- 1Biomarkers in Cancer Unit, Biocruces Bizkaia Health Research Institute, Barakaldo, Spain
- 2Department of Tumor Biology, Institute for Cancer Research, Oslo University Hospital Radiumhospitalet, Oslo, Norway
- 3Department of Pathology, Cruces University Hospital, Barakaldo, Spain
- 4Pediatric Oncology and Hematology, Cruces University Hospital, Barakaldo, Spain
- 5Unit of Pediatric Oncohematology, Donostia University Hospital, San Sebastian, Spain
- 6Department of Pathology, Donostia University Hospital, San Sebastian, Spain
- 7IKERBASQUE, Basque Foundation for Science, Bilbao, Spain
Neuroblastoma is a type of cancer intimately related with early development and differentiation of neuroendocrine cells, and constitutes one of the pediatric cancers with higher incidence and mortality. Protein tyrosine phosphatases (PTPs) are key regulators of cell growth and differentiation by their direct effect on tyrosine dephosphorylation of specific protein substrates, exerting major functions in the modulation of intracellular signaling during neuron development in response to external cues driving cell proliferation, survival, and differentiation. We review here the current knowledge on the role of PTPs in neuroblastoma cell growth, survival, and differentiation. The potential of PTPs as biomarkers and molecular targets for inhibition in neuroblastoma therapies is discussed.
Introduction
Neuroblastoma is the most common extracranial solid tumor diagnosed in infants and the paediatric cancer with higher risk of death, with high-risk neuroblastoma (about 50% of neuroblastoma cases) showing a survival rate of about 50% (Ward et al., 2014; Siegel et al., 2020). Neuroblastomas display high clinical heterogeneity, from tumors that spontaneously regress to metastatic tumors refractory to multi-therapies (Matthay et al., 2016; Brodeur, 2018; Kholodenko et al., 2018; Tsubota and Kadomatsu, 2018). Neuroblastoma tumors arise from endocrine neural crest precursor cells during aberrant development of sympathetic neuronal cells early in life. This makes neuroblastoma potentially actionable from the perspective of the developmental biology of neuroendocrine cells (Cheung and Dyer, 2013; Fletcher et al., 2018). However, the low number of mutations found in neuroblastoma tumors at diagnosis, their high diversity, and the paediatric nature of the patients, have been handicaps for the identification and validation of actionable molecular targets. In this context, anti-disialoganglioside GD2 monoclonal antibody immunotherapy is the only current targeted therapy for high-risk neuroblastoma (Pastor and Mousa, 2019; Moreno et al., 2020). Protein phosphorylation/dephosphorylation plays an important role in the control of neuroblastoma cell growth and transformation, and inhibition of oncogenic protein kinases is actively being tested in paediatric neuroblastoma clinical trials (Stafman and Beierle, 2016; Applebaum et al., 2017; Berlanga et al., 2017; Pacenta and Macy, 2018; Moreno et al., 2020). Distinct groups of protein phosphatases exist that play relevant roles in human disease (Chen et al., 2017), and their significance as molecular targets in cancer, including paediatric cancers, is gaining attention. For instance, the modulation of the activity of the serine/threonine phosphatase PP2A is being explored as an intervention in neuroblastoma cell survival and tumor growth (Williams et al., 2019).
Protein tyrosine phosphatases (PTPs) dephosphorylate key homeostatic phospho-proteins and are major regulators of developmental processes, including neuronal survival and differentiation (Hendriks et al., 2013; Hendriks and Pulido, 2013; Tonks, 2013; Hale et al., 2017). Accordingly, specific inhibitors of PTP catalytic activity are under scrutiny for their therapeutic application in human cancer (Ríos et al., 2014; Frankson et al., 2017; Lazo et al., 2018). In neuroblastoma cells, general inhibition of PTPs by vanadium derivatives limits cell growth and triggers apoptosis (Clark et al., 2013; Clark et al., 2015). The PTP superfamily includes two major subgroups, the classical PTPs, which specifically dephosphorylate phospho-tyrosine residues from proteins; and the dual-specificity PTPs (DUSPs), which dephosphorylate phospho-serine/threonine/tyrosine residues, in many cases from the activation loop of MAP kinases, as well as non-protein substrates (Alonso et al., 2004; Julien et al., 2011; Nunes-Xavier et al., 2011; Alonso et al., 2016). The involvement of DUSPs in neuroblastoma cell growth and differentiation has been recently reviewed (Nunes-Xavier et al., 2019a). Here, we provide an update on the role of the classic family of PTPs in neuroblastoma. We have focused on those PTPs reported to regulate neuroblastoma cell growth, survival, or differentiation, or whose expression it has been reported to be affected in neuroblastoma cells upon different cell growth conditions. Insights are made on specific PTPs as potential neuroblastoma biomarkers and molecular therapeutic targets.
Molecular Signaling Pathways in Neuroblastoma
The amplification of MYCN gene is the most frequent genomic alteration in neuroblastoma, in association with poor cell differentiation and bad prognosis, which occurs in about 25% of primary neuroblastoma tumors and constitutes the major hallmark of high-risk neuroblastoma (Huang and Weiss, 2013; Tolbert and Matthay, 2018; Westermark et al., 2011). MYCN gene encodes the MYCN (N-Myc) transcription factor, whose expression and functional activity is highly regulated in the nervous system during embryonic and early-life development, playing an important role in the maintenance of neural stem cell pluripotency and in the differentiation of neural progenitors (Hurlin, 2005; Ruiz-Pérez et al., 2017). High expression of MYCN protein associates with highly aggressive neuroblastoma in a group of patients, and overexpression of MYCN in developing neuroblasts spontaneously triggers the growth of aggressive undifferentiated neuroblastoma tumors (Kamili et al., 2020; Wang et al., 2015). Thus, MYCN activity drives a major gene expression-regulatory node which is under the control of the major neuroblastoma cell signaling pathways. These signal transduction pathways are often activated upon binding of Trk- and ALK-receptor tyrosine kinases (RTKs) to their ligands, and mainly include the RAS/MAPK, PI3K/AKT, and JAK/STAT pro-oncogenic pathways (Figure 1). Accordingly, pharmacological inhibitors of Trk and ALK RTKs are being tested in clinical trials in neuroblastoma (Lange and Lo, 2018; Pacenta and Macy, 2018; Higashi et al., 2019).
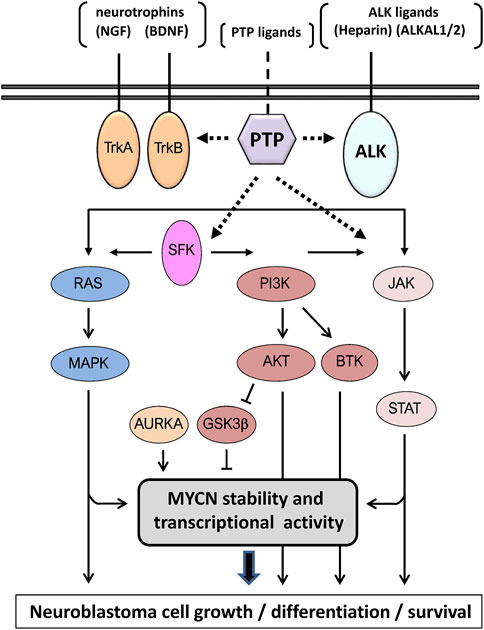
FIGURE 1. Schematic depiction of the major signaling pathways in neuroblastoma cells driven by Trk and ALK receptors upon ligand binding. The potential participation of classical protein tyrosine phosphatases (PTPs) is indicated by dashed lines. Note that the same depiction is used for both receptor-like and non-receptor PTPs. The pathways converge in the regulation of MYCN (N-Myc) functions. See text for more details.
Trks constitute a family of neurotrophin receptors with multiple and complementary roles in the nervous system. Trk family members include TrkA (NTRK1), TrkB (NTRK2), and TrkC (NTRK3), whose neurotrophin binding preference is nerve growth factor (NGF), brain-derived neurotrophic factor and neurotrophin-4/5 (BDNF, NT4/5), and neurotrophin-3 (NT3), respectively (Barbacid, 1994; Brodeur et al., 2009; Cocco et al., 2018). No relevant incidence of mutations has been found in neuroblastoma for NTRK1, NTRK2, or NTRK3 genes (https://cancer.sanger.ac.uk; https://pecan.stjude.cloud). TrkA and TrkC high expression are favorable prognostic markers in neuroblastoma, in association with a differentiated phenotype and absence of MYCN amplification, whereas high TrkB expression associates with aggressive neuroblastomas and chemotherapy resistance (Nakagawara et al., 1993; Nakagawara et al., 1994; Yamashiro et al., 1997; Ho et al., 2002; Jaboin et al., 2002). This relates with the differential roles of these RTKs in neuronal function: NGF/TrkA signaling stimulates sympathetic neuron differentiation, whereas BDNF/TrkB signaling facilitates neuronal plasticity, survival and angiogenesis (Schramm et al., 2005). In this regard, expression of TrkA and TrkC in the absence of their ligands triggers neuronal apoptosis, which has been associated with spontaneous neuroblastoma regression (Bouzas-Rodriguez et al., 2010; Nikoletopoulou et al., 2010; Brodeur, 2018), although it should be mentioned that the expression of the alternative TrkA splice variant TrkAIII associates with advanced neuroblastoma, and TrkAIII displays oncogenic properties in neuroblastoma cell models (Farina et al., 2018). A complex scenario emerges in which the temporal relative expression and regulation of the function of each Trk and neurotrophin ligand selectively drive pro-oncogenic or anti-oncogenic intracellular signaling during neuroblastoma progression.
ALK is the gene more frequently mutated in sporadic neuroblastoma, as well as in the germline of familial neuroblastoma patients. Most of these mutations are gain-of-function missense mutations which target the intracellular catalytic domain of ALK and generate a constitutively active RTK (Ogawa et al., 2011; Carpenter and Mossé, 2012; Janoueix-Lerosey et al., 2018; Trigg and Turner, 2018). ALK mutations have been associated with poorer survival in high-risk neuroblastoma, and ALK gene is amplified in a subgroup of MYCN-amplified neuroblastoma tumors due to the proximity of the two loci (2p23-24) at the 2p-gain region associated with high-risk neuroblastoma (De Brouwer et al., 2010; Bresler et al., 2014). This, together with the findings that MYCN and ALK participate in a positive feedback transcriptional regulatory loop (Schönherr et al., 2012; Hasan et al., 2013), sustains a cooperative pro-oncogenic effect of MYCN and ALK in human neuroblastoma. ALK activating ligands include heparin and ALKAL1/2 (FAM150A/B; AUGβ/α) (Guan et al., 2015; Murray et al., 2015; Reshetnyak et al., 2015), and ALKAL2 gene is also located within the 2p-gain region, specifically at 2p25 (Javanmardi et al., 2019). In the absence of ALK mutations, overexpression of ALKL2 potentiates MYCN-driven neuroblastoma in mice, emphasizing the importance of ALK as a therapeutic neuroblastoma target (Borenäs et al., 2021).
As other tyrosine kinase receptors, Trk and ALK are subjected to phosphorylation at multiple tyrosine residues at their intracellular catalytic domains, mainly due to auto- and trans-phosphorylation mechanisms, which regulate their biological activity (Reichardt, 2006; Palmer et al., 2009; Roskoski, 2013). However, specific tyrosine dephosphorylation of Trk and ALK receptors in neuroblastoma is poorly documented. Other relevant tyrosine-phosphorylated effectors in the propagation of the oncogenic cell signaling in neuroblastoma cells, which also constitute potential therapeutic targets and PTP direct substrates, include Src-family kinases (SFK) (Bieerkehazhi et al., 2017; Molinari et al., 2018), JAK and STAT kinases (Yan et al., 2013; Yogev et al., 2019), Bruton tyrosine kinase (BTK) (Li et al., 2018; Pikatan et al., 2020), and MYCN stabilizing Aurora-A kinase (AURKA) (Brockmann et al., 2013; Roeschert et al., 2021), among others (Figure 1).
Classical Protein Tyrosine Phosphatases in Neuroblastoma
Classical PTPs are defined by the presence of a classical PTP catalytic domain, which displays the conserved HCxxGxxR signature motif containing the catalytic cysteine residue, and they can be broadly classified into two major groups: receptor-like (RPTP, PTPR) and non-receptor enzymes (NRPTP, PTPN). Receptor-like PTPs (20 genes in human genome) harbor a transmembrane and an extracellular region capable of ligand binding, and possess in most of the cases two classic PTP domains located in the cytoplasm and positioned in tandem, one with catalytic activity (D1) and one with regulatory activity (D2). Non-receptor PTPs (17 genes in human genome) are non-transmembrane proteins harboring a single classic PTP cytoplasmic domain, as well as additional regulatory domains important for function and subcellular location (Hendriks et al., 2013; Alonso and Pulido, 2016; Mohebiany et al., 2013; Tonks, 2006). The mRNA expression profiles of the two groups of human classical PTPs in the adrenal gland and in the SH-SY5Y human neuroblastoma cell line are shown in Figures 2A,B, as retrieved from public databases. Retinoic acid induces differentiation of neuroblastoma cells, and it is used as a maintenance therapy agent in high-risk neuroblastoma patients (Bayeva et al., 2021). Figure 2C shows the changes of mRNA expression of human classical PTPs from the human neuroblastoma cell lines SH-SY5Y, SMS-KCNR, and IMR-32 undergoing retinoic acid-induced differentiation. Substantial alterations in the mRNA expression levels of several classical PTPs are observed, arguing for the involvement of members of this group of enzymes in the modulation of neuroblastoma growth and differentiation. A summary of the expression, alterations, and functional effects of selected classical PTPs in neuroblastoma is provided in Table 1, and schematic depictions of classical PTPs with relevance in neuroblastoma are illustrated in Figure 3. Kaplan-Meier plots of neuroblastoma patient survival in relation with mRNA expression of PTPs from Table 1 is shown in Figure 4, as retrieved from R2: Genomics Analysis and Visualization Platform (http://r2.amc.nl). As illustrated, high mRNA expression of a group of PTPs associates with low patient survival, suggesting pro-oncogenic roles for the product of these genes. Conversely, several PTPs display low mRNA expression in association with low patient survival, suggesting tumor suppressive roles for them in neuroblastoma. Protein expression analysis of the distinct PTPs in neuroblastoma tumor samples is required for further validation of these observations.
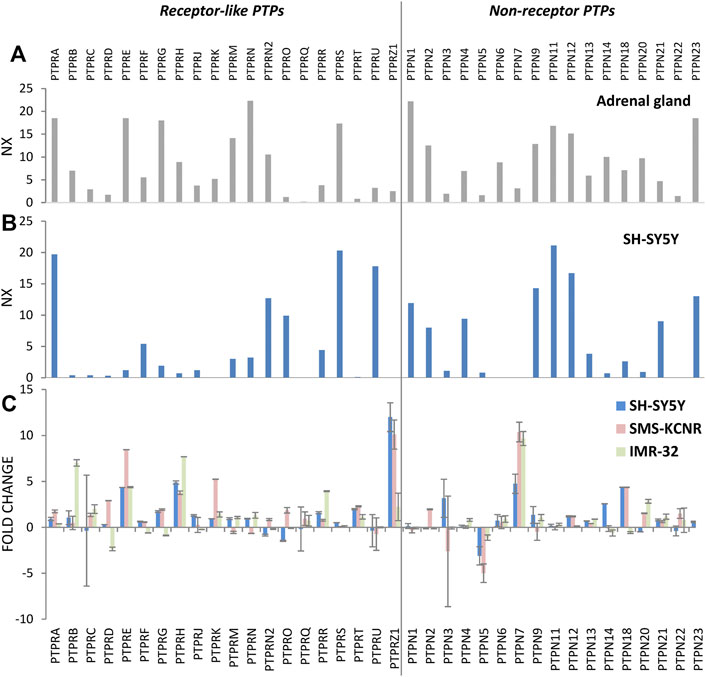
FIGURE 2. mRNA expression of classical protein tyrosine phosphatases (PTPs) in adrenal gland and in neuroblastoma cell lines. (A) mRNA expression in adrenal gland. RNA-seq data is shown as consensus normalized eXpression (NX) by combining HPA, GTEx and FANTOM5 datasets (https://www.proteinatlas.org/). (B) mRNA expression in SH-SY5Y human neuroblastoma cells. RNA-seq data is shown as consensus normalized eXpression (NX) by combining HPA, GTEx and FANTOM5 datasets (https://www.proteinatlas.org/). (C) mRNA expression analysis from SH-SY5Y, SMS-KCNR, and IMR-32 human neuroblastoma cells treated with retinoic acid (RA). Cell lines were kept untreated or were treated for 10 days with 10 μM RA, mRNA was purified and RT-qPCR was performed using a set of classical PTPs primers, as described in (Nunes-Xavier and Pulido, 2016). Relative mRNA expression values are shown in Log2 as fold change ±S.D. of treated cells versus untreated cells, from at least two independent experiments. Quantifications were normalized to the HPRT1 reference gene data, and mean fold change above 2 or below -2 was considered significant using Pearson Chi square analysis, as reported (Nunes-Xavier et al., 2019b).
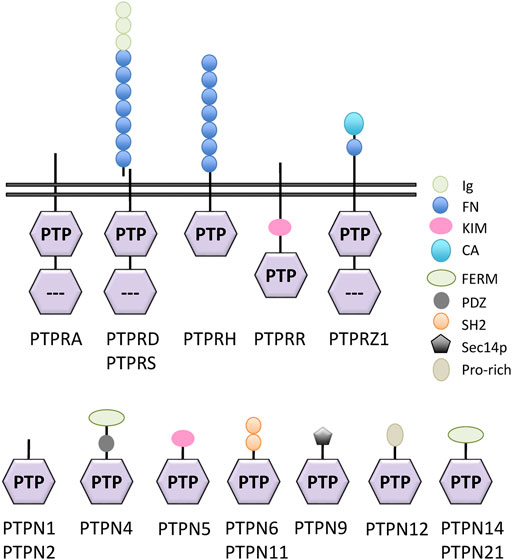
FIGURE 3. Schematic depiction of classical protein tyrosine phosphatases (PTPs) with potential involvement in neuroblastoma. In the top, receptor-like PTPs are depicted; in the bottom, non-receptor PTPs are depicted. Note that both PTPRR and PTPN5 have receptor-like and non-receptor isoforms (not depicted). The domain composition of each protein is indicated. CA, carbonic anhydrase-like; FERM, band 4.1/ezrin/radixin/moesin homology; FN, Fibronectin III-like; IG, immunoglobulin-like; KIM, kinase interaction motif; PDZ, postsynaptic density-95/discs large/ZO1 homology; Pro, proline-rich; PTP, catalytically active protein tyrosine phosphatase; Sec14, lipid-binding Sec14p homology; SH2, Src-homology 2. The C-terminal PTP domain from receptor-like PTPs containing two PTP domains either has low activity or is inactive, and it is crossed with dots.
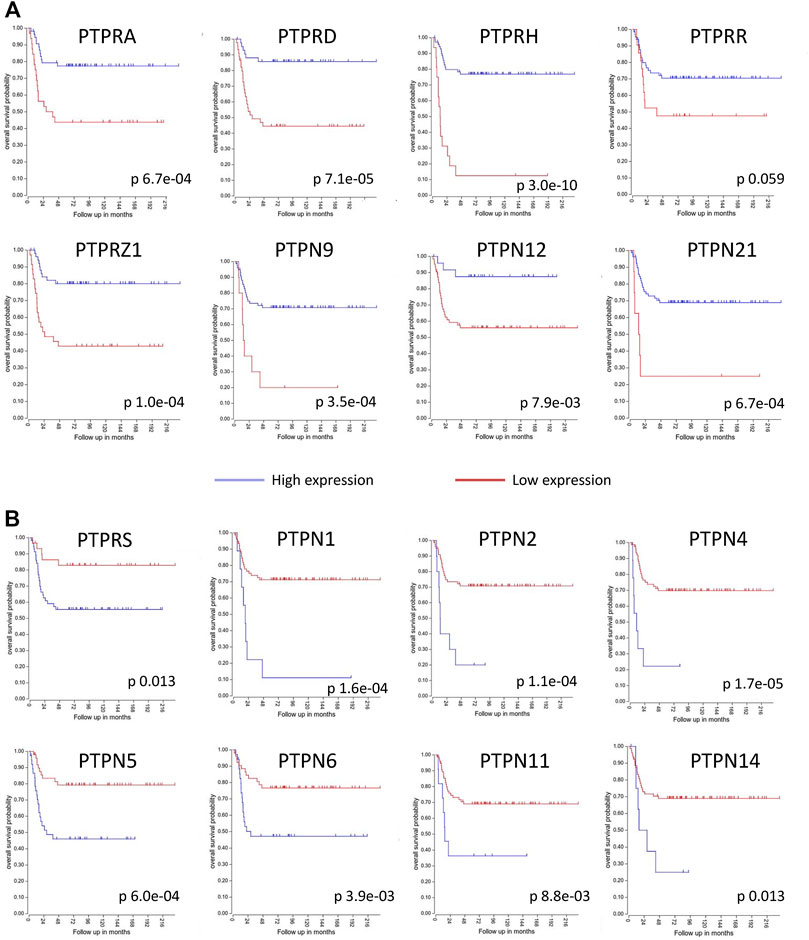
FIGURE 4. Kaplan-Meier plots of neuroblastoma patient overall survival in relation with protein tyrosine phosphatases (PTPs) mRNA expression. PTPs listed in Table 1 are included. Blue and red lines indicate high expression and low expression, respectively. In x axis, overall survival probability; in y axis, follow up in months. Data is from study Tumor Neuroblastoma public - Versteeg - 88 - MAS5.0 - u133p2 (number of patients = 88). (A) PTPs whose low expression associates with lower overall survival (p < 0.05, except for PTPRR). (B) PTPs whose high expression associates with lower overall survival (p < 0.05). Plots are from R2: Genomics Analysis and Visualization Platform (http://r2.amc.nl).
Receptor-Like Protein Tyrosine Phosphatases in Neuroblastoma
PTPRA (RPTPα) has been documented to be involved in neuronal differentiation, as well as in neuroblastoma cell motility, in association with dephosphorylation and activation of Src (den Hertog et al., 1993; Wu and Song, 2018), a PTPRA substrate shared by other cell types (Pallen, 2003). PTPRA is highly expressed in SH-SY5Y neuroblastoma cells, whereas the related PTPRE (RPTPε) is expressed at low levels but induced upon retinoic acid differentiation (Figure 2). The possibility exists that the use of PTPRA/PTPRE inhibitors could complement the use of Src inhibitors in neuroblastoma therapies (Navarra et al., 2010; Kratimenos et al., 2014; Tintori et al., 2015). Although PTPRA gene mutations in neuroblastoma are uncommon, a missense variant in the PTPRA PTP D1 domain has been associated with low survival of patients (Esposito et al., 2018).
PTPRD (RPTPδ) and PTPRS (RPTPσ), together with PTPRF (LAR) belong to a subfamily of classical receptor-like PTPs which display large extracellular regions susceptible to proteolytic shedding and containing tandem repeats of fibronectin type III- and immunoglobulin-like domains (Figure 3) (Chagnon et al., 2004; Pulido et al., 1995). A major physiologic role for these PTPs involves the regulation of synapsis formation and function (Han et al., 2016; Um and Ko, 2013). In the context of cancer disease, PTPRD is absent or inactive in a variety of human tumor types, playing a tumor suppressor role linked with the downregulation of STAT3 activity (Lin et al., 2021; Ortiz et al., 2014; Solomon et al., 2008; Veeriah et al., 2009). A tumor suppressor role has also been proposed for PTPRD in neuroblastoma, with a relatively high incidence of gene losses and gene fusions targeting the PTPRD gene in neuroblastoma samples (https://pecan.stjude.cloud) (Stallings et al., 2006; Nair et al., 2008). Low expression of PTPRD mRNA associates with poor disease progression and neuroblastoma patient survival, and a direct role has been proposed for PTPRD in AURKA dephosphorylation and destabilization in neuroblastoma cells (Nair et al., 2008; Meehan et al., 2012). However, no evidence of PTPRD tumor suppressor activity in neuroblastoma cells has also been reported (Clark et al., 2012). It should be interesting to test the potential regulatory role of PTPRD in the JAK/STAT pathway in neuroblastoma cells.
Distinctly to PTPRD, the related PTPRS enzyme is highly expressed in neuroblastoma cells (Clark et al., 2012) (Figure 2). A role for PTPRS in tyrosine dephosphorylation of Trk receptors has been shown in primary sensory neurons (Faux et al., 2007), and synapse signaling-inducing binding between the extracellular regions of PTPRS and TrkC has been reported (Takahashi et al., 2011). A mAb against the ectodomain of PTPRS promotes neurite outgrowth in SH-SY5Y cells (Wu et al., 2017), and PTPRS pharmacological inhibitors revert the inhibitory neurite outgrowth effect of chondroitin sulfate proteoglycan in PC12 cells (Lee et al., 2016). Conversely, overexpression of PTPRS inhibited NGF-induced PC12 axonal outgrowth (Chagnon et al., 2010). These findings support a negative role for PTPRS in neurite extension/neuroblastoma cell differentiation. PTPRF has also been functionally associated with TrkB and neurotrophic signaling in embryonic neurons (Yang et al., 2006). PTPRF gene maps at 1p34, within a region frequently deleted in high-risk neuroblastoma (Bown, 2001). Whether PTPRS or PTPRF may directly regulate the tyrosine phosphorylation of Trk receptors or other specific substrates in neuroblastoma cells, deserves experimental analysis.
PTPRH (SAP-1) belongs to a subfamily of receptor like-PTPs with a large extracellular region enriched in fibronectin type III-like domains and a single intracellular catalytic classical PTP domain (Figure 3). Other members of this subfamily include PTPRB, PTPRJ, PTPRO, and PTPRQ (Chicote et al., 2017; Jeon and Zinn, 2015; Matozaki et al., 2010). PTPRH protein is highly expressed in neuroblastomas, in association with tumor low stage and patient low risk, and PTPRH mRNA is induced in neuroblastoma cells upon retinoic acid differentiation (Nunes-Xavier et al., 2019b) (Figure 2). These findings suggest a role for PTPRH in differentiation programs limiting neuroblastoma cell growth, and are consistent with the upregulation of PTPRH expression upon differentiation of other cell types (Nunes-Xavier et al., 2012; Nunes-Xavier et al., 2013), as well as with its downregulation in some cancer types (Nagano et al., 2003; Bujko et al., 2017). Remarkably, PTPRH was selected in a siRNA screening as a potential STAT3 regulator (Parri et al., 2020), and it has also been found to associate with and dephosphorylate several RTK, including EGFR and IR (Shintani et al., 2015; Yao et al., 2017). Since the related PTPRO enzyme, which is mainly expressed in the developing nervous system, dephosphorylates Trk receptors (Hower et al., 2009; Gatto et al., 2013), a role is possible for PTPRH in the direct regulation of Trk signaling in neuroblastoma cells. PTPRQ is a unique classical PTP because it specifically dephosphorylates phosphatidylinositide substrates (Oganesian et al., 2003). It is of interest that the PTPRQ gene is among the few PTP genes that have been found mutated with relative high frequency in neuroblastoma tumor samples (https://pecan.stjude.cloud), although the functional consequences of these mutations need to be evaluated.
PTPRR (PCPTP1, PTP-SL), PTPN5 (STEP), and PTPN7 (HePTP, LC-PTP), belong to the subgroup of kinase interaction motif (KIM)-containing classical PTPs, whose major substrates are the MAPK ERK1/2 and p38s (Barr and Knapp, 2006; Pulido et al., 1998; Torres et al., 2004). A major negative regulatory mechanism of these PTPs is PKA-mediated phosphorylation of their KIM, which abrogates substrate binding (Blanco-Aparicio et al., 1999). The PTPRR and PTPN5 genes are mainly expressed in the brain and encode transmembrane and non-transmembrane PTP isoforms, although PTPN5 was originally classified as a non-transmembrane PTP (Hendriks et al., 2009; Karasawa and Lombroso, 2014). PTPN7 is a non-transmembrane PTP expressed predominantly in hemopoietic cells (Zanke et al., 1992). PTPRR mRNA (but not PTPN5 or PTPN7 mRNAs) was expressed in PC12 cells, and cytosolic PTPRR expression down-regulated ERK1/2 phosphorylation (Noordman et al., 2006). In SH-SY5Y cells, PTPN5 KIM phosphorylation was shown to be regulated through adenosine A2A receptor and the countereffects of PKA and PP2A (Mallozzi et al., 2020). Interestingly, a distinct pattern of PTPRR, PTPN5, and PTPN7 mRNA expression was observed in human neuroblastoma cell lines upon retinoic acid differentiation, with down-regulation of PTPN5 and up-regulation of PTPN7 and, to a lesser extent, PTPRR (Figure 2). These observations support the notion that MAPK activation status is finely tuned during neuroblastoma cells differentiation by the action of specific PTPs, which could impact on tumor development. In line with this, the expression of the dual-specificity MAPK phosphatase DUSP5 associates with neuroblastoma relapse and poor prognosis (Aurtenetxe et al., 2018). Since activating mutations in components of the RAS/MAPK pathway are frequent in relapsed neuroblastoma (Huang et al., 2013), therapeutic targeting of this pathway is currently being tested in neuroblastoma patients (Mlakar et al., 2021). Whether the KIM-containing classical PTPs may play a physiologic role in the control of MAPK activity in neuroblastomas deserves dedicated analysis.
PTPRZ1 (RPTPζ, RPTPβ/ζ) is a receptor-like PTP predominantly expressed in the central nervous system and in endothelial tumor cells. Its extracellular domains include a carbonic anhydrase-like domain, a fibronectin type III-like domain, and a carbohydrate-rich region (Figure 3). Alternative splicing generates a shorter transmembrane protein lacking the glycosylated region, as well as two soluble extracellular isoforms, the chondroitin sulfate proteoglycans phosphacans (Pantazaka and Papadimitriou, 2014; Papadimitriou et al., 2016). A wide array of ligands and substrates have been described for PTPRZ1 in a variety of cell types, which could account for the many cell-cell adhesion and communication, cell migration, and cell growth functions attributed to this PTP. Major PTPRZ1 ligands include the heparin-binding growth factors pleiotrophin (PTN) and midkine (MK), the extracellular matrix proteins tenascins (TN), and cell adhesion contactin (CNTN) family members, among others. Several PDZ domain-containing proteins, such as MAGIs and DLGs, associate intracellularly with PTPRZ1 through its PDZ-binding motif. PTPRZ1 substrates with potential relevance in neuroblastoma include ALK, TrkA, p190 RhoGAP, β-catenin, and SFK, among others (Herradon and Ezquerra, 2009; Mohebiany et al., 2013; Xia et al., 2019). PTN binding to PTPRZ1 inhibits its catalytic activity by triggering PTPRZ1 oligomerization. In neuroblastoma cells, this leads to increased tyrosine phosphorylation of p190 RhoGAP, GIT1, and DNER (Kawachi et al., 2001; Tamura et al., 2006; Fukazawa et al., 2008), and it has been proposed that the PTN inhibitory effect on PTPRZ1 acts as a major ALK indirect activating mechanism (Deuel, 2013). In addition, regulated glycosylation of PTPRZ1 in neuroblastoma cells also induces its dimerization and catalytic inactivation (Abbott et al., 2008), suggesting a tight physiologic control of constitutive PTPRZ1 catalytic activity. In this regard, PTPRZ1 protein is abundantly expressed in neuroblastoma tumors, and PTPRZ1 mRNA expression is induced in neuroblastoma cell lines upon cell differentiation, although its expression in adrenal gland or in neuroblastoma cells under normal cell growth conditions seems to be very low, making difficult a quantitative comparison (Nunes-Xavier et al., 2019b). PTN mRNA expression in neuroblastoma correlates with favorable prognosis (Nakagawara et al., 1995), and in an in vivo neuroblastoma xenograft model, PTN gene expression was found down-regulated in tumors resistant to irinotecan therapy, as compared to sensitive tumors (Calvet et al., 2006). Small-molecule inhibitors of PTPRZ1 have been proposed as suitable therapeutic alternatives for glioblastoma and central nervous system disorders (Fujikawa et al., 2016; Fujikawa et al., 2017; Pastor et al., 2018), and pharmacological inhibition of PTPRZ1 resulted in increased ALK and TrkA phosphorylation in SH-SY5Y neuroblastoma cells (Fernández-Calle et al., 2018). Modulation of PTPRZ1 activity using specific ligands or pharmacological inhibitors could be an alternative treatment for neuroblastoma that needs to be explored.
Non-Receptor Protein Tyrosine Phosphatases in Neuroblastoma
PTPN1 (PTP1B), the founding member of the PTP gene family (Tonks, 2013), is an ubiquitously expressed negative regulator of insulin signaling, and it plays both oncogenic and tumor suppressor roles in human cancer (Bakke and Haj, 2015; Feldhammer et al., 2013). This makes PTPN1 a potential therapeutic target for human disease, including human malignancies (Kostrzewa et al., 2019; Sharma et al., 2020). A hydrophobic C-terminal sequence targets PTPN1 to the cytoplasmic face of the endoplasmic reticulum, which determines PTPN1 access to protein substrates during biosynthetic, endocytic, or cell signaling pathways. Potential PTPN1 substrates relevant in neuroblastoma include, among others, RTK, JAK/STAT, SFK, p130Cas, and PERK. PTPN1 has been linked to tyrosine dephosphorylation of the intracellular pool of ALK in mouse fibroblasts (Boutterin et al., 2013), raising the possibility that PTPN1 may dephosphorylate ALK in neuroblastoma cells. Knock-down of PTPN1 in SH-SY5Y cells increased the protein phospho-tyrosine content upon EGF stimulation, as well as cell proliferation, whereas high PTPN1 protein expression in neuroblastoma tumors associated with poor prognosis (Nunes-Xavier et al., 2019b). This suggests a regulatory role for PTPN1 in transformation of neuroblastoma cells. Using different in vitro SH-SY5Y cellular models, a role for PTPN1 in the dephosphorylation of JAK2, STAT3, ERK1/2, TrkB, and AKT has been proposed (Benomar et al., 2009; Jeon et al., 2017). It will be important to define the direct substrates of PTPN1 in neuroblastoma cells upon different conditions of growth and differentiation. PTPN2 (TC-PTP) is highly related to PTPN1. It also displays a wide tissue distribution and exists in humans as two isoforms, one nuclear and one associated to the endoplasmic reticulum (Stuible et al., 2008; Tiganis, 2013). PTPN2 has been involved in different human cancers, with a wide implication in lymphoid malignancies. This, together with its negative role in anti-tumor immunity, make PTPN2 a suitable target in oncology (Pike et al., 2016; Wiede et al., 2020). PTPN2 has been found to dephosphorylate the Rap1guanine nucleotide exchange factor C3G in SH-SY5Y cells, and to inhibit the neurite outgrowth triggered by the adenylate cyclase activator forskolin (Mitra et al., 2011). C3G is a positive regulator of differentiation and survival of neuroblastoma cells, through signaling pathways involving ALK (Radha et al., 2008; Schönherr et al., 2010). Additional PTPN2 substrates related with neuroblastoma cell growth are expected, although the expression of PTPN2 in neuroblastoma cells is poorly documented. In this regard, both PTPN1 and PTPN2 mRNA expression is detected in SH-SY5Y cells (Figure 2). In addition, PTPN1 and PTPN2 have been shown to dephosphorylate ALK and PTPN11 in anaplastic large cell lymphomas, and gene deletion of either PTPN1 or PTPN2 induced resistance to ALK inhibitors (Karaca Atabay et al., 2021). The possibility exists that ALK and PTPN11 are direct substrates of PTPN1 and PTPN2 in neuroblastoma cells.
PTPN6 (SHP1) and PTPN11 (SHP2) are structurally related PTPs which harbour two tandem regulatory Src homology 2 (SH2) phospho-tyrosine binding domains preceding the catalytic PTP domain (Figure 3). Intramolecular interaction of the N-terminal SH2 (N-SH2) domain with the PTP domain occludes the enzyme active site and keeps the protein catalytically inactive. Upon SH2-mediated binding to defined phospho-tyrosine residues in receptor or adaptor proteins, PTPN6 and PTPN11 are activated to dephosphorylate specific protein substrates. This makes these two PTPs major regulators of intracellular signaling in response to growth and differentiation factors. PTPN6 is mainly expressed in hematopoietic cells and, to a lesser extent, in endothelial cells, whereas the tissue expression of PTPN11 is ubiquitous (Chong and Maiese, 2007; Lorenz, 2009; Dempke et al., 2018). PTPN6 overexpression in P19 cells has been linked to a decrease in neuronal differentiation and an increase in proliferation, whereas PTPN6 inhibition has been found to facilitate apoptosis of splice variant TrkAIII-expressing SH-SY5Y cells in a Src-mediated process (Mizuno et al., 1997; Gneo et al., 2016). This is in accordance with the association found between good patient prognosis and PTPN6 low expression and tyrosine phosphorylated TrkA (Tyr674/675) expression in neuroblastoma tumors (Youssef et al., 2019). In this regard, PTPN6 has been proposed to negatively regulate TrkA in neurons and in breast cancer cells by dephosphorylation of TrkA Tyr674/675 residues (Marsh et al., 2003; Montano, 2009).
PTPN11 acts as an oncogenic phosphatase in several cancer types by dephosphorylation of a wide variety of effector and regulatory signaling proteins, mainly acting downstream of RTKs to promote activation of the RAS/MAPK pathway. PTPN11 direct substrates include RTKs, RAS proteins and RAS negative regulators, as well as SFKs and SFK regulators, among others (Buday and Vas, 2020; Chan et al., 2008; Grossmann et al., 2010; Matozaki et al., 2009). Accordingly, pharmacological inhibitors of PTPN11 catalysis have emerged as potential anti-cancer drugs (Shen et al., 2020; Song et al., 2021; Yuan et al., 2020), making the elucidation of the cancer type-specific PTPN11 substrates an important demand. In addition, phosphatase-independent functions, in some cases mediated by protein-protein interactions, also contribute to PTPN11 regulation of oncogenic signaling (Guo and Xu, 2020). Thus, both the catalytic activity and the expression levels of PTPN11 are relevant in human cancer. Although a wide diversity in the functional output of PTPN11 gene disease-associated mutations exists, gain-of-function PTPN11 mutations are relatively frequent in human cancer, mainly targeting two hot-spots encoding specific regions at the N-SH2 and PTP domains of the enzyme (https://cancer.sanger.ac.uk). In addition, PTPN11 is mutated in the germline of patients with several developmental disorders (Noonan Syndrome [NS], and NS-related syndromes) and hematological malignancies (Juvenile Myelomonocytic Leukemia [JMML], and other childhood leukemias) (Huang et al., 2014; Tajan et al., 2015). PTPN11 is among the genes more frequently mutated in neuroblastoma, especially in relapsed tumors, with a mutation distribution pattern similar to the one found in other human cancers (Gröbner et al., 2018; Ma et al., 2018; Pugh et al., 2013; Eleveld et al., 2015) (https://cancer.sanger.ac.uk; https://pecan.stjude.cloud). In addition, high expression of PTPN11 mRNA associates with poorer survival of high-risk neuroblastoma patients with MYCN amplification (Zhang et al., 2017). This supports the notion that high expression and gain-of-function mutations at PTPN11 enable therapy resistance and recurrence in neuroblastoma. In this regard, pharmacological inhibition of PTPN11 in neuroblastoma cells causes RAS/MAPK pathway- and cell growth inhibition, in a manner dependent on the RAS mutational status and synergistic with RAF/MEK/ERK inhibitors (Valencia-Sama et al., 2020; Pedersen et al., 2021; Uçkun et al., 2021). This highlights the therapeutic potential in high-risk neuroblastoma of combination therapies targeting different effectors in the RAS/MAPK pathway. PTPN11 has been functionally related with TrkB and ALK in neuroblastoma (Chitranshi et al., 2017; Uçkun et al., 2021), making possible that these RTKs are PTPN11 neuroblastoma substrates.
PTPN14 has been shown to be involved in migration, invasion, and proliferation of neuroblastoma cells, as well as in the regulation of the nuclear translocation of the transcription coactivator YAP (Schramm et al., 2015; Po’uha et al., 2020). Mutations at PTPN14 gene have been found specifically in relapsed neuroblastoma tumors, suggesting the existence of an operative functional axis PTPN14-YAP in neuroblastoma relapse (Schramm et al., 2015). YAP interacts with the PPxY motifs from PTPN14, which causes YAP cytoplasmic sequestration independently of PTPN14 catalytic activity. In addition, it has also been reported that YAP is a substrate of PTPN14 (Huang et al., 2013; Liu et al., 2013; Michaloglou et al., 2013). PTPN21 is structurally related to PTPN14, and it has been shown to be involved in negative regulation of apoptosis in PC12 cells in association with ERK1/2 activation (Cui et al., 2017). The identification of PTPN14 and PTPN21 direct substrates is necessary to fully understand the role of these non-receptor PTPs in neuroblastoma.
Other non-receptor PTPs related with neuroblastoma include PTPN4, PTPN9, and PTPN12. PTPN4 possesses a FERM and a PDZ protein domain specialized in protein-protein interactions, and the binding of PTPN4 PDZ domain with protein partners has been postulated to positively regulate cell survival in cancer cells, including neuroblastoma cells (Préhaud et al., 2010). PTPN9 harbors a lipid-binding Sec14 domain that target the enzyme to secretory vesicles, regulating vesicle size and fusion, and PTPN9 has been shown to directly dephosphorylate TrkA in P19 neuroblastoma cells, which would regulate its transport to the plasma membrane (Zhang et al., 2016). Finally, PTPN12 is highly expressed in SH-SY5Y cells (Figure 2), where it has been proposed to regulate cell differentiation by dephosphorylation of p130Cas, FAK, and TrkB (Ambjørn et al., 2013).
Concluding Remarks
The involvement of PTPs in the regulation of neuroblastoma cell signaling and development mediated by the distinct RTK/MYCN axes argues for PTPs as relevant biomarkers and potential therapeutic targets in this type of cancer. This is reinforced by the differential association found between neuroblastoma patient outcome and expression of specific PTP genes in neuroblastoma tumor samples, as illustrated along this review. A prominent example of pro-oncogenic PTP in neuroblastoma is PTPN11, whose gene is mutated with relative frequency in high-risk neuroblastoma tumors and whose inhibition by both allosteric and catalytic inhibitors is under intense scrutiny in neuroblastoma experimental models. PTPs are difficult to target specifically using small molecule inhibitors. In this regard, the diversity of regulatory and protein interaction domains present in PTPs offers a wide variety of potential intervention points in addition to direct modulation of catalysis by compounds targeting the enzyme active site (Stanford and Bottini, 2017; Turdo et al., 2021). Since dual pro- and anti-oncogenic properties are proposed for several PTPs in neuroblastoma, dedicated studies are necessary that address the expression, subcellular location, and substrate specificity of individual PTPs in different neuroblastoma scenarios. The deep understanding of PTP biology during neuroblastoma cell growth and differentiation will facilitate the testing of modulation of PTP catalysis, as well as the interference with catalytically-independent PTP biological functions, as helpful strategies in the setting of novel neuroblastoma targeted therapies.
Author Contributions
Conceptualization, draft writing, and design of Figures: CN-X and RP; revision of the manuscript: CN-X, LZ, LM, RL-A, NG, PA, ME, LT, JL, and RP. All authors approved the submitted manuscript.
Funding
This work has been partially supported by grants BIO20/CI/004 from BIOEF (EITB maratoia), Basque Country, Spain (to CN-X and RP). CN-X is funded by Instituto de Salud Carlos III (Spain and the European Social Fund+, grant number: CP20/00,008). LT is the recipient of a predoctoral fellowship from Asociación Española Contra el Cáncer (AECC, Junta Provincial de Bizkaia, Spain, PREDO17JPVIZ0003).
Conflict of Interest
The authors declare that the research was conducted in the absence of any commercial or financial relationships that could be construed as a potential conflict of interest.
Publisher’s Note
All claims expressed in this article are solely those of the authors and do not necessarily represent those of their affiliated organizations, or those of the publisher, the editors and the reviewers. Any product that may be evaluated in this article, or claim that may be made by its manufacturer, is not guaranteed or endorsed by the publisher.
References
Abbott, K. L., Matthews, R. T., and Pierce, M. (2008). Receptor Tyrosine Phosphatase β (RPTPβ) Activity and Signaling Are Attenuated by Glycosylation and Subsequent Cell Surface Galectin-1 Binding. J. Biol. Chem. 283, 33026–33035. doi:10.1074/jbc.m803646200
Alonso, A., Nunes-Xavier, C. E., Bayón, Y., and Pulido, R. (2016). The Extended Family of Protein Tyrosine Phosphatases. Methods Mol. Biol. 1447, 1–23. doi:10.1007/978-1-4939-3746-2_1
Alonso, A., and Pulido, R. (2016). The Extended Human PTPome: a Growing Tyrosine Phosphatase Family. Febs J. 283, 1404–1429. doi:10.1111/febs.13600
Alonso, A., Sasin, J., Bottini, N., Friedberg, I., Friedberg, I., Osterman, A., et al. (2004). Protein Tyrosine Phosphatases in the Human Genome. Cell 117, 699–711. doi:10.1016/j.cell.2004.05.018
Ambjørn, M., Dubreuil, V., Miozzo, F., Nigon, F., Møller, B., Issazadeh-Navikas, S., et al. (2013). A Loss-Of-Function Screen for Phosphatases that Regulate Neurite Outgrowth Identifies PTPN12 as a Negative Regulator of TrkB Tyrosine Phosphorylation. PloS one 8, e65371. doi:10.1371/journal.pone.0065371
Applebaum, M. A., Desai, A. V., Glade Bender, J. L., and Cohn, S. L. (2017). Emerging and Investigational Therapies for Neuroblastoma. Expert Opin. Orphan Drugs 5, 355–368. doi:10.1080/21678707.2017.1304212
Aurtenetxe, O., Zaldumbide, L., Erramuzpe, A., López, R., López, J. I., Cortés, J. M., et al. (2018). DUSP5 Expression Associates with Poor Prognosis in Human Neuroblastoma. Exp. Mol. Pathol. 105, 272–278. doi:10.1016/j.yexmp.2018.08.008
Bakke, J., and Haj, F. G. (2015). Protein-tyrosine Phosphatase 1B Substrates and Metabolic Regulation. Semin. Cel Developmental Biol. 37, 58–65. doi:10.1016/j.semcdb.2014.09.020
Barbacid, M. (1994). The Trk Family of Neurotrophin Receptors. J. Neurobiol. 25, 1386–1403. doi:10.1002/neu.480251107
Barr, A. J., and Knapp, S. (2006). MAPK-specific Tyrosine Phosphatases: New Targets for Drug Discovery? Trends Pharmacological Sciences 27, 525–530. doi:10.1016/j.tips.2006.08.005
Bayeva, N., Coll, E., and Piskareva, O. (2021). Differentiating Neuroblastoma: A Systematic Review of the Retinoic Acid, its Derivatives, and Synergistic Interactions. J. Pers Med. 11, 211. doi:10.3390/jpm11030211
Bedecs, K., Elbaz, N., Sutren, M., Masson, M., Susini, C., Strosberg, A. D., et al. (1997). Angiotensin II Type 2 Receptors Mediate Inhibition of Mitogen-Activated Protein Kinase cascade and Functional Activation of SHP-1 Tyrosine Phosphatase. Biochem. J. 325 ( Pt 2) (Pt 2), 449–454. doi:10.1042/bj3250449
Benomar, Y., Berthou, F., Vacher, C.-M., Bailleux, V., Gertler, A., Djiane, J., et al. (2009). Leptin but Not Ciliary Neurotrophic Factor (CNTF) Induces Phosphotyrosine phosphatase-1B Expression in Human Neuronal Cells (SH-Sy5y): Putative Explanation of CNTF Efficacy in Leptin-Resistant State. Endocrinology 150, 1182–1191. doi:10.1210/en.2008-1097
Bentires-Alj, M., Paez, J. G., David, F. S., Keilhack, H., Halmos, B., Naoki, K., et al. (2004). Activating Mutations of the noonan Syndrome-Associated SHP2/PTPN11 Gene in Human Solid Tumors and Adult Acute Myelogenous Leukemia. Cancer Res. 64, 8816–8820. doi:10.1158/0008-5472.can-04-1923
Berlanga, P., Cañete, A., and Castel, V. (2017). Advances in Emerging Drugs for the Treatment of Neuroblastoma. Expert Opin. emerging Drugs 22, 63–75. doi:10.1080/14728214.2017.1294159
Bieerkehazhi, S., Chen, Z., Zhao, Y., Yu, Y., Zhang, H., Vasudevan, S. A., et al. (2017). Novel Src/Abl Tyrosine Kinase Inhibitor Bosutinib Suppresses Neuroblastoma Growth via Inhibiting Src/Abl Signaling. Oncotarget 8, 1469–1480. doi:10.18632/oncotarget.13643
Blanco-Aparicio, C., Torres, J., and Pulido, R. (1999). A Novel Regulatory Mechanism of MAP Kinases Activation and Nuclear Translocation Mediated by PKA and the PTP-SL Tyrosine Phosphatase. J. Cel. Biol. 147, 1129–1136. doi:10.1083/jcb.147.6.1129
Borenäs, M., Umapathy, G., Lai, W. Y., Lind, D. E., Witek, B., Guan, J., et al. (2021). ALK Ligand ALKAL2 Potentiates MYCN-Driven Neuroblastoma in the Absence of ALK Mutation. EMBO J. 40, e105784. doi:10.15252/embj.2020105784
Boutterin, M. C., Mazot, P., Faure, C., Doly, S., Gervasi, N., Tremblay, M. L., et al. (2013). Control of ALK (Wild Type and Mutated Forms) Phosphorylation: Specific Role of the Phosphatase PTP1B. Cell Signal. 25, 1505–1513. doi:10.1016/j.cellsig.2013.02.020
Bouzas-Rodriguez, J., Cabrera, J. R., Delloye-Bourgeois, C., Ichim, G., Delcros, J.-G., Raquin, M.-A., et al. (2010). Neurotrophin-3 Production Promotes Human Neuroblastoma Cell Survival by Inhibiting TrkC-Induced Apoptosis. J. Clin. Invest. 120, 850–858. doi:10.1172/jci41013
Bown, N. (2001). Neuroblastoma Tumour Genetics: Clinical and Biological Aspects. J. Clin. Pathol. 54, 897–910. doi:10.1136/jcp.54.12.897
Bresler, S. C., Weiser, D. A., Huwe, P. J., Park, J. H., Krytska, K., Ryles, H., et al. (2014). ALK Mutations Confer Differential Oncogenic Activation and Sensitivity to ALK Inhibition Therapy in Neuroblastoma. Cancer cell 26, 682–694. doi:10.1016/j.ccell.2014.09.019
Brockmann, M., Poon, E., Berry, T., Carstensen, A., Deubzer, H. E., Rycak, L., et al. (2013). Small Molecule Inhibitors of aurora-a Induce Proteasomal Degradation of N-Myc in Childhood Neuroblastoma. Cancer cell 24, 75–89. doi:10.1016/j.ccr.2013.05.005
Brodeur, G. M., Minturn, J. E., Ho, R., Simpson, A. M., Iyer, R., Varela, C. R., et al. (2009). Trk Receptor Expression and Inhibition in Neuroblastomas. Clin. Cancer Res. 15, 3244–3250. doi:10.1158/1078-0432.ccr-08-1815
Brodeur, G. M. (2018). Spontaneous Regression of Neuroblastoma. Cell Tissue Res 372, 277–286. doi:10.1007/s00441-017-2761-2
Buday, L., and Vas, V. (2020). Novel Regulation of Ras Proteins by Direct Tyrosine Phosphorylation and Dephosphorylation. Cancer Metastasis Rev. 39, 1067–1073. doi:10.1007/s10555-020-09918-2
Bujko, M., Kober, P., Statkiewicz, M., Mikula, M., Grecka, E., Rusetska, N., et al. (2017). Downregulation of PTPRH (Sap-1) in Colorectal Tumors. Int. J. Oncol. 51, 841–850. doi:10.3892/ijo.2017.4068
Calvet, L., Geoerger, B., Regairaz, M., Opolon, P., Machet, L., Morizet, J., et al. (2006). Pleiotrophin, a Candidate Gene for Poor Tumor Vasculature and In Vivo Neuroblastoma Sensitivity to Irinotecan. Oncogene 25, 3150–3159. doi:10.1038/sj.onc.1209348
Carotenuto, M., Pedone, E., Diana, D., de Antonellis, P., Džeroski, S., Marino, N., et al. (2013). Neuroblastoma Tumorigenesis Is Regulated through the Nm23-H1/h-Prune C-Terminal Interaction. Sci. Rep. 3, 1351. doi:10.1038/srep01351
Carpenter, E. L., and Mossé, Y. P. (2012). Targeting ALK in Neuroblastoma-Preclinical and Clinical Advancements. Nat. Rev. Clin. Oncol. 9, 391–399. doi:10.1038/nrclinonc.2012.72
Chagnon, M. J., Uetani, N., and Tremblay, M. L. (2004). Functional Significance of the LAR Receptor Protein Tyrosine Phosphatase Family in Development and Diseases. Biochem. Cel Biol. 82, 664–675. doi:10.1139/o04-120
Chagnon, M. J., Wu, C.-L., Nakazawa, T., Yamamoto, T., Noda, M., Blanchetot, C., et al. (2010). Receptor Tyrosine Phosphatase Sigma (RPTPσ) Regulates, p250GAP, a Novel Substrate that Attenuates Rac Signaling. Cell Signal. 22, 1626–1633. doi:10.1016/j.cellsig.2010.06.001
Chan, G., Kalaitzidis, D., and Neel, B. G. (2008). The Tyrosine Phosphatase Shp2 (PTPN11) in Cancer. Cancer Metastasis Rev. 27, 179–192. doi:10.1007/s10555-008-9126-y
Chen, M. J., Dixon, J. E., and Manning, G. (2017). Genomics and Evolution of Protein Phosphatases. Sci. Signal. 10, eaag1796. doi:10.1126/scisignal.aag1796
Cheung, N.-K. V., and Dyer, M. A. (2013). Neuroblastoma: Developmental Biology, Cancer Genomics and Immunotherapy. Nat. Rev. Cancer 13, 397–411. doi:10.1038/nrc3526
Chicote, J. U., DeSalle, R., and García-España, A. (2017). Phosphotyrosine Phosphatase R3 Receptors: Origin, Evolution and Structural Diversification. PloS one 12, e0172887. doi:10.1371/journal.pone.0172887
Chitranshi, N., Dheer, Y., Gupta, V., Abbasi, M., Mirzaei, M., You, Y., et al. (2017). PTPN11 Induces Endoplasmic Stress and Apoptosis in SH-Sy5y Cells. Neuroscience 364, 175–189. doi:10.1016/j.neuroscience.2017.09.028
Chong, Z. Z., and Maiese, K. (2007). The Src Homology 2 Domain Tyrosine Phosphatases SHP-1 and SHP-2: Diversified Control of Cell Growth, Inflammation, and Injury. Histol. Histopathol 22, 1251–1267. doi:10.14670/HH-22.1251
Clark, O., Daga, S., and Stoker, A. W. (2013). Tyrosine Phosphatase Inhibitors Combined with Retinoic Acid Can Enhance Differentiation of Neuroblastoma Cells and Trigger ERK- and AKT-dependent, P53-independent Senescence. Cancer Lett. 328, 44–54. doi:10.1016/j.canlet.2012.09.014
Clark, O., Park, I., Di Florio, A., Cichon, A.-C., Rustin, S., Jugov, R., et al. (2015). Oxovanadium-based Inhibitors Can Drive Redox-Sensitive Cytotoxicity in Neuroblastoma Cells and Synergise Strongly with Buthionine Sulfoximine. Cancer Lett. 357, 316–327. doi:10.1016/j.canlet.2014.11.039
Clark, O., Schmidt, F., Coles, C. H., Tchetchelnitski, V., and Stoker, A. W. (2012). Functional Analysis of the Putative Tumor Suppressor PTPRD in Neuroblastoma Cells. Cancer Invest. 30, 422–432. doi:10.3109/07357907.2012.675383
Cocco, E., Scaltriti, M., and Drilon, A. (2018). NTRK Fusion-Positive Cancers and TRK Inhibitor Therapy. Nat. Rev. Clin. Oncol. 15, 731–747. doi:10.1038/s41571-018-0113-0
Cui, N., Lu, H., Li, M., and Yan, Q. (2017). PTPN21 Protects PC12 Cell against Oxygen-Glucose Deprivation by Activating Cdk5 through ERK1/2 Signaling Pathway. Eur. J. Pharmacol. 814, 226–231. doi:10.1016/j.ejphar.2017.08.021
De Brouwer, S., De Preter, K., Kumps, C., Zabrocki, P., Porcu, M., Westerhout, E. M., et al. (2010). Meta-analysis of Neuroblastomas Reveals a Skewed ALK Mutation Spectrum in Tumors with MYCN Amplification. Clin. Cancer Res. 16, 4353–4362. doi:10.1158/1078-0432.ccr-09-2660
Del Campo, M., Fernandez-Calle, R., Vicente-Rodriguez, M., Martin Martinez, S., Gramage, E., Zapico, J. M., et al. (2021). Role of Receptor Protein Tyrosine Phosphatase Beta/zeta in Neuron-Microglia Communication in a Cellular Model of Parkinson's Disease. Int. J. Mol. Sci. 22. doi:10.3390/ijms22136646
Dempke, W. C. M., Uciechowski, P., Fenchel, K., and Chevassut, T. (2018). Targeting SHP-1, 2 and SHIP Pathways: A Novel Strategy for Cancer Treatment? Oncology 95, 257–269. doi:10.1159/000490106
den Hertog, J., Pals, C. E., Peppelenbosch, M. P., Tertoolen, L. G., de Laat, S. W., and Kruijer, W. (1993). Receptor Protein Tyrosine Phosphatase Alpha Activates Pp60c-Src and Is Involved in Neuronal Differentiation. EMBO J. 12, 3789–3798. doi:10.1002/j.1460-2075.1993.tb06057.x
Deuel, T. F. (2013). Anaplastic Lymphoma Kinase: "Ligand Independent Activation" Mediated by the PTN/RPTPβ/ζ Signaling Pathway. Biochim. Biophys. Acta (Bba) - Proteins Proteomics 1834, 2219–2223. doi:10.1016/j.bbapap.2013.06.004
Eleveld, T. F., Oldridge, D. A., Bernard, V., Koster, J., Daage, L. C., Diskin, S. J., et al. (2015). Relapsed Neuroblastomas Show Frequent RAS-MAPK Pathway Mutations. Nat. Genet. 47, 864–871. doi:10.1038/ng.3333
Esposito, M. R., Binatti, A., Pantile, M., Coppe, A., Mazzocco, K., Longo, L., et al. (2018). Somatic Mutations in Specific and Connected Subpathways Are Associated with Short Neuroblastoma Patients' Survival and Indicate Proteins Targetable at Onset of Disease. Int. J. Cancer 143, 2525–2536. doi:10.1002/ijc.31748
Farina, A. R., Cappabianca, L., Ruggeri, P., Gneo, L., Pellegrini, C., Fargnoli, M.-C., et al. (2018). The Oncogenic Neurotrophin Receptor Tropomyosin-Related Kinase Variant, TrkAIII. J. Exp. Clin. Cancer Res. 37, 119. doi:10.1186/s13046-018-0786-3
Faux, C., Hawadle, M., Nixon, J., Wallace, A., Lee, S., Murray, S., et al. (2007). PTPσ Binds and Dephosphorylates Neurotrophin Receptors and Can Suppress NGF-dependent Neurite Outgrowth from Sensory Neurons. Biochim. Biophys. Acta (Bba) - Mol. Cel Res. 1773, 1689–1700. doi:10.1016/j.bbamcr.2007.06.008
Feldhammer, M., Uetani, N., Miranda-Saavedra, D., and Tremblay, M. L. (2013). PTP1B: a Simple Enzyme for a Complex World. Crit. Rev. Biochem. Mol. Biol. 48, 430–445. doi:10.3109/10409238.2013.819830
Fernández-Calle, R., Gramage, E., Zapico, J. M., de Pascual-Teresa, B., Ramos, A., and Herradón, G. (2019). Inhibition of RPTPβ/ζ Blocks Ethanol-Induced Conditioned Place Preference in Pleiotrophin Knockout Mice. Behav. Brain Res. 369, 111933. doi:10.1016/j.bbr.2019.111933
Fernández-Calle, R., Vicente-Rodríguez, M., Pastor, M., Gramage, E., Di Geronimo, B., Zapico, J. M., et al. (2018). Pharmacological Inhibition of Receptor Protein Tyrosine Phosphatase β/ζ (PTPRZ1) Modulates Behavioral Responses to Ethanol. Neuropharmacology 137, 86–95. doi:10.1016/j.neuropharm.2018.04.027
Fletcher, J. I., Ziegler, D. S., Trahair, T. N., Marshall, G. M., Haber, M., and Norris, M. D. (2018). Too many Targets, Not Enough Patients: Rethinking Neuroblastoma Clinical Trials. Nat. Rev. Cancercancer 18, 389–400. doi:10.1038/s41568-018-0003-x
Frankson, R., Yu, Z.-H., Bai, Y., Li, Q., Zhang, R.-Y., and Zhang, Z.-Y. (2017). Therapeutic Targeting of Oncogenic Tyrosine Phosphatases. Cancer Res. 77, 5701–5705. doi:10.1158/0008-5472.can-17-1510
Fujikawa, A., Nagahira, A., Sugawara, H., Ishii, K., Imajo, S., Matsumoto, M., et al. (2016). Small-molecule Inhibition of PTPRZ Reduces Tumor Growth in a Rat Model of Glioblastoma. Sci. Rep. 6, 20473. doi:10.1038/srep20473
Fujikawa, A., Sugawara, H., Tanaka, T., Matsumoto, M., Kuboyama, K., Suzuki, R., et al. (2017). Targeting PTPRZ Inhibits Stem Cell-like Properties and Tumorigenicity in Glioblastoma Cells. Sci. Rep. 7, 5609. doi:10.1038/s41598-017-05931-8
Fukazawa, N., Yokoyama, S., Eiraku, M., Kengaku, M., and Maeda, N. (2008). Receptor Type Protein Tyrosine Phosphatase ζ-Pleiotrophin Signaling Controls Endocytic Trafficking of DNER that Regulates Neuritogenesis. Mol. Cel Biol 28, 4494–4506. doi:10.1128/mcb.00074-08
Gatto, G., Dudanova, I., Suetterlin, P., Davies, A. M., Drescher, U., Bixby, J. L., et al. (2013). Protein Tyrosine Phosphatase Receptor Type O Inhibits Trigeminal Axon Growth and Branching by Repressing TrkB and Ret Signaling. J. Neurosci. 33, 5399–5410. doi:10.1523/jneurosci.4707-12.2013
Gneo, L., Ruggeri, P., Cappabianca, L., Farina, A. R., Ianni, N. D., and Mackay, A. R. (2016). TRAIL Induces Pro-apoptotic Crosstalk between the TRAIL-Receptor Signaling Pathway and TrkAIII in SH-Sy5y Cells, Unveiling a Potential Therapeutic "Achilles Heel" for the TrkAIII Oncoprotein in Neuroblastoma. Oncotarget 7, 80820–80841. doi:10.18632/oncotarget.13098
Gröbner, S. N., Worst, B. C., Worst, B. C., Weischenfeldt, J., Buchhalter, I., Kleinheinz, K., et al. (2018). The Landscape of Genomic Alterations across Childhood Cancers. Nature 555, 321–327. doi:10.1038/nature25480
Grossmann, K. S., Rosário, M., Birchmeier, C., and Birchmeier, W. (2010). The Tyrosine Phosphatase Shp2 in Development and Cancer. Adv. Cancer Res. 106, 53–89. doi:10.1016/s0065-230x(10)06002-1
Guan, J., Umapathy, G., Yamazaki, Y., Wolfstetter, G., Mendoza, P., Pfeifer, K., et al. (2015). FAM150A and FAM150B Are Activating Ligands for Anaplastic Lymphoma Kinase. eLife 4, e09811. doi:10.7554/eLife.09811
Guo, W., and Xu, Q. (2020). Phosphatase-independent Functions of SHP2 and its Regulation by Small Molecule Compounds. J. Pharmacol. Sci. 144, 139–146. doi:10.1016/j.jphs.2020.06.002
Hale, A. J., Ter Steege, E., and den Hertog, J. (2017). Recent Advances in Understanding the Role of Protein-Tyrosine Phosphatases in Development and Disease. Developmental Biol. 428, 283–292. doi:10.1016/j.ydbio.2017.03.023
Han, K. A., Jeon, S., Um, J. W., and Ko, J. (2016). Emergent Synapse Organizers: LAR-RPTPs and Their Companions. Int. Rev. Cel. Mol. Biol. 324, 39–65. doi:10.1016/bs.ircmb.2016.01.002
Hasan, M. K., Nafady, A., Takatori, A., Kishida, S., Ohira, M., Suenaga, Y., et al. (2013). ALK Is a MYCN Target Gene and Regulates Cell Migration and Invasion in Neuroblastoma. Sci. Rep. 3, 3450. doi:10.1038/srep03450
Hendriks, W. J. A. J., Dilaver, G., Noordman, Y. E., Kremer, B., and Fransen, J. A. M. (2009). PTPRR Protein Tyrosine Phosphatase Isoforms and Locomotion of Vesicles and Mice. Cerebellum 8, 80–88. doi:10.1007/s12311-008-0088-y
Hendriks, W. J. A. J., Elson, A., Harroch, S., Pulido, R., Stoker, A., and den Hertog, J. (2013). Protein Tyrosine Phosphatases in Health and Disease. FEBS J. 280, 708–730. doi:10.1111/febs.12000
Hendriks, W. J. A. J., and Pulido, R. (2013). Protein Tyrosine Phosphatase Variants in Human Hereditary Disorders and Disease Susceptibilities. Biochim. Biophys. Acta (Bba) - Mol. Basis Dis. 1832, 1673–1696. doi:10.1016/j.bbadis.2013.05.022
Herradon, G., and Ezquerra, L. (2009). Blocking Receptor Protein Tyrosine Phosphatase β/ζ: A Potential Therapeutic Strategy for Parkinsons Disease. Cmc 16, 3322–3329. doi:10.2174/092986709788803240
Higashi, M., Sakai, K., Fumino, S., Aoi, S., Furukawa, T., and Tajiri, T. (2019). The Roles Played by the MYCN, Trk, and ALK Genes in Neuroblastoma and Neural Development. Surg. Today 49, 721–727. doi:10.1007/s00595-019-01790-0
Ho, R., Eggert, A., Hishiki, T., Minturn, J. E., Ikegaki, N., Foster, P., et al. (2002). Resistance to Chemotherapy Mediated by TrkB in Neuroblastomas. Cancer Res. 62, 6462–6466.
Hower, A. E., Beltran, P. J., and Bixby, J. L. (2009). Dimerization of Tyrosine Phosphatase PTPRO Decreases its Activity and Ability to Inactivate TrkC. J. Neurochem. 110, 1635–1647. doi:10.1111/j.1471-4159.2009.06261.x
Huang, J.-M., Nagatomo, I., Suzuki, E., Mizuno, T., Kumagai, T., Berezov, A., et al. (2013). YAP Modifies Cancer Cell Sensitivity to EGFR and Survivin Inhibitors and Is Negatively Regulated by the Non-receptor Type Protein Tyrosine Phosphatase 14. Oncogene 32, 2220–2229. doi:10.1038/onc.2012.231
Huang, M., and Weiss, W. A. (2013). Neuroblastoma and MYCN. Cold Spring Harbor Perspect. Med. 3, a014415. doi:10.1101/cshperspect.a014415
Huang, W.-Q., Lin, Q., Zhuang, X., Cai, L.-L., Ruan, R.-S., Lu, Z.-X., et al. (2014). Structure, Function, and Pathogenesis of SHP2 in Developmental Disorders and Tumorigenesis. Ccdt 14, 567–588. doi:10.2174/1568009614666140717105001
Hurlin, P. J. (2005). N-myc Functions in Transcription and Development. Birth Defect Res. C 75, 340–352. doi:10.1002/bdrc.20059
Jaboin, J., Kim, C. J., Kaplan, D. R., and Thiele, C. J. (2002). Brain-derived Neurotrophic Factor Activation of TrkB Protects Neuroblastoma Cells from Chemotherapy-Induced Apoptosis via Phosphatidylinositol 3'-kinase Pathway. Cancer Res. 62, 6756–6763.
Janoueix-Lerosey, I., Lopez-Delisle, L., Delattre, O., and Rohrer, H. (2018). The ALK Receptor in Sympathetic Neuron Development and Neuroblastoma. Cel Tissue Res 372, 325–337. doi:10.1007/s00441-017-2784-8
Javanmardi, N., Fransson, S., Djos, A., Umapathy, G., Ostensson, M., Milosevic, J., et al. (2019). Analysis of ALK, MYCN, and the ALK Ligand ALKAL2 (FAM150B/AUGalpha) in Neuroblastoma Patient Samples with Chromosome Arm 2p Rearrangements. Genes Chromosomes Cancer. doi:10.1002/gcc.22790
Jeon, M., and Zinn, K. (2015). R3 Receptor Tyrosine Phosphatases: Conserved Regulators of Receptor Tyrosine Kinase Signaling and Tubular Organ Development. Semin. Cel Developmental Biol. 37, 119–126. doi:10.1016/j.semcdb.2014.09.005
Jeon, Y.-M., Lee, S., Kim, S., Kwon, Y., Kim, K., Chung, C. G., et al. (2017). Neuroprotective Effects of Protein Tyrosine Phosphatase 1B Inhibition against ER Stress-Induced Toxicity. Mol. Cell 40, 280–290. doi:10.14348/molcells.2017.2320
Julien, S. G., Dubé, N., Hardy, S., and Tremblay, M. L. (2011). Inside the Human Cancer Tyrosine Phosphatome. Nat. Rev. Cancer 11, 35–49. doi:10.1038/nrc2980
Kamili, A., Atkinson, C., Trahair, T. N., and Fletcher, J. I. (2020). Mouse Models of High-Risk Neuroblastoma. Cancer Metastasis Rev. 39, 261–274. doi:10.1007/s10555-020-09855-0
Karaca Atabay, E., Mecca, C., Wang, Q., Ambrogio, C., Mota, I., Prokoph, N., et al. (2021). Tyrosine Phosphatases Regulate Resistance to ALK Inhibitors in ALK+ Anaplastic Large Cell Lymphoma. Blood 2020008136. doi:10.1182/blood.2020008136
Karasawa, T., and Lombroso, P. J. (2014). Disruption of Striatal-Enriched Protein Tyrosine Phosphatase (STEP) Function in Neuropsychiatric Disorders. Neurosci. Res. 89, 1–9. doi:10.1016/j.neures.2014.08.018
Kawachi, H., Fujikawa, A., Maeda, N., and Noda, M. (2001). Identification of GIT1/Cat-1 as a Substrate Molecule of Protein Tyrosine Phosphatase/by the Yeast Substrate-Trapping System. Proc. Natl. Acad. Sci. 98, 6593–6598. doi:10.1073/pnas.041608698
Kholodenko, I. V., Kalinovsky, D. V., Doronin, , Deyev, S. M., and Kholodenko, R. V. (2018). Neuroblastoma Origin and Therapeutic Targets for Immunotherapy. J. Immunol. Res. 2018, 7394268. doi:10.1155/2018/7394268
Kostrzewa, T., Styszko, J., Gorska-Ponikowska, M., Sledzinski, T., and Kuban-Jankowska, A. (2019). Inhibitors of Protein Tyrosine Phosphatase PTP1B with Anticancer Potential. Anticancer Res. 39, 3379–3384. doi:10.21873/anticanres.13481
Kratimenos, P., Koutroulis, I., Marconi, D., Syriopoulou, V., Delivoria-Papadopoulos, M., Chrousos, G. P., et al. (2014). Multi-targeted Molecular Therapeutic Approach in Aggressive Neuroblastoma: the Effect of Focal Adhesion Kinase-Src-Paxillin System. Expert Opin. Ther. Targets 18, 1395–1406. doi:10.1517/14728222.2014.952280
Lange, A. M., and Lo, H. W. (2018). Inhibiting TRK Proteins in Clinical Cancer Therapy. Cancers (Basel) 10, 105. doi:10.3390/cancers10040105
Lazo, J. S., McQueeney, K. E., Burnett, J. C., Wipf, P., and Sharlow, E. R. (2018). Small Molecule Targeting of PTPs in Cancer. Int. J. Biochem. Cel Biol. 96, 171–181. doi:10.1016/j.biocel.2017.09.011
Lee, H. S., Ku, B., Park, T. H., Park, H., Choi, J.-K., Chang, K.-T., et al. (2016). Identification of Novel Protein Tyrosine Phosphatase Sigma Inhibitors Promoting Neurite Extension. Bioorg. Med. Chem. Lett. 26, 87–93. doi:10.1016/j.bmcl.2015.11.026
Li, T., Deng, Y., Shi, Y., Tian, R., Chen, Y., Zou, L., et al. (2018). Bruton's Tyrosine Kinase Potentiates ALK Signaling and Serves as a Potential Therapeutic Target of Neuroblastoma. Oncogene 37, 6180–6194. doi:10.1038/s41388-018-0397-7
Lin, Y., Zhou, X., Yang, K., Chen, Y., Wang, L., Luo, W., et al. (2021). Protein Tyrosine Phosphatase Receptor Type D Gene Promotes Radiosensitivity via STAT3 Dephosphorylation in Nasopharyngeal Carcinoma. Oncogene 40, 3101–3117. doi:10.1038/s41388-021-01768-8
Liu, X., Yang, N., Figel, S. A., Wilson, K. E., Morrison, C. D., Gelman, I. H., et al. (2013). PTPN14 Interacts with and Negatively Regulates the Oncogenic Function of YAP. Oncogene 32, 1266–1273. doi:10.1038/onc.2012.147
Lorenz, U. (2009). SHP-1 and SHP-2 in T Cells: Two Phosphatases Functioning at many Levels. Immunological Rev. 228, 342–359. doi:10.1111/j.1600-065x.2008.00760.x
Ma, X., Liu, Y., Liu, Y., Alexandrov, L. B., Edmonson, M. N., Gawad, C., et al. (2018). Pan-cancer Genome and Transcriptome Analyses of 1,699 Paediatric Leukaemias and Solid Tumours. Nature 555, 371–376. doi:10.1038/nature25795
Mallozzi, C., Pepponi, R., Visentin, S., Chiodi, V., Lombroso, P. J., Bader, M., et al. (2020). The Activity of the Striatal‐enriched Protein Tyrosine Phosphatase in Neuronal Cells Is Modulated by Adenosine A 2A Receptor. J. Neurochem. 152, 284–298. doi:10.1111/jnc.14866
Marsh, H. N., Dubreuil, C. I., Quevedo, C., Lee, A., Majdan, M., Walsh, G. S., et al. (2003). SHP-1 Negatively Regulates Neuronal Survival by Functioning as a TrkA Phosphatase. J. Cel. Biol. 163, 999–1010. doi:10.1083/jcb.200309036
Matozaki, T., Murata, Y., Mori, M., Kotani, T., Okazawa, H., and Ohnishi, H. (2010). Expression, Localization, and Biological Function of the R3 Subtype of Receptor-type Protein Tyrosine Phosphatases in Mammals. Cell Signal. 22, 1811–1817. doi:10.1016/j.cellsig.2010.07.001
Matozaki, T., Murata, Y., Saito, Y., Okazawa, H., and Ohnishi, H. (2009). Protein Tyrosine Phosphatase SHP-2: a Proto-Oncogene Product that Promotes Ras Activation. Cancer Sci. 100, 1786–1793. doi:10.1111/j.1349-7006.2009.01257.x
Matthay, K. K., Maris, J. M., Schleiermacher, G., Nakagawara, A., Mackall, C. L., Diller, L., et al. (2016). Neuroblastoma. Nat. Rev. Dis. Primers 2, 16078. doi:10.1038/nrdp.2016.78
Meehan, M., Parthasarathi, L., Moran, N., Jefferies, C. A., Foley, N., Lazzari, E., et al. (2012). Protein Tyrosine Phosphatase Receptor delta Acts as a Neuroblastoma Tumor Suppressor by Destabilizing the aurora Kinase A Oncogene. Mol. Cancer 11, 6. doi:10.1186/1476-4598-11-6
Michaloglou, C., Lehmann, W., Martin, T., Delaunay, C., Hueber, A., Barys, L., et al. (2013). The Tyrosine Phosphatase PTPN14 Is a Negative Regulator of YAP Activity. PloS one 8, e61916. doi:10.1371/journal.pone.0061916
Mitra, A., Kalayarasan, S., Gupta, V., and Radha, V. (2011). TC-PTP Dephosphorylates the Guanine Nucleotide Exchange Factor C3G (RapGEF1) and Negatively Regulates Differentiation of Human Neuroblastoma Cells. PloS one 6, e23681. doi:10.1371/journal.pone.0023681
Mizuno, K., Katagiri, T., Maruyama, E., Hasegawa, K., Ogimoto, M., and Yakura, H. (1997). SHP-1 Is Involved in Neuronal Differentiation of P19 Embryonic Carcinoma Cells. FEBS Lett. 417, 6–12. doi:10.1016/s0014-5793(97)01234-9
Mlakar, V., Morel, E., Mlakar, S. J., Ansari, M., and Gumy-Pause, F. (2021). A Review of the Biological and Clinical Implications of RAS-MAPK Pathway Alterations in Neuroblastoma. J. Exp. Clin. Cancer Res. 40, 189. doi:10.1186/s13046-021-01967-x
Mohebiany, A. N., Nikolaienko, R. M., Bouyain, S., and Harroch, S. (2013). Receptor-type Tyrosine Phosphatase Ligands: Looking for the Needle in the Haystack. FEBS J. 280, 388–400. doi:10.1111/j.1742-4658.2012.08653.x
Molinari, A., Fallacara, A. L., Di Maria, S., Zamperini, C., Poggialini, F., Musumeci, F., et al. (2018). Efficient Optimization of Pyrazolo[3,4-D]pyrimidines Derivatives as C-Src Kinase Inhibitors in Neuroblastoma Treatment. Bioorg. Med. Chem. Lett. 28, 3454–3457. doi:10.1016/j.bmcl.2018.09.024
Montano, X. (2009). Repression of SHP-1 Expression by P53 Leads to trkA Tyrosine Phosphorylation and Suppression of Breast Cancer Cell Proliferation. Oncogene 28, 3787–3800. doi:10.1038/onc.2009.143
Moreno, L., Barone, G., DuBois, S. G., Molenaar, J., Fischer, M., Schulte, J., et al. (2020). Accelerating Drug Development for Neuroblastoma: Summary of the Second Neuroblastoma Drug Development Strategy Forum from Innovative Therapies for Children with Cancer and International Society of Paediatric Oncology Europe Neuroblastoma. Eur. J. Cancer 136, 52–68. doi:10.1016/j.ejca.2020.05.010
Murray, P. B., Lax, I., Reshetnyak, A., Ligon, G. F., Lillquist, J. S., Natoli, E. J., et al. (2015). Heparin Is an Activating Ligand of the Orphan Receptor Tyrosine Kinase ALK. Sci. Signal. 8, ra6. doi:10.1126/scisignal.2005916
Nagano, H., Noguchi, T., Inagaki, K., Yoon, S., Matozaki, T., Itoh, H., et al. (2003). Downregulation of Stomach Cancer-Associated Protein Tyrosine Phosphatase-1 (SAP-1) in Advanced Human Hepatocellular Carcinoma. Oncogene 22, 4656–4663. doi:10.1038/sj.onc.1206588
Nair, P., DePreter, K., Vandesompele, J., Speleman, F., and Stallings, R. L. (2008). Aberrant Splicing of the PTPRD gene Mimics Microdeletions Identified at This Locus in Neuroblastomas. Genes Chromosom. Cancer 47, 197–202. doi:10.1002/gcc.20521
Nakagawara, A., Milbrandt, J., Muramatsu, T., Deuel, T. F., Zhao, H., Cnaan, A., et al. (1995). Differential Expression of Pleiotrophin and Midkine in Advanced Neuroblastomas. Cancer Res. 55, 1792–1797.
Nakagawara, A., Arima-Nakagawara, M., Scavarda, N. J., Azar, C. G., Cantor, A. B., and Brodeur, G. M. (1993). Association between High Levels of Expression of the TRK Gene and Favorable Outcome in Human Neuroblastoma. N. Engl. J. Med. 328, 847–854. doi:10.1056/nejm199303253281205
Nakagawara, A., Azar, C. G., Scavarda, N. J., and Brodeur, G. M. (1994). Expression and Function of TRK-B and BDNF in Human Neuroblastomas. Mol. Cel. Biol. 14, 759–767. doi:10.1128/mcb.14.1.759
Navarra, M., Celano, M., Maiuolo, J., Schenone, S., Botta, M., Angelucci, A., et al. (2010). Antiproliferative and Pro-apoptotic Effects Afforded by Novel Src-Kinase Inhibitors in Human Neuroblastoma Cells. BMC cancer 10, 602. doi:10.1186/1471-2407-10-602
Nikoletopoulou, V., Lickert, H., Frade, J. M., Rencurel, C., Giallonardo, P., Zhang, L., et al. (2010). Neurotrophin Receptors TrkA and TrkC Cause Neuronal Death whereas TrkB Does Not. Nature 467, 59–63. doi:10.1038/nature09336
Noordman, Y. E., Jansen, P. A. M., and Hendriks, W. J. A. J. (2006). Tyrosine-specific MAPK Phosphatases and the Control of ERK Signaling in PC12 Cells. Jms 1, 4. doi:10.1186/1750-2187-1-4
Nunes-Xavier, C. E., Zaldumbide, L., Aurtenetxe, O., López-Almaraz, R., López, J. I., and Pulido, R. (2019). Dual-Specificity Phosphatases in Neuroblastoma Cell Growth and Differentiation. Int. J. Mol. Sci. 20. doi:10.3390/ijms20051170
Nunes-Xavier, C. E., Aurtenetxe, O., Zaldumbide, L., López-Almaraz, R., Erramuzpe, A., Cortés, J. M., et al. (2019). Protein Tyrosine Phosphatase PTPN1 Modulates Cell Growth and Associates with Poor Outcome in Human Neuroblastoma. Diagn. Pathol. 14, 134. doi:10.1186/s13000-019-0919-9
Nunes-Xavier, C. E., Elson, A., and Pulido, R. (2012). Epidermal Growth Factor Receptor (EGFR)-mediated Positive Feedback of Protein-Tyrosine Phosphatase ϵ (PTPϵ) on ERK1/2 and AKT Protein Pathways Is Required for Survival of Human Breast Cancer Cells. J. Biol. Chem. 287, 3433–3444. doi:10.1074/jbc.m111.293928
Nunes-Xavier, C. E., Martín-Pérez, J., Elson, A., and Pulido, R. (2013). Protein Tyrosine Phosphatases as Novel Targets in Breast Cancer Therapy. Biochim. Biophys. Acta (Bba) - Rev. Cancer 1836, 211–226. doi:10.1016/j.bbcan.2013.06.001
Nunes-Xavier, C. E., and Pulido, R. (2016). Global RT-PCR and RT-qPCR Analysis of the mRNA Expression of the Human PTPome. Methods Mol. Biol. 1447, 25–37. doi:10.1007/978-1-4939-3746-2_2
Nunes-Xavier, C., Roma-Mateo, C., Rios, P., Tarrega, C., Cejudo-Marin, R., Tabernero, L., et al. (2011). Dual-specificity MAP Kinase Phosphatases as Targets of Cancer Treatment. Acamc 11, 109–132. doi:10.2174/187152011794941190
Oganesian, A., Poot, M., Daum, G., Coats, S. A., Wright, M. B., Seifert, R. A., et al. (2003). Protein Tyrosine Phosphatase RQ Is a Phosphatidylinositol Phosphatase that Can Regulate Cell Survival and Proliferation. Proc. Natl. Acad. Sci. 100, 7563–7568. doi:10.1073/pnas.1336511100
Ogawa, S., Takita, J., Sanada, M., and Hayashi, Y. (2011). Oncogenic Mutations of ALK in Neuroblastoma. Cancer Sci. 102, 302–308. doi:10.1111/j.1349-7006.2010.01825.x
Ortiz, B., Fabius, A. W. M., Wu, W. H., Pedraza, A., Brennan, C. W., Schultz, N., et al. (2014). Loss of the Tyrosine Phosphatase PTPRD Leads to Aberrant STAT3 Activation and Promotes Gliomagenesis. Proc. Natl. Acad. Sci. 111, 8149–8154. doi:10.1073/pnas.1401952111
Ozek, C., Kanoski, S. E., Zhang, Z.-Y., Grill, H. J., and Bence, K. K. (2014). Protein-tyrosine Phosphatase 1B (PTP1B) Is a Novel Regulator of central Brain-Derived Neurotrophic Factor and Tropomyosin Receptor Kinase B (TrkB) Signaling. J. Biol. Chem. 289, 31682–31692. doi:10.1074/jbc.m114.603621
Pacenta, H. L., and Macy, M. E. (2018). Entrectinib and Other ALK/TRK Inhibitors for the Treatment of Neuroblastoma. Dddt Vol. 12, 3549–3561. doi:10.2147/dddt.s147384
Pallen, C. (2003). Protein Tyrosine Phosphatase α (PTPα): A Src Family Kinase Activator and Mediator of Multiple Biological Effects. Ctmc 3, 821–835. doi:10.2174/1568026033452320
Palmer, R. H., Vernersson, E., Grabbe, C., and Hallberg, B. (2009). Anaplastic Lymphoma Kinase: Signalling in Development and Disease. Biochem. J. 420, 345–361. doi:10.1042/bj20090387
Pantazaka, E., and Papadimitriou, E. (2014). Chondroitin Sulfate-Cell Membrane Effectors as Regulators of Growth Factor-Mediated Vascular and Cancer Cell Migration. Biochim. Biophys. Acta (Bba) - Gen. Subjects 1840, 2643–2650. doi:10.1016/j.bbagen.2014.01.009
Papadimitriou, E., Pantazaka, E., Castana, P., Tsalios, T., Polyzos, A., and Beis, D. (2016). Pleiotrophin and its Receptor Protein Tyrosine Phosphatase Beta/zeta as Regulators of Angiogenesis and Cancer. Biochim. Biophys. Acta (Bba) - Rev. Cancer 1866, 252–265. doi:10.1016/j.bbcan.2016.09.007
Parri, E., Kuusanmäki, H., van Adrichem, A. J., Kaustio, M., and Wennerberg, K. (2020). Identification of Novel Regulators of STAT3 Activity. PloS one 15, e0230819. doi:10.1371/journal.pone.0230819
Pastor, E. R., and Mousa, S. A. (2019). Current Management of Neuroblastoma and Future Direction. Crit. Rev. oncology/hematology 138, 38–43. doi:10.1016/j.critrevonc.2019.03.013
Pastor, M., Fernández-Calle, R., Di Geronimo, B., Vicente-Rodríguez, M., Zapico, J. M., Gramage, E., et al. (2018). Development of Inhibitors of Receptor Protein Tyrosine Phosphatase β/ζ (PTPRZ1) as Candidates for CNS Disorders. Eur. J. Med. Chem. 144, 318–329. doi:10.1016/j.ejmech.2017.11.080
Pedersen, A.-K., Pfeiffer, A., Karemore, G., Akimov, V., Bekker-Jensen, D. B., Blagoev, B., et al. (2021). Proteomic Investigation of Cbl and Cbl-B in Neuroblastoma Cell Differentiation Highlights Roles for SHP-2 and CDK16. iScience 24, 102321. doi:10.1016/j.isci.2021.102321
Pikatan, N. W., Liu, Y.-L., Bamodu, O. A., Hsiao, M., Hsu, W.-M., Haryana, S. M., et al. (2020). Aberrantly Expressed Bruton's Tyrosine Kinase Preferentially Drives Metastatic and Stem Cell-like Phenotypes in Neuroblastoma Cells. Cell Oncol. 43, 1067–1084. doi:10.1007/s13402-020-00541-5
Pike, K. A., Tremblay, M. L., and Ptp1B, (2016). TC-PTP and PTP1B: Regulating JAK-STAT Signaling, Controlling Lymphoid Malignancies. Cytokine 82, 52–57. doi:10.1016/j.cyto.2015.12.025
Po'uha, S. T., Le Grand, M., Brandl, M. B., Gifford, A. J., Goodall, G. J., Khew-Goodall, Y., et al. (2020). Stathmin Levels Alter PTPN14 Expression and Impact Neuroblastoma Cell Migration. Br. J. Cancer 122, 434–444. doi:10.1038/s41416-019-0669-1
Préhaud, C., Wolff, N., Terrien, E., Lafage, M., Mégret, F., Babault, N., et al. (2010). Attenuation of Rabies Virulence: Takeover by the Cytoplasmic Domain of its Envelope Protein. Sci. Signal. 3, ra5. doi:10.1126/scisignal.2000510
Pugh, T. J., Morozova, O., Attiyeh, E. F., Asgharzadeh, S., Wei, J. S., Auclair, D., et al. (2013). The Genetic Landscape of High-Risk Neuroblastoma. Nat. Genet. 45, 279–284. doi:10.1038/ng.2529
Pulido, R., Serra-Pages, C., Tang, M., and Streuli, M. (1995). The LAR/PTP delta/PTP Sigma Subfamily of Transmembrane Protein-Tyrosine-Phosphatases: Multiple Human LAR, PTP delta, and PTP Sigma Isoforms Are Expressed in a Tissue-specific Manner and Associate with the LAR-Interacting Protein LIP.1. Proc. Natl. Acad. Sci. 92, 11686–11690. doi:10.1073/pnas.92.25.11686
Pulido, R., Zuniga, A., and Ullrich, A. (1998). PTP-SL and STEP Protein Tyrosine Phosphatases Regulate the Activation of the Extracellular Signal-Regulated Kinases ERK1 and ERK2 by Association through a Kinase Interaction Motif. EMBO J. 17, 7337–7350. doi:10.1093/emboj/17.24.7337
Radha, V., Rajanna, A., Gupta, R. K., Dayma, K., and Raman, T. (2008). The Guanine Nucleotide Exchange Factor, C3G Regulates Differentiation and Survival of Human Neuroblastoma Cells. J. Neurochem. 107, 1424–1435. doi:10.1111/j.1471-4159.2008.05710.x
Reichardt, L. F. (2006). Neurotrophin-regulated Signalling Pathways. Phil. Trans. R. Soc. B 361, 1545–1564. doi:10.1098/rstb.2006.1894
Reshetnyak, A. V., Murray, P. B., Shi, X., Mo, E. S., Mohanty, J., Tome, F., et al. (2015). Augmentor α and β (FAM150) Are Ligands of the Receptor Tyrosine Kinases ALK and LTK: Hierarchy and Specificity of Ligand-Receptor Interactions. Proc. Natl. Acad. Sci. USA 112, 15862–15867. doi:10.1073/pnas.1520099112
Ríos, P., Nunes-Xavier, C. E., Tabernero, L., Köhn, M., and Pulido, R. (2014). Dual-specificity Phosphatases as Molecular Targets for Inhibition in Human Disease. Antioxid. Redox Signaling 20, 2251–2273. doi:10.1089/ars.2013.5709
Roeschert, I., Poon, E., Henssen, A. G., Dorado Garcia, H., Gatti, M., Giansanti, C., et al. (2021). Combined Inhibition of Aurora-A and ATR Kinases Results in Regression of MYCN-Amplified Neuroblastoma. Nat. Cancer 2, 312–326. doi:10.1038/s43018-020-00171-8
Roskoski, R. (2013). Anaplastic Lymphoma Kinase (ALK): Structure, Oncogenic Activation, and Pharmacological Inhibition. Pharmacol. Res. 68, 68–94. doi:10.1016/j.phrs.2012.11.007
Ruiz-Pérez, M. V., Henley, A. B., and Arsenian-Henriksson, M. (2017). The MYCN Protein in Health and Disease. Genes (Basel) 8. doi:10.3390/genes8040113
Schönherr, C., Ruuth, K., Kamaraj, S., Wang, C.-L., Yang, H.-L., Combaret, V., et al. (2012). Anaplastic Lymphoma Kinase (ALK) Regulates Initiation of Transcription of MYCN in Neuroblastoma Cells. Oncogene 31, 5193–5200. doi:10.1038/onc.2012.12
Schönherr, C., Yang, H.-L., Vigny, M., Palmer, R. H., and Hallberg, B. (2010). Anaplastic Lymphoma Kinase Activates the Small GTPase Rap1 via the Rap1-specific GEF C3G in Both Neuroblastoma and PC12 Cells. Oncogene 29, 2817–2830. doi:10.1038/onc.2010.27
Schramm, A., Köster, J., Assenov, Y., Althoff, K., Peifer, M., Mahlow, E., et al. (2015). Mutational Dynamics between Primary and Relapse Neuroblastomas. Nat. Genet. 47, 872–877. doi:10.1038/ng.3349
Schramm, A., Schulte, J. H., Astrahantseff, K., Apostolov, O., Limpt, V. v., Sieverts, H., et al. (2005). Biological Effects of TrkA and TrkB Receptor Signaling in Neuroblastoma. Cancer Lett. 228, 143–153. doi:10.1016/j.canlet.2005.02.051
Servidei, T., Aoki, Y., Lewis, S. E., Symes, A., Fink, J. S., and Reeves, S. A. (1998). Coordinate Regulation of STAT Signaling and C-fosExpression by the Tyrosine Phosphatase SHP-2. J. Biol. Chem. 273, 6233–6241. doi:10.1074/jbc.273.11.6233
Sharma, B., Xie, L., Yang, F., Wang, W., Zhou, Q., Xiang, M., et al. (2020). Recent advance on PTP1B Inhibitors and Their Biomedical Applications. Eur. J. Med. Chem. 199, 112376. doi:10.1016/j.ejmech.2020.112376
Shen, D., Chen, W., Zhu, J., Wu, G., Shen, R., Xi, M., et al. (2020). Therapeutic Potential of Targeting SHP2 in Human Developmental Disorders and Cancers. Eur. J. Med. Chem. 190, 112117. doi:10.1016/j.ejmech.2020.112117
Shintani, T., Higashi, S., Takeuchi, Y., Gaudio, E., Trapasso, F., Fusco, A., et al. (2015). The R3 Receptor-like Protein Tyrosine Phosphatase Subfamily Inhibits Insulin Signalling by Dephosphorylating the Insulin Receptor at Specific Sites. J. Biochem. 158, 235–243. doi:10.1093/jb/mvv045
Shintani, T., and Noda, M. (2008). Protein Tyrosine Phosphatase Receptor Type Z Dephosphorylates TrkA Receptors and Attenuates NGF-dependent Neurite Outgrowth of PC12 Cells. J. Biochem. 144, 259–266. doi:10.1093/jb/mvn064
Siegel, R. L., Miller, K. D., and Jemal, A. (2020). Cancer Statistics, 2020. CA A. Cancer J. Clin. 70, 7–30. doi:10.3322/caac.21590
Solomon, D. A., Kim, J.-S., Cronin, J. C., Sibenaller, Z., Ryken, T., Rosenberg, S. A., et al. (2008). Mutational Inactivation of PTPRD in Glioblastoma Multiforme and Malignant Melanoma. Cancer Res. 68, 10300–10306. doi:10.1158/0008-5472.can-08-3272
Song, Y., Zhao, M., Zhang, H., and Yu, B. (2021). Double-edged Roles of Protein Tyrosine Phosphatase SHP2 in Cancer and its Inhibitors in Clinical Trials. Pharmacology & therapeutics, 107966. doi:10.1016/j.pharmthera.2021.107966
Stafman, L. L., and Beierle, E. A. (2016). Cell Proliferation in Neuroblastoma. Cancers (Basel) 8. doi:10.3390/cancers8010013
Stallings, R. L., Nair, P., Maris, J. M., Catchpoole, D., McDermott, M., O'Meara, A., et al. (2006). High-resolution Analysis of Chromosomal Breakpoints and Genomic Instability Identifies PTPRD as a Candidate Tumor Suppressor Gene in Neuroblastoma. Cancer Res. 66, 3673–3680. doi:10.1158/0008-5472.can-05-4154
Stanford, S. M., and Bottini, N. (2017). Targeting Tyrosine Phosphatases: Time to End the Stigma. Trends Pharmacological Sciences 38, 524–540. doi:10.1016/j.tips.2017.03.004
Stuible, M., Doody, K. M., and Tremblay, M. L. (2008). PTP1B and TC-PTP: Regulators of Transformation and Tumorigenesis. Cancer Metastasis Rev. 27, 215–230. doi:10.1007/s10555-008-9115-1
Tajan, M., de Rocca Serra, A., Valet, P., Edouard, T., and Yart, A. (2015). SHP2 Sails from Physiology to Pathology. Eur. J. Med. Genet. 58, 509–525. doi:10.1016/j.ejmg.2015.08.005
Takahashi, H., Arstikaitis, P., Prasad, T., Bartlett, T. E., Wang, Y. T., Murphy, T. H., et al. (2011). Postsynaptic TrkC and Presynaptic PTPσ Function as a Bidirectional Excitatory Synaptic Organizing Complex. Neuron 69, 287–303. doi:10.1016/j.neuron.2010.12.024
Tamura, H., Fukada, M., Fujikawa, A., and Noda, M. (2006). Protein Tyrosine Phosphatase Receptor Type Z Is Involved in Hippocampus-dependent Memory Formation through Dephosphorylation at Y1105 on P190 RhoGAP. Neurosci. Lett. 399, 33–38. doi:10.1016/j.neulet.2006.01.045
Tiganis, T. (2013). PTP1B and TCPTP - Nonredundant Phosphatases in Insulin Signaling and Glucose Homeostasis. FEBS J. 280, 445–458. doi:10.1111/j.1742-4658.2012.08563.x
Tintori, C., Fallacara, A. L., Radi, M., Zamperini, C., Dreassi, E., Crespan, E., et al. (2015). Combining X-ray Crystallography and Molecular Modeling toward the Optimization of Pyrazolo[3,4-D]pyrimidines as Potent C-Src Inhibitors Active In Vivo against Neuroblastoma. J. Med. Chem. 58, 347–361. doi:10.1021/jm5013159
Tolbert, V. P., and Matthay, K. K. (2018). Neuroblastoma: Clinical and Biological Approach to Risk Stratification and Treatment. Cel Tissue Res 372, 195–209. doi:10.1007/s00441-018-2821-2
Tonks, N. K. (2013). Protein Tyrosine Phosphatases - from Housekeeping Enzymes to Master Regulators of Signal Transduction. FEBS J. 280, 346–378. doi:10.1111/febs.12077
Tonks, N. K. (2006). Protein Tyrosine Phosphatases: from Genes, to Function, to Disease. Nat. Rev. Mol. Cel Biol 7, 833–846. doi:10.1038/nrm2039
Torres, J., Blanco-Aparicio, C., and Pulido, R. (2004). Regulation of MAPK Cascades by Protein Tyrosine Phosphatases. Methods Mol. Biol. 250, 103–112. doi:10.1385/1-59259-671-1:103
Trigg, R. M., and Turner, S. D. (2018). ALK in Neuroblastoma: Biological and Therapeutic Implications. Cancers (Basel) 10. doi:10.3390/cancers10040113
Tsubota, S., and Kadomatsu, K. (2018). Origin and Initiation Mechanisms of Neuroblastoma. Cel Tissue Res 372, 211–221. doi:10.1007/s00441-018-2796-z
Turdo, A., D’Accardo, C., Glaviano, A., Porcelli, G., Colarossi, C., Colarossi, L., et al. (2021). Targeting Phosphatases and Kinases: How to Checkmate Cancer. Front. Cel Dev. Biol. 9, 690306. doi:10.3389/fcell.2021.690306
Um, J. W., and Ko, J. (2013). LAR-RPTPs: Synaptic Adhesion Molecules that Shape Synapse Development. Trends Cell Biology 23, 465–475. doi:10.1016/j.tcb.2013.07.004
Uçkun, E., Siaw, J. T., Guan, J., Anthonydhason, V., Fuchs, J., Wolfstetter, G., et al. (2021). BioID-Screening Identifies PEAK1 and SHP2 as Components of the ALK Proximitome in Neuroblastoma Cells. J. Mol. Biol. 433, 167158. doi:10.1016/j.jmb.2021.167158
Valencia-Sama, I., Ladumor, Y., Kee, L., Adderley, T., Christopher, G., Robinson, C. M., et al. (2020). NRAS Status Determines Sensitivity to SHP2 Inhibitor Combination Therapies Targeting the RAS-MAPK Pathway in Neuroblastoma. Cancer Res. 80, 3413–3423. doi:10.1158/0008-5472.can-19-3822
van Ham, M., Kemperman, L., Wijers, M., Fransen, J., and Hendriks, W. (2005). Subcellular Localization and Differentiation-Induced Redistribution of the Protein Tyrosine Phosphatase PTP-BL in Neuroblastoma Cells. Cell Mol Neurobiol 25, 1225–1244. doi:10.1007/s10571-005-8500-3
Veeriah, S., Brennan, C., Meng, S., Singh, B., Fagin, J. A., Solit, D. B., et al. (2009). The Tyrosine Phosphatase PTPRD Is a Tumor Suppressor that Is Frequently Inactivated and Mutated in Glioblastoma and Other Human Cancers. Proc. Natl. Acad. Sci. 106, 9435–9440. doi:10.1073/pnas.0900571106
Wang, L. L., Teshiba, R., Ikegaki, N., Tang, X. X., Naranjo, A., London, W. B., et al. (2015). Augmented Expression of MYC And/or MYCN Protein Defines Highly Aggressive MYC-Driven Neuroblastoma: a Children's Oncology Group Study. Br. J. Cancer 113, 57–63. doi:10.1038/bjc.2015.188
Ward, E., DeSantis, C., Robbins, A., Kohler, B., and Jemal, A. (2014). Childhood and Adolescent Cancer Statistics, 2014. CA A Cancer J. Clinicians 64, 83–103. doi:10.3322/caac.21219
Westermark, U. K., Wilhelm, M., Frenzel, A., and Henriksson, M. A. (2011). The MYCN Oncogene and Differentiation in Neuroblastoma. Semin. Cancer Biol. 21, 256–266. doi:10.1016/j.semcancer.2011.08.001
Wiede, F., Lu, K. H., Du, X., Liang, S., Hochheiser, K., Dodd, G. T., et al. (2020). PTPN2 Phosphatase Deletion in T Cells Promotes Anti-tumour Immunity and CAR T-Cell Efficacy in Solid Tumours. EMBO J. 39, e103637. doi:10.15252/embj.2019103637
Williams, A. P., Garner, E. F., Waters, A. M., Stafman, L. L., Aye, J. M., Markert, H., et al. (2019). Investigation of PP2A and its Endogenous Inhibitors in Neuroblastoma Cell Survival and Tumor Growth. Translational Oncol. 12, 84–95. doi:10.1016/j.tranon.2018.09.011
Wu, C.-L., Hardy, S., Aubry, I., Landry, M., Haggarty, A., Saragovi, H. U., et al. (2017). Identification of Function-Regulating Antibodies Targeting the Receptor Protein Tyrosine Phosphatase Sigma Ectodomain. PloS one 12, e0178489. doi:10.1371/journal.pone.0178489
Wu, J., and Song, Y. (2018). Expression and Clinical Significance of Serum MMP-7 and PTEN Levels in Patients with Acute Myeloid Leukemia. Oncol. Lett. 15, 3447–3452. doi:10.3892/ol.2018.7799
Xia, Z., Ouyang, D., Li, Q., Li, M., Zou, Q., Li, L., et al. (2019). The Expression, Functions, Interactions and Prognostic Values of PTPRZ1: A Review and Bioinformatic Analysis. J. Cancer 10, 1663–1674. doi:10.7150/jca.28231
Yamashiro, D. J., Liu, X.-G., Lee, C. P., Nakagawara, A., Ikegaki, N., McGregor, L. M., et al. (1997). Expression and Function of Trk-C in Favourable Human Neuroblastomas. Eur. J. Cancer 33, 2054–2057. doi:10.1016/s0959-8049(97)00309-2
Yan, S., Li, Z., and Thiele, C. J. (2013). Inhibition of STAT3 with Orally Active JAK Inhibitor, AZD1480, Decreases Tumor Growth in Neuroblastoma and Pediatric SarcomasIn vitroandIn Vivo. Oncotarget 4, 433–445. doi:10.18632/oncotarget.930
Yang, T., Massa, S. M., and Longo, F. M. (2006). LAR Protein Tyrosine Phosphatase Receptor Associates with TrkB and Modulates Neurotrophic Signaling Pathways. J. Neurobiol. 66, 1420–1436. doi:10.1002/neu.20291
Yao, Z., Darowski, K., St-Denis, N., Wong, V., Offensperger, F., Villedieu, A., et al. (2017). A Global Analysis of the Receptor Tyrosine Kinase-Protein Phosphatase Interactome. Mol. Cel. 65, 347–360. doi:10.1016/j.molcel.2016.12.004
Yogev, O., Almeida, G. S., Barker, K. T., George, S. L., Kwok, C., Campbell, J., et al. (2019). In Vivo Modeling of Chemoresistant Neuroblastoma Provides New Insights into Chemorefractory Disease and Metastasis. Cancer Res. 79, 5382–5393. doi:10.1158/0008-5472.can-18-2759
Youssef, G., Gillett, C., Rampling, D., Chagtai, T., Virasami, A., Barton, J., et al. (2019). The Presence of Y674/Y675 Phosphorylated NTRK1 via TP53 Repression of PTPN6 Expression as a Potential Prognostic Marker in Neuroblastoma. Hum. Pathol. 86, 182–192. doi:10.1016/j.humpath.2018.12.003
Yuan, X., Bu, H., Zhou, J., Yang, C.-Y., and Zhang, H. (2020). Recent Advances of SHP2 Inhibitors in Cancer Therapy: Current Development and Clinical Application. J. Med. Chem. 63, 11368–11396. doi:10.1021/acs.jmedchem.0c00249
Zanke, B., Suzuki, H., Kishihara, K., Mizzen, L., Minden, M., Pawson, A., et al. (1992). Cloning and Expression of an Inducible Lymphoid-specific, Protein Tyrosine Phosphatase (HePTPase). Eur. J. Immunol. 22, 235–239. doi:10.1002/eji.1830220134
Zhang, D., Marlin, M. C., Liang, Z., Ahmad, M., Ashpole, N. M., Sonntag, W. E., et al. (2016). The Protein Tyrosine Phosphatase MEG2 Regulates the Transport and Signal Transduction of Tropomyosin Receptor Kinase A. J. Biol. Chem. 291, 23895–23905. doi:10.1074/jbc.m116.728550
Keywords: protein tyrosine phosphatases, neuroblastoma, biomarker, cell signaling, phosphorylation, dephosphorylation
Citation: Nunes-Xavier CE, Zaldumbide L, Mosteiro L, López-Almaraz R, García de Andoin N, Aguirre P, Emaldi M, Torices L, López JI and Pulido R (2021) Protein Tyrosine Phosphatases in Neuroblastoma: Emerging Roles as Biomarkers and Therapeutic Targets. Front. Cell Dev. Biol. 9:811297. doi: 10.3389/fcell.2021.811297
Received: 08 November 2021; Accepted: 23 November 2021;
Published: 08 December 2021.
Edited by:
Pedro A. Lazo, Spanish National Research Council (CSIC), SpainReviewed by:
Jeroen Den Hertog, Hubrecht Institute (KNAW), NetherlandsFabio Luis Forti, University of São Paulo, Brazil
Michel L. Tremblay, McGill University, Canada
Copyright © 2021 Nunes-Xavier, Zaldumbide, Mosteiro, López-Almaraz, García de Andoin, Aguirre, Emaldi, Torices, López and Pulido. This is an open-access article distributed under the terms of the Creative Commons Attribution License (CC BY). The use, distribution or reproduction in other forums is permitted, provided the original author(s) and the copyright owner(s) are credited and that the original publication in this journal is cited, in accordance with accepted academic practice. No use, distribution or reproduction is permitted which does not comply with these terms.
*Correspondence: Caroline E. Nunes-Xavier, Y2Fyb2xpbmVudW5lc3hhdmllckBnbWFpbC5jb20=; Rafael Pulido, cnB1bGlkb211cmlsbG9AZ21haWwuY29t