- 1National Clinical Research Center for Metabolic Disease, Hunan Provincial Key Laboratory of Metabolic Bone Diseases, Department of Endocrinology and Metabolism, The Second Xiangya Hospital, Central South University, Changsha, China
- 2Department of Radiology, The Second Xiangya Hospital, Central South University, Changsha, China
Ferroptosis is classified as an iron-dependent form of regulated cell death (RCD) attributed to the accumulation of lipid hydroperoxides and redox imbalance. In recent years, accumulating researches have suggested that ferroptosis may play a vital role in the development of diverse metabolic diseases, for example, diabetes and its complications (e.g., diabetic nephropathy, diabetic cardiomyopathy, diabetic myocardial ischemia/reperfusion injury and atherosclerosis [AS]), metabolic bone disease and adrenal injury. However, the specific physiopathological mechanism and precise therapeutic effect is still not clear. In this review, we summarized recent advances about the development of ferroptosis, focused on its potential character as the therapeutic target in metabolic diseases, and put forward our insights on this topic, largely to offer some help to forecast further directions.
Introduction
Cell death is pivotal for regular growth and development, homeostasis and, to some extent, prevention of diseases (Fuchs and Steller, 2011; Bedoui et al., 2020). Traditionally, cell death is classified into two typical forms, termed as accidental cell death (ACD) (e.g., necrosis) and regulated cell death (RCD) (e.g., apoptosis). RCD is a normal phenomenon that involves exact signaling cascades and mechanisms, taking place in a certain stage of cell life. In comparison, ACD is the process in which cells passively die as a result of infection or injury in most circumstances (Hotchkiss et al., 2009; Fuchs and Steller, 2011; Tang et al., 2019). Along with the thorough study, some other types of RCD have been found gradually over the years, such as necroptosis and autophagy, which are radically different lethal pathways characterized by their distinct features in morphology, biochemistry, molecular mechanisms, among others (Hotchkiss et al., 2009; Denton and Kumar, 2019; Frank and Vince, 2019; Green, 2019; Tang et al., 2020). In-depth research in 2012 defined a new concept known as ‘ferroptosis’ after screening out two compounds, erastin and RSL3, which had a lethal effect on RAS-mutant tumor cells. As a non-apoptotic form of cell death, ferroptosis has its unique trait in morphological, biochemical and genetic aspects, classified as an iron-dependent form of RCD attributed to the accumulation of lipid hydroperoxides and redox imbalance (Dixon et al., 2012; Stockwell et al., 2017). This discovery has become a hot research topic in recent years, and therefore has shed light on the new territory of the progression of diseases, such as neurodegenerative diseases (i.e., Alzheimer’s and Parkinson’s diseases), cardiovascular disease, cancer, ischemia-reperfusion injury, damage of liver and metabolic diseases (Figure 1) and challenges the search for more ways of prevention and treatment (Bruni et al., 2018b; Liang et al., 2019; Weiland et al., 2019; Capelletti et al., 2020; Han et al., 2020; Li J. et al., 2020; Stockwell et al., 2020; Viktorinova and Durfinova, 2021).
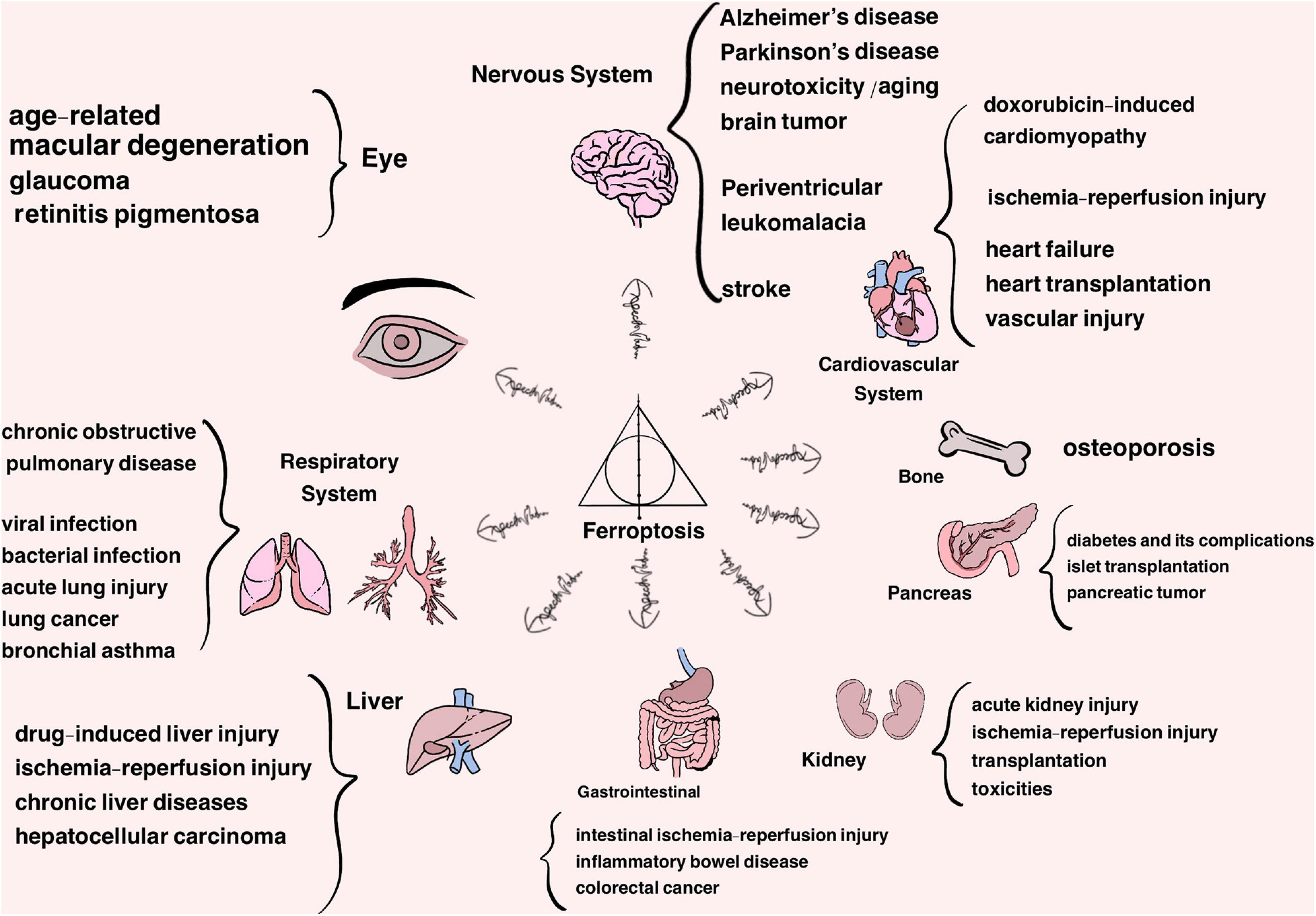
Figure 1. Ferroptosis is approved to participate in the progression of a variety of organs and system diseases, listed above.
Emerging evidence demonstrates that ferroptosis may be part and parcel of the metabolic diseases, or, more specifically, inducing or inhibiting ferroptosis might significant impact these diseases. Thus, in this review, we summarized the recent achievement regarding the pathways controlling ferroptosis and the function of ferroptosis in a series of metabolic diseases.
Overview of Ferroptosis – Magic of The Deathly Hallows
Initially, the compounds erastin and RSL3 were found to induce a diverse phenotype of cell death that can be suppressed by iron-chelating agents (Dolma et al., 2003; Yagoda et al., 2007; Yang and Stockwell, 2008). In 2012, Dixon et al. was the first team ever to denominate the unprecedented topic of ferroptosis, the death type carried out in cells with earmarks of shrinkable mitochondrial volume with lessened mitochondrial crista and denser mitochondrial membrane, but paradoxically with unaltered cell membrane, nucleus and chromatin, on par with other RCD (Yang and Stockwell, 2008; Dixon et al., 2012; Friedmann Angeli et al., 2014). To illustrate, there is an enrichment of reactive oxygen species (ROS) and ferrous ion (Fe2+), accompanied by a biochemical activation of mitogen-activated protein kinases (MAPKs). The bulk of studies to date have clarified that the conventional mechanism of ferroptosis involved the glutathione-glutathione peroxidase 4 (GPX4) axis, namely, restraining the system Xc–, which decreases the ingestion of cysteine, resulting in the deficiency of glutathione (GSH) that leads to the deposition of lipid hydroperoxides, reaching a lethal level (Stockwell et al., 2017, 2020; Chen X. et al., 2020; Tang et al., 2021). Except for the classical channel mentioned above, a significant number of studies mushroomed, revealing that some other pathways also act on the progress of ferroptosis. To date, the requirements for the occurrence of ferroptosis have proven to include iron overload, oxidation of free polyunsaturated fatty acids (PUFAs) and impaired redox pathways, which can be graphically compared to the Elder Wand, the Cloak of Invisibility and the Resurrection Stone, which together make up the Deathly Hallows-Ferroptosis, eventually leading to cell death. Moreover, ferroptosis is regulated by manifold genes, which have already been identified by the shRNA library, but the elaborate procedures still need to be developed (Dixon et al., 2012; Galluzzi et al., 2018; Lei et al., 2019).
Labile Iron Pool–Powerful Strength of the Elder Wand
Ferroptosis, as the name suggests, is characterized by the need for iron. In the body, Fe2+ can be transformed to Fe3+ by ceruloplasmin, resulting in the combination of Fe3+ with transferrin (TF) as a complex endocytosed through membrane protein TF receptor 1 (TFR1) (Li J. et al., 2020; Zheng and Conrad, 2020). Intracellular Fe3+ is reduced to Fe2+, either used to compose iron-dependent enzymes (Anderson and Vulpe, 2009) or stored in the labile iron pool (LIP) and ferritin (Bogdan et al., 2016), and the redundancy exported by ferroportin 1 (FPN1), multi-copper ferroxidase (e.g., ceruloplasmin) and ion transporter, lipocalin 2 (LCN2) (Bogdan et al., 2016; Anderson and Frazer, 2017; Li J. et al., 2020; Liu J. et al., 2021). Ultimately, the increased iron released to the LIP by ferritin-targeted autophagy, depletion of ferritin or some other circumstances is the essential condition for ferroptosis, just like a wizard’s wand, waiting for a killing curse to taste death (Figure 2).
Ferroptosis can be obstructed by iron chelators (Friedmann Angeli et al., 2014), knockdown of TFR1 (Gao et al., 2015; Torii et al., 2016) (identified as a ferroptosis marker) (Feng et al., 2020) and eliminating autophagy-related genes or the selective cargo receptor nuclear receptor coactivator 4 (NCOA4) for blocking ferritinophagy (Gao et al., 2016; Zheng and Conrad, 2020). The stress response gene, transcriptional regulator (NUPR1), targeted LCN2 as an effector gene to inhibit iron-dependent ferroptosis and LCN2 can retard acute pancreatitis by lowering the iron level in the cytoplasm (Liu J. et al., 2021), which help us draw inspiration from this pathway to explore the relationship between iron metabolism and ferroptosis on the gene level. Lately, an unanticipated axis of ATM (Ataxia-Telangiectasia)-MTF1 (metal-regulatory transcription factor 1)-Ferritin/FPN1 contributed to lessen labile iron via inhibiting ATM, which was detected by P. H. Chen et al., discovering a new dimension in ferroptosis through kinome screen (Chen P. H. et al., 2020). In contrast, decreasing the expression of ferritin (Chen P. H. et al., 2020), FPN1 (Bao et al., 2020; Chen P. H. et al., 2020) and ceruloplasmin (Shang et al., 2020) have testified to be sensitized to ferroptosis. Other iron-related proteins, such as poly-(rC)-binding protein 1 (PCBP1) (Protchenko et al., 2020; Zheng and Conrad, 2020) and heme oxygenase-1 (HO-1) (Adedoyin et al., 2018; Fang et al., 2019), have been found to be involved in ferroptosis as well. A recent study supported that ferritin-containing multivesicular bodies and exosomes are qualified to discharge iron driven by prominin2, illuminating a new mode to prevent ferroptosis (Brown et al., 2019). What merits attention is that the endothelial cell-secreted exosome has proven to throw a wrench in the glucocorticoid-induced osteoporosis process by abating ferritinophagy (Yang et al., 2021). Dysregulation of iron homeostasis and metabolism is substantiated to have a close connection to diverse metabolic diseases, such as diabetes (Dubey et al., 2020), obesity, metabolic syndrome (Gonzalez-Dominguez et al., 2020), osteoporosis (Che et al., 2020; Sato et al., 2020) and AS (Wunderer et al., 2020). Thereby, we forecast that the link between exosome and iron metastasis in ferroptosis may be a heated discussion in the near future. Likewise, exosomes as a therapy method against ferroptosis in varied metabolic diseases may also soon invigorate a conversation.
Oxidation of PUFAs – Death Clothed in the Cloak of Invisibility
According to a series of studies, free PUFAs are known to be the imperative substrates of lipid oxidation, which are esterified into membrane phospholipids and result in an oxidation that triggers ferroptosis (Yang and Stockwell, 2016; Doll et al., 2019; Zheng and Conrad, 2020), likened to the attire in the Cloak of Invisibility toward death. Phosphatidylethanolamine (PE) containing arachidonic acid (AA) and adrenaline is certified to initially be this kind of phospholipid (Kagan et al., 2017). The substrates mentioned above are catalyzed by Acyl-CoA synthetase long-chain family member 4 (ACSL4) (Kuch et al., 2014) and lysophosphatidylcholine acyltransferase 3 (LPCAT3) (Kagan et al., 2017; Zheng and Conrad, 2020) into their acyl-CoA esters and lysophospholipids. The next steps in the pathway of lipid oxidation-induced ferroptosis are still not yet interpreted perspicuously, in that lipoxygenase (LOX), especially ALOX15, has been widely reported to actuate lipid oxidation (Yang et al., 2016; Kagan et al., 2017; Wenzel et al., 2017; Zheng and Conrad, 2020). On the other hand, several researches have developed, explaining that the knockdown Alox15 gene cannot succeed to reverse ferroptosis induced by GPX4 deficiency in multiple types of cells (Brutsch et al., 2015; Matsushita et al., 2015) and the expression of ALOX (mRNA level) remains at a low content in many cancer cells (Zou et al., 2020b). Clearly speaking, lipid autoxidation may be the primary cause of ferroptosis (Shah et al., 2018). Nonetheless, it is worth noting that cytochrome P450 oxidoreductase (POR), located in the endoplasmic reticulum, conduces to phospholipid peroxidation (Zou et al., 2020b) and leads to membrane damage, with the participation of cytochrome b5 reductase (CYB5R1) (Yan et al., 2021). This evidence may highlight the importance of enzymatic reactions in ferroptosis, given that with the effect of enzymes, the phospholipid hydroperoxide (PLOOH) threshold may be reached physiologically, in spite of the antioxidant systems (Shah et al., 2018).
Apart from the process discussed above, another pathway of lipid metabolism in ferroptosis has emerged, which is an ACSL4/LPCAT3-independent way that relies on peroxisomes, whose function is to synthesize plasmalogens, an alternative substrate of lipid oxidation classified as a subclass of ether phospholipids (Zou et al., 2020a). This pathway is indebted to the peroxisomal enzymes involved: alkylglycerone phosphate synthase (AGPS), fatty acyl-CoA reductase 1 (FAR1), and glyceronephosphate O-acyltransferase (GNPAT), under which the precursor 1-O-alkyl-glycerol-3-phosphate (AGP) is synthesized and subsequently transported to the endoplasmic reticulum to form PUFA-plasmalogen by 1-acylglycerol-3-phosphate O-acyltransferase 3 (AGPAT3) and endoplasmic reticulum(ER)-resident enzyme plasmanylethanolamine desaturase 1 (PEDS1) (Tang and Kroemer, 2020; Zou et al., 2020a). Therefore, insights into the peroxisomes may provide a newly built framework of the development of ferroptosis, which may guide a direction to treatment; meanwhile, complete access to this pathway and the other roles the peroxisomes play in ferroptosis remain to be tested.
Three Pathways – The Repair Network of the Resurrection Stone
There are three predominant pathways, similar to the resurrection stone in Harry Potter, which manage to repair the peroxide state, contributing to prevent ferroptosis and reviving cells in the body. By blocking these procedures, cells may be exposed to the sensitivity of ferroptosis.
System Xc-GSH-GPX4 Axis
The amino acid system Xc-GSH-GPX4 axis is an indispensable part of lipid peroxidation elimination. Comprised of SLC3A2 and SLC7A11, system Xc– imports cystine into cells by reversing glutamate transport (Wang et al., 2020b; Li N. et al., 2021). Moreover, cystine transforms into cysteine, performing as raw material in the synthesis of GSH. As the glutathione peroxidase, the selenoprotein GPX4 catalyzes the combination of lipid hydroperoxides (L-OOH) with the sulfhydryl group of reduced glutathione, converting the harmful substances into non-toxic lipid alcohols (L-OH) and hence blocking the ROS chain reaction, avoiding ferroptosis (Yang et al., 2014; Ingold et al., 2018; Seibt et al., 2019). The aforementioned contents are the main framework of the system Xc-GSH-GPX4 axis, and plenty of molecules are certified to be mediators of the above substances, which primely enrich the pathway described below.
To regulate system Xc–. (Erythoid-derived)-like 2 (Nrf2) belongs to the cap-n-collar subfamily of transcription factors, proven to be conjugated with Kelch-like ECH-associated protein 1 (Keap1) in non-oxidizing states and while the oxidative stress was aggrandized, it may separate from Keap1, acting as a key member in oxidation-reduction reactions. According to manifold studies, the Nrf2-Keap1 pathway is able to give an impulse to system Xc–, enhancing the resistibility of ferroptosis via Nrf2 overexpression or Keap1 drawdown, which was further confirmed to occur in the development of retinopathy in Type 1 diabetes (T1DM) (Fan et al., 2017; Carpi-Santos and Calaza, 2018). Notably, active Nrf2 is in a position to ease delayed gastric emptying in obesity-induced diabetic (Type 2 diabetes, T2DM) mice, implying a potent role of this pathway in diabetes (Sampath et al., 2019). In addition, an alternative reading frame product of the CDKN2A locus (ARF) can weaken the course of Nrf2-controlled sensitization of SLC7A11, taking a role in facilitating ferroptosis by relying or not relying on tumor suppressor gene p53 in cancer cells (Chen et al., 2017). Based on previous data, p53 is crucial for ferroptosis by performing double- action work: boost ferroptosis on SLC7A11 expression (L. Jiang et al., 2015; Liu J. et al., 2020) and suppress ferroptosis by declining susceptibility, which may need a share with its target gene, P21 (Liu J. et al., 2020; Venkatesh et al., 2020b). Furthermore, activating transcription factor 3 (ATF3) is also proven to couple with a SLC7A11 promoter to advance ferroptosis in a p53-independent way, as with the tumor suppressor BRCA1-associated protein 1 (BAP1) (Zhang Y. et al., 2018; Zhang et al., 2019a; Wang et al., 2020b). Several factors, such as glutamate, sorafenib, sulfasalazine, imidazole ketone erastin, diaryl-isoxazoles, and INF-γ, are also approved to be inhibitors of system Xc–, competitively or not (Dixon et al., 2012, 2014; Newell et al., 2014; Leu et al., 2019; Zhang X. et al., 2019; Zitvogel and Kroemer, 2019).
In metabolic and endocrine diseases, eliminating SLC7A11 is related to inhibiting the growth of pancreatic ductal adenocarcinoma (Badgley et al., 2020). In addition, researchers have identified a gene, pyruvate dehydrogenase kinase 4 (PDK4), that is obligated to alter ferroptosis sensitivity in human pancreatic ductal carcinoma cells through the system Xc–, influenced by glucose metabolism, implicating a new view of cancer therapy (Song et al., 2021). Of note, lowering the level of CD44 variant (CD44v), which can steady the system Xc–, increases insulin secretion and contributes to the amino acid transport regulated by L-type amino acid transporter LAT1 in pancreatic β cells; thus guiding a new therapeutic target in diabetes (Kobayashi et al., 2018).
To regulate GPX4. GPX4 is designated as a determinant upstream mediator of ferroptosis, extensively existing in cytoplasm, the cell nucleus, mitochondria and other cellular organs (Arai et al., 1996; Friedmann Angeli et al., 2014; Seibt et al., 2019; Li N. et al., 2021). RSL3 was discovered to be an inhibitor of GPX4 to induce ferroptosis sufficiently and early (Yang et al., 2014). Subsequently, compounds FINO2, FIN56, ML162, ML210, DPI7, DPI10 and buthionine sulfoximine, which have the same effect as RSL3, have been discovered as well (Gaschler et al., 2018; Seibt et al., 2019; Li N. et al., 2021). The latest research depicted that folate-vectorized exosomes loaded with erastin were capable of inhibiting GPX4 in triple-negative breast cancer cells (Yu et al., 2019), also emphasizing the crosstalk between exosome and ferroptosis. Currently, the molecular mechanism that selenium takes a part of in the synthesis of GPX4 by shaping into the 21st amino acid, selenocysteine (Sec), has been enucleated. This gives insight into the point that using selenium is necessary to avoid ferroptosis due to its ability to activate the transcription factors TFAP2c and Sp1, causing the reinforcement of GPX4, and resulting in the safeguarding of neurons (Ingold et al., 2018; Alim et al., 2019). Analogously, the mevalonate (MVA) pathway subserves the selenocysteine tRNA in order to participate in the synthesis of GPX4, and isopentenyl pyrophosphate (IPP) and COQ10 are the main products of this process (Warner et al., 2000). Notably, the heat shock protein family also affects GPX4; specifically, HSPA5 attenuates erastin-induced GPX4 evanishment while the chaperone-mediated autophagy on the strength of HSP90 antagonizes that matter, possibly hastened by legumain (Zhu et al., 2017; Wu Z. et al., 2019; Chen et al., 2021).
Intriguingly, as the micronutrient, selenium (Se) and selenoprotein are integral in several metabolic and endocrine diseases, such as thyroid disease and diabetes, and raising their level has been relevant to insulin-induced, hydrogen peroxide (H2O2)-dependent signaling impairment, resulting in insulin resistance and hyperglycemia (Wang et al., 2016; Kim et al., 2019; Schomburg, 2020). Meanwhile, in the Se-deficient population, serum Se positively correlates with glucose, indicating that Se supply is basilical for glucose homeostasis (Wang et al., 2020g). It is conceivable, but not yet demonstrated penetratingly, that ferroptosis could be significantly diminished by mediating GPX4 with a supplement of Se, playing a role in insulin regulation and diabetes.
NADPH-FSP1-CoQ10 Axis
Ferroptosis suppressor protein 1 (FSP1) (previously referred to as apoptosis-inducing factor mitochondrial 2, AIFM2) was one of anti-ferroptotic genes identified relatively recently (Stockwell et al., 2020). By means of its catalytic action with nicotinamide adenine dinucleotide phosphate (NADPH), CoQ10 (also referred to as ubiquinone) regenerates so as to exhibit oxidation resistance, capturing lipid peroxyl radicals to suppress ferroptosis. Correlation studies examined this pathway, giving rise to an unaided parallel route of ferroptosis distinct from the foregone system Xc-GSH-GPX4 axis. FSP1-induced ferroptosis resistance is corroborated to take place in a number of cancer cell lines, elucidating a new anticancer target (Elguindy and Nakamaru-Ogiso, 2015; Bersuker et al., 2019; Doll et al., 2019). However, whether upregulating FSP1 can really enshield cells not to suffer from ferroptosis remains to be clarified in the near future (Santoro, 2020). It is worth noting that a study recently revealed that FSP1 could solely depress erastin-, RSL3-, and sorafenib-induced ferroptosis without CoQ10, dealing with the endosomal sorting complexes required for transport (ESCRT)-III–dependent membrane repair (Dai et al., 2020). Nonetheless, this research did not discover the downstream target of FSP1, and this still awaits us and requires a deeper investigation into the mechanism.
To regulate this axis. Murine double minute 2 (MDM2) family (MDM2 and MDMX) are regarded as negative adjusters of p53. Inhibiting the MDM2-MDMX complex was clarified to heighten the levels of FSP1 protein by remodeling peroxisome proliferator-activated receptor α (PPARα) activity (Venkatesh et al., 2020a). It is noteworthy that FIN56 initiates ferroptosis by way of depleting GPX4 and the mevalonate-derived CoQ10 (Shimada et al., 2016; Stockwell et al., 2020). By the same token, statins could bridle CoQ10 for being a hindrance of the enzyme HMG CoA reductase (Shimada et al., 2016). Strikingly, the long non-coding RNA maternally-expressed gene 3 (MEG3)-microRNA-214 (miR-214) axis may negatively regulate FSP1, which was known to direct against activating transcription factor 4 (ATF4, a SLC7A11 promoter) (Bai et al., 2020b). cAMP-response-element-binding protein (Nguyen et al., 2020) and P53 (Bersuker et al., 2019; Doll et al., 2019) also have a share in FSP modulation.
In cellular metabolism, FSP1 was estimated to accelerate glycolysis via oxidizing NADH in the outer side of the mitochondrial inner membrane in brown adipose tissue (BAT), resulting in retarding diet-induced obesity and insulin resistance (Nguyen et al., 2020). Replenishing mitochondrial CoQ has been showed to ameliorate insulin resistance in adipocytes, principally by decreasing superoxide/H2O2 production via complex II (Fazakerley et al., 2018). An interesting research reported there might be a marginal coenzyme Q10 deficiency in athletes, which was associated with blood glucose and antioxidant capacity (Ho et al., 2020) and then through recharging ubiquinol, resulting in exercise performance upgrades with a raised level of free fatty acids (FFA) (Chen et al., 2019). Besides, CoQ10 united with pioglitazone modified the mRNA expression of adipocytokines and oxidative stress parameters in diabetic rats (Maheshwari et al., 2020); meanwhile, CoQ10 with alpha lipoic acid (ALA) could decrease degeneration and apoptosis of dorsal root ganglion (DRG) neurons of diabetic neuropathy (DN), seemingly by mediating the expression of caspase 3 and uncouplingprotein 2 (UCP2) proteins (Sadeghiyan Galeshkalami et al., 2019). In summary, abundant investigations have documented that supplementation of CoQ may have a beneficial effect in metabolic syndrome (Raygan et al., 2016; Mazza et al., 2018), diabetes (Zhang S. Y. et al., 2018) and its complications (Mantle, 2017), such as diabetic retinopathy (Zhang X. et al., 2017; Derosa et al., 2019), macroangiopathy (disorder of lipid metabolism and AS) (Montano et al., 2015; Zhang P. et al., 2018; Suarez-Rivero et al., 2019), and diabetic kidney diseases (Zhang X. et al., 2019). Yet, there is a requirement for more participant-involved studies and clinical trials to ravel the molecular mechanism and ensure the therapeutic effect is meeting the clinical needs. We speculate that ferroptosis could be attached to these morbid processes, and its inducers and inhibitors targeted to the NADPH–FSP1-CoQ10 axis may be useful for treatment, although further study is required to test this possibility.
GCH1-BH4-Phospholipid Axis
Recently, with a method of genome-wide activation screen, Kraft et al. (2020) documented that GTP cyclohydrolase-1 (GCH1) and its metabolic derivatives tetrahydrobiopterin/dihydrobiopterin (BH4/BH2) partake in developing ferroptosis resistance. Mechanistically, BH4 is capable of selectively deterring autoxidation of phospholipids with two polyunsaturated fatty acyl tails and expediting the generation of CoQ10 (Kraft et al., 2020), in order to endowBH4 with emerging lipophilic radical-trapping antioxidants (RTAs) requiring dihydrofolate reductase (DHFR) for rebirth (Soula et al., 2020). Until recently, the discussions about this axis were incomplete; therefore, more in-depth exploration of its pathophysiological role in ferroptosis needed to be conducted.
Accumulating evidence illustrate that the GCH1-BH4-phospholipid axis plays a part in energy metabolism and metabolic diseases (Kim and Han, 2020). Concretely, BH4 is signalized for being a cofactor involving in the enzymatic conversion of amino acids; for instance, tyrosine and phenylalanine to precursors of dopamine and serotonin, as well as the formation of nitric oxide (NO) demand for BH4 combining with nitric oxide synthase (NOS) (Mayer and Werner, 1995; Kim and Han, 2020). All of the biological functions are the cornerstone for BH4’s role in glycolipid metabolism disorder, endothelial injury and inflammation. BH4 decreased due to oxidative stress, opening the door for BAT dysfunction, mainly through NO and noradrenaline signaling, contributing to higher obesity, insulin resistance, and glucose intolerance (Oguri et al., 2017). In addition, in hypercholesterolemia, oxidized low-density lipoproteins (ox-LDLs) reduce mRNA expression of GCH1 and BH4, leading to a decline of NO damaging the endothelium to induce vascular injury (i.e., AS) (Douglas et al., 2018; Kim and Han, 2020), which may be accelerated by nicotine (Li et al., 2018). Interestingly, more studies were performed to show that GCH1/BH4 may act as a therapeutic target for diabetic cardiomyopathy (Wu et al., 2016; Kim et al., 2020; Carnicer et al., 2021). Also, CTRP13 (Wang et al., 2020f), zinc (Liu P. et al., 2020), and curcumin nanoparticles (Abu-Taweel et al., 2020) have been utilized to exalt the level of GCH1 (Alp et al., 2004) or BH4 (Shah et al., 2017), resulting in observation of a relief of endothelial dysfunction. These results furnished a clue that the ferroptosis appears to assist the metabolic and vascular diseases mentioned before dependency on GCH1/BH4, and repressed ferroptosis may protect cells from the harm of those pathological processes, which may be a hotspot for the further investigation. Strikingly, a new study unveiled that glucose starvation-induced energy stress significantly attenuated erastin- and cysteine/GPX4 depletion-induced ferroptosis due to AMP-activated protein kinase (AMPK) in immortalized mouse embryonic fibroblasts (Lee et al., 2020); whereas, activation of AMPK is verified to recede T2DM-induced BH4 reduction by preventing GCH1 degradation, further intimating about the potential role of ferroptosis in metabolic diseases (Day et al., 2017).
Ferroptosis Cast Spells to Metabolic and Endocrine Diseases
Ferroptosis and Diabetes and Its Complications
According to the investigation of the International Diabetes Federation, under half a billion people are suffering from diabetes worldwide and this number is increasing rapidly (Saeedi et al., 2019). Diabetes is classified into two types: T1DM, caused by damnification of pancreatic β cells, along with insufficient insulin secretion, and T2DM, which is mainly induced by insulin resistance (Saberzadeh-Ardestani et al., 2018; Galicia-Garcia et al., 2020). Both types contribute to the building of a high glucose state, which may inflict SLC7A11 and SLC3A2L impairment, leading to system Xc– dysfunction (Koppula et al., 2017). It has been affirmed that patients with T2DM are devoid of GSH, especially if microvascular complications are present (Lutchmansingh et al., 2018). Substantial researches have called attention to the close relationship between iron and glucose metabolism; that is, iron deficiency and excess may affect glucose regulation (Fernandez-Real et al., 2015), and high glucose may give rise to iron overload (Shu et al., 2019), which is known to trigger ferroptosis. Meanwhile, under iron overload condition, insulin resistance might be brought on by oxidative stress (Sung et al., 2019) and the pancreatic fat fraction has proven to be associated with glucose dysregulation (Shur et al., 2020). Evidence has shown that ferroptosis arises in patients with diabetes and its complications and inhibiting it may become a welcome sign in treatment.
Diabetes and Pancreatic Dysfunction
The damage of β cells in T1DM mainly occurs due to the negative effect of proinflammatory cytokines and its products: reactive oxygen and nitrogen species (RNS) (Lenzen, 2017). A study conducted in 2018 has indicated that ferroptosis-inducing agents (FIA), such as erastin or RSL3, have the ability to impact islet function in vitro (Bruni et al., 2018b) and ferroptosis may be involved in islet isolation and transplantation (Bruni et al., 2018a). β cells often have decreased levels of H2O2-detoxifying enzymes, i.e., catalase, glutathione peroxidase 1 (Lenzen, 2008), GPX7 and GPX8 (Mehmeti et al., 2017), as well as the generation of ROS and iron accumulation (Krummel et al., 2021), owing to proinflammatory cytokines (Lortz et al., 2014). Krummel et al. (2021) determined that β cells may have a higher sensitivity to ferroptosis and confirmed that GPX4 distributes throughout the β cells to a large extent, eliminating that GPX4 induces ferroptosis. Interestingly, proinflammatory cytokine-induced death is independent of ferroptosis, probably because the offspring of toxic cytokines, nitric oxide, can wipe out generated lipid radicals in the membrane.
Although the phenotype and mechanism of ferroptosis developed in β cells have been pressed for in-depth exploration, some substances directed against ferroptosis have been found to ameliorate impairment of transplanted islet cells and treat diabetes. Bilirubin may protect the islet by raising GPX4, upregulating Nrf2/HO-1 and chelating iron to interdict ferroptosis (Yao et al., 2020). Cryptochlorogenic acid (CCA), the active constituent of the mulberry leaf, was reported to target some major regulators (system Xc-GPX4, Nrf2 and NCOA4) to inhibit ferroptosis in a concentration-dependent manner in a diabetic model (Zhou, 2020). Similarly, in T2DM, a natural iron chelator, quercetin, may also exert a positive effect to reverse pancreatic iron deposition as an inhibitor of ferroptosis (Li D. et al., 2020). Additionally, as a high-risk factor for pancreatic dysfunction and T2DM (Grau-Perez et al., 2017), chronic arsenic exposure may induce islet autophagy (Wu et al., 2018) and impact insulin secretion in pancreatic β cells for its destructive effect in mitochondrial metabolism (Dover et al., 2018; Carmean et al., 2019; Zhang Q. et al., 2019). One research study demonstrated that ferroptosis can be triggered by arsenic, which causes mitochondrial ROS-dependent autophagy via regulating iron level in a MIN6 cell model (Wei et al., 2020). All results clearly showed that inhibiting ferroptosis may expect to improve islet viability, and propose new therapeutic targets. However, many questions remained unanswered: can blocking ferroptosis truly increase the lifespan of a transplanted islet?; which factors or pathways may trigger ferroptosis under physiopathologic conditions?; and what is the integrated molecular mechanism? The answers to these questions still need to be worked out, but will not obstruct expectations of the underlying role of ferroptosis in diabetes.
Diabetic Nephropathy (DN)
Until fairly recently, researchers demonstrated that kidney tubular cell death in DN is related to ferroptosis based on the observation of declining expression of system Xc– and GPX4 mRNA and the enhancive ROS and lipid oxidation in vivo and in vitro respectively, which can be ameliorated by ferrostatin-1 (Fer-1) (Wang et al., 2020e; Kim et al., 2021; Li S. et al., 2021), implicating the positive curative effect to hinder ferroptosis. A recent study revealed the mechanism, which may have evinced HO-1 regulated by hypoxia-inducible factor (HIF), administering to iron accumulation by decomposing heme, resulting in the induction of ferroptosis to harm renal tubular in db/db mice (Feng et al., 2021), but detailing the pathophysiological process merits further investigation. High-mobility group box-1 (HMGB1) is a transcription factor enriched in the cell nucleus, involved in DNA repair and synthesis of inflammatory factors by activating the NF-κB signaling pathway (Xu T. et al., 2019). It exerted an effective effect to impede the development of DN via suppressing HMGB1 (Chen et al., 2018), by which it may inhibit high glucose-induced activation of TLR4/NF-κB and ferroptosis in mesangial cells through the Nrf2 pathway (Wu et al., 2021). Controversially, Nrf2 has a dual effect in diabetes and its complications, such as DN (Xu J. et al., 2012; Uruno et al., 2013), and upregulation of Nrf2 appears to block ferroptosis to delay the progression of DN (Li S. et al., 2021) while it may also attenuate DN by augmenting the expression of intrarenal angiotensin-converting enzyme-2 and angiotensin 1–7 receptor (Zhao et al., 2018). These present researches indicate the significance of Nrf2 preliminarily; therefore, the ferroptosis-specific role of Nrf2 in DN should be further elucidated.
Diabetic Myocardial Dysfunction
Myocardial oxidative stress and fibrosis produced by high glucose arethe major causes of diabetic cardiomyopathy (DCM) (Zang et al., 2020). Emerging evidence has manifested that suppressing ferroptosis is ofbenefit in delaying the progression of DCM. Firstly, GPX4 has beenobserved to ameliorate streptozotocin-associated cardiac injury(Baseler et al., 2013) and its shortage, related to mitochondrial lipidperoxidation and resulting in cardiac hypertrophy in mice under a diet high in sugar and fat (Behring et al., 2014). In addition, ferroptosis inhibitors, vitamin E (Shirpoor et al., 2009) and CoQ10 (Huynh et al., 2012), have been reported to improve cardiac diastolic dysfunction in diabetic models successively. Heat shock factor 1 (HSF1) has recently been shown to be able to mitigate palmitic acid-induced lipid peroxidation in obesity and T2DM-involved cardiomyopathy and further mediate the transcription of iron metabolism-related genes (e.g., Tfrc, Fth1, and Slc40a1) to maintain iron homeostasis in H9c2 cardiomyoblasts (Wang et al., 2021). We therefore believe that links between ferroptosis and diabetic cardiomyopathy should exist. Remarkably, and similar to the previous section on DN, Nrf2 also became a central issue by academicians for its protective role to cardiac cells in both T1DM and T2DM, principally via the Nrf2-Keap1 pathway (Ge et al., 2019) and its detrimental role noticed in fibroblast growth factor 21-knockout mice (Yan et al., 2015). A novel experiment uncovered that in diabetic setting, cardiac autophagy is disserved, causing cell death and myocardial damage, mainly due to ferroptosis triggered by the activation of Nrf2 (Zang et al., 2020). Thus far, there have been many drugs found to target the key regulators of ferroptosis to resist ROS generation and lipid peroxidation in DCM. Wang et al. (2020f) noted that exogenous spermine may upregulate calcium-sensitive receptor (CaSR) expression by blocking the Nrf2-ROS-p53-muscle-specific ring finger protein 1 (MuRF1) in T1DM rats, ultimately recuperating calcium homeostasis and decreasing oxidative stress. Sulforaphane (SFN) has been found to be capable of activating Nrf2 through AMPK/AKT/GSK-3β signaling pathways in order to upregulate its downstream metallothionein (MT), which is a set of low molecular weight proteins enriched with cysteine, resulting in reversing oxidative damage and fibrosis (Gu et al., 2017). Confirmed recently, this entire protective process depends on the AMPK partaking (Sun et al., 2020). Other compounds that play similar roles are listed in Table 1. Despite this, it remains to be clarified whether these compounds exert a protective effect by interrupting ferroptosis in diabetic cardiac cells.
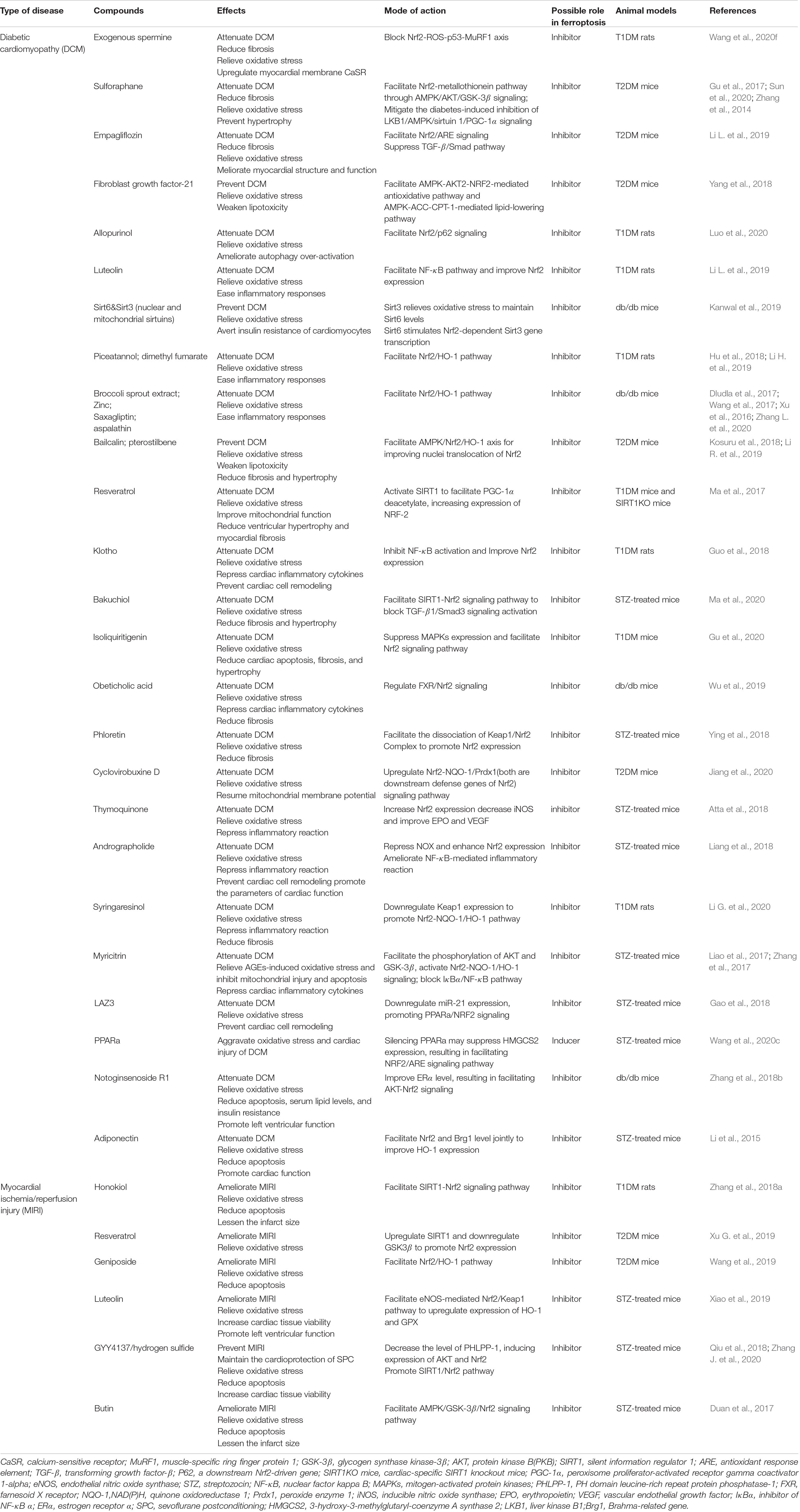
Table 1. Emerging compounds targeted key regulators of ferroptosis to attenuate diabetic myocardial dysfunction.
Myocardial ischemia/reperfusion injury (MIRI) was more likely occur in diabetics, in addition to a worse prognosis, leading to myocardial vulnerability (Palomer et al., 2013). Under diabetic conditions, oxidative stress and programmed cell death were observed and supposed to turn down AMPK expression, conducing to a higher level of NADPH oxidase (Nox), whose main function is to supply ROS (Wang et al., 2020b). Also demonstrated is that suppressing ferroptosis may alleviate endoplasmic reticulum stress (ERS), which was triggered by ATF4-C/EBP homologous protein (CHOP) pathway and played an critical role in rat myocardial I/RI (Li W. et al., 2020). Some compounds have been approved to palliate MIRI; for example, honokiol (Zhang Y. et al., 2018), resveratrol (Xu G. et al., 2019), and luteolin (Xiao et al., 2019; Table 1), offering strong evidence of ferroptosis-dependent MIRI, which undoubtedly deserve further studies.
Vascular Injury
Current studies have shown that hyperglycemia-induced oxidative stress and incremental generation of ROS, two typical ferroptotic hallmarks, play capital roles in the development of endothelial dysfunction (Brandes and Kreuzer, 2005), which is a key contributor to diabetic vascular complications. The complications can be impressed by depressed production of NO, along with a growing level of inflammatory factors, endothelial repair dysfunction, and can contribute to the pathogenesis of thrombosis and AS (Dhananjayan et al., 2016; Luo et al., 2021). Past research identified particulate matter 2.5 (PM2.5) as a potential initiator of ferroptosis in endothelial cells due to its function of inducing ROS production and iron overload (Wang and Tang, 2019). New evidence has suggested that high glucose (HG) and interleukin-1β (IL-1β) treated with human umbilical vein endothelial cells (HUVECs) have ferroptosis-related characteristics, which were induced by augmenting the p53-system Xc-GSH pathway, and the same manifestation was aroused in the aorta of db/db mice as well (Luo et al., 2021). This evidence corroborates that ferroptosis is involved in endothelial dysfunction, though more experiments should be performed in vivo. Moreover, lipid oxidation is also linked to vascular smooth muscle cell (VSMC) injury, leading to vascular calcification. Studies have certified that increased FFAs can induce endothelial dysfunction and insulin resistance, prompting an intimate relationship with ferroptosis and VSMC calcification in patients with diabetes. It has been enunciated that metformin (Met) exerts a protective effect on VSMC calcification via its anti-ferroptotic role by boosting Nrf2 expression, and intriguingly, periostin (POSTN), known as an upregulated protein in AS, downregulates p53 to sensitize VSMCs to ferroptosis in this process (Ma et al., 2021). As previously discussed, anti-ferroptosis has a potential role against vascular calcification; meanwhile, both POSTN and p53 may become novel targets, revealing the secrets of prevention and treatment of vascular injury. Additionally, cigarette smoke extract (CSE) has the ability to trigger ferroptosis in VSMCs by consuming GSH, which cannot be reversed via increased GPX4; resulting in the fact alarming people that smoking is a high-risk factor of vascular injury (Sampilvanjil et al., 2020).
The accumulation of ROS, disturbance in lipid and glucose metabolism, endothelial dysfunction and vascular calcification are all-important pathogenic factors involved in AS (Gimbrone and Garcia-Cardena, 2016; Poznyak et al., 2020), which is characterized by lipid overload and the establishment of atherosclerotic plaques in the arterial wall (Falk, 2006). In addition, an enhancive level of iron has been examined in AS (Xu, 2019), and in a situation of iron overload, oxidative stress and endothelial dysfunction may aggravate the state of AS in apolipoprotein E knockout mice (Marques et al., 2019). This information motivated a research group to evaluate the inhibition of ferroptosis in AS with ferrostatin-1, in which they observed a positive therapeutic effect in both high-fat diet-fed mice and ox-LDL-treated mouse aortic endothelial cells (MAECs) (Bai et al., 2020a), thus unveiling the mysterious character of ferroptosis in AS. Generally speaking, prospective therapy for treating AS might be more attentive for the repression of ferroptosis and more comprehensive exploration of ferroptosis-related vascular injury is required.
Ferroptosis and Metabolic Dysfunction-Associated Fatty Liver Disease (MAFLD)
To meet the demand of drug development and accurately reflect its mechanism, experts reached consensus to use the new terminology, metabolic dysfunction-associated fatty liver disease (MAFLD), to replace non-alcoholic fatty liver disease (NAFLD), which clearly put finger on the close link between this kind of chronic liver disease and metabolic disorder, such as diabetes, obesity and the metabolic syndrome (Eslam et al., 2020). It has been pointed out that free fatty acid accumulation, oxidative stress and inflammatory responses are all associated with MAFLD progression, however, the detailed mechanism propelling the simple steatosis in the directions to non-alcoholic steatohepatitis (NASH) still need to be enucleated (Mao et al., 2020). Of note, 4-hydroxy-2,3-non-anal (4-HNE), produced by lipid peroxidation reactions, may cause a damage to liver cells via Fenton reaction (Zhao et al., 2021) and it can be regarded as oxidative stress markers of NASH, so as the malondialdehyde (MDA) (Loguercio et al., 2001). Moreover, among all kinds of necrotic cell death, ferroptosis was verified to be the primary reason for the initiation of inflammation in NASH (Tsurusaki et al., 2019). Experiments have been carried out that interfering with FeCl3, specific responses of distinct liver-derived cells (rat primary hepatocytes (RPH), mouse primary hepatocytes (MPH), HepG2 human hepatoma cells and Hepa1-6 mouse hepatoma cells) were observed as a consequence of iron overload (Chen H. J. et al., 2020). Subsequently, Zhao et al. (2021) have described at length that GPX4 plays a key role in the protection of liver cells, indicating that targeting ferroptosis may be evolved into a new therapeutic options for MAFLD. Exerting a positive effect of preventing lipotoxicity, poly-(rC)-binding protein 1 (PCBP1), as a cytosolic iron chaperonin, delay the progression of ferroprosis in mouse liver (Protchenko et al., 2020). Sestrin2 (Park et al., 2019), Ginkgolide B (GB) (Yang et al., 2020) and dehydroabietic acid (DA) (Gao et al., 2021) became operative in relieving MAFLD mainly through activating Nrf2 pathway to obstruct ferroptosis while enoyl coenzyme A hydratase 1 (ECH1) (considered as an ingredient in mitochondrial fatty acid β-oxidation) seemingly play a role of mediating the Erk expression (Liu B. et al., 2021). Conducted in clinical trials, several antioxidants, such as Vitamin E and pioglitazone, are also proved to ameliorate oxidation levels resulting in the improvement of steatosis, inflammation, ballooning and fibrosis in NASH patients (Sanyal et al., 2010; Bril et al., 2019).
Remarkably, iron metabolism appears to have an intimate relationship with the disease development. Due to the metabolic disturbance, NASH is exacerbated as a result of hepatic iron deposition (Nelson et al., 2011) and can be improved by using deferoxamine mesylate salt (an iron chelator) and liproxstatin-1 (a ferroptosis inhibitor) in the methionine/choline-deficient diet (MCD) fed mice (Qi et al., 2020). In addition, the divalent metal transporter 1 (DMT-1) protein located in the small intestine is in charge of absorbing Fe2+ which is stored in cells subsequently and then exported by FPN1. Based on the level of iron and the saturation of FPN, hepatocytes take participated in modulating iron homeostasis through secreting hepcidin which can attenuate the production of DMT-1 in order to regulate the absorption of Fe2+. A research showing that serum hepcidin level and expression of DMT1 messenger RNA were upregulated depending on the enhancive activity of iron regulatory protein (IRP) in the NASH patients during an oral iron absorption test (OIAT) (Hoki et al., 2015), which was consistent with the observations of previous studies (Senates et al., 2011). Most recently, Yu et al. (2020) have clarified that ferroptosis-induced liver fibrosis may easily develop in hepatocyte-specific Trf (encoding transferrin) knockout mice (Trf-LKO) with a high-iron diet, which can be reversed with the treatment of reducing Slc39a14 expression in liver or applying ferrostatin-1.
Ferroptosis and Osteoporosis
Accumulating researches have demonstrated that inordinate iron metabolism can destroy bone homeostasis inducing osteoporosis, which is a systemic metabolic disease characterized by reduced bone mass, augmented bone fragility and increased risk of fracture (Che et al., 2020). Conducted within certain iron overload models, the phenotype of diminished bone density and trabecular thickness was observed due to heightened osteoclast differentiation and osteoblast apoptosis, leading to intense bone dissolution and abating bone formation due to decreased osteoblast specific genes, i.e., alkaline phosphatase (ALP), runt-related transcription factor 2 (Runx2) and type I collagen (Tsay et al., 2010; Li et al., 2012; Chen et al., 2014; Cheng et al., 2017). Redundant iron stimulates osteoclasts and osteoblasts to yield a generous amount of ROS, engendering maladjustment of the intracellular antioxidant/peroxidation balance system by activating the MAPKs and NF-κB pathways, resulting in osteoblast death (Ray et al., 2012; Che et al., 2020). All of the above discoveries foreshadow that ferroptosis may participate in the development of osteoporosis, especially the devitalization of osteoblasts. Recently, a study indicated that extracellular vesicles derived from endothelial progenitor cells (EPC-EVs) satisfied the need of retarding the progress of steroid-induced osteoporosis (SIOP) by inhibiting ferroptosis, specifically by reversing the deactivation of GPX4, system Xc– and decreased cysteine levels, which is initiated by dexamethasone (Lu et al., 2019). Since these speculations are based on bioinformatics evidence, the detailed signal pathways and in vitro and in vivo experiments warrant further investigation. Another study sustained these results. Due to the ferroptosis-induced role played by glucocorticoids in osteoblasts (Lu et al., 2019), Yang et al. (2021) originally found that exosomes derived from vascular endothelial cells (EC-Exos) counteract this process both in vitro and in vivo by decreasing NCOA4 expression to suppress ferritinophagy and simultaneously targeting the Keap1-Nrf2-HO-1/NQO-1 pathway to inhibit lipid oxidation, though the specific signal has not yet been elucidated.
Some drugs that target osteoporosis may actualize a ferroptotic role during the therapeutic mechanism, which, in our perspective, is reasonable to surmise, but not yet visible. Artemisinin (ARS) and its related compounds, which are already known as inhibitors of osteoporosis due to their suppression of osteoclast differentiation by blocking the receptor activator of nuclear factor kappa-B ligand (RANKL) pathway, may induce ferroptosis in osteoclasts due to the high levels of ferritin and LIP in osteoclasts (Zhang, 2020). Furthermore, andrographolide (AP), an herbal medicine, was found to have the ability to regulate the osteoprotegerin (OPG)/RANKL signal (Tantikanlayaporn et al., 2020) and stifle the NF-κB pathway activated by TNFα (Yongyun et al., 2019) so as to hasten osteoblast differentiation. It may also weaken the extracellular signal-regulated kinase (ERK)/MAPK and NF-κB signals in order to block RANKL-induced osteoclast differentiation (Yongyun et al., 2019). Engagingly, those signal ways are also targeted in the protection of diabetic myocardial dysfunction (Table 1), which naturally evokes reflections about whether a drug can work on the Nrf2/ARE pathway as well exert an antioxidant effect to inhibit ferroptosis in osteoporosis. Compounds such as luteolin (Jing et al., 2019), isoliquiritigenin (Jeong et al., 2020), and myricitrin (Huang et al., 2014) may play a corresponding role as andrographolide, which may promote or inhibit ferroptosis in osteoclasts and osteoblasts alike. Remarkably, Ma H. et al. (2020) first reported that melatonin is capable of restraining ferroptosis in T2DM-induced osteoporosis, mainly by accelerating the Nrf2/HO-1 pathway, and provided convincing evidence to motivate extensive work to be done in the future.
In conclusion, the stimulation of ferroptosis in osteoclasts and its converse repression in osteoblasts in the prevention and treatment of osteoporosis may be a potential field of exploration. Likewise, some drugs affecting osteoporosis appear to have the latent target to Nrf2 or to the other key mediators of ferroptosis, which have maintained undetected.
Ferroptosis and Other Metabolic and Endocrine Disorders
There also exist some researches of the role of ferroptosis in endocrine gland disorders. Some studies, which examined the relationship between adrenal gland and ferroptosis, suggested that a adrenocortical cell-producing steroid presents a higher sensitivity to ferroptosis, compared to the non-steroidogenic adrenal medulla, when the GPX4 is suppressed, mainly because of its elevated expression of GPX4, ACSL4, adrenic and arachidonic acid, which are key regulators of ferroptosis (Weigand et al., 2020). Adrenocortical carcinomas (ACCs) are also sensitive to the induction of ferroptosis, and based on this result, a new therapeutic target to ACCs may present beyond the mitotane who cannot energize the ferroptosis pathway (Belavgeni et al., 2019). Additionally, due to prostate cancer cells’ high sensitivity to iron toxicity, activating ferroptosis appears to be a new strategy for treating advanced prostate cancer, with an option to include second-generation anti-androgens (Bordini et al., 2020; Ghoochani et al., 2021). As an FDA-approved anthelmintic, flubendazole was found to target P53 to induce ferroptosis in an effort to delay the development of prostate cancer (Zhou et al., 2021). At present, ferroptosis-related work in endocrine gland disorders mostly focus on cancer cells; the link between other types of diseases and ferroptosis is still unknown.
Interestingly, level of hormone is closely correlated to regulations of cell growth. A recent study suggested that hyperandrogenism and insulin resistance may trigger the gravid uterine and placental ferroptosis in rats with polycystic ovary syndrome (PCOS), leading to fetal loss (Zhang Y. et al., 2020). Actually, it is acknowledged that a variety of hormones, such as melatonin (Dehdashtian et al., 2018), thyroid hormone (Liu et al., 2019), glucocorticoid, estrogens and androgens (Billig et al., 1996), among others, could bring about an effect to control cell death, causing autophagy, inflammation, oxidative stress and cancer progression. Questions remain; for instance: does ferroptosis have a share in this process?; will a curative effect result if using inhibitors against ferroptosis in cells and tissues undergoing hormone hypersecretion? All of these issues need to be researched further. Ferroptosis was also supposed to act on some specific inherited metabolic diseases such as lysosomal storage diseases (LSD), which was reviewed integrally by Pierzynowska et al. (2021) from a perspective of mechanism in autophagy-dependent ferroptosis. Moreover, with the discovery of transferrin receptor 1 downregulating in satellite cells of old mice, researchers caught sight of the relationship of ferroptosis and age related impairment of skeletal muscle regeneration gradually (Ding et al., 2021). Overall, to date, many illuminating reviews on ferroptosis from different perspectives have emerged, providing a much wider view for researchers to its mechanism and treatment for the related diseases (Jiang et al., 2021). Despite some of the way ahead still shrouding in mist, the research into ferroptosis is in full swing and a rapid progress has been made as more and more metabolic diseases having been found to possess ferroptotic-feature (Conrad et al., 2021).
Conclusion and Outlooks
In this review, we summarized three essential conditions and specific mechanisms of ferroptosis development, including three major regulated pathways that repaired lipid oxidation disturb, which may cause ROS accumulation, oxidation of PUFAs and excessive iron. We concluded the emerging regulators targeted the key mediators of ferroptosis systematically and from all sides, assisting readers to comprehend the latest investigations in the field of ferroptosis. Moreover, we placed emphasis on the ferroptotic role in some metabolic disorders, offering an intimate portrait of diabetes and its complications and osteoporosis.
In recent years, there has been a heated discussion regarding ferroptosis in the academic world, since it is a novel type of RCD involved in many diseases. Seldom could anyone deny the importance of ferroptosis in cell growth and its role in the prevention and treatment of diverse disorders. However, from our perspective, there are still some problems pressed for solutions. Firstly, is there a synergetic or antagonistic effect between ferroptosis and the other forms of RCD as they share some critical regulators in the pathway (such as P53)? Secondly, the detailed molecular mechanisms that triggered ferroptosis still present with some void. For instance, the downstream effectors of lipid oxidation have not yet been identified and the level of iron and ROS that should be achieved to initiate ferroptosis remain unknown. Besides, the study about the GCH1-BH4-phospholipid axis that was recently published is still in its infancy. The other mediators targeted by this pathway should be investigated. Some of the existing studies results are ambivalent; for example, the precise role of Nrf2 in the induction of ferroptosis; specifically, whether activating or blocking Nrf2 appears to have a dual effect on diabetes and its complication, which are conducted with same method in different laboratories. In addition, does ferroptosis definitely occur in cells possessing the three necessary conditions? What is the alternative requirement of cells to improve or reduce the sensitivity to ferroptosis? Thirdly, as previously mentioned, exosomes may participate in the regulation of ferroptosis, not only exporting iron out of cells to prevent ferroptosis, but also reversing peroxidation through the delivered effect. This effect is performed by the exosomes derived from some certain types of cells, such as endothelial progenitor cells. Therefore, in the long run, the crosstalk between exosomes and ferroptosis may be a future hot topic. Last but not least, with the shortage of human evidence, there is a long way to go in terms of applying basic research results of ferroptosis to clinical applications. This brings into question if making use of ferroptotic inhibitors will do harm to other cells and tissues that rely on the metabolism of iron and ROS, or from a different angle, if anti-ferroptotic compounds present as the antioxidant agents to be applied to some metabolic diseases caused by oxidative stress.
In summary, ferroptosis is undoubtedly regarded as a promising target to treat metabolic diseases, however, the complete molecular mechanism and its underlying role in metabolic diseases still warrant further examination.
Author Contributions
L-QY wrote the manuscript and approved the final version of the manuscript. J-YD contributed to study conduct, data analysis, and manuscript writing. XL, FX, S-KS, BG, F-X-ZL, M-HZ, YW, Q-SX, L-ML, W-LO-Y, Y-YW, and K-XT contributed to data analysis. All authors reviewed the manuscript.
Funding
This work was supported by funding from the National Natural Science Foundation of China (Nos. 81770881 and 82070910) and Key R&D Plan of Hunan Province (2020SK2078).
Conflict of Interest
The authors declare that the research was conducted in the absence of any commercial or financial relationships that could be construed as a potential conflict of interest.
Abbreviations
RCD, regulated cell death; AS, atherosclerosis; ACD, accidental cell death; ROS, reactive oxygen species; MAPKs, mitogen-activated protein kinases; GPX4, glutathione-glutathione peroxidase 4; GSH, glutathione; PUFAs, polyunsaturated fatty acids; TF, transferrin; TFR1, transferrin receptor 1; LIP, labile iron pool; FPN1, ferroportin 1; LCN2, lipocalin 2; NCOA4, nuclear receptor coactivator 4; NUPR1, transcriptional regulator; PCBP1, poly-(rC)-binding protein 1; HO-1, heme oxygenase-1; PE, phosphatidylethanolamine; AA, arachidonic acid; ACSL4, Acyl-CoA synthetase long-chain family member 4; LPCAT3, lysophosphatidylcholine acyltransferase 3; LOX, lipoxygenase; POR, cytochrome P450 oxidoreductase; CYB5R1, cytochrome b5 reductase; PLOOH, phospholipid hydroperoxide; AGPS, alkylglycerone phosphate synthase; FAR1, fatty acyl-CoA reductase 1; GNPAT, glyceronephosphate O-acyltransferase; AGP, 1-O-alkyl-glycerol-3-phosphate; AGPAT3, 1-acylglycerol-3-phosphate O-acyltransferase 3; PEDS1, plasmanylethanolamine desaturase 1; L-OOH, lipid hydroperoxides; L-OH, lipid alcohols; Nrf2, (Erythoid-derived)-like 2; Keap1, Kelch-like ECH-associated protein 1; T1DM, Type 1 diabetes; T2DM, Type 2 diabetes; ARF, CDKN2A locus; ATF3, transcription factor 3; BAP1, BRCA1-associated protein 1; PDK4, pyruvate dehydrogenase kinase 4; CD44v, CD44 variant; LAT1, L-type amino acid transporter 1; Sec, selenocysteine; IPP, isopentenyl pyrophosphate; HSPA5, heat shock protein family 5; Se, selenium; H2O2, hydrogen peroxide; FSP1, ferroptosis suppressor protein 1; AIFM2, apoptosis-inducing factor mitochondrial 2; NADPH, nicotinamide adenine dinucleotide phosphate; CoQ10, ubiquinone; MDM2, murine double minute 2; PPAR α, peroxisome proliferator-activated receptor α; MEG3, maternally-expressed gene 3; ATF4, transcription factor 4; BAT, brown adipose tissue; FFA, free fatty acids; ALA, alpha lipoic acid; DRG, dorsal root ganglion; DN, diabetic neuropathy; UCP2, uncouplingprotein 2; GCH1, GTP cyclohydrolase-1; BH4/BH2, tetrahydrobiopterin/dihydrobiopterin; RTAs, radical-trapping antioxidants; DHFR, dihydrofolate reductase; NOS, nitric oxide synthase; Ox-LDLs, oxidized low-density lipoproteins; AMPK, AMP-activated protein kinase; RNS, reactive oxygen and nitrogen species; FIA, ferroptosis-inducing agents; CCA, cryptochlorogenic acid; Fer-1, ferrostatin-1; HIF, hypoxia-inducible factor; HMGB1, high-mobility group box-1; DCM, diabetic cardiomyopathy; HSF1, heat shock factor 1; CaSR, calcium-sensitive receptor; MuRF1, muscle-specific ring finger protein 1; SFN, sulforaphane; MT, metallothionein; MIRI, myocardial ischemia/reperfusion injury; Nox, NADPH oxidase; ERS, endoplasmic reticulum stress; CHOP, ATF4-C/EBP homologous protein; PM2.5, particulate matter 2.5; IL-1 β, interleukin-1 β; HG, high glucose; HUVECs, human umbilical vein endothelial cells; VSMC, vascular smooth muscle cell; Met, metformin; POSTN, periostin; CSE, cigarette smoke extract; MAECs, mouse aortic endothelial cells; ALP, alkaline phosphatase; Runx2, runt-related transcription factor 2; SIOP, steroid-induced osteoporosis; EPC-EVs, endothelial progenitor cells derived extracellular vesicles; EC-Exos, endothelial cells derived exosomes; ARS, artemisinin; RANKL, nuclear factor kappa- B ligand; AP, andrographolide; OPG, osteoprotegerin; ERK, extracellular signal-regulated kinase; ACCs, adrenocortical carcinomas; PCOS, polycystic ovary syndrome; ATM, Ataxia-Telangiectasia; MTF1, metal-regulatory transcription factor 1; MAFLD, metabolic dysfunction-associated fatty liver disease; NAFLD, non-alcoholic fatty liver disease; NASH, non-alcoholic steatohepatitis; 4-HNE, 4-hydroxy-2,3-non-anal; MDA, malondialdehyde; RPH, rat primary hepatocytes; MPH, mouse primary hepatocytes; GB, Ginkgolide B; ECH1, enoyl coenzyme A hydratase 1; MCD, methionine/choline-deficient diet; DMT-1, divalent metal transporter 1; IRP, iron regulatory protein; OIAT, oral iron absorption test; LSD, lysosomal storage diseases.
References
Abu-Taweel, G. M., Attia, M. F., Hussein, J., Mekawi, E. M., Galal, H. M., Ahmed, E. I., et al. (2020). Curcumin nanoparticles have potential antioxidant effect and restore tetrahydrobiopterin levels in experimental diabetes. Biomed. Pharmacother. 131:110688. doi: 10.1016/j.biopha.2020.110688
Adedoyin, O., Boddu, R., Traylor, A., Lever, J. M., Bolisetty, S., George, J. F., et al. (2018). Heme oxygenase-1 mitigates ferroptosis in renal proximal tubule cells. Am. J. Physiol. Renal Physiol. 314, F702–F714. doi: 10.1152/ajprenal.00044.2017
Alim, I., Caulfield, J. T., Chen, Y., Swarup, V., Geschwind, D. H., Ivanova, E., et al. (2019). Selenium Drives a Transcriptional Adaptive Program to Block Ferroptosis and Treat Stroke. Cell 177, 1262–1279e1225. doi: 10.1016/j.cell.2019.03.032
Alp, N. J., McAteer, M. A., Khoo, J., Choudhury, R. P., and Channon, K. M. (2004). Increased endothelial tetrahydrobiopterin synthesis by targeted transgenic GTP-cyclohydrolase I overexpression reduces endothelial dysfunction and atherosclerosis in ApoE-knockout mice. Arterioscler. Thromb. Vasc. Biol. 24, 445–450. doi: 10.1161/01.ATV.0000115637.48689.77
Anderson, G. J., and Frazer, D. M. (2017). Current understanding of iron homeostasis. Am. J. Clin. Nutr. 106, (Suppl. 6), 1559S–1566S. doi: 10.3945/ajcn.117.155804
Anderson, G. J., and Vulpe, C. D. (2009). Mammalian iron transport. Cell Mol. Life Sci. 66, 3241–3261. doi: 10.1007/s00018-009-0051-1
Arai, M., Imai, H., Sumi, D., Imanaka, T., Takano, T., Chiba, N., et al. (1996). Import into mitochondria of phospholipid hydroperoxide glutathione peroxidase requires a leader sequence. Biochem. Biophys. Res. Commun. 227, 433–439. doi: 10.1006/bbrc.1996.1525
Atta, M. S., El-Far, A. H., Farrag, F. A., Abdel-Daim, M. M., Al Jaouni, S. K., and Mousa, S. A. (2018). Thymoquinone Attenuates Cardiomyopathy in Streptozotocin-Treated Diabetic Rats. Oxid. Med. Cell Longev. 2018:7845681. doi: 10.1155/2018/7845681
Badgley, M. A., Kremer, D. M., Maurer, H. C., DelGiorno, K. E., Lee, H. J., Purohit, V., et al. (2020). Cysteine depletion induces pancreatic tumor ferroptosis in mice. Science 368, 85–89. doi: 10.1126/science.aaw9872
Bai, T., Li, M., Liu, Y., Qiao, Z., and Wang, Z. (2020a). Inhibition of ferroptosis alleviates atherosclerosis through attenuating lipid peroxidation and endothelial dysfunction in mouse aortic endothelial cell. Free Radic. Biol. Med. 160, 92–102. doi: 10.1016/j.freeradbiomed.2020.07.026
Bai, T., Liang, R., Zhu, R., Wang, W., Zhou, L., and Sun, Y. (2020b). MicroRNA-214-3p enhances erastin-induced ferroptosis by targeting ATF4 in hepatoma cells. J. Cell Physiol. 235, 5637–5648. doi: 10.1002/jcp.29496
Bao, W. D., Zhou, X. T., Zhou, L. T., Wang, F., Yin, X., Lu, Y., et al. (2020). Targeting miR-124/Ferroportin signaling ameliorated neuronal cell death through inhibiting apoptosis and ferroptosis in aged intracerebral hemorrhage murine model. Aging Cell 19:e13235. doi: 10.1111/acel.13235
Baseler, W. A., Dabkowski, E. R., Jagannathan, R., Thapa, D., Nichols, C. E., Shepherd, D. L., et al. (2013). Reversal of mitochondrial proteomic loss in Type 1 diabetic heart with overexpression of phospholipid hydroperoxide glutathione peroxidase. Am. J. Physiol. Regul. Integr. Comp. Physiol. 304, R553–R565. doi: 10.1152/ajpregu.00249.2012
Bedoui, S., Herold, M. J., and Strasser, A. (2020). Emerging connectivity of programmed cell death pathways and its physiological implications. Nat. Rev. Mol. Cell Biol. 21, 678–695. doi: 10.1038/s41580-020-0270-8
Behring, J. B., Kumar, V., Whelan, S. A., Chauhan, P., Siwik, D. A., Costello, C. E., et al. (2014). Does reversible cysteine oxidation link the Western diet to cardiac dysfunction? FASEB J. 28, 1975–1987. doi: 10.1096/fj.13-233445
Belavgeni, A., Bornstein, S. R., von Massenhausen, A., Tonnus, W., Stumpf, J., Meyer, C., et al. (2019). Exquisite sensitivity of adrenocortical carcinomas to induction of ferroptosis. Proc. Natl. Acad. Sci. U S A. 116, 22269–22274. doi: 10.1073/pnas.1912700116
Bersuker, K., Hendricks, J. M., Li, Z., Magtanong, L., Ford, B., Tang, P. H., et al. (2019). The CoQ oxidoreductase FSP1 acts parallel to GPX4 to inhibit ferroptosis. Nature 575, 688–692. doi: 10.1038/s41586-019-1705-2
Billig, H., Chun, S. Y., Eisenhauer, K., and Hsueh, A. J. (1996). Gonadal cell apoptosis: hormone-regulated cell demise. Hum. Reprod. Update 2, 103–117. doi: 10.1093/humupd/2.2.103
Bogdan, A. R., Miyazawa, M., Hashimoto, K., and Tsuji, Y. (2016). Regulators of Iron Homeostasis: New Players in Metabolism, Cell Death, and Disease. Trends Biochem. Sci. 41, 274–286. doi: 10.1016/j.tibs.2015.11.012
Bordini, J., Morisi, F., Elia, A. R., Santambrogio, P., Pagani, A., Cucchiara, V., et al. (2020). Iron Induces Cell Death and Strengthens the Efficacy of Antiandrogen Therapy in Prostate Cancer Models. Clin. Cancer Res. 26, 6387–6398. doi: 10.1158/1078-0432.CCR-20-3182
Brandes, R. P., and Kreuzer, J. (2005). Vascular NADPH oxidases: molecular mechanisms of activation. Cardiovasc. Res. 65, 16–27. doi: 10.1016/j.cardiores.2004.08.007
Bril, F., Biernacki, D. M., Kalavalapalli, S., Lomonaco, R., Subbarayan, S. K., Lai, J., et al. (2019). Role of Vitamin E for Nonalcoholic Steatohepatitis in Patients With Type 2 Diabetes: A Randomized Controlled Trial. Diabetes Care 42, 1481–1488. doi: 10.2337/dc19-0167
Brown, C. W., Amante, J. J., Chhoy, P., Elaimy, A. L., Liu, H., Zhu, L. J., et al. (2019). Prominin2 Drives Ferroptosis Resistance by Stimulating Iron Export. Dev. Cell 51, 575–586e574. doi: 10.1016/j.devcel.2019.10.007
Bruni, A., Bornstein, S., Linkermann, A., and Shapiro, A. M. J. (2018a). Regulated Cell Death Seen through the Lens of Islet Transplantation. Cell Transplant. 27, 890–901. doi: 10.1177/0963689718766323
Bruni, A., Pepper, A. R., Pawlick, R. L., Gala-Lopez, B., Gamble, A. F., Kin, T., et al. (2018b). Ferroptosis-inducing agents compromise in vitro human islet viability and function. Cell Death Dis. 9:595. doi: 10.1038/s41419-018-0506-0
Brutsch, S. H., Wang, C. C., Li, L., Stender, H., Neziroglu, N., Richter, C., et al. (2015). Expression of inactive glutathione peroxidase 4 leads to embryonic lethality, and inactivation of the Alox15 gene does not rescue such knock-in mice. Antioxid Redox. Signal. 22, 281–293. doi: 10.1089/ars.2014.5967
Capelletti, M. M., Manceau, H., Puy, H., and Peoc’h, K. (2020). Ferroptosis in Liver Diseases: An Overview. Int. J. Mol. Sci. 21:ijms21144908. doi: 10.3390/ijms21144908
Carmean, C. M., Yokoi, N., Takahashi, H., Oduori, O. S., Kang, C., Kanagawa, A., et al. (2019). Arsenic modifies serotonin metabolism through glucuronidation in pancreatic beta-cells. Am. J. Physiol. Endocrinol. Metab. 316, E464–E474. doi: 10.1152/ajpendo.00302.2018
Carnicer, R., Duglan, D., Ziberna, K., Recalde, A., Reilly, S., Simon, J. N., et al. (2021). BH4 Increases nNOS Activity and Preserves Left Ventricular Function in Diabetes. Circ. Res. 128, 585–601. doi: 10.1161/CIRCRESAHA.120.316656
Carpi-Santos, R., and Calaza, K. C. (2018). Alterations in System xc(-) Expression in the Retina of Type 1 Diabetic Rats and the Role of Nrf2. Mol. Neurobiol. 55, 7941–7948. doi: 10.1007/s12035-018-0961-8
Che, J., Yang, J., Zhao, B., Zhang, G., Wang, L., Peng, S., et al. (2020). The Effect of Abnormal Iron Metabolism on Osteoporosis. Biol. Trace Elem. Res. 195, 353–365. doi: 10.1007/s12011-019-01867-4
Chen, B., Yan, Y. L., Liu, C., Bo, L., Li, G. F., Wang, H., et al. (2014). Therapeutic effect of deferoxamine on iron overload-induced inhibition of osteogenesis in a zebrafish model. Calcif Tissue Int. 94, 353–360. doi: 10.1007/s00223-013-9817-4
Chen, C., Wang, D., Yu, Y., Zhao, T., Min, N., Wu, Y., et al. (2021). Legumain promotes tubular ferroptosis by facilitating chaperone-mediated autophagy of GPX4 in AKI. Cell Death Dis. 12:65. doi: 10.1038/s41419-020-03362-4
Chen, D., Tavana, O., Chu, B., Erber, L., Chen, Y., Baer, R., et al. (2017). NRF2 Is a Major Target of ARF in p53-Independent Tumor Suppression. Mol. Cell 68, 224–232e224. doi: 10.1016/j.molcel.2017.09.009
Chen, H. C., Huang, C. C., Lin, T. J., Hsu, M. C., and Hsu, Y. J. (2019). Ubiquinol Supplementation Alters Exercise Induced Fatigue by Increasing Lipid Utilization in Mice. Nutrients 11:nu11112550. doi: 10.3390/nu11112550
Chen, H. J., Sugiyama, M., Shimokawa, F., Murakami, M., Hashimoto, O., Matsui, T., et al. (2020). Response to iron overload in cultured hepatocytes. Sci. Rep. 10:21184. doi: 10.1038/s41598-020-78026-6
Chen, P. H., Wu, J., Ding, C. C., Lin, C. C., Pan, S., Bossa, N., et al. (2020). Kinome screen of ferroptosis reveals a novel role of ATM in regulating iron metabolism. Cell Death Differ. 27, 1008–1022. doi: 10.1038/s41418-019-0393-7
Chen, X., Li, J., Kang, R., Klionsky, D. J., and Tang, D. (2020). Ferroptosis: machinery and regulation. Autophagy 2020, 1–28. doi: 10.1080/15548627.2020.1810918
Chen, X., Ma, J., Kwan, T., Stribos, E. G. D., Messchendorp, A. L., Loh, Y. W., et al. (2018). Blockade of HMGB1 Attenuates Diabetic Nephropathy in Mice. Sci. Rep. 8:8319. doi: 10.1038/s41598-018-26637-5
Cheng, Q., Zhang, X., Jiang, J., Zhao, G., Wang, Y., Xu, Y., et al. (2017). Postmenopausal Iron Overload Exacerbated Bone Loss by Promoting the Degradation of Type I Collagen. Biomed. Res. Int. 2017:1345193. doi: 10.1155/2017/1345193
Conrad, M., Lorenz, S. M., and Proneth, B. (2021). Targeting Ferroptosis: New Hope for As-Yet-Incurable Diseases. Trends Mol. Med. 27, 113–122. doi: 10.1016/j.molmed.2020.08.010
Dai, E., Zhang, W., Cong, D., Kang, R., Wang, J., and Tang, D. (2020). AIFM2 blocks ferroptosis independent of ubiquinol metabolism. Biochem. Biophys. Res. Commun. 523, 966–971. doi: 10.1016/j.bbrc.2020.01.066
Day, E. A., Ford, R. J., and Steinberg, G. R. (2017). AMPK as a Therapeutic Target for Treating Metabolic Diseases. Trends Endocrinol. Metab. 28, 545–560. doi: 10.1016/j.tem.2017.05.004
Dehdashtian, E., Mehrzadi, S., Yousefi, B., Hosseinzadeh, A., Reiter, R. J., Safa, M., et al. (2018). Diabetic retinopathy pathogenesis and the ameliorating effects of melatonin; involvement of autophagy, inflammation and oxidative stress. Life Sci. 193, 20–33. doi: 10.1016/j.lfs.2017.12.001
Denton, D., and Kumar, S. (2019). Autophagy-dependent cell death. Cell Death Differ. 26, 605–616. doi: 10.1038/s41418-018-0252-y
Derosa, G., D’Angelo, A., and Maffioli, P. (2019). Coenzyme q10 liquid supplementation in dyslipidemic subjects with statin-related clinical symptoms: a double-blind, randomized, placebo-controlled study. Drug Des. Devel. Ther. 13, 3647–3655. doi: 10.2147/DDDT.S223153
Dhananjayan, R., Koundinya, K. S., Malati, T., and Kutala, V. K. (2016). Endothelial Dysfunction in Type 2 Diabetes Mellitus. Ind. J. Clin. Biochem. 31, 372–379. doi: 10.1007/s12291-015-0516-y
Ding, H., Chen, S., Pan, X., Dai, X., Pan, G., Li, Z., et al. (2021). Transferrin receptor 1 ablation in satellite cells impedes skeletal muscle regeneration through activation of ferroptosis. J. Cachexia Sarcopenia Muscle 2021:12700. doi: 10.1002/jcsm.12700
Dixon, S. J., Lemberg, K. M., Lamprecht, M. R., Skouta, R., Zaitsev, E. M., Gleason, C. E., et al. (2012). Ferroptosis: an iron-dependent form of nonapoptotic cell death. Cell 149, 1060–1072. doi: 10.1016/j.cell.2012.03.042
Dixon, S. J., Patel, D. N., Welsch, M., Skouta, R., Lee, E. D., Hayano, M., et al. (2014). Pharmacological inhibition of cystine-glutamate exchange induces endoplasmic reticulum stress and ferroptosis. Elife 3:e02523. doi: 10.7554/eLife.02523
Dludla, P. V., Muller, C. J., Joubert, E., Louw, J., Essop, M. F., Gabuza, K. B., et al. (2017). Aspalathin Protects the Heart against Hyperglycemia-Induced Oxidative Damage by Up-Regulating Nrf2 Expression. Molecules 22:molecules22010129. doi: 10.3390/molecules22010129
Doll, S., Freitas, F. P., Shah, R., Aldrovandi, M., da Silva, M. C., Ingold, I., et al. (2019). FSP1 is a glutathione-independent ferroptosis suppressor. Nature 575, 693–698. doi: 10.1038/s41586-019-1707-0
Dolma, S., Lessnick, S. L., Hahn, W. C., and Stockwell, B. R. (2003). Identification of genotype-selective antitumor agents using synthetic lethal chemical screening in engineered human tumor cells. Cancer Cell 3, 285–296. doi: 10.1016/s1535-6108(03)00050-3
Douglas, G., Hale, A. B., Patel, J., Chuaiphichai, S., Al Haj, Zen, A., et al. (2018). Roles for endothelial cell and macrophage Gch1 and tetrahydrobiopterin in atherosclerosis progression. Cardiovasc. Res. 114, 1385–1399. doi: 10.1093/cvr/cvy078
Dover, E. N., Beck, R., Huang, M. C., Douillet, C., Wang, Z., Klett, E. L., et al. (2018). Arsenite and methylarsonite inhibit mitochondrial metabolism and glucose-stimulated insulin secretion in INS-1 832/13 beta cells. Arch. Toxicol. 92, 693–704. doi: 10.1007/s00204-017-2074-y
Duan, J., Guan, Y., Mu, F., Guo, C., Zhang, E., Yin, Y., et al. (2017). Protective effect of butin against ischemia/reperfusion-induced myocardial injury in diabetic mice: involvement of the AMPK/GSK-3beta/Nrf2 signaling pathway. Sci. Rep. 7:41491. doi: 10.1038/srep41491
Dubey, P., Thakur, V., and Chattopadhyay, M. (2020). Role of Minerals and Trace Elements in Diabetes and Insulin Resistance. Nutrients 12:nu12061864. doi: 10.3390/nu12061864
Elguindy, M. M., and Nakamaru-Ogiso, E. (2015). Apoptosis-inducing Factor (AIF) and Its Family Member Protein, AMID, Are Rotenone-sensitive NADH:Ubiquinone Oxidoreductases (NDH-2). J. Biol. Chem. 290, 20815–20826. doi: 10.1074/jbc.M115.641498
Eslam, M., Sanyal, A. J., George, J., and International Consensus, P. (2020). MAFLD: A Consensus-Driven Proposed Nomenclature for Metabolic Associated Fatty Liver Disease. Gastroenterology 158:e1991.
Falk, E. (2006). Pathogenesis of atherosclerosis. J. Am. Coll. Cardiol. 47(8 Suppl.), C7–C12. doi: 10.1016/j.jacc.2005.09.068
Fan, Z., Wirth, A. K., Chen, D., Wruck, C. J., Rauh, M., Buchfelder, M., et al. (2017). Nrf2-Keap1 pathway promotes cell proliferation and diminishes ferroptosis. Oncogenesis 6:e371. doi: 10.1038/oncsis.2017.65
Fang, X., Wang, H., Han, D., Xie, E., Yang, X., Wei, J., et al. (2019). Ferroptosis as a target for protection against cardiomyopathy. Proc. Natl. Acad. Sci. U S A. 116, 2672–2680. doi: 10.1073/pnas.1821022116
Fazakerley, D. J., Chaudhuri, R., Yang, P., Maghzal, G. J., Thomas, K. C., Krycer, J. R., et al. (2018). Mitochondrial CoQ deficiency is a common driver of mitochondrial oxidants and insulin resistance. Elife 7:32111. doi: 10.7554/eLife.32111
Feng, H., Schorpp, K., Jin, J., Yozwiak, C. E., Hoffstrom, B. G., Decker, A. M., et al. (2020). Transferrin Receptor Is a Specific Ferroptosis Marker. Cell Rep. 30, 3411–3423e3417. doi: 10.1016/j.celrep.2020.02.049
Feng, X., Wang, S., Sun, Z., Dong, H., Yu, H., Huang, M., et al. (2021). Ferroptosis Enhanced Diabetic Renal Tubular Injury via HIF-1alpha/HO-1 Pathway in db/db Mice. Front. Endocrinol. 12:626390. doi: 10.3389/fendo.2021.626390
Fernandez-Real, J. M., McClain, D., and Manco, M. (2015). Mechanisms Linking Glucose Homeostasis and Iron Metabolism Toward the Onset and Progression of Type 2 Diabetes. Diabetes Care 38, 2169–2176. doi: 10.2337/dc14-3082
Frank, D., and Vince, J. E. (2019). Pyroptosis versus necroptosis: similarities, differences, and crosstalk. Cell Death Differ. 26, 99–114. doi: 10.1038/s41418-018-0212-6
Friedmann Angeli, J. P., Schneider, M., Proneth, B., Tyurina, Y. Y., Tyurin, V. A., Hammond, V. J., et al. (2014). Inactivation of the ferroptosis regulator Gpx4 triggers acute renal failure in mice. Nat. Cell Biol. 16, 1180–1191. doi: 10.1038/ncb3064
Fuchs, Y., and Steller, H. (2011). Programmed cell death in animal development and disease. Cell 147, 742–758. doi: 10.1016/j.cell.2011.10.033
Galicia-Garcia, U., Benito-Vicente, A., Jebari, S., Larrea-Sebal, A., Siddiqi, H., Uribe, K. B., et al. (2020). Pathophysiology of Type 2 Diabetes Mellitus. Int. J. Mol. Sci. 21:ijms21176275. doi: 10.3390/ijms21176275
Galluzzi, L., Vitale, I., Aaronson, S. A., Abrams, J. M., Adam, D., Agostinis, P., et al. (2018). Molecular mechanisms of cell death: recommendations of the Nomenclature Committee on Cell Death 2018. Cell Death Differ. 25, 486–541. doi: 10.1038/s41418-017-0012-4
Gao, G., Xie, Z., Li, E. W., Yuan, Y., Fu, Y., Wang, P., et al. (2021). Dehydroabietic acid improves nonalcoholic fatty liver disease through activating the Keap1/Nrf2-ARE signaling pathway to reduce ferroptosis. J. Nat. Med. 2021, 1491–1494. doi: 10.1007/s11418-021-01491-4
Gao, L., Liu, Y., Guo, S., Xiao, L., Wu, L., Wang, Z., et al. (2018). LAZ3 protects cardiac remodeling in diabetic cardiomyopathy via regulating miR-21/PPARa signaling. Biochim. Biophys. Acta Mol. Basis Dis. 1864, 3322–3338. doi: 10.1016/j.bbadis.2018.07.019
Gao, M., Monian, P., Pan, Q., Zhang, W., Xiang, J., and Jiang, X. (2016). Ferroptosis is an autophagic cell death process. Cell Res. 26, 1021–1032. doi: 10.1038/cr.2016.95
Gao, M., Monian, P., Quadri, N., Ramasamy, R., and Jiang, X. (2015). Glutaminolysis and Transferrin Regulate Ferroptosis. Mol. Cell 59, 298–308. doi: 10.1016/j.molcel.2015.06.011
Gaschler, M. M., Andia, A. A., Liu, H., Csuka, J. M., Hurlocker, B., Vaiana, C. A., et al. (2018). FINO2 initiates ferroptosis through GPX4 inactivation and iron oxidation. Nat. Chem. Biol. 14, 507–515. doi: 10.1038/s41589-018-0031-6
Ge, Z. D., Lian, Q., Mao, X., and Xia, Z. (2019). Current Status and Challenges of NRF2 as a Potential Therapeutic Target for Diabetic Cardiomyopathy. Int. Heart J. 60, 512–520. doi: 10.1536/ihj.18-476
Ghoochani, A., Hsu, E. C., Aslan, M., Rice, M. A., Nguyen, H. M., Brooks, J. D., et al. (2021). Ferroptosis Inducers Are a Novel Therapeutic Approach for Advanced Prostate Cancer. Cancer Res. 81, 1583–1594. doi: 10.1158/0008-5472.CAN-20-3477
Gimbrone, M. A. Jr., and Garcia-Cardena, G. (2016). Endothelial Cell Dysfunction and the Pathobiology of Atherosclerosis. Circ. Res. 118, 620–636. doi: 10.1161/CIRCRESAHA.115.306301
Gonzalez-Dominguez, A., Visiedo-Garcia, F. M., Dominguez-Riscart, J., Gonzalez-Dominguez, R., Mateos, R. M., and Lechuga-Sancho, A. M. (2020). Iron Metabolism in Obesity and Metabolic Syndrome. Int. J. Mol. Sci. 21:ijms21155529. doi: 10.3390/ijms21155529
Grau-Perez, M., Kuo, C. C., Spratlen, M., Thayer, K. A., Mendez, M. A., Hamman, R. F., et al. (2017). The Association of Arsenic Exposure and Metabolism With Type 1 and Type 2 Diabetes in Youth: The SEARCH Case-Control Study. Diabetes Care 40, 46–53. doi: 10.2337/dc16-0810
Green, D. R. (2019). The Coming Decade of Cell Death Research: Five Riddles. Cell 177, 1094–1107. doi: 10.1016/j.cell.2019.04.024
Gu, J., Cheng, Y., Wu, H., Kong, L., Wang, S., Xu, Z., et al. (2017). Metallothionein Is Downstream of Nrf2 and Partially Mediates Sulforaphane Prevention of Diabetic Cardiomyopathy. Diabetes 66, 529–542. doi: 10.2337/db15-1274
Gu, X., Shi, Y., Chen, X., Sun, Z., Luo, W., Hu, X., et al. (2020). Isoliquiritigenin attenuates diabetic cardiomyopathy via inhibition of hyperglycemia-induced inflammatory response and oxidative stress. Phytomedicine 78:153319. doi: 10.1016/j.phymed.2020.153319
Guo, Y., Zhuang, X., Huang, Z., Zou, J., Yang, D., Hu, X., et al. (2018). Klotho protects the heart from hyperglycemia-induced injury by inactivating ROS and NF-kappaB-mediated inflammation both in vitro and in vivo. Biochim. Biophys. Acta Mol. Basis Dis. 1864, 238–251. doi: 10.1016/j.bbadis.2017.09.029
Han, C., Liu, Y., Dai, R., Ismail, N., Su, W., and Li, B. (2020). Ferroptosis and Its Potential Role in Human Diseases. Front. Pharmacol. 11:239. doi: 10.3389/fphar.2020.00239
Ho, C. C., Tseng, C. Y., Chen, H. W., Chiu, Y. W., Tsai, M. C., Chang, P. S., et al. (2020). Coenzyme Q10 status, glucose parameters, and antioxidative capacity in college athletes. J. Int. Soc. Sports Nutr. 17:5. doi: 10.1186/s12970-020-0334-3
Hoki, T., Miyanishi, K., Tanaka, S., Takada, K., Kawano, Y., Sakurada, A., et al. (2015). Increased duodenal iron absorption through up-regulation of divalent metal transporter 1 from enhancement of iron regulatory protein 1 activity in patients with nonalcoholic steatohepatitis. Hepatology 62, 751–761. doi: 10.1002/hep.27774
Hotchkiss, R. S., Strasser, A., McDunn, J. E., and Swanson, P. E. (2009). Cell death. N. Engl. J. Med. 361, 1570–1583. doi: 10.1056/NEJMra0901217
Hu, X., Rajesh, M., Zhang, J., Zhou, S., Wang, S., Sun, J., et al. (2018). Protection by dimethyl fumarate against diabetic cardiomyopathy in type 1 diabetic mice likely via activation of nuclear factor erythroid-2 related factor 2. Toxicol. Lett. 287, 131–141. doi: 10.1016/j.toxlet.2018.01.020
Huang, Q., Gao, B., Wang, L., Hu, Y. Q., Lu, W. G., Yang, L., et al. (2014). Protective effects of myricitrin against osteoporosis via reducing reactive oxygen species and bone-resorbing cytokines. Toxicol. Appl. Pharmacol. 280, 550–560. doi: 10.1016/j.taap.2014.08.004
Huynh, K., Kiriazis, H., Du, X. J., Love, J. E., Jandeleit-Dahm, K. A., Forbes, J. M., et al. (2012). Coenzyme Q10 attenuates diastolic dysfunction, cardiomyocyte hypertrophy and cardiac fibrosis in the db/db mouse model of type 2 diabetes. Diabetologia 55, 1544–1553. doi: 10.1007/s00125-012-2495-3
Ingold, I., Berndt, C., Schmitt, S., Doll, S., Poschmann, G., Buday, K., et al. (2018). Selenium Utilization by GPX4 Is Required to Prevent Hydroperoxide-Induced Ferroptosis. Cell 172, 409–422e421. doi: 10.1016/j.cell.2017.11.048
Jeong, S., Lee, S., Kim, K., Lee, Y., Lee, J., Oh, S., et al. (2020). Isoliquiritigenin Derivatives Inhibit RANKL-Induced Osteoclastogenesis by Regulating p38 and NF-kappaB Activation in RAW 264.7 Cells. Molecules 25:molecules25173908. doi: 10.3390/molecules25173908
Jiang, L., Kon, N., Li, T., Wang, S. J., Su, T., Hibshoosh, H., et al. (2015). Ferroptosis as a p53-mediated activity during tumour suppression. Nature 520, 57–62. doi: 10.1038/nature14344
Jiang, X., Stockwell, B. R., and Conrad, M. (2021). Ferroptosis: mechanisms, biology and role in disease. Nat. Rev. Mol. Cell Biol. 22, 266–282. doi: 10.1038/s41580-020-00324-8
Jiang, Z., Fu, L., Xu, Y., Hu, X., Yang, H., Zhang, Y., et al. (2020). Cyclovirobuxine D protects against diabetic cardiomyopathy by activating Nrf2-mediated antioxidant responses. Sci. Rep. 10:6427. doi: 10.1038/s41598-020-63498-3
Jing, Z., Wang, C., Yang, Q., Wei, X., Jin, Y., Meng, Q., et al. (2019). Luteolin attenuates glucocorticoid-induced osteoporosis by regulating ERK/Lrp-5/GSK-3beta signaling pathway in vivo and in vitro. J. Cell Physiol. 234, 4472–4490. doi: 10.1002/jcp.27252
Kagan, V. E., Mao, G., Qu, F., Angeli, J. P., Doll, S., Croix, C. S., et al. (2017). Oxidized arachidonic and adrenic PEs navigate cells to ferroptosis. Nat. Chem. Biol. 13, 81–90. doi: 10.1038/nchembio.2238
Kanwal, A., Pillai, V. B., Samant, S., Gupta, M., and Gupta, M. P. (2019). The nuclear and mitochondrial sirtuins, Sirt6 and Sirt3, regulate each other’s activity and protect the heart from developing obesity-mediated diabetic cardiomyopathy. FASEB J. 33, 10872–10888. doi: 10.1096/fj.201900767R
Kim, H. K., and Han, J. (2020). Tetrahydrobiopterin in energy metabolism and metabolic diseases. Pharmacol. Res. 157:104827. doi: 10.1016/j.phrs.2020.104827
Kim, H. K., Ko, T. H., Song, I. S., Jeong, Y. J., Heo, H. J., Jeong, S. H., et al. (2020). BH4 activates CaMKK2 and rescues the cardiomyopathic phenotype in rodent models of diabetes. Life Sci. Alliance 3:201900619. doi: 10.26508/lsa.201900619
Kim, J., Chung, H. S., Choi, M. K., Roh, Y. K., Yoo, H. J., Park, J. H., et al. (2019). Association between Serum Selenium Level and the Presence of Diabetes Mellitus: A Meta-Analysis of Observational Studies. Diabetes Metab. J. 43, 447–460. doi: 10.4093/dmj.2018.0123
Kim, S., Kang, S. W., Joo, J., Han, S. H., Shin, H., Nam, B. Y., et al. (2021). Characterization of ferroptosis in kidney tubular cell death under diabetic conditions. Cell Death Dis. 12:160. doi: 10.1038/s41419-021-03452-x
Kobayashi, N., Okazaki, S., Sampetrean, O., Irie, J., Itoh, H., and Saya, H. (2018). CD44 variant inhibits insulin secretion in pancreatic beta cells by attenuating LAT1-mediated amino acid uptake. Sci. Rep. 8:2785. doi: 10.1038/s41598-018-20973-2
Koppula, P., Zhang, Y., Shi, J., Li, W., and Gan, B. (2017). The glutamate/cystine antiporter SLC7A11/xCT enhances cancer cell dependency on glucose by exporting glutamate. J. Biol. Chem. 292, 14240–14249. doi: 10.1074/jbc.M117.798405
Kosuru, R., Kandula, V., Rai, U., Prakash, S., Xia, Z., and Singh, S. (2018). Pterostilbene Decreases Cardiac Oxidative Stress and Inflammation via Activation of AMPK/Nrf2/HO-1 Pathway in Fructose-Fed Diabetic Rats. Cardiovasc. Drugs Ther. 32, 147–163. doi: 10.1007/s10557-018-6780-3
Kraft, V. A. N., Bezjian, C. T., Pfeiffer, S., Ringelstetter, L., Muller, C., Zandkarimi, F., et al. (2020). GTP Cyclohydrolase 1/Tetrahydrobiopterin Counteract Ferroptosis through Lipid Remodeling. ACS Cent. Sci. 6, 41–53. doi: 10.1021/acscentsci.9b01063
Krummel, B., Plotz, T., Jorns, A., Lenzen, S., and Mehmeti, I. (2021). The central role of glutathione peroxidase 4 in the regulation of ferroptosis and its implications for pro-inflammatory cytokine-mediated beta-cell death. Biochim. Biophys. Acta Mol. Basis Dis. 1867:166114. doi: 10.1016/j.bbadis.2021.166114
Kuch, E. M., Vellaramkalayil, R., Zhang, I., Lehnen, D., Brugger, B., Sreemmel, W., et al. (2014). Differentially localized acyl-CoA synthetase 4 isoenzymes mediate the metabolic channeling of fatty acids towards phosphatidylinositol. Biochim. Biophys. Acta 1841, 227–239. doi: 10.1016/j.bbalip.2013.10.018
Lee, H., Zandkarimi, F., Zhang, Y., Meena, J. K., Kim, J., Zhuang, L., et al. (2020). Energy-stress-mediated AMPK activation inhibits ferroptosis. Nat. Cell Biol. 22, 225–234. doi: 10.1038/s41556-020-0461-8
Lei, P., Bai, T., and Sun, Y. (2019). Mechanisms of Ferroptosis and Relations With Regulated Cell Death: A Review. Front. Physiol. 10:139. doi: 10.3389/fphys.2019.00139
Lenzen, S. (2008). Oxidative stress: the vulnerable beta-cell. Biochem. Soc. Trans. 36(Pt 3), 343–347. doi: 10.1042/BST0360343
Lenzen, S. (2017). Chemistry and biology of reactive species with special reference to the antioxidative defence status in pancreatic beta-cells. Biochim. Biophys. Acta Gen. Subj. 1861, 1929–1942. doi: 10.1016/j.bbagen.2017.05.013
Leu, J. I., Murphy, M. E., and George, D. L. (2019). Mechanistic basis for impaired ferroptosis in cells expressing the African-centric S47 variant of p53. Proc. Natl. Acad. Sci. U S A. 116, 8390–8396. doi: 10.1073/pnas.1821277116
Li, C., Zhang, J., Xue, M., Li, X., Han, F., Liu, X., et al. (2019). SGLT2 inhibition with empagliflozin attenuates myocardial oxidative stress and fibrosis in diabetic mice heart. Cardiovasc. Diabetol. 18:15. doi: 10.1186/s12933-019-0816-2
Li, L., Luo, W., Qian, Y., Zhu, W., Qian, J., Li, J., et al. (2019). Luteolin protects against diabetic cardiomyopathy by inhibiting NF-kappaB-mediated inflammation and activating the Nrf2-mediated antioxidant responses. Phytomedicine 59:152774. doi: 10.1016/j.phymed.2018.11.034
Li, H., Shi, Y., Wang, X., Li, P., Zhang, S., Wu, T., et al. (2019). Piceatannol alleviates inflammation and oxidative stress via modulation of the Nrf2/HO-1 and NF-kappaB pathways in diabetic cardiomyopathy. Chem. Biol. Interact. 310:108754. doi: 10.1016/j.cbi.2019.108754
Li, R., Liu, Y., Shan, Y. G., Gao, L., Wang, F., and Qiu, C. G. (2019). Bailcalin Protects against Diabetic Cardiomyopathy through Keap1/Nrf2/AMPK-Mediated Antioxidative and Lipid-Lowering Effects. Oxid. Med. Cell Longev. 2019:3206542. doi: 10.1155/2019/3206542
Li, D., Jiang, C., Mei, G., Zhao, Y., Chen, L., Liu, J., et al. (2020). Quercetin Alleviates Ferroptosis of Pancreatic beta Cells in Type 2 Diabetes. Nutrients 12:nu12102954. doi: 10.3390/nu12102954
Li, W., Li, W., Leng, Y., Xiong, Y., and Xia, Z. (2020). Ferroptosis Is Involved in Diabetes Myocardial Ischemia/Reperfusion Injury Through Endoplasmic Reticulum Stress. DNA Cell Biol. 39, 210–225. doi: 10.1089/dna.2019.5097
Li, G., Yang, L., Feng, L., Yang, J., Li, Y., An, J., et al. (2020). Syringaresinol Protects against Type 1 Diabetic Cardiomyopathy by Alleviating Inflammation Responses, Cardiac Fibrosis, and Oxidative Stress. Mol. Nutr. Food Res. 64:e2000231. doi: 10.1002/mnfr.202000231
Li, J., Cao, F., Yin, H. L., Huang, Z. J., Lin, Z. T., Mao, N., et al. (2020). Ferroptosis: past, present and future. Cell Death Dis. 11:88. doi: 10.1038/s41419-020-2298-2
Li, G. F., Pan, Y. Z., Sirois, P., Li, K., and Xu, Y. J. (2012). Iron homeostasis in osteoporosis and its clinical implications. Osteoporos Int. 23, 2403–2408. doi: 10.1007/s00198-012-1982-1
Li, H., Yao, W., Irwin, M. G., Wang, T., Wang, S., Zhang, L., et al. (2015). Adiponectin ameliorates hyperglycemia-induced cardiac hypertrophy and dysfunction by concomitantly activating Nrf2 and Brg1. Free Radic. Biol. Med. 84, 311–321. doi: 10.1016/j.freeradbiomed.2015.03.007
Li, J., Liu, S., Cao, G., Sun, Y., Chen, W., Dong, F., et al. (2018). Nicotine induces endothelial dysfunction and promotes atherosclerosis via GTPCH1. J. Cell Mol. Med. 22, 5406–5417. doi: 10.1111/jcmm.13812
Li, N., Jiang, W., Wang, W., Xiong, R., Wu, X., and Geng, Q. (2021). Ferroptosis and its emerging roles in cardiovascular diseases. Pharmacol. Res. 166:105466. doi: 10.1016/j.phrs.2021.105466
Li, S., Zheng, L., Zhang, J., Liu, X., and Wu, Z. (2021). Inhibition of ferroptosis by up-regulating Nrf2 delayed the progression of diabetic nephropathy. Free Radic Biol. Med. 162, 435–449. doi: 10.1016/j.freeradbiomed.2020.10.323
Liang, C., Zhang, X., Yang, M., and Dong, X. (2019). Recent Progress in Ferroptosis Inducers for Cancer Therapy. Adv. Mater. 31:e1904197. doi: 10.1002/adma.201904197
Liang, E., Liu, X., Du, Z., Yang, R., and Zhao, Y. (2018). Andrographolide Ameliorates Diabetic Cardiomyopathy in Mice by Blockage of Oxidative Damage and NF-kappaB-Mediated Inflammation. Oxid. Med. Cell Longev. 2018:9086747. doi: 10.1155/2018/9086747
Liao, H. H., Zhu, J. X., Feng, H., Ni, J., Zhang, N., Chen, S., et al. (2017). Myricetin Possesses Potential Protective Effects on Diabetic Cardiomyopathy through Inhibiting IkappaBalpha/NFkappaB and Enhancing Nrf2/HO-1. Oxid. Med. Cell Longev. 2017:8370593. doi: 10.1155/2017/8370593
Liu, B., Yi, W., Mao, X., Yang, L., and Rao, C. (2021). Enoyl coenzyme A hydratase 1 alleviates nonalcoholic steatohepatitis in mice by suppressing hepatic ferroptosis. Am. J. Physiol. Endocrinol. Metab. 320, E925–E937. doi: 10.1152/ajpendo.00614.2020
Liu, J., Song, X., Kuang, F., Zhang, Q., Xie, Y., Kang, R., et al. (2021). NUPR1 is a critical repressor of ferroptosis. Nat. Commun. 12:647. doi: 10.1038/s41467-021-20904-2
Liu, J., Zhang, C., Wang, J., Hu, W., and Feng, Z. (2020). The Regulation of Ferroptosis by Tumor Suppressor p53 and its Pathway. Int. J. Mol. Sci. 21:ijms21218387. doi: 10.3390/ijms21218387
Liu, P., Liu, J., Wu, Y., Xi, W., Wei, Y., Yuan, Z., et al. (2020). Zinc supplementation protects against diabetic endothelial dysfunction via GTP cyclohydrolase 1 restoration. Biochem. Biophys. Res. Commun. 521, 1049–1054. doi: 10.1016/j.bbrc.2019.11.046
Liu, Y. C., Yeh, C. T., and Lin, K. H. (2019). Molecular Functions of Thyroid Hormone Signaling in Regulation of Cancer Progression and Anti-Apoptosis. Int. J. Mol. Sci. 20:ijms20204986. doi: 10.3390/ijms20204986
Loguercio, C., De Girolamo, V., de Sio, I., Tuccillo, C., Ascione, A., Baldi, F., et al. (2001). Non-alcoholic fatty liver disease in an area of southern Italy: main clinical, histological, and pathophysiological aspects. J. Hepatol. 35, 568–574. doi: 10.1016/s0168-8278(01)00192-1
Lortz, S., Schroter, S., Stuckemann, V., Mehmeti, I., and Lenzen, S. (2014). Influence of cytokines on Dmt1 iron transporter and ferritin expression in insulin-secreting cells. J. Mol. Endocrinol. 52, 301–310. doi: 10.1530/JME-13-0261
Lu, J., Yang, J., Zheng, Y., Chen, X., and Fang, S. (2019). Extracellular vesicles from endothelial progenitor cells prevent steroid-induced osteoporosis by suppressing the ferroptotic pathway in mouse osteoblasts based on bioinformatics evidence. Sci. Rep. 9:16130. doi: 10.1038/s41598-019-52513-x
Luo, E. F., Li, H. X., Qin, Y. H., Qiao, Y., Yan, G. L., Yao, Y. Y., et al. (2021). Role of ferroptosis in the process of diabetes-induced endothelial dysfunction. World J. Diabetes 12, 124–137. doi: 10.4239/wjd.v12.i2.124
Luo, J., Yan, D., Li, S., Liu, S., Zeng, F., Cheung, C. W., et al. (2020). Allopurinol reduces oxidative stress and activates Nrf2/p62 to attenuate diabetic cardiomyopathy in rats. J. Cell Mol. Med. 24, 1760–1773. doi: 10.1111/jcmm.14870
Lutchmansingh, F. K., Hsu, J. W., Bennett, F. I., Badaloo, A. V., McFarlane-Anderson, N., Gordon-Strachan, G. M., et al. (2018). Glutathione metabolism in type 2 diabetes and its relationship with microvascular complications and glycemia. PLoS One 13:e0198626. doi: 10.1371/journal.pone.0198626
Ma, H., Wang, X., Zhang, W., Li, H., Zhao, W., Sun, J., et al. (2020). Melatonin Suppresses Ferroptosis Induced by High Glucose via Activation of the Nrf2/HO-1 Signaling Pathway in Type 2 Diabetic Osteoporosis. Oxid. Med. Cell Longev. 2020:9067610. doi: 10.1155/2020/9067610
Ma, S., Feng, J., Zhang, R., Chen, J., Han, D., Li, X., et al. (2017). SIRT1 Activation by Resveratrol Alleviates Cardiac Dysfunction via Mitochondrial Regulation in Diabetic Cardiomyopathy Mice. Oxid. Med. Cell Longev. 2017:4602715. doi: 10.1155/2017/4602715
Ma, W. Q., Sun, X. J., Zhu, Y., and Liu, N. F. (2021). Metformin attenuates hyperlipidaemia-associated vascular calcification through anti-ferroptotic effects. Free Radic. Biol. Med. 165, 229–242. doi: 10.1016/j.freeradbiomed.2021.01.033
Ma, W., Guo, W., Shang, F., Li, Y., Li, W., Liu, J., et al. (2020). Bakuchiol Alleviates Hyperglycemia-Induced Diabetic Cardiomyopathy by Reducing Myocardial Oxidative Stress via Activating the SIRT1/Nrf2 Signaling Pathway. Oxid. Med. Cell Longev. 2020:3732718. doi: 10.1155/2020/3732718
Maheshwari, R. A., Parmar, G. R., Hinsu, D., Seth, A. K., and Balaraman, R. (2020). Novel therapeutic intervention of coenzyme Q10 and its combination with pioglitazone on the mRNA expression level of adipocytokines in diabetic rats. Life Sci. 258:118155. doi: 10.1016/j.lfs.2020.118155
Mantle, D. (2017). Coenzyme Q10 supplementation for diabetes and its complications: an overview. Br. J. Diabet. 17:149.
Mao, L., Zhao, T., Song, Y., Lin, L., Fan, X., Cui, B., et al. (2020). The emerging role of ferroptosis in non-cancer liver diseases: hype or increasing hope? Cell Death Dis. 11:518. doi: 10.1038/s41419-020-2732-5
Marques, V. B., Leal, M. A. S., Mageski, J. G. A., Fidelis, H. G., Nogueira, B. V., Vasquez, E. C., et al. (2019). Chronic iron overload intensifies atherosclerosis in apolipoprotein E deficient mice: Role of oxidative stress and endothelial dysfunction. Life Sci. 233:116702. doi: 10.1016/j.lfs.2019.116702
Matsushita, M., Freigang, S., Schneider, C., Conrad, M., Bornkamm, G. W., and Kopf, M. (2015). T cell lipid peroxidation induces ferroptosis and prevents immunity to infection. J. Exp. Med. 212, 555–568. doi: 10.1084/jem.20140857
Mayer, B., and Werner, E. R. (1995). In search of a function for tetrahydrobiopterin in the biosynthesis of nitric oxide. Naunyn Schmiedebergs Arch. Pharmacol. 351, 453–463. doi: 10.1007/BF00171035
Mazza, A., Lenti, S., Schiavon, L., Di Giacomo, E., Tomasi, M., Manunta, R., et al. (2018). Effect of Monacolin K and COQ10 supplementation in hypertensive and hypercholesterolemic subjects with metabolic syndrome. Biomed. Pharmacother. 105, 992–996. doi: 10.1016/j.biopha.2018.06.076
Mehmeti, I., Lortz, S., Avezov, E., Jorns, A., and Lenzen, S. (2017). ER-resident antioxidative GPx7 and GPx8 enzyme isoforms protect insulin-secreting INS-1E beta-cells against lipotoxicity by improving the ER antioxidative capacity. Free Radic. Biol. Med. 112, 121–130. doi: 10.1016/j.freeradbiomed.2017.07.021
Montano, S. J., Grunler, J., Nair, D., Tekle, M., Fernandes, A. P., Hua, X., et al. (2015). Glutaredoxin mediated redox effects of coenzyme Q10 treatment in type 1 and type 2 diabetes patients. BBA Clin. 4, 14–20. doi: 10.1016/j.bbacli.2015.06.001
Nelson, J. E., Wilson, L., Brunt, E. M., Yeh, M. M., Kleiner, D. E., Unalp-Arida, A., et al. (2011). Relationship between the pattern of hepatic iron deposition and histological severity in nonalcoholic fatty liver disease. Hepatology 53, 448–457. doi: 10.1002/hep.24038
Newell, J. L., Keyari, C. M., McDaniel, S. W., Diaz, P. J., Natale, N. R., Patel, S. A., et al. (2014). Novel di-aryl-substituted isoxazoles act as noncompetitive inhibitors of the system Xc(-) cystine/glutamate exchanger. Neurochem. Int. 73, 132–138. doi: 10.1016/j.neuint.2013.11.012
Nguyen, H. P., Yi, D., Lin, F., Viscarra, J. A., Tabuchi, C., Ngo, K., et al. (2020). Aifm2, a NADH Oxidase, Supports Robust Glycolysis and Is Required for Cold- and Diet-Induced Thermogenesis. Mol. Cell 77, 600–617e604. doi: 10.1016/j.molcel.2019.12.002
Oguri, Y., Fujita, Y., Abudukadier, A., Ohashi, A., Goto, T., Furuya, F., et al. (2017). Tetrahydrobiopterin activates brown adipose tissue and regulates systemic energy metabolism. JCI Insight 2:91981. doi: 10.1172/jci.insight.91981
Palomer, X., Salvado, L., Barroso, E., and Vazquez-Carrera, M. (2013). An overview of the crosstalk between inflammatory processes and metabolic dysregulation during diabetic cardiomyopathy. Int. J. Cardiol. 168, 3160–3172. doi: 10.1016/j.ijcard.2013.07.150
Park, S. J., Cho, S. S., Kim, K. M., Yang, J. H., Kim, J. H., Jeong, E. H., et al. (2019). Protective effect of sestrin2 against iron overload and ferroptosis-induced liver injury. Toxicol. Appl. Pharmacol. 379:114665. doi: 10.1016/j.taap.2019.114665
Pierzynowska, K., Rintz, E., Gaffke, L., and Wegrzyn, G. (2021). Ferroptosis and Its Modulation by Autophagy in Light of the Pathogenesis of Lysosomal Storage Diseases. Cells 10:cells10020365. doi: 10.3390/cells10020365
Poznyak, A., Grechko, A. V., Poggio, P., Myasoedova, V. A., Alfieri, V., and Orekhov, A. N. (2020). The Diabetes Mellitus-Atherosclerosis Connection: The Role of Lipid and Glucose Metabolism and Chronic Inflammation. Int. J. Mol. Sci. 21:ijms21051835. doi: 10.3390/ijms21051835
Protchenko, O., Baratz, E., Jadhav, S., Li, F., Shakoury-Elizeh, M., Gavrilova, O., et al. (2020). Iron Chaperone Poly rC Binding Protein 1 Protects Mouse Liver From Lipid Peroxidation and Steatosis. Hepatology 2020:31328. doi: 10.1002/hep.31328
Qi, J., Kim, J. W., Zhou, Z., Lim, C. W., and Kim, B. (2020). Ferroptosis Affects the Progression of Nonalcoholic Steatohepatitis via the Modulation of Lipid Peroxidation-Mediated Cell Death in Mice. Am. J. Pathol. 190, 68–81. doi: 10.1016/j.ajpath.2019.09.011
Qiu, Y., Wu, Y., Meng, M., Luo, M., Zhao, H., Sun, H., et al. (2018). GYY4137 protects against myocardial ischemia/reperfusion injury via activation of the PHLPP-1/Akt/Nrf2 signaling pathway in diabetic mice. J. Surg. Res. 225, 29–39. doi: 10.1016/j.jss.2017.12.030
Ray, P. D., Huang, B. W., and Tsuji, Y. (2012). Reactive oxygen species (ROS) homeostasis and redox regulation in cellular signaling. Cell Signal 24, 981–990. doi: 10.1016/j.cellsig.2012.01.008
Raygan, F., Rezavandi, Z., Dadkhah Tehrani, S., Farrokhian, A., and Asemi, Z. (2016). The effects of coenzyme Q10 administration on glucose homeostasis parameters, lipid profiles, biomarkers of inflammation and oxidative stress in patients with metabolic syndrome. Eur. J. Nutr. 55, 2357–2364. doi: 10.1007/s00394-015-1042-7
Saberzadeh-Ardestani, B., Karamzadeh, R., Basiri, M., Hajizadeh-Saffar, E., Farhadi, A., Shapiro, A. M. J., et al. (2018). Type 1 Diabetes Mellitus: Cellular and Molecular Pathophysiology at A Glance. Cell J. 20, 294–301. doi: 10.22074/cellj.2018.5513
Sadeghiyan Galeshkalami, N., Abdollahi, M., Najafi, R., Baeeri, M., Jamshidzade, A., Falak, R., et al. (2019). Alpha-lipoic acid and coenzyme Q10 combination ameliorates experimental diabetic neuropathy by modulating oxidative stress and apoptosis. Life Sci. 216, 101–110. doi: 10.1016/j.lfs.2018.10.055
Saeedi, P., Petersohn, I., Salpea, P., Malanda, B., Karuranga, S., Unwin, N., et al. (2019). Global and regional diabetes prevalence estimates for 2019 and projections for 2030 and 2045: Results from the International Diabetes Federation Diabetes Atlas, 9(th) edition. Diabet. Res. Clin. Pract. 157:107843. doi: 10.1016/j.diabres.2019.107843
Sampath, C., Sprouse, J. C., Freeman, M. L., and Gangula, P. R. (2019). Activation of Nrf2 attenuates delayed gastric emptying in obesity induced diabetic (T2DM) female mice. Free Radic. Biol. Med. 135, 132–143. doi: 10.1016/j.freeradbiomed.2019.02.029
Sampilvanjil, A., Karasawa, T., Yamada, N., Komada, T., Higashi, T., Baatarjav, C., et al. (2020). Cigarette smoke extract induces ferroptosis in vascular smooth muscle cells. Am. J. Physiol. Heart Circ. Physiol. 318, H508–H518. doi: 10.1152/ajpheart.00559.2019
Santoro, M. M. (2020). The Antioxidant Role of Non-mitochondrial CoQ10: Mystery Solved! Cell Metab. 31, 13–15. doi: 10.1016/j.cmet.2019.12.007
Sanyal, A. J., Chalasani, N., Kowdley, K. V., McCullough, A., Diehl, A. M., Bass, N. M., et al. (2010). Pioglitazone, vitamin E, or placebo for nonalcoholic steatohepatitis. N. Engl. J. Med. 362, 1675–1685. doi: 10.1056/NEJMoa0907929
Sato, H., Takai, C., Kazama, J. J., Wakamatsu, A., Hasegawa, E., Kobayashi, D., et al. (2020). Serum hepcidin level, iron metabolism and osteoporosis in patients with rheumatoid arthritis. Sci. Rep. 10:9882. doi: 10.1038/s41598-020-66945-3
Schomburg, L. (2020). The other view: the trace element selenium as a micronutrient in thyroid disease, diabetes, and beyond. Hormones 19, 15–24. doi: 10.1007/s42000-019-00150-4
Seibt, T. M., Proneth, B., and Conrad, M. (2019). Role of GPX4 in ferroptosis and its pharmacological implication. Free Radic. Biol. Med. 133, 144–152. doi: 10.1016/j.freeradbiomed.2018.09.014
Senates, E., Yilmaz, Y., Colak, Y., Ozturk, O., Altunoz, M. E., Kurt, R., et al. (2011). Serum levels of hepcidin in patients with biopsy-proven nonalcoholic fatty liver disease. Metab. Syndr. Relat. Disord. 9, 287–290. doi: 10.1089/met.2010.0121
Shah, R., Shchepinov, M. S., and Pratt, D. A. (2018). Resolving the Role of Lipoxygenases in the Initiation and Execution of Ferroptosis. ACS Cent Sci. 4, 387–396. doi: 10.1021/acscentsci.7b00589
Shah, Y., Bass, L., Davison, G. W., Seigler, N., Pollock, J. S., Thomas, J., et al. (2017). BH4 improves postprandial endothelial function after a high-fat meal in men and postmenopausal women. Menopause 24, 555–562. doi: 10.1097/GME.0000000000000785
Shang, Y., Luo, M., Yao, F., Wang, S., Yuan, Z., and Yang, Y. (2020). Ceruloplasmin suppresses ferroptosis by regulating iron homeostasis in hepatocellular carcinoma cells. Cell Signal 72:109633. doi: 10.1016/j.cellsig.2020.109633
Shimada, K., Skouta, R., Kaplan, A., Yang, W. S., Hayano, M., Dixon, S. J., et al. (2016). Global survey of cell death mechanisms reveals metabolic regulation of ferroptosis. Nat. Chem. Biol. 12, 497–503. doi: 10.1038/nchembio.2079
Shirpoor, A., Salami, S., Khadem-Ansari, M. H., Ilkhanizadeh, B., Pakdel, F. G., and Khademvatani, K. (2009). Cardioprotective effect of vitamin E: rescues of diabetes-induced cardiac malfunction, oxidative stress, and apoptosis in rat. J. Diabetes Complicat. 23, 310–316. doi: 10.1016/j.jdiacomp.2008.02.009
Shu, T., Lv, Z., Xie, Y., Tang, J., and Mao, X. (2019). Hepcidin as a key iron regulator mediates glucotoxicity-induced pancreatic beta-cell dysfunction. Endocr. Connect 8, 150–161. doi: 10.1530/EC-18-0516
Shur, J., Kannengiesser, S. A. R., Menezes, R., Ward, R., Kuo, K., and Jhaveri, K. (2020). Glucose dysregulation in patients with iron overload: is there a relationship with quantitative pancreas and liver iron and fat content measured by MRI? Eur. Radiol. 30, 1616–1623. doi: 10.1007/s00330-019-06487-z
Song, X., Liu, J., Kuang, F., Chen, X., Zeh, H. J. III, Kang, R., et al. (2021). PDK4 dictates metabolic resistance to ferroptosis by suppressing pyruvate oxidation and fatty acid synthesis. Cell Rep. 34:108767. doi: 10.1016/j.celrep.2021.108767
Soula, M., Weber, R. A., Zilka, O., Alwaseem, H., La, K., Yen, F., et al. (2020). Metabolic determinants of cancer cell sensitivity to canonical ferroptosis inducers. Nat. Chem. Biol. 16, 1351–1360. doi: 10.1038/s41589-020-0613-y
Stockwell, B. R., Friedmann Angeli, J. P., Bayir, H., Bush, A. I., Conrad, M., Dixon, S. J., et al. (2017). Ferroptosis: A Regulated Cell Death Nexus Linking Metabolism, Redox Biology, and Disease. Cell 171, 273–285. doi: 10.1016/j.cell.2017.09.021
Stockwell, B. R., Jiang, X., and Gu, W. (2020). Emerging Mechanisms and Disease Relevance of Ferroptosis. Trends Cell Biol. 30, 478–490. doi: 10.1016/j.tcb.2020.02.009
Suarez-Rivero, J. M., Pastor-Maldonado, C. J., de la Mata, M., Villanueva-Paz, M., Povea-Cabello, S., Alvarez-Cordoba, M., et al. (2019). Atherosclerosis and Coenzyme Q10. Int. J. Mol. Sci. 20:ijms20205195. doi: 10.3390/ijms20205195
Sun, Y., Zhou, S., Guo, H., Zhang, J., Ma, T., Zheng, Y., et al. (2020). Protective effects of sulforaphane on type 2 diabetes-induced cardiomyopathy via AMPK-mediated activation of lipid metabolic pathways and NRF2 function. Metabolism 102:154002. doi: 10.1016/j.metabol.2019.154002
Sung, H. K., Song, E., Jahng, J. W. S., Pantopoulos, K., and Sweeney, G. (2019). Iron induces insulin resistance in cardiomyocytes via regulation of oxidative stress. Sci. Rep. 9:4668. doi: 10.1038/s41598-019-41111-6
Tang, D., and Kroemer, G. (2020). Peroxisome: the new player in ferroptosis. Signal Transduct. Target Ther. 5:273. doi: 10.1038/s41392-020-00404-3
Tang, D., Chen, X., Kang, R., and Kroemer, G. (2021). Ferroptosis: molecular mechanisms and health implications. Cell Res. 31, 107–125. doi: 10.1038/s41422-020-00441-1
Tang, D., Kang, R., Berghe, T. V., Vandenabeele, P., and Kroemer, G. (2019). The molecular machinery of regulated cell death. Cell Res. 29, 347–364. doi: 10.1038/s41422-019-0164-5
Tang, R., Xu, J., Zhang, B., Liu, J., Liang, C., Hua, J., et al. (2020). Ferroptosis, necroptosis, and pyroptosis in anticancer immunity. J. Hematol. Oncol. 13:110. doi: 10.1186/s13045-020-00946-7
Tantikanlayaporn, D., Wichit, P., Suksen, K., Suksamrarn, A., and Piyachaturawat, P. (2020). Andrographolide modulates OPG/RANKL axis to promote osteoblastic differentiation in MC3T3-E1 cells and protects bone loss during estrogen deficiency in rats. Biomed. Pharmacother. 131:110763. doi: 10.1016/j.biopha.2020.110763
Torii, S., Shintoku, R., Kubota, C., Yaegashi, M., Torii, R., Sasaki, M., et al. (2016). An essential role for functional lysosomes in ferroptosis of cancer cells. Biochem. J. 473, 769–777. doi: 10.1042/BJ20150658
Tsay, J., Yang, Z., Ross, F. P., Cunningham-Rundles, S., Lin, H., Coleman, R., et al. (2010). Bone loss caused by iron overload in a murine model: importance of oxidative stress. Blood 116, 2582–2589. doi: 10.1182/blood-2009-12-260083
Tsurusaki, S., Tsuchiya, Y., Koumura, T., Nakasone, M., Sakamoto, T., Matsuoka, M., et al. (2019). Hepatic ferroptosis plays an important role as the trigger for initiating inflammation in nonalcoholic steatohepatitis. Cell Death Dis. 10:449. doi: 10.1038/s41419-019-1678-y
Uruno, A., Furusawa, Y., Yagishita, Y., Fukutomi, T., Muramatsu, H., Negishi, T., et al. (2013). The Keap1-Nrf2 system prevents onset of diabetes mellitus. Mol. Cell Biol. 33, 2996–3010. doi: 10.1128/MCB.00225-13
Venkatesh, D., O’Brien, N. A., Zandkarimi, F., Tong, D. R., Stokes, M. E., Dunn, D. E., et al. (2020a). MDM2 and MDMX promote ferroptosis by PPARalpha-mediated lipid remodeling. Genes Dev. 34, 526–543. doi: 10.1101/gad.334219.119
Venkatesh, D., Stockwell, B. R., and Prives, C. (2020b). p21 can be a barrier to ferroptosis independent of p53. Aging 12, 17800–17814. doi: 10.18632/aging.103961
Viktorinova, A., and Durfinova, M. (2021). Mini-Review: Is iron-mediated cell death (ferroptosis) an identical factor contributing to the pathogenesis of some neurodegenerative diseases? Neurosci. Lett. 745:135627. doi: 10.1016/j.neulet.2021.135627
Wang, C., Chao, Y., Xu, W., Liang, M., Deng, S., Zhang, D., et al. (2020a). CTRP13 Preserves Endothelial Function by Targeting GTP Cyclohydrolase 1 in Diabetes. Diabetes 69, 99–111. doi: 10.2337/db19-0635
Wang, C., Zhu, L., Yuan, W., Sun, L., Xia, Z., Zhang, Z., et al. (2020b). Diabetes aggravates myocardial ischaemia reperfusion injury via activating Nox2-related programmed cell death in an AMPK-dependent manner. J. Cell Mol. Med. 24, 6670–6679. doi: 10.1111/jcmm.15318
Wang, J., De-Qiong, X., Hong, D. Q., Zhang, Q. Q., and Zhang, J. (2019). Attenuation of Myocardial ischemia reperfusion injury by Geniposide preconditioning in diabetic rats. Curr. Res. Transl. Med. 67, 35–40. doi: 10.1016/j.retram.2019.03.002
Wang, L., Bi, X., and Han, J. (2020c). Silencing of peroxisome proliferator-activated receptor-alpha alleviates myocardial injury in diabetic cardiomyopathy by downregulating 3-hydroxy-3-methylglutaryl-coenzyme A synthase 2 expression. IUBMB Life 72, 1997–2009. doi: 10.1002/iub.2337
Wang, L., Liu, Y., Du, T., Yang, H., Lei, L., Guo, M., et al. (2020d). ATF3 promotes erastin-induced ferroptosis by suppressing system Xc(.). Cell Death Differ. 27, 662–675. doi: 10.1038/s41418-019-0380-z
Wang, N., Ma, H., Li, J., Meng, C., Zou, J., Wang, H., et al. (2021). HSF1 functions as a key defender against palmitic acid-induced ferroptosis in cardiomyocytes. J. Mol. Cell Cardiol. 150, 65–76. doi: 10.1016/j.yjmcc.2020.10.010
Wang, S., Wang, B., Wang, Y., Tong, Q., Liu, Q., Sun, J., et al. (2017). Zinc Prevents the Development of Diabetic Cardiomyopathy in db/db Mice. Int. J. Mol. Sci. 18:ijms18030580. doi: 10.3390/ijms18030580
Wang, X. L., Yang, T. B., Wei, J., Lei, G. H., and Zeng, C. (2016). Association between serum selenium level and type 2 diabetes mellitus: a non-linear dose-response meta-analysis of observational studies. Nutr. J. 15:48. doi: 10.1186/s12937-016-0169-6
Wang, Y., and Tang, M. (2019). PM2.5 induces ferroptosis in human endothelial cells through iron overload and redox imbalance. Environ. Pollut. 254(Pt A):112937. doi: 10.1016/j.envpol.2019.07.105
Wang, Y., Bi, R., Quan, F., Cao, Q., Lin, Y., Yue, C., et al. (2020e). Ferroptosis involves in renal tubular cell death in diabetic nephropathy. Eur. J. Pharmacol. 888:173574. doi: 10.1016/j.ejphar.2020.173574
Wang, Y., Chen, J., Li, S., Zhang, X., Guo, Z., Hu, J., et al. (2020f). Exogenous spermine attenuates rat diabetic cardiomyopathy via suppressing ROS-p53 mediated downregulation of calcium-sensitive receptor. Redox Biol. 32:101514. doi: 10.1016/j.redox.2020.101514
Wang, Y., Rijntjes, E., Wu, Q., Lv, H., Gao, C., Shi, B., et al. (2020g). Selenium deficiency is linearly associated with hypoglycemia in healthy adults. Redox Biol. 37:101709. doi: 10.1016/j.redox.2020.101709
Warner, G. J., Berry, M. J., Moustafa, M. E., Carlson, B. A., Hatfield, D. L., and Faust, J. R. (2000). Inhibition of selenoprotein synthesis by selenocysteine tRNA[Ser]Sec lacking isopentenyladenosine. J. Biol. Chem. 275, 28110–28119. doi: 10.1074/jbc.M001280200
Wei, S., Qiu, T., Yao, X., Wang, N., Jiang, L., Jia, X., et al. (2020). Arsenic induces pancreatic dysfunction and ferroptosis via mitochondrial ROS-autophagy-lysosomal pathway. J. Hazard. Mater. 384:121390. doi: 10.1016/j.jhazmat.2019.121390
Weigand, I., Schreiner, J., Rohrig, F., Sun, N., Landwehr, L. S., Urlaub, H., et al. (2020). Active steroid hormone synthesis renders adrenocortical cells highly susceptible to type II ferroptosis induction. Cell Death Dis. 11:192. doi: 10.1038/s41419-020-2385-4
Weiland, A., Wang, Y., Wu, W., Lan, X., Han, X., Li, Q., et al. (2019). Ferroptosis and Its Role in Diverse Brain Diseases. Mol. Neurobiol. 56, 4880–4893. doi: 10.1007/s12035-018-1403-3
Wenzel, S. E., Tyurina, Y. Y., Zhao, J., St Croix, C. M., Dar, H. H., Mao, G., et al. (2017). PEBP1 Wardens Ferroptosis by Enabling Lipoxygenase Generation of Lipid Death Signals. Cell 171, 628–641e626. doi: 10.1016/j.cell.2017.09.044
Wu, H. E., Baumgardt, S. L., Fang, J., Paterson, M., Liu, Y., Du, J., et al. (2016). Cardiomyocyte GTP Cyclohydrolase 1 Protects the Heart Against Diabetic Cardiomyopathy. Sci. Rep. 6:27925. doi: 10.1038/srep27925
Wu, H., Liu, G., He, Y., Da, J., and Xie, B. (2019). Obeticholic acid protects against diabetic cardiomyopathy by activation of FXR/Nrf2 signaling in db/db mice. Eur. J. Pharmacol. 858:172393. doi: 10.1016/j.ejphar.2019.05.022
Wu, W., Yao, X., Jiang, L., Zhang, Q., Bai, J., Qiu, T., et al. (2018). Pancreatic islet-autonomous effect of arsenic on insulin secretion through endoplasmic reticulum stress-autophagy pathway. Food Chem. Toxicol. 111, 19–26. doi: 10.1016/j.fct.2017.10.043
Wu, Y., Zhao, Y., Yang, H. Z., Wang, Y. J., and Chen, Y. (2021). HMGB1 regulates ferroptosis through Nrf2 pathway in mesangial cells in response to high glucose. Biosci. Rep. 41:BSR20202924. doi: 10.1042/BSR20202924
Wu, Z., Geng, Y., Lu, X., Shi, Y., Wu, G., Zhang, M., et al. (2019). Chaperone-mediated autophagy is involved in the execution of ferroptosis. Proc. Natl. Acad. Sci. U S A. 116, 2996–3005. doi: 10.1073/pnas.1819728116
Wunderer, F., Traeger, L., Sigurslid, H. H., Meybohm, P., Bloch, D. B., and Malhotra, R. (2020). The role of hepcidin and iron homeostasis in atherosclerosis. Pharmacol. Res. 153:104664. doi: 10.1016/j.phrs.2020.104664
Xiao, C., Xia, M. L., Wang, J., Zhou, X. R., Lou, Y. Y., Tang, L. H., et al. (2019). Luteolin Attenuates Cardiac Ischemia/Reperfusion Injury in Diabetic Rats by Modulating Nrf2 Antioxidative Function. Oxid. Med. Cell Longev. 2019:2719252. doi: 10.1155/2019/2719252
Xu, G., Zhao, X., Fu, J., and Wang, X. (2019). Resveratrol increase myocardial Nrf2 expression in type 2 diabetic rats and alleviate myocardial ischemia/reperfusion injury (MIRI). Ann. Palliat. Med. 8, 565–575. doi: 10.21037/apm.2019.11.25
Xu, J., Kulkarni, S. R., Donepudi, A. C., More, V. R., and Slitt, A. L. (2012). Enhanced Nrf2 activity worsens insulin resistance, impairs lipid accumulation in adipose tissue, and increases hepatic steatosis in leptin-deficient mice. Diabetes 61, 3208–3218. doi: 10.2337/db11-1716
Xu, S. (2019). Iron and Atherosclerosis: The Link Revisited. Trends Mol. Med. 25, 659–661. doi: 10.1016/j.molmed.2019.05.012
Xu, T., Jiang, L., and Wang, Z. (2019). The progression of HMGB1-induced autophagy in cancer biology. Onco Targets Ther. 12, 365–377. doi: 10.2147/OTT.S185876
Xu, Z., Wang, S., Ji, H., Zhang, Z., Chen, J., Tan, Y., et al. (2016). Broccoli sprout extract prevents diabetic cardiomyopathy via Nrf2 activation in db/db T2DM mice. Sci. Rep. 6:30252. doi: 10.1038/srep30252
Yagoda, N., von Rechenberg, M., Zaganjor, E., Bauer, A. J., Yang, W. S., Fridman, D. J., et al. (2007). RAS-RAF-MEK-dependent oxidative cell death involving voltage-dependent anion channels. Nature 447, 864–868. doi: 10.1038/nature05859
Yan, B., Ai, Y., Sun, Q., Ma, Y., Cao, Y., Wang, J., et al. (2021). Membrane Damage during Ferroptosis Is Caused by Oxidation of Phospholipids Catalyzed by the Oxidoreductases POR and CYB5R1. Mol. Cell 81, 355–369e310. doi: 10.1016/j.molcel.2020.11.024
Yan, X., Chen, J., Zhang, C., Zhou, S., Zhang, Z., Chen, J., et al. (2015). FGF21 deletion exacerbates diabetic cardiomyopathy by aggravating cardiac lipid accumulation. J. Cell Mol. Med. 19, 1557–1568. doi: 10.1111/jcmm.12530
Yang, H., Feng, A., Lin, S., Yu, L., Lin, X., Yan, X., et al. (2018). Fibroblast growth factor-21 prevents diabetic cardiomyopathy via AMPK-mediated antioxidation and lipid-lowering effects in the heart. Cell Death Dis. 9:227. doi: 10.1038/s41419-018-0307-5
Yang, R. Z., Xu, W. N., Zheng, H. L., Zheng, X. F., Li, B., Jiang, L. S., et al. (2021). Exosomes derived from vascular endothelial cells antagonize glucocorticoid-induced osteoporosis by inhibiting ferritinophagy with resultant limited ferroptosis of osteoblasts. J. Cell Physiol. 2021:30331. doi: 10.1002/jcp.30331
Yang, W. S., and Stockwell, B. R. (2008). Synthetic lethal screening identifies compounds activating iron-dependent, nonapoptotic cell death in oncogenic-RAS-harboring cancer cells. Chem. Biol. 15, 234–245. doi: 10.1016/j.chembiol.2008.02.010
Yang, W. S., and Stockwell, B. R. (2016). Ferroptosis: Death by Lipid Peroxidation. Trends Cell Biol. 26, 165–176. doi: 10.1016/j.tcb.2015.10.014
Yang, W. S., Kim, K. J., Gaschler, M. M., Patel, M., Shchepinov, M. S., and Stockwell, B. R. (2016). Peroxidation of polyunsaturated fatty acids by lipoxygenases drives ferroptosis. Proc. Natl. Acad. Sci. U S A. 113, E4966–E4975. doi: 10.1073/pnas.1603244113
Yang, W. S., SriRamaratnam, R., Welsch, M. E., Shimada, K., Skouta, R., Viswanathan, V. S., et al. (2014). Regulation of ferroptotic cancer cell death by GPX4. Cell 156, 317–331. doi: 10.1016/j.cell.2013.12.010
Yang, Y., Chen, J., Gao, Q., Shan, X., Wang, J., and Lv, Z. (2020). Study on the attenuated effect of Ginkgolide B on ferroptosis in high fat diet induced nonalcoholic fatty liver disease. Toxicology 445, 152599. doi: 10.1016/j.tox.2020.152599
Yao, Q., Sun, R., Bao, S., Chen, R., and Kou, L. (2020). Bilirubin Protects Transplanted Islets by Targeting Ferroptosis. Front. Pharmacol. 11:907. doi: 10.3389/fphar.2020.00907
Ying, Y., Jin, J., Ye, L., Sun, P., Wang, H., and Wang, X. (2018). Phloretin Prevents Diabetic Cardiomyopathy by Dissociating Keap1/Nrf2 Complex and Inhibiting Oxidative Stress. Front. Endocrinol. 9:774. doi: 10.3389/fendo.2018.00774
Yongyun, C., Jingwei, Z., Zhiqing, L., Wenxiang, C., and Huiwu, L. (2019). Andrographolide stimulates osteoblastogenesis and bone formation by inhibiting nuclear factor kappa-Beta signaling both in vivo and in vitro. J. Orthop. Translat. 19, 47–57. doi: 10.1016/j.jot.2019.02.001
Yu, M., Gai, C., Li, Z., Ding, D., Zheng, J., Zhang, W., et al. (2019). Targeted exosome-encapsulated erastin induced ferroptosis in triple negative breast cancer cells. Cancer Sci. 110, 3173–3182. doi: 10.1111/cas.14181
Yu, Y., Jiang, L., Wang, H., Shen, Z., Cheng, Q., Zhang, P., et al. (2020). Hepatic transferrin plays a role in systemic iron homeostasis and liver ferroptosis. Blood 136, 726–739. doi: 10.1182/blood.2019002907
Zang, H., Wu, W., Qi, L., Tan, W., Nagarkatti, P., Nagarkatti, M., et al. (2020). Autophagy Inhibition Enables Nrf2 to Exaggerate the Progression of Diabetic Cardiomyopathy in Mice. Diabetes 69, 2720–2734. doi: 10.2337/db19-1176
Zhang, B., Shen, Q., Chen, Y., Pan, R., Kuang, S., Liu, G., et al. (2017). Myricitrin Alleviates Oxidative Stress-induced Inflammation and Apoptosis and Protects Mice against Diabetic Cardiomyopathy. Sci. Rep. 7:44239. doi: 10.1038/srep44239
Zhang, B., Zhai, M., Li, B., Liu, Z., Li, K., Jiang, L., et al. (2018a). Honokiol Ameliorates Myocardial Ischemia/Reperfusion Injury in Type 1 Diabetic Rats by Reducing Oxidative Stress and Apoptosis through Activating the SIRT1-Nrf2 Signaling Pathway. Oxid. Med. Cell Longev. 2018:3159801. doi: 10.1155/2018/3159801
Zhang, B., Zhang, J., Zhang, C., Zhang, X., Ye, J., Kuang, S., et al. (2018b). Notoginsenoside R1 Protects Against Diabetic Cardiomyopathy Through Activating Estrogen Receptor alpha and Its Downstream Signaling. Front. Pharmacol. 9:1227. doi: 10.3389/fphar.2018.01227
Zhang, J. (2020). The osteoprotective effects of artemisinin compounds and the possible mechanisms associated with intracellular iron: A review of in vivo and in vitro studies. Environ. Toxicol. Pharmacol. 76:103358. doi: 10.1016/j.etap.2020.103358
Zhang, J., Cai, X., Zhang, Q., Li, X., Li, S., Ma, J., et al. (2020). Hydrogen sulfide restores sevoflurane postconditioning mediated cardioprotection in diabetic rats: Role of SIRT1/Nrf2 signaling-modulated mitochondrial dysfunction and oxidative stress. J. Cell Physiol. 2020:30214. doi: 10.1002/jcp.30214
Zhang, L., Qi, X., Zhang, G., Zhang, Y., and Tian, J. (2020). Saxagliptin protects against hypoxia-induced damage in H9c2 cells. Chem. Biol. Interact. 315:108864. doi: 10.1016/j.cbi.2019.108864
Zhang, P., Yang, C., Guo, H., Wang, J., Lin, S., Li, H., et al. (2018). Treatment of coenzyme Q10 for 24 weeks improves lipid and glycemic profile in dyslipidemic individuals. J. Clin. Lipidol. 12, 417–427e415. doi: 10.1016/j.jacl.2017.12.006
Zhang, Q., Bai, J., Yao, X., Jiang, L., Wu, W., Yang, L., et al. (2019). Taurine rescues the arsenic-induced injury in the pancreas of rat offsprings and in the INS-1 cells. Biomed. Pharmacother. 109, 815–822. doi: 10.1016/j.biopha.2018.10.134
Zhang, S. Y., Yang, K. L., Zeng, L. T., Wu, X. H., and Huang, H. Y. (2018). Effectiveness of Coenzyme Q10 Supplementation for Type 2 Diabetes Mellitus: A Systematic Review and Meta-Analysis. Int. J. Endocrinol. 2018:6484839. doi: 10.1155/2018/6484839
Zhang, X., Shi, Z., Liu, Q., Quan, H., and Cheng, X. (2019). Effects of coenzyme Q10 intervention on diabetic kidney disease: A systematic review and meta-analysis. Medicine 98:e15850. doi: 10.1097/MD.0000000000015850
Zhang, X., Tohari, A. M., Marcheggiani, F., Zhou, X., Reilly, J., Tiano, L., et al. (2017). Therapeutic Potential of Co-enzyme Q10 in Retinal Diseases. Curr. Med. Chem. 24, 4329–4339. doi: 10.2174/0929867324666170801100516
Zhang, Y., Hu, M., Jia, W., Liu, G., Zhang, J., Wang, B., et al. (2020). Hyperandrogenism and insulin resistance modulate gravid uterine and placental ferroptosis in PCOS-like rats. J. Endocrinol. 246, 247–263. doi: 10.1530/JOE-20-0155
Zhang, Y., Koppula, P., and Gan, B. (2019a). Regulation of H2A ubiquitination and SLC7A11 expression by BAP1 and PRC1. Cell Cycle 18, 773–783. doi: 10.1080/15384101.2019.1597506
Zhang, Y., Shi, J., Liu, X., Feng, L., Gong, Z., Koppula, P., et al. (2018). BAP1 links metabolic regulation of ferroptosis to tumour suppression. Nat. Cell Biol. 20, 1181–1192. doi: 10.1038/s41556-018-0178-0
Zhang, Y., Tan, H., Daniels, J. D., Zandkarimi, F., Liu, H., Brown, L. M., et al. (2019b). Imidazole Ketone Erastin Induces Ferroptosis and Slows Tumor Growth in a Mouse Lymphoma Model. Cell Chem. Biol. 26, 623–633e629. doi: 10.1016/j.chembiol.2019.01.008
Zhang, Z., Wang, S., Zhou, S., Yan, X., Wang, Y., Chen, J., et al. (2014). Sulforaphane prevents the development of cardiomyopathy in type 2 diabetic mice probably by reversing oxidative stress-induced inhibition of LKB1/AMPK pathway. J. Mol. Cell Cardiol. 77, 42–52. doi: 10.1016/j.yjmcc.2014.09.022
Zhao, J., Hu, Y., and Peng, J. (2021). Targeting programmed cell death in metabolic dysfunction-associated fatty liver disease (MAFLD): a promising new therapy. Cell Mol. Biol. Lett. 26:17. doi: 10.1186/s11658-021-00254-z
Zhao, S., Ghosh, A., Lo, C. S., Chenier, I., Scholey, J. W., Filep, J. G., et al. (2018). Nrf2 Deficiency Upregulates Intrarenal Angiotensin-Converting Enzyme-2 and Angiotensin 1-7 Receptor Expression and Attenuates Hypertension and Nephropathy in Diabetic Mice. Endocrinology 159, 836–852. doi: 10.1210/en.2017-00752
Zheng, J., and Conrad, M. (2020). The Metabolic Underpinnings of Ferroptosis. Cell Metab. 32, 920–937. doi: 10.1016/j.cmet.2020.10.011
Zhou, X., Zou, L., Chen, W., Yang, T., Luo, J., Wu, K., et al. (2021). Flubendazole, FDA-approved anthelmintic, elicits valid antitumor effects by targeting P53 and promoting ferroptosis in castration-resistant prostate cancer. Pharmacol. Res. 164:105305. doi: 10.1016/j.phrs.2020.105305
Zhou, Y. (2020). The Protective Effects of Cryptochlorogenic Acid on beta-Cells Function in Diabetes in vivo and vitro via Inhibition of Ferroptosis. Diabetes Metab. Syndr. Obes. 13, 1921–1931. doi: 10.2147/DMSO.S249382
Zhu, S., Zhang, Q., Sun, X., Zeh, H. J. III, Lotze, M. T., Kang, R., et al. (2017). HSPA5 Regulates Ferroptotic Cell Death in Cancer Cells. Cancer Res. 77, 2064–2077. doi: 10.1158/0008-5472.CAN-16-1979
Zitvogel, L., and Kroemer, G. (2019). Interferon-gamma induces cancer cell ferroptosis. Cell Res. 29, 692–693. doi: 10.1038/s41422-019-0186-z
Zou, Y., Henry, W. S., Ricq, E. L., Graham, E. T., Phadnis, V. V., Maretich, P., et al. (2020a). Plasticity of ether lipids promotes ferroptosis susceptibility and evasion. Nature 585, 603–608. doi: 10.1038/s41586-020-2732-8
Keywords: ferroptosis, iron, metabolic diseases, reactive oxygen species, lipid peroxidation
Citation: Duan J-Y, Lin X, Xu F, Shan S-K, Guo B, Li F-X-Z, Wang Y, Zheng M-H, Xu Q-S, Lei L-M, Ou-Yang W-L, Wu Y-Y, Tang K-X and Yuan L-Q (2021) Ferroptosis and Its Potential Role in Metabolic Diseases: A Curse or Revitalization? Front. Cell Dev. Biol. 9:701788. doi: 10.3389/fcell.2021.701788
Received: 28 April 2021; Accepted: 04 June 2021;
Published: 09 July 2021.
Edited by:
Gustavo P. Amarante-Mendes, University of São Paulo, BrazilReviewed by:
Ivarne L. S. Tersariol, Federal University of São Paulo, BrazilGrzegorz Wegrzyn, University of Gdańsk, Poland
Copyright © 2021 Duan, Lin, Xu, Shan, Guo, Li, Wang, Zheng, Xu, Lei, Ou-Yang, Wu, Tang and Yuan. This is an open-access article distributed under the terms of the Creative Commons Attribution License (CC BY). The use, distribution or reproduction in other forums is permitted, provided the original author(s) and the copyright owner(s) are credited and that the original publication in this journal is cited, in accordance with accepted academic practice. No use, distribution or reproduction is permitted which does not comply with these terms.
*Correspondence: Ling-Qing Yuan, YWxsZW55bHFAY3N1LmVkdS5jbg==