- 1Hospital of Stomatology, Guanghua School of Stomatology, Sun Yat-sen University, Guangzhou, China
- 2Guangdong Provincial Key Laboratory of Stomatology, Guangzhou, China
- 3The Forsyth Institute, Cambridge, MA, United States
- 4Department of Oral Medicine, Infection and Immunity, Harvard School of Dental Medicine, Boston, MA, United States
Streptococcus mutans is an oral species closely associated with dental caries. As an early oral colonizer, S. mutans utilizes interspecies coaggregation to promote the colonization of subsequent species and affect polymicrobial pathogenesis. Previous studies have confirmed several adhering partner species of S. mutans, including Candida albicans and Fusobacterium nucleatum. In this study, we discovered new intergeneric co-adherence between S. mutans and the saliva isolate Streptococcus agalactiae (GBS-SI101). Research shows that GBS typically colonizes the human gastrointestinal and vaginal tracts. It is responsible for adverse pregnancy outcomes and life-threatening infections in neonates and immunocompromised people. Our results revealed that GtfB and GtfC of S. mutans, which contributed to extracellular polysaccharide synthesis, promoted coaggregation of S. mutans with GBS-SI101. In addition, oral streptococci, including Streptococcus sanguinis, Streptococcus gordonii and S. mutans, barely inhibited the growth of GBS-SI101. This study indicated that S. mutans could help GBS integrate into the Streptococcus-associated oral polymicrobial community and become a resident species in the oral cavity, increasing the risk of oral infections.
Introduction
The oral microbiome consists of ~700 species of bacteria (Paster et al., 2010). The disparity of oral bacteria among different individuals is relatively low (The Human Microbiome Project Consortium, 2012). In contrast, the complexity of the oral microbiota in a single individual is the highest compared to that of other body sites (Aas et al., 2005). Oral microbes engage in extensive cell-cell interactions. Early colonizers adhere to accessible host surfaces and facilitate the colonization of subsequent species via intergeneric coaggregation, forming highly structured polymicrobial oral biofilms (Kolenbrander et al., 2010). In addition, intergeneric cell-cell communication can trigger signaling cascades and induce changes in the gene expression of the attached species, influencing the expression of virulence factors of microorganisms in the oral cavity (Krzysciak et al., 2014).
Oral streptococci, including Streptococcus mutans (S. mutans), are the earliest oral colonizers, which can be acquired immediately after birth (Abranches et al., 2018). S. mutans is a prevalent etiological bacterial species associated with early childhood caries (ECC) (Van Houte et al., 1982; Tanzer et al., 2001; Agnello et al., 2017). Accumulative reports show that S. mutans has been detected in predentate infants, and the colonization rate of S. mutans increases rapidly as primary teeth erupt (Wan et al., 2001, 2003; Plonka et al., 2012). Infants usually acquire S. mutans from their mothers, and maternal salivary bacterial abundance is closely associated with early oral infections among children (Chaffee et al., 2014). S. mutans possesses multiple virulence factors that contribute to tooth decay. Potent in synthesizing extracellular polysaccharides (EPS), S. mutans adheres to enamel surfaces and forms intercellular clustering within dental plaques. S. mutans can metabolize digestible carbohydrates to create an acidic microenvironment (acidogenicity), which leads to enamel demineralization, meanwhile, S. mutans can thrive under low pH conditions (aciduricity) (Lemos and Burne, 2008; Forssten et al., 2010).
The ability of primary oral inhabitants to bind to various subsequent species has been well established (Hojo et al., 2009), but there are limited reports on the interspecies co-adherence between S. mutans and other species. Previous studies show that S. mutans adheres to Candida albicans (C. albicans), and the presence of C. albicans enhances the production of the EPS-rich matrix in dual-species biofilms (Barbieri et al., 2007; Metwalli et al., 2013; Falsetta et al., 2014). Guo et al. (2017) discovered that S. mutans, utilizing its adhesin SpaP, specifically binds to RadD of Fusobacterium nucleatum ssp. polymorphum (Fnp). The interplay between S. mutans and Fnp improves both species' abilities to effectively colonize the oral cavity (Guo et al., 2017). In this study, using a pull-down assay, we identified a Streptococcus agalactiae (S. agalactiae, also known as Group B Streptococcus, GBS) strain from human saliva samples, which displayed direct physical interactions with S. mutans.
GBS is a facultative anaerobe and a leading cause of infections in pregnant women and neonates (Gibbs et al., 2004; Kim, 2010). Half of GBS infections in pregnant women affect the upper genital tract, placenta, or amniotic sac, increasing the risk of fetal death (Phares et al., 2008). A mother who carries GBS can transmit it to her infant during delivery. GBS infections in neonates often cause meningitis and can lead to long-term neurodevelopmental impairment after recovery (Phares et al., 2008; Kohli-Lynch et al., 2017). GBS infections are also common among immunocompromised individuals and the elderly, causing bacteremia, soft tissue infections, or pneumonia (Farley and Strasbaugh, 2001; High et al., 2005). The gastrointestinal and vaginal tracts are the main reservoirs for GBS (Meyn et al., 2009). Some studies have reported the presence of GBS in the oropharynx (Hickman et al., 1999; Van der Mee-Marquet et al., 2008).
While adherence via pili to salivary pellicles could promote GBS colonization within the oral cavity (Brittan and Nobbs, 2015), an alternative mechanism allowing GBS to persist within oral cavity could be due to its ability to integrate into existing biofilms through intergeneric coaggregation, as has been well documented in many other bacteria (Jakubovics et al., 2014). However, there is scarce evidence to demonstrate the direct physical interactions between GBS and oral bacteria. Thus, little is known regarding the role of oral species in GBS's survival and persistence within the oral microbial community.
This study aimed to investigate the interactions between S. mutans and GBS and evaluate the potential for S. mutans to facilitate GBS integration into the Streptococcus-associated oral multispecies community.
Materials and Methods
Bacterial Strains and Growth Conditions
S. mutans UA140, Streptococcus sanguinis (S. sanguinis) ATCC 10,556 and Streptococcus gordonii (S. gordonii) DL1 were grown in Todd-Hewitt broth (THB) (BD Difco, Detroit, MI, USA) under anaerobic conditions (10% H2, 10% CO2, 80% N2) at 37°C. Professor Wenyuan Shi's laboratory has preserved gtfB-, gtfC-, gtfD-, gtfBC-, and gtfBCD-deficient strains of S. mutans (kindly provided by H. Kuramitsu, University at Buffalo, State University of New York, NY, USA).
SHI medium, which can support the growth of a highly diverse oral microbial community in vitro (Tian et al., 2010), was adopted to cultivate the saliva microbiota. SHI medium has the following composition (Tian et al., 2010): proteose peptone (Difco) 10 g/L; trypticase peptone (Difco) 5.0 g/L; yeast extract (Difco) 5.0 g/L; KCl 2.5 g/L; sucrose 5 g/L; haemin 5 mg/L; VitK 1 mg/L; urea 0.06 g/L, arginine 0.174 g/L; mucin (type III, porcine, gastric, Sigma Chemical Co., St. Louis, Mo) 2.5 g/L; sheep blood (Colorado serum company) 5% and N-acetylmuramic acid (NAM) 10 mg/L.
Saliva Collection
Saliva samples were collected from 10 healthy participants aged 25~40. None of the participants was being treated for any systemic disease or dental disease or taking any medication. Participants were asked to refrain from any food or drink 2 h before saliva donation. At 10 a.m., participants were asked to spit directly into the saliva collection tubes, and 5 ml of saliva was collected from each participant.
S. mutans Biofilm Formation
One milliliter of saliva from each participant was pooled together and centrifuged at 14,000 × g for 10 min at 4°C to remove saliva microorganisms. The supernatant, referred to as pooled saliva proteins, was collected to coat sterile 6-well flat-bottomed polystyrene microtiter plates (Corning, New York, NY). The 6-well culture plates were dried and sterilized under UV light for 1 h before bacterial inoculation. Overnight culture (OD600~ 0.7) of S. mutans was diluted 1:100 into THB containing 0.5% (w/v) sucrose, with a final concentration of approximately 2 × 105 cells/ml. A total of 400 μl of this suspension was inoculated into each well and incubated overnight under anaerobic conditions for biofilm formation. The wells were washed three times with phosphate-buffered saline (PBS) to remove planktonic and loosely bound S. mutans cells.
Cultivating Human Saliva-Derived Microbiota (S-Mix)
Pooled saliva was centrifuged at 2,600 × g for 10 min at 20°C to spin down large debris and eukaryotic cells. The supernatant was inoculated into 5 ml of SHI medium and incubated anaerobically overnight to obtain saliva-derived microbiota (S-mix). S-mix was used in the following experiments in this study.
Pull-Down Assay
To identify microbial species from saliva samples that could directly adhere to S. mutans, we performed a pull-down assay. The S-mix was resuspended in sterile coaggregation buffer (CAB) (OD600~1). CAB consists of 150 mM NaCl, 1 mM Tris, 0.1 mM CaCl2, and 0.1 mM MgCl2 (Cisar et al., 1979). A total of 400 μl of S-mix was overlaid onto the 6-well culture plate with pre-existing S. mutans biofilms and incubated under anaerobic conditions for 1, 2, and 3 h, respectively. The wells were then rinsed three times with PBS to remove cells that failed to adhere to S. mutans biofilms. Biofilm S. mutans cells with the remaining binding species (co-adhering mixtures) were carefully scraped off. The co-adhering mixtures, obtained after each incubation time, were divided into two portions. One portion was immediately subjected to extraction of total bacterial genomic DNA. The other portion was regrown in fresh SHI medium anaerobically overnight, followed by DNA extraction. Four hundred microliters of sterile CAB were added to 6-well culture plates with pre-coated pooled saliva proteins or pre-existing S. mutans biofilms to serve as control.
PCR-DGGE Analysis
The co-adhering mixtures were added directly into a 0.5-mL screw cap microtube containing lysis buffer (MasterPureTM DNA Purification Kit, Epicenter) and 0.1-mm silica beads. The samples were treated with bead beating for 30 s three times at 1-min intervals at 4°C. After centrifugation of the samples at 13,000 × g for 5 min, the supernatant was transferred to a fresh tube and incubated with Proteinase K for 1 h at 56°C. The total genomic DNA of samples from each group was isolated using the MasterPureTM DNA Purification Kit according to the manufacturer's instructions. DNA quality and quantity were determined using a Spectronic Genesys UV spectrophotometer at 260 nm and 280 nm (Spectronic Instrument, Inc. Rochester, New York, USA). Amplification of bacterial 16S rRNA genes by PCR was carried out as described previously (Li et al., 2005; He et al., 2011). Bac1 (5′- CGCCCGCCGCGCCCCGCGCCCGTCCCGCCGCCCCCGCCCGAC
TACGTGCCAGCA GCC-3) and Bac2 (5′-GGACTACCAGGGTATCTAATCC-3′) (Sheffield et al., 1989) were used as the universal primer set to amplify an approximately 300-bp internal fragment of the 16S rRNA gene. Each 50 μl PCR contained 100 ng of genomic DNA, 200 μm of each dNTP, 40 pmol of each primer, 4.0 mM MgCl2, 5 μl 10× PCR buffer, and 2.5 U Taq DNA polymerase (Invitrogen). Cycling conditions were set as follows: 94°C/3 min, 30 cycles of 94°C/1 min, 56°C/1 min and 72°C/1 min, and 72°C/5 min (final extension period).
Polyacrylamide gels (8%) with a denaturing gradient between 40 and 70% (urea/formamide) were prepared as previously described (Tian et al., 2010). Each well was loaded with 300 ng of the PCR product. The gels were submerged in 1 × TAE (Tris-acetate-EDTA) buffer, and PCR products were separated by electrophoresis for 16 h at 60°C using a fixed voltage of 60 V (Bio-Rad DCode System, Bio-Rad, Hercules, CA, USA). After electrophoresis, the gels were rinsed and stained for 15 min in 1 × TAE buffer containing ethidium bromide (0.5 μg/ml), followed by destaining in 1 × TAE buffer for 10 min. DGGE profile images were recorded using the Molecular Imager Gel Documentation system (Bio-Rad, Hercules, CA, USA). Diversity Database Software (Bio-Rad, Hercules, CA, USA) was used to assess the intensity of bands of interest.
Identification of Bacterial Species From DGGE Gel
The DNA bands, which only existed in co-adhering mixtures or S-mix but not in the control, were excised from the DGGE gels, transferred to 1.5 ml microfuge tubes containing 20 μl of sterile H2O, and kept overnight at 4°C to allow DNA recovery.
Procedures for species identification were performed as described previously with modifications (He et al., 2011). DNA samples were reamplified with Bac1 and Bac2, purified using the QIAquick PCR purification kit (Qiagen), and sequenced. The obtained sequences were matched to nucleotide BLAST searches against the NCBI (http://blast.ncbi.nlm.nih.gov/) and Human Oral Microbiome Database (http://www.homd.org/index.php). The saliva isolate S. agalactiae, designated GBS-SI101, was identified by sequencing.
Isolation and Identification of Bacterial Species Pulled Down by Biofilm S. mutans Cells
A 100-μl aliquot of co-adhering mixtures was taken, subjected to serial dilution, and seeded onto SHI agar plates supplemented with sheep blood, which helped detect β-hemolytic strains. The plates were incubated for 48 h under anaerobic conditions. Based on the sequencing result from DGGE gels, colonies that were β-hemolytic and grayish-white on a sheep blood-containing plate (Cools and Melin, 2017) were picked and grown in fresh SHI medium under anaerobic conditions until turbid. The bacterial genomic DNA was prepared using the MasterPureTM DNA Purification Kit (Epicenter).
For species identification, the universal bacterial 16S rDNA primer pair 27F (5′- AGAGTTTGATCCTGGCTCAG-3′) and 1492R (5′-GGTTACCTTGTTACGACTT-3′) (Martinlaurent et al., 2001) was used to generate an approximately 1,500-bp amplicon. Procedures described previously were used to proceed with PCRs and sequence purified PCR products (He et al., 2011). Sequences with over 98% identity to those deposited in the databases were considered to be positive for taxa identification.
Establishment of Dual-Species Biofilms
Overnight culture (OD600~ 0.7) of S. mutans was diluted 1:200 into THB containing 0.5% (w/v) sucrose. Four hundred microliters of cell suspension were inoculated onto 6-well culture plates with pre-coated pooled saliva proteins, and the plates were incubated anaerobically overnight to form S. mutans biofilms. Overnight culture (OD600~ 0.8) of GBS-SI101 was diluted 1:100 into THB, and 400 μl of GBS-SI101 was overlaid onto 6-well culture plates with pre-existing S. mutans biofilms or pre-coated pooled saliva proteins, followed by 16 h incubation under anaerobic conditions.
Confocal Laser Scanning Microscopy (CLSM) and Image Analysis
Overnight dual-species biofilms were rinsed three times with PBS to remove the unattached bacteria. The average cell sizes for S. mutans bacterium and GBS bacterium are 0.5–0.75 μm and 0.6–1.0 μm, respectively (Nagao, 2015; Zhou and Li, 2015). For visualization, biofilms were stained with 1.67 μM SYTO 9 green-fluorescent nucleic acid stain (Life Technologies) according to the manufacturer's protocol. S. mutans was further labeled with a specific anti-S. mutans monoclonal antibody SWLA1–IgG2a, as described previously (Fang et al., 2005). The far-red dye Alexa Fluor 633-conjugated goat anti-mouse IgG (Sigma, St. Louis, MO) was used as a secondary antibody.
The biofilms were monitored through a 40× oil-immersion lens with a PASCAL LSM5 CLSM (Zeiss, Germany). Image stacks of five randomly chosen spots were collected for each experimental sample, and representative images are shown in the results section. The excitation/emission maxima for SYTO 9 staining were set at 488 nm/500 nm. A 560 nm and 650 nm long-pass emission filter was utilized to reveal Alexa Fluor 633-labeled cells within the biofilms. CLSM images were analyzed using the computer program COMSTAT.
Fluorescence-Based Coaggregation Assay
To achieve a visual and sensitive readout, a fluorescence-based coaggregation assay was used with minor modifications (Wu et al., 2015). GBS-SI101 cells were grown in THB anaerobically, collected from exponential-phase culture, washed, and adjusted to an OD600 of 10 in CAB. Two hundred microliters of cell suspension were anaerobically stained with 1 μl of 10 μM SYTO 9 (Life Technologies) for 30 min at room temperature. Cells were washed 10 times with PBS and resuspended in CAB to a final OD600 of 1. In parallel, gtfB-, gtfC-, gtfD-, gtfBC-, and gtfBCD-deficient strains of S. mutans and the parent strain were collected from exponential-phase culture, washed, and resuspended in CAB to a final OD600 of 1.
For the coaggregation assay, S. mutans and GBS-SI101 were mixed with equal volumes of 250 μl in reaction tubes, vigorously vortexed for 30 s, and allowed to stand at room temperature for 10 min under anaerobic conditions. The coaggregation pellet was collected at 100 g for 1 min, and the supernatant with unbound bacterial cells was discarded carefully using a micropipette (Research Plus, Eppendorf, Germany). The coaggregation pellet was added to 5 ml of PBS, followed by 5 min of vortexing. Next, 250 μl of the solution in each group was evaluated for its fluorescence intensity (GOLMAX-MULTI PLUS, Promega, USA).
For data presentation, the coaggregation results of S. mutans derivatives/GBS-SI101 groups and GBS-SI101 only group are shown as the percentage of the fluorescence signal compared with the fluorescence intensity of the wild-type S. mutans/GBS-SI101 control group. Three replicates were performed for this assay.
Growth Competition Assay
Growth competition assay was performed as described previously with modifications (Tong et al., 2007). Overnight cultures of Streptococcus species, including S. mutans, S. sanguinis, and S. gordonii, and GBS-SI101 were adjusted to the same optical density at 600 nm (OD600 ~ 1). Ten-microliter aliquots of GBS-SI101 and 10-μl aliquots of each Streptococcus species were, sequentially or simultaneously, inoculated adjacently on SHI agar plates such that the bacterial spots almost touched each other. In the sequential inoculation groups, species that were inoculated first were grown anaerobically overnight, followed by the inoculation of the other species. The plates were incubated anaerobically for 24 h. Growth inhibition was assessed by the presence of a proximal zone of inhibition.
Viability Assay in Spent Culture Media (Spent Medium Assay)
GBS-SI101 and Streptococcus species, including S. mutans, S. sanguinis, and S. gordonii, were cultured independently in THB. After overnight cultivation under anaerobic conditions, the supernatants were collected as spent media and filter-sterilized.
First, we examined whether diffusible metabolites of oral streptococci affected the viability of GBS-SI101. GBS-SI101 was grown in different media, including PBS (control), GBS-SI101 spent medium (control), S. mutans spent medium, S. sanguinis spent medium, and S. gordonii spent medium. Each group was supplemented with an equal volume of fresh THB. Viability counts (CFU/ml) were monitored for GBS-SI101 in different media after 24 h incubation under anaerobic conditions. Three replicates were performed for this assay.
Second, we examined whether diffusible metabolites of GBS-SI101 affected the growth of Streptococcus species. Each of the Streptococcus species was inoculated into its own spent medium and GBS-SI101 spent medium. Each group was supplemented with an equal volume of fresh THB. Viability counts (CFU/ml) were monitored for each of the Streptococcus species in each spent medium after 24 h incubation under anaerobic conditions. Three replicates were performed for this assay.
Statistical Analysis
Statistical significance (p <0.05) of differences was analyzed by One-Way analysis of variance (ANOVA) with post-hoc Dunnett's test.
Results
GBS From Saliva Microbiota Adhered to S. mutans Biofilm
The pull-down assay has been used successfully to identify bacterial species that exhibit physical interactions with oral bacteria (Guo et al., 2015). In this study, we used this assay, together with the PCR-DGGE technique, to detect saliva isolates that could physically bind to S. mutans. Bands were relatively blurry in co-adhering mixtures, possibly due to the short incubation time and the low abundance of bacteria. Bands were of higher intensity in the co-adhering mixtures that were regrown overnight. DNA samples were recovered from the DGGE bands of interest, and GBS-SI101 was identified by sequencing. Our results showed that GBS-SI101 could be pulled down by S. mutans biofilms in vitro (Figure 1).
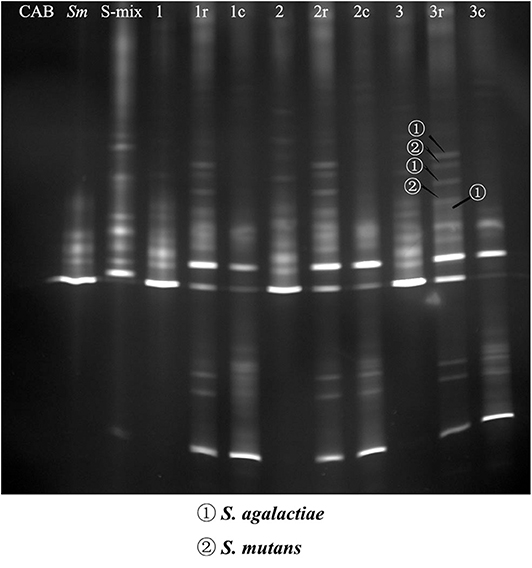
Figure 1. PCR-DGGE profiles of co-adhering mixtures. S-mix was overlaid onto S. mutans biofilms and allowed to stand for 1 h (Group 1), 2 h (Group 2), and 3 h (Group 3). Unbound cells were washed. Then, the co-adhering mixtures were collected either immediately or after overnight regrowth in fresh SHI medium under anaerobic conditions (Groups 1r, 2r, and 3r). In the control groups, CAB, instead of S-mix, was overlaid onto S. mutans biofilm, and the biofilm was collected after incubation times of 1 h (Group 1c), 2 h (Group 2c), and 3 h (Group 3c). The first three lanes on the left represent the blank control (CAB), S. mutans (Sm) and species profile of S-mix (S-mix), respectively. DNA bands of interest were excised, and DNA samples were recovered and sequenced. S. agalactiae and S. mutans were identified by sequencing and are indicated with arrows.
CLSM imaging (Figure 2A) showed that S. mutans formed monospecies biofilms. The S. mutans cells, labeled with specific antibodies, are displayed in red. The far-red dye failed to penetrate some dense microcolonies in S. mutans biofilms. The enlarged images in Figures 2A,B showed that the cell sizes of GBS-SI101 were visually larger than those of S. mutans. CLSM imaging of the dual-species biofilm (Figure 2C) confirmed GBS integration into the S. mutans biofilm.
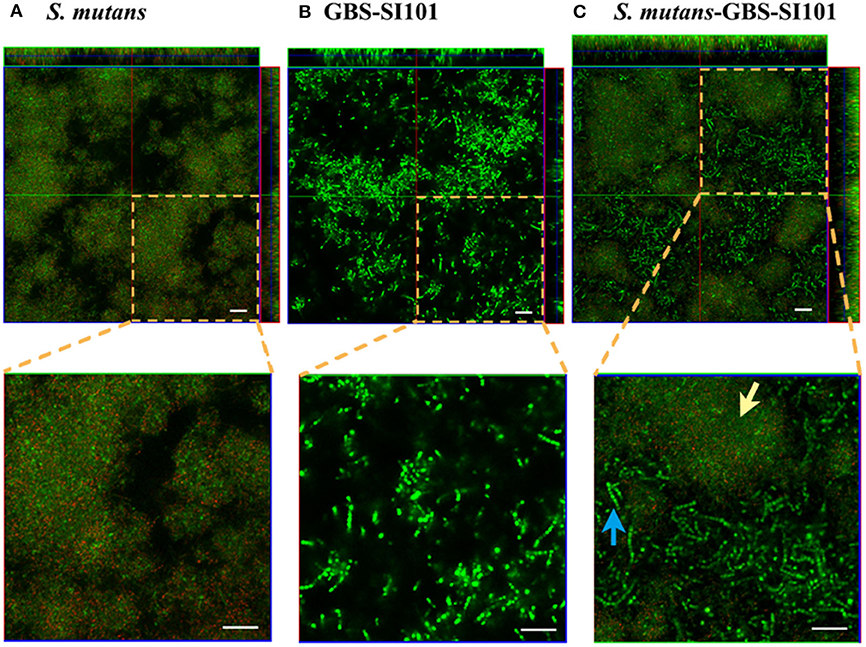
Figure 2. Monospecies and dual-species biofilms visualized by CLSM. GBS-SI101 was overlaid onto 6-well culture plates with pre-coated pooled saliva proteins or pre-existing S. mutans biofilms. The plates were incubated anaerobically overnight. Biofilms were labeled with SYTO 9 displaying green in CLSM imaging. Biofilm S. mutans cells were further specifically labeled with an anti-S. mutans monoclonal antibody SWLA1–IgG2a, which was later attached with Alexa Fluor 633-conjugated goat anti-mouse IgG, displaying red in CLSM imaging. (A) S. mutans biofilm; (B) GBS-SI101 biofilm; (C) S. mutans and GBS-SI101 dual-species biofilm. The green cluster (yellow arrow) represents S. mutans microcolonies where monoclonal antibody SWLA1–IgG2a failed to penetrate. The blue arrow points to GBS-SI101 within the dual-species biofilm. The scale bar represents 20 μm.
GtfB and GtfC of S. mutans Were Involved in Coaggregation With GBS-SI101
Glucosyltransferases (Gtfs) are mainly responsible for synthesizing EPS in S. mutans. S. mutans utilizes EPS to facilitate intercellular and interspecies adhesion (Koo et al., 2010). Three gtf genes expressing Gtfs activity in S. mutans, including gtfB, gtfC, and gtfD, have been identified (Kuramitsu, 1993). The results from the fluorescence-based coaggregation assay showed that, compared with S. mutans parent strain, gtfB-, gtfC-, gtfBC-, and gtfBCD-deficient strains displayed significantly reduced levels of interspecies binding with GBS-SI101 (Figure 3; p < 0.05). The gtfD-deficient strain of S. mutans, similar to the parent strain, demonstrated high levels of interspecies coaggregation with GBS-SI101 (Figure 3). The results indicated that lack of either gtfB- or gtfC-encoded functions impaired S. mutans' ability to physically bind to GBS-SI101.
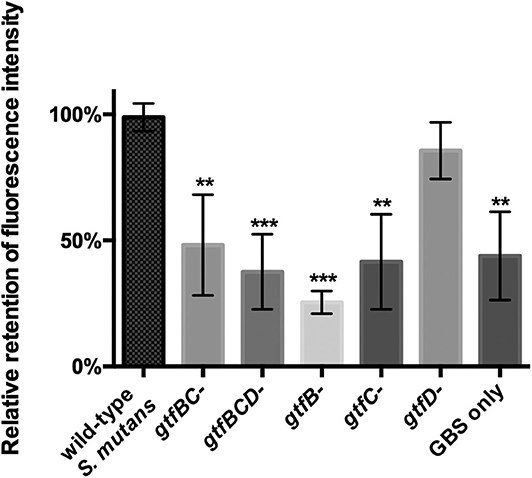
Figure 3. Coaggregation between GBS-SI101 and S. mutans gtf-deficient derivatives. gtfB-, gtfC-, gtfD-, gtfBC-, and gtfBCD-deficient strains, as well as the parent strain of S. mutans, were used to coaggregate with SYTO 9-labeled GBS-SI101. The coaggregation result was shown as the ratio of fluorescence intensity retained in co-aggregates to that in the wild-type S. mutans/GBS-SI101 pairs. Data represent the mean ± S.D. of the results of three independent experiments. The asterisk indicates a significant difference between each testing group and the parent strain S. mutans/GBS-SI101 group (p < 0.05).
The Growth of GBS-SI101 Was Not Affected by Oral Streptococci
Oral streptococci are predominant microorganisms in the oral microbiota (Jakubovics et al., 2014). Our study further assessed the potential growth competition between GBS-SI101 and common oral streptococci. GBS-SI101 was inoculated close to different streptococci species on SHI agar plates and grown anaerobically for 24 h. A clear growth-inhibition zone of S. sanguinis was observed when GBS-SI101 was inoculated first, however, no inhibition on GBS-SI101 was monitored when S. sanguinis was inoculated first. Meanwhile, no inhibition zone was observed for either strain when they were inoculated simultaneously. The results suggested that the sequence of inoculation influenced the growth antagonism of GBS-SI101 to S. sanguinis. Growth-inhibition zones were not observed in other Streptococcus spp./GBS-SI101 pairs, indicating that S. mutans and S. gordonii did not inhibit the growth of GBS-SI101 on SHI agar plates, and vice versa (Figure 4). We further investigated the effects of diffusible metabolites on bacterial growth by adopting the spent medium assay. The results showed that metabolites produced by tested Streptococcus species had nonsignificant effects on the viability of GBS-SI101 (Figure 5). Similarly, GBS-SI101 spent media did not significantly affect the viability of S. mutans, S. gordonii, and S. sanguinis compared to their own spent media control (Figure 6).
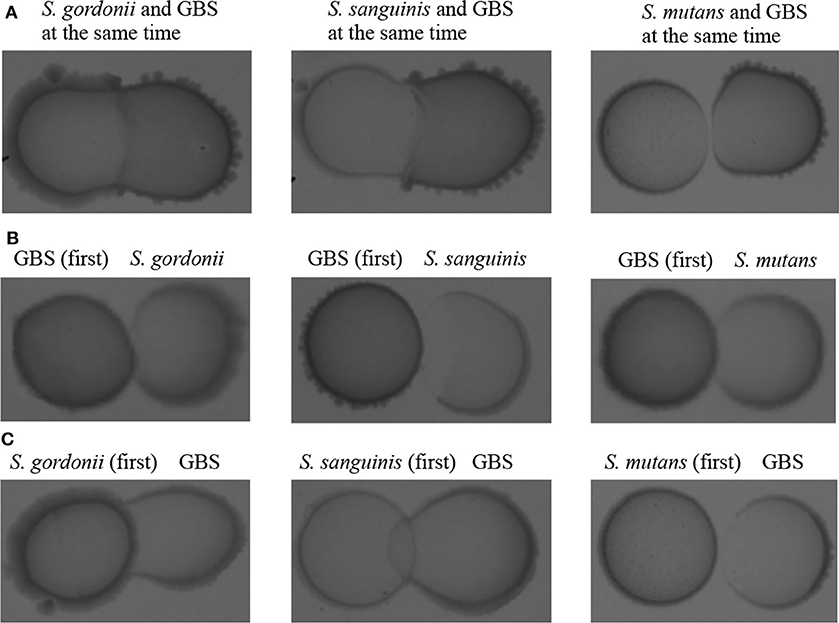
Figure 4. Growth competition between GBS-SI101 and three different Streptococcus species. (A) GBS-SI101 and oral streptococci were inoculated simultaneously under anaerobic conditions. (B) GBS-SI101 was inoculated first and allowed to grow anaerobically overnight. (C) Oral streptococci were inoculated first and allowed to grow anaerobically overnight.
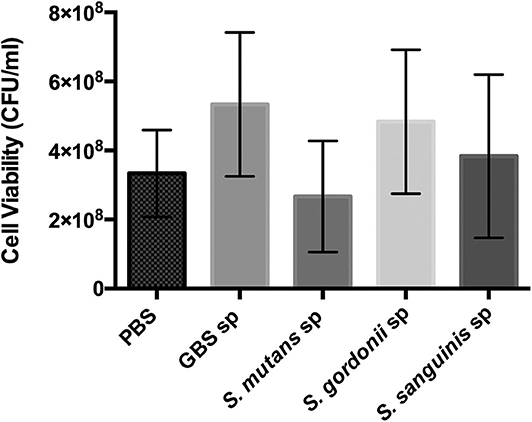
Figure 5. Viability of GBS-SI101 in Streptococci-spent media. GBS-SI101 was inoculated into PBS (control), and the spent medium (sp) of GBS-SI101 (control), S. mutans, S. gordonii, and S. sanguinis, respectively. Each group was supplemented with an equal volume of fresh THB. Viability counts (CFU/ml) were monitored for GBS-SI101 in all six groups after 24 h incubation under anaerobic conditions. Data represent the mean ± S.D. of the results of three independent experiments.
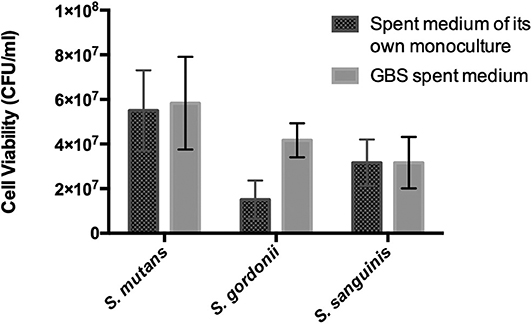
Figure 6. Viability of Streptococcus species in GBS-spent media. Each Streptococcus species was cultured in the spent medium of its own and GBS spent medium. An equal volume of fresh THB was added to each spent medium. Viability counts (CFU/ml) of the Streptococcus species in their own spent medium or the GBS spent medium were monitored after 24 h incubation under anaerobic conditions. Data represent the mean ± S.D. of the results of three independent experiments.
Discussion
In the complex oral microbial community, interspecies coaggregation creates close spatial proximity, promoting close intercellular communication and impacting polymicrobial pathogenesis (Hansen et al., 2007). In addition, intergeneric interactions may influence the establishment of highly structured multispecies oral biofilms (Rickard et al., 2003). In this study, our results showed that GBS-SI101, derived from saliva samples, adhered to S. mutans directly. Enzymes associated with EPS biosynthesis, including GtfB and GtfC, could play a significant role in the physical interplay between S. mutans and GBS-SI101.
S. mutans forms stable bonds with other oral bacteria or salivary pellicles via sucrose-dependent or sucrose-independent mechanism (Koga et al., 1986; Cvitkovitch et al., 2003). Gtfs are responsible for converting sucrose to glucans and play a critical role in sucrose-dependent adhesion (Krzysciak et al., 2014). Our results showed that GtfB and GtfC of S. mutans, which were mainly responsible for the synthesis of water-insoluble glucans (Aoki et al., 1986; Hanada and Kuramitsu, 1988), were involved in dual-species adhesion between S. mutans and GBS-SI101. Loss of either gtfB- or gtfC-encoded functions impaired the binding of S. mutans with GBS-SI101, which is consistent with a previous study associating GtfB and GtfC with microbial adherence and biofilm formation in S. mutans (Koo et al., 2010).
EPS production by Gtfs enhances the formation of a spatially distinct 3-dimensional matrix, increasing the creation of highly cohesive S. mutans biofilms (Xiao et al., 2012). In parallel, S. mutans cells within biofilms can convert fermentable carbohydrates (such as sucrose) into acids, resulting in a low pH microenvironment (Xiao et al., 2012). The continuous low pH below a critical point (5.5) will promote enamel demineralization and consequent development of cavitation (Takahashi and Nyvad, 2008). Low pH environments also limit the growth of many acid-sensitive oral commensals, promoting the development of S. mutans-dominated biofilms (Abranches et al., 2018).
When GBS adheres to S. mutans, GBS will likely be exposed to acidic microenvironments. Our results showed that neither the close dual-species interactions on SHI agar plates nor the diffusible metabolites of planktonic S. mutans interfered with the growth of GBS-SI101. GBS is a commensal bacterium of gastrointestinal and vaginal tracts and favors colonization in the acidic vaginal mucosa (pH 4.0 ± 0.5) (Verani et al., 2010; Shabayek and Spellerberg, 2017). Since GBS can survive and cause infections in the acidic genital tracts, it would not be surprising that GBS could thrive in the S. mutans-dominant acidic environment. Early studies verified enhanced adherence of GBS to alveolar epithelial cells (A549 cell line) at low pH conditions (Zawaneh et al., 1979; Tamura et al., 1994). D'Urzo et al. (2014) studied 389 GBS isolates and found that acidified culture media can increase GBS biofilm formation. To survive the acidic microenvironments, GBS has possessed several mechanisms similar to S. mutans, such as arginine deiminase system (ADI), proton pumps, and acid tolerance response (ATR) (Shabayek and Spellerberg, 2017; Lemos et al., 2019). Hence, the acid-generating S. mutans might offer a favorable niche for GBS to survive in the oral cavity.
In addition to generating acids, S. mutans can produce bacteriocins (mutacins) to impair the growth of nearby bacterial species (Qi et al., 2001). Bacteriocins are antimicrobial peptides (AMPs) produced by bacteria and are classified as non-lantibiotics or lantibiotics (Riley and Wertz, 2002). Lantibiotics are post-translationally modified cationic AMPs and contain unusual amino acids (dehydroalanine and dehydrobutyrine) (Draper et al., 2015).
Under planktonic conditions, S. mutans UA140 produces nonlantibiotic mutacin IV, which mainly targets group A streptococci and mitis group streptococci (Qi et al., 2001). The results of the spent medium assay showed that metabolites in the S. mutans spent medium nonsignificantly affected the growth of GBS-SI101. Planktonic GBS cells are faced with various challenges, but they have developed certain coping strategies. One possible approach for GBS to address toxic diffusible metabolites such as mutacins might be the production of capsular polysaccharides (CPSs). The CPSs of GBS attach to the cell wall peptidoglycan and form a capsule layer covering the cell surface (Deng et al., 2000). Studies have suggested that surface-bound CPSs may endow bacterial species with resistance to AMPs (Campos et al., 2004; Spinosa et al., 2007; Llobet et al., 2008). Campos et al. (2004) showed that CPSs protect Klebsiella pneumoniae by limiting interactions between AMPs and the cell surface. Llobet et al. (2008) also demonstrated that anionic CPSs attract cationic AMPs to reduce the number of peptides binding to the bacterial surface.
When grown on a culture plate, S. mutans UA140 produces the cationic lantibiotic mutacin I, which is active against various gram-positive bacteria (Qi et al., 2001; Nicolas et al., 2007). Khosa et al. (2016) discovered that GBS expresses a resistance protein called SaNSR. By cleaving off specific amino acids, SaNSR can reduce the antimicrobial activity of a lantibiotic produced by Lactococcus lactis (Khosa et al., 2016). In addition, the GBS cell wall comprises a thick peptidoglycan layer as well as glycerol-phosphate polymers called lipoteichoic acid (LTA). Saar-Dover et al. (2012) showed that D-alanylation of LTAs in GBS results in reduced flexibility and permeability of the cell wall, leading to enhanced resistance to cationic AMPs. GBS has the potential to counteract the antimicrobial effects of lantibiotics, but further studies are needed to elucidate the specific responses of GBS to lantibiotics produced by S. mutans.
A healthy and balanced oral microbial community is often associated with greater proportions of commensal streptococci from mitis and sanguinis groups and lower proportions of S. mutans (Abranches et al., 2018). S. gordonii and S. sanguinis are both predominant oral colonizers that modulate the balanced structure in the oral microbiota (Abranches et al., 2018). The generation of oxidative stress gives these species a competitive edge over some oral pathogens. Kreth et al. (2008) reported that both S. gordonii and S. sanguinis produced significant amounts of hydrogen peroxide (H2O2) to repress the growth of S. mutans, although the production of H2O2 was reduced under anaerobic conditions. Liu et al. (2011) showed that S. gordonii biofilms grown under aerobic conditions generated a steady level of 1.4 mM H2O2 at 100 μm above the biofilm surface, and the concentration of H2O2 decreased at a greater distance.
GBS, which binds to S. mutans, might also experience selective pressure from dominant commensal streptococci. Our results showed that S. gordonii or S. sanguinis imposed few inhibitory effects on the growth of GBS. Poyart et al. (2001) demonstrated that superoxide dismutase (SodA) of GBS is crucial in GBS resistance to high levels of H2O2 (up to 20 mM). Other studies also showed that GBS, utilizing MntH (Mn21/Fe21 transporter) and NADH oxidase, can survive exposure to high levels of oxidative stress (Shabayek et al., 2016; Korir et al., 2018). Therefore, GBS might be able to endure oxidative stress from these oral commensals. Interestingly, in the growth competition assay, we found that GBS-SI101 could inhibit S. sanguinis in an inoculation sequence-dependent manner, and further studies are warranted to explore the mechanism of this phenomenon.
Conclusion
The focus of this study was to discover a new binding partner of S. mutans and investigate interactions between them. We identified for the first time the interspecies cell-cell contact between S. mutans and GBS-SI101, which typically resides in the gastrointestinal and vaginal tracts. GtfB and GtfC of S. mutans, which are responsible for EPS biosynthesis, played essential roles in this interspecies coaggregation. In addition, several oral streptococcus species used in this study barely impaired the growth of GBS-SI101. Instead of being a transient species in the oral cavity, GBS-SI101 might bind to S. mutans and overcome interspecies growth competition from commensal streptococci, integrating into the Streptococcus-associated polymicrobial community.
Given the limitation of an in vitro study, our results cannot fully represent the sophisticated situations in the oral microbial community. The real process in vivo during the integration of GBS into Streptococcus-associated oral multispecies community will be far more complex and may involve extensive intergeneric and host-GBS interactions. Nevertheless, our study investigated the S. mutans-GBS-SI101 interactions and provided evidence for a possible strategy used by GBS to colonize and persist within the oral cavity. Synergistic interactions between the two opportunistic pathogens might affect the balanced oral microbiota and increase the risk of oral infections.
Data Availability Statement
All datasets generated for this study are included in the article/supplementary material.
Ethics Statement
The studies involving human participants were reviewed and approved by Ethical Committee of Guanghua School of Stomatology, Hospital of Stomatology, Sun Yat-Sen University, Guangzhou, PR China. The patients/participants provided their written informed consent to participate in this study.
Author Contributions
WS and LG designed and performed the experiments. LG, JiaL, and TL analyzed the data. LG and TL wrote the manuscript. All authors revised the manuscript critically and approved the final manuscript.
Funding
This work was supported financially by grants from the National Natural Science Foundation of China to LG (funding number: 81670982) and Pearl River S&T Nova Program of Guangzhou for JiaL (funding number: 201806010158). The funding institutions were not involved in the design of the study, collection, analysis, or interpretation of data, or in the writing of the manuscript.
Conflict of Interest
The authors declare that the research was conducted in the absence of any commercial or financial relationships that could be construed as a potential conflict of interest.
Acknowledgments
We thank Howard Kuramitsu (University at Buffalo, State University of New York, NY, USA) for kindly providing the different S. mutans UA140 mutant derivatives.
Abbreviations
AMPs, Antimicrobial peptides; C. albicans, Candida albicans; CPSs, capsular polysaccharides; GBS-SI101, saliva isolate Streptococcus agalactia; CAB, coaggregation buffer; CLSM, confocal laser scanning microscopy; ECC, early childhood caries; EPS, extracellular polysaccharides; Fnp, Fusobacterium nucleatum ssp. polymorphum; Gtfs, glucosyltransferases; LTA, lipoteichoic acid; NAM, N -acetylmuramic acid; PBS, phosphate-buffered saline; S-mix, saliva-derived microbiota; S. agalactiae, Streptococcus agalactiae; GBS, also known as Group B Streptococcus; S. gordonii, Streptococcus gordonii; S. mutans, Streptococcus mutans; S. sanguinis, Streptococcus sanguinis; SodA, superoxide dismutase; THB, Todd-Hewitt Broth.
References
Aas, J. A., Paster, B. J., Stokes, L. N., Olsen, I., and Dewhirst, F. E. (2005). Defining the normal bacterial flora of the oral cavity. J. Clin. Microbiol. 43, 5721–5732. doi: 10.1128/JCM.43.11.5721-5732.2005
Abranches, J., Zeng, L., Kajfasz, J. K., Palmer, S. R., Chakraborty, B., Wen, Z. T., et al. (2018). Biology of oral streptococci. Microbiol. Spectr. 6:5. doi: 10.1128/microbiolspec.GPP3-0042-2018
Agnello, M., Marques, J., Cen, L., Mittermuller, B., Huang, A., Tran, N. C., et al. (2017). Microbiome associated with severe caries in Canadian first nations children. J. Dent. Res. 96, 1378–1385. doi: 10.1177/0022034517718819
Aoki, H., Shiroza, T., Hayakawa, M., Sato, S., and Kuramitsu, H. K. (1986). Cloning of a Streptococcus mutans glucosyltransferase gene coding for insoluble glucan synthesis. Infect. Immun. 53, 587–594. doi: 10.1128/IAI.53.3.587-594.1986
Barbieri, D. D. S. A. V., Vicente, V. A., Fraiz, F. C., Lavoranti, O. J., Svidzinski, T. I. E., and Pinheiro, R. L. (2007). Analysis of the in vitro adherence of Streptococcus mutans and Candida albicans. Braz. J. Microbiol. 38, 624–631. doi: 10.1590/S1517-83822007000400009
Brittan, J. L., and Nobbs, A. H. (2015). Group B Streptococcus pili mediate adherence to salivary glycoproteins. Microbes Infect. 17, 360–368. doi: 10.1016/j.micinf.2014.12.013
Campos, M. A., Vargas, M. A., Regueiro, V., Llompart, C. M., Alberti, S., and Bengoechea, J. A. (2004). Capsule polysaccharide mediates bacterial resistance to antimicrobial peptides. Infect. Immun. 72, 7107–7114. doi: 10.1128/IAI.72.12.7107-7114.2004
Chaffee, B. W., Gansky, S. A., Weintraub, J. A., Featherstone, J. D., and Ramos-Gomez, F. J. (2014). Maternal oral bacterial levels predict early childhood caries development. J. Dent. Res. 93, 238–244. doi: 10.1177/0022034513517713
Cisar, J. O., Kolenbrander, P. E., and Mcintire, F. C. (1979). Specificity of coaggregation reactions between human oral streptococci and strains of Actinomyces viscosus or Actinomyces naeslundii. Infect. Immun. 24, 742–752. doi: 10.1128/IAI.24.3.742-752.1979
Cools, P., and Melin, P. (2017). Group B Streptococcus and perinatal mortality. Res. Microbiol. 168, 793–801. doi: 10.1016/j.resmic.2017.04.002
Cvitkovitch, D. G., Li, Y. H., and Ellen, R. P. (2003). Quorum sensing and biofilm formation in Streptococcal infections. J. Clin. Invest. 112, 1626–1632. doi: 10.1172/JCI200320430
Deng, L., Kasper, D. L., Krick, T. P., and Wessels, M. R. (2000). Characterization of the linkage between the type III capsular polysaccharide and the bacterial cell wall of group B Streptococcus. J. Biol. Chem. 275, 7497–7504. doi: 10.1074/jbc.275.11.7497
Draper, L. A., Cotter, P. D., Hill, C., and Ross, R. P. (2015). Lantibiotic resistance. Microbiol. Mol. Biol. Rev. 79, 171–191. doi: 10.1128/MMBR.00051-14
D'Urzo, N., Martinelli, M., Pezzicoli, A., De Cesare, V., Pinto, V., Margarit, I., et al. (2014). Acidic pH strongly enhances in vitro biofilm formation by a subset of hypervirulent ST-17 Streptococcus agalactiae strains. Appl. Environ. Microbiol. 80, 2176–2185. doi: 10.1128/AEM.03627-13
Falsetta, M. L., Klein, M. I., Colonne, P. M., Scott-Anne, K., Gregoire, S., Pai, C. H., et al. (2014). Symbiotic relationship between Streptococcus mutans and Candida albicans synergizes virulence of plaque biofilms in vivo. Infect. Immun. 82, 1968–1981. doi: 10.1128/IAI.00087-14
Fang, G., Lux, R., Du-Thumm, L., Stokes, I., Kreth, J., Anderson, M. H., et al. (2005). In situ and non-invasive detection of specific bacterial species in oral biofilms using fluorescently labeled monoclonal antibodies. J. Microbiol. Methods 62, 145–160. doi: 10.1016/j.mimet.2005.02.013
Farley, M. M., and Strasbaugh, L. J. (2001). Group B streptococcal disease in nonpregnant adults. Clin. Infect. Dis. 33, 556–561. doi: 10.1086/322696
Forssten, S. D., Bjorklund, M., and Ouwehand, A. C. (2010). Streptococcus mutans, caries and simulation models. Nutrients 2, 290–298. doi: 10.3390/nu2030290
Gibbs, R. S., Schrag, S., and Schuchat, A. (2004). Perinatal infections due to group B streptococci. Obstet. Gynecol. 104, 1062–1076. doi: 10.1097/01.AOG.0000144128.03913.c2
Guo, L., McLean, J. S., Yang, Y., Eckert, R., Kaplan, C. W., Kyme, P., et al. (2015). Precision-guided antimicrobial peptide as a targeted modulator of human microbial ecology. Proc. Natl. Acad. Sci. U.S.A 112:7569. doi: 10.1073/pnas.1506207112
Guo, L., Shokeen, B., He, X., Shi, W., and Lux, R. (2017). Streptococcus mutans SpaP binds to RadD of Fusobacterium nucleatum ssp. polymorphum. Mol. Oral Microbiol. 32, 355–364. doi: 10.1111/omi.12177
Hanada, N., and Kuramitsu, H. K. (1988). Isolation and characterization of the Streptococcus mutans gtfC gene, coding for synthesis of both soluble and insoluble glucans. Infect. Immun. 56, 1999–2005. doi: 10.1128/IAI.56.8.1999-2005.1988
Hansen, S. K., Rainey, P. B., Haagensen, J. A., and Molin, S. (2007). Evolution of species interactions in a biofilm community. Nature 445, 533–536. doi: 10.1038/nature05514
He, X., Hu, W., He, J., Guo, L., Lux, R., and Shi, W. (2011). Community-based interference against integration of Pseudomonas aeruginosa into human salivary microbial biofilm. Mol. Oral Microbiol. 26, 337–352. doi: 10.1111/j.2041-1014.2011.00622.x
Hickman, M. E., Rench, M. A., Ferrieri, P., and Baker, C. J. (1999). Changing epidemiology of group B streptococcal colonization. Pediatrics 104, 203–209. doi: 10.1542/peds.104.2.203
High, K. P., Edwards, M. S., and Baker, C. J. (2005). Group B streptococcal infections in elderly adults. Clin. Infect. Dis. 41, 839–847. doi: 10.1086/432804
Hojo, K., Nagaoka, S., Ohshima, T., and Maeda, N. (2009). Bacterial interactions in dental biofilm development. J. Dent. Res. 88, 982–990. doi: 10.1177/0022034509346811
Jakubovics, N. S., Yassin, S. A., and Rickard, A. H. (2014). Community interactions of oral streptococci. Adv. Appl. Microbiol. 87, 43–110. doi: 10.1016/B978-0-12-800261-2.00002-5
Khosa, S., Lagedroste, M., and Smits, S. H. (2016). Protein defense systems against the lantibiotic nisin: function of the immunity protein NisI and the resistance protein NSR. Front. Microbiol. 7:504. doi: 10.3389/fmicb.2016.00504
Kim, K. S. (2010). Acute bacterial meningitis in infants and children. Lancet Infect. Dis. 10, 32–42. doi: 10.1016/S1473-3099(09)70306-8
Koga, T., Asakawa, H., Okahashi, N., and Hamada, S. (1986). Sucrose-dependent cell adherence and cariogenicity of serotype C Streptococcus mutans. J. Gen. Microbiol. 132, 2873–2883. doi: 10.1099/00221287-132-10-2873
Kohli-Lynch, M., Russell, N. J., Seale, A. C., Dangor, Z., Tann, C. J., Baker, C. J., et al. (2017). Neurodevelopmental impairment in children after group B streptococcal disease worldwide: Systematic review and meta-analyses. Clin. Infect. Dis. 65, S190–S199. doi: 10.1093/cid/cix663
Kolenbrander, P. E., Palmer, R. J., Periasamy, S., and Jakubovics, N. S. (2010). Oral multispecies biofilm development and the key role of cell-cell distance. Nat. Rev. Microbiol. 8, 471–480. doi: 10.1038/nrmicro2381
Koo, H., Xiao, J., Klein, M. I., and Jeon, J. G. (2010). Exopolysaccharides produced by Streptococcus mutans glucosyltransferases modulate the establishment of microcolonies within multispecies biofilms. J. Bacteriol. 192, 3024–3032. doi: 10.1128/JB.01649-09
Korir, M. L., Flaherty, R. A., Rogers, L. M., Gaddy, J. A., Aronoff, D. M., and Manning, S. D. (2018). Investigation of the role that NADH peroxidase plays in oxidative stress survival in group B Streptococcus. Front. Microbiol. 9:2786. doi: 10.3389/fmicb.2018.02786
Kreth, J., Zhang, Y., and Herzberg, M. C. (2008). Streptococcal antagonism in oral biofilms: Streptococcus sanguinis and Streptococcus gordonii interference with Streptococcus mutans. J. Bacteriol. 190, 4632–4640. doi: 10.1128/JB.00276-08
Krzysciak, W., Jurczak, A., Koscielniak, D., Bystrowska, B., and Skalniak, A. (2014). The virulence of Streptococcus mutans and the ability to form biofilms. Eur. J. Clin. Microbiol. Infect. Dis. 33, 499–515. doi: 10.1007/s10096-013-1993-7
Kuramitsu, H. K. (1993). Virulence factors of mutans streptococci: role of molecular genetics. Crit. Rev. Oral Biol. Med. 4, 159–176. doi: 10.1177/10454411930040020201
Lemos, J. A., and Burne, R. A. (2008). A model of efficiency: stress tolerance by Streptococcus mutans. Microbiology 154, 3247–3255. doi: 10.1099/mic.0.2008/023770-0
Lemos, J. A., Palmer, S. R., Zeng, L., Wen, Z. T., Kajfasz, J. K., Freires, I. A., et al. (2019). The biology of Streptococcus mutans. Microbiol. Spectr. 7:ch27. doi: 10.1128/9781683670131.ch27
Li, Y., Ku, C. Y., Xu, J., Saxena, D., and Caufield, P. W. (2005). Survey of oral microbial diversity using PCR-based denaturing gradient gel electrophoresis. J. Dent. Res. 84, 559–564. doi: 10.1177/154405910508400614
Liu, X., Ramsey, M. M., Chen, X., Koley, D., Whiteley, M., and Bard, A. J. (2011). Real-time mapping of a hydrogen peroxide concentration profile across a polymicrobial bacterial biofilm using scanning electrochemical microscopy. Proc. Natl. Acad. Sci. U.S.A. 108, 2668–2673. doi: 10.1073/pnas.1018391108
Llobet, E., Tomas, J. M., and Bengoechea, J. A. (2008). Capsule polysaccharide is a bacterial decoy for antimicrobial peptides. Microbiology 154, 3877–3886. doi: 10.1099/mic.0.2008/022301-0
Martinlaurent, F., Philippot, L., Hallet, S., Chaussod, R., Germon, J. C., Soulas, G., et al. (2001). DNA extraction from soils: old bias for new microbial diversity analysis methods. Appl. Environ. Microbiol. 67, 2354–2359. doi: 10.1128/AEM.67.5.2354-2359.2001
Metwalli, K. H., Khan, S. A., Krom, B. P., and Jabra-Rizk, M. A. (2013). Streptococcus mutans, Candida albicans, and the human mouth: a sticky situation. PLoS Pathog. 9:e1003616. doi: 10.1371/journal.ppat.1003616
Meyn, L. A., Krohn, M. A., and Hillier, S. L. (2009). Rectal colonization by group B Streptococcus as a predictor of vaginal colonization. Am. J. Obstet. Gynecol. 201, e71–e76. doi: 10.1016/j.ajog.2009.02.011
Nagao, P. E. (2015). “Streptococcus agalactiae (Group B streptococci),” in Molecular Medical Microbiology, eds Y. W. Tang, M. Sussman, D. Liu, I. Poxton, and J. Schwartzman (Boston, MA: Academic Press), 1751–1767.
Nicolas, G., Lavoie, M., and LaPointe, G. (2007). Molecular genetics, genomics and biochemistry of mutacins. Genes Genomes Genom. 1, 193–208. Available online at: https://scholar.google.com/scholar_lookup?journal=G3&title=Molecular+genetics,+genomics+and+biochemistry+of+mutacins&author=GG+Nicolas&author=MC+Lavoie&author=G+LaPointe&volume=1&publication_year=2007&pages=193-208&
Paster, B. J., Olsen, I., Aas, J. A., and Dewhirst, F. E. (2010). The breadth of bacterial diversity in the human periodontal pocket and other oral sites. Periodontol 42, 80–87. doi: 10.1111/j.1600-0757.2006.00174.x
Phares, C. R., Lynfield, R., Farley, M. M., Mohle-Boetani, J., Harrison, L. H., Petit, S., et al. (2008). Epidemiology of invasive group B Streptococcal disease in the United States, 1999-2005. JAMA 299, 2056–2065. doi: 10.1001/jama.299.17.2056
Plonka, K. A., Pukallus, M. L., Barnett, A. G., Walsh, L. J., Holcombe, T. H., and Seow, W. K. (2012). Mutans streptococci and lactobacilli colonization in predentate children from the neonatal period to seven months of age. Caries Res. 46, 213–220. doi: 10.1159/000337353
Poyart, C., Pellegrini, E., Gaillot, O., Boumaila, C., Baptista, M., and Trieu-Cuot, P. (2001). Contribution of Mn-cofactored superoxide dismutase (SodA) to the virulence of Streptococcus agalactiae. Infect. Immun. 69, 5098–5106. doi: 10.1128/IAI.69.8.5098-5106.2001
Qi, F., Chen, P., and Caufield, P. W. (2001). The group I strain of Streptococcus mutans, UA140, produces both the lantibiotic mutacin I and a nonlantibiotic bacteriocin, mutacin IV. Appl. Environ. Microbiol. 67, 15–21. doi: 10.1128/AEM.67.1.15-21.2001
Rickard, A. H., Gilbert, P., High, N. J., Kolenbrander, P. E., and Handley, P. S. (2003). Bacterial coaggregation: an integral process in the development of multi-species biofilms. Trends Microbiol. 11, 94–100. doi: 10.1016/S0966-842X(02)00034-3
Riley, M. A., and Wertz, J. E. (2002). Bacteriocins: evolution, ecology, and application. Annu. Rev. Microbiol. 56, 117–137. doi: 10.1146/annurev.micro.56.012302.161024
Saar-Dover, R., Bitler, A., Nezer, R., Shmuel-Galia, L., Firon, A., Shimoni, E., et al. (2012). D-alanylation of lipoteichoic acids confers resistance to cationic peptides in group B streptococcus by increasing the cell wall density. PLoS Pathog. 8:e1002891. doi: 10.1371/journal.ppat.1002891
Shabayek, S., Bauer, R., Mauerer, S., Mizaikoff, B., and Spellerberg, B. (2016). A streptococcal NRAMP homologue is crucial for the survival of Streptococcus agalactiae under low pH conditions. Mol. Microbiol. 100, 589–606. doi: 10.1111/mmi.13335
Shabayek, S., and Spellerberg, B. (2017). Acid stress response mechanisms of group B streptococci. Front. Cell. Infect. Microbiol. 7:395. doi: 10.3389/fcimb.2017.00395
Sheffield, V. C., Cox, D. R., Lerman, L. S., and Myers, R. M. (1989). Attachment of a 40-base-pair G + C-rich sequence (GC-clamp) to genomic DNA fragments by the polymerase chain reaction results in improved detection of single-base changes. Proc. Natl. Acad. Sci. U.S.A. 86, 232–236. doi: 10.1073/pnas.86.1.232
Spinosa, M. R., Progida, C., Tala, A., Cogli, L., Alifano, P., and Bucci, C. (2007). The Neisseria meningitidis capsule is important for intracellular survival in human cells. Infect. Immun. 75, 3594–3603. doi: 10.1128/IAI.01945-06
Takahashi, N., and Nyvad, B. (2008). Caries ecology revisited: microbial dynamics and the caries process. Caries Res. 42, 409–418. doi: 10.1159/000159604
Tamura, G. S., Kuypers, J. M., Smith, S., Raff, H., and Rubens, C. E. (1994). Adherence of group B streptococci to cultured epithelial cells: roles of environmental factors and bacterial surface components. Infect. Immun. 62, 2450–2458. doi: 10.1128/IAI.62.6.2450-2458.1994
Tanzer, J. M., Livingston, J., and Thompson, A. M. (2001). The microbiology of primary dental caries in humans. J. Dent. Educ. 65, 1028–1037. doi: 10.1002/j.0022-0337.2001.65.10.tb03446.x
The Human Microbiome Project Consortium (2012). Structure, function and diversity of the healthy human microbiome. Nature 486, 207–214. doi: 10.1038/nature11234
Tian, Y., He, X., Torralba, M., Yooseph, S., Nelson, K. E., Lux, R., et al. (2010). Using DGGE profiling to develop a novel culture medium suitable for oral microbial communities. Mol. Oral Microbiol. 25, 357–367. doi: 10.1111/j.2041-1014.2010.00585.x
Tong, H., Chen, W., Merritt, J., Qi, F., Shi, W., and Dong, X. (2007). Streptococcus oligofermentans inhibits Streptococcus mutans through conversion of lactic acid into inhibitory H2O2: a possible counteroffensive strategy for interspecies competition. Mol. Microbiol. 63, 872–880. doi: 10.1111/j.1365-2958.2006.05546.x
Van der Mee-Marquet, N., Fourny, L., Arnault, L., Domelier, A. S., Salloum, M., Lartigue, M. F., et al. (2008). Molecular characterization of human-colonizing Streptococcus agalactiae strains isolated from throat, skin, anal margin, and genital body sites. J. Clin. Microbiol. 46, 2906. doi: 10.1128/JCM.00421-08
Van Houte, J., Gibbs, G., and Butera, C. (1982). Oral flora of children with “nursing bottle caries”. J. Dent. Res. 61, 382–385. doi: 10.1177/00220345820610020201
Verani, J. R., McGee, L., and Schrag, S. J. (2010). Division of bacterial diseases national center for immunization and respiratory diseases and centers for disease control prevention. Prevention of perinatal group B streptococcal disease–revised guidelines from CDC, 2010. MMWR Recomm. Rep. 59, 1–36. Available online at: https://www.cdc.gov/mmwr/preview/mmwrhtml/rr5910a1.htm
Wan, A. K., Seow, W. K., Purdie, D. M., Bird, P. S., Walsh, L. J., and Tudehope, D. I. (2003). A longitudinal study of Streptococcus mutans colonization in infants after tooth eruption. J. Dent. Res. 82, 504–508. doi: 10.1177/154405910308200703
Wan, A. K., Seow, W. K., Walsh, L. J., Bird, P., Tudehope, D. L., and Purdie, D. M. (2001). Association of Streptococcus mutans infection and oral developmental nodules in pre-dentate infants. J. Dent. Res. 80, 1945–1948. doi: 10.1177/00220345010800101601
Wu, T., Cen, L., Kaplan, C., Zhou, X., Lux, R., Shi, W., et al. (2015). Cellular components mediating coadherence of Candida albicans and Fusobacterium nucleatum. J. Dent. Res. 94, 1432–1438. doi: 10.1177/0022034515593706
Xiao, J., Klein, M. I., Falsetta, M. L., Lu, B., Delahunty, C. M., Yates, J. R. III., et al. (2012). The exopolysaccharide matrix modulates the interaction between 3D architecture and virulence of a mixed-species oral biofilm. PLoS Pathog. 8:e1002623. doi: 10.1371/journal.ppat.1002623
Zawaneh, S. M., Ayoub, E. M., Baer, H., Cruz, A. C., and Spellacy, W. N. (1979). Factors influencing adherence of group B streptococci to human vaginal epithelial cells. Infect. Immun. 26, 441–447. doi: 10.1128/IAI.26.2.441-447.1979
Keywords: group B Streptococcus, coaggregation, Streptococcus mutans, interspecies interactions, Streptococcus agalactiae
Citation: Liu T, Liu J, Liu J, Yang R, Lu X, He X, Shi W and Guo L (2020) Interspecies Interactions Between Streptococcus Mutans and Streptococcus Agalactiae in vitro. Front. Cell. Infect. Microbiol. 10:344. doi: 10.3389/fcimb.2020.00344
Received: 13 March 2020; Accepted: 04 June 2020;
Published: 07 July 2020.
Edited by:
Zezhang Tom Wen, Louisiana State University, United StatesReviewed by:
Ping Xu, Virginia Commonwealth University, United StatesLei Cheng, Sichuan University, China
Copyright © 2020 Liu, Liu, Liu, Yang, Lu, He, Shi and Guo. This is an open-access article distributed under the terms of the Creative Commons Attribution License (CC BY). The use, distribution or reproduction in other forums is permitted, provided the original author(s) and the copyright owner(s) are credited and that the original publication in this journal is cited, in accordance with accepted academic practice. No use, distribution or reproduction is permitted which does not comply with these terms.
*Correspondence: Lihong Guo, Z3VvbGg1QG1haWwuc3lzdS5lZHUuY24=