- 1Department of Radiation Oncology, The First Affiliated Hospital of Zhengzhou University, Zhengzhou, China
- 2Arbuzov Institute of Organic and Physical Chemistry, FRC Kazan Scientific Center, Russian Academy of Sciences, Kazan, Russia
- 3Institute of Physiologically Active Compounds at Federal Research Center of Problems of Chemical Physics and Medicinal Chemistry, Russian Academy of Sciences, Chernogolovka, Russia
- 4Department of Hepatology, Royal Adelaide Hospital, CALHN, Adelaide, SA, Australia
- 5Sechenov First Moscow State Medical University, Sechenov University, Moscow, Russia
Successful clinical methods for tumor elimination include a combination of surgical resection, radiotherapy, and chemotherapy. Radiotherapy is one of the crucial components of the cancer treatment regimens which allow to extend patient life expectancy. Current cutting-edge radiotherapy research is focused on the identification of methods that should increase cancer cell sensitivity to radiation and activate anti-cancer immunity mechanisms. Radiation treatment activates various cells of the tumor microenvironment (TME) and impacts tumor growth, angiogenesis, and anti-cancer immunity. Radiotherapy was shown to regulate signaling and anti-cancer functions of various TME immune and vasculature cell components, including tumor-associated macrophages, dendritic cells, endothelial cells, cancer-associated fibroblasts (CAFs), natural killers, and other T cell subsets. Dual effects of radiation, including metastasis-promoting effects and activation of oxidative stress, have been detected, suggesting that radiotherapy triggers heterogeneous targets. In this review, we critically discuss the activation of TME and angiogenesis during radiotherapy which is used to strengthen the effects of novel immunotherapy. Intracellular, genetic, and epigenetic mechanisms of signaling and clinical manipulations of immune responses and oxidative stress by radiotherapy are accented. Current findings indicate that radiotherapy should be considered as a supporting instrument for immunotherapy to limit the cancer-promoting effects of TME. To increase cancer-free survival rates, it is recommended to combine personalized radiation therapy methods with TME-targeting drugs, including immune checkpoint inhibitors.
1 Introduction
The tumor microenvironment (TME) is a complex, dynamic, and cancer-orchestrated system of cells and cell-secreted components, including heterogeneous tumor cells and cancer stem cells (CSCs), cancer-associated fibroblasts (CAFs), various subsets of pro-inflammatory immune effectors, tumor-associated macrophages (TAM), endothelial components of blood and lymphatic vasculature, extracellular matrix and numerous cytokines and chemokines (Figure 1) (Liotta and Kohn, 2001; Lu et al., 2022; Xie and Su, 2022; Zhang et al., 2023a). TME is often subdivided into at least two major categories, such as a non-immune microenvironment (dominated by fibroblasts) and an immune microenvironment (dominated by immune T cells and TAM). Tumor cells and TME represent a functionally and highly dynamic structure, where tumor cells are regarded as “seeds” submerged in cancer-promoting and supporting TME (“soil”) modified during cancer immunoediting (Talbot et al., 2012; Cao et al., 2022; Lu et al., 2022; Xie and Su, 2022). According to the proposed model, tumor cells mutually interact with components of TME which is shaped to promote tumorigenesis and metastasis (Weber and Kuo, 2012).
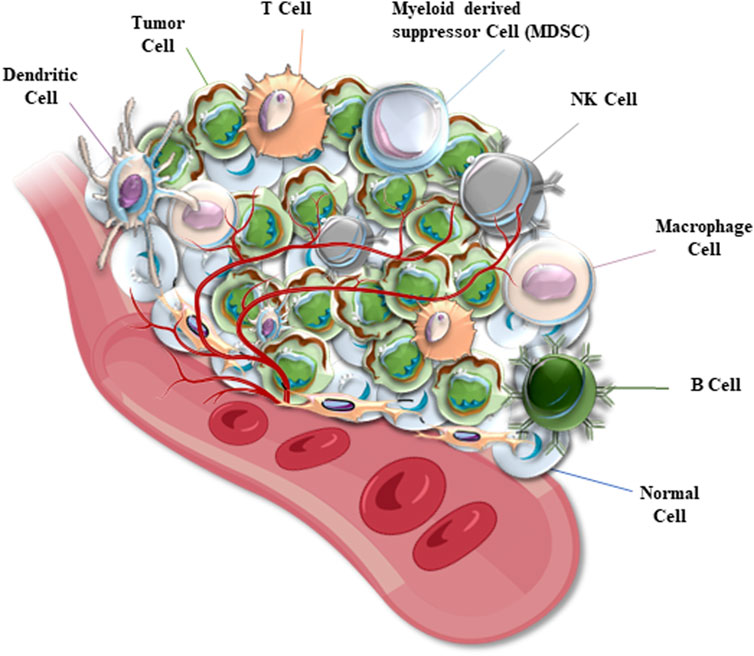
FIGURE 1. A diagram represents the structure of a solid tumor microenvironment (TME). Cancer cells are mixed with TME cells which include normal tissue cells, different immune cells, and microvessels. TEM immune cells are represented by dendritic cells (DCs), macrophages, natural killer (NK) and other T cells, B cells, myeloid-derived suppressor (MDS) cells, and others (depending on the cancer type).
In 2020, the number of cancer patients worldwide reached nearly twenty million, of which Asia accounted for nearly half of all cases (9,503,710 cases; 49.3%). The number of cancer cases also highly increased in China, reaching 4,568,754, compared to 2018 statistical data (Bray et al., 2018; Sung et al., 2021). The analysis of cancer therapy worldwide indicated that almost half of cancer patients received radiation treatment (Bernier, 2016; Zakeri et al., 2018). Radiotherapy is designed to kill tumor cells using high-energy ionizing radiation, such as gamma rays and X-rays, and electron and proton beams. Certain radiation doses are calculated to deliver the most efficient killing of tumor cells while sparing the normal surrounding tissues (Bernier et al., 2004; Glicksman, 2006). In sensitized cancers, radiotherapy delivers a highly desirable elimination of cancer cells, although the cancer-free outcome is hard to reach. During radiotherapy treatment, TME impacts the successful elimination of tumor cells and may negatively contribute the treatment and patient survival (Zakeri et al., 2018; Pontoriero et al., 2023). Research data confirmed that radiotherapy triggers various anti-cancer changes in TME and affects anti-tumor responses. The favorable effects of radiotherapy deliver a rationale for the combined therapeutic strategy. Application of complex treatment methods, including the use of radiotherapy as an activator of internal anti-cancer mechanisms in support of chemotherapy and immunotherapy, is recommended (Zhang et al., 2023b; Pontoriero et al., 2023; Shannon et al., 2023; Viswanath et al., 2023). In this study, we discuss the role of radiotherapy in the regulation of TME-related cancer-promoting effects. The success of targeted anti-cancer immunotherapy, combined with radiotherapy, is also discussed.
2 Radiation therapy effects in tumor vasculature
2.1 An unexpected turn: radiotherapy may promote angiogenesis and metastasis
High-energy radiation, which is required to initiate cancer cell death, provokes endothelial cell dysfunction, characterized by increased permeability, endothelial cell detachment from the basement membrane, and apoptosis, which often promotes inflammation and fibrosis during and after radiotherapy (Paris et al., 2001). Radiation may cause microthrombosis and increased adhesion of inflammatory immune cells to endothelial cells in the vasculature which is followed by extravasation of blood cells into the perivascular space (Wang et al., 2007). Following this, the intimal layer of the irradiated blood vessel was reported to thicken, leading to irreversible change in vasculature wall (Gujral et al., 2014; Mohammadkarim et al., 2022). Accordingly, controversial findings were reported about the impact of radiation on the angiogenesis and metastasis. Different studies indicated that damaged blood vessels may promote angiogenesis, migration of cancer cells, metastasis, and exacerbate the prognosis outcome (Girinsky, 2000; Quail and Joyce, 2013; Chaurasia et al., 2016; Hu et al., 2016; Manier et al., 2016; Viallard and Larrivee, 2017; Yadav et al., 2018; Yuan et al., 2018; Schulz et al., 2019). Despite this negative side effects in endothelium, efficient cancer elimination and better overall survival were observed during radiation treatment in many different cancers (Chaurasia et al., 2016; Hu et al., 2016; Yuan et al., 2018), suggesting that the balance towards complete cancer elimination is under control of specific component(s) in TME.
Tumor metastasis and recurrence are the main causes of death in patients with clinically advanced cancers. Metastasis and recurrence are ultimately associated with the induction of angiogenesis, which can be affected by radiation therapy (Viallard and Larrivee, 2017). Growing tumor cells require a constant supply of nutrients and oxygen, delivered through the activated growth of vasculature. A unique tumor-associated vasculature is activated through a variety of mechanisms, including an increased level of reactive oxygen spices (ROS) and activation of mechanistic target of rapamycin (mTOR) pathway (Quail and Joyce, 2013; Chaurasia et al., 2016; Hu et al., 2016; Manier et al., 2016; Schulz et al., 2019). Therefore, radiation therapy was found to stimulate not only the autophagy/apoptosis in cancer cells, but also proliferation of blood vessels near the treated tumor, increasing the degree of blood supply to tumor cells and promoting tumor metastasis (Girinsky, 2000; Yadav et al., 2018; Yuan et al., 2018).
Preliminary studies have confirmed that radiation increases the expression of vascular endothelial growth factor (VEGF) and activates pro-angiogenic signal transduction pathways (Song and Levitt, 1970; Sonveaux et al., 2003). Experimental data confirmed the association between angiogenesis and levels of VEGF. For instance, the xenografted squamous cell carcinoma cell line A431, which survived multiple rounds of irradiation (Pueyo et al., 2010), showed faster growth compared to controls in vivo (Pueyo et al., 2010). Significantly increased levels of VEGF, epidermal growth factor receptor (EGFR), and transforming growth factor-α (TGF-α) were detected in this study and linked to enhanced angiogenesis (Pueyo et al., 2010).
Radiation treatment may also promote metastasis in patients with squamous cell head and neck cancer (SCHNC). Conventional fractionated radiotherapy is routinely administered to stop the growth of SCHNC. The reported results showed that the microvessel density (MVD) in active SCHNC tissues after radiotherapy was significantly higher than that before radiotherapy (Zhang et al., 2003; Svagzdys et al., 2009; Harmankaya et al., 2022). The data indicated abundant angiogenesis during radiotherapy, an unexpected adverse effect. A similar finding was reported in patients with stage II/III colorectal cancers (CRCs), who received preoperative chemoradiotherapy (Zhang et al., 2017). In these patients assessed before radiotherapy, high vascular density (VD) values were found to be associated with poorer local relapse-free survival (LRFS) (Abramyuk et al., 2015; Zhang et al., 2017; Chen et al., 2021a). Following radiotherapy treatment, the proliferative index Ki-67 of the patients increased, and the VD value also exceeded the level before treatment, leading to a lower LRFS. This finding was closely related to the failure of radiotherapy in CRC patients (Koukourakis et al., 2019).
Another study assessed gene expression profiling in the paracancerous tissues of rectal cancer patients who received radiotherapy. The study reported that the expression of angiogenesis-promoting genes and MVD were significantly increased, indicating activation of angiogenesis by radiation (Gil Marques et al., 2019). In lung cancer tissues, radiotherapy triggered the hypoxia-inducible factor 1α (HIF-1α) and VEGF pathway. Alternatively, inhibition of this pathway reduced radiotherapy-induced tumor angiogenesis, indicating the importance of the HIF-1α/VEGF pathway for radiation-related stimulation of vascular growth (Zhu and Zhang, 2018). Conclusively, the tumor cells that were not eliminated by radiation, may progress towards a more advanced malignancy which is supported by abundant blood vessel formation. Therefore, the undesirable side-effects of radiotherapy were ultimately linked to tumor recurrence and metastasis.
Irradiation was also found to activate various cell membrane tyrosine kinase receptors, such as insulin-like growth factor-1 receptor 1 (IGFR-1) (Husain et al., 2010; Owens et al., 2013; Kilpatrick and Hill, 2021). The receptor is actively involved into autocrine signaling processes and was shown to promote cell-to-cell communication, cell growth, and division (Husain et al., 2010; Owens et al., 2013; Kilpatrick and Hill, 2021). Activated IGFR-1 transmits signals through multiple downstream effectors, including transcription factor NF-κB and associated signaling networks, such as rat sarcoma (Ras) pathway, phosphoinositide 3-kinase (PI3K), mitogen-activated protein kinase (MAPK), c-Jun N-terminal kinase (JNK), Fas cell surface death receptor (Fas-R), and tumor necrosis factor-α (TNF-α) signaling network (Sorolla et al., 2020). Many of radiation-induced mediators directly or indirectly promote the expression of VEGF/IGFR-1 and angiogenesis (Figure 2). For instance, radiation was found to activate cyclooxygenase-2 (COX-2), nitric oxide synthase (NOS), EGFR, and IGFR-1 pathways (Eldesoky et al., 2011; Banys-Paluchowski et al., 2018). The pathways are represented by complex networks and can stimulate angiogenesis via intermediate signaling molecules. Radiation-activated COX-2 can induce the production of prostaglandin H2 (PGH2). Following this, PGH2 triggers thromboxane A2 (TXA2) and promotes vascular growth (Mitryayeva et al., 2019; Anoopkumar-Dukie et al., 2020). Radiotherapy was also shown to activate production of prostaglandin E2 (PGE2) which promotes the expression of HIF-1α and VEGF (Kovacs et al., 2019). PGE2 can also bind to prostaglandin E2 receptor subtype 2 (EP2) and reinforce VEGF signaling (Figure 2) (Li et al., 2019).
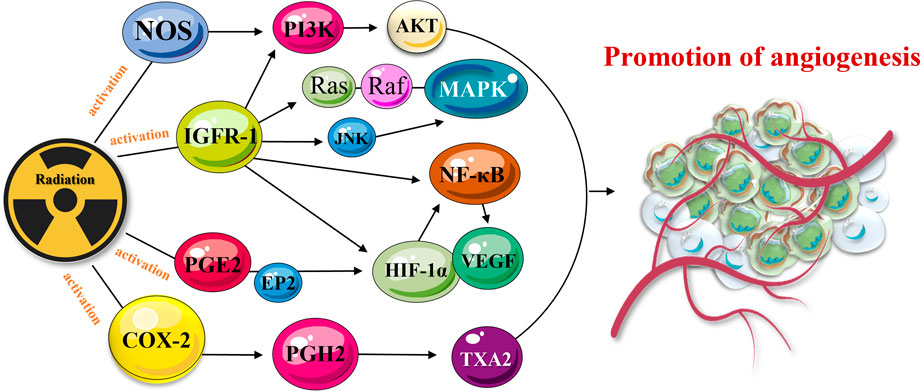
FIGURE 2. TME-activated COX-2 and PGE2 signaling pathways mediate cancer progression. The pathway is linked to angiogenesis via a complex molecular network. Radiation-activated COX-2 and secreted PGH2 cause the production of TXA2 and promote angiogenesis. Released PGE2 activates the expression of HIF-1α and VEGF which also promote angiogenesis and cancer resistance/metastasis.
Radiation was also found to activate NOS (Holotiuk et al., 2019; Wei et al., 2019), which is responsible for the generation of nitric oxide (NO), induction of HIF-1α expression (Masoud and Li, 2015), activation of PI3K/Akt/FRAP or PI3K/Akt/mTOR pathways, and angiogenesis (Sharifpanah et al., 2019). Similar effects (activation of angiogenesis and cancer recurrence/metastasis) were achieved when radiotherapy activated the EGFR network (Alique et al., 2020). EGFRs are coupled to the induction of VEGF expression via Ras/MEK/MAPK, Ras/MEK/ERK/MNK, PI3K/Akt/FRAP, PI3K/Akt/mTOR and other kinase signaling pathways (Zhu et al., 2020). The signaling pathways, including PI3K/Akt/FRAP, PI3K/Akt/mTOR, and MEK/ERK/MNK, can be also activated by radiation and IGFR-1. HIF-1α and VEGF are also triggered by above-mentioned networks, leading to blood vessel growth (Masoud and Li, 2015) (Figure 2). Furthermore, in the hypoxic microenvironment, which is commonly detected within solid tumor tissues (Alique et al., 2020), low-dose irradiation activates VEGF receptor 2 (VEGFR2) (Marques et al., 2020). The cell damage, caused by radiation, increases the degree of hypoxia, and stimulates the secretion of VEGF-A by tumor cells; thereby, stimulating angiogenesis via VEGF pathway (Jabbari et al., 2019).
Aside from VEGF, TGF-β also connects multiple signaling pathways, participates in the promotion of tumor angiogenesis, stimulates CAF activation, activates HIF-1 signaling, and is also involved in the activation of T cells and dendritic cells (DCs) in TME (Barcellos-Hoff, 2022). Radiation was found to upregulate TGF-β expression in tumor cells. TGF-β indirectly affects angiogenesis through induced expression of HIF-1α/VEGF (Rodriguez-Ruiz et al., 2020). In conclusion, radiation may stimulate and promote angiogenesis, employing HIF-1α/VEGF and other signaling pathways.
2.2 Combined application of radiotherapy and targeted anti-tumor vascular therapy
Progressive growth of solid tumors leads to the generation of hypoxic TME areas due to insufficient vascular distribution. The hypoxic TME was associated with tumor radioresistance (Chen et al., 2016). To increase the radiosensitivity of tumor cells, several effective anti-angiogenic agents (vascular inhibitors) were generated. These substances can specifically target and inhibit the growth of new blood vessels, regulate the blood flow and oxygenation of cancer tissues (Chen and Xu, 2015; Siemann et al., 2017; Uribesalgo et al., 2019). Vascular inhibitor therapy can block tumor growth to a certain extent. The therapy was limited by the development of drug resistance, increased levels of hypoxia, and various obstructions to effective drug delivery. Unfortunately, the overall survival after treatment with vascular inhibitors in clinical practice is relatively poor (Lin et al., 2016). However, combined treatment with angiogenesis inhibitors and radiotherapy were found to enhance the radiosensitivity of endothelial cells (Blomberg et al., 2020), attenuate radiotherapy-induced angiogenesis, and prevent tumor recurrence. Experimental and clinical studies have shown that radiotherapy combined with vascular inhibitors has significantly better anti-cancer effect (Kanthou and Tozer, 2019).
Several molecular targets have been selected to improve effects of radiotherapy, including purine/pyrimidine endonuclease 1 (apurinic/apyrimidinic endonuclease 1, APE1), the enzyme with DNA repair functions (Teicher et al., 1995; Kidoikhammouan et al., 2012; Gu et al., 2013; Xi et al., 2013). It has been found that X-rays can induce both the expression of APE1 and VEGF in A549 cells (Gu et al., 2013; Xi et al., 2013). Inhibition of APE1 expression significantly attenuated radiation-induced endothelial cell migration and greatly reduced the formation of capillary-like structures (Gu et al., 2013). The sensitivity of the tumor to radiotherapy was significantly enhanced by co-treatment with another anti-angiogenic agent TNP-470 (minocycline; methionine aminopeptidase-2 inhibitor) (Kidoikhammouan et al., 2012). TNP-470 demonstrated efficient anti-angiogenetic properties in rat subcutaneous gliosarcoma model in vivo (Teicher et al., 1995).
Bevacizumab, a VEGF inhibitor (a recombinant humanized monoclonal antibody against VEGF), has been used in clinical trials for the treatment of head and neck cancer (HNC), where it has significantly improved radiation therapy (Ahn et al., 2018). Combined regimens with bevacizumab and erlotinib (EGFR blocker) were used during chemoradiotherapy (CRT) protocols to treat patients with advanced HNC. The survival rate of these HNC patients with complete remission (cure rate, CR) reached 96%, while 3-year locoregional control rate was 85%, distant metastasis-free survival rate was 93%, and overall survival rate was 86% (Yoo et al., 2012). Postoperative chemotherapy and pelvic IMRT plus bevacizumab in patients with high-risk endometrial cancer were well tolerated and improved 2-year overall survival (Viswanathan et al., 2015). A similar combined regimen was also tested in patients with extremity soft tissue sarcomas (ESTS). The patients were treated with pazopanib (another monoclonal antibody against VEGF), combined with radiotherapy. The results showed that pathological complete remission was achieved in 4 out of 10 patients (Haas et al., 2015). Drug toxicity and side effects were acceptable when conventional fractionated radiotherapy was combined with sorafenib (VEGF pathway inhibitor; small molecule tyrosine kinase inhibitor) (Zhao et al., 2010; Chen et al., 2014).
Endostatin, an endogenous inhibitor of angiogenesis, is a 20-kD proteolytic fragment of type XVIII collagen (Digtyar et al., 2007). Endostatin can bind the integrin α5β1 in the endothelial cell membranes, leading to the activation of Src-kinase signaling. Combined with concurrent chemoradiotherapy (CCRT), endostatin was used for the treatment of patients with unresectable stage III Non-Small Cell Lung Cancer (NSCLC). The results showed considerable improvements in survival and local cancer control rates (Bao et al., 2015). A recent study with PEGylated recombinant human endostatin indicated the potential of this anti-angiogenic substance for the treatment of pancreatic and gastric cancers (Guo L. et al., 2023). Endostatin can not only block cancer-induced VEGF expression, but also demonstrated a better safety profile (compared to bevacizumab) in small clinical studies (Mendez-Valdes et al., 2023), although further testing with combined radiotherapy is required.
Erlotinib (also known as OSI-774 or Tarceva), is an anti-EGFR antibody that was approved as an anticancer drug by the US-FDA and tested as a treatment for NSCLC and pancreatic cancer (Feng et al., 2014). For patients with esophageal squamous cell carcinoma who cannot tolerate chemoradiotherapy, the administration of erlotinib combined with radiotherapy was more tolerable and effective (Zhai et al., 2013). The targeted search for improved inhibition of angiogenesis continues and resulted in the design of more efficient inhibitors of EGFR signaling (Shah et al., 2023), although clinical testing is required before those drugs can be implemented. Conclusively, the previous studies showed that local control rate and overall survival rate of patients who were treated with angiogenesis inhibitor combined with radiotherapy are significantly improved compared with radiotherapy alone. The application of vascular inhibitors also reduced radiation-induced damage to normal tissues and improved patients’ treatment tolerance. Moreover, the combined treatment protocol significantly reduced tumor recurrence, although further testing is warranted. It is foreseeable that combined radiotherapy and adjuvant therapy with anti-angiogenic substances will play an increasingly important role in tumor therapy.
3 Effects of radiation therapy on cancer-associated fibroblasts (CAF)
3.1 Radiotherapy affects CAF-regulated network
CAFs are represented by highly heterogeneous cell populations which account for about 50% of the total number of all TME cells (Butti et al., 2023; Wieder, 2023). Therefore, CAFs are often defined as stromal TME cells (De et al., 2021; Kochetkova and Samuel, 2021; Mor et al., 2022). It has been suggested that quiescent fibroblasts can be transformed into CAFs in tumor tissue. Undergoing epithelial/endothelial-mesenchymal transition (EMT), bone marrow-derived differentiated mesenchymal stem cells, and epithelial or endothelial cells were also linked to the generation of CAFs (Kamali Zonouzi et al., 2021; Kochetkova and Samuel, 2021; Loh and Ma, 2021; Simon and Salhia, 2021). CAFs were shown to play an important regulatory role in the TME, where these cells promoted cancer occurrence, metastasis, and recurrence (Barbazán and Matic Vignjevic, 2019; Kashima et al., 2019). CAFs can express and secrete a variety of cytokines that promote tumor cell proliferation, invasion, and metastasis (Peng et al., 2021; Chen et al., 2022; Li et al., 2023; Morgan et al., 2023). For instance, isolated from tumor-stromal junctions CAFs were able to activate EMT in tumor cells, leading to increases in cancer cell migration (Asif et al., 2021; Majidpoor and Mortezaee, 2021; Stuelten and Zhang, 2021; Taeb et al., 2022; Pei et al., 2023). Numerous pro-angiogenic factors and matrix metalloproteinases (MMPs), which activate angiogenesis and lymphangiogenesis, can be secreted by CAFs (Wei et al., 2017; Cadamuro et al., 2019; Chen et al., 2021b; Ambrosetti et al., 2022; Chiavarina et al., 2022; Watanabe et al., 2022). Notably, CAFs can also secrete anti-inflammatory chemokines and modulate immune anti-cancer responses, thus, promoting carcinogenesis (Zheng et al., 2021a; Zheng et al., 2021b; Kobayashi et al., 2021). The presence of CAFs in TME was linked to the poor prognosis and development of drug resistance (Chen et al., 2021b; Dong et al., 2021; Kamali Zonouzi et al., 2021; Ambrosetti et al., 2022).
A very limited number of studies addressed the impact of radiotherapy on the level of CAFs in TME. It has been demonstrated that CAFs, which were isolated and cultured from lung cancer tissue, can survive radiation exposure, but their invasive ability may be lost (Hellevik et al., 2012). The CAF-based analysis suggested that radiation activates the overexpression of integrins α2 and β1, thereby attenuating the migratory ability of CAFs (Park et al., 2008; Nam et al., 2013). Subsequent clinical studies confirmed the important role of β1 integrin expression in tumor cells surviving after radiotherapy (Park et al., 2008). Further studies have shown that tumor stroma mediates the development of radioresistance in cancers, and β1 integrin is a key link involved in this process (Carracedo et al., 2010; Eke et al., 2012; Ahmed et al., 2013; Broustas and Lieberman, 2014; Wu et al., 2015; Zhang et al., 2018; Yokosaki and Nishimichi, 2021; Park et al., 2023).
Radiation was suggested to enhance the secretion of growth-promoting growth factors and ECM regulators (TGF-β and MMPs) from CAFs (Figure 3).
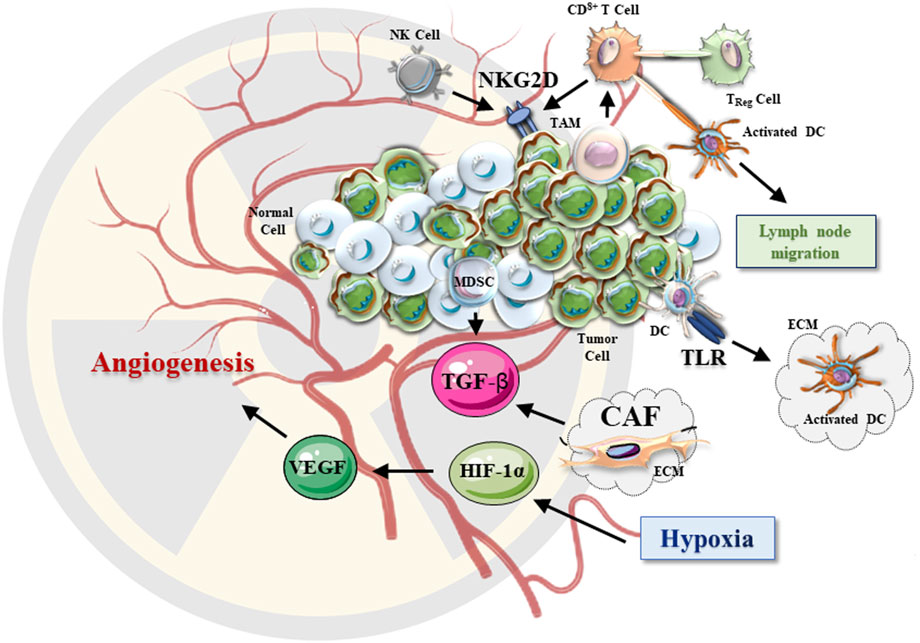
FIGURE 3. The effects of radiotherapy on TME. After irradiation, TME undergoes a series of changes. The hypoxic environment of irradiated solid tumors triggers the HIF-1 signaling pathway. The secreted VEGF and TGF-β promote tumor growth and angiogenesis. CAFs in the tumor extracellular matrix are activated upon irradiation and lead to changes in the secretion of various growth factors. After irradiation, NKG2D signaling is also enhanced, resulting in improved (anti-cancer) cytotoxic effects of NK and CD8+ T cells. Increased proinflammatory factors, DAMP-related TLRs may activate DCs, which further activate the anti-cancer effect of T cells in TME.
TGF-β can be secreted by CAFs and directly stimulate tumor immune evasion and activate the HIF-1 signaling pathway. MMPs were also shown to promote angiogenesis and tumor cell invasion and metastasis (Dancea et al., 2009; Hneino et al., 2012; Neuzillet et al., 2015). Considering that radiotherapy was shown to promote EMT, tumor invasion, and migration, it is logical to suggest that CAFs may be induced by radiation. For instance, radiotherapy promoted the expression of MMP-2 (via activated EGFR/Akt pathway) and enhanced the invasive ability of glioma cells (Park et al., 2006). Increased MMP-9 expression by was also associated with higher invasive ability of hepatocellular carcinoma cells (Cheng et al., 2006). However, it remains to confirm whether CAFs are involved in this process and activated by radiotherapy.
3.2 Combined effects of radiotherapy and CAF-targeting therapy
According to the mutation analysis, CAFs are genetically more stable than tumor cells, making them a better target for therapy. CAF genes, which are responsible for the secretion of TGF-β, MMPs, hepatocyte growth factor (HGF), and tenascin-C (Tn-C), were identified as promising therapeutic targets (Guo S. et al., 2023; Greimelmaier et al., 2023; Li and Mu, 2023; Yang et al., 2023). The combined treatment protocol with radiotherapy and TGF-β inhibitors chemotherapy was found to be effective for attenuation of tumor cell proliferation and spreading (Bouquet et al., 2011). It was also found that MET (mesenchymal epithelial transition factor) inhibitors enhanced the efficacy of radiotherapy and attenuated the invasive activity of tumor cells. This additive effect was mediated by HGF secretion (De Bacco et al., 2011). However, there have been no clinical trials that tested the use of HGF and MET inhibitors combined with radiotherapy. Therefore, it is warranted to verify this novel approach in cancer therapy.
Other CAF-linked molecular targets were also identified, although not completely tested in clinics. For instance, during the activation of CAF, the high expression of Tn-C in the cell matrix may allow the iodine-131-labeled anti-Tn-C antibody to enter the matrix precisely into cancer cells. It was suggested that this cell-targeted approach should be tested in clinical settings. (Reardon et al., 2002). Treatment effects of monotherapy clinical trials were found to be suboptimal, possibly due to the broad-spectrum activity of MMP inhibitors. Relevant preclinical studies have found that MMP inhibitors with higher specificity can improve the efficacy of radiotherapy (Waas et al., 2005; Heath et al., 2006; Olivares-Urbano et al., 2020; Waller and Pruschy, 2021), but further research is needed.
4 Radiation therapy and cancer-associated exosomes
4.1 Effects of radiotherapy on the secretion and composition of exosomes
Tumor cells maintain the development and stability of their TME by secreting various growth-promoting factors and extracellular matrix filaments. Vesicle-encapsulated structures (exosomes) with a diameter of up to 50–150 nm are often released by cancer cells (Gyorgy et al., 2011). The exosomes were found to contain various microRNAs, proteins, and lipids (Malla et al., 2017). The released structures are generated by the initiation membrane, undergo endocytosis to form polycystic bodies, and are secreted to the extracellular liquids after maturation. The released exosome may be absorbed by recipient cells (phagocytosis or membrane fusion), leading to the activation of various physiological processes in the targeted cells and tissues. Accordingly, cancer-released exosomes were shown to direct intercellular signal transmission (Bang and Thum, 2012), and immune response (Robbins and Morelli, 2014), and facilitate the cancer escape from apoptosis (Miksa et al., 2009).
The two significant factors, which affected the release of exosomes, were linked to radiation-induced DNA damage and activation of p53-related pathways (Tarasov et al., 2021; Tan et al., 2023). Radiotherapy is designed to cause DNA damage in cancer tissues. Moreover, it also activates many related pathways, including the p53 network. The tumor suppressor protein p53 regulates the transcription of many genes, including the genes responsible for the communication between adjacent cells. The activation of p53 pathway was found associated with the regulation of endocytic trafficking and the exosome secretion during apoptosis (Yu et al., 2006; Lakoduk et al., 2021). Increased cancer cell invasion and resistance were observed in cells with mutant p53 which influenced expression and function of genes responsible for vesicle trafficking and recycling of growth factor receptors. Formation and secretion of exosomes are also regulated by p53 in the TME (Yu et al., 2006; Lakoduk et al., 2021).
The composition of exosomes is defined by the cell type and is strongly affected by radiation. Several experimental studies confirmed that tumor cells treated with ionizing radiation generate exosomes with various and complex content. For instance, human brain astrocytoma cell U-87MG, irradiated with 4 Gy for 48 h, released exosomes with connective tissue growth factor and IGF-binding proteins (Arscott et al., 2013). Prostate cancer cells 22Rv1, exposed to 4 Gy radiation (for 96 h), released a higher amount of B7-H3 proteins (Lehmann et al., 2008). In samples from prostate cancer patients, the content of heat shock protein 72 was found to increase after radiotherapy (Hurwitz et al., 2010). After irradiation of human head and neck squamous cell carcinoma cells using the Feature Aggregate Depth Utility (FaDu) (2 Gy, 18 h) approach, it was registered that levels of proteins involved in the regulation of translation and transcription (cell cycle regulators) were increased (Chung et al., 2021). Alternatively, the expression levels of apolipoproteins and immunoglobulins decreased (Jelonek et al., 2015). These findings indicated that the effects of ionizing radiation can be reflected in changes in the exosome content, which may be used in the post-treatment diagnostics.
4.2 Exosome functions and radiotherapy
Exosomes are functional elements of normal physiology and can be released by normal cells. Moreover, exosomes mediate the activation of immune responses. For instance, the major histocompatibility complex (MHC)-peptide complexes carried by exosomes can bind to cognate T cell receptors and activate CD4+/CD8+ T lymphocytes (Nolte-'t Hoen et al., 2009; Xie et al., 2010; Admyre et al., 2006). This is the common mechanism of antigen presentation which initiates the body’s immune response. Exosomes may also interfere with the body’s immune system and promote tumor growth. Several recent studies have shown that cancer-released exosomes can slow down the proliferation of NK cells (Liu et al., 2006) or CD4+/CD8+ T cells (Clayton et al., 2007), promote the mutation of myeloid cells (Valenti et al., 2006) and regulatory T cells (Szajnik et al., 2010).
The ability of exosomes to activate immune responses relies on the expression of membrane antigens they carry and the physiological state of the target cells (Segura et al., 2005; Bhatnagar et al., 2007). Exosomes secreted by tumor cells may also carry immunosuppressive peptide complexes. Accordingly, tumor-released exosomes have also been implicated in tumor spreading. Irradiation-induced exosome-specific changes were found to trigger nerve growth factor (NGF)-tyrosine protein kinase (Trk) A and focal adhesion kinase (FAK) signaling pathways in brain cancer patients (Wang H. et al., 2021). Activation of Trk ultimately promotes brain glial plasma cell migration and metastasis (Arscott et al., 2013). Trk is also involved in the regulation of intracellular trafficking (Scott-Solomon et al., 2018), required for the establishment of cell-to-cell communications in TME (Okimoto and Bivona, 2016).
Exosomes regulate the information exchange between tumor cells and TME by transmitting unique signals related to apoptosis, division, and growth to target cells (Ludw et al., 2012). Ionizing radiation can significantly affect the exchange of information between cells through the conformation of different signal transduction systems. The release of specific exosomes with pro-survival cargo affects the biological behavior and therapeutic effects in tumors and TME. Radiation-related changes in cell-to-cell communications have been shown to regulate radiotherapy responses in the directly irradiated cells and surrounding unirradiated cells via the employment of exosomes and their cargo (Moore et al., 2005; Facoetti et al., 2006; Burr et al., 2010). Experimental data demonstrated the increased gene instability which is translated into changed content of exosomes (modified RNAs, microRNAs, and protein cargo) in irradiated breast cancer (Al-Mayah et al., 2012; Al-Mayah et al., 2015) and other cells (Malla et al., 2017). Furthermore, radiation may promote the release of exosomes with specific cargo (microRNAs) which facilitate the development of radioresistance and radiation-induced bystander effects (RIBEs) (Yang et al., 2022). Additionally, the uptake of exosomes by irradiated cells was found to be also affected by radiation. It has been shown (Hazawa et al., 2014) that human bone marrow-derived cells exposed to ionizing radiation increased exosome uptake through CD29/CD81 complex formation and p38 mitogen-activated protein kinase-dependent endocytosis and pinocytosis. In conclusion, considering the role of exosomes and their cargo in the regulation of cancer cell growth and survival, it has been suggested to employ exosomes as a target for anti-cancer treatment (Ni et al., 2019).
5 Radiotherapy effects on cellular components of TME
5.1 Radiotherapy enhances the anti-cancer immune responses in TME
Tumor immunity is designed to deliver anti-tumor effects via mobilization of the host’s natural defense mechanism and secretion of endogenous anti-cancer substances (Wattenberg et al., 2014). Accordingly, tumor immunotherapy, which was introduced in the 1980s, has become the fourth tumor treatment method following surgery, radiotherapy, and chemotherapy. In 2013, it was indicated as the leading technological breakthrough achievement in cancer therapy (Couzin-Frankel, 2013). Currently, a large number of drugs have been developed and tested in clinics (Table 1), although only some of them have been tested in combination with radiotherapy. Notably, radiotherapy can promote the body’s natural anti-tumor immunity. Irradiation causes DNA damage and apoptosis in malignant cells (Golden et al., 2012). The triggering of apoptosis and necrosis can induce the innate immune response in the TME, causing the release of cytokines and chemokines. The released products can recruit macrophages, NK T cells, regulatory T cells, and myeloid suppressor cells (MDSC) (Er et al., 2007; Gallo and Gallucci, 2013). The reported anti-tumor effect in the remote non-radiation area induced by radiation (abscopal radiation effect) indicated that radiotherapy promoted immune anti-cancer responses (Lhuillier et al., 2021). Accordingly, radiation treatment of immunodeficient tumor-bearing nude mice did not activate the abscopal radiation effect, indicating the need for robust immune effectors to trigger the response (Demaria et al., 2004; Lumniczky and Safrany, 2015).
There is increasing evidence that radiotherapy can re-activate cancer surveillance and enhance innate and adaptive immune responses against tumors. A common hypothetical theory is that local radiotherapy induces immunogenic cell death, resulting in the activation of pro-inflammatory TME. The pro-inflammatory condition is characterized by the release of tumor antigens and damage-associated pattern molecules (DAMPs) from the dead cells (Ahmed et al., 2014; Weichselbaum et al., 2017). Radiation therapy can also induce the expression of various chemokines, leading to the enrichment of T cells in the TME. These factors promote the activation of antigen-presenting cells (Oh et al., 2020). Several in vitro and in vivo experiments indicated that radiation-induced the production of pro-inflammatory cytokines IL-1β and tumor necrosis factor (TNF-α). The secretion of these cytokines promoted dendritic cell (DC) maturation (Shi et al., 2003). DCs are bone marrow-derived cells that are greatly affected by radiation-induced alterations in the TME (Kim et al., 2004). Radiation has been shown to increase the amount of tumor-associated DCs, enhance the homing of these cells to lymph nodes, and induce the maturation of DCs and their ability to present antigens (Cummings et al., 2009).
Preclinical studies have demonstrated that radiation-induced tumor antigen release promote antigen-presenting cells (APCs) to migrate to lymph nodes, where T cells are primed to initiate systemic responses (Aggarwal, 2023). The National Cancer Institute (NCI) report shows that radiation can alter the phenotype of tumor cells, leading to upregulation of cell surface molecules, thereby broadening the antigens available for presentation and making tumors more susceptible to T cell-mediated anti-tumor effect (anti-cancer surveillance) (Roses et al., 2014). Aside from T lymphocytes, NK cells are also an important part of tumor surveillance. Involvement of the NK Group 2 member D (NKG2D) receptor was reported (Figure 3). Radiation increases the expression of NKG2D ligands in several human tumor cell lines, suggesting that NK cell-based therapies should be developed and explored in clinical trials (Sayitoglu et al., 2020; Wang J. et al., 2021; Khan et al., 2021).
However, some factors may impede the radiotherapy effects and promote resistance. For instance, changes in the level of TGF-β in patients with esophageal cancer after radiotherapy were associated with the development of resistance and were suggested to be explored as a predictor of pulmonary fibrosis after radiotherapy. Accordingly, the inhibition of TGF-β is crucial to improve the efficacy of radiotherapy (Biswas et al., 2007; Biswas et al., 2017). The dual role of radiotherapy and the development of resistance will be described below.
5.2 Undesirable effects of radiotherapy: immunosuppression
A variety of inhibitory immune cells has been found in TME and are associated with the development of resistance and cancer progression. The inhibitory cells are often represented by the regulatory T cells (Treg), macrophages, and MDSCs (Fridman et al., 2012). The Treg cells group includes T cells with significant immunosuppressive effects, characterized by the expression of Foxp3, CD25, and CD4 (Hariyanto et al., 2022). Those Tregs can suppress the immune response of other immune cells and are the main mediators of self-tolerance (Sakaguchi et al., 2020). Under normal conditions, the low levels, or dysfunctions in Tregs result in the development of autoimmune diseases. The majority of immune cells, such as T cells, B cells, and NK cells, can recognize and protect the body from the “non-self” agents, generate an immune response, and remove harmful substances. Treg cells are destined to regulate immune responses, stop the propagation of exaggerated immune signals, regulate immune homeostasis, and prevent autoimmune diseases (Collison et al., 2007; Haddadi and Negahdari, 2022). Specifically, Treg cells can secrete anti-inflammatory cytokines TGF-β and IL-10 in the TME. Those cytokines inhibit the activities of effector T cells and activate other suppressor cells, including MDSCs (Facciabene et al., 2012; Bu et al., 2013). Therefore, Treg cells may serve as immune system suppressors.
It has been observed that the number of Treg cells in tumor tissues and immune organs is often significantly increased in patients receiving clinical radiation therapy (Kachikwu et al., 2011). Experiments showed that the expression of the cytotoxic T-lymphocyte-associated antigen 4 (CTLA-4) in Treg cells increased within 72 h after whole-body irradiation. Simultaneously, the production of TGF-β (Moreau et al., 2022) and IL-10 by Treg cells was also increased (Balogh et al., 2013). This data suggested that Treg cells may enhance immunosuppressive TME after irradiation (Persa et al., 2015). Therefore, to improve anti-cancer therapy, it was suggested to target Treg cells and the associated immunosuppressive molecules (TGF-β and CTLA-4) (Whiteside, 2012; Sanjabi et al., 2017). Aside from Tregs, MDSCs and tumor-associated macrophages (TAMs) play an important role in promoting tumor angiogenesis which provides substantial pro-survival support to the irradiated cancer tissues (Figure 3) (Sica et al., 2008). MDSCs may also promote the development of radiotherapy resistance (Persa et al., 2015). After irradiation, the accumulation of MDSCs in the tumor stroma was found to be elevated (Lehmann et al., 2008). Furthermore, radiation altered the expression levels of TAM-produced chemokines, thereby altering the regulation of T-cell infiltration in cancer tissues (Inoue et al., 2004; Beach et al., 2022). However, it is warranted to clarify how the TME is involved in the regulation of radiation-induced TAM recruitment and which MDSC and TAM cell populations should be targeted to improve the anti-cancer treatment.
5.3 Radiotherapy and immune checkpoint inhibitors: a combined approach
The immune system has multiple checkpoints that can be engaged to stimulate or inhibit certain T-cell functions. Immune checkpoint inhibitors (ICIs) were shown to play an important role in overcoming tumor immune tolerance, providing a new treatment method in cancer therapy (Bagchi et al., 2021). The application and development of ICIs represent an attractive method in the current cancer immunotherapy (Galluzzi et al., 2020). During the last decade, ICI-based drug research has made fruitful progress (Gubin et al., 2014; Powles et al., 2014). The immune system is a key component of the abscopal radiation effect of radiotherapy (Postow et al., 2012). Local radiotherapy is tightly linked to the activation of various immune system responses, including T cell priming and activation, immunogenic cell death, T cell trafficking, and tissue infiltration, and cancer recognition and elimination (Figure 4).
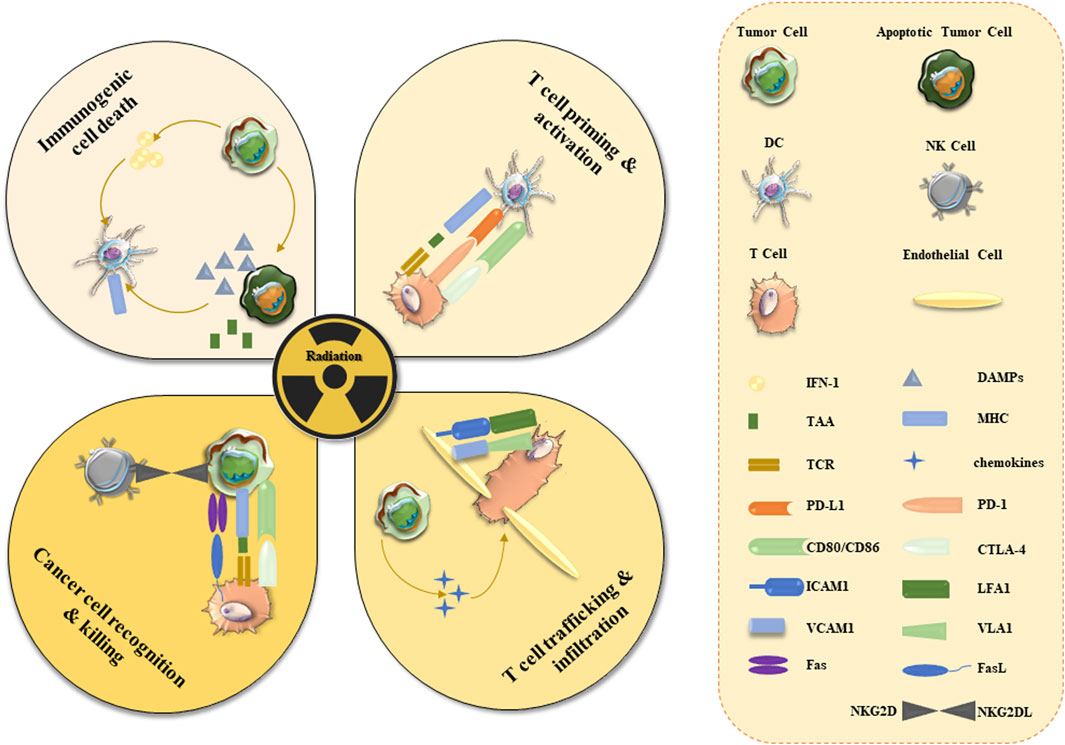
FIGURE 4. Activation of anti-cancer immune responses in TME by radiotherapy. Radiotherapy induces cancer cell apoptosis and necrosis which are followed by the release of cancer cell antigens. Apoptosis leads to the increased expression of the damage-related molecular pattern (DAMPs) proteins and interferon-1 (INF-1), which can activate various immune cells (DC, NK, and T cells). Tumor-derived antigens trigger NK/T cell recognition and elimination of tumor cells. Expression of inhibitory effectors (PD-1 and CTLA-4) can prevent tumor elimination and should be targeted by ICIs. Angiogenesis (marked by the expression of VCAM) may slow down cancer eradication. Radiotherapy stimulates immune surveillance and triggers the expression of MHC, Fas ligands/receptors, and NKG2D ligands. Abbreviations: CD - cluster of differentiation; ICAM1—intracellular adhesion molecule 1; L-ligand; LFA1 - leukocyte function-associated antigen-1; TAA-tumor-associated antigens; TCR - T cell receptor; VCAM1 - vascular adhesion molecule 1; VLA1-vascular integrin alpha 1.
Radiation induces immunogenic cell death of tumor cells, which activates the immune system by presenting tumor antigens to cytotoxic T lymphocytes (Krysko et al., 2012). For instance, radiotherapy can promote the migration of effector CD8+ T cells and enhance the function of effector T cells by inducing the TME-linked production of pro-inflammatory chemokines and cytokines (Burnette et al., 2011). Antigen expression and presentation, and enhanced effector T cell function provide a plausible underlying rationale for immune-mediated radiation abscission effects (Lee et al., 2020). Therefore, the combined application of radiotherapy and ICIs may produce a better outcome, although it remains to be confirmed in clinical trials.
At present, ICI drugs were approved and are being clinically tested as a treatment for solid tumors, including melanoma and non-small cell lung cancer (Borghaei et al., 2015; Larkin et al., 2015). ICIs for CTLA-4 and programmed death (PD) ligand-1 (PD-1/PD-L1) targets are the most studied substances which indicated promising results. For instance, the CTLA-4 pathway is an immunosuppressor for CD8+ T cells. Combined with radiation therapy, CTLA-4 inhibitor drugs, such as ipilimumab, were found to be highly effective (Demaria et al., 2013). Experimental studies have shown that combined radiation and CTLA-4 inhibitor treatment effectively inhibited lung metastasis of primary breast cancer in mice (Demaria et al., 2005). Notably, radiotherapy combined with CTLA-4 mAb was more effective than either modality used alone (Dewan et al., 2009). It has been suggested that combination therapy is more effective in preclinical model studies because radiation can increase the expression level of the NKG2D protein in tumor cells, making the cancer cells more vulnerable to NK cell attack (Demaria et al., 2013). Recently, several studies have explored and summarized the progress and challenges of ICI drug application during immunotherapy. Novel immunosuppressive pathways were targeted by various therapeutic molecules, such as LAG-3, TIM-3, TIGIT, VISTA, and B7-H3 (Franzin et al., 2020; Marin-Acevedo et al., 2021). Application of novel substances and/or use of novel methods have both specific benefits and challenges, including increased toxicity, which should not be neglected (Marin-Acevedo et al., 2021). For instance, the serious renal side effects of ICI drug application, such as acute kidney injury (AKI) and acute tubulointerstitial nephritis (ATIN), were reported. Several strategies for prevention and management of these complications were proposed (Franzin et al., 2020) and requires future assessment.
PD-L1, whose expression is undetectable in most normal tissues, can be induced in various cell types by inflammatory cytokines (Minn, 2015). PD-L1 is expressed in various solid tumors, and its presence is an important indicator of whether a patient responds to PD-1/PD-L1 inhibitors (Ribas, 2012; Topalian et al., 2012; Postow et al., 2015; National Library of Medicine, 2018). Using tumor-bearing mice as a model, combined treatment with radiotherapy and a PD-L1 inhibitor led to significant tumor regression (Lan et al., 2021). It was further observed that the infiltration of CD8+ T cells resulted in a significant decrease in the number of MDSCs in the tumor tissues treated with the combined therapy method (Raskov et al., 2021; Reina-Campos et al., 2021). It has been noted that the level of CD8+ T cells in the host decreases when the level of MDSCs increases. PD-L1 inhibitors can restore anti-cancer T-cell function and increase sensitivity to apoptosis in tumors (Gabrilovich et al., 2012; Kumar et al., 2016). Stereotactic radiotherapy combined with a PD-1 inhibitor significantly improved survival compared with monotherapy in a mouse model of brain tumors (Zeng et al., 2013; Stessin et al., 2020). A higher level of CD8+ T cells and a lower degree of Treg cell infiltration were also detected in the tumors treated with combination therapy (Zeng et al., 2013). Research data indicated that radiotherapy combined with PD-1 inhibitors significantly increased the number of memory CD8+ T cells (Sharabi et al., 2015). In conclusion, radiotherapy can activate local and systemic immune responses required for tumor elimination. Therefore, the combination of radiotherapy and immunotherapy can enhance the body’s innate and adaptive anti-cancer immunity essential for patient survival. However, it remains to understand how to minimize the side effects of combination therapy and design the best optimal protocol for clinical applications.
6 Conclusion and future perspectives
Anti-tumor combined therapies are the current focus in the development of effective cancer treatment for advanced malignancies. This study provides insights into the mechanisms of radiotherapy effects on TME and the effectiveness of radiation therapy in the regulation of tumor-related immune responses, angiogenesis, activation of CAFs in the tumor stroma, generation, and composition of tumor-secreted exosomes. The substantial amount of data indicates that radiotherapy impacts major components of TME, resulting in the activation of dual pro- and anti-inflammatory effects.
To improve overall survival and tumor eradication, radiotherapy may need to be combined with ICIs to target TME in some cancer patients. A combined approach may also prevent the development of radiotherapy resistance caused by the anti-inflammatory TME components. The strategy to use complex treatment protocols (radiotherapy combined with targeted anti-tumor drugs, including ICIs) has been successfully implemented in clinical research, although not all promising drugs have been tested using the combined protocol (Table 1). Recently obtained clinical trial data shows that combined therapies have substantial treatment advantages, although further testing is required.
Author contributions
DZ: Software, Visualization, Writing–original draft. YM: Software, Visualization, Writing–original draft, Writing–review and editing. MN: Funding acquisition, Investigation, Software, Validation, Visualization, Writing–original draft. YA: Investigation, Software, Validation, Visualization, Writing–original draft. ET: Conceptualization, Supervision, Writing–review and editing, Project administration. VC: Conceptualization, Data curation, Investigation, Supervision, Validation, Writing–original draft. RF: Conceptualization, Data curation, Funding acquisition, Project administration, Resources, Supervision, Writing–review and editing. OS: Conceptualization, Data curation, Investigation, Project administration, Supervision, Validation, Writing–original draft, Writing–review and editing. JL: Conceptualization, Data curation, Funding acquisition, Investigation, Project administration, Resources, Supervision, Validation, Writing–review and editing.
Funding
The author(s) declare financial support was received for the research, authorship, and/or publication of this article. This research was supported by the Henan Natural Science Foundation of China (No. 222300420534), and Henan Medical Research Program (SBGJ202003027); and the Ministry of Science and Higher Education of the Russian Federation at FRC Kazan Scientific Center (grant No. 075-15-2022-1128).
Acknowledgments
We apologize to those authors whose work was not cited due to space restrictions.
Conflict of interest
The authors declare that the research was conducted in the absence of any commercial or financial relationships that could be construed as a potential conflict of interest.
Publisher’s note
All claims expressed in this article are solely those of the authors and do not necessarily represent those of their affiliated organizations, or those of the publisher, the editors and the reviewers. Any product that may be evaluated in this article, or claim that may be made by its manufacturer, is not guaranteed or endorsed by the publisher.
References
Abramyuk, A., Hietschold, V., Appold, S., von Kummer, R., and Abolmaali, N. (2015). Radiochemotherapy-induced changes of tumour vascularity and blood supply estimated by dynamic contrast-enhanced CT and fractal analysis in malignant head and neck tumours. Br. J. Radiol. 88 (1045), 20140412. doi:10.1259/bjr.20140412
Admyre, C., Johansson, S. M., Paulie, S., and Gabrielsson, S. (2006). Direct exosome stimulation of peripheral human T cells detected by ELISPOT. Eur. J. Immunol. 36 (7), 1772–1781. doi:10.1002/eji.200535615
Aggarwal, D., Yang, J., Salam, M. A., Sengupta, S., Al-Amin, M. Y., and Mustafa, S. (2023). Antibody-drug conjugates: the paradigm shifts in the targeted cancer therapy. Front. Immunol. 14, 1203073. doi:10.3389/fimmu.2023.1203073
Ahmed, K. M., Zhang, H., and Park, C. C. (2013). NF-κB regulates radioresistance mediated by β1-integrin in three-dimensional culture of breast cancer cells. Cancer Res. 73 (12), 3737–3748. doi:10.1158/0008-5472.CAN-12-3537
Ahmed, M. M., Guha, C., Hodge, J. W., and Jaffee, E. (2014). Immunobiology of radiotherapy: new paradigms. Radiat. Res. 182 (2), 123–125. doi:10.1667/RR13849.1
Ahn, P. H., Machtay, M., Anne, P. R., Cognetti, D., Keane, W. M., Wuthrick, E., et al. (2018). Phase I trial using induction ciplatin, docetaxel, 5-FU and erlotinib followed by cisplatin, bevacizumab and erlotinib with concurrent radiotherapy for advanced head and neck cancer. Am. J. Clin. Oncol. 41 (5), 441–446. doi:10.1097/COC.0000000000000317
Al-Mayah, A., Bright, S., Chapman, K., Irons, S., Luo, P., Carter, D., et al. (2015). The non-targeted effects of radiation are perpetuated by exosomes. Mutat. Res. 772, 38–45. doi:10.1016/j.mrfmmm.2014.12.007
Al-Mayah, A. H., Irons, S. L., Pink, R. C., Carter, D. R. F., and Kadhim, M. A. (2012). Possible role of exosomes containing RNA in mediating nontargeted effect of ionizing radiation. Radiat. Res. 177 (5), 539–545. doi:10.1667/rr2868.1
Alique, M., Sánchez-López, E., Bodega, G., Giannarelli, C., Carracedo, J., and Ramírez, R. (2020). Hypoxia-inducible factor-1α: the master regulator of endothelial cell senescence in vascular aging. Cells 9 (1), 195. doi:10.3390/cells9010195
Ambrosetti, D., Coutts, M., Paoli, C., Durand, M., Borchiellini, D., Montemagno, C., et al. (2022). Cancer-associated fibroblasts in renal cell carcinoma: implication in prognosis and resistance to anti-angiogenic therapy. BJU Int. 129 (1), 80–92. doi:10.1111/bju.15506
Anoopkumar-Dukie, S., Conere, T., Houston, A., King, L., Christie, D., McDermott, C., et al. (2020). The COX-2 inhibitor NS398 selectively sensitizes hypoxic HeLa cells to ionising radiation by mechanisms both dependent and independent of COX-2. Prostagl. Other Lipid Mediat 148, 106422. doi:10.1016/j.prostaglandins.2020.106422
Arscott, W. T., Tandle, A. T., Zhao, S., Shabason, J. E., Gordon, I. K., Schlaff, C. D., et al. (2013). Ionizing radiation and glioblastoma exosomes: implications in tumor biology and cell migration. Transl. Oncol. 6 (6), 638–648. doi:10.1593/tlo.13640
Asif, P. J., Longobardi, C., Hahne, M., and Medema, J. P. (2021). The role of cancer-associated fibroblasts in cancer invasion and metastasis. Cancers (Basel) 13 (18), 4720. doi:10.3390/cancers13184720
Bagchi, S., Yuan, R., and Engleman, E. G. (2021). Immune checkpoint inhibitors for the treatment of cancer: clinical impact and mechanisms of response and resistance. Annu. Rev. Pathol. 16, 223–249. doi:10.1146/annurev-pathol-042020-042741
Balogh, A., Persa, E., Bogdándi, E. N., Benedek, A., Hegyesi, H., Sáfrány, G., et al. (2013). The effect of ionizing radiation on the homeostasis and functional integrity of murine splenic regulatory T cells. Inflamm. Res. 62 (2), 201–212. doi:10.1007/s00011-012-0567-y
Bang, C., and Thum, T. (2012). Exosomes: new players in cell-cell communication. Int. J. Biochem. Cell Biol. 44 (11), 2060–2064. doi:10.1016/j.biocel.2012.08.007
Banys-Paluchowski, M., Witzel, I., Riethdorf, S., Pantel, K., Rack, B., Janni, W., et al. (2018). The clinical relevance of serum vascular endothelial growth factor (VEGF) in correlation to circulating tumor cells and other serum biomarkers in patients with metastatic breast cancer. Breast Cancer Res. Treat. 172 (1), 93–104. doi:10.1007/s10549-018-4882-z
Bao, Y., Peng, F., Zhou, Q. C., Yu, Z. H., Li, J. C., Cheng, Z. B., et al. (2015). Phase II trial of recombinant human endostatin in combination with concurrent chemoradiotherapy in patients with stage III non-small-cell lung cancer. Radiother. Oncol. 114 (2), 161–166. doi:10.1016/j.radonc.2014.11.039
Barbazán, J., and Matic Vignjevic, D. (2019). Cancer associated fibroblasts: is the force the path to the dark side? Curr. Opin. Cell Biol. 56, 71–79. doi:10.1016/j.ceb.2018.09.002
Barcellos-Hoff, M. H. (2022). The radiobiology of TGFβ. Semin. Cancer Biol. 86 (3), 857–867. doi:10.1016/j.semcancer.2022.02.001
Beach, C., MacLean, D., Majorova, D., Arnold, J. N., and Olcina, M. M. (2022). The effects of radiation therapy on the macrophage response in cancer. Front. Oncol. 12, 1020606. doi:10.3389/fonc.2022.1020606
Bernier, J., Hall, E. J., and Giaccia, A. (2004). Radiation oncology: A century of achievements. Nat. Rev. Cancer 4 (9), 737–747. doi:10.1038/nrc1451
Bernier, J. (2016). Immuno-oncology: Allying forces of radio- and immuno-therapy to enhance cancer cell killing. Crit. Rev. Oncol. Hematol. 108, 97–108. doi:10.1016/j.critrevonc.2016.11.001
Bhatnagar, S., Shinagawa, K., Castellino, F. J., and Schorey, J. S. (2007). Exosomes released from macrophages infected with intracellular pathogens stimulate a proinflammatory response in vitro and in vivo. Blood 110 (9), 3234–3244. doi:10.1182/blood-2007-03-079152
Biswas, S., Guix, M., Rinehart, C., Dugger, T. C., Chytil, A., Moses, H. L., et al. (2007). Inhibition of TGF-beta with neutralizing antibodies prevents radiation-induced acceleration of metastatic cancer progression. J. Clin. Invest. 117 (5), 1305–1313. doi:10.1172/JCI30740
Biswas, S., Guix, M., Rinehart, C., Dugger, T. C., Chytil, A., Moses, H. L., et al. (2017). Inhibition of TGF-β with neutralizing antibodies prevents radiation-induced acceleration of metastatic cancer progression. J. Clin. Invest. 127 (3), 1116. doi:10.1172/JCI93333
Blomberg, A. J., Nyhan, M. M., Bind, M. A., Vokonas, P., Coull, B. A., Schwartz, J., et al. (2020). The role of ambient particle radioactivity in inflammation and endothelial function in an elderly cohort. Epidemiology 31 (4), 499–508. doi:10.1097/EDE.0000000000001197
Borghaei, H., Paz-Ares, L., Horn, L., Spigel, D. R., Steins, M., Ready, N. E., et al. (2015). Nivolumab versus docetaxel in advanced nonsquamous non-small-cell lung cancer. N. Engl. J. Med. 373 (17), 1627–1639. doi:10.1056/NEJMoa1507643
Bouquet, F., Pal, A., Pilones, K. A., Demaria, S., Hann, B., Akhurst, R. J., et al. (2011). TGFβ1 inhibition increases the radiosensitivity of breast cancer cells in vitro and promotes tumor control by radiation in vivo. Clin. Cancer Res. 17 (21), 6754–6765. doi:10.1158/1078-0432.CCR-11-0544
Bray, F., Ferlay, J., Soerjomataram, I., Siegel, R. L., Torre, L. A., and Jemal, A. (2018). Global cancer statistics 2018: GLOBOCAN estimates of incidence and mortality worldwide for 36 cancers in 185 countries. CA Cancer J. Clin. 68 (6), 394–424. doi:10.3322/caac.21492
Broustas, C. G., and Lieberman, H. B. (2014). RAD9 enhances radioresistance of human prostate cancer cells through regulation of ITGB1 protein levels. Prostate 74 (14), 1359–1370. doi:10.1002/pros.22842
Burnette, B., and Weichselbaum, R. R. (2013). Radiation as an immune modulator. Semin. Radiat. Oncol. 23 (4), 273–280. doi:10.1016/j.semradonc.2013.05.009
Burnette, B. C., Liang, H., Lee, Y., Chlewicki, L., Khodarev, N. N., Weichselbaum, R. R., et al. (2011). The efficacy of radiotherapy relies upon induction of type i interferon-dependent innate and adaptive immunity. Cancer Res. 71 (7), 2488–2496. doi:10.1158/0008-5472.CAN-10-2820
Burr, K. L., Robinson, J. I., Rastogi, S., Boylan, M. T., Coates, P. J., Lorimore, S. A., et al. (2010). Radiation-induced delayed bystander-type effects mediated by hemopoietic cells. Radiat. Res. 173 (6), 760–768. doi:10.1667/RR1937.1
Butti, R., Khaladkar, A., Bhardwaj, P., and Prakasam, G. (2023). Heterotypic signaling of cancer-associated fibroblasts in shaping the cancer cell drug resistance. Cancer Drug Resist 6 (1), 182–204. doi:10.20517/cdr.2022.72
Cadamuro, M., Brivio, S., Mertens, J., Vismara, M., Moncsek, A., Milani, C., et al. (2019). Platelet-derived growth factor-D enables liver myofibroblasts to promote tumor lymphangiogenesis in cholangiocarcinoma. J. Hepatol. 70 (4), 700–709. doi:10.1016/j.jhep.2018.12.004
Cao, Y. H., Ding, J., Tang, Q. H., Zhang, J., Huang, Z. Y., Tang, X. M., et al. (2022). Deciphering cell-cell interactions and communication in the tumor microenvironment and unraveling intratumoral genetic heterogeneity via single-cell genomic sequencing. Bioengineered 13 (7-12), 14974–14986. doi:10.1080/21655979.2023.2185434
Carracedo, S., Lu, N., Popova, S. N., Jonsson, R., Eckes, B., and Gullberg, D. (2010). The fibroblast integrin alpha11beta1 is induced in a mechanosensitive manner involving activin A and regulates myofibroblast differentiation. J. Biol. Chem. 285 (14), 10434–10445. doi:10.1074/jbc.M109.078766
Chaurasia, M., Bhatt, A. N., Das, A., Dwarakanath, B. S., and Sharma, K. (2016). Radiation-induced autophagy: mechanisms and consequences. Free Radic. Res. 50 (3), 273–290. doi:10.3109/10715762.2015.1129534
Chen, J., Zhu, H., Yin, Y., Jia, S., and Luo, X. (2022). Colorectal cancer: metabolic interactions reshape the tumor microenvironment. Biochim. Biophys. Acta Rev. Cancer 1877 (5), 188797. doi:10.1016/j.bbcan.2022.188797
Chen, S. W., Lin, L. C., Kuo, Y. C., Liang, J. A., Kuo, C. C., and Chiou, J. F. (2014). Phase 2 study of combined sorafenib and radiation therapy in patients with advanced hepatocellular carcinoma. Int. J. Radiat. Oncol. Biol. Phys. 88 (5), 1041–1047. doi:10.1016/j.ijrobp.2014.01.017
Chen, X., Zhang, H., Zhu, H., Yang, X., Yang, Y., Yang, Y., et al. (2016). Endostatin combined with radiotherapy suppresses vasculogenic mimicry formation through inhibition of epithelial-mesenchymal transition in esophageal cancer. Tumour Biol. 37 (4), 4679–4688. doi:10.1007/s13277-015-4284-3
Chen, Y., McAndrews, K. M., and Kalluri, R. (2021b). Clinical and therapeutic relevance of cancer-associated fibroblasts. Nat. Rev. Clin. Oncol. 18 (12), 792–804. doi:10.1038/s41571-021-00546-5
Chen, Y., Zheng, X., and Wu, C. (2021a). The role of the tumor microenvironment and treatment strategies in colorectal cancer. Front. Immunol. 12, 792691. doi:10.3389/fimmu.2021.792691
Chen, Z., and Xu, X. H. (2015). Combining antiangiogenic therapy and radiation in nasopharyngeal carcinoma. Saudi Med. J. 36 (6), 659–664. doi:10.15537/smj.2015.6.11460
Cheng, J. C., Chou, C. H., Kuo, M. L., and Hsieh, C. Y. (2006). Radiation-enhanced hepatocellular carcinoma cell invasion with MMP-9 expression through PI3K/Akt/NF-kappaB signal transduction pathway. Oncogene 25 (53), 7009–7018. doi:10.1038/sj.onc.1209706
Chiavarina, B., Ronca, R., Otaka, Y., Sutton, R. B., Rezzola, S., Yokobori, T., et al. (2022). Fibroblast-derived prolargin is a tumor suppressor in hepatocellular carcinoma. Oncogene 41, 1410–1420. doi:10.1038/s41388-021-02171-z
Chung, M., Adkins, R. S., Mattick, J. S. A., Bradwell, K. R., Shetty, A. C., Sadzewicz, L., et al. (2021). Fadu: A quantification tool for prokaryotic transcriptomic analyses. mSystems 6 (1), e00917-20. doi:10.1128/mSystems.00917-20
Clayton, A., Mitchell, J. P., Court, J., Mason, M. D., and Tabi, Z. (2007). Human tumor-derived exosomes selectively impair lymphocyte responses to interleukin-2. Cancer Res. 67 (15), 7458–7466. doi:10.1158/0008-5472.CAN-06-3456
Collison, L. W., Workman, C. J., Kuo, T. T., Boyd, K., Wang, Y., Vignali, K. M., et al. (2007). The inhibitory cytokine IL-35 contributes to regulatory T-cell function. Nature 450 (7169), 566–569. doi:10.1038/nature06306
Couzin-Frankel, J. (2013). Breakthrough of the year 2013. Cancer immunotherapy. Science 342 (6165), 1432–1433. doi:10.1126/science.342.6165.1432
Cummings, R. J., Mitra, S., Foster, T. H., and Lord, E. M. (2009). Migration of skin dendritic cells in response to ionizing radiation exposure. Radiat. Res. 171 (6), 687–697. doi:10.1667/RR1600.1
Dancea, H. C., Shareef, M. M., and Ahmed, M. M. (2009). Role of radiation-induced TGF-beta signaling in cancer therapy. Mol. Cell Pharmacol. 1 (1), 44–56. doi:10.4255/mcpharmacol.09.06
De Bacco, F., Luraghi, P., Medico, E., Reato, G., Girolami, F., Perera, T., et al. (2011). Induction of MET by ionizing radiation and its role in radioresistance and invasive growth of cancer. J. Natl. Cancer Inst. 103 (8), 645–661. doi:10.1093/jnci/djr093
De, P., Aske, J., and Dey, N. (2021). Cancer-associated fibroblast functions as a road-block in cancer therapy. Cancers (Basel) 13 (20), 5246. doi:10.3390/cancers13205246
Demaria, S., Kawashima, N., Yang, A. M., Devitt, M. L., Babb, J. S., Allison, J. P., et al. (2005). Immune-mediated inhibition of metastases after treatment with local radiation and CTLA-4 blockade in a mouse model of breast cancer. Clin. Cancer Res. 11 (2-1), 728–734. doi:10.1158/1078-0432.728.11.2
Demaria, S., Ng, B., Devitt, M. L., Babb, J. S., Kawashima, N., Liebes, L., et al. (2004). Ionizing radiation inhibition of distant untreated tumors (abscopal effect) is immune mediated. Int. J. Radiat. Oncol. Biol. Phys. 58 (3), 862–870. doi:10.1016/j.ijrobp.2003.09.012
Demaria, S., Pilones, K. A., Formenti, S. C., and Dustin, M. L. (2013). Exploiting the stress response to radiation to sensitize poorly immunogenic tumors to anti-CTLA-4 treatment. Oncoimmunology 2 (3), e23127. doi:10.4161/onci.23127
Dewan, M. Z., Galloway, A. E., Kawashima, N., Dewyngaert, J. K., Babb, J. S., Formenti, S. C., et al. (2009). Fractionated but not single-dose radiotherapy induces an immune-mediated abscopal effect when combined with anti-CTLA-4 antibody. Clin. Cancer Res. 15 (17), 5379–5388. doi:10.1158/1078-0432.CCR-09-0265
Digtyar, A. V., Pozdnyakova, N. V., Feldman, N. B., Lutsenko, S. V., and Severin, S. E. (2007). Endostatin: current concepts about its biological role and mechanisms of action. Biochem. (Mosc) 72 (3), 235–246. doi:10.1134/s0006297907030017
Dong, X., Yang, Y., Yuan, Q., Hou, J., and Wu, G. (2021). High expression of CEMIP correlates poor prognosis and the tumur microenvironment in breast cancer as a promisingly prognostic biomarker. Front. Genet. 12, 768140. doi:10.3389/fgene.2021.768140
Eke, I., Dickreuter, E., and Cordes, N. (2012). Enhanced radiosensitivity of head and neck squamous cell carcinoma cells by β1 integrin inhibition. Radiother. Oncol. 104 (2), 235–242. doi:10.1016/j.radonc.2012.05.009
Eldesoky, A., Shouma, A., Mosaad, Y., and Elhawary, A. (2011). Clinical relevance of serum vascular endothelial growth factor and interleukin-6 in patients with colorectal cancer. Saudi J. Gastroenterol. 17 (3), 170–173. doi:10.4103/1319-3767.80378
Eriksson, D., Löfroth, P. O., Johansson, L., Riklund, K. A., and Stigbrand, T. (2007). Cell cycle disturbances and mitotic catastrophes in HeLa Hep2 cells following 2.5 to 10 Gy of ionizing radiation. Clin. Cancer Res. 13 (18), 5501s–5508s. doi:10.1158/1078-0432.CCR-07-0980
Facciabene, A., Motz, G. T., and Coukos, G. (2012). T-Regulatory cells: key players in tumor immune escape and angiogenesis. Cancer Res. 72 (9), 2162–2171. doi:10.1158/0008-5472.CAN-11-3687
Facoetti, A., Ballarini, F., Cherubini, R., Gerardi, S., Nano, R., Ottolenghi, A., et al. (2006). Gamma ray-induced bystander effect in tumour glioblastoma cells: A specific study on cell survival, cytokine release and cytokine receptors. Radiat. Prot. Dosim. 122 (1-4), 271–274. doi:10.1093/rpd/ncl431
Feng, Q. Y., Wei, Y., Chen, J. W., Chang, W. J., Ye, L. C., Zhu, D. X., et al. (2014). Anti-EGFR and anti-VEGF agents: important targeted therapies of colorectal liver metastases. World J. Gastroenterol. 20 (15), 4263–4275. doi:10.3748/wjg.v20.i15.4263
Franzin, R., Netti, G. S., Spadaccino, F., Porta, C., Gesualdo, L., Stallone, G., et al. (2020). The use of immune checkpoint inhibitors in oncology and the occurrence of AKI: where do we stand? Front. Immunol. 11, 574271. doi:10.3389/fimmu.2020.574271
Fridman, W. H., Pagès, F., Sautès-Fridman, C., and Galon, J. (2012). The immune contexture in human tumours: impact on clinical outcome. Nat. Rev. Cancer 12 (4), 298–306. doi:10.1038/nrc3245
Gabrilovich, D. I., Ostrand-Rosenberg, S., and Bronte, V. (2012). Coordinated regulation of myeloid cells by tumours. Nat. Rev. Immunol. 12 (4), 253–268. doi:10.1038/nri3175
Gallo, P. M., and Gallucci, S. (2013). The dendritic cell response to classic, emerging, and homeostatic danger signals. Implications for autoimmunity. Front. Immunol. 4, 138. doi:10.3389/fimmu.2013.00138
Galluzzi, L., Humeau, J., Buqué, A., Zitvogel, L., and Kroemer, G. (2020). Immunostimulation with chemotherapy in the era of immune checkpoint inhibitors. Nat. Rev. Clin. Oncol. 17 (12), 725–741. doi:10.1038/s41571-020-0413-z
Gil Marques, F., Poli, E., Malaquias, J., Carvalho, T., Portêlo, A., Ramires, A., et al. (2019). Low doses of ionizing radiation activate endothelial cells and induce angiogenesis in peritumoral tissues. Radiother. Oncol. 141, 256–261. doi:10.1016/j.radonc.2019.06.035
Girinsky, T. (2000). Effects of ionizing radiation on the blood vessel wall. J. Mal. Vasc. 25 (5), 321–324.
Glicksman, A. S. (2006). Introduction: radiation oncology for the 21st century--the best is yet to be. Med. Health R. I. 89 (2), 54.
Golden, E. B., Pellicciotta, I., Demaria, S., Barcellos-Hoff, M. H., and Formenti, S. C. (2012). The convergence of radiation and immunogenic cell death signaling pathways. Front. Oncol. 2, 88. doi:10.3389/fonc.2012.00088
Greimelmaier, K., Klopp, N., Mairinger, E., Wessolly, M., Borchert, S., Steinborn, J., et al. (2023). Fibroblast activation protein-α expression in fibroblasts is common in the tumor microenvironment of colorectal cancer and may serve as a therapeutic target. Pathol. Oncol. Res. 29, 1611163. doi:10.3389/pore.2023.1611163
Gu, X., Cun, Y., Li, M., Qing, Y., Jin, F., Zhong, Z., et al. (2013). Human apurinic/apyrimidinic endonuclease siRNA inhibits the angiogenesis induced by X-ray irradiation in lung cancer cells. Int. J. Med. Sci. 10 (7), 870–882. doi:10.7150/ijms.5727
Gubin, M. M., Zhang, X., Schuster, H., Caron, E., Ward, J. P., Noguchi, T., et al. (2014). Checkpoint blockade cancer immunotherapy targets tumour-specific mutant antigens. Nature 515 (7528), 577–581. doi:10.1038/nature13988
Gujral, D. M., Chahal, N., Senior, R., Harrington, K. J., and Nutting, C. M. (2014). Radiation-induced carotid artery atherosclerosis. Radiother. Oncol. 110 (1), 31–38. doi:10.1016/j.radonc.2013.08.009
Guo, L., Hua, L., Hu, B., and Wang, J. (2023a). Pre-clinical efficacy and safety pharmacology of PEGylated recombinant human endostatin. Curr. Mol. Med. 23. doi:10.2174/1566524023666230331091757
Guo, S., Yuan, J., Meng, X., Feng, X., Ma, D., Han, Y., et al. (2023b). Cancer-associated fibroblasts: just on the opposite side of antitumour immunity? Int. Immunopharmacol. 122, 110601. doi:10.1016/j.intimp.2023.110601
Gyorgy, B., Szabó, T. G., Pásztói, M., Pál, Z., Misják, P., Aradi, B., et al. (2011). Membrane vesicles, current state-of-the-art: emerging role of extracellular vesicles. Cell Mol. Life Sci. 68 (16), 2667–2688. doi:10.1007/s00018-011-0689-3
Haas, R. L., Gelderblom, H., Sleijfer, S., van Boven, H. H., Scholten, A., Dewit, L., et al. (2015). A phase I study on the combination of neoadjuvant radiotherapy plus pazopanib in patients with locally advanced soft tissue sarcoma of the extremities. Acta Oncol. 54 (8), 1195–1201. doi:10.3109/0284186X.2015.1037404
Haddadi, M. H., and Negahdari, B. (2022). Clinical and diagnostic potential of regulatory T cell markers: from bench to bedside. Transpl. Immunol. 70, 101518. doi:10.1016/j.trim.2021.101518
Hariyanto, A. D., Permata, T. B. M., and Gondhowiardjo, S. A. (2022). Role of CD4(+)CD25(+)FOXP3(+) T(Reg) cells on tumor immunity. Immunol. Med. 45 (2), 94–107. doi:10.1080/25785826.2021.1975228
Harmankaya, I., Çaloğlu, V. Y., Taştekin, E., Türkkan, G., Çaloğlu, M., and Uzal, C. (2022). Prognostic importance of microvessel density, VEGF expression and perineural invasion in laryngeal cancer treated with adjuvant radiotherapy. Indian J. Pathol. Microbiol. 65 (3), 521–526. doi:10.4103/ijpm.ijpm_655_21
Hazawa, M., Tomiyama, K., Saotome-Nakamura, A., Obara, C., Yasuda, T., Gotoh, T., et al. (2014). Radiation increases the cellular uptake of exosomes through CD29/CD81 complex formation. Biochem. Biophys. Res. Commun. 446 (4), 1165–1171. doi:10.1016/j.bbrc.2014.03.067
Heath, E. I., Burtness, B. A., Kleinberg, L., Salem, R. R., Yang, S. C., Heitmiller, R. F., et al. (2006). Phase II, parallel-design study of preoperative combined modality therapy and the matrix metalloprotease (mmp) inhibitor prinomastat in patients with esophageal adenocarcinoma. Invest. New Drugs 24 (2), 135–140. doi:10.1007/s10637-006-5934-5
Hellevik, T., Pettersen, I., Berg, V., Winberg, J. O., Moe, B. T., Bartnes, K., et al. (2012). Cancer-associated fibroblasts from human NSCLC survive ablative doses of radiation but their invasive capacity is reduced. Radiat. Oncol. 7, 59. doi:10.1186/1748-717X-7-59
Hneino, M., François, A., Buard, V., Tarlet, G., Abderrahmani, R., Blirando, K., et al. (2012). The TGF-β/Smad repressor TG-interacting factor 1 (TGIF1) plays a role in radiation-induced intestinal injury independently of a Smad signaling pathway. PLoS One 7 (5), e35672. doi:10.1371/journal.pone.0035672
Holotiuk, V. V., Kryzhanivska, A. Y., Churpiy, I. K., Tataryn, B. B., and Ivasiutyn, D. Y. (2019). Role of nitric oxide in pathogenesis of tumor growth and its possible application in cancer treatment. Exp. Oncol. 41 (3), 210–215. doi:10.32471/exp-oncology.2312-8852.vol-41-no-3.13515
Hu, L., Wang, H., Huang, L., Zhao, Y., and Wang, J. (2016). Crosstalk between autophagy and intracellular radiation response (Review). Int. J. Oncol. 49 (6), 2217–2226. doi:10.3892/ijo.2016.3719
Hurwitz, M. D., Kaur, P., Nagaraja, G. M., Bausero, M. A., Manola, J., and Asea, A. (2010). Radiation therapy induces circulating serum Hsp72 in patients with prostate cancer. Radiother. Oncol. 95 (3), 350–358. doi:10.1016/j.radonc.2010.03.024
Husain, H., Hann, C., and Hales, R. (2010). Radiotherapy in small-cell lung cancer. Oncol. Willist. Park) 24 (11), 1047–1049.
Inoue, T., Fujishima, S., Ikeda, E., Yoshie, O., Tsukamoto, N., Aiso, S., et al. (2004). CCL22 and CCL17 in rat radiation pneumonitis and in human idiopathic pulmonary fibrosis. Eur. Respir. J. 24 (1), 49–56. doi:10.1183/09031936.04.00110203
Jabbari, N., Nawaz, M., and Rezaie, J. (2019). Bystander effects of ionizing radiation: conditioned media from X-ray irradiated MCF-7 cells increases the angiogenic ability of endothelial cells. Cell Commun. Signal 17 (1), 165. doi:10.1186/s12964-019-0474-8
Jelonek, K., Wojakowska, A., Marczak, L., Muer, A., Tinhofer-Keilholz, I., Lysek-Gladysinska, M., et al. (2015). Ionizing radiation affects protein composition of exosomes secreted in vitro from head and neck squamous cell carcinoma. Acta Biochim. Pol. 62 (2), 265–272. doi:10.18388/abp.2015_970
Kachikwu, E. L., Iwamoto, K. S., Liao, Y. P., DeMarco, J. J., Agazaryan, N., Economou, J. S., et al. (2011). Radiation enhances regulatory T cell representation. Int. J. Radiat. Oncol. Biol. Phys. 81 (4), 1128–1135. doi:10.1016/j.ijrobp.2010.09.034
Kamali Zonouzi, S., Pezeshki, P. S., Razi, S., and Rezaei, N. (2021). Cancer-associated fibroblasts in colorectal cancer. Clin. Transl. Oncol. 24, 757–769. doi:10.1007/s12094-021-02734-2
Kanthou, C., and Tozer, G. (2019). Targeting the vasculature of tumours: combining VEGF pathway inhibitors with radiotherapy. Br. J. Radiol. 92 (1093), 20180405. doi:10.1259/bjr.20180405
Kashima, H., Noma, K., Ohara, T., Kato, T., Katsura, Y., Komoto, S., et al. (2019). Cancer-associated fibroblasts (CAFs) promote the lymph node metastasis of esophageal squamous cell carcinoma. Int. J. Cancer 144 (4), 828–840. doi:10.1002/ijc.31953
Khan, A. U. H., Blimkie, M., Yang, D. S., Serran, M., Pack, T., Wu, J., et al. (2021). Effects of chronic low-dose internal radiation on immune-stimulatory responses in mice. Int. J. Mol. Sci. 22 (14), 7303. doi:10.3390/ijms22147303
Kidoikhammouan, S., Seubwai, W., Tantapotinan, N., Silsirivanit, A., Wongkham, S., Sawanyawisuth, K., et al. (2012). TNP-470, a methionine aminopeptidase-2 inhibitor, inhibits cell proliferation, migration and invasion of human cholangiocarcinoma cells in vitro. Asian Pac J. Cancer Prev. 13, 155–160. doi:10.7314/APJCP.2012.13.KKSuppl.155
Kilpatrick, L. E., and Hill, S. J. (2021). Transactivation of G protein-coupled receptors (GPCRs) and receptor tyrosine kinases (RTKs): recent insights using luminescence and fluorescence technologies. Curr. Opin. Endocr. Metab. Res. 16, 102–112. doi:10.1016/j.coemr.2020.10.003
Kim, K. W., Kim, S. H., Shin, J. G., Kim, G. S., Son, Y. O., Park, S. W., et al. (2004). Direct injection of immature dendritic cells into irradiated tumor induces efficient antitumor immunity. Int. J. Cancer 109 (5), 685–690. doi:10.1002/ijc.20036
Kobayashi, H., Gieniec, K. A., Lannagan, T. R. M., Wang, T., Asai, N., Mizutani, Y., et al. (2021). The origin and contribution of cancer-associated fibroblasts in colorectal carcinogenesis. Gastroenterology 162, 890–906. doi:10.1053/j.gastro.2021.11.037
Kochetkova, M., and Samuel, M. S. (2021). Differentiation of the tumor microenvironment: are CAFs the organizer? Trends Cell Biol. 32, 285–294. doi:10.1016/j.tcb.2021.11.008
Koukourakis, M. I., Koukourakis, I. M., Arelaki, S., Kouroupi, M., Domoxoudis, S., and Giatromanolaki, A. (2019). Angiogenic regeneration defines loco-regional recurrence following pre-operative radio-chemotherapy for rectal cancer: A pilot study. Mol. Biol. Rep. 46 (2), 2147–2152. doi:10.1007/s11033-019-04668-z
Kovacs, B., Vajda, E., and Nagy, E. E. (2019). Regulatory effects and interactions of the wnt and OPG-RANKL-RANK signaling at the bone-cartilage interface in osteoarthritis. Int. J. Mol. Sci. 20 (18), 4653. doi:10.3390/ijms20184653
Krysko, D. V., Garg, A. D., Kaczmarek, A., Krysko, O., Agostinis, P., and Vandenabeele, P. (2012). Immunogenic cell death and DAMPs in cancer therapy. Nat. Rev. Cancer 12 (12), 860–875. doi:10.1038/nrc3380
Kumar, V., Patel, S., Tcyganov, E., and Gabrilovich, D. I. (2016). The nature of myeloid-derived suppressor cells in the tumor microenvironment. Trends Immunol. 37 (3), 208–220. doi:10.1016/j.it.2016.01.004
Lakoduk, A. M., Lee, C. F., and Chen, P. H. (2021). Gain-of-"endocytic' function in mutant p53 cancer cells. Int. J. Biochem. Cell Biol. 131, 105905. doi:10.1016/j.biocel.2020.105905
Lan, Y., Moustafa, M., Knoll, M., Xu, C., Furkel, J., Lazorchak, A., et al. (2021). Simultaneous targeting of TGF-β/PD-L1 synergizes with radiotherapy by reprogramming the tumor microenvironment to overcome immune evasion. Cancer Cell 39 (10), 1388–1403 e10. doi:10.1016/j.ccell.2021.08.008
Larkin, J., Chiarion-Sileni, V., Gonzalez, R., Grob, J. J., Cowey, C. L., Lao, C. D., et al. (2015). Combined nivolumab and ipilimumab or monotherapy in untreated melanoma. N. Engl. J. Med. 373 (1), 23–34. doi:10.1056/NEJMoa1504030
Lee, Y. H., Tai, D., Yip, C., Choo, S. P., and Chew, V. (2020). Combinational immunotherapy for hepatocellular carcinoma: radiotherapy, immune checkpoint blockade and beyond. Front. Immunol. 11, 568759. doi:10.3389/fimmu.2020.568759
Lehmann, B. D., Paine, M. S., Brooks, A. M., McCubrey, J. A., Renegar, R. H., Wang, R., et al. (2008). Senescence-associated exosome release from human prostate cancer cells. Cancer Res. 68 (19), 7864–7871. doi:10.1158/0008-5472.CAN-07-6538
Lhuillier, C., Rudqvist, N. P., Yamazaki, T., Zhang, T., Charpentier, M., Galluzzi, L., et al. (2021). Radiotherapy-exposed CD8+ and CD4+ neoantigens enhance tumor control. J. Clin. Invest. 131 (5), e138740. doi:10.1172/JCI138740
Li, X., and Mu, P. (2023). The critical interplay of CAF plasticity and resistance in prostate cancer. Cancer Res. 83, 2990–2992. doi:10.1158/0008-5472.CAN-23-2260
Li, Y., Sun, R., Zou, J., Ying, Y., and Luo, Z. (2019). Dual roles of the AMP-activated protein kinase pathway in angiogenesis. Cells 8 (7), 752. doi:10.3390/cells8070752
Li, Y., Wang, C., Huang, T., Yu, X., and Tian, B. (2023). The role of cancer-associated fibroblasts in breast cancer metastasis. Front. Oncol. 13, 1194835. doi:10.3389/fonc.2023.1194835
Lin, Z., Zhang, Q., and Luo, W. (2016). Angiogenesis inhibitors as therapeutic agents in cancer: challenges and future directions. Eur. J. Pharmacol. 793, 76–81. doi:10.1016/j.ejphar.2016.10.039
Liotta, L. A., and Kohn, E. C. (2001). The microenvironment of the tumour-host interface. Nature 411 (6835), 375–379. doi:10.1038/35077241
Liu, C., Yu, S., Zinn, K., Wang, J., Zhang, L., Jia, Y., et al. (2006). Murine mammary carcinoma exosomes promote tumor growth by suppression of NK cell function. J. Immunol. 176 (3), 1375–1385. doi:10.4049/jimmunol.176.3.1375
Loh, J. J., and Ma, S. (2021). The role of cancer-associated fibroblast as a dynamic player in mediating cancer stemness in the tumor microenvironment. Front. Cell Dev. Biol. 9, 727640. doi:10.3389/fcell.2021.727640
Lu, C., Liu, Y., Ali, N. M., Zhang, B., and Cui, X. (2022). The role of innate immune cells in the tumor microenvironment and research progress in anti-tumor therapy. Front. Immunol. 13, 1039260. doi:10.3389/fimmu.2022.1039260
Ludwig, A. K., and Giebel, B. (2012). Exosomes: small vesicles participating in intercellular communication. Int. J. Biochem. Cell Biol. 44 (1), 11–15. doi:10.1016/j.biocel.2011.10.005
Lumniczky, K., and Safrany, G. (2015). The impact of radiation therapy on the antitumor immunity: local effects and systemic consequences. Cancer Lett. 356 (1), 114–125. doi:10.1016/j.canlet.2013.08.024
Majidpoor, J., and Mortezaee, K. (2021). Steps in metastasis: an updated review. Med. Oncol. 38 (1), 3. doi:10.1007/s12032-020-01447-w
Malla, B., Zaugg, K., Vassella, E., Aebersold, D. M., and Dal Pra, A. (2017). Exosomes and exosomal MicroRNAs in prostate cancer radiation therapy. Int. J. Radiat. Oncol. Biol. Phys. 98 (5), 982–995. doi:10.1016/j.ijrobp.2017.03.031
Manier, S., Kawano, Y., Bianchi, G., Roccaro, A. M., and Ghobrial, I. M. (2016). Cell autonomous and microenvironmental regulation of tumor progression in precursor states of multiple myeloma. Curr. Opin. Hematol. 23 (4), 426–433. doi:10.1097/MOH.0000000000000259
Marin-Acevedo, J. A., Kimbrough, E. O., and Lou, Y. (2021). Next generation of immune checkpoint inhibitors and beyond. J. Hematol. Oncol. 14 (1), 45. doi:10.1186/s13045-021-01056-8
Marques, F. G., Carvalho, L., Sousa, J. S., Rino, J., Diegues, I., Poli, E., et al. (2020). Low doses of ionizing radiation enhance angiogenesis and consequently accelerate post-embryonic development but not regeneration in zebrafish. Sci. Rep. 10 (1), 3137. doi:10.1038/s41598-020-60129-9
Masoud, G. N., and Li, W. (2015). HIF-1α pathway: role, regulation and intervention for cancer therapy. Acta Pharm. Sin. B 5 (5), 378–389. doi:10.1016/j.apsb.2015.05.007
Mendez-Valdes, G., Gómez-Hevia, F., Lillo-Moya, J., González-Fernández, T., Abelli, J., Cereceda-Cornejo, A., et al. (2023). Endostatin and cancer therapy: A novel potential alternative to anti-VEGF monoclonal antibodies. Biomedicines 11 (3), 718. doi:10.3390/biomedicines11030718
Miksa, M., Wu, R., Dong, W., Komura, H., Amin, D., Ji, Y., et al. (2009). Immature dendritic cell-derived exosomes rescue septic animals via milk fat globule epidermal growth factor-factor VIII [corrected]. J. Immunol. 183 (9), 5983–5990. doi:10.4049/jimmunol.0802994
Minn, A. J. (2015). Interferons and the immunogenic effects of cancer therapy. Trends Immunol. 36 (11), 725–737. doi:10.1016/j.it.2015.09.007
Mitryayeva, N. A., Grebinyk, L. V., and Uzlenkova, N. E. (2019). Influence of combined action of X-radiation and cyclooxygenase-2 - meloxivet inhibitor on vegf and pge-2 content in blood of rat-tumor carriers. Probl. Radiac. Med. Radiobiol. 24, 261–269. doi:10.33145/2304-8336-2019-24-261-269
Mohammadkarim, A., Mokhtari-Dizaji, M., Kazemian, A., Saberi, H., Yazdi, N. A., and Esfehani, M. (2022). Assessing the short-term effects of radiotherapy on the shear modulus of the common carotid artery as a new biomarker of radiation-induced atherosclerosis. Ultrasonography 41 (1), 114–123. doi:10.14366/usg.21032
Moore, S. R., Marsden, S., Macdonald, D., Mitchell, S., Folkard, M., Michael, B., et al. (2005). Genomic instability in human lymphocytes irradiated with individual charged particles: involvement of tumor necrosis factor alpha in irradiated cells but not bystander cells. Radiat. Res. 163 (2), 183–190. doi:10.1667/rr3298
Mortezaee, K., and Majidpoor, J. (2022). Key promoters of tumor hallmarks. Int. J. Clin. Oncol. 27 (1), 45–58. doi:10.1007/s10147-021-02074-9
Moreau, J. M., Velegraki, M., Bolyard, C., Rosenblum, M. D., and Li, Z. (2022). Transforming growth factor-β1 in regulatory T cell biology. Sci. Immunol. 7 (69), eabi4613. doi:10.1126/sciimmunol.abi4613
Morgan, A., Griffin, M., Kameni, L., Wan, D. C., Longaker, M. T., and Norton, J. A. (2023). Medical biology of cancer-associated fibroblasts in pancreatic cancer. Biol. (Basel) 12 (8), 1044. doi:10.3390/biology12081044
Nam, J. M., Ahmed, K. M., Costes, S., Zhang, H., Onodera, Y., Olshen, A. B., et al. (2013). β1-Integrin via NF-κB signaling is essential for acquisition of invasiveness in a model of radiation treated in situ breast cancer. Breast Cancer Res. 15 (4), R60. doi:10.1186/bcr3454
National Library of Medicine (2018). Neoadjuvant PD-1 blockade in resectable lung cancer; nivolumab and ipilimumab in advanced melanoma; overall survival with combined nivolumab and ipilimumab in advanced melanoma; prolonged survival in stage III melanoma with ipilimumab adjuvant therapy; combined nivolumab and ipilimumab or monotherapy in untreated melanoma; combined nivolumab and ipilimumab or monotherapy in untreated melanoma; nivolumab and ipilimumab versus ipilimumab in untreated melanoma; rapid eradication of a bulky melanoma mass with one dose of immunotherapy; genetic basis for clinical response to CTLA-4 blockade; genetic basis for clinical response to CTLA-4 blockade in melanoma; nivolumab plus ipilimumab in advanced melanoma; safety and tumor responses with lambrolizumab (Anti-PD-1) in melanoma; hepatotoxicity with combination of vemurafenib and ipilimumab. N. Engl. J. Med. 379 (22), 2185. doi:10.1056/NEJMx180040
Neuzillet, C., Tijeras-Raballand, A., Cohen, R., Cros, J., Faivre, S., Raymond, E., et al. (2015). Targeting the TGFβ pathway for cancer therapy. Pharmacol. Ther. 147, 22–31. doi:10.1016/j.pharmthera.2014.11.001
Ni, J., Bucci, J., Malouf, D., Knox, M., Graham, P., and Li, Y. (2019). Exosomes in cancer radioresistance. Front. Oncol. 9, 869. doi:10.3389/fonc.2019.00869
Nolte-'t Hoen, E. N., Buschow, S. I., Anderton, S. M., Stoorvogel, W., and Wauben, M. H. M. (2009). Activated T cells recruit exosomes secreted by dendritic cells via LFA-1. Blood 113 (9), 1977–1981. doi:10.1182/blood-2008-08-174094
Oh, D. Y., Kwek, S. S., Raju, S. S., Li, T., McCarthy, E., Chow, E., et al. (2020). Intratumoral CD4(+) T cells mediate anti-tumor cytotoxicity in human bladder cancer. Cell 181 (7), 1612–1625. doi:10.1016/j.cell.2020.05.017
Okimoto, R. A., and Bivona, T. G. (2016). Tracking down response and resistance to TRK inhibitors. Cancer Discov. 6 (1), 14–16. doi:10.1158/2159-8290.CD-15-1352
Olivares-Urbano, M. A., Griñán-Lisón, C., Zurita, M., Del Moral, R., Ríos-Arrabal, S., Artacho-Cordón, F., et al. (2020). Matrix metalloproteases and TIMPs as prognostic biomarkers in breast cancer patients treated with radiotherapy: A pilot study. J. Cell Mol. Med. 24 (1), 139–148. doi:10.1111/jcmm.14671
Owens, C., Abbott, L. S., and Gupta, A. A. (2013). Optimal management of ewing sarcoma family of tumors: recent developments in systemic therapy. Paediatr. Drugs 15 (6), 473–492. doi:10.1007/s40272-013-0037-1
Paris, F., Fuks, Z., Kang, A., Capodieci, P., Juan, G., Ehleiter, D., et al. (2001). Endothelial apoptosis as the primary lesion initiating intestinal radiation damage in mice. Science 293 (5528), 293–297. doi:10.1126/science.1060191
Park, C. C., Zhang, H. J., Yao, E. S., and Bissell, M. J. (2008). Beta1 integrin inhibition dramatically enhances radiotherapy efficacy in human breast cancer xenografts. Cancer Res. 68 (11), 4398–4405. doi:10.1158/0008-5472.CAN-07-6390
Park, C. M., Park, M. J., Kwak, H. J., Lee, H. C., Kim, M. S., Lee, S. H., et al. (2006). Ionizing radiation enhances matrix metalloproteinase-2 secretion and invasion of glioma cells through Src/epidermal growth factor receptor-mediated p38/Akt and phosphatidylinositol 3-kinase/Akt signaling pathways. Cancer Res. 66 (17), 8511–8519. doi:10.1158/0008-5472.CAN-05-4340
Park, S. J., Min, H. J., Yoon, C., Kim, S. H., Kim, J. H., and Lee, S. Y. (2023). Integrin β1 regulates the perineural invasion and radioresistance of oral squamous carcinoma cells by modulating cancer cell stemness. Cell Signal 110, 110808. doi:10.1016/j.cellsig.2023.110808
Pei, L., Liu, Y., Liu, L., Gao, S., Gao, X., Feng, Y., et al. (2023). Roles of cancer-associated fibroblasts (CAFs) in anti- PD-1/PD-L1 immunotherapy for solid cancers. Mol. Cancer 22 (1), 29. doi:10.1186/s12943-023-01731-z
Peng, L., Wang, D., Han, Y., Huang, T., He, X., Wang, J., et al. (2021). Emerging role of cancer-associated fibroblasts-derived exosomes in tumorigenesis. Front. Immunol. 12, 795372. doi:10.3389/fimmu.2021.795372
Persa, E., Balogh, A., Sáfrány, G., and Lumniczky, K. (2015). The effect of ionizing radiation on regulatory T cells in health and disease. Cancer Lett. 368 (2), 252–261. doi:10.1016/j.canlet.2015.03.003
Pontoriero, A., Critelli, P., Chillari, F., Ferrantelli, G., Sciacca, M., Brogna, A., et al. (2023). Modulation of radiation doses and chimeric antigen receptor T cells: A promising new weapon in solid tumors-A narrative review. J. Pers. Med. 13 (8), 1261. doi:10.3390/jpm13081261
Postow, M. A., Callahan, M. K., Barker, C. A., Yamada, Y., Yuan, J., Kitano, S., et al. (2012). Immunologic correlates of the abscopal effect in a patient with melanoma. N. Engl. J. Med. 366 (10), 925–931. doi:10.1056/NEJMoa1112824
Postow, M. A., Chesney, J., Pavlick, A. C., Robert, C., Grossmann, K., McDermott, D., et al. (2015). Nivolumab and ipilimumab versus ipilimumab in untreated melanoma. N. Engl. J. Med. 372 (21), 2006–2017. doi:10.1056/NEJMoa1414428
Powles, T., Eder, J. P., Fine, G. D., Braiteh, F. S., Loriot, Y., Cruz, C., et al. (2014). MPDL3280A (anti-PD-L1) treatment leads to clinical activity in metastatic bladder cancer. Nature 515 (7528), 558–562. doi:10.1038/nature13904
Pueyo, G., Mesia, R., Figueras, A., Lozano, A., Baro, M., Vazquez, S., et al. (2010). Cetuximab may inhibit tumor growth and angiogenesis induced by ionizing radiation: A preclinical rationale for maintenance treatment after radiotherapy. Oncologist 15 (9), 976–986. doi:10.1634/theoncologist.2008-0290
Quail, D. F., and Joyce, J. A. (2013). Microenvironmental regulation of tumor progression and metastasis. Nat. Med. 19 (11), 1423–1437. doi:10.1038/nm.3394
Raskov, H., Orhan, A., Christensen, J. P., and Gögenur, I. (2021). Cytotoxic CD8(+) T cells in cancer and cancer immunotherapy. Br. J. Cancer 124 (2), 359–367. doi:10.1038/s41416-020-01048-4
Reardon, D. A., Akabani, G., Coleman, R. E., Friedman, A. H., Friedman, H. S., Herndon, J. E., et al. (2002). Phase II trial of murine (131)I-labeled antitenascin monoclonal antibody 81C6 administered into surgically created resection cavities of patients with newly diagnosed malignant gliomas. J. Clin. Oncol. 20 (5), 1389–1397. doi:10.1200/JCO.2002.20.5.1389
Reina-Campos, M., Scharping, N. E., and Goldrath, A. W. (2021). CD8(+) T cell metabolism in infection and cancer. Nat. Rev. Immunol. 21 (11), 718–738. doi:10.1038/s41577-021-00537-8
Ribas, A. (2012). Tumor immunotherapy directed at PD-1. N. Engl. J. Med. 366 (26), 2517–2519. doi:10.1056/NEJMe1205943
Robbins, P. D., and Morelli, A. E. (2014). Regulation of immune responses by extracellular vesicles. Nat. Rev. Immunol. 14 (3), 195–208. doi:10.1038/nri3622
Rodriguez-Ruiz, M. E., Vitale, I., Harrington, K. J., Melero, I., and Galluzzi, L. (2020). Immunological impact of cell death signaling driven by radiation on the tumor microenvironment. Nat. Immunol. 21 (2), 120–134. doi:10.1038/s41590-019-0561-4
Roses, R. E., Datta, J., and Czerniecki, B. J. (2014). Radiation as immunomodulator: implications for dendritic cell-based immunotherapy. Radiat. Res. 182 (2), 211–218. doi:10.1667/RR13495.1
Sakaguchi, S., Mikami, N., Wing, J. B., Tanaka, A., Ichiyama, K., and Ohkura, N. (2020). Regulatory T cells and human disease. Annu. Rev. Immunol. 38, 541–566. doi:10.1146/annurev-immunol-042718-041717
Sanjabi, S., Oh, S. A., and Li, M. O. (2017). Regulation of the immune response by TGF-β: from conception to autoimmunity and infection. Cold Spring Harb. Perspect. Biol. 9 (6), a022236. doi:10.1101/cshperspect.a022236
Sayitoglu, E. C., Georgoudaki, A. M., Chrobok, M., Ozkazanc, D., Josey, B. J., Arif, M., et al. (2020). Boosting natural killer cell-mediated targeting of sarcoma through DNAM-1 and NKG2D. Front. Immunol. 11, 40. doi:10.3389/fimmu.2020.00040
Schulz, M., Salamero-Boix, A., Niesel, K., Alekseeva, T., and Sevenich, L. (2019). Microenvironmental regulation of tumor progression and therapeutic response in brain metastasis. Front. Immunol. 10, 1713. doi:10.3389/fimmu.2019.01713
Scott-Solomon, E., and Kuruvilla, R. (2018). Mechanisms of neurotrophin trafficking via Trk receptors. Mol. Cell Neurosci. 91, 25–33. doi:10.1016/j.mcn.2018.03.013
Segura, E., Nicco, C., Lombard, B., Véron, P., Raposo, G., Batteux, F., et al. (2005). ICAM-1 on exosomes from mature dendritic cells is critical for efficient naive T-cell priming. Blood 106 (1), 216–223. doi:10.1182/blood-2005-01-0220
Shah, A. A., Ahmad, S., Yadav, M. K., Raza, K., Kamal, M. A., and Akhtar, S. (2023). Structure-based virtual screening, molecular docking, molecular dynamics simulation, and metabolic reactivity studies of quinazoline derivatives for their anti-EGFR activity against tumor angiogenesis. Curr. Med. Chem. doi:10.2174/0929867330666230309143711
Shannon, A. H., Manne, A., Diaz Pardo, D. A., and Pawlik, T. M. (2023). Combined radiotherapy and immune checkpoint inhibition for the treatment of advanced hepatocellular carcinoma. Front. Oncol. 13, 1193762. doi:10.3389/fonc.2023.1193762
Sharabi, A. B., Nirschl, C. J., Kochel, C. M., Nirschl, T. R., Francica, B. J., Velarde, E., et al. (2015). Stereotactic radiation therapy augments antigen-specific PD-1-mediated antitumor immune responses via cross-presentation of tumor antigen. Cancer Immunol. Res. 3 (4), 345–355. doi:10.1158/2326-6066.CIR-14-0196
Sharifpanah, F., Ali, E. H., Wartenberg, M., and Sauer, H. (2019). The milk thistle (Silybum marianum) compound Silibinin stimulates leukopoiesis from mouse embryonic stem cells. Phytother. Res. 33 (2), 452–460. doi:10.1002/ptr.6241
Shi, Y., Evans, J. E., and Rock, K. L. (2003). Molecular identification of a danger signal that alerts the immune system to dying cells. Nature 425 (6957), 516–521. doi:10.1038/nature01991
Sica, A., Larghi, P., Mancino, A., Rubino, L., Porta, C., Totaro, M. G., et al. (2008). Macrophage polarization in tumour progression. Semin. Cancer Biol. 18 (5), 349–355. doi:10.1016/j.semcancer.2008.03.004
Siemann, D. W., Chaplin, D. J., and Horsman, M. R. (2017). Realizing the potential of vascular targeted therapy: the rationale for combining vascular disrupting agents and anti-angiogenic agents to treat cancer. Cancer Invest. 35 (8), 519–534. doi:10.1080/07357907.2017.1364745
Simon, T., and Salhia, B. (2021). Cancer-associated fibroblast subpopulations with diverse and dynamic roles in the tumor microenvironment. Mol. Cancer Res. 20, 183–192. doi:10.1158/1541-7786.MCR-21-0282
Song, C. W., and Levitt, S. H. (1970). Effect of x irradiation on vascularity of normal tissues and experimental tumor. Radiology 94 (2), 445–447. doi:10.1148/94.2.445
Sonveaux, P., Brouet, A., Havaux, X., Grégoire, V., Dessy, C., Balligand, J. L., et al. (2003). Irradiation-induced angiogenesis through the up-regulation of the nitric oxide pathway: implications for tumor radiotherapy. Cancer Res. 63 (5), 1012–1019.
Sorolla, M. A., Parisi, E., and Sorolla, A. (2020). Determinants of sensitivity to radiotherapy in endometrial cancer. Cancers (Basel) 12 (7), 1906. doi:10.3390/cancers12071906
Stessin, A. M., Clausi, M. G., Zhao, Z., Lin, H., Hou, W., Jiang, Z., et al. (2020). Repolarized macrophages, induced by intermediate stereotactic dose radiotherapy and immune checkpoint blockade, contribute to long-term survival in glioma-bearing mice. J. Neurooncol 147 (3), 547–555. doi:10.1007/s11060-020-03459-y
Stuelten, C. H., and Zhang, Y. E. (2021). Transforming growth factor-β: an agent of change in the tumor microenvironment. Front. Cell Dev. Biol. 9, 764727. doi:10.3389/fcell.2021.764727
Sung, H., Ferlay, J., Siegel, R. L., Laversanne, M., Soerjomataram, I., Jemal, A., et al. (2021). Global cancer statistics 2020: GLOBOCAN estimates of incidence and mortality worldwide for 36 cancers in 185 countries. CA Cancer J. Clin. 71, 209–249. doi:10.3322/caac.21660
Svagzdys, S., Lesauskaite, V., Pavalkis, D., Nedzelskiene, I., Pranys, D., and Tamelis, A. (2009). Microvessel density as new prognostic marker after radiotherapy in rectal cancer. BMC Cancer 9, 95. doi:10.1186/1471-2407-9-95
Szajnik, M., Czystowska, M., Szczepanski, M. J., Mandapathil, M., and Whiteside, T. L. (2010). Tumor-derived microvesicles induce, expand and up-regulate biological activities of human regulatory T cells (Treg). PLoS One 5 (7), e11469. doi:10.1371/journal.pone.0011469
Taeb, S., Ashrafizadeh, M., Zarrabi, A., Rezapoor, S., Musa, A. E., Farhood, B., et al. (2022). Role of tumor microenvironment in cancer stem cells resistance to radiotherapy. Curr. Cancer Drug Targets 22 (1), 18–30. doi:10.2174/1568009622666211224154952
Talbot, L. J., Bhattacharya, S. D., and Kuo, P. C. (2012). Epithelial-mesenchymal transition, the tumor microenvironment, and metastatic behavior of epithelial malignancies. Int. J. Biochem. Mol. Biol. 3 (2), 117–136.
Tan, S., Yang, Y., Yang, W., Han, Y., Huang, L., Yang, R., et al. (2023). Exosomal cargos-mediated metabolic reprogramming in tumor microenvironment. J. Exp. Clin. Cancer Res. 42 (1), 59. doi:10.1186/s13046-023-02634-z
Tarasov, V. V., Svistunov, A. A., Chubarev, V. N., Dostdar, S. A., Sokolov, A. V., Brzecka, A., et al. (2021). Extracellular vesicles in cancer nanomedicine. Semin. Cancer Biol. 69, 212–225. doi:10.1016/j.semcancer.2019.08.017
Teicher, B. A., Holden, S. A., Ara, G., Dupuis, N. P., Liu, F., Yuan, J., et al. (1995). Influence of an anti-angiogenic treatment on 9L gliosarcoma: oxygenation and response to cytotoxic therapy. Int. J. Cancer 61 (5), 732–737. doi:10.1002/ijc.2910610523
Topalian, S. L., Hodi, F. S., Brahmer, J. R., Gettinger, S. N., Smith, D. C., McDermott, D. F., et al. (2012). Safety, activity, and immune correlates of anti-PD-1 antibody in cancer. N. Engl. J. Med. 366 (26), 2443–2454. doi:10.1056/NEJMoa1200690
Uribesalgo, I., Hoffmann, D., Zhang, Y., Kavirayani, A., Lazovic, J., Berta, J., et al. (2019). Apelin inhibition prevents resistance and metastasis associated with anti-angiogenic therapy. EMBO Mol. Med. 11 (8), e9266. doi:10.15252/emmm.201809266
Valenti, R., Huber, V., Filipazzi, P., Pilla, L., Sovena, G., Villa, A., et al. (2006). Human tumor-released microvesicles promote the differentiation of myeloid cells with transforming growth factor-beta-mediated suppressive activity on T lymphocytes. Cancer Res. 66 (18), 9290–9298. doi:10.1158/0008-5472.CAN-06-1819
Viallard, C., and Larrivee, B. (2017). Tumor angiogenesis and vascular normalization: alternative therapeutic targets. Angiogenesis 20 (4), 409–426. doi:10.1007/s10456-017-9562-9
Viswanath, D., Park, J., Misra, R., Pizzuti, V. J., Shin, S. H., Doh, J., et al. (2023). Nanotechnology-enhanced radiotherapy and the abscopal effect: current status and challenges of nanomaterial-based radio-immunotherapy. Wiley Interdiscip. Rev. Nanomed Nanobiotechnol, e1924. doi:10.1002/wnan.1924
Viswanathan, A. N., Moughan, J., Miller, B. E., Xiao, Y., Jhingran, A., Portelance, L., et al. (2015). NRG oncology/RTOG 0921: A phase 2 study of postoperative intensity-modulated radiotherapy with concurrent cisplatin and bevacizumab followed by carboplatin and paclitaxel for patients with endometrial cancer. Cancer 121 (13), 2156–2163. doi:10.1002/cncr.29337
Waas, E. T., Hendriks, T., Lomme, R. M. L. M., and Wobbes, T. (2005). Plasma levels of matrix metalloproteinase-2 and tissue inhibitor of metalloproteinase-1 correlate with disease stage and survival in colorectal cancer patients. Dis. Colon Rectum 48 (4), 700–710. doi:10.1007/s10350-004-0854-y
Waller, V., and Pruschy, M. (2021). Combined radiochemotherapy: metalloproteinases revisited. Front. Oncol. 11, 676583. doi:10.3389/fonc.2021.676583
Wang, H., Zheng, Q., Lu, Z., Wang, L., Ding, L., Xia, L., et al. (2021a). Role of the nervous system in cancers: A review. Cell Death Discov. 7 (1), 76. doi:10.1038/s41420-021-00450-y
Wang, J., Boerma, M., Fu, Q., and Hauer-Jensen, M. (2007). Significance of endothelial dysfunction in the pathogenesis of early and delayed radiation enteropathy. World J. Gastroenterol. 13 (22), 3047–3055. doi:10.3748/wjg.v13.i22.3047
Wang, J., Dai, Z., Miao, Y., Zhao, T., Gan, J., Zhao, C., et al. (2021b). Carbon ion ((12)C(6+)) irradiation induces the expression of Klrk1 in lung cancer and optimizes the tumor microenvironment based on the NKG2D/NKG2D-Ls pathway. Cancer Lett. 521, 178–195. doi:10.1016/j.canlet.2021.09.003
Watanabe, K., Shiga, K., Maeda, A., Harata, S., Yanagita, T., Suzuki, T., et al. (2022). Chitinase 3-like 1 secreted from cancer-associated fibroblasts promotes tumor angiogenesis via interleukin-8 secretion in colorectal cancer. Int. J. Oncol. 60 (1), 3. doi:10.3892/ijo.2021.5293
Wattenberg, M. M., Fahim, A., Ahmed, M. M., and Hodge, J. W. (2014). Unlocking the combination: potentiation of radiation-induced antitumor responses with immunotherapy. Radiat. Res. 182 (2), 126–138. doi:10.1667/RR13374.1
Weber, C. E., and Kuo, P. C. (2012). The tumor microenvironment. Surg. Oncol. 21 (3), 172–177. doi:10.1016/j.suronc.2011.09.001
Wei, J., Wang, B., Wang, H., Meng, L., Zhao, Q., Li, X., et al. (2019). Radiation-induced normal tissue damage: oxidative stress and epigenetic mechanisms. Oxid. Med. Cell Longev. 2019, 3010342. doi:10.1155/2019/3010342
Wei, R., Lv, M., Li, F., Cheng, T., Zhang, Z., Jiang, G., et al. (2017). Human CAFs promote lymphangiogenesis in ovarian cancer via the Hh-VEGF-C signaling axis. Oncotarget 8 (40), 67315–67328. doi:10.18632/oncotarget.18621
Weichselbaum, R. R., Liang, H., Deng, L., and Fu, Y. X. (2017). Radiotherapy and immunotherapy: A beneficial liaison? Nat. Rev. Clin. Oncol. 14 (6), 365–379. doi:10.1038/nrclinonc.2016.211
Whiteside, T. L. (2012). What are regulatory T cells (Treg) regulating in cancer and why? Semin. Cancer Biol. 22 (4), 327–334. doi:10.1016/j.semcancer.2012.03.004
Wieder, R. (2023). Fibroblasts as turned agents in cancer progression. Cancers (Basel) 15 (7), 2014. doi:10.3390/cancers15072014
Wu, J., Li, Y., Dang, Y. Z., Gao, H. X., Jiang, J. L., and Chen, Z. N. (2015). HAb18G/CD147 promotes radioresistance in hepatocellular carcinoma cells: A potential role for integrin β1 signaling. Mol. Cancer Ther. 14 (2), 553–563. doi:10.1158/1535-7163.MCT-14-0618
Xia, L., Guan, W., Wang, D., Zhang, Y. S., Zeng, L. L., Li, Z. P., et al. (2013). Killing effect of Ad5/F35-APE1 siRNA recombinant adenovirus in combination with hematoporphrphyrin derivative-mediated photodynamic therapy on human nonsmall cell lung cancer. Biomed. Res. Int. 2013, 957913. doi:10.1155/2013/957913
Xie, M., and Su, C. (2022). Microenvironment and the progress of immunotherapy in clinical practice of NSCLC brain metastasis. Front. Oncol. 12, 1006284. doi:10.3389/fonc.2022.1006284
Xie, Y., Zhang, H., Li, W., Deng, Y., Munegowda, M. A., Chibbar, R., et al. (2010). Dendritic cells recruit T cell exosomes via exosomal LFA-1 leading to inhibition of CD8+ CTL responses through downregulation of peptide/MHC class I and Fas ligand-mediated cytotoxicity. J. Immunol. 185 (9), 5268–5278. doi:10.4049/jimmunol.1000386
Yadav, V. N., Altshuler, D., Kadiyala, P., Zamler, D., Comba, A., Appelman, H., et al. (2018). Molecular ablation of tumor blood vessels inhibits therapeutic effects of radiation and bevacizumab. Neuro Oncol. 20 (10), 1356–1367. doi:10.1093/neuonc/noy055
Yang, D., Liu, J., Qian, H., and Zhuang, Q. (2023). Cancer-associated fibroblasts: from basic science to anticancer therapy. Exp. Mol. Med. 55 (7), 1322–1332. doi:10.1038/s12276-023-01013-0
Yang, Z., Zhong, W., Yang, L., Wen, P., Luo, Y., and Wu, C. (2022). The emerging role of exosomes in radiotherapy. Cell Commun. Signal 20 (1), 171. doi:10.1186/s12964-022-00986-1
Yokosaki, Y., and Nishimichi, N. (2021). New therapeutic targets for hepatic fibrosis in the integrin family, α8β1 and α11β1, induced specifically on activated stellate cells. Int. J. Mol. Sci. 22 (23), 12794. doi:10.3390/ijms222312794
Yoo, D. S., Kirkpatrick, J. P., Craciunescu, O., Broadwater, G., Peterson, B. L., Carroll, M. D., et al. (2012). Prospective trial of synchronous bevacizumab, erlotinib, and concurrent chemoradiation in locally advanced head and neck cancer. Clin. Cancer Res. 18 (5), 1404–1414. doi:10.1158/1078-0432.CCR-11-1982
Yu, X., Harris, S. L., and Levine, A. J. (2006). The regulation of exosome secretion: A novel function of the p53 protein. Cancer Res. 66 (9), 4795–4801. doi:10.1158/0008-5472.CAN-05-4579
Yuan, L., Shu, C., Zhou, X., Li, J., Wang, L., Li, X., et al. (2018). Radiation suppresses neointimal hyperplasia through affecting proliferation and apoptosis of vascular smooth muscle cells. J. Vasc. Access 19 (2), 153–161. doi:10.5301/jva.5000804
Zakeri, K., Coleman, C. N., and Vikram, B. (2018). Radiation oncology in the 21st century: prospective randomized trials that changed practice. Or didn't!. Front. Oncol. 8, 130. doi:10.3389/fonc.2018.00130
Zeng, J., See, A. P., Phallen, J., Jackson, C. M., Belcaid, Z., Ruzevick, J., et al. (2013). Anti-PD-1 blockade and stereotactic radiation produce long-term survival in mice with intracranial gliomas. Int. J. Radiat. Oncol. Biol. Phys. 86 (2), 343–349. doi:10.1016/j.ijrobp.2012.12.025
Zhai, Y., Hui, Z., Wang, J., Zou, S., Liang, J., Wang, X., et al. (2013). Concurrent erlotinib and radiotherapy for chemoradiotherapy-intolerant esophageal squamous cell carcinoma patients: results of a pilot study. Dis. Esophagus 26 (5), 503–509. doi:10.1111/j.1442-2050.2012.01380.x
Zhang, C., Deng, X., Qiu, L., Peng, F., Geng, S., Shen, L., et al. (2018). Knockdown of C1GalT1 inhibits radioresistance of human esophageal cancer cells through modifying β1-integrin glycosylation. J. Cancer 9 (15), 2666–2677. doi:10.7150/jca.25252
Zhang, K., Wang, Y., Yu, X., Shi, Y., Yao, Y., Wei, X., et al. (2017). Recombinant human endostatin combined with radiotherapy inhibits colorectal cancer growth. BMC Cancer 17 (1), 899. doi:10.1186/s12885-017-3903-3
Zhang, S. C., Miyamoto, S. i., Kamijo, T., Hayashi, R., Hasebe, T., Ishii, G., et al. (2003). Intratumor microvessel density in biopsy specimens predicts local response of hypopharyngeal cancer to radiotherapy. Jpn. J. Clin. Oncol. 33 (12), 613–619. doi:10.1093/jjco/hyg121
Zhang, Y., Lv, N., Li, M., Liu, M., and Wu, C. (2023b). Cancer-associated fibroblasts: tumor defenders in radiation therapy. Cell Death Dis. 14 (8), 541. doi:10.1038/s41419-023-06060-z
Zhang, Y., Peng, Q., Zheng, J., Yang, Y., Zhang, X., Ma, A., et al. (2023a). The function and mechanism of lactate and lactylation in tumor metabolism and microenvironment. Genes Dis. 10 (5), 2029–2037. doi:10.1016/j.gendis.2022.10.006
Zhao, J. D., Liu, J., Ren, Z. G., Gu, K., Zhou, Z. H., Li, W. T., et al. (2010). Maintenance of sorafenib following combined therapy of three-dimensional conformal radiation therapy/intensity-modulated radiation therapy and transcatheter arterial chemoembolization in patients with locally advanced hepatocellular carcinoma: A phase I/II study. Radiat. Oncol. 5, 12. doi:10.1186/1748-717X-5-12
Zheng, H., Liu, H., Ge, Y., and Wang, X. (2021a). Integrated single-cell and bulk RNA sequencing analysis identifies a cancer associated fibroblast-related signature for predicting prognosis and therapeutic responses in colorectal cancer. Cancer Cell Int. 21 (1), 552. doi:10.1186/s12935-021-02252-9
Zheng, H., Liu, H., Li, H., Dou, W., and Wang, X. (2021b). Weighted gene Co-expression network analysis identifies a cancer-associated fibroblast signature for predicting prognosis and therapeutic responses in gastric cancer. Front. Mol. Biosci. 8, 744677. doi:10.3389/fmolb.2021.744677
Zhu, H., and Zhang, S. (2018). Hypoxia inducible factor-1α/vascular endothelial growth factor signaling activation correlates with response to radiotherapy and its inhibition reduces hypoxia-induced angiogenesis in lung cancer. J. Cell Biochem. 119 (9), 7707–7718. doi:10.1002/jcb.27120
Keywords: drug resistance, radiotherapy, tumor microenvironment, angiogenesis, cancer-associated fibroblasts, exosome, anti-cancer immunity
Citation: Zhao D, Mo Y, Neganova ME, Aleksandrova Y, Tse E, Chubarev VN, Fan R, Sukocheva OA and Liu J (2023) Dual effects of radiotherapy on tumor microenvironment and its contribution towards the development of resistance to immunotherapy in gastrointestinal and thoracic cancers. Front. Cell Dev. Biol. 11:1266537. doi: 10.3389/fcell.2023.1266537
Received: 25 July 2023; Accepted: 19 September 2023;
Published: 03 October 2023.
Edited by:
Cailian Wang, Southeast University, ChinaReviewed by:
Lingxiang Jiang, Indiana University, United StatesMichele Bernasconi, University Children’s Hospital Bern, Switzerland
Copyright © 2023 Zhao, Mo, Neganova, Aleksandrova, Tse, Chubarev, Fan, Sukocheva and Liu. This is an open-access article distributed under the terms of the Creative Commons Attribution License (CC BY). The use, distribution or reproduction in other forums is permitted, provided the original author(s) and the copyright owner(s) are credited and that the original publication in this journal is cited, in accordance with accepted academic practice. No use, distribution or reproduction is permitted which does not comply with these terms.
*Correspondence: Olga A. Sukocheva, b2xnYS5zdWtvY2hldmFAc2EuZ292LmF1; Junqi Liu, ZmNjbGl1anFAenp1LmVkdS5jbg==