Fine-tuning of regulatory T cells is indispensable for the metabolic steatosis-related hepatocellular carcinoma: A review
- 1Center for Cancer Immunology, Shenzhen Institutes of Advanced Technology, Chinese Academy of Sciences, Shenzhen, China
- 2Chongqing Key Laboratory of Pediatrics, Department of otolaryngology, Children’s Hospital of Chongqing Medical University, National Clinical Research Center for Child Health and Disorders, Ministry of Education Key Laboratory of Child Development and Disorders, Chongqing, China
The majority of chronic hepatic diseases are caused by nutritional imbalance. These nutritional inequities include excessive intake of alcohol and fat, which causes alcoholic liver disease (ALD) and non-alcoholic fatty liver disease (NAFLD), respectively. The pathogenesis of hepatic diseases is mainly dependent on oxidative stress, autophagy, DNA damage, and gut microbiota and their metabolites. These factors influence the normal physiology of the liver and impact the hepatic microenvironment. The hepatic microenvironment contains several immune cells and inflammatory cytokines which interact with each other and contribute to the progression of chronic hepatic diseases. Among these immune cells, Foxp3+ CD4+ regulatory T cells (Tregs) are the crucial subset of CD4+ T cells that create an immunosuppressive environment. This review emphasizes the function of Tregs in the pathogenesis of ALD and NAFLD and their role in the progression of NAFLD-associated hepatocellular carcinoma (HCC). Briefly, Tregs establish an immunosuppressive landscape in the liver by interacting with the innate immune cells and gut microbiota and their metabolites. Meanwhile, with the advancement of steatosis, these Tregs inhibit the proliferation, activation and functions of other cytotoxic T cells and support the progression of simple steatosis to HCC. Briefly, it can be suggested that targeting Tregs can act as a favourable prognostic indicator by modulating steatosis and insulin resistance during the pathogenesis of hepatic steatosis and NAFLD-associated HCC.
Introduction
Human beings acquire a sophisticated immune system that actively exists with complex biological mechanisms to defend the host by attacking and destroying the foreign substances (antigen) and transformed or infected cells. Simultaneously, numerous immune regulatory processes are projected to dodge the auto-immune mechanisms resulting against the body’s own tissues. The immune system retains a distinct CD4+ cells population, regulatory T cells (Tregs), known to serve essential modulatory roles in immune homeostasis by maintaining peripheral tolerance and controlling the pathological and physiological immune response (Vignali et al., 2008; Lu et al., 2017). These Treg cells assist in limiting the inflammatory responses and abolish autoreactive T cells (Peterson, 2012; Scheinecker et al., 2020).
In 1970, it was first proposed that the presence of thymic-derived suppressor T cells, other than helper T (Th) cells, plays a role in self-tolerance by restricting the effector immune reactions (Gershon and Kondo, 1970). These suppressor T cells were later found responsible for the over-production of immunosuppressive cytokines, such as transforming growth factor β (TGF-β) and interleukin 10 (IL-10), which take part in the immune suppression (O'Garra and Murphy, 1994; Cottrez et al., 2000). Afterward, Sakaguchi et al. (1995), identified the IL-2 receptor α-chain (CD25 molecule) on the surface of these suppressive T cells and named Treg cells. It has been estimated that 5% to 50% of CD4+ T cells in the human peripheral blood are comprised of naturally arising CD25+ Treg cells that suppress immune activity (Sakaguchi et al., 1995; Gregg et al., 2005). Moreover, CD25+CD4+ Tregs were determined to express forkhead box P3 (FOXP3), a transcriptional factor which regulated the development and proper functioning of Treg cells (Hori et al., 2003). Therefore, these suppressor T cells can be characterized as CD4+CD25+FOXP3+ Treg cells in murine and humans (Ziegler, 2006).
Multiple mechanisms have been defined for the proper functioning of Treg cells. These include the secretion of cytokines and soluble factors, cell-to-cell contact, and changes in the extracellular milieu to target a diverse population of immune cell populations, such as antigen-presenting cells (APCs), CD8+ T killers and cell counterparts of conventional CD4+ T cells (Vignali et al., 2008). Emerging evidence suggests the presence of these Treg cells population residing or infiltrating in numerous peripheral organs where they mediate tissue-specific functions. For instance, peroxisome proliferator‐activated receptor‐γ (PPAR-γ) expressing visceral adipose tissue-specific Treg population execute highly distinct functions, including the regulation of numerous genes known to have crucial functions in lipid and glucose metabolism (Cipolletta et al., 2012). Similarly, in muscle and lung, the presence of amphiregulin, a ligand of epidermal growth factor receptor, expressing Treg cells population can directly expedite the tissue repairing process (Burzyn et al., 2013; Arpaia et al., 2015). Foxp3+ Tregs play various roles in liver homeostasis and pathogenesis by interacting with other hepatic immune cells and hepatocytes. Hepatocytes have exhibited enclysis ability to engulf CD4+ T cells, predominantly Tregs, during hepatic inflammation to regulate T cell population (Davies et al., 2019).
Although the non-lymphoid tissue-specific Tregs molecular signature and functions have been interrogated in numerous investigations, our knowledge of the functions and fundamental biology of these liver-specific Treg cells and how they vary from other non-lymphoid Treg cells and immune cells is yet overlooked. Here, we reviewed the currently available studies about Treg cells that infiltrate the liver, emphasizing the mechanisms in the progression of chronic liver diseases from simple steatosis to hepatocellular carcinoma (HCC).
Treg cells in hepatic microenvironment
One of the largest internal tissues, the liver, connects with the gastrointestinal tract through the portal vein, which delivers multiple pathogenic and non-pathogenic antigens derived from the gastrointestinal tract (Jenne and Kubes, 2013; Kubes and Jenne, 2018). Therefore, besides enduring non-pathogenic organisms, it plays frontline immunological functions by establishing and escalating specific immune responses (Jenne and Kubes, 2013). As a vast organ, liver retains a predominant population of cells, including T lymphocytes, which are immunologically active and maintain essential immunological functions (Parker and Picut, 2012). The phenotypic characteristics and function of Treg cells differ between intrahepatic and circulatory compartments. This difference is due to the residence of Tregs in the hepatic microenvironment, which are deprived of sufficient oxygen while supplemented with inflammatory cytokines, hormones and metabolic products (Jeffery et al., 2016).
As the gut and liver are connected via the portal vein, several environmental factors, including dietary nutrients and metabolites, impact the functionality of Treg cells (Zeng and Chi, 2015). It is evident that gut microbiota and their metabolites strongly affect immune responses (Wu and Wu, 2012). Several microbial metabolites and bile acids have been linked with the expansion and stability of Tregs (Hang et al., 2019; Campbell et al., 2020; Song et al., 2020; Wiechers et al., 2021). Increasing evidence suggests that intake of short-chain fatty acids (SCFAs), metabolites produced by numerous symbionts, improves the proportion of Treg cells in mice treated with antibiotics (Arpaia et al., 2013). Similarly, oral gavage of microbes or SCFAs also activates Treg cells in response to several diseases (Ben Ya’acov et al., 2015; Smith et al., 2013). These gut microbiota and their metabolites also influence the normal physiology and immune response in the liver (Visekruna and Luu, 2021). It can be suggested that microbiota and their metabolites can also play a vital role in stabilizing the Treg cells.
Bile acids, cholesterol metabolites, are immensely produced in the liver through a multiple-step complex process that involves peroxisomal, mitochondrial, and cytosolic enzymes (Russell, 2003). These bile acids are best known to regulate metabolic processes, cellular processes, and the immune system (Chiang and Ferrell, 2019; Fiorucci et al., 2021). Bile acids exert their function by activating numerous receptors, including RAR-related orphan receptor γt (RORγt), liver X receptors α/β (LXRα/β), farnesoid X receptor (FXR), vitamin D receptor (VDR), constitutive androstane receptor (CAR), pregnane X receptor (PXR), and membrane-bound G protein-coupled receptors Takeda G protein-coupled receptor 5 (TGR5) (Keitel et al., 2019; Shin and Wang, 2019; Cai et al., 2021). Among these receptors, FXR and TGR5 have been acknowledged as legitimate targets for treating metabolic-associated NAFLD (Arab et al., 2017). Activation of these receptors also influences and shapes the innate immune response, thus playing critical roles in the progression and development of NAFLD-related HCC (Schubert et al., 2017). Increasing evidence indicates that bile acids and their receptors also influence the adaptive immune system (Campbell et al., 2020; Song et al., 2020); however, the role of these receptors in adaptive immune response, especially in liver resident Tregs, is not studied well. A recent study identified isoallo-LCA and 3-oxo-LCA as metabolites of lithocholic acid (LCA). Among these, 3-oxo-LCA directly binds to the RORγt and suppresses the differentiation of Th17 cells. Meanwhile, isoallo-LCA stimulates mitochondrial ROS production and promotes Treg differentiation by increasing FOXP3 expression (Hang et al., 2019). Interestingly, obeticholic acid (OCA), FXR agonist, was approved by FDA for the treatment of primary sclerosing (Ali and Lindor, 2016). On the other hand, OCA ameliorates fibrosis and NASH; thus, it exerts beneficial effects by reducing hepatic cirrhosis (Shah and Kowdley, 2020). However, the effect of OCA and other bile acid molecules on the population and function of FOXP3+CD4+ cells is not studied.
It has been documented that fat-soluble vitamins are highly prevalent in the liver (Stacchiotti et al., 2021). A metabolite of vitamin A, all-trans retinoic acid (RA), plays a role in the growth, differentiation and proper functioning of immune cells, including Treg cells (Liu et al., 2015). In the liver, RA is produced by stellate cells, which increases the Foxp3 in CD4+ T cells and takes part in the development (Dunham et al., 2013), functioning and stability of the Treg cells during the inflammatory microenvironment (Zhou et al., 2010; Lu et al., 2014). TGF-β is considered one of the essential immunosuppressive cytokines that activate the population of CD4+ CD25+ FoxP3+ Treg cells (Kanamori et al., 2016). RA can directly influence and promote the TGF-β-dependent differentiation of naive T cells into the FoxP3+ Treg cells (Mucida et al., 2009; Martínez-Blanco et al., 2021).
Besides the TGF-β in an inflammatory hepatic microenvironment, several other pro-inflammatory cytokines, including IL-1, IL-12, IL-6, IL-8, and TNFα, are also present. However, it was reported that IL-2 is deficient, which is necessary for the overall survival of Treg cells (Chen et al., 2016a). Activation of APCs is known to secrete these pro-inflammatory cytokines (Blanco et al., 2008). These intrahepatic resident Treg cells also rely on the APCs for their differentiation, proper functioning, and survival. Tregs interact with APCs by binding with CD80/86 through CD28/CTLA-4, engaging with MHC Class II through TCR in the presence of antigen, and responding to APC secretory cytokines through cytokine receptors (Goddard et al., 2004; Lai et al., 2007). However, TGF-β, in combination with higher IL-6, generates IL-17 producing Th17 cells from naïve T cells, which further suppresses the induction of Treg cells (Bettelli et al., 2006). In addition, hepatocytes also play an important role in the differentiation of Foxp3+ Tregs upon TCR stimulation from the CD4+ T cells via Notch-signaling (Burghardt et al., 2014). Resident Treg cells, even present in slightly lower regulatory potential, acquire an intact functional ability and affirm short-term lineage during culturing conditions that imitate and maintain the intrahepatic microenvironment (Chen et al., 2016a).
Immune metabolic pathways have been well studied for the activation, differentiation, survival, and proliferation of the immune cells, including metabolically active Treg cells (O'Neill et al., 2016; Wang et al., 2019). The expression of Foxp3, a pivotal transcription factor of Treg, is known to involve in metabolic pathways, such as glycolysis and fatty-acid oxidation (Fontenot et al., 2003; Gerriets et al., 2016; Angelin et al., 2017). During inflammatory circumstances, the ratios of metabolic supply-and-demand dramatically alter. Inflammatory lesions induce the level of hypoxia-inducible factor-1α (HIF-1α) in the tissues deficient in enough oxygen. HIF-1α plays a vital role in enhancing the population and suppressive roles of thymic Treg (Ben-Shoshan et al., 2008; Clambey et al., 2012) by directly binding the promoter region of FOXP3 (Clambey et al., 2012). However, during an oxygen-deficient environment, the ablation of mTOR signaling is found to be involved in the elimination of the HIF-1α functions (Land and Tee, 2007). Activating transcription factor 3 (ATF3), a member of ATF/CREB transcriptional family, is induced at early inflammatory responses by cytokines, i.e., IFN-α, IFN-β, IFN-γ and IL-4 (Farber, 1992; Drysdale et al., 1996). The absence of ATF3 escalated the mTOR-dependent induction of the HIF-1α, which minimized the Foxp3+ Treg cells (Zhu et al., 2018). Therefore, the hypoxic anti-inflammatory process induces HIF-1α that improves the population and functions of Treg cells by strengthening their effectiveness and reducing the proliferation of effector cells (Ben-Shoshan et al., 2008).
Conclusively, it is evident that hepatic immune responses and Treg population and functionality are greatly dependent on the hepatic microenvironment (Figure 1). This hepatic microenvironment has an excessive hypoxic atmosphere due to higher blood supply, especially around zone 3 (Jeffery et al., 2016). Meanwhile, other environmental signallings, including environmental factors, microbial metabolites and metabolic pathways, also influence the transcriptional programming of Foxp3 and the functional plasticity of Treg cells.
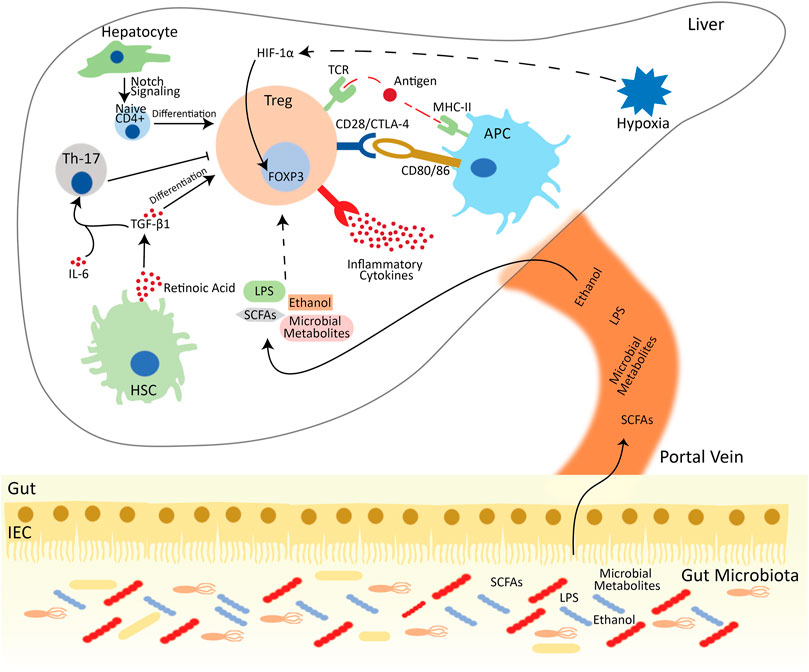
FIGURE 1. Treg mediated immune suppression in the hepatic microenvironment. Tregs are activated by interacting with the MHC-II on the surface of APCs in the presence of antigen. Tregs also suppress conventional T cells by interacting with pro-inflammatory cytokines and pairing with CD80/86 via CTL-4/CD28 and deprive the co-stimulatory signal to responder T cells. Besides the APC, Tregs also interact with other hepatic cells. Hepatocytes differentiate the naïve CD4+ cells into FOXP3+ Tregs via notch signaling. HSCs release retinoic acid, which activates the TGF-β signaling and aids Tregs differentiation. Meanwhile, in the presence of IL-6, TGF-β activates Th-17 cells, which reduces the activation and development of Tregs. Additionally, in oxygen-deprived environment, HIF-1α directly interacts with the FOXP3 and enhance the population of Tregs. Besides the intrahepatic regulation of Treg development and functions, gut microbiota and their metabolites also influence the Treg functions. Abbreviations: APC, Antigen presenting cell; Treg, regulatory T cell; Th-17, T helper 17 cell; HSC, hepatic stellate cell; IL, interleukin; CTLA4, cytotoxic T lymphocyte-associated antigen 4; DC, dendritic cell; CD, cluster of differentiation; TGF-β1, transforming growth factor-beta 1; MHC, major histocompatibility complex; IEC, intestinal epithelial cells; LPS, lipopolysaccharide; SCFA, Short-chain fatty acids.
Functions of Tregs in metabolic-associated chronic hepatic diseases
As mentioned earlier, the human liver acquires a large amount of blood supply, ∼70%–80%, from the portal vein, which is intensified with numerous metabolites and nutrients (Balmer et al., 2014). Therefore, the liver-resident immune cells, e.g., APCs, Tregs and T effector cells (Teffs), are continuously exposed to numerous signals which significantly impact their activation and alter their functions. Besides the effects of metabolites and nutrients on Tregs, innate immune cells and pro-inflammatory cytokines also impact the Tregs (Figure 2). Reported data on the immunosuppressive functions of Tregs suggest that intrahepatic suppression/overexpression or inadequate regulation of Tregs contribute to the onset of various diseases, including chronic hepatitis B&C virus (Cabrera et al., 2004), HCC (Unitt et al., 2005), autoimmune hepatitis (Longhi et al., 2004), alcoholic liver disease (ALD) (Matos et al., 2013), non-alcoholic liver disease (NAFLD) (Rau et al., 2016), primary biliary cirrhosis (Lan et al., 2006), acute rejection after liver transplant (Demirkiran et al., 2006). Here, we will be focusing on the function of Tregs in metabolic-associated chronic hepatic diseases, including ALD, NAFLD, NASH, and NAFLD-associated HCC.
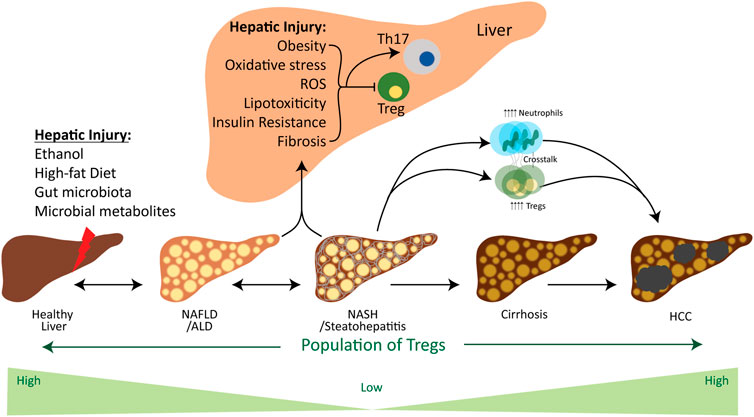
FIGURE 2. Influence of factors involved in the chronic liver disease spectrum on the Treg cells. Hepatic injury is caused by various environmental stressors, including ethanol, a high-fat diet and gut microbiota and their metabolites. These stressors initiate the simple steatosis and lead to steatohepatitis and cirrhosis in the liver, which finally progresses towards the tumor development. In the beginning, environmental stressors induce hepatic oxidative stress, ROS, lipotoxicity, insulin resistance, fibrosis and obesity, which are the pathogenic factors for ALD, NAFLD, NASH and HCC. These factors considerably contribute in downregulating the activation of Tregs and their population for the development of steatosis and fibrosis. However, the progression of NASH towards the tumor development increases the population of Tregs to establish a pro-tumor microenvironment. Meanwhile, adaptive and innate immune systems communicate with each other through Tregs and neutrophils, which aid in the progression of NAFLD-associated HCC. Abbreviations: Treg, regulatory T cell; Th-17, T helper 17 cell; NAFLD, Non-alcoholic fatty liver disease; ALD, alcoholic liver disease; NASH, non-alcoholic steatohepatitis; HCC, hepatocellular carcinoma.
Role of Treg in alcoholic liver disease
Alcohol abuse, an emerging and alarming health concern with high mortality worldwide, encompasses a broad range of injuries. These include alcoholic liver disease (ALD) with mild steatosis to steatohepatitis, fibrosis, and cirrhosis, leading to hepatocellular cancer (O'Shea et al., 2010; Breitkopf et al., 2009). It has been estimated that excessive alcohol intake is responsible for up to 4% of the yearly deaths (Singal and Anand, 2013). Ample evidence suggests that innate and adaptive immune responses are entailed in the development, pathogenesis and progression of ALD (Mille r et al., 2011; Albano, 2012; Gao et al., 2019). Alcoholic hepatic injury recruits peripheral immune-related cells, including infiltrating monocytes, neutrophils and T lymphocytes (Chedid et al., 1993; Nagy, 2015). Excessive exposure to alcohol or chronic ALD leads to the dysregulation of the balance between these immune cells and disruption of the immune activity. It contributes to the development of the unresolved inflammation features of ALD.
The role of inflammatory response activation and aberrant immune responses in worsening the ALD and its outcome has been extensively studied. It is generally believed that mild or excessive ethanol abuse may modify the immune responses and functions (Szabo et al., 2011; Azizov et al., 2020; Luck et al., 2021). Ethanol impairs the functions of APCs and monocytes, decreases the proliferation of T cells and interferes with the expression of adhesion molecules (Szabo and Mandrekar, 2009). During the progression of ALD, there is an increase in the production of pro-inflammatory chemokines/cytokines by the liver resident macrophages, i.e., kupffer cells (KC) (Slevin et al., 2020). Meanwhile, damaged hepatocytes may produce several antigens to initiate the intrahepatic immune responses, resulting in massive intrahepatic inflammatory cell recruitment, including Treg cells (Viitala et al., 2000).
With the advancement in experimental techniques, multiple T cell subtypes have been identified. It has been recognized that alcohol exposure impacts the T cells phenotypes and Treg cells population (Matos et al., 2013). Earlier studies elucidating the molecular mechanism underlying the progression and development of ALD illustrated the presence of CD4+ and CD8+ cells in the liver biopsies from ALD patients (Chedid et al., 1993). The population of peripheral blood CD4+/CD25+ Treg cells is not altered (non-significant increase) in chronic alcoholic patients. Still, it significantly decreases with the increased inflammatory cytokines in patients with alcoholic hepatitis (AH) (Almeida et al., 2013). A similar relative and absolute Treg population in AH, regardless of chronic hepatopathy symptoms, concluded that decreased CD4+/CD25+ Treg cells in AH depend on the acute hepatic inflammation (Almeida et al., 2013).
Similarly, Treg cells are most likely to participate in the pathogenesis of viral hepatitis in individuals with alcohol abuse. It was evident by the enhanced CD4+ FOXP3+ and CD25+ FOXP3+ Treg cell subtypes which were prompted in mice immunized with DCs, isolated from ethanol-fed mice, and loaded with HCV core (Ortiz and Wands, 2014). In contrast to decreased circulatory CD4+/CD25+ Treg cells in alcoholic patients (Almeida et al., 2013), another study reported an increased population of circulatory CD4+/CD25+ Treg cells in alcoholic patients (Ribeiro et al., 2017), suggested the role of Treg cells in reducing the detrimental effects of excessive alcohol intake on the liver. Meanwhile, it has been investigated that inflammatory immune response in ALD has resulted from the increased Th17 population (Kasztelan-Szczerbińska et al., 2015), and Treg cells reduce the development and differentiation of Th17 cells by modulating the levels of anti-inflammatory IL-10 and TGF-β cytokines (Chaudhry et al., 2011; O'Garra and Vieira, 2004; Lee, 2018). Likewise, the increased distribution of Treg cells close to the portal tract in the inflamed human liver demonstrates the close association with the suppression of immune response (Oo et al., 2010). Therefore, it can be suggested that an increased Treg cells population in patients with extreme ethanol might reduce the alcoholic hepatic inflammatory responses.
As previously described, the liver is directly connected to the gut and is continuously susceptible to harmless antigens and byproducts of gut bacteria. Currently available studies have discovered that alterations in the intestinal microbiome serve an important regulatory role in initiating ALD in humans and murine (Yan et al., 2011; Mutlu et al., 2012). Likewise, excessive alcohol intake may damage microbiome balance, distort the intestinal barrier, and lead to a dysfunctional liver and other vital organs (Bishehsari et al., 2017). Alcohol-induced intestinal barrier disruption helps the endotoxins, including lipopolysaccharide (LPS), and cytokines, including IL-6 and TNFα, to translocate into the liver and interact with hepatocytes and immune cells (Bala et al., 2014; Bishehsari et al., 2017; Zheng and Wang, 2021), which play pivotal roles in ALD and hepatic inflammation. Evidence of the ethanol-dependent modifications in the expression of CD4+ T Cell subsets in LPS-stimulated peripheral blood mononuclear cells suggests that ethanol impedes the Foxp3 kinetics and the production of IL-1 and TNF-α after the LPS challenge, thereby affecting the Treg/Th17 cells balance (von Haefen et al., 2011).
Ethanol intake damages the liver by reducing the population of Treg cells while increasing the Th17 cells population along with the production of IL-17 and increasing the intestinal permeability by reducing the expression of tight junction proteins (Wang et al., 2011; Chen et al., 2016b). Probiotic supplementation is well known to improve the functions of the intestinal barrier by improving the expression of tight junction proteins and exerting protective effects in response to damaging factors, including alcohol abuse (Rao and Samak, 2013). Lactobacillus rhamnosus GG (LGG) is a probiotic that strives to treat and prevent various diseases by stimulating immune responses (Segers and Lebeer, 2014). A recent study showed that LGG supernatant supplementation ameliorates ALD by improving the population of Treg cells and decreasing the Th17 population (Chen et al., 2016b).
Alcohol consumption, either acute or chronic, lowers the antigen presentation by DCs, and reduces the T-cell proliferation and activation by affecting the levels of costimulatory molecules (Eken et al., 2011). Hepatic resident APCs activate the Treg cells from naive CD4+ precursors (Bamboat et al., 2009). In ALD-cirrhosis patients, diminished levels of circulatory IL-1β, IL-6, IL-12, and TNF-α inflammatory cytokines, along with fewer number of circulatory DCs have been observed (Laso et al., 2007). Excessive alcohol consumption impairs the production of cytokines IL-6, IL-12, IL-17A and IFN-γ from APCs which are basically involved in initiating the adaptive immune response (Heinz and Waltenbaugh, 2007). Generally, ethanol diminishes the expression of stimulatory molecules on the surface of DCs, impairs their ability to prime T cells, affects the activation of naive T cells, and restricts the development of allogeneic T cells. Upon CpG stimulation, hepatic DCs collected from ethanol-fed mice exhibited reduced functional maturation (Lau et al., 2006). It implies that the alcohol-induced dysfunctional DCs might serve as a protective mechanism for immunosuppression by excessive alcohol drinking (Szabo, 1999; Lau et al., 2006) and may impair the Treg population.
Meanwhile, cell-cell interaction between DCs and Treg cells depends on the MHC-II for activation of Treg cells. Individuals with chronic alcohol intake may show lower MHC II-dependent T cell response (Chang and Norman, 1999). It can be advocated that besides the exclusive effects of alcohol on the cells responsible for innate immune response, early consequences on the adaptive immune system by alcohol contribute to the AH and ALD. Similarly, dysfunctional Treg cells are well known to contribute to the development and progression of these alcoholic diseases. However, the influence of alcohol on the roles of liver resident Treg cells is not studied well. Therefore, further investigations are necessary to improve our understanding of mechanisms underlying ALD and provide aid in finding novel therapeutic targets to treat AH and ALD.
Role of Treg in non-alcoholic fatty liver disease
Non-alcoholic fatty liver disease (NAFLD), affecting one-third of the population, is known for the presence of hepatic steatosis, which accelerates a series of hepatic diseases ranging from non-alcoholic fatty liver (NAFL) to non-alcoholic steatohepatitis (NASH) and progress to cirrhosis and hepatocellular carcinoma (HCC) (Younossi et al., 2016; Younossi et al., 2018). Hypothetically, the progression of NAFLD has been illustrated in a “multi-hit” manner, which initiates the accumulation of lipids in hepatocytes. Afterward, an increase in free fatty acids secretion from adipocytes, oxidative stress, decreased adiponectin, and increased pro-inflammatory cytokines (resistin, leptin, TNFα, and IL-6) collectively prompt the development of hepatic steatosis and inflammation (Starley et al., 2010). Meanwhile, several other factors, including TGF-β1-dependent collagen deposition, macrophage activation, hepatic reactive oxygen species (ROS), metabolically active natural killer T cells (NK-T) and CD8+ T cells, and imbalance between Th17 and Treg, take part in the disease progression beyond NAFL (Wolf et al., 2014; Paquissi, 2016).
Increasing evidence suggested the close association of NASH with activated innate immune response in mice (Tosello-Trampont et al., 2012) and humans (Malehmir et al., 2019). However, the role of adaptive immunity and Treg cells in NASH hasn’t been studied well. A recent clinical study showed the low population of resting Tregs in the peripheral blood of NASH patients and an increase in intrahepatic Th17 cells (Rau et al., 2016), suggesting that higher Th17/rTreg is engaged in NAFL to NASH progression. Another study indicated that NAFLD-related severe hepatic inflammation in children was linked with higher intralobular Foxp3+ lymphocytes, while adults exhibited decreased Foxp3+ and higher IL-17A+ lymphocytes in portal/periportal (P/P) tracts (Cairoli et al., 2021). Similarly, the high-fat diet (HFD)-induced NAFLD model showed an elevated population of intrahepatic Th17 cells. This increased Th17 and IL-17 are linked with the development of steatosis and the expression of pro-inflammatory cytokines (Tang et al., 2011). Subsequently, increased Th17 in liver during the chronic liver injuries exhibits a decreased proportion of Treg cells with the increased IL-6, IL-17 and IL-23 (He et al., 2017; Khanam et al., 2019).
Insulin resistance (IR), lipotoxicity and adipose inflammation are the hallmarks of NAFLD. Generally, lipotoxicity accelerates the pathogenesis of NAFLD by aggravating hepatic inflammation, adipose tissue inflammation and IR (Manco, 2017). It has been implicated that CD4+ T cells, especially Tregs, play critical roles in the regulation of IR, adipose inflammation and obesity. For instance, Tregs repress the immune response, whereas Th1 and Th17 cells enhance adipose inflammation (Bluestone et al., 2009; Newton et al., 2016). Upon activation, resting naive CD4+ T lymphocytes are differentiated into Tregs and Teffs to and employ immunological responses. Obesity and IR affect the Tregs cells by suppressing their differentiation or impairing their functions (Feuerer et al., 2009; Cipolletta et al., 2012; Wagner et al., 2013). It was found that maintenance of Tregs in VATs of HFD-induced obese animal model significantly reduces adipocyte size and decreases the body weight gain and visceral adipose tissue weight; thus, impairing Tregs worsens HFD-induced obesity and IR in mice (Tian et al., 2011; Cipolletta et al., 2012).
Several cellular metabolic activities, including inflammation, stress responses, and cell survival, are responsible for the oxidative stress and production of ROS within the cells (Pizzino et al., 2017). ROS production promotes hepatic inflammation, fibrogenesis and lipotoxicity in NAFLD (Delli Bovi et al., 2021). Lack of fatty acid β-oxidation along with intensive lipogenesis cause the excessive accumulation of triglycerides within the hepatocytes. During the process of NASH, increased ROS combines triglycerides and leads to IR and hepatic steatosis (Mansouri et al., 2018). Oxidative stress in the liver exerts detrimental effects on the hepatic Tregs. It was observed that oxidative stress induces Treg apoptosis and leads to their deletion within the steatotic liver (Ma et al., 2007), and consequently increases hepatic inflammation and avitivates TNF-α signaling pathway. This results in further hepatic injury, including the progression of simple steatosis to steatohepatitis, especially when liver is exposed to endotoxins, e.g., LPS, which can be delivered to liver or endogenously produced by gut microbiota (Ma et al., 2007; An et al., 2021). Moreover, adoptive transfer of Tregs have decreased HFD-induced intrahepatic TNF-α signaling and diminished the LPS-induced hepatotoxicity (Ma et al., 2007).
As mentioned earlier, activation of Tregs and their ability to perform suppressive functions are somehow dependent on the MHC-II. Liver sinusoidal endothelial cells (LSECs) and KCs are the key MHC-II expressing hepatic APC populations, which display antigen to Tregs and other CD4+ T cells (Wiegard et al., 2005). These Treg cells stimulated by KC and LSEC suppress the other CD4+ T cells proliferation (Wiegard et al., 2005). Similarly, hepatocytes can express MHC-II during hepatic inflammation, which helps them contribute to inflammatory immune responses by overcoming Treg suppressive functions during microbial antigenic signals (Herkel et al., 2003; Wiegard et al., 2005). The inflammatory hepatic microenvironment, i.e., TNF-α, IFN-γ and oxidative stress induced by KCs and DCs impair the survival and induce the apoptosis of Foxp3+ Treg cells during the apoptosis of hepatocytes and NASH (Ma et al., 2007; Roh et al., 2018). However, another study reported that the population of hepatic Tregs enhanced during the NASH, and depletion of Tregs can significantly inhibit the development of HCC from choline-deficient, high-fat diet feeding and diethylnitrosamine injection-induced NASH model (Wang et al., 2021). These opposing findings describing the functions of Tregs in NASH could have resulted from the different NASH models, or there is a probability that Tregs exert contrasting functions during the early and late NASH.
KLF10 is a well-known responsive transcription factor of TGF-β1, which regulates the functions and differentiation of Teffs and Tregs (Cao et al., 2009). A recent study concluded that the expression of KLF10 is significantly reduced in Teffs and Tregs isolated from peripheral blood and spleen of HFD-induced and obese mice (Wara et al., 2020). It is known that diet-induced obesity and liver diseases increase the immune cell accumulation in the liver and aggravate hepatic inflammation and lipid metabolic dysfunction (Dallio et al., 2021). CD4+ T cell-specific KLF10 deficiency leads to inflammation in adipose tissue, IR, obesity and the onset of NAFLD with impaired Treg accumulation (Wara et al., 2020). However, adoptive transfer of Tregs in CD4+ T cell-specific KLF10 deficient mice impede obesity, IR, adipose tissue inflammation and fatty liver phenotype (Wara et al., 2020). Overall, Foxp3+ Tregs exert protective roles during the progression of NAFLD from simple steatosis to steatohepatitis. Therefore, it can be concluded that despite the activation and marginal clonal expansion of hepatic T cells in NASH, the increased population of Treg counterbalance these effects. Meanwhile, the adoptive transfer of Treg in NASH aggravates the disease severity (Dywicki et al., 2022). Overall, the current understandings of the protective function of Tregs are still limited and need additional investigations to provide aid in treating NAFLD and NASH through Treg targeted therapy.
Role of Treg in NAFLD-associated HCC
HCC is deemed a major histological type of primary liver cancer which accounts for approximately 75% of all hepatic cancers (McGlynn et al., 2015). Leading evidence suggests that a high incidence of NAFLD and the ultimate progression of liver diseases are the leading causes of HCC. NASH leads to the hepatocytes’ death and compensatory proliferation, and converts the mild fibrosis to advanced fibrosis with elevated levels of TNFα, TGF-β1 and IL-18, which increase the risks of HCC (Anstee et al., 2019). The tumor microenvironment comprises cancer cells, immune cells and their mediators, and pro-inflammatory cytokines and chemokines (Gallimore and Simon, 2008). In the liver, the pathogenesis of NASH-associated HCC exclusively depends on the intrahepatic inflammatory and immune responses, autophagy, oxidative stress and DNA damage (Wolf et al., 2014; Anstee et al., 2019). Immune evasion of cancer cells is regulated by various immune suppressor mechanisms, which involve different subsets of immune cells and contribute to the initiation and progression of HCC (Greten et al., 2015). Meanwhile, lymphocytic infiltrate at the tumor site decreases the risk of a recurrent tumor and increases the overall survival rate (Greten et al., 2013). However, the role of T cells, especially Tregs, in NASH-associated HCC is not understood well. Striking evidence advocated that the Tregs population increases in peripheral blood and tumor tissues collected from HCC individuals (Guo et al., 2014). Therefore, researchers believe that increase in Tregs may exert adverse effects on the HCC disease prognosis (Wilke et al., 2010), and an augmented ratio of effector CD4+/Treg cells represent a better prognosis for HCC (Kalathil et al., 2019).
Several studies have determined the critical role of CD4+ T cells in the initiation and progression of HCC. CD4+ T cells generally impede the HCC initiation and progression, thereby contributing to the tumor regression (Rakhra et al., 2010). Tregs adversely affect the local immune microenvironment (Nishida and Kudo, 2017). FOXP3+ Tregs exert immunosuppressive functions in the tumor settings by restricting the development and activation of anti-tumor effector cells and facilitating the tumor immune escape (Khazaie and von Boehmer, 2006). Thus, elevated population of CD4+ CD25+ FoxP3+ Tregs promotes the disease initiation and progression by impairing the functions of effector CD8+ cells (Fu et al., 2007; Gao et al., 2007; Shi et al., 2018). Earlier studies have indicated that Tregs could regulate the differentiation and development of T cells by secreting anti-inflammatory IL-10 and TGF-β1 or repressing the IFN-γ and the T cells proliferation, thereby inhibiting their immune function (Bergmann et al., 2011). Meanwhile, HCC tumors themselves secret TGF-β1, which serves as the foremost vital source of TGF-β1 in HCC patients (Wang et al., 2016). This TGF-β1 could be a major factor in activating the regulatory phenotype of Tregs and maintaining their biological functions (Marie et al., 2005).
The functional heterogenicity of CD4+ T cells embraces Teff and Treg cell functions depending on their differentiation (Sallusto and Lanzavecchia, 2009). HCC pathogenesis greatly depends on the selective loss of hepatic resident CD4+ T cells, which accelerates the progression of HCC from NAFLD liver (Ma et al., 2016). It is obvious that IFN-γ secreting cytotoxic CD4+ Th1 cells monitor and clear the premalignant senescent hepatocytes (Kang et al., 2011). However, FOXP3+ Tregs inhibit the proliferation and function of Th1 and other Teffs. Therefore, despite the decreased population of total CD4+ in NASH liver, an enhanced population of Tregs has been observed, which aggravates the inflammation in NASH by establishing the pro-tumorigenic settings and leading to the initiation of the NASH-HCC malignant process (Wang et al., 2021).
Yes-associated protein-1 (YAP1) is a transcriptional coactivator and downstream effector of the Hippo signaling pathway (Manmadhan and Ehmer, 2019). Several studies have reported the positive correlation of YAP1 with the severity of hepatocyte injury and the progression of NAFLD and NASH (Chen et al., 2018; Salloum et al., 2021). Moreover, inhibiting the YAP1, reported as an independent prognostic marker and associated with the disease-free survival HCC patients (Xu et al., 2009), restores hepatocyte differentiation, and reduces the tumor development and the advancement of HCC (Fitamant et al., 2015). Besides the direct evidence of YAP in the development of NAFLD-HCC, it was investigated that YAP is necessary for the differentiation and immunosuppressive functions of Treg cells (Fan et al., 2017; Ni et al., 2018).
Mounting evidence indicated the potential role of gut microbiota in modulating T-cell immunity directly or via their metabolites, including SCFAs (Asarat et al., 2016). Microbial dysbiosis leads to the generation of excessive amounts of SCFA, especially butyrate, which aid in setting the tumor microenvironment (Singh et al., 2018). An ex-vivo investigation showed a positive correlation between Treg and butyrate. It indicated that gut microbiota in the NAFLD-HCC model expands the population of total and effector IL-10+ Tregs while decreasing the expansion of CD8+ cells (Behary et al., 2021). Similarly, it has been verified that IL-2 plays important role in the activation and expansion of CD8+ T cells (Chinen et al., 2016). The presence of peripheral Tregs consumes the IL-2, thereby attenuating the functions of CD8+ T cells (Chinen et al., 2016). Previous ex-vivo investigation implied that this T cell expression profile is triggered by the gut-microbiota isolated from NAFLD-HCC patients, but not cirrhosis, and demonstrated the microbiota and metabolites-specific regulatory effects on T cells in NAFLD-HCC (Behary et al., 2021). Together, all these investigations highlight the role of Treg in the onset of NAFLD-HCC. However, detailed mechanistic studies are still required to thoroughly understand the functions of Tregs in the pre-tumor process from NAFLD to HCC.
Conclusion
Chronic hepatic diseases, such as ALD and NAFLD, are emerging as the foremost cause of liver cancer and morbidity worldwide. It has been estimated that the prevalence of NAFLD-associated HCC will drastically increase (up to 45%–130%) in a decade (Grgurevic et al., 2021). The progression of hepatic diseases largely depends on several factors, such as ROS, oxidative stress, lipotoxicity, IR and gut microbiota. In the hepatic microenvironment, numerous hepatic parenchymal, non-parenchymal, innate immune cells, adaptive immune cells, and inflammatory cytokines and chemokines interact with each other to maintain immune homeostasis. However, factors contributing to metabolic steatosis disrupt this homeostasis which greatly affects the population of T cells in the hepatic microenvironment and leads to the CD4+ T cell infiltration in the liver. Numerous CD4+ T cell subsets participate in regulating the ALD, NAFLD, and NAFLD-related HCC disease progression. Among these T cells, FOXP3+ Tregs play pivotal roles in the progression of steatohepatitis and in creating pre-tumor microenvironment settings. It was suggested that during the hepatic injury, factors contributing to the disease progression reduce the activation and development of FOXP3+ Tregs. However, an increase in Tregs is involved in tempering the features of ALD and NAFLD by reducing the steatohepatitis and fibrosis, and exerting the immunosuppressive effects by hindering the inflammatory cellular immunity (Albano, 2012; Ikeno et al., 2020). Meanwhile, an increased population of Tregs promotes tumor development by setting a premalignant stage for the progression of HCC in the NASH-associated liver (Wang et al., 2021). It is noteworthy that various parenchymal and non-parenchymal cells in the liver interact to induce an immune response. Increasing evidence demonstrates that various factors influence the activity and function of Tregs. However, studies reporting the impacts of Tregs on the hepatic parenchymal and non-parenchymal cells are not illustrated.
Tregs-targeted therapy is regarded as a prospective HCC therapeutic strategy. So far, numerous innovative therapeutic strategies have been reported to target Tregs in clinical trials. These strategies, including using small molecules or antibodies, disrupt the function or differentiation of Tregs (Tsung and Norton, 2015). Despite advancements in biological and cancer research, little attention has been paid to developing strategies targeting the Tregs, especially in NAFLD-associated HCC. Various effective therapies, including monoclonal antibodies (anti-PD-1, anti-PD-L1, and anti-CTLA-4), have shown favorable prognosis and improved overall survival rates in patients with solid tumous (Saleh and Elkord, 2020a). However, studies demonstrating the effect of these monoclonal antibody usages in treating NAFLD-associated HCC are limited and need attention. More importantly, considering the side effects and low efficacy of these monoclonal antibodies, it is important to identify new anti-tumor drugs and validate the efficacy of newly identified drugs alone or in combination with other immune checkpoint inhibitors. Treg-targeted therapy represses tumor growth by enhancing the infiltration of CD8+ cells at the site of the tumor, improving the functions of APCs and minimizing the infiltration of myeloid suppressive cells in TME (Saleh and Elkord, 2019; Saleh and Elkord, 2020b). Thus, realizing the significant effects of the adoptive transfer of Tregs in the NAFLD and NAFLD-related HCC (Van Herck et al., 2020), it is important to evaluate the efficacy of natural or engineered Tregs along with other immune checkpoint inhibitors in NAFLD, premalignant NAFLD and NAFLD-related HCC. Meanwhile, it is worthwhile to understand the effects and function of Tregs in HCC tumors induced by different causative agents and varying disease stages and histological grades.
In conclusion, the cell-cell interactions, production of inflammatory cytokines and chemokines, and antigens in the tumor microenvironment diversify the functional studies of Tregs in HCC tumors. A comprehensive and clear mechanistic understanding of the biology of hepatic Treg cells in the steatosis and premalignant process may lead to inventing novel therapeutic approaches that target Tregs to restrict and treat chronic hepatic diseases, metabolic steatosis and NAFLD-associated HCC.
Author contributions
FR and PW prepared the draft. FP outlined the topic and edited it.
Funding
This work was supported by the National Key R&D Program of China (2021YFC2400500), the National Natural Science Foundation of China (Grant 32170925), and the startup fund of SIAT, CAS.
Conflict of interest
The authors declare that the research was conducted in the absence of any commercial or financial relationships that could be construed as a potential conflict of interest.
Publisher’s note
All claims expressed in this article are solely those of the authors and do not necessarily represent those of their affiliated organizations, or those of the publisher, the editors and the reviewers. Any product that may be evaluated in this article, or claim that may be made by its manufacturer, is not guaranteed or endorsed by the publisher.
References
Albano, E. (2012). Role of adaptive immunity in alcoholic liver disease. Int. J. Hepatol. 2012, 893026. doi:10.1155/2012/893026
Ali, A. H., and Lindor, K. D. (2016). Obeticholic acid for the treatment of primary biliary cholangitis. Expert Opin. Pharmacother. 17 (13), 1809–1815. doi:10.1080/14656566.2016.1218471
Almeida, J., Polvorosa, M. A., Gonzalez-Quintela, A., Marcos, M., Pastor, I., Hernandez Cerceno, M. L., et al. (2013). Decreased peripheral blood CD4+/CD25+ regulatory T cells in patients with alcoholic hepatitis. Alcohol. Clin. Exp. Res. 37 (8), 1361–1369. doi:10.1111/acer.12095
An, L., Wirth, U., Koch, D., Schirren, M., Drefs, M., Koliogiannis, D., et al. (2021). The role of gut-derived lipopolysaccharides and the intestinal barrier in fatty liver diseases. J. Gastrointest. Surg. 26, 671–683. doi:10.1007/s11605-021-05188-7
Angelin, A., Gil-de-Gomez, L., Dahiya, S., Jiao, J., Guo, L., Levine, M. H., et al. (2017). Foxp3 reprograms T cell metabolism to function in low-glucose, high-lactate environments. Cell. Metab. 25 (6), 1282–1293. e7. doi:10.1016/j.cmet.2016.12.018
Anstee, Q. M., Reeves, H. L., Kotsiliti, E., Govaere, O., and Heikenwalder, M. (2019). From NASH to HCC: Current concepts and future challenges. Nat. Rev. Gastroenterol. Hepatol. 16 (7), 411–428. doi:10.1038/s41575-019-0145-7
Arab, J. P., Karpen, S. J., Dawson, P. A., Arrese, M., and Trauner, M. (2017). Bile acids and nonalcoholic fatty liver disease: Molecular insights and therapeutic perspectives. Hepatology 65 (1), 350–362. doi:10.1002/hep.28709
Arpaia, N., Campbell, C., Fan, X., Dikiy, S., van der Veeken, J., deRoos, P., et al. (2013). Metabolites produced by commensal bacteria promote peripheral regulatory T-cell generation. Nature 504 (7480), 451–455. doi:10.1038/nature12726
Arpaia, N., Green, J. A., Moltedo, B., Arvey, A., Hemmers, S., Yuan, S., et al. (2015). A distinct function of regulatory T cells in tissue protection. Cell. 162 (5), 1078–1089. doi:10.1016/j.cell.2015.08.021
Asarat, M., Apostolopoulos, V., Vasiljevic, T., and DOnkOr, O. (2016). Short-chain fatty acids regulate cytokines and Th17/treg cells in human peripheral blood mononuclear cells in vitro. Immunol. Investig. 45 (3), 205–222. doi:10.3109/08820139.2015.1122613
Azizov, V., Dietel, K., Steffen, F., Durholz, K., Meidenbauer, J., Lucas, S., et al. (2020). Ethanol consumption inhibits T(FH) cell responses and the development of autoimmune arthritis. Nat. Commun. 11 (1), 1998. doi:10.1038/s41467-020-15855-z
Bala, S., Marcos, M., Gattu, A., Catalano, D., and Szabo, G. (2014). Acute binge drinking increases serum endotoxin and bacterial DNA levels in healthy individuals. PloS one 9 (5), e96864. doi:10.1371/journal.pone.0096864
Balmer, M. L., Slack, E., de Gottardi, A., Lawson, M. A. E., Hapfelmeier, S., Miele, L., et al. (2014). The liver may act as a firewall mediating mutualism between the host and its gut commensal microbiota. Sci. Transl. Med. 6 (237), 237ra66. doi:10.1126/scitranslmed.3008618
Bamboat, Z. M., Stableford, J. A., Plitas, G., Burt, B. M., Nguyen, H. M., Welles, A. P., et al. (2009). Human liver dendritic cells promote T cell hyporesponsiveness. J. Immunol. 182 (4), 1901–1911. doi:10.4049/jimmunol.0803404
Behary, J., Amorim, N., Jiang, X. T., Raposo, A., Gong, L., McGovern, E., et al. (2021). Gut microbiota impact on the peripheral immune response in non-alcoholic fatty liver disease related hepatocellular carcinoma. Nat. Commun. 12 (1), 187. doi:10.1038/s41467-020-20422-7
Ben Ya'acov, A., Lichtenstein, Y., Zolotarov, L., and Ilan, Y. (2015). The gut microbiome as a target for regulatory T cell-based immunotherapy: Induction of regulatory lymphocytes by oral administration of anti-LPS enriched colostrum alleviates immune mediated colitis. BMC Gastroenterol. 15, 154. doi:10.1186/s12876-015-0388-x
Ben-Shoshan, J., Maysel-Auslender, S., Mor, A., Keren, G., and George, J. (2008). Hypoxia controls CD4+CD25+ regulatory T-cell homeostasis via hypoxia-inducible factor-1alpha. Eur. J. Immunol. 38 (9), 2412–2418. doi:10.1002/eji.200838318
Bergmann, C., Wild, C. A., Narwan, M., Lotfi, R., Lang, S., and Brandau, S. (2011). Human tumor-induced and naturally occurring Treg cells differentially affect NK cells activated by either IL-2 or target cells. Eur. J. Immunol. 41 (12), 3564–3573. doi:10.1002/eji.201141532
Bettelli, E., Carrier, Y., Gao, W., Korn, T., Strom, T. B., Oukka, M., et al. (2006). Reciprocal developmental pathways for the generation of pathogenic effector TH17 and regulatory T cells. Nature 441 (7090), 235–238. doi:10.1038/nature04753
Bishehsari, F., Magno, E., Swanson, G., Desai, V., Voigt, R. M., Forsyth, C. B., et al. (2017). Alcohol and gut-derived inflammation. Alcohol Res. 38 (2), 163–171.
Blanco, P., Palucka, A. K., Pascual, V., and Banchereau, J. (2008). Dendritic cells and cytokines in human inflammatory and autoimmune diseases. Cytokine Growth Factor Rev. 19 (1), 41–52. doi:10.1016/j.cytogfr.2007.10.004
Bluestone, J. A., Mackay, C. R., O'Shea, J. J., and Stockinger, B. (2009). The functional plasticity of T cell subsets. Nat. Rev. Immunol. 9 (11), 811–816. doi:10.1038/nri2654
Breitkopf, K., Nagy, L. E., Beier, J. I., Mueller, S., Weng, H., and Dooley, S. (2009). Current experimental perspectives on the clinical progression of alcoholic liver disease. Alcohol. Clin. Exp. Res. 33 (10), 1647–1655. doi:10.1111/j.1530-0277.2009.01015.x
Burghardt, S., Claass, B., Erhardt, A., Karimi, K., and Tiegs, G. (2014). Hepatocytes induce Foxp3⁺ regulatory T cells by Notch signaling. J. Leukoc. Biol. 96 (4), 571–577. doi:10.1189/jlb.2AB0613-342RR
Burzyn, D., Kuswanto, W., Kolodin, D., Shadrach, J. L., Cerletti, M., Jang, Y., et al. (2013). A special population of regulatory T cells potentiates muscle repair. Cell. 155 (6), 1282–1295. doi:10.1016/j.cell.2013.10.054
Cabrera, R., Tu, Z., Xu, Y., Firpi, R. J., Rosen, H. R., Liu, C., et al. (2004). An immunomodulatory role for CD4(+)CD25(+) regulatory T lymphocytes in hepatitis C virus infection. Hepatology 40 (5), 1062–1071. doi:10.1002/hep.20454
Cai, X., Young, G. M., and Xie, W. (2021). The xenobiotic receptors PXR and CAR in liver physiology, an update. Biochim. Biophys. Acta. Mol. Basis Dis. 1867 (6), 166101. doi:10.1016/j.bbadis.2021.166101
Cairoli, V., De Matteo, E., Rios, D., Lezama, C., Galoppo, M., Casciato, P., et al. (2021). Hepatic lymphocytes involved in the pathogenesis of pediatric and adult non-alcoholic fatty liver disease. Sci. Rep. 11 (1), 5129. doi:10.1038/s41598-021-84674-z
Campbell, C., McKenney, P. T., Konstantinovsky, D., Isaeva, O. I., Schizas, M., Verter, J., et al. (2020). Bacterial metabolism of bile acids promotes generation of peripheral regulatory T cells. Nature 581 (7809), 475–479. doi:10.1038/s41586-020-2193-0
Cao, Z., Wara, A. K., Icli, B., Sun, X., Packard, R. R. S., Esen, F., et al. (2009). Kruppel-like factor KLF10 targets transforming growth factor-beta1 to regulate CD4(+)CD25(-) T cells and T regulatory cells. J. Biol. Chem. 284 (37), 24914–24924. doi:10.1074/jbc.M109.000059
Chang, M. P., and Norman, D. C. (1999). Ethanol impairs major histocompatibility complex (MHC) class II molecule-mediated but not MHC class I molecule-mediated T cell response in alcohol-consuming mice. Immunopharmacol. Immunotoxicol. 21 (1), 65–87. doi:10.3109/08923979909016395
Chaudhry, A., Samstein, R. M., Treuting, P., Liang, Y., Pils, M. C., Heinrich, J. M., et al. (2011). Interleukin-10 signaling in regulatory T cells is required for suppression of Th17 cell-mediated inflammation. Immunity 34 (4), 566–578. doi:10.1016/j.immuni.2011.03.018
Chedid, A., Mendenhall, C. L., Moritz, T. E., French, S. W., Chen, T. S., Morgan, T. R., et al. (1993). Cell-mediated hepatic injury in alcoholic liver disease. Veterans Affairs Cooperative Study Group 275. Gastroenterology 105 (1), 254–266. doi:10.1016/0016-5085(93)90034-a
Chen, P., Luo, Q., Huang, C., Gao, Q., Li, L., Chen, J., et al. (2018). Pathogenesis of non-alcoholic fatty liver disease mediated by YAP. Hepatol. Int. 12 (1), 26–36. doi:10.1007/s12072-017-9841-y
Chen, R. C., Xu, L. M., Du, S. J., Huang, S. S., Wu, H., Dong, J. J., et al. (2016). Lactobacillus rhamnosus GG supernatant promotes intestinal barrier function, balances Treg and TH17 cells and ameliorates hepatic injury in a mouse model of chronic-binge alcohol feeding. Toxicol. Lett. 241, 103–110. doi:10.1016/j.toxlet.2015.11.019
Chen, Y. Y., Jeffery, H. C., Hunter, S., Bhogal, R., Birtwistle, J., Braitch, M. K., et al. (2016). Human intrahepatic regulatory T cells are functional, require IL-2 from effector cells for survival, and are susceptible to Fas ligand-mediated apoptosis. Hepatology 64(1), 138–150. doi:10.1002/hep.28517
Chiang, J. Y. L., and Ferrell, J. M. (2019). Bile acids as metabolic regulators and nutrient sensors. Annu. Rev. Nutr. 39, 175–200. doi:10.1146/annurev-nutr-082018-124344
Chinen, T., Kannan, A. K., Levine, A. G., Fan, X., Klein, U., Zheng, Y., et al. (2016). An essential role for the IL-2 receptor in T(reg) cell function. Nat. Immunol. 17 (11), 1322–1333. doi:10.1038/ni.3540
Cipolletta, D., Feuerer, M., Li, A., Kamei, N., Lee, J., Shoelson, S. E., et al. (2012). PPAR-γ is a major driver of the accumulation and phenotype of adipose tissue Treg cells. Nature 486 (7404), 549–553. doi:10.1038/nature11132
Clambey, E. T., McNamee, E. N., Westrich, J. A., Glover, L. E., Campbell, E. L., Jedlicka, P., et al. (2012). Hypoxia-inducible factor-1 alpha-dependent induction of FoxP3 drives regulatory T-cell abundance and function during inflammatory hypoxia of the mucosa. Proc. Natl. Acad. Sci. U. S. A. 109 (41), E2784–E2793. doi:10.1073/pnas.1202366109
Cottrez, F., Hurst, S. D., Coffman, R. L., and Groux, H. (2000). T regulatory cells 1 inhibit a Th2-specific response in vivo. J. Immunol. 165 (9), 4848–4853. doi:10.4049/jimmunol.165.9.4848
Dallio, M., Sangineto, M., Romeo, M., Villani, R., Romano, A. D., Loguercio, C., et al. (2021). Immunity as cornerstone of non-alcoholic fatty liver disease: The contribution of oxidative stress in the disease progression. Int. J. Mol. Sci. 22 (1), E436. doi:10.3390/ijms22010436
Davies, S. P., Reynolds, G. M., Wilkinson, A. L., Li, X., Rose, R., Leekha, M., et al. (2019). Hepatocytes delete regulatory T cells by enclysis, a CD4(+) T cell engulfment process. Cell. Rep. 29 (6), 1610–1620. doi:10.1016/j.celrep.2019.09.068
Delli Bovi, A. P., Marciano, F., Mandato, C., Siano, M. A., Savoia, M., and Vajro, P. (2021). Oxidative stress in non-alcoholic fatty liver disease. An updated mini review. Front. Med. 8, 595371. doi:10.3389/fmed.2021.595371
Demirkiran, A., Kok, A., Kwekkeboom, J., Kusters, J. G., Metselaar, H. J., Tilanus, H. W., et al. (2006). Low circulating regulatory T-cell levels after acute rejection in liver transplantation. Liver Transpl. 12 (2), 277–284. doi:10.1002/lt.20612
Drysdale, B. E., Howard, D. L., and Johnson, R. J. (1996). Identification of a lipopolysaccharide inducible transcription factor in murine macrophages. Mol. Immunol. 33 (11-12), 989–998. doi:10.1016/s0161-5890(96)00043-0
Dunham, R. M., Thapa, M., Velazquez, V. M., Elrod, E. J., Denning, T. L., Pulendran, B., et al. (2013). Hepatic stellate cells preferentially induce Foxp3+ regulatory T cells by production of retinoic acidJ. Immunol. 190 (5), 2009–2016. doi:10.4049/jimmunol.1201937
Dywicki, J., Buitrago-Molina, L. E., Noyan, F., Davalos-Misslitz, A. C., Hupa-Breier, K. L., Lieber, M., et al. (2022). The detrimental role of regulatory T cells in nonalcoholic steatohepatitis. Hepatol. Commun. 6 (2), 320–333. doi:10.1002/hep4.1807
Eken, A., Ortiz, V., and Wands, J. R. (2011). Ethanol inhibits antigen presentation by dendritic cells. Clin. Vaccine Immunol. 18 (7), 1157–1166. doi:10.1128/CVI.05029-11
Fan, Y., Gao, Y., Rao, J., Wang, K., Zhang, F., and Zhang, C. (2017). YAP-1 promotes Tregs differentiation in hepatocellular carcinoma by enhancing TGFBR2 transcription. Cell. Physiol. biochem. 41 (3), 1189–1198. doi:10.1159/000464380
Farber, J. M. (1992). A collection of mRNA species that are inducible in the RAW 264.7 mouse macrophage cell line by gamma interferon and other agents. Mol. Cell. Biol. 12 (4), 1535–1545. doi:10.1128/mcb.12.4.1535
Feuerer, M., Herrero, L., Cipolletta, D., Naaz, A., Wong, J., Nayer, A., et al. (2009). Lean, but not obese, fat is enriched for a unique population of regulatory T cells that affect metabolic parameters. Nat. Med. 15 (8), 930–939. doi:10.1038/nm.2002
Fiorucci, S., Distrutti, E., Carino, A., Zampella, A., and Biagioli, M. (2021). Bile acids and their receptors in metabolic disorders. Prog. Lipid Res. 82, 101094. doi:10.1016/j.plipres.2021.101094
Fitamant, J., Kottakis, F., Benhamouche, S., Tian, H. S., Chuvin, N., Parachoniak, C. A., et al. (2015). YAP inhibition restores hepatocyte differentiation in advanced HCC, leading to tumor regression. Cell. Rep. 10 (10), 1692–1707. doi:10.1016/j.celrep.2015.02.027
Fontenot, J. D., Gavin, M. A., and Rudensky, A. Y. (2003). Foxp3 programs the development and function of CD4+CD25+ regulatory T cells. Nat. Immunol. 4 (4), 330–336. doi:10.1038/ni904
Fu, J., Xu, D., Liu, Z., Shi, M., Zhao, P., Fu, B., et al. (2007). Increased regulatory T cells correlate with CD8 T-cell impairment and poor survival in hepatocellular carcinoma patients. Gastroenterology 132 (7), 2328–2339. doi:10.1053/j.gastro.2007.03.102
Gallimore, A. M., and Simon, A. K. (2008). Positive and negative influences of regulatory T cells on tumour immunity. Oncogene 27 (45), 5886–5893. doi:10.1038/onc.2008.269
Gao, B., Ahmad, M. F., Nagy, L. E., and Tsukamoto, H. (2019). Inflammatory pathways in alcoholic steatohepatitis. J. Hepatol. 70 (2), 249–259. doi:10.1016/j.jhep.2018.10.023
Gao, Q., Qiu, S. J., Fan, J., Zhou, J., Wang, X. Y., Xiao, Y. S., et al. (2007). Intratumoral balance of regulatory and cytotoxic T cells is associated with prognosis of hepatocellular carcinoma after resection. J. Clin. Oncol. 25 (18), 2586–2593. doi:10.1200/JCO.2006.09.4565
Gerriets, V. A., Kishton, R. J., Johnson, M. O., Cohen, S., Siska, P. J., Nichols, A. G., et al. (2016). Foxp3 and Toll-like receptor signaling balance T(reg) cell anabolic metabolism for suppression. Nat. Immunol. 17 (12), 1459–1466. doi:10.1038/ni.3577
Gershon, R. K., and Kondo, K. (1970). Cell interactions in the induction of tolerance: The role of thymic lymphocytes. Immunology 18 (5), 723–737.
Goddard, S., Youster, J., Morgan, E., and Adams, D. H. (2004). Interleukin-10 secretion differentiates dendritic cells from human liver and skin. Am. J. Pathol. 164 (2), 511–519. doi:10.1016/S0002-9440(10)63141-0
Gregg, R., Smith, C. M., Clark, F. J., Dunnion, D., Kha, N. N., Chak, R. R., et al. (2005). The number of human peripheral blood CD4+ CD25high regulatory T cells increases with age. Clin. Exp. Immunol. 140 (3), 540–546. doi:10.1111/j.1365-2249.2005.02798.x
Greten, T. F., Duffy, A. G., and Korangy, F. (2013). Hepatocellular carcinoma from an immunologic perspective. Clin. Cancer Res. 19 (24), 6678–6685. doi:10.1158/1078-0432.CCR-13-1721
Greten, T. F., Wang, X. W., and Korangy, F. (2015). Current concepts of immune based treatments for patients with HCC: From basic science to novel treatment approaches. Gut 64 (5), 842–848. doi:10.1136/gutjnl-2014-307990
Grgurevic, I., Bozin, T., Mikus, M., Kukla, M., and O'Beirne, J. (2021). Hepatocellular carcinoma in non-alcoholic fatty liver disease: From epidemiology to diagnostic approach. Cancers 13 (22), 5844. doi:10.3390/cancers13225844
Guo, C. L., Yang, X. H., Cheng, W., Xu, Y., Li, J. B., Sun, Y. X., et al. (2014). Expression of Fas/FasL in CD8+ T and CD3+ Foxp3+ Treg cells--relationship with apoptosis of circulating CD8+ T cells in hepatocellular carcinoma patients. Asian pac. J. Cancer Prev. 15 (6), 2613–2618. doi:10.7314/apjcp.2014.15.6.2613
Hang, S., Paik, D., Yao, L., Kim, E., Trinath, J., Lu, J., et al. (2019). Bile acid metabolites control T(H)17 and T(reg) cell differentiation. Nature 576 (7785), 143–148. doi:10.1038/s41586-019-1785-z
He, B., Wu, L., Xie, W., Shao, Y., Jiang, J., Zhao, Z., et al. (2017). The imbalance of Th17/Treg cells is involved in the progression of nonalcoholic fatty liver disease in mice. BMC Immunol. 18 (1), 33. doi:10.1186/s12865-017-0215-y
Heinz, R., and Waltenbaugh, C. (2007). Ethanol consumption modifies dendritic cell antigen presentation in mice. Alcohol. Clin. Exp. Res. 31 (10), 1759–1771. doi:10.1111/j.1530-0277.2007.00479.x
Herkel, J., Jagemann, B., Wiegard, C., Lazaro, J. F. G., Lueth, S., Kanzler, S., et al. (2003). MHC class II-expressing hepatocytes function as antigen-presenting cells and activate specific CD4 T lymphocyutes. Hepatology 37 (5), 1079–1085. doi:10.1053/jhep.2003.50191
Hori, S., Nomura, T., and Sakaguchi, S. (2003). Control of regulatory T cell development by the transcription factor Foxp3. Science 299 (5609), 1057–1061. doi:10.1126/science.1079490
Ikeno, Y., Ohara, D., Takeuchi, Y., Watanabe, H., Kondoh, G., Taura, K., et al. (2020). Foxp3+ regulatory T cells inhibit CCl(4)-induced liver inflammation and fibrosis by regulating tissue cellular immunity. Front. Immunol. 11, 584048. doi:10.3389/fimmu.2020.584048
Jeffery, H. C., Braitch, M. K., Brown, S., and Oo, Y. H. (2016). Clinical potential of regulatory T cell therapy in liver diseases: An overview and current perspectives. Front. Immunol. 7, 334. doi:10.3389/fimmu.2016.00334
Jenne, C. N., and Kubes, P. (2013). Immune surveillance by the liver. Nat. Immunol. 14 (10), 996–1006. doi:10.1038/ni.2691
Kalathil, S. G., Hutson, A., Barbi, J., Iyer, R., and Thanavala, Y. (2019). Augmentation of IFN-γ+ CD8+ T cell responses correlates with survival of HCC patients on sorafenib therapy. JCI Insight 4 (15), 130116. doi:10.1172/jci.insight.130116
Kanamori, M., Nakatsukasa, H., Okada, M., Lu, Q., and Yoshimura, A. (2016). Induced regulatory T cells: Their development, stability, and applications. Trends Immunol. 37 (11), 803–811. doi:10.1016/j.it.2016.08.012
Kang, T. W., Yevsa, T., Woller, N., Hoenicke, L., Wuestefeld, T., Dauch, D., et al. (2011). Senescence surveillance of pre-malignant hepatocytes limits liver cancer development. Nature 479 (7374), 547–551. doi:10.1038/nature10599
Kasztelan-Szczerbińska, B., Surdacka, A., Celinski, K., Rolinski, J., Zwolak, A., Miacz, S., et al. (2015). Prognostic significance of the systemic inflammatory and immune balance in alcoholic liver disease with a focus on gender-related differences. PLoS One 10 (6), e0128347. doi:10.1371/journal.pone.0128347
Keitel, V., Stindt, J., and Häussinger, D. (2019). Bile acid-activated receptors: GPBAR1 (TGR5) and other G protein-coupled receptors. Handb. Exp. Pharmacol. 256, 19–49. doi:10.1007/164_2019_230
Khanam, A., Trehanpati, N., and Sarin, S. K. (2019). Increased interleukin-23 receptor (IL-23R) expression is associated with disease severity in acute-on-chronic liver failure. Liver Int. 39 (6), 1062–1070. doi:10.1111/liv.14015
Khazaie, K., and von Boehmer, H. (2006). The impact of CD4+CD25+ Treg on tumor specific CD8+ T cell cytotoxicity and cancer. Semin. Cancer Biol. 16 (2), 124–136. doi:10.1016/j.semcancer.2005.11.006
Kubes, P., and Jenne, C. (2018). Immune responses in the liver. Annu. Rev. Immunol. 36, 247–277. doi:10.1146/annurev-immunol-051116-052415
Lai, W. K., Curbishley, S. M., Goddard, S., Alabraba, E., Shaw, J., Youster, J., et al. (2007). Hepatitis C is associated with perturbation of intrahepatic myeloid and plasmacytoid dendritic cell function. J. Hepatol. 47 (3), 338–347. doi:10.1016/j.jhep.2007.03.024
Lan, R. Y., Cheng, C., Lian, Z. X., Tsuneyama, K., Yang, G. X., Moritoki, Y., et al. (2006). Liver-targeted and peripheral blood alterations of regulatory T cells in primary biliary cirrhosis. Hepatology 43 (4), 729–737. doi:10.1002/hep.21123
Land, S. C., and Tee, A. R. (2007). Hypoxia-inducible factor 1alpha is regulated by the mammalian target of rapamycin (mTOR) via an mTOR signaling motif. J. Biol. Chem. 282 (28), 20534–20543. doi:10.1074/jbc.M611782200
Laso, F. J., Vaquero, J. M., Almeida, J., Marcos, M., and Orfao, A. (2007). Chronic alcohol consumption is associated with changes in the distribution, immunophenotype, and the inflammatory cytokine secretion profile of circulating dendritic cells. Alcohol. Clin. Exp. Res. 31 (5), 846–854. doi:10.1111/j.1530-0277.2007.00377.x
Lau, A. H., Abe, M., and Thomson, A. W. (2006). Ethanol affects the generation, cosignaling molecule expression, and function of plasmacytoid and myeloid dendritic cell subsets in vitro and in vivo. J. Leukoc. Biol. 79 (5), 941–953. doi:10.1189/jlb.0905517
Lee, G. R. (2018). The balance of Th17 versus Treg cells in autoimmunity. Int. J. Mol. Sci. 19 (3), 730. doi:10.3390/ijms19030730
Liu, Z.-M., Wang, K. P., Ma, J., and Guo Zheng, S. (2015). The role of all-trans retinoic acid in the biology of Foxp3+ regulatory T cells. Cell. Mol. Immunol. 12 (5), 553–557. doi:10.1038/cmi.2014.133
Longhi, M. S., Ma, Y., Bogdanos, D. P., Cheeseman, P., Mieli-Vergani, G., and Vergani, D. (2004). Impairment of CD4(+)CD25(+) regulatory T-cells in autoimmune liver disease. J. Hepatol. 41 (1), 31–37. doi:10.1016/j.jhep.2004.03.008
Lu, L., Barbi, J., and Pan, F. (2017). The regulation of immune tolerance by FOXP3. Nat. Rev. Immunol. 17 (11), 703–717. doi:10.1038/nri.2017.75
Lu, L., Lan, Q., Li, Z., Zhou, X., Gu, J., Li, Q., et al. (2014). Critical role of all-trans retinoic acid in stabilizing human natural regulatory T cells under inflammatory conditions. Proc. Natl. Acad. Sci. U. S. A. 111 (33), E3432–E3440. doi:10.1073/pnas.1408780111
Luck, M. E., Li, X., Herrnreiter, C. J., and Choudhry, M. A. (2021). Ethanol intoxication and burn injury increases intestinal regulatory T cell population and regulatory T cell suppressive capability. Shock 57, 230. doi:10.1097/SHK.0000000000001853
Ma, C., Kesarwala, A. H., Eggert, T., Medina-Echeverz, J., Kleiner, D. E., Jin, P., et al. (2016). NAFLD causes selective CD4(+) T lymphocyte loss and promotes hepatocarcinogenesis. Nature 531 (7593), 253–257. doi:10.1038/nature16969
Ma, X., Hua, J., Mohamood, A. R., Hamad, A. R. A., Ravi, R., and Li, Z. (2007). A high-fat diet and regulatory T cells influence susceptibility to endotoxin-induced liver injury. Hepatology 46 (5), 1519–1529. doi:10.1002/hep.21823
Malehmir, M., Pfister, D., Gallage, S., Szydlowska, M., Inverso, D., Kotsiliti, E., et al. (2019). Platelet GPIbα is a mediator and potential interventional target for NASH and subsequent liver cancer. Nat. Med. 25 (4), 641–655. doi:10.1038/s41591-019-0379-5
Manco, M. (2017). Insulin resistance and NAFLD: A dangerous liaison beyond the genetics. Child. (Basel, Switz. 4 (8), 74. doi:10.3390/children4080074
Manmadhan, S., and Ehmer, U. (2019). Hippo signaling in the liver - a long and ever-expanding story. Front. Cell. Dev. Biol. 7, 33. doi:10.3389/fcell.2019.00033
Mansouri, A., Gattolliat, C. H., and Asselah, T. (2018). Mitochondrial dysfunction and signaling in chronic liver diseases. Gastroenterology 155 (3), 629–647. doi:10.1053/j.gastro.2018.06.083
Marie, J. C., Letterio, J. J., Gavin, M., and Rudensky, A. Y. (2005). TGF-beta1 maintains suppressor function and Foxp3 expression in CD4+CD25+ regulatory T cells. J. Exp. Med. 201 (7), 1061–1067. doi:10.1084/jem.20042276
Martínez-Blanco, M., Lozano-Ojalvo, D., Perez-Rodriguez, L., Benede, S., Molina, E., and Lopez-Fandino, R. (2021). Retinoic acid induces functionally suppressive Foxp3(+)RORγt(+) T cells in vitro. Front. Immunol. 12, 675733. doi:10.3389/fimmu.2021.675733
Matos, L. C., Batista, P., Monteiro, N., Ribeiro, J., Cipriano, M. A., Henriques, P., et al. (2013). Lymphocyte subsets in alcoholic liver disease. World J. Hepatol. 5 (2), 46–55. doi:10.4254/wjh.v5.i2.46
McGlynn, K. A., Petrick, J. L., and London, W. T. (2015). Global epidemiology of hepatocellular carcinoma: An emphasis on demographic and regional variability. Clin. Liver Dis. 19 (2), 223–238. doi:10.1016/j.cld.2015.01.001
Miller, A. M., Horiguchi, N., Jeong, W. I., Radaeva, S., and Gao, B. (2011). Molecular mechanisms of alcoholic liver disease: Innate immunity and cytokines. Alcohol. Clin. Exp. Res. 35 (5), 787–793. doi:10.1111/j.1530-0277.2010.01399.x
Mucida, D., Pino-Lagos, K., Kim, G., Nowak, E., Benson, M. J., Kronenberg, M., et al. (2009). Retinoic acid can directly promote TGF-beta-mediated Foxp3(+) Treg cell conversion of naive T cells. Immunity 30 (4), 471–472. author reply 472-3. doi:10.1016/j.immuni.2009.03.008
Mutlu, E. A., Gillevet, P. M., Rangwala, H., Sikaroodi, M., Naqvi, A., Engen, P. A., et al. (2012). Colonic microbiome is altered in alcoholism. Am. J. Physiol. Gastrointest. Liver Physiol. 302 (9), G966–G978. doi:10.1152/ajpgi.00380.2011
Nagy, L. E. (2015). The role of innate immunity in alcoholic liver disease. Alcohol Res. 37 (2), 237–250.
Newton, R., Priyadharshini, B., and Turka, L. A. (2016). Immunometabolism of regulatory T cells. Nat. Immunol. 17 (6), 618–625. doi:10.1038/ni.3466
Ni, X., Tao, J., Barbi, J., Chen, Q., Park, B. V., Li, Z., et al. (2018). YAP is essential for treg-mediated suppression of antitumor immunity. Cancer Discov. 8 (8), 1026–1043. doi:10.1158/2159-8290.CD-17-1124
Nishida, N., and Kudo, M. (2017). Immunological microenvironment of hepatocellular carcinoma and its clinical implication. Oncology 92 (Suppl. 1), 40–49. doi:10.1159/000451015
O'Garra, A., and Murphy, K. (1994). Role of cytokines in determining T-lymphocyte function. Curr. Opin. Immunol. 6 (3), 458–466. doi:10.1016/0952-7915(94)90128-7
O'Garra, A., and Vieira, P. (2004). Regulatory T cells and mechanisms of immune system control. Nat. Med. 10 (8), 801–805. doi:10.1038/nm0804-801
O'Neill, L. A., Kishton, R. J., and Rathmell, J. (2016). A guide to immunometabolism for immunologists. Nat. Rev. Immunol. 16 (9), 553–565. doi:10.1038/nri.2016.70
O'Shea, R. S., Dasarathy, S., and McCullough, A. J. (2010). Alcoholic liver disease. Hepatology 51 (1), 307–328. doi:10.1002/hep.23258
Oo, Y. H., Weston, C. J., Lalor, P. F., Curbishley, S. M., Withers, D. R., Reynolds, G. M., et al. (2010). Distinct roles for CCR4 and CXCR3 in the recruitment and positioning of regulatory T cells in the inflamed human liver. J. Immunol. 184 (6), 2886–2898. doi:10.4049/jimmunol.0901216
Ortiz, V., and Wands, J. R. (2014). Chronic ethanol diet increases regulatory T-cell activity and inhibits hepatitis C virus core-specific cellular immune responses in mice. Hepatol. Res. 44 (7), 788–797. doi:10.1111/hepr.12173
Paquissi, F. C. (2016). Immune imbalances in non-alcoholic fatty liver disease: From general biomarkers and neutrophils to interleukin-17 Axis Activation and new therapeutic targets. Front. Immunol. 7, 490. doi:10.3389/fimmu.2016.00490
Parker, G. A., and Picut, C. A. (2012). Immune functioning in non lymphoid organs: The liver. Toxicol. Pathol. 40 (2), 237–247. doi:10.1177/0192623311428475
Peterson, R. A. (2012). Regulatory T-cells: Diverse phenotypes integral to immune homeostasis and suppression. Toxicol. Pathol. 40 (2), 186–204. doi:10.1177/0192623311430693
Pizzino, G., Irrera, N., Cucinotta, M., Pallio, G., Mannino, F., Arcoraci, V., et al. (2017). Oxidative stress: Harms and benefits for human health. Oxidative Med. Cell. Longev. 2017, 8416763.
Rakhra, K., Bachireddy, P., Zabuawala, T., Zeiser, R., Xu, L., Kopelman, A., et al. (2010). CD4(+) T cells contribute to the remodeling of the microenvironment required for sustained tumor regression upon oncogene inactivation. Cancer Cell. 18 (5), 485–498. doi:10.1016/j.ccr.2010.10.002
Rao, R. K., and Samak, G. (2013). Protection and restitution of gut barrier by probiotics: Nutritional and clinical implications. Curr. Nutr. Food Sci. 9 (2), 99–107. doi:10.2174/1573401311309020004
Rau, M., Schilling, A. K., Meertens, J., Hering, I., Weiss, J., Jurowich, C., et al. (2016). Progression from nonalcoholic fatty liver to nonalcoholic steatohepatitis is marked by a higher frequency of Th17 cells in the liver and an increased Th17/resting regulatory T cell ratio in peripheral blood and in the liver. J. Immunol. 196 (1), 97–105. doi:10.4049/jimmunol.1501175
Ribeiro, S. R., Covre, L. P., Stringari, L. L., da Penha Zago-Gomes, M., Gomes, D. C. O., and Pereira, F. E. L. (2017). Peripheral blood CD4(+)/CD25(+) regulatory T cells in alcoholic patients with Strongyloides stercoralis infection. Parasitol. Res. 116 (3), 1071–1074. doi:10.1007/s00436-016-5355-0
Roh, Y. S., Kim, J. W., Park, S., Shon, C., Kim, S., Eo, S. K., et al. (2018). Toll-Like receptor-7 signaling promotes nonalcoholic steatohepatitis by inhibiting regulatory T cells in mice. Am. J. Pathol. 188 (11), 2574–2588. doi:10.1016/j.ajpath.2018.07.011
Russell, D. W. (2003). The enzymes, regulation, and genetics of bile acid synthesis. Annu. Rev. Biochem. 72, 137–174. doi:10.1146/annurev.biochem.72.121801.161712
Sakaguchi, S., Sakaguchi, N., Asano, M., Itoh, M., and Toda, M. (1995). Immunologic self-tolerance maintained by activated T cells expressing IL-2 receptor alpha-chains (CD25). Breakdown of a single mechanism of self-tolerance causes various autoimmune diseases. J. Immunol. 155 (3), 1151–1164.
Saleh, R., and Elkord, E. (2020). Acquired resistance to cancer immunotherapy: Role of tumor-mediated immunosuppression. Semin. Cancer Biol. 65, 13–27. doi:10.1016/j.semcancer.2019.07.017
Saleh, R., and Elkord, E. (2020). FoxP3(+) T regulatory cells in cancer: Prognostic biomarkers and therapeutic targets. Cancer Lett. 490, 174–185. doi:10.1016/j.canlet.2020.07.022
Saleh, R., and Elkord, E. (2019). Treg-mediated acquired resistance to immune checkpoint inhibitors. Cancer Lett. 457, 168–179. doi:10.1016/j.canlet.2019.05.003
Salloum, S., Jeyarajan, A. J., Kruger, A. J., Holmes, J. A., Shao, T., Sojoodi, M., et al. (2021). Fatty acids activate the transcriptional coactivator YAP1 to promote liver fibrosis via p38 mitogen-activated protein kinase. Cell. Mol. Gastroenterol. Hepatol. 12 (4), 1297–1310. doi:10.1016/j.jcmgh.2021.06.003
Sallusto, F., and Lanzavecchia, A. (2009). Heterogeneity of CD4+ memory T cells: Functional modules for tailored immunity. Eur. J. Immunol. 39 (8), 2076–2082. doi:10.1002/eji.200939722
Scheinecker, C., Göschl, L., and Bonelli, M. (2020). Treg cells in health and autoimmune diseases: New insights from single cell analysis. J. Autoimmun. 110, 102376. doi:10.1016/j.jaut.2019.102376
Schubert, K., Olde Damink, S. W. M., von Bergen, M., and Schaap, F. G. (2017). Interactions between bile salts, gut microbiota, and hepatic innate immunity. Immunol. Rev. 279 (1), 23–35. doi:10.1111/imr.12579
Segers, M. E., and Lebeer, S. (2014). Towards a better understanding of Lactobacillus rhamnosus GG--host interactions. Microb. Cell. Fact. 13 (Suppl. 1), S7. doi:10.1186/1475-2859-13-S1-S7
Shah, R. A., and Kowdley, K. V. (2020). Obeticholic acid for the treatment of nonalcoholic steatohepatitis. Expert Rev. Gastroenterol. Hepatol. 14 (5), 311–321. doi:10.1080/17474124.2020.1748498
Shi, C., Chen, Y., Chen, Y., Yang, Y., Bing, W., and Qi, J. (2018). CD4(+) CD25(+) regulatory T cells promote hepatocellular carcinoma invasion via TGF-β1-induced epithelial-mesenchymal transition. Onco. Targets. Ther. 12, 279–289. doi:10.2147/OTT.S172417
Shin, D. J., and Wang, L. (2019). Bile acid-activated receptors: A review on FXR and other nuclear receptors. Handb. Exp. Pharmacol. 256, 51–72. doi:10.1007/164_2019_236
Singal, A. K., and Anand, B. S. (2013). Recent trends in the epidemiology of alcoholic liver disease. Clin. Liver Dis. 2 (2), 53–56. doi:10.1002/cld.168
Singh, V., Yeoh, B. S., Chassaing, B., Xiao, X., Saha, P., Aguilera Olvera, R., et al. (2018). Dysregulated microbial fermentation of soluble fiber induces cholestatic liver cancer. Cell. 175 (3), 679–694. e22. doi:10.1016/j.cell.2018.09.004
Slevin, E., Baiocchi, L., Wu, N., Ekser, B., Sato, K., Lin, E., et al. (2020). Kupffer cells: Inflammation pathways and cell-cell interactions in alcohol-associated liver disease. Am. J. Pathol. 190 (11), 2185–2193. doi:10.1016/j.ajpath.2020.08.014
Smith, P. M., Howitt, M. R., Panikov, N., Michaud, M., Gallini, C. A., Bohlooly-Y, M., et al. (2013). The microbial metabolites, short-chain fatty acids, regulate colonic Treg cell homeostasis. Sci. (New York, N.Y.) 341 (6145), 569–573. doi:10.1126/science.1241165
Song, X., Sun, X., Oh, S. F., Wu, M., Zhang, Y., Zheng, W., et al. (2020). Microbial bile acid metabolites modulate gut RORγ(+) regulatory T cell homeostasis. Nature 577 (7790), 410–415. doi:10.1038/s41586-019-1865-0
Stacchiotti, V., Rezzi, S., Eggersdorfer, M., and Galli, F. (2021). Metabolic and functional interplay between gut microbiota and fat-soluble vitamins. Crit. Rev. Food Sci. Nutr. 61 (19), 3211–3232. doi:10.1080/10408398.2020.1793728
Starley, B. Q., Calcagno, C. J., and Harrison, S. A. (2010). Nonalcoholic fatty liver disease and hepatocellular carcinoma: A weighty connection. Hepatology 51 (5), 1820–1832. doi:10.1002/hep.23594
Szabo, G. (1999). Consequences of alcohol consumption on host defence. Alcohol Alcohol 34 (6), 830–841. doi:10.1093/alcalc/34.6.830
Szabo, G., and Mandrekar, P. (2009). A recent perspective on alcohol, immunity, and host defense. Alcohol. Clin. Exp. Res. 33 (2), 220–232. doi:10.1111/j.1530-0277.2008.00842.x
Szabo, G., Mandrekar, P., Petrasek, J., and Catalano, D. (2011). The unfolding web of innate immune dysregulation in alcoholic liver injury. Alcohol. Clin. Exp. Res. 35 (5), 782–786. doi:10.1111/j.1530-0277.2010.01398.x
Tang, Y., Bian, Z., Zhao, L., Liu, Y., Liang, S., Wang, Q., et al. (2011). Interleukin-17 exacerbates hepatic steatosis and inflammation in non-alcoholic fatty liver disease. Clin. Exp. Immunol. 166 (2), 281–290. doi:10.1111/j.1365-2249.2011.04471.x
Tian, J., Dang, H. N., Yong, J., Chui, W. S., Dizon, M. P. G., Yaw, C. K. Y., et al. (2011). Oral treatment with γ-aminobutyric acid improves glucose tolerance and insulin sensitivity by inhibiting inflammation in high fat diet-fed mice. PLoS One 6 (9), e25338. doi:10.1371/journal.pone.0025338
Tosello-Trampont, A. C., Landes, S. G., Nguyen, V., Novobrantseva, T. I., and Hahn, Y. S. (2012). Kuppfer cells trigger nonalcoholic steatohepatitis development in diet-induced mouse model through tumor necrosis factor-α production. J. Biol. Chem. 287 (48), 40161–40172. doi:10.1074/jbc.M112.417014
Tsung, K., and Norton, J. A. (2015). An immunological view of chemotherapy. Immunotherapy 7 (9), 941–943. doi:10.2217/imt.15.62
Unitt, E., Rushbrook, S. M., Marshall, A., Davies, S., Gibbs, P., Morris, L. S., et al. (2005). Compromised lymphocytes infiltrate hepatocellular carcinoma: The role of T-regulatory cells. Hepatology 41 (4), 722–730. doi:10.1002/hep.20644
Van Herck, M. A., Vonghia, L., Kwanten, W. J., Vanwolleghem, T., Ebo, D. G., Michielsen, P. P., et al. (2020). Adoptive cell transfer of regulatory T cells exacerbates hepatic steatosis in high-fat high-fructose diet-fed mice. Front. Immunol. 11, 1711. doi:10.3389/fimmu.2020.01711
Vignali, D. A., Collison, L. W., and Workman, C. J. (2008). How regulatory T cells work. Nat. Rev. Immunol. 8 (7), 523–532. doi:10.1038/nri2343
Viitala, K., MaKKonen, K., Israel, Y., LehTimaki, T., JaakkOla, O., Koivula, T., et al. (2000). Autoimmune responses against oxidant stress and acetaldehyde-derived epitopes in human alcohol consumers. Alcohol. Clin. Exp. Res. 24 (7), 1103–1109. doi:10.1111/j.1530-0277.2000.tb04656.x
Visekruna, A., and Luu, M. (2021). The role of short-chain fatty acids and bile acids in intestinal and liver function, inflammation, and carcinogenesis. Front. Cell. Dev. Biol. 9, 703218. doi:10.3389/fcell.2021.703218
von Haefen, C., Mei, W., Menk, M., Klemz, R., Jones, A., Wernecke, K. D., et al. (2011). Ethanol changes gene expression of transcription factors and cytokine production of CD4+ T-cell subsets in PBMCs stimulated with LPS. Alcohol. Clin. Exp. Res. 35 (4), 621–631. doi:10.1111/j.1530-0277.2010.01376.x
Wagner, N. M., Brandhorst, G., Czepluch, F., Lankeit, M., Eberle, C., Herzberg, S., et al. (2013). Circulating regulatory T cells are reduced in obesity and may identify subjects at increased metabolic and cardiovascular risk. Obes. (Silver Spring) 21 (3), 461–468. doi:10.1002/oby.20087
Wang, A., Luan, H. H., and Medzhitov, R. (2019). An evolutionary perspective on immunometabolism. Science 363 (6423), eaar3932. doi:10.1126/science.aar3932
Wang, H., Zhang, H., Wang, Y., Brown, Z. J., Xia, Y., Huang, Z., et al. (2021). Regulatory T-cell and neutrophil extracellular trap interaction contributes to carcinogenesis in non-alcoholic steatohepatitis. J. Hepatol. 75 (6), 1271–1283. doi:10.1016/j.jhep.2021.07.032
Wang, Y., Kirpich, I., Liu, Y., Ma, Z., Barve, S., McClain, C. J., et al. (2011). Lactobacillus rhamnosus GG treatment potentiates intestinal hypoxia-inducible factor, promotes intestinal integrity and ameliorates alcohol-induced liver injury. Am. J. Pathol. 179 (6), 2866–2875. doi:10.1016/j.ajpath.2011.08.039
Wang, Y., Liu, T., Tang, W., Deng, B., Chen, Y., Zhu, J., et al. (2016). Hepatocellular carcinoma cells induce regulatory T cells and lead to poor prognosis via production of transforming growth factor-β1. Cell. Physiol. biochem. 38 (1), 306–318. doi:10.1159/000438631
Wara, A. K., Wang, S., Wu, C., Fang, F., Haemmig, S., Weber, B. N., et al. (2020). KLF10 deficiency in CD4(+) T cells triggers obesity, insulin resistance, and fatty liver. Cell. Rep. 33 (13), 108550. doi:10.1016/j.celrep.2020.108550
Wiechers, C., Zou, M., Galvez, E., Beckstette, M., Ebel, M., Strowig, T., et al. (2021). The microbiota is dispensable for the early stages of peripheral regulatory T cell induction within mesenteric lymph nodes. Cell. Mol. Immunol. 18 (5), 1211–1221. doi:10.1038/s41423-021-00647-2
Wiegard, C., Frenzel, C., Herkel, J., Kallen, K. J., Schmitt, E., and Lohse, A. W. (2005). Murine liver antigen presenting cells control suppressor activity of CD4+CD25+ regulatory T cells. Hepatology 42 (1), 193–199. doi:10.1002/hep.20756
Wilke, C. M., Wu, K., Zhao, E., Wang, G., and Zou, W. (2010). Prognostic significance of regulatory T cells in tumor. Int. J. Cancer 127 (4), 748–758. doi:10.1002/ijc.25464
Wolf, M. J., Adili, A., Piotrowitz, K., Abdullah, Z., Boege, Y., Stemmer, K., et al. (2014). Metabolic activation of intrahepatic CD8+ T cells and NKT cells causes nonalcoholic steatohepatitis and liver cancer via cross-talk with hepatocytes. Cancer Cell. 26 (4), 549–564. doi:10.1016/j.ccell.2014.09.003
Wu, H.-J., and Wu, E. (2012). The role of gut microbiota in immune homeostasis and autoimmunity. Gut microbes 3 (1), 4–14. doi:10.4161/gmic.19320
Xu, M. Z., Yao, T. J., Lee, N. P. Y., Ng, I. O. L., Chan, Y. T., Zender, L., et al. (2009). Yes-associated protein is an independent prognostic marker in hepatocellular carcinoma. Cancer 115 (19), 4576–4585. doi:10.1002/cncr.24495
Yan, A. W., Fouts, D. E., Brandl, J., Starkel, P., Torralba, M., Schott, E., et al. (2011). Enteric dysbiosis associated with a mouse model of alcoholic liver disease. Hepatology 53 (1), 96–105. doi:10.1002/hep.24018
Younossi, Z., Anstee, Q. M., Marietti, M., Hardy, T., Henry, L., Eslam, M., et al. (2018). Global burden of NAFLD and NASH: Trends, predictions, risk factors and prevention. Nat. Rev. Gastroenterol. Hepatol. 15 (1), 11–20. doi:10.1038/nrgastro.2017.109
Younossi, Z. M., Koenig, A. B., Abdelatif, D., Fazel, Y., Henry, L., and Wymer, M. (2016). Global epidemiology of nonalcoholic fatty liver disease-Meta-analytic assessment of prevalence, incidence, and outcomes. Hepatology 64 (1), 73–84. doi:10.1002/hep.28431
Zeng, H., and Chi, H. (2015). Metabolic control of regulatory T cell development and function. Trends Immunol. 36 (1), 3–12. doi:10.1016/j.it.2014.08.003
Zheng, Z., and Wang, B. (2021). The gut-liver Axis in health and disease: The role of gut microbiota-derived signals in liver injury and regeneration. Front. Immunol. 12, 775526. doi:10.3389/fimmu.2021.775526
Zhou, X., Kong, N., Wang, J., Fan, H., Zou, H., Horwitz, D., et al. (2010). Cutting edge: All-trans retinoic acid sustains the stability and function of natural regulatory T cells in an inflammatory milieu. J. Immunol. 185 (5), 2675–2679. doi:10.4049/jimmunol.1000598
Zhu, Q., Wang, H., Jiang, B., Ni, X., Jiang, L., Li, C., et al. (2018). Loss of ATF3 exacerbates liver damage through the activation of mTOR/p70S6K/HIF-1α signaling pathway in liver inflammatory injury. Cell. Death Dis. 9 (9), 910. doi:10.1038/s41419-018-0894-1
Keywords: regulatory (Treg) cell, forkhead box P3 (FOXP3), hepatocellular carcinoma, metabolic liver diseases, nonalcoholic fatty liver disease -NAFLD, alcoholic liver disease -ALD, NAFLD-associated HCC
Citation: Riaz F, Wei P and Pan F (2022) Fine-tuning of regulatory T cells is indispensable for the metabolic steatosis-related hepatocellular carcinoma: A review. Front. Cell Dev. Biol. 10:949603. doi: 10.3389/fcell.2022.949603
Received: 21 May 2022; Accepted: 28 June 2022;
Published: 15 July 2022.
Edited by:
Wu Qi, Tongji University, ChinaReviewed by:
Hanqing Chen, Guangzhou First People’s Hospital, ChinaIkhwan Rinaldi, RSUPN Dr. Cipto Mangunkusumo, Indonesia
Copyright © 2022 Riaz, Wei and Pan. This is an open-access article distributed under the terms of the Creative Commons Attribution License (CC BY). The use, distribution or reproduction in other forums is permitted, provided the original author(s) and the copyright owner(s) are credited and that the original publication in this journal is cited, in accordance with accepted academic practice. No use, distribution or reproduction is permitted which does not comply with these terms.
*Correspondence: Fan Pan, fan.pan@siat.ac.cn