- Institut Pasteur, Université Paris Cité, CNRS UMR3738, Structure and Signals in the Neurogenic Niche, Paris, France
Neural stem/progenitor cells live in an intricate cellular environment, the neurogenic niche, which supports their function and enables neurogenesis. The niche is made of a diversity of cell types, including neurons, glia and the vasculature, which are able to signal to and are structurally organised around neural stem/progenitor cells. While the focus has been on how individual cell types signal to and influence the behaviour of neural stem/progenitor cells, very little is actually known on how the niche is assembled during development from multiple cellular origins, and on the role of the resulting topology on these cells. This review proposes to draw a state-of-the art picture of this emerging field of research, with the aim to expose our knowledge on niche architecture and formation from different animal models (mouse, zebrafish and fruit fly). We will span its multiple aspects, from the existence and importance of local, adhesive interactions to the potential emergence of larger-scale topological properties through the careful assembly of diverse cellular and acellular components.
1 Introduction
Our central nervous system (CNS) is an exquisitely complex organ which allows us to live in and interact with our environment by receiving, integrating and sending information (Purves et al., 2019). It relies on the functions of highly specialized cells, the neurons, which form intricate cellular connections and ultimately networks to handle these exchanges. Alongside, glial cells (astrocytes and oligodendrocytes) are key players ensuring structural support and insulation of neuronal fibers, as well as a diversity of regulatory roles. Both cell types must be produced and integrated in time and space to assemble into a functional community.
The complete process of generating new functional neurons is called neurogenesis and encompasses cell division, migration, differentiation, maturation, and integration into circuits (Binder et al., 2009; Squire et al., 2009; Purves et al., 2019). Similarly, gliogenesis produces functional glial cells. In this review, we will use neuro/gliogenesis when we do not wish to specifically restrict or distinguish between the two.
Developmental neuro/gliogenesis supports the formation of a functional nervous system in animals, whether centralized or diffuse (Bayraktar et al., 2014; Paridaen and Huttner, 2014; Holguera and Desplan, 2018; Villalba et al., 2021). It is unsurprisingly high, taking place throughout the tissue at a sustained pace, and often extends for some time after birth/hatching. Throughout this period, an extensive range of neuronal and glial subtypes is generated.
At later stages (from infancy to adulthood and into ageing), neuro/gliogenesis still happens in many organisms, with variable extents of outputs and locations, defying a long-held notion that there was little capacity for de novo production of neurons and glia outside of development (Colucci-D’Amato et al., 2006; Bond et al., 2015). Evidence of adult neuro/gliogenesis has now been reported for teleost fishes (Byrd and Brunjes, 2001), cephalopods (Bertapelle et al., 2017), amphibians (Bernocchi et al., 1990) and reptiles (Marchioro et al., 2005), song birds (Goldman and Nottebohm, 1983), rats (Altman and Das, 1965) and mice (Reynolds and Weiss, 1992), non-human primates (Gould et al., 1998) and humans (Eriksson et al., 1998) -a non-extensive list (for an illuminating comparative view, see (Lindsey and Tropepe, 2006)).
Both in a developing or adult nervous system (we will focus here on centralized systems), decades of study have shown that neuro/gliogenesis is initiated and supported by cells endowed with remarkable properties, the neural stem cells.
2 Building a nervous system from stem cells
Tissue stem cells are responsible for the formation and maintenance of the tissue/organ they reside in. To do so, they balance self-renewal with differentiation to maintain their pool while generating specialized cells. They can also shuttle between proliferation and quiescence, a reversible, mitotically dormant phase. A precise regulation of stem cell identity, fate and features (stemness; self-renewal; survival; proliferation; differentiation) is thus crucial to the health of their tissue of residence (for an inspiring discussion on the definition of stem cell, see (Potten and Loeffler, 1990)).
The stem cells of the CNS are called neural stem cells (NSCs). NSCs are traditionally defined as multipotent progenitors able to self-renew through their lifetime to generate the diversity of neuron and glial cells which makes up the nervous system ((Temple, 2012) and see (Breunig et al., 2011) for an historical perspective). Thus far, NSCs have been discovered in many species, and their identification keeps increasing thanks to advancements in single-cell sequencing technologies. Best developed experimental models comprise rodents (mouse, and rat to a lesser extent), fishes (zebrafish, and medaka and killifish to a lesser extent), amphibians (Xenopus), birds (especially songbirds) and invertebrates (mainly Drosophila melanogaster). Newer or on the rise models include octopus (Styfhals et al., 2022), hydra (Galliot and Quiquand, 2011) and axolotl (Lust et al., 2022).
During development, NSCs, from ectodermal origin, are found throughout the CNS. They actively proliferate, mixing expansion, through symmetric division, with differentiation, through asymmetric division (Schmidt et al., 2013; Paridaen and Huttner, 2014; Mira and Morante, 2020), to ensure that the correct amount of progeny is generated while preserving their pool. Asymmetric division can be directly neurogliogenic, but often rather produce progenitors with more restricted potential, and which further divide to generate neurons and/or glia.
In adults, NSCs are also present and active to support neuro/gliogenesis, whose extent varies between species. In most teleost fishes, for example, the CNS keeps growing along with the skull, and NSCs are found in extensive locations, providing constant neuro/gliogenesis (reviewed in (Zupanc, 2021)). Conversely, in mammals, NSCs are found in restricted locations, where they provide both basal and adaptive neuro/gliogenesis (reviewed in (Gage, 2019; Obernier and Alvarez-Buylla, 2019). In humans in particular, adult neuro/gliogenesis also happens in restricted places, and potentially declining with age, with its extent still debated (Ghibaudi and Bonfanti, 2022). Nevertheless, a common trait of adult neuro/gliogenesis is the balance between proliferative and quiescent states to provide homeostatic control of the NSC population and/or long-term NSC maintenance and protection. Quiescence is proposed to exist in multiple shades, from deep to shallow, with an active on-going research to find discriminating markers and crucial regulators (Urbán et al., 2019).
It is worth noting that the frontier between NSCs and neural progenitors can sometimes be hazy, and during development, the overarching term of neural stem/progenitor cells (NSPCs) is often used. In the developing mouse for example, NSCs stricto sensu are the neuroepithelial cells and the radial glia, yet at the single cell level these exist in a range of identity, fate and time restriction with no differentiating markers. The advent of single-cell technologies has indeed brought forward the idea that cell identity might be a continuum rather than a series of discrete states (Marcy and Raineteau, 2019). Live-imaging also revealed an unexpected range of individual cellular behaviour and fate, with some restricted progenitors (transient amplifying) able of prolonged symmetric divisions while stem cells (radial glia) can directly differentiate into two neurons (Pilz et al., 2018). Earlier studies already showed that most radial glia primarily produce neurons, while some are specialized in generating glial cells (Malatesta et al., 2000; Pinto et al., 2008). This raised questions about their multipotency and suggested that many radial glia may already have lineage restrictions, making them better suited to a progenitor classification. An interesting discussion on stemness, potency and generally cell individuality in the CNS can be found in (Petrik et al., 2022).
3 What is a neurogenic niche?
3.1 Emergence and evolution of the concept of stem cell niche
While a well-defined genetic program instructs stem cell behaviour during development and homeostasis, they are also exposed and sensitive to the extrinsic signals coming from their cellular microenvironment. Indeed, tissue stem cells are not isolated but rather embedded within a complex and specific neighbourhood made up by cellular as well as acellular cues. From this observation rose the concept of the stem cell niche.
Shortly after the discovery of tissue stem cells in the hematopoietic system, and pioneering work on their potential regulation by their cellular context (Lord et al., 1975; Dexter et al., 1977), Schofield indeed proposed that stem cells were found in and regulated by a unique localized microenvironment he termed stem cell niche (Schofield, 1978). This initial definition was drawn from the ecological field and also within the experimental context of the mammalian haemopoietic stem cells, one of the flourishing models at the time. Stem cells were believed to lose their characteristics like self-renewal or ability to differentiate into other cell types if taken away from their residency site. Conversely, a cell could become stem cell if transplanted in such niche. Other examples of niche, for different stem cells and in different biological systems, followed to confirm the importance of such microenvironment in stem cell maintenance. In mammals, tissue stem cells and their niches were also uncovered in the intestine (Cheng and Leblond, 1974), skin (Cotsarelis et al., 1990) and brain (Eriksson et al., 1998; Doetsch et al., 1999). Experimental evidence from Caenorhabditis elegans identified stem cells in the germline in constant communication with nearby somatic cells to maintain their features (Kimble and White, 1981). Similarly, a landmark paper showed in Drosophila melanogaster that somatic cells in the female ovariole organise into a cellular microenvironment that maintains and controls germline stem cells (Xie and Spradling, 2000). Stem cell niches were also identified in plants, starting with the shoot apical meristem of Arabidopsis thaliana (Clark et al., 1997).
Yet, the identification over the years of more and more stem cell types, both during developmental and adult stages, and in a diversity of organisms, slowly challenged the initial, strict definition of the stem cell niche (Lander et al., 2012). NSCs can keep their stemness and proliferate outside of their normal cellular microenvironment (Temple, 1989), forming neurospheres. Not every cell can become stem cell once implanted in a stem cell niche. The conversion of transplanted cells into tissue-specific stem cells might also not only depend on inductive properties of the niche, but rather be in combination with the plasticity/reprogramming potential of these cells themselves; for example, NSCs transplanted in irradiated mice produce a variety of blood cell types, acquiring an hematopoietic identity (Bjornson et al., 1999).
3.2 The stem cell niche nowadays
So, what defines a stem cell niche nowadays? Our opinion is that a combination of functional and spatial attributes could hold the answer.
First, we propose that the niche enables and supports the physiological roles of tissue stem cells. These roles can encompass survival and maintenance of stemness as initially proposed, but also finer, yet crucial, characteristics, such as the temporal generation of diverse progeny, cell fitness or the balance between proliferative and quiescent phases. As such, the underlying idea here is not that tissue stem cells cannot exist without a niche, but rather that they cannot perform their normal homeostatic program and response to perturbations.
Second, the niche retains a proximity element, encompassing cells, cell parts (i.e., cytoplasmic extensions) and acellular components in direct contact or very close vicinity of the stem cells. As such, neighbouring stem cells can also be considered part of one stem cell’s niche, and a stem cell progeny is an automatic component, at least in the timescale following its generation. While we propose that the concept of a “distant” niche, regulating stem cells from afar, can also be brought in, it can be redefined as a special case in which even though the initial component might be remote, its acting element(s) (soluble molecule, cytoplasmic extensions) are able to reach the stem cells. As illustration, adult NSCs are regulated by the innervation of neurons from the distant hypothalamic region (Paul et al., 2017) and also by circulating factors produced from remote sources and carried by the blood (Horowitz et al., 2020) and the cerebro-spinal fluid (CSF) (Silva-Vargas et al., 2016). While the cell bodies generating this innervation and factors are far, their products are ultimately in contact with the stem cells. Linked to this proximity aspect is also the concept that the niche is not merely a collection of juxtaposed components but harbours a stereotypical architecture, organising stem cells spatially and poising them for specific interactions.
Third, the niche acts as a signalling hub for the stem cells. It can produce and exchange its own paracrine signals, be the local receiver of further cues, and it is also an interface and mediator of systemic and environmental cues. For instance, Paneth cells of the intestinal stem cell niche secrete crucial signalling molecules for stem cell function and protection (Cui et al., 2023). An extension of its signalling role is the capacity of the niche to coordinate signals, between different tissue compartments and with environmental cues. For instance, in the Drosophila testis, the niche coordinates responses to extrinsic stress signals like high temperature or toxin exposure by activating the JAK-STAT pathway in the germline stem cells, ensuring the appropriate production of sperm in changing conditions (Tulina and Matunis, 2001).
3.3 The neurogenic niche: definition and core components
The neurogenic niche is the niche of the NSCs, and of neural progenitors in a larger extent. It is predicted to co-exist with NSPCs, yet so far has only been properly characterized for few species and at given stages. From these different models, and in line with what we discussed above, a current, prevailing view of the neurogenic niche brings the proximity of cellular and molecular components with their ability to influence NSPC behaviour while conveying informational cues (Binder et al., 2009; Bjornsson et al., 2015; Llorente et al., 2022). The neurogenic niche is typically a supportive and specialized microenvironment in which NSPCs reside. A core set of common cellular components has also emerged from these models, comprising the NSPCs themselves, different types of neurons and glia, a local circulatory system and finally cells with some immune or cell clearance capacity. Moreover, a “distant” niche often exists as a secretory organ. Around this definition and features exist declinations in space, time, composition and roles depending on the species.
This review aims to discuss how such a neurogenic niche is built during development, specifically how its different components come together and interact to form its architecture, comparing the three current main systems for the study of neuro/gliogenesis: the mouse, the zebrafish and the fruit fly. This implies the choice of a well-defined neurogenic niche as a destination, what we will call here a mature niche. Before delving into niche formation, we will thus first introduce the composition and architecture of these selected mature niches. It will correspond to the adult ventricular-subventricular zone in the mouse (end of the timeline in Figure 1), the adult pallium in the zebrafish (end of the timeline in Figure 2) and the late larval ventral nerve cord in the fruit fly (end of the timeline in Figure 3). We will then describe how a rollercoaster of events during development generate these mature niches as a result of spatial and temporal continuity.
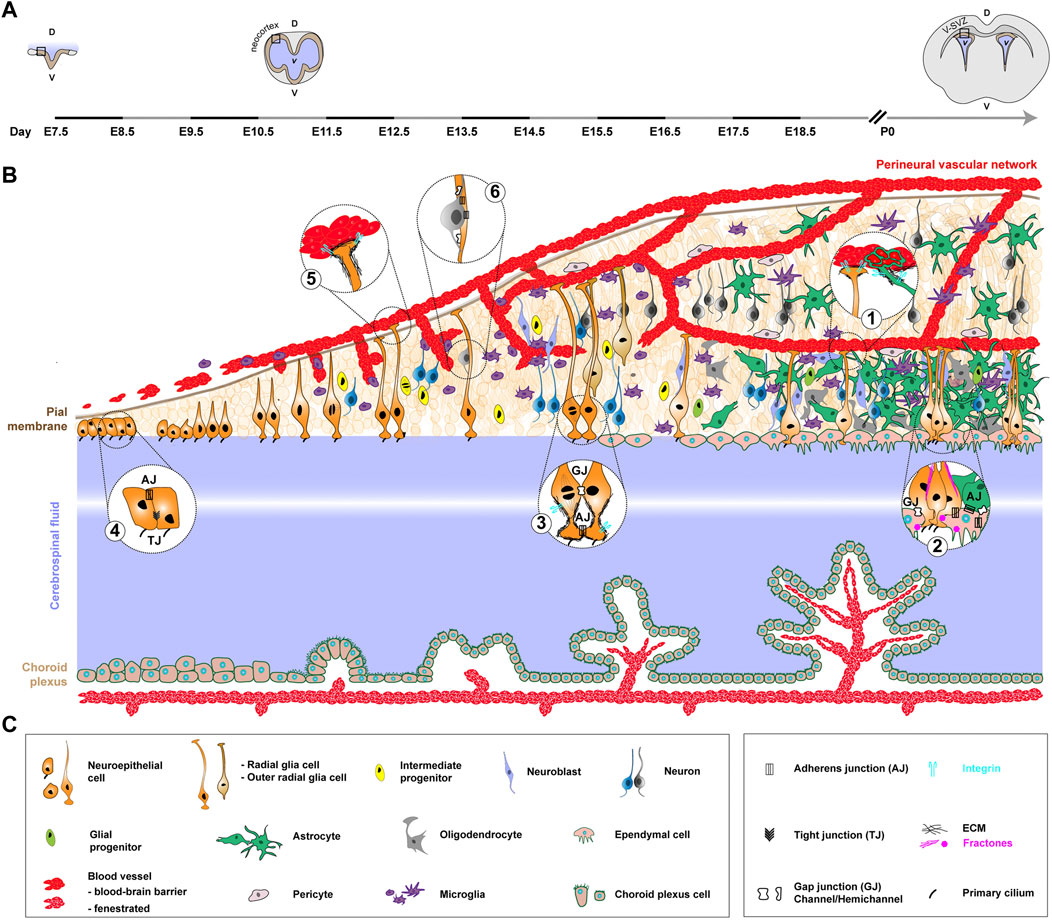
FIGURE 1. Formation of the neurogenic niche in the mouse, from early embryonic development to adulthood. This figure illustrates ventricular neurogenesis, which occurs along the wall of the CSF-filled ventricles. It serves as a model for understanding the core principles governing the formation and organization of the neurogenic niche in mice. It starts from the ventricular zone of the developing neocortex in embryonic stages and reaches the restricted location of the V-SVZ of the lateral walls (mature niche) by early postnatal period and then adulthood. (A). Coronal view of the developing mouse CNS at different stages and associated timeline. The timeline, left to right, covers early embryonic development (embryonic day 7.5, E7.5) to early postnatal period. The stages depicted are neural groove (E7.5), closed and developed neural tube at the onset of neurogenesis (E11.5) and post-birth/young adult (P0, pups 0 h). The black rectangle highlights the zone represented in (B). D, dorsal. V, ventral. v, ventricle. (B). NSPCs mostly comprise the neuroepithelial cells and RGCs they convert into (orange), with also a contribution of outer RGCs (light brown). The apical processes of RGCs lie in the ventricular zone, whereas their elongated basal processes extend to the outer surface of the brain, connecting to the pial surface and blood vessels. NSPCs divide both symmetrically to amplify their pool and asymmetrically to give rise to intermediate neural (yellow) progenitors, further leading to the production of neuroblasts (light purple) and neurons (blue or grey, colour-coded to show temporal series). The basal process of RGCs guides newborn neurons to migrate away from the V-SVZ niche into the outer surface of the CNS to differentiate and function by forming connections. The neuronal subtypes which are produced, and their destinations, are different between embryonic and adult neurogenesis, yet the general principles of generation and migration are preserved. Late during embryonic development and during the early postnatal period, neural progenitors largely produce glial cells, including astrocytes (green) and oligodendrocytes (grey), either through the generation of glial progenitors (aniseed colour) or direct conversion. They also convert into ependymal cells (beige) which will separate the niche from the CSF (in light blue/purple, with a central white hue to represent greater distance). In parallel, three cell populations from external origin will contribute to the neurogenic niche. First, microglia (purple), generated from precursors in the yolk sac, enter the CNS during early development, where they will amplify and mature. A complex network of ventricular vasculature (red) is also built from sprouting and invading vessels coming from a peri-CNS plexus (perineural vascular network). Pericytes (light grey), from heterogeneous origin, are recruited to blood vessels to help forming the blood-brain barrier. Finally, the choroid plexus (beige), in continuity with the ependymal layer, is formed in remote locations from folding epithelial sheet, encloses blood vessels as they form and offers an example of a distant neurogenic niche. Bubbles contain magnification of specific structural features of the niche, completed by relevant adhesions and other molecular complexes (not depicted on the cellular timeline). (C). Legends of the cellular and molecular features depicted in (B).
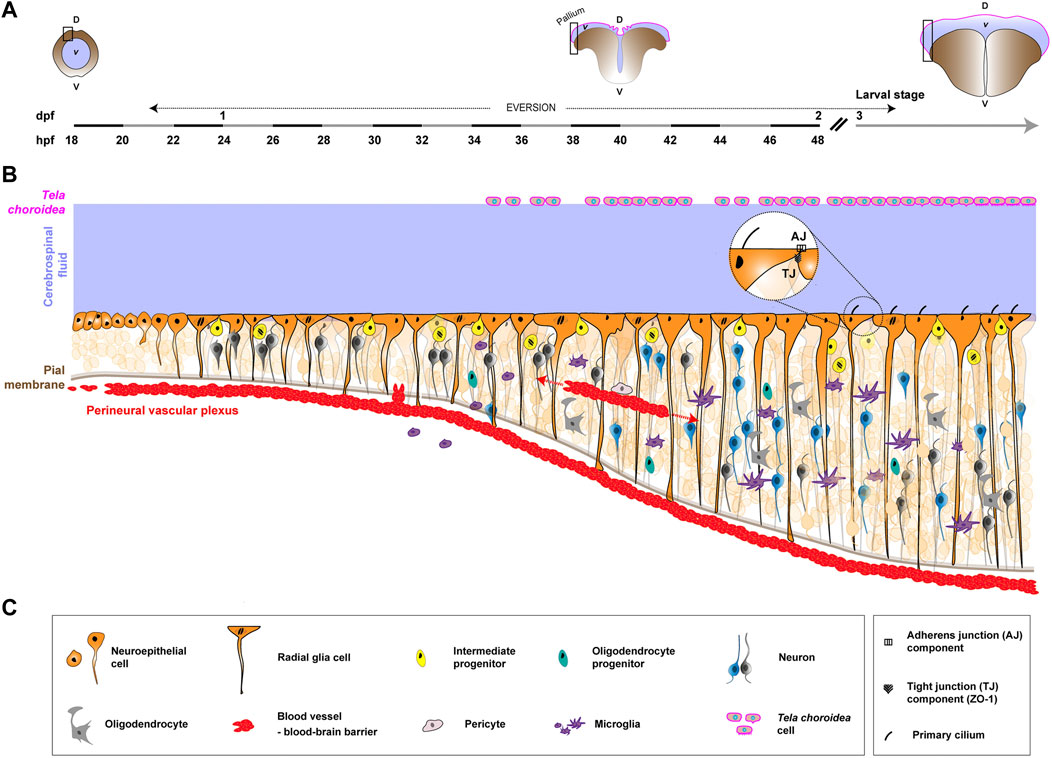
FIGURE 2. Formation of the neurogenic niche in the zebrafish pallium, from early embryonic development to larval/adulthood stage. Pallial neuro/gliogenesis happens in a continuous fashion from embryonic to adult stages and offers a straightforward link between developing and mature neurogenic niches. (A). Coronal view of the developing zebrafish CNS at different stages and associated timeline. The timeline, left to right, covers early embryonic development (18 h post-fertilization, 18 hpf) into the larval stage (3 days post-fertilization, 3 dpf). The stages depicted are newly-formed neural tube (18–20 hpf), during telencephalon eversion (18 hpf to 5 dpf) and post-hatching/larval stage (from 3 dpf). The black rectangle highlights the zone represented in (B). D, dorsal. V, ventral. v, ventricle. While a neural tube filled with CSF (light blue/purple) is originally formed, this canal structure is transient. The neuroepithelium undergoes eversion, exposing proliferative zones on the outer surface of the telencephalon. The tela choroidea (magenta line) covers the ventricle dorsally, encasing the CSF. (B). Schematics of the formation of the neurogenic niche of the zebrafish pallium. Similarly to mammals, NSPCs (orange) are neuroepithelial cells and RGCs, successively. The later exhibit a radial, apico-basal polarity, with their basal process reaching the pial surface. Asymmetric cell divisions occur, with a Notch-driven signaling between daughter cells determining their fate and producing more restricted progenitors (yellow) which generate neurons (dark grey and blue) from 24 hpf. Some Oligo2+ progenitors (duck egg) give rise to oligodendrocytes (grey) around 30 hpf, which further mature and myelinate. External components also make the zebrafish neurogenic niche. First, microglia (purple), derived from the yolk sac, begin to colonize the CNS during mid-embryogenesis. They shift from an ameboid-like shape to a ramified morphology. Vascularization (red) starts with vasculogenesis and angiogenesis, forming the perineural vascular plexus around the brain and spinal cord. In different CNS regions, angiogenic endothelial tips sprout and connect to create a dense intraneural network. Blood-brain barrier function is established around 2.5–3 days post fertilization (dpf), coinciding with angiogenesis, and is supported by pericytes (light grey). Yet, most of the knowledge on CNS vascularization is derived from other regions, and much is left to understand in the pallium. Finally, the tela choroidea (magenta) covers the pallial sheet of radial glial cells in the zebrafish. Its morphogenetic events and role are little documented. (C). Legends of the cellular and molecular features depicted in (B).
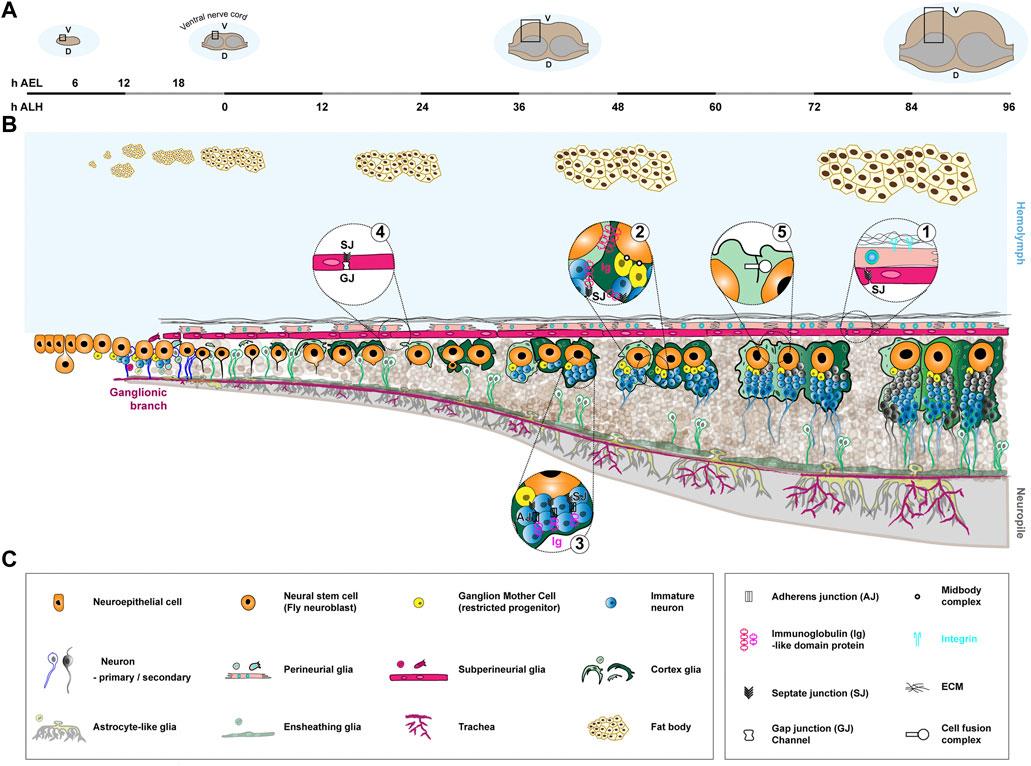
FIGURE 3. Formation of the neurogenic niche in the Drosophila larval ventral nerve cord. Neuro/gliogenesis in the ventral nerve cord is sustained by Type I NSCs from the embryonic stage to late larval (mature) stages, with a clear spatial and temporal continuity allowing to follow the formation of the corresponding neurogenic niche. (A). Coronal view of the Drosophila CNS (ventral nerve cord) at different stages and associated timeline. The timeline, left to right, covers the embryonic (0–22 h after egg laying (AEL) at 25°C) and larval stages (0–96 h after larval hatching (ALH) at 25°C). The stages depicted are early embryo (6 h AEL), just hatched larva (0 h ALH), when NSCs have reactivated (36–48 h ALH) and at the end of larval stage, when NSCs prepare to exit the cell cycle (96 h ALH). The black rectangle highlights the zone represented in (B). D, dorsal. V, ventral. (B). Schematics of the formation of the neurogenic niche of the Drosophila ventral nerve cord. NSCs (orange) are generated by delamination from a neuroectodermal layer during embryogenesis and persist during the larval stage. They actively proliferate during embryogenesis, relying on asymmetric division to self-renew and generate a restricted precursor, the Ganglion Mother Cell (yellow), which will divide once more to give birth to two primary neurons (white body and blue outline). NSCs then enter a quiescence phase around 16 h AEL, which lasts until the beginning of larval stages. They then reactivate upon nutritional cues and divide asymmetrically to generate secondary neurons (blue and grey bodies and outlines). Gliogenesis mostly happens during embryogenesis, during which cortex glia (light and dark green) are born. Cortex glia are in close association with NSCs and progeny. They also encapsulate mature primary, embryonic (white body and green outline) and secondary (blue and grey bodies and green outlines) neurons. Cortex glia undergo morphogenetic processes during larval stages: expansion (growth, 0–36 h ALH), chamber formation around neural stem cell (24–48 h ALH), and extension (growth to adapt to lineage size, 48–96 h ALH). Cortex glia growth is sustained by different replicative strategies, making them polyploid (represented through bigger nuclei) and multinucleated (represented through smaller nuclei in higher number). Chamber formation involves the encasing of individual NSC and secondary lineage within a continuous layer of cortex glia membrane. Neurons from the same lineage remain together within a cortex glia chamber during maturation and extend fasciculated axons into the neuropile (light grey), where axons meet and synapses form. One cortex glia cell can encase several individual neural stem cell lineages, forming a regional mosaic structure (illustrated by different colours of cortex glia). In addition, cellular fusion occurs between cortex glia cells, leading to changing boundaries. The blood-brain barrier consists of the neural lamella (black mesh), perineurial glia (beige), and subperineurial glia (pink), and separates the CNS from the hemolymph (light blue). The ECM of the neural lamella is deposited early on and is remodeled during larval stages. Perineurial and subperineurial glia both are generated during embryogenesis and migrate to the CNS surface. Perineurial glia start as a squamous-like epithelium, then form a continuous monolayer between the neural lamella and subperineurial glia following growth mechanisms. The subperineurial glia already seal the barrier through septate junctions at late embryogenesis and undergo endoreplicative growth during larval stages to adapt to CNS development. Both ensheathing (green) and astrocyte-like (lime green) glia are positioned at the interface between cortex glia and the neuropile and regulate axonal and synaptic properties. They are generated during embryogenesis and grow during larval stage to accommodate CNS expansion. They have not been characterized as bona fide niche cells yet, and thus are represented in transparency. An external component of the niche is the tracheal system (dark pink), responsible for supplying oxygen. It originates from embryonic ectodermal cell clusters along the body, undergoing invagination and branching processes to send tracheal tracts to the different organs. Ganglionic branches target the ventral nerve cord around 10 h AEL and by the beginning of the larval stage form a rudimentary tracheal plexus which will grow and undergo branching throughout larval stages. Finally, the fat body (light yellow cells with brown outlines) is known to regulate NSC reactivation from quiescence and forms an example of a distant neurogenic niche. It starts as individual cell clusters during embryonic stages, which will divide, fuse and then further proliferate to perform diverse metabolic and immune functions. (C). Legends of the cellular and molecular features depicted in (B).
4 The mature neurogenic niche across species
4.1 Neurogenic niches in the adult mouse
The neurogenic niche was initially primarily studied, and as such defined, in the adult mouse (Mus musculus). It is owing to the restricted locations of bona fide NSCs in the adult, making it easier to delineate and describe their microenvironment. Two main neurogenic zones were identified in the adult mouse: the ventricular-subventricular zone of the lateral ventricle (V-SVZ), and the subgranular zone of the dentate gyrus of the hippocampus (SGZ). There, NSCs (named Type B cells in the SVZ (Doetsch et al., 1999) and Radial glia-like 1, RGL, in the SGZ (Eriksson et al., 1998)) exist mostly in a quiescent state (Type B cells can be quiescent for months (Obernier et al., 2018)), to which some awake to produce more restricted progenitor cells (transient amplifying progenitors in the V-SVZ and intermediate progenitor cells in the SGZ). These progenitors will go through a limited round of proliferation before generating neuroblasts, which will migrate to their respective destination (the olfactory bulb for the V-SVZ and the dentate gyrus of the hippocampus for the SGZ) and differentiate into neurons. Despite this extended quiescence, adult NSCs thus actively produce new neurons fated to these CNS regions and supporting fine odor discrimination and odor-reward association (V-SVZ) and learning, memory and spatial pattern separation (SGZ). Several excellent reviews (Bond et al., 2015; Obernier and Alvarez-Buylla, 2019) already exist on adult NSC/progenitors cells, which are not the central focus of this review.
Both the NSCs of the V-SVZ and SGZ are found embedded in the heterogeneous, complex and dense arrangement of cellular and acellular components of their niche (Silva-Vargas et al., 2013; Conover and Todd, 2017). As introduced earlier, common cellular components comprise other NSCs and progenitors, their neuronal and glia progeny, other neurons, different glial subtypes (astrocytes and oligodendrocytes), blood vessels and associated cells (supporting mural cells/pericytes) and resident immune cells (microglia). An additional component of the V-SVZ are ependymal cells lining the surface of the ventricle. Here, we will use the V-SVZ (Figure 1) as a model for the mouse mature niche, with much of this information being relevant to the SGZ as well.
In this niche, multiple tight interactions are spatially arranged between the different cellular components and are supported by a diversity of molecular interactions involving a range of adhesion complexes (reviewed in (Morante-Redolat and Porlan, 2019)). In the V-SVZ, NSCs send a radial basal process to directly contact blood vessels at specific places lacking astrocyte and pericyte coverage (Mirzadeh et al., 2008; Tavazoie et al., 2008) (Figure 1, bubble 1). Parenchymal astrocytes indeed also contact the blood vessels, enwrapping them in a glial sheet involved in blood-brain barrier maintenance and regulation of blood flow throughout the brain ((Mathiisen et al., 2010; Mishra et al., 2016) and reviewed in (Benz and Liebner, 2022)). The mature blood-barrier is indeed nowadays seen as a tripartite structure, with the endothelial cells at its core, and astrocytes and pericytes as supporting yet essential elements ensuring this function (Daneman and Prat, 2015). Astrocytic end-feet of long cytoplasmic extensions contact endothelial cells through the basement membrane, forming rosette-like structures at the surface of CNS blood vessels (Figure 1, bubble 1) (Kacem et al., 1998). In the V-SVZ however, gaps exist in this coverage, allowing some permeability and direct contact with other cells (including the NSCs), thus making the SVZ vasculature apart (Tavazoie et al., 2008). Contact with the vasculature is crucial for NSCs in balancing quiescence and proliferation (Ottone et al., 2014). On the apical side, gap junctions connect NSCs with ependymal cells, which they also attach to via N-cadherin mediated adherens junctions (Figure 1, bubble 2), a key adhesion regulating their quiescent state (Porlan et al., 2014). Meanwhile a single primary cilium per NSC inserts itself within ependymal rosettes to reach the CSF (Figure 1, bubble 2) (Mirzadeh et al., 2008; Shen et al., 2008). NSCs are themselves coupled to each other through gap junctions (Figure 1, bubble 3) (Lacar et al., 2011; Malmersjö et al., 2013). In addition, the neurogenic niche is rich in neurons and innervations coming from further, which form synapses and fill the niche with neurotransmitters. The latter can directly regulate NSC function, as exemplified with GABA maintaining their quiescent state (Song et al., 2012). Finally, microglia extend dynamic processes that can contact other niche populations (Nimmerjahn et al., 2005) and perform multiple roles, from immune surveillance to synaptic pruning, which have been covered by in-depth reviews (Wu et al., 2015; Sirerol-Piquer et al., 2019). This paints a picture of a neurogenic niche abundant in intricate cellular and molecular interactions.
Acellular components also participate in making the niche. First, NSCs are embedded in a basement membrane of extracellular matrix (ECM) (Figure 1, bubble 3). The ECM is composed of a number of core molecules, including collagen(s), elastin, laminin(s), fibronectin, proteoglycans (e.g., perlecan) and tenascin, all present in the adult niche and tightly interacting with NSCs (Kazanis and ffrench-Constant, 2011). In addition, basement membrane-associated fractones (Figure 1, bubble 2) are specialized ECM structures which extend from the blood vessels and can contact NSCs and progenitors (Mercier et al., 2002; Kerever et al., 2007). So far they have been clearly identified only in the V-SVZ, where most of these long and thin, finger-like extensions reach the ependymal surface, establishing direct contact with NSCs and the CSF-filled ventricle (Kerever et al., 2007; Shen et al., 2008). Fractones can also have a bulbar morphology and originate from the ependymal cells themselves, providing anchoring spots and signalling molecules (Nascimento et al., 2018). Finally, interactions between NSCs’ basal processes and blood vessels are mediated by a perivascular basement membrane. This basement membrane is itself made of two layers, the inner vascular layer secreted by endothelial cells and pericytes, and the outer glial layer built by astrocytes, which as such are also critical in providing a structural and signalling belt to blood vessels (Zhao et al., 2015). Therefore, NSCs are in contact with (at least) three different sources of ECM: interstitial, at the vasculature’s attachment and from fractones. Interactions between NSCs and the different ECM layers and structures can be mediated by diverse receptors, with so far most studies on integrins and dystroglycan (reviewed in (Faissner and Reinhard, 2015; Morante-Redolat and Porlan, 2019)). For example, NSCs adhere to blood vessels through the binding of endogenous α6β1 integrin to perivascular laminin, an interaction required for NSCs’ positioning and proliferation (Shen et al., 2008), and fractones can interact with NSCs through α6 integrin (Sato et al., 2019).
Interestingly, choroid plexuses have emerged as an integral component of the adult neurogenic niche (Stolp and Molnár, 2015). They are vascularized epithelial fold-like sacs budding from the ventricular ependymal wall, protruding in the brain ventricles and bathed by the CSF. They also form the blood-CSF barrier. They can be seen as a “distant niche” (Silva-Vargas et al., 2016). Indeed, they are not part of the physical structure embedding the NSCs, and their roles on neuro/gliogenesis go through the CSF, which they produce the bulk of, with the addition of their own, dynamically-regulated secreted factors and of what they pass on from the bloodstream (Ghersi-Egea et al., 2018). As such, considering the angle of this review, the formation of choroid plexuses will be more briefly addressed, yet included to emphasize the development, and possibly coordination, of niche component structures across (distant) space.
4.2 Neurogenic niches in the adult zebrafish
One prominent model which has been instrumental in gaining knowledge on NSCs and the process of neuro/gliogenesis in vertebrates is the teleost fish Danio rerio, or zebrafish (reviewed in (Barbosa and Ninkovic, 2016; Diotel et al., 2020; Jurisch-Yaksi et al., 2020; Labusch et al., 2020)). In contrast to mammals (and Drosophila), the zebrafish displays sustained, extensive and life-long neuro/gliogenesis, supported by NSCs broadly distributed in the CNS and kept from embryogenesis. Many neurogenic zones, and corresponding putative niches, exist in the zebrafish, with varying cellular compositions (Zupanc et al., 2005; Adolf et al., 2006; Grandel et al., 2006; Lindsey et al., 2012). In particular, the zebrafish telencephalon contains regions equivalent to the two main neurogenic niches of adult rodents (V-SZV and SGZ). We will here choose as an example of mature niche the highly-characterized pallium (dorsal region) of the telencephalon (from now referred simply as pallium; Figure 2) (Mueller et al., 2011).
In the adult pallium, NSCs appear as radial glia cells (RGCs), with an apico-basal polarity overtly displayed by polarized cytoplasmic processes, and expressing conserved astroglial markers (Than-Trong and Bally-Cuif, 2015). They also display a single primary cilium peeking in the CSF (Kishimoto et al., 2011) and express components of adherens and tight junction complexes (Figure 2, bubble) (Obermann et al., 2019). They are organised in a continuous epithelial-like sheet, with basal processes contacting blood vessels at the pial surface and with apical processes in the ventricular zone, in contact with the CSF. NSCs are mostly quiescent, a state they exit to maintain population homeostasis (Dray et al., 2021b) and upon specific stimuli, such as regeneration after injury (Alunni and Bally-Cuif, 2016; Zambusi and Ninkovic, 2020). They can follow three different modes of division (Rothenaigner et al., 2011; Than-Trong et al., 2020; Mancini et al., 2023): asymmetric gliogenic, the predominant mode, which generate one RGC and one intermediate (neural, non-gliogenic) progenitor; symmetric gliogenic, which gives birth to two identical RGCs, both having gliogenic fate and typical radial process; and symmetric neurogenic, the least frequent, which generates two intermediate neural progenitors or two neurons. Intermediate neural progenitors appear spatially intermingled with RGCs, which they attach to by tight junctions, and further divide (once to twice) to generate newborn neurons, a process leading to their detachment from the ventricular surface (Mancini et al., 2023). Neurons can also be generated by direct conversion of RGCs, without division or an intermediate progenitor stage (Barbosa et al., 2015; Dray et al., 2021a). Newborn neurons are deposited below the ventricular surface in sequential layers (Furlan et al., 2017), without extensive cell death nor migration happening (with the exception of those produced at the palatial-subpallial boundary and fated for the olfactory bulb, in a process akin to mouse neurons emanating from the V-SVZ (Kishimoto et al., 2011)). The zebrafish CNS does not contain bona fide parenchymal astrocytes, except in the optic region (Grupp et al., 2010). Interestingly though, adult pallial RGCs express multiple markers of astrocyte-specific functions (Morizet et al., 2023), which might be fulfilled by the RGCs, a role yet to be demonstrated. A population of oligodendrocytes exist in the pallium (März et al., 2010), and can respond to injury (Baumgart et al., 2012), although their origin remains to be ascertained. Mature ependymal cells are also not widespread in lining ventricular surfaces. Until recently, ependymal-like cells, identified by molecular expression and the presence of beating cilia, were mostly detected in restricted ventricular zones (spinal cord and forebrain diencephalon) (Kishimoto et al., 2011). However, a flurry of recent studies has characterized multi-ciliated cells in multiple CNS regions, including the dorsal and ventral telencephalon (Olstad et al., 2019; Ringers et al., 2020), some formed during development (D’Gama et al., 2021) and some appearing in aged adults (Ogino et al., 2016). These cells are able to move the CSF (Fame et al., 2016; Grimes et al., 2016; Olstad et al., 2019). Yet there are no mature ependymal-like cells in the close structural vicinity of the pallial RGCs. Microglia however are found in the adult pallium (Wu et al., 2020; Pandey et al., 2023), where they can activate in response to injury (März et al., 2011). Their role in homeostatic conditions have been poorly documented.
There is little description of a potential vascular network in the pallium. Blood vessels were detected through electron microscopy deep within the pallial subventricular zone (Lindsey et al., 2012). Blood vessels are also present at the pial surface, contacted by the RGCs (Diotel et al., 2010; 2018) and connected to the host vasculature. It is nonetheless clear that a protective blood-brain barrier exists in the zebrafish (Jeong et al., 2008; Dunton et al., 2021). It is very similar to the mammalian blood-brain barrier, with endothelial walls blocking transcellular permeability through tight junctions, surrounded by a basement membrane, and contacted by perivascular/mural cells (Galanternik et al., 2017). The impact of the vasculature on adult RGCs and neurogenesis is so far unknown.
Finally, zebrafish have choroid plexuses in charge of producing and modulating the CSF (Van Leeuwen et al., 2018; Korzh, 2023), which itself is in direct contact with the apical side of the RGCs. The specific roles of theses choroid plexuses and the CSF on adult neurogenesis also remain to be uncovered.
4.3 Neurogenic niches in the Drosophila larva
Drosophila melanogaster has risen as a powerful system for NSC biology thanks to the conservation of molecular and cellular pathway, its ease of manipulation and the versatility and tractability of its genetics (Homem and Knoblich, 2012). Larval Drosophila NSCs (historically called neuroblasts, not to be confused with the mammalian restricted precursors) exist throughout the CNS, in different flavours. Type I NSCS are found both in the central brain and the ventral nerve cord (the equivalent of the vertebrate spinal cord), and divide asymmetrically to produce one intermediate progenitor, the Ganglion Mother Cell (GMC), which will divide once to produce mostly neurons (Truman and Bate, 1988; Pollington et al., 2023). Type II NSCs are found in the central brain as two ventral clusters of eight cells of each side of the midline (Bello et al., 2008; Boone and Doe, 2008; Bowman et al., 2008; Walsh and Doe, 2017). They divide asymmetrically to produce one Intermediate Neural Progenitor (INP), which itself will cycle asymmetrically a few times to give birth to GMCs. As such, Type II lineages are bigger than Type I. In the optic lobe, another CNS region corresponding to the visual processing center, NSCs are produced later, after transitioning from localized neuroepithelia, and follow specific division and proliferation patterns (reviewed in (Nériec and Desplan, 2016; Contreras et al., 2019)). In this review, we will adopt the ventral nerve cord (Type I NSCs only) in late larval stage (Figure 3) as the mature neurogenic niche of Drosophila, with however most findings also applicable to the central brain, either for Type II or Type II NSCs. We will explicitly mention when these regions or NSC types diverge in behaviour.
Following a period of quiescence started in late embyrogenesis, larval NSCs cycle actively from mid-larval stage. They are found in a niche made of a diversity of glial cell types (reviewed in (Freeman, 2015; Yildirim et al., 2019; Corty and Coutinho-Budd, 2023)) organised in layers. From the outside, the first niche component is the blood-brain barrier. It is itself composite, made of the neural lamella, a layer of ECM; the perineurial glia, a relatively squamous layer; and the subperineurial glia, which bears the barrier function (Stork et al., 2008; Hindle and Bainton, 2014). The perineurial glia membrane is mechanically linked to the neural lamella through integrin-based focal adhesion complexes, paired with the adhesion molecule Basigin/CD147/EMMPRIN (Figure 3, bubble 1) (Hunter et al., 2020). The subperineurial glia exhibit tight-like junctions, the septate junctions, which provide a physical barrier to paracellular diffusion (Figure 3, bubble 1). In addition, they are equipped with many conserved transporters ensuring chemical filtering (Mayer et al., 2009), while being more permissive for lipid and lipoprotein entry (Brankatschk and Eaton, 2010). As such, the Drosophila blood-brain barrier is a neuroprotective barrier, using chemical and physical strategies like in mammals (Juang and Carlson, 1994; Hindle and Bainton, 2014).
Below the subperineurial glia lay the cortex glia. The cortex glia form a striking, dual membrane structure around cycling NSC lineages. It is both a seemingly continuous network infiltrating the whole CNS and encompassing the NSC population; and an individual encasing, called chamber, around each NSC and their whole larval (secondary) lineage (Pereanu et al., 2005; Spéder and Brand, 2018). Mature secondary (larval) neurons and primary (embryonic) neurons display their own individual encasing (shown as a green outline for neurons in Figure 3). Cortex glia have been shown to sustain neurogenesis in multiple fashion, including support of NSC proliferation (Dong et al., 2021), NSC protection from stress (Cheng et al., 2011; Bailey et al., 2015), neuron protection from apoptosis (Coutinho-Budd et al., 2017; Spéder and Brand, 2018; Plazaola-Sasieta et al., 2019) and neuron positioning and features (Plazaola-Sasieta et al., 2019; Banach-Latapy et al., 2023). In addition, secreted cues (netrins and Slit) from the cortex glia bind to their receptors on the NSC membrane, signalling to and regulating the asymmetric division machinery (de Torres-Jurado et al., 2022). Adhesions between cortex glia and NSC membranes also exist, and are at least partially mediated by a Wrapper to Neurexin-IV (homologous to human Caspr/Paranodin) binding (Figure 3, bubble 2) (Banach-Latapy et al., 2023). In addition, several cell adhesion molecules are present within the developing, still immature neuronal lineages contained within a cortex glia chamber, including components of adherens junctions, components of occluding junctions and Neuroglian, the fly homolog of Neurofascin-155 (Figure 3, bubble 3) (Dumstrei et al., 2003; Banach-Latapy et al., 2023). Some sort of physical adhesion seems to also exist between NSCs and their GMC through the midbody generated during asymmetric division (Figure 3, bubble 2) (Loyer and Januschke, 2018).
Below the cortex glia, other types of glial cells are in close association with axonal and synaptic structures. The ensheathing glia, abutting the cortex glia, are found at the interface with the neuropile, the cell body-devoided region where axons meet and synapses forms. While the cortex glia surround the cell bodies and axons of newborn secondary neurons outside of the neuropile, ensheathing glia wrap these axons once they enter this compartment and their function appear important for proper axonal tracts (Spindler et al., 2009). Ensheathing glia also form a diffusion barrier between the cellular and neuropile compartments (Pogodalla et al., 2021). The astrocyte-like glia, with their cell bodies also at the interface between the cortex glia and the neuropile, display a highly-ramified morphology and infiltrate the latter through cytoplasmic extensions with stereotyped tiled locations (Stork et al., 2014; Peco et al., 2016). Astrocyte-like glia are important for axonal pruning and synaptogenesis during pupal metamorphosis (Tasdemir-Yilmaz and Freeman, 2014). So far, there is no evidence that ensheathing and astrocyte-like glia influence neuro/gliogenesis, although we believe they might be revealed to do so, at least through the regulation of newborn neurons. As such, we will not develop them further in this review.
Last but not least, trachea, the branched network of epithelial tubules in charge of supplying oxygen to the tissues, and functioning as a respiratory system, are also present within the CNS. A key question is the importance of oxygen levels in the neurogenic niche. Interestingly, NSCs appear to be more sensitive than neurons, glia or GMCs to oxygen levels, which correlate with the distance to the closest trachea (Baccino-Calace et al., 2020).
4.4 State of the field and outstanding questions
So far studies have primarily focused on the signalling side of the niche, investigating the nature and cellular source of the signals modulating NSPC function, both in physiological and stress conditions. While our knowledge has been steadily increasing and has uncovered a tremendous diversity of cues and impacts on NSCs, much is still left to do to map the whole signalome of the niche, how it changes under a range of stress/pathological conditions, and the interplay between the different signals.
Yet, the architectural aspect of the NSC niche is even less understood and still very poorly explored. Many central questions are left to be investigated.
1. What is the developmental origin of the adult niche: contribution of components; plasticity?
2. How does the niche architecture form: timescale and dynamics; cell types involved; cellular and molecular mechanisms?
3. What are the different scales of the niche: local, regional, population-wide? What are the associated topological rules?
4. Which roles does niche architecture perform on NSC and niche functions? What is the relationship with the different scales?
5 Building a neurogenic niche
All in all, the (local) neurogenic niche is a dense microenvironment, rich in a diversity of cellular and molecular interactions, and formed by components of different origins yet brought together in one place. We will now describe the developmental events building the architecture of the mature niche from the start of neuro/gliogenesis, when “a” niche cannot actually be properly localised. We will also discuss some functions of these niches when they appear tied to architectural features.
5.1 The developing mouse neocortex
The adult V-SVZ niche originates from a highly proliferative neuroepithelium lining the brain’s ventricular system during development, but which becomes significantly limited later, localized along the lateral wall of the lateral ventricles. As such it can be regarded as a spatially restricted continuation of the embryonic V-SVZ. We will thus focus on the V-SVZ of the developing neocortex (in the dorsal telencephalon) to convey the main principles behind the formation of a neurogenic niche (Figure 1). Of note, an outstanding review from (Bjornsson et al., 2015) has described, and bridged, the developing and adult niche in the mouse (composition and signalling).
5.1.1 Neural progenitors
The first step of developmental neuro/gliogenesis is neuroectodermal specification (or neural induction (Lumsden and Krumlauf, 1996)) between embryonic day (E)6.0 and E8.5, leading to the formation of a neuroepithelial sheet. Developmental cortical neuro/gliogenesis (reviewed in (Paridaen and Huttner, 2014; Villalba et al., 2021) starts around embryonic day E9.5, with the neuroepithelial cells as primary neural progenitors. Neuroepithelial cells create a tube structure with a central canal and exhibit characteristics of epithelial cells, forming lateral connections through adherens and tight junctions and displaying apicobasal polarity (Figure 1, bubble 4). They are organised as a pseudo-stratified neuroepithelium, a result of interkinetic nuclear migration (Noctor et al., 2001). After several initial rounds of division to amplify their pool, neuroepithelial cells transform into radial glia cells (RGCs) around E10.5. RGCs’ cell bodies are still mechanically linked to each other by adherens junctions (but no more tight junctions) at the apical side, an interaction critical to their behaviour and to the integrity of the ventricular zone (Rašin et al., 2007). RGCs are bipolar, displaying both apical and basal processes (Noctor et al., 2002). Apical processes, harbouring a primary cilium, peek into the ventricle, filled with the CSF. Primary cilia are immotile microtubule-based organelles, present in most cells, and which serve as a signalling plateform, hence gaining the nickname of “cell’ antenna”. They are abundantly present in the mammalian developing CNS, in most of the niche cell types, and as such their alteration has been associated with many neurodevelopmental disorders (Liu et al., 2021). In embryonic RGCs, the primary cilium is disassembled then re-assembled during each cell division, in a tight relationship with centriolar inheritance between the daughter cells. Primary cilium’s ablation in RGCs disrupts mitotic axis and the cellular outcome of division (Foerster et al., 2017), while disruption of some of its signalling roles alters apico-basal polarity (Higginbotham et al., 2013). The elongated basal processes of RGCs extend towards the outer (pial) surface of the brain, attaching to the basement membrane of pial vasculature or to the pial membrane itself (Figure 1, bubble 5). RGCs undergo both symmetric and asymmetric divisions. Self-renewal through asymmetric division can either generate a neuron, a glial cell or an intermediate progenitor, which itself will divide further to produce neurons. Of note, in the developing neocortex, two other (and smaller in the mouse) populations of NSPCs exist: the short neural precursor cells, which lack basal attachment and exclusively produce neurons through differentiative divisions; and the outer/basal RGCs which lack apical attachment and generate basal intermediate progenitors (reviewed in (Agirman et al., 2017; Kalebic and Huttner, 2020)). Basal RGCs are proposed to be a key support for cortical expansion during evolution.
Gliogenesis tends to happen later than neurogenesis at the level of the population (reviewed in (Clavreul et al., 2022)). In particular, in early postnatal development, progenitor cells primarily generate glial cells. The remaining RGCs mostly differentiate into ependymal cells, or into various types of glial cells, such as astrocytes. A restricted number of RGCs still persist (Fuentealba et al., 2015; Berg et al., 2019), remaining in localised microenvironments which will become the adult neurogenic niches. The basis of these adult niches is thus made by the diverse components assembled during development, and which will be remodelled later on.
5.1.2 Extracellular matrix
One niche component in direct contact with NSPCs is the ECM, from the basement membrane or from fractones. The ECM is composed of a number of core molecules and can also sequester diverse growth factors and cytokines. All these can work as signalling molecules (ligands of cellular receptors) per se, and together also provide a mechanical scaffold to the cells. In the embryonic CNS, the core ECM components all appear expressed (reviewed in (Kazanis and ffrench-Constant, 2011)), as well as many of their cellular receptors (integrins; syndecans; dystroglycan) (Lathia et al., 2007). The cellular sources of the different ECM components have not been precisely characterized, except for the perivascular basement membrane (see further) and the expectation that some should come from the cells they surround. Whether the composition of the ECM varies during developmental neuro/gliogenesis, and between the developing and adult neurogenic niches, remain poorly understood.
The study of ECM function in the developing CNS has uncovered both signalling and mechanical roles, roles often difficult to disentangle. Several studies have assessed the effect of removing the integrin subunits serving as laminin’s receptor in NSPCs, whose apical and basal processes are both anchored to basement membranes via integrin complexes (Figure 1, bubbles 3 and 5). These removals all resulted in the disruption of basal process attachment, yet with different outputs on NSPC function, leading to either death (Radakovits et al., 2009) and alteration of proliferation axis (Loulier et al., 2009) for integrin ß1, or to no detectable impact for integrin α6 (Haubst et al., 2006). Altered expression of the heparan sulfate proteoglycan Perlecan both led to structural disruption of the neuroepithelium by disorganizing the ventricular basement membrane (Costell et al., 1999), and to decreased NSPC proliferation through its control on Sonic Hedgehog signalling (Girós et al., 2007). The knockout during embryonic development of another ECM component, Tenascin C, also affects NSPC proliferation (Garcion et al., 2004). Fractones represent another form of ECM structures which is already present early on during CNS development, at the neuroepithelium stage in the V-SVZ (Mercier, 2016). Little is known yet on their roles at this stage and how they relate to adult fractones.
In addition to ECM production, several cell types start to populate the developing neocortex, progressively assembling a cellular niche structure.
5.1.3 Neurons
Newborn neurons migrate away towards the pial surface (/cortical plate), where they fully differentiate and establish connections. Neurons generated directly from RGCs must initially detach from the ventricular surface by breaking N-cadherin-based adherens junctions (Rousso et al., 2012). In contrast, neurons born by indirect neurogenesis from intermediate progenitors, which have themselves already detached and migrated basally, do not require this detachment step. Newborn neurons then use RGCs’ basal processes as a guiding scaffold (Figure 1, bubble 6), which they attach to through gap junction hemichannels (Elias et al., 2007) and/or by relying on a dynamic, Rab GTPases-regulated binding to N-cadherin (Kawauchi et al., 2010; Shikanai et al., 2011). These neurons, arranged in consecutive subtype-specific layers depending on their time of birth (early-born in deep layer and late-born in superficial layer) can in turn regulate the behaviour and functions of NSPCs through paracrine mechanisms (Xing and Huttner, 2020).
5.1.4 Vasculature and the blood-brain barrier
A key component of the mouse neurogenic niche is a complex network of blood vessels which develop and grow alongside its emergence and expansion (for extensive reviews on neurodevelopmental angiogenesis, see (Saunders et al., 2012; Paredes et al., 2018; Biswas et al., 2020; Peguera et al., 2021; Vogenstahl et al., 2022)). Angioblasts, the vascular progenitors of the endothelial cells, are recruited to the outside of the neural tube, where they form the perineural vascular plexus (see top vessel on Figure 1) around E8.5-E9.5. They then initiate angiogenesis at around E9.5-E10, at the time neuroepithelial cells convert into RGCs. Vasculogenesis and neuro/gliogenesis thus appear temporally coupled. Endothelial tip cells lead vascular sprouts from the perineural vascular plexus, breaking through the pial basement membrane. These primitive vessels then infiltrate the CNS tissue and, guided by NSPC-derived VEGF, quickly extend radially towards the ventricle. There, they form branches which connect with neighbouring ones to create the intraneural vascular plexus (Engelhardt and Liebner, 2014). The intraneural vascular plexus further extends, also developing additional capillary meshes reinforcing this early vascular framework, and becomes the periventricular vascular plexus. The periventricular vascular plexus basically corresponds to the dense, grid-like vascular network spanning the neurogenic niche, connected to the perineural vascular plexus. This entire process occurs rapidly (Ziegler et al., 2014), and is vital for delivering oxygen as the brain expands.
The formation of the blood-brain barrier happens concomitantly to angiogenesis and vascularization of the CNS, with endothelial cells setting up tight junctions and expressing specialized transporters. A primitive blood-brain barrier is already present at E15, then matures and is stabilized by the addition of cellular components (Daneman et al., 2010; Ben-Zvi et al., 2014). Pericytes in particular are recruited to forming blood vessels during vascularization of the developing CNS, where they are critical for the formation and maintenance of the blood-brain barrier (Daneman et al., 2010). While pericytes are also associated with the vasculature in other tissues, they are more abundant along the neurovasculature than in any other part of the body. These contractile mural cells (Armulik et al., 2011), of heterogeneous origin including the neural crest (Dias Moura Prazeres et al., 2017), wrap around endothelial cells through long cytoplasmic processes.
Blood vessels and NSPCs mutually influence each other’s development and architecture. First, correct vasculature growth and topography are crucial for defining the neurogenic niches during CNS development. NSPCs are indeed preferentially found in the close vicinity of blood vessels, in particular close to tip cells during vasculature branching, which contact them through filopodial extensions (Javaherian and Kriegstein, 2009; Di Marco et al., 2020). Disturbing blood vessel growth in the embryonic brain or in embryonic brain cultures transfers NSPCs to non-neurogenic regions (Javaherian and Kriegstein, 2009; Li et al., 2013), a striking observation suggesting that the vasculature directs niche location. In a contrasting picture, neurogenic niches tend to be hypoxic, a feature supporting RGC proliferation (Mohyeldin et al., 2010). Blood-dependent oxygen levels in the developing CNS indeed seem to participate in balancing proliferation and differentiation of NSPCs, with hypoxia favouring the former while oxygenation promotes the latter in a HIF1a-dependent manner (Lange et al., 2016). Even more, progenitor identities can depend on oxygen level (Wagenführ et al., 2015). The timing and pattern of vascularization of the developing CNS is thus a driver of niche morphogenesis.
While the influence of the vascular niche on neuro/gliogenesis depends on its role of an interface with blood-borne factors, it is also a structural scaffold, bearing direct physical interaction. It is indeed able to regulate RGC proliferation through direct cell-cell contacts. Apical end-feet of RGCs anchor to blood vessels of the periventricular plexus through integrin-based adhesions (Figure 1, bubble 5), an interaction regulating RGC proliferation (Tan et al., 2016). Of note, different subtypes of progenitors in different CNS regions are more or less dependent on this association, showing that not all niches are equal. Direct contact of RGCs by endothelial tip cell filopodia can also modulate RGC proliferation by extending their mitotic phase, what ultimately triggers earlier neural differentiation while limiting pool amplification (Di Marco et al., 2020). In addition, RGCs’ attachment to pia vessels via their basal processes and integrin/ECM interactions influences the positioning of newborn neurons (Figure 1, bubble 5) (Graus-Porta et al., 2001; Haubst et al., 2006; Radakovits et al., 2009; Segarra et al., 2018).
Reciprocally, NSPCs and progeny are important for the extent and patterning of CNS vascularization. First, the key angiogenic factor VEGF is expressed by NSPCs (Hogan et al., 2004; James et al., 2009) and neurons (Himmels et al., 2017), and is essential for the different steps of CNS vascularization, including the formation of the perineural vascular plexus and the ingression of blood vessels into the periventricular zone. Earlier on, neuroepithelial cells also secrete Wnt7a/b, activating the corresponding pathway in endothelial cells, what in turn ensures proper angiogenesis and blood-brain barrier function (Daneman et al., 2009). A delicate balance in Wnt signalling in endothelial cells, controlled by the NSPCs, is actually important for the proper growth and stabilization of periventricular blood vessels (Stenman et al., 2008; Ma et al., 2013). Astrocytes also play a key role in regulating developmental angiogenesis, both for the vascularization process (Ma et al., 2012) but also, more prominently, for blood-brain barrier function, through their direct interaction with endothelial cells’ basement membrane.
5.1.5 Glia: astrocytes and oligodendrocytes
Astrocytes indeed are another pivotal component of the neurogenic niche. They appear relatively late in the process, following gliogenesis (reviewed in (Clavreul et al., 2022)). Around E16.5, RGCs switch to generate astrocytes and oligodendrocytes at the expense of neuronal lineages, producing glial rather than neural (intermediate) progenitors. This switch is seen at the population level, while RGCs’ potential is divided into neurogenic-only, gliogenic-only or bipotent (nicely synthesized in (Kriegstein and Alvarez-Buylla, 2009)). RGCs can also directly convert into astrocytes perinatally. Astrocytes are born immature and migrate to their final position before acquiring markers of fully mature astrocytes, a process occurring up to perinatal development. Astrocytes are a heterogeneous cell population, with gene expression, functional and spatial differences (Molofsky and Deneen, 2015). In addition to their role in supporting neuronal functions, which have been covered before in (Durkee and Araque, 2019; Bonvento and Bolaños, 2021), they are in tight relationship with the developing vasculature. Not only do they participate in angiogenesis (Ma et al., 2012) and express several angiogenic factors, they are also an integral part of the blood-brain barrier.
5.1.6 Microglia
In contrast, microglia are an early component of the neurogenic niche and are recruited from the outside. Microglia are tissue-resident macrophages, born during development in the yolk sac and derived mostly from erythro myeloid progenitors, with a potential contribution from primitive macrophage (Alliot et al., 1999; Ginhoux et al., 2010; Schulz et al., 2012; Perdiguero et al., 2014; Ferrero et al., 2018). In the mouse, erythro myeloid progenitors form in the yolk sac at E8.5 and microglia precursors then migrate to their tissue of residence, entering the CNS around E9.5, when angiogenesis starts. Primitive blood vessels and meninges have been proposed to serve as entry points (Navascués et al., 2000; Ginhoux et al., 2010), and neuronal apoptosis as one attracting cue. There microglia follow a stepwise developmental program (early microliga > pre-microglia > adult microglia) allowing them to adapt to their novel environment, and leading to their adult form (Matcovitch-Natan et al., 2016). They first proliferate until the post-natal period and colonize the entire CNS, while showing preferential, dynamic localizations, with transient hotspots on regulated migratory paths (Swinnen et al., 2013). Some of these paths might be influenced by RGCs, which directly interact with microglia during early development (Rigato et al., 2011; Rosin et al., 2021) and also appear to be important for their recruitment (Arnò et al., 2014). They might also use the expanding periventricular vasculature as a migratory track, with half of the microglia found closely associated with blood vessels at E14 (Hattori et al., 2022). In a reciprocal fashion, microglia appear to support angiogenesis (Fantin et al., 2010).
Microglia are very diverse in shapes and functions (from amoeboid-like to highly ramified), one linked to the other, and are also dependent on their state of maturation and to their location within the CNS. During development, microglia have been involved in regulating NSC proliferation and differentiation. While microglia are difficult to specifically deplete, several studies using complementary genetic or chemical ablations have been able to assess how their loss of function impacts CNS development. Conditional depletions of microglia in the mouse developing neocortex showed that microglia support stem cell differentiation into intermediate progenitors (Arnò et al., 2014; Hattori and Miyata, 2018). Chromatin-dependent regulation of microglial features (shape, metabolism) is also important for correct NSPC self-renewal and differentiation during embryonic development, through modulation of the Wnt/β-catenin signalling pathway (Su et al., 2022). In the rat developing brain, microglia activation in the ventricular zone promotes neuro/gliogenesis (Shigemoto-Mogami et al., 2014). Microglia also help building and refining neuronal circuits, using signalling as well as their pruning and phagocytic abilities. In particular, an excessive number of neurons are generated during early brain development, and many of them undergo programmed cell death before birth (Blaschke et al., 1998; Yeo and Gautier, 2004). Microglia are found to concentrate in zone of neuronal death in various CNS regions and respond to death signals by engulfing dying neurons with their processes (Ferrer et al., 1990; Wakselman et al., 2008; Rigato et al., 2011). Microglia can also establish cell contacts with neuronal cell bodies to shape their wiring (Squarzoni et al., 2014).
5.1.7 Ependymal cells
Ependymal cells are a very late player in niche morphogenesis, and present in the SVZ in contrast to the non-ventricular SGZ. They are multiciliated epithelial cells which line all brain ventricles, forming a mostly continuous cellular barrier with the CSF. The coordinated and oriented beating of their cilia moves this fluid through the ventricles. Ependymal cells are post-mitotic and come from the differentiation of RGCs at late developmental stages (E14-16), arising from terminal symmetric divisions (Spassky et al., 2005; Ortiz-Álvarez et al., 2019; Redmond et al., 2019). They further need to mature post-birth to adopt their adult profile (Del Bigio, 2010). Ependymal cells are linked to each other by adherens junctions, but do not display tight junctions except at few restricted locations. In addition, they assemble in rosette structures around grouped apical processed of several RGCs, similar to the pinwheel structures of the adult neurogenic niche (Figure 1, bubble 2) (Mirzadeh et al., 2008; Codega et al., 2014). These pinwheel structures provide restricted breaks in the ependymal barrier, offering exchange points with the CSF. Different subtypes of ependymal cells exist (tanycytes, radial and cuboidal), classified based on their morphology, location and molecular markers, and potentially fulfilling different functions. The role of ependymal cells in the developing niche is not characterized, and possibly limited due to their late arrival. In the adult niche, they are essential in regulating neuro/gliogenesis, both as a source of signalling molecules which influence the behaviour and fate of nearby NSCs, and indirectly as a main controller of the CSF (reviewed in (Quaresima et al., 2022)).
5.1.8 Choroid plexus: an example of a distant niche
There are four choroid plexuses, one in each brain ventricle (two lateral, third and fourth). While they diverge in morphology and function, they share a similar cellular structure, made by two major cell types. The first element is a monolayer of cuboidal epithelial cells of neuroectodermal origin and in continuity with the ependymal cells of the ventricular wall. They are also ciliated, but, in contrast to the latter, they are connected by tight junctions, providing a barrier to paracellular diffusion, and exhibit microvilli structures to increase their total surface. The second element is a network of fenestrated capillary blood vessels connected to the brain circulation system. Pericytes and immune cells are found in the space between capillaries and epithelial layer.
The formation of the different choroid plexuses is a complex process bringing different cell types (epithelium and blood vessels), from different origins (neuroectoderm and mesoderm respectively) together. Presumptive choroid plexuses’ territories in the neuroepithelium can be identified from as early as E.8.5 (Thomas and Dziadek, 1993; Awatramanil et al., 2003), specified through the coordinated action of signalling patways (Notch, BMP) to repress neural fate. Choroid plexuses’ epithelia then enter a maturation and differentiation stage, with their structures becoming apparent around E11.5 following morphological changes (Sturrock, 1979). In particular, the epithelium undergoes thinning, transitioning from pseudostratified to columnar and eventually cuboidal, forming a proper single layer as it starts to extrude into the ventricles (Dziegielewska et al., 2001). Mature choroid plexus epithelial cells are mostly post-mitotic, and as such growth during development occurs by incorporating cells from the proliferative zone at its base. During these morphogenetic changes, the underlying, developing vascular network in the mesenchyme ends up being enveloped by the growing epithelium. This is not only a result of physical topological constraints, as the development of the two components of the choroid plexuses appears coordinated: signalling from the neuroepithelium is proposed to trigger vascular differentiation (Broom et al., 2012), and growth (Nielsen and Dymecki, 2010).
While still maturing and growing, choroid plexuses already influence neuro/gliogenesis during development by modulating CSF composition (reviewed in (Zappaterra and Lehtinen, 2012; Johansson, 2014)). The early CSF indeed carries, in a dynamic fashion, a range of signalling molecules, including, and not restricted to, growth factors, BMP ligands, Wnt ligands, and retinoic acid. All these factors have the potential to regulate NSPC function and neuro/gliogenesis. As examples, CSF-borne Sonic Hedgehog supports RGC division in the cerebellar ventricular zone (Huang et al., 2010), and in the developing cortex Insulin-like growth factor 2 binds to its receptor on the apical process of the RGCs, stimulating their proliferation (Lehtinen et al., 2011).
5.2 The zebrafish pallium
The zebrafish pallium displays continuous neurogenesis, and a neurogenic niche, which can be observed and studied throughout life, simplifying the link between developmental and adult phases. Well-described components of the neurogenic niche in the zebrafish pallium are the RGCs themselves and their neuronal progeny. Other identified, but poorly characterized, components are an oligodendrocyte population, the microglia and some vascular system. In this light, we propose here to mostly focus on the formation of the neurogenic cells (RGCs) during development (embryonic (0–48 h post-fertilization, hpf) and larval (3–90 days post-fertilization, dpf) stages, with hatching (day 2-3 post-fertilization) in between) (Figure 2).
5.2.1 Neural progenitors
Following neuroectodermal specification, the neuroepithelium, which will shortly progress into RGCs, experiences a number of morphogenetic events (formation of a neural keel then rod) to ultimately generate a neural tube (around 18–20 hpf (Araya et al., 2016)), with a typical central canal filled with CSF. However, this canal structure is transient, and the neuropithelium in the telencephalon undergoes an eversion process, resulting in the exposure of the proliferative zones on the outer surface of the telencephalon (Nieuwenhuys, 2011). As such, and in contrast to mammals, the CSF is not encased in a canal but flows at the surface of the brain, lined on the other side by the tela choroidea, an epithelial-like sheet formed during eversion. Zebrafish telencephalic eversion is a complex multi-step morphogenetic process, starting shortly before 2 dpf (18 hpf) and completed at 5 dpf, and thus covering the beginning of neurogenesis during embryogenesis (Folgueira et al., 2012). Neuroepithelial cells, which are first organised as a pseudo-stratified epithelium (Hiscock et al., 2018), indeed convert into proper RGCs, with a radial, apico-basal polarity around mi-embryogenesis (22 hpf) (Dong et al., 2012). They are at least morphologically detectable at this stage, with some molecular markers expressed earlier. At this early stage, RGCs already perform a majority of asymmetric cell divisions along with some symmetric divisions (generating either two RGCs or two restricted neural progenitors) or differentiation into neurons. Interestingly, the daughters issued from asymmetric division adopt different positions along the apico-basal axis of the RGCs (with the basal daughter keeping the self-renewal ability), highlighting some degree of relationship between topography and fate. Ultimately, differential Notch-driven signalling between the daughter cells will decide of their fate (Dong et al., 2012). Some progenitors (Oligo2+), appearing from 30 hpf, give birth to oligodendrocytes, which start maturing at 48 hpf, and fully myelinate around 72 hpf (Ackerman and Monk, 2016). From this time, RGCs will keep proliferating to sustain the continuous growth of the zebrafish pallium, albeit with a shift towards a mostly quiescent state in adult (Dirian et al., 2014; Furlan et al., 2017).
5.2.2 Microglia
Embryonic zebrafish microglia derive from the yolk sac (Herbomel et al., 1999), like in mammals, yet with a unique origin from primitive macrophage (Ferrero et al., 2018). They start to spread as microglial precursors from around mid-embryogenesis (22 hpf) and slowly start to colonize the brain (including the telencephalon) from 35 hpf by crossing cellular barriers rather than using the blood circulation (Herbomel et al., 2001). There, they undergo a phenotypic shift, from an ameboid-like shape to a ramified morphology. Of note, a second wave of microglial colonization, derived from the hematopoietic stem cells, happens later at 14 dpf and participates to the adult pool (Xu et al., 2015; Ferrero et al., 2021).
In a variety of locations in the developing zebrafish brain, microglia have been shown to phagocytose dying neurons (Peri and Nüsslein-Volhard, 2008), which recruit them through the release of lysophosphatidylcholine and its binding to its microglial receptor (Casano et al., 2016; Xu et al., 2016). Cytokine signalling also seems to be an attractant, in conjunction or not with apoptotic cues (Herbomel et al., 1999; Wu et al., 2018). Beyond the regulation of neuronal number through their phagocytic role, no studies have so far uncovered a role for microglia in developmental neurogenesis.
5.2.3 Vasculature and the blood-brain barrier
To our knowledge, there is no description of vasculogenesis and angiogenesis specifically in the developing telencephalon. We will briefly describe the general process of brain vascularization in other regions (reviewed in (Gore et al., 2012; Quiñonez-Silvero et al., 2020)), and its interactions with neurogenesis. After angioblast specification and their migration to the midline, vasculogenesis starts and gives rise to the perineural vascular plexuses (Hogan et al., 2004) surrounding the brain and spinal cord, around 20 hpf. Angiogenic endothelial tips sprout from these plexuses and invade the brain (30–32 hpf for midbrain and hindbrain). There, they will go into rounds of angiogenesis, branching and connecting to other vessels to form a dense intraneural network inside the brain (Fujita et al., 2011; Ulrich et al., 2011). These events are highly dependent on VEGF signalling. The establishment of the blood-brain barrier function is temporally coupled to angiogenesis, with CNS protected from 2.5-3 dpf (Quiñonez-Silvero et al., 2020). Pericytes are also present in the zebrafish, and support blood-brain barrier establishment and function (Galanternik et al., 2017; Stratman et al., 2017; Bahrami and Childs, 2018). The whole process is thus very similar to the sequence of events in the mouse. Using a mutant (cloche) in which most of the vasculature is absent, studies have found that neurogenesis and/or formation of neuronal networks was impaired in some regions (cerebellum and optic tectum) but not others (hindbrain) (Ulrich et al., 2011; Taberner et al., 2020). In a reciprocal fashion, RGCs’ activity seems important for brain vascularization. Developing blood vessels appear closely associated with RGCs, whose presence is required for their growth (Umans et al., 2021). Of note, in the developing spinal cord, RGCs regulate the extent of sprouting from venous trunk vessels, in a VEGF-dependent manner (Matsuoka et al., 2016).
5.2.4 Tela choroidea
In the telencephalic region, the development of a choroid plexus-like tissue start with the formation of the tela choroidea during eversion (Folgueira et al., 2012; Turner et al., 2022). Around 18 to 22 hpf, an out-pocketing of the ventricular surface between the telencephalon and diencephalon creates a fold with a diamond-shaped roof of neuroepithelial origin. Between 2 to 5 dpf, accompanying pallial growth, this roof expands forward over the pallium to cover it, forming the wide and thin tela choroidea. Tela choroidea cells might become ciliated from around 14 dpf (D’Gama et al., 2021). A folded and more conventionally structured choroid plexus is found posterior to the telencephalon. There is still limited documentation regarding its formation and function during zebrafish development (Bill et al., 2008; García-Lecea et al., 2008; Henson et al., 2014; Van Leeuwen et al., 2018).
5.3 The Drosophila ventral nerve cord
The mature Drosophila neurogenic niche in larval stages is articulated around NSC lineages and their neuronal progeny, with the blood-brain barrier and the cortex glia as main known structures and regulators. We will follow the formation of this niche around Type I NSCs from early embryo to late larva, in spatial and temporal continuity within the ventral nerve cord. As mentioned earlier, most findings also apply to central brain Type I and Type II NSCs.
5.3.1 Neural stem cells and neurons
Drosophila Type I NSCs appear at embryonic stages following delamination from the neuroectoderm (Campos-Ortega, 1993; Bossing et al., 1996). They proliferate extensively through rounds of asymmetric division, producing embryogenesis-born neurons (called primary neurons) as well as glial cells, which will form the larval CNS at hatching. NSCs come in various types, producing both neurons and glia (“neuroglioblast”), only neurons (“neuroblast”) or only glia (“glioblast”) (Udolph et al., 1993; Bossing et al., 1996; Bernardoni et al., 1997; Schmidt et al., 1997; Schmid et al., 1999; Altenhein et al., 2015), hinting that some fate restriction might already be in place early. At the end of embryogenesis, they slow down their proliferation, shrink in size (3–5 μm), and ultimately enter a quiescent stage, characterized by their mitotic silence and the existence of a primary actin-rich and microtubule-rich (Li et al., 2017) membrane extension which reaches the neuropile (Truman and Bate, 1988; Chell and Brand, 2010). They stay quiescent until early larval stage, when they reactivate and start proliferating again (Prokop and Technau, 1991; Ding et al., 2020). Larval feeding triggers their reactivation. More precisely, circulating amino acids are sensed by the fat body (the invertebrate equivalent on the liver and adipose tissue), which sends a yet-to-be identified signal to the CNS (Britton and Edgar, 1998). As such, the fat body behaves as a “distant” niche for fly NSCs. In turn, insulin signalling is activated in NSCs, initiating the whole reactivation process (Chell and Brand, 2010; Sousa-Nunes et al., 2011). Reactivation encompasses their growth back to their original size (10–12 μm) and their re-entry into mitosis. They also lose their membrane extension, which appears retained only for the first division (Gujar et al., 2023). Then, NSCs will actively divide in an asymmetric fashion to produce secondary neuronal lineages, which will mature later on to form the majority (90%) of the adult CNS (White and Kankel, 1978; Truman and Bate, 1988). NSCs then appear to differentiate or die (Bello et al., 2003; Maurange et al., 2008) during late larval/early pupal stage. Of note, neuro/gliogenesis still happens in the adult fly, primarily within the visual system, with the identity, and link to developmental stages, of dividing cells yet to be ascertained (Kato et al., 2009; von Trotha et al., 2009; Fernández-Hernández et al., 2013; Simões et al., 2022). Both during primary and secondary neurogenesis, Type I and Type II NCS undergo a series of temporal transitions which, in combination with spatial identity, will generate neuronal diversity (reviewed in (Doe, 2017)). Most of the NSCs generated during embryogenesis persist during larval stage, with the exception of an apoptotic wave in the abdominal part of the ventral nerve cord (Maurange et al., 2008).
NSCs are relatively regularly spaced within the central brain or ventral nerve cord. No specific adhesion or interaction has been found so far between NSCs, in embryonic or larval stage, in accordance at least with their separation by the cortex glia during the latter. Very little is also known regarding neurons’ role as a niche for NSCs. Primary neurons are scattered in between NSCs, and individually encapsulated by the cortex glia membrane (Coutinho-Budd et al., 2017). Secondary neurons from the same lineage stay together with their mother NSC within a cortex glia chamber until they mature (Pereanu et al., 2005). This clustering happens, at least in part, through a mechanism of differential adhesion using complexes containing immunoglobulin-like domains (Banach-Latapy et al., 2023). It involves intra-lineage homophilic interactions by Neuroglian, and a weaker interaction between Neurexin-IV in the NSC lineages and Wrapper in the cortex glia (Figure 3, bubble 3). While these adhesions appear to impact axonal features of the secondary neurons, no apparent phenotype is detected at the level of the NSCs themselves. Components of the septate junctions also appear enriched in developing NSC lineages with no further role identified so far. Similarily, adherens junctions are detected between cells from the same NSC lineage. Conflicting results exist on the importance of these adherens junctions. One study using a dominant negative form for Shotgun, the fly E-cadherin, observed strong alterations of the cortex glia structure when clonally expressed in the CNS, including in the cortex glia (Dumstrei et al., 2003). In contrast, another study found no effect when using cell type-specific RNAi knockdown or null mutant clones in the NSC lineages (Banach-Latapy et al., 2023). All in all, still little is known about the complexity of physical interactions between newborn neurons, and their potential impact on neurogenesis.
5.3.2 The blood-brain barrier
The blood-brain barrier includes the neural lamella, the perineurial glia and the subperineurial glia.
Little is known on the ECM of the neural lamella, either the source(s) of its components and its functions towards NCSs and neurogenesis. The ECM sheath is present from embryonic stage 16 (13–16 h after egg laying (AEL)) (Stork et al., 2008) and remodels to accommodate CNS growth during development, a remodelling depending on the expression of matrix metalloproteases in the underlying perineurial glia layer (Meyer et al., 2014). The heparan sulfate proteoglycan Perlecan, a core ECM component, appears important for NSC reactivation and for the overall shape of the CNS (Datta, 1995; Voigt et al., 2002; Park et al., 2003). Collagen-IV (referred to as Viking in Drosophila) in the neural lamella appears to have, at least in part, an external origin, being produced and secreted by the fat body (Pastor-Pareja and Xu, 2011). How the different components are assembled, and their dynamics along the development of the CNS at larval stages, are unknown.
Perineurial glia cells, in direct contact with the neural lamella, are generated during late embryogenesis and must migrate to the CNS surface, although to our knowledge their exact origin and the migratory process have not be characterized. They do not touch each other at the beginning of larval stages, but do so later one, following rounds of proliferation (Ito et al., 1995; Awasaki et al., 2008; Stork et al., 2008). At late larval stage, when NSCs proliferate, they form a complete monolayer sandwiched between the neural lamella and the subperineurial glia. Perineurial glia are understudied, and little is known on their function and interactions with other components of the niche. One study showed that perineurial glia participate in NSC reactivation through the expression and function of the heparan sulfate proteoglycan Dally-like (Kanai et al., 2018). Reciprocally, perineurial glia depend on BMP signalling from NSCs for their survival. Curiously, the same study shows that direct contact seems to be possible between NSCs and perineurial glia at reactivation time, during early larval stage, despite an interleaved subperineurial glia layer.
Subperineurial glia cells themselves are generated during embryogenesis from specific NSCs (amongst which NBs 1-1A, 2-2T and 5–6A) (Beckervordersandforth et al., 2008). They migrate to the surface of the CNS and grow laterally until they touch each other and form occluding/septate junctions at the point of contact (Schwabe et al., 2017). The subperineurial glia seal and provide a barrier to paracellular diffusion by late embryonic stages (stage 17, from 15 h after egg laying) (Bainton et al., 2005; Schwabe et al., 2005). During larval stage, to accommodate the expanding volume of the CNS driven by NSC proliferation, subperineurial glia grow through endoreplicative mechanisms (mainly endomitosis), without cell division, a strategy crucial for the maintenance of its barrier function (Unhavaithaya and Orr-Weaver, 2012). Rather, they remodel their septate junctions through stretching to keep up with their growing surface (Babatz et al., 2018). Part of their growth seem to be fueled by the nutrition-dependent activation of the PI3K/Akt at early stages, during CG expansion (Yuan et al., 2020). The subperineurial glia is also a sensing interface of this nutritional signal, mediating its impact on NSC reactivation (Chell and Brand, 2010; Spéder and Brand, 2014). Such sensing triggers the production and release of Drosophila Insulin-like peptides (dIlps), a process dependent on gap junction coupling between subperineurial glial cells (Figure 3, bubble 4). More precisely, nutritional cues trigger calcium oscillations which are synchronized across the entire subperineurial layer through gap junction channels. This is proposed to result in an en masse regulated secretion of dIlps, required for synchronous NSC reactivation. dIlps further bind to the insulin receptor at the surface of the NSCs, resulting in the activation of the conserved PI3k/Akt pathway (Chell and Brand, 2010; Sousa-Nunes et al., 2011). PI3k/Akt signalling in the NSC is necessary and sufficient for reactivation. Interestingly the assembly of heteromeric gap junctions (made by the innexins 1 and 2) is lost after reactivation (Spéder and Brand, 2014), suggesting a coordination between subperineurial glia architecture and metabolic cues. So far, study of the subperineurial glia morphogenesis has mainly focused on intrinsic mechanisms. There is no well-characterized physical association between perineurial and subperineurial glia, nor between the subperineurial and the underlying cortex glia in the CNS, except for one documented instance of gap and adherens junctions in the latter case of interaction (Pereanu et al., 2005). How these cells talk to each other, at the signalling and adhesion levels, remain to be uncovered.
5.3.3 Cortex glia
Cortex glia correspond to the glial layer with the closest association to NSCs and progeny when larval NSCs start cycling. They are generated during embryogenesis, from the activity of specific NSC lineages (NB6-4, NB6-4T and NB7-4) (Ito et al., 1995; Awasaki et al., 2008; Beckervordersandforth et al., 2008). There, their functions and structure are little known. At larval hatching, when NSCs are still quiescent, the dual structure of the cortex glia around NSC lineages is not present, and cortex glia rather appear as a discontinuous meshwork with no individual encasing of NSCs. Cortex glia structure indeed remodels during larval life (Pereanu et al., 2005). Recent works have started elucidating the morphogenetic processes supporting the progressive formation of this elaborate glial structure in later larval stages. This process can be divided in three main steps (Spéder and Brand, 2018): expansion, during which the cortex glia grow parallel to NSC reactivation and the chamber is still not formed; chamber formation, when individual NSC are encased around the time they perform their first division; and extension, during which the cortex glia keep adapting to the growth of NSC lineages while maintaining their chamber organization. The expansion phase corresponds to a massive production of cortex glia membranes and, like NSC reactivation, is nutritionally-regulated (Spéder and Brand, 2018; Yuan et al., 2020). More precisely, the production and secretion of dILPs in the subperineurial glia leads to the autonomous activation of the conserved PI3K-Akt signalling pathway in the cortex glia. Starvation, preventing dIlps production and release from the subperineurial glia, and blocking the activation of the PI3K/Akt pathway in the cortex glia all prevent cortex glia expansion. Interestingly, PI3K/Akt-dependent growth of the cortex glia during the expansion phase itself seems to promote NSC reactivation, pinpointing some coordination between the two cell types (Yuan et al., 2020). The nutrition-fueled cellular growth of the CG is supported by a number of proliferative strategies (Pereanu et al., 2005; Avet-Rochex et al., 2012; Rujano et al., 2022) to match its extent. CG first undergo endoreplicative cycles, seemingly both endocyles (an alternance of S and G2 phases) and endomitosis (displaying some mitotic hallmarks but without nuclear division), leading to their polyploidy. CG growth appears mostly autonomous, at least partly regulated by the homeodomain transcription factor Cut (Yadav et al., 2023), yet with some moderate input from NSC reactivation (Spéder and Brand, 2018; Yuan et al., 2020). In contrast, chamber formation and individual NSC encasing are highly dependent of NSC reactivation, and timely-correlated with NSC re-entry into the cell cycle (Spéder and Brand, 2018). The cues sent by reactivating NSCs to recruit cortex glia membrane and trigger their encasing are so far unknown, and likely to combine signalling and adhesion processes. Interestingly, a NSC (Pvf ligand secretion) to cortex glia (Pvr receptor signalling) interaction is also crucial for proper morphogenesis of the cortex glia (Read, 2018), and might be a good candidate if the temporal window of its requirement fits. Other cortex glia to NSC physical interactions are also set up during development through Neurexin to Wrapper interaction (Banach-Latapy et al., 2023), and septate junctions have been detected between the two cell types (Pereanu et al., 2005).
Chamber formation is a critical morphogenetic event matching a tipping point in NSC behaviour. First, reactivation is complete, and active proliferation by asymmetric division starts. NSCs are also becoming independent from nutrition-induced PI3K/Akt signalling and their proliferation is rather sustained by the Alk pathway, whose ligand Jelly Belly appears produced by the now fully enwrapping cortex glia (Cheng et al., 2011). Their access to external factor also changes, as their encasing by the cortex glia inserts an extra layer between NSCs and the blood-brain barrier/the environment. Indeed this cortex glia layer appears to provide some permeability barrier for solute (Yuan et al., 2020) and, while it needs to be demonstrated, is likely to regulate the passage of many signalling molecules. In this light, it is striking that NSC dependence on subperineurial glia-secreted dIlps ends when the cortex glia chamber forms (Cheng et al., 2011; Spéder and Brand, 2018).
Cortex glia behaviour also seems to change after chamber formation, during the extension phase. While they still need to grow to accommodate the extensive generation of newborn neurons by actively cycling NSCs, endoreplication slowly dwindles down, and another replicative strategy, acytokinetic miosis, takes place (Rujano et al., 2022; Yadav et al., 2023). Acytokinetic mitosis leads to nuclear division without cellular separation, thus forming multinucleated, syncytial cells. Much like endoreplication, this strategy ensures that one cortex glia cell will be equipped with several genome copies supporting important protein production and cellular growth, and that such growth will not disrupt cellular architecture.
An important feature of cortex glia organization is indeed the combination of cell size and cell arrangement. After expansion and from the time of encasing, one cortex glia cell spans several NSCs, while forming a membranous chamber around each (Rujano et al., 2022). These cellular units thus physically partition the NSC population in subgroups, while organising themselves as a cellular mosaic tiling the whole CNS. These NSC subgroups varies between individuals and as such do not seem to be predetermined. Whether the process is fully stochastic remains to be assessed. In addition to the population (network) and the individual (chamber) scales, the discovery of such organization identified a third, regional (unit) scale of the cortex glia niche. From early larval stage, cortex glia cells thus appear to grow, enwrapping neighbouring NSCs, until they meet each other and set cellular boundaries. The picture is still more complex, as cortex glia units can undergo cellular fusion, sharing membrane, cytoplasm, and organelle’s compartments (Figure 3, bubble 5). These fusion events vary in time and space. Even more strikingly, they appear transient, as compartments once connected can lose this ability to exchange (Rujano et al., 2022). The existence of cellular fusion between CG units, combined with its variability and transientness, results in changing boundaries between the units, making the regional structure of the niche plastic around NSCs and progeny. What regulates the time, place, frequency and duration of cell fusion in the cortex glia remains a mystery.
5.3.4 Trachea
The Drosophila tracheal system comprises a complex network of epithelial tubes responsible for transporting oxygen throughout the body and generated through a series of complex morphogenetic events (described in (Hartenstein, 1993) and reviewed in (Ehrhardt et al., 2022)). This intricate structure originates from 20 ectodermal cell clusters, each composed of approximately 80 cells, and visible very early during development (5–6 h after egg laying). These clusters undergo a process of invagination, forming sac-like epithelial structures, one in each segment. Primary, secondary and terminal branches sequentially bud from these sacs, contributing to the formation of the tracheal network through cell migration and fusion (Samakovlis et al., 1996; Sutherland et al., 1996). Ganglionic branches (represented in Figure 3) target the ventral nerve cord and cerebral branches target the central brain (Hartenstein, 1993; Pereanu et al., 2007). A ganglionic branch (one per segment) is a primary epithelial tube made by seven cells (Samakovlis et al., 1996). During budding and elongation, a single cell at the tip of the emerging structure leads the migratory process towards the CNS. This process starts during embryogenesis around 10 h after egg laying and is guided by the presence of clusters of epidermal cells expressing branchless, a member of the Fibroblast Growth Factor family (Sutherland et al., 1996). Within the next two hours, the leading cell at the tip of the ganglionic branch reaches, migrates along and finally enters the ventral nerve cord laterally and ventrally (Samakovlis et al., 1996; Englund et al., 1999). By the beginning of the larval stage, a ring-like rudimentary tracheal plexus (much like the mammalian perineural vascular plexus) is set up around the neuropile by the invasion, extension/migration and fusion of segmental ganglionic branches. From there, this plexus will grow and branch throughout larval stages to form a complex network mostly localized close to the neuropile (Pereanu et al., 2007; Baccino-Calace et al., 2020). Their growth appears constrained by glial cells (Pereanu et al., 2007) and involves some proliferative mechanisms at late stages (Rao et al., 2015). Little is known about the coordination between trachea growth, function and interplay with other cell types of the niche. One common sensitivity is to nutrition-induced PI3K/Akt signalling at early stage, which fuels tracheal growth. In turn, tracheal growth seems to favour NSC reactivation (Yuan et al., 2020). Whether this role is linked to the supply of oxygen or of other signalling factors is not known. Interestingly, the distance between the tracheal network and NSCs increases during larval stage along with NSC lineage growth (Bailey et al., 2015).
5.3.5 The fat body: an example of a distant niche
The fat body is a crucial player in NSC reactivation, secreting nutrition-responsive factors, yet unknown, which reach the CNS and trigger insulin signalling from the glial cells (Britton and Edgar, 1998; Chell and Brand, 2010; Sousa-Nunes et al., 2011; Spéder and Brand, 2014). Fat body development (reviewed in (Zheng et al., 2016; Li et al., 2019; Parra-Peralbo et al., 2021)) starts with the progressive apparition of mesodermal cell clusters between embryonic stages 10-14 (5–10 h after egg laying). These fat body primordia undergo mitosis to reach around 2,200 cells and shortly afterwards at stage 16 (13–16 h after egg laying) fuse to generate one continuous monolayer of fat cells. These cells keep growing through endoreplication, until entering quiescence in late embryogenesis. Fat cells will resume endoreplication around 8 h after larval hatching, paralleling the timing of NSC reactivation, and grow throughout larval stages without a change in number, while storing various nutrients.
6 Conclusion
6.1 Take home messages across all model systems
Niche morphogenesis is a coordinated process between different cell types and acellular components to ensure that the needs of the tissue to develop and acquire specialized functions are met in the right time and place. The timing of growth, adhesion and inter-cellular communication will generate a specific, yet dynamic, niche architecture, which in turn is a powerful means to regulate the reach of signalling.
The scales of both niche morphogenesis and niche architecture are multifold. Niche morphogenesis spans time (from early embryonic development to early post-natal growth, and even adulthood) and tissues (neural, hematopoietic, and immune compartments). Niche architecture is built on cell-to-cell adhesions (ECM, adherens junctions, tight junctions, surface immunoglobulins, gap junctions), which will act to generate local structures (e.g., astrocyte/blood vessel/RGC basal process unit; cortex glia chamber) and signalling (e.g., primary cilia of RGCs; ECM/integrin signalling), but which also can reach to farther regions of the tissue (e.g., gap junction coupling in the subperineurial glia; attachment of RGCs’ basal process to blood vessels; ECM as a mechanical constraint). Some of these molecular and cellular processes can also be regional (e.g., ependymal pinwheel structure grouping few RGCs together; mosaic of cortex glia), producing “mini-niches” within the niche.
6.2 Where the neurogenic niche field should go next
Stem cell niches have emerged as powerful and plastic regulators of stem cell behaviour. The neurogenic niche is no exception yet distinguishes itself by its especially heterogeneous and complex composition, the inclusion of components with unique role at the organism level (i.e., the blood-brain barrier) and its multiscale architecture.
While the field has made critical progress in the signalling aspect of the neurogenic niche, and despite the growing understanding that mechanical cues and physical constraints have powerful implications, little is still known on niche architecture.
The first question which has been explored in recent years is how the niche forms and acquired its “final” (mature) architecture (at least in homeostatic conditions), since such understanding is a prerequisite to its manipulation for probing its function. Deciphering niche formation includes the identification of the morphogenetic processes at play, their cellular and molecular players, and the adhesive properties eventually set in place. The mouse and the fruit fly have been leading this aspect, with different focuses, and with still much left to comprehend. Niche morphogenesis is pretty much unknown in the CNS of other organisms, and one of the further challenges of the field is to bring this comparative understanding to the table.
Nearly all is left to achieve on the other aspects of niche architecture, covering scales, rules, and functions. Our own perception of the neurogenic niche, and its relationship with other fields of biological research, would suggest concentrating on the following questions.
1. Interplay between architecture and signalling, including physical/mechanical cues both as a signal per se and as a modulator of signalling range
2. Relationship between local, regional and tissue scales, especially in relationship with the cellular coordination or independence within the NSPC population
3. Plasticity in response to time (from development to ageing), signals (metabolic status), and conditions (pathologies)
The answers to all these questions bid exciting times for stem cell biology, converging with other outstanding questions in the field touching their regulation under stress and their double-edged potential for curative (e.g., regeneration) and pathological (e.g., tumourigenesis) outcomes.
Author contributions
GV: Writing–original draft, Writing–review and editing. PS: Funding acquisition, Writing–original draft, Writing–review and editing,
Funding
The author(s) declare financial support was received for the research, authorship, and/or publication of this article. This work has been funded by a core funding from Institut Pasteur to PS. GV was supported by a post-doctoral fellowship from the LabEx Revive (ANR-10-LABX-0073).
Acknowledgments
We deeply thank Laure Bally-Cuif, Alice Davy and Elisa Gomez Perdiguero for critical and helpful comments on this review. We are very grateful to Mónica Folgueira for discussion about the zebrafish tela choroidea and telencephalic eversion.
Conflict of interest
The authors declare that the research was conducted in the absence of any commercial or financial relationships that could be construed as a potential conflict of interest.
Publisher’s note
All claims expressed in this article are solely those of the authors and do not necessarily represent those of their affiliated organizations, or those of the publisher, the editors and the reviewers. Any product that may be evaluated in this article, or claim that may be made by its manufacturer, is not guaranteed or endorsed by the publisher.
References
Ackerman, S. D., and Monk, K. R. (2016). The scales and tales of myelination: using zebrafish and mouse to study myelinating glia. Brain Res. 1641, 79–91. doi:10.1016/J.BRAINRES.2015.10.011
Adolf, B., Chapouton, P., Lam, C. S., Topp, S., Tannhäuser, B., Strähle, U., et al. (2006). Conserved and acquired features of adult neurogenesis in the zebrafish telencephalon. Dev. Biol. 295, 278–293. doi:10.1016/J.YDBIO.2006.03.023
Agirman, G., Broix, L., and Nguyen, L. (2017). Cerebral cortex development: an outside-in perspective. FEBS Lett. 591, 3978–3992. doi:10.1002/1873-3468.12924
Alliot, F., Godin, I., and Pessac, B. (1999). Microglia derive from progenitors, originating from the yolk sac, and which proliferate in the brain. Dev. Brain Res. 117, 145–152. doi:10.1016/S0165-3806(99)00113-3
Altenhein, B., Cattenoz, P. B., and Giangrande, A. (2015). The early life of a fly glial cell. Wiley Interdiscip. Rev. Dev. Biol. 5, 67–84. doi:10.1002/wdev.200
Altman, J., and Das, G. D. (1965). Autoradiographic and histological evidence of postnatal hippocampal neurogenesis in rats. J. Comp. Neurol. 124, 319–335. doi:10.1002/CNE.901240303
Alunni, A., and Bally-Cuif, L. (2016). A comparative view of regenerative neurogenesis in vertebrates. Development 143, 741–753. doi:10.1242/dev.122796
Araya, C., Ward, L. C., Girdler, G. C., and Miranda, M. (2016). Coordinating cell and tissue behavior during zebrafish neural tube morphogenesis. Dev. Dyn. 245, 197–208. doi:10.1002/DVDY.24304
Armulik, A., Genové, G., and Betsholtz, C. (2011). Pericytes: developmental, physiological, and pathological perspectives, problems, and promises. Dev. Cell 21, 193–215. doi:10.1016/J.DEVCEL.2011.07.001
Arnò, B., Grassivaro, F., Rossi, C., Bergamaschi, A., Castiglioni, V., Furlan, R., et al. (2014). Neural progenitor cells orchestrate microglia migration and positioning into the developing cortex. Nat. Commun. 5, 5611. doi:10.1038/NCOMMS6611
Avet-Rochex, A., Kaul, A. K., Gatt, A. P., McNeill, H., and Bateman, J. M. (2012). Concerted control of gliogenesis by InR/TOR and FGF signalling in the Drosophila post-embryonic brain. Development 139, 2763–2772. doi:10.1242/dev.074179
Awasaki, T., Lai, S.-L., Ito, K., and Lee, T. (2008). Organization and postembryonic development of glial cells in the adult central brain of Drosophila. J. Neurosci. 28, 13742–13753. doi:10.1523/JNEUROSCI.4844-08.2008
Awatramanil, R., Soriano, P., Rodriguez, C., Mai, J. J., and Dymecki, S. M. (2003). Cryptic boundaries in roof plate and choroid plexus identified by intersectional gene activation. Nat. Genet. 35, 70–75. doi:10.1038/NG1228
Babatz, F., Naffin, E., and Klämbt, C. (2018). The Drosophila blood-brain barrier adapts to cell growth by unfolding of pre-existing septate junctions. Dev. Cell 47, 697–710. doi:10.1016/j.devcel.2018.10.002
Baccino-Calace, M., Prieto, D., Cantera, R., and Egger, B. (2020). Compartment and cell-type specific hypoxia responses in the developing Drosophila brain. Biol. Open 9, bio053629. doi:10.1242/bio.053629
Bahrami, N., and Childs, S. J. (2018). Pericyte biology in zebrafish. Adv. Exp. Med. Biol. 1109, 33–51. doi:10.1007/978-3-030-02601-1_4
Bailey, A. P., Koster, G., Guillermier, C., Hirst, E. M. A., MacRae, J. I., Lechene, C. P., et al. (2015). Antioxidant role for lipid droplets in a stem cell niche of Drosophila. Cell 163, 340–353. doi:10.1016/j.cell.2015.09.020
Bainton, R. J., Tsai, L. T.-Y., Schwabe, T., DeSalvo, M., Gaul, U., and Heberlein, U. (2005). Moody encodes two GPCRs that regulate cocaine behaviors and blood-brain barrier permeability in Drosophila. Cell 123, 145–156. doi:10.1016/j.cell.2005.07.029
Banach-Latapy, A., Rincheval, V., Briand, D., Guénal, I., and Spéder, P. (2023). Differential adhesion during development establishes individual neural stem cell niches and shapes adult behaviour in Drosophila. PLoS Biol. 21, e3002352. doi:10.1371/journal.pbio.3002352
Barbosa, J. S., and Ninkovic, J. (2016). Adult neural stem cell behavior underlying constitutive and restorative neurogenesis in zebrafish. Neurogenesis 3, e1148101–e1148109. doi:10.1080/23262133.2016.1148101
Barbosa, J. S., Sanchez-Gonzalez, R., Di Giaimo, R., Baumgart, E. V., Theis, F. J., Götz, M., et al. (2015). Neurodevelopment. Live imaging of adult neural stem cell behavior in the intact and injured zebrafish brain. Science 348, 789–793. doi:10.1126/SCIENCE.AAA2729
Baumgart, E. V., Barbosa, J. S., Bally-cuif, L., Götz, M., and Ninkovic, J. (2012). Stab wound injury of the zebrafish telencephalon: a model for comparative analysis of reactive gliosis. Glia 60, 343–357. doi:10.1002/GLIA.22269
Bayraktar, O. A., Fuentealba, L. C., Alvarez-Buylla, A., and Rowitch, D. H. (2014). Astrocyte development and heterogeneity. Cold Spring Harb. Perspect. Biol. 7, a020362. doi:10.1101/CSHPERSPECT.A020362
Beckervordersandforth, R. M., Rickert, C., Altenhein, B., and Technau, G. M. (2008). Subtypes of glial cells in the Drosophila embryonic ventral nerve cord as related to lineage and gene expression. Mech. Dev. 125, 542–557. doi:10.1016/j.mod.2007.12.004
Bello, B. C., Hirth, F., and Gould, A. P. (2003). A pulse of the Drosophila Hox protein Abdominal-A schedules the end of neural proliferation via neuroblast apoptosis. Neuron 37, 209–219. doi:10.1016/S0896-6273(02)01181-9
Bello, B. C., Izergina, N., Caussinus, E., and Reichert, H. (2008). Amplification of neural stem cell proliferation by intermediate progenitor cells in Drosophila brain development. Neural Dev. 3, 5. doi:10.1186/1749-8104-3-5
Benz, F., and Liebner, S. (2022). Structure and function of the blood–brain barrier (BBB). Handb. Exp. Pharmacol. 273, 3–31. doi:10.1007/164_2020_404
Ben-Zvi, A., Lacoste, B., Kur, E., Andreone, B. J., Mayshar, Y., Yan, H., et al. (2014). Mfsd2a is critical for the formation and function of the blood–brain barrier. Nat 509, 507–511. doi:10.1038/nature13324
Berg, D. A., Su, Y., Jimenez-Cyrus, D., Patel, A., Huang, N., Morizet, D., et al. (2019). A common embryonic origin of stem cells drives developmental and adult neurogenesis. Cell 177, 654–668. doi:10.1016/J.CELL.2019.02.010
Bernardoni, R., Vivancos, V., and Giangrande, A. (1997). glide/gcm is expressed and required in the scavenger cell lineage. Dev. Biol. 191, 118–130. doi:10.1006/dbio.1997.8702
Bernocchi, G., Scherini, E., Giacometti, S., and Mareš, V. (1990). Premitotic DNA synthesis in the brain of the adult frog (Rana esculenta L.): an autoradiographic 3H-thymidine study. Anat. Rec. 228, 461–470. doi:10.1002/AR.1092280413
Bertapelle, C., Polese, G., and Di Cosmo, A. (2017). Enriched environment increases PCNA and PARP1 levels in Octopus vulgaris central nervous system: first evidence of adult neurogenesis in lophotrochozoa. J. Exp. Zool. B. Mol. Dev. Evol. 328, 347–359. doi:10.1002/JEZ.B.22735
Bill, B. R., Balciunas, D., McCarra, J. A., Young, E. D., Xiong, T., Spahn, A. M., et al. (2008). Development and Notch signaling requirements of the zebrafish choroid plexus. PLoS One 3, e3114. doi:10.1371/JOURNAL.PONE.0003114
Binder, M. D., Hirokawa, N., and Windhorst, U. (2009). Encyclopedia of neuroscience. Available at: https://books.google.com/books/about/Encyclopedia_of_Neuroscience.html?id=mcvhAAAACAAJ (Accessed November 4, 2023).4393.
Biswas, S., Cottarelli, A., and Agalliu, D. (2020). Neuronal and glial regulation of CNS angiogenesis and barriergenesis. Dev 147, dev182279. doi:10.1242/dev.182279
Bjornson, C. R., Rietze, R. L., Reynolds, B. A., Magli, M. C., and Vescovi, A. L. (1999). Turning brain into blood: a hematopoietic fate adopted by adult neural stem cells in vivo. Science 283 (5401). New York, NY: JPEG, 534–537. doi:10.1126/science.283.5401.534
Bjornsson, C. S., Apostolopoulou, M., Tian, Y., and Temple, S. (2015). It takes a village: constructing the neurogenic niche. Dev. Cell 32, 435–446. doi:10.1016/j.devcel.2015.01.010
Blaschke, A. J., Weiner, J. A., and Chun, J. (1998). Programmed cell death is a universal feature of embryonic and postnatal neuroproliferative regions throughout the central nervous system. J. Comp. Neurol. 396, 39–50. doi:10.1002/(sici)1096-9861(19980622)396:1<39::aid-cne4>3.0.co;2-j(19980622)
Bond, A. M., Ming, G. L., and Song, H. (2015). Adult mammalian neural stem cells and neurogenesis: five decades later. Cell Stem Cell 17, 385–395. doi:10.1016/j.stem.2015.09.003
Bonvento, G., and Bolaños, J. P. (2021). Astrocyte-neuron metabolic cooperation shapes brain activity. Cell Metab. 33, 1546–1564. doi:10.1016/J.CMET.2021.07.006
Boone, J. Q., and Doe, C. Q. (2008). Identification of Drosophila type II neuroblast lineages containing transit amplifying ganglion mother cells. Dev. Neurobiol. 68, 1185–1195. doi:10.1002/dneu.20648
Bossing, T., Udolph, G., Doe, C. Q., and Technau, G. M. (1996). The embryonic central nervous system lineages of Drosophila melanogaster. I. Neuroblast lineages derived from the ventral half of the neuroectoderm. Dev. Biol. 179, 41–64. doi:10.1006/dbio.1996.0240
Bowman, S. K., Rolland, V., Betschinger, J., Kinsey, K. a., Emery, G., and Knoblich, J. a. (2008). The tumor suppressors Brat and Numb regulate transit-amplifying neuroblast lineages in Drosophila. Dev. Cell 14, 535–546. doi:10.1016/j.devcel.2008.03.004
Brankatschk, M., and Eaton, S. (2010). Lipoprotein particles cross the blood-brain barrier in Drosophila. J. Neurosci. 30, 10441–10447. doi:10.1523/JNEUROSCI.5943-09.2010
Breunig, J. J., Haydar, T. F., and Rakic, P. (2011). Neural stem cells: historical perspective and future prospects age of rationalism: origin of neural stem cell research. doi:10.1016/j.neuron.2011.05.005
Britton, J. S., and Edgar, B. A. (1998). Environmental control of the cell cycle in Drosophila: nutrition activates mitotic and endoreplicative cells by distinct mechanisms. Development 125, 2149–2158. doi:10.1242/dev.125.11
Broom, E. R., Gilthorpe, J. D., Butts, T., Campo-Paysaa, F., and Wingate, R. J. T. (2012). The roof plate boundary is a bi-directional organiser of dorsal neural tube and choroid plexus development. Development 139, 4261–4270. doi:10.1242/DEV.082255
Byrd, C. A., and Brunjes, P. C. (2001). Neurogenesis in the olfactory bulb of adult zebrafish. Neuroscience 105, 793–801. doi:10.1016/S0306-4522(01)00215-9
Campos-Ortega, J. A. (1993). Mechanisms of early neurogenesis in Drosophila melanogaster. J. Neurobiol. 24, 1305–1327. doi:10.1002/NEU.480241005
Casano, A. M., Albert, M., and Peri, F. (2016). Developmental apoptosis mediates entry and positioning of microglia in the zebrafish brain. Cell Rep. 16, 897–906. doi:10.1016/J.CELREP.2016.06.033
Chell, J. M., and Brand, A. H. (2010). Nutrition-responsive glia control exit of neural stem cells from quiescence. Cell 143, 1161–1173. doi:10.1016/j.cell.2010.12.007
Cheng, H., and Leblond, C. P. (1974). Origin, differentiation and renewal of the four main epithelial cell types in the mouse small intestine. V. Unitarian Theory of the origin of the four epithelial cell types. Am. J. Anat. 141, 537–561. doi:10.1002/AJA.1001410407
Cheng, L. Y., Bailey, A. P., Leevers, S. J., Ragan, T. J., Driscoll, P. C., and Gould, A. P. (2011). Anaplastic lymphoma kinase spares organ growth during nutrient restriction in Drosophila. Cell 146, 435–447. doi:10.1016/j.cell.2011.06.040
Clark, S. E., Williams, R. W., and Meyerowitz, E. M. (1997). The CLAVATA1 gene encodes a putative receptor kinase that controls shoot and floral meristem size in Arabidopsis. Cell 89, 575–585. doi:10.1016/S0092-8674(00)80239-1
Clavreul, S., Dumas, L., and Loulier, K. (2022). Astrocyte development in the cerebral cortex: complexity of their origin, genesis, and maturation. Front. Neurosci. 16, 916055. doi:10.3389/FNINS.2022.916055
Codega, P., Silva-Vargas, V., Paul, A., Maldonado-Soto, A. R., Deleo, A. M., Pastrana, E., et al. (2014). Prospective identification and purification of quiescent adult neural stem cells from their in vivo niche. Neuron 82, 545–559. doi:10.1016/j.neuron.2014.02.039
Colucci-D’Amato, L., Bonavita, V., and Porzio, U. (2006). The end of the central dogma of neurobiology: stem cells and neurogenesis in adult CNS. Neurol. Sci. 27, 266–270. doi:10.1007/S10072-006-0682-Z
Conover, J. C., and Todd, K. L. (2017). Neuronal stem cell niches of the brain. Biol. Eng. Stem Cell Niches, 75–91. doi:10.1016/B978-0-12-802734-9.00006-8
Contreras, E. G., Sierralta, J., and Oliva, C. (2019). Novel strategies for the generation of neuronal diversity: lessons from the fly visual system. Front. Mol. Neurosci. 12, 140. doi:10.3389/FNMOL.2019.00140
Corty, M. M., and Coutinho-Budd, J. (2023). Drosophila glia take shape to sculpt the nervous system. Curr. Opin. Neurobiol. 79, 102689. doi:10.1016/J.CONB.2023.102689
Costell, M., Gustafsson, E., Aszódi, A., Mörgelin, M., Bloch, W., Hunziker, E., et al. (1999). Perlecan maintains the integrity of cartilage and some basement membranes. J. Cell Biol. 147, 1109–1122. doi:10.1083/JCB.147.5.1109
Cotsarelis, G., Sun, T. T., and Lavker, R. M. (1990). Label-retaining cells reside in the bulge area of pilosebaceous unit: implications for follicular stem cells, hair cycle, and skin carcinogenesis. Cell 61, 1329–1337. doi:10.1016/0092-8674(90)90696-C
Coutinho-Budd, J. C., Sheehan, A. E., and Freeman, M. R. (2017). The secreted neurotrophin spätzle 3 promotes glial morphogenesis and supports neuronal survival and function. Genes Dev. 31, 2023–2038. doi:10.1101/gad.305888.117
Cui, C., Wang, F., Zheng, Y., Wei, H., and Peng, J. (2023). From birth to death: the hardworking life of Paneth cell in the small intestine. Front. Immunol. 14, 1122258. doi:10.3389/FIMMU.2023.1122258
Daneman, R., Agalliu, D., Zhou, L., Kuhnert, F., Kuo, C. J., and Barres, B. A. (2009). Wnt/beta-catenin signaling is required for CNS, but not non-CNS, angiogenesisProc. Natl. Acad. Sci. U. S. A. 106 (2), 641–646. doi:10.1073/pnas.0805165106
Daneman, R., Zhou, L., Kebede, A. A., and Barres, B. A. (2010). Pericytes are required for blood–brain barrier integrity during embryogenesis. Nat 468, 562–566. doi:10.1038/nature09513
Datta, S. (1995). Control of proliferation activation in quiescent neuroblasts of the Drosophila central nervous system. Development 121, 1173–1182. doi:10.1242/dev.121.4.1173
Del Bigio, M. R. (2010). Ependymal cells: biology and pathology. Acta Neuropathol. 119, 55–73. doi:10.1007/s00401-009-0624-y
de Torres-Jurado, A., Manzanero-Ortiz, S., and Carmena, A. (2022). Glial-secreted Netrins regulate Robo1/Rac1-Cdc42 signaling threshold levels during Drosophila asymmetric neural stem/progenitor cell division. Curr. Biol. 32, 2174–2188.e3. doi:10.1016/J.CUB.2022.04.001
Dexter, T. M., Allen, T. D., and Lajtha, L. G. (1977). Conditions controlling the proliferation of haemopoietic stem cells in vitro. J. Cell. Physiol. 91, 335–344. doi:10.1002/JCP.1040910303
D’Gama, P. P., Qiu, T., Cosacak, M. I., Rayamajhi, D., Konac, A., Hansen, J. N., et al. (2021). Diversity and function of motile ciliated cell types within ependymal lineages of the zebrafish brain. Cell Rep. 37, 109775. doi:10.1016/J.CELREP.2021.109775
Dias Moura Prazeres, P. H., Sena, I. F. G., Borges, I. da T., de Azevedo, P. O., Andreotti, J. P. P., de Paiva, A. E., et al. (2017). PERICYTES ARE HETEROGENEOUS IN THEIR ORIGIN WITHIN THE SAME TISSUE. Dev. Biol. 427, 6–11. doi:10.1016/J.YDBIO.2017.05.001
Di Marco, B., Crouch, E. E., Shah, B., Duman, C., Paredes, M. F., Ruiz de Almodovar, C., et al. (2020). Reciprocal interaction between vascular filopodia and neural stem cells shapes neurogenesis in the ventral telencephalon. Cell Rep. 33, 108256. doi:10.1016/J.CELREP.2020.108256
Ding, W. Y., Huang, J., and Wang, H. (2020). Waking up quiescent neural stem cells: molecular mechanisms and implications in neurodevelopmental disorders. PLoS Genet. 16, e1008653. doi:10.1371/journal.pgen.1008653
Diotel, N., Charlier, T. D., Lefebvre d’Hellencourt, C., Couret, D., Trudeau, V. L., Nicolau, J. C., et al. (2018). Steroid transport, local synthesis, and signaling within the brain: roles in neurogenesis, neuroprotection, and sexual behaviors. Front. Neurosci. 12, 84. doi:10.3389/FNINS.2018.00084
Diotel, N., Lübke, L., Strähle, U., and Rastegar, S. (2020). Common and distinct features of adult neurogenesis and regeneration in the telencephalon of zebrafish and mammals. Front. Neurosci. 14, 568930. doi:10.3389/FNINS.2020.568930
Diotel, N., Vaillant, C., Gueguen, M. M., Mironov, S., Anglade, I., Servili, A., et al. (2010). Cxcr4 and Cxcl12 expression in radial glial cells of the brain of adult zebrafish. J. Comp. Neurol. 518, 4855–4876. doi:10.1002/CNE.22492
Dirian, L., Galant, S., Coolen, M., Chen, W., Bedu, S., Houart, C., et al. (2014). Spatial regionalization and heterochrony in the formation of adult pallial neural stem cells. Dev. Cell 30, 123–136. doi:10.1016/J.DEVCEL.2014.05.012
Doe, C. Q. (2017). Temporal patterning in the Drosophila CNS. Annu. Rev. Cell Dev. Biol. 33, 219–240. doi:10.1146/ANNUREV-CELLBIO-111315-125210
Doetsch, F., Caillé, I., Lim, D. A., García-Verdugo, J. M., and Alvarez-Buylla, A. (1999). Subventricular zone astrocytes are neural stem cells in the adult mammalian brain. Cell 97, 703–716. doi:10.1016/s0092-8674(00)80783-7
Dong, Q., Zavortink, M., Froldi, F., Golenkina, S., Lam, T., and Cheng, L. Y. (2021). Glial Hedgehog signalling and lipid metabolism regulate neural stem cell proliferation in Drosophila. EMBO Rep. 22, e52130. doi:10.15252/embr.202052130
Dong, Z., Yang, N., Yeo, S. Y., Chitnis, A., and Guo, S. (2012). Intralineage directional Notch signaling regulates self-renewal and differentiation of asymmetrically dividing radial glia. Neuron 74, 65–78. doi:10.1016/J.NEURON.2012.01.031
Dray, N., Mancini, L., Binshtok, U., Cheysson, F., Supatto, W., Mahou, P., et al. (2021a). Dynamic spatiotemporal coordination of neural stem cell fate decisions occurs through local feedback in the adult vertebrate brain. Cell Stem Cell 28, 1457–1472.e12. doi:10.1016/j.stem.2021.03.014
Dray, N., Than-Trong, E., and Bally-Cuif, L. (2021b). Neural stem cell pools in the vertebrate adult brain: homeostasis from cell-autonomous decisions or community rules? Bioessays 43, e2000228. doi:10.1002/BIES.202000228
Dumstrei, K., Wang, F., and Hartenstein, V. (2003). Role of DE-cadherin in neuroblast proliferation, neural morphogenesis, and axon tract formation in Drosophila larval brain development. J. Neurosci. 23, 3325–3335. doi:10.1523/JNEUROSCI.23-08-03325.2003
Dunton, A. D., Göpel, T., Ho, D. H., and Burggren, W. (2021). Form and function of the vertebrate and invertebrate blood-brain barriers. Int. J. Mol. Sci. 22, 12111. doi:10.3390/IJMS222212111
Durkee, C. A., and Araque, A. (2019). Diversity and specificity of astrocyte-neuron communication. Neuroscience 396, 73–78. doi:10.1016/J.NEUROSCIENCE.2018.11.010
Dziegielewska, K. M., Ek, J., Habgood, M. D., and Saunders, N. R. (2001). Dev. Choroid Plexus. doi:10.1002/1097-0029
Ehrhardt, B., El-Merhie, N., Kovacevic, D., Schramm, J., Bossen, J., Roeder, T., et al. (2022). Airway remodeling: the Drosophila model permits a purely epithelial perspective. Front. Allergy 3, 876673. doi:10.3389/FALGY.2022.876673
Elias, L. a. B., Wang, D. D., and Kriegstein, A. R. (2007). Gap junction adhesion is necessary for radial migration in the neocortex. Nature 448, 901–907. doi:10.1038/nature06063
Englund, C., Uv, A. E., Cantera, R., Mathies, L. D., Krasnow, M. A., and Samakovlis, C. (1999). adrift, a novel bnl-induced Drosophila gene, required for tracheal pathfinding into the CNS. Development 126, 1505–1514. doi:10.1242/DEV.126.7.1505
Engelhardt, B., and Liebner, S. (2014). Novel insights into the development and maintenance of the blood-brain barrier. Cell Tissue Res. 355 (3), 687–699. doi:10.1007/s00441-014-1811-2
Eriksson, P. S., Perfilieva, E., Björk-Eriksson, T., Alborn, A. M., Nordborg, C., Peterson, D. A., et al. (1998). Neurogenesis in the adult human hippocampus. Nat. Med. 4, 1313–1317. doi:10.1038/3305
Faissner, A., and Reinhard, J. (2015). The extracellular matrix compartment of neural stem and glial progenitor cells. Glia 63, 1330–1349. doi:10.1002/GLIA.22839
Fame, R. M., Chang, J. T., Hong, A., Aponte-Santiago, N. A., and Sive, H. (2016). Directional cerebrospinal fluid movement between brain ventricles in larval zebrafish. Fluids Barriers CNS 13, 11. doi:10.1186/S12987-016-0036-Z
Fantin, A., Vieira, J. M., Gestri, G., Denti, L., Schwarz, Q., Prykhozhij, S., et al. (2010). Tissue macrophages act as cellular chaperones for vascular anastomosis downstream of VEGF-mediated endothelial tip cell induction. Blood 116, 829–840. doi:10.1182/BLOOD-2009-12-257832
Fernández-Hernández, I., Rhiner, C., and Moreno, E. (2013). Adult neurogenesis in Drosophila. Cell Rep. 3, 1857–1865. doi:10.1016/j.celrep.2013.05.034
Ferrer, I., Bernet, E., Soriano, E., Del Rio, T., and Fonseca, M. (1990). Naturally occurring cell death in the cerebral cortex of the rat and removal of dead cells by transitory phagocytes. Neuroscience 39, 451–458. doi:10.1016/0306-4522(90)90281-8
Ferrero, G., Mahony, C. B., Dupuis, E., Yvernogeau, L., Di Ruggiero, E., Miserocchi, M., et al. (2018). Embryonic microglia derive from primitive macrophages and are replaced by cmyb-dependent definitive microglia in zebrafish. Cell Rep. 24, 130–141. doi:10.1016/J.CELREP.2018.05.066
Ferrero, G., Miserocchi, M., Di Ruggiero, E., and Wittamer, V. (2021). A c sf1rb mutation uncouples two waves of microglia development in zebrafish. Development 148, dev194241. doi:10.1242/DEV.194241
Foerster, P., Daclin, M., Asm, S., Faucourt, M., Boletta, A., Genovesio, A., et al. (2017). mTORC1 signaling and primary cilia are required for brain ventricle morphogenesis. Development 144, 201–210. doi:10.1242/DEV.138271
Folgueira, M., Bayley, P., Navratilova, P., Becker, T. S., Wilson, S. W., and Clarke, J. D. W. (2012). Morphogenesis underlying the development of the everted teleost telencephalon. Neural Dev. 7, 32. doi:10.1186/1749-8104-7-32
Freeman, M. R. (2015). Drosophila central nervous system glia. Cold Spring Harb. Perspect. Biol. 7, a020552. doi:10.1101/cshperspect.a020552
Fuentealba, L. C., Rompani, S. B., Parraguez, J. I., Obernier, K., Romero, R., Cepko, C. L., et al. (2015). Embryonic origin of postnatal neural stem cells. Cell 161, 1644–1655. doi:10.1016/J.CELL.2015.05.041
Fujita, M., Cha, Y. R., Pham, V. N., Sakurai, A., Roman, B. L., Silvio Gutkind, J., et al. (2011). Assembly and patterning of the vascular network of the vertebrate hindbrain. Development 138, 1705–1715. doi:10.1242/DEV.058776
Furlan, G., Cuccioli, V., Vuillemin, N., Dirian, L., Muntasell, A. J., Coolen, M., et al. (2017). Life-long neurogenic activity of individual neural stem cells and continuous growth establish an outside-in architecture in the teleost pallium. Curr. Biol. 27, 3288–3301. doi:10.1016/J.CUB.2017.09.052
Gage, F. H. (2019). Adult neurogenesis in mammals. Science 364, 827–828. doi:10.1126/SCIENCE.AAV6885
Galanternik, M. V., Castranova, D., Gore, A. V., Blewett, N. H., Jung, H. M., Stratman, A. N., et al. (2017). A novel perivascular cell population in the zebrafish brain. Elife 6, e24369. doi:10.7554/ELIFE.24369
Galliot, B., and Quiquand, M. (2011). A two-step process in the emergence of neurogenesis. Eur. J. Neurosci. 34, 847–862. doi:10.1111/J.1460-9568.2011.07829.X
García-Lecea, M., Kondrychyn, I., Fong, S. H., Ye, Z. R., and Korzh, V. (2008). In vivo analysis of choroid plexus morphogenesis in zebrafish. PLoS One 3, e3090. doi:10.1371/JOURNAL.PONE.0003090
Garcion, E., Halilagic, A., Faissner, A., and Ffrench-Constant, C. (2004). Generation of an environmental niche for neural stem cell development by the extracellular matrix molecule tenascin C. Development 131, 3423–3432. doi:10.1242/DEV.01202
Ghersi-Egea, J. F., Strazielle, N., Catala, M., Silva-Vargas, V., Doetsch, F., and Engelhardt, B. (2018). Molecular anatomy and functions of the choroidal blood-cerebrospinal fluid barrier in health and disease. Acta Neuropathol. 1353 (135), 337–361. doi:10.1007/S00401-018-1807-1
Ghibaudi, M., and Bonfanti, L. (2022). How widespread are the “young” neurons of the mammalian brain? Front. Neurosci. 16, 918616. doi:10.3389/fnins.2022.918616
Ginhoux, F., Greter, M., Leboeuf, M., Nandi, S., See, P., Gokhan, S., et al. (2010). Fate mapping analysis reveals that adult microglia derive from primitive macrophages. Science 330, 841–845. doi:10.1126/SCIENCE.1194637
Girós, A., Morante, J., Gil-Sanz, C., Fairén, A., and Costell, M. (2007). Perlecan controls neurogenesis in the developing telencephalon. BMC Dev. Biol. 7, 29. doi:10.1186/1471-213X-7-29
Goldman, S. A., and Nottebohm, F. (1983). Neuronal production, migration, and differentiation in a vocal control nucleus of the adult female canary brain. Proc. Natl. Acad. Sci. U. S. A. 80, 2390–2394. doi:10.1073/PNAS.80.8.2390
Gore, A. V., Monzo, K., Cha, Y. R., Pan, W., and Weinstein, B. M. (2012). Vascular development in the zebrafish. Cold Spring Harb. Perspect. Med. 2, a006684. doi:10.1101/CSHPERSPECT.A006684
Gould, E., Tanapat, P., Mcewen, B. S., Flügge, G., and Fuchs, E. (1998). Proliferation of granule cell precursors in the dentate gyrus of adult monkeys is diminished by stress. Proc. Natl. Acad. Sci. U. S. A. 95, 3168–3171. doi:10.1073/PNAS.95.6.3168
Grandel, H., Kaslin, J., Ganz, J., Wenzel, I., and Brand, M. (2006). Neural stem cells and neurogenesis in the adult zebrafish brain: origin, proliferation dynamics, migration and cell fate. Dev. Biol. 295, 263–277. doi:10.1016/J.YDBIO.2006.03.040
Graus-Porta, D., Blaess, S., Senften, M., Littlewood-Evans, A., Damsky, C., Huang, Z., et al. (2001). Beta1-class integrins regulate the development of laminae and folia in the cerebral and cerebellar cortex. Neuron 31, 367–379. doi:10.1016/S0896-6273(01)00374-9
Grimes, D. T., Boswell, C. W., Morante, N. F. C., Henkelman, R. M., Burdine, R. D., and Ciruna, B. (2016). Zebrafish models of idiopathic scoliosis link cerebrospinal fluid flow defects to spine curvature. Science 352, 1341–1344. doi:10.1126/SCIENCE.AAF6419
Grupp, L., Wolburg, H., and Mack, A. F. (2010). Astroglial structures in the zebrafish brain. J. Comp. Neurol. 518, 4277–4287. doi:10.1002/CNE.22481
Gujar, M. R., Gao, Y., Teng, X., Ding, W. Y., Lin, J., Tan, Y. S., et al. (2023). Patronin/CAMSAP promotes reactivation and regeneration of Drosophila quiescent neural stem cells. EMBO Rep. 24, e56624. doi:10.15252/EMBR.202256624
Hartenstein, V. (1993). Atlas of Drosophila development. Cold Spring Harbor. New York, NY: Cold Spring Harbor Laboratory Press.
Hattori, Y., Itoh, H., Tsugawa, Y., Nishida, Y., Kurata, K., Uemura, A., et al. (2022). Embryonic pericytes promote microglial homeostasis and their effects on neural progenitors in the developing cerebral cortex. J. Neurosci. 42, 362–376. doi:10.1523/JNEUROSCI.1201-21.2021
Hattori, Y., and Miyata, T. (2018). Microglia extensively survey the developing cortex via the CXCL12/CXCR4 system to help neural progenitors to acquire differentiated properties. Genes cells. 23, 915–922. doi:10.1111/GTC.12632
Haubst, N., Georges-Labouesse, E., De Arcangelis, A., Mayer, U., and Götz, M. (2006). Basement membrane attachment is dispensable for radial glial cell fate and for proliferation, but affects positioning of neuronal subtypes. Development 133, 3245–3254. doi:10.1242/DEV.02486
Henson, H. E., Parupalli, C., Ju, B., and Taylor, M. R. (2014). Functional and genetic analysis of choroid plexus development in zebrafish. Front. Neurosci. 8, 364. doi:10.3389/fnins.2014.00364
Herbomel, P., Thisse, B., and Thisse, C. (1999). Ontogeny and behaviour of early macrophages in the zebrafish embryo. Development 126, 3735–3745. doi:10.1242/DEV.126.17.3735
Herbomel, P., Thisse, B., and Thisse, C. (2001). Zebrafish early macrophages colonize cephalic mesenchyme and developing brain, retina, and epidermis through a M-CSF receptor-dependent invasive process. Dev. Biol. 238, 274–288. doi:10.1006/DBIO.2001.0393
Higginbotham, H., Guo, J., Yokota, Y., Umberger, N. L., Su, C. Y., Li, J., et al. (2013). Arl13b-regulated cilia activities are essential for polarized radial glial scaffold formation. Nat. Neurosci. 16, 1000–1007. doi:10.1038/NN.3451
Himmels, P., Paredes, I., Adler, H., Karakatsani, A., Luck, R., Marti, H. H., et al. (2017). Motor neurons control blood vessel patterning in the developing spinal cord. Nat. Commun. 81, 14583–14616. doi:10.1038/ncomms14583
Hindle, S. J., and Bainton, R. J. (2014). Barrier mechanisms in the Drosophila blood-brain barrier. Front. Neurosci. 8, 414. doi:10.3389/fnins.2014.00414
Hiscock, T. W., Miesfeld, J. B., Mosaliganti, K. R., Link, B. A., and Megason, S. G. (2018). Feedback between tissue packing and neurogenesis in the zebrafish neural tube. Dev 145, dev157040. doi:10.1242/dev.157040
Hogan, K. A., Ambler, C. A., Chapman, D. L., and Bautch, V. L. (2004). The neural tube patterns vessels developmentally using the VEGF signaling pathway. Development 131, 1503–1513. doi:10.1242/DEV.01039
Holguera, I., and Desplan, C. (2018). Neuronal specification in space and time. Sci. (80- 362, 176–180. doi:10.1126/science.aas9435
Homem, C. C. F., and Knoblich, J. a. (2012). Drosophila neuroblasts: a model for stem cell biology. Development 139, 4297–4310. doi:10.1242/dev.080515
Horowitz, A. M., Fan, X., Bieri, G., Smith, L. K., Sanchez-Diaz, C. I., Schroer, A. B., et al. (2020). Blood factors transfer beneficial effects of exercise on neurogenesis and cognition to the aged brain. Science 369, 167–173. doi:10.1126/SCIENCE.AAW2622
Huang, X., Liu, J., Ketova, T., Fleming, J. T., Grover, V. K., Cooper, M. K., et al. (2010). Transventricular delivery of sonic hedgehog is essential to cerebellar ventricular zone development. Proc. Natl. Acad. Sci. U. S. A. 107, 8422–8427. doi:10.1073/pnas.0911838107
Hunter, A. C., Petley-Ragan, L. M., Das, M., and Auld, V. J. (2020). Basigin associates with integrin in order to regulate perineurial glia and Drosophila nervous system morphology. J. Neurosci. 40, 3360–3373. doi:10.1523/JNEUROSCI.1397-19.2020
Ito, K., Urban, J., and Technau, G. (1995). Distribution, classification, and development ofDrosophila glial cells in the late embryonic and early larval ventral nerve cord. Dev. Genes Evol. 204, 284–307. doi:10.1007/BF02179499
James, J. M., Gewolb, C., and Bautch, V. L. (2009). Neurovascular development uses VEGF-A signaling to regulate blood vessel ingression into the neural tube. Development 136, 833–841. doi:10.1242/DEV.028845
Javaherian, A., and Kriegstein, A. (2009). A stem cell niche for intermediate progenitor cells of the embryonic cortex. Cereb. Cortex 19, i70–i77. doi:10.1093/CERCOR/BHP029
Jeong, J. Y., Kwon, H. B., Ahn, J. C., Kang, D., Kwon, S. H., Park, J. A., et al. (2008). Functional and developmental analysis of the blood-brain barrier in zebrafish. Brain Res. Bull. 75, 619–628. doi:10.1016/J.BRAINRESBULL.2007.10.043
Johansson, P. A. (2014). The choroid plexuses and their impact on developmental neurogenesis. Front. Neurosci. 8, 340. doi:10.3389/fnins.2014.00340
Juang, J. L., and Carlson, S. D. (1994). Analog of vertebrate anionic sites in blood-brain interface of larval Drosophila. Cell Tissue Res. 277, 87–95. doi:10.1007/BF00303084
Jurisch-Yaksi, N., Yaksi, E., and Kizil, C. (2020). Radial glia in the zebrafish brain: functional, structural, and physiological comparison with the mammalian glia. Glia 68, 2451–2470. doi:10.1002/GLIA.23849
Kacem, K., Lacombe, P., Seylaz, J., and Bonvento, G. (1998). Structural organization of the perivascular astrocyte endfeet and their relationship with the endothelial glucose transporter: a confocal microscopy study. Glia 23, 1–10. doi:10.1002/(sici)1098-1136(199805)23:1<1::aid-glia1>3.0.co;2-b
Kalebic, N., and Huttner, W. B. (2020). Basal progenitor morphology and neocortex evolution. Trends Neurosci. 43, 843–853. doi:10.1016/J.TINS.2020.07.009
Kanai, M. I., Kim, M.-J., Akiyama, T., Takemura, M., Wharton, K., O’Connor, M. B., et al. (2018). Regulation of neuroblast proliferation by surface glia in the Drosophila larval brain. Sci. Rep. 8, 3730. doi:10.1038/s41598-018-22028-y
Kato, K., Awasaki, T., and Ito, K. (2009). Neuronal programmed cell death induces glial cell division in the adult Drosophila brain. Development 136, 51–59. doi:10.1242/dev.023366
Kawauchi, T., Sekine, K., Shikanai, M., Chihama, K., Tomita, K., Kubo, K. ichiro, et al. (2010). Rab GTPases-dependent endocytic pathways regulate neuronal migration and maturation through N-cadherin trafficking. Neuron 67, 588–602. doi:10.1016/J.NEURON.2010.07.007
Kazanis, I., and ffrench-Constant, C. (2011). Extracellular matrix and the neural stem cell niche. Dev. Neurobiol. 71, 1006–1017. doi:10.1002/dneu.20970
Kerever, A., Schnack, J., Vellinga, D., Ichikawa, N., Moon, C., Arikawa-Hirasawa, E., et al. (2007). Novel extracellular matrix structures in the neural stem cell niche capture the neurogenic factor fibroblast growth factor 2 from the extracellular milieu. Stem Cells 25, 2146–2157. doi:10.1634/STEMCELLS.2007-0082
Kimble, J. E., and White, J. G. (1981). On the control of germ cell development in Caenorhabditis elegans. Dev. Biol. 81, 208–219. doi:10.1016/0012-1606(81)90284-0
Kishimoto, N., Alfaro-Cervello, C., Shimizu, K., Asakawa, K., Urasaki, A., Nonaka, S., et al. (2011). Migration of neuronal precursors from the telencephalic ventricular zone into the olfactory bulb in adult zebrafish. J. Comp. Neurol. 519, 3549–3565. doi:10.1002/CNE.22722
Korzh, V. (2023). Development of the brain ventricular system from a comparative perspective. Clin. Anat. 36, 320–334. doi:10.1002/CA.23994
Kriegstein, A., and Alvarez-Buylla, A. (2009). The glial nature of embryonic and adult neural stem cells. Annu. Rev. Neurosci. 32, 149–184. doi:10.1146/ANNUREV.NEURO.051508.135600
Labusch, M., Mancini, L., Morizet, D., and Bally-Cuif, L. (2020). Conserved and divergent features of adult neurogenesis in zebrafish. Front. Cell Dev. Biol. 8, 525. doi:10.3389/fcell.2020.00525
Lacar, B., Young, S. Z., Platel, J.-C., and Bordey, A. (2011). Gap junction-mediated calcium waves define communication networks among murine postnatal neural progenitor cells. Eur. J. Neurosci. 34, 1895–1905. doi:10.1111/j.1460-9568.2011.07901.x
Lander, A. D., Kimble, J., Clevers, H., Fuchs, E., Montarras, D., Buckingham, M., et al. (2012). What does the concept of the stem cell niche really mean today? BMC Biol. 10, 19. doi:10.1186/1741-7007-10-19
Lange, C., Turrero Garcia, M., Decimo, I., Bifari, F., Eelen, G., Quaegebeur, A., et al. (2016). Relief of hypoxia by angiogenesis promotes neural stem cell differentiation by targeting glycolysis. EMBO J. 35, 924–941. doi:10.15252/EMBJ.201592372
Lathia, J. D., Patton, B., Eckley, D. M., Magnus, T., Mughal, M. R., Sasaki, T., et al. (2007). Patterns of laminins and integrins in the embryonic ventricular zone of the CNS. J. Comp. Neurol. 505, 630–643. doi:10.1002/CNE.21520
Lehtinen, M. K., Zappaterra, M. W., Chen, X., Yang, Y. J., Hill, A. D., Lun, M., et al. (2011). The cerebrospinal fluid provides a proliferative niche for neural progenitor cells. Neuron 69, 893–905. doi:10.1016/J.NEURON.2011.01.023
Li, S., Haigh, K., Haigh, J. J., and Vasudevan, A. (2013). Endothelial VEGF sculpts cortical cytoarchitecture. J. Neurosci. 33, 14809–14815. doi:10.1523/JNEUROSCI.1368-13.2013
Li, S., Koe, C. T., Tay, S. T., Tan, A. L. K., Zhang, S., Zhang, Y., et al. (2017). An intrinsic mechanism controls reactivation of neural stem cells by spindle matrix proteins. Nat. Commun. 8, 122. doi:10.1038/s41467-017-00172-9
Li, S., Yu, X., and Feng, Q. (2019). Fat body biology in the last decade, 315–333. doi:10.1146/ANNUREV-ENTO-011118-112007
Lindsey, B. W., Darabie, A., and Tropepe, V. (2012). The cellular composition of neurogenic periventricular zones in the adult zebrafish forebrain. J. Comp. Neurol. 520, 2275–2316. doi:10.1002/CNE.23065
Lindsey, B. W., and Tropepe, V. (2006). A comparative framework for understanding the biological principles of adult neurogenesis. Prog. Neurobiol. 80, 281–307. doi:10.1016/J.PNEUROBIO.2006.11.007
Liu, S., Trupiano, M. X., Simon, J., Guo, J., and Anton, E. S. (2021). The essential role of primary cilia in cerebral cortical development and disorders. Curr. Top. Dev. Biol. 142, 99–146. doi:10.1016/BS.CTDB.2020.11.003
Llorente, V., Velarde, P., Desco, M., and Gómez-Gaviro, M. V. (2022). Current understanding of the neural stem cell niches. Cells 11, 3002. doi:10.3390/CELLS11193002
Lord, B. I., Testa, N. G., and Hendry, J. H. (1975). The relative spatial distributions of CFUs and CFUc in the normal mouse femur. Blood 46, 65–72. doi:10.1182/BLOOD.V46.1.65.65
Loulier, K., Lathia, J. D., Marthiens, V., Relucio, J., Mughal, M. R., Tang, S. C., et al. (2009). beta1 integrin maintains integrity of the embryonic neocortical stem cell niche. PLoS Biol. 7, 1000176. doi:10.1371/JOURNAL.PBIO.1000176
Loyer, N., and Januschke, J. (2018). The last-born daughter cell contributes to division orientation of Drosophila larval neuroblasts. Nat. Commun. 9, 3745. doi:10.1038/s41467-018-06276-0
Lumsden, A., and Krumlauf, R. (1996). Patterning the vertebrate neuraxis. Science 274, 1109–1115. doi:10.1126/SCIENCE.274.5290.1109
Lust, K., Maynard, A., Gomes, T., Fleck, J. S., Camp, J. G., Tanaka, E. M., et al. (2022). Single-cell analyses of axolotl telencephalon organization, neurogenesis, and regeneration. Science 377, eabp9262. doi:10.1126/SCIENCE.ABP9262
Ma, S., Kwon, H. J., and Huang, Z. (2012). A functional requirement for astroglia in promoting blood vessel development in the early postnatal brain. PLoS One 7, e48001. doi:10.1371/JOURNAL.PONE.0048001
Ma, S., Kwon, H. J., Johng, H., Zang, K., and Huang, Z. (2013). Radial glial neural progenitors regulate nascent brain vascular network stabilization via inhibition of Wnt signaling. PLOS Biol. 11, e1001469. doi:10.1371/JOURNAL.PBIO.1001469
Malatesta, P., Hartfuss, E., and Götz, M. (2000). Isolation of radial glial cells by fluorescent-activated cell sorting reveals a neuronal lineage. Development 127, 5253–5263. doi:10.1242/DEV.127.24.5253
Malmersjö, S., Rebellato, P., Smedler, E., Planert, H., Kanatani, S., Liste, I., et al. (2013). Neural progenitors organize in small-world networks to promote cell proliferation. Proc. Natl. Acad. Sci. U. S. A. 110, E1524–E1532. –9. doi:10.1073/pnas.1220179110
Mancini, L., Guirao, B., Ortica, S., Labusch, M., Cheysson, F., Bonnet, V., et al. (2023). Apical size and deltaA expression predict adult neural stem cell decisions along lineage progression. Sci. Adv. 9, eadg7519. doi:10.1126/SCIADV.ADG7519
Marchioro, M., De Azevedo Mota Nunes, J. M., Rabelo Ramalho, A. M., Molowny, A., Perez-Martinez, E., Ponsoda, X., et al. (2005). Postnatal neurogenesis in the medial cortex of the tropical lizard Tropidurus hispidus. Neuroscience 134, 407–413. doi:10.1016/J.NEUROSCIENCE.2005.04.014
Marcy, G., and Raineteau, O. (2019). Contributions of single-cell approaches for probing heterogeneity and dynamics of neural progenitors throughout life: concise review. Stem Cells 37, 1381–1388. doi:10.1002/STEM.3071
März, M., Schmidt, R., Rastegar, S., and Strähle, U. (2010). Expression of the transcription factor Olig2 in proliferating cells in the adult zebrafish telencephalon. Dev. Dyn. 239, 3336–3349. doi:10.1002/DVDY.22455
März, M., Schmidt, R., Rastegar, S., and Strahle, U. (2011). Regenerative response following stab injury in the adult zebrafish telencephalon. Dev. Dyn. 240, 2221–2231. doi:10.1002/DVDY.22710
Matcovitch-Natan, O., Winter, D. R., Giladi, A., Aguilar, S. V., Spinrad, A., Sarrazin, S., et al. (2016). Microglia development follows a stepwise program to regulate brain homeostasis. Science 353, aad8670. doi:10.1126/SCIENCE.AAD8670
Mathiisen, T. M., Lehre, K. P., Danbolt, N. C., and Ottersen, O. P. (2010). The perivascular astroglial sheath provides a complete covering of the brain microvessels: an electron microscopic 3D reconstruction. Glia 58, 1094–1103. doi:10.1002/GLIA.20990
Matsuoka, R. L., Marass, M., Avdesh, A., Helker, C. S. M., Maischein, H. M., Grosse, A. S., et al. (2016). Radial glia regulate vascular patterning around the developing spinal cord. Elife 5, e20253. doi:10.7554/ELIFE.20253
Maurange, C., Cheng, L., and Gould, A. P. (2008). Temporal transcription factors and their targets schedule the end of neural proliferation in Drosophila. Cell 133, 891–902. doi:10.1016/j.cell.2008.03.034
Mayer, F., Mayer, N., Chinn, L., Pinsonneault, R. L., Kroetz, D., and Bainton, R. J. (2009). Evolutionary conservation of vertebrate blood-brain barrier chemoprotective mechanisms in Drosophila. J. Neurosci. 29, 3538–3550. doi:10.1523/JNEUROSCI.5564-08.2009
Mercier, F. (2016). Fractones: extracellular matrix niche controlling stem cell fate and growth factor activity in the brain in health and disease. Cell. Mol. Life Sci. 73, 4661–4674. doi:10.1007/s00018-016-2314-y
Mercier, F., Kitasako, J. T., and Hatton, G. I. (2002). Anatomy of the brain neurogenic zones revisited: fractones and the fibroblast/macrophage network. J. Comp. Neurol. 451, 170–188. doi:10.1002/CNE.10342
Meyer, S., Schmidt, I., and Klämbt, C. (2014). Glia ECM interactions are required to shape the Drosophila nervous system. Mech. Dev. 133, 105–116. doi:10.1016/J.MOD.2014.05.003
Mira, H., and Morante, J. (2020). Neurogenesis from embryo to adult – lessons from flies and mice. Front. Cell Dev. Biol. 8, 533. doi:10.3389/fcell.2020.00533
Mirzadeh, Z., Merkle, F. T., Soriano-Navarro, M., Garcia-Verdugo, J. M., and Alvarez-Buylla, A. (2008). Neural stem cells confer unique pinwheel architecture to the ventricular surface in neurogenic regions of the adult brain. Cell Stem Cell 3, 265–278. doi:10.1016/J.STEM.2008.07.004
Mishra, A., Reynolds, J. P., Chen, Y., Gourine, A. V., Rusakov, D. A., and Attwell, D. (2016). Astrocytes mediate neurovascular signaling to capillary pericytes but not to arterioles. Nat. Neurosci. 19, 1619–1627. doi:10.1038/NN.4428
Mohyeldin, A., Garzón-Muvdi, T., and Quiñones-Hinojosa, A. (2010). Oxygen in stem cell biology: a critical component of the stem cell niche. Cell Stem Cell 7, 150–161. doi:10.1016/J.STEM.2010.07.007
Molofsky, A. V., and Deneen, B. (2015). Astrocyte development: a guide for the perplexed. Glia 63, 1320–1329. doi:10.1002/GLIA.22836
Morante-Redolat, J. M., and Porlan, E. (2019). Neural stem cell regulation by adhesion molecules within the subependymal niche. doi:10.3389/fcell.2019.00102
Morizet, D., Foucher, I., Alunni, A., and Bally-Cuif, L. (2023). Integrative single-cell transcriptomics clarifies adult neurogenesis and macroglia evolution. bioRxiv 02 (27), 530203. doi:10.1101/2023.02.27.530203
Mueller, T., Dong, Z., Berberoglu, M. A., and Guo, S. (2011). The dorsal pallium in zebrafish, Danio rerio (cyprinidae, teleostei). Brain Res. 1381, 95–105. doi:10.1016/J.BRAINRES.2010.12.089
Nascimento, M. A., Sorokin, L., and Coelho-Sampaio, T. (2018). Fractone bulbs derive from ependymal cells and their laminin composition influence the stem cell niche in the subventricular zone. J. Neurosci. 38, 3880–3889. doi:10.1523/JNEUROSCI.3064-17.2018
Navascués, J., Calvente, R., Marín-Teva, J. L., and Cuadros, M. A. (2000). Entry, dispersion and differentiation of microglia in the developing central nervous system. An. Acad. Bras. Cienc. 72, 91–102. doi:10.1590/S0001-37652000000100013
Nériec, N., and Desplan, C. (2016). “From the eye to the brain. Development of the Drosophila visual system,” in Current topics in developmental biology (Academic Press Inc.), 247–271. doi:10.1016/bs.ctdb.2015.11.032
Nielsen, C. M., and Dymecki, S. M. (2010). Sonic hedgehog is required for vascular outgrowth in the hindbrain choroid plexus. Dev. Biol. 340, 430–437. doi:10.1016/J.YDBIO.2010.01.032
Nieuwenhuys, R. (2011). The development and general morphology of the telencephalon of actinopterygian fishes: synopsis, documentation and commentary. Brain Struct. Funct. 215, 141–157. doi:10.1007/S00429-010-0285-6
Nimmerjahn, A., Kirchhoff, F., and Helmchen, F. (2005). Resting microglial cells are highly dynamic surveillants of brain parenchyma in vivo. Science 308, 1314–1318. doi:10.1126/science.1110647
Noctor, S. C., Flint, A. C., Weissman, T. A., Dammerman, R. S., and Kriegstein, A. R. (2001). Neurons derived from radial glial cells establish radial units in neocortex. Nature 409, 714–720. doi:10.1038/35055553
Noctor, S. C., Flint, A. C., Weissman, T. A., Wong, W. S., Clinton, B. K., and Kriegstein, A. R. (2002). Dividing precursor cells of the embryonic cortical ventricular zone have morphological and molecular characteristics of radial glia. J. Neurosci. 22, 3161–3173. doi:10.1523/JNEUROSCI.22-08-03161.2002
Obermann, J., Wagner, F., Kociaj, A., Zambusi, A., Ninkovic, J., Hauck, S. M., et al. (2019). The surface proteome of adult neural stem cells in zebrafish unveils long-range cell-cell connections and age-related changes in responsiveness to IGF. Stem Cell Rep. 12, 258–273. doi:10.1016/J.STEMCR.2018.12.005
Obernier, K., and Alvarez-Buylla, A. (2019). Neural stem cells: origin, heterogeneity and regulation in the adult mammalian brain. Dev 146, dev156059. doi:10.1242/dev.156059
Obernier, K., Cebrian-Silla, A., Thomson, M., Parraguez, J. I., Anderson, R., Guinto, C., et al. (2018). Adult neurogenesis is sustained by symmetric self-renewal and differentiation. Cell Stem Cell 22, 221–234. doi:10.1016/J.STEM.2018.01.003
Ogino, T., Sawada, M., Takase, H., Nakai, C., Herranz-Pérez, V., Cebrián-Silla, A., et al. (2016). Characterization of multiciliated ependymal cells that emerge in the neurogenic niche of the aged zebrafish brain. J. Comp. Neurol. 524, 2982–2992. doi:10.1002/CNE.24001
Olstad, E. W., Ringers, C., Hansen, J. N., Wens, A., Brandt, C., Wachten, D., et al. (2019). Ciliary beating compartmentalizes cerebrospinal fluid flow in the brain and regulates ventricular development. Curr. Biol. 29, 229–241. doi:10.1016/J.CUB.2018.11.059
Ortiz-Álvarez, G., Daclin, M., Shihavuddin, A., Lansade, P., Fortoul, A., Faucourt, M., et al. (2019). Adult neural stem cells and multiciliated ependymal cells share a common lineage regulated by the geminin family members. Neuron 102, 159–172. doi:10.1016/J.NEURON.2019.01.051
Ottone, C., Krusche, B., Whitby, A., Clements, M., Quadrato, G., Pitulescu, M. E., et al. (2014). Direct cell-cell contact with the vascular niche maintains quiescent neural stem cells. Nat. Cell Biol. 16, 1045–1056. doi:10.1038/ncb3045
Pandey, S., Moyer, A. J., and Thyme, S. B. (2023). A single-cell transcriptome atlas of the maturing zebrafish telencephalon. Genome Res. 33, 658–671. doi:10.1101/gr.277278.122
Paredes, I., Himmels, P., and Ruiz de Almodóvar, C. (2018). Neurovascular communication during CNS development. Dev. Cell 45, 10–32. doi:10.1016/J.DEVCEL.2018.01.023
Paridaen, J. T. M. L., and Huttner, W. B. (2014). Neurogenesis during development of the vertebrate central nervous system. EMBO Rep. 15, 351–364. doi:10.1002/embr.201438447
Park, Y., Rangel, C., Reynolds, M. M., Caldwell, M. C., Johns, M., Nayak, M., et al. (2003). Drosophila perlecan modulates FGF and hedgehog signals to activate neural stem cell division. Dev. Biol. 253, 247–257. doi:10.1016/s0012-1606(02)00019-2
Parra-Peralbo, E., Talamillo, A., and Barrio, R. (2021). Origin and development of the adipose tissue, a key organ in physiology and disease. Front. Cell Dev. Biol. 9, 786129. doi:10.3389/FCELL.2021.786129
Pastor-Pareja, J. C., and Xu, T. (2011). Shaping cells and organs in Drosophila by opposing roles of fat body-secreted collagen IV and perlecan. Dev. Cell 21, 245–256. doi:10.1016/j.devcel.2011.06.026
Paul, A., Chaker, Z., and Doetsch, F. (2017). Hypothalamic regulation of regionally distinct adult neural stem cells and neurogenesis. Sci. (80- 356, 1383–1386. doi:10.1126/science.aal3839
Peco, E., Davla, S., Camp, D., Stacey, S. M., Landgraf, M., and van Meyel, D. J. (2016). Drosophila astrocytes cover specific territories of the CNS neuropil and are instructed to differentiate by Prospero, a key effector of Notch. Development 143, 1170–1181. doi:10.1242/DEV.133165
Peguera, B., Segarra, M., and Acker-Palmer, A. (2021). Neurovascular crosstalk coordinates the central nervous system development. Curr. Opin. Neurobiol. 69, 202–213. doi:10.1016/J.CONB.2021.04.005
Perdiguero, E. G., Klapproth, K., Schulz, C., Busch, K., Azzoni, E., Crozet, L., et al. (2014). Tissue-resident macrophages originate from yolk-sac-derived erythro-myeloid progenitors. Nature 518, 547–551. doi:10.1038/nature13989
Pereanu, W., Shy, D., and Hartenstein, V. (2005). Morphogenesis and proliferation of the larval brain glia in Drosophila. Dev. Biol. 283, 191–203. doi:10.1016/j.ydbio.2005.04.024
Pereanu, W., Spindler, S., Cruz, L., and Hartenstein, V. (2007). Tracheal development in the Drosophila brain is constrained by glial cells. Dev. Biol. 302, 169–180. doi:10.1016/J.YDBIO.2006.09.022
Peri, F., and Nüsslein-Volhard, C. (2008). Live imaging of neuronal degradation by microglia reveals a role for v0-ATPase a1 in phagosomal fusion in vivo. Cell 133, 916–927. doi:10.1016/J.CELL.2008.04.037
Petrik, D., Jörgensen, S., Eftychidis, V., and Siebzehnrubl, F. A. (2022). Singular adult neural stem cells do not exist. Cells 11, 722. doi:10.3390/CELLS11040722
Pilz, G. A., Bottes, S., Betizeau, M., Jörg, D. J., Carta, S., Simons, B. D., et al. (2018). Live imaging of neurogenesis in the adult mouse hippocampus. Science 359, 658–662. doi:10.1126/SCIENCE.AAO5056
Pinto, L., Mader, M. T., Irmler, M., Gentilini, M., Santoni, F., Drechsel, D., et al. (2008). Prospective isolation of functionally distinct radial glial subtypes--lineage and transcriptome analysis. Mol. Cell. Neurosci. 38, 15–42. doi:10.1016/J.MCN.2008.01.012
Plazaola-Sasieta, H., Zhu, Q., Gaitán-Peñas, H., Rios, M., Estévez, R., and Morey, M. (2019). Drosophila ClC-a is required in glia of the stem cell niche for proper neurogenesis and wiring of neural circuits. Glia 67, 2374–2398. doi:10.1002/glia.23691
Pogodalla, N., Kranenburg, H., Rey, S., Rodrigues, S., Cardona, A., and Klämbt, C. (2021). Drosophila ßHeavy-Spectrin is required in polarized ensheathing glia that form a diffusion-barrier around the neuropil. Nat. Commun. 12, 6357. doi:10.1038/S41467-021-26462-X
Pollington, H. Q., Seroka, A. Q., and Doe, C. Q. (2023). From temporal patterning to neuronal connectivity in Drosophila type I neuroblast lineages. Semin. Cell Dev. Biol. 142, 4–12. doi:10.1016/J.SEMCDB.2022.05.022
Potten, C. S., and Loeffler, M. (1990). Stem cells: attributes, cycles, spirals, pitfalls and uncertainties Lessons for and from the Crypt. Development 110, 1001–1020. doi:10.1242/DEV.110.4.1001
Prokop, A., and Technau, G. M. (1991). The origin of postembryonic neuroblasts in the ventral nerve cord of Drosophila melanogaster. Development 111, 79–88. doi:10.1242/DEV.111.1.79
Purves, D., Augustine, G. J., Fitzpatrick, D., Hall, W., LaMantia, A. S., White, L., et al. (2019). Neurosciences. De boeck supérieur. Available at: https://books.google.fr/books?id=JDSZDwAAQBAJ.
Quaresima, S., Istiaq, A., Jono, H., Cacci, E., Ohta, K., and Lupo, G. (2022). Assessing the role of ependymal and vascular cells as sources of extracellular cues regulating the mouse ventricular-subventricular zone neurogenic niche. Front. Cell Dev. Biol. 10, 845567. doi:10.3389/fcell.2022.845567
Quiñonez-Silvero, C., Hübner, K., and Herzog, W. (2020). Development of the brain vasculature and the blood-brain barrier in zebrafish. Dev. Biol. 457, 181–190. doi:10.1016/J.YDBIO.2019.03.005
Radakovits, R., Barros, C. S., Belvindrah, R., Patton, B., and Müller, U. (2009). Regulation of radial glial survival by signals from the meninges. J. Neurosci. 29, 7694–7705. doi:10.1523/JNEUROSCI.5537-08.2009
Rao, P. R., Lin, L., Huang, H., Guha, A., Roy, S., and Kornberg, T. B. (2015). Developmental compartments in the larval trachea of Drosophila. Elife 4, e08666. doi:10.7554/ELIFE.08666
Rašin, M. R., Gazula, V. R., Breunig, J. J., Kwan, K. Y., Johnson, M. B., Liu-Chen, S., et al. (2007). Numb and Numbl are required for maintenance of cadherin-based adhesion and polarity of neural progenitors. Nat. Neurosci. 10, 819–827. doi:10.1038/NN1924
Read, R. D. (2018). Pvr receptor tyrosine kinase signaling promotes post-embryonic morphogenesis, and survival of glia and neural progenitor cells in drosophila. Dev 145, dev164285. doi:10.1242/dev.164285
Redmond, S. A., Figueres-Oñate, M., Obernier, K., Nascimento, M. A., Parraguez, J. I., López-Mascaraque, L., et al. (2019). Development of ependymal and postnatal neural stem cells and their origin from a common embryonic progenitor. Cell Rep. 27, 429–441. doi:10.1016/J.CELREP.2019.01.088
Reynolds, B. A., and Weiss, S. (1992). Generation of neurons and astrocytes from isolated cells of the adult mammalian central nervous system. Science 255, 1707–1710. doi:10.1126/science.1553558
Rigato, C., Buckinx, R., Le-Corronc, H., Rigo, J. M., and Legendre, P. (2011). Pattern of invasion of the embryonic mouse spinal cord by microglial cells at the time of the onset of functional neuronal networks. Glia 59, 675–695. doi:10.1002/GLIA.21140
Ringers, C., Olstad, E. W., and Jurisch-Yaksi, N. (2020). The role of motile cilia in the development and physiology of the nervous system. Philos. Trans. R. Soc. B 375, 20190156. doi:10.1098/RSTB.2019.0156
Rosin, J. M., Marsters, C. M., Malik, F., Far, R., Adnani, L., Schuurmans, C., et al. (2021). Embryonic microglia interact with hypothalamic radial glia during development and upregulate the TAM receptors MERTK and AXL following an insult. Cell Rep. 34, 108587. doi:10.1016/J.CELREP.2020.108587
Rothenaigner, I., Krecsmarik, M., Hayes, J. A., Bahn, B., Lepier, A., Fortin, G., et al. (2011). Clonal analysis by distinct viral vectors identifies bona fide neural stem cells in the adult zebrafish telencephalon and characterizes their division properties and fate. Development 138, 1459–1469. doi:10.1242/DEV.058156
Rousso, D. L., Pearson, C. A., Gaber, Z. B., Miquelajauregui, A., Li, S., Portera-Cailliau, C., et al. (2012). Foxp-mediated suppression of N-cadherin regulates neuroepithelial character and progenitor maintenance in the CNS. Neuron 74, 314–330. doi:10.1016/J.NEURON.2012.02.024
Rujano, M. A., Briand, D., Ðelić, B., Marc, J., and Spéder, P. (2022). An interplay between cellular growth and atypical fusion defines morphogenesis of a modular glial niche in Drosophila. Nat. Commun. 13, 4999. doi:10.1038/S41467-022-32685-3
Samakovlis, C., Hacohen, N., Manning, G., Sutherland, D. C., Guillemin, K., and Krasnow, M. A. (1996). Development of the Drosophila tracheal system occurs by a series of morphologically distinct but genetically coupled branching events. Development 122, 1395–1407. doi:10.1242/DEV.122.5.1395
Sato, Y., Kiyozumi, D., Futaki, S., Nakano, I., Shimono, C., Kaneko, N., et al. (2019). Ventricular–subventricular zone fractones are speckled basement membranes that function as a neural stem cell niche. Mol. Biol. Cell 30, 56–68. doi:10.1091/MBC.E18-05-0286
Saunders, N. R., Liddelow, S. A., and Dziegielewska, K. M. (2012). Barrier mechanisms in the developing brain. Front. Pharmacol. 3, 46. doi:10.3389/fphar.2012.00046
Schmid, a, Chiba, A., and Doe, C. Q. (1999). Clonal analysis of Drosophila embryonic neuroblasts: neural cell types, axon projections and muscle targets. Development 126, 4653–4689. doi:10.1242/dev.126.21.4653
Schmidt, H., Rickert, C., Bossing, T., Vef, O., Urban, J., and Technau, G. M. (1997). The embryonic central nervous system lineages of Drosophila melanogaster. II. Neuroblast lineages derived from the dorsal part of the neuroectoderm. Dev. Biol. 189, 186–204. doi:10.1006/DBIO.1997.8660
Schmidt, R., Strähle, U., and Scholpp, S. (2013). Neurogenesis in zebrafish – from embryo to adult. Neural Dev. 8, 3. doi:10.1186/1749-8104-8-3
Schofield, R. (1978). The relationship between the spleen colony-forming cell and the haemopoietic stem cell. Blood Cells 4, 7–25.
Schulz, C., Perdiguero, E. G., Chorro, L., Szabo-Rogers, H., Cagnard, N., Kierdorf, K., et al. (2012). A lineage of myeloid cells independent of Myb and hematopoietic stem cells. Science 336, 86–90. doi:10.1126/SCIENCE.1219179
Schwabe, T., Bainton, R. J., Fetter, R. D., Heberlein, U., and Gaul, U. (2005). GPCR signaling is required for blood-brain barrier formation in drosophila. Cell 123, 133–144. doi:10.1016/j.cell.2005.08.037
Schwabe, T., Li, X., and Gaul, U. (2017). Dynamic analysis of the mesenchymal-epithelial transition of blood-brain barrier forming glia in Drosophila. Biol. Open 6, 232–243. doi:10.1242/bio.020669
Segarra, M., Aburto, M. R., Cop, F., Llaó-Cid, C., Härtl, R., Damm, M., et al. (2018). Endothelial Dab1 signaling orchestrates neuro-glia-vessel communication in the central nervous system. Science 361, eaao2861. doi:10.1126/SCIENCE.AAO2861
Shen, Q., Wang, Y., Kokovay, E., Lin, G., Chuang, S. M., Goderie, S. K., et al. (2008). Adult SVZ stem cells lie in a vascular niche: a quantitative analysis of niche cell-cell interactions. Cell Stem Cell 3, 289–300. doi:10.1016/j.stem.2008.07.026
Shigemoto-Mogami, Y., Hoshikawa, K., Goldman, J. E., Sekino, Y., and Sato, K. (2014). Microglia enhance neurogenesis and oligodendrogenesis in the early postnatal subventricular zone. J. Neurosci. 34, 2231–2243. doi:10.1523/JNEUROSCI.1619-13.2014
Shikanai, M., Nakajima, K., and Kawauchi, T. (2011). N-cadherin regulates radial glial fiber-dependent migration of cortical locomoting neurons. Commun. Integr. Biol. 4, 326–330. doi:10.4161/CIB.4.3.14886
Silva-Vargas, V., Crouch, E. E., and Doetsch, F. (2013). Adult neural stem cells and their niche: a dynamic duo during homeostasis, regeneration, and aging. Curr. Opin. Neurobiol. 23, 935–942. doi:10.1016/j.conb.2013.09.004
Silva-Vargas, V., Maldonado-Soto, A. R., Mizrak, D., Codega, P., and Doetsch, F. (2016). Age-dependent niche signals from the choroid plexus regulate adult neural stem cells. Cell Stem Cell 19, 643–652. doi:10.1016/J.STEM.2016.06.013
Simões, A. R., Neto, M., Alves, C. S., Santos, M. B., Fernández-Hernández, I., Veiga-Fernandes, H., et al. (2022). Damage-responsive neuro-glial clusters coordinate the recruitment of dormant neural stem cells in Drosophila. Dev. Cell 57, 1661–1675.e7. doi:10.1016/J.DEVCEL.2022.05.015
Sirerol-Piquer, M. S., Belenguer, G., Morante-Redolat, J. M., Duart-Abadia, P., Perez-Villalba, A., and Fariñas, I. (2019). Physiological interactions between microglia and neural stem cells in the adult subependymal niche. Neuroscience 405, 77–91. doi:10.1016/J.NEUROSCIENCE.2019.01.009
Song, J., Zhong, C., Bonaguidi, M. A., Sun, G. J., Hsu, D., Gu, Y., et al. (2012). Neuronal circuitry mechanism regulating adult quiescent neural stem-cell fate decision. Nature 489, 150–154. doi:10.1038/nature11306
Sousa-Nunes, R., Yee, L. L., and Gould, A. P. (2011). Fat cells reactivate quiescent neuroblasts via TOR and glial insulin relays in Drosophila. Nature 471, 508–512. doi:10.1038/nature09867
Spassky, N., Merkle, F. T., Flames, N., Tramontin, A. D., García-Verdugo, J. M., and Alvarez-Buylla, A. (2005). Adult ependymal cells are postmitotic and are derived from radial glial cells during embryogenesis. J. Neurosci. 25, 10–18. doi:10.1523/JNEUROSCI.1108-04.2005
Spéder, P., and Brand, A. H. (2014). Gap junction proteins in the blood-brain barrier control nutrient-dependent reactivation of Drosophila neural stem cells. Dev. Cell 30, 309–321. doi:10.1016/j.devcel.2014.05.021
Spéder, P., and Brand, A. H. (2018). Systemic and local cues drive neural stem cell niche remodelling during neurogenesis in Drosophila. Elife 7, e30413. doi:10.7554/eLife.30413
Spindler, S. R., Ortiz, I., Fung, S., Takashima, S., and Hartenstein, V. (2009). Drosophila cortex and neuropile glia influence secondary axon tract growth, pathfinding, and fasciculation in the developing larval brain. Dev. Biol. 334, 355–368. doi:10.1016/J.YDBIO.2009.07.035
Squarzoni, P., Oller, G., Hoeffel, G., Pont-Lezica, L., Rostaing, P., Low, D., et al. (2014). Microglia modulate wiring of the embryonic forebrain. Cell Rep. 8, 1271–1279. doi:10.1016/J.CELREP.2014.07.042
Squire, L. R., Bloom, F. E., Spitzer, N. C., Gage, F., and Albright, T. (2009). Encyclopedia of neuroscience. Elsevier Science.
Stenman, J. M., Rajagopal, J., Carroll, T. J., Ishibashi, M., McMahon, J., McMahon, A. P., et al. (2008). Canonical Wnt signaling regulates organ-specific assembly and differentiation of CNS vasculature 322 (5905). New York, NY: Science, 1247–1250. doi:10.1126/science.1164594
Stolp, H. B., and Molnár, Z. (2015). Neurogenic niches in the brain: help and hindrance of the barrier systems. Front. Neurosci. 9, 20. doi:10.3389/fnins.2015.00020
Stork, T., Engelen, D., Krudewig, A., Silies, M., Bainton, R. J., and Klämbt, C. (2008). Organization and function of the blood-brain barrier in Drosophila. J. Neurosci. 28, 587–597. doi:10.1523/JNEUROSCI.4367-07.2008
Stork, T., Sheehan, A., Tasdemir-Yilmaz, O. E., and Freeman, M. R. (2014). Neuron-glia interactions through the Heartless FGF receptor signaling pathway mediate morphogenesis of Drosophila astrocytes. Neuron 83, 388–403. doi:10.1016/J.NEURON.2014.06.026
Stratman, A. N., Pezoa, S. A., Farrelly, O. M., Castranova, D., Dye, L. E., Butler, M. G., et al. (2017). Interactions between mural cells and endothelial cells stabilize the developing zebrafish dorsal aorta. Development 144, 115–127. doi:10.1242/DEV.143131
Sturrock, R. R. (1979). A morphological study of the development of the mouse choroid plexus. J. Anat. 129, 777–793.
Styfhals, R., Zolotarov, G., Hulselmans, G., Spanier, K. I., Poovathingal, S., Elagoz, A. M., et al. (2022). Cell type diversity in a developing octopus brain. Nat. Commun. 13, 7392. doi:10.1038/S41467-022-35198-1
Su, L., Zhang, M., Ji, F., Zhao, J., Wang, Y., Wang, W., et al. (2022). Microglia homeostasis mediated by epigenetic ARID1A regulates neural progenitor cells response and leads to autism-like behaviors. Mol. Psychiatry. doi:10.1038/S41380-022-01703-7
Sutherland, D., Samakovlis, C., and Krasnow, M. A. (1996). Branchless encodes a Drosophila FGF homolog that controls tracheal cell migration and the pattern of branching. Cell 87, 1091–1101. doi:10.1016/S0092-8674(00)81803-6
Swinnen, N., Smolders, S., Avila, A., Notelaers, K., Paesen, R., Ameloot, M., et al. (2013). Complex invasion pattern of the cerebral cortex bymicroglial cells during development of the mouse embryo. Glia 61, 150–163. doi:10.1002/GLIA.22421
Taberner, L., Bañón, A., and Alsina, B. (2020). Sensory neuroblast quiescence depends on vascular cytoneme contacts and sensory neuronal differentiation requires initiation of blood flow. Cell Rep. 32, 107903. doi:10.1016/J.CELREP.2020.107903
Tan, X., Liu, W. A., Zhang, X. J., Shi, W., Ren, S. Q., Li, Z., et al. (2016). Vascular influence on ventral telencephalic progenitors and neocortical interneuron production. Dev. Cell 36, 624–638. doi:10.1016/J.DEVCEL.2016.02.023
Tasdemir-Yilmaz, O. E., and Freeman, M. R. (2014). Astrocytes engage unique molecular programs to engulf pruned neuronal debris from distinct subsets of neurons. Genes Dev. 28, 20–33. doi:10.1101/gad.229518.113
Tavazoie, M., Van der Veken, L., Silva-Vargas, V., Louissaint, M., Colonna, L., Zaidi, B., et al. (2008). A specialized vascular niche for adult neural stem cells. Cell Stem Cell 3, 279–288. doi:10.1016/j.stem.2008.07.025
Temple, S. (1989). Division and differentiation of isolated CNS blast cells in microculture. Nat 340, 471–473. doi:10.1038/340471a0
Temple, S. (2012). Defining neural stem cells and their role in normal development of the nervous system. Neural Dev. Stem Cells, 1–30. doi:10.1007/978-1-4614-3801-4_1/FIGURES/3
Than-Trong, E., and Bally-Cuif, L. (2015). Radial glia and neural progenitors in the adult zebrafish central nervous system. Glia 63, 1406–1428. doi:10.1002/GLIA.22856
Than-Trong, E., Kiani, B., Dray, N., Ortica, S., Simons, B., Rulands, S., et al. (2020). Lineage hierarchies and stochasticity ensure the long-term maintenance of adult neural stem cells. Sci. Adv. 6, eaaz5424. doi:10.1126/SCIADV.AAZ5424
Thomas, T., and Dziadek, M. (1993). Capacity to form choroid plexus-like cells in vitro is restricted to specific regions of the mouse neural ectoderm. Development 117, 253–262. doi:10.1242/DEV.117.1.253
Truman, J. W., and Bate, M. (1988). Spatial and temporal patterns of neurogenesis in the central nervous system of Drosophila melanogaster. Dev. Biol. 125, 145–157. doi:10.1016/0012-1606(88)90067-x
Tulina, N., and Matunis, E. (2001). Control of stem cell self-renewal in Drosophila spermatogenesis by JAK-STAT signaling. Science 294, 2546–2549. doi:10.1126/SCIENCE.1066700
Turner, K. J., Hawkins, T. A., Henriques, P. M., Valdivia, L. E., Bianco, I. H., Wilson, S. W., et al. (2022). A structural atlas of the developing zebrafish telencephalon based on spatially-restricted transgene expression. Front. Neuroanat. 16, 840924. doi:10.3389/fnana.2022.840924
Udolph, G., Prokop, A., Bossing, T., Technau, G. M., and Urban, J. (1993). A common precursor for glia and neurons in the embryonic CNS of Drosophila gives rise to segment-specific lineage variants. Development 118, 765–775. doi:10.1242/dev.118.3.765
Ulrich, F., Ma, L. H., Baker, R. G., and Torres-Vázquez, J. (2011). Neurovascular development in the embryonic zebrafish hindbrain. Dev. Biol. 357, 134–151. doi:10.1016/J.YDBIO.2011.06.037
Umans, R. A., Pollock, C., Mills, W. A., Clark, K. C., Pan, Y. A., and Sontheimer, H. (2021). Using zebrafish to elucidate glial-vascular interactions during CNS development. Front. Cell Dev. Biol. 9, 654338. doi:10.3389/fcell.2021.654338
Unhavaithaya, Y., and Orr-Weaver, T. L. (2012). Polyploidization of glia in neural development links tissue growth to blood-brain barrier integrity. Genes Dev. 26, 31–36. doi:10.1101/gad.177436.111
Urbán, N., Blomfield, I. M., and Guillemot, F. (2019). Quiescence of adult mammalian neural stem cells: a highly regulated rest. Neuron 104, 834–848. doi:10.1016/j.neuron.2019.09.026
Van Leeuwen, L. M., Evans, R. J., Jim, K. K., Verboom, T., Fang, X., Bojarczuk, A., et al. (2018). A transgenic zebrafish model for the in vivo study of the blood and choroid plexus brain barriers using claudin 5. Biol. Open 7, bio030494. doi:10.1242/bio.030494
Villalba, A., Götz, M., and Borrell, V. (2021). The regulation of cortical neurogenesis. Curr. Top. Dev. Biol. 142, 1–66. doi:10.1016/BS.CTDB.2020.10.003
Vogenstahl, J., Parrilla, M., Acker-Palmer, A., and Segarra, M. (2022). Vascular regulation of developmental neurogenesis. Front. Cell Dev. Biol. 10, 890852. doi:10.3389/FCELL.2022.890852
Voigt, A., Pflanz, R., Schäfer, U., and Jäckle, H. (2002). Perlecan participates in proliferation activation of quiescent Drosophila neuroblasts. Dev. Dyn. 224, 403–412. doi:10.1002/dvdy.10120
von Trotha, J. W., Egger, B., and Brand, A. H. (2009). Cell proliferation in the Drosophila adult brain revealed by clonal analysis and bromodeoxyuridine labelling. Neural Dev. 4, 9. doi:10.1186/1749-8104-4-9
Wagenführ, L., Meyer, A. K., Braunschweig, L., Marrone, L., and Storch, A. (2015). Brain oxygen tension controls the expansion of outer subventricular zone-like basal progenitors in the developing mouse brain. Development 142, 2904–2915. doi:10.1242/DEV.121939
Wakselman, S., Béchade, C., Roumier, A., Bernard, D., Triller, A., and Bessis, A. (2008). Developmental neuronal death in hippocampus requires the microglial CD11b integrin and DAP12 immunoreceptor. J. Neurosci. 28, 8138–8143. doi:10.1523/JNEUROSCI.1006-08.2008
Walsh, K. T., and Doe, C. Q. (2017). Drosophila embryonic type II neuroblasts: origin, temporal patterning, and contribution to the adult central complex. Development 144, 4552–4562. doi:10.1242/dev.157826
White, K., and Kankel, D. R. (1978). Patterns of cell division and cell movement in the formation of the imaginal nervous system in Drosophila melanogaster. Dev. Biol. 65, 296–321. doi:10.1016/0012-1606(78)90029-5
Wu, S., Nguyen, L. T. M., Pan, H., Hassan, S., Dai, Y., Xu, J., et al. (2020). Two phenotypically and functionally distinct microglial populations in adult zebrafish. Sci. Adv. 6, eabd1160. doi:10.1126/sciadv.abd1160
Wu, S., Xue, R., Hassan, S., Nguyen, T. M. L., Wang, T., Pan, H., et al. (2018). Il34-Csf1r pathway regulates the migration and colonization of microglial precursors. Dev. Cell 46, 552–563. doi:10.1016/j.devcel.2018.08.005
Wu, Y., Dissing-Olesen, L., MacVicar, B. A., and Stevens, B. (2015). Microglia: dynamic mediators of synapse development and plasticity. Trends Immunol. 36, 605–613. doi:10.1016/j.it.2015.08.008
Xie, T., and Spradling, A. C. (2000). A niche maintaining germ line stem cells in the Drosophila ovary. Science 290, 328–330. doi:10.1126/SCIENCE.290.5490.328
Xing, L., and Huttner, W. B. (2020). Neurotransmitters as modulators of neural progenitor cell proliferation during mammalian neocortex development. Front. Cell Dev. Biol. 8, 548200. doi:10.3389/fcell.2020.00391
Xu, J., Wang, T., Wu, Y., Jin, W., and Wen, Z. (2016). Microglia colonization of developing zebrafish midbrain is promoted by apoptotic neuron and lysophosphatidylcholine. Dev. Cell 38, 214–222. doi:10.1016/j.devcel.2016.06.018
Xu, J., Zhu, L., He, S., Wu, Y., Jin, W., Yu, T., et al. (2015). Temporal-spatial resolution fate mapping reveals distinct origins for embryonic and adult microglia in zebrafish. Dev. Cell 34, 632–641. doi:10.1016/J.DEVCEL.2015.08.018
Yadav, V., Mishra, R., Das, P., and Arya, R. (2023). Cut homeodomain transcription factor is a novel regulator of growth and morphogenesis of cortex glia niche around neural cells. Genetics, iyad173. doi:10.1093/GENETICS/IYAD173
Yeo, W., and Gautier, J. (2004). Early neural cell death: dying to become neurons. Dev. Biol. 274, 233–244. doi:10.1016/J.YDBIO.2004.07.026
Yildirim, K., Petri, J., Kottmeier, R., and Klämbt, C. (2019). Drosophila glia: few cell types and many conserved functions. Glia 67, 5–26. doi:10.1002/glia.23459
Yuan, X., Sipe, C. W., Suzawa, M., Bland, M. L., and Siegrist, S. E. (2020). Dilp-2-mediated PI3-kinase activation coordinates reactivation of quiescent neuroblasts with growth of their glial stem cell niche. PLoS Biol. 18, e3000721–e3000724. doi:10.1371/journal.pbio.3000721
Zambusi, A., and Ninkovic, J. (2020). Regeneration of the central nervous system-principles from brain regeneration in adult zebrafish. World J. Stem Cells 12, 8–24. doi:10.4252/WJSC.V12.I1.8
Zappaterra, M. W., and Lehtinen, M. K. (2012). The cerebrospinal fluid: regulator of neurogenesis, behavior, and beyond. Cell. Mol. Life Sci. 69, 2863–2878. doi:10.1007/S00018-012-0957-X
Zhao, Z., Nelson, A. R., Betsholtz, C., and Zlokovic, B. V. (2015). Establishment and dysfunction of the blood-brain barrier. Cell 163, 1064–1078. doi:10.1016/J.CELL.2015.10.067
Zheng, H., Yang, X., and Xi, Y. (2016). Fat body remodeling and homeostasis control in Drosophila. Life Sci. 167, 22–31. doi:10.1016/J.LFS.2016.10.019
Zupanc, G. K. H. (2021). Adult neurogenesis in the central nervous system of teleost fish: from stem cells to function and evolution. J. Exp. Biol. 224, jeb226357. doi:10.1242/jeb.226357
Keywords: neural stem cell (NSC), niche, architecture, development, mouse, zebrafish, Drosophila
Citation: Valamparamban GF and Spéder P (2023) Homemade: building the structure of the neurogenic niche. Front. Cell Dev. Biol. 11:1275963. doi: 10.3389/fcell.2023.1275963
Received: 10 August 2023; Accepted: 16 November 2023;
Published: 01 December 2023.
Edited by:
Kunimasa Ohta, Kyushu University, JapanReviewed by:
Yuki Hirota, Keio University, JapanSilvia Olivera-Bravo, Instituto de Investigaciones Biológicas Clemente Estable (IIBCE), Uruguay
Copyright © 2023 Valamparamban and Spéder. This is an open-access article distributed under the terms of the Creative Commons Attribution License (CC BY). The use, distribution or reproduction in other forums is permitted, provided the original author(s) and the copyright owner(s) are credited and that the original publication in this journal is cited, in accordance with accepted academic practice. No use, distribution or reproduction is permitted which does not comply with these terms.
*Correspondence: Pauline Spéder, cGF1bGluZS5zcGVkZXJAcGFzdGV1ci5mcg==