Look who’s TORking: mTOR-mediated integration of cell status and external signals during limb development and endochondral bone growth
- 1Australian Regenerative Medicine Institute, Monash University, Clayton, VIC, Australia
- 2Department of Biochemistry, Central University of Hyderabad, Hyderabad, India
- 3Department of Physiology, Development and Neuroscience, University of Cambridge, Cambridge, United Kingdom
The balance of cell proliferation and size is key for the control of organ development and repair. Moreover, this balance has to be coordinated within tissues and between tissues to achieve robustness in the organ’s pattern and size. The tetrapod limb has been used to study these topics during development and repair, and several conserved pathways have emerged. Among them, mechanistic target of rapamycin (mTOR) signaling, despite being active in several cell types and developmental stages, is one of the least understood in limb development, perhaps because of its multiple potential roles and interactions with other pathways. In the body of this review, we have collated and integrated what is known about the role of mTOR signaling in three aspects of tetrapod limb development: 1) limb outgrowth; 2) chondrocyte differentiation after mesenchymal condensation and 3) endochondral ossification-driven longitudinal bone growth. We conclude that, given its ability to interact with the most common signaling pathways, its presence in multiple cell types, and its ability to influence cell proliferation, size and differentiation, the mTOR pathway is a critical integrator of external stimuli and internal status, coordinating developmental transitions as complex as those taking place during limb development. This suggests that the study of the signaling pathways and transcription factors involved in limb patterning, morphogenesis and growth could benefit from probing the interaction of these pathways with mTOR components.
1 The coordination of cell growth and proliferation during limb development
Development of the tetrapod limb requires the exquisite coordination of growth between multiple tissues (cartilage, muscle, bone, tendons, dermis, nerves, diffuse connective tissue, etc.). In most of these tissues, controlling the balance between cell proliferation and size is critical to achieve a proper size and function, and is one of the main cellular processes that are regulated by the mechanisms controlling limb development. The coupling between proliferation and cell size has been extensively studied in multiple systems, and several highly conserved pathways have been identified that link cell size to proliferative capacity. One of such pathways is the mTOR pathway (Gu et al., 2011). This review will focus on the known roles of mTOR during limb development, with a special focus on the long bones. From this review, we conclude that, besides its well-known role in controlling cell size via insulin-like growth factor (IGF) signaling, mTOR activity can crosstalk with many other pathways, such as bone morphogenetic protein (BMP), wingless/int1 (WNT), hypoxia inducible factor 1α (HIF-1α), fibroblast growth factor (FGF) and hedgehog (HH). Through these pleiotropic effects, mTOR may play important roles in limb outgrowth, chondrocyte proliferation, differentiation and metabolism, and overall in long-bone growth.
2 mTOR, regulators and effectors
mTOR stands for mechanistic (formerly mammalian) target of rapamycin, a macrolide produced by Streptomyces Hygroscopicus bacteria. Rapamycin was named after the island of Rapa Nui, where it was discovered in the early 1990 s during a genetic screen in the budding yeast, where TOR1 and TOR2 were identified as the toxic agents of rapamycin (Cafferkey et al., 1993; Kunz et al., 1993). Biochemical approaches in mammals allowed purification of mTOR and confirmed it as the target of rapamycin (Brown et al., 1994; Sabatini et al., 1994; Sabers et al., 1995).
mTOR is a Ser/Thr protein kinase closely associated with cell growth, survival, proliferation and tumorigenesis, especially in the abundance of amino acids, growth factors and energy. mTOR needs to form complexes with other proteins in order to exert its biological activities, named mTOR complex 1 and 2 (mTORC1 and 2, respectively). The sections below will elaborate on the known regulators and downstream effectors of both complexes (Figures 1, 2).
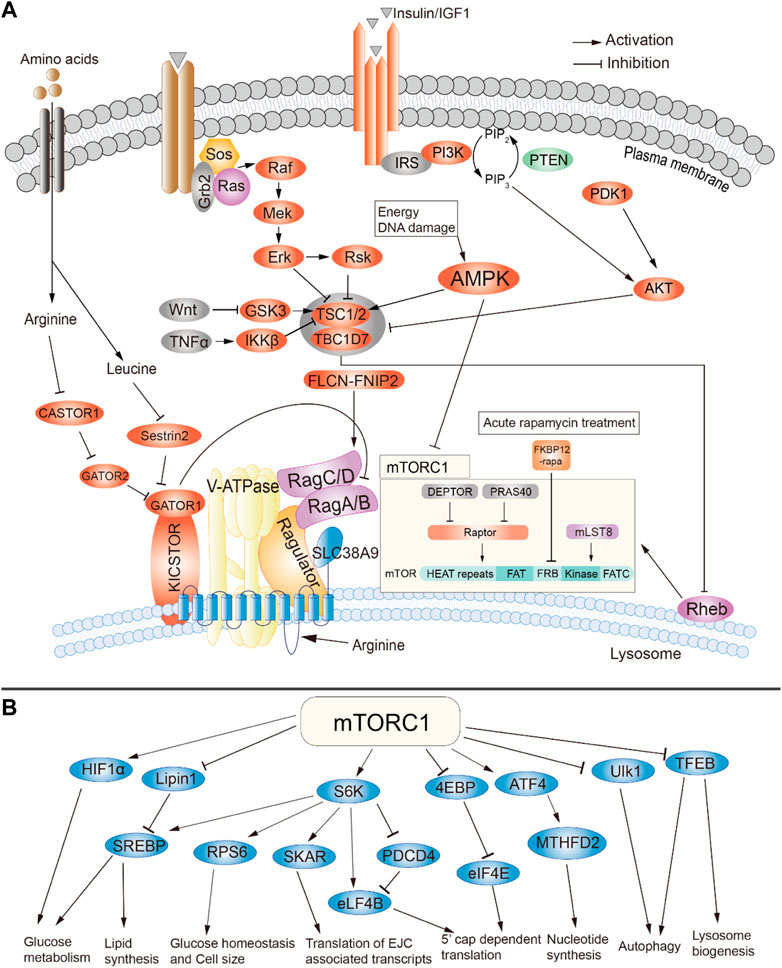
FIGURE 1. Summary of signaling through mTOR complex 1. (A) Activators of mTORC1. (B) Downstream targets and cellular effects of mTORC1. Adapted from (Wei et al., 2019), available through an CC BY license http://creativecommons.org/licenses/by/4.0/.
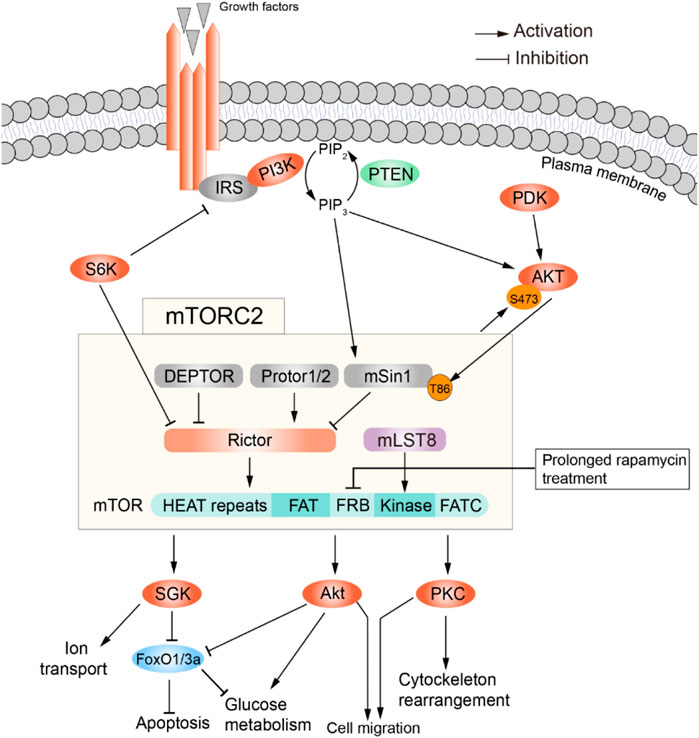
FIGURE 2. Summary of signaling through mTOR complex 2. Activators (top) and effectors (bottom) of mTORC2. Adapted from (Wei et al., 2019), available via a CC license http://creativecommons.org/licenses/by/4.0/.
2.1 mTORC1
2.1.1 Upstream signals and regulators of mTORC1
mTORC1 is composed of three core components: mTOR, Raptor, and mLST8. In addition, it contains two inhibitory subunits, PRAS40 and DEPTOR. As elaborated below, stress, growth factors, amino acid bioavailability, oxygen and other metabolites status have been identified as the upstream regulators of mTORC1 pathway (Figure 1A). At the molecular level, the activation of mTORC1 is mainly dependent on Rheb, a small GTPase that localizes to the lysosomal membrane and is negatively regulated by the tuberous sclerosis protein complex (TSC) 1 and 2 (Figure 1A) (Inoki et al., 2003; Tee et al., 2003). Growth factors, such as IGF1, can stimulate the PI3K and Ras pathways, leading to phosphorylation and thus inactivation of TSC1/2 through AKT/PKB, ERK1/2 and RSK1 kinases (Figure 1A). TSC1/2 then dissociate from the lysosomal membrane, leading to activation of mTORC1 (Inoki et al., 2002; Manning et al., 2002; Potter et al., 2002; Roux et al., 2004; Ma et al., 2005; Menon et al., 2014). This process can be further compounded by the abundance of amino acids, energy in the form of glucose and other nutrients. It has been shown that both the nutrients and the growth factors are necessary for the full activation of mTORC1 which contributes to the Rag-GTPase mediated amino acid-sensing regulation of mTORC1 (Beugnet et al., 2003; Kim et al., 2008; Sancak et al., 2008). In addition, pro-inflammatory cytokine tumor necrosis factor alpha (TNFα) also activates mTORC1 through phosphorylation-mediated inactivation of TSC1 (Lee et al., 2007). It was also shown that the canonical WNT pathway activates mTORC1 through inhibition of GSK3-β, which phosphorylates and promotes TSC2 activity (Inoki et al., 2006). This crosstalk with other signaling pathways will be further discussed in other sections.
2.1.2 Downstream effectors of mTORC1
As mTORC1 is activated in the presence of nutrients, its downstream effectors are mainly associated with pathways related to protein and lipid biogenesis, cell growth and the accumulation of cell mass. mTORC1 control protein synthesis through phosphorylation of 4E-BP1 and S6K1 (Figure 1B). 4E-BP1 phosphorylation attenuates binding to eukaryotic initiation factor 4 E (eIF4E), thus allowing formation of the eIF4F complex required for protein translation. In contrast, S6K1 phosphorylation results in both increased transcription and translation. Moreover, mTORC1 also promotes protein synthesis through the regulation of TIF-1A and MAF1 (Mayer et al., 2004; Kantidakis et al., 2010; Shor et al., 2010).
mTORC1 also plays a major role in lipid synthesis, which is required for cell membrane synthesis during cell proliferation (Duvel et al., 2010; Vaidyanathan et al., 2022). mTORC1 regulates fatty acid and cholesterol synthesis via Sterol Responsive Element Binding Protein 1 and 2 (SREBP1/2). mTORC1 is required for cleavage and nuclear translocation of SREBP1/2, which leads to the expression of lipogenic genes (Porstmann et al., 2008; Duvel et al., 2010; Li et al., 2010; Wang et al., 2011). In Drosophila, silencing of dSREBP caused a reduction in cell and organ size and impaired the stimulation of cell growth by dPI3K (Porstmann et al., 2008). Genetic studies in mice have shown that SREBP1 preferentially regulates fatty acid biosynthesis while SREBP2 mainly controls the expression of genes related to cholesterol synthesis (Horton et al., 2003). S6K1 and/or Lipin-1 have been shown to be the mediators between mTORC1 and SREBP1/2 (Duvel et al., 2010; Li et al., 2011; Peterson et al., 2011; Wang et al., 2011). Moreover, mTORC1 also promotes expression of the master regulator of adipogenesis, PPAR-γ (Kim and Chen, 2004; Zhang et al., 2009).
2.2 mTORC2
2.2.1 Upstream regulators of mTORC2
mTORC2 is comprised of mTOR, DEPTOR, PRAS40, mLST8, mSin1, Protor1/2, and Rictor, with DEPTOR being the only inhibitory subunit. In addition, rapamycin treatment affects mTORC1 and mTORC2 differently: acute treatment suffices to inhibit mTORC1, while mTORC2 requires prolonged treatment to get inhibited (Sarbassov et al., 2006). As shown in Figure 2, growth factors (Insulin/IGF) are the main activators of this complex. In fact, mTORC2 was identified in 2004 as the elusive ‘second’ insulin-responsive AKT kinase. The most accepted model is as follows: PI3K, activated by growth factor-receptor signaling, phosphorylates the plasma membrane phospholipid PIP2 to generate PIP3 (phosphatidylinositol 3,4,5-trisphosphate); PIP3 interacts with the pleckstrin homology (PH) domains within AKT and PDK1, triggering their translocation from the cytosol to the plasma membrane; there, PDK1 phosphorylates AKT in Thr308, partially activating it. This is sufficient to catalyze Sin1 phosphorylation within mTORC2, which then phosphorylates AKT at Ser473 to fully activate it. However, this model was challenged by a study reporting that phosphorylation of Sin1 impairs mTORC2 activity in mouse embryonic fibroblasts (Liu et al., 2013). There are also growth factor-independent activation mechanisms (Figure 2). For example, in specific cell types mTORC2 is involved in mechanically induced activation of β-catenin, such that inhibition of Rictor disrupts mechanically induced cytoskeletal reorganization (Lewis et al., 2020). Moreover, mTORC2 can be activated by association with ribosomes (Oh et al., 2010; Zinzalla et al., 2011). Lastly, p300-mediated acetylation of Rictor seems to potentiate IGF-1-induced mTORC2 kinase activity at Ser473 of AKT (Glidden et al., 2012), and is in general a positive regulator of mTORC2 activity (Singh et al., 2016). Intriguingly, mTORC2 location inside the cell shows broad heterogeneity depending on how it is activated. Indeed, it has been found at the plasma membrane, mitochondria, and sub-endosomal vesicles, by tracing the localization of mSin1 (Ebner et al., 2017). In summary, more work is needed to further elucidate the mechanisms of mTORC2 activation, and how this relates to its subcellular location.
2.2.2 Downstream effectors of mTORC2
Once activated, mTORC2 regulates cell survival, cell migration, cytoskeletal rearrangements and glucose metabolism by mediating the phosphorylation of conserved motifs in AGC kinases (i.e., PKA, PKC, PKG). This promotes their maturation, stability and allosteric activation (Cameron et al., 2011; Baffi et al., 2021). Another likely phosphorylation target is Serum- and Glucocorticoid-induced Kinase 1 (SGK1), which in turn regulates sodium transport in kidney epithelial cells (Lang and Pearce, 2016). SGK also decreases nuclear localization of the transcription factor FOXO3a and its DNA-binding activity (Brunet et al., 2001), thus inhibiting apoptosis and promoting survival.
3 Role of mTOR in limb outgrowth, morphogenesis and skeletal development
For the purposes of this review, tetrapod limb development will be roughly divided into three processes (Figure 3): 1) limb outgrowth and patterning; 2) Chondrocyte differentiation after the formation of mesenchymal condensations; 3) Bone growth driven by endochondral ossification.
3.1 mTOR interactions with the limb outgrowth and patterning programs
The limb primordia, or limb buds, arise as outgrowths from the lateral plate mesoderm, due to epithelial-to-mesenchymal transition of the coelomic epithelium (Gros and Tabin, 2014). The limb mesenchyme then expands in response to its interactions with the overlying epithelium (Figure 4A), A key event is the induction of an epithelial signaling center at the distal tip, the apical ectodermal ridge (AER), due to BMP4 signaling from the mesenchyme to BMP receptor IA in the epithelium (Ahn et al., 2001; Pizette et al., 2001). The AER is necessary (Saunders, 1948) and sufficient (Rosello-Diez and Torres, 2011) to promote the outgrowth of the limb. Also critical for limb outgrowth is the concomitant stabilization of Fgf10 expression in the limb field, which then signals to the overlying AER and activates WNT3A/β-catenin signaling, in turn inducing Fgf8 expression, which maintains Fgf10 expression in the mesenchyme and is critical for limb outgrowth (Ahn et al., 2001; Kawakami et al., 2001; Pizette et al., 2001).
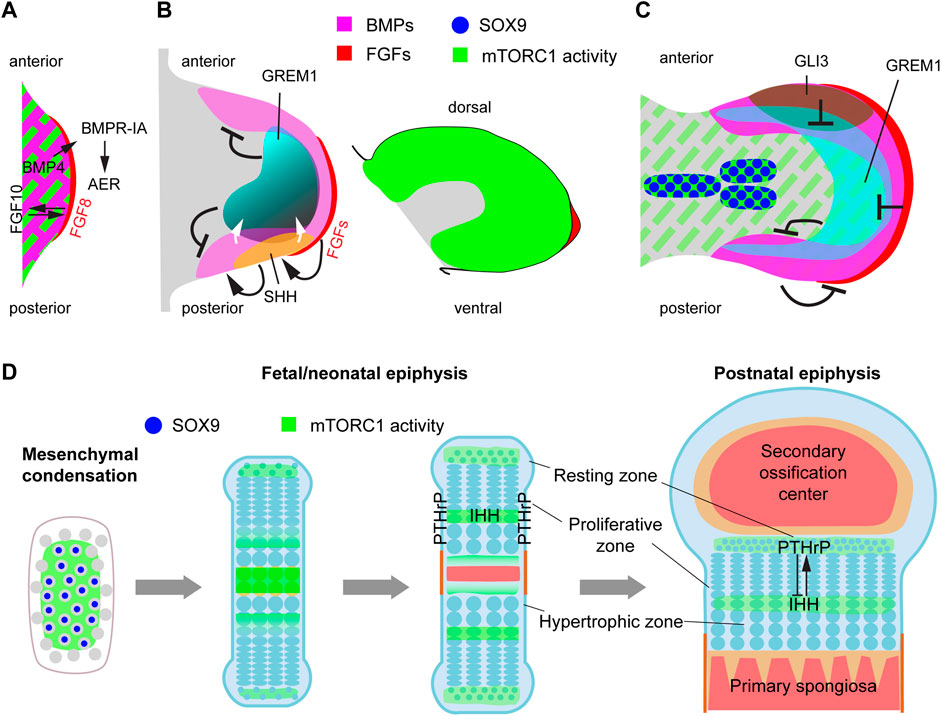
FIGURE 4. mTORC1 interacts with multiple signaling pathways and transcription factors involved in limb outgrowth, progression of skeletal condensations and bone growth. (A) Schematic representation of the signaling events involved in early limb-bud induction. We propose that mTORC1 could be involved in the EMT process that gives rise to the limb mesenchyme (Gros and Tabin, 2014). (B) During the patterning phase, mTORC1 activity is high in most of the limb mesenchyme, but seems to be only required for cell growth and timely progression of limb development (Jiang et al., 2017). (C) Interacting with BMP and HIF-1α pathways, mTORC1 is required for the transition of the initial condensations towards proliferative cartilage (Jiang et al., 2017; Iezaki et al., 2018; Lee et al., 2018). (D) Endochondral ossification process, including growth plate dynamics and main sites of mTORC1 action.
The role of mTOR in the limb outgrowth process has not been specifically addressed, as the earliest genetic conditional deletion of key pathway components such as Raptor were done via Prrx1-Cre, which is only active once the limb bud induction process is finalized (Logan et al., 2002). However, given the role of mTORC1 and 2 in mediating EMT in organogenesis and cancer (Gulhati et al., 2011; Karimi Roshan et al., 2019), and that the mTORC1 readout p-S6 is present in most of the mesenchyme of the early limb bud (Figures 4A,B), we propose that mTOR (or at least mTORC1) may play a role in the EMT process that leads to limb bud initiation. Supporting this possibility, artificial overexpression of RhoA represses mTORC1 signaling in mammalian cells (Gordon et al., 2014) and interferes with EMT-initiated limb outgrowth in chicken (Gros and Tabin, 2014).
After induction, the limb bud undergoes significant growth. Deletion of Raptor with Prrx1-Cre showed that mTORC1 signaling in the limb mesenchyme is required for the normal size of both the limb bud and its individual cells, but relatively dispensable for skeletal patterning (Jiang et al., 2017). A potential link between mTOR and limb growth is the FGF-IGF connection. It has been shown that the maintenance of limb growth by AER-FGFs requires the induction and signaling of IGF1 in the subridge mesenchyme, at least in the chicken embryo (Dealy et al., 1996). Moreover, insulin/IGF signaling in the limb mesenchyme is mediated by PI3K/AKT (Knobloch et al., 2008), which, as mentioned above, is an upstream regulator of mTORC1. Since mTORC1 activity is detected in the limb mesenchyme (Figure 4B), it is possible that mTORC1 mediates part of the effects of AER-FGFs on limb growth. Although this possibility has not been directly explored, there are studies in which one copy of Rps6 (encoding ribosomal protein S6, a mediator of mTORC1 activity) was removed in mouse limb buds using Prrx1-Cre, leading to smaller limb buds by embryonic day (E) 11.5, and agenesis of the humerus, radius and most anterior digit at E17.5 (Tiu et al., 2021). It is noteworthy that the hindlimb, where Prrx1-Cre is activated later and more sparsely than in the forelimb (Logan et al., 2002), was mostly unaffected in this study, suggesting that Rps6 full dosage is only needed in early limb growth.
3.2 mTOR role in chondrocyte differentiation after mesenchymal condensation
During the limb growth process, mesenchymal cells far from the AER start coalescing into the so-called mesenchymal condensations (Figure 3), which will give rise to the skeletal elements in a complex but stereotypical manner (Markman et al., 2023). In this differentiation process, the extracellular space between cells collapses, increasing the packing density. This requires partial replacement of the extracellular matrix (ECM) with new ECM components. Also, as density increases, cells become rounder and lose their filopodia (Thorogood and Hinchliffe, 1975). Subsequently, the condensations enlarge through cell proliferation, and a layer of elongated cells surrounds the condensation, forming the perichondrium. BMP signaling induces condensation and proliferation of mesenchymal cells and promotes their survival (Yoon et al., 2005). Moreover, these condensations become hypoxic, and the HIF-1α pathway is required for their progression towards cartilage cells or chondrocytes (Provot et al., 2007). Interestingly, levels of the mTORC1 readout p-S6 are high in the condensations, while they decrease in the rest of the mesenchyme (Figure 4C), suggesting a role in their formation/progression and potential interactions with the BMP and HIF1-α pathways. Indeed, BMPs have been shown to induce mTORC1 activation via the ALK3 receptor and Smad4-mediated inhibition of PTEN (Lim et al., 2016; Lee et al., 2018). mTORC1, in turn, is required for the translational control of SOX9, a key transcription factor in the progression towards cartilage (Iezaki et al., 2018). Moreover, mTORC1 has been shown to upregulate HIF-1α protein levels in the cartilage, which is critical for the control of glucose metabolism, proliferation and differentiation in chondrocytes (Lee et al., 2018).
3.3 mTOR and endochondral ossification
The process of endochondral ossification plays a predominant role in long-bone growth. In this process, a cartilage template (formed when mesenchymal cells in the condensations transition to chondrocytes) is progressively replaced by bone. From the ends towards the center of the bone, chondrocytes undergo subsequent differentiation stages (Figures 4D, 5A). Round resting chondrocytes constitute a reserve pool that with a certain frequency gives rise to proliferative chondrocytes arrayed in columns. Proliferating chondrocytes eventually undergo hypertrophy by increasing protein synthesis and osmotic swelling (Cooper et al., 2013), while they lay down the ECM that forms the cartilage scaffold. While some hypertrophic chondrocytes die, others transdifferentiate to osteoblasts (Yang et al., 2014; Zhou et al., 2014; Park et al., 2015). Osteoblasts can also derive from precursors located in the perichondrium (Maes et al., 2010), and regardless of their origin, lay down the bone matrix, forming the primary ossification center in the middle region of the skeletal element. This divides the cartilage template into two parts (growth plates), located at both ends, where the process continues (Figure 4D). Eventually, a secondary ossification center forms within each cartilage pole (Figure 4D). Key transcription factors like SOX9, RUNX2, MEF2C, and cell signaling pathways such as WNT, BMP, FGF, indian hedgehog (IHH) and parathyroid hormone-related peptide (PTHrP) affect chondrocyte proliferation and differentiation and osteoblast differentiation, often interacting with mTORC1 and 2 (Figures 5A, B).
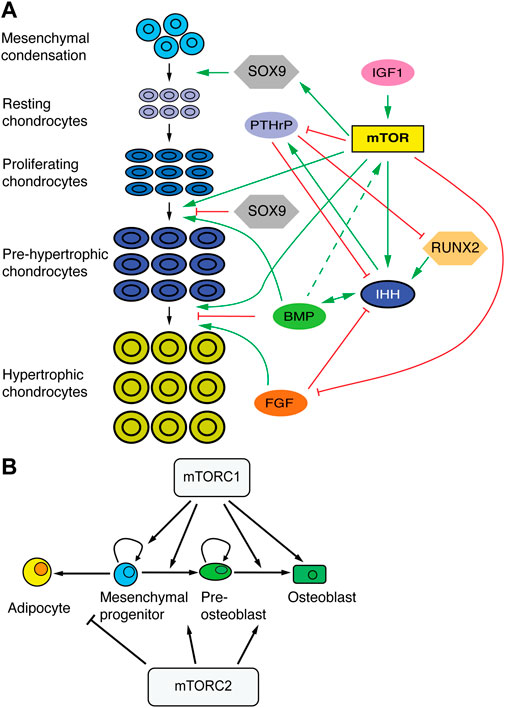
FIGURE 5. mTOR in skeletal development. (A) Schematic of chondrocyte transitions during cartilage growth and their regulators. mTOR plays an integrative role at the intersection of progenitor/stem cell proliferation, chondrocyte differentiation and cell size control. (B) Effects of mTORC1 and 2 in the differentiation of mesenchymal progenitors. Based on (Chen and Long, 2018).
3.3.1 Role of mTOR in chondrocyte proliferation and hypertrophic differentiation
As a first approach to studying the role of mTORC1 in endochondral ossification, rapamycin was used to treat fetal rat metatarsal cultures or pregnant rats in the last day of gestation, finding that mTORC1 inhibition impaired fetal chondrocyte differentiation and response to insulin, but not proliferation (Phornphutkul et al., 2008). Similarly, genetic deletion of either Mtor or Raptor in the mouse cartilage impaired skeletal growth through reduced matrix production, decreased chondrocyte size and delayed chondrocyte hypertrophy (Chen and Long, 2014). While these landmark studies were important first steps, given the multiple signaling interactions and roles that mTOR can participate in, as well as the multiple states and complex spatiotemporal dynamics that chondrocytes go through, it is worth considering more recent studies as well, focused on specific aspects of chondrocyte regulation by mTOR.
Due to the continuous cartilage destruction near the bone, maintenance of the cartilage regions, and hence bone growth, requires de novo production of cartilage at roughly the same rate. At fetal/early postnatal stages, cartilage progenitors located in the resting zone do not self-renew and have limited proliferative potential, so that when they are recruited into the proliferative pool, they deplete the pool of progenitors and give rise to short clonal columns of chondrocytes (Newton et al., 2019). Some time after formation of the secondary ossification center (SOC), however, some resting chondrocytes start to self-renew and show higher clonogenic potential, so that the pool of resting chondrocytes is not depleted as fast, and long clonal columns of chondrocytes are formed (Newton et al., 2019). Signals from the SOC (most prominently sonic hedgehog, Shh) seem to be critical for this change of behavior, as was the activation of the mTORC1 pathway in these so-called cartilage stem cells (Newton et al., 2019). Importantly, pharmacological abrogation of HH signaling reduced proliferation in the resting zone, while ectopic activation of mTORC1 via Tsc1 deletion led to a bias towards symmetric division in the resting zone, disorganizing cartilage structure (Newton et al., 2018; Newton et al., 2019). There is no consensus about this phenotype, however, as other group reported drastically different results (Yan et al., 2016), warranting further analyses.
Besides the control of cartilage progenitors, balanced cartilage growth requires maintenance of a relatively constant height of the different regions of the growth plate. The size of the proliferative zone is controlled by a well characterized negative feedback loop between IHH and PTHrP (Lee et al., 1996; Vortkamp et al., 1996; St-Jacques et al., 1999; Minina et al., 2001). In this loop, IHH produced by pre-hypertrophic chondrocytes induces PTHrP expression in resting chondrocytes, whereas PTHrP secreted from the resting zone promotes chondrocyte proliferation and delays differentiation, including Ihh expression (Figures 4D, 5A). mTOR is likely involved in this feedback loop in two different ways: via mechanotransduction-dependent Ihh expression, and via regulation of PTHrP signaling. Regarding the former, mechanical loading is an important regulator of chondrocyte maturation, and experiments in chicken embryos showed that elimination of muscle contraction results in mTOR inhibition in the cartilaginous growth plate (Guan et al., 2014). This led to significant inhibition of chondrocyte proliferation and reduced expression of Ihh. Conversely, mechanical stimulation of chondrocytes in vitro led to mTORC1-dependent activation of Ihh expression (Guan et al., 2014). Moreover, one of us (AR-D) showed that in vivo inhibition of SHP2, an antagonist of mTORC1-mediated mechanotransduction, leads to increased Ihh expression (Rosello-Diez et al., 2017). Along these lines, it was recently shown in chicken and alligator embryos that limb proportions can change in response to embryo movement, an effect due to mTOR-mediated changes in chondrocyte proliferation and specific of certain growth plates (Pollard et al., 2017).
On the other hand, mTORC1 activation has been shown to reduce expression of the PTHrP receptor in articular cartilage (Zhang et al., 2017), which could potentially happen in the growth plate cartilage as well. Moreover, Yan et al. showed that S6K1, a downstream effector of mTORC1, phosphorylates and allows nuclear translocation of HH-signaling transducer GLI2, leading to transcription of Pthlh, encoding PTHrP (Yan et al., 2016). The mTOR/PTHrP interaction also works in reverse. Studies of skeletal dysplasia syndromes characterized by constitutive activation of PTH/PTHrP showed reduced activities of salt inducible kinase 3 (SIK3), which caused accumulation of DEPTOR, in turn inhibiting mTORC1 and 2 activity, biasing skeletal progenitor differentiation towards fat instead of bone (Csukasi et al., 2018). This new PTH/PTHrP-SIK3-mTOR axis has been recently explored further, showing that, in the presence of nutrients, DEPTOR directly interacts with PTH1R to regulate PTH/PTHrP signaling, whereas in the absence of nutrients it forms a complex with TAZ (an effector of the Hippo pathway), to prevent its translocation to the nucleus and therefore inhibit its transcriptional activity (Csukasi et al., 2022).
Another potential signaling interaction of mTORC1 is with the canonical WNT pathway. Although related to calvarial bone (not long bones), it was shown that Wnt10b overexpression causes enlargement of calvarial tissue and phosphorylation of S6, both of which effects were abrogated by rapamycin (Inoki et al., 2006). Similarly, mice with reduced expression of LRP1 (a WNT receptor) had smaller than normal calvarias and reduced mTORC1 signaling. The connection seems to be mediated by WNT-dependent inhibition of GSK-3β, which via its interdependence with AMPK phosphorylates TSC2, acting as an mTORC1 inhibitor (Inoki et al., 2006). In the future, it would be interesting to test this crosstalk during endochondral ossification in the long bones.
A key signal transducer that interacts with the mTOR pathway during cartilage and skeletal growth is AKT (aka protein kinase B). AKT is the collective name of three Ser/Thr protein kinases that play key roles in cellular processes such as cell proliferation, migration, apoptosis, glucose metabolism and transcription. The functions of AKT and its downstream molecular mechanisms were explored during skeletal development using transgenic mouse embryos that expressed constitutively active AKT (Rokutanda et al., 2009). AKT positively regulated ECM production, chondrocyte growth, proliferation and maturation; associated with increased phosphorylation of FoxO3a, S6K (i.e., mTORC1 signaling) and GSK-3β in the growth plates of the AKT transgenic mice. Further examination of the downstream signaling pathways by ex vivo culture revealed that the AKT-mTOR pathway positively regulated proliferation, maturation and ECM production (Rokutanda et al., 2009).
mTORC2 and mTORC2-mediated activation of mTORC1 are also crucial in skeletal development. It was found that genetic deletion of Rictor (encoding an essential component of mTORC2) in the limb mesenchyme led to a smaller skeleton in mice (Chen et al., 2015). This phenotype is caused by delayed chondrocyte hypertrophy and not changes in cell size, proliferation, apoptosis, or ECM production (Chen et al., 2015). Furthermore, RUNX2, a master regulator of skeletal development, has been shown to activate PI3K/AKT signaling via mTORC2 in breast cancer cells (Tandon et al., 2014). However, the question of whether the RUNX2/PI3K/AKT/mTORC2 axis is conserved in skeletal development remains to be examined.
3.3.2 Role of mTOR in the osteoblast/osteocyte lineage
Besides its roles in chondrocytes, mTORC1 is required for the transition of pre-osteoblasts to mature osteoblasts (Figure 5B). Indeed, Mtor or Raptor deletion (and thus perturbation of mTORC1 function) in preosteoblasts (using Osx-Cre) or in calvarial cultures caused reduced osteogenic capacity and delayed bone formation (Chen and Long, 2015; Dai et al., 2017; Fitter et al., 2017). Mechanistically, this was caused by reduced expression of Runx2, a master regulator of osteogenesis (Dai et al., 2017).
Regarding mTORC2, its inactivation via Prrx1-Cre mediated deletion of Rictor in the limb mesenchyme did not affect chondrocyte proliferation, apoptosis, cell size or matrix production, but instead caused a delay in osteoblast differentiation (Figure 5B) (Chen et al., 2015). Moreover, Rictor deletion in late-stage osteoblasts/osteocytes with Dmp1-Cre hampered load-induced bone formation, causing a reduced bone mass phenotype (Lewis et al., 2020).
mTORC2 can also interact with other signaling pathways. Through its tyrosine kinase activity, mTORC2 is involved in the activation of IGF-1R/InsR signaling, which plays an essential role in osteoblasts proliferation, survival, and differentiation during skeletal growth. Indeed, a study reported that mTORC2 is recruited to the IGF-1R/InsR via SIN1 interaction with insulin receptor substrate (IRS) (Yin et al., 2016). Another study showed that IGF-activated AKT and PI3-kinase are crucial for BMP2-stimulated Runx2 expression and therefore osteoblast differentiation, maturation, and function in mouse metatarsal bones (Mukherjee and Rotwein, 2009) (Figure 5B).
WNT signaling has been shown to play a role in both prenatal and postnatal skeletal formation, in part interacting with mTORC1 and 2. Prenatal induction of WNT7B in the osteoblast lineage (Col1a1-Cre) increased bone mass via increased osteoblast number during bone formation (Chen et al., 2014). Deletion of Raptor in the osteoblast lineage reduced the WNT7B-induced phenotype. In addition, induction of WNT7B postnatally in Runx2+ cells also enhanced bone formation (Chen et al., 2014). Moreover, WNT3A signaling via LRP5, independent of β-catenin, has been shown to activate mTORC2-AKT downstream of RAC1 and promote a metabolic reprogramming that is critical for osteoblast differentiation (Esen et al., 2013).
Therefore, all together, it can be inferred that mTORC1 and 2, activated by IGFs, BMPs and WNTs are involved in the regulation of osteoblast activity to maintain skeletal growth.
4 Conclusion. mTOR and limb development: a TORrent of possibilities
In this review, we have addressed the role and regulation of the mTOR pathway during multiple steps of tetrapod limb development, with a focus on the long bones. mTOR, as an integrator of intrinsic cellular status (e.g., metabolism, stress) and extrinsic signals (e.g., growth factors, nutrients), is in a unique position to coordinate multiple inputs into a robust growth program. In fact, its widespread expression, pleiotropic effects and ability to interact with multiple signaling pathways and transcription factors suggest that it could be involved in more processes than the ones studied so far. For example, we have already mentioned that, while no study has found a clear role for mTORC1 or 2 in limb initiation, most genetic interventions have been performed once the limb bud is already present, precluding a clear conclusion. There is also the possibility of synergies. Since mTORC1 and 2 can modulate/interact with many of the signaling pathways involved in limb patterning (BMP, FGF, HH, WNT, Figures 4B, C), it is reasonable to think that heterozygous deletions of mTORC components may synergize with genetic defects impacting said pathways. This could also have implications in other fields. For example, in the context of cancer it has been recently proposed that the interaction between HH and mTORC1, happening at the level of the primary cilium, is more widespread than currently thought (Larsen and Moller, 2020). We submit that disentangling these interactions could open new avenues to understanding the control of limb size and proportions during development and evolution, and treating limb growth disorders.
Author contributions
CHH wrote the first drafts of the manuscript and the rebuttal. AR-D designed the layout of the review, prepared the figures and finalized the manuscript. AK drafted sub-sections of the manuscript. All authors read and approved the submitted version.
Funding
HFSP CDA00021/2019-C to AR-D and NHMRC 2002084 to CHH and AR-D.
Acknowledgments
We thank Edwina McGlinn, Jan Manent, Jan Kaslin and all members of the Rosello-Diez lab for interesting discussions about mTOR in development and regeneration. We also thank the reviewers for their suggestions to improve the manuscript.
Conflict of interest
The authors declare that the research was conducted in the absence of any commercial or financial relationships that could be construed as a potential conflict of interest.
Publisher’s note
All claims expressed in this article are solely those of the authors and do not necessarily represent those of their affiliated organizations, or those of the publisher, the editors and the reviewers. Any product that may be evaluated in this article, or claim that may be made by its manufacturer, is not guaranteed or endorsed by the publisher.
References
Ahn, K., Mishina, Y., Hanks, M. C., Behringer, R. R., and Crenshaw, E. B. (2001). BMPR-IA signaling is required for the formation of the apical ectodermal ridge and dorsal-ventral patterning of the limb. Development 128 (22), 4449–4461. doi:10.1242/dev.128.22.4449
Baffi, T. R., Lorden, G., Wozniak, J. M., Feichtner, A., Yeung, W., Kornev, A. P., et al. (2021). mTORC2 controls the activity of PKC and Akt by phosphorylating a conserved TOR interaction motif. Sci. Signal 14 (678), eabe4509. doi:10.1126/scisignal.abe4509
Beugnet, A., Tee, A. R., Taylor, P. M., and Proud, C. G. (2003). Regulation of targets of mTOR (mammalian target of rapamycin) signalling by intracellular amino acid availability. Biochem. J. 372 (2), 555–566. doi:10.1042/bj20021266
Brown, E. J., Albers, M. W., Shin, T. B., Ichikawa, K., Keith, C. T., Lane, W. S., et al. (1994). A mammalian protein targeted by G1-arresting rapamycin-receptor complex. Nature 369 (6483), 756–758. doi:10.1038/369756a0
Brunet, A., Park, J., Tran, H., Hu, L. S., Hemmings, B. A., and Greenberg, M. E. (2001). Protein kinase SGK mediates survival signals by phosphorylating the forkhead transcription factor FKHRL1 (FOXO3a). Mol. Cell Biol. 21 (3), 952–965. doi:10.1128/MCB.21.3.952-965.2001
Cafferkey, R., Young, P. R., McLaughlin, M. M., Bergsma, D. J., Koltin, Y., Sathe, G. M., et al. (1993). Dominant missense mutations in a novel yeast protein related to mammalian phosphatidylinositol 3-kinase and VPS34 abrogate rapamycin cytotoxicity. Mol. Cell Biol. 13 (10), 6012–6023. doi:10.1128/mcb.13.10.6012-6023.1993
Cameron, A. J., Linch, M. D., Saurin, A. T., Escribano, C., and Parker, P. J. (2011). mTORC2 targets AGC kinases through Sin1-dependent recruitment. Biochem. J. 439 (2), 287–297. doi:10.1042/BJ20110678
Chen, J., Holguin, N., Shi, Y., Silva, M. J., and Long, F. (2015). mTORC2 signaling promotes skeletal growth and bone formation in mice. J. Bone Min. Res. 30 (2), 369–378. doi:10.1002/jbmr.2348
Chen, J., and Long, F. (2018). mTOR signaling in skeletal development and disease. Bone Res. 6, 1. doi:10.1038/s41413-017-0004-5
Chen, J., and Long, F. (2014). mTORC1 signaling controls mammalian skeletal growth through stimulation of protein synthesis. Development 141 (14), 2848–2854. doi:10.1242/dev.108811
Chen, J., and Long, F. (2015). mTORC1 signaling promotes osteoblast differentiation from preosteoblasts. PLoS One 10 (6), e0130627. doi:10.1371/journal.pone.0130627
Chen, J., Tu, X., Esen, E., Joeng, K. S., Lin, C., Arbeit, J. M., et al. (2014). WNT7B promotes bone formation in part through mTORC1. PLoS Genet. 10 (1), e1004145. doi:10.1371/journal.pgen.1004145
Cooper, K. L., Oh, S., Sung, Y., Dasari, R. R., Kirschner, M. W., and Tabin, C. J. (2013). Multiple phases of chondrocyte enlargement underlie differences in skeletal proportions. Nature 495 (7441), 375–378. doi:10.1038/nature11940
Csukasi, F., Bosakova, M., Barta, T., Martin, J. H., Arcedo, J., Barad, M., et al. (2022). Skeletal diseases caused by mutations in PTH1R show aberrant differentiation of skeletal progenitors due to dysregulation of DEPTOR. Front. Cell Dev. Biol. 10, 963389. doi:10.3389/fcell.2022.963389
Csukasi, F., Duran, I., Barad, M., Barta, T., Gudernova, I., Trantirek, L., et al. (2018). The PTH/PTHrP-SIK3 pathway affects skeletogenesis through altered mTOR signaling. Sci. Transl. Med. 10 (459), eaat9356. doi:10.1126/scitranslmed.aat9356
Dai, Q., Xu, Z., Ma, X., Niu, N., Zhou, S., Xie, F., et al. (2017). mTOR/Raptor signaling is critical for skeletogenesis in mice through the regulation of Runx2 expression. Cell Death Differ. 24 (11), 1886–1899. doi:10.1038/cdd.2017.110
Dealy, C. N., Clarke, K., and Scranton, V. (1996). Ability of FGFs to promote the outgrowth and proliferation of limb mesoderm is dependent on IGF-I activity. Dev. Dyn. 206 (4), 463–469. doi:10.1002/(SICI)1097-0177(199608)206:4<463::AID-AJA12>3.0.CO;2-Y
Duvel, K., Yecies, J. L., Menon, S., Raman, P., Lipovsky, A. I., Souza, A. L., et al. (2010). Activation of a metabolic gene regulatory network downstream of mTOR complex 1. Mol. Cell 39 (2), 171–183. doi:10.1016/j.molcel.2010.06.022
Ebner, M., Sinkovics, B., Szczygiel, M., Ribeiro, D. W., and Yudushkin, I. (2017). Localization of mTORC2 activity inside cells. J. Cell Biol. 216 (2), 343–353. doi:10.1083/jcb.201610060
Esen, E., Chen, J., Karner, C. M., Okunade, A. L., Patterson, B. W., and Long, F. (2013). WNT-LRP5 signaling induces Warburg effect through mTORC2 activation during osteoblast differentiation. Cell Metab. 17 (5), 745–755. doi:10.1016/j.cmet.2013.03.017
Fitter, S., Matthews, M. P., Martin, S. K., Xie, J., Ooi, S. S., Walkley, C. R., et al. (2017). mTORC1 plays an important role in skeletal development by controlling preosteoblast differentiation. Mol. Cell Biol. 37 (7), e00668. doi:10.1128/MCB.00668-16
Glidden, E. J., Gray, L. G., Vemuru, S., Li, D., Harris, T. E., and Mayo, M. W. (2012). Multiple site acetylation of Rictor stimulates mammalian target of rapamycin complex 2 (mTORC2)-dependent phosphorylation of Akt protein. J. Biol. Chem. 287 (1), 581–588. doi:10.1074/jbc.M111.304337
Gordon, B. S., Kazi, A. A., Coleman, C. S., Dennis, M. D., Chau, V., Jefferson, L. S., et al. (2014). RhoA modulates signaling through the mechanistic target of rapamycin complex 1 (mTORC1) in mammalian cells. Cell Signal. 26 (3), 461–467. doi:10.1016/j.cellsig.2013.11.035
Gros, J., and Tabin, C. J. (2014). Vertebrate limb bud formation is initiated by localized epithelial-to-mesenchymal transition. Science 343 (6176), 1253–1256. doi:10.1126/science.1248228
Gu, Y., Lindner, J., Kumar, A., Yuan, W., and Magnuson, M. A. (2011). Rictor/mTORC2 is essential for maintaining a balance between beta-cell proliferation and cell size. Diabetes 60 (3), 827–837. doi:10.2337/db10-1194
Guan, Y., Yang, X., Yang, W., Charbonneau, C., and Chen, Q. (2014). Mechanical activation of mammalian target of rapamycin pathway is required for cartilage development. FASEB J. 28 (10), 4470–4481. doi:10.1096/fj.14-252783
Gulhati, P., Bowen, K. A., Liu, J., Stevens, P. D., Rychahou, P. G., Chen, M., et al. (2011). mTORC1 and mTORC2 regulate EMT, motility, and metastasis of colorectal cancer via RhoA and Rac1 signaling pathways. Cancer Res. 71 (9), 3246–3256. doi:10.1158/0008-5472.CAN-10-4058
Horton, J. D., Shah, N. A., Warrington, J. A., Anderson, N. N., Park, S. W., Brown, M. S., et al. (2003). Combined analysis of oligonucleotide microarray data from transgenic and knockout mice identifies direct SREBP target genes. Proc. Natl. Acad. Sci. U. S. A. 100 (21), 12027–12032. doi:10.1073/pnas.1534923100
Iezaki, T., Horie, T., Fukasawa, K., Kitabatake, M., Nakamura, Y., Park, G., et al. (2018). Translational control of Sox9 RNA by mTORC1 contributes to skeletogenesis. Stem Cell Rep. 11 (1), 228–241. doi:10.1016/j.stemcr.2018.05.020
Inoki, K., Li, Y., Xu, T., and Guan, K. L. (2003). Rheb GTPase is a direct target of TSC2 GAP activity and regulates mTOR signaling. Genes Dev. 17 (15), 1829–1834. doi:10.1101/gad.1110003
Inoki, K., Li, Y., Zhu, T., Wu, J., and Guan, K. L. (2002). TSC2 is phosphorylated and inhibited by Akt and suppresses mTOR signalling. Nat. Cell Biol. 4 (9), 648–657. doi:10.1038/ncb839
Inoki, K., Ouyang, H., Zhu, T., Lindvall, C., Wang, Y., Zhang, X., et al. (2006). TSC2 integrates Wnt and energy signals via a coordinated phosphorylation by AMPK and GSK3 to regulate cell growth. Cell 126 (5), 955–968. doi:10.1016/j.cell.2006.06.055
Jiang, M., Fu, X., Yang, H., Long, F., and Chen, J. (2017). mTORC1 signaling promotes limb bud cell growth and chondrogenesis. J. Cell Biochem. 118 (4), 748–753. doi:10.1002/jcb.25728
Kantidakis, T., Ramsbottom, B. A., Birch, J. L., Dowding, S. N., and White, R. J. (2010). mTOR associates with TFIIIC, is found at tRNA and 5S rRNA genes, and targets their repressor Maf1. Proc. Natl. Acad. Sci. U. S. A. 107 (26), 11823–11828. doi:10.1073/pnas.1005188107
Karimi Roshan, M., Soltani, A., Soleimani, A., Rezaie Kahkhaie, K., Afshari, A. R., and Soukhtanloo, M. (2019). Role of AKT and mTOR signaling pathways in the induction of epithelial-mesenchymal transition (EMT) process. Biochimie 165, 229–234. doi:10.1016/j.biochi.2019.08.003
Kawakami, Y., Capdevila, J., Buscher, D., Itoh, T., Rodriguez Esteban, C., and Izpisua Belmonte, J. C. (2001). WNT signals control FGF-dependent limb initiation and AER induction in the chick embryo. Cell 104 (6), 891–900. doi:10.1016/s0092-8674(01)00285-9
Kim, E., Goraksha-Hicks, P., Li, L., Neufeld, T. P., and Guan, K. L. (2008). Regulation of TORC1 by Rag GTPases in nutrient response. Nat. Cell Biol. 10 (8), 935–945. doi:10.1038/ncb1753
Kim, J. E., and Chen, J. (2004). Regulation of peroxisome proliferator-activated receptor-gamma activity by mammalian target of rapamycin and amino acids in adipogenesis. Diabetes 53 (11), 2748–2756. doi:10.2337/diabetes.53.11.2748
Knobloch, J., Schmitz, I., Gotz, K., Schulze-Osthoff, K., and Ruther, U. (2008). Thalidomide induces limb anomalies by PTEN stabilization, Akt suppression, and stimulation of caspase-dependent cell death. Mol. Cell Biol. 28 (2), 529–538. doi:10.1128/MCB.00553-07
Kunz, J., Henriquez, R., Schneider, U., Deuter-Reinhard, M., Movva, N. R., and Hall, M. N. (1993). Target of rapamycin in yeast, TOR2, is an essential phosphatidylinositol kinase homolog required for G1 progression. Cell 73 (3), 585–596. doi:10.1016/0092-8674(93)90144-f
Lang, F., and Pearce, D. (2016). Regulation of the epithelial Na+ channel by the mTORC2/SGK1 pathway. Nephrol. Dial. Transpl. 31 (2), 200–205. doi:10.1093/ndt/gfv270
Larsen, L. J., and Moller, L. B. (2020). Crosstalk of hedgehog and mTORC1 pathways. Cells 9 (10), 2316. doi:10.3390/cells9102316
Lee, D. F., Kuo, H. P., Chen, C. T., Hsu, J. M., Chou, C. K., Wei, Y., et al. (2007). IKK beta suppression of TSC1 links inflammation and tumor angiogenesis via the mTOR pathway. Cell 130 (3), 440–455. doi:10.1016/j.cell.2007.05.058
Lee, K., Lanske, B., Karaplis, A. C., Deeds, J. D., Kohno, H., Nissenson, R. A., et al. (1996). Parathyroid hormone-related peptide delays terminal differentiation of chondrocytes during endochondral bone development. Endocrinology 137 (11), 5109–5118. doi:10.1210/endo.137.11.8895385
Lee, S. Y., Abel, E. D., and Long, F. (2018). Glucose metabolism induced by Bmp signaling is essential for murine skeletal development. Nat. Commun. 9 (1), 4831. doi:10.1038/s41467-018-07316-5
Lewis, K. J., Yi, X., Wright, C. S., Pemberton, E. Z., Bullock, W. A., Thompson, W. R., et al. (2020). The mTORC2 component rictor is required for load-induced bone formation in late-stage skeletal cells. JBMR Plus 4 (7), e10366. doi:10.1002/jbm4.10366
Li, S., Brown, M. S., and Goldstein, J. L. (2010). Bifurcation of insulin signaling pathway in rat liver: mTORC1 required for stimulation of lipogenesis, but not inhibition of gluconeogenesis. Proc. Natl. Acad. Sci. U. S. A. 107 (8), 3441–3446. doi:10.1073/pnas.0914798107
Li, S., Ogawa, W., Emi, A., Hayashi, K., Senga, Y., Nomura, K., et al. (2011). Role of S6K1 in regulation of SREBP1c expression in the liver. Biochem. Biophys. Res. Commun. 412 (2), 197–202. doi:10.1016/j.bbrc.2011.07.038
Lim, J., Shi, Y., Karner, C. M., Lee, S. Y., Lee, W. C., He, G., et al. (2016). Dual function of Bmpr1a signaling in restricting preosteoblast proliferation and stimulating osteoblast activity in mouse. Development 143 (2), 339–347. doi:10.1242/dev.126227
Liu, P., Gan, W., Inuzuka, H., Lazorchak, A. S., Gao, D., Arojo, O., et al. (2013). Sin1 phosphorylation impairs mTORC2 complex integrity and inhibits downstream Akt signalling to suppress tumorigenesis. Nat. Cell Biol. 15 (11), 1340–1350. doi:10.1038/ncb2860
Logan, M., Martin, J. F., Nagy, A., Lobe, C., Olson, E. N., and Tabin, C. J. (2002). Expression of Cre Recombinase in the developing mouse limb bud driven by a Prxl enhancer. Genesis 33 (2), 77–80. doi:10.1002/gene.10092
Ma, L., Chen, Z., Erdjument-Bromage, H., Tempst, P., and Pandolfi, P. P. (2005). Phosphorylation and functional inactivation of TSC2 by Erk implications for tuberous sclerosis and cancer pathogenesis. Cell 121 (2), 179–193. doi:10.1016/j.cell.2005.02.031
Maes, C., Kobayashi, T., Selig, M. K., Torrekens, S., Roth, S. I., Mackem, S., et al. (2010). Osteoblast precursors, but not mature osteoblasts, move into developing and fractured bones along with invading blood vessels. Dev. Cell 19 (2), 329–344. doi:10.1016/j.devcel.2010.07.010
Manning, B. D., Tee, A. R., Logsdon, M. N., Blenis, J., and Cantley, L. C. (2002). Identification of the tuberous sclerosis complex-2 tumor suppressor gene product tuberin as a target of the phosphoinositide 3-kinase/akt pathway. Mol. Cell 10 (1), 151–162. doi:10.1016/s1097-2765(02)00568-3
Markman, S., Zada, M., David, E., Giladi, A., Amit, I., and Zelzer, E. (2023). A single-cell census of mouse limb development identifies complex spatiotemporal dynamics of skeleton formation. Dev. Cell S1534-5807, 00072. doi:10.1016/j.devcel.2023.02.013
Mayer, C., Zhao, J., Yuan, X., and Grummt, I. (2004). mTOR-dependent activation of the transcription factor TIF-IA links rRNA synthesis to nutrient availability. Genes Dev. 18 (4), 423–434. doi:10.1101/gad.285504
Menon, S., Dibble, C. C., Talbott, G., Hoxhaj, G., Valvezan, A. J., Takahashi, H., et al. (2014). Spatial control of the TSC complex integrates insulin and nutrient regulation of mTORC1 at the lysosome. Cell 156 (4), 771–785. doi:10.1016/j.cell.2013.11.049
Minina, E., Wenzel, H. M., Kreschel, C., Karp, S., Gaffield, W., McMahon, A. P., et al. (2001). BMP and Ihh/PTHrP signaling interact to coordinate chondrocyte proliferation and differentiation. Development 128 (22), 4523–4534. doi:10.1242/dev.128.22.4523
Mukherjee, A., and Rotwein, P. (2009). Akt promotes BMP2-mediated osteoblast differentiation and bone development. J. Cell Sci. 122 (5), 716–726. doi:10.1242/jcs.042770
Newton, P. T., Li, L., Zhou, B., Schweingruber, C., Hovorakova, M., Xie, M., et al. (2019). A radical switch in clonality reveals a stem cell niche in the epiphyseal growth plate. Nature 567 (7747), 234–238. doi:10.1038/s41586-019-0989-6
Newton, P. T., Xie, M., Medvedeva, E. V., Savendahl, L., and Chagin, A. S. (2018). Activation of mTORC1 in chondrocytes does not affect proliferation or differentiation, but causes the resting zone of the growth plate to become disordered. Bone Rep. 8, 64–71. doi:10.1016/j.bonr.2018.02.006
Oh, W. J., Wu, C. C., Kim, S. J., Facchinetti, V., Julien, L. A., Finlan, M., et al. (2010). mTORC2 can associate with ribosomes to promote cotranslational phosphorylation and stability of nascent Akt polypeptide. EMBO J. 29 (23), 3939–3951. doi:10.1038/emboj.2010.271
Park, J., Gebhardt, M., Golovchenko, S., Perez-Branguli, F., Hattori, T., Hartmann, C., et al. (2015). Dual pathways to endochondral osteoblasts: A novel chondrocyte-derived osteoprogenitor cell identified in hypertrophic cartilage. Biol. Open 4 (5), 608–621. doi:10.1242/bio.201411031
Peterson, T. R., Sengupta, S. S., Harris, T. E., Carmack, A. E., Kang, S. A., Balderas, E., et al. (2011). mTOR complex 1 regulates lipin 1 localization to control the SREBP pathway. Cell 146 (3), 408–420. doi:10.1016/j.cell.2011.06.034
Phornphutkul, C., Wu, K.-Y., Auyeung, V., Chen, Q., and Gruppuso, P. A. (2008). mTOR signaling contributes to chondrocyte differentiation. Dev. Dyn. 237 (3), 702–712. doi:10.1002/dvdy.21464
Pizette, S., Abate-Shen, C., and Niswander, L. (2001). BMP controls proximodistal outgrowth, via induction of the apical ectodermal ridge, and dorsoventral patterning in the vertebrate limb. Development 128 (22), 4463–4474. doi:10.1242/dev.128.22.4463
Pollard, A. S., Charlton, B. G., Hutchinson, J. R., Gustafsson, T., McGonnell, I. M., Timmons, J. A., et al. (2017). Limb proportions show developmental plasticity in response to embryo movement. Sci. Rep. 7, 41926. doi:10.1038/srep41926
Porstmann, T., Santos, C. R., Griffiths, B., Cully, M., Wu, M., Leevers, S., et al. (2008). SREBP activity is regulated by mTORC1 and contributes to Akt-dependent cell growth. Cell Metab. 8 (3), 224–236. doi:10.1016/j.cmet.2008.07.007
Potter, C. J., Pedraza, L. G., and Xu, T. (2002). Akt regulates growth by directly phosphorylating Tsc2. Nat. Cell Biol. 4 (9), 658–665. doi:10.1038/ncb840
Provot, S., Zinyk, D., Gunes, Y., Kathri, R., Le, Q., Kronenberg, H. M., et al. (2007). Hif-1alpha regulates differentiation of limb bud mesenchyme and joint development. J. Cell Biol. 177 (3), 451–464. doi:10.1083/jcb.200612023
Rokutanda, S., Fujita, T., Kanatani, N., Yoshida, C. A., Komori, H., Liu, W., et al. (2009). Akt regulates skeletal development through GSK3, mTOR, and FoxOs. Dev. Biol. 328 (1), 78–93. doi:10.1016/j.ydbio.2009.01.009
Rosello-Diez, A., Stephen, D., and Joyner, A. L. (2017). Altered paracrine signaling from the injured knee joint impairs postnatal long bone growth. Elife 6, e27210. doi:10.7554/eLife.27210
Rosello-Diez, A., and Torres, M. (2011). Regulative patterning in limb bud transplants is induced by distalizing activity of apical ectodermal ridge signals on host limb cells. Dev. Dyn. 240 (5), 1203–1211. doi:10.1002/dvdy.22635
Roux, P. P., Ballif, B. A., Anjum, R., Gygi, S. P., and Blenis, J. (2004). Tumor-promoting phorbol esters and activated Ras inactivate the tuberous sclerosis tumor suppressor complex via p90 ribosomal S6 kinase. Proc. Natl. Acad. Sci. U. S. A. 101 (37), 13489–13494. doi:10.1073/pnas.0405659101
Sabatini, D. M., Erdjument-Bromage, H., Lui, M., Tempst, P., and Snyder, S. H. (1994). RAFT1: A mammalian protein that binds to FKBP12 in a rapamycin-dependent fashion and is homologous to yeast TORs. Cell 78 (1), 35–43. doi:10.1016/0092-8674(94)90570-3
Sabers, C. J., Martin, M. M., Brunn, G. J., Williams, J. M., Dumont, F. J., Wiederrecht, G., et al. (1995). Isolation of a protein target of the FKBP12-rapamycin complex in mammalian cells. J. Biol. Chem. 270 (2), 815–822. doi:10.1074/jbc.270.2.815
Sancak, Y., Peterson, T. R., Shaul, Y. D., Lindquist, R. A., Thoreen, C. C., Bar-Peled, L., et al. (2008). The Rag GTPases bind raptor and mediate amino acid signaling to mTORC1. Science 320 (5882), 1496–1501. doi:10.1126/science.1157535
Sarbassov, D. D., Ali, S. M., Sengupta, S., Sheen, J. H., Hsu, P. P., Bagley, A. F., et al. (2006). Prolonged rapamycin treatment inhibits mTORC2 assembly and Akt/PKB. Mol. Cell 22 (2), 159–168. doi:10.1016/j.molcel.2006.03.029
Saunders, J. W. (1948). The proximo-distal sequence of origin of the parts of the chick wing and the role of the ectoderm. J. Exp. Zool. 108 (3), 363–403. doi:10.1002/jez.1401080304
Shor, B., Wu, J., Shakey, Q., Toral-Barza, L., Shi, C., Follettie, M., et al. (2010). Requirement of the mTOR kinase for the regulation of Maf1 phosphorylation and control of RNA polymerase III-dependent transcription in cancer cells. J. Biol. Chem. 285 (20), 15380–15392. doi:10.1074/jbc.M109.071639
Singh, B. K., Sinha, R. A., Zhou, J., Tripathi, M., Ohba, K., Wang, M. E., et al. (2016). Hepatic FOXO1 target genes are Co-regulated by thyroid hormone via RICTOR protein deacetylation and MTORC2-AKT protein inhibition. J. Biol. Chem. 291 (1), 198–214. doi:10.1074/jbc.M115.668673
St-Jacques, B., Hammerschmidt, M., and McMahon, A. P. (1999). Indian hedgehog signaling regulates proliferation and differentiation of chondrocytes and is essential for bone formation. Genes Dev. 13 (16), 2072–2086. doi:10.1101/gad.13.16.2072
Tandon, M., Chen, Z., and Pratap, J. (2014). Runx2 activates PI3K/Akt signaling via mTORC2 regulation in invasive breast cancer cells. Breast Cancer Res. 16 (1), R16. doi:10.1186/bcr3611
Tee, A. R., Manning, B. D., Roux, P. P., Cantley, L. C., and Blenis, J. (2003). Tuberous sclerosis complex gene products, Tuberin and Hamartin, control mTOR signaling by acting as a GTPase-activating protein complex toward Rheb. Curr. Biol. 13 (15), 1259–1268. doi:10.1016/s0960-9822(03)00506-2
Thorogood, P. V., and Hinchliffe, J. R. (1975). An analysis of the condensation process during chondrogenesis in the embryonic chick hind limb. J. Embryol. Exp. Morphol. 33 (3), 581–606. doi:10.1242/dev.33.3.581
Tiu, G. C., Kerr, C. H., Forester, C. M., Krishnarao, P. S., Rosenblatt, H. D., Raj, N., et al. (2021). A p53-dependent translational program directs tissue-selective phenotypes in a model of ribosomopathies. Dev. Cell 56 (14), 2089–2102.e11. doi:10.1016/j.devcel.2021.06.013
Vaidyanathan, S., Salmi, T. M., Sathiqu, R. M., McConville, M. J., Cox, A. G., and Brown, K. K. (2022). YAP regulates an SGK1/mTORC1/SREBP-dependent lipogenic program to support proliferation and tissue growth. Dev. Cell 57, 719–731.e8. doi:10.1016/j.devcel.2022.02.004
Vortkamp, A., Lee, K., Lanske, B., Segre, G. V., Kronenberg, H. M., and Tabin, C. J. (1996). Regulation of rate of cartilage differentiation by Indian hedgehog and PTH-related protein. Science 273 (5275), 613–622. doi:10.1126/science.273.5275.613
Wang, B. T., Ducker, G. S., Barczak, A. J., Barbeau, R., Erle, D. J., and Shokat, K. M. (2011). The mammalian target of rapamycin regulates cholesterol biosynthetic gene expression and exhibits a rapamycin-resistant transcriptional profile. Proc. Natl. Acad. Sci. U. S. A. 108 (37), 15201–15206. doi:10.1073/pnas.1103746108
Wei, X., Luo, L., and Chen, J. (2019). Roles of mTOR signaling in tissue regeneration. Cells 8 (9), 1075. doi:10.3390/cells8091075
Yan, B., Zhang, Z., Jin, D., Cai, C., Jia, C., Liu, W., et al. (2016). mTORC1 regulates PTHrP to coordinate chondrocyte growth, proliferation and differentiation. Nat. Commun. 7, 11151. doi:10.1038/ncomms11151
Yang, G., Zhu, L., Hou, N., Lan, Y., Wu, X. M., Zhou, B., et al. (2014). Osteogenic fate of hypertrophic chondrocytes. Cell Res. 24 (10), 1266–1269. doi:10.1038/cr.2014.111
Yin, Y., Hua, H., Li, M., Liu, S., Kong, Q., Shao, T., et al. (2016). mTORC2 promotes type I insulin-like growth factor receptor and insulin receptor activation through the tyrosine kinase activity of mTOR. Cell Res. 26 (1), 46–65. doi:10.1038/cr.2015.133
Yoon, B. S., Ovchinnikov, D. A., Yoshii, I., Mishina, Y., Behringer, R. R., and Lyons, K. M. (2005). Bmpr1a and Bmpr1b have overlapping functions and are essential for chondrogenesis in vivo. Proc. Natl. Acad. Sci. U. S. A. 102 (14), 5062–5067. doi:10.1073/pnas.0500031102
Zhang, H. H., Huang, J., Duvel, K., Boback, B., Wu, S., Squillace, R. M., et al. (2009). Insulin stimulates adipogenesis through the Akt-TSC2-mTORC1 pathway. PLoS One 4 (7), e6189. doi:10.1371/journal.pone.0006189
Zhang, H., Wang, H., Zeng, C., Yan, B., Ouyang, J., Liu, X., et al. (2017). mTORC1 activation downregulates FGFR3 and PTH/PTHrP receptor in articular chondrocytes to initiate osteoarthritis. Osteoarthr. Cartil. 25 (6), 952–963. doi:10.1016/j.joca.2016.12.024
Zhou, X., von der Mark, K., Henry, S., Norton, W., Adams, H., and de Crombrugghe, B. (2014). Chondrocytes transdifferentiate into osteoblasts in endochondral bone during development, postnatal growth and fracture healing in mice. PLoS Genet. 10 (12), e1004820. doi:10.1371/journal.pgen.1004820
Keywords: mTOR, limb development, skeletal, appendicular, cartilage, endochondral ossification
Citation: H’ng CH, Khaladkar A and Rosello-Diez A (2023) Look who’s TORking: mTOR-mediated integration of cell status and external signals during limb development and endochondral bone growth. Front. Cell Dev. Biol. 11:1153473. doi: 10.3389/fcell.2023.1153473
Received: 29 January 2023; Accepted: 03 April 2023;
Published: 19 April 2023.
Edited by:
John Young, Simmons University, United StatesReviewed by:
Mimi C. Sammarco, Tulane University, United StatesJessika Bertacchini, University of Modena and Reggio Emilia, Italy
Copyright © 2023 H’ng, Khaladkar and Rosello-Diez. This is an open-access article distributed under the terms of the Creative Commons Attribution License (CC BY). The use, distribution or reproduction in other forums is permitted, provided the original author(s) and the copyright owner(s) are credited and that the original publication in this journal is cited, in accordance with accepted academic practice. No use, distribution or reproduction is permitted which does not comply with these terms.
*Correspondence: Alberto Rosello-Diez, alberto.rosellodiez@monash.edu, ar2204@cam.ac.uk