- 1Department of Animal Morphology, Physiology and Genetics, Faculty of AgriSciences, Mendel University in Brno, Brno, Czech Republic
- 2NPPC, Research Institute for Animal Production in Nitra, Luzianky, Slovak Republic
Three-dimensional (3D) cell culture is attracting increasing attention today because it can mimic tissue environments and provide more realistic results than do conventional cell cultures. On the other hand, very little attention has been given to using 3D cell cultures in the field of avian cell biology. Although mimicking the bone marrow niche is a classic challenge of mammalian stem cell research, experiments have never been conducted in poultry on preparing in vitro the bone marrow niche. It is well known, however, that all diseases cause immunosuppression and target immune cells and their development. Hematopoietic stem cells (HSC) reside in the bone marrow and constitute a source for immune cells of lymphoid and myeloid origins. Disease prevention and control in poultry are facing new challenges, such as greater use of alternative breeding systems and expanding production of eggs and chicken meat in developing countries. Moreover, the COVID-19 pandemic will draw greater attention to the importance of disease management in poultry because poultry constitutes a rich source of zoonotic diseases. For these reasons, and because they will lead to a better understanding of disease pathogenesis, in vivo HSC niches for studying disease pathogenesis can be valuable tools for developing more effective disease prevention, diagnosis, and control. The main goal of this review is to summarize knowledge about avian hematopoietic cells, HSC niches, avian immunosuppressive diseases, and isolation of HSC, and the main part of the review is dedicated to using 3D cell cultures and their possible use for studying disease pathogenesis with practical examples. Therefore, this review can serve as a practical guide to support further preparation of 3D avian HSC niches to study the pathogenesis of avian diseases.
Introduction
As a precursor of immune cells, hematopoietic stem cells (HSC) play an indispensable role in the immune response against the main avian diseases (Cui et al., 2009; Gurung et al., 2017; Hosokawa et al., 2020). HSC reside in a unique bone marrow environment, where they interact with other cells and molecules to create a bone marrow niche (Zhang P. et al., 2019). Hematopoietic colonization of the bone marrow starts at 13 days of embryonic development. During embryonic life, the bone marrow, yolk sac, and liver contribute temporarily to hematopoiesis. Subsequently, the bone marrow serves as the major site for hematopoiesis in adult chickens (Guedes et al., 2014). HSC fate, migration, and differentiation are influenced by many factors, and these circumstances complicate the preparation of realistic in vitro HSC culture. Current approaches in mimicking bone marrow environments comprise scaffold-based systems using hydrogels, and macroporous and nanofiber scaffolds (Bello et al., 2018). These scaffolds can be embedded in perfused chambers and through microfluidic technology, further enhancing the biocompatibility of cell culture (Bhatia and Ingber, 2014). Nowadays, increasing interest is given to using these culture systems to study interactions of immune cells with bacteria and other pathogens (Lee et al., 2021). In vitro studies constitute the gold standard for researching disease pathogenesis; it is interesting that there exists a lack of studies involving pathogens' interactions with HSC to evaluate impacts of disease causative agents on the fate and differentiation of HSC and on immune cell development. In human medicine, relatively many studies have been conducted to describe immunosuppressive mechanisms of human viruses in three-dimensional (3D) cell culture, as reviewed by He et al. (2016). In chickens, there have been no experiments with 3D cell culture to study disease pathogenesis. The main goal of this review, therefore, is to provide information about 3D cell cultures that will be useful for in vivo avian HSC 3D culture preparation and further conceiving of host–pathogen studies.
Hematopoietic Stem Cells and Hematopoiesis
Hematopoiesis is a group of processes giving rise to blood cells. The para-aortic foci comprise a source of HSC in embryos. From there, HSC colonize the developing lymphoid organs (Yvernogeau and Robin, 2017). CD45+ progenitors of immune cells from the bone marrow colonize the bursa of Fabricius, thymus, and spleen already during embryonic development and continue in this function throughout the life of the bird (Fellah et al., 2014). A simplified scheme showing the development of avian immune cells is shown in Figure 1.
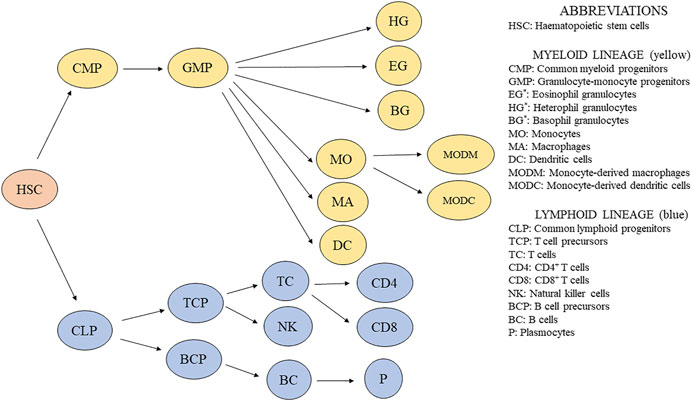
FIGURE 1. Simplified scheme of immune cell development in chickens. *Granulocytes are usually distinguished by light microscopy using blood smears, and their percentage is counted. Additionally, granulocytes are recognized based on their granularity by flow cytometry (Bilkova et al., 2017).
Growth factors and cytokines stimulate immune cell differentiation from hematopoietic progenitors, so they can be used in vitro for cell differentiation studies. Stem cell factor (SCF) is the main cytokine responsible for the self-renewal, proliferation, and differentiation of stem cells and their progenitors. SCF is a ligand for c-kit receptor (Siatskas and Boyd, 2000).
Differentiation of myeloid lineage is ensured by colony-stimulating factors (CSFs). Macrophage CSF elicits macrophage differentiation from bone marrow progenitors. The same effect has been shown in IL-34 (Garceau et al., 2015; Wu et al., 2020). Granulocyte CSF stimulates monocyte growth and mobilizes heterophils from the bone marrow when inflammation occurs (Kogut et al., 1997; Gibson et al., 2009). The final member of the CSF family in chickens is granulocyte-macrophage CSF, which by itself is responsible for macrophage differentiation from monocytes (Peng et al., 2020) as well as the proliferation of tissue macrophages and increasing responsiveness to macrophage CSF (Chen et al., 1988). Together with IL-4, granulocyte-macrophage CSF can stimulate dendritic cells differentiation from bone marrow progenitors (Wu et al., 2010) and blood monocytes (Kalaiyarasu et al., 2016). Chicken myelomonocytic growth factor has a function similar to that of CSF because it stimulates proliferation and differentiation of granulocytes and macrophages in vitro (Siatskas and Boyd, 2000). The chicken homolog of CAAT, NF-M, has a proven ability to stimulate the differentiation of eosinophils in the bone marrow (Müller et al., 1995).
T-cell colonization of the thymus begins in waves during embryonal development when HEMCAM+ and c-kit+ bone marrow precursors colonize the cortex of the thymic lobules (Vainio et al., 1996). Both beta 2-microglobulin (Dunon et al., 1990) and CCL21 chemokine (Annamalai and Selvaraj, 2010; Kozai et al., 2017) seem to be involved in this process. Additional differentiation of naïve T cells occurs under the regulation of cytokines based on immune response against pathogens, as reviewed by Kogut (2000) and Wigley and Kaiser (2003).
B-cell precursors from the bone marrow colonize bursal anlage from 10 days of embryogenesis (Mansikka et al., 1990). Based on the expression of CXCR4 on B cells and their precursors, it can be assumed that CXCL12 chemokine plays a role in the migration of B-cell precursors (Nagy et al., 2020). Interestingly, B-cell precursors can be found in the bone marrow during embryogenesis but not after hatching, presumably because the colonization of bursal anlage by the bone marrow precursor occurs only in embryos (Weber and Foglia, 1980). In a more recent study, B-cell precursors were sorted based on cell size, and larger precursors were observed to proliferate and differentiate during development more than did smaller precursors (Ko et al., 2018). After antigen stimulation, naïve B cells can differentiate into plasmocytes and stimulate the production of specific antibodies. B-cell biology in chicken has been reviewed in detail by Sayegh et al. (2000). Relevant distinctive markers of avian immune cells for phenotype analysis are shown in Table 1.
Avian Bone Marrow
In birds, the bone marrow distribution is highly correlated with the existence of medullary bones; therefore, the major sites of bone marrow hematopoiesis in adult birds are the femur and tibiotarsus (Canoville et al., 2019). Brandon et al. (2000) optimized conditions to obtain a full range of blood cells from hematopoietic precursors in quail. They isolated bone marrow cells by gradient centrifugation and separated adherent cells by overnight adherence selection. Obtained blast-like cells were cultured on methylcellulose and fibrin gel cultures and differentiated in 6 morphologically different colonies. The most abundant were granulocyte-macrophage, erythroid, and macrophage cell colonies. After 6 days of culture, cells achieved maximal differentiation, and May–Grunwald–Giemsa staining was performed to recognize cells phenotype. Based on the visual evaluation, erythroblasts, erythrocytes, aggregated macrophages, monocytes, heterophils, basophils, eosinophils, and thrombocytes were recognized; therefore, they successfully imitate the avian hematopoiesis in vitro. Additionally, Nazifi et al. (1999) provided a detailed composition of quail bone marrow. Erythroid cells were the most prevalent (almost 70%), and myeloid cells comprised 25% of the whole cell population. Myeloid/erythroid ratios were described in various avian species, such as 1 for ducks (Tadjalli et al., 1997), 1.24 for pheasants (Tadjalli et al., 2013), and 1:14.6 for chickens at 4 weeks (Glick and Rosse, 1981). Glick (1987) showed that cellular composition is constant in chicken femur and tibiotarsus, but with increasing age, the numbers of immature granulocytes decreased. Based on light microscopy evaluation, the cellular composition of the bone marrow from various avian species was described, including chicken (Glick, 1987), partridge (Tadjalli et al., 2012), duck (Tadjalli et al., 1997), black-headed gull (Tadjalli and Hadipoor, 2002), pheasant (Tadjalli et al., 2013), Japanese quail (Nazifi et al., 1999), and parrots (Schwartz et al., 2019).
Bone Marrow Hematopoietic Stem Cell Niche
In order properly to imitate the bone marrow environment, it is necessary to understand the morphological structure of the avian HSC bone marrow niche. Two main subniches can be distinguished in the bone marrow. The endosteal niche is located close to the osteoblasts in the endosteum (Bello et al., 2018). In the endosteal niche, HSC prevalently reside in a quiescent state due to high concentrations of Ca2+ and hypoxia. The second subniche is termed the vascular niche (Figure 2A) where, by contrast, HSC can easily proliferate and differentiate because there is a higher level of oxygen and lower concentration of Ca2+ in comparison with the endosteal niche (Ferreira and Mousavi, 2018). Niche physical properties strongly influence HSC fate. Young’s modulus (YM) is an indicator of materials’ stiffness. In the case of the endosteal region, values of about 40–50 kPa have been determined, but areas near the blood vessels are much softer, with YM < 3 kPa (Shrestha and Yoo, 2019). Because greater tissue stiffness directly impairs HSC differentiation, in an endosteal niche, HSC are losing their stemness (Wen et al., 2014). In the endosteal niche, HSC reside in a quiescent state that is necessary for sustaining long-term hematopoiesis. Through signaling and adhesion molecules, osteoblasts regulate quiescence in HSC and ensure maintenance of quiescent HSC (Arai and Suda, 2007).
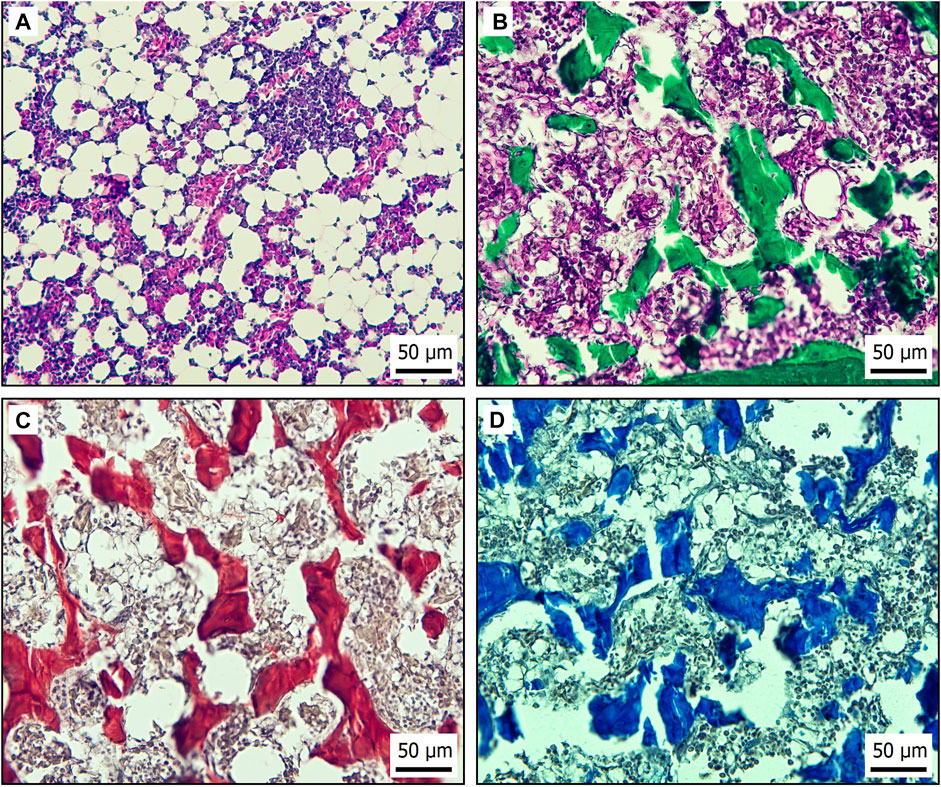
FIGURE 2. Histological bone marrow specimens from laying hen aged 21 weeks. (A) H&E staining of vascular niche in bone marrow. (B) Endosteal region of bone marrow stained by Masson’s green trichrome to demonstrate connective tissue based on collagen (green). (C) Sirius red staining of collagen type I (red) in endosteal niche of bone marrow. (D) Endosteal niche of bone marrow stained by Alcian blue to recognize hyaluronic acid (blue) in extracellular matrix.
Quiescent HSC from the endosteal niche eventually travel through the vascular niche, where they undergo differentiation and expansion. The vascular niche allows HSC to enter the peripheral blood through blood vessels (Tamma and Ribatti, 2017). More specifically, the vascular niche is divided into arterial and sinusoidal niches (Zhang P. et al., 2019). In avian species, CD45+ HSC have been described outside the bone marrow sinuses in extravascular regions near the arteries, where lymphopoiesis and myelopoiesis occur (Olah et al., 2014). Many cell types influence HSC fate within the (sub)niches, but some of these seem to be more important in creating those (sub)niches. Endothelial cells line the border of arteries and express high levels of VCAM-1 adhesion molecule, which ensures retention of HSC (Ulyanova et al., 2005). On the other hand, HSC homing and proliferation are supported by E selectin molecules expressed on sinusoidal endothelial cells (SEC) (Winkler et al., 2012). Mesenchymal stem cells (MSC), often termed mesenchymal stromal cells, play an important role in creating a stroma for the bone marrow niche and influencing HSC by cell-to-cell contact and production of active molecules (Walenda et al., 2010; Walenda et al., 2011). Coculture of HSC and MSC in collagenous hydrogel has revealed some important findings. HSC were observed to have a higher capacity for self-renewal in 3D cell culture, and coculture with MSC further supports HSC proliferation. MSC produce fibronectin and osteopontin that form an extracellular matrix (ECM) component, which, in turn, allows HSC easier migration due to the presence of cell attachment sites (Leisten et al., 2012). MSC further produce IL-6, granulocyte-macrophage CSF, SCF, and adhesion molecules such as VCAM-1 and E-selectin. By means of all these molecules, MSC regulate homing, proliferation, and differentiation of HSC (Li and Wu, 2011). Chicken MSC express CD73 and CD44 and can easily be isolated from the bone marrow and used to coculture with HSC to mimic HSC niche environment (Adhikari et al., 2019).
Moreover, an HSC niche is composed of other cellular components that produce molecules involved in HSC self-renewal, quiescence, or proliferation. Endothelial cells are further divided into SEC and arteriolar endothelial cells (AEC), and the biggest difference between them is in the production of SCF, which ensures the maintenance of HSC. AEC are more effective producers of SCF than SEC (Ding et al., 2012; Xu et al., 2018). The rich population of bone marrow adipocytes is another producer of SCF, which plays an indispensable role in the maintenance of HSC (Zhou et al., 2017). Megakaryocytes give rise to thrombocytes in blood. On the other hand, they directly influence hematopoiesis by cytokine production. They can affect HSC in various ways. Firstly, megakaryocytes ensure quiescence of myeloid-based HSC in megakaryocyte-dependent regions by the production of CXCL4 (Pinho et al., 2018) and TGF-β (Gong et al., 2018). Secondly, megakaryocytes can promote the proliferation of HSC by the production of fibroblast growth factors (Zhao et al., 2014). TGF-β is known as the inducer of quiescence, but it was proven that TGF-β in low concentration can promote the proliferation of myeloid HSC. However, the quiescence-promoting effect of a high concentration of TGF-β is undisputed (Blank and Karlsson, 2015). Various cell types are further involved in TGF-β production, for instance, Schwann cells (Yamazaki et al., 2011) and macrophages (Hur et al., 2016). Interferons (IFN-α, IFN-γ) are known inducers of an antiviral state in a cell, but they can cause loss of quiescence and promote the proliferation of HSC. TNF-α as a major pro-inflammatory cytokine was found to suppress proliferation of HSC by induction of apoptosis, but some studies described positive effects of TNF-α treatment on the expansion of lymphoid progenitors and bone marrow granulocytes; therefore, the influence of TNF-α on HSC fate is controversial (Baldridge et al., 2011).
Various immune cells influence HSC in direct or indirect ways, and these cells are the target for most pathogens. Therefore, there is another way in which pathogens influence HSC in their niche. The most known influencers of HSC are regulatory T cells and subtypes of macrophages (Man et al., 2021). Marek’s disease in susceptible chicken lines triggers the production of TGF-β+ regulator T cells, and subsequently high amounts of TGF-β released into the bloodstream dysregulate hematopoiesis (Gurung et al., 2017). The same CD4+ CD25+ regulatory T cells are induced in cecal tonsil of Salmonella-infected chickens (Shanmugasundaram et al., 2015). Regulatory T cells, via the production of adenosine, can ensure further quiescence of allogeneic HSC (Hirata et al., 2018). Many viral pathogens replicate in bone marrow macrophages (von Bülow and Klasen, 1983a), and these phagocytic cells influence HSC in a direct way. They can provide retention sites for HSC through VCAM-1 (Dutta et al., 2015) and induction of expression of CXCL12 in MSC (Chow et al., 2011). Therefore, bone marrow macrophage’s function seems to be promoting retention of HSC by regulating osteoblast and MSC to maintain retention of HSC, because treatment with granulocyte CSF caused rapid depletion of osteoblast and endosteal region macrophages. Due to the loss of binding sites and factors responsible for the retention, high numbers of HSC were released into the blood (Winkler et al., 2010).
Non-cellular HSC niche components such as ECM proteins not only play supporting roles but also have the ability to greatly influence HSC functions. Furthermore, they can be easily incorporated into various 3D cell culture scaffolds (Caliari and Burdick, 2016). The most abundant molecules in HSC niches are proteoglycans, which ensure signal delivery between cells (Redondo et al., 2017) and fibrous proteins (Figure 2B) (Frantz et al., 2010). Collagen I, a major ECM fibrous protein of the bone marrow, causes a decrease in CD34+ HSC expansion (Oswald et al., 2006) and an increase in the numbers of cells in a quiescent state. That is because collagen I is associated with the endosteal region (Figure 2C), where HSC quiescence occurs (Chitteti et al., 2015). Also in the endosteal region, hyaluronic acid supports osteogenesis while ensuring viscoelasticity and compressive strength of the bone marrow (Figure 2D). Moreover, hyaluronic acid provides attachment sites through ICAM-1 and CD44 expressed on chicken MSC (Adhikari et al., 2019; Zhai et al., 2020). The additional fibrous proteins fibronectin and laminin express tri-amino acid sequence (arginine–glycine–aspartate) and are termed RGD peptides (Bellis, 2011). Integrins are RGD peptide ligands with numerous functions in HSC (Coulombel et al., 1997). For instance, β7 integrin activation has been found to regulate HSC homing and engraftment (Murakami et al., 2016). HSC in a presence of laminin and fibronectin within ex vivo culture improved bone marrow engrafting ability and stem cell expansion, probably due to integrin-induced pathways (Sagar et al., 2006).
The Notch signaling pathway is involved in the proliferation of HSC. Through stimulation of Notch receptors, expansion of HSC in the niche can be achieved (Mendelson and Frenette, 2014). On the other hand, the Wnt signaling pathway is involved in HSC proliferation and differentiation based on the level of activation. Only mild activation of the Wnt pathway increased HSC proliferation. Subsequently, by increasing Wnt activation, differentiation into myeloid precursors and then into lymphoid precursors occurs. If the Wnt pathway is highly stimulated, hematopoiesis impairment occurs (Luis et al., 2011).
An HSC niche is a complex system, where many factors affect HSC function. Because specific information about chicken bone marrow is lacking in this area, it is necessary to apply information about mammalian HSC niches to the preparation of an ex vivo chicken HSC niche.
Ensuring Quiescence in In Vitro Studies
In in vitro models, achieving the quiescent and active states of HSC is critical for studying the process of hematopoiesis. In a recent study, Kobayashi et al. (2019) defined key factors responsible for maintaining a quiescent state in vitro HSC cultures. The first factor is low oxygen concentration, which is about 1.3% (Spencer et al., 2014). In mice, it was found that over 80% of quiescent HSC had low adenosine triphosphate levels and utilize cytoplasmic glycolysis (Simsek et al., 2010). Secondly, attenuation of metabolic processes necessary for maintaining quiescent stadium is achieved by relatively high fatty acid concentration (Kobayashi et al., 2019). Lastly, the crucial factor is low cytokines concentration because it is well known that cytokines and growth factors ensure mobilization and activation of HSC (Jahandideh et al., 2020). Under these conditions, Kobayashi et al. (2019) were able to maintain engraftable quiescent HSC for 1 month. To study the influence of pathogens on activation of HSC and further differentiation, it must be achieved by changes during the HSC culture, which can be ensured by perfusion systems with the flow of the medium. Furthermore, the system must allow the incorporation of nutrients and cytokines. 3D HSC culture system prepared by Rödling et al. (2017) was a bioreactor filled with macroporous hydrogel with niche-mimicking properties, and the flow of the medium was powered by a peristaltic pump. Their attention was focused on mimicking steady-state and activation conditions in HSC culture. They measured cytokine levels in static and dynamic cultures, and it is not surprising that levels of cytokines were much lower in dynamic culture because they washed out the 3D culture. In static culture, strong upregulation of IGFBP2 and MIF cytokines was found, and both were described to support proliferation and expansion of HSC. Hypoxia in cell culture can be easily achieved by packing the cell culture chambers with vacuum-sealing machines (Matthiesen et al., 2021). In hypoxic cells is a characteristic high expression of hypoxia-inducing factor (HIF), and quiescent HSC express high levels of HIF-1α (Takubo et al., 2010).
Impacts of Avian Immunosuppressive Diseases on Bone Marrow-Derived Cells
Most diseases cause immunosuppression because disease agents must overcome the organism’s immune barrier. In vitro studies in this area generally have been conducted with individual bone marrow-derived cells, but we lack information about direct impacts on HSC and hematopoiesis as a whole. A better understanding of immunosuppression is important for determining better preventive and diagnostic mechanisms for avian diseases (Gimeno and Schat, 2018). Avian immunosuppressive diseases have been described in detail in reviews by Schat and Skinner (2014) and by Gimeno and Schat (2018), so we have narrowed our focus to summarizing information about avian diseases and their impacts on bone marrow-derived cells cultured in cell culture wells and flasks. To date, a methodology for generating cells from the bone marrow in chickens has been described only for dendritic cells (Wu et al., 2010). Therefore, most studies in this area have been focused on the main antigen-presenting cells (Table 2).
Deriving Chicken Stem Cells From Bone Marrow
Standard methods for avian stem cells culture are described in a review by Farzaneh et al. (2017). Derivation of HSC niche cells is the first step in cell culture preparation. In chickens, methods have been described for isolating HSC and MSC. In the case of avian HSC, the usual sources are chicken femur and tibiotarsus. The ends (epiphysis) of the bones are cut, and their content is flushed with sterile Dulbecco’s phosphate-buffered saline without Ca and Mg (DPBS). Cell aggregates in the flushed content of the bones must be disaggregated by pipetting and sieved through a 40-μm cell strainer. Subsequently, bone content diluted in DPBS is loaded onto an equal volume of Histopaque®-1,119 (1.119 g/ml at 25°C) and centrifuged at 1,200 g for 30 min. Cells at the interface are collected and then washed two times with PBS. Cells are cultured in complete RPMI-1640 medium with 10% of chicken serum at 41°C and 5% CO2 (Wu et al., 2010). Anti-chicken CD45 phycoerythrin (PE)-conjugated antibody (SouthernBiotech, Birmingham, AL, USA) can be used in combination with anti-PE microbeads (Miltenyi Biotech, Bergisch Gladbach, Germany) to isolate HSC by magnetic-activated cell sorting.
MSC can be embedded simultaneously with HSC into 3D cell culture to improve the biocompatibility of the culture. MSC can be isolated from compact bones of the femurs and tibiotarsus of day-old chicks. The bone marrow must be completely washed out, and bones are then chopped into small pieces. Bone fragments are disaggregated in Dulbecco’s modified Eagle’s Medium (DMEM) with 0.25% collagenase and then incubated in a shaking bath for 60 min at 37°C and 180 rpm. Suspension with bone fragments is filtered through a 40-μm cell strainer. Subsequently, the cell suspension is washed in DMEM, then placed in complete DMEM, and transferred into a humidified incubator at 37°C and 5% CO2. After 24 h of incubation, non-adherent cells are removed, and adherent cells are considered as MSC (Adhikari et al., 2019; Adhikari et al., 2020).
Three-Dimensional Cell Cultures
In vitro studies are the gold standard for studying various cell types and for understanding the pathogenesis of diseases. Classic culture using plastic culture wells or dishes, where cells have adhered to the surface, does not imitate cell niche microenvironment inside the body. Very little attention has been given to the use of 3D cell cultures in avian models. It has been studied predominantly in embryonal cells, such as primordial germ cells (Chen et al., 2018), intestinal epithelial cells (Pierzchalska et al., 2019), or chicken bone marrow cells as support for osteogenesis of human stromal cells (Yang et al., 2020). Therefore, our current knowledge about 3D stem cell cultures comes from studies in mammals. An in vitro HSC niche cell culture model has never been studied in avian species.
Two-dimensional (2D) cell culture has some disadvantages, as explained by Kapałczyńska et al. (2018). Such cell cultures do not imitate the natural structure of tissue and do not provide cell-to-cell and cell-to-microenvironment interactions. Adherence to the plastic surface causes cells to take on an unnatural flattened shape and changes their morphology. In contrast to tissue, cells in 2D cultures have unlimited access to oxygen, nutrients, metabolites, and signaling molecules. Finally, 2D cell cultures cause changes in gene expression and topography of the cells in comparison with the in vivo environment.
Hydrogel Scaffolds
All limitations of 2D cell cultures hinder the study of cell proliferation and differentiation in ways that are similar to doing so in tissue. For these reasons, scaffolds based on hydrogels have been developed for 3D cell culture, and the scaffolds should mitigate these limitations. Caliari and Burdick prepared a practical, focused review as a hydrogel selection guide (Caliari and Burdick, 2016).
Three-dimensional HSC culture requires biocompatible materials that ensure imitation of bone marrow niche elements as influencers of HSC fate. ECM of the bone marrow is composed of the most prevalent collagen structural proteins (Kramer et al., 2017) and then the important laminin and fibronectin proteins (Zhang P. et al., 2019) that are necessary for the growth, development, proliferation, and differentiation of HSC. These proteins express integrin-binding domains termed RGD. HSC express on their surfaces such RGD-binding integrins as α4, α6, α7, α9, and β1 that play important roles in cell development and proliferation (Zhang P. et al., 2019). Collagen I, collagen IV, laminin, and fibronectin together have been able significantly to increase HSC expansion and induce greater myeloid progenitor cell expansion (Celebi et al., 2011). Not only ECM but also niche cells contribute to the production of molecules that build a hematopoietic niche. The MSC-based scaffold has been shown to provide signaling molecules for HSC, because αIIb, αV, and β3 integrins were induced in HSC within decellularized ECM scaffolds derived from SCP-1+ MSC. These findings support the contribution of MSC to the modulation of HSC function by cell-to-cell contact (Kräter et al., 2017).
Leisten et al. (2012) performed a coculture of HSC with MSC in collagen-based hydrogel and revealed some useful findings. A collagenous matrix is optimal for HSC migration and proliferation. Proliferation marker Ki67 was highly expressed, suggesting high self-renewal in HSC. MSC in coculture produce fibronectin and osteopontin, and they support collagen I synthesis to form a hematopoietic niche. MSC isolated from the bone marrow in collagen-based hydrogel stimulated higher levels of CD34+ HSC. This ability was proven only in the case of MSC derived from the bone marrow, so coculture with bone marrow-derived HSC is necessary for the applicability of bone marrow MSC (Leisten et al., 2012). Collagen-based hydrogels seem to be a promising platform for HSC 3D cell culture, but naturally based hydrogels have shortcomings in terms of their mechanical properties. These hydrogels have limitations with regard to controlling the material stiffness and elasticity. Holst et al. (2010) found that the addition of tropoelastin, which changed stiffness and elasticity, caused greater expansion of hematopoietic progenitor cells. For this reason, synthetic hydrogels with easier controllability of stiffness have been developed.
Polyacrylamide (PAM) has a uniquely tunable mechanical character that allows setting up optimal stiffness and elasticity (Kandow et al., 2007). As indicated by YM, tensile stiffness of the bone marrow has been established to be in the range 0.25–24.7 kPa at the physiological temperature (Jansen et al., 2015). In a recent study with ECM ligand-coated PAM, it was suggested that biophysical elements combined with ligands (laminin or fibronectin) of the HSC niches directly modulate HSC fate decisions (Choi and Harley, 2017).
Synthetic hydrogels based upon polyethylene glycol (PEG) present another choice for culture with tunable stiffness (Carthew et al., 2018). The biggest advantage of PEG is its enormous biocompatibility (Tsou et al., 2016). Macroporous PEG-based hydrogel is indicated as a great approach for mimicking an HSC niche because it reflects 3D bone marrow regions. Coculture with bone marrow-derived MSC in PEG-based hydrogel led to the much higher proliferation and self-renewing capacity of HSC (as in the natural environment) compared with ordinary 2D cell culture (Raic et al., 2014). PAM- and PEG-based hydrogels or scaffolds do not provide cell adhesion and support proliferation, but this disadvantage can easily be overcome by conjugation with RGD peptides. This treatment ensures MSC (Sawyer et al., 2005) and HSC adhesion and proliferation (Raic et al., 2014; Tsou et al., 2016).
Hydrogels with synthetic crosslinkers can be prepared based on hyaluronic acid, which allows for easier stiffness tunability. On the other hand, fully synthetic hydrogels are composed of chemically defined components that ensure easy stiffness tunability, administration of adhesive proteins, and cell recovery, but biocompatibility is lower (Tibbitt and Anseth, 2009). The main components of these hydrogels consist of polymers, such as polyvinyl alcohol or dextran with crosslinkers such as PEG that connect polymer chains (Caliari and Burdick, 2016). Hyaluronic acid-based hydrogels can be enriched with carbon nanotubes to ensure antioxidant properties, thereby supporting HSC proliferation and pluripotency and protecting against oxidative stress (Zhang Y. et al, 2019). Results from testing these hydrogels in mammalian models show that this technique is promising for application in avian models. Host–pathogen interactions can be conducted simply on hydrogels embedded in cell culture. The hydrogels also can be included in advanced cell culture systems, such as organoids and organ-on-a-chip (OCM), where interactions with pathogens can be studied (Liu et al., 2019; Feaugas and Sauvonnet, 2021).
Nanofiber Scaffolds
Several synthetic materials have been used to mimic the bone marrow niche. Among these are polycaprolactone (PCL), polylactic acid, polyurethane, and polyethylene terephthalate. These polymers have several limitations, including lower biocompatibility, hydrophobicity (compared with cells niche), and lack of binding sites for cell adhesion (Ferreira and Mousavi, 2018). On the other hand, synthetic materials have good mechanical properties, are highly reproducible, can be produced at a low cost, and do not stimulate the activation of immune cells (Tallawi et al., 2015; Kim T. E. et al., 2016).
The absence of biocompatibility and binding sites can be overcome relatively easily by blending polymers with ECM proteins and coating them with adhesion molecules. Ma et al. (2008) blended poly(dl-lactide-co-glycolide) polymer with collagen I to create nanofibers by electrospinning method and then coated nanofibers with E-selectin. This nanofiber scaffold increased HSC capture by 44% within 30 min and by 40% within 60 min. Biocompatibility can be increased by the inclusion of other HSC niche cells. HSC coculture with MSC on poly-l-lactic acid nanofiber scaffold led to greater expansion, purity, viability, and clonogenicity of HSC (Darvish et al., 2019).
PCL is widely used in tissue engineering and drug delivery (Chen and Lin, 2020). It is likewise often used in 3D cell cultures, where it promotes the proliferation and differentiation of various kinds of cells (Kim T. E. et al., 2016). PCL is a biocompatible and biodegradable polymer with adjustable hydrophobicity and cell adhesion abilities. Hydrophilicity can be enhanced by simple sodium hydroxide treatment (Bosworth et al., 2019). Cell adhesion and biocompatibility may be improved by coating with several of the aforementioned proteins (collagens, fibronectin, laminin, and RGD peptides) to mimic an HSC niche (Tallawi et al., 2015). In recent years, some experiments have been performed that lent support to the importance of PCL coating with proteins. Mousavi et al. (2019) coated PCL nanofibers with collagen I. Coating caused higher total cell counts (58 × 38-fold) and higher numbers of CD34+ cells (20-fold × 2.6-fold) compared with 2D cell culture. The ability to form colonies was significantly stronger in 3D cell culture based on the colony-forming assay. Fibronectin coating also has been shown to have a significant effect on HSC expansion. HSC cultured on fibronectin-coated PCL nanofibers have significantly greater expansion and expression of genes related to self-renewal. Greater expression of CD34 and CD45 markers in cells cultured on fibronectin-coated PCL nanofibers has been observed (Mousavi et al., 2018). Based on these findings, it can be assumed that PCL nanofiber coating promoted interactions between cells and provided a larger cell attachment area for cell expansion. In another comparative study, fibronectin-coated surface proved to have higher expansion potential in HSC and a higher percentage of CD34+ and CD45+ cells compared with when collagen I coating was used. Probably this is because fibronectin provided a larger surface for cell attachment and stronger adhesion forces in HSC (Kang et al., 2016). A supporting role of MSC with HSC has been proven in many cocultures on various materials. Total cell counts and percentages of CD34+ cells were significantly higher in coculture of HSC with MSC on PCL nanofibers compared with culture without MSC. The authors explained that the greater adhesion surface provided by MSC and thus higher proliferation rate of HSC contributed to greater HSC expansion (Ferreira et al., 2012). Moreover, the differentiation potential of HSC cultured on PCL nanofibers was also improved in comparison with 2D cell culture (Dehdilani et al., 2016). The results of current studies with PCL nanofibers point to positive effects on HSC self-renewal, differentiation, and migration. It is necessary, however, to coat PCL nanofibers with proteins and peptides, as well as to evaluate nanofiber thickness and scaffold pore size to ensure better biocompatibility.
Microfluidic cell cultures have been described for studying host–pathogen interactions (Barrila et al., 2018), and these systems can be enriched by PCL nanofibers scaffold to mimic various tissues. Based on this approach, pathogen-infected cells or pathogens with uninfected cells can be cultured to study host–pathogen interactions (Kim et al., 2019).
Rotating Wall Vessel Culture
A rotating wall vessel (RWV) is a rotating cylinder filled with a cell culture medium where cells are constantly falling through the medium. In contrast to scaffold-based cell cultures, therefore, RWV cell culture allows cells to be in constant movement. Cells grown on culture plastic surfaces are collected and incubated with microcarrier beads for attachment (Nickerson et al., 2007). Microcarrier beads can be coated with ECM compounds, such as collagen (Radtke and Herbst-Kralovetz, 2012) or hyaluronic acid (Skardal et al., 2010). Cells attached to the beads are replaced with the RWV, and rotation is initiated. Within the RWV, cells can respond to chemical gradients and react with active molecules and microorganisms, and that means RWV cell culture can be used for cell differentiation and host–pathogen interaction studies. After an experiment, cells can easily be removed from the microbead carriers for further culture or evaluation (Nickerson et al., 2007). Pathogens can be added directly to the RWV or to the cell culture after recovery from RWV (Barrila et al., 2018).
Organ-on-a-Chip Cell Culture
Dynamic processes in the bone marrow can be imitated by microfluidic technology based upon OCM. OCM consists of micro channels with a flowing medium separated by porous membranes that allow cells to remain in neighboring chambers (Barrila et al., 2018). In the case of HSC 3D culture preparation, the chambers can be filled with materials having a structure similar to that of the bone marrow. Furthermore, MSC can be precultured on chambers where they create an HSC niche by producing a stroma and such ECM components as fibronectin. HSC cultured on OCM have been found to remain in a primitive CD34+ state and be capable of differentiation and long-term culture for 28 days (Sieber et al., 2018). Similarly, microfluidic technology has been used to create a multigradient hydrogel system for HSC proliferation and differentiation assays within a 3D environment where surrounding ECM components and niche cells can be manipulated (Mahadik et al., 2014). For studying disease pathogenesis, disease causative agents can flow into the microchannels, and then cultured cells can be recovered and evaluated by morphological, genetic, and biochemical analyses (Kim H. J. et al., 2016). Figure 3 provides a schematic presentation as to the possible use of 3D HSC cell culture to study interactions with pathogens.
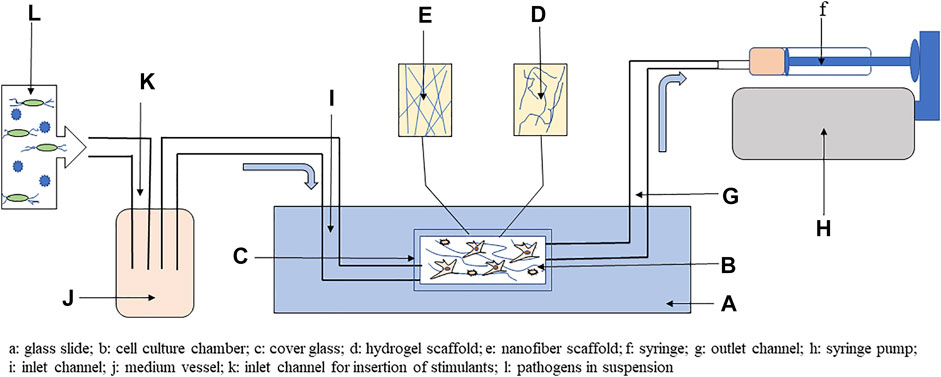
FIGURE 3. Simplified scheme using 3D cell culture with microfluidic system to study host–pathogen interactions. The main part of the system is a glass slide with chamber covered by glass coverslip, where hydrogels or nanofibers scaffold can be included. In the cell culture chamber, mesenchymal stem cells (MSC) and hematopoietic stem cells (HSC) can be cocultured on scaffold. The chamber is connected to a syringe by outlet channel, and flow of medium is produced by syringe pump through negative pressure. Cell culture medium is introduced into the chamber by inlet channel from the vessel. To study host–pathogen interactions, the medium vessel can be enriched via the inlet for inclusion of pathogens or other stimulants, such as metabolites, toxins, vitamins, or minerals. Adapted from Kim et al. (2019).
Polydimethylsiloxane (PDMS) is a commonly used polymer for OCM preparation and based on PDMS was developed 3D bone marrow on a chip with precultured MSC composed of two microchambers. In the top chamber, a coculture of MSC and HSC was performed, and the bottom chamber was separated by a porous membrane where the medium flows (Kefallinou et al., 2020). These membrane systems can be used to study host–pathogen interactions. Staphylococcus aureus infection model was used to study interactions with neutrophils, macrophages, and dendritic cells on poly(ε-caprolactone) nanofiber membrane. In this culture system, immune cells secreted TNF-α and IL-1α in comparison with 2D cell culture; therefore, 3D culture mimics standard inflammatory response as in the organism (Lee et al., 2021). The OCM culture can use scaffold-based cultures. Di Maggio et al. (2011) described a ceramic scaffold-based perfusion system with embedded MSC to mimic an HSC niche. Additionally, the platform allowed easy insertion of cytokines, growth factors, and potentially disease causative agents.
OCM cultures to study host–pathogen interactions were used mainly for mimicking respiratory and digestive tract disease pathogenesis. The importance of fluidic-based systems showed the study of Sunuwar et al. (2020). Luminal flow in their jejunal enteroid chip was able to stimulate the production of cyclic guanosine monophosphate upon exposure to heat-stable enterotoxin A from enterotoxigenic Escherichia coli. Similarly, Villenave et al. (2017) prepared microfluidic-based gut on chip and continuous flow enhanced viral replication and subsequently enterocyte damage. Mimicking physiological stretching of the gut caused by peristalsis can be an important factor for the increased invasion of some bacteria. For instance, Shigella human bacteria causing severe intestinal damage uses the peristaltic movement of the intestine wall and luminal flow to enhance their invasion potential (Grassart et al., 2019). The liver represents the most important organ for metabolic processes, and the liver on a chip is a valuable tool for studying the pathogenesis of hepatic diseases. Ortega-Prieto et al. (2018) prepared a microfluidic collagen scaffold-based liver on a chip with polarized primary human hepatocytes to examine the pathogenesis of hepatitis B virus, and they successfully imitated the production of cytokines and innate immune response as in the case of patients infected by hepatitis B virus.
OCM cultures were successfully used to imitate respiratory disease pathogenesis. Superinfection of influenza virus and S. aureus was observed in a virus–bacteria coculture on lung alveolus on chip (Deinhardt-Emmer et al., 2020). OCM cultures can play a role in models where it is impossible to study interactions in vivo. Surfactants on the surface of the respiratory tract create a part of a protective response against respiratory pathogens. Surfactant-deficient animals have high lethality; therefore, it is impossible to study pathogenesis in these animals. For this reason, Thacker et al. (2020) developed a lung on a chip with three layers, with the top layer composed of alveolar epithelial cells with macrophages with the bottom composed of endothelial cells and air–liquid interface. Through time-lapse imaging, they can reveal the dynamics of the initial phases of Mycobacterium tuberculosis infection and describe the host protective role of surfactants. The usability of a lung on a chip was also proved in viral infection models. Additionally, the impact of a lung on a chip devise on increased virulence of several serotypes of influenza virus was also proved (Si et al., 2019).
In a recent year, OCM is being developed to ensure that several probiotic bacteria strains are maintained for longer periods of time of more than 1 week; therefore, it can be used for studying the long-term effects of pathogens with the chronic progression of pathogenesis (Kim T. E. et al., 2016). Additionally, host–pathogen interactions using OCM cultures can be performed with various oxygen levels or under hypoxia, which is necessary for HSC quiescence (Shah et al., 2016).
Using Three-Dimensional Cell Cultures to Study Host–Pathogen Interactions
The multipotency of HSC enables to differentiate them into various immune cells and study how pathogens interfere with hematopoiesis. The process of preparation includes cell seeding, the inclusion of cocultured cells, and growth factors and cytokines, which ensure HSC differentiation. Inclusion of pathogens can be performed in every step of the process, so this enables to study the disruption of hematopoiesis. The scheme of workflow for host–pathogen study using scaffold-based 3D stem cell cultures is described in Figure 4.
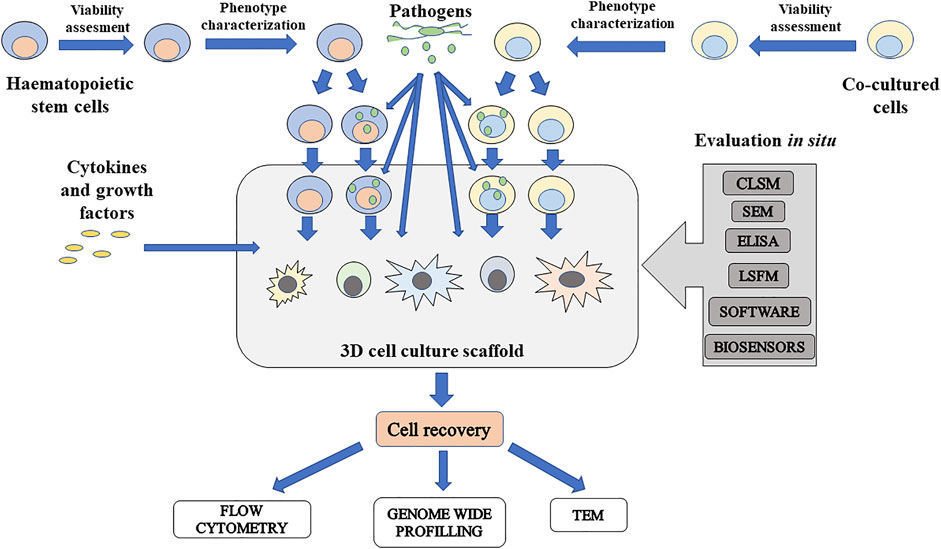
FIGURE 4. Schematic presentation of scaffold-based 3D cell culture to study influence of pathogens on hematopoiesis. Isolated hematopoietic stem cells (HSC) and cocultured cells can be infected before insertion to culture or after culturing in 3D culture. To study differentiation potential of infected HSC, cytokines and growth factors can be added and can initiate differentiation in various immune cells. At the same time, pathogens can be included to study how these disease causative agents can disrupt differentiation in immune cells. For in situ evaluation, there are some usable techniques. Imaging techniques include confocal laser scanning microscopy (CLSM), scanning electron microscopy (SEM), and light sheet fluorescence microscopy (LSFM). Content of molecules released by infected cells can be measured by standard ELISA method. Software-based techniques such as FluoroCellTrack can be used for evaluation of cells in flow, in microfluidic systems, or embedded cells in a scaffold. For monitoring of culture conditions, microchips for electrochemical detection of important measurable parameters such as oxygen, carbon dioxide, nutrients, and metabolite levels can be included. If it is possible to recover cells from the cultured scaffold, cells can be evaluated by antibody-based techniques, genome-wide profiling, and microscopy techniques such as transmission electron microscopy (TEM) for intracellular structure imaging.
Seeding of Cells
Cells can be seeded sequentially or simultaneously. It is better to seed them simultaneously because bone marrow HSC and MSC are located right next to each other. This system of cell seeding ensures homogenous localization (Baldwin et al., 2014). To mimic a bone marrow environment, scaffold-based cultures should be preferred. Then it is possible to seed cells on the upper sides of hydrogels or nanofiber scaffolds for cell migration studies. For the achievement of homogenous localization, cells can be mixed in liquid hydrogels. After the addition of crosslinker or through temperature-induced solidification, the creation of solid hydrogels is achieved (Rizwan et al., 2021). In the case of nanofiber scaffolds, cell localization is highly affected by pore size. Therefore, it is important to evaluate scaffold structure by scanning electron microscopy; otherwise, cell infiltration can be improved by sonication (Lee et al., 2011).
Medium Exchange
Culture medium exchange is an important factor to mimic the bone marrow environment. After the exchange of the medium, cell culture losses cytokines and other substances produced by cocultured cells and pathogenic agents. On the other hand, the medium brings new nutrients to the culture. Low fluctuation in amounts of endogenic and exogenic factors entering the culture can be achieved by the exchange of low amounts of media in static cultures or a proper setup of a pump in microfluidic systems (Baldwin et al., 2014). The evaluation of stability in cell culture can be measured by biosensors in the culture because they can be used for the evaluation of oxygen, nutrients, pH, and metabolites. The problems of the current approaches in electrochemical sensing in 3D cultures are reviewed by Oliveira et al. (2021). In a dynamic microfluidic system, channel volumes and dilution requirements must be determined to obtain reliable results by detection systems (Castiaux et al., 2019). ELISA is used for the detection of molecules in a culture medium; therefore, it can be used for measurements of changes in metabolites (Kim et al., 2019).
Evaluation In Situ
Standard morphological viability assays can be performed by fluorescence microscopy using confocal laser scanning microscopy (CLSM). CLSM can easily evaluate the location of cells and migration of cells in a scaffold by stacking images (Kim T. E. et al., 2016). The combination of CLSM with matrix-assisted laser desorption ionization-time (MALDI) proposes a complex analysis method. Machalkova et al. (2019) used CLSM to localize cells with fluorescence markers of apoptosis and proliferation. They also used MALDI for the detection of drugs of interest on tissue sections obtained from 3D cell culture. The principle of MALDI and usability in pathogen detection was reviewed by Singhal et al. (2015). Using CLSM can be problematic for scaffolds with higher thickness because of the loss of fluorescence signals of labelled molecules from deeper layers of more than 100 µm. On the other hand, light sheet fluorescence microscopy was able to create complete 3D tomography of tumor spheroids (Lazzari et al., 2019). Samples with thicknesses of more than 1 cm can be analyzed (Agrawal et al., 2021). After high-resolution images are obtained, the captured pictures can be analyzed by appropriate software equipment. Usable software-based analyses for 3D cell culture were reviewed by Agrawal et al. (2021). For instance, FluoroCellTrack is usable for high-throughput analyses of fluorescently labeled cells in a microfluidic system. Therefore, FluoroCellTrack can detect cells or droplets in a flow in a similar manner to flow cytometry but for longer periods of time (Vaithiyanathan et al., 2019). On the other hand, real-time monitoring of cells embedded in a scaffold can be performed by MetaXpress Software and ImageXpress Micro System, which allows to study the migration of cells toward the gradients (Agrawal et al., 2021).
Cell Recovery
Another group of analytical methods comprises techniques after recovery of cells from 3D cell culture. However, the crucial step is cell recovery, which can cause serious problems, because the cell can be destroyed due to inappropriate treatment. Enzymatic cell recovery is a classic method for cell liberation from the hydrogel and nanofiber scaffolds. However, it must be taken with great care to avoid the degradation of cell receptors. The enzyme used for cell recovery is selected based on scaffold material hyaluronic acid (hyaluronidase), collagen (collagenase), et cetera (Caliari and Burdick, 2016). Cells cultured on nanofibers are usually collected by trypsinization (Kim T. E. et al., 2016; Kim et al., 2018). Enzyme concentration seems to be critical for achieving optimal cell liberation. Virumbrales-Muñoz et al. (2019) dealt with the optimization of cell recovery from collagen-based hydrogel in a microfluidic system. They tracked enzymatic degradation by confocal reflection microscopy, and the degradation rate was highly correlated with the concentration of the collagenase. For further experiments, collagenase’s highest concentration (8 mg/ml) was used and applied to the microfluidic system, and the scaffold was degraded. For 10 min, the majority of cells (80%) was extracted. This recovery method did not negatively affect the viability (above 90%) after cell recovery, and it was possible to reseed cells into another hydrogel. Cells were then used for gene expression analyses, and additionally cells were usable for image flow cytometry analyses, which support the usability of this approach as a gentle method to obtain cells from hydrogel scaffolds. Photodegradable PEG-based hydrogels propose more than easily degradable material for cell recovery. Shin et al. (2014) prepared photogel functionalized with anti-CD4 and anti-CD8 antibodies to isolate lymphocytes from a heterogenous cell population. Then, cell attachment sites were visualized by fluorescent microscopy; and then through site-specific exposure to UV light, CD4+ and CD8+ lymphocytes were successfully released. Isolating individual cells allows novel microscopy techniques, such as laser capture microdissection, which cut off the block from the scaffold, and subsequently, appropriate enzymes are used to digest scaffold and release cells (Oldenhof et al., 2020).
Post-Harvest Evaluation of Cells
Post-harvest evaluation of cells in disease models comprises flow cytometry analyses of apoptotic and necrotic cells, phenotype markers, and activation markers in antigen-presenting cells. However, more comprehensive analyses can be performed through gene expression analyses of pro-inflammatory and anti-inflammatory cytokines, chemokines, receptors, proteins involved in programmed cell death, and pathogens (Kalaiyarasu et al., 2016). Genome-wide profiling of infected cells can provide deeper information about pathways involved in pathogen-induced immunosuppression (Lin et al., 2016). Various microscopy techniques can be used for the detection of pathogens, but precisely, the infection can be analyzed by transmission electron microscopy, which provides analyses of intracellular changes in response to infection (Rajput et al., 2014). The high-content single-cell technologies provide wide spectrum techniques to study host–pathogen interactions in different points of view, and all of them and their usability are reviewed by Chattopadhyay et al. (2018).
Conclusion and Future Developments
The bone marrow as a source of immune cells in adult birds is disrupted by numerous diseases. Very little is known about these impacts in avian models. Immunosuppression caused by many avian diseases can be mitigated if we will have a better understanding of their pathogeneses, and therefore, we can create more effective vaccines and vaccination programs. That, in turn, will facilitate the realization of the genetic potential of poultry for maximum production while improving welfare in flocks. Poultry flocks are also a source of zoonotic diseases, and so preventing avian disease is very important to ensure human health globally. For these purposes, the creation of in vitro avian HSC niches for studying diseases’ pathogeneses can provide a valuable tool for improving global poultry health. Moreover, it can be used for studying pharmacokinetics and the effects of metabolites and additives on hematopoiesis. First, however, it is necessary to create an HSC niche in vitro. This review is a source of knowledge obtained from mammalian models that can be applied to achieve that objective.
Author Contributions
VZ designed the structure of the review, collected the literature, and wrote the article. AS prepared the graphical schemes and collected the literature. PS revised and formatted the manuscript. AB prepared the histological specimens.
Funding
This research was funded by the Technology Agency of the Czech Republic (TJ04000511).
Conflict of Interest
The authors declare that the research was conducted in the absence of any commercial or financial relationships that could be construed as a potential conflict of interest.
Publisher’s Note
All claims expressed in this article are solely those of the authors and do not necessarily represent those of their affiliated organizations, or those of the publisher, the editors, and the reviewers. Any product that may be evaluated in this article, or claim that may be made by its manufacturer, is not guaranteed or endorsed by the publisher.
Acknowledgments
The authors are thankful to the Technology Agency of the Czech Republic for the support.
References
Adhikari, R., Chen, C., and Kim, W. K. (2020). Effect of 20(S)-hydroxycholesterol on Multilineage Differentiation of Mesenchymal Stem Cells Isolated from Compact Bones in Chicken. Genes 11, 1360. doi:10.3390/genes11111360
Adhikari, R., Chen, C., Waters, E., West, F. D., and Kim, W. K. (2019). Isolation and Differentiation of Mesenchymal Stem Cells from Broiler Chicken Compact Bones. Front. Physiol. 9, 1892. doi:10.3389/fphys.2018.01892
Agrawal, G., Ramesh, A., Aishwarya, P., Sally, J., and Ravi, M. (2021). Devices and Techniques Used to Obtain and Analyze Three‐dimensional Cell Cultures. Biotechnol. Prog. 37, e3126. doi:10.1002/btpr.3126
Annamalai, T., and Selvaraj, R. K. (2010). Chicken Chemokine Receptors in T Cells Isolated from Lymphoid Organs and in Splenocytes Cultured with Concanavalin A. Poult. Sci. 89, 2419–2425. doi:10.3382/ps.2010-00968
Arai, F., and Suda, T. (2007). Maintenance of Quiescent Hematopoietic Stem Cells in the Osteoblastic Niche. Ann. N.Y Acad. Sci. 1106, 41–53. doi:10.1196/annals.1392.005
Baldridge, M. T., King, K. Y., and Goodell, M. A. (2011). Inflammatory Signals Regulate Hematopoietic Stem Cells. Trends Immunol. 32, 57–65. doi:10.1016/j.it.2010.12.003
Baldwin, J., Antille, M., Bonda, U., De-Juan-Pardo, E. M., Khosrotehrani, K., Ivanovski, S., et al. (2014). In Vitro pre-vascularisation of Tissue-Engineered Constructs A Co-culture Perspective. Vasc. Cel 6, 13. doi:10.1186/2045-824X-6-13
Barrila, J., Crabbé, A., Yang, J., Franco, K., Nydam, S. D., Forsyth, R. J., et al. (2018). Modeling Host-Pathogen Interactions in the Context of the Microenvironment: Three-Dimensional Cell Culture Comes of Age. Infect. Immun. 86, e00282–18. doi:10.1128/IAI.00282-18
Bellis, S. L. (2011). Advantages of RGD Peptides for Directing Cell Association with Biomaterials. Biomaterials 32 (18), 4205–4210. doi:10.1016/j.biomaterials.2011.02.029
Bello, A. B., Park, H., and Lee, S.-H. (2018). Current Approaches in Biomaterial-Based Hematopoietic Stem Cell Niches. Acta Biomater. 72, 1–15. doi:10.1016/j.actbio.2018.03.028
Bhatia, S. N., and Ingber, D. E. (2014). Microfluidic Organs-On-Chips. Nat. Biotechnol. 32, 760–772. doi:10.1038/nbt.2989
Bílková, B., Bainová, Z., Janda, J., Zita, L., and Vinkler, M. (2017). Different Breeds, Different Blood: Cytometric Analysis of Whole Blood Cellular Composition in Chicken Breeds. Vet. Immunol. Immunopathology 188, 71–77. doi:10.1016/j.vetimm.2017.05.001
Blank, U., and Karlsson, S. (2015). TGF-β Signaling in the Control of Hematopoietic Stem Cells. Blood 125, 3542–3550. doi:10.1182/blood-2014-12-618090
Bosworth, L. A., HuShi, W. Y., Shi, Y., and Cartmell, S. H. (2019). Enhancing Biocompatibility without Compromising Material Properties: an Optimised Naoh Treatment for Electrospun Polycaprolactone Fibres. J. Nanomater. 2019, 1–11. doi:10.1155/2019/4605092
Brandon, C., Eisenberg, L. M., and Eisenberg, C. A. (2000). WNT Signaling Modulates the Diversification of Hematopoietic Cells. Blood 96, 4132–4141. doi:10.1182/blood.v96.13.4132
Caliari, S. R., and Burdick, J. A. (2016). A Practical Guide to Hydrogels for Cell Culture. Nat. Methods 13, 405–414. doi:10.1038/nmeth.3839
Canoville, A., Schweitzer, M. H., and Zanno, L. E. (2019). Systemic Distribution of Medullary Bone in the Avian Skeleton: Ground Truthing Criteria for the Identification of Reproductive Tissues in Extinct Avemetatarsalia. BMC Evol. Biol. 19, 71. doi:10.1186/s12862-019-1402-7
Carthew, J., Frith, J. E., Forsythe, J. S., and Truong, V. X. (2018). Polyethylene Glycol-Gelatin Hydrogels with Tuneable Stiffness Prepared by Horseradish Peroxidase-Activated Tetrazine-Norbornene Ligation. J. Mater. Chem. B 6, 1394–1401. doi:10.1039/c7tb02764h
Castiaux, A. D., Spence, D. M., and Martin, R. S. (2019). Review of 3D Cell Culture with Analysis in Microfluidic Systems. Anal. Methods 11, 4220–4232. doi:10.1039/c9ay01328h
Çelebi, B., Mantovani, D., and Pineault, N. (2011). Effects of Extracellular Matrix Proteins on the Growth of Haematopoietic Progenitor Cells. Biomed. Mater. 6, 055011. doi:10.1088/1748-6041/6/5/055011
Chattopadhyay, P. K., Roederer, M., and Bolton, D. L. (2018). A Deadly Dance: the Choreography of Host-Pathogen Interactions, as Revealed by Single-Cell Technologies. Nat. Commun. 9, 4638. doi:10.1038/s41467-018-06214-0
Chen, B., Clark, C., and Chou, T. (1988). Granulocyte/macrophage colony-stimulating Factor Stimulates Monocyte and Tissue Macrophage Proliferation and Enhances Their Responsiveness to Macrophage colony-stimulating Factor. Blood 71, 997–1002. doi:10.1182/blood.v71.4.997.bloodjournal714997
Chen, C. H., Göbel, T. W. F., Kubota, T., and Cooper, M. D. (1994). T Cell Development in the Chicken. Poult. Sci. 73, 1012–1018. doi:10.3382/ps.0731012
Chen, H.-W., and Lin, M.-F. (2020). Characterization, Biocompatibility, and Optimization of Electrospun SF/PCL/CS Composite Nanofibers. Polymers 12, 1439. doi:10.3390/polym12071439
Chen, Y.-C., Chang, W.-C., Lin, S.-P., Minami, M., Jean, C., Hayashi, H., et al. (2018). Three-dimensional Culture of Chicken Primordial Germ Cells (cPGCs) in Defined media Containing the Functional Polymer FP003. PLoS One 13, e0200515. doi:10.1371/journal.pone.0200515
Chitteti, B. R., Kacena, M. A., Voytik-Harbin, S. L., and Srour, E. F. (2015). Modulation of Hematopoietic Progenitor Cell Fate In Vitro by Varying Collagen Oligomer Matrix Stiffness in the Presence or Absence of Osteoblasts. J. Immunological Methods 425, 108–113. doi:10.1016/j.jim.2015.07.001
Choi, J. S., and Harley, B. A. C. (2017). Marrow-inspired Matrix Cues Rapidly Affect Early Fate Decisions of Hematopoietic Stem and Progenitor Cells. Sci. Adv. 3, e1600455. doi:10.1126/sciadv.1600455
Chow, A., Lucas, D., Hidalgo, A., Méndez-Ferrer, S., Hashimoto, D., Scheiermann, C., et al. (2011). Bone Marrow CD169+ Macrophages Promote the Retention of Hematopoietic Stem and Progenitor Cells in the Mesenchymal Stem Cell Niche. J. Exp. Med. 208, 261–271. doi:10.1084/jem.20101688
Coulombel, L., Auffray, I., Gaugler, M.-H., and Rosemblatt, M. (1997). Expression and Function of Integrins on Hematopoietic Progenitor Cells. Acta Haematol. 97, 13–21. doi:10.1159/000203655
Cui, Z., Sun, S., Zhang, Z., and Meng, S. (2009). Simultaneous Endemic Infections with Subgroup J Avian Leukosis Virus and Reticuloendotheliosis Virus in Commercial and Local Breeds of Chickens. Avian Pathol. 38, 443–448. doi:10.1080/03079450903349188
Darvish, M., Payandeh, Z., Soleimanifar, F., Taheri, B., Soleimani, M., and Islami, M. (2019). Umbilical Cord Blood Mesenchymal Stem Cells Application in Hematopoietic Stem Cells Expansion on Nanofiber Three‐dimensional Scaffold. J. Cel Biochem 120, 12018–12026. doi:10.1002/jcb.28487
Dehdilani, N., Shamsasenjan, K., Movassaghpour, A., Akbarzadehlaleh, P., Amoughli Tabrizi, B., Parsa, H., et al. (2016). Improved Survival and Hematopoietic Differentiation of Murine Embryonic Stem Cells on Electrospun Polycaprolactone Nanofiber. Cell J 17, 629–638. doi:10.22074/cellj.2016.3835
Deinhardt-Emmer, S., Rennert, K., Schicke, E., Cseresnyés, Z., Windolph, M., Nietzsche, S., et al. (2020). Co-infection with Staphylococcus aureus after Primary Influenza Virus Infection Leads to Damage of the Endothelium in a Human Alveolus-On-A-Chip Model. Biofabrication 12, 025012. doi:10.1088/1758-5090/ab7073
Di Maggio, N., Piccinini, E., Jaworski, M., Trumpp, A., Wendt, D. J., and Martin, I. (2011). Toward Modeling the Bone Marrow Niche Using Scaffold-Based 3D Culture Systems. Biomaterials 32, 321–329. doi:10.1016/j.biomaterials.2010.09.041
Ding, L., Saunders, T. L., Enikolopov, G., and Morrison, S. J. (2012). Endothelial and Perivascular Cells Maintain Haematopoietic Stem Cells. Nature 481, 457–462. doi:10.1038/nature10783
Dunon, D., Kaufman, J., Salomonsen, J., Skjoedt, K., Vainio, O., Thiery, J. P., et al. (1990). T Cell Precursor Migration towards Beta 2-microglobulin Is Involved in Thymus Colonization of Chicken Embryos. EMBO J. 9, 3315–3322. doi:10.1002/j.1460-2075.1990.tb07531.x
Dutta, P., Hoyer, F. F., Grigoryeva, L. S., Sager, H. B., Leuschner, F., Courties, G., et al. (2015). Macrophages Retain Hematopoietic Stem Cells in the Spleen via VCAM-1. J. Exp. Med. 212, 497–512. doi:10.1084/jem.20141642
Farzaneh, M., Attari, F., Mozdziak, P. E., and Khoshnam, S. E. (2017). The Evolution of Chicken Stem Cell Culture Methods. Br. Poult. Sci. 58, 681–686. doi:10.1080/00071668.2017.1365354
Feaugas, T., and Sauvonnet, N. (2021). Organ‐on‐chip to Investigate Host‐pathogens Interactions. Cell Microbiol. 23, e13336. doi:10.1111/cmi.13336
Fellah, J. S., Jaffredo, T., Nagy, N., and Dunon, D. (2014). “Development of the Avian Immune System,” in Avian Immunology. Editors K. Schat, B. Kaspers, and P. Kaiser (London: Elsevier), 45–63. doi:10.1016/b978-0-12-396965-1.00003-0
Ferreira, M. S. V., and Mousavi, S. H. (2018). Nanofiber Technology in the Ex Vivo Expansion of Cord Blood-Derived Hematopoietic Stem Cells. Nanomedicine: Nanotechnology, Biol. Med. 14, 1707–1718. doi:10.1016/j.nano.2018.04.017
Frantz, C., Stewart, K. M., and Weaver, V. M. (2010). The Extracellular Matrix at a Glance. J. Cel Sci 123, 4195–4200. doi:10.1242/jcs.023820
Garceau, V., Balic, A., Garcia-Morales, C., Sauter, K. A., McGrew, M. J., Smith, J., et al. (2015). The Development and Maintenance of the Mononuclear Phagocyte System of the Chick Is Controlled by Signals from the Macrophage colony-stimulating Factor Receptor. BMC Biol. 13, 12. doi:10.1186/s12915-015-0121-9
Garcia-Morales, C., Rothwell, L., Moffat, L., Garceau, V., Balic, A., Sang, H. M., et al. (2014). Production and Characterisation of a Monoclonal Antibody that Recognises the Chicken CSF1 Receptor and Confirms that Expression Is Restricted to Macrophage-Lineage Cells. Develop. Comp. Immunol. 42, 278–285. doi:10.1016/j.dci.2013.09.011
Gibson, M. S., Kaiser, P., and Fife, M. (2009). Identification of Chicken Granulocyte colony-stimulating Factor (G-CSF/CSF3): the Previously Described Myelomonocytic Growth Factor Is Actually CSF3. J. Interferon Cytokine Res. 29, 339–344. doi:10.1089/jir.2008.0103
Gimeno, I. M., and Schat, K. A. (2018). Virus-induced Immunosuppression in Chickens. Avian Dis. 62, 272–285. doi:10.1637/11841-041318-Review.1
Glick, B. (1987). Cellular Composition of the Bone Marrow in the Chicken, a Comparison of Femur, Tibia and Humerus. Comp. Biochem. Physiol. A: Physiol. 86, 709–712. doi:10.1016/0300-9629(87)90629-3
Glick, B., and Rosse, C. (1981). Cellular Composition of the Bone Marrow in the Chicken: II. The Effect of Age and the Influence of the Bursa of Fabricius on the Size of Cellular Compartments. Anat. Rec. 200, 471–479. doi:10.1002/ar.1092000410
Gong, Y., Zhao, M., Yang, W., Gao, A., Yin, X., Hu, L., et al. (2018). Megakaryocyte-derived Excessive Transforming Growth Factor β1 Inhibits Proliferation of normal Hematopoietic Stem Cells in Acute Myeloid Leukemia. Exp. Hematol. 60, 40–46. e2. doi:10.1016/j.exphem.2017.12.010
Grassart, A., Malardé, V., Gobaa, S., Sartori-Rupp, A., Kerns, J., Karalis, K., et al. (2019). Bioengineered Human Organ-On-Chip Reveals Intestinal Microenvironment and Mechanical Forces Impacting Shigella Infection. Cell Host & Microbe 26, 435–444. e4. doi:10.1016/j.chom.2019.08.007
Guedes, P. T., Oliveira, B. C. E. P. D. d., Manso, P. P. d. A., Caputo, L. F. G., Cotta-Pereira, G., and Pelajo-Machado, M. (2014). Histological Analyses Demonstrate the Temporary Contribution of Yolk Sac, Liver, and Bone Marrow to Hematopoiesis during Chicken Development. PLoS One 9, e90975. doi:10.1371/journal.pone.0090975
Gurung, A., Kamble, N., Kaufer, B. B., Pathan, A., and Behboudi, S. (2017). Association of Marek's Disease Induced Immunosuppression with Activation of a Novel Regulatory T Cells in Chickens. Plos Pathog. 13, e1006745. doi:10.1371/journal.ppat.1006745
Hao, X., Li, S., Chen, L., Dong, M., Wang, J., Hu, J., et al. (2020). Establishing a Multicolor Flow Cytometry to Characterize Cellular Immune Response in Chickens Following H7N9 Avian Influenza Virus Infection. Viruses 12, 1396. doi:10.3390/v12121396
He, B., Chen, G., and Zeng, Y. (2016). Three-dimensional Cell Culture Models for Investigating Human Viruses. Virol. Sin. 31, 363–379. doi:10.1007/s12250-016-3889-z
Hirata, Y., Furuhashi, K., Ishii, H., Li, H. W., Pinho, S., Ding, L., et al. (2018). CD150high Bone Marrow Tregs Maintain Hematopoietic Stem Cell Quiescence and Immune Privilege via Adenosine. Cell stem cell 22, 445–453. e5. doi:10.1016/j.stem.2018.01.017
Holst, J., Watson, S., Lord, M. S., Eamegdool, S. S., Bax, D. V., Nivison-Smith, L. B., et al. (2010). Substrate Elasticity Provides Mechanical Signals for the Expansion of Hemopoietic Stem and Progenitor Cells. Nat. Biotechnol. 28, 1123–1128. doi:10.1038/nbt.1687
Hosokawa, K., Imai, K., Dong, H. V., Ogawa, H., Suzutou, M., Linn, S. H., et al. (2020). Pathological and Virological Analysis of Concurrent Disease of Chicken Anemia Virus Infection and Infectious Bronchitis in Japanese Native Chicks. J. Vet. Med. Sci. 82, 422–430. doi:10.1292/jvms.20-0006
Huang, X., Ma, S., Wang, L., Zhou, H., Jiang, Y., Cui, W., et al. (2020). Lactobacillus Johnsonii-Activated Chicken Bone Marrow-Derived Dendritic Cells Exhibit Maturation and Increased Expression of Cytokines and Chemokines In Vitro. Cytokine 136, 155269. doi:10.1016/j.cyto.2020.155269
Hur, J., Choi, J.-I., Lee, H., Nham, P., Kim, T.-W., Chae, C.-W., et al. (2016). CD82/KAI1 Maintains the Dormancy of Long-Term Hematopoietic Stem Cells through Interaction with DARC-Expressing Macrophages. Cell stem cell 18, 508–521. doi:10.1016/j.stem.2016.01.013
Jahandideh, B., Derakhshani, M., Abbaszadeh, H., Akbar Movassaghpour, A., Mehdizadeh, A., Talebi, M., et al. (2020). The Pro-inflammatory Cytokines Effects on Mobilization, Self-Renewal and Differentiation of Hematopoietic Stem Cells. Hum. Immunol. 81, 206–217. doi:10.1016/j.humimm.2020.01.004
Jansen, C. A., van de Haar, P. M., van Haarlem, D., van Kooten, P., de Wit, S., van Eden, W., et al. (2010). Identification of New Populations of Chicken Natural Killer (NK) Cells. Develop. Comp. Immunol. 34, 759–767. doi:10.1016/j.dci.2010.02.009
Jansen, L. E., Birch, N. P., Schiffman, J. D., Crosby, A. J., and Peyton, S. R. (2015). Mechanics of Intact Bone Marrow. J. Mech. Behav. Biomed. Mater. 50, 299–307. doi:10.1016/j.jmbbm.2015.06.023
Kalaiyarasu, S., Bhatia, S., Mishra, N., Sood, R., Kumar, M., SenthilKumar, D., et al. (2016). Elevated Level of Pro Inflammatory Cytokine and Chemokine Expression in Chicken Bone Marrow and Monocyte Derived Dendritic Cells Following LPS Induced Maturation. Cytokine 85, 140–147. doi:10.1016/j.cyto.2016.06.022
Kamble, N. M., Jawale, C. V., and Lee, J. H. (2016a). Activation of Chicken Bone Marrow-Derived Dendritic Cells Induced by a Salmonella Enteritidis Ghost Vaccine Candidate. Poult. Sci. 95, 2274–2280. doi:10.3382/ps/pew158
Kamble, N. M., Jawale, C. V., and Lee, J. H. (2016b). Interaction of a Live attenuatedSalmonellaGallinarum Vaccine Candidate with Chicken Bone Marrow-Derived Dendritic Cells. Avian Pathol. 45, 235–243. doi:10.1080/03079457.2016.1144919
Kandow, C. E., Georges, P. C., Janmey, P. A., and Beningo, K. A. (2007). Polyacrylamide Hydrogels for Cell Mechanics: Steps toward Optimization and Alternative Uses. Methods Cel Biol 83, 29–46. doi:10.1016/S0091-679X(07)83002-0
Kang, Y. G., Shin, J. W., Park, S. H., Kim, Y. M., Gu, S. R., Wu, Y., et al. (2016). A Three-Dimensional Hierarchical Scaffold Fabricated by a Combined Rapid Prototyping Technique and Electrospinning Process to Expand Hematopoietic Stem/progenitor Cells. Biotechnol. Lett. 38, 175–181. doi:10.1007/s10529-015-1952-8
Kapałczyńska, M., Kolenda, T., Przybyła, W., Zajączkowska, M., Teresiak, A., Filas, V., et al. (2018). 2D and 3D Cell Cultures - a Comparison of Different Types of Cancer Cell Cultures. aoms 14, 910–919. doi:10.5114/aoms.2016.63743
Kefallinou, D., Grigoriou, M., Boumpas, D. T., Gogolides, E., and Tserepi, A. (2020). Fabrication of a 3D Microfluidic Cell Culture Device for Bone Marrow-On-A-Chip. Micro Nano Eng. 9, 100075. doi:10.1016/j.mne.2020.100075
Khatri, M., and Sharma, J. M. (2009). Susceptibility of Chicken Mesenchymal Stem Cells to Infectious Bursal Disease Virus. J. Virol. Methods 160, 197–199. doi:10.1016/j.jviromet.2009.05.008
Kim, H. J., Oh, D. X., ChoyNguyen, S. H. L., Nguyen, H.-L., Cha, H. J., and Hwang, D. S. (2018). 3D Cellulose Nanofiber Scaffold with Homogeneous Cell Population and Long-Term Proliferation. Cellulose 25, 7299–7314. doi:10.1007/s10570-018-2058-y
Kim, J. H., Park, J. Y., Jin, S., Yoon, S., Kwak, J.-Y., and Jeong, Y. H. (2019). A Microfluidic Chip Embracing a Nanofiber Scaffold for 3D Cell Culture and Real-Time Monitoring. Nanomaterials 9, 588. doi:10.3390/nano9040588
Kim, T. E., Kim, C. G., Kim, J. S., Jin, S., Yoon, S., Bae, H. R., et al. (2016). Three-dimensional Culture and Interaction of Cancer Cells and Dendritic Cells in an Electrospun Nano-Submicron Hybrid Fibrous Scaffold. Int. J. Nanomedicine 11, 823–835. doi:10.2147/IJN.S101846
Kim, H. J., Li, H., Collins, J. J., and Ingber, D. E. (2016). Contributions of Microbiome and Mechanical Deformation to Intestinal Bacterial Overgrowth and Inflammation in a Human Gut-On-A-Chip. Proc. Natl. Acad. Sci. USA 113, E7–E15. doi:10.1073/pnas.1522193112
Ko, K. H., Jeong, Y. H., Kwak, J. Y., Gu, M. J., Kim, H. Y., Park, B. C., et al. (2018). Changes in Bursal B Cells in Chicken during Embryonic Development and Early Life after Hatching. Sci. Rep. 8, 16905. doi:10.1038/s41598-018-34897-4
Kobayashi, H., Morikawa, T., Okinaga, A., Hamano, F., Hashidate-Yoshida, T., Watanuki, S., et al. (2019). Environmental Optimization Enables Maintenance of Quiescent Hematopoietic Stem Cells Ex Vivo. Cel Rep. 28, 145–158. e9. doi:10.1016/j.celrep.2019.06.008
Kogut, M. H. (2000). Cytokines and Prevention of Infectious Diseases in Poultry: a Review. Avian Pathol. 29, 395–404. doi:10.1080/030794500750047135
Kogut, M. H., Moyes, R., and Deloach, J. R. (1997). Neutralization of G-CSF Inhibits ILK-Induced Heterophil Influx: Granulocyte-colony Stimulating Factor Mediates the Salmonella Enteritidis-Immune Lymphokine Potentiation of the Acute Avian Inflammatory Response. Inflammation 21, 9–25. doi:10.1023/a:1027382523535
Kozai, M., Kubo, Y., Katakai, T., Kondo, H., Kiyonari, H., Schaeuble, K., et al. (2017). Essential Role of CCL21 in Establishment of central Self-Tolerance in T Cells. J. Exp. Med. 214, 1925–1935. doi:10.1084/jem.20161864
Kramer, A. C., Blake, A. L., Taisto, M. E., Lehrke, M. J., Webber, B. R., and Lund, T. C. (2017). Dermatopontin in Bone Marrow Extracellular Matrix Regulates Adherence but Is Dispensable for Murine Hematopoietic Cell Maintenance. Stem Cel Rep. 9, 770–778. doi:10.1016/j.stemcr.2017.07.021
Kräter, M., Jacobi, A., Otto, O., Tietze, S., Müller, K., Poitz, D. M., et al. (2017). Bone Marrow Niche-Mimetics Modulate HSPC Function via Integrin Signaling. Sci. Rep. 7, 2549. doi:10.1038/s41598-017-02352-5
Lampisuo, M., Katevuo, K., and Lassila, O. (1998). Antigenic Phenotype of Early Intra‐Embryonic Lymphoid Progenitors in the Chicken. Scand. J. Immunol. 48, 52–58. doi:10.1046/j.1365-3083.1998.00361.x
Larsen, F. T., Guldbrandtsen, B., Christensen, D., Pitcovski, J., Kjærup, R. B., and Dalgaard, T. S. (2020). Pustulan Activates Chicken Bone Marrow-Derived Dendritic Cells In Vitro and Promotes Ex Vivo CD4+ T Cell Recall Response to Infectious Bronchitis Virus. Vaccines 8, 226. doi:10.3390/vaccines8020226
Lazzari, G., Vinciguerra, D., Balasso, A., Nicolas, V., Goudin, N., Garfa-Traore, M., et al. (2019). Light Sheet Fluorescence Microscopy versus Confocal Microscopy: in Quest of a Suitable Tool to Assess Drug and Nanomedicine Penetration into Multicellular Tumor Spheroids. Eur. J. Pharmaceutics Biopharmaceutics 142, 195–203. doi:10.1016/j.ejpb.2019.06.019
Lee, J. B., Jeong, S. I., Bae, M. S., Yang, D. H., Heo, D. N., Kim, C. H., et al. (2011). Highly Porous Electrospun Nanofibers Enhanced by Ultrasonication for Improved Cellular Infiltration. Tissue Eng. A 17, 2695–2702. doi:10.1089/ten.TEA.2010.0709
Lee, S.-J., Maza, P. A. M. A., Sun, G.-M., Slama, P., Lee, I.-J., and Kwak, J.-Y. (2021). Bacterial Infection-Mimicking Three-Dimensional Phagocytosis and Chemotaxis in Electrospun Poly(ε-Caprolactone) Nanofibrous Membrane. Membranes 11, 569. doi:10.3390/membranes11080569
Leisten, I., Kramann, R., Ventura Ferreira, M. S., Bovi, M., Neuss, S., Ziegler, P., et al. (2012). 3D Co-culture of Hematopoietic Stem and Progenitor Cells and Mesenchymal Stem Cells in Collagen Scaffolds as a Model of the Hematopoietic Niche. Biomaterials 33, 1736–1747. doi:10.1016/j.biomaterials.2011.11.034
Li, T., and Wu, Y. (2011). Paracrine Molecules of Mesenchymal Stem Cells for Hematopoietic Stem Cell Niche. Bone Marrow Res. 2011, 1–8. doi:10.1155/2011/353878
Liang, J., Yin, Y., Qin, T., and Yang, Q. (2015). Chicken Bone Marrow-Derived Dendritic Cells Maturation in Response to Infectious Bursal Disease Virus. Vet. Immunol. Immunopathology 164, 51–55. doi:10.1016/j.vetimm.2014.12.012
Lin, J., Xia, J., Zhang, K., and Yang, Q. (2016). Genome-wide Profiling of Chicken Dendritic Cell Response to Infectious Bursal Disease. BMC Genomics 17, 878. doi:10.1186/s12864-016-3157-5
Liu, D., Qiu, Q., Zhang, X., Dai, M., Qin, J., Hao, J., et al. (2016a). Infection of Chicken Bone Marrow Mononuclear Cells with Subgroup J Avian Leukosis Virus Inhibits Dendritic Cell Differentiation and Alters Cytokine Expression. Infect. Genet. Evol. 44, 130–136. doi:10.1016/j.meegid.2016.06.045
Liu, D., Dai, M., Zhang, X., Cao, W., and Liao, M. (2016b). Subgroup J Avian Leukosis Virus Infection of Chicken Dendritic Cells Induces Apoptosis via the Aberrant Expression of microRNAs. Sci. Rep. 6, 20188. doi:10.1038/srep20188
Liu, H., Wang, Y., Cui, K., Guo, Y., Zhang, X., and Qin, J. (2019). Advances in Hydrogels in Organoids and Organs‐on‐a‐Chip. Adv. Mater. 31, 1902042. doi:10.1002/adma.201902042
Liu, Q., Yang, J., Huang, X., Liu, Y., Han, K., Zhao, D., et al. (2020). Transcriptomic Profile of Chicken Bone Marrow-Derive Dendritic Cells in Response to H9N2 Avian Influenza A Virus. Vet. Immunol. Immunopathology 220, 109992. doi:10.1016/j.vetimm.2019.109992
Luis, T. C., Naber, B. A. E., Roozen, P. P. C., Brugman, M. H., de Haas, E. F. E., Ghazvini, M., et al. (2011). Canonical Wnt Signaling Regulates Hematopoiesis in a Dosage-dependent Fashion. Cell Stem Cell 9, 345–356. doi:10.1016/j.stem.2011.07.017
Ma, K., Chan, C. K., Liao, S., Hwang, W. Y. K., Feng, Q., and Ramakrishna, S. (2008). Electrospun Nanofiber Scaffolds for Rapid and Rich Capture of Bone Marrow-Derived Hematopoietic Stem Cells. Biomaterials 29, 2096–2103. doi:10.1016/j.biomaterials.2008.01.024
Machálková, M., Pavlatovská, B., Michálek, J., Pruška, A., Štěpka, K., Nečasová, T., et al. (2019). Drug Penetration Analysis in 3D Cell Cultures Using Fiducial-Based Semiautomatic Coregistration of MALDI MSI and Immunofluorescence Images. Anal. Chem. 91, 13475–13484. doi:10.1021/acs.analchem.9b02462
Mahadik, B. P., Wheeler, T. D., Skertich, L. J., Kenis, P. J. A., and Harley, B. A. C. (2014). Microfluidic Generation of Gradient Hydrogels to Modulate Hematopoietic Stem Cell Culture Environment. Adv. Healthc. Mater. 3 (3), 449–458. doi:10.1002/adhm.201300263
Man, Y., Yao, X., Yang, T., and Wang, Y. (2021). Hematopoietic Stem Cell Niche during Homeostasis, Malignancy, and Bone Marrow Transplantation. Front. Cel Dev. Biol. 9, 621214. doi:10.3389/fcell.2021.621214
Mansikka, A., Sandberg, M., Lassila, O., and Toivanen, P. (1990). Rearrangement of Immunoglobulin Light Chain Genes in the Chicken Occurs Prior to Colonization of the Embryonic Bursa of Fabricius. Proc. Natl. Acad. Sci. 87, 9416–9420. doi:10.1073/pnas.87.23.9416
Mast, J., and Goddeeris, B. M. (1997). CD57, a Marker for B-Cell Activation and Splenic Ellipsoid-Associated Reticular Cells of the Chicken. Cel Tissue Res. 291, 107–115. doi:10.1007/s004410050984
Matthiesen, S., Jahnke, R., and Knittler, M. R. (2021). A Straightforward Hypoxic Cell Culture Method Suitable for Standard Incubators. MPs 4, 25. doi:10.3390/mps4020025
McNeilly, F., Adair, B. M., and McNulty, M. S. (1994). In Vitroinfection of Mononuclear Cells Derived from Various Chicken Lymphoid Tissues by Chicken Anaemia Virus. Avian Pathol. 23, 547–556. doi:10.1080/03079459408419024
Mendelson, A., and Frenette, P. S. (2014). Hematopoietic Stem Cell Niche Maintenance during Homeostasis and Regeneration. Nat. Med. 20, 833–846. doi:10.1038/nm.3647
Mousavi, S. H., Abroun, S., Soleimani, M., and Mowla, S. J. (2018). 3-Dimensional Nano-Fibre Scaffold for Ex Vivo Expansion of Cord Blood Haematopoietic Stem Cells. Artif. Cell Nanomedicine, Biotechnol. 46, 740–748. doi:10.1080/21691401.2017.1337026
Müller, C., Kowenz-Leutz, E., Grieser-Ade, S., Graf, T., and Leutz, A. (1995). NF-M (Chicken C/EBP Beta) Induces Eosinophilic Differentiation and Apoptosis in a Hematopoietic Progenitor Cell Line. EMBO J. 14, 6127–6135.
Murakami, J. L., Xu, B., Franco, C. B., Hu, X., Galli, S. J., Weissman, I. L., et al. (2016). Evidence that β7 Integrin Regulates Hematopoietic Stem Cell Homing and Engraftment through Interaction with MAdCAM-1. Stem Cell Develop. 25, 18–26. doi:10.1089/scd.2014.0551
Nagy, N., Bódi, I., and Oláh, I. (2016). Avian Dendritic Cells: Phenotype and Ontogeny in Lymphoid Organs. Develop. Comp. Immunol. 58, 47–59. doi:10.1016/j.dci.2015.12.020
Nagy, N., Busalt, F., Halasy, V., Kohn, M., Schmieder, S., Fejszak, N., et al. (2020). In and Out of the Bursa-The Role of CXCR4 in Chicken B Cell Development. Front. Immunol. 11, 1468. doi:10.3389/fimmu.2020.01468
Nazifi, S., Tadjalli, M., and Mohaghgheghzadeh, M. (1999). Normal Haematopoiesis Cellular Components and M/E Ratio in the Bone Marrow of Japanese Quail (Coturnix coturnix Japonica). Comp. Haematol. Int. 9, 188–192. doi:10.1007/BF02585504
Nickerson, C. A., Richter, E. G., and Ott, C. M. (2007). Studying Host-Pathogen Interactions in 3-D: Organotypic Models for Infectious Disease and Drug Development. Jrnl Neuroimmune Pharm. 2, 26–31. doi:10.1007/s11481-006-9047-x
Oláh, I., Nagy, N., and Vervelde, L. (2014). “Structure of the Avian Lymphoid System,” in Avian Immunology. Editors K. Schat, B. Kaspers, and P. Kaiser (London: Elsevier), 11–44. doi:10.1016/b978-0-12-396965-1.00002-9
Oldenhof, S., Mytnyk, S., Arranja, A., de Puit, M., and van Esch, J. H. (2020). Imaging-assisted Hydrogel Formation for Single Cell Isolation. Sci. Rep. 10, 6595. doi:10.1038/s41598-020-62623-6
Oliveira, M., Conceição, P., Kant, K., Ainla, A., and Diéguez, L. (2021). Electrochemical Sensing in 3D Cell Culture Models: New Tools for Developing Better Cancer Diagnostics and Treatments. Cancers 13, 1381. doi:10.3390/cancers13061381
Ortega-Prieto, A. M., Skelton, J. K., Wai, S. N., Large, E., Lussignol, M., Vizcay-Barrena, G., et al. (2018). 3D Microfluidic Liver Cultures as a Physiological Preclinical Tool for Hepatitis B Virus Infection. Nat. Commun. 9, 682. doi:10.1038/s41467-018-02969-8
Oswald, J., Steudel, C., Salchert, K., Joergensen, B., Thiede, C., Ehninger, G., et al. (2006). Gene‐Expression Profiling of CD34 + Hematopoietic Cells Expanded in a Collagen I Matrix. Stem Cells 24, 494–500. doi:10.1634/stemcells.2005-0276
Peng, L., van den Biggelaar, R. H. G. A., Jansen, C. A., Haagsman, H. P., and Veldhuizen, E. J. A. (2020). A Method to Differentiate Chicken Monocytes into Macrophages with Proinflammatory Properties. Immunobiology 225, 152004. doi:10.1016/j.imbio.2020.152004
Pierzchalska, M., Panek, M., Czyrnek, M., and Grabacka, M. (2016). The Three-Dimensional Culture of Epithelial Organoids Derived from Embryonic Chicken Intestine. Methods Mol. Biol. 1576, 135–144. doi:10.1007/7651_2016_15
Pinho, S., Marchand, T., Yang, E., Wei, Q., Nerlov, C., and Frenette, P. S. (2018). Lineage-Biased Hematopoietic Stem Cells Are Regulated by Distinct Niches. Develop. Cel 44, 634–641. e4. doi:10.1016/j.devcel.2018.01.016
Radtke, A. L., and Herbst-Kralovetz, M. M. (2012). Culturing and Applications of Rotating wall Vessel Bioreactor Derived 3D Epithelial Cell Models. JoVE 62, 3868. doi:10.3791/3868
Raic, A., Rödling, L., Kalbacher, H., and Lee-Thedieck, C. (2014). Biomimetic Macroporous PEG Hydrogels as 3D Scaffolds for the Multiplication of Human Hematopoietic Stem and Progenitor Cells. Biomaterials 35, 929–940. doi:10.1016/j.biomaterials.2013.10.038
Rajput, I. R., Hussain, A., Li, Y. L., Zhang, X., Xu, X., Long, M. Y., et al. (2014). Saccharomyces boulardiiandBacillus subtilisB10 Modulate TLRs Mediated Signaling to Induce Immunity by Chicken BMDCs. J. Cel. Biochem. 115, 189–198. doi:10.1002/jcb.24650
Redondo, P. A., Pavlou, M., Loizidou, M., and Cheema, U. (2017). Elements of the Niche for Adult Stem Cell Expansion. J. Tissue Eng. 8, 204173141772546. doi:10.1177/2041731417725464
Rizwan, M., Baker, A. E. G., and Shoichet, M. S. (2021). Designing Hydrogels for 3D Cell Culture Using Dynamic Covalent Crosslinking. Adv. Healthc. Mater. 10, 2100234. doi:10.1002/adhm.202100234
Rödling, L., Schwedhelm, I., Kraus, S., Bieback, K., Hansmann, J., and Lee-Thedieck, C. (2017). 3D Models of the Hematopoietic Stem Cell Niche under Steady-State and Active Conditions. Sci. Rep. 7, 4625. doi:10.1038/s41598-017-04808-0
Sagar, B. M. M., Rentala, S., Gopal, P. N. V., Sharma, S., and Mukhopadhyay, A. (2006). Fibronectin and Laminin Enhance Engraftibility of Cultured Hematopoietic Stem Cells. Biochem. Biophysical Res. Commun. 350, 1000–1005. doi:10.1016/j.bbrc.2006.09.140
Sawyer, A. A., Hennessy, K. M., and Bellis, S. L. (2005). Regulation of Mesenchymal Stem Cell Attachment and Spreading on Hydroxyapatite by RGD Peptides and Adsorbed Serum Proteins. Biomaterials 26, 1467–1475. doi:10.1016/j.biomaterials.2004.05.008
Sayegh, C. E., Demaries, S. L., Pike, K. A., Friedman, J. E., and Ratcliffe, M. J. H. (2000). The Chicken B-Cell Receptor Complex and its Role in Avian B-Cell Development. Immunol. Rev. 175, 187–200. doi:10.1111/j.1600-065x.2000.imr017507.x
Schat, K. A., and Skinner, M. A. (2014). “Avian Immunosuppressive Diseases and Immunoevasion,” in The Avian Immunology. Editors K. Schat, B. Kaspers, and P. Kaiser (London: Elsevier), 275–297. doi:10.1016/b978-0-12-396965-1.00016-9
Schwartz, D., Guzman, D. S. M., Beaufrere, H., Ammersbach, M., Paul‐Murphy, J., Tully, T. N., et al. (2019). Morphologic and Quantitative Evaluation of Bone Marrow Aspirates from Hispaniolan Amazon Parrots ( Amazona ventralis ). Vet. Clin. Pathol. 48, 645–651. doi:10.1111/vcp.12799
Sekelova, Z., Stepanova, H., Polansky, O., Varmuzova, K., Faldynova, M., Fedr, R., et al. (2017). Differential Protein Expression in Chicken Macrophages and Heterophils In Vivo Following Infection with Salmonella Enteritidis. Vet. Res. 48, 35. doi:10.1186/s13567-017-0439-0
Mousavi, S. H., Saeid, A., Masoud, S., and Seyed Javad, M. (2019). Potential of Polycaprolactone Nanofiber Scaffold for Ex Vivo Expansion of Cord Blood-Derived CD34+ Hematopoietic Stem Cells. Int. J. Stem Cel Res. Ther. 6, 1–8. doi:10.23937/2469-570X/1410059
Shah, P., Fritz, J. V., Glaab, E., Desai, M. S., Greenhalgh, K., Frachet, A., et al. (2016). A Microfluidics-Based In Vitro Model of the Gastrointestinal Human-Microbe Interface. Nat. Commun. 7, 11535. doi:10.1038/ncomms11535
Shanmugasundaram, R., Kogut, M. H., Arsenault, R. J., Swaggerty, C. L., Cole, K., Reddish, J. M., et al. (2015). Effect of Salmonella Infection on Cecal Tonsil Regulatory T Cell Properties in Chickens. Poult. Sci. 94, 1828–1835. doi:10.3382/ps/pev161
Shin, D.-S., You, J., Rahimian, A., Vu, T., Siltanen, C., Ehsanipour, A., et al. (2014). Photodegradable Hydrogels for Capture, Detection, and Release of Live Cells. Angew. Chem. Int. Ed. 53, 8221–8224. doi:10.1002/anie.201404323
Shrestha, K. R., and Yoo, S. Y. (2019). Phage-based Artificial Niche: the Recent Progress and Future Opportunities in Stem Cell Therapy. Stem Cell Int. 2019, 1–14. doi:10.1155/2019/4038560
Si, L., Prantil-Baun, R., Benam, K. H., Bai, H., Rodas, M., Burt, M., et al. (2019). Discovery of Influenza Drug Resistance Mutations and Host Therapeutic Targets Using a Human Airway Chip. bioRxiv, 685552. doi:10.1101/685552
Siatskas, C., and Boyd, R. (2000). Regulation of Chicken Haemopoiesis by Cytokines. Develop. Comp. Immunol. 24, 37–59. doi:10.1016/s0145-305x(99)00051-8
Sieber, S., Wirth, L., Cavak, N., Koenigsmark, M., Marx, U., Lauster, R., et al. (2018). Bone Marrow‐on‐a‐chip: Long‐term Culture of Human Haematopoietic Stem Cells in a Three‐dimensional Microfluidic Environment. J. Tissue Eng. Regen. Med. 12, 479–489. doi:10.1002/term.2507
Simsek, T., Kocabas, F., Zheng, J., Deberardinis, R. J., Mahmoud, A. I., Olson, E. N., et al. (2010). The Distinct Metabolic Profile of Hematopoietic Stem Cells Reflects Their Location in a Hypoxic Niche. Cell stem cell 7, 380–390. doi:10.1016/j.stem.2010.07.011
Singhal, N., Kumar, M., Kanaujia, P. K., and Virdi, J. S. (2015). MALDI-TOF Mass Spectrometry: an Emerging Technology for Microbial Identification and Diagnosis. Front. Microbiol. 6, 791. doi:10.3389/fmicb.2015.00791
Skardal, A., Sarker, S. F., Crabbé, A., Nickerson, C. A., and Prestwich, G. D. (2010). The Generation of 3-D Tissue Models Based on Hyaluronan Hydrogel-Coated Microcarriers within a Rotating wall Vessel Bioreactor. Biomaterials 31, 8426–8435. doi:10.1016/j.biomaterials.2010.07.047
Spencer, J. A., Ferraro, F., Roussakis, E., Klein, A., Wu, J., Runnels, J. M., et al. (2014). Direct Measurement of Local Oxygen Concentration in the Bone Marrow of Live Animals. Nature 508, 269–273. doi:10.1038/nature13034
Sunuwar, L., Yin, J., Kasendra, M., Karalis, K., Kaper, J., Fleckenstein, J., et al. (2020). Mechanical Stimuli Affect Escherichia coli Heat-Stable Enterotoxin-Cyclic GMP Signaling in a Human Enteroid Intestine-Chip Model. Infect. Immun. 88, e00866–19. doi:10.1128/IAI.00866-19
Tadjalli, M., Nazifi, S., and Haghjoo, R. (2013). Evaluation of Hematopoietic Cells and Myeloid/erythroid Ratio in the Bone Marrow of the Pheasant (Phasianus colchicus). Vet. Res. Forum 4, 119–122.
Tadjalli, M., and Hadipoor, M. M. (2002). Haematopoiesis N. Cellular Components and M/E Ratio in the Bone Marrow of the Black‐headed Gull (Larus Ridibundus). Comp. Clin. Pathol. 11, 6. doi:10.1007/s005800200022
Tadjalli, M., Nazifi, S., and Saedi, M. S. (1997). Morphological Study and Determination of M/E Ratio of the Haematopoietic Cells of the Duck. Comp. Haematol. Int. 7, 117–121. doi:10.1007/bf02652579
Tadjalli, M., Nazifi, S., and Haghjoo, R. (2011). Evaluation of Haematopoietic Cells and M/E Ratio in the Bone Marrow of the Partridge (Alectoris chukar). Int. J. Poult. Sci. 11, 23–27. doi:10.3923/ijps.2012.23.27
Takubo, K., Goda, N., Yamada, W., Iriuchishima, H., Ikeda, E., Kubota, Y., et al. (2010). Regulation of the HIF-1α Level Is Essential for Hematopoietic Stem Cells. Cell stem cell 7, 391–402. doi:10.1016/j.stem.2010.06.020
Tallawi, M., Rosellini, E., Barbani, N., Cascone, M. G., Rai, R., Saint-Pierre, G., et al. (2015). Strategies for the Chemical and Biological Functionalization of Scaffolds for Cardiac Tissue Engineering: a Review. J. R. Soc. Interf. 12, 20150254. doi:10.1098/rsif.2015.0254
Tamma, R., and Ribatti, D. (2017). Bone Niches, Hematopoietic Stem Cells, and Vessel Formation. Ijms 18, 151. doi:10.3390/ijms18010151
Thacker, V. V., Dhar, N., Sharma, K., Barrile, R., Karalis, K., and McKinney, J. D. (2020). A Lung-On-Chip Model of Early Mycobacterium tuberculosis Infection Reveals an Essential Role for Alveolar Epithelial Cells in Controlling Bacterial Growth. eLife 9, e59961. doi:10.7554/eLife.59961
Tibbitt, M. W., and Anseth, K. S. (2009). Hydrogels as Extracellular Matrix Mimics for 3D Cell Culture. Biotechnol. Bioeng. 103, 655–663. doi:10.1002/bit.22361
Tsou, Y.-H., Khoneisser, J., Huang, P.-C., and Xu, X. (2016). Hydrogel as a Bioactive Material to Regulate Stem Cell Fate. Bioactive Mater. 1, 39–55. doi:10.1016/j.bioactmat.2016.05.001
Ulyanova, T., Scott, L. M., Priestley, G. V., Jiang, Y., Nakamoto, B., Koni, P. A., et al. (2005). VCAM-1 Expression in Adult Hematopoietic and Nonhematopoietic Cells Is Controlled by Tissue-Inductive Signals and Reflects Their Developmental Origin. Blood 106, 86–94. doi:10.1182/blood-2004-09-3417
Vainio, O., Dunon, D., Aïssi, F., Dangy, J. P., McNagny, K. M., and Imhof, B. A. (1996). HEMCAM, an Adhesion Molecule Expressed by C-Kit+ Hemopoietic Progenitors. J. Cel Biol 135, 1655–1668. doi:10.1083/jcb.135.6.1655
Vaithiyanathan, M., Safa, N., and Melvin, A. T. (2019). FluoroCellTrack: An Algorithm for Automated Analysis of High-Throughput Droplet Microfluidic Data. PloS one 14, e0215337. doi:10.1371/journal.pone.0215337
Ferreira, M. S. V., Jahnen-Dechent, W., Labude, N., Bovi, M., Hieronymus, T., Zenke, M., et al. (2012). Cord Blood-Hematopoietic Stem Cell Expansion in 3D Fibrin Scaffolds with Stromal Support. Biomaterials 33, 6987–6997. doi:10.1016/j.biomaterials.2012.06.029
Vervelde, L., Reemers, S. S., van Haarlem, D. A., Post, J., Claassen, E., Rebel, J. M. J., et al. (2013). Chicken Dendritic Cells Are Susceptible to Highly Pathogenic Avian Influenza Viruses Which Induce strong Cytokine Responses. Develop. Comp. Immunol. 39, 198–206. doi:10.1016/j.dci.2012.10.011
Villenave, R., Wales, S. Q., Hamkins-Indik, T., Papafragkou, E., Weaver, J. C., Ferrante, T. C., et al. (2017). Human Gut-On-A-Chip Supports Polarized Infection of Coxsackie B1 Virus In Vitro. PloS one 12, e0169412. doi:10.1371/journal.pone.0169412
Virumbrales-Muñoz, M., Ayuso, J. M., Lacueva, A., Randelovic, T., Livingston, M. K., Beebe, D. J., et al. (2019). Enabling Cell Recovery from 3D Cell Culture Microfluidic Devices for Tumour Microenvironment Biomarker Profiling. Sci. Rep. 9, 6199. doi:10.1038/s41598-019-42529-8
von Bülow, V., and Klasen, A. (1983a). Effects of Avian Viruses on Cultured Chicken Bone‐marrow‐derived Macrophages. Avian Pathol. 12, 179–198. doi:10.1080/03079458308436162
von Bülow, V., and Klasen, A. (1983b). Growth Inhibition of Marek's Disease T‐lymphoblastoid Cell Lines by Chicken Bone‐marrow‐derived Macrophages Activated In Vitro. Avian Pathol. 12, 161–178. doi:10.1080/03079458308436161
Walenda, T., Bokermann, G., Ventura Ferreira, M. S., Piroth, D. M., Hieronymus, T., Neuss, S., et al. (2011). Synergistic Effects of Growth Factors and Mesenchymal Stromal Cells for Expansion of Hematopoietic Stem and Progenitor Cells. Exp. Hematol. 39, 617–628. doi:10.1016/j.exphem.2011.02.011
Walenda, T., Bork, S., Horn, P., Wein, F., Saffrich, R., Diehlmann, A., et al. (2010). Co-culture with Mesenchymal Stromal Cells Increases Proliferation and Maintenance of Haematopoietic Progenitor Cells. J. Cel Mol Med 14, 337–350. doi:10.1111/j.1582-4934.2009.00776.x
Weber, W. T., and Foglia, L. M. (1980). Evidence for the Presence of Precursor B Cells in normal and in Hormonally Bursectomized Chick Embryos. Cell Immunol. 52, 84–94. doi:10.1016/0008-8749(80)90402-5
Wen, J. H., Vincent, L. G., Fuhrmann, A., Choi, Y. S., Hribar, K. C., Taylor-Weiner, H., et al. (2014). Interplay of Matrix Stiffness and Protein Tethering in Stem Cell Differentiation. Nat. Mater 13, 979–987. doi:10.1038/nmat4051
Wigley, P., and Kaiser, P. (2003). Avian Cytokines in Health and Disease. Rev. Bras. Cienc. Avic. 5, 1–14. doi:10.1590/S1516-635X2003000100001
Winkler, I. G., Barbier, V., Nowlan, B., Jacobsen, R. N., Forristal, C. E., Patton, J. T., et al. (2012). Vascular Niche E-Selectin Regulates Hematopoietic Stem Cell Dormancy, Self Renewal and Chemoresistance. Nat. Med. 18, 1651–1657. doi:10.1038/nm.2969
Winkler, I. G., Sims, N. A., Pettit, A. R., Barbier, V., Nowlan, B., Helwani, F., et al. (2010). Bone Marrow Macrophages Maintain Hematopoietic Stem Cell (HSC) Niches and Their Depletion Mobilizes HSCs. Blood 116, 4815–4828. doi:10.1182/blood-2009-11-253534
Wu, Z., Harne, R., Chintoan-Uta, C., Hu, T.-J., Wallace, R., MacCallum, A., et al. (2020). Regulation and Function of Macrophage colony-stimulating Factor (CSF1) in the Chicken Immune System. Develop. Comp. Immunol. 105, 103586. doi:10.1016/j.dci.2019.103586
Wu, Z., Rothwell, L., Young, J. R., Kaufman, J., Butter, C., and Kaiser, P. (2010). Generation and Characterization of Chicken Bone Marrow‐derived Dendritic Cells. Immunology 129, 133–145. doi:10.1111/j.1365-2567.2009.03129.x
Xiang, B., Zhu, W., Li, Y., Gao, P., Liang, J., Liu, D., et al. (2018). Immune Responses of Mature Chicken Bone-Marrow-Derived Dendritic Cells Infected with Newcastle Disease Virus Strains with Differing Pathogenicity. Arch. Virol. 163, 1407–1417. doi:10.1007/s00705-018-3745-6
Xu, C., Gao, X., Wei, Q., Nakahara, F., Zimmerman, S. E., Mar, J., et al. (2018). Stem Cell Factor Is Selectively Secreted by Arterial Endothelial Cells in Bone Marrow. Nat. Commun. 9, 2449. doi:10.1038/s41467-018-04726-3
Yamazaki, S., Ema, H., Karlsson, G., Yamaguchi, T., Miyoshi, H., Shioda, S., et al. (2011). Nonmyelinating Schwann Cells Maintain Hematopoietic Stem Cell Hibernation in the Bone Marrow Niche. Cell 147, 1146–1158. doi:10.1016/j.cell.2011.09.053
Yang, W. S., Kim, W. J., Ahn, J. Y., Lee, J., Ko, D. W., Park, S., et al. (2020). New Bioink Derived from Neonatal Chicken Bone Marrow Cells and its 3D-Bioprinted Niche for Osteogenic Stimulators. ACS Appl. Mater. Inter. 12, 49386–49397. doi:10.1021/acsami.0c13905
Yasmin, A. R., Yeap, S. K., Tan, S. W., Hair-Bejo, M., Fakurazi, S., Kaiser, P., et al. (2015). In Vitrocharacterization of Chicken Bone Marrow-Derived Dendritic Cells Following Infection with Very Virulent Infectious Bursal Disease Virus. Avian Pathol. 44, 452–462. doi:10.1080/03079457.2015.1084997
Yvernogeau, L., and Robin, C. (2017). Restricted Intra-embryonic Origin of Bona Fide Hematopoietic Stem Cells in the Chicken. Development 144, 2352–2363. doi:10.1242/dev.151613
Zhai, P., Peng, X., Li, B., Liu, Y., Sun, H., and Li, X. (2020). The Application of Hyaluronic Acid in Bone Regeneration. Int. J. Biol. Macromolecules 151, 1224–1239. doi:10.1016/j.ijbiomac.2019.10.169
Zhang, P., Zhang, C., Li, J., Han, J., Liu, X., and Yang, H. (2019). The Physical Microenvironment of Hematopoietic Stem Cells and its Emerging Roles in Engineering Applications. Stem Cel Res Ther 10, 327. doi:10.1186/s13287-019-1422-7
Zhang, Y., Tong, Y., Pan, X., Cai, H., Gao, Y., and Zhang, W. (2019). Promoted Proliferation of Hematopoietic Stem Cells Enabled by a Hyaluronic Acid/carbon Nanotubes Antioxidant Hydrogel. Macromol. Mater. Eng. 304, 1800630. doi:10.1002/mame.201800630
Zhao, M., Perry, J. M., Marshall, H., Venkatraman, A., Qian, P., He, X. C., et al. (2014). Megakaryocytes Maintain Homeostatic Quiescence and Promote post-injury Regeneration of Hematopoietic Stem Cells. Nat. Med. 20, 1321–1326. doi:10.1038/nm.3706
Zhou, B. O., Yu, H., Yue, R., Zhao, Z., Rios, J. J., Naveiras, O., et al. (2017). Bone Marrow Adipocytes Promote the Regeneration of Stem Cells and Haematopoiesis by Secreting SCF. Nat. Cel Biol 19, 891–903. doi:10.1038/ncb3570
Glossary
2D Two-dimensional
3D Three-dimensional
Arteriolar endothelial cells
BC B cells
BCP B cell precursors
BG Basophil granulocytes
CD4 CD4+ T cells
CD8 CD8+ T cells
CLP Common lymphoid progenitors
CMP Common myeloid progenitors
CLSM Confocal laser scanning microscopy
CSF Colony-stimulating factor
DC Dendritic cells
DMEM Dulbecco’s Modified Eagle’s Medium
DPBS Dulbecco’s phosphate-buffered saline without Ca and Mg
ECM Extracellular matrix
EG Eosinophil granulocytes
GMP Granulocyte-monocyte progenitors
HG Heterophil granulocytes
HSC Hematopoietic stem cells
HIF Hypoxia-inducing factor
MA Macrophages
MALDI Matrix-assisted laser desorption ionization-time
MO Monocytes
MODC Monocyte-derived dendritic cells
MODM Monocyte-derived macrophages
MSC Mesenchymal stem cells
NK Natural killer cells
OCM Organ-on-a-chip
P Plasmocytes
PAM Polyacrylamide
PCL Polycaprolactone
PDMS Polydimethylsiloxane
PE Phycoerythrin
PEG Polyethylene glycol
RWV Rotating wall vessel
SCF Stem cell factor
SEC Sinusoidal endothelial cells
TC T cells
TCP T cell precursors
YM Young’s modulus
Keywords: bone marrow niche, disease prevention, hematopoietic stem cell, poultry, three-dimensional cell culture
Citation: Zmrhal V, Svoradova A, Batik A and Slama P (2022) Three-Dimensional Avian Hematopoietic Stem Cell Cultures as a Model for Studying Disease Pathogenesis. Front. Cell Dev. Biol. 9:730804. doi: 10.3389/fcell.2021.730804
Received: 25 June 2021; Accepted: 17 December 2021;
Published: 20 January 2022.
Edited by:
Ming Li, Osaka University, JapanReviewed by:
Fengchao Wang, Third Military Medical University, ChinaAnjali P. Kusumbe, University of Oxford, United Kingdom
Copyright © 2022 Zmrhal, Svoradova, Batik and Slama. This is an open-access article distributed under the terms of the Creative Commons Attribution License (CC BY). The use, distribution or reproduction in other forums is permitted, provided the original author(s) and the copyright owner(s) are credited and that the original publication in this journal is cited, in accordance with accepted academic practice. No use, distribution or reproduction is permitted which does not comply with these terms.
*Correspondence: Petr Slama, cGV0ci5zbGFtYUBtZW5kZWx1LmN6