- 1Aarhus Institute of Advanced Studies, Aarhus University, Aarhus, Denmark
- 2Department of Biomedicine, Aarhus University, Aarhus, Denmark
Dendritic cells (DCs) play a critical role in mediating innate and adaptive immune responses. Since their discovery in the late 1970's, DCs have been recognized as the most potent antigen-presenting cells (APCs). DCs have a superior capacity for acquiring, processing, and presenting antigens to T cells and they express costimulatory or coinhibitory molecules that determine immune activation or anergy. For these reasons, cell-based therapeutic approaches using DCs have been explored in cancer and infectious diseases but with limited success. In humans, DCs are divided into heterogeneous subsets with distinct characteristics. Two major subsets are CD11c+ myeloid (m)DCs and CD11c− plasmacytoid (p)DCs. pDCs are different from mDCs and play an essential role in the innate immune system via the production of type I interferons (IFN). However, pDCs are also able to take-up antigens and effectively cross present them. Given the rarity of pDCs in blood and technical difficulties in obtaining them from human blood samples, the understanding of human pDC biology and their potential in immunotherapeutic approaches (e.g. cell-based vaccines) is limited. However, due to the recent advancements in cell culturing systems that allow for the generation of functional pDCs from CD34+ hematopoietic stem and progenitor cells (HSPC), studying pDCs has become easier. In this mini-review, we hypothesize about the use of pDCs as a cell-based therapy to treat HIV by enhancing anti-HIV-immune responses of the adaptive immune system and enhancing the anti-viral responses of the innate immune system. Additionally, we discuss obstacles to overcome before this approach becomes clinically applicable.
Introduction
Antiretroviral therapy (ART) successfully suppresses human immunodeficiency virus (HIV) replication but it does not cure an individual from having the infection (Finzi et al., 1997). The major barrier to clearing the virus is the persistence of a latent reservoir in long-lived resting and proliferating memory CD4+ T cells (Chun et al., 1997; Wong et al., 1997; Hosmane et al., 2017). Different molecular mechanisms have been described that contribute to HIV latency, reviewed in Dahabieh et al. (2015) and Vanhamel et al. (2019). Accordingly, different strategies that aim at HIV clearance are investigated, reviewed in Margolis et al. (2020). One strategy is to induce HIV expression, combined with enhanced immune function to clear infected cells. Here, we hypothesize if therapeutic vaccination with pDCs can enhance immune function to clear HIV infected cells.
Plasmacytoid DCs: A Small but Significant Cell Population
Dendritic cells (DCs) play a pivotal role in mediating innate and adaptive immune responses by various cellular mechanisms. In humans, DCs are categorized into heterogeneous subsets with distinct characteristics. Two major subsets are CD11c+ myeloid (m)DCs and CD11c− plasmacytoid (p)DCs. Although both subsets are classified as DCs, they are in fact very different cell types. Initially, the primary role of pDCs was defined as type I Interferon (IFN)-producing cells in response to a viral infection (Cella et al., 1999; Siegal et al., 1999). PDCs were described as a cell type that effectively senses viral RNA or DNA via toll-like receptors (TLRs) 7 and 9 and subsequently produce vast amounts of type I and type III IFNs (Jarrossay et al., 2001; Kadowaki et al., 2001; Yin et al., 2012). However, the function of pDCs is more diverse, and amongst other functions, pDCs have been described to serve as antigen presenting cells (APC) that stimulate CD8+ and CD4+ T cells, or become “killer-pDCs” with cytotoxic properties, reviewed in Swiecki and Colonna (2015), Musumeci et al. (2019), and Reizis (2019).
DC-based vaccination has been of interest in the fields of oncology and infectious diseases for two decades. It is safe with few adverse effects but despite inducing favorable immune responses in preclinical studies, DC-based immunotherapies have not successfully induced significant clinical responses, reviewed in Mastelic-Gavillet et al. (2019) and da Silva et al. (2018). Because pDCs are key mediators for both innate and adaptive immune cells, interest in using pDCs for cell-based therapeutics is increasing. In this mini-review, we will discuss if therapeutic vaccination with pDCs could be beneficial for the treatment of HIV infection and focus on literature describing observations with pDCs. As the available studies are based on cells from several different species, we will try to simplify it by primarily referring to observations with human pDCs where possible. In doing so, we aim to answer the question: Can therapeutic vaccination with pDCs achieve a functional cure for HIV?
pDC Vaccination in Humans
To this date, only two studies have tested peripheral blood-derived pDCs as cell-based cancer therapy. In the first trial, Tel et al., explored pDCs activated with inactivated Frühsommer-Meningoenzephalitis (FSME) and loaded with tumor antigen-associated peptides ex vivo, followed by administration to patients with metastatic melanoma (Tel et al., 2013a). The pDCs distributed over multiple lymph nodes (LNs), mounted both CD4+ and CD8+ T cell responses, and an IFN response was observed after each vaccination. This study showed that vaccination with pDCs was safe and induced favorable immune responses. The second study by Westdorp et al., investigated patients with chemo-naïve castration-resistant prostate cancer receiving vaccinations with mature CD1c+ mDCs, pDCs or a combination of the two, that were stimulated with protamine/RNA and loaded with tumor-associated antigens (Westdorp et al., 2019). The immunotherapy was feasible, well-tolerated with low-grade toxicity and induced functional antigen-specific T cells, which correlated with improved progression-free survival. However, no difference in T cell functionality was observed between the administration of mDCs, pDCs, or mDCs plus pDCs. This strategy is currently under evaluation in a phase I/II clinical trial for stage III melanoma patients (clinicaltrials.gov Identifier: NCT02574377). Additionally, the combined administration of mDCs and pDCs in metastatic endometrial cancer patients is under evaluation (NCT04212377). See Table 1 for an overview of the clinical trials.
A limiting factor in using peripheral blood-derived pDCs is the number of pDCs available for vaccination. Only a small fraction of peripheral blood mononuclear cells (PBMCs) consists of pDCs (<1%). In the two before-mentioned clinical trials, leukapheresis was used to obtain pDCs and a maximum of three million pDCs could be administered per infusion, which was repeated on three occasions (Tel et al., 2013a; Westdorp et al., 2019). Overcoming the limitation in pDC numbers would greatly improve the application of pDCs as cell-based therapy. One way to get around this is to use an allogeneic pDC cell line. Allogeneic HLA-A*02:01 pDCs can induce melanoma antigen-specific and functional cytotoxic T cell responses ex vivo and have been shown to inhibit tumor growth in a humanized mouse model (Aspord et al., 2010, 2012). The safety and tolerability of using the irradiated HLA-A*02:01 pDC cell line loaded with four melanoma peptides (GeniusVac-Mel4) is currently under evaluation in a phase I clinical trial (NCT01863108). Similarly, a pDC cell line (PDC*lung01, PDC*line Pharma) is currently in a phase I/II study for the treatment of non-small-cell lung cancer (NCT03970746). However, the allogeneic pDC vaccine approach has some challenges; it is restricted to HLA-A2 patients and irradiation of the cells impairs the possibility to initiate an innate immune response via the secretion of IFN.
One possibility to obtain a continuous source of pDCs applicable for vaccination is to generate them ex vivo from hematopoietic stem cells. Cord blood CD34+ hematopoietic stem and progenitor cells (HSPC) have been shown to be suitable for the differentiation into functional pDCs in vitro (Blom et al., 2000; Chen et al., 2004; Olivier et al., 2006; Demoulin et al., 2012; Thordardottir et al., 2014) and can yield clinically relevant cell numbers: up to 81 (±20) pDCs per single HSPC (Laustsen et al., 2018). CD34+ stem cells can also be isolated from peripheral blood after mobilization with G-CSF and the generated pDCs can induce Ag-specific activation of autologous CD8+ memory T cells ex vivo (Thordardottir et al., 2017). Although using autologous stem cell-derived pDCs for vaccination is a promising avenue for personalized pDC therapeutics, the HSPC differentiation into pDCs still requires long-term culturing, implying that the field still needs to make several advancements before it has clinical potential.
pDCs as Therapeutic Vaccine for the Treatment of Infectious Diseases
As of today, there are two reports that describe the use of pDCs as therapeutic vaccine for the treatment of an infectious disease. In the first study, the HLA-A*02:01 pDC line was used for the treatment of Hepatitis B Virus (HBV) (Martinet et al., 2012). Immunodeficient NOD/SCID β2m−/− mice, reconstituted with HBV patient's PBMCs and xenotransplanted with human HBV-transfected hepatocytes, received two vaccinations of irradiated HBV-peptide pulsed pDCs per treatment. Vaccination elicited HBV-specific T cells that were able to lyse the transfected hepatocytes and reduce systemic viral load. In the second study, pDCs were used to vaccinate BALB/c mice to provide protection against the parasitic infection Leishmania major (L. major) (Remer et al., 2007). Mice received a single dose of splenic murine pDCs that were pulsed with L. major lysate. Vaccination provided complete protection when mice were challenged 1 or 4 weeks after vaccination. Additionally, adoptive T cell transfer of protected mice onto naïve susceptible mice provided complete protection to L. major challenge.
These studies show that pDC vaccination can provide protection against two different infectious diseases in murine models.
HIV Infection Negatively Impacts pDC Numbers and Functionality
Similar to the decline in CD4+ T cell counts following acute HIV infection, a decline in circulating pDCs can be observed. Upon initiation of antiretroviral therapy (ART) the pDC numbers can increase but are not fully restored (Donaghy et al., 2001; Chehimi et al., 2002; Barron et al., 2003; Finke et al., 2004; Almeida et al., 2005; Kamga et al., 2005; Lichtner et al., 2008; Centlivre et al., 2011; Boichuk et al., 2015; Marquez-Coello et al., 2019). Additionally, pDCs that remain in circulation seem to be dysfunctional (further discussed below).
The reason for the decline in pDC numbers is not fully understood. PDCs express CD4, CCR5 and CXCR4, which theoretically makes them susceptible to infection with HIV. When isolated from peripheral blood or the thymus, pDCs can indeed be infected with both X4 and R5-tropic HIV in vitro (Patterson et al., 2001; Fong et al., 2002; Yonezawa et al., 2003; Lore et al., 2005; Smed-Sorensen et al., 2005; Evans et al., 2011). Whether this occurs in vivo is not clear, as conflicting observations have been reported. HIV DNA has been detected in pDCs isolated from people living with HIV (PLWH) and pDCs from the tonsil have been found to be positive for the CA-p24 protein (Fong et al., 2002; Donaghy et al., 2003; Centlivre et al., 2011). In contrast, others have not been able to demonstrate the presence of HIV RNA or DNA in peripheral blood pDCs, although this has been explored in samples from PLWH on suppressive ART (Otero et al., 2003) whereas in the other studies the PLWH were therapy naïve.
Yet, the infection of pDCs by HIV seems counterintuitive since they are the major producers of type I IFNs, and IFNs are well-known to have a potent anti-viral effect by increasing the expression of virus restriction and inhibition factors (Kluge et al., 2015; Colomer-Lluch et al., 2018). However, the HIV-induced IFN production by pDCs is delayed and is up to 20-fold lower than IFN production induced by Influenza, Sendai, and HIV-2 (Lo et al., 2012). Furthermore, pDCs do express the restriction factors SAMHD1 (Bloch et al., 2014), tetherin/CD317 (Tavano et al., 2013), and APOBEC3G (Wang et al., 2008) but, similar to other cell types, the expression of these is enhanced by IFN signaling. Thus, it remains a possibility that pDCs can be infected with HIV prior to this delay in IFN production and subsequent delay in viral restriction factor expression. PDC depletion may also occur in people living with HIV-2 in the absence of detectable viremia (Cavaleiro et al., 2009). This indicates that mechanisms other than direct viral infection determine the pDC depletion during persistent infections. A second potential mechanism is that type I IFN negatively controls pDC cell numbers as this has been observed using mice. Viral infection induced the upregulation of pro-apoptotic molecules in pDCs in a type I IFN-dependent manner, resulting in caspase activation and subsequent pDC death (Swiecki et al., 2011). A third possibility to explain the decrease in pDC cell numbers in the periphery is that pDCs no longer circulate. HIV-activated pDCs enhance expression of the lymphoid homing receptor CCR7 and during progressive infection or in the absence of ART, they have been reported to accumulate in LNs and lymphoid tonsillar tissue (Fonteneau et al., 2004; Schmidt et al., 2005; Herbeuval et al., 2006; Lehmann et al., 2010). Another study reported that in the presence of ART, pDCs also home to the LNs but compared to people living without HIV, the pDC numbers in LNs were significantly reduced (Biancotto et al., 2007). This indicates that both circulatory and LN pDC numbers decline, but that the decrease of pDCs in circulation is not necessarily due to homing to lymphoid tissue.
Besides reduced cell numbers, pDCs are also dysfunctional in PLWH and persistently produce low levels of IFNs (O'Brien et al., 2011). During ART, the TLR7/8 response appears to remain intact (Chang et al., 2012; Bam et al., 2017; Tsai et al., 2017) but IFN production upon TLR9 signaling is reduced, potentially due to continuous HIV-induced activation of pDCs in vivo or the interaction of CD40 with CD40L (Feldman et al., 2001; Tilton et al., 2008; Cavaleiro et al., 2009; Donhauser et al., 2012). Additionally, pDCs in the blood of PLWH display an exhausted phenotype and this may interfere with TLR signaling and subsequent IFN production (Cavaleiro et al., 2009; Schwartz et al., 2017; Font-Haro et al., 2018). However, administration of the TLR9 agonist MGN1703 to PLWH on ART enhances pDC activation, as measured by activation markers CD86 and CD40. Plasma IFNα-2a levels increased as well, indicating that some TLR9 responsiveness remains or that other cells may produce IFN in response to MGN1703 (Vibholm et al., 2017).
Would There Be Benefit in Restoring pDC Cell Numbers With a Functional IFN Response?
There are observations that indicate a beneficial role of maintaining pDC numbers during HIV infection. First, PLWH on ART with a low baseline pDC blood count are more likely to have an increase of HIV-RNA compared to individuals with high pDC counts during a 30 months follow-up (Lichtner et al., 2008). Second, elite controllers and long-term non-progressors (LTNPs) have preserved pDC counts and functionality (Almeida et al., 2005; Machmach et al., 2012). It is unclear whether the pDCs from elite controllers are better equipped to suppress viral replication or if the pDC cell numbers are maintained because the viral load is suppressed by other immune cells, like CTLs or natural killer (NK) cells. Third, expansion of pDCs during acute infection delays onset of viremia and reduces HIV replication in humanized mice (Pham et al., 2019).
pDCs Can Potentially Control HIV via Three Mechanisms
PDCs can contribute to suppression of virus replication by fulfilling three different functions: (i) as a professional IFN-producing cell; (ii) as a professional APC and; (iii) as a “killer-pDC” with cytotoxic properties (Figure 1). Each mechanism is discussed individually below.
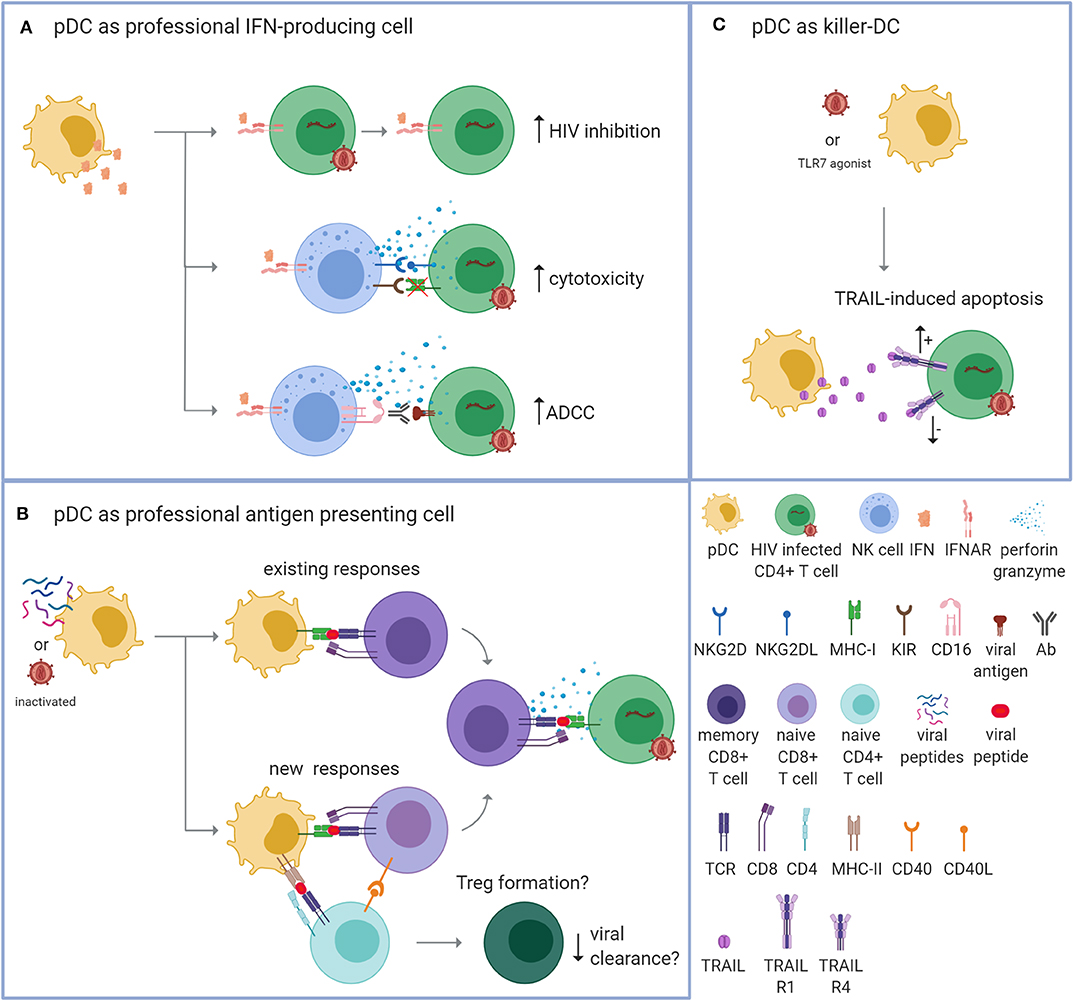
Figure 1. Plasmacytoid dendritic cells can potentially control HIV via three mechanisms. Plasmacytoid dendritic cells (pDCs) can contribute to the suppression of virus replication by fulfilling three different functions. (A) As a professional interferon (IFN)-producing cell. PDCs can produce vast amounts of IFNs upon TLR7/9 signaling. IFNs can directly inhibit HIV replication by enhancing the expression of virus restriction and inhibition factors within the CD4+ T cell (top; increased HIV inhibition). Additionally, IFNs can provide an immunostimulatory environment that enhances the cytotoxic function of natural killer (NK) cells. Apoptosis of HIV-infected cells can be induced by the release of cytotoxic perforins and granzymes after detecting reduced expression of MHC-I molecules in combination with activating signals, such as the binding of NKG2D to NKG2DL (middle; enhanced cytotoxicity). Apoptosis can also be induced by detecting the Fc tail of an antibody (Ab) that is bound to a viral antigen on the surface of the infected cell [bottom; enhanced antibody-dependent cellular cytotoxicity (ADCC)]. (B) As a professional antigen presenting cell (APC). PDCs can process endogenous and exogenous antigens for the antigen-specific stimulation of T cells. PDCs could be loaded with autologous inactivated HIV or HIV peptides that are presented on MHC-I molecules to activate existing HIV-specific memory CD8+ T cells (top; existing responses). Upon repeated exposure, this strategy could be applied to induce new responses by activating naïve CD8+ T cells with the help of CD4+ T cells (bottom; new responses). The activated HIV-specific CD8+ T cells are then able to recognize and eliminate HIV-infected cells via a cognate TCR-MHC interaction. PDCs may also induce the formation of regulatory T cells (Tregs) that could potentially suppress immune activation and counteract viral clearance. (C) As a “killer-pDC.” TLR7-stimulated or HIV-exposed pDCs can obtain cytotoxic properties through the expression and secretion of TNF-related apoptosis-inducing ligand (TRAIL) that can bind to the apoptosis-transmitting TRAIL receptor 1 (R1). However, HIV-infected CD4+ T cells from PLWH who receive antiretroviral treatment express the TRIAL R1 receptor and the decoy receptor TRAIL R4, which makes the T cells likely resistant to pDC-mediated killing.
Controlling HIV as a Professional IFN-Producing Cell
PDCs produce abundant type I IFNs (Kadowaki et al., 2000) and type III IFNs (Yin et al., 2012) when sensing viral products such as single stranded RNA by TLR7 or unmethylated DNA molecules by TLR9, reviewed in Swiecki and Colonna (2015). In the context of HIV, pDCs produce type I IFNs in response to cell-free HIV and to HIV infected cells; both require endocytosis and likely initiate the TLR7 signaling pathway (Beignon et al., 2005; Schmidt et al., 2005). IFNs function as the first line of defense against viruses because they have a broad antiviral effect. IFNs can induce inflammation, activate specific immune cells including NK cells, CD8+ T cells, and macrophages, and prime antigen-specific responses, reviewed in Hoffmann et al. (2015) and Kotenko and Durbin (2017). IFNs can also directly inhibit HIV replication by enhancing the expression of virus restriction and inhibition factors, reviewed in Colomer-Lluch et al. (2018) (Figure 1A).
However, chronic inflammation and long-term exposure to IFNs have clear detrimental effects (Acchioni et al., 2015). In the context of HIV, the timing of IFN seems to be critical, as demonstrated in a study with SIV-infected rhesus macaques. Blocking type I IFN signaling during acute SIV infection enhanced virus replication and CD4+ T cell loss, whereas administering IFNα-2a during acute SIV infection induced an antiviral state and limited viral spread. However, when IFNα-2a treatment continued, cells became unresponsive to IFN, resulting in decreased viral inhibition and subsequent enhanced CD4+ T cell loss (Sandler et al., 2014). Thus, ideally pDCs should produce IFNs for a limited time only.
In addition to upregulating virus restriction and inhibition factors, IFNs provide an immunostimulatory environment for other immune cells (Gonzalez-Navajas et al., 2012). This is particularly apparent for the cytolytic capacity of natural killer (NK) cells (Figure 1A). IFNs produced by stem-cell derived pDCs can trigger NK cell activation and increase the capacity of NK cell-mediated killing of acute lymphoblastic leukemia (Diaz-Rodriguez et al., 2017). Similarly, IFN produced by TLR9-stimulated peripheral blood pDCs can enhance NK-mediated lysis of autologous CD4+ T cells infected with HIV in vitro (Tomescu et al., 2007). Additionally, NK-mediated antibody-dependent cellular cytotoxicity (ADCC) via autologous and heterologous HIV serum antibodies can be enhanced by IFNα (Lee et al., 2015; Tomescu et al., 2017). ADCC is a mechanism of cell-mediated cytotoxicity where the effector cell, here the NK cell, lyses a target cell via the recognition of an antibody that is bound to a viral antigen on the target cell. Via CD16, the effector cell binds the Fc portion of the antibody followed by the release of cytotoxic factors (Perussia and Trinchieri, 1984; Perussia et al., 1984).
Controlling HIV as an Antigen-Presenting Cell
Multiple studies have shown that pDCs can process and present endogenous and exogenous antigens on MHC I and II molecules and induce antigen-specific activation of both CD8+ and CD4+ T cells (Fonteneau et al., 2003; Benitez-Ribas et al., 2006; Young et al., 2008; Tel et al., 2010, 2013b). Important for therapeutic purposes, pDCs can be loaded with synthetic long and short peptides to trigger CD4+ and CD8+ T cell responses in vitro (Aspord et al., 2014) and in vivo (Tel et al., 2013a; Westdorp et al., 2019). This suggests that, similar to monocyte-derived DCs (moDCs), pDCs could be loaded with HIV antigens in vitro to enhance T cell responses in vivo (Figure 1B). To date, 17 clinical trials using moDC vaccination for HIV infection have been published and the clinical outcomes were variable, reviewed in da Silva et al. (2018). The studies all had in common the use of moDCs, but differed regarding moDC preparation and maturation, HIV antigen, route of administration, the number of cells administered per dose, and the number of doses administered. Regardless of these variations, all trials were safe and well tolerated. Seven of the seventeen studies demonstrated a prolonged suppression of HIV RNA in plasma and this correlated with HIV-specific T cell responses. In these studies the moDCs were exposed to autologous aldrithiol-2-inactivated HIV (Lu et al., 2004), autologous heat-inactivated HIV (Garcia et al., 2005, 2011, 2013), autologous inactivated HIV-infected apoptotic cells (Macatangay et al., 2016), or HIV peptides (Kloverpris et al., 2009; Levy et al., 2014). If these strategies are appropriate for pDC vaccination remains to be determined.
Besides inducing inflammatory T cells, pDCs have been reported to induce the formation of regulatory T cells (Tregs) and expression of indoleamine 2,3-dioxygenase (IDO) by pDCs is one of the reported mechanisms (Chen et al., 2008). Additionally, HIV-stimulated pDCs have been reported to differentiate naïve CD4+ T cells into Tregs (Manches et al., 2008). Tregs suppress immune activation and limit viral clearance, reviewed in Kleinman et al. (2018). Thus, in the context of using pDCs as a therapy to induce elimination of HIV-infected cells, the induction of Tregs would likely be undesirable. However, whether pDCs exposed to inactivated HIV or HIV peptides also induce Treg formation remains to be determined.
Controlling HIV as a Killer-pDC
In a mouse model for melanoma, TRL7-stimulated pDCs have been shown to directly kill tumor cells via the secretion of TNF-related apoptosis-inducing ligand (TRAIL) and granzymes (Drobits et al., 2012). Similarly, HIV-exposed pDCs also gain cytotoxic properties, thereby creating so-called “killer-pDCs,” which can induce apoptosis of CD4+ T cell lines via the TRAIL pathway (Hardy et al., 2007; Chehimi et al., 2010; Barblu et al., 2012; Figure 1C). This seems to have great potential for eliminating virus-infected cells but expression of the apoptosis-transmitting TRAIL receptor 1 (R1) is not restricted to HIV-infected cells and seems to be the result of chronic immune activation, possibly resulting in the killing of bystander T cells as well (Stary et al., 2009). CD4+ T cells express TRAIL R1 during detectable viremia but initiation of ART enhances expression of the decoy receptor TRAIL R4, making the T cells resistant to pDC-mediated killing (Stary et al., 2009; Chehimi et al., 2010). Unless expression of the decoy TRAIL R4 can be downregulated on HIV-infected cells, the TRAIL-expressing pDC approach seems like a rather unattractive strategy to treat HIV infection because it would require pausing therapy and would not be limited to HIV-infected cells only.
Conclusions and Future Directions
In summary, pDCs are multifaceted cells and could have the potential to enhance immune function to inhibit HIV replication and clear infected cells (Figure 1). Similar to moDCs, pDCs have the capacity to function as APC and activate existing memory T cells or induce the activation of naïve T cells. Whether pDCs can achieve this in vivo after exposure to HIV Ag needs to be evaluated. Additionally, a key point to address in this regard is the pDCs' capacity to induce Tregs. If pDCs were to be used as therapeutic vaccine, it would be critical to evaluate the formation of Tregs as these anti-inflammatory cells could limit viral clearance.
IFNs are key mediators of an anti-viral response and although pDCs are potent producers of type I IFNα it is important to emphasize that IFNα comprises 13 different subtypes. They all bind to the same receptor but have distinct biological activities, and display differences in their HIV inhibiting properties (Gibbert et al., 2013; Harper et al., 2015; Lavender et al., 2016). Depending on the type of TLR stimulation, pDCs can produce a broad range of IFNα subtypes (Puig et al., 2012; Harper et al., 2015). There may be a benefit in using pDCs as a tool to deliver a broad range of IFNs, rather than administering a single subtype, which is the current approach with pegylated (peg)IFNα-2a. An additional benefit of using pDCs for the production of IFNs could be the local delivery of IFNs. As pDC can travel to lymphoid organs and other peripheral tissues, this may allow for more local and targeted delivery of IFNs rather than systemic administration via intravenous injection.
Lastly, there are currently three possibilities to obtain pDCs for therapeutic vaccination. pDCs can be obtained from peripheral blood via leukapheresis, generated from stem cells obtained from peripheral blood after mobilization, or provided as irradiated pDC cell lines. Each source has its advantages and disadvantages, and the choice may depend on the function that the pDCs are required to fulfill in order for the therapy to be effective. As APCs, pDCs would ideally be from an autologous source, but could also be from an allogeneic HLA-matched source.
However, as professional IFN-producing cells there would be no requirement for HLA-matching and it would perhaps be possible to generate a pDC vaccine from allogeneic origin. While this is an intriguing avenue of research, there are several advancements required within the field before pDC vaccination is able to reach its clinical potential for the treatment of HIV.
Author Contributions
RS and MJ conceptualized the idea for this manuscript. RS outlined the manuscript and wrote the first draft. JE conceptualized Table 1 and contributed together with MJ to editing the manuscript. All authors approved the final version.
Funding
RS was an AIAS fellow and this project has received funding from the European Union's Horizon 2020 research and innovation programme under the Marie Sklodowska-Curie grant agreement No 754513 and The Aarhus University Research Foundation. JE was supported by a Ph.D. fellowship from the Graduate School of Health, Aarhus University. MJ was funded by the Lundbeck Foundation (R238–2016–2708).
Conflict of Interest
The authors declare that the research was conducted in the absence of any commercial or financial relationships that could be construed as a potential conflict of interest.
Acknowledgments
We thank Rasmus O. Bak, Christian Krapp, and Luke Ring for critical reading of the manuscript. The figure in this manuscript was created using BioRender 2020 software.
References
Acchioni, C., Marsili, G., Perrotti, E., Remoli, A. L., Sgarbanti, M., and Battistini, A. (2015). Type I IFN–a blunt spear in fighting HIV-1 infection. Cytokine Growth Factor Rev. 26, 143–158. doi: 10.1016/j.cytogfr.2014.10.004
Almeida, M., Cordero, M., Almeida, J., and Orfao, A. (2005). Different subsets of peripheral blood dendritic cells show distinct phenotypic and functional abnormalities in HIV-1 infection. AIDS 19, 261–271.
Aspord, C., Charles, J., Leccia, M. T., Laurin, D., Richard, M. J., Chaperot, L., et al. (2010). A novel cancer vaccine strategy based on HLA-A*0201 matched allogeneic plasmacytoid dendritic cells. PLoS ONE 5:e10458. doi: 10.1371/journal.pone.0010458
Aspord, C., Leccia, M. T., Salameire, D., Laurin, D., Chaperot, L., Charles, J., et al. (2012). HLA-A(*)0201(+) plasmacytoid dendritic cells provide a cell-based immunotherapy for melanoma patients. J. Invest. Dermatol. 132, 2395–2406. doi: 10.1038/jid.2012.152
Aspord, C., Leloup, C., Reche, S., and Plumas, J. (2014). pDCs efficiently process synthetic long peptides to induce functional virus- and tumour-specific T-cell responses. Eur. J. Immunol. 44, 2880–2892. doi: 10.1002/eji.201444588
Bam, R. A., Hansen, D., Irrinki, A., Mulato, A., Jones, G. S., Hesselgesser, J., et al. (2017). TLR7 agonist GS-9620 is a potent inhibitor of acute HIV-1 infection in human peripheral blood mononuclear cells. Antimicrob. Agents Chemother. 61:e01369–16. doi: 10.1128/AAC.01369-16
Barblu, L., Machmach, K., Gras, C., Delfraissy, J. F., Boufassa, F., Leal, M., et al. (2012). Plasmacytoid dendritic cells (pDCs) from HIV controllers produce interferon-α and differentiate into functional killer pDCs under HIV activation. J. Infect. Dis. 206, 790–801. doi: 10.1093/infdis/jis384
Barron, M. A., Blyveis, N., Palmer, B. E., Mawhinney, S., and Wilson, C. C. (2003). Influence of plasma viremia on defects in number and immunophenotype of blood dendritic cell subsets in human immunodeficiency virus 1-infected individuals. J. Infect. Dis. 187, 26–37. doi: 10.1086/345957
Beignon, A. S., Mckenna, K., Skoberne, M., Manches, O., Dasilva, I., Kavanagh, D. G., et al. (2005). Endocytosis of HIV-1 activates plasmacytoid dendritic cells via toll-like receptor-viral RNA interactions. J. Clin. Invest. 115, 3265–3275. doi: 10.1172/JCI26032
Benitez-Ribas, D., Adema, G. J., Winkels, G., Klasen, I. S., Punt, C. J., Figdor, C. G., et al. (2006). Plasmacytoid dendritic cells of melanoma patients present exogenous proteins to CD4+ T cells after Fc gamma RII-mediated uptake. J. Exp. Med. 203, 1629–1635. doi: 10.1084/jem.20052364
Biancotto, A., Grivel, J. C., Iglehart, S. J., Vanpouille, C., Lisco, A., Sieg, S. F., et al. (2007). Abnormal activation and cytokine spectra in lymph nodes of people chronically infected with HIV-1. Blood 109, 4272–4279. doi: 10.1182/blood-2006-11-055764
Bloch, N., O'Brien, M., Norton, T. D., Polsky, S. B., Bhardwaj, N., and Landau, N. R. (2014). HIV type 1 infection of plasmacytoid and myeloid dendritic cells is restricted by high levels of SAMHD1 and cannot be counteracted by Vpx. AIDS Res. Hum. Retroviruses 30, 195–203. doi: 10.1089/aid.2013.0119
Blom, B., Ho, S., Antonenko, S., and Liu, Y. J. (2000). Generation of interferon alpha-producing predendritic cell (Pre-DC)2 from human CD34(+) hematopoietic stem cells. J. Exp. Med. 192, 1785–1796. doi: 10.1084/jem.192.12.1785
Boichuk, S. V., Khaiboullina, S. F., Ramazanov, B. R., Khasanova, G. R., Ivanovskaya, K. A., Nizamutdinov, E. Z., et al. (2015). Gut-associated plasmacytoid dendritic cells display an immature phenotype and upregulated granzyme B in subjects with HIV/AIDS. Front. Immunol. 6:485. doi: 10.3389/fimmu.2015.00485
Cavaleiro, R., Baptista, A. P., Soares, R. S., Tendeiro, R., Foxall, R. B., Gomes, P., et al. (2009). Major depletion of plasmacytoid dendritic cells in HIV-2 infection, an attenuated form of HIV disease. PLoS Pathog. 5:e1000667. doi: 10.1371/journal.ppat.1000667
Cella, M., Jarrossay, D., Facchetti, F., Alebardi, O., Nakajima, H., Lanzavecchia, A., et al. (1999). Plasmacytoid monocytes migrate to inflamed lymph nodes and produce large amounts of type I interferon. Nat. Med. 5, 919–923. doi: 10.1038/11360
Centlivre, M., Legrand, N., Steingrover, R., Van Der Sluis, R., Grijsen, M. L., Bakker, M., et al. (2011). Altered dynamics and differential infection profiles of lymphoid and myeloid cell subsets during acute and chronic HIV-1 infection. J. Leukoc. Biol. 89, 785–795. doi: 10.1189/jlb.0410231
Chang, J. J., Lacas, A., Lindsay, R. J., Doyle, E. H., Axten, K. L., Pereyra, F., et al. (2012). Differential regulation of toll-like receptor pathways in acute and chronic HIV-1 infection. AIDS 26, 533–541. doi: 10.1097/QAD.0b013e32834f3167
Chehimi, J., Campbell, D. E., Azzoni, L., Bacheller, D., Papasavvas, E., Jerandi, G., et al. (2002). Persistent decreases in blood plasmacytoid dendritic cell number and function despite effective highly active antiretroviral therapy and increased blood myeloid dendritic cells in HIV-infected individuals. J. Immunol. 168, 4796–4801. doi: 10.4049/jimmunol.168.9.4796
Chehimi, J., Papasavvas, E., Tomescu, C., Gekonge, B., Abdulhaqq, S., Raymond, A., et al. (2010). Inability of plasmacytoid dendritic cells to directly lyse HIV-infected autologous CD4+ T cells despite induction of tumor necrosis factor-related apoptosis-inducing ligand. J. Virol. 84, 2762–2773. doi: 10.1128/JVI.01350-09
Chen, W., Antonenko, S., Sederstrom, J. M., Liang, X., Chan, A. S., Kanzler, H., et al. (2004). Thrombopoietin cooperates with FLT3-ligand in the generation of plasmacytoid dendritic cell precursors from human hematopoietic progenitors. Blood 103, 2547–2553. doi: 10.1182/blood-2003-09-3058
Chen, W., Liang, X., Peterson, A. J., Munn, D. H., and Blazar, B. R. (2008). The indoleamine 2,3-dioxygenase pathway is essential for human plasmacytoid dendritic cell-induced adaptive T regulatory cell generation. J. Immunol. 181, 5396–5404. doi: 10.4049/jimmunol.181.8.5396
Chun, T. W., Carruth, L., Finzi, D., Shen, X., Digiuseppe, J. A., Taylor, H., et al. (1997). Quantification of latent tissue reservoirs and total body viral load in HIV-1 infection. Nature 387, 183–188. doi: 10.1038/387183a0
Colomer-Lluch, M., Ruiz, A., Moris, A., and Prado, J. G. (2018). Restriction factors: from intrinsic viral restriction to shaping cellular immunity against HIV-1. Front. Immunol. 9:2876. doi: 10.3389/fimmu.2018.02876
da Silva, L. T., Santillo, B. T., De Almeida, A., Duarte, A., and Oshiro, T. M. (2018). Using dendritic cell-based immunotherapy to treat HIV: how can this strategy be improved? Front. Immunol. 9:2993. doi: 10.3389/fimmu.2018.02993
Dahabieh, M. S., Battivelli, E., and Verdin, E. (2015). Understanding HIV latency: the road to an HIV cure. Annu. Rev. Med. 66, 407–421. doi: 10.1146/annurev-med-092112-152941
Demoulin, S., Roncarati, P., Delvenne, P., and Hubert, P. (2012). Production of large numbers of plasmacytoid dendritic cells with functional activities from CD34(+) hematopoietic progenitor cells: use of interleukin-3. Exp. Hematol. 40, 268–278. doi: 10.1016/j.exphem.2012.01.002
Diaz-Rodriguez, Y., Cordeiro, P., Belounis, A., Herblot, S., and Duval, M. (2017). In vitro differentiated plasmacytoid dendritic cells as a tool to induce anti-leukemia activity of natural killer cells. Cancer Immunol. Immunother. 66, 1307–1320. doi: 10.1007/s00262-017-2022-y
Donaghy, H., Gazzard, B., Gotch, F., and Patterson, S. (2003). Dysfunction and infection of freshly isolated blood myeloid and plasmacytoid dendritic cells in patients infected with HIV-1. Blood 101, 4505–4511. doi: 10.1182/blood-2002-10-3189
Donaghy, H., Pozniak, A., Gazzard, B., Qazi, N., Gilmour, J., Gotch, F., et al. (2001). Loss of blood CD11c(+) myeloid and CD11c(-) plasmacytoid dendritic cells in patients with HIV-1 infection correlates with HIV-1 RNA virus load. Blood 98, 2574–2576. doi: 10.1182/blood.V98.8.2574
Donhauser, N., Pritschet, K., Helm, M., Harrer, T., Schuster, P., Ries, M., et al. (2012). Chronic immune activation in HIV-1 infection contributes to reduced interferon alpha production via enhanced CD40:CD40 ligand interaction. PLoS ONE 7:e33925. doi: 10.1371/journal.pone.0033925
Drobits, B., Holcmann, M., Amberg, N., Swiecki, M., Grundtner, R., Hammer, M., et al. (2012). Imiquimod clears tumors in mice independent of adaptive immunity by converting pDCs into tumor-killing effector cells. J. Clin. Invest. 122, 575–585. doi: 10.1172/JCI61034
Evans, V. A., Lal, L., Akkina, R., Solomon, A., Wright, E., Lewin, S. R., et al. (2011). Thymic plasmacytoid dendritic cells are susceptible to productive HIV-1 infection and efficiently transfer R5 HIV-1 to thymocytes in vitro. Retrovirology 8:43. doi: 10.1186/1742-4690-8-43
Feldman, S., Stein, D., Amrute, S., Denny, T., Garcia, Z., Kloser, P., et al. (2001). Decreased interferon-alpha production in HIV-infected patients correlates with numerical and functional deficiencies in circulating type 2 dendritic cell precursors. Clin. Immunol. 101, 201–210. doi: 10.1006/clim.2001.5111
Finke, J. S., Shodell, M., Shah, K., Siegal, F. P., and Steinman, R. M. (2004). Dendritic cell numbers in the blood of HIV-1 infected patients before and after changes in antiretroviral therapy. J. Clin. Immunol. 24, 647–652. doi: 10.1007/s10875-004-6250-5
Finzi, D., Hermankova, M., Pierson, T., Carruth, L. M., Buck, C., Chaisson, R. E., et al. (1997). Identification of a reservoir for HIV-1 in patients on highly active antiretroviral therapy. Science 278, 1295–1300. doi: 10.1126/science.278.5341.1295
Fong, L., Mengozzi, M., Abbey, N. W., Herndier, B. G., and Engleman, E. G. (2002). Productive infection of plasmacytoid dendritic cells with human immunodeficiency virus type 1 is triggered by CD40 ligation. J. Virol. 76, 11033–11041. doi: 10.1128/JVI.76.21.11033-11041.2002
Fonteneau, J. F., Gilliet, M., Larsson, M., Dasilva, I., Munz, C., Liu, Y. J., et al. (2003). Activation of influenza virus-specific CD4+ and CD8+ T cells: a new role for plasmacytoid dendritic cells in adaptive immunity. Blood 101, 3520–3526. doi: 10.1182/blood-2002-10-3063
Fonteneau, J. F., Larsson, M., Beignon, A. S., Mckenna, K., Dasilva, I., Amara, A., et al. (2004). Human immunodeficiency virus type 1 activates plasmacytoid dendritic cells and concomitantly induces the bystander maturation of myeloid dendritic cells. J. Virol. 78, 5223–5232. doi: 10.1128/JVI.78.10.5223-5232.2004
Font-Haro, A., Janovec, V., Hofman, T., Machala, L., Jilich, D., Melkova, Z., et al. (2018). Expression of TIM-3 on plasmacytoid dendritic cells as a predictive biomarker of decline in HIV-1 RNA level during ART. Viruses 10:154. doi: 10.3390/v10040154
Garcia, F., Climent, N., Assoumou, L., Gil, C., Gonzalez, N., Alcami, J., et al. (2011). A therapeutic dendritic cell-based vaccine for HIV-1 infection. J. Infect. Dis. 203, 473–478. doi: 10.1093/infdis/jiq077
Garcia, F., Climent, N., Guardo, A. C., Gil, C., Leon, A., Autran, B., et al. (2013). A dendritic cell-based vaccine elicits T cell responses associated with control of HIV-1 replication. Sci. Transl. Med. 5:166ra2. doi: 10.1126/scitranslmed.3004682
Garcia, F., Lejeune, M., Climent, N., Gil, C., Alcami, J., Morente, V., et al. (2005). Therapeutic immunization with dendritic cells loaded with heat-inactivated autologous HIV-1 in patients with chronic HIV-1 infection. J. Infect. Dis. 191, 1680–1685. doi: 10.1086/429340
Gibbert, K., Schlaak, J. F., Yang, D., and Dittmer, U. (2013). IFN-α subtypes: distinct biological activities in anti-viral therapy. Br. J. Pharmacol. 168, 1048–1058. doi: 10.1111/bph.12010
Gonzalez-Navajas, J. M., Lee, J., David, M., and Raz, E. (2012). Immunomodulatory functions of type I interferons. Nat. Rev. Immunol. 12, 125–135. doi: 10.1038/nri3133
Hardy, A. W., Graham, D. R., Shearer, G. M., and Herbeuval, J. P. (2007). HIV turns plasmacytoid dendritic cells (pDC) into TRAIL-expressing killer pDC and down-regulates HIV coreceptors by toll-like receptor 7-induced IFN-alpha. Proc. Natl. Acad. Sci. U.S.A. 104, 17453–17458. doi: 10.1073/pnas.0707244104
Harper, M. S., Guo, K., Gibbert, K., Lee, E. J., Dillon, S. M., Barrett, B. S., et al. (2015). Interferon-alpha subtypes in an ex vivo model of acute HIV-1 infection: expression, potency and effector mechanisms. PLoS Pathog. 11:e1005254. doi: 10.1371/journal.ppat.1005254
Herbeuval, J. P., Nilsson, J., Boasso, A., Hardy, A. W., Kruhlak, M. J., Anderson, S. A., et al. (2006). Differential expression of IFN-alpha and TRAIL/DR5 in lymphoid tissue of progressor versus nonprogressor HIV-1-infected patients. Proc. Natl. Acad. Sci. U.S.A. 103, 7000–7005. doi: 10.1073/pnas.0600363103
Hoffmann, H. H., Schneider, W. M., and Rice, C. M. (2015). Interferons and viruses: an evolutionary arms race of molecular interactions. Trends Immunol. 36, 124–138. doi: 10.1016/j.it.2015.01.004
Hosmane, N. N., Kwon, K. J., Bruner, K. M., Capoferri, A. A., Beg, S., Rosenbloom, D. I., et al. (2017). Proliferation of latently infected CD4+ T cells carrying replication-competent HIV-1: potential role in latent reservoir dynamics. J. Exp. Med. 214, 959–972. doi: 10.1084/jem.20170193
Jarrossay, D., Napolitani, G., Colonna, M., Sallusto, F., and Lanzavecchia, A. (2001). Specialization and complementarity in microbial molecule recognition by human myeloid and plasmacytoid dendritic cells. Eur. J. Immunol. 31, 3388–3393. doi: 10.1002/1521-4141(200111)31:11<3388::aid-immu3388>3.0.co
Kadowaki, N., Antonenko, S., Lau, J. Y., and Liu, Y. J. (2000). Natural interferon alpha/beta-producing cells link innate and adaptive immunity. J. Exp. Med. 192, 219–226. doi: 10.1084/jem.192.2.219
Kadowaki, N., Ho, S., Antonenko, S., Malefyt, R. W., Kastelein, R. A., Bazan, F., et al. (2001). Subsets of human dendritic cell precursors express different toll-like receptors and respond to different microbial antigens. J. Exp. Med. 194, 863–869. doi: 10.1084/jem.194.6.863
Kamga, I., Kahi, S., Develioglu, L., Lichtner, M., Maranon, C., Deveau, C., et al. (2005). Type I interferon production is profoundly and transiently impaired in primary HIV-1 infection. J. Infect. Dis. 192, 303–310. doi: 10.1086/430931
Kleinman, A. J., Sivanandham, R., Pandrea, I., Chougnet, C. A., and Apetrei, C. (2018). Regulatory T cells as potential targets for HIV cure research. Front. Immunol. 9:734. doi: 10.3389/fimmu.2018.00734
Kloverpris, H., Karlsson, I., Bonde, J., Thorn, M., Vinner, L., Pedersen, A. E., et al. (2009). Induction of novel CD8+ T-cell responses during chronic untreated HIV-1 infection by immunization with subdominant cytotoxic T-lymphocyte epitopes. AIDS 23, 1329–1340. doi: 10.1097/QAD.0b013e32832d9b00
Kluge, S. F., Sauter, D., and Kirchhoff, F. (2015). SnapShot: antiviral restriction factors. Cell 163, 774–774.e1. doi: 10.1016/j.cell.2015.10.019
Kotenko, S. V., and Durbin, J. E. (2017). Contribution of type III interferons to antiviral immunity: location, location, location. J. Biol. Chem. 292, 7295–7303. doi: 10.1074/jbc.R117.777102
Laustsen, A., Bak, R. O., Krapp, C., Kjaer, L., Egedahl, J. H., Petersen, C. C., et al. (2018). Interferon priming is essential for human CD34+ cell-derived plasmacytoid dendritic cell maturation and function. Nat. Commun. 9:3525. doi: 10.1038/s41467-018-05816-y
Lavender, K. J., Gibbert, K., Peterson, K. E., Van Dis, E., Francois, S., Woods, T., et al. (2016). Interferon alpha subtype-specific suppression of HIV-1 infection in vivo. J. Virol. 90, 6001–6013. doi: 10.1128/JVI.00451-16
Lee, W. S., Richard, J., Lichtfuss, M., Smith, A. B., 3rd Park, J., Courter, J. R., et al. (2015). Antibody-dependent cellular cytotoxicity against reactivated HIV-1-infected cells. J. Virol. 90, 2021–2030. doi: 10.1128/JVI.02717-15
Lehmann, C., Lafferty, M., Garzino-Demo, A., Jung, N., Hartmann, P., Fatkenheuer, G., et al. (2010). Plasmacytoid dendritic cells accumulate and secrete interferon alpha in lymph nodes of HIV-1 patients. PLoS ONE 5:e11110. doi: 10.1371/journal.pone.0011110
Levy, Y., Thiebaut, R., Montes, M., Lacabaratz, C., Sloan, L., King, B., et al. (2014). Dendritic cell-based therapeutic vaccine elicits polyfunctional HIV-specific T-cell immunity associated with control of viral load. Eur. J. Immunol. 44, 2802–2810. doi: 10.1002/eji.201344433
Lichtner, M., Rossi, R., Rizza, M. C., Mengoni, F., Sauzullo, I., Massetti, A. P., et al. (2008). Plasmacytoid dendritic cells count in antiretroviral-treated patients is predictive of HIV load control independent of CD4+ T-cell count. Curr. HIV Res. 6, 19–27. doi: 10.2174/157016208783571937
Lo, C. C., Schwartz, J. A., Johnson, D. J., Yu, M., Aidarus, N., Mujib, S., et al. (2012). HIV delays IFN-α production from human plasmacytoid dendritic cells and is associated with SYK phosphorylation. PLoS ONE 7:e37052. doi: 10.1371/journal.pone.0037052
Lore, K., Smed-Sorensen, A., Vasudevan, J., Mascola, J. R., and Koup, R. A. (2005). Myeloid and plasmacytoid dendritic cells transfer HIV-1 preferentially to antigen-specific CD4+ T cells. J. Exp. Med. 201, 2023–2033. doi: 10.1084/jem.20042413
Lu, W., Arraes, L. C., Ferreira, W. T., and Andrieu, J. M. (2004). Therapeutic dendritic-cell vaccine for chronic HIV-1 infection. Nat. Med. 10, 1359–1365. doi: 10.1038/nm1147
Macatangay, B. J., Riddler, S. A., Wheeler, N. D., Spindler, J., Lawani, M., Hong, F., et al. (2016). Therapeutic vaccination with dendritic cells loaded with autologous HIV type 1-infected apoptotic cells. J. Infect. Dis. 213, 1400–1409. doi: 10.1093/infdis/jiv582
Machmach, K., Leal, M., Gras, C., Viciana, P., Genebat, M., Franco, E., et al. (2012). Plasmacytoid dendritic cells reduce HIV production in elite controllers. J. Virol. 86, 4245–4252. doi: 10.1128/JVI.07114-11
Manches, O., Munn, D., Fallahi, A., Lifson, J., Chaperot, L., Plumas, J., et al. (2008). HIV-activated human plasmacytoid DCs induce tregs through an indoleamine 2,3-dioxygenase-dependent mechanism. J. Clin. Invest. 118, 3431–3439. doi: 10.1172/JCI34823
Margolis, D. M., Archin, N. M., Cohen, M. S., Eron, J. J., Ferrari, G., Garcia, J. V., et al. (2020). Curing HIV: seeking to target and clear persistent infection. Cell 181, 189–206. doi: 10.1016/j.cell.2020.03.005
Marquez-Coello, M., Montes De Oca Arjona, M., Martin-Aspas, A., Guerrero Sanchez, F., Fernandez-Gutierrez Del Alamo, C., and Giron-Gonzalez, J. A. (2019). Antiretroviral therapy partially improves the abnormalities of dendritic cells and lymphoid and myeloid regulatory populations in recently infected HIV patients. Sci. Rep. 9:11654. doi: 10.1038/s41598-019-48185-2
Martinet, J., Leroy, V., Dufeu-Duchesne, T., Larrat, S., Richard, M. J., Zoulim, F., et al. (2012). Plasmacytoid dendritic cells induce efficient stimulation of antiviral immunity in the context of chronic hepatitis B virus infection. Hepatology 56, 1706–1718. doi: 10.1002/hep.25879
Mastelic-Gavillet, B., Balint, K., Boudousquie, C., Gannon, P. O., and Kandalaft, L. E. (2019). Personalized dendritic cell vaccines-recent breakthroughs and encouraging clinical results. Front. Immunol. 10:766. doi: 10.3389/fimmu.2019.00766
Musumeci, A., Lutz, K., Winheim, E., and Krug, A. B. (2019). What makes a pDC: recent advances in understanding plasmacytoid DC development and heterogeneity. Front. Immunol. 10:1222. doi: 10.3389/fimmu.2019.01222
O'Brien, M., Manches, O., Sabado, R. L., Baranda, S. J., Wang, Y., Marie, I., et al. (2011). Spatiotemporal trafficking of HIV in human plasmacytoid dendritic cells defines a persistently IFN-α-producing and partially matured phenotype. J. Clin. Invest. 121, 1088–1101. doi: 10.1172/JCI44960
Olivier, A., Lauret, E., Gonin, P., and Galy, A. (2006). The notch ligand delta-1 is a hematopoietic development cofactor for plasmacytoid dendritic cells. Blood 107, 2694–2701. doi: 10.1182/blood-2005-03-0970
Otero, M., Nunnari, G., Leto, D., Sullivan, J., Wang, F. X., Frank, I., et al. (2003). Peripheral blood dendritic cells are not a major reservoir for HIV type 1 in infected individuals on virally suppressive HAART. AIDS Res. Hum. Retroviruses 19, 1097–1103. doi: 10.1089/088922203771881194
Patterson, S., Rae, A., Hockey, N., Gilmour, J., and Gotch, F. (2001). Plasmacytoid dendritic cells are highly susceptible to human immunodeficiency virus type 1 infection and release infectious virus. J. Virol. 75, 6710–6713. doi: 10.1128/JVI.75.14.6710-6713.2001
Perussia, B., and Trinchieri, G. (1984). Antibody 3G8, specific for the human neutrophil Fc receptor, reacts with natural killer cells. J. Immunol. 132, 1410–1415.
Perussia, B., Trinchieri, G., Jackson, A., Warner, N. L., Faust, J., Rumpold, H., et al. (1984). The Fc receptor for IgG on human natural killer cells: phenotypic, functional, and comparative studies with monoclonal antibodies. J. Immunol. 133, 180–189.
Pham, T. N. Q., Meziane, O., Miah, M. A., Volodina, O., Colas, C., Beland, K., et al. (2019). Flt3L-mediated expansion of plasmacytoid dendritic cells suppresses HIV infection in humanized mice. Cell Rep. 29, 2770–2782.e5. doi: 10.1016/j.celrep.2019.10.094
Puig, M., Tosh, K. W., Schramm, L. M., Grajkowska, L. T., Kirschman, K. D., Tami, C., et al. (2012). TLR9 and TLR7 agonists mediate distinct type I IFN responses in humans and nonhuman primates in vitro and in vivo. J. Leukoc. Biol. 91, 147–158. doi: 10.1189/jlb.0711371
Reizis, B. (2019). Plasmacytoid dendritic cells: development, regulation, and function. Immunity 50, 37–50. doi: 10.1016/j.immuni.2018.12.027
Remer, K. A., Apetrei, C., Schwarz, T., Linden, C., and Moll, H. (2007). Vaccination with plasmacytoid dendritic cells induces protection against infection with Leishmania major in mice. Eur. J. Immunol. 37, 2463–2473. doi: 10.1002/eji.200636780
Sandler, N. G., Bosinger, S. E., Estes, J. D., Zhu, R. T., Tharp, G. K., Boritz, E., et al. (2014). Type I interferon responses in rhesus macaques prevent SIV infection and slow disease progression. Nature 511, 601–605. doi: 10.1038/nature13554
Schmidt, B., Ashlock, B. M., Foster, H., Fujimura, S. H., and Levy, J. A. (2005). HIV-infected cells are major inducers of plasmacytoid dendritic cell interferon production, maturation, and migration. Virology 343, 256–266. doi: 10.1016/j.virol.2005.09.059
Schwartz, J. A., Clayton, K. L., Mujib, S., Zhang, H., Rahman, A. K., Liu, J., et al. (2017). Tim-3 is a marker of plasmacytoid dendritic cell dysfunction during HIV infection and is associated with the recruitment of IRF7 and p85 into lysosomes and with the submembrane displacement of TLR9. J. Immunol. 198, 3181–3194. doi: 10.4049/jimmunol.1601298
Siegal, F. P., Kadowaki, N., Shodell, M., Fitzgerald-Bocarsly, P. A., Shah, K., Ho, S., et al. (1999). The nature of the principal type 1 interferon-producing cells in human blood. Science 284, 1835–1837. doi: 10.1126/science.284.5421.1835
Smed-Sorensen, A., Lore, K., Vasudevan, J., Louder, M. K., Andersson, J., Mascola, J. R., et al. (2005). Differential susceptibility to human immunodeficiency virus type 1 infection of myeloid and plasmacytoid dendritic cells. J. Virol. 79, 8861–8869. doi: 10.1128/JVI.79.14.8861-8869.2005
Stary, G., Klein, I., Kohlhofer, S., Koszik, F., Scherzer, T., Mullauer, L., et al. (2009). Plasmacytoid dendritic cells express TRAIL and induce CD4+ T-cell apoptosis in HIV-1 viremic patients. Blood 114, 3854–3863. doi: 10.1182/blood-2009-04-217927
Swiecki, M., and Colonna, M. (2015). The multifaceted biology of plasmacytoid dendritic cells. Nat. Rev. Immunol. 15, 471–85. doi: 10.1038/nri3865
Swiecki, M., Wang, Y., Vermi, W., Gilfillan, S., Schreiber, R. D., and Colonna, M. (2011). Type I interferon negatively controls plasmacytoid dendritic cell numbers in vivo. J. Exp. Med. 208, 2367–2374. doi: 10.1084/jem.20110654
Tavano, B., Galao, R. P., Graham, D. R., Neil, S. J., Aquino, V. N., Fuchs, D., et al. (2013). Ig-like transcript 7, but not bone marrow stromal cell antigen 2 (also known as HM1.24, tetherin, or CD317), modulates plasmacytoid dendritic cell function in primary human blood leukocytes. J. Immunol. 190, 2622–2630. doi: 10.4049/jimmunol.1202391
Tel, J., Aarntzen, E. H., Baba, T., Schreibelt, G., Schulte, B. M., Benitez-Ribas, D., et al. (2013a). Natural human plasmacytoid dendritic cells induce antigen-specific T-cell responses in melanoma patients. Cancer Res. 3, 1063–1075. doi: 10.1158/0008-5472.CAN-12-2583
Tel, J., Lambeck, A. J., Cruz, L. J., Tacken, P. J., De Vries, I. J., and Figdor, C. G. (2010). Human plasmacytoid dendritic cells phagocytose, process, and present exogenous particulate antigen. J. Immunol. 184, 4276–4283. doi: 10.4049/jimmunol.0903286
Tel, J., Schreibelt, G., Sittig, S. P., Mathan, T. S., Buschow, S. I., Cruz, L. J., et al. (2013b). Human plasmacytoid dendritic cells efficiently cross-present exogenous Ags to CD8+ T cells despite lower Ag uptake than myeloid dendritic cell subsets. Blood 121, 459–467. doi: 10.1182/blood-2012-06-435644
Thordardottir, S., Hangalapura, B. N., Hutten, T., Cossu, M., Spanholtz, J., Schaap, N., et al. (2014). The aryl hydrocarbon receptor antagonist StemRegenin 1 promotes human plasmacytoid and myeloid dendritic cell development from CD34+ hematopoietic progenitor cells. Stem Cells Dev. 23, 955–967. doi: 10.1089/scd.2013.0521
Thordardottir, S., Schaap, N., Louer, E., Kester, M. G., Falkenburg, J. H., Jansen, J., et al. (2017). Hematopoietic stem cell-derived myeloid and plasmacytoid DC-based vaccines are highly potent inducers of tumor-reactive T cell and NK cell responses ex vivo. Oncoimmunology 6:e1285991. doi: 10.1080/2162402X.2017.1285991
Tilton, J. C., Manion, M. M., Luskin, M. R., Johnson, A. J., Patamawenu, A. A., Hallahan, C. W., et al. (2008). Human immunodeficiency virus viremia induces plasmacytoid dendritic cell activation in vivo and diminished alpha interferon production in vitro. J. Virol. 82, 3997–4006. doi: 10.1128/JVI.01545-07
Tomescu, C., Chehimi, J., Maino, V. C., and Montaner, L. J. (2007). NK cell lysis of HIV-1-infected autologous CD4 primary T cells: requirement for IFN-mediated NK activation by plasmacytoid dendritic cells. J. Immunol. 179, 2097–2104. doi: 10.4049/jimmunol.179.4.2097
Tomescu, C., Tebas, P., and Montaner, L. J. (2017). IFN-alpha augments natural killer-mediated antibody-dependent cellular cytotoxicity of HIV-1-infected autologous CD4+ T cells regardless of major histocompatibility complex class 1 downregulation. AIDS 31, 613–622. doi: 10.1097/QAD.0000000000001380
Tsai, A., Irrinki, A., Kaur, J., Cihlar, T., Kukolj, G., Sloan, D. D., et al. (2017). Toll-like receptor 7 agonist GS-9620 induces HIV expression and HIV-specific immunity in cells from HIV-infected individuals on suppressive antiretroviral therapy. J. Virol. 91:e02166–16. doi: 10.1128/JVI.02166-16
Vanhamel, J., Bruggemans, A., and Debyser, Z. (2019). Establishment of latent HIV-1 reservoirs: what do we really know? J. Virus Erad. 5, 3–9.
Vibholm, L., Schleimann, M. H., Hojen, J. F., Benfield, T., Offersen, R., Rasmussen, K., et al. (2017). Short-course toll-like receptor 9 agonist treatment impacts innate immunity and plasma viremia in individuals with human immunodeficiency virus infection. Clin. Infect. Dis. 64, 1686–1695. doi: 10.1093/cid/cix201
Wang, F. X., Huang, J., Zhang, H., Ma, X., and Zhang, H. (2008). APOBEC3G upregulation by alpha interferon restricts human immunodeficiency virus type 1 infection in human peripheral plasmacytoid dendritic cells. J. Gen. Virol. 89, 722–730. doi: 10.1099/vir.0.83530-0
Westdorp, H., Creemers, J. H. A., Van Oort, I. M., Schreibelt, G., Gorris, M. A. J., Mehra, N., et al. (2019). Blood-derived dendritic cell vaccinations induce immune responses that correlate with clinical outcome in patients with chemo-naive castration-resistant prostate cancer. J. Immunother. Cancer 7:302. doi: 10.1186/s40425-019-0787-6
Wong, J. K., Hezareh, M., Gunthard, H. F., Havlir, D. V., Ignacio, C. C., Spina, C. A., et al. (1997). Recovery of replication-competent HIV despite prolonged suppression of plasma viremia. Science 278, 1291–1295. doi: 10.1126/science.278.5341.1291
Yin, Z., Dai, J., Deng, J., Sheikh, F., Natalia, M., Shih, T., et al. (2012). Type III IFNs are produced by and stimulate human plasmacytoid dendritic cells. J. Immunol. 189, 2735–2745. doi: 10.4049/jimmunol.1102038
Yonezawa, A., Morita, R., Takaori-Kondo, A., Kadowaki, N., Kitawaki, T., Hori, T., et al. (2003). Natural alpha interferon-producing cells respond to human immunodeficiency virus type 1 with alpha interferon production and maturation into dendritic cells. J. Virol. 77, 3777–3784. doi: 10.1128/JVI.77.6.3777-3784.2003
Young, L. J., Wilson, N. S., Schnorrer, P., Proietto, A., Ten Broeke, T., Matsuki, Y., et al. (2008). Differential MHC class II synthesis and ubiquitination confers distinct antigen-presenting properties on conventional and plasmacytoid dendritic cells. Nat. Immunol. 9, 1244–1252. doi: 10.1038/ni.1665
Keywords: HIV, HIV latency, dendritic cells, DC vaccine, plasmacytoid DC, pDC, CD8+ T cells, NK cells
Citation: van der Sluis RM, Egedal JH and Jakobsen MR (2020) Plasmacytoid Dendritic Cells as Cell-Based Therapeutics: A Novel Immunotherapy to Treat Human Immunodeficiency Virus Infection? Front. Cell. Infect. Microbiol. 10:249. doi: 10.3389/fcimb.2020.00249
Received: 09 February 2020; Accepted: 29 April 2020;
Published: 26 May 2020.
Edited by:
Heather Shannon Smallwood, The University of Tennessee Health Sciences Center, United StatesReviewed by:
Victor Hugo Aquino, University of São Paulo, BrazilDamien Ferhadian, National Institutes of Health (NIH), United States
Christopher Lupfer, Missouri State University, United States
Copyright © 2020 van der Sluis, Egedal and Jakobsen. This is an open-access article distributed under the terms of the Creative Commons Attribution License (CC BY). The use, distribution or reproduction in other forums is permitted, provided the original author(s) and the copyright owner(s) are credited and that the original publication in this journal is cited, in accordance with accepted academic practice. No use, distribution or reproduction is permitted which does not comply with these terms.
*Correspondence: Martin R. Jakobsen, bXJqQGJpb21lZC5hdS5kaw==