- 1Department of Nephrology, Beijing Friendship Hospital, Capital Medical University, Beijing, China
- 2Department of Radiology, Fudan University Shanghai Cancer Center, Shanghai, China
- 3Department of Orthopaedic Surgery, University of Pittsburgh School of Medicine, Pittsburgh, PA, United States
- 4McGowan Institute for Regenerative Medicine, University of Pittsburgh School of Medicine, Pittsburgh, PA, United States
- 5Department of Orthopedic Surgery, Shanghai Sixth People’s Hospital, Shanghai Jiaotong University, Shanghai, China
In the past 3 decades, the cartilage repair potential of mesenchymal stromal cells, or mesenchymal stem cells (MSCs), has been widely examined in animal studies. Unfortunately, the phenotype and physical properties of MSC-derived cartilage tissue are not comparable to native hyaline cartilage. In particular, chondrocytic hypertrophy, a phenotype that is not observed in healthy hyaline cartilage, is concomitant with MSC chondrogenesis. Given that hypertrophic chondrocytes potentially undergo apoptosis or convert into osteoblasts, this undesired phenotype needs to be prevented or minimized before MSCs can be used to repair cartilage injuries in the clinic. In this review, we first provide an overview of chondrocytic hypertrophy and briefly summarize current methods for suppressing hypertrophy in MSC-derived cartilage. We then highlight recent progress on modulating the canonical Wnt/β-catenin pathway for inhibiting hypertrophy. Specially, we discuss the potential crosstalk between Wnt/β-catenin with other pathways in regulating hypertrophy. Lastly, we explore future perspectives to further understand the role of Wnt/β-catenin in chondrocytic hypertrophy.
Introduction
Articular cartilage, an essential component of the joint organ, helps maintain stability, distribute force and reduce friction during physical activity. Once damaged by trauma, disease, or aging, it is unable to fully self-heal due to its avascular nature (Shi et al., 2016). Consequently, focal cartilage defects may gradually destroy adjacent cartilage and negatively influence other joint components, eventually leading to the onset of osteoarthritis (OA) (Chen et al., 2006; Deng et al., 2019b).
Currently, several strategies are clinically used to treat focal cartilage defects, including autograft and allograft transplantation, microfracture, and autologous chondrocyte transplantation (ACI) (Chen et al., 2015). However, each of these techniques has its own limitations (Ahmed and Hincke, 2010). For example, autograft transplantation relies on the transfer of osteochondral tissue from a non-weight-bearing or lesser weight-bearing region to the defective area, for which the donor tissue may not be mechanically suited (Kon et al., 2013). The use of allografts may induce immunological rejection (Bugbee et al., 2012). The core technology of microfracture is drilling into the subchondral bone to release bone marrow, allowing marrow-derived stem cells to migrate into the defective area to facilitate the healing process. Unfortunately, the neo-cartilage generated after microfracture consists mainly of fibrocartilage, not hyaline cartilage (Mithoefer et al., 2009). Consequently, the neo-cartilage degrades in several years. ACI involves the extraction of chondrocytes from a non-weight-bearing area, expansion of the cells in vitro, and re-introduction into the defect area. This technique eliminates the possibility of an immune response, but the proliferation process is always accompanied by the loss of chondrocytic phenotype and function, known as dedifferentiation (Kon et al., 2009). In addition, the quality control for chondrocytes before implantation has not been established, which likely results in highly variable reparative outcomes (Pietschmann et al., 2012).
In the past 3 decades, the chondroinduction of mesenchymal stem/stromal cells (MSCs) has become a popular method to generate cartilage tissue for the repair of cartilage injury in animal models (Caplan, 1991). Furthermore, some clinical trials have shown the potential of MSC-based cartilage tissue engineering in the repair of chondral defects in humans (Gupta et al., 2016; Park et al., 2017; Qiao et al., 2020), but a greater sample size is required to confirm the reparative results and phenotype of newly formed cartilage. In spite of this exciting progress, extensive in vitro data and animal studies have shown that MSC-derived cartilage does not fully recapitulate the structure or composition of hyaline cartilage matrix (Bian et al., 2013; Watts et al., 2013; Yang et al., 2018). In particular, chondrocytic hypertrophy-relevant molecules, such as collagen type X (COL10) and Indian hedgehog (IHH), are found to be highly expressed in MSCs-derived cartilage (Chen et al., 2015), which are not observed in healthy hyaline cartilage. This undesired phenotype represents one of the major obstacles to the clinical application of MSCs for repairing articular cartilage. In this review, we first provide an overview of hypertrophy in MSC-derived cartilage, and briefly summarize current methods used in suppressing hypertrophy. Second, we focus on recent progress in the modulation of the canonical Wnt/β-catenin pathway for inhibiting hypertrophy. Lastly, we discuss the crosstalk between Wnt/β-catenin and other pathways in regulating hypertrophy.
Hypertrophic Chondrocytes in Growth Plate
During endochondral ossification in skeletal development, several sequential events are observed, including cellular condensation of mesenchymal precursor cells, chondrogenic differentiation, hypertrophy and vascularization/osteogenesis (Sun and Beier, 2014). These stages are also clearly observed within the fetal growth plate, in which resting zone, proliferating zone, hypertrophic zone and osteogenic zones are clearly and simultaneously observed (Figure 1A). Hypertrophic chondrocytes contribute to skeletal development by increasing cell volume and tissue size. These cells also remodel the matrix to support osteogenesis, which is mainly characterized by the expression of runt-related transcription factor 2 (RUNX2), matrix metalloproteinase (MMP)-13, COL10, alkaline phosphatase (ALP) and osteogenic growth factors, such as bone morphogenetic protein (BMPs), IHH, and vascular endothelial growth factor (VEGF) among others (Sun and Beier, 2014).
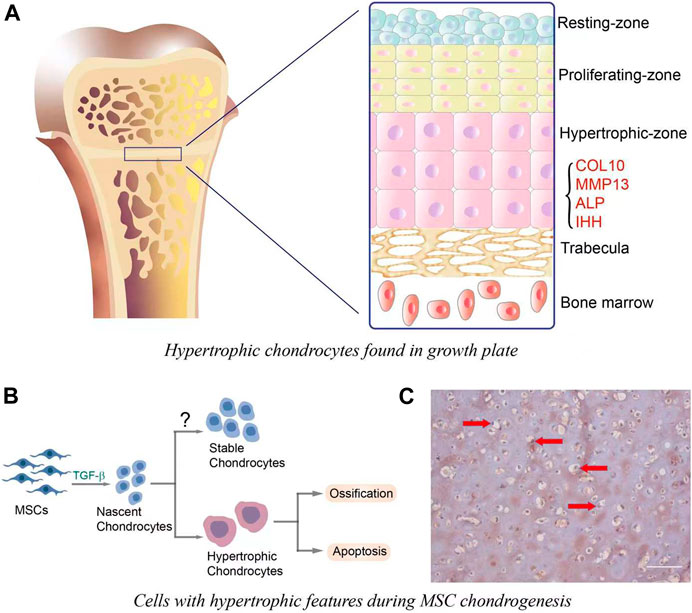
FIGURE 1. (A). Hypertrophic chondrocytes observed in growth plate. Cells in the resting-zone, located at the end of bone, are round and small. Proliferating zone is adjacent to the resting-zone, and chondrocytes in this zone have the ability to proliferate and differentiate. The differentiation is also accompanied by the increase of cell size and the acquisition of hypertrophic phenotype, which is mainly characterized by the expression of hypertrophic markers, such as COL10, MMP-13, ALP, and IHH. (B). In vitro MSC chondrogenesis and hypertrophic transition. In the initiation stage (∼7 days) of chondrogenesis, MSCs are differentiated into nascent chondrocytes, which then undergo hypertrophy and the following ossification or apoptosis. It is important to note that nascent MSCs-derived chondrocytes may have the potential to become stable chondrocytes, yet this has not been completely achieved. (C). COL10 immunostaining for MSCs-laden hyaluronic acid (HA) construct that was subjected to 21 days of chondrogenic culture. The hypertrophic chondrocytes were characterized by large cell volume and the deposition of COL10 (brown staining, red arrows). Scale bar = 100 μm.
Cellular Hypertrophy in MSC Chondrogenesis
When MSCs are induced to undergo chondrogenesis with medium containing transforming growth factor (TGF-β), the newly formed cartilage recapitulates many features observed in hypertrophic chondrocytes in bone development, such as large cell size and high expression of hypertrophy-relevant markers (Chen et al., 2015) (Figures 1B,C). Therefore, the term “hypertrophy” was adapted to describe the phenotype of chondroinduced MSCs. Of note, the health states of donors and tissue sources affect MSC hypertrophy levels in both naïve and chondroinduced conditions. For example, the basal COL10 protein level in MSCs from donors with osteoarthritis (OA) was systematically (4.9 ± 1.2-fold) higher than that in normal MSCs (Mwale et al., 2010). After chondroinduction, articular cartilage-derived MSCs from osteoarthritis patients (OA-MSC) displayed higher expression of RUNX2 when compared to the cells cultured in the growth medium (Hu et al., 2019). In comparison, the level of RUNX2 in MSCs from bone marrow (BMSCs) did not change after chondrogenic culture. Moreover, the peak of COL10A1 expression in chondroinduced OA-MSCs appeared earlier (on day 7) than BMSCs (on day 14), implying their intrinsic difference in hypertrophy potential (Liu et al., 2020). Therefore, the selection of MSC for cartilage repair requires careful considerations, which may significantly influence the quality of regenerated cartilage.
Currently, the mechanistic or physiologic sameness of in vivo and in vitro hypertrophy is not clear. A comprehensive and in-depth comparison of hypertrophy between these two processes is lacking. It has been demonstrated that hypertrophic transition in bone development is spatiotemporally regulated. For instance, IHH, released by hypertrophic chondrocytes, stimulates the production of Parathyroid hormone-related peptide (PTHrP), which not only induces resting chondrocytes into proliferating chondrocytes, but also negatively suppresses hypertrophy (Kronenberg, 2003). Panexxin3 and CCAAT enhancer binding protein beta (C/EBPβ), generated by pre-hypertrophic chondrocytes, and plays an essential role in promoting the transition of proliferative chondrocytes to hypertrophic chondrocytes (Marino, 2011). This well-orchestrated regulation and feedback network does not exist in MSC-derived chondrogenesis and hypertrophic transition.
As of now, it is not clear if MSCs can form stable chondrocytes with features similar to cells in hyaline cartilage. Many studies have shown that the hypertrophic chondrocytes derived form TGFβ-stimulated MSCs undergo ossification and apoptosis when tested in murine subcutaneous or intramuscular implantation experiments (Mueller and Tuan, 2008; Bian et al., 2013; Lee et al., 2013; Zhu et al., 2017). However, whether intraarticularly implanted MSCs-derived chondrocytes also undergo osteogenesis and/or apoptosis in humans is not conclusively known.
Currently, the exact mechanism underlying hypertrophy is not clear. Based on current evidence, it is hypothesized that chondrocytic hypertrophy is associated with high level of RUNX2 and activation of Smad1/5 signaling upon TGF-β treatment (Mueller and Tuan, 2008; Chen et al., 2015). Several pathways, including the BMP, TGF-β, fibroblast growth factor (FGF) and wingless-related integration site (Wnt) pathways (Yang et al., 2001; Yu et al., 2010; Shen et al., 2013; Wang et al., 2014; Zhong et al., 2015), were shown to regulate this process. In particular, low TGFβ type I receptor/BMP type I receptor ratio was shown to account for the high hypertrophy potential of naïve OA-MSCs (Liu et al., 2020). In the past two decades, numerous strategies have been tested to suppress hypertrophy during MSC chondrogenesis. For example, supplementing fibroblast growth factor (FGF)-9 or 18 after the initiation of chondrogenesis (≥14 days) suppressed hypertrophy-related changes, which functioned through FGF receptor-3 (Correa et al., 2015). Browe et al., found that hypoxia culture attenuated the hypertrophy phenotype during MSCs chondrogenesis through modulating the parathyroid hormone-related peptide (PTHrP)-Myocyte Enhancer Factor 2C (MEF2C) pathway (Browe et al., 2019). Curcumin also suppressed MSC hypertrophy through inhibiting IHH and Notch signaling (Cao et al., 2017). Among these methods, treatments that suppress Wnt/β-catenin signaling pathway have showed the most promising results in reducing hypertrophy level (Yang et al., 2012; Narcisi et al., 2015; Zhong et al., 2016), which are specifically discussed in this review.
Canonical Wnt/β-Catenin Pathway in Chondrogenesis
The expansion of MSCs is partially driven by the Wnt pathway, which also maintains their potential (ten Berge et al., 2008; Cooper et al., 2011). Of note, the regulation of Wnt signaling depends not only on the presence of ligands but also on the action of endogenous antagonists of Wnt signaling (Usami et al., 2016). Numerous studies have shown that β-catenin-mediated canonical Wnt signaling exhibits the suppression of chondrogenesis (Day et al., 2005; Dong et al., 2007; Churchman et al., 2012; Wang et al., 2021). However, Deng et al. showed that de novo inhibiting β-catenin with XAV939 throughout a 21-days MSC chondrogenic pellet culture completely blocked the deposition of cartilage matrix and expression of chondrogenic markers (Deng et al., 2019a). Therefore, the exact role of the canonical Wnt/β-catenin pathway in MSCs chondrogenesis needs further investigation.
Inhibition of Hypertrophy Through Modulating Wnt/β-Catenin Pathway
Extensive evidence has shown that Wnt pathway participates in chondrocytic hypertrophy during MSC chondrogenesis. For instance, the study from Yang et al. found that transient stimulation of the Wnt pathway promoted the expression of hypertrophic phenotype during the chondrogenic differentiation of MSCs in pellet culture (Yang et al., 2012). Melatonin induced chondrogenic hypertrophy, which resulted from upregulated Wnt/β-catenin signaling (Wang et al., 2021). Interestingly, the basal level of β-catenin protein in BMSCs isolated from OA patients was also significantly higher than BMSCs from healthy donors (Tornero-Esteban et al., 2015), suggesting Wnt/β-catenin may also account for the high hypertrophy level in native OA MSCs. However, the relevant studies have not been reported yet.
In contrast, inhibiting Wnt signaling with IWP2 (Narcisi et al., 2015; Diederichs et al., 2019b), DKK1 (Rojas et al., 2019), XAV939 (Deng et al., 2019a; Wang et al., 2021), PKF (Huang et al., 2018) prevented hypertrophic maturation of MSC-derived cartilage. It is important to note that the timing of introducing Wnt inhibitors needs to be carefully considered. Deng et al. found that the addition of XAV939 from the beginning of chondrogenic culture blocked both hypertrophy and chondrogenesis (Deng et al., 2019a). In this study, MSCs were encapsulated in hyaluronic acid-based scaffolds, so it is not clear whether such a phenomenon translates to other culture systems.
Regarding the mechanism, there are not too many relevant studies. The study by Deng et al. demonstrated that XAV939 reduced hypertrophy level by inhibiting the Smad1/5-RUNX2 pathway (Deng et al., 2019a). In another study, IWP-2 was found to suppress hypertrophy through inhibiting the gene expression of BMP7 and Gli1, which are associated with BMP and IHH pathways (Diederichs et al., 2019b). Dreher et al. also reported a reduction in MSC-derived chondrocyte hypertrophy following IWP-2 inhibition of Wnt signaling. However, the mechanism discovered was different (Dreher et al., 2020). Specifically, IWP-2 was shown to inhibit hypertrophy through decreasing levels of myocyte enhancer factor 2C (MEF2C) and RUNX3. Interestingly, this study also showed that the absence of RUNX2 did not prevent hypertrophy. Therefore, Wnt inhibitors suppress hypertrophy through different mechanisms, which highly depend on their respective direct target(s). The resulting inhibition is also dependent on the cell types and culture platforms, such as two-dimensional versus three-dimensional cultures, and pellet versus scaffold cultures.
Interaction of Wnt/β-Catenin Signaling With Other Pathways in Hypertrophy
Currently, the network between Wnt signaling and other pathways during hypertrophy has not been established. In this review, we would like to summarize current findings from different studies and map the network. In the study of Bouaziz et al., it was found that the hypoxia-inducible factor 1α (HIF1α)-β-catenin interaction was a negative regulator of Wnt signaling and MMP-13 transcription (Bouaziz et al., 2016). Co-transfection of lymphoid enhancer binding factor 1 (LEF1) and β-catenin in chicken sternal chondrocytes induced hypertrophy through activating RUNX2, which was inhibited by high-mobility group box 2 (HMGB2) (Taniguchi et al., 2011). Zhu et al. found that downregulation of α-B-crystallin (CRYAB) reduced RUNX2 level through canonical Wnt/β-catenin pathway, resulting in a lower potential level of hypertrophy/osteogenesis. They also determined that CRYAB can interact with β-catenin and protect it from ubiquitination and degradation (Zhu et al., 2020). Recently, Riedl et al. reported that MSC-based pellet cultures underwent hypertrophy when retinoic acid receptor (RAR) pathway was activated. As a result of RAR activation, there was increased expression of Wnt2b and Wnt5a, which subsequently increased Wnt/β-catenin pathway activity (Riedl et al., 2020).
Yes-associated protein (YAP) and transcriptional coactivator with PDZ-binding motif (TAZ) signaling are important in the development of cartilage (Vanyai et al., 2020). Specifically, low level of yes-associated protein (YAP), a transcription factor of the Hippo pathway, was associated with the formation of articular cartilage from MSCs (Lee et al., 2020). Using murine models, Deng et al. demonstrated that Yap1 overexpression significantly reduced COL10A1 level, which however was at the expense of reduced chondrogenesis. In contrast, suppressing YAP with siRNA promoted the expression of both COL2A1 and COL10A1 without selection (Deng et al., 2016). Recently, mechanical loading, under certain conditions, was shown to suppress hypertrophy (Aisenbrey et al., 2019). In the study by Lee et al., MSCs cultured in 3D chondrogenic combinatorial system with 40% strain showed a trend toward hypertrophic chondrocytes, indicated by the high level of RUNX2, which was also accompanied with more YAP expression (Lee et al., 2020). Of note, the association between mechanical loading and Wnt/β-catenin has been previously demonstrated (Sen et al., 2011; He et al., 2020). These observations raise the question, how does the YAP pathway interact with Wnt/β-catenin in hypertrophy? To the best of our knowledge, no relevant study has been published that directly addresses this question. However, interactions between YAP and Wnt/β-catenin have been previously explored in other cell types. In studies of colon cancer, the inhibition of Wnt signaling suppressed the expression of YAP. Conversely, YAP could be upregulated by activating Wnt pathway (Konsavage et al., 2012; Park et al., 2015). During the fibrosis process, stimulation of Wnt pathway caused dissociation of YAP from β-catenin destruction complex after they translocate to nucleus and modulate downstream target genes (Piersma et al., 2015). Unfortunately, the Wnt/β-catenin pathway was not simultaneously investigated in this study. Based on the findings above, we speculate that Wnt/β-catenin is upstream of the YAP pathway in regulating chondrocytic hypertrophy, which should be further explored in the future.
The molecules related to histone modification were also shown to participate in hypertrophy through Wnt pathways. Cornelis et al. found that Disruptor of telomeric silencing 1-like (Dot1L)-deficient mice could present Wnt signaling hyper-activation and ectopic chondrocyte hypertrophy (Cornelis et al., 2019). The study by Chen et al. demonstrated that inhibition of histone methyltransferase enhancer of zeste homologue 2 (EZH2) inhibited IHH, MMP-13, ADAMTS-5 and COL10A1 expression, thus ameliorating OA development (Chen et al., 2016). Interestingly, this study also showed that inhibition of EZH2 silenced β-catenin signaling. A genome-wide analysis has shown that the Wnt/β-catenin transcriptome was inhibited after regulating of HDAC (Chen et al., 2011). Moreover, in the study of Smith et al., the Wnt-related pathway’s transcription levels were restricted by overexpression of HDAC (Smith and Frenkel, 2005). In light of these findings, we summarized the potential interaction of Wnt pathways and other molecules and pathways controlling hypertrophy in Figure 2. In brief, when TGF-β binds to its receptor Activin-receptor like kinase (ALK) 1 accompanied with its co-receptor CD105, it phosphorylates Smad1/5 and stimulates the transcription of RUNX2, RUNX3 and MEF2, initiating the hypertrophy process. The activation of Smad1/5 is also regulated by Wnt-β-catenin, YAP (Gabriel et al., 2016) and IHH (Handorf et al., 2015). Particularly, the Wnt/β-catenin pathway plays an essential role in regulating chondrocytic hypertrophy, which is activated by EZH2 and inhibited by DOT1L, HDAC4, DKK1. XAV939 and other β-catenin inhibitors, such as IWP-2 and PKF, eventually inhibit Smad1/5, IHH and YAP, which collectively reduce chondrocytic hypertrophy.
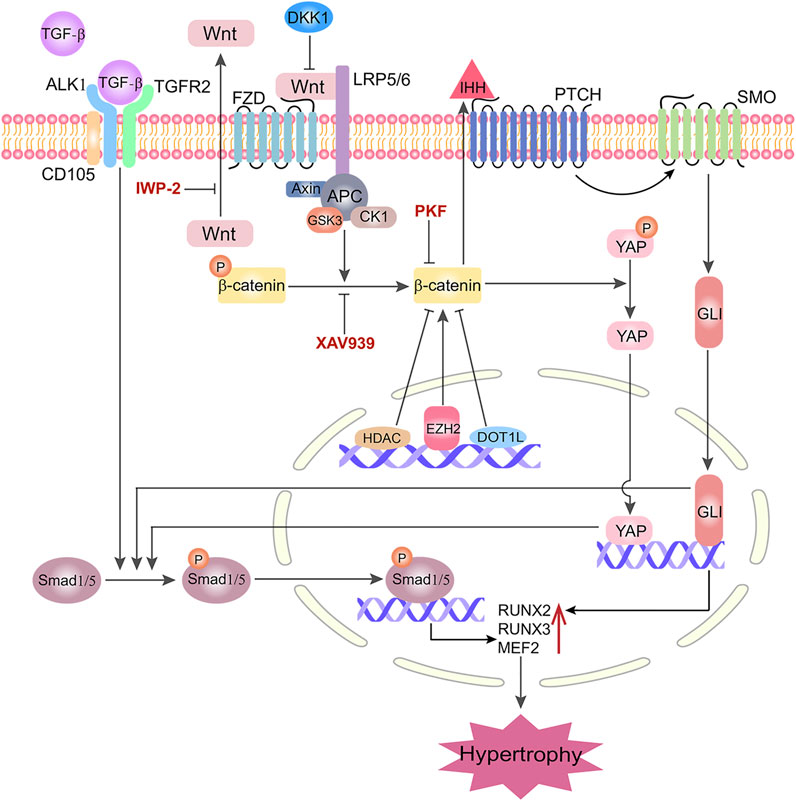
FIGURE 2. Interactions of Wnt/β-catenin signaling with other molecules/pathways in governing chondrogenic hypertrophy in MSC chondrogenesis. LRP5/6: Lipoprotein Receptor-Related Protein 5/6; FZD: Frizzled; ALK1: Activin Receptor-Like kinase 1; CD105: Endoglin; TGFR2: TGF-Receptor 2; PTCH: Patched; SMO: Smoothened; GlI: Cubitus interruptus; MEF2: Myocyte enhancer factor; DC: Destruction Complex; APC: Adenomatous Polyposis Coli; CK1: Casein kinase 1; GSK3: Glycogen synthase kinase 3; EZH2: Zeste homologue 2; DOT1L: Disruptor of telomeric silencing 1-like; HDAC4: Histone deacetylase 4; IHH: Indian Hedgehog; YAP: Yes-associated protein; SIRT1: Silent mating type information regulator 2 homolog 1; XAV939, IWP-2, PKF and DKK1 are representative Wnt/β-catenin inhibitors in suppressing hypertrophy.
Conclusion and Future Perspectives
Chondrocytic hypertrophy remains a crucial obstacle when developing MSC-based therapy for the repair of hyaline cartilage injury. The hypertrophic phenotype in MSC-derived cartilage is most identified and characterized in vitro, or in animal models that are often used to assess bone formation, such as subcutaneous or intramuscular implantation. Whether MSC-derived chondrocytes also maintain hypertrophic features after long-term intraarticular implantation in humans is not clear. Of note, the concentration of active TGF-β in native synovial is around 60 pg/ml (de Sousa et al., 2019), which is significantly lower than that are used in inducing MSC chondrogenesis in vitro (1–10 ng/ml). In addition, the hypoxic environment found in the knee joint may also suppress hypertrophy. Therefore, the potential to generate hyaline cartilage from MSCs within native joints is still possible, which however needs to be validated in clinical studies.
The critical role of Wnt/β-catenin in promoting hypertrophy has been reviewed above. Largely due to our limited knowledge about the pathways controlling hypertrophy, the molecular mediation of Wnt and hypertrophic gene expression need further study. Current evidence suggests that RUNX2 may account for the hypertrophy phenotype. However, knocking down RUNX2 did not entirely suppress COL10A1 expression (Chen et al., 2020), which suggests that RUNX2 may not be the only transcriptional factor controlling COL10A1 expression (Dreher et al., 2020). As described above, activation of the IHH pathway and YAP upregulated RUNX2 expression and function (Shimoyama et al., 2007). Studying the interactions of the Wnt pathway, IHH, and YAP in the context of MSC hypertrophy will shed light on the network that dictates hypertrophy. Recently, iPSCs-derived multipotent cells displayed lower hypertrophy potential than MSCs after chondroinduction (Diederichs et al., 2019a). However, the underlying mechanism is unknown. Comparison of these two types of cells through an omics-based method, such as RNA-Sequencing, may enable the identification of new molecules that dictate the hypertrophy process.
Although the similarity of these two processes requires further investigation, knowledge from cells within the epiphyseal growth plate should still be informative for future study of in vitro hypertrophy of chondroinduced MSCs. The comparison between resting and hypertrophic chondrocytes in the growth plate confirmed that the cells in the resting zone are maintained in a Wnt-inhibitory environment (Hallett et al., 2021).
The use of spatial transcriptomics and/or proteomics in the growth plate can precisely define gene and protein levels in these 2 cell types. These findings can potentially identify the molecules involved in the network that governs hypertrophy. Presently, similar studies have not been reported.
Another strategy is to mine publicly available data, in particular omics data. Current studies associated with these datasets only investigated a small fraction of data points. We can use machine learning and other bioinformatic strategies to analyze large quantities of data that may assist in improved mapping of the hypertrophic development network. For example, Ochsner et al. developed a web knowledgebase, which incorporates the nodes of signaling pathways (receptors, enzymes, transcription factors and co-nodes) and their cognate bioactive small molecules (Ochsner et al., 2019). This tool enabled the prediction of pathway node-gene target transcriptional regulatory relationships.
Currently, extensive Wnt inhibitors that target different components in this pathway have been reported. Screening Wnt inhibitors that displayed high hypertrophy-suppressing potential with low side-effect is also critical. Recently, SM04690, a kind of small-molecule Wnt pathway inhibitor, was shown to be effective in treating OA. In particular, SM04690 may protect chondrocytes from dedifferentiation and hypertrophic chondrocyte conversion (Deshmukh et al., 2018). Given the demonstrated safety of SM04690, this new type of Wnt inhibitor is ready for clinical trials to enhance MSCs-based therapy for repairing articular cartilage in humans.
Lastly, the Wnt pathway is critical in maintaining cell function and viability. For example, long-term treatment with PKF, a Wnt inhibitor, induced cell apoptosis in MSCs-derived cartilage (Huang et al., 2018). Therefore, the timing and duration of suppressing Wnt/β-catenin pathway to reduce hypertrophy require careful investigation.
Author Contributions
All authors listed above have made a substantial, direct, and intellectual contribution to the work, and approved for publication.
Funding
This work was sponsored by Department of Orthopaedic Surgery at the University of Pittsburgh School of Medicine (to HL), Shanghai Sailing Program (21YF1422800, to YD), and Beijing Friendship Hospital The Seed Program (YYZZ202044, to XW).
Conflict of Interest
The authors declare that the research was conducted in the absence of any commercial or financial relationships that could be construed as a potential conflict of interest.
Publisher’s Note
All claims expressed in this article are solely those of the authors and do not necessarily represent those of their affiliated organizations, or those of the publisher, the editors and the reviewers. Any product that may be evaluated in this article, or claim that may be made by its manufacturer, is not guaranteed or endorsed by the publisher.
References
Ahmed, T. A. E., and Hincke, M. T. (2010). Strategies for Articular Cartilage Lesion Repair and Functional Restoration. Tissue Eng. B: Rev. 16, 305–329. doi:10.1089/ten.teb.2009.0590
Aisenbrey, E. A., Bilousova, G., Payne, K., and Bryant, S. J. (2019). Dynamic Mechanical Loading and Growth Factors Influence Chondrogenesis of Induced Pluripotent Mesenchymal Progenitor Cells in a Cartilage-Mimetic Hydrogel. Biomater. Sci. 7, 5388–5403. doi:10.1039/c9bm01081e
Bian, L., Hou, C., Tous, E., Rai, R., Mauck, R. L., and Burdick, J. A. (2013). The Influence of Hyaluronic Acid Hydrogel Crosslinking Density and Macromolecular Diffusivity on Human MSC Chondrogenesis and Hypertrophy. Biomaterials 34, 413–421. doi:10.1016/j.biomaterials.2012.09.052
Bouaziz, W., Sigaux, J., Modrowski, D., Devignes, C.-S., Funck-Brentano, T., Richette, P., et al. (2016). Interaction of HIF1α and β-catenin Inhibits Matrix Metalloproteinase 13 Expression and Prevents Cartilage Damage in Mice. Proc. Natl. Acad. Sci. USA 113, 5453–5458. doi:10.1073/pnas.1514854113
Browe, D. C., Coleman, C. M., Barry, F. P., and Elliman, S. J. (2019). Hypoxia Activates the PTHrP -MEF2C Pathway to Attenuate Hypertrophy in Mesenchymal Stem Cell Derived Cartilage. Sci. Rep. 9, 13274. doi:10.1038/s41598-019-49499-x
Bugbee, W., Cavallo, M., and Giannini, S. (2012). Osteochondral Allograft Transplantation in the Knee. J. Knee Surg. 25, 109–116. doi:10.1055/s-0032-1313743
Cao, Z., Dou, C., and Dong, S. (2017). Curcumin Inhibits Chondrocyte Hypertrophy of Mesenchymal Stem Cells through IHH and Notch Signaling Pathways. Chem. Pharm. Bull. 65, 762–767. doi:10.1248/cpb.c17-00225
Caplan, A. I. (1991). Mesenchymal Stem Cells. J. Orthop. Res. 9, 641–650. doi:10.1002/jor.1100090504
Chen, D., Kim, D. J., Shen, J., Zou, Z., and O'keefe, R. J. (2020). Runx2 Plays a central Role in Osteoarthritis Development. J. Orthopaedic Translation 23, 132–139. doi:10.1016/j.jot.2019.11.008
Chen, F. H., Rousche, K. T., and Tuan, R. S. (2006). Technology Insight: Adult Stem Cells in Cartilage Regeneration and Tissue Engineering. Nat. Rev. Rheumatol. 2, 373–382. doi:10.1038/ncprheum0216
Chen, L., Wu, Y., Wu, Y., Wang, Y., Sun, L., and Li, F. (2016). The Inhibition of EZH2 Ameliorates Osteoarthritis Development through the Wnt/β-Catenin Pathway. Sci. Rep. 6, 29176. doi:10.1038/srep29176
Chen, S., Bellew, C., Yao, X., Stefkova, J., Dipp, S., Saifudeen, Z., et al. (2011). Histone Deacetylase (HDAC) Activity Is Critical for Embryonic Kidney Gene Expression, Growth, and Differentiation. J. Biol. Chem. 286, 32775–32789. doi:10.1074/jbc.m111.248278
Chen, S., Fu, P., Cong, R., Wu, H., and Pei, M. (2015). Strategies to Minimize Hypertrophy in Cartilage Engineering and Regeneration. Genes Dis. 2, 76–95. doi:10.1016/j.gendis.2014.12.003
Churchman, S. M., Ponchel, F., Boxall, S. A., Cuthbert, R., Kouroupis, D., Roshdy, T., et al. (2012). Transcriptional Profile of Native CD271+ Multipotential Stromal Cells: Evidence for Multiple Fates, with Prominent Osteogenic and Wnt Pathway Signaling Activity. Arthritis Rheum. 64, 2632–2643. doi:10.1002/art.34434
Cooper, K. L., Hu, J. K.-H., Ten Berge, D., Fernandez-Teran, M., Ros, M. A., and Tabin, C. J. (2011). Initiation of Proximal-Distal Patterning in the Vertebrate Limb by Signals and Growth. Science 332, 1083–1086. doi:10.1126/science.1199499
Cornelis, F. M. F., De Roover, A., Storms, L., Hens, A., Lories, R. J., and Monteagudo, S. (2019). Increased Susceptibility to Develop Spontaneous and post-traumatic Osteoarthritis in Dot1l-Deficient Mice. Osteoarthritis and Cartilage 27, 513–525. doi:10.1016/j.joca.2018.11.008
Correa, D., Somoza, R. A., Lin, P., Greenberg, S., Rom, E., Duesler, L., et al. (2015). Sequential Exposure to Fibroblast Growth Factors (FGF) 2, 9 and 18 Enhances hMSC Chondrogenic Differentiation. Osteoarthritis and Cartilage 23, 443–453. doi:10.1016/j.joca.2014.11.013
Day, T. F., Guo, X., Garrett-Beal, L., and Yang, Y. (2005). Wnt/β-Catenin Signaling in Mesenchymal Progenitors Controls Osteoblast and Chondrocyte Differentiation during Vertebrate Skeletogenesis. Develop. Cel 8, 739–750. doi:10.1016/j.devcel.2005.03.016
De Sousa, E. B., Dos Santos Junior, G. C., Aguiar, R. P., Da Costa Sartore, R., De Oliveira, A. C. L., Almeida, F. C. L., et al. (2019). Osteoarthritic Synovial Fluid Modulates Cell Phenotype and Metabolic Behavior In Vitro. Stem Cell Int 2019, 8169172. doi:10.1155/2019/8169172
Deng, Y., Lei, G., Lin, Z., Yang, Y., Lin, H., and Tuan, R. S. (2019a). Engineering Hyaline Cartilage from Mesenchymal Stem Cells with Low Hypertrophy Potential via Modulation of Culture Conditions and Wnt/β-Catenin Pathway. Biomaterials 192, 569–578. doi:10.1016/j.biomaterials.2018.11.036
Deng, Y., Sun, A. X., Overholt, K. J., Yu, G. Z., Fritch, M. R., Alexander, P. G., et al. (2019b). Enhancing Chondrogenesis and Mechanical Strength Retention in Physiologically Relevant Hydrogels with Incorporation of Hyaluronic Acid and Direct Loading of TGF-β. Acta Biomater. 83, 167–176. doi:10.1016/j.actbio.2018.11.022
Deng, Y., Wu, A., Li, P., Li, G., Qin, L., Song, H., et al. (2016). Yap1 Regulates Multiple Steps of Chondrocyte Differentiation during Skeletal Development and Bone Repair. Cell Rep. 14, 2224–2237. doi:10.1016/j.celrep.2016.02.021
Deshmukh, V., Hu, H., Barroga, C., Bossard, C., Kc, S., Dellamary, L., et al. (2018). A Small-Molecule Inhibitor of the Wnt Pathway (SM04690) as a Potential Disease Modifying Agent for the Treatment of Osteoarthritis of the Knee. Osteoarthritis and Cartilage 26, 18–27. doi:10.1016/j.joca.2017.08.015
Diederichs, S., Klampfleuthner, F. A. M., Moradi, B., and Richter, W. (2019a). Chondral Differentiation of Induced Pluripotent Stem Cells without Progression into the Endochondral Pathway. Front. Cel Dev. Biol. 7, 270. doi:10.3389/fcell.2019.00270
Diederichs, S., Tonnier, V., März, M., Dreher, S. I., Geisbüsch, A., and Richter, W. (2019b). Regulation of WNT5A and WNT11 during MSC In Vitro Chondrogenesis: WNT Inhibition Lowers BMP and Hedgehog Activity, and Reduces Hypertrophy. Cell. Mol. Life Sci. 76, 3875–3889. doi:10.1007/s00018-019-03099-0
Dong, Y.-F., Soung, D. Y., Chang, Y., Enomoto-Iwamoto, M., Paris, M., O’Keefe, R. J., et al. (2007). Transforming Growth Factor-β and Wnt Signals Regulate Chondrocyte Differentiation through Twist1 in a Stage-specific Manner. Mol. Endocrinol. 21, 2805–2820. doi:10.1210/me.2007-0199
Dreher, S. I., Fischer, J., Walker, T., Diederichs, S., and Richter, W. (2020). Significance of MEF2C and RUNX3 Regulation for Endochondral Differentiation of Human Mesenchymal Progenitor Cells. Front. Cel Dev. Biol. 8, 81. doi:10.3389/fcell.2020.00081
Gabriel, B. M., Hamilton, D. L., Tremblay, A. M., and Wackerhage, H. (20161985). The Hippo Signal Transduction Network for Exercise Physiologists. J. Appl. Physiol. 120, 1105–1117. doi:10.1152/japplphysiol.01076.2015
Gupta, P. K., Chullikana, A., Rengasamy, M., Shetty, N., Pandey, V., Agarwal, V., et al. (2016). Efficacy and Safety of Adult Human Bone Marrow-Derived, Cultured, Pooled, Allogeneic Mesenchymal Stromal Cells (Stempeucel): Preclinical and Clinical Trial in Osteoarthritis of the Knee Joint. Arthritis Res. Ther. 18, 301. doi:10.1186/s13075-016-1195-7
Hallett, S. A., Matsushita, Y., Ono, W., Sakagami, N., Mizuhashi, K., Tokavanich, N., et al. (2021). Chondrocytes in the Resting Zone of the Growth Plate Are Maintained in a Wnt-Inhibitory Environment. Elife 10, e64513. doi:10.7554/eLife.64513
Handorf, A. M., Chamberlain, C. S., and Li, W.-J. (2015). Endogenously Produced Indian Hedgehog Regulates TGFβ-Driven Chondrogenesis of Human Bone Marrow Stromal/Stem Cells. Stem Cell Develop. 24, 995–1007. doi:10.1089/scd.2014.0266
He, Y., Makarczyk, M. J., and Lin, H. (2020). Role of Mitochondria in Mediating Chondrocyte Response to Mechanical Stimuli. Life Sci. 263, 118602. doi:10.1016/j.lfs.2020.118602
Hu, N., Gao, Y., Jayasuriya, C. T., Liu, W., Du, H., Ding, J., et al. (2019). Chondrogenic Induction of Human Osteoarthritic Cartilage-Derived Mesenchymal Stem Cells Activates Mineralization and Hypertrophic and Osteogenic Gene Expression through a mechanomiR. Arthritis Res. Ther. 21, 167. doi:10.1186/s13075-019-1949-0
Huang, X., Zhong, L., Hendriks, J., Post, J. N., and Karperien, M. (2018). The Effects of the WNT-Signaling Modulators BIO and PKF118-310 on the Chondrogenic Differentiation of Human Mesenchymal Stem Cells. Int. J. Mol. Sci. 19, 561. doi:10.3390/ijms19020561
Kon, E., Verdonk, P., Condello, V., Delcogliano, M., Dhollander, A., Filardo, G., et al. (2009). Matrix-assisted Autologous Chondrocyte Transplantation for the Repair of Cartilage Defects of the Knee: Systematic Clinical Data Review and Study Quality Analysis. Am. J. Sports Med. 37 (Suppl. 1), 156s–66S. doi:10.1177/0363546509351649
Kon, E., Filardo, G., Di Matteo, B., Perdisa, F., and Marcacci, M. (2013). Matrix Assisted Autologous Chondrocyte Transplantation for Cartilage Treatment. Bone Jt. Res. 2, 18–25. doi:10.1302/2046-3758.22.2000092
Konsavage, W. M., Kyler, S. L., Rennoll, S. A., Jin, G., and Yochum, G. S. (2012). Wnt/β-Catenin Signaling Regulates Yes-Associated Protein (YAP) Gene Expression in Colorectal Carcinoma Cells. J. Biol. Chem. 287, 11730–11739. doi:10.1074/jbc.m111.327767
Kronenberg, H. M. (2003). Developmental Regulation of the Growth Plate. Nature 423, 332–336. doi:10.1038/nature01657
Lee, H.-H., Chang, C.-C., Shieh, M.-J., Wang, J.-P., Chen, Y.-T., Young, T.-H., et al. (2013). Hypoxia Enhances Chondrogenesis and Prevents Terminal Differentiation through PI3K/Akt/FoxO Dependent Anti-apoptotic Effect. Sci. Rep. 3, 2683. doi:10.1038/srep02683
Lee, J., Jeon, O., Kong, M., Abdeen, A. A., Shin, J. Y., Lee, H. N., et al. (2020). Combinatorial Screening of Biochemical and Physical Signals for Phenotypic Regulation of Stem Cell-Based Cartilage Tissue Engineering. Sci. Adv. 6, eaaz5913. doi:10.1126/sciadv.aaz5913
Liu, W., Feng, M., Jayasuriya, C. T., Peng, H., Zhang, L., Guan, Y., et al. (2020). Human Osteoarthritis Cartilage‐derived Stromal Cells Activate Joint Degeneration through TGF‐beta Lateral Signaling. FASEB j. 34, 16552–16566. doi:10.1096/fj.202001448r
Marino, R. (2011). Growth Plate Biology: New Insights. Curr. Opin. Endocrinol. Diabetes Obes. 18, 9–13. doi:10.1097/med.0b013e3283423df9
Mithoefer, K., Mcadams, T., Williams, R. J., Kreuz, P. C., and Mandelbaum, B. R. (2009). Clinical Efficacy of the Microfracture Technique for Articular Cartilage Repair in the Knee. Am. J. Sports Med. 37, 2053–2063. doi:10.1177/0363546508328414
Mueller, M. B., and Tuan, R. S. (2008). Functional Characterization of Hypertrophy in Chondrogenesis of Human Mesenchymal Stem Cells. Arthritis Rheum. 58, 1377–1388. doi:10.1002/art.23370
Mwale, F., Yao, G., Ouellet, J. A., Petit, A., and Antoniou, J. (2010). Effect of Parathyroid Hormone on Type X and Type II Collagen Expression in Mesenchymal Stem Cells from Osteoarthritic Patients. Tissue Eng. A 16, 3449–3455. doi:10.1089/ten.tea.2010.0091
Narcisi, R., Cleary, M. A., Brama, P. A. J., Hoogduijn, M. J., Tüysüz, N., Ten Berge, D., et al. (2015). Long-term Expansion, Enhanced Chondrogenic Potential, and Suppression of Endochondral Ossification of Adult Human MSCs via WNT Signaling Modulation. Stem Cell Rep. 4, 459–472. doi:10.1016/j.stemcr.2015.01.017
Ochsner, S. A., Abraham, D., Martin, K., Ding, W., Mcowiti, A., Kankanamge, W., et al. (2019). The Signaling Pathways Project, an Integrated 'omics Knowledgebase for Mammalian Cellular Signaling Pathways. Sci. Data 6, 252. doi:10.1038/s41597-019-0193-4
Park, H. W., Kim, Y. C., Yu, B., Moroishi, T., Mo, J.-S., Plouffe, S. W., et al. (2015). Alternative Wnt Signaling Activates YAP/TAZ. Cell 162, 780–794. doi:10.1016/j.cell.2015.07.013
Park, Y.-B., Ha, C.-W., Lee, C.-H., Yoon, Y. C., and Park, Y.-G. (2017). Cartilage Regeneration in Osteoarthritic Patients by a Composite of Allogeneic Umbilical Cord Blood-Derived Mesenchymal Stem Cells and Hyaluronate Hydrogel: Results from a Clinical Trial for Safety and Proof-Of-Concept with 7 Years of Extended Follow-Up. Stem Cell Transl Med 6, 613–621. doi:10.5966/sctm.2016-0157
Piersma, B., Bank, R. A., and Boersema, M. (2015). Signaling in Fibrosis: TGF-β, WNT, and YAP/TAZ Converge. Front. Med. 2, 59. doi:10.3389/fmed.2015.00059
Pietschmann, M. F., Niethammer, T. R., Horng, A., Gülecyüz, M. F., Feist-Pagenstert, I., Jansson, V., et al. (2012). The Incidence and Clinical Relevance of Graft Hypertrophy after Matrix-Based Autologous Chondrocyte Implantation. Am. J. Sports Med. 40, 68–74. doi:10.1177/0363546511424396
Qiao, Z., Tang, J., Yue, B., Wang, J., Zhang, J., Xuan, L., et al. (2020). Human Adipose-Derived Mesenchymal Progenitor Cells Plus Microfracture and Hyaluronic Acid for Cartilage Repair: a Phase IIa Trial. Regenerative Med. 15, 1193–1214. doi:10.2217/rme-2019-0068
Riedl, M., Witzmann, C., Koch, M., Lang, S., Kerschbaum, M., Baumann, F., et al. (2020). Attenuation of Hypertrophy in Human MSCs via Treatment with a Retinoic Acid Receptor Inverse Agonist. Int. J. Mol. Sci. 21. doi:10.3390/ijms21041444
Rojas, A., Mardones, R., Pritzker, K., Van Wijnen, A. J., Galindo, M. A., and Las Heras, F. (2019). Dickkopf-1 Reduces Hypertrophic Changes in Human Chondrocytes Derived from Bone Marrow Stem Cells. Gene 687, 228–237. doi:10.1016/j.gene.2018.11.037
Sen, B., Guilluy, C., Xie, Z., Case, N., Styner, M., Thomas, J., et al. (2011). Mechanically Induced Focal Adhesion Assembly Amplifies Anti‐Adipogenic Pathways in Mesenchymal Stem Cells. Stem Cells 29, 1829–1836. doi:10.1002/stem.732
Shen, J., Li, J., Wang, B., Jin, H., Wang, M., Zhang, Y., et al. (2013). Deletion of the Transforming Growth Factor β Receptor Type II Gene in Articular Chondrocytes Leads to a Progressive Osteoarthritis-like Phenotype in Mice. Arthritis Rheum. 65, 3107–3119. doi:10.1002/art.38122
Shi, D., Xu, X., Ye, Y., Song, K., Cheng, Y., Di, J., et al. (2016). Photo-Cross-Linked Scaffold with Kartogenin-Encapsulated Nanoparticles for Cartilage Regeneration. ACS Nano 10, 1292–1299. doi:10.1021/acsnano.5b06663
Shimoyama, A., Wada, M., Ikeda, F., Hata, K., Matsubara, T., Nifuji, A., et al. (2007). Ihh/Gli2 Signaling Promotes Osteoblast Differentiation by Regulating Runx2 Expression and Function. MBoC 18, 2411–2418. doi:10.1091/mbc.e06-08-0743
Smith, E., and Frenkel, B. (2005). Glucocorticoids Inhibit the Transcriptional Activity of LEF/TCF in Differentiating Osteoblasts in a Glycogen Synthase Kinase-3β-dependent and -independent Manner. J. Biol. Chem. 280, 2388–2394. doi:10.1074/jbc.m406294200
Sun, M. M.-G., and Beier, F. (2014). Chondrocyte Hypertrophy in Skeletal Development, Growth, and Disease. Birth Defect Res. C 102, 74–82. doi:10.1002/bdrc.21062
Taniguchi, N., Caramés, B., Hsu, E., Cherqui, S., Kawakami, Y., and Lotz, M. (2011). Expression Patterns and Function of Chromatin Protein HMGB2 during Mesenchymal Stem Cell Differentiation*. J. Biol. Chem. 286, 41489–41498. doi:10.1074/jbc.m111.236984
Ten Berge, D., Brugmann, S. A., Helms, J. A., and Nusse, R. (2008). Wnt and FGF Signals Interact to Coordinate Growth with Cell Fate Specification during Limb Development. Development 135, 3247–3257. doi:10.1242/dev.023176
Tornero-Esteban, P., Peralta-Sastre, A., Herranz, E., Rodríguez-Rodríguez, L., Mucientes, A., Abásolo, L., et al. (2015). Altered Expression of Wnt Signaling Pathway Components in Osteogenesis of Mesenchymal Stem Cells in Osteoarthritis Patients. PLoS One 10, e0137170. doi:10.1371/journal.pone.0137170
Usami, Y., Gunawardena, A. T., Iwamoto, M., and Enomoto-Iwamoto, M. (2016). Wnt Signaling in Cartilage Development and Diseases: Lessons from Animal Studies. Lab. Invest. 96, 186–196. doi:10.1038/labinvest.2015.142
Vanyai, H. K., Prin, F., Guillermin, O., Marzook, B., Boeing, S., Howson, A., et al. (2020). Control of Skeletal Morphogenesis by the Hippo-YAP/TAZ Pathway. Development 147.
Wang, W., Rigueur, D., and Lyons, K. M. (2014). TGFβ Signaling in Cartilage Development and Maintenance. Birth Defect Res. C 102, 37–51. doi:10.1002/bdrc.21058
Wang, X., He, T., He, L., Yang, B., Liu, Z., Pang, M., et al. (2021). Melatonin Contributes to the Hypertrophic Differentiation of Mesenchymal Stem Cell-Derived Chondrocytes via Activation of the Wnt/β-Catenin Signaling Pathway. Stem Cel Res Ther 12, 467. doi:10.1186/s13287-021-02536-x
Watts, A. E., Ackerman-Yost, J. C., and Nixon, A. J. (2013). A Comparison of Three-Dimensional Culture Systems to Evaluate In Vitro Chondrogenesis of Equine Bone Marrow-Derived Mesenchymal Stem Cells. Tissue Eng. Part A 19, 2275–2283. doi:10.1089/ten.tea.2012.0479
Yang, X., Chen, L., Xu, X., Li, C., Huang, C., and Deng, C.-X. (2001). TGF-β/Smad3 Signals Repress Chondrocyte Hypertrophic Differentiation and Are Required for Maintaining Articular Cartilage. J. Cel Biol 153, 35–46. doi:10.1083/jcb.153.1.35
Yang, Y., Lin, H., Shen, H., Wang, B., Lei, G., and Tuan, R. S. (2018). Mesenchymal Stem Cell-Derived Extracellular Matrix Enhances Chondrogenic Phenotype of and Cartilage Formation by Encapsulated Chondrocytes In Vitro and In Vivo. Acta Biomater. 69, 71–82. doi:10.1016/j.actbio.2017.12.043
Yang, Z., Zou, Y., Guo, X. M., Tan, H. S., Denslin, V., Yeow, C. H., et al. (2012). Temporal Activation of β-Catenin Signaling in the Chondrogenic Process of Mesenchymal Stem Cells Affects the Phenotype of the Cartilage Generated. Stem Cell Develop. 21, 1966–1976. doi:10.1089/scd.2011.0376
Yu, Y. Y., Lieu, S., Lu, C., Miclau, T., Marcucio, R. S., and Colnot, C. (2010). Immunolocalization of BMPs, BMP Antagonists, Receptors, and Effectors during Fracture Repair. Bone 46, 841–851. doi:10.1016/j.bone.2009.11.005
Zhong, L., Huang, X., Karperien, M., and Post, J. (2015). The Regulatory Role of Signaling Crosstalk in Hypertrophy of MSCs and Human Articular Chondrocytes. Ijms 16, 19225–19247. doi:10.3390/ijms160819225
Zhong, L., Huang, X., Rodrigues, E. D., Leijten, J. C. H., Verrips, T., El Khattabi, M., et al. (2016). Endogenous DKK1 and FRZB Regulate Chondrogenesis and Hypertrophy in Three-Dimensional Cultures of Human Chondrocytes and Human Mesenchymal Stem Cells. Stem Cell Develop. 25, 1808–1817. doi:10.1089/scd.2016.0222
Zhu, B., Xue, F., Li, G., and Zhang, C. (2020). CRYAB Promotes Osteogenic Differentiation of Human Bone Marrow Stem Cells via Stabilizing β-catenin and Promoting the Wnt Signalling. Cell Prolif 53, e12709. doi:10.1111/cpr.12709
Keywords: mesenchymal stem cells, hyaline cartilage, chondrocytic hypertrophy, chondrogenesis, cartilage tissue engineering
Citation: Wang X, Guan Y, Xiang S, Clark KL, Alexander PG, Simonian LE, Deng Y and Lin H (2022) Role of Canonical Wnt/β-Catenin Pathway in Regulating Chondrocytic Hypertrophy in Mesenchymal Stem Cell-Based Cartilage Tissue Engineering. Front. Cell Dev. Biol. 10:812081. doi: 10.3389/fcell.2022.812081
Received: 09 November 2021; Accepted: 06 January 2022;
Published: 24 January 2022.
Edited by:
Marcela F. Bolontrade, CONICET Instituto de Medicina Traslacional e Ingeniería Biomédica (IMTIB), ArgentinaReviewed by:
Adetola B. Adesida, University of Alberta, CanadaCopyright © 2022 Wang, Guan, Xiang, Clark, Alexander, Simonian, Deng and Lin. This is an open-access article distributed under the terms of the Creative Commons Attribution License (CC BY). The use, distribution or reproduction in other forums is permitted, provided the original author(s) and the copyright owner(s) are credited and that the original publication in this journal is cited, in accordance with accepted academic practice. No use, distribution or reproduction is permitted which does not comply with these terms.
*Correspondence: Hang Lin, aGFsNDZAcGl0dC5lZHU=; Yuhao Deng, ZHloMTMyNDJAMTYzLmNvbQ==