- The Ohio State University Comprehensive Cancer Center, Columbus, OH, United States
Aging is associated with various hematological disorders and a higher risk of myeloproliferative disorders. An aged hematopoietic system can be characterized by decreased immune function and increased myeloid cell production. Hematopoietic stem cells (HSCs) regulate the production of blood cells throughout life. The self-renewal and regenerative potential of HSCs determine the quality and quantity of the peripheral blood cells. External signals from the microenvironment under different conditions determine the fate of the HSCs to proliferate, self-renew, differentiate, or remain quiescent. HSCs respond impromptu to a vast array of extracellular signaling cascades such as cytokines, growth factors, or nutrients, which are crucial in the regulation of HSCs. Early growth response factor 1 (EGR1) is one of the key transcription factors controlling HSC proliferation and their localization in the bone marrow (BM) niche. Downregulation of Egr1 activates and recruits HSCs for their proliferation and differentiation to produce mature blood cells. Increased expression of Egr1 is implicated in immuno-aging of HSCs. However, dysregulation of Egr1 is associated with hematological malignancies such as acute myeloid leukemia (AML), acute lymphoblastic leukemia (ALL), and chronic myelogenous leukemia (CML). Here, we summarize the current understanding of the role of EGR1 in the regulation of HSC functionality and the manifestation of leukemia. We also discuss the alternative strategies to rejuvenate the aged HSCs by targeting EGR1 in different settings.
Introduction
Hematopoietic stem cells (HSCs) maintain the peripheral blood pool by regulating the delicate balance between self-renewal, proliferation, and differentiation. Quiescent—long-term HSCs reside at the apex position of the differentiation pyramid, thus holding prime importance in the development of the entire hematopoietic system. These cells give rise to the activated—short-term HSCs which have higher proliferative and differentiation capability. Further, the short-term activated HSCs form the multipotent as well as the lineage-committed progenitor cells that eventually differentiate into various types of functional and mature blood cells (Orkin and Zon, 2008).
HSCs respond to various intrinsic and extrinsic signals and regulate their fate decisions accordingly (Ross and Li, 2006; Zon, 2008). Aging brings about drastic changes in the HSC microenvironment (Latchney and Calvi, 2017; Comazzetto et al., 2021), thereby altering the normal BM niche - HSC inter-communication and affecting the HSC functionality. Moreover, the aged stem cells may lose their ability to sense nutrients and respond to microenvironmental stimuli (López-Otín et al., 2013).
Extrinsic stimuli largely govern the cellular responses and determine cell fate. The quest to understand the immediate effector molecules responding to a wide array of mitogens led to the discovery of a zinc finger transcription factor- early growth response factor 1 (EGR1) (Sukhatme et al., 1987). Egr1 expression is induced during cell growth, differentiation, and cellular depolarization in a similar manner as governed by c-myc and c-fos and it is co-regulated with c-fos (Sukhatme et al., 1988; Tsai-Morris et al., 1988; Seyfert et al., 1990). The potential targets of EGR1 are tumor necrosis factors (TNFs), interleukin 2 (IL-2), CD44, and Intercellular adhesion molecule 1 (ICAM-1) (McMahon and Monroe, 1996). EGR1 regulates the transcription of more than 30 genes involved in growth, development, and differentiation. It has been also reported to have a transformation suppression activity. The EGR1 molecule has three zinc finger motifs at the C-terminal end, which confer the ability to bind DNA and various promotors having GC rich elements GCEs, (Liu et al., 1996). Thus, EGR1 regulates the expression of various growth factors upon immediate induction by the mitogenic stimulus. Similarly, EGR1 is also reported to have TGF-ß mediated growth and transformation suppression activity (Liu et al., 1996). As EGR1 is prominently involved in establishing early cell proliferative responses to the extrinsic signaling molecules, understanding its role in the regulation of HSCs and hematopoietic disorders becomes essential.
EGR1 in Hematopoiesis
Earlier studies with the interleukin 3 (IL-3) dependent hematopoietic precursor 32Dcl3 cells, showed that the ectopic overexpression of Egr1 in them blocked the granulocyte differentiation despite the presence of granulocyte colony-stimulating factor (G-CSF). These cells initiate their terminal differentiation solely into macrophage lineage in presence of granulocyte-macrophage colony-stimulating factor (GM-CSF) (Krishnaraju et al., 1995). Further, the same group studied the effect of overexpression of Egr1 in stem cell-enriched bone marrow (BM) cells and myeloid enriched BM cells. The study confirmed the shift in the differentiation pattern of stem cell-enriched BM cells from granulocyte or erythroid lineage towards the macrophage lineage upon enhanced EGR1 activity. Moreover, even the myeloid enriched BM cells population in the study differentiated toward macrophage lineage despite the presence of erythropoietin (EPO) and IL-3 (Krishnaraju et al., 2001). Hematopoietic progenitors overexpressing Egr1 failed to establish the BM engraftment upon transplantation in lethally irradiated mice. This resulted in reduced survival of the recipients due to excessive differentiation towards the macrophage lineage (Krishnaraju et al., 2001). The antisense oligomers targeting Egr1 RNA block the macrophage differentiation while its constitutive expression specifically induced macrophage differentiation in myeloblastic cell lines. Thus, the Egr1 gene was identified to positively regulate myeloid differentiation. (Nguyen et al., 1993).
Mice with disrupted Egr1 alleles show no prominent differences in macrophage differentiation or various macrophage compartments when compared with the wild-type mice. Under normal culture conditions, the BM cells from these mice have similar differentiation responses to various cytokines required for inducing myeloid differentiation, such as macrophage colony-stimulating factor (M-CSF), GM-CSF, or G-CSF. The Egr1−/− macrophages demonstrate unaltered activation potential and have a comparable expression of major histocompatibility complex molecules class-II (MHC class-II). These mice have normal pathogen responses with a comparable life span to that of wild-type mice (Lee et al., 1996). This evidence suggests that Egr1 expression can induce a differentiation bias towards the macrophage lineage, but it is dispensable for normal hematopoiesis and differentiation. It advocates the compensatory effect of structurally similar Egr family members such as Egr2, Egr3, and Egr4 in absence of Egr1 (Beckmann and Wilce, 1997; Tourtellote et al., 2000). Egr1 and Egr2 are redundant in promoting macrophage differentiation and neutrophil repression. However, simultaneous loss of both, Egr1 and Egr2 is embryonically lethal. Interestingly, Egr1−/− Egr2−/+ compound mutant mice are viable but show reduced weight and size. Egr1−/− Egr2−/+ compound mutant mice also show a reduced number of BM HSCs along with a reduced number of functionalities of macrophage progenitors evident from the decreased size of colony-forming units- macrophage (CFU-M) in methylcellulose assay. Their differentiation into macrophages upon GM-CSF or M-CSF stimulation is also reduced, which underscores the role of Egr1 and Egr2 in the lineage commitment of progenitor cells. EGR1 and EGR2 do so by counteracting with the neutrophil priming regulator, GIF-1, and positive regulation of macrophage-specific gene expression (Laslo et al., 2006).
In B-lymphocytes, EGR1 is known to regulate the expression of the homing and migration molecule- CD44. Increased Cd44 expression seen after increased nuclear Egr1 transcription is a result of binding of newly synthesized EGR to the Cd44 promotor ∼300 bps before the transcription start point (Maltzman et al., 1996). Similarly, overexpression of Egr1 in murine B-cell line K46 leads to an increase in Cd44 expression and downregulation of Fas/Apo-1 and Cd23 molecules which reduce the apoptosis mediated cell death (Dinkel et al., 1997). Studies with transgenic mice overexpressing Egr1 confirm that the EGR1 promotes B and T cells maturation as evident by an increase in the number of mature cells and a concomitant decrease in cells at the immature stage (Miyazaki, 1997; Dinkel et al., 1998). Further findings in Egr1−/− mice validate that Egr1 is dispensable for B cell maturation. However, RGR1 is important for the differentiation of B cells into plasma cells (Oh et al., 2015). The role of Egr1 and Egr2 expression is implicated in NKT cell differentiation in response to T cell receptor (TCR) signaling (Seiler et al., 2012). Enforced expression of Egr1 also increases the megakaryocyte differentiation evident by increased expression of megakaryocyte marker CD41a on K562 cells (Cheng et al., 1994). Thus, EGR1 is well established as the regulator of hematopoietic cell differentiation and maturation.
EGR1 in Aging Hematopoietic Stem Cells
Aging, in general, is characterized by the progressive loss in functionality of various vital organs, tissues, and cells. This cellular and molecular deterioration at the functional level ultimately results in increased vulnerability to death. Stem cell exhaustion increased cellular senescence and altered intercellular communication are the major hallmarks of aging (López-Otín et al., 2013). Aging alters immune system compositions and impairs lymphopoiesis (Linton and Dorshkind, 2004). The altered differentiation capacity of the aged HSCs increases myeloid cell frequencies and decreases lymphoid cell frequencies in the peripheral blood (Geiger and Van Zant, 2002). The frequency of myeloid biased HSCs also increases during aging (Gekas and Graf, 2013). Aged HSCs demonstrate increased quiescence (Beerman et al., 2014), and delayed cell cycle progression (Noda et al., 2009), with an increase in the senescent cell population (Chen, 2004). Quiescent aged HSCs are capable of repairing their accumulated DNA damage upon re-entry into the cell cycle (Beerman et al., 2014). Aging-related changes in HSCs are the implications of intrinsic dysregulations (Mejia-Ramirez, and Florian, 2020) as well as the contributions of an altered HSC microenvironment (Kulkarni et al., 2018; Ho and Méndez-Ferrer, 2020; Kulkarni and Kale, 2020). As these mechanisms have a proven relation to Egr1 expression, it is of utmost interest to explore its link with HSC aging.
Overexpression of Egr1 in Caenorhabditis elegans promotes longevity and improves stress resistance to heat and ultraviolet light. EGR1 modulates the insulin signaling cascade and eventually increases the lifespan. While decreased EGR1 signaling in C. elegans results in the reduced lifespan of these worms. The same study reports that the expression of Egr1 increases with age and protects the organism from aging-related dysfunctions (Zimmerman and Kim, 2014). Another study shows that the deletion of the Egr1 results in a striking phenotype with complete bypass of senescence and induced immortal cell growth ability with a concomitant decrease in p53 and p21Cip1/Waf1 signaling (Krones-Herzig et al., 2003). This highlights the role of Egr1 in the regulation of senescence and cell death. EGR1 is also a well-known regulator of aging-related genes (Baron et al., 2005) such as transforming growth factor β1 (Tgf- β1) (Valletta et al., 2020), phosphatase and tensin homolog (Pten) (Chen et al., 2009), p53 (Dumble et al., 2007). A detailed study with the Egr1−/− mice model validates the significance of EGR1 in HSC proliferation and the HSCs localization in the BM niche. Egr1−/− mice show a significant increase in HSC cycling with about a two-fold increase in the HSC percentages present in the S-G2-M phase. These HSCs also exhibit significant downregulation of p21Cip1/Waf1 expression along with an increase in Cdk4 expression in them. Loss of Egr1 does not alter the long-term reconstitution potential of the HSCs but results in premature exhaustion of stem cells due to the high proliferation rate upon serial transplantation. G-CSF-induced mobilization of HSCs also downregulates Egr1 expression, signifying its role in HSC localization under a steady state. On the other hand, Egr1−/− mice demonstrate spontaneous mobilization of HSCs from the BM, confirming the importance of EGR1 in HSC localization (Min et al., 2008).
Recently, Desterke et al. analyzed the single-cell transcriptomics of the aged human HSCs. They found that the EGR1 is substantially upregulated in aged Lineage- CD34- CD38- cells. These cells exhibit dysregulation of the cell cycle with a reduction in cell population present in the G2-M phase and a reduction in CCND2 expression during the S phase. Aged HSCs also show induced expression of the other molecules which are generally implicated in the hematopoietic and immune disorders, AP-1, and HSC quiescence regulators such as BTG, JUNB, and NR41A. Aged human HSCs present dysregulation of CDH4/6/D type cyclin complex and CDK6-EGR1 axis, which has an important implication in the activation of quiescent HSCs (Desterke et al., 2021). CDK6 suppresses the transcription of Egr1 during the recruitment of quiescent HSCs for activation. Loss of Cdk6 in HSCs induces an increase in the frequency of quiescent HSCs resulting in their failure to repopulate bone marrow after transplantation. Consistent Egr1 expression despite repeated 5-FU treatment leads to the inability of Cdk6−/− mice to recruit HSCs for activation. This significantly hampers the survival of the mice due to reduced hematopoietic activity under induced stress (Scheicher et al., 2015). Single-cell RNA sequencing analysis and bulk RNA sequencing strategies have demonstrated a notable increase in EGR1 expression in aged human HSCs (Adelman et al., 2019). Another report on single-cell sequencing for combined analysis of epigenetic, transcriptomic, and functional data identified the effect of Egr1 expression in fetal HSCs. The report showed a correlation between DNA hypermethylation with decreased expression of Egr1 transcription network genes including SOCS3, KLF2, and JUNB. These changes are associated with decreased activation of HSCs and the inability to respond to the stimulation (Pelletier et al., 2021). Thes data suggest the importance of EGR1 in HSC cell cycle regulation, activation, localization, and differentiation.
Bone marrow stromal cells (BMSCs) regulate HSC functionality in-vivo (Garcia-Garcia et al., 2015) and have been used to support the HSCs for in-vitro expansion cultures (Jing et al., 2010). A recent study confirms the role of Egr1 in BMSC proliferation. Egr1 expression levels are found to be high in the freshly isolated BMSCs but are rapidly downregulated upon proliferation under culturing conditions. However, Egr1 expression levels are also seen to be reduced in the colony-forming BM MSCs. Enforced expression of Egr1 decreases proliferation in stromal cells but upon culturing for HSC expansion, their HSC support potential increases, evident through the elevated number of transplantable HSCs (Li et al., 2020). Netrin-1, expressed on arteriolar endothelial and periarteriolar stromal cells, regulates niche-dependent HSC self-renewal and quiescence through its interaction with Neogenin-1 by triggering Egr1 expression. The aged niche shows a decline in Netrin-1, which induces compensatory overexpression of Neogenin-1 in HSCs, inducing Egr1 expression further (Renders et al., 2021). These findings suggest that Egr1 expression during aging is amendable by extrinsic modulations and can be a potential target for HSC rejuvenation therapies.
Differential Role of EGR1 in Mature and Immature Cell Types
EGR1 is a master transcription factor that can regulate various genes by acting as an activator or a suppressor, depending on distinct cofactors associated with it (Thiel and Cibelli, 2002). Importantly, EGR1 regulates immune cells through its target genes such as Il-2, Cd44, Icam, and Tnf (McMahon and Monroe, 1996). In the case of the cancer cells, Wang et al. have reported that high glucose levels induce cell proliferation via increased Tgf- β1 levels, mediated by increased binding of EGR1 to the Tgf- β1 promoter site (Wang et al., 2015).
While in HSCs, increased TGF- β signaling induces cell hibernation via inhibition of cytokine-mediated lipid raft clustering, which is required for re-entering the cell cycle (Yamazaki et al., 2009). Egr1 promotor also has EGF response elements and serum response elements which induce Egr1 expression through the EGF-ERK-Elk1 signaling cascade (Gregg and Fraizer, 2011). Though ERK is also known to induce the proliferation of cells, the ERK-dependent feedback mechanism prevents HSC exhaustion during hematopoiesis (Baumgartner et al., 2018). Cyclin D1 transcription during progression through the G1 to S phase of the cell cycle requires MAPK-induced Egr1 expression and its binding to the promotor (Xiao et al., 2005). On the other hand, maintenance of self-renewal in HSCs requires balanced MAPK signaling (Geest and Coffer, 2009). As discussed earlier, CDK6 acts as the transcriptional suppressor of Egr1 in HSCs, which is required for their activation (Scheicher et al., 2015). Moreover, Egr−/− HSCs show increased cycling with a significant increase in Cdk4 expression without any significant change in the cyclin D1 expression levels (Min et al., 2008). These reports suggest that EGR1 can play different roles in mature and stem cell populations depending on the upstream and downstream signaling cascades and regulation of EGR1 expression levels.
EGR1 in Leukemia
Alongside the role of Egr1 in normal hematopoiesis discussed in the previous section, its role in mature or leukemic hematopoietic cells has also been studied extensively. Ectopic overexpression of EGR1 in M1 myeloblastic leukemia cells abrogates the G0/G1 block imparted by oncogenic c-Myc or E2F-1 in these cells. Further, EGR1 commits them to terminal differentiation under the effect of extrinsic IL-6 treatment and diminishes the aggression of the M1 myeloblastic leukemic cells (Gibbs et al., 2008). In acute lymphoblastic leukemia (ALL) EGR1 induction via suppression of HSP70 induces apoptosis and inhibits cell proliferation, whereas loss of EGR1 in these cells increased the proliferation and reduces apoptosis of ALL cells (Guo et al., 2019). Upregulation of EGR1 in leukemic cells suppresses invasion and migration of the leukemic cell (Liu et al., 2009). Tumor suppressor activity of EGR1 is well proven in Chronic Myelogenous Leukemia (CML) as well (Maifrede et al., 2014). Importantly, the EGR1 gene is located on chromosome 5, in a region that is often found to be deleted in acute myeloid leukemia (AML) cells (Sukhatme et al., 1988). In the AML settings, upregulation of EGR1 has been shown to down-regulate Survivin, an inhibitor of apoptosis, and regulator of cell division, which also sensitizes AML cells to TRAIL-induced apoptosis (Tamm et al., 2006).
In multiple myeloma cells, JUN overexpression induces cell death and growth inhibition by upregulation of the EGR1, which in turn downregulates Survivin and triggers caspase signaling. Interestingly improved outcome for the bortezomib treatment is associated with increased JUN or Egr1 expression, while JUN or EGR1 knockdown increases the resistance of myeloma cells against bortezomib (Chen et al., 2010). In lymphoblastic leukemia, miR-181a can target and downregulate EGR1 which increases the proliferation and survival of leukemic cells leading to negative outcomes (Verduci et al., 2015). EGR1 expression in the tumor microenvironment has been also proven to inhibit tumor growth. Ectopic expression of EGR1 in thymic lymphoma stromal cells downregulates matrix metalloproteinase-9 (MMP-9) expression in them and inhibits the lymphoma growth (Bouchard et al., 2010). Overall, Egr1 ability to induce terminal differentiation of various mature hematopoietic cells and its tumor-suppressive activity have been explored as potential anti-leukemia therapies.
Discussion
EGR1 being the regulator of HSC quiescence, proliferation, and localization is the key factor in determining HSC function and fate. Egr1 deletion also inhibits cellular senescence and induces proliferation in senescent cells. Recent evidence suggests dysregulation of EGR1 in the aged HSCs. Aged HSCs have increased quiescence and senescence which results in decreased HSC functionality. In the scenario of being enforced to enter the cell cycle, aged HSCs repair the accumulated DNA damage. Egr1 downregulation might decrease senescence and induce their activation in aged HSCs, in turn improving their functionality. Thus, studying the effect of extrinsic modulation of EGR1 in aged HSCs needs to be investigated for potential strategies for rejuvenation of the aged HSCs. Some of these promising approaches include- niche-mediated modulatory mechanisms, targeted vesicle-mediated antisense oligonucleotide delivery, and the development of EGR1 inhibitors (Figure 1).
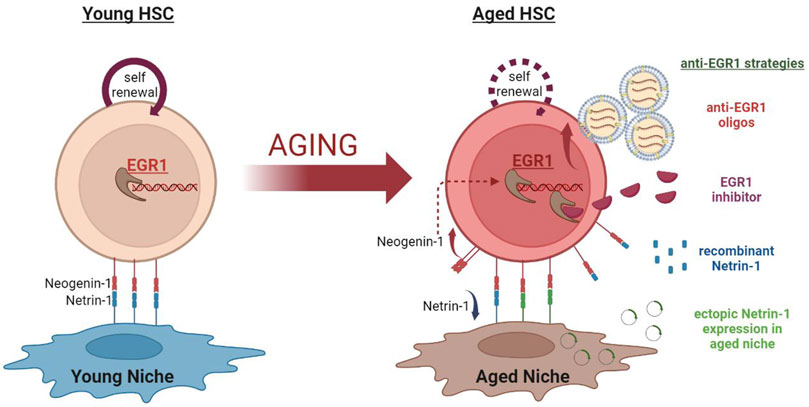
FIGURE 1. EGR1 targeting strategies for rejuvenation of aged Hematopoietic Stem Cells. The expression of the zinc finger transcription factor - EGR1 is significantly increased in aged HSCs. This is, at least partially, mediated by the aging-associated changes in the BM niche. Increased EGR1 activation in the aged HSCs hampers their self-renewal activity and functions. EGR1 targeting strategies may be used to explore the possibility to rejuvenate aged HSCs. These strategies include - (A) Using the vesicle-mediated transfer of anti-Egr1 oligos specifically targeting aged HSCs. (B) Developing pharmacological EGR1 inhibitors. (C) Treating aged HSCs with recombinant Netrin-1 protein to reduce EGR1 activation in them. (D) Ectopic expression of Netrin-1 in aged BM niche cells to rescue Netrin-1 -Neogenin-1 interaction to modulate downstream EGR1 levels in the aged HSCs.
Author Contributions
RK contributed to the conceptualization, research of primary literature, and writing of the article.
Conflict of Interest
The author declares that the research was conducted in the absence of any commercial or financial relationships that could be construed as a potential conflict of interest.
Publisher’s Note
All claims expressed in this article are solely those of the authors and do not necessarily represent those of their affiliated organizations, or those of the publisher, the editors and the reviewers. Any product that may be evaluated in this article, or claim that may be made by its manufacturer, is not guaranteed or endorsed by the publisher.
Acknowledgments
I thank Dr. Anushree Kogje for helping with article editing, proofreading, and critical scientific suggestions for writing the article.
References
Adelman, E. R., Huang, H.-T., Roisman, A., Olsson, A., Colaprico, A., Qin, T., et al. (2019). Aging Human Hematopoietic Stem Cells Manifest Profound Epigenetic Reprogramming of Enhancers that May Predispose to Leukemia. Cancer Discov. 9 (8), 1080–1101. doi:10.1158/2159-8290.cd-18-1474
Baron, V., Vo, C., and Mercola, D. (2005). A Combination Study of Egr-1 Antisense with Chemotherapeutic Drugs in Human Prostate Cancer Cells, 65. United States: American Association for Cancer Research, 140.
Baumgartner, C., Toifl, S., Farlik, M., Halbritter, F., Scheicher, R., Fischer, I., et al. (2018). An ERK-dependent Feedback Mechanism Prevents Hematopoietic Stem Cell Exhaustion. Cell stem Cell 22 (6), 879–892. doi:10.1016/j.stem.2018.05.003
Beckmann, A. M., and Wilce, P. A. (1997). Egr Transcription Factors in the Nervous System. Neurochem. Int. 31 (4), 477–510. doi:10.1016/s0197-0186(96)00136-2
Beerman, I., Seita, J., Inlay, M. A., Weissman, I. L., and Rossi, D. J. (2014). Quiescent Hematopoietic Stem Cells Accumulate DNA Damage during Aging that Is Repaired upon Entry into Cell Cycle. Cell stem Cell 15 (1), 37–50. doi:10.1016/j.stem.2014.04.016
Bouchard, F., Bélanger, S. D., Biron-Pain, K., and St-Pierre, Y. (2010). EGR-1 Activation by EGF Inhibits MMP-9 Expression and Lymphoma Growth. Blood, J. Am. Soc. Hematol. 116 (5), 759–766. doi:10.1182/blood-2009-12-257030
Chen, C., Liu, Y., Liu, Y., and Zheng, P. (2009). mTOR Regulation and Therapeutic Rejuvenation of Aging Hematopoietic Stem Cells. Sci. Signal 2 (98), ra75. doi:10.1126/scisignal.2000559
Chen, J. (2004). Senescence and Functional Failure in Hematopoietic Stem Cells. Exp. Hematol. 32 (11), 1025–1032. doi:10.1016/j.exphem.2004.08.001
Chen, L., Wang, S., Zhou, Y., Wu, X., Entin, I., Epstein, J., et al. (2010). Identification of Early Growth Response Protein 1 (EGR-1) as a Novel Target for JUN-Induced Apoptosis in Multiple Myeloma. Blood, J. Am. Soc. Hematol. 115 (1), 61–70. doi:10.1182/blood-2009-03-210526
Cheng, T., Wang, Y., and Dai, W. (1994). Transcription Factor Egr-1 Is Involved in Phorbol 12-myristate 13-Acetate-Induced Megakaryocytic Differentiation of K562 Cells. J. Biol. Chem. 269 (49), 30848–30853. doi:10.1016/s0021-9258(18)47359-0
Comazzetto, S., Shen, B., and Morrison, S. J. (2021). Niches that Regulate Stem Cells and Hematopoiesis in Adult Bone Marrow. Dev. Cell 56 (13), 1848–1860. doi:10.1016/j.devcel.2021.05.018
Desterke, C., Bennaceur-Griscelli, A., and Turhan, A. G. (2021). EGR1 Dysregulation Defines an Inflammatory and Leukemic Program in Cell Trajectory of Human-Aged Hematopoietic Stem Cells (HSC). Stem cell Res. Ther. 12 (1), 1–20.
Dinkel, A., Aicher, W. K., Haas, C., Zipfel, P. F., Peter, H. H., and Eibel, H. (1997). Transcription Factor Egr-1 Activity Down-Regulates Fas and CD23 Expression in B Cells. J. Immunol. 159 (6), 2678–2684.
Dinkel, A., Warnatz, K., Ledermann, B., Rolink, A., Zipfel, P. F., Bürki, K., et al. (1998). The Transcription Factor Early Growth Response 1 (Egr-1) Advances Differentiation of Pre-B and Immature B Cells. J. Exp. Med. 188 (12), 2215–2224. doi:10.1084/jem.188.12.2215
Dumble, M., Moore, L., Chambers, S. M., Geiger, H., Van Zant, G., Goodell, M. A., et al. (2007). The Impact of Altered P53 Dosage on Hematopoietic Stem Cell Dynamics during Aging. Blood 109 (4), 1736–1742. doi:10.1182/blood-2006-03-010413
García-García, A., de Castillejo, C. L. F., and Méndez-Ferrer, S. (2015). BMSCs and Hematopoiesis. Immunol. Lett. 168 (2), 129–135. doi:10.1016/j.imlet.2015.06.020
Geest, C. R., and Coffer, P. J. (2009). MAPK Signaling Pathways in the Regulation of Hematopoiesis. J. Leukoc. Biol. 86 (2), 237–250. doi:10.1189/jlb.0209097
Geiger, H., and Van Zant, G. (2002). The Aging of Lympho-Hematopoietic Stem Cells. Nat. Immunol. 3 (4), 329–333. doi:10.1038/ni0402-329
Gekas, C., and Graf, T. (2013). CD41 Expression Marks Myeloid-Biased Adult Hematopoietic Stem Cells and Increases with Age. J. Am. Soc. Hematol. 121 (22), 4463–4472. doi:10.1182/blood-2012-09-457929
Gibbs, J. D., Liebermann, D. A., and Hoffman, B. (2008). Egr-1 Abrogates the E2F-1 Block in Terminal Myeloid Differentiation and Suppresses Leukemia. Oncogene 27 (1), 98–106. doi:10.1038/sj.onc.1210627
Gregg, J., and Fraizer, G. (2011). Transcriptional Regulation of EGR1 by EGF and the ERK Signaling Pathway in Prostate Cancer Cells. Genes & cancer 2 (9), 900–909. doi:10.1177/1947601911431885
Guo, D., Zhang, A., Huang, J., Suo, M., Zhong, Y., and Liang, Y. (2019). Suppression of HSP70 Inhibits the Development of Acute Lymphoblastic Leukemia via TAK1/Egr-1. Biomed. Pharmacother. 119, 109399. doi:10.1016/j.biopha.2019.109399
Ho, Y.-H., and Méndez-Ferrer, S. (2020). Microenvironmental Contributions to Hematopoietic Stem Cell Aging. Haematologica 105 (1), 38–46. doi:10.3324/haematol.2018.211334
Jing, D., Fonseca, A. V., Alakel, N., Fierro, F. A., Muller, K., Bornhauser, M., et al. (2010). Hematopoietic Stem Cells in Co-culture with Mesenchymal Stromal Cells - Modeling the Niche Compartments In Vitro. haematologica 95 (4), 542–550. doi:10.3324/haematol.2009.010736
Krones-Herzig, A., Adamson, E., and Mercola, D. (2003). Early Growth Response 1 Protein, an Upstream Gatekeeper of the P53 Tumor Suppressor, Controls Replicative Senescence. Proc. Natl. Acad. Sci. U.S.A. 100 (6), 3233–3238. doi:10.1073/pnas.2628034100
Krishnaraju, K., Nguyen, H. Q., Liebermann, D. A., and Hoffman, B. (1995). The Zinc Finger Transcription Factor Egr-1 Potentiates Macrophage Differentiation of Hematopoietic Cells. Molecul. Cellul. Biol. 15 (10), 5499–5507.
Krishnaraju, K., Hoffman, B., and Liebermann, D. A. (2001). Early Growth Response Gene 1 Stimulates Development of Hematopoietic Progenitor Cells Along the Macrophage Lineage at the Expense of the Granulocyte and Erythroid Lineages. Blood, J. Amer. Soc. Hematol. 97 (5), 1298–1305.
Kulkarni, R., Bajaj, M., Ghode, S., Jalnapurkar, S., Limaye, L., and Kale, V. P. (2018). Intercellular Transfer of Microvesicles from Young Mesenchymal Stromal Cells Rejuvenates Aged Murine Hematopoietic Stem Cells. Stem Cells 36 (3), 420–433. doi:10.1002/stem.2756
Kulkarni, R., and Kale, V. (2020). Physiological Cues Involved in the Regulation of Adhesion Mechanisms in Hematopoietic Stem Cell Fate Decision. Front. Cell Dev. Biol. 8, 611. doi:10.3389/fcell.2020.00611
Laslo, P., Spooner, C. J., Warmflash, A., Lancki, D. W., Lee, H.-J., Sciammas, R., et al. (2006). Multilineage Transcriptional Priming and Determination of Alternate Hematopoietic Cell Fates. Cell 126 (4), 755–766. doi:10.1016/j.cell.2006.06.052
Latchney, S. E., and Calvi, L. M. (2017). The Aging Hematopoietic Stem Cell Niche: Phenotypic and Functional Changes and Mechanisms that Contribute to Hematopoietic Aging. Seminors Hematol 54, 25–32. WB Saunders. doi:10.1053/j.seminhematol.2016.10.001
Lee, S. L., Wang, Y., and Milbrandt, J. (1996). Unimpaired Macrophage Differentiation and Activation in Mice Lacking the Zinc Finger Transplantation Factor NGFI-A (EGR1). Mol. Cell Biol. 16 (8), 4566–4572. doi:10.1128/mcb.16.8.4566
Li, H., Lim, H.-C., Zacharaki, D., Xian, X., Kenswil, K. J. G., Bräunig, S., et al. (2020). Early Growth Response 1 Regulates Hematopoietic Support and Proliferation in Human Primary Bone Marrow Stromal Cells. haematologica 105 (5), 1206–1215. doi:10.3324/haematol.2019.216648
Linton, P. J., and Dorshkind, K. (2004). Age-related Changes in Lymphocyte Development and Function. Nat. Immunol. 5 (2), 133–139. doi:10.1038/ni1033
Liu, C., Adamson, E., and Mercola, D. (1996). Transcription Factor EGR-1 Suppresses the Growth and Transformation of Human HT-1080 Fibrosarcoma Cells by Induction of Transforming Growth Factor Beta 1. Proc. Natl. Acad. Sci. U.S.A. 93 (21), 11831–11836. doi:10.1073/pnas.93.21.11831
Liu, P., Li, J., Lu, H., and Xu, B. (2009). Thalidomide Inhibits Leukemia Cell Invasion and Migration by Upregulation of Early Growth Response Gene 1. Leukemia lymphoma 50 (1), 109–113. doi:10.1080/10428190802588352
López-Otín, C., Blasco, M. A., Partridge, L., Serrano, M., and Kroemer, G. (2013). The Hallmarks of Aging. Cell 153 (6), 1194–1217. doi:10.1016/j.cell.2013.05.039
Maifrede, S., Liebermann, D., and Hoffman, B. (2014). Egr-1, a Stress Response Transcription Factor and Myeloid Differentiation Primary Response Gene, Behaves as Tumor Suppressor in CML. Blood 124 (21), 2211. doi:10.1182/blood.v124.21.2211.2211
Maltzman, J. S., Carman, J. A., and Monroe, J. G. (1996). Role of EGR1 in Regulation of Stimulus-dependent CD44 Transcription in B Lymphocytes. Mol. Cell Biol. 16 (5), 2283–2294. doi:10.1128/mcb.16.5.2283
McMahon, S. B., and Monroe, J. G. (1996). The Role of Early Growth Response Gene 1 (Egr -1) in Regulation of the Immune Response. J. Leukoc. Biol. 60 (2), 159–166. doi:10.1002/jlb.60.2.159
Mejia-Ramirez, E., and Florian, M. C. (2020). Understanding Intrinsic Hematopoietic Stem Cell Aging. haematologica 105 (1), 22–37. doi:10.3324/haematol.2018.211342
Min, I. M., Pietramaggiori, G., Kim, F. S., Passegué, E., Stevenson, K. E., and Wagers, A. J. (2008). The Transcription Factor EGR1 Controls Both the Proliferation and Localization of Hematopoietic Stem Cells. Cell stem Cell 2 (4), 380–391. doi:10.1016/j.stem.2008.01.015
Miyazaki, T. (1997). Two Distinct Steps during Thymocyte Maturation from CD4−CD8− to CD4+CD8+ Distinguished in the Early Growth Response (Egr)-1 Transgenic Mice with a Recombinase-Activating Gene-Deficient Background. J. Exp. Med. 186 (6), 877–885. doi:10.1084/jem.186.6.877
Nguyen, H. Q., Hoffman-Liebermann, B., and Liebermann, D. A. (1993). The Zinc Finger Transcription Factor Egr-1 Is Essential for and Restricts Differentiation along the Macrophage Lineage. Cell 72 (2), 197–209. doi:10.1016/0092-8674(93)90660-i
Noda, S., Ichikawa, H., and Miyoshi, H. (2009). Hematopoietic Stem Cell Aging is Associated With Functional Decline and Delayed Cell Cycle Progression. Biochem. Biophys. Res. Communicat. 383 (2), 210–215.
Oh, Y.-K., Jang, E., Paik, D.-J., and Youn, J. (2015). Early Growth Response-1 Plays a Non-redundant Role in the Differentiation of B Cells into Plasma Cells. Immune Netw. 15 (3), 161–166. doi:10.4110/in.2015.15.3.161
Orkin, S. H., and Zon, L. I. (2008). Hematopoiesis: an Evolving Paradigm for Stem Cell Biology. Cell 132 (4), 631–644. doi:10.1016/j.cell.2008.01.025
Pelletier, A., Carrier, A., Zhao, Y. M., Canouil, M., Derhourhi, M., Durand, E., et al. (2021). Excessive Fetal Growth Affects HSC Quiescence Maintenance through Epigenetic Programming of EGR1 Transcriptional Network. NY: bioRxiv.
Renders, S., Svendsen, A. F., Panten, J., Rama, N., Maryanovich, M., Sommerkamp, P., et al. (2021). Niche Derived Netrin-1 Regulates Hematopoietic Stem Cell Dormancy via its Receptor Neogenin-1. Nat. communicat. 12 (1), 1–15.
Ross, J., and Li, L. (2006). Recent Advances in Understanding Extrinsic Control of Hematopoietic Stem Cell Fate. Curr. Opin. Hematol. 13 (4), 237–242. doi:10.1097/01.moh.0000231420.92782.8f
Scheicher, R., Hoelbl-Kovacic, A., Bellutti, F., Tigan, A.-S., Prchal-Murphy, M., Heller, G., et al. (2015). CDK6 as a Key Regulator of Hematopoietic and Leukemic Stem Cell Activation. J. Am. Soc. Hematol. 125 (1), 90–101. doi:10.1182/blood-2014-06-584417
Seiler, M. P., Mathew, R., Liszewski, M. K., Spooner, C. J., Barr, K., Meng, F., et al. (2012). Elevated and Sustained Expression of the Transcription Factors Egr1 and Egr2 Controls NKT Lineage Differentiation in Response to TCR Signaling. Nat. Immunol. 13 (3), 264–271. doi:10.1038/ni.2230
Seyfert, V. L., McMahon, S., Glenn, W., Cao, X. M., Sukhatme, V. P., and Monroe, J. G. (1990). Egr-1 Expression in Surface Ig-Mediated B Cell Activation. Kinetics and Association with Protein Kinase C Activation. J. Immunol. 145 (11), 3647–3653.
Sukhatme, V. P., Kartha, S., Toback, F. G., Taub, R., Hoover, R. G., and Tsai-Morris, C. H. (1987). A Novel Early Growth Response Gene Rapidly Induced by Fibroblast, Epithelial Cell and Lymphocyte Mitogens. Oncogene Res. 1 (4), 343–355.
Sukhatme, V. P., Cao, X., Chang, L. C., Tsai-Morris, C.-H., Stamenkovich, D., Ferreira, P. C. P., et al. (1988). A Zinc Finger-Encoding Gene Coregulated with C-Fos during Growth and Differentiation, and after Cellular Depolarization. Cell 53 (1), 37–43. doi:10.1016/0092-8674(88)90485-0
Tamm, I., Schmelz, K., Dörken, B., and Wagner, M. (2006). Survivin Is Negatively Regulated by Early Growth Response (Egr)-1 Transcription Factor in Acute Myeloid Leukemia. Blood 108 (11), 1943. doi:10.1182/blood.v108.11.1943.1943
Thiel, G., and Cibelli, G. (2002). Regulation of Life and Death by the Zinc Finger Transcription Factor Egr-1. J. Cell. Physiol. 193 (3), 287–292. doi:10.1002/jcp.10178
Tourtellotte, W. G., Nagarajan, R., Bartke, A., and Milbrandt, J. (2000). Functional Compensation by Egr4 in Egr1-Dependent Luteinizing Hormone Regulation and Leydig Cell Steroidogenesis. Mole. Cellul. Biol. 20 (14), 5261–5268.
Tsai-Morris, C.-H., Cao, X., and Sukhatme, V. P. (1988). 5' Flanking Sequence and Genomic Structure of Egr-1, a Murine Mitogen Inducible Zinc Finger Encoding Gene. Nucleic acids Res. 16 (18), 8835–8846. doi:10.1093/nar/16.18.8835
Valletta, S., Thomas, A., Meng, Y., Ren, X., Drissen, R., Sengül, H., et al. (2020). Micro-environmental Sensing by Bone Marrow Stroma Identifies IL-6 and TGFβ1 as Regulators of Hematopoietic Ageing. Nat. Commun. 11 (1), 4075–4113. doi:10.1038/s41467-020-17942-7
Verduci, L., Azzalin, G., Gioiosa, S., Carissimi, C., Laudadio, I., Fulci, V., et al. (2015). microRNA-181a Enhances Cell Proliferation in Acute Lymphoblastic Leukemia by Targeting EGR1. Leukemia Res. 39 (4), 479–485. doi:10.1016/j.leukres.2015.01.010
Wang, D., Guan, M.-P., Zheng, Z.-J., Li, W.-Q., Lyv, F.-P., Pang, R.-Y., et al. (2015). Transcription Factor Egr1 Is Involved in High Glucose-Induced Proliferation and Fibrosis in Rat Glomerular Mesangial Cells. Cell Physiol. Biochem. 36 (6), 2093–2107. doi:10.1159/000430177
Xiao, D., Chinnappan, D., Pestell, R., Albanese, C., and Weber, H. C. (2005). Bombesin Regulates Cyclin D1 Expression through the Early Growth Response Protein Egr-1 in Prostate Cancer Cells. Cancer Res. 65 (21), 9934–9942. doi:10.1158/0008-5472.can-05-1830
Yamazaki, S., Iwama, A., Takayanagi, S.-i., Eto, K., Ema, H., and Nakauchi, H. (2009). TGF-β as a Candidate Bone Marrow Niche Signal to Induce Hematopoietic Stem Cell Hibernation. Blood, J. Am. Soc. Hematol. 113 (6), 1250–1256. doi:10.1182/blood-2008-04-146480
Zimmerman, S. M., and Kim, S. K. (2014). TheGATAtranscription factor/MTA‐1 Homologegr‐1promotes Longevity and Stress Resistance inCaenorhabditis Elegans. Aging Cell 13 (2), 329–339. doi:10.1111/acel.12179
Keywords: HSC rejuvenation, leukemia, HSC activation, aged HSCs, hematopoitic stem cells, early growth response 1 (EGR1)
Citation: Kulkarni R (2022) Early Growth Response Factor 1 in Aging Hematopoietic Stem Cells and Leukemia. Front. Cell Dev. Biol. 10:925761. doi: 10.3389/fcell.2022.925761
Received: 21 April 2022; Accepted: 14 June 2022;
Published: 18 July 2022.
Edited by:
Anuradha Vaidya, Symbiosis International University, IndiaReviewed by:
Marie-Dominique Filippi, Cincinnati Children’s Research Foundation, United StatesCopyright © 2022 Kulkarni. This is an open-access article distributed under the terms of the Creative Commons Attribution License (CC BY). The use, distribution or reproduction in other forums is permitted, provided the original author(s) and the copyright owner(s) are credited and that the original publication in this journal is cited, in accordance with accepted academic practice. No use, distribution or reproduction is permitted which does not comply with these terms.
*Correspondence: Rohan Kulkarni, cm9zdWt1NDdAZ21haWwuY29t