- 1Department of Chemistry, Lehman College, City University of New York, Bronx, NY, United States
- 2Graduate Center, City University of New York, New York, NY, United States
Per- and polyfluoroalkyl substances (PFAS) are emerging contaminants with increasing health concern due to their persistence, widespread presence, and adverse health effects. Short-chain PFAS, in particular, are more challenging to remove using conventional water treatment technologies. Hydrogel adsorbents have shown as a promising solution for short-chain PFAS removal, offering high adsorption capacity, rapid kinetics, and tunable material properties. However, biofouling contamination which is easier to happen on wet hydrogels adsorbents compared with conventional adsorbents in water treatment process, could significantly reduce adsorption efficiency, shorten operational lifespan, and increase overall costs. Antibiofouling modifications present a viable strategy to enhance hydrogel functionality in drinking water treatment applications. This review summarizes recent advancements of hydrogel in antibiofouling and short-chain PFAS removal applications through functional group modifications. Furthermore, it highlights gaps in the current literature, particularly the lack of studies on the development and evaluation of hydrogels with both biofouling resistance and short-chain PFAS removal capabilities for drinking water treatment applications.
1 Introduction
Promoting access to safe drinking water remains a critical global challenge, with the presence of emerging contaminants such as per- and polyfluoroalkyl substances (PFAS) posing significant risks to water quality and public health as a significant factor contributing to this issue (Stoiber et al., 2020; UNESCO, 2023). PFAS are persistent organic pollutants widely detected in drinking water due to industrial discharges, the use of firefighting foams and the disposal of consumer product waste into the environment (Stoiber et al., 2020; Munoz et al., 2023; Hu et al., 2016; Wang et al., 2020). Most PFAS are resistant to chemical degradation due to their strong C-F bonds (Bao et al., 2018; Verma et al., 2023; Ye et al., 2023). Short-chain PFAS (carbon chain ≤7), with a shorter fluorinated tail, were introduced as safer alternatives to their long-chain counterparts (carbon chain ≥7) in industry applications in the past decades (Jensen and Warming, 2015). However, the smaller molecular size and increased hydrophilicity make short-chain PFAS difficult to remove (Brendel et al., 2018; Li et al., 2020), posing new challenges for water treatment. Moreover, short-chain PFAS have been widely detected in groundwater and treated drinking water with Liquid Chromatography-Mass Spectrometry and High-Resolution Mass Spectrometry through either direct injection or solid-phase extraction to concentrate the PFAS and clean the matrix background before injection (Ateia et al., 2019; Li et al., 2011; Wang et al., 2024a). And short-chain PFAS have been found to pose significant health risks, including lipid metabolism disruption, infertility, reproductive issues, and endocrine dysfunction (Sheng et al., 2018; Chen et al., 2018; Feng et al., 2017; Nian et al., 2020). Short-chain PFAS ammonium salt of hexafluoropropylene oxide dimer acid (GenX) has also been shown to induce liver toxicity in animal studies (Solan et al., 2023).
Advanced treatment process is an additional step incorporated in novel technologies to remove emerging contaminants like PFAS during water treatment processes, and has shown better PFAS removal effects from water than other treatment processes such as coagulation, flocculation, sedimentation, and filtration (Figure 1A; Seven seas water, 2023; Jafarinejad, 2025). Advanced treatment process is normally implemented before the disinfection process (Figure 1A). PFAS removal from drinking water primarily relies on activated carbon (GAC) (Kempisty et al., 2022) and ion exchange resins (IER) from advanced treatment process (Boyer et al., 2021). However, both adsorbents are more effective in removing long-chain PFAS than short-chain PFAS (Jafarinejad, 2025). Furthermore, short-chain PFAS adsorbed onto GAC can be displaced by longer-chain PFAS and other more hydrophobic compounds over time (Newcombe, 1994; Park et al., 2020), and the presence of other natural organic contaminants and ions in the water matrix could reduce the performance of these adsorbents due to competitive adsorption (Ateia et al., 2019).
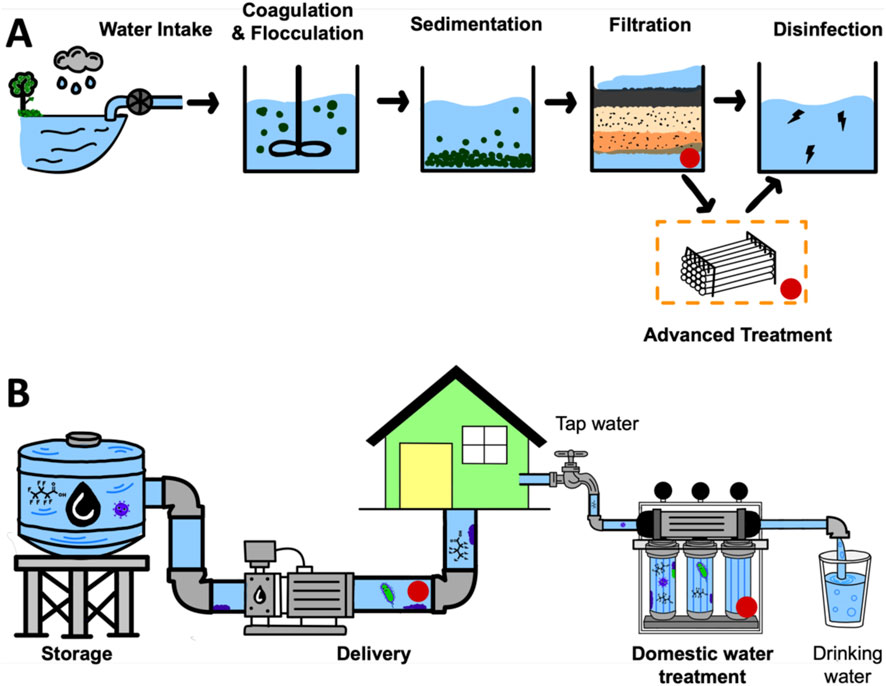
Figure 1. (A) Schematic graph of the drinking water treatment process includes water intake, coagulation, sedimentation, filtration, disinfection, and distribution. Advanced treatment technologies are used in some drinking water treatment systems to remove emerging contaminants like PFAS. Red spots indicate vulnerable stages exposed to potential biofouling risks. (B) Diagram of water distribution from storage to household use, highlighting potential biofouling risks in domestic water treatment systems and tap water supply, marked by red dots.
In addition, biofouling is another challenge for most materials used for water treatment (LeChevallier et al., 1984; Wells and Sytsma, 2009; Nguyen et al., 2012; Abdelsalam et al., 2017). Although disinfection methods such as chlorination, ultraviolet irradiation, and advanced oxidation processes are widely employed to control bacterial growth, some bacteria can withstand these conventional treatments and remain in treated water (Sharma and Bhattacharya, 2017). Microorganisms present in flowing water can adhere to surfaces within drinking water treatment materials, distribution piping systems, as well as domestic treatment adsorbents or membranes (Bachmann and Edyvean, 2005; Sójka et al., 2023; Zuo et al., 2022; Daschner et al., 1996), where they utilize accumulated nutrients to grow and form biofilms over time, ultimately leading to biofouling (Figure 1B). Biofilm formed on the surface of adsorbents can occupy the active sites on adsorbent materials (Flemming and Geesey, 1991) and clog membranes (Razali et al., 2023), thereby reducing the adsorption efficiency of adsorbents and compromising system performance by decreasing flux, permeability, and usage life over time. Despite this, effective antibiofouling strategies for these materials remain lacking for short-chain PFAS control applications. Hydrogel based adsorbents have emerged as a promising alternative to conventional adsorbents and filtration membrane for removing anion short-chain PFAS (Xu et al., 2024; Huang et al., 2018; Verduzco and Wong, 2020; Naim Shaikh and Nawaz, 2024). Comparing with GAC and IER, the hydrophilic, and porous nature of hydrogel and its tunable surface property makes it not only possible to be effective for short-chain PFAS removal but also with dual function of biofouling resistance after modification. These three-dimensional, cross-linked polymeric materials offer high water content, tunable porosity, and surface functionality, enabling them to interact effectively with water-soluble PFAS molecules through mechanisms like hydrophobicity, electrostatic interactions, hydrogen bonding, and size exclusion (Xu et al., 2024; Wang et al., 2025).
Although hydrogel-based adsorbents have been extensively studied for contaminant removal and antibiofouling separately, their potential to integrate both properties for efficient short-chain PFAS removal remains underexplored. However, this approach holds significant promise. For instance, hydrogel coatings on filtration membranes have been shown to impart antibiofouling properties in addition to contaminant removal (Sójka et al., 2023; Li et al., 2022).Additionally, graphene oxide-incorporated hydrogels have been reported to be effective for short-chain PFAS removal in one study (Becanova et al., 2021) and for antibiofouling in another (Zhang et al., 2018).
This review explores hydrogel adsorbents for short-chain PFAS removal and the challenges they face in engineering applications due to insufficient antibiofouling properties. Furthermore, it examines advancements in antibiofouling hydrogels for water treatment and the potential for enhanced performance by integrating antibiofouling features with effective PFAS removal components. It also highlights key insights and future directions for developing antibiofouling hydrogel technologies to improve short-chain PFAS removal in water treatment applications.
2 Hydrogels for short-chain PFAS removal
Hydrogel based adsorbents emerges as a highly effective alternative to traditional adsorbents for short-chain PFAS removal in water treatment, offering benefits as shown in Supplementary Table S1 such as faster kinetics, high affinity for short-chain PFAS, easy regeneration, and reusability (Ateia et al., 2019; Huang et al., 2018; Verduzco and Wong, 2020; Alsaka et al., 2025) comparing with GAC (Boyer et al., 2021; Zhang et al., 2021). Additionally, IER, the other conventional adsorbent, exhibits good adsorption capacity over multiple short-chain PFAS, however, it is costly and can experience performance declines in the presence of organic matter and competing ions in the water matrix (Wang et al., 2024a). Additionally, IER has a low regeneration rate of 27.5% and degrades over time, limiting its long-term applicability (Wang et al., 2024a; Liu et al., 2022).
2.1 Interaction mechanisms of reported functional groups on hydrogels for short-chain PFAS removal
2.1.1 Electrostatic interactions
Electrostatic interactions are the dominant mechanism for short-chain PFAS removal improvement (Xu et al., 2024; Huang et al., 2018; Kumarasamy et al., 2020). Positively charged aminated functional groups, such as quaternary ammonium ([NR4]+) (Huang et al., 2018; Kumarasamy et al., 2020), and amine(-NH2) (Xu et al., 2024) have been incorporated into hydrogels, imparting a positive charge to the adsorbent and facilitating strong electrostatic interactions with anionic short-chain PFAS (Supplementary Table S2). The hydrophilic property of hydrogels reduces this kind of adsorbents’ diffusion resistance to short-chain PFAS, enhancing adsorption kinetics and affinity (Xu et al., 2024; Verduzco and Wong, 2020) and their porous structure exposes a higher density of charged polymers to PFAS compared to IER, leading to increased adsorption capacity (Ateia et al., 2019). Huang et al. (2018) developed aminated poly (ethylene glycol) diacrylate hydrogels with adsorption capacities 8 to 63 times higher than conventional adsorbents, particularly for short-chain PFAS such as perfluorobutanesulfonic acid (PFBS), perfluorobutanoic acid (PFBA), and GenX (Huang et al., 2018). Similarly, aminated polyacrylamide hydrogel foam in another study (Xu et al., 2024) demonstrated a rapid equilibration within 15 min and a high affinity for perfluorohexanoic acid (PFHxA), perfluoroheptanoic acid (PFHpA), and GenX, achieving removal rates exceeding 90%, while PFBA removal reached 82%. This was attributed to the synergistic effects of electrostatic interactions, hydrogen bonding, and hydrophobic interactions (Xu et al., 2024). All studies have also demonstrated that aminated hydrogels have stronger binding affinities for PFBS over PFBA (Ateia et al., 2019; Xu et al., 2024; Huang et al., 2018). The versatility of hydrogel material allows hydrogel to be fabricated into beads (McCarty, 2016), porous membrane (Cai et al., 2023), foams (Li et al., 2022), or coatings on various surfaces (Wibisono et al., 2015) depending on application requirements.
2.1.2 Hydrophobic interactions
In contrast to electrostatic interactions, hydrophobic interactions via fluorination have been explored as another strategy to enhance PFAS adsorption, by leveraging the like-like affinity between hydrogel’s fluorinated surfaces and the perfluoroalkyl chains of PFAS, but this approach is less effective for short-chain PFAS (Huang et al., 2018; Verduzco and Wong, 2020; Koda et al., 2014). Koda et al. (2014) developed perfluoroalkane-functionalized star polymer microgels, which captured only 23% of short-chain PFAS like PFHxA, while the hydrogel adsorbent modified with amination alone achieved over 97% removal efficiency. Notably, the combination of fluorination with cationic functional groups on hydrogel adsorbents (Wang et al., 2025; Huang et al., 2018; Verduzco and Wong, 2020) showed no obvious enhancement in short-chain PFAS adsorption compared to adsorbents modified with cationic functional groups alone. Huang et al. (2018) observed that aminated hydrogels exhibited similar adsorption efficiencies to those containing both amination and fluorination, achieving 100% removal of PFBS and >95% removal of GenX, while non-fluorinated hydrogels performed better for PFBA removal. Given the limited effectiveness of fluorinated materials for short-chain PFAS removal and the potential release of PFAS from degraded fluorinated materials, fluorination strategy is not suggested for hydrogel based short-chain PFAS adsorbent development. Hence, electrostatic interactions remain the dominant mechanism for short-chain PFAS removal, particularly for compounds like PFBA and PFBS.
2.2 Selectivity and reusability of functionalized hydrogels
In addition, functionalized hydrogels with high reusability offers a higher practical advantage over GAC and IER (Supplementary Table S1), improving its practicality, scalability, and cost-effectiveness for future drinking water treatment applications. For example, Huang et al. (2018) demonstrated that their hydrogels could be easily regenerated in 70% methanol containing 1% NaCl and reused for up to 5 cycles before disposal, while mostly GAC were dumped to landfills for disposal or recycled for less than 3 times (Boyer et al., 2021). A report suggests potential for up to 10 cycles through optimizing material (Ateia et al., 2019), underscoring the hydrogels’ potential for cost-effective, and sustainable use.
3 Antibiofouling hydrogels development for drinking water treatment
Nonetheless, biofouling contamination caused by colonization of attached bacteria on both conventional and emerging adsorbents is an inevitable challenge that decrease efficiency (LeChevallier et al., 1984; Abdelsalam et al., 2017; Flemming and Geesey, 1991; Wang et al., 2024b). Biofouling on hydrogel obstructs active adsorption sites and increases diffusion resistance for contaminants such as PFAS, degrades materials via enzymatic activity, ultimately shortening the operational lifespan of adsorbents and increasing maintenance costs (Flemming and Geesey, 1991; Vuong et al., 2023). Wang P. et al. (2022) found that PFAS passive sampling hydrogels exhibited significantly reduced adsorption performance after 21 days in surface water due to biofouling. Endowing hydrogel based materials with antibiofouling properties is crucial for ensuring their long-term efficacy while preventing secondary bacterial contamination to treated water.
Various antibiofouling strategies including polymers (Sójka et al., 2023; Li et al., 2022; Peng et al., 2022; Xu et al., 2023; Wen et al., 2021; Chen et al., 2024), nanoparticles (Zhang et al., 2018; Baek et al., 2015), quorum-quenching bacteria (Lee et al., 2018; Yi et al., 2024), and enzymes (Ye et al., 2019; Li et al., 2021; Zanoni et al., 2016) can be integrated into hydrogels designed to address this issue. These components inhibit biofilm formation through multiple mechanisms (Supplementary Table S3; Figure 2). The dominant antibiofouling mechanism is through electrostatic interactions, which causes bacterial membrane damage through the interaction between the oppositely charged polymer adsorbent and bacteria (Li et al., 2022; Chen et al., 2024; Wang J. et al., 2022). Hydrophilic protection is another mechanism that prevents biofouling contamination by forming a hydrophilic water layer on the adsorbent surface, thereby preventing bacterial attachment (Sójka et al., 2023; Zhang et al., 2018; Wibisono et al., 2015; Peng et al., 2022; Xu et al., 2023; Wen et al., 2021; Chen et al., 2024). Nanoparticles inhibit the formation of biofilm through physically breaking bacterial cells and chemically damaging bacteria membrane with generated reactive oxygen species (ROS) (Guo et al., 2021; Ye et al., 2017) or released toxic silver ions (Baek et al., 2015). In contrast, quorum-quenching bacteria and enzymes offer a more sustainable antibiofouling strategy (Lee et al., 2018; Yi et al., 2024; Ye et al., 2019; Li et al., 2021; Zanoni et al., 2016). Quorum-sensing bacteria suppress bacterial growth and biofilm development by disrupting bacterial communication (Lee et al., 2018; Yi et al., 2024). Whereas, enzymatic approaches can either hydrolyze biofilm components to prevent their formation or mechanically detach established biofilms (Figure 2; Ye et al., 2019; Li et al., 2021; Zanoni et al., 2016).
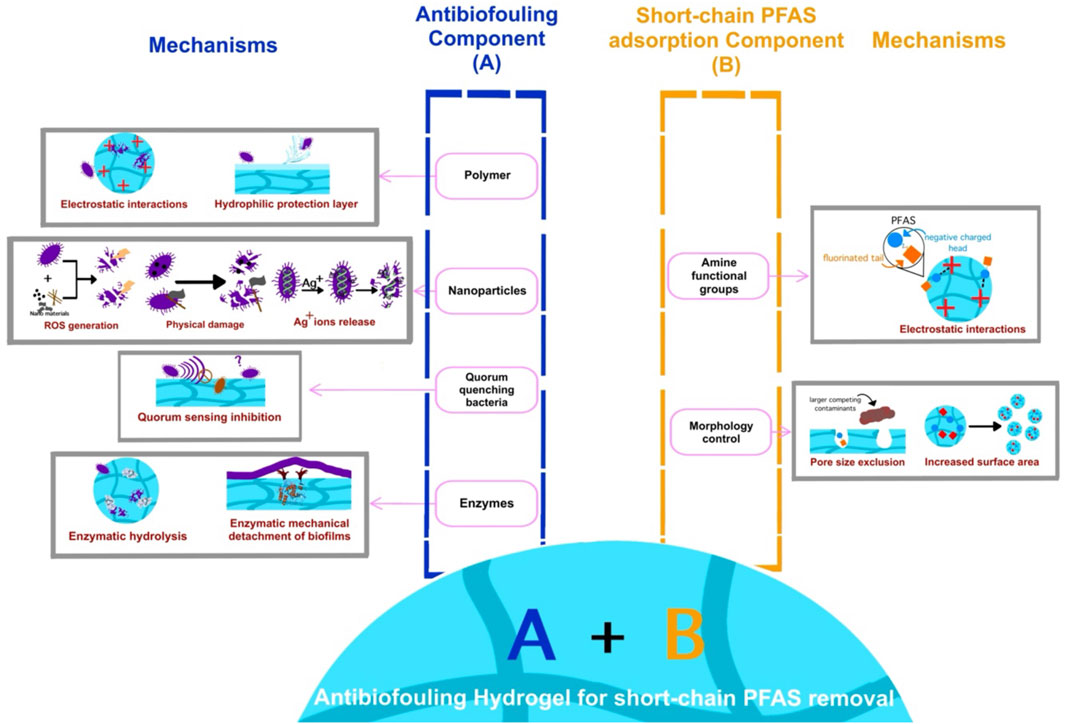
Figure 2. Schematic diagram of hydrogel modification strategies designed to impart both short-chain PFAS removal and antibiofouling properties, along with the underlying mechanisms of each strategy.
3.1 Customized antibiofouling polymers in hydrogels
Antibacterial polymers are widely used in the synthesis of anti-biofouling hydrogels due to their sustainability, ease of production, and broad availability. As summarized in Supplementary Table S2, various positively charged nitrogen-substituted polymers interact with negatively charged bacterial components inhibiting the biofouling on the surface of the material through electrostatic interactions. The presence of cationic charges on these polymer hydrogels is crucial for the antibacterial properties. Poly (imidazolium) (Wang J. et al., 2022) exhibited 99% antibiofouling performance within 1 h against E. coli (Escherichia coli) and S. aureus (Staphylococcus aureus). Chitosan, a cationic natural polymer with inherent amine functionality is a sustainable option (Li et al., 2022), has demonstrated 95% antibiofouling efficiency in a 15-day test (Supplementary Table S3; Li et al., 2022).
3.2 Antibiofouling hydrogels with microbial adhesion resistance and bactericidal properties
Biofouling occurs on hydrogel in two key steps: the initial adhesion of microorganisms to the surface of hydrogel and colonization after that (Roberts et al., 2012; AlSawaftah et al., 2022). Hence, antibiofouling mechanisms of hydrogels are primarily driven by two factors: improving resistance to microbial adhesion and delivering bactericidal effects through various physicochemical properties. One key mechanism of microbial adhesion resistance is the creation of a hydrophilic protection layer on the hydrogel’s surface, which attracts water molecules, forming a layer of water on the surface of the material (Sójka et al., 2023; Zhang et al., 2018; Wibisono et al., 2015; Peng et al., 2022; Xu et al., 2023; Wen et al., 2021). This water layer acts as a barrier, preventing bacteria from adhering to the materials surface, thereby reducing biofilm and colony formation. Notably, combining cationic and hydrophilic functionalities strengthens electrostatic interactions with water while further enhancing microbial resistance (Wen et al., 2021; Zhou et al., 2019; Yang et al., 2018). These polymer hydrogels demonstrate strong antibacterial performance in water treatment applications. Polymers such as poly-sulfobetaine methacrylate (Sójka et al., 2023) and carboxybetaine acrylamide (Wen et al., 2021) facilitate the formation of a protective hydration layer, thereby preventing bacterial adhesion with 90%–96% effectiveness against E. coli (Supplementary Table S3; Li et al., 2022; Peng et al., 2022; Wen et al., 2021) This is further supported by studies showing hydrogels containing hydrophilic surfactants like benzalkonium chloride and dicocoalkyldimethyl ammonium chloride which have demonstrated extended fouling-free periods of 10–14 weeks in marine environments (Supplementary Table S3; Cowie et al., 2006)
3.3 Integration of nanoparticles into hydrogels for enhanced antibiofouling properties
Nanoparticles inhibit biofilm formation through biocidal ion release or ROS generation (Zhang et al., 2018; Baek et al., 2015; Wang J. et al., 2022; Guo et al., 2021). Silver nanoparticles (AgNPs) are widely studied for their antimicrobial and antibiofouling properties in water treatment (Baek et al., 2015), as hydrogels incorporating AgNPs release Ag+ ions, which disrupt bacterial cell walls, proteins, and genetic material, leading to bacterial death. AgNPs have been shown to lyse approximately 80% of E. coli cells after prolonged exposure (Supplementary Table S3; Baek et al., 2015). However, AgNPs could induce oxidative stress in non-target organisms (Luoma SN, 2008; Asharani et al., 2008), disrupt microbial ecosystems essential for nutrient cycling (León-Silva et al., 2016), and contribute to antimicrobial resistance, posing public health risks (Luoma SN, 2008). To mitigate these risks, alternative antimicrobial nanoparticles have been explored. Saleque et al. (2023) Embedded tantalum telluride quantum dots in hydrogels has suppressed the growth of S. aureus and E. coli cultures by 26% and 34%, respectively through physical disrupting bacterial membranes and interference with deoxyribonucleic acid replication in bacteria (Supplementary Table S3). Additionally, graphene oxide nanosheets, when loaded onto zwitterionic polyampholyte hydrogels grafted onto ultrafiltration polyethersulfone membranes, led to an 80% reduction in E. coli colonies by combining biocidal activity with a hydrophilic protection layer (Supplementary Table S3; Zhang et al., 2018)
ROS-based mechanisms can further enhance the antibiofouling performance of hydrogel. ROS, such as hydrogen peroxide and hydroxyl radicals, disrupt bacterial membranes, proteins, and DNA. Quinone-modified activated carbon membranes, for example, generate ROS, achieving 99% bacterial inactivation within an hour while maintaining mechanical stability and preventing biofouling for up to 5 days (Supplementary Table S3; Guo et al., 2021) Despite these advancements, the toxicity of these nanoparticles remains largely unexplored for large-scale applications. Further research is needed to assess their biocompatibility and long-term environmental impact.
3.4 Antibiofouling hydrogels formation with sustainable approaches
Quorum-quenching bacteria and enzymes are considered effective and sustainable antibiofouling agents due to their natural origin and environmentally benign properties (Supplementary Table S3; Lee et al., 2018; Yi et al., 2024; Ye et al., 2019; Li et al., 2021; Zanoni et al., 2016) Hydrogels incorporating quorum-quenching bacteria have been shown to inhibit bacterial growth by disrupting quorum sensing, a critical bacterial communication system that regulates biofilm formation (Lee et al., 2018; Yi et al., 2024). Lee et al. (2018) embedded Acinetobacter sp. into hydrogels, which degraded AI-2 quorum-sensing molecules and reduced biofilm formation in Aeromonas sp., Enterobacter sp., E. coli K-12, and B. subtilis by 51%, 70%, 33%, and 33%, respectively. When encapsulated in membrane bioreactors, these hydrogels delayed biofouling and extended operational lifespan by 4.8 times. Similarly, Yi et al. (2024) developed microcapsules containing Rhodococcus sp. in a semi-interpenetrating hydrogel network, achieving over 99% quorum-sensing molecule degradation within 8 h and maintaining efficiency for 3 months. Enzyme-based antibiofouling hydrogels offer another assuring strategy. Li et al. (2021) designed a lysozyme-encapsulated hydrogel with a dual antibacterial mechanism: its negatively charged surface repelled bacteria to reduce adhesion, while it released lysozyme in response to acidic conditions or glutathione, breaking down bacterial cell walls. This system increased E. coli and S. aureus mortality by 85%. Ye et al. (2019) further improved lysozyme stability by immobilizing it within a hydrogel, enhancing temperature tolerance and retaining activity for 55 days. This porous hydrogel structure captured bacteria and catalyzed lysis, achieving 99.4% inhibition of E. coli within an hour and maintaining over 96% inhibition for 30 h. In another approach, Zanoni et al. (2016) functionalized silica nanobeads with Proteinase K, which physically disrupted P. fluorescens biofilms and enzymatically degraded their protein matrix. This method reduced biofilm coverage and thickness while preserving bacterial viability, offering an alternative to conventional bactericidal strategies. Integrating such quorum-quenching bacteria and enzymes into hydrogels not only enhances antibiofouling performance but also allows for material reuse by immobilizing active agents within a hydrated polymer network. Additionally, hydrogels can trap contaminants within their matrix, further improving antibacterial properties.
Despite these advantages, several trade-offs must be considered. Antibacterial nanoparticles, while effective, raise concerns about cytotoxicity and environmental impact due to the release of toxic ions that can disrupt microbial ecosystems and contribute to antimicrobial resistance. Additionally, while hydrophilic polymer coatings enhance biofouling resistance, they may compromise mechanical strength and long-term durability, limiting practical use. Quorum-quenching bacteria and enzyme-based approaches offer a sustainable alternative but present challenges related to stability, scalability, and potential loss of activity over time. Balancing efficacy, longevity, and environmental impact remains a key challenge in developing hydrogel-based water treatment materials.
4 Antibiofouling component modifications to improve short-chain PFAS removal
Unlike conventional water treatment materials with limited tunability, hydrogels offer customizable functionality, allowing their properties to be tailored for specific applications. By modifying surface functional groups to enhance both antibiofouling and short-chain PFAS removal, hydrogel-based materials can achieve high adsorption efficiency while preventing microbial growth, making them ideal for long-term water treatment (Figure 2). This approach includes incorporating positively charged or hydrophobic groups to facilitate short-chain PFAS adsorption, while also introducing additional functional elements that discourage microbial attachment and inhibit biofilm formation, ensuring sustained filtration performance (Wang P. et al., 2022; Chaix et al., 2024). For example, quaternary ammonium-modified hydrogels, which exhibit a strong binding affinity for short-chain PFAS due to robust electrostatic interactions (Ateia et al., 2019), and antimicrobial properties effectively disrupt bacterial membranes, making them highly effective against biofilm-forming microorganisms such as E. coli and S. aureus (Li et al., 2022; Peng et al., 2022; Chen et al., 2024). Predictably, the combination of quaternary ammonium and antibiofouling components in hydrogels demonstrate high effectiveness for short-chain PFAS removal for long term with resistance to biofouling.
Furthermore, these obtained materials can also be designed to optimize PFAS adsorption through size-exclusion mechanisms within porous adsorbents (Ateia et al., 2019). Advanced fabrication techniques such as 3D printing and electrospinning enable the development of hydrogels with intricate geometries and expanded surface areas, ultimately improving adsorption efficiency and long-term performance in water treatment applications (Chaix et al., 2024).
5 Challenges and future directions on antibiofouling hydrogel-based water treatment material for short-chain PFAS removal
Hydrogel-based materials have shown significant potential for short-chain PFAS removal and biofouling resistance in water treatment applications. However, several challenges remain (Supplementary Table S1). First, further studies are needed to investigate the compatibility and performance of antibiofouling components integrated into hydrogels to enhance both short-chain PFAS removal and biofouling resistance (Figure 2). The performance of these hydrogels should be evaluated for antibacterial activity, biofouling resistance, and PFAS removal, with further data required to validate their long-term effectiveness in short-chain PFAS removal applications. This includes both advanced treatment processes for drinking water treatment and domestic water treatment products.
Moreover, hydrogel materials as emerging adsorbents are still under lab-scale study, therefore, more pilot-scale studies or full application studies are expected to further validate the performance of promising hydrogels. Unlike conventional adsorbents, which are highly commercialized, the cost of hydrogels for water treatment is still unknown. Finding more low-cost raw materials for hydrogel preparation have the potential to reduce production costs by utilizing bio-based materials and demonstrate promising scalability.
The engineering application of hydrogel adsorbents is limited by factors such as gel strength and degradation under extreme conditions. Further optimization is needed for field use, with the ability to be customized for both short- and long-chain PFAS. Additionally, the long-term effectiveness, environmental impact, and potential toxicity of these materials remain concerns, necessitating life cycle assessments to evaluate their full environmental footprint—from production to disposal—to ensure that their benefits outweigh any ecological risks before large-scale implementation (Verduzco and Wong, 2020). Future research should focus on enhancing the stability, selectivity, and environmental safety of hydrogel-based systems, as well as exploring advanced fabrication techniques and more sustainable material options to ensure their feasibility for large-scale water treatment applications. With ongoing research and optimization, hydrogel-based systems have significant potential to become a sustainable, efficient solution for addressing short-chain PFAS contamination in water, providing both enhanced performance and long-term applicability in real-world water treatment scenarios.
Author contributions
KD: Writing – original draft, Writing – review and editing, Visualization. GF: Writing – original draft, Writing – review and editing. IV: Writing – original draft, Writing – review and editing. SS: Writing – original draft. RP: Visualization, Writing – review and editing. RD: Writing – review and editing. YY: Writing – original draft, Funding acquisition, Project administration, Supervision, Visualization, Writing – review and editing.
Funding
The author(s) declare that financial support was received for the research and/or publication of this article. This work is supported by Lehman College startup support, PSC-CUNY Research Award (# 67244-00 55), and CUNY ASRC SEED-AWARD (#92994-00 01). Co-author Genesis T. Fermin is supported by the NIH Undergraduate Research Training Initiative for Student Enhancement (U-RISE) Scholarships (5T34GM149385-02) to conduct research activity in YeEnvLab (https://www.yeenvlab.com/).
Conflict of interest
The authors declare that the research was conducted in the absence of any commercial or financial relationships that could be construed as a potential conflict of interest.
Generative AI statement
The author(s) declare that Generative AI was used in the creation of this manuscript. Used AI technology to check my grammar error and reorganize my sentences in manuscript.
Publisher’s note
All claims expressed in this article are solely those of the authors and do not necessarily represent those of their affiliated organizations, or those of the publisher, the editors and the reviewers. Any product that may be evaluated in this article, or claim that may be made by its manufacturer, is not guaranteed or endorsed by the publisher.
Supplementary material
The Supplementary Material for this article can be found online at: https://www.frontiersin.org/articles/10.3389/fceng.2025.1565754/full#supplementary-material
References
Abdelsalam, S., Kheirallaa, Z. M., Abo-Seif, F. A., and Asker, S. (2017). Abiotic factors and microbial communities fouling anion exchange resin causing performance deficiency in electric power plants. Egypt J. Microbiol. 52, 17–28. doi:10.21608/ejm.2017.812.1017
Alsaka, L., Alsaka, L., Altaee, A., Zaidi, S. J., Zhou, J., and Kazwini, T. (2025). A review of hydrogel application in wastewater purification. Separations 12, 51. doi:10.3390/separations12020051
AlSawaftah, N., Abuwatfa, W., Darwish, N., and Husseini, G. A. (2022). A review on membrane biofouling: prediction, characterization, and mitigation. Membranes 12, 1271. doi:10.3390/membranes12121271
Asharani, P., Wu, Y. L., Gong, Z., and Valiyaveettil, S. (2008). Toxicity of silver nanoparticles in zebrafish models. Nanotechnology 19, 255102. doi:10.1088/0957-4484/19/25/255102
Ateia, M., Arifuzzaman, M., Pellizzeri, S., Attia, M. F., Tharayil, N., Anker, J. N., et al. (2019). Cationic polymer for selective removal of GenX and short-chain PFAS from surface waters and wastewaters at ng/L levels. Water Res. 163, 114874. doi:10.1016/j.watres.2019.114874
Bachmann, R., and Edyvean, R. (2005). Biofouling: an historic and contemporary review of its causes, consequences and control in drinking water distribution systems. Biofilms 2, 197–227. doi:10.1017/S1479050506001979
Baek, K., Liang, J., Lim, W. T., Zhao, H., Kim, D. H., and Kong, H. (2015). In situ assembly of antifouling/bacterial silver nanoparticle-hydrogel composites with controlled particle release and matrix softening. ACS Appl. Mater Interfaces 7, 15359–15367. doi:10.1021/acsami.5b03313
Bao, Y., Deng, S., Jiang, X., Qu, Y., He, Y., Liu, L., et al. (2018). Degradation of PFOA substitute: GenX (HFPO–DA ammonium salt): oxidation with UV/persulfate or reduction with UV/sulfite? Environ. Sci. Technol. 52, 11728–11734. doi:10.1021/acs.est.8b02172
Becanova, J., Saleeba, Z. S., Stone, A., Robuck, A. R., Hurt, R. H., and Lohmann, R. (2021). A graphene-based hydrogel monolith with tailored surface chemistry for PFAS passive sampling. Environ. Sci. Nano 8, 2894–2907. doi:10.1039/D1EN00517K
Boyer, T. H., Ellis, A., Fang, Y., Schaefer, C. E., Higgins, C. P., and Strathmann, T. J. (2021). Life cycle environmental impacts of regeneration options for anion exchange resin remediation of PFAS impacted water. Water Res. 207, 117798. doi:10.1016/j.watres.2021.117798
Brendel, S., Fetter, É., Staude, C., Vierke, L., and Biegel-Engler, A. (2018). Short-chain perfluoroalkyl acids: environmental concerns and a regulatory strategy under REACH. Environ. Sci. Eur. 30, 9. doi:10.1186/s12302-018-0134-4
Cai, R., Chen, Y., Hu, J., Xiong, J., Lu, J., Liu, J., et al. (2023). A self-supported sodium alginate composite hydrogel membrane and its performance in filtering heavy metal ions. Carbohydr. Polym. 300, 120278. doi:10.1016/j.carbpol.2022.120278
Chaix, A., Gomri, C., Benkhaled, B. T., Habib, M., Dupuis, R., Petit, E., et al. (2024). Efficient PFAS removal using reusable and non-toxic 3D printed porous trianglamine hydrogels. Adv. Mater 2024, 2410720. doi:10.1002/adma.202410720
Chen, F., Wei, C., Chen, Q., Zhang, J., Wang, L., Zhou, Z., et al. (2018). Internal concentrations of perfluorobutane sulfonate (PFBS) comparable to those of perfluorooctane sulfonate (PFOS) induce reproductive toxicity in Caenorhabditis elegans. Ecotoxicol. Environ. Saf. 158, 223–229. doi:10.1016/j.ecoenv.2018.04.032
Chen, M., Wang, P., Jiang, H., Yan, J., Qiu, S., Zhang, Z., et al. (2024). Inhibition of biofouling by in-situ grown zwitterionic hydrogel nanolayer on membrane surface in ultralow-pressurized ultrafiltration process. Water Res. 253, 121263. doi:10.1016/j.watres.2024.121263
Cowie, P. R., Smith, M. J., Hannah, F., Cowling, M. J., and Hodgkeiss, T. (2006). The prevention of microfouling and macrofouling on hydrogels impregnated with either Arquad 2C-75® or benzalkonium chloride. Biofouling 22, 195–207. doi:10.1080/08927010600783296
Daschner, F., Rüden, H., Simon, R., and Clotten, J. (1996). Microbiological contamination of drinking water in a commercial household water filter system. Eur. J. Clin. Microbiol. Infect. Dis. 15, 233–237. doi:10.1007/BF01591360
Feng, X., Cao, X., Zhao, S., Wang, X., Hua, X., Chen, L., et al. (2017). Exposure of pregnant mice to perfluorobutanesulfonate causes hypothyroxinemia and developmental abnormalities in female offspring. Toxicol. Sci. 155, 409–419. doi:10.1093/toxsci/kfw219
Flemming, H.-C., and Geesey, G. G. (1991). “Biofouling and biocorrosion in industrial water systems,” in Proceedings of the international workshop on industrial biofouling and biocorrosion, stuttgart, september 13–14, 1990 (Springer Berlin, Heidelberg: Springer Science and Business Media; 2012), 29–46.
Guo, Y., Dundas, C. M., Zhou, X., Johnston, K. P., and Yu, G. (2021). Molecular engineering of hydrogels for rapid water disinfection and sustainable solar vapor generation. Adv. Mat. 33, 2102994. doi:10.1002/adma.202102994
Hu, X. C., Andrews, D. Q., Lindstrom, A. B., Bruton, T. A., Schaider, L. A., Grandjean, P., et al. (2016). Detection of poly-and perfluoroalkyl substances (PFASs) in US drinking water linked to industrial sites, military fire training areas, and wastewater treatment plants. Environ. Sci. Technol. Lett. 3, 344–350. doi:10.1021/acs.estlett.6b00260
Huang, P.-J., Hwangbo, M., Chen, Z., Liu, Y., Kameoka, J., and Chu, K.-H. (2018). Reusable functionalized hydrogel sorbents for removing long-and short-chain perfluoroalkyl acids (PFAAs) and GenX from aqueous solution. ACS Omega 3, 17447–17455. doi:10.1021/acsomega.8b02279
Jafarinejad, S. (2025). A mini-review of full-scale drinking water treatment plants for per-and polyfluoroalkyl substances (PFAS) removal: possible solutions and future directions. Sustainability 17, 451. doi:10.3390/su17020451
Jensen, A. A., and Warming, M. (2015). Short-chain polyfluoroalkyl substances (PFAS). Copenhagen, Denmark: The Danish Environmental Protection Agency. Available online at: https://www2.mst.dk/Udgiv/publications/2015/05/978-87-93352-15-5.pdf.
Kempisty, D. M., Arevalo, E., Spinelli, A. M., Edeback, V., Dickenson, E. R., Husted, C., et al. (2022). Granular activated carbon adsorption of perfluoroalkyl acids from ground and surface water. AWS 4, e1269. doi:10.1002/aws2.1269
Koda, Y., Terashima, T., and Sawamoto, M. (2014). Fluorous microgel star polymers: selective recognition and separation of polyfluorinated surfactants and compounds in water. J. Am. Chem. Soc. 136, 15742–15748. doi:10.1021/ja508818j
Kumarasamy, E., Manning, I. M., Collins, L. B., Coronell, O., and Leibfarth, F. A. (2020). Ionic fluorogels for remediation of per-and polyfluorinated alkyl substances from water. ACS Cent. Sci. 6, 487–492. doi:10.1021/acscentsci.9b01224
LeChevallier, M. W., Hassenauer, T. S., Camper, A. K., and McFETERS, G. A. (1984). Disinfection of bacteria attached to granular activated carbon. Appl. Environ. Microbiol. 48, 918–923. doi:10.1128/aem.48.5.918-923.1984
Lee, K., Kim, Y.-W., Lee, S., Lee, S. H., Nahm, C. H., Kwon, H., et al. (2018). Stopping autoinducer-2 chatter by means of an indigenous bacterium (Acinetobacter sp. DKY-1): a new antibiofouling strategy in a membrane bioreactor for wastewater treatment. Environ. Sci. Technol. 52, 6237–6245. doi:10.1021/acs.est.7b05824
León-Silva, S., Fernández-Luqueño, F., and López-Valdez, F. (2016). Silver nanoparticles (AgNP) in the environment: a review of potential risks on human and environmental health. Water Air Soil Pollut. 227, 306. doi:10.1007/s11270-016-3022-9
Li, F., Duan, J., Tian, S., Ji, H., Zhu, Y., Wei, Z., et al. (2020). Short-chain per-and polyfluoroalkyl substances in aquatic systems: occurrence, impacts and treatment. Chem. Eng. J. 380, 122506. doi:10.1016/j.cej.2019.122506
Li, F., Zhang, C., Qu, Y., Chen, J., Hu, X., and Zhou, Q. (2011). Method development for analysis of short-and long-chain perfluorinated acids in solid matrices. Int. J. Environ. Anal. Chem. 91, 1117–1134. doi:10.1080/03067310903191721
Li, N., Luo, L., Guo, C., He, J., Wang, S., Yu, L., et al. (2022). Shape-controlled fabrication of cost-effective, scalable and anti-biofouling hydrogel foams for solar-powered clean water production. Chem. Eng. J. 431, 134144. doi:10.1016/j.cej.2021.134144
Li, S., Zhao, S., Pei, J., Wang, H., Meng, H., Vrouwenvelder, J. S., et al. (2021). Stimuli-responsive lysozyme nanocapsule engineered microfiltration membranes with a dual-function of anti-adhesion and antibacteria for biofouling mitigation. ACS Appl. Mater Interfaces 13, 32205–32216. doi:10.1021/acsami.1c07445
Liu, Y., Li, T., Bao, J., Hu, X., Zhao, X., Shao, L., et al. (2022). A review of treatment techniques for short-chain perfluoroalkyl substances. Appl. Sci. 12, 1941. doi:10.3390/app12041941
McCarty, M. W. (2016). Development of a novel perfluoroalkyl substance sequestration scheme using alginate macrobeads and common water treatment polymers. Master's thesis. Minneapolis, MN: University of Minnesota.
Munoz, G., Liu, M., Duy, S. V., Liu, J., and Sauvé, S. (2023). Target and nontarget screening of PFAS in drinking water for a large-scale survey of urban and rural communities in Québec, Canada. Water Res. 233, 119750. doi:10.1016/j.watres.2023.119750
Naim Shaikh, M. A., and Nawaz, T. (2024). Highly efficient cationic surfactant functionalized alginate hydrogel for perfluorooctanoic acid adsorption: optimization through response surface methodology and performance evaluation for aqueous media. ACS ES&T Water 4, 3078–3088. doi:10.1021/acsestwater.4c00319
Newcombe, G. (1994). Activated carbon and soluble humic substances: adsorption, desorption, and surface charge effects. J. Colloid Interface Sci. 164, 452–462. doi:10.1006/jcis.1994.1188
Nguyen, T., Roddick, F. A., and Fan, L. (2012). Biofouling of water treatment membranes: a review of the underlying causes, monitoring techniques and control measures. Membranes 2, 804–840. doi:10.3390/membranes2040804
Nian, M., Luo, K., Luo, F., Aimuzi, R., Huo, X., Chen, Q., et al. (2020). Association between prenatal exposure to PFAS and fetal sex hormones: are the short-chain PFAS safer? Environ. Sci. Technol. 54, 8291–8299. doi:10.1021/acs.est.0c02444
Park, M., Wu, S., Lopez, I. J., Chang, J. Y., Karanfil, T., and Snyder, S. A. (2020). Adsorption of perfluoroalkyl substances (PFAS) in groundwater by granular activated carbons: roles of hydrophobicity of PFAS and carbon characteristics. Water Res. 170, 115364. doi:10.1016/j.watres.2019.115364
Peng, B., Lyu, Q., Gao, Y., Li, M., Xie, G., Xie, Z., et al. (2022). Composite polyelectrolyte photothermal hydrogel with anti-biofouling and antibacterial properties for the real-world application of solar steam generation. ACS Appl. Mater Interfaces 14, 16546–16557. doi:10.1021/acsami.2c02464
Razali, M. C., Wahab, N. A., Sunar, N., and Shamsudin, N. H. (2023). Existing filtration treatment on drinking water process and concerns issues. Membranes 13, 285. doi:10.3390/membranes13030285
Roberts, J. R., Park, J., Helton, K., Wisniewski, N., and McShane, M. J. (2012). Biofouling of polymer hydrogel materials and its effect on diffusion and enzyme-based luminescent glucose sensor functional characteristics. J. Diabetes Sci. Technol. 6, 1267–1275. doi:10.1177/193229681200600605
Saleque, A. M., Ivan, MNAS, Ahmed, S., and Tsang, Y. H. (2023). Light-trapping texture bio-hydrogel with anti-biofouling and antibacterial properties for efficient solar desalination. Chem. Eng. J. 458, 141430. doi:10.1016/j.cej.2023.141430
Seven seas water (2023). The role of municipal drinking water treatment systems. Available online at: https://sevenseaswater.com/role-of-municipal-water-treatment-systems/ (Accessed December, 2024).
Sharma, S., and Bhattacharya, A. (2017). Drinking water contamination and treatment techniques. Appl. Water Sci. 7, 1043–1067. doi:10.1007/s13201-016-0455-7
Sheng, N., Cui, R., Wang, J., Guo, Y., Wang, J., and Dai, J. (2018). Cytotoxicity of novel fluorinated alternatives to long-chain perfluoroalkyl substances to human liver cell line and their binding capacity to human liver fatty acid binding protein. Arch. Toxicol. 92, 359–369. doi:10.1007/s00204-017-2055-1
Sójka, O., van der Mei, H. C., van Rijn, P., and Gagliano, M. C. (2023). Zwitterionic poly (sulfobetaine methacrylate)-based hydrogel coating for drinking water distribution systems to inhibit adhesion of waterborne bacteria. Front. Bioeng. Biotechnol. 11, 1066126. doi:10.3389/fbioe.2023.1066126
Solan, M. E., Schackmuth, B., Bruce, E. D., Pradhan, S., Sayes, C. M., and Lavado, R. (2023). Effects of short-chain per-and polyfluoroalkyl substances (PFAS) on toxicologically relevant gene expression profiles in a liver-on-a-chip model. Environ. Pollut. 337, 122610. doi:10.1016/j.envpol.2023.122610
Stoiber, T., Evans, S., Temkin, A. M., Andrews, D. Q., and Naidenko, O. V. (2020). PFAS in drinking water: an emergent water quality threat. Water Solutions 1, e49 Available online at: https://www.ewg.org/sites/default/files/u352/Stoiber_Evans_WaterSolutions_2020.pdf (Accessed December, 2024).
UNESCO (2023). Imminent risk of a global water crisis, warns the UN World Water Development Report. Available online at: https://www.unesco.org/en/articles/imminent-risk-global-water-crisis-warns-un-world-water-development-report-2023 (Accessed December, 2025).
Verduzco, R., and Wong, M. S. (2020). Fighting PFAS with PFAS. ACS Cent. Sci. 6, 453–455. doi:10.1021/acscentsci.0c00164
Verma, S., Lee, T., Sahle-Demessie, E., Ateia, M., and Nadagouda, M. N. (2023). Recent advances on PFAS degradation via thermal and nonthermal methods. Chem. Eng. J. Adv. 13, 100421. doi:10.1016/j.ceja.2022.100421
Vuong, P., McKinley, A., and Kaur, P. (2023). Understanding biofouling and contaminant accretion on submerged marine structures. npj Mater. Degrad. 7, 50. doi:10.1038/s41529-023-00370-5
Wang, B., Yao, Y., Chen, H., Chang, S., Tian, Y., and Sun, H. (2020). Per-and polyfluoroalkyl substances and the contribution of unknown precursors and short-chain (C2–C3) perfluoroalkyl carboxylic acids at solid waste disposal facilities. Sci. Total Environ. 705, 135832. doi:10.1016/j.scitotenv.2019.135832
Wang, J., Ning, J., Li, S., Jia, M., Liu, L., Lu, J., et al. (2022b). Multipurpose of zwitterionic poly (imidazolium)-based hydrogel coating for oil/water separation with long-term antibiofouling property. Sep. Purif. Technol. 295, 121353. doi:10.1016/j.seppur.2022.121353
Wang, J., Qi, Q., Ji, Y., Liu, X., and Shi, Q. (2025). Thermosensitive hydrogels for efficient removal of perfluorooctanesulfonic acid. Mater. Lett. 382, 137886. doi:10.1016/j.matlet.2024.137886
Wang, P., Challis, J. K., He, Z.-X., Wong, C. S., and Zeng, E. Y. (2022a). Effects of biofouling on the uptake of perfluorinated alkyl acids by organic-diffusive gradients in thin films passive samplers. Environ. Sci. Process. Impacts 24, 242–251. doi:10.1039/D1EM00436K
Wang, Y., Liu, M., Duy, S. V., Munoz, G., Sauvé, S., and Liu, J. (2024a). Fast analysis of short-chain and ultra-short-chain fluorinated organics in water by on-line extraction coupled to HPLC-HRMS. Sci. Total Environ. 943, 173682. doi:10.1016/j.scitotenv.2024.173682
Wang, Y., Zhou, X., He, L., Zhou, X., Wang, Y., and Zhou, P. (2024b). Research progress on using modified hydrogel coatings as marine antifouling materials. Mar. Drugs 22, 546. doi:10.3390/md22120546
Wells, S., and Sytsma, M. (2009). A review of the use of coatings to mitigate biofouling in freshwater. Center for Lakes and Reservoirs. Portland, OR: Portland State University.
Wen, C., Guo, H., Yang, J., Li, Q., Zhang, X., Sui, X., et al. (2021). Zwitterionic hydrogel coated superhydrophilic hierarchical antifouling floater enables unimpeded interfacial steam generation and multi-contamination resistance in complex conditions. Chem. Eng. J. 421, 130344. doi:10.1016/j.cej.2021.130344
Wibisono, Y., Yandi, W., Golabi, M., Nugraha, R., Cornelissen, E. R., Kemperman, A. J., et al. (2015). Hydrogel-coated feed spacers in two-phase flow cleaning in spiral wound membrane elements: a novel platform for eco-friendly biofouling mitigation. Water Res. 71, 171–186. doi:10.1016/j.watres.2014.12.030
Xu, X., Guillomaitre, N., Christie, K. S., Rkn, B., Bizmark, N., Datta, S. S., et al. (2023). Quick-release antifouling hydrogels for solar-driven water purification. ACS Cent. Sci. 9, 177–185. doi:10.1021/acscentsci.2c01245
Xu, Y., Yu, X., Wang, X., Song, Y., Wang, W., Zhang, M., et al. (2024). Efficient separation of per-and polyfluoroalkyl substances (PFAS) from water by aminated polyacrylamide hydrogel foam. Chem. Eng. J. 501, 157833. doi:10.1016/j.cej.2024.157833
Yang, Z., Saeki, D., and Matsuyama, H. (2018). Zwitterionic polymer modification of polyamide reverse-osmosis membranes via surface amination and atom transfer radical polymerization for anti-biofouling. J. Membr. Sci. 550, 332–339. doi:10.1016/j.memsci.2018.01.001
Ye, Y., Klimchuk, S., Shang, M., and Niu, J. (2019). Improved antibacterial performance using hydrogel-immobilized lysozyme as a catalyst in water. RSC Adv. 9, 20169–20173. doi:10.1039/C9RA02464F
Ye, Y., Steigerwald, J. M., Bang, H., Jones, V., Dennehy, K., and Ray, J. R. (2023). H2O2-catalyzed defluorination of perfluorooctanesulfonate (PFOS) by oxidized vanadium carbide MXene nanosheets. J. Mater Chem. A 11, 16803–16814. doi:10.1039/D3TA02073H
Ye, Y., Xiao, L., He, B., Zhang, Q., Nie, T., Yang, X., et al. (2017). Oxygen-tuned nanozyme polymerization for the preparation of hydrogels with printable and antibacterial properties. J. Mater Chem. B 5, 1518–1524. doi:10.1039/C6TB03317B
Yi, K., Huang, J., Pang, H., Li, S., Liu, Z., Wang, X., et al. (2024). Semi-interpenetrating network hydrogels-based microcapsule for quorum quenching bacteria biocontainment to enhance biofouling control in membrane bioreactor. Chem. Eng. J. 486, 150103. doi:10.1016/j.cej.2024.150103
Zanoni, M., Habimana, O., Amadio, J., and Casey, E. (2016). Antifouling activity of enzyme-functionalized silica nanobeads. Biotechnol. Bioeng. 113, 501–512. doi:10.1002/bit.25835
Zhang, D., He, Q., Wang, M., Zhang, W., and Liang, Y. (2021). Sorption of perfluoroalkylated substances (PFASs) onto granular activated carbon and biochar. Environ. Technol. 42, 1798–1809. doi:10.1080/09593330.2019.1680744
Zhang, W., Cheng, W., Ziemann, E., Be’er, A., Lu, X., Elimelech, M., et al. (2018). Functionalization of ultrafiltration membrane with polyampholyte hydrogel and graphene oxide to achieve dual antifouling and antibacterial properties. J. Membr. Sci. 565, 293–302. doi:10.1016/j.memsci.2018.08.017
Zhou, X., Guo, Y., Zhao, F., and Yu, G. (2019). Hydrogels as an emerging material platform for solar water purification. Acc. Chem. Res. 52, 3244–3253. doi:10.1021/acs.accounts.9b00455
Keywords: antibiofouling hydrogel, drinking water treatment, short-chain PFAS removal, long-term usage, domestic water treatment
Citation: Donovan KK-A, Fermin GT, Vigil I, Shaker S, Paulino RA, Difo R and Ye Y (2025) Advancements in antibiofouling hydrogel-based approaches for the removal of short-chain per- and polyfluoroalkyl substances in drinking water treatment. Front. Chem. Eng. 7:1565754. doi: 10.3389/fceng.2025.1565754
Received: 23 January 2025; Accepted: 10 April 2025;
Published: 30 April 2025.
Edited by:
Xunhao Wang, Michigan State University, United StatesReviewed by:
Kiyan Sorgog, Jackson State University, United StatesZhuorui Zhang, Massachusetts Institute of Technology, United States
Yiting Xiao, University of Michigan, United States
Copyright © 2025 Donovan, Fermin, Vigil, Shaker, Paulino, Difo and Ye. This is an open-access article distributed under the terms of the Creative Commons Attribution License (CC BY). The use, distribution or reproduction in other forums is permitted, provided the original author(s) and the copyright owner(s) are credited and that the original publication in this journal is cited, in accordance with accepted academic practice. No use, distribution or reproduction is permitted which does not comply with these terms.
*Correspondence: Yuemei Ye, eXVlbWVpLnllQGxlaG1hbi5jdW55LmVkdQ==