All Roads Lead to Cytosol: Trypanosoma cruzi Multi-Strategic Approach to Invasion
- 1CONICET-Universidad de Buenos Aires, IQUIBICEN, Ciudad de Buenos Aires, Argentina
- 2Laboratorio de Biología Molecular de Trypanosoma, Departamento de Química Biológica, Facultad de Ciencias Exactas y Naturales, Universidad de Buenos, Ciudad de Buenos Aires, Argentina
- 3Department of Pharmacology and Chemical Biology, School of Medicine, University of Pittsburgh, Pittsburgh, PA, United States
T. cruzi has a complex life cycle involving four developmental stages namely, epimastigotes, metacyclic trypomastigotes, amastigotes and bloodstream trypomastigotes. Although trypomastigotes are the infective forms, extracellular amastigotes have also shown the ability to invade host cells. Both stages can invade a broad spectrum of host tissues, in fact, almost any nucleated cell can be the target of infection. To add complexity, the parasite presents high genetic variability with differential characteristics such as infectivity. In this review, we address the several strategies T. cruzi has developed to subvert the host cell signaling machinery in order to gain access to the host cell cytoplasm. Special attention is made to the numerous parasite/host protein interactions and to the set of signaling cascades activated during the formation of a parasite-containing vesicle, the parasitophorous vacuole, from which the parasite escapes to the cytosol, where differentiation and replication take place.
Introduction
Chagas Disease is a serious life-threatening disease caused by the protozoan parasite Trypanosoma cruzi and transmitted by blood-sucking triatomine insects from the Reduviidae family. In addition to an estimated of 6–8 million infected people and an alarming 50,000 deaths per year, 65–100 million people are living in areas at risk for infection (Lidani et al., 2019). This regional issue is now becoming global due to the migration of infected people to non-endemic countries, resulting in an estimated global economic burden of $7.19 billion (Lee et al., 2013).
T. cruzi has a complex life cycle, involving an insect vector and a mammalian host. Typically, metacyclic trypomastigotes (MTs) gain access to the mammalian host through feces contamination at the insect bite wound. Upon internalization by the host cells close to the site of entry, MTs initially reside in a parasite-containing vesicle, the parasitophorous vacuole (TcPV), from which they escape to the host cell cytoplasm and differentiate into the proliferative amastigote form. After several rounds of replication, amastigotes differentiate into motile flagellated trypomastigotes, bloodstream (BSTs), or tissue culture-derived (TCTs) trypomastigotes, that are released into the bloodstream, from where they could disseminate by infecting distant tissues or taken up by the triatomine vector during a bloodmeal (Monteón et al., 1996). Interestingly, although the parasite could potentially infect any nucleated cells, it has been demonstrated that different strains exhibit distinct tropism, measured as parasite load, for organs such as esophagus, liver, spleen, intestine, heart, and skeletal muscle, during acute phase of the infection, while tropism in the chronic phase has shown to be more homogeneous and restricted to intestine, skeletal muscle, and heart (Santi-Rocca et al., 2017). In this regard, it is worth to mention that adipocytes are also an important target cell during the acute phase of the disease, and may represent an important long-term reservoir for parasites during chronic infection (Combs et al., 2005). Additionally, it has been shown that amastigotes represent 10% of the parasites circulating in the blood of infected animals during the acute phase of infection (Andrews et al., 1987). Extracellular amastigotes (EAs), originated from premature rupture of infected cells or transformed from BSTs, are also infective and can disseminate in the infected hosts (Walker et al., 2014; Bonfim-Melo et al., 2018a).
In addition to a complex life cycle, T. cruzi has shown to be a remarkably heterogeneous taxon, that presents multiple strains with a high degree of genetic variability. This immense genetic diversity has been classified into six Discrete Typing Units (DTUs): the ancestral strains DTU-I and II, homozygote-derived hybrids DTU-III and IV, and heterozygote hybrids DTU-V and VI (Zingales et al., 2009). T. cruzi’s genome presents a conserved core of genes and extremely variable multigene surface proteins families (Berná et al., 2018). These multigene families are expanded in the genome, accordingly to its repetitive structure, and there is a rich source of diversity between different strains (De Pablos and Osuna, 2012). Among these families are the Trans-sialidase (TS) superfamily (about 1400 genes), the mucin family (about 860 genes), the Dispersed Gene Family-1 (DGF-1) family (565 genes) and the Mucin-Associated Surface Proteins (MASPs) family, which comprises around 1370 genes (El-Sayed et al., 2005; Kawashita et al., 2009). This incredible number of genes, coupled to tightly regulated post-transcriptional control of gene expression, are key players in the specific stage expression of the main surface constituents (Herreros-Cabello et al., 2020). As a consequence of the great expansion of surface protein families, the parasite is able to interact with a large number of surface receptors on the different host cells, a fundamental requirement for invasion.
In the process of invasion, the parasite hijacks the host cellular functions with the ultimate goal of establishing the replicative niche. Several pathways, converging in the formation of the TcPV, have been implicated in host cell invasion (Barrias et al., 2013). In general, T. cruzi invasion can be divided into four major steps: 1) host cell recognition and adhesion, 2) parasite internalization, 3) TcPVformation and maturation, and 4) escape to the cytosol. In this review, we highlight the different host cell signaling pathways that the parasite exploits to promote internalization, TcPV formation and the establishment of a productive intracellular infection.
The focus will be first placed on three strategies that T. cruzi uses to hijack host cell signaling pathways to facilitate invasion: 1) Engagement of host cell surface receptors (Alba Soto and González Cappa, 2019); 2) Protein and molecule shedding, including microvesicles and other vesicles, such as exosomes (Borges et al., 2016; Watanabe Costa et al., 2016); and 3) Host cell plasma membrane mechanical wounding (Fernandes and Andrews, 2012). These events converge in preparing the cell for subsequent invasion. The display of redundant strategies is crucial because it guarantees an effective invasion by T. cruzi.
Second, attention will be placed on the strategies that lead to the internalization of the parasite. T. cruzi exploits three main mechanisms in the host cell to facilitate internalization: a) Ca2+-dependent recruitment of lysosomes, b) Endocytosis, and c) Autophagy. As a result of the activation of these pathways, invading trypomastigotes end up localizing inside the TcPV. The mechanism for vacuolar escape is known to be lysosome- and pH- dependent, involving secretion of a porin-like/complement 9-related factor TcTOX (Andrews, 1994). As an obligate intracellular parasite, ensuring cell integrity is essential for the establishment of a productive infection. Accordingly, signaling pathways are also manipulated to avoid apoptosis (Stahl et al., 2014).
Bidirectional signaling pathways are activated in both the parasite and the host cell during invasion. T. cruzi specific signal transduction pathways have recently been reviewed elsewhere (Schoijet et al., 2019). This review provides a general overview of the key parasite/host interactions and signaling pathways activated in the host cell during T. cruzi invasion, which are summarized in Table 1 and Figure 1.
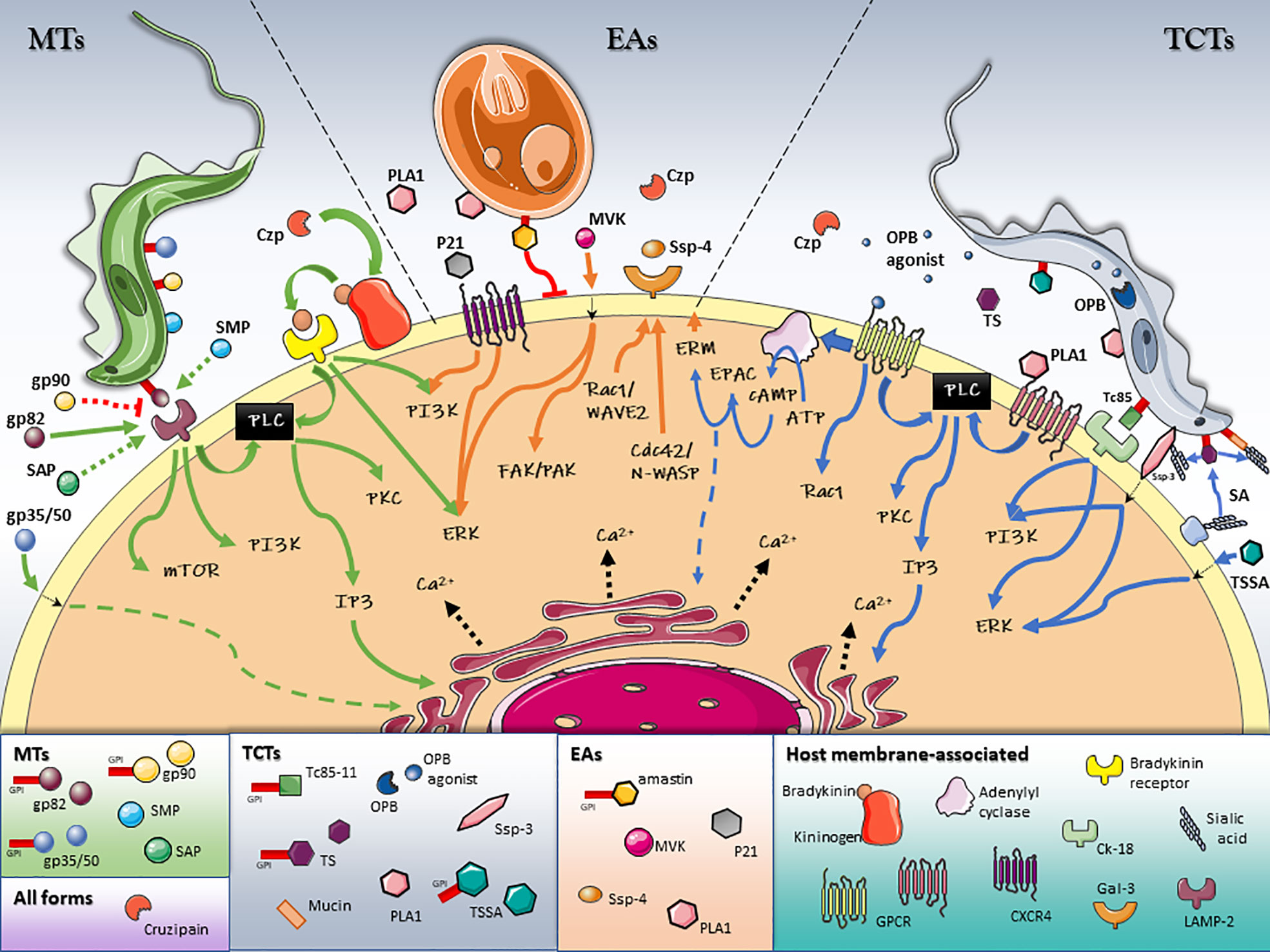
Figure 1 Schematic model of T. cruzi/host cell protein interactions and activated signaling pathways during invasion. Stage-specific Surface Molecules expressed on the membrane of the parasite or shed into the extracellular medium, play an essential role in the recognition, adhesion and activation of signaling pathways that lead to a successful invasion of the host cell. Figures were created using images from Servier Medical Art Commons Attribution 3.0 Unported License. (http://smart.servier.com). Servier Medical Art by Servier is licensed under a Creative Commons Attribution 3.0 Unported License.
The Prelude to Invasion: Host Cell Recognition, Adhesion, and Activation
Stage-Specific Surface Molecules
Early studies have shown that proteolytic treatment of trypomastigotes resulted in 90% inhibition of invasion, establishing a clear correlation between parasite surface proteins and infectivity (Andrews et al., 1984). Since then, several surface proteins have been identified and characterized. More recently, the first mass spectrometry-based exhaustive glycoproteome analysis of T. cruzi was completed, allowing the identification of 690 glycoproteins. Among them, 170 were exclusively identified in epimastigotes and 334 in trypomastigotes (Alves et al., 2017). In addition, it has been well established that every infective form of T. cruzi (MTs, TCTs and EAs) expresses on its surface a distinct set of stage-specific glycoproteins (Alba Soto and González Cappa, 2019). Several parasite stage-specific glycoprotein/host cell receptor interactions, and the corresponding signaling cascade activated in the host, are currently known (Figure 1 and Table 1).
Metacyclic Trypomastigotes
- gp82
Among surface glycoproteins involved in the adhesion of T. cruzi to the host cell is gp82, a MT-specific virulence factor, member of the gp85/TS family (Teixeira and Yoshida, 1986; Cortez et al., 2012; Maeda et al., 2012). Gp82 is attached to the outer cell membrane of the parasite by a glycosylphosphatidylinositol (GPI) anchor, which is susceptible to cleavage by an endogenous phosphatidylinositol-specific phospholipase C (PI-PLC) and released into the extracellular medium (Bayer-Santos et al., 2013a). During the MTs invasion process, secreted, and/or surface-anchored gp82 molecules interact with a host receptor and trigger signaling pathways leading to intracellular Ca2+ and lysosome mobilization in the host cell (Manque et al., 2003). Gp82 mediates the mobilization of Ca2+ from thapsigargin-sensitive intracellular stores (Yoshida et al., 2000). The activation of PLC in the host cell generats diacylglycerol (DAG) and inositol 1,4,5-trisphosphate (IP3), which induces protein kinase C (PKC) and promotes the release of Ca2+ from IP3-sensitive compartments (Maeda et al., 2012). Mammalian target of rapamycin (mTOR) and phosphatidylinositol 3-kinase (PI3K) are also activated during MTs invasion. The elevation in the cytosolic Ca2+ concentration triggered by these pathways, promotes actin cytoskeleton disruption and lysosome mobilization to the cell periphery, both events promoting the internalization of the parasite (Martins et al., 2011; Cortez et al., 2014). The lysosome-associated membrane protein 2 (LAMP‐2) has been recently identified as the host cell receptor for gp82 (Rodrigues et al., 2019). In this work, Rodrigues et al. have shown that antibodies directed against LAMP‐2, but not to LAMP‐1, significantly inhibit MTs internalization. Moreover, co‐immunoprecipitation assays demonstrated that gp82 binds to LAMP‐2 protein in a receptor‐mediated manner (Rodrigues et al., 2019).
- gp90
Another key MTs surface glycoprotein is gp90, also a member of the gp85/TS superfamily, which in opposition to gp82, has a negative effect on parasite invasion (Bubis et al., 2018). Early studies have shown an inverse correlation between gp90 expression levels and MTs infectivity (Málaga and Yoshida, 2001). Moreover, monoclonal antibodies confirmed low levels of gp90 in a highly invasive strain (CL), while high expression of gp90 was observed in a poor invasive strain (G) (Ruiz et al., 1998). Although gp90 binds to the host cell, it fails to trigger cytosolic Ca2+ mobilization (Ruiz et al., 1998). The inhibition of host cell lysosome spreading has been recently proposed as the mechanism by which gp90 exerts its down-regulatory role (Rodrigues et al., 2017).
- gp35/50
Gp35/50 mucins are highly glycosylated proteins expressed by MTs forms of T. cruzi (Ramirez et al., 1993). Like gp82, gp35/50 has the ability to trigger intracellular Ca2+ elevation when binding to host cell (Dorta et al., 1995; Ruiz et al., 1998). However, gp35/50-medited invasion induces actin recuitment, in contrast to gp82, that triggers signaling pathways leading to disassembly of F-actin (Ferreira et al., 2006). In addition, high level expression of gp35/50 was found to be inversely correlated to infectivity (Ruiz et al., 1998), although, when treatment of MTs with neuraminidase was applied before invasion assays, an increase in infectivity was observed, probably due to the fact that desialylated gp35/50 can interact with the host cell (Yoshida et al., 1997).
Cell-Derived Trypomastigotes
- Trans-sialidase
Among the different surface molecules involved in TCTs invasion is the unique T. cruzi trans-sialidase (TS), an important parasite virulence factor. Unable to synthesize sialic acid (SA), TS enables TCTs to transfer terminal SA residues linked α2,3 to terminal β-galactopyranoses from host cell donor macromolecules to glycans of mucin-type proteins displayed on the parasite membrane (Schenkman et al., 1991; da Fonseca et al., 2019; Campetella et al., 2020). The generation of a sialylated surface plays a central role in promoting the evasion of immune responses, favoring survival and the establishment of the chronic disease (Nardy et al., 2016). In addition, the transference of SA to the parasite surface creates the Stage-Specific Epitope 3 (Ssp-3) that promotes invasion of the host cell (Schenkman et al., 1991). TS has also been postulated as counter-receptor for TCTs binding to α2,3-sialyl receptors on the host cell, as a prelude to T. cruzi invasion (Ming et al., 1993). Signaling pathways implicated in TS mediated promotion of invasion includes the PI3K/AKT (Chuenkova et al., 2001; Butler et al., 2013) and the MAPK/ERK (mitogen-activated protein kinase/extracellular regulated kinase) pathways (Chuenkova and Pereira, 2001). Furthermore, shedding of TS into the bloodstream allows T. cruzi to manipulate the surface sialylation pattern of the target cell and different cell types distant from the site of infection. This soluble form of TS has been involved, among other processes, in host immunomodulation and haematological alterations, mainly by disruption of cell surface sialyl homeostasis (Campetella et al., 2020). Moreover, differential TS expression and gene dosage between different T. cruzi strains, have been reported. In a murine model, highly virulent strains of the parasite, belonging to DTU-VI, expressed and shed high amounts of TS, whereas the opposite was observed in mice infected by the low-virulence DTU-I strains (Risso et al., 2004; Burgos et al., 2013). Intriguingly, a naturally occurring point mutation, the Y342H substitution, accounts for the lack of trans-sialylation activity that generates an inactive form of TS (iTS) (Cremona et al., 1995). Still, iTS behaves as a lectin-like protein, that maintains the ability to bind SA and β-galactose residues (Cremona et al., 1999). Experimental data strongly suggest that iTS confers alternative and/or complementary roles to TS in the parasite virulence and pathogenesis (Campetella et al., 2020).
- Tc85
Another TCTs surface molecule with affinity for the extracellular matrix is the Tc85 family (Giordano et al., 1999). Belonging to the gp85/TS superfamily, Tc85 proteins lack enzymatic activity and, although unable to transfer SA, they have been implicated in cell adhesion and invasion (Mattos et al., 2014). A Laminin-G like domain (LamG) at the C-terminus of gp85/TS seems to be responsible for binding different receptors present in the extracellular matrix and host cell surface (Teixeira A. A. R. et al., 2015). Two motifs in the LamG domain have been described: The FLY motif (VTVxNVxLYNRPLN), present at the C-terminus of Tc85 proteins, mediates the interaction with cytokeratins (Tonelli et al., 2010), and the TS9 motif that showed significant cell binding capacity (Teixeira A. A. R. et al., 2015). In particular, FLY has been implicated in cytokeratin remodeling, ERK1/2 signaling pathway activation and increased internalization (Magdesian et al., 2007). It was shown that the FLY interacts with the endothelium in an organ-dependent manner with significantly higher avidity for the heart vasculature (Tonelli et al., 2010). These results, and the fact that TS9 and FLY are separated from each other by approximately 100 amino acids in the primary sequence of the gp85/TS proteins, are in agreement with the idea that TS9 and FLY comprise a non-linear conformational binding site (Teixeira A. A. R. et al., 2015).
- TSSA
The trypomastigote small surface antigen (TSSA) is a small mucin-like protein from the TcMUC family of T. cruzi mucin genes, the main mucins on the surface of TCTs and the scaffolds of the Ssp-3 epitope (Buscaglia et al., 2004; Campo et al., 2006). Although TSSA is not a SA acceptor, it binds to mammalian cells and induces Ca2+ signaling (Cánepa et al., 2012a). There are four allelic variants (TSSA I-IV), each one corresponding to an ancestral DTU (I-IV), while in hybrid genomes (DTU V-VI) TSSA isoforms II and III can be found (Balouz et al., 2021). TSSAII showed higher adhesion to host cells than TSSAI. Furthermore, TSSAII elicited a much more rapid and sustained increase in intracellular Ca2+ and promoted a stronger stimulation of the ERK1/2 pathway, than TSSA I (Cánepa et al., 2012a). Mapping experiments and cell-binding assays revealed that at least two peptidic motifs are critical for the interaction of the “adhesive” TSSA variant to host cell surface receptor(s) prior to trypomastigote internalization. These observations were supported by the fact that transgenic trypomastigotes over-expressing the ‘adhesive’ TSSA displayed improved adhesion and infectivity towards non-macrophagic cell lines (Cámara et al., 2017).
Extracellular Amastigotes
- δ-Amastin
The amastin multi-gene family was originally identified by screening an amastigote cDNA library (Teixeira et al., 1994). In particular, δ-Amastin, a transmembrane glycoprotein highly expressed on the surface of intracellular amastigotes, has been implicated in EAs cell invasion and differentiation (Cruz et al., 2012). Although amastin is present in all sequenced T. cruzi strains (Cerqueira et al., 2008), transcript levels were found to be down-regulated in amastigotes of the G strain (Cruz et al., 2012; Kangussu-Marcolino et al., 2013). It was shown that recombinant δ-amastin binds to cells in a saturable and dose-dependent manner and was able to inhibit parasite internalization, suggesting a role for amastin in T. cruzi invasion (Cruz et al., 2012). Moreover, in transgenic EAs, the overexpression of amastin promoted liver tropism during in vivo infections in mice and accelerated amastigogenesis (Cruz et al., 2012). The involvement of amastins in T. cruzi virulence was also supported by knocking down δ-amastins in Leshmania braziliensis, which resulted in a decrease in survival and proliferation of intracellular parasites after in vitro macrophage infection and no detectable parasites after in vivo infections (de Paiva et al., 2015).
Protein Secretion and Extracellular Vesicles Cargo
Trypomastigotes (MTs and TCTs) and EAs shed a wide number of GPI-anchored surface proteins/glycoproteins such as members of the gp85/TS family, mucins and MASPs (Trocoli Torrecilhas et al., 2009; Cánepa et al., 2012b; Bayer-Santos et al., 2013b; Lantos et al., 2016; Watanabe Costa et al., 2016). These proteins are not secreted by the classical endoplasmic reticulum (ER)/Golgi-dependent secretion pathway, but instead, gradually released into milieu by the action of an endogenous PI-PLC (Andrews et al., 1988), or associated to extracellular vesicles (EVs) involved in host cell invasion, immunomodulation and pathogenesis (Borges et al., 2016; Torrecilhas et al., 2020).
EVs can be divided into: microvesicles or ectosomes (100 nm to1 μM), directly originated by budding from plasma membrane, and exosomes (30–100 nM), that are secreted following the fusion of multivesicular endosomes with the membrane at the flagellar pocket (Evans-Osses et al., 2015). Quantitative proteomic analysis revealed differences in protein content between these two populations of EVs (Bayer-Santos et al., 2013a). An interesting case is the trypomastigote excreted/secreted antigens (TESA), around 80 parasite proteins with the majority being highly immunogenic gp85s, associated with exosomal vesicles shed by MTs, TCTs, and intracellular amastigotes, used as a reagent in the diagnosis of the disease (Berrizbeitia et al., 2006; Bautista-López et al., 2017). Although EVs are secreted by all forms of T. cruzi, only those shed by infective forms are able to enhance internalization of host cells, by inducing intracellular Ca2+ mobilization (Moreira et al., 2019). Inoculation of EVs before infection in mice produced an increment of parasitemia in early days post-infection and more amastigote nests in mice hearts (Lovo-Martins et al., 2018). Moreover, it has been shown that vesicles from TCTs from the T. cruzi strains Colombiana (DTU I), YuYu (DTU I), Y (DTU II), and CL-14 (DTU VI) presented differences in their protein and α-galactosyl contents and were able to differentially modulate host’s immune responses and parasite invasion (Nogueira et al., 2015). Although all strains were capable of activating MAPKs like p38, JNK, and ERK 1/2, CL-14, and YuYu activated MAPKs via TLR2, while EVs from Colombiana and Y strains needed to be internalized to activate the MAPK pathway (Nogueira et al., 2015). Thus, the composition and effects of EVs on host cell seems to be strain-dependent.
In addition to glycoproteins, a substantial number of other molecules are released into the extracellular medium, like complement regulatory proteins (CRPs), cruzipain (Czp), peptidyl-prolyl cis-trans-isomerases, oligopeptidases and proteases, phospholipases A1 and C, P21, and amastigote specific proteins (Torrecilhas et al., 2012; Watanabe Costa et al., 2016).
Interesting examples are SAP (serine-, alanine-, and proline-rich protein) and TcSMP (Trypanosoma cruzi Surface Membrane Proteins), which have been involved in MTs invasion by binding to host cells and triggering Ca2+ signaling and lysosome mobilization (Baida et al., 2006; Zanforlin et al., 2013; Martins et al., 2015).
- SAP
Diverse paralogs of SAPs, with different cellular localization, are expressed in the different development stages of the parasite. In particular, SAP peptides were identified by by mass spectrometry in vesicle and soluble-protein fractions from epimastigotes and MTs conditioned medium. Although, SAP transcript levels and protein expression in MTs were found to be twice as high as in epimastigotes, in agreement with their proposed role in cell adhesion and invasion (Zanforlin et al., 2013). In this regard, the fact that gp82 and SAP share the ability to induce Ca2+ signaling and lysosome mobilization, led to the hypothesis that both molecules display a synergistic effect in the process of MTs host-cell invasion (Baida et al., 2006; Zanforlin et al., 2013).
- TcSMP
Recently described, the TcSMP family, possesses two main features typical of surface proteins, an N-terminal signal peptide and a C-terminal hydrophobic sequence, predicted to be a transmembrane domain, rather than the most prevalent GPI anchoring (Martins et al., 2015). TcSMPs are expressed in all T. cruzi developmental stages, located at the surface and present in the secretome of epimastigotes and MTs. Similarly to SAP, TcSMPs have been shown to promote a weaker lysosome mobilization and parasite internalization than gp82, suggesting an auxiliary role in parasite invasion (Martins et al., 2015).
- TcPLA1
The membrane-associated phospholipase A1 (TcPLA1) can be also found in the extracellular medium of TCTs and EAs (Belaunzarán et al., 2007). Host cells exposed to the conditioned medium of EAs, TCTs, or recombinant TcPLA1, showed modified lipid profiles, with increased cellular concentrations of free fatty acids, diacylglycerol and lysophosphatidylcholine, that contributed to the concomitant activation of the PKC pathway (Belaunzarán et al., 2013). Remarkably, PKC has been previously implicated in parasite invasion, suggesting that Tc-PLA1 would participate in the events preceding host cell invasion (Watanabe Costa et al., 2016).
Peptidases
Peptidases, a class of hydrolytic enzymes responsible for breaking peptide bonds, has attracted the attention of distinct research groups because of their role in several crucial steps of the life cycle of the trypanosomatid parasites. The T. cruzi genome contains several families of peptidases that play central roles in diverse processes, such as adhesion and cell invasion (Alvarez et al., 2012; Rawlings et al., 2014).
- Cruzipain
Cruzipain (Czp), the most notorious cysteine peptidase, is expressed as a complex mixture of isoforms in all forms of T. cruzi and mainly located in lysosome-related organelles (Lima et al., 2012), have been shown to be required but not essential for invasion (San Francisco et al., 2017). Czp released by trypomastigote promotes invasion through its cysteine protease activity by producing bradykinin from membrane-bound kininogen on the surface of the host cell and triggering IP3-mediated Ca2+ signaling upon recognition by bradykinin B2 receptor (B2R) (Scharfstein et al., 2000). More recently, a second cruzipain-mediated route, blocked by a cysteine protease inhibitor, thapsigargin and immunodepletion of Czp, but not by kinin receptor antagonists, was described for TCTs (Aparicio et al., 2004). Experimental data evidenced that this effect is mediated by a soluble trypomastigote-associated factor released by Czp (Aparicio et al., 2004).
- Oligopeptidase B
Oligopeptidase B (OPB), a serine endopeptidase from the prolyl oligopeptidase family, is conserved in trypanosomatids but not present in any mammalian genome (Motta et al., 2019). OPB has a cytosolic localization and there is not any strong evidence suggesting its secretion by the parasite. Instead, it has been involved in the cytoplasmatic processing of a trypomastigote-specific precursor that generates a soluble factor of unknown structure which is shed by TCTs (Burleigh et al., 1997).
Upon binding to the host cell receptor, the OPB-agonist induces PLC activation and an IP3-dependent release of Ca2+ from intracellular stores. This Ca2+ mobilization promotes lysosomal recruitment to the entry site and F-actin filaments disruption, both events associated with an increased parasite invasion (Burleigh et al., 1997; Caler et al., 1998). Surprisingly, even today, with genomes of several T. cruzi strains available, the identity of the precursor it is still unknown. However, the secretion of OPB cannot be ruled out since OPB activity has been found in trypomastigotes supernatants (Fernandes et al., 2005; Motta et al., 2019). Trypanosoma brucei and Trypanosoma evansi OPBs, are released into the extracellular milieu and contribute to pathogenesis by hydrolyzing host circulating factors (Motta et al., 2019). In the case of T. cruzi, hydrolyzed peptides would mimics ligands capable of activating GPCR and/or RTK (Motta et al., 2019).
EAs Specific Proteins
EAs are capable of invading mammalian cells in an actin-dependent mechanism, forming a phagocytic cup that engulfs the parasite (Mortara et al., 2005). Secreted proteins from EAs, such as P21, mevalonate kinase (TcMVK) and specific-surface protein 4 (Ssp-4), mediate host cell signaling during the phagocytosis-like mechanism of invasion (Rodrigues et al., 2012; Ferreira et al., 2016; Florentino et al., 2018).
- P21
P21 is a 21kDa protein expressed in all developmental stages of T. cruzi and secreted by EAs to induce host cell invasion (da Silva et al., 2009). Evidence for this observation came from the use of a recombinant version of P21 (rP21) that bound to the CXCR4 chemokine receptor and promoted phagocytosis by induction of actin cytoskeleton polymerization and the modulation of the expression of actin-related genes in a PI3K-dependent manner (Rodrigues et al., 2012; Teixeira et al., 2017). In addition, in mice infections with the T. cruzi naturally attenuated TCC strain, rP21 lead to an exacerbated infection and parasite load in target organs (Brandán et al., 2019).
- TcMVK
MVK is a key enzyme involved in the early steps of the sterol isoprenoids biosynthesis pathway (Ferreira et al., 2016). In T. cruzi, TcMVK localizes to glycosomes, and may be also secreted into the extracellular milieu where it modulate host cell invasion, independently of its catalytic function. More precisely, TcMVK activates the actin-related kinases FAK (focal adhesion kinase) and PAK (p21-activated kinase), and the MAPK pathway components, ERK, and p38 to promote EAs internalization (Ferreira et al., 2016).
- Ssp-4
Ssp4 is a major surface GPI-anchored glycoprotein that is secreted by the EAs (Andrews et al., 1987). Although EAs Ssp-4 expression does not correlate with infectivity, glycosylation of Ssp-4 was associated with host cell invasion. It has been shown that only EAs from highly infective strains secreted a differentially glycosylated Ssp-4 into vesicle trails at the site of entry, contributing to Galectin-3 (Gal-3) recruitment and establishing a physical link between the parasite and the host cell surface (Florentino et al., 2018). Gal-3, a 31kDa β-galactoside-binding protein, is recruited to the site of EAs entry during cell invasion and participates in the intracellular trafficking of the parasite (Machado et al., 2014).
Plasma Membrane Damage
It has been proposed that flagellar motility of trypomastigotes strongly attached to the host cells surface through their posterior end produces membrane damage in the host cell. An active gliding motility of parasites firmly attached to host cells was evidenced using time-lapse phase-contrast live images of trypomastigotes interacting with a HeLa cells (Fernandes et al., 2011). Supporting evidence was also obtained from the analysis of scanning electron microscopy images of T. cruzi during early steps of invasion, showing parasites gliding under cells or in close contact with the plasma membrane at the cell periphery (Fernandes et al., 2011). Parasite-mediated membrane damage triggers Ca2+-dependent fusion of lysosomes and internalization through Plasma Membrane Repair Mechanism (PMR), that will be discussed below.
Hijacking Host’s Signaling Machinery
To maintain homeostasis, host cells have a complex vesicular transport system, that consist of multiple connected networks with different levels of cross-talk (Salimi et al., 2020). T. cruzi has developed the ability of subverting and exploiting the most suitable mechanism at the time of invasion to gain access to the host cell (Figure 2). Three main mechanisms of internalization, involving several coordinated and integrated pathways, are used by the parasite to gain access to the target cell: 1) Ca2+-mediated recruitment and fusion of lysosomes to the entry site (Figures 2.1–3), 2) Endocytosis of plasma membrane (Figures 2.4–7) and 3) Autophagy (Figure 2.8).
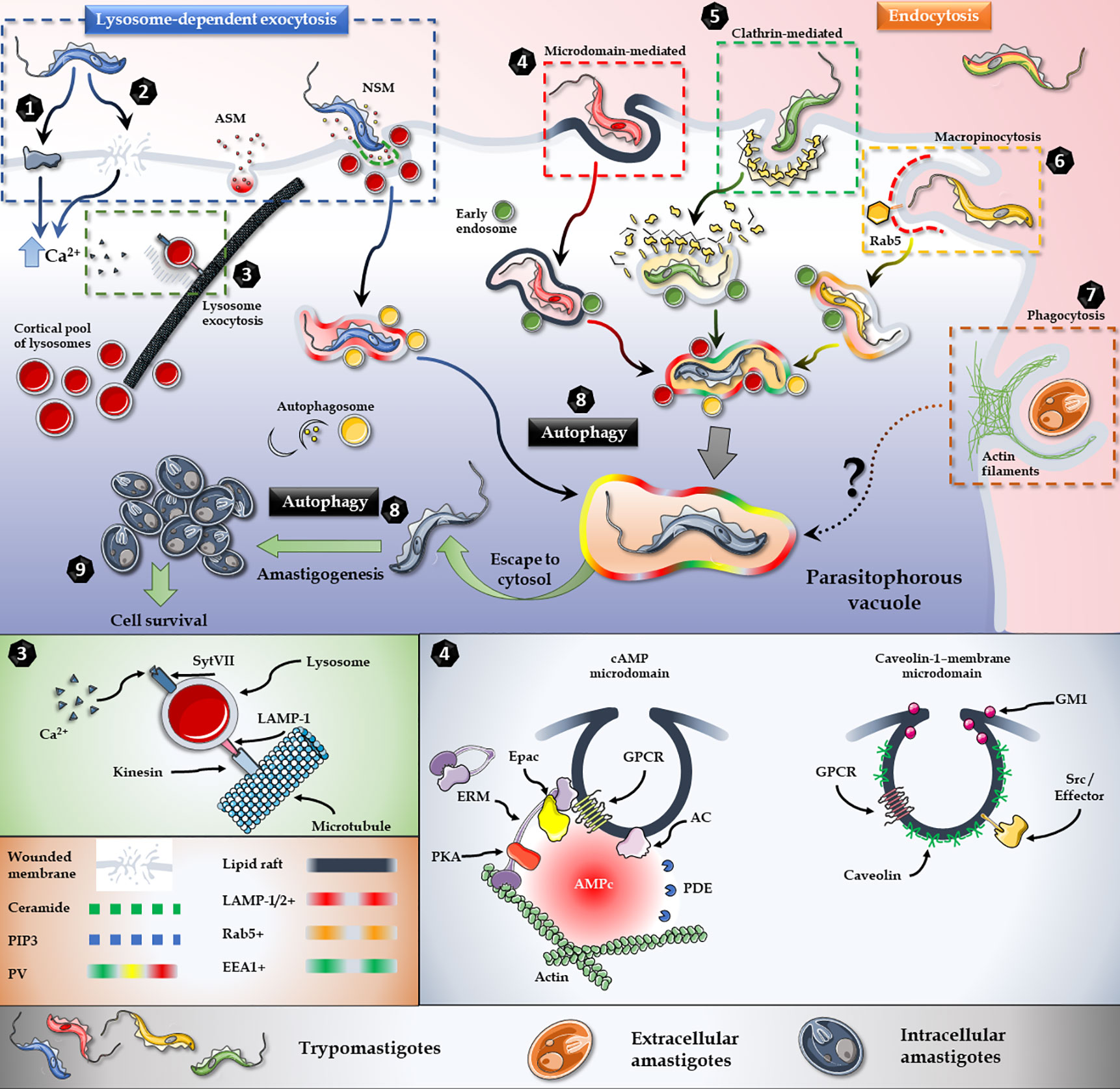
Figure 2 T. cruzi invasion model. Lysosome exocytosis involves surface/secreted proteins (1) or micro-injuries (2) that trigger an elevation in the intracellular levels of Ca2+ and the microtubule/kinesin-mediated recruitment of lysosomes from surrounding areas to the parasite entry site (3). Host’s Acid Sphingomyelinase (ASM) and a parasite Neutral Sphingomyelinase (NSM) are secreted to the extracellular milieu and participates in the breakdown of sphingomyelin to ceramide and phosphorylcholine in the outer leaflet of the plasma membrane (3). Endocytic mechanisms, such as lipid raft-dependent endocytosis (4), clathrin-mediated endocytosis (5) and macropinocytosis (6) also converge in the internalization of the parasite. Extracellular amastigotes, on the other hand, employ a phagocytosis-like mechanism for invasion (7). Moreover, autophagy is a key player during the invasion and also promotes trypomastigotes to amastigotes differentiation (8). In brief, regardless of the parasite stage, or the activated cascade, all internalization pathways culminate in the parasitophorous vacuole, from which parasite escapes to the cytoplasm and differentiates into amastigotes and proliferates (9). Figures were created using images from Servier Medical Art Commons Attribution 3.0 Unported License. (http://smart.servier.com). Servier Medical Art by Servier is licensed under a Creative Commons Attribution 3.0 Unported License.
It is important to note that regardless of the parasite strain, the developmental stage, the repertoire of surface/secreted molecules expressed and the signaling cascades activated to prepare the host cell for invasion, all the internalization mechanisms lead to the biogenesis of TcPV (Batista et al., 2020). Moreover, despite the invasion mechanism as well, the acquisition of lysosome markers by the TcPV during the process of internalization has shown to be essential for intracellular retention of the parasite and the establishment of a successful infection (Andrade and Andrews, 2004).
Plasma Membrane Repair Mechanism
In order to maintain cellular homeostasis, membrane disruptions are rapidly resealed by a conserved PMR. Upon wounding, toxic levels of Ca2+ and oxidants from the extracellular milieu enter the cell. To avoid cell death, the damage is rapidly repaired by an extracellular Ca2+-induced recruitment of intracellular vesicles (Blazek et al., 2015) (Figures 2.1–3). It has been shown that conventional lysosomal exocytosis mediates the resealing in primary skin fibroblasts (Reddy et al., 2001). After wounding, Ca2+-dependent fusion of lysosomes to the host cell membrane was evidenced by the exposure of the luminal domain of lysosomal LAMP-1 and Synaptotagmin isoform VII (SytVII), a putative Ca2+ sensor in exocytosis (Sugita et al., 2001).
Moreover, a SytVII regulation of lysosome Ca2+-dependent exocytosis was evident by a dramatic inhibitory effect on plasma membrane resealing by antibodies directed against the cytosolic domain of this protein (Reddy et al., 2001). In addition to the exposure of lysosomal luminal proteins on the surface of the cell, the lysosomal enzyme Acid Sphingomyelinase (ASM) is secreted during cell injury and promotes plasma membrane repair (Tam et al., 2010) (Figures 2.1–3).
Invasion assays in the presence the pore-forming bacterial toxin Streptolysin O (SLO) increased parasite internalization, while bromoenol lactone (BEL), a lysosomal exocytosis inhibitor, strongly restrained invasion by T. cruzi trypomastigotes. Moreover, PMR and T. cruzi internalization have been shown to depend on the secretion of ASM, the lysosomal enzyme responsible for catalysing the breakdown of sphingomyelin to ceramide and phosphorylcholine in the outer leaflet of the plasma membrane (Tam et al., 2010; Fernandes et al., 2011). Lysosomal cysteine proteases cathepsin B and L are also secreted and may participate in the repair process by facilitating membrane access of ASM (Castro-Gomes et al., 2016). As a result, surface staining with anti-ceramide monoclonal antibodies and EEA1(early endosome-associated protein)-positive vesicles increased after treatement with extracellular ASM, suggesting that ceramide-enriched endocytic vesicles formation can facilitate trypomastigote entry. In the same line, inhibition of ASM reduced trypomastigote entry and this inhibition was reverted by the addition of extracellular sphingomyelinase (Fernandes et al., 2011). Proteomics studies have shown that trypomastigotes express and shed a neutral sphingomyelinase (Brossas et al., 2017) that might be contributing to the production of the required ceramide in the outer leaflet of the plasma membrane during host cell invasion by T. cruzi, although this hypothesis has not been yet explored. In accordance with the PMR-mediated invasion model, it was recently reported that the parasite could modulate the expression of plasma membrane repair-related proteins and the fold of change depends on the number of parasites interacting with the host cell (Brígido et al., 2017) (Figures 2.1–3).
Ca2+-Dependent Lysosome Exocytosis
Originally considered terminal degradative organelles, lysosomes have been found to participate in many other cellular processes (Pu et al., 2016). The involvement of lysosomes in these different processes depends on their sub-cellular distribution and their ability to move throughout the cytoplasm (Pu et al., 2016). Lysosomes distribute in a rather immobile perinuclear pool and a more dynamic pool in the cell periphery (Cabukusta and Neefjes, 2018). Living cells video microscopy during TCTs host cell invasion showed a directional microtubule/kinesin-mediated migration of lysosomes from surrounding areas to the parasite entry site (Rodríguez et al., 1996). It was later demonstrated that TCTs uses the cortical pool of lysosomes in the invasion process (Hissa and Andrade, 2015) (Figures 2.1–3).
Membrane-associated rafts enriched in cholesterol and ganglioside GM1 have been also implicated in adhesion and internalization of all infective forms of T. cruzi (Barrias et al., 2007; Fernandes et al., 2007). Immunofluorescence analysis demonstrated a colocalization of GM1, flotillin 1, and caveolin 1 in the nascent TcPV, supporting the fact that membrane rafts participate in T. cruzi invasion (Barrias et al., 2007). Consistently, cholesterol involvement in the recruitment of lysosomes was evidenced using methyl-beta cyclodextrin (MβCD), a cholesterol-removing agent used for lipid raft disruption. Noteworthy, LAMP-2 have shown to play a major role in cholesterol and caveolin traffic, membrane repair and T. cruzi invasion. Cells lacking LAMP-2 showed deficiency in cholesterol delivered to the plasma membrane and an altered caveolin-1 distribution, both phenomena being refractory to TCTs invasion (do Couto et al., 2020). Similarly, MTs internalization was significantly reduced in LAMP-2-depleted HeLa cells (Cortez et al., 2016).
In MTs invasion of Hela cells, lysosome biogenesis/scattering was stimulated upon interaction of the parasites with the host cell and a reduction in the number of cortical lysosomes negatively affected MTs invasion (Cortez et al., 2016), as previously reported for TCTs invasion of cardiomyocytes (Hissa et al., 2012; Hissa and Andrade, 2015). However, in the HeLa model, the stimulation of lysosome biogenesis/scattering diminished TCTs ability for invasion, whereas rapamycin-promoted lysosome accumulation at the perinuclear region led to a higher TCTs invasion (Cortez et al., 2016). While these observations may result contradictory, it is important to consider that different parasite strains have been used in order to establish the different models of invasion. T. cruzi invasion, already showed to be a complex process when only taking into account the different stages of the invading parasite. This complexity gets even higher when considering different DTUs, strains, the repertoire of surface/secreted molecules, and signaling pathways activated in the host cell. In this regard, differential infectivity has been reported for trypomastigotes of different strains (Cortez et al., 2012; Santi-Rocca et al., 2017); thus, it is not unlikely that a particular mechanism of invasion is exploited depending on the strain and host cell/tissue. For example, in contrast to the results reported for TCTs, the absence of extracellular Ca2+ had no effect on MTs invasion, while the presence of the pore‐forming bacterial toxin SLO decreased MTs internalization (Rodrigues et al., 2019), strongly suggesting a PMR-independent mechanism of invasion for MTs.
Ca2+ release from cellular compartments, such as the endoplasmic reticulum, is accompanied by an activation of PLC and an elevation of intracellular cAMP levels. It has been shown that cAMP is able to potentiate the Ca2+-dependent exocytosis of lysosomes and lysosome-mediated cell invasion by T. cruzi (Rodríguez et al., 1999). In mammalian cells, both cAMP effector pathways, i.e., Protein Kinase A (PKA) and Exchange protein activated directly by cAMP (Epac), are involved in Ca2+-triggered exocytic events (Seino and Shibasaki, 2005). Moreover, members of this latter pathway, including Rap1, have been localized to late endosomes/lysosomes (Pizon et al., 1994), and Epac-mediated Rap activation has been involved in regulated exocytosis in human sperm (Miro-Moran et al., 2012), insulin secretion (Tengholm and Gylfe, 2017) and pancreatic amylase release (Sabbatini et al., 2008). Accordingly, it was recently shown that Epac1-mediated signaling represents the main mechanism for cAMP-dependent host cell invasion by T. cruzi (Musikant et al., 2017). Additionally, ERM proteins (ezrin, radixin and moesin), which are essential for the cell cortex function and architecture by linking plasma membrane to the underneath actin cytoskeleton (McClatchey, 2014), have been associated to T. cruzi invasion (Ferreira et al., 2017). Confocal microscopy studies have shown that ERM proteins are recruited to the EA invasion site, where they co-localize with F-actin, and that depletion of host ERM proteins inhibited T. cruzi invasion in HeLa cells (Ferreira et al., 2017). Remarkably, radixin was identified as a scaffolding unit for cAMP effectors in the spatial regulation of cAMP-Epac1-Rap-mediated signaling (Gloerich et al., 2010; Hochbaum et al., 2011). A link between radixin and cAMP-Epac-mediated TCTs invasion was recently evidenced by blocking invasion in pre-treated NRK host cells with a 15 amino acid permeable peptide spanning Epac’s minimal ERM-biding domain (Musikant et al., 2017). This observation was consistent with a co-localization of a pool of Epac1 and radixin, as a requirement for invasion. Also, F-actin regulation is in part due to the activity of the focal adhesion kinase (FAK), a cytoplasmic protein tyrosine kinase (PTK) that participates during invasion by T. cruzi (Melo et al., 2014) (Figure 2.4). Both, inhibition of FAK autophosphorylation or knockdown of FAK expression by siRNA in cardiomyocytes, led to a reduction in T. cruzi internalization, hence showing a key role of the FAK-mediated pathway in this process (Melo et al., 2014). FAK inhibition was associated with ERK1/2 dephosphorylation and F-actin rearrangement, suggesting a crosstalk between this signaling cascade and the MEK/ERK pathway (Onofre et al., 2019). Likewise, the interaction between HeLa cells and EAs induced N-WASP-dependent actin polymerization via PI3K/AKT and ERK but not SFK (Src family kinases) (Bonfim-Melo et al., 2018a). In opposition, previous works on cardiomyocytes have shown that Src was required for TCTs internalization (Melo et al., 2014). However, observations are not conclusive since internalization experiments were done using PP1 as Src inhibitor, which also blocks TGF-β-mediated cellular responses in a Src-independent fashion (Ming et al., 1995; Yoshida and Cortez, 2008; Ferrão et al., 2015; Silva et al., 2019).
Endocytic Pathways
Endocytic processes can be divided into different classes: clathrin-mediated, caveolae-mediated, membrane microdomain-mediated, macropinocytosis and phagocytosis (Chou et al., 2011). Several of these endocytic pathways are exploited by T. cruzi for invasion (Barrias et al., 2013) (Figures 2.4–7). Moreover, lysosome-independent endocytosis has been proposed to be the main entry mechanism for TCTs (Burleigh, 2005; Cortez et al., 2016).
- Phagocytosis
Experimental evidence showed that approximately 20 to 25% of the internalized trypomastigotes were associated to lysosomes, while 50% of the invading parasites exploited an alternative PI3-kinase-dependent mechanism of invasion, involving a host cell plasma membrane-derived vacuole enriched in the lipid products of class I PI3-kinases, Phosphatidylinositol 3-Phosphate PI3P/Phosphatidylinositol 3,4-bisphosphate (P3,4P2) (Woolsey et al., 2003). In this endocytic mechanism of internalization, downstream of cell entry the EEA1 marker was never associated to the parasite-containing vacuoles, instead, a gradual lysosomal fusion was revealed by the acquisition of lysosomal markers such as LAMP-1 and fluid-phase endocytic tracers from the lysosomal compartment (Woolsey et al., 2003). However, Rab5, a marker for early endosomes, was found to associate to a fraction of T. cruzi-containing vacuoles during and immediately following internalization. Likewise, the remaining 20% to 30% of the T. cruzi-containing vacuoles were positive for EEA1, the Rab5 effector, indicating that early endocytic pathway of internalization took place as well. Interestingly, it has been shown that the Toll‐Like Receptor 2 (TLR2) was required to activate PI3K and Rab5 binding to early endosomes in the Rab5/Rab7-endosome-dependent invasion mechanism (Maganto-Garcia et al., 2008). Accordingly, a strong activation of PI3K and PKB/AKT was detected when cells were incubated with trypomastigotes or their isolated membranes (Wilkowsky et al., 2001). Noteworthy, T. cruzi-infected human macrophages shed EVs that enhance host cell TLR-2-mediated invasion (Cronemberger-Andrade et al., 2020).
EAs employ a phagocytosis-like mechanism when invading non-professional phagocytic cells (Fernandes et al., 2013), with positive participation of Cdc42, N-WASP, WAVE2, and Rac1, and negative regulation of RhoA (Bonfim-Melo et al., 2018b) (Figure 2.7). Furthermore, EAs interaction with HeLa cells produced an increase in ERK1/2 phosphorylation, while pre-treatment of HeLa cells with an ERK1/2 inhibitor had a negative effect on internalization. This results demonstrated a key role for that PI3K/AKT and ERK pathway during T. cruzi EA invasion (Ferreira et al., 2019), probably through activation of proteins that regulate microfilament remodelling such as calpain, FAK and cortactin (Bonfim-Melo et al., 2018a).
- Clathrin-Mediated Endocytosis
Clathrin-mediated endocytosis in T. cruzi internalization was recently evidenced (Barrias et al., 2019) (Figure 2.5). Clathrin-containing vesicles and actin filaments were localized at sites of parasites attachment and internalization and around the nascent TcPV. Accordingly, specific inhibition of clathrin-coated pit formation impaired T. cruzi internalization (Barrias et al., 2019).
- Macropinocytosis
Macropinocytosis, an actin-driven process originally described as a mechanism of non-specific uptake of fluid into large cytoplasmic vesicles, has also been implicated in host cell invasion by T. cruzi is (King and Kay, 2019) (Figure 2.6). The nascent macropinosome accumulates PI3P and active Rab5, that regulates the fusion of membranous organelles at early stages of endocytosis (Feliciano et al., 2011). Signaling patches involving PIP3, Ras, and Rac direct actin polymerization to the periphery of the macropinocytic cup (Kay et al., 2018). The involvement of macropinocytosis as a mechanism of entry for T. cruzi was demonstrated by blocking parasite internalization using macropinocytosis inhibitors, such as amiloride, rottlerin and IPA3 (Barrias et al., 2012). In accordance, the stimulation of macropinocytic activity through activation of PKC by PMA, showed an increased internalization of parasites (Barrias et al., 2012). Moreover, colocalization at entry sites of trypomastigotes with the Rab5 effector rabankyrin 5, tyrosine kinases, Pak1 and actin microfilaments, confirmed macropinosomes formation (Barrias et al., 2012).
Autophagic Pathway
Autophagy as an alternative pathway of internalization for T. cruzi was evidenced in starved cells, where the induction of autophagy was a positive modulator of invasion (Figure 2.8). On the other hand, the disruption of mammalian autophagy led to a reduction in infectivity (Salassa and Romano, 2019). The autophagy pathway consists of several coordinated and consecutive events: initiation, elongation, maturation, and fusion of lysosomes to the autophagosome. Upon activation, autophagosome biogenesis is initiated with the induction and nucleation of the phagophore, a double-membrane structure that grows to engulf the autophagic cargo, and the recruitment of the core autophagymachinery (Dikic and Elazar, 2018). Lipidated LC3-II is required in autophagosome biogenesis, and since it forms a stable association with the membrane of autophagosomes it is used as a marker for autophagy (Tanida et al., 2008). The presence of LC3 in the membrane of the TcPV during the internalization process showed a connection between the TCTs and the host-cell autophagic pathway (Romano et al., 2009). Accordingly, infection was reduced in the absence of specific autophagy genes Atg5 or Beclin1, confirming the requirement of an autophagic-derived compartment in autophagy-mediated invasion (Romano et al., 2009). Moreover, starvation and rapamycin treatment induced an increase of LAMP-1 in T. cruzi-containing vesicles, indicating lysosomal association to TcPV and the consequent autolysosome formation were required for an increased internalization (Romano et al., 2009).
TcPV Maturation and Escape to Cytosol
Several proteins are recruited to the TcPV at different times during the biogenesis and maturation process (Batista et al., 2020). Within these proteins are the SNAREs, fusion proteins that regulate docking of granules and vesicles to target membranes including the plasma membrane (Wang et al., 2017). Vesicle associated membrane proteins 3 (VAMP3) and VAMP7, are consequtively recruited to the TcPV. VAMP3, usually present in recycling or early endosomes, is not essential for invasion, whereas SNARE complexes involving VAMP7, required for late endosome/lysosome fusion, are crucial in the establishment of T. cruzi infection (Cueto et al., 2017). Besides, early (Rab5, Rab22a, and Rab21 positive vesicles) and late (Rab7 and Rab39a) endocytic compartments, also recruited to the TcPV at early times post internalization, regulate the transit of the TcPV and promote fusion with lysosomes (Salassa et al., 2020). TcPV maturation is characterized by an initial interaction with Rab5 and VAMP3‐positive vesicles, followed by the recruitment of Rab7 and VAMP7, to finally fuse with lysosomes (Cueto et al., 2017; Salassa et al., 2020).
It is well established that fusion of lysosomes to the TcPV induces acidification that triggers the vacuole disruption and subsequent release of T. cruzi into the host cell cytosol (Ley et al., 1990). T. cruzi viability in the TcPV depends on a highly effective antioxidant defense machinery involving specialized antioxidant enzymes, such as peroxidases and superoxide dismutases (SODs), that protects the parasite against reactive oxygen and nitrogen species (Cardoso et al., 2016). Interestingly, oxidative stress has been shown to be an enhancer of T. cruzi infection in macrophages (Paiva et al., 2012). Although, a plausible hypothesis is that T. cruzi needs minimal levels of ROS, signaling for replication, while high levels of ROS are deleterious (Goes et al., 2016). Heavily sialylated LAMP-1 and 2, located in the inner coat of the TcPV, have been shown to protect the TcPV from lysis (Rubin-de-Celis et al., 2006). Additionally, LAMP-1 and 2 are essential to retain the intracellular parasite (Albertti et al., 2010) and avoid reversible invasion (Caradonna and Burleigh, 2011).
Under acidic conditions, the disruption of de TcPV occurs through the several T. cruzi proteins, such as secreted TS (Hall et al., 1992) and two pore-forming proteins, TcTOX (Andrews et al., 1990) and LYT1 (Manning-Cela et al., 2001). In TCTs, the expression of TS induces the escape from the TcPV by desialylation of LAMP-1 and 2, making membranes more susceptible to disruption by pore-forming proteins (Hall et al., 1992; Rubin-de-Celis et al., 2006). Pores are then formed by TcTOX and LYT1. Interestingly, TcTOX and LYT1 share similar characteristics: both are secreted, present cross-reactivity with C9 antibodies and have hemolytic activity at low PH. In fact, the molecular identity of TcTOX still remains unknown, and all available data suggest that LYT1 is TcTOX, or a TcTOX‐like protein (Benabdellah et al., 2007; Friedrich et al., 2012).
Once in the cytoplasm, host cellular and metabolic pathways will be targeted by amastigotes in order to successfully replicate. Four to seven days post-invasion, amastigotes differentiate into the non-replicative infective trypomastigote form, that is released into the bloodstream (Caradonna et al., 2013; Li et al., 2016; Oliveira et al., 2020)
Concluding Remarks
Numerous works have endeavored to comprehend the molecular basis of T. cruzi invasion. Although some mechanisms involved in parasite/host interaction have been already described, a thorough understanding off the process would contribute to find new key players and provide a more diverse set of potential molecular targets against the disease. However, the evolution of this parasite has provided it with redundant and diverse molecular tools, able to interfere with multiple host cell pathways, to achieve a successful invasion.
The process of invasion begins with the recognition and adhesion of the parasite to the target cell. This interaction, reinforced by EVs and secreted proteins, leads to the activation of signaling pathways in the host cell that promote parasite internalization into an encasing vacuole, from which the parasite escapes to the cytosol where differentiation and replication take place.
Invasion has a pyramidal structure, in the base the diverse parasite/host protein interactions involved in internalization converge in the activation of a smaller set of signaling cascades and, regardless of the parasite strategy of internalization, all pathways end at the top of the pyramid with a parasite-containing vesicle to which lysosomes fuse to generate the parasitophorous vacuole. Little is known about the events that occur after TcPV is established, and a better understanding of this crucial mechanism may be the key to define new therapeutic targets against Chagas disease.
In this review, we address the several strategies Trypanosoma cruzi, the etiological agent of Chagas disease, has developed to subvert the host cell signaling pathways in order to gain access to the host cell cytoplasm, where replication and differentiation. Special attention is made to the numerous parasite/host protein interactions and the set of signaling cascades interfered during the formation of the parasitophorous vacuole. We first discuss the three strategies that T. cruzi exploits to trigger host cell signaling pathways to facilitate invasion: 1) Parasite surface/secreted proteins/host cell receptor interactions, 2) Protein shedding and 3) Host plasma membrane wounding. Later, strategies that lead to the internalization of the parasite, involving three main mechanisms: 1) Ca2+-dependent recruitment of lysosomes, 2) Endocytosis, and 3) Autophagy, are discussed. Finally, we examine the mechanisms by which the parasite escapes from the parasitophorous vacuole to establish a successful invasion. The topics discussed in this work were partially covered by other authors, however, we present a bigger picture, describing the complexity of the process considering genetic variability, strains, parasite/host interactions, signaling pathways activated and host cell. To our knowledge, this would be the more complete and updated review currently available.
Author Contributions
GF and MME conceived, designed, and wrote the article. All authors contributed to the article and approved the submitted version.
Funding
This work was partially supported by the FIC-NIH award number R03TW009001.
Conflict of Interest
The authors declare that the research was conducted in the absence of any commercial or financial relationships that could be construed as a potential conflict of interest
Acknowledgments
Authors would like to thank Dr. Daniel Altschuler from the University of Pittsburgh for his constant support.
References
Alba Soto C. D., González Cappa S. M. (2019). “Trypanosoma cruzi Journey from the Insect Vector to the Host Cell,” in Chagas Disease: A Clinical Approach. Eds. J. M. Altcheh and H. Freilij (Cham, Switzerland: Springer International Publishing), 25–59. doi: 10.1007/978-3-030-00054-7_2
Albertti L. A. G., Macedo A. M., Chiari E., Andrews N. W., Andrade L. O. (2010). Role of Host Lysosomal Associated Membrane Protein (LAMP) in Trypanosoma Cruzi Invasion and Intracellular Development. Microbes Infect. 12 (10), 784–789. doi: 10.1016/j.micinf.2010.05.015
Alvarez V. E., Niemirowicz G. T., Cazzulo J. J. (2012). The Peptidases of Trypanosoma Cruzi: Digestive Enzymes, Virulence Factors, and Mediators of Autophagy and Programmed Cell Death. Biochim. Biophys. Acta - Proteins Proteomics 1824, 195–206. doi: 10.1016/j.bbapap.2011.05.011
Alves M. J. M., Kawahara R., Viner R., Colli W., Mattos E. C., Thaysen-Andersen M., et al. (2017). Comprehensive Glycoprofiling of the Epimastigote and Trypomastigote Stages of Trypanosoma Cruzi. J. Proteomics 151, 182–192. doi: 10.1016/j.jprot.2016.05.034
Andrade L. O., Andrews N. W. (2004). Lysosomal Fusion Is Essential for the Retention of Trypanosoma Cruzi inside Host Cells. J. Exp. Med. 200 (9), 1135–1143. doi: 10.1084/jem.20041408
Andrews N. W., Katzin A. M., Colli W. (1984). Mapping of Surface Glycoproteins of Trypanosoma Cruzi by Two-dimensional Electrophoresis: A Correlation with the Cell Invasion Capacity. Eur. J. Biochem 140, 599–604. doi: 10.1111/j.1432-1033.1984.tb08144.x
Andrews N. W., Hong K. S., Robbins E. S., Nussenzweig V. (1987). Stage-Specific Surface Antigens Expressed during the Morphogenesis of Vertebrate Forms of Trypanosoma Cruzi. Exp. Parasitol. 64, 474–484. doi: 10.1016/0014-4894(87)90062-2
Andrews N. W., Robbins E., Ley V., Nussenzweig V. (1988). Stage-Specific Surface Antigens during the Morphogenesis of Trypanosoma Cruzi: Developmentally Regulated Expression of a Glycosyl-Phosphatidylinositol Anchored Glycoprotein of Amastigotes. Em Inst. Oswaldo Cruz 83 (Suppl 1), 561–562. doi: 10.1590/s0074-02761988000500067
Andrews N. W., Abrams C. K., Slatin S. L., Griffiths G. (1990). A T. Cruzi-Secreted Protein Immunologically Related to the Complement Component C9: Evidence for Membrane Pore-Forming Activity at Low PH. Cell 61, 1277–1287. doi: 10.1016/0092-8674(90)90692-8
Andrews N. W. (1994). From Lysosomes into the Cytosol: The Intracellular Pathway of Trypanosoma Cruzi. Braz. J. Med. Biol. Res. 27 (2), 471–475.
Aparicio I. M., Scharfstein J., Lima A. P. C. A. (2004). A New Cruzipain-Mediated Pathway of Human Cell Invasion by Trypanosoma Cruzi Requires Trypomastigote Membranes. Infect. Immunity 72, 5892–5902. doi: 10.1128/IAI.72.10.5892-5902.2004
Baida R. C. P., Carmo M. S., Yoshida N., Ferreira D., Ferreira A. T., El Sayed N. M., et al. (2006). Molecular Characterization of Serine-, Alanine-, and Proline-Rich Proteins Of. Society 74 (3), 1537–1546. doi: 10.1128/IAI.74.3.1537
Balouz V., Bracco L., Romer G., Ricci A. D., Agüero F., Buscaglia C. (2021). Serological Approaches for Trypanosoma Cruzi Strain Typing. Trends Parasitol. 37, 214–225. doi: 10.1016/j.pt.2020.12.002
Barrias E. S., Dutra J. M. F., De Souza W., Carvalho T. M. U. (2007). Participation of Macrophage Membrane Rafts in Trypanosoma Cruzi Invasion Process. Biochem. Biophys. Res. Commun. 363 (3), 828–834. doi: 10.1016/j.bbrc.2007.09.068
Barrias E. S., Reignault L. C., De Souza W., Carvalho T. M. U. (2012). Trypanosoma Cruzi Uses Macropinocytosis as an Additional Entry Pathway into Mammalian Host Cell. Microbes Infect. 14 (14), 1340–1351. doi: 10.1016/j.micinf.2012.08.003
Barrias E. S., de Carvalho T. M. U., Souza W. De (2013). Trypanosoma Cruzi: Entry into Mammalian Host Cells and Parasitophorous Vacuole Formation. Front. Immunol 4, 186. doi: 10.3389/fimmu.2013.00186
Barrias E., Reignault L., de Carvalho T. M. U., de Souza W. (2019). Clathrin Coated Pit Dependent Pathway for Trypanosoma Cruzi Internalization into Host Cells. Acta Tropica 199 (June), 105057. doi: 10.1016/j.actatropica.2019.105057
Batista M. F., Nájera C. A., Meneghelli I., Bahia D. (2020). The Parasitic Intracellular Lifestyle of Trypanosomatids: Parasitophorous Vacuole Development and Survival. Front. Cell Dev. Biol 8, 396. doi: 10.3389/fcell.2020.00396
Bautista-López N. L., Ndao M., Camargo F. V., Nara T., Annoura T., Hardie D. B., et al. (2017). Characterization and Diagnostic Application of Trypanosoma Cruzi Trypomastigote Excreted-Secreted Antigens Shed in Extracellular Vesicles Released from Infected Mammalian Cells. J. Clin. Microbiol 55, 744–758. doi: 10.1128/JCM.01649-16
Bayer-Santos E., Aguilar-Bonavides C., Rodrigues S. P., Maurício Cordero E., Ferreira Marques A., Varela-Ramirez A., et al. (2013a). Proteomic Analysis of Trypanosoma Cruzi Secretome: Characterization of Two Populations of Extracellular Vesicles and Soluble Proteins. J. Proteome Res. 12 (2), 883–897. doi: 10.1021/pr300947g
Bayer-Santos E., Cunha-E-Silva N. L., Yoshida N., Silveira J. F. Da (2013b). Expression and Cellular Trafficking of GP82 and GP90 Glycoproteins during Trypanosoma Cruzi Metacyclogenesis. Parasites Vectors 6 (1), 1–10. doi: 10.1186/1756-3305-6-127
Belaunzarán M. L., Wainszelbaum M. J., Lammel E. M., Gimenez G., Aloise M. M., Florin-Christensen J., et al. (2007). Phospholipase A1 from Trypanosoma Cruzi Infective Stages Generates Lipid Messengers That Activate Host Cell Protein Kinase C. Parasitology 134 (4), 491–502. doi: 10.1017/S0031182006001740
Belaunzarán M. L., Wilkowsky S. E., Lammel E. M., Giménez G., Bott E., Barbieri M. A., et al. (2013). Phospholipase A1: A Novel Virulence Factor in Trypanosoma Cruzi. Mol. Biochem. Parasitol. 187, 77–86. doi: 10.1016/j.molbiopara.2012.12.004
Benabdellah K., González-Rey E., González A. (2007). Alternative Trans-Splicing of the Trypanosoma Cruzi LYT1 Gene Transcript Results in Compartmental and Functional Switch for the Encoded Protein. Mol. Microbiol. 65 (6), 1559–1567. doi: 10.1111/j.1365-2958.2007.05892.x
Berná L., Rodriguez M., Chiribao M. L., Parodi-Talice A., Pita S., Rijo G., et al. (2018). Expanding an Expanded Genome: Long-Read Sequencing of Trypanosoma Cruzi. Microbial Genomics 4, e000177. doi: 10.1099/mgen.0.000177
Berrizbeitia M., Ndao M., Bubis J., Gottschalk M., Aché A., Lacouture S., et al. (2006). Purified Excreted-Secreted Antigens from Trypanosoma Cruzi Trypomastigotes as Tools for Diagnosis of Chagas’ Disease. J. Clin. Microbiol. 44, 291–296. doi: 10.1128/JCM.44.2.291-296.2006
Blazek A. D., Paleo B. J., Weisleder N. (2015). Plasma Membrane Repair: A Central Process for Maintaining Cellular Homeostasis. Physiology 30, 438–448. doi: 10.1152/physiol.00019.2015
Bonfim-Melo A., Ferreira E. R., Florentino P. T. V., Mortara R. A. (2018a). Amastigote Synapse: The Tricks of Trypanosoma Cruzi Extracellular Amastigotes. Front. Microbiol. 9, 2018.01341 (JUN). doi: 10.3389/fmicb.2018.01341
Bonfim-Melo A., Ferreira É. R., Mortara R. A. (2018b). Rac1/WAVE2 and Cdc42/N-WASP Participation in Actin-Dependent Host Cell Invasion by Extracellular Amastigotes of Trypanosoma Cruzi. Front. Microbiol. 9, 2018.00360 (FEB). doi: 10.3389/fmicb.2018.00360
Borges B. C., Uehara I. A., Dias L. O. S., Brígido P. C., da Silva C. V., Silva M. J. B. (2016). Mechanisms of Infectivity and Evasion Derived from Microvesicles Cargo Produced by Trypanosoma Cruzi. Front. Cell. Infect. Microbiol 6, 161. doi: 10.3389/fcimb.2016.00161
Brandán C. P., Mesias A. C., Acuña L., Teixeira T. L., Silva C. V. Da (2019). Evaluation of Pathogen P21 Protein as a Potential Modulator of the Protective Immunity Induced by Trypanosoma Cruzi Attenuated Parasites. Memorias Do Instituto Oswaldo Cruz 114 (4), 1–7. doi: 10.1590/0074-02760180571
Brígido R. T. e., Tavares P. C. B., dos Santos M. A., de Gouveia Santos J., de Souza M. A., Goulart I. M. B., et al. (2017). Trypanosoma Cruzi Modulates Gene Expression of Plasma Membrane Repair-Related Proteins. Acta Tropica 153–157. doi: 10.1016/j.actatropica.2016.06.008
Brossas J. Y., Gulin J. E. N., Bisio M. M. C., Chapelle M., Marinach-Patrice C., Bordessoules M., et al. (2017). Secretome Analysis of Trypanosoma Cruzi by Proteomics Studies. PLoS One 12 (10), 1–16. doi: 10.1371/journal.pone.0185504
Bubis J., Martínez J. C., Calabokis M., Ferreira J., Sanz-Rodríguez C. E., Navas V., et al. (2018). The Gene Product of a Trypanosoma Equiperdum Ortholog of the CAMP-Dependent Protein Kinase Regulatory Subunit Is a Monomeric Protein That Is Not Capable of Binding Cyclic Nucleotides. Biochimie 146, 166–180. doi: 10.1016/j.biochi.2017.12.010
Burgos J. M., Risso M. G., Brenière S. F., Barnabé C., Campetella O., Leguizamón M. S. (2013). Differential Distribution of Genes Encoding the Virulence Factor Trans-Sialidase along Trypanosoma Cruzi Discrete Typing Units. PLoS One 8 (3), 9–11. doi: 10.1371/journal.pone.0058967
Burleigh B. A., Caler E. V., Webster P., Andrews N. W. (1997). A Cytosolic Serine Endopeptidase from Trypanosoma Cruzi Is Required for the Generation of Ca2+ Signaling in Mammalian Cells. J. Cell Biol. 136 (3), 609–620. doi: 10.1083/jcb.136.3.609
Burleigh B. A. (2005). Host Cell Signaling and Trypanosoma Cruzi Invasion: Do All Roads Lead to Lysosomes? Sci. STKE 2005, pe36. doi: 10.1126/stke.2932005pe36
Buscaglia C. A., Campo V. A., Noia J. M. Di, Torrecilhas A. C. T., De Marchi C. R., Ferguson M. A. J., et al. (2004). The Surface Coat of the Mammal-Dwelling Infective Trypomastigote Stage of Trypanosoma Cruzi Is Formed by Highly Diverse Immunogenic Mucins. J. Biol. Chem. 279, 15860–15869 . doi: 10.1074/jbc.M314051200
Butler C. E., de Carvalho T. M. U., Grisard E. C., Field R. A., Tyler K. M. (2013). Trans-Sialidase Stimulates Eat Me Response from Epithelial Cells. Traffic 14 (7), 853–869. doi: 10.1111/tra.12078
Cabukusta B., Neefjes J. (2018). Mechanisms of Lysosomal Positioning and Movement. Traffic 19, 761–769. doi: 10.1111/tra.12587
Caler E. V., Avalos S. V. De, Haynes P. A., Andrews N. W., Burleigh B. A. (1998). Oligopeptidase B-Dependent Signaling Mediates Host Cell Invasion by Trypanosoma Cruzi. EMBO J. 17 (17), 4975–4986. doi: 10.1093/emboj/17.17.4975
Cámara M. de l. M., Cánepa G. E., Lantos A. B., Balouz V., Yu H., Chen X., et al. (2017). The Trypomastigote Small Surface Antigen (TSSA) Regulates Trypanosoma Cruzi Infectivity and Differentiation. PLoS Neglect. Trop. Dis. 11 (8), 1–21. doi: 10.1371/journal.pntd.0005856
Campetella O., Buscaglia C. A., Mucci J., Leguizamón M. S. (2020). Parasite-Host Glycan Interactions during Trypanosoma Cruzi Infection: Trans-Sialidase Rides the Show. Biochim. Biophys. Acta - Mol. Basis Dis. 1866 (5), 165692. doi: 10.1016/j.bbadis.2020.165692
Campo V. A., Buscaglia C. A., Di Noia J. M., Frasch A. C. C. (2006). Immunocharacterization of the Mucin-Type Proteins from the Intracellular Stage of Trypanosoma Cruzi. Microbes Infect. 8, 401–409. doi: 10.1016/j.micinf.2005.07.008
Cánepa G. E., Degese M. S., Budu A., Garcia C. R. S., Buscaglia C. A., Canepa G. E., et al. (2012a). Involvement of TSSA (Trypomastigote Small Surface Antigen) in Trypanosoma Cruzi Invasion of Mammalian Cells. Biochem. J. 444 (2), 211–218. doi: 10.1042/BJ20120074
Cánepa G. E., Mesías A. C., Yu H., Chen X., Buscaglia C. A., Canepa G. E., et al. (2012b). Structural Features Affecting Trafficking, Processing, and Secretion of Trypanosoma Cruzi Mucins. J. Biol. Chem. 287 (31), 26365–26376. doi: 10.1074/jbc.M112.354696
Caradonna K. L., Engel J. C., Jacobi D., Lee C. H., Burleigh B. A. (2013). Host Metabolism Regulates Intracellular Growth of Trypanosoma Cruzi. Cell Host Microbe 13, 108–117. doi: 10.1016/j.chom.2012.11.011
Caradonna K. L., Burleigh B. A. (2011). Mechanisms of Host Cell Invasion by Trypanosoma Cruzi. Adv. Parasitol. 76, 33–61. doi: 10.1016/B978-0-12-385895-5.00002-5
Cardoso M. S., Reis-Cunha J. L., Bartholomeu D. C. (2016). Evasion of the Immune Response by Trypanosoma Cruzi during Acute Infection. Front. Immunol. 6, 2015.00659 (JAN). doi: 10.3389/fimmu.2015.00659
Castro-Gomes T., Corrotte M., Tam C., Andrews N. W. (2016). Plasma Membrane Repair Is Regulated Extracellularly by Proteases Released from Lysosomes. PLoS One 11, e0152583. doi: 10.1371/journal.pone.0152583
Cerqueira G. C., Bartholomeu D. C., DaRocha W. D., Hou L., Freitas-Silva D. M., Renato Machado C., et al. (2008). Sequence Diversity and Evolution of Multigene Families in Trypanosoma Cruzi. Mol. Biochem. Parasitol. 157, 65–72. doi: 10.1016/j.molbiopara.2007.10.002
Chou L. Y. T., Ming K., Chan W. C. W. (2011). Strategies for the Intracellular Delivery of Nanoparticles. Chem. Soc. Rev. 40, 233–245. doi: 10.1039/c0cs00003e
Chuenkova M. V., Furnari F. B., Cavenee W. K., Pereira M. A. (2001). Trypanosoma Cruzi Trans-Sialidase: A Potent and Specific Survival Factor for Human Schwann Cells by Means of Phosphatidylinositol 3-Kinase/Akt Signaling. Proc. Natl. Acad. Sci. U. S. A. 98 (17), 9936–9941. doi: 10.1073/pnas.161298398
Chuenkova M. V., Pereira M. A. (2001). The T. Cruzi Trans-Sialidase Induces PC12 Cell Differentiation via MAPK/ERK Pathway. NeuroReport 12 (17), 3715–3718. doi: 10.1097/00001756-200112040-00022
Combs T. P., Shankar Mukherjee N., De Almeida C. J. G., Jelicks L. A., Schubert W., Lin Y., et al. (2005). The Adipocyte as an Important Target Cell for Trypanosoma Cruzi Infection. J. Biol. Chem. 280, 24085‐24094. doi: 10.1074/jbc.M412802200
Cordero E. M., Gentil L. G., Crisante G., Ramírez J. L., Yoshida N., Añez N., et al. (2008). Expression of GP82 and GP90 Surface Glycoprotein Genes of Trypanosoma Cruzi during in Vivo Metacyclogenesis in the Insect Vector Rhodnius Prolixus. Acta Tropica 105 (1), 87–91. doi: 10.1016/j.actatropica.2007.08.004
Cortez C., Martins R. M., Alves R. M., Silva R. C., Bilches L. C., Macedo S., et al. (2012). Differential Infectivity by the Oral Route of Trypanosoma Cruzi Lineages Derived from Y Strain. PLoS Neglect. Trop. Dis. 6 (10), e1804. doi: 10.1371/journal.pntd.0001804
Cortez C., Sobreira T. J., Maeda F. Y., Yoshida N. (2014). The Gp82 Surface Molecule of Trypanosoma Cruzi Metacyclic Forms. Subcellular Biochem. 74, 137–150. doi: 10.1007/978-94-007-7305-9
Cortez C., Real F., Yoshida N. (2016). Lysosome Biogenesis/Scattering Increases Host Cell Susceptibility to Invasion by Trypanosoma Cruzi Metacyclic Forms and Resistance to Tissue Culture Trypomastigotes. Cell. Microbiol. 18 (5), 748–760. doi: 10.1111/cmi.12548
Cremona M. L., Sánchez D. O., Frasch A. C. C., Campetella O. (1995). A Single Tyrosine Differentiates Active and Inactive Trypanosoma Cruzi Trans-Sialidases. Gene 160, 123–128. doi: 10.1016/0378-1119(95)00175-6
Cremona M. L., Campetella O., Sánchez D. O., Frasch A. C. C. (1999). Enzymically Inactive Members of the Trans-Sialidase Family from Trypanosoma Cruzi Display β-Galactose Binding Activity. Glycobiology 9, 581–587. doi: 10.1093/glycob/9.6.581
Cronemberger-Andrade A., Xander P. , Soares R. P., Pessoa N. L., Campos M. A., Ellis C. C., et al. (2020). Trypanosoma Cruzi-Infected Human Macrophages Shed Proinflammatory Extracellular Vesicles That Enhance Host-Cell Invasion via Toll-Like Receptor 2. Front. Cell. Infect. Microbiol. 10:2020.00099 (March). doi: 10.3389/fcimb.2020.00099
Cruz M. C., Souza-Melo N., da Silva C. V., DaRocha W. D., Bahia D., Araújo P. R., et al. (2012). Trypanosoma Cruzi: Role of δ-Amastin on Extracellular Amastigote Cell Invasion and Differentiation. PLoS One 7 (12), e51804. doi: 10.1371/journal.pone.0051804
Cueto J. A., Vanrell M. C., Salassa B. N., Nola S., Galli T., Colombo M. I., et al. (2017). Soluble N-Ethylmaleimide-Sensitive Factor Attachment Protein Receptors Required during Trypanosoma Cruzi Parasitophorous Vacuole Development. Cell. Microbiol. 19 (6), e12713. doi: 10.1111/cmi.12713
da Fonseca L. M., da Costa K. M., de Sousa Chaves V., Freire-De-Lima C. G., Morrot A., Mendonça-Previato L., et al. (2019). Theft and Reception of Host Cell’s Sialic Acid: Dynamics of Trypanosoma Cruzi Trans-Sialidases and Mucin-like Molecules on Chagas’ Disease Immunomodulation. Front. Immunol. 10, 2019.00164 (February). doi: 10.3389/fimmu.2019.00164
da Silva C. V., Kawashita S. Y., Probst C. M., Dallagiovanna B., Cruz M. C., da Silva E. A., et al. (2009). Characterization of a 21 KDa Protein from Trypanosoma Cruzi Associated with Mammalian Cell Invasion. Microbes Infect. 11, 563–570. doi: 10.1016/j.micinf.2009.03.007
De Pablos L. M., Osuna A. (2012). Multigene Families in Trypanosoma Cruzi and Their Role in Infectivity. Infect. Immun. 80 (7), 2258–2264. doi: 10.1128/IAI.06225-11
de Paiva R. M. C., Grazielle-Silva V., Cardoso M. S., Nakagaki B. N., Mendonça-Neto R. P., Canavaci A. M. C., et al. (2015). Amastin Knockdown in Leishmania Braziliensis Affects Parasite-Macrophage Interaction and Results in Impaired Viability of Intracellular Amastigotes. PLoS Pathog. 11 (12), e1005296. doi: 10.1371/journal.ppat.1005296
Dikic I., Elazar Z. (2018). Mechanism and Medical Implications of Mammalian Autophagy. Nat. Rev. Mol. Cell Biol. 19, 349–364. doi: 10.1038/s41580-018-0003-4
do Couto N. F., Pedersane D., Rezende L., Dias P. P., Corbani T. L., Bentini L. C., et al. (2020). Correction: LAMP-2 Absence Interferes with Plasma Membrane Repair and Decreases T. Cruzi Host Cell Invasion. PLoS Neglect. Trop. Dis. 14 (9), e0008724. doi: 10.1371/journal.pntd.0008724
Dorta M. L., Ferreira A. T., Oshiro M. E. M., Yoshida N. (1995). Ca2+ Signal Induced by Trypanosoma Cruzi Metacyclic Trypomastigote Surface Molecules Implicated in Mammalian Cell Invasion. Mol. Biochem. Parasitol. 73 (1–2), 285–289. doi: 10.1016/0166-6851(94)00123-5
El-Sayed N. M., Myler P. J., Bartholomeu D. C., Nilsson D., Aggarwal G., Tran A. N., et al. (2005). The Genome Sequence of Trypanosoma Cruzi, Etiologic Agent of Chagas Disease. Science 309 (5733), 409–415. doi: 10.1126/science.1112631
Evans-Osses I., Reichembach L. H., Ramirez M. I. (2015). Exosomes or Microvesicles? Two Kinds of Extracellular Vesicles with Different Routes to Modify Protozoan-Host Cell Interaction. Parasitol. Res. 114, 3567–3575. doi: 10.1007/s00436-015-4659-9
Feliciano W. D., Yoshida S., Straight S. W., Swanson J. A. (2011). Coordination of the Rab5 Cycle on Macropinosomes. Traffic 12, 1911–1922. doi: 10.1111/j.1600-0854.2011.01280.x
Fernandes L. C., Bastos I. M. D., Lauria-Pires L., Rosa A. C. O., Teixeira A. R. L., Grellier P., et al. (2005). Specific Human Antibodies Do Not Inhibit Trypanosoma Cruzi Oligopeptidase B and Cathepsin B, and Immunoglobulin G Enhances the Activity of Trypomastigote-Secreted Oligopeptidase B. Microbes Infect. 7, 375–384. doi: 10.1016/j.micinf.2004.11.009
Fernandes M. C., Cortez M., Yoneyama K. A. G., Straus A. H., Yoshida N., Mortara R. A. (2007). Novel Strategy in Trypanosoma Cruzi Cell Invasion: Implication of Cholesterol and Host Cell Microdomains. Int. J. Parasitol. 37 (13), 1431–1441. doi: 10.1016/j.ijpara.2007.04.025
Fernandes M. C., Cortez M., Flannery A. R., Tam C., Mortara R. A., Andrews N. W. (2011). Trypanosoma Cruzi Subverts the Sphingomyelinase-Mediated Plasma Membrane Repair Pathway for Cell Invasion. J. Exp. Med. 208 (5), 909–921. doi: 10.1084/jem.20102518
Fernandes M. C., Flannery A. R., Andrews N., Mortara R. A. (2013). Extracellular Amastigotes of Trypanosoma Cruzi Are Potent Inducers of Phagocytosis in Mammalian Cells. Cell. Microbiol. 15 (6), 977–991. doi: 10.1111/cmi.12090
Fernandes M. C., Andrews N. W. (2012). Host Cell Invasion by Trypanosoma Cruzi: A Unique Strategy That Promotes Persistence. FEMS Microbiol. Rev. 36, 734–747. doi: 10.1111/j.1574-6976.2012.00333.x
Ferrão P. M., D’Avila-Levy C. M., Araujo-Jorge T. C., Degrave W. M., Gonçalves A. Da S., Garzoni L. R., et al. (2015). Cruzipain Activates Latent TGF-β from Host Cells during T. Cruzi Invasion. PLoS One 10 (5), 1–15. doi: 10.1371/journal.pone.0124832
Ferreira D., Cortez M., Atayde V. D., Yoshida N. (2006). Actin Cytoskeleton-Dependent and -Independent Host Cell Invasion by Trypanosoma Cruzi Is Mediated by Distinct Parasite Surface Molecules. Infect. Immun. 74 (10), 5522–5528. doi: 10.1128/IAI.00518-06
Ferreira É. R., Horjales E., Bonfim-Melo A., Cortez C., Da Silva C. V., De Groote M., et al. (2016). Unique Behavior of Trypanosoma Cruzi Mevalonate Kinase: A Conserved Glycosomal Enzyme Involved in Host Cell Invasion and Signaling. Sci. Rep. 6 (April), 1–13. doi: 10.1038/srep24610
Ferreira E. R., Bonfim-Melo A., Cordero E. M., Mortara R. A. (2017). ERM Proteins Play Distinct Roles in Cell Invasion by Extracellular Amastigotes of Trypanosoma Cruzi. Front. Microbiol. 8, 2017.02230 (NOV). doi: 10.3389/fmicb.2017.02230
Ferreira B. L., Ferreira E. R., Bonfim-Melo A., Mortara R. A., Bahia D. (2019). Trypanosoma Cruzi Extracellular Amastigotes Selectively Trigger the PI3K/Akt and Erk Pathways during HeLa Cell Invasion. Microbes Infect. 21 (10), 485–489. doi: 10.1016/j.micinf.2019.06.003
Florentino P. T. V., Real F., Orikaza C. M., da Cunha J. P. C., Vitorino F. N. L., Cordero E. M., et al. (2018). A Carbohydrate Moiety of Secreted Stage-Specific Glycoprotein 4 Participates in Host Cell Invasion by Trypanosoma Cruzi Extracellular Amastigotes. Front. Microbiol. 9, 2018.00693 (APR). doi: 10.3389/fmicb.2018.00693
Friedrich N., Hagedorn M., Soldati-Favre D., Soldati T. (2012). Prison Break: Pathogens’ Strategies To Egress from Host Cells. Microbiol. Mol. Biol. Rev. 76, 707–720. doi: 10.1128/mmbr.00024-12
Giordano R., Fouts D. L., Tewari D., Colli W., Manning J. E., Alves M. J. M. (1999). Cloning of a Surface Membrane Glycoprotein Specific for the Infective Form of Trypanosoma Cruzi Having Adhesive Properties to Laminin. J. Biol. Chem. 274 (6), 3461–3468. doi: 10.1074/jbc.274.6.3461
Gloerich M., Ponsioen B., Vliem M. J., Zhang Z., Zhao J., Kooistra M. R., et al. (2010). Spatial Regulation of Cyclic AMP-Epac1 Signaling in Cell Adhesion by ERM Proteins. Mol. Cell. Biol. 30 (22), 5421–5431. doi: 10.1128/mcb.00463-10
Goes G. R., Rocha P. S., Diniz A. R. S., Aguiar P. H. N., Machado C. R., Vieira L. Q. (2016). Trypanosoma Cruzi Needs a Signal Provided by Reactive Oxygen Species to Infect Macrophages. PLoS Negl. Trop. Dis. 10 (4), e0004555. doi: 10.1371/journal.pntd.0004555
Hall B.F., Webster P., Ma A. K., Joiner K. A., Andrews N. W. (1992). Desialylation of Lysosomal Membrane Glycoproteins by Trypanosoma Cruzi: A Role for the Surface Neuraminidase in Facilitating Parasite Entry into the Host Cell Cytoplasm. J. Exp. Med. 176 (2), 313–325. doi: 10.1084/jem.176.2.313
Herreros-Cabello A., Callejas-Hernández F., Gironès N., Fresno M. (2020). Trypanosoma cruzi genome: Organization, multi-gene families, transcription, and biological implications. Genes (Basel) 11, 1196. doi: 10.3390/genes11101196
Hissa B., Duarte J. G., Kelles L. F., Santos F. P., del Puerto H. L., Gazzinelli-Guimarães P. H., et al. (2012). Membrane Cholesterol Regulates Lysosome-Plasma Membrane Fusion Events and Modulates Trypanosoma Cruzi Invasion of Host Cells. PLoS Neglect. Trop. Diseases 6, e1583. doi: 10.1371/journal.pntd.0001583
Hissa B., Andrade L. de O. (2015). Trypasonoma Cruzi Uses a Specific Subset of Host Cell Lysosomes for Cell Invasion. Parasitol. Int. 64 (2), 135–138. doi: 10.1016/j.parint.2014.11.005
Hochbaum D., Barila G., Ribeiro-Neto F., Altschuler D. L. (2011). Radixin Assembles CAMP Effectors Epac and PKA into a Functional CAMP Compartment: Role in CAMP-Dependent Cell Proliferation. J. Biol. Chem. 286 (1), 859–866. doi: 10.1074/jbc.M110.163816
Kangussu-Marcolino M. M., Paiva R. M. C. De, Araújo P. R., De Mendonça-Neto R. P., Lemos L., Bartholomeu D. C., et al. (2013). Distinct Genomic Organization, MRNA Expression and Cellular Localization of Members of Two Amastin Sub-Families Present in Trypanosoma Cruzi. BMC Microbiol. 13, 10. doi: 10.1186/1471-2180-13-10
Kawashita S. Y., da Silva C. V., Mortara R. A., Burleigh B. A., Briones M. R. S. (2009). Homology, Paralogy and Function of DGF-1, a Highly Dispersed Trypanosoma Cruzi Specific Gene Family and Its Implications for Information Entropy of Its Encoded Proteins. Mol. Biochem. Parasitol. 165 (1), 19–31. doi: 10.1016/j.molbiopara.2008.12.010
Kay R. R., Williams T. D., Paschke P. (2018). Amplification of Pip3 Signalling by Macropinocytic Cups. Biochem. J. 475, 643–648. doi: 10.1042/BCJ20170785
King J. S., Kay R. R. (2019). The Origins and Evolution of Macropinocytosis. Philos. Trans. R. Soc. B: Biol. Sci. 374, 20180158. doi: 10.1098/rstb.2018.0158
Lantos A. B., Carlevaro G., Araoz B., Diaz P. R., de los Milagros Camara M., Buscaglia C. A., et al. (2016). Sialic Acid Glycobiology Unveils Trypanosoma Cruzi Trypomastigote Membrane Physiology. PLoS Pathog. 12 (4), e1005559. doi: 10.1371/journal.ppat.1005559
Lee B. Y., Bacon K. M., Bottazzi M. E., Hotez P. J. (2013). Global Economic Burden of Chagas Disease: A Computational Simulation Model. Lancet Infect. Dis. 13 (4), 342–348. doi: 10.1016/S1473-3099(13)70002-1
Ley V., Robbins E. S., Nussenzweig V., Andrews N. W. (1990). The Exit of Trypanosoma Cruzi from the Phagosome Is Inhibited by Raising the PH of Acidic Compartments. J. Exp. Med. 171 (2), 401–413. doi: 10.1084/jem.171.2.401
Li Y., Shah-Simpson S., Okrah K., Trey Belew A., Choi J., Caradonna K. L., et al. (2016). Transcriptome Remodeling in Trypanosoma Cruzi and Human Cells during Intracellular Infection. PLoS Pathog. 12, e1005511. doi: 10.1371/journal.ppat.1005511
Lidani K. C. F., Andrade F. A., Bavia L., Damasceno F. S., Beltrame M. H., Messias-Reason I. J., et al. (2019). Chagas Disease: From Discovery to a Worldwide Health Problem. Front. Public Health 7 (6), 166. doi: 10.3389/fpubh.2019.00166
Lima L., Ortiz P. A., da Silva F. M., Alves J. M. P., Serrano M. G., Cortez A. P., et al. (2012). Repertoire, Genealogy and Genomic Organization of Cruzipain and Homologous Genes in Trypanosoma Cruzi, T. Cruzi-like and Other Trypanosome Species. PLoS One 7, e38385. doi: 10.1371/journal.pone.0038385
Lovo-Martins M. I., Malvezi A. D., Zanluqui N. G., Lucchetti B. F. C., Tatakihara V. L. H., Mörking P. A., et al. (2018). Extracellular Vesicles Shed By Trypanosoma Cruzi Potentiate Infection and Elicit Lipid Body Formation and PGE2 Production in Murine Macrophages. Front. Immunol. 9, 896. doi: 10.3389/fimmu.2018.00896
Machado F. C., Cruz L., Da Silva A. A., Cruz M. C., Mortara R. A., Roque-Barreira M. C., et al. (2014). Recruitment of Galectin-3 during Cell Invasion and Intracellular Trafficking of Trypanosoma Cruzi Extracellular Amastigotes. Glycobiology 24 (2), 179–184. doi: 10.1093/glycob/cwt097
Maeda F. Y., Cortez C., Yoshida N. (2012). Cell Signaling during Trypanosoma Cruzi Invasion. Front. Immunol. 3361. doi: 10.3389/fimmu.2012.00361
Maganto-Garcia E., Punzon C., Terhorst C., Fresno M. (2008). Rab5 Activation by Toll-Like Receptor 2 Is Required for Trypanosoma Cruzi Internalization and Replication in Macrophages. Traffic 9, 1299–1315. doi: 10.1111/j.1600-0854.2008.00760.x
Magdesian M. H., Tonelli R. R., Fessel M. R., Silveira M. S., Schumacher R. I., Linden R., et al. (2007). A Conserved Domain of the Gp85/Trans-Sialidase Family Activates Host Cell Extracellular Signal-Regulated Kinase and Facilitates Trypanosoma Cruzi Infection. Exp. Cell Res. 313 (1), 210–218. doi: 10.1016/j.yexcr.2006.10.008
Málaga S., Yoshida N. (2001). Targeted Reduction in Expression of Trypanosoma Cruzi Surface Glycoprotein Gp90 Increases Parasite Infectivity. Infect. Immun. 69 (1), 353–359. doi: 10.1128/IAI.69.1.353-359.2001
Manning-Cela R., Cortés A., González-Rey E., Van Voorhis W. C., Swindle J., González A. (2001). LYT1 Protein Is Required for Efficient In Vitro Infection by Trypanosoma Cruzi. Infect. Immun. 69 (6), 3916–3235. doi: 10.1128/IAI.69.6.3916
Manque P. M., Neira I., Atayde V. D., Cordero E., Ferreira A. T., Da Silveira J. F., et al. (2003). Cell Adhesion and Ca2+ Signaling Activity in Stably Transfected Trypanosoma Cruzi Epimastigotes Expressing the Metacyclic Stage-Specific Surface Molecule Gp82. Infect. Immun. 71, 1561–1565. doi: 10.1128/IAI.71.3.1561-1565.2003
Martins R. M., Covarrubias C., Rojas R. G., Silber A. M., Yoshida N. (2009). Use of L-Proline and ATP Production by Trypanosoma Cruzi Metacyclic Forms as Requirements for Host Cell Invasion. Infect. Immun. 77 (7), 3023–3032. doi: 10.1128/IAI.00138-09
Martins R. M., Alves R. M., Macedo S., Yoshida N. (2011). Starvation and Rapamycin Differentially Regulate Host Cell Lysosome Exocytosis and Invasion by Trypanosoma Cruzi Metacyclic Forms. Cell. Microbiol. 13 (7), 943–954. doi: 10.1111/j.1462-5822.2011.01590.x
Martins N. O., de Souza R. T., Cordero E. M., Maldonado D. C., Cortez C., Marini M. M., et al. (2015). Molecular Characterization of a Novel Family of Trypanosoma Cruzi Surface Membrane Proteins (TcSMP) Involved in Mammalian Host Cell Invasion. PLoS Neglect. Trop. Dis. 9 (11), 1–28. doi: 10.1371/journal.pntd.0004216
Mattos E. C., Tonelli R. R., Colli W., Alves M. J. M. (2014). The Gp85 Surface Glycoproteins from Trypanosoma Cruzi. Sub Cellular Biochem. 74, 151–180. doi: 10.1007/978-94-007-7305-9_7
McClatchey A. I. (2014). ERM Proteins at a Glance. J. Cell Sci. 127 (15), 3199–3204. doi: 10.1242/jcs.098343
Melo T. G., Tucci A. R., Nogueira A. R., Meirelles M. de N. S. L., Pereira M. C. S. (2014). The Involvement of FAK and Src in the Invasion of Cardiomyocytes by Trypanosoma Cruzi. Exp. Parasitol. 139 (1), 49–57. doi: 10.1016/j.exppara.2014.02.008
Ming M., Chuenkova M., Ortega-Barria E., Pereira M. E. A. (1993). Mediation of Trypanosoma Cruzi Invasion by Sialic Acid on the Host Cell and Trans-Sialidase on the Trypanosome. Mol. Biochem. Parasitol. 59, 243–252. doi: 10.1016/0166-6851(93)90222-J
Ming M., Ewen M. E., Pereira M. E. A. (1995). Trypanosome Invasion of Mammalian Cells Requires Activation of the TGFβ Signaling Pathway. Cell 82 (2), 287–296. doi: 10.1016/0092-8674(95)90316-X
Miro-Moran A., Jardin I., Ortega-Ferrusola C., Salido G. M., Peña F. J., Tapia J. A., et al. (2012). Identification and Function of Exchange Proteins Activated Directly by Cyclic AMP (Epac) in Mammalian Spermatozoa. PLoS One 7, e37713. doi: 10.1371/journal.pone.0037713
Monteón V. M., Furuzawa-Carballeda J., Alejandre-Aguilar R., Aranda-Fraustro A., Rosales-Encina J. L., Reyes P. A. (1996). American Trypanosomosis: In Situ and Generalized Features of Parasitism and Inflammation Kinetics in a Murine Model. Exp. Parasitol. 83 (3), 267–274. doi: 10.1006/expr.1996.0074
Moreira L. R., Serrano F. R., Osuna A. (2019). Extracellular Vesicles of Trypanosoma Cruzi Tissue-Culture Cell-Derived Trypomastigotes: Induction of Physiological Changes in Non-Parasitized Culture Cells. PLoS Neglect. Trop. Dis. 13 (2), 1–26. doi: 10.1371/journal.pntd.0007163
Mortara R. A., Andreoli W. K., Tantwaki N. N., Fernandes A. B., Silva C. V. Da, Fernandes M. C. D. C., et al. (2005). Mammalian Cell Invasion and Intracellular Trafficking by Trypanosoma Cruzi Infective Forms. Anais Da Academia Bras. Cienc. 77, 77–94. doi: 10.1590/s0001-37652005000100006
Motta F. N., Azevedo C. S., Neves B. P., de Araújo C. N., Grellier P., de Santana J. M., et al. (2019). Oligopeptidase B, a Missing Enzyme in Mammals and a Potential Drug Target for Trypanosomatid Diseases. Biochimie 167, 207–216. doi: 10.1016/j.biochi.2019.10.006
Musikant D., Ferri G., Durante I. M., Buscaglia C. A., Altschuler D. L., Edreira M. M. (2017). Host Epac1 Is Required for CAMP-Mediated Invasion by Trypanosoma Cruzi. Mol. Biochem. Parasitol. 211 (January), 67–70. doi: 10.1016/j.molbiopara.2016.10.003
Nardy A. F. F. R., Freire-de-Lima C. G., Pérez A. R., Morrot A. (2016). Role of Trypanosoma Cruzi Trans-Sialidase on the Escape from Host Immune Surveillance. Front. Microbiol. 7, 348. doi: 10.3389/fmicb.2016.00348
Nogueira P. M., Ribeiro K., Silveira A. C. O., Campos J. H., Martins-Filho O. A., Bela S. R., et al. (2015). Vesicles from Different Trypanosoma Cruzi Strains Trigger Differential Innate and Chronic Immune Responses. J. Extracellular Vesicles 4 (1), 1–16. doi: 10.3402/jev.v4.28734
Oliveira A. E. R., Grazielle-Silva V., Ferreira L. R. P., Teixeira S. M. R. (2020). Close Encounters between Trypanosoma Cruzi and the Host Mammalian Cell: Lessons from Genome-Wide Expression Studies. Genomics 112, 990–997. doi: 10.1016/j.ygeno.2019.06.015
Onofre T. S., Rodrigues J. P. F., Yoshida N. (2019). Depletion of Host Cell Focal Adhesion Kinase Increases the Susceptibility to Invasion by Trypanosoma Cruzi Metacyclic Forms. Front. Cell. Infect. Microbiol. 9:2019.00231 (JUN). doi: 10.3389/fcimb.2019.00231
Paiva C. N., Feijó D. F., Dutra F. F., Carneiro V. C., Freitas G. B., Alves L. S., et al. (2012). Oxidative Stress Fuels Trypanosoma Cruzi Infection in Mice. J. Clin. Invest. 122 (7), 2531–2542. doi: 10.1172/JCI58525
Pizon V., Desjardins M., Bucci C., Parton R. G., Zerial M. (1994). Association of Rap1a and Rap1b Proteins with Late Endocytic/Phagocytic Compartments and Rap2a with the Golgi Complex. J. Cell Sci. 107, 1661–1670.
Pu J., Guardia C. M., Keren-Kaplan T., Bonifacino J. S. (2016). Mechanisms and Functions of Lysosome Positioning. J. Cell Sci. 129, 4329–4339. doi: 10.1242/jcs.196287
Ramirez M. I., De Cassia Ruiz R., Araya J. E., Da Silveira J. F., Yoshida N. (1993). Involvement of the Stage-Specific 82-Kilodalton Adhesion Molecule of Trypanosoma Cruzi Metacyclic Trypomastigotes in Host Cell Invasion. Infect. Immun. 61 (9), 3636–3641. doi: 10.1128/iai.61.9.3636-3641.1993
Rawlings N. D., Waller M., Barrett A. J., Bateman A. (2014). MEROPS: The Database of Proteolytic Enzymes, Their Substrates and Inhibitors. Nucleic Acids Res. 42, D503–D509. doi: 10.1093/nar/gkt953
Reddy A., Caler E. V., Andrews N. W. (2001). Plasma Membrane Repair Is Mediated by Ca2+-Regulated Exocytosis of Lysosomes. Cell 106, 157–169. doi: 10.1016/S0092-8674(01)00421-4
Risso M. G., Garbarino G. B., Mocetti E., Campetella O., Cappa S. M. G., Buscaglia C. A., et al. (2004). Differential Expression of a Virulence Factor, the Trans-Sialidase, by the Main Trypanosoma Cruzi Phylogenetic Lineages. J. Infect. Dis. 189 (12), 2250–2259. doi: 10.1086/420831
Rodrigues A. A., Clemente T. M., dos Santos M. A., Machado F. C., Gomes R. G. B., Moreira H. H. T., et al. (2012). A Recombinant Protein Based on Trypanosoma Cruzi P21 Enhances Phagocytosis. PLoS One 7 (12), 1–9. doi: 10.1371/journal.pone.0051384
Rodrigues J. P. F., Santana G. H. T., Juliano M. A., Yoshida N. (2017). Inhibition of Host Cell Lysosome Spreading by Trypanosoma Cruzi Metacyclic Stage-Specific Surface Molecule Gp90 Downregulates Parasite Invasion. Infect. Immun. 85 (9), 1–10. doi: 10.1128/IAI.00302-17
Rodrigues J. P. F., Onofre T. S., Barbosa B. C., Ferreira E. R., Bonfim-Melo A., Yoshida N. (2019). Host Cell Protein LAMP-2 Is the Receptor for Trypanosoma Cruzi Surface Molecule Gp82 That Mediates Invasion. Cell. Microbiol. 21 (5), 1–11. doi: 10.1111/cmi.13003
Rodríguez A., Samoff E., Rioult M. G., Chung A., Andrews N. W. (1996). Host Cell Invasion by Trypanosomes Requires Lysosomes and Microtubule/Kinesin-Mediated Transport. J. Cell Biol. 134 (2), 349–362. doi: 10.1083/jcb.134.2.349
Rodríguez A., Martinez I., Chung A., Berlot C. H., Andrews N. W. (1999). CAMP Regulates Ca2+-Dependent Exocytosis of Lysosomes and Lysosome- Mediated Cell Invasion by Trypanosomes. J. Biol. Chem. 274 (24), 16754–16759. doi: 10.1074/jbc.274.24.16754
Romano P. S., Arboit M. A., Vázquez C. L., Colombo M. I. (2009). The Autophagic Pathway Is a Key Component in the Lysosomal Dependent Entry of Trypanosoma Cruzi into the Host Cell. Autophagy 5 (1), 6–18. doi: 10.4161/auto.5.1.7160
Rubin-de-Celis S. S. C., Uemura H., Yoshida N., Schenkman S. (2006). Expression of Trypomastigote Trans-Sialidase in Metacyclic Forms of Trypanosoma Cruzi Increases Parasite Escape from Its Parasitophorous Vacuole. Cell. Microbiol. 8 (12), 1888–1898. doi: 10.1111/j.1462-5822.2006.00755.x
Ruiz R. C., Favoreto S., Dorta M. L., Oshiro M. E. M., Ferreira A. T., Manque P. M., et al. (1998). Infectivity of Trypanosoma Cruzi Strains Is Associated with Differential Expression of Surface Glycoproteins with Differential Ca2+ Signalling Activity. Biochem. J. 330 (1), 505–511. doi: 10.1042/bj3300505
Sabbatini M. E., Chen X., Ernst S. A., Williams J. A. (2008). Rap1 Activation Plays a Regulatory Role in Pancreatic Amylase Secretion. J. Biol. Chem. 283, 23884–23894. doi: 10.1074/jbc.M800754200
Salassa B. N., Cueto J. A., Tudela J.G., Romano P. S. (2020). Endocytic Rabs Are Recruited to the Trypanosoma Cruzi Parasitophorous Vacuole and Contribute to the Process of Infection in Non-Professional Phagocytic Cells. Front. Cell. Infect. Microbiol. 10, 2020.536985 (October). doi: 10.3389/fcimb.2020.536985
Salassa B. N., Romano P. S. (2019). Autophagy: A Necessary Process during the Trypanosoma Cruzi Life-Cycle. Virulence 10 (1), 460–469. doi: 10.1080/21505594.2018.1543517
Salimi L., Akbari A., Jabbari N., Mojarad B., Vahhabi A., Szafert S., et al. (2020). Synergies in Exosomes and Autophagy Pathways for Cellular Homeostasis and Metastasis of Tumor Cells. Cell Biosci. 10, 64. doi: 10.1186/s13578-020-00426-y
San Francisco J., Barría I., Gutiérrez B., Neira I., Muñoz C., Sagua H., et al. (2017). Decreased Cruzipain and Gp85/Trans-Sialidase Family Protein Expression Contributes to Loss of Trypanosoma Cruzi Trypomastigote Virulence. Microbes Infect. 19 (1), 55–61. doi: 10.1016/j.micinf.2016.08.003
Santi-Rocca J., Fernandez-Cortes F., Chillón-Marinas C., González-Rubio M. L., Martin D., Fresno M. (2017). A Multi-Parametric Analysis of Trypanosoma Cruzi Infection: Common Pathophysiologic Patterns beyond Extreme Heterogeneity of Host Responses. Sci. Rep. 7, 1–12. doi: 10.1038/s41598-017-08086-8
Scharfstein J., Schmitz V., Morandi V., Capella M. M. A., Lima A. P. C. A., Morrot A., et al. (2000). Host Cell Invasion by Trypanosoma Cruzi Is Potentiated by Activation of Bradykinin B2 Receptors. J. Exp. Med. 192, 1289–1300. doi: 10.1084/jem.192.9.1289
Schenkman S., Jiang M. S., Hart G. W., Nussenzweig V. (1991). A Novel Cell Surface Trans-Sialidase of Trypanosoma Cruzi Generates a Stage-Specific Epitope Required for Invasion of Mammalian Cells. Cell 65, 1117–1125. doi: 10.1016/0092-8674(91)90008-M
Schoijet A. C., Sternlieb T., Alonso G. D. (2019). Signal Transduction Pathways as Therapeutic Target for Chagas Disease. Curr. Medicinal Chem. 26, 6572–6589. doi: 10.2174/0929867326666190620093029
Seino S., Shibasaki T. (2005). PKA-Dependent and PKA-Independent Pathways for CAMP-Regulated Exocytosis. Physiol. Rev. 85 (4), 1303–1342. doi: 10.1152/physrev.00001.2005
Silva T. A., Ferreira L. F. de C., de Souza Pereira M. C., Calvet C. M. (2019). Differential Role of TGF-B in Extracellular Matrix Regulation during Trypanosoma Cruzi-Host Cell Interaction. Int. J. Mol. Sci. 20 (19), 4836. doi: 10.3390/ijms20194836
Stahl P., Schwarz R. T., Debierre-Grockiego F., Meyer T. (2014). Trypanosoma Cruzi Parasites Fight for Control of the JAK-STAT Pathway by Disarming Their Host. Jak-Stat 3 (4), e1012964. doi: 10.1080/21623996.2015.1012964
Sugita S., Han W., Butz S., Liu X., Fernández-Chacón R., Lao Y., et al. (2001). Synaptotagmin VII as a Plasma Membrane Ca2+ Sensor in Exocytosis. Neuron 30, 459–473. doi: 10.1016/S0896-6273(01)00290-2
Taketo M., Yokoyama S., Kimura Y., Higashida H. (1997). Ca2+ Release and Ca2+ Influx in Chinese Hamster Ovary Cells Expressing the Cloned Mouse B2 Bradykinin Receptor: Tyrosine Kinase Inhibitor-Sensitive and -Insensitive Processes. Biochim. Biophys. Acta - Mol. Cell Res. 1355 (1), 89–98. doi: 10.1016/S0167-4889(96)00126-7
Tam C., Idone V., Devlin C., Fernandes M. C., Flannery A., He X., et al. (2010). Exocytosis of Acid Sphingomyelinase by Wounded Cells Promotes Endocytosis and Plasma Membrane Repair. J. Cell Biol. 189 (6), 1027–1038. doi: 10.1083/jcb.201003053
Tanida I., Ueno T., Kominami E. (2008). LC3 and Autophagy. Methods Mol. Biol. 445, 77–88. doi: 10.1007/978-1-59745-157-4_4
Teixeira A. A. R., de Cássia Sardinha de Vasconcelos V., Colli W., Alves M. J. M., Giordano R. J. (2015). Trypanosoma Cruzi Binds to Cytokeratin through Conserved Peptide Motifs Found in the Laminin-G-Like Domain of the Gp85/Trans-Sialidase Proteins. PLoS Neglect. Trop. Dis. 9 (9), e0004099. doi: 10.1371/journal.pntd.0004099
Teixeira S. M. R., Russell D. G., Kirchhoff L. V., Donelson J. E. (1994). A Differentially Expressed Gene Family Encoding ‘amastin,’ a Surface Protein of Trypanosoma Cruzi Amastigotes. J. Biol. Chem. 269 (32), 20509–20516. doi: 10.1016/S0021-9258(17)32022-7
Teixeira S. C., Lopes D. S., Gimenes S. N. C., Teixeira T. L., Da Silva M. S., Silva Brígido R. T. E., et al. (2017). Mechanistic Insights into the Anti-Angiogenic Activity of Trypanosoma Cruzi Protein 21 and Its Potential Impact on the Onset of Chagasic Cardiomyopathy. Sci. Rep. 7, 44978. doi: 10.1038/srep44978
Teixeira T. L., Machado F. C., Da Silva A. A., Teixeira S. C., Borges B. C., Dos Santos M. A., et al. (2015). Trypanosoma Cruzi P21: A Potential Novel Target for Chagasic Cardiomyopathy Therapy. Sci. Rep. 5, 1–10. doi: 10.1038/srep16877
Teixeira M. M. G., Yoshida N. (1986). Stage-Specific Surface Antigens of Metacyclic Trypomastigotes of Trypanosoma Cruzi Identified by Monoclonal Antibodies. Mol. Biochem. Parasitol. 18 (3), 271–282. doi: 10.1016/0166-6851(86)90085-X
Tengholm A., Gylfe E. (2017). CAMP Signalling in Insulin and Glucagon Secretion. Diabetes Obes. Metab. 19, 42–53. doi: 10.1111/dom.12993
Tonelli R. R., Giordano R. J., Barbu E. M., Torrecilhas A. C., Kobayashi G. S., Langley R. R., et al. (2010). Role of the Gp85/Trans-Sialidases in Trypanosoma Cruzi Tissue Tropism: Preferential Binding of a Conserved Peptide Motif to the Vasculature in Vivo. PLoS Neglect. Trop. Dis. 4 (11), e864. doi: 10.1371/journal.pntd.0000864
Torrecilhas A. C., Schumacher R. I., Alves M. J. M., Colli W. (2012). Vesicles as Carriers of Virulence Factors in Parasitic Protozoan Diseases. Microbes Infect. 14 (15), 1465–1474. doi: 10.1016/j.micinf.2012.07.008
Torrecilhas A. C., Soares R. P., Schenkman S., Fernández-Prada C., Olivier M. (2020). Extracellular Vesicles in Trypanosomatids: Host Cell Communication. Front. Cell. Infect. Microbiol. 10, 2020.602502. doi: 10.3389/fcimb.2020.602502
Trocoli Torrecilhas A. C., Tonelli R. R., Pavanelli W. R., da Silva J. S., Schumacher R. I., de Souza W., et al. (2009). Trypanosoma Cruzi: Parasite Shed Vesicles Increase Heart Parasitism and Generate an Intense Inflammatory Response. Microbes Infect. 11 (1), 29–39. doi: 10.1016/j.micinf.2008.10.003
Wainszelbaum M., Isola E., Wilkowsky S., Cannata J. J. B., Florin-Christensen J., Florin-Christensen M. (2001). Lysosomal Phospholipase A1 in Trypanosoma Cruzi: An Enzyme with a Possible Role in the Pathogenesis of Chagas’ Disease. Biochem. J. 355 (3), 765–770. doi: 10.1042/bj3550765
Walker D. M., Oghumu S., Gupta G., Mcgwire B. S., Drew M. E., Satoskar A. R. (2014). Mechanisms of Cellular Invasion by Intracellular Parasites Mechanisms of Host Cell Invasion in Leishmania. Cell Mol. Life Sci. 71 (7), 1245–1263. doi: 10.1007/s00018-013-1491-1
Wang T., Li L., Hong W. (2017). SNARE Proteins in Membrane Trafficking. Traffic 18, 767–775. doi: 10.1111/tra.12524
Watanabe Costa R., da Silveira J. F., Bahia D. (2016). Interactions between Trypanosoma Cruzi Secreted Proteins and Host Cell Signaling Pathways. Front. Microbiol. 7:2016.00388 (MAR). doi: 10.3389/fmicb.2016.00388
Wilkowsky S. E., Barbieri M. A., Stahl P., Isola E. L. D. (2001). Trypanosoma Cruzi: Phosphatidylinositol 3-Kinase and Protein Kinase B Activation Is Associated with Parasite Invasion. Exp. Cell Res. 264 (2), 211–218. doi: 10.1006/excr.2000.5123
Woolsey A. M., Sunwoo L., Petersen C. A., Brachmann S. M., Cantley L. C., Burleigh B. A. (2003). Novel Pl 3-Kinase-Dependent Mechanisms of Trypanosome Invasion and Vacuole Maturation. J. Cell Sci. 116 (17), 3611–3622. doi: 10.1242/jcs.00666
Yoshida N., Dorta M. L., Ferreira A. T., Oshiro M. E. M., Mortara R. A., Acosta-Serrano A., et al. (1997). Removal of Sialic Acid from Mucin-like Surface Molecules of Trypanosoma Cruzi Metacyclic Trypomastigotes Enhances Parasite-Host Cell Interaction. Mol. Biochem. Parasitol. 84 (1), 57–67. doi: 10.1016/S0166-6851(96)02783-1
Yoshida N., Favoreto S., Ferreira A. T., Manque P. M. (2000). Signal Transduction Induced in Trypanosoma Cruzi Metacyclic Trypomastigotes during the Invasion of Mammalian Cells. Braz. J. Med. Biol. Res. 33, 269–278. doi: 10.1590/S0100-879X2000000300003
Yoshida N., Cortez M. (2008). Parasite and Host Cell Signaling during the Invasion Process. Mol. Mech. Parasite Invasion 47, 82–91. doi: 10.1007/978-0-387-78267-6_6
Zanforlin T., Bayer-Santos E., Cortez C., Almeida I. C., Yoshida N., Silveira J. F. Da. (2013). Molecular Characterization of Trypanosoma Cruzi SAP Proteins with Host-Cell Lysosome Exocytosis-Inducing Activity Required for Parasite Invasion. PLoS One 8 (12), e83864. doi: 10.1371/journal.pone.0083864
Zingales B., Andrade S. G., Briones M. R. S., Campbell D. A., Chiari E., Fernandes O., et al. (2009). A New Consensus for Trypanosoma Cruzi Intraspecific Nomenclature: Second Revision Meeting Recommends TcI to TcVI. Memorias Do Instituto Oswaldo Cruz 104 (7), 1051–1054. doi: 10.1590/S0074-02762009000700021
Keywords: invasion, internalization, lysosome-mediated invasion, exocytic pathway, autophagic pathway, host signaling, host/parasite interaction
Citation: Ferri G and Edreira MM (2021) All Roads Lead to Cytosol: Trypanosoma cruzi Multi-Strategic Approach to Invasion. Front. Cell. Infect. Microbiol. 11:634793. doi: 10.3389/fcimb.2021.634793
Received: 28 November 2020; Accepted: 27 January 2021;
Published: 05 March 2021.
Edited by:
Gustavo Benaim, Fundación Instituto de Estudios Avanzados (IDEA), VenezuelaReviewed by:
Marcel I. Ramirez, Oswaldo Cruz Foundation (Fiocruz), BrazilCarlos A. Buscaglia, Consejo Nacional de Investigaciones Científicas y Técnicas (CONICET) y la Universidad Nacional de General San Martín, Argentina
Copyright © 2021 Ferri and Edreira. This is an open-access article distributed under the terms of the Creative Commons Attribution License (CC BY). The use, distribution or reproduction in other forums is permitted, provided the original author(s) and the copyright owner(s) are credited and that the original publication in this journal is cited, in accordance with accepted academic practice. No use, distribution or reproduction is permitted which does not comply with these terms.
*Correspondence: Martin M. Edreira, mme2@pitt.edu