining the self-renewal of AT2 stem cells. LIF have been proposed to transdifferentiate into Myofibroblast (MYF) following injury to AT2 cells, thereby contributing to fibrosis. We hypothesized that LIF’s activity could be impacted by DM or obesity in an age- and gender-dependent manner, rendering them more prone to transition toward the profibrotic MYF status in the context of severe COVID-19 pneumonia. Understanding the cumulative effects of DM and/or obesity in the context of SARS-CoV-2 infection at the cellular level will be crucial for efficient therapeutic solutions.
Introduction
Severe acute respiratory syndrome coronavirus 2 (SARS-CoV-2) is responsible for the ongoing global outbreak of the so-called “coronavirus disease 2019 (COVID-19)”-related pneumonia (Chen N. et al., 2020). In general, respiratory viruses boost lung injury by activating the cytokine storm, inflammation, massive epithelial/endothelial cell death, vascular leakage, and other pathological conditions (Khomich et al., 2018). Overt immune reactions trigger the surge in production of numerous cytokines causing respiratory diseases like pneumonitis and acute respiratory distress syndrome (ARDS). Overtime, repeated events of exacerbated immune responses, result in organ failure and death (Wang and Ma, 2008; Matthay et al., 2012). Following respiratory infections, activated alveolar macrophages release proinflammatory cytokines, leading to increased expression of cell adhesion molecules (CAMs) and vascular endothelial growth factor (VEGF) accompanied by increased permeability of the lung endothelium (Hiraiwa and van Eeden, 2014). Lung specimens from infected patients showed desquamation of pneumocytes, pulmonary edema and proteinaceous exudates, hyaline membrane formation, and alveolar wall thickening due to fibroblast proliferation accompanied by type II pneumocyte hyperplasia (Leng et al., 2020).
Among the pre-existing risk factors know to exacerbate COVID-19-induced pathogenic mechanisms in humans, diabetes mellitus (DM), and obesity (in relation with aging and gender) have been highlighted as predisposing factors for complications associated with sever COVID-19 pneumonia (summarized in Table 1; Cai et al., 2020; Muniyappa and Gubbi, 2020; Zhang et al., 2020). DM and obesity are clear manifestations of metabolic dysfunctions associated with cardiovascular diseases and hypertension (Gao et al., 2020; Stefan et al., 2020). Moreover, induction of inflammation in obese people through imbalanced production of adipokines, especially adiponectin (anti-inflammatory adipokine) and leptin (pro-inflammatory), by the adipose tissue increases the obesity-associated risk of severe COVID-19 complications (Assad and Sood, 2012; Messina et al., 2020; Salvator et al., 2020). Diabetic patients with uncontrolled glucose homeostasis have been reported to display poor immunity against viral infection (Hodgson et al., 2015). Indeed, the severity of COVID-19 infection in DM and obese patients correlates with unbalanced immune response, increased sensitivity to hyperinflammation and cytokine storm, as well as low level of viral clearance (Muniyappa and Gubbi, 2020).
Recruitment and normal functions of neutrophil and macrophage can be impaired in patients with DM (Hodgson et al., 2015). It is well established that innate and adaptive immune responses to viral infections and the subsequent bacterial infections in the lungs are disrupted by poor glycemic control in multiple ways (Carey et al., 2018; Ferlita et al., 2019). DM/obesity exerts a detrimental impact on the host immune system, increasing susceptibility to infections and extending their clinical manifestations (Talbot et al., 2012; Frydrych et al., 2018). These defects, such as T cell dysfunction, defective natural killer (NK) cells, and abnormal complement activity impair viral clearance (Nyambuya et al., 2020). Indeed, metabolic changes caused by persistent hyperglycemia in diabetic individuals (Brownlee, 2001; Tiwari et al., 2011) result in generation of higher amounts of superoxide and activate inflammatory pathways, which lead to immune system dysfunction (Hameed et al., 2015). A study on patients with type 2 diabetes demonstrated that neutrophils and monocytes release excessive proinflammatory cytokines such as TNF-α, IL-1β, and IL-8, which provide suitable conditions for pathogen invasions (Hatanaka et al., 2006). Besides alteration of the phenotypes and activity of NK cells, there is also downregulation of receptors on NK cells which recognize viruses (Berrou et al., 2013). In addition, it has been reported that serum level of inflammatory cytokines, including IL-6, in COVID-19 patients with diabetes is higher than those patients without diabetes resulting in increased susceptibility to COVID-19-related infections in diabetic patients (Guo et al., 2020). Interestingly, exposure of pulmonary epithelial cells to high level of glucose concentrations significantly elevated influenza virus replication and infection, suggesting that elevated levels of glucose can facilitate respiratory virus replication. To further complicate further the clinical situation, diabetic patients have a delay in clearance of SARS-CoV-2 which may lead to further injuries (Wang J. et al., 2020).
In addition, DM/obesity can increase the expression of angiotensin-converting enzyme 2 (ACE2, the main cell receptor for SARS-CoV-2) (Rao et al., 2020). It has been found that high fat diet-induced obesity in mouse increases ACE2 expression in lung epithelial cells, which can enhance the infection of AT2 cells by COVID-19 (Al Heialy et al., 2020). AT2 cells represent the main functional cells in the alveolar region by virtue of producing surfactant, critical for lowering/reducing surface tension to prevent alveolar collapse (Shao et al., 2020) as well as by serving as stem cells (Barkauskas et al., 2013). Therefore, massive AT2 injury leads to impaired lung function, and eventually cell death, especially in the context of pre-existing metabolic dysregulation (obesity/diabetes), gender (males versus females), and age (old versus young). However, the molecular mechanism(s) and the cell type(s) involved in the exacerbated response to SARS-CoV-2 in patients with pre-existing conditions are still largely unknown.
A better vision of the cumulative effects of DM/obesity and COVID-19 in the induction of the pathogenic mechanisms at the cellular level in the lung, such as in depth characterization of the destructive effects on the resident mesenchymal niche cells associated with AT2 cells, is essential for understanding the role of DM/obesity in increasing the risk of COVID-19-related complications and for further treatments and prevention strategies, and the detailed investigation of the destructive effects on the resident mesenchymal niche cells which are associated with AT2 cells.
Therefore, in the next paragraphs of this review, we first focus on the signaling pathways/molecules that have a role in the sensitivity of diabetic/obese patients to COVID-19-related infections. We then review the latest knowledge about the pathogenic mechanisms-related to COVID-19 at the cellular level. Finally, we discuss the AT2-LIF interactions in this condition and introduce new cell- and organoid based models, which can be utilized, to better characterized the status of such interactions at the cellular and molecular level (Bode et al., 2020).
Destructive Pathways Triggered by SARS-CoV-2 in the Context of DM/Obesity
General Pathways Triggered by SARS-CoV-2/DM
Diabetes mellitus/obesity can impact lung function as well as the integrity of the capillary endothelium (Goldman, 2003; Zheng et al., 2017; Roberts et al., 2018). Increased thickness of the capillary and epithelial basal lamina of the alveoli has been reported in the patients with DM (Pitocco et al., 2012). These damages are illustrated by the reduction in the pulmonary diffusing capacity for carbon monoxide, a reduction in the total lung capacity, and a lower forced vital capacity (Pitocco et al., 2012; Kolahian et al., 2019). Poorly controlled blood glucose levels and abnormalities in insulin function can cause damage in the lung through different mechanisms including inflammation and oxidative stress (Yang et al., 2011; Wu et al., 2017). On the other hand, infection of lung cells with respiratory viruses can lead to the activation of apoptotic or pyroptosis pathways, inflammation as well as enhanced reactive oxygen species (ROS) production (Khomich et al., 2018).
Induced Oxidative Stress in Infected Diabetic Lung Cells
Hyperglycemia can induce oxidative stress, resulting in damage to the lipids, proteins and DNA. Experiments showed that in diabetic lung, content of malondialdehyde (MDA) was significantly increased, but the activity of superoxide dismutase (SOD), one of the key antioxidant enzymes, was decreased (Forgiarini Junior et al., 2010; Zhang et al., 2015). Hyperglycemia can induce different abnormalities in the lung, and vascular endothelial cell that can provoke virus-related damage (Goldman, 2003). These abnormalities include mitochondrial dysfunction, advanced glycation end products (AGEs) accumulation as well as the activation of the polyol pathway and the protein kinase C pathway (PKC) (Zheng et al., 2017). These abnormalities can result in the formation of ROS thereby inducing lung damage (Yan, 2018). Recent studies reported that mitochondrial ROS and excessive amount of NADH in the lung that likely contributed to polyol pathway activation in a diabetic rat model (Wu et al., 2017). In addition, interaction of AGEs with their receptors (RAGEs) can affect various pathways and induce oxidative stress, inflammation, fibrosis, and cell death in different cells including endothelial cells, AT2 cells, bronchial epithelial cells, alveolar macrophages, and endothelial cells (Morbini et al., 2006; Byun et al., 2017). Binding of AGES to extracellular matrix (ECM) proteins can create anchoring sites for proteins such as albumin, collagen, and elastin that lead to ECM thickening and damage to endothelial cells. Indeed, the clinical severity of COVID-19 is associated with both endothelial damage and pulmonary microvascular thrombosis (Ciceri et al., 2020; Gattinoni et al., 2020; Pitocco et al., 2020; Varga et al., 2020). So, pre-existing complications in DM patients including increased ROS production in diabetic cells and endothelial cell damages could be a risk factor for adverse outcomes in patients with COVID-19 (Pitocco et al., 2020; Whyte et al., 2020). Viral infection similar to DM can induce ROS in infected cells and pre-existing ROS in diabetic cells can provoke virus-induced ROS production (Chernyak et al., 2020). It has been reported that imbalance in redox homeostasis in respiratory viruses-infected cells can trigger different pathological pathways in these cells and induce tissue damage (Khomich et al., 2018). In general, appropriate levels of ROS are required for immunological responses and viral clearance. However, excessive ROS levels have destructive effects, not only on virus-infected cells but also on healthy cells in the lung by oxidizing lipid membranes, cellular proteins, and nucleic acids (Wu et al., 2013; Wang J. Z. et al., 2020). Respiratory viruses can induce enzyme activities that have a role in ROS generation including xanthine oxidase and dinucleotide phosphate oxidases. In addition, antioxidant enzymes activity such as SOD, glutathione (GSH), and catalase (CAT) can be affected during respiratory viruses infection (Khomich et al., 2018). Therefore, ROS production in diabetic cells infected by viruses is significantly higher compared to non-diabetic infected cells (Oliveira et al., 2020). As described previously, in diabetic cells compared to non-diabetic cells, increased expression of ACE2, endothelial cells dysfunction, and high glucose level in diabetic cells can facilitate virus entry and replication, as well as low antioxidant level and high ROS level (Oliveira et al., 2020).
Induced Inflammation in Infected Diabetic Lung Cells
In addition to oxidative stress, DM/obesity can induce inflammation in the lung. It has been demonstrated that both in chemically induced diabetes and in spontaneously type 1 diabetic mouse model, DM can promote inflammatory cell infiltration into the lung interstitium (Yang et al., 2011). Additionally, expression of tumor necrosis factor (TNF)-α, a robust pro-inflammatory cytokine, was significantly higher in diabetic lung compared to normal lung. Another study showed that TNF-α, IL-1β, and IL-6 levels are higher in the lung tissue of diabetic rats compared to that of healthy rats. Moreover, the NF-κB pathway was activated in the lung tissue of diabetic rat (Zhang et al., 2015). The NF-κB pathway can be activated by hyperglycemia and oxidative stress, and induce expression of pro-inflammatory genes (Evans et al., 2002; Baker et al., 2011). It has been reported that the level of inflammatory cytokines including IL-6, TNF-α, and IL-1β is higher in the lung of patients with DM and/or obesity (Talakatta et al., 2018). Pre-existing DM-induced inflammation can therefore exacerbate virus-induced inflammation in diabetic/infected cells compared to non-diabetic and healthy cells, thereby contributing to the cytokine storm observed in severe COVID-19 manifestations (Zhou et al., 2021).
It has been shown that respiratory viruses induced-ROS can also induce inflammation through activation of the NF-κB pathway or through induction of cell death and subsequently trigger macrophage activity and inflammation (Khomich et al., 2018). Pre-existing immune and endothelial cell dysfunction, high level of ROS, and elevated inflammatory cytokines in diabetic cells may set the stage for exacerbated inflammatory cytokines production following virus infection (Oliveira et al., 2020). Moreover, active viral replication would drive primary inflammatory responses, which lead to apoptosis or pyroptosis as a result of cytokine/chemokine production and cellular damage (Fu et al., 2020). Since the plasma level of IL-1β, an essential cytokine during pyroptosis is elevated in SARS-CoV-2 patients (Huang et al., 2020), pyroptosis has been suggested as another pathogenic mechanism involved in COVID-19 infected lung cells (Yang, 2020). When a pathogen like a virus invades the cell, pattern-recognition receptors (PRRs) such as NOD-like receptors protein 3 (NLRP3) on the cell membrane, recognize pathogen-associated molecular patterns (PAMPs) on the surface of the pathogen and bind to the precursor of caspase-1 (pro-caspase-1) through the adaptor protein ASC (Apoptosis-associated speck-like protein containing a caspase recruitment domain) in the cell. The newly formed multiprotein complex can activate caspase-1, which participates in innate immunity by activating pro-inflammatory cytokines like pro-IL-1β and pro-IL-18. Caspase-1 also plays an essential role in cell perforation by incising and polymerizing Gasdermin family members such as GSDMD. Consequently, the cellular content would be released into the extracellular space due to extensive cell perforation, resulting in inflammation (Yang, 2020). It has been reported that DM/obesity may activate the NLRP3 inflammasome cascade in diabetic cells and exacerbate COVID-19 symptoms. So, pre-existing NLRP3 inflammasome activation and increased release of inflammatory cytokines in diabetic cells may be one of the mechanisms that can provoke virus-related damages (Lambadiari et al., 2020). Secondary inflammatory responses occur when adaptive immunity appears and when the neutralizing antibodies are generated. Binding of the virus-anti-S-IgG complex to Fc receptors present on monocytes/macrophages membranes (Fu et al., 2020) would promote the production and secretion of pro-inflammatory cytokines and chemokines like IL-6, IFNγ, MCP-1, and IP-10 into the blood stream of patients (Huang et al., 2020; Zhang et al., 2020).
Cumulative Effects of Destructive Mechanisms at the Cellular Level
As described earlier, COVID-19 symptoms can be exacerbated in patients with DM and obesity (Guan et al., 2020; Huang et al., 2020; Yang et al., 2020). DM through different mechanisms including mitochondrial dysfunction, polyol pathway, and AGEs formation can cause cell damage and induce oxidative stress and inflammation (Figure 1; Zheng et al., 2017). DM can affect SOD, CAT, and GSH enzymatic activities and facilitate ROS production (Forgiarini Junior et al., 2010). DM can induce inflammation through activation of the NF-κB pathway (Baker et al., 2011). It has been reported that the level of inflammatory cytokines including IL-6, TNF-α, and IL-1β is higher in the lung of patients with DM and/or obesity (Talakatta et al., 2018). Virus infection can induce oxidative stress and inflammation in the cells through different mechanisms. Virus replication in infected cells can induce ROS (Cecchini and Cecchini, 2020). Similar to what is observed for DM-induced oxidative stress, antioxidant enzyme activities including CAT, SOD, and GSH are decreased during the virus infection. Induced-ROS in infected cells can induce inflammation through activation of the NF-κB pathway (Fernandes et al., 2020). However, cumulative effects of two main pathogenic events including oxidative stress and inflammation in infected/diabetic cells may contribute to increased cell damage and tissue injury. So far, it is not clear whether DM-induced oxidative stress and inflammation have direct effects on the initiation and/or amplitude of virus-dependent pathogenic mechanisms. During virus infection in patients with DM/obesity, interactions between deregulated antioxidant enzymes activity and activated inflammatory pathways such as the NF-κB pathway may be the likely cause for the reported virus-dependent complications. Inhibition of oxidative stress by quercetin and inflammation by cortisone in diabetic patients play indeed a crucial role in attenuating COVID-19 complications (Azimi et al., 2020; Colunga Biancatelli et al., 2020).
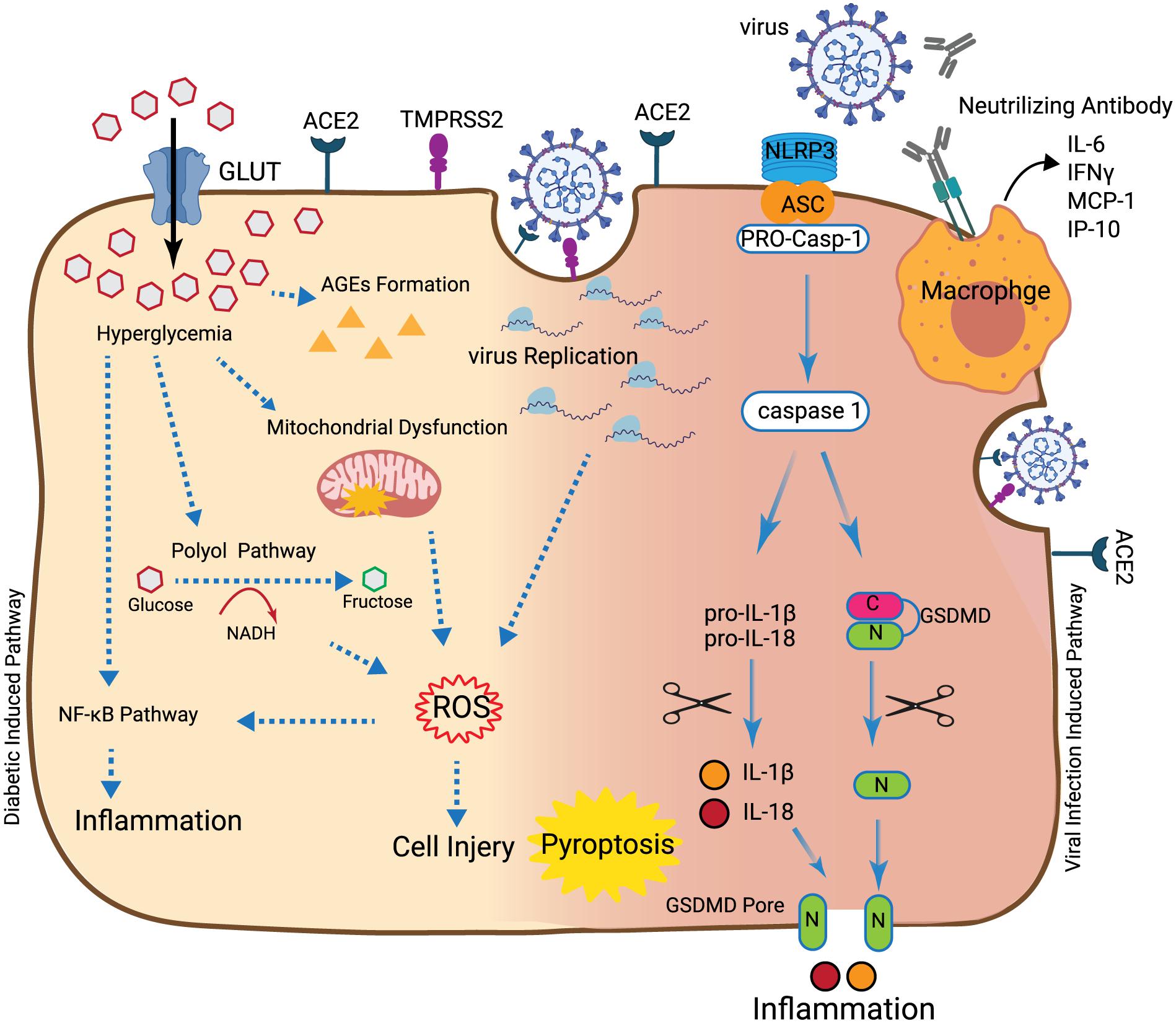
Figure 1. Synergic destructive effects of diabetes and SARS-CoV-2. In the hyperglycemia situation, accumulation of glucose into the cell can induce mitochondrial dysfunction, production of AGEs and polyol pathway activation that can induce ROS. Production of ROS can lead to cell injury and also inflammation through activation of the NFκB pathway. Also, after the virus enters into the cell through ACE2, the initial virus replication can lead to ROS induction. Once more, ROS can induce inflammation through activation of the NFκB pathway or induction of macrophage activity. Recognition of the virus by NLRP3 at the cell surface leads to binding of NLRP3 to the pro-caspase-1 through the ASC. This connection activates caspase-1 which leads to the cleavage of pro-inflammatory cytokines like pro-IL-1β, pro-IL-18, and GSMD. This results in the production of mature IL-1β and IL-18 and N-terminal products, respectively. N-terminal products located into cell membranes result in the creation of the GSMD pore. Following cell perforation and release of the cellular content into the extracellular space, inflammation, cell swelling, and pyroptosis can occur. After appearance of the adaptive immune response, generation of neutralizing antibodies and binding of macrophage virus receptors to the virus, cytokines, and chemokines such as IL-6, IFNγ, MCP-1, and IP-10 are produced and secreted into the bloodstream of patients.
Further studies need to be carried out to determine the role of pre-existing oxidative stress and inflammation present in the lung of diabetic patients in the context of SARS-CoV-2-infection. To that end, it is important to understand the interaction of AT2 cells, the target of SARS-CoV-2, and their tightly associated mesenchymal cells which play the role of a niche for AT2 stem cells; this title is developed and discussed through following section.
Adult Lung Regeneration in Terms of Cell-Cell Interactions
An Overview of AT2-Resident Stromal Cells Interaction
Lipofibroblasts (LIFs) are lipid-droplet-containing interstitial fibroblast which functions to transfer triglycerides to the AT2s. AT2 cells are also considered as stem/progenitor cells capable of self-renewal and differentiation toward alveolar epithelial type 1 (AT1) cells (Youk et al., 2020). They interact with resident lung mesenchymal cells which control the maintenance of these stem cell properties. Therefore, LIFs are proposed to constitute the mesenchymal niche for AT2 stem cells. However, LIFs are heterogeneous populations which have been isolated based on the expression of different markers. Platelet derived growth factor receptor alpha (PdgfrαPos), Axin2Pos and Fibroblast growth factor 10 (Fgf10Pos) are three sub-lineages reported to be located close to AT2 cells. However, these three markers do not label clearly distinct populations of mesenchymal cells raising the possibility that these three populations may at least partially overlap. For example, 74% of resident stromal Axin2Pos cells in the alveolar region are also expressing Pdgfrα. Axin2Pos PdgfrαPos mesenchymal cells are called mesenchymal alveolar niche cells (MANC) and are located close to AT2 cells (Zepp et al., 2017). The interaction between AT2 and stromal cells has been functionally tested using the alveolosphere model, which is a co-culture of AT2s with MANC in growth factor reduced Matrigel. In this assay, the MANCs have been reported to support AT2 self-renewal and differentiation toward the AT1 lineage (Barkauskas et al., 2013; Zepp et al., 2017).
Fgf10Pos Cells Maintain the Self-Renewal and Differentiation of AT2 Stem Cells
Fgf10 is expressed early during lung development in the distal mesenchyme (Bellusci et al., 1997). Fgf10 acts in a paracrine fashion on the adjacent epithelium expressing Fgfr2b. Fgf10 promotes epithelial survival and branching morphogenesis. A subpopulation of Fgf10-expressing cells labeled at embryonic day 12.5 serve as progenitor for LIFs at later stages of lung development. Additionally, in postnatal lungs, around 30% of LIFs express Fgf10 (Al Alam et al., 2015; Chu et al., 2021). Fgf10 itself, through an autocrine effect, appears to contribute to their differentiation (Bellusci et al., 1997). Using the alveolosphere assay, Fgf10Pos cells maintain the self-renewal and differentiation of AT2 stem cells and appear to be distinct from the previously described MANCs (Taghizadeh et al., 2021).
The Resident Mesenchymal Niche Is Impacted Following Massive AT2 Injury
Repetitive AT2 damage results in scarring and accumulation of activated MYF in the lung, which is the main characteristic of lung fibrosis (Figure 2). Activated myofibroblasts originate from multiple sources (Phillips et al., 2004; Kim et al., 2006; Rock et al., 2011; El Agha et al., 2014) and express high level of smooth muscle actin (SMA) as well as extracellular matrix proteins. Lung fibrosis is a chronic, progressive, and lethal disease considered as a long-term complication of COVID-19. It has been reported, using the bleomycin-induced fibrosis mouse model, that LIF transdifferentiate to activate MYF (El Agha et al., 2017). The contribution of the LIF-Fgf10Pos cells to fibrosis formation is still unclear and will require further investigation using our recently developed Fgf10CreERT2 knock in mouse line (Chu et al., 2021).
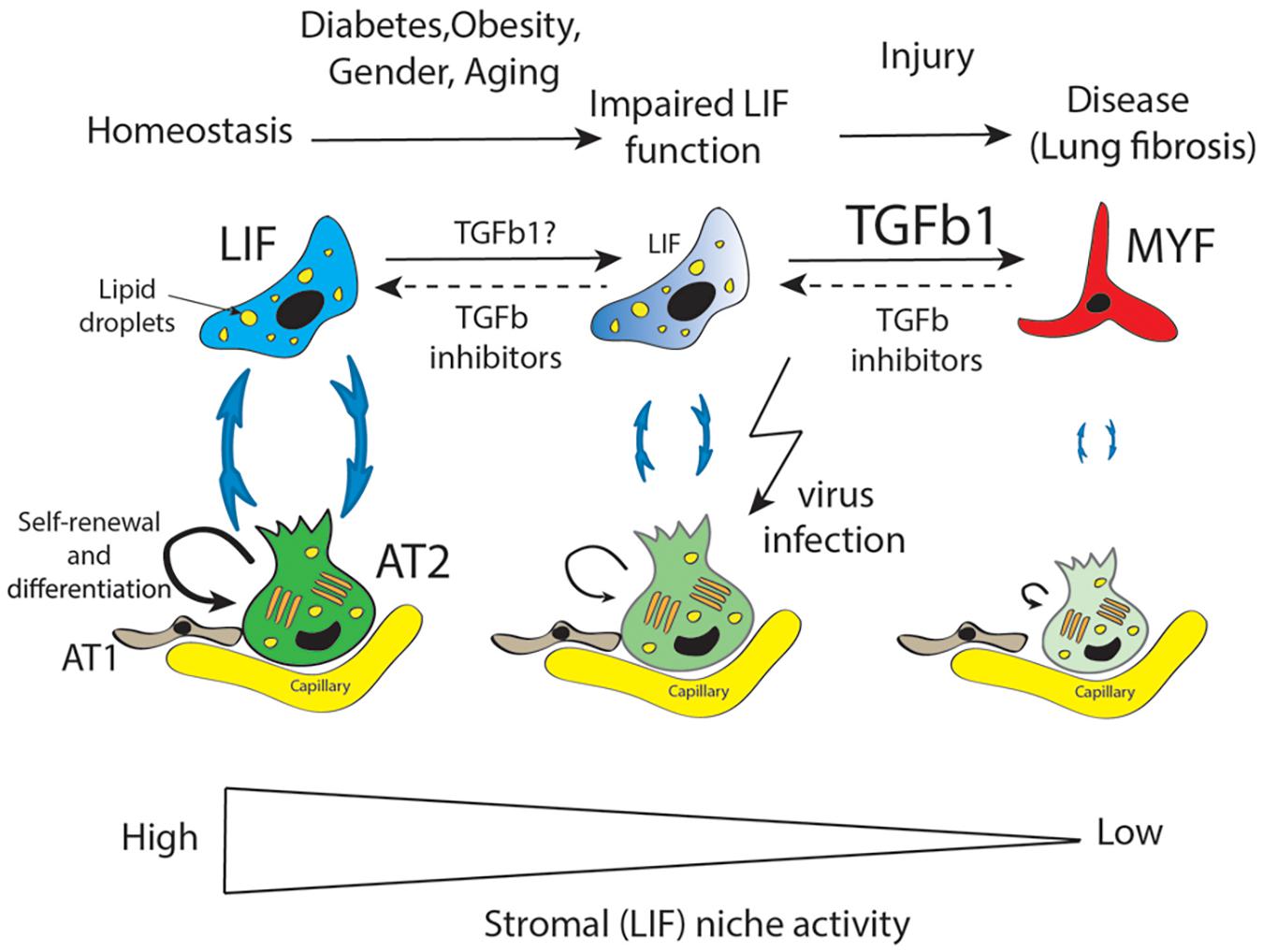
Figure 2. LIF is an important mesenchymal population supporting AT2 stem cells in terms of self-renewal and differentiation to AT1 cells. LIF cells provide triglycerides to AT2 for the elaboration of surfactant. Diabetes, obesity, aging, and gender are proposed to be the main factors impacting negatively the mesenchymal/stromal (LIF) niche activity resulting in decreased AT2-LIF interaction. Against this background, further injury to the lung leads the impaired LIF to transdifferentiate to activate myofibroblast (MYF). Accumulation of MYF is a main characteristic of lung fibrosis which over time, in particular in old males, leads to failure of lung function. Moreover, AT2 cells are the main target of virus infection which drastically reduces their function in terms of surfactant protein production. TGFβ is a causative growth factor for fibrosis induction and maintenance. We propose that TGFβ inhibitors could be instrumental in restoring impaired LIF function in the context of diabetes, obesity, gender, and aging as well as in the MYF to LIF transdifferentiation in the context of fibrosis.
AT2 expressing ACE2 are thought to be the main target of COVID-19. However, there is also the possibility that the LIFs themselves may be a target for COVID-19 (Kruglikov and Scherer, 2020). Adipocytes (from the white adipose tissue) in COVID-19 patients express higher level of ACE2 compared non-infected patients. In addition, obesity enhances ACE2 expression in adipocytes. As LIFs share commonalities with adipocytes (for example, their high lipid droplet content, their dependence on Fgf10 signaling for their formation), it is possible that LIFs also represent cellular targets for COVID-19. In this situation, both the AT2 stem cells and their niche would be impacted, resulting in irreversible lung damage and lethality (Figure 2; Lv et al., 2021).
From a mechanistic point of view, it was reported that COVID-19 induces the expression of transforming growth factor β (Tgf-β), a growth factor causative for fibrosis formation and maintenance (Lal, 2010). On the therapeutic side, two drugs, Pirfenidone (TGFβ1 inhibitor) and nintedanib (pan-tyrosine kinase FGF receptor inhibitor) have been approved for patients suffering from idiopathic pulmonary fibrosis (IPF), a form of fibrosis which does not resolve and develops in old age, mostly in men. In addition, it has been demonstrated that DM and obesity were associated with IPF (Kruglikov and Scherer, 2020). Therefore, at least two of the three aggravating conditions described in the context of COVID-19 are also met in the context of IPFs raising the possibility that Pirfenidone and nintedanib could be instrumental to limit fibrosis progression post-COVID-19 infection.
Interestingly metformin, a first line antidiabetic drug, has been proposed to enhance MYF to LIF transition in the bleomycin-induced fibrosis murine model as well as in vitro using primary IPF fibroblasts (Kheirollahi et al., 2019). This drug may be useful for COVID-19 patients who are not suffering from diabetes.
Linking Metabolic Dysfunction, Gender and Age With the Activity of the Resident Mesenchymal Niche
It was previously reported that aging is impacting the capacity of the stromal niche to support AT2 cell proliferation following pneumonectomy in mice (Paxson et al., 2011). Using the alveolosphere assay, it has been reported that obesity and gender also impact the capacity of resident mesenchymal cells defined as (Cd31Cd45Epcam)NegSca1Pos, isolated from Ob/Ob and Wild type male and female mice, to support AT2 stem cell self-renewal and differentiation (Taghizadeh et al., 2021). Resident stromal cells from Ob/Ob mice were less capable to support organoid formation compared to resident mesenchymal cells from wild type mice. It has been found that resident mesenchymal cells from male mice were less capable to support organoid formation compared to resident mesenchymal cells from female mice. The combined presence of obesity and male gender led to the complete loss of resident mesenchymal cells functionality. This is strikingly identical to what is observed in the human population, where males and obese/diabetic patients are the most impacted by COVID-19. To our knowledge, this observation represents the first clear link between metabolic diseases, gender and aging and a critical mesenchymal cell type needed for the proper maintenance of AT2 stem cells. How aging, gender and obesity can impact the resident mesenchymal cells is completely unknown and will be important to define per se using state of the art techniques. In the future, we propose that the use of the in vitro alveolosphere assay to test drugs capable of activating resident mesenchymal cells from Ob/Ob male mice will allow to designing new therapies to treat fibrosis complications in male, aged and diabetic patients who survived COVID-19-infection.
Conclusion
Diabetes mellitus/obesity may exacerbate SARS-CoVID-2 infection through different mechanisms. Immune cell dysfunction, increased expression of ACE2 on lung cells, induction of virus replication, delay in virus clearance after infection, and high glucose concentration are just some of the mechanisms that can exacerbate SARS-CoVID-2-related pathogenic mechanisms in diabetic cells compared to non-diabetic cells (Oliveira et al., 2020). DM/obesity as a pre-existing risk factor can induce different pathogenic mechanisms including oxidative stress and inflammation at the cellular level and promote virus-related damages in infected cells. Oxidative stress and inflammation are the two key linking pathogenic cascades in DM/obesity and SARS-CoVID-2-induced mechanisms (Albulescu et al., 2020). So, potential therapies for the reduction of inflammation and oxidative stress in patients with DM may have a critical role in attenuating COVID-19-related infections and mortality. In addition, DM/obesity can negatively impact the ability of the lung mesenchymal niche to support AT2 stem cells. We propose that this failure is causative for fibrosis formation in surviving COVID-19 patients. Moreover, in vitro lung models such as alveolar organoids can be instrumental to investigate the impact of DM, obesity and gender on lung mesenchymal niche and provide a platform for testing new drugs capable of restoring their function.
Author Contributions
MN-K, ST, AF, and AE wrote the manuscript. SV prepared the table and read the manuscript. RH, MT, AK, CC, and J-SZ read the manuscript and revised it. SB and YT designed the manuscript, read the manuscript, revised it, and approved the final version. All authors contributed to the article and approved the submitted version.
Funding
YT was supported by the Royan Institute for Stem Cell Biology and Technology, Royan’s Lotus Charity Investment Foundation, and by grant #97014846 from Iran National Science Foundation. SB was supported by Cardio-Pulmonary Institute and by grants from the Deutsche Forschungsgemeinschaft (DFG; BE4443/4-1, BE4443/6-1, KFO309 P7, and SFB CRC1213-projects A02 and A04).
Conflict of Interest
The authors declare that the research was conducted in the absence of any commercial or financial relationships that could be construed as a potential conflict of interest.
Abbreviations
DM, Diabetes mellitus; SARS-CoV-2, severe acute respiratory syndrome coronavirus 2; COVID-19, coronavirus disease 2019; ARDS, acute respiratory distress syndrome; CAMs, cell adhesion molecules; VEGF, vascular endothelial growth factor; NK, natural killer; ACE2, angiotensin-converting enzyme 2; AT2, alveolar epithelial type 2; GSH, glutathione; CAT, catalase; PRRs, pattern-recognition receptors; NLRP3, NOD-like receptors protein 3; PAMPs, pathogen-associated molecular patterns; MDA, malondialdehyde; SOD, superoxide dismutase; AGEs, advanced glycation end products; PKC, protein kinase C; ROS, reactive oxygen species; TNF- α, tumor necrosis factor; ECM, extracellular matrix proteins; LIFs, Lipofibroblasts; AT1, alveolar epithelial type 1; IPF, idiopathic pulmonary fibrosis; MANC, mesenchymal alveolar niche cells; SMA, smooth muscle actin.
References
Al Alam, D., El Agha, E., Sakurai, R., Kheirollahi, V., Moiseenko, A., Danopoulos, S., et al. (2015). Evidence for the involvement of fibroblast growth factor 10 in lipofibroblast formation during embryonic lung development. Development 142, 4139–4150.
Al Heialy, S., Hachim, M. Y., Senok, A., Tayoun, A. A., Hamoudi, R., Alsheikh-Ali, A., et al. (2020). Regulation of angiotensin converting enzyme 2 (ACE2) in obesity: implications for COVID-19. bioRxiv [Preprint]. doi: 10.1101/2020.04.17.046938
Albulescu, R., Dima, S. O., Florea, I. R., Lixandru, D., Serban, A. M., Aspritoiu, V. M., et al. (2020). COVID-19 and diabetes mellitus: unraveling the hypotheses that worsen the prognosis. Exp. Ther. Med. 20:194.
Assad, N. A., and Sood, A. (2012). Leptin, adiponectin and pulmonary diseases. Biochimie 94, 2180–2189. doi: 10.1016/j.biochi.2012.03.006
Azimi, S., Sahebnasagh, A., Sharifnia, H., and Najmeddin, F. (2020). Corticosteroids administration following COVID-19-induced acute respiratory distress syndrome. Is it harmful or Life-saving? Adv. J. Emerg. Med. 4:e43.
Baker, R. G., Hayden, M. S., and Ghosh, S. (2011). NF-κB, inflammation, and metabolic disease. Cell Metab. 13, 11–22. doi: 10.1016/j.cmet.2010.12.008
Barkauskas, C. E., Cronce, M. J., Rackley, C. R., Bowie, E. J., Keene, D. R., Stripp, B. R., et al. (2013). Type 2 alveolar cells are stem cells in adult lung. J. Clin. Invest. 123, 3025–3036.
Barron, E., Bakhai, C., Kar, P., Weaver, A., Bradley, D., Ismail, H., et al. (2020). Associations of type 1 and type 2 diabetes with COVID-19-related mortality in England: a whole-population study. Lancet Diabetes Endocrinol. 8, 813–822. doi: 10.1016/s2213-8587(20)30272-2
Bellusci, S., Grindley, J., Emoto, H., Itoh, N., and Hogan, B. (1997). Fibroblast growth factor 10 (FGF10) and branching morphogenesis in the embryonic mouse lung. Development 124, 4867–4878. doi: 10.1242/dev.124.23.4867
Berrou, J., Fougeray, S., Venot, M., Chardiny, V., Gautier, J.-F., Dulphy, N., et al. (2013). Natural killer cell function, an important target for infection and tumor protection, is impaired in type 2 diabetes. PloS one 8:e62418. doi: 10.1371/journal.pone.0062418
Bode, B., Garrett, V., Messler, J., Mcfarland, R., Crowe, J., Booth, R., et al. (2020). Glycemic characteristics and clinical outcomes of COVID-19 patients hospitalized in the United States. J. Diabetes Sci. Technol. 4, 813–821. doi: 10.1177/1932296820924469
Brownlee, M. (2001). Biochemistry and molecular cell biology of diabetic complications. Nature 414, 813–820. doi: 10.1038/414813a
Byun, K., Yoo, Y., Son, M., Lee, J., Jeong, G. B., Park, Y. M., et al. (2017). Advanced glycation end-products produced systemically and by macrophages: a common contributor to inflammation and degenerative diseases. Pharmacol. Ther. 177, 44–55. doi: 10.1016/j.pharmthera.2017.02.030
Cai, Q., Chen, F., Wang, T., Luo, F., Liu, X., Wu, Q., et al. (2020). Obesity and COVID-19 severity in a designated hospital in Shenzhen, China. Diabetes Care 43, 1392–1398. doi: 10.2337/dc20-0576
Carey, I. M., Critchley, J. A., Dewilde, S., Harris, T., Hosking, F. J., and Cook, D. G. (2018). Risk of infection in type 1 and type 2 diabetes compared with the general population: a matched cohort study. Diabetes Care 41, 513–521. doi: 10.2337/dc17-2131
Cecchini, R., and Cecchini, A. L. (2020). SARS-CoV-2 infection pathogenesis is related to oxidative stress as a response to aggression. Med. Hypotheses 143:110102. doi: 10.1016/j.mehy.2020.110102
Chen, N., Zhou, M., Dong, X., Qu, J., Gong, F., Han, Y., et al. (2020). Epidemiological and clinical characteristics of 99 cases of 2019 novel coronavirus pneumonia in Wuhan, China: a descriptive study. Lancet 395, 507–513. doi: 10.1016/s0140-6736(20)30211-7
Chen, Y., Chen, J., Gong, X., Rong, X., Ye, D., Jin, Y., et al. (2020). Clinical characteristics and outcomes of type 2 diabetes patients infected with COVID-19: a retrospective study. Engineering (Beijing) 6, 1170–1177. doi: 10.1016/j.eng.2020.05.017
Chernyak, B., Popova, E., Prikhodko, A., Grebenchikov, O., Zinovkina, L., and Zinovkin, R. (2020). COVID-19 and oxidative stress. Biochemistry (Mosc.) 85, 1543–1553.
Chu, X., Taghizadeh, S., Vasquez-Armendariz, A. I., Herold, S., Lei, C., San Zhang, J., et al. (2021). Characterization of a novel Fgf10CreERT2 knock-in mouse line targeting postnatal lung Fgf10 lineages. bioRxiv [Preprint]. doi: 10.1101/2021.02.05.429562
Ciceri, F., Beretta, L., Scandroglio, A. M., Colombo, S., Landoni, G., Ruggeri, A., et al. (2020). Microvascular COVID-19 lung vessels obstructive thromboinflammatory syndrome (MicroCLOTS): an atypical acute respiratory distress syndrome working hypothesis. Crit. Care Resusc. 15, 95–97.
Colunga Biancatelli, R. M. L., Berrill, M., Catravas, J. D., and Marik, P. E. (2020). Quercetin and vitamin C: an experimental, synergistic therapy for the prevention and treatment of SARS-CoV-2 related disease (COVID-19). Front. Immunol. 11:1451. doi: 10.3389/fimmu.2020.01451
El Agha, E., Herold, S., Al Alam, D., Quantius, J., Mackenzie, B., Carraro, G., et al. (2014). Fgf10-positive cells represent a progenitor cell population during lung development and postnatally. Development 141, 296–306. doi: 10.1242/dev.099747
El Agha, E., Moiseenko, A., Kheirollahi, V., De Langhe, S., Crnkovic, S., Kwapiszewska, G., et al. (2017). Two-way conversion between lipogenic and myogenic fibroblastic phenotypes marks the progression and resolution of lung fibrosis. Cell Stem Cell 20, 261–273.e3.
Evans, J. L., Goldfine, I. D., Maddux, B. A., and Grodsky, G. M. (2002). Oxidative stress and stress-activated signaling pathways: a unifying hypothesis of type 2 diabetes. Endocr. Rev. 23, 599–622. doi: 10.1210/er.2001-0039
Ferlita, S., Yegiazaryan, A., Noori, N., Lal, G., Nguyen, T., To, K., et al. (2019). Type 2 diabetes mellitus and altered immune system leading to susceptibility to pathogens, especially Mycobacterium tuberculosis. J. Clin. Med. 8:2219. doi: 10.3390/jcm8122219
Fernandes, I. G., De Brito, C. A., Dos Reis, V. M. S., Sato, M. N., and Pereira, N. Z. (2020). SARS-CoV-2 and other respiratory viruses: what does oxidative stress have to do with it? Oxid. Med. Cell Longev. 2020:8844280.
Forgiarini Junior, L. A., Kretzmann, N. A., Tieppo, J., Picada, J. N., Dias, A. S., and Marroni, N. A. (2010). Lung alterations in a rat model of diabetes mellitus: effects of antioxidant therapy. J. Bras. Pneumol. 36, 579–587.
Frydrych, L. M., Bian, G., O’lone, D. E., Ward, P. A., and Delano, M. J. (2018). Obesity and type 2 diabetes mellitus drive immune dysfunction, infection development, and sepsis mortality. J. Leukoc. Biol. 104, 525–534. doi: 10.1002/jlb.5vmr0118-021rr
Fu, Y., Cheng, Y., and Wu, Y. (2020). Understanding SARS-CoV-2-mediated inflammatory responses: from mechanisms to potential therapeutic tools. Virol. Sin. 35, 266–271. doi: 10.1007/s12250-020-00207-4
Gao, F., Zheng, K. I., Wang, X. B., Sun, Q. F., Pan, K. H., Wang, T. Y., et al. (2020). Obesity is a risk factor for greater COVID-19 severity. Diabetes Care 43, e72–e74.
Gattinoni, L., Coppola, S., Cressoni, M., Busana, M., Rossi, S., and Chiumello, D. (2020). Covid-19 does not lead to a “typical” acute respiratory distress syndrome. Am. J. Resp. Crit. Care Med. 201, 1299–1300. doi: 10.1164/rccm.202003-0817le
Goldman, M. D. (2003). Lung dysfunction in diabetes. Diabetes Care 26, 1915–1918. doi: 10.2337/diacare.26.6.1915
Guan, W.-J., Ni, Z.-Y., Hu, Y., Liang, W.-H., Ou, C.-Q., He, J.-X., et al. (2020). Clinical characteristics of 2019 novel coronavirus infection in China. MedRxiv [Preprint]. doi: 10.1101/2020.02.06.20020974
Guo, W., Li, M., Dong, Y., Zhou, H., Zhang, Z., Tian, C., et al. (2020). Diabetes is a risk factor for the progression and prognosis of COVID-19. Diabetes Metab. Res. Rev. 36:e3319.
Hameed, I., Masoodi, S. R., Mir, S. A., Nabi, M., Ghazanfar, K., and Ganai, B. A. (2015). Type 2 diabetes mellitus: from a metabolic disorder to an inflammatory condition. World J. Diabetes 6:598. doi: 10.4239/wjd.v6.i4.598
Hatanaka, E., Monteagudo, P., Marrocos, M., and Campa, A. (2006). Neutrophils and monocytes as potentially important sources of proinflammatory cytokines in diabetes. Clin. Exp. Immunol. 146, 443–447. doi: 10.1111/j.1365-2249.2006.03229.x
Hiraiwa, K., and van Eeden, S. F. (2014). “Nature and consequences of the systemic inflammatory response induced by lung inflammation,” in Lung Inflammation (London: Intechopen), 79.
Hodgson, K., Morris, J., Bridson, T., Govan, B., Rush, C., and Ketheesan, N. (2015). Immunological mechanisms contributing to the double burden of diabetes and intracellular bacterial infections. Immunology 144, 171–185. doi: 10.1111/imm.12394
Huang, C., Wang, Y., Li, X., Ren, L., Zhao, J., Hu, Y., et al. (2020). Clinical features of patients infected with 2019 novel coronavirus in Wuhan, China. Lancet 395, 497–506.
Hussain, S., Baxi, H., Jamali, M. C., Nisar, N., and Hussain, M. S. (2020). Burden of diabetes mellitus and its impact on COVID-19 patients: a meta-analysis of real-world evidence. Diabetes Metab. Syndr. 14, 1595–1602. doi: 10.1016/j.dsx.2020.08.014
Kalligeros, M., Shehadeh, F., Mylona, E. K., Benitez, G., Beckwith, C. G., Chan, P. A., et al. (2020). Association of obesity with disease severity among patients with COVID-19. Obesity (Silver Spring) 28, 1200–1204.
Kheirollahi, V., Wasnick, R. M., Biasin, V., Vazquez-Armendariz, A. I., Chu, X., Moiseenko, A., et al. (2019). Metformin induces lipogenic differentiation in myofibroblasts to reverse lung fibrosis. Nat. Commun. 10:2987.
Khomich, O. A., Kochetkov, S. N., Bartosch, B., and Ivanov, A. V. (2018). Redox biology of respiratory viral infections. Viruses 10:392. doi: 10.3390/v10080392
Kim, K. K., Kugler, M. C., Wolters, P. J., Robillard, L., Galvez, M. G., Brumwell, A. N., et al. (2006). Alveolar epithelial cell mesenchymal transition develops in vivo during pulmonary fibrosis and is regulated by the extracellular matrix. Proc. Natl. Acad. Sci. U.S.A. 103, 13180–13185. doi: 10.1073/pnas.0605669103
Kolahian, S., Leiss, V., and Nurnberg, B. (2019). Diabetic lung disease: fact or fiction? Rev. Endocr. Metab. Disord. 20, 303–319. doi: 10.1007/s11154-019-09516-w
Kruglikov, I. L., and Scherer, P. E. (2020). The role of adipocytes and adipocyte-like cells in the severity of COVID-19 infections. Obesity (Silver Spring) 28, 1187–1190. doi: 10.1002/oby.22856
Lambadiari, V., Kousathana, F., Raptis, A., Katogiannis, K., Kokkinos, A., and Ikonomidis, I. (2020). Pre-existing cytokine and NLRP3 inflammasome activation and increased vascular permeability in diabetes: a possible fatal link with worst COVID-19 infection outcomes? Front. Immunol. 11:557235. doi: 10.3389/fimmu.2020.557235
Leng, L., Cao, R., Ma, J., Mou, D., Zhu, Y., Li, W., et al. (2020). Pathological features of COVID-19-associated lung injury: a preliminary proteomics report based on clinical samples. Signal. Transduct. Target Ther. 5:240.
Lv, Y., Dhlamini, Q., Chen, C., Li, X., Bellusci, S., and Zhang, J.-S. (2021). FGF10 and lipofibroblasts in lung homeostasis and disease: insights gained from the adipocytes. Front. Cell Dev. Biol. 9:645400. doi: 10.3389/fcell.2021.645400
Malik, P., Patel, U., Patel, K., Martin, M., Shah, C., Mehta, D., et al. (2020). Obesity a predictor of outcomes of COVID-19 hospitalized patients-A systematic review and meta-analysis. J. Med. Virol. 93, 1188–1193. doi: 10.1002/jmv.26555
Mantovani, A., Byrne, C. D., Zheng, M.-H., and Targher, G. (2020). Diabetes as a risk factor for greater COVID-19 severity and in-hospital death: a meta-analysis of observational studies. Nutr. Metab. Cardiovasc. Dis. 30, 1236–1248. doi: 10.1016/j.numecd.2020.05.014
Matthay, M. A., Ware, L. B., and Zimmerman, G. A. (2012). The acute respiratory distress syndrome. J. Clin. Investig. 122, 2731–2740.
Messina, G., Polito, R., Monda, V., Cipolloni, L., Di Nunno, N., Di Mizio, G., et al. (2020). Functional role of dietary intervention to improve the outcome of COVID-19: a hypothesis of work. Int. J. Mol. Sci. 21:3104. doi: 10.3390/ijms21093104
Morbini, P., Villa, C., Campo, I., Zorzetto, M., Inghilleri, S., and Luisetti, M. (2006). The receptor for advanced glycation end products and its ligands: a new inflammatory pathway in lung disease? Mod. Pathol. 19, 1437–1445. doi: 10.1038/modpathol.3800661
Muniyappa, R., and Gubbi, S. (2020). COVID-19 Pandemic, Corona Viruses, and Diabetes Mellitus. Rockville, MD: American Physiological Society.
Nyambuya, T. M., Dludla, P. V., Mxinwa, V., and Nkambule, B. B. (2020). T-cell activation and cardiovascular risk in adults with type 2 diabetes mellitus: a systematic review and meta-analysis. Clin. Immunol. 210:108313. doi: 10.1016/j.clim.2019.108313
Oliveira, T. L., Melo, I. S., Cardoso-Sousa, L., Santos, I. A., El Zoghbi, M. B., Shimoura, C. G., et al. (2020). Pathophysiology of SARS-CoV-2 in lung of diabetic patients. Front. Physiol. 11:587013. doi: 10.3389/fphys.2020.587013
Paxson, J. A., Gruntman, A., Parkin, C. D., Mazan, M. R., Davis, A., Ingenito, E. P., et al. (2011). Age-dependent decline in mouse lung regeneration with loss of lung fibroblast clonogenicity and increased myofibroblastic differentiation. PLoS One 6:e23232. doi: 10.1371/journal.pone.0023232
Phillips, R. J., Burdick, M. D., Hong, K., Lutz, M. A., Murray, L. A., Xue, Y. Y., et al. (2004). Circulating fibrocytes traffic to the lungs in response to CXCL12 and mediate fibrosis. J. Clin. Invest. 114, 438–446. doi: 10.1172/jci200420997
Pitocco, D., Fuso, L., Conte, E. G., Zaccardi, F., Condoluci, C., Scavone, G., et al. (2012). The diabetic lung–a new target organ? Rev. Diabet. Stud. 9, 23–35. doi: 10.1900/rds.2012.9.23
Pitocco, D., Viti, L., Tartaglione, L., Di Leo, M., Rizzo, G., Manto, A., et al. (2020). Diabetes and severity of COVID-19: what is the link? Med. Hypotheses 143:109923. doi: 10.1016/j.mehy.2020.109923
Popkin, B. M., Du, S., Green, W. D., Beck, M. A., Algaith, T., Herbst, C. H., et al. (2020). Individuals with obesity and COVID-19: a global perspective on the epidemiology and biological relationships. Obes. Rev. 21:e13128.
Rao, S., Lau, A., and So, H. C. (2020). Exploring diseases/traits and blood proteins causally related to expression of ACE2, the putative receptor of SARS-CoV-2: a mendelian randomization analysis highlights tentative relevance of diabetes-related traits. Diabetes Care 43, 1416–1426. doi: 10.2337/dc20-0643
Roberts, T. J., Burns, A. T., Macisaac, R. J., Macisaac, A. I., Prior, D. L., and La Gerche, A. (2018). Diagnosis and significance of pulmonary microvascular disease in diabetes. Diabetes Care 41, 854–861. doi: 10.2337/dc17-1904
Rock, J. R., Barkauskas, C. E., Cronce, M. J., Xue, Y., Harris, J. R., Liang, J., et al. (2011). Multiple stromal populations contribute to pulmonary fibrosis without evidence for epithelial to mesenchymal transition. Proc. Natl. Acad. Sci. U.S.A. 108, E1475–E1483.
Rottoli, M., Bernante, P., Belvedere, A., Tonetti, T., Garelli, S., Giannella, M., et al. (2020). Obesity is one of the strongest risk factor for respiratory failure and death in COVID-19 patients: a retrospective multicentric cohort study. SSRN Electron. J. [Preprint]. doi: 10.2139/ssrn.3578779
Salvator, H., Grassin-Delyle, S., Naline, E., Brollo, M., Fournier, C., Couderc, L.-J., et al. (2020). Contrasting effects of adipokines on the cytokine production by primary human bronchial epithelial cells: inhibitory effects of adiponectin. Front. Pharmacol. 11:56. doi: 10.3389/fphar.2020.00056
Shao, H., Qin, Z., Geng, B., Wu, J., Zhang, L., Zhang, Q., et al. (2020). Impaired lung regeneration after SARS-CoV-2 infection. Cell Prolif. 53:e12927.
Shi, Q., Zhang, X., Jiang, F., Zhang, X., Hu, N., Bimu, C., et al. (2020). Clinical characteristics and risk factors for mortality of COVID-19 patients with diabetes in Wuhan, China: a two-center, retrospective study. Diabetes Care 43, 1382–1391. doi: 10.2337/dc20-0598
Simonnet, A., Chetboun, M., Poissy, J., Raverdy, V., Noulette, J., Duhamel, A., et al. (2020). High prevalence of obesity in severe acute respiratory syndrome coronavirus-2 (SARS-CoV-2) requiring invasive mechanical ventilation. Obesity (Silver Spring) 28, 1195–1199.
Stefan, N., Birkenfeld, A. L., Schulze, M. B., and Ludwig, D. S. (2020). Obesity and impaired metabolic health in patients with COVID-19. Nat. Rev. Endocrinol. 16, 341–342. doi: 10.1038/s41574-020-0364-6
Taghizadeh, S., Heiner, M., Vazquez-Amendariz, A., Wilhelm, J., Herold, S., et al. (2021). Characterization in mice of the resident mesenchymal niche maintaining AT2 stem cell proliferation in homeostasis and disease. Stem Cell. 2021, 1–13. doi: 10.1002/stem.3423
Talakatta, G., Sarikhani, M., Muhamed, J., Dhanya, K., Somashekar, B. S., Mahesh, P. A., et al. (2018). Diabetes induces fibrotic changes in the lung through the activation of TGF-β signaling pathways. Sci. Rep. 8:11920.
Talbot, H., Coleman, L., Crimin, K., Zhu, Y., Rock, M., Meece, J., et al. (2012). Association between obesity and vulnerability and serologic response to influenza vaccination in older adults. Vaccine 30, 3937–3943. doi: 10.1016/j.vaccine.2012.03.071
Tiwari, S., Pratyush, D. D., Gahlot, A., and Singh, S. K. (2011). Sepsis in diabetes: a bad duo. Diabetes Metab. Syndr. Clin. Res. Rev. 5, 222–227. doi: 10.1016/j.dsx.2012.02.026
Varga, Z., Flammer, A. J., Steiger, P., Haberecker, M., Andermatt, R., Zinkernagel, A. S., et al. (2020). Endothelial cell infection and endotheliitis in COVID-19. Lancet 395, 1417–1418. doi: 10.1016/s0140-6736(20)30937-5
Wang, H., and Ma, S. (2008). The cytokine storm and factors determining the sequence and severity of organ dysfunction in multiple organ dysfunction syndrome. Am. J. Emerg. Med. 26, 711–715. doi: 10.1016/j.ajem.2007.10.031
Wang, J., Zhu, L., Liu, L., Zhao, X. A., Zhang, Z., Xue, L., et al. (2020). Overweight and obesity are risks factors of severe illness in patients with COVID-19. Obesity (Silver Spring) 28, 2049–2055. doi: 10.1002/oby.22979
Wang, J.-Z., Zhang, R.-Y., and Bai, J. (2020). An anti-oxidative therapy for ameliorating cardiac injuries of critically ill COVID-19-infected patients. Int. J. Cardiol. 312, 137–138. doi: 10.1016/j.ijcard.2020.04.009
Whyte, M. B., Vas, P., Heiss, C., and Feher, M. D. (2020). The contribution of diabetic micro-angiopathy to adverse outcomes in COVID-19. Diabetes Res. Clin. Pract. 164:108217. doi: 10.1016/j.diabres.2020.108217
Wu, J., Jin, Z., and Yan, L. J. (2017). Redox imbalance and mitochondrial abnormalities in the diabetic lung. Redox Biol. 11, 51–59. doi: 10.1016/j.redox.2016.11.003
Wu, J. Q., Kosten, T. R., and Zhang, X. Y. (2013). Free radicals, antioxidant defense systems, and schizophrenia. Prog. Neuro Psychopharmacol. Biol. Psychiatry 46, 200–206. doi: 10.1016/j.pnpbp.2013.02.015
Yan, L. J. (2018). Redox imbalance stress in diabetes mellitus: role of the polyol pathway. Anim. Models Exp. Med. 1, 7–13. doi: 10.1002/ame2.12001
Yang, J., Tan, Y., Zhao, F., Ma, Z., Wang, Y., Zheng, S., et al. (2011). Angiotensin II plays a critical role in diabetic pulmonary fibrosis most likely via activation of NADPH oxidase-mediated nitrosative damage. Am. J. Physiol. Endocrinol. Metab. 301, E132–E144.
Yang, M. (2020). Cell Pyroptosis, A Potential Pathogenic Mechanism of 2019-nCoV Infection. Available online at SSRN: https://ssrn.com/abstract=3527420 (accessed January 29, 2020).
Yang, X., Yu, Y., Xu, J., Shu, H., Liu, H., Wu, Y., et al. (2020). Clinical course and outcomes of critically ill patients with SARS-CoV-2 pneumonia in Wuhan, China: a single-centered, retrospective, observational study. Lancet Resp. Med. 8, 475–481. doi: 10.1016/s2213-2600(20)30079-5
Youk, J., Kim, T., Evans, K. V., Jeong, Y.-I., Hur, Y., Hong, S. P., et al. (2020). Three-dimensional human alveolar stem cell culture models reveal infection response to SARS-CoV-2. Cell Stem Cell 27, 905–919.e10.
Zepp, J. A., Zacharias, W. J., Frank, D. B., Cavanaugh, C. A., Zhou, S., Morley, M. P., et al. (2017). Distinct mesenchymal lineages and niches promote epithelial self-renewal and myofibrogenesis in the lung. Cell 170, 1134–1148.e10.
Zhang, B., Zhou, X., Qiu, Y., Feng, F., Feng, J., Jia, Y., et al. (2020). Clinical characteristics of 82 death cases with COVID-19. medRxiv [Preprint]. doi: 10.1101/2020.02.26.20028191
Zhang, F., Yang, F., Zhao, H., and An, Y. (2015). Curcumin alleviates lung injury in diabetic rats by inhibiting nuclear factor-kappaB pathway. Clin. Exp. Pharmacol. Physiol. 42, 956–963. doi: 10.1111/1440-1681.12438
Zheng, H., Wu, J., Jin, Z., and Yan, L. J. (2017). Potential biochemical mechanisms of lung injury in diabetes. Aging Dis. 8, 7–16. doi: 10.14336/ad.2016.0627
Keywords: SARS-CoV-2, diabetes mellitus, obesity, inflammation, Lipofibroblasts, angiotensin-converting enzyme 2, alveolar epithelial type 2 cells, myofibroblast
Citation: Nouri-Keshtkar M, Taghizadeh S, Farhadi A, Ezaddoustdar A, Vesali S, Hosseini R, Totonchi M, Kouhkan A, Chen C, Zhang J-S, Bellusci S and Tahamtani Y (2021) Potential Impact of Diabetes and Obesity on Alveolar Type 2 (AT2)-Lipofibroblast (LIF) Interactions After COVID-19 Infection. Front. Cell Dev. Biol. 9:676150. doi: 10.3389/fcell.2021.676150
Received: 04 March 2021; Accepted: 11 June 2021;
Published: 08 July 2021.
Edited by:
Jorge Bernardino De La Serna, Imperial College London, United KingdomReviewed by:
Valeria D’Argenio, CEINGE Advanced Biotechnologies, University of Naples Federico II, ItalyMargarita Dominguez-Villar, Imperial College London, United Kingdom
Copyright © 2021 Nouri-Keshtkar, Taghizadeh, Farhadi, Ezaddoustdar, Vesali, Hosseini, Totonchi, Kouhkan, Chen, Zhang, Bellusci and Tahamtani. This is an open-access article distributed under the terms of the Creative Commons Attribution License (CC BY). The use, distribution or reproduction in other forums is permitted, provided the original author(s) and the copyright owner(s) are credited and that the original publication in this journal is cited, in accordance with accepted academic practice. No use, distribution or reproduction is permitted which does not comply with these terms.
*Correspondence: Saverio Bellusci, saverio.bellusci@innere.med.uni-giessen.de; Yaser Tahamtani, y.tahamtani@royan-rc.ac.ir
†These authors have contributed equally to this work