Scoping carbon dioxide removal options for Germany–What is their potential contribution to Net-Zero CO2?
- 1Department of Bioenergy, Helmholtz Centre for Environmental Research GmbH (UFZ), Leipzig, Germany
- 2Deutsches Biomasseforschungszentrum gGmbH (DBFZ), Leipzig, Germany
- 3Institute of Catalysis Research and Technology, Karlsruhe Institute of Technology (KIT), Eggenstein-Leopoldshafen, Germany
- 4Institute for Micro Process Engineering, Karlsruhe Institute of Technology (KIT), Eggenstein-Leopoldshafen, Germany
- 5Wadden Sea Station Sylt, Alfred Wegener Institute Helmholtz Centre for Polar and Marine Research (AWI), List, Germany
- 6Department of Agroecology, Aarhus University, Tjele, Denmark
- 7Department of Environmental Politics, Helmholtz Centre for Environmental Research (UFZ), Leipzig, Germany
- 8Institute for Bio- and Geosciences - Agrosphere (IBG-3), Forschungszentrum Jülich GmbH (FZJ), Jülich, Germany
- 9Remote Sensing and Geoinformatics, GFZ German Research Centre for Geosciences, Potsdam, Germany
- 10Biogeochemical Modelling, GEOMAR Helmholtz Centre for Ocean Research Kiel, Kiel, Germany
- 11Marine Evolutionary Ecology, GEOMAR Helmholtz Centre for Ocean Research Kiel, Kiel, Germany
- 12Institute of Energy and Climate Research–Systems Analysis and Technology Evaluation (IEK-STE), Forschungszentrum Jülich GmbH (FZJ), Jülich, Germany
- 13Geoenergy, GFZ German Research Centre for Geosciences, Potsdam, Germany
In its latest assessment report the IPCC stresses the need for carbon dioxide removal (CDR) to counterbalance residual emissions to achieve net zero carbon dioxide or greenhouse gas emissions. There are currently a wide variety of CDR measures available. Their potential and feasibility, however, depends on context specific conditions, as among others biophysical site characteristics, or availability of infrastructure and resources. In our study, we selected 13 CDR concepts which we present in the form of exemplary CDR units described in dedicated fact sheets. They cover technical CO2 removal (two concepts of direct air carbon capture), hybrid solutions (six bioenergy with carbon capture technologies) and five options for natural sink enhancement. Our estimates for their CO2 removal potentials in 2050 range from 0.06 to 30 million tons of CO2, depending on the option. Ten of the 13 CDR concepts provide technical removal potentials higher than 1 million tons of CO2 per year. To better understand the potential contribution of analyzed CDR options to reaching net-zero CO2 emissions, we compare our results with the current CO2 emissions and potential residual CO2 emissions in 2050 in Germany. To complement the necessary information on technology-based and hybrid options, we also provide an overview on possible solutions for CO2 storage for Germany. Taking biophysical conditions and infrastructure into account, northern Germany seems a preferable area for deployment of many concepts. However, for their successful implementation further socio-economic analysis, clear regulations, and policy incentives are necessary.
Introduction
In order to meet the climate protection goals of the Paris Agreement (PA) and to keep global warming well-below 2°C, global action to reduce greenhouse gas (GHG) emissions to zero (or net-zero) is needed (UNFCCC, 2015). To limit global warming to 1.5°C, the remaining carbon budget from 2020 onwards would allow us to emit another 440 (230, 670) gigatonnes (Gt) CO2 globally (Matthews et al., 2021). The median amount of Carbon Dioxide Removal (CDR) included in the IPCC's 1.5° scenarios with limited overshoot amounts to 7 Gt CO2 per year in 2050, high overshoot scenarios are at 9 Gt CO2 per year in 2050 (Rogelj et al., 2018). The CDR measures in these scenarios have two functions: (1) they compensate for emissions up until the point net-zero is reached, potentially allowing more time to transition to a carbon neutral system, and (2) reaching substantial net-negative emissions (especially in overshoot scenarios) by removing previously emitted carbon dioxide. However, as stated by Zickfeld et al. (2021), one ton emitted might not equate to one ton removed.
Next to far-reaching mitigation actions, which would require systems transformation in the areas of energy, industry, land use, and transportation, CDR measures would compensate for remaining, hard-to-abate emissions to reach net-zero. However, the implementation of such CDR measures would require its very own infrastructure. To achieve the global goals of the PA, countries need to fulfill ambitious GHG reduction targets in line with their national carbon budgets. The revised Germany's Climate Change Act (KSG, 2021) pushes the climate neutrality goal to 2045 and strives for negative emissions after 2050. The permissible emission budget per sector for the years 2020–2030 has been set to 5.53 Gt CO2 − eq. For the years beyond 2030 the annual reduction targets will be announced in 2024 for the period 2031–2040, and no later than in 2032 for the period 2041–2045 (KSG, 2021).
Allocation of emission quota is a pivotal element of a country's emission reduction strategy. This distribution at the national level is a balance of many factors, including environmental effectiveness, equity, political feasibility, economic efficiency and technical requirements (Höhne et al., 2003, 2014; Gignac and Matthews, 2015). Given Germany's capacities, abilities and responsibilities, a remaining CO2 budget of 6.9 Gt (1st January 2021 until net-zero) seems to be a reasonable option (Mengis et al., 2021). It takes into account the infrastructural lock-in in Germany that needs to be overcome and reaches equal-per-capita emissions by 2035 (Mengis et al., 2021). Nevertheless, to stay within the assumed carbon budget of 6.9 Gt CO2 for Germany, net-zero CO2 emissions have to be achieved. This implies that supportive mitigation efforts such as the deployment of CDR measures are inevitable as some residual emissions sources, e.g., agriculture, industrial processes, or aviation, will be hard to completely eliminate (cf. Geden and Schenuit, 2020). The amount of CDR required will depend on the progress in reducing emissions. In case we succeed in reducing fossil fuel and industry emissions by 85% in 2050, 1.9 Gt CO2/year would need to be removed through CDR in Germany (Mengis et al., 2021). Other studies range between 36 and 63 megatonnes (Mt) of CO2 per year for remaining, hard-to-abate emissions in Germany (Ariadne Report, 2021; Mengis et al., 2022), which in turn translates into the CDR needed for reaching net-zero. Pozo et al. (2020) allocate a cumulative CDR quota of 5–18 Gt CO2 between 2018 and 2100 to Germany based on responsibility, capability and equality considerations.
In contrast to “conventional” mitigation where emissions from the use of fossil fuels or industrial processes are reduced or avoided, CDR measures, sometimes referred to as “unconventional” climate change mitigation measures (Geden and Schenuit, 2020), take CO2 directly out of the atmosphere. Such atmospheric CO2 removal can occur by enhancing natural sinks of ecosystems or through technological processes. If anthropogenic carbon sinks exceed anthropogenic emissions to the atmosphere, so-called net negative CO2 emissions can be achieved.
Natural sink enhancement (NSE), also called nature-based solutions or natural climate solutions, encompasses a variety of ecosystem-based options, which focus on ecosystem restoration or the increase of naturally occurring processes that sequester carbon. Those measures may relate to forests (re- and afforestation), wetlands (e.g., peatland rewetting), seas (seagrass meadows, salt marshes), or soils (restorative agriculture). Depending on the measure the captured CO2 is then sequestered in above ground biomass, top-soils, peats or sediments, which influence the permanence of the CO2 storage.
Technology-based solutions [or geochemical-based CDR (Schenuit et al., 2021)], like direct air carbon capture and storage (DACCS), remove CO2 by using either chemical or physical processes to separate CO2 from the ambient air. Unlike NSE (and bioenergy with carbon capture and storage–BECCS), DACCS requires large amounts of energy and is thus a costly CDR measure. While the plants are expected to become cheaper in the future due to economies of scale and mass fabrication, energy demand and associated costs may remain high. However, in case of DACCS, the area demand per ton of CO2 captured is smaller compared to other CDR options.
Bioenergy with carbon capture and storage is a hybrid approach of the two previously mentioned concepts (UNEP, 2017; Bellamy and Osaka, 2020). In this process, CO2 is captured from the atmosphere by biological net primary production (nature-based capture) which is further used to generate bioenergy. The CO2 which could be released in this conversion process is captured (technology-based capture) and stored underground. If the biomass life cycle emissions are lower than the carbon captured, BECCS may provide negative emissions. BECCS is not a one-technology concept, it encompasses a wide portfolio of technological options, which follow the same principle: they convert biomass into energy (electricity, heat, fuels), capture CO2 generated in this process, and store it under the ground or in long-lived products. The most common BECCS technology applied to date is bioethanol production with CCS, since it delivers high-purity fermentation CO2 and thus provides a cost-competitive CDR solution with sequestration cost of $60 or less per ton of CO2 (Sanchez et al., 2018). At such rate this option may compete with some natural sink enhancement solutions, such as afforestation, biochar, soil carbon sequestration or enhanced weathering (Minx et al., 2018). Other promising bioenergy technologies to be coupled with CCS include direct combustion of biomass for generation of heat and/or power (CHP), biomass gasification or pyrolysis for biofuels production, or anaerobic digestion for biogas production with CHP or for upgrading to biomethane. Unlike DACCS, BECCS produces energy, but its land demand (and related impacts) is higher.
Although CDR provides a wide range of highly differentiated solutions, none of them can be treated like a silver bullet for addressing climate change (Minx et al., 2018). They need to become a part of national GHG removal strategies and be incorporated into existing national infrastructures, among further development of renewable energies or improvement of energy efficiency. Only in such combinations they can enable countries to achieve their national emission targets.
CDR options are at different stages of maturity, including both well-researched and widely deployed options (e.g., afforestation) as well as options in a relatively early stage of development (e.g., ocean alkalinity enhancement). These differences between the various CDR option maturity levels lead to many uncertainties around the feasibility of their deployment, especially at larger scales (Low and Schäfer, 2020).
There is no “one-fits-all” solution. Thus, the deployment of CDR measures in each country or region needs to be tailor-made, both in terms of selecting best-fitting CDR options and defining their individual parameters and implementation aspects. This information needs to cover more than just techno-economic elements and be coherent among different CDR concepts. Therefore, in this study we present a portfolio of CDR options (technology-based, hybrid and NSE), which we embed specifically into the German context (e.g., biophysical and techno-economic). Each option is presented along a possible implementation example and assessed with regard to the following two analytical questions:
• How do experts envision and describe exemplary CDR concepts in Germany?
• What is the technical potential of the CDR concepts and their individual contribution to a net-zero carbon dioxide emission in Germany?
Our aim is to provide a harmonized dataset on different CDR options. These structured considerations can further serve as a basis for comparison and deeper evaluations of different CDR options as well as indicate pathways with the highest potential to support achieving net-zero CO2 emissions in Germany.
Materials and methods
In the following section we will describe how we selected CDR options that we identified for Germany, how we collected and checked the data for the CDR concepts, and how we finally calculated CO2 removal potentials for Germany.
Our methodological approach is presented in the following steps:
1. Selection of CDR options available in Germany.
2. CDR concepts development and description.
3. Data collection review and quality control.
4. Calculation of CO2 removal potential.
Selection of CDR options available in Germany
There are many CDR options already identified and described in the scientific literature (e.g., Oschlies and Klepper, 2017; Fuss et al., 2018; IPCC, 2018, 2021). In this paper we selected and described options that are or likely will be available for deployment in Germany.
To provide a possibly wide portfolio of options, our selection was based on their general availability:
• Spatial availability–occurrence of geographical conditions which enable or facilitate their deployment, e.g., natural presence of seagrass meadows or areas suitable for peatlands, availability of biomass (type and amount), etc.
• Technology and market availability–maturity of CDR option in terms of its readiness to be deployed, e.g., state-of-the-art technologies.
However, our selection does not aim to provide an exhaustive list of options but rather is targeted to present possible examples.
CDR concepts development
There is vast information available on individual CDR options (Minx et al., 2018). Yet, it is scattered across many different research fields and levels of assessment (from laboratory to global scale). Putting this information into context by designing dedicated CDR concepts makes it not only more manageable but also increases the relevance of their assessment for decision making since national (German) conditions (such as land availability, biomass potentials, existing infrastructure, or priorities in energy policy) shape and influence their prospective implementation.
On the basis of a literature review, experts' knowledge on CDR options and an analysis of German conditions, we prepared exemplary concepts of selected CDR options, which can be implemented in reality. For each CDR concept, we created a tabular fact sheet with a unified set of indicators (see Supplementary materials) that serves as a tool for organizing the collected information. This allowed us to achieve a coherent description across selected CDR options.
In the fact sheets, information on the CDR concepts is further classified into eight categories which cover different aspects of the CDR systems:
• Concept characteristics–addresses general parameters of the concept.
• Input–specifies the option's resources requirements.
• Output–specifies expected CO2 removal and co-products generated by the CDR.
• Environmental parameters–address impacts on, among others, soils, water system and biodiversity.
• Economic parameters–address the economic performance of the option.
• Systemic parameters–consider potentials, permanence and verifiability.
• Permission–addresses compliance with regulations.
• Scalability–expresses reproducibility of the CDR unit.
Each above mentioned category comprises a set of specific parameters which are listed and described in Table 1.
A limitation of the current study is that we have not considered socio-political parameters for the development of the CDR concepts. The main reasons behind this choice is that (1) there is relatively little socio-political information available on CDR options in Germany, (2) for this paper we focus on quantitative information whereas much of the available information about socio-political parameters comes from qualitative studies. Within the overarching research project (Cluster I of the Helmholtz Climate Initiative1), broader assessments including socio-political parameters are carried out. In other words, the focus on quantitative information about the different CDR options is a delimitation of scope rather than a normative statement about what parameters are most important. Clearly, socio-political parameters matter for the deployment of CDR options (see e.g., Buck, 2016; Honegger et al., 2020; Morrow et al., 2020; Waller et al., 2020).
Data collection review and quality control
The data collection process has been conducted on each CDR option by dedicated assessment teams and CDR experts. Diverse data channels have been used by CDR experts including peer-reviewed literature, reports, results of simulation exercises and modeling and expert assessment for the data collection. For a literature review, a standard literature surveying (hand-selection process) as well as a systematic review using the search engine of Scopus has been carried out.
Since the data collected concerned different CDR options, it was essential to ensure a coherent integration of data into the fact sheets. Therefore, the units used in individual parameters have been standardized across all options and their integrity and quality has been checked with the respective experts.
Calculation of CO2 removal potential
The ability of CDR options to avoid or remove CO2 from the atmosphere is a key parameter which describes their CO2 emission mitigation potential. In our study, we adopted the approach of Mengis et al. (2022), and focused on “(...) CO2 rather than CO2-equivalent emissions, following the scientific reasoning of (net) zero CO2 targets based on the transient climate response to cumulative CO2 emissions (Matthews et al., 2009; Rogelj et al., 2018)” as a necessary condition for temperature stabilization.
Avoided emissions express the amount of CO2 that has not been emitted to the atmosphere as a result of actions which lower or prevent CO2 emissions, i.e., shift to the renewable energies use, change in land-use practices or human behavior. Removed emissions express the amount of CO2 removed from the atmosphere and durably stored in geological, terrestrial, or ocean reservoirs. These individual storage options provide a certain bandwidth for the storage durability as, e.g., for soil carbon sequestration a minimum 100 years is reached (Dynarski et al., 2020), whereas for geological CO2 storage, several thousands of years may be achieved (Kempka et al., 2014). Such removal may be realized through enhancement of biological or biochemical sinks and DACCS. The natural CO2 uptake, which is not caused by human intervention, is not regarded here as CO2 removal (IPCC, 2018). If the overall process removes more emissions from the atmosphere than it emits, it can be addressed as a negative emissions technology (NET). In this paper we quantify the CO2 removal potential of selected CDR options by multiplying the emissions removed by one CDR unit by a number of possible units deployed by 2050 (Equation 1):
Apart from the option's emission reduction potential, we also estimate its technical implementation potential by taking into consideration the option's maturity level, availability of infrastructure, biophysical conditions, and scalability. Such a combination allows both the assessment of the possible dynamics of option implementation and the designation of the general readiness of the system for deployment.
The maturity level of a certain technology is often expressed as the Technology Readiness Level (TRL) (DOE, 2011). The TRL categorizes technologies into nine levels according to their maturity and scale of deployment: from being formulated (TRL1-2), through laboratory tests (TRL 3-4), demonstration (TRL 5-6), and pilots in a relevant environment (TRL 7-8) to market rollout (TRL 9). Progression to a higher TRL comes with an effort of further research, financial investment and/or policy support (Bui et al., 2018). Estimating a TRL of a CDR measure is not a straightforward task. First, not all concepts are strictly technology-based, and second, sometimes separate components of individual measures differ in their development stage (Hepburn et al., 2019). For this reason, in this study we do not adhere strictly to the common TRL classification (e.g., as defined by EC in HORIZON 2020 Work Programme2). Instead, we express the maturity of a certain concept by indicating the maturity of its components and level of their integration (see Annex 1 in Supplementary materials).
Taking these factors into consideration, 13 CDR exemplary options were selected for this study as being prospective for deployment in Germany by 2050. The options are shortly described in Section Results, whereas the details are displayed in the fact sheets (see Supplementary materials). Additionally, a fact sheet on CO2 storage solutions has been created, complementing the information for the hybrid and technology-based options. This separation of the carbon storage component was dictated by the current legal situation in Germany, which does not allow underground storage of CO2 and thus limits the full application of BECCS and DACCS in Germany [see Section Geological carbon dioxide storage solutions (concept 14)]. Because of this separation, we refer to BECC and DACC when we talk about exemplary CDR concepts presented in our study, which do not have an integrated CO2 storage component, and to BECCS and DACCS when we talk about the general concepts with the storage component. We use expert knowledge from different CDR fields to further identify some of the key characteristics of these options (e.g., concerning their economic parameters, permanence of storage, or scalability) and to develop the fact sheets and dedicated CDR concepts (see Section CDR concepts portfolio).
Results
CDR concepts portfolio
Following selection criteria (Section Selection of CDR options available in Germany), our portfolio includes six technology options for BECC, two concepts of DACC, and five NSE options. Storage solutions were described as an additional concept (Figure 1).
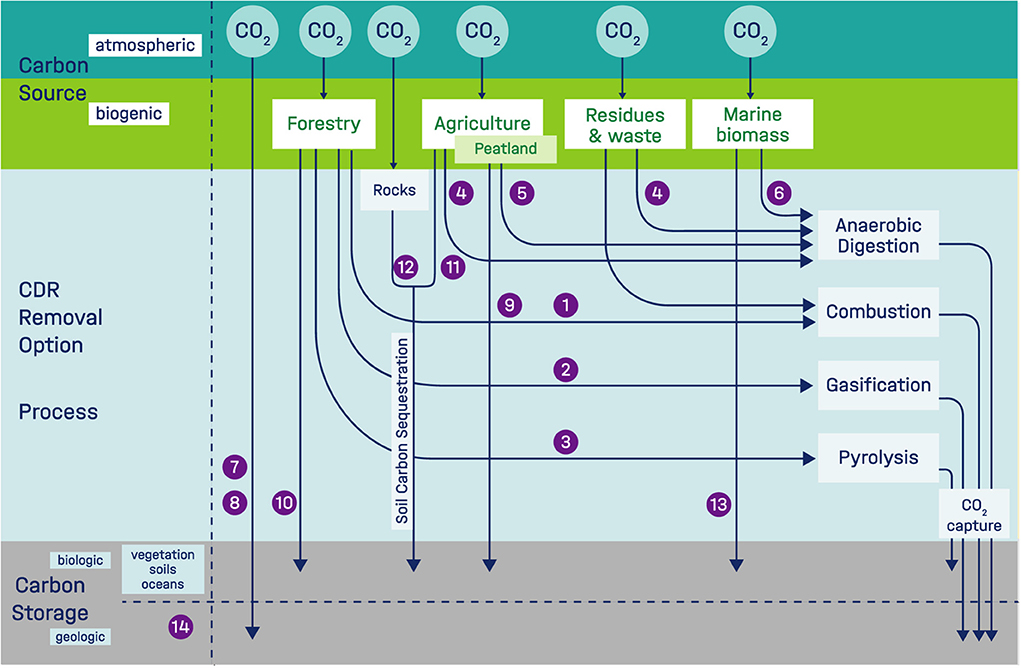
Figure 1. Overview of the selected CDR options. Circled numbers correspond to the numbering of CDR concepts in the paper and represent following options: 1–BECC: biomass combustion CHP; 2–BECC: biogas CHP; 3–BECC: paludiculture for biogas CHP; 4–BECC: macroalgae for biogas CHP; 5–BECC: pyrolysis for biocoal production; 6–BECC: gasification for biofuels production; 7–DACC: centralized; 8–DACC: decentralized; 9–NSE: peatland rewetting; 10–NSE: afforestation of cropland; 11–NSE: SOC, cover crops; 12–NSE: SOC, ERW; 13–NSE: seagrass meadows Baltic Sea; 14–geological storage solutions (source: UFZ/Malgorzata Borchers, Helmholtz Climate Initiative // Julia Blenn, Creative Commons CC-BY NC 4.0 license).
BECC is considered as one of the most viable and cost-effective options to achieve negative emissions (Babin et al., 2021), but there are concerns related to its possible negative impacts on land use (competition for arable land and water) and biodiversity (IPBES-IPCC, 2021; IPCC, 2021). To avoid negative interactions with the environment, only sustainable sources of biomass should be taken into account. This can include considerations such as favoring the usage of by-products, residues and waste, sourcing the biomass from sustainable agricultural and forestry practices, including novel approaches like paludiculture, or growing biomass in marine environments (e.g., macroalgae farming). In Germany, thanks to favorable geographical conditions and supporting funding programs for bioenergy provision [e.g., Renewable Energy Act (EEG, 2000 and later modifications), Biofuels Quota Act (BioKraftQu, 2006 and later modifications)], various types of biomass are already available and used for energy production (e.g., waste and residues, woody and agricultural biomass, energy crops). However, the German natural conditions also show a potential for growing new types of biomass, i.e., macroalgae or paludiculture biomass, and therefore, they have also been included in our portfolio. We also describe four terrestrial and one marine NSE (so-called “Blue Carbon,” Nellemann et al., 2009) options–all based on ecosystems that naturally occur in Germany. They include practices which either restore (e.g., peatland rewetting) or manage (e.g., soil carbon sequestration) existing ecosystems to increase their CO2 capture and storage properties. Considering the coastal regions of Germany, we also examined coastal vegetation systems like seagrass meadows and salt marshes due to their ability to capture CO2 from the air and store it as organic carbon in biomass and below the ground. In our portfolio we also include DACC technologies, which are geographically independent with prerequisites being free space and access to energy sources.
Because climate mitigation requires immediate action, our portfolio consists exclusively of options whose maturity level is high enough to ensure possible implementation within a decade or sooner (assuming no social and legal constraints/barriers).
We shortly describe our CDR concepts below and enclose their details in the fact sheets provided in the Supplementary materials.
BECC–Combustion of woody biomass for heat and power co-generation (concept 1)
As Germany planned a coal phase-out by 2038 (KVBG, 2020), the conversion of coal-fired power plants to biomass may not only make a substantial contribution to the rapid reduction of CO2 emissions but also support on multiple levels (e.g., economic, social) a system with largely existing infrastructure. Our concept assumes the use of a 500 megawatts electric (MWel) CHP plant fueled by wood pellets equipped with post-combustion, monoethanolamine (MEA)-based capture units, which could remove 2.99 Mt CO2 per year. If 10 out of 18 currently operating coal-fired CHP plants (with minimum 500 MWel capacity) (BNetzA, 2021) would be converted to biomass and retrofitted with CCS units, approximately 30 Mt of CO2 could be removed annually (Donnison et al., 2020). However, the use of woody biomass in large power plants is likely to significantly increase the overall demand for biomass including the need for biomass imports (Erlach et al., 2019). This is likely to cause direct and indirect land use impacts with negative environmental effects on forest ecosystems within and outside of Germany including additional carbon emissions resulting from land use change (Birdsey et al., 2018; Schlesinger, 2018).
BECC–Gasification of woody biomass for biofuels production (concept 2)
Gasification is a well-developed technology for the conversion of fossil fuels and waste. Biomass as feedstock has been investigated intensively and large pilot and demonstration plants have been erected and operated worldwide (Rauch et al., 2014). The technology is mature for market implementation. Small scale facilities for CHP applications in the kilowatt (kW)-range are already commercialized (Patuzzi et al., 2021). Large scale conversion plants would aim at the production of several hundred thousand tons of synthetic hydrocarbon fuels per year. CO2 is an inevitable by-product in autothermal gasification but can be separated by common means as already conducted in existing plants with fossil fuels. Our concept - a gasification plant of 100 megawatts thermal (MWth) capacity - could remove 60 kilotonnes (kt) CO2 per year. If scaled up, it could provide removal of 3.72 Mt CO2 by 2050. However, if this application leads to an increase in the use of woody biomass, it can also lead to direct and indirect land use impacts with negative environmental effects on ecosystems and additional carbon emissions from land use change (Birdsey et al., 2018; Schlesinger, 2018).
BECC–Pyrolysis of woody biomass for biocoal production (concept 3)
Pyrolysis is well-established for fossil fuels, but also for biomass feedstocks. Fast pyrolysis aims at the production of liquid fuels, which are used as heating oil today. CO2 is a by-product here as well as in slow pyrolysis, which favors the production of biocoal (Tripathi et al., 2016). This product can be used energetically, but also for carbon storage in soil applications. The impact of biocoal additions to soil have been investigated in a number of studies, considering a variety of soil-physical factors like dry density, water and nutrient exchange, pore volume and distribution and the like (e.g., Lehmann and Joseph, 2015). Naturally, long term investigations have not been finished so far. Also the number of studies investigating biocoal stability is constantly increasing (Lehmann et al., 2006; Leng et al., 2019). Biocoal stability can serve as an indicator to assess the carbon sequestration potential of biocoal. There are several methods for estimating the stability of biocoal, one of which is pyrolysis temperature (Leng et al., 2019). According to a rule of thumb, biocoal produced at higher temperature is expected to be more stable and thus persistent then low temperature char like hydrochar. While for the latter some decades have been derived as medium life time, for pyrochar average life times between 100 and over 1,000 years have been estimated, depending on a number of parameters (environmental conditions, type of coal and feedstock used, soil use/treatment, investigation method). The presence of volatile, accessible organic molecules favors microbial attack, consequently leading to faster decomposition than mineralization by slow oxidation of the carbon by air. Derived from the existing studies, correlations for the mean residence times were suggested. As an example, Lehmann et al. (2009) estimated a carbon loss by around 20% in 500 years when 10 % of labile carbon was contained in the pyrochar. Other studies gave similar results (Kuzyakov et al., 2009, 2014) using biochar from grass pyrolysis (mean residence time > 400 years). Interestingly it was found that incubation test in a laboratory environment led to higher biocoal degradation rates than open air tests. However, comparison and interpretation of the existing studies is difficult and for sure further investigations are required. Anyhow, in terms of carbon storage related to climate effects, biocoal stabilites of several hundred years can be reasonably assumed or need to be assured by appropriate processing and soil application.
Several technology providers entered the market already offering small to medium size conversion technologies. Our concept–a 50 MWth plant–could provide removal of 100 kt CO2 per year. Assuming the use of 10 Mt of wood per year, which equals 15.5% of 66.8 Mt of wood used energetically today (FNR, 2018), 12.2 Mt CO2 could be removed annually if this technology would be implemented. Both the gasification and pyrolysis CDR numbers are based on the assumption that around 10 Mt of wood used energetically today in Germany are utilized. The lignocellulose feedstock potential is around twice as much, if non-woody biomass is included.
BECC–Biogas production for co-generation of heat and power (concept 4)
With around 9 000 operating biogas plants (FNR, 2020), Germany has the biggest potential to explore this technology as a prospective option for bio-CCS in Europe. As estimated by Billig et al. (2019), in 2050 around 11.3 Mt of biogenic CO2 could be captured from both biogas CHP and biogas upgrading plants based on wastes and residues. However, due to the upcoming termination of feed-in tariffs, limitations of support for “post-subsidy plants” and no long-term alternative support mechanisms (EEG, 2021), sustaining this potential can be possible only in a short term period (until ~2024–2027), and the future is highly uncertain (Scheftelowitz and Thrän, 2016). Our concept uses a medium sized, 500 kilowatts electric (kWel), biogas CHP plant which generates biogas from a mixture of feedstock, i.e., 50% wastes and residues, 20% cow manure and 30% energy crops, as described by Thrän (2019). CO2 is separated in a post-combustion, MEA-based process. The annual removal per plant equals 3.15 kt CO2. Assuming that until 2050 ~4,000 biogas plants with minimal capacity of 500 kWel would be retrofitted with carbon capture units, around 12.6 Mt CO2 could be removed. The technical parameters of the biogas plant and capture unit described in this section have also been adapted for further two biogas-based concepts which differ in the type and amount of biomass used (concepts 5 and 6, Sections BECC–Paludiculture-sourced biomass for biogas production for co-generation of heat and power (concept 5) and BECC–Macroalgae farming for biogas production for co-generation of heat and power (concept 6), respectively).
BECC–Paludiculture-sourced biomass for biogas production for co-generation of heat and power (concept 5)
Paludiculture is the use of wet ecosystems such as wetlands and peatlands for multiple purposes, including biomass and bioenergy production (Wichtmann et al., 2015). In our concept, we use it as a feedstock for biogas production with heat and power co-generation. Restored wetlands and peatlands can be used for paludiculture including the production of biomass for bioenergy, which is already practiced in a pilot site (Agrotherm GmbH3). Combining bioenergy production from paludiculture with CCS has not been done yet. However, using biomass from paludiculture could generate multiple benefits not only for generating negative emissions but also for CO2 mitigation, climate change adaptation and biodiversity conservation. In Germany, large areas of wetlands and peatlands have been drained in the past for agricultural production. These drained areas are causing large carbon emissions−43 Mt CO2 per year in Germany alone (Tanneberger et al., 2021), and 2 Gt globally (Joosten, 2009; Joosten et al., 2016)–due to the ongoing degradation of the peat soils. Restoring these peatlands and wetlands by reversing the drainage and increasing the water table to the soil surface reduces carbon emissions substantially (Günther et al., 2020). In addition, establishing paludiculture on restored wetlands has considerable benefits for biodiversity by resembling more natural habitats, restoring the natural water cycle benefiting climate change adaptation (buffering impacts of droughts and floods) and retaining nutrients with benefits for water quality (Tanneberger et al., 2020, 2022). Given the substantial benefits of paludiculture for addressing multiple challenges related to climate change, biodiversity and water quality, the use of paludiculture for BECCS could be considered a sustainable biomass source and a low-risk/low-regret option for CDR. In Germany paludiculture is estimated to be applicable on an area of at least 215,000 hectares (Scholwin and Siegert, 2020). Such an area could provide feedstock for ~156 biogas CHP plants with 500 kWel capacity each, which would provide a removal of 0.49 Mt CO2 per year.
BECC–Macroalgae farming for biogas production for co-generation of heat and power (concept 6)
A BECC feedstock which could bypass the problem of competition for land is macroalgae (seaweed) biomass cultivated in the marine environment. Seaweeds have proved suitability for bioenergy production because of their notably high growth rates and high polysaccharide and negligible lignin content (Chung et al., 2013; Fernand et al., 2017; Kim et al., 2017). Thus, macroalgae biomass, together with microalgae biomass, are regarded as a feedstock for the third generation biofuels production (Montingelli et al., 2015). The macroalgae breeding and cultivation technologies have been well-developed and matured with successful deployment, especially in Asian countries (e.g., China, Japan and South Korea). In Germany, a new structure for mariculture of brown macroalgae (Saccharina latissima) has been developed and tested off the North Sea coast (so-called “Offshore Ring,” e.g., Buck and Buchholz, 2004). A preference for offshore locations for macroalgae cultivation in Germany have also been suggested by Fernand et al. (2017). The German North Sea exclusive economic zone (EEZ) covers 20,600 km2, which could potentially be utilized. Meanwhile, the increasing number of offshore wind farms in the German North Sea EEZ could expand the feasible area for anchoring cultivation infrastructures (Deutsche WindGuard GmbH, 2020). Cultivation of macroalgae does not require use of arable land or freshwater. Combined with ecological engineering aquaculture (e.g., the Integrated Multi-Trophic Aquaculture, IMTA), macroalgae cultivation can bring further ecological and socioeconomic benefits by alleviating eutrophication and increasing bioremediation capability and aquaculture productivity (Wartenberg et al., 2017; Gao et al., 2020). There are ~250 biogas power plants with >400 kWel capacity each operating in the North Sea coast area in Germany (coastal districts in the states of Lower Saxony and Schleswig-Holstein). If those plants would be retrofitted with CCS units, it could provide removal of approximately 0.79 Mt CO2/year.
DACC: Centralized and decentralized options (concepts 7 and 8)
Processes called Direct Air Carbon Capture, or short DACC, are technological solutions to filter CO2 from the atmosphere. The systems use specific chemical interactions of the CO2 with special materials to bind it and therefore remove the greenhouse gas from the air. DACC-processes generally function as a two-step process: capture and regeneration (Heß et al., 2020). While captured, air is moved along the specialized material, called sorbent. This sorbent can be either a strong alkaline solution or a solid. Only the CO2 reacts with the sorbent and forms covalent bonds, while most other components of the air are inert to the sorbent. Only water vapor also bonds to the sorbent. The CO2-depleted air leaves the DACC-unit and the loaded sorbent is left behind. When sufficient loading is reached, the DACC changes to the regeneration step and the flow of air is shut down (Fasihi et al., 2019). To release the CO2, the strong bonds must be broken by heating the sorbent. There are two different designs: on the one hand the Low Temperature (LT)-DACC uses solid amine sorbents, which are regenerated using steam at around 100°C (Deutz and Bardow, 2021), and on the other hand, High Temperature (HT)-DACC uses an alkaline solution, which must be regenerated at 900°C (Keith et al., 2018). While LT-DACC can use numerous heat sources, the high temperatures for HT-DACC can only be reached by combustion processes. The amount of heat needed is very similar for the two systems with roughly 1,500 kilowatt-hour per ton of CO2 removed (Heß et al., 2020). In addition, DACC needs to be supplied with 300 kilowatt-hour electric power per ton of CO2 removed, mainly for the fans, driving air through the absorber and the vacuum pumps in case of LT-DACC and the combined power demand of the process equipment at HT-DACC plants (such as pumps, fans, stirred tanks, etc.) (Heß et al., 2020). The application of DACC units is, therefore, heavily dependent on external constraints like energy supply or available construction land. While HT-systems are usually only feasible at large scales (~1 Mt of CO2 per year), LT-DACC could potentially be fit into small scale systems and deployed in a decentralized application, e.g., into ventilation systems of buildings, reducing the required area (Dittmeyer et al., 2019).
Since the deployment of DACC is heavily dependent on external factors, giving a potential is rather difficult. In the case of a decentralized implementation, an approximation via the current installed ventilation rate is possible (HICAM, 2020). For this parameter there are only numbers for the EU available (Kemna and Moreno Acedo, 2014). If calculated per capita, it would be possible to filter up to 100 Mt of CO2 per year in Germany (Kemna and Moreno Acedo, 2014; Eurostat Press Office, 2015). If only large buildings would be equipped (over 2,500 m2 of floor space), the number would shrink to 15 Mt CO2 a year (Stottrop and Flüshöh, 2007). Other constraints such as space requirements or lower capture rates could further decrease the potential, yet, if compared to the DACC needed in Germany, it could play a significant role. Prognos (2021) calculate the demand for DACC in Germany to be somewhere between 20 and 600 Mt CO2 a year, depending on the decarbonisation scenario. The remaining DACC would have to be installed in big DACC farms, which are, large centralized DACC plants with several adsorber/absorber units and a large combined capture capacity in the Mt range. Considering the typical size of such a farm of ~1 Mt CO2 captured per year and the associated energy demand, it is questionable if this technology is possible within the German territorial boundaries.
NSE–Peatland rewetting (concept 9)
Peatlands are areas with naturally accumulated thick layers of dead organic material (peat) (Joosten and Clarke, 2002). Undrained peatlands, situated between upland and water as a subclass of wetlands, accumulate peat in soils owing to incomplete decomposition of plant material and animal remains under water-saturated conditions (Rydin and Jeglum, 2013). Degraded peatlands, largely caused by drainage for agriculture and forestry, are responsible for ~5% of the total 2014 global anthropogenic CO2 emissions despite their mere ~0.4% coverage of global land surface (Joosten et al., 2016). In Germany, more than 5 % (47 Mt CO2-eq per year) of GHG emissions originate from drained organic soils. Across the globe, both public and private efforts to restore degraded peatlands are increasing as their benefits for providing multiple ecosystem services including carbon storage and the avoidance of CO2 emissions are increasingly recognized and acknowledged to outweigh the needs for drainage-based agriculture and forestry on peatlands (Bonn et al., 2016). In Germany, more than 98% of the organic soils (about 1.8 Mha) are drained mainly for agricultural use (Trepel et al., 2017; Tanneberger et al., 2021). With appropriate planning to reconcile with the demands of agricultural land use and water use, rewetting these drained peatlands offers cost-effective low-hanging fruits to avoid up to 43 Mt of CO2 emissions in the agricultural sector alone with additional multiple benefits for biodiversity and ecosystem services.
NSE–Afforestation of cropland (concept 10)
Forests are land areas >0.5 ha with trees >5 m height and canopy cover >10% and without predominant agricultural or urban use (MacDicken, 2013). The anthropogenic land use change (LUC) of forests substantially affected carbon emissions over the last centuries. It was estimated that LUC from natural forests to cropland and pastures globally emitted on average 1,569 Gt CO2-eq between 1765 and 2005 (Kim and Kirschbaum, 2015). Afforestation is the LUC to forest by active seeding/planting or natural rejuvenation of trees (MacDicken, 2013). The focus here is on afforestation of cropland because this LUC has reportedly the highest sequestration rates (Kim and Kirschbaum, 2015). The afforestation of cropland to secondary forests will sequester atmospheric carbon as soil organic carbon (SOC) and in biomass at rates of 2.2 and 2.8 tons of CO2 per ha per year, respectively (Kim and Kirschbaum, 2015). The focus for afforestation measures should lie on marginal agricultural land, which has been subjected to land use change in the past years (Meyer and Früh-Müller, 2020). There is an estimated 3.3 Mha of marginal land in Germany suitable for plant production (Gerwin et al., 2018), of which a fraction may be also appropriate for afforestation. This estimate may also include non-cropland, however, this is outside the scope of this proposed concept. Winter cereals and winter rapeseed, apart from maize, are the most common field crops in Germany, covering 6.97 Mha of agricultural land (Griffiths et al., 2019). Winter crops can often not be combined with cover crops [see Section NSE–Soil organic carbon sequestration–cover crops (concept 11)], and are considered here for afforestation. A proposed conversion of 10% of the area at a rate of 0.5% per year to secondary forests could sequester 72 Mt CO2 between 2020 and 2050 in Germany.
NSE–Soil organic carbon sequestration–cover crops (concept 11)
Soil organic carbon sequestration as a natural sink enhancement measure for atmospheric CO2 capture has gained interest in the past years. Especially cropland soils have been of interest, because these soils (1) are highly managed, (2) are generally SOC depleted compared to natural ecosystems, and (3) cover vast areas on the planet. Including cover crops into the cropping cycle can reportedly increase SOC with a mean sequestration rate of 1.2 tons CO2 ha/year (Poeplau and Don, 2015). In Germany, ~2.2 Mha cropland are already grown with cover crops (DESTATIS, 2018; Griffiths et al., 2019), meaning that growers have implemented this management option. Another 2 Mha of cropland (potato, sugar beet, summer cereals, and corn) may be suited for cover crops in crop rotations. Assuming that an additional 2 Mha of cropland are seeded with cover crops by 2050 with an annual 2% increase in cover crops, ~44 Mt CO2 can be removed from the atmosphere.
NSE–Terrestrial enhanced weathering (concept 12)
Increased weathering, both on land and on the seafloor, is one of the major natural responses of the Earth system to elevated atmospheric CO2 concentrations or temperature (Archer, 2005). This naturally occurring weathering process is the main drawdown of anthropogenic CO2 emissions over time scales of thousands to hundreds of thousands years (Archer and Brovkin, 2008). Enhanced rock weathering as a CDR measure accelerates this process to capture CO2 by weathering carbonate and silicate minerals that are spread in powdered form on terrestrial surfaces like agricultural soils. In principle, a variety of natural or artificial sources of alkalinity can be used, but the focus here is on limestone or silicate rocks, like basalt. These rocks release positively charged magnesium or calcium ions when weathered. During this reaction, CO2 is converted into carbonate and bicarbonate ions by consuming protons (i.e., “acid”), allowing the soils to absorb atmospheric CO2 (Beerling et al., 2020). Basalt is mined mainly in the central part of Germany (BGR, 2017), with annual production rates per quarry of between 0.1 and 4.9 Mt rock with a maximum mining capacity of 32 Mt. There is substantial electric energy demand associated with this CDR option from extraction (18.8 MJ/t), crushing (5–10 MJ/t), and grinding (0.6–2 GJ/t) of silicate rocks. Taking one of the larger quarries as a reference, 4 Mt of basalt spread over cropland in Germany would allow for 0.727 Mt CO2 to be removed from the atmosphere, at a cost of about 120–140 million $US and an energy demand of 2.492–8.112*106 GJ (calculations based on Moosdorf et al., 2014 and Beerling et al., 2020). If scaled up to maximum mining capacity (i.e., 32 Mt silicate rocks), approximately 5.82 Mt CO2/year could be removed by enhanced rock weathering applied on 0.6 Mha of cropland by 2050.
NSE–Blue carbon: Seagrass meadows and salt marshes (concept 13)
In addition to increasing associated biodiversity, seagrass meadows and salt marshes are already making an important contribution to mitigating man-made climate change. In Germany, these ecosystems absorb an estimated amount of around 132 kt of CO2 annually. As natural CO2 sinks, the existing ecosystems are valuable resources for Germany.
Salt marshes are among the most efficient carbon sequestering ecosystems in the world and occur naturally along the German Wadden Sea coast on an area of about 23,250 ha. Salt marsh sediments provide an environment that allows long-term storage of organic carbon derived either from autochthonous primary production in the salt marsh or from imported allochthonous organic material from adjacent ecosystems (Müller et al., 2019).
Similarly, seagrass can sequester significant amounts of carbon. Seagrasses are found in the southeastern North Sea (area about 18,000 ha), where they grow on tidal flats, and on the German Baltic coast, where sublittoral seagrasses occur (area about 28,500 ha). A large part of the CO2 is absorbed by the seagrass during photosynthesis. Another part comes from organisms that live in these systems and absorb CO2 via respiration. When seagrasses die, they sink to the sea floor where the CO2 is stored as carbon. Still another portion comes from particles such as plankton that the dense seagrass canopy trap.
The expansion of natural CO2 sinks is considered a carbon removal measure and can make an additional contribution to achieving climate goals. However, almost the entire Wadden Sea is under nature protection, and thus active measures aiming at the reestablishment or new establishment of seagrass beds or salt marshes are not allowed. By improving the general conditions, e.g., water quality through sewage treatment plants or adjustments to regulations of fertilizer use on agricultural land, the seagrass bed area can increase and the natural CO2 uptake by seagrass could amount to a total 19.1 kt in the North Sea each year.
In the Baltic Sea, natural seagrass meadow expansion of up to 5,672 ha is also possible through consistent implementation of EU-wide nutrient reduction targets (defined in the Baltic Sea Action Plan, BSAP) (Bobsien et al., 2021). In order to accelerate the slow recolonization, it is also possible to actively renaturalize such habitats in the Baltic Sea. The goal of these measures is also to increase the size of the existing seagrass meadows nearby, and essentially restore an area that once had seagrass. Already today one can implement such activities to increase the natural CO2 sink. Assuming an additional 42,750 ha would be colonized with seagrass by 2050, doubling the existing area, about 103 kt of CO2 could be sequestered from the atmosphere annually. However, these values are still subject to great uncertainty because there is little data on the rate of carbon sequestration, in stark contrast to information available on carbon pools. It is important to understand that seagrass beds reach their full potential after ~18 years (Marbà et al., 2015; Eriander et al., 2016; Infantes et al., 2016; Infantes and Moksnes, 2018; Moksnes et al., 2018). This means that the sooner we implement this measure, the sooner we can realize the full potential of seagrasses.
Seagrass meadows and salt marshes in the North Sea and Baltic Sea hold the seafloor together because their complex roots and root stems prevent water movement from stirring it up. This creates fine-grained sediments that are virtually devoid of oxygen. These inhibit microorganisms from breaking down the carbon stored in the soil into its component parts, which would produce CO2 that would be released into the ocean.
As a result, the carbon in the sediment and biomass is basically stored for several centuries to millennia. However, the actual storage capacity of seagrass beds depends on multiple factors. Temperature and depth are generally the most important limiting factors for seagrass beds. Thus, warming waters and sea level rise may affect their distribution and reduce their range. In addition, increased wave action and storm surges due to extreme weather events associated with climate change are detrimental to seagrass habitats. Strong movements due to storms also stir up the soil and expose buried carbon fixed in the sediment.
Assuming that by 2050 the entire area that can potentially sustain seagrass beds is vegetated (97,750 ha) and healthy seagrass beds absorb CO2 according to their maximum, mature sequestration potential, ~122 kt of CO2 could be removed from the atmosphere annually. As the development of salt marshes and their CO2 uptake potential depend on many factors and as it is unknown whether erosion predominates or they increase in area, we assume that salt marshes absorb by 2050 as much CO2 as they do today. Altogether, a total of 200 kt CO2 could be removed from the atmosphere at the coasts of Germany every year.
Geological carbon dioxide storage solutions (concept 14)
Geological storage at an industrial scale (i.e., captured CO2 >400,000 tons/year) offers the capacity to lock CO2 outside of the atmosphere within the vast porous space of the Earth's underground. Technological CDR solutions, such as BECCS and DACCS, are associated with this CO2 storage option and enable large-scale removal of CO2 from the atmosphere as a significant negative emission contribution to climate mitigation efforts. In this context, the chapter only deals with geological CO2 storage.
Captured CO2 can be permanently stored into a deep underground geological reservoir of porous rock (e.g., sandstone) overlaid by an impermeable layer of cap rocks, sealing the reservoir and preventing the CO2 from upward migration out of the storage formation. Worldwide, there are several types of suitable CO2 storage reservoirs available, e.g., deep saline aquifer formations, depleted oil and gas reservoirs, unmineable coal beds and basalt rock formations (IPCC, 2005). Deep saline aquifer formations, offering the largest storage capacity, are layers of porous and permeable rocks saturated with saline formation water (brine). They are widely distributed across the globe in onshore and offshore sedimentary basins, and in Germany, the North East German Basin provides the major part of the country's saline aquifer assets. When CO2 is injected into a reservoir, it flows through the rock pores, filling the pore space and displaces the saline formation water. Usually, the captured CO2 is compressed into a liquid of increased density for efficient transport, ease of injection and reduced storage space requirement. The reservoir must be located at depths >800 meters to retain the CO2 in a dense liquid (supercritical) state (IPCC, 2005). The injected CO2 is permanently trapped in the reservoir through several mechanisms: (i) structural trapping by the geological formation and its seal, (ii) solubility trapping in the formation water, (iii) residual trapping as gas in the rock pores, and (iv) mineral trapping by reacting with the reservoir rocks to form carbonate minerals. The shares of trapping mechanisms and their dominance depend on the individual storage site's characteristics and contribute to a safe, reliable and effective CO2 storage over the years (Kempka et al., 2014). Environmental parameters are required to be controlled by a long-term monitoring program along the whole storage life-cycle, and energy and economic parameters depend on the location, size and site specific characterization of the storage project. Depending on the regional conditions and the technical parameters of the project, the resulting additional expenses for CO2 conditioning and storage are indicated in the literature within cost bandwidths (Chen and Morosuk, 2021; Smith et al., 2021).
Until the end of 2019, a number of 19 large-scale CCS facilities with permanent CO2 storage were in operation worldwide (GCCSI Report, 2019). The most prominent and longest running project is the Sleipner CCS facility with off-shore storage in the North Sea since 1996 and with about 20 Mt of CO2 stored so far, offering a technology readiness level of TRL 9 (Kearns et al., 2021). A comparable on-shore CO2 storage in a saline aquifer formation is presented by the Quest project, with a storage of about 4.8 Mt CO2 since 2015 (Shell Canada Ltd. Report, 2019).
In Kearns et al. (2017), a total global storage capacity has been estimated at between 8,000 Gt and 55,000 Gt, based on a methodology of estimated average CO2 storage capacity per cubic kilometer of sedimentary rock. For Germany, Knopf and May (2017) used an updated volumetric CO2 storage capacity approach which provided an estimate of about 40 Gt of regional aquifer based capacity assessment.
Due to the given constraints of the national act of carbon dioxide storage (KSpG, 2012) in Germany, the only currently available option to permanently store larger amounts of CO2 is seen in transportation to the North Sea fields, which is offered by the industrial-scale offshore projects, e.g., Northern Lights (Norway), Porthos (The Netherlands), and Net-Zero Teesside (UK) - also known as projects of common interest (PCI).
Another option would be an intermediate storage of CO2 together with its subsequent usage as part of a decarburization cycle. For this application, the already injected CO2 is back-produced on demand from the storage reservoir and returned into a chemical cycle as raw material for further chemical reactions, e.g., together with hydrogen for a methanation process toward synthetic fuels (Graves et al., 2011). In Germany the demonstration of efficient CO2 back-production has been successfully conducted at the Ketzin CO2 pilot storage site (Martens et al., 2015).
Data collection
The collected data for the 13 CDR and storage concepts is presented in tabular fact sheets included in Supplementary materials.
Technical potential of options deployment
Options readiness for deployment
CDR options, whether technology- or ecosystem-based, come at different stages of development–some have been demonstrated as viable and are already widely deployed (e.g., afforestation), some may need more research and/or investment to advance and be put on the market (e.g., decentralized DACC).
In our portfolio, we present options which have been largely successfully demonstrated at different scales in deployment environments - e.g., from small projects to commercial-scale projects (Table 2). All selected ecosystem-based options are proven practices which could be implemented and/or expanded in Germany immediately. On the other hand, most technology-based options would require a few years to be successfully deployed (Table 2). This is related to the fact that some of their elements, even though they are mature, still need to be integrated (e.g., retrofitting bioenergy plants with CCS). Only DACC and ERW would require more time for implementation, due to a need for their further development. In case of DACC, a pilot application in real ventilation systems would be necessary, and ERW would need to be further tested to deliver conclusive results.
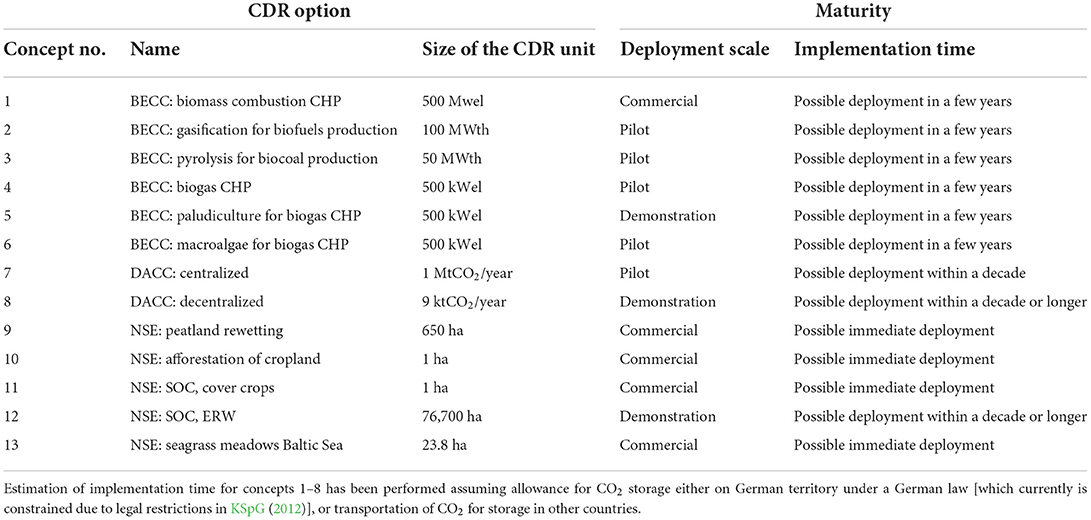
Table 2. Overview of the CDR concepts considered in the study with current scale and estimated time of deployment of CDR options.
However, an option's implementation time is not only a function of their maturity, but is also influenced by societal factors such as public acceptance and/or political support. Those factors may either speed up or slow down their deployment (see Section Discussion).
Carbon dioxide removal potential of options for Germany
The amount of CDR that will be deployed in the next 30 years is highly uncertain. Estimates as to what would be the appropriate amount of CDR deployment in Germany range from 3 to 18 Gt CO2 between today and 2100, depending on the historical responsibility, capability and global equity (Pozo et al., 2020). In addition, annual CO2 removal demand to reach net-zero CO2 emissions strongly depends on the actions to reduce and avoid emission in the coming years. In the following paragraph we will accordingly discuss the CDR options through the perspective of their respective system utility, i.e., how much CO2 can they remove to achieve national net-zero targets.
With regards to annual CO2 removal potential, our concepts differ widely, ranging from 34 tons to 2.9 million tons per year per concept (Figure 2). The by far highest specific removal potential of a single CDR concept can be realized with BECC, specifically with biomass combustion for CHP in former coal-fired power plants, followed by centralized DACC (1 Mt CO2/year) assuming that effective CO2 storage can be implemented. Other BECC and DACC options present smaller CO2 capture potential which ranges between 3,150 and 100,000 tons CO2 per unit. For NSE, the potential is expressed in the amount of CO2 removed per hectare per year, and varies from 1.5 to 9.5 tCO2/ha/year, with enhanced rock weathering having the highest removal potential per area.
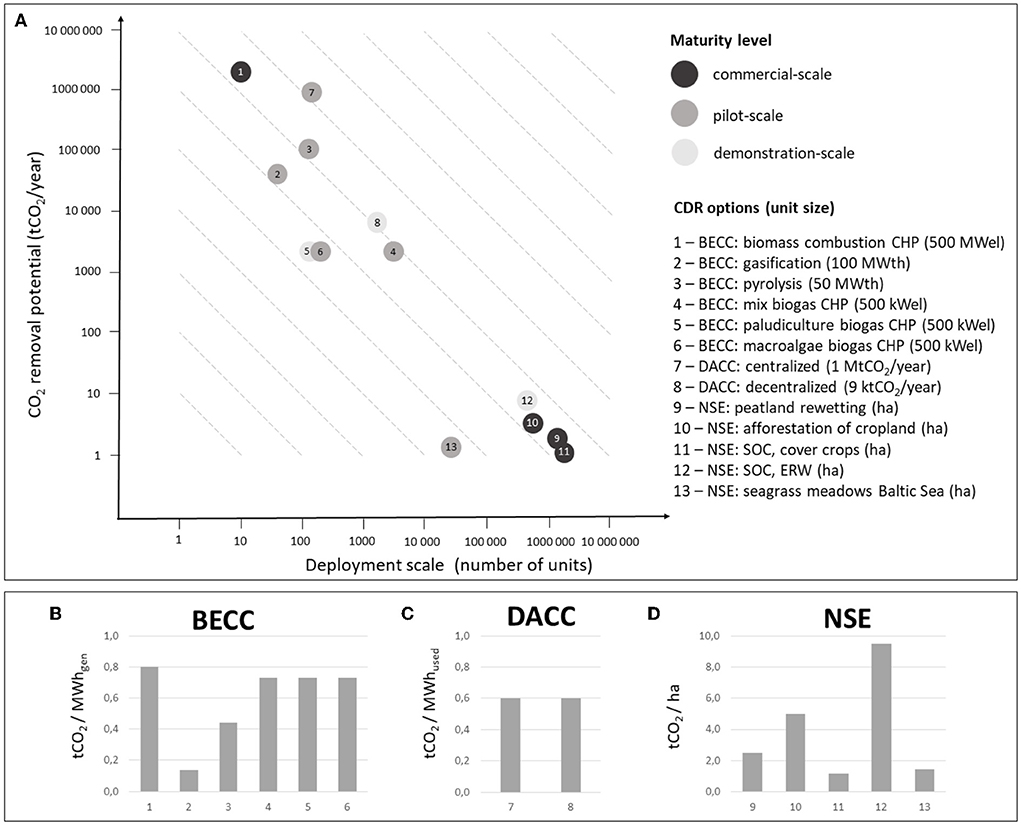
Figure 2. Overview of CO2 removal potential of selected options. (A) Scalability of options expressed as a number of units available for deployment by 2050 and CO2 removal potential. (B) Amount of CO2 removed per unit of energy generated by BECC technologies. (C) Amount of CO2 removed per unit of energy used by DACC technologies. (D) Amount of CO2 removed per hectare by NSE options.
It is also important to consider the near-term deployment potentials of the different options. While NSE are partly already deployed today, and could potentially be expanded, most of the high-tech options would need 5–10 years to reach market readiness (see Section Options readiness for deployment). This lower maturity level is an important characteristic considering the cumulative CDR potential between 2020 and 2050, as delays in the deployment of CDR options cause reduced cumulative CDR potential.
Considering scalability of the CDR options, a more comprehensive picture of CDR potential can be drawn. Large-scale BECC deployment is limited by the amount of available biomass and number of bioenergy plants that would be available for retrofitting. DACC is mostly limited by its cost and access to renewable energy supply. Most of the NSE are limited by the available area. Taken each of these limiting factors into account, the BECC potentials range from 0.5, 0.8, 3.72, 12.6, 14, 29.9 Mt CO2/year for paludi- and macroalgae-fed biogas CHP, gasification, mixed feedstock biogas CHP, pyrolysis and biomass combustion CHP, respectively. For the DACC options the potential ranges between 15 and 150 Mt CO2/year, for the decentralized and centralized approach, respectively. The potential is based on the assumption that 15% of the largest buildings in Germany can be equipped with DACC units and an area one hundredth as big as the agricultural area (115,100 ha) can be utilized to install DACC farms. And finally, the projected CDR potentials for the NSE measures are found to be between 0.06, 2.3, 3.3, 3.5 and 5.8 Mt CO2/year for seagrass restoration, cover crops, peatland rewetting, afforestation and enhanced rock weathering, respectively. In Figure 2 we present the efficiency of the different CDR concepts to remove CO2 with regard to their potential to scale up, energy provision and demand (for the hybrid and technical solutions) and land/water area (for NSE).
Discussion
Bringing CDR options closer to deployment-bottlenecks and open questions
There are many different options for CDR available today – from technological to hybrid and natural solutions. The principles of many options are already well-researched. However, their real-life implementation is a process which cannot be based only on general assumptions. Our CDR concepts combine German framework conditions with their respective performance parameters to help bring CDR from the general conceptual level to the ground. They show not only the possible methods of CDR in Germany but also how they could look like in reality, in a more manageable, one-unit scale (i.e., easier to locate, run necessary calculations, evaluations and scaling).
In principle, German natural conditions and technology availability enable the implementation of diverse CDR options. While we are aware of the fact that some, if not all, of the CDR options discussed here will cause various side-effects, e.g., GHG generation in peatlands and short-lived halocarbons from macroalgae farming (Leedham et al., 2013; Ziska et al., 2013; Tanneberger et al., 2021), we wanted to concentrate on the technological readiness and potentials of these options within the German context. More comprehensive assessments will need to follow (see Thoni et al., 2020). Using our CDR concepts in combination with the analysis of German geophysical conditions, we determined an approximate area on which a given option could be applied and how much CO2 per hectare it could remove. Such information represents a crucial first step to understanding the feasibility of CDR deployment and can be understood as a measurement for maximum potential. To better understand the actual removal potential, social, political, and legal parameters need to be considered as well, as these may represent hurdles to deployment and thus reduce the actual removal potential (cf. Boysen et al., 2017; Geden et al., 2018; Wieding et al., 2020).
Economic viability of CDR options is also highly variable and dependent on the technologies and their characteristics. Regarding renewable energy-based options, the Budget Scenario by Simon et al. (2022), which aims at implementing a 100% renewable energy system by 2050 for Germany, requires considerable investment in technologies and infrastructure. In the short term, fuel costs determine overall costs to two thirds, whereas their share decreases from 2030, while power exchange costs increase. This is due to CO2 emission costs being eliminated while phasing out fossil fuels. Considering energy security, increasing costs for energy imports also highlight that different energy systems are subject to dynamic changes of the economic circumstances. Future viability and reliability of CDR options are therefore sensitive and must be considered in a holistic approach, which, as a downside, increases the number of uncertainty parameters in modeling and for interpretation of feasible outcomes.
In this section, we highlight some of the most important bottlenecks and open questions that need further consideration, as highlighted in previous literature, focusing on the German context (Hahn et al., 2020). In other words, we see that the CDR potential of a single approach is limited under incremental implementation of the CDR concepts within an existing landscape and infrastructure. Hence, in addition to technological feasibility, a portfolio of concepts needs to be demonstrated and tested with regard to market implementation as well as socio-political acceptance. For example, the most mature option with an additionally high CDR potential is biomass combustion with CHP – an option which assumes retrofitting a coal-fired plant to burn biomass, which for certain industry actors can be attractive, especially in the light of the upcoming coal-phase out (KVBG, 2020). Such concepts can thus build on pre-existing infrastructure, as well as already tested technology, e.g., in the UK (Drax Group, 2018) or Denmark (Orsted Media Relations, 2016). However, if deployed at scale, their demand for resources (e.g., wooden pellets) may be a limiting factor, if no imports are taken into account (Thrän and Schindler, 2021). Moreover, previous research indicates that model assumptions for future biomass production for BECCS rely on optimistic views on increased agricultural efficiency, as well as land-use changes that may interfere with other societal goals such as food security, biodiversity, and cultural services, and could cause, inter alia, loss of ecosystems, replacement of natural ecosystems for monocultures, and pollution (Dooley and Kartha, 2018; Daggash et al., 2019; Dooley et al., 2020). These are all important factors that would need to be critically reviewed if BECCS options were to be moved closer to deployment in Germany. If such risks are deemed insurmountable and the impacts socially and ecologically unacceptable, they will, consequently, reduce the potential for BECCS. However, in comparison with the other CDR options, the integrated energy provision of BECCS is a significant advantage, especially in the light of ongoing energy transition and related strong need for renewables.
Scaling is not only an issue in sustainable biomass sourcing for large scale BECCS application. NSE are typically implemented in smaller units and therefore need different support and permission schemes than technical facilities. Some practices are already in use or tested in Germany, especially in urban areas (e.g., Kabisch et al., 2016) but the scale on which they could be implemented to maximize CO2 capture has not been evaluated yet.
The CDR concept approach clarifies the options for CO2 removal for Germany with regard to BECC, DACC and NSE under the consideration of technical, infrastructural and biophysical conditions. However, the relevant bottleneck for long term storage, which enables BECC to BECCS and DACC to DACCS is carbon storage, which is still not fully addressed in Germany. Theoretically, Germany has large potential for permanent CO2 storage as well as multiple storage scenarios–local storage, offshore storage or a combination of these may all be envisaged. The choice of storage options is in turn closely tied to the organization of CO2 emitters and CO2 transportation networks around the storage sites. Indeed, the establishment of a CO2 transportation network and CO2 source clustering could represent a strategic opportunity for economic development. For instance, proximity to a storage site or even a CO2 transportation network may offer an easy path to carbon neutrality for hard-to-abate industries such as cement or lime (Hills et al., 2017). Emitters may be clustered regionally, as spatial clusters in view of a local onshore storage site, or instead form a unique national network with a large unique storage location (or neighboring storage locations). While both scenarios are technically feasible, varying costs, political will and social acceptance will determine the chosen implementation. CO2 storage is currently restricted to pilot-scale research endeavors and no new storage sites can currently be proposed (KSpG, 2012), although some emerging signs seem to indicate that politicians are reconsidering German CO2 subsurface storage (Drucksache 19/25295, 2020; Drucksache 19/30724, 2021). Offshore storage in a select few, large North Sea storage sites may reduce geological prospection and storage costs but a significant CO2 transport network will be required. On the other hand, onshore regional storage may require less infrastructure investment but storage availability is not uniform among German federal states and there is no legal support for CO2 storage in Germany (KSpG, 2012). Moreover, public acceptance of CO2 storage in Germany is currently low (Tcvetkov et al., 2019) while acceptance for CCU is higher (Linzenich et al., 2019). In addition to low public support for CCS, the public's knowledge about CCS is also low (Merk et al., 2019). Hence, all options which rely on CCS may face a delay of unknown duration or insurmountable hurdles if acceptance is never generated. Early CCS projects generally faced limited public acceptance or outright public opposition for underground storage and transportation of CO2 in pipelines, although research indicates that public acceptance is higher for Bio-CCS than CCS of fossil fuel emissions (Dütschke, 2011; Wallquist et al., 2012; Otto and Gross, 2021). Previous research suggests that reintroducing CO2 storage firmly within the public debate may require a fine balancing of communication and lobbying to inform of the low risks and numerous opportunities associated with CO2 storage, while avoiding association with the failed CCS impetus in Germany of the late 2000's (Linzenich et al., 2019).
When it comes to public acceptance of CDR in general, interfering with nature is often seen as problematic, and thus less technology heavy options could be more acceptable (Wallquist et al., 2012; Wolske et al., 2019, e.g., afforestation preferred over BECCS and DACCS). For instance, in addition to perceived risks of storage especially related to leakage, data from Germany shows that BECCS-options are also seen as problematic by the public because of concerns with regards to biodiversity impacts4. Similarly, data from the US and UK shows that for enhanced weathering people are generally concerned about downstream effects on oceans (Spence et al., 2021). That being said, also NSE like rewetting peatlands can be perceived as interfering with nature, if areas have been drained for a long time and thus perceived as naturally dry (Ziegler et al., 2021). In general, many CDR options could potentially cause trade-offs to other societal goals, such as energy- and food security, or cause health implications, or land-use conflicts (Honegger et al., 2020). This includes NSE like afforestation, which can have negative social and ecological impacts, such as loss of biodiversity, land-use conflicts, and high resource use, in particular, if forest plantations are established in previously non-forested areas, replace natural ecosystems and are established without the consent of local communities (e.g., Smith and Torn, 2013; Honegger et al., 2020). Societal impacts are, however, highly dependent on scale of implementation and need to be understood within their specific local context (Honegger et al., 2020). Acceptance is often associated with trust and seen as something that is built over time (e.g., Waller et al., 2020). Public participation in the decision making process has been highlighted as important for building trust and acceptance (e.g., Dütschke, 2011; Merk et al., 2019; Cox et al., 2021). According to Honegger et al. (2021), while Germany has well developed participatory governance processes in general, not enough has been done for public engagement around CDR, which in turn makes it more challenging to build social acceptance around these measures.
Distribution patterns
The location of different CDR options depends to a large extent on biophysical conditions occurring in the country of deployment. This parameter is inextricably linked to NSE, as these options are based directly on the occurrence of certain natural conditions and ecosystems. However, hybrid options like BECC and purely technology-driven options like DACC also rely to a different degree on natural conditions (e.g., proper conditions to grow different types of biomass in case of BECC, and intensity of insolation and wind for production of electricity for DACC).
Apart from biophysical conditions, some CDR options are accompanied by additional energy demand (especially DACC), and some generate energy (like BECC). Therefore, the energy supply also plays an important role. The required transformation of the energy system on the path to achieve net zero CO2 emissions by 2050 will further accelerate extensive societal and economic changes. The extension of the electrical grid, pipeline siting, various storage potential to be exploited and resistance against the phase out of coal-fired power plants affect different regions of Germany to varying degrees and scales. This, together with diverse levels of acceptance for decarbonization measures across Germany, could lead to strong regional differences in application potentials of technologies, which in turn could restrict a speedy transition. For instance, divergences can result from different resource allocation, which is especially important considering renewable energy. German photovoltaic (PV) power potential is allocated mostly in the South (The World Bank, 2019), whereas the northern regions can provide less of this resource. Contrastingly, the mean wind power density is located mostly in the North-West coastal regions and in few mountainous areas (except the South of Germany) (DTU Wind Energy, 2021). These natural resource occurrences are determining CDR options availability as well as future changes in energy supply by illustrating requirements for expansion in installations and infrastructure (Simon et al., 2022). Corresponding to PV potential, storage options needed for CO2 are mostly allocated in the northern half of the country, with some locations also in the center and South of Germany (Müller and Reinhold, 2011).
The energy intensive industries are located mostly in the West and South of Germany and in several highly agglomerated regions, which coincide with the density of population (GTAI, 2020; BBSR, 2021). These agglomerations also correspond to the highest emissions, which is where CO2 storage should be located to minimize transport. However, capacities for that are not matching the geographical location of emitters (Emissionshandelsstelle, 2018).
For the CDR concepts presented in the preceding sections, a regional allocation scheme that illustrates explicitly the implementation potential in Germany can be developed (Table 3). This scheme is based on the current status of biomass and renewable energy availability, type of area (terrestrial or marine) and degree of urbanization. In this table, concerning the geographic distinction, the country has been split into five main areas: the north, east, south, west, and center. Generally, the cardinal direction provided by the regional classification and the distinction according to federal states should be understood only as a fluid concept and not as a fixed distinction, since resources and potentials cannot be measured by administrative boundaries. Assigning the CDR options to the mentioned regions further depends on technological flexibility, scale of the options deployment, degree of decentralization, and the nature of the concept itself (e.g., marine options are not implementable on land). The latter also informs the additional distinction of the area type in Table 3, as urban and rural areas are characterized by different implementation abilities (e.g., concepts with need for agricultural products are relatively infeasible to implement in highly urbanized regions). Additionally, marine areas are introduced to capture the needs of concepts which use offshore-based technologies or marine CDR processes.
The applicability of the regional disaggregation is marked by either a ‘+', which stands for moderate implementation in the specific area that may be limited on certain conditions, ‘++' stands for high implementation potential.
For BECCS, different options depend strongly on suitable biomass availability. As agricultural and forestry residues and waste are widely occurring feedstocks, it is difficult to set clear regional restrictions for implementation of technologies based on these resources. Among BECC concepts, an exception can be macroalgae- and paludiculture-based concepts, which have stronger limitations as they can only work in marine or rural areas with specific biophysical conditions (e.g., suitability for restoring peatland). DACC implementation is connected to the specific renewable energy potential and thus follows along the power potentials of either wind (relatively higher potential in the north) or PV (relatively higher potential in the south). For larger, centralized DACC-facilities one of the most important location factors is the availability of renewable energy in sufficient quantities. Therefore, we assume these plants will follow the potential of renewable energy sources, with a secondary preference for less expensive construction land in rural areas (Heß et al., 2020). On the other hand, decentralized HVAC-integrated DACC-systems primarily require suitable buildings in which they can be implemented. The selection of renewable energy supply must be decided on a more local, case-by-case basis. On-site generation of renewables has to be complemented with other energy sources, such as industrial waste heat, which is more readily available near industrial and urban centers (Dittmeyer et al., 2019).
If there are further specifications of the type of region where a concept can be implemented, it is noted in brackets in Table 3. For instance, with biogas for CHP, regions of deployment in Germany are generally not restricted to either north, south, etc., but in urban areas the option depends on bio-waste, and in rural regions, agriculture is a prerequisite for implementation.
Overall, it is important to account for the different perception of concepts that are implementable and spatially diffused (Rhoden et al., 2021). Especially considering the expansion of renewable energy, and depending on the context of a concept, the regional effect on the landscape, and thus on the need of environmental conditions, can differ strongly.
Setting the CO2 removal potential in context
Beyond the different bottlenecks and hurdles for implementation, in order to understand the potential contribution of CDR options to reaching net-zero CO2 emissions, the estimated CO2 removal potentials should be compared with the actual CO2 emissions in Germany. In 2020, Germany emitted 644 Mt CO2, mostly coming from the energy sector (211 Mt CO2), industry sector (163 Mt CO2), and transport sector (144 Mt CO2) (UBA, 2021). Noteworthy in this context is that energy production by fossil fuel combustion across sectors amounts to over 90% of current CO2 emissions in Germany.
If Germany would achieve its 88% reduction in emissions relative to 1990 by 2040, this amounts to annual emission of 130 Mt CO2, which would have to be entirely eliminated or compensated for within 5 years (KSG, 2021). While central DACC units could theoretically provide 150 Mt CO2 capture per year if limited by area demand alone, constraining DACC by energy provision limits its potential to about 16 Mt CO2 (Ariadne Report, 2021). No other CDR option alone can provide the necessary order of magnitude of carbon removal to compensate for 130 Mt CO2/year. In total, BECC options could provide about 62 MtCO2, half of which would be provided by biomass CHP. This option however, would need large amounts of woody biomass as feedstock. As for the enhancement of natural carbon sinks, we find that enhanced rock weathering, afforestation and peatland rewetting are the options which show the highest CDR potentials (5.8, 3.5 and 3.4 Mt CO2/year, respectively). In total, we assess the CDR potential of the five selected NSE options in Germany to be in the order of magnitude of 15 Mt CO2/year. Our overall estimated CDR potential in the year 2050 amounts to about 240 Mt CO2/year, which is reduced to 91 Mt CO2/year if the central DACC option were constrained by energy supply. While single CDR options are constrained differently and therefore single potentials vary, our estimate of the overall CDR potential agrees well with the order of magnitude of 103–116 Mt CO2/year identified in the Ariadne Report (2021).
This conclusion clearly illustrates that CO2 removal can only compensate for a very small share of today's emissions in Germany. Moreover, the maturity of the options illustrates that most of the CDR options might only be deployed in 5–10 years' time. In other words, in the coming decade, the focus should be laid on mitigating emissions in the first place rather than relying on negative emission technologies for removing emissions after they have been emitted. In Figure 3, we present the potentials of analyzed CDR concepts, along with their relation to current German CO2 emissions and projected residual emissions in 2050.
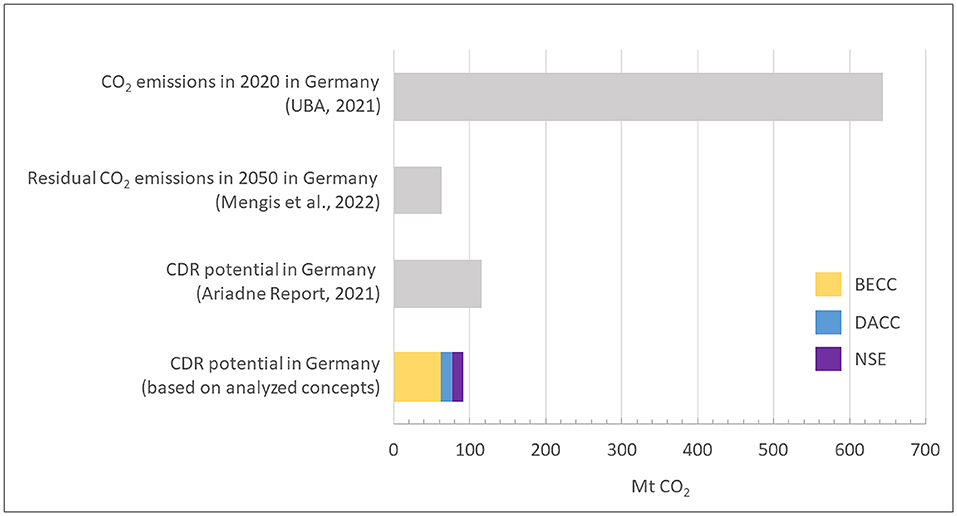
Figure 3. CDR potential of analyzed concepts in comparison with estimates of current CO2 emissions in Germany (UBA 2021), projected residual emissions for 2050 (Mengis et al., 2022) and CDR potential in Germany (Ariadne Report, 2021). BECC: based on all analyzed BECC concepts, likely requiring imports of woody biomass; DACC: based on decentralized DACC concept, excluding centralized DACC due to energy constraints; NSE: based on all analyzed NSE concepts. The indicated potentials for BECC and DACC do not include possible constraints due to legal restrictions for geological CO2 storage in Germany.
Limitations and further research demand
The definition and description of exemplary CDR concepts was performed with experts in the different fields, using the–to our knowledge–best available information. However, we know that also within the expert fields there are uncertainties in the optimized design and performance of the concepts, which is also affecting the data quality of our fact sheets. Larger data collections can help to reduce these uncertainties in the future, and we propose to use this study as a starting point to broaden the database of CDR concepts for CO2 removal in Germany.
Additionally, in our assessment we focused mainly on technical and CO2 removal related criteria. For implementation of CDR concepts, a broader view on societal and political aspects is necessary. With regard to economic, environmental or social aspects, the fact sheets will need more harmonization, e.g., by commonly agreed indicators (see Fridahl et al., 2020; Förster et al., 2022).
We also did not consider interactions between the CDR concepts, especially when it comes to their upscaling. In general, for each concept, competition by increased market potential is facilitating market penetration and ensures cost-efficiency. In technologies, further innovation support enables efficiency gains and cost reductions (Hepburn et al., 2019). Among that, concepts can compete for land and resources, and the deployment of one option may reduce the potential of implementation of another concept. This makes it essential that a regionally specific CDR portfolios are developed in order to avoid possibly conflicting solutions.
Conclusion
CDR is discussed as a relevant measure to reach the temperature goals of the Paris Agreement not only globally but also in national contexts. Different CDR measures are available and their implementation depends on site specific conditions, such as biophysical conditions, existing infrastructure and regulations. In our study, we investigated near-to-market-options for CDR in Germany, which we depicted in 13 concepts and systematically described in fact sheets (see Supplementary materials). These concepts are based on the current situation and allow incremental implementation of CDR options during the next decade. The CDR concepts cover technical, hybrid, and natural sink enhancement options with CO2 removal in a range from 34 tons (Baltic Sea seagrass meadows) to 3 megatonnes CO2 (biomass combustion CHP) per concept, and with costs ranging from −45 (minimal estimate for cover crops; Fuss et al., 2018) to 800 € (maximal estimate for DACC; Heß et al., 2020) per ton of CO2 removed (see Supplementary materials for details). With their elaboration in fact sheets, a substantial database has been generated which can be further used in climate research and policy actions, such as research and demonstration of CDR options, as well as climate and energy scenario development.
With regard to the overall CO2 removal potential, 10 of the 13 CDR concepts provide technical removal potential of at least 1 million tons CO2 per year, so a combination of concepts is necessary to achieve the necessary impact for the climate targets. Options with the highest CO2 removal potentials are: DACC farms (150 Mt CO2/year), BECC: biomass combustion with CHP (29.9 Mt CO2/year), DACC combined with HVAC systems (15 Mt CO2/year), BECC: pyrolysis (14 Mt CO2/year), BECC: biogas CHP (12.6 Mt CO2/year), NSE: enhanced rock weathering (5.82 Mt CO2/year), NSE: afforestation of cropland (3.49 Mt CO2/year), NSE: peatland rewetting (3.35 Mt CO2/year), and NSE: cover crops (2.34 Mt CO2/year). Our overall, maximized estimation of CDR potential of analyzed options in 2050 varies from 91 to 240 Mt CO2/year, mostly depending on the scale of deployment of DACC farms. However, it should be noted that this potential can further decline, e.g., if the future availability of biomass for BECC application decreases, there are further constraints in renewable energy supply for DACC or limitations in CO2 storage. Compared to Germany's total actual CO2 emissions of 644 Mt CO2 (data from 2020; UBA, 2021) and the estimated hard-to-abate emissions of 36–63 Mt CO2 in 2050 (Ariadne Report, 2021; Mengis et al., 2022), it seems that CDR could theoretically provide sufficient potential to counterbalance the residual emissions. However, it should not be considered as a primary mitigation measure, but as a complementary option following emission reductions and avoidance.
Taking biophysical conditions and infrastructure into account, northern Germany seems preferable for many concepts. Necessary next steps needed to enable their successful implementation and include consideration of economic aspects, societal perception and political frameworks (Cherry et al., 2018; Nemet et al., 2018). Therefore, in general, a comprehensive framework like the SDGs can foster political action to deployment and give guidance to a German approach to bring technology concepts to the market (see also Honegger et al., 2020). Participatory processes and a focus on good governance can relieve some of the barriers to implementation (Honegger et al., 2018). They need to be applied highly region-specific as the federal structure of the political background and the geographical feasibility potentials of technologies (centralized or decentralized) differ strongly and require careful place-based consideration. Specifically for the hybrid and technical carbon dioxide removal options (BECCS and DACCS), implementation strongly depends on the availability of carbon storage options, which are currently constrained by laws in Germany and can only be unlocked by changing the current regulations on carbon storage.
Data availability statement
The original contributions presented in the study are included in the article/Supplementary material, further inquiries can be directed to the corresponding author.
Author contributions
DT, MB, and NM conceived the idea of the paper, which was then drafted and further developed by MB and DT, with contributions from all co-authors. MB, DT, and NM designed the fact sheets. DT and MB designed Figure 1 and provided input to BECC–combustion of woody biomass for heat and power co-generation (concept 1) and BECC–biogas production for co-generation of heat and power (concept 4). MB designed all tables and Figure 2. MB and JF designed Figure 3. ND and YC contributed to sections BECC–gasification of woody biomass for biofuels production (concept 2) and BECC–pyrolysis of woody biomass for biocoal production (concept 3). JF contributed to section BECC–paludiculture-sourced biomass for biogas production for co-generation of heat and power (concept 5). JW and NM contributed to section BECC–macroalgae farming for biogas production for co-generation of heat and power (concept 6) and associated content about utilization of macroalgae. DH and RD contributed to section DACC–centralized and decentralized options (concepts 7 and 8). AK, ZL, and TS contributed to section NSE–peatland rewetting (concept 9). CD and MH provided the information connected to sections NSE–afforestation of cropland (concept 10), NSE–soil organic carbon sequestration–cover crops (concept 11), and NSE–terrestrial enhanced weathering (concept 12), with contributions to the latter by NM. TD, K-KJ, AS, and TR provided information for section NSE–blue carbon: seagrass meadows and salt marshes (concept 13). CS-H and CY provided information on CO2 storage and contributed to sections BECC–pyrolysis of woody biomass for biocoal production (concept 3) and bringing CDR options closer to deployment–bottlenecks and open questions. TT contributed primarily to sections NSE–blue carbon: seagrass meadows and salt marshes (concept 13) and section discussion. IR provided insights on the economic scope of the concepts and contributed to section distribution patterns. NM contributed to section limitations and further research demand. All authors approved the submitted version.
Funding
The Helmholtz-Climate-Initiative (HI-CAM) was funded by the Helmholtz Associations Initiative and Networking Fund. The authors are responsible for the content of this publication.
Acknowledgments
We would like to thank Juliane El Zhobi and Swantje Preuschmann (Helmholtz-Zentrum Hereon) for a useful discussion on the status of natural sink enhancement options in Germany. We also would like to thank Alberto Bezama and Forrest Kluson (Helmholtz Center for Environmental Research) for proofreading of the manuscript.
Conflict of interest
Authors MB, DT, JF, TT, MH, and IR were employed by GmbH.
The remaining authors declare that the research was conducted in the absence of any commercial or financial relationships that could be construed as a potential conflict of interest.
Publisher's note
All claims expressed in this article are solely those of the authors and do not necessarily represent those of their affiliated organizations, or those of the publisher, the editors and the reviewers. Any product that may be evaluated in this article, or claim that may be made by its manufacturer, is not guaranteed or endorsed by the publisher.
Supplementary material
The Supplementary Material for this article can be found online at: https://www.frontiersin.org/articles/10.3389/fclim.2022.810343/full#supplementary-material
Footnotes
1. ^https://www.netto-null.org/index.php.en (accessed November 2, 2021).
2. ^https://ec.europa.eu/research/participants/data/ref/h2020/other/wp/2016_2017/annexes/h2020-wp1617-annex-g-trl_en.pdf (accessed on: November 3, 2021).
3. ^http://www.niedermoor-nutzen.de/ (accessed on: November 3, 2021).
4. ^https://www.spp-climate-engineering.de/index.php/buergerforum.html (accessed November 4, 2021).
References
Archer, D. (2005). Fate of fossil fuel CO2 in geologic time. J. Geophys. Res. Oceans 110, C9. doi: 10.1029/2004JC002625
Archer, D., and Brovkin, V. (2008). The millennial atmospheric lifetime of anthropogenic CO2. Clim. Change 90, 283–297. doi: 10.1007/s10584-008-9413-1
Ariadne Report. (2021). “Deutschland auf dem Weg zur Klimaneutralität 2045,” in Szenarien und Pfade im Modellvergleich, eds G. Luderer, C. Kost, and D. Sörgel (Potsdam: Kopernikus-Projekt Ariadne, Potsdam-Institut für Klimafolgenforschung). doi: 10.48485/pik.2021.006
Babin, A., Vaneeckhaute, C., and Iliuta, M. C. (2021). Potential and challenges of bioenergy with carbon capture and storage as a carbon-negative energy source: a review. Biomass Bioenergy 146, 105968. doi: 10.1016/j.biombioe.2021.105968
BBSR (2021). INKAR – Indikatoren und Karten zur Raum- und Stadtentwicklung. Bundesinstitut für Bau-, Stadt- und Raumforschung (BBSR), Bonn. Available online at: https://www.inkar.de/ (accessed November 2, 2021).
Beerling, D. J., Kantzas, E. P., Lomas, M. R., Wade, P., Eufrasio, R. M., Renforth, P., et al. (2020). Potential for large-scale CO2 removal via enhanced rock weathering with croplands. Nature 583, 242–248. doi: 10.1038/s41586-020-2448-9
Bellamy, R., and Osaka, S. (2020). Unnatural climate solutions? Nat. Clim. Chang. 10, 98–99. doi: 10.1038/s41558-019-0661-z
BGR. (2017). Karte der Bergbau- und Speicherbetriebe 1:2000000 (BergSP), Stand 31.12.2017, 48. Auflage, zusammengestellt vom Referat L 2.7 des Landesamt für Bergbau, Energie und Geologie Hannover, veröffentlicht von den Bergbehörden der Länder und der Bundesanstalt für Geowissenschaften und Rohstoffe.
Billig, E., Decker, M., Benzinger, W., Ketelsen, F., Pfeifer, P., Peters, R., et al. (2019). Non-fossil CO2 recycling - the technical potential for the present and future utilization for fuels in Germany. J. CO2 Util. 30, 130–141. doi: 10.1016/j.jcou.2019.01.012
BioKraftQu, G. (2006). Biofuels Quota Act. Gesetz zur Einführung einer Biokraftstoffquote durch Änderung des Bundes-Immissionsschutzgesetzes und zur Änderung energie- und stromsteuerrechtlicher Vorschriften (Biokraftstoffquotengesetz). BGBI I, 3180. Available online at: https://www.bgbl.de/xaver/bgbl/start.xav?start=//*%5B@attr_id=%27bgbl106s3180.pdf%27%5D#__bgbl__%2F%2F*%5B%40attr_id%3D%27bgbl106s3180.pdf%27%5D__1658772578431 (accessed July 28, 2022).
Birdsey, R., Duffy, P., Smyth, C., Kurz, W. A., Dugan, A. J., and Houghton, R. (2018). Climate, economic, and environmental impacts of producing wood for bioenergy. Environ. Res. Lett. 13, 050201. doi: 10.1088/1748-9326/aab9d5
BNetzA (2021). Kraftwerksliste Bundesnetzagentur, Stand 19.01.2021. Available online at: https://www.bundesnetzagentur.de/SharedDocs/Downloads/DE/Sachgebiete/Energie/Unternehmen_Institutionen/Versorgungssicherheit/Erzeugungskapazitaeten/Kraftwerksliste/Kraftwerksliste_2021_1.xlsx?__blob=publicationFile&v=4 (accessed November 2, 2021).
Bobsien, I. C., Hukriede, W., Schlamkow, C., Friedland, R., Dreier, N., Schubert, P. R., et al. (2021). Modeling eelgrass spatial response to nutrient abatement measures in a changing climate. Ambio 50, 400–412. doi: 10.1007/s13280-020-01364-2
Bonn, A., Allott, T., Evans, M., Joosten, H., and Stoneman, R. (2016). “Peatland restoration and ecosystem services: an introduction,” in Peatland Restoration and Ecosystem Services: Science, Policy and Practice, Ecological Reviews, eds A. Bonn, H. Joosten, M. Evans, R. Stoneman, and T. Allott, T (Cambridge: Cambridge University Press), 1–16.
Boysen, L. R., Lucht, W., Gerten, D., Heck, V., Lenton, T. M., and Schellnhuber, H. J. (2017). The limits to global-warming mitigation by terrestrial carbon removal. Earths Fut. 5, 463–474. doi: 10.1002/2016EF000469
Buck, B. H., and Buchholz, C. M. (2004). The offshore-ring: a new system design for the open ocean aquaculture of macroalgae. J. Appl. Phycol. 16, 355–368. doi: 10.1023/B:JAPH.0000047947.96231.ea
Buck, H. J. (2016). Rapid scale-up of negative emissions technologies: social barriers and social implications. Clim. Change 139, 155–167. doi: 10.1007/s10584-016-1770-6
Bui, M., Adjiman, C. S., Bardow, A., Anthony, E. J., Boston, A., Brown, S., et al. (2018). Carbon capture and storage (CCS): the way forward. Energy Environ. Sci. 11,1062–1176, doi: 10.1039/C7EE02342A
Chen, F., and Morosuk, T. (2021). Exergetic and economic evaluation of CO2 liquefaction processes. Energies 14, 7174. doi: 10.3390/en14217174
Cherry, C., Scott, K., Barrett, J., and Pidgeon, N. (2018). Public acceptance of resource-efficiency strategies to mitigate climate change. Nat. Clim. Change 8, 1007–1012. doi: 10.1038/s41558-018-0298-3
Chung, I. K., Oak, J. H., Lee, J. A., Shin, J. A., Kim, J. G., and Park, K. S. (2013). Installing kelp forests/seaweed beds for mitigation and adaptation against global warming: Korean project overview. ICES J. Mar. Sci. 70, 1038–1044. doi: 10.1093/icesjms/fss206
Cox, E., Pidgeon, N., and Spence, E. (2021). But they told us it was safe! carbon dioxide removal, fracking, and ripple effects in risk perceptions. Risk Anal. 42, 1472–1487. doi: 10.1111/risa.13717
Daggash, H. A., Fajardy, M., Heptonstall, P., Mac Dowell, N., and Gross, R. (2019). Bioenergy with Carbon Capture and Storage, and Direct Air Carbon Capture and Storage: Examining the Evidence on Deployment Potential and Costs in the UK. UKERC Technology and Policy Assessment, April 2019. Available online at: https://d2e1qxpsswcpgz.cloudfront.net/uploads/2020/05/UKERC-TPA-Negative-Emissions-V3-Final.pdf (accessed November 2, 2021).
DESTATIS (2018). Land- und Forstwirtschaft, Fischerei - Bodennutzung der Betriebe (Struktur der Bodennutzung), Fachserie 3 Reihe 2.1.2. Retrieved from Wiesbaden, Germany. Available online at: https://www.statistischebibliothek.de/mir/receive/DEHeft_mods_00070848 (accessed November 2, 2021).
Deutsche WindGuard GmbH (2020). Status des Offshore-Windenergieausbaus in Deutschland – Erstes Halbjahr 2020. Available online at: https://www.windguard.de/id-1-halbjahr-2020.html?file=files/cto_layout/img/unternehmen/windenergiestatistik/2020/Status%20des%20Offshore-Windenergieausbaus_Halbjahr%202020.pdf (accessed November 2, 2021).
Deutz, S., and Bardow, A. (2021). Life-cycle assessment of an industrial direct air capture process based on temperature–vacuum swing adsorption. Nat. Energy 6, 203–213. doi: 10.1038/s41560-020-00771-9
Dittmeyer, R., Klumpp, M., Kant, P., and Ozin, G. (2019). Crowd oil not crude oil. Nat. Commun. 10, 1818. doi: 10.1038/s41467-019-09685-x
DOE (2011). Technology Readiness Assessment Guide. Department of Energy (DOE). Washington, DC. U.S. Available online at: https://www.directives.doe.gov/directives-documents/400-series/0413.3-EGuide-04 (accessed November 3, 2021).
Donnison, C., Holland, R. A., Hastings, A., Armstrong, L. M., Eigenbrod, F., and Taylor, G. (2020). Bioenergy with carbon capture and storage (BECCS): finding the win-wins for energy, negative emissions and ecosystem services-size matters. GCB Bioenergy 2020, 1–19. doi: 10.1111/gcbb.12695
Dooley, K., Harrould-Kolieb, E., and Talberg, A. (2020). Carbon-dioxide removal and biodiversity: a threat identification framework. Glob. Policy 12, 34–44. doi: 10.1111/1758-5899.12828
Dooley, K., and Kartha, S. (2018). Land-based negative emissions: risks for climate mitigation and impacts on sustainable development. Int. Environ. Agreements 18, 79–98. doi: 10.1007/s10784-017-9382-9
Drax Group (2018). Drax Closer to Coal-Free Future with Fourth Biomass Unit Conversion. Available online at: https://www.drax.com/press_release/drax-closer-coal-free-future-fourth-biomass-unit-conversion/ (accessed October 19, 2021).
Drucksache 19/25295 (2020). Antrag der Abgeordneten Dr. Lukas Köhler, Frank Sitta, Grigorios Aggelidis, Olaf in der Beek, Judith Skudelny, et al. und der Fraktion der FDP. 55+5 - Ein ambitioniertes EU-Klimaziel mit Negativemissionstechnologien ermöglichen. Available online at: https://dserver.bundestag.de/btd/19/252/1925295.pdf (accessed November 2, 2021).
Drucksache 19/30724 (2021). Antwort der Bundesregierung auf die Kleine Anfrage der Abgeoordneten Dr. Lukas Köhler, Frank Sitta, Grigorios Aggelidis et al. und der Fraktion der FDP. CO2-SPeicherung als Voraussetzung für Klimaneutralität. Available online at: https://dserver.bundestag.de/btd/19/307/1930724.pdf (accessed November 2, 2021).
DTU Wind Energy The World Bank Vortex. (2021). Global Wind Atlas. Available online at: https://globalwindatlas.info/area/Germany (accessed November 2, 2021).
Dütschke, E. (2011). What drives local public acceptance–Comparing two cases from Germany. Energy Procedia 4, 6234–6240. doi: 10.1016/j.egypro.2011.02.636
Dynarski, K. A., Bossio, D. A., and Scow, K. M. (2020). Dynamic stability of soil carbon: Reassessing the “permanence” of soil carbon sequestration. Front. Environ. Sci. 8, 514701. doi: 10.3389/fenvs.2020.514701
EEG (2000). German Renewable Energy Act. Gesetz für den Vorrang Erneuerbarer Energien, Erneuerbare-Energien-Gesetz. BGBI I 2000, 305. Available online at: https://www.bgbl.de/xaver/bgbl/start.xav?start=%2F%2F*%5B%40attr_id%3D%27bgbl100s0305.pdf%27%5D#__bgbl__%2F%2F*%5B%40attr_id%3D%27bgbl100s0305.pdf%27%5D__1658772437646 (accessed July 28, 2022).
EEG (2021). German Renewable Energy Act. Available online at: https://www.gesetze-im-internet.de/eeg_2014/ (accessed November 3, 2021).
Emissionshandelsstelle (2018). Installations covered by ETS in Germany 2018, Deutsche Emissionshandelsstelle. Available online at: https://www.dehst.de/SharedDocs/downloads/EN/installation_lists/2018.pdf (accessed November 2, 2021).
Eriander, L., Infantes, E., Olofsson, M., Olsen, J. L., and Moksnes, P. O. (2016). Assessing methods for restoration of eelgrass (Zostera marina L.) in a cold temperate region. J. Exp. Mar. Biol. Ecol. 479, 76–88. doi: 10.1016/j.jembe.2016.03.005
Erlach, B., Hennig, C., and Schünemann, F. (2019). Biomasse im Spannungsfeld zwischen Energie- und Klimapolitik. Strategien für eine nachhaltige Bioenergienutzung. Stellungnahme Februar 2019. Herausgeber: Nationale Akademie der Wissenschaften Leopoldina, acatech – Deutsche Akademie der Technikwissenschaften, Union der deutschen Akademien der Wissenschaften.
Eurostat Press Office (2015). Erste Bevölkerungsschätzungen. EU-Bevölkerung zum 1. Januar 2015 auf 508,2 Millionen gestiegen. Available online at: https://ec.europa.eu/eurostat/documents/2995521/6903514/3-10072015-AP-DE.pdf/1dc02177-b1d7-47ed-8928-66fec35e2e36#:~:text=Am%201.,Januar%202014 (accessed October 5, 2021).
Fasihi, M., Efimova, O., and Breyer, C. (2019). Techno-economic assessment of CO2 direct air capture plants. J. Clean. Prod. 224, 957–980. doi: 10.1016/j.jclepro.2019.03.086
Fernand, F., Israel, A., Skjermo, J., Wichard, T., Timmermans, K. R., and Golberg, A. (2017). Offshore macroalgae biomass for bioenergy production: environmental aspects, technological achievements and challenges. Renew. Sustain. Energy Rev. 75, 35–45. doi: 10.1016/j.rser.2016.10.046
FNR (2018). Rohstoffmonitoring Holz. Fachagentur Nachwachsende Rohstoffe (FNR). Available online at: https://www.fnr.de/fileadmin/allgemein/pdf/broschueren/Broschuere_Kurzfassung_Rohstoffmonitoring_Web.pdf (accessed November 3, 2021).
FNR (2020). Basisdaten Bioenergie Deutschland 2021. Fachagentur Nachwachsende, ed Rohstoffe. Gülzow, 2021. Available online at: https://www.fnr.de/fileadmin/Projekte/2020/Mediathek/broschuere_basisdaten_bioenergie_2020_web.pdf (accessed November 4, 2021).
Förster, J., Beck, S., Borchers, M., Gawel, E., Korte, K., Markus, T., et al. (2022). Framework for assessing the feasibility of carbon dioxide removal options within the national context of Germany. Front. Clim. 4, 758628. doi: 10.3389/fclim.2022.758628
Fridahl, M., Hansson, A., and Haikola, S. (2020). Towards indicators for a negative emission climate stabilization index: problems and prospects. Climate 8, 75. doi: 10.3390/cli8060075
Fuss, S., Lamb, W. F., Callaghan, M. W., Hilaire, J., Creutzig, F., Amann, T., et al. (2018). Negative emissions—part 2: costs, potentials and side effects. Environ. Res. Lett. 13, 063002. doi: 10.1088/1748-9326/aabf9f
Gao, G., Burgess, J., Wu, M., Wang, S., and Gao, K. (2020). Using macroalgae as biofuel: current opportunities and challenges. Bot. Mar. 63, 355–370. doi: 10.1515/bot-2019-0065
GCCSI Report (2019). Global Status of CCS 2019. Available online at: https://www.globalccsinstitute.com/resources/publications-reports-research/global-status-of-ccs-report-2019/ (accessed November 2, 2021).
Geden, O., and Schenuit, F. (2020). Unconventional Mitigation. Carbon Dioxide Removal as a New Approach in EU Climate Policy. SWP Research Paper 2020/RP 08. Berlin: Stiftung Wissenschaft und Politik; German Institute for International and Security Affairs. doi: 10.18449/2020RP08
Geden, O., Scott, V., and Palmer, J. (2018). Integrating carbon dioxide removal into EU climate policy: prospects for a paradigm shift. WIREs Clim. Change 9, e521. doi: 10.1002/wcc.521
Gerwin, W., Repmann, F., Galatsidas, S., Vlachaki, D., Gounaris, N., Baumgarten, et al. (2018). Assessment and quantification of marginal lands for biomass production in Europe using soil-quality indicators. Soil 4, 267–290. doi: 10.5194/soil-4-267-2018
Gignac, R., and Matthews, H. D. (2015). Allocating a 2°C cumulative carbon budget to countries. Environ. Res. Lett. 10, 7. doi: 10.1088/1748-9326/10/7/075004
Graves, C., Sune, D., Ebbesen, S. D., Mogensen, M., and Lackner, K. S. (2011). Sustainable hydrocarbon fuels by recycling CO2 and H2O with renewable or nuclear energy. Renew. Sust. Energ. Rev. 15, 1–23. doi: 10.1016/j.rser.2010.07.014
Griffiths, P., Nendel, C., and Hostert, P. (2019). Intra-annual reflectance composites from sentinel-2 and landsat for national-scale crop and land cover mapping. Remote Sens. Environ. 220, 135–151. doi: 10.1016/j.rse.2018.10.031
GTAI (2020). The Automotive Industry in Germany. Germany Trade and Invest (GTAI), Berlin. Available online at: https://www.gtai.de/resource/blob/64100/07f5613dd96b786a118a106788e3b988/20200812_IO_Automotive_Web%20(1).pdf (accessed November 2, 2021).
Günther, A., Barthelmes, A., Huth, V., Joosten, H., Jurasinski, G., Koebsch, F., et al. (2020). Prompt rewetting of drained peatlands reduces climate warming despite methane emissions. Nat. Commun. 11, 1644. doi: 10.1038/s41467-020-15499-z
Hahn, A., Szarka, N., and Thrän, D. (2020). German energy and decarbonization scenarios: “blind spots” with respect to biomass-based carbon removal options. Front. Energy Res. 8, 130. doi: 10.3389/fenrg.2020.00130
Hepburn, C., Adlen, E., Beddington, J., Carter, E. A., Fuss, S., Mac Dowell, N., et al. (2019). The technological and economic prospects for CO2 utilization and removal. Nature 575, 87–97. doi: 10.1038/s41586-019-1681-6
Heß, D., Klumpp, M., and Dittmeyer, R. (2020). Nutzung von CO2 aus Luft als Rohstoff für synthetische Kraftstoffe und Chemikalien. Verkehrsmininsterium Baden-Württemberg. Karlsruher Institut für Technologie. Available online at: https://vm.baden-wuerttemberg.de/fileadmin/redaktion/m-mvi/intern/Dateien/PDF/29-01-2021-DAC-Studie.pdf (accessed March 1, 2021).
HICAM (2020). Factsheet No. 04. Direct Air Capture. Helmholtz Klima Initiative. Hermann von Helmholtz-Gemeinschaft Deutscher Forschungszentren e.V. Berlin. Available online at: https://www.helmholtz-klima.de/sites/default/files/medien/dokumente/Factsheet%2004_Direct%20Air%20Capture.pdf (accessed November 2, 2021).
Hills, T. P., Sceats, M., Rennie, D., and Fennell, P. (2017). LEILAC: low cost CO2 capture for the cement and lime industries. Energy Procedia 114, 6166–6170, doi: 10.1016/j.egypro.2017.03.1753
Höhne, N., den Elzen, M., and Escalante, D. (2014). Regional GHG reduction targets based on effort sharing: a comparison of studies. Clim. Policy 14, 122–147. doi: 10.1080/14693062.2014.849452
Höhne, N., Galleguillos, C., Kornelis, B., Harnisch, J., and Phylipson, D. (2003). Evolution of Commitments Under the UNFCCC: Involving Newly Industrialized Economies and Developing Countries (Berlin, Germany: Ecofys/Federal Environmental Agency). Available online at: https://www.umweltbundesamt.de/sites/default/files/medien/publikation/long/2235.pdf (accessed November 3, 2021).
Honegger, M., Derwent, H., Harrison, N., Michaelowa, A., and Schäfer, S. (2018). Carbon Removal and Solar Geoengineering: Potential Implications for Delivery of the Sustainable Development Goals. (New York, Carnegie Climate Geoengineering Governance Initiative). Available online at: https://www.c2g2.net/wp-content/uploads/C2G2-Geoeng-SDGs_20180521.pdf (accessed November 3, 2021).
Honegger, M., Michaelowa, A., and Roy, J. (2020). Potential implications of carbon dioxide removal for the sustainable development goals. Clim. Policy 21, 678–698. doi: 10.1080/14693062.2020.1843388
Honegger, M., Poralla, M., Michaelowa, A., and Ahonen, H.-M. (2021). Who is paying for carbon dioxide removal? designing policy instruments for mobilizing negative emissions technologies. Front. Clim. 3, 50. doi: 10.3389/fclim.2021.672996
Infantes, E., Eriander, L., and Moksnes, P. O. (2016). Eelgrass (Zostera marina) restoration on the west coast of Sweden using seeds. Mar. Ecol. Prog. Ser. 546, 31–45. doi: 10.3354/meps11615
Infantes, E., and Moksnes, P.O. (2018). Eelgrass seed harvesting: Flowering shoots development and 1293 restoration on the Swedish west coast. Aquat. Bot. 144, 9–19. doi: 10.1016/j.aquabot.2017.10.002
IPBES-IPCC (2021). Scientific Outcome of the IPBES-IPCC Co-Sponsored Workshop on Biodiversity and Climate Change. Available online at: https://ipbes.net/sites/default/files/2021-06/2021_IPCC-IPBES_scientific_outcome_20210612.pdf (accessed November 3, 2021).
IPCC (2005). IPCC Special Report on Carbon Dioxide Capture and Storage. Prepared by Working Group III of the Intergovernmental Panel on Climate Change, eds B. Metz, O. Davidson, H. C. de Coninck, M. Loos, and L. A. Meyer (Cambridge University Press, Cambridge), 442.
IPCC (2018). Global Warming of 1.5°C. An IPCC Special Report on the Impacts of Global Warming of 1.5°C Above Pre-industrial Levels and Related Global Greenhouse Gas Emission Pathways, in the Context of Strengthening the Global Response to the Threat of Climate Change, Sustainable Development, and Efforts to Eradicate Poverty, eds, V. Masson-Delmotte, P. Zhai, H. O. Pörtner, D. Roberts, J. Skea, P. R. Shukla, et al. Global warming of 1.5 °C, (IPCC Special Report) (Geneva: Intergovernmental Panel on Climate Change, 93–174.
IPCC (2021). Climate Change 2021: The Physical Science Basis. Contribution of Working Group I to the Sixth Assessment Report of the Intergovernmental Panel on Climate Change, eds V. Masson-Delmotte, P. Zhai, A. Pirani, S.L. Connors, C. Péan, S. Berger (Cambridge; New York, NY: Cambridge University Press). doi: 10.1017/9781009157896
Joosten, H. (2009). The Global Peatland CO2 Picture. Peatland Status and Emissions in All Countries of the World. Draft. Available online at: https://unfccc.int/sites/default/files/draftpeatlandco2report.pdf (accessed November 2, 2021).
Joosten, H., and Clarke, D. (2002). Wise Use of Mires and Peatlands - Background and Principles Including a Framework for Decision-Making. International Mire Conservation Group and International Peat Society.
Joosten, H., Sirin, A., Couwenberg, J., Laine, J., and Smith, P. (2016). “The role of peatlands in climate regulation,” in Peatland Restoration and Ecosystem Service, eds A. Bonn, T. Allott, M. Evans, H. Joosten, R. Stoneman. (Cambridge: Cambridge University Press), 63–76.
Kabisch, N., Stadlet, J., Korn, H., and Bonn, A. (2016). Nature-Based Solutions to Climate Change Mitigation and Adaptation in Urban Areas. Bonn: Bundesamt für Naturschutz (BfN); Federal Agency for Nature Conservation. Available online at: https://www.bfn.de/sites/default/files/BfN/service/Dokumente/skripten/skript446.pdf (accessed September 28, 2022).
Kearns, J., Teletzke, G., Palmer, J., Thomann, H., Kheshgi, H., Chen, Y.-H. H., et al. (2017). Developing a consistent database for regional geologic CO2 storage capacity worldwide. Energy Procedia 114, 4697–4709. doi: 10.1016/j.egypro.2017.03.1603
Kearns, K., Liu, H., and Consoli, C. (2021). GCCSI Report: Technology Readiness and Costs of CCS. Available online at: https://www.globalccsinstitute.com/resources/publications-reports-research/technology-readiness-and-costs-of-ccs/ (accessed November 2, 2021).
Keith, D. W., Holmes, G., St. Angelo, D., and Heidel, K. (2018). A process for capturing CO2 from the atmosphere. Joule 2, 1573–1594. doi: 10.1016/j.joule.2018.05.006
Kemna, R., and Moreno Acedo, J. (2014). Average EU Building Heat Load for HVAC Equipment. VHK. Available online at: https://ec.europa.eu/energy/sites/ener/files/documents/2014_final_report_eu_building_heat_demand.pdf (accessed October 28, 2020).
Kempka, T., de Lucia, M., and Kühn, M. (2014). Geomechanical integrity verification and mineral trapping quantification for the Ketzin CO2 storage pilot site by coupled numerical simulations. Energy Procedia 63, 3330–3338. doi: 10.1016/j.egypro.2014.11.361
Kim, D. G., and Kirschbaum, M. U. F. (2015). The effect of land-use change on the net exchange rates of greenhouse gases: a compilation of estimates. Agric. Ecosyst. Environ. 208, 114–126. doi: 10.1016/j.agee.2015.04.026
Kim, J. K., Yarish, C., Hwang, E. K., Park, M., and Kim, Y. (2017). Seaweed aquaculture: cultivation technologies, challenges and its ecosystem services. Algae 32, 1–13. doi: 10.4490/algae.2017.32.3.3
Knopf, S., and May, F. (2017). Comparing methods for the estimation of CO2 storage capacity in saline aquifers in Germany: regional aquifer based vs. structural trap based assessments. Energy Procedia 114, 4710–4721. doi: 10.1016/j.egypro.2017.03.1605
KSG (2021). Bundes-Klimaschutzgesetz (KSG). Available online at: https://www.bmu.de/fileadmin/Daten_BMU/Download_PDF/Klimaschutz/ksg_aendg_2021_bf.pdf (accessed November 2, 2021).
KSpG (2012). Gesetz zur Demonstration der dauerhaften Speicherung von Kohlendioxid (Kohlendioxid-Speicherungsgesetz - KSpG). Available online at: https://ra.de/gesetze/kspg (accessed November 3, 2021).
Kuzyakov, Y., Bogomolova, I., and Glaser, B. (2014). Biochar stability in soil: Decomposition during eight years and transformation as assessed by compound-specific 14C analysis. Soil Biol. Biochem. 70, 229–236. doi: 10.1016/j.soilbio.2013.12.021
Kuzyakov, Y., Subbotina, I., Chen, H., Bogomolova, I., and Xu, X. (2009). Black carbon decomposition and incorporation into soil microbial biomass estimated by 14C labeling. Soil Biol. Biochem. 41, 210–219. doi: 10.1016/j.soilbio.2008.10.016
KVBG (2020). Kohleausstiegsgesetz Gesetz zur Reduzierung und zur Beendigung der Kohleverstromung und zur Änderung weiterer Gesetze. Available online at: https://www.bundesrat.de/SharedDocs/drucksachen/2020/0301-0400/392-20.pdf?__blob=publicationFile&v=1 (accessed November 3, 2021).
Leedham, E. C., Hughes, C., Keng, F. S. L., Phang, S.-M., Malin, G., and Sturges, W. T. (2013). Emission of atmospherically significant halocarbons by naturally occurring and farmed tropical macroalgae. Biogeosciences 10, 3615–3633. doi: 10.5194/bg-10-3615-2013
Lehmann, J., Czimczik, C., Laird, D., and Sohi, S. (2009). “Stability of biochar in soil,” in Biochar for Environmental Management - Science and Technology, eds J. Lehmann and S. Joseph (London: Routledge), 183–205.
Lehmann, J., Gaunt, J., and Rondon, M. (2006). Bio-char sequestration in terrestrial ecosystems - a review. Mitig. Adapt. Strateg. Glob. Chang. 11, 403–427. doi: 10.1007/s11027-005-9006-5
Lehmann, J., and Joseph, S. (2015). Biochar for Environmental Management: Science, Technology and Implementation, 2nd ed. London and New York, NY: Routledge.
Leng, L., Huang, H., Li, H., Li, J., and Zhou, W. (2019). Biochar stability assessment methods: a review. Sci. Total Environ. 647, 210–222. doi: 10.1016/j.scitotenv.2018.07.402
Linzenich, A., Arning, K., Offermann-van Heek, J., and Ziefle, M. (2019). Uncovering attitudes towards carbon capture storage and utilization technologies in Germany: Insights into affective-cognitive evaluations of benefits and risks. Energy Res. Soc. Sci. 48, 205–218. doi: 10.1016/j.erss.2018.09.017
Low, S., and Schäfer, S. (2020). Is bio-energy carbon capture and storage (BECCS) feasible? The contested authority of integrated assessment modeling. Energy Res. Soc. Sci. 60, 101326. doi: 10.1016/j.erss.2019.101326
MacDicken, K. (2013). Forest Resources Assessment Working Paper 180. FAO: Rome. Available online at: http://www.fao.org/docrep/017/ap862e/ap862e00.pdf (accessed November 3, 202).
Marbà, N., Arias-Ortiz, A., Masqué, P., Kendrick, G. A., Mazarrasa, I., Bastyan, G. R., et al. (2015). Impact of seagrass loss and subsequent revegetation on carbon sequestration and stocks. J. Ecol. 103, 296–302. doi: 10.1111/1365-2745.12370
Martens, S., Kempka, T., Liebscher, A., Möller, F., Schmidt-Hattenberger, C., Streibel, M., et al. (2015). Field experiment on CO2 back-production at the ketzin pilot site. Energy Procedia 76, 519–527. doi: 10.1016/j.egypro.2015.07.902
Matthews, H., Gillett, N., Stott, P., and Zickfeld, K. (2009). The proportionality of global warming to cumulative carbon emissions. Nature 459, 829–832. doi: 10.1038/nature08047
Matthews, H. D., Tokarska, K. B., Rogelj, J., et al. (2021). An integrated approach to quantifying uncertainties in the remaining carbon budget. Commun. Earth Environ. 2, 7. doi: 10.1038/s43247-020-00064-9
Mengis, N., Kalhori, A., Simon, S., Harpprecht, C., Baetcke, L., Prats-Salvado, E., et al. (2022). Net-zero CO2 Germany - A retrospect from the year 2050. Earths Future 10, e2021EF002324. doi: 10.1029/2021EF002324
Mengis, N., Simon, S., Thoni, T., Stevenson, A., Goerl, K., Steuri, B., et al. (2021). Net-Zero-2050 Cluster: Definig the German carbon budget. Project briefing #2, ver. 2, October 2021. Available online at: https://www.netto-null.org/imperia/md/assets/net_zero/dokumente/2_carbonbudget_2021_10_web.pdf (accessed November 3, 2021).
Merk, C., Klaus, G., Pohlers, J., Ernst, A., Ott, K., and Rehdanz, K. (2019). Public perceptions of climate engineering: laypersons' acceptance at different levels of knowledge and intensities of deliberation. GAIA 28, 348–355. doi: 10.14512/gaia.28.4.6
Meyer, M. A., and Früh-Müller, A. (2020). Patterns and drivers of recent agricultural land-use change in Southern Germany. Land Use Policy 99, 104959. doi: 10.1016/j.landusepol.2020.104959
Minx, J. C., Lamb, W. F., Callaghan, M. W., Fuss, S., Hilaire, J., Creutzig, F., et al. (2018). Negative emissions - part 1: research landscape and synthesis. Environ. Res. Lett. 13, 063001. doi: 10.1088/1748-9326/aabf9b
Moksnes, P. O., Eriander, L., Infantes, E., and Holmer, M. (2018). Local regime shifts prevent natural recovery and restoration of lost eelgrass beds along the Swedish west coast. Estuaries Coast. 41, 1712–1731. doi: 10.1007/s12237-018-0382-y
Montingelli, M. E., Tedesco, S., and Olabi, A. G. (2015). Biogas production from algal biomass: a review. Renew. Sust. Energ. Rev. 43, 961–972. doi: 10.1016/j.rser.2014.11.052
Moosdorf, N., Renforth, P., and Hartmann, J. (2014). Carbon dioxide efficiency of terrestrial enhanced weathering. Environ. Sci. Technol. 48, 4809–4816. doi: 10.1021/es4052022
Morrow, D. R., Thompson, M. S., Anderson, A., Batres, M., Buck, H. J., Dooley, K., et al. (2020). Principles for thinking about carbon dioxide removal in just climate policy. One Earth 3, 150–153. doi: 10.1016/j.oneear.2020.07.015
Müller, C., and Reinhold, K. (2011). Geologische Charakterisierung tiefliegender Speicher- und Barrierehorizonte in Deutschland – Speicher-Kataster Deutschland – Schriftenr. dt. Ges. Geowiss., Heft 74; Hannover.
Müller, P., Ladiges, N., Jack, A., Schmiedl, G., Kutzbach, L., Jensen, K., et al. (2019). Assessing the long-term carbon-sequestration potential of the semi natural salt marshes in the European wadden sea. Ecosphere 10, e02556. doi: 10.1002/ecs2.2556
Nellemann, C., Corcoran, E., Duarte, C. M., Valdés, L., De Young, C., Fonseca, L., et al. (2009). Blue Carbon. A Rapid Response Assessment. United Nations Environment Programme, GRID-Arendal. Available online at: https://www.grida.no/publications/145 (accessed November 3, 2021).
Nemet, G. F., Callaghan, M. W., Creutzig, F., Fuss, S., Hartmann, J., Hilaire, J., et al. (2018). Negative emissions - part 3: innovation and upscaling. Environ. Res. Lett. 13, 063003. doi: 10.1088/1748-9326/aabff4
Orsted Media Relations (2016). Denmark's Largest Power Station Replaces Coal with Wood Pellets. Available online at: https://orsted.com/en/media/newsroom/news/2017/10/denmarks-largest-power- station-replaces-coal-with-wood-pellets (accessed October 19, 2021).
Oschlies, A., and Klepper, G. (2017). Research for assessment, not deployment, of climate engineering: the German research foundation's priority program SPP 1689. Earth's Future 5, 128–134. doi: 10.1002/2016EF000446
Otto, D., and Gross, M. (2021). Stuck on coal and persuasion? A critical review of carbon capture and storage communication. Energy Res. Soc. Sci. 82, 102306. doi: 10.1016/j.erss.2021.102306
Patuzzi, F., Basso, D., Vakalis, S., Antolini, D., Piazzi, S., Benedetti, V., et al. (2021). State-of-the-art of small-scale biomass gasification systems: an extensive and unique monitoring review. Energy 223, 120039. doi: 10.1016/j.energy.2021.120039
Poeplau, C., and Don, A. (2015). Carbon sequestration in agricultural soils via cultivation of cover crops – a meta-analysis. Agric. Ecosyst. Environ. 200, 33–41. doi: 10.1016/j.agee.2014.10.024
Pozo, C., Galán-Martín, Á., Reiner, D. M., et al. (2020). Equity in allocating carbon dioxide removal quotas. Nat. Clim. Chang. 10, 640–646. doi: 10.1038/s41558-020-0802-4
Prognos, Öko-Institut, Wuppertal-Institut. (2021). Klimaneutrales Deutschland 2045. Wie Deutschland seine Klimaziele schon vor 2050 erreichen kann. Langfassung. Hg. v. Stiftung Klimaneutralität, Agora Energiewende, Agora Verkehrswende. Available online at: https://static.agora-energiewende.de/fileadmin/Projekte/2021/2021_04_KNDE45/A-EW_209_KNDE2045_Zusammenfassung_DE_WEB.pdf (accessed November 3, 2021).
Rauch, R., Hrbek, J., and Hofbauer, H. (2014). Biomass gasification for synthesis gas production and application. Wiley Interdiscip. Rev. Energy Environ. 3, 343–362. doi: 10.1002/wene.97
Rhoden, I., Vögele, S., Ball, C., Simon, S., Mengis, N., Baetcke, L., et al. (2021). Spatial Heterogeneity - Challenge and Opportunity for Net-Zero Germany. Report, in Press. Helmholtz Climate Initiative. Available online at: https://www.netto-null.org/imperia/md/assets/net_zero/dokumente/2021_netto-null_spatial-heterogeneity.pdf (accessed July 28, 2022).
Rogelj, J., Shindell, D., Jiang, K., Fifita, S., Forster, P., Ginzburg, V., et al. (2018). “Mitigation pathways compatible with 1.5°c in the context of sustainable development,” in Global warming of 1.5 °C, (IPCC Special Report), eds V. Masson-Delmotte, P. Zhai, H. O. Pörtner, D. Roberts, J. Skea, P. R. Shukla, et al. (Geneva, Intergovernmental Panel on Climate Change), 93–174.
Rydin, H., and Jeglum, J. K. (2013). “Peatland habitats,” in The Biology of Peatlands (New York, NY: Oxford University Press), 1–17.
Sanchez, D. L., Johnson, N., McCoy, S. T., Turner, P. A., and Mach, K. J. (2018). Near-term deployment of carbon capture and sequestration from biorefineries in the United States. Proc. Natl. Acad. Sci. U. S. A., 115, 4875–4880. doi: 10.1073/pnas.1719695115
Scheftelowitz, M., and Thrän, D. (2016). Biomasse im EEG 2016: Hintergrundpapier zur Situation der Bestandsanlagen in den verschiedenen Bundesländern. Available online at: https://www.dbfz.de/fileadmin/user_upload/Referenzen/Statements/Hintergundpapier_Biomasse_EEG2016.pdf (accessed November 3, 2021).
Schenuit, F., Colvin, R., Fridahl, M., McMullin, B., Reisinger, A., Sanchez, D. L., et al. (2021). Carbon dioxide removal policy in the making: assessing developments in 9 OECD cases. Front. Clim. 3, 638805. doi: 10.3389/fclim.2021.638805
Schlesinger, W. H. (2018). Are wood pellets a green fuel? Science 359, 1328–1329. doi: 10.1126/science.aat2305
Scholwin, F., and Siegert, G. (2020). Biogas aus Paludikulturen. Produktionsweg, Hintergründe, Klimaschutzwirkung. Institut für Biogas, Kreislaufwirtschaft und Energie. Greifswald Moor Centrum. Greenpeace Energy eG. Available online at: https://green-planet-energy.de/fileadmin/docs/publikationen/Studien/201109_GPE-Studie_zu_Paludi_final.pdf (accessed November 3, 2021).
Shell Canada Ltd. Report (2019). Quest Carbon Capture and Storage Project, 2019 Annual Status Report. Available online at: https://open.alberta.ca/dataset/f74375f3-3c73-4b9c-af2b-ef44e59b7890/resource/92e474c0-b043-4c8f-8693-1336447933d0/download/energy-quest-annual-status-report-alberta-energy-regulator-2019.pdf (accessed November 3, 2021).
Simon, S., Xiao, M., Harpprecht, C., Sasanpour, S., Gardian, H., and Pregger, T. A. (2022). Pathway for the German energy sector compatible with a 1.5°C carbon budget. Sustainability 14, 1025. doi: 10.3390/su14021025
Smith, E., Morris, J., Kheshgi, H., Teletzke, G., Herzog, H., and Paltsev, S. (2021). The cost of CO2 transport and storage in global integrated assessment modeling. Int. J. Greenh. Gas Control 109, 103367. doi: 10.1016/j.ijggc.2021.103367
Smith, L. J., and Torn, M. S. (2013). Ecological limits to terrestrial biological carbon dioxide removal. Clim. Change 118, 89–103. doi: 10.1007/s10584-012-0682-3
Spence, E., Cox, E., and Pidgeon, N. (2021). Exploring cross-national public support for the use of enhanced weathering as a land-based carbon dioxide removal strategy. Clim. Change 165, 23. doi: 10.1007/s10584-021-03050-y
Stottrop, D., and Flüshöh, C. (2007). Büroflächenbestand-Grundlagen, Daten und Methoden. Hg. v. Prof. Dr. Karl-Werner Schulte und Prof. Dr. Stephan Bone-Winkel. International Real Estate Business School (IREBS), Universität Regensburg. Regensburg.
Tanneberger, F., Able, S., Couwenberg, J., Dahms, T., Gauding, G., Günther, A., et al. (2021). Towards net-zero CO2 in 2050: An emission reduction pathway for organic soils in Germany. Mires Peat 27:5, 17. doi: 10.19189/MaP.2020.SNPG.StA.1951
Tanneberger, F., Appulo, L., Ewert, S., Lakner, S. Ó., Brolcháin, N., Peters, J., et al. (2020). The power of nature-based solutions: how peatlands can help us to achieve key eu sustainability objectives. Adv. Sustain. Syst. 5, 2000146. doi: 10.1002/adsu.202000146
Tanneberger, F., Birr, F., Couwenberg, J., Kaiser, M., Luthardt, V., Nerger, M., et al. (2022). Saving soil carbon, greenhouse gas emissions, biodiversity and the economy: paludiculture as sustainable land use option in German fen peatlands. Reg. Environ. Change 22, 69. doi: 10.1007/s10113-022-01900-8
Tcvetkov, P., Cherepovitsyn, A., and Fedoseev, S. (2019). Public perception of carbon capture and storage: a state-of-the-art overview. Heliyon 5, e02845. doi: 10.1016/j.heliyon.2019.e02845
The World Bank (2019). Global Solar Atlas 2.0, Solar resource data: Solargis. Available online at: https://solargis.com/maps-and-gis-data/download/germany (accessed November 3, 2021).
Thoni, T., Beck, S., Borchers, M., Förster, J., Görl, K., Hahn, A., et al. (2020). Deployment of negative emissions technologies at the national level: a need for holistic feasibility assessments. Front. Clim. 2, 590305. doi: 10.3389/fclim.2020.590305
Thrän, D. (2019). Interdisziplinäres Bewertungsinstrument für Bioenergie-Entwicklungspfade. Materialien zur Analyse Biomasse im Spannungsfeld zwischen Energie- und Klimapolitik. Potenziale – Technologien – Zielkonflikte. Schriftenreihe Energiesysteme der Zukunft, München. Available online at: https://www.leopoldina.org/uploads/tx_leopublication/2019_ESYS_Materialien_Bioenergie.pdf (accessed November 4, 2021).
Thrän, D., and Schindler, H. (2021). Umrüstung von Kohlekraftwerken auf Biomasse. DBFZ Positionspapier, Deutsches Biomasseforschungszentrum gGmbH (DBFZ), Juni 2021. Available online at: https://www.dbfz.de/fileadmin/user_upload/Referenzen/Statements/2021_Position_Kohlekraftwerke.pdf (accessed November 2, 2021).
Trepel, M., Pfadenhauer, J., Zeitz, J., and Jeschke, L. (2017). “Part II: Germany,” in Mires and Peatlands of Europe: Status, Distribution and Conservation, eds H. Joosten, F. Tanneberger, and A. Moen (Stuttgart: Schweizerbart Science Publishers), 413–424.
Tripathi, M., Sahu, J., and Ganesan, P. (2016). Effect of process parameters on production of biochar from biomass waste through pyrolysis. Renew. Sust. Energ. Rev. 55, 467–481. doi: 10.1016/j.rser.2015.10.122
UBA (2021). Previous Year's Estimate of German Greenhouse Gas Emissions for 2020. Umweltbundesamt (UBA) Dessau, 15.03.2021. Available online at: https://www.umweltbundesamt.de/sites/default/files/medien/361/dokumente/2021_03_10_trendtabellen_thg_nach_sektoren_v1.0.xlsx (accessed November 2, 2021).
UNEP (2017). The Emissions Gap Report 2017: A UN Environment Synthesis Report. Nairobi: United Nations Environmental Program. Available online at: https://wedocs.unep.org/bitstream/handle/20.500.11822/22070/EGR_2017.pdf?sequence=1&isAllowed=y (accessed November 3, 2021).
UNFCCC (2015). Paris Agreement. Available online at: https://unfccc.int/process-and-meetings/the-paris-agreement/the-paris-agreement (accessed February 19, 2021).
Waller, L., Rayner, T., Chilvers, J., Gough, C. A., Lorenzoni, I., Jordan, A., et al. (2020). Contested framings of greenhouse gas removal and its feasibility: social and political dimensions. Wiley Interdiscip. Rev. Clim. Change 11, e649. doi: 10.1002/wcc.649
Wallquist, L., Seigo, S. L. O., Visschers, V. H. M., and Siegrist, M. (2012). Public acceptance of CCS system elements: a conjoint measurement. Int. J. Greenh. Gas Control. 6, 77–83. doi: 10.1016/j.ijggc.2011.11.008
Wartenberg, R., Feng, L., Wu, J. J., Mak, Y. L., Chan, L. L., Trefler, T. C., et al. (2017). The impacts of suspended mariculture on coastal zones in China and the scope for integrated multi-trophic aquaculture. Ecosyst. Health Sustain. 3, 1340268. doi: 10.1080/20964129.2017.1340268
Wichtmann, W., Schröder, C., and Joosten, H. (2015). Paludikultur — Bewirtschaftung nasser Moore für regionale Wertschöpfung, Klimaschutz und Biodiversität. Schweizerbart Science Publishers, Stuttgart.
Wieding, J., Stubenrauch, J., and Ekardt, F. (2020). Human rights and precautionary principle: limits to geoengineering, SRM, and IPCC scenarios. Sustainability 12, 8858. doi: 10.3390/su12218858
Wolske, K. S., Raimi, K. T., Campbell-Arvai, V., and Hart, P. S. (2019). Public support for carbon dioxide removal strategies: the role of tampering with nature perceptions. Clim. Change 152, 345–361. doi: 10.1007/s10584-019-02375-z
Zickfeld, K., Azevedo, D., Mathesius, S., and Matthews, H. D. (2021). Asymmetry in the climate–carbon cycle response to positive and negative CO2 emissions. Nat. Clim. Chang. 11, 613–617. doi: 10.1038/s41558-021-01061-2
Ziegler, R., Wichtmann, W., Abel, S., Kemp, R., Simard, M., and Joosten, H. (2021). Wet peatland utilisation for climate protection – an international survey of paludiculture innovation. Clean. Eng. Technol. 5, 100305. doi: 10.1016/j.clet.2021.100305
Keywords: carbon dioxide removal (CDR), BECC(S), DACC(S), natural sink enhancement (NSE), climate mitigation, CO2 storage
Citation: Borchers M, Thrän D, Chi Y, Dahmen N, Dittmeyer R, Dolch T, Dold C, Förster J, Herbst M, Heß D, Kalhori A, Koop-Jakobsen K, Li Z, Mengis N, Reusch TBH, Rhoden I, Sachs T, Schmidt-Hattenberger C, Stevenson A, Thoni T, Wu J and Yeates C (2022) Scoping carbon dioxide removal options for Germany–What is their potential contribution to Net-Zero CO2? Front. Clim. 4:810343. doi: 10.3389/fclim.2022.810343
Received: 06 November 2021; Accepted: 07 September 2022;
Published: 31 October 2022.
Edited by:
Patrick Lamers, National Renewable Energy Laboratory (DOE), United StatesReviewed by:
Ludger Eltrop, University of Stuttgart, GermanyElliott Thomas Campbell, Maryland Department of Natural Resources, United States
Copyright © 2022 Borchers, Thrän, Chi, Dahmen, Dittmeyer, Dolch, Dold, Förster, Herbst, Heß, Kalhori, Koop-Jakobsen, Li, Mengis, Reusch, Rhoden, Sachs, Schmidt-Hattenberger, Stevenson, Thoni, Wu and Yeates. This is an open-access article distributed under the terms of the Creative Commons Attribution License (CC BY). The use, distribution or reproduction in other forums is permitted, provided the original author(s) and the copyright owner(s) are credited and that the original publication in this journal is cited, in accordance with accepted academic practice. No use, distribution or reproduction is permitted which does not comply with these terms.
*Correspondence: Malgorzata Borchers, malgorzata.borchers@ufz.de
†These authors have contributed equally to this work and share first authorship