- Colorectal Surgery, Third Affiliated Hospital of Kunming Medical University, Tumor Hospital of Yunnan Province, Kunming, China
Aging is a major risk factor for cancer development. As dysfunction in protein homeostasis, or proteostasis, is a universal hallmark of both the aging process and cancer, a comprehensive understanding of the proteostasis system and its roles in aging and cancer will shed new light on how we can improve health and quality of life for older individuals. In this review, we summarize the regulatory mechanisms of proteostasis and discuss the relationship between proteostasis and aging and age-related diseases, including cancer. Furthermore, we highlight the clinical application value of proteostasis maintenance in delaying the aging process and promoting long-term health.
Introduction
Aging is a complex biological process characterized by gradual and progressive cellular and functional decline. Aging thus remains the greatest risk factor for most chronic disorders, including cardiovascular disease, neurodegenerative disease, and cancer. Protein homeostasis (proteostasis) is essential for preserving normal cellular metabolism and safeguarding physiological function through the proper biosynthesis, folding, trafficking, and degradation of proteins (Morimoto and Cuervo, 2014; Li et al., 2018). Growing evidence indicates that a progressive decline in the capacity to maintain a stable and functional proteome occurs with organismal aging (Vilchez et al., 2014; Kaushik and Cuervo, 2015). Consequently, increased intracellular accumulation of abnormal proteins (e.g., damaged, misfolded, or aggregated proteins) is regarded as an almost universal hallmark of aging, with chronic expression of abnormal proteins resulting in disruption of various biological processes that drive multiple age-related diseases (e.g., Alzheimer’s disease (AD)) (López-Otín et al., 2013). Therefore, ensuring proteostasis is tightly associated with elderly health.
To achieve protein homeostasis, cells have evolved sophisticated quality control mechanisms, primarily consisting of molecular chaperones, ubiquitin-proteasome system, and autophagy-lysosomal system, to promote successful protein folding and eliminate abnormal or misfolded proteins, and thereby adapt to dynamic stress conditions (Kaushik and Cuervo, 2015). Typically, these systems can restore basal homeostasis by rapidly sensing and rectifying the disturbances in proteome; however, long-term chronic stress (e.g., oxidative stress) makes cells difficult to maintain protein homeostasis and proteotoxicity can develop (Figure 1). Various studies have identified functional decline in protein quality control (PQC), including impaired function of the cellular proteolytic mechanisms (i.e., ubiquitin-proteasome and autophagy-lysosome), during aging in different mammals (e.g., human and rat) (reviewed in ref. (Vilchez et al., 2014)). For example, age-related accumulation of intralysosomal lipofuscin (age pigment), likely due to iron-catalyzed oxidative processes, can reduce the degradative function of lysosomes (Brunk and Terman, 2002; Jung et al., 2007). In turn, evidence has also shown that an increase in autophagy-lysosome and/or proteasome activity can extend longevity in diverse organisms, including humans (Chondrogianni et al., 2000; Pérez et al., 2009; Xiao et al., 2018).
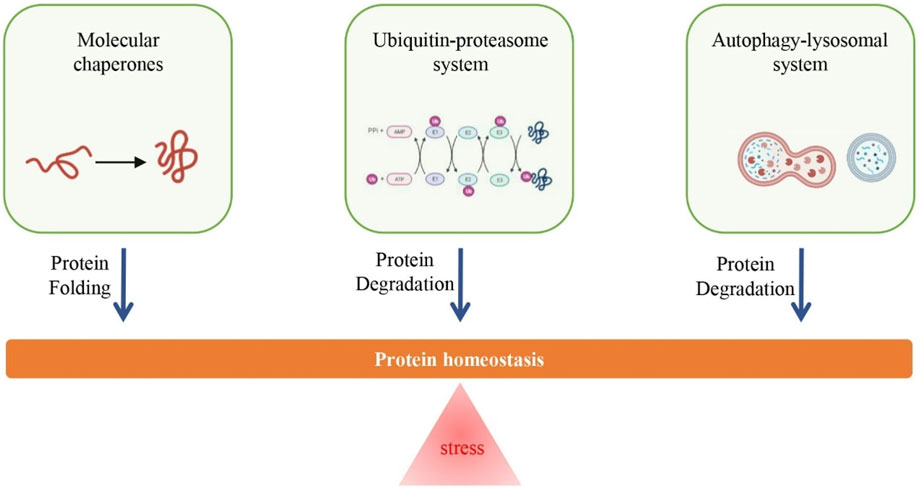
FIGURE 1. Overview of regulatory mechanisms of protein homeostasis. Long-term chronic stress (e.g., oxidative stress) is an important cause for the loss of protein homeostasis, however, the increase in the function of proteolytic system (i.e., ubiquitin-proteasome system and autophagy-lysosomal system) and repair system (i.e., molecular chaperones) can promote the maintenance of protein homeostasis.
Importantly, cancer is considered a disease of aging, but involves an integrated functional network of biological processes related to the regulation of protein homeostasis that dynamically responds to the needs of cancer cells. Cancer cells must adapt to a wide variety of chronic stresses, especially high misfolded protein burdens due to genomic aberrations, and therefore require sustained PQC for survival and proliferation (Chen et al., 2017; Bastola et al., 2018). Thus, modulation of the protein homeostasis network can promote longevity, but at the potential cost of cancer progression. In this review, we summarize the intracellular PQC system and discuss how protein homeostasis functions as a double-edged sword in aging and tumorigenesis. We also highlight the potential of targeting protein homeostasis as a therapeutic strategy for age-related pathologies, including cancer.
Intracellular regulation mechanisms of protein homeostasis
Molecular chaperones
Molecular chaperones are structurally diverse and highly conserved ubiquitous proteins that function to maintain protein homeostasis in cells (Arslan et al., 2006). Molecular chaperones, also known as heat shock proteins (HSPs), account for 5%–10% of total proteins in most normal cells (Pockley, 2003). They can specifically and non-covalently bind to the surfaces of interactive proteins and are usually classified according to their functional properties and molecular weight, including major HSP families such as HSP40 (J-proteins), HSP60 (chaperonins), HSP70 (68–78 kDa), HSP90 (85–96 kDa), HSP100 (Clp proteins), and small HSPs (sHSPs, 10–30 kDa) (Hartl et al., 2011). These proteins play important roles in de novo protein folding and refolding, protein-complex assembly and disassembly, protein transport across membranes, and protein degradation (Kim et al., 2013; Brandvold and Morimoto, 2015; Shemesh et al., 2021). For example, as an abundant molecular chaperone, HSP90 participates in the folding of a variety of proteins (viz. so-called “clients”) involved in protein trafficking, signal transduction, transcriptional regulation, and immunity, utilizing energy generated by adenosine triphosphate (ATP) binding and hydrolysis and interacting with various co-chaperones (Frydman, 2001; Brown et al., 2007; Taipale et al., 2010); moreover, HSP90 can also enhance protein degradation (e.g., oxidized proteins) through the proteasome (Whittier et al., 2004). Likewise, HSP70 also participates in the maintenance of protein homeostasis by interacting with many proteins to facilitate the prevention of protein misfolding or the degradation of damaged proteins (Seo et al., 2016; Garbuz et al., 2019). Taken together, HSPs play important roles in responding to various stresses (e.g., high temperature) and facilitating cellular survival during life.
Ubiquitin-proteasome system
The ubiquitin-proteasome system is a key player in intracellular protein degradation and turnover, and thus plays an essential role in cellular protein homeostasis (Glickman and Ciechanover, 2002; Raaben et al., 2010; Gong et al., 2016). Approximately 80% of cellular proteins can be degraded by the ubiquitin-proteasome system (Meyer-Schwesinger, 2019). These proteins are associated with many biological processes, including cell cycle progression, apoptosis, gene transcription and translation, cell survival, and antigen presentation (Raaben et al., 2010; Park et al., 2020). This degradation system requires the conjugation of ubiquitin (small, highly conserved protein of 76 amino acids) to target proteins by the sequential action of three enzymes: ubiquitin-activating enzyme (E1), ubiquitin-conjugating enzyme (E2), and ubiquitin ligase enzyme (E3) (Shu and Yang, 2017). Briefly, this degradation process is initiated by the formation of E1-ubiquitin thioester bonds between the active Cys residue site of E1 and C-terminal Gly carboxyl group of ubiquitin through ATP-dependent reactions. Thioester-linked ubiquitin is then transferred to the catalytic Cys in E2, resulting in the formation of an E2-ubiquitin thioester-linked conjugate. After this, E3 promotes the transfer of ubiquitin from the E2-ubiquitin conjugate to the Lys residues within the different substrate proteins by recognizing their specific motifs. Finally, the targeted proteins with polyubiquitin chains are recognized and degraded by the proteasome (Haas et al., 1982; Gong et al., 2016; Shu and Yang, 2017). During this progress, ubiquitin is the “signal” for protease cleavage of the protein, with various chains of ubiquitin molecules labeling different abnormal proteins. Furthermore, ubiquitylation is a reversible process, catalyzed by a series of deubiquitylating enzymes, in which ubiquitin molecules removed from protein substrates can be released and recycled (Finley, 2009; Qiu et al., 2022).
Autophagy-lysosomal system
The autophagy-lysosomal system is another important mechanism in cellular homeostasis for the degradation and recycling of cytoplasmic components, such as defective proteins and organelles (Mariño et al., 2011; Li et al., 2018). This system participates in the regulation of multiple biological processes, including cell growth, differentiation, remodeling, and senescence (Schuck, 2020). Depending on how excess or damaged cytoplasmic material is delivered to the lysosomes, autophagy can be classified as macroautophagy, microautophagy, or chaperone-mediated autophagy (CMA) (Mariño et al., 2011). Macroautophagy is a dominant form of autophagy (Miller and Thorburn, 2021; Griffey and Yamamoto, 2022), whereby superfluous and damaged proteins/organelles as sequestered in nascent double-membrane autophagosomes that fuse with lysosomes for degradation (Feng et al., 2014). The degradation products are released from the lysosomes into the cytosol, with the macromolecular constituents recycled into metabolic and biosynthetic pathways to maintain cell viability under unfavorable conditions and to protect the cell during stress (Feng et al., 2014; White et al., 2015). Unlike macroautophagy, microautophagy involves the direct engulfment of material by lysosomes through invaginations or protrusions of the lysosomal membrane (Schuck, 2020). Microautophagy is responsible for the maintenance of organelle size, membrane composition, cell survival under nitrogen restriction, and transition from starvation-induced growth arrest to the logarithmic growth phase (Roberts et al., 2003). CMA is a form of selective autophagy responsible for the degradation of 30% of cytosolic proteins under prolonged nutrient deprivation (Dice, 2007; Bourdenx et al., 2021). CMA is distinct from other types of autophagy in that the substrate protein is directly translocated across the lysosomal membrane for degradation (Schneider et al., 2015). In CMA, substrate proteins are selectively targeted to lysosomes and translocated into the lysosomal lumen through the coordinated action of chaperones located on both sides of the membrane and the dedicated protein translocation complex lysosomal-associated membrane protein 2A (LAMP2A) (Cuervo and Wong, 2014; Bourdenx et al., 2021).
Protein homeostasis in aging and longevity
Impaired protein homeostasis, characterized by the accumulation of protein aggregates, is a crucial hallmark of aging and age-related diseases, including neurodegeneration (López-Otín et al., 2013; Hipp et al., 2019). Various internal and external stresses that persist throughout life can disrupt protein homeostasis in organisms. Here, we mainly discuss oxidative stress, generated by redox imbalance between the production of reactive oxygen and nitrogen species (ROS and RNS, respectively) and antioxidant defenses, given its importance as a driver of oxidized protein accumulation in senescent cells and aged organisms (Squier, 2001). Multiple studies have observed increases in oxidized proteins in different tissues (e.g., brain and heart) of aged animals, including humans (Granold et al., 2015). Oxidative modification of proteins leads to changes in protein structure, including oligomerization, protein misfolding, and protein backbone fragmentation (Zavadskiy et al., 2022). In turn, oxidative damage to proteins plays a crucial role in accelerating aging (Nyström, 2005; Kim et al., 2012). For example, oxidized low-density lipoprotein plays an important role in promoting retinal pigment epithelial cell senescence (Kim et al., 2012).
In general, oxidized proteins can be eliminated by degradation systems and repair mechanisms in younger cells and organisms, with an extensive network involving molecular chaperones, ubiquitin-proteasome system, and autophagy-lysosomal system. However, protein homeostasis network capacity declines significantly with age (Ben-Zvi et al., 2009; Morimoto and Cuervo, 2014). Age-related intralysosomal lipofuscin accumulation and impaired acidification (i.e., pH) are two important causes of reduced lysosomal degradation activity (Brunk and Terman, 2002; Colacurcio and Nixon, 2016). Age-related reductions in certain key regulatory factors, such as LAMP2A, can also disrupt the autophagy-lysosomal process (Cuervo and Dice, 2000). In addition, decreased proteasomal degradation activity can also result from age-related factors, including decreased expression of both non-catalytic and catalytic subunits of the proteasome with aging (Ponnappan et al., 2007). Studies have also linked the accumulation of oxidatively modified proteins to different age-related diseases, such as neurodegenerative disorder and cardiovascular disease (Barnham et al., 2004; Dunlop et al., 2009; Luna et al., 2016). For example, based on redox proteomics, several studies have reported the presence of oxidized/misfolded proteins in different brain regions of patients with AD (Sultana et al., 2006), with oxidization of glycolytic and TCA enzymes leading to a decrease in ATP production and progression of AD (Tramutola et al., 2017). There are also studies supporting that the aberrant expression of genes involving to the maintenance of protein homeostasis plays an important role in the deposition of Aβ peptide and tau protein in the brains of AD patients (Freer et al., 2016; Rishika et al., 2017). In addition, oxidized albumin can induce endothelial injury and increase the risk of cardiovascular disease in elderly individuals (Luna et al., 2016). Taken together, these findings highlight the crucial role of cellular homeostasis maintenance, especially the elimination of oxidized proteins, in healthy aging organisms.
Accordingly, increasing evidence suggests that enhancement of protein homeostasis network capacity can extend lifespan or promote longevity in various species, such as yeast, worms, flies, mice, and humans (Pyo et al., 2013; Schumpert et al., 2014; Chondrogianni et al., 2015; Madeo et al., 2015; Xiao et al., 2018). For example, overexpression of molecular chaperones (e.g., HSP70, HSP16) can lead to an increase in lifespan (Walker and Lithgow, 2003; Schumpert et al., 2014). Likewise, upregulation of certain autophagy-lysosomal pathway genes is linked to lifespan extension (or longevity promotion). Activation of transcription factor EB (TFEB), a key regulator driving autophagy and lysosomal gene expression, is associated with healthy longevity (Lapierre et al., 2013). Overexpression of the Atg5 gene, which is essential for autophagosome formation, can extend the median lifespan of mice (Pyo et al., 2013). In addition, a summary of key genes required for protein homeostasis maintenance and longevity promotion is provided in Table 1. Furthermore, centenarian-based evidence suggests that increased autophagy-lysosomal activity is an important mechanism of healthy aging and longevity in humans (Xiao et al., 2018). Studies have also shown that overexpression of proteasome subunits can increase lifespan (Chondrogianni et al., 2015). Interestingly, a growing body of research suggests that protein homeostasis is a key mechanism linking certain interventions to longevity promotion and health improvement, with most demonstrating autophagy-activating properties (Kaushik and Cuervo, 2015). For example, calorie restriction, physical exercise, mTORC1 inhibition, sirtuin 1 (SIRT1) activation, spermidine treatment, and p53 suppression, interventions known to extend lifespan and/or healthspan, can enhance protein homeostasis network capacity, although probably through different mechanisms (Mizushima and Komatsu, 2011; Madeo et al., 2015; Ulbricht et al., 2015; Plaza-Zabala et al., 2017). Thus, exploring how to mitigate age-related decline in PQC capacity should provide new perspectives for achieving healthy aging and longevity.
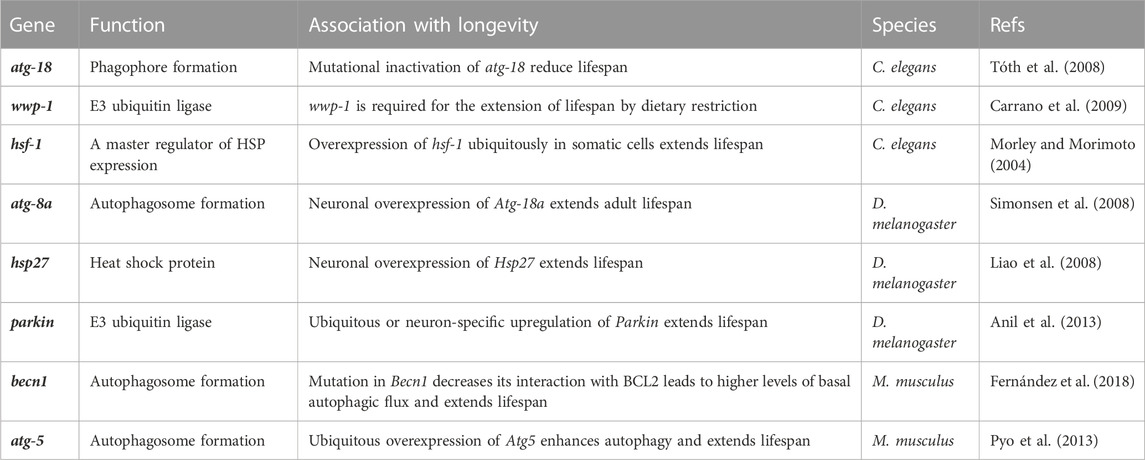
TABLE 1. Examples of proteostasis-related genes linked to organismal longevity. (All gene names were in lower case and only one representative study for each gene was listed here).
Protein homeostasis and cancer
Although cancer is also an age-related disease, its biological underpinnings are tightly associated with protein homeostasis. Genomic instability and oxidative stress can lead to increased production of damaged and/or dysregulated proteins in cancer cells (Wu et al., 2014; Bastola et al., 2018). To resolve the overwhelming proteotoxic stress, cancer cells require sophisticated PQC mechanisms to maintain a proper protein homeostasis for survival and growth. Accordingly, there is a growing body of evidence supporting the dual roles of PQC mechanisms in the pathogenesis of human cancers through the building and turnover of tumor-promoting/suppressing proteins. Here we mainly summarized the findings on the crucial functions of the three PQC systems (viz., molecular chaperones, ubiquitin-proteasome system, and autophagy-lysosomal system) in cancer progression.
First, HSPs function as the molecular chaperones to mediate proper protein folding, with more likely being oncogenic function. Numerous HSPs (e.g., HSP60, HSP70, HSP90) have been reported to be overexpressed in a wide range of cancers and are indicative of poor patient prognosis (e.g., gastric, liver and breast cancer) (Ciocca and Calderwood, 2005; Zagouri et al., 2012; Li et al., 2014; Wang et al., 2021). HSPs can promote cancer progression via different pathways. For example, overexpression of HSP90 can downregulate E-cadherin and promote epithelial-mesenchymal transition (EMT), a key step in tumor metastasis (Hance et al., 2012). HSP90 can also stabilize vascular endothelial growth factor and nitric oxide synthetase in endothelial cells to induce tumor angiogenesis (Sun and Liao, 2004).
Second, as one of the major proteolytic system, autophagy-lysosomal system is tightly associated with cancer development and progression (Rosenfeldt and Ryan, 2009; Yun and Lee, 2018). On the one hand, autophagy is thought to play an important role in promoting cancer cell survival and growth in advanced cancers (Luo et al., 2016; Liu et al., 2018), as it can provide the substrates (e.g., amino acid) for metabolism through the intracellular recycling of damaged or superfluous proteins and then elicit the formation of an adaptive protein homeostasis in cancer cells (White, 2012; 2015). Furthermore, autophagy may contribute to tumor progression by decreasing the levels of some proteins with tumor-suppressing function. For example, the ATG7 gene, which is overexpressed in invasive bladder cancer tissue, can promote autophagic degradation of the HNRNPD (ARE/poly(U)-binding/degradation factor 1) protein, which, in turn, increases ARHGDIB mRNA stability and bladder cancer cell invasion (Zhu et al., 2019). Study shows that autophagy participates in the degradation of tumor suppressor PP6 (protein phosphatase 6), the level of which correlates with poor prognosis in glioblastoma (Fujiwara et al., 2020). However, on the other hand, autophagy is also considered to be a tumor suppressor mechanism in the early phages of tumorigenesis as it can inhibit tumors by removing oncogenic protein substrates, toxic unfolded proteins, and damaged organelles, thereby maintaining genomic stability (White, 2012; 2015; Xu et al., 2015). Important evidence for the role of autophagy in tumor suppression comes from the depletion of the essential autophagy regulator BECN1 (Beclin 1, also known as autophagy-related gene 6 (ATG6)) in human breast, prostate, and ovarian cancers (Aita et al., 1999; Liang et al., 1999; Choi et al., 2013). Loss of BECN1 can lead to a reduction in autophagy and increase in cell proliferation (Liang et al., 1999; Qu et al., 2003; Shen et al., 2008). In addition, there is a study showing that autophagy induction can attenuates the Wnt signalling by promoting Dishevelled degradation, which further inhibits the formation of colon cancer (Gao et al., 2010). Autophagy is also required to suppress the accumulation of oncogenic p62 protein aggregates and prevent tumor initiation (White, 2012).
Third, it has been reported that the increased rate of protein turnover in cancer cells also requires the ubiquitin-proteasome system, which subsequently regulates the “quantity” and “quality” of various proteins (Crunkhorn, 2018; Deng et al., 2020; Zhang et al., 2020). That is, in order to adapt the oxidative and proteotoxic stresses during tumorigenesis, cancer cells rely on the ubiquitinating and deubiquitinating enzymes to maintain protein homeostasis and cell viability (Hyer et al., 2018; Harris et al., 2019). In addition, accumulating evidence suggests that proteins encoded by oncogenes and tumor suppressor genes may be targets of ubiquitination, that is, ubiquitin-mediated proteasomal degradation could either activate or deactivate the tumorigenic pathways. For example, there are studies showing that the protein level of tumor suppressor p53 can be reduced by its ubiquitination and proteasome degradation, resulting in poor survival and prognosis in cancer patients (e.g., colorectal cancer) (Zeng et al., 2018; Liu et al., 2020). In addition, studies also show that multiple proto-oncogenic proteins (e.g., MYC and JUN) can be degraded by the ubiquitin-proteasome system, and then function in suppressing cancer growth and progression (e.g., glioma) (Welcker and Clurman, 2008; Kim et al., 2017).
Concluding remarks
In this review, we discuss the molecular mechanisms involved in protecting the stability and functional properties of the proteome, including molecular chaperones, ubiquitin-proteasome system, and autophagy-lysosomal system. We also describe the causes of accumulation of damaged/misfolded protein aggregates during aging, such as long-term chronic stress (e.g., oxidative stress) and dysfunctional proteolytic and repair systems, and the subsequent detrimental effects on organismal health. Current evidence suggests that improvements in cellular protein homeostasis capacity can prolong lifespan or promote healthy aging and longevity, but with a potential increase in the risk of cancer. Thus, a comprehensive understanding of the protein homeostasis network will not only shed light on the fundamental biology of aging and anti-aging, but also provide new avenues for context-dependent therapeutic interventions in various age-related diseases, including neurodegeneration and cancer.
Author contributions
X-QC and Y-FL conceived the concept of the review. X-QC, TS, S-JF drafted the manuscript. X-MS and G-YL reviewed the manuscript. All authors read and approved the final manuscript.
Funding
This work was supported by a grant from Scientific Research Fund Project of Yunnan Provincial Department of Education (2023Y0657).
Conflict of interest
The authors declare that the research was conducted in the absence of any commercial or financial relationships that could be construed as a potential conflict of interest.
Publisher’s note
All claims expressed in this article are solely those of the authors and do not necessarily represent those of their affiliated organizations, or those of the publisher, the editors and the reviewers. Any product that may be evaluated in this article, or claim that may be made by its manufacturer, is not guaranteed or endorsed by the publisher.
References
Aita, V. M., Liang, X. H., Murty, V. V. V. S., Pincus, D. L., Yu, W., Cayanis, E., et al. (1999). Cloning and genomic organization of beclin 1, a candidate tumor suppressor gene on chromosome 17q21. Genomics 59, 59–65. doi:10.1006/geno.1999.5851
Anil, R., Michael, R., and David, W. W. (2013). Parkin overexpression during aging reduces proteotoxicity, alters mitochondrial dynamics, and extends lifespan. Proc. Natl. Acad. Sci. U. S. A. 110 (21), 8638–8643. doi:10.1073/pnas.1216197110
Arslan, M. A., Csermely, P., and Sőti, C. (2006). Protein homeostasis and molecular chaperones in aging. Biogerontology 7, 383–389. doi:10.1007/s10522-006-9053-7
Barnham, K. J., Masters, C. L., and Bush, A. I. (2004). Neurodegenerative diseases and oxidative stress. Nat. Rev. Drug Discov. 3, 205–214. doi:10.1038/nrd1330
Bastola, P., Oien, D. B., Cooley, M., and Chien, J. (2018). Emerging cancer therapeutic targets in protein homeostasis. AAPS J. 20, 94. doi:10.1208/s12248-018-0254-1
Ben-Zvi, A., Miller, E. A., and Morimoto, R. I. (2009). Collapse of proteostasis represents an early molecular event in Caenorhabditis elegans aging. Proc. Natl. Acad. Sci. U. S. A. 106, 14914–14919. doi:10.1073/pnas.0902882106
Bourdenx, M., Martín-Segura, A., Scrivo, A., Rodriguez-Navarro, J. A., Kaushik, S., Tasset, I., et al. (2021). Chaperone-mediated autophagy prevents collapse of the neuronal metastable proteome. Cell 184, 2696–2714.e25. doi:10.1016/j.cell.2021.03.048
Brandvold, K. R., and Morimoto, R. I. (2015). The chemical biology of molecular chaperones—implications for modulation of proteostasis. J. Mol. Biol. 427, 2931–2947. doi:10.1016/j.jmb.2015.05.010
Brown, M. A., Zhu, L., Schmidt, C., and Tucker, P. W. (2007). Hsp90—from signal transduction to cell transformation. Biochem. Biophys. Res. Commun. 363, 241–246. doi:10.1016/j.bbrc.2007.08.054
Brunk, U. T., and Terman, A. (2002). Lipofuscin: Mechanisms of age-related accumulation and influence on cell function. Free Radic. Biol. Med. 33, 611–619. doi:10.1016/S0891-5849(02)00959-0
Carrano, A. C., Liu, Z., Dillin, A., and Hunter, T. (2009). A conserved ubiquitination pathway determines longevity in response to diet restriction. Nature 460, 396–399. doi:10.1038/nature08130
Chen, L., Brewer, M. D., Guo, L., Wang, R., Jiang, P., and Yang, X. (2017). Enhanced degradation of misfolded proteins promotes tumorigenesis. Cell Rep. 18, 3143–3154. doi:10.1016/j.celrep.2017.03.010
Choi, A. M. K., Ryter, S. W., and Levine, B. (2013). Autophagy in human health and disease. N. Engl. J. Med. 368, 651–662. doi:10.1056/NEJMra1205406
Chondrogianni, N., Georgila, K., Kourtis, N., Tavernarakis, N., and Gonos, E. S. (2015). 20S proteasome activation promotes life span extension and resistance to proteotoxicity in Caenorhabditis elegans. FASEB J. 29, 611–622. doi:10.1096/fj.14-252189
Chondrogianni, N., Petropoulos, I., Franceschi, C., Friguet, B., and Gonos, E. S. (2000). Fibroblast cultures from healthy centenarians have an active proteasome. Exp. Gerontol. 35, 721–728. doi:10.1016/s0531-5565(00)00137-6
Ciocca, D. R., and Calderwood, S. K. (2005). Heat shock proteins in cancer: Diagnostic, prognostic, predictive, and treatment implications. Cell Stress Chaperon 10, 86–103. doi:10.1379/csc-99r.1
Colacurcio, D. J., and Nixon, R. A. (2016). Disorders of lysosomal acidification—the emerging role of v-ATPase in aging and neurodegenerative disease. Ageing Res. Rev. 32, 75–88. doi:10.1016/j.arr.2016.05.004
Crunkhorn, S. (2018). Cancer: Targeting the ubiquitin pathway. Nat. Rev. Drug Discov. 17, 166. doi:10.1038/nrd.2018.33
Cuervo, A. M., and Dice, J. F. (2000). Age-related decline in chaperone-mediated autophagy. J. Biol. Chem. 275, 31505–31513. doi:10.1074/jbc.M002102200
Cuervo, A. M., and Wong, E. (2014). Chaperone-mediated autophagy: Roles in disease and aging. Cell Res. 24, 92–104. doi:10.1038/cr.2013.153
Deng, L., Meng, T., Chen, L., Wei, W., and Wang, P. (2020). The role of ubiquitination in tumorigenesis and targeted drug discovery. Sig Transduct. Target Ther. 5, 11–28. doi:10.1038/s41392-020-0107-0
Dunlop, R. A., Brunk, U. T., and Rodgers, K. J. (2009). Oxidized proteins: Mechanisms of removal and consequences of accumulation. IUBMB Life 61, 522–527. doi:10.1002/iub.189
Feng, Y., He, D., Yao, Z., and Klionsky, D. J. (2014). The machinery of macroautophagy. Cell Res. 24, 24–41. doi:10.1038/cr.2013.168
Fernández, Á. F., Sebti, S., Wei, Y., Zou, Z., Shi, M., McMillan, K. L., et al. (2018). Disruption of the beclin 1–BCL2 autophagy regulatory complex promotes longevity in mice. Nature 558, 136–140. doi:10.1038/s41586-018-0162-7
Finley, D. (2009). Recognition and processing of ubiquitin-protein conjugates by the proteasome. Annu. Rev. Biochem. 78, 477–513. doi:10.1146/annurev.biochem.78.081507.101607
Freer, R., Sormanni, P., Vecchi, G., Ciryam, P., Dobson, C. M., and Vendruscolo, M. (2016). A protein homeostasis signature in healthy brains recapitulates tissue vulnerability to Alzheimer’s disease. Sci. Adv. 2, e1600947. doi:10.1126/sciadv.1600947
Frydman, J. (2001). Folding of newly translated proteins in vivo: The role of molecular chaperones. Annu. Rev. Biochem. 70, 603–647. doi:10.1146/annurev.biochem.70.1.603
Fujiwara, N., Shibutani, S., Sakai, Y., Watanabe, T., Kitabayashi, I., Oshima, H., et al. (2020). Autophagy regulates levels of tumor suppressor enzyme protein phosphatase 6. Cancer Sci. 111, 4371–4380. doi:10.1111/cas.14662
Gao, C., Cao, W., Bao, L., Zuo, W., Xie, G., Cai, T., et al. (2010). Autophagy negatively regulates Wnt signalling by promoting Dishevelled degradation. Nat. Cell Biol. 12, 781–790. doi:10.1038/ncb2082
Garbuz, D. G., Zatsepina, O. G., and Evgen’ev, M. B. (2019). The major human stress protein Hsp70 as a factor of protein homeostasis and a cytokine-like regulator. Mol. Biol. 53, 176–191. doi:10.1134/S0026893319020055
Glickman, M. H., and Ciechanover, A. (2002). The ubiquitin-proteasome proteolytic pathway: Destruction for the sake of construction. Physiol. Rev. 82, 373–428. doi:10.1152/physrev.00027.2001
Gong, B., Radulovic, M., Figueiredo-Pereira, M. E., and Cardozo, C. (2016). The ubiquitin-proteasome system: Potential therapeutic targets for Alzheimer’s disease and spinal cord injury. Front. Mol. Neurosci. 9, 4. doi:10.3389/fnmol.2016.00004
Granold, M., Moosmann, B., Staib-Lasarzik, I., Arendt, T., del Rey, A., Engelhard, K., et al. (2015). High membrane protein oxidation in the human cerebral cortex. Redox Biol. 4, 200–207. doi:10.1016/j.redox.2014.12.013
Griffey, C. J., and Yamamoto, A. (2022). Macroautophagy in CNS health and disease. Nat. Rev. Neurosci. 23, 411–427. doi:10.1038/s41583-022-00588-3
Haas, A. L., Warms, J. V., Hershko, A., and Rose, I. A. (1982). Ubiquitin-activating enzyme. Mechanism and role in protein-ubiquitin conjugation. J. Biol. Chem. 257, 2543–2548. doi:10.1016/S0021-9258(18)34958-5
Hance, M. W., Dole, K., Gopal, U., Bohonowych, J. E., Jezierska-Drutel, A., Neumann, C. A., et al. (2012). Secreted Hsp90 is a novel regulator of the epithelial to mesenchymal transition (EMT) in prostate cancer. J. Biol. Chem. 287, 37732–37744. doi:10.1074/jbc.M112.389015
Harris, I. S., Endress, J. E., Coloff, J. L., Selfors, L. M., McBrayer, S. K., Rosenbluth, J. M., et al. (2019). Deubiquitinases maintain protein homeostasis and survival of cancer cells upon glutathione depletion. Cell Metab. 29, 1166–1181.e6. doi:10.1016/j.cmet.2019.01.020
Hartl, F. U., Bracher, A., and Hayer-Hartl, M. (2011). Molecular chaperones in protein folding and proteostasis. Nature 475, 324–332. doi:10.1038/nature10317
Hipp, M. S., Kasturi, P., and Hartl, F. U. (2019). The proteostasis network and its decline in ageing. Nat. Rev. Mol. Cell Biol. 20, 421–435. doi:10.1038/s41580-019-0101-y
Hyer, M. L., Milhollen, M. A., Ciavarri, J., Fleming, P., Traore, T., Sappal, D., et al. (2018). A small-molecule inhibitor of the ubiquitin activating enzyme for cancer treatment. Nat. Med. 24, 186–193. doi:10.1038/nm.4474
Jung, T., Bader, N., and Grune, T. (2007). Lipofuscin: Formation, distribution, and metabolic consequences. Ann. N. Y. Acad. Sci. 1119, 97–111. doi:10.1196/annals.1404.008
Kaushik, S., and Cuervo, A. M. (2015). Proteostasis and aging. Nat. Med. 21, 1406–1415. doi:10.1038/nm.4001
Kim, E.-J., Kim, S.-H., Jin, X., Jin, X., and Kim, H. (2017). KCTD2, an adaptor of Cullin3 E3 ubiquitin ligase, suppresses gliomagenesis by destabilizing c-Myc. Cell Death Differ. 24, 649–659. doi:10.1038/cdd.2016.151
Kim, J. H., Lee, S.-J., Kim, K.-W., Yu, Y. S., and Kim, J. H. (2012). Oxidized low density lipoprotein-induced senescence of retinal pigment epithelial cells is followed by outer blood-retinal barrier dysfunction. Int. J. Biochem. Cell Biol. 44, 808–814. doi:10.1016/j.biocel.2012.02.005
Kim, Y. E., Hipp, M. S., Bracher, A., Hayer-Hartl, M., and Ulrich Hartl, F. (2013). Molecular chaperone functions in protein folding and proteostasis. Annu. Rev. Biochem. 82, 323–355. doi:10.1146/annurev-biochem-060208-092442
Lapierre, L. R., De Magalhaes Filho, C. D., McQuary, P. R., Chu, C.-C., Visvikis, O., Chang, J. T., et al. (2013). The TFEB orthologue HLH-30 regulates autophagy and modulates longevity in Caenorhabditis elegans. Nat. Commun. 4, 2267. doi:10.1038/ncomms3267
Li, J., Zhang, D., Wiersma, M., and Brundel, B. J. J. M. (2018). Role of autophagy in proteostasis: Friend and foe in cardiac diseases. Cells 7, 279. doi:10.3390/cells7120279
Li, X., Xu, Q., Fu, X., and Luo, W. (2014). Heat shock protein 60 overexpression is associated with the progression and prognosis in gastric cancer. PLoS One 9, e107507. doi:10.1371/journal.pone.0107507
Liang, X. H., Jackson, S., Seaman, M., Brown, K., Kempkes, B., Hibshoosh, H., et al. (1999). Induction of autophagy and inhibition of tumorigenesis by beclin 1. Nature 402, 672–676. doi:10.1038/45257
Liao, P.-C., Lin, H.-Y., Yuh, C.-H., Yu, L.-K., and Wang, H.-D. (2008). The effect of neuronal expression of heat shock proteins 26 and 27 on lifespan, neurodegeneration, and apoptosis in Drosophila. Biochem. Biophys. Res. Commun. 376, 637–641. doi:10.1016/j.bbrc.2008.08.161
Liu, J., Guan, D., Dong, M., Yang, J., Wei, H., Liang, Q., et al. (2020). UFMylation maintains tumour suppressor p53 stability by antagonizing its ubiquitination. Nat. Cell Biol. 22, 1056–1063. doi:10.1038/s41556-020-0559-z
Liu, M., Jiang, L., Fu, X., Wang, W., Ma, J., Tian, T., et al. (2018). Cytoplasmic liver kinase B1 promotes the growth of human lung adenocarcinoma by enhancing autophagy. Cancer Sci. 109, 3055–3067. doi:10.1111/cas.13746
López-Otín, C., Blasco, M. A., Partridge, L., Serrano, M., and Kroemer, G. (2013). The hallmarks of aging. Cell 153, 1194–1217. doi:10.1016/j.cell.2013.05.039
Luna, C., Alique, M., Navalmoral, E., Noci, M.-V., Bohorquez-Magro, L., Carracedo, J., et al. (2016). Aging-associated oxidized albumin promotes cellular senescence and endothelial damage. Clin. Interv. Aging 11, 225–236. doi:10.2147/CIA.S91453
Luo, T., Fu, J., Xu, A., Su, B., Ren, Y., Li, N., et al. (2016). PSMD10/gankyrin induces autophagy to promote tumor progression through cytoplasmic interaction with ATG7 and nuclear transactivation of ATG7 expression. Autophagy 12, 1355–1371. doi:10.1080/15548627.2015.1034405
Madeo, F., Zimmermann, A., Maiuri, M. C., and Kroemer, G. (2015). Essential role for autophagy in life span extension. J. Clin. Invest. 125, 85–93. doi:10.1172/JCI73946
Mariño, G., Madeo, F., and Kroemer, G. (2011). Autophagy for tissue homeostasis and neuroprotection. Curr. Opin. Cell Biol. 23, 198–206. doi:10.1016/j.ceb.2010.10.001
Meyer-Schwesinger, C. (2019). The ubiquitin–proteasome system in kidney physiology and disease. Nat. Rev. Nephrol. 15, 393–411. doi:10.1038/s41581-019-0148-1
Miller, D. R., and Thorburn, A. (2021). Autophagy and organelle homeostasis in cancer. Dev. Cell 56, 906–918. doi:10.1016/j.devcel.2021.02.010
Mizushima, N., and Komatsu, M. (2011). Autophagy: Renovation of cells and tissues. Cell 147, 728–741. doi:10.1016/j.cell.2011.10.026
Morimoto, R. I., and Cuervo, A. M. (2014). Proteostasis and the aging proteome in health and disease. J. Gerontol. A Biol. Sci. Med. Sci. 69, S33–S38. doi:10.1093/gerona/glu049
Morley, J. F., and Morimoto, R. I. (2004). Regulation of longevity in Caenorhabditis elegans by heat shock factor and molecular chaperones. Mol. Biol. Cell 15, 657–664. doi:10.1091/mbc.e03-07-0532
Nyström, T. (2005). Role of oxidative carbonylation in protein quality control and senescence. EMBO J. 24, 1311–1317. doi:10.1038/sj.emboj.7600599
Park, J., Cho, J., and Song, E. J. (2020). Ubiquitin–proteasome system (UPS) as a target for anticancer treatment. Arch. Pharm. Res. 43, 1144–1161. doi:10.1007/s12272-020-01281-8
Pérez, V. I., Buffenstein, R., Masamsetti, V., Leonard, S., Salmon, A. B., Mele, J., et al. (2009). Protein stability and resistance to oxidative stress are determinants of longevity in the longest-living rodent, the naked mole-rat. Proc. Natl. Acad. Sci. U. S. A. 106, 3059–3064. doi:10.1073/pnas.0809620106
Plaza-Zabala, A., Sierra-Torre, V., and Sierra, A. (2017). Autophagy and microglia: Novel partners in neurodegeneration and aging. IJMS 18, 598. doi:10.3390/ijms18030598
Pockley, A. G. (2003). Heat shock proteins as regulators of the immune response. Lancet 362, 469–476. doi:10.1016/S0140-6736(03)14075-5
Ponnappan, S., Ovaa, H., and Ponnappan, U. (2007). Lower expression of catalytic and structural subunits of the proteasome contributes to decreased proteolysis in peripheral blood T lymphocytes during aging. Int. J. Biochem. Cell Biol. 39, 799–809. doi:10.1016/j.biocel.2007.01.002
Pyo, J.-O., Yoo, S.-M., Ahn, H.-H., Nah, J., Hong, S.-H., Kam, T.-I., et al. (2013). Overexpression of Atg5 in mice activates autophagy and extends lifespan. Nat. Commun. 4, 2300. doi:10.1038/ncomms3300
Qiu, M., Chen, J., Li, X., and Zhuang, J. (2022). Intersection of the ubiquitin–proteasome system with oxidative stress in cardiovascular disease. IJMS 23, 12197. doi:10.3390/ijms232012197
Qu, X., Yu, J., Bhagat, G., Furuya, N., Hibshoosh, H., Troxel, A., et al. (2003). Promotion of tumorigenesis by heterozygous disruption of the beclin 1 autophagy gene. J. Clin. Invest. 112, 1809–1820. doi:10.1172/JCI20039
Raaben, M., Posthuma, C. C., Verheije, M. H., te Lintelo, E. G., Kikkert, M., Drijfhout, J. W., et al. (2010). The ubiquitin-proteasome system plays an important role during various stages of the coronavirus infection cycle. J. Virol. 84, 7869–7879. doi:10.1128/JVI.00485-10
Rishika, K., Prajwal, C., Richard, M., Christopher, D., and Michele, V. (2017). Protein homeostasis of a metastable subproteome associated with Alzheimer’s disease. Proc. Natl. Acad. Sci. U. S. A. 114, E5703–E5711. doi:10.1073/pnas.1618417114
Roberts, P., Moshitch-Moshkovitz, S., Kvam, E., O’Toole, E., Winey, M., and Goldfarb, D. S. (2003). Piecemeal microautophagy of nucleus in Saccharomyces cerevisiae. MBoC 14, 129–141. doi:10.1091/mbc.e02-08-0483
Rosenfeldt, M. T., and Ryan, K. M. (2009). The role of autophagy in tumour development and cancer therapy. Expert Rev. Mol. Med. 11, e36. doi:10.1017/S1462399409001306
Schneider, J. L., Villarroya, J., Diaz-Carretero, A., Patel, B., Urbanska, A. M., Thi, M. M., et al. (2015). Loss of hepatic chaperone-mediated autophagy accelerates proteostasis failure in aging. Aging Cell 14, 249–264. doi:10.1111/acel.12310
Schuck, S. (2020). Microautophagy – distinct molecular mechanisms handle cargoes of many sizes. J. Cell Sci. 133, jcs246322. doi:10.1242/jcs.246322
Schumpert, C., Handy, I., Dudycha, J. L., and Patel, R. C. (2014). Relationship between heat shock protein 70 expression and life span in Daphnia. Mech. Ageing Dev. 139, 1–10. doi:10.1016/j.mad.2014.04.001
Seo, J. H., Park, J.-H., Lee, E. J., Vo, T. T. L., Choi, H., Kim, J. Y., et al. (2016). ARD1-mediated Hsp70 acetylation balances stress-induced protein refolding and degradation. Nat. Commun. 7, 12882. doi:10.1038/ncomms12882
Shemesh, N., Jubran, J., Dror, S., Simonovsky, E., Basha, O., Argov, C., et al. (2021). The landscape of molecular chaperones across human tissues reveals a layered architecture of core and variable chaperones. Nat. Commun. 12, 2180. doi:10.1038/s41467-021-22369-9
Shen, Y., Li, D.-D., Wang, L.-L., Deng, R., and Zhu, X.-F. (2008). Decreased expression of autophagy-related proteins in malignant epithelial ovarian cancer. Autophagy 4, 1067–1068. doi:10.4161/auto.6827
Shu, K., and Yang, W. (2017). E3 ubiquitin ligases: Ubiquitous actors in plant development and abiotic stress responses. Plant Cell Physiol. 58, 1461–1476. doi:10.1093/pcp/pcx071
Simonsen, A., Cumming, R. C., Brech, A., Isakson, P., Schubert, D. R., and Finley, K. D. (2008). Promoting basal levels of autophagy in the nervous system enhances longevity and oxidant resistance in adult Drosophila. Autophagy 4, 176–184. doi:10.4161/auto.5269
Squier, T. C. (2001). Oxidative stress and protein aggregation during biological aging. Exp. Gerontol. 36, 1539–1550. doi:10.1016/s0531-5565(01)00139-5
Sultana, R., Boyd-Kimball, D., Poon, H. F., Cai, J., Pierce, W. M., Klein, J. B., et al. (2006). Redox proteomics identification of oxidized proteins in Alzheimer’s disease hippocampus and cerebellum: An approach to understand pathological and biochemical alterations in AD. Neurobiol. Aging 27, 1564–1576. doi:10.1016/j.neurobiolaging.2005.09.021
Sun, J., and Liao, J. K. (2004). Induction of angiogenesis by heat shock protein 90 mediated by protein kinase akt and endothelial nitric oxide synthase. ATVB 24, 2238–2244. doi:10.1161/01.ATV.0000147894.22300.4c
Taipale, M., Jarosz, D. F., and Lindquist, S. (2010). HSP90 at the hub of protein homeostasis: Emerging mechanistic insights. Nat. Rev. Mol. Cell Biol. 11, 515–528. doi:10.1038/nrm2918
Tóth, M. L., Sigmond, T., Borsos, E., Barna, J., Erdélyi, P., Takács-Vellai, K., et al. (2008). Longevity pathways converge on autophagy genes to regulate life span in Caenorhabditis elegans. Autophagy 4, 330–338. doi:10.4161/auto.5618
Tramutola, A., Lanzillotta, C., Perluigi, M., and Butterfield, D. A. (2017). Oxidative stress, protein modification and Alzheimer disease. Brain Res. Bull. 133, 88–96. doi:10.1016/j.brainresbull.2016.06.005
Ulbricht, A., Gehlert, S., Leciejewski, B., Schiffer, T., Bloch, W., and Höhfeld, J. (2015). Induction and adaptation of chaperone-assisted selective autophagy CASA in response to resistance exercise in human skeletal muscle. Autophagy 11, 538–546. doi:10.1080/15548627.2015.1017186
Vilchez, D., Saez, I., and Dillin, A. (2014). The role of protein clearance mechanisms in organismal ageing and age-related diseases. Nat. Commun. 5, 5659. doi:10.1038/ncomms6659
Walker, G. A., and Lithgow, G. J. (2003). Lifespan extension in C. elegans by a molecular chaperone dependent upon insulin-like signals. Aging Cell 2, 131–139. doi:10.1046/j.1474-9728.2003.00045.x
Wang, X., Xie, L., and Zhu, L. (2021). Clinicopathological significance of HSP70 expression in gastric cancer: A systematic review and meta-analysis. BMC Gastroenterol. 21, 437. doi:10.1186/s12876-021-01990-4
Welcker, M., and Clurman, B. E. (2008). FBW7 ubiquitin ligase: A tumour suppressor at the crossroads of cell division, growth and differentiation. Nat. Rev. Cancer 8, 83–93. doi:10.1038/nrc2290
White, E. (2012). Deconvoluting the context-dependent role for autophagy in cancer. Nat. Rev. Cancer 12, 401–410. doi:10.1038/nrc3262
White, E., Mehnert, J. M., and Chan, C. S. (2015). Autophagy, metabolism, and cancer. Clin. Cancer Res. 21, 5037–5046. doi:10.1158/1078-0432.CCR-15-0490
White, E. (2015). The role for autophagy in cancer. J. Clin. Invest. 125, 42–46. doi:10.1172/JCI73941
Whittier, J. E., Xiong, Y., Rechsteiner, M. C., and Squier, T. C. (2004). Hsp90 enhances degradation of oxidized calmodulin by the 20 S proteasome. J. Bioll Chem. 279, 46135–46142. doi:10.1074/jbc.M406048200
Wu, L., Chou, M., and Zhu, S. (2014). Unfolded protein response and cancer. Discov. (Craiova) 2, e10. doi:10.15190/d.2014.2
Xiao, F.-H., Chen, X.-Q., Yu, Q., Ye, Y., Liu, Y.-W., Yan, D., et al. (2018). Transcriptome evidence reveals enhanced autophagy-lysosomal function in centenarians. Genome Res. 28, 1601–1610. doi:10.1101/gr.220780.117
Xu, D.-W., Zhang, G.-Q., Wang, Z.-W., Xu, X.-Y., and Liu, T.-X. (2015). Autophagy in tumorigenesis and cancer treatment. Asian Pac J. Cancer Prev. 16, 2167–2175. doi:10.7314/apjcp.2015.16.6.2167
Yun, C., and Lee, S. (2018). The roles of autophagy in cancer. IJMS 19, 3466. doi:10.3390/ijms19113466
Zagouri, F., Bournakis, E., Koutsoukos, K., and Papadimitriou, C. A. (2012). Heat shock protein 90 (hsp90) expression and breast cancer. Pharm. (Basel) 5, 1008–1020. doi:10.3390/ph5091008
Zavadskiy, S., Sologova, S., and Moldogazieva, N. (2022). Oxidative distress in aging and age-related diseases: Spatiotemporal dysregulation of protein oxidation and degradation. Biochimie 195, 114–134. doi:10.1016/j.biochi.2021.12.002
Zeng, K., Chen, X., Hu, X., Liu, X., Xu, T., Sun, H., et al. (2018). LACTB, a novel epigenetic silenced tumor suppressor, inhibits colorectal cancer progression by attenuating MDM2-mediated p53 ubiquitination and degradation. Oncogene 37, 5534–5551. doi:10.1038/s41388-018-0352-7
Zhang, X., Linder, S., and Bazzaro, M. (2020). Drug development targeting the ubiquitin-proteasome system (UPS) for the treatment of human cancers. Cancers (Basel) 12, 902. doi:10.3390/cancers12040902
Keywords: protein homeostasis, molecular chaperones, ubiquitin-proteasome system, autophagy-lysosomal system, aging, cancer
Citation: Chen X-Q, Shen T, Fang S-J, Sun X-M, Li G-Y and Li Y-F (2023) Protein homeostasis in aging and cancer. Front. Cell Dev. Biol. 11:1143532. doi: 10.3389/fcell.2023.1143532
Received: 13 January 2023; Accepted: 09 February 2023;
Published: 16 February 2023.
Edited by:
Ji-Xin Tang, Guangdong Medical University, ChinaReviewed by:
Jie Song, Chinese Academy of Medical Sciences and Peking Union Medical College, ChinaXia Zhou, University of Science and Technology of China, China
Copyright © 2023 Chen, Shen, Fang, Sun, Li and Li. This is an open-access article distributed under the terms of the Creative Commons Attribution License (CC BY). The use, distribution or reproduction in other forums is permitted, provided the original author(s) and the copyright owner(s) are credited and that the original publication in this journal is cited, in accordance with accepted academic practice. No use, distribution or reproduction is permitted which does not comply with these terms.
*Correspondence: Xiao-Qiong Chen, Y2hlbnhpYW9kdW95Y2NAMTI2LmNvbQ==; Yun-Feng Li, bGl5dW5mZW5nMDAxQGZveG1haWwuY29t, bGl5dW5mZW5nQG1lZG1haWwuY29tLmNu
†These authors have contributed equally to this work