Influence of the Host and Parasite Strain on the Immune Response During Toxoplasma Infection
- Department of Pathology, Microbiology & Immunology, School of Veterinary Medicine, University of California, Davis, Davis, CA, United States
Toxoplasma gondii is an exceptionally successful parasite that infects a very broad host range, including humans, across the globe. The outcome of infection differs remarkably between hosts, ranging from acute death to sterile infection. These differential disease patterns are strongly influenced by both host- and parasite-specific genetic factors. In this review, we discuss how the clinical outcome of toxoplasmosis varies between hosts and the role of different immune genes and parasite virulence factors, with a special emphasis on Toxoplasma-induced ileitis and encephalitis.
Introduction
Toxoplasma gondii is an obligate intracellular parasite and the causative agent of toxoplasmosis, a worldwide zoonotic disease that can affect virtually all mammals and birds, including approximately one third of humans (Montoya and Liesenfeld, 2004; Pappas et al., 2009). Toxoplasma has a complex life cycle with sexual multiplication taking place exclusively in the intestine of the definitive host (members of the felidae family) and asexual multiplication in intermediate hosts (warm-blooded animals). Infection of intermediate hosts occurs by ingestion of food or water contaminated with oocysts, which are secreted with feline feces, or meat containing tissue cysts. Upon ingestion, sporozoites within oocysts or bradyzoites within tissue cysts are released in the small intestine and invade intestinal epithelial cells. Here they transform into tachyzoites, the fast-replicating stage responsible for symptoms during the acute phase of the disease, which can vary in severity depending on the host and parasite strain. After invasion of the host cell, Toxoplasma establishes its niche inside the host cytosol by forming a parasitophorous vacuole (PV), inside which it replicates (Blader et al., 2015). Toxoplasma modulates host pathways through the secretion and delivery of GRA and ROP effectors from its dense granule and rhoptry organelles, respectively, to the host cell cytoplasm. Although some Toxoplasma secreted GRAs and ROPs help to evade host immunity (Hunter and Sibley, 2012; Butcher et al., 2014; Hakimi and Bougdour, 2015; Hakimi et al., 2017; Boothroyd and Hakimi, 2020), others activate it (Melo et al., 2011; Hunter and Sibley, 2012; Hakimi et al., 2017; Boothroyd and Hakimi, 2020). This dynamic balance is required by the parasite to convert to bradyzoites and form tissue cysts, which are orally infectious to other intermediate hosts, thus favoring further transmission. Hence, a complete evasion of host immunity leading to uncontrolled parasite proliferation would be fatal for the host, while a too strong host immune response would be fatal for the parasite, either of which would limit the parasite's transmission.
Most hosts with competent immune responses develop only mild flu-like symptoms or remain asymptomatic, as they are capable of controlling parasite multiplication. By contrast, susceptible host species or individuals with immunosuppressed conditions develop clinical toxoplasmosis that may manifest as encephalitis, myocarditis or pneumonia, among others. In addition, infection during gestation can result in abortion, neonatal mortality or congenital infection (Dubey, 2016).
Susceptibility to the Disease Is Determined by Both Parasite Strain and Host Species
The immune response against Toxoplasma varies depending on the genetic background and immune status of the host, as well as on the parasite strain. In Europe and North America, the population of Toxoplasma is dominated by four major clonal types, I, II, III, and XII, although the majority of human and livestock infections in these regions are caused by type II strains (Howe and Sibley, 1995; Ajzenberg et al., 2002; Sibley and Ajioka, 2008; Lorenzi et al., 2016). However, in South America Toxoplasma shows an extreme genetic diversity with more than 150 strains (Sibley and Ajioka, 2008; Su et al., 2012). Toxoplasma virulence is defined by the number of tachyzoites that are needed to kill 50% of the mice upon inoculation (LD50). In the house mouse (Mus musculus -M.m.- domesticus) type I strains are very virulent (LD100 of 1 parasite), whereas type II and III are less virulent strains (LD50 ~103 and ~105 parasites, respectively) (Sibley and Boothroyd, 1992; Saeij et al., 2006). However, little is known about the correlation between Toxoplasma strain virulence in mice compared to other animal species and humans (Saeij et al., 2005). For instance, while type I strains are highly virulent in most inbred murine hosts, they fail to produce observable disease in other animals such as rats or cows.
Generally, there seems to be a trend for Toxoplasma strains that are abundant in specific regions to not be virulent for the native mouse subspecies from those regions. For example, type I strains are more prevalent in North and Southeast Asia (Shwab et al., 2014), where M. m. castaneus and M.m. musculus are also more abundant. Type I strains do not kill these mice but are extremely virulent to M.m. domesticus, which is the dominant subspecies in Europe and North-America. By contrast, type II and III strains, which predominate in Europe and North-America, generally do not kill M.m. domesticus. South-American Toxoplasma strains are mostly virulent in mice, which are not indigenous to the continent, and these strains might have adapted to South-American rodents (Lilue et al., 2013; Hassan et al., 2019; Murillo-León et al., 2019). The difference in susceptibility against Toxoplasma in these different mouse (sub)species has been mapped to chromosome 11, which contains the immunity related GTPases (IRGs), a family of proteins that are induced by interferon gamma (IFNγ) and which coat and subsequently vesiculate the parasitophorous vacuole membrane (PVM), leading to the death of the parasite (Lilue et al., 2013; Hassan et al., 2019; Murillo-León et al., 2019). These IRG proteins are highly polymorphic and their function is blocked by the Toxoplasma effectors ROP5 and ROP18 (Fentress et al., 2010; Fleckenstein et al., 2012; Niedelman et al., 2012; Lilue et al., 2013). Thus, an evolutionary arms race of reciprocal selection pressure might drive the polymorphisms of IRGs in mice vs. ROP5 and ROP18 in Toxoplasma. Several inbred and outbred murine models have also been shown to have different degrees of susceptibility, which has been mostly investigated after infection with type II strains (Johnson, 1984; Shirahata et al., 1986; McLeod et al., 1989a,b, 1993; Schade and Fischer, 2001; Lee and Kasper, 2004). For instance, A/J inbred mice are relatively resistant regardless of the route of infection, showing very low cyst numbers in the brain and minimal inflammation and necrosis in the small intestine upon oral infection (McLeod et al., 1989a,b; Jensen et al., 2013). This is possibly due to the increased parasite killing ability of A/J derived macrophages compared to C57BL/6 (Hassan et al., 2015), although the differences in cyst numbers are also influenced by the Histocompatibility system 2 (H-2) locus (H-2a for A/J and H-2b for C57BL/6) (McLeod et al., 1989b). Other wild rodents, such as voles, are common in certain areas and are more resistant than mice (Sedlák et al., 2001). Similarly, guinea pigs show only a moderate susceptibility to type I and type II Toxoplasma infections (Flori et al., 2002).
Evidence from other animals also suggests a species-dependent susceptibility to toxoplasmosis. For instance, Australian marsupials, especially wallabies, and new world monkeys are particularly susceptible to toxoplasmosis, which often proves fatal (Innes, 1997). These species have either largely evolved in the absence of felines or their habitat is high in the canopies of trees, thus preventing them from being exposed to oocysts on the ground, which may explain their high vulnerability. Other animals are more resistant to Toxoplasma infection, with the infection usually being inapparent or producing only transient mild symptoms during the acute phase, although the host remains chronically infected for its lifetime. This group includes sheep, pigs, and humans, among others. Bovines are more resistant than other farm animals (Innes, 1997; Stelzer et al., 2019). In addition, although most types of rats are resistant to the acute phase of infection by the major Toxoplasma clonal types, rats often develop brain cysts (Kempf et al., 1999; Sergent et al., 2005). However, Lewis rats have sterile immunity against most Toxoplasma strains, which is a dominant trait mapped to the Toxo1 locus (Cavaillès et al., 2006, 2014). This locus contains a nucleotide-binding oligomerization domain, leucine-rich repeat protein 1 (NLRP1), which encodes for the NLRP1 inflammasome sensor that causes rapid cell death and disruption of the replicative niche of the parasite upon activation (Cirelli et al., 2014). One exception is the South American strain GUY008-ABE, which can establish a chronic infection in Lewis rats and is fatal to F334 rats (Loeuillet et al., 2019). Thus, although it is in the parasite's best interest to cause a lifelong chronic infection, it is clear that not every parasite strain can produce an optimal infection in every animal.
Arguably, one of the most critical factors influencing susceptibility to Toxoplasma is the host immune system and its interplay with the parasite. For example, it is possible that in highly susceptible species the induction or function of critical components of protective immunity is less efficient than in more resistant species. As natural infection in immunocompetent humans is mostly asymptomatic, studies addressing the human immune response in primary toxoplasmosis are challenging. Furthermore, the immune response in humans also varies with the clinical presentation, ranging from toxoplasmic ileitis to toxoplasmic encephalitis (TE), ocular toxoplasmosis (OT) or congenital toxoplasmosis (Montoya and Liesenfeld, 2004). To study different clinical manifestations of toxoplasmosis in the laboratory with relative ease, various rodent models have been developed and provided much of our understanding of toxoplasmosis immunobiology (Munoz et al., 2011; Dupont et al., 2012). In this review we will discuss the immune response against Toxoplasma, focusing on parasite and host factors that influence Toxoplasma-induced ileitis and encephalitis.
The Immune Response During Toxoplasmosis
The immune response against invading microorganisms starts with the sensing of pathogens via microbe associated molecular patterns (PAMPs/MAMPs) by receptors known as pattern recognition receptors (PRRs). The toll-like receptors (TLRs) and nucleotide-binding oligomerization domain-like receptors (NLRs) are the most extensively studied (Akira et al., 2006; O'Neill and Bowie, 2007; Mogensen, 2009). Upon engagement of TLRs or NLRs with MAMPs, signaling pathways are triggered. Downstream signal transduction pathways via phosphorylation, ubiquitination, or protein-protein interactions, activate host cell transcription factors which in turn upregulate the expression of genes involved in inflammation and antimicrobial host defenses (Akira et al., 2006; O'Neill and Bowie, 2007; Mogensen, 2009). Three major signaling pathways are responsible for mediating TLR or NLR-induced immune responses: (i) nuclear factor κB (NF-κB), (ii) mitogen-activated protein kinases (MAPKs), and (iii) IFN regulatory factors (IRFs). These three pathways play central roles in the induction of interleukin (IL)12, IL1β, tumor necrosis factor α (TNFα) and interferons (IFN) type I and type II (Akira et al., 2006; O'Neill and Bowie, 2007; Mogensen, 2009). The activation of these pathways leads to the recruitment of immune cells to the site of infection via the secretion of chemokines, and these recruited cells can kill Toxoplasma via the upregulation of effector molecules. There are important differences in how the innate immune system of different host species detect Toxoplasma and, once it is detected, how it is eliminated via IFNγ-induced anti-parasitic activities.
Host Differences in the Innate Sensing of Toxoplasma
Role of Toll Like Receptors (TLRs)
In murine hosts, Toxoplasma is primarily detected by endosomal TLR11 and TLR12, which bind to the bona-fide Toxoplasma MAMP actin-binding protein, profilin in uninfected dendritic cells (DCs) (Yarovinsky, 2005; Andrade et al., 2013; Raetz et al., 2013). This interaction signals via myeloid differentiation factor 88 (MyD88) and activates the IRF8 transcription factor, resulting in the secretion of large amounts of interleukin 12 (IL12) (Yarovinsky, 2005, 2014; Andrade et al., 2013; Raetz et al., 2013) (Figure 1). However, the TLR11/12-profilin interaction is largely restricted to the CD8α subset of DCs, and by what means other immune cells, like macrophages, sense the pathogen remains elusive. In addition, the endosomal RNA and DNA sensing TLR7 and TLR9 are also important for the secretion of IL12, as 3d mice (which lack the chaperone UNC93B1 that is important for the correct localization of TLR 3/7/9/11/12) and Tlr3/7/9/11−/− quadruple knockout mice (Melo et al., 2010; Andrade et al., 2013) are very susceptible to infection (Figure 1).
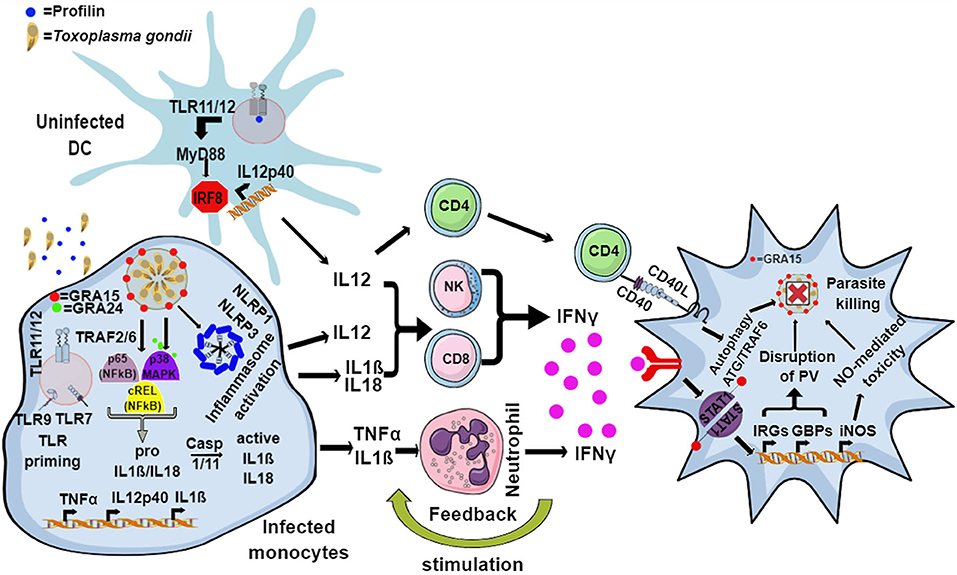
Figure 1. Model for the murine immune response to Toxoplasma. The endosomal TLR11 and TLR12 heterodimeric complex in uninfected DCs recognizes Toxoplasma profilin, resulting in MyD88 and IRF8-mediated production of IL12. Infected monocytes and macrophages produce IL1β and IL18 through the activation of the NLRP1 and NLRP3 inflammasome, while secretion of IL12 and TNFα is induced by GRA15- and GRA24-mediated activation of p65-NFκB, cREL-NFκB, and p38 MAPK pathways. Additionally, priming through nucleic acid sensing TLRs also leads to induction of IL1β. IL12, along with IL18 and IL1β, activates NK, CD4, and CD8 T cells to produce host-protective IFNγ. IL1β and TNFα act together to activate incoming neutrophils to secrete IFNγ, in turn inducing the expression of a battery of effector molecules including the immunity related GTPases (IRGs), guanylate binding proteins (GBPs), reactive oxygen species (ROS), nitric oxide (NO), and autophagy pathways inside the infected cells that eliminate the parasite.
Many other hosts, including humans, lack functional TLR11/12 (Gazzinelli et al., 2014) (Table 1) suggesting that these hosts have other mechanisms to detect Toxoplasma. Most hosts express TLR7, TLR9 and other nucleic acid sensors such as “absent in melanoma protein 2” (AIM2), cyclic GMP-AMP synthase (cGAS) or retinoic acid-inducible gene I (RIG-I). It is likely that only when the PVM is destroyed, parasite-derived nucleic acids are released and detected by cytoplasmic nucleic acid sensors. Indeed, human peripheral blood mononuclear cells (PBMCs) do not secrete cytokines upon treatment with Toxoplasma derived RNA or DNA unless pre-stimulated with IFNγ (Andrade et al., 2013). Cell surface TLR2 can sense the glycosylphosphatidylinositol (GPI) anchors on the parasite surface; however it does not seem to be essential for cytokine production by DC (Scanga et al., 2002; Debierre-Grockiego et al., 2007). Nevertheless, TLR2 was shown to be essential for the survival of infected mice, especially at high doses of infection, a scenario also observed in Mycobacterium tuberculosis (Reiling et al., 2002; Mun et al., 2003). Therefore, further studies are needed to underpin the role of TLR2 in Toxoplasma infection. Human and bovine TLR5 are closely related to murine TLR11; however, the response triggered by engagement of Toxoplasma-derived profilin with bovine and human TLR5 is controversial. While one study showed that human TLR5 binds to profilin and is able to stimulate the cells to secrete pro-inflammatory cytokines (Salazar Gonzalez et al., 2014), another study showed that human and porcine TLR5 failed to trigger cytokine secretion from cells upon stimulation with profilin (Tombácz et al., 2018). The discrepancy might be due to the purity of the profilin used. Although information from humans is scarce, a recent study showed that human monocytes can sense Toxoplasma in an indirect way (Safronova et al., 2018). Lysis of the infected cells releases the alarmin S100A11 through the inflammatory caspase 1 dependent pathway, which is sensed by monocytes through the receptor of advanced glycation end product (RAGE). This results in the production of the monocyte chemoattractant protein 1(MCP1), also called C-C motif chemokine ligand 2 or CCL2, which in turn recruits more monocytes, DCs and T cells to the site of infection (Safronova et al., 2018).
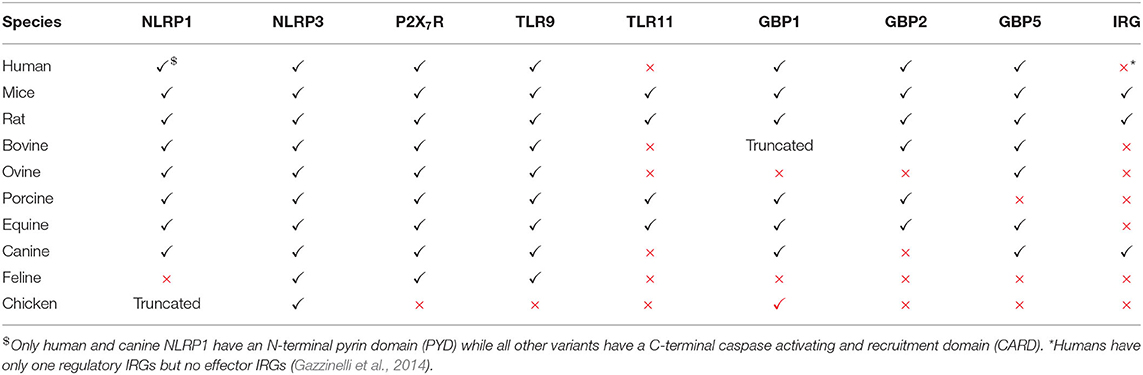
Table 1. Immune genes that can induce toxoplasmacidal mechanisms and their functional status in different hosts.
Role of Inflammasomes in Sensing Parasites
In mammals or mice lacking TLR11, activation of the immune system upon Toxoplasma infection is dependent on cytoplasmic sensors such as NLRP1/3, the adaptor protein apoptosis associated speck-like protein containing a CARD (ASC) and the caspases 1 and 4, all of which lead to the secretion of IL1β and IL18, a process known as inflammasome activation (Witola et al., 2011; Gov et al., 2013, 2017; Mukhopadhyay et al., 2020a). In mice lacking TLR11, and therefore DC-derived IL12, a large amount of IFNγ is secreted by neutrophils and CD8 T cells via pro-inflammatory monocyte and macrophage dependent inflammasome driven secretion of IL1β and IL18 (Sturge et al., 2013; López-Yglesias et al., 2019) (Figure 1). Indeed, mice deficient in components of the inflammasome sensors NLRP1, NLRP3, ASC, caspase 1 and caspase 11, or downstream cytokine IL18 or its receptor (IL18R) all showed increased susceptibility to Toxoplasma infection (Gorfu et al., 2014). In line with this, in human PBMCs, both IL18 and IL1β secreted through the activation of the NLRP3 inflammasome pathway are responsible for the secretion of IFNγ and IL12 (Mukhopadhyay et al., 2020a). Furthermore, apart from murine models, inflammasome activation is also important to restrict parasite growth in human and rat macrophages (Sergent et al., 2005; Witola et al., 2011; Cavaillès et al., 2014; Cirelli et al., 2014; Gov et al., 2017; Fisch et al., 2019a). Polymorphisms in NLRP1 determine susceptibility to toxoplasmosis in rats and humans but not in mice (Witola et al., 2011; Ewald et al., 2014). However, other hosts such as felines and chickens do not possess NLRP1 and are not susceptible to the disease (Table 1). This highlights the fact that other PRRs may also elicit an effective immune response against Toxoplasma. NLRP3 and the purinergic receptor P2X7R, which also leads to inflammasome activation, are widely expressed in rodents, humans and other animals (Table 1). These receptors play an important role in the control of toxoplasmosis, as their deficiency or polymorphisms produce susceptibility to the disease (Corrêa et al., 2010; Lees et al., 2010; Miller et al., 2011; Gorfu et al., 2014) (Figure 1).
Role of Cytokines and Chemokines in Recruitment and Activation of Immune Cells
Central to the host defense against toxoplasmosis is the generation of IL12 by DCs and macrophages, as it primes NK and T cells (CD4 and CD8) to secrete IFNγ. Although DC-derived IL12 is important for secretion of IFNγ in vivo, it alone is not sufficient to protect the host, as Myd88−/− mice that were administered recombinant IL12 post-infection did not survive infection (Hou et al., 2011). The pro-inflammatory cytokines IL18 and IL1β secreted by monocytes and macrophages upon inflammasome activation also enhance IFNγ production and, together with IL12, can thereby contribute to host resistance against Toxoplasma (Hunter et al., 1995; Cai et al., 2000; Gov et al., 2013, 2017; Mukhopadhyay et al., 2020a). Furthermore, while mice lacking either MyD88 or IL12p40 succumb rapidly to infection, mice that lack Myd88 specifically in DCs or macrophage lineages do not, indicating that there are other factors and cytokines that regulate IL12 and IFNγ secretion (Scanga et al., 2002; Hou et al., 2011). As a matter of fact, neutrophils recruited to the site of infection secrete IL12 and IFNγ, while monocytes contribute by secreting IL1β and TNFα, which in turn induce the secretion of IFNγ by neutrophils, NK cells and T cells in a MyD88-dependent way (LaRosa et al., 2008; Sturge et al., 2013; Sturge and Yarovinsky, 2014). Therefore, recruitment of immune cells to the site of infection via chemokine secretion is considered an essential step in the control of the parasite. For instance, NK cells are recruited to the site of infection through the CC chemokine receptor 5 (CCR5) and are among the first cells to secrete IFNγ, while neutrophils and monocytes are recruited through CCR1 and CCR2, respectively (Khan et al., 2001, 2006; Robben et al., 2005). The importance of immune cell recruitment was revealed by the acute susceptibility of mice lacking either CCR5, CCR1, or CCR2 (Khan et al., 2001, 2006; Robben et al., 2005; Dunay et al., 2008). Cooperation between DCs and NK cells further stimulates IL12 and IFNγ production by each cell type (Khan et al., 2006; Guan et al., 2007). The importance of CD8α DCs and NK cells was revealed from experiments conducted in mice lacking CCR5, IRF8, or basic leucine zipper transcription factor ATF-like 3 (BATF3), a transcription factor required for the development of CD8α DCs, as all of them succumb quickly to the infection (Scharton-Kersten et al., 1997; Khan et al., 2006; Mashayekhi et al., 2011).
Elimination of Parasites Within the Host
IFNγ, alone and together with TNFα, induces the secretion of effector molecules in both hematopoietic and non-hematopoietic cells, eliciting a direct anti-parasitic activity that will be discussed in the next section. Although IFNγ is indispensable for mouse immunity to Toxoplasma (Suzuki et al., 1988; Scharton-Kersten et al., 1996; Yap and Sher, 1999), humans with IFNγ-receptor deficiency do not show an increased susceptibility. This could be possibly due to TNFα and CD40-CD40L-mediated (Janssen et al., 2002; Andrade et al., 2003) induction of host protective autophagy in infected macrophages and non-hematopoietic cells, leading to parasite elimination (Andrade et al., 2003, 2006; Subauste and Wessendarp, 2006; Van Grol et al., 2013) (Figure 1). Neutrophils, the most abundant cells at the site of infection, can form neutrophil extracellular traps, or NETs. These consist of extracellular masses of nuclear DNA fibers associated with histones and enzymes of cytoplasmic granules and anti-microbial peptides, such as myeloperoxidase (MPO) or lactoperoxidase, that are able to kill various microorganisms (Brinkmann et al., 2004; Díaz-Godínez and Carrero, 2019). In the case of Toxoplasma, NETs cause the immobilization of entrapped parasites, leading to their death (Abdallah et al., 2012; Yildiz et al., 2017). This mechanism is conserved in mice, humans, farm animals and marine mammals (Abdallah et al., 2012; Yildiz et al., 2017; Imlau et al., 2020).
After the initial sensing, immune activation and clearance of parasites within macrophages and other antigen presenting cells (APCs), the CD8 T cell response is initiated when they encounter epitopes presented by major histocompatibility complex (MHC) I on APCs. The degree of CD8 T cell activation depends on the effective killing of intracellular parasites within the APCs, after which the immunodominant epitopes derived from Toxoplasma proteins GRA6, Tgd_057, ROP7 and GRA4 are presented to CD8 T cells (Blanchard et al., 2008; Feliu et al., 2013; Lee et al., 2015). Furthermore, in vitro NLRP3 is reported to be crucial for this CD8 T cell mediated immune response (Kongsomboonvech et al., 2020). Mice depleted of CD8 T cells show increased susceptibility in the chronic, but not acute, phase of infection (Denkers et al., 1997). Finally, CD4 and CD8 T cells restrain parasite growth by producing IFNγ, by contact dependent CD40-CD40L interaction mediated autophagy or by perforin-mediated lysis of infected cells (Gazzinelli et al., 1992; Denkers et al., 1997; Reichmann et al., 2000).
Species Differences in IFNγ-Mediated Parasite Elimination
The primary mechanism of IFNγ-induced cell autonomous immunity against Toxoplasma in murine cells is the induction of members of the p47 and p65 family of GTPases (also known as immunity related GTPases, or IRGs, and guanylate binding proteins, or GBPs, respectively). By acting cooperatively, these cause the disruption of the PV, leaving the parasites exposed to the host cell cytoplasm (Taylor et al., 2000; Collazo et al., 2001; Pawlowski et al., 2011; Yamamoto et al., 2012). This, in turn induces autophagy through ubiquitin and autophagy related proteins (ATG) dependent mechanisms (Ling et al., 2006; Choi et al., 2014), as well as the production of reactive oxygen and nitrogen species (Langermans et al., 1992; Arsenijevic et al., 2001) (Figure 1). Detailed mechanisms by which these pathways eliminate Toxoplasma have been reviewed elsewhere (Saeij and Frickel, 2017; Evans et al., 2018; Sasai and Yamamoto, 2019). The mechanism of IFNγ-induced cell autonomous immunity varies between murine and other hosts (Gazzinelli et al., 2014; Saeij and Frickel, 2017; Sasai and Yamamoto, 2019). For instance, while in murine cells the IRGs play a dominant role in killing Toxoplasma, humans and other animals do not have IFNγ-inducible IRGs (Hunn et al., 2011; Gazzinelli et al., 2014; Müller and Howard, 2016). Furthermore, in murine cells GBPs are recruited and cause the disruption of the PVM, while in human cells GBPs are not recruited to the PVM in most cell types except in mesenchymal stromal cells (MSCs), and are hypothesized to “work from a distance” (Yamamoto et al., 2012; Niedelman et al., 2013; Haldar et al., 2015; Selleck et al., 2015; Johnston et al., 2016; Qin et al., 2017; Saeij and Frickel, 2017; Fisch et al., 2019a). In many IFNγ-stimulated human and murine cell types, ubiquitin and its downstream adaptor proteins p62 and LC3B are recruited to the PVM resulting in parasite growth restriction either by endolysosomal fusion, inhibition of parasite replication or through further GBP-mediated parasite killing (Degrandi et al., 2013; Selleck et al., 2013, 2015; Choi et al., 2014; Haldar et al., 2015; Lee et al., 2015; Clough et al., 2016; Kravets et al., 2016; Foltz et al., 2017; Mukhopadhyay et al., 2020b). Furthermore, in human primary fibroblasts and macrophages, IFNγ-induced cell death can also restrict Toxoplasma growth (Niedelman et al., 2013; Fisch et al., 2019a). Additionally, in many human and bovine cell types, IFNγ induces indoleamine 2,3 dioxygenase 1 (IDO1)-mediated L-Tryptophan (L-Trp) breakdown, thus restricting Toxoplasma growth, since the parasite is auxotroph for this amino acid (Pfefferkorn, 1984; Pfefferkorn et al., 1986; Dai et al., 1994; Nagineni et al., 1996; Heseler et al., 2008; Schmidt et al., 2009; Spekker et al., 2009; Niedelman et al., 2013; Qin et al., 2017; Bando et al., 2018, 2019). By contrast, this pathway did not play any role in parasite growth restriction in murine cells (Schwartzman et al., 1990; Meisel et al., 2011). Nevertheless, IDO1 was induced in the acute phase of disease in murine models (Divanovic et al., 2012; Ufermann et al., 2019). Furthermore, in mice chronically infected with Toxoplasma, inhibition of IDO1 leads to encephalitis (Divanovic et al., 2012). This is possibly due to the fact that L-Trp acts as an essential element for T cell proliferation; indeed regulatory T cells (Tregs) often induce IDO to control excessive T cell proliferation (Puccetti and Grohmann, 2007; Yan et al., 2010). In rat enterocytes, parasite growth is inhibited in an iron-dependent way (Dimier and Bout, 1998). Some of these toxoplasmacidal mechanisms could be especially important in hosts that do not express functional GBP1, GBP2, or GBP5 (Table 1).
Toxoplasma Effectors
Toxoplasma strains differ remarkably in virulence and in the immune response they trigger (Sibley and Boothroyd, 1992; Melo et al., 2011; Hunter and Sibley, 2012; Su et al., 2012). Toxoplasma differences in attraction of particular cell types might explain variations in their capacity to disseminate, replicate or survive. This, in turn could directly contribute to strain-specific access to immunoprivileged sites such as the central nervous system or the fetus (Saeij et al., 2005). For example, type I strains exhibit a superior extracellular migratory capacity compared to type II or III strains (Barragan and Sibley, 2002). However, type II and, to a lesser extent, type III strains have an increased ability to use DCs as Trojan horses for dissemination compared to type I (Lambert et al., 2009). The difference in virulence between the major strains of Toxoplasma is due to multiple polymorphic effectors that act in concert to modulate murine immune responses at different steps, a subject that has been reviewed in-depth elsewhere (Melo et al., 2011; Boothroyd and Hakimi, 2020). The strain variation in specific phenotypes is not only limited to clonal types but is also present within the same haplogroups, possibly due to the presence of polymorphic effectors or differential expression of effector proteins (Khan et al., 2009; Melo et al., 2013; Yang et al., 2013). For instance, while the lab adapted type I RH strain does not activate NFκB due to the lack of a functional version of the polymorphic effector GRA15, another type I strain, GT1, does activate NFκB, as it expresses a functional GRA15 (Khan et al., 2009; Yang et al., 2013). RH also exhibits greater extracellular viability, faster replication and increased resistance against IFNγ compared to GT1, in which GRA15 recruits more p62 and LC3B on the PVM and promotes autophagy by interacting with TRAF6 (Khan et al., 2009; Mukhopadhyay et al., 2020b). As most studies use RH as the prototype for type I strains, one should be careful when extrapolating results to other type I strains (Khan et al., 2009; Yang et al., 2013). Similarly, these intra-clonal group variations are also observed within type II strains; for example the ME49 and Pru strains differ in the induction of CD40 on macrophages due to different GRA15 expression levels likely caused by polymorphisms in its promoter region (Morgado et al., 2014) (see Table 2 for other strain-specific effectors).
It is well-known that type II strains induce a much stronger pro-inflammatory response compared to type I and III strains (Robben et al., 2004; Jensen et al., 2011). Quantitative trait locus (QTL) analysis of host gene expression levels after infection with F1 progenies derived from IIxIII crosses led to the discovery that ROP16 (encoding a secreted kinase) (Saeij et al., 2007) and GRA15 (Rosowski et al., 2011) determine most Toxoplasma strain differences in the modulation of the inflammatory response in mice. Through the NFκB pathway, type II GRA15 (GRA15II) induces the production of inflammatory cytokines such as IL12 and IL1β, and elicits classically activated macrophages (M1) (Jensen et al., 2011; Gov et al., 2013; Mukhopadhyay et al., 2020a). These M1 macrophages are generally good at killing intracellular pathogens but their secretion of pro-inflammatory cytokines can cause pathology through an excessive activation of NK, Th1, and Th17 cells, and by inducing pro-apoptotic pathways (Murray et al., 2014). On the other hand, type I/III ROP16 (ROP6I/III) phosphorylates the transcription factors STAT3/6, driving macrophages toward an alternatively activated (or M2) state that is associated with increased IL10 expression and a Th2-polarized response (Yamamoto et al., 2009; Butcher et al., 2011; Jensen et al., 2013; Chen et al., 2020). These M2 macrophages are less efficient in killing intracellular pathogens (Murray et al., 2014). In addition, ROP16 can also inhibit GRA15-mediated activation of NFκB (Jensen et al., 2013). GRA24 binds to p38 mitogen activated kinase (p38 MAPK), leading to its phosphorylation, nuclear translocation and persistent activation (Braun et al., 2013). GRA24 induces inflammatory cytokine and chemokine secretion from macrophages, resulting in immune activation. All three major clonal populations of Toxoplasma express GRA24, however, it is in type II strains where GRA24, together with GRA15 and in the absence of active ROP16, can induce a heightened inflammatory immune response. GRA15 and GRA24-induced IL12 secretion by macrophages is achieved through the activation of the cREL NFκB pathway (Mukhopadhyay et al., 2020a). GRA15 and GRA24 together induce the secretion of IL18 and IL12, resulting in higher IFNγ secretion and lower parasite burden, while infection with parasites lacking these two effectors results in significantly lower IFNγ secretion and concomitantly higher peritoneal parasite burden in comparison to wild-type parasites (Mercer et al., 2020; Mukhopadhyay et al., 2020a). Thus, by inducing the IL12-IL18-IFNγ axis of host immunity, type II strains keep parasite numbers controlled, enabling persistence and further transmission to other hosts. Notably, while type I strains cause mortality primarily due to faster replication and uncontrolled parasite proliferation, type II strains can also cause disease due to their ability to induce an excessive pro-inflammatory response in the host that may lead to ileitis or encephalitis.
Toxoplasmic Ileitis in Mice
Within 8 days of oral ingestion of Toxoplasma tissue cysts or oocysts, susceptible mouse strains such as C57BL/6 develop severe ileitis resulting in necrosis of mucosal villi and tissue destruction. This is not limited to murine models, as several other animals infected naturally or experimentally with Toxoplasma have been reported to develop ileitis (Schreiner and Liesenfeld, 2009). This ileitis is not associated with high parasite burdens but rather with a strong Th1-biased immune response characterized by an overproduction of pro-inflammatory mediators including IFNγ, TNFα, IL1β, IL18, and NO, which is commonly known as “cytokine storm” (Liesenfeld et al., 1996, 1999). The initiation of the immune response starts within the small intestine, where the parasites first invade enterocytes. These cells secrete a variety of chemokines and cytokines, such as CCL2, CCL3, CCL4,and IL15, that attract immune cells including intraepithelial lymphocytes (IELs) (Khan and Kasper, 1996; Buzoni-Gatel et al., 1997; Mennechet et al., 2002) (Figure 2). Intestinal innate lymphoid cells (ILCs) also contribute to the immune response upon oral infection. Mice lacking the transcription factor T-bet have impaired production of IFNγ due to a deficiency of ILC1, failing to control parasite growth despite having NK cells (Klose et al., 2014). Furthermore, while macrophages and DCs mostly produce IL12 and IL18, in turn activating cytotoxic NK cells, CD8 T cells and IELs produce more IFNγ (Egan et al., 2009, 2011) (Figure 2). The effects of IL15 involve DC-mediated production of IL12 and subsequent priming and activation of CD4 and CD8 T cells (Khan et al., 2002; Combe et al., 2006). Different CD4 helper T cell subsets play a dominant role in this Th1-biased immunopathology during ileitis by secreting the inflammatory cytokines IL17 and IL22 (Muñoz et al., 2009; Guiton et al., 2010).
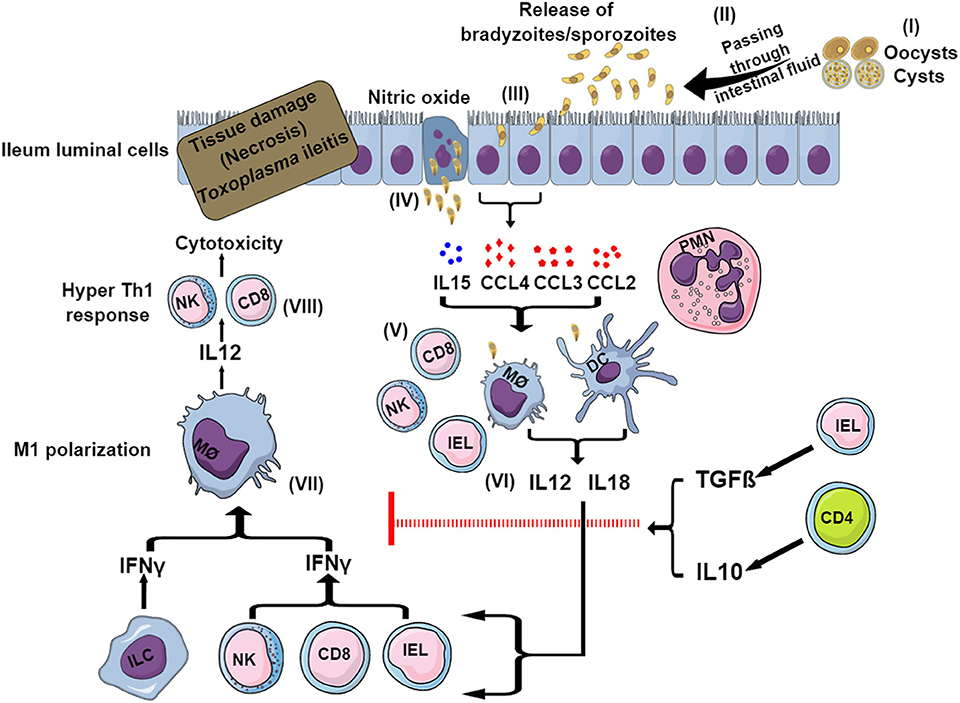
Figure 2. Model for sequential events during Toxoplasma-induced ileitis. Following ingestion of oocysts or tissue cysts, digestive enzymes break their wall and sporozoites or bradyzoites, respectively, are released (I). After invading intestinal epithelial cells, bradyzoites and sporozoites convert to tachyzoites (II) (III). Infected enterocytes release cytokines, chemokines and nitric oxides (IV) that attract and recruit neutrophils, macrophages, DCs, CD4, and CD8 T cells, NK cells, IELs, and ILCs (V). DCs and macrophages produce IL12 and IL18 (VI) that in turn stimulates ILCs, IELs, CD8 T cells and NK cells to secrete IFNγ (VII). This IFNγ causes the polarization of macrophages to the pro-inflammatory M1 phenotype. M1 macrophages release more IL12, which exacerbates the Th1-induced immunity (VIII), resulting in necrosis and tissue damage ultimately leading to ileitis. To control this exacerbated immune response, in certain murine strains (BALB/c) IELs and CD4 T cells are able to produce IL10 and TGFβ, which limit the inflammation and thus prevents the development of ileitis.
During Toxoplasma-induced ileitis, dramatic changes in the commensal gut flora have been observed, bearing a resemblance to inflammatory bowel disease (IBD) (Liesenfeld, 2002). The disturbance of the gut flora has been observed from day 5 post-infection (p.i.) when the epithelial barrier of the intestine shows morphological changes (Heimesaat et al., 2006). High abundance of gram negative bacteria in the intestine and their translocation into subepithelial tissues after oral infection with Toxoplasma increased TLR2- and TLR4-mediated sensing and amplified inflammation (Heimesaat et al., 2006, 2007). Thus, this breaching of the intestine by the gut flora acts as a molecular adjuvant that exacerbates the Th1 response regardless of the parasites. The role of the gut flora in Toxoplasma-induced ileitis was further demonstrated by two additional findings; firstly, prophylactic or therapeutic administration of antibiotics (ciprofloxacin and metronidazole) against the overgrowing gram negative bacteria improves the immunopathology by reducing the cytokine storm (Heimesaat et al., 2006); secondly, gnotobiotic mice do not develop ileitis following oral infection with Toxoplasma (Heimesaat et al., 2006). Furthermore, TLR11 deficient mice display no detectable pathology in the small intestine and other tissues, suggesting that TLR11 is responsible for the immunopathological response during toxoplasmosis (Benson et al., 2009). Additionally, when Tlr11−/− mice were infected with increasing doses of Toxoplasma, a significantly lower mortality was observed in comparison to wild-type mice (Benson et al., 2009). Nevertheless, how TLR11 contributes to toxoplasmic ileitis immunopathology remains elusive, as intraperitoneal infection of Tlr11−/− mice leads to severe pancreatitis, fat necrosis and systemic increase of inflammatory cytokines, which is at least partially related to Caspase1-mediated inflammasome driven IL18 secretion (Yarovinsky et al., 2008). The underlying mechanism of the opposing behaviors inTlr11−/− mice infected orally or intraperitoneally deserves further investigation. Apart from TLR2, TLR4, and TLR11, TLR9 also contributes to the ileitis immunopathology after oral infection with Toxoplasma (Minns et al., 2006; Benson et al., 2009). This is not surprising, as both parasite and bacterial DNA can activate the TLR9-signaling pathway. However, contradictory reports indicate that although wild-type and Tlr9−/− mice exhibit a comparable degree of ileitis upon oral Toxoplasma infection, the latter develops a higher parasite burden and higher IFNγ and NO levels (Bereswill et al., 2014). Although, the reasons for this discrepancy are not known, it is possible that an altered gut flora is present in mice bred at different colonies or acquired from different vendors, as previously observed by others (Ericsson et al., 2015; Alegre, 2019).
Control of Toxoplasmic Ileitis Related Immunopathology
To curb the hyper-inflammatory milieu generated by the gut flora and TLR-induced signaling, CD4 T cells play an important role by producing regulatory IL10, which causes a downregulation of IFNγ-induced responses (Figure 2). As a matter of fact, CD4 T cell and IL10 knockout mice succumb rapidly to oral cyst infection (Gazzinelli et al., 1996). Furthermore, IELs produce transforming growth factor (TGF)β, which downregulates the IFNγ response and inhibits chemokine production from enterocytes, thereby limiting the recruitment of more immune cells and reducing inflammation (Buzoni-Gatel et al., 2001; Mennechet et al., 2004) (Figure 2). IL27, another anti-inflammatory cytokine, also helps in reducing the inflammation. This was evident in WSX-1 (the IL27 receptor) deficient mice which displayed more severe immunopathology after Toxoplasma oral infection despite having a similar parasite burden compared to wild-type mice (Villarino et al., 2003). IL27 controls immunopathology by promoting IL10 production, by inhibiting CD4 T cell proliferation, by decreasing IL2 production and possibly also by increasing the inhibitory ligand programmed cell death ligand 1 (PD-L1) on CD4 T cells (Dupont et al., 2012).
Toxoplasma-Induced Ileitis Is Dependent on the Mouse Genetic Background
The aforementioned gut immunopathology has been observed in susceptible C57BL/6 mice, while BALB/c mice are in general resistant (Johnson, 1984; McLeod et al., 1989a; Liesenfeld et al., 1996; Subauste, 2012). This could be explained by the fact that T cells from C57BL/6 mice preferentially secrete Th1 cytokines with high IFNγ and low IL4 production, whereas those from BALB/c mice favor Th2 cytokine production with low IFNγ and high IL4 secretion (Hsieh et al., 1995). Moreover, BALB/c mice produce more anti-inflammatory IL10 to dampen the Th1 immunity (Suzuki et al., 2000). On the other hand, the fatal outcome upon oral infection in C57BL/6 mice is mainly caused by an excessive intestinal tissue damage produced by an hyper-inflammatory immune reaction and lower IL10 production that leads to a leakage of gut flora and a cytokine storm (Suzuki et al., 2000). Furthermore, macrophages from BALB/c mice produce less IL12 and TNFα than those from C57BL/6 mice when stimulated with Toxoplasma antigen or live parasites, as well as other microbial ligands (Schade and Fischer, 2001; Watanabe et al., 2004). BALB/c mice also produce significantly less IFNγ than C57BL/6 mice upon Toxoplasma infection (Shirahata et al., 1986). However, whether this also applies to Toxoplasma-induced ileitis is currently unknown and warrants further investigation.
Toxoplasmic Encephalitis
During the chronic phase, Toxoplasma cysts are controlled, but not eliminated, remaining largely quiescent for the life of the host. In immunocompromised patients, such as those with advanced AIDS, transplanted or under chemotherapy, cysts can reactivate and cause toxoplasmic encephalitis (TE). This progressive inflammatory disease is characterized by cyst rupture, tachyzoite conversion and parasite replication within the CNS, which often results in a fatal outcome in the absence of treatment (Blanchard et al., 2015; Schlüter and Barragan, 2019).
Clinical Signs
Clinical manifestations include headaches, fever, hemiparesis, ataxia, and seizures, among others (Navia et al., 1986; Porter and Sande, 1992; Marra, 2018). Moreover, one of the most intriguing effects of Toxoplasma chronic infection is the behavioral changes produced in various hosts (Berdoy et al., 2000; Webster, 2007; Webster et al., 2013; Boillat et al., 2020). This has been mainly investigated in mice, in which a variety of effects have been described, ranging from impaired movements, deficits in spatial learning and memory, higher activity and explorative behavior, reduced anxiety, and, most notably, reduced aversion to feline odors (Vyas, 2015; Tedford and McConkey, 2017; Schlüter and Barragan, 2019). This later phenomenon is commonly referred to as “fatal attraction” and in nature it is thought to enhance the likelihood of Toxoplasma transmission to its definitive feline host (Berdoy et al., 2000; Giacomini et al., 2007). Although it has been long assumed that this attraction was specific to felids, a recent report showed that Toxoplasma-infected mice lose their innate fear to several other animal odors and unknown objects as well (Boillat et al., 2020). Notwithstanding, the decreased aversion seems to be caused by complex mechanisms altering the perception of the host. Local neural tissue destruction caused directly through parasite multiplication or indirectly by an excessively inflammatory immune response may produce adverse effects. This is usually considered marginal, given the low density of cysts and the fact that they are randomly scattered in different areas of the CNS (Vyas and Sapolsky, 2010; Berenreiterová et al., 2011; Tedford and McConkey, 2017). The absence of a specific tropism for brain regions points toward a behavioral manipulation mediated by neuroinflammation rather than by direct interference of the parasite itself with specific areas of the brain (Martynowicz et al., 2019). In support of this, a positive correlation has been observed between the severity of behavioral alterations and the level of inflammation, which in turn is reflected by cyst loads (Boillat et al., 2020).
The cells and effectors involved in an adequate control of Toxoplasma and tissue damage in the brain are discussed in detail below. Nevertheless, the production of pro-inflammatory cytokines may alter the levels of several neuromodulators, including dopamine, glutamate and serotonin, thereby affecting the behavior (Webster and McConkey, 2010; Berenreiterová et al., 2011; David et al., 2016; Babaie et al., 2017). Other studies have also described that the parasite itself can influence neurotransmitter levels such as dopamine, likely contributing to the hyperactivity and enhanced novelty seeking in infected rodents (Skallová et al., 2006; Prandovszky et al., 2011). It is important to remark that data obtained in murine models do not necessarily apply to humans or other animals, since the immune response against Toxoplasma greatly differs as outlined earlier. Although it is out of the scope of this review, a considerable number of epidemiological studies have linked Toxoplasma infection with several mental illnesses such as schizophrenia, Parkinson's and bipolar disorders (Fabiani et al., 2015). However, there is no consensus as to whether Toxoplasma is a risk factor to mental illnesses or mental illnesses are risk factors to Toxoplasma infection. Moreover, these mental illnesses in humans are surely influenced by many other factors, which is also reflected by the lack of correlation between the incidence of mental illnesses and Toxoplasma prevalence in different regions of the world.
Blood-Brain Barrier, Cyst Location, and Cell Tropism
How Toxoplasma reaches the brain and invades neural tissues in the infected host is not completely understood. It is assumed that during the acute phase of infection tachyzoites are transported in the bloodstream/lymph and disseminate through the body either directly as extracellular parasites or by exploiting infected leukocytes as Trojan horses. In this process, the parasite TgWIP rhoptry protein enhances motility and transmigration abilities of infected DCs (Sangaré et al., 2019a). This effector is essential for the parasite to reach the brain, as mice infected with knockout parasites do not have cysts in the brain, at least after infection with the type II ME49 ΔTgwip strain (Sangaré et al., 2019a). Whether this applies to other strains or hosts, and the possible role of other virulence factors deserves further research. Regardless, once the parasite reaches the brain vasculature, it is believed that it can cross the blood-brain barrier (BBB) either as intracellular parasites using transmigrating infected cells as deliverers or directly as extracellular tachyzoites by transcytosis or paracytosis (Blanchard et al., 2015; Konradt et al., 2016; Wohlfert et al., 2017). A growing body of evidence suggests that rather than using infected leukocytes as true Trojan horses to cross the BBB, Toxoplasma uses them to reach the cerebral vasculature, subsequently egressing to contact endothelial cells and finally extracellular parasites directly cross the BBB following paracellular mechanisms (Drewry and Sibley, 2019). Indeed, it seems that parasites need to invade, replicate and lyse cerebral endothelial cells in order to reach the brain parenchyma (Konradt et al., 2016). In addition, cells infected with the non-replicating CPS strain were not observed in the brain after intravenous injection, supporting the notion that although the trojan horse mechanism is important for dissemination, it may not contribute to the crossing of the BBB. The ROP17 kinase promotes an integrin-independent interstitial migration of infected monocytes while inhibiting the integrin-dependent cross of biological barriers such as the BBB (Drewry et al., 2019). Hence, it is likely that, besides TgWIP, ROP17 is also important in type II and III strains (which have reduced extracellular dissemination abilities and rely mostly on the Trojan host mechanism) to reach the brain or other distant organs (Lambert et al., 2009). In support of this possibility, a recent study showed that the formation of tissue cysts in the brain of infected mice is almost completely abrogated in the type II Pru Δrop17 strain, which also had a decreased virulence (Fox et al., 2016). Taken together, these results suggest that the integrin-dependent crossing of the BBB (naturally inhibited by ROP17) is not the main mechanism used for type II strains, as otherwise it would be expected that in the absence of ROP17 the parasite had an enhanced transmigration through the BBB. However, it is also possible that the parasites are not even able to reach the brain because of the lack of ROP17-induced promotion of monocyte migration, or that other factors or mechanisms are also involved. For instance, the early egress phenotype observed in vitro for Pru Δrop17 parasites may preclude bradyzoite differentiation in vivo (Fox et al., 2016). Be that as it may, since these mechanisms are likely influenced by the host and strain involved in the infection (Barragan and Sibley, 2002; Lambert et al., 2009), further studies are warranted to investigate how Toxoplasma crosses the BBB.
Even under the same experimental conditions, the number and location of cysts largely varies among individuals (Berenreiterová et al., 2011; Boillat et al., 2020). However, a number of facts are consistently reported. Firstly, cyst numbers decline with time, likely due to reactivation and destruction by the immune system (Melzer et al., 2010; Watts et al., 2015), although the frequent observation of clusters of cysts of varying sizes also indicate that new or second-generation cysts can be formed during chronic infections (Melzer et al., 2010; Berenreiterová et al., 2011). Secondly, the distribution of cysts is bilateral, widespread and non-homogeneous, indicating an absence of targeted areas. Although there is no clear consensus on which regions are usually more affected, generally the gray matter and the cerebral cortex, especially the frontal cortex, harbors the great majority of cysts, while consistently low numbers are seen in the cerebellum (Melzer et al., 2010; Berenreiterová et al., 2011; Pittman et al., 2014; Boillat et al., 2020). Since the olfactory bulbs are contained in the frontal cortex region, the higher presence of the parasite in this area could explain the loss of feline odor aversion in infected mice (Berdoy et al., 1995; Torres et al., 2018). However, it appears that the accumulation of cysts in this region is not sufficient to account for this effect, but rather it seems to be a consequence of complex mechanisms altering the perception of the host (Boillat et al., 2020). Finally, cysts are found almost exclusively in neurons, and these are located in the neuronal processes rather than in the cell body (Ferguson and Hutchison, 1987a,b; Melzer et al., 2010; Cabral et al., 2016). Numerous in vitro studies using mouse and human brain cells have shown that, apart from neurons, the parasite is able to infect and encyst in astrocytes, microglia and Purkinje cells (Jones et al., 1986; Fischer et al., 1997; Halonen et al., 1998; Carruthers and Suzuki, 2007). Despite this, studies performed in chronically infected patients have described that, although it is possible to observe cysts in different brain cells, the great majority are found within neurons (Powell et al., 1978; Bertoli et al., 1995). Likewise, practically only neurons harbored cysts in chronically infected mice (Melzer et al., 2010; Cabral et al., 2016; Schlüter and Barragan, 2019). Why Toxoplasma, which is able to virtually invade all nucleated cells, has this predilection for neurons remains unclear. It is possible that the limited capacity of neurons for antigen presentation and responsiveness to IFNγ stimulation, together with their extensive size compared to other cells, provides the parasite with a safe niche to avoid the immune response and form large cysts, as opposed to astrocytes, which efficiently destroy the parasite (Schlüter et al., 2001; Blanchard et al., 2015; Cabral et al., 2016; Wohlfert et al., 2017).
Immune Response in the Brain During Chronic Infection
During the chronic phase of infection, a continuous and effective immune response is required to keep the parasite under control and prevent the reactivation of cysts (Wohlfert et al., 2017). Hence, under immunocompromised circumstances the periodic cyst rupture and parasite conversion back to tachyzoites are uncontrolled, leading to massive tissue destruction and inflammation of the brain (Carruthers and Suzuki, 2007). This life-threatening TE can also occur in immunocompetent patients in some instances, such as in congenitally infected individuals or infections produced by non-archetypal strains, where an exacerbated immune response produces excessive inflammation leading to tissue damage and neurological signs (Carme et al., 2009; Hamilton et al., 2019).
Mouse models, as well as observations made in AIDS patients, show that the type of immune response required to prevent reactivation in the brain and to control acute infections are similar. Indeed, IFNγ and other pro-inflammatory cytokines are needed to control parasite burden; however without regulatory and anti-inflammatory cytokines the highly activated immune cells filtering into the brain could produce encephalitis regardless of parasite burden (Landrith et al., 2015). CD8 and, to a lesser extent, CD4 T cells play a critical role in controlling infection in the brain during chronic toxoplasmosis (Gazzinelli et al., 1992; Luft and Remington, 1992). CD8 T cells secrete cytokines involved in parasiticidal effects, in particular IFNγ and TNFα (Fischer et al., 2000; Schlüter and Barragan, 2019), and can directly control cyst burdens in the CNS via perforin-mediated cell lysis, although this effect seems to be limited and independent of IFNγ (Denkers et al., 1997; Wang et al., 2004; Suzuki et al., 2010, 2011; Landrith et al., 2015). In the course of TE with ongoing inflammation, the permeability of the BBB is likely high and accompanied by a continuous influx of immune cells from the periphery (Blanchard et al., 2015). These inflammatory infiltrates are mainly composed of CD4 and CD8 T cells, as well as macrophages, CD11c+ DCs, and Ly6Chigh monocytes (Schlüter and Barragan, 2019). Immune cells may cross the BBB through a process involving slowing, adhesion and extravasation (diapedesis) into the perivascular space. This is achieved by their interaction with several molecules expressed on endothelial cells of the cerebral vasculature, including adhesins, integrins, selectins, or metalloproteinases (Wilson et al., 2010; Clark et al., 2011; Suzuki et al., 2011; Landrith et al., 2015). Among them, the vascular cell adhesion molecule 1 (VCAM-1) plays a pivotal role in the recruitment of T-cells to the CNS by interacting with its main ligand VLA-4, an α4β1 integrin that is expressed on activated T-cells (Carrithers et al., 2000; Wang et al., 2007). Moreover, increased expression of VCAM-1 in cerebral vessels is partially dependent on IFNγ (and possibly TNFα and IL1β) levels, acting as a pro-inflammatory feedback mechanism (Wang et al., 2007; Suzuki et al., 2011). Leukocytes can also migrate to the interstitium using integrin-independent mechanisms, such as blebbing, deformation-based movement and membrane flow (Fackler and Grosse, 2008; Renkawitz and Sixt, 2010; Paluch et al., 2016). Different studies have shown that this integrin-independent migration occurs for DCs, neutrophils and T-cells (Woolf et al., 2007; Lämmermann et al., 2008, 2013). Apart from the aforementioned role in the recruitment of T-cells into the brain through the increased expression of VCAM-1 in endothelial cells, IFN-γ can activate other non-T cells such as microglia, astrocytes and macrophages, in turn developing different mechanisms to control the proliferation of the parasite (Suzuki et al., 2005, 2011). Moreover, astrocytes, microglia and neurons residing in the brain contribute to the control of TE by the production of cytokines, chemokines and by expressing immunoregulatory cell surface molecules (Blanchard et al., 2015).
Several in vitro and in vivo studies have shown that IFNγ-activated astrocytes control TE in a STAT1-dependent manner and are able to produce different cytokines and chemokines such as IL1β, IL-6, granulocyte-macrophage colony-stimulating factor (GM-CSF), CXCL10 or CCL2 (Fischer et al., 1997; Strack et al., 2002; Hidano et al., 2016; Wohlfert et al., 2017; Schlüter and Barragan, 2019). Astrocytes have also a role in limiting the lesions size and necrosis by encircling inflammatory infiltrates around cysts (Wilson and Hunter, 2004; Drögemüller et al., 2008; Melzer et al., 2010). Other in vitro reports have observed that different mediators act on astrocytes depending on the host. For example, in human astrocytes and glioblastoma cells, tachyzoites are killed through nitric oxide (NO) and its growth is inhibited by the upregulation of IDO through the synergistic action of IFNγ and TNFα (Däubener et al., 1996; Suzuki et al., 2011; Bando et al., 2019). In mice, on the other hand, is seems that the Toxoplasma inhibitory effects of astrocytes are mediated by IRGM3 (IGTP) and IRGA6 (IIGP1), which disrupt the PV (Halonen et al., 1998, 2001; Martens et al., 2005; Melzer et al., 2008). Brain resident macrophages, also known as microglia, are also important in the control of TE, as these are the only brain cells that express MHC-II, thus acting as professional APCs that tune T-cell protective responses (Blanchard et al., 2015). Similar to astrocytes, both NO-dependent and independent mechanisms appear to be involved in the inhibitory effects of microglia on TE, at least in mice (Chao et al., 1993; Deckert-Schlüter et al., 1999; Schlüter et al., 1999; Rozenfeld et al., 2005). IFNγ-activated microglia also produce TNFα that exerts an important effect on the resistance against parasite reactivation during chronic toxoplasmosis (Blanchard et al., 2015). Moreover, a distinct population of alternatively activated brain macrophages/microglia are able to destroy Toxoplasma cysts by secreting the acidic mammalian chitinase (AMCase), an enzyme produced in response to the structural chitin present in the cyst wall (Nance et al., 2012). The chitin-mediated attack by these M2 macrophages releases parasites from latent cysts, providing a constant antigenic stimulation for the immune response and likely explaining the continuous recruitment of T cells into the brain during chronic toxoplasmosis. Microglia are also critical during TE for their immunoregulatory properties mediated by GM-CSF and TGFβ to avoid excessive inflammation and tissue damage (Hunter et al., 1992; Chao et al., 1993, 1994; Fischer et al., 1993). Finally, infiltrating DCs and NK cells are also important to control TE (Landrith et al., 2015). The former are the main producers of IL12, which is crucial for the maintenance of IFNγ production by T-cells, and may also act as APCs to further activate T-cells (Fischer et al., 2000; John et al., 2011; Blanchard et al., 2015). The role of NK cells in Toxoplasma chronic infection and TE is not well-understood, although it is possible that they are recruited to the brain and represent an important source of IFNγ (Suzuki, 2002; Pittman et al., 2014).
To prevent immunopathology while controlling parasite reactivation, a delicate balance between a protective pro-inflammatory and a dampening regulatory response is needed. In this sense, immunosuppressive and regulatory cytokines are important for the downregulation of inflammatory reactions during the chronic stage of Toxoplasma infection. For instance, recruited monocytes secrete IL10 to prevent an excessive immunopathological response in the inflamed brain during Toxoplasma chronic stage in mice (Deckert-Schlüter et al., 1997; Biswas et al., 2015). Similarly, astrocytes have been shown to produce IL27, which is critical to suppress the development of Th17 cells and inhibit the toxic effects of IL17 occurring in TE (Stumhofer et al., 2006; Schlüter and Barragan, 2019). TGFβ signaling activation in astrocytes and microglia is also important to prevent neuroinflammation during TE (Cekanaviciute et al., 2014). This regulatory cytokine seems to be secreted by infected neurons (Schlüter et al., 2001; Händel et al., 2012). Other studies have described the role of IL33 and Lipoxin A4 (LXA4) in the downregulation of IFNγ and IL12 production, respectively, during Toxoplasma chronic infection (Aliberti et al., 2002; Machado and Aliberti, 2006; Jones et al., 2010).
Influence of the Host on Toxoplasmic Encephalitis
As mentioned above, the cytokine-induced mechanisms taking place during TE differ between animal species. Most studies have been conducted in mice and humans, and even within mice, the functional relevance of anti-parasitic effector molecules depends on the mouse strain (Schlüter and Barragan, 2019). For example, while NO is essential for the control of intracerebral Toxoplasma in susceptible C57BL/6 mice, deletion of iNOS in the resistant BALB/c mice does not exacerbate TE (Schlüter et al., 1999). Moreover, the resistance to development of TE in mice is influenced by the genetic background of the strain and was mapped almost 30 years ago to the Ld region of the MHC class I H-2 gene (Suzuki et al., 1991, 1994; Brown et al., 1995). Mice with b (e.g., C57BL/6) or k (e.g., CBA/Ca) alleles at the H-2 region develop severe encephalitis during the chronic stage of Toxoplasma infection. By contrast, those mice strains with H-2a (e.g., A/J) or H-2d (e.g., BALB/c) haplotypes are resistant to the infection, harbor fewer cysts in the brain and rarely show signs or excessive inflammation (Suzuki et al., 1991). Hence, mice with the H-2d haplotype would be the most appropriate models to study human chronic toxoplasmosis in immunocompetent patients. The molecular basis underlying this mouse strain difference is that the immunodominant decamer epitope (HF10) located at the C-terminus of GRA6 elicits a strong and protective CD8 immune response through its presentation exclusively by the H-2 Ld (Blanchard et al., 2008; Feliu et al., 2013). Besides being very immunogenic, this region of GRA6 presents a high polymorphism between strains; in fact, peptides derived from this region have been successfully used for serotyping techniques (e.g., Kong et al., 2003; Arranz-Solís et al., 2019). Only the type II specific HF10 sequence (HPGSVNEFDF) binds to H-2d in CD8 T-cells, providing protection by controlling parasite burden. This does not occur in the GRA6 type I/III version of the epitope (HY10, HPERVNVFDY) or with the H-2b or k alleles in susceptible mice. Hence, the recognition of the GRA6 HF10 peptide by CD8 T-cells may account for the differences in virulence among mouse strains during chronic toxoplasmosis, and might also explain why subdominant responses, such as CD4 T-cells, could not be sufficient to control the parasite. In humans, some studies pointed out that the MHC II Human Leukocyte Antigen (HLA)-DQ3 and DQ1 could be genetic markers of susceptibility and resistance, respectively, to cerebral toxoplasmosis in AIDS and congenitally infected patients (Suzuki et al., 1996; Mack et al., 1999). Therefore, both in mice and humans the regulation of the immune response by MHC genes appears to be important for the fate of the host during chronic toxoplasmosis.
Influence of the Parasite Strain on Toxoplasmic Encephalitis
The parasite strain causing the infection plays a major role in the final outcome of chronic toxoplasmosis. The difference in virulence and immune response elicited during the acute phase strongly influences the fate of the host during the chronic infection. Although few studies have directly investigated how specific virulence factors affect Toxoplasma infection in the brain, ROP proteins seem to exert a strong effect on the outcome of infection. In a recent study, the role of several ROPs was investigated by infecting mice with individual knockouts in the Pru type II strain and comparing virulence and cyst formation (Fox et al., 2016). ROP16 deletion increased the parasite burden in the brain, while ROP5, ROP17, and ROP18 deletion produced an almost complete ablation of cyst formation. However, one has to be careful with the interpretation of these results, as reduced cyst numbers could be due to fewer parasites reaching the brain, because of increased susceptibility to immune clearance, or because of reduced dissemination to the brain. For example, the ~100-fold decrease in virulence of Δrop5 and Δrop18 type II strains likely led to fewer parasites reaching the brain (Fox et al., 2016). Similarly, as ROP17 plays an important role in parasite dissemination by increasing infected monocyte migratory capacities (Fox et al., 2016; Drewry et al., 2019) and in the export of GRA effectors beyond the PVM (Panas et al., 2019), it is likely that the defect in cyst formation of Δrop17 parasites was due to decreased dissemination and virulence. In another study, compared to type II Pru-infected mice, type III CEP-infected mice showed a larger number of macrophages/microglia and infiltrating T cells, as well as increased levels of pro-inflammatory cytokines in the brain, while the number of M2 macrophages and Treg cells was lower (Tuladhar et al., 2019). This is in clear contrast to other reports analyzing the acute infection both in vitro and in vivo, where macrophages infected with type III parasites show M2-polarized phenotypes (Jensen et al., 2011, 2013; Chen et al., 2020). This suggests that the immune response against Toxoplasma evolves over time, as previously reported in type I strains (Mordue et al., 2001). Nonetheless, caution is needed when comparing these studies, as Tuladhar et al. (2019) did not directly analyze the phenotype of infected macrophages but rather the entire population.
In addition, macrophages have been shown to play a dual role in initiating and subsequently resolving inflammation with the coexistence of M1 and M2 activation programs in response to Toxoplasma infection (Patil et al., 2014). Early after infection, type III-infected mice have more M2 macrophages compared to type II-infected mice, which induce a stronger acute pro-inflammatory response. In CEP-infected mice, on the other hand, the initial response switches to a more pro-inflammatory profile after 21 days p.i., when the chronic infection is starting to establish (Tuladhar et al., 2019). ROP16 was the factor responsible for this change in the immune response over time, as the CEP Δrop16 strain showed a typical type II immune response profile in the brain, with fewer T-cell and macrophage infiltrates and an increased M2 phenotype. Furthermore, CEP Δrop16-infected animals showed a mixed acute immune response with increased pro-inflammatory macrophages but also M2 macrophages and Tregs, hence its rapid clearance and lowered cyst burden compared to the wild-type (Tuladhar et al., 2019). Since type III strains do not express ROP18, they are very sensitive to mouse IRGs (Fentress et al., 2010; Niedelman et al., 2012), which makes ROP16 crucial in these strains to dampen the initial immune response early after infection and thus avoid rapid clearance. In contrast to ROP16I/III, the inactive version of ROP16 expressed by type II strains (ROP16II) does not seem to be important for the chronic stage of infection in these strains (Fox et al., 2016).
In addition to ROPs, GRA proteins have also been described to play an important role in the chronic phase of infection, some of them in a strain-specific manner. For example, Bando et al., showed that type II GRA15 suppresses IFNγ-mediated anti-Toxoplasma responses in several human liver and brain cell lines (including neurons, glioblastoma, and neuroblastoma cell lines) when co-cultured with monocytes. Namely, GRA15 induces the expression of IL1β by monocytes that in turn promotes iNOS expression in the brain cell lines, which inhibits IDO1-mediated tryptophan degradation, thus facilitating parasite proliferation (Bando et al., 2019). Two other factors involved in parasite dissemination in a strain-specific manner are GRA6 and GRA25 via their effect on CCL2 induction (Ma et al., 2014; Shastri et al., 2014). Only the C-terminus GRA6 epitope from type I and III strains elicit NFAT4 activation leading to CCL2 secretion (Ma et al., 2014). However, as mentioned above, this polymorphic epitope is also crucial in the induction of CD8 T cell responses, but in this case the type II version is the one exerting this effect (Blanchard et al., 2008; Feliu et al., 2013). Therefore, it seems that type II strains have high CD8 T cell activation but a reduced NFAT4-dependent attraction of other immune cells. In place of GRA6, type II strains might elicit a production of chemokines and attraction of monocytes through GRA25 (Shastri et al., 2014). Since GRA25 is equally expressed by tachyzoites and bradyzoites, it is possible that it plays an important role in the control of the parasite in the brain during the chronic phase of infection.
In summary, an effective control of TE depends on IFNγ and CD8 T-cells, while a correct immunoregulation is critical to control excessive inflammatory response in the brain. Of note, since human studies cannot be performed in vivo, most research on the mechanisms of intracerebral Toxoplasma control and immune response have been conducted in vitro or in mice. Thus, caution is needed when extrapolating results obtained with these models, as there are significantly different genetic backgrounds and immune mechanisms involved. Moreover, given the strong influence of both the host and the parasite strain on the outcome of chronic toxoplasmosis, it is possible that specific combinations can uniquely affect the course and pathology of the disease. Nevertheless, a further understanding of the association between strain, immune response and clinical manifestation is needed, especially in severe TE or ocular toxoplasmosis. This, together with an accurate diagnosis would in turn allow an individualized monitorization and improved treatments in risk groups.
Concluding Remarks and Future Directions
Toxoplasmosis is a disease that varies enormously in its clinical form, ranging from asymptomatic cases to lethal consequences. In addition to the importance of Toxoplasma infections in livestock that causes important economic losses, it also poses a risk to humans by direct exposure to contaminated animal products. The variable outcome of infection is strongly dependent on the Toxoplasma strain producing the infection, the host and its immune response. In the last decades advancements have been made to understand the mechanisms underlying these differences. Although in this review we focused on the current knowledge of host and parasite factors affecting the outcome of the disease and the control of the parasite, most studies have been performed in murine models and using laboratory adapted strains that do not reflect natural conditions of infection. In addition, most effectors that are important in mice for the control of Toxoplasma play no role in other animal species, including humans; thus, the results obtained in these models have to be carefully considered and extrapolations are not always possible.
There are still many questions to be answered: for instance, why is there an intrinsic variability between individuals from the same species? Why are some Toxoplasma strains extremely virulent in some hosts and totally controlled in others? What is the most critical component of the immune response that makes some hosts resistant to Toxoplasma infection? Arguably, many other factors besides the parasite strain and the host also play an important role and account for these differences, such as the infective dose, the immunological status or genetic individual differences. Moreover, although we have focused on toxoplasmic ileitis and encephalitis, Toxoplasma also causes other important diseases in humans such as ocular toxoplasmosis, congenital infection or abortions in pregnant women. Each one of the clinical forms deserve individual investigation to uncover the most relevant host and parasite factors affecting the outcome of infection. Finally, experimental models beyond the murine ones would greatly improve our understanding of the host-parasite relation in toxoplasmosis and, more importantly, would help to elucidate the mechanisms of resistance in humans, with potential applications in terms of new vaccine or drug targets.
Author Contributions
DM, DA-S, and JPJS have contributed to the conceptualization, writing, and editing of this manuscript. All authors contributed to the article and approved the submitted version.
Funding
This study was supported by the National Institutes of Health (R01-AI080621) awarded to JPJS. DM was supported by the American Heart Association Post-doctoral fellowship (18POST34030036). The funders had no role in study design, data collection and analysis, decision to publish, or preparation of the manuscript.
Conflict of Interest
The authors declare that the research was conducted in the absence of any commercial or financial relationships that could be construed as a potential conflict of interest.
References
Abdallah, D. S. A., Lin, C., Ball, C. J., King, M. R., Duhamel, G. E., and Denkers, E. Y. (2012). Toxoplasma gondii triggers release of human and mouse neutrophil extracellular traps. Infect. Immun. 80, 768–777. doi: 10.1128/IAI.05730-11
Ajzenberg, D., Cogné, N., Paris, L., Bessières, M., Thulliez, P., Filisetti, D., et al. (2002). Genotype of 86 Toxoplasma gondii isolates associated with human congenital toxoplasmosis, and correlation with clinical findings. J. Infect. Dis. 186, 684–689. doi: 10.1086/342663
Akira, S., Uematsu, S., and Takeuchi, O. (2006). Pathogen recognition and innate immunity. Cell 124, 783–801. doi: 10.1016/j.cell.2006.02.015
Alegre, M.-L. (2019). Mouse microbiomes: overlooked culprits of experimental variability. Genome Biol. 20:108. doi: 10.1186/s13059-019-1723-2
Aliberti, J., Serhan, C., and Sher, A. (2002). Parasite-induced lipoxin A4 is an endogenous regulator of IL-12 production and immunopathology in Toxoplasma gondii infection. J. Exp. Med. 196, 1253–1262. doi: 10.1084/jem.20021183
Andrade, R. M., Wessendarp, M., Gubbels, M. J., Striepen, B., and Subauste, C. S. (2006). CD40 induces macrophage anti–Toxoplasma gondii activity by triggering autophagy-dependent fusion of pathogen-containing vacuoles and lysosomes. J. Clin. Invest.116, 2366–2377. doi: 10.1172/JCI28796
Andrade, R. M., Wessendarp, M., and Subauste, C. S. (2003). CD154 activates macrophage antimicrobial activity in the absence of IFN-gamma through a TNF-alpha-dependent mechanism. J. Immunol. 171, 6750–6756. doi: 10.4049/jimmunol.171.12.6750
Andrade, W. A., Souza, M., Do, C., Ramos-Martinez, E., Nagpal, K., Dutra, M. S., et al. (2013). Combined action of nucleic acid-sensing toll-like receptors and TLR11/TLR12 heterodimers imparts resistance to Toxoplasma gondii in mice. Cell. Host. Microbe 13, 42–53. doi: 10.1016/j.chom.2012.12.003
Arranz-Solís, D., Cordeiro, C., Young, L. H., Dardé, M. L., Commodaro, A. G., Grigg, M. E., et al. (2019). Serotyping of Toxoplasma gondii infection using peptide membrane arrays. Front. Cell. Infect. Microbiol. 9:408. doi: 10.3389/fcimb.2019.00408
Arsenijevic, D., Bilbao, F. D., Giannakopoulos, P., Girardier, L., Samec, S., and Richard, D. (2001). A role for interferon-gamma in the hypermetabolic response to murine toxoplasmosis. Eur. Cytok. Netw. 12, 518–527.
Babaie, J., Sayyah, M., Fard-Esfahani, P., Golkar, M., and Gharagozli, K. (2017). Contribution of dopamine neurotransmission in proconvulsant effect of Toxoplasma gondii infection in male mice. J. Neurosci. Res. 95, 1894–1905. doi: 10.1002/jnr.24036
Bando, H., Lee, Y., Sakaguchi, N., Pradipta, A., Sakamoto, R., Tanaka, S., et al. (2019). Toxoplasma effector GRA15-dependent suppression of IFN-γ-induced antiparasitic response in human neurons. Front. Cell Infect. Microbiol. 9:140. doi: 10.3389/fcimb.2019.00140
Bando, H., Sakaguchi, N., Lee, Y., Pradipta, A., Ma, J. S., Tanaka, S., et al. (2018). Toxoplasma effector TgIST targets host IDO1 to antagonize the IFN-γ-induced anti-parasitic response in human cells. Front. Immunol. 9:2073. doi: 10.3389/fimmu.2018.02073
Barragan, A., and Sibley, L. D. (2002). Transepithelial migration of Toxoplasma gondii is linked to parasite motility and virulence. J. Exp. Med. 195, 1625–1633. doi: 10.1084/jem.20020258
Behnke, M. S., Fentress, S. J., Mashayekhi, M., Li, L. X., Taylor, G. A., and Sibley, L. D. (2012). The polymorphic pseudokinase ROP5 controls virulence in Toxoplasma gondii by regulating the active kinase ROP18. PLoS Pathog. 8:e1002992. doi: 10.1371/journal.ppat.1002992
Behnke, M. S., Khan, A., Wootton, J. C., Dubey, J. P., Tang, K., and Sibley, L. D. (2011). Virulence differences in Toxoplasma mediated by amplification of a family of polymorphic pseudokinases. Proc. Natl. Acad. Sci. U.S.A. 108, 9631–9636. doi: 10.1073/pnas.1015338108
Benson, A., Pifer, R., Behrendt, C. L., Hooper, L. V., and Yarovinsky, F. (2009). Gut commensal bacteria direct a protective immune response against Toxoplasma gondii. Cell. Host. Microbe 6, 187–196. doi: 10.1016/j.chom.2009.06.005
Berdoy, M., Webster, J. P., and Macdonald, D. W. (1995). Parasite-altered behaviour: is the effect of Toxoplasma gondii on Rattus norvegicus specific? Parasitology 111, 403–409. doi: 10.1017/S0031182000065902
Berdoy, M., Webster, J. P., and Macdonald, D. W. (2000). Fatal attraction in rats infected with Toxoplasma gondii. Proc. Roy. Soc. Lond. Ser. B Biol. Sci. 267, 1591–1594. doi: 10.1098/rspb.2000.1182
Berenreiterová, M., Flegr, J., Kuběna, A. A., and Němec, P. (2011). The distribution of Toxoplasma gondii cysts in the brain of a mouse with latent toxoplasmosis: Implications for the behavioral manipulation hypothesis. PLoS ONE 6:28925. doi: 10.1371/journal.pone.0028925
Bereswill, S., Kühl, A. A., Alutis, M., Fischer, A., Möhle, L., Struck, D., et al. (2014). The impact of Toll-like-receptor-9 on intestinal microbiota composition and extra-intestinal sequelae in experimental Toxoplasma gondii induced ileitis. Gut Pathog. 6:19. doi: 10.1186/1757-4749-6-19
Bertoli, F., Espino, M., Arosemena, J. R. V., Fishback, J. L., and Frenkel, J. K. (1995). A spectrum in the pathology of toxoplasmosis in patients with acquired immunodeficiency syndrome. Arch. Pathol. Lab. Med. 119, 214–224.
Biswas, A., Bruder, D., Wolf, S. A., Jeron, A., Mack, M., Heimesaat, M. M., et al. (2015). Ly6C(high) monocytes control cerebral toxoplasmosis. J. Immunol. 194, 3223–3235. doi: 10.4049/jimmunol.1402037
Blader, I. J., Coleman, B. I., Chen, C. T., and Gubbels, M.-J. (2015). Lytic cycle of Toxoplasma gondii : 15 years later. Annu. Rev. Microbiol. 69, 463–485. doi: 10.1146/annurev-micro-091014-104100
Blanchard, N., Dunay, I. R., and Schlüter, D. (2015). Persistence of Toxoplasma gondii in the central nervous system: a fine-tuned balance between the parasite, the brain and the immune system. Parasite Immunol. 37, 150–158. doi: 10.1111/pim.12173
Blanchard, N., Gonzalez, F., Schaeffer, M., Joncker, N. T., Cheng, T., Shastri, A. J., et al. (2008). Immunodominant, protective response to the parasite Toxoplasma gondii requires antigen processing in the endoplasmic reticulum. Nat. Immunol. 9, 937–944. doi: 10.1038/ni.1629
Boillat, M., Hammoudi, P.-M., Dogga, S. K., Pagès, S., Goubran, M., Rodriguez, I., et al. (2020). Neuroinflammation-associated aspecific manipulation of mouse predator fear by Toxoplasma gondii. Cell Rep. 30, 320–334.e6. doi: 10.1016/j.celrep.2019.12.019
Boothroyd, J. C., and Hakimi, M. -,A. (2020). Effectors produced by rhoptries and dense granules: an intense conversation between parasite and host in many languages. Toxoplasma gondii 2000, 789–806. doi: 10.1016/B978-0-12-815041-2.00017-7
Braun, L., Brenier-Pinchart, M.-P., Yogavel, M., Curt-Varesano, A., Curt-Bertini, R.-L., Hussain, T., et al. (2013). A Toxoplasma dense granule protein, GRA24, modulates the early immune response to infection by promoting a direct and sustained host p38 MAPK activation. J. Exp. Med. 210, 2071–2086. doi: 10.1084/jem.20130103
Brinkmann, V., Reichard, U., Goosmann, C., Fauler, B., Uhlemann, Y., Weiss, D. S., et al. (2004). Neutrophil extracellular traps kill bacteria. Science 303, 1532–1535. doi: 10.1126/science.1092385
Brown, C. R., Hunter, C. A., Estes, R. G., Beckmann, E., Forman, J., David, C., et al. (1995). Definitive identification of a gene that confers resistance against Toxoplasma cyst burden and encephalitis. Immunology 85, 419–428.
Butcher, B. A., Fox, B. A., Rommereim, L. M., Kim, S. G., Maurer, K. J., Yarovinsky, F., et al. (2011). Toxoplasma gondii rhoptry kinase ROP16 activates STAT3 and STAT6 resulting in cytokine inhibition and arginase-1-dependent growth control. PLoS Pathog. 7:1002236. doi: 10.1371/journal.ppat.1002236
Butcher, B. A., Reese, M. L., Boothroyd, J. C., and Denkers, E. Y. (2014). Interactions between toxoplasma effectors and host immune responses. Toxoplasma gondii 2008, 505–519. doi: 10.1016/B978-0-12-396481-6.00014-3
Buzoni-Gatel, D., Debbabi, H., Mennechet, F. J. D., and Martin, V. (2001). Murine ileitis after intracellular parasite infection is controlled by TGF-β-producing intraepithelial lymphocytes. Gastroenterology. 20, 914–924. doi: 10.1053/gast.2001.22432a
Buzoni-Gatel, D., Lepage, A. C., Dimier-Poisson, I. H., Bout, D. T., and Kasper, L. H. (1997). Adoptive transfer of gut intraepithelial lymphocytes protects against murine infection with Toxoplasma gondii. J. Immunol. 158, 5883–5889.
Cabral, C. M., Tuladhar, S., Dietrich, H. K., Nguyen, E., MacDonald, W. R., Trivedi, T., et al. (2016). Neurons are the primary target cell for the brain-tropic intracellular parasite Toxoplasma gondii. PLoS Pathog. 12:e1005447. doi: 10.1371/journal.ppat.1005447
Cai, G., Kastelein, R., and Hunter, C. A. (2000). Interleukin-18 (IL-18) enhances innate IL-12-mediated resistance to Toxoplasma gondii. Infect. Immun. 68, 6932–6938. doi: 10.1128/IAI.68.12.6932-6938.2000
Carme, B., Demar, M., Ajzenberg, D., Dardé, M. L., Darde, M. L., Dardé, M. L., et al. (2009). Severe acquired toxoplasmosis caused by wild cycle of Toxoplasma gondii, French Guiana. Emerg. Infect. Dis. 15, 656–658. doi: 10.3201/eid1504.081306
Carrithers, M. D., Visintin, I., Kang, S. J., and Janeway, C. A. (2000). Differential adhesion molecule requirements for immune surveillance and inflammatory recruitment. Brain 123, 1092–1101. doi: 10.1093/brain/123.6.1092
Carruthers, V. B., and Suzuki, Y. (2007). Effects of Toxoplasma gondii infection on the brain. Schizophr. Bull. 33, 745–751. doi: 10.1093/schbul/sbm008
Cavaillès, P., Flori, P., Papapietro, O., Bisanz, C., Lagrange, D., Pilloux, L., et al. (2014). A highly conserved Toxo1 haplotype directs resistance to toxoplasmosis and its associated caspase-1 dependent killing of parasite and host macrophage. PLoS Pathog. 10:e1004005. doi: 10.1371/journal.ppat.1004005
Cavaillès, P., Sergent, V., Bisanz, C., Papapietro, O., Colacios, C., Mas, M., et al. (2006). The rat Toxo1 locus directs toxoplasmosis outcome and controls parasite proliferation and spreading by macrophage-dependent mechanisms. Proc. Natl. Acad. Sci. U.S.A. 103, 744–749. doi: 10.1073/pnas.0506643103
Cekanaviciute, E., Dietrich, H. K., Axtell, R. C., Williams, A. M., Egusquiza, R., Wai, K. M., et al. (2014). Astrocytic TGF-β signaling limits inflammation and reduces neuronal damage during central nervous system Toxoplasma infection. J. Immunol. 193, 139–149. doi: 10.4049/jimmunol.1303284
Chao, C. C., Anderson, W. R., Hu, S., Gekker, G., Martella, A., and Peterson, P. K. (1993). Activated microglia inhibit multiplication of Toxoplasma gondii via a nitric oxide mechanism. Clin. Immunol. Immunopathol. 67, 178–183. doi: 10.1006/clin.1993.1062
Chao, C. C., Gekker, G., Hu, S., and Peterson, P. K. (1994). Human microglial cell defense against Toxoplasma gondii. The role of cytokines. J. Immunol. 152, 1246–1252.
Chen, L., Christian, D. A., Kochanowsky, J. A., Phan, A. T., Clark, J. T., Wang, S., et al. (2020). The Toxoplasma gondii virulence factor ROP16 acts in cis and trans, and suppresses T cell responses. J. Exp. Med. 217:e20181757. doi: 10.1084/jem.20181757
Choi, J., Park, S., Biering, S. B., Selleck, E., Liu, C. Y., Zhang, X., et al. (2014). The Parasitophorous vacuole membrane of Toxoplasma gondii is targeted for disruption by ubiquitin-like conjugation systems of autophagy. Immunity 40, 924–935. doi: 10.1016/j.immuni.2014.05.006
Cirelli, K. M., Gorfu, G., Hassan, M. A., Printz, M., Crown, D., Leppla, S. H., et al. (2014). Inflammasome sensor NLRP1 controls rat macrophage susceptibility to Toxoplasma gondii. PLoS Pathog. 10:e1003927. doi: 10.1371/journal.ppat.1003927
Clark, R. T., Nance, J. P., Noor, S., and Wilson, E. H. (2011). T-cell production of matrix metalloproteinases and inhibition of parasite clearance by TIMP-1 during chronic Toxoplasma infection in the brain. ASN Neuro 3:e00049. doi: 10.1042/AN20100027
Clough, B., Wright, J. D., Pereira, P. M., Hirst, E. M., Johnston, A. C., Henriques, R., et al. (2016). K63-linked ubiquitination targets Toxoplasma gondii for endo-lysosomal destruction in IFNγ-stimulated human cells. PLoS Pathog. 12:e1006027. doi: 10.1371/journal.ppat.1006027
Collazo, C. M., Yap, G. S., Sempowski, G. D., Lusby, K. C., Tessarollo, L., Woude, G. F. V., et al. (2001). Inactivation of Lrg-47 and Irg-47 reveals a family of interferon γ-inducible genes with essential, pathogen-specific roles in resistance to infection. J. Exp. Med. 194, 181–188. doi: 10.1084/jem.194.2.181
Combe, C. L., Moretto, M. M., Schwartzman, J. D., Gigley, J. P., Bzik, D. J., and Khan, I. A. (2006). Lack of IL-15 results in the suboptimal priming of CD4+ T cell response against an intracellular parasite. Proc. Natl. Acad. Sci. U.S.A. 103, 6635–6640. doi: 10.1073/pnas.0506180103
Corrêa, G., Marques da Silva, C., de Abreu Moreira-Souza, A. C., Vommaro, R. C., and Coutinho-Silva, R. (2010). Activation of the P2X7 receptor triggers the elimination of Toxoplasma gondii tachyzoites from infected macrophages. Microbes Infect. 12, 497–504. doi: 10.1016/j.micinf.2010.03.004
Dai, W., Pan, H., Kwok, O., and Dubey, J. P. (1994). Human indoleamine 2,3-dioxygenase inhibits Toxoplasma gondii growth in fibroblast cells. J. Interferon Res. 14, 313–317. doi: 10.1089/jir.1994.14.313
Däubener, W., Remscheid, C., Nockemann, S., Pilz, K., Seghrouchni, S., Mackenzie, C., et al. (1996). Anti-parasitic effector mechanisms in human brain tumor cells: role of interferon-γ and tumor necrosis factor-α. Eur. J. Immunol. 26, 487–492. doi: 10.1002/eji.1830260231
David, C. N., Frias, E. S., Szu, J. I., Vieira, P. A., Hubbard, J. A., Lovelace, J., et al. (2016). GLT-1-dependent disruption of CNS glutamate homeostasis and neuronal function by the protozoan parasite Toxoplasma gondii. PLoS Pathog. 12:e1005643. doi: 10.1371/journal.ppat.1005643
Debierre-Grockiego, F., Campos, M. A., Azzouz, N., Schmidt, J., Bieker, U., Resende, M. G., et al. (2007). Activation of TLR2 and TLR4 by glycosylphosphatidylinositols derived from Toxoplasma gondii. J. Immunol. 179, 1129–1137. doi: 10.4049/jimmunol.179.2.1129
Deckert-Schlüter, M., Bluethmann, H., Kaefer, N., Rang, A., and Schlüter, D. (1999). Interferon-gamma receptor-mediated but not tumor necrosis factor receptor type 1- or type 2-mediated signaling is crucial for the activation of cerebral blood vessel endothelial cells and microglia in murine Toxoplasma encephalitis. Am. J. Pathol. 154, 1549–1561. doi: 10.1016/S0002-9440(10)65408-9
Deckert-Schlüter, M., Buck, C., Weiner, D., Kaefer, N., Rang, A., Hof, H., et al. (1997). Interleukin-10 downregulates the intracerebral immune response in chronic Toxoplasma encephalitis. J. Neuroimmunol. 76, 167–176. doi: 10.1016/S0165-5728(97)00047-7
Degrandi, D., Kravets, E., Konermann, C., Beuter-Gunia, C., Klumpers, V., Lahme, S., et al. (2013). Murine guanylate binding protein 2 (mGBP2) controls Toxoplasma gondii replication. Proc. Natl. Acad. Sci. U.S.A. 110, 294–299. doi: 10.1073/pnas.1205635110
Denkers, E. Y., Yap, G., Scharton-Kersten, T., Charest, H., Butcher, B. A., Caspar, P., et al. (1997). Perforin-mediated cytolysis plays a limited role in host resistance to Toxoplasma gondii. J. Immunol. 159, 1903–1908.
Díaz-Godínez, C., and Carrero, J. C. (2019). The state of art of neutrophil extracellular traps in protozoan and helminthic infections. Biosci. Rep. 39:916. doi: 10.1042/BSR20180916
Dimier, I. H., and Bout, D. T. (1998). Interferon-gamma-activated primary enterocytes inhibit Toxoplasma gondii replication: a role for intracellular iron. Immunology 94, 488–495. doi: 10.1046/j.1365-2567.1998.00553.x
Divanovic, S., Sawtell, N. M., Trompette, A., Warning, J. I., Dias, A., Cooper, A. M., et al. (2012). Opposing biological functions of tryptophan catabolizing enzymes during intracellular infection. J. Infect. Dis. 205, 152–161. doi: 10.1093/infdis/jir621
Drewry, L. L., Jones, N. G., Wang, Q., Onken, M. D., Miller, M. J., and Sibley, L. D. (2019). The secreted kinase ROP17 promotes Toxoplasma gondii dissemination by hijacking monocyte tissue migration. Nat. Microbiol. 4, 1951–1963. doi: 10.1038/s41564-019-0504-8
Drewry, L. L., and Sibley, L. D. (2019). The hitchhiker's guide to parasite dissemination. Cell. Microbiol. 21:e13070. doi: 10.1111/cmi.13070
Drögemüller, K., Helmuth, U., Brunn, A., Sakowicz-Burkiewicz, M., Gutmann, D. H., Mueller, W., et al. (2008). Astrocyte gp130 expression is critical for the control of toxoplasma encephalitis. J. Immunol. 181, 2683–2693. doi: 10.4049/jimmunol.181.4.2683
Dubey, J. P. (2016). Toxoplasmosis of Animals and Humans. CRC Press. Available online at: https://play.google.com/store/books/details?id=5Nm7t5p9APAC
Dunay, I. R., DaMatta, R. A., Fux, B., Presti, R., Greco, S., Colonna, M., et al. (2008). Gr1+ Inflammatory monocytes are required for mucosal resistance to the pathogen Toxoplasma gondii. Immunity 29, 306–317. doi: 10.1016/j.immuni.2008.05.019
Dupont, C. D., Christian, D. A., and Hunter, C. A. (2012). Immune response and immunopathology during toxoplasmosis. Semin. Immunopathol. 34, 793–813. doi: 10.1007/s00281-012-0339-3
Egan, C. E., Craven, M. D., Leng, J., Mack, M., Simpson, K. W., and Denkers, E. Y. (2009). CCR2-dependent intraepithelial lymphocytes mediate inflammatory gut pathology during Toxoplasma gondii infection. Mucosal Immunol. 2, 527–535. doi: 10.1038/mi.2009.105
Egan, C. E., Maurer, K. J., Cohen, S. B., Mack, M., Simpson, K. W., and Denkers, E. Y. (2011). Synergy between intraepithelial lymphocytes and lamina propria T cells drives intestinal inflammation during infection. Mucosal Immunol. 4, 658–670. doi: 10.1038/mi.2011.31
Ericsson, A. C., Davis, J. W., Spollen, W., Bivens, N., Givan, S., Hagan, C. E., et al. (2015). Effects of vendor and genetic background on the composition of the fecal microbiota of inbred mice. PLoS ONE 10:e0116704. doi: 10.1371/journal.pone.0116704
Evans, R. J., Sundaramurthy, V., and Frickel, E.-M. (2018). The interplay of host autophagy and eukaryotic pathogens. Front. Cell. Dev. Biol. 6:118. doi: 10.3389/fcell.2018.00118
Ewald, S. E., Chavarria-Smith, J., and Boothroyd, J. C. (2014). NLRP1 is an inflammasome sensor for Toxoplasma gondii. Infect. Immun. 82, 460–468. doi: 10.1128/IAI.01170-13
Fabiani, S., Pinto, B., Bonuccelli, U., and Bruschi, F. (2015). Neurobiological studies on the relationship between toxoplasmosis and neuropsychiatric diseases. J. Neurol. Sci. 351, 3–8. doi: 10.1016/j.jns.2015.02.028
Fackler, O. T., and Grosse, R. (2008). Cell motility through plasma membrane blebbing. Int. J. Cell Biol. 181, 879–884. doi: 10.1083/jcb.200802081
Feliu, V., Vasseur, V., Grover, H. S., Chu, H. H., Brown, M. J., Wang, J., et al. (2013). Location of the CD8 T cell epitope within the antigenic precursor determines immunogenicity and protection against the Toxoplasma gondii parasite. PLoS Pathog. 9:e1003449. doi: 10.1371/journal.ppat.1003449
Fentress, S. J., Behnke, M. S., Dunay, I. R., Mashayekhi, M., Rommereim, L. M., Fox, B. A., et al. (2010). Phosphorylation of immunity-related GTPases by a Toxoplasma gondii-secreted kinase promotes macrophage survival and virulence. Cell. Host. Microbe 8, 484–495. doi: 10.1016/j.chom.2010.11.005
Ferguson, D. J., and Hutchison, W. M. (1987a). An ultrastructural study of the early development and tissue cyst formation of Toxoplasma gondii in the brains of mice. Parasitol. Res. 73, 483–491. doi: 10.1007/BF00535321
Ferguson, D. J., and Hutchison, W. M. (1987b). The host-parasite relationship of Toxoplasma gondii in the brains of chronically infected mice. Virchows Arch. A Pathol. Anat. Histopathol. 411, 39–43. doi: 10.1007/BF00734512
Fisch, D., Bando, H., Clough, B., Hornung, V., Yamamoto, M., Shenoy, A. R., et al. (2019a). Human GBP1 is a microbe-specific gatekeeper of macrophage apoptosis and pyroptosis. EMBO J. 38:e100926. doi: 10.15252/embj.2018100926
Fisch, D., Yakimovich, A., Clough, B., Wright, J., Bunyan, M., Howell, M., et al. (2019b). Defining host-pathogen interactions employing an artificial intelligence workflow. Elife 8:e40560. doi: 10.7554/eLife.40560
Fischer, H.-G., Bonifas, U., and Reichmann, G. (2000). Phenotype and functions of brain dendritic cells emerging during chronic infection of mice with Toxoplasma gondii. J. Immunol. 164, 4826–4834. doi: 10.4049/jimmunol.164.9.4826
Fischer, H. G., Bielinsky, A. K., Nitzgen, B., Däubener, W., and Hadding, U. (1993). Functional dichotomy of mouse microglia developed in vitro: differential effects of macrophage and granulocyte/macrophage colony-stimulating factor on cytokine secretion and antitoxoplasmic activity. J. Neuroimmunol. 45, 193–201. doi: 10.1016/0165-5728(93)90180-7
Fischer, H. G., Nitzgen, B., Reichmann, G., Gross, U., and Hadding, U. (1997). Host cells of Toxoplasma gondii encystation in infected primary culture from mouse brain. Parasitol. Res. 83, 637–641. doi: 10.1007/s004360050311
Fleckenstein, M. C., Reese, M. L., Konen-Waisman, S., Boothroyd, J. C., Howard, J. C., and Steinfeldt, T. (2012). A Toxoplasma gondii pseudokinase inhibits host IRG resistance proteins. PLoS Biol. 10:e1001358. doi: 10.1371/journal.pbio.1001358
Flori, P., Hafid, J., Bourlet, T., Raberin, H., Genin, C., and Tran Manh Sung, R. (2002). Experimental model of congenital toxoplasmosis in guinea-pigs: use of quantitative and qualitative PCR for the study of maternofetal transmission. J. Med. Microbiol. 51, 871–878. doi: 10.1099/0022-1317-51-10-871
Foltz, C., Napolitano, A., Khan, R., Clough, B., Hirst, E. M., and Frickel, E.-M. (2017). TRIM21 is critical for survival of Toxoplasma gondii infection and localises to GBP-positive parasite vacuoles. Sci. Rep. 7:5209. doi: 10.1038/s41598-017-05487-7
Fox, B. A., Rommereim, L. M., Guevara, R. B., Falla, A., Hortua Triana, M. A., Sun, Y., et al. (2016). The Toxoplasma gondii rhoptry kinome is essential for chronic infection. MBio 7:e00193-16. doi: 10.1128/mBio.00193-16
Gazzinelli, R., Xu, Y., Hieny, S., Cheever, A., and Sher, A. (1992). Simultaneous depletion of CD4+ and CD8+ T lymphocytes is required to reactivate chronic infection with Toxoplasma gondii. J. Immunol. 149, 175–180.
Gazzinelli, R. T., Mendonça-Neto, R., Lilue, J., Howard, J., and Sher, A. (2014). Innate resistance against Toxoplasma gondii: an evolutionary tale of mice, cats, and men. Cell. Host. Microbe 15, 132–138. doi: 10.1016/j.chom.2014.01.004
Gazzinelli, R. T., Wysocka, M., and Hieny, S. (1996). In the absence of endogenous IL-10, mice acutely infected with Toxoplasma gondii succumb to a lethal immune response dependent on CD4+ T cells and accompanied by overproduction of IL-12, IFN-gamma and TNF-alpha. J. Immunol. 157, 798–805.
Giacomini, N., Vyas, A., Kim, S.-K., Sapolsky, R. M., and Boothroyd, J. C. (2007). Behavioral changes induced by Toxoplasma infection of rodents are highly specific to aversion of cat odors. Proc. Natl. Acad. Sci. U.S.A. 104, 6442–6447. doi: 10.1073/pnas.0608310104
Gorfu, G., Cirelli, K. M., Melo, M. B., Mayer-Barber, K., Crown, D., Koller, B. H., et al. (2014). Dual role for inflammasome sensors NLRP1 and NLRP3 in murine resistance to Toxoplasma gondii. mBio 5:13. doi: 10.1128/mBio.01117-13
Gov, L., Karimzadeh, A., Ueno, N., and Lodoen, M. B. (2013). Human innate immunity to Toxoplasma gondii is mediated by host caspase-1 and ASC and parasite GRA15. mBio 4:13. doi: 10.1128/mBio.00255-13
Gov, L., Schneider, C. A., Lima, T. S., Pandori, W., and Lodoen, M. B. (2017). NLRP3 and potassium efflux drive rapid IL-1β release from primary human monocytes during Toxoplasma gondii infection. J. Immunol. 199, 2855–2864. doi: 10.4049/jimmunol.1700245
Guan, H., Moretto, M., Bzik, D. J., Gigley, J., and Khan, I. A. (2007). NK cells enhance dendritic cell response against parasite antigens via NKG2D pathway. J. Immunol. 179, 590–596. doi: 10.4049/jimmunol.179.1.590
Guiton, R., Vasseur, V., Charron, S., Arias, M. T., Van Langendonck, N., Buzoni-Gatel, D., et al. (2010). Interleukin 17 receptor signaling is deleterious during Toxoplasma gondii infection in susceptible BL6 mice. J. Infect. Dis. 202, 427–435. doi: 10.1086/653738
Hakimi, M.-A., Olias, P., and Sibley, L. D. (2017). Toxoplasma effectors targeting host signaling and transcription. Clin. Microbiol. Rev. 30, 615–645. doi: 10.1128/CMR.00005-17
Hakimi, M. A., and Bougdour, A. (2015). Toxoplasma's ways of manipulating the host transcriptome via secreted effectors. Curr. Opin. Microbiol. 26, 24–31. doi: 10.1016/j.mib.2015.04.003
Haldar, A. K., Foltz, C., Finethy, R., Piro, A. S., Feeley, E. M., Pilla-Moffett, D. M., et al. (2015). Ubiquitin systems mark pathogen-containing vacuoles as targets for host defense by guanylate binding proteins. Proc. Natl. Acad. Sci. U.S.A. 112, E5628–E5637. doi: 10.1073/pnas.1515966112
Halonen, S. K., Taylor, G. A., and Weiss, L. M. (2001). Gamma interferon-induced inhibition of Toxoplasma gondii in astrocytes is mediated by IGTP. Infect. Immun. 69, 5573–5576. doi: 10.1128/IAI.69.9.5573-5576.2001
Halonen, S. K., Weiss, L. M., and Chiu, F.-C. (1998). Association of host cell intermediate filaments with Toxoplasma gondii cysts in murine astrocytes in vitro. Int. J. Parasitol. 28, 815–823. doi: 10.1016/S0020-7519(98)00035-6
Hamilton, C. M., Black, L., Oliveira, S., Burrells, A., Bartley, P. M., Melo, R. P. B., et al. (2019). Comparative virulence of Caribbean, Brazilian and European isolates of Toxoplasma gondii. Parasit. Vectors 12:104. doi: 10.1186/s13071-019-3372-4
Händel, U., Brunn, A., Drögemüller, K., Müller, W., Deckert, M., and Schlüter, D. (2012). Neuronal gp130 expression is crucial to prevent neuronal loss, hyperinflammation, and lethal course of murine Toxoplasma encephalitis. Am. J. Pathol. 181, 163–173. doi: 10.1016/j.ajpath.2012.03.029
Hassan, M. A., Jensen, K. D., Butty, V., Hu, K., Boedec, E., Prins, P., et al. (2015). Transcriptional and linkage analyses identify loci that mediate the differential macrophage response to inflammatory stimuli and infection. PLoS Genet. 11:e1005619. doi: 10.1371/journal.pgen.1005619
Hassan, M. A., Olijnik, A.-A., Frickel, E.-M., and Saeij, J. P. (2019). Clonal and atypical Toxoplasma strain differences in virulence vary with mouse sub-species. Int. J. Parasitol. 49, 63–70. doi: 10.1016/j.ijpara.2018.08.007
Heimesaat, M. M., Bereswill, S., Fischer, A., Fuchs, D., Struck, D., Niebergall, J., et al. (2006). Gram-negative bacteria aggravate murine small intestinal Th1-type immunopathology following oral infection with Toxoplasma gondii. J. Immunol. 177, 8785–8795. doi: 10.4049/jimmunol.177.12.8785
Heimesaat, M. M., Fischer, A., Jahn, H.-K., Niebergall, J., Freudenberg, M., Blaut, M., et al. (2007). Exacerbation of murine ileitis by Toll-like receptor 4 mediated sensing of lipopolysaccharide from commensal Escherichia coli. Gut 56, 941–948. doi: 10.1136/gut.2006.104497
Heseler, K., Spekker, K., Schmidt, S. K., MacKenzie, C. R., and Däubener, W. (2008). Antimicrobial and immunoregulatory effects mediated by human lung cells: role of IFN-gamma-induced tryptophan degradation. FEMS Immunol. Med. Microbiol. 52, 273–281. doi: 10.1111/j.1574-695X.2007.00374.x
Hidano, S., Randall, L. M., Dawson, L., Dietrich, H. K., Konradt, C., Klover, P. J., et al. (2016). STAT1 Signaling in astrocytes is essential for control of infection in the central nervous system. mBio 7:16. doi: 10.1128/mBio.01881-16
Hou, B., Benson, A., Kuzmich, L., DeFranco, A. L., and Yarovinsky, F. (2011). Critical coordination of innate immune defense against Toxoplasma gondii by dendritic cells responding via their Toll-like receptors. Proc. Natl. Acad. Sci. U.S.A. 108, 278–283. doi: 10.1073/pnas.1011549108
Howe, D. K., and Sibley, L. D. (1995). Toxoplasma gondii comprises three clonal lineages: correlation of parasite genotype with human disease. J. Infect. Dis. 172, 1561–1566. doi: 10.1093/infdis/172.6.1561
Hsieh, C. S., Macatonia, S. E., O'Garra, A., and Murphy, K. M. (1995). T cell genetic background determines default T helper phenotype development in vitro. J. Exp. Med. 181, 713–721. doi: 10.1084/jem.181.2.713
Hunn, J. P., Feng, C. G., Sher, A., and Howard, J. C. (2011). The immunity-related GTPases in mammals: a fast-evolving cell-autonomous resistance system against intracellular pathogens. Mamm. Genome 22, 43–54. doi: 10.1007/s00335-010-9293-3
Hunter, C. A., Chizzonite, R., and Remington, J. S. (1995). IL-1 beta is required for IL-12 to induce production of IFN-gamma by NK cells. A role for IL-1 beta in the T cell-independent mechanism of resistance against intracellular pathogens. J. Immunol. 155, 4347–4354.
Hunter, C. A., Roberts, C. W., and Alexander, J. (1992). Kinetics of cytokine mRNA production in the brains of mice with progressive toxoplasmic encephalitis. Eur. J. Immunol. 22, 2317–2322. doi: 10.1002/eji.1830220921
Hunter, C. A., and Sibley, L. D. (2012). Modulation of innate immunity by Toxoplasma gondii virulence effectors. Nat. Rev. Microbiol. 10, 766–778. doi: 10.1038/nrmicro2858
Imlau, M., Conejeros, I., Muñoz-Caro, T., Zhou, E., Gärtner, U., Ternes, K., et al. (2020). Dolphin-derived NETosis results in rapid Toxoplasma gondii tachyzoite ensnarement and different phenotypes of NETs. Dev. Comp. Immunol. 103:103527. doi: 10.1016/j.dci.2019.103527
Innes, E. A. (1997). Toxoplasmosis: comparative species susceptibility and host immune response. Comp. Immunol. Microbiol. Infect. Dis. 20, 131–138. doi: 10.1016/S0147-9571(96)00038-0
Janssen, R., van Wengen, A., Verhard, E., de Boer, T., Zomerdijk, T., Ottenhoff, T. H. M., et al. (2002). Divergent role for TNF-α in IFN-γ-induced killing of Toxoplasma gondii and Salmonella typhimurium contributes to selective susceptibility of patients with partial IFN-γ receptor 1 deficiency. J. Immunol. 169, 3900–3907. doi: 10.4049/jimmunol.169.7.3900
Jensen, K. D., Hu, K., Whitmarsh, R. J., Hassan, M. A., Julien, L., Lu, D., et al. (2013). Toxoplasma gondii rhoptry 16 kinase promotes host resistance to oral infection and intestinal inflammation only in the context of the dense granule protein GRA15. Infect. Immun. 81, 2156–2167. doi: 10.1128/IAI.01185-12
Jensen, K. D., Wang, Y., Wojno, E. D. T., Shastri, A. J., Hu, K., Cornel, L., et al. (2011). Toxoplasma polymorphic effectors determine macrophage polarization and intestinal inflammation. Cell. Host. Microbe 9, 472–483. doi: 10.1016/j.chom.2011.04.015
John, B., Ricart, B., Tait Wojno, E. D., Harris, T. H., Randall, L. M., Christian, D. A., et al. (2011). Analysis of behavior and trafficking of dendritic cells within the brain during toxoplasmic encephalitis. PLoS Pathog. 7:e1002246. doi: 10.1371/journal.ppat.1002246
Johnson, A. M. (1984). Strain-dependent, route of challenge-dependent, murine susceptibility to toxoplasmosis. Z. Parasitenkd 70, 303–309. doi: 10.1007/BF00927816
Johnston, A. C., Piro, A., Clough, B., Siew, M., Virreira Winter, S., Coers, J., et al. (2016). Human GBP1 does not localize to pathogen vacuoles but restricts Toxoplasma gondii. Cell. Microbiol. 18, 1056–1064. doi: 10.1111/cmi.12579
Jones, L. A., Roberts, F., Nickdel, M. B., Brombacher, F., McKenzie, A. N. J., Henriquez, F. L., et al. (2010). IL-33 receptor (T1/ST2) signaling is necessary to prevent the development of encephalitis in mice infected with Toxoplasma gondii. Eur. J. Immunol. 40, 426–436. doi: 10.1002/eji.200939705
Jones, T. C., Bienz, K. A., and Erb, P. (1986). In vitro cultivation of Toxoplasma gondii cysts in astrocytes in the presence of gamma interferon. Infect. Immun. 51, 147–156. doi: 10.1128/IAI.51.1.147-156.1986
Kempf, M. C., Cesbron-Delauw, M. F., Deslee, D., Gross, U., Herrmann, T., and Sutton, P. (1999). Different manifestations of Toxoplasma gondii infection in F344 and LEW rats. Med. Microbiol. Immunol. 187, 137–142. doi: 10.1007/s004300050085
Khaminets, A., Hunn, J. P., Konen-Waisman, S., Zhao, Y. O., Preukschat, D., Coers, J., et al. (2010). Coordinated loading of IRG resistance GTPases on to the Toxoplasma gondii parasitophorous vacuole. Cell. Microbiol. 12, 939–961. doi: 10.1111/j.1462-5822.2010.01443.x
Khan, A., Behnke, M. S., Dunay, I. R., White, M. W., and Sibley, L. D. (2009). Phenotypic and gene expression changes among clonal type I strains of Toxoplasma gondii. Eukaryot. Cell 8, 1828–1836. doi: 10.1128/EC.00150-09
Khan, I. A., and Kasper, L. H. (1996). IL-15 augments CD8+ T cell-mediated immunity against Toxoplasma gondii infection in mice. J. Immunol. 157, 2103–2108.
Khan, I. A., Moretto, M., Wei, X.-Q., Williams, M., Schwartzman, J. D., and Liew, F. Y. (2002). Treatment with soluble interleukin-15Ralpha exacerbates intracellular parasitic infection by blocking the development of memory CD8+ T cell response. J. Exp. Med. 195, 1463–1470. doi: 10.1084/jem.20011915
Khan, I. A., Murphy, P. M., Casciotti, L., Schwartzman, J. D., Collins, J., Gao, J. L., et al. (2001). Mice lacking the chemokine receptor CCR1 show increased susceptibility to Toxoplasma gondii infection. J. Immunol. 166, 1930–1937. doi: 10.4049/jimmunol.166.3.1930
Khan, I. A., Thomas, S. Y., Moretto, M. M., Lee, F. S., Islam, S. A., Combe, C., et al. (2006). CCR5 is essential for NK cell trafficking and host survival following Toxoplasma gondii infection. PLoS Pathog. 2:e49. doi: 10.1371/journal.ppat.0020049
Klose, C. S. N., Flach, M., Möhle, L., Rogell, L., Hoyler, T., Ebert, K., et al. (2014). Differentiation of type 1 ILCs from a common progenitor to all helper-like innate lymphoid cell lineages. Cell 157, 340–356. doi: 10.1016/j.cell.2014.03.030
Kong, J., Grigg, M. E., Uyetake, L., Parmley, S., and Boothroyd, J. C. (2003). Serotyping of Toxoplasma gondii infections in humans using synthetic peptides. J. Infect. Dis. 187, 1484–1495. doi: 10.1086/374647
Kongsomboonvech, A. K., Rodriguez, F., Diep, A. L., Justice, B. M., Castallanos, B. E., Camejo, A., et al. (2020). Naïve CD8 T cell IFNγ responses to a vacuolar antigen are regulated by an inflammasome-independent NLRP3 pathway and Toxoplasma gondii ROP5. PLoS Pathog. 16:e912568. doi: 10.1101/2020.01.20.912568
Konradt, C., Ueno, N., Christian, D. A., Delong, J. H., Pritchard, G. H., Herz, J., et al. (2016). Endothelial cells are a replicative niche for entry of Toxoplasma gondii to the central nervous system. Nat. Microbiol. 1:16001. doi: 10.1038/nmicrobiol.2016.1
Kravets, E., Degrandi, D., Ma, Q., Peulen, T.-O., Klumpers, V., Felekyan, S., et al. (2016). Guanylate binding proteins directly attack Toxoplasma gondii via supramolecular complexes. Elife 5:e11479. doi: 10.7554/eLife.11479
Lambert, H., Vutova, P. P., Adams, W. C., Lore, K., and Barragan, A. (2009). The Toxoplasma gondii-shuttling function of dendritic cells is linked to the parasite genotype. Infect. Immun. 77, 1679–1688. doi: 10.1128/IAI.01289-08
Lämmermann, T., Afonso, P. V., Angermann, B. R., Wang, J. M., Kastenmüller, W., Parent, C. A., et al. (2013). Neutrophil swarms require LTB4 and integrins at sites of cell death in vivo. Nature 498, 371–375. doi: 10.1038/nature12175
Lämmermann, T., Bader, B. L., Monkley, S. J., Worbs, T., Wedlich-Söldner, R., Hirsch, K., et al. (2008). Rapid leukocyte migration by integrin-independent flowing and squeezing. Nature 453, 51–55. doi: 10.1038/nature06887
Landrith, T. A., Harris, T. H., and Wilson, E. H. (2015). Characteristics and critical function of CD8 T cells in the Toxoplasma-infected brain. Semin. Immunopathol. 37, 261–270. doi: 10.1007/s00281-015-0487-3
Langermans, J. A., Van der Hulst, M. E., Nibbering, P. H., Hiemstra, P. S., Fransen, L., and Van Furth, R. (1992). IFN-gamma-induced L-arginine-dependent Toxoplasmastatic activity in murine peritoneal macrophages is mediated by endogenous tumor necrosis factor-alpha. J. Immunol. 148, 568–574.
LaRosa, D. F., Stumhofer, J. S., Gelman, A. E., Rahman, A. H., Taylor, D. K., Hunter, C. A., et al. (2008). T cell expression of MyD88 is required for resistance to Toxoplasma gondii. Proc. Natl. Acad. Sci. U.S.A. 105, 3855–3860. doi: 10.1073/pnas.0706663105
Lee, Y., Sasai, M., Ma, J. S., Sakaguchi, N., Ohshima, J., Bando, H., et al. (2015). P62 Plays a specific role in interferon-γ-induced presentation of a Toxoplasma vacuolar antigen. Cell Rep. 13, 223–233. doi: 10.1016/j.celrep.2015.09.005
Lee, Y. H., and Kasper, L. H. (2004). Immune responses of different mouse strains after challenge with equivalent lethal doses of Toxoplasma gondii. Parasite 11, 89–97. doi: 10.1051/parasite/200411189
Lees, M. P., Fuller, S. J., McLeod, R., Boulter, N. R., Miller, C. M., Zakrzewski, A. M., et al. (2010). P2X7 receptor-mediated killing of an intracellular parasite, Toxoplasma gondii, by human and murine macrophages. J. Immunol. 184, 7040–7046. doi: 10.4049/jimmunol.1000012
Liesenfeld, O. (2002). Oral infection of C57BL/6 mice with Toxoplasma gondii: a new model of inflammatory bowel disease? J. Infect. Dis. 185(Suppl 1), S96–101. doi: 10.1086/338006
Liesenfeld, O., Kang, H., Park, D., Nguyen, T. A., Parkhe, C. V., Watanabe, H., et al. (1999). TNF-α, nitric oxide and IFN-γ are all critical for development of necrosis in the small intestine and early mortality in genetically susceptible mice infected perorally with Toxoplasma gondii. Parasite Immunol. 21, 365–376. doi: 10.1046/j.1365-3024.1999.00237.x
Liesenfeld, O., Kosek, J., Remington, J. S., and Suzuki, Y. (1996). Association of CD4+ T cell-dependent, interferon-gamma-mediated necrosis of the small intestine with genetic susceptibility of mice to peroral infection with Toxoplasma gondii. J. Exp. Med. 184, 597–607. doi: 10.1084/jem.184.2.597
Lilue, J., Müller, U. B., Steinfeldt, T., and Howard, J. C. (2013). Reciprocal virulence and resistance polymorphism in the relationship between Toxoplasma gondii and the house mouse. Elife 2:e1298. doi: 10.7554/eLife.01298
Ling, Y. M., Shaw, M. H., Ayala, C., Coppens, I., Taylor, G. A., Ferguson, D. J. P., et al. (2006). Vacuolar and plasma membrane stripping and autophagic elimination of Toxoplasma gondii in primed effector macrophages. J. Exp. Med. 203, 2063–2071. doi: 10.1084/jem.20061318
Loeuillet, C., Mondon, A., Kamche, S., Curri, V., Boutonnat, J., Cavaillès, P., et al. (2019). Toxoplasma hypervirulence in the rat model parallels human infection and is modulated by the toxo1 locus. Front. Cell. Infect. Microbiol. 9:134. doi: 10.3389/fcimb.2019.00134
López-Yglesias, A. H., Camanzo, E., Martin, A. T., Araujo, A. M., and Yarovinsky, F. (2019). TLR11-independent inflammasome activation is critical for CD4+ T cell-derived IFN-γ production and host resistance to Toxoplasma gondii. PLoS Pathog. 15:e1007872. doi: 10.1371/journal.ppat.1007872
Lorenzi, H., Khan, A., Behnke, M. S., Namasivayam, S., Swapna, L. S., Hadjithomas, M., et al. (2016). Local admixture of amplified and diversified secreted pathogenesis determinants shapes mosaic Toxoplasma gondii genomes. Nat. Commun. 7:10147. doi: 10.1038/ncomms10147
Luft, B. J., and Remington, J. S. (1992). Toxoplasmic encephalitis in AIDS. Clin. Infect. Dis. 15, 211–222. doi: 10.1093/clinids/15.2.211
Ma, J. S., Sasai, M., Ohshima, J., Lee, Y., Bando, H., Takeda, K., et al. (2014). Selective and strain-specific NFAT4 activation by the Toxoplasma gondii polymorphic dense granule protein GRA6. J. Exp. Med. 211, 2013–2032. doi: 10.1084/jem.20131272
Machado, F. S., and Aliberti, J. (2006). Impact of lipoxin-mediated regulation on immune response to infectious disease. Immunol. Res. 35, 209–218. doi: 10.1385/IR:35:3:209
Mack, D. G., Johnson, J. J., Roberts, F., Roberts, C. W., Estes, R. G., David, C., et al. (1999). HLA-class II genes modify outcome of Toxoplasma gondii infection. Int. J. Parasitol. 29, 1351–1358. doi: 10.1016/S0020-7519(99)00152-6
Marra, C. M. (2018). Central nervous system infection with Toxoplasma gondii. Handb. Clin. Neurol. 152, 117–122. doi: 10.1016/B978-0-444-63849-6.00009-8
Martens, S., Parvanova, I., Zerrahn, J., Griffiths, G., Schell, G., Reichmann, G., et al. (2005). Disruption of Toxoplasma gondii parasitophorous vacuoles by the mouse p47-resistance GTPases. PLoS Pathog. 1:e24. doi: 10.1371/journal.ppat.0010024
Martynowicz, J., Augusto, L., Wek, R. C., Boehm, S. L., and Sullivan, W. J. (2019). Guanabenz reverses a key behavioral change caused by latent toxoplasmosis in mice by reducing neuroinflammation. mBio 10:19. doi: 10.1128/mBio.00381-19
Mashayekhi, M., Sandau, M. M., Dunay, I. R., Frickel, E. M., Khan, A., Goldszmid, R. S., et al. (2011). CD8α+ dendritic cells are the critical source of interleukin-12 that controls acute infection by Toxoplasma gondii tachyzoites. Immunity 35, 249–259. doi: 10.1016/j.immuni.2011.08.008
McLeod, R., Brown, C., and Mack, D. (1993). Immunogenetics influence outcome of Toxoplasma gondii infection. Res. Immunol. 144, 61–65. doi: 10.1016/S0923-2494(05)80101-4
McLeod, R., Eisenhauer, P., Mack, D., Brown, C., Filice, G., and Spitalny, G. (1989a). Immune responses associated with early survival after peroral infection with Toxoplasma gondii. J. Immunol. 142, 3247–3255.
McLeod, R., Skamene, E., Brown, C. R., Eisenhauer, P. B., and Mack, D. G. (1989b). Genetic regulation of early survival and cyst number after peroral Toxoplasma gondii infection of A x B/B x a recombinant inbred and B10 congenic mice. J. Immunol. 143, 3031–3034.
Meisel, R., Brockers, S., Heseler, K., Degistirici, O., Bülle, H., Woite, C., et al. (2011). Human but not murine multipotent mesenchymal stromal cells exhibit broad-spectrum antimicrobial effector function mediated by indoleamine 2,3-dioxygenase. Leukemia 25, 648–654. doi: 10.1038/leu.2010.310
Melo, M. B., Jensen, K. D. C., and Saeij, J. P. J. (2011). Toxoplasma gondii effectors are master regulators of the inflammatory response. Trends Parasitol. 27, 487–495. doi: 10.1016/j.pt.2011.08.001
Melo, M. B., Kasperkovitz, P., Cerny, A., Könen-Waisman, S., Kurt-Jones, E. A., Lien, E., et al. (2010). UNC93B1 mediates host resistance to infection with Toxoplasma gondii. PLoS Pathog. 6:e1001071. doi: 10.1371/journal.ppat.1001071
Melo, M. B., Nguyen, Q. P., Cordeiro, C., Hassan, M. A., Yang, N., McKell, R., et al. (2013). Transcriptional analysis of murine macrophages infected with different toxoplasma strains identifies novel regulation of host signaling pathways. PLoS Pathog. 9:e1003779. doi: 10.1371/journal.ppat.1003779
Melzer, T., Duffy, A., Weiss, L. M., and Halonen, S. K. (2008). The gamma interferon (IFN-gamma)-inducible GTP-binding protein IGTP is necessary for Toxoplasma vacuolar disruption and induces parasite egression in IFN-gamma-stimulated astrocytes. Infect. Immun. 76, 4883–4894. doi: 10.1128/IAI.01288-07
Melzer, T. C., Cranston, H. J., Weiss, L. M., and Halonen, S. K. (2010). Host cell preference of Toxoplasma gondii cysts in murine brain: a confocal study. J. Neuroparasitol. 1, 1–6. doi: 10.4303/jnp/N100505
Mennechet, F. J. D., Kasper, L. H., Rachinel, N., Li, W., Vandewalle, A., and Buzoni-Gatel, D. (2002). Lamina propria CD4+ T lymphocytes synergize with murine intestinal epithelial cells to enhance proinflammatory response against an intracellular pathogen. J. Immunol. 168, 2988–2996. doi: 10.4049/jimmunol.168.6.2988
Mennechet, F. J. D., Kasper, L. H., Rachinel, N., Minns, L. A., Luangsay, S., Vandewalle, A., et al. (2004). Intestinal intraepithelial lymphocytes prevent pathogen-driven inflammation and regulate the Smad/T-bet pathway of lamina propria CD4+ T cells. Eur. J. Immunol. 34, 1059–1067. doi: 10.1002/eji.200324416
Mercer, H. L., Snyder, L. M., Doherty, C. M., Fox, B. A., Bzik, D. J., and Denkers, E. Y. (2020). Toxoplasma gondii dense granule protein GRA24 drives MyD88-independent p38 MAPK activation, IL-12 production and induction of protective immunity. PLoS Pathog. 16:e1008572. doi: 10.1371/journal.ppat.1008572
Miller, C. M., Zakrzewski, A. M., Ikin, R. J., Boulter, N. R., Katrib, M., Lees, M. P., et al. (2011). Dysregulation of the inflammatory response to the parasite, Toxoplasma gondii, in P2X7 receptor-deficient mice. Int. J. Parasitol. 41, 301–308. doi: 10.1016/j.ijpara.2010.10.001
Minns, L. A., Menard, L. C., Foureau, D. M., Darche, S., Ronet, C., Mielcarz, D. W., et al. (2006). TLR9 is required for the gut-associated lymphoid tissue response following oral infection of Toxoplasma gondii. J. Immunol. 176, 7589–7597. doi: 10.4049/jimmunol.176.12.7589
Mogensen, T. H. (2009). Pathogen recognition and inflammatory signaling in innate immune defenses. Clin. Microbiol. Rev. 22, 240–273. doi: 10.1128/CMR.00046-08
Montoya, J. G., and Liesenfeld, O. (2004). Toxoplasmosis. Lancet 363, 1965–1976. doi: 10.1016/S0140-6736(04)16412-X
Mordue, D. G., Monroy, F., La Regina, M., Dinarello, C. A., and Sibley, L. D. (2001). Acute toxoplasmosis leads to lethal overproduction of Th1 cytokines. J. Immunol. 167, 4574–4584. doi: 10.4049/jimmunol.167.8.4574
Morgado, P., Sudarshana, D. M., Gov, L., Harker, K. S., Lam, T., Casali, P., et al. (2014). Type II Toxoplasma gondii induction of CD40 on infected macrophages enhances interleukin-12 responses. Infect. Immun. 82, 4047–4055. doi: 10.1128/IAI.01615-14
Mukhopadhyay, D., Arranz-Solís, D., and Saeij, J. P. J. (2020a). Toxoplasma GRA15 and GRA24 are important activators of the host innate immune response in the absence of TLR11. PLoS Pathog. 16:e1008586. doi: 10.1371/journal.ppat.1008586
Mukhopadhyay, D., Sangaré, L. O., Braun, L., Hakimi, M.-A., and Saeij, J. P. (2020b). Toxoplasma GRA15 limits parasite growth in IFNγ-activated fibroblasts through TRAF ubiquitin ligases. EMBO J. 39:e103758. doi: 10.1101/2020.02.24.963496
Müller, U. B., and Howard, J. C. (2016). The impact of Toxoplasma gondii on the mammalian genome. Curr. Opin. Microbiol. 32, 19–25. doi: 10.1016/j.mib.2016.04.009
Mun, H.-S., Aosai, F., Norose, K., Chen, M., Piao, L.-X., Takeuchi, O., et al. (2003). TLR2 as an essential molecule for protective immunity against Toxoplasma gondii infection. Int. Immunol. 15, 1081–1087. doi: 10.1093/intimm/dxg108
Muñoz, M., Heimesaat, M. M., Danker, K., and Struck, D. (2009). Interleukin (IL)-23 mediates Toxoplasma gondii–induced immunopathology in the gut via matrixmetalloproteinase-2 and IL-22 but independent of IL-17. J. Exp. Med. 206, 3047–3059. doi: 10.1084/jem.20090900
Munoz, M., Liesenfeld, O., and Heimesaat, M. M. (2011). Immunology of Toxoplasma gondii. Immunol. Rev. 240, 269–285. doi: 10.1111/j.1600-065X.2010.00992.x
Murillo-León, M., Müller, U. B., Zimmermann, I., Singh, S., Widdershooven, P., Campos, C., et al. (2019). Molecular mechanism for the control of virulent Toxoplasma gondii infections in wild-derived mice. Nat. Commun. 10:1233. doi: 10.1038/s41467-019-09200-2
Murray, P. J., Allen, J. E., Biswas, S. K., Fisher, E. A., Gilroy, D. W., Goerdt, S., et al. (2014). Macrophage activation and polarization: nomenclature and experimental guidelines. Immunity 41, 14–20. doi: 10.1016/j.immuni.2014.06.008
Nagineni, C. N., Pardhasaradhi, K., Martins, M. C., Detrick, B., and Hooks, J. J. (1996). Mechanisms of interferon-induced inhibition of Toxoplasma gondii replication in human retinal pigment epithelial cells. Infect. Immun. 64, 4188–4196. doi: 10.1128/IAI.64.10.4188-4196.1996
Nance, J. P., Philip Nance, J., Vannella, K. M., Worth, D., David, C., Carter, D., et al. (2012). Chitinase dependent control of protozoan cyst burden in the brain. PLoS Pathog. 8:e1002990. doi: 10.1371/journal.ppat.1002990
Navia, B. A., Petito, C. K., Gold, J. W. M., Cho, E.-S., Jordan, B. D., and Price, R. W. (1986). Cerebral toxoplasmosis complicating the acquired immune deficiency syndrome: clinical and neuropathological findings in 27 patients. Ann. Neurol. 19, 224–238. doi: 10.1002/ana.410190303
Niedelman, W., Gold, D. A., Rosowski, E. E., Sprokholt, J. K., Lim, D., Arenas, A. F., et al. (2012). The rhoptry proteins ROP18 and ROP5 mediate Toxoplasma gondii evasion of the murine, but not the human, interferon-gamma response. PLoS Pathog. 8:e1002784. doi: 10.1371/journal.ppat.1002784
Niedelman, W., Sprokholt, J. K., Clough, B., Frickel, E. M., and Saeij, J. P. J. (2013). Cell death of gamma interferon-stimulated human fibroblasts upon Toxoplasma gondii infection induces early parasite egress and limits parasite replication. Infect. Immun. 81, 4341–4349. doi: 10.1128/IAI.00416-13
O'Neill, L. A. J., and Bowie, A. G. (2007). The family of five: TIR-domain-containing adaptors in Toll-like receptor signalling. Nat. Rev. Immunol. 7, 353–364. doi: 10.1038/nri2079
Ong, Y.-C., Reese, M. L., and Boothroyd, J. C. (2010). Toxoplasma rhoptry protein 16 (ROP16) subverts host function by direct tyrosine phosphorylation of STAT6. J. Biol. Chem. 285, 28731–28740. doi: 10.1074/jbc.M110.112359
Paluch, E. K., Aspalter, I. M., and Sixt, M. (2016). Focal adhesion–independent cell migration. Annu. Rev.Cell Dev. Bi. 32, 469–490. doi: 10.1146/annurev-cellbio-111315-125341
Panas, M. W., Ferrel, A., Naor, A., Tenborg, E., Lorenzi, H. A., and Boothroyd, J. C. (2019). Translocation of dense granule effectors across the parasitophorous vacuole membrane in Toxoplasma-infected cells requires the activity of ROP17, a rhoptry protein kinase. mSphere 4:e00276-19. doi: 10.1101/613208
Pappas, G., Roussos, N., and Falagas, M. E. (2009). Toxoplasmosis snapshots: global status of Toxoplasma gondii seroprevalence and implications for pregnancy and congenital toxoplasmosis. Int. J. Parasitol. 39, 1385–1394. doi: 10.1016/j.ijpara.2009.04.003
Patil, V., Zhao, Y., Shah, S., Fox, B. A., Rommereim, L. M., Bzik, D. J., et al. (2014). Co-existence of classical and alternative activation programs in macrophages responding to Toxoplasma gondii. Int. J. Parasitol. 44, 161–164. doi: 10.1016/j.ijpara.2013.08.003
Pawlowski, N., Khaminets, A., Hunn, J. P., Papic, N., Schmidt, A., Uthaiah, R. C., et al. (2011). The activation mechanism of Irga6, an interferon-inducible GTPase contributing to mouse resistance against Toxoplasma gondii. BMC Biol. 9:7. doi: 10.1186/1741-7007-9-7
Peixoto, L., Chen, F., Harb, O. S., Davis, P. H., Beiting, D. P., Brownback, C. S., et al. (2010). Integrative genomic approaches highlight a family of parasite-specific kinases that regulate host responses. Cell Host Microbe 8, 208–218. doi: 10.1016/j.chom.2010.07.004
Pernas, L., Adomako-Ankomah, Y., Shastri, A. J., Ewald, S. E., Treeck, M., Boyle, J. P., et al. (2014). Toxoplasma effector MAF1 mediates recruitment of host mitochondria and impacts the host response. PLoS Biol. 12:e1001845. doi: 10.1371/journal.pbio.1001845
Pfefferkorn, E. R. (1984). Interferon gamma blocks the growth of Toxoplasma gondii in human fibroblasts by inducing the host cells to degrade tryptophan. Proc. Natl. Acad. Sci. U.S.A. 81, 908–912. doi: 10.1073/pnas.81.3.908
Pfefferkorn, E. R., Eckel, M., and Rebhun, S. (1986). Interferon-γ suppresses the growth of Toxoplasma gondii in human fibroblasts through starvation for tryptophan. Mol. Biochem. Parasitol. 20, 215–224. doi: 10.1016/0166-6851(86)90101-5
Pittman, K. J., Aliota, M. T., and Knoll, L. J. (2014). Dual transcriptional profiling of mice and Toxoplasma gondii during acute and chronic infection. BMC Genomics 15:806. doi: 10.1186/1471-2164-15-806
Porter, S. B., and Sande, M. A. (1992). Toxoplasmosis of the central nervous system in the acquired immunodeficiency syndrome. N. Engl. J. Med. 327, 1643–1648. doi: 10.1056/NEJM199212033272306
Powell, H. C., Gibbs, C. J. Jr, Lorenzo, A. M., Lampert, P. W., and Gajdusek, D. C. (1978). Toxoplasmosis of the central nervous system in the adult. Electron microscopic observations. Acta Neuropathol. 41, 211–216. doi: 10.1007/BF00690438
Prandovszky, E., Gaskell, E., Martin, H., Dubey, J. P., Webster, J. P., and McConkey, G. A. (2011). The neurotropic parasite Toxoplasma gondii increases dopamine metabolism. PLoS ONE 6:e23866. doi: 10.1371/journal.pone.0023866
Puccetti, P., and Grohmann, U. (2007). IDO and regulatory T cells: a role for reverse signalling and non-canonical NF-kappaB activation. Nat. Rev. Immunol. 7, 817–823. doi: 10.1038/nri2163
Qin, A., Lai, D.-H., Liu, Q., Huang, W., Wu, Y.-P., Chen, X., et al. (2017). Guanylate-binding protein 1 (GBP1) contributes to the immunity of human mesenchymal stromal cells against Toxoplasma gondii. Proc. Natl. Acad. Sci. U.S.A. 114, 1365–1370. doi: 10.1073/pnas.1619665114
Raetz, M., Kibardin, A., Sturge, C. R., Pifer, R., Li, H., Burstein, E., et al. (2013). Cooperation of TLR12 and TLR11 in the IRF8-dependent IL-12 response to Toxoplasma gondii profilin. J. Immunol. 191, 4818–4827. doi: 10.4049/jimmunol.1301301
Reese, M. L., Zeiner, G. M., Saeij, J. P. J., Boothroyd, J. C., and Boyle, J. P. (2011). Polymorphic family of injected pseudokinases is paramount in Toxoplasma virulence. Proc. Natl. Acad. Sci. U.S.A. 108, 9625–9630. doi: 10.1073/pnas.1015980108
Reichmann, G., Walker, W., Villegas, E. N., Craig, L., Cai, G., Alexander, J., et al. (2000). The CD40/CD40 ligand interaction is required for resistance to toxoplasmic encephalitis. Infect. Immun. 68, 1312–1318. doi: 10.1128/IAI.68.3.1312-1318.2000
Reiling, N., Hölscher, C., Fehrenbach, A., Kröger, S., Kirschning, C. J., Goyert, S., et al. (2002). Cutting edge: Toll-like receptor (TLR) 2-and TLR4-mediated pathogen recognition in resistance to airborne infection with Mycobacterium tuberculosis. J. Immunol. 169, 3480–3484. doi: 10.4049/jimmunol.169.7.3480
Renkawitz, J., and Sixt, M. (2010). Mechanisms of force generation and force transmission during interstitial leukocyte migration. EMBO Rep. 11, 744–750. doi: 10.1038/embor.2010.147
Robben, P. M., LaRegina, M., Kuziel, W. A., and Sibley, L. D. (2005). Recruitment of Gr-1+ monocytes is essential for control of acute toxoplasmosis. J. Exp. Med. 201, 1761–1769. doi: 10.1084/jem.20050054
Robben, P. M., Mordue, D. G., Truscott, S. M., Takeda, K., Akira, S., and Sibley, L. D. (2004). Production of IL-12 by macrophages infected with Toxoplasma gondii depends on the parasite genotype. J Immunol. 172, 3686–3694. doi: 10.4049/jimmunol.172.6.3686
Rosowski, E. E., Lu, D., Julien, L., Rodda, L., Gaiser, R. A., Jensen, K. D., et al. (2011). Strain-specific activation of the NF-κB pathway by GRA15, a novel Toxoplasma gondii dense granule protein. J. Exp. Med. 208, 195–212. doi: 10.1084/jem.20100717
Rozenfeld, C., Martinez, R., Seabra, S., Sant'Anna, C., Gonçalves, J. G. R., Bozza, M., et al. (2005). Toxoplasma gondii prevents neuron degeneration by interferon-γ-activated microglia in a mechanism involving inhibition of inducible nitric oxide synthase and transforming growth factor-β1 production by infected microglia. Am J. Pathol. 167, 1021–1031. doi: 10.1016/S0002-9440(10)61191-1
Saeij, J. P., Boyle, J. P., Coller, S., Taylor, S., Sibley, L. D., Brooke-Powell, E. T., et al. (2006). Polymorphic secreted kinases are key virulence factors in toxoplasmosis. Science 314, 1780–1783. doi: 10.1126/science.1133690
Saeij, J. P., Boyle, J. P. P., and Boothroyd, J. C. (2005). Differences among the three major strains of Toxoplasma gondii and their specific interactions with the infected host. Trends Parasitol. 21, 476–481. doi: 10.1016/j.pt.2005.08.001
Saeij, J. P., and Frickel, E. M. (2017). Exposing Toxoplasma gondii hiding inside the vacuole: a role for GBPs, autophagy and host cell death. Curr. Opin. Microbiol. 40, 72–80. doi: 10.1016/j.mib.2017.10.021
Saeij, J. P. J., Coller, S., Boyle, J. P., Jerome, M. E., White, M. W., and Boothroyd, J. C. (2007). Toxoplasma co-opts host gene expression by injection of a polymorphic kinase homologue. Nature 445, 324–327. doi: 10.1038/nature05395
Safronova, A., Araujo, A., Camanzo, E. T., Moon, T. J., Elliott, M. R., Beiting, D. P., et al. (2018). Alarmin S100A11 initiates a chemokine response to the human pathogen Toxoplasma gondii. Nat. Immunol. 20, 64–72. doi: 10.1038/s41590-018-0250-8
Salazar Gonzalez, M. R., Shehata, H., Connell, M. J. O., Yang, Y., Chougnet, C. A., and Aliberti, J. (2014). Toxoplasma gondii-derived profilin triggers Human TLR5- dependent cytokine production. J. Innate Immun. 6, 685–694. doi: 10.1159/000362367
Sangaré, L. O., Ólafsson, E. B., Wang, Y., Yang, N., Julien, L., Camejo, A., et al. (2019a). In vivo CRISPR screen identifies TgWIP as a Toxoplasma modulator of dendritic cell migration. Cell. Host. Microbe 26, 478–492.e8. doi: 10.1016/j.chom.2019.09.008
Sangaré, L. O., Yang, N., Konstantinou, E. K., Lu, D., Mukhopadhyay, D., Young, L. H., et al. (2019b). Toxoplasma GRA15 activates the NF-κB pathway through interactions with TNF receptor-associated factors. MBio 10:19. doi: 10.1128/mBio.00808-19
Sasai, M., and Yamamoto, M. (2019). Innate, adaptive, and cell-autonomous immunity against Toxoplasma gondii infection. Exp. Mol. Med. 51, 1–10. doi: 10.1038/s12276-019-0353-9
Scanga, C. A., Aliberti, J., Jankovic, D., Tilloy, F., Bennouna, S., Denkers, E. Y., et al. (2002). Cutting edge: MyD88 is required for resistance to Toxoplasma gondii infection and regulates parasite-induced IL-12 production by dendritic cells. J. Immunol. 168, 5997–6001. doi: 10.4049/jimmunol.168.12.5997
Schade, B., and Fischer, H. G. (2001). Toxoplasma gondii induction of interleukin-12 is associated with acute virulence in mice and depends on the host genotype. Vet. Parasitol. 100, 63–74. doi: 10.1016/S0304-4017(01)00484-8
Scharton-Kersten, T., Contursi, C., Masumi, A., Sher, A., and Ozato, K. (1997). Interferon consensus sequence binding protein–deficient mice display impaired resistance to intracellular infection due to a primary defect in interleukin 12 p40 induction. J. Exp. Med. 186, 1523–1534. doi: 10.1084/jem.186.9.1523
Scharton-Kersten, T. M., Wynn, T. A., Denkers, E. Y., Bala, S., Grunvald, E., Hieny, S., et al. (1996). In the absence of endogenous IFN-gamma, mice develop unimpaired IL-12 responses to Toxoplasma gondii while failing to control acute infection. J. Immunol. 157, 4045–4054.
Schlüter, D., and Barragan, A. (2019). Advances and challenges in understanding cerebral toxoplasmosis. Front. Immunol. 10:242. doi: 10.3389/fimmu.2019.00242
Schlüter, D., Deckert, M., Hof, H., and Frei, K. (2001). Toxoplasma gondii infection of neurons induces neuronal cytokine and chemokine production, but gamma interferon- and tumor necrosis factor-stimulated neurons fail to inhibit the invasion and growth of T. gondii. Inf. Immun. 69, 7889–7893. doi: 10.1128/IAI.69.12.7889-7893.2001
Schlüter, D., Deckert-Schlüter, M., Lorenz, E., Meyer, T., Röllinghoff, M., and Bogdan, C. (1999). Inhibition of inducible nitric oxide synthase exacerbates chronic cerebral toxoplasmosis in Toxoplasma gondii-susceptible C57BL/6 mice but does not reactivate the latent disease in T. gondii-resistant BALB/c mice. J. Immunol. 162, 3512–3518.
Schmidt, S. K., Müller, A., Heseler, K., Woite, C., Spekker, K., MacKenzie, C. R., et al. (2009). Antimicrobial and immunoregulatory properties of human tryptophan 2,3-dioxygenase. Eur. J. Immunol. 39, 2755–2764. doi: 10.1002/eji.200939535
Schreiner, M., and Liesenfeld, O. (2009). Small intestinal inflammation following oral infection with Toxoplasma gondii does not occur exclusively in C57BL/6 mice: review of 70 reports from the literature. Mem. Inst. Oswaldo Cruz 104, 221–233. doi: 10.1590/S0074-02762009000200015
Schwartzman, J. D., Gonias, S. L., and Pfefferkorn, E. R. (1990). Murine gamma interferon fails to inhibit Toxoplasma gondii growth in murine fibroblasts. Infect. Immun. 58, 833–834. doi: 10.1128/IAI.58.3.833-834.1990
Sedlák, K., Literák, I., Pavlásek, I., and Benák, J. (2001). Susceptibility of common voles to experimental toxoplasmosis. J. Wildl. Dis. 37, 640–642. doi: 10.7589/0090-3558-37.3.640
Selleck, E. M., Fentress, S. J., Beatty, W. L., Degrandi, D., Pfeffer, K., Virgin, H. W. IV, et al. (2013). Guanylate-binding protein 1 (Gbp1) contributes to cell-autonomous immunity against Toxoplasma gondii. PLoS Pathog. 9:e1003320. doi: 10.1371/journal.ppat.1003320
Selleck, E. M., Orchard, R. C., Lassen, K. G., Beatty, W. L., Xavier, R. J., Levine, B., et al. (2015). A noncanonical autophagy pathway restricts Toxoplasma gondii growth in a strain-specific manner in ifn-gamma-activated human cells. MBio 6, e01157–e01115. doi: 10.1128/mBio.01157-15
Sergent, V., Cautain, B., Khalife, J., Deslée, D., Bastien, P., Dao, A., et al. (2005). Innate refractoriness of the Lewis rat to toxoplasmosis is a dominant trait that is intrinsic to bone marrow-derived cells. Infect. Immun. 73, 6990–6997. doi: 10.1128/IAI.73.10.6990-6997.2005
Shastri, A. J., Marino, N. D., Franco, M., Lodoen, M. B., and Boothroyd, J. C. (2014). GRA25 is a novel virulence factor of Toxoplasma gondii and influences the host immune response. Infect. Immun. 82, 2595–2605. doi: 10.1128/IAI.01339-13
Shirahata, T., Mori, A., Ishikawa, H., and Goto, H. (1986). Strain differences of interferon-generating capacity and resistance in Toxoplasma-infected mice. Microbiol. Immunol. 30, 1307–1316. doi: 10.1111/j.1348-0421.1986.tb03051.x
Shwab, E. K., Zhu, X. Q., Majumdar, D., Pena, H. F., Gennari, S. M., Dubey, J. P., et al. (2014). Geographical patterns of Toxoplasma gondii genetic diversity revealed by multilocus PCR-RFLP genotyping. Parasitology 141, 453–461. doi: 10.1017/S0031182013001844
Sibley, L. D., and Ajioka, J. W. (2008). Population structure of Toxoplasma gondii : clonal expansion driven by infrequent recombination and selective sweeps. Annu. Rev. Microbiol. 62, 329–351. doi: 10.1146/annurev.micro.62.081307.162925
Sibley, L. D., and Boothroyd, J. C. (1992). Virulent strains of Toxoplasma gondii comprise a single clonal lineage. Nature 359, 82–85. doi: 10.1038/359082a0
Skallová, A., Kodym, P., Frynta, D., and Flegr, J. (2006). The role of dopamine in Toxoplasma-induced behavioural alterations in mice: an ethological and ethopharmacological study. Parasitology 133:525. doi: 10.1017/S0031182006000886
Spekker, K., Czesla, M., Ince, V., Heseler, K., Schmidt, S. K., Schares, G., et al. (2009). Indoleamine 2,3-dioxygenase is involved in defense against Neospora caninum in human and bovine cells. Infect. Immun. 77, 4496–4501. doi: 10.1128/IAI.00310-09
Steinfeldt, T., Konen-Waisman, S., Tong, L., Pawlowski, N., Lamkemeyer, T., Sibley, L. D., et al. (2010). Phosphorylation of mouse immunity-related GTPase (IRG) resistance proteins is an evasion strategy for virulent Toxoplasma gondii. PLoS Biol. 8:e1000576. doi: 10.1371/journal.pbio.1000576
Stelzer, S., Basso, W., Benavides Silván, J., Ortega-Mora, L. M., Maksimov, P., Gethmann, J., et al. (2019). Toxoplasma gondii infection and toxoplasmosis in farm animals: risk factors and economic impact. Food Waterborne Parasitol 15:e00037. doi: 10.1016/j.fawpar.2019.e00037
Strack, A., Asensio, V., Campbell, I., Schlüter, D., and Deckert, M. (2002). Chemokines are differentially expressed by astrocytes, microglia and inflammatory leukocytes in Toxoplasma encephalitis and critically regulated by interferon-γ. Acta Neuropathol. 103, 458–468. doi: 10.1007/s00401-001-0491-7
Stumhofer, J. S., Laurence, A., Wilson, E. H., Huang, E., Tato, C. M., Johnson, L. M., et al. (2006). Interleukin 27 negatively regulates the development of interleukin 17-producing T helper cells during chronic inflammation of the central nervous system. Nat. Immunol. 7, 937–945. doi: 10.1038/ni1376
Sturge, C. R., Benson, A., Raetz, M., Wilhelm, C. L., Mirpuri, J., Vitetta, E. S., et al. (2013). TLR-independent neutrophil-derived IFN- is important for host resistance to intracellular pathogens. Proc. Natl. Acad. Sci. U.S.A. 110, 10711–10716. doi: 10.1073/pnas.1307868110
Sturge, C. R., and Yarovinsky, F. (2014). Complex immune cell interplay in the gamma interferon response during Toxoplasma gondii infection. Infect. Immun. 82, 3090–3097. doi: 10.1128/IAI.01722-14
Su, C., Dubey, J. P., Ajzenberg, D., Khan, A., Ajioka, J. W., Rosenthal, B. M., et al. (2012). Globally diverse Toxoplasma gondii isolates comprise six major clades originating from a small number of distinct ancestral lineages. Proc. Natl. Acad. Sci. U.S.A. 109, 5844–5849. doi: 10.1073/pnas.1203190109
Subauste, C. (2012). Animal models for Toxoplasma gondii infection. Curr. Protoc. Immunol. Chapter 19, Unit 19.3.1–23. doi: 10.1002/0471142735.im1903s96
Subauste, C. S., and Wessendarp, M. (2006). CD40 restrains in vivo growth of Toxoplasma gondii independently of gamma interferon. Infect. Immun. 74, 1573–1579. doi: 10.1128/IAI.74.3.1573-1579.2006
Suzuki, Y. (2002). Host resistance in the brain against Toxoplasma gondii. J. Infect. Dis. 185, S58–S65. doi: 10.1086/337999
Suzuki, Y., Claflin, J., Wang, X., Lengi, A., and Kikuchi, T. (2005). Microglia and macrophages as innate producers of interferon-gamma in the brain following infection with Toxoplasma gondii. Int. J. Parasitol. 35, 83–90. doi: 10.1016/j.ijpara.2004.10.020
Suzuki, Y., Joh, K., Kwon, O. C., Yang, Q., Conley, F. K., and Remington, J. S. (1994). MHC class I gene(s) in the D/L region but not the TNF-alpha gene determines development of toxoplasmic encephalitis in mice. J. Immunol. 153, 4649–4654.
Suzuki, Y., Joh, K., Orellana, M. A., Conley, F. K., and Remington, J. S. (1991). A gene(s) within the H-2D region determines the development of toxoplasmic encephalitis in mice. Immunology 74, 732–739.
Suzuki, Y., Orellana, M. A., Schreiber, R. D., and Remington, J. S. (1988). Interferon-gamma: the major mediator of resistance against Toxoplasma gondii. Science 240, 516–518. doi: 10.1126/science.3128869
Suzuki, Y., Sa, Q., Gehman, M., and Ochiai, E. (2011). Interferon-gamma- and perforin-mediated immune responses for resistance against Toxoplasma gondii in the brain. Expert Rev. Mol. Med. 13:e31. doi: 10.1017/S1462399411002018
Suzuki, Y., Sher, A., Yap, G., Park, D., Neyer, L. E., Liesenfeld, O., et al. (2000). IL-10 is required for prevention of necrosis in the small intestine and mortality in both genetically resistant BALB/c and susceptible C57BL/6 mice following peroral infection with Toxoplasma gondii. J. Immunol. 164, 5375–5382. doi: 10.4049/jimmunol.164.10.5375
Suzuki, Y., Wang, X., Jortner, B. S., Payne, L., Ni, Y., Michie, S. A., et al. (2010). Removal of Toxoplasma gondii cysts from the brain by perforin-mediated activity of CD8 T Cells. Am. J. Pathol. 176, 1607–1613. doi: 10.2353/ajpath.2010.090825
Suzuki, Y., Wong, S. Y., Grumet, F. C., Fessel, J., Montoya, J. G., Zolopa, A. R., et al. (1996). Evidence for genetic regulation of susceptibility to toxoplasmic encephalitis in AIDS patients. J. Infect. Dis. 173, 265–268. doi: 10.1093/infdis/173.1.265
Taylor, G. A., Collazo, C. M., Yap, G. S., Nguyen, K., Gregorio, T. A., Taylor, L. S., et al. (2000). Pathogen-specific loss of host resistance in mice lacking the IFN-γ-inducible gene IGTP. Proc. Natl. Acad. Sci. U.S.A. 97, 751–755. doi: 10.1073/pnas.97.2.751
Taylor, S., Barragan, A., Su, C., Fux, B., Fentress, S. J., Tang, K., et al. (2006). A secreted serine-threonine kinase determines virulence in the eukaryotic pathogen Toxoplasma gondii. Science 314, 1776–1780. doi: 10.1126/science.1133643
Tedford, E., and McConkey, G. (2017). Neurophysiological changes induced by chronic Toxoplasma gondii infection. Pathogens 6:19. doi: 10.20944/preprints201705.0071.v1
Tombácz, K., Burgess, G., Holder, A., Werners, A., and Werling, D. (2018). Toxoplasma gondii profilin does not stimulate an innate immune response through bovine or human TLR5. Innate Immun. 24, 422–429. doi: 10.1177/1753425918798450
Torres, L., Robinson, S.-A., Kim, D.-G., Yan, A., Cleland, T. A., and Bynoe, M. S. (2018). Toxoplasma gondii alters NMDAR signaling and induces signs of Alzheimer's disease in wild-type, C57BL/6 mice. J Neuroinflam. 15:57. doi: 10.1186/s12974-018-1086-8
Tuladhar, S., Kochanowsky, J. A., Bhaskara, A., Ghotmi, Y., Chandrasekaran, S., and Koshy, A. A. (2019). The ROP16III-dependent early immune response determines the subacute CNS immune response and type III Toxoplasma gondii survival. PLoS Pathog. 15:e645390. doi: 10.1101/645390
Ufermann, C.-M., Domröse, A., Babel, T., Tersteegen, A., Cengiz, S. C., Eller, S. K., et al. (2019). Indoleamine 2,3-dioxygenase activity during acute toxoplasmosis and the suppressed T cell proliferation in mice. Front. Cell.Inf. Microbiol. 9:e184. doi: 10.3389/fcimb.2019.00184
Van Grol, J., Muniz-Feliciano, L., Portillo, J. A. C., Bonilha, V. L., and Subaustea, C. S. (2013). CD40 induces anti-Toxoplasma gondii activity in nonhematopoietic cells dependent on autophagy proteins. Infect. Immun. 81, 2002–2011. doi: 10.1128/IAI.01145-12
Villarino, A., Hibbert, L., Lieberman, L., Wilson, E., Mak, T., Yoshida, H., et al. (2003). The IL-27R (WSX-1) is required to suppress T cell hyperactivity during infection. Immunity 19, 645–655. doi: 10.1016/S1074-7613(03)00300-5
Virreira Winter, S., Niedelman, W., Jensen, K. D., Rosowski, E. E., Julien, L., Spooner, E., et al. (2011). Determinants of GBP recruitment to Toxoplasma gondii vacuoles and the parasitic factors that control it. PLoS ONE 6:e24434. doi: 10.1371/journal.pone.0024434
Vyas, A. (2015). Mechanisms of host behavioral change in Toxoplasma gondii rodent association. PLoS Pathog. 11:e1004935. doi: 10.1371/journal.ppat.1004935
Vyas, A., and Sapolsky, R. (2010). Manipulation of host behaviour by Toxoplasma gondii: what is the minimum a proposed proximate mechanism should explain? Folia Parasit. 57, 88–94. doi: 10.14411/fp.2010.011
Wang, X., Kang, H., Kikuchi, T., and Suzuki, Y. (2004). Gamma interferon production, but not perforin-mediated cytolytic activity, of t cells is required for prevention of toxoplasmic encephalitis in balb/c mice genetically resistant to the disease. Infect. Immun. 72, 4432–4438. doi: 10.1128/IAI.72.8.4432-4438.2004
Wang, X., Michie, S. A., Xu, B., and Suzuki, Y. (2007). Importance of IFN-gamma-mediated expression of endothelial VCAM-1 on recruitment of CD8+ T cells into the brain during chronic infection with Toxoplasma gondii. J. Interferon Cytok. Res. 27, 329–338. doi: 10.1089/jir.2006.0154
Watanabe, H., Numata, K., Ito, T., Takagi, K., and Matsukawa, A. (2004). Innate immune response in Th1- and Th2-dominant mouse strains. Shock 22, 460–466. doi: 10.1097/01.shk.0000142249.08135.e9
Watts, E., Zhao, Y., Dhara, A., Eller, B., Patwardhan, A., and Sinai, A. P. (2015). Novel Approaches reveal that Toxoplasma gondii bradyzoites within tissue cysts are dynamic and replicating entities in vivo. mBio 6:e01155. doi: 10.1128/mBio.01155-15
Webster, J. P. (2007). The effect of Toxoplasma gondii on animal behavior: playing cat and mouse. Schizophr. Bull. 33, 752–756. doi: 10.1093/schbul/sbl073
Webster, J. P., Kaushik, M., Bristow, G. C., and McConkey, G. A. (2013). Toxoplasma gondii infection, from predation to schizophrenia: can animal behaviour help us understand human behaviour? J. Exp. Biol. 216, 99–112. doi: 10.1242/jeb.074716
Webster, J. P., and McConkey, G. A. (2010). Toxoplasma gondii-altered host behaviour: clues as to mechanism of action. Folia Parasit. 57, 95–104. doi: 10.14411/fp.2010.012
Wilson, E. H., and Hunter, C. A. (2004). The role of astrocytes in the immunopathogenesis of toxoplasmic encephalitis. Int. J. Parasitol. 34, 543–548. doi: 10.1016/j.ijpara.2003.12.010
Wilson, E. H., Weninger, W., and Hunter, C. A. (2010). Trafficking of immune cells in the central nervous system. J. Clin. Invest. 120, 1368–1379. doi: 10.1172/JCI41911
Witola, W. H., Mui, E., Hargrave, A., Liu, S., Hypolite, M., Montpetit, A., et al. (2011). NALP1 influences susceptibility to human congenital toxoplasmosis, proinflammatory cytokine response, and fate of Toxoplasma gondii-infected monocytic cells. Infect. Immun. 79, 756–766. doi: 10.1128/IAI.00898-10
Wohlfert, E. A., Blader, I. J., and Wilson, E. H. (2017). Brains and brawn: Toxoplasma infections of the central nervous system and skeletal muscle. Trends Parasitol. 33, 519–531. doi: 10.1016/j.pt.2017.04.001
Woolf, E., Grigorova, I., Sagiv, A., Grabovsky, V., Feigelson, S. W., Shulman, Z., et al. (2007). Lymph node chemokines promote sustained T lymphocyte motility without triggering stable integrin adhesiveness in the absence of shear forces. Nat. Immunol. 8, 1076–1085. doi: 10.1038/ni1499
Xu, Y., Wang, X., Liu, J., Fu, Y., Xu, J., and Liu, Q. (2018). Toxoplasma gondii rhoptry protein38 (TgROP38) affects parasite invasion, egress, and induces IL-18 secretion during early infection. Acta Biochim. Biophys. Sin. 50, 766–775. doi: 10.1093/abbs/gmy075
Yamamoto, M., Ma, J. S., Mueller, C., Kamiyama, N., Saiga, H., Kubo, E., et al. (2011). ATF6β is a host cellular target of the Toxoplasma gondii virulence factor ROP18. J. Exp. Med. 208, 1533–1546. doi: 10.1084/jem.20101660
Yamamoto, M., Okuyama, M., Ma, J. S., Kimura, T., Kamiyama, N., Saiga, H., et al. (2012). A Cluster of interferon-γ-inducible p65 gtpases plays a critical role in host defense against Toxoplasma gondii. Immunity 37, 302–313. doi: 10.1016/j.immuni.2012.06.009
Yamamoto, M., Standley, D. M., Takashima, S., Saiga, H., Okuyama, M., Kayama, H., et al. (2009). A single polymorphic amino acid on Toxoplasma gondii kinase ROP16 determines the direct and strain-specific activation of Stat3. J. Exp. Med. 206, 2747–2760. doi: 10.1084/jem.20091703
Yan, Y., Zhang, G.-X., Gran, B., Fallarino, F., Yu, S., Li, H., et al. (2010). IDO upregulates regulatory T cells via tryptophan catabolite and suppresses encephalitogenic T cell responses in experimental autoimmune encephalomyelitis. J. Immunol. 185, 5953–5961. doi: 10.4049/jimmunol.1001628
Yang, N., Farrell, A., Niedelman, W., Melo, M., Lu, D., Julien, L., et al. (2013). Genetic basis for phenotypic differences between different Toxoplasma gondii type I strains. BMC Genomics 14:467. doi: 10.1186/1471-2164-14-467
Yap, G. S., and Sher, A. (1999). Effector cells of both nonhemopoietic and hemopoietic origin are required for interferon (IFN)-gamma- and tumor necrosis factor (TNF)-alpha-dependent host resistance to the intracellular pathogen, Toxoplasma gondii. J. Exp. Med. 189, 1083–1092. doi: 10.1084/jem.189.7.1083
Yarovinsky, F. (2005). tlr11 activation of dendritic cells by a protozoan profilin-like protein. Science 308, 1626–1629. doi: 10.1126/science.1109893
Yarovinsky, F. (2014). Innate immunity to Toxoplasma gondii infection. Nat. Rev. Immunol. 14:109. doi: 10.1038/nri3598
Yarovinsky, F., Hieny, S., and Sher, A. (2008). Recognition of Toxoplasma gondii by TLR11 prevents parasite-induced immunopathology. J. Immunol. 181, 8478–8484. doi: 10.4049/jimmunol.181.12.8478
Yildiz, K., Gokpinar, S., Gazyagci, A. N., Babur, C., Sursal, N., and Azkur, A. K. (2017). Role of NETs in the difference in host susceptibility to Toxoplasma gondii between sheep and cattle. Vet. Immunol. Immunopathol. 189, 1–10. doi: 10.1016/j.vetimm.2017.05.005
Keywords: Toxoplasma, immune response, strain, virulence factor, ileitis, encephalitis
Citation: Mukhopadhyay D, Arranz-Solís D and Saeij JPJ (2020) Influence of the Host and Parasite Strain on the Immune Response During Toxoplasma Infection. Front. Cell. Infect. Microbiol. 10:580425. doi: 10.3389/fcimb.2020.580425
Received: 06 July 2020; Accepted: 11 September 2020;
Published: 15 October 2020.
Edited by:
Tiago W. P. Mineo, Federal University of Uberlandia, BrazilReviewed by:
Tajie Harris, University of Virginia, United StatesMattie Christine Pawlowic, University of Dundee, United Kingdom
Copyright © 2020 Mukhopadhyay, Arranz-Solís and Saeij. This is an open-access article distributed under the terms of the Creative Commons Attribution License (CC BY). The use, distribution or reproduction in other forums is permitted, provided the original author(s) and the copyright owner(s) are credited and that the original publication in this journal is cited, in accordance with accepted academic practice. No use, distribution or reproduction is permitted which does not comply with these terms.
*Correspondence: Jeroen P. J. Saeij, jsaeij@ucdavis.edu
†These authors have contributed equally to this work