- 1Exopharm Ltd., Melbourne, VIC, Australia
- 2Department of Biochemistry and Pharmacology, University of Melbourne, Parkville, VIC, Australia
Mesenchymal stromal cells (MSCs) are multipotent cells obtained from many tissues including bone marrow, adipose tissue, umbilical cord, amniotic fluid, and placenta. MSCs are the leading cell source for stem cell therapy due to their regenerative and immunomodulatory properties, their low risk of tumorigenesis and lack of ethical constraints. However, clinical applications of MSCs remain limited. MSC therapeutic development continues to pose challenges in terms of preparation, purity, consistency, efficiency, reproducibility, processing time and scalability. Additionally, there are issues with their poor engraftment and survival in sites of disease or damage that limit their capacity to directly replace damaged cells. A key recent development in MSC research, however, is the now widely accepted view that MSCs primarily exert therapeutic effects via paracrine factor secretion. One of the major paracrine effectors are extracellular vesicles (EVs). EVs represent a potential cell-free alternative to stem cell therapy but are also rapidly emerging as a novel therapeutic platform in their own right, particularly in the form of engineered EVs (EEVs) tailored to target a broad range of clinical indications. However, the development of EVs and EEVs for therapeutic application still faces a number of hurdles, including the establishment of a consistent, scalable cell source, and the development of robust GMP-compliant upstream and downstream manufacturing processes. In this review we will highlight the clinical challenges of MSC therapeutic development and discuss how EVs and EEVs can overcome the challenges faced in the clinical application of MSCs.
Introduction
Mesenchymal stromal cells (MSCs) were identified more than 5 decades ago and have captured substantial attention among the scientific community since then due to their potential clinical applications (Friedenstein et al., 1970; Haynesworth et al., 1992). The ability of MSCs to differentiate into various cell types, and to support tissue function and repair, was quickly recognized. Since their initial discovery in bone marrow, MSCs from many other organs and tissues including adipose tissue, placenta, umbilical cord, Wharton’s Jelly, peripheral blood, skeletal muscle, skin, heart, liver, and the brain have also been isolated and studied (Baksh et al., 2004).
Mesenchymal stromal cells’ particular biological properties, including their self-renewal, proliferation and differentiation potential, make them attractive stem cell resources for pre-clinical and clinical studies that focus on the repair and/or regeneration of damaged tissue (Uccelli et al., 2008). The beneficial attributes of MSCs include their high resistance to oxidative stress, potent differentiation capacity, unique immunophenotypic characteristics, limited ethical constraints, low risk of tumor formation and powerful immunomodulatory activity (Trounson and McDonald, 2015). The medical interest in MSCs in indications ranging from cardiac conditions to neurodegenerative diseases has so far led to more than 1200 clinical trials being listed on clinicaltrials.gov.
The path from pre-clinical promise into clinical use has not been smooth, however. Multiple MSC therapeutics have failed to demonstrate efficacy in early or late clinical trials, in indications ranging from amyotrophic lateral sclerosis to stroke (Levy et al., 2020; FDA, 2021). Despite more than 30 years of work very few MSC therapeutic products have been approved for clinical use in any jurisdiction, indicating the significant pre-clinical and clinical challenges that remain to be resolved.
Despite the setbacks the field has experienced, the scale of effort still being applied to MSC-based therapeutics development is testament to the clinical promise that MSCs and their derivatives still hold. MSCs continue to show an excellent safety profile and remain the most studied stem cell population, particularly in the context of therapeutic use.
One of the most significant shifts in the MSC research field over the past decade has been the growing recognition that, rather than acting by engrafting and differentiating in sites of disease or injury to directly replace damaged cells, MSCs primarily exert their therapeutic effects via the release of paracrine factors (Yang et al., 2013). One of the major paracrine effectors are extracellular vesicles (EVs); nanoscale lipid-wrapped packages of lipids, proteins and nucleic acids that possess the same therapeutic properties as their parent cells (Bruno et al., 2009; Lai et al., 2010). As a cell-free MSC product, EV therapeutics pose few of the complications hampering the development of MSC cell therapies.
This review aims to highlight the ongoing issues associated with the development of MSC therapeutics and also to discuss how EVs isolated from MSCs overcome these issues to replace or complement cell-based therapies.
Challenges in the Clinical Application of MSCs
Most MSC clinical studies have produced disappointing findings despite encouraging results in pre-clinical animal studies. Although 10 MSC therapeutics have received market approval in some jurisdictions (Table 1), in general low efficacy continues to blight MSC clinical trials. Some of the reasons proposed for this low efficacy are discussed below.
MSC Diversity
One factor that can lead to unexpected clinical outcomes is the significant heterogeneity that can exist between MSCs from different sources. MSCs isolated from different tissues show differences in their proliferative behavior and differentiation capacity in both in vitro and in vivo studies (Hass et al., 2011). Even when isolated from the same tissue type, significant differences in MSC populations have been observed between individual donors, with the characteristics of MSCs varying according to factors such as the donor’s age, health, sex, and body weight. For example, the age-associated deficits observed for MSCs include loss of key attributes such as proliferation and differentiation potential (Zhou et al., 2008). A study of aged bone marrow derived MSCs (BM-MSCs) recorded increased senescence, and a loss of bone formation capability (Stolzing et al., 2008). The decline of MSC function with age has significant implications for autologous use–particularly when considering that ill health itself can impair MSC function (van Rhijn-Brouwer et al., 2018).
Donor sex can also have an impact on the characteristics and function of harvested MSCs. In a rat model of lung inflammation, Female BM-MSCs reduced inflammation more effectively than BM-male MSCs (Sammour et al., 2016). A meta-analysis of human adipose tissue derived MSCs showed significant differences in the gene expression of cells from males and females, with the changes predicted to affect processes including inflammation, differentiation and cell communication (Bianconi et al., 2020).
MSC Manufacturing Challenges
Once harvested, MSCs often need to be expanded to generate sufficient cells to be formulated into therapeutic doses. Treating a condition such as graft-versus-host disease may require tens of millions of cells per dose (Introna et al., 2014). Low cell harvest yield is particularly acute for BM-MSCs (Pittenger et al., 1999). Scale-up to a cell number sufficient for clinical use usually involves their proliferation in a large batch culture system. This process is lengthy and costly and therefore commercially unattractive. Additionally, MSC expansion and long-term culture to generate sufficient MSCs for clinical studies is often associated with increasing cell senescence and decreasing potency (Wagner et al., 2009).
Cost of MSC product manufacture and delivery is a significant barrier to its commercial viability. Depending on production scale and dose size, the cost of goods (COG) per dose varies dramatically, from US$485 to US$111,488 (Chilima et al., 2018). Technological advances such as bioreactors have been proposed to alleviate COG issues. This development may have the potential to improve MSC manufacturing output, and lower production costs (Chilima et al., 2018) but may not sufficiently address the COG issues. For example, hollow-fiber bioreactors were recently shown to be the least cost-effective manufacturing method due to high consumables and equipment costs with a COG almost double that required for a product to be commercially viable (Mizukami et al., 2018).
Culture medium development is another challenge for MSC production at the clinical level. Culture and expansion of MSCs has traditionally required media enriched with serum, but the complex and variable nature of this mixture of nutrients, growth factors and other constituents poses further challenges for maintaining product consistency. The development of serum-free media or chemically defined media is encouraging but they generally do not perform as well, especially for longer passages and scaling-up. They also put upward pressure on COG (Jung et al., 2012).
Bioengineering to Boost the Clinical Potential of MSCs
The potential for clinical-scale MSC manufacture has been restricted by limited yield of donated cells, quality variation, and biosafety concerns regarding potential transmission of pathogens. An ideal cell source for industrial-scale MSC product manufacture should offer easy and unrestricted availability, have regulatorily acceptable provenance, present no biological safety risks, and be amenable to unlimited expansion while retaining its original “as harvested” phenotype. In a step toward this ideal, bioengineering approaches to increase both the yield and the homogeneity of MSCs are now being explored. For example, differentiation of induced pluripotent stem cells (iPSCs) to MSCs can be expanded to produce large quantities of cells thereby generating large quantities of highly homogenous MSCs (Ozay et al., 2019). Although concerns have been raised regarding the teratogenic potential of iPSC-derived cells, MSCs produced from iPSCs have been shown not to form teratomas or to show pro-tumor potential (Qingguo et al., 2015). After a series of pre-clinical studies, in indications including critical limb ischemia, asthma and organ transplant rejection, this approach was recently assessed in a Phase I clinical trial for acute steroid-resistant graft-versus-host disease (Bloor et al., 2020). No adverse events were reported during this study. In a related process, researchers have used a CRISPR/Cas9-based strategy to temporarily immortalize BM-MSCs, to readily expand these cells in long-term culture without the phenotypic changes that typically accumulate in high passage MSCs, before reversing the immortalization for potential therapeutic use (Hu et al., 2017). The safety of these reversibly immortalized cells now needs to be investigated before being used in clinical trials.
Despite the potential of engineered MSC sources to overcome specific limitations in MSC production, additional issues associated with MSC therapeutics persist. Immortalized live cell therapies may retain the issues associated with potential immunogenicity and tumorigenicity, and their engraftment qualities post-administration remain to be elucidated.
EVs as a Novel Class of Therapeutic
Although it was previously believed that the therapeutic potential of MSCs was due to cell-to-cell contact or engraftment and differentiation of transplanted MSCs, it has become increasingly clear that MSC therapeutics’ prime mode of action is to release paracrine factors (Timmers et al., 2008; Caplan and Correa, 2011). In one non-human primate study of MSC infusion following total body irradiation, MSC engraftment levels were found to be below 3% (Devine et al., 2003). Similarly, in a mouse model of emphysema, Katsha et al. (2011) found that intratracheal injection with fluorescent labeled MSCs restored lung function, yet few engrafted MSCs and no differentiated cells could be detected in the lung beyond day 7 post-injection. The observed therapeutic effect was attributed to MSC paracrine factor release. Seminal papers reported that administration of conditioned media from cultured MSCs exerted the same beneficial effects as with whole cells (Gnecchi et al., 2006; Lai et al., 2010). Paracrine factors within the media were shown to be taken up by cells in the damaged tissue or by immune cells to promote cellular rejuvenation and restoration of tissue function. Prominent among the paracrine factors exerting these effects are EVs. Isolated MSC-EVs have shown to possess the same therapeutic potential as their parent cells (Bruno et al., 2009). This discovery has contributed to the interest in, and development of, EVs as medicines. As next generation, cell-free, MSC-based therapeutics, EVs have significant advantages in overcoming the limitations and risks associated with MSC-based cell therapy, as discussed below.
EVs as a Cell-Free Application of MSCs
Extracellular vesicles are lipid bilayer-wrapped vesicles approximately 30–200 nanometers in size, released by virtually every cell type in the body. Once thought to be a mechanism for cellular refuse disposal, EVs are now known to be key mediators of cell-to-cell communication, delivering a cargo of lipids, proteins and nucleic acids that reflects their cell of origin. Although significantly simpler than live MSCs, EVs are still highly complex in composition and exert their effects by either transferring bioactive cargo such as functional protein and miRNAs that alter cell fate, or by modulating cell surface receptors and triggering intracellular signaling pathways (Xin et al., 2012; Guo et al., 2017).
In the case of MSC-derived EVs, they have been found to share many attributes such as content, immunophenotype and unique homing ability (tropism) as their parental MSCs (Wei et al., 2021). As a result, their intrinsic therapeutic ability has been heavily investigated in recent years. MSC-EVs have been demonstrated as a potential treatment for several clinical indications including graft-versus-host disease (Kordelas et al., 2014), kidney injury (Nassar et al., 2016), myocardial infarction (Lai et al., 2010), systemic lupus erythematosus (Liu et al., 2015) and wound healing (Guo et al., 2017), and represent a novel therapeutic modality that could overcome roadblocks faced by MSC-based cell therapies (Figure 1).
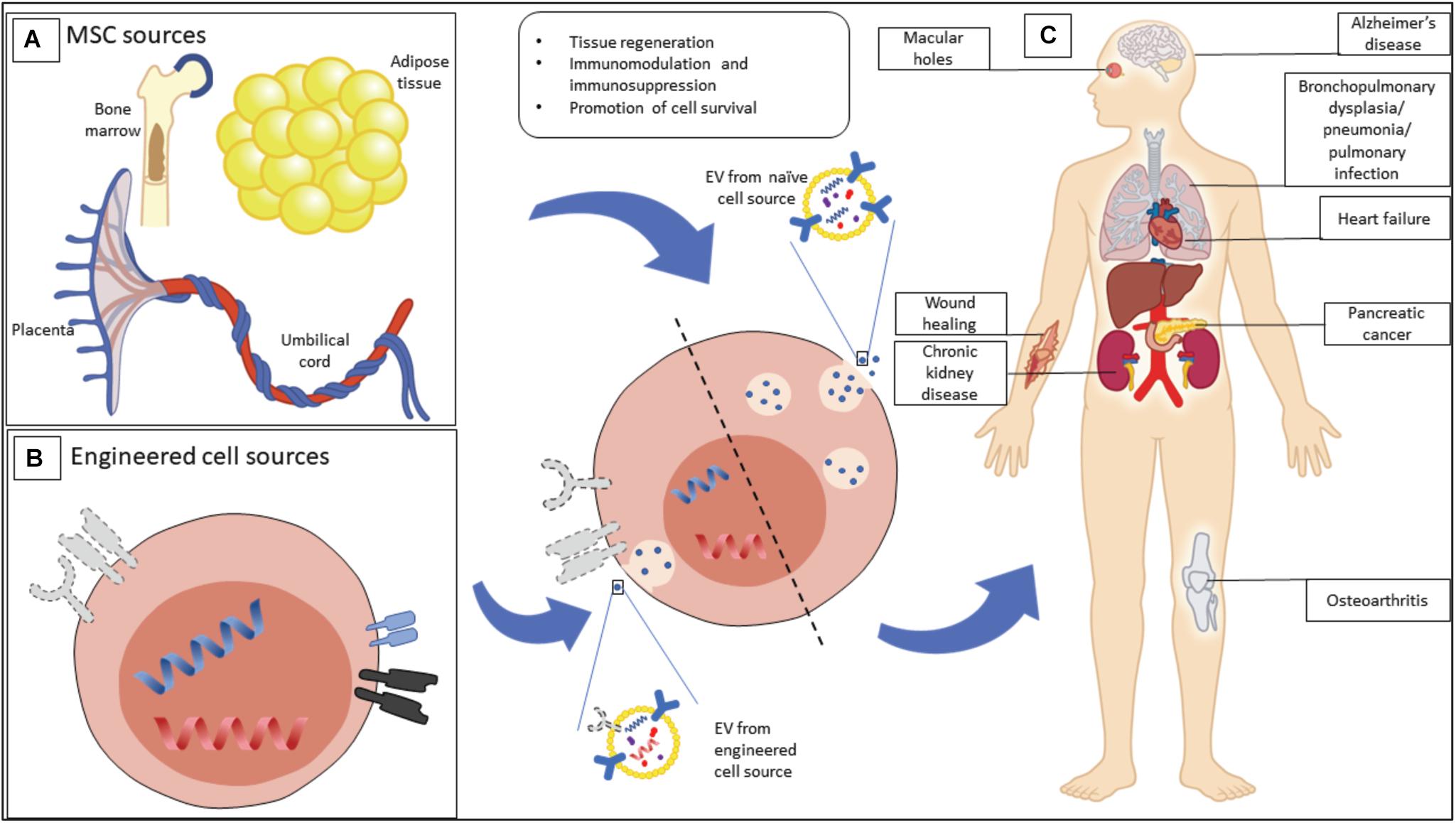
Figure 1. Mesenchymal stromal cell (MSC) EVs have therapeutic potential for a range of possible indications. (A) MSCs, as a source of MSC-EVs, can be isolated from a wide range of tissues, including bone marrow, adipose tissue, and birth-associated tissue. (B) MSCs can be engineered in order to enhance their output of EVs, or to produce EVs with particular properties. (C) Beneficial properties of EVs from naive and engineered sources are being explored for a range of clinical indications.
The Advantages of EVs Compared to Cell-Based Therapies
The nature of EVs helps overcome several technological challenges faced by MSC-based therapy. Firstly, due to their phospholipid bilayer EVs are more resistant to damage caused by freeze-thaw cycles (Jeyaram and Jay, 2017) and possess high in vivo stability (Dang et al., 2020). In mice, intravenously injected MSC-EVs labeled with a bioluminescent dye were found to have an EV plasma half-life of 1.2–1.3 min due to their rapid uptake into tissues, where they were still detectable 24 h post-injection (Shelke et al., 2018). Secondly, as EVs do not self-replicate, they bypass the risk of endogenous tumor-formation that accompanies MSC-based therapy (Volarevic et al., 2018). Moreover, their small size as well as low or lack of expression of membrane histocompatibility complexes reduces the risk of inducing immune responses (Reis et al., 2016).
Extracellular vesicles also represent a novel strategy for hard-to-treat diseases for which there is a high unmet medical need. For example, in the treatment of neurological disorders some studies have shown that MSCs have difficulty transmigrating across the blood–brain barrier (BBB). In one study, MSCs were found to be retained in the lungs (Wang et al., 2010), while another reported that MSCs required a permeabilizing agent to cross the BBB (Cerri et al., 2015). Conversely, MSC-EVs have been shown to cross the BBB offering solutions in terms of route of administration and dosage (Moon et al., 2019). An example of their potential utility demonstrates that they can induce immunomodulatory and neuroprotective effects in a 3xTg model of Alzheimer’s disease (Losurdo et al., 2020) after systemic administration. MSC-EVs can also regulate neuronal cell apoptosis in vitro (Wei et al., 2020).
As EVs can be continually harvested from a MSC population, they can potentially overcome some of the issues of limited supply associated with MSCs themselves. As MSCs are highly amenable to modification, EV production can potentially be boosted to improve scalability. For example, the application of various stimuli to the parent cells, including physical stressors such as hypoxia or mechanical forces, or the addition of various small molecule modulators, can increase EV release (Zhu et al., 2017; Wang et al., 2020). MSCs have also been shown to produce significantly higher yields of EVs when grown in three-dimensional bioreactors rather than two-dimensional cell culture, and the EVs produced in these reactors can show enhanced therapeutic properties (Yan and Wu, 2020).
From the perspective of drug manufacturing, EVs offer several logistical advantages over cell-based therapies. These includes the possible addition of sterile filtration of the drug substance before aseptic filling, unfeasible for whole cells (Gimona et al., 2017). EVs are also suited to a wider range of storage conditions including flexibility in storage buffers as well as preservation techniques (Kusuma et al., 2018). Methods such as lyophilisation has been successfully utilized to store EVs (Frank et al., 2018) leading to an extended shelf-life and reduced costs due to a simplified cold chain (Kusuma et al., 2018). These characteristics suggest EV therapeutics could be developed as “off-the-shelf” products.
Bioengineering to Broaden EV Therapeutic Utility
The therapeutic application of MSC-derived EVs is not limited to naïve, i.e., naturally secreted, EVs. The parent cell or its secreted EVs can be bioengineered to generate an EV product with enhanced or altered therapeutic properties. EV engineering strategies can be divided into two broad categories. Firstly, EVs can be engineered to alter their tropism and bias their uptake toward a target cell type. Alternatively, or in addition, EVs can be loaded with a particular therapeutic cargo.
Engineering EVs for Altered Tropism
A key factor determining the potential therapeutic utility of MSC-EVs is their fate once infused into the body. Several biodistribution studies have used dye- or bioluminescent-labeled naïve EVs to observe EV uptake by different tissues following infusion into animals (Wiklander et al., 2015; Shelke et al., 2018).
In a study on MSC-EVs labeled with a near-infrared lipophilic dye, more than 70% of EVs were found to accumulate in the liver when systemically injected into mice (Wiklander et al., 2015). Other animal studies have analyzed labeled naive EVs from a range of cell sources and shown that the EVs distribute widely through the body but accumulate primarily in the liver and spleen (Charoenviriyakul et al., 2017; Shelke et al., 2018). This natural tropism may be exploited to treat conditions associated with these organs. However, to enhance EV accumulation in other organs, EV engineering may be considered. This approach has been received favorably by some biopharma companies who are incorporating it into their developmental pipeline for “hard-to-treat disease,” e.g., cancer (Lewis et al., 2021).
Engineered EV (EEV) in vivo tropism may be altered by incorporating selected surface proteins onto their surface. One method for conferring targeting capabilities onto EVs is to manipulate the parent cell. One route is to create fusion proteins, in which the protein tail attaches or inserts into the EV membrane, and the head binds a receptor on the target cell type. In one early example, a fusion protein created between EV membrane protein Lamp2b and the rabies viral glycoprotein (RVG) peptide which binds the acetylcholine receptor on brain cells. These EEVs were used to target neurons, microglia and oligodendrocytes in the brain after systemic injection (Lydia et al., 2011). More recently, Lu et al. showed that a glycoprotein-g originally identified on the vesicular stomatitis virus, VSV-G, can be readily incorporated into nascent EV’s membrane by the parent cell. In addition, a large section of the VSV-G protein can be substituted for a “tropic” protein (Meyer et al., 2017). This approach has been shown to target EEVs to specific cell types including B cells, neurons and tumor cells (Yang et al., 2018).
Targeting proteins can also be incorporated onto EVs after their isolation from cell culture. Tian et al. (2021) recently showed that fusion proteins that bind the phosphatidylserine component of the EV lipid membrane can be used to target EVs to ischemic brain tissue after systemic injection, relieving inflammation. The targeting protein was attached to EVs by simple incubation. A similar approach has also been used to target tumor cells in a mouse model of glioblastoma (Zhilan et al., 2018).
As well as altering EV tropism, proteins can be added to the vesicle surface for a range of other applications. For example, EEVs with protein receptors on their surface have been developed as decoys to capture target molecules such as the pro-inflammatory cytokine IL-6, as a potential therapeutic for chronic inflammatory diseases (Conceição et al., 2021). Another study demonstrated that EEVs engineered to express CD47 on their surface have reduced systemic clearance, which extends circulation time to improve targeting of pancreatic cancer cells (Kamerkar et al., 2017).
Engineering EVs as Therapeutic Delivery Vehicles
Mesenchymal stromal cell-EVs are known to possess a cargo that can promote regeneration in damaged tissues, for example by lowering inflammation and inhibiting apoptosis to promote healing. EV engineering allows the possibility of loading EVs with a defined therapeutic cargo that could enhance this capability. MSCs have been engineered to overexpress microRNA-let7c, to generate EEVs which contain elevated levels of this microRNA. Infusion of these MSC-derived EEVs attenuated renal fibrosis in a mouse model of unilateral ureteral obstruction (Wang et al., 2016). More recently, MSC engineered to overexpress bone morphogenetic protein 2 produced EEVs with an enhanced capacity to promote bone regeneration (Chun-Chieh et al., 2020).
However, EV drug loading methodologies also enable the potential development of EEV therapeutics for a wide range of indications beyond tissue regeneration and healing. The key characteristics of EVs–non-immunogenic capsules able to deliver cargo to a target cell type–appear to make them ideal as drug delivery vehicles, such as for targeting tumor cells.
Many therapeutics, from small molecule drugs to RNAs, need to be shepherded to their site of action, either to overcome unfavorable pharmacokinetics, as protection from metabolic breakdown, or to reduce off-target effects. Synthetic delivery systems such as liposomes or nanoparticles have been limited by poor stability in storage, toxicity, poor efficacy and a limited capability to deliver cargo to target tissues other than the liver (Setten et al., 2019).
As knowledge surrounding EV biogenesis and mechanisms of cargo sorting has increased, new strategies have emerged to generate EEVs that contain a defined therapeutic cargo. The techniques associated with the generation of EEVs include incubation with drugs for delivery (Pascucci et al., 2014) or transfection of the parent cells to allow specific small RNA and small molecules to be incorporated into their EVs. For example, MSCs have been engineered to produce EVs enriched in the microRNA miR-379, a potent tumor suppressor. In a mouse model of breast cancer, systemic administration of the miR-379 EEVs significantly reduced tumor growth–whereas administration of the engineered parental MSCs themselves had no impact on tumor growth (O’Brien et al., 2018).
In head-to-head testing, EVs were recently shown to be superior to liposomes for RNA transfer, delivering a cargo of RNA several orders of magnitude more efficiently into cells (Murphy et al., 2021). The biggest hurdle, the researchers noted, was to efficiently load the RNA cargo into EVs–but emerging technologies can offer highly effective ways to accomplish EV RNA loading. Nguyen and Ferguson at the University at Buffalo identified RNA sequences that are specifically loaded by cells into EVs. By attaching therapeutic RNA to these sequences, up to 100-fold gains in loading of the therapeutic RNA into EVs was achieved (Nguyen and Ferguson, 2018).
Extracellular vesicle loading can also take place after EV isolation from cell culture. Techniques including freeze-thawing, sonication, electroporation, osmotic shock and saponin permeabilization have been developed to temporarily disrupt the EV membrane sufficient for uptake of a therapeutic cargo. However, as the cargo loading method itself may alter the biophysical characteristics or biological function of treated EVs, and the loading efficiency can vary between different types of cargo, care must be taken to determine the appropriate cargo-loading methodology in each case (Franziska et al., 2021).
Clinical Application of EVs and EEVs
The progress made in EV pre-clinical studies and the additional advantages of EVs relative to whole cell therapeutics has led to a growing number of MSC-EV human clinical trials. Over 80 studies have been registered at the www.ClinicalTrials.gov database to assess the therapeutic effects of EVs in several therapeutic arenas. MSC-EVs have demonstrated acceptable safety and tolerability profiles, and promising signs of therapeutic efficacy (Table 2).
In one early study, for example, repeated injections of MSC-EVs demonstrated significant improvement in a patient with severe therapy-refractory acute graft-versus-host disease. The patient’s pro-inflammatory cytokine levels and disease symptoms improved markedly after MSC-EVs therapy, and the patient’s improvement remained stable for at least 4 months after treatment (Kordelas et al., 2014). In a Phase II/III placebo-controlled clinical trial, umbilical cord blood derived MSC-EVs administration was shown to be safe, and to modulate inflammation and improve kidney function, in patients with chronic kidney disease (Nassar et al., 2016).
Studies currently underway include a safety study of the use of bone marrow MSC-EVs to treat bronchopulmonary dysplasia, a chronic lung disease that mainly affects premature babies (NCT03857841); a Phase I study of MSC-EVs loaded with a siRNA therapeutic for patients with pancreatic cancer (NCT03608631); and a study to assess MSC-EV administration for improvement of disability in patients with acute ischemic stroke (NCT03384433).
There are also studies underway to test inhaled MSC-EVs in patients with acute respiratory distress syndrome (ARDS) associated with severe cases of COVID-19 (NCT04602442; NCT04602104). Table 2 summarizes clinical trials that have been performed using EVs isolated from MSCs.
Extracellular vesicles from other sources than MSCs have also been used in clinical trials. Significantly, in addition to the early phase trials conducted by academic groups, several trials of experimental EV therapeutic products developed by biopharmaceutical companies are now underway, reflecting the increasing maturity of the therapeutic EV field. These trials include platelet derived EVs tested by Exopharm in two Phase I clinical trials for wound healing applications (ANZCTR, 2019, 2020). Codiak Biosciences is currently investigating engineering the HEK293 cell line for their clinical applications, and in late 2020 commenced two Phase I clinical trials testing the safety and efficacy of EEVs for certain cancers (Clinicaltrials.gov, 2020).
Despite rapid development in the EV field and the commencement of clinical trials, several challenges remain and should be resolved before EVs can achieve widespread clinical utility. These challenges are discussed in the following section.
Challenges in the Clinical Application of EVs
Although regulatory approval and safety of clinical application of EVs appears feasible, and may be simpler than cell-based therapy, important barriers that need to be addressed for clinical GMP-grade production of EVs include selection of the starting cellular material and optimizing cell culture, purification, quantification, and quality control (Gowen et al., 2020). Understanding and determining the quantity, purity and potency of EVs, by the development of appropriate assays, will be an important part of the quality control criteria (Gimona et al., 2021). Further challenges include issues of EV preservation and long-term stability, as we also go on to describe below.
Identifying Ideal EV Cell Sources
As EV are a secreted product of cells, their manufacture is heavily dependent on the ability to produce large quantities of cells in ways that do not alter their phenotype. However, the opportunities for producing large quantities of stem-cell based conditioned medium with which to undertake meaningful scale-up of EV production are limited.
Generally, for stem cell derived EVs, the potential cell sources for EV production are either MSCs, or pluripotent stem cells (PSCs) such as embryonic stem cells (ESCs) or iPSCs derived lineages. In each case there are advantages and disadvantages associated with their clinical use, which are summarized in Table 3.
Primary or somatic MSCs may not be an ideal cell source for EV manufacture at clinical scale due to their limited lifespan, heterogeneity and batch-to-batch or donor-to-donor variations. One approach to increase the yield of EVs from MSCs is to immortalize MSCs using the hTERT method or a CRISPR/Cas9-based strategy, to enable long term cell culture and scaled up EV production. Recently, EVs from immortalized MSCs were shown to be non-tumorigenic both in vitro and in vivo, neither promoting nor inhibiting tumor growth (Tan et al., 2021).
Alternatively, pluripotent cells could be exploited as source cells. As stated previously, the ideal cell source for industrial-scale EV production should have unrestricted availability, regulatorily acceptable provenance, present no biological safety risks, and be amenable to unlimited expansion while retaining its original “as harvested” phenotype. The only cell types which can have most or least some of these characteristics are ESCs and iPSCs. The main advantage of these cells is their unlimited capacity and growth. The main disadvantages of these cells are the ethical concern in the case of ESCs, and the likelihood of immune responses and risk of teratoma for iPSCs.
An approach to exploit the benefits of pluripotent cells, but navigate their disadvantages, could be to incorporate iPSCs as a source of MSCs for EV manufacture. This approach might increase the yield and the homogeneity of the cell source for industrial scale production of EVs. The same scenario can also be applied to ESCs, by directly differentiating ESCs to MSCs. The MSCs derived from these sources have shown similar properties to adult MSCs, including their differentiation potential and immunomodulatory effect (Bloor et al., 2020). Several studies have demonstrated that these cell lines have superior efficacy over MSCs derived from somatic tissues (Jiang et al., 2019). The unique advantages of PSC derived MSCs over adult MSCs include unlimited supply, high purity, lower cost and most importantly, scalable production. The process of manufacturing clinical GMP-grade MSCs involves donor identification, screening, tissue harvest, cell isolation, purification and expansion which require a theater and clinician involvement as well as GMP facilities each time. Since this whole process must be repeated several times due to the limited expansion of adult MSCs compared with iPSC derived MSCs, this can increase the cost of GMP-grade production of adult MSCs compared with iPSC derived MSC line. These factors are all important for the development of a cell system for MSC-EV manufacture.
Alternate EV Cell Sources for EEV Applications
For therapeutic applications in which EVs are employed for their drug delivery capabilities rather than for their inherent regenerative properties, a broader range of EV cell sources can be considered. One potentially important cell source being explored for the mass production of EEVs are human stable cell lines such as HEK293. Currently, HEK293 cells are predominantly used for recombinant protein production and vector transfection. HEK293 cell derived EVs are a promising platform from which to produce EEVs modified specifically for the clinical indication of interest.
The main advantages of HEK293 are that this cell line proliferates rapidly, can be easily grown in serum-free suspension culture, and importantly is FDA-approved as a human cell line for recombinant protein production. As such, this cell type has become a particular focus for biotechnology companies developing EEV therapeutics. For instance, Codiak Bioscience is using HEK293 to produce engineered EVs as a potential cancer therapy and is conducting clinical trials using HEK293 engineered cells as an EEV source for the treatment of cutaneous T cell lymphoma and solid tumors (Dooley et al., 2021). In addition, ILIAS Biologics researchers and their collaborators have used HEK239-derived EEVs as a platform for several experimental therapeutics. These EEVs were recently used to deliver an inhibitor of transcription factor NF-κB, to prolong pregnancy in a mouse model of pre-term birth (Sheller-Miller et al., 2021).
Although the HEK293-derived EEV production system may offer potential manufacturing advantages, the potency, efficacy and associated biological risk factor of EV generated from this cell line need to be evaluated in both in vitro and in vivo systems.
Meeting GMP-Grade Processing Requirements for Clinical EV Production
Once a reliable cell source for industrial-scale EV production is identified, issues remain in the upstream and downstream processing and quality control of the resulting EVs.
Purity is one significant issue that needs to be addressed for GMP-grade production of EVs. Research labs engaged in EV research, development and pre-clinical studies predominantly use media formulations containing fetal bovine serum (FBS) for cell culture and EV collection, which cannot be applied for clinical application (Gottipamula et al., 2013; Pachler et al., 2017). Although ultracentrifuged, EV-depleted FBS is often used by researchers and academician, not all the EV contaminants can be removed (Zhiyun et al., 2016; Shelke et al., 2018). The use of human platelet lysate (hPL) instead of FBS may solve the problem (Torreggiani et al., 2014). Ideally when manufacturing GMP-grade EVs, serum-free and chemically defined media are safest for EV collection, preventing the inadvertent introduction of contaminating EVs in the source serum (Pachler et al., 2017). However, switching from serum to serum-free cell culture media causes cell stress which in turn may lead to production of EVs with different cargo and profile. Therefore, cell culture conditions must be carefully considered. Large-scale culture systems that align with GMP requirements for the production of master and working cell banks also need to be adopted. Hollow fiber and stirred-tank bioreactors are the more promising approaches because they are closed, scalable, GMP-compatible systems that provide a high surface-to-volume ratio for cell growth (Mendt et al., 2018).
Large-scale isolation and purification of EVs is one of the most significant challenges in downstream processing for clinical GMP-grade EV production. Ultracentrifugation (UC) remains the most popular method of EV isolation, despite ongoing concerns over product purity, and that UC protocols are not directly scalable for clinical-scale manufacture (Casulli et al., 2018; Colao et al., 2018). Alternative purification methods such as those based on scalable and high throughput ion exchange protocols and size exclusion chromatography with membrane filtration such as tangential flow filtration (TFF), are being investigated as a promising way around this significant roadblock (Law et al., 2021). EV purification should ideally utilize methods such as filtration and chromatography that are currently available for the manufacture of biologics, as these methods are already demonstrated to be GMP-compliant.
As different isolation and purification methods have an impact on EV characteristics and produce different population of EVs, the need for standardized GMP is emphasized (Agnes et al., 2018). MISEV guidelines lay out basic standards for EV markers, but specific standardized universal markers are still lacking for EV characterization, and further investigation is required to fully characterize EV populations to establish their identity.
Quantification of EVs is another fundamental issue which remains challenging. How to accurately measure and assess EV purity is a critical issue when evaluating EV dosage for both pre-clinical and clinical applications. At present, there is no single method that can accurately measure EVs. One of the most common approaches to measuring EVs is determining the total amount of proteins and particle numbers. There are several methods and instruments measuring EV numbers including nanoparticle tracking analysis (NTA), resistive pulse sensing (RPS), and dynamic light scattering (DLS). However, copurification of other proteins by measuring total protein concentration and current particle tracking approaches might be biased toward a designated EV size range which does not discriminate EVs from other nanoparticulate materials. Recent developments in this field include nanoflow cytometry, imaging flow cytometry, and ExoCounter with optic disk technology to quantify EVs (Sotiris et al., 2018; Hartjes et al., 2019). A combination of flow cytometry-based methods for analyzing the membrane surface markers and quantification of EVs may represent improvements in the assessment of EV purity and yield.
For GMP compliance, fill and finish of the final product should be performed in a closed system. This might present as a logistical challenge for small biotech companies and academic labs operating at research scale (Rohde et al., 2019). Overall, the currently available methods and technologies for purification, quantification and characterization of EVs are inconsistent and GMP standards need to be developed that are reproducible, practical and scalable for clinical GMP-grade EV application. However, with better know-how and strategic decisions early on in development, GMP-grade EV preparations can be successfully produced for clinical administration (Mendt et al., 2018).
Finally, storage and long-term stability of EVs is another important issue if EVs are to be used as a third party “off the shelf” product. EV quantity decreased in a dose-dependent manner at room temperature, at 4°C, −20°C, but not when frozen in −80°C, therefore most protocols store EVs at −80°C long term without any reported changes in EV profile and structure (Lõrincz et al., 2014). Preservation condition and storage solution buffer is also very important as EVs are sensitive to shifts in pH. The majority of published studies have used phosphate-buffered saline (PBS) and some have used sucrose and trehalose buffers (Steffi et al., 2016; Busatto et al., 2018; Li et al., 2019). Preservation conditions need to be further optimized and validated in both in vitro and in vivo assays to ensure post-thaw potency of the EVs product is retained (Chung et al., 2021). Alternatively, EV lyophilization has been successfully utilized to store EVs and may offer a simpler option for transport and storage (Frank et al., 2018; Kusuma et al., 2018). Standardized GMP-grade methods to define specific storage condition including temperature, buffer solution, pH and duration must be addressed for clinical applications of EVs to be advanced.
Conclusion
Experimental MSC therapies continue to show promise in pre-clinical research. This promise, however, is still to translate into widespread clinical use. The inherent complexity of a live cell product presents considerable challenges to successful therapeutic translation. Although cell engineering approaches may address some of these challenges, the potential risks associated with cell-based therapeutics, such as tumorigenicity and undesired differentiation, and limitations such as rejection of cells and poor engraftment, will remain.
Mesenchymal stromal cell-EVs, as a cell-free product, offer a potential pathway through these challenges. An increasing number of studies have shown that the therapeutic effects of MSC-EVs are equal to those of the MSCs. The use of EVs rather than MSCs protects against issues of tumorigenicity, immunogenicity, and poor engraftment. In addition, EV-based therapeutic manufacture, as well as transport and storage, promises to be more readily scalable at lower cost than live cell MSC therapeutics. However, to achieve their full clinical potential and to avoid some of the pitfalls that have contributed to clinical trial failures of MSC therapeutics, EVs need to be thoroughly investigated in terms of purification, quantification, characterization and potency before their clinical use. Genetic modification of the source cells for large scale manufacture of MSC-EVs may serve as an effective strategy to further improve the therapeutic effect of MSC derived cell-free products. The increasing sophistication with which EVs from a variety of cell sources can be engineered, to enhance their homing capabilities and bolster their therapeutic cargo, further demonstrates the considerable potential of this versatile therapeutic platform.
Author Contributions
RK, JJ, JMC, and MS designed and wrote the content, and revised the manuscript. All authors contributed to the article and approved the submitted version.
Funding
The authors declare that this study received funding from Exopharm Ltd. Exopharm Ltd. was involved in supporting the salary of all the authors and provided the facilities to authors for conducting and submitting this study.
Conflict of Interest
MS, JJ, and RK are employed by Exopharm Ltd. JMC is a contractor to Exopharm Ltd.
Acknowledgments
The authors would like to thank Balarka Banerjee, Gregor Lichtfuss, and Andrew Coley for their helpful input during the preparation of this manuscript.
References
Agnes, H. R., Rachael, M., Matteo, S., Yves, Y. S., Andrew, C., Marie-Cecile, D., et al. (2018). Exosomes produced from 3D cultures of MSCs by tangential flow filtration show higher yield and improved activity. Mol. Ther. 26:2838. doi: 10.1016/j.ymthe.2018.09.015
Akira, N., Makoto, I., Takuya, Y., Akira, W., Yonghui, J., Yoshihisa, M., et al. (2013). Genetically matched human IPS cells reveal that propensity for cartilage and bone differentiation differs with clones, not cell type of origin. PLoS One 8:e53771. doi: 10.1371/journal.pone.0053771
ANZCTR (2019). A Phase I Clinical Study to Evaluate Safety, Tolerability and Biological Activity of Platelet-Derived Extracellular Vesicles on Wound Healing in Healthy Adults. Available online at: http://anzctr.org.au/Trial/Registration/TrialReview.aspx?id=378212. (accessed April 6, 2021).
ANZCTR (2020). Phase I Study to Assess the Safety of a Human Non-Autologous Platelet Derived Extracellular Vesicle Therapy in Wound Healing. Available online at: http://anzctr.org.au/Trial/Registration/TrialReview.aspx?id=380050 (accessed April 6, 2021).
Baksh, D., Song, L., and Tuan, R. S. (2004). Adult mesenchymal stem cells: characterization, differentiation, and application in cell and gene therapy. J. Cell. Mol. Med. 8, 301–316. doi: 10.1111/j.1582-4934.2004.tb00320.x
Bianconi, E., Casadei, R., Frabetti, F., Ventura, C., Facchin, F., and Canaider, S. (2020). Sex-specific transcriptome differences in human adipose mesenchymal stem cells. Genes Basel 11:909. doi: 10.3390/genes11080909
Bloor, A. J. C., Patel, A., Griffin, J. E., Gilleece, M. H., Radia, R., Yeung, D. T., et al. (2020). Production, safety and efficacy of iPSC-derived mesenchymal stromal cells in acute steroid-resistant graft versus host disease: a phase I, multicenter, open-label, dose-escalation study. Nat. Med. 26, 1720–1725. doi: 10.1038/s41591-020-1050-x
Bruno, S., Grange, C., Deregibus, M. C., Calogero, R. A., Saviozzi, S., Collino, F., et al. (2009). Mesenchymal stem cell-derived microvesicles protect against acute tubular injury. J. Am. Soc. Nephrol. 20, 1053–1067. doi: 10.1681/asn.2008070798
Busatto, S., Vilanilam, G., Ticer, T., Lin, W.-L., Dickson, D. W., Shapiro, S., et al. (2018). Tangential flow filtration for highly efficient concentration of extracellular vesicles from large volumes of fluid. Cells 7:273. doi: 10.3390/cells7120273
Caplan, A. I., and Correa, D. (2011). The MSC: an injury drugstore. Cell Stem Cell 9, 11–15. doi: 10.1016/j.stem.2011.06.008
Casulli, A., Konoshenko, M. Yu, Lekchnov, E. A., Vlassov, A. V., and Laktionov, P. P. (2018). Isolation of extracellular vesicles: general methodologies and latest trends. Biomed. Res. Int. 2018:8545347. doi: 10.1155/2018/8545347
Cerri, S., Greco, R., Levandis, G., Ghezzi, C., Mangione, A. S., Fuzzati-Armentero, M.-T., et al. (2015). Intracarotid infusion of mesenchymal stem cells in an animal model of parkinson’s disease, focusing on cell distribution and neuroprotective and behavioral effects. Stem Cell Transl. Med. 4, 1073–1085. doi: 10.5966/sctm.2015-0023
Charoenviriyakul, C., Takahashi, Y., and Morishita, M. (2017). Cell type-specific and common characteristics of exosomes derived from mouse cell lines: yield, physicochemical properties, and pharmacokinetics. Eur. J. Pharm. Sci. 96, 316–322. doi: 10.1016/j.ejps.2016.10.009
Chilima, T. D. P., Moncaubeig, F., and Farid, S. S. (2018). Impact of allogeneic stem cell manufacturing decisions on cost of goods, process robustness and reimbursement. Biochem. Eng. J. 137, 132–151.
Chun-Chieh, H., Miya, K., Yu, L., Sajjad, S., Iriarte-Diaz, J., Lyndon, F. C., et al. (2020). Functionally engineered extracellular vesicles improve bone regeneration. Acta Biomater. 109:182. doi: 10.1016/j.actbio.2020.04.017
Chung, J. J., Kim, S. T., Zaman, S., Helmers, M. R., Arisi, M. F., Li, E. C., et al. (2021). Therapeutic efficacy of cryopreserved, allogeneic extracellular vesicles for treatment of acute myocardial infarction. Int. Heart J. 62, 381–389. doi: 10.1536/ihj.20-224
Clinicaltrials.gov (2020). A First-in-Human Study of CDK-002 (exoSTING) in Subjects With Advanced/Metastatic, Recurrent, Injectable Solid Tumors. Available online at: https://clinicaltrials.gov/ct2/show/NCT04592484 (accessed July 6, 2021).
Colao, I. L., Corteling, R., Bracewell, D., and Wall, I. (2018). Manufacturing exosomes: a promising therapeutic platform. Trends Mol. Med. 24, 242–256. doi: 10.1016/j.molmed.2018.01.006
Conceição, M., Forcina, L., Wiklander, O. P. B., Gupta, D., Nordin, J. Z., Vrellaku, B., et al. (2021). Engineered extracellular vesicle decoy receptor-mediated modulation of the IL6 trans-signalling pathway in muscle. Biomaterials 266:120435.
Dang, X. T. T., Kavishka, J. M., Zhang, D. X., Pirisinu, M., and Le, M. T. N. (2020). Extracellular vesicles as an efficient and versatile system for drug delivery. Cells 9:2191. doi: 10.3390/cells9102191
Devine, S. M., Cobbs, C., Jennings, M., Bartholomew, A., and Hoffman, R. (2003). Mesenchymal stem cells distribute to a wide range of tissues following systemic infusion into nonhuman primates. Blood 101, 2999–3001. doi: 10.1182/blood-2002-06-1830
Dooley, K., McConnell, R. E., Xu, K., Lewis, N. D., Haupt, S., Youniss, M. R., et al. (2021). A versatile platform for generating engineered extracellular vesicles with defined therapeutic properties. Mol. Ther. 29, 1729–1743. doi: 10.1016/j.ymthe.2021.01.020
FDA (2021). Update on Amyotrophic Lateral Sclerosis (ALS) Product Development. Available online at: https://www.fda.gov/vaccines-blood-biologics/cellular-gene-therapy-products/update-amyotrophic-lateral-sclerosis-als-product-development (accessed June 16, 2021).
Frank, J., Richter, M., de Rossi, C., Lehr, C.-M., Fuhrmann, K., and Fuhrmann, G. (2018). Extracellular vesicles protect glucuronidase model enzymes during freeze-drying. Sci. Rep. UK 8:12377. doi: 10.1038/s41598-018-30786-y
Franziska, H. B., Josua, B. J., and Jean-Christophe, L. (2021). Encapsulation of hydrophilic compounds in small extracellular vesicles: loading capacity and impact on vesicle functions. Adv. Healthc. Mater. n/a:2100047. doi: 10.1002/adhm.202100047
Friedenstein, A. J., Chailakhjan, R. K., and Lalykina, K. S. (1970). The development of fibroblast colonies in monolayer cultures of guinea-pig bone marrow and spleen cells. Cell Prolif. 3, 393–403. doi: 10.1111/j.1365-2184.1970.tb00347.x
Gimona, M., Brizzi, M. F., Choo, A. B. H., Dominici, M., Davidson, S. M., Grillari, J., et al. (2021). Critical considerations for the development of potency tests for therapeutic applications of mesenchymal stromal cell-derived small extracellular vesicles. Cytotherapy 23, 373–380.
Gimona, M., Pachler, K., Laner-Plamberger, S., Schallmoser, K., and Rohde, E. (2017). Manufacturing of human extracellular vesicle-based therapeutics for clinical use. Int. J. Mol. Sci. 18:1190. doi: 10.3390/ijms18061190
Gnecchi, M., He, H., Noiseux, N., Liang, O. D., Zhang, L., Morello, F., et al. (2006). Evidence supporting paracrine hypothesis for Akt-modified mesenchymal stem cell-mediated cardiac protection and functional improvement. FASEB J. 20, 661–669. doi: 10.1096/fj.05-5211com
Gottipamula, S., Muttigi, M. S., Kolkundkar, U., and Seetharam, R. N. (2013). Serum-free media for the production of human mesenchymal stromal cells: a review. Cell Prolif. 46, 608–627. doi: 10.1111/cpr.12063
Gowen, A., Shahjin, F., Chand, S., Odegaard, K. E., and Yelamanchili, S. V. (2020). Mesenchymal stem cell-derived extracellular vesicles: challenges in clinical applications. Front. Cell Dev. Biol. 8:149. doi: 10.3389/fcell.2020.00149
Guo, S., Tao, S., Yin, W., Qi, X., Yuan, T., and Zhang, C. (2017). Exosomes derived from platelet-rich plasma promote the re-epithelization of chronic cutaneous wounds via activation of YAP in a diabetic rat model. Theranostics 7, 81–96. doi: 10.7150/thno.16803
Hartjes, T. A., Mytnyk, S., Jenster, G. W., van Steijn, V., and van Royen, M. E. (2019). Extracellular vesicle quantification and characterization: common methods and emerging approaches. Bioengineering 6:7. doi: 10.3390/bioengineering6010007
Hass, R., Kasper, C., Böhm, S., and Jacobs, R. (2011). Different populations and sources of human mesenchymal stem cells (MSC): a comparison of adult and neonatal tissue-derived MSC. Cell Commun. Signal. 9, 12–12. doi: 10.1186/1478-811x-9-12
Haynesworth, S. E., Goshima, J., Goldberg, V. M., and Caplan, A. I. (1992). Characterization of cells with osteogenic potential from human marrow. Bone 13, 81–88. doi: 10.1016/8756-3282(92)90364-3
Hu, X., Li, L., Yu, X., Zhang, R., Yan, S., Zeng, Z., et al. (2017). CRISPR/Cas9-mediated reversibly immortalized mouse bone marrow stromal stem cells (BMSCs) retain multipotent features of mesenchymal stem cells (MSCs). Oncotarget 8, 111847–111865. doi: 10.18632/oncotarget.22915
Introna, M., Lucchini, G., Dander, E., Galimberti, S., Rovelli, A., Balduzzi, A., et al. (2014). Treatment of graft versus host disease with mesenchymal stromal cells: a phase I study on 40 adult and pediatric patients. Biol. Blood Marrow Transplant. 20, 375–381. doi: 10.1016/j.bbmt.2013.11.033
Jeyaram, A., and Jay, S. M. (2017). Preservation and storage stability of extracellular vesicles for therapeutic applications. AAPS J. 20:1. doi: 10.1208/s12248-017-0160-y
Jiang, B., Yan, L., Wang, X., Li, E., Murphy, K., Vaccaro, K., et al. (2019). Concise review: mesenchymal stem cells derived from human pluripotent cells, an unlimited and quality-controllable source for therapeutic applications. Stem Cells 37, 572–581. doi: 10.1002/stem.2964
Jing, L., Xiulan, C., Jiao, Y., Yuchen, L., Dameng, L., Jifeng, W., et al. (2016). Identification and characterization of 293T cell-derived exosomes by profiling the protein, mRNA and MicroRNA components. PLoS One 11:e0163043. doi: 10.1371/journal.pone.0163043
Jung, S., Panchalingam, K. M., Rosenberg, L., and Behie, L. A. (2012). Ex vivo expansion of human mesenchymal stem cells in defined serum-free media. Stem Cells Int 2012:123030. doi: 10.1155/2012/123030
Kamerkar, S., LeBleu, V. S., Sugimoto, H., Yang, S., Ruivo, C. F., Melo, S. A., et al. (2017). Exosomes facilitate therapeutic targeting of oncogenic KRAS in pancreatic cancer. Nature 546, 498–503. doi: 10.1038/nature22341
Katsha, A. M., Ohkouchi, S., Xin, H., Kanehira, M., Sun, R., Nukiwa, T., et al. (2011). Paracrine factors of multipotent stromal cells ameliorate lung injury in an elastase-induced emphysema model. Mol. Ther. 19, 196–203. doi: 10.1038/mt.2010.192
Keisuke, O., Tomoko, I., and Shinya, Y. (2007). Generation of germline-competent induced pluripotent stem cells. Nature 448:313. doi: 10.1038/nature05934
Kordelas, L., Rebmann, V., Ludwig, A.-K., Radtke, S., Ruesing, J., Doeppner, T. R., et al. (2014). MSC-derived exosomes: a novel tool to treat therapy-refractory graft-versus-host disease. Leukemia 28, 970–973. doi: 10.1038/leu.2014.41
Kusuma, G. D., Barabadi, M., Tan, J. L., Morton, D. A. V., Frith, J. E., and Lim, R. (2018). To protect and to preserve: novel preservation strategies for extracellular vesicles. Front. Pharmacol. 9:1199. doi: 10.3389/fphar.2018.01199
Lai, R. C., Arslan, F., Lee, M. M., Sze, N. S. K., Choo, A., Chen, T. S., et al. (2010). Exosome secreted by MSC reduces myocardial ischemia/reperfusion injury. Stem Cell Res. 4, 214–222.
Law, S., Johnson, J., James, P. F., Whitmore, M., Silva, A., Kong, K., et al. (2021). Ligand-based Exosome Affinity Purification (LEAP): a scalable solution to the extracellular vesicle downstream bottleneck. BioProcess. Int. 19, 28–35. in press.
Levy, O., Kuai, R., Siren, E. M. J., Bhere, D., Milton, Y., Nissar, N., et al. (2020). Shattering barriers toward clinically meaningful MSC therapies. Sci. Adv. 6:eaba6884. doi: 10.1126/sciadv.aba6884
Lewis, N. D., Sia, C. L., Kirwin, K., Haupt, S., Mahimkar, G., Zi, T., et al. (2021). Exosome surface display of IL12 results in tumor-retained pharmacology with superior potency and limited systemic exposure compared with recombinant IL12. Mol. Cancer Ther. 20, 523–534. doi: 10.1158/1535-7163.mct-20-0484
Li, X., Corbett, A. L., Taatizadeh, E., Tasnim, N., Little, J. P., Garnis, C., et al. (2019). Challenges and opportunities in exosome research—perspectives from biology, engineering, and cancer therapy. Apl. Bioeng. 3:011503. doi: 10.1063/1.5087122
Liu, S., Liu, D., Chen, C., Hamamura, K., Moshaverinia, A., Yang, R., et al. (2015). MSC transplantation improves osteopenia via epigenetic regulation of notch signaling in lupus. Cell Metab. 22, 606–618. doi: 10.1016/j.cmet.2015.08.018
Lõrincz, ÁM., Timár, C. I., Marosvári, K. A., Veres, D. S., Otrokocsi, L., and Kittel, Á, et al. (2014). Effect of storage on physical and functional properties of extracellular vesicles derived from neutrophilic granulocytes. J. Extracell. Vesicles 3:25465. doi: 10.3402/jev.v3.25465
Losurdo, M., Pedrazzoli, M., D’Agostino, C., Elia, C. A., Massenzio, F., Lonati, E., et al. (2020). Intranasal delivery of mesenchymal stem cell-derived extracellular vesicles exerts immunomodulatory and neuroprotective effects in a 3xTg model of Alzheimer’s disease. Stem Cells Transl. Med. 9, 1068–1084. doi: 10.1002/sctm.19-0327
Lydia, A.-E., Yiqi, S., HaiFang, Y., Corinne, B., Samira, L., and Matthew, J. A. W. (2011). Delivery of siRNA to the mouse brain by systemic injection of targeted exosomes. Nat. Biotechnol. 29:341. doi: 10.1038/nbt.1807
Magdalena, M., Rasool, S., Magnus, L., Marco, G., Veronique, C., Ray, F., et al. (2020). Evolution from adherent to suspension: systems biology of HEK293 cell line development. Sci Rep UK 10:18996. doi: 10.1038/s41598-020-76137-8
Mastrolia, I., Foppiani, E. M., Murgia, A., Candini, O., Samarelli, A. V., Grisendi, G., et al. (2019). Challenges in clinical development of mesenchymal stromal/stem cells: concise review. Stem Cells Transl. Med. 8, 1135–1148.
Mendt, M., Kamerkar, S., Sugimoto, H., McAndrews, K. M., Wu, C.-C., Gagea, M., et al. (2018). Generation and testing of clinical-grade exosomes for pancreatic cancer. JCI Insight 3:e99263. doi: 10.1172/jci.insight.99263
Meyer, C., Losacco, J., Stickney, Z., Li, L., Marriott, G., and Lu, B. (2017). Pseudotyping exosomes for enhanced protein delivery in mammalian cells. Int. J. Nanomed. 12, 3153–3170. doi: 10.2147/ijn.s133430
Mizukami, A., Chilima, T. D. P., Orellana, M. D., Neto, M. A., Covas, D. T., Farid, S. S., et al. (2018). Technologies for large-scale umbilical cord-derived MSC expansion: experimental performance and cost of goods analysis. Biochem. Eng. J. 135, 36–48.
Moon, G. J., Sung, J. H., Kim, D. H., Kim, E. H., Cho, Y. H., Son, J. P., et al. (2019). Application of mesenchymal stem cell-derived extracellular vesicles for stroke: biodistribution and MicroRNA study. Transl. Stroke Res. 10, 509–521. doi: 10.1007/s12975-018-0668-1
Murphy, D. E., de Jong, O. G., Evers, M. J. W., Nurazizah, M., Schiffelers, R. M., and Vader, P. (2021). Natural or synthetic RNA delivery: a stoichiometric comparison of extracellular vesicles and synthetic nanoparticles. Nano Lett. 21, 1888–1895. doi: 10.1021/acs.nanolett.1c00094
Nassar, W., El-Ansary, M., Sabry, D., Mostafa, M. A., Fayad, T., Kotb, E., et al. (2016). Umbilical cord mesenchymal stem cells derived extracellular vesicles can safely ameliorate the progression of chronic kidney diseases. Biomater. Res. 20:21. doi: 10.1186/s40824-016-0068-0
Nguyen, J., and Ferguson, S. (2018). “Compositions and methods for loading extracellular vesicles with chemical and biological agents/molecules,” in U.S. Patent Application No 20200080092, (Washington, DC: U.S. Patent and Trademark Office).
O’Brien, K. P., Khan, S., Gilligan, K. E., Zafar, H., Lalor, P., Glynn, C., et al. (2018). Employing mesenchymal stem cells to support tumor-targeted delivery of extracellular vesicle (EV)-encapsulated microRNA-379. Oncogene 37, 2137–2149. doi: 10.1038/s41388-017-0116-9
Ozay, E. I., Vijayaraghavan, J., Gonzalez-Perez, G., Shanthalingam, S., Sherman, H. L., Garrigan, D. T., et al. (2019). CymerusTM iPSC-MSCs significantly prolong survival in a pre-clinical, humanized mouse model of Graft-vs-host disease. Stem Cell Res. 35:101401.
Pachler, K., Lener, T., Streif, D., Dunai, Z. A., Desgeorges, A., Feichtner, M., et al. (2017). A good manufacturing practice–grade standard protocol for exclusively human mesenchymal stromal cell–derived extracellular vesicles. Cytotherapy 19, 458–472. doi: 10.1016/j.jcyt.2017.01.001
Pascucci, L., Coccè, V., Bonomi, A., Ami, D., Ceccarelli, P., Ciusani, E., et al. (2014). Paclitaxel is incorporated by mesenchymal stromal cells and released in exosomes that inhibit in vitro tumor growth: a new approach for drug delivery. J. Control. Release 192, 262–270. doi: 10.1016/j.jconrel.2014.07.042
Pittenger, M. F., Mackay, A. M., Beck, S. C., Jaiswal, R. K., Douglas, R., Mosca, J. D., et al. (1999). Multilineage potential of adult human mesenchymal stem cells. Science 284, 143–147. doi: 10.1126/science.284.5411.143
Qingguo, Z., Carl, A. G., Hwa, L. R., Roxanne, L. R., Lizheng, Q., Bo, H., et al. (2015). MSCs derived from iPSCs with a modified protocol are tumor-tropic but have much less potential to promote tumors than bone marrow MSCs. Proc. Natl. Acad. Sci. U.S.A. 112:530–535. doi: 10.1073/pnas.1423008112
Reis, M., Ogonek, J., Qesari, M., Borges, N. M., Nicholson, L., Preußner, L., et al. (2016). Recent developments in cellular immunotherapy for HSCT-associated complications. Front. Immunol. 7:500. doi: 10.3389/fimmu.2016.00500
Rohde, E., Pachler, K., and Gimona, M. (2019). Manufacturing and characterization of extracellular vesicles from umbilical cord–derived mesenchymal stromal cells for clinical testing. Cytotherapy 21, 581–592. doi: 10.1016/j.jcyt.2018.12.006
Sammour, I., Somashekar, S., Huang, J., Batlahally, S., Breton, M., Valasaki, K., et al. (2016). The effect of gender on mesenchymal stem cell (MSC) efficacy in neonatal hyperoxia-induced lung injury. PLoS One 11:e0164269. doi: 10.1371/journal.pone.0164269
Setten, R. L., Rossi, J. J., and Han, S. (2019). The current state and future directions of RNAi-based therapeutics. Nat. Rev. Drug Discov. 18, 421–446. doi: 10.1038/s41573-019-0017-4
Shelke, G. V., Lässer, C., Gho, Y. S., and Lötvall, J. (2018). Importance of exosome depletion protocols to eliminate functional and RNA-containing extracellular vesicles from fetal bovine serum. J. Extracell. Vesicles 3:24783. doi: 10.3402/jev.v3.24783
Sheller-Miller, S., Radnaa, E., Yoo, J.-K., Choi, K., Kim, Y., Kim, Y. N., et al. (2021). Exosomal delivery of NF-κB inhibitor delays LPS-induced preterm birth and modulates fetal immune cell profile in mouse models. Sci. Adv. 7:eabd3865. doi: 10.1126/sciadv.abd3865
Sotiris, M., Minani, G. B., Gavin, W., Francesco, D., Alberto, S.-F., and Marc, M.-L. (2018). Multiparametric analysis of circulating exosomes and other small extracellular vesicles by advanced imaging flow cytometry. Front. Immunol. 9:1583. doi: 10.3389/fimmu.2018.01583
Steffi, B., Laurence, d. B, Marie, A., Mathilde, M., Claire, H., Denis, C., et al. (2016). Trehalose prevents aggregation of exosomes and cryodamage. Sci. Rep. UK 6:36162. doi: 10.1038/srep36162
Stolzing, A., Jones, E., McGonagle, D., and Scutt, A. (2008). Age-related changes in human bone marrow-derived mesenchymal stem cells: consequences for cell therapies. Mech. Ageing Dev. 129, 163–173.
Tan, T. T., Lai, R. C., Padmanabhan, J., Sim, W. K., Choo, A. B. H., and Lim, S. K. (2021). Assessment of tumorigenic potential in mesenchymal-stem/stromal-cell-derived small extracellular vesicles (MSC-sEV). Pharm 14:345. doi: 10.3390/ph14040345
Thomson, J. A., Itskovitz-Eldor, J., Shapiro, S. S., Waknitz, M. A., Swiergiel, J. J., Marshall, V. S., et al. (1998). Embryonic stem cell lines derived from human blastocysts. Science 282, 1145–1147. doi: 10.1126/science.282.5391.1145
Tian, T., Cao, L., He, C., Ye, Q., Liang, R., You, W., et al. (2021). Targeted delivery of neural progenitor cell-derived extracellular vesicles for anti-inflammation after cerebral ischemia. Theranostics 11, 6507–6521. doi: 10.7150/thno.56367
Timmers, L., Lim, S. K., Arslan, F., Armstrong, J. S., Hoefer, I. E., Doevendans, P. A., et al. (2008). Reduction of myocardial infarct size by human mesenchymal stem cell conditioned medium. Stem Cell Res. 1, 129–137.
Torreggiani, E., Perut, F., Roncuzzi, L., Zini, N., Baglìo, S., and Baldini, N. (2014). Exosomes: novel effectors of human platelet lysate activity. Eur. Cell. Mater. 28, 137–151.
Trounson, A., and McDonald, C. (2015). Stem cell therapies in clinical trials: progress and challenges. Cell Stem Cell 17, 11–22. doi: 10.1016/j.stem.2015.06.007
Uccelli, A., Moretta, L., and Pistoia, V. (2008). Mesenchymal stem cells in health and disease. Nat. Rev. Immunol. 8, 726–736. doi: 10.1038/nri2395
van Rhijn-Brouwer, F. C. C., Gremmels, H., Fledderus, J. O., and Verhaar, M. C. (2018). Mesenchymal stromal cell characteristics and regenerative potential in cardiovascular disease: implications for cellular therapy. Cell Transplant. 27, 765–785. doi: 10.1177/0963689717738257
Volarevic, V., Markovic, B. S., Gazdic, M., Volarevic, A., Jovicic, N., Arsenijevic, N., et al. (2018). Ethical and safety issues of stem cell-based therapy. Int. J. Med. Sci. 15, 36–45. doi: 10.7150/ijms.21666
Wagner, W., Bork, S., Horn, P., Krunic, D., Walenda, T., Diehlmann, A., et al. (2009). Aging and replicative senescence have related effects on human stem and progenitor cells. PLoS One 4:e5846. doi: 10.1371/journal.pone.0005846
Wang, B., Yao, K., Huuskes, B. M., Shen, H.-H., Zhuang, J., Godson, C., et al. (2016). Mesenchymal stem cells deliver exogenous microRNA-let7c via exosomes to attenuate renal fibrosis. Mol. Ther. 24, 1290–1301. doi: 10.1038/mt.2016.90
Wang, F., Yasuhara, T., Shingo, T., Kameda, M., Tajiri, N., Yuan, W. J., et al. (2010). Intravenous administration of mesenchymal stem cells exerts therapeutic effects on parkinsonian model of rats: focusing on neuroprotective effects of stromal cell-derived factor-1α. BMC Neurosci. 11:52. doi: 10.1186/1471-2202-11-52
Wang, J., Bonacquisti, E. E., Brown, A. D., and Nguyen, J. (2020). Boosting the biogenesis and secretion of mesenchymal stem cell-derived exosomes. Cells 9:660. doi: 10.3390/cells9030660
Wei, H., Xu, Y., Chen, Q., Chen, H., Zhu, X., and Li, Y. (2020). Mesenchymal stem cell-derived exosomal miR-223 regulates neuronal cell apoptosis. Cell Death Dis. 11:290. doi: 10.1038/s41419-020-2490-4
Wei, W., Ao, Q., Wang, X., Cao, Y., Liu, Y., Zheng, S. G., et al. (2021). Mesenchymal stem cell–derived exosomes: a promising biological tool in nanomedicine. Front. Pharmacol. 11:1954. doi: 10.3389/fphar.2020.590470
Wiklander, O. P., Nordin, J. Z., O’Loughlin, A., Gustafsson, Y., Corso, G., Mäger, I., et al. (2015). Extracellular vesicle in vivo biodistribution is determined by cell source, route of administration and targeting. J. Extracell. Vesicles 4:26316. doi: 10.3402/jev.v4.26316
Xin, H., Li, Y., Buller, B., Katakowski, M., Zhang, Y., Wang, X., et al. (2012). Exosome-mediated transfer of miR-133b from multipotent mesenchymal stromal cells to neural cells contributes to neurite outgrowth. Stem Cells 30, 1556–1564. doi: 10.1002/stem.1129
Xing-Liang, F., Qing-Xiang, Z., Xin, L., Cheng-Lin, L., Zhi-Bin, X., Xue-Quan, D., et al. (2018). Induced pluripotent stem cell-derived mesenchymal stem cells activate quiescent T cells and elevate regulatory T cell response via NF-κB in allergic rhinitis patients. Stem Cell Res. Ther. 9:170. doi: 10.1186/s13287-018-0896-z
Yan, L., and Wu, X. (2020). Exosomes produced from 3D cultures of umbilical cord mesenchymal stem cells in a hollow-fiber bioreactor show improved osteochondral regeneration activity. Cell Biol. Toxicol. 36, 165–178. doi: 10.1007/s10565-019-09504-5
Yang, D., Wang, W., Li, L., Peng, Y., Chen, P., Huang, H., et al. (2013). The relative contribution of paracine effect versus direct differentiation on adipose-derived stem cell transplantation mediated cardiac repair. PLoS One 8:e59020. doi: 10.1371/journal.pone.0059020
Yang, Y., Hong, Y., Cho, E., Kim, G. B., and Kim, I.-S. (2018). Extracellular vesicles as a platform for membrane-associated therapeutic protein delivery. J. Extracell. Vesicles 7:1440131. doi: 10.1080/20013078.2018.1440131
Zhilan, Y., Tao, Z., Wenshan, H., Honglin, J., Cuiwei, L., Zhe, Y., et al. (2018). Methotrexate-loaded extracellular vesicles functionalized with therapeutic and targeted peptides for the treatment of glioblastoma multiforme. ACS Appl. Mater. Interfaces 10:12341. doi: 10.1021/acsami.7b18135
Zhiyun, W., Arsen, O. B., David, F. C. R., and Anna, M. K. (2016). Fetal bovine serum RNA interferes with the cell culture derived extracellular RNA. Sci. Rep. UK 6:31175. doi: 10.1038/srep31175
Zhou, S., Greenberger, J. S., Epperly, M. W., Goff, J. P., Adler, C., LeBoff, M. S., et al. (2008). Age-related intrinsic changes in human bone-marrow-derived mesenchymal stem cells and their differentiation to osteoblasts. Aging Cell 7, 335–343. doi: 10.1111/j.1474-9726.2008.00377.x
Zhu, J., Lu, K., Zhang, N., Zhao, Y., Ma, Q., Shen, J., et al. (2017). Myocardial reparative functions of exosomes from mesenchymal stem cells are enhanced by hypoxia treatment of the cells via transferring microRNA-210 in an nSMase2-dependent way. Artif. Cells Nanomed. Biotechnol. 46, 1659–1670. doi: 10.1080/21691401.2017.1388249
Keywords: mesenchymal stromal cells, MSCs, extracellular vesicles, MSC-EVs, EV therapeutics, engineered EVs
Citation: Johnson J, Shojaee M, Mitchell Crow J and Khanabdali R (2021) From Mesenchymal Stromal Cells to Engineered Extracellular Vesicles: A New Therapeutic Paradigm. Front. Cell Dev. Biol. 9:705676. doi: 10.3389/fcell.2021.705676
Received: 06 May 2021; Accepted: 29 June 2021;
Published: 20 July 2021.
Edited by:
Mayasari Lim, FUJIFILM Irvine Scientific, Inc., United StatesReviewed by:
Vaijayanti Prakash Kale, Symbiosis International University, IndiaPavel Makarevich, Lomonosov Moscow State University, Russia
Copyright © 2021 Johnson, Shojaee, Mitchell Crow and Khanabdali. This is an open-access article distributed under the terms of the Creative Commons Attribution License (CC BY). The use, distribution or reproduction in other forums is permitted, provided the original author(s) and the copyright owner(s) are credited and that the original publication in this journal is cited, in accordance with accepted academic practice. No use, distribution or reproduction is permitted which does not comply with these terms.
*Correspondence: Ramin Khanabdali, cmFtaW4ua2hhbmFiZGFsaUBleG9waGFybS5jb20=
†These authors have contributed equally to this work