- 1Senior Department of Ophthalmology, The Third Medical Center of Chinese People’s Liberation Army General Hospital, Beijing, China
- 2Department of Endocrinology, The Second Medical Center and National Clinical Research Center for Geriatric Diseases, Chinese People’s Liberation Army General Hospital, Beijing, China
Retinal degenerative disease (RDD) refers to a group of diseases with retinal degeneration that cause vision loss and affect people’s daily lives. Various therapies have been proposed, among which stem cell therapy (SCT) holds great promise for the treatment of RDDs. Microglia are immune cells in the retina that have two activation phenotypes, namely, pro-inflammatory M1 and anti-inflammatory M2 phenotypes. These cells play an important role in the pathological progression of RDDs, especially in terms of retinal inflammation. Recent studies have extensively investigated the therapeutic potential of stem cell therapy in treating RDDs, including the immunomodulatory effects targeting microglia. In this review, we substantially summarized the characteristics of RDDs and microglia, discussed the microglial changes and phenotypic transformation of M1 microglia to M2 microglia after SCT, and proposed future directions for SCT in treating RDDs.
1 Introduction
The retina is a stratiform sensory tissue that consists of various cell types, including retinal pigment epithelium (RPE) cells, photoreceptors, intermediate neurons, retinal ganglion cells (RGCs) and glial cells (Malhotra et al., 2011; Madeira et al., 2015). Three distinct glial cell types are present within the retina: Müller cells, astrocytes, and microglia (Vecino et al., 2016). Müller cells are responsible for providing metabolic support to retinal neurons and regulating synaptic activity (Reichenbach and Bringmann, 2013). Together with Müller cells, astrocytes integrate the vascular and neuronal activity of the retina (Kolb, 1995). Microglia, the third type of retinal glial cell, are regarded as resident tissue macrophages and play important roles in retinal homeostasis (Langmann, 2007). Generally, microglia are proposed to originate from the yolk sac and are distributed widely in the whole retina. The main functions of microglia are phagocytosis and regulation of tissue inflammation. Two phenotypes of microglia have been identified: M1 microglia and M2 microglia. The former phenotype is generally considered pro-inflammatory, while the latter phenotype is anti-inflammatory (Tang and Le, 2016; Jiang et al., 2020).
Retinal degenerative diseases (RDDs) are a group of irreversible diseases characterized by the progressive degeneration of retinal cells, which eventually culminate in cell death. Certain conditions lead to an imbalance in the retinal microenvironment, which in turn causes retinal degeneration (Gorbatyuk and Gorbatyuk, 2013). The chronic inflammatory response is a nonnegligible part, where microglia serve as the culprit. Different therapeutic approaches have focused on controlling the activity of microglia to inhibit retinal inflammation (Karali et al., 2020; Lew et al., 2020), including stem cell therapy (SCT). The “control” was aimed at alleviating the functions of activated microglia, which normally refer to M1 microglia. However, recent studies also confirmed that SCT was likely to modulate microglial polarization toward the anti-inflammatory M2 phenotype (Jha et al., 2018). This review therefore offers a comprehensive overview of the interrelationship among RDDs, microglial modulation, and SCT. In addition, assumptions that SCT may be better used to treat RDDs by targeting microglial polarization are also discussed.
2 Characteristics of Retinal Degenerative Diseases
RDDs are a common form of neural degenerative diseases worldwide. They affect approximately 3.4 million people in the United States alone and are considered the dominant health issue. The number of patients continues to increase due to the aging of the population in industrialized countries. People with RDDs may suffer from a substantial loss of quality of life when their vision decreases to a certain extent (Wert et al., 2014). Additionally, RDDs have become a heavy burden for patients and society (Brown et al., 2006; Sapieha et al., 2010). For instance, over 250 billion dollars per year are spent on care for patients with age-related macular degeneration (AMD) in the United States (DeAngelis et al., 2017). A group of retinal diseases, such as retinitis pigmentosa (RP), diabetic retinopathy (DR), AMD, glaucoma, and Alzheimer’s disease (AD)-related retinal degeneration, are collectively known as RDDs (Yang et al., 2013; Madeira et al., 2015; Jin et al., 2019; Nashine et al., 2019). As substantial genetic and allelic heterogeneity exist among different RDDs, the specific classification of these diseases can be ambiguous (Wert et al., 2014). Considering the genetic perspectives, RDDs are classified as inherited retinal degeneration and noninherited retinal degeneration. For example, RP belongs to the former and DR to the latter. Currently, a cure for RDDs is unavailable. However, various therapies have been proposed, including pharmacotherapy (e.g., 9-cis-retinyl acetate), neuroprotection (application of neurotrophic factors), gene replacement (e.g., RPE65), retinal prostheses (restore visual function with devices), and SCT (Cideciyan et al., 2008; Zrenner et al., 2011; Trifunovic et al., 2012; Scholl et al., 2015; da Cruz et al., 2018). Although all current therapies have limitations in controlling the progression of RDDs, SCT is still one of the promising treatments. The implementation and related benefits of SCT will be discussed in the next sections.
The pathological characteristics of RDDs are similar but different. For example, loss of retinal neurons occurs in all RDDs, while neovascularization is a unique feature of AMD and DR. More importantly, a certain RDD itself can also be highly variable. Patients with RP can develop symptomatic visual loss both in childhood and in middle age (Hamel, 2006). Many patients suffer from nyctalopia in adolescence and loss of the mid-peripheral visual field in early adulthood (Hartong et al., 2006). The corresponding pathogenic process in RP is the gradual degeneration of two photoreceptor cells: the primary atrophy of rod cells and the subsequent death of cone cells (Wert et al., 2014). DR is a common and specific microvascular complication of diabetes. In the early stages, DR is largely asymptomatic. However, it can result in retinal detachment and sudden loss of vision as the disease progresses (Lechner et al., 2017). Chronic exposure to hyperglycemia and other risk factors (e.g., hypertension) is postulated to enhance the biochemical and physiological changes that lead to microvascular damage of the retina (Cheung et al., 2010). Vasculopathy subsequently leads to retinal hypoxia and harmful neovascularization (Grossniklaus et al., 2010). Besides, DR exhibits characteristics of low-grade chronic inflammation. Increased expression of inflammatory cytokines, such as TNF and IL-1β, subsequently increases the endothelial cell permeability, promotes the breakdown of blood-retinal barrier (BRB), and induces the adhesion of leukocytes (Madeira et al., 2015). Affecting the macula, AMD compromises the central, fine vision of patients. It has become the leading cause of visual impairment in the aging population, especially in those over 55 years of age (DeAngelis et al., 2017). Two major forms of this disease have been identified. “Dry AMD” is the most prevalent form related to slow progressive degeneration of the RPE and loss of photoreceptors. “Wet AMD” is the less frequent but more symptomatic form characterized by the formation of choroidal neovascularization (CNV). Similar to harmful neovascularization in DR, CNV causes intraretinal or subretinal leakage, hemorrhage, and RPE detachment (Salvi et al., 2006; Velez-Montoya et al., 2013). Macula-affected CNV is the primary cause of vision loss in patients with wet AMD (Ambati and Fowler, 2012). The two AMD forms are not mutually exclusive, as one patient can present both pathological changes (Ashraf and Souka, 2017). Glaucoma is often divided into two major subtypes, open angle and angle closure. Open-angle glaucoma is a chronic process. Patients are often asymptomatic until vision loss has progressed significantly. Angle-closure glaucoma can be an acute process with more immediate symptoms and tends to be more destructive (Mantravadi and Vadhar, 2015). Both subtypes have typical structural and functional defects characterized by the death of a substantial number of RGCs in the inner retina and the loss of their axons in the optic nerve (Quigley, 2011). The loss of RGCs in patients with glaucoma is closely related to the level of intraocular pressure (Weinreb et al., 2014). AD also causes retinal degeneration, which becomes a prominent feature of AD pathology (Koronyo-Hamaoui et al., 2011; Mirzaei et al., 2020). AD patients may suffer from various visual impairments such as loss of contrast and color sensitivity, limited visual field, compromised visual attention and reduced stereopsis (Hart et al., 2016). The hallmark pathology in ocular tissues of AD patients is the deposition of amyloid β (Aβ) and phosphorylated tau protein aggregates, which lead to the RGC degeneration and thinning of retinal nerve fiber layer (Gao et al., 2015; Ashok et al., 2020).
In addition to the abovementioned differences, chronic inflammatory responses also play important roles in the development of RDDs (Madeira et al., 2015). A group of main immune cells within the central nervous system (CNS) and the retina, namely, microglia, plays major roles in chronic inflammation (Li et al., 2015; Rashid et al., 2019). Damage to retinal cells activates microglia to restrict injuries and eliminate cellular debris. However, the overactivation of microglia results in the excessive production of inflammatory factors, which damages retinal cells and aggravates other harmful processes, such as enhancing Aβ-induced toxicity (Qin et al., 2002; Madeira et al., 2015). Therefore, microglia play leading roles in the initiation and persistence of inflammation within RDDs, which subsequently traps RDDs into vicious cycles. More detailed descriptions of the conditions are provided below.
3 Microglia and the Retina
3.1 Origin, Maintenance, and Morphology of Microglia
Microglia were first declared a population in the CNS different from neurons and astrocytes by del Río-Hortega (1993). Previously, microglia in the brain were presumed to have a hemopoietic origin, with monocytes serving as their precursor cells (Imamoto and Leblond, 1978). In contrast, an authoritative study reported that microglia were mainly derived from primitive macrophages in the yolk sac (Ginhoux et al., 2010). Hoeffel et al. (2015) used a fate mapping system to reveal two waves of erythromyeloid precursors (EMPs) in the yolk sac of mice. The first wave of E7.5 progenitors gave rise to early EMPs and subsequently differentiated into primitive macrophages. The second wave of EMPs generated other hematopoietic progenitors and differentiated into hematopoietic stem cells (HSCs) that colonize the fetal liver. Between these two waves, the first wave of EMPs is the origin wave for microglia (Hoeffel et al., 2015). Consistent with these findings, another in vitro study confirmed that the vast majority of mouse microglia in the brain originated from EMPs distinct from HSCs (Gomez Perdiguero et al., 2015). A study of human tissue indicated that microglia migrated to the retina mainly from two sources: The retinal margin and the optic disc (Diaz-Araya et al., 1995). In summary, microglia are generally considered to originate from the yolk sac and invade the retina later. Afterwards, the production of microglia is different from the process occurring in the developmental period. Although the origin of microglia in vertebrate model animals is clear, the ontogeny of human microglia is still a matter of debate due to the lack of direct evidence.
Microglia exhibit a self-renewal pattern under both physiological and pathological conditions (Bruttger et al., 2015). After comparing cases, the density of microglia was shown to be remarkably stable in young and aged brains of both mice and humans in one study. Coupled proliferation and apoptosis maintained the turnover of microglia, while no extra infiltration of monocytes was involved. Additionally, an average of 0.69% of microglia were in S phase at a particular time, which allowed researchers to estimate that the microglia population in the mouse brain is renewed every ∼95 days (Askew et al., 2017). Another study used a special strategy to retrospectively analyze the birth date of microglia isolated from human adults and found that the age of microglia can be long to 6 decades. The majority of microglia in the healthy human cortex were replaced by newly produced cells at a median rate of 28% per year (or 0.08% per day) and the average age was 4.2 years (Reu et al., 2017). These results established that the microglial population in the human brain is sustained by continuous slow turnover throughout life. However, researchers have not clearly determined whether microglia in the retina have the same self-renewal pattern. In addition to self-proliferation, bone marrow (BM)-derived macrophages and monocytes invade the CNS and contribute to the microglial pool under specific conditions, such as irradiation (Jin et al., 2017).
Normally, microglia adopt a quiescent phenotype characterized by very small stomata and extensively ramified filopodia-like processes. They monitor the entire CNS, including the retina, by continuously moving their processes (Karlstetter et al., 2015). Microglia in the CNS are activated when encountering acute damage and adopt an amoeboid shape. Amoeboid microglia differ from ramified microglia with spherical shapes because they lack processes and have numerous phagocytic vacuoles (Boche et al., 2013). The label “amoeboid” implies that such cells are capable of motility. In addition, several other morphological states of microglia have been identified, such as rod cells, multinucleated cells, and “dystrophic” microglia. Rod cells have elongated nuclei, scant cytoplasm, few processes and are most notable in chronic disorders. Multinucleated cells form as a reaction to indigestible material and are commonly observed in mycobacterial infection. “Dystrophic” microglia are cells with dysfunction due to aging (Boche et al., 2013).
3.2 Microglial Polarization Toward Different Phenotypes
Activated macrophages have consistently been shown to present different phenotypes in several inflammation-induced human diseases (Shapouri-Moghaddam et al., 2018). Microglia are recognized as a specialized macrophage population within the CNS (McMenamin et al., 2019). Similar to macrophages, microglia have two activation phenotypes, the M1 phenotype and the M2 phenotype (Tang and Le, 2016), which represent simplified models to describe two polar states of inflammatory responses, namely, pro- and anti-inflammatory responses. Polarization refers to the activation of microglia toward a specific phenotype (Kobashi et al., 2020).
Classical M1 microglia contribute to the release of pro-inflammatory substances such as TNF-α, IL-1β, IL-6, superoxide, inducible nitric oxide synthase (iNOS), reactive oxygen species, and proteases (Le et al., 2001; Indaram et al., 2015; Collmann et al., 2019). These substances promote neuroinflammation and result in a poor outcome. For example, the secretion of pro-inflammatory molecules such as TNF-α and IL-1β by microglia cause chronic inflammation and lead to the damage of the BRB in the diabetic retina (Kinuthia et al., 2020). Several markers, including CD11b, CD16, CD32, CD68, and CD86, are used to identify M1 microglia (Jiang et al., 2020). This phenotype is typically induced by stimuli such as lipopolysaccharide (LPS) or granulocyte-macrophage colony-stimulating factor (GM-CSF) (Liu et al., 2019; Kobashi et al., 2020). In contrast, M2 microglia dampen the inflammatory response by producing four major anti-inflammatory cytokines: IL-4, IL-13, IL-10, and TGF-β (Butovsky et al., 2005; Zhou et al., 2012). For example, IL-4 has been shown to decrease the production of several pro-inflammatory mediators, and IL-10 inhibits the activity of many pro-inflammatory factors (Vanderwall and Milligan, 2019). In addition, these cytokines promote the release of neurotrophic factors such as insulin-like growth factor 1 (IGF-1) to increase neuron survival (Suh et al., 2013). M2 microglia also play a beneficial role in CNS diseases. By secreting chitinase-3-like protein 3, IL-10, and TGF-β, M2 microglia promote angiogenesis and ultimately mitigate blood-brain barrier (BBB) leakage (Zhu et al., 2019). In addition, M2 microglia-derived exosomes attenuate ischemic brain injury and promote neuronal survival (Song et al., 2019). M2 microglia can be identified by markers such as CD206, CCL22 and arginase-1 (Arg-1) (Jiang et al., 2020), and can be induced by IL-4 (Kobashi et al., 2020).
3.3 Roles of Microglia in the Normal Retina
3.3.1 Microglia Play a Supporting Role in the Development of the Retina
During the developmental period, the proliferation, survival, and neurite outgrowth of embryonic neurons are promoted by microglia-mediated trophic factors (Morgan et al., 2004). More recently, microglia have been shown to play an important role in the postnatal maturation of retinal photoreceptors (Jobling et al., 2018). They also participate in the retention of selected neurons and the elimination of unwanted cells, a process that is achieved by the microglia-mediated phagocytosis of cellular debris, pruning of weak presynaptic terminals of RGCs, and decrease in costly neural connections deemed to be unfit for proper function (Bodeutsch and Thanos, 2000; Schafer et al., 2012). In addition, microglia are important in the process of retinal vascularization, which comprises two phases. The first phase is that hyaloid vessels extend from the optic disk to the lens and supply blood and nutrients to the developing eye. In the second phase, hyaloid vessels regress and the retina develops its own independent vascular network (Li et al., 2019a). Microglia-mediated apoptosis of vascular endothelial cells contributes to the main step in the first phase (Lobov et al., 2005), the failure of which can cause persistent hyperplastic primary vitreous in the postnatal period. In the next phase, microglia are closely apposed to endothelial tip cell filopodia, which guide blood vessel growth through the tissue (Checchin et al., 2006).
3.3.2 Microglia Keep Silent and Supervise the Healthy Retina
After development, microglia maintain the ramified morphology with small cell bodies and long cellular protrusions and form a non-overlapping microglia network, which provides a comprehensive surveillance coverage of the entire retina (Damani et al., 2011). Some researchers propose that there are mechanisms of inhibiting microglial activation in the healthy retina to prevent deleterious effects. Cell types including photoreceptors, vascular endothelial cells, ganglion cells, and Müller cells are involved in this process (Dick et al., 2003; Langmann, 2007). Especially, microglia perform an active cross-talk with Müller cells. On the one hand, Microglia can directly trigger the release of several neurotrophic factors from Müller cells. On the other hand, Müller cells can limit microglial reactivity and potentially transform activated microglia into their ramified surveillance state (Karlstetter et al., 2015). However, these mechanisms lose control of microglial behavior in pathological conditions where microglia become activated (Rathnasamy et al., 2019).
3.4 Roles of Microglia in Retinal Degenerative Diseases
3.4.1 Microglia Phagocytose Both Wastes and Living Cells
Phagocytosis by microglia has been extensively studied in adult individuals. The main phagocytic targets of microglia in the brain and retina include pathogens, dead cells, dying cells and protein aggregates (McMenamin et al., 2019). Following interventions such as axotomy, microglia that removed debris of neuronal cells in the postnatal retina were reactivated later in life to phagocytose damaged neurons (Thanos, 1991). Nonetheless, phagocytosis by microglia is a double-edged sword. In a mouse model of RP, activated microglia phagocytosed not only cell debris (Gupta et al., 2003) but also living neurons and accelerated retinal lesions (Zhao et al., 2015). In addition, microglia contributed to the leakage of the BRB by phagocytosing endothelial cells in another rat model of DR (Xie et al., 2021). The molecular mechanisms involved in microglial phagocytosis are still under investigation. Retinal microglia promote the clearance of infectious microbes by expressing receptors such as Toll-like receptors (TLRs) and dectin-1 (Maneu et al., 2011; Kochan et al., 2012). The expression of TLR4 in microglia contributes to their activation and phagocytosis of photoreceptor proteins (Kohno et al., 2013). In addition, phagocytosis is mediated by triggering receptor expressed on myeloid cells 2 (TREM2) and Mer receptor tyrosine kinase (MerTK) in the brain (Neher et al., 2013; Kim et al., 2017). However, whether they participate in microglial phagocytosis of apoptotic retinal neurons is still unknown.
3.4.2 Microglia Promote the Neovascularization
As mentioned above, microglia play an important role in retinal vascularization during development. It is noted that microglia also contribute to the retinal neovascularization in retinal diseases, which is associated with the microglia migration and microglia-related inflammation (Usui-Ouchi et al., 2020). In AMD, microglia contribute to the formation of CNV through accumulating in the subretinal space, releasing inflammatory cytokines (IL-1β, TNF-α, IL-6 and TGF-β), producing pro-angiogenic cytokines and growth factors (VEGF and PGF), and activating microglial VEGF receptors (VEGFR1 and VEGFR2) (Alves et al., 2020). In DR, angiogenesis and inflammation are not independent. Microglia might induce the neovascularization by releasing pro-angiogenic mediators, including cytokines, growth factors, and proteases (Altmann and Schmidt, 2018).
3.4.3 Microglia Aggravate Retinal Inflammation
Regarding immune surveillance and regulatory functions, microglia act as surveillants with their processes continuously extending and retracting in all directions in a random and repeated manner. These cells can sense subtle changes in the retinal microenvironment through various surface receptors and rapidly react to these changes (Nimmerjahn et al., 2005). During the rapid phase of RDDs, microglia are activated immediately and proliferate and migrate to degenerative sites (Zhou et al., 2017). The morphology of microglia also changes to an amoeboid shape (Zhou et al., 2017). Simultaneously, by secreting TNF-α and IL-1β, microglia participate in retinal inflammation and function as a “double sword” again (Krady et al., 2005; Sivakumar et al., 2011). On the one hand, these cytokines initiate immune defenses. On the other hand, they aggravate the death of retinal neurons and damage the integrity of the BRB (Claudio et al., 1994; Tezel and Yang, 2004; Abcouwer et al., 2008). In addition, microglia release pro-inflammatory cytokines, including IL-3, IL-6, IL-8, IL-10, IL-12, and IL-18 (Liu et al., 2012; Grigsby et al., 2014). Moreover, microglia express major histocompatibility complex class II (MHC II) and share phenotypic characteristics with professional antigen-presenting cells (Penfold et al., 1993). Normally, quiescent microglia express low levels of MHC II proteins (Kreutzberg, 1996). Once activated, microglia upregulate MHC II molecules that are required for antigen presentation to T cells. This feature suggests the capability of microglia to reactivate primed T cells entering the CNS (Rawji and Yong, 2013). In addition, microglia are related to the activation of the complement system, an innate immune response that protects host tissue from immunological stimuli. Luo et al. identified microglia and RPE cells as the main sources of retinal complement gene expression (Luo et al., 2011). Unfortunately, in DR models, microglia contribute to the deposition of complement C3 and C1qa, which promote the apoptosis of photoreceptors and RGCs (Howell et al., 2011; Rutar et al., 2011).
It can be seen that microglia exert M1 phenotype due to the release of pro-inflammatory factors. However, some studies propose that M2 microglia also exist in the retina of RDDs. The polarization tendency of microglia in the retina is an intricate process, which is a topic of extensive debate. According to Arroba and Valverde (2017), M2 microglia and M1 microglia both appear at the early stage of DR; however, during the progression of DR, the M1 phenotype is maintained, whereas the M2 phenotype decreases. Different results were obtained for the polarization of microglia in a model of retinal degeneration. Initially, most of the activated microglia (CD86+, CD16/32+, CD40+) tended to exhibit the M1 phenotype and release pro-inflammatory cytokines. Although no M2 microglia (CD206+) were observed at this stage, many microglia were colabeled with CD86 and CD206, indicating an intermediate state of microglial polarization (Zhou et al., 2017). Another study examining oxygen-induced retinopathy models found that M1 microglia dominated during the initial period, but M2 microglial activity predominated during the late phase (Li et al., 2021a). Consistent with these findings, in a study of light-induced retinal damage, pro-inflammatory M1 macrophages/microglia dominated in the early phase, while the chronic postexposure period was accompanied by persistent upregulation of the M2 phenotype. The authors speculated that resident macrophages/microglia might switch to the M1 phenotype in response to light damage; however, infiltrated BM-derived macrophages/microglia mainly contributed to M2 polarization (Jiao et al., 2015). Therefore, more research is needed to better understand the spatiotemporal cadence of microglial polarization in the retina.
Taken together, in the diseased retina, microglia exert both beneficial and detrimental effects. However, the function of promoting inflammation is widely considered pernicious. Many treatments are currently focusing on the regulation of microglial behavior patterns. The question is whether all microglia should be eliminated. As the two phenotypes of microglia are closely linked to the progression of retinal inflammation, microglia polarization may be a vital target for the treatment of RDDs. Since SCT plays a beneficial role in treating retinal diseases, we propose that the modulation of microglia phenotype following SCT is also valuable, which will be discussed in the next sections.
4 Basic Approaches of Stem Cell Therapy to Develop Therapeutic Effects
Stem cells are a population of undifferentiated cells characterized by the ability to extensively proliferate and differentiate into different types of cells and tissues (Kolios and Moodley, 2013). Recent years, SCT has been extensively applied in the treatment of various diseases, including neurological disorders (Alessandrini et al., 2019), heart diseases (Muller et al., 2018), and discogenic back pain (Barakat et al., 2019). The eye is “immune privileged” due to the protection of the BBB and BRB (Forrester et al., 2018). In addition, the high operability and convenient observability make the eye an ideal target organ for stem cell transplantation. Currently, numerous studies have transplanted RPE cells, photoreceptors and RGCs derived from induced pluripotent stem cells (iPSCs), embryonic stem cells (ESCs) and retinal progenitor cells (RPCs), and some of them have advanced to clinical trials (Liu et al., 2017; Mandai et al., 2017; Zhang et al., 2020b). Previously, we used human ESCs (hESCs), human mesenchymal stem cells (MSCs), human RPCs (hRPCs) and neural stem cells (NSCs) to treat retinal degeneration in animal models (Qu et al., 2017; Zhai et al., 2020; Li et al., 2021b). Stem cells are directly transplanted or differentiated into anticipated cell types prior to transplantation. Basically, stem cells contribute to the retinal recovery through two approaches: cell replacement and secretome (Figure 1). Here, we will briefly discuss these two approaches.
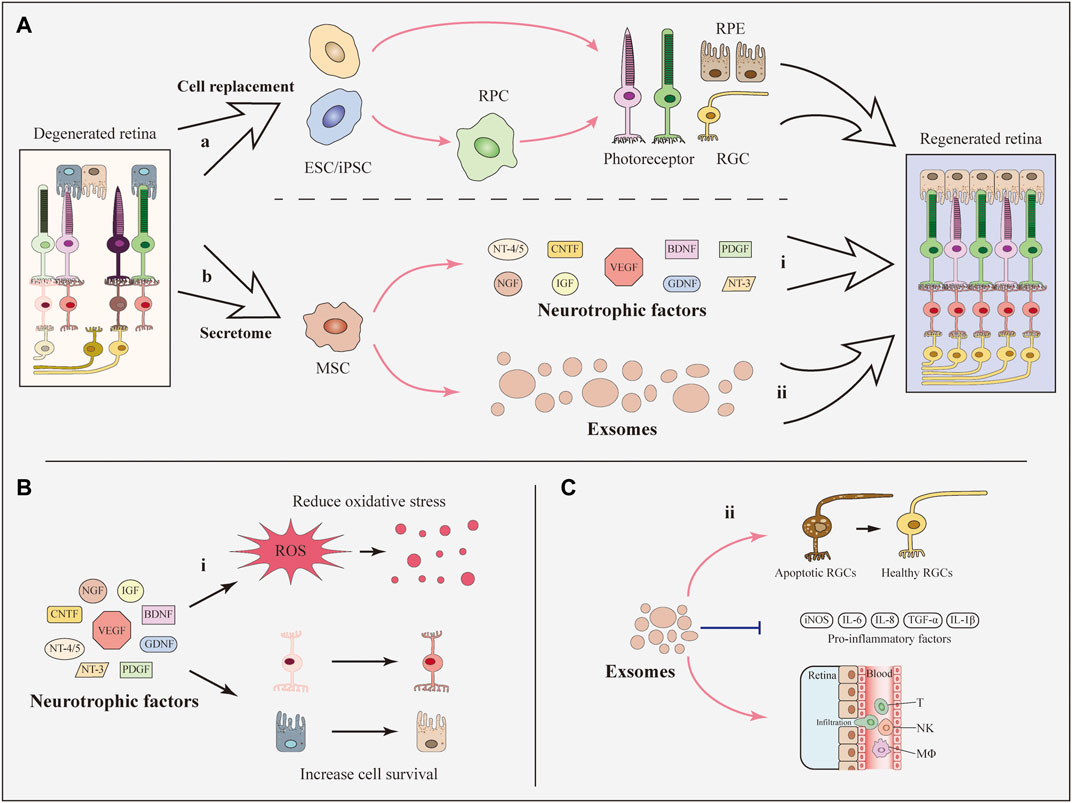
FIGURE 1. Basic approaches of SCT to develop therapeutic effects. (A) Basically, stem cells contribute to the retinal recovery through two approaches: Cell replacement and the secretome. (i) iPSCs, ESCs, and RPCs can differentiate into retinal cells including RGCs and photoreceptors and can be used for cell replacement. (ii) MSCs secret neurotrophic factors and exosomes which are beneficial for the recovery of regenerated retina. (B) Neurotrophic factors contribute to the reduction of oxidative stress and the survival of retinal cells. (C) MSC-derived exosomes prevent the decline of RGCs and reduce the expression of inflammatory factors as well as the infiltration of inflammatory cells.
4.1 Cell Replacement
To date, SCT has been widely studied in animal models for cell replacement in RDDs. ESCs and iPSCs have the greatest potential for cell replacement in RDDs while MSCs and NSCs are sparsely reported to differentiate into retinal cells after transplantation (Mead et al., 2015). As the loss of RPE cells and photoreceptors primarily contributes to visual impairments in these RDDs, studies have more frequently focused on replacing these two cell types.
RPE cells are a layer of pigment cells that transport nutrients from the blood to photoreceptors and digest deciduous disks from the outer segment of photoreceptors (Ma et al., 2019). Both hESCs and human iPSCs (hiPSCs) can be induced to generate RPE cells (Figure 1) (Klimanskaya et al., 2004; Buchholz et al., 2009). In 2018, successful delivery of hESC-derived RPE cells in two patients was reported in a clinical trial and indicated the feasibility and safety of hESC-derived RPE transplantation (da Cruz et al., 2018). Additionally, clinical trials of cell replacement based on RPCs and iPSCs have been conducted in recent years (Liu et al., 2017; Mandai et al., 2017). A scalable protocol was published that facilitated the production of high-quality RPE cells in a short time span and convenient application for both investigative and clinical use to solve the problem of high cost and low purity when generating RPE cells. Pure functional RPE monolayers have been derived from hiPSCs within 90 days using simplified 2D cultures (Buchholz et al., 2013; Maruotti et al., 2015; Michelet et al., 2020). However, no further studies have adopted this protocol to date.
Photoreceptors are irreplaceable in sensing light signals and visual cues by converting exogenous cues into bioelectrical signals (Gollisch and Meister, 2010). Photoreceptors have been generated from hESCs, iPSCs and RPCs (Figure 1) (Qiu et al., 2007; Lamba et al., 2009; Lamba et al., 2010). The replacement of photoreceptors differentiated from iPSCs has been studied in a mouse model. Human iPSCs differentiated into photoreceptors and subsequently survived and integrated into the host retina (Lamba et al., 2010). Recently, hRPCs transplanted in patients with RP successfully differentiated into photoreceptors in a clinical trial. The results showed the long-term safety and feasibility of vision repair following hRPC transplantation (Liu et al., 2017). One important obstacle to the clinical use of photoreceptors is that an appropriate source of precursor cells is required (Schmeer et al., 2012). Lately, an optimized protocol for generating labeled and transplantable photoreceptor precursors from hESCs was published, which was an advance for future research (Markus et al., 2019).
Although cell replacement therapy has been implemented in many animal studies, limitations still confine its further applications in clinical trials due to several unsolved challenges. The low survival rate of transplanted cells and potential tumorigenicity risks have been widely studied (Ballios et al., 2015; Wang et al., 2020). Moreover, the immune rejection of allografts (Baker and Brown, 2009), which is partially associated with the pro-inflammatory stimuli caused by M1 microglia, also must be resolved in future studies.
4.2 Secretome
The secretome of stem cells is defined as the set of molecules and biological factors secreted by stem cells into the extracellular space by mechanisms including protein translocation, exocytosis, and vesicle or exosome encapsulation (Xia et al., 2019; Daneshmandi et al., 2020). Among these molecules and factors, neurotrophic factors and exosomes are widely reported to play beneficial roles in retinal diseases (Wen et al., 2012; Xiao and Le, 2016; Hu et al., 2017; Mead et al., 2018). Although paracrine effects have been discovered in many types of stem cells, including MSC, NSC, ESC, and iPSC (Khan et al., 2015; Bakondi et al., 2017; Mead et al., 2018; Taheri et al., 2019; Zhang et al., 2020a), MSC is the major force of secreting neurotrophic factors and exosomes (Mead et al., 2015; Mead and Tomarev, 2020). Thus, we will focus on the secretome of MSCs and related therapeutic effects in the following sections.
4.2.1 Neurotrophic Factors
Neurotrophic factors are growth factors that nourish neurons and promote the survival and regeneration of neurons, including photoreceptors, RGCs and RPE cells (Wen et al., 2012; Xiao and Le, 2016). They are classified into three families based on their structures, receptors, and signaling pathways: 1) the neurotrophin family, including nerve growth factor (NGF), brain-derived growth factor (BDNF), neurotrophin-3 (NT-3), and NT-4/5; 2) the glial cell-derived neurotrophic factor (GDNF) family, including GDNF, neurturin, persephin, and artemin; and 3) neuropoietic cytokines, including ciliary neurotrophic factor (CNTF) and cardiotrophin-1 (Boyd and Gordon, 2003). Transplantation of stem cells can play positive roles of secreting neurotrophic factors which further improve the survival of retinal neurons.
MSCs secrete neurotrophic factors via a paracrine mechanism (Figure 1), which dampens retinal degeneration instead of replacing damaged cells (Bakondi et al., 2017). MSCs derived from different sources secret similar neurotrophic factors. To be specific, bone marrow derived MSCs (BMSCs) secret an array of neurotrophic factors involving all the three families including CNTF, BDNF, GDNF, platelet derived growth factor (PDGF), NGF, NT-3, NT-4/5 (Mead et al., 2015), IGF-1, basic fibroblast growth factor 2 (FGF2), pigment epithelium-derived factor, and erythropoietin (Usategui-Martin et al., 2020). Analogously, adipose derived MSCs (ADMSCs) contribute to the release of hepatocyte growth factor, CNTF, IGF (Fontanilla et al., 2015), FGF2, VEGF, NGF, BDNF, GDNF, NT-3, and PDGF (Mead et al., 2014; Ezquer et al., 2016).
Many studies have confirmed the protective effects of MSC-secreted neurotrophic factors on eye diseases (Figure 1). For example, in an experimental optic nerve crush model, intravitreal transplantation of BMSCs that secrete GDNF and BDNF resulted in a greater number of living RGCs than in the control group (Hu et al., 2017). In addition, intravitreal administration of murine ADMSCs prevented RGC loss and reduced oxidative stress in the retina with increasing levels of NGF, FGF2 and GDNF in a diabetic mouse model (Ezquer et al., 2016). As another option, neurotrophic factors have also been delivered through direct intravitreal injections (Daly et al., 2018). However, researchers have not clearly determined whether the paracrine effects of neurotrophic factors observed following SCT are more efficient than direct injection of neurotrophic factors. More research is required to expand the function of stem cell-secreted neurotrophic factors and explore their neuroprotective effects on retinal cells.
4.2.2 Exosomes
Exosomes are cell-derived nanovesicles that have low toxicity, exquisite target-homing specificity and the potential for drug/gene delivery (Kalluri and LeBleu, 2020). The secretion of exosomes plays positive roles in SCT treating RDDs. Among several types of MSCs, adipose, BM and umbilical derived-MSCs are the main sources of secreted exosomes (Mead and Tomarev, 2020).
MSC-derived exosomes have many functions, such as neuroprotection and immunoregulation. In a mouse model of glaucoma, exosomes derived from BMSCs significantly reduced the number of degenerating axons in the optic nerve. Meanwhile, exosomes prevented the decrease in RGC function in the early phase (Figure 1) (Mead et al., 2018). Regarding immunoregulation, MSC-derived exosomes substantially suppressed the progression of autoimmune uveoretinitis in a rat model by reducing the infiltration of inflammatory cells, such as T cell subsets (Figure 1) (Bai et al., 2017), and alleviated the expression of inflammatory mediators in the injured retina, including TNF-α, monocyte chemoattractant protein-1 and intercellular adhesion molecule 1 (Harrell et al., 2018). However, the key component of exosomes remains unknown. More importantly, the production of highly purified exosomes with stable long-term functional efficacy for clinical trials is a great challenge (Zhang et al., 2021).
5 Immunomodulatory Effects of Stem Cell Therapy Targeting Microglia
SCT has been proven to possess broad immunomodulatory potential in neurodegenerative diseases by regulating inflammatory responses. As mentioned above, microglia play a leading role in the immune system of the retina through polarization toward two phenotypes. Here, we emphatically discuss the immunomodulatory effects of SCT on regulating the microglial polarization and microglia-mediated inflammation.
5.1 Stem Cell Therapy Inhibit M1 Microglial Polarization
In pathological states, microglia proliferate rapidly with a distinctly increased number of cells. At the same time, they migrate to degenerative sites upon activation. In many studies of RDDs, microglia present the M1 phenotype by releasing pro-inflammatory factors (Yuan and Neufeld, 2001; Krady et al., 2005; Zeng et al., 2005). To date, many stem cell treatments have been shown to exert regulatory effects on M1 microglia both in vitro and in vivo. By coculturing the retinas of adult rats with human BMSCs (hBMSCs) in vitro, researchers found that the number of CD68+ ameboid microglia decreased and the loss of RGCs was prevented (Teixeira-Pinheiro et al., 2020). Consistently, the activation of CD11b+ M1 microglia was inhibited by coculturing with MSC-derived microvesicles in an in-vitro model. Meanwhile, the production of pro-inflammatory molecules by M1 microglia, such as TNF-α, IL-6, IL-1β and iNOS, was reduced (Jaimes et al., 2017). In our previous in-vivo study, by transplanting organoid-derived hRPCs into the subretinal space of rat RDD models (RCS rats), we found that the number of Iba1+/CD68+ phagocytic M1 microglia was significantly lower in the transplantation group than that in the control group (Zou et al., 2019). In addition, we performed a combined transplantation of human MSCs (hMSCs) and hRPCs into the subretinal space of RCS rats. The number of Iba1+ retinal microglia was significantly reduced following transplantation, especially in the combined transplantation group. The expression levels of TNF-α and IL-1β were decreased while the expression levels of neurotrophic NGF and BDNF were increased (Qu et al., 2017).
All these results confirmed the inhibitory effects of SCT on M1 microglia (Figure 2). Along with the inhibition of M1 microglia activation, the expression levels of inflammatory factors and cytotoxic molecules were decreased, which can alleviate the chronic injury of the retina.
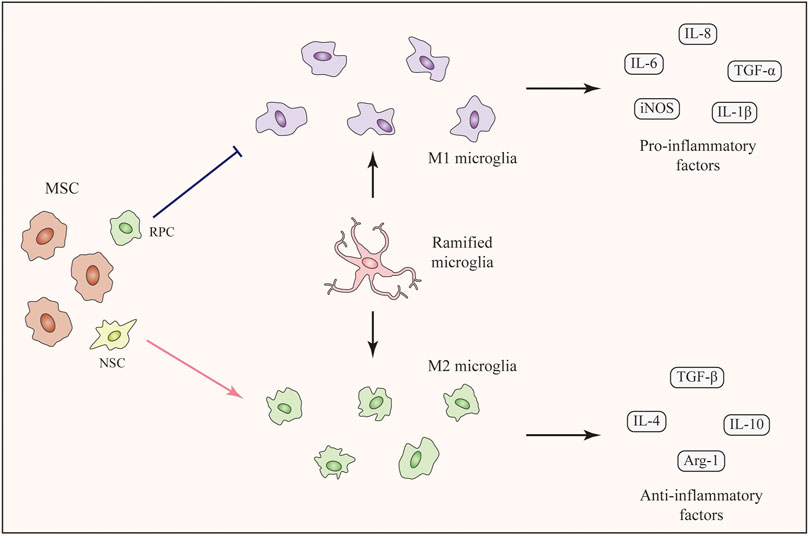
FIGURE 2. Immunomodulatory effects of SCT targeting microglia. Ramified microglia can be activated with two phenotypes, namely M1 phenotype and M2 phenotype. Stem cells including MSCs, RPCs, and NSCs can modulate microglial polarization (M1-M2), among which MSCs are the major force. MSCs inhibit M1 microglial polarization and decrease the expression level of pro-inflammatory factors. On the contrary, M2 microglial polarization is enhanced and the expression levels of anti-inflammatory factors are increased. The transition of microglial phenotype alleviates the inflammation and improves the living-conditions of neuronal cells.
5.2 Stem Cell Therapy Enhance M2 Microglial Polarization
As mentioned above, polarization of microglia to the M2 phenotype is beneficial for treating RDDs. Several studies have shown that SCT can promote M2 microglial polarization and MSCs are the major force of this function (Zanier et al., 2014; Park et al., 2016; Jha et al., 2018). In an in-vitro study of LPS-stimulated microglia, treatment with ADMSCs or ADMSC-derived GDNF enhanced the expression of the M2 marker CD206. The expression levels of anti-inflammatory IL-10 and TGF-β were upregulated (Zhong et al., 2020b). As for in-vivo studies, the intravitreal injection of concentrated conditioned media from ADMSCs restored the M1-M2 balance in a mouse model of visual deficits following mild traumatic brain injury. This treatment increased the number of Arg-1+ M2 microglia along with the increased expression of anti-inflammatory cytokines. As a result, the inflammation-related loss of endothelial barrier integrity and retinal cells was suppressed (Jha et al., 2018). Unfortunately, the number of studies which discovered M2 polarization-promotion effect following SCT in the retina are limited. However, many studies focusing on brain injury have confirmed the effects of MSCs on enhancing M2 microglial polarization. In a mouse model of neurodegeneration, the intracerebral transplantation of MSCs promoted M2 polarization and increased the expression of anti-inflammatory TGF-β and IL-10 (Liu et al., 2019). In addition, BMSCs promoted the M2 microglial polarization in a rat middle cerebral artery occlusion (MCAO) model and suppressed neuroinflammation (Yang et al., 2020). According to some recent studies, MSCs from human exfoliated deciduous teeth reduced neuroinflammation by shifting microglial polarization (M1 to M2) through exosome secretion and paracrine effects (Li et al., 2017; Kitase et al., 2020). In addition to MSCs, transplantation of NSCs showed the function of promoting microglial phenotypic transition from M1 toward M2 phenotype in a study of traumatic brain injury. This study showed the possibility of other stem cells rather than MSCs to induce M2 microglial polarization following SCT (Gao et al., 2016).
Taken together, SCT, especially MSC therapy, are capable of enhancing M2 microglial polarization (Figure 2). The secretion of anti-inflammatory factors by M2 microglia help to alleviate the inflammation and improve the living-conditions of neuronal cells respectively. Related pathways and factors of MSC regulating microglia polarization can be seen in Table 1. Unfortunately, these pathways related to the regulation of microglial polarization have all been identified in studies of the brain rather than the retina. More specific further studies aiming at discovering the effects and related mechanisms of microglial polarization in the retina following SCT are urgently needed.
6 Future Directions
6.1 Cotransplantation of Therapeutic Cells With Mesenchymal Stem Cells-Derived Exosomes May be a Better Solution
Injury-induced inflammation is detrimental to the regeneration of retinal cells (Silva et al., 2020). Moreover, the survival and integration of transplanted cells are substantially influenced by microglia and inflammation (Burns and Stevens, 2018). As mentioned above, MSC-derived exosomes are capable of suppressing retinal inflammation by reducing the expression of inflammatory mediators. Additionally, MSC-derived exosomes can enhance the M2 polarization of microglia (Li et al., 2019c). Therefore, we speculate that the cotransplantation of the anticipated cells with MSC-derived exosomes might be a better choice to achieve therapeutic goals (Figure 3). In addition, the application of MSC-derived exosomes remarkably does not cause vitreous opacity, immunologic rejection, or proliferative vitreous retinopathy (Zhang et al., 2018; Mathew et al., 2019). As combined transplantation traditionally requires the injection of at least two cell types, transplantation of a single cell type together with MSC-derived exosomes may decrease the safety risk.
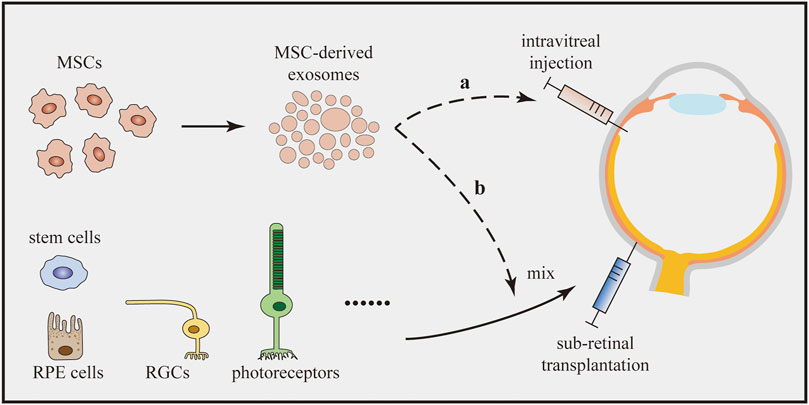
FIGURE 3. Cotransplantation of therapeutic cells with MSC-derived exosomes. MSC-derived exosomes enhance the M2 microglial polarization and suppress retinal inflammation. The cotransplantation can be implemented by two options: (a) The cotransplantation can combine the intravitreal injection of MSC-derived exosomes with subretinal transplantation of therapeutic cells. (b) MSC-derived exosomes and therapeutic cells can be mixed in advance and subsequently be transplanted into the subretinal space together.
No documents have reported the use of cell-exosome combined therapy to treat retinal diseases. However, recent studies have reported successful paradigms of cotransplantation of exosomes and stem cells and are worth continuing in the field of RDDs. In a rat model of acute ischemic stroke, combined transplantation of ADMSCs and ADMSC-derived exosomes was confirmed to be superior to either ADMSC or ADMSC-derived exosome transplantation alone at reducing the brain infarct zone and improving the recovery of neurological function (Chen et al., 2016). In one study of acute myocardial infarction, the sequential delivery of exosomes and MSCs enhanced the survival of MSCs and reduced their apoptosis both in vitro and in vivo. Additionally, cardiac function was improved to a greater extent in the cotransplantation group than in the other groups treated with exosomes or MSCs alone (Huang et al., 2019). In addition, Lin et al. demonstrated that the combined treatment of ADMSCs and ADMSC-derived exosomes resulted in the most significant preservation of kidney function and the integrity of kidney architecture compared to the single transplantation groups (Lin et al., 2016).
Regarding the implementation of the new treatment, we assume that two options exist (Figure 3). As MSC-derived exosomes are usually administered by intravitreal injection in studies of eye diseases (Harrell et al., 2018), cotransplantation can combine the intravitreal injection of MSC-derived exosomes and subretinal transplantation of other cells. However, researchers have not clearly determined whether the treatment would work since the two effectors are separately transplanted at different sites. As an alternative, MSC-derived exosomes and other cells could be mixed in advance and subsequently transplanted into the subretinal space. Although these two methods seem to be effective, studies should be carried out to confirm their efficacy. Meanwhile, the underlying cellular interactions and molecular mechanisms also require further research.
6.2 Transplantation of Induced Pluripotent Stem Cells-Derived M2 Microglia as a Potential Tool for Retinal Degenerative Disease Treatment
As M2 microglia are more beneficial than M1 microglia in RDDs, the question of whether direct transplantation of M2 microglia into the retina is a feasible approach to modulate the immune microenvironment and alleviate retinal inflammation has been proposed. Unfortunately, no related research has been published yet. However, studies have used this strategy to treat other neurological injuries. Recently, M1 and M2 microglia were transplanted into mouse models of spinal cord injury induced by GM-CSF and IL-4 and marked with CD86 and CD206, respectively. Compared with the control groups, significant recovery was observed in the M2 group, while deterioration was found in the M1 group (Kobashi et al., 2020). Similar outcomes have been reported in studies of brain injuries where transplantation of M2 macrophages improved cognitive impairment in a rat model of AD (Zhu et al., 2016) and transplantation of M2 microglia promoted axonal outgrowth and angiogenesis in a rat model of stroke (Kanazawa et al., 2017).
An obvious limitation is the difficulty of collecting a sufficient number of primary microglia directly from human tissues. Fortunately, recent advances in iPSCs have provided exciting new approaches to overcome this obstacle. Many protocols for generating microglia from hiPSCs have been published (Douvaras et al., 2017; Haenseler et al., 2017; Pandya et al., 2017). Hasselmann and Blurton-Jones (2020) concluded that three techniques are useful to generate iPSC-derived microglia, generally named “in-vitro microglia,” “organoid microglia,” and “xenotransplanted microglia” (Figure 4). Each technique features unique routes and has its own benefits and limitations. The “in-vitro microglia” technique, as the name implies, differentiates iPSCs into microglia in vitro. This technique is superior in terms of high throughput but limited by transcriptomic deficiencies (McQuade et al., 2018). “Organoid microglia” means that microglia are innately generated from iPSC-derived organoids. However, the branching pattern of organoid microglia is still different from that of adult human microglia (Ormel et al., 2018). Two steps are needed to generate “xenotransplanted microglia”. Microglial precursors are first differentiated from iPSCs and then transplanted into the brains of immune-deficient mice carrying the human allele for the colony stimulating factor 1 protein. In one study, microglia produced by this technique showed a better microglial morphology and gene expression signatures that closely resembled those of adult human microglia (Svoboda et al., 2019).
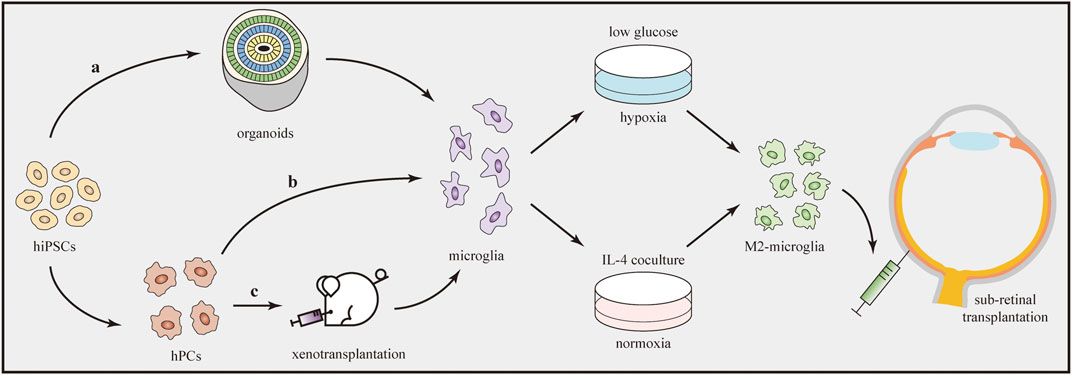
FIGURE 4. Transplantation of iPSC-derived M2 microglia. Microglia can be generated from hiPSCs through three techniques (a–c). Afterwards, microglia are induced to the M2 phenotype by using oxygen–glucose deprivation technique or IL-4 co-culture treatment. Direct transplantation of M2 microglia into the subretinal space can influence the immune microenvironment and alleviate retinal inflammation.
After the production of microglial cells, the next step is to drive them to transform into the M2 phenotype. Several methods have been developed to induce polarization in vitro (Figure 4). IL-4 is a cytokine that promotes tissue repair and M2 microglial polarization. According to one study using different concentrations (20 or 40 ng/ml) of IL-4, Arg1 (the M2 marker) expression significantly increased to similar levels in both groups, indicating the dose-independent characteristic of the M2 polarization-stimulating effect of IL-4 (Kobashi et al., 2020). In another study, after preconditioning by oxygen–glucose deprivation, microglia polarized to the M2 phenotype. Under this condition, cultures containing low-glucose medium were first placed in a hypoxia chamber (95% N2 + 5% CO2) for 1 h and then closed for 18 h (Kanazawa et al., 2017). In addition, several drugs, such as minocycline (Ahmed et al., 2017) and Lycium barbarum (Li et al., 2019b), were reported to modulate microglial polarization from the M1 to M2 phenotype in some in-vivo models. However, further studies are needed to determine whether they can be used to induce M2 polarization of microglia in vitro.
Although current studies are still in their infancy, the development of iPSC-derived microglia has provided insights into the production of abundant M2 microglia for transplantation. As studies in the brain have proven the feasibility and advantages of M2 microglial transplantation, we predict that the transplantation of M2 microglia will also become a proper and effective method to treat RDDs.
7 Conclusion
In recent years, SCT in subjects with other neurological disorders has been proven to exert positive effects of switching the polarization of microglia from the M1 to M2 phenotype, providing good examples for the application of SCT in the treatment of RDDs by targeting microglial polarization. However, the function of regulating microglial polarization by SCT in the retina is rarely reported and limited in the field of MSCs. Thus, we wondered if solutions were available to improve this situation and proposed some expectations for SCT to be a better treatment for RDDs, which may be helpful for future research. We postulate that the therapeutic effects of SCT will be improved by cotransplantation therapy with MSC-derived exosomes, which enhance M2 microglial polarization and create an optimized environment for the survival of transplanted cells to achieve better results. Besides, advances in iPSC-derived microglia may promote the development of transplantation of M2 microglia to treat RDDs and alleviate retinal inflammation.
Author Contributions
LG and NJ prepared the manuscript. LG generated the figures. WS drew the table. All authors contributed to the article and approved the submitted manuscript.
Funding
This work was supported by the National Nature Science Foundation of China (No. 82000923) and the Innovation Cultivating Foundation of 6th Medical center of PLA General Hospital (No. CXPY201823).
Conflict of Interest
The authors declare that the research was conducted in the absence of any commercial or financial relationships that could be construed as a potential conflict of interest.
Publisher’s Note
All claims expressed in this article are solely those of the authors and do not necessarily represent those of their affiliated organizations, or those of the publisher, the editors and the reviewers. Any product that may be evaluated in this article, or claim that may be made by its manufacturer, is not guaranteed or endorsed by the publisher.
References
Abcouwer, S. F., Shanmugam, S., Gomez, P. F., Shushanov, S., Barber, A. J., Lanoue, K. F., et al. (2008). Effect of IL-1β on Survival and Energy Metabolism of R28 and RGC-5 Retinal Neurons. Invest. Ophthalmol. Vis. Sci. 49 (12), 5581–5592. doi:10.1167/iovs.07-1032
Ahmed, A., Wang, L.-L., Abdelmaksoud, S., Aboelgheit, A., Saeed, S., and Zhang, C.-L. (2017). Minocycline Modulates Microglia Polarization in Ischemia-Reperfusion Model of Retinal Degeneration and Induces Neuroprotection. Sci. Rep. 7 (1), 14065. doi:10.1038/s41598-017-14450-5
Alessandrini, M., Preynat-Seauve, O., De Briun, K., and Pepper, M. S. (2019). Stem Cell Therapy for Neurological Disorders. S Afr. Med. J. 109 (8b), 70–77. doi:10.7196/SAMJ.2019.v109i8b.14009
Altmann, C., and Schmidt, M. (2018). The Role of Microglia in Diabetic Retinopathy: Inflammation, Microvasculature Defects and Neurodegeneration. Ijms 19 (1), 110. doi:10.3390/ijms19010110
Alves, C. H., Fernandes, R., Santiago, A. R., and Ambrósio, A. F. (2020). Microglia Contribution to the Regulation of the Retinal and Choroidal Vasculature in Age-Related Macular Degeneration. Cells 9 (5), 1217. doi:10.3390/cells9051217
Ambati, J., and Fowler, B. J. (2012). Mechanisms of Age-Related Macular Degeneration. Neuron 75 (1), 26–39. doi:10.1016/j.neuron.2012.06.018
Arroba, A. I., and Valverde, Á. M. (2017). Modulation of Microglia in the Retina: New Insights into Diabetic Retinopathy. Acta Diabetol. 54 (6), 527–533. doi:10.1007/s00592-017-0984-z
Ashok, A., Singh, N., Chaudhary, S., Bellamkonda, V., Kritikos, A. E., Wise, A. S., et al. (2020). Retinal Degeneration and Alzheimer's Disease: An Evolving Link. Ijms 21 (19), 7290. doi:10.3390/ijms21197290
Ashraf, M., and Souka, A. A. R. (2017). Aflibercept in Age-Related Macular Degeneration: Evaluating its Role as a Primary Therapeutic Option. Eye 31 (11), 1523–1536. doi:10.1038/eye.2017.81
Askew, K., Li, K., Olmos-Alonso, A., Garcia-Moreno, F., Liang, Y., Richardson, P., et al. (2017). Coupled Proliferation and Apoptosis Maintain the Rapid Turnover of Microglia in the Adult Brain. Cel Rep. 18 (2), 391–405. doi:10.1016/j.celrep.2016.12.041
Bai, L., Shao, H., Wang, H., Zhang, Z., Su, C., Dong, L., et al. (2017). Effects of Mesenchymal Stem Cell-Derived Exosomes on Experimental Autoimmune Uveitis. Sci. Rep. 7 (1), 4323. doi:10.1038/s41598-017-04559-y
Baker, P. S., and Brown, G. C. (2009). Stem-cell Therapy in Retinal Disease. Curr. Opin. Ophthalmol. 20 (3), 175–181. doi:10.1097/icu.0b013e328329b5f2
Bakondi, B., Girman, S., Lu, B., and Wang, S. (2017). Multimodal Delivery of Isogenic Mesenchymal Stem Cells Yields Synergistic Protection from Retinal Degeneration and Vision Loss. STEM CELLS Translational Med. 6 (2), 444–457. doi:10.5966/sctm.2016-0181
Ballios, B. G., Cooke, M. J., Donaldson, L., Coles, B. L. K., Morshead, C. M., van der Kooy, D., et al. (2015). A Hyaluronan-Based Injectable Hydrogel Improves the Survival and Integration of Stem Cell Progeny Following Transplantation. Stem Cel Rep. 4 (6), 1031–1045. doi:10.1016/j.stemcr.2015.04.008
Barakat, A. H., Elwell, V. A., and Lam, K. S. (2019). Stem Cell Therapy in Discogenic Back Pain. J. Spine Surg. 5 (4), 561–583. doi:10.21037/jss.2019.09.22
Boche, D., Perry, V. H., and Nicoll, J. A. R. (2013). Review: Activation Patterns of Microglia and Their Identification in the Human Brain. Neuropathol. Appl. Neurobiol. 39 (1), 3–18. doi:10.1111/nan.12011
Bodeutsch, N., and Thanos, S. (2000). Migration of Phagocytotic Cells and Development of the Murine Intraretinal Microglial Network: an In Vivo Study Using Fluorescent Dyes. Glia 32 (1), 91–101. doi:10.1002/1098-1136(200010)32:1<91::aid-glia90>3.0.co;2-x
Boyd, J. G., and Gordon, T. (2003). Neurotrophic Factors and Their Receptors in Axonal Regeneration and Functional Recovery after Peripheral Nerve Injury. Mn 27 (3), 277–324. doi:10.1385/MN:27:3:277
Brown, M. M., Brown, G. C., Sharma, S., Stein, J. D., Roth, Z., Campanella, J., et al. (2006). The burden of Age-Related Macular Degeneration: a Value-Based Analysis. Curr. Opin. Ophthalmol. 17 (3), 257–266. doi:10.1097/01.icu.0000193079.55240.18
Bruttger, J., Karram, K., Wörtge, S., Regen, T., Marini, F., Hoppmann, N., et al. (2015). Genetic Cell Ablation Reveals Clusters of Local Self-Renewing Microglia in the Mammalian Central Nervous System. Immunity 43 (1), 92–106. doi:10.1016/j.immuni.2015.06.012
Buchholz, D. E., Hikita, S. T., Rowland, T. J., Friedrich, A. M., Hinman, C. R., Johnson, L. V., et al. (2009). Derivation of Functional Retinal Pigmented Epithelium from Induced Pluripotent Stem Cells. Stem Cells 27 (10), 2427–2434. doi:10.1002/stem.189
Buchholz, D. E., Pennington, B. O., Croze, R. H., Hinman, C. R., Coffey, P. J., and Clegg, D. O. (2013). Rapid and Efficient Directed Differentiation of Human Pluripotent Stem Cells into Retinal Pigmented Epithelium. Stem Cell Transl. Med. 2 (5), 384–393. doi:10.5966/sctm.2012-0163
Burns, M. E., and Stevens, B. (2018). Report on the National Eye Institute's Audacious Goals Initiative: Creating a Cellular Environment for Neuroregeneration. eNeuro 5 (2), ENEURO.0035–18. doi:10.1523/ENEURO.0035-18.2018
Butovsky, O., Talpalar, A. E., Ben-Yaakov, K., and Schwartz, M. (2005). Activation of Microglia by Aggregated β-amyloid or Lipopolysaccharide Impairs MHC-II Expression and Renders Them Cytotoxic whereas IFN-γ and IL-4 Render Them Protective. Mol. Cell Neurosci. 29 (3), 381–393. doi:10.1016/j.mcn.2005.03.005
Checchin, D., Sennlaub, F., Levavasseur, E., Leduc, M., and Chemtob, S. (2006). Potential Role of Microglia in Retinal Blood Vessel Formation. Invest. Ophthalmol. Vis. Sci. 47 (8), 3595–3602. doi:10.1167/iovs.05-1522
Chen, K.-H., Chen, C.-H., Wallace, C. G., Yuen, C.-M., Kao, G.-S., Chen, Y.-L., et al. (2016). Intravenous Administration of Xenogenic Adipose-Derived Mesenchymal Stem Cells (ADMSC) and ADMSC-Derived Exosomes Markedly Reduced Brain Infarct Volume and Preserved Neurological Function in Rat after Acute Ischemic Stroke. Oncotarget 7 (46), 74537–74556. doi:10.18632/oncotarget.12902
Cheung, N., Mitchell, P., and Wong, T. Y. (2010). Diabetic Retinopathy. The Lancet 376 (9735), 124–136. doi:10.1016/S0140-6736(09)62124-3
Cideciyan, A. V., Aleman, T. S., Boye, S. L., Schwartz, S. B., Kaushal, S., Roman, A. J., et al. (2008). Human Gene Therapy for RPE65 Isomerase Deficiency Activates the Retinoid Cycle of Vision but with Slow Rod Kinetics. Proc. Natl. Acad. Sci. 105 (39), 15112–15117. doi:10.1073/pnas.0807027105
Claudio, L., Martiney, J. A., and Brosnan, C. F. (1994). Ultrastructural Studies of the Blood-Retina Barrier after Exposure to Interleukin-1 Beta or Tumor Necrosis Factor-Alpha. Lab. Invest. 70 (6), 850–861.
Collmann, F. M., Pijnenburg, R., Hamzei-Taj, S., Minassian, A., Folz-Donahue, K., Kukat, C., et al. (2019). Individual In Vivo Profiles of Microglia Polarization after Stroke, Represented by the Genes iNOS and Ym1. Front. Immunol. 10, 1236. doi:10.3389/fimmu.2019.01236
da Cruz, L., Fynes, K., Georgiadis, O., Kerby, J., Luo, Y. H., Ahmado, A., et al. (2018). Phase 1 Clinical Study of an Embryonic Stem Cell-Derived Retinal Pigment Epithelium Patch in Age-Related Macular Degeneration. Nat. Biotechnol. 36 (4), 328–337. doi:10.1038/nbt.4114
Daly, C., Ward, R., Reynolds, A. L., Galvin, O., Collery, R. F., and Kennedy, B. N. (2018). Brain-Derived Neurotrophic Factor as a Treatment Option for Retinal Degeneration. Adv. Exp. Med. Biol. 1074, 465–471. doi:10.1007/978-3-319-75402-4_57
Damani, M. R., Zhao, L., Fontainhas, A. M., Amaral, J., Fariss, R. N., and Wong, W. T. (2011). Age-related Alterations in the Dynamic Behavior of Microglia. Aging Cell 10 (2), 263–276. doi:10.1111/j.1474-9726.2010.00660.x
Daneshmandi, L., Shah, S., Jafari, T., Bhattacharjee, M., Momah, D., Saveh-Shemshaki, N., et al. (2020). Emergence of the Stem Cell Secretome in Regenerative Engineering. Trends Biotechnol. 38 (12), 1373–1384. doi:10.1016/j.tibtech.2020.04.013
DeAngelis, M. M., Owen, L. A., Morrison, M. A., Morgan, D. J., Li, M., Shakoor, A., et al. (2017). Genetics of Age-Related Macular Degeneration (AMD). Hum. Mol. Genet. 26 (R1), R45–R50. doi:10.1093/hmg/ddx228
Diaz-Araya, C. M., Provis, J. M., Penfold, P. L., and Billson, F. A. (1995). Development of Microglial Topography in Human Retina. J. Comp. Neurol. 363 (1), 53–68. doi:10.1002/cne.903630106
Dick, A. D., Carter, D., Robertson, M., Broderick, C., Hughes, E., Forrester, J. V., et al. (2003). Control of Myeloid Activity during Retinal Inflammation. J. Leukoc. Biol. 74 (2), 161–166. doi:10.1189/jlb.1102535
Douvaras, P., Sun, B., Wang, M., Kruglikov, I., Lallos, G., Zimmer, M., et al. (2017). Directed Differentiation of Human Pluripotent Stem Cells to Microglia. Stem Cel Rep. 8 (6), 1516–1524. doi:10.1016/j.stemcr.2017.04.023
Ezquer, M., Urzua, C. A., Montecino, S., Leal, K., Conget, P., and Ezquer, F. (2016). Intravitreal Administration of Multipotent Mesenchymal Stromal Cells Triggers a Cytoprotective Microenvironment in the Retina of Diabetic Mice. Stem Cel Res. Ther. 7, 42. doi:10.1186/s13287-016-0299-y
Fontanilla, C. V., Gu, H., Liu, Q., Zhu, T. Z., Zhou, C., Johnstone, B. H., et al. (2015). Adipose-derived Stem Cell Conditioned Media Extends Survival Time of a Mouse Model of Amyotrophic Lateral Sclerosis. Sci. Rep. 5, 16953. doi:10.1038/srep16953
Forrester, J. V., McMenamin, P. G., and Dando, S. J. (2018). CNS Infection and Immune Privilege. Nat. Rev. Neurosci. 19 (11), 655–671. doi:10.1038/s41583-018-0070-8
Gao, J., Grill, R. J., Dunn, T. J., Bedi, S., Labastida, J. A., Hetz, R. A., et al. (2016). Human Neural Stem Cell Transplantation-Mediated Alteration of Microglial/Macrophage Phenotypes after Traumatic Brain Injury. Cel Transpl. 25 (10), 1863–1877. doi:10.3727/096368916X691150
Gao, L., Chen, X., Tang, Y., Zhao, J., Li, Q., Fan, X., et al. (2015). Neuroprotective Effect of Memantine on the Retinal Ganglion Cells of APPswe/PS1ΔE9 Mice and its Immunomodulatory Mechanisms. Exp. Eye Res. 135, 47–58. doi:10.1016/j.exer.2015.04.013
Ginhoux, F., Greter, M., Leboeuf, M., Nandi, S., See, P., Gokhan, S., et al. (2010). Fate Mapping Analysis Reveals that Adult Microglia Derive from Primitive Macrophages. Science 330 (6005), 841–845. doi:10.1126/science.1194637
Gollisch, T., and Meister, M. (2010). Eye Smarter Than Scientists Believed: Neural Computations in Circuits of the Retina. Neuron 65 (2), 150–164. doi:10.1016/j.neuron.2009.12.009
Gomez Perdiguero, E., Klapproth, K., Schulz, C., Busch, K., Azzoni, E., Crozet, L., et al. (2015). Tissue-resident Macrophages Originate from Yolk-Sac-Derived Erythro-Myeloid Progenitors. Nature 518 (7540), 547–551. doi:10.1038/nature13989
Gorbatyuk, M., and Gorbatyuk, O. (2013). Review: Retinal Degeneration: Focus on the Unfolded Protein Response. Mol. Vis. 19, 1985–1998.
Grigsby, J. G., Cardona, S. M., Pouw, C. E., Muniz, A., Mendiola, A. S., Tsin, A. T. C., et al. (2014). The Role of Microglia in Diabetic Retinopathy. J. Ophthalmol. 2014, 1–15. doi:10.1155/2014/705783
Grossniklaus, H. E., Kang, S. J., and Berglin, L. (2010). Animal Models of Choroidal and Retinal Neovascularization. Prog. Retin. Eye Res. 29 (6), 500–519. doi:10.1016/j.preteyeres.2010.05.003
Gupta, N., Brown, K. E., and Milam, A. H. (2003). Activated Microglia in Human Retinitis Pigmentosa, Late-Onset Retinal Degeneration, and Age-Related Macular Degeneration. Exp. Eye Res. 76 (4), 463–471. doi:10.1016/s0014-4835(02)00332-9
Haenseler, W., Sansom, S. N., Buchrieser, J., Newey, S. E., Moore, C. S., Nicholls, F. J., et al. (2017). A Highly Efficient Human Pluripotent Stem Cell Microglia Model Displays a Neuronal-co-culture-specific Expression Profile and Inflammatory Response. Stem Cel Rep. 8 (6), 1727–1742. doi:10.1016/j.stemcr.2017.05.017
Han, M., Cao, Y., Guo, X., Chu, X., Li, T., Xue, H., et al. (2021). Mesenchymal Stem Cell-Derived Extracellular Vesicles Promote Microglial M2 Polarization after Subarachnoid Hemorrhage in Rats and Involve the AMPK/NF-κB Signaling Pathway. Biomed. Pharmacother. 133, 111048. doi:10.1016/j.biopha.2020.111048
Harrell, C. R., Simovic Markovic, B., Fellabaum, C., Arsenijevic, A., Djonov, V., Arsenijevic, N., et al. (2018). Therapeutic Potential of Mesenchymal Stem Cell-Derived Exosomes in the Treatment of Eye Diseases. Adv. Exp. Med. Biol. 1089, 47–57. doi:10.1007/5584_2018_219
Hart, N. J., Koronyo, Y., Black, K. L., and Koronyo-Hamaoui, M. (2016). Ocular Indicators of Alzheimer's: Exploring Disease in the Retina. Acta Neuropathol. 132 (6), 767–787. doi:10.1007/s00401-016-1613-6
Hartong, D. T., Berson, E. L., and Dryja, T. P. (2006). Retinitis Pigmentosa. The Lancet 368 (9549), 1795–1809. doi:10.1016/S0140-6736(06)69740-7
Hasselmann, J., and Blurton‐Jones, M. (2020). Human iPSC‐derived Microglia: A Growing Toolset to Study the Brain's Innate Immune Cells. Glia 68 (4), 721–739. doi:10.1002/glia.23781
Hoeffel, G., Chen, J., Lavin, Y., Low, D., Almeida, F. F., See, P., et al. (2015). C-Myb+ Erythro-Myeloid Progenitor-Derived Fetal Monocytes Give Rise to Adult Tissue-Resident Macrophages. Immunity 42 (4), 665–678. doi:10.1016/j.immuni.2015.03.011
Howell, G. R., Macalinao, D. G., Sousa, G. L., Walden, M., Soto, I., Kneeland, S. C., et al. (2011). Molecular Clustering Identifies Complement and Endothelin Induction as Early Events in a Mouse Model of Glaucoma. J. Clin. Invest. 121 (4), 1429–1444. doi:10.1172/JCI44646
Hu, Z. L., Li, N., Wei, X., Tang, L., Wang, T. H., and Chen, X. M. (2017). Neuroprotective Effects of BDNF and GDNF in Intravitreally Transplanted Mesenchymal Stem Cells after Optic Nerve Crush in Mice. Int. J. Ophthalmol. 10 (1), 35–42. doi:10.18240/ijo.2017.01.06
Huang, P., Wang, L., Li, Q., Xu, J., Xu, J., Xiong, Y., et al. (2019). Combinatorial Treatment of Acute Myocardial Infarction Using Stem Cells and Their Derived Exosomes Resulted in Improved Heart Performance. Stem Cel Res. Ther. 10 (1), 300. doi:10.1186/s13287-019-1353-3
Imamoto, K., and Leblond, C. P. (1978). Radioautographic Investigation of Gliogenesis in the Corpus Callosum of Young Rats II. Origin of Microglial Cells. J. Comp. Neurol. 180 (1), 139–163. doi:10.1002/cne.901800109
Indaram, M., Ma, W., Zhao, L., Fariss, R. N., Rodriguez, I. R., and Wong, W. T. (2015). 7-Ketocholesterol Increases Retinal Microglial Migration, Activation and Angiogenicity: A Potential Pathogenic Mechanism Underlying Age-Related Macular Degeneration. Sci. Rep. 5, 9144. doi:10.1038/srep09144
Jaimes, Y., Naaldijk, Y., Wenk, K., Leovsky, C., and Emmrich, F. (2017). Mesenchymal Stem Cell‐Derived Microvesicles Modulate Lipopolysaccharides‐Induced Inflammatory Responses to Microglia Cells. Stem Cells 35 (3), 812–823. doi:10.1002/stem.2541
Jha, K., Pentecost, M., Lenin, R., Klaic, L., Elshaer, S., Gentry, J., et al. (2018). Concentrated Conditioned Media from Adipose Tissue Derived Mesenchymal Stem Cells Mitigates Visual Deficits and Retinal Inflammation Following Mild Traumatic Brain Injury. Ijms 19 (7), 2016. doi:10.3390/ijms19072016
Jiang, C. T., Wu, W. F., Deng, Y. H., and Ge, J. W. (2020). Modulators of Microglia Activation and Polarization in Ischemic Stroke (Review). Mol. Med. Rep. 21 (5), 2006–2018. doi:10.3892/mmr.2020.11003
Jiao, H., Natoli, R., Valter, K., Provis, J. M., and Rutar, M. (2015). Spatiotemporal Cadence of Macrophage Polarisation in a Model of Light-Induced Retinal Degeneration. PLoS One 10 (12), e0143952. doi:10.1371/journal.pone.0143952
Jin, N., Gao, L., Fan, X., and Xu, H. (2017). Friend or Foe? Resident Microglia vs Bone Marrow-Derived Microglia and Their Roles in the Retinal Degeneration. Mol. Neurobiol. 54 (6), 4094–4112. doi:10.1007/s12035-016-9960-9
Jin, Z.-B., Gao, M.-L., Deng, W.-L., Wu, K.-C., Sugita, S., Mandai, M., et al. (2019). Stemming Retinal Regeneration with Pluripotent Stem Cells. Prog. Retin. Eye Res. 69, 38–56. doi:10.1016/j.preteyeres.2018.11.003
Jobling, A. I., Waugh, M., Vessey, K. A., Phipps, J. A., Trogrlic, L., Greferath, U., et al. (2018). The Role of the Microglial Cx3cr1 Pathway in the Postnatal Maturation of Retinal Photoreceptors. J. Neurosci. 38 (20), 4708–4723. doi:10.1523/JNEUROSCI.2368-17.2018
Kalluri, R., and LeBleu, V. S. (2020). The Biology, Function, and Biomedical Applications of Exosomes. Science 367 (6478), eaau6977. doi:10.1126/science.aau6977
Kanazawa, M., Miura, M., Toriyabe, M., Koyama, M., Hatakeyama, M., Ishikawa, M., et al. (2017). Microglia Preconditioned by Oxygen-Glucose Deprivation Promote Functional Recovery in Ischemic Rats. Sci. Rep. 7, 42582. doi:10.1038/srep42582
Karali, M., Guadagnino, I., Marrocco, E., De Cegli, R., Carissimo, A., Pizzo, M., et al. (2020). AAV-miR-204 Protects from Retinal Degeneration by Attenuation of Microglia Activation and Photoreceptor Cell Death. Mol. Ther. - Nucleic Acids 19, 144–156. doi:10.1016/j.omtn.2019.11.005
Karlstetter, M., Scholz, R., Rutar, M., Wong, W. T., Provis, J. M., and Langmann, T. (2015). Retinal Microglia: Just Bystander or Target for Therapy? Prog. Retin. Eye Res. 45, 30–57. doi:10.1016/j.preteyeres.2014.11.004
Khan, M., Nickoloff, E., Abramova, T., Johnson, J., Verma, S. K., Krishnamurthy, P., et al. (2015). Embryonic Stem Cell-Derived Exosomes Promote Endogenous Repair Mechanisms and Enhance Cardiac Function Following Myocardial Infarction. Circ. Res. 117 (1), 52–64. doi:10.1161/CIRCRESAHA.117.305990
Kim, S.-M., Mun, B.-R., Lee, S.-J., Joh, Y., Lee, H.-Y., Ji, K.-Y., et al. (2017). TREM2 Promotes Aβ Phagocytosis by Upregulating C/EBPα-dependent CD36 Expression in Microglia. Sci. Rep. 7 (1), 11118. doi:10.1038/s41598-017-11634-x
Kinuthia, U. M., Wolf, A., and Langmann, T. (2020). Microglia and Inflammatory Responses in Diabetic Retinopathy. Front. Immunol. 11, 564077. doi:10.3389/fimmu.2020.564077
Kitase, Y., Sato, Y., Ueda, K., Suzuki, T., Mikrogeorgiou, A., Sugiyama, Y., et al. (2020). A Novel Treatment with Stem Cells from Human Exfoliated Deciduous Teeth for Hypoxic-Ischemic Encephalopathy in Neonatal Rats. Stem Cell Dev. 29 (2), 63–74. doi:10.1089/scd.2019.0221
Klimanskaya, I., Hipp, J., Rezai, K. A., West, M., Atala, A., and Lanza, R. (2004). Derivation and Comparative Assessment of Retinal Pigment Epithelium from Human Embryonic Stem Cells Using Transcriptomics. Cloning and Stem Cells 6 (3), 217–245. doi:10.1089/clo.2004.6.217
Kobashi, S., Terashima, T., Katagi, M., Nakae, Y., Okano, J., Suzuki, Y., et al. (2020). Transplantation of M2-Deviated Microglia Promotes Recovery of Motor Function after Spinal Cord Injury in Mice. Mol. Ther. 28 (1), 254–265. doi:10.1016/j.ymthe.2019.09.004
Kochan, T., Singla, A., Tosi, J., and Kumar, A. (2012). Toll-like Receptor 2 Ligand Pretreatment Attenuates Retinal Microglial Inflammatory Response but Enhances Phagocytic Activity toward Staphylococcus aureus. Infect. Immun. 80 (6), 2076–2088. doi:10.1128/IAI.00149-12
Kohno, H., Chen, Y., Kevany, B. M., Pearlman, E., Miyagi, M., Maeda, T., et al. (2013). Photoreceptor Proteins Initiate Microglial Activation via Toll-like Receptor 4 in Retinal Degeneration Mediated by All-Trans-Retinal. J. Biol. Chem. 288 (21), 15326–15341. doi:10.1074/jbc.M112.448712
Kolb, H. (1995). “Glial Cells of the Retina,” in Webvision: The Organization of the Retina and Visual System. Editors H. Kolb, E. Fernandez, and R. Nelson. Salt Lake City (UT): University of Utah Health Sciences Center.
Kolios, G., and Moodley, Y. (2013). Introduction to Stem Cells and Regenerative Medicine. Respiration 85 (1), 3–10. doi:10.1159/000345615
Koronyo-Hamaoui, M., Koronyo, Y., Ljubimov, A. V., Miller, C. A., Ko, M. K., Black, K. L., et al. (2011). Identification of Amyloid Plaques in Retinas from Alzheimer's Patients and Noninvasive In Vivo Optical Imaging of Retinal Plaques in a Mouse Model. Neuroimage 54 (Suppl. 1), S204–S217. doi:10.1016/j.neuroimage.2010.06.020
Krady, J. K., Basu, A., Allen, C. M., Xu, Y., LaNoue, K. F., Gardner, T. W., et al. (2005). Minocycline Reduces Proinflammatory Cytokine Expression, Microglial Activation, and Caspase-3 Activation in a Rodent Model of Diabetic Retinopathy. Diabetes 54 (5), 1559–1565. doi:10.2337/diabetes.54.5.1559
Kreutzberg, G. W. (1996). Microglia: a Sensor for Pathological Events in the CNS. Trends Neurosciences 19 (8), 312–318. doi:10.1016/0166-2236(96)10049-7
Lamba, D. A., Gust, J., and Reh, T. A. (2009). Transplantation of Human Embryonic Stem Cell-Derived Photoreceptors Restores Some Visual Function in Crx-Deficient Mice. Cell Stem Cell 4 (1), 73–79. doi:10.1016/j.stem.2008.10.015
Lamba, D. A., McUsic, A., Hirata, R. K., Wang, P.-R., Russell, D., and Reh, T. A. (2010). Generation, Purification and Transplantation of Photoreceptors Derived from Human Induced Pluripotent Stem Cells. PLoS One 5 (1), e8763. doi:10.1371/journal.pone.0008763
Langmann, T. (2007). Microglia Activation in Retinal Degeneration. J. Leukoc. Biol. 81 (6), 1345–1351. doi:10.1189/jlb.0207114
Le, W.-d., Rowe, D., Xie, W., Ortiz, I., He, Y., and Appel, S. H. (2001). Microglial Activation and Dopaminergic Cell Injury: AnIn VitroModel Relevant to Parkinson's Disease. J. Neurosci. 21 (21), 8447–8455. doi:10.1523/jneurosci.21-21-08447.2001
Lechner, J., O'Leary, O. E., and Stitt, A. W. (2017). The Pathology Associated with Diabetic Retinopathy. Vis. Res. 139, 7–14. doi:10.1016/j.visres.2017.04.003
Lew, D. S., Mazzoni, F., and Finnemann, S. C. (2020). Microglia Inhibition Delays Retinal Degeneration Due to MerTK Phagocytosis Receptor Deficiency. Front. Immunol. 11, 1463. doi:10.3389/fimmu.2020.01463
Li, F., Jiang, D., and Samuel, M. A. (2019a). Microglia in the Developing Retina. Neural Dev. 14 (1), 12. doi:10.1186/s13064-019-0137-x
Li, H.-Y., Huang, M., Luo, Q.-Y., Hong, X., Ramakrishna, S., and So, K.-F. (2019b). Lycium Barbarum (Wolfberry) Increases Retinal Ganglion Cell Survival and Affects Both Microglia/Macrophage Polarization and Autophagy after Rat Partial Optic Nerve Transection. Cel Transpl. 28 (5), 607–618. doi:10.1177/0963689719835181
Li, J., Wang, H., Du, C., Jin, X., Geng, Y., Han, B., et al. (2020). hUC-MSCs Ameliorated CUMS-Induced Depression by Modulating Complement C3 Signaling-Mediated Microglial Polarization during Astrocyte-Microglia Crosstalk. Brain Res. Bull. 163, 109–119. doi:10.1016/j.brainresbull.2020.07.004
Li, J., Yu, S., Lu, X., Cui, K., Tang, X., Xu, Y., et al. (2021a). The Phase Changes of M1/M2 Phenotype of Microglia/macrophage Following Oxygen-Induced Retinopathy in Mice. Inflamm. Res. 70 (2), 183–192. doi:10.1007/s00011-020-01427-w
Li, L., Eter, N., and Heiduschka, P. (2015). The Microglia in Healthy and Diseased Retina. Exp. Eye Res. 136, 116–130. doi:10.1016/j.exer.2015.04.020
Li, Q.-Y., Zou, T., Gong, Y., Chen, S.-Y., Zeng, Y.-X., Gao, L.-X., et al. (2021b). Functional Assessment of Cryopreserved Clinical Grade hESC-RPE Cells as a Qualified Cell Source for Stem Cell Therapy of Retinal Degenerative Diseases. Exp. Eye Res. 202, 108305. doi:10.1016/j.exer.2020.108305
Li, Y., Yang, Y.-Y., Ren, J.-L., Xu, F., Chen, F.-M., and Li, A. (2017). Exosomes Secreted by Stem Cells from Human Exfoliated Deciduous Teeth Contribute to Functional Recovery after Traumatic Brain Injury by Shifting Microglia M1/M2 Polarization in Rats. Stem Cel Res. Ther. 8 (1), 198. doi:10.1186/s13287-017-0648-5
Li, Z., Liu, F., He, X., Yang, X., Shan, F., and Feng, J. (2019c). Exosomes Derived from Mesenchymal Stem Cells Attenuate Inflammation and Demyelination of the central Nervous System in EAE Rats by Regulating the Polarization of Microglia. Int. Immunopharmacology 67, 268–280. doi:10.1016/j.intimp.2018.12.001
Lin, K.-C., Yip, H.-K., Shao, P.-L., Wu, S.-C., Chen, K.-H., Chen, Y.-T., et al. (2016). Combination of Adipose-Derived Mesenchymal Stem Cells (ADMSC) and ADMSC-Derived Exosomes for Protecting Kidney from Acute Ischemia-Reperfusion Injury. Int. J. Cardiol. 216, 173–185. doi:10.1016/j.ijcard.2016.04.061
Liu, Y., Chen, S. J., Li, S. Y., Qu, L. H., Meng, X. H., Wang, Y., et al. (2017). Long-term Safety of Human Retinal Progenitor Cell Transplantation in Retinitis Pigmentosa Patients. Stem Cel Res. Ther. 8 (1), 209. doi:10.1186/s13287-017-0661-8
Liu, Y., Leo, L. F., McGregor, C., Grivitishvili, A., Barnstable, C. J., and Tombran-Tink, J. (2012). Pigment Epithelium-Derived Factor (PEDF) Peptide Eye Drops Reduce Inflammation, Cell Death and Vascular Leakage in Diabetic Retinopathy in Ins2Akita Mice. Mol. Med. 18, 1387–1401. doi:10.2119/molmed.2012.00008
Liu, Y., Zeng, R., Wang, Y., Huang, W., Hu, B., Zhu, G., et al. (2019). Mesenchymal Stem Cells Enhance Microglia M2 Polarization and Attenuate Neuroinflammation through TSG-6. Brain Res. 1724, 146422. doi:10.1016/j.brainres.2019.146422
Lobov, I. B., Rao, S., Carroll, T. J., Vallance, J. E., Ito, M., Ondr, J. K., et al. (2005). WNT7b Mediates Macrophage-Induced Programmed Cell Death in Patterning of the Vasculature. Nature 437 (7057), 417–421. doi:10.1038/nature03928
Luo, C., Chen, M., and Xu, H. (2011). Complement Gene Expression and Regulation in Mouse Retina and Retinal Pigment Epithelium/choroid. Mol. Vis. 17, 1588–1597.
Ma, X., Li, H., Chen, Y., Yang, J., Chen, H., Arnheiter, H., et al. (2019). The Transcription Factor MITF in RPE Function and Dysfunction. Prog. Retin. Eye Res. 73, 100766. doi:10.1016/j.preteyeres.2019.06.002
Madeira, M. H., Boia, R., Santos, P. F., Ambrósio, A. F., and Santiago, A. R. (2015). Contribution of Microglia-Mediated Neuroinflammation to Retinal Degenerative Diseases. Mediators Inflamm. 2015, 1–15. doi:10.1155/2015/673090
Malhotra, A., Minja, F. J., Crum, A., and Burrowes, D. (2011). Ocular Anatomy and Cross-Sectional Imaging of the Eye. Semin. Ultrasound CT MRI 32 (1), 2–13. doi:10.1053/j.sult.2010.10.009
Mandai, M., Watanabe, A., Kurimoto, Y., Hirami, Y., Morinaga, C., Daimon, T., et al. (2017). Autologous Induced Stem-Cell-Derived Retinal Cells for Macular Degeneration. N. Engl. J. Med. 376 (11), 1038–1046. doi:10.1056/NEJMoa1608368
Maneu, V., Yáñez, A., Murciano, C., Molina, A., Gil, M. L., and Gozalbo, D. (2011). Dectin-1 Mediatesin Vitrophagocytosis ofCandida Albicansyeast Cells by Retinal Microglia: Figure 1. FEMS Immunol. Med. Microbiol. 63 (1), 148–150. doi:10.1111/j.1574-695X.2011.00829.x
Mantravadi, A. V., and Vadhar, N. (2015). Glaucoma. Prim. Care Clin. Off. Pract. 42 (3), 437–449. doi:10.1016/j.pop.2015.05.008
Markus, A., Shamul, A., Chemla, Y., Farah, N., Shaham, L., Goldstein, R. S., et al. (2019). An Optimized Protocol for Generating Labeled and Transplantable Photoreceptor Precursors from Human Embryonic Stem Cells. Exp. Eye Res. 180, 29–38. doi:10.1016/j.exer.2018.11.013
Maruotti, J., Sripathi, S. R., Bharti, K., Fuller, J., Wahlin, K. J., Ranganathan, V., et al. (2015). Small-molecule-directed, Efficient Generation of Retinal Pigment Epithelium from Human Pluripotent Stem Cells. Proc. Natl. Acad. Sci. USA 112 (35), 10950–10955. doi:10.1073/pnas.1422818112
Mathew, B., Ravindran, S., Liu, X., Torres, L., Chennakesavalu, M., Huang, C.-C., et al. (2019). Mesenchymal Stem Cell-Derived Extracellular Vesicles and Retinal Ischemia-Reperfusion. Biomaterials 197, 146–160. doi:10.1016/j.biomaterials.2019.01.016
McMenamin, P. G., Saban, D. R., and Dando, S. J. (2019). Immune Cells in the Retina and Choroid: Two Different Tissue Environments that Require Different Defenses and Surveillance. Prog. Retin. Eye Res. 70, 85–98. doi:10.1016/j.preteyeres.2018.12.002
McQuade, A., Coburn, M., Tu, C. H., Hasselmann, J., Davtyan, H., and Blurton-Jones, M. (2018). Development and Validation of a Simplified Method to Generate Human Microglia from Pluripotent Stem Cells. Mol. Neurodegeneration 13 (1), 67. doi:10.1186/s13024-018-0297-x
Mead, B., Ahmed, Z., and Tomarev, S. (2018). Mesenchymal Stem Cell-Derived Small Extracellular Vesicles Promote Neuroprotection in a Genetic DBA/2J Mouse Model of Glaucoma. Invest. Ophthalmol. Vis. Sci. 59 (13), 5473–5480. doi:10.1167/iovs.18-25310
Mead, B., Berry, M., Logan, A., Scott, R. A. H., Leadbeater, W., and Scheven, B. A. (2015). Stem Cell Treatment of Degenerative Eye Disease. Stem Cel Res. 14 (3), 243–257. doi:10.1016/j.scr.2015.02.003
Mead, B., Logan, A., Berry, M., Leadbeater, W., and Scheven, B. A. (2014). Paracrine-mediated Neuroprotection and Neuritogenesis of Axotomised Retinal Ganglion Cells by Human Dental Pulp Stem Cells: Comparison with Human Bone Marrow and Adipose-Derived Mesenchymal Stem Cells. PLoS One 9 (10), e109305. doi:10.1371/journal.pone.0109305
Mead, B., and Tomarev, S. (2020). Extracellular Vesicle Therapy for Retinal Diseases. Prog. Retin. Eye Res. 79, 100849. doi:10.1016/j.preteyeres.2020.100849
Michelet, F., Balasankar, A., Teo, N., Stanton, L. W., and Singhal, S. (2020). Rapid Generation of Purified Human RPE from Pluripotent Stem Cells Using 2D Cultures and Lipoprotein Uptake-Based Sorting. Stem Cel Res. Ther. 11 (1), 47. doi:10.1186/s13287-020-1568-3
Mirzaei, N., Shi, H., Oviatt, M., Doustar, J., Rentsendorj, A., Fuchs, D.-T., et al. (2020). Alzheimer's Retinopathy: Seeing Disease in the Eyes. Front. Neurosci. 14, 921. doi:10.3389/fnins.2020.00921
Morgan, S. C., Taylor, D. L., and Pocock, J. M. (2004). Microglia Release Activators of Neuronal Proliferation Mediated by Activation of Mitogen-Activated Protein Kinase, Phosphatidylinositol-3-kinase/Akt and delta-Notch Signalling Cascades. J. Neurochem. 90 (1), 89–101. doi:10.1111/j.1471-4159.2004.02461.x
Müller, P., Lemcke, H., and David, R. (2018). Stem Cell Therapy in Heart Diseases - Cell Types, Mechanisms and Improvement Strategies. Cell. Physiol. Biochem. 48 (6), 2607–2655. doi:10.1159/000492704
Nashine, S., Nesburn, A. B., Kuppermann, B. D., and Kenney, M. C. (2019). Age-related Macular Degeneration (AMD) Mitochondria Modulate Epigenetic Mechanisms in Retinal Pigment Epithelial Cells. Exp. Eye Res. 189, 107701. doi:10.1016/j.exer.2019.107701
Neher, J. J., Emmrich, J. V., Fricker, M., Mander, P. K., Thery, C., and Brown, G. C. (2013). Phagocytosis Executes Delayed Neuronal Death after Focal Brain Ischemia. Proc. Natl. Acad. Sci. 110 (43), E4098–E4107. doi:10.1073/pnas.1308679110
Nimmerjahn, A., Kirchhoff, F., and Helmchen, F. (2005). Resting Microglial Cells Are Highly Dynamic Surveillants of Brain Parenchyma In Vivo. Science 308 (5726), 1314–1318. doi:10.1126/science.1110647
Ormel, P. R., Vieira de Sá, R., van Bodegraven, E. J., Karst, H., Harschnitz, O., Sneeboer, M. A. M., et al. (2018). Microglia Innately Develop within Cerebral Organoids. Nat. Commun. 9 (1), 4167. doi:10.1038/s41467-018-06684-2
Pandya, H., Shen, M. J., Ichikawa, D. M., Sedlock, A. B., Choi, Y., Johnson, K. R., et al. (2017). Differentiation of Human and Murine Induced Pluripotent Stem Cells to Microglia-like Cells. Nat. Neurosci. 20 (5), 753–759. doi:10.1038/nn.4534
Park, H. J., Oh, S. H., Kim, H. N., Jung, Y. J., and Lee, P. H. (2016). Mesenchymal Stem Cells Enhance α-synuclein Clearance via M2 Microglia Polarization in Experimental and Human Parkinsonian Disorder. Acta Neuropathol. 132 (5), 685–701. doi:10.1007/s00401-016-1605-6
Penfold, P. L., Provis, J. M., and Liew, S. C. (1993). Human Retinal Microglia Express Phenotypic Characteristics in Common with Dendritic Antigen-Presenting Cells. J. Neuroimmunol. 45 (1-2), 183–191. doi:10.1016/0165-5728(93)90179-3
Qin, L., Liu, Y., Cooper, C., Liu, B., Wilson, B., and Hong, J.-S. (2002). Microglia Enhance β-amyloid Peptide-Induced Toxicity in Cortical and Mesencephalic Neurons by Producing Reactive Oxygen Species. J. Neurochem. 83 (4), 973–983. doi:10.1046/j.1471-4159.2002.01210.x
Qiu, G., Seiler, M. J., Thomas, B. B., Wu, K., Radosevich, M., and Sadda, S. R. (2007). Revisiting Nestin Expression in Retinal Progenitor Cells In Vitro and after Transplantation In Vivo. Exp. Eye Res. 84 (6), 1047–1059. doi:10.1016/j.exer.2007.01.014
Qu, L., Gao, L., Xu, H., Duan, P., Zeng, Y., Liu, Y., et al. (2017). Combined Transplantation of Human Mesenchymal Stem Cells and Human Retinal Progenitor Cells into the Subretinal Space of RCS Rats. Sci. Rep. 7 (1), 199. doi:10.1038/s41598-017-00241-5
Quigley, H. A. (2011). Glaucoma. The Lancet 377 (9774), 1367–1377. doi:10.1016/S0140-6736(10)61423-7
Rashid, K., Akhtar-Schaefer, I., and Langmann, T. (2019). Microglia in Retinal Degeneration. Front. Immunol. 10, 1975. doi:10.3389/fimmu.2019.01975
Rathnasamy, G., Foulds, W. S., Ling, E.-A., and Kaur, C. (2019). Retinal Microglia - A Key Player in Healthy and Diseased Retina. Prog. Neurobiol. 173, 18–40. doi:10.1016/j.pneurobio.2018.05.006
Rawji, K. S., and Yong, V. W. (2013). The Benefits and Detriments of Macrophages/microglia in Models of Multiple Sclerosis. Clin. Dev. Immunol. 2013, 1–13. doi:10.1155/2013/948976
Reichenbach, A., and Bringmann, A. (2013). New Functions of Müller Cells. Glia 61 (5), 651–678. doi:10.1002/glia.22477
Réu, P., Khosravi, A., Bernard, S., Mold, J. E., Salehpour, M., Alkass, K., et al. (2017). The Lifespan and Turnover of Microglia in the Human Brain. Cel Rep. 20 (4), 779–784. doi:10.1016/j.celrep.2017.07.004
Rio-hortega, P. D. (1993). Art and Artifice in the Science of Histology. Histopathology 22 (6), 515–525. doi:10.1111/j.1365-2559.1993.tb00171.x
Rutar, M., Natoli, R., Kozulin, P., Valter, K., Gatenby, P., and Provis, J. M. (2011). Analysis of Complement Expression in Light-Induced Retinal Degeneration: Synthesis and Deposition of C3 by Microglia/macrophages Is Associated with Focal Photoreceptor Degeneration. Invest. Ophthalmol. Vis. Sci. 52 (8), 5347–5358. doi:10.1167/iovs.10-7119
Salvi, S. M., Akhtar, S., and Currie, Z. (2006). Ageing Changes in the Eye. Postgrad. Med. J. 82 (971), 581–587. doi:10.1136/pgmj.2005.040857
Sapieha, P., Hamel, D., Shao, Z., Rivera, J. C., Zaniolo, K., Joyal, J. S., et al. (2010). Proliferative Retinopathies: Angiogenesis that Blinds. Int. J. Biochem. Cel Biol. 42 (1), 5–12. doi:10.1016/j.biocel.2009.10.006
Schafer, D. P., Lehrman, E. K., Kautzman, A. G., Koyama, R., Mardinly, A. R., Yamasaki, R., et al. (2012). Microglia Sculpt Postnatal Neural Circuits in an Activity and Complement-dependent Manner. Neuron 74 (4), 691–705. doi:10.1016/j.neuron.2012.03.026
Schmeer, C. W., Wohl, S. G., and Isenmann, S. (2012). Cell-replacement Therapy and Neural Repair in the Retina. Cell Tissue Res 349 (1), 363–374. doi:10.1007/s00441-012-1335-6
Scholl, H. P. N., Moore, A. T., Koenekoop, R. K., Wen, Y., Fishman, G. A., van den Born, L. I., et al. (2015). Safety and Proof-Of-Concept Study of Oral QLT091001 in Retinitis Pigmentosa Due to Inherited Deficiencies of Retinal Pigment Epithelial 65 Protein (RPE65) or Lecithin:Retinol Acyltransferase (LRAT). PLoS One 10 (12), e0143846. doi:10.1371/journal.pone.0143846
Shapouri‐Moghaddam, A., Mohammadian, S., Vazini, H., Taghadosi, M., Esmaeili, S. A., Mardani, F., et al. (2018). Macrophage Plasticity, Polarization, and Function in Health and Disease. J. Cel. Physiol. 233 (9), 6425–6440. doi:10.1002/jcp.26429
Silva, N. J., Nagashima, M., Li, J., Kakuk‐Atkins, L., Ashrafzadeh, M., Hyde, D. R., et al. (2020). Inflammation and Matrix Metalloproteinase 9 (Mmp‐9) Regulate Photoreceptor Regeneration in Adult Zebrafish. Glia 68 (7), 1445–1465. doi:10.1002/glia.23792
Sivakumar, V., Foulds, W. S., Luu, C. D., Ling, E.-A., and Kaur, C. (2011). Retinal Ganglion Cell Death Is Induced by Microglia Derived Pro-inflammatory Cytokines in the Hypoxic Neonatal Retina. J. Pathol. 224 (2), 245–260. doi:10.1002/path.2858
Song, Y., Li, Z., He, T., Qu, M., Jiang, L., Li, W., et al. (2019). M2 Microglia-Derived Exosomes Protect the Mouse Brain from Ischemia-Reperfusion Injury via Exosomal miR-124. Theranostics 9 (10), 2910–2923. doi:10.7150/thno.30879
Suh, H.-S., Zhao, M.-L., Derico, L., Choi, N., and Lee, S. C. (2013). Insulin-like Growth Factor 1 and 2 (IGF1, IGF2) Expression in Human Microglia: Differential Regulation by Inflammatory Mediators. J. Neuroinflammation 10, 37. doi:10.1186/1742-2094-10-37
Svoboda, D. S., Barrasa, M. I., Shu, J., Rietjens, R., Zhang, S., Mitalipova, M., et al. (2019). Human iPSC-Derived Microglia Assume a Primary Microglia-like State after Transplantation into the Neonatal Mouse Brain. Proc. Natl. Acad. Sci. USA 116 (50), 25293–25303. doi:10.1073/pnas.1913541116
Taheri, B., Soleimani, M., Fekri Aval, S., Esmaeili, E., Bazi, Z., and Zarghami, N. (2019). Induced Pluripotent Stem Cell‐derived Extracellular Vesicles: A Novel Approach for Cell‐free Regenerative Medicine. J. Cel. Physiol. 234 (6), 8455–8464. doi:10.1002/jcp.27775
Tang, Y., and Le, W. (2016). Differential Roles of M1 and M2 Microglia in Neurodegenerative Diseases. Mol. Neurobiol. 53 (2), 1181–1194. doi:10.1007/s12035-014-9070-5
Teixeira-Pinheiro, L. C., Toledo, M. F., Nascimento-Dos-Santos, G., Mendez-Otero, R., Mesentier-Louro, L. A., and Santiago, M. F. (2020). Paracrine Signaling of Human Mesenchymal Stem Cell Modulates Retinal Microglia Population Number and Phenotype In Vitro. Exp. Eye Res. 200, 108212. doi:10.1016/j.exer.2020.108212
Tezel, G. l. n., and Yang, X. (2004). Caspase-independent Component of Retinal Ganglion Cell Death, In Vitro. Invest. Ophthalmol. Vis. Sci. 45 (11), 4049–4059. doi:10.1167/iovs.04-0490
Thanos, S. (1991). The Relationship of Microglial Cells to Dying Neurons during Natural Neuronal Cell Death and Axotomy-Induced Degeneration of the Rat Retina. Eur. J. Neurosci. 3 (12), 1189–1207. doi:10.1111/j.1460-9568.1991.tb00054.x
Trifunovic, D., Sahaboglu, A., Kaur, J., Mencl, S., Zrenner, E., Ueffing, M., et al. (2012). Neuroprotective Strategies for the Treatment of Inherited Photoreceptor Degeneration. Cmm 12 (5), 598–612. doi:10.2174/156652412800620048
Usategui-Martín, R., Puertas-Neyra, K., García-Gutiérrez, M.-T., Fuentes, M., Pastor, J. C., and Fernandez-Bueno, I. (2020). Human Mesenchymal Stem Cell Secretome Exhibits a Neuroprotective Effect over In Vitro Retinal Photoreceptor Degeneration. Mol. Ther. - Methods Clin. Dev. 17, 1155–1166. doi:10.1016/j.omtm.2020.05.003
Usui-Ouchi, A., Usui, Y., Kurihara, T., Aguilar, E., Dorrell, M. I., Ideguchi, Y., et al. (2020). Retinal Microglia Are Critical for Subretinal Neovascular Formation. JCI Insight 5 (12), e137317. doi:10.1172/jci.insight.137317
Vanderwall, A. G., and Milligan, E. D. (2019). Cytokines in Pain: Harnessing Endogenous Anti-inflammatory Signaling for Improved Pain Management. Front. Immunol. 10, 3009. doi:10.3389/fimmu.2019.03009
Vecino, E., Rodriguez, F. D., Ruzafa, N., Pereiro, X., and Sharma, S. C. (2016). Glia-neuron Interactions in the Mammalian Retina. Prog. Retin. Eye Res. 51, 1–40. doi:10.1016/j.preteyeres.2015.06.003
Velez-Montoya, R., Oliver, S. C. N., Olson, J. L., Fine, S. L., Mandava, N., and Quiroz-Mercado, H. (2013). Current Knowledge and Trends in Age-Related Macular Degeneration. Retina 33 (8), 1487–1502. doi:10.1097/IAE.0b013e318271f265
Wang, Y., Tang, Z., and Gu, P. (2020). Stem/progenitor Cell-Based Transplantation for Retinal Degeneration: a Review of Clinical Trials. Cell Death Dis 11 (9), 793. doi:10.1038/s41419-020-02955-3
Weinreb, R. N., Aung, T., and Medeiros, F. A. (2014). The Pathophysiology and Treatment of Glaucoma. JAMA 311 (18), 1901–1911. doi:10.1001/jama.2014.3192
Wen, R., Tao, W., Li, Y., and Sieving, P. A. (2012). CNTF and Retina. Prog. Retin. Eye Res. 31 (2), 136–151. doi:10.1016/j.preteyeres.2011.11.005
Wert, K. J., Lin, J. H., and Tsang, S. H. (2014). General Pathophysiology in Retinal Degeneration. Dev. Ophthalmol. 53, 33–43. doi:10.1159/000357294
Xia, J., Minamino, S., Kuwabara, K., and Arai, S. (2019). Stem Cell Secretome as a New Booster for Regenerative Medicine. Bst 13 (4), 299–307. doi:10.5582/bst.2019.01226
Xiao, N., and Le, Q.-T. (2016). Neurotrophic Factors and Their Potential Applications in Tissue Regeneration. Arch. Immunol. Ther. Exp. 64 (2), 89–99. doi:10.1007/s00005-015-0376-4
Xie, H., Zhang, C., Liu, D., Yang, Q., Tang, L., Wang, T., et al. (2021). Erythropoietin Protects the Inner Blood-Retinal Barrier by Inhibiting Microglia Phagocytosis via Src/Akt/cofilin Signalling in Experimental Diabetic Retinopathy. Diabetologia 64 (1), 211–225. doi:10.1007/s00125-020-05299-x
Yang, F., Li, W.-B., Qu, Y.-W., Gao, J.-X., Tang, Y.-S., Wang, D.-J., et al. (2020). Bone Marrow Mesenchymal Stem Cells Induce M2 Microglia Polarization through PDGF-AA/MANF Signaling. Wjsc 12 (7), 633–658. doi:10.4252/wjsc.v12.i7.633
Yang, Y., Shiao, C., Hemingway, J. F., Jorstad, N. L., Shalloway, B. R., Chang, R., et al. (2013). Suppressed Retinal Degeneration in Aged Wild Type and APPswe/PS1ΔE9 Mice by Bone Marrow Transplantation. PLoS One 8 (6), e64246. doi:10.1371/journal.pone.0064246
Yuan, L., and Neufeld, A. H. (2001). Activated Microglia in the Human Glaucomatous Optic Nerve Head. J. Neurosci. Res. 64 (5), 523–532. doi:10.1002/jnr.1104
Zanier, E. R., Pischiutta, F., Riganti, L., Marchesi, F., Turola, E., Fumagalli, S., et al. (2014). Bone Marrow Mesenchymal Stromal Cells Drive Protective M2 Microglia Polarization after Brain Trauma. Neurotherapeutics 11 (3), 679–695. doi:10.1007/s13311-014-0277-y
Zeng, H.-y., Zhu, X.-a., Zhang, C., Yang, L.-P., Wu, L.-m., and Tso, M. O. M. (2005). Identification of Sequential Events and Factors Associated with Microglial Activation, Migration, and Cytotoxicity in Retinal Degeneration inrdMice. Invest. Ophthalmol. Vis. Sci. 46 (8), 2992–2999. doi:10.1167/iovs.05-0118
Zhai, W., Gao, L., Qu, L., Li, Y., Zeng, Y., Li, Q., et al. (2020). Combined Transplantation of Olfactory Ensheathing Cells with Rat Neural Stem Cells Enhanced the Therapeutic Effect in the Retina of RCS Rats. Front. Cel. Neurosci. 14, 52. doi:10.3389/fncel.2020.00052
Zhang, Q., Li, J., An, W., Fan, Y., and Cao, Q. (2020a). Neural Stem Cell Secretome and its Role in the Treatment of Neurodegenerative Disorders. J. Integr. Neurosci. 19 (1), 179–185. doi:10.31083/j.jin.2020.01.1142
Zhang, X., Liu, J., Yu, B., Ma, F., Ren, X., and Li, X. (2018). Effects of Mesenchymal Stem Cells and Their Exosomes on the Healing of Large and Refractory Macular Holes. Graefes Arch. Clin. Exp. Ophthalmol. 256 (11), 2041–2052. doi:10.1007/s00417-018-4097-3
Zhang, X., Tenerelli, K., Wu, S., Xia, X., Yokota, S., Sun, C., et al. (2020b). Cell Transplantation of Retinal Ganglion Cells Derived from hESCs. Rnn 38 (2), 131–140. doi:10.3233/RNN-190941
Zhang, Z., Mugisha, A., Fransisca, S., Liu, Q., Xie, P., and Hu, Z. (2021). Emerging Role of Exosomes in Retinal Diseases. Front. Cel Dev. Biol. 9, 643680. doi:10.3389/fcell.2021.643680
Zhao, L., Zabel, M. K., Wang, X., Ma, W., Shah, P., Fariss, R. N., et al. (2015). Microglial Phagocytosis of Living Photoreceptors Contributes to Inherited Retinal Degeneration. EMBO Mol. Med. 7 (9), 1179–1197. doi:10.15252/emmm.201505298
Zhao, Y., Gan, Y., Xu, G., Yin, G., and Liu, D. (2020). MSCs-Derived Exosomes Attenuate Acute Brain Injury and Inhibit Microglial Inflammation by Reversing CysLT2R-Erk1/2 Mediated Microglia M1 Polarization. Neurochem. Res. 45 (5), 1180–1190. doi:10.1007/s11064-020-02998-0
Zhong, Z., Chen, A., Fa, Z., Ding, Z., Xiao, L., Wu, G., et al. (2020a). Bone Marrow Mesenchymal Stem Cells Upregulate PI3K/AKT Pathway and Down-Regulate NF-Κb Pathway by Secreting Glial Cell-Derived Neurotrophic Factors to Regulate Microglial Polarization and Alleviate Deafferentation Pain in Rats. Neurobiol. Dis. 143, 104945. doi:10.1016/j.nbd.2020.104945
Zhong, Z., Chen, A., Fa, Z., Ding, Z., Xie, J., Sun, Y., et al. (2020b). Adipose-Derived Stem Cells Modulate BV2 Microglial M1/M2 Polarization by Producing GDNF. Stem Cell Dev. 29 (11), 714–727. doi:10.1089/scd.2019.0235
Zhou, T., Huang, Z., Sun, X., Zhu, X., Zhou, L., Li, M., et al. (2017). Microglia Polarization with M1/M2 Phenotype Changes in Rd1 Mouse Model of Retinal Degeneration. Front. Neuroanat. 11, 77. doi:10.3389/fnana.2017.00077
Zhou, X., Spittau, B., and Krieglstein, K. (2012). TGFβ Signalling Plays an Important Role in IL4-induced Alternative Activation of Microglia. J. Neuroinflammation 9, 210. doi:10.1186/1742-2094-9-210
Zhu, D., Yang, N., Liu, Y.-Y., Zheng, J., Ji, C., and Zuo, P.-P. (2016). M2 Macrophage Transplantation Ameliorates Cognitive Dysfunction in Amyloid-β-Treated Rats through Regulation of Microglial Polarization. Jad 52 (2), 483–495. doi:10.3233/JAD-151090
Zhu, J., Cao, D., Guo, C., Liu, M., Tao, Y., Zhou, J., et al. (2019). Berberine Facilitates Angiogenesis against Ischemic Stroke through Modulating Microglial Polarization via AMPK Signaling. Cell. Mol. Neurobiol. 39 (6), 751–768. doi:10.1007/s10571-019-00675-7
Zou, T., Gao, L., Zeng, Y., Li, Q., Li, Y., Chen, S., et al. (2019). Organoid-derived C-Kit+/SSEA4− Human Retinal Progenitor Cells Promote a Protective Retinal Microenvironment during Transplantation in Rodents. Nat. Commun. 10 (1), 1205. doi:10.1038/s41467-019-08961-0
Keywords: microglia, stem cell therapy, retinal degenerative disease, microglial polarization, retinal inflammation
Citation: Jin N, Sha W and Gao L (2021) Shaping the Microglia in Retinal Degenerative Diseases Using Stem Cell Therapy: Practice and Prospects. Front. Cell Dev. Biol. 9:741368. doi: 10.3389/fcell.2021.741368
Received: 14 July 2021; Accepted: 29 November 2021;
Published: 13 December 2021.
Edited by:
Zi-Bing Jin, Capital Medical University, ChinaReviewed by:
Akiko Maeda, Kobe City Eye Hospital, JapanAna Isabel Arroba, Fundación Para la Gestión de la Investigación Biomédica de Cádiz, Spain
Copyright © 2021 Jin, Sha and Gao. This is an open-access article distributed under the terms of the Creative Commons Attribution License (CC BY). The use, distribution or reproduction in other forums is permitted, provided the original author(s) and the copyright owner(s) are credited and that the original publication in this journal is cited, in accordance with accepted academic practice. No use, distribution or reproduction is permitted which does not comply with these terms.
*Correspondence: Lixiong Gao, Z2x4OTE5QDE2My5jb20=