- 1Immunology of Aging, Leibniz Institute on Aging – Fritz Lipmann Institute, Jena, Germany
- 2School of Medicine, Department of Internal Medicine III, Technical University of Munich, Munich, Germany
- 3Proteomics of Aging, Leibniz Institute on Aging – Fritz Lipmann Institute, Jena, Germany
- 4Department of Hematology and Oncology, Medical Faculty Mannheim, Heidelberg University, Mannheim, Germany
- 5Heidelberg Institute for Stem Cell Technology and Experimental Medicine (HI-STEM gGmbH), Heidelberg, Germany
- 6Division Inflammatory Stress in Stem Cells, German Cancer Research Center (DKFZ), Heidelberg, Germany
- 7Institute of Biochemistry and Biophysics, Faculty of Biological Sciences, Friedrich Schiller University Jena, Jena, Germany
- 8Department of Medicine III, Technical University Dresden, Dresden, Germany
The bone marrow (BM) microenvironment, also called the BM niche, is essential for the maintenance of fully functional blood cell formation (hematopoiesis) throughout life. Under physiologic conditions the niche protects hematopoietic stem cells (HSCs) from sustained or overstimulation. Acute or chronic stress deregulates hematopoiesis and some of these alterations occur indirectly via the niche. Effects on niche cells include skewing of its cellular composition, specific localization and molecular signals that differentially regulate the function of HSCs and their progeny. Importantly, while acute insults display only transient effects, repeated or chronic insults lead to sustained alterations of the niche, resulting in HSC deregulation. We here describe how changes in BM niche composition (ecosystem) and structure (remodeling) modulate activation of HSCs in situ. Current knowledge has revealed that upon chronic stimulation, BM remodeling is more extensive and otherwise quiescent HSCs may be lost due to diminished cellular maintenance processes, such as autophagy, ER stress response, and DNA repair. Features of aging in the BM ecology may be the consequence of intermittent stress responses, ultimately resulting in the degeneration of the supportive stem cell microenvironment. Both chronic stress and aging impair the functionality of HSCs and increase the overall susceptibility to development of diseases, including malignant transformation. To understand functional degeneration, an important prerequisite is to define distinguishing features of unperturbed niche homeostasis in different settings. A unique setting in this respect is xenotransplantation, in which human cells depend on niche factors produced by other species, some of which we will review. These insights should help to assess deviations from the steady state to actively protect and improve recovery of the niche ecosystem in situ to optimally sustain healthy hematopoiesis in experimental and clinical settings.
Introduction: Scope of This Review
The bone marrow (BM) niche is an important gate-keeper in the maintenance of the blood cell system, which is unique in its circulation throughout the body. Blood cells are produced from a limited number of hematopoietic stem cells (HSCs), which reside in the bone marrow. It is here, that the BM microenvironment exerts its regulatory activity on HSCs and their progeny. The BM comprises a complex microenvironment of different cell types (hematopoietic and non-hematopoietic), together with extracellular components such as the extracellular matrix (ECM), chemical, and physical factors. The cells and factors coordinately regulate the balance between HSC quiescence and activation and the subsequent processes of cell fate determination, proliferation, self-renewal, and differentiation. Consequently, deregulation of BM niche cells is sufficient to interfere with normal stem cell behavior, which may even result in malignant transformation (Walkley et al., 2007a, b; Raaijmakers et al., 2010; Kode et al., 2014). Malignant cells further aggravate deregulation of normal hematopoiesis by creating their own environment which does not support efficient normal hematopoiesis (Colmone et al., 2008; Waclawiczek et al., 2020).
Deregulation of the bone marrow architecture occurs in both acute and chronic infections as well as aging (Mawatari et al., 1999; Chan and Duque, 2002; Polzer et al., 2010; Hirche et al., 2017). Such morphologic age-related changes are further observed in chronic malignancies, such as myelodysplastic syndrome (MDS), and myeloproliferative neoplasms (MPNs), which are consistently found together with ineffective normal hematopoiesis (Bartl et al., 1992). During ageing as well as in chronic malignancies these morphological changes sometimes co-inside with a prominent increase in an inflammatory milieu (Bartl et al., 1992; Hasselbalch, 2013), underscoring the role for inflammatory processes in the development of the changes. Still, it remains poorly understood which precise cells or cellular units within the niche are responsible for the deregulation of quiescent, healthy HSCs (Waclawiczek et al., 2020), and promote survival or accumulation of malignant clones (Lutzny et al., 2013).
Together, these studies not only highlight the complexity of the BM niche, they also provide opportunities for the BM microenvironment as a therapeutic target to interfere with microenvironmental support in pathological states, or to improve healthy hematopoiesis. Furthermore, these studies illustrate that the BM niche can only be understood through integrated approaches looking at the tissue as an adaptable ecosystem (Schmitt-Graeff et al., 2015).
Here, we will review how the BM maintains HSCs by approaching the BM, HSCs and their progeny as such an ecosystem. This means that we will not discuss the wealth of data gathered from in vitro cell culture studies, for which we would like to refer to a bioinformatic integration of bulk RNAseq analyses of different cell lines and sorted BM cells known to maintain HSCs in in vitro co-cultures (Desterke et al., 2020). Bearing in mind the wealth of data concerning in vivo BM niche dependence in normal, stressed, and malignant hematopoiesis, but the scarcity of precise data about the numbers and behavior of the exact cell types involved, we will review how stress stimuli or malignant disease alters niche heterogeneity in experimental systems and patients in brief summaries. We will first focus on the different cell types and then highlight some secreted factors that have been shown to be important for human hematopoiesis in experimental xenogeneic systems, highlighting how the murine BM niche supports hematopoiesis across species barriers.
The Steady-State and Stress of the Bone Marrow Niche
In order to understand changes from the norm, it is critical that hallmarks of the homeostatic steady state of the healthy niche are defined. The hematopoietic niche consists of hematopoietic cells intertwined by different types of supportive cells of the microenvironment. In mammals, this cellular network is localized mainly in the BM. The cellular composition of the BM under steady-state as well as in different “stress states” has been assessed by bulk analyses and single cell approaches to investigate the heterogeneity in niche cells, by assessing their presence at defined anatomical sites, by identifying cell-cell interactions and communication based on ligand-receptor pairing, and through exploring interactions of niche cells with the ECM. In the future, these studies will help to identify a “bench mark” for what could be defined as the steady-state of a healthy BM niche in terms of which cells are present, in which numbers, and what is their steady-state transcriptome, proteome, or secretome.
Recently, single cell analyses have been instrumental in elucidating the composition of the niche as an ecology of different interdependent cell types. Analyses aimed at deciphering the composition of the murine BM niche in mice have identified over twenty different subpopulations of cells (Baryawno et al., 2019; Tikhonova et al., 2019; Baccin et al., 2020; Zhong et al., 2020) with distinct lineage relationships [designated populations P1 to P7 by Wolock et al. (2019)]. An integrated analysis combining all of the datasets in these studies defined fourteen meta-clusters of cell subpopulations characterized by the expression of pro-hematopoietic factors (Dolgalev and Tikhonova, 2021). These fourteen clusters comprise endothelial cells [ECs: arterial (AEC), arteriolar, and sinusoidal (SEC), 32% of all cells], mesenchymal stem and progenitor cells [adipogenic and osteogenic MSPCs, also designated as CXCL12-abundant reticular (CAR) cells, 27%], osteoblasts (mature and immature, 5%), chondrocytes (16%), fibroblasts (18%), pericytes (1%), smooth muscle cells (0.1%), and Schwann cells (0.3%)1 (Dolgalev and Tikhonova, 2021).
Since the earliest description of BM-derived mesenchymal stromal cells (MSCs), it has been recognized that stromal cells with the ability to form fibroblast-like colonies show differentiation into adipo-, chondro- and osteogenic lineages (Friedenstein et al., 1974; Pittenger et al., 1999; Bianco et al., 2008), indicating the close relationship between mesenchymal populations in the BM niche. The different progeny cells may, however, have different effects on HSCs, and it was shown that although adipocytes are an important source of SCF and thus, indispensable for maintaining HSCs (Zhou et al., 2017), they may be negative regulators in some skeletal tissues (Naveiras et al., 2009), because they inhibit formation of sinusoidal vasculature (Zhou et al., 2017). On the other hand, osteoblasts appear to be indispensable for HSC function (Calvi et al., 2003; Zhang et al., 2003). Both, adipo- and osteogenic lineages, are thought to arise from a common precursor, and, indeed, recent single cell studies show a consistent pattern of differentiation (Wolock et al., 2019), in which a multipotent subpopulation of osteoblastic markers corresponding to OsteoCAR cells (Dolgalev and Tikhonova, 2021), differentiates into apparently committed adipo-, osteo-, and chondrogenic precursors. Lineage fate decisions toward the osteogenic lineage may well depend on epigenetic KDM4B-dependent regulation as deletion of this methyltransferase leads to bone loss and osteoporosis (Deng et al., 2021). In addition, the osteogenic factor osteolectin (CLEC11A), is required for osteogenesis (Yue et al., 2016), and promotes skeletal maintenance through its osteoblast-specific receptor ITGA11 (Kaltz et al., 2010; Shen et al., 2019). Adipogenesis, on the other hand, is regulated by estrogens, which may lead to gender- and age-specific differences in BM homeostasis (Bragdon et al., 2015). These studies strongly suggest that a disbalance between fat and bone, not only affects skeletal tissues, but also profoundly impacts on the hematopoietic niche.
Knowledge on the cellular composition and factors released in the steady-state BM niche are helpful in a number of issues that are still being intensely debated.
First, the currently accumulating data helps in assessing and understanding the remodeling response of the niche to acute or chronic stress, such as cytotoxic treatment, irradiation, inflammatory stress, stress caused by microbial infections, as well as aging or the presence of malignant disease. Such states may induce the release of pro-inflammatory cytokines such as Interferon alpha in infections, which rapidly recruit quiescent HSCs into cell cycle (Essers et al., 2009) and induce remodeling of the vascular niche (Prendergast et al., 2017).
Second, it is still unresolved whether niche remodeling is reversible after resolving stress, or whether, similar to observations in HSCs (Flach et al., 2014; Walter et al., 2015), initial damage is not completely reversible and reduces niche health and function over time. Observations in telomere dysfunctional Terc–/– mice strongly suggest that niche function declines over time (Ju et al., 2007). In addition, in aging and leukemia, the relative contribution of the Lepr+ Adipoq+ Kitl+ AdipoCAR population changes [down in leukemia (Baryawno et al., 2019), up in aging (Zhong et al., 2020)], thereby changing the factors to which HSCs are exposed, such as varying concentrations of ANG-1/2, CXCL12 (Stromal-Derived Factor 1: SDF1), IL7, KITL (Stem Cell Factor: SCF), and SFRP1. Observations that deletion of the Angpt1, Kitl, or Sfrp1 genes have a strong negative impact on HSC maintenance (Renström et al., 2009; Ding et al., 2012; Zhou et al., 2015) underline the importance of AdipoCAR cells and their secreted factors in maintaining HSCs. Thus, a better understanding of the steady state niche may help to define targets useful for restoring the compromised niche in leukemia back to steady state.
Third, even under steady-state conditions, levels of HSC and the release of blood cells from the marrow shows circadian day-night oscillations, which at least in part depends on oscillating production of CXCL12 by the niche (Méndez-Ferrer et al., 2008). Interestingly, additional circadian oscillations were noted in norepinephrine and TNF levels, which appear to drive temporary increases in melatonin and ROS levels in the hematopoietic compartment (Golan et al., 2018). The melatonin not only promotes HSC self-renewal (Golan et al., 2018), but also protects stromal niche cells against toxic side-effects of ROS (Mehrzadi et al., 2017). These observations show that hematopoiesis and the niche reversibly oscillate between stressed (increases in TNF, ROS) and non-stressed states on a daily basis and suggest an important role for melatonin in protecting the niche from ROS-dependent stress.
Fourth, the above data may help to resolve the puzzling observation that induced niche remodeling in which specific niche cell populations are deleted by using iDTR/diphtheria toxin targeting, such as mesenchymal Nes+, Lepr+ or Cspg4+ (NG2+) cells (Méndez-Ferrer et al., 2008; Kunisaki et al., 2013; Acar et al., 2015), Cxcl4+ megakaryocytes (Bruns et al., 2014; Zhao et al., 2014), or Gfap+ Schwann cells (Yamazaki et al., 2011), all lead to a declining support of hematopoiesis and, over time, to HSC attrition and BM failure. These observations indicate that different populations within the niche are collectively responsible for maintaining HSC function over time.
Finally, although the precise architecture of the niche ecosystem is still a matter of debate, it is clear that the bone marrow architecture is essential for the integrity of HSC behavior. Indeed, precise localization within the marrow with respect to different niche cell types has a strong impact on HSC behavior, particularly on HSC quiescence (Lo Celso et al., 2009; Fujisaki et al., 2011; Haltalli et al., 2020). Importantly, recent evidence shows that HSCs localizing to perisinusoidal niches, which include megakaryocytes (Bruns et al., 2014; Zhao et al., 2014) and AdipoCAR cells (Baccin et al., 2020), are protected from aging-associated attrition (Saçma et al., 2019). This suggests that even under adverse conditions, there are anatomical regions, such as the perisinuses, which preserve HSCs and their function.
The above studies include markers that can be used to monitor and predict the extent of adverse changes from the steady-state norm and may document shifts in the pools of BM niche subpopulations and their transcriptomes/secretomes. These will be key tools to diagnose changes in niche fidelity and follow therapeutic success and prognosis upon targeting the niche.
Long-Term HSC Maintenance by Balancing Niche Fitness and Cellular Collaboration
The mechanisms governing stem cell activation and return to quiescence still need to be resolved in detail. For instance, it is not understood why depletion of different niche cell populations all lead to the same outcome: a loss of quiescent HSCs (Méndez-Ferrer et al., 2008; Yamazaki et al., 2011; Kunisaki et al., 2013; Bruns et al., 2014; Zhao et al., 2014; Acar et al., 2015). A recently proposed competition model of a niche ecology may offer new approaches to this problem. The mathematical model predicts that changes in the composition of niche cells by default also change the degree of cooperation between those cells. As a result, the fitness of the ecosystem as a whole decreases and aging-like degeneration of the system is inevitable (Nelson and Masel, 2017). This fitness/cooperation model also provides an explanation of why there appears to be no redundancy between different BM niche subpopulations.
What hampers the study of fitness and cooperation in the BM niche is that current knowledge about non-hematopoietic populations of the hematopoietic niche is insufficient to determine how stress responses affect the fitness of these subpopulations or their ability to cooperate with other cells. Indeed, in most hematopoietic conditions, bulk and single cell analyses using different “omics” (transcript-, prote-, methyl-, or metabolomics, etc.), focus on the study of the hematopoietic cells, not on the niche cells. Thus, in order to attempt restoration of the steady state situation within the BM niche, several questions need to be answered. First, what is the optimal steady state configuration of the BM microenvironment favoring maintenance of healthy hematopoiesis. Second, niche-intrinsic factors determining fitness of different niche cell subpopulations need to be defined. Third, cellular communication between niche subpopulations need to be assessed, and finally, the degree of deviation from the steady state condition in situations of chronic stress, aging or in the presence of age-related chronic malignancies should be addressed in future studies.
The Response of the BM Niche to Acute Stress
It is becoming clear that different stressful challenges such as LPS or polyInosine- polyCytosine (pIpC), which simulate bacterial or viral infections, respectively, lead to different outcomes. Both treatments are known to rapidly recruit quiescent HSCs into cell cycle (Walter et al., 2015; Takizawa et al., 2017), but their effect on different niche cell populations was unclear. Upon LPS treatment, HSCs start proliferation and neutrophils depart the BM, which is accompanied by remodeling of the sinusoidal vasculature (Vandoorne et al., 2018). Stromal cells are instrumental in the activation of HSCs, for example via transfer of mitochondria from stromal cells (Mistry et al., 2019). RNAseq analyses of Sca1– CAR cells, PDGFRa+Sca1+ PaS cells, and either CD31+CD105hi sinusoidal or CD31hiCD105+ arterial endothelial cells (SECs and AECs) from LPS and pIpC challenged mice, demonstrate a shift of the transcriptome of different cell types toward a proinflammatory signature, characterized by upregulation of cytokines and chemokines. Thus, IL6, CCL5, and CXCL10 are upregulated in CAR cells and CCN1 and −2, CCL2 and −7 as well as IL15 are expressed at a higher level in SECs (Helbling et al., 2019).
The question of stress-induced changes in the niche can be answered in single cell analyses, which not only show transcriptional changes, but also whether remodeling of the abundance in different niche cell populations occurs. For instance, treatment with 5-fluorouracil (5FU), which targets cells in S-phase and is frequently used to activate recruitment of quiescent HSCs into cell cycle, has long since been known to increase the frequency of niche cells with bone nodule-forming potential (Falla et al., 1993). Tikhonova and coworkers showed similar increases in AdipoCAR cells, which may perhaps be induced to differentiate along the osteogenic lineage (Wolock et al., 2019), and mature Col16a1+Tnn+ osteoblasts, while immature Bglap+Car3+ osteoblasts were decreased (Tikhonova et al., 2019). With ongoing or chronic stress, such as in mice with leukemia, most stromal cell populations are diminished, with loss of expression of quiescence-promoting genes (Cxcl12, Angpt1, Vcam1) in Lepr+ AdipoCAR cells (Baryawno et al., 2019). At the same time, sinusoid vessels show significantly increased diameter with reduced EC cellularity (Saçma et al., 2019), thus altering how they affect HSCs through their production of KITL [SCF (Waskow et al., 2009)], DLL1/4 or JAG2 (Saçma et al., 2019; Tikhonova et al., 2019).
Stromal cells of the BM niche are very sensitive to total body irradiation (Chamberlin et al., 1974; Abbuehl et al., 2017), which was recently supported by single cell studies (Severe et al., 2019). Intriguingly, the stromal compartment also shows a remarkably rapid recovery with an increase in osteoblast-lineage cells driven by the replacement of surviving megakaryocytes to the endosteal surface (Dominici et al., 2009; Olson et al., 2013). The expanding osteoblastic cells then promote engraftment of subsequently transplanted HSCs (Marino et al., 2013). However, the early recovery of osteoblastic cells comes at the expense of “sinusoid dilatation and congestion,” followed by a progressive decrease in cellularity and later fat degeneration (Huang et al., 2009). Although irradiation targets S-phase cells like 5FU treatment, irradiation appears to target different niche cell populations, as most niche subpopulations show severe reductions in numbers, including AdipoCAR cells and mature osteoblasts. Interestingly, however, a small CD73+NGFR+ chondrocytic population is preserved (Severe et al., 2019). Subsequent experiments with Cd73–/– mice strongly suggest that these stress-primed cells are critical in recovering from stress responses through high expression of several pro-hematopoietic cytokines (CXCL12, IL7, KITL, SPP1, and TGFb), suggesting that monitoring CD73+NGFR+ cells may be useful in predicting recovery after irradiation.
Viral infections and pIpC both rapidly and strongly upregulate production of interferon alpha (IFNa), which, in in vivo stimulation studies recruit HSCs into cell cycle in both autonomous and non-autonomous mechanisms (Essers et al., 2009; Uckelmann et al., 2016; Hirche et al., 2017; Prendergast et al., 2017). Whether the non-autonomous effects involve non-hematopoietic cells remains to be established. It is clear, however, that IFNa and plpC treatments increase Sca1-dependent signaling in HSCs and the relative numbers of ECs in the BM, again through both direct and indirect mechanisms (Prendergast et al., 2017). Gene expression shows that IFNa also increases VEGF expression, suggesting the EC proliferation noted may be stimulated through this growth factor. RNAseq of SECs after pIpC treatment show a particular upregulation of Ifnar2 and Ifngr1, suggesting heightened sensitivity toward interferons (Abbuehl et al., 2017). In infections with either vesicular stomatitis virus or murine cytomegalovirus HSC activation occurs, but involvement of BM niche cells remains to be elucidated (Hirche et al., 2017).
Together, these studies show that different stressors induce HSC activation and emergency hematopoiesis, which appears to depend on both changes in numbers of different niche cell subpopulations (remodeling) as well as alterations in their transcriptomes in the BM niche ecosystem (Figure 1). Most studies also show that upon a single challenge with a stressor such as LPS, pIpC, 5FU or irradiation both remodeling and transcriptome changes seem to be reversible. However, the impact of chronic inflammatory stress on niche remodeling as well as reversibility of these changes needs to be further investigated.
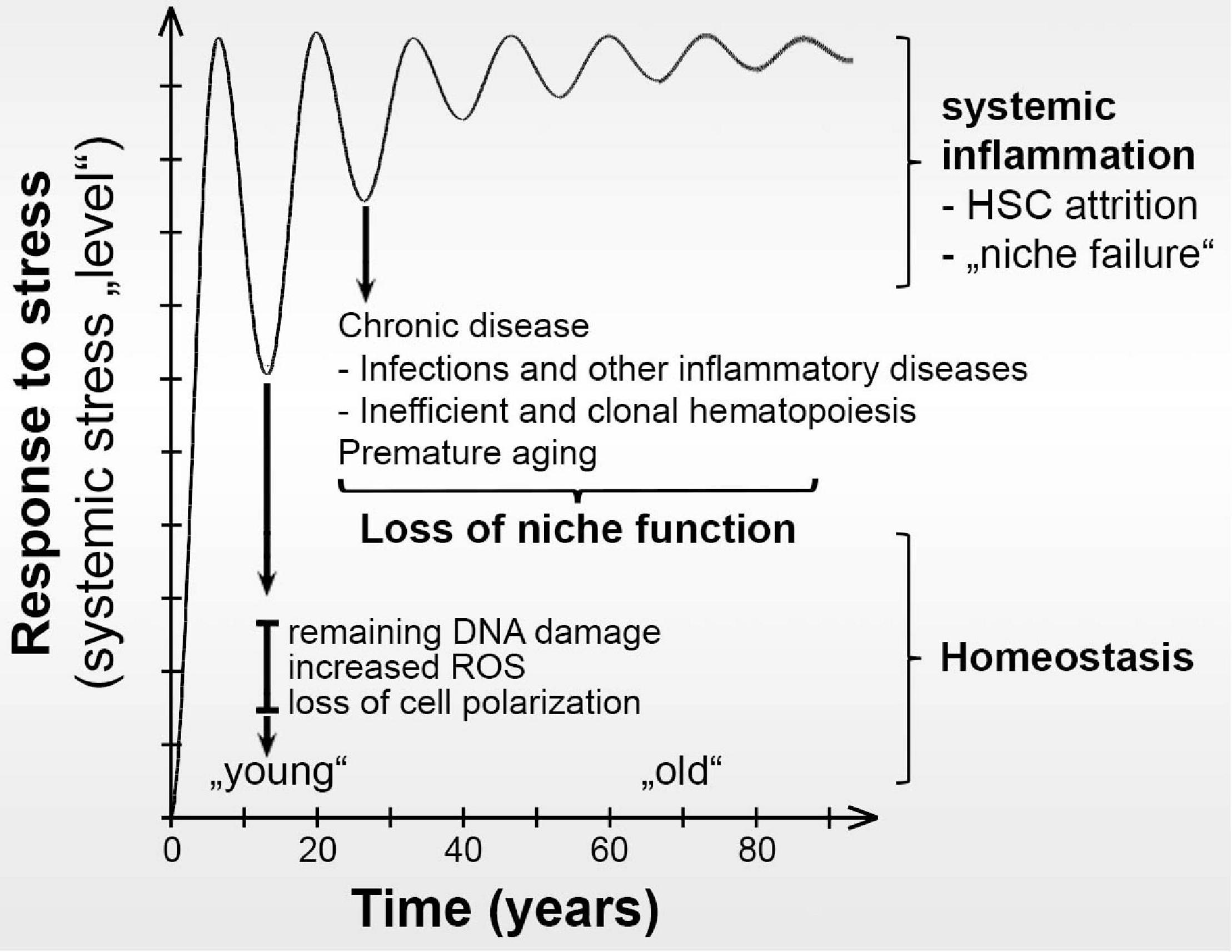
Figure 1. Hypothesis of how repeated stress may progressively change the fitness of BM niche cells. After each cycle of stress, some damage remains. This low frequency changes the transcriptional, proteomic, and ecological BM niche cellular composition landscapes over time. Eventually, aging-like changes in the BM niche result in loss of functional HSCs, systemic inflammation, and development of chronic alterations with loss of niche support for healthy hematopoiesis in the BM. Instead, the altered niche supports the development of chronic malignancies.
Age-Related Changes in the BM Niche Ecosystem
Aging is a natural process of slow deterioration of cells and tissues over time. Aging is the main risk factor for functional decline of HSCs, defects in immunity, and increased occurrence of hematological abnormalities. Two striking features of hematopoiesis in aging humans are a relative increase in myeloid cells, and the occurrence of clonal hematopoiesis in 10–20% of healthy individuals aged 70 years or more. Clonal hematopoiesis is significantly associated with an increased risk of overall mortality, the development of cardiovascular diseases and tumors of the blood (Genovese et al., 2014; Jaiswal et al., 2014; Xie et al., 2014).
Recent studies have further shown that aging is also associated with chronic remodeling of the niche, which involves an increase in adipogenic cells (Sanchez-Aguilera et al., 2014). This fatty degeneration has been attributed to a decline in osteogenic cell fate determination (Nishikawa et al., 2010; Yu et al., 2018; Kato et al., 2019; Lacava et al., 2019) which switches mesenchymal precursors to an adipogenic state, further associated with an increase in stromal senescence (Gnani et al., 2019), loss of niche-forming endomucin-positive (EMCN+) CD31hi “Type H” vessels (Kusumbe et al., 2014), and b3-adrenergic innervation (Maryanovich et al., 2018; Ho et al., 2019). Added complexity of this system is that niche remodeling is associated with an altered lineage output, including increased myelopoiesis, thrombopoiesis, and clonal hematopoiesis. These changes further result in reduction of myelopoiesis-suppressing osteocytes (Fulzele et al., 2013) and influx of myelopoiesis-promoting plasma cells (Pioli et al., 2019) as well as b2-adrenergic stimulated megakaryocytes (Ho et al., 2019) and increased platelet production (Davizon-Castillo et al., 2019; Frisch et al., 2019). In addition, telomere shortening in stromal cells is associated with reduced B-lymphopoiesis and reduced HSC function (Ju et al., 2007).
Thus, age-related processes support remodeling of the niche, which is associated with chronic malignancies (Figure 1), that often transform into aggressive myeloid leukemias for which there is currently no reliable cure. However, the link between niche remodeling, myeloid expansion, clonal hematopoiesis and increased susceptibility to chronic hematologic malignancies remains underexplored.
In single cell studies, in line with the observed increased adipogenesis, AdipoCAR numbers increase tremendously in 16-month-old mice and subdivide into three distinguishable Adipoq+Cxcl12+ populations. Furthermore, and osteogenic fate degeneration is accompanied by an almost complete loss of mature Tnn+ Postn+ osteoblasts (Zhong et al., 2020). Imaging of BM in old mice show remodeling of SECs and sinusoidal vessels with increased vessel diameter, while at the same time the total number of SECs reduces (Saçma et al., 2019). A surprising finding was that the vascular remodeling of the sinuses housed the most potent HSCs, showing that sinusoidal remodeling produces HSC-protective areas in the aging BM niche.
Changes in the BM Niche and the Development of Chronic Malignancies
Chronic hematologic malignancies evolve over a period of several years showing clonal (pre-) oncogenic mutations. These mutations improve fitness through proliferative and/or anti-apoptotic advantages or through improving homing to the BM niche (Godavarthy et al., 2020). But, during the chronic stages, the mutations are not sufficient to allow cell-autonomous growth as pre-malignant cells depend on the niche for their survival. Over time, the microarchitecture of the BM niche distorts with associated osteopenic changes (Mawatari et al., 1999; Chan and Duque, 2002; Polzer et al., 2010) as shown in histological studies of MDS (Bartl et al., 1992), and MPN (Chan and Duque, 2002). The underlying mechanisms of microarchitectural distortion of the BM are not entirely clear, however, the niche has been shown to support malignant cells through inflammatory NFkB-driven stromal activation and the expression of proinflammatory factors, such as IL6 and TGFb1 (Krause et al., 2013). Indeed, cultured MSCs from MDS patients show inflammatory changes compared to healthy MSCs (Medyouf et al., 2014). Prospectively isolated non-cultured CD271+CD105+ MDS-MSCs also show a significant inflammatory signature (Chen et al., 2016) with increased adipogenic potential (Weickert et al., 2021), showing that stromal inflammation does not depend on cell culture and may be the result of BM niche remodeling.
MPN-MSCs differ from MDS-MSCs and show less senescence, increased cell cycling and also increased cell death with reduced differentiation into both adipogenic and osteogenic lineages (Sun et al., 2020). Although both observations point toward niche remodeling with proinflammatory features in MDS and MPN, the outcome of the remodeling is not easily predictable. Similar proinflammatory changes in other niche cells have not been studied in detail. But, ECs, for example, localize close to the endosteum and were shown to reduce proinflammatory transformation of stromal cells thereby improving maintenance of healthy hematopoiesis (Duarte et al., 2018). In addition, vascular permeability is strongly increased in AML, and targeting their NO/ROS production improves chemotherapeutic responses (Passaro et al., 2017). These studies support the view that understanding deregulation of specific subpopulations of the BM niche facilitates the development of relevant strategies to restore healthy hematopoiesis in chronic malignancies.
Age-related systemic inflammation through increased expression of IL1b and TNF (Davizon-Castillo et al., 2019) is a driving force in MDS (Chen et al., 2016), and MPN (Hasselbalch, 2013). For instance, in MDS, increased inflammation-associated and S100A8/A9-mediated genotoxic stress (Zambetti et al., 2016), ineffective hematopoiesis (Cheng et al., 2019), fatty degeneration with a decline of osteoblastic cells (Krevvata et al., 2014; Wenk et al., 2018), myelofibrosis (Leimkühler et al., 2021), and loss of niche-forming EMCNhi vessels all potentially contribute to the disease. Systemic inflammation also drives stromal remodeling in MPN with downregulation of collagen genes and Sparc (Tripodo et al., 2017), neuroglial damage, and a decline in Nes+ MSCs in myelofibrotic MPN (Schmitt-Graeff et al., 2015). The extent of functional and numerical BM microenvironment remodeling in vivo is still to be elucidated. Age-related changes in Sp7+ (osterix) osteoprogenitors have been attributed to accumulation of DNA damage, pro-inflammatory changes, and senescent cells. These changes then lead to functional deterioration and depletion of these progenitors from the BM niche over time (Kim et al., 2017).
In MPN, constitutive activation of the NFkB pathway in niche cells promotes survival of oncogenic cells (Kleppe et al., 2018). Direct targeting of NFkB or its deregulated targets, such as PKC may thus induce cell death of malignant cells. Similarly, age-related denervation promotes development of AML and three sympathicomimetics improve both niche function and block progression of JAK2(V617F) MPN (Arranz et al., 2014). Interestingly, upregulation of S100a8 and a9 appears to be a general feature of proinflammatory changes in (pre-)malignant disease, and in a murine JAK2V16F model of myelofibrosis, the S100A8/9 inhibitor tasquinimod, ameliorates development of malignancies, suggesting that targeting this arm of proinflammatory molecules are tangible targets in malignancies such as MPN (Leimkühler et al., 2021).
Other age-related changes in the BM microenvironment are fatty degeneration, and the loss of niche-forming EMCNhi vessels. Both characteristics are also found in MDS (Wenk et al., 2018) and AML (Duarte et al., 2018), respectively. These observations suggest that anti-adipogenic therapy, such as through inhibition of PPAR or PIM kinases may be of benefit for MDS and other chronic malignancies. The observed vessel remodeling in AML can be rescued using deferoxamine, which besides targeting iron overload also inhibits the collagen biosynthesis modulator prolyl-4-hydroxylase (P4H or PHD) (Duarte et al., 2018). Interestingly, deferoxamine is already used to treat patients with iron overload in MDS. Thus, deferoxamine may have a dual effect through normalizing effects on iron overload as well as restoring remodeling of niche-forming vessels.
The BM Niche in Hematopoietic Transplantation and Graft-Versus-Host Disease
In transplantation protocols, the host is not only conditioned through irradiation or cytotoxic drug treatment, but also infused with donor hematopoietic cells. Conditioning reduces the residual cancer load, dampens the immune response to the graft, and is thought to “create space” for the incoming graft cells to grow in. Since both irradiation and cytotoxic treatments damage the BM niche, it remains poorly understood through which mechanisms the microenvironment affects the long-term success-rate in hematopoietic transplantation. It is clear, however, that several niche factors which do not seem to play a major role in steady-state hematopoiesis, such as, Ptn, Sfrp1/2, and Wnt5a, are required for successful regeneration of quiescent HSCs (Renström et al., 2009; Istvanffy et al., 2011; Ruf et al., 2016; Schreck et al., 2017). This observation indicates that the process of regeneration requires a different set of factors than maintaining HSCs during steady-state conditions. As mentioned above, it has long been thought that irradiation or cytotoxic conditioning depletes existing niches, which can then be occupied by incoming donor cells. However, evidence is accumulating that conditioning also stimulates the expression of engraftment-promoting factors. An example of this is the secretion of PTN, which, under steady-state conditions is mostly expressed by mature osteoblasts and Lepr+ cells, but after irradiation its expression also increases in expanding Cdh5+ ECs (Himburg et al., 2012, 2018). It was already established that engraftment was poorer in Ptn–/– mice (Himburg et al., 2010; Istvanffy et al., 2011). Later studies showed that although HSC maintenance mainly depends on PTN from Lepr+ cells under steady-state conditions, expression from both cellular sources is required for successful engraftment (Himburg et al., 2018).
Another issue important for successful engraftment of HSCs is the presence of the donor cells, whose function depends on the cooperation of the graft with the BM niche. Indeed, recipient-dependent parameters, such as gender (Notta et al., 2010) and aging (McCurdy et al., 2018) have been shown to have a critical impact on engraftment. Gender-specific steroid-signaling through the estrogen receptors promotes engraftment of human HSC in immunodeficient female mice (Notta et al., 2010), possibly by promoting HSC self-renewal in female mice (Nakada et al., 2014). Estrogen signals differentially affect HSCs and progenitor cells, as it was shown that the estrogen modulator Tamoxifen induces apoptosis in more mature HSPCs (Sanchez-Aguilera et al., 2014). The effects of estrogens on the niche have not been described in detail. It is clear, however, that the estrogen receptor Esr1 is expressed by both Adipo- and OsteoCAR cells, as well as fibroblasts (Dolgalev and Tikhonova, 2021). Thus, estrogen-directed signals affecting these cells of the niche may be expected in steady-state hematopoiesis and in transplantations.
Moreover, serious transplant-related complications, such as capillary leak syndrome or thrombotic angiopathy, affect the microenvironment through endothelial dysfunction (Pagliuca et al., 2019).
It remains to be studied to what extent donor hematopoietic cells help in restoring the niche function in transplantation. In in vitro co-culture experiments of HSPCs with stromal cells, it was shown that upon contact of the hematopoietic cells, the stromal cells produce Ctgf (Ccn2) which promotes HSC self-renewal (Istvanffy et al., 2015). It is unclear whether donor cells similarly alter gene expression of BM niche cells upon transplantation. Other studies have demonstrated that co-transplantation of recipient mice with either MSCs or ECs in murine transplantation models limit niche damage and prevent premature exhaustion of normal HSCs (Abbuehl et al., 2017; Poulos et al., 2017) or facilitate engraftment of HSCs from MDS patients (Medyouf et al., 2014).
One way of improving clinical outcomes is physical exercise during the revalidation phase after hematopoietic transplantation (Prins et al., 2021). Recent studies strongly suggest that the benefit of exercise is at least partly due to effects on the niche, since exercise increases the levels of CXCL12 and KITL (SCF) while lowering Leptin (Frodermann et al., 2019), an effect that is also observed in exercising middle-aged or obese patients (Rostás et al., 2017). In addition, the lowering of leptin levels reduces body fat, exercise increases a population of osteogenesis-committed Clec11a+Lepr+ cells which promote bone formation, most probably by stimulating signaling through the PIEZO1 cation channel in these cells (Shen et al., 2021).
A major complication in transplant procedures is the development of graft-versus-host disease (GvHD), which accounts for 10–20% mortality amongst transplant recipients. Acute GvHD is initiated by systemic inflammation associated with IL-1, IFN, and TNF signaling. These early events are preceded by angiogenesis with metabolic activation of ECs (Riesner et al., 2017; Furukawa et al., 2019), which could lead to EC activation syndromes (Pagliuca et al., 2019). This phase is followed by development of recipient-reactivity of donor T cells. Prolonged IFN and TNF signaling is further known to promote apoptosis of ECs. In addition, the inflammatory cytokines upregulate MHC class II, thus rendering different cell types targets for recipient-reactive donor T cells. This may have a severe effect on the composition of the BM niche in GvHD, as is clear by the finding that the number of CFU-F-forming MSCs reduces with the grade of GvHD (Okamoto et al., 1991). Such a damaged environment is not conducive to either maintaining host HSCs or engraftment by donor HSCs, which results in poor graft function, or graft failure (Masouridi-Levrat et al., 2016).
Xenograft Models: Human HSC Engraftment in the Murine BM Niche
To link cell biological modifications to functional alterations in human HSCs (huHSCs) or to determine the contribution of niche cells to normal and malignant human hematopoiesis in vivo, a surrogate microenvironment is required. Thus, an interesting approach is the study of the BM niche in xenograft models. In such models, engraftment of human cells depends on the murine niche. But, not all factors expressed and produced by the host (murine) environment (cytokines, adhesion molecules, extracellular matrix) act on the (human) donor cells. Thus, xenograft models enable the definition of species-dependent and -independent cellular and molecular pathways as targets to improve niche formation/restoration as well as transplantation protocols. To prevent xenogenic donor-cell rejection and to allow for their settlement in the bone marrow niche, immune deficient animals are used to prevent graft rejection, and recipient mice need to be conditioned using irradiation or chemical treatment before transplantation similar to syn- and congenic transplantation models. However, as described above, such conditioning is toxic for sinusoidal blood vessels and MSCs in the murine stem cell niche (Yin et al., 2020) and, importantly, it impairs the function of xenogenic donor HSCs promoting their senescence and apoptosis by increasing ROS levels and mitochondrial damage (Hu et al., 2021).
To overcome this problem, we have established recipient mouse models that allow for transplantation of huHSCs in the absence of a toxic conditioning regimen. In such models, endogenous murine HSCs have a competitive disadvantage compared to donor huHSCs, and as a consequence, donor stem cells stably engraft in the murine stem cell niche (Cosgun et al., 2014; Rahmig et al., 2016). Furthermore, reduced competitiveness of endogenous murine HSCs is conferred by loss of function mutations in the receptor tyrosine kinase Kit. We have shown before that mutant Kit combined with immunodeficiency mediated by null mutations in the recombination activating gene 2 (Rag2) and interleukin 2 receptor gamma chain gene (Il2rgc) facilitates near-complete engraftment of murine syngeneic but also histoincompatible allogeneic donor mouse HSCs in the absence of any further conditioning therapy (Waskow et al., 2009). The C57BL/6 genetic background is not suitable for the transplantation of huHSCs because donor cells are subject to clearance by phagocytes (Takenaka et al., 2007). The introduction of mutant Kit onto immune deficient mice of different genetic backgrounds including BALB/c and NOD allows for efficient engraftment of xenogenic human donor HSCs and multilineage reconstitution without any need of physical conditioning therapy (Cosgun et al., 2014; Rahmig et al., 2016). The mouse lines generated are BRgWv [Balb/c.Rag2–/–Il2rgc–/–KitWv/Wv; (Cosgun et al., 2014)], BRgSK [C57BL/6.Rag2–/–Il2rgc–/–NOD-Sirpa KitWv/Wv (Yurino et al., 2016)], and NSGW41 [NOD. PrkdcScidIl2rg–/–KitW41/W41 (Cosgun et al., 2014; Rahmig et al., 2016)], and NBSGW [NOD/B6.PrkdcScidIl2rg–/–KitW41/W41 (McIntosh et al., 2015)].
The Factors Supporting Human HSCs in the Murine BM Niche
It remains unclear which murine niche cell types and molecular interactions promote huHSC engraftment and continued human hematopoiesis in the murine microenvironment. This is further complicated by the fact that many factors are produced by a set of distinct niche cell types and that many defined niche cells provide more than one important factor (Figure 2). We focus on factors produced by murine non-hematopoietic niche cells that have been investigated also in the context of huHSC engraftment in mice.
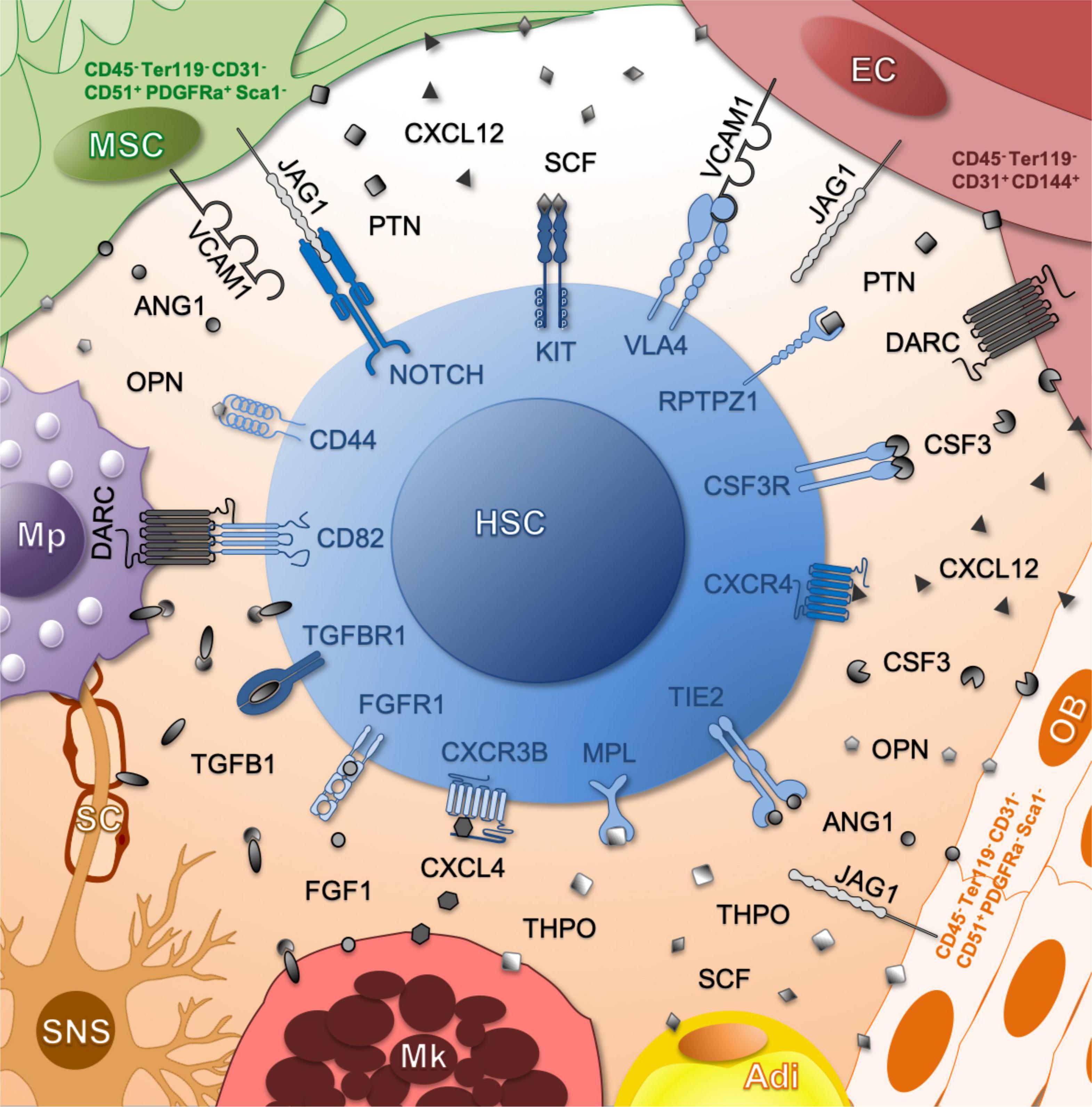
Figure 2. Schematic representation of molecular communication partners between HSCs and their niche. The ligands produced by each niche cell are indicated. Color of receptors correlates to the number of ligand-secreting cell types (dark – many producers, light – few producers). Adi, adipocyte; EC, endothelial cell; Mk, megakaryocyte; Mp, macrophage; MSC, mesenchymal stromal cell; Ob, osteoblast; SC, Schwann cell; SNS, sympathetic nervous system.
One of the most important and earliest identified receptor/ligand interactions crucial for hematopoietic stem and progenitor cell function is the KITL (SCF) - KIT signaling axis (McCarthy et al., 1977; Broxmeyer et al., 1991; Ikuta et al., 1991; Waskow et al., 2002). In single cell studies from steady-state BM cells, expression of Kitl is mostly found in Lepr+ AdipoCAR cells, AECs, some pericytes and OsteoCAR cells, whereas Kit is expressed in scattered AdipoCAR cells and SECs (Dolgalev and Tikhonova, 2021). Elegant, cell-type-specific depletion experiments have shown that mSCF provided by distinct niche cells can affect the function of different HSC and progenitor populations. Kitl-encoding transcripts are primarily detected in MSCs (Table 1) and perivascular cells are consistently labeled in Scf-gfp mice (Ding et al., 2012). Depletion of Scf in Lepr+ cells, a population that is included in CD51+PDGFRa+Sca1– MSCs (Mende et al., 2019), and CD105+Tie2+ ECs results in the loss of murine HSCs in situ (Ding et al., 2012). Further, deletion of Kitl from Lepr+ cells also depletes myelo-erythroid but no other hematopoietic progenitor cells, suggesting specialization of vascular niches in supporting HSC and myeloid progenitors harboring defined differentiation propensity, particularly toward the erythroid lineage (Comazzetto et al., 2019; Zhang et al., 2021). In line with this idea, lack of mSCF produced by ECs results in the depletion of stem but not progenitor cells, further indicating spatial separation of functional niches (Comazzetto et al., 2019; Zhang et al., 2021). Consistent with a role for mSCF derived from Lepr+ cells in erythroid differentiation, the specific support of erythroid differentiation mediates survival of Kit-null mice where hematopoietic progenitor cells cannot respond to mSCF signals (Waskow et al., 2004), further pointing at a crucial role of mSCF-mediated signals in supporting erythroid progenitor function. Along this line, it was shown that soluble mSCF plays a significant role in myeloid progenitor production whereas its membrane-bound isoform has more importance for the erythroid lineage (Kapur et al., 1998). Further, mSCF-mediated signals have distinct effects on maintenance and proliferation of HSCs that express different levels of mKIT (Grinenko et al., 2014; Shin et al., 2014), suggesting that not only the cellular source and isoform, but also receptor density on the target cell population shapes the mutual niche/HSC communication.
In xenotransplantation experiments, we have shown that hKIT-proficient human donor HSCs engraft best into mKIT-mutant mouse recipients in the steady state (Cosgun et al., 2014). This result suggests that functional signaling through the KIT receptor provides a competitive advantage to human HSCs in the murine BM niche. Furthermore, engraftment of human erythroid and megakaryocyte progenitor populations in mKIT-mutant recipients is significantly increased compared to conventional, mKIT-proficient recipient mice (Rahmig et al., 2016). Considering the existence of a vascular Lepr+ erythropoiesis-promoting niche (Comazzetto et al., 2019), this either suggests improved central engraftment of huHSCs in the stem cell niche, or an advantage of KIT-proficient human donor progenitor cells over endogenous mKIT-mutant precursors, or a combination of both. Taken together, the data suggests that human HSCs and HPCs show better engraftment when the endogenous counterparts have diminished mKIT activity. Together with the high protein identity between human and murine KIT and SCF (Table 1), we predict that mSCF produced by murine Lepr+ MSCs is crucial for the maintenance of human hematopoietic stem and defined progenitor cells in mice.
The chemokine CXCL12 (SDF1) is the ligand of CXCR4 and the main chemoattractant for murine HSC homing and maintenance (Sugiyama et al., 2006). Cxcl12 is expressed by various non-hematopoietic niche cells such as AECs (Ding and Morrison, 2013), osteoblasts (Ding and Morrison, 2013), and MSCs (Greenbaum et al., 2013; Asada et al., 2017; Table 1). Cxcl12 shows the highest transcript abundance of niche factors discussed (Table 1), especially in the subset of Lepr+Adipoq+ AdipoCAR cells (Dolgalev and Tikhonova, 2021). More than 90% of murine HSCs in the BM are in direct contact to CAR-MSCs that surround the sinusoidal endothelium (Sugiyama et al., 2006). Constitutive deletion of Cxcr4 (Sugiyama et al., 2006) or Cxcl12 (Tzeng et al., 2011) results in the depletion of HSCs from the BM. Conditional depletion mutants of Cxcl12 from distinct niche cells in mice revealed that mCXCL12 expressed by Tie2+ endothelial cells and Lepr+ MSCs is required for both HSC retention and maintenance (Ding and Morrison, 2013; Greenbaum et al., 2013; Asada et al., 2017), whereas Col2.3+ osteoblast-derived mCXCL12 supports the function of early lymphoid progenitors (Ding and Morrison, 2013). Interestingly, sympathetic nerves regulating circadian oscillations mediate daily fluctuations of HSC numbers mobilized from the bone marrow into the circulation in a Cxcl12-dependent manner (Katayama et al., 2006; Méndez-Ferrer et al., 2008; Golan et al., 2018).
CXCL12 is highly conserved between species, and human and mouse CXCL12 and its receptor CXCR4 both show a protein identity of 92.5 and 90.1% (Table 1), respectively. The proteins from both species differ in only one amino acid, suggesting cross-species compatibility. The administration of the CXCL12 antagonist AMD3100 (plerixafor), efficiently mobilizes murine and human HSPCs (Broxmeyer et al., 2005), as well as human HSCs in humanized mice (Samuelson et al., 2021). A role for the mCXCL12 - hCXCR4 signaling axis in homing of human HSCs in mice was further shown by reduced engraftment of human HSCs in NOD/SCID mice after pretreatment of donor cells with anti-CXCR4 antibodies (Peled et al., 1999). Consistently, overexpression of human CXCR4 on huHSPCs significantly improves their engraftment in NOD/SCID mice (Brenner et al., 2004; Kahn et al., 2004) and corrected the immediate loss of human HSPCs derived from pluripotent stem cells in NSG mice (Reid et al., 2018). Recently, a knock-in of human CXCR4 into the mouse gene locus showed competent mouse CXCL12 to human CXCR4 signaling across species (Costa et al., 2018).
Transforming growth factor beta1 (TGFB1), a pleiotropic cytokine that is produced in a latent complexed form by HSCs and progenitor cells (HSPCs), hematopoietic and several different non-hematopoietic niche cells (Table 1), indirectly regulates the responsiveness of mouse HSPCs to mSCF through the decrease of mKIT expression (Dubois et al., 1994). Surgical denervation of Gfap-GFP+ non-myelinating Schwann cells results in loss of HSCs (Yamazaki et al., 2011). These Schwann cells support HSC maintenance most probably through conversion of latent to bioactive mTGFB1 via cell-to-cell contact in situ (Yamazaki et al., 2011). Since different concentrations of the three isoforms mTGFB1, −2 and −3 differentially affect differentiation and self-renewal (Langer et al., 2004), it is thought that mTGFB is important in the fine-tuning of HSC cell division (Sitnicka et al., 1996b). Murine and human TGFB1 and its receptor TGFBR1 show a high protein identity between mouse and human (89.7 and 95.7%, Table 1) suggesting possible interspecies cross-reactivity. Indeed, murine TGFB1 and −3 were shown to stimulate hematopoietic specification of differentiating human embryonic stem cells (Ledran et al., 2008). Consistently, in a xenotransplant model treatment of recipient mice with anti-human TGFB1 antibody GC1008 lead to higher engraftment of the human donor cells (Zhang et al., 2016) confirming murine TGFB1 to play a role in huHSPC maintenance.
Another pathway crucial for HSC maintenance and differentiation is the JAGGED – NOTCH interaction. The receptor NOTCH1 is expressed by mouse and human HSPCs (Varnum-Finney et al., 1998) and the ligand JAG1 is expressed by MSCs (Varnum-Finney et al., 1998), osteoblasts (Calvi et al., 2003), and endothelial cells (Poulos et al., 2013; Table 1). In the integrated analysis of single cell RNAseq data (Dolgalev and Tikhonova, 2021), Jag1 is mostly expressed in AdipoCAR cells, Jag2 in AECs, Notch1 in ECs, Notch2 to -4 mostly in mesenchymal cells, whereas Notch3 has the highest expression in AdipoCAR cells. The precise role of NOTCH for murine and human HSC function is still controversially discussed (Lampreia et al., 2017). Among other possibilities it was suggested that NOTCH signaling is exclusively important for lymphoid differentiation (Radtke et al., 1999; Wilson et al., 2001). Consistent with this idea, Notch1 is found expressed to low levels by purified mouse HSCs (Table 1), whereas committed progenitors, especially common lymphoid progenitors (CLP), express high levels of Notch12. NOTCH1 and JAG1 share 90.2 and 96.6% protein sequence identity between mice and humans (Table 1) and NOTCH signaling is highly conserved across species (Artavanis-Tsakonas, 1999). Thus, the JAG1 - NOTCH1 signaling axis presents a promising candidate to mediate huHSPC – mouse niche interactions. HuHSCs treated with hJAG1 show a moderately enhanced engraftment after transplantation into NOD/SCID mice (Karanu et al., 2000). Consistently, huHSCs that bind a JAG1-FLAG protein show higher engraftment levels compared to huHSCs that don’t bind JAG1-FLAG protein (Guezguez et al., 2013). Inhibition of NOTCH signaling in xeno-HSC-engrafted mice led to a reduction of overall chimerism and huHSC frequency (Guezguez et al., 2013). This finding suggests a role for mNOTCH in maintaining huHSCs in the endosteal niche in a xenotransplantation setting. To improve NOTCH signaling in humanized mice, human JAG1 was expressed by osteoblasts in NOG mice [hJ1-NOG (Negishi et al., 2014)]. An increased frequency of huHSCs was found in hJAG1-transgenic recipient mice, suggesting that the maintenance of phenotypic huHSCs is improved through the NOTCH1-hJAG1 signaling axis.
Thrombopoietin (THPO) is a cytokine mainly involved in the expansion and differentiation of megakaryocytes (Kaushansky et al., 1994). Beyond that, THPO was one of the first cytokines identified to support HSPC proliferation in vitro (Ku et al., 1996; Sitnicka et al., 1996a) and is still an important component of the cytokine mix used to expand functional HSCs in culture (Wilkinson et al., 2019). Constitutive deletion of Thpo or its receptor myeloproliferative leukemia protein (Mpl) results in reduced numbers of HSPCs in mice (Qian et al., 2007). Murine THPO maintains adult HSCs in quiescence (Qian et al., 2007; Yoshihara et al., 2007). Initially, it was thought that mTHPO is produced by osteoblasts (Yoshihara et al., 2007), however, recent analysis suggests that mTHPO rather is provided by parenchymal liver cells (Alb-cre+ hepatocytes) but not by hematopoietic cells, osteoblasts, or Lepr+ MSCs in mice (Decker et al., 2018). THPO and MPL show considerable low protein identity between mouse and human (71.6 and 80.6%, respectively, Table 1), which is why they may act cross-reactive between species only when used at supraphysiological doses in vitro (Rongvaux et al., 2011). Consistently, endogenous mTHPO provides only insufficient support for the engraftment of donor human HSCs, and the injection of hTHPO improves the engraftment of huHSCs (Verstegen et al., 2003). Further, the knock-in of human THPO into BRg mice (BRg-TPOh/h) induces increased huHSC engraftment on the expense of the maintenance of endogenous muHSCs and megakaryopoiesis (Rongvaux et al., 2011), confirming suboptimal interspecies cross-reactivity.
TEK (TIE2) is a receptor tyrosine kinase mainly expressed by endothelial cells (Sato et al., 1993) and HSCs (Iwama et al., 1993). Constitutive depletion of mTEK results in embryonic lethality with lack of HSPCs and decreased vascular networks, displaying the importance of mTEK-mediated signals during development (Takakura et al., 1998). TEK is specifically expressed by quiescent HSCs (Arai et al., 2004) that contain the majority of the repopulation potential compared to TEK-negative HSCs of murine (Ito et al., 2016) or human (Yuasa et al., 2002) origin. Angiopoietin-1 (ANG1) and ANG2 bind to TEK and treatment of mouse HSCs with ANG1 improves their engraftment, whereas ANG2, in contrast, antagonizes the beneficial effect of ANG1 (Gomei et al., 2010). ANG1 is moderately expressed by murine osteoblasts (Arai et al., 2004) and to higher levels by Lepr+ MSCs (Zhou et al., 2015; Table 1), but also by HSCs and megakaryocytes (Zhou et al., 2015). TEK+ HSCs are found in close proximity to ANG1+ osteoblasts (Arai et al., 2004). Protein identity between mouse and human TEK and ANG1 is 92.7 and 97.4% (Table 1), respectively, suggesting a molecular communication between murine osteoblasts and huHSC via this axis. However, conditional depletion of mANG1 from MSCs or osteoblasts has no effect on HSC numbers and function (Zhou et al., 2015). Furthermore, HSC-, megakaryocyte-specific, or even constitutive deletion of Ang1 has also no effect on HSC biology, suggesting functional compensation through alternative ligands (Zhou et al., 2015). Taken together, although the direct functional importance for TEK on murine and human HSCs remains to be shown, TEK expression corresponds with improved human and mouse HSC repopulation activity independent of ANG1 mediated communication in vivo.
The phosphorylated glycoprotein Osteopontin (OPN or secreted phosphoprotein 1, SPP1), is expressed by MSCs, osteoblasts, and endothelial cells (Table 1) with the highest expression by AdipoCAR cells (Dolgalev and Tikhonova, 2021). After cleavage by thrombin (Grassinger et al., 2009) it binds to a variety of matrix receptors, including CD44 and very late antigen-4 (VLA4, integrin a4b1, both highly expressed on HSCs, Table 1). VLA4 on HSPCs further binds vascular cell adhesion molecule 1 (VCAM1) that is highly expressed by stromal reticular cells and endothelial cells lining bone marrow sinusoids (Jacobsen et al., 1996; Table 1) and integrin engagement mediates intimate cell-to-cell contact required for the support or HSPC function (Jung et al., 2005). Consistently, the conditional deletion of Itga4 from adult HSPCs (Scott et al., 2003) or Vcam1 from endothelial cells (Koni et al., 2001) mobilizes HSPCs to the blood and impairs homing to the bone marrow niche, critically delaying short-term engraftment after transplantation (Koni et al., 2001; Scott et al., 2003). Constitutive deletion of Opn in mice results in elevated HSC numbers and better engraftment of mOPN-deficient donor BM cells after transplantation (Stier et al., 2005). Consistently, the transfer of wild type cells into an mOPN-deficient microenvironment impairs donor HSC engraftment (Nilsson et al., 2005), which may be based on the efficient chemoattractant function of mOPN (Grassinger et al., 2009). However, OPN (63.3%) and its receptors (CD44 67.2%, VLA4 85%) show moderate protein identity between mouse and human (Table 1), suggesting a minor contribution to the maintenance of human HSPCs in the murine niche.
Fibroblast growth factors (FGFs) make up an extensive family of 23 growth factors that regulate a wide range of biological processes during development and in the adult organism (Turner and Grose, 2010). Two members, FGF1 and FGF2, are suggested to play a role in hematopoiesis. In vitro, FGF1 supports expansion of functional murine HSPCs (de Haan et al., 2003; Yeoh et al., 2006). Further, the corresponding receptor, FGFR1, is a marker enriching long-term repopulating activity in mice (de Haan et al., 2003). Deletion of Fgfr1 on HSPCs renders HSCs intact in the steady state but impairs the recovery of HSPCs after cytotoxic insult and delays repopulation of irradiated recipient mice (Zhao et al., 2012). Megakaryocytes are producers of FGF1 during post-injury regeneration and, consistently, conditional deletion of Fgf1 from megakaryocytes impairs HSC regeneration after 5FU treatment (Zhao et al., 2014). There is high protein identity between human and mouse FGF1 and FGFR1, 95.5 and 98.4%, respectively, indicating possible cross-species reactivity (Table 1). Together with transcript detection in endothelial cells and MSCs (Table 1), the interaction of mFGF1 and hFGFR1 might be of importance during engraftment of human HSPCs in the murine bone marrow. FGF2 exerts indirect effects on HSPCs by acting on other niche cell populations. FGF2 expands HSPCs within bone marrow cultures but fails to do so when sorted HSPCs are cultured “alone” (Zhao et al., 2012). The anabolic effects of PTH (Calvi et al., 2003), including expansion of osteoblasts and MSCs are highly dependent on FGF2 (Sabbieti et al., 2009). Treatment of mice with FGF2 leads to an expansion of Nestin+ and other stromal cells, which in turn produce increased levels of SCF mediating an expansion of HSPCs (Itkin et al., 2012). FGF2 levels are also increased after challenges including chemotherapy or irradiation, indicating a role in emergency hematopoiesis. These data indicate that FGF2 affects HSPCs by remodeling the HSC niche, however, Fgf2 is not expressed by non-hematopoietic niche cells analyzed in this review.
Consistent with its pleiotrophic functions, the small cytokine pleiotrophin (PTN, also known as osteoblast-specific factor-1, OSF-1, or heparin-binding growth-associated molecule, HB-GAM) plays important roles in the proliferation and differentiation in various cell types. While its absence does not affect HSCs in steady-state hematopoiesis, PTN was shown to improve hematopoietic regeneration after sublethal irradiation (Himburg et al., 2010; Istvanffy et al., 2011). As discussed before for SCF, the specific niche cell type producing PTN is of great importance for its functional effects (Himburg et al., 2018). Lepr+ MSCs are the key source of mPTN during steady-state, express it constitutively and Ptn deletion from MSCs reduces HSC numbers. mPTN is also expressed by ECs but endothelial cell-specific depletion has no effect on the HSC pool. However, PTN production by endothelial cells is massively increased after myeloablation and its deletion impairs hematopoietic regeneration that is unaffected by MSC-specific Ptn deletion (Himburg et al., 2018). Further, overexpression of hPTN in mice increases bone formation, fracture healing, and bone repair (Li et al., 2005). hPTN produced by human tonsil-derived MSCs as well as recombinant hPTN was shown to enhance engraftment of mouse HSCs after prior cultivation (Kim et al., 2020). Engraftment capacity of ex vivo hPTN-treated huHSPCs in NSG mice was also greatly increased compared to cultivation with SCF, FLT3L, and TPO alone (Himburg et al., 2010). These findings, together with the very high protein identity between mouse and human PTN (97.5%, Table 1) point toward possible cross-species support of huHSCs by murine niche PTN.
The interaction between Granulocyte-Colony Stimulating Factor (GCSF, CSF) and CSF3 receptor (CSF3R, GCSFR) is important for granulocyte differentiation, and the constitutive deletion of either the ligand [(Lieschke et al., 1994) mouse; (Dong et al., 1994) human] or its receptor (Liu et al., 1996; Mitsui et al., 2003) shows the crucial role in neutrophil production. In mice, CSF3 secreted by endothelial cells (Boettcher et al., 2014; Table 1) is important for emergency granulopoiesis in response to bacterial challenges through the increase of CSF3-responsive progenitors (Boettcher et al., 2014). In humans, the proliferative response of CD34+ HSPCs to osteoblast-derived CSF3 also suggests an expansion on the level of stem or progenitor cells (Taichman and Emerson, 1994). Exogenous CSF3 administration mobilizes murine (Molineux et al., 1990) and human (Uyl-de Groot et al., 1994; Lévesque et al., 2003) HSCs to the periphery through the remodeling of the stem cell niche reducing the number of osteoblasts and macrophages (Winkler et al., 2012). CSF3 also regulates HSC activity through the activation of toll-like receptor signaling (Schuettpelz et al., 2014). While human recombinant CSF3 efficiently activates huHSCs in NOD/SCID mice (Broxmeyer et al., 2005), the precise role of endothelial cell-derived murine CSF3 in huHSC engraftment is unknown. Based on the low protein identity between human and mouse CSF3 and CSF3R (Table 1) we hypothesize that this interaction is of minor importance for the maintenance of huHSCs in mice.
Duffy antigen receptor for chemokines (DARC or CD234, encoded by atypical chemokine receptor 1, Ackr1) is a transmembrane protein produced by sinusoidal ECs (Bandyopadhyay et al., 2006; Table 1), macrophages (Hur et al., 2016) and (nucleated) erythroid precursors (Duchene et al., 2017). DARC directly interacts with CD82 that is predominantly expressed by LT-HSCs (Hur et al., 2016). DARC-deficiency in erythroid precursors decreases the myeloid output of murine HSPCs and leads to phenotypically distinct neutrophils that leave the circulation and cause neutropenia in mice and human (Duchene et al., 2017). Further, macrophage-derived mDARC promotes HSC quiescence via TGFB1 signaling (Hur et al., 2016). This mechanism also seems to support huHSC maintenance in vitro (Hur et al., 2016). Given that RBC but not leukocyte transfusions prevent bleeding-dependent HSC expansion in mice (Cheshier et al., 2007) it is tempting to speculate that DARC on RBC precursors may also induce quiescence in mHSCs. However, given the low protein sequence identity between mouse and human DARC it remains to be shown whether mDARC produced by murine macrophages or erythroid cells can support huCD82-positive huHSCs in humanized mice.
Concluding Remarks
It is an exciting time for research about the interrelationship of HSCs and their BM niche. We have here attempted to review decades of studies describing how changes in BM niche composition (ecology) and structure (remodeling) modulate hematopoiesis. With the technical advancements of the last decade in RNAseq, genomic targeting, and imaging techniques and how these types of data are analyzed it is slowly becoming apparent which populations in the BM are important for early and late hematopoiesis and which factors they produce for their hematopoiesis-promoting activity. It would be too simple to attribute support of in vivo hematopoiesis solely to AdipoCAR (MSPC-Adipo, Lepr+Adipoq+Cxcl12+ cells) and ECs (either sinusoidal, arterial, or arteriolar). Indeed, whereas these two cell types produce the bulk of the known hematopoiesis promoting factors (Table 1), other factors known to be required for HSC maintenance in steady-state, aging, or transplantation settings are clearly expressed by other cell types, such as OsteoCAR cells and other osteoblasts, chondrocytes and fibroblasts. Interestingly, the above reviewed studies also reveal the cross-species reactive factors which enable engraftment and maintenance of human HSPCs in mice, thus giving unique insights in the requirements for species-specific and -cross-reactive activities. The most important development surrounding BM niche composition and remodeling is that the in-depth analyses start to reveal the secrets of the BM niche enabling the identification of possible targets to maintain or restore the microenvironment required for long-term maintenance of healthy HSC, as well as ways to interrupt and perhaps even eradicate their malignant counterparts.
Author Contributions
JF, CS, ME, CW, and RO edited the manuscript. JF, CW, and RO prepared the figures and table. JF, TL, GP, CS, SR, AO, DN, ME, CW, and RO wrote parts of the manuscript. All authors contributed to the article and approved the submitted version.
Funding
This work was funded by the Research Unit FOR2033 from the German Research Foundation (Deutsche Forschungsgemeinschaft, DFG). This work was further supported by grants from the DFG TRR127-A5, WA2837/6-1, and WA2837/7-1, through the project EDISCIDPROG funded by the EU ERA-Net for Research Programmes on Rare Diseases, E-Rare, and the SAW program on collaborative excellence of the Leibniz Association (K243/2019) to CW, and grants from the DFG: OO8/16-1 and OO8/18-1 to RO.
Conflict of Interest
The authors declare that the research was conducted in the absence of any commercial or financial relationships that could be construed as a potential conflict of interest.
Abbreviations
5FU, 5-fluorouracil; AEC, arterial endothelial cell; AML, acute myeloid leukemia; BM, bone marrow; CAR, CXCL12-abundant reticular (cell); DTR, diphtheria toxin receptor; EC, endothelial cell; ECM, extracellular matrix; EMCN, endomucin; ER, endoplasmic reticulum; GvHD, graft-versus-host disease; HPC, hematopoietic progenitor cell; HPSC, hematopoietic stem and progenitor cell; HSC, hematopoietic stem cell; huHSC, human HSC; LPS, lipopolysaccharide; MDS, myelodysplastic syndrome; MPN, myeloproliferative neoplasm; MSC, mesenchymal stromal cell; MSPC, mesenchymal stem and progenitor cell; P4H, prolyl-4-hydroxylase; PaS, PDGFRa+ Sca-1+ (cell); pIpC, polyInosine-polyCytosine; ROS, reactive oxygen species; SEC, sinusoidal endothelial cell.
Footnotes
References
Abbuehl, J.-P., Tatarova, Z., Held, W., and Huelsken, J. (2017). Long-term engraftment of primary bone marrow stromal cells repairs niche damage and improves hematopoietic stem cell transplantation. Cell Stem Cell 21, 241–255.e6. doi: 10.1016/j.stem.2017.07.004
Acar, M., Kocherlakota, K. S., Murphy, M. M., Peyer, J. G., Oguro, H., Inra, C. N., et al. (2015). Deep imaging of bone marrow shows non-dividing stem cells are mainly perisinusoidal. Nature 526, 126–130. doi: 10.1038/nature15250
Arai, F., Hirao, A., Ohmura, M., Sato, H., Matsuoka, S., Takubo, K., et al. (2004). Tie2/Angiopoietin-1 signaling regulates hematopoietic stem cell quiescence in the bone marrow niche. Cell 118, 149–161. doi: 10.1016/j.cell.2004.07.004
Arranz, L., Sánchez-Aguilera, A., Martín-Pérez, D., Isern, J., Langa, X., Tzankov, A., et al. (2014). Neuropathy of haematopoietic stem cell niche is essential for Myeloproliferative Neoplasms. Nature 512, 78–81. doi: 10.1038/nature13383
Artavanis-Tsakonas, S. (1999). Notch signaling: cell fate control and signal integration in development. Science 284, 770–776. doi: 10.1126/science.284.5415.770
Asada, N., Kunisaki, Y., Pierce, H., Wang, Z., Fernandez, N. F., Birbrair, A., et al. (2017). Differential cytokine contributions of perivascular haematopoietic stem cell niches. Nat. Cell Biol. 19, 214–223. doi: 10.1038/ncb3475
Baccin, C., Al-Sabah, J., Velten, L., Helbling, P. M., Grünschläger, F., Hernández-Malmierca, P., et al. (2020). Combined single-cell and spatial transcriptomics reveal the molecular, cellular and spatial bone marrow niche organization. Nat. Cell Biol. 22, 38–48. doi: 10.1038/s41556-019-0439-6
Bandyopadhyay, S., Zhan, R., Chaudhuri, A., Watabe, M., Pai, S. K., Hirota, S., et al. (2006). Interaction of KAI1 on tumor cells with DARC on vascular endothelium leads to metastasis suppression. Nat. Med. 12, 933–938. doi: 10.1038/nm1444
Bartl, R., Frisch, B., and Baumgart, R. (1992). Morphologic classification of the myelodysplastic syndromes (MDS): combined utilization of bone marrow aspirates and trephine biopsies. Leuk. Res. 16, 15–33. doi: 10.1016/0145-2126(92)90096-p
Baryawno, N., Przybylski, D., Kowalczyk, M. S., Kfoury, Y., Severe, N., Gustafsson, K., et al. (2019). A Cellular taxonomy of the bone marrow stroma in homeostasis and leukemia. Cell 177, 1915–1932.e16. doi: 10.1016/j.cell.2019.04.040
Bianco, P., Robey, P. G., and Simmons, P. J. (2008). Mesenchymal stem cells: revisiting history. Concepts, and Assays. Cell Stem Cell 2, 313–319. doi: 10.1016/j.stem.2008.03.002
Boettcher, S., Gerosa, R. C., Radpour, R., Bauer, J., Ampenberger, F., Heikenwalder, M., et al. (2014). Endothelial cells translate pathogen signals into G-CSF–driven emergency granulopoiesis. Blood 124, 1393–1403. doi: 10.1182/blood-2014-04-570762
Bragdon, B., Burns, R., Baker, A. H., Belkina, A. C., Morgan, E. F., Denis, G. V., et al. (2015). Intrinsic sex-linked variations in osteogenic and adipogenic differentiation potential of bone marrow multipotent stromal cells. J. Cell. Physiol. 230, 296–307. doi: 10.1002/jcp.24705
Brenner, S., Whiting-Theobald, N., Kawai, T., Linton, G. F., Rudikoff, A. G., Choi, U., et al. (2004). CXCR4-transgene expression significantly improves marrow engraftment of cultured hematopoietic stem cells. Stem Cells 22, 1128–1133. doi: 10.1634/stemcells.2003-0196
Broxmeyer, H. E., Maze, R., Miyazawa, K., Carow, C., Hendrie, P. C., Cooper, S., et al. (1991). The kit receptor and its ligand, steel factor, as regulators of hemopoiesis. Cancer Cells Cold Spring Harb. N 1989, 480–487.
Broxmeyer, H. E., Orschell, C. M., Clapp, D. W., Hangoc, G., Cooper, S., Plett, P. A., et al. (2005). Rapid mobilization of murine and human hematopoietic stem and progenitor cells with AMD3100, a CXCR4 antagonist. J. Exp. Med. 201, 1307–1318. doi: 10.1084/jem.20041385
Bruns, I., Lucas, D., Pinho, S., Ahmed, J., Lambert, M. P., Kunisaki, Y., et al. (2014). Megakaryocytes regulate hematopoietic stem cell quiescence through CXCL4 secretion. Nat. Med. 20, 1315–1320. doi: 10.1038/nm.3707
Calvi, L. M., Adams, G. B., Weibrecht, K. W., Weber, J. M., Olson, D. P., Knight, M. C., et al. (2003). Osteoblastic cells regulate the Haematopoietic stem cell niche. Nature 425, 841–846. doi: 10.1038/nature02040
Chamberlin, W., Barone, J., Kedo, A., and Fried, W. (1974). Lack of recovery of murine hematopoietic stromal cells after irradiation-induced damage. Blood 44, 385–392.
Chan, G. K., and Duque, G. (2002). Age-related bone loss: old bone, new facts. Gerontology 48, 62–71. doi: 10.1159/000048929
Chen, S., Zambetti, N. A., Bindels, E. M. J., Kenswill, K., Mylona, A. M., Adisty, N. M., et al. (2016). Massive parallel RNA sequencing of highly purified mesenchymal elements in low-risk MDS reveals tissue-context-dependent activation of inflammatory programs. Leukemia 30, 1938–1942. doi: 10.1038/leu.2016.91
Cheng, P., Eksioglu, E. A., Chen, X., Kandell, W., Le Trinh, T., Cen, L., et al. (2019). S100A9-induced overexpression of PD-1/PD-L1 contributes to ineffective hematopoiesis in myelodysplastic syndromes. Leukemia 33, 2034–2046. doi: 10.1038/s41375-019-0397-9
Cheshier, S. H., Prohaska, S. S., and Weissman, I. L. (2007). The effect of bleeding on hematopoietic stem cell cycling and self-renewal. Stem Cells Dev. 16, 707–717. doi: 10.1089/scd.2007.0017
Colmone, A., Amorim, M., Pontier, A. L., Wang, S., Jablonski, E., and Sipkins, D. A. (2008). Leukemic cells create bone marrow niches that disrupt the behavior of normal hematopoietic progenitor cells. Science 322, 1861–1865. doi: 10.1126/science.1164390
Comazzetto, S., Murphy, M. M., Berto, S., Jeffery, E., Zhao, Z., and Morrison, S. J. (2019). Restricted Hematopoietic progenitors and erythropoiesis require SCF from leptin Receptor+ Niche cells in the bone marrow. Cell Stem Cell 24, 477–486.e6. doi: 10.1016/j.stem.2018.11.022
Cosgun, K. N., Rahmig, S., Mende, N., Reinke, S., Hauber, I., Schäfer, C., et al. (2014). Kit regulates HSC engraftment across the human-mouse species barrier. Cell Stem Cell 15, 227–238. doi: 10.1016/j.stem.2014.06.001
Costa, M. J., Kudaravalli, J., Liu, W.-H., Stock, J., Kong, S., and Liu, S.-H. (2018). A mouse model for evaluation of efficacy and concomitant toxicity of anti-human CXCR4 therapeutics. PLoS One 13:e0194688. doi: 10.1371/journal.pone.0194688
Davizon-Castillo, P., McMahon, B., Aguila, S., Bark, D., Ashworth, K., Allawzi, A., et al. (2019). TNF-α-driven inflammation and mitochondrial dysfunction define the platelet hyperreactivity of aging. Blood 134, 727–740. doi: 10.1182/blood.2019000200
de Haan, G., Weersing, E., Dontje, B., van Os, R., Bystrykh, L. V., Vellenga, E., et al. (2003). In vitro generation of long-term repopulating hematopoietic stem cells by fibroblast growth factor-1. Dev. Cell 4, 241–251. doi: 10.1016/s1534-5807(03)00018-2
Decker, M., Leslie, J., Liu, Q., and Ding, L. (2018). Hepatic thrombopoietin is required for bone marrow hematopoietic stem cell maintenance. Science 360, 106–110. doi: 10.1126/science.aap8861
Deng, P., Yuan, Q., Cheng, Y., Li, J., Liu, Z., Liu, Y., et al. (2021). Loss of KDM4B exacerbates bone-fat imbalance and mesenchymal stromal cell exhaustion in skeletal aging. Cell Stem Cell. 28, 1057–1073.e7. doi: 10.1016/j.stem.2021.01.010
Desterke, C., Petit, L., Sella, N., Chevallier, N., Cabeli, V., Coquelin, L., et al. (2020). Inferring gene networks in bone marrow hematopoietic stem cell-supporting stromal niche populations. iScience 23, 101222. doi: 10.1016/j.isci.2020.101222
Ding, L., and Morrison, S. J. (2013). Haematopoietic stem cells and early lymphoid progenitors occupy distinct bone marrow niches. Nature 495, 231–235. doi: 10.1038/nature11885
Ding, L., Saunders, T. L., Enikolopov, G., and Morrison, S. J. (2012). Endothelial and perivascular cells maintain haematopoietic stem cells. Nature 481, 457–462. doi: 10.1038/nature10783
Dolgalev, I., and Tikhonova, A. N. (2021). Connecting the dots: resolving the bone marrow niche heterogeneity. Front. Cell Dev. Biol. 9:622519. doi: 10.3389/fcell.2021.622519
Dominici, M., Rasini, V., Bussolari, R., Chen, X., Hofmann, T. J., Spano, C., et al. (2009). Restoration and reversible expansion of the Osteoblastic hematopoietic stem cell niche after marrow radioablation. Blood 114, 2333–2343. doi: 10.1182/blood-2008-10-183459
Dong, F., Hoefsloot, L. H., Schelen, A. M., Broeders, C. A., Meijer, Y., Veerman, A. J., et al. (1994). Identification of a nonsense mutation in the granulocyte-colony-stimulating factor receptor in severe congenital neutropenia. Proc. Natl. Acad. Sci. U.S.A. 91, 4480–4484. doi: 10.1073/pnas.91.10.4480
Duarte, D., Hawkins, E. D., Akinduro, O., Ang, H., De Filippo, K., Kong, I. Y., et al. (2018). Inhibition of endosteal vascular niche remodeling rescues hematopoietic stem cell loss in AML. Cell Stem Cell 22, 64–77.e6. doi: 10.1016/j.stem.2017.11.006
Dubois, C., Ruscetti, F., Stankova, J., and Keller, J. (1994). Transforming growth factor-beta regulates c-kit message stability and cell-surface protein expression in hematopoietic progenitors. Blood 83, 3138–3145. doi: 10.1182/blood.V83.11.3138.3138
Duchene, J., Novitzky-Basso, I., Thiriot, A., Casanova-Acebes, M., Bianchini, M., Etheridge, S. L., et al. (2017). Atypical chemokine receptor 1 on nucleated erythroid cells regulates hematopoiesis. Nat. Immunol. 18, 753–761. doi: 10.1038/ni.3763
Essers, M. A. G., Offner, S., Blanco-Bose, W. E., Waibler, Z., Kalinke, U., Duchosal, M. A., et al. (2009). IFNalpha activates dormant haematopoietic stem cells in vivo. Nature 458, 904–908. doi: 10.1038/nature07815
Falla, N., Van Vlasselaer, N., Bierkens, J., Borremans, B., Schoeters, G., and Van Gorp, U. (1993). Characterization of a 5-fluorouracil-enriched osteoprogenitor population of the murine bone marrow. Blood 82, 3580–3591.
Flach, J., Bakker, S. T., Mohrin, M., Conroy, P. C., Pietras, E. M., Reynaud, D., et al. (2014). Replication stress is a potent driver of functional decline in ageing haematopoietic stem cells. Nature 512, 198–202. doi: 10.1038/nature13619
Friedenstein, A. J., Chailakhyan, R. K., Latsinik, N. V., Panasyuk, A. F., and Keiliss-Borok, I. V. (1974). Stromal cells responsible for transferring the microenvironment of the hemopoietic tissues. Cloning in vitro and retransplantation in vivo. Transplantation 17, 331–340. doi: 10.1097/00007890-197404000-00001
Frisch, B. J., Hoffman, C. M., Latchney, S. E., LaMere, M. W., Myers, J., Ashton, J., et al. (2019). Aged marrow macrophages expand platelet-biased hematopoietic stem cells via Interleukin1B. JCI Insight 5:e124213. doi: 10.1172/jci.insight.124213
Frodermann, V., Rohde, D., Courties, G., Severe, N., Schloss, M. J., Amatullah, H., et al. (2019). Exercise reduces inflammatory cell production and cardiovascular inflammation via instruction of hematopoietic progenitor cells. Nat. Med. 25, 1761–1771. doi: 10.1038/s41591-019-0633-x
Fujisaki, J., Wu, J., Carlson, A. L., Silberstein, L., Putheti, P., Larocca, R., et al. (2011). In vivo imaging of Treg cells providing immune privilege to the haematopoietic stem-cell niche. Nature 474, 216–219. doi: 10.1038/nature10160
Fulzele, K., Krause, D. S., Panaroni, C., Saini, V., Barry, K. J., Liu, X., et al. (2013). Myelopoiesis is regulated by osteocytes through Gsα-dependent signaling. Blood 121, 930–939. doi: 10.1182/blood-2012-06-437160
Furukawa, M., Wang, X., Ohkawara, H., Fukatsu, M., Alkebsi, L., Takahashi, H., et al. (2019). A critical role of the Gas6-Mer axis in endothelial dysfunction contributing to TA-TMA associated with GVHD. Blood Adv. 3, 2128–2143. doi: 10.1182/bloodadvances.2019000222
Genovese, G., Kähler, A. K., Handsaker, R. E., Lindberg, J., Rose, S. A., Bakhoum, S. F., et al. (2014). Clonal hematopoiesis and blood-cancer risk inferred from blood DNA sequence. N. Engl. J. Med. 371, 2477–2487. doi: 10.1056/NEJMoa1409405
Gnani, D., Crippa, S., Della Volpe, L., Rossella, V., Conti, A., Lettera, E., et al. (2019). An early-senescence state in aged mesenchymal stromal cells contributes to hematopoietic stem and progenitor cell clonogenic impairment through the activation of a pro-inflammatory program. Aging Cell 18:e12933. doi: 10.1111/acel.12933
Godavarthy, P. S., Kumar, R., Herkt, S. C., Pereira, R. S., Hayduk, N., Weissenberger, E. S., et al. (2020). The vascular bone marrow niche influences outcome in chronic myeloid leukemia via the E-selectin - SCL/TAL1 - CD44 axis. Haematologica 105, 136–147. doi: 10.3324/haematol.2018.212365
Golan, K., Kumari, A., Kollet, O., Khatib-Massalha, E., Subramaniam, M. D., Ferreira, Z. S., et al. (2018). Daily onset of light and darkness differentially controls Hematopoietic stem cell differentiation and maintenance. Cell Stem Cell 23, 572–585.e7. doi: 10.1016/j.stem.2018.08.002
Gomei, Y., Nakamura, Y., Yoshihara, H., Hosokawa, K., Iwasaki, H., Suda, T., et al. (2010). Functional differences between two Tie2 ligands, angiopoietin-1 and -2, in regulation of adult bone marrow hematopoietic stem cells. Exp. Hematol. 38, 82–89. doi: 10.1016/j.exphem.2009.11.007
Grassinger, J., Haylock, D. N., Storan, M. J., Haines, G. O., Williams, B., Whitty, G. A., et al. (2009). Thrombin-cleaved osteopontin regulates hemopoietic stem and progenitor cell functions through interactions with α9β1 and α4β1 integrins. Blood 114, 49–59. doi: 10.1182/blood-2009-01-197988
Greenbaum, A., Hsu, Y.-M. S., Day, R. B., Schuettpelz, L. G., Christopher, M. J., Borgerding, J. N., et al. (2013). CXCL12 in early mesenchymal progenitors is required for haematopoietic stem-cell maintenance. Nature 495, 227–230. doi: 10.1038/nature11926
Grinenko, T., Arndt, K., Portz, M., Mende, N., Günther, M., Cosgun, K. N., et al. (2014). Clonal expansion capacity defines two consecutive developmental stages of long-term hematopoietic stem cells. J. Exp. Med. 211, 209–215. doi: 10.1084/jem.20131115
Guezguez, B., Campbell, C. J. V., Boyd, A. L., Karanu, F., Casado, F. L., Di Cresce, C., et al. (2013). Regional localization within the bone marrow influences the functional capacity of human HSCs. Cell Stem Cell 13, 175–189. doi: 10.1016/j.stem.2013.06.015
Haltalli, M. L. R., Watcham, S., Wilson, N. K., Eilers, K., Lipien, A., Ang, H., et al. (2020). Manipulating niche composition limits damage to haematopoietic stem cells during Plasmodium infection. Nat. Cell Biol. 22, 1399–1410. doi: 10.1038/s41556-020-00601-w
Hasselbalch, H. C. (2013). Chronic inflammation as a promotor of mutagenesis in essential thrombocythemia, polycythemia vera and myelofibrosis. A human inflammation model for cancer development? Leuk. Res. 37, 214–220. doi: 10.1016/j.leukres.2012.10.020
Helbling, P. M., Piñeiro-Yáñez, E., Gerosa, R., Boettcher, S., Al-Shahrour, F., Manz, M. G., et al. (2019). Global transcriptomic profiling of the bone marrow stromal microenvironment during postnatal development, aging, and inflammation. Cell Rep. 29, 3313–3330.e4. doi: 10.1016/j.celrep.2019.11.004
Himburg, H. A., Harris, J. R., Ito, T., Daher, P., Russell, J. L., Quarmyne, M., et al. (2012). Pleiotrophin regulates the retention and self-renewal of hematopoietic stem cells in the bone marrow vascular niche. Cell Rep. 2, 964–975. doi: 10.1016/j.celrep.2012.09.002
Himburg, H. A., Muramoto, G. G., Daher, P., Meadows, S. K., Russell, J. L., Doan, P., et al. (2010). Pleiotrophin regulates the expansion and regeneration of hematopoietic stem cells. Nat. Med. 16, 475–482. doi: 10.1038/nm.2119
Himburg, H. A., Termini, C. M., Schlussel, L., Kan, J., Li, M., Zhao, L., et al. (2018). Distinct bone marrow sources of pleiotrophin control hematopoietic stem cell maintenance and regeneration. Cell Stem Cell 23, 370–381.e5. doi: 10.1016/j.stem.2018.07.003
Hirche, C., Frenz, T., Haas, S. F., Döring, M., Borst, K., Tegtmeyer, P.-K., et al. (2017). Systemic virus infections differentially modulate cell cycle state and functionality of long-term Hematopoietic stem cells in vivo. Cell Rep. 19, 2345–2356. doi: 10.1016/j.celrep.2017.05.063
Ho, Y.-H., Del Toro, R., Rivera-Torres, J., Rak, J., Korn, C., García-García, A., et al. (2019). Remodeling of bone marrow Hematopoietic stem cell niches promotes myeloid cell expansion during premature or physiological aging. Cell Stem Cell 25, 407–418.e6. doi: 10.1016/j.stem.2019.06.007
Hu, L., Yin, X., Zhang, Y., Pang, A., Xie, X., Yang, S., et al. (2021). Radiation-induced bystander effects impair transplanted human hematopoietic stem cells via oxidative DNA damage. Blood 137, 3339–3350. doi: 10.1182/blood.2020007362
Huang, W., Yang, Y., Sun, Z., and Zeng, X. (2009). Early radiation-induced bone marrow injury: serial MR imaging during initial 4 weeks after irradiation. Acad. Radiol. 16, 733–738. doi: 10.1016/j.acra.2008.12.008
Hur, J., Choi, J.-I., Lee, H., Nham, P., Kim, T.-W., Chae, C.-W., et al. (2016). CD82/KAI1 Maintains the Dormancy of Long-Term Hematopoietic Stem Cells through Interaction with DARC-Expressing Macrophages. Cell Stem Cell 18, 508–521. doi: 10.1016/j.stem.2016.01.013
Ikuta, K., Ingolia, D. E., Friedman, J., Heimfeld, S., and Weissman, I. L. (1991). Mouse hematopoietic stem cells and the interaction of c-kit receptor and steel factor. Int. J. Cell Cloning 9, 451–460. doi: 10.1002/stem.1991.5530090503
Istvanffy, R., Kröger, M., Eckl, C., Gitzelmann, S., Vilne, B., Bock, F., et al. (2011). Stromal pleiotrophin regulates repopulation behavior of hematopoietic stem cells. Blood 118, 2712–2722. doi: 10.1182/blood-2010-05-287235
Istvanffy, R., Vilne, B., Schreck, C., Ruf, F., Pagel, C., Grziwok, S., et al. (2015). Stroma-derived connective tissue growth factor maintains cell cycle progression and repopulation activity of hematopoietic stem cells in vitro. Stem Cell Rep. 5, 702–715. doi: 10.1016/j.stemcr.2015.09.018
Itkin, T., Ludin, A., Gradus, B., Gur-Cohen, S., Kalinkovich, A., Schajnovitz, A., et al. (2012). FGF-2 expands murine hematopoietic stem and progenitor cells via proliferation of stromal cells, c-Kit activation, and CXCL12 down-regulation. Blood 120, 1843–1855. doi: 10.1182/blood-2011-11-394692
Ito, K., Turcotte, R., Cui, J., Zimmerman, S. E., Pinho, S., Mizoguchi, T., et al. (2016). Self-renewal of a purified Tie2+ hematopoietic stem cell population relies on mitochondrial clearance. Science 354, 1156–1160. doi: 10.1126/science.aaf5530
Iwama, A., Hamaguchi, I., Hashiyama, M., Murayama, Y., Yasunaga, K., and Suda, T. (1993). Molecular cloning and characterization of mouse TIE and TEK receptor tyrosine kinase genes and their expression in hematopoietic stem cells. Biochem. Biophys. Res. Commun. 195, 301–309. doi: 10.1006/bbrc.1993.2045
Jacobsen, K., Kravitz, J., Kincade, P. W., and Osmond, D. G. (1996). Adhesion receptors on bone marrow stromal cells: in vivo expression of vascular cell adhesion molecule-1 by reticular cells and sinusoidal endothelium in normal and gamma-irradiated mice. Blood 87, 73–82.
Jaiswal, S., Fontanillas, P., Flannick, J., Manning, A., Grauman, P. V., Mar, B. G., et al. (2014). Age-related clonal hematopoiesis associated with adverse outcomes. N. Engl. J. Med. 371, 2488–2498. doi: 10.1056/NEJMoa1408617
Ju, Z., Jiang, H., Jaworski, M., Rathinam, C., Gompf, A., Klein, C., et al. (2007). Telomere dysfunction induces environmental alterations limiting hematopoietic stem cell function and engraftment. Nat. Med. 13, 742–747. doi: 10.1038/nm1578
Jung, Y., Wang, J., Havens, A., Sun, Y., Wang, J., Jin, T., et al. (2005). Cell-to-cell contact is critical for the survival of hematopoietic progenitor cells on osteoblasts. Cytokine 32, 155–162. doi: 10.1016/j.cyto.2005.09.001
Kahn, J., Byk, T., Jansson-Sjostrand, L., Petit, I., Shivtiel, S., Nagler, A., et al. (2004). Overexpression of CXCR4 on human CD34+ progenitors increases their proliferation, migration, and NOD/SCID repopulation. Blood 103, 2942–2949. doi: 10.1182/blood-2003-07-2607
Kaltz, N., Ringe, J., Holzwarth, C., Charbord, P., Niemeyer, M., Jacobs, V. R., et al. (2010). Novel markers of mesenchymal stem cells defined by genome-wide gene expression analysis of stromal cells from different sources. Exp. Cell Res. 316, 2609–2617. doi: 10.1016/j.yexcr.2010.06.002
Kapur, R., Majumdar, M., Xiao, X., McAndrews-Hill, M., Schindler, K., and Williams, D. A. (1998). Signaling through the interaction of membrane-restricted stem cell factor and c-kit receptor tyrosine kinase: genetic evidence for a differential role in erythropoiesis. Blood 91, 879–889.
Karanu, F. N., Murdoch, B., Gallacher, L., Wu, D. M., Koremoto, M., Sakano, S., et al. (2000). The notch ligand Jagged-1 represents a novel growth factor of human hematopoietic stem cells. J. Exp. Med. 192, 1365–1372. doi: 10.1084/jem.192.9.1365
Katayama, Y., Battista, M., Kao, W.-M., Hidalgo, A., Peired, A. J., Thomas, S. A., et al. (2006). Signals from the sympathetic nervous system regulate Hematopoietic stem cell egress from bone marrow. Cell 124, 407–421. doi: 10.1016/j.cell.2005.10.041
Kato, Y., Hou, L.-B., Miyagi, S., Nitta, E., Aoyama, K., Shinoda, D., et al. (2019). Bmi1 restricts the adipogenic differentiation of bone marrow stromal cells to maintain the integrity of the hematopoietic stem cell niche. Exp. Hematol. 76, 24–37. doi: 10.1016/j.exphem.2019.07.006
Kaushansky, K., Lok, S., Holly, R. D., Broudy, V. C., Lin, N., Bailey, M. C., et al. (1994). Promotion of megakaryocyte progenitor expansion and differentiation by the c-Mpl ligand thrombopoietin. Nature 369, 568–571. doi: 10.1038/369568a0
Kim, H.-N., Chang, J., Shao, L., Han, L., Iyer, S., Manolagas, S. C., et al. (2017). DNA damage and senescence in osteoprogenitors expressing Osx1 may cause their decrease with age. Aging Cell 16, 693–703. doi: 10.1111/acel.12597
Kim, Y.-H., Cho, K.-A., Lee, H.-J., Park, M., Shin, S.-J., Park, J.-W., et al. (2020). Conditioned Medium from Human Tonsil-derived mesenchymal stem cells enhances bone marrow engraftment via endothelial cell restoration by Pleiotrophin. Cells 9:E221. doi: 10.3390/cells9010221
Kleppe, M., Koche, R., Zou, L., van Galen, P., Hill, C. E., Dong, L., et al. (2018). Dual targeting of oncogenic activation and inflammatory signaling increases therapeutic efficacy in Myeloproliferative neoplasms. Cancer Cell 33, 29–43.e7. doi: 10.1016/j.ccell.2017.11.009
Kode, A., Manavalan, J. S., Mosialou, I., Bhagat, G., Rathinam, C. V., Luo, N., et al. (2014). Leukaemogenesis induced by an activating β-catenin mutation in osteoblasts. Nature 506, 240–244. doi: 10.1038/nature12883
Koni, P. A., Joshi, S. K., Temann, U.-A., Olson, D., Burkly, L., and Flavell, R. A. (2001). Conditional vascular cell adhesion molecule 1 deletion in mice. J. Exp. Med. 193, 741–754. doi: 10.1084/jem.193.6.741
Krause, D. S., Fulzele, K., Catic, A., Sun, C. C., Dombkowski, D., Hurley, M. P., et al. (2013). Differential regulation of myeloid leukemias by the bone marrow microenvironment. Nat. Med. 19, 1513–1517. doi: 10.1038/nm.3364
Krevvata, M., Silva, B. C., Manavalan, J. S., Galan-Diez, M., Kode, A., Matthews, B. G., et al. (2014). Inhibition of leukemia cell engraftment and disease progression in mice by osteoblasts. Blood 124, 2834–2846. doi: 10.1182/blood-2013-07-517219
Ku, H., Yonemura, Y., Kaushansky, K., and Ogawa, M. (1996). Thrombopoietin, the ligand for the Mpl receptor, synergizes with steel factor and other early acting cytokines in supporting proliferation of primitive hematopoietic progenitors of mice. Blood 87, 4544–4551.
Kunisaki, Y., Bruns, I., Scheiermann, C., Ahmed, J., Pinho, S., Zhang, D., et al. (2013). Arteriolar niches maintain haematopoietic stem cell quiescence. Nature 502, 637–643. doi: 10.1038/nature12612
Kusumbe, A. P., Ramasamy, S. K., and Adams, R. H. (2014). Coupling of angiogenesis and osteogenesis by a specific vessel subtype in bone. Nature 507, 323–328. doi: 10.1038/nature13145
Lacava, G., Laus, F., Amaroli, A., Marchegiani, A., Censi, R., Di Martino, P., et al. (2019). P62 deficiency shifts mesenchymal/stromal stem cell commitment toward adipogenesis and disrupts bone marrow homeostasis in aged mice. J. Cell. Physiol. 234, 16338–16347. doi: 10.1002/jcp.28299
Lampreia, F. P., Carmelo, J. G., and Anjos-Afonso, F. (2017). Notch signaling in the regulation of hematopoietic stem cell. Curr. Stem Cell Rep. 3, 202–209. doi: 10.1007/s40778-017-0090-8
Langer, J. C., Henckaerts, E., Orenstein, J., and Snoeck, H.-W. (2004). Quantitative trait analysis reveals transforming growth factor-beta2 as a positive regulator of early hematopoietic progenitor and stem cell function. J. Exp. Med. 199, 5–14. doi: 10.1084/jem.20030980
Ledran, M. H., Krassowska, A., Armstrong, L., Dimmick, I., Renström, J., Lang, R., et al. (2008). Efficient hematopoietic differentiation of human embryonic stem cells on stromal cells derived from hematopoietic niches. Cell Stem Cell 3, 85–98. doi: 10.1016/j.stem.2008.06.001
Leimkühler, N. B., Gleitz, H. F. E., Ronghui, L., Snoeren, I. A. M., Fuchs, S. N. R., Nagai, J. S., et al. (2021). Heterogeneous bone-marrow stromal progenitors drive myelofibrosis via a druggable alarmin axis. Cell Stem Cell 28, 637–652.e8. doi: 10.1016/j.stem.2020.11.004
Lévesque, J.-P., Hendy, J., Winkler, I. G., Takamatsu, Y., and Simmons, P. J. (2003). Granulocyte colony-stimulating factor induces the release in the bone marrow of proteases that cleave c-KIT receptor (CD117) from the surface of hematopoietic progenitor cells. Exp. Hematol. 31, 109–117. doi: 10.1016/s0301-472x(02)01028-7
Li, G., Bunn, J. R., Mushipe, M. T., He, Q., and Chen, X. (2005). Effects of pleiotrophin (PTN) over-expression on mouse long bone development, fracture healing and bone repair. Calcif. Tissue Int. 76, 299–306. doi: 10.1007/s00223-004-0145-6
Lieschke, G. J., Grail, D., Hodgson, G., Metcalf, D., Stanley, E., Cheers, C., et al. (1994). Mice lacking granulocyte colony-stimulating factor have chronic neutropenia, granulocyte and macrophage progenitor cell deficiency, and impaired neutrophil mobilization. Blood 84, 1737–1746.
Liu, F., Wu, H. Y., Wesselschmidt, R., Kornaga, T., and Link, D. C. (1996). Impaired production and increased apoptosis of neutrophils in granulocyte colony-stimulating factor receptor-deficient mice. Immunity 5, 491–501. doi: 10.1016/s1074-7613(00)80504-x
Lo Celso, C., Fleming, H. E., Wu, J. W., Zhao, C. X., Miake-Lye, S., Fujisaki, J., et al. (2009). Live-animal tracking of individual haematopoietic stem/progenitor cells in their niche. Nature 457, 92–96. doi: 10.1038/nature07434
Lutzny, G., Kocher, T., Schmidt-Supprian, M., Rudelius, M., Klein-Hitpass, L., Finch, A. J., et al. (2013). Protein kinase c-β-dependent activation of NF-κB in stromal cells is indispensable for the survival of chronic lymphocytic leukemia B cells in vivo. Cancer Cell 23, 77–92. doi: 10.1016/j.ccr.2012.12.003
Marino, R., Otsuru, S., Hofmann, T. J., Olson, T. S., Rasini, V., Veronesi, E., et al. (2013). Delayed marrow infusion in mice enhances hematopoietic and osteopoietic engraftment by facilitating transient expansion of the osteoblastic niche. Biol. Blood Marr. Transpl. J. Am. Soc. Blood Marrow Transpl. 19, 1566–1573. doi: 10.1016/j.bbmt.2013.07.025
Maryanovich, M., Zahalka, A. H., Pierce, H., Pinho, S., Nakahara, F., Asada, N., et al. (2018). Adrenergic nerve degeneration in bone marrow drives aging of the hematopoietic stem cell niche. Nat. Med. 24, 782–791. doi: 10.1038/s41591-018-0030-x
Masouridi-Levrat, S., Simonetta, F., and Chalandon, Y. (2016). Immunological basis of bone marrow failure after allogeneic hematopoietic stem cell transplantation. Front. Immunol. 7:362. doi: 10.3389/fimmu.2016.00362
Mawatari, T., Miura, H., Higaki, H., Kurata, K., Moro-oka, T., Murakami, T., et al. (1999). Quantitative analysis of three-dimensional complexity and connectivity changes in trabecular microarchitecture in relation to aging, menopause, and inflammation. J. Orthop. Sci. Off. J. Jpn. Orthop. Assoc. 4, 431–438. doi: 10.1007/s007760050126
McCarthy, K. F., Ledney, G. D., and Mitchell, R. (1977). A deficiency of hematopoietic stem cells in steel mice. Cell Tissue Kinet. 10, 121–126. doi: 10.1111/j.1365-2184.1977.tb00137.x
McCurdy, S. R., Zhang, M.-J., St Martin, A., Al Malki, M. M., Bashey, A., Gaballa, S., et al. (2018). Effect of donor characteristics on haploidentical transplantation with posttransplantation cyclophosphamide. Blood Adv. 2, 299–307. doi: 10.1182/bloodadvances.2017014829
McIntosh, B. E., Brown, M. E., Duffin, B. M., Maufort, J. P., Vereide, D. T., Slukvin, I. I., et al. (2015). Nonirradiated NOD,B6.SCID Il2rγ−/− KitW41/W41 (NBSGW) Mice Support Multilineage Engraftment of Human Hematopoietic Cells. Stem Cell Rep. 4, 171–180. doi: 10.1016/j.stemcr.2014.12.005
Medyouf, H., Mossner, M., Jann, J.-C., Nolte, F., Raffel, S., Herrmann, C., et al. (2014). Myelodysplastic cells in patients reprogram mesenchymal stromal cells to establish a transplantable stem cell niche disease unit. Cell Stem Cell 14, 824–837. doi: 10.1016/j.stem.2014.02.014
Mehrzadi, S., Safa, M., Kamrava, S. K., Darabi, R., Hayat, P., and Motevalian, M. (2017). Protective mechanisms of melatonin against hydrogen-peroxide-induced toxicity in human bone-marrow-derived mesenchymal stem cells. Can. J. Physiol. Pharmacol. 95, 773–786. doi: 10.1139/cjpp-2016-0409
Mende, N., Jolly, A., Percin, G. I., Günther, M., Rostovskaya, M., Krishnan, S. M., et al. (2019). Prospective isolation of nonhematopoietic cells of the niche and their differential molecular interactions with HSCs. Blood 134, 1214–1226. doi: 10.1182/blood.2019000176
Méndez-Ferrer, S., Lucas, D., Battista, M., and Frenette, P. S. (2008). Haematopoietic stem cell release is regulated by circadian oscillations. Nature 452, 442–447. doi: 10.1038/nature06685
Mistry, J. J., Marlein, C. R., Moore, J. A., Hellmich, C., Wojtowicz, E. E., Smith, J. G. W., et al. (2019). ROS-mediated PI3K activation drives mitochondrial transfer from stromal cells to hematopoietic stem cells in response to infection. Proc. Natl. Acad. Sci. U.S.A. 116, 24610–24619. doi: 10.1073/pnas.1913278116
Mitsui, T., Watanabe, S., Taniguchi, Y., Hanada, S., Ebihara, Y., Sato, T., et al. (2003). Impaired neutrophil maturation in truncated murine G-CSF receptor-transgenic mice. Blood 101, 2990–2995. doi: 10.1182/blood.V101.8.2990
Molineux, G., Pojda, Z., Hampson, I. N., Lord, B. I., and Dexter, T. M. (1990). Transplantation potential of peripheral blood stem cells induced by granulocyte colony-stimulating factor. Blood 76, 2153–2158.
Nakada, D., Oguro, H., Levi, B. P., Ryan, N., Kitano, A., Saitoh, Y., et al. (2014). Oestrogen increases haematopoietic stem-cell self-renewal in females and during pregnancy. Nature 505, 555–558. doi: 10.1038/nature12932
Naveiras, O., Nardi, V., Wenzel, P. L., Hauschka, P. V., Fahey, F., and Daley, G. Q. (2009). Bone-marrow adipocytes as negative regulators of the haematopoietic microenvironment. Nature 460, 259–263. doi: 10.1038/nature08099
Negishi, N., Suzuki, D., Ito, R., Irie, N., Matsuo, K., Yahata, T., et al. (2014). Effective expansion of engrafted human hematopoietic stem cells in bone marrow of mice expressing human Jagged1. Exp. Hematol. 42, 487–494.e1. doi: 10.1016/j.exphem.2014.02.001
Nelson, P., and Masel, J. (2017). Intercellular competition and the inevitability of multicellular aging. Proc. Natl. Acad. Sci. U.S.A. 114, 12982–12987. doi: 10.1073/pnas.1618854114
Nilsson, S. K., Johnston, H. M., Whitty, G. A., Williams, B., Webb, R. J., Denhardt, D. T., et al. (2005). Osteopontin, a key component of the hematopoietic stem cell niche and regulator of primitive hematopoietic progenitor cells. Blood 106, 1232–1239. doi: 10.1182/blood-2004-11-4422
Nishikawa, K., Nakashima, T., Takeda, S., Isogai, M., Hamada, M., Kimura, A., et al. (2010). Maf promotes osteoblast differentiation in mice by mediating the age-related switch in mesenchymal cell differentiation. J. Clin. Invest. 120, 3455–3465. doi: 10.1172/JCI42528
Notta, F., Doulatov, S., and Dick, J. E. (2010). Engraftment of human hematopoietic stem cells is more efficient in female NOD/SCID/IL-2Rgc-null recipients. Blood 115, 3704–3707. doi: 10.1182/blood-2009-10-249326
Okamoto, T., Kanamaru, A., Kakishita, E., and Nagai, K. (1991). Stromal fibroblastic and hematopoietic progenitors in patients with graft-versus-host disease (GVHD). Int. J. Hematol. 54, 299–306.
Olson, T. S., Caselli, A., Otsuru, S., Hofmann, T. J., Williams, R., Paolucci, P., et al. (2013). Megakaryocytes promote murine osteoblastic HSC niche expansion and stem cell engraftment after radioablative conditioning. Blood 121, 5238–5249. doi: 10.1182/blood-2012-10-463414
Pagliuca, S., Michonneau, D., Sicre de Fontbrune, F., Sutra Del Galy, A., Xhaard, A., Robin, M., et al. (2019). Allogeneic reactivity-mediated endothelial cell complications after HSCT: a plea for consensual definitions. Blood Adv. 3, 2424–2435. doi: 10.1182/bloodadvances.2019000143
Passaro, D., Abarrategi, A., Foster, K., Ariza-McNaughton, L., and Bonnet, D. (2017). Bioengineering of humanized bone marrow microenvironments in mouse and their visualization by live imaging. J. Vis. Exp. 2017:55914. doi: 10.3791/55914
Peled, A., Petit, I., Kollet, O., Magid, M., Ponomaryov, T., Byk, T., et al. (1999). Dependence of human stem cell engraftment and repopulation of NOD/SCID mice on CXCR4. Science 283, 845–848. doi: 10.1126/science.283.5403.845
Pioli, P. D., Casero, D., Montecino-Rodriguez, E., Morrison, S. L., and Dorshkind, K. (2019). Plasma cells are obligate effectors of enhanced myelopoiesis in aging bone marrow. Immunity 51, 351–366.e6. doi: 10.1016/j.immuni.2019.06.006
Pittenger, M. F., Mackay, A. M., Beck, S. C., Jaiswal, R. K., Douglas, R., Mosca, J. D., et al. (1999). Multilineage potential of adult human mesenchymal stem cells. Science 284, 143–147. doi: 10.1126/science.284.5411.143
Polzer, K., Joosten, L., Gasser, J., Distler, J. H., Ruiz, G., Baum, W., et al. (2010). Interleukin-1 is essential for systemic inflammatory bone loss. Ann. Rheum. Dis. 69, 284–290. doi: 10.1136/ard.2008.104786
Poulos, M. G., Guo, P., Kofler, N. M., Pinho, S., Gutkin, M. C., Tikhonova, A., et al. (2013). Endothelial Jagged-1 is necessary for homeostatic and regenerative hematopoiesis. Cell Rep. 4, 1022–1034. doi: 10.1016/j.celrep.2013.07.048
Poulos, M. G., Ramalingam, P., Gutkin, M. C., Llanos, P., Gilleran, K., Rabbany, S. Y., et al. (2017). Endothelial transplantation rejuvenates aged hematopoietic stem cell function. J. Clin. Invest. 127, 4163–4178. doi: 10.1172/JCI93940
Prendergast, ÁM., Kuck, A., van Essen, M., Haas, S., Blaszkiewicz, S., and Essers, M. A. G. (2017). IFNα-mediated remodeling of endothelial cells in the bone marrow niche. Haematologica 102, 445–453. doi: 10.3324/haematol.2016.151209
Prins, M. C., van Hinte, G., Koenders, N., Rondel, A. L., Blijlevens, N. M. A., and van den Berg, M. G. A. (2021). The effect of exercise and nutrition interventions on physical functioning in patients undergoing haematopoietic stem cell transplantation: a systematic review and meta-analysis. Support. Care Cancer Off. J. Multinatl. Assoc. Support. Care Cancer doi: 10.1007/s00520-021-06334-2 [Epub ahead of print].
Qian, H., Buza-Vidas, N., Hyland, C. D., Jensen, C. T., Antonchuk, J., Månsson, R., et al. (2007). Critical Role of thrombopoietin in maintaining adult quiescent hematopoietic stem cells. Cell Stem Cell 1, 671–684. doi: 10.1016/j.stem.2007.10.008
Raaijmakers, M. H. G. P., Mukherjee, S., Guo, S., Zhang, S., Kobayashi, T., Schoonmaker, J. A., et al. (2010). Bone progenitor dysfunction induces myelodysplasia and secondary Leukaemia. Nature 464, 852–857. doi: 10.1038/nature08851
Radtke, F., Wilson, A., Stark, G., Bauer, M., van Meerwijk, J., MacDonald, H. R., et al. (1999). Deficient T cell fate specification in mice with an induced inactivation of Notch1. Immunity 10, 547–558. doi: 10.1016/s1074-7613(00)80054-0
Rahmig, S., Kronstein-Wiedemann, R., Fohgrub, J., Kronstein, N., Nevmerzhitskaya, A., Bornhäuser, M., et al. (2016). Improved Human Erythropoiesis and Platelet Formation in Humanized NSGW41 Mice. Stem Cell Rep. 7, 591–601. doi: 10.1016/j.stemcr.2016.08.005
Reid, J. C., Tanasijevic, B., Golubeva, D., Boyd, A. L., Porras, D. P., Collins, T. J., et al. (2018). CXCL12/CXCR4 signaling enhances human PSC-derived hematopoietic progenitor function and overcomes early in vivo transplantation failure. Stem Cell Rep. 10, 1625–1641. doi: 10.1016/j.stemcr.2018.04.003
Renström, J., Istvanffy, R., Gauthier, K., Shimono, A., Mages, J., Jardon-Alvarez, A., et al. (2009). Secreted frizzled-related protein 1 extrinsically regulates cycling activity and maintenance of hematopoietic stem cells. Cell Stem Cell 5, 157–167. doi: 10.1016/j.stem.2009.05.020
Riesner, K., Shi, Y., Jacobi, A., Kräter, M., Kalupa, M., McGearey, A., et al. (2017). Initiation of acute graft-versus-host disease by angiogenesis. Blood 129, 2021–2032. doi: 10.1182/blood-2016-08-736314
Rongvaux, A., Willinger, T., Takizawa, H., Rathinam, C., Auerbach, W., Murphy, A. J., et al. (2011). Human thrombopoietin knockin mice efficiently support human hematopoiesis in vivo. Proc. Natl. Acad. Sci. U.S.A. 108, 2378–2383. doi: 10.1073/pnas.1019524108
Rostás, I., Pótó, L., Mátrai, P., Hegyi, P., Tenk, J., Garami, A., et al. (2017). In middle-aged and old obese patients, training intervention reduces leptin level: a meta-analysis. PLoS One 12:e0182801. doi: 10.1371/journal.pone.0182801
Ruf, F., Schreck, C., Wagner, A., Grziwok, S., Pagel, C., Romero, S., et al. (2016). Loss of Sfrp2 in the Niche amplifies stress-induced cellular responses, and impairs the in vivo regeneration of the hematopoietic stem cell Pool. Stem Cells 34, 2381–2392. doi: 10.1002/stem.2416
Sabbieti, M. G., Agas, D., Xiao, L., Marchetti, L., Coffin, J. D., Doetschman, T., et al. (2009). Endogenous FGF-2 is critically important in PTH anabolic effects on bone. J. Cell. Physiol. 219, 143–151. doi: 10.1002/jcp.21661
Saçma, M., Pospiech, J., Bogeska, R., de Back, W., Mallm, J.-P., Sakk, V., et al. (2019). Haematopoietic stem cells in perisinusoidal niches are protected from ageing. Nat. Cell Biol. 21, 1309–1320. doi: 10.1038/s41556-019-0418-y
Samuelson, C., Radtke, S., Cui, M., Perez, A., Kiem, H.-P., and Humbert, O. (2021). AMD3100 redosing fails to repeatedly mobilize hematopoietic stem cells in the nonhuman primate and humanized mouse. Exp. Hematol. 93, 52–60.e1. doi: 10.1016/j.exphem.2020.11.001
Sanchez-Aguilera, A., Arranz, L., Martin-Perez, D., Garcia-Garcia, A., Stavropoulou, V., Kubovcakova, L., et al. (2014). Estrogen signaling selectively induces apoptosis of hematopoietic progenitors and myeloid neoplasms without harming steady-state hematopoiesis. Cell Stem Cell 15, 791–804. doi: 10.1016/j.stem.2014.11.002
Sato, T. N., Qin, Y., Kozak, C. A., and Audus, K. L. (1993). Tie-1 and tie-2 define another class of putative receptor tyrosine kinase genes expressed in early embryonic vascular system. Proc. Natl. Acad. Sci. U.S.A. 90, 9355–9358. doi: 10.1073/pnas.90.20.9355
Schmitt-Graeff, A. H., Nitschke, R., and Zeiser, R. (2015). The hematopoietic niche in myeloproliferative neoplasms. Med. Inflamm. 2015:347270. doi: 10.1155/2015/347270
Schreck, C., Istvanffy, R., Ziegenhain, C., Sippenauer, T., Ruf, F., Henkel, L., et al. (2017). Niche WNT5A regulates the actin cytoskeleton during regeneration of hematopoietic stem cells. J. Exp. Med. 214, 165–181. doi: 10.1084/jem.20151414
Schuettpelz, L. G., Borgerding, J. N., Christopher, M. J., Gopalan, P. K., Romine, M. P., Herman, A. C., et al. (2014). G-CSF regulates hematopoietic stem cell activity, in part, through activation of Toll-like receptor signaling. Leukemia 28, 1851–1860. doi: 10.1038/leu.2014.68
Scott, L. M., Priestley, G. V., and Papayannopoulou, T. (2003). Deletion of alpha4 integrins from adult hematopoietic cells reveals roles in homeostasis, regeneration, and homing. Mol. Cell. Biol. 23, 9349–9360. doi: 10.1128/mcb.23.24.9349-9360.2003
Severe, N., Karabacak, N. M., Gustafsson, K., Baryawno, N., Courties, G., Kfoury, Y., et al. (2019). Stress-induced changes in bone marrow stromal cell populations revealed through single-cell protein expression mapping. Cell Stem Cell 25, 570–583.e7. doi: 10.1016/j.stem.2019.06.003
Shen, B., Tasdogan, A., Ubellacker, J. M., Zhang, J., Nosyreva, E. D., Du, L., et al. (2021). A mechanosensitive peri-arteriolar niche for osteogenesis and lymphopoiesis. Nature 591, 438–444. doi: 10.1038/s41586-021-03298-5
Shen, B., Vardy, K., Hughes, P., Tasdogan, A., Zhao, Z., Yue, R., et al. (2019). Integrin alpha11 is an Osteolectin receptor and is required for the maintenance of adult skeletal bone mass. eLife 8:e42274. doi: 10.7554/eLife.42274
Shin, J. Y., Hu, W., Naramura, M., and Park, C. Y. (2014). High c-Kit expression identifies hematopoietic stem cells with impaired self-renewal and megakaryocytic bias. J. Exp. Med. 211, 217–231. doi: 10.1084/jem.20131128
Sitnicka, E., Lin, N., Priestley, G. V., Fox, N., Broudy, V. C., Wolf, N. S., et al. (1996a). The effect of thrombopoietin on the proliferation and differentiation of murine hematopoietic stem cells. Blood 87, 4998–5005.
Sitnicka, E., Ruscetti, F. W., Priestley, G. V., Wolf, N. S., and Bartelmez, S. H. (1996b). Transforming growth factor beta 1 directly and reversibly inhibits the initial cell divisions of long-term repopulating hematopoietic stem cells. Blood 88, 82–88.
Stier, S., Ko, Y., Forkert, R., Lutz, C., Neuhaus, T., Grünewald, E., et al. (2005). Osteopontin is a hematopoietic stem cell niche component that negatively regulates stem cell pool size. J. Exp. Med. 201, 1781–1791. doi: 10.1084/jem.20041992
Sugiyama, T., Kohara, H., Noda, M., and Nagasawa, T. (2006). Maintenance of the hematopoietic stem cell Pool by CXCL12-CXCR4 chemokine signaling in bone marrow stromal cell niches. Immunity 25, 977–988. doi: 10.1016/j.immuni.2006.10.016
Sun, T., Ju, M., Dai, X., Dong, H., Gu, W., Gao, Y., et al. (2020). Multilevel defects in the hematopoietic niche in essential thrombocythemia. Haematologica 105, 661–673. doi: 10.3324/haematol.2018.213686
Taichman, R. S., and Emerson, S. G. (1994). Human osteoblasts support hematopoiesis through the production of granulocyte colony-stimulating factor. J. Exp. Med. 179, 1677–1682. doi: 10.1084/jem.179.5.1677
Takakura, N., Huang, X. L., Naruse, T., Hamaguchi, I., Dumont, D. J., Yancopoulos, G. D., et al. (1998). Critical role of the TIE2 endothelial cell receptor in the development of definitive hematopoiesis. Immunity 9, 677–686. doi: 10.1016/s1074-7613(00)80665-2
Takenaka, K., Prasolava, T. K., Wang, J. C. Y., Mortin-Toth, S. M., Khalouei, S., Gan, O. I., et al. (2007). Polymorphism in Sirpa modulates engraftment of human hematopoietic stem cells. Nat. Immunol. 8, 1313–1323. doi: 10.1038/ni1527
Takizawa, H., Fritsch, K., Kovtonyuk, L. V., Saito, Y., Yakkala, C., Jacobs, K., et al. (2017). Pathogen-Induced TLR4-TRIF innate immune signaling in hematopoietic stem cells promotes proliferation but reduces competitive fitness. Cell Stem Cell 21, 225–240.e5. doi: 10.1016/j.stem.2017.06.013
Tikhonova, A. N., Dolgalev, I., Hu, H., Sivaraj, K. K., Hoxha, E., and Cuesta-Domínguez, Á (2019). The bone marrow microenvironment at single-cell resolution. Nature 569, 222–228. doi: 10.1038/s41586-019-1104-8
Tripodo, C., Burocchi, A., Piccaluga, P. P., Chiodoni, C., Portararo, P., Cappetti, B., et al. (2017). Persistent immune stimulation exacerbates genetically driven myeloproliferative disorders via stromal remodeling. Cancer Res. 77, 3685–3699. doi: 10.1158/0008-5472.CAN-17-1098
Turner, N., and Grose, R. (2010). Fibroblast growth factor signalling: from development to cancer. Nat. Rev. Cancer 10, 116–129. doi: 10.1038/nrc2780
Tzeng, Y.-S., Li, H., Kang, Y.-L., Chen, W.-C., Cheng, W.-C., and Lai, D.-M. (2011). Loss of Cxcl12/Sdf-1 in adult mice decreases the quiescent state of hematopoietic stem/progenitor cells and alters the pattern of hematopoietic regeneration after myelosuppression. Blood 117, 429–439. doi: 10.1182/blood-2010-01-266833
Uckelmann, H., Blaszkiewicz, S., Nicolae, C., Haas, S., Schnell, A., Wurzer, S., et al. (2016). Extracellular matrix protein Matrilin-4 regulates stress-induced HSC proliferation via CXCR4. J. Exp. Med. 213, 1961–1971. doi: 10.1084/jem.20151713
UniProt Consortium (2021). UniProt: the universal protein knowledgebase in 2021. Nucleic Acids Res. 49, D480–D489. doi: 10.1093/nar/gkaa1100
Uyl-de Groot, C. A., Richel, D. J., and Rutten, F. F. (1994). Peripheral blood progenitor cell transplantation mobilised by r-metHuG-CSF (filgrastim); a less costly alternative to autologous bone marrow transplantation. Eur. J. Cancer Oxf. Engl. 1990, 1631–1635. doi: 10.1016/0959-8049(94)00328-3
Vandoorne, K., Rohde, D., Kim, H.-Y., Courties, G., Wojtkiewicz, G., Honold, L., et al. (2018). Imaging the vascular bone marrow niche during inflammatory stress. Circ. Res. 123, 415–427. doi: 10.1161/CIRCRESAHA.118.313302
Varnum-Finney, B., Purton, L. E., Yu, M., Brashem-Stein, C., Flowers, D., Staats, S., et al. (1998). The Notch ligand, Jagged-1, influences the development of primitive hematopoietic precursor cells. Blood 91, 4084–4091.
Verstegen, M. M. A., Wognum, A. W., and Wagemaker, G. (2003). Thrombopoietin is a major limiting factor for selective outgrowth of human umbilical cord blood cells in non-obese diabetic/severe combined immunodeficient recipient mice: TPO in NOD/SCID Mouse Recipients of Human CD34 + Cells. Br. J. Haematol. 122, 837–846. doi: 10.1046/j.1365-2141.2003.04498.x
Waclawiczek, A., Hamilton, A., Rouault-Pierre, K., Abarrategi, A., Albornoz, M. G., Miraki-Moud, F., et al. (2020). Mesenchymal niche remodeling impairs hematopoiesis via stanniocalcin 1 in acute myeloid leukemia. J. Clin. Invest. 130, 3038–3050. doi: 10.1172/JCI133187
Walkley, C. R., Olsen, G. H., Dworkin, S., Fabb, S. A., Swann, J., McArthur, G. A., et al. (2007a). A microenvironment-induced myeloproliferative syndrome caused by retinoic acid receptor gamma deficiency. Cell 129, 1097–1110. doi: 10.1016/j.cell.2007.05.014
Walkley, C. R., Shea, J. M., Sims, N. A., Purton, L. E., and Orkin, S. H. (2007b). Rb regulates interactions between hematopoietic stem cells and their bone marrow microenvironment. Cell 129, 1081–1095. doi: 10.1016/j.cell.2007.03.055
Walter, D., Lier, A., Geiselhart, A., Thalheimer, F. B., Huntscha, S., Sobotta, M. C., et al. (2015). Exit from dormancy provokes DNA-damage-induced attrition in haematopoietic stem cells. Nature 520, 549–552. doi: 10.1038/nature14131
Waskow, C., Madan, V., Bartels, S., Costa, C., Blasig, R., and Rodewald, H.-R. (2009). Hematopoietic stem cell transplantation without irradiation. Nat. Methods 6, 267–269. doi: 10.1038/nmeth.1309
Waskow, C., Paul, S., Haller, C., Gassmann, M., and Rodewald, H.-R. (2002). Viable c-KitW/W Mutants Reveal Pivotal Role for c-Kit in the Maintenance of Lymphopoiesis. Immunity 17, 277–288. doi: 10.1016/S1074-7613(02)00386-2
Waskow, C., Terszowski, G., Costa, C., Gassmann, M., and Rodewald, H.-R. (2004). Rescue of lethal c-KitW/W mice by erythropoietin. Blood 104, 1688–1695. doi: 10.1182/blood-2004-04-1247
Weickert, M.-T., Hecker, J. S., Buck, M. C., Schreck, C., Rivière, J., Schiemann, M., et al. (2021). Bone marrow stromal cells from MDS and AML patients show increased adipogenic potential with reduced Delta-like-1 expression. Sci. Rep. 11:5944. doi: 10.1038/s41598-021-85122-8
Wenk, C., Garz, A.-K., Grath, S., Huberle, C., Witham, D., Weickert, M., et al. (2018). Direct modulation of the bone marrow mesenchymal stromal cell compartment by azacitidine enhances healthy hematopoiesis. Blood Adv. 2, 3447–3461. doi: 10.1182/bloodadvances.2018022053
Wilkinson, A. C., Ishida, R., Kikuchi, M., Sudo, K., Morita, M., Crisostomo, R. V., et al. (2019). Long-term ex vivo haematopoietic-stem-cell expansion allows nonconditioned transplantation. Nature 571, 117–121. doi: 10.1038/s41586-019-1244-x
Wilson, A., MacDonald, H. R., and Radtke, F. (2001). Notch 1-deficient common lymphoid precursors adopt a B cell fate in the thymus. J. Exp. Med. 194, 1003–1012. doi: 10.1084/jem.194.7.1003
Winkler, I. G., Pettit, A. R., Raggatt, L. J., Jacobsen, R. N., Forristal, C. E., Barbier, V., et al. (2012). Hematopoietic stem cell mobilizing agents G-CSF, cyclophosphamide or AMD3100 have distinct mechanisms of action on bone marrow HSC niches and bone formation. Leukemia 26, 1594–1601. doi: 10.1038/leu.2012.17
Wolock, S. L., Krishnan, I., Tenen, D. E., Matkins, V., Camacho, V., Patel, S., et al. (2019). Mapping distinct bone marrow niche populations and their differentiation paths. Cell Rep. 28, 302–311.e5. doi: 10.1016/j.celrep.2019.06.031
Xie, M., Lu, C., Wang, J., McLellan, M. D., Johnson, K. J., Wendl, M. C., et al. (2014). Age-related mutations associated with clonal hematopoietic expansion and malignancies. Nat. Med. 20, 1472–1478. doi: 10.1038/nm.3733
Yamazaki, S., Ema, H., Karlsson, G., Yamaguchi, T., Miyoshi, H., Shioda, S., et al. (2011). Nonmyelinating schwann cells maintain hematopoietic stem cell hibernation in the bone marrow niche. Cell 147, 1146–1158. doi: 10.1016/j.cell.2011.09.053
Yeoh, J. S. G., van Os, R., Weersing, E., Ausema, A., Dontje, B., Vellenga, E., et al. (2006). Fibroblast growth factor-1 and -2 preserve long-term repopulating ability of hematopoietic stem cells in serum-free cultures. Stem Cells Dayt. Ohio 24, 1564–1572. doi: 10.1634/stemcells.2005-0439
Yin, X., Hu, L., Zhang, Y., Zhu, C., Cheng, H., Xie, X., et al. (2020). PDGFB-expressing mesenchymal stem cells improve human hematopoietic stem cell engraftment in immunodeficient mice. Bone Marrow Transpl. 55, 1029–1040. doi: 10.1038/s41409-019-0766-z
Yoshihara, H., Arai, F., Hosokawa, K., Hagiwara, T., Takubo, K., Nakamura, Y., et al. (2007). Thrombopoietin/MPL signaling regulates hematopoietic stem cell quiescence and interaction with the osteoblastic niche. Cell Stem Cell 1, 685–697. doi: 10.1016/j.stem.2007.10.020
Yu, B., Huo, L., Liu, Y., Deng, P., Szymanski, J., Li, J., et al. (2018). PGC-1α controls skeletal stem cell fate and bone-fat balance in osteoporosis and skeletal aging by inducing TAZ. Cell Stem Cell 23, 193–209.e5. doi: 10.1016/j.stem.2018.06.009
Yuasa, H., Takakura, N., Shimomura, T., Suenobu, S., Yamada, T., Nagayama, H., et al. (2002). Analysis of human TIE2 function on hematopoietic stem cells in umbilical cord blood. Biochem. Biophys. Res. Commun. 298, 731–737. doi: 10.1016/s0006-291x(02)02524-x
Yue, R., Shen, B., and Morrison, S. J. (2016). Clec11a/osteolectin is an osteogenic growth factor that promotes the maintenance of the adult skeleton. eLife 5:e18782. doi: 10.7554/eLife.18782
Yurino, A., Takenaka, K., Yamauchi, T., Nunomura, T., Uehara, Y., Jinnouchi, F., et al. (2016). Enhanced Reconstitution of human Erythropoiesis and Thrombopoiesis in an immunodeficient mouse model with Kit Wv mutations. Stem Cell Rep. 7, 425–438. doi: 10.1016/j.stemcr.2016.07.002
Zambetti, N. A., Ping, Z., Chen, S., Kenswil, K. J. G., Mylona, M. A., Sanders, M. A., et al. (2016). Mesenchymal inflammation drives Genotoxic stress in hematopoietic stem cells and predicts disease evolution in human pre-leukemia. Cell Stem Cell 19, 613–627. doi: 10.1016/j.stem.2016.08.021
Zhang, H., Kozono, D. E., O’Connor, K. W., Vidal-Cardenas, S., Rousseau, A., Hamilton, A., et al. (2016). TGF-β inhibition rescues hematopoietic stem cell defects and bone marrow failure in fanconi anemia. Cell Stem Cell 18, 668–681. doi: 10.1016/j.stem.2016.03.002
Zhang, J., Niu, C., Ye, L., Huang, H., He, X., Tong, W.-G., et al. (2003). Identification of the haematopoietic stem cell niche and control of the niche size. Nature 425, 836–841. doi: 10.1038/nature02041
Zhang, J., Wu, Q., Johnson, C. B., Pham, G., Kinder, J. M., Olsson, A., et al. (2021). In situ mapping identifies distinct vascular niches for myelopoiesis. Nature 590, 457–462. doi: 10.1038/s41586-021-03201-2
Zhao, M., Perry, J. M., Marshall, H., Venkatraman, A., Qian, P., He, X. C., et al. (2014). Megakaryocytes maintain homeostatic quiescence and promote post-injury regeneration of hematopoietic stem cells. Nat. Med. 20, 1321–1326. doi: 10.1038/nm.3706
Zhao, M., Ross, J. T., Itkin, T., Perry, J. M., Venkatraman, A., Haug, J. S., et al. (2012). FGF signaling facilitates postinjury recovery of mouse hematopoietic system. Blood 120, 1831–1842. doi: 10.1182/blood-2011-11-393991
Zhong, L., Yao, L., Tower, R. J., Wei, Y., Miao, Z., Park, J., et al. (2020). Single cell transcriptomics identifies a unique adipose lineage cell population that regulates bone marrow environment. eLife 9:e54695. doi: 10.7554/eLife.54695
Zhou, B. O., Ding, L., and Morrison, S. J. (2015). Hematopoietic stem and progenitor cells regulate the regeneration of their niche by secreting Angiopoietin-1. eLife 4:e05521. doi: 10.7554/eLife.05521
Keywords: niche, microenvironment, bone marrow, hematopoiesis, leukemia, aging, transplantation, xenograft
Citation: Fröbel J, Landspersky T, Percin G, Schreck C, Rahmig S, Ori A, Nowak D, Essers M, Waskow C and Oostendorp RAJ (2021) The Hematopoietic Bone Marrow Niche Ecosystem. Front. Cell Dev. Biol. 9:705410. doi: 10.3389/fcell.2021.705410
Received: 05 May 2021; Accepted: 28 June 2021;
Published: 22 July 2021.
Edited by:
Aldo Roccaro, Civil Hospital of Brescia, ItalyReviewed by:
Hannes Klump, Essen University Hospital, GermanyBorhane Guezguez, German Cancer Research Center (DKFZ), Germany
Copyright © 2021 Fröbel, Landspersky, Percin, Schreck, Rahmig, Ori, Nowak, Essers, Waskow and Oostendorp. This is an open-access article distributed under the terms of the Creative Commons Attribution License (CC BY). The use, distribution or reproduction in other forums is permitted, provided the original author(s) and the copyright owner(s) are credited and that the original publication in this journal is cited, in accordance with accepted academic practice. No use, distribution or reproduction is permitted which does not comply with these terms.
*Correspondence: Claudia Waskow, Y2xhdWRpYS53YXNrb3dAbGVpYm5pei1mbGkuZGU=; Robert A. J. Oostendorp, cm9iZXJ0Lm9vc3RlbmRvcnBAdHVtLmRl